- 1Changchun University of Chinese Medicine, Changchun, China
- 2School of Pharmacy, Changchun University of Chinese Medicine, Changchun, China
- 3The First People’s Hospital of Guangzhou, Department of Hepatobiliary and Pancreatic Surgery, Guangzhou, China
The mucosal barrier serves as a crucial defense against external pathogens and allergens, being widely distributed across the respiratory, gastrointestinal, urogenital tracts, and oral cavity. Its disruption can lead to various diseases, including inflammatory bowel disease, asthma, urinary tract infections, and oral inflammation. Current mainstream treatments for mucosa-associated diseases primarily involve glucocorticoids and immunosuppressants, but their long-term use may cause adverse effects. Therefore, the development of safer and more effective therapeutic strategies has become a focus of research. Natural products, with their multi-target and multi-system regulatory advantages, offer a promising avenue for the treatment of mucosal diseases. This review summarizes the potential applications of natural products in diseases of mucosal barrier dysfunction through mechanisms such as immune modulation, inflammation inhibition, tight junction protein restoration, and gut microbiota regulation, with the aim of providing insights for the exploration of novel therapeutic strategies.
1 Introduction
The mucosa serves as the body’s first line of defense against external pathogens and allergens and is widely distributed throughout the respiratory, gastrointestinal, genitourinary, and oral cavities (1). Far beyond a passive physical barrier, mucosa harbors a sophisticated immune network, including mucosa-associated lymphoid tissue (MALT) and diverse immune cells, which collectively regulate both innate and adaptive immune responses. Under homeostatic conditions, the structural integrity and immunological function of the mucosa are critical for maintaining host health and internal environmental stability (2).
However, various internal and external factors—such as infections, inflammatory responses, oxidative stress, medication use, and poor dietary habits—can impair mucosal barrier function, potentially leading to a range of chronic diseases. In the digestive system, intestinal mucosal damage can increase permeability, disrupt the microbiota, and trigger immune activation, all of which contribute to the pathogenesis of inflammatory bowel disease and food allergies. In the respiratory tract, mucosal damage increases the risk of asthma and chronic obstructive pulmonary disease and impairs respiratory function (3). In the urinary tract, mucosal damage predisposes individuals to urinary tract infections and impairs excretory function (4). Similarly, in the oral cavity, damage to the mucosal microenvironment is closely associated with conditions such as candidiasis and recurrent oral ulcers (5). Currently, commonly used clinical treatments—such as aminosalicylates, corticosteroids, and immunosuppressants—may alleviate symptoms in the short term, but long-term use often leads to drug resistance, hormone dependence, or systemic side effects, thereby limiting their therapeutic potential (6). Therefore, the development of novel, safe and effective therapeutics for mucosal diseases is urgently needed.
Natural products have unique advantages, including multi-system, multi-target, and multi-mechanism effects, providing new research directions for the treatment of mucosa-associated diseases (7). Numerous studies have demonstrated that natural products such as polysaccharides, alkaloids, and polyphenols can promote mucosal barrier repair through various mechanisms, including regulation of immune cell function, reduction of inflammatory cytokine levels, and enhancement of tight junction protein expression (8). In addition, there is increasing evidence of interactions between natural products and the host microbiota (9). Many natural products can modulate the composition of the gut microbiota, increase the abundance of short chain fatty acid (SCFA)-producing bacteria, and increase butyrate levels, thereby providing essential nutrients to intestinal epithelial cells and facilitating mucosal barrier repair. In addition, some metabolites of natural products exert protective effects on the mucosal barrier through mechanisms similar to those of their parent compounds.
These findings highlight the potential of natural products in the treatment of mucosal diseases. Although significant progress has been made in exploring the use of natural products for mucosa-related disease interventions, their mechanisms of action remain incompletely understood. Target specificity in different disease models, structure-function relationships, and interactions with host microecology require further investigation. This review aims to systematically summarize the latest advances in the application of natural products to various mucosa-associated diseases, with a focus on their core mechanisms in modulating mucosal immunity, repairing mucosal structures, and maintaining microecological balance, and discusses their clinical significance.
2 Immune system and mucosal barriers
2.1 Intestinal mucosal barrier
2.1.1 Composition and barrier function of intestinal mucosa
The intestinal mucosa serves as a critical immune barrier, not only facilitating nutrient absorption and providing an interactive surface for commensal microbes, but also preventing the invasion of harmful substances (10). The intestinal microbiota is comprised of several components, including an outer mucus layer containing commensal microbes, antimicrobial proteins (AMPs), and secretory immunoglobulin A (sIgA); a central monolayer of specialized epithelial cells; and an inner lamina propria housing innate and adaptive immune cells such as T cells, B cells, macrophages, and dendritic cells (11, 12) (Figure 1).
The mucus layer serves as the first physical barrier against external molecules entering the intestinal lumen (11), Its primary components are highly glycosylated mucins (13), which play a crucial role in protecting the immune system and intestinal epithelial cells from antigen exposure. In both the colon and small intestine, mucin 2 (MUC2) secreted by goblet cells is the most abundant mucin. MUC2 reduces antigen exposure to the immune system and intestinal epithelial cells, which is essential for disease prevention (13). Studies have shown that MUC2-deficient mice spontaneously develop colitis, and their mucus layer is more susceptible to bacterial penetration (14). Moreover, mucus defects are associated with the occurrence of neonatal Escherichia coli meningitis (15)and necrotizing enterocolitis (16), indicating that damage to the mucus layer can promote inflammatory responses, thereby inducing acute and chronic colitis as well as colorectal cancer (17). Beneath the mucus layer, intestinal epithelial cells (IECs) play a critical role in maintaining intestinal barrier integrity. Multipotent stem cells within the intestinal crypts differentiate into five cell types: absorptive enterocytes, goblet cells, enteroendocrine cells, Paneth cells, and microfold (M) cells (18). These cells form a continuous polarized monolayer that separates the lumen from the lamina propria. Molecular transport between IECs is regulated by intercellular junction complexes, primarily including tight junctions (TJs), adherens junctions (AJs), and desmosomes (19). TJs and AJs are connected to the surrounding actin-myosin network, thereby regulating intercellular adhesion through the cytoskeleton (11) (19),. Additionally, IECs express a variety of innate immune signaling molecules on their surface and within their cytoplasm (20), including intracellular peptidoglycan receptors NOD1 and NOD2, as well as surface and intracellular Toll-like receptors (TLRs), which recognize bacterial, fungal, and viral structures (21, 22) and activate immune responses via the NF-κB pathway (23). The lamina propria serves as the final line of defense in the intestinal mucosa, where immune cells interact with epithelial cells to collectively maintain intestinal barrier function. For instance, goblet cells deliver antigens to CD11c+/CD103+ dendritic cell (DC) subsets in the lamina propria (24), followed by cross-presentation of antigens by DCs, inducing the differentiation of Foxp3+ regulatory T cells (Tregs) (25, 26).
2.1.2 Immunomodulation of the intestinal mucosa
Interferon-gamma (IFN-γ) and tumor necrosis factor-alpha (TNF-α), derived from T cells, are key mediators of intestinal inflammatory diseases, including inflammatory bowel disease (IBD). TJs and increase intestinal permeability by regulating the expression of claudin and occludin (27–30). Zolotarevsky et al. demonstrated that IFN-γ and TNF-α promote the redistribution of TJ proteins (ZO-1, JAM-A, occludin, claudin-1, and claudin-4) in intestinal epithelial cells (Caco-2 and T84), leading to impaired barrier function (31). This mechanism is likely mediated by myosin light chain kinase (MLCK), which facilitates TJ disruption through myosin light chain (MLC) phosphorylation. Inhibition of MLC phosphorylation can restore barrier function.
Th2 cell cytokines also play a role in regulating intestinal barrier function. Studies have shown that stimulation of colonic epithelial cell lines T84 and HT-29/B6 with IL-4 or IL-13 increases intestinal permeability (32–34). The underlying mechanism involves epithelial cell apoptosis and upregulation of claudin-2 expression. The PI3K pathway plays a critical role in this process (33, 35), as blocking IL-4/IL-13-mediated PI3K activation can prevent barrier dysfunction (33). IL-10, as an anti-inflammatory cytokine, also modulates intestinal barrier function (36, 37). Treatment with IL-10 can prevent IFN-γ-induced increases in epithelial permeability (38). Immune cells likewise contribute to the regulation of the intestinal mucosal barrier. For instance, CD3 stimulation leads to CD4+ T cell activation, resulting in increased permeability and enhanced secretion of IFN-γ and TNF-α (39, 40). Mice lacking intraepithelial lymphocytes (iIELγδ+) exhibit abnormal localization of claudin-3, occludin, and ZO-1, impaired TJ formation, and consequently, barrier dysfunction (41). Additionally, mast cells are widely distributed throughout the gastrointestinal tract (42). Upon activation, they release a variety of potent inflammatory mediators, including histamine, serotonin (5-HT), neutral proteases, prostaglandins, leukotrienes, platelet-activating factor, and cytokines such as TNF-α, IL-3, and IL-4 (43–45). Research indicates that mast cells participate in intestinal barrier regulation through models of food allergy or parasitic infection (46).
2.1.3 Intestinal mucosal injury and related diseases
Intestinal mucosal injury can lead to diseases such as IBD, food allergies, celiac disease, and diabetes, with immune regulation being a key factor influencing its function (47). IL-10-deficient mice exhibit increased permeability and spontaneously develop chronic colitis (36, 48), indicating its protective role in barrier function. IL-10 may regulate intestinal permeability through the claudin/claudin receptor pathway and TNF-α-related mechanisms, and inhibition of this pathway has been shown to improve permeability and reduce the risk of colitis in IL-10-deficient mice (37). The zonulin/zonulin receptor pathway is believed to regulate TJ formation via PKC-dependent actin cytoskeleton remodeling (49).
Eosinophils and their granular proteins, such as major basic protein (MBP), eosinophil peroxidase, and eosinophil cationic protein (ECP), are increased in IBD and functional bowel disorders (50–53). In vitro co-culture experiments have shown that eosinophils or their major basic protein can reduce transepithelial electrical resistance (TER) in T84 cells, increase permeability, and downregulate occludin expression (54).
In summary, the function of the intestinal mucosal barrier is regulated by various immune cells and cytokines. Its damage can lead to a variety of intestinal diseases. A deeper understanding of its immune regulatory mechanisms will contribute to the development of related therapeutic strategies.
2.2 Respiratory mucosal barrier
2.2.1 Composition and function of respiratory barrier
The respiratory tract is divided into the upper respiratory tract (URT) and lower respiratory tract (LRT). The URT includes the nasal cavity, pharynx, and larynx, which together form the pathway for air flow and contain associated lymphoid tissues such as the nasal-associated lymphoid tissue and cervical lymph nodes. The LRT includes the trachea, as well as the bronchi and bronchioles within the lungs (55). As a crucial barrier against external pathogens and particulate matter, the respiratory tract relies on the synergistic action of the mucous layer and epithelial cells to maintain functional homeostasis (Figure 2).
The respiratory mucosal barrier consists of a mucus layer and epithelial cells. The mucus layer is composed of an upper gel-like mucus layer and a lower ciliary surrounding layer (55), which forms a bottlebrush structure. Its main component is mucin, which is crucial for protecting the respiratory tract from pathogen infections (56). The respiratory epithelium is primarily composed of ciliated epithelial cells, goblet cells, basal cells, and club cells (57), with airway epithelial cells being central to the pathogenesis of major lung diseases, including COPD, asthma, and bronchial cancer (58). In these diseases, localized inflammation and immune signaling further impact the function of airway epithelium (59, 60).
2.2.2 Regulation and function of epithelial cell differentiation
Ciliated epithelial cells are the predominant cell type in the airways, and their differentiation is strictly regulated by the conserved Notch signaling pathway (57). Inhibition of Notch signaling promotes the differentiation of basal cells into ciliated epithelial cells, while high levels of Notch signaling drive their differentiation into goblet cells (61, 62). Goblet cell hyperplasia and excessive mucus secretion are common pathological features of asthma, COPD, and cystic fibrosis. Research by Agrawal et al. suggests that blocking excessive mucus secretion in an asthma mouse model can alleviate airway obstruction (63). Although airway epithelial cell proliferation was not observed in the asthma mouse model, the data support the hypothesis of transdifferentiation from ciliated cells to goblet cells (64).
Goblet cells primarily secrete mucins, such as Muc5AC and Muc5B, to capture foreign molecules (65, 66).Under normal conditions, the production and clearance of these mucins maintain a dynamic balance. However, excessive differentiation of goblet cells driven by IL-4 and IL-13 disrupts this balance, leading to the development of asthma, allergic rhinitis (AR), and chronic rhinosinusitis (CRS) (67).
Basal cells are stem cell-like progenitors in the upper and lower airways. Under the regulation of the Notch signaling pathway, they can differentiate into ciliated epithelial cells, goblet cells, and other epithelial cells (68). They are tightly attached to the basement membrane and provide structural support through hemidesmosomes (69). Under homeostatic conditions, basal cells remain quiescent, but after barrier injury, they are rapidly activated and migrate to the damaged area to form a temporary barrier (70). Basal cell proliferation and differentiation are observed in chronic airway diseases. For example, studies on ex vivo basal cell cultures from nasal polyps in CRSwNP patients and bronchial biopsies from asthma patients have shown reduced proliferative capacity of basal cells (65). A recent study by Ordovas-Montanes et al. revealed that in CRS, the IL-4/IL-13 signaling signature persists in basal cells and their progeny, keeping airway epithelial cells in an undifferentiated state and reducing cellular and functional diversity. Blocking the IL-4 receptor α subunit can restore basal cell function (71).Additionally, basal cells can mediate innate immune responses. For instance, in cigarette-induced epithelial injury, they secrete antimicrobial protein RNase7 while upregulating innate immune mediators such as β-defensin-2, lipid-binding protein 2, IL-6, IL-8, and CCL20 (72, 73).
2.2.3 Impaired barrier function and disease
Although the mucus layer plays an important role in the respiratory barrier, the true physical barrier is maintained by the adhesive complexes between epithelial cells, including TJs, adherens junctions, and desmosomes (65). TJs are composed of transmembrane proteins such as occludin, claudins, and the immunoglobulin-like (IgG) family members of JAMs, which are connected to cytoplasmic scaffold proteins like ZO-1, ZO-2, and ZO-3 (74). Adherens junctions are located beneath TJs and are formed by the E-cadherin-catenin complex (75). Desmosomes ensure the attachment of basal and other epithelial cells to the basement membrane (76). The epithelial cell barrier is closely associated with the development and progression of various respiratory diseases. Disruption of the epithelial barrier in allergic asthma is linked to TJ defects and reductions in adherens junctions and desmosomes (77). Compared to healthy controls, AR patients show reduced expression of occludin and ZO-1, which correlates with disease severity (78). Increased epithelial permeability and irregular, decreased expression of TJ molecules such as occludin and ZO-1 were found in the in vivo specimens of CRSwNP patients (79). Various cytokines have been shown to interfere with TJs, such as the typical Th2 cytokines IL-4 and IL-13, which are released upon allergen exposure and lead to epithelial barrier dysfunction (80). Steelant et al.’s study indicated that IL-4 and IL-13 disrupt the barrier integrity of nasal epithelial cells in both AR patients and healthy controls, resulting in a vicious cycle of increased epithelial permeability (81). In contrast, strengthening the epithelial cell barrier effectively reduces inflammation in various in vitro and in vivo models of Th2-mediated respiratory inflammation (82). Additionally, mediators released by mast cells can also increase epithelial permeability, making it easier for allergens to penetrate the host (83).
2.3 Urinary mucosal barrier
The urinary system consists of the bladder, ureters, and kidneys, primarily responsible for filtering and excreting waste while maintaining systemic homeostasis (84). The inner surface of the urinary tract is lined with a mucosal barrier composed of the urothelium, basement membrane, and lamina propria (LP) (85). The urothelium is a tightly packed transitional epithelium, with its surface covered by a glycocalyx composed of mucopolysaccharides, providing both physical and chemical protection to epithelial cells (86). Compared to the intestinal mucosa, the glycocalyx layer of the urothelium is thinner (87–90). Its main components include membrane-bound glycoproteins, glycolipids, and soluble factors such as galectins and proteoglycans (91). Glycosaminoglycans (GAGs) are linked to core proteins to form proteoglycans, with chondroitin sulfate and hyaluronic acid being the major components of the GAG layer, playing a crucial role in barrier formation and antimicrobial defense (91).
2.3.1 Structure and function of the urothelium
The urothelium consists of three layers: the basal cell layer attached to the basement membrane, the intermediate layer, and the superficial or apical layer composed of “umbrella cells” (92). Umbrella cells are hexagonal and interconnect to form a dense barrier, strictly regulating the permeability of solutes and water through TJs, thereby effectively preventing harmful substances in urine from entering the tissue (92). Additionally, adherens junctions (AJs) and desmosomes between urothelial cells play a crucial role in maintaining epithelial integrity (93). Beneath the urothelium lies the LP, which is composed of an extracellular matrix and contains various cell types, including fibroblasts, interstitial cells, as well as afferent and efferent nerve endings (94). This structure not only provides mechanical support but also participates in signal transduction and immune regulation.
2.3.2 Urinary tract microbiota and the infection mechanism
Urothelial injury and exposure to harmful substances may be associated with the pathology of spinal cord injury and are often accompanied by irritative lower urinary tract symptoms (95). Due to its anatomical proximity to the gastrointestinal tract, the bladder mucosa is frequently exposed to microorganisms. Additionally, in females, the urethral opening is close to the vaginal mucosa, resulting in a unique microbiota composition (96–98).
Urinary tract infection (UTI) is the most common infection worldwide, affecting either the upper urinary tract (pyelonephritis) or the lower urinary tract (cystitis). Uropathogenic Escherichia coli (UPEC) is the primary causative agent of UTIs (99). UPEC colonizes the lower gastrointestinal tract and can migrate across the perineum to the urethra, entering the urinary tract. It initiates UTI by covalently binding to the UPK1A protein expressed on the apical surface of umbrella cells via the adhesin FimH, located at the tip of type 1 fimbriae (100). Moreover, FimH can interact with urothelial cells to induce the exfoliation of umbrella cells, thereby promoting urothelial cell proliferation (101).
2.3.3 Injury and repair of the urothelial barrier
Under homeostatic conditions, the mitotic activity of urothelial cells is largely quiescent, with a very slow cell cycle (102, 103). However, in response to acute injury caused by chemical exposure, surgical trauma, or urinary tract infection (UTI), urothelial cells rapidly proliferate to facilitate repair and regeneration (104). The urothelium expresses multiple Toll-like receptors (TLRs), which recognize pathogen-associated molecular patterns and damage-associated molecular patterns, thereby activating inflammatory responses and promoting the clearance of infected cells (105). The urothelial barrier plays a critical role in maintaining urinary tract homeostasis, preventing pathogen invasion, and facilitating tissue repair. Its barrier function primarily relies on tight junctions, the glycocalyx layer, and the structural support of the underlying lamina propria. Following UTI or injury, urothelial cells can swiftly initiate repair mechanisms, while TLR signaling pathways play a central role in inflammatory responses and pathogen clearance. Elucidating the regulatory mechanisms of the urothelial barrier will contribute to the development of effective therapeutic interventions for UTIs and related urinary tract disorders.
2.4 Oral mucosal barrier
The oral mucosa represents the initial segment of the gastrointestinal (GI) tract and shares anatomical and histological similarities with the GI system (106, 107). In addition to mucus secretion, the oral cavity produces saliva through the salivary glands (108, 109). Saliva is initially derived from serous exudates and is further enriched with a diverse array of molecules originating from mucosal cells, immune cells, and resident microbiota (109). The dynamic secretion and swallowing of saliva facilitate the mechanical clearance of pathogens (109). Moreover, saliva contains immunoglobulins (such as secretory IgA), antimicrobial peptides (such as defensins), and enzymes secreted by the salivary glands, collectively mediating innate and adaptive humoral immunity (109).
2.4.1 Structure and immune function of the oral mucosa
Similar to other mucosal tissues, the oral mucosa consists of three structural layers: the epithelial layer, the lamina propria (LP), and specialized lymphoid tissue (106). However, unlike the single-layer columnar epithelium of the gastrointestinal tract, the oral mucosa is composed of stratified squamous epithelium, forming a thicker and denser mechanical barrier (106). The LP is a loose connective tissue rich in blood vessels and lymphatic vessels, serving as a primary site for the induction and effector functions of immune cells (110). Under homeostatic conditions, dendritic cells (DCs) are present throughout the lamina propria, freely migrating between self and foreign antigens (111) and playing a critical role in tolerogenic immune responses. Oral DCs typically express low levels of maturation markers (CD80, CD83, CD86) and exhibit high tolerance to stimuli from the oral microenvironment (112, 113). Upon encountering pathogens or injury, DCs become activated and migrate to lymphoid tissues, initiating T-cell immune responses (110). In mouse models, different subsets of CD11c+ DCs, along with Langerhans cells (LCs), are distributed within the epithelium of the oral, sublingual, and gingival mucosa. These antigen-presenting cells (APCs) capture antigens and deliver immune signals to T cells (114, 115).
2.4.2 Oral mucosa-related diseases and immune regulation
Clinical studies have shown that the oral mucosa can be affected by various pathological factors, including viral infections, Candida infections, and OLP (110). Among these, immune deficiency and imbalance in the oral immune system are major predisposing factors for these diseases. Cases of mucosal immune dysfunction have been observed in individuals infected with human immunodeficiency virus (HIV). HIV infection leads to a reduction in CD4+ T cell levels, resulting in immune deficiency and making patients more susceptible to infections by commensal microorganisms in the oral and pharyngeal regions, such as Candida albicans (116).
Another case involves hyper-IgE syndrome, which is caused by mutations in STAT3 (117–119). Patients with hyper-IgE syndrome are highly prone to oral candidiasis due to the absence of TH17 cells, a finding consistent with animal studies: IL-17 receptor-deficient mice (IL-17RA-/-) and IL-23p19-deficient mice exhibit significantly increased susceptibility to C. albicans infection (120). Although TH17 cells are critical for combating oral fungal infections, their excessive activation may trigger chronic inflammation and even lead to autoimmune diseases (118, 121).
2.4.3 Inflammation and autoimmune diseases of the oral mucosa
Under normal conditions, the immune response of the oral mucosa to food antigens and commensal bacteria does not induce inflammation but rather promotes immune tolerance. However, aberrant activation of the immune system may lead to inflammation and autoimmune diseases such as periodontitis, Sjögren’s syndrome, and OLP. Periodontitis is triggered by bacterial plaque accumulation, associated tissue damage, and bone loss due to the host immune response and inappropriate inflammation. T helper (TH) cells play a crucial role in the recruitment of neutrophils and osteoclasts, contributing to alveolar bone and gingival barrier destruction (122, 123).OLP is a chronic inflammatory disease characterized by massive lymphocyte infiltration in the LP and chronic destruction of the epithelial basal layer (124–126). Scully et al. demonstrated that TH1 and TH2 cells contribute to OLP-associated inflammation and mucosal lesion formation, with increased levels of pro-inflammatory cytokines, including IL-6, IL-17, and TNF-α, in the saliva and serum of OLP patients (125, 127–129). In contrast, serum levels of TGF-β are lower in OLP patients compared to healthy individuals (130, 131). A single nucleotide polymorphism (SNP) study on IL-10 polymorphisms revealed a higher frequency of four haplotypes (-1082 G/A, -819 C/T, and -592 C/A polymorphisms) in the peripheral blood of OLP patients, which is associated with reduced serum IL-10 levels (132).
Based on these findings, several reports suggest that T cells may be involved in the development of OLP (133). However, given that various immune cell types can produce these cytokines, the precise role of T cells in OLP pathogenesis remains to be determined.
3 Potential applications of natural products in mucosal barrier-related diseases
In recent years, natural products have received much attention in the study of mucosa-associated diseases due to their excellent safety, biocompatibility, and therapeutic potential (134, 135). Natural products can attach to mucosal surfaces, inhibit inflammation, modulate microflora, and enhance the expression of tight junction proteins (TJPs), thereby restoring intestinal barrier function (Table 1). Numerous studies have confirmed that polysaccharides can alleviate dextran sulfate sodium (DSS)-induced UC (136, 137).
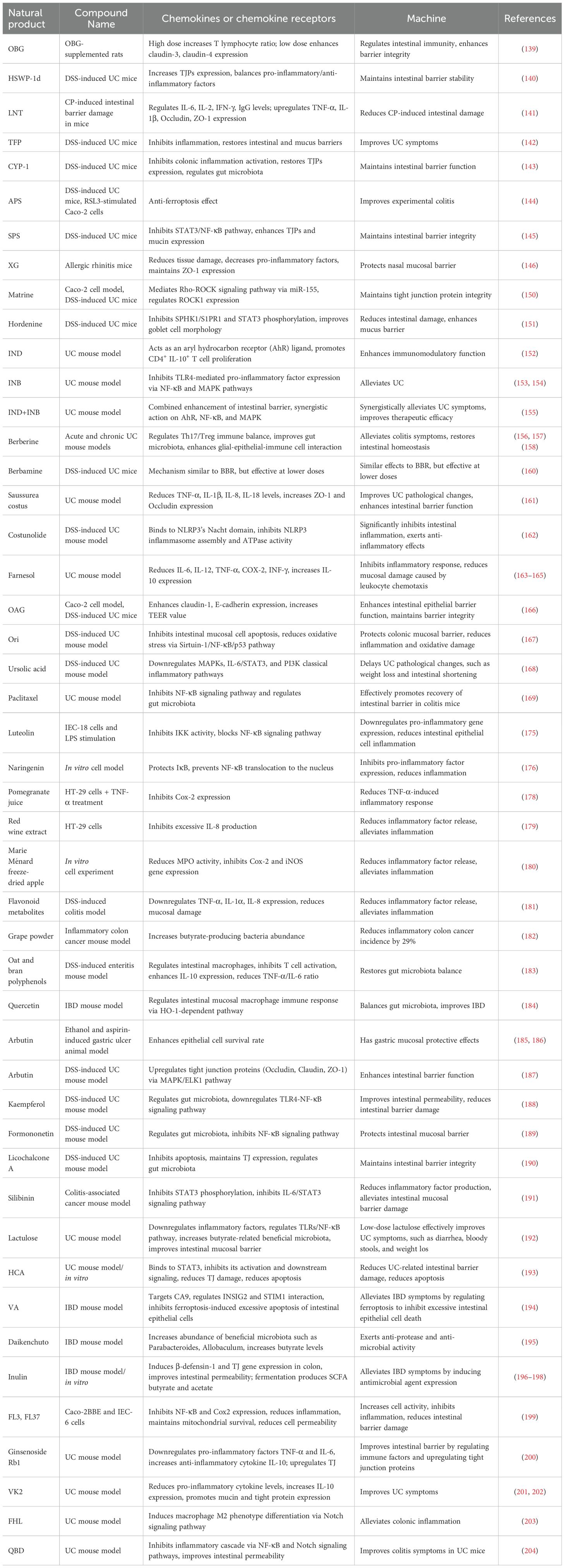
Table 1. Mechanism of natural products and their extracts in the treatment of mucosal barrier related diseases.
3.1 Polysaccharides
Magdalena et al. found that dietary oat β-glucan (OBG) supplementation modulated gut immune responses and barrier integrity. Rats receiving high-dose (3%) OBG exhibited a higher percentage of LP T lymphocytes, whereas those receiving low-dose (1%) OBG showed significantly increased expression of intestinal barrier proteins claudin-3 and claudin-4 (138, 139).
Ni et al. extracted a mannose-glucan (HSWP-1d) from Hirsutella sinensis, which effectively improved DSS-induced colitis symptoms in mice and maintained intestinal barrier stability by enhancing TJP expression and regulating the balance of pro-inflammatory and anti-inflammatory factors (140). Likewise, Jin et al. evaluated the protective effects of lentinan (LNT) on cyclophosphamide (CP)-induced intestinal barrier injury by assessing serological markers, histopathological changes in ileal tissues, TJP expression, and cytokine levels. The results indicated that LNT significantly alleviated CP-induced abnormalities in body weight, immune organ index, and serum IL-6, IL-2, IFN-γ, and IgG levels (p<0.05), while increasing the mRNA levels of TNF-α, IL-1β, IFN-γ, occludin, and ZO-1 (p<0.05), thereby mitigating CP-induced intestinal barrier damage (141).
Furthermore, Tremella fuciformis polysaccharides (TFP) have been shown to exert therapeutic effects in DSS-induced UC models by suppressing inflammation and restoring intestinal and mucus barrier functions (142). A water-soluble polysaccharide (CYP-1) from Dioscorea opposita inhibited the activation of colonic inflammation, restored TJP expression, and regulated gut microbiota in UC mice (143). Astragalus polysaccharides (APS) have been demonstrated to ameliorate experimental colitis in DSS-challenged mice and RSL3-stimulated Caco-2 cells, significantly inhibiting ferroptosis (144). Additionally, safflower polysaccharides (SPS) alleviated intestinal inflammation in UC models by suppressing the STAT3/NF-κB signaling pathway, protecting goblet cells, and enhancing the expression of TJPs and mucins, thereby improving intestinal barrier integrity (145).
Moreover, polysaccharides offer advantages in restoring barrier integrity with fewer adverse effects, ultimately improving patients’ quality of life. Marika et al. evaluated the therapeutic efficacy of xyloglucan (XG) nasal spray compared to several standard treatments (corticosteroid sprays, oral mast cell stabilizers, and oral antihistamines) for AR. The results indicated that XG exhibited significant efficacy in reducing histological damage in AR mice, suppressing pro-inflammatory cytokines, and maintaining ZO-1 expression (146). Additionally, xyloglucan has been shown to possess barrier-forming protective properties in adult and pediatric gastroenteritis and dry eye symptoms, making it a safe, non-pharmacological alternative for various diseases (147). Its potential role in other mucosal barrier disorders, such as dermatological diseases, warrants further investigation.
3.2 Alkaloid
Alkaloids are important active compounds in natural herbal medicines, characterized by highly diversified heterocyclic structures (148). Among them, plant alkaloids have attracted attention in traditional Chinese medicine due to their anti-inflammatory properties, which can suppress the expression of pro-inflammatory cytokines, lipid mediators, histamine, and inflammation-related enzymes (149). Based on this, alkaloids are considered important candidate drugs for repairing the mucosal barrier. Yu et al. studied the protective effect of matrine on the intestinal barrier through miR-155 in the Caco-2 cell line, DSS-induced colitis in mice, and clinical samples from patients with obstructive sterility. The results indicated that matrine could promote the expression of ROCK1, a protein associated with the Rho-Rock pathway, in Caco-2 cells and maintain tight junctions (150). Xu et al. treated DSS-induced ulcerative colitis (UC) mice with hordenine, and the histological examination showed that intestinal damage in the treatment group was significantly improved. Additionally, compared to the control group, the hordenine-treated group exhibited a more regular arrangement of goblet cells, more complete cell shapes, and a greater surface coverage of glycoproteins and other mucous substances, showing a certain dose-dependent effect. At the molecular level, hordenine inhibited the increase in sphingosine kinase-1 (SPHK1) and sphingosine-1-phosphate receptor-1 (S1PR1) expression, as well as the phosphorylation of STAT3 in the colon tissue of DSS-induced mice (151). Indirubin (IND) and indirubin-3’-monoxime (INB) are isomers and active molecules of natural indigo in traditional Chinese medicine, with therapeutic activity against UC. IND is a ligand for the aryl hydrocarbon receptor (Ahr), which can promote the proliferation of CD4+ IL-10+ T cells (152). INB reduces the expression of inflammatory factors such as TNF-α and IFN-γ through the NF-κB and MAPK signaling pathways mediated by TLR4, thereby eliminating inflammation (153, 154). Xie et al. explored the therapeutic effect of combined IND and INB treatment for UC, and the results showed that this combination could synergistically enhance the function of the intestinal barrier (155). Previous experiments have shown that berberine (BBR) can alleviate acute and chronic experimental colitis by regulating the T17/Treg balance (156), gut microbiota balance and metabolism (157), and the interactions between gut glial cells, epithelial cells, and immune cells (158). Dong et al.’s proteomics study indicated that the Wnt/β-catenin pathway was significantly enhanced in the colon tissue of mice treated with BBR, and the therapeutic effect of BBR was lost after intervention with the Wnt pathway inhibitor FH535, suggesting that BBR protects the mucosal barrier through the Wnt/β-catenin pathway (159). Interestingly, berberine’s main active metabolite, berberrubine (BB), has also been shown to exert a similar effect in attenuating DSS-induced UC, with a similar mechanism but at much lower doses (160).
3.3 Terpenoid
Terpenoid compounds are widely present in various traditional Chinese medicines and have been shown to repair intestinal barrier function by downregulating inflammatory factors and increasing the expression of tight junction proteins. Pang et al. revealed that Saussurea costus could reduce the levels of TNF-α, IL-1β, IL-8, and IL-18, while enhancing the expression of ZO-1 and Occludin, thereby improving the pathological characteristics of ulcerative colitis (UC) (161). They identified its main components, including proline, phenylalanine, isoleucine, ganoderic acid M, and pyroglutamic acid. Xu et al. demonstrated that the main active ingredient of Saussurea lappa, the sesquiterpene lactone Costunolide (COS), exerted a potent anti-inflammatory effect in a UC mouse model by inhibiting the NLRP3 inflammasome. Although the specific mechanism is not fully elucidated, the study suggested that COS could bind to the Nacht domain of NLRP3, altering its ATPase activity and inflammasome assembly (162). Farnesol (FAR), one of the main volatile oil components of grapefruit flowers, is a natural sesquiterpene alcohol (163). FAR alleviates intestinal inflammation and reduces intestinal mucosal damage caused by leukocyte chemotaxis by lowering the levels of IL-6, IL-12, TNF-α, COX-2, and IFN-γ, while increasing the expression of IL-10 (164, 165). Wang et al. demonstrated in Caco-2 cell models and DSS-induced mouse models that oleanolic acid 28-O-β-D-glucopyranoside (OAG), a naturally occurring pentacyclic triterpene, enhances intestinal epithelial barrier function by increasing the expression of tight junction proteins (claudin-1 and E-cadherin) and raising TEER values (166). Wang et al.’s research also showed that oridonin (Ori) could alleviate DSS-induced UC inflammation in mice and reduce oxidative stress levels, while inhibiting intestinal mucosal cell apoptosis through the Sirtuin-1/NF-κB/p53 pathway, thereby protecting the integrity of the colonic mucosal barrier (167). Sheng et al. demonstrated that ursolic acid (UA) downregulated three classical inflammatory signaling pathways—MAPKs, IL-6/STAT3, and PI3K—effectively delaying weight loss and intestinal shortening in mice (168). Hou et al. showed that dietary paclitaxel effectively enhanced the recovery of the intestinal barrier in colitis mice by inhibiting the NF-κB signaling pathway and regulating the gut microbiota (169).
3.4 Flavonoid
Flavonoids have been shown to exert protective effects on the epithelial barrier (170). Studies suggest that the underlying mechanisms may be closely related to the regulation of tight junction proteins (TJs) and the balance of the gut microbiota. For example, cranberry extract, which is rich in flavonoids, significantly increases the proportion of Akkermansia spp. in the mouse gut (171). Akkermansia, a mucin-degrading bacterium in the intestinal mucus layer, has been confirmed to be crucial for maintaining epithelial integrity (172). Luteolin, a flavonoid abundant in plants such as carrots, peppers, and celery (173, 174), has been shown by Jin et al. to inhibit the activity of IκB kinase (IKK) in LPS-induced IEC-18 cells (175), thus blocking the NF-κB signaling pathway and reducing the expression of pro-inflammatory genes. Additionally, flavonoids such as naringenin can protect IκB from degradation, preventing the translocation of NF-κB to the nucleus and further inhibiting the expression of pro-inflammatory factors (176). Activated NF-κB is involved in the transcription and activation of genes related to immune and inflammatory responses, such as pro-inflammatory cytokines (TNF-α, IL-1β, and IL-6) (177) and inflammation-related enzymes (Cox-2 and iNOS). Flavonoids play a significant role in suppressing intestinal inflammation and the expression of pro-inflammatory enzymes. In one study, pretreatment of HT-29 cells with pomegranate juice rich in anthocyanins and catechins resulted in a reduction of TNF-α-induced Cox-2 expression (178). Nunes et al. observed that pretreatment with red wine extract containing catechins, oligomeric procyanidins, and anthocyanins effectively suppressed excessive IL-8 production in HT-29 cells (179). Furthermore, naringenin can downregulate adhesion molecules (ICAM-1), chemokines (MCP-1), iNOS, Cox-2, TNF-α, and IL-6 (176). Marie Ménard’s freeze-dried apples, rich in flavonols and flavan-3-ols, reduce myeloperoxidase (MPO) activity and inhibit the expression of Cox-2 and iNOS genes (180). MPO is considered a marker of disease activity in intestinal inflammation, further supporting the regulatory role of flavonoids in intestinal inflammation.
It is important to note that the anti-inflammatory properties of flavonoids in the intestine may be mediated by their metabolites. After entering the intestine, flavonoids are metabolized by intestinal cells and the microbiota, resulting in a series of bioactive metabolites. A study by Larrosa et al. demonstrated that certain flavonoid-derived metabolites significantly inhibit DSS-induced colonic mucosal damage and downregulate the expression of TNF-α, IL-1β, and IL-8 (181). However, research on flavonoid metabolites is still limited, and it is expected that this will become a key direction for elucidating their mechanisms of action and developing novel anti-inflammatory drugs in the future.
3.5 Polyphenols
Polyphenolic compounds are primarily found in plant-based foods and regulate the gut microbiome through dynamic interactions with intestinal microbes, alleviating intestinal inflammation and enhancing gut barrier function. Zhao et al. discovered that, compared to a normal diet, the inclusion of polyphenol-rich grape powder in the diet reduced the incidence of inflammatory colon cancer in mice by 29%, with the mechanism related to the increased abundance of butyrate-producing bacteria in the gut (182). Duan et al. demonstrated that the polyphenols in oats and wheat bran could regulate intestinal macrophages, inhibit T-cell activation, enhance IL-10 expression, and significantly reduce the TNF-α/IL-6 ratio, thereby restoring gut microbiota balance (183). Quercetin (QCN) can regulate the immune response of intestinal mucosal macrophages through a heme oxygenase-1 (HO-1)-dependent pathway, improving gut microbiota imbalance and alleviating IBD (184). Studies have shown that arbutin protects against ethanol- and aspirin-induced gastric ulcers in animal models (185) and increases epithelial cell viability (186). Zhang et al. demonstrated that arbutin mediates the expression levels of tight junction proteins (Occludin, Claudin, and ZO-1) through the MAPK/ELK1 signaling pathway (187). Qu et al. used a UC mouse model to show that kaempferol improves intestinal permeability and significantly prevents DSS-induced intestinal barrier disruption by regulating gut microbiota and downregulating the TLR4-NF-κB signaling pathway (188). Similarly, Peng et al. demonstrated that astragaloside can improve the intestinal mucosal barrier function in DSS mice by reducing gut microbiota dysbiosis and inhibiting the NF-κB pathway (189). Zhang et al. revealed that Glycyrrhiza chalcone A (LA) maintains intestinal barrier integrity by inhibiting cell apoptosis and maintaining TJ expression. Additionally, 16S rRNA analysis indicated that LA also regulates gut barrier-associated microbiota (190). Zheng et al. found that silymarin significantly inhibited the phosphorylation of STAT3 in colitis-associated cancer (CAC) mice, thereby suppressing the IL-6/STAT3i signaling pathway to reduce the production of inflammatory cytokines and alleviate damage to the intestinal mucosal barrier (191).
3.6 Other extracts from natural products
The study by Cui et al. demonstrated that low-dose lactulose effectively alleviates symptoms of UC, including diarrhea, hematochezia, and weight loss. Its mechanism involves downregulation of inflammatory factors, modulation of TLRs/NF-κB signaling pathways, and reduction of cecal pH, promoting the proliferation of beneficial microbiota such as SCFAs, thereby improving the intestinal mucosal barrier (192). Chen et al. discovered that the natural product 2-hydroxycinnamaldehyde (HCA), isolated from cinnamon bark, directly interacts with STAT3 to inhibit its activation and downstream signaling. This compound effectively mitigated UC-induced disruption of intestinal barrier tight junctions both in vitro and in vivo, reducing apoptosis and improving intestinal inflammation (193). Ni et al. showed that vanillic acid (VA) targets carbonic anhydrase IX (CA9), facilitating the interaction between INSIG2 and STIM1, which promotes SCAP-SREBP1 translocation and activates SREBP1. This enhances SCD1 transcription, inhibits ferroptosis, and prevents excessive intestinal epithelial cell death (194). Some natural products exert anti-inflammatory effects by modulating the gut microbiota and regulating microbial metabolites. Daikenchuto (DKT) prevents IBD by exhibiting anti-protease and anti-microbial activity via secretory leukocyte protease inhibitor (SLPI), increasing the abundance of butyrate-producing bacteria such as Parabacteroides, Allobaculum, and Akkermansia, thereby enhancing butyrate levels (195). Inulin and sodium butyrate also improve intestinal permeability by inducing β-defensin-1 and tight junction proteins. SCFAs generated from inulin fermentation further stimulate antimicrobial peptide expression by Paneth cells (196–198). In vitro results indicated that flavonoids FL3 and FL37 increased basal activity of Caco-2BBE and IEC-6 cells, reduced apoptosis, and decreased epithelial monolayer permeability. Their mechanism involved the suppression of TNF-α and IFN-γ induced NF-κB and COX-2 expression to alleviate inflammation. Additionally, they preserved mitochondrial survival by maintaining complex I activity and inhibiting TNF-αinduced mitochondrial superoxide generation (199). Zhou et al. used RNA-seq and network pharmacology to study ginsenoside Rb1 in UC, finding that it downregulated TNF-α and IL-6 while increasing IL-10 and tight junction proteins (ZO-1, Occludin, E-cadherin). These effects may be linked to VDR, PPARγ, and NF-κB signaling pathways (200). Vitamin K2 (VK2), a naphthoquinone derivative, has been shown to worsen UC symptoms in mice fed a vitamin K-deficient diet (201). Hu et al. demonstrated that VK2 reduces pro-inflammatory cytokine levels, increases IL-10 levels, and promotes the expression of mucins and tight junction proteins to restore mucosal barrier function (202). Additionally, traditional Chinese medicine formulas have shown potential efficacy. For example, Huaihua Decoction regulates the Notch signaling pathway in mice, inducing macrophage M2 phenotype differentiation, thus enhancing intestinal barrier function in DSS-induced colitis (203). Qingbai Decoction (QBD) similarly modulates NF-κB and Notch signaling to inhibit inflammatory cascades and enhances the mucus and epithelial cell barriers, improving intestinal permeability in colitis mice (204).
The gut microbiota plays a key role in mucosal repair. UC patients often show reduced levels of SCFA-producing bacteria. Zhao et al. found that supplementing mice with SCFA-producing bacterial supernatants restored SCFA levels and inactivated the JAK/STAT3/FOXO3 axis, leading to M2 macrophage polarization and improved colonic health (205). Zhuang et al. demonstrated that extracellular vesicles from O. splanchnicus effectively alleviated weight loss, colon shortening, disease activity index, and histological damage in a DSS-induced IBD mouse model. These effects were associated with the downregulation of IL-1β, TNF-α, and IL-6 expression, the upregulation of IL-10, and the blockade of NLRP3 inflammasome activation, thus ameliorating intestinal barrier dysfunction and colonic apoptosis (206). Yue et al. reported that cytoplasmic membrane vesicles (CMVs) secreted by L. reuteri, which interact with host cells, were taken up by intestinal epithelial cells. This uptake enhanced the expression of ZO-1, E-cadherin, and occludin, reduced intestinal permeability, and improved tight junction function, thereby alleviating DSS-induced colitis in mice (207). Pan et al. evaluated the preventive effect and mechanisms of Lactobacillus fermentum 016 (LF) in a DSS-induced UC mouse model, showing that LF improved intestinal mucosal barrier function through the Nrf2-Keap1 signaling pathway and modulation of systemic inflammatory factors such as IL-1β, IL-6, TNF-α, IFN-γ, IL-4, and IL-10 (207). Cui et al. also showed that HnAg (membrane shell antigen) intervention in UC mice increased goblet cell numbers and elevated mucin and tight junction protein expression. These effects were likely mediated through activation of the AhR/IL-22 pathway (208).
4 Discussion
The mucosal barrier is widely present in various organ systems, including the gastrointestinal tract, respiratory tract, urinary tract, and oral cavity. As an important defense line against harmful external substances, it performs multiple functions such as physical barrier, immune defense, and microbial balance. The integrity of the barrier is maintained by structures such as tight junctions, adherens junctions, and desmosomes between epithelial cells, and it works in conjunction with abundant immune cell populations to form the mucosal immune system, which regulates inflammatory responses and immune tolerance (209).
However, various pathological factors, such as infections, inflammation, autoimmune abnormalities, environmental toxins, drugs (such as NSAIDs and antibiotics), and poor dietary habits, can disrupt the mucosal barrier, leading to increased barrier permeability (leaky mucosa), which in turn triggers IBD, ulcerative colitis (UC), Crohn’s disease (CD), respiratory diseases (such as asthma and chronic obstructive pulmonary disease, COPD), gastric ulcers, oral ulcers, and other conditions (210). These diseases are typically accompanied by chronic inflammation and may further progress to cancer, such as colorectal cancer (CRC) and esophageal cancer. Therefore, repairing the damaged mucosal barrier has become a crucial strategy in the treatment of these diseases (211).
Currently, conventional treatment options for mucosal barrier injury-related diseases mainly rely on chemical drugs, including aminosalicylates, corticosteroids, immunosuppressants (such as azathioprine and cyclosporine), and biologics (such as TNF-α inhibitors). Although these drugs can effectively control inflammation and alleviate symptoms in the short term, long-term use may lead to a range of side effects, such as immunosuppression, osteoporosis, hyperglycemia, and liver and kidney damage. Furthermore, these treatments typically target a single pathway and are insufficient in comprehensively addressing the complex pathophysiology of the diseases. Additionally, some patients may develop drug resistance or poor therapeutic response. Therefore, identifying safer treatment strategies with broader mechanisms of action has become a research focus.
In recent years, extensive studies have shown that natural products offer advantages in improving mucosal barrier damage, with multiple targets, high safety, and fewer side effects, showing promising therapeutic effects in refractory diseases (210). Moreover, the wide variety of traditional Chinese medicine provides abundant sources for treating mucosal barrier diseases. The main mechanisms of natural products in mucosal barrier injury diseases include the regulation of immune cell functions, reduction of inflammatory factor levels, and promotion of tight junction protein expression to aid in mucosal barrier repair and restore its function (212).
With the deepening research on microbiota, the modulatory effects of natural products on the microbiome are gradually being revealed. For instance, some natural products can increase the abundance of short-chain fatty acid-producing bacteria, thereby enhancing the levels of butyrate in the gut (213). Butyrate can be absorbed by intestinal epithelial cells and assist in mucosal barrier repair. Microbial-targeted therapies have become a novel strategy for mucosal barrier repair. Additionally, combination therapy with natural products exhibiting complementary mechanisms of action can improve therapeutic efficacy, providing ideas for exploring new treatment options.
Although natural products demonstrate significant potential in the treatment of mucosal barrier diseases, several challenges remain. For example, current research mainly focuses on gastrointestinal mucosal repair, while studies on mucosal tissues in other organs, such as the respiratory, oral, and urinary systems, remain limited. Furthermore, some traditional Chinese medicines or formulations, although showing good efficacy, still lack a clear understanding of their specific mechanisms of action and active components, requiring further investigation. The interactions between natural products and the microbiome also warrant deeper research to fully elucidate their mechanisms and enhance clinical applications.
Author contributions
WL: Writing – original draft. XR: Writing – review & editing. XC: Writing – original draft. LH: Writing – original draft. HX: Writing – original draft. YJ: Writing – original draft. LM: Writing – original draft. ZS: Writing – original draft. ZM: Writing – original draft. LD: Writing – review & editing. FX: Writing – review & editing.
Funding
The author(s) declare that financial support was received for the research and/or publication of this article. This work was supported by the National Natural Science Foundation of China (Grant number: 82304913, 82204785 and 82474141), the Jilin Province Science and Technology Development Plan Item (Grant Numbers: YDZJ202501ZYTS803, YDZJ202401110ZYTS, 20240601005RC, 20230401074YY, YDZJ202401442ZYTS, 202104011055YY and 20220204001YY), the Guangzhou Science and Technology Program (Grant number: 2023A03J0952 and 2023A03J0953).
Conflict of interest
The authors declare that the research was conducted in the absence of any commercial or financial relationships that could be construed as a potential conflict of interest.
Generative AI statement
The author(s) declare that no Generative AI was used in the creation of this manuscript.
Publisher’s note
All claims expressed in this article are solely those of the authors and do not necessarily represent those of their affiliated organizations, or those of the publisher, the editors and the reviewers. Any product that may be evaluated in this article, or claim that may be made by its manufacturer, is not guaranteed or endorsed by the publisher.
References
1. Karkhanis LU, Ross TM. Mucosal vaccine vectors: replication-competent versus replicationDeficient poxviruses. Curr Pharmaceut Design. (2007) 13:2015–23. doi: 10.2174/138161207781039832
2. Corthésy B. Recombinant secretory IgA for immune intervention against mucosal pathogens. Biochem Soc Trans. (1997) 25:471–5. doi: 10.1042/bst0250471
3. Birru RL, Bein K, Bondarchuk N, Wells H, Lin Q, Di YP, et al. Antimicrobial and anti-inflammatory activity of apple polyphenol phloretin on respiratory pathogens associated with chronic obstructive pulmonary disease. Front Cell Infect Microbiol. (2021) 11:11. doi: 10.3389/fcimb.2021.652944
4. Edyedu I, Ugwu OP-C, Ugwu CN, Alum EU, Eze VHU, Basajja M, et al. The role of pharmacological interventions in managing urological complications during pregnancy and childbirth: A review. Medicine. (2025) 104:e41381. doi: 10.1097/MD.0000000000041381
5. Kirchner FR, Littringer K, Altmeier S, Tran VDT, Schönherr F, Lemberg C, et al. Persistence of candida albicans in the oral mucosa induces a curbed inflammatory host response that is independent of immunosuppression. Front Immunol. (2019) 10:10. doi: 10.3389/fimmu.2019.00330
6. Li L, Chen W, Xiang L. Therapeutic efficacy of the combination therapy of corticosteroids and 5-aminosalicylic acid for treatment of pyoderma gangrenosum with ulcerative colitis. Indian J Dermatol. (2020) 65:38. doi: 10.4103/ijd.IJD_505_18
7. Gao Y, Guo Y. Research progress in the development of natural-product-based mucosal vaccine adjuvants. Front Immunol. (2023) 14:1152855. doi: 10.3389/fimmu.2023.1152855
8. Long D, Mao C, Zhang W, Zhu Y, Xu Y. Natural products for the treatment of ulcerative colitis: focus on the JAK/STAT pathway. Front Immunol. (2025) 16:1538302. doi: 10.3389/fimmu.2025.1538302
9. Hou Q, Huang J, Zhao L, Pan X, Liao C, Jiang Q, et al. Dietary genistein increases microbiota-derived short chain fatty acid levels, modulates homeostasis of the aging gut, and extends healthspan and lifespan. Pharmacol Res. (2023) 188:106676. doi: 10.1016/j.phrs.2023.106676
10. Vancamelbeke M, Vermeire S. The intestinal barrier: a fundamental role in health and disease. Expert Rev gastroenterol hepatol. (2017) 11:821–34. doi: 10.1080/17474124.2017.1343143
11. Turner JR. Intestinal mucosal barrier function in health and disease. Nat Rev Immunol. (2009) 9:799–809. doi: 10.1038/nri2653
12. Helander HF, Fändriks L. Surface area of the digestive tract - revisited. Scandinavian J gastroenterol. (2014) 49:681–9. doi: 10.3109/00365521.2014.898326
13. Pelaseyed T, Bergström JH, Gustafsson JK, Ermund A, Birchenough GM, Schütte A, et al. The mucus and mucins of the goblet cells and enterocytes provide the first defense line of the gastrointestinal tract and interact with the immune system. Immunol Rev. (2014) 260:8–20. doi: 10.1111/imr.2014.260.issue-1
14. Van der Sluis M, De Koning BA, De Bruijn AC, Velcich A, Meijerink JP, Van Goudoever JB, et al. Muc2-deficient mice spontaneously develop colitis, indicating that MUC2 is critical for colonic protection. Gastroenterology. (2006) 131:117–29. doi: 10.1053/j.gastro.2006.04.020
15. Birchenough GM, Johansson ME, Stabler RA, Dalgakiran F, Hansson GC, Wren BW, et al. Altered innate defenses in the neonatal gastrointestinal tract in response to colonization by neuropathogenic Escherichia coli. Infect immun. (2013) 81:3264–75. doi: 10.1128/IAI.00268-13
16. McElroy SJ, Prince LS, Weitkamp JH, Reese J, Slaughter JC, Polk DB. Tumor necrosis factor receptor 1-dependent depletion of mucus in immature small intestine: a potential role in neonatal necrotizing enterocolitis. Am J Physiol Gastrointest liver Physiol. (2011) 301:G656–666. doi: 10.1152/ajpgi.00550.2010
17. Johansson ME, Sjövall H, Hansson GC. The gastrointestinal mucus system in health and disease. Nat Rev Gastroenterol hepatol. (2013) 10:352–61. doi: 10.1038/nrgastro.2013.35
18. Salim SY, Söderholm JD. Importance of disrupted intestinal barrier in inflammatory bowel diseases. Inflamm bowel dis. (2011) 17:362–81. doi: 10.1002/ibd.21403
19. Groschwitz KR, Hogan SP. Intestinal barrier function: molecular regulation and disease pathogenesis. J Allergy Clin Immunol. (2009) 124:3–20. doi: 10.1016/j.jaci.2009.05.038
20. Gill N, Wlodarska M, Finlay BB. The future of mucosal immunology: studying an integrated system-wide organ. Nat Immunol. (2010) 11:558–60. doi: 10.1038/ni0710-558
21. Philpott DJ, Sorbara MT, Robertson SJ, Croitoru K, Girardin SE. NOD proteins: regulators of inflammation in health and disease. Nat Rev Immunol. (2014) 14:9–23. doi: 10.1038/nri3565
22. Song DH, Lee JO. Sensing of microbial molecular patterns by Toll-like receptors. Immunol Rev. (2012) 250:216–29. doi: 10.1111/j.1600-065X.2012.01167.x
23. Swamy M, Jamora C, Havran W, Hayday A. Epithelial decision makers: in search of the ‘epimmunome’. Nat Immunol. (2010) 11:656–65. doi: 10.1038/ni.1905
24. McDole JR, Wheeler LW, McDonald KG, Wang B, Konjufca V, Knoop KA, et al. Goblet cells deliver luminal antigen to CD103+ dendritic cells in the small intestine. Nature. (2012) 483:345–9. doi: 10.1038/nature10863
25. Jaensson E, Uronen-Hansson H, Pabst O, Eksteen B, Tian J, Coombes JL, et al. Small intestinal CD103+ dendritic cells display unique functional properties that are conserved between mice and humans. J Exp med. (2008) 205:2139–49. doi: 10.1084/jem.20080414
26. Burzyn D, Benoist C, Mathis D. Regulatory T cells in nonlymphoid tissues. Nat Immunol. (2013) 14:1007–13. doi: 10.1038/ni.2683
27. MacDonald TT, Hutchings P, Choy MY, Murch S, Cooke A. Tumour necrosis factor-alpha and interferon-gamma production measured at the single cell level in normal and inflamed human intestine. Clin Exp Immunol. (1990) 81:301–5. doi: 10.1111/j.1365-2249.1990.tb03334.x
28. Fais S, Capobianchi MR, Silvestri M, Mercuri F, Pallone F, Dianzani F. Interferon expression in Crohn’s disease patients: increased interferon-gamma and -alpha mRNA in the intestinal lamina propria mononuclear cells. J interfer Res. (1994) 14:235–8. doi: 10.1089/jir.1994.14.235
29. Ye D, Ma I, Ma TY. Molecular mechanism of tumor necrosis factor-alpha modulation of intestinal epithelial tight junction barrier. Am J Physiol Gastrointest liver Physiol. (2006) 290:G496–504. doi: 10.1152/ajpgi.00318.2005
30. Mankertz J, Tavalali S, Schmitz H, Mankertz A, Riecken EO, Fromm M, et al. Expression from the human occludin promoter is affected by tumor necrosis factor alpha and interferon gamma. J Cell Sci. (2000) 113:2085–90. doi: 10.1242/jcs.113.11.2085
31. Zolotarevsky Y, Hecht G, Koutsouris A, Gonzalez DE, Quan C, Tom J, et al. A membrane-permeant peptide that inhibits MLC kinase restores barrier function in in vitro models of intestinal disease. Gastroenterology. (2002) 123:163–72. doi: 10.1053/gast.2002.34235
32. Zünd G, Madara JL, Dzus AL, Awtrey CS, Colgan SP. Interleukin-4 and interleukin-13 differentially regulate epithelial chloride secretion. J Biol Chem. (1996) 271:7460–4. doi: 10.1074/jbc.271.13.7460
33. Ceponis PJ, Botelho F, Richards CD, McKay DM. Interleukins 4 and 13 increase intestinal epithelial permeability by a phosphatidylinositol 3-kinase pathway. Lack evidence Stat 6 involve J Biol Chem. (2000) 275:29132–7. doi: 10.1074/jbc.M003516200
34. Berin MC, Yang PC, Ciok L, Waserman S, Perdue MH. Role for IL-4 in macromolecular transport across human intestinal epithelium. Am J Physiol. (1999) 276:C1046–1052. doi: 10.1152/ajpcell.1999.276.5.C1046
35. Prasad S, Mingrino R, Kaukinen K, Hayes KL, Powell RM, MacDonald TT, et al. Inflammatory processes have differential effects on claudins 2, 3 and 4 in colonic epithelial cells. Lab investigation; A J Tech Methods pathol. (2005) 85:1139–62. doi: 10.1038/labinvest.3700316
36. Madsen KL, Malfair D, Gray D, Doyle JS, Jewell LD, Fedorak RN. Interleukin-10 gene-deficient mice develop a primary intestinal permeability defect in response to enteric microflora. Inflamm bowel dis. (1999) 5:262–70. doi: 10.1097/00054725-199911000-00004
37. Arrieta MC, Madsen K, Doyle J, Meddings J. Reducing small intestinal permeability attenuates colitis in the IL10 gene-deficient mouse. Gut. (2009) 58:41–8. doi: 10.1136/gut.2008.150888
38. Madsen KL, Lewis SA, Tavernini MM, Hibbard J, Fedorak RN. Interleukin 10 prevents cytokine-induced disruption of T84 monolayer barrier integrity and limits chloride secretion. Gastroenterology. (1997) 113:151–9. doi: 10.1016/S0016-5085(97)70090-8
39. Musch MW, Clarke LL, Mamah D, Gawenis LR, Zhang Z, Ellsworth W, et al. T cell activation causes diarrhea by increasing intestinal permeability and inhibiting epithelial Na+/K+-ATPase. J Clin investig. (2002) 110:1739–47. doi: 10.1172/JCI0215695
40. Clayburgh DR, Barrett TA, Tang Y, Meddings JB, Van Eldik LJ, Watterson DM, et al. Epithelial myosin light chain kinase-dependent barrier dysfunction mediates T cell activation-induced diarrhea. vivo J Clin investig. (2005) 115:2702–15. doi: 10.1172/JCI24970
41. Dalton JE, Cruickshank SM, Egan CE, Mears R, Newton DJ, Andrew EM, et al. Intraepithelial gammadelta+ lymphocytes maintain the integrity of intestinal epithelial tight junctions in response to infection. Gastroenterology. (2006) 131:818–29. doi: 10.1053/j.gastro.2006.06.003
42. Yu LC, Perdue MH. Role of mast cells in intestinal mucosal function: studies in models of hypersensitivity and stress. Immunol Rev. (2001) 179:61–73. doi: 10.1034/j.1600-065X.2001.790107.x
43. Galli SJ, Grimbaldeston M, Tsai M. Immunomodulatory mast cells: negative, as well as positive, regulators of immunity. Nat Rev Immunol. (2008) 8:478–86. doi: 10.1038/nri2327
44. Bischoff SC, Krämer S. Human mast cells, bacteria, and intestinal immunity. Immunol Rev. (2007) 217:329–37. doi: 10.1111/j.1600-065X.2007.00523.x
45. Bischoff SC. Role of mast cells in allergic and non-allergic immune responses: comparison of human and murine data. Nat Rev Immunol. (2007) 7:93–104. doi: 10.1038/nri2018
46. Pennock JL, Grencis RK. The mast cell and gut nematodes: damage and defence. Chem Immunol Allergy. (2006) 90:128–40. doi: 10.1159/000088885
47. Meddings JB, Jarand J, Urbanski SJ, Hardin J, Gall DG. Increased gastrointestinal permeability is an early lesion in the spontaneously diabetic BB rat. Am J Physiol. (1999) 276:G951–957. doi: 10.1152/ajpgi.1999.276.4.G951
48. Kühn R, Löhler J, Rennick D, Rajewsky K, Müller W. Interleukin-10-deficient mice develop chronic enterocolitis. Cell. (1993) 75:263–74. doi: 10.1016/0092-8674(93)80068-P
49. Fasano A, Shea-Donohue T. Mechanisms of disease: the role of intestinal barrier function in the pathogenesis of gastrointestinal autoimmune diseases. Nat Clin pract Gastroenterol hepatol. (2005) 2:416–22. doi: 10.1038/ncpgasthep0259
50. Jeziorska M, Haboubi N, Schofield P, Woolley DE. Distribution and activation of eosinophils in inflammatory bowel disease using an improved immunohistochemical technique. J pathol. (2001) 194:484–92. doi: 10.1002/1096-9896(200108)194:4<>1.0.CO;2-W
51. Levy AM, Gleich GJ, Sandborn WJ, Tremaine WJ, Steiner BL, Phillips SF. Increased eosinophil granule proteins in gut lavage fluid from patients with inflammatory bowel disease. Mayo Clinic Proc. (1997) 72:117–23. doi: 10.4065/72.2.117
52. Carvalho AT, Elia CC, de Souza HS, Elias PR, Pontes EL, Lukashok HP, et al. Immunohistochemical study of intestinal eosinophils in inflammatory bowel disease. J Clin gastroenterol. (2003) 36:120–5. doi: 10.1097/00004836-200302000-00006
53. Laukoetter MG, Nava P, Nusrat A. Role of the intestinal barrier in inflammatory bowel disease. World J gastroenterol. (2008) 14:401–7. doi: 10.3748/wjg.14.401
54. Furuta GT, Nieuwenhuis EE, Karhausen J, Gleich G, Blumberg RS, Lee JJ, et al. Eosinophils alter colonic epithelial barrier function: role for major basic protein. Am J Physiol Gastrointest liver Physiol. (2005) 289:G890–897. doi: 10.1152/ajpgi.00015.2005
55. Park SC, Wiest MJ, Yan V, Wong PT, Schotsaert M. Induction of protective immune responses at respiratory mucosal sites. Hum vaccines immunother. (2024) 20:2368288. doi: 10.1080/21645515.2024.2368288
56. Button B, Cai LH, Ehre C, Kesimer M, Hill DB, Sheehan JK, et al. A periciliary brush promotes the lung health by separating the mucus layer from airway epithelia. Sci (New York N.Y.). (2012) 337:937–41. doi: 10.1126/science.1223012
57. Knight DA, Holgate ST. The airway epithelium: structural and functional properties in health and disease. Respirol (Carlton Vic.). (2003) 8:432–46. doi: 10.1046/j.1440-1843.2003.00493.x
58. Crystal RG, Randell SH, Engelhardt JF, Voynow J, Sunday ME. Airway epithelial cells: current concepts and challenges. Proc Am Thoracic Soc. (2008) 5:772–7. doi: 10.1513/pats.200805-041HR
59. Thompson AB, Robbins RA, Romberger DJ, Sisson JH, Spurzem JR, Teschler H, et al. Immunological functions of the pulmonary epithelium. Eur resp J. (1995) 8:127–49. doi: 10.1183/09031936.95.08010127
60. Puchelle E, Zahm JM, Tournier JM, Coraux C. Airway epithelial repair, regeneration, and remodeling after injury in chronic obstructive pulmonary disease. Proc Am Thoracic Soc. (2006) 3:726–33. doi: 10.1513/pats.200605-126SF
61. Heguy A, Harvey BG, Leopold PL, Dolgalev I, Raman T, Crystal RG. Responses of the human airway epithelium transcriptome to in vivo injury. Physiol Genomics. (2007) 29:139–48. doi: 10.1152/physiolgenomics.00167.2006
62. Kim CF, Jackson EL, Woolfenden AE, Lawrence S, Babar I, Vogel S, et al. Identification of bronchioalveolar stem cells in normal lung and lung cancer. Cell. (2005) 121:823–35. doi: 10.1016/j.cell.2005.03.032
63. Agrawal A, Rengarajan S, Adler KB, Ram A, Ghosh B, Fahim M, et al. Inhibition of mucin secretion with MARCKS-related peptide improves airway obstruction in a mouse model of asthma. J Appl Physiol (Bethesda Md.: 1985). (2007) 102:399–405. doi: 10.1152/japplphysiol.00630.2006
64. Tyner JW, Kim EY, Ide K, Pelletier MR, Roswit WT, Morton JD, et al. Blocking airway mucous cell metaplasia by inhibiting EGFR antiapoptosis and IL-13 transdifferentiation signals. J Clin investig. (2006) 116:309–21. doi: 10.1172/JCI25167
65. Hellings PW, Steelant B. Epithelial barriers in allergy and asthma. J Allergy Clin Immunol. (2020) 145:1499–509. doi: 10.1016/j.jaci.2020.04.010
66. Rogers DF. The airway goblet cell. Int J Biochem Cell Biol. (2003) 35:1–6. doi: 10.1016/S1357-2725(02)00083-3
67. Bonser LR, Erle DJ. Airway mucus and asthma: the role of MUC5AC and MUC5B. J Clin Med. (2017) 6:112. doi: 10.20944/preprints201711.0010.v1
68. Rock JR, Randell SH, Hogan BL. Airway basal stem cells: a perspective on their roles in epithelial homeostasis and remodeling. Dis Models mechan. (2010) 3:545–56. doi: 10.1242/dmm.006031
69. Evans MJ, Cox RA, Shami SG, Wilson B, Plopper CG. The role of basal cells in attachment of columnar cells to the basal lamina of the trachea. Am J resp Cell Mol Biol. (1989) 1:463–9. doi: 10.1165/ajrcmb/1.6.463
70. Rock JR, Onaitis MW, Rawlins EL, Lu Y, Clark CP, Xue Y, et al. Basal cells as stem cells of the mouse trachea and human airway epithelium. Proc Natl Acad Sci United States America. (2009) 106:12771–5. doi: 10.1073/pnas.0906850106
71. Ordovas-Montanes J, Dwyer DF, Nyquist SK, Buchheit KM, Vukovic M, Deb C, et al. Allergic inflammatory memory in human respiratory epithelial progenitor cells. Nature. (2018) 560:649–54. doi: 10.1038/s41586-018-0449-8
72. Kim SH, Matthay MA, Mostov K, Hunt CA. Simulation of lung alveolar epithelial wound healing. vitro J R Soc Interf. (2010) 7:1157–70. doi: 10.1098/rsif.2010.0041
73. Amatngalim GD, van Wijck Y, de Mooij-Eijk Y, Verhoosel RM, Harder J, Lekkerkerker AN, et al. Basal cells contribute to innate immunity of the airway epithelium through production of the antimicrobial protein RNase 7. J Immunol (Baltimore Md.: 1950). (2015) 194:3340–50. doi: 10.4049/jimmunol.1402169
74. Steelant B, Seys SF, Boeckxstaens G, Akdis CA, Ceuppens JL, Hellings PW. Restoring airway epithelial barrier dysfunction: a new therapeutic challenge in allergic airway disease. Rhinology. (2016) 54:195–205. doi: 10.4193/Rhino15.376
75. Steelant B. Epithelial dysfunction in chronic respiratory diseases, a shared endotype? Curr Opin pulmonary med. (2020) 26:20–6. doi: 10.1097/MCP.0000000000000638
76. Hartsock A, Nelson WJ. Adherens and tight junctions: structure, function and connections to the actin cytoskeleton. Biochim Biophys Acta. (2008) 1778:660–9. doi: 10.1016/j.bbamem.2007.07.012
77. Xiao C, Puddicombe SM, Field S, Haywood J, Broughton-Head V, Puxeddu I, et al. Defective epithelial barrier function in asthma. J Allergy Clin Immunol. (2011) 128:549–556.e541-512. doi: 10.1164/ajrccm-conference.2011.A065
78. Hackett TL, Singhera GK, Shaheen F, Hayden P, Jackson GR, Hegele RG, et al. Intrinsic phenotypic differences of asthmatic epithelium and its inflammatory responses to respiratory syncytial virus and air pollution. Am J resp Cell Mol Biol. (2011) 45:1090–100. doi: 10.1165/rcmb.2011-0031OC
79. Soyka MB, Wawrzyniak P, Eiwegger T, Holzmann D, Treis A, Wanke K, et al. Defective epithelial barrier in chronic rhinosinusitis: the regulation of tight junctions by IFN-γ and IL-4. J Allergy Clin Immunol. (2012) 130:1087–1096.e1010. doi: 10.1016/j.jaci.2012.05.052
80. Capaldo CT, Nusrat A. Cytokine regulation of tight junctions. Biochim Biophys Acta. (2009) 1788:864–71. doi: 10.1016/j.bbamem.2008.08.027
81. Steelant B, Seys SF, Van Gerven L, Van Woensel M, Farré R, Wawrzyniak P, et al. Histamine and T helper cytokine-driven epithelial barrier dysfunction in allergic rhinitis. J Allergy Clin Immunol. (2018) 141:951–963.e958. doi: 10.1016/j.jaci.2017.08.039
82. Saatian B, Rezaee F, Desando S, Emo J, Chapman T, Knowlden S, et al. Interleukin-4 and interleukin-13 cause barrier dysfunction in human airway epithelial cells. Tissue barriers. (2013) 1:e24333. doi: 10.4161/tisb.24333
83. Kortekaas Krohn I, Seys SF, Lund G, Jonckheere AC, Dierckx de Casterlé I, Ceuppens JL, et al. Nasal epithelial barrier dysfunction increases sensitization and mast cell degranulation in the absence of allergic inflammation. Allergy. (2020) 75:1155–64. doi: 10.1111/all.14132
84. Jones-Freeman B, Chonwerawong M, Marcelino VR, Deshpande AV, Forster SC, Starkey MR. The microbiome and host mucosal interactions in urinary tract diseases. Mucosal Immunol. (2021) 14:779–92. doi: 10.1038/s41385-020-00372-5
85. Fry CH, Vahabi B. The role of the mucosa in normal and abnormal bladder function. Basic Clin Pharmacol toxicol. (2016) 119 Suppl 3:57–62. doi: 10.1111/bcpt.12626
86. Acharya P, Beckel J, Ruiz WG, Wang E, Rojas R, Birder L, et al. Distribution of the tight junction proteins ZO-1, occludin, and claudin-4, -8, and -12 in bladder epithelium. Am J Physiol Renal Physiol. (2004) 287:F305–318. doi: 10.1152/ajprenal.00341.2003
87. Hicks RM. The mammalian urinary bladder: an accommodating organ. Biol Rev Cambridge Philos Soc. (1975) 50:215–46. doi: 10.1111/j.1469-185X.1975.tb01057.x
88. Truschel ST, Wang E, Ruiz WG, Leung SM, Rojas R, Lavelle J, et al. Stretch-regulated exocytosis/endocytosis in bladder umbrella cells. Mol Biol Cell. (2002) 13:830–46. doi: 10.1091/mbc.01-09-0435
89. Levin S, Richter WR. Ultrastructure of cell surface coat (glycocalyx) in rat urinary bladder epithelium. Cell Tissue Res. (1975) 158:281–3. doi: 10.1007/BF00219966
90. Monis B, Dorfman HD. Some histochemical observations on transitional epithelium of man. J Histochem Cytochem. (1967) 15:475–81. doi: 10.1177/15.8.475
91. Klingler CH. Glycosaminoglycans: how much do we know about their role in the bladder? Urologia. (2016) 83 Suppl 1:11–4. doi: 10.5301/uro.5000184
92. Lewis SA. Everything you wanted to know about the bladder epithelium but were afraid to ask. Am J Physiol Renal Physiol. (2000) 278:F867–874. doi: 10.1152/ajprenal.2000.278.6.F867
93. Jafari NV, Rohn JL. The urothelium: a multi-faceted barrier against a harsh environment. Mucosal Immunol. (2022) 15:1127–42. doi: 10.1038/s41385-022-00565-0
94. Kanai A, Andersson KE. Bladder afferent signaling: recent findings. J urol. (2010) 183:1288–95. doi: 10.1016/j.juro.2009.12.060
95. Birder LA. Role of the urothelium in urinary bladder dysfunction following spinal cord injury. Prog Brain Res. (2006) 152:135–46. doi: 10.1016/S0079-6123(05)52009-0
96. Coolen MJ, Post E, Davis CC, Forney LJ. Characterization of microbial communities found in the human vagina by analysis of terminal restriction fragment length polymorphisms of 16S rRNA genes. Appl Environ microbiol. (2005) 71:8729–37. doi: 10.1128/AEM.71.12.8729-8737.2005
97. Ravel J, Gajer P, Abdo Z, Schneider GM, Koenig SS, McCulle SL, et al. Vaginal microbiome of reproductive-age women. Proc Natl Acad Sci United States America. (2011) 108 Suppl 1:4680–7. doi: 10.1073/pnas.1002611107
98. Pfau A, Sacks T. The bacterial flora of the vaginal vestibule, urethra and vagina in the normal premenopausal woman. J urol. (1977) 118:292–5. doi: 10.1016/S0022-5347(17)57976-8
99. Flores-Mireles AL, Walker JN, Caparon M, Hultgren SJ. Urinary tract infections: epidemiology, mechanisms of infection and treatment options. Nat Rev Microbiol. (2015) 13:269–84. doi: 10.1038/nrmicro3432
100. Min G, Stolz M, Zhou G, Liang F, Sebbel P, Stoffler D, et al. Localization of uroplakin Ia, the urothelial receptor for bacterial adhesin FimH, on the six inner domains of the 16 nm urothelial plaque particle. J Mol Biol. (2002) 317:697–706. doi: 10.1006/jmbi.2002.5442
101. Mysorekar IU, Mulvey MA, Hultgren SJ, Gordon JI. Molecular regulation of urothelial renewal and host defenses during infection with uropathogenic Escherichia coli. J Biol Chem. (2002) 277:7412–9. doi: 10.1074/jbc.M110560200
102. Jost SP, Potten CS. Urothelial proliferation in growing mice. Cell Tissue kinetics. (1986) 19:155–60. doi: 10.1111/j.1365-2184.1986.tb00725.x
103. Jost SP. Cell cycle of normal bladder urothelium in developing and adult mice. Virchows Archiv B Cell Pathol including Mol pathol. (1989) 57:27–36. doi: 10.1007/BF02899062
104. Gandhi D, Molotkov A, Batourina E, Schneider K, Dan H, Reiley M, et al. Retinoid signaling in progenitors controls specification and regeneration of the urothelium. Dev Cell. (2013) 26:469–82. doi: 10.1016/j.devcel.2013.07.017
105. LaRue H, Ayari C, Bergeron A, Fradet Y. Toll-like receptors in urothelial cells–targets for cancer immunotherapy. Nat Rev Urol. (2013) 10:537–45. doi: 10.1038/nrurol.2013.153
106. Suárez LJ, Arboleda S, Angelov N, Arce RM. Oral versus gastrointestinal mucosal immune niches in homeostasis and allostasis. Front Immunol. (2021) 12:705206. doi: 10.3389/fimmu.2021.705206
107. Moutsopoulos NM, Konkel JE. Tissue-specific immunity at the oral mucosal barrier. Trends Immunol. (2018) 39:276–87. doi: 10.1016/j.it.2017.08.005
108. Russell MW, Mestecky J. Mucosal immunity: The missing link in comprehending SARS-CoV-2 infection and transmission. Front Immunol. (2022) 13:957107. doi: 10.3389/fimmu.2022.957107
109. Fábián TK, Hermann P, Beck A, Fejérdy P, Fábián G. Salivary defense proteins: their network and role in innate and acquired oral immunity. Int J Mol Sci. (2012) 13:4295–320. doi: 10.3390/ijms13044295
110. Wu RQ, Zhang DF, Tu E, Chen QM, Chen W. The mucosal immune system in the oral cavity-an orchestra of T cell diversity. Int J sci. (2014) 6:125–32. doi: 10.1038/ijos.2014.48
111. Meghil MM, Cutler CW. Oral microbes and mucosal dendritic cells, “Spark and flame” of local and distant inflammatory diseases. Int J Mol Sci. (2020) 21:1643. doi: 10.3390/ijms21051643
112. Pelaez-Prestel HF, Sanchez-Trincado JL, Lafuente EM, Reche PA. Immune tolerance in the oral mucosa. Int J Mol Sci. (2021) 22:12149. doi: 10.3390/ijms222212149
113. Audiger C, Rahman MJ, Yun TJ, Tarbell KV, Lesage S. The importance of dendritic cells in maintaining immune tolerance. J Immunol (Baltimore Md.: 1950). (2017) 198:2223–31. doi: 10.4049/jimmunol.1601629
114. Novak N, Haberstok J, Bieber T, Allam JP. The immune privilege of the oral mucosa. Trends Mol med. (2008) 14:191–8. doi: 10.1016/j.molmed.2008.03.001
115. Hovav AH. Dendritic cells of the oral mucosa. Mucosal Immunol. (2014) 7:27–37. doi: 10.1038/mi.2013.42
116. Cohen MS, Shaw GM, McMichael AJ, Haynes BF. Acute HIV-1 infection. New Engl J med. (2011) 364:1943–54. doi: 10.1056/NEJMra1011874
117. Lü FX, Jacobson RS. Oral mucosal immunity and HIV/SIV infection. J Dental Res. (2007) 86:216–26. doi: 10.1177/154405910708600305
118. Romani L. Immunity to fungal infections. Nat Rev Immunol. (2011) 11:275–88. doi: 10.1038/nri2939
119. Huang W, Na L, Fidel PL, Schwarzenberger P. Requirement of interleukin-17A for systemic anti-Candida albicans host defense in mice. J Infect dis. (2004) 190:624–31. doi: 10.1086/jid.2004.190.issue-3
120. Conti HR, Baker O, Freeman AF, Jang WS, Holland SM, Li RA, et al. New mechanism of oral immunity to mucosal candidiasis in hyper-IgE syndrome. Mucosal Immunol. (2011) 4:448–55. doi: 10.1038/mi.2011.5
121. Cheng SC, van de Veerdonk F, Smeekens S, Joosten LA, van der Meer JW, Kullberg BJ, et al. Candida albicans dampens host defense by downregulating IL-17 production. J Immunol (Baltimore Md.: 1950). (2010) 185:2450–7. doi: 10.4049/jimmunol.1000756
122. Cochran DL. Inflammation and bone loss in periodontal disease. J periodontol. (2008) 79:1569–76. doi: 10.1902/jop.2008.080233
123. Gemmell E, Yamazaki K, Seymour GJ. Destructive periodontitis lesions are determined by the nature of the lymphocytic response. Crit Rev Biol Med. (2002) 13:17–34. doi: 10.1177/154411130201300104
124. Wu RQ, Zhao XF, Wang ZY, Zhou M, Chen QM. Novel molecular events in oral carcinogenesis via integrative approaches. J Dental Res. (2011) 90:561–72. doi: 10.1177/0022034510383691
125. Scully C, Carrozzo M. Oral mucosal disease: Lichen planus. Br J maxillofacial surge. (2008) 46:15–21. doi: 10.1016/j.bjoms.2007.07.199
126. Dan H, Liu W, Zhou Y, Wang J, Chen Q, Zeng X. Association of interleukin-8 gene polymorphisms and haplotypes with oral lichen planus in a Chinese population. Inflammation. (2010) 33:76–81. doi: 10.1007/s10753-009-9160-0
127. Rhodus NL, Cheng B, Ondrey F. Th1/Th2 cytokine ratio in tissue transudates from patients with oral lichen planus. Mediators inflamm. (2007) 2007:19854. doi: 10.1155/2007/19854
128. Bai J, Lin M, Zeng X, Zhang Y, Wang Z, Shen J, et al. Association of polymorphisms in the human IFN-gamma and IL-4 gene with oral lichen planus: a study in an ethnic Chinese cohort. J interfer cytokine Res. (2008) 28:351–8. doi: 10.1089/jir.2007.0056
129. Walton LJ, Macey MG, Thornhill MH, Farthing PM. Intra-epithelial subpopulations of T lymphocytes and Langerhans cells in oral lichen planus. J Pathol med: Off Publ Int Assoc Pathol Am Acad Pathol. (1998) 27:116–23. doi: 10.1111/j.1600-0714.1998.tb01926.x
130. Zhang Y, Lin M, Zhang S, Wang Z, Jiang L, Shen J, et al. NF-kappaB-dependent cytokines in saliva and serum from patients with oral lichen planus: a study in an ethnic Chinese population. Cytokine. (2008) 41:144–9. doi: 10.1016/j.cyto.2007.11.004
131. Zhang Y, Liu W, Zhang S, Dan H, Lu R, Wang F, et al. Salivary and serum interleukin-18 in patients with oral lichen planus: a study in an ethnic Chinese population. Inflammation. (2012) 35:399–404. doi: 10.1007/s10753-011-9327-3
132. Taghavi Zenouz A, Pouralibaba F, Babaloo Z, Mehdipour M, Jamali Z. Evaluation of serum TNF-α and TGF-β in patients with oral lichen planus. J Dental res Dental clinics Dental prospects. (2012) 6:143–7. doi: 10.5681/joddd.2012.029
133. Bai J, Jiang L, Lin M, Zeng X, Wang Z, Chen Q. Association of polymorphisms in the tumor necrosis factor-alpha and interleukin-10 genes with oral lichen planus: a study in a chinese cohort with Han ethnicity. J interfer cytokine Res. (2009) 29:381–8. doi: 10.1089/jir.2008.0089
134. Wang M-X, Lin L, Chen Y-D, Zhong Y-P, Lin Y-X, Li P, et al. Evodiamine has therapeutic efficacy in ulcerative colitis by increasing Lactobacillus acidophilus levels and acetate production. Pharmacol Res. (2020) 159:104978. doi: 10.1016/j.phrs.2020.104978
135. Wang Y-J, Li Q-M, Zha X-Q, Luo J-P. Intervention and potential mechanism of non-starch polysaccharides from natural resources on ulcerative colitis: A review. Int J Biol Macromol. (2022) 210:545–64. doi: 10.1016/j.ijbiomac.2022.04.208
136. Niu W, Chen X, Xu R, Dong H, Yang F, Wang Y, et al. Polysaccharides from natural resources exhibit great potential in the treatment of ulcerative colitis: A review. Carbohydr Polymers. (2021) 254:117189. doi: 10.1016/j.carbpol.2020.117189
137. Lu H, Shen M, Chen Y, Yu Q, Chen T, Xie J. Alleviative effects of natural plant polysaccharides against DSS-induced ulcerative colitis via inhibiting inflammation and modulating gut microbiota. Food Res Int. (2023) 167:112630. doi: 10.1016/j.foodres.2023.112630
138. Zhang L, Fu X, Li J, Xiao W, Xiong X, Lv H, et al. Treatment of acute ulcerative colitis with zinc hyaluronate in mice. J Microbiol Biotechnol. (2025) 35:e2408050. doi: 10.4014/jmb.2408.08050
139. Guzowska M, Dziendzikowska K, Kopiasz Ł, Gajewska M, Wilczak J, Harasym J, et al. Oat beta-glucans modulate the gut microbiome, barrier function, and immune responses in an in vivo model of early-stage colorectal cancer. Int J Mol Sci. (2024) 25:13586. doi: 10.3390/ijms252413586
140. Ni W, Li Y, Feng J, Liu B, Yuan H, Tai G, et al. Therapeutic efficacy and underlying mechanisms of a mannoglucan from hirsutella sinensis mycelium on dextran sulfate sodium-induced inflammatory bowel disease in mice: modulation of the intestinal barrier, oxidative stress and gut microbiota. Int J Mol Sci. (2024) 25(23):13100. doi: 10.3390/ijms252313100
141. Jin X, Wu Z, Chen H, Liu W, Gu F, Li J. Extraction and identification of polysaccharide from lentinus edodes and its effect on immunosuppression and intestinal barrier injury induced by cyclophosphamide. Int J Mol Sci. (2024) 25:12432. doi: 10.3390/ijms252212432
142. Xiao H, Li H, Wen Y, Jiang D, Zhu S, He X, et al. Tremella fuciformis polysaccharides ameliorated ulcerative colitis via inhibiting inflammation and enhancing intestinal epithelial barrier function. Int J Biol Macromol. (2021) 180:633–42. doi: 10.1016/j.ijbiomac.2021.03.083
143. Li P, Xiao N, Zeng L, Xiao J, Huang J, Xu Y, et al. Structural characteristics of a mannoglucan isolated from Chinese yam and its treatment effects against gut microbiota dysbiosis and DSS-induced colitis in mice. Carbohydr Polymers. (2020) 250:116958. doi: 10.1016/j.carbpol.2020.116958
144. Chen Y, Wang J, Li J, Zhu J, Wang R, Xi Q, et al. Astragalus polysaccharide prevents ferroptosis in a murine model of experimental colitis and human Caco-2 cells via inhibiting NRF2/HO-1 pathway. Eur J Pharmacol. (2021) 911:174518. doi: 10.1016/j.ejphar.2021.174518
145. Qi M, Chu S, Wang W, Fu X, Jiang C, Zhang L, et al. Safflower polysaccharide ameliorates acute ulcerative colitis by regulating STAT3/NF-κB signaling pathways and repairing intestinal barrier function. Biomed pharmacother = Biomed pharmacother. (2024) 174:116553. doi: 10.1016/j.biopha.2024.116553
146. Lanza M, Casili G, Filippone A, Campolo M, Paterniti I, Cuzzocrea S, et al. Evaluating the protective properties of a xyloglucan-based nasal spray in a mouse model of allergic rhinitis. Int J Mol Sci. (2021) 22:10472. doi: 10.3390/ijms221910472
147. Piqué N, Gómez-Guillén MDC, Montero MP. Xyloglucan, a plant polymer with barrier protective properties over the mucous membranes: an overview. Int J Mol Sci. (2018) 19:673. doi: 10.3390/ijms19030673
148. Patel A, Vanecha R, Patel J, Patel D, Shah U, Bambharoliya T. Development of natural bioactive alkaloids: anticancer perspective. Mini Rev med Chem. (2022) 22:200–12. doi: 10.2174/1389557521666210712111331
149. Peng J, Zheng TT, Li X, Liang Y, Wang LJ, Huang YC, et al. Plant-derived alkaloids: the promising disease-modifying agents for inflammatory bowel disease. Front Pharmacol. (2019) 10:351. doi: 10.3389/fphar.2019.00351
150. Yu D, Su D, Liu Z. Matrine protects intestinal barrier function via microRNA-155 through ROCK1-signaling pathway. Turkish J gastroenterol. (2023) 34:831–8. doi: 10.5152/tjg.2023.21884
151. Xu Z, Zhang Q, Ding C, Wen F, Sun F, Liu Y, et al. Beneficial effects of hordenine on a model of ulcerative colitis. Mol (Basel Switzerland). (2023) 28:2834. doi: 10.3390/molecules28062834
152. Kawai S, Iijima H, Shinzaki S, Hiyama S, Yamaguchi T, Araki M, et al. Indigo Naturalis ameliorates murine dextran sodium sulfate-induced colitis via aryl hydrocarbon receptor activation. J gastroenterol. (2017) 52:904–19. doi: 10.1007/s00535-016-1292-z
153. Gao W, Guo Y, Wang C, Lin Y, Yu L, Sheng T, et al. Indirubin ameliorates dextran sulfate sodium-induced ulcerative colitis in mice through the inhibition of inflammation and the induction of Foxp3-expressing regulatory T cells. Acta histochem. (2016) 118:606–14. doi: 10.1016/j.acthis.2016.06.004
154. Lai JL, Liu YH, Liu C, Qi MP, Liu RN, Zhu XF, et al. Indirubin inhibits LPS-induced inflammation via TLR4 abrogation mediated by the NF-kB and MAPK signaling pathways. Inflammation. (2017) 40:1–12. doi: 10.1007/s10753-016-0447-7
155. Xie J, Tian S, Liu J, Huang S, Yang M, Yang X, et al. Combination therapy with indigo and indirubin for ulcerative colitis via reinforcing intestinal barrier function. Oxid Med Cell longevity. (2023) 2023:2894695. doi: 10.1155/2023/2894695
156. Kumar A, Ekavali, Chopra K, Mukherjee M, Pottabathini R, Dhull DK. Current knowledge and pharmacological profile of berberine: An update. Eur J Pharmacol. (2015) 761:288–97. doi: 10.1016/j.ejphar.2015.05.068
157. Li L, Chang L, Zhang X, Ning Z, Mayne J, Ye Y, et al. Berberine and its structural analogs have differing effects on functional profiles of individual gut microbiomes. Gut microbes. (2020) 11:1348–61. doi: 10.1080/19490976.2020.1755413
158. Li H, Fan C, Lu H, Feng C, He P, Yang X, et al. Protective role of berberine on ulcerative colitis through modulating enteric glial cells-intestinal epithelial cells-immune cells interactions. Acta Pharm Sinica B. (2020) 10:447–61. doi: 10.1016/j.apsb.2019.08.006
159. Dong Y, Fan H, Zhang Z, Jiang F, Li M, Zhou H, et al. Berberine ameliorates DSS-induced intestinal mucosal barrier dysfunction through microbiota-dependence and Wnt/β-catenin pathway. Int J Biol Sci. (2022) 18:1381–97. doi: 10.7150/ijbs.65476
160. Yu XT, Xu YF, Huang YF, Qu C, Xu LQ, Su ZR, et al. Berberrubine attenuates mucosal lesions and inflammation in dextran sodium sulfate-induced colitis in mice. PloS One. (2018) 13:e0194069. doi: 10.1371/journal.pone.0194069
161. Pang WL, Li TG, Wang YY, Song LY, Li L, Li XY, et al. Saussurea costus alleviates ulcerative colitis by regulating the gut microbiota and improving intestinal barrier integrity. Front Cell infect microbiol. (2025) 15:1528578. doi: 10.3389/fcimb.2025.1528578
162. Xu H, Chen J, Chen P, Li W, Shao J, Hong S, et al. Costunolide covalently targets NACHT domain of NLRP3 to inhibit inflammasome activation and alleviate NLRP3-driven inflammatory diseases. Acta Pharm Sinica B. (2023) 13:678–93. doi: 10.1016/j.apsb.2022.09.014
163. Jabalpurwala FA, Smoot JM, Rouseff RL. A comparison of citrus blossom volatiles. Phytochemistry. (2009) 70:1428–34. doi: 10.1016/j.phytochem.2009.07.031
164. Alruhaimi RS, Hassanein EHM, Ahmeda AF, Atwa AM, Alnasser SM, Sayed GA, et al. Farnesol attenuates cadmium-induced kidney injury by mitigating oxidative stress, inflammation and necroptosis and upregulating cytoglobin and PPARγ in rats. Tissue Cell. (2024) 90:102526. doi: 10.1016/j.tice.2024.102526
165. Yuan Y, Wu D, Chen H, Ma Z, Peng X, Li X, et al. Farnesol ameliorates DSS-induced IBD by regulating inflammatory cytokines, repairing the intestinal barrier, reversing the gut microbiota imbalance, and influencing fecal metabolome in C57BL/6 mice. Biomed Pharmacother. (2024) 180:117518. doi: 10.1016/j.biopha.2024.117518
166. Wang C, Liu H, Li Z, Yang Q, Wang Q, Yang T, et al. Oleanolic acid 28-O-β-D-glucopyranoside: A novel therapeutic agent against ulcerative colitis via anti-inflammatory, barrier-preservation, and gut microbiota-modulation. Biomed pharmacother = Biomed pharmacother. (2024) 180:117534. doi: 10.1016/j.biopha.2024.117534
167. Wang M, Xu B, Liu L, Wang D. Oridonin attenuates dextran sulfate sodium−induced ulcerative colitis in mice via the Sirt1/NF−κB/p53 pathway. Mol Med Rep. (2022) 26:312. doi: 10.3892/mmr.2022.12828
168. Sheng Q, Li F, Chen G, Li J, Li J, Wang Y, et al. Ursolic acid regulates intestinal microbiota and inflammatory cell infiltration to prevent ulcerative colitis. J Immunol Res. (2021) 2021:6679316. doi: 10.1155/2021/6679316
169. Hou J, Hu M, Zhang L, Gao Y, Ma L, Xu Q. Dietary taxifolin protects against dextran sulfate sodium-induced colitis via NF-κB signaling, enhancing intestinal barrier and modulating gut microbiota. Front Immunol. (2020) 11:631809. doi: 10.3389/fimmu.2020.631809
170. Gil-Cardoso K, Ginés I, Pinent M, Ardévol A, Blay M, Terra X. Effects of flavonoids on intestinal inflammation, barrier integrity and changes in gut microbiota during diet-induced obesity. Nutr Res Rev. (2016) 29:234–48. doi: 10.1017/S0954422416000159
171. Anhê FF, Roy D, Pilon G, Dudonné S, Matamoros S, Varin TV, et al. A polyphenol-rich cranberry extract protects from diet-induced obesity, insulin resistance and intestinal inflammation in association with increased Akkermansia spp. population in the gut microbiota of mice. Gut. (2015) 64:872–83. doi: 10.1136/gutjnl-2014-307142
172. Everard A, Belzer C, Geurts L, Ouwerkerk JP, Druart C, Bindels LB, et al. Cross-talk between Akkermansia muciniphila and intestinal epithelium controls diet-induced obesity. Proc Natl Acad Sci United States America. (2013) 110:9066–71. doi: 10.1073/pnas.1219451110
173. Nepali S, Son JS, Poudel B, Lee JH, Lee YM, Kim DK. Luteolin is a bioflavonoid that attenuates adipocyte-derived inflammatory responses via suppression of nuclear factor-κB/mitogen-activated protein kinases pathway. Pharmacogn mag. (2015) 11:627–35. doi: 10.4103/0973-1296.160470
174. Lin P, Tian XH, Yi YS, Jiang WS, Zhou YJ, Cheng WJ. Luteolin-induced protection of H2O2-induced apoptosis in PC12 cells and the associated pathway. Mol Med reports. (2015) 12:7699–704. doi: 10.3892/mmr.2015.4400
175. Kim JS, Jobin C. The flavonoid luteolin prevents lipopolysaccharide-induced NF-kappaB signalling and gene expression by blocking IkappaB kinase activity in intestinal epithelial cells and bone-marrow derived dendritic cells. Immunology. (2005) 115:375–87. doi: 10.1111/j.1365-2567.2005.02156.x
176. Dou W, Zhang J, Sun A, Zhang E, Ding L, Mukherjee S, et al. Protective effect of naringenin against experimental colitis via suppression of Toll-like receptor 4/NF-κB signalling. Br J nutrition. (2013) 110:599–608. doi: 10.1017/S0007114512005594
177. Terra X, Valls J, Vitrac X, Mérrillon JM, Arola L, Ardèvol A, et al. Grape-seed procyanidins act as antiinflammatory agents in endotoxin-stimulated RAW 264.7 macrophages by inhibiting NFkB signaling pathway. J Agric Food Chem. (2007) 55:4357–65. doi: 10.1021/jf0633185
178. Adams LS, Seeram NP, Aggarwal BB, Takada Y, Sand D, Heber D. Pomegranate juice, total pomegranate ellagitannins, and punicalagin suppress inflammatory cell signaling in colon cancer cells. J Agric Food Chem. (2006) 54:980–5. doi: 10.1021/jf052005r
179. Nunes C, Ferreira E, Freitas V, Almeida L, Barbosa RM, Laranjinha J. Intestinal anti-inflammatory activity of red wine extract: unveiling the mechanisms in colonic epithelial cells. Food Funct. (2013) 4:373–83. doi: 10.1039/C2FO30233K
180. Castagnini C, Luceri C, Toti S, Bigagli E, Caderni G, Femia AP, et al. Reduction of colonic inflammation in HLA-B27 transgenic rats by feeding Marie Ménard apples, rich in polyphenols. Br J nutrition. (2009) 102:1620–8. doi: 10.1017/S0007114509990936
181. Larrosa M, Luceri C, Vivoli E, Pagliuca C, Lodovici M, Moneti G, et al. Polyphenol metabolites from colonic microbiota exert anti-inflammatory activity on different inflammation models. Mol Nutr Food Res. (2009) 53:1044–54. doi: 10.1002/mnfr.200800446
182. Zhao Y, Nakatsu C, Jones-Hall Y, Jiang Q. Supplementation of polyphenol-rich grapes attenuates colitis, colitis-associated colon cancer, and disease-associated dysbiosis in mice, but fails to mitigate colitis in antibiotic-treated mice. J Nutr Biochem. (2022) 109:109124. doi: 10.1016/j.jnutbio.2022.109124
183. Duan W, Zheng B, Li T, Liu R. Gut microbiota and metabolites mediate health benefits of oat and oat bran consumption in IBD mice. Nutrients. (2024) 16:4365. doi: 10.3390/nu16244365
184. Ju S, Ge Y, Li P, Tian X, Wang H, Zheng X, et al. Dietary quercetin ameliorates experimental colitis in mouse by remodeling the function of colonic macrophages via a heme oxygenase-1-dependent pathway. Cell Cycle (Georgetown Tex.). (2018) 17:53–63. doi: 10.4103/0973-1296.160470
185. Taha MM, Salga MS, Ali HM, Abdulla MA, Abdelwahab SI, Hadi AH. Gastroprotective activities of Turnera diffusa Willd. ex Schult. revisited: Role of arbutin. J ethnopharmacol. (2012) 141:273–81. doi: 10.1016/j.jep.2012.02.030
186. Zhou W, Chen K, Lu Q, Luo Y, Zhang C, Zheng Y, et al. The protective effect of rosavin from rhodiola rosea on radiation-induced intestinal injury. Chem biodivers. (2020) 17:e2000652. doi: 10.1002/cbdv.202000652
187. Zhang C, Zhu H, Jie H, Ding H, Sun H. Arbutin ameliorated ulcerative colitis of mice induced by dextran sodium sulfate (DSS). Bioengineered. (2021) 12:11707–15. doi: 10.1080/21655979.2021.2005746
188. Qu Y, Li X, Xu F, Zhao S, Wu X, Wang Y, et al. Kaempferol alleviates murine experimental colitis by restoring gut microbiota and inhibiting the LPS-TLR4-NF-κB axis. Front Immunol. (2021) 12:679897. doi: 10.3389/fimmu.2021.679897
189. Peng L, Gao X, Nie L, Xie J, Dai T, Shi C, et al. Astragalin attenuates dextran sulfate sodium (DSS)-induced acute experimental colitis by alleviating gut microbiota dysbiosis and inhibiting NF-κB activation in mice. Front Immunol. (2020) 11:2058. doi: 10.3389/fimmu.2020.02058
190. Zhang J, Cao L, Sun Y, Qing DG, Xu XQ, Wang JC, et al. The regulatory effects of licochalcone A on the intestinal epithelium and gut microbiota in murine colitis. Mol (Basel Switzerland). (2021) 26:4149. doi: 10.3390/molecules26144149
191. Zheng R, Ma J, Wang D, Dong W, Wang S, Liu T, et al. Chemopreventive effects of silibinin on colitis-associated tumorigenesis by inhibiting IL-6/STAT3 signaling pathway. Mediators inflamm. (2018) 2018:1562010. doi: 10.1155/2018/1562010
192. Cui M, Yang WM, Yao P. Protective effect of low-dose lactulose in dextran sulfate sodium induced ulcerative colitis model of rats. Sci reports. (2025) 15:2760. doi: 10.1038/s41598-025-93098-y
193. Chen M, Wei S, Wu X, Xiang Z, Li X, He H, et al. 2’-hydroxycinnamaldehyde alleviates intestinal inflammation by attenuating intestinal mucosal barrier damage via directly inhibiting STAT3. Inflamm bowel dis. (2024) 30:992–1008. doi: 10.1093/ibd/izad283
194. Ni J, Zhang L, Feng G, Bao W, Wang Y, Huang Y, et al. Vanillic acid restores homeostasis of intestinal epithelium in colitis through inhibiting CA9/STIM1-mediated ferroptosis. Pharmacol Res. (2024) 202:107128. doi: 10.1016/j.phrs.2024.107128
195. Ozaka S, Sonoda A, Kudo Y, Ito K, Kamiyama N, Sachi N, et al. Daikenchuto, a Japanese herbal medicine, ameliorates experimental colitis in a murine model by inducing secretory leukocyte protease inhibitor and modulating the gut microbiota. Front Immunol. (2024) 15:1457562. doi: 10.3389/fimmu.2024.1457562
196. Beisner J, Filipe Rosa L, Kaden-Volynets V, Stolzer I, Günther C, Bischoff SC. Prebiotic inulin and sodium butyrate attenuate obesity-induced intestinal barrier dysfunction by induction of antimicrobial peptides. Front Immunol. (2021) 12:678360. doi: 10.3389/fimmu.2021.678360
197. Akram W, Garud N, Joshi R. Role of inulin as prebiotics on inflammatory bowel disease. Drug discover Ther. (2019) 13:1–8. doi: 10.5582/ddt.2019.01000
198. Myhill LJ, Stolzenbach S, Hansen TVA, Skovgaard K, Stensvold CR, Andersen LO, et al. Mucosal barrier and th2 immune responses are enhanced by dietary inulin in pigs infected with trichuris suis. Front Immunol. (2018) 9:2557. doi: 10.3389/fimmu.2018.02557
199. Han J, Zhao Q, Basmadjian C, Désaubry L, Theiss AL. Flavaglines ameliorate experimental colitis and protect against intestinal epithelial cell apoptosis and mitochondrial dysfunction. Inflamm bowel dis. (2016) 22:55–67. doi: 10.1097/MIB.0000000000000592
200. Zhou Y, Xiong X, Cheng Z, Chen Z, Wu S, Yu Y, et al. Ginsenoside rb1 alleviates DSS-induced ulcerative colitis by protecting the intestinal barrier through the signal network of VDR, PPARγ and NF-κB. Drug design Dev Ther. (2024) 18:4825–38. doi: 10.2147/DDDT.S481769
201. Shiraishi E, Iijima H, Shinzaki S, Nakajima S, Inoue T, Hiyama S, et al. Vitamin K deficiency leads to exacerbation of murine dextran sulfate sodium-induced colitis. J gastroenterol. (2016) 51:346–56. doi: 10.1007/s00535-015-1112-x
202. Hu S, Ma Y, Xiong K, Wang Y, Liu Y, Sun Y, et al. Ameliorating effects of vitamin K2 on dextran sulfate sodium-induced ulcerative colitis in mice. Int J Mol Sci. (2023) 24:1986. doi: 10.3390/ijms24032986
203. Wu H, Chen QY, Wang WZ, Chu S, Liu XX, Liu YJ, et al. Compound sophorae decoction enhances intestinal barrier function of dextran sodium sulfate induced colitis via regulating notch signaling pathway in mice. Biomed pharmacother = Biomed pharmacother. (2021) 133:110937. doi: 10.1016/j.biopha.2020.110937
204. Lin JC, Wu JQ, Wang F, Tang FY, Sun J, Xu B, et al. QingBai decoction regulates intestinal permeability of dextran sulphate sodium-induced colitis through the modulation of notch and NF-κB signalling. Cell prolifer. (2019) 52:e12547. doi: 10.1111/cpr.2019.52.issue-2
205. Zhao H, Zhou Y, Xu J, Zhang Y, Wang H, Zhao C, et al. Short-chain fatty acid-producing bacterial strains attenuate experimental ulcerative colitis by promoting M2 macrophage polarization via JAK/STAT3/FOXO3 axis inactivation. J Trans med. (2024) 22:369. doi: 10.1186/s12967-024-05122-w
206. Zhuang J, Zhuang Z, Chen B, Yang Y, Chen H, Guan G. Odoribacter splanchnicus-derived extracellular vesicles alleviate inflammatory bowel disease by modulating gastrointestinal inflammation and intestinal barrier function via the NLRP3 inflammasome suppression. Mol Med (Cambridge Mass.). (2025) 31:56. doi: 10.1186/s10020-025-01063-2
207. Yue N, Zhao H, Hu P, Zhang Y, Tian C, Kong C, et al. Real-world of Limosilactobacillus reuteri in mitigation of acute experimental colitis. J nanobiotechnol. (2025) 23:65. doi: 10.1186/s12951-025-03158-8
208. Cui X, Cheng Y, Wang H, Li X, Li J, Zhang K, et al. Hymenolepis nana antigens alleviate ulcerative colitis by promoting intestinal stem cell proliferation and differentiation via AhR/IL-22 signaling pathway. PloS neglect Trop dis. (2024) 18:e0012714. doi: 10.1371/journal.pntd.0012714
209. Mo LH, Luo XQ, Yang G, Liu JQ, Yang LT, Liu ZQ, et al. Epithelial cell-derived CD83 restores immune tolerance in the airway mucosa by inducing regulatory T-cell differentiation. Immunology. (2021) 163:310–22. doi: 10.1111/imm.v163.3
210. Ferreira AS, Macedo C, Silva AM, Delerue-Matos C, Costa P, Rodrigues F. Natural products for the prevention and treatment of oral mucositis—A review. Int J Mol Sci. (2022) 23:4385. doi: 10.3390/ijms23084385
211. Dineen R, Stewart PM, Sherlock M. Factors impacting on the action of glucocorticoids in patients receiving glucocorticoid therapy. Clin Endocrinol. (2018) 90:3–14. doi: 10.1111/cen.2019.90.issue-1
212. Dey P. Targeting gut barrier dysfunction with phytotherapies.pdf. Pharmacol Res. (2020) 161:105135. doi: 10.1016/j.phrs.2020.105135
Keywords: mucous barrier, natural products, immune regulation, inflammation inhibition, gut microbiota modulation
Citation: Lin W, Ruishi X, Caijiao X, Haoming L, Xuefeng H, Jiyou Y, Minqiang L, Shuo Z, Ming Z, Dongyang L and Xiaoxue F (2025) Potential applications and mechanisms of natural products in mucosal-related diseases. Front. Immunol. 16:1594224. doi: 10.3389/fimmu.2025.1594224
Received: 15 March 2025; Accepted: 09 April 2025;
Published: 30 April 2025.
Edited by:
Yi Wu, Yunnan Agricultural University, ChinaReviewed by:
Haifeng Wang, Shenyang Pharmaceutical University, ChinaLili Yang, Nanjing University of Chinese Medicine, China
Copyright © 2025 Lin, Ruishi, Caijiao, Haoming, Xuefeng, Jiyou, Minqiang, Shuo, Ming, Dongyang and Xiaoxue. This is an open-access article distributed under the terms of the Creative Commons Attribution License (CC BY). The use, distribution or reproduction in other forums is permitted, provided the original author(s) and the copyright owner(s) are credited and that the original publication in this journal is cited, in accordance with accepted academic practice. No use, distribution or reproduction is permitted which does not comply with these terms.
*Correspondence: Xie Ruishi, MTM2MzAzMDUwOTZAMTYzLmNvbQ==; Li Dongyang, bDE1MTg5ODAwODAzQDEyNi5jb20=; Fang Xiaoxue, ZmFuZ3hpYW94dWUxOTk2QDE2My5jb20=