- 1Department of Pathology, Affiliated Cancer Hospital of Dalian University of Technology (Liaoning Cancer Hospital and Institute, Cancer Hospital of China Medical University), Shenyang, China
- 2Department of Gastric Surgery, Affiliated Cancer Hospital of Dalian University of Technology (Liaoning Cancer Hospital and Institute, Cancer Hospital of China Medical University), Shenyang, China
- 3Department of Gynecology, Affiliated Cancer Hospital of Dalian University of Technology (Liaoning Cancer Hospital and Institute, Cancer Hospital of China Medical University), Shenyang, China
- 4Graduate School, Dalian Medical University, Dalian, China
- 5Department of Gastroenterology, Affiliated Cancer Hospital of Dalian University of Technology (Liaoning Cancer Hospital and Institute, Cancer Hospital of China Medical University), Shenyang, China
Gastric cancer (GC) is one of the primary contributors to cancer-related mortality on a global scale. It holds a position within the top five most prevalent malignancies both in terms of occurrence and fatality rates. Immunotherapy, as a breakthrough cancer treatment, brings new hope for GC patients. Various biomarkers, such as the expression of programmed death ligand-1 (PD-L1), the microsatellite instability (MSI) status, tumor mutational burden (TMB), and Epstein–Barr virus (EBV) infection, demonstrate potential to predict the effectiveness of immunotherapy in treating GC. Nevertheless, each biomarker has its own limitations, which leads to a significant portion of patients continue to be unresponsive to immunotherapy. With the understanding of the tumor immune microenvironment (TIME), genome sequencing technology, and recent advances in molecular biology, new molecular markers, such as POLE/POLD1mutations, circulating tumor DNA, intestinal flora, lymphocyte activation gene 3 (LAG-3), and lipid metabolism have emerged. This review aims to consolidate clinical evidence to offer a thorough comprehension of the existing and emerging biomarkers. We discuss the mechanisms, prospects of application, and limitations of each biomarker. We anticipate that this review will open avenues for fresh perspectives in the investigation of GC immunotherapy biomarkers and promote the precise choice of treatment modalities for gastric cancer patients, thereby advancing precision immuno-oncology endeavors.
1 Introduction
Gastric cancer (GC) is prevalent worldwide, consistently ranking among the top five most lethal cancers in terms of both occurrence and fatality rates (1). National cancer statistics released by China’s National Cancer Centre show that in the year 2022, GC accounted for 358,700 new cases, with a mortality rate of 26.04 per 100,000 in China (2). Early GC can be effectively treated after surgery and the five-year survival rate can reach 90 to 100%. Nonetheless, owing to the subtle onset and rapid progression of the illness, almost all GC patients are diagnosed at an advanced stage. Traditional therapy for advanced GC typically involves chemotherapy. However, the clinical benefits of these therapies remain severely constrained. The median overall survival (OS) with conventional chemotherapy in advanced GC is merely 8 months (3).
The advent of immune checkpoint inhibitors (ICIs) presents novel therapeutic avenues for solid tumors that have notably enhanced cure rates. This strategy finds strong support in the scientific community as it is based on the principle that ICIs primarily activate the body’s immune cells to target tumor cells, thereby acting to either remove these or reduce the tumor cell load (4). Currently, immune combination therapy is gradually becoming a treatment modality for GC, of which the most commonly used is the combination of chemotherapy and ICIs. Research has reported the safety and efficacy of ICIs in neoadjuvant therapy for GC (5); their application is gradually expanding from back-line to first-line therapy. Currently, biomarkers, including programmed death ligand 1 (PD-L1), the microsatellite instability (MSI) status, Epstein–Barr virus (EBV) infection and tumor mutational burden (TMB), are often applied clinically to predict immunotherapy efficacy (Figure 1). However, a considerable portion of patients fail to derive benefits from immunotherapy and encounter adverse effects linked with its use. Therefore, further research on biomarkers related to GC immunotherapy is particularly important for the accurate selection of appropriate cohorts for this treatment.
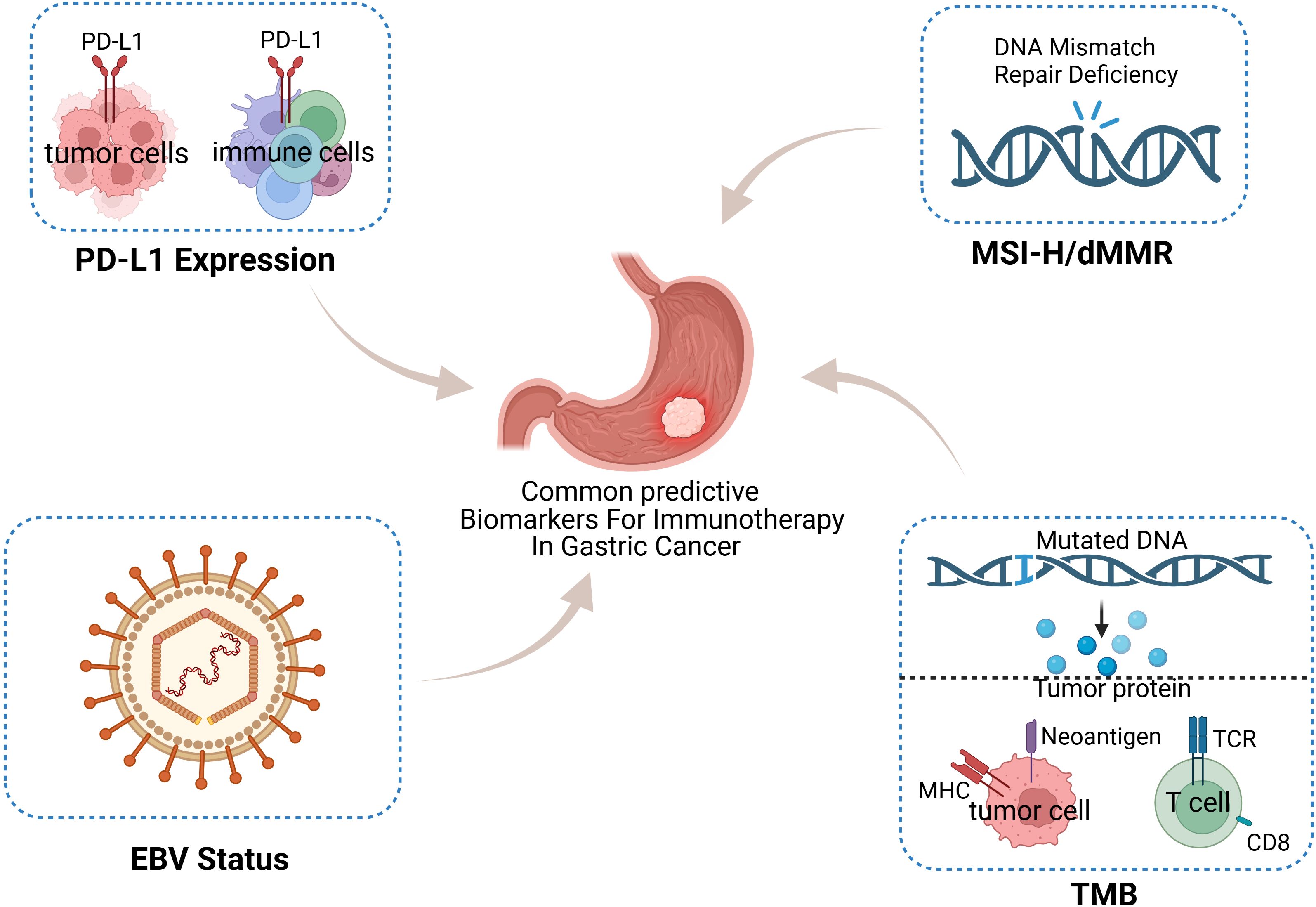
Figure 1. Classic biomarkers predicting the efficacy of neoadjuvant immunotherapy for gastric cancer (GC) include programmed death ligand 1 (PD-L1), the microsatellite instability (MSI) status, Epstein–Barr virus (EBV) infection and tumor mutational burden (TMB).
The current clinical evidence, mechanisms and limitations of existing biomarkers for predicting immunotherapy efficacy are discussed. This review aims to open avenues for new directions in research on biomarkers for GC immunotherapy.
2 Positive biomarkers
2.1 Programmed death ligand 1
PD-L1 is overexpressed in 25-65% of GC. In general, the PD-L1/PD-1 axis is recognized as an important factor for the poor prognosis of patients with different GC types. Firstly, PD-L1 expressed on GC cell surfaces inhibit anti-tumor immunity via multiple pathways. For example, over-expression of PD-L1 prevents the body’s immune system from recognizing GC cells; effector T cells are unable to target GC cells, leading to suppressing the anti-tumor immune response (6–8). Secondly, PD-L1 also binds to the epidermal growth factor receptor (EGFR) and activates it to promote GC progression (9). In addition to GC cells, PD-L1 is highly expressed on immune cell surfaces in the tumor immune microenvironment, including lymphocytes, neutrophils, macrophages, and mast cells (10).
2.1.1 PD-L1 as an effective predictive marker
CPS (combined positive score) is defined as the ratio of PD-L1-positive tumor cells and immune cells to the total number of viable tumor cells. TAP (tumor area positivity) refers to the proportion of PD-L1-positive tumor and immune cell area relative to the total tumor area (viable tumor cells + stroma) (11). These two metrics are currently the most widely used quantitative indicators for assessing PD-L1 expression levels in clinical and research settings. While PD-L1 expression demonstrates a positive association with response to ICIs, the optimal predictive thresholds (CPS/TAP) for clinical benefit continue to be debated. For example, in the KEYNOTE-059 Cohort 1 trial, patients with PD-L1-positive (CPS ≥ 1) G/GEJ adenocarcinoma who received pembrolizumab monotherapy showed significantly higher objective response rates (ORR) and improved overall survival (OS) compared to PD-L1-negative patients (CPS < 1) (12). Promising follow-up data from the CheckMate-649 trial were presented at the 2024 ASCO GI Conference: patients with CPS ≥ 5 who received nivolumab in combination with chemotherapy continued to demonstrate clinically meaningful improvements in OS and progression-free survival (PFS) compared to those receiving chemotherapy alone in the randomized cohort (13). Additionally, for GC patients with TAP ≥ 5%, those treated with tislelizumab in combination with chemotherapy achieved longer median PFS, median OS, and duration of response (DoR) compared to those receiving placebo plus chemotherapy (14). Furthermore, in the KEYNOTE-062 trial, in patients with CPS ≥ 10, pembrolizumab monotherapy demonstrated significantly longer median OS compared to chemotherapy alone. However, when using a CPS cutoff of 1, no significant survival benefit was observed between the treatment groups (15). PD-1 inhibitors used in Phase III clinical trials are shown (12–20) (Table 1). Phase II and I clinical trials are shown (12, 21–38) (Table 2).
Initial studies have demonstrated the potential predictive value of PD-L1 expression thresholds (CPS ≥ 1, CPS ≥ 5, TAP ≥ 5%, and CPS ≥ 10) for immunotherapy efficacy. However, due to variations in patient cohort sizes and differences in study endpoint definitions across clinical trials, further large-scale prospective studies are needed to optimize these cutoff values and improve their predictive accuracy and consistency.
2.1.2 Mechanism
The mechanism by which PD-1 inhibitors can effectively kill tumor cells is closely related to the TIME. Wei et al. found that patients with GC treated with chemotherapy combination and neoadjuvant PD-1 inhibitors had increased CD8+ T-cell counts and M1/M2 macrophage ratios (39). Using a GC mouse model, Tang et al. demonstrated that mice achieving a major response to neoadjuvant PD-1 inhibitors plus chemotherapy exhibited significant infiltration of anti-tumor immune cells, including CD8+ CD44+ CD62L- effector T cells and CD8+ T cells, along with a high M1/M2 macrophage ratio (40). Avgustinovich et al. found that GC patients with CPS ≥ 10 who were sensitive to neoadjuvant immune-combination chemotherapy showed activation of autophagy, which is involved in the activation of tumor immunity (41, 42). Autophagy can not only directly down-regulate PD-L1 through the p62/SQSTM1-NF-κB pathway (43), but also down-regulate PD-L1 by reducing the expression of histone deacetylase (HDAC) (44), participating in the activation of tumor immunity. Therefore, activation of autophagy may be an important factor for their sensitivity to immunotherapy.
While most clinical trials have demonstrated that gastric/gastroesophageal junction cancer (G/GEJC) patients with high PD-L1 expression benefit from immunotherapy, a minority of studies have failed to achieve satisfactory outcomes. In KEYNOTE-061 (CPS ≥ 1), pembrolizumab failed to improve OS as second-line therapy for advanced G/GEJC than paclitaxel (19). Similarly, avelumab (JAVELIN Gastric 100) (18)and nivolumab (ATTRACTION-4) (17)showed no OS/PFS benefits over chemotherapy in later-line settings. Therefore, it is essential to investigate the mechanisms underlying resistance to ICIs in GC.
There is little exploration on the mechanism of resistance to PD-1 inhibitor, and it has been clear that differences in immune cells in TME are related to PD-1 inhibitor resistance. In high-CPS G/GEJ adenocarcinoma patients failing to respond to therapy, Verschoor et al. found a low degree of CD8+ PD-1+ T-cell infiltration, as well as a TME with high regulatory T (Treg) cell infiltration, which may be the cause of immune checkpoint blocking (ICB) treatment failure (21). Of course, there are even worse cases. Approximately 10% of patients with GC receiving PD-1 blockade progress rapidly (45), to hyper-progressive disease, which is related to Treg cell proliferation in tumor tissue (46), an increase in immunosuppressive CD4+ T cell subset that hinders effective anti-tumor immunity (47–52). In addition to differences in immune-infiltrating cells in TME, the spatiotemporal heterogeneity of PD-L1 expression (53, 54), the accuracy of PD-L1 assessment, including sample collection and assay methodology (55), and the complexity of the immunotherapy mechanism (56) can lead to the failure of PD-L1 to effectively predict immunotherapy efficacy. Therefore, more research is required to explore immunotherapy resistance mechanisms in GC patients and identify suitable alternative strategies. Current studies have identified USP7 inhibitors, which are new anti-proliferative agents that inhibit GC cell proliferation and down-regulate PD-L1 expression both in vivo and in vitro (57). Thus, with more research, more targets could be developed to overcome immunotherapy-related drug resistance (Figure 2).
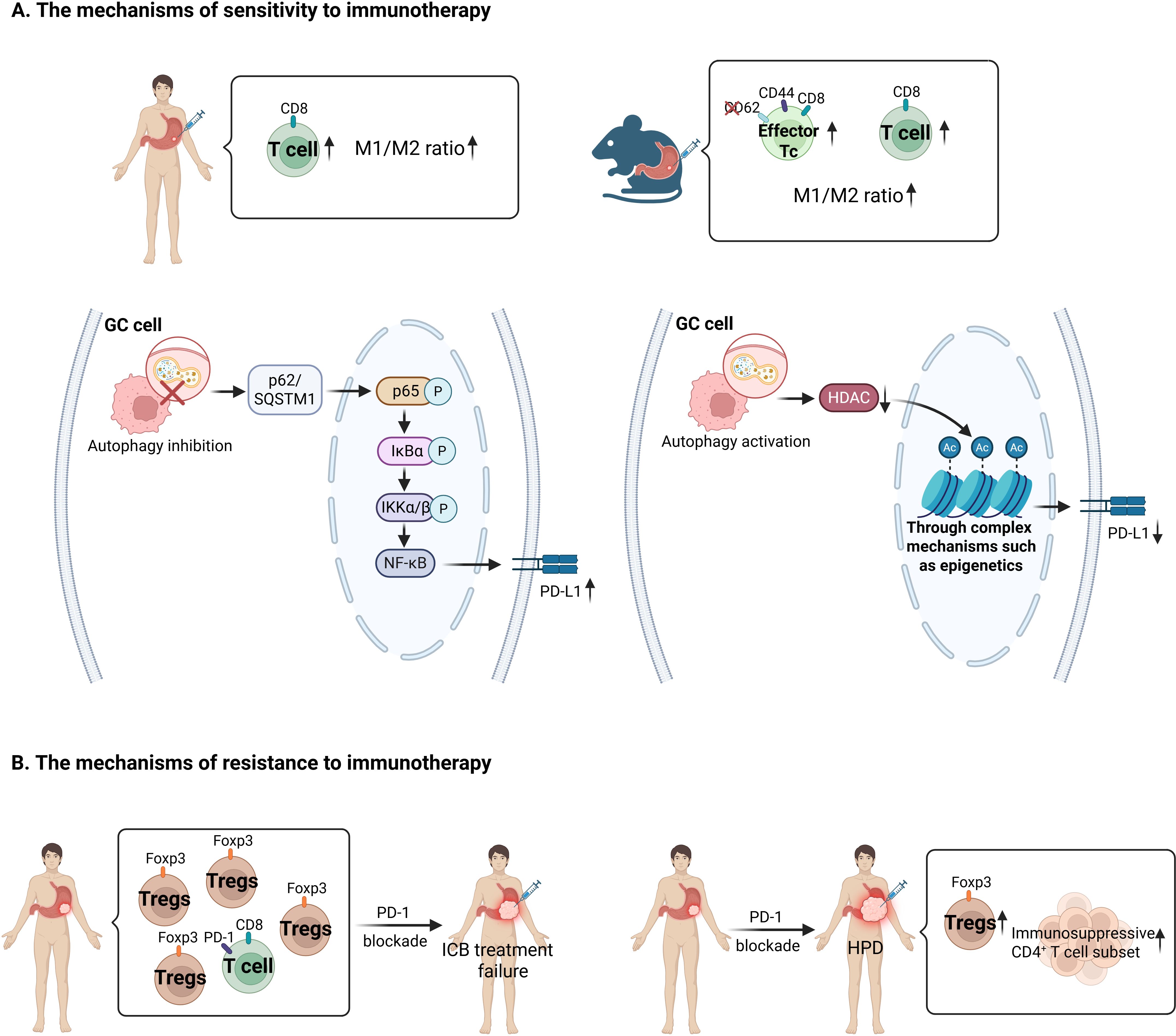
Figure 2. A summary diagram illustrating PD-L1-related immune sensitivity and resistance mechanisms in GC. (A) The mechanisms of sensitivity to immunotherapy. A favorable response to neoadjuvant PD-1 inhibitor combined with chemotherapy is associated with increased infiltration of CD8+ T cells and CD8+ CD44+ CD62L- effector T cells, as well as an elevated M1/M2 macrophage ratio. Autophagy regulates PD-L1 expression in cancer cells. Autophagy inhibition leads to upregulation of p62/SQSTM1, which translocates into the nucleus and then activates NF-κB signaling pathway, ultimately upregulating PD-L1 expression. Autophagy activation attenuates PD-L1 expression via histone deacetylase (HDAC) downregulation, with subsequent complex mechanism such as epigenetic remodeling. (B) The mechanisms of resistance to immunotherapy. Low pre-treatment levels of CD8+ PD-1+ T-cell infiltration and high levels of Treg cell infiltration may contribute to immunotherapy failure. Patients exhibiting hyper-progressive disease (HPD) following immunotherapy demonstrate increased Treg cell infiltration and expansion of immunosuppressive CD4+ T-cell subsets within tumor tissues.
2.2 Microsatellite instability–high/deficient mismatch repair
Microsatellite instability–high/deficient mismatch repair (MSI-H/dMMR) represents a specific subtype of tumor that accounts for 8–10% of GC characterized by high tumor immunogenicity and dense immune cell infiltration (58, 59). Given relatively low MSI-H GC incidences, no targeted Phase III clinical trials have been conducted. As ICIs have revolutionized the therapy of MSI-H/dMMR malignancies, their potential in neoadjuvant therapy is receiving more attention. MSI-H GC, characterized by defective DNA mismatch repair, exhibits a high frequency of somatic mutations, leading to the generation of abundant neoantigens (60). These neoantigens can activate robust anti-tumor T-cell responses and are associated with increased infiltration of anti-tumor immune cells within the tumor microenvironment, thereby rendering MSI-H tumors particularly sensitive to ICIs (61).
2.2.1 Advanced unresectable G/GEJ adenocarcinoma
In the KEYNOTE-062 trial, a subgroup analysis of 7% of patients with MSI-H G/GEJ adenocarcinoma (15) showed that ORR and PFS outcomes in patients receiving pembrolizumab alone and pembrolizumab combined chemotherapy were significantly better than those receiving chemotherapy alone (62). An analysis of 3% of 1581 advanced unresectable HER2-negative GC/GEJC patients (with MSI-H status) in the CheckMate649 study showed that regardless of PD-L1 expression status, MSI-H subgroup patients had higher OS benefits when compared to patients who had chemotherapy alone (63). KEYNOTE-061 is a phase III clinical trial where randomized patients with advanced unresectable G/GEJC were evaluated in terms of pembrolizumab effectiveness and safety as a second-line treatment. An analysis of 27 patients (5.3%) with MSI-H status showed that (62) when compared to the chemotherapy group, median PFS and ORR outcomes in the pembrolizumab monotherapy group were significantly improved. By comparing early survival curves in patients with MSI-H tumors treated with pembrolizumab with patients with MSI-H tumors treated with chemotherapy alone in KEYNOTE-061 and KEYNOTE-062 trials, respectively (15), early pembrolizumab administration in GC patients with the MSI-H subtype gained benefits. Marabelle et al., examined the efficacy and safety profiles of second-line pembrolizumab in 223 patients with advanced MSI-H/dMMR cancer and failed to respond to chemotherapy, of whom, 10.3% had GC. The ORR in patients with MSI-H/dMMR GC was 45.8%, which was higher than the overall 34.3% ORR; the number of patients with complete responses (4) was second only to endometrial cancer. More importantly, tumor responses were long-lasting, with > 75% of respondents showing durable responses of ≥ 24 months in Kaplan–Meier analyses (30). In terms of third-line treatment results in patients with MSI-H G/GEJ adenocarcinoma: 7/259 patients registered in the KEYNOTE-059 cohort 1 had MSI-H GC and received pembrolizumab monotherapy (62). When compared to non-MSI-H patients, the ORR was higher in these seven patients (57.1% versus 9.0%) (64). One patient in Cohort 3 had an MSI-H tumor and experienced a partial response (12). In the CheckMate032 trial, the proportion of patients with MSI-H status (28%) was higher in the NIV03 group when compared to any combination group (NIVO1 + IPI3: MSI-H, 9% or NIV03 + IPI1: MSI-H, 8%). This was possibly why the median OS across groups was not very different. Also, by comparing MSI-H with non-MSI-H patients in groups, the results found that whether it is ORR, 12-month OS rate, or 18-month OS rate, the values of MSI-H patients are better (24).
2.2.2 Locally progressive dMMR/MSI-H G/GEJ adenocarcinoma (neoadjuvant)
Pembrolizumab, ipilimumab, nivolumab, and atezolizumab also demonstrated favorable clinical activity in patients with resectable dMMR/MSI-H G/GEJ adenocarcinoma. Building on previous KEYNOTE-062 data, Liu et al., incorporated pembrolizumab into neoadjuvant chemotherapy to treat six patients with advanced non-metastatic MSI-H GC, and all patients showed good pathological responses (65). In another Phase II clinical trial (NCT02918162), three patients with advanced resectable G/GEJ who received pembrolizumab in combination with chemotherapy had an MSI-H status (33). One patient achieved pathological complete response (pCR). Based on previous CheckMate649 data, the recent NEONIPIGA trial treated patients with locally advanced dMMR/MSI-H G/GEJ adenocarcinoma with neoadjuvant ipilimumab + nivolumab during the perioperative period (27). Of the 29 patients who received an R0 resection, 17 (58.6%) achieved pCR (pathological T0N0) and had no unexpected immune-related adverse events, postoperative morbidity, or death. The other four with locally advanced resectable MSI-H G/GEJ adenocarcinoma who received neoadjuvant ipilimumab + nivolumab had pathological reactions, three achieved pCR, and the other was confirmed with a reduced the tumor-node-metastasis (TNM) stage (27). In the DANTE study (26), the pCR rate in patients with MSI-H GC treated with the 5-fluorouracil + leucovorin + oxaliplatin + docetaxel (FLOT) and atezolizumab (ATZ) (63%) combination was significantly better versus patients treated with FLOT (27%). In the PANDA trial, patients with treatment-naïve resectable G/GEJ tumors (n = 20) with neoadjuvant treatment with atezolizumab in combination with chemotherapy and subsequent surgical resection. Both dMMR patients in this trial achieved pCR (21).
A number of other PD-1 inhibitors are available as combinations of neoadjuvant chemotherapy for people with resectable G/GEJ adenocarcinoma with an MSI-H/dMMR status. Two (5.6%) patients with a dMMR were included in a phase II clinical trial (NCT04065282) aimed to evaluate sintilimab plus chemotherapy (CapeOx) as a neoadjuvant therapy protocol for people with advanced resectable G/GEJ adenocarcinomas (35). In one case their CPS = 68; this patient received a pCR. Another individual, whose PD-L1 expression status was unknown, did not exhibit major pathological response (MPR). A phase II trial investigating the efficacy of camrelizumab + FLOFOX (oxaliplatin+calcium folinate+5-fluorouracil) (NCT03939962) included cases with locally advanced resectable GC/GEJ adenocarcinoma, including one patient with an MSI-H status who achieved a pCR (66).
Limited research exists on the mechanisms of efficacy of immunotherapy for MSI-H tumors. One study applied an exploratory multiple fluorescence analysis to show that in patients with MSI-H, immune-combination chemotherapy increased tumor CD3+ and CD8+ T-cell densities, and that the degree of a pathological response was associated with increased clustering of CD3+ cells to panCK+ cells (33). Kwon et al., reported that increased PD-1+ CD8+ T cells were associated with lasting therapeutic benefit in patients with MSI-HGC (67). Additionally, patients with up-regulated PD-L1 expression in MSI-H GC showed a good clinical prognosis (68–72). But approximately 50% of patients with MSI-H tumors also exhibit intrinsic resistance to PD-1 inhibitors. Ongoing research is actively investigating primary resistance to ICIs in people with advanced MSI-H GC/GEJC. Evidence from the KEYNOTE-059, -061, and -062 trials indicates that the loss of heterogeneity in mismatch repair enzymes within tumors may underlie diminished response to treatment with pembrolizumab alone (73). Future studies should integrate multi-omics approaches (e.g., single-cell sequencing, spatial transcriptomics) with functional experiments to systematically uncover immunotherapy resistance mechanisms in MSI-H GC.
2.3 EBV
Epstein-Barr virus–associated GC (EBVaGC) accounts for 2-10% of all GC cases (74). Epstein-Barr virus (EBV) can cause a local immune response (75, 76). Analysis of tumor genomic profiles shows that EBV+ tumors are often microsatellite-stable (MSS) (77). Evidence exists of a low tumor mutational burden and stronger immune infiltration in EBV+ tumors compared to MSI-H tumors. In addition, EBV+ tumors showed higher expression of immune checkpoint pathway (PD-1, CTLA-4) genes and higher infiltration of lymphocytes (e.g., follicular helper T cells and CD8+ T cells (78)) in their RNA sequence data compared to MSS tumors. Therefore, the use of anti-PD-1 and anti–CTLA-4 monoclonal antibodies in a neoadjuvant setting is possible for patients with EBV+ GC.
2.3.1 Unresectable advanced G/GEJ adenocarcinoma
A phase II trial by An et al. involved 47 treatment-naïve patients with advanced gastroesophageal adenocarcinoma (GEA) who were treated with first-line pembrolizumab in combination with chemotherapy, including two (4.3%) EBV+ patients who achieved a CR and PR, respectively. Bai et al. analyzed 66 people with unresectable GC managed with ICB at a proficient mismatch repair and showed that compared to EBV- patients, 22 EBV+ patients exhibited a better ORR. Survival analysis revealed that EBV+ cases had better PFS and OS compared to EBV- cases. The EBV status is a strong prognostic factor for PFS in patients with GC after ICB (79). What is clear is that, as with performance in neoadjuvant therapy, patients with advanced EBVaGC have varying rates of efficacy for immunotherapy. Based on the results of the above clinical trials, re-screening of patients with EBVaGC before applying immunotherapy appears to be crucial.
Kim et al. analyzed patients with metastatic GC treated with second-line pembrolizumab monotherapy following first-line chemotherapy failure. It was found that six patients who were EBV+ GC had an ORR of 100% and were all positive for PD-L1 expression (CPS ≥ 1) (73). Wang et al. conducted a phase Ib/II clinical trial aimed at assessing the safety and effectiveness of toripalimab for treating advanced GC. Four EBV+ patients participated in this trial, of which one patient with a positive CPS ≥ 1 achieved a PR; the other three EBV+ patients who did not achieve a PR were all negative for PD-L1 expression (32). These results demonstrate that testing for PD-L1 in patients with EBVaGC is necessary and that patients with positive CPS values (CPS ≥ 1) may be better suited for immunotherapy.
2.3.2 Untreated, resectable locally advanced EBVaGC
The Neo-PLANET phase II study evaluated first-line neoadjuvant camrelizumab in combination with chemotherapy to 36 patients with resectable T3-4N+M0 G/GEJ adenocarcinoma. One of the patients with EBV+ had 80% residual tumor cells (38). One of the six patients in the PANDA trial who did not respond to neoadjuvant atezolizumab was EBV+, and this patient had up to 60-70% residual tumor cells (21). The expression of PD-L1 in the two EBV+ patients in these two clinical trials is undetermined. In an analysis of 77 patients with locally advanced GC who underwent neoadjuvant therapy followed by D2 radical surgery, three EBV+ patients were in the PD-1 blockade plus chemotherapy group; one patient with a CPS of 70 achieved a pCR, and two others with CPSs of 10 and 1 achieved an MPR (80). In view of these results, Wei et al. included 159 cases with EBVaGC for PD-L1 immunohistochemical analysis in order to explore the effect of PD-L1 expression in EBVaGC on patient outcomes and prognosis. Patients with CPS ≥ 1 had a greater objective efficacy rate than those who were negative (P = 0.001); ORR values of 83.3% and 100.0% were found for patients with CPS ≥ 10 and CPS ≥ 50, respectively. In terms of prognosis, cases with CPS ≥ 1 showed greater survival (P ≤ 0.001) and longer disease-free survival compared to cases with CPS < 1 (81). These results suggest that PD-L1 is an effective marker for screening the benefit of immunotherapy in EBVaGC.
In addition to PD-L1, Bai et al. analyzed differences in the TME and genomic characteristics distinguishing patients with EBVaGC who responded to ICB therapy from those who did not respond to screen people for EBVaGC immunotherapy. In the group of responders, a notably higher frequency of SMARCA4 gene mutations were found compared to the non-responder group, which may be linked with higher TMB levels in cases with SMARCA4 variants compared to those with wild-type SMARCA4 (79). These findings indicate that TMB and mutations in SMARCA4 may serve as promising predictive biomarkers for the effectiveness of ICB therapy in EBVaGC.
Studies have also shown that EBV+ GC cells not only promoted CD274 (the gene that encodes PD-L1) amplification by activating the IRF3/CD274 axis, but also up-regulated constitutive PD-L1 expression (82). EBV infection stimulated increased interferon (IFN)-γ levels in GC and induced adaptive PD-L1 expression (82–84). In addition, Bai et al. analyzed the TME differences between ICB responders and non-responders in EBVaGC and found that the level of intratumoral CTLA-4 and the density of T-cell immunoglobulin-3 (TIM-3)+ cells exhibited a notable elevation in the group of patients who did not respond to ICB therapy, compared to those who showed a positive response. The above studies can explain to some extent the mechanism by which EBVaGC benefits from immunotherapy, but there are still many EBVaGC patients who are ineffective to neoadjuvant immunotherapy. Therefore, it is necessary to clarify the exact impact and potential mechanisms of EBV infection on the efficacy of ICB.
2.4 TMB
The TMB was defined as the count of non-synonymous mutations per megabase of the tumor genome reflecting tumor immunogenicity (85). The somatic TMB may lead to the formation of new antigens, and the presence of additional antigens enhances the probability that tumor cells will be detected by immune cells that infiltrate the tumor (86), thereby activating T-cell-mediated anti-tumor responses (87). Thus, medications stimulating T-cell activation, such as monoclonal antibodies targeting PD-1 or PD-L1, might offer potent anticancer treatment, especially for individuals with an elevated TMB. Several studies have demonstrated a favorable association between TMB levels and response to ICIs in different types of tumors (88–90). As a result, in June 2020, pembrolizumab was approved by the FDA for all solid tumors with high tumor mutational load (TMB-H). However, the TMB threshold used to screen for immunotherapy efficacy has led to different results in different clinical trials.
A TMB ≥ 10 mutations/megabase is a clinically significant cut-off point (91). In the Keynote-158 study, investigators used pembrolizumab to treat people with solid tumors showing an advanced high-TMB (TMB ≥ 10 mut/Mb). Twenty-four (10.3%) patients with GC were included. The results of the study found an ORR of 29% in patients with TMB-H tumors, compared to only 6% in patients who were non-TMB-H. Exploratory outcome analysis showed a correlation between TMB, and the clinical results of first-line pembrolizumab treatment and pembrolizumab combination chemotherapy after adjusting for CPS (ORR, PFS, and OS; all P < 0.05); those cases that had a TMB ≥ 10 mut/Mb and were managed with pembrolizumab demonstrated better clinical benefits (ORR, PFS, and OS) (92). Two additional studies reached the same conclusion, but with different TMB thresholds of 12 mut/Mb and 20 mut/Mb, respectively. A phase Ib/II clinical trial conducted by F. Wang et al. revealed that for AGC patients who failed first-line chemotherapy receiving Toripalimab monotherapy, regardless of the expression level of PD-L1, the OS of patients with ≥ 12 mut/Mb was significantly better than that of patients with < 12 mut/Mb (32). The findings of Zhang et al. showed that in advanced, resectable MSI-H gastrointestinal tumors, patients with a high TMB (TMB ≥ 20 mut/Mb) responded well to neoadjuvant ICIs, regardless of PD-L1 levels. In the KEYNOTE-061 trial, pembrolizumab improved outcomes in patients with TMB ≥ 175 mutations/exome (whole exome sequencing assessment) compared to chemotherapy (19). The TMB exhibited significant associations with ORR, PFS, and OS (all, P < 0.05) in the pembrolizumab group, whereas no notable correlations were detected in the chemotherapy group.
The above experimental data suggest that the TMB status in GC may serve as a predictive biomarker for pembrolizumab efficacy. However, several factors limit its reliability. Heterogeneity: it should be noted that the TMB cutoff value varies across different tumor types. Therefore, it is necessary to determine the TMB cutoff in GC to establish its value as a predictive biomarker. Gene fusion: conventional TMB assays, such as whole exome sequencing (WES) or targeted panels, may not effectively capture gene fusion events, especially those in non-coding regions or involving complex rearrangements. Gene fusions may serve as oncogenic drivers in tumor progression (e.g., CLDN18-ARHGAP fusion in GC) (93) and can generate neoantigens. However, the impact of gene fusions on immunotherapy response is not captured by TMB (94), which may lead to an underestimation of immunogenic potential. Moreover, fusion-driven tumors (e.g., NTRK fusions) exhibit a distinct immune microenvironment, where high TMB may not reliably predict treatment efficacy but instead shows stronger correlation with targeted therapy response. Clonality: TMB only reflects the total mutational burden, encompassing both clonal (truncal) and subclonal mutations, yet clonal mutations alone may more reliably indicate immunogenicity. Subclonal mutations may contribute to immune escape due to spatial heterogeneity. The heterogeneity of mutational profiles between primary and metastatic sites may compromise the accuracy of TMB assessment based on a single biopsy. Quality of mutations: not all mutations are capable of generating effective immunogenicity. Both nonsynonymous mutations (which may generate neoantigens) and synonymous mutations (which do not alter the amino acid sequence and lack immunogenicity) are counted in TMB, yet only the former may influence immunotherapy response (95). Driver mutations (e.g., TP53, KRAS) may indirectly modulate immune response by altering the tumor microenvironment (96, 97). Although passenger mutations inflate TMB values, most fail to elicit effective immune responses due to issues such as protein stability or impaired MHC binding. TMB cannot distinguish their respective contributions. Mutation type: indels and frameshift mutations may generate highly immunogenic neoantigens, but certain detection methods (e.g., targeted panels) exhibit limited sensitivity for these mutation types. Logistic issue: the TMB is also affected by a variety of factors, such as tumor type, detection method, and analysis technique. For instance, WES covers the entire exonic region but is costly, while targeted panels only interrogate a limited set of genes (98), potentially leading to TMB underestimation. In bioinformatics analysis, both variant calling algorithms and germline mutation filtering criteria can significantly impact the final TMB calculation.
Currently, research is gradually emerging on combining TMB with other biomarkers to predict the effectiveness of immunotherapy. For instance, recent studies suggest that combining TMB with T-cell-inflamed gene expression profile (GEP) can achieve superior predictive performance. In the KEYNOTE clinical dataset, it has been demonstrated that patients with solid tumors, including GC, who exhibit both high TMB (TMB-H) and high GEP (GEP-H), achieve the highest ORR (99). The combination of TMB and TIDE has demonstrated robust predictive efficacy in breast cancer, lung adenocarcinoma, and hepatocellular carcinoma (100–103), with future studies planned to explore its predictive potential for immunotherapy response in GC. Moreover, genomic and transcriptomic factors, such as novel antigen presentation by MHC-I and II complexes, can predict ICI outcomes (87, 104). Currently, there is a lack of validation for such models in GC-specific cohorts. For GC patients who have completed immunotherapy clinical trials (e.g., ATTRACTION-4, KEYNOTE-062), retrospective extraction of transcriptomic data from archived samples can be performed to validate the predictive efficacy of the aforementioned models. Additionally, prospective randomized controlled clinical trials can be conducted to further evaluate the predictive capability of these models. It is important to note that the molecular heterogeneity of GC (e.g., EBV-positive type, genomically stable type) may affect the generalizability of the models. Subgroup analyses (e.g., stratified by PD-L1 CPS, MSI, and EBV status) can be conducted to validate the universality of the models. The results are promising, as they may provide new directions for the development of biomarkers for GC immunotherapy.
3 Novel biomarkers
A lack of reliable predictive biomarkers is one of the biggest issues in ICI therapeutics. In the past, a single immune-specific marker has been the focus of biomarker research, but the approved PD-L1, TMB and MSI-H cannot completely screen out all people who benefit from new adjuvant immunotherapy. Therefore, novel and reliable biomarkers for population screening are urgently required (Figure 3).
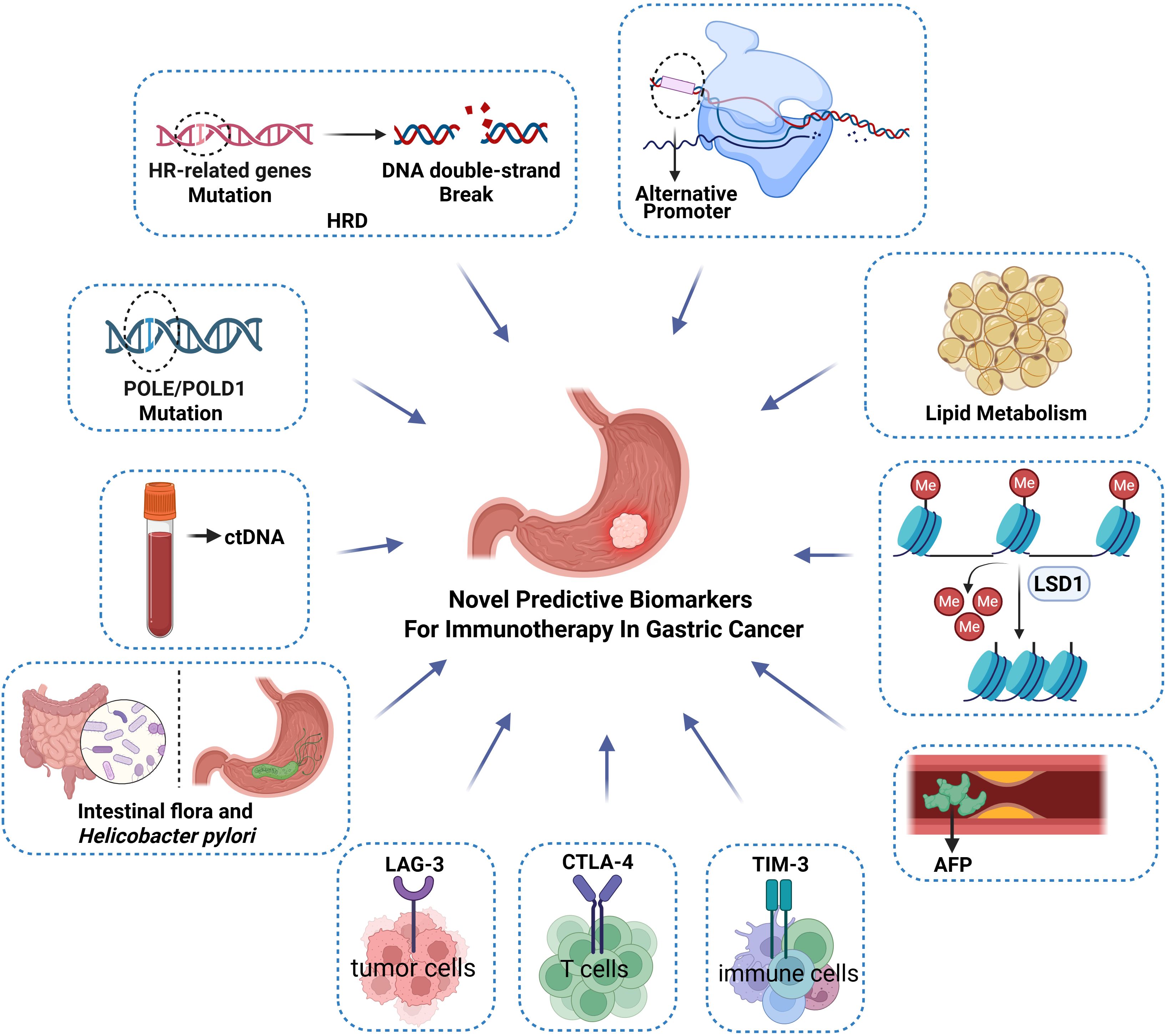
Figure 3. Novel biomarkers possibly predicting the efficacy of neoadjuvant immunotherapy for GC include POLE/POLD1 mutations, circulating tumor DNA (ctDNA), intestinal flora/Helicobacter pylori, cytotoxic T lymphocyte antigen-4 (CTLA-4), T cell immunoglobulin and mucin domain-containing protein 3 (TIM3), alpha fetoprotein (AFP), lysine-specific histone demethylase 1 (LSD-1), lipid metabolism and alternate promoters (AP) and homologous recombination deficiency (HRD).
3.1 POLE/POLD1mutations
POLE/POLD1 mutations are thought to be linked to a high TMB, an enhanced tumor immune reaction and a better response to ICIs (105). It has been shown that POLE and POLD1 mutations are able to be used as stand-alone biomarkers for anticipating the efficacy of pan-cancer immunotherapy, and that they are also markers of a poor prognosis (106–108). Zhu et al. found that patients with GC and POLE/POLD1 mutations typically exhibit an acquired immune resistance to the TME, with an increase in PD-L1 expression and an elevation of TMB (109). The above suggests that mutations of the POLE/POLD1 gene can be used as a biomarker to improve the clinical efficacy of neoadjuvant immunotherapy in people with GC; identical results were obtained in NCT03012581. In this trial, Rousseau et al. prospectively evaluated the efficacy of nivolumab monotherapy in patients with advanced POLE/POLD1 mutated solid tumors. Two patients (9%) with GC were included in this trial. It was found that only tumors with selective pathogenic mutations in the catalytic site of the DNA-binding or nucleic acid exonuclease structural domains exhibited high mutational loads, high T-cell infiltration, and high response rates to anti-PD1 monotherapy (110).
3.2 Circulating tumor DNA
Given the variation in time and space of GC, blood-based predictive biomarkers from liquid biopsies have surfaced as a hopeful strategy for anticipating responses to immunotherapy (111). Circulating tumor DNA (ctDNA) is one widely studied biomarker in liquid biopsies (112). Jin et al. performed next-generation sequencing testing on 46 patients with metastatic GC treated with neoadjuvant PD-1 inhibitor immunotherapy. They showed that patients with a > 25% decrease in the frequency of the largest variant allele in the ctDNA assay had a longer median PFS (7.3 m vs. 3.6 m; P = 0.0011) and higher ORR (53.3% vs. 13.3%). The study also found that patients with TGFBR2, RHOA, and PREX2 mutations in the GC had significantly shorter PFS than those without mutations (113). Results from another study that included 61 patients with metastatic GC receiving neoadjuvant therapy with pembrolizumab showed that a decrease in the concentration of ctDNA in the sixth week of continuous ctDNA monitoring can be used to predict a benefit from neoadjuvant immunotherapy (73). Qiao et al. treated patients with unresectable advanced GC using neoadjuvant dendritic cells mixed with cytokine-induced killer cells (DC-CIK; an immune cell therapy) in combination with chemotherapy. They found that the frequency and number of ctDNA mutations were reduced in 19 patients (63.3%) after DC-CIK infusion. Reduced ctDNA mutation frequency was associated with improved PFS and OS (P = 0.001) (114). The above suggests that ctDNA testing is useful in anticipating the performance of individualized neoadjuvant immunotherapy in patients with GC. A prospective phase II clinical trial (NCT05594381) is currently underway to investigate the feasibility of ctDNA for evaluating the performance of PD-1 inhibitors in combination with a SOX regimen as a neoadjuvant therapy for locally progressive GC. Another aim of this study is to construct a model for evaluating the efficacy of treatment so as to clarify the applicability of the neoadjuvant immunotherapy for locally progressive GC. The results are eagerly anticipated.
Liquid biopsy offers advantages. Compared to other emerging biomarkers (such as TMB, PD-L1, or immune gene signatures), ctDNA testing exhibits higher sensitivity, enabling the detection of minimal residual disease (MRD) tumor burden and early recurrence. Moreover, from a practical standpoint, ctDNA’s blood-based detection offers dual advantages: enhanced clinical accessibility and serial monitoring capacity for tracking disease progression. However, it also has limitations as a screening approach for predictive biomarkers in GC immunotherapy. First of all, technical methods and sample quality limit the sensitivity of liquid biopsies, which may fail to detect tumor biomarkers that are at low concentration levels. Second, compared to other biomarkers such as TMB, PD-L1, or immune gene signature in tumor specimen, biomarkers in the blood may not be tumor-specific, leading to false-positive results. Therefore, given the significant clinical potential of ctDNA, more studies are needed to further improve the reliability of liquid biopsy, thus contributing to the screening of a GC population sensitive to immunotherapy.
3.3 Intestinal flora and Helicobacter pylori
Recent studies have begun to focus on the relationship between the gut microbiota, and tumor progression and treatment outcomes. This has been proposed as a potential biomarker for participating in the outcome of immunotherapy in solid tumors (115, 116). Although specific gut microbes play a role in GC progression and immunomodulation, insufficient evidence exists to support their potential as efficacy biomarkers in neoadjuvant immunotherapy for GC.
Recent research indicates that H. pylori infection may hinder the growth and anti-tumor functions of CD8+ T cells, foster the transformation of naive T cells into Tregs, and modulate the production of inflammatory mediators. These actions influence the TIME, dampen host immune reactions, and diminish the effectiveness of immunotherapy for GC (117–119). Therefore, patients with locally advanced GC should be aware of their H. pylori infection status before receiving neoadjuvant immunotherapy. However, forward-looking research exploring the relationship between H. pylori and prognosis in GC immunotherapy is lacking. In addition, the use of H. pylori as a predictive marker of immunotherapy efficacy needs to be thoroughly demonstrated (120).
3.4 Lymphocyte activation gene 3
LAG-3 is an inhibitory receptor on cell surfaces, which negatively regulates both CD8+ and CD4+T cell activity. It maintains immune system homeostasis under normal physiological conditions (121, 122), while LAG-3 and Treg cell interactions stimulate Treg activity, strengthen immune tolerance, and indirectly suppress dendritic cell (DC) function, thereby facilitating tumor cell immune escape. Consequently, LAG-3 is a promising therapeutic target in cancer immunotherapy, complementing the PD-1/PD-L1 pathway (123). Currently, the joint application of LAG-3 and PD-1 inhibitors is a topical research field. Kelly et al., in their innovative study, combined PD-1 and LAG-3 inhibitors with chemoradiotherapy for the neoadjuvant treatment of patients with gastroesophageal cancer (124). The authors reported that higher baseline PD-L1 (CPS ≥ 5) and LAG-3 expression was associated with superior pathological responses, with a Phase Ib clinical study also providing safety insights on combining PD-1 and LAG-3 for gastroesophageal cancer. But, in a Phase II study (RELATIVITY-060), combined nivolumab and the LAG-3 inhibitor relatlimab with chemotherapy for advanced G/GEJC failed to reach its primary endpoint (125). Therefore, more research is required to test the safety and effectiveness of PD-1 inhibitors when combined with LAG-3 inhibitors as an immunotherapy for patients with GC.
3.5 Cytotoxic T lymphocyte antigen-4
CTLA-4 is a co-inhibitory molecule on activated T and regulatory T cell surfaces (Tregs). It interacts with B7-1/B7–2 ligands on antigen-presenting cells, thereby inhibiting CD28-mediated signaling, which is responsible for T cell activation. Monoclonal antibodies targeting CTLA-4 have been shown to block its competition with CD28 for binding to B7, which in turn activates the CD28 signaling cascade and reduces immunosuppressive Treg cell populations in the TME (126). As the world’s first PD-1/CTLA-4 bispecific antibody, in the COMPASSION-15 (AK104-302) Phase III trial, cadonilimab showed significant efficacy in patients with different PD-L1 expression levels. In the trial, the proportion of individuals with PD-L1 CPS < 5 and CPS < 1 reached 49.8% and 23%, respectively, which was better reflected real-world patients. Therefore, these data confirmed the unique advantages of cadonilimab in individuals with low PD-L1 expression, and reflecting this, on September 30th, 2024, the National Drug Administration officially approved its combination with XELOX for the first-line treatment of unresectable locally advanced recurrent or metastatic G/GEJ adenocarcinoma.
3.6 T cell immunoglobulin and mucin domain-containing protein 3
TIM-3 is encoded by HARVCR2 (127) and is an emerging target for cancer immunotherapy (128). Studies have shown that TIM-3 expression levels in GC tissue are significantly higher than in normal gastric mucosa tissue (129, 130), and positively correlated with PD-1/PD-L1 expression levels in GC tissue (131). Chen et al., reported that TIM-3 potentially promoted CD8+ T cell dysfunction in GC, manifested by decreased IFN-γ, perforin, and Granzyme B (GzmB) levels, but increased PD-1 and CTLA-4 levels (132). Although HARVCR2 mRNA is elevated in most GC subtypes, it is more highly expressed in EBV-positive and MSI subtypes, as characterized by increased immune characteristics and higher immunotherapy responsiveness (73). Further research is required to examine the potential benefits of anti-TIM-3 inhibitors in patients with GC, and investigate their potential in combination with anti-PD-1/PD-L1 inhibitors.
3.7 Alpha fetoprotein
AFP has a wide range of biological functions, with previous research showing that it directly stimulated cancer cell proliferation and growth while also inhibiting apoptosis (133). AFP also inhibited monocyte differentiation to fully functional DCs and prevented them presenting foreign antigens to CD8+ lymphocytes via MHC signaling (134, 135). Furthermore, AFP down-regulated Toll-like receptor 4 expression on DCs and inhibited pro-inflammatory cytokine secretion, including interleukin-12 and tumor necrosis factor-α. These cytokines were shown to stimulate CD4+ and CD8+ lymphocyte production in immunotherapy (136). Additionally, AFP also induced ThCD4+ lymphocytes to differentiate into Tregs, thus negatively regulating immunotherapy. Zhang et al., reported that AFP levels predicted ICI efficacy in treating advanced GC, i.e., high baseline AFP levels were associated with reduced disease control rate (DCR) during ICI treatment and also shortened PFS and OS (137). However, the exact mechanisms whereby AFP levels affect ICI efficacy in patients with GC remain unclear and further research is needed.
3.8 Lysine-specific histone demethylase 1
In 2004, LSD-1 was characterized as the first histone demethylase (138); its expression was significantly elevated in GC and it promoted GC proliferation and metastasis (139–142). LSD-1 also mediates epithelial-mesenchymal transition (143) in GC via H3K4me2 demethylation, thereby promoting drug resistance, disease recurrence, and disease invasion and metastasis (144). Shen et al., reported that LSD-1 deletion offset its immunosuppressive functions by reducing PD-L1 levels in exosomes and inhibiting its transport to other cancer cells, thereby restoring T cell killing functions in the GC microenvironment (145). Therefore, LSD-1 may function as a new immunotherapy target against GC, with the new LSD-1 inhibitor 5ac inhibiting mouse GC cell growth (146).
3.9 Lipid metabolism
An increasing body of evidence now indicates that reprogrammed energy metabolism has critical roles in GC progression (147). Therefore, more in-depth research on the metabolic changes in the GC TME may provide new markers/therapeutic targets for neoadjuvant GC immunotherapy. Yang et al., used database resources to identify eight genes associated with fatty acid metabolism, which correlated with GC prognosis outcomes. The authors developed ‘FRAS’, a model whereby FRAS scores effectively identified patients with GC who were likely to benefit from anti-CTLA-4 antibody immunotherapy. Among the eight genes, RGS2 was significantly correlated with the TMB and CD8+ T cell infiltration in GC, suggesting that RGS2 may be a potential target for future immunotherapy strategies toward GC (148).
3.10 Alternate promoters
On the one hand, the results obtained in previous clinical trials of PD-1/PD-L1 and TMB as screening indicators of whether GC patients can undergo immunotherapy have not been satisfactory. On the other hand, MSI-H and EBV GC patient population only accounts for a small proportion of all GC patients. Therefore, finding new positive predictive biomarkers and exploring negative biomarkers (that identify subjects with clear lack of benefit from specific therapy) are necessary. Promoters are cis-regulatory elements found upstream of transcription start sites, with > 50% of human genes having multiple promoters, i.e., AP (149). AP allow transcription to start at different transcription start sites, which then produces different 5’ untranslated regions and first exons, thereby enhancing mRNA and protein subtype diversity (150). Sundar et al., reported that patients with metastatic GC with high AP use expressed lower CD8A, GZMA, and PFR1 levels (151) (cytolytic T-cell activity marker (152, 153)), indicating that AP use in metastatic GC was inversely correlated with anti-tumor immunity. Subsequent studies reported that patients with advanced GC with higher AP activity showed higher CD8A and PRF1 levels (CTL surface markers) and lower LAG-3 and TIM-3 levels, thus creating an inhibitory immune microenvironment and a mechanism for GC immune escape (154).
3.11 Homologous recombination deficiency
Homologous recombination (HR) is a highly accurate DNA repair mechanism (155). On one hand, HRD induces DNA repair defects, leading to the accumulation of more mutations and the generation of neoantigens, which enhances tumor response to ICI (156). On the other hand, HRD may promote the formation of an anti-tumor immune microenvironment by increasing tumor-infiltrating lymphocytes (TILs) (157), suggesting its potential as a biomarker for predicting immunotherapy response. The study by Fan et al. revealed that HRD-positive GC patients exhibited significantly longer OS following ICI treatment compared to other GC patients. More importantly, the study also demonstrated positive correlations between HRD status and both TMB-H and MSI-H. Additionally, HRD-positive GC patients showed increased CD8+ T cell infiltration after ICIs treatment (158). These findings suggest that HRD has the potential to serve as a predictive biomarker for immunotherapy efficacy in GC.
4 Prospects
In this comprehensive review, we summarized some of the diverse markers used to evaluate immunotherapy efficacy, as documented in several clinical studies, among which, microsatellite instability and EBV associations were recognized as GC subtypes that could benefit the most from ICIs. However, few studies have investigated immunotherapy responses involving two other GC categories in the GC landscape, namely, chromosomal instability and genomic stability; therefore, more studies in these areas are warranted.
Nevertheless, each epitope marker also has its strengths and limitations. For example, temporal or spatial heterogeneity exists in PD-L1, delineating risk thresholds for markers whose measurements are continuous variables (e.g., TMB, PD-L1, etc.) is problematic, and the standardization of marker measurements or calculation methods is required. Therefore, it is difficult for a single biomarker to anticipate the performance of immunotherapy. The development of a mathematical model that includes all the key procedures of anti-tumor immunity to assess the immune status of an individual is a future trend.
One of the key reasons limiting the efficacy of ICIs in patients with GC is the immunosuppressive component of their TIME and the complex immune escape mechanisms involved. However, to date, little research has been conducted on the relationship between biomarkers and TIME, and their dynamic evolution during immunotherapy. An in-depth exploration of the effect of immunotherapy on GC TIME, an understanding of drug resistance mechanisms, the discovery of specific regulatory targets of GC TIME, and searching for more reliable, comprehensive, and dynamic biomarkers for population screening in an effort to bring immunotherapy for GC into the era of precision therapy, are required for the continued development of immunotherapy.
Author contributions
FS: Writing – original draft. XG: Writing – review & editing, Data curation, Methodology. WW: Writing – review & editing, Data curation, Methodology. XZ: Writing – review & editing. JZ: Writing – review & editing, Supervision. YZ: Writing – review & editing, Conceptualization, Investigation.
Funding
The author(s) declare that financial support was received for the research and/or publication of this article. This study was supported by “the Fundamental Research Funds for the Central Universities” (LD2023017, LD2023010, LD2023019) and Liaoning Provincial Natural Science Foundation (2024-MS-271).
Conflict of interest
The authors declare that the research was conducted in the absence of any commercial or financial relationships that could be construed as a potential conflict of interest.
Generative AI statement
The author(s) declare that no Generative AI was used in the creation of this manuscript.
Publisher’s note
All claims expressed in this article are solely those of the authors and do not necessarily represent those of their affiliated organizations, or those of the publisher, the editors and the reviewers. Any product that may be evaluated in this article, or claim that may be made by its manufacturer, is not guaranteed or endorsed by the publisher.
References
1. Bray F, Laversanne M, Sung H, Ferlay J, Siegel RL, Soerjomataram I, et al. Global cancer statistics 2022: GLOBOCAN estimates of incidence and mortality worldwide for 36 cancers in 185 countries. CA Cancer J Clin. (2024) 74:229–63. doi: 10.3322/caac.21834
2. Han B, Zheng R, Zeng H, Wang S, Sun K, Chen R, et al. Cancer incidence and mortality in China, 2022. J Natl Cancer Cent. (2024) 4:47–53. doi: 10.1016/j.jncc.2024.01.006
3. Glimelius B, Ekström K, Hoffman K, Graf W, Sjödén PO, Haglund U, et al. Randomized comparison between chemotherapy plus best supportive care with best supportive care in advanced gastric cancer. Ann Oncol. (1997) 8:163–8. doi: 10.1023/a:1008243606668
4. Petricevic B, Kabiljo J, Zirnbauer R, Walczak H, Laengle J, Bergmann M. Neoadjuvant immunotherapy in gastrointestinal cancers - The new standard of care? Semin Cancer Biol. (2022) 86:834–50. doi: 10.1016/j.semcancer.2022.05.015
5. Eso Y, Seno H. Current status of treatment with immune checkpoint inhibitors for gastrointestinal, hepatobiliary, and pancreatic cancers. Therap Adv Gastroenterol. (2020) 13:1756284820948773. doi: 10.1177/1756284820948773
6. Sinha D, Moseley P, Lu X, Wright Q, Gabrielli B, Frazer IH, et al. Repurposing of commercially existing molecular target therapies to boost the clinical efficacy of immune checkpoint blockade. Cancers (Basel). (2022) 14:6150. doi: 10.3390/cancers14246150
7. Keir ME, Butte MJ, Freeman GJ, Sharpe AH. PD-1 and its ligands in tolerance and immunity. Annu Rev Immunol. (2008) 26:677–704. doi: 10.1146/annurev.immunol.26.021607.090331
8. Pardoll DM. The blockade of immune checkpoints in cancer immunotherapy. Nat Rev Cancer. (2012) 12:252–64. doi: 10.1038/nrc3239
9. Lv J, Guo T, Qu X, Che X, Li C, Wang S, et al. PD-L1 under regulation of miR-429 influences the sensitivity of gastric cancer cells to TRAIL by binding of EGFR. Front Oncol. (2020) 10:1067. doi: 10.3389/fonc.2020.01067
10. Sun C, Mezzadra R, Schumacher TN. Regulation and function of the PD-L1 checkpoint. Immunity. (2018) 48:434–52. doi: 10.1016/j.immuni.2018.03.014
11. Liu C, Fang F, Kong Y, ElGabry EA. Tumor Area Positivity (TAP) score of programmed death-ligand 1 (PD-L1): a novel visual estimation method for combined tumor cell and immune cell scoring. Diagn Pathol. (2023) 18:48. doi: 10.1186/s13000-023-01318-8
12. Bang YJ, Kang YK, Catenacci DV, Muro K, Fuchs CS, Geva R, et al. Pembrolizumab alone or in combination with chemotherapy as first-line therapy for patients with advanced gastric or gastroesophageal junction adenocarcinoma: results from the phase II nonrandomized KEYNOTE-059 study. Gastric Cancer. (2019) 22:828–37. doi: 10.1007/s10120-018-00909-5
13. Janjigian YY, Ajani JA, Moehler M, Shen L, Garrido M, Gallardo C, et al. First-line nivolumab plus chemotherapy for advanced gastric, gastroesophageal junction, and esophageal adenocarcinoma: 3-year follow-up of the phase III checkMate 649 trial. J Clin Oncol. (2024) 42:2012–20. doi: 10.1200/jco.23.01601
14. Qiu MZ, Oh DY, Kato K, Arkenau T, Tabernero J, Correa MC, et al. Tislelizumab plus chemotherapy versus placebo plus chemotherapy as first line treatment for advanced gastric or gastro-oesophageal junction adenocarcinoma: RATIONALE-305 randomised, double blind, phase 3 trial. BMJ. (2024) 385:e078876. doi: 10.1136/bmj-2023-078876
15. Shitara K, Van Cutsem E, Bang YJ, Fuchs C, Wyrwicz L, Lee KW, et al. Efficacy and safety of pembrolizumab or pembrolizumab plus chemotherapy vs chemotherapy alone for patients with first-line, advanced gastric cancer: the KEYNOTE-062 phase 3 randomized clinical trial. JAMA Oncol. (2020) 6:1571–80. doi: 10.1001/jamaoncol.2020.3370
16. Kang YK, Boku N, Satoh T, Ryu MH, Chao Y, Kato K, et al. Nivolumab in patients with advanced gastric or gastro-oesophageal junction cancer refractory to, or intolerant of, at least two previous chemotherapy regimens (ONO-4538-12, ATTRACTION-2): a randomised, double-blind, placebo-controlled, phase 3 trial. Lancet. (2017) 390:2461–71. doi: 10.1016/s0140-6736(17)31827-5
17. Kang YK, Chen LT, Ryu MH, Oh DY, Oh SC, Chung HC, et al. Nivolumab plus chemotherapy versus placebo plus chemotherapy in patients with HER2-negative, untreated, unresectable advanced or recurrent gastric or gastro-oesophageal junction cancer (ATTRACTION-4): a randomised, multicentre, double-blind, placebo-controlled, phase 3 trial. Lancet Oncol. (2022) 23:234–47. doi: 10.1016/s1470-2045(21)00692-6
18. Moehler M, Dvorkin M, Boku N, Özgüroğlu M, Ryu MH, Muntean AS, et al. Phase III trial of avelumab maintenance after first-line induction chemotherapy versus continuation of chemotherapy in patients with gastric cancers: results from JAVELIN gastric 100. J Clin Oncol. (2021) 39:966–77. doi: 10.1200/jco.20.00892
19. Shitara K, Özgüroğlu M, Bang YJ, Di Bartolomeo M, Mandalà M, Ryu MH, et al. Pembrolizumab versus paclitaxel for previously treated, advanced gastric or gastro-oesophageal junction cancer (KEYNOTE-061): a randomised, open-label, controlled, phase 3 trial. Lancet. (2018) 392:123–33. doi: 10.1016/S0140-6736(18)31257-1
20. Rha SY, Oh DY, Yañez P, Bai Y, Ryu MH, Lee J, et al. Pembrolizumab plus chemotherapy versus placebo plus chemotherapy for HER2-negative advanced gastric cancer (KEYNOTE-859): a multicentre, randomised, double-blind, phase 3 trial. Lancet Oncol. (2023) 24:1181–95. doi: 10.1016/S1470-2045(23)00515-6
21. Verschoor YL, van de Haar J, van den Berg JG, van Sandick JW, Kodach LL, van Dieren JM, et al. Neoadjuvant atezolizumab plus chemotherapy in gastric and gastroesophageal junction adenocarcinoma: the phase 2 PANDA trial. Nat Med. (2024) 30:519–30. doi: 10.1038/s41591-024-02898-8
22. Zhao Y, Li D, Zhuang J, Li Z, Xia Q, Li Z, et al. Comprehensive multi-omics analysis of resectable locally advanced gastric cancer: Assessing response to neoadjuvant camrelizumab and chemotherapy in a single-center, open-label, single-arm phase II trial. Clin Transl Med. (2024) 14:e1674. doi: 10.1002/ctm2.1674
23. Kawazoe A, Yamaguchi K, Yasui H, Negoro Y, Azuma M, Amagai K, et al. Safety and efficacy of pembrolizumab in combination with S-1 plus oxaliplatin as a first-line treatment in patients with advanced gastric/gastroesophageal junction cancer: Cohort 1 data from the KEYNOTE-659 phase IIb study. Eur J Cancer. (2020) 129:97–106. doi: 10.1016/j.ejca.2020.02.002
24. Janjigian YY, Bendell J, Calvo E, Kim JW, Ascierto PA, Sharma P, et al. CheckMate-032 study: efficacy and safety of nivolumab and nivolumab plus ipilimumab in patients with metastatic esophagogastric cancer. J Clin Oncol. (2018) 36:2836–44. doi: 10.1200/jco.2017.76.6212
25. Guo H, Ding P, Sun C, Yang P, Tian Y, Liu Y, et al. Efficacy and safety of sintilimab plus XELOX as a neoadjuvant regimen in patients with locally advanced gastric cancer: A single-arm, open-label, phase II trial. Front Oncol. (2022) 12:927781. doi: 10.3389/fonc.2022.927781
26. Lorenzen S, Götze TO, Thuss-Patience P, Biebl M, Homann N, Schenk M, et al. Perioperative atezolizumab plus fluorouracil, leucovorin, oxaliplatin, and docetaxel for resectable esophagogastric cancer: interim results from the randomized, multicenter, phase II/III DANTE/IKF-s633 trial. J Clin Oncol. (2024) 42:410–20. doi: 10.1200/jco.23.00975
27. André T, Tougeron D, Piessen G, de la Fouchardière C, Louvet C, Adenis A, et al. Neoadjuvant nivolumab plus ipilimumab and adjuvant nivolumab in localized deficient mismatch repair/microsatellite instability-high gastric or esophagogastric junction adenocarcinoma: the GERCOR NEONIPIGA phase II study. J Clin Oncol. (2023) 41:255–65. doi: 10.1200/jco.22.00686
28. Gonzales E, Hardikar W, Stormon M, Baker A, Hierro L, Gliwicz D, et al. Efficacy and safety of maralixibat treatment in patients with Alagille syndrome and cholestatic pruritus (ICONIC): a randomised phase 2 study. Lancet. (2021) 398:1581–92. doi: 10.1016/s0140-6736(21)01256-3
29. Muro K, Chung HC, Shankaran V, Geva R, Catenacci D, Gupta S, et al. Pembrolizumab for patients with PD-L1-positive advanced gastric cancer (KEYNOTE-012): a multicentre, open-label, phase 1b trial. Lancet Oncol. (2016) 17:717–26. doi: 10.1016/s1470-2045(16)00175-3
30. Marabelle A, Le DT, Ascierto PA, Di Giacomo AM, De Jesus-Acosta A, Delord JP, et al. Efficacy of pembrolizumab in patients with noncolorectal high microsatellite instability/mismatch repair-deficient cancer: results from the phase II KEYNOTE-158 study. J Clin Oncol. (2020) 38:1–10. doi: 10.1200/jco.19.02105
31. Yamaguchi K, Minashi K, Sakai D, Nishina T, Omuro Y, Tsuda M, et al. Phase IIb study of pembrolizumab combined with S-1 + oxaliplatin or S-1 + cisplatin as first-line chemotherapy for gastric cancer. Cancer Sci. (2022) 113:2814–27. doi: 10.1111/cas.15462
32. Wang F, Wei XL, Wang FH, Xu N, Shen L, Dai GH, et al. Safety, efficacy and tumor mutational burden as a biomarker of overall survival benefit in chemo-refractory gastric cancer treated with toripalimab, a PD-1 antibody in phase Ib/II clinical trial NCT02915432. Ann Oncol. (2019) 30:1479–86. doi: 10.1093/annonc/mdz197
33. Manji GA, Lee S, Del Portillo A, May M, Ana SS, Alouani E, et al. Chemotherapy and immune checkpoint blockade for gastric and gastroesophageal junction adenocarcinoma. JAMA Oncol. (2023) 9:1702–7. doi: 10.1001/jamaoncol.2023.4423
34. Qiu HB. Safety and efficacy of tislelizumab plus chemotherapy for first-line treatment of advanced esophageal squamous cell carcinoma and gastric/gastroesophageal junction adenocarcinoma. Thorac Cancer. (2020) 11:3419–21. doi: 10.1111/1759-7714.13690
35. Jiang H, Yu X, Li N, Kong M, Ma Z, Zhou D, et al. Efficacy and safety of neoadjuvant sintilimab, oxaliplatin and capecitabine in patients with locally advanced, resectable gastric or gastroesophageal junction adenocarcinoma: early results of a phase 2 study. J Immunother Cancer. (2022) 10:e003635. doi: 10.1136/jitc-2021-003635
36. Yuan SQ, Nie RC, Jin Y, Liang CC, Li YF, Jian R, et al. Perioperative toripalimab and chemotherapy in locally advanced gastric or gastro-esophageal junction cancer: a randomized phase 2 trial. Nat Med. (2024) 30:552–9. doi: 10.1038/s41591-023-02721-w
37. Yin Y, Lin Y, Yang M, Lv J, Liu J, Wu K, et al. Neoadjuvant tislelizumab and tegafur/gimeracil/octeracil (S-1) plus oxaliplatin in patients with locally advanced gastric or gastroesophageal junction cancer: Early results of a phase 2, single-arm trial. Front Oncol. (2022) 12:959295. doi: 10.3389/fonc.2022.959295
38. Tang Z, Wang Y, Liu D, Wang X, Xu C, Yu Y, et al. The Neo-PLANET phase II trial of neoadjuvant camrelizumab plus concurrent chemoradiotherapy in locally advanced adenocarcinoma of stomach or gastroesophageal junction. Nat Commun. (2022) 13:6807. doi: 10.1038/s41467-022-34403-5
39. Wei J, Lu X, Liu Q, Li L, Liu S, Liu F, et al. Case report: neoadjuvant PD-1 blockade plus concurrent chemoradiotherapy in unresectable locally advanced gastric cancer patients. Front Oncol. (2020) 10:554040. doi: 10.3389/fonc.2020.554040
40. Tang X, Li M, Wu X, Guo T, Zhang L, Tang L, et al. Neoadjuvant PD-1 blockade plus chemotherapy induces a high pathological complete response rate and anti-tumor immune subsets in clinical stage III gastric cancer. Oncoimmunology. (2022) 11:2135819. doi: 10.1080/2162402x.2022.2135819
41. Dong W, Gong M, Shi Z, Xiao J, Zhang J, Peng J. Programmed cell death-1 polymorphisms decrease the cancer risk: A meta-analysis involving twelve case-control studies. PLoS One. (2016) 11:e0152448. doi: 10.1371/journal.pone.0152448
42. Avgustinovich AV, Bakina OV, Afanas’ev SG, Spirina LV, Volkov AM. Safety and efficacy of neoadjuvant chemoimmunotherapy in gastric cancer patients with a PD-L1 positive status: A case report. Curr Issues Mol Biol. (2023) 45:7642–9. doi: 10.3390/cimb45090481
43. Wang X, Wu WKK, Gao J, Li Z, Dong B, Lin X, et al. Autophagy inhibition enhances PD-L1 expression in gastric cancer. J Exp Clin Cancer Res. (2019) 38:140. doi: 10.1186/s13046-019-1148-5
44. Booth L, Roberts JL, West C, Von Hoff D, Dent P. GZ17-6.02 initiates DNA damage causing autophagosome-dependent HDAC degradation resulting in enhanced anti-PD1 checkpoint inhibitory antibody efficacy. J Cell Physiol. (2020) 235:8098–113. doi: 10.1002/jcp.29464
45. Champiat S, Dercle L, Ammari S, Massard C, Hollebecque A, Postel-Vinay S, et al. Hyperprogressive disease is a new pattern of progression in cancer patients treated by anti-PD-1/PD-L1. Clin Cancer Res. (2017) 23:1920–8. doi: 10.1158/1078-0432.ccr-16-1741
46. Kamada T, Togashi Y, Tay C, Ha D, Sasaki A, Nakamura Y, et al. PD-1(+) regulatory T cells amplified by PD-1 blockade promote hyperprogression of cancer. Proc Natl Acad Sci U S A. (2019) 116:9999–10008. doi: 10.1073/pnas.1822001116
47. Wing JB, Tanaka A, Sakaguchi S. Human FOXP3(+) regulatory T cell heterogeneity and function in autoimmunity and cancer. Immunity. (2019) 50:302–16. doi: 10.1016/j.immuni.2019.01.020
48. Onizuka S, Tawara I, Shimizu J, Sakaguchi S, Fujita T, Nakayama E. Tumor rejection by in vivo administration of anti-CD25 (interleukin-2 receptor alpha) monoclonal antibody. Cancer Res. (1999) 59:3128–33.
49. Shimizu J, Yamazaki S, Sakaguchi S. Induction of tumor immunity by removing CD25+CD4+ T cells: a common basis between tumor immunity and autoimmunity. J Immunol. (1999) 163:5211–8. doi: 10.4049/jimmunol.163.10.5211
50. Curiel TJ, Coukos G, Zou L, Alvarez X, Cheng P, Mottram P, et al. Specific recruitment of regulatory T cells in ovarian carcinoma fosters immune privilege and predicts reduced survival. Nat Med. (2004) 10:942–9. doi: 10.1038/nm1093
51. Saito T, Nishikawa H, Wada H, Nagano Y, Sugiyama D, Atarashi K, et al. Two FOXP3(+)CD4(+) T cell subpopulations distinctly control the prognosis of colorectal cancers. Nat Med. (2016) 22:679–84. doi: 10.1038/nm.4086
52. Togashi Y, Nishikawa H. Regulatory T cells: molecular and cellular basis for immunoregulation. Curr Top Microbiol Immunol. (2017) 410:3–27. doi: 10.1007/82_2017_58
53. Schoemig-Markiefka B, Eschbach J, Scheel AH, Pamuk A, Rueschoff J, Zander T, et al. Optimized PD-L1 scoring of gastric cancer. Gastric Cancer. (2021) 24:1115–22. doi: 10.1007/s10120-021-01195-4
54. Yamashita K, Iwatsuki M, Harada K, Eto K, Hiyoshi Y, Ishimoto T, et al. Prognostic impacts of the combined positive score and the tumor proportion score for programmed death ligand-1 expression by double immunohistochemical staining in patients with advanced gastric cancer. Gastric Cancer. (2020) 23:95–104. doi: 10.1007/s10120-019-00999-9
55. Doroshow DB, Bhalla S, Beasley MB, Sholl LM, Kerr KM, Gnjatic S, et al. PD-L1 as a biomarker of response to immune-checkpoint inhibitors. Nat Rev Clin Oncol. (2021) 18:345–62. doi: 10.1038/s41571-021-00473-5
56. Davis AA, Patel VG. The role of PD-L1 expression as a predictive biomarker: an analysis of all US Food and Drug Administration (FDA) approvals of immune checkpoint inhibitors. J Immunother Cancer. (2019) 7:278. doi: 10.1186/s40425-019-0768-9
57. Wang Z, Kang W, Li O, Qi F, Wang J, You Y, et al. Abrogation of USP7 is an alternative strategy to downregulate PD-L1 and sensitize gastric cancer cells to T cells killing. Acta Pharm Sin B. (2021) 11:694–707. doi: 10.1016/j.apsb.2020.11.005
58. Oliveira AF, Bretes L, Furtado I. Review of PD-1/PD-L1 inhibitors in metastatic dMMR/MSI-H colorectal cancer. Front Oncol. (2019) 9:396. doi: 10.3389/fonc.2019.00396
59. Pietrantonio F, Miceli R, Raimondi A, Kim YW, Kang WK, Langley RE, et al. Individual patient data meta-analysis of the value of microsatellite instability as a biomarker in gastric cancer. J Clin Oncol. (2019) 37:3392–400. doi: 10.1200/jco.19.01124
60. Germano G, Lamba S, Rospo G, Barault L, Magrì A, Maione F, et al. Inactivation of DNA repair triggers neoantigen generation and impairs tumour growth. Nature. (2017) 552:116–20. doi: 10.1038/nature24673
61. Mestrallet G, Brown M, Bozkus CC, Bhardwaj N. Immune escape and resistance to immunotherapy in mismatch repair deficient tumors. Front Immunol. (2023) 14:1210164. doi: 10.3389/fimmu.2023.1210164
62. Chao J, Fuchs CS, Shitara K, Tabernero J, Muro K, Van Cutsem E, et al. Assessment of pembrolizumab therapy for the treatment of microsatellite instability-high gastric or gastroesophageal junction cancer among patients in the KEYNOTE-059, KEYNOTE-061, and KEYNOTE-062 clinical trials. JAMA Oncol. (2021) 7:895–902. doi: 10.1001/jamaoncol.2021.0275
63. Shitara K, Ajani JA, Moehler M, Garrido M, Gallardo C, Shen L, et al. Nivolumab plus chemotherapy or ipilimumab in gastro-oesophageal cancer. Nature. (2022) 603:942–8. doi: 10.1038/s41586-022-04508-4
64. Fuchs CS, Doi T, Jang RW, Muro K, Satoh T, MaChado M, et al. Safety and efficacy of pembrolizumab monotherapy in patients with previously treated advanced gastric and gastroesophageal junction cancer: phase 2 clinical KEYNOTE-059 trial. JAMA Oncol. (2018) 4:e180013. doi: 10.1001/jamaoncol.2018.0013
65. Liu L, Woo Y, D’Apuzzo M, Melstrom L, Raoof M, Liang Y, et al. Immunotherapy-based neoadjuvant treatment of advanced microsatellite instability-high gastric cancer: A case series. J Natl Compr Canc Netw. (2022) 20:857–65. doi: 10.6004/jnccn.2022.7023
66. Liu Y, Han G, Li H, Zhao Y, Li Z, Zhuang J, et al. Camrelizumab combined with FLOFOX as neoadjuvant therapy for resectable locally advanced gastric and gastroesophageal junction adenocarcinoma: Updated results of efficacy and safety. J Clin Oncol. (2021) 39. doi: 10.1200/jco.2021.39.15_suppl.4036
67. Kwon M, An M, Klempner SJ, Lee H, Kim KM, Sa JK, et al. Determinants of response and intrinsic resistance to PD-1 blockade in microsatellite instability-high gastric cancer. Cancer Discovery. (2021) 11:2168–85. doi: 10.1158/2159-8290.cd-21-0219
68. Kim H, An JY, Noh SH, Shin SK, Lee YC, Kim H. High microsatellite instability predicts good prognosis in intestinal-type gastric cancers. J Gastroenterol Hepatol. (2011) 26:585–92. doi: 10.1111/j.1440-1746.2010.06487.x
69. Fang WL, Chang SC, Lan YT, Huang KH, Chen JH, Lo SS, et al. Microsatellite instability is associated with a better prognosis for gastric cancer patients after curative surgery. World J Surg. (2012) 36:2131–8. doi: 10.1007/s00268-012-1652-7
70. Beghelli S, de Manzoni G, Barbi S, Tomezzoli A, Roviello F, Di Gregorio C, et al. Microsatellite instability in gastric cancer is associated with better prognosis in only stage II cancers. Surgery. (2006) 139:347–56. doi: 10.1016/j.surg.2005.08.021
71. Kim SY, Choi YY, An JY, Shin HB, Jo A, Choi H, et al. The benefit of microsatellite instability is attenuated by chemotherapy in stage II and stage III gastric cancer: Results from a large cohort with subgroup analyses. Int J Cancer. (2015) 137:819–25. doi: 10.1002/ijc.29449
72. An JY, Kim H, Cheong JH, Hyung WJ, Kim H, Noh SH. Microsatellite instability in sporadic gastric cancer: its prognostic role and guidance for 5-FU based chemotherapy after R0 resection. Int J Cancer. (2012) 131:505–11. doi: 10.1002/ijc.26399
73. Kim ST, Cristescu R, Bass AJ, Kim KM, Odegaard JI, Kim K, et al. Comprehensive molecular characterization of clinical responses to PD-1 inhibition in metastatic gastric cancer. Nat Med. (2018) 24:1449–58. doi: 10.1038/s41591-018-0101-z
74. Rocken C. Predictive biomarkers in gastric cancer. J Cancer Res Clin Oncol. (2023) 149:467–81. doi: 10.1007/s00432-022-04408-0
75. Grogg KL, Lohse CM, Pankratz VS, Halling KC, Smyrk TC. Lymphocyte-rich gastric cancer: associations with Epstein-Barr virus, microsatellite instability, histology, and survival. Mod Pathol. (2003) 16:641–51. doi: 10.1097/01.mp.0000076980.73826.c0
76. Chiaravalli AM, Feltri M, Bertolini V, Bagnoli E, Furlan D, Cerutti R, et al. Intratumour T cells, their activation status and survival in gastric carcinomas characterised for microsatellite instability and Epstein-Barr virus infection. Virchows Arch. (2006) 448:344–53. doi: 10.1007/s00428-005-0066-4
77. Panda A, Mehnert JM, Hirshfield KM, Riedlinger G, Damare S, Saunders T, et al. Immune activation and benefit from avelumab in EBV-positive gastric cancer. J Natl Cancer Inst. (2018) 110:316–20. doi: 10.1093/jnci/djx213
78. Newman AM, Liu CL, Green MR, Gentles AJ, Feng W, Xu Y, et al. Robust enumeration of cell subsets from tissue expression profiles. Nat Methods. (2015) 12:453–7. doi: 10.1038/nmeth.3337
79. Bai Y, Xie T, Wang Z, Tong S, Zhao X, Zhao F, et al. Efficacy and predictive biomarkers of immunotherapy in Epstein-Barr virus-associated gastric cancer. J Immunother Cancer. (2022) 10:e004080. doi: 10.1136/jitc-2021-004080
80. Zhang X, Zhang C, Hou H, Zhang Y, Jiang P, Zhou H, et al. Neoadjuvant PD-1 blockade plus chemotherapy versus chemotherapy alone in locally advanced stage II-III gastric cancer: A single-centre retrospective study. Transl Oncol. (2023) 31:101657. doi: 10.1016/j.tranon.2023.101657
81. Wei XL, Liu QW, Liu FR, Yuan SS, Li XF, Li JN, et al. The clinicopathological significance and predictive value for immunotherapy of programmed death ligand-1 expression in Epstein-Barr virus-associated gastric cancer. Oncoimmunology. (2021) 10:1938381. doi: 10.1080/2162402x.2021.1938381
82. Nakano H, Saito M, Nakajima S, Saito K, Nakayama Y, Kase K, et al. PD-L1 overexpression in EBV-positive gastric cancer is caused by unique genomic or epigenomic mechanisms. Sci Rep. (2021) 11:1982. doi: 10.1038/s41598-021-81667-w
83. Shinozaki-Ushiku A, Kunita A, Fukayama M. Update on Epstein-Barr virus and gastric cancer (review). Int J Oncol. (2015) 46:1421–34. doi: 10.3892/ijo.2015.2856
84. Casey SC, Tong L, Li Y, Do R, Walz S, Fitzgerald KN, et al. MYC regulates the antitumor immune response through CD47 and PD-L1. Science. (2016) 352:227–31. doi: 10.1126/science.aac9935
85. Goodman AM, Kato S, Bazhenova L, Patel SP, Frampton GM, Miller V, et al. Tumor mutational burden as an independent predictor of response to immunotherapy in diverse cancers. Mol Cancer Ther. (2017) 16:2598–608. doi: 10.1158/1535-7163.mct-17-0386
86. Schumacher TN, Schreiber RD. Neoantigens in cancer immunotherapy. Science. (2015) 348:69–74. doi: 10.1126/science.aaa4971
87. Jardim DL, Goodman A, de Melo Gagliato D, Kurzrock R. The challenges of tumor mutational burden as an immunotherapy biomarker. Cancer Cell. (2021) 39:154–73. doi: 10.1016/j.ccell.2020.10.001
88. Yarchoan M, Hopkins A, Jaffee EM. Tumor mutational burden and response rate to PD-1 inhibition. N Engl J Med. (2017) 377:2500–1. doi: 10.1056/nejmc1713444
89. Kim JY, Kronbichler A, Eisenhut M, Hong SH, van der Vliet HJ, Kang J, et al. Tumor mutational burden and efficacy of immune checkpoint inhibitors: A systematic review and meta-analysis. Cancers. (2019) 11:1798. doi: 10.3390/cancers11111798
90. Osipov A, Lim SJ, Popovic A, Azad NS, Laheru DA, Zheng L, et al. Tumor mutational burden, toxicity, and response of immune checkpoint inhibitors targeting PD(L)1, CTLA-4, and combination: A meta-regression analysis. Clin Cancer Res. (2020) 26:4842–51. doi: 10.1158/1078-0432.ccr-20-0458
91. Hellmann MD, Ciuleanu TE, Pluzanski A, Lee JS, Otterson GA, Audigier-Valette C, et al. Nivolumab plus ipilimumab in lung cancer with a high tumor mutational burden. N Engl J Med. (2018) 378:2093–104. doi: 10.1056/nejmoa1801946
92. Lee KW, Van Cutsem E, Bang YJ, Fuchs CS, Kudaba I, Garrido M, et al. Association of tumor mutational burden with efficacy of pembrolizumab ± Chemotherapy as first-line therapy for gastric cancer in the phase III KEYNOTE-062 study. Clin Cancer Res. (2022) 28:3489–98. doi: 10.1158/1078-0432.CCR-22-0121
93. Hashimoto I, Oshima T. Claudins and gastric cancer: an overview. Cancers (Basel). (2022) 14:290. doi: 10.3390/cancers14020290
94. Wang Y, Wang H, Shi T, Song X, Zhang X, Zhang Y, et al. Immunotherapies targeting the oncogenic fusion gene CLDN18-ARHGAP in gastric cancer. EMBO Mol Med. (2024) 16:2170–87. doi: 10.1038/s44321-024-00120-3
95. Peng M, Mo Y, Wang Y, Wu P, Zhang Y, Xiong F, et al. Neoantigen vaccine: an emerging tumor immunotherapy. Mol Cancer. (2019) 18:128. doi: 10.1186/s12943-019-1055-6
96. Mahadevan KK, McAndrews KM, LeBleu VS, Yang S, Lyu H, Li B, et al. KRAS(G12D) inhibition reprograms the microenvironment of early and advanced pancreatic cancer to promote FAS-mediated killing by CD8(+) T cells. Cancer Cell. (2023) 41:1606–20.e8. doi: 10.1016/j.ccell.2023.07.002
97. Shi Y, Ren X, Cao S, Chen X, Yuan B, Brasil da Costa FH, et al. TP53 gain-of-function mutation modulates the immunosuppressive microenvironment in non-HPV-associated oral squamous cell carcinoma. J Immunother Cancer. (2023) 11:e006666. doi: 10.1136/jitc-2023-006666
98. Li Y, Luo Y. Optimizing the evaluation of gene-targeted panels for tumor mutational burden estimation. Sci Rep. (2021) 11:21072. doi: 10.1038/s41598-021-00626-7
99. Cristescu R, Mogg R, Ayers M, Albright A, Murphy E, Yearley J, et al. Pan-tumor genomic biomarkers for PD-1 checkpoint blockade-based immunotherapy. Science. (2018) 362:eaar3593. doi: 10.1126/science.aar3593
100. Zhang L, Zhang X, Liu H, Yang C, Yu J, Zhao W, et al. MTFR2-dependent mitochondrial fission promotes HCC progression. J Transl Med. (2024) 22:73. doi: 10.1186/s12967-023-04845-6
101. Li J, Zhang W, Ma X, Wei Y, Zhou F, Li J, et al. Cuproptosis/ferroptosis-related gene signature is correlated with immune infiltration and predict the prognosis for patients with breast cancer. Front Pharmacol. (2023) 14:1192434. doi: 10.3389/fphar.2023.1192434
102. Ding D, Wang L, Zhang Y, Shi K, Shen Y. Machine learning developed a programmed cell death signature for predicting prognosis and immunotherapy benefits in lung adenocarcinoma. Transl Oncol. (2023) 38:101784. doi: 10.1016/j.tranon.2023.101784
103. Zhou Q, Xu J, Xu Y, Sun S, Chen J. Role of ICAM1 in tumor immunity and prognosis of triple-negative breast cancer. Front Immunol. (2023) 14:1176647. doi: 10.3389/fimmu.2023.1176647
104. Budczies J, Kazdal D, Menzel M, Beck S, Kluck K, Altbürger C, et al. Tumour mutational burden: clinical utility, challenges and emerging improvements. Nat Rev Clin Oncol. (2024) 21:725–42. doi: 10.1038/s41571-024-00932-9
105. Nebot-Bral L, Brandao D, Verlingue L, Rouleau E, Caron O, Despras E, et al. Hypermutated tumours in the era of immunotherapy: The paradigm of personalised medicine. Eur J Cancer. (2017) 84:290–303. doi: 10.1016/j.ejca.2017.07.026
106. Knoll MA, Griffith KA, Jones RD, Jagsi R. Association of gender and parenthood with conference attendance among early career oncologists. JAMA Oncol. (2019) 5:1503–4. doi: 10.1001/jamaoncol.2019.1864
107. Ma X, Riaz N, Samstein RM, Lee M, Makarov V, Valero C, et al. Functional landscapes of POLE and POLD1 mutations in checkpoint blockade-dependent antitumor immunity. Nat Genet. (2022) 54:996–1012. doi: 10.1038/s41588-022-01108-w
108. Yao J, Gong Y, Zhao W, Han Z, Guo S, Liu H, et al. Comprehensive analysis of POLE and POLD1 Gene Variations identifies cancer patients potentially benefit from immunotherapy in Chinese population. Sci Rep. (2019) 9:15767. doi: 10.1038/s41598-019-52414-z
109. Zhu M, Cui H, Zhang L, Zhao K, Jia X, Jin H. Assessment of POLE and POLD1 mutations as prognosis and immunotherapy biomarkers for stomach adenocarcinoma. Transl Cancer Res. (2022) 11:193–205. doi: 10.21037/tcr-21-1601
110. Rousseau B, Bieche I, Pasmant E, Hamzaoui N, Leulliot N, Michon L, et al. PD-1 blockade in solid tumors with defects in polymerase epsilon. Cancer Discov. (2022) 12:1435–48. doi: 10.1158/2159-8290.CD-21-0521
111. Hou W, Zhao Y, Zhu H. Predictive biomarkers for immunotherapy in gastric cancer: current status and emerging prospects. Int J Mol Sci. (2023) 24:15321. doi: 10.3390/ijms242015321
112. Lengyel CG, Hussain S, Trapani D, El Bairi K, Altuna SC, Seeber A, et al. The emerging role of liquid biopsy in gastric cancer. J Clin Med. (2021) 10:2108. doi: 10.3390/jcm10102108
113. Jin Y, Chen DL, Wang F, Yang CP, Chen XX, You JQ, et al. The predicting role of circulating tumor DNA landscape in gastric cancer patients treated with immune checkpoint inhibitors. Mol cancer. (2020) 19:154. doi: 10.1186/s12943-020-01274-7
114. Qiao G, Wang X, Zhou L, Zhou X, Song Y, Wang S, et al. Autologous dendritic cell-cytokine induced killer cell immunotherapy combined with S-1 plus cisplatin in patients with advanced gastric cancer: A prospective study. Clin Cancer Res. (2019) 25:1494–504. doi: 10.1158/1078-0432.ccr-18-2360
115. Lee PC, Wu CJ, Hung YW, Lee CJ, Chi CT, Lee IC, et al. Gut microbiota and metabolites associate with outcomes of immune checkpoint inhibitor-treated unresectable hepatocellular carcinoma. J Immunother Cancer. (2022) 10:e004779. doi: 10.1136/jitc-2022-004779
116. Thomas AM, Fidelle M, Routy B, Kroemer G, Wargo JA, Segata N, et al. Gut OncoMicrobiome Signatures (GOMS) as next-generation biomarkers for cancer immunotherapy. Nat Rev Clin Oncol. (2023) 20:583–603. doi: 10.1038/s41571-023-00785-8
117. Geng H, Dong Z, Zhang L, Yang C, Li T, Lin Y, et al. An immune signature for risk stratification and therapeutic prediction in helicobacter pylori-infected gastric cancer. Cancers. (2022) 14:3276. doi: 10.3390/cancers14133276
118. Oster P, Vaillant L, Riva E, McMillan B, Begka C, Truntzer C, et al. Helicobacter pylori infection has a detrimental impact on the efficacy of cancer immunotherapies. Gut. (2022) 71:457–66. doi: 10.1136/gutjnl-2020-323392
119. Shi Y, Zheng H, Wang M, Ding S. Influence of Helicobacter pylori infection on PD-1/PD-L1 blockade therapy needs more attention. Helicobacter. (2022) 27:e12878. doi: 10.1111/hel.12878
120. Jiang Q, Liu W, Zeng X, Zhang C, Du Y, Zeng L, et al. Safety and efficacy of tislelizumab plus chemotherapy versus chemotherapy alone as neoadjuvant treatment for patients with locally advanced gastric cancer: real-world experience with a consecutive patient cohort. Front Immunol. (2023) 14. doi: 10.3389/fimmu.2023.1122121
121. Prigent P, El Mir S, Dréano M, Triebel F. Lymphocyte activation gene-3 induces tumor regression and antitumor immune responses. Eur J Immunol. (1999) 29:3867–76. doi: 10.1002/(sici)1521-4141(199912)29:12<3867::aid-immu3867>3.3.co;2-5
122. Chocarro L, Blanco E, Zuazo M, Arasanz H, Bocanegra A, Fernández-Rubio L, et al. Understanding LAG-3 signaling. Int J Mol Sci. (2021) 22:5282. doi: 10.3390/ijms22105282
123. Liang B, Workman C, Lee J, Chew C, Dale BM, Colonna L, et al. Regulatory T cells inhibit dendritic cells by lymphocyte activation gene-3 engagement of MHC class II. J Immunol. (2008) 180:5916–26. doi: 10.4049/jimmunol.180.9.5916
124. Kelly RJ, Landon BV, Zaidi AH, Singh D, Canzoniero JV, Balan A, et al. Neoadjuvant nivolumab or nivolumab plus LAG-3 inhibitor relatlimab in resectable esophageal/gastroesophageal junction cancer: a phase Ib trial and ctDNA analyses. Nat Med. (2024) 30:1023–34. doi: 10.1038/s41591-024-02877-z
125. Hegewisch-Becker S, Mendez G, Chao J, Nemecek R, Feeney K, Van Cutsem E, et al. First-line nivolumab and relatlimab plus chemotherapy for gastric or gastroesophageal junction adenocarcinoma: the phase II RELATIVITY-060 study. J Clin Oncol. (2024) 42:2080–93. doi: 10.1200/jco.23.01636
126. Hosseini A, Gharibi T, Marofi F, Babaloo Z, Baradaran B. CTLA-4: From mechanism to autoimmune therapy. Int Immunopharmacol. (2020) 80:106221. doi: 10.1016/j.intimp.2020.106221
127. Wolf Y, Anderson AC, Kuchroo VK. TIM3 comes of age as an inhibitory receptor. Nat Rev Immunol. (2020) 20:173–85. doi: 10.1038/s41577-019-0224-6
128. Friedlaender A, Addeo A, Banna G. New emerging targets in cancer immunotherapy: the role of TIM3. ESMO Open. (2019) 4:e000497. doi: 10.1136/esmoopen-2019-000497
129. Cheng G, Li M, Wu J, Ji M, Fang C, Shi H, et al. Expression of Tim-3 in gastric cancer tissue and its relationship with prognosis. Int J Clin Exp Pathol. (2015) 8:9452–7.
130. Yu J, Zhang H, Sun S, Sun S, Li L. The effects of Tim-3 activation on T-cells in gastric cancer progression. Oncol Lett. (2019) 17:1461–6. doi: 10.3892/ol.2018.9743
131. Park Y, Seo AN, Koh J, Nam SK, Kwak Y, Ahn SH, et al. Expression of the immune checkpoint receptors PD-1, LAG3, and TIM3 in the immune context of stage II and III gastric cancer by using single and chromogenic multiplex immunohistochemistry. Oncoimmunology. (2021) 10:1954761. doi: 10.1080/2162402x.2021.1954761
132. Chen K, Gu Y, Cao Y, Fang H, Lv K, Liu X, et al. TIM3(+) cells in gastric cancer: clinical correlates and association with immune context. Br J Cancer. (2022) 126:100–8. doi: 10.1038/s41416-021-01607-3
133. Chen D, Lin X, Zhang C, An G, Li Z, Dong B, et al. Activated Wnt signaling promotes growth and progression of AFP-producing gastric cancer in preclinical models. Cancer Manag Res. (2019) 11:1349–62. doi: 10.2147/CMAR.S187219
134. Kim HI, Lim J, Shim JH. Role of the alpha-fetoprotein response in immune checkpoint inhibitor-based treatment of patients with hepatocellular carcinoma. J Cancer Res Clin Oncol. (2022) 148:2069–77. doi: 10.1007/s00432-021-03727-y
135. Shao YY, Liu TH, Hsu C, Lu LC, Shen YC, Lin ZZ, et al. Early alpha-foetoprotein response associated with treatment efficacy of immune checkpoint inhibitors for advanced hepatocellular carcinoma. Liver Int. (2019) 39:2184–9. doi: 10.1111/liv.14210
136. Munson PV, Adamik J, Butterfield LH. Immunomodulatory impact of α-fetoprotein. Trends Immunol. (2022) 43:438–48. doi: 10.1016/j.it.2022.04.001
137. Zhang J, Wang L, Zhang S, Cao R, Zhao Y, Zhao Y, et al. Alpha-fetoprotein predicts the treatment efficacy of immune checkpoint inhibitors for gastric cancer patients. BMC Cancer. (2024) 24:266. doi: 10.1186/s12885-024-11999-z
138. Shi YJ, Matson C, Lan F, Iwase S, Baba T, Shi Y. Regulation of LSD1 histone demethylase activity by its associated factors. Mol Cell. (2005) 19:857–64. doi: 10.1016/j.molcel.2005.08.027
139. Zheng YC, Duan YC, Ma JL, Xu RM, Zi X, Lv WL, et al. Triazole-dithiocarbamate based selective lysine specific demethylase 1 (LSD1) inactivators inhibit gastric cancer cell growth, invasion, and migration. J Med Chem. (2013) 56:8543–60. doi: 10.1021/jm401002r
140. Li ZH, Liu XQ, Geng PF, Suo FZ, Ma JL, Yu B, et al. Discovery of [1,2,3]Triazolo[4,5-d]pyrimidine derivatives as novel LSD1 inhibitors. ACS Med Chem Lett. (2017) 8:384–9. doi: 10.1021/acsmedchemlett.6b00423
141. Ma L, Wang H, You Y, Ma C, Liu Y, Yang F, et al. Exploration of 5-cyano-6-phenylpyrimidin derivatives containing an 1,2,3-triazole moiety as potent FAD-based LSD1 inhibitors. Acta Pharm Sin B. (2020) 10:1658–68. doi: 10.1016/j.apsb.2020.02.006
142. Dai XJ, Liu Y, Xiong XP, Xue LP, Zheng YC, Liu HM. Tranylcypromine based lysine-specific demethylase 1 inhibitor: summary and perspective. J Med Chem. (2020) 63:14197–215. doi: 10.1021/acs.jmedchem.0c00919
143. Zhang J, Zhao D, Li Q, Du X, Liu Y, Dai X, et al. Upregulation of LSD1 promotes migration and invasion in gastric cancer through facilitating EMT. Cancer Manag Res. (2019) 11:4481–91. doi: 10.2147/CMAR.S186649
144. De Craene B, Berx G. Regulatory networks defining EMT during cancer initiation and progression. Nat Rev Cancer. (2013) 13:97–110. doi: 10.1038/nrc3447
145. Shen DD, Pang JR, Bi YP, Zhao LF, Li YR, Zhao LJ, et al. LSD1 deletion decreases exosomal PD-L1 and restores T-cell response in gastric cancer. Mol Cancer. (2022) 21:75. doi: 10.1186/s12943-022-01557-1
146. Dai XJ, Liu Y, Wang N, Chen HX, Wu JW, Xiong XP, et al. Novel acridine-based LSD1 inhibitors enhance immune response in gastric cancer. Eur J Med Chem. (2023) 259:115684. doi: 10.1016/j.ejmech.2023.115684
147. Zhang L, Li S. Lactic acid promotes macrophage polarization through MCT-HIF1α signaling in gastric cancer. Exp Cell Res. (2020) 388:111846. doi: 10.1016/j.yexcr.2020.111846
148. Yang S, Sun B, Li W, Yang H, Li N, Zhang X. Fatty acid metabolism is related to the immune microenvironment changes of gastric cancer and RGS2 is a new tumor biomarker. Front Immunol. (2022) 13:1065927. doi: 10.3389/fimmu.2022.1065927
149. Davuluri RV, Suzuki Y, Sugano S, Plass C, Huang TH. The functional consequences of alternative promoter use in mammalian genomes. Trends Genet. (2008) 24:167–77. doi: 10.1016/j.tig.2008.01.008
150. Bieberstein NI, Carrillo Oesterreich F, Straube K, Neugebauer KM. First exon length controls active chromatin signatures and transcription. Cell Rep. (2012) 2:62–8. doi: 10.1016/j.celrep.2012.05.019
151. Sundar R, Huang KK, Qamra A, Kim KM, Kim ST, Kang WK, et al. Epigenomic promoter alterations predict for benefit from immune checkpoint inhibition in metastatic gastric cancer. Ann Oncol. (2019) 30:424–30. doi: 10.1093/annonc/mdy550
152. Johnson BJ, Costelloe EO, Fitzpatrick DR, Haanen JB, Schumacher TN, Brown LE, et al. Single-cell perforin and granzyme expression reveals the anatomical localization of effector CD8+ T cells in influenza virus-infected mice. Proc Natl Acad Sci U S A. (2003) 100:2657–62. doi: 10.1073/pnas.0538056100
153. Herbst RS, Soria JC, Kowanetz M, Fine GD, Hamid O, Gordon MS, et al. Predictive correlates of response to the anti-PD-L1 antibody MPDL3280A in cancer patients. Nature. (2014) 515:563–7. doi: 10.1038/nature14011
154. Sundar R, Huang KK, Kumar V, Ramnarayanan K, Demircioglu D, Her Z, et al. Epigenetic promoter alterations in GI tumour immune-editing and resistance to immune checkpoint inhibition. Gut. (2022) 71:1277–88. doi: 10.1136/gutjnl-2021-324420
155. Toh M, Ngeow J. Homologous recombination deficiency: cancer predispositions and treatment implications. Oncologist. (2021) 26:e1526–e37. doi: 10.1002/onco.13829
156. Zhou Z, Ding Z, Yuan J, Shen S, Jian H, Tan Q, et al. Homologous recombination deficiency (HRD) can predict the therapeutic outcomes of immuno-neoadjuvant therapy in NSCLC patients. J Hematol Oncol. (2022) 15:62. doi: 10.1186/s13045-022-01283-7
157. Morse CB, Toukatly MN, Kilgore MR, Agnew KJ, Bernards SS, Norquist BM, et al. Tumor infiltrating lymphocytes and homologous recombination deficiency are independently associated with improved survival in ovarian carcinoma. Gynecol Oncol. (2019) 153:217–22. doi: 10.1016/j.ygyno.2019.02.011
Keywords: biomarker, gastric cancer, immune checkpoint inhibitor, immunotherapy, predictive value
Citation: Sun F, Gao X, Wang W, Zhao X, Zhang J and Zhu Y (2025) Predictive biomarkers in the era of immunotherapy for gastric cancer: current achievements and future perspectives. Front. Immunol. 16:1599908. doi: 10.3389/fimmu.2025.1599908
Received: 25 March 2025; Accepted: 24 April 2025;
Published: 14 May 2025.
Edited by:
Ines Zidi, Tunis El Manar University, TunisiaReviewed by:
Dipendra Khadka, Wonkwang University School of Medicine, Republic of KoreaDaniele Caracciolo, Magna Græcia University, Italy
Copyright © 2025 Sun, Gao, Wang, Zhao, Zhang and Zhu. This is an open-access article distributed under the terms of the Creative Commons Attribution License (CC BY). The use, distribution or reproduction in other forums is permitted, provided the original author(s) and the copyright owner(s) are credited and that the original publication in this journal is cited, in accordance with accepted academic practice. No use, distribution or reproduction is permitted which does not comply with these terms.
*Correspondence: Yanmei Zhu, emh1eWFubWVpQGNhbmNlcmhvc3AtbG4tY211LmNvbQ==; Jingdong Zhang, amR6aGFuZ0BjYW5jZXJob3NwLWxuLWNtdS5jb20=
†These authors have contributed equally to this work