- Department of Civil and Environmental Engineering, University of Washington, Seattle, WA, United States
Terrestrial microbial communities drive many soil processes and can be pushed into a state of dysbiosis upon disturbance. This dysregulation negatively impacts soil biogeochemical cycles, which threatens plant and soil health. Effective treatment of soil dysbiosis requires simultaneous restoration of multiple system components, addressing both the physical structure of soil and its microbial communities. Hydrogels with microbial consortia simultaneously remedy soil hydrodynamics while promoting microbial reestablishment. The purpose of this review is to shed light on soil management practices through the lens of soil dysbiosis. This is important to address not only for soil health and crop productivity, but also to mitigate climate change through improved soil carbon sequestration and reduced greenhouse gas emissions. This review positions hydrogels and microbes as tools for the treatment of soil dysbiosis, contributing to agricultural and climate resilience.
1 Introduction
Microbial organisms (e.g., bacteria, archaea, and fungi) operate in a network of multifactional communities that drive biogeochemical soil processes (Philippot et al., 2024; Sokol et al., 2022; van der Heijden et al., 2008). Anthropogenic activities can disturb the equilibrium of these communities, pushing them into a state of dysbiosis, which is characterized by an imbalance in soil microbial functionality. When soils are in a dysbiotic state, abiotic and biotic processes are negatively impacted. These altered processes push the ecosystem out of balance, hampering naturally occurring healthy plant-soil feedback loops, which directly and indirectly influence aquatic and atmospheric systems (Fra̧c et al., 2022; Giovannetti et al., 2023; Weller et al., 2002). Over the last decade, the increased use of the term “dysbiosis” in environmental studies has warranted the consideration of what defines a balanced state in agricultural settings. Both homeostasis and eubiosis are terms originating from the medical field and describe a healthy state of a person or their microbiome, respectively. They are both set relative to the definition of dysbiosis, which describes a diseased state (Iebba et al., 2016). The term homeostasis is more broad and can be used when describing the health of the ecosystem at scale (e.g., a balanced equilibrium among the kingdoms of life, such as plants, bacteria, and fungi), while eubiosis can be used when viewing soil health through the lens of microbial community composition (e.g., a balance of healthy bacteria, fungi, and archaea that promote healthy processes) (Dyke and Weaver, 2013). Therefore, when soils are in a homeostatic state their biogeochemical processes move closer to a state of equilibrium, which creates a more temporally stable system. An example of soils that have been far removed from homeostasis are ailing agricultural soils, which often have multiple issues, such as displaced microbial communities (i.e., dysbiosis), high salinity, low nitrogen (N), phosphorus (P), and potassium (K) availability, and altered pH (Giovannetti et al., 2023). The combination of these issues directly impacts the equilibrium of the ecosystem.
For example, a major reason why there has been a global decline in crop yields over the last several decades is because soils are in dysbiosis, and biogeochemical cycles are unbalanced. This decline has resulted in a critical conundrum regarding global food security and bioenergy supply (Klock et al., 2024; Wing et al., 2021), as food demand is projected to increase by 50% to support the global population in 2050 (Falcon et al., 2022). Often, management strategies prioritize increasing yields from a plant-centric above-ground perspective; for instance, by focusing solely on solutions that boost crop yield without considering other potential repercussions. However, without a shift to more holistic management approaches that focus on soil eubiosis to balance soil microbial processes, food and energy security will be increasingly harder to achieve (Amelung et al., 2020; Lal et al., 2011; Moinet et al., 2023). Therefore, we are entering a part of the Anthropocene where human impact on natural systems is so great that current management practices are not viable solutions for a sustainable future (Currie et al., 2023; Ramos and Timmis, 2021).
Due to these concerns, the focus has started to shift toward managing ecosystems through the perspective of soil dysbiosis, as it is a critical factor to be addressed in the search for climate-smart agricultural solutions (Purohit et al., 2024; Sofo et al., 2024). However, it is still not a term that is commonly used or considered amongst the broad interdisciplinary scientific community when considering the future management of ecosystems. A search for “soil” and “dysbiosis” (i.e., ((soil) AND (dysbiosis))) among peer-reviewed research articles and reviews in the JSTOR database over the last 30 years (1995–2025) returned 133 results, which narrowed to 64 articles when “agriculture” was included. However, no results were achieved when the search was refined to pair the term “soil” with “dysbiosis” (i.e., ((soil dysbiosis) AND (agriculture))), reflecting the limited attention this term has received (in some cases, the imbalance is referred to as “soil microbial dysbiosis”, but only 16 results are returned when this term was paired with “agriculture”). Therefore, the main driver of this review is to shed light on soil management practices through the framework of soil dysbiosis while emphasizing the importance of practices that promote eubiosis by strengthening soil microbial processes that can help move closer to a net state of soil and ecosystems homeostasis. Therefore, management practices that focus on addressing soil dysbiosis represent a powerful leverage point for addressing these interconnected challenges in agriculture. Certain sustainable agricultural management practices have turned to trials that apply biofertilizers with microbial consortia, many of which show promise in restoring microbial function while promoting plant health (Waltz, 2023). Biofertilizer formulations consisting of microbial consortia can address several aspects of soil geochemistry and nutrient availability, such as P and K solubilization and other plant growth promoting aspects such as phytohormone production (Zuluaga et al., 2024; Olaniyan et al., 2022; Poveda and González-Andrés, 2021). However, these products face several challenges with market adoption due to inconsistent viability, short shelf life, and unknown interactions with plants and native soil microbes (Fadiji et al., 2024; Vejan et al., 2019). Therefore, holistic solutions that aim to address soil dysbiosis via the reestablishment of eubiotic microbial communities through biofertilizer application must also consider the overall impact on the balance of surrounding systems and how practices can foster long-term resiliency.
2 Soil dysbiosis mechanisms and impacts
Soil microbial communities are diverse and interconnected, comprising bacteria, archaea, fungi, protozoa, and nematodes; all of which can form complex networks with plants. These complex networks exist in either balanced (eubiotic) or imbalanced (dysbiotic) states (Fra̧c et al., 2022), and this can significantly impact ecosystem services (Banerjee et al., 2018). Approximately 38% of the earth's terrestrial surface is agricultural land (Paustian et al., 2019). In these soil systems, practices such as tilling, fertilization, and pesticide use negatively impact biodiversity (Kepler et al., 2020; Wipf et al., 2021), shifting soil functional and interactive profiles, which can disrupt processes that are crucial to the balance of the system. There are many biological interactions that occur within the soil's geochemical context, where microbial activities can influence different nutrient pools, such as carbon (C) and N pools, through extrapolysaccharide secretion and necromass formation while also contributing to soil aggregation (Costa et al., 2018; Wang et al., 2021). Below, we highlight various examples of how anthropogenically induced soil dysbiosis has shifted geochemical processes out of equilibrium and the aftermath that it has on societal and planetary health.
One biogeochemical cycle that is clearly in an unbalanced state is the N cycle, and how soils are managed plays a crucial role, as soil dysbiosis causes “leaky” N systems which cause a plateau in agricultural yields. Part of this can be attributed to the fact that intensive agricultural practices disrupt key genetic functions like nitrogenase activity (which regulates biological N-fixation via bacteria), while simultaneously increasing N loading to the environment via fertilizer application. This has increased nutrient leaching, water pollution, and greenhouse gas (GHG) emissions (Daisley et al., 2022; Zhang et al., 2021; Yang et al., 2024; Wipf et al., 2021; Suman et al., 2022; Wan et al., 2025). This is of particular concern with nitrous oxide (N2O) emissions, which is ~300x more potent than carbon dioxide (CO2) on a per-molecule basis (Tian et al., 2020). Overfertilized soils are the biggest contributor to global anthropogenic N2O emissions (Ramzan et al., 2020). It has been shown that the application of N fertilizer can alter soil pH and N and P availability, which subsequently impacts the ammonia-oxidizing archaea (AOA) and bacterial (AOB) communities. These functional groups are vital intermediates in soil N processes (Ma et al., 2023), as AOB communities produce significantly more N2O than AOA (Hink et al., 2017). Further, specialized processes like N-fixation and nitrification undertaken by certain bacterial taxa are particularly vulnerable to N loading, as healthy communities that can promote closed-loop N-cycling are typically displaced under heavy fertilization (Calderón et al., 2017). This impaired biological cycling of N paired with copious amounts of N additions increases losses to aquatic systems (through leaching of nitrate) and the atmosphere (as N2O) (Menegat et al., 2022; Shanmugavel et al., 2023).
Agricultural practices and the induction of soil dysbiosis also negatively impairs the C cycle, which is directly linked to the N cycle (Osler and Sommerkorn, 2007). It is widely known that microbial communities play a critical role in decomposition processes, which influence soil organic matter accumulation and C-sequestration (Tao et al., 2023). Agricultural soils are typically depleted in soil C due to reduced net primary productivity, biomass export, nutrient depletion, and anthropogenic disturbances. Sustainable management practices that aim to shift an ecosystem closer to equilibrium by addressing soil dysbiosis could reverse this trend, enabling soils to act as a significant C sink with a global potential to store up to 1.85 Pg C/yr (Zomer et al., 2017). If atmospheric C could be more efficiently sequestered and soils better managed for long-term storage, climate-change mitigation efforts could be greatly advanced.
In addition to N and C cycling, water availability and P resources are strained when soils are managed in a way that promotes dysbiosis. From a water resource perspective, growing crops on a global scale is extremely water-intensive, and the recent increase in wide-spread drought has raised concern on how to increase crop yield while minimizing the use of finite water resources (Chen et al., 2016; Poudel et al., 2021). From a nutrient perspective, P is an essential nutrient for plant growth and a common fertilizer component. However, P is a nonrenewable resource derived from mined rock phosphate and is facing rising costs and geopolitical instability (Cordell et al., 2009). Therefore, how to manage ecosystems in a way that returns soil communities to balanced state is challenging because there are microorganisms that can increase soil water holding capacity through soil microaggregation (Zheng et al., 2018; Pauwels et al., 2023), others that can solubilize residual P to plant available P, and many have multi-functional benefits, such as increasing plant water uptake and resiliency, while providing plants essential nutrients.
An example of this can be viewed with arbuscular mycorrhizal fungi (AMF), which are an obligate plant mutualist and form in extensive belowground networks. AMF associate with plants, exchanging water and nutrients, such as P and N, for plant derived photosynthates (Lanfranco et al., 2018). This mutualism is ancient and broad, dating back to the terrestrial expansion of plants. Today, ~85% of land plant families associate with AMF (Strullu-Derrien et al., 2018). AMF can enhance plant productivity, as well as increase resiliency to salinity, pollution, and disease (Begum et al., 2019). Recent research has illuminated how they act as “fungal highways” and orchestrate bacterial diversity around their hyphae (Warmink et al., 2011; Zhang et al., 2022). They are also key components of the global soil C pool, storing ~13 Gt of the CO2 fixed by plants (Hawkins et al., 2023). To add to the complexity, plants can actively influence subterranean communities by releasing root exudates that recruit specific microbes, for example, during nutrient limitations (low N and P) or drought conditions (Williams and de Vries, 2020; Pascale et al., 2019). However, AMF abundance and network connections are disrupted by intensifying agricultural practices (particularly tilling), sending them into a dysbiotic state which can have a cascade effect on other interactive microbial communities (Wipf et al., 2021). For instance, the disruption of AMF communities can negatively impact plant nutrient allocation, soil structure, and water retention while limiting plant root exploration (Hartman and Tringe, 2019). Additionally, the impact that this has on AMF-associated microbial communities and the repercussions those impacts hold for soil biogeochemical cycling remains widely unexplored. Given these complex interactions, effective treatment of soil dysbiosis requires simultaneous restoration of multiple system components, including microbial communities, soil structure, and nutrient dynamics. Though the use of biofertilizers has started to expand with sustainability goals as a focus, there are various things that need to be considered for efficient delivery systems. For example, shifting focus away from practices that aim to only increase plant yield at the expense of soil eubiosis.
3 Hydrogel-based delivery systems
Hydrogel-based delivery systems hold high potential as carrying agents for biofertilizers that aim to address soil dysbiosis. Hydrogels have been studied for biological use for over 50 years (Wichterle and Lím, 1960). They can be created from natural or synthetic materials (Liu et al., 2022), but organic polymers are the most common (Patra et al., 2022; Tariq et al., 2023). The hydrogel structure consists of matrices created through either chemical methods, such as cross-linking, or physical processes, like the hydrophobic interactions among polar groups (Antunes et al., 2024). Various encapsulation technologies, including spray drying, emulsification, and ionic gelation for cross-linking are used to produce hydrogels (Vejan et al., 2019), and they can be shaped into various forms (e.g., spheres/beads). The hydrophilic matrices in a hydrogel construct can absorb and desorb water into the soil, hence regulating soil moisture (Patra et al., 2022). These structures contain micropores and allow for the encapsulation and immobilization of various agrichemicals, beneficial microbes, or a combination of both. While agrochemicals have been a primary focus, there is growing interest in co-immobilizing multiple microbial strains for synergistic effects (Antunes et al., 2024; Mafune and Winkler, 2024). Once applied, these materials degrade within 12 weeks, with degradation rates influenced by several environmental and material factors (Kurowiak et al., 2020; Wu et al., 2016).
In the context of microbial consortia, hydrogels shield microbes from environmental fluctuations and dehydration (Antunes et al., 2024; Liu et al., 2022). This protection enhances microbial survival and facilitates controlled release dynamics for nutrients, water, and microbes (Chaparro-Rodríguez et al., 2023). When applied to soil, hydrogels can induce controlled delivery of nutrients such as N, P, and K, as well as water (Hamed et al., 2024; Hu et al., 2021). In addition to prokaryotic microbes (i.e., bacteria and archaea), hydrogels can also house fungal spores, hyphae, and propagules. For instance, recent compatibility tests have demonstrated variation in AMF spore germination when co-immobilized with diazotrophic bacteria in alginate beads (Mafune et al., 2024). Combined, the versatility of hydrogels as moisture-regulators and carriers for diverse microbial consortia, chemicals, and physical components (e.g., zeolites or biochar) may offer a multi-functional competitive advantage in commercializing technology that aims to restore soils to a eubiotic state with the goal of returning ecosystem processes to equilibrium.
4 Restoration mechanisms
The restoration of soils in a state of dysbiosis requires the integration of practices that aim to improve soil physical, chemical, and biological properties to improve soil health/homeostasis. As this review has been emphasizing, soil dysbiosis is directly and indirectly linked to soil abiotic processes. This is why approaching the core issue of soil health through the lens of ecosystem homeostasis is important, as holistic frameworks are integral to decision making that results in restoration success (Wyant et al., 1995; Heneghan et al., 2008). By focusing in on how balanced microbial communities can promote beneficial soil aggregation, balanced pH, plant growth, C accumulation and long-term storage, and “non-leaky” nutrient cycles, scientists and engineers can hopefully be inspired to innovate restorative soil solutions through a broadened perspective.
When considering restoration practices that can have multi-functional attributes, microbial-embedded hydrogels with a mixed consortium can simultaneously address all three areas of soil health (chemical, physical, and biological), which are all intrinsically linked to some extent in soils. For instance, in terms of soil physical properties, hydrogels undergo significant volume changes as they absorb and release water, regulating soil moisture through gradual diffusion. This leads to improved water hydrodynamics, including water holding capacity and reduced soil compaction (e.g., a decrease in soil bulk density so roots have more exploration accessibility) (Patra et al., 2022). The physical changes induced by hydrogels create conditions that support both plant root development and microbial re-establishment (Figure 1). Further, these conditions also can drive nutrient transformation and translocation, which links the hydrogels impact on soil physical properties to soil biological and chemical properties.
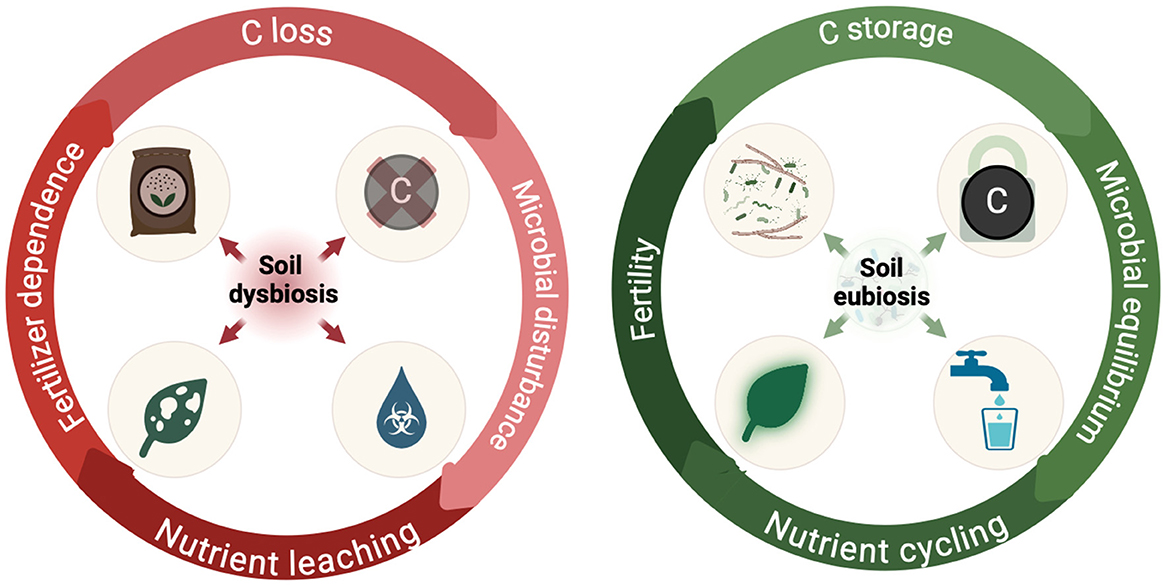
Figure 1. Contrasting states of soil health: dysbiosis drives nutrient leaching and depletion while eubiosis promotes nutritional increases through microbial flourishing. Inspired by concepts conveyed in Fra̧c et al. (2022), Giovannetti et al. (2023), and Purohit et al. (2024).
Another example of the hydrogel construct as a multifunctional biotechnology for soil restoration can be demonstrated from the viewpoint of soil chemistry. The hydrogel can biodegrade slow enough to support microbial activities that may drive soil organic C formation. The high-water content of the hydrogel (approximately 75% at capacity) also creates an environment that facilitates plant-microbe interactions (Aung et al., 2018; Gajić et al., 2023). Hydrogel-encased consortia can include a variety of beneficial organisms, including, but not limited to, diazotrophic (N-fixing) bacteria, phosphate solubilizing bacteria, and AMF that together drive nutrient availability and exchange. Outside of the microbial players in the hydrogel, AMF can also orchestrate a bacterial community in the rhizosphere that improves nutrient availability, e.g. through increased P mineralization (Wang et al., 2023; DiLegge et al., 2022). AMF also play an important role in soil macroaggregation, impacting soil structure, which can further support microbial growth and reestablishment (Hestrin et al., 2021). This demonstrates how a complex biological community within a hydrogel construct could benefit soil biological, physical, and chemical properties.
Further studies would be needed to shed light on how hydrogel consortia assemble, and how this assembly is influenced by soil chemical and physical properties. Hydrogels have potential to enhance synergistic community assembly by protecting microbes from environmental stressors including dehydration, pH changes, temperature fluctuations, and washout. This is particularly important for plant growth promoting bacteria (PGPB), which are often gram-negative (meaning that they have thinner cell walls) and more sensitive to environmental stressors during biofertilizer production (Chaparro-Rodríguez et al., 2023). The potential uses and applications of hydrogels with microbes and/or consortia go beyond nutrient restoration and mineralization processes. Some calcium-alginate hydrogel bead systems incorporate the entomopathogenic (insect pathogen) fungus Metarhizium spp. for pest control (Antunes et al., 2024). Alginate beads also have been tested in bioremediation practices, for instance, Pseudomonas fluorescens was encased in alginate beads to explore their efficacy on PCB degradation (Power et al., 2011).
5 Future directions
Biodegradable hydrogels with microbial consortia offer a sustainable solution for soil health due to their customizable nature and broad applications. However, several key knowledge gaps need to be addressed. The first priority is optimizing microbial consortia composition, and understanding how different players interact to influence soil dynamics and plant growth. Ideally, hydrogel consortia should be selected based on the ecosystem they are intended to be applied to (Mafune and Winkler, 2024), and more research is needed. PGPB serve diverse functions in soil, from N-fixation to P-solubilization and organic matter decomposition. Therefore, some species like Azospirillium brasilense, are versatile and often used in commercial biofertilizers (Rodriguez et al., 2004). These rhizospheric “multitaskers” are particularly valuable for inclusion in hydrogel formulations. However, repercussions of their application could be different across ecosystem types, and native communities could potentially be better suited to promote soil eubiosis. This is because interactions between bacterial species can create beneficial synergies—for instance, certain Pseudomonas strains can enhance the N-fixing ability of Azospirillum brasilense, while increased N availability in turn promotes Pseudomonas aeruginosa growth (Sanow et al., 2023; Marogi et al., 2024). Understanding these metabolic relationships across different soil types and in ecosystems is important for designing and engineering hydrogel consortia and synthetic communities.
An interesting pursuit in search of these solutions would be to understand how hydrogel encased communities derive C from the surrounding environment and hydrogel material for either growth or respiration, since this determines how much soil organic C can be accumulated and sequestered (Tao et al., 2023). These further impacts physical parameters such as aggregate stability and water repellency (Blanco-Canqui and Benjamin, 2013). The addition of particles to the mixed-microbial hydrogel construct (such as biochar) can also enhance how physical, chemical, and biological processes are benefitted. For example, biochars have porous surfaces that provide additional habitat for microbes while contributing to potentially long-term C sequestration (Yang et al., 2020). Spatial patterns show clustering around specific microniches, such as root and biochar micropores (Schmidt et al., 2018). Within these microniches, various levels of resource exchange and interkingdom signaling occur, highlighting the importance of a healthy rhizosphere microbiota (DiLegge et al., 2022).
To date, most research on microbial based hydrogels has been limited to greenhouse, pot, and other in vitro studies, though a couple of studies have applied microbial hydrogels in the field (Table 1). This demonstrates that long-term hydrogel application studies are important to evaluate real-world performance over multiple growing seasons. These studies would help determine optimal application rates and treatment durability; however, it is emphasized that major consideration should be given when selecting what microbial consortia is to be applied. This is because there is little knowledge on what repercussions are associated with applying non-native or genetically modified microbes into different soil communities (Mafune and Winkler, 2024). The degradation process of hydrogels in soils also requires further investigation. While two studies have addressed the biodegradation of alginate beads (Kurowiak et al., 2020; Song et al., 2020), to our knowledge, none have fully characterized the products of degradation, which may have further benefits in soil. For example, oligomers from alginate, chitosan, and carrageenan can support plant growth and disease protection through various pathways and mechanisms (Moenne and González, 2021). Downstream degradation products could provide additional benefits, with C-rich monomers serving as nutrients for microbes, fungi, and plants.
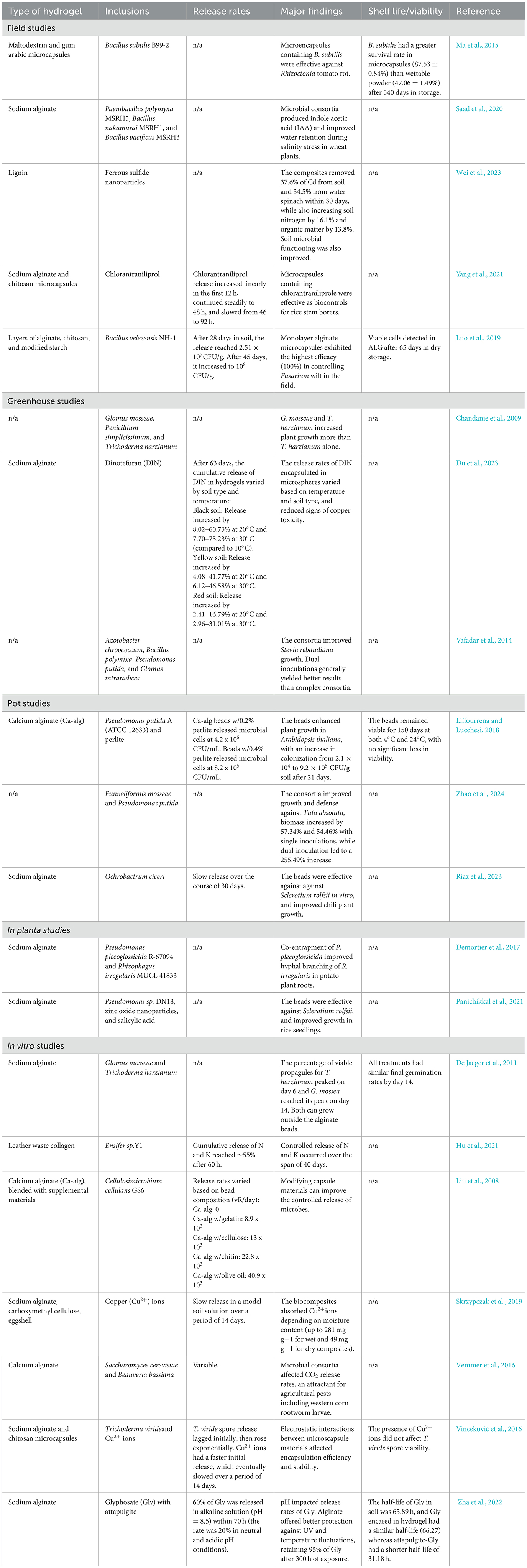
Table 1. Summary of hydrogel-based or microbial consortia agricultural studies, grouped by study type.
From a commercial perspective, several factors will influence market adoption of biodegradable hydrogels with microbial consortia. The plant growth-promoting properties of microbial-encased hydrogels suggest they be used as a biofertilizer, either as supplement or replacement for conventional fertilizers. However, successful commercialization will depend on achieving cost-effective manufacturing at scale, while concurrently balancing chemical inputs to soils. The cost of fertilizers has been rising and when paired with the concern of widespread soil dysbiosis, farmers face challenges sustaining profitable yields. For example, ammonia production has been steadily increasing over the last few decades, and costs fluctuate between 400 and 1600 USD/t because of transportation costs from centralized manufacturing centers, as well as natural gas prices and sources (Fernández et al., 2024; Zhang et al., 2020). Additionally, the production of conventional N fertilizers is energy-intensive and accounts for approximately 2% of global CO2 emissions (Menegat et al., 2022). P is a non-renewable resource and comes with its own set of rising costs and geopolitical instabilities (Brownlie et al., 2023). This combination not only puts stakeholder livelihoods at risk, but it also contributes to the looming concern of global food security. Therefore, there is a need to determine the energy, production, and application costs of hydrogels at scale. There is also a need to understand the potential for this biotechnology in the Carbon Dioxide Removal (CDR) market, while also including emissions other than CO2, such as N2O. As N2O is ~300x more potent than CO2, is emitted largely from fertilized soils, and if the reduction is prioritized, it could greatly benefit the environment, as well as boost CDR market efforts and reach (Tian et al., 2020).
As high-throughput culturing technology advances, there may be a viable niche for cost-effective and labor-efficient customization of enrichment, isolation, and reapplication of site-specific, well-adapted microbial consortia. These would have the added benefit of being non-invasive and naturally evolved to dominate and adapt to local conditions. It could provide an avenue to introduce high-performing strains into the field at higher concentrations, further accelerating N and C translocation and boosting plant yield.
Overall, in vitro and greenhouse studies have provided a foundation for understanding microbial consortia encased in a hydrogel construct. Moving forward, research into microbial synergies, field application methods, ecological impacts, and biodegradation profiles are key. Efforts along these lines will not only advance the scientific understanding of hydrogel-microbe interactions, but also provide the evidence needed to accelerate adoption of this promising biotechnology for sustainable agriculture and restoration practices beyond the agricultural sector.
Author contributions
RD: Conceptualization, Visualization, Writing – original draft, Writing – review & editing. KM: Conceptualization, Visualization, Writing – original draft, Writing – review & editing. MW: Conceptualization, Supervision, Writing – original draft, Writing – review & editing.
Funding
The author(s) declare that financial support was received for the research and/or publication of this article. This research was funded by the Washington Research Foundation (Phase 1) and an award from the United States Department of Agriculture's National Institute of Food and Agriculture (2023-67013-40171).
Acknowledgments
Figure 1 was created with BioRender.com.
Conflict of interest
The authors declare that the research was conducted in the absence of any commercial or financial relationships that could be construed as a potential conflict of interest.
Generative AI statement
The author(s) declare that no Gen AI was used in the creation of this manuscript.
Publisher's note
All claims expressed in this article are solely those of the authors and do not necessarily represent those of their affiliated organizations, or those of the publisher, the editors and the reviewers. Any product that may be evaluated in this article, or claim that may be made by its manufacturer, is not guaranteed or endorsed by the publisher.
References
Amelung, W., Bossio, D., de Vries, W., Kögel-Knabner, I., Lehmann, J., Amundson, R., et al. (2020). Towards a global-scale soil climate mitigation strategy. Nat. Commun. 11:5427. doi: 10.1038/s41467-020-18887-7
Antunes, D. R., Forini, M. M. L. H., Biscalchim, É. R., Lima, P. H. C., Cavalcante, L. A. F., Teixeira Filho, M. C. M., et al. (2024). Polysaccharide-based sustainable hydrogel spheres for controlled release of agricultural inputs. Int. J. Biol. Macromol. 279:135202. doi: 10.1016/j.ijbiomac.2024.135202
Aung, K., Jiang, Y., and He, S. Y. (2018). The role of water in plant-microbe interactions. Plant J. 93, 771–780. doi: 10.1111/tpj.13795
Banerjee, S., Schlaeppi, K., and van der Heijden, M. G. A. (2018). Keystone taxa as drivers of microbiome structure and functioning. Nat. Rev. Microbiol. 16, 567–576. doi: 10.1038/s41579-018-0024-1
Begum, N., Qin, C., Ahanger, M. A., Raza, S., Khan, M. I., Ashraf, M., et al. (2019). Role of arbuscular mycorrhizal fungi in plant growth regulation: implications in abiotic stress tolerance. Front. Plant Sci. 10:1068. doi: 10.3389/fpls.2019.01068
Blanco-Canqui, H., and Benjamin, J. G. (2013). Impacts of soil organic carbon on soil physical behavior. Quantif. Mode. Soil Struct. Dynam. 3, 11–40. doi: 10.2134/advagricsystmodel3.c2
Brownlie, W. J., Sutton, M. A., Cordell, D., Reay, D. S., Heal, K. V., Withers, P. J. A., et al. (2023). Phosphorus price spikes: a wake-up call for phosphorus resilience. Front. Sustain. Food Syst. 7:1088776. doi: 10.3389/fsufs.2023.1088776
Calderón, K., Spor, A., Breuil, M.-C., Bru, D., Bizouard, F., Violle, C., et al. (2017). Effectiveness of ecological rescue for altered soil microbial communities and functions. ISME J. 11, 272–283. doi: 10.1038/ismej.2016.86
Chandanie, W. A., Kubota, M., and Hyakumachi, M. (2009). Interactions between the arbuscular mycorrhizal fungus Glomus mosseae and plant growth-promoting fungi and their significance for enhancing plant growth and suppressing damping-off of cucumber (Cucumis sativus L.). Appl. Soil Ecol. 41, 336–341. doi: 10.1016/j.apsoil.2008.12.006
Chaparro-Rodríguez, M., Estrada-Bonilla, G., Rosas-Pérez, J., Gómez-Álvarez, M., and Cruz-Barrera, M. (2023). Hydrogel capsules as new approach for increasing drying survival of plant biostimulant gram-negative consortium. Appl. Microbiol. Biotechnol. 107:6671–6682. doi: 10.1007/s00253-023-12699-7
Chen, J., Shi, H., Sivakumar, B., and Peart, M. R. (2016). Population, water, food, energy and dams. Renew Sustain Energy Rev. 56, 18–28. doi: 10.1016/j.rser.2015.11.043
Cordell, D., Drangert, J.-O., and White, S. (2009). The story of phosphorus: Global food security and food for thought. GEC. 19, 292–305. doi: 10.1016/j.gloenvcha.2008.10.009
Costa, O. Y. A., Raaijmakers, J. M., and Kuramae, E. E. (2018). Microbial extracellular polymeric substances: ecological function and impact on soil aggregation. Front. Microbiol. 9:1636. doi: 10.3389/fmicb.2018.01636
Currie, T. E., Borgerhoff Mulder, M., Fogarty, L., Schlüter, M., Folke, C., Haider, L. J., et al. (2023). Integrating evolutionary theory and social–ecological systems research to address the sustainability challenges of the Anthropocene. Philos. Trans. R. Soc. B Bio. Sci. 379:20220262. doi: 10.1098/rstb.2022.0262
Daisley, B. A., Chernyshova, A. M., Thompson, G. J., and Allen-Vercoe, E. (2022). Deteriorating microbiomes in agriculture - the unintended effects of pesticides on microbial life. Microbiome Res. Rep. 1:6. doi: 10.20517/mrr.2021.08
De Jaeger, N., De La Providencia, I. E., Rouhier, H., and Declerck, S. (2011). Co-entrapment of Trichoderma harzianum and Glomus sp. within alginate beads: impact on the arbuscular mycorrhizal fungi life cycle: Co-entrapment of beneficial fungi. J. Appl. Microbiol. 111, 125–135. doi: 10.1111/j.1365-2672.2011.05035.x
Demortier, M., Loján, P., Velivelli, S. L. S., Pfeiffer, S., Suárez, J. P., De Vos, P., et al. (2017). Impact of plant growth-promoting rhizobacteria on root colonization potential and life cycle of Rhizophagus irregularis following co-entrapment into alginate beads. J. Appl. Microbiol. 122, 429–440. doi: 10.1111/jam.13355
DiLegge, M. J., Manter, D. K., and Vivanco, J. M. (2022). Soil microbiome disruption reveals specific and general plant-bacterial relationships in three agroecosystem soils. PLoS ONE. 17:e0277529. doi: 10.1371/journal.pone.0277529
Du, Y., Zhang, Q., Yu, M., Jiao, B., Chen, F., and Yin, M. (2023). Sodium alginate-based composite microspheres for controlled release of pesticides and reduction of adverse effects of copper in agricultural soils. Chemosphere 313:137539. doi: 10.1016/j.chemosphere.2022.137539
Dyke, J. G., and Weaver, I. S. (2013). The emergence of environmental homeostasis in complex ecosystems. PLOS Comput. Biol. 9:e1003050. doi: 10.1371/journal.pcbi.1003050
Fadiji, A. E., Xiong, C., Egidi, E., and Singh, B. K. (2024). Formulation challenges associated with microbial biofertilizers in sustainable agriculture and paths forward. J. Sustain. Agricult. Environm. 3:e70006. doi: 10.1002/sae2.70006
Falcon, W. P., Naylor, R. L., and Shakar, N. D. (2022). Rethinking global food demand for 2050. Popul. Dev. Rev. 48:921–957. doi: 10.1111/padr.12508
Fernández, C. A., Chapman, O., Brown, M. A., Alvarez-Pugliese, C. E., and Hatzell, M. C. (2024). Achieving decentralized, electrified, and decarbonized ammonia production. Environ. Sci. Technol. 58, 6964–6977. doi: 10.1021/acs.est.3c10751
Fra̧c, M., Hannula, E. S., Bełka, M., Salles, J. F., and Jedryczka, M. (2022). Soil mycobiome in sustainable agriculture. Front. Microbiol. 13:1033824. doi: 10.3389/fmicb.2022.1033824
Gajić, I. M., Savić, I. M., and Svirčev, Z. (2023). Preparation and characterization of alginate hydrogels with high water-retaining capacity. Polymers 15:2592. doi: 10.3390/polym15122592
Giovannetti, M., Salvioli di Fossalunga, A., Stringlis, I. A., Proietti, S., and Fiorilli, V. (2023). Unearthing soil-plant-microbiota crosstalk: looking back to move forward. Front. Plant Sci. 13:1082752. doi: 10.3389/fpls.2022.1082752
Hamed, R., Jodeh, S., and Alkowni, R. (2024). Nano bio fertilizer capsules for sustainable agriculture. Sci. Rep. 14:13646. doi: 10.1038/s41598-024-62973-5
Hartman, K., and Tringe, S. G. (2019). Interactions between plants and soil shaping the root microbiome under abiotic stress. Biochem. J. 476, 2705–2724. doi: 10.1042/BCJ20180615
Hawkins, H. J., Cargill, R. I. M., van Nuland, M. E., Hagen, S. C., Field, K. J., Sheldrake, M., et al. (2023). Mycorrhizal mycelium as a global carbon pool. Curr. Biol. 33, R560–R573. doi: 10.1016/j.cub.2023.02.027
Heneghan, L., Miller, S. P., Baer, S., Callaham, M. A. Jr, Montgomery, J., Pavao-Zuckerman, M., et al. (2008). Integrating soil ecological knowledge into restoration management. Restorat. Ecol. 16, 608–617. doi: 10.1111/j.1526-100X.2008.00477.x
Hestrin, R., Weber, P. K., Pett - Ridge, J., and Lehmann, J. (2021). Plants and mycorrhizal symbionts acquire substantial soil nitrogen from gaseous ammonia transport. New Phytol. 231, 1746–1757. doi: 10.1111/nph.17527
Hink, L., Nicol, G. W., and Prosser, J. I. (2017). Archaea produce lower yields of N2O than bacteria during aerobic ammonia oxidation in soil. Environ. Microbiol. 19, 4829–4837. doi: 10.1111/1462-2920.13282
Hu, Z.-Y., Chen, G., Yi, S.-H., Wang, Y., Liu, Q., and Wang, R. (2021). Multifunctional porous hydrogel with nutrient controlled-release and excellent biodegradation. J. Environ. Chem. Eng. 9:106146. doi: 10.1016/j.jece.2021.106146
Iebba, V., Totino, V., Gagliardi, A., Santangelo, F., Cacciotti, F., Trancassini, M., et al. (2016). Eubiosis and dysbiosis: the two sides of the microbiota. New Microbiol. 39, 1–12.
Kepler, R. M., Epp Schmidt, D. J., Yarwood, S. A., Cavigelli, M. A., Reddy, K. N., Duke, S. O., et al. (2020). Soil microbial communities in diverse agroecosystems exposed to the herbicide glyphosate. Appl. Environ. Microbiol. 86, e01744–e01719. doi: 10.1128/AEM.01744-19
Klock, A. M., Banerjee, A., Vogt, K. A., Mafune, K. K., Vogt, D. J., and Gordon, J. C. (2024). An ecological framework to index crop yields using productivity and Ecosystem Fit: A case study from India. PLOS Sustain. Transform. 3:e0000122. doi: 10.1371/journal.pstr.0000122
Kurowiak, J., Kaczmarek-Pawelska, A., Mackiewicz, A. G., and Bedzinski, R. (2020). Analysis of the degradation process of alginate-based hydrogels in artificial urine for use as a bioresorbable material in the treatment of urethral injuries. Processes. 8:304. doi: 10.3390/pr8030304
Lal, R., Delgado, J. A., Groffman, P. M., Millar, N., Dell, C., and Rotz, A. (2011). Management to mitigate and adapt to climate change. JSWC. 66, 276–285. doi: 10.2489/jswc.66.4.276
Lanfranco, L., Fiorilli, V., and Gutjahr, C. (2018). Partner communication and role of nutrients in the arbuscular mycorrhizal symbiosis. New Phytol. 220, 1031–1046. doi: 10.1111/nph.15230
Liffourrena, A. S., and Lucchesi, G. I. (2018). Alginate-perlite encapsulated Pseudomonas putida A (ATCC 12633) cells: preparation, characterization and potential use as plant inoculants. J. Biotechnol. 278, 28–33. doi: 10.1016/j.jbiotec.2018.04.019
Liu, C.-H., Wu, J.-Y., and Chang, J.-S. (2008). Diffusion characteristics and controlled release of bacterial fertilizers from modified calcium alginate capsules. Bioresour. Technol. 99, 1904–1910. doi: 10.1016/j.biortech.2007.03.029
Liu, X., Inda, M. E., Lai, Y., Lu, T. K., and Zhao, X. (2022). Engineered living hydrogels. Adv. Mater. Weinheim. 34:e2201326. doi: 10.1002/adma.202201326
Luo, W., Liu, L., Qi, G., Yang, F., Shi, X., and Zhao, X. (2019). Embedding Bacillus velezensis NH-1 in microcapsules for biocontrol of cucumber fusarium wilt. Appl. Environ. Microbiol. 85, e03128–e03118. doi: 10.1128/AEM.03128-18
Ma, M., Zhao, Y., Jiang, X., Guan, D., Yuan, M., Cao, F., et al. (2023). Fertilization altered co-occurrence patterns and microbial assembly process of ammonia-oxidizing microorganisms. Sci. Rep. 13:8234. doi: 10.1038/s41598-022-26293-w
Ma, X., Wang, X., Cheng, J., Nie, X., Yu, X., Zhao, Y., et al. (2015). Microencapsulation of Bacillus subtilis B99-2 and its biocontrol efficiency against Rhizoctonia solani in tomato. Biol. Control. 90, 34–41. doi: 10.1016/j.biocontrol.2015.05.013
Mafune, K., Kasson, M., and Winkler, M.-K. H. (2024). Building blocks towards sustainable biofertilizers: variation in arbuscular mycorrhizal spore germination when immobilized with diazotrophic bacteria in biodegradable hydrogel beads. J. Appl. Microbiol. 135:lxae167. doi: 10.1093/jambio/lxae167
Mafune, K. K., and Winkler, M. K. (2024). The expansion of fungal organisms in environmental biotechnology. COBIOT. 90:103217. doi: 10.1016/j.copbio.2024.103217
Marogi, J. G., Murphy, C. T., Myhrvold, C., and Gitai, Z. (2024). Pseudomonas aeruginosa modulates both Caenorhabditis elegans attraction and pathogenesis by regulating nitrogen assimilation. Nat. Commun. 15:7927. doi: 10.1038/s41467-024-52227-3
Menegat, S., Ledo, A., and Tirado, R. (2022). Greenhouse gas emissions from global production and use of nitrogen synthetic fertilisers in agriculture. Sci. Rep. 12:14490. doi: 10.1038/s41598-022-18773-w
Moenne, A., and González, A. (2021). Chitosan-, alginate- carrageenan-derived oligosaccharides stimulate defense against biotic and abiotic stresses, and growth in plants: a historical perspective. Carbohydr. Res. 503:108298. doi: 10.1016/j.carres.2021.108298
Moinet, G. Y. K., Hijbeek, R., van Vuuren, D. P., and Giller, K. E. (2023). Carbon for soils, not soils for carbon. Glob. Chang. Biol. 29, 2384–2398. doi: 10.1111/gcb.16570
Olaniyan, F. T., Alori, E. T., Adekiya, A. O., Ayorinde, B. B., Daramola, F. Y., Osemwegie, O. O., et al. (2022). The use of soil microbial potassium solubilizers in potassium nutrient availability in soil and its dynamics. Ann. Microbiol. 72:45. doi: 10.1186/s13213-022-01701-8
Osler, G. H., and Sommerkorn, M. (2007). Toward a complete soil C and N cycle: incorporating the soil fauna. Ecology 88, 1611–1621. doi: 10.1890/06-1357.1
Panichikkal, J., Prathap, G., Nair, R. A., and Krishnankutty, R. E. (2021). Evaluation of plant probiotic performance of Pseudomonas sp. encapsulated in alginate supplemented with salicylic acid and zinc oxide nanoparticles. Int. J. Biol. Macromol. 166, 138–143. doi: 10.1016/j.ijbiomac.2020.10.110
Pascale, A., Proietti, S., Pantelides, I. S., and Stringlis, I. A. (2019). Modulation of the root microbiome by plant molecules: the basis for targeted disease suppression and plant growth promotion. Front. Plant Sci. 10:1741. doi: 10.3389/fpls.2019.01741
Patra, S. K., Poddar, R., Brestic, M., Acharjee, P. U., Bhattacharya, P., Sengupta, S., et al. (2022). Prospects of hydrogels in agriculture for enhancing crop and water productivity under water deficit condition. Int. J. Polym. Sci. 2022:4914836. doi: 10.1155/2022/4914836
Paustian, K., Larson, E., Kent, J., Marx, E., and Swan, A. (2019). Soil C sequestration as a biological negative emission strategy. Front. Clim. 1:8. doi: 10.3389/fclim.2019.00008
Pauwels, R., Graefe, J., and Bitterlich, M. (2023). An arbuscular mycorrhizal fungus alters soil water retention and hydraulic conductivity in a soil texture specific way. Mycorrhiza 33, 165–179. doi: 10.1007/s00572-023-01106-8
Philippot, L., Chenu, C., Kappler, A., Rillig, M. C., and Fierer, N. (2024). The interplay between microbial communities and soil properties. Nat. Rev. Microbiol. 22, 226–239. doi: 10.1038/s41579-023-00980-5
Poudel, M., Mendes, R., Costa, L. A. S., Bueno, C. G., Meng, Y., Folimonova, S. Y., et al. (2021). The role of plant-associated bacteria, fungi, and viruses in drought stress mitigation. Front. Microbiol. 12:743512. doi: 10.3389/fmicb.2021.743512
Poveda, J., and González-Andrés, F. (2021). Bacillus as a source of phytohormones for use in agriculture. Appl. Microbiol. Biotechnol. 105:8629–8645. doi: 10.1007/s00253-021-11492-8
Power, B., Liu, X., Germaine, K. J., Ryan, D., Brazil, D., and Dowling, D. N. (2011). Alginate beads as a storage, delivery and containment system for genetically modified PCB degrader and PCB biosensor derivatives of Pseudomonas fluorescens F113. J. Appl. Microbiol. 110, 1351–2358. doi: 10.1111/j.1365-2672.2011.04993.x
Purohit, H. J., Pandit, P., Pal, R., Warke, R., and Warke, G. M. (2024). Soil microbiome: An intrinsic driver for climate smart agriculture. J. Agri. Food Res. 18:101433. doi: 10.1016/j.jafr.2024.101433
Ramos, J.-L., and Timmis, K. N. (2021). The contribution of microbiology toward attainment of sustainable development goals: the need to conserve soil health while maximizing its productivity. Environ. Microbiol. Rep. 13, 425–427. doi: 10.1111/1758-2229.12893
Ramzan, S., Rasool, T., Bhat, R. A., Ahmad, P., Ashraf, I., Rashid, N., et al. (2020). Agricultural soils a trigger to nitrous oxide: a persuasive greenhouse gas and its management. Environ. Monit. Assess. 192:436. doi: 10.1007/s10661-020-08410-2
Riaz, G., Shoaib, A., Javed, S., Perveen, S., Ahmed, W., El-Sheikh, M. A., et al. (2023). Formulation of the encapsulated rhizospheric Ochrobactrum ciceri supplemented with alginate for potential antifungal activity against the chili collar rot pathogen. South African J. Botany 161, 586–598. doi: 10.1016/j.sajb.2023.08.048
Rodriguez, H., Gonzalez, T., Goire, I., and Bashan, Y. (2004). Gluconic acid production and phosphate solubilization by the plant growth-promoting bacterium Azospirillum spp. Naturwissenschaften. 91, 552–555. doi: 10.1007/s00114-004-0566-0
Saad, M., Abo-Koura, H. A., Bishara, M. M., and Gomaa, I. M. (2020). Microencapsulation: toward the reduction of the salinity stress effect on wheat plants using NPK rhizobacteria. BJI. 23:1–18. doi: 10.9734/bji/2019/v23i430091
Sanow, S., Kuang, W., Schaaf, G., Huesgen, P., Schurr, U., Roessner, U., et al. (2023). Molecular mechanisms of pseudomonas -assisted plant nitrogen uptake: opportunities for modern agriculture. MPMI. 36, 536–548. doi: 10.1094/MPMI-10-22-0223-CR
Schmidt, H., Nunan, N., Höck, A., Eickhorst, T., Kaiser, C., Woebken, D., et al. (2018). Recognizing patterns: spatial analysis of observed microbial colonization on root surfaces. Front Environ Sci. 6:61. doi: 10.3389/fenvs.2018.00061
Shanmugavel, D., Rusyn, I., Solorza-Feria, O., and Kamaraj, S.-K. (2023). Sustainable SMART fertilizers in agriculture systems: a review on fundamentals to in-field applications. Sci. Total Environ. 904:166729. doi: 10.1016/j.scitotenv.2023.166729
Skrzypczak, D., Witek-Krowiak, A., Dawiec-Liśniewska, A., Podstawczyk, D., Mikula, K., and Chojnacka, K. (2019). Immobilization of biosorbent in hydrogel as a new environmentally friendly fertilizer for micronutrients delivery. J. Clean. Prod. 241:118387. doi: 10.1016/j.jclepro.2019.118387
Sofo, A., Dichio, B., Elshafie, H. S., Camele, I., Calabritto, M., Tomasi, I., et al. (2024). Enhancing soil properties through sustainable agronomic practices reduced the occurrence of kiwifruit vine decline syndrome. Soil Use Manage. 40:e13052. doi: 10.1111/sum.13052
Sokol, N. W., Slessarev, E., Marschmann, G. L., Nicolas, A., Blazewicz, S. J., Brodie, E. L., et al. (2022). Life and death in the soil microbiome: how ecological processes influence biogeochemistry. Nat. Rev. Microbiol. 20, 415–430. doi: 10.1038/s41579-022-00695-z
Song, B., Liang, H., Sun, R., Peng, P., Jiang, Y., and She, D. (2020). Hydrogel synthesis based on lignin/sodium alginate and application in agriculture. Int. J. Biol. Macromol. 144, 219–230. doi: 10.1016/j.ijbiomac.2019.12.082
Strullu-Derrien, C., Selosse, M.-A., Kenrick, P., and Martin, F. M. (2018). The origin and evolution of mycorrhizal symbioses: from palaeomycology to phylogenomics. New Phytol. 220, 1012–1030. doi: 10.1111/nph.15076
Suman, J., Rakshit, A., Ogireddy, S. D., Singh, S., Gupta, C., and Chandrakala, J. (2022). Microbiome as a key player in sustainable agriculture and human health. Front. Soil Sci. 2:821589. doi: 10.3389/fsoil.2022.821589
Tao, F., Huang, Y., Hungate, B. A., Manzoni, S., Frey, S. D., Schmidt, M. W. I., et al. (2023). Microbial carbon use efficiency promotes global soil carbon storage. Nature 618, 981–985. doi: 10.1038/s41586-023-06042-3
Tariq, Z., Najaf, I. D., Rizwan, M., Ahmad, M., Faheem, M., and Ahmed, M. (2023). Significance of biopolymer-based hydrogels and their applications in agriculture: a review in perspective of synthesis and their degree of swelling for water holding. RSC Adv. 13:24731–24754. doi: 10.1039/D3RA03472K
Tian, H., Xu, R., Canadell, J. G., Thompson, R. L., Winiwarter, W., Suntharalingam, P., et al. (2020). A comprehensive quantification of global nitrous oxide sources and sinks. Nature 586, 248–256. doi: 10.1038/s41586-020-2780-0
Vafadar, F., Amooaghaie, R., and Otroshy, M. (2014). Effects of plant-growth-promoting rhizobacteria and arbuscular mycorrhizal fungus on plant growth, stevioside, NPK, and chlorophyll content of Stevia rebaudiana. J. Plant Interact. 9, 128–136. doi: 10.1080/17429145.2013.779035
van der Heijden, M. G. A., Bardgett, R. D., and van Straalen, N. M. (2008). The unseen majority: soil microbes as drivers of plant diversity and productivity in terrestrial ecosystems. Ecol. Lett. 11, 296–310. doi: 10.1111/j.1461-0248.2007.01139.x
Vejan, P., Khadiran, T., Abdullah, R., Ismail, S., and Dadrasnia, A. (2019). Encapsulation of plant growth promoting Rhizobacteria—prospects and potential in agricultural sector: a review. J. Plant Nutr. 42, 2600–2623. doi: 10.1080/01904167.2019.1659330
Vemmer, M., Schumann, M., Beitzen-Heineke, W., French, B. W., Vidal, S., and Patel, A. V. (2016). Development of a CO-releasing coformulation based on starch, Saccharomyces cerevisiae and Beauveria bassiana attractive towards western corn rootworm larvae. Pest Manag. Sci. 72, 2136–2145. doi: 10.1002/ps.4245
Vinceković, M., Jalšenjak, N., Topolovec-Pintarić, S., Đermić, E., Bujan, M., and Jurić, S. (2016). Encapsulation of biological and chemical agents for plant nutrition and protection: chitosan/alginate microcapsules loaded with copper cations and Trichoderma viride. J. Agric. Food Chem. 64, 8073–8083. doi: 10.1021/acs.jafc.6b02879
Waltz, E. (2023). Small innovators advance microbes as alternatives to chemical crop sprays. Nat. Biotechnol. 41, 162–164. doi: 10.1038/s41587-023-01670-6
Wan, N.-F., Fu, L., Dainese, M., Kiær, L. P., Hu, Y.-Q., Xin, F., et al. (2025). Pesticides have negative effects on non-target organisms. Nat. Commun. 16:1360. doi: 10.1038/s41467-025-56732-x
Wang, B., An, S., Liang, C., Liu, Y., and Kuzyakov, Y. (2021). Microbial necromass as the source of soil organic carbon in global ecosystems. Soil Biol. Biochem. 162:108422. doi: 10.1016/j.soilbio.2021.108422
Wang, L., Zhang, L., George, T. S., and Feng, G. (2023). A core microbiome in the hyphosphere of arbuscular mycorrhizal fungi has functional significance in organic phosphorus mineralization. New Phytol. 238, 859–873. doi: 10.1111/nph.18642
Warmink, J. A., Nazir, R., Corten, B., and van Elsas, J. D. (2011). Hitchhikers on the fungal highway: the helper effect for bacterial migration via fungal hyphae. Soil Biol. Biochem. 43, 760–765. doi: 10.1016/j.soilbio.2010.12.009
Wei, X., Chen, H., Lin, D., Xu, H., Wang, J., Zhang, J., et al. (2023). A field study of nano-FeS loaded lignin hydrogel application for Cd reduction, nutrient enhancement, and microbiological shift in a polluted paddy soil. Chem. Eng. J. 451:138647. doi: 10.1016/j.cej.2022.138647
Weller, D. M., Raaijmakers, J. M., Gardener, B. B. M., and Thomashow, L. S. (2002). Microbial populations responsible for specific soil suppressiveness to plant pathogens. A Rev. of Phytopath. 40:309–348. doi: 10.1146/annurev.phyto.40.030402.110010
Wichterle, O., and Lím, D. (1960). Hydrophilic gels for biological use. Nature 185, 117–118. doi: 10.1038/185117a0
Williams, A., and de Vries, F. T. (2020). Plant root exudation under drought: implications for ecosystem functioning. New Phytol. 225, 1899–1905. doi: 10.1111/nph.16223
Wing, I. S., De Cian, E., and Mistry, M. N. (2021). Global vulnerability of crop yields to climate change. J Environ Econ Manag. 109:102462. doi: 10.1016/j.jeem.2021.102462
Wipf, H. M.-L., Xu, L., Gao, C., Spinner, H. B., Taylor, J., Lemaux, P., et al. (2021). Agricultural soil management practices differentially shape the bacterial and fungal microbiomes of Sorghum bicolor. Appl. Environ. Microbiol. 87, e02345–e02320. doi: 10.1128/AEM.02345-20
Wu, Z., Su, X., Xu, Y., Kong, B., Sun, W., and Mi, S. (2016). Bioprinting three-dimensional cell-laden tissue constructs with controllable degradation. Sci. Rep. 6:24474. doi: 10.1038/srep24474
Wyant, J. G., Meganck, R. A., and Ham, S. H. (1995). A planning and decision-making framework for ecological restoration. Environ. Manage. 19, 789–796. doi: 10.1007/BF02471932
Yang, L., Wang, S., Wang, R., Zheng, Q., Ma, Q., Huang, S., et al. (2021). Floating chitosan-alginate microspheres loaded with chlorantraniliprole effectively control Chilo suppressalis (Walker) and Sesamia inferens (Walker) in rice fields. Sci. Total Environm. 783:147088. doi: 10.1016/j.scitotenv.2021.147088
Yang, S., Chen, X., Jiang, Z., Ding, J., Sun, X., and Xu, J. (2020). Effects of biochar application on soil organic carbon composition and enzyme activity in paddy soil under water-saving irrigation. Int. J. Environ. Res. Public Health 17, 333. doi: 10.3390/ijerph17010333
Yang, Y., Tilman, D., Jin, Z., Smith, P., Barrett, C. B., Zhu, Y.-G., et al. (2024). Climate change exacerbates the environmental impacts of agriculture. Science 385:eadn3747. doi: 10.1126/science.adn3747
Zha, X., Hou, X., Li, Q., Nan, H., Ge, F., Liu, Y., et al. (2022). Loading glyphosate in attapulgite and sodium alginate hydrogels to construct pH-responsive controlled release microsphere for enhanced soil sustained release. ACS Agric Sci Technol. 2, 1090–1100. doi: 10.1021/acsagscitech.2c00195
Zhang, H., Wang, L., Van herle, J., Maréchal, F., and Desideri, U. (2020). Techno-economic comparison of green ammonia production processes. Appl. Energy 259:114135. doi: 10.1016/j.apenergy.2019.114135
Zhang, L., Zhou, J., George, T. S., Limpens, E., and Feng, G. (2022). Arbuscular mycorrhizal fungi conducting the hyphosphere bacterial orchestra. Trends Plant Sci. 27:402–411. doi: 10.1016/j.tplants.2021.10.008
Zhang, Y., Hu, T., Wang, H., Jin, H., Liu, Q., Lin, Z., et al. (2021). How do different nitrogen application levels and irrigation practices impact biological nitrogen fixation and its distribution in paddy system? Plant Soil. 467, 329–344. doi: 10.1007/s11104-021-05093-7
Zhao, W., Du, E., Luo, R., Chen, Y., Sun, Z., and Gui, F. (2024). Arbuscular mycorrhizal fungus and Pseudomonas bacteria affect tomato response to Tuta absoluta (Lepidoptera: Gelechiidae) herbivory. BMC Plant Biol. 24:1236. doi: 10.1186/s12870-024-05952-2
Zheng, W., Zeng, S., Bais, H., LaManna, J. M., Hussey, D. S., Jacobson, D. L., et al. (2018). Plant growth-promoting rhizobacteria (PGPR) reduce evaporation and increase soil water retention. Water Resour. Res. 54, 3673–3687. doi: 10.1029/2018WR022656
Zomer, R. J., Bossio, D. A., Sommer, R., and Verchot, L. V. (2017). Global sequestration potential of increased organic carbon in cropland soils. Sci. Rep. 7:15554. doi: 10.1038/s41598-017-15794-8
Keywords: crop health, environmental biotechnology, fungal-prokaryotic interactions, soil dysbiosis, soil microbial ecology, sustainable agriculture
Citation: Davis RA, Mafune KK and Winkler MKH (2025) Biodegradable hydrogels and microbial consortia as a treatment for soil dysbiosis. Front. Microbiol. 16:1565940. doi: 10.3389/fmicb.2025.1565940
Received: 24 January 2025; Accepted: 11 April 2025;
Published: 01 May 2025.
Edited by:
John Jacob Parnell, Food and Agriculture Organization of the United Nations, ItalyReviewed by:
Hongchen Jiang, China University of Geosciences Wuhan, ChinaCopyright © 2025 Davis, Mafune and Winkler. This is an open-access article distributed under the terms of the Creative Commons Attribution License (CC BY). The use, distribution or reproduction in other forums is permitted, provided the original author(s) and the copyright owner(s) are credited and that the original publication in this journal is cited, in accordance with accepted academic practice. No use, distribution or reproduction is permitted which does not comply with these terms.
*Correspondence: Korena K. Mafune, a21hZnVuZUB1dy5lZHU=
†ORCID: Renee A. Davis orcid.org/0000-0001-7526-7656
Korena K. Mafune orcid.org/0000-0002-9673-5049
Mari K. H. Winkler orcid.org/0000-0002-8366-3293