- 1Center of Clinical Laboratory Medicine, Zhongda Hospital, Southeast University, Nanjing, Jiangsu, China
- 2Department of Laboratory Medicine, Medical School of Southeast University, Nanjing, Jiangsu, China
Sepsis, a life-threatening organ dysfunction resulting from a dysregulated host response to infection, initiates a complex immune response that varies over time, characterized by sustained excessive inflammation and immunosuppression. Sepsis-induced immunosuppression is now recognized as a major cause of septic death, and identifying effective strategies to counteract it poses a significant challenge. This immunosuppression results from the disruption of immune homeostasis, characterized by the abnormal death of immune effector cells, hyperproliferation of immune suppressor cells, release of anti-inflammatory cytokines, and expression of immune checkpoints. Preclinical studies targeting immunosuppression, particularly with immune checkpoint inhibitors, have shown promise in reversing immunocyte dysfunctions and establishing host resistance to pathogens. Here, our review highlights the mechanisms of sepsis-induced immunosuppression and current diagnostic biomarkers, as well as immune-enhancing strategies evaluated in septic patients and therapeutics under investigation.
1 Introduction
Sepsis is a life-threatening organ dysfunction resulting from a dysregulated host response to infection (1). About 48.9 million cases of sepsis were recorded globally in 2017, of which 11 million patients died of sepsis, accounting for 19.7% of global deaths (2). Although the mortality rate of sepsis has declined globally in recent years, it remains one of the most significant medical problems worldwide (3). In addition, the pathogenesis of sepsis has not been fully clarified, and clinical treatment still relies on physical therapies such as controlling infection and maintaining organ function, which results in a long treatment process and poor prognosis. Therefore, understanding the immune regulatory mechanisms of sepsis are crucial for the prevention and treatment of the disease.
The immune status of septic patients changes dynamically at different stages, involving complex interactions between multiple immune cells and molecular mechanisms (4). When invade, pathogens or pathogen-associated molecular patterns (PAMPs), damage-associated molecular patterns (DAMPs) may recognize pattern recognition receptors (PRRs) like RIG-I-like receptors (RLRs), Toll-like receptors (TLRs), and NOD-like receptors (NLRs), et al, on innate immune cells, triggering the innate immune responses characterized by the secretion of amounts of cytokines and chemokines, accompanied by the activation of the coagulation and complement system. An uncontrolled inflammatory response (cytokine storm) or worse still, multiple organ dysfunction syndrome (MODs) may occur as a consequence (5). If the immune system clears pathogens promptly during the early systemic inflammatory response, immune balance can be rapidly restored. However, if pathogens are not removed in time, they can lead to long-term immunosuppression, immune collapse, and even physical disabilities in patients, known as immune-suppression (6) (Figure 1). In the context of sepsis, the immune status alterations across various stages are intricate. A complex interplay between hyperinflammation and immunosuppression can manifest either sequentially or concurrently. The conventional theory posits that the early phase of sepsis is dominated by a pro-inflammatory response characterized by cytokine storm, and the early-stage mortality is attributed predominantly to MODs due to this hyper-inflammation. After the hyper-inflammation stage, the patients are either gradually recovered or transitioned into persistent immunosuppressive state characterized by immune cell exhaustion whereas this late-stage mortality is frequently associated with secondary infection. If the immunosuppression persist, patients would then suffer long-term death as a result of immune dysfunction and chronic catabolism (4, 7, 8) (Figure 2).
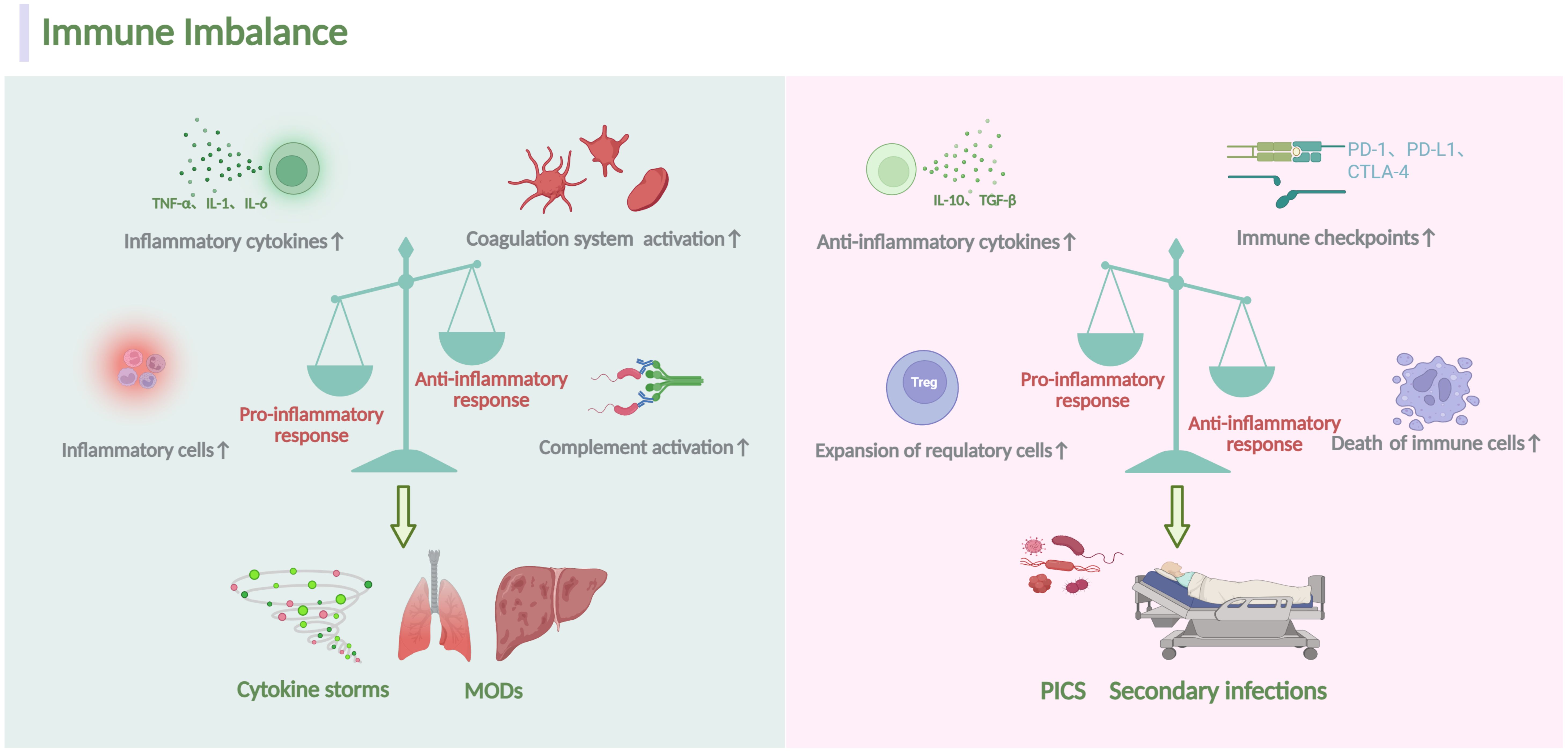
Figure 1. Overview of homeostatic disturbance in sepsis. During sepsis, the immune response is initiated when the host recognizes PAMPs and DAMPs involving both innate and adaptive immune system. Several mechanisms, including the proliferation of inflammatory cells, secretion of inflammatory cytokines and activation of the complement system, can escalate pro-inflammatory responses. Conversely, heightened abnormal death of immune effectors cells, production of anti-inflammatory cytokines, expansion of immunosuppressive cells and expression of specific immune checkpoints can exacerbate anti-inflammatory responses. Dominance of the pro-inflammatory response often correlates with a massive cytokine storm, leading to MODs. Conversely, the dominance of the anti-inflammatory response typically results in secondary infections and a poor prognosis. The figure was created via BioRender (https://BioRender.com).
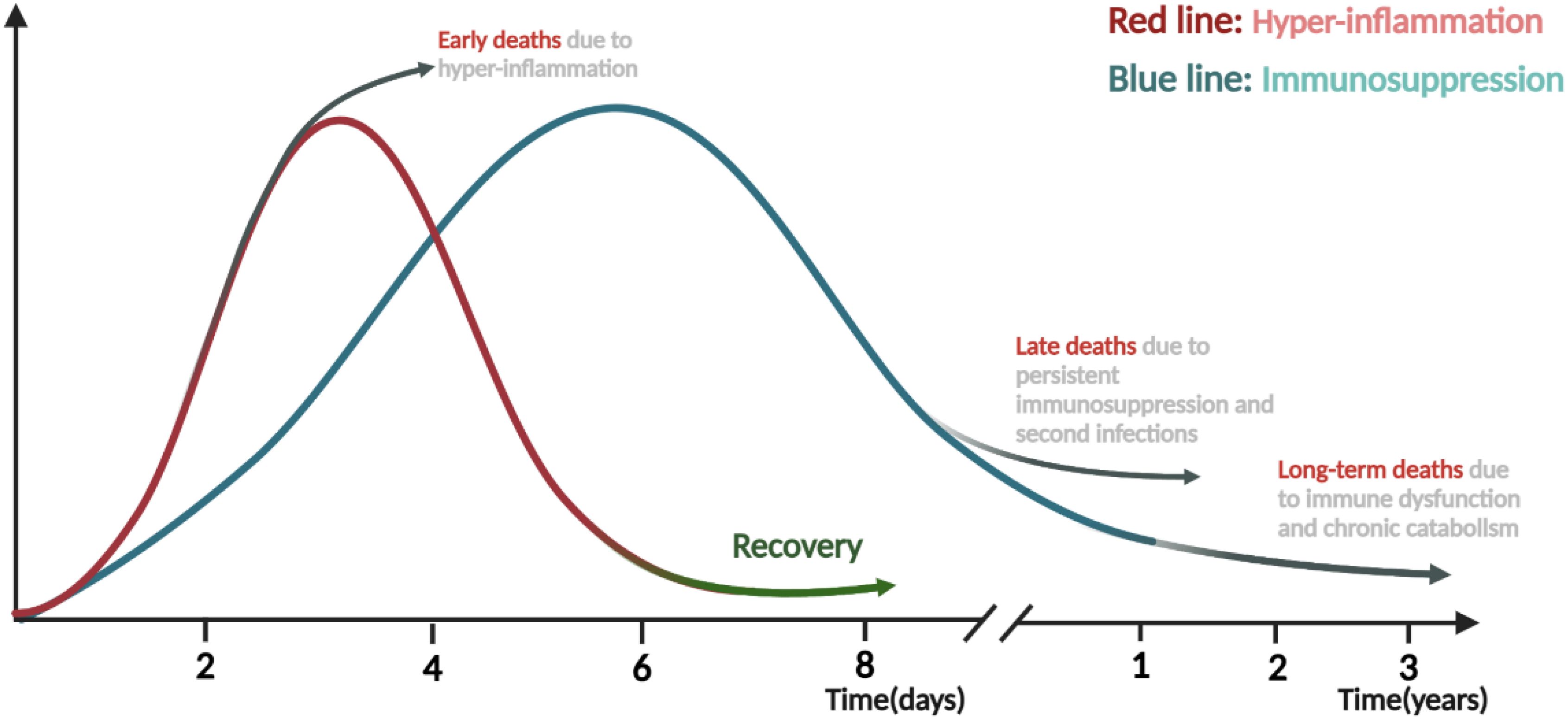
Figure 2. Time course and transition from hyper-inflammation to immunosuppression phase in sepsis. Both pro-inflammatory and anti-inflammatory responses are rapidly triggered after the onset of sepsis, and the transition from immune homeostasis to immune imbalance plays vital roles in the pathogenesis and progression of sepsis. The early stage is mainly dominated by the hyper-inflammation, during which the excessive “cytokine storm” often lead to early death of patients. On the contrary, if the body can restore normal immunity and rebalance, patients will enter the recovery stage or be gradually dominated by immunosuppression and suffer late or long-term death due to secondary infections, immune dysfunction and chronic catabolism. The figure was created via BioRender (https://BioRender.com).
The excessive inflammatory responses and cytokine storms have long been considered primary contributors to the high mortality of sepsis, while therapeutic interventions targeting TNF-α or IL-6, et al, have not yielded the anticipated improvements in patient survival in the past decades (9, 10). Additionally, the use of antibiotics, fluid resuscitation, and organ support therapy have limited prognostic impact in patients with sepsis. This discrepancy between the theoretical understanding of sepsis pathophysiology and the practical outcomes of targeted therapies underscores the complexity of the disease and the need for a more nuanced approach to treatment. In recent years, the role of immunosuppression in sepsis has been increasingly emphasized. Persistent immunosuppression is characterized by excessive and aberrant immune cell death, sustained release of anti-inflammatory mediators, expansion of immunomodulatory cell populations, and upregulation of immune checkpoint molecules, which collectively compromise host defenses against pathogens (11–14). This immunosuppressive state not only increases the risk of secondary infections but also diminishes the efficacy of therapeutic interventions such as immunotherapy and antibiotics (15, 16). Clinical studies have demonstrated that persistent lymphopenia in sepsis patients is strongly associated with 28-day mortality, prolonged hospitalization, and a higher incidence of secondary infections. Notably, sepsis-associated immunosuppression is recognized as a major contributor to mortality through its role in facilitating secondary infections (4, 17). Therefore, understanding and coping with sepsis immunosuppression is crucial for improving patient prognosis and therapeutic outcomes.
Here, we summarize current advances in the mechanisms underlying sepsis-induced immunosuppression, including the dysfunction of immune effector cells, expansion of immune suppressive cells, overproduction of anti-inflammatory cytokines, and dysregulation of immune checkpoint pathways. We also comprehensively summarize the diagnostic and therapeutic biomarkers related to sepsis immunosuppression, highlighting their translational potential in guiding the development of precision therapies for septic patients. Finally, we discuss new insights in immunosuppression-targeted therapies for sepsis, offering a framework to refine clinical staging and advance evidence-based management strategies.
2 Mechanisms of sepsis induced-immunosuppression
The phenomenon of “septic immunosuppression” was first identified by Volk et al. in 1996 (18), and studies on the mechanisms of “sepsis immunosuppression” emerged since then. Over the past decades, research into sepsis-induced immunosuppression has mainly focused on the dysregulation of immune effector cells and their failure to defend against infection, leading to increased susceptibility to secondary infections and death (5, 19). In recent years, with deeper understanding of the mechanisms, numerous studies have shown that sepsis-induced immunosuppression originates from the disorder of innate and adaptive immunity, characterized by the death and dysfunction of immune effector cells, overproduction of immunosuppressive cells, and release of anti-inflammatory cytokines. Increased expression of immune checkpoint molecules further aggravates immunosuppression (4, 20). Below, we comprehensively summarize the well-known existing studies on the mechanisms of sepsis-induced immunosuppression, with an overview shown in Figure 3.
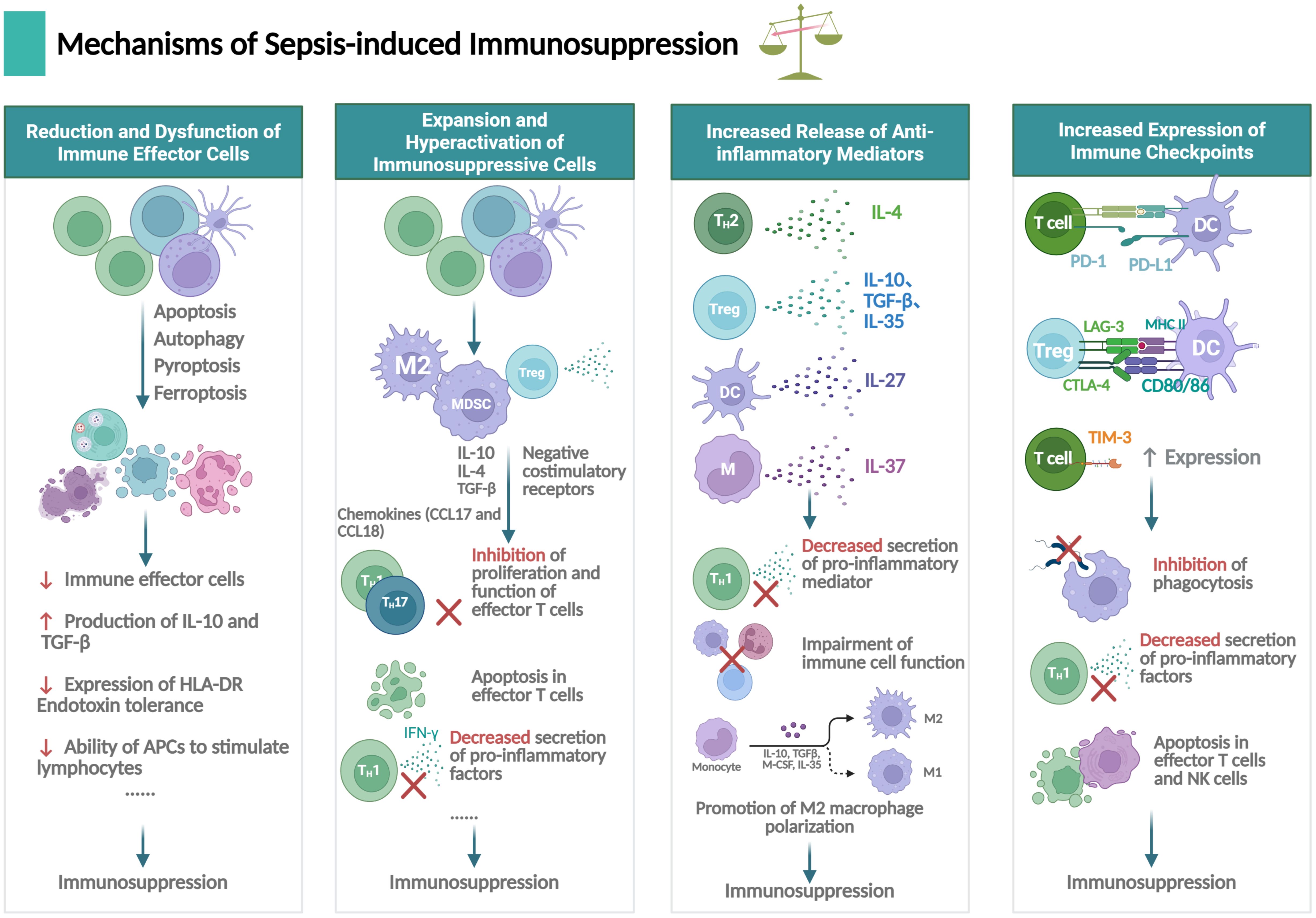
Figure 3. Mechanisms underlying immunosuppression in sepsis. Sepsis-induced immunosuppression involves dysfunction of immune effector cells, expansion of immune suppressive cells, secretion of anti-inflammatory mediators and expression of immune checkpoints, primarily concerning neutrophils, T lymphocytes, B lymphocytes, macrophages and dendritic cells (DCs), et al. The figure was created via BioRender (https://BioRender.com).
2.1 Reduction and dysfunction of immune effector cells
In sepsis, the number of immune effector cells is often severely reduced, leading to immunosuppression and exacerbation of infections (21), which mainly involves apoptosis, autophagy, pyroptosis and ferroptosis.
Apoptosis is a regulated form of cell death that removes damaged cells and maintains homeostasis under physiological conditions (22), which is particularly prominent in CD4+ T cells, CD8+ T cells, B cells, natural killer (NK) cells, and follicular DCs. In the past decades, apoptosis in sepsis-related immunosuppression has gained much attention. Lymphopenia due to apoptotic loss is a common characteristic of septic patients (23). Postmortem analyses reveal profound splenic T cell depletion with concurrent PD-L1 upregulation in sepsis fatalities compared to non-infectious mortality controls (24), which may be attributed to the increased levels of cytochrome C, Bim, caspase-3, caspase-8, and caspase-9, while decreased expression of B-cell lymphoma/leukemia-2 (Bcl-2) (25). Likewise, studies have shown that macrophage apoptosis is significantly increased in sepsis, accompanied by the activation of signaling pathways involving Fas/FasL and TNF-related pathways (26). Consequently, these apoptotic cells are engulfed by macrophages and cleared from the inflamed area, triggering the production of IL-10 and TGF-β, decreased expression of HLA-DR, and endotoxin tolerance, all of which impair the ability of antigen-presenting cells (APCs) to stimulate lymphocytes (27, 28). Currently, the anti-apoptotic strategies have been successfully proven to reduce mortality after sepsis in mice (29), indicating that inhibiting apoptosis may be a strategy to restore immune function against infection.
Autophagy, a catabolic process involving lysosomal degradation and recycling of cytoplasmic components, can be either cytoprotective or induce cell death (30, 31). In sepsis, lipopolysaccharide (LPS) activates selective autophagy through TLR4-MyD88-dependent or MyD88-independent as well as NF-kB pathways (32). Autophagy plays a role in packaging pathogen components, while excessive autophagy can reduce inflammation, and prevent DAMP and PAMPs from binding to PRRs, thereby suppressing immune activation during sepsis (33). Additionally, autophagy can promote sepsis-induced apoptosis of T lymphocytes and reduce the inflammatory response by negatively regulating the abnormal activation of macrophages (34, 35). However, mice with a reduced autophagy capacity in lymphocytes due to cell-specific deletion of Atg5 or Atg7 showed an increased mortality together with higher release of the anti-inflammatory IL-10 and immune dysfunction in abdominal sepsis, suggesting that impaired autophagy can whereas contribute to immunosuppression (36). Therefore, the effect of autophagy on the immune status of sepsis may vary according to the stage.
In recent years, newly identified programmed cell death such as pyroptosis and ferroptosis, have also been found to be induced during sepsis and to play pivotal roles in sepsis-induced immunosuppression (37). Pyroptosis is a form of inflammatory cell death primarily mediated by caspase-dependent gasdermin-D (GSDMD) activation (38), characterized by loss of membrane integrity, cell swelling, rupture, and subsequent release of pro-inflammatory mediators (22). During sepsis, immunocyte pyroptosis may exert biological effects through classical pathways: Caspase-1 is activated, recognizes and cleaves GSDMD, after which the cleaved GSDMD forms pores in the cell membrane, ultimately triggering pyroptosis. Additionally, pyroptosis can occur through non-classical pathways involving caspase-4/5 in humans or caspase-11 in mice, which activate GSDMD to induce pyroptosis (39, 40). Pyroptosis may lead to the release of IL-1β, IL-18, and High Mobility Group Box-1 (HMGB1), recruiting immune cells to aggregate the inflammatory response, which are correlated with the hyperinflammation and subsequent immunosuppression (41). Notably, septic patients exhibit elevated caspase-1 expression in immune cells compared to healthy individuals (41). Furthermore, the costimulatory molecule GITR has been shown to promote immunosuppression in sepsis by enhancing NLRP3 inflammasome-mediated macrophage pyroptosis (42), whereas inhibition of the TMEM173-GSDMD-F3 pathway improves septic mice survival by blocking disseminated intravascular coagulation (DIC) (43), providing further evidence for pyroptosis in sepsis immunosuppression.
Similarly, ferroptosis is an iron-dependent cell death process driven by lipid peroxidation, involving iron overload, reactive oxygen species (ROS) generation, and accumulation of polyunsaturated fatty acids in phospholipids (44). During sepsis, infection induces upregulation of nuclear receptor coactivator 4 (NCOA4), which mediates selective ferritin autophagy, resulting in Fe³+ release and iron-dependent cell death (45). Emerging evidence indicates that ferroptotic cells release DAMPs, activating downstream signaling pathways that exacerbate sepsis-associated organ failure (46). Importantly, elevated serum iron levels, infection markers, and lipid peroxidation have been significantly correlated with increased long-term mortality and cognitive impairment in sepsis patients (47). Together, these evidences support the pivotal roles of ferroptosis in sepsis-induced immunosuppression.
2.2 Expansion and hyperactivation of immunosuppressive cells
2.2.1 Regulatory T cells
The expansion of Tregs during sepsis suppresses the immune system by releasing anti-inflammatory cytokines, upregulating co-inhibitory receptors, and metabolic reprogramming from glycolysis to oxidative phosphorylation, which not only enhances their suppressive capacity but also correlates with long-term mortality in septic patients (48, 49). Mechanistically, Tregs inhibit effector T cell proliferation and function via direct secretion of inhibitory cytokines (e.g., IL-10 and TGF-β) and cell-contact-dependent suppression (50). Notably, Tregs can directly induce effector T cell apoptosis while impairing their antimicrobial activity, potentially mediated by Smad2/Smad3 signaling (51, 52). Furthermore, Treg-derived cytokines drive a feedforward immunosuppressive loop by promoting expansion of myeloid-derived suppressor cells (MDSCs) and establishing an immunosuppressive microenvironment, thereby exacerbating systemic immune paralysis in sepsis (53, 54).
2.2.2 MDSCs
MDSCs constitute a heterogeneous cell population predominantly composed of immature myeloid cells with broad immunosuppressive activity targeting both innate and adaptive immunity (55). The pathological expansion of MDSCs in sepsis is driven by sustained production of inflammatory factors like IL-6 and TNF-α, and the activation of signaling pathways such as STAT3 and NF-κB (56, 57). The expansion of MDSCs is closely linked to chronic immune suppression in septic patients, affecting the severity and progression of sepsis and the incidence of secondary nosocomial infections due to their inhibitory effects on T cell proliferation and function (58, 59). Co-culture of MDSCs isolated from septic patients with T cells showed the impaired ability of antigen-driven T cells to proliferate and secrete proinflammatory factors such as IFN-γ (58). Notably, while immature myeloid cell numbers remain elevated for ≥ 6 weeks post-sepsis onset, only MDSCs acquired after the 2-week post-onset window exhibit potent inhibition of T lymphocyte proliferation and IL-2 synthesis, suggesting their pivotal role in maintaining chronic immunosuppression during the late phase of sepsis (60).
2.2.3 M2 macrophages
Macrophages are functionally polarized into classically activated (M1) and alternatively activated (M2) subtypes, defined by their divergent roles in inflammation regulation. M1 macrophages exhibit pro-inflammatory phenotypes critical for pathogen clearance, whereas M2 macrophages display anti-inflammatory properties that facilitate tissue repair and resolution of inflammation (61). Notably, M2 macrophage polarization has emerged as a key driver of sepsis-induced immunosuppression. Mechanistically, during the late immunosuppressive phase of sepsis, M2 macrophages are activated by Th2 cytokines (IL-4 and IL-13), LPS, glucocorticoids, IL-10, IL-6, or TGF-β (62). In turn, these polarized M2 macrophages establish a self-reinforcing immunosuppressive cascade through massive secretion of IL-10 and TGF-β, thereby inducing host immune paralysis and predisposing to recurrent infections (63). Moreover, M2 macrophages express high levels of C-type lectins (CD206) and scavenger receptors (CD163), promoting the secretion of chemokines (CCL17 and CCL18) to recruit eosinophils, basophils, Th2, and Tregs, exhibiting an “anti-inflammatory cytokine profile” (64). Further research is needed to elucidate their complex regulatory network and functional mechanisms during sepsis.
2.3 Increased release of anti-inflammatory mediators
Anti-inflammatory mediators play pivotal roles in the immunosuppression of sepsis, with specific actions including the inhibition of pro-inflammatory mediator secretion, the impairment of immune cell function, the promotion of M2 macrophage polarization, and the increase of immunosuppressive Tregs, etc. (65, 66). The most prominent cytokines in sepsis related immunosuppressive were IL-4, IL-10, IL-27, IL-37, IL-38 and TGF-β (28) (Figure 4).
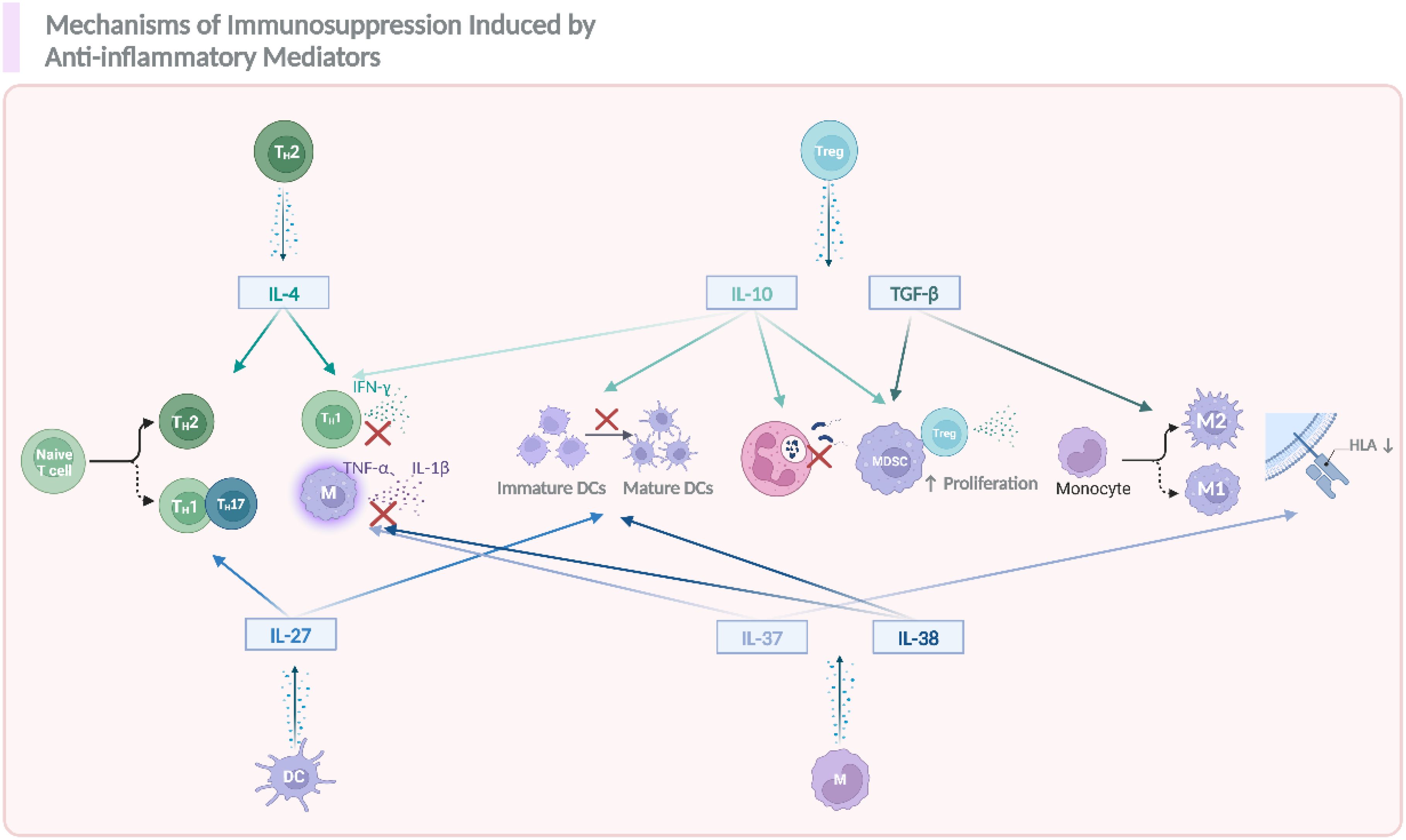
Figure 4. The role of anti-inflammatory cytokines in modulating immune responses and contributing to sepsis related immunosuppression. During the occurrence and progression of immunosuppression in sepsis, IL-4, IL-10, IL-27, TGF-β, IL-37, and IL-38 act as key anti-inflammatory mediators, promoting the differentiation of naïve T cells into T helper (Th) subsets, including Th2, and Th17. These mediators also suppress the activation of immune cells such as DCs, monocytes, and promote the polarization of macrophages toward an anti-inflammatory M2 phenotype. Additionally, they enhance the proliferation of Tregs and MDSCs, which further contribute to the suppression of inflammation. Notably, the reduction in HLA expression on immune cells also happened as a result of the activation of these anti-inflammatory signals. The figure was created via BioRender (https://BioRender.com).
IL-4 and IL-10 are crucial anti-inflammatory cytokines mainly secreted by Th2 cells, monocytes/macrophages and Tregs, et al, upon activation (67, 68). The release of IL-4 and IL-10 in sepsis suppress Th1 inflammatory response by reducing IFN-γ, TNF-α and IL-1β secretion by Th1 cells and inhibit Th1 cells polarization (65, 69). Specifically, IL-4 drives naive T cell differentiation toward Th2 lymphocytes, a process that can be blocked by anti-IL-4 antibodies (70); whereas IL-10 can exacerbate immunosuppression by promoting Treg and MDSCs production, inhibiting DCs maturation and neutrophil function, leading to impaired antigen presentation and pathogen clearance (71–73). Clinically, elevated plasma IL-10 levels demonstrate strong correlations with increased nosocomial infections and mortality rates, validating its central role in sepsis-associated immunosuppression (74). Collectively, these functions promote a Th2-biased immune response and weaken resistance to bacteria and viruses. TGF-β is a multifunctional cytokine belonging to the transforming growth factor superfamily. As for its anti-inflammatory properties, it plays a role in the induction of induced Treg cells (iTreg cells) from CD4+ T cells, alternative macrophage activation which maintain an anti-inflammatory phenotype, and act as an up-regulator of anti-inflammatory response (75, 76). IL-27, a dual-functional member of the IL-12 cytokine family, modulates sepsis pathogenesis through cell-type-specific actions on macrophages, DCs, and lymphocytes. While enhancing early inflammatory responses, IL-27 paradoxically suppresses Th1/Th17 differentiation and potentiates IL-10 production from Tregs and type 1 regulatory T (Tr1) cells during late-phase sepsis (77–79).
In recent years, the novel anti-inflammatory IL-35, IL-37, and IL-38 have also been implicated in sepsis immunosuppression, partly by inhibiting the release of pro-inflammatory mediators (80). Notably, Study have shown that IL-37 could significantly downregulate the expression of HLA-DR and CD86 in septic mice, inhibiting antigen presentation and indicating an immunosuppressive effect in sepsis (81). Clinically, septic patients exhibit elevated IL-37 levels that inversely correlate with inflammatory cytokine production and positively associate with immunosuppression severity (82). Previously, we have also shown that in COVID-19 patients developed into sepsis, IL-38 was increased and correlated with disease severity (83, 84), which consistent with mechanistic studies regarding IL-38 in promoting Treg expansion, while alleviating macrophage related pro-inflammatory responses in sepsis (85, 86).
2.4 Increased expression of immune checkpoints
Cell surface inhibitory immune checkpoints (also known as negative costimulatory molecules), including PD-1, PD-L1, CTLA-4, TIM-3, et al, are implicated in the immunosuppression of sepsis, by functionally inhibit phagocytosis and cytokine release of immune cells and promote T-cell exhaustion (87). As early as 2011, studies have reported increased expression of PD1-related molecules in sepsis patients, accompanied by decreased cytokine production, HLA-DR and CD28 expression, while increased activation of immunosuppressive Tregs (88, 89), providing direct evidence that PD1 is essential to the poor prognosis of sepsis. This concept is further supported by the finding that inhibition of PD-1 improved survival in septic patients (90). In terms of detailed mechanisms, upregulated PD-1 and PD-L1 in sepsis lead to suppression of T-cell function, and this interaction between PD-1 on T cells and PD-L1 on APCs (e.g., DCs) leads to T-cell exhaustion (87, 91). The PD-1 and PD-L1 interaction may also diminish myeloid cell function during sepsis, as indicated by decreased phagocytic capacity of both neutrophils and macrophages in sepsis patients (92). CTLA-4, an inhibitory receptor mainly expressed on activated T cells, inhibits T cell activity and play an immunosuppressive role in sepsis by binding to B7 molecules and CD80/CD86 (93). Recently, it was suggested that the upregulation of TIM-3, an immune checkpoint molecule in NKT cells, can promote NKT cell apoptosis, contributing to sepsis-related immunosuppression (68). TIM-3+CD4+ T cells show reduced proliferative ability and elevated expression of inhibitory markers compared to TIM-3-CD4+ T cells, and conditional deletion of TIM-3 in CD4+ T cells reduces mortality in response to sepsis (94). Additionally, TIGIT, an inhibitory receptor with Ig and ITIM domains, is predominantly expressed on T cells and NK cells; high expression of TIGIT has also been associated with immune dysfunction in sepsis (95). LAG-3 acts as an immunosuppressor in sepsis by inhibiting the activation and proliferation of T cells through binding to MHC II (96). However, so far, different studies have varying interpretations of the specific mechanisms of action of immune checkpoint molecules in sepsis, and the regulatory mechanism remains unclear, especially in patients with different types of sepsis.
2.5 Heterogeneity of immunosuppression in sepsis in terms of infection types
Sepsis is a dysregulated host response to infections caused by bacteria, fungi, viruses, or other pathogens, with distinct pathogenic mechanisms across pathogen types. In bacterial sepsis, endotoxins such as LPS bind to Toll-like receptor 4 (TLR4), thereby activating the NF-κB signaling pathway and driving the overproduction of anti-inflammatory mediators, a key contributor to immunosuppression (97). Bacterial pathogens may also evade immune clearance through biofilm formation, antioxidant enzyme synthesis, and exotoxin secretion, exacerbating immunosuppression (98).For fungal sepsis, beta-glucans on fungal cell walls activate the Dectin-1 receptor, triggering Syk and CARD9 signaling pathways that induce the production of both inflammatory and immunosuppressive cytokines (99). Concurrently, fungal infections impair antigen presentation by DCs and macrophages, while suppressing T cell and B cell activation (100). In viral sepsis, pathogens (e.g., SARS-CoV-2 or cytomegalovirus) activate RLRs and TLRs to initiate type I interferon (IFN-I) release (101). However, when evading the host immune system, virus also inhibits antigen presentation, cause a decrease in the activity of NK cells and cytotoxic T lymphocytes (CTLs), as well as blocking antibody production by B cells (102). Despite pathogen-specific mechanisms, bacterial, fungal, and viral sepsis share common immunosuppressive pathways, such as immune cell apoptosis induction and antigen presentation blockade. While our understanding of the pathogenesis of sepsis has significantly advanced in recent years, there is still much work to do to translate these new insights into effective treatments.
3 Impacts of immunosuppression on immune functions
Sepsis directly or indirectly impairs the function of virtually all types of immune cells, involving macrophages, neutrophils, lymphocytes, NK cells, as well as intrinsic lymphoid cells (ILCs), the dysfunction of which may further aggravate immunosuppression of sepsis, forming positive feedbacks (Figure 5).
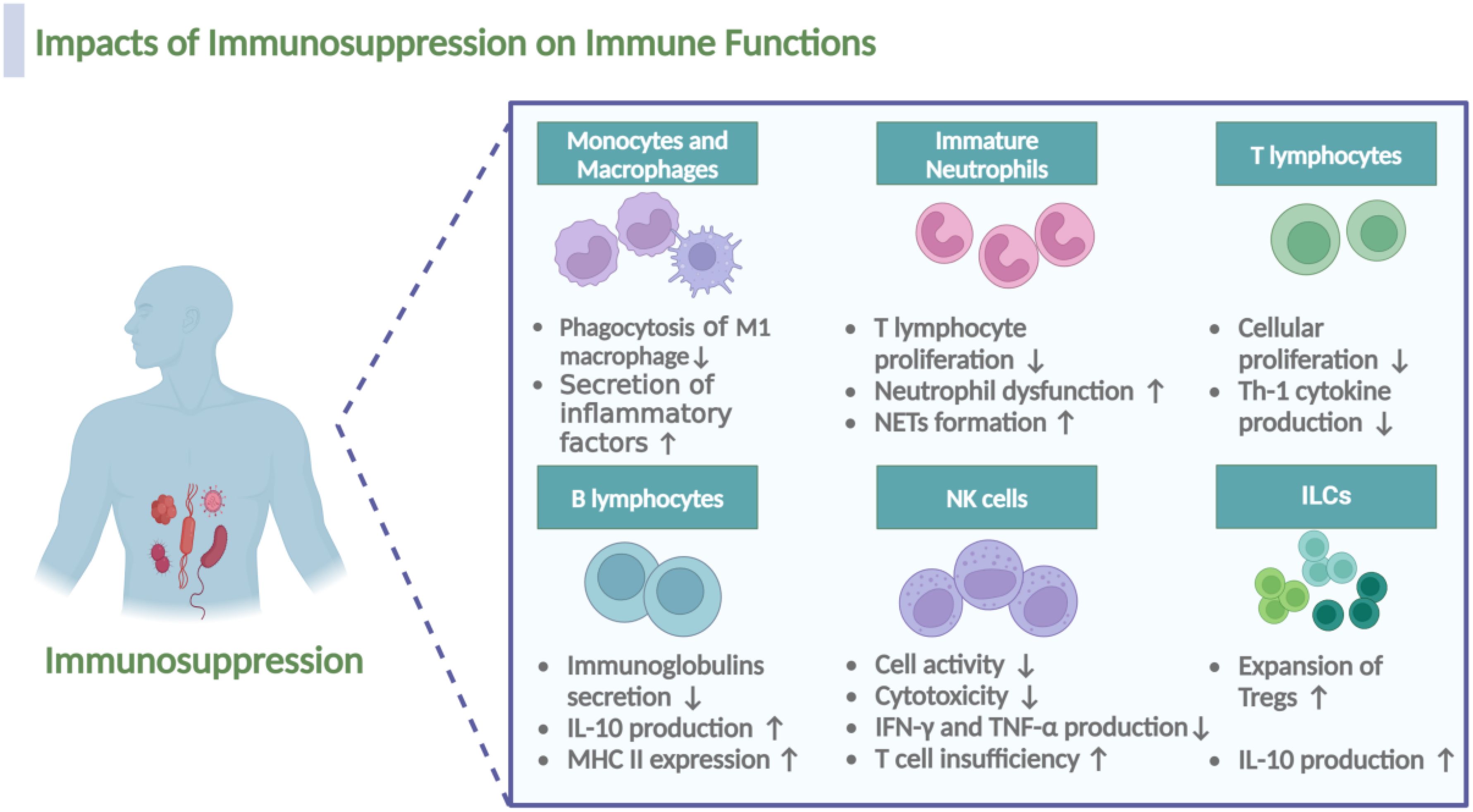
Figure 5. Impacts of immunosuppression on the immune cell functionality during sepsis. Immunosuppression during sepsis induces multifaceted dysfunction across immune cell populations. In macrophages, M1 subsets demonstrate impaired phagocytic capacity, while M2 macrophages exhibit upregulated secretion of anti-inflammatory mediators. Immature neutrophils acquire pathological functions, including suppression of T lymphocyte proliferation, exacerbation of neutrophil dysfunction, and potentiation of neutrophil extracellular traps (NETs) formation. T cells show reduced proliferative activity and diminished Th1 cytokine production, whereas B cells display decreased immunoglobulin secretion alongside elevated IL-10 production and MHC II expression. NK cells manifest compromised cytotoxicity coupled with reduced IFN-γ and TNF-α expression, collectively exacerbating T cell insufficiency. Notably, regulatory cell populations, including Tregs and the recently characterized ILCs, undergo expansion with concomitant increases in IL-10 production. The figure was created via BioRender (https://BioRender.com).
Monocytes and Macrophages. As a result of immunosuppression, the polarization of macrophage transitions from M1-type to M2-type, resulting in impaired antigen presentation and phagocytic dysfunction of M1 macrophages and increased secretion of anti-inflammatory factors of M2 macrophages, which partly depends on the decreased expression of MHC molecules, Tim4 and NALP-3 and the lack of costimulatory molecules (26, 103).
Immature Neutrophils. Notably, the expansion of immature neutrophils is also a characteristic and a result of sepsis related immunosuppression (104). In the first week following sepsis, patients exhibit profound changes in neutrophil characteristics; immature neutrophils, identified phenotypically by low expression of CD10 and CD16 (CD10dimCD16dim), are associated with increased early mortality after sepsis due to their immunosuppressive function (105, 106). Consistent with this observation, the existence of a subset of CD10-CD64+CD16low/−CD123+ immature neutrophils, that could be used for early identification of sepsis in patients was proposed (107). In murine models of sepsis, high expression of HMGB1 contributes to neutrophil dysfunction by limiting the activation of NADPH oxidase; neutrophils from septic patients, when cultured with plasma and anti-HMGB1, demonstrate a higher capacity to activate NADPH oxidase (108). Additionally, under the immunosuppressive stage, neutrophils also form NETs in sepsis, which, while trapping pathogens, also contribute to immunosuppression by interacting with Tregs (109).
T lymphocytes. In the early stages of sepsis, T lymphocytes typically exhibit an over-activated state, leading to the release of substantial amounts of inflammatory factors. As sepsis progresses in to the phase of immunosuppression, T cells gradually transition into an immunosuppressive state (48). Studies have revealed marked alterations in the mTOR pathway of T lymphocytes from septic patients, leading to a catabolic state characterized by a failure to induce glycolysis, oxidative phosphorylation, ATP production, or glucose uptake, ultimately impairing cellular proliferation (110). Notably, sepsis impairs the CD4+ T cell recall response and post-septic CD4+ T cells are highly glycolytic and exhibit a Th17 phenotype (111).
B lymphocytes. Regarding B lymphocytes, Manu Shankar-Hari et al. confirmed the hypothesis that B lymphocyte loss is a consequence of sepsis induced immunosuppression, involving different subsets, with the depletion and dysfunction of the memory B-cell population being the primary outcome of sepsis-induced immunosuppression (112). Notably, the impaired function of memory B cells and plasma cells results in an inability to secrete immunoglobulins in response to antigens (113). Additionally, surviving B lymphocytes exhibit an exhausted phenotype, characterized by increased IL-10 production and decreased MHC II expression (114).
NK cells. Impaired function of effector NK cells may also occur as a result of immunosuppression during sepsis. Secondary infections in patients who survived sepsis are often due to dysfunction in neutrophils, NK cells, and the inhibition of T-cells in the lung (115). As mentioned by Tang et al., NK cell activity is significantly impaired in patients with sepsis, potentially leading to a weakened immune response to infection and an increased risk of secondary infection (116). In septic patients, cytotoxicity of NK cells dramatically decreased, likely due to the reduction of CD3-CD56+NK cells; after stimulation with phorbol-12 myristate 13-acetate (PMA) and ionomycin, the production of IFN-γ and TNF-α by CD3-CD56+ NK cells in septic patients is also impaired (117).
ILCs. Notably, in recent years, the function of ILCs during sepsis induced immunosuppression has gained much attention. ILCs are a distinct class of lymphocyte subpopulations from T and B cells, including ILC1, ILC2, ILC3, and ILCreg. Helper ILC1s require T-bet for development and produce IFN-γ as their main effector cytokine; ILC2s depend on GATA-3 and produce “Th2” cytokines (IL-4, IL-5, IL-9, and IL-13); ILC3s depend on RORγt and secrete “Th17” cytokines (IL-17), or “Th22” cytokines (IL-22) (118, 119). It was found that ILC2 and ILC3 in the peripheral blood of sepsis patients was significantly reduced via apoptosis (120), and the reduction of ILCs may lead to immune escape of pathogens and deterioration of sepsis outcomes (119). A study showed that rhIL-7 immunotherapy could ameliorate sepsis-induced lung ILC loss, thereby ameliorating immunosuppression and survival in mice (121), providing further evidence for the role of ILCs in sepsis-related immunosuppression. Additionally, it was reported that IL-33 activates ILC2s, which produce IL-4 and IL-13 and drive M2 polarization of macrophages, resulting in the expansion of Tregs and immunosuppression via the production of IL-10 (122).
4 Immunosuppression-guided diagnostic biomarkers in sepsis
Over the past decades, numerous studies have identified biomarkers associated with sepsis-induced immunosuppression, significantly contributing to the diagnosis and treatment of sepsis. This paper aims to provide a comprehensive overview of both established and emerging immunosuppression-related biomarkers in sepsis, highlighting their clinical utility with actionable thresholds and assessing their clinical relevance. The relevant content is summarized in Table 1.
4.1 HLA-DR
HLA-DR expression serves as a robust indicator of monocyte antigen presentation capacity. In clinical studies, monocyte HLA-DR (mHLA-DR) has been employed as a marker for innate immunity. Notably, mHLA-DR levels in septic patients are significantly lower than those in healthy individuals (137). Reduced mHLA-DR levels are linked to immune dysfunction and poor prognosis in septic patients and critically ill COVID-19 Patients (138, 139). Clinically, mHLA-DR quantification using flow cytometry is recommended within 3–8 days after sepsis onset to guide immunomodulatory interventions (140). A significant correlation between SOFA score at admission and a decreased mHLA-DR expression at days 3–4 and 6–8 after onset was observed (141), with mHLA-DR < 15,000 AB/C or a percentage < 60% serving as a validated threshold for defining immunosuppression and predicting secondary infection risk (123). Furthermore, studies have demonstrated that mHLA-DR levels in 30-day non-survivors were significantly lower than in survivors, and septic patients with mHLA-DR ≥ 52.29% had a 6.798 times higher 30-day survival rate (142). This threshold may help stratify patients for targeted therapies. Importantly, septic patients with decreased mHLA-DR levels often do not return to normal until six months post-discharge, a pattern observed in patients with poor prognoses (143). Taken together, consensus now exists for considering low-monocyte mHLA-DR as a surrogate for sepsis-induced immunosuppression, making it the most extensively studied and validated biomarker in this domain.
4.2 Immune checkpoints
In sepsis, numerous studies have reported associations between increased PD-1 expression on T cells and PD-L1 expression on APCs with lymphopenia, T cell apoptosis, and mortality in sepsis patients. In 2016, a multivariate analysis found that increased monocyte PD-L1 expression was an independent predictor of mortality after sepsis, highlighting the significance of the PD-1 pathway in sepsis-induced immune dysregulation (124). Elevated PD-1 expression is also indicative of poor clinical outcomes in septic patients (125). The expression levels of PD-1 and PD-L1 in neutrophils and monocytes of septic shock patients are significantly higher than those in non-infected ICU patients and are positively correlated with sepsis severity and mortality (92). Therefore, dynamic monitoring of PD-1/PD-L1 expression may guide immunotherapy trials targeting these checkpoints in patients with sustained immunosuppression. Similarly, high CTLA-4 expression in sepsis is associated with immunosuppression, making it another biomarker for this condition (144). Actually, Cheng et al. demonstrated that CTLA-4 expression > 25% on CD4+ T cells was associated with a 3.2-fold increased risk of 28-day mortality, supporting its role in patient stratification for anti-CTLA-4 therapies for sepsis related immunosuppression (126). Through an RNA sequencing assay, Huang et al. found that the expression of TIM-3 on CD4+ T cells in septic patients with immunosuppression was significantly elevated and has been proposed as a prognostic marker for immune paralysis (94). Recently, Filippo Mearelli et al. examined 12 immune checkpoint markers in 113 patients with bacterial sepsis, and revealed that patients exhibiting elevated serum levels of IRAK-M and Galectin-1 (cutoff values: 2.32 ng/mL and 4.44 ng/mL, respectively) displayed clinical features of immunosuppression and poorer prognosis, with a 7-day mortality rate of 26% and an in-hospital mortality rate of 49% (127). Therefore, IRAK-M and Galectin-1, as inhibitory immune checkpoint biomarkers, could help identify a high-risk sepsis phenotype that might be suitable for enrollment in future checkpoint inhibitor trials.
4.3 Immunocytes
Immunocyte lymphopenia, characterized by reduced peripheral blood T and B lymphocyte counts, is a hallmark of acquired immune dysfunction in sepsis (145). Studies demonstrate that non-surviving sepsis patients exhibit a progressive decline in peripheral blood lymphocytes between days 2–7 post-onset, associated with a 3.5-fold increased mortality risk compared to survivors (146). Persistent lymphocyte counts below 1.0×109/L serves as a clinical indicator of immune dysregulation, correlating with higher mortality rates and susceptibility to chronic infections (123). A retrospective observational study further revealed that a neutrophil-to-lymphocyte ratio (NLR) > 4.18 on day 7 predicts 28-day mortality with 81.94% specificity (128).
Additionally, patients with septic shock frequently develop immune collapse, characterized by reduced HLA-DR expression and elevated Tregs (119). Persistently high Treg levels are strongly linked to sepsis-induced immunosuppression and poor outcomes (147), with both Treg counts and the Th1/Th2 ratio proposed as biomarkers of immune suppression (123). Notably, aberrant Treg expansion often coincides with an inverted Th17/Treg ratio, indicating immunosuppression progression (148). This Th17/Treg imbalance is strongly correlated with disease severity with a ratio < 0.69 predicting mortality (AUC = 0.766) (129). Therefore, dynamic monitoring of lymphocyte counts can provide insights into the balance between innate and adaptive immunity, predict risks of secondary infections and mortality, and guide clinical interventions. Incorporating these thresholds into routine clinical laboratory panels could help identify high-risk patients requiring intensified surveillance.
Neutrophil dysfunction in sepsis includes reduced chemiluminescence intensity, while specific protein activations (e.g., CD88, TREM-1) may serve as diagnostic biomarkers for sepsis. Notably, diminished neutrophil CD88 expression predicts subsequent secondary infections and immunosuppression of sepsis (149). Recent study by Liao et al. identified Siglec-F+ neutrophils as immunosuppressive markers, with targeted depletion of this subset enhancing function of T lymphocytes and improving survival in preclinical models (14). The S100A8/A9 heterodimer, predominantly expressed in neutrophils and monocytes, emerges as a key immunosuppressive mediator in sepsis via impairing DCs maturation and promotes MDSCs accumulation (150). Transcriptomic analyses of monocytes from 29 sepsis patients and 15 healthy donors revealed upregulated S100A8 and S100A9 expressions linked to MDSC recruitment (59). Concurrently, a distinct monocyte subset (HLA-DRlowS100A9high) was further identified as a driver of immunosuppression during sepsis; inhibition of this subset can markedly mitigate sepsis-induced immune depression, thereby providing a novel therapeutic strategy for the management of sepsis (130).
4.4 Cytokines
The immunosuppressive state in sepsis involves dysregulation of multiple cytokines, whose dynamic levels reflect disease severity and serve as diagnostic/prognostic biomarkers (151). During the immunosuppressive phase of sepsis, anti-inflammatory mediators typically demonstrate progressive elevation. Clinical evidence suggests that longitudinal IL-10 monitoring from day 1 to day 3 post-onset shows prognostic value for septic outcomes (131), while IL-10/lymphocyte ratio (IL-10LCR) at a cutoff of 23.39 ng/mL predicts 28-day mortality with 70.0% sensitivity and 74.4% specificity (132). In addition, using a cutoff of 5.0 ng/mL, the specificity of IL-27 to diagnose bacterial infection in immunocompromised septic patients reached 94% (133); and a cutoff value of IL-37 for predicting 28-day mortality in septic patients has been suggested as 107.05 pg/mL (134). Conversely, monocytes isolated from immunosuppressed sepsis patients exhibit markedly reduced production of pro-inflammatory cytokines, including TNF-α, IL-1β, IL-6, and IL-12 (135). Notably, Ploder et al. revealed persistent TNF-α suppression in circulating monocytes from non-surviving sepsis patients identifies it as a reliable immunosuppression marker (136). Mechanistically, LPS-stimulated monocytes from immunosuppressed sepsis patients show significantly attenuated TNF-α secretion than controls, making monocytic TNF-α production below 200 ng/L under LPS stimulation serving as a diagnostic threshold for immunosuppression in sepsis patients (135). Notably, single-cytokine assessment was inadequate to fully diagnosis sepsis immunosuppression due to the lack of specificity, serial monitoring using combined panels (IL-10, TGF-β, IL-37, et al) is therefore recommended for serial monitoring to track immune trajectory in the future.
5 Advances in targeted immunomodulation for sepsis-induced immunosuppression
Despite the failure of numerous clinical trials, some promise likely still exists in using anti-inflammatory treatment strategies in the early hours after the onset of sepsis such as IL-1 receptor blockade or anti-TNF treatments based on the identification of those that could survive from anti-inflammatory therapies in combination with antibiotics and resuscitation (152, 153). Nevertheless, most patients who survive the critical stage may eventually die of immunosuppression, and for those who developed into immunosuppressive conditions, immune-stimulation is therefore promising to revitalize the immune system to allow clearance of initial infectious foci or to fight secondary infections (123), and the field now increasingly focuses on reversing immunosuppression in later stages, a critical frontier where immune checkpoint inhibitors, cytokine therapies, as well as cell therapy like mesenchymal stem cells (MSCs), et al. show transformative potential. Here, we focus on the research of immunomodulatory therapy and provide a detailed summary of developments over the past decades, as depicted in Figure 6.
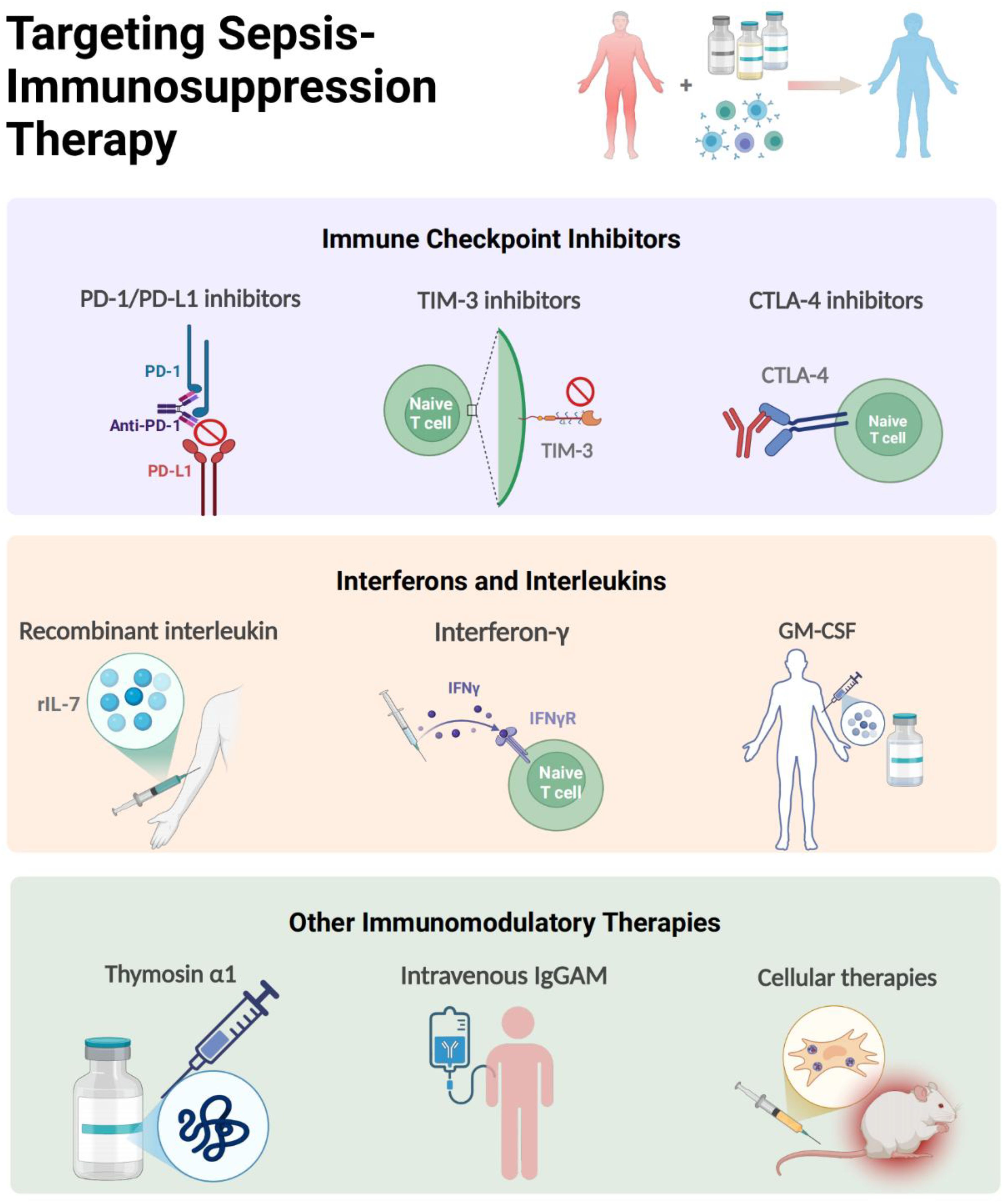
Figure 6. Targeting Immunomodulatory Therapy for Sepsis-immunosuppression. The immunomodulatory strategies of sepsis-induced immunosuppression mainly include immune checkpoint inhibitors, interferons and interleukins (IL-7, GM-CSF, IFN-γ, Thymosin alpha 1), MSCs and intravenous immunoglobulin. The figure was created via BioRender (https://BioRender.com).
At the vanguard of this revolution are PD-1/PD-L1 axis inhibitors, which have progressed from murine sepsis models demonstrating restored T-cell functionality to pioneering human trials. In tested septic mice, 4-octyl itaconic acid modulates immune homeostasis in sepsis by activating Nrf2 and negatively regulating PD-L1, which may provide new therapeutic strategies (154). The landmark phase 1b randomized study by Hotchkiss et al. established the safety profile of the PD-1 inhibitor nivolumab and the PD-L1 inhibitor BMS-936559 in septic patients (90, 155), with ex vivo analyses revealing anti-PD-1 antibodies rescue exhausted CD8+ T cells by restoring IFN-γ production (156), a finding corroborated by parallel discoveries in TIM-3 and LAG-3 knockout mice models, showing that knockout of TIM-3 and LAG-3 reduces immunosuppression-related mortality of septic mice (94, 96). Notably, the use of TIGIT antibodies can restore the function of T cells from sepsis patients ex vivo, suggesting that blocking TIGIT may be a new approach for the immunotherapy of sepsis (157). However, these immunoadjuvant therapies are still under investigation, warranting large scale clinical trials, and whether they are promising in septic patients still has a long way to go.
Complementing checkpoint inhibitors are cytokine-based strategies undergoing clinical validation. Recombinant IL-7 emerges as a particularly promising candidate, demonstrating capacity to reverse sepsis-associated lymphopenia through mTOR pathway modulation (110), while showing excellent tolerability in randomized trials (158). In a prospective, randomized, double-blind, placebo-controlled trial involving 27 patients with septic shock and severe lymphopenia, rhIL-7 induced a three- to fourfold increase in absolute lymphocyte counts and circulating CD4+ and CD8+ T cells that persisted for weeks (159). Therefore, for those septic patients with severe lymphocyte alterations, rhIL-7 therapy is recommended to be one of the most promising potential adjuvant treatments. Moreover, in a recent completed multicenter and multinational, double-blind, double-dummy randomized clinical trial involving 280 patients with sepsis, the rhIL-7 was used to monitor the individualized immunomodulation therapy for those with immunoparalysis (160). The therapeutic effect of IFN-γ in septic patients has been recently validated. As early as in 2002, clinical studies has shown that inhaled IFN-γ treatment led to recovery of HLA-DR expression in alveolar macrophages and decreased incidence of ventilator-associated pneumonia (161). Later, a case report found that IFN-γ therapy combined with nivolumab (an anti-PD1 antibody) was effective in restoring immune function and eliminating invasive infection (162); and it was also being investigated in a phase 2 clinical trial to test whether rhIFN-γ would reverse the hypoinflammation and restoration of immune dysfunction of septic patients (163), and but no randomized, controlled trials have tested IFN-γ therapy in ICU patients to date. Similarly, GM-CSF (sargramostim) has transitioned from early clinical trial in 2002 and 2009 showing improved monocyte HLA-DR expression (164, 165), to recent multicenter trials in reporting reduced ICU acquired infections of immunosuppressed patients (166), proving further evidence that GM-CSF thus represents a promising immunoadjuvant therapy in patients with sepsis, although larger randomized controlled trials are now warranted to confirm these initial results. Notably, the ongoing SepTIC trial that includes investigation of sargramostim for improving outcomes in a high-risk subset of patients admitted to the ICU with sepsis starting in 2023 (167), would help exemplify the field’s growing sophistication in targeting immune paralysis across diverse populations.
The therapeutic arsenal continues expanding with novel modalities like thymosin α1 (Tα1) and IgGAM. A randomized controlled study showed that patients treated with Tα1 exhibited reduce mortality by 18% than the control group, accompanied with reduced mechanical ventilation time and ICU stay (168). In addition, IgGAM, a class of multi-component immunoglobulins rich in IgA and IgM, exerts therapeutic effects in sepsis primarily through mediating opsonization, neutralizing antigens, and regulating Fc receptor expression. The related drug Pentaglobin has demonstrated beneficial effects on sepsis in clinical trials (169, 170). However, the use of IgGAM is still controversial, with risks of various adverse effects and a lack of clear biomarkers to guide its accurate administration. Currently, a clinical trial based on serum IgM titers for IgGAM treatment in patients with septic shock is ongoing (NCT04182737) (171). Hopefully, as an adjuvant therapy, IgGAM may be a potentially effective immunotherapy for treating sepsis patients with immune-paralysis. Perhaps most intriguing therapies in the immunomodulation of sepsis are cellular therapies. MSCs not only mitigate organ damage via NLRP3 inflammasome suppression (172), but also synergize with antimicrobials through extracellular vesicle-mediated immunomodulation (173, 174). A Phase I open-label dose-escalation safety trial evaluating the efficacy of advanced MSC-based therapy in septic patients has enrolled 11 participants, though results remain unpublished (175), this multimodal approach epitomizes the field’s progression from single-target interventions to systems-level immune reprogramming.
6 Conclusions and perspectives
Over the past decade, researchers have made substantial progress in elucidating the mechanisms underlying sepsis-induced immunosuppression, including the identification of novel biomarkers for immune monitoring (e.g., dynamic changes in HLA-DR expression and lymphocyte function) and the development of immunomodulatory therapies aimed at improving clinical outcomes. Nonetheless, there are still limitations in the current studies. A primary concern is the continued reliance on animal models that inadequately replicate the biphasic immunological transition from initial hyperinflammation to subsequent immunosuppression observed in human beings. This fundamental discrepancy likely underlies the recurrent translational failures of immunotherapies that showed efficacy in rodent models but proved ineffective in human trials, such as early TNF-α inhibition strategies. Furthermore, while observational studies have established associations between immunosuppression and secondary infections in sepsis patients, the absence of controlled intervention trials leaves a pivotal question unresolved: Do immunostimulants directly mitigate infection risks, or are they merely markers of preserved immune competence? Compounding this uncertainty, current diagnostic approaches relying on static biomarker measurements may provide incomplete assessments of immune status. These limitations have direct clinical implications, as negative trial results may reflect suboptimal treatment timing rather than inherent therapeutic inefficacy.
To address these challenges, future investigations should prioritize three key domains. First, future research must focus on addressing the pathophysiological heterogeneity of sepsis through multi-omics approaches integrating genomic, metabolic, and immune signatures, as the high dimension of multi-omics profiling has been used to reveal the complexity of sepsis immunity and inflammation, enabling simultaneous analysis of multiple levels of RNA, proteins, lipids, and metabolites (176). Second, to enhance the clinical relevance of preclinical findings for sepsis, studies should focus on elucidating the temporal evolution of immunosuppression using longitudinal bio sampling and advanced functional assays. Third, in the past years, AI-enhanced learning is gradually used to determine individualized treatment strategies for septic patients. Although the system is under development for bedside use in a prospective randomized clinical trial in the ICU (177), the tool has help physicians make decisions. Therefore, implementing machine learning-driven predictive models that synthesize real-time biomarker data with electronic health records may enable precision stratification of septic patients for time-targeted immunotherapies.
In summary, this review synthesizes current understanding of immunopathological cascade of sepsis-induced immunosuppression, encompassing mechanistic insights, biomarker discovery, and emerging therapeutic interventions. We propose that understanding the heterogeneity of sepsis, dynamic monitoring of disease progression, and applying precise and individualized therapy are goals of future research to improve the survival of immunocompromised septic patients.
Author contributions
XG: Conceptualization, Funding acquisition, Project administration, Resources, Supervision, Writing – original draft, Writing – review & editing. SC: Writing – original draft. XL: Writing – original draft. GW: Writing – review & editing.
Funding
The author(s) declare that financial support was received for the research and/or publication of this article. This research project was supported by the National Natural Science Foundation of China (project codes: 82302609); Natural Science Foundation of Jiangsu Province (project code: BK20230840); the Research Personnel Cultivation Program of Zhongda Hospital, Southeast University (project code: CZXM-GSP-RC97); Zhongda Hospital Affiliated to Southeast University, Jiangsu Province High-Level Hospital Construction Funds (project code: GSP-LCYJFH27); and the Special Funds for Research in Laboratory Medicine, Jiangsu Medical Association (project code: SYH-3201160-0054 (2023001)). The founders of the study had no involvement in the study design, data collection, data analysis, interpretation, writing of the report, or decision to submit the paper for publication.
Conflict of interest
The authors declare that the research was conducted in the absence of any commercial or financial relationships that could be constructed as a potential conflict of interest.
Generative AI statement
The author(s) declare that no Generative AI was used in the creation of this manuscript.
Publisher’s note
All claims expressed in this article are solely those of the authors and do not necessarily represent those of their affiliated organizations, or those of the publisher, the editors and the reviewers. Any product that may be evaluated in this article, or claim that may be made by its manufacturer, is not guaranteed or endorsed by the publisher.
References
1. Singer M, Deutschman CS, Seymour CW, Shankar-Hari M, Annane D, Bauer M, et al. The third international consensus definitions for sepsis and septic shock (Sepsis-3). JAMA. (2016) 315:801–10. doi: 10.1001/jama.2016.0287
2. Rudd KE, Johnson SC, Agesa KM, Shackelford KA, Tsoi D, Kievlan DR, et al. Global, regional, and national sepsis incidence and mortality, 1990-2017: analysis for the Global Burden of Disease Study. Lancet (London England). (2020) 395:200–11. doi: 10.1016/S0140-6736(19)32989-7
3. Seymour CW, Kennedy JN, Wang S, Chang C-CH, Elliott CF, Xu Z, et al. Derivation, validation, and potential treatment implications of novel clinical phenotypes for sepsis. JAMA. (2019) 321:2003–17. doi: 10.1001/jama.2019.5791
4. Wiersinga WJ, van der Poll T. Immunopathophysiology of human sepsis. eBioMedicine. (2022) 86:104363. doi: 10.1016/j.ebiom.2022.104363
5. Foley NM, Wang J, Redmond HP, Wang JH. Current knowledge and future directions of TLR and NOD signaling in sepsis. Mil Med Res. (2015) 2:1. doi: 10.1186/s40779-014-0029-7
6. Pandharipande PP, Girard TD, Ely EW. Long-term cognitive impairment after critical illness. New Engl J Med. (2014) 370:185–6. doi: 10.1097/SA.0000000000000071
7. Cao M, Wang G, Xie J. Immune dysregulation in sepsis: experiences, lessons and perspectives. Cell Death Discov. (2023) 9:465. doi: 10.1038/s41420-023-01766-7
8. Bain CR, Myles PS, Corcoran T, Dieleman JM. Postoperative systemic inflammatory dysregulation and corticosteroids: a narrative review. Anaesthesia. (2023) 78:356–70. doi: 10.1111/anae.15896
9. Angus DC. The search for effective therapy for sepsis: back to the drawing board? Jama. (2011) 306:2614–5. doi: 10.1001/jama.2011.1853
10. Daoudlarian D, Segot A, Latifyan S, Bartolini R, Joo V, Mederos N, et al. Tocilizumab and immune signatures for targeted management of cytokine release syndrome in immune checkpoint therapy. Ann Oncol. (2024) 36:444–459. doi: 10.1016/j.annonc.2024.12.004
11. Zhao G, Xie Y, Lei X, Guo R, Cui N. mTOR aggravated CD4+ T cell pyroptosis by regulating the PPARγ–Nrf2 pathway in sepsis. Int Immunopharmacol. (2024) 140:112822. doi: 10.1016/j.intimp.2024.112822
12. Liu J, Song K, Lin B, Chen Z, Zuo Z, Fang Y, et al. HMGB1 promotes neutrophil PD-L1 expression through TLR2 and mediates T cell apoptosis leading to immunosuppression in sepsis. Int Immunopharmacol. (2024) 133:112130. doi: 10.1016/j.intimp.2024.112130
13. Zhao J, Dai RS, Chen YZ, Zhuang YG. Prognostic significance of lymphocyte subpopulations for ICU-acquired infections in patients with sepsis: a retrospective study. J Hosp Infect. (2023) 140:40–5. doi: 10.1016/j.jhin.2023.05.022
14. Liao C, Luo S, Liu X, Zhang L, Xie P, Zhou W, et al. Siglec-F+ neutrophils in the spleen induce immunosuppression following acute infection. Theranost. (2024) 14:2589–604. doi: 10.7150/thno.93812
15. Rubio I, Osuchowski MF, Shankar-Hari M, Skirecki T, Winkler MS, Lachmann G, et al. Current gaps in sepsis immunology: new opportunities for translational research. Lancet Infect Dis. (2019) 19:e422–36. doi: 10.1016/S1473-3099(19)30567-5
16. Hotchkiss RS, Monneret G, Payen D. Sepsis-induced immunosuppression: from cellular dysfunctions to immunotherapy. Nat Rev Immunol. (2013) 13:862–74. doi: 10.1038/nri3552
17. Jiang J, Du H, Su Y, Li X, Zhang J, Chen M, et al. Nonviral infection-related lymphocytopenia for the prediction of adult sepsis and its persistence indicates a higher mortality. Med (Baltimore). (2019) 98:e16535. doi: 10.1097/MD.0000000000016535
18. Volk H-D, Reinke P, Krausch D, Zuckermann H, Asadullah K, Müller JM, et al. Monocyte deactivation-rationale for a new therapeutic strategy in sepsis. Intens Care Med. (1996) 22:S474–81. doi: 10.1007/BF01743727
19. van der Poll T, Shankar-Hari M, Wiersinga WJ. The immunology of sepsis. Immunity. (2021) 54:2450–64. doi: 10.1016/j.immuni.2021.10.012
20. Doernberg SB, Arias CA, Altman DR, Babiker A, Boucher HW, Creech CB, et al. Priorities and progress in gram-positive bacterial infection research by the antibacterial resistance leadership group: A narrative review. Clin Infect Dis. (2023) 77:S295–304. doi: 10.1093/cid/ciad565
21. Liu Z, Ting Y, Li M, Li Y, Tan Y, Long Y. From immune dysregulation to organ dysfunction: understanding the enigma of Sepsis. Front Microbiol. (2024) 15:1415274. doi: 10.3389/fmicb.2024.1415274
22. Newton K, Strasser A, Kayagaki N, Dixit VM. Cell death. Cell. (2024) 187:235–56. doi: 10.1016/j.cell.2023.11.044
23. Venet F, Davin F, Guignant C, Larue A, Cazalis M-A, Darbon R, et al. Early assessment of leukocyte alterations at diagnosis of septic shock. Shock (Augusta Ga.). (2010) 34:358–63. doi: 10.1097/SHK.0b013e3181dc0977
24. Boomer JS, To K, Chang KC, Takasu O, Osborne DF, Walton AH, et al. Immunosuppression in patients who die of sepsis and multiple organ failure. Jama. (2011) 306:2594–605. doi: 10.1001/jama.2011.1829
25. Luan Y-y, Yin C-F, Qin Q-H, Dong N, Zhu X-M, Sheng Z-Y, et al. Effect of regulatory T cells on promoting apoptosis of T lymphocyte and its regulatory mechanism in sepsis. J Interf Cytokine Res. (2015) 35:969–80. doi: 10.1089/jir.2014.0235
26. Gu B, Jiang Y, Huang Z, Li H, Yu W, Li T, et al. MRG15 aggravates sepsis-related liver injury by promoting PCSK9 synthesis and secretion. Int Immunopharmacol. (2024) 140:112898. doi: 10.1016/j.intimp.2024.112898
27. Xu J, Li J, Xiao K, Zou S, Yan P, Xie X, et al. Dynamic changes in human HLA-DRA gene expression and Th cell subsets in sepsis: Indications of immunosuppression and associated outcomes. Scand J Immunol. (2020) 91:e12813. doi: 10.1111/sji.12813
28. Fu X, Liu Z, Wang Y. Advances in the study of immunosuppressive mechanisms in sepsis. J Inflammation Res. (2023) 16:3967–81. doi: 10.2147/JIR.S426007
29. Ayala A, Perl M, Venet F, Lomas-Neira J, Swan R, Chung C-S. Apoptosis in sepsis: mechanisms, clinical impact and potential therapeutic targets. Curr Pharm Design. (2008) 14:1853–9. doi: 10.2174/138161208784980617
30. Hampton T. Autophagy genes linked to sepsis survival in mice. JAMA. (2019) 322:1244–5. doi: 10.1001/jama.2019.14766
31. Cabrera-Perez J, Condotta SA, Badovinac VP, Griffith TS. Impact of sepsis on CD4 T cell immunity. J Leukocyte Biol. (2014) 96:767–77. doi: 10.1189/jlb.5MR0114-067R
32. Su Y, Qu Y, Zhao F, Li H, Mu D, Li X. Regulation of autophagy by the nuclear factor κB signaling pathway in the hippocampus of rats with sepsis. J Neuroinflamm. (2015) 12:116. doi: 10.1186/s12974-015-0336-2
33. Zhang Z, Huang S, Wu S, Qi J, Li W, Liu S, et al. Clearance of apoptotic cells by mesenchymal stem cells contributes to immunosuppression via PGE2. EBioMedicine. (2019) 45:341–50. doi: 10.1016/j.ebiom.2019.06.016
34. Wang Z, Li Y, Yang X, Zhang L, Shen H, Xu W, et al. Protective effects of rapamycin induced autophagy on CLP septic mice. Comp Immunol Microbiol Infect Dis. (2019) 64:47–52. doi: 10.1016/j.cimid.2019.01.009
35. Lin C-W, Lo S, Hsu C, Hsieh C-H, Chang Y-F, Hou B-S, et al. T-cell autophagy deficiency increases mortality and suppresses immune responses after sepsis. PloS One. (2014) 9:e102066. doi: 10.1371/journal.pone.0102066
36. Seeley JJ, Ghosh S. Molecular mechanisms of innate memory and tolerance to LPS. J Leukocyte Biol. (2017) 101:107–19. doi: 10.1189/jlb.3MR0316-118RR
37. Jorgensen I, Rayamajhi M, Miao EA. Programmed cell death as a defence against infection. Nat Rev Immunol. (2017) 17:151–64. doi: 10.1038/nri.2016.147
38. Man SM, Karki R, Kanneganti T-D. Molecular mechanisms and functions of pyroptosis, inflammatory caspases and inflammasomes in infectious diseases. Immunol Rev. (2017) 277:61–75. doi: 10.1111/imr.2017.277.issue-1
39. Chen R, Zeng L, Zhu S, Liu J, Zeh HJ, Kroemer G, et al. cAMP metabolism controls caspase-11 inflammasome activation and pyroptosis in sepsis. Sci Adv. (2019) 5:eaav5562. doi: 10.1126/sciadv.aav5562
40. Rathkey JK, Zhao J, Liu Z, Chen Y, Yang J, Kondolf HC, et al. Chemical disruption of the pyroptotic pore-forming protein gasdermin D inhibits inflammatory cell death and sepsis. Sci Immunol. (2018) 3:eaat2738. doi: 10.1126/sciimmunol.aat2738
41. Wang Y-C, Liu Q-X, Liu T, Xu X-E, Gao W, Bai X-J, et al. Caspase-1-dependent pyroptosis of peripheral blood mononuclear cells predicts the development of sepsis in severe trauma patients: A prospective observational study. Medicine. (2018) 97:e9859. doi: 10.1097/MD.0000000000009859
42. Liang S, Zhou J, Cao C, Liu Y, Ming S, Liu X, et al. GITR exacerbates lysophosphatidylcholine-induced macrophage pyroptosis in sepsis via posttranslational regulation of NLRP3. Cell Mol Immunol. (2024) 21:674–88. doi: 10.1038/s41423-024-01170-w
43. Zhang H, Zeng L, Xie M, Liu J, Zhou B, Wu R, et al. TMEM173 drives lethal coagulation in sepsis. Cell Host Microbe. (2020) 27:556–570.e6. doi: 10.1016/j.chom.2020.02.004
44. Jiang X, Stockwell BR, Conrad M. Ferroptosis: mechanisms, biology and role in disease. Nat Rev Mol Cell Biol. (2021) 22:266–82. doi: 10.1038/s41580-020-00324-8
45. Wu J, Liu Q, Zhang X, Tan M, Li X, Liu P, et al. The interaction between STING and NCOA4 exacerbates lethal sepsis by orchestrating ferroptosis and inflammatory responses in macrophages. Cell Death Dis. (2022) 13:653. doi: 10.1038/s41419-022-05115-x
46. Olonisakin TF, Suber T, Gonzalez-Ferrer S, Xiong Z, Peñaloza HF, van der Geest R, et al. Stressed erythrophagocytosis induces immunosuppression during sepsis through heme-mediated STAT1 dysregulation. J Clin Invest. (2021) 131:e137468. doi: 10.1172/JCI137468
47. Lan P, Pan K-H, Wang S-J, Shi Q-C, Yu Y-X, Fu Y, et al. High serum iron level is associated with increased mortality in patients with sepsis. Sci Rep. (2018) 8:11072. doi: 10.1038/s41598-018-29353-2
48. Liu D, Huang SY, Sun JH, Zhang HC, Cai QL, Gao C, et al. Sepsis-induced immunosuppression: mechanisms, diagnosis and current treatment options. Mil Med Res. (2022) 9:56. doi: 10.1186/s40779-022-00422-y
49. Venet F, Pachot A, Debard AL, Bohé J, Bienvenu J, Lepape A, et al. Increased percentage of CD4+CD25+ regulatory T cells during septic shock is due to the decrease of CD4+CD25- lymphocytes. Crit Care Med. (2004) 32:2329–31. doi: 10.1097/01.CCM.0000145999.42971.4B
50. Gaborit BJ, Chaumette T, Chauveau M, Asquier-Khati A, Roquilly A, Boutoille D, et al. Circulating regulatory T cells expressing tumor necrosis factor receptor type 2 contribute to sepsis-induced immunosuppression in patients during septic shock. J Infect Dis. (2021) 224:2160–9. doi: 10.1093/infdis/jiab276
51. Guo R, Zhao G, Bai G, Chen J, Han W, Cui N, et al. Depletion of mTOR ameliorates CD4+ T cell pyroptosis by promoting autophagy activity in septic mice. Int Immunopharmacol. (2023) 124:110964. doi: 10.1016/j.intimp.2023.110964
52. Goswami TK, Singh M, Dhawan M, Mitra S, Emran TB, Rabaan AA, et al. Regulatory T cells (Tregs) and their therapeutic potential against autoimmune disorders - Advances and challenges. Hum Vaccin Immunother. (2022) 18:2035117. doi: 10.1080/21645515.2022.2035117
53. Sehgal R, Maiwall R, Rajan V, Islam M, Baweja S, Kaur N, et al. Granulocyte-macrophage colony-stimulating factor modulates myeloid-derived suppressor cells and treg activity in decompensated cirrhotic patients with sepsis. Front Immunol. (2022) 13. doi: 10.3389/fimmu.2022.828949
54. Durand M, Hagimont E, Louis H, Asfar P, Frippiat J-P, Singer M, et al. The β 1 -adrenergic receptor contributes to sepsis-induced immunosuppression through modulation of regulatory T-cell inhibitory function. Crit Care Med. (2022) 50:e707–e718. doi: 10.1097/CCM.0000000000005503
55. Singh A, Peschel A, Mehling R, Rieber N, Hartl D. Myeloid-derived suppressor cells in bacterial infections. Front In Cell Infect Microbiol. (2016) 6:37. doi: 10.3389/fcimb.2016.00037
56. Millrud CR, Bergenfelz C, Leandersson K. On the origin of myeloid-derived suppressor cells. Oncotarget. (2017) 8:3649–65. doi: 10.18632/oncotarget.12278
57. Veglia F, Sanseviero E, Gabrilovich DI. Myeloid-derived suppressor cells in the era of increasing myeloid cell diversity. Nat Rev Immunol. (2021) 21:485–98. doi: 10.1038/s41577-020-00490-y
58. Mathias B, Delmas AL, Ozrazgat-Baslanti T, Vanzant EL, Szpila BE, Mohr AM, et al. Human myeloid-derived suppressor cells are associated with chronic immune suppression after severe sepsis/septic shock. Ann Surg. (2017) 265:827–34. doi: 10.1097/SLA.0000000000001783
59. Uhel F, Azzaoui I, Grégoire M, Pangault C, Dulong J, Tadié J-M, et al. Early expansion of circulating granulocytic myeloid-derived suppressor cells predicts development of nosocomial infections in patients with sepsis. Am J Respir Crit Care Med. (2017) 196:315–27. doi: 10.1164/rccm.201606-1143OC
60. Mira JC, Gentile LF, Mathias BJ, Efron PA, Brakenridge SC, Mohr AM, et al. Sepsis pathophysiology, chronic critical illness, and persistent inflammation-immunosuppression and catabolism syndrome. Crit Care Med. (2017) 45:253–62. doi: 10.1097/CCM.0000000000002074
61. Zhang K, Jagannath C. Crosstalk between metabolism and epigenetics during macrophage polarization. Epigenet Chromatin. (2025) 18:16. doi: 10.1186/s13072-025-00575-9
62. Huang X, Li Y, Fu M, Xin H-B. Polarizing macrophages in vitro. Methods In Mol Biol (Clifton N.J.). (2018) 1784:119–26. doi: 10.1007/978-1-4939-7837-3_12
63. Wang Z, Wang Z. The role of macrophages polarization in sepsis-induced acute lung injury. Front Immunol. (2023) 14:1209438. doi: 10.3389/fimmu.2023.1209438
64. Shrivastava R, Shukla N. Attributes of alternatively activated (M2) macrophages. Life Sci. (2019) 224:222–31. doi: 10.1016/j.lfs.2019.03.062
65. Su J, Chen W, Zhou F, Li R, Tong Z, Wu S, et al. Inhibitory mechanisms of decoy receptor 3 in cecal ligation and puncture-induced sepsis. mBio. (2024) 15:e00521–24. doi: 10.1128/mbio.00521-24
66. Wu D, Wang L, Hong D, Zheng C, Zeng Y, Ma H, et al. Interleukin 35 contributes to immunosuppression by regulating inflammatory cytokines and T cell populations in the acute phase of sepsis. Clin Immunol. (2022) 235:108915. doi: 10.1016/j.clim.2021.108915
67. Schuijs MJ, Brenis Gomez CM, Bick F, Van Moorleghem J, Vanheerswynghels M, van Loo G, et al. Interleukin-33–activated basophils promote asthma by regulating Th2 cell entry into lung tissue. J Exp Med. (2024) 221:e20240103. doi: 10.1084/jem.20240103
68. Wu H, Tang T, Deng H, Chen D, Zhang C, Luo J, et al. Immune checkpoint molecule Tim-3 promotes NKT cell apoptosis and predicts poorer prognosis in Sepsis. Clin Immunol (Orlando Fla.). (2023) 254:109249. doi: 10.1016/j.clim.2023.109249
69. Duan M, Jie J, Li C, Bai X, Hua S, Tang M, et al. EChinatin alleviates sepsis severity through modulation of the NF-κB and MEK/ERK signaling pathways. Biomed Pharmacother. (2024) 179:117359. doi: 10.1016/j.biopha.2024.117359
70. Gao K, Jin J, Huang C, Li J, Luo H, Li L, et al. Exosomes derived from septic mouse serum modulate immune responses via exosome-associated cytokines. Front In Immunol. (2019) 10:1560. doi: 10.3389/fimmu.2019.01560
71. Cheng X, Li Y, Wang H. Activation of Wnt/β-catenin signal induces DCs to differentiate into immune tolerant regDCs in septic mice. Mol Immunol. (2024) 172:38–46. doi: 10.1016/j.molimm.2024.04.015
72. Zhang H, Wu T, Ren C, Dong N, Wu Y, Yao Y. p53 promotes the expansion of regulatory T cells via DNMT3a- and TET2- mediated Foxp3 expression in sepsis. Burns Trauma. (2023) 11:tkad021. doi: 10.1093/burnst/tkad021
73. Bah I, Kumbhare A, Nguyen L, McCall CE, Gazzar ME. IL-10 induces an immune repressor pathway in sepsis by promoting S100A9 nuclear localization and MDSC development. Cell Immunol. (2018) 332:32–8. doi: 10.1016/j.cellimm.2018.07.003
74. van Vught LA, Wiewel MA, Hoogendijk AJ, Frencken JF, Scicluna BP, Klouwenberg Klein PMC, et al. The host response in patients with sepsis developing intensive care unit-acquired secondary infections. Am J Respir Crit Care Med. (2017) 196:458–70. doi: 10.1164/rccm.201606-1225OC
75. Massagué J, Sheppard D. TGF-β signaling in health and disease. Cell. (2023) 186:4007–37. doi: 10.1016/j.cell.2023.07.036
76. Deng Z, Fan T, Xiao C, Tian H, Zheng Y, Li C, et al. TGF-β signaling in health, disease, and therapeutics. Signal Transduct Target Ther. (2024) 9:61. doi: 10.1038/s41392-024-01764-w
77. Morita Y, Masters EA, Schwarz EM, Muthukrishnan G. Interleukin-27 and its diverse effects on bacterial infections. Front Immunol. (2021) 12. doi: 10.3389/fimmu.2021.678515
78. Yoshida H, Hunter CA. The immunobiology of interleukin-27. Annu Rev Immunol. (2015) 33:417–43. doi: 10.1146/annurev-immunol-032414-112134
79. Aparicio-Siegmund S, Garbers C. The biology of interleukin-27 reveals unique pro- and anti-inflammatory functions in immunity. Cytokine Growth Factor Rev. (2015) 26:579–86. doi: 10.1016/j.cytogfr.2015.07.008
80. Boomer JS, Green JM, Hotchkiss RS. The changing immune system in sepsis: is individualized immuno-modulatory therapy the answer? Virulence. (2014) 5:45–56. doi: 10.4161/viru.26516
81. Ge Y, Huang M, Yao Y-M. Recent advances in the biology of IL-1 family cytokines and their potential roles in development of sepsis. Cytokine Growth Factor Rev. (2019) 45:24–34. doi: 10.1016/j.cytogfr.2018.12.004
82. Wang Y-C, Weng G-P, Liu J-P, Li L, Cheng Q-H. Elevated serum IL-37 concentrations in patients with sepsis. Medicine. (2019) 98:e14756. doi: 10.1097/MD.0000000000014756
83. Gao X, Wu G, Tsang MS, Huang D, Lam CW, Wong CK. Novel insights into the role of anti-inflammatory IL-38 in immunity against infection. Cell Mol Immunol. (2022) 19:1322–4. doi: 10.1038/s41423-022-00876-z
84. Gao X, Chan PKS, Lui GCY, Hui DSC, Chu IM, Sun X, et al. Interleukin-38 ameliorates poly(I:C) induced lung inflammation: therapeutic implications in respiratory viral infections. Cell Death Dis. (2021) 12:53. doi: 10.1038/s41419-020-03283-2
85. Xu F, Lin S, Yan X, Wang C, Tu H, Yin Y, et al. Interleukin 38 protects against lethal sepsis. J Infect Dis. (2018) 218:1175–84. doi: 10.1093/infdis/jiy289
86. Ge Y, Chen J, Hu Y, Chen X, Huang M. IL-38 alleviates inflammation in sepsis in mice by inhibiting macrophage apoptosis and activation of the NLRP3 inflammasome. Mediators Inflammation. (2021) 2021:6370911. doi: 10.1155/2021/6370911
87. McBride MA, Patil TK, Bohannon JK, Hernandez A, Sherwood ER, Patil NK. Immune checkpoints: novel therapeutic targets to attenuate sepsis-induced immunosuppression. Front Immunol. (2020) 11:624272. doi: 10.3389/fimmu.2020.624272
88. Zhang Y, Li J, Lou J, Zhou Y, Bo L, Zhu J, et al. Upregulation of programmed death-1 on T cells and programmed death ligand-1 on monocytes in septic shock patients. Crit Care. (2011) 15:R70. doi: 10.1186/cc10059
89. Venet F, Rimmelé T, Monneret G. Management of sepsis-induced immunosuppression. Crit Care Clin. (2018) 34:97–106. doi: 10.1016/j.ccc.2017.08.007
90. Hotchkiss RS, Colston E, Yende S, Crouser ED, Martin GS, Albertson T, et al. Immune checkpoint inhibition in sepsis: a Phase 1b randomized study to evaluate the safety, tolerability, pharmacokinetics, and pharmacodynamics of nivolumab. Intens Care Med. (2019) 45:1360–71. doi: 10.1007/s00134-019-05704-z
91. Zhang T, Yu-Jing L, Ma T. Role of regulation of PD-1 and PD-L1 expression in sepsis. Front Immunol. (2023) 14:1029438. doi: 10.3389/fimmu.2023.1029438
92. Patera AC, Drewry AM, Chang K, Beiter ER, Osborne D, Hotchkiss RS. Frontline Science: Defects in immune function in patients with sepsis are associated with PD-1 or PD-L1 expression and can be restored by antibodies targeting PD-1 or PD-L1. J Leukocyte Biol. (2016) 100:1239–54. doi: 10.1189/jlb.4HI0616-255R
93. Wurster S, Watowich SS, Kontoyiannis DP. Checkpoint inhibitors as immunotherapy for fungal infections: Promises, challenges, and unanswered questions. Front Immunol. (2022) 13:1018202. doi: 10.3389/fimmu.2022.1018202
94. Huang S, Liu D, Sun J, Zhang H, Zhang J, Wang Q, et al. Tim-3 regulates sepsis-induced immunosuppression by inhibiting the NF-κB signaling pathway in CD4 T cells. Mol Ther. (2022) 30:1227–38. doi: 10.1016/j.ymthe.2021.12.013
95. Zhong X, Xie H, Wang S, Ren T, Chen J, Huang Y, et al. TIGIT regulates CD4+ T cell immunity against polymicrobial sepsis. Front Immunol. (2024) 15:1290564. doi: 10.3389/fimmu.2024.1290564
96. Lou J-S, Wang J-F, Fei M-M, Zhang Y, Wang J, Guo Y, et al. Targeting lymphocyte activation gene 3 to reverse T-lymphocyte dysfunction and improve survival in murine polymicrobial sepsis. J Infect Dis. (2020) 222:1051–61. doi: 10.1093/infdis/jiaa191
97. Raju SM, Kumar AP, Yadav AN, Rajkumar K, Mvs S, Burgula S. Haptoglobin improves acute phase response and endotoxin tolerance in response to bacterial LPS. Immunol Lett. (2019) 207:17–27. doi: 10.1016/j.imlet.2019.01.002
98. Volk CF, Proctor RA, Rose WE. The complex intracellular lifecycle of staphylococcus aureus contributes to reduced antibiotic efficacy and persistent bacteremia. Int J Mol Sci. (2024) 25:6486. doi: 10.3390/ijms25126486
99. Goh KGK, Desai D, Thapa R, Prince D, Acharya D, Sullivan MJ, et al. An opportunistic pathogen under stress: how Group B Streptococcus responds to cytotoxic reactive species and conditions of metal ion imbalance to survive. FEMS Microbiol Rev. (2024) 48:fuae009. doi: 10.1093/femsre/fuae009
100. Ryan TAJ, Hooftman A, Rehill AM, Johansen MD, Brien ECO, Toller-Kawahisa JE, et al. Dimethyl fumarate and 4-octyl itaconate are anticoagulants that suppress Tissue Factor in macrophages via inhibition of Type I Interferon. Nat Commun. (2023) 14:3513. doi: 10.1038/s41467-023-39174-1
101. Geanes ES, McLennan R, Pierce SH, Menden HL, Paul O, Sampath V, et al. SARS-CoV-2 envelope protein regulates innate immune tolerance. iScience. (2024) 27:109975. doi: 10.1016/j.isci.2024.109975
102. Xu JQ, Zhang WY, Fu JJ, Fang XZ, Gao CG, Li C, et al. Viral sepsis: diagnosis, clinical features, pathogenesis, and clinical considerations. Mil Med Res. (2024) 11:78. doi: 10.1186/s40779-024-00581-0
103. Liu Y, Freed DC, Li L, Tang A, Li F, Murray EM, et al. A replication-defective human cytomegalovirus vaccine elicits humoral immune responses analogous to those with natural infection. J Virol. (2019) 93:e00747-19. doi: 10.1128/JVI.00747-19
104. Shen X, Cao K, Zhao Y, Du J. Targeting neutrophils in sepsis: from mechanism to translation. Front Pharmacol. (2021) 12:644270. doi: 10.3389/fphar.2021.644270
105. Demaret J, Venet F, Friggeri A, Cazalis M-A, Plassais J, Jallades L, et al. Marked alterations of neutrophil functions during sepsis-induced immunosuppression. J Leukocyte Biol. (2015) 98:1081–90. doi: 10.1189/jlb.4A0415-168RR
106. Guérin E, Orabona M, Raquil M-A, Giraudeau B, Bellier R, Gibot S, et al. Circulating immature granulocytes with T-cell killing functions predict sepsis deterioration*. Crit Care Med. (2014) 42:2007–18. doi: 10.1097/CCM.0000000000000344
107. Meghraoui-Kheddar A, Chousterman BG, Guillou N, Barone SM, Granjeaud S, Vallet H, et al. Two new neutrophil subsets define a discriminating sepsis signature. Am J Respir Crit Care Med. (2022) 205:46–59. doi: 10.1164/rccm.202104-1027OC
108. Grégoire M, Tadié J-M, Uhel F, Gacouin A, Piau C, Bone N, et al. Frontline Science: HMGB1 induces neutrophil dysfunction in experimental sepsis and in patients who survive septic shock. J Leukocyte Biol. (2017) 101:1281–7. doi: 10.1189/jlb.5HI0316-128RR
109. Stiel L, Mayeur-Rousse C, Helms J, Meziani F, Mauvieux L. First visualization of circulating neutrophil extracellular traps using cell fluorescence during human septic shock-induced disseminated intravascular coagulation. Thromb Res. (2019) 183:153–8. doi: 10.1016/j.thromres.2019.09.036
110. Venet F, Demaret J, Blaise BJ, Rouget C, Girardot T, Idealisoa E, et al. IL-7 Restores T Lymphocyte Immunometabolic Failure in Septic Shock Patients through mTOR Activation. J Immunol. (2017) 199:1606–15. doi: 10.4049/jimmunol.1700127
111. Assis PA, Allen RM, Schaller MA, Kunkel SL, Bermick JR. Metabolic reprogramming and dysregulated IL-17 production impairs CD4 T cell function post sepsis. iScience. (2024) 27:110114. doi: 10.1016/j.isci.2024.110114
112. Shankar-Hari M, Fear D, Lavender P, Mare T, Beale R, Swanson C, et al. Activation-associated accelerated apoptosis of memory B cells in critically ill patients with sepsis. Crit Care Med. (2017) 45:875–82. doi: 10.1097/CCM.0000000000002380
113. Kashimura M. Blood defense system - Proposal for a new concept of an immune system against blood borne pathogens comprising the liver, spleen and bone marrow. Scand J Immunol. (2024) 99:e13363. doi: 10.1111/sji.13363
114. Gustave C-A, Gossez M, Demaret J, Rimmelé T, Lepape A, Malcus C, et al. Septic shock shapes B cell response toward an exhausted-like/immunoregulatory profile in patients. J Immunol (Baltimore Md: 1950). (2018) 200:2418–25. doi: 10.4049/jimmunol.1700929
115. Vázquez AC, Arriaga-Pizano L, Ferat-Osorio E. Cellular markers of immunosuppression in sepsis. Arch Med Res. (2021) 52:828–35. doi: 10.1016/j.arcmed.2021.10.001
116. Tang J, Shang C, Chang Y, Jiang W, Xu J, Zhang L, et al. Peripheral PD-1+NK cells could predict the 28-day mortality in sepsis patients. Front Immunol. (2024) 15:1426064. doi: 10.3389/fimmu.2024.1426064
117. Feng T, Liao X, Yang X, Yang C, Lin F, Guo Y, et al. A shift toward inhibitory receptors and impaired effector functions on NK cells contribute to immunosuppression during sepsis. J Leukocyte Biol. (2020) 107:57–67. doi: 10.1002/JLB.4A0818-313RR
118. Vivier E, Artis D, Colonna M, Diefenbach A, Di Santo JP, Eberl G, et al. Innate lymphoid cells: 10 years on. Cell. (2018) 174:1054–66. doi: 10.1016/j.cell.2018.07.017
119. Carvelli J, Piperoglou C, Bourenne J, Farnarier C, Banzet N, Demerlé C, et al. Imbalance of circulating innate lymphoid cell subpopulations in patients with septic shock. Front Immunol. (2019) 10:2179. doi: 10.3389/fimmu.2019.02179
120. Cruz-Zárate D, Cabrera-Rivera GL, Ruiz-Sánchez BP, Serafín-López J, Chacón-Salinas R, López-Macías C, et al. Innate Lymphoid Cells Have Decreased HLA-DR Expression but Retain Their Responsiveness to TLR Ligands during Sepsis. J Immunol. (2018) 201:3401–10. doi: 10.4049/jimmunol.1800735
121. Shindo Y, Fuchs AG, Davis CG, Eitas T, Unsinger J, Burnham C-AD, et al. Interleukin 7 immunotherapy improves host immunity and survival in a two-hit model of Pseudomonas aeruginosa pneumonia. J Leukocyte Biol. (2017) 101:543–54. doi: 10.1189/jlb.4A1215-581R
122. Nascimento DC, Melo PH, Piñeros AR, Ferreira RG, Colón DF, Donate PB, et al. IL-33 contributes to sepsis-induced long-term immunosuppression by expanding the regulatory T cell population. Nat Commun. (2017) 8:14919. doi: 10.1038/ncomms14919
123. Pei F, Yao R-Q, Ren C, Bahrami S, Billiar TR, Chaudry IH, et al. Expert consensus on the monitoring and treatment of sepsis-induced immunosuppression. Military Med Res. (2022) 9:74. doi: 10.1186/s40779-022-00430-y
124. Shao R, Fang Y, Yu H, Zhao L, Jiang Z, Li C-S. Monocyte programmed death ligand-1 expression after 3–4 days of sepsis is associated with risk stratification and mortality in septic patients: a prospective cohort study. Crit Care (London England). (2016) 20:124. doi: 10.1186/s13054-016-1301-x
125. Liu Q, Xue M, Song Q, Xie J, Yang Y, Liu S. Expression of PD-1 on memory T lymphocytes predicts 28-day mortality of patients with sepsis: A prospective observational study. J Inflammation Res. (2022) 15:5043–52. doi: 10.2147/JIR.S376897
126. Cheng W, Zhang J, Li D, Lei X, Wang H, Cui N. CTLA-4 expression on CD4+ lymphocytes in patients with sepsis-associated immunosuppression and its relationship to mTOR mediated autophagic-lysosomal disorder. Front In Immunol. (2024) 15:1396157. doi: 10.3389/fimmu.2024.1396157
127. Mearelli F, Nunnari A, Rombini A, Chitti F, Spagnol F, Casarsa C, et al. Inhibitory immune checkpoints predict 7-day, in-hospital, and 1-year mortality of internal medicine patients admitted with bacterial sepsis. J Infect Dis. (2025) 231:706–15. doi: 10.1093/infdis/jiae370
128. Liu S, Li Y, She F, Zhao X, Yao Y. Predictive value of immune cell counts and neutrophil-to-lymphocyte ratio for 28-day mortality in patients with sepsis caused by intra-abdominal infection. Burns Trauma. (2021) 9:tkaa040. doi: 10.1093/burnst/tkaa040
129. Wu Y, Wu G, Li M, Chang Y, Yu M, Meng Y, et al. Prediction of Th17/Treg cell balance on length of stay in intensive care units of patients with sepsis. J Intens Med. (2024) 4:240–6. doi: 10.1016/j.jointm.2023.09.005
130. Yao R-Q, Zhao P-Y, Li Z-X, Liu Y-Y, Zheng L-Y, Duan Y, et al. Single-cell transcriptome profiling of sepsis identifies HLA-DRlowS100Ahigh monocytes with immunosuppressive function. Military Med Res. (2023) 10:27. doi: 10.1186/s40779-023-00462-y
131. Yin J, Chen Y, Huang J-L, Yan L, Kuang Z-S, Xue M-M, et al. Prognosis-related classification and dynamic monitoring of immune status in patients with sepsis: A prospective observational study. World J Emergency Med. (2021) 12:185–91. doi: 10.5847/wjem.j.1920-8642.2021.03.004
132. Li X, Xu Z, Pang X, Huang Y, Yang B, Yang Y, et al. Interleukin-10/lymphocyte ratio predicts mortality in severe septic patients. PloS One. (2017) 12:e0179050. doi: 10.1371/journal.pone.0179050
133. Wang Y, Zhao J, Yao Y, Zhao D, Liu S. Interleukin-27 as a diagnostic biomarker for patients with sepsis: A meta-analysis. BioMed Res Int. (2021) 2021:5516940. doi: 10.1155/2021/5516940
134. Wu C, Ma J, Yang H, Zhang J, Sun C, Lei Y, et al. Interleukin-37 as a biomarker of mortality risk in patients with sepsis. J Infect. (2021) 82:346–54. doi: 10.1016/j.jinf.2021.01.019
135. Hall MW, Knatz NL, Vetterly C, Tomarello S, Wewers MD, Volk HD, et al. Immunoparalysis and nosocomial infection in children with multiple organ dysfunction syndrome. Intens Care Med. (2011) 37:525–32. doi: 10.1007/s00134-010-2088-x
136. Ploder M, Pelinka L, Schmuckenschlager C, Wessner B, Ankersmit HJ, Fuerst W, et al. Lipopolysaccharide-induced tumor necrosis factor alpha production and not monocyte human leukocyte antigen-DR expression is correlated with survival in septic trauma patients. Shock (Augusta Ga.). (2006) 25:129–34. doi: 10.1097/01.shk.0000191379.62897.1d
137. Winkler MS, Rissiek A, Priefler M, Schwedhelm E, Robbe L, Bauer A, et al. Human leucocyte antigen (HLA-DR) gene expression is reduced in sepsis and correlates with impaired TNFα response: A diagnostic tool for immunosuppression? PloS One. (2017) 12:e0182427. doi: 10.1371/journal.pone.0182427
138. Ansari MA, Das S, Rai G, Singh PK, Lahan S, Tyagi A, et al. Low monocytic HLA-DR expression in critically ill patients of sepsis: An indicator for antimicrobial and/or immunomodulatory intervention. Transpl Immunol. (2023) 81:101942. doi: 10.1016/j.trim.2023.101942
139. Spinetti T, Hirzel C, Fux M, Walti LN, Schober P, Stueber F, et al. Reduced monocytic human leukocyte antigen-DR expression indicates immunosuppression in critically ill COVID-19 patients. Anesth Analg. (2020) 131:993–9. doi: 10.1213/ANE.0000000000005044
140. de Roquetaillade C, Dupuis C, Faivre V, Lukaszewicz AC, Brumpt C, Payen D. Monitoring of circulating monocyte HLA-DR expression in a large cohort of intensive care patients: relation with secondary infections. Ann Intens Care. (2022) 12:39. doi: 10.1186/s13613-022-01010-y
141. Leijte GP, Rimmelé T, Kox M, Bruse N, Monard C, Gossez M, et al. Monocytic HLA-DR expression kinetics in septic shock patients with different pathogens, sites of infection and adverse outcomes. Crit Care. (2020) 24:110. doi: 10.1186/s13054-020-2830-x
142. Cui J, Cai W, Zhang L, Wu Y, Huang Y, Zhao W. Decreased monocytic HLA-DR in patients with sepsis: Prediction of diagnosis, severity and prognosis. Clin Biochem. (2025) 135:110851. doi: 10.1016/j.clinbiochem.2024.110851
143. Zorio V, Venet F, Delwarde B, Floccard B, Marcotte G, Textoris J, et al. Assessment of sepsis-induced immunosuppression at ICU discharge and 6 months after ICU discharge. Ann Intens Care. (2017) 7:80. doi: 10.1186/s13613-017-0304-3
144. Harper J, Gordon S, Chan CN, Wang H, Lindemuth E, Galardi C, et al. CTLA-4 and PD-1 dual blockade induces SIV reactivation without control of rebound after antiretroviral therapy interruption. Nat Med. (2020) 26:519–28. doi: 10.1038/s41591-020-0782-y
145. Wang Z, Zhang W, Chen L, Lu X, Tu Y. Lymphopenia in sepsis: a narrative review. Crit Care (London England). (2024) 28:315. doi: 10.1186/s13054-024-05099-4
146. Vulliamy PE, Perkins ZB, Brohi K, Manson J. Persistent lymphopenia is an independent predictor of mortality in critically ill emergency general surgical patients. Eur J Trauma Emerg Surg. (2016) 42:755–60. doi: 10.1007/s00068-015-0585-x
147. Huang S, Liu D, Han L, Deng J, Wang Z, Jiang J, et al. Decoding the potential role of regulatory T cells in sepsis-induced immunosuppression. Eur J Immunol. (2024) 54:e2350730. doi: 10.1002/eji.202350730
148. Roy D, Bose S, Pati S, Guin A, Banerjee K, Saha S, et al. GFI1/HDAC1-axis differentially regulates immunosuppressive CD73 in human tumor-associated FOXP3+ Th17 and inflammation-linked Th17 cells. Eur J Immunol. (2021) 51:1206–17. doi: 10.1002/eji.202048892
149. Conway Morris A, Datta D, Shankar-Hari M, Stephen J, Weir CJ, Rennie J, et al. Cell-surface signatures of immune dysfunction risk-stratify critically ill patients: INFECT study. Intens Care Med. (2018) 44:627–35. doi: 10.1007/s00134-018-5247-0
150. Wang Q, Long G, Luo H, Zhu X, Han Y, Shang Y, et al. S100A8/A9: An emerging player in sepsis and sepsis-induced organ injury. Biomed Pharmacother. (2023) 168:115674. doi: 10.1016/j.biopha.2023.115674
151. Zhang X, Zhang Y, Yuan S, Zhang J. The potential immunological mechanisms of sepsis. Front Immunol. (2024) 15:1434688. doi: 10.3389/fimmu.2024.1434688
152. Shakoory B, Carcillo JA, Chatham WW, Amdur RL, Zhao H, Dinarello CA, et al. Interleukin-1 receptor blockade is associated with reduced mortality in sepsis patients with features of macrophage activation syndrome: reanalysis of a prior phase III trial. Crit Care Med. (2016) 44:275–81. doi: 10.1097/CCM.0000000000001402
153. Panacek EA, Marshall JC, Albertson TE, Johnson DH, Johnson S, MacArthur RD, et al. Efficacy and safety of the monoclonal anti-tumor necrosis factor antibody F(ab’)2 fragment afelimomab in patients with severe sepsis and elevated interleukin-6 levels. Crit Care Med. (2004) 32:2173–82. doi: 10.1097/01.CCM.0000145229.59014.6C
154. Zhang P, Wang Y, Yang W, Yin Y, Li C, Ma X, et al. 4-Octyl itaconate regulates immune balance by activating Nrf2 and negatively regulating PD-L1 in a mouse model of sepsis. Int J Biol Sci. (2022) 18:6189–209. doi: 10.7150/ijbs.74456
155. Hotchkiss RS, Colston E, Yende S, Angus DC, Moldawer LL, Crouser ED, et al. Immune checkpoint inhibition in sepsis: A phase 1b randomized, placebo-controlled, single ascending dose study of antiprogrammed cell death-ligand 1 antibody (BMS-936559). Crit Care Med. (2019) 47:632–42. doi: 10.1097/CCM.0000000000003685
156. Kamphorst AO, Wieland A, Nasti T, Yang S, Zhang R, Barber DL, et al. Rescue of exhausted CD8 T cells by PD-1-targeted therapies is CD28-dependent. Science. (2017) 355:1423–7. doi: 10.1126/science.aaf0683
157. Sun Y, Ding R, Chang Y, Li J, Ma X. Immune checkpoint molecule TIGIT manipulates T cell dysfunction in septic patients. Int Immunopharmacol. (2021) 101:108205. doi: 10.1016/j.intimp.2021.108205
158. Unsinger J, McGlynn M, Kasten KR, Hoekzema AS, Watanabe E, Muenzer JT, et al. IL-7 promotes T cell viability, trafficking, and functionality and improves survival in sepsis. J Immunol (Baltimore Md: 1950). (2010) 184:3768–79. doi: 10.4049/jimmunol.0903151
159. Francois B, Jeannet R, Daix T, Walton AH, Shotwell MS, Unsinger J, et al. Interleukin-7 restores lymphocytes in septic shock: the IRIS-7 randomized clinical trial. JCI Insight. (2018) 3:e98960. doi: 10.1172/jci.insight.98960
160. Sepsis, H.I.f.t.S.o. Personalized Immunotherapy in Sepsis: a Multicentre and Multinational, Double-blind, Double-dummy Randomized Clinical Trial (2021). Available online at: https://clinicaltrials.gov/study/NCT04990232 (Accessed April 4, 2025).
161. Nakos G, Malamou-Mitsi VD, Lachana A, Karassavoglou A, Kitsiouli E, Agnandi N, et al. Immunoparalysis in patients with severe trauma and the effect of inhaled interferon-gamma. Crit Care Med. (2002) 30:1488–94. doi: 10.1097/00003246-200207000-00015
162. Grimaldi D, Pradier O, Hotchkiss RS, Vincent J-L. Nivolumab plus interferon-γ in the treatment of intractable mucormycosis. Lancet Infect Dis. (2017) 17:18. doi: 10.1016/S1473-3099(16)30541-2
163. Sepsis, H.I.f.t.S.o. A Personalized Randomized Trial of Validation and Restoration of Immune Dysfunction in Severe Infections and Sepsis (2017). Available online at: https://clinicaltrials.gov/study/NCT03332225?a=6.
164. Drossou-Agakidou V, Kanakoudi-Tsakalidou F, Sarafidis K, Tzimouli V, Taparkou A, Kremenopoulos G, et al. In vivo effect of rhGM-CSF And rhG-CSF on monocyte HLA-DR expression of septic neonates. Cytokine. (2002) 18:260–5. doi: 10.1006/cyto.2002.1037
165. Meisel C, Schefold JC, Pschowski R, Baumann T, Hetzger K, Gregor J, et al. Granulocyte-macrophage colony-stimulating factor to reverse sepsis-associated immunosuppression: a double-blind, randomized, placebo-controlled multicenter trial. Am J Respir Crit Care Med. (2009) 180:640–8. doi: 10.1164/rccm.200903-0363OC
166. Lyon H. A Double-Blind, Randomized, Placebo-controlled Multicenter Trial of GRanulocyte-Macrophage Colony-stimulating Factor Administration to Decrease ICU Acquired Infections in Sepsis-induced ImmunoDepression (2015). Available online at: https://clinicaltrials.gov/study/NCT02361528 (Accessed April 4, 2025).
167. (NIHR), N.I.f.H.a.C.R. Funding and awards: Sepsis trials in critical care (SepTIC) (2022). Available online at: https://fundingawards.nihr.ac.uk/award/17/136/02 (Accessed April 4, 2025).
168. Pei F, Guan X, Wu J. Thymosin alpha 1 treatment for patients with sepsis. Expert Opin On Biol Ther. (2018) 18:71–6. doi: 10.1080/14712598.2018.1484104
169. Cui J, Wei X, Lv H, Li Y, Li P, Chen Z, et al. The clinical efficacy of intravenous IgM-enriched immunoglobulin (pentaglobin) in sepsis or septic shock: a meta-analysis with trial sequential analysis. Ann Intens Care. (2019) 9:27. doi: 10.1186/s13613-019-0501-3
170. Nierhaus A, Berlot G, Kindgen-Milles D, Müller E, Girardis M. Best-practice IgM- and IgA-enriched immunoglobulin use in patients with sepsis. Ann Intens Care. (2020) 10:132. doi: 10.1186/s13613-020-00740-1
171. Biagioni E, Tosi M, Berlot G, Castiglione G, Corona A, Cristofaro De MG, et al. Adjunctive IgM-enriched immunoglobulin therapy with a personalised dose based on serum IgM-titres versus standard dose in the treatment of septic shock: a randomised controlled trial (IgM-fat trial). BMJ Open. (2021) 11:e036616. doi: 10.1136/bmjopen-2019-036616
172. Khosrojerdi A, Soudi S, Hosseini AZ, Eshghi F, Shafiee A, Hashemi SM. Immunomodulatory and therapeutic effects of mesenchymal stem cells on organ dysfunction in sepsis. Shock (Augusta Ga.). (2021) 55:423–40. doi: 10.1097/SHK.0000000000001644
173. Keane C, Jerkic M, Laffey JG. Stem cell-based therapies for sepsis. Anesthesiology. (2017) 127:1017–34. doi: 10.1097/ALN.0000000000001882
174. Ahangari F, Soudi S, Khaligh Ghaffari S, Mirsanei Z, Soufihasanabad S, Asl Ebadi P, et al. Combinational therapy of mesenchymal stem cell-derived extracellular vesicles and azithromycin improves clinical and histopathological recovery in CLP sepsis model. Int Immunopharmacol. (2024) 139:112732. doi: 10.1016/j.intimp.2024.112732
175. Therapeutics N. Advanced mesenchymal enhanced cell THerapY for sepTic patients. (2021). Available online at: https://clinicaltrials.gov/study/NCT04961658
176. Schuurman AR, Reijnders TDY, Kullberg RFJ, Butler JM, van der Poll T, Wiersinga WJ. Sepsis: deriving biological meaning and clinical applications from high-dimensional data. Intens Care Med Exp. (2021) 9:27. doi: 10.1186/s40635-021-00383-x
Keywords: sepsis, immunosuppression, biomarkers, therapy, immunology
Citation: Gao X, Cai S, Li X and Wu G (2025) Sepsis-induced immunosuppression: mechanisms, biomarkers and immunotherapy. Front. Immunol. 16:1577105. doi: 10.3389/fimmu.2025.1577105
Received: 15 February 2025; Accepted: 07 April 2025;
Published: 29 April 2025.
Edited by:
Nikunj Umedbhai Tandel, Centre for Cellular & Molecular Biology (CCMB), IndiaReviewed by:
Yan-wei Cheng, Henan Provincial People’s Hospital, ChinaKingsley Yin, Rowan University School of Osteopathic Medicine, United States
Copyright © 2025 Gao, Cai, Li and Wu. This is an open-access article distributed under the terms of the Creative Commons Attribution License (CC BY). The use, distribution or reproduction in other forums is permitted, provided the original author(s) and the copyright owner(s) are credited and that the original publication in this journal is cited, in accordance with accepted academic practice. No use, distribution or reproduction is permitted which does not comply with these terms.
*Correspondence: Xun Gao, Z2FveHVuMTAyMUAxNjMuY29t