- Dendritic Cells in Infection and Cancer, German Cancer Research Center, Heidelberg, Germany
Multiple myeloma (MM) is a type of hematologic cancer characterized by the uncontrolled clonal expansion of plasma cells in the bone marrow (BM). This leads to significant dysfunction and suppression of the immune system in affected patients. Myeloma cells employ sophisticated strategies to manipulate immune and non-immune cells, evading immune surveillance and enhancing their survival. One key factor in this evasion is the disruption of dendritic cell (DC)-mediated immune mechanisms. Extensive evidence indicates that in the presence of myeloma cells, DC numbers are notably reduced, and their phenotype and function are altered, impairing their ability to present antigens and activate robust T-cell responses effectively. Despite rapid advances in MM treatment, with promising strategies such as DC-based vaccines being already achieved, DC dysfunction remains a substantial hurdle, associated with or contributing to poor therapeutic outcomes, disease relapse, and MM’s persistence as an incurable disease. To address these challenges, it is essential to understand the intricate mechanisms through which myeloma cells transform DCs into their “accomplices,” undermining immune responses. This review comprehensively summarizes the current understanding of the role of DCs in MM. Additionally, it evaluates the potential of DCs in anti-MM immunotherapy, discussing persistent challenges and highlighting emerging perspectives that may lead to promising breakthroughs for improved patient outcomes.
1 Introduction
MM is a B-cell malignancy characterized by the uncontrolled proliferation of plasma cells, primarily within the BM. It is the second most common hematologic cancer, accounting for 10–17% of cases, and predominantly affects individuals over 40, with a median diagnosis age of 65. Men are at a higher risk than women (1).
MM often progresses from two asymptomatic precursor stages: monoclonal gammopathy of undetermined significance (MGUS) and smoldering MM (SMM). MGUS has <10% clonal plasma cells in the BM with a 1% annual progression risk (2), while SMM has >10% clonal plasma cells and a 10% annual progression risk (3). Clinical symptoms are summarized by the CRAB criteria: hypercalcemia, renal dysfunction, anemia, and bone lesions (4).
A hallmark of MM is severe immunodeficiency. Myeloma cells evade immune responses by impairing DCs, critical for activating T cells. This immune suppression facilitates disease progression and limits the efficacy of therapies, including DC-based vaccines. Despite advancements in treatment, MM remains incurable, with a median survival of 5–7 years (5). Understanding DC interactions within the tumor microenvironment is essential for developing effective immune-based therapies.
2 DC in health & disease: a brief overview
DCs are pivotal antigen-presenting cells that link innate and adaptive immunity by activating T-cell responses. They process and present antigens via MHC molecules, enabling T-cell priming and differentiation. Human DCs are classified into three main subsets: conventional DCs (cDCs), plasmacytoid DCs (pDCs), and monocyte-derived DCs (moDCs) (6).
cDCs are divided into cDC1s, cDC2s, and DC3s according to transcriptional and functional properties. cDC1s express markers such as XCR1, DNGR-1 (CLEC9A), CD141 (BDCA3), and CADM1 and are specialized in cross-presenting antigens to CD8+ T cells, promoting cytotoxic responses (6). Ex vivo studies demonstrated their ability to stimulate naïve CD4 T cells into Th1 and Th2 cells (7–9).
cDC2s are more heterogeneous and express CD11b, CD1c (BDCA1), and SIRPα (CD172a). cDC2s promote CD4+ T cell activation and differentiation into Th1, Th2, Th17 and T follicular helper cells (10).
Subsets of cDC2s include DC2s and DC3s, the latter sharing features with monocytes but developing differently (11, 12).
pDCs play a critical role in antiviral immunity by producing type I interferons and expressing markers such as CD123, BDCA2 (CD303), and BDCA4 (CD304) (13). MoDCs arise during inflammation and share phenotypic similarities with cDCs, but they can be distinguished by CCR2 and Fcγ receptors.
All DC subsets originate from hematopoietic stem cells in the BM. They develop through granulocyte-monocyte DC precursors (GMDPs) into common DC progenitors (CDPs) before differentiating into pre-cDCs or pre-pDCs. While pre-cDCs migrate to peripheral tissues to mature into cDCs, pDCs complete their differentiation in the BM (14).
Upon activation by inflammatory signals or pathogens, immature DCs mature, upregulating MHC and co-stimulatory molecules like CD80, CD86, and CD40. Mature DCs migrate to lymphoid organs to initiate immune responses (15). However, under oncogenic or septic conditions, DC functions can be impaired, promoting disease progression. Understanding these subsets is crucial for advancing immunotherapy and cancer treatment (16, 17).
3 Myeloma immune escape: role of DCs
Immune surveillance is crucial for the immune system to recognize and eliminate cancer cells before tumor formation. This concept ties into immunoediting, which outlines the dynamic between the immune system and tumors. Cancer immunoediting includes three phases: elimination, equilibrium, and escape, each marking a specific interaction stage (reviewed in detail in (18–20)).
In the premalignant stages of MM, particularly during MGUS and SMM, the immune system initially targets emerging malignant clones. However, as MM progresses, specific genetic alterations may allow certain tumor subclones to persist. This persistence creates a fragile balance where some myeloma cells are eradicated while more resilient cells remain in a dormant state maintained by the immune system. It is proposed that when the immune system can no longer sustain this delicate equilibrium, it leads to the uncontrolled proliferation of malignant cells (21), marking the transition from MGUS and SMM to active MM (reviewed in detail in (5)). MM genetic events include chromosomal abnormalities like trisomies, IgH translocations, somatic mutations, and secondary cytogenetic abnormalities like Del(17p) (22).
3.1 The tumor microenvironment
The tumor microenvironment (TME) is a complex ecosystem around a tumor, comprising cellular elements like cancer, stromal, and immune cells and non-cellular components like blood vessels, the extracellular matrix (ECM), and secreted factors. It is dynamic and crucial for tumor growth, progression, metastasis, and treatment response (23). Evidence shows that myeloma cells employ various mechanisms to suppress antitumor immunity while aiding their survival (24). These mechanisms primarily involve interactions with immune and non-immune cells in the BM microenvironment (BMME), which are related to MM risk and severity (25–29). While the overall composition influences MM progression, the specific roles of individual immune cells need further exploration, though DCs are recognized as key players in MM’s progression (30).
3.2 DCs in the MM-microenvironment
DCs activate T cells and infiltrate tumors, making them vital for immune responses in various cancers, including MM. However, the TME often impedes their functions, weakening anti-tumor responses. In the TME, DCs become dysfunctional, compromising immune responses due to immunosuppressive TME traits that affect maturation, migration, and effector functions (31, 32). This dysfunction impairs antigen capture and downregulates costimulatory molecule expression. Conventional type 1 DCs (cDC1s) are essential for generating anti-tumor responses by cross-presenting tumor antigens from necrotic cells (33). Also, stimulatory DC infiltration correlates with better prognosis and immunotherapy response (34). Recent studies found a universal mature DC population within the TME, but their development and functions are unclear. This group, called “mregDCs” or “LAMP3+ DCs,” shows similarities and heterogeneity due to environmental factors (35).
Extensive evidence emphasizes DCs’ central role in MM pathophysiology. Instead of fostering effective immune responses, DCs often become impaired (36–38), promoting MM cell survival, tumor growth, and immune evasion (24, 30). DC dysfunction stems from various molecular mechanisms involving direct interactions between myeloma cells and DCs or high levels of cytokines, especially IL-6, in the TME (37). This results in the aberrant activation of key signaling pathways and advances disease progression.
3.3 Myeloma-DC cellular interaction
Previous studies show that DCs enhance myeloma cell survival in the BMME through direct interactions that activate APRIL and BAFF signaling pathways, essential for plasma cell growth. APRIL and BAFF, produced by myeloid cells like DCs and monocytes, regulate normal B lymphocyte and plasma cell survival through BCMA or TACI receptors. APRIL and BAFF levels rise significantly in MM, with their receptors overexpressed on myeloma cells (39, 40). While BCMA is typically expressed more than TACI on these cells (41), Kukreja et al. and Chauhan et al. found that moDC/pDC-myeloma interactions mainly promote BAFF/APRIL signaling via TACI, aiding malignant plasma cell growth, respectively (24, 30). Although mechanisms are not fully understood, studies confirm blocking the BAFF/APRIL–TACI interaction inhibits DC-mediated tumor clonogenicity in myeloma cells (24, 30). However, newer studies show that pDCs from MM patients show higher BCMA levels than myeloma cells, suggesting BCMA on pDCs may be vital for supporting myeloma cell survival (42).
3.4 Cytokine-mediated effects on DCs
In addition to directly interacting with MM cells, DCs facilitate immune evasion in MM, stimulated through immunosuppressive cytokines, including transforming growth factor-β1 (TGF-β1), vascular endothelial growth factor (VEGF), IL-6, and IL-10. These are primarily secreted by myeloma and BM stromal cells during their interactions (43). The cytokine signaling activates key cell growth and survival pathways, impairing DCs (44). For instance, IL-6 binding to its receptors activates Janus kinases (JAKs) and STAT proteins, particularly STAT3, which diminishes DC function (45–48).
4 DC impairments in MM
Strong evidence shows DCs are impaired in MM, prompting numerous studies on their numbers, phenotype, and function throughout progression (36). Despite some contradictions in findings, consistent observations include a significant decrease in DCs and cytokine-mediated changes impacting DC differentiation, maturation, and function (Figure 1).
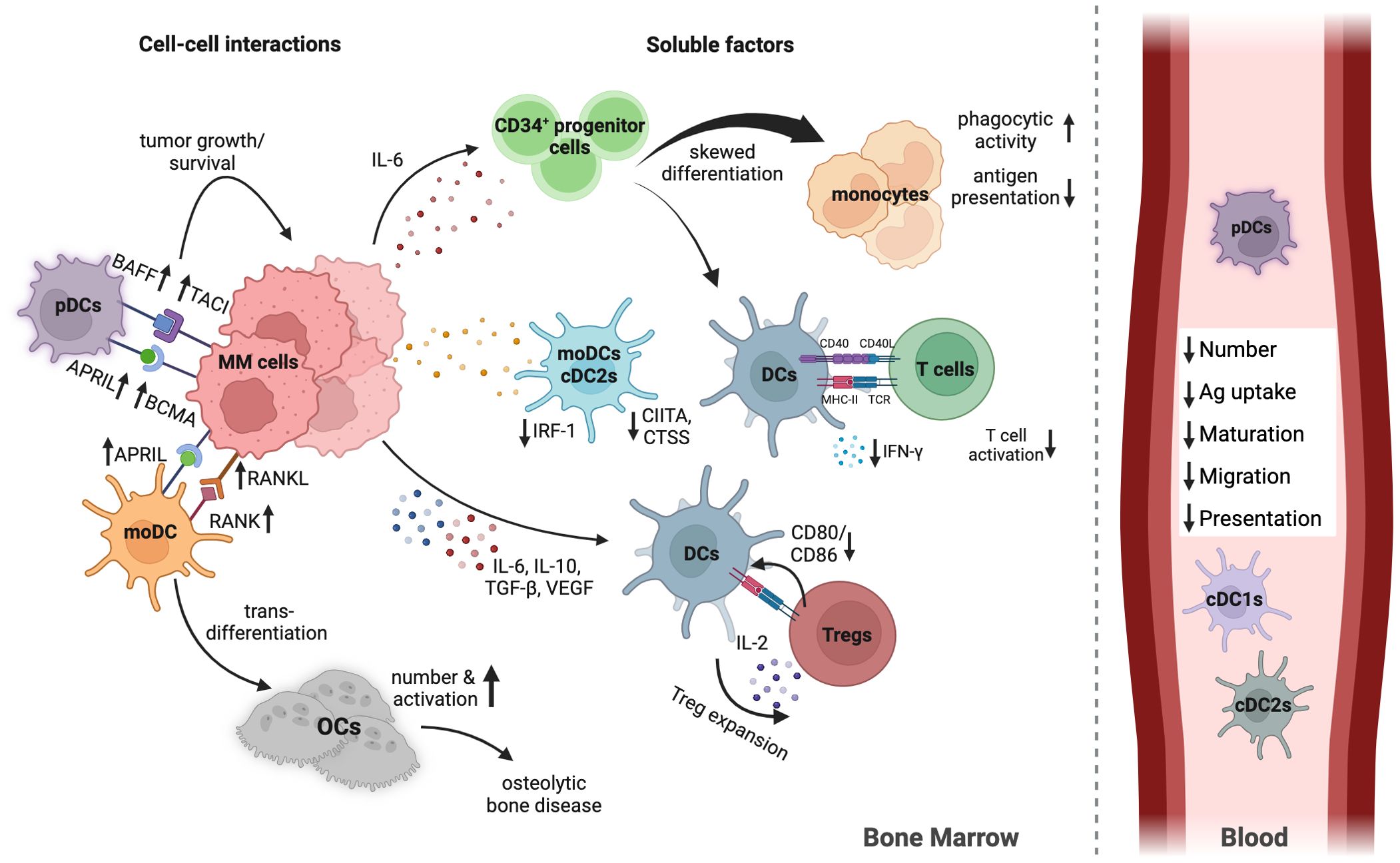
Figure 1. Human DC in MM BMME. This overview outlines the main mechanisms of DC impairment in the MM TME. Not all mechanisms are detailed for simplicity. MM cells limit the number, differentiation, maturation, and function of various DC subsets by secreting inhibiting cytokines and engaging directly with DCs. Elevated IL-6 shifts differentiation from CD34+ progenitor cells to monocytes rather than DCs. IL-10, VEGF, and TGF-β impair DC migration and maturation. DCs and MM cells communicate via TACI and APRIL, promoting tumor growth. The interaction between them increases RANKL expression on MM cells, while DCs express RANK, triggering DC transdifferentiation into osteoclasts, contributing to MM-related bone disease. mRNA expression of IRF-1, CIITA, and CTSS in moDCs and cDC2s decreases in MM, impairing maturation and antigen presentation pathways. pDCs are less effective at activating T cells and stimulating IFN-γ due to immunosuppressive factors in the TME. Additionally, DCs facilitate Treg expansion through direct contact with T cells and low CD80/CD86 co-stimulatory molecule expression. In the peripheral blood DC subsets are reduced in numbers, combined with less expression of migration and maturation markers, and reduced antigen uptake and presentation capacity. Created with Biorender.com.
4.1 Reduced DC numbers in the periphery of MM patients
DCs comprise 0.1-2.0% of peripheral blood mononuclear cells. Still, studies show a significant decrease in both cDCs and pDCs in MM patients compared to healthy individuals (49, 50). For instance, Do et al. noted a 50% reduction in blood DCs among MM patients (51). Similar DC depletion has been observed in other cancers and infections (52–57). Ratta et al. found that peripheral blood DCs are stable regardless of disease stage, with a trend for lower PBDCs in patients with more significant tumor burdens (37). Studies show fewer DCs in advanced disease stages in MM patients. While the reasons for these discrepancies are unclear, factors like sample collection variability and chemotherapy could affect DC counts. Nevertheless, there is a clear trend of declining DC numbers as MM progresses, yet studies on specific DC subsets in untreated MM patients are lacking.
During DC development, they differentiate from CD34+ hematopoietic stem cells through several stages. High IL-6 levels in the TME may skew differentiation towards monocytes instead of DCs, at least in GM-CSF cultures, potentially explaining reduced blood DC numbers in MM patients (37).
Research indicates increased pDC abundance in the BMME (24) but diminished in the PB (36). Moreover, MM progression from MGUS correlates with increased cDCs and pDCs in the BM, which can contribute to immunosuppression and tumor growth. However, they did not distinguish cDC1s from cDC2s, focusing only on CD141+ cDC1s, which have distinct roles and are fewer than cDC2s. Consequently, their response in MM may vary significantly (50).
A recent analysis of DC subsets post-BCMA CAR-T therapy revealed increased proportions of all cDC types except pDCs in the BMME (58). However, the variability in the patient cohort limits the findings. Overall, studies confirm significant reductions in conventional and pDCs in MM patients. These DCs impact T-cell responses and foster immune tolerance through immature DC interactions with myeloma cells.
4.2 Impaired DC activation and maturation in MM
DC number alterations due to immunosuppressive cytokines IL-6, IL-10, VEGF, and TGF-β1 in the TME of MM link to significant phenotypic changes in these cells. These changes indicate functional deficiencies affecting DC maturation, antigen presentation, and T-cell priming. Key markers like HLA-DR, CD40, CD80, and CD86 show lower expression on PB myeloid and pDCs from MM patients than healthy donors (36–38). Despite high phagocytic activity, antigen presentation capacity is low (37). Blocking IL-6 with anti-IL-6 antibodies does not fully restore this function, suggesting additional factors impair DC functions in MM (37). Brown et al. showed that IL-10 and TGF-β from myeloma cells hinder DC maturation by affecting CD80 and CD86 expression, which can be reverted using either IL-12 or IFN-γ (59, 60). DC maturation involves complex processes for efficient tumor antigen handling, regulated by interferon regulatory factor 1 (IRF-1). IRF-1 controls MHC class II transactivator (CIITA) for HLA class II expression and cathepsin S (CTSS) activity that facilitates antigen peptide binding (61). Recent findings by Jiang et al. indicate IRF-1 expression is low in cDC2s and mo-DCs of MM patients, leading to decreased CIITA and CTSS levels, harming maturation and antigen presentation capacity (62).
Additionally, Brimnes et al. reported reduced CCR5 and CCR7 expression in cDCs and pDCs during MM (36). CCR5 mediates immature DC migration to inflammation sites, while CCR7 indicates DC maturation, guiding them to lymph nodes for T cell priming (63). Impaired CCR5 and CCR7 expression further highlights defective DC maturation in MM. Shinde et al. linked impaired CCR7 migration to increased IL-6 levels and p38 activation in the MM TME. These observations reveal dysregulation in DC maturation in MM, suggesting complex mechanisms behind the immature DC phenotype warranting further exploration (64).
4.3 Impaired T cell priming by pDCs and moDCs in MM
DCs are essential for the immune system to effectively target and eliminate myeloma cells. They must maintain a balance of hematopoiesis, migration, maturation, and antigen processing to prime T-cell responses and expand T-cell clones that recognize and destroy malignant cells. However, studies show deficiencies in MM’s DC differentiation, maturation, and migration. This leads to a loss of tumor antigenicity and reduced T-cell priming, allowing MM to evade the immune response (24, 36, 37, 51, 60). In human MM models, pDCs and moDCs play critical roles, while cDCs remain underexplored. In mixed lymphocyte reactions, PB myeloid and pDCs from MM patients show reduced T cell proliferation. Brimnes et al. first note that they stimulate IFN-γ production by T cells much less than DCs from healthy individuals (36).
Similarly, moDCs from MM patients exhibit reduced co-stimulatory marker expression and impaired cytokine expression, like less IL-12p70 and increased IL-10, leading to reduced T cell activation and proliferation (64), indicating a tolerogenic DC phenotype.
Studies also highlight the DC-T cell interaction fostering Treg differentiation in the MM TME (65). In inflammatory conditions, DCs co-cultured with T cells expand functional Tregs, dampening T-cell responses (66, 67). Tregs may inhibit co-stimulatory molecule expression on DCs and reduce their pro-inflammatory cytokine release (68). These interactions suggest that expanding Tregs might exacerbate DC dysfunction in MM, as also shown in a mouse model of MM (69), although the precise mechanisms require further investigation.
Taken together, these studies indicate a tolerogenic DC phenotype in MM. MM-DCs exhibit functional traits similar to mregDCs (e.g., immunosuppressive cytokine profiles, impaired T cell priming), suggesting possible parallels. However, no direct evidence exists regarding the involvement of mregDCs or Lamp3+DCs in MM. Additional studies are necessary to assess these subsets in MM.
5 Role of DCs in MM-induced osteolytic bone disease
Most MM patients develop bone lesions due to excessive BM osteoclast (OC) formation, resulting from myeloma cell interactions with BM components (70, 71). This impacts OC and DC processes (72). Research shows that OC hyperactivity relates to DC dysfunction (73–76), stemming from their myeloid precursor relationship (77). Although some studies suggest OC precursors arise from monocyte/DC progenitors (MDPs), their exact nature is unclear (78). OC differentiation, or osteoclastogenesis, relies on macrophage colony-stimulating factor (M-CSF) and receptor activator of nuclear factor κB ligand (RANKL), activating T cells and DCs (45, 79). In MM, myeloma cells hinder normal osteoclastogenesis by recruiting, differentiating, and activating OC precursors in the BM (45, 73).
Additionally, interactions with immature DCs release cytokines like IL-6, increasing RANK-L and M-CSF production by stromal cells, osteoblasts, and myeloma cells (30, 45, 73, 75, 80). Elevated RANK-L in the BMME can convert DCs into OC-like cells via RANK-RANK-L interactions (76, 79). Supporting this, Tucci and colleagues confirmed that myeloma cells induce OC-like changes in moDCs (45). DCs also promote MM-induced osteolysis by overexpressing IL-17, produced by OCs and myeloma cells, as Th17 cells expand due to mature DCs (45, 81). This IL-17-rich environment enhances RANK-L activity, further stimulating osteoclastogenesis and osteolytic bone disease in MM and other cancers (81–84).
6 DC-based immunotherapies in MM
6.1 Current MM therapy
MM therapy advancements have significantly improved survival rates, with over 50% of patients surviving five years and 30% reaching ten years. Current standard treatment involves high-dose chemotherapy and hematopoietic stem-cell transplantation (HSCT). For newly diagnosed MM, a four-drug regimen including an anti-CD38 antibody, proteasome inhibitor, lenalidomide, and dexamethasone is widely used (85).
CAR T-cell therapy has revolutionized treatment for relapsed/refractory MM, mainly targeting BCMA (86, 87). Bispecific antibodies and immunocytokine therapies are emerging as promising options for relapsed cases. Bone-modifying agents like bisphosphonates and denosumab manage bone complications, while proteasome inhibitors and monoclonal antibodies remain essential (85).
For high-risk SMM, treatments such as daratumumab monotherapy or lenalidomide-based regimens delay disease progression (88, 89).
Many preclinical and clinical studies are investigating immunotherapeutic strategies, including DC-based vaccines, to enhance or restore the function of DCs in tumors (31, 32, 90).
6.2 DC-based vaccination
DC- based vaccines are promising in cancer immunotherapy. They activate tumor-specific T-cells to enhance cancer cell destruction (91). Their personalized nature allows customization based on individual tumor characteristics, offering a favorable safety profile with fewer immune-related adverse events than other immunotherapies (92). DC vaccines also induce long-lasting antitumor immunity, potentially reducing cancer recurrence and improving long-term outcomes. Additionally, they can convert “cold” tumors into “hot” ones, increasing T-cell infiltration and boosting the efficacy of subsequent treatments (91, 93, 94).
Despite these advantages, DC vaccines have shown limited clinical success due to challenges such as the immunosuppressive tumor microenvironment, manufacturing complexities, and suboptimal activation states of ex vivo-generated DCs (93). Most current vaccines rely on moDCs loaded with tumor-associated antigens (TAAs) or RNA (95). While moDCs are less efficient than natural DCs, they remain widely used due to their ease of production and scalability (96–98). Innovative strategies, such as loading DCs with a broad spectrum of antigens via tumor lysates or RNA electroporation, aim to enhance immune responses and minimize antigenic escape (99–102). However, as described above, mo-DCs derived from MM patients, exhibit a compromised functionality resembling tolerogenic DCs (64). This situation may hinder the efficacy of MM immunotherapy by fostering tolerance instead of immunity. To counteract this effect, strategies aimed at targeting tolerogenic DC-like dysfunction in MM—such as enhancing IL-12 production or inhibiting IL-10—could reflect tactics used against mregDCs in other cancers (103, 104).
Research is also exploring alternatives like DC-derived exosomes, which can carry cancer-specific antigens and stimulate robust immune responses while addressing some limitations of traditional DC vaccines (105, 106).
Despite the promise of DC-based vaccines, clinical results have varied, with only 5–15% of patients exhibiting significant immune responses and most experiencing relapses (94, 107). This limited efficacy stems from various immune escape and immunosuppressive mechanisms that foster therapy resistance.
6.3 Combinatory therapy
Consequently, considerable effort has been dedicated to transforming traditional DC vaccines into ‘next-generation’ versions that can counteract TME-related immunosuppression and bolster antitumor activity responses (108). Combination therapies have garnered significant interest in enhancing therapeutic effectiveness and tackling resistance by integrating DC vaccines with immune checkpoint inhibitors, CAR-T treatments, chemotherapy, and immunomodulatory agents (109, 110). Research indicates that modifying immune checkpoint molecules on DCs in vitro through siRNA, lentivirus, adenovirus, or CRISPR-Cas-9 boosts T-cell activation T cell activation (111). Similarly, Chu et al. demonstrated that combining DC vaccines with PD-L1 blockade effectively hinders tumor growth in MM models (112). Moreover, integrating DC vaccines with prior stem cell transplantation has led to an overall survival increase of nearly two years compared to patients who did not receive DC therapy (113, 114). Nonetheless, the optimal timing for DC immunotherapy continues to be debated, although evidence suggests that administering it following HSCT, when the disease burden is low, is preferable (115).
Efforts are ongoing to refine vaccine formulations, optimize antigen-loading techniques, and improve the in vitro generation of cDCs to mimic their natural counterparts better. These advancements aim to enhance the clinical effectiveness of DC-based vaccines and establish them as a cornerstone in cancer immunotherapy.
7 Discussion
This review explores DCs in MM, emphasizing their dual role in immune regulation and cancer progression. DCs, essential for antigen presentation and T-cell priming, are impaired in the MM tumor microenvironment (TME), contributing to immune tolerance and tumor growth. Understanding these dysfunctions and leveraging DCs as therapeutic targets remains a critical area of research.
DC dysfunction in MM involves reduced numbers, altered phenotypes, and impaired T-cell activation capacity. However, the molecular mechanisms driving these changes remain unclear, necessitating further investigation to identify therapeutic targets and restore DC function. Additionally, the heterogeneity of DC subsets—cDC1s, cDC2s, DC3s, pDCs, and moDCs—and their specific roles in MM progression require deeper exploration to develop targeted therapies.
The immunosuppressive nature of the MM TME poses significant challenges for DC-based therapies. Future strategies could include combination treatments targeting multiple immune pathways or enhancing DC-based vaccines’ efficacy through improved design and delivery methods. Personalized medicine approaches also promise to tailor DC vaccines to individual tumor profiles and immune statuses for better outcomes. This approach would require biomarker discovery and validation advancements to predict response to DC-based therapies and guide treatment decisions.
Another critical focus is long-term efficacy. Research should assess the durability of immune responses induced by DC therapies and explore maintenance strategies to sustain remission. Enhancing immunological memory against MM cells is key to improving survival rates.
Improving ex vivo DC manufacturing processes is vital for scaling up therapies. Alternatively, in vivo, approaches targeting DC activation directly in patients may overcome current limitations and improve treatment effectiveness.
In conclusion, while challenges remain, advancing our understanding of DC biology and MM pathogenesis could unlock the full potential of DC-based immunotherapies, offering hope for better outcomes in MM patients.
Author contributions
MJ: Writing – original draft, Visualization. JM: Visualization, Writing – review & editing. SA: Conceptualization, Funding acquisition, Resources, Writing – original draft, Writing – review & editing.
Funding
The author(s) declare that financial support was received for the research and/or publication of this article. This study was funded by the Deutsche José Carreras Leukämie-Stiftung (DJCLS 13 R/2021).
Conflict of interest
The authors declare that the research was conducted in the absence of any commercial or financial relationships that could be construed as a potential conflict of interest.
Generative AI statement
The author(s) declare that Generative AI was used in the creation of this manuscript.
Grammarly was used to edit and spell-check the manuscript.
Publisher’s note
All claims expressed in this article are solely those of the authors and do not necessarily represent those of their affiliated organizations, or those of the publisher, the editors and the reviewers. Any product that may be evaluated in this article, or claim that may be made by its manufacturer, is not guaranteed or endorsed by the publisher.
References
1. Rajkumar S. Multiple myeloma: 2024 update on diagnosis, risk-stratification, and management. Am J Hematol. (2024) 99:1802–24. doi: 10.1002/ajh.27422
2. Kyle RA, Therneau TM, Rajkumar SV, Larson DR, Plevak MF, Offord JR, et al. Prevalence of monoclonal gammopathy of undetermined significance. N Engl J Med. (2006) 354:1362–9. doi: 10.1056/nejmoa054494
3. Kyle RA, Remstein ED, Therneau TM, Dispenzieri A, Kurtin PJ, Hodnefield JM, et al. Clinical course and prognosis of smoldering (Asymptomatic) multiple myeloma. N Engl J Med. (2007) 356:2582–90. doi: 10.1056/nejmoa070389
4. Hemminki K, Försti A, Houlston R, Sud A. Epidemiology, genetics and treatment of multiple myeloma and precursor diseases. Int J Cancer. (2021) 149:1980–96. doi: 10.1002/ijc.v149.12
5. Wang C, Wang W, Wang M, Deng J, Sun C, Hu Y, et al. Different evasion strategies in multiple myeloma. Front Immunol. (2024) 15:1346211. doi: 10.3389/fimmu.2024.1346211
6. Collin M, Ginhoux F. Human dendritic cells. Semin Cell Dev Biol. (2019) 86:1–2. doi: 10.1016/j.semcdb.2018.04.015
7. Yu CI, Becker C, Metang P, Marches F, Wang Y, Toshiyuki H, et al. Human CD141+ Dendritic cells induce CD4+ T cells to produce type 2 cytokines. J Immunol. (2014) 193:4335–43. doi: 10.4049/jimmunol.1401159
8. Jongbloed SL, Kassianos AJ, McDonald KJ, Clark GJ, Ju X, Angel CE, et al. Human CD141+ (BDCA-3)+ dendritic cells (DCs) represent a unique myeloid DC subset that cross-presents necrotic cell antigens. J Exp Med. (2010) 207:1247–60. doi: 10.1084/jem.20092140
9. Segura E, Valladeau-Guilemond J, Donnadieu M-H, Sastre-Garau X, Soumelis V, Amigorena S. Characterization of resident and migratory dendritic cells in human lymph nodes. J Exp Med. (2012) 209:653–60. doi: 10.1084/jem.20111457
10. Rojas IML, Mok W-H, Pearson FE, Minoda Y, Kenna TJ, Barnard RT, et al. Human blood CD1c+ Dendritic cells promote th1 and th17 effector function in memory CD4+ T cells. Front Immunol. (2017) 8:971. doi: 10.3389/fimmu.2017.00971
11. Liu Z, Wang H, Li Z, Dress RJ, Zhu Y, Zhang S, et al. Dendritic cell type 3 arises from Ly6C+ monocyte-dendritic cell progenitors. Immunity. (2023) 56:1761–1777.e6. doi: 10.1016/j.immuni.2023.07.001
12. Dutertre CA, Becht E, Irac SE, Khalilnezhad A, Narang V, Khalilnezhad S, et al. Single-cell analysis of human mononuclear phagocytes reveals subset-defining markers and identifies circulating inflammatory dendritic cells. Immunity. (2019) 51:573–589.e8. doi: 10.1016/j.immuni.2019.08.008
13. Reizis B. Plasmacytoid dendritic cells: Development, regulation, and function. Immunity. (2019) 50:37–50. doi: 10.1016/j.immuni.2018.12.027
14. Cabeza-Cabrerizo M, Cardoso A, Minutti CM, Pereira da Costa M, Reis e Sousa C. Dendritic cells revisited. Annu Rev Immunol. (2021) 39:131–66. doi: 10.1146/annurev-immunol-061020-053707
15. Liu J, Zhang X, Cheng Y, Cao X. Dendritic cell migration in inflammation and immunity. Cell Mol Immunol. (2021) 18:2461–71. doi: 10.1038/s41423-021-00726-4
16. Prete AD, Salvi V, Soriani A, Laffranchi M, Sozio F, Bosisio D, et al. Dendritic cell subsets in cancer immunity and tumor antigen sensing. Cell Mol Immunol. (2023) 20:432–47. doi: 10.1038/s41423-023-00990-6
17. Zheng L, Duan Y, He P, Wu M, Wei S, Du X, et al. Dysregulated dendritic cells in sepsis: Functional impairment and regulated cell death. Cell Mol Biol Lett. (2024) 29:81. doi: 10.1186/s11658-024-00602-9
18. Gubin MM, Vesely MD. Cancer immunoediting in the era of immuno-oncology. Clin Cancer Res. (2022) 28:3917–28. doi: 10.1158/1078-0432.ccr-21-1804
19. O’Donnell JS, Teng MWL, Smyth MJ. Cancer immunoediting and resistance to T cell-based immunotherapy. Nat Rev Clin Oncol. (2019) 16:151–67. doi: 10.1038/s41571-018-0142-8
20. McCoach CE, Bivona TG. The evolving understanding of immunoediting and the clinical impact of immune escape. J Thorac Dis. (2018) 10:1248–52. doi: 10.21037/jtd.2018.03.60
21. Koebel CM, Vermi W, Swann JB, Zerafa N, Rodig SJ, Old LJ, et al. Adaptive immunity maintains occult cancer in an equilibrium state. Nature. (2007) 450:903–7. doi: 10.1038/nature06309
22. Chng WJ, Santana-Dávila R, Wier SAV, Ahmann GJ, Jalal SM, Bergsagel PL, et al. Prognostic factors for hyperdiploid-myeloma: Effects of chromosome 13 deletions and IgH translocations. Leukemia. (2006) 20:807–13. doi: 10.1038/sj.leu.2404172
23. Anderson NM, Simon MC. The tumor microenvironment. Curr Biol. (2020) 30:R921–r925. doi: 10.1016/j.cub.2020.06.081
24. Chauhan D, Singh AV, Brahmandam M, Carrasco R, Bandi M, Hideshima T, et al. Functional interaction of plasmacytoid dendritic cells with multiple myeloma cells: A therapeutic target. Cancer Cell. (2009) 16:309–23. doi: 10.1016/j.ccr.2009.08.019
25. Tibullo D, Longo A, Vicario N, Romano A, Barbato A, Rosa MD, et al. Ixazomib Improves Bone Remodeling and Counteracts sonic Hedgehog signaling Inhibition Mediated by Myeloma Cells. Cancers (Basel). (2020) 12:323. doi: 10.3390/cancers12020323
26. Noll JE, Williams SA, Tong CM, Wang H, Quach JM, Purton LE, et al. Myeloma plasma cells alter the bone marrow microenvironment by stimulating the proliferation of mesenchymal stromal cells. Haematologica. (2014) 99:163–71. doi: 10.3324/haematol.2013.090977
27. Perez C, Botta C, Zabaleta A, Puig N, Cedena M-T, Goicoechea I, et al. Immunogenomic identification and characterization of granulocytic myeloid-derived suppressor cells in multiple myeloma. Blood. (2020) 136:199–209. doi: 10.1182/blood.2019004537
28. Holthof LC, Mutis T. Challenges for immunotherapy in multiple myeloma: Bone marrow microenvironment-mediated immune suppression and immune resistance. Cancers (Basel). (2020) 12:988. doi: 10.3390/cancers12040988
29. Lopes R, Caetano J, Ferreira B, Barahona F, Carneiro EA, João C. The immune microenvironment in multiple myeloma: Friend or foe? Cancers (Basel). (2021) 13:625. doi: 10.3390/cancers13040625
30. Kukreja A, Hutchinson A, Dhodapkar K, Mazumder A, Vesole D, Angitapalli R, et al. Enhancement of clonogenicity of human multiple myeloma by dendritic cells. J Exp Med. (2006) 203:1859–65. doi: 10.1084/jem.20052136
31. Xiao Z, Wang R, Wang X, Yang H, Dong J, He X, et al. Impaired function of dendritic cells within the tumor microenvironment. Front Immunol. (2023) 14:1213629. doi: 10.3389/fimmu.2023.1213629
32. Chen J, Duan Y, Che J, Zhu J. Dysfunction of dendritic cells in tumor microenvironment and immunotherapy. Cancer Commun. (2024) 44:1047–70. doi: 10.1002/cac2.12596
33. Zelenay S, Keller AM, Whitney PG, Schraml BU, Deddouche S, Rogers NC, et al. The dendritic cell receptor DNGR-1 controls endocytic handling of necrotic cell antigens to favor cross-priming of CTLs in virus-infected mice. J Clin Invest. (2012) 122:1615–27. doi: 10.1172/JCI60644
34. Janco JMT, Lamichhane P, Karyampudi L, Knutson KL. Tumor-infiltrating dendritic cells in cancer pathogenesis. J Immunol. (2015) 194:2985–91. doi: 10.4049/jimmunol.1403134
35. Maier B, Leader AM, Chen ST, Tung N, Chang C, LeBerichel J, et al. A conserved dendritic-cell regulatory program limits antitumor immunity. Nature. (2020) 580:257–62. doi: 10.1038/s41586-020-2134-y
36. Brimnes MK, Svane IM, Johnsen HE. Impaired functionality and phenotypic profile of dendritic cells from patients with multiple myeloma. Clin Exp Immunol. (2006) 144:76–84. doi: 10.1111/j.1365-2249.2006.03037.x
37. Ratta M, Fagnoni F, Curti A, Vescovini R, Sansoni P, Oliviero B, et al. Dendritic cells are functionally defective in multiple myeloma: the role of interleukin-6. Blood. (2002) 100:230–7. doi: 10.1182/blood.v100.1.230
38. Hayashi T. Ex vivo induction of multiple myeloma-specific cytotoxic T lymphocytes. Blood. (2003) 102:1435–42. doi: 10.1182/blood-2002-09-2828
39. Tai Y-T, Dulos J, Guelen L, Lin L, Xing L, Cho S-F, et al. APRIL is significantly elevated at all stages of multiple myeloma (MM) and interferes with anti-bcma monoclonal antibody-mediated cytolysis, supporting the clinical evaluation of bion-1301 as a novel therapeutic approach in MM. Blood. (2018) 132:3209. doi: 10.1182/blood-2018-99-117296
40. Moreaux J, Sprynski A-C, Dillon SR, Mahtouk K, Jourdan M, Ythier A, et al. APRIL and TACI interact with syndecan-1 on the surface of multiple myeloma cells to form an essential survival loop. Eur J Hematol. (2009) 83:119–29. doi: 10.1111/j.1600-0609.2009.01262.x
41. Moreaux J, Cremer FW, Reme T, Raab M, Mahtouk K, Kaukel P, et al. The level of TACI gene expression in myeloma cells is associated with a signature of microenvironment dependence versus a plasmablastic signature. Blood. (2005) 106:1021–30. doi: 10.1182/blood-2004-11-4512
42. Schuh E, Musumeci A, Thaler FS, Laurent S, Ellwart JW, Hohlfeld R, et al. Human plasmacytoid dendritic cells display and shed B cell maturation antigen upon TLR engagement. J Immunol. (2017) 198:3081–8. doi: 10.4049/jimmunol.1601746
43. Musolino C, Allegra A, Innao V, Allegra AG, Pioggia G, Gangemi S. Inflammatory and anti-inflammatory equilibrium, proliferative and antiproliferative balance: the role of cytokines in multiple myeloma. Mediators Inflam. (2017) 2017:1852517. doi: 10.1155/2017/1852517
44. Melaccio A, Reale A, Saltarella I, Desantis V, Lamanuzzi A, Cicco S, et al. Pathways of angiogenic and inflammatory cytokines in multiple myeloma: Role in plasma cell clonal expansion and drug resistance. J Clin Med. (2022) 11:6491. doi: 10.3390/jcm11216491
45. Tucci M, Stucci S, Strippoli S, Dammacco F, Silvestris F. Dendritic cells and Malignant plasma cells: an alliance in multiple myeloma tumor progression? Oncol. (2011) 16:1040–8. doi: 10.1634/theoncologist.2010-0327
46. Kowalczyk A, D’Souza CA, Zhang L. Cell-extrinsic CTLA4-mediated regulation of dendritic cell maturation depends on STAT3. Eur J Immunol. (2014) 44:1143–55. doi: 10.1002/eji.201343601
47. Ohno Y, Kitamura H, Takahashi N, Ohtake J, Kaneumi S, Sumida K, et al. IL-6 down-regulates HLA class II expression and IL-12 production of human dendritic cells to impair activation of antigen-specific CD4+ T cells. Cancer Immunol. IO. (2016) 65:193–204. doi: 10.1007/s00262-015-1791-4
48. Park S-J, Nakagawa T, Kitamura H, Atsumi T, Kamon H, Sawa S, et al. IL-6 regulates in vivo dendritic cell differentiation through STAT3 activation1. J Immunol. (2004) 173:3844–54. doi: 10.4049/jimmunol.173.6.3844
49. Martín-Ayuso M, Almeida J, Pérez-Andrés M, Cuello R, Galende J, González-Fraile MI, et al. Peripheral blood dendritic cell subsets from patients with monoclonal gammopathies show an abnormal distribution and are functionally impaired. Oncol. (2008) 13:82–92. doi: 10.1634/theoncologist.2007-0127
50. Leone P, Berardi S, Frassanito MA, Ria R, Re VD, Cicco S, et al. Dendritic cells accumulate in the bone marrow of myeloma patients where they protect tumor plasma cells from CD8+ T-cell killing. Blood. (2015) 126:1443–51. doi: 10.1182/blood-2015-01-623975
51. Do TH, Johnsen HE, Kjærsgaard E, Taaning E, Svane IM. Impaired circulating myeloid DCs from myeloma patients. Cytotherapy. (2004) 6:196–203. doi: 10.1080/14653240410006004
52. Autenrieth SE, Linzer T-R, Hiller C, Keller B, Warnke P, Köberle M, et al. Immune evasion by yersinia enterocolitica: Differential targeting of dendritic cell subpopulations In Vivo. PLoS Pathog. (2010) 6:e1001212. doi: 10.1371/journal.ppat.1001212
53. Bieber K, Autenrieth SE. Dendritic cell development in infection. Mol Immunol. (2020) 121:111–7. doi: 10.1016/j.molimm.2020.02.015
54. Pérez-Gómez A, Vitallé J, Gasca-Capote C, Gutierrez-Valencia A, Trujillo-Rodriguez M, Serna-Gallego A, et al. Dendritic cell deficiencies persist seven months after SARS-CoV-2 infection. Cell Mol Immunol. (2021) 18:2128–39. doi: 10.1038/s41423-021-00728-2
55. Sawant A, Hensel JA, Chanda D, Harris BA, Siegal GP, Maheshwari A, et al. Depletion of plasmacytoid dendritic cells inhibits tumor growth and prevents bone metastasis of breast cancer cells. J Immunol. (2012) 189:4258–65. doi: 10.4049/jimmunol.1101855
56. Huarte E, Cubillos-Ruiz JR, Nesbeth YC, Scarlett UK, Martinez DG, Buckanovich RJ, et al. Depletion of dendritic cells delays ovarian cancer progression by boosting antitumor immunity. Cancer Res. (2008) 68:7684–91. doi: 10.1158/0008-5472.can-08-1167
57. Teijeira A, Garasa S, Luri-Rey C, de Andrea C, Gato M, Molina C, et al. Depletion of conventional type-1 dendritic cells in established tumors suppresses immunotherapy efficacy. Cancer Res. (2022) 82:4373–85. doi: 10.1158/0008-5472.can-22-1046
58. Yang Y, Qin S, Yang M, Wang T, Feng R, Zhang C, et al. Reconstitution of the multiple myeloma microenvironment following lymphodepletion with BCMA CAR-T therapy. Clin Cancer Res. (2024) 30:4201–14. doi: 10.1158/1078-0432.ccr-24-0352
59. Brown R, Murray A, Pope B, Sze DM, Gibson J, Ho PJ, et al. Either interleukin-12 or interferon-γ can correct the dendritic cell defect induced by transforming growth factor β1 in patients with myeloma. Br J Hematol. (2004) 125:743–8. doi: 10.1111/j.1365-2141.2004.04984.x
60. Brown RD, Pope B, Murray A, Esdale W, Sze DM, Gibson J, et al. Dendritic cells from patients with myeloma are numerically normal but functionally defective as they fail to up-regulate CD80 (B7-1) expression after huCD40LT stimulation because of inhibition by transforming growth factor-β1 and interleukin-10. Blood. (2001) 98:2992–8. doi: 10.1182/blood.v98.10.2992
61. Smyth P, Sasiwachirangkul J, Williams R, Scott CJ. Cathepsin S (CTSS) activity in health and disease - A treasure trove of untapped clinical potential. Mol Asp Med. (2022) 88:101106. doi: 10.1016/j.mam.2022.101106
62. Jiang J, Xiang J, Chen M, Wan Y, Zhong L, Han X, et al. Distinct mechanisms of dysfunctional antigen-presenting DCs and monocytes by single-cell sequencing in multiple myeloma. Cancer Sci. (2023) 114:2750–60. doi: 10.1111/cas.15800
63. Worbs T, Hammerschmidt SI, Förster R. Dendritic cell migration in health and disease. Nat Rev Immunol. (2017) 17:30–48. doi: 10.1038/nri.2016.116
64. Shinde P, Fernandes S, Melinkeri S, Kale V, Limaye L. Compromised functionality of monocyte-derived dendritic cells in multiple myeloma patients may limit their use in cancer immunotherapy. Sci Rep. (2018) 8:5705. doi: 10.1038/s41598-018-23943-w
65. Beyer M, Kochanek M, Giese T, Endl E, Weihrauch MR, Knolle PA, et al. In vivo peripheral expansion of naive CD4+CD25high FoxP3 + regulatory T cells in patients with multiple myeloma. Blood. (2006) 107:3940–9. doi: 10.1182/blood-2005-09-3671
66. Ghiringhelli F, Puig PE, Roux S, Parcellier A, Schmitt E, Solary E, et al. Tumor cells convert immature myeloid dendritic cells into TGF-beta-secreting cells inducing CD4+CD25+ regulatory T cell proliferation. J Exp Med. (2005) 202:919–29. doi: 10.1084/jem.20050463
67. Banerjee DK, Dhodapkar MV, Matayeva E, Steinman RM, Dhodapkar KM. Expansion of FOXP3high regulatory T cells by human dendritic cells (DCs) in vitro and after injection of cytokine-matured DCs in myeloma patients. Blood. (2006) 108:2655–61. doi: 10.1182/blood-2006-03-011353
68. Chen X, Du Y, Hu Q, Huang Z. Tumor-derived CD4+CD25+regulatory T cells inhibit dendritic cells function by CTLA-4. Pathol - Res Pract. (2017) 213:245–9. doi: 10.1016/j.prp.2016.12.008
69. Suzuki S, Komiya K, Tsuda S, Yoshino M, Kaisho T, Bergsagel PL, et al. Type I-conventional dendritic cells support the progression of multiple myeloma in the bone marrow. Front Immunol. (2024) 15:1444821. doi: 10.3389/fimmu.2024.1444821
70. Lambert L, Ourednicek P, Meckova Z, Gavelli G, Straub J, Spicka I. Whole-body low-dose computed tomography in multiple myeloma staging: Superior diagnostic performance in the detection of bone lesions, vertebral compression fractures, rib fractures and extraskeletal findings compared to radiography with similar radiation exposure. Oncol Lett. (2017) 13:2490–4. doi: 10.3892/ol.2017.5723
71. Wolf MB, Murray F, Kilk K, Hillengass J, Delorme S, Heiss C, et al. Sensitivity of whole-body CT and MRI versus projection radiography in the detection of osteolyses in patients with monoclonal plasma cell disease. Eur J Radiol. (2014) 83:1222–30. doi: 10.1016/j.ejrad.2014.02.008
72. Ormond Filho AG, Carneiro BC, Pastore D, Silva IP, Yamashita SR, Consolo FD, et al. Whole-body imaging of multiple myeloma: Diagnostic criteria. RadioGraphics. (2019) 39:1077–97. doi: 10.1148/rg.2019180096
73. Mundy GR. Myeloma bone disease. Eur J Cancer. (1998) 34:246–51. doi: 10.1016/s0959-8049(97)10133-2
74. Alnaeeli M, Park J, Mahamed D, Penninger JM, Teng YA. Dendritic cells at the osteo-immune interface: Implications for inflammation-induced bone loss1*. J Bone Mineral Res. (2009) 22:775–80. doi: 10.1359/jbmr.070314
75. Kukreja A, Radfar S, Sun B-H, Insogna K, Dhodapkar MV. Dominant role of CD47–thrombospondin-1 interactions in myeloma-induced fusion of human dendritic cells: Implications for bone disease. Blood. (2009) 114:3413–21. doi: 10.1182/blood-2009-03-211920
76. Tucci M, Stucci S, Savonarola A, Ciavarella S, Cafforio P, Dammacco F, et al. Immature dendritic cells in multiple myeloma are prone to osteoclast-like differentiation through interleukin-17A stimulation. Br J Hematol. (2013) 161:821–31. doi: 10.1111/bjh.12333
77. Charles JF, Hsu LY, Niemi EC, Weiss A, Aliprantis AO, Nakamura MC. Inflammatory arthritis increases mouse osteoclast precursors with myeloid suppressor function. J Clin Invest. (2012) 122:4592–605. doi: 10.1172/jci60920
78. Tsai J, Kaneko K, Suh AJ, Bockman R, Park-Min K-H. Origin of osteoclasts: Osteoclast precursor cells. J Bone Metab. (2023) 30:127–40. doi: 10.11005/jbm.2023.30.2.127
79. Rivollier A, Mazzorana M, Tebib J, Piperno M, Aitsiselmi T, Rabourdin-Combe C, et al. Immature dendritic cell transdifferentiation into osteoclasts: a novel pathway sustained by the rheumatoid arthritis microenvironment. Blood. (2004) 104:4029–37. doi: 10.1182/blood-2004-01-0041
80. Abe M, Hiura K, Wilde J, Shioyasono A, Moriyama K, Hashimoto T, et al. Osteoclasts enhance myeloma cell growth and survival via cell-cell contact: a vicious cycle between bone destruction and myeloma expansion. Blood. (2004) 104:2484–91. doi: 10.1182/blood-2003-11-3839
81. Dhodapkar KM, Barbuto S, Matthews P, Kukreja A, Mazumder A, Vesole D, et al. Dendritic cells mediate the induction of polyfunctional human IL17-producing cells (Th17-1 cells) enriched in the bone marrow of patients with myeloma. Blood. (2008) 112:2878–85. doi: 10.1182/blood-2008-03-143222
82. Kotake S, Udagawa N, Takahashi N, Matsuzaki K, Itoh K, Ishiyama S, et al. IL-17 in synovial fluids from patients with rheumatoid arthritis is a potent stimulator of osteoclastogenesis. J Clin Invest. (1999) 103:1345–52. doi: 10.1172/jci5703
83. Kryczek I, Banerjee M, Cheng P, Vatan L, Szeliga W, Wei S, et al. Phenotype, distribution, generation, and functional and clinical relevance of Th17 cells in the human tumor environments. Blood. (2009) 114:1141–9. doi: 10.1182/blood-2009-03-208249
84. Wang X, Sun B, Wang Y, Gao P, Song J, Chang W, et al. Research progress of targeted therapy regulating Th17/Treg balance in bone immune diseases. Front Immunol. (2024) 15:1333993. doi: 10.3389/fimmu.2024.1333993
85. Khatib HHE, Abdulla K, Nassar LK, Ellabban MG, Kakarougkas A. Advancements in multiple myeloma therapies: A comprehensive review by disease stage. Lymphatics. (2025) 3:2. doi: 10.3390/lymphatics3010002
86. Sheykhhasan M, Ahmadieh-Yazdi A, Vicidomini R, Poondla N, Tanzadehpanah H, Dirbaziyan A, et al. CAR T therapies in multiple myeloma: Unleashing the future. Cancer Gene Ther. (2024) 31:667–86. doi: 10.1038/s41417-024-00750-2
87. Rasche L, Hudecek M, Einsele H. CAR T-cell therapy in multiple myeloma: Mission accomplished? Blood. (2024) 143:305–10. doi: 10.1182/blood.2023021221
88. Rajkumar SV, Kumar S, Lonial S, Mateos MV. Smoldering multiple myeloma current treatment algorithms. Blood Cancer J. (2022) 12:129. doi: 10.1038/s41408-022-00719-0
89. Dimopoulos MA, Voorhees PM, Schjesvold F, Cohen YC, Hungria V, Sandhu I, et al. Daratumumab or active monitoring for high-risk smoldering multiple myeloma. N Engl J Med. (2024) 0. doi: 10.1056/nejmoa2409029
90. Han J, Wang H. Cytokine-overexpressing dendritic cells for cancer immunotherapy. Exp Mol Med. (2024) 56:2559–68. doi: 10.1038/s12276-024-01353-5
91. Bol KF, Schreibelt G, Gerritsen WR, de Vries IJM, Figdor CG. Dendritic cell–based immunotherapy: State of the art and beyond. Clin Cancer Res. (2016) 22:1897–906. doi: 10.1158/1078-0432.ccr-15-1399
92. Kamigaki T, Takimoto R, Okada S, Ibe H, Oguma ERI, Goto S. Personalized dendritic-cell-based vaccines targeting cancer neoantigens. Anticancer Res. (2024) 44:3713. doi: 10.21873/anticanres.17196
93. Sánchez-León ML, Jiménez-Cortegana C, Cabrera G, Vermeulen EM, de la Cruz-Merino L, Sánchez-Margalet V. The effects of dendritic cell-based vaccines in the tumor microenvironment: Impact on myeloid-derived suppressor cells. Front Immunol. (2022) 13:1050484. doi: 10.3389/fimmu.2022.1050484
94. Fu C, Zhou L, Mi QS, Jiang A. DC-based vaccines for cancer immunotherapy. Vaccines (Basel). (2020) 8:706. doi: 10.3390/vaccines8040706
95. Hopewell EL, Cox C. Manufacturing dendritic cells for immunotherapy: Monocyte enrichment. Mol Ther Methods Clin Dev. (2020) 16:155–60. doi: 10.1016/j.omtm.2019.12.017
96. Massa C, Thomas C, Wang E, Marincola F, Seliger B. Different maturation cocktails provide dendritic cells with different chemoattractive properties. J Trans Med. (2015) 13:175. doi: 10.1186/s12967-015-0528-7
97. Laureano RS, Sprooten J, Vanmeerbeerk I, Borras DM, Govaerts J, Naulaerts S, et al. Trial watch: Dendritic cell (DC)-based immunotherapy for cancer. OncoImmunology. (2022) 11:2096363. doi: 10.1080/2162402x.2022.2096363
98. Lee KW, Yam JWP, Mao X. Dendritic cell vaccines: A shift from conventional approach to new generations. Cells. (2023) 12:2147. doi: 10.3390/cells12172147
99. Verheye E, Melgar JB, Deschoemaeker S, Raes G, Maes A, Bruyne ED, et al. Dendritic cell-based immunotherapy in multiple myeloma: Challenges, opportunities, and future directions. Int J Mol Sci. (2022) 23:904. doi: 10.3390/ijms23020904
100. Zahradova L, Mollova K, Ocadlikova D, Kovarova L, Adam Z, Krejci M, et al. Efficacy and safety of Id-protein-loaded dendritic cell vaccine in patients with multiple myeloma–phase II study results. Neoplasma. (2012) 59:440–9. doi: 10.4149/neo_2012_057
101. Vasileiou S, Baltadakis I, Delimpasi S, Karatza M-H, Liapis K, Garofalaki M, et al. Ex vivo induction of multiple myeloma-specific immune responses by monocyte-derived dendritic cells following stimulation by whole-tumor antigen of autologous myeloma cells. J Immunother. (2017) 40:253–64. doi: 10.1097/cji.0000000000000182
102. Rosenblatt J, Avivi I, Vasir B, Uhl L, Munshi NC, Katz T, et al. Vaccination with dendritic cell/tumor fusions following autologous stem cell transplant induces immunologic and clinical responses in multiple myeloma patients. Clin Cancer Res. (2013) 19:3640–8. doi: 10.1158/1078-0432.ccr-13-0282
103. Shinde P, Melinkeri S, Santra MK, Kale V, Limaye L. Autologous hematopoietic stem cells are a preferred source to generate dendritic cells for immunotherapy in multiple myeloma patients. Front Immunol. (2019) 10:1079. doi: 10.3389/fimmu.2019.01079
104. Li J, Zhou J, Huang H, Jiang J, Zhang T, Ni C. Mature dendritic cells enriched in immunoregulatory molecules (mregDCs): A novel population in the tumor microenvironment and immunotherapy target. Clin Trans Med. (2023) 13:e1199. doi: 10.1002/ctm2.1199
105. Zitvogel L, Regnault A, Lozier A, Wolfers J, Flament C, Tenza D, et al. Eradication of established murine tumors using a novel cell-free vaccine: Dendritic cell derived exosomes. Nat Med. (1998) 4:594–600. doi: 10.1038/nm0598-594
106. Tran T-H, Mattheolabakis G, Aldawsari H, Amiji M. Exosomes as nanocarriers for immunotherapy of cancer and inflammatory diseases. Clin Immunol. (2015) 160:46–58. doi: 10.1016/j.clim.2015.03.021
107. Fu C, Ma T, Zhou L, Mi Q-S, Jiang A. Dendritic cell-based vaccines against cancer: Challenges, advances and future opportunities. Immunol Investig. (2022) 51:2133–58. doi: 10.1080/08820139.2022.2109486
108. Sprooten J, Garg AD. Next-generation DC vaccines with an immunogenic trajectory against cancer: Therapeutic opportunities vs. resistance mechanisms. Genes Immun. (2024). doi: 10.1038/s41435-024-00294-3
109. Zhang Y, Tang W, Li Y, Yi Y, Yu Z, Liu X, et al. A systematic review on performance analysis of critical time points in multiple myeloma treated by CAR-T cell immunotherapy. Int Immunopharmacol. (2023) 114:109592. doi: 10.1016/j.intimp.2022.109592
110. Ghorbaninezhad F, Asadzadeh Z, Masoumi J, Mokhtarzadeh A, Kazemi T, Aghebati-Maleki L, et al. Dendritic cell-based cancer immunotherapy in the era of immune checkpoint inhibitors: From bench to bedside. Life Sci. (2022) 297:120466. doi: 10.1016/j.lfs.2022.120466
111. Ding J, Zheng Y, Wang G, Zheng J, Chai D. The performance and perspectives of dendritic cell vaccines modified by immune checkpoint inhibitors or stimulants. Biochim Biophys Acta (BBA) - Rev Cancer. (1877) 2022):188763. doi: 10.1016/j.bbcan.2022.188763
112. Chu T-H, Vo M-C, Park H-S, Lakshmi TJ, Jung S-H, Kim H-J, et al. Potent anti-myeloma efficacy of dendritic cell therapy in combination with pomalidomide and programmed death-ligand 1 blockade in a preclinical model of multiple myeloma. Cancer Immunol Immunother. (2021) 70:31–45. doi: 10.1007/s00262-020-02654-0
113. Rosenblatt J, Vasir B, Uhl L, Blotta S, MacNamara C, Somaiya P, et al. Vaccination with dendritic cell/tumor fusion cells results in cellular and humoral antitumor immune responses in patients with multiple myeloma. Blood. (2011) 117:393–402. doi: 10.1182/blood-2010-04-277137
114. Lacy MQ, Mandrekar S, Dispenzieri A, Hayman S, Kumar S, Buadi F, et al. Idiotype-pulsed antigen presenting cells following autologous transplantation for multiple myeloma may be associated with prolonged survival. Am J Hematol. (2009) 84:799–802. doi: 10.1002/ajh.21560
Keywords: dendritic cells, multiple myeloma, immune evasion, DC vaccine therapy, tumor microenvironment
Citation: Jordan MA, Morschl J and Autenrieth SE (2025) Dendritic cells in multiple myeloma: from immune evasion to therapeutic potential. Front. Immunol. 16:1575509. doi: 10.3389/fimmu.2025.1575509
Received: 12 February 2025; Accepted: 02 April 2025;
Published: 17 April 2025.
Edited by:
Domenico Galati, IRCCS, Fondazione G. Pascale, ItalyReviewed by:
Rao Prabhala, Harvard Medical School, United StatesKoji Tokoyoda, Tottori University, Japan
Copyright © 2025 Jordan, Morschl and Autenrieth. This is an open-access article distributed under the terms of the Creative Commons Attribution License (CC BY). The use, distribution or reproduction in other forums is permitted, provided the original author(s) and the copyright owner(s) are credited and that the original publication in this journal is cited, in accordance with accepted academic practice. No use, distribution or reproduction is permitted which does not comply with these terms.
*Correspondence: Stella E. Autenrieth, c3RlbGxhLmF1dGVucmlldGhAZGtmei5kZQ==
†ORCID: Melanie Andrea, orcid.org/0009-0005-2992-2943
Johannes Morschl, orcid.org/0009-0009-2449-1864
Stella E. Autenrieth, orcid.org/0000-0003-4054-9041