- 1Drexel University College of Medicine, Department of Microbiology and Immunology, Philadelphia, PA, United States
- 2Drexel University College of Medicine, Department of Medicine, Division of Infectious Diseases and HIV Medicine, Philadelphia, PA, United States
For more than two centuries, the field of vaccine development has progressed through the adaptation of novel platforms in parallel with technological developments. Building off the advantages and shortcomings of first and second-generation vaccine platforms, the advent of third-generation nucleic acid vaccines has enabled new approaches to tackle emerging infectious diseases, cancers, and pathogens where vaccines remain unavailable. Unlike traditional vaccine platforms, nucleic acid vaccines offer several new advantages, including their lower cost and rapid production, which was widely demonstrated during the COVID-19 pandemic. Beyond production, DNA and mRNA vaccines can elicit unique and targeted responses through specialized design and delivery approaches. Considering the growth of nucleic acid vaccine research over the past two decades, the evaluation of their efficacy in at-risk populations is paramount for refining and improving vaccine design. Importantly, the aging population represents a significant portion of individuals highly susceptible to infection and disease. This review seeks to outline the major impairments in vaccine-induced responses due to aging that may be targeted for improvement with design and delivery components encompassing mRNA and DNA vaccine formulations. Results of pre-clinical and clinical applications of these vaccines in aged animal models and humans will also be evaluated to outline current successes and limitations observed in these platforms.
Introduction
Traditional vaccine platforms, including whole virus, viral vector, and protein-based vaccines have been used for numerous infectious diseases and cancers. Vaccine development is vital globally for preventing infectious diseases and mitigating the risk and economic burden of outbreaks. Recent estimates suggest that bringing a novel vaccine to market in the United States costs approximately $886.8 million and requires 10–15 years of laboratory research, highlighting the extensive and expensive nature of vaccine development (1). Beyond preventative vaccines, therapeutic vaccines for autoimmune diseases and cancers have also been of significant interest. Technological advancement has played a major role in shaping the history of the vaccine development pipeline, leading to the most recent progression of next-generation vaccine platforms. Over the past 20–30 years, nucleic acid-based vaccines (DNA and mRNA) have gained substantial attention. Since their inception, nucleic acid vaccines have evolved significantly. The emergence of DNA vaccines began in the 1990s when investigators discovered injection of plasmid DNA had immunostimulatory properties, subsequently leading to the first DNA vaccine clinical trials (2). Although early preclinical results held promise, clinical trials revealed limited efficacy due to weak immune stimulation and delivery, thereafter sparking advancements in delivery technologies including electroporation and lipid nanoparticles to improve performance. In parallel, beginning in the late 1980s, landmark experiments by Robert Malone served as the foundation for the use of mRNA as a potential drug target (3). From this point, massive developments have been made on the lipid nanoparticle technology necessary to deliver mRNA transcripts. Importantly, the COVID-19 pandemic showcased the rapid development and promising efficacy of mRNA vaccines in humans through its accelerated development. Nucleic acid vaccines offer several potential advantages over traditional vaccine platforms, including rapid and cost-effective production, high antigen specificity compared to whole-organism vaccines, versatile applications across infectious disease, cancer, and genetic disorders, and potent induction of both humoral and cellular responses. Nonetheless, as with any new therapeutic, further investigation into the safety and efficacy of nucleic acid vaccines is still necessary.
While vaccines are essential for the general population to protect against emerging diseases and reduce their spread, the induction of strong vaccine-induced responses in at-risk populations is of paramount interest. Notably, elderly individuals (65+ years) constitute the largest at-risk group, comprising 10% of the world population in 2024, with this percentage expected to more than double by 2050 (4, 5). Given the significant age-related decline in immune responses and the rapidly growing elderly population, enhancing vaccine-induced immunity is crucial. For example, in 2022, mortalities for those 65 and older reached almost 2.5 million with COVID-19 and cancer as two of the top three causes of death (6). This data emphasizes the burden of infectious diseases and cancer on elderly mortality rates and, therefore, the necessity to progress and enhance vaccination strategies in aging populations.
This review will outline age-related impairments in immune cells associated with vaccination responses. Considering the growing efforts in investigating next-generation nucleic acid vaccine formulations, this review will also explore the mechanisms, design, delivery, advantages, and disadvantages of mRNA and DNA vaccines to further our understanding of these platforms. Pre-clinical and clinical studies emphasizing responses elicited in aged animal models and humans will be discussed to highlight the potential of these platforms for vaccinating the elderly and their shortcomings. Finally, as adjuvants and immune modulators are critical components for enhancing vaccine-induced responses in the elderly, previously investigated adjuvants and adjuvant-like properties of nucleic acid vaccines will be examined.
Immunosenescence and challenges of vaccination in the elderly
Aging is a biological process that causes a gradual deterioration in the function of multiple systems of the body, including the immune system. This process of impaired immune function with aging is also known as immunosenescence. With an increase in life expectancy of humans over the past century, the consequential growth of elderly populations has resulted in higher rates of age-related diseases such as cancer in addition to failure of therapeutics for cancers and infectious diseases. Several aspects of immune function at both the innate and adaptive levels decline because of immunosenescence. One key factor influencing immunosenescence is thymic involution, or the progressive reduction in size of the thymus, which acts as a primary lymphoid organ important for T cell maturation. Similarly, aging correlates with an increase in pro-inflammatory status, a phenomenon known as “inflammaging”, representing another hallmark of immunosenescence. Beyond this, aging-mediated hematopoietic stem cell dysfunction, altered T/B cell ratios, impaired antigen responses, accumulation of senescent cells, mitochondrial dysfunction, and genomic instability also underly immunosenescence. This section will focus on age-associated changes to innate antigen-presenting cells (APCs) and adaptive T and B cell function as it relates to vaccine responses (Figure 1).
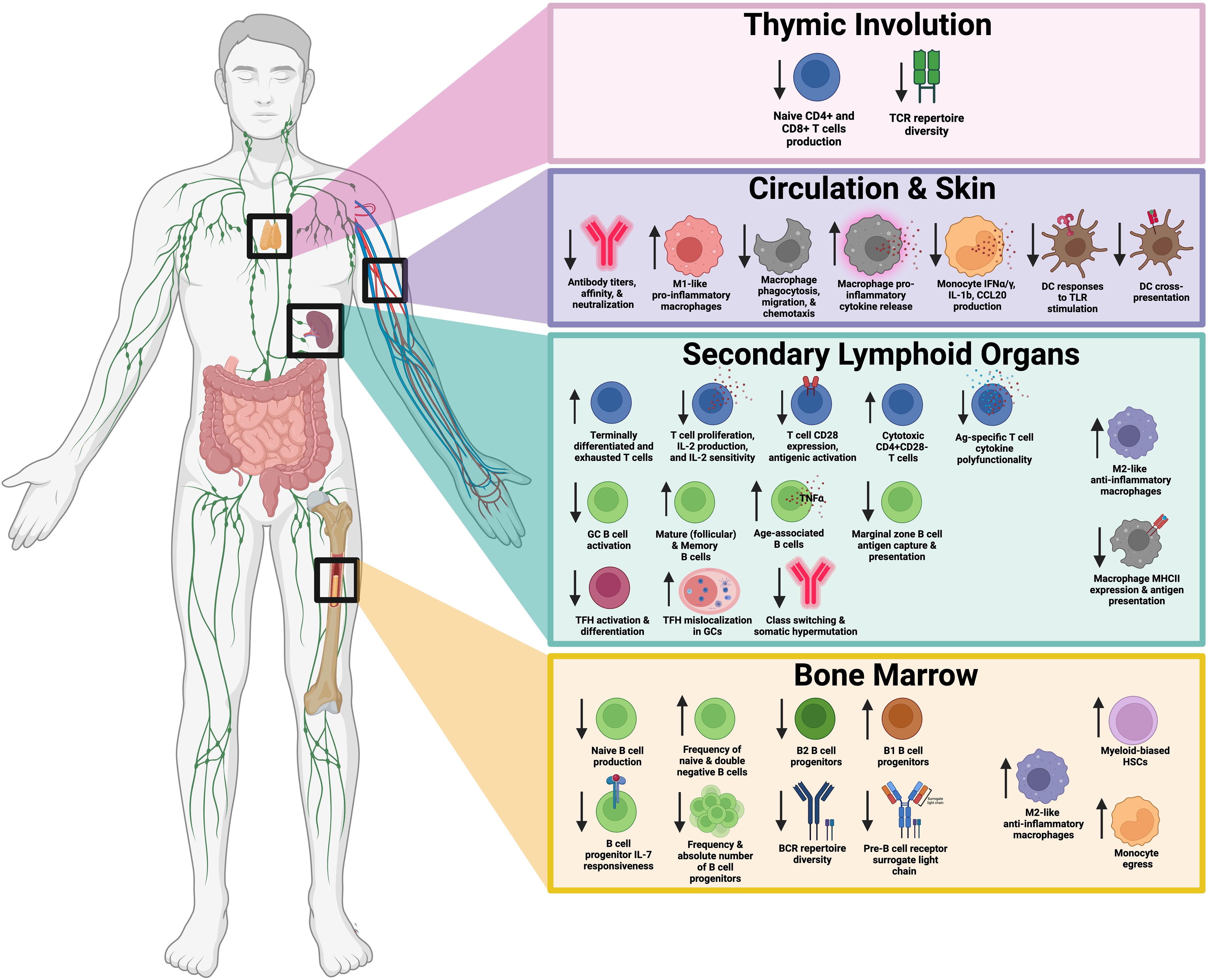
Figure 1. Summary of age-related impairments in T cells, B cells, and antigen presenting cells responsible for vaccine-mediated responses. This schematic illustrates key changes in immune function with age, broken down into four primary regions: the thymus, circulation and skin, secondary lymphoid organs, and bone marrow. Thymic Involution (top panel) leads to decreased production of naïve CD4+ and CD8+ T cells and reduced T cell receptor (TCR) repertoire diversity. In circulation and skin (second panel), altered immune cell populations and function, including changes in antibody titers, macrophage pro-inflammatory cytokine release, and macrophage polarization are observed. Secondary lymphoid organs (third panel), including the spleen and lymph nodes, exhibit changes in T cell subsets, B cell function, and antigen-presentation. Bone marrow (bottom panel) experiences shifts in hematopoietic stem cell populations, reduced B cell and T cell progenitors, and altered macrophage signaling. Downward arrows indicate decreased function or numbers, while upward arrows indicate increased activity or numbers. Created in BioRender.
Immunosenescence associated with antigen presenting cell function
Modern vaccines function by prompting immunological memory through the induction of innate (macrophages, dendritic cells) and adaptive (T, B cells) arms of the immune system by introducing antigen. The development of immunologic memory is initially mediated by innate cells that activate T and B lymphocytes through antigen presentation and the secretion of cytokines. This process enables the differentiation of antigen-specific lymphocytes into memory T and B cells that can be stimulated rapidly upon a subsequent encounter with the same antigen.
The three professional APCs—macrophages, dendritic cells, and B cells—play a crucial role in initiating antigen-specific immune responses and are susceptible to immunosenescence. First, age-related dysregulation of macrophage populations is one of the many alterations responsible for impaired immune function. Generally, macrophages can be grouped into two major types, including M1 (pro-inflammatory) and M2 (anti-inflammatory). In mice, aging is associated with increased M1-like macrophage populations in hepatic and adipose tissues, while M2-like macrophage populations expand in the bone marrow, lymphoid tissues, and muscle, among others (7–9). Furthermore, aged human macrophages were found to exhibit decreased antigen presentation because of lower co-receptor and MHCII expression (10–12). A recent study further identified age-related decreases in the transcription factors MYC and USF1 correlates with reduced phagocytosis, migration, and chemotaxis observed in aged human and murine macrophages (13). With decreased antigen presentation, the induction of antigen-specific adaptive immune responses, particularly through T cells, is impaired in aged individuals, resulting in poor acquired immunity from vaccination and infection. Although elevated M2-like macrophages and decreased antigen presentation are observed with aging, non-specific release of pro-inflammatory cytokines from aged macrophages is commonly attributed to the persistent low-grade inflammation, or inflammaging, seen in aged individuals (14). This increased basal inflammation is likely, in part, due to the ineffective clearance of pathogens by macrophages, resulting in persistent activation. Increased basal inflammation in aged macrophages can further promote T cell immunosenescence, which will be discussed later in this review. Targeting macrophage phagocytosis, antigen presentation, and trafficking through novel optimized vaccine antigens and adjuvants could offer promising approaches to improve vaccine responses in the elderly.
Monocytes are precursors to both macrophages and dendritic cells (DCs). Monocytes have been shown to exhibit weakened expression of notable cytokines including IFNα/γ, IL-1β, and CCL20 with advanced age (15). Although monocytes exhibit reduced pro-inflammatory cytokine secretion, increased egress of monocytes from the bone marrow–due to elevated circulating TNFα–can further contribute to inflammaging upon activation by bacterial products (16). Dendritic cells are the second professional APC and exhibit similar age-related changes to monocytes and macrophages. For example, myeloid and plasmacytoid DCs, which are critical for inducing Th1 and CD8+ T cells (via IL-12) and interferon responses to infection, respectively, show diminished TNFα, IL-6, and IL-12 production following TLR stimulation. These age-related changes to DCs have been associated with impaired vaccination responses to influenza (17). Additionally, aged murine DCs exhibit diminished cross-presentation and subsequent CD8+ T cell priming compared to young mice, in part due to increased production of reactive oxygen species (18).
The third class of professional APCs, which will be discussed further, are B cells. While B cells are critical for antibody production in response to infection and vaccination, their APC capabilities assist in T cell-mediated responses. In humans and animals, aging is associated with the expansion of antigen-experienced aged-associated B cells (ABCs) (19). In contrast to macrophages, monocytes, and DCs, ABCs are considered more efficient APCs than follicular B cells and are heavily associated with autoimmunity development (20). On the other hand, marginal zone B cells, which are important APCs for Th1 effector cell differentiation, exhibit defective antigen capture and presentation with increased age, resulting in impaired T cell-independent immune responses (21).
Immunosenescence associated with T cell function
T cells are essential for adaptive immunity, as evidenced by severe combined immunodeficiency (SCID), where genetic defects impair T cell development, leading to profound immune dysfunction and vulnerability to infections. Without T cells, the immune system is incapable of producing acquired immunity to infectious diseases, resulting in life-threatening infections and eventual death in humans as well as other animal models of SCID. Within the classification of T cells, several subsets with a diverse range of functions exist, including CD4+ Th1, Th2, Th17, Treg, Tfh, CD8+ cytotoxic T cells, and more. Unfortunately, as individuals age, T cells are among the immune cell types highly impacted by immunosenescence with several hallmarks representing key factors of T cell aging. Evidence of T cell immunosenescence has been widely studied in humans and animal models. First, as previously discussed, thymic involution with age leads to a gradual but impactful decrease in T cell production and maturation. Thymic involution results in the production of fewer naïve CD4+ and CD8+ T cells, with the most notable decrease in CD8+ T cells (22). The diversity of the naïve CD4+ T cell repertoire is maintained up to the age of 65 years, at which point it begins to collapse (23), whereas the CD8+ T cell repertoire decline is observed even earlier in the onset of aging (24). Lower T cell receptor (TCR) clonal diversity and accumulation of terminally differentiated T cells exhibiting dysfunction or exhaustion have also been observed as a result (22). Such age-related changes to naïve T cell populations are correlated with a greater risk for severe infections like COVID-19 (25).
Age associated thymic involution reduces the TCR repertoire and leads to accumulation of mature T cells that are susceptible to repeat antigen activation and recurrent stimulation. Loss of proliferative capacity (26), telomerase activity (27), reduced IL-2 production and sensitivity (28–30), decreased CD28 expression (31, 32), and elevated IFNγ production (33) are among the many phenotypes observed in aging CD4+ and CD8+ T cells. One study found that increased IL-2Rα expression and phosphorylated STAT5 in aged CD4+ T cells direct their differentiation into short-lived effector cells. These changes in IL-2Rα expression were linked to diminished HELIOS expression, a transcriptional repressor (34). Furthermore, CD28 is an important costimulatory molecule for T cells that diminishes with age. As a result of reduced CD28 expression, aged T cells exhibit qualities of cellular senescence including poor antigenic activation and response. The accumulation of CD8+CD28- T cells is associated with a reduced overall immune response to pathogens and vaccines in the elderly (35). Moreover, expansion of CD4+CD28- T cells in the elderly, which exhibit natural killer cell and CD8 T cell-like cytotoxic properties (36) elevate IFNγ production, creating a more pro-inflammatory environment. In a study using single-cell RNA sequencing of CD4+ T cells from young and aged mice, an accumulation of exhausted, cytotoxic, and activated regulatory T cells in aged mice was observed (37). In activated regulatory T cells and cytotoxic CD4+ T cells, enhanced regulatory and pro-inflammatory phenotypes were observed, respectively, indicating their contribution to the decline in immune function. Similarly, an elevated ratio of regulatory T cells to effector T cells in the CD4+ compartment occurs with age and has been correlated to poorer Influenza vaccine responses in aged humans (38, 39). Additional post-vaccination analyses in humans indicate a diminished magnitude of antigen-specific CD8+ T cell responses and CD4+ T cell polyfunctionality in aged individuals (40).
Beyond these changes to T cell populations and function, a specific subset of T cells, known as T follicular helper (Tfh) cells, represent a critical cell type altered with age that are necessary for protective humoral responses to infection and vaccination. Increased age correlates with decreased antibody titers, class switching, somatic hypermutation, affinity, and neutralization, all of which correlate with Tfh function, demonstrated in both research and clinical studies (41–44). Tfh cell activation and differentiation into mature GC Tfh declines with age, leading to impaired antigen-specific immune responses (42). A study in aged mice further revealed Tfh upregulation of CXCR4 in aging leads to spatial mislocalization of GC Tfh cells to the dark zone of germinal centers where they cannot properly interact with GC B cells for induction of somatic hypermutation and affinity maturation (45). Likewise, overall GC response magnitude, volume, and number are reported to decline with age in several mouse immunization models (46–48). With these age-related impairments of T cells in mind, additional considerations for T cell-targeted stimulation must be considered in vaccine design for improved responses in elderly populations. As such, next-generation nucleic acid vaccines have shown great potential at inducing potent T-cell responses compared to traditional vaccine platforms and will be discussed further.
Immunosenescence associated with B cell function
Humoral responses from aging B cells in response to infection and vaccination also decline with age. As a result, aged individuals are often at higher risk for severe disease and lower protection and durability from vaccination. B cell immunosenescence is associated with reduced total antibody production, and poor-quality antibodies demonstrated with reduced neutralization and affinity. This phenomenon is a result of impaired germinal center B cell reactions necessary for somatic hypermutation and affinity maturation (49). Increasing age is further linked to decreased B cell differentiation within the bone marrow as well as increased aberrant production of mature B cells. Aging correlates with a shift in B cell frequencies as naïve B cells are displaced by memory B cells reducing B cell receptor (BCR) repertoire diversity (50–52), which is associated with poor antigen-specific responses to infection and vaccination. Increased frequency of pro-inflammatory B cells and decreased expression of molecules necessary for immunoglobulin class-switching and somatic hypermutation has also been observed (53, 54).
In an aged mice, the generation of conventional B2 B cells, necessary for antigen-specific humoral responses, is diminished, while B1 progenitors are maintained. This is corroborated by the finding that the frequency and absolute number of B cell lineage precursor populations are decreased in aged mice and this is, in part, due to suboptimal IL-7 responsiveness from these progenitors (55). An additional factor responsible for the decrease of B cell progenitors is the accumulation of myeloid-biased hematopoietic stem cell populations in the bone marrow of aged mice and humans (56, 57). At the molecular level, aging has been associated with decreased expression of E2A and PAX5 transcription factors as well as the pre-B cell receptor surrogate light chain necessary for B cell development and humoral responses (58–60).
When considering age-related changes to peripheral B cell subsets, studies have found that aged humans exhibit a decrease in switched memory B cells and an increased frequency of naïve and double-negative B cells (61, 62). Given the importance of long-lived switched memory B cells for timely antibody responses to repeat antigen exposure, it is clear that aging associated reduced frequencies contribute to an elevated risk for severe infections and poor vaccine responses in the elderly. Additionally, an increase in pro-inflammatory TNFα secretion from memory and double-native B cells in aged individuals negatively correlates with B cell function and vaccine-specific antibody responses (61, 63). Aged-associated B cells (ABCs), separate from follicular (FO) and marginal zone (MZ) B cells (64), are considerably higher in aged humans and mice with nearly 50% of splenic B cells being ABCs in 24+ month old mice (65). More specifically, ABCs were found to be refractory to BCR/CD40 stimulation, whereas innate TLR9/7 stimulation combined with BCR signaling induce Ig secretion and cytokine production (64). The same study also found ABCs favor T cell polarization to the pro-inflammatory Th17 subtype which may further promote inflammaging. In aged humans and mice, ABCs inhibit pro-B cell generation due to elevated TNF secretion (66–68). This increase in ABCs coincides with a decline in the FO B cell pool (67), with studies suggesting that the transition of FO B cells to ABCs may contribute to this shift (62). These age-related changes in B cell function highlight the need for targeted strategies to enhance immune responses in the elderly and improve vaccine efficacy.
Immunosenescence associated with long-lived immunity
Failure of vaccines to induce long-term protective immune responses in the elderly coincides with the cellular immunosenescence described above. Pre-clinical and clinical findings support the observation that aging leads to a more rapid decline in vaccine-induced antibody titers, often requiring more frequent booster doses to reach and maintain protective levels (69, 70). The combination of reduced bone marrow niches, impaired T cell function, and reduced germinal center responses observed in age-related impairments to vaccination play a role in the reduction of long-lived plasma cell (LLPC) production and survival which represent critical mediators of long-term immunity (71). Therefore, advancements in nucleic acid vaccine technology and adjuvants to target these compartments could offer significant improvement in LLPC production and overall protection induced by fewer doses in the elderly. Critical analysis of these long-term vaccine responses in the context of aging are necessary to progress the development of optimized vaccine formulations.
As shown in Figure 1, key changes in immune function associated with age can be broken down into four primary regions: the thymus, circulation and skin, secondary lymphoid organs, and bone marrow. Thymic Involution (top panel) leads to decreased production of naïve CD4+ and CD8+ T cells and reduced TCR repertoire diversity. Circulation & Skin (second panel) exhibit altered immune cell populations and function, including changes in antibody titers, macrophage pro-inflammatory cytokine release, and macrophage polarization. Secondary lymphoid organs (third panel), including the spleen and lymph nodes, exhibit changes in T cell subsets, B cell function, and APC activity. The Bone Marrow (bottom panel) experiences shifts in hematopoietic stem cell populations, reduced B cell and T cell progenitors, and altered macrophage signaling. Taken together, age-related impairments in T cells, B cells, and APCs play a role in reduced immunity to vaccination.
Nucleic acid vaccines
Nucleic acid vaccines offer unique properties differentiating them from first- and second-generation vaccine platforms. For example, unlike protein-based vaccines, nucleic acid vaccines introduce mRNA or DNA transcripts that guide cells to produce a protein of interest for subsequent immune responses. In contrast to viral vector vaccines, the genetic material delivered is not accompanied by replication machinery. Furthermore, compared to inactivated virus vaccines which risk virulence reversion, nucleic acid vaccines are safe for administration to immunocompromised individuals. Lastly, across all vaccine platforms, nucleic acid vaccines offer the most rapid production and scalability. This section will cover the mechanism, design, and delivery mRNA and DNA vaccines (Figure 2), highlighting their advantages, disadvantages, and relevant pre-clinical and clinical studies in aging.
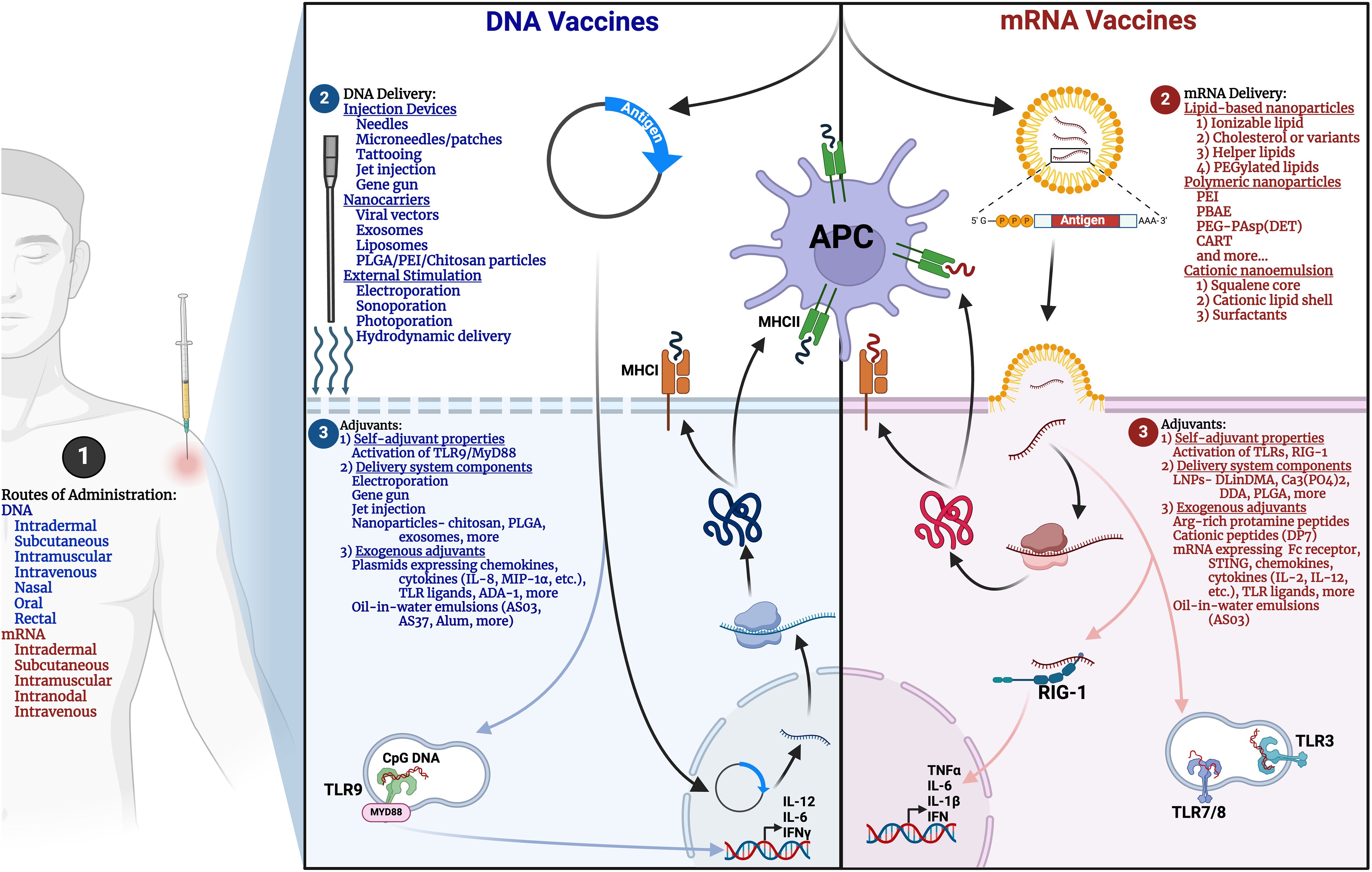
Figure 2. Comparative overview of mRNA and DNA vaccine design. Methods for (1) Routes of administration, (2) Delivery, and (3) Adjuvants for mRNA (right panel, red) and DNA (left panel, blue) vaccines are illustrated. APC, antigen-presenting cell; MHC, Major histocompatibility complex; PLGA, Poly(lactic-co-glycolic acid); PEI, Polyethylenimine; PBAE, poly(β-amino ester); CART, charge-altering releasable transporters; DLinDMA, 1,2-dilinoleyloxy-N,N-dimethyl-3-aminopropane; DDA, dimethyl-dioctadecyl ammonium; ADA-1, Adenosine deaminase-1. Created in BioRender.
mRNA vaccine mechanism and design
mRNA is a single-stranded molecule created during the process of transcription from a DNA template and is responsible for the subsequent translation of encoded proteins. Depending on the encoded protein(s), signal sequences can direct the protein to remain within the cell, traffic to the cellular membrane, or be secreted. Over 30 years ago, the ability of mRNA to be administered to produce vaccine antigens rapidly and cost-effectively was envisioned. Compared to other vaccine platforms such as viral vectors and DNA vaccines, mRNA vaccines circumvent potential risks of genome integration and overall exhibit favorable safety profiles (72, 73). Furthermore, mRNA can encode multiple antigens, allowing for vaccination against multiple pathogens and variants. When administered, mRNA will enter the cytoplasm of cells where it can be directly translated into antigenic proteins, enabling a cascade of subsequent innate and adaptive immune responses. This is an advantage compared to DNA vaccines that require access to the nucleus, and require the additional step of transcription, before effective translation of protein antigen. To bring mRNA vaccines to fruition, several considerations must be made for successful administration and induction of immune responses.
In terms of vector design, synthetic mRNA molecules encoding one or multiple proteins can be designed and produced rapidly in a cell-free setting (74). Similar to endogenous mRNA, synthetic mRNA is comprised of a 3’ UTR, poly(A) tail, antigen-encoding region, 5’ UTR, and 5’ cap. On the 5’ end, the 2’-O-methylation must be retained to prevent detection and innate responses by mammalian cytosolic sensors of RNA. Further changes can be made to the 5’ and 3’ UTRs, including the removal of micro-RNA binding sites and AU-rich sites to delay degradation. Additionally, the protein-encoding region of synthetic mRNA can be optimized by incorporating more common codons to enhance protein production. N1-methylpseudouridine, pseudouridine, and other modified nucleosides are also used to optimize protein translation and prevent immune recognition (74).
The delivery of mRNA vaccines has been widely investigated since the 1990s but were first broadly deployed in humans with the release of the SARS-CoV-2 spike mRNA vaccine. To deliver mRNA, it must breach the barrier of cellular membranes to enter the cytosol where it can be translated to protein. Due to the negatively charged membrane, negatively charged mRNA must be encapsulated to avoid repulsion. Three main nanoparticle delivery systems have been used in mRNA vaccine design, including lipid-based nanoparticles (LNPs), polymeric nanoparticles, and cationic nanoemulsion. Besides encapsulating the mRNA within their core, these delivery systems also share cationic or ionizable molecules. In the case of LNPs, these nanoparticles often contain four major components, including ionizable lipids (DLin-MC3-DMA (75), SM-102 (76), A6 (77), and more), cholesterol or cholesterol variants (β-sitosterol (78) and 20α-hydroxycholesterol (79)), helper lipids (DSPC (80) and DOPE (81)), and PEGylated lipids (ALC-0159 (82) and PEG-DMG (82)). The cationic lipid facilitates encapsulation of the negatively charged mRNA but may induce toxic pro-inflammatory responses (83). The addition of an ionizable lipid can improve the safety profile, extend circulation time (84), and promote endosomal release of the mRNA (85). Investigation into other ionizable lipids for mRNA delivery has expanded rapidly and includes lipids targeting immune cells such as DCs (86) and T cells (87) to improve immunogenicity. Furthermore, inclusion of cholesterol or its derivatives improves LNP stability and endosomal fusion (86), whereas helper lipids influence fluidity, endosomal fusion (88), and can enable organ specificity (89). Finally, PEGylated lipids can be used to alter the size, circulation time, and efficacy of LNPs (90, 91).
Polymeric nanoparticles like PEI (92), PBAE (93), PEG-PAsp(DET) (94), and charge-altering releasable transporters (CART) (95) can be used alternatively to form condensed polymer-mRNA complexes for effective mRNA delivery. Similar to LNPs, different polymers can be chosen to influence organ specificity (96), pH responsiveness (97), and more. Modifications such as the introduction of disulfide linkages and PEGylation can further modulate toxicity (98) and organ specificity (94, 99–101). Lastly, cationic nanoemulsions have also been studied for mRNA delivery. This delivery system contains a squalene core, a cationic lipid shell, and surfactants like Tween 80 for the electrostatic binding and adsorption of mRNA (102). Investigation into peptide-based nanocomplexes for mRNA delivery are also under investigation and has shown promising results for eliciting T-cell mediated immunity (103). The continued advancement of mRNA vaccine technology presents exciting opportunities for improving immune responses in aging populations, with potential strategies focusing on optimizing delivery systems and enhancing antigen-specific immunity to address age-related immune decline.
Pre-clinical testing of mRNA vaccines related to aging
mRNA vaccines are being developed for a broad range of infectious diseases (e.g., Influenza, Clostridioides difficile, Norovirus, Tuberculosis, Herpes Simplex Virus, Hepatitis C), genetic disorders, and cancers, that disproportionately affect elderly individuals, however there is further research necessary to evaluate their efficacy pre-clinically, in aged models (104). To review our current understanding of mRNA vaccine efficacy in aged individuals, we investigated the most up-to-date preclinical studies using aged models for COVID-19 and Influenza A vaccination. Evaluating the efficacy of mRNA vaccines in elderly populations requires a critical analysis of overall protection, humoral immune responses, and cellular immune responses.
The use of aged mouse models has proven to be a useful tool to further our understanding of the impact of aging on the immune response and level of protection conferred by mRNA vaccines specifically in the context of COVID-19 mRNA vaccines. Brooke et al., combined an aged mouse model with human clinical data to characterize an impaired Th1 response to the COVID-19 vaccine in vivo (105). Chen et al. utilized a similar aged model to conduct rechallenge experiments with COVID-19 to better characterize breakthrough infection, finding that even with 2 doses of the vaccine, aged mice were more susceptible to infection (106). Taken together, these in vivo models allow us to identify additional opportunities for optimized treatments and prevention strategies against SARS-CoV-2 among older individuals (107). Similarly, preclinical studies investigated the efficacy of mRNA vaccines for Influenza A in aged models. Specifically, one group has developed an mRNA vaccine capable of inducing long-lived protective immunity to Influenza A in very young and very old mice, as evidenced by the effective humoral and cellular responses elicited (108). These findings highlight the critical role of aged models in advancing the development and optimization of mRNA vaccines, providing valuable insights and opportunities for improving vaccine efficacy and durability in older populations across a range of infectious diseases.
Clinical testing of mRNA vaccines related to aging
A variety of mRNA vaccines are currently undergoing clinical trials targeting infectious diseases beyond COVID-19, including Human immunodeficiency virus (HIV), Zika virus, Nipah virus, and Respiratory syncytial virus (RSV)—as well as genetic disorders and various cancers (109), however there are limited trials that look at the efficacy of these mRNA vaccines specifically in aged populations. While randomized clinical trials typically exclude elderly populations, the heightened vulnerability of this group to COVID-19 led to their inclusion in licensing trials under the exceptional circumstances of the pandemic which allowed for considerable insight into the efficacy of mRNA vaccines in elderly (109). Here, we will review the key findings from clinical trials evaluating the efficacy of mRNA vaccines in aged populations, focusing on their response to the COVID-19 pandemic, as well as trials for RSV and Influenza A. While we, among others, have characterized the impact of immunosenescence on vaccine efficacy (110, 111), mRNA vaccines demonstrated surprising efficacy in aged individuals during the COVID-19 pandemic and in multiple clinical trials (112). A large-scale matched case–control study of individuals aged 80–83 showed that emergency hospital admissions were 75.6% lower among those fully vaccinated with BNT162b2 compared to unvaccinated controls, and SARS-CoV-2 positivity was reduced by 70.1% in the vaccinated group. These findings, among others, underscore the high efficacy of COVID-19 vaccine in the elderly, providing strong protection against infection and hospitalization (113, 114).
However, beyond high-level efficacy comparing vaccinated vs. unvaccinated groups, it is important to interrogate differences in the immune response between young and older vaccinated adults to form a complete picture of the immune response to mRNA vaccines. Results from previous aging-focused mRNA vaccine clinical trials in response to COVID-19 highlight three critical takeaways (1): the reduced humoral response in aged populations, (2) the importance of repeat dosing to improve the humoral response, and (3) the challenge in driving cellular immunity for aged populations. In the context of the BNT162b2 COVID-19 vaccine (115), the compiled results of many trials demonstrate the importance of repeat dosing to mount a protective humoral response in elderly patients. The first clinical challenge observed was the delayed response/increase in dosing required to mount a protective humoral response. A trial in Greece examining differences in antibody responses after 1 or 2 doses of the BNT162b2 COVID-19 vaccine (115) found that individuals over 85 exhibit a 41.18-fold increase in neutralizing antibodies after the second dose. Furthermore, in the cohort of 400 individuals who received the first dose, the mean antibody response reached 69.75%, whereas among the 297 recipients of the second dose, it increased to 98.99% (116). This was further demonstrated by Collier, et al. when investigating immune responses in elderly individuals against key variants of concern (VOCs) (117). After the first dose, older individuals, particularly those over 80, exhibited reduced serum neutralization, IgG, and IgA levels, with lower neutralization potency against Alpha, Beta, and Gamma variants compared to the wild-type virus. Many individuals over 80 lacked neutralizations against variants of concern (VOCs). However, the second dose restored neutralization against VOCs across all age groups. Elderly responders also showed reduced somatic hypermutation in class-switched cells compared to younger individuals (44). Interestingly, Jergoiv et al. highlighted that while there was robust humoral immunity achieved in the older cohort, it was delayed in onset. More specifically, the older cohort displayed lower neutralizing capacity at 7–14 days following the second dose that equilibrates with the younger cohort after 2–3 months (118). Despite the increase in humoral response after repeat dosing, a decrease in overall durability in aged individuals was displayed by Korosec et al., finding that individuals aged 18–55 are predicted to have a four-fold advantage in humoral response compared to those aged 56–70 and 70+ by 8 months following two doses (119). Taken together, these data highlight that the humoral response in elderly groups in response to the COVID-19 vaccine is delayed and requires additional dosing.
To investigate if increased dosing could close the disparity gap observed between aged and young populations, some groups demonstrated that there was a rescue of the humoral immune response in the elderly after 3–4 doses of the COVID-19 vaccine. To complement the increasing immunity that is conferred with 2 doses of the vaccine, Renia et al. found that older individuals take longer to achieve vaccine-induced immunity but maintain more sustained responses at 6 months. A third dose significantly enhances antibody levels in older adults against the Wuhan strain and, Delta and Omicron variants (120). Shapiro et al. also looked at dose response across various age groups and found that the third dose of vaccine restored functional antibody responses and eliminated disparities caused by sex, age, and frailty in older adults (121). Furthermore, 4 doses of the vaccine enhanced the neutralizing antibodies against the Wuhan Strain and Omicron (122).
T cells are critical in driving the adaptive immune response of mRNA vaccines, as previously described, cellular immunity is impaired by age-mediated reduced thymic activity (123, 124). The composition and status of both naive and memory T cell repertoires are critical in determining the quality of immune responses, including those to SARS-CoV-2 (125). While some groups found that after repeat vaccination, older vaccinees manifest cellular immunity comparable to the younger individuals against early-pandemic SARS-CoV-2 and more recent variants (44, 118, 119), a more comprehensive study that included longitudinal analysis of TCR tracking in conjunction with pre/post-vaccination CD4+T cell analysis argues that it might be more complex (125). Saggau et al. found that the SARS-CoV-2-specific T cell repertoire determines the quality of the immune response to vaccination. They investigated both naïve and memory T cell compartments and found that the T cell expansion in both compartments in response to the mRNA vaccine was severely compromised—calling for a need for alternative strategies to increase the T cell response to mRNA vaccines in the elderly (125). These findings underscore the pivotal role of CD4+ T cells in driving mRNA vaccine responses and highlight the need for alternative strategies to enhance T cell responses in older individuals, given the compromised expansion of both naive and memory T cell compartments with aging.
In addition to insights gained from the COVID-19 vaccine rollout, five completed and five ongoing clinical trials are testing mRNA vaccines for influenza and RSV in older individuals (A list of completed and ongoing clinical trials of mRNA and DNA vaccines involving aged individuals, outside of those tested for COVID-19 is shown in Table 1). Most compelling is a Phase II-III clinical trial with an mRNA vaccine targeting RSV that enrolled older adults over 60, showing high efficacy against RSV-associated lower respiratory tract disease (83.7% with two symptoms and 82.4% with three symptoms) and 68.4% efficacy against acute respiratory disease. The vaccine also protected both RSV A and B. There is additional early promise from 2 Phase I clinical trials that showed increased humoral and cellular responses in aged and young adult groups (126, 127). These findings highlight the potential of mRNA vaccines to significantly improve protection against respiratory infections in older adults, warranting further investigation and development. A significant area for opportunity for future mRNA vaccine development for the elderly is in cancer applications. mRNA vaccines allow for greater precision and specificity in delivering tumor associated antigens at a personalized level, while maintaining effective scalability and low cost. Beyond these advantages, mRNA vaccines are shown to elicit robust T cell responses in younger demographics: making this an optimal platform to consider (128). With the broad increase in the aged population and associated increased incidence of cancer in elderly, mRNA vaccines for cancer must be investigated clinically for elderly individuals.
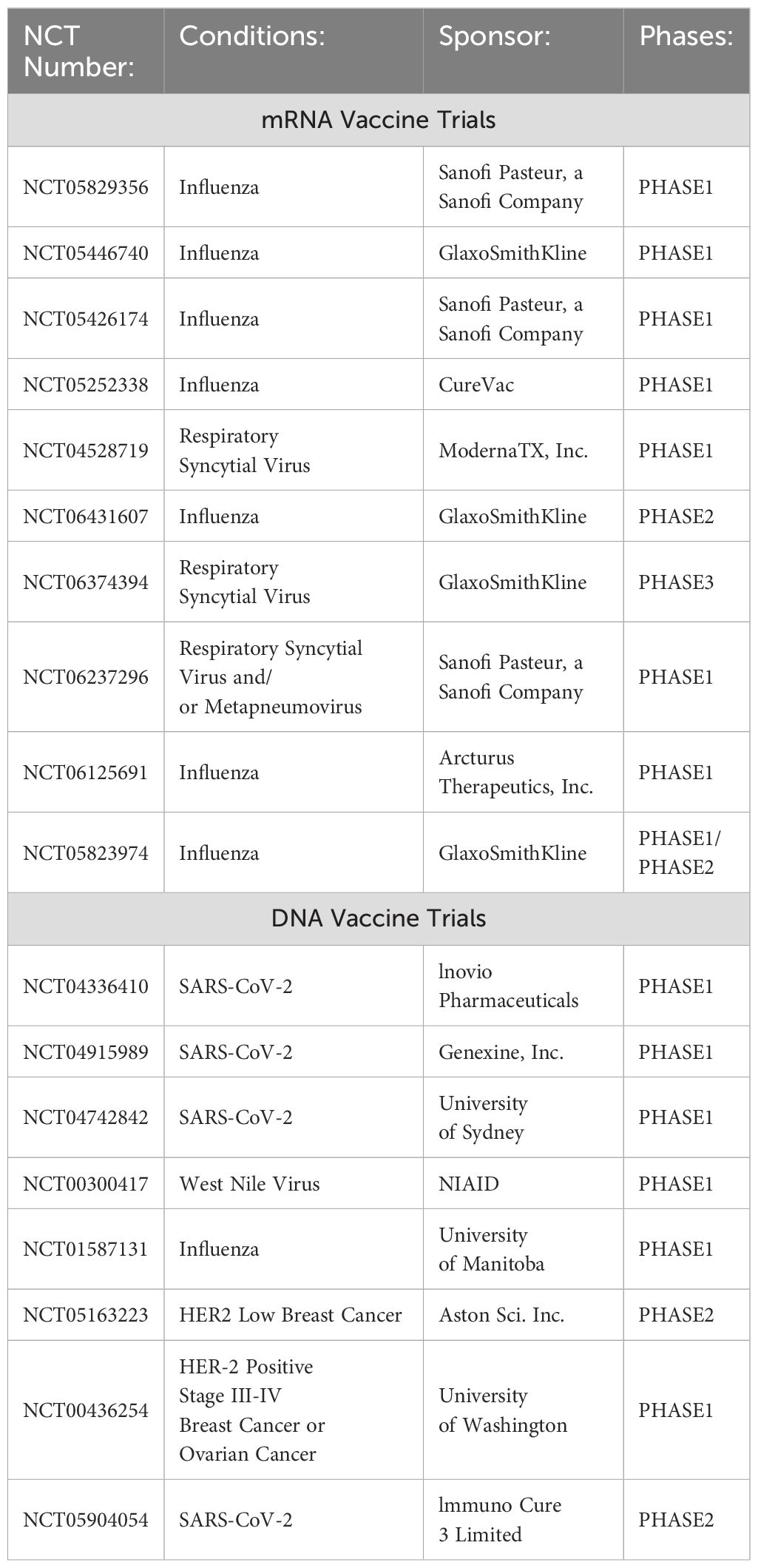
Table 1. List of completed and ongoing clinical trials of mRNA and DNA vaccines involving aged individuals.
Advantages and disadvantages of mRNA vaccines
mRNA vaccines represent a significant advancement in immunization technology, offering several advantages for the elderly. These advantages include rapid development, high efficacy, and a favorable safety profile. The rapid, cost-effective, and scalable production of mRNA vaccines enables swift responses to emerging health threats that disproportionately affect older adults. The efficacy of mRNA vaccines in preventing severe disease outcomes in older populations was demonstrated during the COVID-19 pandemic (112–114). mRNA have improved safety implications for elderly because it is non-infectious compared to viral vector or live attenuated vaccines which potentiate additional risks in aged individuals. Furthermore, the transient expression of mRNA that results from its single stranded nature, may be an additional safety feature for vaccines in the context of targeting aged populations and its application in cancer therapy. While additional clinical trials are needed to consider the safety profile of mRNA vaccines in elderly populations, the previously described clinical trials for mRNA-based RSV vaccines found no evident safety concern (126). Broadly, mRNA vaccines have advantages over alternative traditional approaches such as DNA plasmids, viral vector vaccines or live attenuated vaccines. Compared to DNA vaccines, mRNA vaccines do not require access to the nucleus and does not pose the threat of chromosomal integration (129). Compared to traditional viral vector or live attenuated vaccines mRNA vaccines can be rapidly designed, scaled quickly and at very low cost.
However, like any emerging advancement in medicine, there are existing challenges, limitations and opportunities for improvement for the mRNA vaccine platform for elderly patients. Key limitations of mRNA vaccines for elderly populations include stability, durability and potential adverse immune reactions. First, as mRNA acts as a transient intermediate between DNA and protein, its short half-life in the body is of primary concern. mRNA can be broken down rapidly by deadenylases, the exosome, ribonuclease, and endonucleases, so carrier design is critical to mRNA efficacy. These concerns are also critical for storage and transportation, particularly for countries without access to rapid transportation and devices necessary to proper storage. Second, improving the durability and memory of immune responses to mRNA vaccines is an area that must be further studied in aged individuals. While mRNA vaccine mediated immune response can improved with increased dosing in aged individuals (120–122), there is a lack of clinical evidence showing long lived memory to mRNA vaccines in aging. Increased dosing is a potential strategy to improve durability in elderly, however it could also increases the risk associated with off target, adverse immune reactions for elderly. Many steps have been taken to improve the safety of mRNA, to mitigate adverse immune reactions, such as the 2’-O-methylation strategy to mRNA which prevents detection by innate pattern recognition receptors (PRRs). However, the concern mRNA vaccination and development of autoimmune disease is a concern for elderly. In the context of SARS-COV2, it was concluded that mRNA vaccines were not associated with an increased risk of most autoimmune connective tissue diseases (AI-CTDs) (130), however, understanding long term safety profile and risk associated with mRNA vaccines in elderly requires further investigation. Overall, further advancement and characterization of mRNA vaccine stabilization, potency and durability is necessary, particularly for purposes of distribution and application to individuals with impaired vaccine responses like the elderly.
DNA vaccine mechanism and design
DNA-based vaccines are a third-generation vaccine platform that relies on the use of artificially synthesized plasmids encoding an antigen of interest to be transfected within cells of the body for subsequent production of antigen in vivo. Beginning in the 1990s, it was found that intramuscular injection of plasmid DNA could be performed to induce protein expression and that most of the DNA could be taken up into the nucleus of cells without specialized delivery methods (131). Since this study, investigations of genetically engineered DNA plasmids for vaccine use have expanded, showing the scalable and stable nature of this platform. DNA vaccines are designed to encode an antigen of interest, ranging from cancer-specific molecules to proteins from infectious organisms, that can subsequently induce antigen-specific immune responses. To effectively induce transcription and translation of a DNA vaccine, a delivery system is used to enable the plasmid to enter the nucleus of cells within the body.
Several routes of administration and delivery systems have been investigated over the past several decades. DNA vaccines can be delivered through the skin (intradermal, intramuscular, intravenous, subcutaneous), nasally, orally, or rectally. Depending on the administration method used, injection devices, nanocarriers, or external stimulation can be applied to facilitate DNA delivery to cells. In terms of injection devices, traditional needles and microneedles enable penetration of the epidermis. Microneedles prepared as adhesive patches facilitate delivery to the interstitial fluid. This delivery method was shown to improve DNA vaccine immunogenicity in an influenza model by inducing potent humoral and cellular responses compared to traditional intramuscular injection (132). Tattooing represents a unique method for intradermal vaccine delivery by introducing numerous skin perforations in seconds with controlled vaccine release over a larger area than traditional needles or micropatches. Improved tattooing methods in Ebola and HIV DNA vaccine models show superior T and B cell responses compared to intradermal and intramuscular delivery across several animal species, including mice, rabbits, and non-human primates (133). Needle-free methods for DNA vaccine delivery have also been explored. Jet injection, such as the Biojector (134), is an injection device that forces microdroplets carrying the vaccine of choice into the skin using high pressure for subcutaneous, intradermal, or intramuscular delivery (135). Separately, the gene gun enables gold microparticles to be coated with a DNA vaccine and delivered directly into intradermal or mucosal cells with compressed helium gas (136). In an HPV DNA vaccine model, the gene gun was shown to induce potent CD8+ T cell responses comparable to electroporation (137).
A variety of nanocarriers have been evaluated for DNA vaccine delivery. Nanocarriers are capable of improving antigen delivery by protecting mRNA from degradation and promoting cellular uptake and antigen presentation. They can also be prepared with innate-stimulating properties such as TLR agonists and designed to induce specific cytokine responses. Such carriers include viral vectors (138), exosomes (139), liposomes (140), PLGA (141), PEI (142), and chitosan (143) particles. While each nanocarrier offers different benefits for DNA vaccine delivery, collectively, they enable the protection of DNA plasmids from degradation, increase circulation stability, and offer targeted tissue delivery (136). Finally, external stimulation represents a commonly used form of DNA vaccine delivery. Electroporation applies electrical impulses to transiently permeabilize cellular and nuclear membranes to deliver DNA plasmids to the nucleus of cells for transcription and translation. This method is common for intramuscular DNA vaccination, allowing for versatile delivery of naked DNA or nanocarriers in addition to self-adjuvating properties by inducing minor inflammation and trafficking of APCs and lymphocytes to the site of vaccination (144). Similarly, sonoporation (145), photoporation (146), and hydrodynamic delivery (147) of DNA vaccines use ultrasound waves, lasers, and capillary pressure, respectively, to create small pores in cellular membranes for DNA entry. Although many delivery methods exist for DNA vaccination, the cancer or infectious agent of interest must be considered before vaccine design to ensure optimal immune responses are achieved.
Pre-clinical testing of DNA vaccines related to aging
While several pre-clinical studies of DNA vaccines exist, examination of these vaccines in aging models can help provide evidence for the potential of this platform’s immunostimulatory capabilities and areas for improvement. Few pre-clinical DNA vaccines have been tested in models of aging, possibly in part due to the expense of aged animals. However, those that exist improve our understanding of the benefits and shortcomings of DNA vaccine formulations and, therefore, will be discussed for this review. In the context of infectious diseases, DNA vaccines have shown promising results in aged models. Starting with a current and clinically relevant viral target, the recent COVID-19 pandemic sparked interest in DNA vaccines targeting SARS-CoV-2, which has proven particularly threatening to the aged population (148). In one study of a DNA vaccine encoding chimeric SARS-CoV-2 S1 spike protein fused to a trimerization transmembrane region, aged mice were vaccinated in a two-dose regimen for subsequent analysis of antigen-specific adaptive immune responses (149). Cui et al. found two doses induced strong humoral responses with neutralizing capacity to both Wuhan and Delta spike variants, while a third booster dose after 6 months significantly boosted the magnitude of these responses in aged C57BL/6 mice like young mice. Similarly, spike-specific T cell TNFα responses were induced in aged mice, although to a lesser extent compared to young. In a separate study, DNA vaccines against Influenza A haemagglutinin (HA) and nucleoprotein (NP), another clinically relevant viral pathogen disproportionately impacting elderly populations, were tested in young and aged BALB/c mice (150). Both HA- and NP-DNA plasmids induced antibody responses in aged mice, though to a lesser extent than observed in young mice. Notably, the NP-DNA vaccine induced similar cytotoxic CD8+ T lymphocyte activity in both age groups and protected against low-dose intranasal challenge based on weight loss recovery. However, aged mice were not protected against high-dose A/HR/68 (H3N2) challenge, suggesting vaccine alterations to target improved antibody neutralization capacity in aged mice is necessary. In contrast, investigation of a DNA vaccine targeting the thrombocytopenia syndrome virus (SFTSV) showed that aged ferrets developed strong T cell and antibody responses to SFTSV and were completely protected from lethal challenge (151). Beyond these viral targets, aged models of DNA vaccination have also been tested in the context of malaria. Using a plasmid encoding the circumsporozoite protein of the Plasmodiumyoelii malaria parasite, Klinman et al. found vaccinated aged BALB/c mice exhibited lower humoral and CD8+ T cell responses compared to young mice, with an overall 40% protection from challenge compared to 80% in young mice (152). It should be noted that the aged mice used in this study were of extreme age (26 months compared to standard 18–24 months used for aged models), but these findings, along with the previously described viral DNA vaccine models, indicate improvements can be made to induce greater immunogenicity in aged individuals.
Besides infectious diseases, cancer, autoimmune disorders, and neurodegenerative diseases represent attractive targets for DNA vaccines, particularly in the context of aging. For example, breast cancer is one of many cancers that are significantly more common with increased age. In an attempt to target a tumor antigen commonly detected in metastatic breast cancer, young and aged mice were vaccinated with a plasmid encoding Mage-b intramuscularly followed by challenge with mild (4TO7cg) and aggressive (4T1) syngeneic metastatic mouse breast tumor models (153). Aged mice exhibited lower IL-2 and IFNγ levels in the draining lymph nodes and spleen, along with a reduced frequency of Mage-b-specific CD8+ T cells, compared to young mice. These immunological deficits correlated with a diminished protective response against tumor challenge. These results suggest that there is room for improvement, and tailoring DNA vaccination to improve cytokine and CD8+ T cell responses could enhance cancer vaccination efficacy in aged models. For example, electroporation following intramuscular delivery can improve immune responses, as demonstrated by a pre-clinical study investigating a DNA vaccine against the HER-2/neu antigen expressed in many breast tumors and adenocarcinomas (154). Compared to intramuscular delivery alone, electroporation significantly improved humoral and cellular responses to HER-2/neu and induced complete protection (40% increase) against HER-2/neu overexpressing cancer cell line challenge in aged mice. Beyond cancer, neurodegenerative diseases such as Alzheimer’s disease (AD) heavily impact aged individuals. As a result, studies investigating DNA vaccines targeting amyloid-β (Aβ) plaques that accumulate in the brain of Alzheimer’s patients have been of interest. In one study, DNA immunizations with the amino-terminal Aβ (1-11) fragment exposed on the surface of HBsAg particles resulted in high anti-Aβ antibody titers, reduced Aβ plaques, and reduced cognitive impairments in an aged transgenic mouse model of AD (155). In a separate study, DNA vaccination against recombinant Aβ3–10 similarly reduced Aβ plaques, synaptic and neuron loss, and memory impairment in aged transgenic mice (156). These studies exemplify the promise of DNA vaccines in aged individuals beyond infectious disease targets.
Clinical testing of DNA vaccines related to aging
While pre-clinical evaluations of DNA vaccines in aging models are sparse, fewer clinical trials in elderly humans exist (Table 1). In a more recent clinical trial for the SARS-CoV-2 DNA vaccine INO-4800 (NCT04336410), a 2.0 mg dose induced strong and durable humoral responses in all age groups, including those >65 years, although IFNγ ELISpot and CD8+ T cell analyses suggested poorer, but detectable, T cell cytokine responses to vaccination (157). In a promising clinical trial for a West Nile Virus (WNV) DNA vaccine (NCT00300417), subjects aged 18–50 years and 51–65 years were vaccinated against the premembrane protein and the E glycoproteins of the NY99 strain (158). Following a three-dose regimen, the majority of subjects in both age groups exhibited T cell responses, and the older age group demonstrated antibody responses with similar frequency, magnitude, and durability as those observed in the younger participants. With no licensed vaccine against WNV available for use in humans, this trial indicated promising results for eliciting protection against WNV in young and older individuals using next-generation vaccine platforms where traditional platforms have failed. Furthermore, a phase 1 clinical trial evaluating Inovio’s H1N1 Influenza A DNA vaccine in healthy elderly subjects (NCT01587131) found a single dose in conjugation with the seasonal flu vaccine induced protective immune responses in 40% of subjects compared to 20% in those who received the seasonal flu vaccine alone (159). These results indicated the potential for this DNA platform to promote potent, protective immune responses against influenza, particularly for at-risk elderly populations, which current flu vaccines have failed to achieve.
As previously mentioned for pre-clinical vaccines, DNA vaccines for cancer have also reached the clinical trial phases. In a phase 1 trial for a DNA vaccine encoding HER-2/neu for advanced-stage ERBB2-positive breast cancer (NCT00436254), participants aged 34–77 years were immunized three times with one of three dosages (50, 100, or 500ug) (160). Most subjects developed HER-2-postive Th1 T cell responses with minimal toxicity, and the 100ug dose has progressed to phase 2 trials (NCT05163223). While these studies include elderly participants, details on age-dependent variations in vaccine responses were not evaluated. Similarly, although other cancer DNA vaccines are in clinical trials, age-associated responses have not been examined. Further analyses of DNA vaccine immunogenicity and efficacy in aged participants are necessary and could aid in the optimization of vaccine formulations and delivery for the elderly.
Advantages and disadvantages of DNA vaccines
The DNA vaccine platform offers its own unique set of advantages and disadvantages. Beyond the advantages of low manufacturing costs and rapid production shared between mRNA and DNA vaccines, DNA vaccines offer several additional advantages including stability, safety, flexibility in design, and the ability to induce robust immune responses. The high stability of DNA vaccines makes them convenient to store, transport, and distribute. Furthermore, DNA offers no safety risk of viral transformation like that associated with live attenuated vaccines or other side effects associated with inactivated cell vaccines. In terms of vector design, DNA vaccines are flexible and easily manipulated and offer the potential to encode one or multiple antigens of interest for protection against one or multiple diseases in a single plasmid. Beyond the ability to encode multiple antigens, DNA vaccines enable host cells to produce antigens with the post-translational modifications necessary for the native-like structure (161). Lastly, DNA vaccines can elicit a broad spectrum of immune responses, including humoral and cellular immunity.
Unfortunately, concerns surrounding the use of DNA in vaccine design have prevented widespread development and use clinically. Integration into the host genome is of paramount concern, particularly in the public eye, despite DNA exhibiting a low tendency to integrate when circular in a plasmid (160, 162). Additionally, the amount of DNA necessary for vaccination is small, further lowering risk of integration. Nonetheless, administration of a DNA vaccine, in rare cases, could result in insertional mutagenesis potentially resulting in gene malfunction, inactivation, or even upregulation of gene expression. To help decrease risk for such insertions, the FDA has offered recommendations to increase plasmid DNA supercoiling to greater than 80% and keep the DNA copies administered below 10,000 (163). In addition to DNA integration, another major safety concern of DNA vaccines is the induction of autoimmunity. By injecting foreign DNA encoding an antigen of interest, DNA vaccines have the potential to elicit anti-DNA humoral responses. Although an early animal study suggested DNA immunization induced anti-DNA antibodies in mice (164), other work suggests there is little to no risk for autoimmunity development, particularly when using non-viral delivery methods (165, 166). Other disadvantages also exist. For example, delivery methods such as electroporation can cause discomfort not associated with other vaccine platforms. Further work on DNA vaccine design and delivery, particularly in the field of nanocarriers, may promote the development and distribution of this platform in the future. However, addressing the risk for DNA integration and autoimmunity is paramount for the progression of DNA vaccines to widespread use.
The role of adjuvants in enhancing nucleic acid vaccine responses
Adjuvants represent a critical component of vaccine formulations necessary for enhancing antigen-specific immune responses. Particularly for individuals with impaired immune functions, such as the elderly, adjuvants are necessary to elicit protective immune responses to vaccination. As the number of vaccine adjuvants has grown, their diversity has expanded to include natural extracts, synthetic compounds, oil-in-water emulsions, and more. The discovery of adjuvants and their application to boost vaccine responses first occurred in the 1920s when the immune-enhancing properties of aluminum salts were observed when co-formulated with vaccine antigens (167). After successfully translating aluminum as an adjuvant to human vaccinations in the 1930s, it remained the only licensed vaccine adjuvant for roughly seven decades. Since then, many new adjuvants have been discovered, and their applications to specific vaccine platforms and pathogen types have been investigated closely. For example, aluminum does not function well for vaccine-induced immune responses to intracellular pathogens (168). Importantly, in the context of nucleic acid-based vaccines, the choice of adjuvants varies. For both mRNA (Table 2) and DNA (Table 3) vaccines, these adjuvants can be broken down into three overarching categories, including (1) self-adjuvant (2), delivery system components, and (3) exogenous adjuvants.
Self-adjuvant properties
Both mRNA and DNA vaccine platforms can exhibit self-adjuvating properties without the presence of additional mediators. mRNA alone has the capability of acting as an immunostimulatory molecule. The innate immune system is equipped with pattern recognition receptors that can bind foreign or host RNA and elicit responses. For example, TLR3 and TLR7/8 recognize double-stranded and single-stranded unmodified mRNA, respectively. Detection of poly uracil (U) and short double-stranded RNA with 5’ triphosphate can activate RIG-1 and TLR3, leading to the induction of pro-inflammatory cytokines TNFα, IL-6, IL-1β, and more without impeding the expression of the encoded antigens (234, 235). Although these responses can act to potentially enhance vaccine responses, excessive inflammation from unmodified mRNA can also promote RNA degradation (236). To overcome this, nucleoside-modified mRNAs, including pseudouridine incorporation, can help overcome such excessive responses and are commonly used in mRNA vaccine development (237). Further work is needed to determine the optimal balance of the intrinsic ability of vaccine mRNA to act as an adjuvant.
Similarly, DNA plasmids used in vaccine design have their own adjuvant-like capabilities through the activation of innate immune system DNA sensors. DNA plasmids used in these vaccines to encode an antigen of interest are derived from bacterial species. This bacterial DNA contains unmethylated CpG dinucleotide motifs bordered by two 3’ pyrimidines and 5’ purines, which can trigger the innate immune receptor TLR9 to activate the MyD88 signaling pathway resulting in pro-inflammatory cytokine release (238). As a result, when these plasmids are used for DNA vaccination, innate responses from APCs and other cells that take up the plasmid result in IL-6, IL-12, and IFNγ production. These responses were shown to be a significant factor in promoting DNA vaccine immunogenicity in vivo, indicating the advantageous self-adjuvating properties of DNA vaccines (239).
Delivery system components
The second category of mRNA vaccine adjuvants includes the delivery system components. Because mRNA on its own is susceptible to extracellular degradation within the body and is unable to efficiently enter cells due to its negatively charged backbone, specific delivery systems are necessary. As the most used and optimal delivery platform for mRNA vaccines, lipid nanoparticles (LNPs) have been widely investigated for their immunostimulatory properties to enhance antigen-specific responses. Briefly, LNP-encapsulated mRNA is produced through the combination of cationic and structural lipids that complex with negatively charged mRNA molecules (240). Depending on the LNP, certain lipid components with adjuvating properties can be incorporated. The ionizable cationic lipid DLinDMA, for example, can be incorporated to induce potent germinal center Tfh and B cell responses to an mRNA-encoded antigen, leading to the production of neutralizing antibodies (169, 170, 241). As these responses are impaired in the elderly, targeted enhancement of GC Tfh and B cell responses offers an encouraging approach for enhancing aged vaccine responses. Additionally, a lipid-coated calcium phosphate nanoparticle can be applied to induce CD80/86 upregulation for enhanced DC maturation, further promoting improved antigen-specific responses following mRNA vaccination (172). Separately, the quaternary ammonium lipid DDA can be incorporated to enhance innate immunity and Th1 cell responses to vaccination when tested in a rabies mRNA vaccine model (173). At a higher level, the impact of the LNP component from SARS-CoV-2 mRNA vaccines was investigated in cells from young and aged individuals using an empty LNP (eLNP) (242). eLNP was found to induce maturation of monocyte derived DCs and upregulated CD40 and cytokine production (e.g. IFNγ) from DC and monocyte subsets in cells from both age groups, although to a lesser extent in cells from aged donors. Interestingly, eLNP was able to rescue phagocytic capacity in aged DCs. The aforementioned lipid components of LNPs for mRNA vaccine delivery represent only a fraction of the many components currently being investigated for adjuvant-like properties and offer promising directions for improving mRNA vaccine-elicited responses in elderly populations.
In the context of DNA vaccines, the diverse range of delivery systems includes many adjuvant-like properties. As previously discussed, external stimulation methods for DNA vaccine delivery largely stimulate immune responses through inflammatory induction to improve immunogenicity. For example, electroporation sends electrical pulses that induce danger signals from localized cell death and tissue damage, attracting APCs and lymphocytes to the site of vaccination for effective induction of the immune response, particularly in T cells, against the antigen of interest (186, 187). These local inflammatory responses recruit APCs and T cells through pro-inflammatory cytokine release, including TNFα and IL-1β (144). Electroporation not only improves cellular immune responses but can also lengthen the duration and array of responses to plasmid-encoded antigens (188). Studies have shown electroporation alone can increase immune responses to vaccination by 10- to 1000-fold (189, 190). Delivery devices can also stimulate the immunogenicity of DNA vaccines. The gene gun promotes a more specific Th2-biased response, but inferior cell-mediated immunity and limited capacity of DNA carried on the gold particles has resulted in its limited use (191, 192). Needle-free injections with next-generation jet injection devices have also shown to elicit dose-sparing vaccine responses, indicating improved immunogenicity; however, the specific causes of this have not been fully elucidated (243, 244).
Furthermore, like emerging formulations of mRNA vaccines, nanoparticles for DNA vaccine delivery have shown great potential for adjuvant capabilities outside of their function in DNA delivery. Chitosan, a cationic polymer that can form a protective nanoparticle around DNA vaccine plasmids, can enhance humoral and cellular responses to vaccination (193, 194) in addition to cytokine production (IFNγ), macrophage activation, and T cell responses (245). PLGA, another polymer used in DNA vaccine nanocarriers, has been found to improve MHC-I antigen presentation and T cell cytokine production (246). Additionally, studies of exosomes for DNA delivery have found the potential for deriving cell type-specific exosomes, such as DCs and other immune cells, to elicit intrinsic adjuvant effects following DNA vaccination (247).
Exogenous adjuvants
Lastly, exogenous adjuvants for mRNA-based vaccines represent another method to boost vaccine responses. For example, arginine-rich protamine peptides can be applied to form a complex with mRNA to enhance TLR7/8 activation and promote B and T cell responses in a young and aged mouse model as well as in humans (108, 179). The use of the cholesterol-modified cationic peptide DP7 was found to enhance CD103+ DC maturation for heightened antigen presentation in mice (178). Exogenous adjuvants can also be delivered by encoding them in separate mRNA molecules administered during vaccination. In a proof-of-concept study, mice vaccinated against mRNA-encoded HPV E6 and E7 oncoproteins in combination with mRNA-encoded constitutively active STING showed reduced HPV+ TC-1 tumor growth and extended survival. This was linked to STING-mediated activation of type I interferon responses and enhanced antigen-specific T cell responses (180). Beyond these adjuvants, investigations into encoding additional proteins that are separate or linked to the encoded antigen show promising immunostimulatory properties. For example, the conjugation of human Fc to the receptor binding domain of SARS-CoV-2 encoded within a single mRNA molecule can promote recognition by Fc receptors on APCs to elicit enhanced vaccine responses (248). Interestingly, a more recent study that included mRNA-encoded IL-12p70 in a COVID-19 mRNA vaccine formulation found that the addition of the encoded cytokine significantly improved both humoral and cellular responses in aged mice, comparable to those seen in young (183). Such modifications offer promising new directions for enhancing the adjuvant-like properties of mRNA and their encoded antigens to improve protective responses to mRNA-based vaccines for vulnerable populations, such as the elderly.
Several adjuvants are available for use in both mRNA and DNA vaccine formulations. AS03 is a commercially available oil-in-water emulsion adjuvant that promotes both Th1 and Th2 responses for DNA and mRNA vaccinations (184, 249). Conversely, aluminum salts, or alum, can also be applied to these nucleic acid vaccine platforms for Th2-biased responses by enhancing antigen presentation through the absorption of antigens on their surface, activation of the NLRP3 inflammasome to generate IL-1β and IL-18, and activation of DCs through cytotoxic effects (226).
Both mRNA and DNA vaccines further enable adjuvants, such as cytokines, to be encoded along with the antigen of interest. Depending on the disease of interest, specific cytokines may be chosen to direct responses. For example, cell-mediated responses may be targeted by encoding IL-2, IL-12, IL-15, IL-18, or IFNγ (208, 209, 250). In the context of DNA vaccines, investigation into chemokines such as IL-8 (210), MIP-1α (251, 252), CCL28 (211), CCR10L (212), and others have shown to aid in DNA vaccine effectiveness in vivo. Other plasmid-encoded mediators like PD-1 have been used to promote CD8+ T cell responses to vaccination (220, 221). Beyond this, more recent investigations into plasmid-encoded adenosine deaminase-1 as a molecular adjuvant revealed improved antibody neutralization and durability, antigen-specific T cell cytokine production and polyfunctionality, and induction of Tfh-inducing cytokines, including IL-6 (222–225). While the list of molecular adjuvants for mRNA and DNA vaccines continues to expand, the ease of incorporating and investigating new adjuvants in nucleic acid vaccines highlights the potential for enhancing targeted immune responses to improve protection, particularly in immunocompromised individuals like the elderly. The selection of adjuvants enables the tailoring of immune responses to meet therapeutic requirements for the disease of interest.
Vaccine adjuvants are particularly critical to induce protective immune responses in elderly populations, and although several have been investigated in the context of aging including AS01 and MF59, many of these adjuvants have not been applied to nucleic acid vaccine platforms. With limited work on vaccine adjuvants in this context, it is evident that extensive work remains to be done on mRNA and DNA vaccine adjuvant efficacy and development in the aged.
Future directions of nucleic acid-based vaccines
Further investigations into pre-clinical mRNA and DNA vaccines currently lacking aged models are still necessary to guide vaccine development for the elderly. In the rapidly growing field of mRNA vaccines, there remain several infectious agents not previously discussed that are being targeted in early investigations. The University of Pennsylvania, widely known for its role in successfully developing the first clinically used mRNA vaccine against COVID-19, is at the forefront for many new mRNA vaccine formulations that are undergoing investigation. Although they remain to be tested in aging models, infectious diseases that disproportionately impact elderly individuals including Avian Bird Flu (253), Clostridioides difficile (254), Norovirus, and Tuberculosis are being targeted with novel mRNA formulations (255). Similarly, DNA vaccines targeting C. difficile (256, 257), Streptococcus pneumoniae (258), and RSV (259) exist but have not been tested in aging. Incorporation of aged models, particularly for pathogens that significantly impact elderly populations, could provide insight into the potential for nucleic acid vaccines to induce protective immune responses against targets where previously tested vaccines have fallen short.
Newer developments in self-amplifying mRNA (saRNA) are also paving the way for the future of nucleic acid vaccine design. Despite first being described in 2012 (260), saRNA has gained more attention since the implementation of the COVID-19 mRNA vaccine. This emerging mRNA technology, unlike conventional mRNA vaccines, incorporates encoded viral replicase genes in addition to the antigen of interest to promote self-amplification of the mRNA, thereby rapidly increasing production of antigen. This self-amplifying property overcomes obstacles observed in conventional mRNA vaccines including balancing high doses with adverse side effects, stability, and the need for multiple boosting vaccinations. Based on their potent induction of immune responses at lower vaccination doses (261), saRNA vaccine technology is primed for investigation aging models. In fact, recent approval of the first saRNA vaccine in Japan, which targets COVID-19, highlights the potential of this new mRNA vaccine technology and the need for evaluation of immune responses elicited in elderly populations (262).
Finally, this review highlights the shortcomings of clinical trials examining the efficacy of nucleic acid vaccines and adjuvants in aged individuals. A greater focus on age-related deficits following nucleic acid vaccination are critically needed to identify and correct vaccine design for improved responses in this susceptible population.
Author contributions
EK: Conceptualization, Formal analysis, Project administration, Software, Visualization, Writing – original draft, Writing – review & editing. AE: Conceptualization, Formal analysis, Writing – original draft, Writing – review & editing, Visualization. MK: Conceptualization, Funding acquisition, Project administration, Supervision, Writing – review & editing.
Funding
The author(s) declare that financial support was received for the research and/or publication of this article. This work was funded by the PA Department of Community & Economic Development COVID AID, Relief, and Economic Security (CARES) act to MK; the W.W. Smith Charitable Trust Foundation award to MK; The Pennsylvania Department of Health CURE program; and Merck & Co., Investigator Studies Program.
Conflict of interest
Author MK receives grant funding, participates in industry collaborations funded through sponsored research agreements. MK holds patent licensure through Drexel University and Inovio Pharmaceuticals for molecular adjuvants and DNA vaccine antigens.
The remaining authors that the research was conducted in the absence of any commercial or financial relationships that could be construed as a potential conflict of interest.
Generative AI statement
The author(s) declare that no Generative AI was used in the creation of this manuscript.
Publisher’s note
All claims expressed in this article are solely those of the authors and do not necessarily represent those of their affiliated organizations, or those of the publisher, the editors and the reviewers. Any product that may be evaluated in this article, or claim that may be made by its manufacturer, is not guaranteed or endorsed by the publisher.
References
1. Beleche T, Sertkaya A, Knope C, Ertis MD, Berlind A. Preventive Vaccine Development Final. Washington, DC: Office of the Assistant Secretary for Planning and Evaluation (2022).
2. MacGregor RR, Boyer JD, Ugen KE, Lacy KE, Gluckman SJ, Bagarazzi ML, et al. First human trial of a DNA-based vaccine for treatment of human immunodeficiency virus type 1 infection: safety and host response - PubMed. J Infect Dis. (1998) 178:92–110. doi: 10.1086/515613
3. Dolgin E. The tangled history of mRNA vaccines. Nature. (2021) 597:318–24. doi: 10.1038/d41586-021-02483-w
4. Wilmoth JR, Bas D, Mukherjee S, Hanif N. World social report 2023: Leaving no one behind in an ageing world: UN. New York, NY: United Nations (2023).
5. Bureau PR. 2024 World Population Data Sheet prb.org: Population Reference Bureau (2024). Available online at: https://2024-wpds.prb.org/ (Accessed February 15, 2025).
6. National Vital Statistics System. Mortality 2018–2023. Atlanta, GA: CDC WONDER Online Database (2024). Available at: http://wonder.cdc.gov/ucd-icd10-expanded.html (Accessed February 21, 2025).
7. Fontana L, Zhao E, Amir M, Dong H, Tanaka K, Czaja MJ. Aging promotes the development of diet-induced murine steatohepatitis but not steatosis. Hepatology. (2013) 57:995–1004. doi: 10.1002/hep.26099
8. Lumeng CN, Liu J, Geletka L, Delaney C, Delproposto J, Desai A, et al. Aging is associated with an increase in T cells and inflammatory macrophages in visceral adipose tissue. J Immunol. (2011) 187:6208–16. doi: 10.4049/jimmunol.1102188
9. Wang Y, Wehling-Henricks M, Samengo G, Tidball JG. Increases of M2a macrophages and fibrosis in aging muscle are influenced by bone marrow aging and negatively regulated by muscle-derived nitric oxide. Aging Cell. (2015) 14:678–88. doi: 10.1111/acel.2015.14.issue-4
10. Sobajima J, Ozaki S, Okazaki T, Osakada F, Sumita S, Mori K, et al. Anti-neutrophil cytoplasmic antibodies (ANCA) in ulcerative colitis: anti-cathepsin G and a novel antibody correlate with a refractory type. Clin Exp Immunol. (2003) 105:120–4. doi: 10.1046/j.1365-2249.1996.d01-724.x
11. Plowden J, Renshaw-Hoelscher M, Gangappa S, Engleman C, Katz JM, Sambhara S. Impaired antigen-induced CD8+ T cell clonal expansion in aging is due to defects in antigen presenting cell function. Cell Immunol. (2004) 229:86–92. doi: 10.1016/j.cellimm.2004.07.001
12. van Duin D, Allore HG, Mohanty S, Ginter S, Newman FK, Belshe RB, et al. Prevaccine determination of the expression of costimulatory B7 molecules in activated monocytes predicts influenza vaccine responses in young and older adults. J Infect Diseases. (2007) 195:1590–7. doi: 10.1086/516788
13. Moss CE, Johnston SA, Kimble JV, Clements M, Codd V, Hamby S, et al. Aging-related defects in macrophage function are driven by MYC and USF1 transcriptional programs. Cell Reports. (2024) 43:114073. doi: 10.1016/j.celrep.2024.114073
14. Fulop T, Dupuis G, Baehl S, Le Page A, Bourgade K, Frost E, et al. From inflamm-aging to immune-paralysis: a slippery slope during aging for immune-adaptation. Biogerontology. (2016) 17:147–57. doi: 10.1007/s10522-015-9615-7
15. Metcalf TU, Wilkinson PA, Cameron MJ, Ghneim K, Chiang C, Wertheimer AM, et al. Human monocyte subsets are transcriptionally and functionally altered in aging in response to pattern recognition receptor agonists. J Immunol. (2017) 199:1405–17. doi: 10.4049/jimmunol.1700148
16. Puchta A, Naidoo A, Verschoor CP, Loukov D, Thevaranjan N, Mandur TS, et al. TNF drives monocyte dysfunction with age and results in impaired anti-pneumococcal immunity. PLoS Pathogens. (2016) 12:e1005368. doi: 10.1371/journal.ppat.1005368
17. Panda A, Qian F, Mohanty S, Van Duin D, Newman FK, Zhang L, et al. Age-associated decrease in TLR function in primary human dendritic cells predicts influenza vaccine response. J Immunol. (2010) 184:2518–27. doi: 10.4049/jimmunol.0901022
18. Chougnet CA, Thacker RI, Shehata HM, Hennies CM, Lehn MA, Lages CS, et al. Loss of phagocytic and antigen cross-presenting capacity in aging dendritic cells is associated with mitochondrial dysfunction. J Immunol. (2015) 195:2624–32. doi: 10.4049/jimmunol.1501006
19. Cancro MP. Age-associated B cells. Annu Rev Immunol. (2020) 38:315–40. doi: 10.1146/annurev-immunol-092419-031130
20. Rubtsov AV, Rubtsova K, Kappler JW, Jacobelli J, Friedman RS, Marrack P. CD11c-expressing B cells are located at the T cell/B cell border in spleen and are potent APCs. J Immunol. (2015) 195:71–9. doi: 10.4049/jimmunol.1500055
21. De Mol J, Kuiper J, Tsiantoulas D, Foks AC. The dynamics of B cell aging in health and disease. Front Immunol. (2021) 12. doi: 10.3389/fimmu.2021.733566
22. Rodriguez IJ, Lalinde Ruiz N, Llano León M, Martínez Enríquez L, Montilla Velásquez MDP, Ortiz Aguirre JP, et al. Immunosenescence study of T cells: A systematic review. Front Immunol. (2020) 11:604591. doi: 10.3389/fimmu.2020.604591
23. Naylor K, Li G, Vallejo AN, Lee WW, Koetz K, Bryl E, et al. The influence of age on T cell generation and TCR diversity. J Immunol. (2005) 174:7446–52. doi: 10.4049/jimmunol.174.11.7446
24. Goronzy JJ, Lee W-W, Weyand CM. Aging and T-cell diversity. Exp Gerontology. (2007) 42:400–6. doi: 10.1016/j.exger.2006.11.016
25. Rydyznski Moderbacher C, Ramirez SI, Dan JM, Grifoni A, Hastie KM, Weiskopf D, et al. Antigen-specific adaptive immunity to SARS-coV-2 in acute COVID-19 and associations with age and disease severity. Cell. (2020) 183:996–1012.e19. doi: 10.1016/j.cell.2020.09.038
26. Bryl E, Witkowski JM. Decreased proliferative capability of CD4+ cells of elderly people is associated with faster loss of activation-related antigens and accumulation of regulatory T cells. Exp Gerontology. (2004) 39:587–95. doi: 10.1016/j.exger.2003.10.029
27. Valenzuela HF, Effros RB. Divergent telomerase and CD28 expression patterns in human CD4 and CD8 T cells following repeated encounters with the same antigenic stimulus. Clin Immunol. (2002) 105:117–25. doi: 10.1006/clim.2002.5271
28. Pieren DKJ, Smits NAM, van de Garde MDB, Guichelaar T. Response kinetics reveal novel features of ageing in murine T cells. Sci Reports. (2019) 9:5587. doi: 10.1038/s41598-019-42120-1
29. Bazdar DA, Kalinowska M, Sieg SF. Interleukin-7 receptor signaling is deficient in CD4+ T cells from HIV-infected persons and is inversely associated with aging. J Infect Dis. (2009) 199:1019–28. doi: 10.1086/597210
30. Whisler RL, Beiqing L, Chen M. Age-related decreases in IL-2 production by human T cells are associated with impaired activation of nuclear transcriptional factors AP-1 and NF-AT. Cell Immunol. (1996) 169:185–95. doi: 10.1006/cimm.1996.0109
31. Fagnoni FF, Vescovini R, Mazzola M, Bologna G, Nigro E, Lavagetto G, et al. Expansion of cytotoxic CD8+ CD28- T cells in healthy ageing people, including centenarians. Immunology. (1996) 88:501–7. doi: 10.1046/j.1365-2567.1996.d01-689.x
32. Vallejo AN, Nestel AR, Schirmer M, Weyand CM, Goronzy JJ. Aging-related deficiency of CD28 expression in CD4+ T cells is associated with the loss of gene-specific nuclear factor binding activity. J Biol Chem. (1998) 273:8119–29. doi: 10.1074/jbc.273.14.8119
33. Appay V, Van Lier RAW, Sallusto F, Roederer M. Phenotype and function of human T lymphocyte subsets: Consensus and issues. Cytometry Part A. (2008) 73A:975–83. doi: 10.1002/cyto.a.v73a:11
34. Zhang H, Jadhav RR, Cao W, Goronzy IN, Zhao TV, Jin J, et al. Aging-associated HELIOS deficiency in naive CD4+ T cells alters chromatin remodeling and promotes effector cell responses. Nat Immunol. (2023) 24:96–109. doi: 10.1038/s41590-022-01369-x
35. Saurwein-Teissl M, Lung TL, Marx F, Gschösser C, Asch E, Blasko I, et al. Lack of antibody production following immunization in old age: association with CD8(+)CD28(-) T cell clonal expansions and an imbalance in the production of Th1 and Th2 cytokines. J Immunol. (2002) 168:5893–9. doi: 10.4049/jimmunol.168.11.5893
36. Appay V, Zaunders JJ, Papagno L, Sutton J, Jaramillo A, Waters A, et al. Characterization of CD4+ CTLs ex vivo. J Immunol. (2002) 168:5954–8. doi: 10.4049/jimmunol.168.11.5954
37. Elyahu Y, Hekselman I, Eizenberg-Magar I, Berner O, Strominger I, Schiller M, et al. Aging promotes reorganization of the CD4 T cell landscape toward extreme regulatory and effector phenotypes. Sci Adv. (2019) 5:eaaw8330. doi: 10.1126/sciadv.aaw8330
38. Wen Z, Wang X, Dong K, Zhang H, Bu Z, Ye L, et al. Blockage of regulatory T cells augments induction of protective immune responses by influenza virus-like particles in aged mice. Microbes Infection. (2017) 19:626–34. doi: 10.1016/j.micinf.2017.08.013
39. Geest KSMVD, Abdulahad WH, Tete SM, Lorencetti PG, Horst G, Bos NA, et al. Aging disturbs the balance between effector and regulatory CD4+ T cells. Exp Gerontology. (2014) 60:190–6. doi: 10.1016/j.exger.2014.11.005
40. Schulz AR, Mälzer JN, Domingo C, Jürchott K, Grützkau A, Babel N, et al. Low thymic activity and dendritic cell numbers are associated with the immune response to primary viral infection in elderly humans. J Immunol. (2015) 195:4699–711. doi: 10.4049/jimmunol.1500598
41. Frasca D, Blomberg BB. Aging induces B cell defects and decreased antibody responses to influenza infection and vaccination. Immun Ageing. (2020) 17:37. doi: 10.1186/s12979-020-00210-z
42. Sage PT, Tan CL, Freeman GJ, Haigis M, Sharpe AH. Defective TFH cell function and increased TFR cells contribute to defective antibody production in aging. Cell Rep. (2015) 12:163–71. doi: 10.1016/j.celrep.2015.06.015
43. Bates TA, Leier HC, Lyski ZL, Goodman JR, Curlin ME, Messer WB, et al. Age-dependent neutralization of SARS-CoV-2 and P.1 Variant by Vaccine Immune Serum Samples. JAMA. (2021) 326:868–9. doi: 10.1001/jama.2021.11656
44. Collier DA, Ferreira IATM, Kotagiri P, Datir RP, Lim EY, Touizer E, et al. Age-related immune response heterogeneity to SARS-CoV-2 vaccine BNT162b2. Nature. (2021) 596:417–22. doi: 10.1038/s41586-021-03739-1
45. Silva-Cayetano A, Fra-Bido S, Robert PA, Innocentin S, Burton AR, Watson EM, et al. Spatial dysregulation of T follicular helper cells impairs vaccine responses in aging. Nat Immunol. (2023) 24:1124–37. doi: 10.1038/s41590-023-01519-9
46. Stebegg M, Bignon A, Hill DL, Silva-Cayetano A, Krueger C, Vanderleyden I, et al. Rejuvenating conventional dendritic cells and T follicular helper cell formation after vaccination. eLife. (2020) 9:e52473. doi: 10.7554/eLife.52473
47. Silva-Cayetano A, Foster WS, Innocentin S, Belij-Rammerstorfer S, Spencer AJ, Burton OT, et al. A booster dose enhances immunogenicity of the COVID-19 vaccine candidate ChAdOx1 nCoV-19 in aged mice. Med. (2021) 2:243–62.e8. doi: 10.1016/j.medj.2020.12.006
48. Lefebvre JS, Masters AR, Hopkins JW, Haynes L. Age-related impairment of humoral response to influenza is associated with changes in antigen specific T follicular helper cell responses. Sci Reports. (2016) 6:25051. doi: 10.1038/srep25051
49. Frasca D, Diaz A, Romero M, Garcia D, Blomberg BB. B cell immunosenescence. Annu Rev Cell Dev Biol. (2020) 36:551–74. doi: 10.1146/annurev-cellbio-011620-034148
50. Dunn-Walters DK, Banerjee M, Mehr R. Effects of age on antibody affinity maturation. Biochem Soc Trans. (2003) 31:447–8. doi: 10.1042/bst0310447
51. Wang C, Liu Y, Xu LT, Jackson KJL, Roskin KM, Pham TD, et al. Effects of aging, cytomegalovirus infection, and EBV infection on human B cell repertoires. J Immunol. (2014) 192:603–11. doi: 10.4049/jimmunol.1301384
52. Hoehn KB, Vander Heiden JA, Zhou JQ, Lunter G, Pybus OG, Kleinstein SH. Repertoire-wide phylogenetic models of B cell molecular evolution reveal evolutionary signatures of aging and vaccination. Proc Natl Acad Sci. (2019) 116:22664–72. doi: 10.1073/pnas.1906020116
53. Frasca D, Diaz A, Romero M, Thaller S, Blomberg BB. Metabolic requirements of human pro-inflammatory B cells in aging and obesity. PLoS One. (2019) 14:e0219545. doi: 10.1371/journal.pone.0219545
54. Lee-Chang C, Bodogai M, Moritoh K, Olkhanud PB, Chan AC, Croft M, et al. Accumulation of 4-1BBL+ B cells in the elderly induces the generation of granzyme-B+ CD8+ T cells with potential antitumor activity. Blood. (2014) 124:1450–9. doi: 10.1182/blood-2014-03-563940
55. Miller JP, Allman D. The decline in B lymphopoiesis in aged mice reflects loss of very early B-lineage precursors 1. J Immunol. (2003) 171:2326–30. doi: 10.4049/jimmunol.171.5.2326
56. Muller-Sieburg CE, Sieburg HB, Bernitz JM, Cattarossi G. Stem cell heterogeneity: implications for aging and regenerative medicine. Blood. (2012) 119:3900–7. doi: 10.1182/blood-2011-12-376749
57. McKenna RW, Washington LT, Aquino DB, Picker LJ, Kroft SH. Immunophenotypic analysis of hematogones (B-lymphocyte precursors) in 662 consecutive bone marrow specimens by 4-color flow cytometry. Blood. (2001) 98:2498–507. doi: 10.1182/blood.V98.8.2498
58. Lescale C, Dias S, Maës J, Cumano A, Szabo P, Charron D, et al. Reduced EBF expression underlies loss of B-cell potential of hematopoietic progenitors with age. Aging Cell. (2010) 9:410–9. doi: 10.1111/j.1474-9726.2010.00566.x
59. Riley RL, Blomberg BB, Frasca D. B cells, E2A, and aging. Immunological Rev. (2005) 205:30–47. doi: 10.1111/j.0105-2896.2005.00268.x
60. Anspach J, Poulsen G, Kaattari I, Pollock R, Zwollo P. Reduction in DNA binding activity of the transcription factor pax-5a in B lymphocytes of aged mice1. J Immunol. (2001) 166:2617–26. doi: 10.4049/jimmunol.166.4.2617
61. Frasca D, Diaz A, Romero M, Blomberg BB. Human peripheral late/exhausted memory B cells express a senescent-associated secretory phenotype and preferentially utilize metabolic signaling pathways. Exp Gerontology. (2017) 87:113–20. doi: 10.1016/j.exger.2016.12.001
62. Frasca D, Diaz A, Romero M, D’Eramo F, Blomberg BB. Aging effects on T-bet expression in human B cell subsets. Cell Immunol. (2017) 321:68–73. doi: 10.1016/j.cellimm.2017.04.007
63. Frasca D, Diaz A, Romero M, Landin AM, Blomberg BB. High TNF-α levels in resting B cells negatively correlate with their response. Exp Gerontology. (2014) 54:116–22. doi: 10.1016/j.exger.2014.01.004
64. Hao Y, O’Neill P, Naradikian MS, Scholz JL, Cancro MP. A B-cell subset uniquely responsive to innate stimuli accumulates in aged mice. Blood. (2011) 118:1294–304. doi: 10.1182/blood-2011-01-330530
65. Ratliff M, Alter S, Frasca D, Blomberg BB, Riley RL. In senescence, age-associated B cells secrete TNFα and inhibit survival of B-cell precursors. Aging Cell. (2013) 12:303–11. doi: 10.1111/acel.2013.12.issue-2
66. Keren Z, Naor S, Nussbaum S, Golan K, Itkin T, Sasaki Y, et al. B-cell depletion reactivates B lymphopoiesis in the BM and rejuvenates the B lineage in aging. Blood. (2011) 117:3104–12. doi: 10.1182/blood-2010-09-307983
67. Riley RL, Khomtchouk K, Blomberg BB. Age-associated B cells (ABC) inhibit B lymphopoiesis and alter antibody repertoires in old age. Cell Immunol. (2017) 321:61–7. doi: 10.1016/j.cellimm.2017.04.008
68. Ratliff M, Alter S, Frasca D, Blomberg BB, Riley RL. In senescence, age-associated B cells secrete TNFα and inhibit survival of B-cell precursors*. Aging Cell. (2013) 12:303–11. doi: 10.1111/acel.2013.12.issue-2
69. Coe CL, Lubach GR, Kinnard J. Immune senescence in old and very old rhesus monkeys: reduced antibody response to influenza vaccination. Age. (2012) 34:1169–77. doi: 10.1007/s11357-011-9356-8
70. Kaml M, Weiskirchner I, Keller M, Luft T, Hoster E, Hasford J, et al. Booster vaccination in the elderly: their success depends on the vaccine type applied earlier in life as well as on pre-vaccination antibody titers. Vaccine. (2006) 24:6808–11. doi: 10.1016/j.vaccine.2006.06.037
71. Siegrist C-A, Aspinall R. B-cell responses to vaccination at the extremes of age. Nat Rev Immunol. (2009) 9:185–94. doi: 10.1038/nri2508
72. Pardi N, Hogan MJ, Porter FW, Weissman D. mRNA vaccines - a new era in vaccinology. Nat Rev Drug Discov. (2018) 17:261–79. doi: 10.1038/nrd.2017.243
73. Poveda C, Biter AB, Bottazzi ME, Strych U. Establishing preferred product characterization for the evaluation of RNA vaccine antigens. Vaccines (Basel). (2019) 7:131. doi: 10.3390/vaccines7040131
74. Chaudhary N, Weissman D, Whitehead KA. mRNA vaccines for infectious diseases: principles, delivery and clinical translation. Nat Rev Drug Discovery. (2021) 20:817–38. doi: 10.1038/s41573-021-00283-5
75. Jayaraman M, Ansell SM, Mui BL, Tam YK, Chen J, Du X, et al. Maximizing the potency of siRNA lipid nanoparticles for hepatic gene silencing in vivo. Angew Chem Int Ed Engl. (2012) 51:8529–33. doi: 10.1002/anie.201203263
76. Sabnis S, Kumarasinghe ES, Salerno T, Mihai C, Ketova T, Senn JJ, et al. A novel amino lipid series for mRNA delivery: improved endosomal escape and sustained pharmacology and safety in non-human primates. Mol Ther. (2018) 26:1509–19. doi: 10.1016/j.ymthe.2018.03.010
77. Miao L, Lin J, Huang Y, Li L, Delcassian D, Ge Y, et al. Synergistic lipid compositions for albumin receptor mediated delivery of mRNA to the liver. Nat Commun. (2020) 11:2424. doi: 10.1038/s41467-020-16248-y
78. Patel S, Ashwanikumar N, Robinson E, Xia Y, Mihai C, Griffith JP 3rd, et al. Naturally-occurring cholesterol analogues in lipid nanoparticles induce polymorphic shape and enhance intracellular delivery of mRNA. Nat Commun. (2020) 11:983. doi: 10.1038/s41467-020-14527-2
79. Paunovska K, Da Silva Sanchez AJ, Sago CD, Gan Z, Lokugamage MP, Islam FZ, et al. Nanoparticles Containing Oxidized Cholesterol Deliver mRNA to the Liver Microenvironment at Clinically Relevant Doses. Adv Mater. (2019) 31:e1807748. doi: 10.1002/adma.201807748
80. Ball RL, Hajj KA, Vizelman J, Bajaj P, Whitehead KA. Lipid Nanoparticle Formulations for Enhanced Co-delivery of siRNA and mRNA. Nano Lett. (2018) 18:3814–22. doi: 10.1021/acs.nanolett.8b01101
81. Kauffman KJ, Dorkin JR, Yang JH, Heartlein MW, DeRosa F, Mir FF, et al. Optimization of lipid nanoparticle formulations for mRNA delivery in vivo with fractional factorial and definitive screening designs. Nano Lett. (2015) 15:7300–6. doi: 10.1021/acs.nanolett.5b02497
82. Kim J, Eygeris Y, Gupta M, Sahay G. Self-assembled mRNA vaccines. Adv Drug Delivery Rev. (2021) 170:83–112. doi: 10.1016/j.addr.2020.12.014
83. Cui S, Wang Y, Gong Y, Lin X, Zhao Y, Zhi D, et al. Correlation of the cytotoxic effects of cationic lipids with their headgroups. Toxicol Res (Camb). (2018) 7:473–9. doi: 10.1039/C8TX00005K
84. Cullis PR, Hope MJ. Lipid nanoparticle systems for enabling gene therapies. Mol Ther. (2017) 25:1467–75. doi: 10.1016/j.ymthe.2017.03.013
85. Sahay G, Alakhova DY, Kabanov AV. Endocytosis of nanomedicines. J Control Release. (2010) 145:182–95. doi: 10.1016/j.jconrel.2010.01.036
86. Miao L, Li L, Huang Y, Delcassian D, Chahal J, Han J, et al. Delivery of mRNA vaccines with heterocyclic lipids increases anti-tumor efficacy by STING-mediated immune cell activation. Nat Biotechnol. (2019) 37:1174–85. doi: 10.1038/s41587-019-0247-3
87. Zhao X, Chen J, Qiu M, Li Y, Glass Z, Xu Q. Imidazole-Based Synthetic Lipidoids for In Vivo mRNA Delivery into Primary T Lymphocytes. Angew Chem Int Ed Engl. (2020) 59:20083–9. doi: 10.1002/anie.202008082
88. Cheng X, Lee RJ. The role of helper lipids in lipid nanoparticles (LNPs) designed for oligonucleotide delivery. Adv Drug Delivery Rev. (2016) 99:129–37. doi: 10.1016/j.addr.2016.01.022
89. Liu S, Cheng Q, Wei T, Yu X, Johnson LT, Farbiak L, et al. Membrane-destabilizing ionizable phospholipids for organ-selective mRNA delivery and CRISPR-Cas gene editing. Nat Mater. (2021) 20:701–10. doi: 10.1038/s41563-020-00886-0
90. Kulkarni JA, Darjuan MM, Mercer JE, Chen S, van der Meel R, Thewalt JL, et al. On the formation and morphology of lipid nanoparticles containing ionizable cationic lipids and siRNA. ACS Nano. (2018) 12:4787–95. doi: 10.1021/acsnano.8b01516
91. Kanasty R, Dorkin JR, Vegas A, Anderson D. Delivery materials for siRNA therapeutics. Nat Mater. (2013) 12:967–77. doi: 10.1038/nmat3765
92. Zhao M, Li M, Zhang Z, Gong T, Sun X. Induction of HIV-1 gag specific immune responses by cationic micelles mediated delivery of gag mRNA. Drug Deliv. (2016) 23:2596–607. doi: 10.3109/10717544.2015.1038856
93. Lynn DM, Langer R. Degradable poly(β-amino esters): Synthesis, characterization, and self-assembly with plasmid DNA. J Am Chem Society. (2000) 122:10761–8. doi: 10.1021/ja0015388
94. Matsui A, Uchida S, Ishii T, Itaka K, Kataoka K. Messenger RNA-based therapeutics for the treatment of apoptosis-associated diseases. Sci Rep. (2015) 5:15810. doi: 10.1038/srep15810
95. McKinlay CJ, Vargas JR, Blake TR, Hardy JW, Kanada M, Contag CH, et al. Charge-altering releasable transporters (CARTs) for the delivery and release of mRNA in living animals. Proc Natl Acad Sci U S A. (2017) 114:E448–e56. doi: 10.1073/pnas.1614193114
96. Kaczmarek JC, Kauffman KJ, Fenton OS, Sadtler K, Patel AK, Heartlein MW, et al. Optimization of a degradable polymer-lipid nanoparticle for potent systemic delivery of mRNA to the lung endothelium and immune cells. Nano Lett. (2018) 18:6449–54. doi: 10.1021/acs.nanolett.8b02917
97. Kim HJ, Ogura S, Otabe T, Kamegawa R, Sato M, Kataoka K, et al. Fine-tuning of hydrophobicity in amphiphilic polyaspartamide derivatives for rapid and transient expression of messenger RNA directed toward genome engineering in brain. ACS Cent Sci. (2019) 5:1866–75. doi: 10.1021/acscentsci.9b00843
98. Ulkoski D, Bak A, Wilson JT, Krishnamurthy VR. Recent advances in polymeric materials for the delivery of RNA therapeutics. Expert Opin Drug Deliv. (2019) 16:1149–67. doi: 10.1080/17425247.2019.1663822
99. Lin CY, Perche F, Ikegami M, Uchida S, Kataoka K, Itaka K. Messenger RNA-based therapeutics for brain diseases: An animal study for augmenting clearance of beta-amyloid by intracerebral administration of neprilysin mRNA loaded in polyplex nanomicelles. J Control Release. (2016) 235:268–75. doi: 10.1016/j.jconrel.2016.06.001
100. Crowley ST, Fukushima Y, Uchida S, Kataoka K, Itaka K. Enhancement of Motor Function Recovery after Spinal Cord Injury in Mice by Delivery of Brain-Derived Neurotrophic Factor mRNA. Mol Ther Nucleic Acids. (2019) 17:465–76. doi: 10.1016/j.omtn.2019.06.016
101. Aini H, Itaka K, Fujisawa A, Uchida H, Uchida S, Fukushima S, et al. Messenger RNA delivery of a cartilage-anabolic transcription factor as a disease-modifying strategy for osteoarthritis treatment. Sci Rep. (2016) 6:18743. doi: 10.1038/srep18743
102. Brito LA, Chan M, Shaw CA, Hekele A, Carsillo T, Schaefer M, et al. A cationic nanoemulsion for the delivery of next-generation RNA vaccines. Mol Ther. (2014) 22:2118–29. doi: 10.1038/mt.2014.133
103. McCarthy HO, McCaffrey J, McCrudden CM, Zholobenko A, Ali AA, McBride JW, et al. Development and characterization of self-assembling nanoparticles using a bio-inspired amphipathic peptide for gene delivery. J Control Release. (2014) 189:141–9. doi: 10.1016/j.jconrel.2014.06.048
104. What’s Next? The Future of mRNA Vaccines for “Every Imaginable Infectious Disease” (2022). Available online at: https://www.pennmedicine.org/mrna (Accessed February 24, 2025).
105. Brook B, Fatou B, Kumar Checkervarty A, Barman S, Sweitzer C, Bosco AN, et al. The mRNA vaccine BNT162b2 demonstrates impaired T(H)1 immunogenicity in human elders in vitro and aged mice in vivo. Res Sq. (2022) 27(11):111055. doi: 10.21203/rs.3.rs-2395118/v1
106. Chen Y, Li C, Liu F, Ye Z, Song W, Lee ACY, et al. Age-associated SARS-CoV-2 breakthrough infection and changes in immune response in a mouse model. Emerg Microbes Infect. (2022) 11:368–83. doi: 10.1080/22221751.2022.2026741
107. Shin JH, Gao Y, Moore JH 2nd, Bolick DT, Kolling GL, Wu M, et al. Innate immune response and outcome of clostridium difficile infection are dependent on fecal bacterial composition in the aged host. J Infect Dis. (2018) 217:188–97. doi: 10.1093/infdis/jix414
108. Petsch B, Schnee M, Vogel AB, Lange E, Hoffmann B, Voss D, et al. Protective efficacy of in vitro synthesized, specific mRNA vaccines against influenza A virus infection. Nat Biotechnol. (2012) 30:2012–11-25. doi: 10.1038/nbt.2436
109. Gote V, Bolla PK, Kommineni N, Butreddy A, Nukala PK, Palakurthi SS, et al. A comprehensive review of mRNA vaccines. Int J Mol Sci. (2023) 24:2700. doi: 10.3390/ijms24032700
110. Bell MR, Kutzler MA. An old problem with new solutions: Strategies to improve vaccine efficacy in the elderly. Advanced Drug delivery Rev. (2022) 183:114175. doi: 10.1016/j.addr.2022.114175
111. Crooke SN, Ovsyannikova IG, Poland GA, Kennedy RB. Immunosenescence and human vaccine immune responses. Immun ageing. (2019) 16:1–16. doi: 10.1186/s12979-019-0164-9
112. Pawelec G, McElhaney J, Pawelec G, McElhaney J. Unanticipated efficacy of SARS-CoV-2 vaccination in older adults. Immun Ageing. (2021) 18:7. doi: 10.1186/s12979-021-00219-y
113. Soiza RL, Scicluna C, Thomson EC. Efficacy and safety of COVID-19 vaccines in older people. Age Ageing. (2021) 50:279–83. doi: 10.1093/ageing/afaa274
114. Koirala A, Joo YJ, Khatami A, Chiu C, Britton PN. Vaccines for COVID-19: The current state of play. Paediatric respiratory Rev. (2020) 35:43–49. doi: 10.1016/j.prrv.2020.06.010
115. Polack FP, Thomas SJ, Kitchin N, Absalon J, Gurtman A, Lockhart S, et al. Safety and efficacy of the BNT162b2 mRNA Covid-19 vaccine. New Engl J Med. (2020) 383:2603–15. doi: 10.1056/NEJMoa2034577
116. Kontopoulou K, Nakas CT, Ainatzoglou A, Ifantidou A, Ntotsi P, Katsioulis C, et al. Immunogenicity of the BNT162b2 mRNA Covid-19 vaccine in elderly people over 85 years of age in Greece: the GREVAXIMO study. Aging Clin Exp Res. (2021) 33:3385–89. doi: 10.1007/s40520-021-01997-7
117. Walsh EE, Frenck RW Jr., Falsey AR, Kitchin N, Absalon J, Gurtman A, et al. Safety and immunogenicity of two RNA-based Covid-19 vaccine candidates. New Engl J Medicine. (2020) 383:2439–50. doi: 10.1056/NEJMoa2027906
118. Jergović M, Uhrlaub JL, Watanabe M, Bradshaw CM, White LM, LaFleur BJ, et al. Competent immune responses to SARS-CoV-2 variants in older adults following two doses of mRNA vaccination. Nat Commun. (2022) 13:2891. doi: 10.1038/s41467-022-30617-9
119. Korosec CS, Farhang-Sardroodi S, Dick DW, Gholami S, Ghaemi MS, Moyles IR, et al. Long-term durability of immune responses to the BNT162b2 and mRNA-1273 vaccines based on dosage, age and sex. Sci Rep. (2022) 12:21232. doi: 10.1038/s41598-022-25134-0
120. Renia L, Goh YS, Rouers A, Le Bert N, Chia WN, Chavatte J-M, et al. Lower vaccine-acquired immunity in the elderly population following two-dose BNT162b2 vaccination is alleviated by a third vaccine dose. Nat Commun. (2022) 13:4615. doi: 10.1038/s41467-022-32312-1
121. Shapiro JR, Sitaras I, Park HS, Aytenfisu TY, Caputo C, Li M, et al. Association of frailty, age, and biological sex with severe acute respiratory syndrome coronavirus 2 messenger RNA vaccine–induced immunity in older adults. Clin Infect Dis. (2022) 75:S61–71. doi: 10.1093/cid/ciac397
122. Rouvinski A, Friedman A, Kirillov S, Attal JH, Kumari S, Fahoum J, et al. Antibody response in elderly vaccinated four times with an mRNA anti-COVID-19 vaccine. Sci Rep. (2023) 13:14165. doi: 10.1038/s41598-023-41399-5
123. Cicin-Sain L, Smyk-Pearson S, Currier N, Byrd L, Koudelka C, Robinson T, et al. Loss of naive T cells and repertoire constriction predict poor response to vaccination in old primates. J Of Immunol. (2010) 184:6739. doi: 10.4049/jimmunol.0904193
124. Gustafson CE, Kim C, Weyand CM, Goronzy JJ. Influence of immune aging on vaccine responses. J Allergy Clin Immunol. (2020) 145:1309–21. doi: 10.1016/j.jaci.2020.03.017
125. Saggau C, Martini GR, Rosati E, Meise S, Messner B, Kamps AK, et al. The pre-exposure SARS-CoV-2-specific T cell repertoire determines the quality of the immune response to vaccination. Immunity. (2022) 55:1924–39. doi: 10.1016/j.immuni.2022.08.003
126. Aliprantis AO, Shaw CA, Griffin P, Farinola N, Railkar RA, Cao X, et al. A phase 1, randomized, placebo-controlled study to evaluate the safety and immunogenicity of an mRNA-based RSV prefusion F protein vaccine in healthy younger and older adults. Hum Vaccines Immunotherapeutics. (2021) 17:1248–61. doi: 10.1080/21645515.2020.1829899
127. Chen GL, Mithani R, Kapoor A, Lu S, Asmar LE, Panozzo CA, et al. 234. Safety and Immunogenicity of mRNA-1345, an mRNA-Based RSV Vaccine in Younger and Older Adult Cohorts: Results from a Phase 1, Randomized Clinical Trial. Open Forum Infect Dis. (2022) 9:S164–5. doi: 10.1093/ofid/ofac492.312
128. Liao H-C, Liu S-J. Advances in nucleic acid-based cancer vaccines. J Biomed Sci. (2025) 32:10. doi: 10.1186/s12929-024-01102-w
129. Kuhn AN, Beißert T, Simon P, Vallazza B, Buck J, Davies BP, et al. mRNA as a versatile tool for exogenous protein expression. Curr Gene Ther. (2012) 12:347–61. doi: 10.2174/156652312802762536
130. Jung S-W, Jeon JJ, Kim YH, Choe SJ, Lee S. Long-term risk of autoimmune diseases after mRNA-based SARS-CoV2 vaccination in a Korean, nationwide, population-based cohort study. Nat Commun. (2024) 15:6181. doi: 10.1038/s41467-024-50656-8
131. Wolff JA, Malone RW, Williams P, Chong W, Acsadi G, Jani A, et al. Direct gene transfer into mouse muscle in vivo. Science. (1990) 247:1465–8. doi: 10.1126/science.1690918
132. Song JM, Kim YC OE, Compans RW, Prausnitz MR, Kang SM. DNA vaccination in the skin using microneedles improves protection against influenza. Mol Ther. (2012) 20:1472–80. doi: 10.1038/mt.2012.69
133. Gomez AM, Babuadze G, Plourde-Campagna M-A, Azizi H, Berger A, Kozak R, et al. A novel intradermal tattoo-based injection device enhances the immunogenicity of plasmid DNA vaccines. NPJ Vaccines. (2022) 7:172. doi: 10.1038/s41541-022-00581-y
134. Graham BS, Enama ME, Nason MC, Gordon IJ, Peel SA, Ledgerwood JE, et al. DNA vaccine delivered by a needle-free injection device improves potency of priming for antibody and CD8+ T-cell responses after rAd5 boost in a randomized clinical trial. PLoS One. (2013) 8:e59340. doi: 10.1371/journal.pone.0059340
135. Suschak JJ, Bixler SL, Badger CV, Spik KW, Kwilas SA, Rossi FD, et al. A DNA vaccine targeting VEE virus delivered by needle-free jet-injection protects macaques against aerosol challenge. NPJ Vaccines. (2022) 7:46. doi: 10.1038/s41541-022-00469-x
136. Lu B, Lim JM, Yu B, Song S, Neeli P, Sobhani N, et al. The next-generation DNA vaccine platforms and delivery systems: advances, challenges and prospects. Front Immunol. (2024) 15. doi: 10.3389/fimmu.2024.1332939
137. Best SR, Peng S, Juang CM, Hung CF, Hannaman D, Saunders JR, et al. Administration of HPV DNA vaccine via electroporation elicits the strongest CD8+ T cell immune responses compared to intramuscular injection and intradermal gene gun delivery. Vaccine. (2009) 27:5450–9. doi: 10.1016/j.vaccine.2009.07.005
138. Travieso T, Li J, Mahesh S, Mello JDFRE, Blasi M. The use of viral vectors in vaccine development. NPJ Vaccines. (2022) 7:75. doi: 10.1038/s41541-022-00503-y
139. Pegtel DM, Gould SJ. Exosomes. Annu Rev Biochem. (2019) 88:487–514. doi: 10.1146/annurev-biochem-013118-111902
140. Watson DS, Endsley AN, Huang L. Design considerations for liposomal vaccines: influence of formulation parameters on antibody and cell-mediated immune responses to liposome associated antigens. Vaccine. (2012) 30:2256–72. doi: 10.1016/j.vaccine.2012.01.070
141. Lu Y, Wu F, Duan W, Mu X, Fang S, Lu N, et al. Engineering a “PEG-g-PEI/DNA nanoparticle-in- PLGA microsphere” hybrid controlled release system to enhance immunogenicity of DNA vaccine. Materials Sci Engineering: C. (2020) 106:110294. doi: 10.1016/j.msec.2019.110294
142. Karimov M, Appelhans D, Ewe A, Aigner A. The combined disulfide cross-linking and tyrosine-modification of very low molecular weight linear PEI synergistically enhances transfection efficacies and improves biocompatibility. Eur J Pharmaceutics Biopharmaceutics. (2021) 161:56–65. doi: 10.1016/j.ejpb.2021.02.005
143. Gao Y, Wu Y. Recent advances of chitosan-based nanoparticles for biomedical and biotechnological applications. Int J Biol Macromolecules. (2022) 203:379–88. doi: 10.1016/j.ijbiomac.2022.01.162
144. Chiarella P, Massi E, De Robertis M, Sibilio A, Parrella P, Fazio VM, et al. Electroporation of skeletal muscle induces danger signal release and antigen-presenting cell recruitment independently of DNA vaccine administration. Expert Opin Biol Ther. (2008) 8:1645–57. doi: 10.1517/14712598.8.11.1645
145. Tomizawa M, Shinozaki F, Motoyoshi Y, Sugiyama T, Yamamoto S, Sueishi M. Sonoporation: Gene transfer using ultrasound. World J Methodol. (2013) 3:39–44. doi: 10.5662/wjm.v3.i4.39
146. Wang L, Wei X, Liu H, Fan Y. Nanomaterial-mediated photoporation for intracellular delivery. Acta Biomaterialia. (2023) 157:24–48. doi: 10.1016/j.actbio.2022.12.050
147. Suda T, Liu D. Hydrodynamic gene delivery: its principles and applications. Mol Ther. (2007) 15:2063–9. doi: 10.1038/sj.mt.6300314
148. Farshbafnadi M, Kamali Zonouzi S, Sabahi M, Dolatshahi M, Aarabi MH. Aging & COVID-19 susceptibility, disease severity, and clinical outcomes: The role of entangled risk factors. Exp Gerontol. (2021) 154:111507. doi: 10.1016/j.exger.2021.111507
149. Cui L, Wang J, Orlando F, Giacconi R, Malavolta M, Bartozzi B, et al. Enhancing immune responses against SARS-CoV-2 variants in aged mice with INDUK: A chimeric DNA vaccine encoding the spike S1-TM subunits. ACS Omega. (2024) 9:34624–35. doi: 10.1021/acsomega.4c03285
150. Bender BS, Ulmer JB, DeWitt CM, Cottey R, Taylor SF, Ward AM, et al. Immunogenicity and efficacy of DNA vaccines encoding influenza A proteins in aged mice. Vaccine. (1998) 16:1748–55. doi: 10.1016/S0264-410X(98)00135-2
151. Kwak J-E, Kim Y-I, Park S-J, Yu M-A, Kwon H-I, Eo S, et al. Development of a SFTSV DNA vaccine that confers complete protection against lethal infection in ferrets. Nat Communications. (2019) 10:3836. doi: 10.1038/s41467-019-11815-4
152. Klinman DM, Conover J, Bloom ET, Weiss W. Immunogenicity and efficacy of a DNA vaccine in aged mice. Journals Gerontology. (1998) 53A:B281–B6. doi: 10.1093/gerona/53A.4.B281
153. Castro F, Leal B, Denny A, Bahar R, Lampkin S, Reddick R, et al. Vaccination with Mage-b DNA induces CD8 T-cell responses at young but not old age in mice with metastatic breast cancer. Br J Cancer. (2009) 101:1329–37. doi: 10.1038/sj.bjc.6605329
154. Provinciali M, Barucca A, Pierpaoli E, Orlando F, Pierpaoli S, Smorlesi A. In vivo electroporation restores the low effectiveness of DNA vaccination against HER-2/neu in aging. Cancer Immunology Immunother. (2012) 61:363–71. doi: 10.1007/s00262-011-1107-2
155. Olkhanud PB, Mughal M, Ayukawa K, Malchinkhuu E, Bodogai M, Feldman N, et al. DNA immunization with HBsAg-based particles expressing a B cell epitope of amyloid β-peptide attenuates disease progression and prolongs survival in a mouse model of Alzheimer’s disease. Vaccine. (2012) 30:1650–8. doi: 10.1016/j.vaccine.2011.12.136
156. Qu L, Sha S, Xing X-N, Wang T, Li Y, Zhang R-W, et al. DNA vaccines targeting amyloid-β oligomer ameliorate cognitive deficits of aged APP/PS1/tau triple-transgenic mouse models of Alzheimer’s disease. Neural Regeneration Res. (2022) 17:2305. doi: 10.4103/1673-5374.337054
157. Kraynyak KA, Blackwood E, Agnes J, Tebas P, Giffear M, Amante D, et al. SARS-CoV-2 DNA vaccine INO-4800 induces durable immune responses capable of being boosted in a phase 1 open-label trial. J Infect Diseases. (2022) 225:1923–32. doi: 10.1093/infdis/jiac016
158. Ledgerwood JE, Pierson TC, Hubka SA, Desai N, Rucker S, Gordon IJ, et al. A west nile virus DNA vaccine utilizing a modified promoter induces neutralizing antibody in younger and older healthy adults in a phase I clinical trial. J Infect Diseases. (2011) 203:1396–404. doi: 10.1093/infdis/jir054
159. Rohn J. Inovio: a Pennsylvania company hopes to turn synthetic DNA vaccines into rapid response agents against flu epidemics and cancer. Nat Biotechnol. (2013) 31:98. doi: 10.1038/nbt0213-98
160. Disis MLN, Guthrie KA, Liu Y, Coveler AL, Higgins DM, Childs JS, et al. Safety and outcomes of a plasmid DNA vaccine encoding the ERBB2 intracellular domain in patients with advanced-stage ERBB2-positive breast cancer: A phase 1 nonrandomized clinical trial. JAMA Oncol. (2023) 9:71–8. doi: 10.1001/jamaoncol.2022.5143
161. Li L, Petrovsky N. Molecular mechanisms for enhanced DNA vaccine immunogenicity. Expert Rev Vaccines. (2016) 15:313–29. doi: 10.1586/14760584.2016.1124762
162. Chavda VP, Pandya R, Apostolopoulos V. DNA vaccines for SARS-CoV-2: toward third-generation vaccination era. Expert Rev Vaccines. (2021) 20:1549–60. doi: 10.1080/14760584.2021.1987223
163. Klinman DM, Klaschik S, Tross D, Shirota H, Steinhagen F. FDA guidance on prophylactic DNA vaccines: analysis and recommendations. Vaccine. (2009) 28:2801–5. doi: 10.1016/j.vaccine.2009.11.025
164. Gilkeson GS, Grudier JP, Karounos DG, Pisetsky DS. Induction of anti-double stranded DNA antibodies in normal mice by immunization with bacterial DNA. J Immunol. (1989) 142:1482–6. doi: 10.4049/jimmunol.142.5.1482
165. Mor G, Singla M, Steinberg AD, Hoffman SL, Okuda K, Klinman DM. Do DNA vaccines induce autoimmune disease? (2008). Available online at: https://homeliebertpubcom/hum (Accessed March 31, 2025).
166. Schattner A. Consequence or coincidence? The occurrence, pathogenesis and significance of autoimmune manifestations after viral vaccines. Vaccine. (2005) 23:3876–86. doi: 10.1016/j.vaccine.2005.03.005
167. Glenny AT, Pope CG, Waddington H, Wallace U. Immunological notes. XVII–XXIV. J Pathol Bacteriology. (1926) 29:31–40. doi: 10.1002/path.1700290106
168. Marrack P, McKee AS, Munks MW. Towards an understanding of the adjuvant action of aluminium. Nat Rev Immunol. (2009) 9:287–93. doi: 10.1038/nri2510
169. Alameh MG, Tombácz I, Bettini E, Lederer K, Sittplangkoon C, Wilmore JR, et al. Lipid nanoparticles enhance the efficacy of mRNA and protein subunit vaccines by inducing robust T follicular helper cell and humoral responses. Immunity. (2021) 54:2877–92.e7. doi: 10.1016/j.immuni.2021.11.001
170. Pardi N, Hogan MJ, Naradikian MS, Parkhouse K, Cain DW, Jones L, et al. Nucleoside-modified mRNA vaccines induce potent T follicular helper and germinal center B cell responses. J Exp Med. (2018) 215:1571–88. doi: 10.1084/jem.20171450
171. Morrissey DV, Lockridge JA, Shaw L, Blanchard K, Jensen K, Breen W, et al. Potent and persistent in vivo anti-HBV activity of chemically modified siRNAs. Nat Biotechnol. (2005) 23:1002–7. doi: 10.1038/nbt1122
172. Wang Y, Zhang L, Xu Z, Miao L, Huang L. mRNA vaccine with antigen-specific checkpoint blockade induces an enhanced immune response against established melanoma. Mol Ther. (2018) 26:420–34. doi: 10.1016/j.ymthe.2017.11.009
173. Lou G, Anderluzzi G, Schmidt ST, Woods S, Gallorini S, Brazzoli M, et al. Delivery of self-amplifying mRNA vaccines by cationic lipid nanoparticles: The impact of cationic lipid selection. J Controlled Release. (2020) 325:370–9. doi: 10.1016/j.jconrel.2020.06.027
174. Tahtinen S, Tong A-J, Himmels P, Oh J, Paler-Martinez A, Kim L, et al. IL-1 and IL-1ra are key regulators of the inflammatory response to RNA vaccines. Nat Immunol. (2022) 23:532–42. doi: 10.1038/s41590-022-01160-y
175. Zhang H, You X, Wang X, Cui L, Wang Z, Xu F, et al. Delivery of mRNA vaccine with a lipid-like material potentiates antitumor efficacy through Toll-like receptor 4 signaling. Proc Natl Acad Sci. (2021) 118:e2005191118. doi: 10.1073/pnas.2005191118
176. Han X, Alameh M-G, Butowska K, Knox JJ, Lundgreen K, Ghattas M, et al. Adjuvant lipidoid-substituted lipid nanoparticles augment the immunogenicity of SARS-CoV-2 mRNA vaccines. Nat Nanotechnology. (2023) 18:1105–14. doi: 10.1038/s41565-023-01404-4
177. Zhang Y, Yan J, Hou X, Wang C, Kang DD, Xue Y, et al. STING agonist-derived LNP-mRNA vaccine enhances protective immunity against SARS-CoV-2. Nano Lett. (2023) 23:2593–600. doi: 10.1021/acs.nanolett.2c04883
178. Zhang R, Tang L, Tian Y, Ji X, Hu Q, Zhou B, et al. DP7-C-modified liposomes enhance immune responses and the antitumor effect of a neoantigen-based mRNA vaccine. J Controlled Release. (2020) 328:210–21. doi: 10.1016/j.jconrel.2020.08.023
179. Kallen KJ, Heidenreich R, Schnee M, Petsch B, Schlake T, Thess A, et al. A novel, disruptive vaccination technology: self-adjuvanted RNActive(®) vaccines. Hum Vaccin Immunother. (2013) 9:2263–76. doi: 10.4161/hv.25181
180. Tse SW, McKinney K, Walker W, Nguyen M, Iacovelli J, Small C, et al. mRNA-encoded, constitutively active STING(V155M) is a potent genetic adjuvant of antigen-specific CD8(+) T cell response. Mol Ther. (2021) 29:2227–38. doi: 10.1016/j.ymthe.2021.03.002
181. Islam MA, Rice J, Reesor E, Zope H, Tao W, Lim M, et al. Adjuvant-pulsed mRNA vaccine nanoparticle for immunoprophylactic and therapeutic tumor suppression in mice. Biomaterials. (2020) 266:120431. doi: 10.1016/j.biomaterials.2020.120431
182. Verbeke R, Lentacker I, Breckpot K, Janssens J, Calenbergh SV, Smedt SCD, et al. Broadening the message: A nanovaccine co-loaded with messenger RNA and α-galCer induces antitumor immunity through conventional and natural killer T cells. ACS Nano. (2019) 13(2):1655–69. doi: 10.1021/acsnano.8b07660
183. Brook B, Duval V, Barman S, Speciner L, Sweitzer C, Khanmohammed A, et al. Adjuvantation of a SARS-CoV-2 mRNA vaccine with controlled tissue-specific expression of an mRNA encoding IL-12p70. Sci Trans Med. (2024) 16:eadm8451. doi: 10.1126/scitranslmed.adm8451
184. Neeli P, Chai D, Wang X, Sobhani N, Udeani G, Li Y. Comparison of DNA vaccines with AddaS03 as an adjuvant and an mRNA vaccine against SARS-CoV-2. iScience. (2023) 26:107120. doi: 10.1016/j.isci.2023.107120
185. Grigoryan L, Feng Y, Bellusci L, Lai L, Wali B, Ellis M, et al. AS03 adjuvant enhances the magnitude, persistence, and clonal breadth of memory B cell responses to a plant-based COVID-19 vaccine in humans. Sci Immunol. (2024) 9(94):eadi8039. doi: 10.1126/sciimmunol.adi8039
186. Petkov SP, Heuts F, Krotova OA, Kilpelainen A, Engström G, Starodubova ES, et al. Evaluation of immunogen delivery by DNA immunization using non-invasive bioluminescence imaging. Hum Vaccin Immunother. (2013) 9:2228–36. doi: 10.4161/hv.25561
187. Eusébio D, Neves AR, Costa D, Biswas S, Alves G, Cui Z, et al. Methods to improve the immunogenicity of plasmid DNA vaccines. Drug Discov Today. (2021) 26:2575–92. doi: 10.1016/j.drudis.2021.06.008
188. Vasan S, Hurley A, Schlesinger SJ, Hannaman D, Gardiner DF, Dugin DP, et al. In vivo electroporation enhances the immunogenicity of an HIV-1 DNA vaccine candidate in healthy volunteers. PLoS One. (2011) 6:e19252. doi: 10.1371/journal.pone.0019252
189. van Drunen Littel-van den Hurk S, Hannaman D. Electroporation for DNA immunization: clinical application. Expert Rev Vaccines. (2010) 9:503–17. doi: 10.1586/erv.10.42
190. Sardesai NY, Weiner DB. Electroporation delivery of DNA vaccines: prospects for success. Curr Opin Immunol. (2011) 23:421–9. doi: 10.1016/j.coi.2011.03.008
191. McCluskie MJ, Brazolot Millan CL, Gramzinski RA, Robinson HL, Santoro JC, Fuller JT, et al. Route and method of delivery of DNA vaccine influence immune responses in mice and non-human primates. Mol Med. (1999) 5:287–300. doi: 10.1007/BF03402065
192. Saade F, Petrovsky N. Technologies for enhanced efficacy of DNA vaccines. Expert Rev Vaccines. (2012) 11:189–209. doi: 10.1586/erv.11.188
193. Bueter CL, Lee CK, Wang JP, Ostroff GR, Specht CA, Levitz SM. Spectrum and mechanisms of inflammasome activation by chitosan. J Immunol. (2014) 192:5943–51. doi: 10.4049/jimmunol.1301695
194. Xing L, Fan YT, Zhou TJ, Gong JH, Cui LH, Cho KH, et al. Chemical modification of chitosan for efficient vaccine delivery. Molecules. (2018) 23:229. doi: 10.3390/molecules23020229
195. Boyoglu S, Vig K, Pillai S, Rangari V, Dennis VA, Khazi F, et al. Enhanced delivery and expression of a nanoencapsulated DNA vaccine vector for respiratory syncytial virus. Nanomedicine. (2009) 5:463–72. doi: 10.1016/j.nano.2009.02.004
196. Iqbal M, Lin W, Jabbal-Gill I, Davis SS, Steward MW, Illum L. Nasal delivery of chitosan–DNA plasmid expressing epitopes of respiratory syncytial virus (RSV) induces protective CTL responses in BALB/c mice. Vaccine. (2003) 21:1478–85. doi: 10.1016/S0264-410X(02)00662-X
197. Wang G, Pan L, Zhang Y, Wang Y, Zhang Z, Lü J, et al. Intranasal delivery of cationic PLGA nano/microparticles- loaded FMDV DNA vaccine encoding IL-6 elicited protective immunity against FMDV challenge. PLoS One. (2011) 6:e27605. doi: 10.1371/journal.pone.0027605
198. Kumar MNVR, Bakowsky U, Lehr CM. Preparation and characterization of cationic PLGA nanospheres as DNA carriers. Biomaterials. (2004) 25:1771–7. doi: 10.1016/j.biomaterials.2003.08.069
199. Xu L, Liu Y, Chen Z, Li W, Liu Y, Wang L, et al. Surface-engineered gold nanorods: promising DNA vaccine adjuvant for HIV-1 treatment. Nano Lett. (2012) 12(4):2003–12. doi: 10.1021/nl300027p
200. Shim B-S, Park S-M, Quan J-S, Jere D, Chu H, Song MK, et al. Intranasal immunization with plasmid DNA encoding spike protein of SARS-coronavirus/polyethylenimine nanoparticles elicits antigen-specific humoral and cellular immune responses. BMC Immunol. (2010) 11:65. doi: 10.1186/1471-2172-11-65
201. Torrieri-Dramard L, Lambrecht B, Ferreira HL, TVd B, Klatzmann D, Bellier B. Intranasal DNA vaccination induces potent mucosal and systemic immune responses and cross-protective immunity against influenza viruses. Mol Ther. (2011) 19:1924–39. doi: 10.1038/mt.2010.222
202. Grant EV, Thomas M, Fortune J, Klibanov AM, Letvin NL. Enhancement of plasmid DNA immunogenicity with linear polyethylenimine. Eur J Immunol. (2012) 42:2937–48. doi: 10.1002/eji.201242410
203. D’Souza S, Rosseels V, Denis O, Tanghe A, Smet ND, Jurion F, et al. Improved tuberculosis DNA vaccines by formulation in cationic lipids. Infection Immun. (2002) 70:3681–8. doi: 10.1128/IAI.70.7.3681-3688.2002
204. Rosada RS, de la Torre LG, Frantz FG, Trombone AP, Zárate-Bladés CR, Fonseca DM, et al. Protection against tuberculosis by a single intranasal administration of DNA-hsp65 vaccine complexed with cationic liposomes. BMC Immunol. (2008) 9:38. doi: 10.1186/1471-2172-9-38
205. Cheng J-Y, Huang H-N, Tseng W-C, Li T-L, Chan Y-L, Cheng K-C, et al. Transcutaneous immunization by lipoplex-patch based DNA vaccines is effective vaccination against Japanese encephalitis virus infection. J Controlled Release. (2009) 135:242–9. doi: 10.1016/j.jconrel.2009.01.014
206. Chen R, Lu S-H, Tong Q-B, Lou D, Shi D-Y, Jia B-B, et al. Protective effect of DNA-mediated immunization with liposome-encapsulated GRA4 against infection of Toxoplasma gondii. J Zhejiang Univ Sci B. (2009) 10:512–21. doi: 10.1631/jzus.B0820300
207. Dai Z, Cai R, Zeng H, Zhu H, Dou Y, Sun S. Exosome may be the next generation of promising cell-free vaccines. Hum Vaccines Immunotherapeutics. (2024) 20:2345940. doi: 10.1080/21645515.2024.2345940
208. Kraynyak KA, Kutzler MA, Cisper NJ, Laddy DJ, Morrow MP, Waldmann TA, et al. Plasmid-encoded interleukin-15 receptor α Enhances specific immune responses induced by a DNA vaccine in vivo. Hum Gene Ther. (2009) 20:1143–56. doi: 10.1089/hum.2009.025
209. Chong S-Y, Egan MA, Kutzler MA, Megati S, Masood A, Roopchard V, et al. Comparative ability of plasmid IL-12 and IL-15 to enhance cellular and humoral immune responses elicited by a SIVgag plasmid DNA vaccine and alter disease progression following SHIV89.6P challenge in rhesus macaques. Vaccine. (2007) 25:4967–82. doi: 10.1016/j.vaccine.2006.11.070
210. Sin J-I, Kim Jong J, Pachuk C, Satishchandran C, Weiner David B. DNA vaccines encoding interleukin-8 and RANTES enhance antigen-specific th1-type CD4+ T-cell-mediated protective immunity against herpes simplex virus type 2 in vivo. J Virol. (2000) 74:11173–80. doi: 10.1128/JVI.74.23.11173-11180.2000
211. Gary E, Kathuria N, Makurumidze G, Curatola A, Ramamurthi A, Bernui ME, et al. CCR10 expression is required for the adjuvant activity of the mucosal chemokine CCL28 when delivered in the context of an HIV-1 Env DNA vaccine. Vaccine. (2020) 38:2626–35. doi: 10.1016/j.vaccine.2020.01.023
212. Kutzler MA, Wise MC, Hutnick NA, Moldoveanu Z, Hunter M, Reuter M, et al. Chemokine adjuvanted electroporated-DNA vaccine induces substantial protection from simian immunodeficiency virus vaginal challenge. Mucosal Immunol. (2015) 9:13–23. doi: 10.1038/mi.2015.31
213. Xin K-Q, Hamajima K, Sasaki S, Tsuji T, Watabe S, Okada E, et al. IL-15 expression plasmid enhances cell-mediated immunity induced by an HIV-1 DNA vaccine. Vaccine. (1999) 17:858–66. doi: 10.1016/S0264-410X(98)00271-0
214. Barouch DH, Santra S, Schmitz JE, Kuroda MJ, Fu T-M, Wagner W, et al. Control of viremia and prevention of clinical AIDS in rhesus monkeys by cytokine-augmented DNA vaccination. Science. (2000) 290:486–92. doi: 10.1126/science.290.5491.486
215. Barouch DH, Letvin NL, Seder RA. The role of cytokine DNAs as vaccine adjuvants for optimizing cellular immune responses. Immunological Rev. (2004) 202:266–74. doi: 10.1111/j.0105-2896.2004.00200.x
216. Jeon B-Y, Eoh H, Ha S-J, Bang H, Kim S-C, Sung Y-C, et al. Co-immunization of plasmid DNA encoding IL-12 and IL-18 with bacillus calmette-guérin vaccine against progressive tuberculosis. Yonsei Med J. (2011) 52:1008–15. doi: 10.3349/ymj.2011.52.6.1008
217. Barouch DH, McKay PF, Sumida SM, Santra S, Jackson SS, Gorgone DA, et al. Plasmid chemokines and colony-stimulating factors enhance the immunogenicity of DNA priming-viral vector boosting human immunodeficiency virus type 1 vaccines. J Virol. (2003) 77:8729–35. doi: 10.1128/JVI.77.16.8729-8735.2003
218. Sumida SM, McKay PF, Truitt DM, Kishko MG, Arthur JC, Seaman MS, et al. Recruitment and expansion of dendritic cells in vivo potentiate the immunogenicity of plasmid DNA vaccines. J Clin Invest. (2004) 114:1334–42. doi: 10.1172/JCI200422608
219. Chang DZ, Lomazow W, Somberg CJ, Stan R, Perales M-A. Granulocyte-macrophage colony stimulating factor: an adjuvant for cancer vaccines. Hematology. (2004) 9:207–15. doi: 10.1080/10245330410001701549
220. Song M-Y, Park S-H, Nam HJ, Choi D-H, Sung Y-C. Enhancement of vaccine-induced primary and memory CD8+ T-cell responses by soluble PD-1. J Immunotherapy. (2011) 34:297–306. doi: 10.1097/CJI.0b013e318210ed0e
221. Zhou J, Cheung AKL, Tan Z, Wang H, Yu W, Du Y, et al. PD1-based DNA vaccine amplifies HIV-1 GAG-specific CD8+ T cells in mice. J Clin Investigation. (2013) 123:2629–42. doi: 10.1172/JCI64704
222. Gary E, O’Connor M, Chakhtoura M, Tardif V, Kumova OK, Malherbe DC, et al. Adenosine deaminase-1 enhances germinal center formation and functional antibody responses to HIV-1 Envelope DNA and protein vaccines. Vaccine. (2020) 38:3821–31. doi: 10.1016/j.vaccine.2020.03.047
223. Gary EN, Tursi NJ, Warner BM, Cuismano G, Connors J, Parzych EM, et al. Adenosine deaminase augments SARS-CoV-2 specific cellular and humoral responses in aged mouse models of immunization and challenge. Front Immunol. (2023) 14. doi: 10.3389/fimmu.2023.1138609
224. Kutzler MA, Cusimano G, Joyner D, Konopka E, Muir R, Barnette P, et al. The molecular immune modulator adenosine deaminase-1 enhances HIV specific humoral and cellular responses to a native-like HIV envelope trimer DNA vaccine. Res Square. (2024). doi: 10.21203/rs.3.rs-4139764/v1
225. Cusimano GM, Gary EN, Bell MR, Warner BM, Connors J, Tursi NJ, et al. Improved durability to SARS-CoV-2 vaccine immunity following co-immunization with molecular adjuvant adenosine deaminase-1. J Immunol (Baltimore Md: 1950). (2022) 209:118–27. doi: 10.4049/jimmunol.2200056
226. Hogenesch H. Mechanism of immunopotentiation and safety of aluminum adjuvants. Front Immunol. (2012) 3:406. doi: 10.3389/fimmu.2012.00406
227. Bode C, Zhao G, Steinhagen F, Kinjo T, Klinman DM. CpG DNA as a vaccine adjuvant. Expert Rev Vaccines. (2011) 10:499–511. doi: 10.1586/erv.10.174
228. Sasaki S, Tsuji T, Hamajima K, Fukushima J, Ishii N, Kaneko T, et al. Monophosphoryl lipid A enhances both humoral and cell-mediated immune responses to DNA vaccination against human immunodeficiency virus type 1. Infection Immun. (1997) 65:3520–8. doi: 10.1128/iai.65.9.3520-3528.1997
229. Mata-Haro VN, Cekic C, Martin M, Chilton PM, Casella CR, Mitchell TC. The vaccine adjuvant monophosphoryl lipid A as a TRIF-biased agonist of TLR4. Science. (2007) 316:1628–32. doi: 10.1126/science.1138963
230. Arrington J, Braun RP, Dong L, Fuller DH, Macklin MD, Umlauf SW, et al. Plasmid vectors encoding cholera toxin or the heat-labile enterotoxin from escherichia coli are strong adjuvants for DNA vaccines. J Virol. (2002) 76:4536–46. doi: 10.1128/jvi.76.9.4536-4546.2002
231. Bagley K, Xu R, Ota-Setlik A, Egan M, Schwartz J, Fouts T. The catalytic A1 domains of cholera toxin and heat-labile enterotoxin are potent DNA adjuvants that evoke mixed Th1/Th17 cellular immune responses. Hum Vaccines Immunotherapeutics. (2015) 11:2228–40. doi: 10.1080/21645515.2015.1026498
232. Melkebeek V, Sonck E, Verdonck F, Goddeeris BM, Cox E. Optimized faeG expression and a thermolabile enterotoxin DNA adjuvant enhance priming of an intestinal immune response by an faeG DNA vaccine in pigs. Clin Vaccine Immunol. (2007) 14:28–35. doi: 10.1128/CVI.00268-06
233. Wan Y, Ren X, Ren Y, Wang J, Hu Z, Xie X, et al. As a genetic adjuvant, CTA improves the immunogenicity of DNA vaccines in an ADP-ribosyltransferase activity- and IL-6-dependent manner. Vaccine. (2014) 32:2173–80. doi: 10.1016/j.vaccine.2014.02.056
234. Uchida S, Yoshinaga N, Yanagihara K, Yuba E, Kataoka K, Itaka K. Designing immunostimulatory double stranded messenger RNA with maintained translational activity through hybridization with poly A sequences for effective vaccination. Biomaterials. (2018) 150:162–70. doi: 10.1016/j.biomaterials.2017.09.033
235. Tockary TA, Abbasi S, Matsui-Masai M, Hayashi A, Yoshinaga N, Boonstra E, et al. Comb-structured mRNA vaccine tethered with short double-stranded RNA adjuvants maximizes cellular immunity for cancer treatment. Proc Natl Acad Sci U S A. (2023) 120:e2214320120. doi: 10.1073/pnas.2214320120
236. Karikó K, Muramatsu H, Welsh FA, Ludwig J, Kato H, Akira S, et al. Incorporation of pseudouridine into mRNA yields superior nonimmunogenic vector with increased translational capacity and biological stability. Mol Ther. (2008) 16:1833–40. doi: 10.1038/mt.2008.200
237. Karikó K, Buckstein M, Ni H, Weissman D. Suppression of RNA recognition by toll-like receptors: the impact of nucleoside modification and the evolutionary origin of RNA. Immunity. (2005) 23:165–75. doi: 10.1016/j.immuni.2005.06.008
238. Hemmi H, Takeuchi O, Kawai T, Kaisho T, Sato S, Sanjo H, et al. A Toll-like receptor recognizes bacterial DNA. Nature. (2000) 408:740–5. doi: 10.1038/35047123
239. Klinman DM, Yamshchikov G, Ishigatsubo Y. Contribution of CpG motifs to the immunogenicity of DNA vaccines. J Immunol. (1997) 158:3635–9. doi: 10.4049/jimmunol.158.8.3635
240. Hald Albertsen C, Kulkarni JA, Witzigmann D, Lind M, Petersson K, Simonsen JB. The role of lipid components in lipid nanoparticles for vaccines and gene therapy. Adv Drug Delivery Rev. (2022) 188:114416. doi: 10.1016/j.addr.2022.114416
241. Owens J. Knocked down for the count. Nat Rev Drug Discovery. (2005) 4:717–. doi: 10.1038/nrd1833
242. Connors J, Joyner D, Mege NJ, Cusimano GM, Bell MR, Marcy J, et al. Lipid nanoparticles (LNP) induce activation and maturation of antigen presenting cells in young and aged individuals. Commun Biol. (2023) 6:188. doi: 10.1038/s42003-023-04555-1
243. Alamri SS, Alluhaybi KA, Alhabbab RY, Basabrain M, Algaissi A, Almahboub S, et al. Synthetic SARS-coV-2 spike-based DNA vaccine elicits robust and long-lasting th1 humoral and cellular immunity in mice. Front Microbiol. (2021) 12:727455. doi: 10.3389/fmicb.2021.727455
244. Teixeira L, Medioni J, Garibal J, Adotevi O, Doucet L, Durey M-AD, et al. A first-in-human phase I study of INVAC-1, an optimized human telomerase DNA vaccine in patients with advanced solid tumors. Clin Cancer Res. (2020) 26:588–97. doi: 10.1158/1078-0432.CCR-19-1614
245. Seferian PG, Martinez ML. Immune stimulating activity of two new chitosan containing adjuvant formulations. Vaccine. (2000) 19:661–8. doi: 10.1016/S0264-410X(00)00248-6
246. Shen H, Ackerman AL, Cody V, Giodini A, Hinson ER, Cresswell P, et al. Enhanced and prolonged cross-presentation following endosomal escape of exogenous antigens encapsulated in biodegradable nanoparticles. Immunology. (2006) 117:78–88. doi: 10.1111/j.1365-2567.2005.02268.x
247. Tan D, Li G, Fu W, Lei C. Exosomes: the next frontier in vaccine development and delivery. Front Immunol. (2024) 15:1435426. doi: 10.3389/fimmu.2024.1435426
248. Elia U, Rotem S, Bar-Haim E, Ramishetti S, Naidu GS, Gur D, et al. Lipid Nanoparticle RBD-hFc mRNA Vaccine Protects hACE2 Transgenic Mice against a Lethal SARS-CoV-2 Infection. Nano Letters. (2021) 21:4774–9. doi: 10.1021/acs.nanolett.1c01284
249. Charland N, Gobeil P, Pillet S, Boulay I, Séguin A, Makarkov A, et al. Safety and immunogenicity of an AS03-adjuvanted plant-based SARS-CoV-2 vaccine in Adults with and without Comorbidities. NPJ Vaccines. (2022) 7:142. doi: 10.1038/s41541-022-00561-2
250. Wan YY, Flavell RA. How diverse—CD4 effector T cells and their functions. J Mol Cell Biol. (2009) 1:20–36. doi: 10.1093/jmcb/mjp001
251. Kim JJ, Nottingham LK, Sin JI, Tsai A, Morrison L, Oh J, et al. CD8 positive T cells influence antigen-specific immune responses through the expression of chemokines. J Clin Investigation. (1998) 102:1112–24. doi: 10.1172/JCI3986
252. Song R, Liu S, Leong KW. Effects of MIP-1&x3b1;, MIP-3&x3b1;, and MIP-3&x3b2; on the induction of HIV gag-specific immune response with DNA vaccines. Mol Ther. (2007) 15:1007–15. doi: 10.1038/mt.sj.6300129
253. Furey C, Scher G, Ye N, Kercher L, DeBeauchamp J, Crumpton JC, et al. Development of a nucleoside-modified mRNA vaccine against clade 2.3.4.4b H5 highly pathogenic avian influenza virus. Nat Commun. (2024) 15:4350. doi: 10.1038/s41467-024-48555-z
254. Alameh M-G, Semon A, Bayard NU, Pan Y-G, Dwivedi G, Knox J, et al. A multivalent mRNA-LNP vaccine protects against Clostridioides difficile infection. Science. (2024) 386:69–75. doi: 10.1126/science.adn4955
255. Pardi N, Krammer F. mRNA vaccines for infectious diseases — advances, challenges and opportunities. Nat Rev Drug Discovery. (2024) 23:838–61. doi: 10.1038/s41573-024-01042-y
256. Baliban SM, Michael A, Shammassian B, Mudakha S, Khan AS, Cocklin S, et al. An optimized, synthetic DNA vaccine encoding the toxin A and toxin B receptor binding domains of Clostridium difficile induces protective antibody responses in vivo. Infect Immun. (2014) 82:4080–91. doi: 10.1128/IAI.01950-14
257. Gardiner DF, Rosenberg T, Zaharatos J, Franco D, Ho DD. A DNA vaccine targeting the receptor-binding domain of Clostridium difficile toxin A. Vaccine. (2009) 27:3598–604. doi: 10.1016/j.vaccine.2009.03.058
258. Lesinski GB, Smithson SL, Srivastava N, Chen D, Widera G, Westerink MAJ. A DNA vaccine encoding a peptide mimic of Streptococcus pneumoniae serotype 4 capsular polysaccharide induces specific anti-carbohydrate antibodies in Balb/c mice. Vaccine. (2001) 19:1717–26. doi: 10.1016/S0264-410X(00)00397-2
259. Eroglu E, Singh A, Bawage S, Tiwari PM, Vig K, Pillai SR, et al. Immunogenicity of RSV F DNA vaccine in BALB/c mice. Adv Virology. (2016) 2016:1–10. doi: 10.1155/2016/7971847
260. Geall AJ, Verma A, Otten GR, Shaw CA, Hekele A, Banerjee K, et al. Nonviral delivery of self-amplifying RNA vaccines. Proc Natl Acad Sci United States America. (2012) 109:14604–9. doi: 10.1073/pnas.1209367109
261. Bloom K, van den Berg F, Arbuthnot P. Self-amplifying RNA vaccines for infectious diseases. Gene Ther. (2020) 28:117–29. doi: 10.1038/s41434-020-00204-y
Keywords: nucleic acid vaccines, mRNA vaccine, DNA vaccine, aging, immunosenescence
Citation: Konopka EN, Edgerton AO and Kutzler MA (2025) Nucleic acid vaccines: innovations, efficacy, and applications in at-risk populations. Front. Immunol. 16:1584876. doi: 10.3389/fimmu.2025.1584876
Received: 27 February 2025; Accepted: 09 April 2025;
Published: 14 May 2025.
Edited by:
Ebony Gary, Wistar Institute, United StatesReviewed by:
Arjun Ramamurthi, Children’s Hospital of Philadelphia Research Institute, United StatesDan Wang, Wistar Institute, United States
Copyright © 2025 Konopka, Edgerton and Kutzler. This is an open-access article distributed under the terms of the Creative Commons Attribution License (CC BY). The use, distribution or reproduction in other forums is permitted, provided the original author(s) and the copyright owner(s) are credited and that the original publication in this journal is cited, in accordance with accepted academic practice. No use, distribution or reproduction is permitted which does not comply with these terms.
*Correspondence: Michele A. Kutzler, bWFrOThAZHJleGVsLmVkdQ==
†ORCID: Emily N. Konopka, orcid.org/0009-0007-6039-8857
Arden O. Edgerton, orcid.org/0009-0004-9913-3432
Michele A. Kutzler, orcid.org/0000-0001-5692-5617