- 1Department of Biology, Life Sciences Research Center, United States Air Force Academy, Colorado Springs, CO, United States
- 2National Research Council Research Associateships Program, Washington, DC, United States
- 3Dr. RV House LLC, Harpers Ferry, WV, United States
- 4Appili Therapeutics, Halifax, NS, Canada
Francisella tularensis (Ft) is a Gram negative intracellular bacterial pathogen, commonly transmitted via arthropod bites, but is most lethal when contracted via inhalation. The nature of a Gram-negative intracellular pathogen presents unique challenges to the mammalian immune response, unlike more common viral pathogens and extracellular bacterial pathogens. The current literature on Ft involves numerous variables, including the use of differing research strains and variation in animal models. This review aims to consolidate much of the recent literature on Ft to suggest promising research to better understand the complex immune response to this bacterium.
1 Introduction
Francisella tularensis (Ft) is a highly infectious Gram-negative, facultative intracellular coccobacillus; infection with Ft results in the disease tularemia. Clinical tularemia manifestations depend on the site of infection, with ulceroglandular, glandular, oculoglandular, oropharyngeal, pneumonic, and typhoidal forms described (Cummings et al., 2014). Whereas the ulceroglandular form of the disease is the most common naturally acquired form (usually via arthropod bites), the pneumonic form (infection via inhalation) exhibits the most serious pathology and has one of the highest mortality rates among all tularemia forms. Fortunately, naturally occurring Ft infections are rare, with approximately 200 infections reported annually in the US (Centers for Disease Control and Prevention, 2022).
The genus Francisella has over a dozen species, with Ft being the mammalian pathogenic species (Degabriel et al., 2023). Human pathogenic Ft is further subdivided into three subspecies by virulence and geographic distribution: Ft tularensis (Ftt, most virulent, found in North America), Ft holartica (Fth, moderate virulence, found throughout the northern hemisphere), and Ft mediasiatica (Ftm, lowest virulence, found in central Asia) (Larson et al., 2020). Ft has long been considered an important biological warfare/bioterrorism threat and has been weaponized by both the US and the former Soviet Union (McLendon et al., 2006; Janik et al., 2020). Characteristics of Ft that support its use as a bioweapon include a very low infectious dose, ease of aerosolization, multiple debilitating and potentially fatal clinical syndromes, suboptimal antibiotic countermeasures requiring prolonged therapy, and lack of effective vaccines. In addition, tularemia is an important zoonotic disease that is transmitted by various arthropod vectors (Yeni et al., 2021).
Efforts to develop and license an effective tularemia vaccine have been underway since the 1940s (Griffin et al., 2007). Efforts have included live attenuated vaccines for tularemia, with the preponderance of data using the Live Vaccine Strain (LVS) candidate. The LVS vaccine candidate was generated from an Fth strain in the USSR and tested in human volunteers who were vaccinated and then challenged with virulent Ft via intracutaneous (Saslaw et al., 1961) and respiratory (Saslaw et al., 1961) challenge routes. United States (US) published studies were conducted at the Ohio State Penitentiary on inmates who agreed to participate in the clinical trial. Additional studies were conducted by the US military at Fort Detrick under Operation Whitecoat, where researchers conducted 40 clinical studies of tularemia from 1958 to 1968 (Pittman et al., 2005). These studies used male volunteers on active duty in the Army, including members of the Seventh Day Adventist Church who were conscientious objectors. In these studies, the volunteers were exposed to the SCHU S4 strain of Ft. LVS was shown to protect against aerosol challenge, albeit at low challenge doses (Saslaw et al., 1961). There are extensive data on the history of the immune response to LVS.
LVS has many advantages in the study of Ft, primarily because of its reduced virulence; it is safer for researchers to use and is exempt from select agent regulations. However, as a less virulent subspecies, it may not elicit the same immune response as the more virulent Ftt subspecies. Recently, more laboratories have been using the SCHU S4 strain of Ftt, and there is increasing immunological literature using Ftt. In addition, a SCHU S4 based live attenuated tularemia vaccine is reported to be in development.
It should be noted that extensive literature exists regarding the role of T cells in the immune response to Ft and particularly to a second infection or infection following challenge with a live attenuated strain. Early studies on the need for T cell immunity to protect against a second Ft exposure have shown that BALB/c mice infected intradermally with LVS died, whereas BALB/c nu/nu mice (which lack a thymus and T cells) lived (Elkins et al., 1993). Furthermore, the addition of either CD4+ or CD8+ T cells to BALB/c scid (lymphocyte deficient) mice restored the protection from intradermal LVS infection (Elkins et al., 1996). Similar results were observed with antibody depletion of CD4+ and CD8+ T cells in BALB/c mice infected with LVS (Yee et al., 1996). The extensive role of ɑꞵ T cells in tularemia vaccines has been reviewed recently by others (Roberts et al., 2018; Harrell et al., 2024) and is not discussed here. This is not intended to diminish the role of T cells in Ft infections, as it is substantial, but rather to recognize that this topic is well addressed by other recent reviews.
Another historical aspect of the immune response to Ft is the involvement of B cells and the production of antibodies. It is well known that antibodies are produced in response to Ft infection and these antibodies are used to determine the seroprevalence of Ft in many environmental species. Human antibody production after Ft exposure has been used as a diagnostic tool as well as a tool to measure immune response to LVS vaccination (Maurin, 2020; Koskela, 1985). However, antibodies have been shown to give protection only against strains of reduced virulence (Tarnvik, 1989). The role of antibodies and B cells has been recently reviewed by others (Kubelkova and Macela, 2021) and is not discussed here. As with T cells, this is not to diminish the role of antibodies or B cells, but to focus this review on other parts of the immune response.
While certain aspects of the immune response to Ft infection have been well established, we review other aspects of the immune response to Ft, including molecular responses, innate immune responses and some less-considered aspects of the adaptive immune response (Table 1). Differences in the response to Fth vs. Ftt are described, including differences in toll-like receptors (TLRs), inhibition of reactive oxygen species (ROS), interferon (IFN)-γ and other cytokine responses, cellular depletion, cellular expansion responses, and tissue necrosis. We review the literature to gain more detailed understanding of the immune response to Ft and the differences in responses among the subspecies. The complete immune response, considering all cellular and molecular aspects, has not been fully established. This review summarizes the state of knowledge based on the past decade of research and identifies areas for future research. We examine a variety of experimental models recently used (Figure 1). We conclude that Ft is an inherently immunomodulatory organism, but that differences in animal models, including routes of infection and/or immunization, have resulted in conflicting results. Furthermore, inconsistencies in the Ft subspecies and strain used cause differential activation of various molecular pathways, leading to divergent conclusions from the respective data. Though caveats in the models and bacterial strains are important and should be acknowledged, there remains an abundance of evidence that offers valuable insights into the pathophysiology and mechanisms of Ft infection.
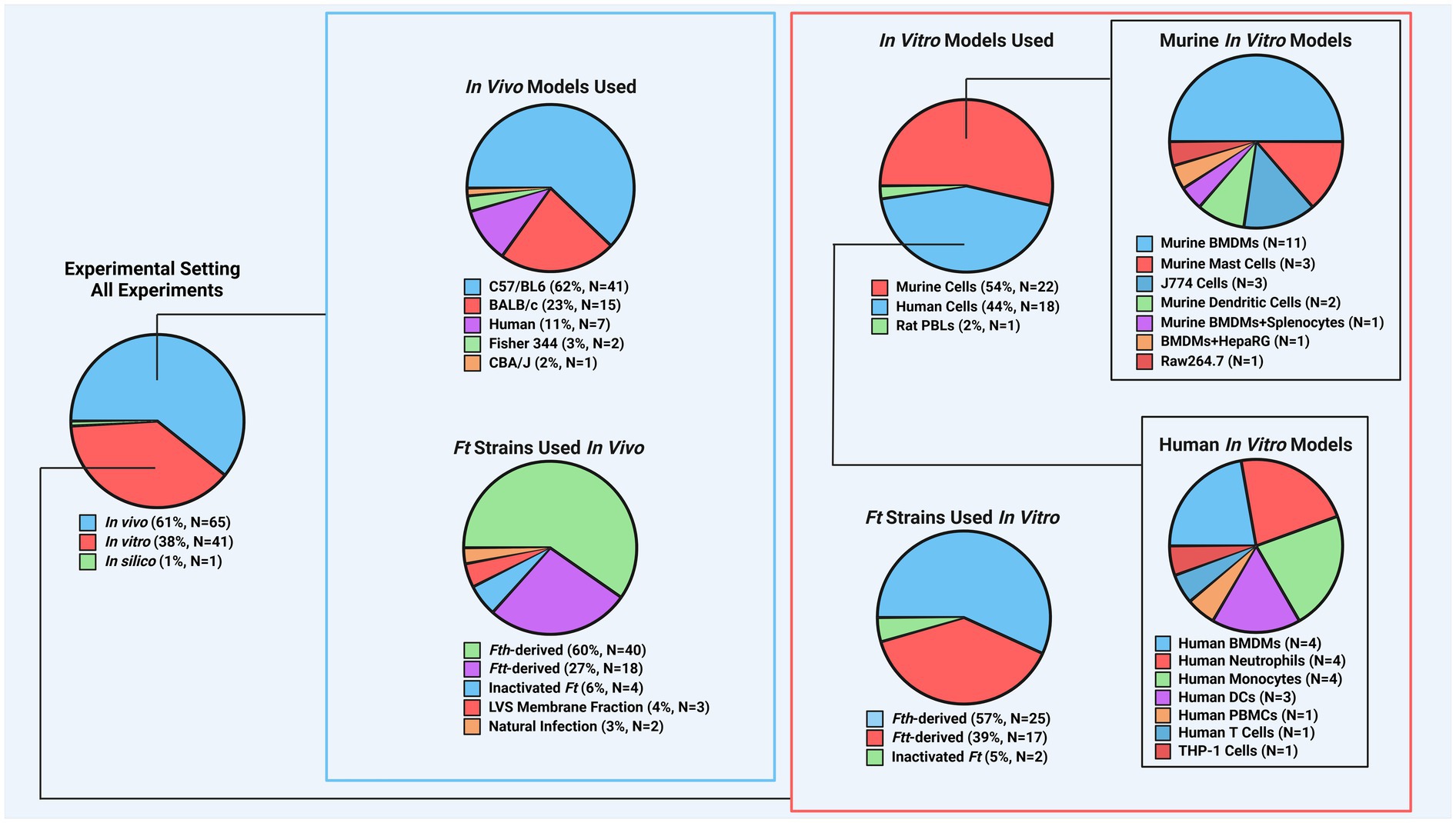
Figure 1. Breakdown of experimental models found in recent Ft literature. To better understand the distribution of experimental models in studies, counts were recorded for each model aspect, including species used for in vivo and in vitro experiments and the frequency of specific Ft strains. For instance, if a publication used Fth, Ftt, BALB/c mice, and C57BL/6 mice, each was counted once. Mouse models dominated in vivo studies (~86%), with Fth-derived strains being the most common. This highlights a need for more research on other animal models. In vitro studies were more balanced, with mouse models comprising ~54% and a more even use of Fth and Ftt strains. (Ft: Francisella tularensis, Fth: Francisella tularensis subsp. holarctica, Ftt: Francisella tularensis subsp. tularensis, LVS: live vaccine strain, PBL: peripheral blood leukocyte, BMDM: bone marrow-derived macrophage, PBMC: peripheral blood mononuclear cell).
2 Methods
Review Criteria
Our systematic review identified not only recent cellular immunity and cytokine-mediated immunity studies, but also molecular changes. First, the molecular changes are reviewed below.
The purpose of this literature-based critical review is to identify changes following Ft exposure; changes associated with the immune system with an interest in those changes that could be assessed to guide development and testing of MCM specific for tularemia. It is crucial to note that the search for useful correlates of protection for Ft is complicated by observations that the nature and course of infection with Ft, as well as mechanisms of protection, varies significantly depending on route of exposure (Nicol et al., 2021). This difference is important when designing an Ft MCM development program, since the indication (i.e., the expected route of exposure) will drive the testing design. More specifically, although naturally occurring tularemia results from arthropod bites (i.d. exposure), these infections are milder and often self-limiting. Respiratory exposure is rare in nature but would be the expected route of exposure if Ft were used as a bioweapon. In this case, the disease would be far more dangerous with an untreated mortality rate of approximately 60% (Hong et al., 2013). Here, we review the immunological literature against the backdrop of a tularemia MCM intended to prevent pneumonic tularemia following a respiratory exposure in humans.
The following strategy was used in the current review:
1. An initial search was conducted in PubMed using search terms” tular* innate” and “tular* adaptive.”
2. The Inclusion/Exclusion criteria and screening process included:
a. Papers published in 2013 or later in scientific journals
b. Relevant to Ft and its mechanisms of infection/evasion once inside the host
c. Only articles in English were reviewed
d. Initial search included only research papers (not reviews)
3. This initial search identified several hundred primary publications that met the search strategy.
4. The publications were collated in Qiqqa (https://bit.ly/45f6a3U, RRID:SCR_004440), an open-source citation management program that employs tags and metadata to organize the publications and allows collation of keywords and topics. Qiqqa identified several common terms including absent in melanoma (AIM)2, Cas9, CRISPR, Caspases, IRF3, macrophages, ROS, T6SS, and TLR2/4. Additional mechanisms were identified during review of the literature.
5. Information from additional resources (reviews and other relevant publications) outside of the studies identified by the initial search was subsequently included for context.
Thirty papers were reviewed and found to refer more to Francisella biology (e.g., virulence factors) than to host immune responses, including six papers on CRISPR/Cas. These papers were not included in the literature findings.
3 Results
Literature findings
3.1 Molecular defense mechanism changes
Our systematic review identified not only recent cellular immunity and cytokine-mediated immunity studies, but also molecular changes. First, the molecular changes are reviewed below.
3.1.1 Genetic mechanisms of Ft immune evasion
SCHU S4 and LVS use genetic methods to evade the immune system, such as leveraging host microRNAs (miRNAs) to inhibit messenger RNA (mRNA) translation. Notably, miR-155 broadly suppresses the host inflammatory response in macrophages, B cells, and T cells. This miRNA, along with miR-150 and miR-146, which target macrophage function, is upregulated in human bone marrow-derived macrophages (BMDMs) after infection with SCHU S4 and LVS. Bioinformatics analysis also identified SH2-containing inositol phosphatase-1 (SHIP-1) and myeloid differentiation primary response 88 (MyD88) as potential targets of miR-155 (Bandyopadhyay et al., 2014). The potential blocking of SHIP-1 by miR-155 is intriguing. SHIP-1 indirectly inhibits the Akt pathway by dephosphorylating phosphatidylinositol triphosphate (PIP3), which is crucial for Akt pathway activity. Thus, SHIP-1 hinders basic cellular functions like growth, proliferation, and survival (Cremer et al., 2011). If miR-155 is induced by infection in non-macrophages, it could enhance these processes, promoting cell survival.
miR-155 targets MyD88, a key player in TLR signaling that triggers inflammatory cytokines like tumor necrosis factor (TNF)-α and interleukin (IL)-1β. SHIP-1 also participates in TLR signaling by inhibiting MyD88 and its counterpart, TIR domain-containing adapter molecule (TIRAM)/TIR domain-containing adapter-inducing INF-β (TRIF). Interestingly, miR-155 blocks both MyD88 and SHIP-1, disinhibiting the MyD88 and TIRAM pathways but ultimately shuts down the MyD88 branch, leaving only the TIRAM pathway active (Figure 2). Bandyopadhyay et al. (2014) noted that miR-155 expression increases over time post-infection, favoring the TIRAM pathway and leading to late-stage production of IFN-α and IFN-β in tularemia (Furuya et al., 2014). The full implications of these interactions in innate immune responses to tularemia remain unclear and need further exploration.
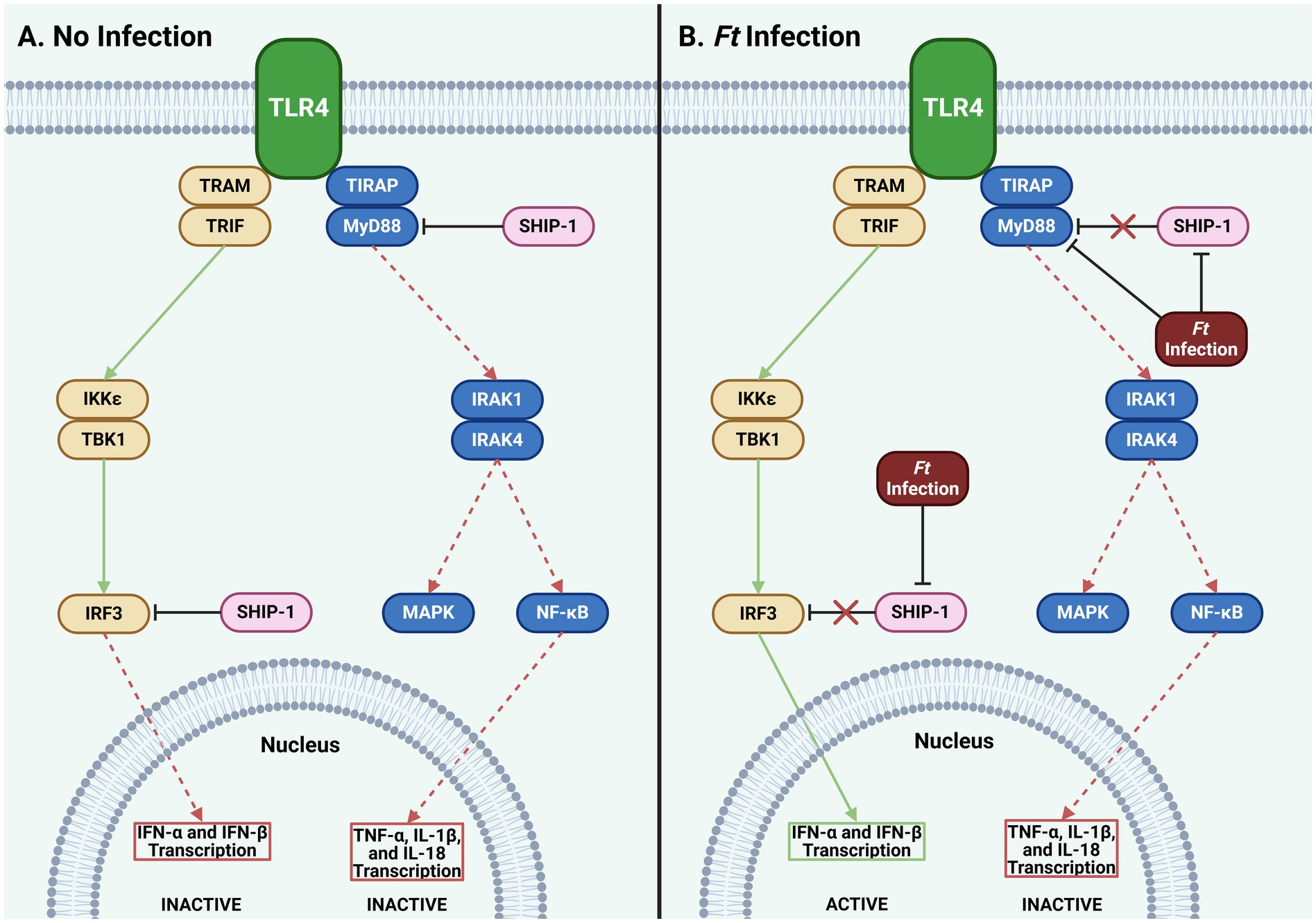
Figure 2. Effect of Ft infection on TLR4 signaling. (A) SHIP-1 inhibits MyD88 and IRF3, blocking transcription of type I IFNs, TNF-α, IL-1β, and IL-18. (B) Ft infection inhibits SHIP-1, typically engaging IRF3 and MyD88. Though a second, unidentified mechanism, Ft simultaneously continues to inhibit MyD88. This results in the transcription of type I IFN genes while the transcription of TNF- α, IL-1β, and IL-18 remains suppressed. While these mechanisms are specific to only a subset of cells, they may provide insights to help accelerate a more robust innate immune response to Ft (Ft: Francisella tularensis, IFN: interferon, IKK: IκB kinase, IL: interleukin, IRAK: interleukin receptor-associated kinase, IRF: interferon regulatory factor, MAPK: mitogen-activated protein kinase, MyD88: myeloid differentiation primary response 88, NF-κB: nuclear factor-κB, SHIP: Src homology 2 domain containing inositol polyphosphate 5-phosphatase, TBK: TANK-binding kinase, TIRAP: toll-interleukin-1 receptor domain-containing adaptor protein, TLR: toll-like receptor, TNF: tumor necrosis factor, TRAM: translocating chain-associating membrane protein, TRIF: TIR-domain-containing adapter-inducing interferon-β).
SHIP-1 is also active in adaptive immune cells like B cells, T cells, mast cells, and natural killer (NK) cells, generally reducing their signaling (Conde et al., 2011). Since these cells are not primary targets for Ft infection, it’s unclear if miR-155 would be upregulated in them through paracrine or other signaling methods. The literature has not explored miR-155 activity in these cells post-tularemia infection, making it a promising research area for understanding Ft’s immune evasion. Future studies should also consider the roles of other miRNAs upregulated after tularemia infection and whether SHIP-2 could compensate for the lack of SHIP-1 activity.
The literature on Ft’s genetic immune evasion is limited compared to studies on protein and cytokine interactions between host and pathogen cells. Future research may reveal important genetic interactions with Ft and its hosts, but for now, most efforts focus on understanding protein interactions during Ft infections.
3.1.2 Inflammasome
Intracellular pathogens like Ft evade extracellular defenses by replicating inside host cells. Hosts counteract these threats by triggering regulated cell death pathways, such as apoptosis, pyroptosis, and necroptosis. Inflammasomes, protein complexes with sensor proteins, detect pathogen virulence traits directly or indirectly and cause proinflammatory cytokine release and cell death (Nozaki et al., 2022). Common inflammasome mechanisms involve cytoplasmic NOD-like receptors (NLR) and absent in melanoma (AIM) 2. NLR pathways, activated by pathogen patterns and secretions or other innate immune pathways like TLR4, are complex (Man and Kanneganti, 2015). Recent literature focuses on AIM2 inflammasomes in Ft infections, are reviewed below.
Researchers have studied the role of the AIM2 inflammasome protein during Ft infection and immunization. AIM2 forms a complex with apoptosis-associated speck-like protein containing a CARD (ASC) to cleave pro-caspase-1 into caspase-1, which then activates pro-IL-1β and pro-IL-18 and induces gasdermin-D (GSDMD) to form pores in the cell membrane, allowing cytokine secretion. This process also triggers pyroptosis, a form of programmed cell death (Kumari et al., 2021) and is crucial for defending against intracellular pathogens, as shown in studies with Ft and Brucella abortus (Costa Franco et al., 2019). These molecular pathways are complex and interwoven with other innate immune signaling pathways, but their downstream effectors are distinct enough to require separate discussions.
Investigating Ft’s relationship with inflammasome mechanisms is challenging. Certain LVS mutants might be compromised structurally and lyse intracellularly, artificially increasing inflammasome signaling as host cytosolic DNA sensors detect the foreign DNA (Costa Franco et al., 2019). Singh et al. (2013) confirmed that immune responses to Ft differ between in vivo and in vitro systems, influenced by bacterial growth conditions and emphasized that not all in vitro findings may be relevant to tularemia pathogenesis in mammals. There is also evidence that ASC can function independently of caspase-1, bypassing canonical inflammasome activation (Cheong et al., 2020; Hughes et al., 2018). Additionally, murine BMDMs lacking ASC supported LVS growth similarly to those with functional ASC, indicating ASC’s limited impact on LVS growth. These findings were consistent across LVS strains with different mutations in the Francisella pathogenicity island (FPI). Interestingly, MyD88−/− cells did not support significant replication of intracellular LVS, except for LVS strains with FPI mutations (Meyer et al., 2015). These redundant pathways downstream of ASC allow host immune cells to initiate the IFN-γ/IL-18 cascade. Further research is needed to understand how Ft evades or inhibits these redundancies in cell death pathways.
Within these pathways, multiple regulatory mechanisms exist, including negative feedback loops and protein complex formations. One such loop involves mature caspase-1 inhibiting AIM2 production. A 2013 study found that sublethal caspase-1 levels may prevent AIM2 from associating with the inflammasome adapter ASC, thus inhibiting AIM2 speck formation and subsequent pyroptosis (Juruj et al., 2013). This inhibition helps Ft evade the immune system and incubate within cells. Additionally, AIM2 activity blocks the stimulator of interferon genes (STING) pathway, which produces IFN-β and induces inflammasome activity. Therefore, as AIM2 inflammasome activity increases, it suppresses the upstream pathways that activate itself (Corrales et al., 2016; Figure 3).
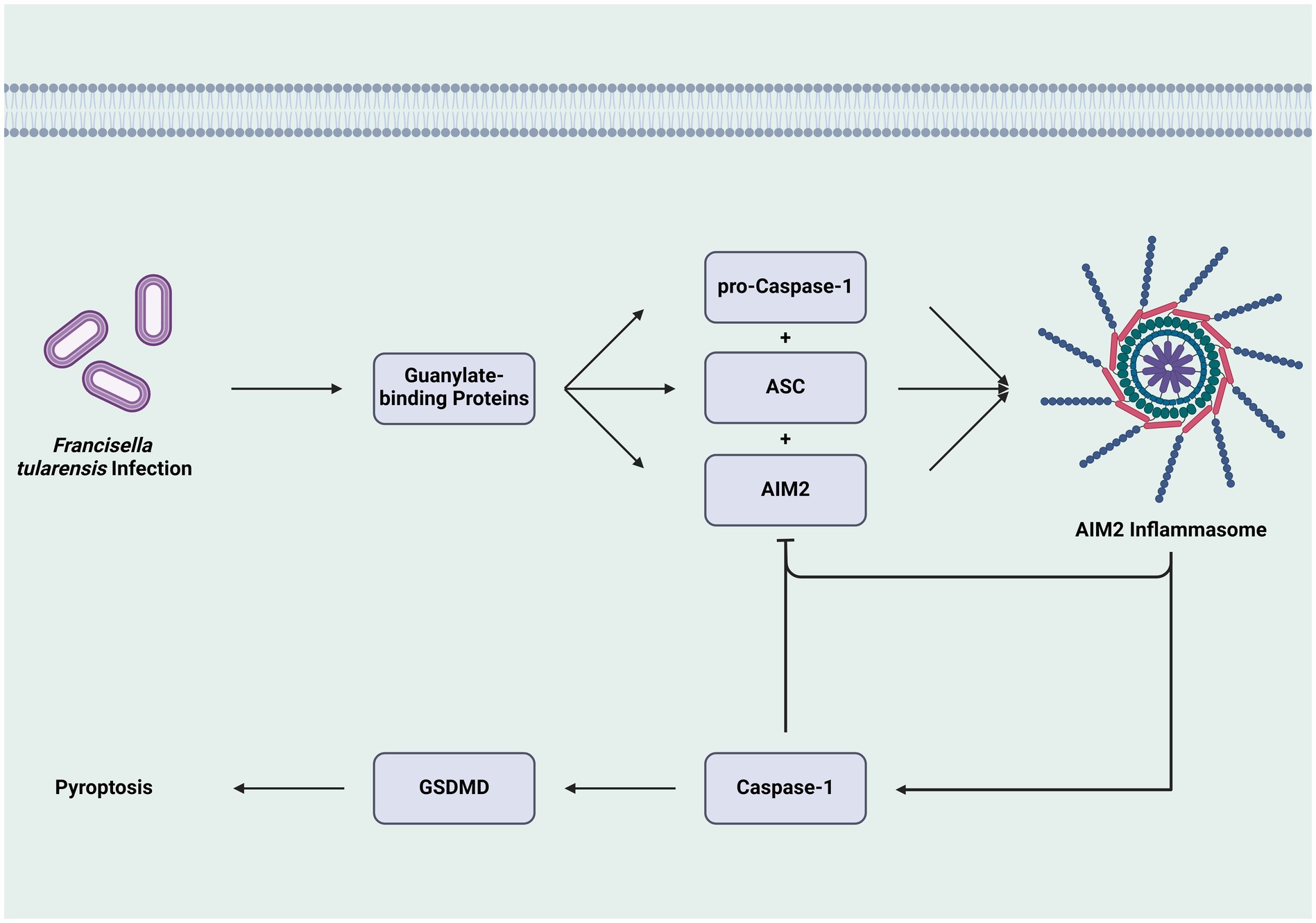
Figure 3. Feedback inhibition of inflammasome formation and caspase-1 activation. The construction of the AIM2 inflammasome through the combination of pro-caspase-1, ASC, and AIM, leads to the activation of caspase-1 and GSDMD, culminating in cell death via pyroptosis. However, the activity of the inflammasome and caspase-1 inhibit the formation of additional inflammasomes, which can attenuate the cell death pathway and allow Ft-infected cells to survive and contribute to the pathogen’s replication. While this pathway is specific to one mechanism, it is one that helps create an intracellular environment that facilitates Ft growth by preventing cell death (AIM2: absent in melanoma 2, ASC: adaptor molecule apoptosis-associated speck-like protein containing a CARD, GSDMD: Gasdermin D).
The nonreceptor tyrosine kinase c-Abl impacts inflammasome signaling by interacting with IFN regulatory factor (IRF3), a key transcriptional regulator in IFN-α and -β production, which feeds into the pyroptosis pathway (Messaoud-Nacer et al., 2022). Typically, c-Abl is involved in cell cycle regulation, apoptosis, and antioxidant responses to hydrogen peroxide (H2O2) (Shaul, 2000; Cao et al., 2003). Though IRF3, c-Abl, and its relative Arg typically function independently, they are interconnected in inflammasome signaling. IRF3-mediated IFN-β production relies on binding and phosphorylation by c-Abl, and since AIM2 inflammasome induction depends on IFN-β, inhibiting c-Abl affects AIM2 activity. C57BL/6 mouse studies show that c-Abl is crucial for protection against LVS; inhibiting c-Abl with AMN-107 significantly reduced mRNA expression of IFN-β and AIM2, protein expression of IL-18, and survival rates. Small interfering RNA (siRNA) targeting c-Abl and Arg also decreased survival and expression levels, highlighting the complex regulatory interactions in the AIM2 inflammasome pathway and its role in Ft infections (Luo et al., 2019).
Ft can manipulate apoptosis pathways in human neutrophils. LVS robustly inhibits apoptosis, regulating 365 host genes related to apoptosis or cell survival. It downregulates the pro-apoptotic BCL2-associated X protein (BAX) and its mRNA 24 h post-infection, while sustaining the anti-apoptotic X-linked inhibitor of apoptosis protein (XIAP) for up to 48 h. Nuclear factor (NF)-κB markers are upregulated, promoting neutrophil survival (Schwartz et al., 2013). Despite these changes, proinflammatory signaling remains intact, consistent with an anti-apoptotic phenotype (Kobayashi et al., 2003). In 2015, it was further shown that LVS inhibits apoptosis markers like BAX, XIAP, and caspases −3 and − 9, and prevents apoptosis induced by R-roscovitine in neutrophils (McCracken et al., 2016).
IFNs can induce guanylate binding proteins (GBPs) in nearby host cells, which lyse the bacteria, exposing bacterial DNA to cytosolic sensors and triggering the AIM2/ASC inflammasome response. This results in the release of IL-1β and IL-18, intensifying the proinflammatory environment post-infection (Snyder et al., 2017). The cyclic GMP-AMP synthase (cGAS)-STING pathway can also be triggered by extracellular vesicles carrying bacterial DNA from nearby cells. These vesicles are produced via a STING-mediated pathway involving TANK-binding kinase 1 (TBK1) and multivesicular body subunit 12b (MVB12b) after Ftt infection. This cell-to-cell signaling activates the cGAS-STING pathway in target cells, leading to proinflammatory and inflammasome triggering. However, this process can aid intracellular pathogens by blocking IL-17A production, which is crucial for neutrophil expansion, thereby limiting aspects of the immune response (Nandakumar et al., 2019).
Ft evades the inflammasome by entering macrophages. Complement receptor 3 (CR3) binds to opsonized Ft, facilitating its uptake into macrophages (Hoang et al., 2018). Dai et al. (2016) found that opsonizing SCHU S4 before uptake reduces extracellular signal-regulated kinase (ERK) signaling, suppressing the inflammasome. This process upregulates Ras-specific GTPase-activating proteins (RasGAP), inhibiting the rapidly accelerated fibrosarcoma (Raf)-mitogen-activated kinase (MEK)-ERK pathway and suppressing innate immune pathways like TLR2. Opsonized Ft exploits these pathways to inhibit inflammasome activity, as shown by decreased mature caspase-1 and IL-18 secretion.
Inflammasome signaling molecules AIM2 and NLRP3 are not essential for vaccine-induced immunity against LVS. Alqahtani et al. (2021) found that AIM2−/− and NLRP3−/− C57BL/6 mice were fully protected against a lethal dose of LVS and showed normal antibody and cell-mediated responses. This suggests that AIM2 and NLRP3 are dispensable for vaccine-induced immunity against respiratory tularemia caused by Ft. However, it is unclear if this would hold true for a virulent strain of Ft. Overall, inflammasome activation is a complex and challenging aspect of tularemia infection, involving intricate regulatory mechanisms and interactions with different Ft strains. Identifying protective correlates is difficult, especially since early evidence suggests this pathway may be unnecessary for immune protection, as indicated by LVS.
3.1.3 Toll-like receptors
Alongside the AIM2 inflammasome system, extracellular receptors like TLRs identify pathogens before they infiltrate cells. TLRs, working with key cytokines, trigger a rapid innate immune response by recognizing pathogen-associated molecular patterns. However, some pathogens evade this recognition, facilitating infection and replication. Ftt is particularly effective at evading the host immune system. A 2021 study showed that SCHU S4 does not trigger TLR2 or TLR4 responses in human embryonic kidney (HEK) 293 cells overexpressing these receptors (Amemiya et al., 2021). While LVS does not trigger TLR4, it robustly triggers TLR2. Unlike LPS from other Gram-negative bacteria, Ft lipopolysaccharide (LPS) does not interact with TLR4 to induce inflammation, contributing to its pathogenesis (Gunn and Ernst, 2007).
These innate immune interactions only partially explain how Ft interacts with its hosts. The pathogen-host interactions are highly complex, requiring significant crosstalk between the innate and adaptive immune systems. A recent study modeled these molecular interactions, identifying many potential targets for future therapies and preventative treatments (Miryala and Ramaiah, 2022). Despite progress in understanding these relationships, much work remains to translate these findings into medical countermeasures (MCMs) for humans.
3.1.4 Suppression of ROS
Production of ROS is an important mechanism for control and elimination of intracellular pathogens; these ROS include microbicidal superoxide radicals (O2−), H2O2, and highly reactive hydroxyl radicals (HO−) produced from H2O2. To survive in this potentially hostile environment, Ft has evolved a variety of mechanisms to inhibit the activity of nicotinamide adenine dinucleotide phosphate (NADPH) oxidase, contributing to the organism’s pathology (Moreland et al., 2009; McCaffrey et al., 2010).
Several Ft proteins are differentially expressed under oxidative stress, notably those associated with translation processes, cellular stress, metabolism, and cell membrane proteins, in addition to changes in the Igl operon in the FPI of Fth. Affected proteins include ribosomal proteins, superoxide dismutase (Sod)B, acetyl CoA carboxylase, LpxD2, and IglA. These factors and others are differentially regulated in Fth so that it can both evade or suppress the host’s immune reaction while also providing necessary nutrients for its survival. Interestingly, the changes in proteins relating to oxidative stress were not induced if the host cells were previously primed with IFN-γ before infection, indicating that the early induction of IFN-γ’s targets more easily trigger an immune response from the host (Pavkova et al., 2013).
In a series of in vitro experiments, Newstead et al. (2014) infected murine MH-S alveolar macrophages and J774A.1 peritoneal macrophages with LVS or SCHU S4 to evaluate the relevance of nitric oxide (NO) induction in resistance to intracellular bacterial infection in a TLR-stimulated context. Cell monolayers were stimulated with bacterial (E. coli) LPS and IFN-γ. Importantly, these studies demonstrated that SCHU S4 appears to have a mechanism which prevents cellular nitrite production, and this mechanism is lacking in LVS. These studies demonstrate that LVS is not directly analogous to SCHU S4, and that caution should be exercised when extrapolating data gained from one strain to the other.
Ma et al. (2016) investigated the role of OxyR in the regulation of oxidative stress responses by LVS. These investigators found that OxyR of Ft regulates oxidative stress responses to promote resistance against ROS, thereby contributing to its intracellular survival and promoting its virulence in C57BL/6 mice. Proteomic analysis revealed that OxyR modulates the level of 128 proteins, indicating a broader regulatory role of OxyR in overcoming oxidative stress. Moreover, OxyR regulated the transcription of the primary antioxidant enzyme genes ahpC and katG by binding directly to their putative promoter regions. This finding was supported by later studies by Honn et al. (2017) using a double deletion mutant of LVS, ΔoxyR/ΔkatG, who demonstrated that Ft lacking OxyR and catalase were more susceptible to control by macrophages than WT Ft.
3.2 Antigen-presenting cells
In addition to identifying intracellular, molecular immune responses to Ft, our systematic review identified many cellular responses.
3.2.1 Dendritic cells
Various cell types in the innate immune system contribute to an appropriate response to a pathogen (Figure 4). One such cell type is the dendritic cell (DC), and Ft seems well-adapted to evade and suppress its immune mechanisms. Ireland et al. (2013) demonstrated that lipids isolated from SCHU S4 were sufficient to suppress an immune response in human DCs, notably suppressing the production of IL-12p40. This suppression extended to secondary exposure to LPS after the application of the SCHU S4 lipids. Additionally, these lipids had the same effect in vivo and prevented NF-κB from translocating to the nucleus of host cells in C57BL/6 J mice. In the same study, it was also shown that SCHU S4 was able to block the activity of IRF1 and IRF8, inhibiting the transcription of IL-12p40 (Ireland et al., 2013).
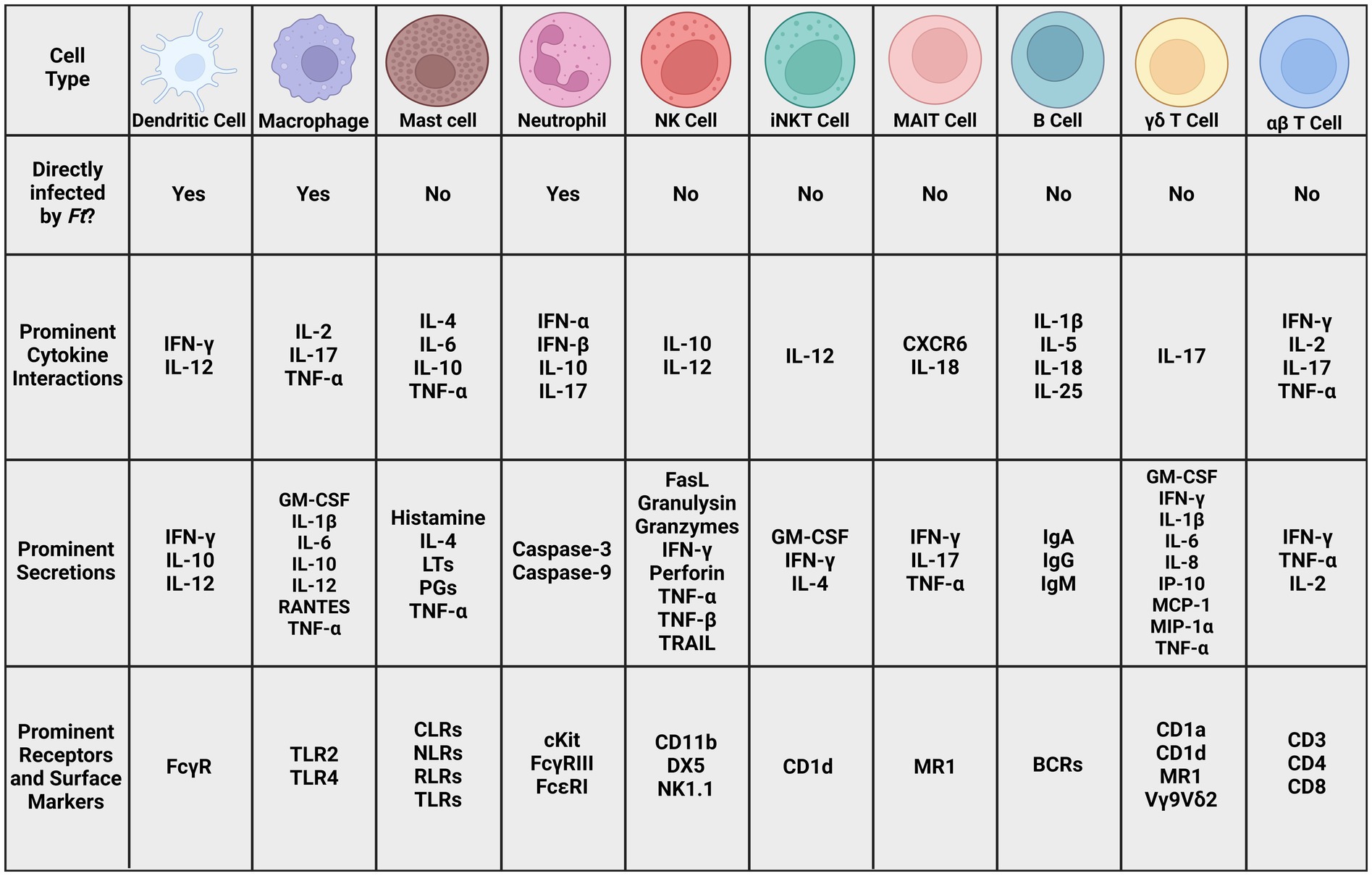
Figure 4. Prominent cell types in Ft literature. Many cell types of both the innate and adaptive immune system, as well as cells that bridge the gap between those systems. The cytokines, secretions, receptors, and surface markers involved in potentially recognizing and fighting tularemia infections. Given the complex interactions between these cells and signaling molecules, it is unlikely that any one will serve as a definitive correlate of protection. More research is necessary to elucidate these interactions and identify ones that could indicate vaccine efficacy (BCR: B cell receptor, CD: cluster of differentiation, CLR: C-type lectin receptor, CXCR: chemokine receptor, DX5: α2 integrin, FasL: Fas ligand, GM-CSF: granulocyte macrophage colony-stimulating factor, IFN: interferon, Ig: immunoglobulin, IL: interleukin, iNKT: innate natural killer T cell, IP: Interferon-gamma-inducible protein, LT: leukotriene, MAIT: mucosal-associated invariant T cell, MCP: monocyte chemoattractant protein, MIP: macrophage inflammatory protein, MR1: MHC class I-related protein, NK: natural killer cell, NK1.1: killer cell lectin-like receptor subfamily B member 1C protein, NLR: Nod-like receptor, PG: prostaglandin, RANTES: regulated on activation normal T cell expressed and secreted, RLR: RIG-I-like receptor, TRAIL: Tumor necrosis factor-related apoptosis-inducing ligand, TLR: toll-like receptor).
Administration of antigen (Ag)-pulsed DCs is an effective strategy for enhancing immunity to tumors and infectious organisms. Studies have also demonstrated that targeting antigens to Fcγ receptors (FcγR) on Ag-presenting cells can enhance humoral and cellular immunity in vitro and in vivo. Studies by Pham et al. (2014) using Ft demonstrated that targeting immunogens to FcγR via intranasal (i.n.) administration of inactivated Ft (iFt) immune complexes enhanced protection against Ft challenge in C57BL/6 and FcγRIIB knockout (KO) mice on a C57BL/6 background. The authors sought to determine if ex vivo targeting of iFt to FcγR on DCs would enhance the potency of iFt-pulsed DCs administered i.n. Bone marrow-derived DCs (BMDCs) were pulsed ex vivo with iFt or monoclonal antibody (mAb)-iFt immune complexes. I.n. administration of these BMDCs enhanced humoral and cellular immune responses, as well as protection against LVS challenge. Increased protection correlated with increased iFt loading on the BMDC surface due to FcγR targeting.
3.2.2 Macrophages
Ft infection of macrophages has been well studied. The early interplay between Ft and the innate immune system is a key facet of infection, as macrophages are some of the earliest targets for infection by the bacterium. This is highlighted in a 2014 study in mice showing that Fn, SCHU S4, and LVS all preferentially infected alveolar macrophages (F4/80high, CD11chigh, CD11bmid, DEC-205mid) in the first 4 h after inhalation in C57BL/6 mice (Roberts et al., 2014).
De Pascalis et al. (2018) investigated a macrophage co-culture assay to identify immune correlates for tularemia vaccines. They infected peripheral blood leukocyte (PBL)-derived macrophages taken from Fischer 344 rats with LVS and less protective LVS variants from LVS-immunized rats, then co-cultured these macrophages with PBLs from vaccinated rats. They found 22 genes were significantly upregulated in PBLs from LVS-vaccinated rats. Combining intramacrophage Ft growth control with specific gene expression levels [IFN-γ, IL-21, nitric oxide synthase (NOS)2, lymphotoxin α (LTA), T-bet, IL-12rβ2, and chemokine ligand (CCL)5] using multivariate analyses distinguished protected from non-protected rats with over 95% sensitivity and specificity. In 2022, they showed that more effective vaccines (e.g., Ft SCHU S4 ΔclpB) increased IFN-γ and nitric oxide levels while reducing bacterial levels in macrophages (De Pascalis et al., 2022). Further investigation into T cell-mediated infection control in macrophages and related cytokines is needed to develop candidates for immune correlates of protection.
In another study evaluating the role of macrophages in immunity provided by Ft vaccines (Babadjanova et al., 2015), C57BL/6 mice were vaccinated with paraformaldehyde-inactivated Ft in a prime-boost strategy. Peritoneal macrophages from immunized mice produced more IL-12p70 and TNF, and less IL-10 than unvaccinated mice. The addition of a mAb to the inactivated Ft to enhance macrophage targeting further enhanced IL-12p70 and TNF production and further reduced IL-10 production. IL-10 production from macrophages was shown to attenuate pro-inflammatory cytokine production.
A 2016 study utilized an in vitro model to simulate macrophages in the human liver by co-culturing HepaRG hepatocytes and BMDMs. The application of LVS, WT Fth, and Ftm caused macrophage cell death and production of TNF, IL-6, IL-1β, and fractalkine (Rennert et al., 2016). This model may have utility in examining Kupfer cell (i.e., liver resident macrophages) contributions to Ft-specific immunity.
Rabadi et al. (2016) found that macrophages infected with LVS produced little IL-6 and TNF and possessed potent anti-inflammatory capabilities. Live, but not UV-killed, LVS suppressed IL-6 and TNF production, consistent with previous SCHU S4 studies (Gillette et al., 2014). Ft SodB and mitogen-activated protein kinase (MAPK) contributed to this suppression by preventing the activation of redox-sensitive MAPK signaling, NF-κB signaling, and pro-inflammatory cytokine production through inhibition of ROS accumulation in infected macrophages.
Golovliov et al. (2016) studied NO as a predictor of Ft vaccine efficacy using an in vitro co-culture model with murine BMDMs and splenocytes from BALB/c and C57BL/6 mice immunized with LVS or the ΔclpB attenuated strain of Ft. Similar to De Pascalis et al. (2018) they found that nitrite levels in the supernatants of splenocyte-BMDM co-cultures infected with LVS strongly correlated with growth inhibition and levels of IFN-γ, granulocyte-macrophage colony-stimulating factor (GM-CSF), IL-6, IL-10, IL-12p40, and CCL5. ΔclpB-immune splenocytes produced more nitrite than LVS-immune splenocytes. When SCHU S4 was the infecting agent, nitrite levels also correlated with Ft growth inhibition, with ΔclpB-immune splenocytes showing higher nitrite levels than LVS-immune splenocytes. These findings suggest a link between cytokine and nitrite production and growth suppression by both LVS and SCHU S4. Similar results were observed in studies using Fischer 344/IcoCrl rat macrophages and splenocytes (Lindgren et al., 2020). Demonstrating this correlation in human cell cultures would be important, though technically challenging to adapt for vaccine development.
Macrophages stimulated with IFN-γ can resist Ft infection in vitro. To determine if this protection requires macrophages receptive to stimulation, Steiner et al. (2017) used alveolar macrophage depletion in WT C57BL/6, BALB/c, and IFN-γ insensitive mice (MIIG model) with sublethal pulmonary LVS infection. Both macrophage depletion and IFN-γ insensitivity worsened the infection. While exogenous IL-12 usually provides protection in mice, this was not seen in MIIG mice, indicating IL-12’s effects are not directly through macrophages. MIIG mice also showed reduced neutrophil recruitment to the lungs after LVS infection.
Richard et al. (2016) discovered that IFN-β enhances macrophage resistance to LVS infection by increasing TLR2’s response to Ft, leading to the production of pro-inflammatory cytokines like IL-6. This enhanced innate recognition and TLR-dependent transcriptional response were observed in primary human monocyte-derived macrophages and primary murine peritoneal macrophages, but not in murine BMDMs. The type I IFN-enhanced response works synergistically with TLR2 transcriptional responses and is partially TLR2-independent but strictly MyD88-dependent. These findings differ from other macrophage studies where IFN-β reduces pro-inflammatory cytokine production, complicating the use of cytokines to measure immune responses to Ft.
All these studies suggest that macrophage cytokine production, as influenced by other immune cell types and Ft virulence factors, contribute to Ft infection outcomes. Macrophage cytokine production, particularly in co-culture with immune lymphocytes has great potential for the development of an immune correlate of protection, but additional work is required.
3.2.3 Dendritic cell cytokines
IFN-γ production in response to Ft infection is known to occur by NK and T cells, but the production of this cytokine by DCs is less-studied. De Pascalis et al. (2020) demonstrated substantial production of IFN-γ by DC isolated from wild-type (WT) C57BL/6 and Rag1 KO mice infected with LVS, as well as hybrid NK-DC, from LVS-infected Rag1 KO mice. This indicates that DC produced IFN-γ independently of B or T cells. These authors demonstrated that the numbers of conventional DC producing IFN-γ increased progressively over the course of 8 days of LVS infection; in contrast, the numbers of conventional NK cells producing IFN-γ, which represented about 40% of non-B/T IFN-γ-producing cells, peaked at Day 4 after LVS infection and declined thereafter. This pattern was similar to that of hybrid NK-DCs. To further confirm IFN-γ production by infected cells, DC and neutrophils were sorted from naive and LVS-infected mice and analyzed for gene expression. Quantification of LVS by polymerase chain reaction (PCR) revealed the presence of Ft DNA not only in macrophages but also in highly purified, IFN-γ producing DCs and neutrophils. Finally, production of IFN-γ by infected DCs was confirmed by immunohistochemistry and confocal microscopy. Notably, IFN-γ production patterns similar to those in wild type C57BL/6 J mice were observed in cells derived from LVS-infected TLR2, TLR4, and TLR2xTLR9 KO mice, but not from MyD88 KO mice. These data add to the complexity of IFN-γ as an indicator of a specific immune response, as IFN-γ can come from multiple sources, dependent and independent of a memory immune response.
IL-12 is produced by DCs, macrophages, and B cells following infection with pathogenic bacteria and stimulates IFN-γ production by T cells. IFN-γ also stimulates IL-12 in antigen-presenting cells, creating a feedback loop that facilitates Th1 cell differentiation; the Th1 response is important for responding to intracellular pathogens including Ft. IL-12 can also facilitate the production of IFN-γ by NK cells (Vignali and Kuchroo, 2012). IL-12 is often designated IL-12p70, since it is a heterodimeric cytokine comprising p40 and p35 subunits.
It has been known for more than two decades that infection with Ft in mice results in a rapid induction of IL-12, along with TNF-α and IFN-γ (Stenmark et al., 1999). To better understand the role of IL-12 in tularemia, Elkins et al. (2002) employed BALB/cByJ and C57BL/6 J murine models of IL-12 deficiency (p40 KO mice, p35 KO mice, and mice treated with IL-12-neutralizing antibodies) infected with LVS. p40 KO mice and mice treated in vivo with anti-IL-12 antibodies survived large doses of primary and secondary LVS infection but never cleared bacteria, instead resulting in a chronic infection. p35 KO mice survived large doses of primary sublethal LVS infection as well as maximal secondary otherwise lethal challenge. LVS-immune lymphocytes from WT mice produced large amounts of IFN-γ, while p35 and p40 KO lymphocytes produced much less. The authors surmised that while IL-12p70 stimulation of IFN-γ production may be important for bacteriostasis, it is not necessary for controlling intracellular bacterial growth and for clearance of primary or secondary LVS infection. Similar results were more recently found in C57BL/6 J mice by Mittereder et al. (2023).
The role of IL-12 in inducing protective immunity in the lungs of BALB/c and C57BL/6 mice against i.n. infection with LVS was further investigated by Duckett et al. (2005). They found that IFN-γ and IL-12 were strictly required for protection, since mice deficient in IFN-γ, IL-12p35, or IL-12p40 all succumbed to LVS doses that were sublethal for WT mice. They further showed that exogenous IL-12 treatment 24 h prior to infection with a lethal dose of LVS significantly decreased bacterial loads in the lungs, livers, and spleens of both WT BALB/c and C57BL/6 mice and allowed the animals to survive infection; this protection was not observed in IFN-γ-deficient mice. The resistance to LVS induced by IL-12 was also observed in NK cell-deficient mice, although not in CD8−/− mice, suggesting that this mechanism is at least partially dependent upon the expression of IFN-γ and CD8+ T cells.
Using in vitro cultures of Ft-infected, human monocyte-derived DCs, Bauler et al. (2011) found that whereas LVS infection quickly induced the production of several cytokines including IL-12p40, SCHU S4 infection did not result in production of inflammatory cytokines. This difference between LVS and SCHU S4 was noted even though both strains proliferated in culture to equivalent degrees. Further evaluation revealed SCHU S4 induced IFN-β, which in turn blocked IL-12p40 production in DCs. Subsequent studies by Ireland et al. (2013) revealed that this activity was mediated by lipids in SCHU S4 (and not LVS) that inhibited IRF1 and IRF8. Nota bene: This clear differential effect between virulent and non-virulent strains of Ft suggests that caution should be exercised when extrapolating results from historical LVS research to more virulent strains.
3.3 B cells, antibodies, and associated cytokines
Antibodies are a key component in control of and protection from many microbial pathogens; however, our understanding of the precise role of antibodies during the immunological response to Ft has a long and often contradictory history. The antibody response to Ft exposure is complex, involving immunoglobulin (Ig)A, IgM, and IgG, and includes the participation of both antigen-specific and natural antibodies (Kubelkova and Macela, 2021; Kubelkova et al., 2021). It should also be noted that several studies have shown that transfer of immune sera or monoclonal antibodies to naive animals did not provide protection against LVS (Rhinehart-Jones et al., 1994; Narayanan et al., 1993; Dreisbach et al., 2000). Much of the response is specific to Ft LPS (Kirimanjeswara et al., 2008), especially the O-antigen (O-polysaccharide component of the LPS complex).
Injection of LVS membrane protein fractions after infection has yielded elevated IgM and IgG in BALB/c murine sera, which, combined with gentamicin regimens, has been efficacious against Ft SCHU S4 infection (Chandler et al., 2015). Secondary computational analysis of the membrane fractions identified a subset of LVS surface proteins likely to elicit immunogenic responses, however it is unclear exactly how these factors can be used for prophylactic or post-exposure immunization (Chandler et al., 2015) or if specific antibody epitopes could be used to predict survival outcomes.
Richard et al. (2014) generated antisera by immunizing C57BL/6 mice with LVS vesicles. Those antisera appeared to confer limited and passive protection against challenge with LVS. This novel vaccine approach used synthetic nanoparticles made from catanionic surfactant vesicles functionalized by the incorporation of either LVS or SCHU S4 components. Vesicles which did not express Ft components partially protected against LVS yet failed to protect against SCHU S4. In contrast, immunization with LVS vesicles (LVS-V) fully protected C57BL/6 mice against intraperitoneal (i.p.) LVS challenge, while immunization of mice with either LVS vesicles or SCHU S4 vesicles partially protected them against an i.n. SCHU S4 challenge and significantly increased the mean time to death for non-survivors. LVS-V immunization, but not immunization with empty vehicles, elicited high levels of IgG against non-LPS epitopes that were increased after LVS challenge and significantly increased early cytokine production.
Many studies of the role of antibodies in control of Ft have focused on IgM and IgG (Kubelkova and Macela, 2021). IgA, the most abundant antibody isotype in mucosal tissues, may have importance in Ft lung infections. IgA−/− mice vaccinated with UV-inactivated LVS plus exogenous IL-12 were not protected from subsequent live LVS challenge, but vaccinated C57BL/6 mice with IgA were protected (Baron et al., 2007). This suggests that in the LVS-sensitive mouse model, IgA may play a role in protection. Kubelkova and Macela (2021) provide a more comprehensive review of the role of antibodies in protection from Ft. However, we found additional points of interest regarding B1b cells.
3.3.1 B1b cells
Several investigators have explored the protective function of B1a cells. Studies in which Ft-LPS was administered to various mouse models (Cole et al., 2009) found that all animals pre-treated with 100 ng Ft-LPS were protected from LVS infection, whereas control mice all died. This protection was long-lived, up to 72 days post-treatment. B cells were required, as demonstrated by the lack of protection against LVS challenge following LPS vaccination in μMT mice (deficient in B cells) and JhD mice (IgH chain deletion). Studies with X-linked immunodeficiency (XID) mice (severely deficient in B1a cells) resulted in animals losing Ft-LPS-induced protection, demonstrating that B1a cells are required for this model. This was further confirmed by noting that Ft-LPS pretreatment induced large numbers of antigen-specific B1a cells, which differentiated into anti-Ft-LPS antibody-secreting plasma cells. Subsequent studies by this group (Cole et al., 2011) with C57BL/6 TLR2−/− mice suggested that Ft-LPS-mediated protection against LVS infection requires two discrete events, the first being the production of Ft-LPS-specific antibody and the second being TLR-mediated macrophage activation.
Recent evidence suggests that innate lymphoid cells (ILCs) of the second (lung mucosal) subset (ILC2s) can contribute to the activation of B1 lymphocytes after C57BL/6 murine immunization with LPS isolated from LVS. This process, traditionally thought to be carried out predominantly by TLRs and MyD88, can operate independently through the secretion of interleukin (IL)-25 by ILC2s. IL-25, a member of the IL-17 family, then stimulates other ILC2s to release IL-5, inducing the migration and differentiation of B1 cells to antibody-secreting cells. This response was seen only with LPS isolated from LVS, not with LVS infection itself (Barbosa et al., 2021).
3.3.2 IL-1β/IL-18
Del Barrio et al. (2015) found that during LVS infection, antibodies were produced by B1a cells that decreased susceptibility in a cytokine-dependent fashion. C57BL/6, IL-1rβ−/−, or IL-18−/− mice infected i.n. with LVS exhibited greater susceptibility to LVS than controls, with mean time to death and bacterial burdens in IL-18 knockout (KO) mice greater than those seen in IL-1β KO mice. The increased susceptibility was not observed with IL-1rα−/− mice. Mice deficient in IL-18 quickly succumbed to infection with LVS, but this was found to be mitigated by administration of exogenous IFN-γ. On the other hand, mice deficient in IL-1β were able to control the infection initially but eventually died from the infection, and this could not be mitigated by exogenous IFN-γ, indicating that IL-1β and IL-18 operate differentially in response to Ft. The IL-1β-deficient mice were found to have significantly reduced serum levels of IgM antibodies specific for Ft LPS. In control mice, these antibodies promoted bacterial agglutination and phagocytosis and were protective in passive immunization experiments. These antibodies were determined to be produced by B1a cells, which were significantly decreased in the spleen and peritoneal cavity of infected IL-1β-deficient mice. The authors concluded that IL-1β and IL-18 activate non-redundant protective responses against tularemia and identify an essential role for IL-1β in the rapid generation of pathogen-specific IgM by B1a cells. While B1b cells may play an important role in murine LVS infections, it is important to note that mouse lung infections may not be reflective of other species (most importantly humans).
3.4 T cells and associated cytokines
T cells have long been known to contribute to protection against tularemia (Tarnvik, 1989) and the production of cytokines from T cells, such as IFN-γ, has also long been known as a key component of this protection (Messaoud-Nacer et al., 2022; Shaul, 2000). In studies by Sjostedt et al. (1994), the responses to four Francisella antigens were shown to be confined mostly to the CD45RO+ memory T-cell subset. To characterize further the phenotype of the responding cells, purified CD4+ and CD8+ T cells were stimulated with the antigens. CD4+ T cells, but not CD8+ T cells, proliferated and produced IFN-γ. However, when conditions permitted or simulated CD4+ T cell help, CD8+ T cells responded similarly to CD4+ T cells. There was a direct quantitative correlation between the proliferative response of CD4+ and CD8+ T cells and their production of IFN-γ. In conclusion, both CD4+ and CD8+ T cells of LVS-vaccinated humans respond with proliferation to various protein antigens of Ft, and the proliferative response was strictly associated with IFN-γ production.
Roberts et al. (2016) evaluated the importance of engaging CD4+ cells by generating Ft strains expressing well-characterized lymphocytic choriomeningitis virus (LCMV) epitopes and using these epitopes to track the T cell response. They found that T cells from vaccinated C57BL/6 J mice could be isolated and could control Ft replication in macrophages cultured in vitro and that prolonged survival time was related to the major histocompatibility complex (MHC) haplotype capable of presenting the LCMV epitope to T cells. Furthermore, the T cells produced IFN-γ, TNF, and IL-2, which adds to the literature showing that T cells producing multiple cytokines are protective against tularemia.
De Pascalis et al. (2012) found that T-bet, a T cell transcription factor, was strongly upregulated in immune lymphocytes. T-bet (also known as TBOX21 or TBX21) is a transcription factor specific to IFN-γ-producing T cells (Szabo et al., 2002; Lazarevic and Glimcher, 2011). T-bet has been increasingly recognized as having multiple functions, particularly as a bridge between the innate and adaptive immune responses (Lazarevic et al., 2013) and as a regulator of mucosal immunity (Mohamed and Lord, 2016). Melillo et al. (2014) found that intradermal (i.d.) or i.n. infection of C57BL/6 J with LVS was lethal at a two log-lower dose in T-bet deficient mice by both i.d. and i.n. dosing. T-bet deficient mice also exhibited significantly increased bacterial burdens in the lung and spleen. These differences were associated with changes in cytokine levels (IFN-γ, TNF, and IL-17) and recruitment of B cells, T cells, neutrophils, macrophages, DCs, and NK cells to the lungs. LVS-vaccinated T-bet-KO mice survived lethal LVS i.p. challenge but not i.n. challenge, regardless of the route of vaccination (i.d. or i.n.). Immune T lymphocytes from the spleens of i.d. LVS-vaccinated WT or T-bet-KO mice controlled intracellular bacterial replication in an in vitro co-culture system (described further in the macrophage section above), but cultures with T-bet-KO splenocyte supernatants contained less IFN-γ and increased amounts of TNF-α. T-bet-KO lung lymphocytes were greatly impaired in controlling intramacrophage growth of LVS. The authors concluded that “...T-bet represents a true, useful correlate for immunity to LVS”; whereas this may be accurate regarding protection against LVS, it remains uncertain whether this is true for virulent strains of Ft.
Numerous studies have investigated the cytokine response following naturally acquired infection with Ft, as well as the immune response induced by various tularemia vaccines (Lindgren et al., 2020; Eneslatt et al., 2012; Cunningham et al., 2020; Goll et al., 2020; Lindgren et al., 2023). Infection with Ft has been shown to induce hypercytokinemia (“cytokine storm”) in which infection results in an uncontrolled upregulation of various inflammatory cytokines with subsequent tissue damage and pathology (Mares et al., 2008; D’Elia et al., 2013; Ramos Muniz et al., 2018). Thus, understanding cytokine responses in Ft infection and immunization is crucial. Some of the cytokines that have been studied in the context of immunity to tularemia are discussed below.
3.4.1 Type II IFNs
Arguably, IFN-γ has been assessed more than any other cytokine in the context of tularemia and responses to vaccination. IFN-γ is a pivotal cytokine with multiple functions and is a key mediator of both innate and adaptive immunity (Kak et al., 2018; Mezouar and Mege, 2020). Production of IFN-γ following exposure to Ft antigens, either by natural infection or immunization, is a characteristic cellular response to Ft. In studies by Karttunen et al. (Karttunen et al., 1987), IL-2 and IFN-γ production were evaluated in subjects naturally infected 2 years previously, subjects vaccinated with LVS 5–6 years previously, and control subjects with no history of infection or vaccination. Subjects with a history of naturally acquired infection synthesized more DNA in both whole-blood and mononuclear cell cultures and secreted more IL-2 and IFN-γ than control subjects did. Vaccinees exhibited somewhat lower responses than the naturally infected subjects did, but in terms of DNA synthesis and IL-2 secretion responded in a manner similar to that of this group, with respect to receptor positivity and IFN-γ secretion.
Given the importance and variability of IFN-γ’s actions in the immune response to Ft, Parsa et al. (2008) hypothesized that Ft could interfere with IFN-γ downstream intracellular signaling. These authors demonstrated that Ft suppresses IFN-γ-induced signal transducer and activator of transcription (STAT) 1 expression and phosphorylation in both human and murine mononuclear phagocytes, and that this suppressive effect was independent of phagosomal escape or replication and was mediated by a heat-stable and constitutively expressed bacterial factor. An analysis of the molecular mechanism of STAT1 inhibition indicated that expression of suppressor of cytokine signaling (SOCS)3, an established negative regulator of IFN-γ signaling, was highly upregulated during infection and suppressed STAT1 phosphorylation. Functional analyses revealed that this interference with IFN-γ signaling was accompanied by the suppression of IFN-γ-induced protein (IP)-10 production and inducible NOS induction, resulting in increased intracellular bacterial survival. It remains unclear if vaccination impacts this Ft-mediated IFN-γ signaling disruption and if it contributes to immune correlates.
3.4.2 IL-17
IL-17 is a key cytokine for host protection against mucosal infections. The IL-17 family comprises six members (IL-17A to IL-17F) that mediate their functions through several receptors. The most studied IL-17 family member is IL-17A, generally referred to simply as IL-17. IL-17 is produced primarily by IL-17-secreting CD4+ T (TH17) cells; it is also produced by CD8+ T cells, gammadelta (γδ) T cells, NK cells, invariant NK T cells, mucosal-associated invariant T (MAIT) cells, mast cells, and others. Whereas T cell receptor (TCR) activation is key for IL-17 production by CD4+ and CD8+ T cells, IL-17 production by innate immune cells is primarily driven by inflammatory cytokines, especially IL-1β and IL-23 (Mills, 2023). One of the functions of IL-17 during Ft infection is regulation of the Th1 immune response. Studies by Lin et al. (2009) in C57BL/6 mice infected intratracheally with LVS demonstrated that IL-17 induced IL-12 production in DCs and thus mediated Th1 responses. In addition, they demonstrated that IL-17 also induces IL-12 and IFN-γ production in macrophages and mediated bacterial killing.
Murine studies utilizing C57BL/6 and BALB/c strains with sub-lethal inhalational doses of LVS revealed IL-17 in lavage fluids of infected lungs with pulmonary γδ and Th17 cells as the IL-17 sources. IL-17 production appeared early during infection and preceded the peak of immune activation, afterward subsiding. The investigators demonstrated that exogenous airway administration of IL-17 (or IL-23, which leads to the development and activity of Th17 cells) had a limited yet consistent effect of delaying the onset of death. The protective role for IL-17 against LVS was directly demonstrated by in vivo neutralization of the cytokine with anti-IL-17 antibodies, resulting in a fatal disease (Markel et al., 2010). Subsequent studies with SCHU S4, however, clearly demonstrated that IL-17 is not involved in resolution of infection (Skyberg et al., 2013). Skyberg et al. (2013) (again using C57BL/6 and BALB/c strains) were able to replicate the protective role of IL-17, with IL-17−/− mice less able to control LVS replication in the lung and spleen, but SCHU S4 infected IL-17−/− mice having similar numbers of bacteria as WT. Importantly, IL-17−/− mice orally vaccinated with LVS were equally protected from a subsequent SCHU S4 challenge, relative to WT mice. These data caution against the overinterpretation of studies based on LVS alone.
Studies (Roberts et al., 2014) have shown that a secondary infection in mice immunized with LVS or LVS clpB did not induce an IL-17 response that was different from control mice, suggesting that the IL-17 cytokine is not required for protection during secondary infection (i.e., immunological memory). These investigators followed up by treating immunized (LVS and LVS clpB) mice with anti-IL-17 antibodies, and then challenging the animals with LVS i.n. The mice were subsequently evaluated for microbial burden and weight loss and were found to not be significantly different from controls. Therefore, while it has been shown that IFN-γ continues to play a major role during a secondary (memory) response, IL-17 was not important. Additional studies have shown the ability of the immunoregulatory cytokine IL-10 to downregulate the activity of IL-17 during Ft infection of C57BL/6 and BALB/c mice, apparently preventing an over-accumulation of neutrophils and thus limiting the inflammatory response (Slight et al., 2013; Metzger et al., 2013). These studies, together with the findings of Skyberg et al. (2013) indicates that while IL-17 figures prominently in tularemia immune responses (though less so for SCHU S4), its role is limited in memory immune responses.
3.4.3 IL-6
IL-6 is a pleiotropic cytokine with key activities in regulating immunity and inflammation (Tanaka et al., 2014). Its production is known to vary during Ft infection as well as during immunization with LVS. For example, Krakauer and colleagues (Krakauer, 1995) found that in human volunteers immunized with LVS, circulating levels of IL-6 (along with TNF) were higher in humans with a positive antibody response to the vaccines compared with non-responders.
In more recent studies, Chiavolini et al. (2008) infected BALB/c mice with LVS and evaluated post-infection cytokine levels in serum, homogenized lung, and homogenized spleen tissues at various time points. Cytokines were measured from both moribund and surviving animals, and levels were compared. Macrophage inflammatory protein (MIP)-2, monocyte chemoattractant protein (MCP)-1, and IL-6 levels were elevated above baseline in lungs and spleens of moribund mice, while in surviving mice, levels were similar to uninfected controls. The splenic increases in MCP-1 and IL-6 levels were highly significant; an IL-6 increase in serum was noted, although this was not statistically significant. The authors cited the previous observation that IL-6 is a known marker of sepsis. Interestingly, in the same year, Ashtekar et al. (2008) demonstrated that purified Ft heat shock protein DnaK induced production of IL-6 and other proinflammatory cytokines in mouse-derived DCs. In studies using in vitro recall stimulation of PBLs from naive, immunized, or convalescent human donors with purified antigen from either LVS or SCHU S4, Eneslatt et al. (2012) demonstrated that a panel of cytokines including IL-6 was able to discriminate between naive and immune donors; moreover, IL-6 was able to discriminate between the immunized and convalescent donors. Using an IL-6 KO C57BL/6 mouse strain, Kurtz et al. (2013) demonstrated that IL-6 is essential to primary resistance to infection (either i.d. or i.n.) with LVS; this protection did not appear to be due to altered splenic immune cell populations during infection or decreased serum antibody production, as IL-6 KO mice had similar compositions of each compared to WT mice. However, studies by Laws et al. (2013) using the virulent SCHU S4 suggested that IL-6 does not have any effect on the progression of this disease. Clearly, continued effort examining IL-6 in the context of Ft infections is warranted.
3.5 Additional cell types
Thus far, we have focused on the major lymphocyte and antigen-presenting cell types. However, many other cell types have been investigated in response to Ft infection and/or vaccination. We discuss recent findings in these cells next.
3.5.1 Innate T cells
We have described the recent work in conventional alpha beta (αβ) T cell subsets. While conventional αβ T cells recognize a broad range of peptide antigens typically presented by MHC I and II complexes, enabled through their highly diverse TCR arrangement. Conversely, innate T cells are a heterogeneous group of αβ and γδ T cells that respond rapidly (<2 h) upon activation and which recognize foreign/self-lipid presented by non-classical MHC molecules such as CD1a, CD1d, and MHC class I-related protein (MR1). Innate T cells are activated during the early stages of bacterial infection and act as a bridge between the innate and adaptive immune systems (Gao and Williams, 2015; Harly et al., 2022). Three types of innate T cells, γδ T cells, MAIT cells, and Invariant Natural Killer T (iNKT) cells have been shown to have roles in tularemia. MAIT and iNKT cells are both restricted αβ T cells. Except for iNKT cells, the role of these various populations in tularemia and vaccines against Ft has been evaluated to varying degrees, as is discussed below.
3.5.2 γδ T cells
Although γδ T cells constitute only a few percent of the circulating lymphocyte population, they represent up to 20% of lymphocytes resident in the lungs (Cheng and Hu, 2017). In one of the earliest examinations of the role of γδ T cells in tularemia, Poquet et al. (1998) measured the ratio of blood circulating αβ and γδ T cells in tularemia-infected and uninfected humans. There was a dramatic increase in γδ T cells in infected patients. This increase was due primarily to the Vγ9Vδ2 phenotype, a γδ T cell subset with specificity for phosphoantigens, and found in primates but not rodents or most other mammals. This was mirrored by in vitro studies in which PBLs were co-cultured with Ft extract, with similar expansion of the Vγ9Vδ2 cells with extracts from either LVS or virulent strains of Ft. Interestingly, a similar expansion was not observed when PBL from individuals immunized with LVS were tested in vitro. These findings were expanded on by Kroca et al. (2000), who found that the expansion of Vγ9Vδ2 did not occur within the first week of infection; however, after the first week of infection the percentage of Vγ9Vδ2 increased dramatically and remained higher than respective controls for 18 months before returning to control levels by 24 months. Rowland et al. (2012) expanded on these findings by culturing human Vγ9Vδ2 T cells with SCHU S4-infected macrophage cells. They found that Vγ9Vδ2 T cells controlled SCHU S4 growth and increased culture levels of GM-CSF, IFN-γ, IL-1ꞵ, IL-6, IL-8, IP-10, MCP-1, MIP-1ɑ, and TNF.
Challenges limit the utility of γδ T cells, namely that the various subsets of γδ T cells that exist in mice and humans do not necessarily have overlapping identifying markers or functionality (Qu et al., 2022). The role of murine γδ T cells was further explored by Henry et al. (2010). In these studies, BALB/cJ mice deficient for type I IFN receptor (IFNAR1−/−) were infected i.n. with SCHU S4. IFNAR1−/− mice presented higher levels of IL-17A in the spleen, but levels were similar to the WT in other organs. This suggests that type I IFNs suppress IL-17A, potentially through changes in γδ T cell activity. However, additional studies are recommended to confirm this connection. Overall, there is promise in γδ T cell activity, however, their limited T cell repertoire makes it unclear how they contribute to Ft-specific immunity.
3.5.3 Invariant natural killer T cells
Mucosal surfaces, including the lungs, are populated by NKT cells, thus representing an important cellular milieu for interacting with Ft. Given their immunoregulatory activity, NKT cells have important roles to play in both immunity and pathology within the lungs (Paget and Trottein, 2013; Kumar and Delovitch, 2014). Perhaps because NK cells and NKT cells are so similar in their function, the few studies that reference NKT cells in the context of tularemia also include NK cells. Also, a major impediment to evaluating the potential role of iNKT cells is posed by the difficulty of experimentally differentiating the roles of NK and iNKT cells, given the high degree of similarity between them. Antibody-mediated depletion is impractical since there are currently no known iNKT-specific surface receptors; this leaves the use of genetically modified mice, in particular CD1d−/− mice. In the only study we identified with focus on NKT cells, Hill and colleagues (Hill et al., 2015) infected WT C57BL/6 and CD1d−/− mice with LVS and evaluated progression of disease. By 4 days post infection (dpi) both WT and CD1d−/− mice began exhibiting signs of disease, including weight loss and ruffled fur. By dpi 5–6, WT mice continued to lose more weight and showed more severe outward signs of disease as indicated by clinical score. While the WT mice continued to lose weight, by dpi 7 nearly all CD1d−/− mice began to recover and a significantly lower percentage of CD1d−/− mice succumbed to LVS infection relative to the WT mice. By dpi 14, fewer than 50% WT and almost all CD1d−/− mice regained weight, showed no signs of disease, and survived the infection. The authors concluded that NKT cells exacerbate pneumonic tularemia. To confirm this finding, the investigators infected Vα14tg mice, which have increased iNKT cell activity. These animals were indeed found to have increased susceptibility to infection with LVS. The authors further found that LVS infection preferentially recruits NKT cells to the lung interstitium. Investigations into the role of NKT cells in infection after vaccination may be of value for further elucidating the complex immune response to Ft.
3.5.4 MAIT cells
MAIT cells are a subset of innate-like T cells that express an evolutionarily conserved T cell receptor α chain that is restricted by the non-polymorphic MR1. They are activated by microbial riboflavin metabolite-derived antigens, and this distinguishes them from all other αβ T cells (Meierovics and Cowley, 2016; Legoux et al., 2020). In humans, the highest concentration of MAIT cells is found in the blood and liver, with approximately 70% of these cells exhibiting a CD8+ phenotype and 15% with a double-negative phenotype. However, MAIT cells are also enriched in the mucosal tissues, with between 40 and 80% having the double-negative phenotype (Nel et al., 2021).
MAIT cells have been extensively studied in tularemia. In a C57BL/6 murine model of pulmonary LVS infection, Meierovics et al. (2013) found that MAIT cells expanded significantly in the lungs during the acute infection phase and accumulated there after peak expansion in the late phase. These cells produced IFN-γ, TNF-α, and IL-17A throughout the infection and required MR1 and IL-12p40 signals from infected antigen-presenting cells to control LVS intracellular growth. In MR1−/− mice (lacking MAIT cells), pulmonary LVS infection showed defects in early mucosal cytokine production, timely recruitment of IFN-γ–producing CD4+ and CD8+ T cells to the lungs, and control of pulmonary LVS growth.
MAIT cells promote early differentiation of CCR2-dependent monocytes into monocyte-derived DCs (Mo-DCs) in the lungs after LVS pulmonary infection. Transferring Mo-DCs to MAIT cell-deficient (MR1−/−) mice corrected their defect in recruiting activated CD4+ T cells to the lungs. MAIT cell-dependent GM-CSF production stimulates monocyte differentiation in vitro, and GM-CSF production was delayed in MR1−/− mice. GM-CSF-deficient mice showed a similar defect in monocyte differentiation to MR1−/− mice. These findings demonstrate that MAIT cells drive early pulmonary GM-CSF production, promoting the differentiation of inflammatory monocytes into Mo-DCs (Meierovics and Cowley, 2016).
Jesteadt et al. (2018) found that LVS-infected macrophages produced little IL-18 and low MAIT cell IFN-γ. IL-18-deficient macrophages also failed to induce substantial IFN-γ in MAIT cells. However, in vivo, IL-18-deficient mice and WT C57BL/6 mice had similar IFN-γ levels in MAIT cells during LVS pulmonary infection. This suggests that while IL-18 is crucial for the MAIT cell IFN-γ response in vitro, other signals drive MAIT cell IFN-γ production in vivo, highlighting the complex cytokine interactions during Ft infections.
Also using C57BL/6 mice infected via the pulmonary route with LVS as a model, Yu et al. (2020) demonstrated that LVS infection resulted in a large number of MAIT cells in the lungs. These MAIT cells were predominantly chemokine receptor 6 (CXCR6)+, although CXCR6 was not required for MAIT cell accumulation in the lungs. CXCR6 was found to contribute to long-term retention of MAIT cells in the airway lumen following resolution of the infection. The authors also found MAIT cells were not recruited from secondary lymphoid organs and largely proliferated in situ in the lungs following infection. This work was built upon by Zhao et al. (2021), using LVS. The authors found that during systemic infection of C57BL/6 mice with LVS, MAIT cells expanded in the liver, lungs, kidney, spleen, and peripheral blood. The responding MAIT cells exhibited a polarized transcription factor and cytokine profile characteristic of a Th1-like MAIT-1 phenotype, which was critical in controlling bacterial numbers. Following resolution of the primary infection, the expanded MAIT cells formed stable memory-like MAIT-1 cell populations. This finding of a MAIT memory cell population suggests a possible correlate of protection following vaccination, although repeating the study with a virulent strain would strengthen this association.
3.5.5 Natural killer cells
NK cells are lymphoid cells that are phenotypically and functionally distinct from T cells and have crucial innate immune activity, operating to bridge innate and adaptive immune functions. They secrete several cytokines, including IFN-γ, TNF-α/β, CD95/FasL, and TNF-related apoptosis-inducing ligand (TRAIL) (Belizario et al., 2018). NK activity is initiated very soon after infection with Ft. Studies by Natrajan et al. (2019) evaluated peripheral blood mononuclear cells (PBMC) from humans vaccinated with either of two sources of LVS (USAMRIID-LVS or DVC-LVS) for a variety of activation signals, including assessment of NK-specific markers (CD56dimCD16-CD69high). These investigators found that NK cell activation increased at Day 1 after immunization, reached peak levels by Day 2, and decreased to pre-vaccination levels by Day 7 post-immunization. There were no differences found in activation between the two vaccine sources. Studies by Lopez et al. (2004) found a dramatic increase in the numbers of cells secreting IFN-γ observed 72 h after i.n. infection of BALB/c and C57BL/6 mice with sublethal (1,000 CFU) or lethal (10,000 CFU) doses of LVS and that the cells primarily responsible for this expression were CD11b+ DX5+ NK cells. The findings were further confirmed in C57BL/6 mice showing that cells responsible for IFN-γ secretion in the lungs were CD11b+ DX5+ NK1.1+. NK cell depletion studies revealed a decrease in the percentage not only of IFN-γ secreting cells, but also a reduced percentage of T cells secreting IFN-γ. The results indicate that NK cells are the early responders responsible for IFN-γ secretion following Ft infection. NK cells may be an important source of IFN-γ early in Ft infection.
There appears to be an important interaction between B1a cells and NK/NKT cells as described in studies utilizing WT CBA/J mice and CBA/CaHN-BtkXID/J (XID due to defective Bruton’s tyrosine kinase) mice. Mice were infected i.n. with SCHU S4 (Crane et al., 2013). XID mice exhibited an enhanced survival as compared to WT mice; furthermore, although the mean time to death was similar between the two strains, lung and spleen burden with SCHU S4 was less in XID mice as compared with WT controls. This difference was determined to be due to the reduced number of IL-10-producing B1a cells in the XID mice; IL-10 is known to downregulate the production of IL-12, which subsequently reduces the production of IFN-γ by NK and NKT cells. These investigators also found an increase in NK/NKT cells in the spleens and lungs of XID mice as compared with WT, and when the XID animals were treated with anti-Asialo GM1 antibodies (which eliminated NK/NKT cells by more than 98%), the animals were less able to control SCHU S4 infection (Crane et al., 2013).
Schmitt et al. (2013) also evaluated the role of NK cells in controlling Ft infection. In their studies, C57BL/6 J mice were NK-depleted using either anti-asialo GM1 or anti-NK1.1 antibodies, and NK cells were augmented by administration of a complex of IL-15 + IL-15Rα. The mice were infected with either LVS or SCHU S4 and cell populations in the lungs were subsequently evaluated over time. Although no significant differences in the frequency or absolute number of T cells or NK cells were observed in LVS-infected mice compared to controls, NK cells and T cells decreased by approximately 90% in the lungs of SCHU S4-infected mice. Also, in LVS-infected mice, IFN-γ was detected at three dpi in the lung and continued to increase until seven dpi. However, IFN-γ levels peaked three dpi with SCHU S4 and declined four dpi. Depletion of NK cells in SCHU S4-infection mice resulted in reduced IFN-γ and granzyme B levels in the lung, although it did not change bacterial burden. Conversely, administration of the IL-15 + IL-15Rα complex, which is anti-apoptotic, was demonstrated to significantly increase the frequency of NK cells and T cells in infected mice as well as to downregulated inflammatory cytokines including IL-6 and IFN-γ; however, this was not accompanied by any alteration in the progression of disease. The authors concluded from these results that NK cells do not appear to contribute to the control of acute type A Francisella infection.
In addition to their ability to secrete immunomodulatory cytokines, NK cells have cytolytic/cytotoxic granules containing perforin, granulysin, and granzymes A and B which they employ in microbial host defense. NK cells have long been studied for their ability to control cancer and viral infections, and it is only more recently that their ability to control bacterial - and especially intracellular bacterial - infection has been investigated. For example, NK cells have been shown to induce programmed cell death in cells hosting intracellular pathogens, using a variety of lytic molecules (Belizario et al., 2018), although there have been relatively few studies evaluating this function relative to Ft infection. In one study conducted to evaluate protection against Ft induced by LVS using different Francisella novicida-based vaccines, WT C57BL/6 and perforin-deficient (PRF−/−) mice were immunized with the vaccines and then challenged with LVS. Although there were no appreciable differences between the ability of WT and perforin-deficient mice to control a lethal infection with LVS, a differential level of protection was afforded by the two different vaccines. The authors followed up the challenge study with mechanistic evaluation and demonstrated the perforin and ADCC activity was responsible for this difference (Sanapala et al., 2012). Although this is an interesting observation, it is unclear whether perforin plays a major role in NK cell control of Ft. To date, there does not appear to be any significant ongoing research in this area, and it seems unlikely that this would be a promising line of investigation for further insights into Ft pathophysiology.
3.5.6 Polymorphonuclear cells (neutrophils)
Neutrophils are crucial for controlling primary Ft infections and resisting reinfection (Sjostedt et al., 1994). While lung macrophages are the principal targets upon respiratory exposure, both LVS and SCHU S4 can infect and replicate within neutrophils to a similar extent (Hall et al., 2008). Early in the infection, neutrophils are heavily recruited, but their bactericidal mechanisms are inhibited. Over time, immature myeloid cells and myeloid-derived suppressor cells dominate, eventually dying in the lungs and causing a necrotizing cascade that damages the lungs and leads to host death. This pattern is seen with both SCHU S4 and LVS, though LVS requires higher doses to replicate SCHU S4’s effects (Periasamy et al., 2016).
In addition to Ft’s ability to subvert inflammation and thus escape destruction by neutrophils, Ft contributes to overall pathology by prolonging survival of neutrophils. Kinkead et al. (2022) employed in vitro cultures of purified human neutrophils infected with LVS to monitor apoptosis in the presence of various inhibitors. The study showed that both ERK2 and p38α were activated in Ft-infected neutrophils, but only p38α MAPK was required for delayed apoptosis, revealing another mechanism whereby Ft enhances its survival in the host while producing significant pathology. Another mechanism of neutrophil pathology was demonstrated by Pulavendran et al. (2020), who showed that BALB/c mice infected with LVS exhibited a large number of neutrophil extracellular traps (NETs), which harbored large numbers of Ft, although without producing any bactericidal activity. While there were changes in ERK2 and p38α in Ft-infected neutrophils, we founded no evidence that this is linked with a memory or recall immune response.
3.5.7 Mast cells
Mast cells have historically been associated with type 1 hypersensitivity; however, their ability to produce a variety of soluble bioactive molecules (histamine, leukotrienes, prostaglandins and a variety of cytokines such as TNF-α and IL-4), their ability to phagocytose microorganisms, their bactericidal capability, and their geographic location in the lungs suggests a potential role in controlling bacterial infections including Ft. To evaluate whether mast cells are important in Ft infections, Ketavarapu et al. (2008) infected C57BL/6 and BALB/c mice i.n. with LVS and subsequently evaluated the lungs and cervical (draining) lymph nodes for inflammatory cells (macrophages, neutrophils, and mast cells) by flow cytometry. The investigators found that mast cells accumulated within the lungs earlier than other inflammatory cell populations. Employing in vitro culture systems with bone marrow-derived cells infected with LVS revealed only limited replication of LVS within mast cells as compared with a robust replication within macrophages. However, when the bone marrow-derived cells were co-cultured and infected with LVS, there was a significant reduction in LVS replication within macrophages. Follow-up in vitro studies demonstrated that IL-4 production by mast cells was a contact-independent mechanism controlling LVS replication in macrophages. Rodriguez et al. (2011) showed this activity to be mediated by increased ATP production and phagosomal acidification. Subsequent studies by this group using in vitro culture of mast cells with either LVS or SCHU S4 demonstrated that mast cells require TLR2 for effective bacterial killing, regulation of the hydrolytic enzyme cathepsin L, and for coordination and trafficking of MHC-II and lysosomal associated membrane protein 2 (LAMP2) (Rodriguez et al., 2012). Hunter et al. (2012) have shown that mucosal mast cells are phenotypically like bone marrow-derived mast cells (as assessed by expression of FcεRI, c-Kit, and MHC-I) and can limit intramacrophage Ft replication via elucidation of IL-4. This is important in that it demonstrates a tissue-relevant function for these cells.
3.5.8 Innate lymphoid cells
Innate lymphoid cells (ILCs) are tissue-resident cells, divided into three groups, ILC1, ILC2, and ILC3. ILCs are defined by high expression of CD90.2 and CD127. Following i.n. infection of C57BL/6 mice with LVS, there was a decrease in ILC2 and bacterial burdens. Enhancing ILC2 numbers with IL-33 resulted in more LVS in lung, liver, and spleen. IFN-γ contributed to the reduction of ILC2s and the ILC2 enhancement of bacterial burden could be reduced by blockade of IL-5 (Dow et al., 2023). A more comprehensive understanding of these ILCs and how they interact during tularemia is needed.
4 Discussion
Historically, research on immunity to Ft has centered on B cell antibody production, T cell functions (particularly relating to cytokine roles), and the contributions of macrophages and antigen-presenting cells in processing antigens and coordinating cellular and humoral responses (Rhinehart-Jones et al., 1994; Koskela and Herva, 1982; Cowley and Elkins, 2011). Whereas these basic components have been studied extensively by many investigators, the full host immune system interaction with Ft is far from established (Bosio, 2011; Jones et al., 2011; Bahuaud et al., 2021). In the present review, we have endeavored to summarize more recently (approximately the past 10 years) studied mechanisms including Ft’s ability to modulate host immunity, the role of the inflammasome in successful (and unsuccessful) immune responses, the panoply of cytokines that modulate the immune and inflammatory responses, and a variety of both innate and adaptive cellular effectors including γδ T cells, NKT cells, MAIT cells, neutrophils and mast cells. Despite this wealth of added information, an overarching understanding of this complex interactions between host and pathogen remains elusive, for numerous reasons summarized below.
4.1 Ft is inherently immunomodulatory
An underappreciated feature of Ft infection is the organism’s ability to deploy a variety of molecular mechanisms, enabling it to evade and survive the host’s immune response. One surprising mechanism is the post-transcriptional regulation of SHIP-1 via miR-155 while Ft simultaneously suppresses MyD88 activity, selectively disinhibiting one of the two pathways downstream of TLR2 (Bandyopadhyay et al., 2014; Cremer et al., 2011). This selective activation and suppression of specific immunological pathways, even for the same receptor, highlight Ft’s sophisticated immune evasion strategies. Inflammasome pathways interact extensively with TLR2 and TLR4, which are intricately linked with Ft pathophysiology. The pathways for these receptors produce IFN-α and -β, activating mechanisms for programmed cell death by necrosis, apoptosis, and pyroptosis. Ft can prevent the activation of pyroptosis receptors AIM2 and NLRP3, which interact with caspase-1 and cytokines like IL-18 to trigger cell death. Redundant regulation mechanisms of these pathways by negative feedback loops and external factors such as c-Abl and IFN-β also suggest that further research into these pathways may be beneficial, given that inflammasome signaling can trigger multiple cell death pathways at once. Ft does not trigger responses from these receptors, causing deficiencies, particularly in macrophage activation and subsequent signaling that trigger the proper response to a pathogen (Juruj et al., 2013; Corrales et al., 2016; McCracken et al., 2016). Finally, Ft also suppresses ROS, which are crucial for destroying intracellular pathogens. Ft can evade ROS destruction through mechanisms like SodB and OxyR activity and by preventing nitrite production in host cells. These strategies neutralize or inhibit host ROS production, creating a favorable environment for Ft’s survival and replication (Ma et al., 2016; Honn et al., 2017; Rabadi et al., 2016; Gillette et al., 2014). Although many of the studies of Ft’s immunomodulatory activities are descriptive, we believe that a mechanistic understanding of Ft’s ability to down-modulate immunity is important to consider as a component of MCM development.
4.2 Animal model differences clearly lead to dissimilar/conflicting results
While mammalian immune systems share many essential features, it is axiomatic that humans and rodents are dissimilar in many ways. These fundamental differences between human and murine immune responses are a key factor that undermines conclusions based solely on studies of murine models (Nauseef, 2001; Zschaler et al., 2014). This impacts responses to Ft and the ability to translate all mouse data to the human clinical outcomes.
The choice of the most appropriate animal models is crucial when developing MCM against Ft that require use of the Animal Rule (Roberts et al., 2018). Our review of the literature revealed that an overwhelming majority of studies have used mice as the animal model (Figure 1); this is unsurprising since mice are a traditional model for immunological studies, their immune systems are well characterized, and they are readily available and affordable for performing in vivo studies. However, there are numerous and often significant differences between the immune response of these species (Mestas and Hughes, 2004), making a direct comparison imprecise at best. Even within mice, there are strain differences. The studies reviewed here showed that BALB/c mice and C57BL/6 mice to be the most common strains used, although it is unclear in many cases why one strain was selected over another. The known BALB/c and C57BL/6 strain differences predispose to certain cytokine profiles, for example, macrophages from C57BL/6 mice produce higher levels of TNF and IL-12 compared to macrophages from BALB/c mice (Watanabe et al., 2004) in an in vitro Ft exposure culture.
Despite the concerns associated with using primates, greater emphasis should be placed on using this model. Cynomolgus macaques appear to more closely approximate human immune responses to Ft than rodents (Glynn et al., 2015; Guina et al., 2018; Frick et al., 2021). In addition, considerable progress has been made with experimental systems including transgenic animals (Oh et al., 2018), cell co-culture systems (De Pascalis et al., 2018; David et al., 2014; Eneslatt et al., 2018; Koppen et al., 2023) and ex vivo organ culture (Rennert et al., 2016). These systems will require further refinement but show promise in understanding Ft’s immune response and may lead to assays for immune correlates of protection and provide valuable bridges between human and nonhuman data. A combination of nonhuman primate data and ex vivo human data, supplemented by well-controlled rodent data, would be an appropriate pathway for Animal Rule compliance.
4.3 Inconsistency in infecting/challenge Ft strain may be problematic
A preponderance of the literature reveals the use of the LVS strain as a challenge model, although for development of MCM this is not an appropriate choice. Although LVS and SCHU S4 exhibit up to 99.8% homology in their genomes (Kudryavtseva TaM, 2022), the fact remains that these strains often produce differing results in both in vitro and in vivo models. Notwithstanding technical challenges associated with high-risk infectious agents, to better support MCM development, more emphasis should be placed on strategies to evaluate the immune response to virulent strains of Ft such as SCHU S4.
4.4 Immunization and challenge routes are important
Inconsistency in route of vaccination and challenge routes may be problematic since disease type and outcome vary with exposure route (Degabriel et al., 2023). As reviewed by Nicol et al. (Nicol et al., 2021), data generated in rodents, rabbits, NHP, and humans reveal differences in immune response following i.n. and i.d. infection. For MCM development, it will be important to standardize both immunization and challenge routes, preferably using aerosol exposure.
4.5 Immunity to Ft depends on far more than T and B cells
Although humans and other animals are known to mount a humoral immune response following exposure to Ft, the historical data remain inconclusive as to the protective value of this response. Likewise with cellular immunity, multiple investigators have developed extensive cytokine panels to understand the role of these mediators (Lindgren et al., 2023), correlations remain elusive, especially between humans and other species. As demonstrated by the present review, effective defense against Ft is likely to require multiple innate and adaptive responses working in concert. For MCM development, what is needed are approaches that incorporate multiple effectors.
Ft’s ability to evade cellular and molecular defenses, combined with its potential for weaponization, underscores the need for additional interventions. Although current antibiotics are effective, the risk of Ft developing antibiotic resistance, either naturally or through genetic engineering, highlights the need for prophylactic solutions as a component of a layered defense strategy. Despite decades of effort, no tularemia prophylactic, vaccine or otherwise has been approved by the FDA. Based on the Animal Rule guidance on data linking the effective dose to human patients and animal models, we recognize immune correlates of protective immunity should be examined across human and animal species. According to Plotkin (Plotkin, 2020; Plotkin, 2022), a correlate of protection is “an immune function that correlates with and may be biologically responsible for vaccine-induced efficacy.” What is needed are concerted efforts to specifically evaluate the multitude of immune effectors that have been identified as contributing to immunity to Ft so that correlates of protection can be incorporated into effective MCM for tularemia.
Author contributions
DB: Data curation, Writing – original draft. RH: Conceptualization, Data curation, Writing – original draft. HCG: Conceptualization, Data curation, Funding acquisition, Writing – review & editing.
Funding
The author(s) declare that financial support was received for the research and/or publication of this article. The development of this manuscript was funded by the United States Air Force Academy Cooperative Agreement FA7000-23-2-00007.
Acknowledgments
All figures were made using BioRender.com. Microsoft Copilot, based on Chat GPT version 4 architecture, developed by OpenAI, and integrated by Microsoft, was used to make several sections of this work more concise to meet maximum word count requirements, and all authors have approved its use for this purpose.
Conflict of interest
RH was employed by Dr. RV House LLC, and was retained as a consultant by Appili Therapeutics.
The remaining authors declare that the research was conducted in the absence of any commercial or financial relationships that could be construed as a potential conflict of interest.
Generative AI statement
The author(s) declare that Gen AI was used in the creation of this manuscript. Microsoft Copilot was used to edit the length of some sections of the manuscript and was confirmed to be accurate by the authors.
Publisher’s note
All claims expressed in this article are solely those of the authors and do not necessarily represent those of their affiliated organizations, or those of the publisher, the editors and the reviewers. Any product that may be evaluated in this article, or claim that may be made by its manufacturer, is not guaranteed or endorsed by the publisher.
References
Centers for Disease Control and Prevention. Tularemia Data and Statistics. (2022). Available online at: https://www.cdc.gov/tularemia/data-research/index.html.
Alqahtani, M., Ma, Z., Fantone, K., Malik, M., and Bakshi, C. S. (2021). Aim2 and Nlrp3 are dispensable for vaccine-induced immunity against Francisella tularensis live vaccine strain. Infect. Immun. 89:e0013421. doi: 10.1128/IAI.00134-21
Amemiya, K., Dankmeyer, J. L., Bernhards, R. C., Fetterer, D. P., Waag, D. M., Worsham, P. L., et al. (2021). Activation of toll-like receptors by live gram-negative bacterial pathogens reveals mitigation of TLR4 responses and activation of TLR5 by flagella. Front. Cell. Infect. Microbiol. 11:745325. doi: 10.3389/fcimb.2021.745325
Ashtekar, A. R., Zhang, P., Katz, J., Deivanayagam, C. C., Rallabhandi, P., Vogel, S. N., et al. (2008). TLR4-mediated activation of dendritic cells by the heat shock protein DnaK from Francisella tularensis. J. Leukoc. Biol. 84, 1434–1446. doi: 10.1189/jlb.0308215
Babadjanova, Z., Wiedinger, K., Gosselin, E. J., and Bitsaktsis, C. (2015). Targeting of a fixed bacterial immunogen to FC receptors reverses the anti-inflammatory properties of the gram-negative bacterium, Francisella tularensis, during the early stages of infection. PLoS One 10:e0129981. doi: 10.1371/journal.pone.0129981
Bahuaud, O., Le Brun, C., and Lemaignen, A. (2021). Host immunity and Francisella tularensis: a review of tularemia in immunocompromised patients. Microorganisms 9. doi: 10.3390/microorganisms9122539
Bandyopadhyay, S., Long, M. E., and Allen, L. A. (2014). Differential expression of microRNAs in Francisella tularensis-infected human macrophages: miR-155-dependent downregulation of MyD88 inhibits the inflammatory response. PLoS One 9:e109525. doi: 10.1371/journal.pone.0109525
Barbosa, C. H. D., Lantier, L., Reynolds, J., Wang, J., and Re, F. (2021). Critical role of IL-25-ILC2-IL-5 axis in the production of anti-Francisella LPS IgM by B1 B cells. PLoS Pathog. 17:e1009905. doi: 10.1371/journal.ppat.1009905
Baron, S. D., Singh, R., and Metzger, D. W. (2007). Inactivated Francisella tularensis live vaccine strain protects against respiratory tularemia by intranasal vaccination in an immunoglobulin A-dependent fashion. Infect. Immun. 75, 2152–2162. doi: 10.1128/IAI.01606-06
Bauler, T. J., Chase, J. C., and Bosio, C. M. (2011). IFN-beta mediates suppression of IL-12p40 in human dendritic cells following infection with virulent Francisella tularensis. J. Immunol. 187, 1845–1855. doi: 10.4049/jimmunol.1100377
Belizario, J. E., Neyra, J. M., and Rodrigues, M. F. S. (2018). When and how NK cell-induced programmed cell death benefits immunological protection against intracellular pathogen infection. Innate Immun. 24, 452–465. doi: 10.1177/1753425918800200
Bosio, C. M. (2011). The subversion of the immune system by francisella tularensis. Front. Microbiol. 2:9. doi: 10.3389/fmicb.2011.00009
Cao, C., Leng, Y., Huang, W., Liu, X., and Kufe, D. (2003). Glutathione peroxidase 1 is regulated by the c-Abl and Arg tyrosine kinases. J. Biol. Chem. 278, 39609–39614. doi: 10.1074/jbc.M305770200
Chandler, J. C., Sutherland, M. D., Harton, M. R., Molins, C. R., Anderson, R. V., Heaslip, D. G., et al. (2015). Francisella tularensis LVS surface and membrane proteins as targets of effective post-exposure immunization for tularemia. J. Proteome Res. 14, 664–675. doi: 10.1021/pr500628k
Cheng, M., and Hu, S. (2017). Lung-resident gammadelta T cells and their roles in lung diseases. Immunology 151, 375–384. doi: 10.1111/imm.12764
Cheong, M., Gartlan, K. H., Lee, J. S., Tey, S. K., Zhang, P., Kuns, R. D., et al. (2020). ASC modulates CTL cytotoxicity and transplant outcome independent of the Inflammasome. Cancer Immunol. Res. 8, 1085–1098. doi: 10.1158/2326-6066.CIR-19-0653
Chiavolini, D., Alroy, J., King, C. A., Jorth, P., Weir, S., Madico, G., et al. (2008). Identification of immunologic and pathologic parameters of death versus survival in respiratory tularemia. Infect. Immun. 76, 486–496. doi: 10.1128/IAI.00862-07
Cole, L. E., Mann, B. J., Shirey, K. A., Richard, K., Yang, Y., Gearhart, P. J., et al. (2011). Role of TLR signaling in Francisella tularensis-LPS-induced, antibody-mediated protection against Francisella tularensis challenge. J. Leukoc. Biol. 90, 787–797. doi: 10.1189/jlb.0111014
Cole, L. E., Yang, Y., Elkins, K. L., Fernandez, E. T., Qureshi, N., Shlomchik, M. J., et al. (2009). Antigen-specific B-1a antibodies induced by Francisella tularensis LPS provide long-term protection against F. tularensis LVS challenge. Proc. Natl. Acad. Sci. U. S. A. 106, 4343–4348. doi: 10.1073/pnas.0813411106
Conde, C., Gloire, G., and Piette, J. (2011). Enzymatic and non-enzymatic activities of SHIP-1 in signal transduction and cancer. Biochem. Pharmacol. 82, 1320–1334. doi: 10.1016/j.bcp.2011.05.031
Corrales, L., Woo, S. R., Williams, J. B., McWhirter, S. M., Dubensky, T. W., and Gajewski, T. F. (2016). Antagonism of the STING pathway via activation of the AIM2 Inflammasome by intracellular DNA. J. Immunol. 196, 3191–3198. doi: 10.4049/jimmunol.1502538
Costa Franco, M. M. S., Marim, F. M., Alves-Silva, J., Cerqueira, D., Rungue, M., Tavares, I. P., et al. (2019). AIM2 senses Brucella abortus DNA in dendritic cells to induce IL-1beta secretion, pyroptosis and resistance to bacterial infection in mice. Microbes Infect. 21, 85–93. doi: 10.1016/j.micinf.2018.09.001
Cowley, S. C., and Elkins, K. L. (2011). Immunity to Francisella. Front. Microbiol. 2:26. doi: 10.3389/fmicb.2011.00026
Crane, D. D., Griffin, A. J., Wehrly, T. D., and Bosio, C. M. (2013). B1a cells enhance susceptibility to infection with virulent Francisella tularensis via modulation of NK/NKT cell responses. J. Immunol. 190, 2756–2766. doi: 10.4049/jimmunol.1202697
Cremer, T. J., Butchar, J. P., and Tridandapani, S. (2011). Francisella subverts innate immune signaling: focus on PI3K/Akt. Front. Microbiol. 5:13. doi: 10.3389/fmicb.2011.00013
Cummings, J. E., Kingry, L. C., Rholl, D. A., Schweizer, H. P., Tonge, P. J., and Slayden, R. A. (2014). The Burkholderia pseudomallei enoyl-acyl carrier protein reductase FabI1 is essential for in vivo growth and is the target of a novel chemotherapeutic with efficacy. Antimicrob. Agents Chemother. 58, 931–935. doi: 10.1128/AAC.00176-13
Cunningham, A. L., Mann, B. J., Qin, A., Santiago, A. E., Grassel, C., Lipsky, M., et al. (2020). Characterization of Schu S4 aro mutants as live attenuated tularemia vaccine candidates. Virulence 11, 283–294. doi: 10.1080/21505594.2020.1746557
Dai, S., Rajaram, M. V., Curry, H. M., Leander, R., and Schlesinger, L. S. (2016). Correction: fine tuning inflammation at the front door: macrophage complement receptor 3-mediates phagocytosis and immune suppression for Francisella tularensis. PLoS Pathog. 12:e1005504. doi: 10.1371/journal.ppat.1005504
David, J., Sayer, N. M., and Sarkar-Tyson, M. (2014). The use of a three-dimensional cell culture model to investigate host-pathogen interactions of Francisella tularensis in human lung epithelial cells. Microbes Infect. 16, 735–745. doi: 10.1016/j.micinf.2014.04.001
De Pascalis, R., Chou, A. Y., Bosio, C. M., Huang, C. Y., Follmann, D. A., and Elkins, K. L. (2012). Development of functional and molecular correlates of vaccine-induced protection for a model intracellular pathogen, F. tularensis LVS. PLoS Pathog. 8:e1002494. doi: 10.1371/journal.ppat.1002494
De Pascalis, R., Frey, B., Rice, H. M., Bhargava, V., Wu, T. H., Peterson, R. L., et al. (2022). Working correlates of protection predict SchuS4-derived-vaccine candidates with improved efficacy against an intracellular bacterium, Francisella tularensis. NPJ Vaccines. 7:95. doi: 10.1038/s41541-022-00506-9
De Pascalis, R., Hahn, A., Brook, H. M., Ryden, P., Donart, N., Mittereder, L., et al. (2018). A panel of correlates predicts vaccine-induced protection of rats against respiratory challenge with virulent Francisella tularensis. PLoS One 13:e0198140. doi: 10.1371/journal.pone.0198140
De Pascalis, R., Rossi, A. P., Mittereder, L., Takeda, K., Akue, A., Kurtz, S. L., et al. (2020). Production of IFN-gamma by splenic dendritic cells during innate immune responses against Francisella tularensis LVS depends on MyD88, but not TLR2, TLR4, or TLR9. PLoS One 15:e0237034. doi: 10.1371/journal.pone.0237034
Degabriel, M., Valeva, S., Boisset, S., and Henry, T. (2023). Pathogenicity and virulence of Francisella tularensis. Virulence 14:2274638. doi: 10.1080/21505594.2023.2274638
Del Barrio, L., Sahoo, M., Lantier, L., Reynolds, J. M., Ceballos-Olvera, I., and Re, F. (2015). Production of anti-LPS IgM by B1a B cells depends on IL-1beta and is protective against lung infection with Francisella tularensis LVS. PLoS Pathog. 11:e1004706. doi: 10.1371/journal.ppat.1004706
D’Elia, R. V., Harrison, K., Oyston, P. C., Lukaszewski, R. A., and Clark, G. C. (2013). Targeting the "cytokine storm" for therapeutic benefit. Clin. Vaccine Immunol. 20, 319–327. doi: 10.1128/CVI.00636-12
Dow, J., Cytlak, U. M., Casulli, J., McEntee, C. P., Smedley, C., Hodge, S. H., et al. (2023). Group 2 innate lymphoid cells are detrimental to the control of infection with Francisella tularensis. J. Immunol. 210, 618–627. doi: 10.4049/jimmunol.2100651
Dreisbach, V. C., Cowley, S., and Elkins, K. L. (2000). Purified lipopolysaccharide from Francisella tularensis live vaccine strain (LVS) induces protective immunity against LVS infection that requires B cells and gamma interferon. Infect. Immun. 68, 1988–1996. doi: 10.1128/IAI.68.4.1988-1996.2000
Duckett, N. S., Olmos, S., Durrant, D. M., and Metzger, D. W. (2005). Intranasal interleukin-12 treatment for protection against respiratory infection with the Francisella tularensis live vaccine strain. Infect. Immun. 73, 2306–2311. doi: 10.1128/IAI.73.4.2306-2311.2005
Elkins, K. L., Cooper, A., Colombini, S. M., Cowley, S. C., and Kieffer, T. L. (2002). In vivo clearance of an intracellular bacterium, Francisella tularensis LVS, is dependent on the p40 subunit of interleukin-12 (IL-12) but not on IL-12 p70. Infect. Immun. 70, 1936–1948. doi: 10.1128/IAI.70.4.1936-1948.2002
Elkins, K. L., Rhinehart-Jones, T. R., Culkin, S. J., Yee, D., and Winegar, R. K. (1996). Minimal requirements for murine resistance to infection with Francisella tularensis LVS. Infect. Immun. 64, 3288–3293
Elkins, K. L., Rhinehart-Jones, T., Nacy, C. A., Winegar, R. K., and Fortier, A. H. (1993). T-cell-independent resistance to infection and generation of immunity to Francisella tularensis. Infect. Immun. 61, 823–829. doi: 10.1128/iai.61.3.823-829.1993
Eneslatt, K., Golovliov, I., Ryden, P., and Sjostedt, A. (2018). Vaccine-mediated mechanisms controlling replication of Francisella tularensis in human peripheral blood mononuclear cells using a co-culture system. Front. Cell. Infect. Microbiol. 8:27. doi: 10.3389/fcimb.2018.00027
Eneslatt, K., Normark, M., Bjork, R., Rietz, C., Zingmark, C., Wolfraim, L. A., et al. (2012). Signatures of T cells as correlates of immunity to Francisella tularensis. PLoS One 7:e32367. doi: 10.1371/journal.pone.0032367
Frick, O. M., Livingston, V. A., Whitehouse, C. A., Norris, S. L., Alves, D. A., Facemire, P. R., et al. (2021). The natural history of aerosolized Francisella tularensis infection in Cynomolgus macaques. Pathogens 10. doi: 10.3390/pathogens10050597
Furuya, Y., Steiner, D., and Metzger, D. W. (2014). Does type I interferon limit protective neutrophil responses during pulmonary Francisella Tularensis infection? Front. Immunol. 5:355. doi: 10.3389/fimmu.2014.00355
Gao, Y., and Williams, A. P. (2015). Role of innate T cells in anti-bacterial immunity. Front. Immunol. 6:302. doi: 10.3389/fimmu.2015.00302
Gillette, D. D., Curry, H. M., Cremer, T., Ravneberg, D., Fatehchand, K., Shah, P. A., et al. (2014). Virulent type a Francisella tularensis actively suppresses cytokine responses in human monocytes. Front. Cell. Infect. Microbiol. 4:45. doi: 10.3389/fcimb.2014.00045
Glynn, A. R., Alves, D. A., Frick, O., Erwin-Cohen, R., Porter, A., Norris, S., et al. (2015). Comparison of experimental respiratory tularemia in three nonhuman primate species. Comp. Immunol. Microbiol. Infect. Dis. 39, 13–24. doi: 10.1016/j.cimid.2015.01.003
Goll, J. B., Li, S., Edwards, J. L., Bosinger, S. E., Jensen, T. L., Wang, Y., et al. (2020). Transcriptomic and metabolic responses to a live-attenuated Francisella tularensis vaccine. Vaccines (Basel) 8. doi: 10.3390/vaccines8030412
Golovliov, I., Lindgren, H., Eneslatt, K., Conlan, W., Mosnier, A., Henry, T., et al. (2016). An in vitro co-culture mouse model demonstrates efficient vaccine-mediated control of Francisella tularensis SCHU S4 and identifies nitric oxide as a predictor of efficacy. Front. Cell. Infect. Microbiol. 6:152. doi: 10.3389/fcimb.2016.00152
Griffin, K. F., Oyston, P. C., and Titball, R. W. (2007). Francisella tularensis vaccines. FEMS Immunol. Med. Microbiol. 49, 315–323. doi: 10.1111/j.1574-695X.2007.00219.x
Guina, T., Lanning, L. L., Omland, K. S., Williams, M. S., Wolfraim, L. A., Heyse, S. P., et al. (2018). The Cynomolgus macaque natural history model of pneumonic tularemia for predicting clinical efficacy under the animal rule. Front. Cell. Infect. Microbiol. 8:99. doi: 10.3389/fcimb.2018.00099
Gunn, J. S., and Ernst, R. K. (2007). The structure and function of Francisella lipopolysaccharide. Ann. N. Y. Acad. Sci. 1105, 202–218. doi: 10.1196/annals.1409.006
Hall, J. D., Woolard, M. D., Gunn, B. M., Craven, R. R., Taft-Benz, S., Frelinger, J. A., et al. (2008). Infected-host-cell repertoire and cellular response in the lung following inhalation of Francisella tularensis Schu S4, LVS, or U112. Infect. Immun. 76, 5843–5852. doi: 10.1128/IAI.01176-08
Harly, C., Robert, J., Legoux, F., and Lantz, O. (2022). Gammadelta T, NKT, and MAIT cells during evolution: redundancy or specialized functions? J. Immunol. 209, 217–225. doi: 10.4049/jimmunol.2200105
Harrell, J. E., Roy, C. J., Gunn, J. S., and McLachlan, J. B. (2024). Current vaccine strategies and novel approaches to combatting Francisella infection. Vaccine 42, 2171–2180. doi: 10.1016/j.vaccine.2024.02.086
Henry, T., Kirimanjeswara, G. S., Ruby, T., Jones, J. W., Peng, K., Perret, M., et al. (2010). Type I IFN signaling constrains IL-17A/F secretion by gammadelta T cells during bacterial infections. J. Immunol. 184, 3755–3767. doi: 10.4049/jimmunol.0902065
Hill, T. M., Gilchuk, P., Cicek, B. B., Osina, M. A., Boyd, K. L., Durrant, D. M., et al. (2015). Border patrol gone awry: lung NKT cell activation by Francisella tularensis exacerbates tularemia-like disease. PLoS Pathog. 11:e1004975. doi: 10.1371/journal.ppat.1004975
Hoang, K. V., Rajaram, M. V. S., Curry, H. M., Gavrilin, M. A., Wewers, M. D., and Schlesinger, L. S. (2018). Complement receptor 3-mediated inhibition of Inflammasome priming by Ras GTPase-activating protein during Francisella tularensis phagocytosis by human mononuclear phagocytes. Front. Immunol. 9:561. doi: 10.3389/fimmu.2018.00561
Hong, K. J., Park, P. G., Seo, S. H., Rhie, G. E., and Hwang, K. J. (2013). Current status of vaccine development for tularemia preparedness. Clin. Exp. Vaccine Res. 2, 34–39. doi: 10.7774/cevr.2013.2.1.34
Honn, M., Lindgren, H., Bharath, G. K., and Sjostedt, A. (2017). Lack of OxyR and KatG results in extreme susceptibility of Francisella tularensis LVS to oxidative stress and marked attenuation in vivo. Front. Cell. Infect. Microbiol. 7:14. doi: 10.3389/fcimb.2017.00014
Hughes, A. J., Knoten, C. A., Morris, A. R., and Hauser, A. R. (2018). ASC acts in a caspase-1-independent manner to worsen acute pneumonia caused by Pseudomonas aeruginosa. J. Med. Microbiol. 67, 1168–1180. doi: 10.1099/jmm.0.000782
Hunter, C., Rodriguez, A., Yu, J. J., Chambers, J., Guentzel, M. N., and Arulanandam, B. (2012). Comparison of bone marrow-derived and mucosal mast cells in controlling intramacrophage Francisella tularensis replication. Exp. Biol. Med. (Maywood) 237, 617–621. doi: 10.1258/ebm.2012.011389
Ireland, R., Wang, R., Alinger, J. B., Small, P., and Bosio, C. M. (2013). Francisella tularensis SchuS4 and SchuS4 lipids inhibit IL-12p40 in primary human dendritic cells by inhibition of IRF1 and IRF8. J. Immunol. 191, 1276–1286. doi: 10.4049/jimmunol.1300867
Janik, E., Ceremuga, M., Niemcewicz, M., and Bijak, M. (2020). Dangerous pathogens as a potential problem for public health. Medicina (Kaunas) 56:591. doi: 10.3390/medicina56110591
Jesteadt, E., Zhang, I., Yu, H., Meierovics, A., Chua Yankelevich, W. J., and Cowley, S. (2018). Interleukin-18 is critical for mucosa-associated invariant T cell gamma interferon responses to Francisella species in vitro but not in vivo. Infect. Immun. 86:e00117. doi: 10.1128/IAI.00117-18
Jones, J. W., Broz, P., and Monack, D. M. (2011). Innate immune recognition of francisella tularensis: activation of type-I interferons and the inflammasome. Front. Microbiol. 2:16. doi: 10.3389/fmicb.2011.00016
Juruj, C., Lelogeais, V., Pierini, R., Perret, M., Py, B. F., Jamilloux, Y., et al. (2013). Caspase-1 activity affects AIM2 speck formation/stability through a negative feedback loop. Front. Cell. Infect. Microbiol. 3:14. doi: 10.3389/fcimb.2013.00014
Kak, G., Raza, M., and Tiwari, B. K. (2018). Interferon-gamma (IFN-gamma): exploring its implications in infectious diseases. Biomol. Concepts 9, 64–79. doi: 10.1515/bmc-2018-0007
Karttunen, R., Andersson, G., Ekre, H. P., Juutinen, K., Surcel, H. M., Syrjala, H., et al. (1987). Interleukin 2 and gamma interferon production, interleukin 2 receptor expression, and DNA synthesis induced by tularemia antigen in vitro after natural infection or vaccination. J. Clin. Microbiol. 25, 1074–1078. doi: 10.1128/jcm.25.6.1074-1078.1987
Ketavarapu, J. M., Rodriguez, A. R., Yu, J. J., Cong, Y., Murthy, A. K., Forsthuber, T. G., et al. (2008). Mast cells inhibit intramacrophage Francisella tularensis replication via contact and secreted products including IL-4. Proc. Natl. Acad. Sci. U. S. A. 105, 9313–9318. doi: 10.1073/pnas.0707636105
Kinkead, L. C., Krysa, S. J., and Allen, L. H. (2022). Neutrophil survival signaling during Francisella tularensis infection. Front. Cell. Infect. Microbiol. 12:889290. doi: 10.3389/fcimb.2022.889290
Kirimanjeswara, G. S., Olmos, S., Bakshi, C. S., and Metzger, D. W. (2008). Humoral and cell-mediated immunity to the intracellular pathogen Francisella tularensis. Immunol. Rev. 225, 244–255. doi: 10.1111/j.1600-065X.2008.00689.x
Kobayashi, S. D., Voyich, J. M., Braughton, K. R., and DeLeo, F. R. (2003). Down-regulation of proinflammatory capacity during apoptosis in human polymorphonuclear leukocytes. J. Immunol. 170, 3357–3368. doi: 10.4049/jimmunol.170.6.3357
Koppen, K., Fatykhova, D., Holland, G., Rauch, J., Tappe, D., Graff, M., et al. (2023). Ex vivo infection model for Francisella using human lung tissue. Front. Cell. Infect. Microbiol. 13:1224356. doi: 10.3389/fcimb.2023.1224356
Koskela, P. (1985). Humoral immunity induced by a live Francisella tularensis vaccine. Complement fixing antibodies determined by an enzyme-linked immunosorbent assay (CF-ELISA). Vaccine 3, 389–391. doi: 10.1016/0264-410X(85)90129-X
Koskela, P., and Herva, E. (1982). Cell-mediated and humoral immunity induced by a live Francisella tularensis vaccine. Infect. Immun. 36, 983–989. doi: 10.1128/iai.36.3.983-989.1982
Krakauer, T. (1995). Levels of interleukin 6 and tumor necrosis factor in serum from humans vaccinated with live, attenuated Francisella tularensis. Clin. Diagn. Lab. Immunol. 2, 487–488. doi: 10.1128/cdli.2.4.487-488.1995
Kroca, M., Tarnvik, A., and Sjostedt, A. (2000). The proportion of circulating gammadelta T cells increases after the first week of onset of tularaemia and remains elevated for more than a year. Clin. Exp. Immunol. 120, 280–284. doi: 10.1046/j.1365-2249.2000.01215.x
Kubelkova, K., Hudcovic, T., Kozakova, H., Pejchal, J., and Macela, A. (2021). Early infection-induced natural antibody response. Sci. Rep. 11:1541. doi: 10.1038/s41598-021-81083-0
Kubelkova, K., and Macela, A. (2021). Francisella and antibodies. Microorganisms 9:10. doi: 10.3390/microorganisms9102136
Kudryavtseva TaM, A. N. (2022). Molecular-genetic bases of differences between Tularaemia pathogen subspecies and Francisella tularensis subsp. holarctica strain typing. Mol. Genet. Microbiol. Virol. 37, 10–18. doi: 10.3103/S0891416822010049
Kumar, V., and Delovitch, T. L. (2014). Different subsets of natural killer T cells may vary in their roles in health and disease. Immunology 142, 321–336. doi: 10.1111/imm.12247
Kumari, P., Russo, A. J., Wright, S. S., Muthupalani, S., and Rathinam, V. A. (2021). Hierarchical cell-type-specific functions of caspase-11 in LPS shock and antibacterial host defense. Cell Rep. 35:109012. doi: 10.1016/j.celrep.2021.109012
Kurtz, S. L., Foreman, O., Bosio, C. M., Anver, M. R., and Elkins, K. L. (2013). Interleukin-6 is essential for primary resistance to Francisella tularensis live vaccine strain infection. Infect. Immun. 81, 585–597. doi: 10.1128/IAI.01249-12
Larson, M. A., Sayood, K., Bartling, A. M., Meyer, J. R., Starr, C., Baldwin, J., et al. (2020). Differentiation of Francisella tularensis subspecies and subtypes. J. Clin. Microbiol. 58. doi: 10.1128/JCM.01495-19
Laws, T. R., Clark, G., and D'Elia, R. V. (2013). Differential role for interleukin-6 during Francisella tularensis infection with virulent and vaccine strains. Infect. Immun. 81, 3055–3056. doi: 10.1128/IAI.00234-13
Lazarevic, V., and Glimcher, L. H. (2011). T-bet in disease. Nat. Immunol. 12, 597–606. doi: 10.1038/ni.2059
Lazarevic, V., Glimcher, L. H., and Lord, G. M. (2013). T-bet: a bridge between innate and adaptive immunity. Nat. Rev. Immunol. 13, 777–789. doi: 10.1038/nri3536
Legoux, F., Salou, M., and Lantz, O. (2020). MAIT cell development and functions: the microbial connection. Immunity 53, 710–723. doi: 10.1016/j.immuni.2020.09.009
Lin, Y., Ritchea, S., Logar, A., Slight, S., Messmer, M., Rangel-Moreno, J., et al. (2009). Interleukin-17 is required for T helper 1 cell immunity and host resistance to the intracellular pathogen Francisella tularensis. Immunity 31, 799–810. doi: 10.1016/j.immuni.2009.08.025
Lindgren, H., Eneslatt, K., Golovliov, I., Gelhaus, C., Ryden, P., Wu, T., et al. (2020). Vaccine-mediated mechanisms controlling Francisella tularensis SCHU S4 growth in a rat co-culture system. Pathogens 9:338. doi: 10.3390/pathogens9050338
Lindgren, H., Eneslatt, K., Golovliov, I., Gelhaus, C., and Sjostedt, A. (2023). Analyses of human immune responses to Francisella tularensis identify correlates of protection. Front. Immunol. 14:1238391. doi: 10.3389/fimmu.2023.1238391
Lopez, M. C., Duckett, N. S., Baron, S. D., and Metzger, D. W. (2004). Early activation of NK cells after lung infection with the intracellular bacterium, Francisella tularensis LVS. Cell Immunol. 232, 75–85. doi: 10.1016/j.cellimm.2005.02.001
Luo, F., Liu, H., Yang, S., Fang, Y., Zhao, Z., Hu, Y., et al. (2019). Nonreceptor tyrosine kinase c-Abl- and Arg-mediated IRF3 phosphorylation regulates innate immune responses by promoting type I IFN production. J. Immunol. 202, 2254–2265. doi: 10.4049/jimmunol.1800461
Ma, Z., Russo, V. C., Rabadi, S. M., Jen, Y., Catlett, S. V., Bakshi, C. S., et al. (2016). Elucidation of a mechanism of oxidative stress regulation in Francisella tularensis live vaccine strain. Mol. Microbiol. 101, 856–878. doi: 10.1111/mmi.13426
Man, S. M., and Kanneganti, T. D. (2015). Regulation of inflammasome activation. Immunol. Rev. 265, 6–21. doi: 10.1111/imr.12296
Mares, C. A., Ojeda, S. S., Morris, E. G., Li, Q., and Teale, J. M. (2008). Initial delay in the immune response to Francisella tularensis is followed by hypercytokinemia characteristic of severe sepsis and correlating with upregulation and release of damage-associated molecular patterns. Infect. Immun. 76, 3001–3010. doi: 10.1128/IAI.00215-08
Markel, G., Bar-Haim, E., Zahavy, E., Cohen, H., Cohen, O., Shafferman, A., et al. (2010). The involvement of IL-17A in the murine response to sub-lethal inhalational infection with Francisella tularensis. PLoS One 5:e11176. doi: 10.1371/journal.pone.0011176
Maurin, M. (2020). Francisella tularensis, Tularemia and Serological Diagnosis. Front. Cell. Infect. Microbiol. 10:512090. doi: 10.3389/fcimb.2020.512090
McCaffrey, R. L., Schwartz, J. T., Lindemann, S. R., Moreland, J. G., Buchan, B. W., Jones, B. D., et al. (2010). Multiple mechanisms of NADPH oxidase inhibition by type a and type B Francisella tularensis. J. Leukoc. Biol. 88, 791–805. doi: 10.1189/jlb.1209811
McCracken, J. M., Kinkead, L. C., McCaffrey, R. L., and Allen, L. A. (2016). Francisella tularensis modulates a distinct subset of regulatory factors and sustains mitochondrial integrity to impair human neutrophil apoptosis. J. Innate Immun. 8, 299–313. doi: 10.1159/000443882
McLendon, M. K., Apicella, M. A., and Allen, L. A. (2006). Francisella tularensis: taxonomy, genetics, and Immunopathogenesis of a potential agent of biowarfare. Ann. Rev. Microbiol. 60, 167–185. doi: 10.1146/annurev.micro.60.080805.142126
Meierovics, A. I., and Cowley, S. C. (2016). MAIT cells promote inflammatory monocyte differentiation into dendritic cells during pulmonary intracellular infection. J. Exp. Med. 213, 2793–2809. doi: 10.1084/jem.20160637
Meierovics, A., Yankelevich, W. J., and Cowley, S. C. (2013). MAIT cells are critical for optimal mucosal immune responses during in vivo pulmonary bacterial infection. Proc. Natl. Acad. Sci. USA 110, E3119–E3128. doi: 10.1073/pnas.1302799110
Melillo, A. A., Foreman, O., Bosio, C. M., and Elkins, K. L. (2014). T-bet regulates immunity to Francisella tularensis live vaccine strain infection, particularly in lungs. Infect. Immun. 82, 1477–1490. doi: 10.1128/IAI.01545-13
Messaoud-Nacer, Y., Culerier, E., Rose, S., Maillet, I., Rouxel, N., Briault, S., et al. (2022). STING agonist diABZI induces PANoptosis and DNA mediated acute respiratory distress syndrome (ARDS). Cell Death Dis. 13:269. doi: 10.1038/s41419-022-04664-5
Mestas, J., and Hughes, C. C. (2004). Of mice and not men: differences between mouse and human immunology. J. Immunol. 172, 2731–2738. doi: 10.4049/jimmunol.172.5.2731
Metzger, D. W., Salmon, S. L., and Kirimanjeswara, G. (2013). Differing effects of interleukin-10 on cutaneous and pulmonary Francisella tularensis live vaccine strain infection. Infect. Immun. 81, 2022–2027. doi: 10.1128/IAI.00024-13
Meyer, L., Broms, J. E., Liu, X., Rottenberg, M. E., and Sjostedt, A. (2015). Microinjection of Francisella tularensis and Listeria monocytogenes reveals the importance of bacterial and host factors for successful replication. Infect. Immun. 83, 3233–3242. doi: 10.1128/IAI.00416-15
Mezouar, S., and Mege, J. L. (2020). Changing the paradigm of IFN-gamma at the interface between innate and adaptive immunity: macrophage-derived IFN-gamma. J. Leukoc. Biol. 108, 419–426. doi: 10.1002/JLB.4MIR0420-619RR
Mills, K. H. G. (2023). IL-17 and IL-17-producing cells in protection versus pathology. Nat. Rev. Immunol. 23, 38–54. doi: 10.1038/s41577-022-00746-9
Miryala, S. K., and Ramaiah, S. (2022). Cellular and molecular level host-pathogen interactions in Francisella tularensis: a microbial gene network study. Comput. Biol. Chem. 96:107601. doi: 10.1016/j.compbiolchem.2021.107601
Mittereder, L. R., Swoboda, J., De Pascalis, R., and Elkins, K. L. (2023). IL-12p40 is essential but not sufficient for Francisella tularensis LVS clearance in chronically infected mice. PLoS One 18:e0283161. doi: 10.1371/journal.pone.0283161
Mohamed, R., and Lord, G. M. (2016). T-bet as a key regulator of mucosal immunity. Immunology 147, 367–376. doi: 10.1111/imm.12575
Moreland, J. G., Hook, J. S., Bailey, G., Ulland, T., and Nauseef, W. M. (2009). Francisella tularensis directly interacts with the endothelium and recruits neutrophils with a blunted inflammatory phenotype. Am. J. Physiol. Lung Cell. Mol. Physiol. 296, L1076–L1084. doi: 10.1152/ajplung.90332.2008
Nandakumar, R., Tschismarov, R., Meissner, F., Prabakaran, T., Krissanaprasit, A., Farahani, E., et al. (2019). Intracellular bacteria engage a STING-TBK1-MVB12b pathway to enable paracrine cGAS-STING signalling. Nat. Microbiol. 4, 701–713. doi: 10.1038/s41564-019-0367-z
Narayanan, R. B., Drabick, J. J., Williams, J. C., Fortier, A. H., Meltzer, M. S., Sadoff, J. C., et al. (1993). Immunotherapy of tularemia: characterization of a monoclonal antibody reactive with Francisella tularensis. J. Leukoc. Biol. 53, 112–116. doi: 10.1002/jlb.53.1.112
Natrajan, M. S., Rouphael, N., Lai, L., Kazmin, D., Jensen, T. L., Weiss, D. S., et al. (2019). Systems vaccinology for a live attenuated tularemia vaccine reveals unique transcriptional signatures that predict humoral and cellular immune responses. Vaccines (Basel) 8:10004. doi: 10.3390/vaccines8010004
Nauseef, W. M. (2001). The proper study of mankind. J. Clin. Invest. 107, 401–403. doi: 10.1172/JCI11713
Nel, I., Bertrand, L., Toubal, A., and Lehuen, A. (2021). MAIT cells, guardians of skin and mucosa? Mucosal Immunol. 14, 803–814. doi: 10.1038/s41385-021-00391-w
Newstead, S. L., Gates, A. J., Hartley, M. G., Rowland, C. A., Williamson, E. D., and Lukaszewski, R. A. (2014). Control of intracellular Francisella tularensis by different cell types and the role of nitric oxide. J Immunol Res 2014:694717, 1–8. doi: 10.1155/2014/694717
Nicol, M. J., Williamson, D. R., Place, D. E., and Kirimanjeswara, G. S. (2021). Differential immune response following intranasal and intradermal infection with Francisella tularensis: implications for vaccine development. Microorganisms 9. doi: 10.3390/microorganisms9050973
Nozaki, K., Li, L., and Miao, E. A. (2022). Innate sensors trigger regulated cell death to combat intracellular infection. Annu. Rev. Immunol. 40, 469–498. doi: 10.1146/annurev-immunol-101320-011235
Oh, H., Kim, C. Y., Kim, C. H., Hur, G. H., Lee, J. M., Chang, S. N., et al. (2018). Humanized mice for the evaluation of Francisella tularensis vaccine candidates. J. Microbiol. Biotechnol. 28, 157–164. doi: 10.4014/jmb.1707.07075
Paget, C., and Trottein, F. (2013). Role of type 1 natural killer T cells in pulmonary immunity. Mucosal Immunol. 6, 1054–1067. doi: 10.1038/mi.2013.59
Parsa, K. V., Butchar, J. P., Rajaram, M. V., Cremer, T. J., Gunn, J. S., Schlesinger, L. S., et al. (2008). Francisella gains a survival advantage within mononuclear phagocytes by suppressing the host IFNgamma response. Mol. Immunol. 45, 3428–3437. doi: 10.1016/j.molimm.2008.04.006
Pavkova, I., Brychta, M., Straskova, A., Schmidt, M., Macela, A., and Stulik, J. (2013). Comparative proteome profiling of host-pathogen interactions: insights into the adaptation mechanisms of Francisella tularensis in the host cell environment. Appl. Microbiol. Biotechnol. 97, 10103–10115. doi: 10.1007/s00253-013-5321-z
Periasamy, S., Avram, D., McCabe, A., MacNamara, K. C., Sellati, T. J., and Harton, J. A. (2016). An immature myeloid/myeloid-suppressor cell response associated with necrotizing inflammation mediates lethal pulmonary tularemia. PLoS Pathog. 12:e1005517. doi: 10.1371/journal.ppat.1005517
Pham, G. H., Iglesias, B. V., and Gosselin, E. J. (2014). Fc receptor-targeting of immunogen as a strategy for enhanced antigen loading, vaccination, and protection using intranasally administered antigen-pulsed dendritic cells. Vaccine 32, 5212–5220. doi: 10.1016/j.vaccine.2014.07.050
Pittman, P. R., Norris, S. L., Coonan, K. M., and McKee, K. T. Jr. (2005). An assessment of health status among medical research volunteers who served in the project Whitecoat program at fort Detrick, Maryland. Mil. Med. 170, 183–187. doi: 10.7205/MILMED.170.3.183
Plotkin, S. A. (2020). Updates on immunologic correlates of vaccine-induced protection. Vaccine 38, 2250–2257. doi: 10.1016/j.vaccine.2019.10.046
Plotkin, S. A. (2022). Recent updates on correlates of vaccine-induced protection. Front. Immunol. 13:1081107. doi: 10.3389/fimmu.2022.1081107
Poquet, Y., Kroca, M., Halary, F., Stenmark, S., Peyrat, M. A., Bonneville, M., et al. (1998). Expansion of Vgamma9 Vdelta2 T cells is triggered by Francisella tularensis-derived phosphoantigens in tularemia but not after tularemia vaccination. Infect. Immun. 66, 2107–2114. doi: 10.1128/IAI.66.5.2107-2114.1998
Pulavendran, S., Prasanthi, M., Ramachandran, A., Grant, R., Snider, T. A., Chow, V. T. K., et al. (2020). Production of neutrophil extracellular traps contributes to the pathogenesis of Francisella tularemia. Front. Immunol. 11:679. doi: 10.3389/fimmu.2020.00679
Qu, G., Wang, S., Zhou, Z., Jiang, D., Liao, A., and Luo, J. (2022). Comparing mouse and human tissue-resident γδ T cells. Front. Immunol. 13:891687. doi: 10.3389/fimmu.2022.891687
Rabadi, S. M., Sanchez, B. C., Varanat, M., Ma, Z., Catlett, S. V., Melendez, J. A., et al. (2016). Antioxidant defenses of Francisella tularensis modulate macrophage function and production of Proinflammatory cytokines. J. Biol. Chem. 291, 5009–5021. doi: 10.1074/jbc.M115.681478
Ramos Muniz, M. G., Palfreeman, M., Setzu, N., Sanchez, M. A., Saenz Portillo, P., Garza, K. M., et al. (2018). Obesity exacerbates the cytokine storm elicited by Francisella tularensis infection of females and is associated with increased mortality. Biomed. Res. Int. 2018:3412732. doi: 10.1155/2018/3412732
Rennert, K., Otto, P., Funke, H., Huber, O., Tomaso, H., and Mosig, A. S. (2016). A human macrophage-hepatocyte co-culture model for comparative studies of infection and replication of Francisella tularensis LVS strain and subspecies holarctica and mediasiatica. BMC Microbiol. 16:2. doi: 10.1186/s12866-015-0621-3
Rhinehart-Jones, T. R., Fortier, A. H., and Elkins, K. L. (1994). Transfer of immunity against lethal murine Francisella infection by specific antibody depends on host gamma interferon and T cells. Infect. Immun. 62, 3129–3137. doi: 10.1128/iai.62.8.3129-3137.1994
Richard, K., Mann, B. J., Stocker, L., Barry, E. M., Qin, A., Cole, L. E., et al. (2014). Novel catanionic surfactant vesicle vaccines protect against Francisella tularensis LVS and confer significant partial protection against F. tularensis Schu S4 strain. Clin. Vaccine Immunol. 21, 212–226. doi: 10.1128/CVI.00738-13
Richard, K., Vogel, S. N., and Perkins, D. J. (2016). Type I interferon licenses enhanced innate recognition and transcriptional responses to Franciscella tularensis live vaccine strain. Innate Immun. 22, 363–372. doi: 10.1177/1753425916650027
Roberts, L. M., Crane, D. D., Wehrly, T. D., Fletcher, J. R., Jones, B. D., and Bosio, C. M. (2016). Inclusion of epitopes that expand high-avidity CD4+ T cells transforms subprotective vaccines to efficacious immunogens against virulent Francisella tularensis. J. Immunol. 197, 2738–2747. doi: 10.4049/jimmunol.1600879
Roberts, L. M., Davies, J. S., Sempowski, G. D., and Frelinger, J. A. (2014). IFN-gamma, but not IL-17A, is required for survival during secondary pulmonary Francisella tularensis live vaccine stain infection. Vaccine 32, 3595–3603. doi: 10.1016/j.vaccine.2014.05.013
Roberts, L. M., Powell, D. A., and Frelinger, J. A. (2018). Adaptive immunity to Francisella tularensis and considerations for vaccine development. Front. Cell. Infect. Microbiol. 8:115. doi: 10.3389/fcimb.2018.00115
Roberts, L. M., Tuladhar, S., Steele, S. P., Riebe, K. J., Chen, C. J., Cumming, R. I., et al. (2014). Identification of early interactions between Francisella and the host. Infect. Immun. 82, 2504–2510. doi: 10.1128/IAI.01654-13
Rodriguez, A. R., Yu, J. J., Guentzel, M. N., Navara, C. S., Klose, K. E., Forsthuber, T. G., et al. (2012). Mast cell TLR2 signaling is crucial for effective killing of Francisella tularensis. J. Immunol. 188, 5604–5611. doi: 10.4049/jimmunol.1200039
Rodriguez, A. R., Yu, J. J., Murthy, A. K., Guentzel, M. N., Klose, K. E., Forsthuber, T. G., et al. (2011). Mast cell/IL-4 control of Francisella tularensis replication and host cell death is associated with increased ATP production and phagosomal acidification. Mucosal Immunol. 4, 217–226. doi: 10.1038/mi.2010.59
Rowland, C. A., Hartley, M. G., Flick-Smith, H., Laws, T. R., Eyles, J. E., and Oyston, P. C. (2012). Peripheral human γδ T cells control growth of both avirulent and highly virulent strains of Francisella tularensis in vitro. Microbes Infect. 14, 584–589. doi: 10.1016/j.micinf.2012.02.001
Sanapala, S., Yu, J. J., Murthy, A. K., Li, W., Guentzel, M. N., Chambers, J. P., et al. (2012). Perforin- and granzyme-mediated cytotoxic effector functions are essential for protection against Francisella tularensis following vaccination by the defined F. tularensis subsp. novicida DeltafopC vaccine strain. Infect. Immun. 80, 2177–2185. doi: 10.1128/IAI.00036-12
Saslaw, S., Eigelsbach, H. T., Prior, J. A., Wilson, H. E., and Carhart, S. (1961). Tularemia vaccine study. II. Respiratory challenge. Arch. Intern. Med. 107, 702–714. doi: 10.1001/archinte.1961.03620050068007
Saslaw, S., Eigelsbach, H. T., Wilson, H. E., Prior, J. A., and Carhart, S. (1961). Tularemia vaccine study. I. Intracutaneous challenge. Arch. Intern. Med. 107, 689–701. doi: 10.1001/archinte.1961.03620050055006
Schmitt, D. M., O'Dee, D. M., Brown, M. J., Horzempa, J., Russo, B. C., Morel, P. A., et al. (2013). Role of NK cells in host defense against pulmonary type a Francisella tularensis infection. Microbes Infect. 15, 201–211. doi: 10.1016/j.micinf.2012.11.008
Schwartz, J. T., Bandyopadhyay, S., Kobayashi, S. D., McCracken, J., Whitney, A. R., Deleo, F. R., et al. (2013). Francisella tularensis alters human neutrophil gene expression: insights into the molecular basis of delayed neutrophil apoptosis. J. Innate Immun. 5, 124–136. doi: 10.1159/000342430
Shaul, Y. (2000). C-Abl: activation and nuclear targets. Cell Death Differ. 7, 10–16. doi: 10.1038/sj.cdd.4400626
Singh, A., Rahman, T., Malik, M., Hickey, A. J., Leifer, C. A., Hazlett, K. R., et al. (2013). Discordant results obtained with Francisella tularensis during in vitro and in vivo immunological studies are attributable to compromised bacterial structural integrity. PLoS One 8:e58513. doi: 10.1371/journal.pone.0058513
Sjostedt, A., Conlan, J. W., and North, R. J. (1994). Neutrophils are critical for host defense against primary infection with the facultative intracellular bacterium Francisella tularensis in mice and participate in defense against reinfection. Infect. Immun. 62, 2779–2783. doi: 10.1128/iai.62.7.2779-2783.1994
Skyberg, J. A., Rollins, M. F., Samuel, J. W., Sutherland, M. D., Belisle, J. T., and Pascual, D. W. (2013). Interleukin-17 protects against the Francisella tularensis live vaccine strain but not against a virulent F. tularensis type a strain. Infect. Immun. 81, 3099–3105. doi: 10.1128/IAI.00203-13
Slight, S. R., Monin, L., Gopal, R., Avery, L., Davis, M., Cleveland, H., et al. (2013). IL-10 restrains IL-17 to limit lung pathology characteristics following pulmonary infection with Francisella tularensis live vaccine strain. Am. J. Pathol. 183, 1397–1404. doi: 10.1016/j.ajpath.2013.07.008
Snyder, D. T., Hedges, J. F., and Jutila, M. A. (2017). Getting "inside" type I IFNs: type I IFNs in intracellular bacterial infections. J Immunol Res 2017, 1–17. doi: 10.1155/2017/9361802
Steiner, D. J., Furuya, Y., Jordan, M. B., and Metzger, D. W. (2017). Protective role for macrophages in respiratory Francisella tularensis infection. Infect. Immun. 85. doi: 10.1128/IAI.00064-17
Stenmark, S., Sunnemark, D., Bucht, A., and Sjostedt, A. (1999). Rapid local expression of interleukin-12, tumor necrosis factor alpha, and gamma interferon after cutaneous Francisella tularensis infection in tularemia-immune mice. Infect. Immun. 67, 1789–1797
Szabo, S. J., Sullivan, B. M., Stemmann, C., Satoskar, A. R., Sleckman, B. P., and Glimcher, L. H. (2002). Distinct effects of T-bet in TH1 lineage commitment and IFN-gamma production in CD4 and CD8 T cells. Science 295, 338–342. doi: 10.1126/science.1065543
Tanaka, T., Narazaki, M., and Kishimoto, T. (2014). IL-6 in inflammation, immunity, and disease. Cold Spring Harb. Perspect. Biol. 6:a016295. doi: 10.1101/cshperspect.a016295
Tarnvik, A. (1989). Nature of protective immunity to Francisella tularensis. Rev. Infect. Dis. 11, 440–451. doi: 10.1093/clinids/11.3.440
Vignali, D. A., and Kuchroo, V. K. (2012). IL-12 family cytokines: immunological playmakers. Nat. Immunol. 13, 722–728. doi: 10.1038/ni.2366
Watanabe, H., Numata, K., Ito, T., Takagi, K., and Matsukawa, A. (2004). Innate immune response in Th1- and Th2-dominant mouse strains. Shock 22, 460–466. doi: 10.1097/01.shk.0000142249.08135.e9
Yee, D., Rhinehart-Jones, T. R., and Elkins, K. L. (1996). Loss of either CD4+ or CD8+ T cells does not affect the magnitude of protective immunity to an intracellular pathogen, Francisella tularensis strain LVS. J. Immunol. 157, 5042–5048. doi: 10.4049/jimmunol.157.11.5042
Yeni, D. K., Buyuk, F., Ashraf, A., and Shah, M. (2021). Tularemia: a re-emerging tick-borne infectious disease. Folia Microbiol. (Praha) 66, 1–14. doi: 10.1007/s12223-020-00827-z
Yu, H., Yang, A., Liu, L., Mak, J. Y. W., Fairlie, D. P., and Cowley, S. (2020). CXCL16 stimulates antigen-induced MAIT cell accumulation but trafficking during lung infection is CXCR6-independent. Front. Immunol. 11:1773. doi: 10.3389/fimmu.2020.01773
Zhao, Z., Wang, H., Shi, M., Zhu, T., Pediongco, T., Lim, X. Y., et al. (2021). Francisella tularensis induces Th1 like MAIT cells conferring protection against systemic and local infection. Nat. Commun. 12:4355. doi: 10.1038/s41467-021-24570-2
Keywords: Francisella tularensis, tularemia, adaptive immunity, innate immunity, correlates of protection, vaccine
Citation: Barthels DA, House RV and Gelhaus HC (2025) The immune response to Francisella tularensis. Front. Microbiol. 16:1549343. doi: 10.3389/fmicb.2025.1549343
Edited by:
Monica Cartelle Gestal, Louisiana State University Health Shreveport, United StatesReviewed by:
Lee-Ann H. Allen, University of Missouri, United StatesRiccardo D’Elia, Defence Science and Technology Laboratory, United Kingdom
Copyright © 2025 Barthels, House and Gelhaus. This is an open-access article distributed under the terms of the Creative Commons Attribution License (CC BY). The use, distribution or reproduction in other forums is permitted, provided the original author(s) and the copyright owner(s) are credited and that the original publication in this journal is cited, in accordance with accepted academic practice. No use, distribution or reproduction is permitted which does not comply with these terms.
*Correspondence: H. Carl Gelhaus, Y2dlbGhhdXNAYXBwaWxpdGhlcmFwZXV0aWNzLmNvbQ==