- 1Programa de Ecología Genómica, Centro de Ciencias Genómicas, Universidad Nacional Autónoma de México, Cuernavaca, Mexico
- 2Programa de Doctorado en Ciencias Biomédicas, Centro de Ciencias Genómicas, Universidad Nacional Autónoma de México, Cuernavaca, Mexico
- 3Programa de Genómica Evolutiva, Centro de Ciencias Genómicas, Universidad Nacional Autónoma de México, Cuernavaca, Mexico
Rhizobium tropici CIAT899 is a nodule-forming α-proteobacterium displaying intrinsic resistance to several abiotic stress conditions such as low pH and high temperatures, which are common in tropical environments. It is a good competitor for Phaseolus vulgaris (common bean) nodule occupancy at low pH values, however little is known about the genetic and physiological basis of the tolerance to acidic conditions. To identify genes in R. tropici involved in pH stress response we combined two different approaches: (1) A Tn5 mutant library of R. tropici CIAT899 was screened and 26 acid-sensitive mutants were identified. For 17 of these mutants, the transposon insertion sites could be identified. (2) We also studied the transcriptomes of cells grown under different pH conditions using RNA-Seq. RNA was extracted from cells grown for several generations in minimal medium at 6.8 or 4.5 (adapted cells). In addition, we acid-shocked cells pre-grown at pH 6.8 for 45 min at pH 4.5. Of the 6,289 protein-coding genes annotated in the genome of R. tropici CIAT 899, 383 were differentially expressed under acidic conditions (pH 4.5) vs. control condition (pH 6.8). Three hundred and fifty one genes were induced and 32 genes were repressed; only 11 genes were induced upon acid shock. The acid stress response of R. tropici CIAT899 is versatile: we found genes encoding response regulators and membrane transporters, enzymes involved in amino acid and carbohydrate metabolism and proton extrusion, in addition to several hypothetical genes. Our findings enhance our understanding of the core genes that are important during the acid stress response in R. tropici.
Introduction
The response to acidic stress conditions is understood best in enterobacteria, and Escherichia coli, S. enterica var. Typhimurium, P. mirabilis and Y. enterocolitica (Castanie-Cornet et al., 1999; Kieboom and Abee, 2006; De Biase and Pennacchietti, 2012), all have effective systems to contend with acid stress. Well-known are the decarboxylation systems, which are composed of two components: a decarboxylase and an antiporter. Protons are consumed in the cytoplasm through the decarboxylation of specific amino acids and the corresponding antiporter exports the decarboxylation product and imports more of the required amino acid (Foster, 2004). The best-studied example is the glutamate decarboxylase (Gad) system depending upon the concerted action of glutamate decarboxylase (GadA/GadB) and of the glutamate/GABA antiporter, GadC (Foster, 1999; Audia et al., 2001; Lund et al., 2014).
Rhizobium tropici CIAT899 is an α-proteobacterium capable of establishing a symbiosis with different leguminous plants including common bean (Phaseolous vulgaris) (Martínez-Romero et al., 1991). During this symbiosis root nodules are formed, which are specialized organs where biological nitrogen fixation (BNF) will take place (Suzaki et al., 2015). The efficiency of this symbiosis can be restrained by different environmental conditions, such as high temperature and low pH (Martínez-Romero et al., 1991; Graham et al., 1994; Hungría et al., 2000; Vinuesa et al., 2003). For example under acidic pH conditions, where survival and persistence of the bacteria are limited, nodulation and BNF can be severely affected. Acidic conditions can be found in the rhizosphere of plants, where the pH is lowered by plant exudates containing protons and organic acids, and inside symbiosomes (Udvardi and Day, 1997). Compared to most other nodule-forming bacteria R. tropici CIAT899 presents an increased resistance to acidic growth conditions.
A few studies trying to identify the genetic determinants of growth at acidic pH have been made in R. tropici (Riccillo et al., 2000; Vinuesa et al., 2003; Rojas-Jiménez et al., 2005; Vences-Guzmán et al., 2011). In 2003, Vinuesa et al. (2003), identified novel rhizobial genes required for acid tolerance. During a screen of a small Tn5 mutant library composed of 1,728 clones, they identified two mutants affected in acid tolerance. In one mutant, the Tn5 was inserted in the sycA-olsC gene cluster and in the second mutant, it was inserted in the lpiA-atvA operon. OlsC catalyzes the hydroxylation at the 2-position of the secondary fatty acid of ornithine lipids (OLs). The presence of this hydroxyl group has been correlated later to an increase tolerance to acidic conditions and high temperatures in CIAT899 (Vinuesa et al., 2003; Rojas-Jiménez et al., 2005; Vences-Guzmán et al., 2011). LpiA is a lysyl-phosphatidylglycerol synthase homologous to MprF from Staphylococcus aureus and the atvA gene is encoding a putative serine lipase homologous to the virulence proteins AcvB and VirJ from Agrobacterium tumefaciens. It was demonstrated that lpiA is induced under acidic conditions, and that LpiA participates in lysyl-phosphatidylglycerol (LPG) biosynthesis, which confers an increased resistance of R. tropici CIAT899 to the cationic peptide polymyxin under acidic growth conditions (Vinuesa et al., 2003; Sohlenkamp et al., 2007). Transcriptional induction of lpiA expression was also induced in Sinorhizobium medicae and Sinorhizobium meliloti as part of the response to low pH (Reeve et al., 2006; Hellweg et al., 2009). GshB participates in glutathione biosynthesis (Riccillo et al., 2000), which is necessary to grow in several environmental conditions like oxidative stress, osmotic stress and acid stress and transcription of gshB is induced under acidic stress conditions as shown by quantitative PCR (Muglia et al., 2007).
In addition, the CIAT899 genome encodes homologs to several proteins that are involved in acid stress response in other bacteria: (1) eptA encodes a putative lipid A phosphoethanolamine transferase, which confers acid resistance in E. coli, Salmonella typhimurium, and Shigella flexneri 2a (Martinić et al., 2011); (2) cfa encodes cyclopropane-fatty-acyl-phospholipid synthase which enhances acid tolerance in E. coli by reducing the permeability of the membrane to H+ (Shabala and Ross, 2008; Sohlenkamp, 2017). Five different copies of cfa genes are encoded in the genome of R. tropici CIAT899; (3) H+/Cl− antiporters are responsible for protons extrusion when E. coli or Helicobacter pylori are exposed to acidic conditions (Inoue et al., 1999; Padan et al., 2004; Padan, 2008). CIAT899 possesses four genes encoding antiporters that possibly help to maintain pH homeostasis; (4) Exopolysaccharide (EPS) biosynthesis has been related to acid tolerance. In S. meliloti it has been shown that genes related to EPS production are expressed under acidic conditions (Cunningham and Munns, 1984; Hellweg et al., 2009) and the genome of CIAT899 encodes several exo genes and EPS production could help the bacteria to resist acid stress. It is not known, if the orthologues to these genes involved in acid stress response in other bacteria have a function during the acid stress response in R. tropici.
Vinuesa et al. (2003) had identified at least two novel genes involved in the acid stress response in R. tropici out of a screen using only 1,728 mutants. As the genome of R. tropici CIAT899 contains more than 6,280 genes (Ormeño-Orrillo et al., 2012), we think that it should be possible to discover further novel genes having a function in acid stress response in this organism. Here we present a study combining random transposon mutagenesis with transcriptomics to obtain a broad catalog of genes important or essential for the acid stress response in R. tropici CIAT899.
Materials and Methods
Bacterial Strains and Culture Conditions
Bacterial strains and plasmids used in this work are listed in Table 1. R. tropici CIAT899 was routinely grown in TY medium (Beringer, 1974) or the minimal medium described by Kingsley and Bohlool (Kingsley and Bohlool, 1992), adjusted to pH 6.8 [MM- buffered to pH 6.8 with 20 mM Hepes (N-(2-Hydroxyethyl)piperazine-N′-(2-ethanesulfonic acid))] or to pH 4.5 (MAM-buffered to pH 4.5 with 25 mM Homopipes (Homopiperazine-N,N′-bis-2-(ethanesulfonic acid))), Research Organics, Cleveland, OH, USA) at 30°C. MM and MAM were solidified with 0.8% gelrite (Carl Roth GmbH, Karlsruhe, Germany). E. coli strains were grown in Luria-Bertani (LB) medium at 37°C. Antibiotics were added at the following final concentrations (μg/mL): kanamycin (Km) 150; carbenicillin (Cb) 100; tetracycline (Tc) 10, and nalidixic acid (Nal) 20.
Tn5 Mutagenesis of Rhizobium tropici CIAT899
A general Tn5 transposon mutagenesis of R. tropici CIAT899 was performed via conjugal transfer of pSUP1021 into CIAT899 utilizing E. coli S17.1 as the donor strain (Simon et al., 1983). Appropriate dilutions were plated on TY medium, supplemented with kanamycin and nalidixic acid to select for Tn5 transconjugants. Individual colonies were transferred to microtiter plate wells with TY medium and were grown overnight at 30°C. Glycerol was added to a final concentration of 30% (w/v) and the mutant library was stored at −80 °C. To check the quality of the library, 10 transconjugants were randomly selected, genomic DNA was extracted, digested with EcoRI (New England Biolabs), and Southern blot hybridization was performed using a digoxigenin-labeled probe hybridizing to a fragment of the Tn5. The probe was synthesized using the oligos Tn5-1 and Tn5-2 (Table 2). This analysis established that the transposon had inserted in each strain only once and into different regions within the genome of R. tropici CIAT899.
Screen for Acid-Sensitive Mutants
The ordered mutant library was replica-plated from the glycerol stock onto TY plates and grown for 3 days. Clones were transferred using a 48-pin replicator onto MM and MAM plates, and grown for 5 days. Mutants that grew as the wildtype under neutral pH conditions (MM), but that were strongly affected or that did not grow at low pH (MAM) were selected for further analysis. The Tn5 insertion sites were mapped using two approaches. Most of the transposon insertion sites were determined using inverse PCR (iPCR) using the oligos described in Table 2. Briefly, 1 μg of genomic DNA of an acid-sensitive mutant is digested with the enzyme BamHI (New England Biolabs) that cuts just in the middle of the Tn5. Following digestion, the reactions were inactivated at 65°C for 15 min. The restricted DNA was circularized overnight at 16°C in 100 μL reactions with 200 U of T4 DNA ligase (New England Biolabs), and then purified with High Pure PCR Product Purification Kit (Roche). The iPCR mix contained 10 μL of 10x PCR buffer, 6 μL of 25 mM MgCl2 (final concentration of 1.5 mM), 2 μL 10 mM dNTP mix (final concentration of 200 μM each dNTP), 0.5 μM (4 μL) of each primer (different combinations like BL-IR1, BL-IR2, or BR-IR1, BR-IR2 were used for the amplification, see Table 2), 10 μL (500 ng) of circularized template and 1.6 U of rTth DNA polymerase XL (Applied Biosystems) and ultrapure water (Milli-Q). The target DNA sequence was amplified using the following program: 94°C for 10 min, then 35 cycles 94°C for 1 min, 68°C for 5 min and 72°C for 10 min, and finally 72 °C for 10 min. PCR products were purified and sequenced (using different combinations of primers (see Table 2) at the Institute of Biotechnology (IBt) of the UNAM and mapped to the reference genome to identify the position of the Tn5 insertions. For the other approach genomic DNA of acid-sensitive mutants was digested with EcoRI (cuts Tn5 sequence without damaging the kanamycin resistance cassette) and then cloned into pUC19. After transformation we selected for kanamycin-resistant clones containing the resistance cassette and flanking sequences from the R. tropici genome. Plasmids were sequenced, and the insertion sites were mapped in the reference genome.
RNA Isolation
R. tropici CIAT899 was pre-grown in TY medium, then cultured in MM (control pH 6.8) (sample AR) or MAM (pH 4.5) (sample BR) until an OD620 of 0.6–0.7. For acid shock treatment cells were cultured in MM medium to mid-log phase, then washed with MAM and incubated another 45 min in MAM (pH 4.5) (sample CR). Cells were harvested by centrifugation and the pellets were immediately frozen in liquid nitrogen. Total RNA was prepared using the RNeasy mini kit (QIAGEN, Hildesheim, Germany) with some modifications. Pellets were resuspended in RLT buffer supplemented with lysozyme (20 mg per mL; QIAGEN, Hildesheim, Germany) and containing Zirconium Oxide Beads (0.5 mm, Next Advance). Cells were disrupted using the Bullet Blender Tissue Homogenizer (Next-Advance) in impact-resistant 2 mL tubes. Genomic DNA was eliminated by digestion with RNase-free DNase (QIAGEN, Hildesheim, Germany) for 20 min at room temperature. Final RNA concentrations were determined using a NanoDrop (Thermo Scientific). The typical OD260 to OD280 ratio of RNA samples was approximately 2.0. The integrity of RNA samples was verified using a TapeStation 2,200 instrument (Agilent Technologies) and the RNA integrity number (RINe) was determined. Three independent total RNA extractions were obtained for each condition and each one was analyzed separately.
RNA-Seq and Data Analysis
RNA-Seq libraries were prepared using the TruSeq RNA sample Prep kit (Illumina) and sequenced (150 nt per read) by HiSeq 2500 instrument (Illumina) at the Beijing Genomics Institute (BGI, China). For the analysis of RNA-Seq data, Bowtie2 was used to align raw reads to the R. tropici CIAT 899 genome (Genbank entry CP004015, CP004016, CP004017, and CP004018) and samtools was used to obtain BAM files. Differentially expressed genes (DEGs) were obtained via NOISeq 2.14.1 Bioconductor package using local fit and betaPrior parameter set to False. NOISeq implements differential expression analysis based on the Negative Binomial distribution. A false discovery rate (FDR) threshold of 0.95 was set for DEG calling. Sample clustering and principal component analyses were performed upon variance stabilizing transformation of expression data (NOISeq package). Transcripts were called as differentially expressed when the FDR-Log2FC adjusted p-values were below 0.05 and fold-changes over 2 (Tarazona et al., 2011, 2015).
Functional Categorization of Genes
The Clusters of Orthologous Groups (COGs) database was used to classify DEGsin R. tropici CIAT899 into functional categories (https://www.ncbi.nlm.nih.gov/COG/).
Results
Insertional Mutagenesis of Rhizobium tropici CIAT899 and Selection of Acid-Sensitive Transposon Mutants
R. tropici CIAT899 has a 6.3 Mb genome in which some 6,280 protein-encoding genes are annotated. The aim was to saturate the genome with transposon insertions or at least to come close to saturation. An ordered library of 18,300 mutants was constructed using Tn5-derived transposons (Simon et al., 1983). For a small subset of mutants, we showed that each one presented a single Tn5-insertion and that they presented different RFLPs, indicating that the insertions were at different sites. Mutants were replica-plated on TY medium from the 96-well plate glycerol stock. After 3 days of growth, cells were replicated to minimal acid medium (MAM) and to neutral minimal medium (MM) to identify and select acid-sensitive mutants. Mutants were grown for 5 days, selecting those that grew at neutral pH as well as the wildtype but did not grow at acidic pH or that showed a drastically reduced growth under the latter condition (Figure 1). We grouped the mutants into two classes, those unable to grow at acid pH (AS: acid-sensitive) and those that presented only residual growth (MAS: mildly acid-sensitive). In total, 26 mutants were identified that showed growth deficiency under acid conditions, of these three are AS and 23 MAS at pH 4.5. We succeeded in identifying 17 Tn5 insertions among these (Table 3). Most mutants presented insertions sites in the chromosome (14 mutants), except JG283 and JG9867, for which the insertion site was located in the megaplasmid pC (locus tag RTCIAT899_RS28660, polysaccharide biosynthesis protein). Megaplasmid pC has the characteristics of a chromid (Harrison et al., 2010), since it harbors essential genes involved in the biosynthesis of vitamins like thiamine and cobalamine. For mutant JG6057 the transposon insertion site was located to the plasmid pA (locus tag RTCIAT899_ RS18385, hypothetical protein). This is the smallest plasmid of R. tropici CIAT899, it is self-transmissible and includes various conjugation systems genes (Figure 2 and Table 3). None of the insertions was localized in the symbiotic plasmid (pSym or pB).
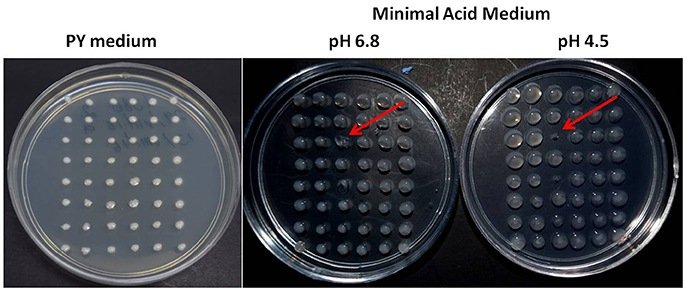
Figure 1. Screening of the R. tropici CIAT899 transposon mutant library for acid-sensitive mutants. A set of 48 Tn5 mutants on complex TY medium, minimal medium (MM) and minimal acid medium (MAM) after 5 days of incubation. The arrow on the MAM plate indicates the position of an acid-sensitive strain (JG9587).
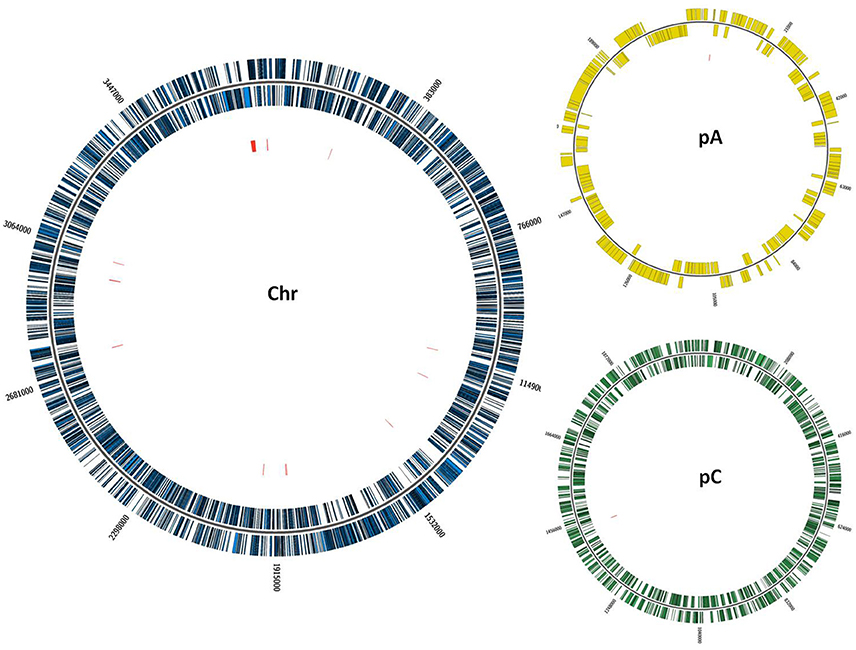
Figure 2. Location of transposon insertions (899::Tn5) in the R. tropici CIAT899 chromosome. Sites of transposon insertions are indicated by the red lines inside the circles. Colors in the three largest rings represent chromosome (blue), the smallest plasmid pRtrCIAT899a (pA) (yellow), and megaplasmid pRtrCIAT899c (pC) (green).
Transcriptomic Analysis Shows That Many Genes Are Expressed Differentially in Response to Acidic Conditions
The principal aim of this study was to identify novel genes important during acid stress in R. tropici. The screening of Tn5 mutants allowed us to identify genes that are important under acid stress, but dispensable under neutral pH conditions. Unfortunately, this approach would not allow for the identification of genes that are important during acid stress, but that are at the same time essential for growth at neutral pH. We guessed that these genes should be either transcriptionally induced or repressed during acid stress. R. tropici CIAT899 cells were grown under control conditions (pH 6.8 adapted, sample AR), in MAM at pH 4.5 (pH 4.5 adapted cells, sample BR), or pre-grown at pH 6.8 until an OD 600 nm of 0.6, washed and then transferred to pH 4.5 for 45 min (acid-shocked, sample CR). Three independent biological experiments were performed for each condition and transcriptomes were analyzed by RNA-Seq. Libraries were sequenced and between 6 and 11 million reads were obtained under each condition. Before subsequent analysis, a normalization process was carried out to eschew statistical deviations due to differences in library sizes (Alexandre et al., 2014). Differentially expressed genes in each condition were identified using the statistical software R (Figure 3). 394 genes were expressed differentially and most of these genes (353) were located in the chromosome, but a few were located in plasmid A (3), plasmid B (8), and plasmid C (30) (Figure 3A). RNA-seq data were submitted to the Sequence Read Archive (SRA) database with the accession number GSE108074.
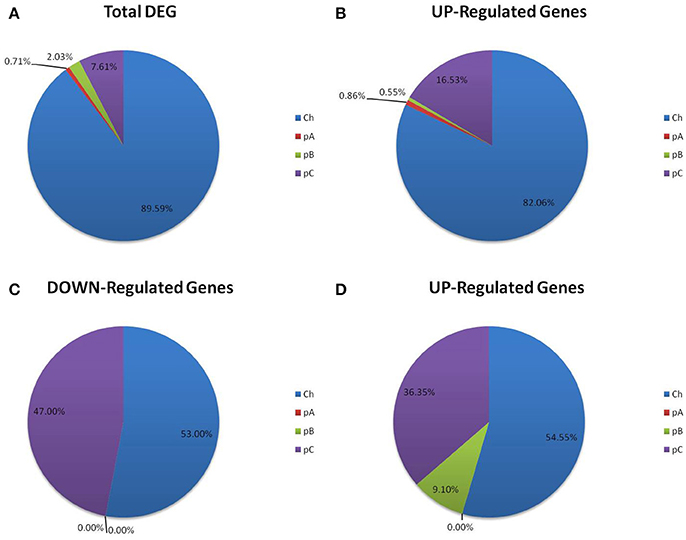
Figure 3. Distribution of differentially expressed genes (DEGs). Location of differentially expressed genes within the multipartite genome of R. tropici CIAT899. Total DEG (A), up-regulated genes at pH 6.8 vs. pH 4.5 (B), down-regulated genes at pH 6.8 vs. pH 4.5 (C) up-regulated genes at pH 6.8 vs. pH 4.5/acid shock (D). Ch, chromosome; pA, pRtrCIAT899a; pB, pRtrCIAT899b; pC, pRtrCIAT899c.
When comparing the transcriptomes of cells grown at pH 6.8 to cells adapted to pH 4.5, we observed that 351 genes were induced (chromosome: 288, pA: 3, pB: 2 and pC:58, respectively) (Figure 4A). The large majority of these genes was located on the chromosome and several others on plasmid C. Only very few of the induced genes were on plasmids A and B. 32 genes were repressed (chromosome: 17 and pC: 15) (Figures 3B,C, 4A). However, when comparing the transcriptomes of cells grown at pH 6.8 to the transcriptome of cells pre-grown at pH 6.8 and then acid-shocked for 45 min, only 11 genes were up-regulated (chromosome: 6, pB: 1 and pC: 4) and no gene was down-regulated under these conditions (Figures 3D, 4).
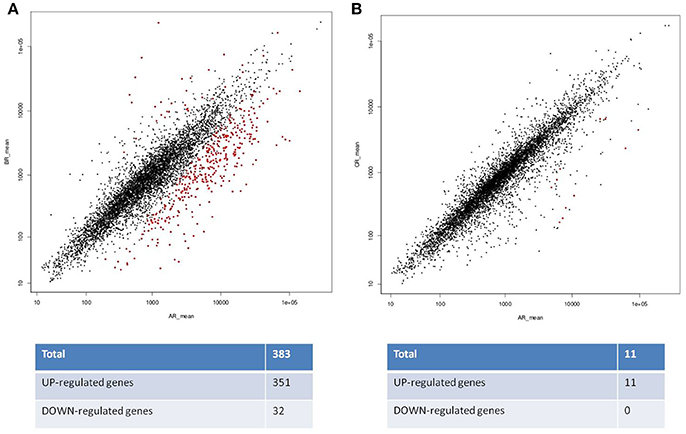
Figure 4. Summary plot of the expression values for both comparisons. Each dot corresponds to an expressed gene, differentially expressed genes are highlighted in red. (A) DEG pH6.8 (AR), vs. pH 4.5 (BR) and (B) DEG pH6.8 (AR) vs. 45 min acid shock at pH 4.5 (CR).
Functional Analysis of Differentially Expressed Genes
Genes that were differentially expressed (DEGs) were grouped according to the COGs protein database (https://www.ncbi.nlm.nih.gov/COG/) and were classified into four principal categories: (1) processes and signaling, (2) information storage and processing, (3) metabolism and (4) poorly characterized (Figure 5). DEGs assigned to these principal categories were further sub-grouped. When comparing the transcriptomes of acid-adapted cells (sample BR) and control cells (sample AR), of the 126 differently expressed genes grouped into the COG category metabolism, 35 DEGs were assigned to amino acid transport and metabolism (E), 26 DEGs to inorganic ion transport and metabolism (P), 17 DEGs to energy production and conversion (C) and to coenzyme transport and metabolism (H), 15 DEGs to carbohydrate transport and metabolism (G), 7 DEGs to lipid transport and metabolism (I), 6 DEGs to nucleotide transport and metabolism (F) and finally 3 DEGs were assigned to the sub-category secondary metabolites biosynthesis, transport, and catabolism (Q). Another principal category that is also over-represented is information storage and processing (99 DEGs). Of these DEGs, 79 DEGs were grouped to the sub-categories translation, ribosome structure and biogenesis (J), 16 DEGs to transcription (K) and 4 DEGs to replication, recombination and repair (L). The third principal category is cellular processes and signaling with 55 DEGs. Of these, 19 DEGs were assigned to post-translational modification, protein turnover, and chaperones (O), 15 DEGs to cell wall/membrane/envelope biogenesis (M), 8 DEGs to intracellular trafficking, secretion, and vesicular transport (U), 6 DEGs to defense mechanisms (V), 5 DEGs to signal transduction mechanism (T) and 2 DEG to cell cycle control, cell division, chromosome partitioning (D). Finally, a few DEGs are poorly characterized and cannot be classified within the other principal categories. This includes General functional prediction only (R, 12 DEGs) and Function unknown (S, 14 DEGs) (Figure 5A). When we analyzed the DEGs that were repressed comparing the transcriptomes of acid-adapted cells and control cells, 8 DEGs were grouped in amino acid transport and metabolism (E), and 3 DEGs in carbohydrate transport and metabolism (G). To a few other subcategories, one DEG each was assigned (M, T, D, J, P, C, F, and S). When comparing the transcriptomes of acid-shocked cells and control cells, we found only 11 DEGs, with 6 DEGs grouped into the principal category metabolism and one DEG grouped into the principal category information storage and processing (Figure 5C). In total, 62 DEGs could not be classified in COGs (for all conditions).
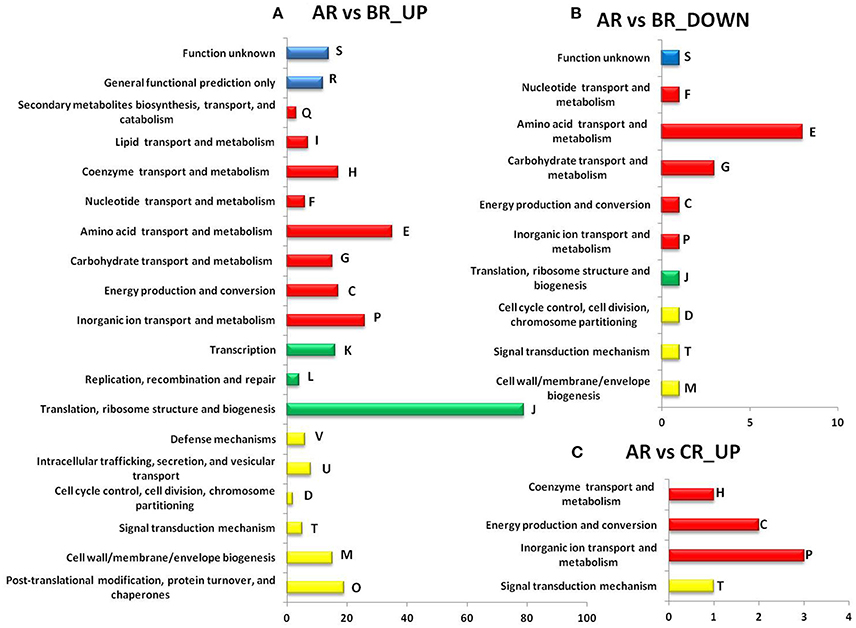
Figure 5. Differentially expressed genes classified in COGs (Clusters of Orthologous Groups) categories. COGs of genes up-regulated (A) and down-regulated (B) genes and COGs up-regulated affected after acid shock (C). General category letter associations by groups and letters (see above); in yellow: cellular processes and signaling (D, M, O, T, U, V), in green: information storage and processing (J, K, L), in red: metabolism (C, E, F, G, H, I, P, Q) and blue: poorly characterized (R and S) (Tatusov et al., 2000, see NCBI COG website). The X axis shows the number of DEGs.
Discussion
The effects of acid stress have been characterized best in Enterobacteriaceae that can be exposed to extreme changes in extracellular pH as they pass through the inhospitable environment of the stomach into the lower digestive tract (Foster, 2004; Lund et al., 2014). Rhizobia show significant variability in their ability to grow under different low pH conditions, and within this family R. tropici CIAT899 is among the most tolerant strains (Martínez-Romero et al., 1991; Graham et al., 1994). The capacity to grow and persist under environmental changes is essential for survival in the rhizosphere and within the nodules of leguminous plants. A Tn5-based transposon library of 18,300 insertional mutants was screened to identify mutants growing as the wildtype at neutral pH, but that did not grow or that showed a drastically reduced growth under low pH conditions. This strategy aimed at identifying genes specifically required for the acid stress response, but not for growth at neutral pH. We isolated 26 Tn5 mutants that were unable to grow under acidic conditions (see Table 3). For 17 of these we could identify the transposon insertion sites, which could be located mainly to the chromosome, but also to the plasmids pA and pC. None of the selected mutants presented an insertion site in the symbiotic plasmid pB (Figure 2).
Vinuesa et al. (2003) had made a similar screening, but on a much smaller scale. One of the mutants identified in the earlier study had an insertion in the sycA/olsC gene cluster (Vinuesa et al., 2003; Rojas-Jiménez et al., 2005). Interestingly, the mutant JG8656 identified in the present study also had an insertion in the gene olsC. This gene codes for the ornithine lipid (OLs) hydroxylase OlsC, which is responsible for the 2-hydroxylation of the secondary fatty acid in OLs. This hydroxylation has been correlated to an increased resistance of R. tropici to acid stress and high temperatures (Vences-Guzmán et al., 2011). One hypothesis is that the presence of the additional hydroxyl group allows the formation of hydrogen bonds between lipid head groups, thereby making the membrane less fluid and less permeable to protons (Nikaido, 2003; Rojas-Jiménez et al., 2005; Vences-Guzmán et al., 2011; Sohlenkamp and Geiger, 2016). Another gene found by Vinuesa et al. was lpiA (low pH inducible) encoding a putative lysyl-phosphatidylglycerol (LPG) synthase, implicated in LPG biosynthesis. LPG is a membrane lipid whose presence confers resistance to various cationic peptides to S. aureus (Peschel et al., 2001). The lpiA gene is found in an operon with atvA (acid tolerance and virulence), which is an orthologue of acvB from A. tumefaciens. Interestingly, in our study we found a mutant (JG163) affected in atvA, which is consistent with the results obtained by Vinuesa and co-workers (Vinuesa et al., 2003). Furthermore, three mutants were affected in a gene whose product is a hypothetical protein (JG1076, JG4655 and JG6057), in this case two of them have an insertion in the same ORF (JG1076, JG4655). Interestingly, two more mutants (JG283 and JG9867) also presented transposon insertions in an identical ORF. This could be indicating that the transposon mutagenesis is close to saturation.
Mutant JG241 presents an insertion in a gene encoding a putative triose phosphate isomerase enzyme (tpiA1). It has been reported that H. pylori, in addition to the genes involved in urea hydrolysis, induces genes at pH 5.5 that are related to carbohydrate metabolism (including the tpiA gene). An explanation suggested was that the cell's energy requirement is increased under this condition (Ang et al., 2001; Wen et al., 2003; Zanotti and Cendron, 2010). Besides, we found other mutants with insertions in genes related to anabolic and energy generation process like ribulose phosphate 3-epimerase (locus tag RTCIAT899_RS09635), prolyl aminopeptidase (RTCIAT899_RS05920) and acetyl-coenzyme A synthetase (Acs) (RTCIAT899_RS18500). Another mutant (JG283) presented an insertion in a gene encoding a polysaccharide biosynthesis protein. Polysaccharide and exopolysaccharide biosynthesis has been reported to help counteract high proton concentrations by preventing protons from passing into the cell (Aarons and Graham, 1991; Graham et al., 1994; Reeve et al., 1997, 1998; Hellweg et al., 2009). Mutant JG9587 has a Tn5 insertion in an ORF encoding a putative antiporter protein, whose function could be to expel protons toward the periplasm under acid stress conditions. In E. coli the Na+/H+ antiporter (NhaA) helps to maintain Na+ and H+ homeostasis and several reports indicate that NhaA activity is increased at high or neutral pH (Padan et al., 2004; Padan, 2008). In contrast, H. pylori has a Na+/H+ antiporter whose activity is high at acidic and neutral pH, indicating that H. pylori employs this antiporter under acidic conditions (Inoue et al., 1999).
In some bacteria an increase of the extracellular proton concentration is sensed through two-component systems (Lund et al., 2014), examples being the PhoPQ system from S. typhimurium which is induced in acidic conditions (Prost et al., 2007) and the ArsRS system from H. pylori, which senses increased proton concentrations and responds by regulating genes of biosynthesis and metabolism of the urease system (Pflock et al., 2006). Mutant JG2646 presented a transposon insertion in a gene encoding a response regulator. This gene is found in an operon with a gene encoding a histidine kinase, and so this two-component system may be sensing the external pH and may be responsible for the activation of a subset of genes under this condition. Two more mutants had transposon insertions in genes encoding transcription factors (JG9477 and JG8654). These genes code for proteins with homology to MarR and FtrA (locus tag RTCIAT899_ RS00995 and RTCIAT899_RS009190), respectively. MarR presents similarity to the transcriptional regulator Rv1404 from Mycobacterium tuberculosis, which regulates the transcription of the genes rv1403c and rv1405c encoding putative S-adenosyl methionine (SAM)-dependent methyltransferases. This activation under acid stress conditions (pH 5.5) also requires phoP (Healy et al., 2016). In the case of FtrA, there are no reports about its role acid stress conditions.
In mutant JG5373 the transposon insertion is probably affecting a gene whose product might be involved in catalyzing the condensation of formaldehyde and glutathione to S-hydroxymethylglutathion (gshA). In the genomic context of R. tropici CIAT899, a glutamate synthase (possibly gshB) is located downstream of gshA. Ricillo et al. reported that a mutant affected in a gene involved in the biosynthesis of tripeptide glutathione (gshB) in R. tropici was affected in growth under acidic conditions (Riccillo et al., 2000). It is possible that the transposon insertion in the mutant JG5373 creates a polar effect affecting glutathione biosynthesis and causing susceptibility to acid stress. Most often an polar effect occurs when the transposon is inserted into the first ORFs of an operon affecting the expression of the downstream genes of the same operon (Zipser, 1969). In our study, we identified three mutants (JG4882, JG163 and JG2646), whose Tn5 insertion occurred in putative operon, however, only the mutant JG2646 has the insertion in the first gene (Supplementary Figure S1) of the operon. The latter transposon insertion can be expected to cause a polar effect on the expression of the downstream genes. This hypothesis was verified by a complementation assay of mutant JG2646: the gene encoding for the response regulator alone was not able to complement the mutant phenotype when provided in trans whereas the complete operon (including the histidine kinase (HK), locus tag RT899_RS05455) complemented the mutant phenotype when provided in trans.
The results obtained by our library screening indicate that outside of certain conserved components of the acid stress response, such as response regulators or antiporters, the genes necessary for an acid stress response vary from species to species. To study the acid stress response from another angle we used RNA-seq to analyze the transcriptomes of R. tropici CIAT899 cells grown at neutral pH (6.8, control condition), of cells grown at pH 4.5 (acid-adapted cells), and of cells pre-grown at pH 6.8 and then acid-shocked for 45 min at pH 4.5. We chose such a short time (45 min) for the acid shock because we were interested in the very first transcriptional responses. Earlier transcriptome studies in S. meliloti 1021 applied a pH shift from pH 7.0 to pH 5.75 (the lowest pH at which S. meliloti grows) for varying times (from 3 to 63 min), observing the maximum induction between 33 and 63 min (Hellweg et al., 2009). Our RNA-seq results exhibit that gene expression of R. tropici CIAT899 was broadly changed by the acid stress. Hundreds of genes (351 up-regulated and 32 down-regulated in acidic conditions and 11 up-regulated in acid shock) were significantly up- or down-regulated under acidic conditions (Table 4 and Supplementary Table S1). These differently expressed genes (DEGs) can be functionally grouped according to the predicted functions of the encoded proteins. We identified several genes associated with metal transport (like RTCIAT899_RS04615, RTCIAT899_RS04620, RTCIAT899_RS04610, RTCIAT899_RS17535, RTCIAT899_RS10385, or RTCIAT899_RS14255). It is known that in tropical acid soils high concentrations of metals like Zn2+, Co2+, Cd2+, Ni2+, and often, Mn2+, Fe2+, Cu2+ and mercury ions are very common. Therefore, these metal transporters could be contributing to the efflux of these metals to avoid the toxic effects of metal ions (Montanini et al., 2007) when the cells encounter high proton and metal concentrations. ABC transporters transport solutes across the membrane utilizing the energy of ATP hydrolysis and they have been over-expressed after acid shock in other bacteria, including S. aureus (Bore et al., 2007). In our study, several of the identified DEGs encoded ABC transporters that were significantly over-expressed under acid stress (Jia et al., 2017) (Figure 5 and Supplementary Table S1). Furthermore, we identified a few putative transcription factors that might be implicated in the regulation of efflux pumps under acid stress (like DeoR, RTCIAT899_RS29995; TetR, RTCIAT899_RS10760, RTCIAT899_RS28830, or YebC/PmpR, RTCIAT899_RS14585). The TetR family of transcriptional regulators has been reported to be involved in the regulation of efflux pumps (Perrone et al., 2017) and drug efflux in mycobacteria (Betts et al., 2003; Wei et al., 2014). Among the DEGs that were up-regulated were also the hyc genes encoding hydrogenases proteins. In E. coli and Salmonella these hydrogenases can reduce acid stress by proton consumption and H2 production. In this study, 10 genes encoding hydrogenases were over-expressed and the transcriptional induction of hydrogenase genes observed in this study is probably involved in the acid stress response, indicating that R. tropici uses this mechanism to resist high proton concentrations (Supplementary Table S1; Hayes et al., 2006; Zbell and Maier, 2009; Noguchi et al., 2010; Jia et al., 2017). F0F1 synthase normally catalyzes the synthesis of ATP from ADP using the energy derived from an electrochemical proton gradient. Under acid stress conditions, hydrolysis of ATP may be used to expel protons from the cytoplasm. This flow of protons through the F0 subunit could contribute to pH homeostasis and therefore contributed to acid stress response like in E. coli or Corynebacterium glutamicum (Diez et al., 2004; Barriuso-Iglesias et al., 2013). In our study we identify two gene clusters encoding several subunits of F0F1 ATPase encompassing nine genes involved in the assembly of the two units of ATPase (F0 and F1), which are up-regulated more than 4 times at pH 4.5 compared to pH 6.8. Sigma factors are subunits of the bacterial RNA polymerase (RNAP) playing important roles in transcription initiation, especially during promoter recognition. Specific sigma factors have functions during the differential expression of genes during abiotic stress, during development or during specific growth phases (Paget, 2015). The sigma factor E (SigE) in M. tuberculosis is up-regulated during acid stress response, and Bansal et al. (2017) demonstrated that PhoP interacts with acid-inducible extra-cytoplasmic SigE to regulate a complex transcriptional of genes. In this study, we observed that three sigma factors (RTCIAT899_RS15835, RTCIAT899_RS13855, and RTCIAT899_RS12250) were induced during the acid stress response. These sigma factors are possibly responsible for regulating other genes important for growth under acid conditions. In addition, forty hypothetical proteins were differentially expressed in acidic conditions (see Table 4 and Supplementary Table S1). Further studies are required to explore the functions of these proteins under acid stress. We also specifically looked at the transcriptome data of orthologues of genes that had been reported to contribute to the acid stress response in other bacteria. For example, the gene glutathione synthase (RTCIAT899_RS01980) and the molecular chaperone DnaK (RTCIAT899_RS00760), have a log2FC of 1 and 1.8 respectively. Genes such as lpiA (RTCIAT899_RS14615) and atvA (RTCIAT899_RS14610) also have a similar log2FC, and most of the orthologues of genes involved in the acid response in other bacteria have very low log2FC values or are even repressed (Table 5). To our surprise only a very small number of genes was induced in response to 45 min of acid shock. We had designed our experiment based on the article published by Hellweg et al. (2009). Maybe the transcription induction responding to acid stress is slower in R. tropici, but there is also the possibility that pH 4.5 is not sufficiently stressful for the bacteria to induce a major short-term response (Figure 6). Although R. tropici grows slower at pH 4.5 than at pH 6.8, there is no clear adaptation phase visible in the growth curve obtained at the lower pH.
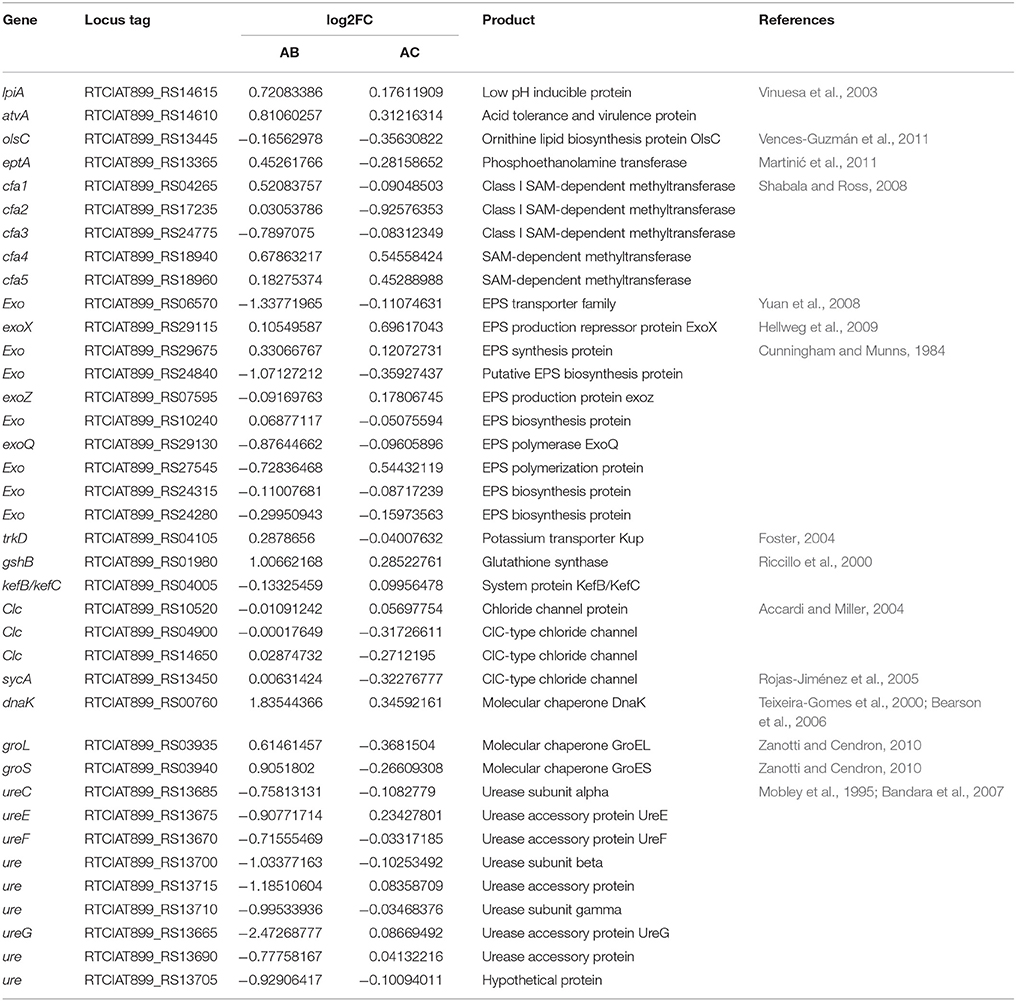
Table 5. Expression of genes whose orthologues are reported to be involved in acid stress response in other bacteria.
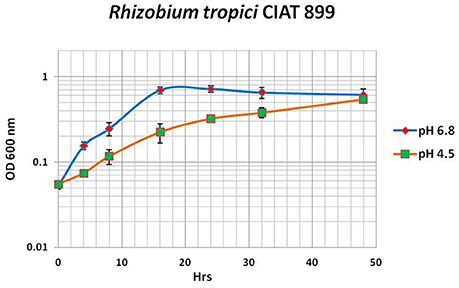
Figure 6. Growth of Rhizobium tropici CIAT 899 in minimal medium adjusted to different pH values. Wild type was grown either in MM (pH 6.8, blue) or MAM (pH 4.5, red). Cultures were grown at 30°C in a gyratory shaker. Growth was observed by measuring the OD 620 and the generation time was calculated (2.2 h at pH 6.8 and 1.9 h at pH 4.5). Mean values for triplicate replicates are shown for each condition ± standard deviation (bars).
As expected, both, the Tn5 mutagenesis and RNA-seq approach, produced complementary sets of results. But, the use of both approaches allows the identification of a wider range of genes involved in the acid stress response. Therefore, with this work, we make an important step toward comprehending the genetic bases to understanding the acid stress response in R. tropici CIAT899. Much works remains to be done to understand how all the identified genes contribute to the acid stress response. This is especially interesting in the case of genes where the correlation to acid stress is not completely obvious.
Author Contributions
JG-C and CS designed the study. JG-C and CS carried out the experiments. JG-C and LL carried out the data analysis. JG-C and CS were involved in drafting the manuscript and all authors read and approved the final manuscript.
Conflict of Interest Statement
The authors declare that the research was conducted in the absence of any commercial or financial relationships that could be construed as a potential conflict of interest.
Acknowledgments
JG-C was a recipient of a Ph.D. scholarship from the Consejo Nacional de Ciencia y Tecnología (CONACyT) and this article is part of his thesis at the Ph.D. Program in Biomedical Sciences (Programa de Doctorado en Ciencias Biomédicas) of the Universidad Nacional Autónoma de México (UNAM). Research in our lab was supported by grants from CONACyT-Mexico (153200, 237713), PAPIIT/UNAM (IN202413 and IN208116), and UC-MEXUS/CONACyT (CN-12-552) to CS.
Supplementary Material
The Supplementary Material for this article can be found online at: https://www.frontiersin.org/articles/10.3389/fmicb.2018.00846/full#supplementary-material
Supplementary Table S1. Complete list of differentially expressed genes. DEGs over-regulated in acid stress conditions. AR_vs_BR_UP (pH 6.8 vs. pH 4.5), AR_vs_BR_DOWN (pH 6.8 vs. pH 4.5) and AR_vs_CR_UP (pH 6.8 vs. acid shock 45 min). Genes differentially expressed in both conditions are highlighted in boldface.
Supplementary Figure S1. Genomic context of Tn5 mutants from R. tropici CIAT899. White arrows represent the genes that were interrupted by the transposon Tn5 (yellow triangles), gray arrows represent neighboring genes to each mutated gene, black lines indicate the genome of R. tropici CIAT899.
References
Aarons, S. R., and Graham, P. H. (1991). Response of Rhizobium leguminosarum bv phaseoli to acidity. Plant Soil 134, 145–151. doi: 10.1007/BF00010727
Accardi, A., and Miller, C. (2004). Secondary active transport mediated by a prokaryotic homologue of ClC Cl- channels. Nature 427, 803–807. doi: 10.1038/nature02314
Alexandre, A., Laranjo, M., and Oliveira, S. (2014). Global transcriptional response to heat shock of the legume symbiont Mesorhizobium loti MAFF303099 comprises extensive gene downregulation. DNA Res. 21, 195–206. doi: 10.1093/dnares/dst050
Ang, S., Lee, C. Z., Peck, K., Sindici, M., Matrubutham, U., Gleeson, M. A., et al. (2001). Acid-induced gene expression in Helicobacter pylori: study in genomic scale by microarray. Infect. Immun. 69, 1679–1686. doi: 10.1128/IAI.69.3.1679-1686.2001
Audia, J. P., Webb, C. C., and Foster, J. W. (2001). Breaking through the acid barrier: an orchestrated response to proton stress by enteric bacteria. Int. J. Med. Microbiol. 291, 97–106. doi: 10.1078/1438-4221-00106
Bandara, A. B., Contreras, A., Contreras-Rodríguez, A., Martins, A. M., Dobrean, V., Poff-Reichow, S., et al. (2007). Brucella suis urease encoded by ure1 but not ure2 is necessary for intestinal infection of BALB/c mice. BMC Microbiol. 7:57. doi: 10.1186/1471-2180-7-57
Bansal, R., Anil Kumar, V., Sevalkar, R. R., Singh, P. R., and Sarkar, D. (2017). Mycobacterium tuberculosis virulence-regulator PhoP interacts with alternative sigma factor SigE during acid-stress response. Mol. Microbiol. 104, 400–411. doi: 10.1111/mmi.13635
Barriuso-Iglesias, M., Barreiro, C., Sola-Landa, A., and Martin, J. F. (2013). Transcriptional control of the F0F1-ATP synthase operon of Corynebacterium glutamicum: sigmaH factor binds to its promoter and regulates its expression at different pH values. Microb. Biotechnol. 6, 178–188. doi: 10.1111/1751-7915.12022
Bearson, S. M., Bearson, B. L., and Rasmussen, M. A. (2006). Identification of Salmonella enterica serovar typhimurium genes important for survival in the swine gastric environment. Appl. Environ. Microbiol. 72, 2829–2836. doi: 10.1128/AEM.72.4.2829-2836.2006
Beringer, J. E. (1974). R factor transfer in Rhizobium leguminosarum. J. Gen. Microbiol. 84, 188–198. doi: 10.1099/00221287-84-1-188
Betts, J. C., McLaren, A., Lennon, M. G., Kelly, F. M., Lukey, P. T., Blakemore, S. J., et al. (2003). Signature gene expression profiles discriminate between isoniazid-, thiolactomycin-, and triclosan-treated Mycobacterium tuberculosis. Antimicrob. Agents Chemother. 47, 2903–2913. doi: 10.1128/AAC.47.9.2903-2913.2003
Bore, E., Langsrud, S., Langsrud, O., Rode, T. M., and Holck, A. (2007). Acid-shock responses in Staphylococcus aureus investigated by global gene expression analysis. Microbiology 153, 2289–2303. doi: 10.1099/mic.0.2007/005942-0
Castanie-Cornet, M. P., Penfound, T. A., Smith, D., Elliott, J. F., and Foster, J. W. (1999). Control of acid resistance in Escherichia coli. J. Bacteriol. 181, 3525–3535.
Cunningham, S. D., and Munns, D. N. (1984). The correlation between extracellular polysaccharide production and acid tolerance in Rhizobium. Soil Sci. Soc. Am. J. 48, 1273–1276. doi: 10.2136/sssaj1984.03615995004800060014x
De Biase, D., and Pennacchietti, E. (2012). Glutamate decarboxylase-dependent acid resistance in orally acquired bacteria: function, distribution and biomedical implications of the gadBC operon. Mol. Microbiol. 86, 770–786. doi: 10.1111/mmi.12020
Diez, M., Zimmermann, B., Borsch, M., Konig, M., Schweinberger, E., Steigmiller, S., et al. (2004). Proton-powered subunit rotation in single membrane-bound F0F1-ATP synthase. Nat. Struct. Mol. Biol. 11, 135–141. doi: 10.1038/nsmb718
Ditta, G., Schmidhauser, T., Yakobson, E., Lu, P., Liang, X. W., Finlay, D. R., et al. (1985). Plasmids related to the broad host range vector, pRK290, useful for gene cloning and for monitoring gene expression. Plasmid 13, 149–153. doi: 10.1016/0147-619X(85)90068-X
Foster, J. W. (1999). When protons attack: microbial strategies of acid adaptation. Curr. Opin. Microbiol. 2, 170–174. doi: 10.1016/S1369-5274(99)80030-7
Foster, J. W. (2004). Escherichia coli acid resistance: tales of an amateur acidophile. Nat. Rev. Microbiol. 2, 898–907. doi: 10.1038/nrmicro1021
Graham, P. H., Draeger, K. J., Ferrey, M. L., Conroy, M. J., Hammer, B. E., Martínez, E., et al. (1994). Acid pH tolerance in strains of Rhizobium and Bradyrhizobium, and initial studies on the basis for acid tolerance of Rhizobium tropici Umr1899. Can. J. Microbiol. 40, 198–207. doi: 10.1139/m94-033
Hanahan, D. (1983). Studies on transformation of Escherichia coli with plasmids. J. Mol. Biol. 166, 557–580. doi: 10.1016/S0022-2836(83)80284-8
Harrison, P. W., Lower, R. P., Kim, N. K., and Young, J. P. (2010). Introducing the bacterial 'chromid': not a chromosome, not a plasmid. Trends Microbiol. 18, 141–148. doi: 10.1016/j.tim.2009.12.010
Hayes, E. T., Wilks, J. C., Sanfilippo, P., Yohannes, E., Tate, D. P., Jones, B. D., et al. (2006). Oxygen limitation modulates pH regulation of catabolism and hydrogenases, multidrug transporters, and envelope composition in Escherichia coli K-12. BMC Microbiol. 6:89. doi: 10.1186/1471-2180-6-89
Healy, C., Golby, P., MacHugh, D. E., and Gordon, S. V. (2016). The MarR family transcription factor Rv1404 coordinates adaptation of Mycobacterium tuberculosis to acid stress via controlled expression of Rv1405c, a virulence-associated methyltransferase. Tuberculosis 97, 154–162. doi: 10.1016/j.tube.2015.10.003
Hellweg, C., Pühler, A., and Weidner, S. (2009). The time course of the transcriptomic response of Sinorhizobium meliloti 1021 following a shift to acidic pH. BMC Microbiol. 9:37. doi: 10.1186/1471-2180-9-37
Huang, G., Zhang, L., and Birch, R. G. (2000). Rapid amplification and cloning of Tn5 flanking fragments by inverse PCR. Lett. Appl. Microbiol. 31, 149–153. doi: 10.1046/j.1365-2672.2000.00781.x
Hungría, M., Andrade, D. D., Chueire, L. M. D., Probanza, A., Guttiérrez-Manero, F. J., and Megías, M. (2000). Isolation and characterization of new efficient and competitive bean (Phaseolus vulgaris L.) rhizobia from Brazil. Soil Biol. Biochem. 32, 1515–1528. doi: 10.1016/S0038-0717(00)00063-8
Inoue, H., Sakurai, T., Ujike, S., Tsuchiya, T., Murakami, H., and Kanazawa, H. (1999). Expression of functional Na+/H+ antiporters of Helicobacter pylori in antiporter-deficient Escherichia coli mutants. FEBS Lett. 443, 11–16. doi: 10.1016/S0014-5793(98)01652-4
Jia, K., Wang, G. Y., Liang, L. J., Wang, M., Wang, H. H., and Xu, X. L. (2017). Preliminary transcriptome analysis of mature biofilm and planktonic cells of Salmonella enteritidis exposure to acid stress. Front. Microbiol. 8:1861. doi: 10.3389/fmicb.2017.01861
Kieboom, J., and Abee, T. (2006). Arginine-dependent acid resistance in Salmonella enterica serovar typhimurium. J. Bacteriol. 188, 5650–5653. doi: 10.1128/JB.00323-06
Kingsley, M. T., and Bohlool, B. (1992). Extracellular polysaccharide is not responsible for aluminum tolerance of Rhizobium leguminosarum bv phaseoli CIAT899. Appl. Environ. Microbiol. 58, 1095–1101.
Kovach, M. E., Phillips, R. W., Elzer, P. H., Roop, R. M., and Peterson, K. M. (1994). pBBR1mcs - a broad-host-range cloning vector. Biotechniques 16, 800–802.
Lund, P., Tramonti, A., and De Biase, D. (2014). Coping with low pH: molecular strategies in neutralophilic bacteria. FEMS Microbiol. Rev. 38, 1091–1125. doi: 10.1111/1574-6976.12076
Martínez-Romero, E., Segovia, L., Mercante, F. M., Franco, A. A., Graham, P., and Pardo, M. A. (1991). Rhizobium tropici, a novel species nodulating Phaseolus vulgaris L. beans and Leucaena sp. trees. Int. J. Syst. Bacteriol. 41, 417–426. doi: 10.1099/00207713-41-3-417
Martinić, M., Hoare, A., Contreras, I., and Alvarez, S. A. (2011). Contribution of the lipopolysaccharide to resistance of Shigella flexneri 2a to extreme acidity. PLoS ONE 6:e25557. doi: 10.1371/journal.pone.0025557
Mobley, H. L., Island, M. D., and Hausinger, R. P. (1995). Molecular biology of microbial ureases. Microbiol. Rev. 59, 451–480.
Montanini, B., Blaudez, D., Jeandroz, S., Sanders, D., and Chalot, M. (2007). Phylogenetic and functional analysis of the Cation Diffusion Facilitator (CDF) family: improved signature and prediction of substrate specificity. BMC Genomics 8:107. doi: 10.1186/1471-2164-8-107
Muglia, C. I., Grasso, D. H., and Aguilar, O. M. (2007). Rhizobium tropici response to acidity involves activation of glutathione synthesis. Microbiology 153, 1286–1296. doi: 10.1099/mic.0.2006/003483-0
Nikaido, H. (2003). Molecular basis of bacterial outer membrane permeability revisited. Microbiol. Mol. Biol. Rev. 67, 593–656. doi: 10.1128/MMBR.67.4.593-656.2003
Noguchi, K., Riggins, D. P., Eldahan, K. C., Kitko, R. D., and Slonczewski, J. L. (2010). Hydrogenase-3 contributes to anaerobic acid resistance of Escherichia coli. PLoS ONE 5:e10132. doi: 10.1371/journal.pone.0010132
Ormeño-Orrillo, E., Menna, P., Almeida, L. G. P., Ollero, F. J., Nicolas, M. F., Rodrigues, E. P., et al. (2012). Genomic basis of broad host range and environmental adaptability of Rhizobium tropici CIAT 899 and Rhizobium sp PRF 81 which are used in inoculants for common bean (Phaseolus vulgaris L.). BMC Genomics 13:735. doi: 10.1186/1471-2164-13-735
Padan, E. (2008). The enlightening encounter between structure and function in the NhaA Na+-H+ antiporter. Trends Biochem. Sci. 33, 435–443. doi: 10.1016/j.tibs.2008.06.007
Padan, E., Tzubery, T., Herz, K., Kozachkov, L., Rimon, A., and Galili, L. (2004). NhaA of Escherichia coli, as a model of a pH-regulated Na+/H+ antiporter. Biochim. Biophys. Acta 1658, 2–13. doi: 10.1016/j.bbabio.2004.04.018
Paget, M. S. (2015). Bacterial sigma factors and anti-sigma factors: structure, function and distribution. Biomolecules 5, 1245–1265. doi: 10.3390/biom5031245
Perrone, F., De Siena, B., Muscariello, L., Kendall, S. L., Waddell, S. J., and Sacco, M. (2017). A novel TetR-Like transcriptional regulator is induced in acid-nitrosative stress and controls expression of an efflux pump in mycobacteria. Front. Microbiol. 8:2039. doi: 10.3389/fmicb.2017.02039
Peschel, A., Jack, R. W., Otto, M., Collins, L. V., Staubitz, P., Nicholson, G., et al. (2001). Staphylococcus aureus resistance to human defensins and evasion of neutrophil killing via the novel virulence factor MprF is based on modification of membrane lipids with L-lysine. J. Exp. Med. 193, 1067–1076. doi: 10.1084/jem.193.9.1067
Pflock, M., Finsterer, N., Joseph, B., Mollenkop, H., Meyer, T. F., and Beier, D. (2006). Characterization of the ArsRS regulon of Helicobacter pylori, involved in acid adaptation. J. Bacteriol. 188, 3449–3462. doi: 10.1128/JB.188.10.3449-3462.2006
Prost, L. R., Daley, M. E., Le Sage, V., Bader, M. W., Le Moual, H., Klevit, R. E., et al. (2007). Activation of the bacterial sensor kinase PhoQ by acidic pH. Mol. Cell 26, 165–174. doi: 10.1016/j.molcel.2007.03.008
Reeve, W. G., Brau, L., Castelli, J., Garau, G., Sohlenkamp, C., Geiger, O., et al. (2006). The Sinorhizobium medicae WSM419 IpiA gene is transcriptionally activated by FsrR and required to enhance survival in lethal acid conditions. Microbiology 152, 3049–3059. doi: 10.1099/mic.0.28764-0
Reeve, W. G., Dilworth, M. J., Tiwari, R. P., and Glenn, A. R. (1997). Regulation of exopolysaccharide production in Rhizobium leguminosarum biovar viciae WSM710 involves exoR. Microbiology 143, 1951–1958. doi: 10.1099/00221287-143-6-1951
Reeve, W. G., Tiwari, R. P., Wong, C. M., Dilworth, M. J., and Glenn, A. R. (1998). The transcriptional regulator gene phrR in Sinorhizobium meliloti WSM419 is regulated by low pH and other stresses. Microbiology 144, 3335–3342. doi: 10.1099/00221287-144-12-3335
Riccillo, P. M., Muglia, C. I., de Bruijn, F. J., Roe, A. J., Booth, I. R., and Aguilar, O. M. (2000). Glutathione is involved in environmental stress responses in Rhizobium tropici, including acid tolerance. J. Bacteriol. 182, 1748–1753. doi: 10.1128/JB.182.6.1748-1753.2000
Rojas-Jiménez, K., Sohlenkamp, C., Geiger, O., Martínez-Romero, E., Werner, D., and Vinuesa, P. (2005). A CIC chloride channel homolog and ornithine-containing membrane lipids of Rhizobium tropici CIAT899 are involved in symbiotic efficiency and acid tolerance. Mol. Plant-Microbe Interact. 18, 1175–1185. doi: 10.1094/MPMI-18-1175
Schäfer, A., Tauch, A., Jäger, W., Kalinowski, J., Thierbach, G., and Pühler, A. (1994). Small mobilizable multipurpose cloning vectors derived from the Escherichia coli plasmids pK18 and pK19 - selection of defined deletions in the chromosome of Corynebacterium glutamicum. Gene 145, 69–73. doi: 10.1016/0378-1119(94)90324-7
Shabala, L., and Ross, T. (2008). Cyclopropane fatty acids improve Escherichia coli survival in acidified minimal media by reducing membrane permeability to H+ and enhanced ability to extrude H+. Res. Microbiol. 159, 458–461. doi: 10.1016/j.resmic.2008.04.011
Simon, R., Priefer, U., and Pühler, A. (1983). A broad host range mobilization system for in vivo genetic engineering - transposon mutagenesis in gram-negative bacteria. Bio-Technology 1, 784–791. doi: 10.1038/nbt1183-784
Sohlenkamp, C. (2017). “Membrane homeostasis in bacteria upon pH challenge,” in Biogenesis of Fatty Acids, Lipids and Membranes, ed O. Geiger (Cham: Springer International Publishing), 1–13.
Sohlenkamp, C., Galindo-Lagunas, K. A., Guan, Z. Q., Vinuesa, P., Robinson, S., Thomas-Oates, J., et al. (2007). The lipid lysyl-phosphatidylglycerol is present in membranes of Rhizobium tropici CIAT899 and confers increased resistance to polymyxin B under acidic growth conditions. Mol. Plant Microbe Interact. 20, 1421–1430. doi: 10.1094/MPMI-20-11-1421
Sohlenkamp, C., and Geiger, O. (2016). Bacterial membrane lipids: diversity in structures and pathways. FEMS Microbiol. Rev. 40, 133–159. doi: 10.1093/femsre/fuv008
Studier, F. W. (1991). Use of bacteriophage-T7 lysozyme to improve an inducible T7 expression system. J. Mol. Biol. 219, 37–44. doi: 10.1016/0022-2836(91)90855-Z
Suzaki, T., Yoro, E., and Kawaguchi, M. (2015). “Chapter three - leguminous plants: inventors of root nodules to accommodate symbiotic bacteria,” in International Review of Cell and Molecular Biology, ed K. W. Jeon (Okazaki: Academic Press), 111–158.
Tarazona, S., Furio-Tari, P., Turra, D., Di Pietro, A., Nueda, M. J., Ferrer, A., et al. (2015). Data quality aware analysis of differential expression in RNA-seq with NOISeq R/Bioc package. Nucleic Acids Res. 43:e140. doi: 10.1093/nar/gkv711
Tarazona, S., Garcia-Alcalde, F., Dopazo, J., Ferrer, A., and Conesa, A. (2011). Differential expression in RNA-seq: a matter of depth. Genome Res. 21, 2213–2223. doi: 10.1101/gr.124321.111
Tatusov, R. L., Galperin, M. Y., Natale, D. A., and Koonin, E. V. (2000). The COG database: a tool for genome-scale analysis of protein functions and evolution. Nucleic Acids Res. 28, 33–36. doi: 10.1093/nar/28.1.33
Teixeira-Gomes, A. P., Cloeckaert, A., and Zygmunt, M. S. (2000). Characterization of heat, oxidative, and acid stress responses in Brucella melitensis. Infect. Immun. 68, 2954–2961. doi: 10.1128/IAI.68.5.2954-2961.2000
Udvardi, M. K., and Day, D. A. (1997). Metabolite transport across symbiotic membranes of legume nodules. Annu. Rev. Plant Physiol. Plant Mol. Biol. 48, 493–523. doi: 10.1146/annurev.arplant.48.1.493
Vences-Guzmán, M. A., Guan, Z., Ormeno-Orrillo, E., González-Silva, N., López-Lara, I. M., Martínez-Romero, E., et al. (2011). Hydroxylated ornithine lipids increase stress tolerance in Rhizobium tropici CIAT899. Mol. Microbiol. 79, 1496–1514. doi: 10.1111/j.1365-2958.2011.07535.x
Vinuesa, P., Neumann-Silkow, F., Pacios-Bras, C., Spaink, H. P., Martínez-Romero, E., and Werner, D. (2003). Genetic analysis of a pH-regulated operon from Rhizobium tropici CIAT899 involved in acid tolerance and nodulation competitiveness. Mol. Plant-Microbe Interact. 16, 159–168. doi: 10.1094/MPMI.2003.16.2.159
Wei, J., Liang, J. C., Shi, Q. Y., Yuan, P., Meng, R. Z., Tang, X. D., et al. (2014). Genome-wide transcription analyses in Mycobacterium tuberculosis treated with lupulone. Braz. J. Microbiol. 45, 333–341. doi: 10.1590/S1517-83822014005000032
Wen, Y., Marcus, E. A., Matrubutham, U., Gleeson, M. A., Scott, D. R., and Sachs, G. (2003). Acid-adaptive genes of Helicobacter pylori. Infect. Immun. 71, 5921–5939. doi: 10.1128/IAI.71.10.5921-5939.2003
Yanisch-Perron, C., Vieira, J., and Messing, J. (1985). Improved M13 phage cloning vectors and host strains - nucleotide-sequences of the M13mp18 and pUC19 vectors. Gene 33, 103–119. doi: 10.1016/0378-1119(85)90120-9
Yuan, Z. C., Liu, P., Saenkham, P., Kerr, K., and Nester, E. W. (2008). Transcriptome profiling and functional analysis of Agrobacterium tumefaciens reveals a general conserved response to acidic conditions (pH 5.5) and a complex acid-mediated signaling involved in Agrobacterium-plant interactions. J. Bacteriol. 190, 494–507. doi: 10.1128/JB.01387-07
Zanotti, G., and Cendron, L. (2010). Functional and structural aspects of Helicobacter pylori acidic stress response factors. IUBMB Life 62, 715–723. doi: 10.1002/iub.382
Zbell, A. L., and Maier, R. J. (2009). Role of the hya hydrogenase in recycling of anaerobically produced H-2 in Salmonella enterica serovar Typhimurium. Appl. Environ. Microbiol. 75, 1456–1459. doi: 10.1128/AEM.02064-08
Keywords: Rhizobium tropici CIAT899, pH, acid stress response, Tn5, transcriptome, RNA-Seq
Citation: Guerrero-Castro J, Lozano L and Sohlenkamp C (2018) Dissecting the Acid Stress Response of Rhizobium tropici CIAT 899. Front. Microbiol. 9:846. doi: 10.3389/fmicb.2018.00846
Received: 22 December 2017; Accepted: 12 April 2018;
Published: 30 April 2018.
Edited by:
Marc Strous, University of Calgary, CanadaReviewed by:
Ernesto Ormeño-Orrillo, National Agrarian University, PeruAmy Michele Grunden, North Carolina State University, United States
Copyright © 2018 Guerrero-Castro, Lozano and Sohlenkamp. This is an open-access article distributed under the terms of the Creative Commons Attribution License (CC BY). The use, distribution or reproduction in other forums is permitted, provided the original author(s) and the copyright owner are credited and that the original publication in this journal is cited, in accordance with accepted academic practice. No use, distribution or reproduction is permitted which does not comply with these terms.
*Correspondence: Christian Sohlenkamp, Y2hzb2hsZW5AY2NnLnVuYW0ubXg=