- 1Department of Botany, School of Life Sciences, Periyar University, Salem, India
- 2Department of Plant Protection, College of Agriculture and Food, Qassim University, Buraidah, Saudi Arabia
- 3Faculty of Health and Life Sciences (FHLS), Inti International University, Nilai, Malaysia
- 4Innovation Centre in Agritechnology for Advanced Bioprocessing (ICA), Universiti Teknologi Malaysia, Johor Bahru, Malaysia
- 5Faculty of Chemical and Energy Engineering, Universiti Teknologi Malaysia (UTM), Johor Bahru, Malaysia
- 6PG and Research Department of Botany, Padmavani Arts and Science College for Women, Salem, India
- 7Key Laboratory of Integrated Crop Pest Management of Anhui Province, College of Plant Protection, Anhui Agricultural University, Hefei, Anhui, China
- 8Department of Biological Sciences and Chemistry, University of Nizwa, Nizwa, Oman
Introduction: Salt stress is a major global issue that negatively affects plant growth and physiological processes. Plant growth-promoting rhizobacteria (PGPR) are known to alleviate salt stress and promote plant growth. This study aimed to isolate and characterize salt-tolerant PGPR from salinity-affected soils in Tamil Nadu, India, and assess their potential to enhance growth and salt tolerance in sesame (Sesamum indicum L.).
Methods: Salt-tolerant PGPR were isolated and screened for plant growth-promoting traits. One isolate, designated PAS1, demonstrated significant capabilities, including the production of indole-3-acetic acid (IAA; 48.56 μg ml−1), siderophore production (89.20 ± 0.65%), phosphate solubilization (7.8 mm zone of clearance), ammonia, and hydrogen cyanide (HCN) production. PAS1 was identified as Bacillus flexus. Sesame plants were inoculated with B. flexus and grown under different salt concentrations (0, 100, and 200 mM NaCl) for 45 days.
Results: Inoculation with B. flexus significantly improved the biochemical parameters of sesame plants under salt stress, including increased chlorophyll content (4.4 mg g−1), proline (0.0017 mg g−1), soluble sugars (61.34 mg g−1), amino acids (1.10 mg g−1), and proteins (3.31 mg g−1). Additionally, antioxidant enzyme activities were enhanced, as indicated by DPPH scavenging activity (60.25%), superoxide dismutase (231.29 U mg g−1 protein), peroxidase (6.21 U mg g−1 protein), catalase (3.38 U mg g−1 protein), and a reduction in malondialdehyde (23.32 μmol g−1).
Discussion: The study demonstrates that inoculation with salt-tolerant B. flexus can effectively improve sesame plant growth and enhance tolerance to salt stress. These findings suggest that halo-tolerant PGPR strains like B. flexus could serve as promising biofertilizers to improve crop productivity in salt-affected agricultural soils.
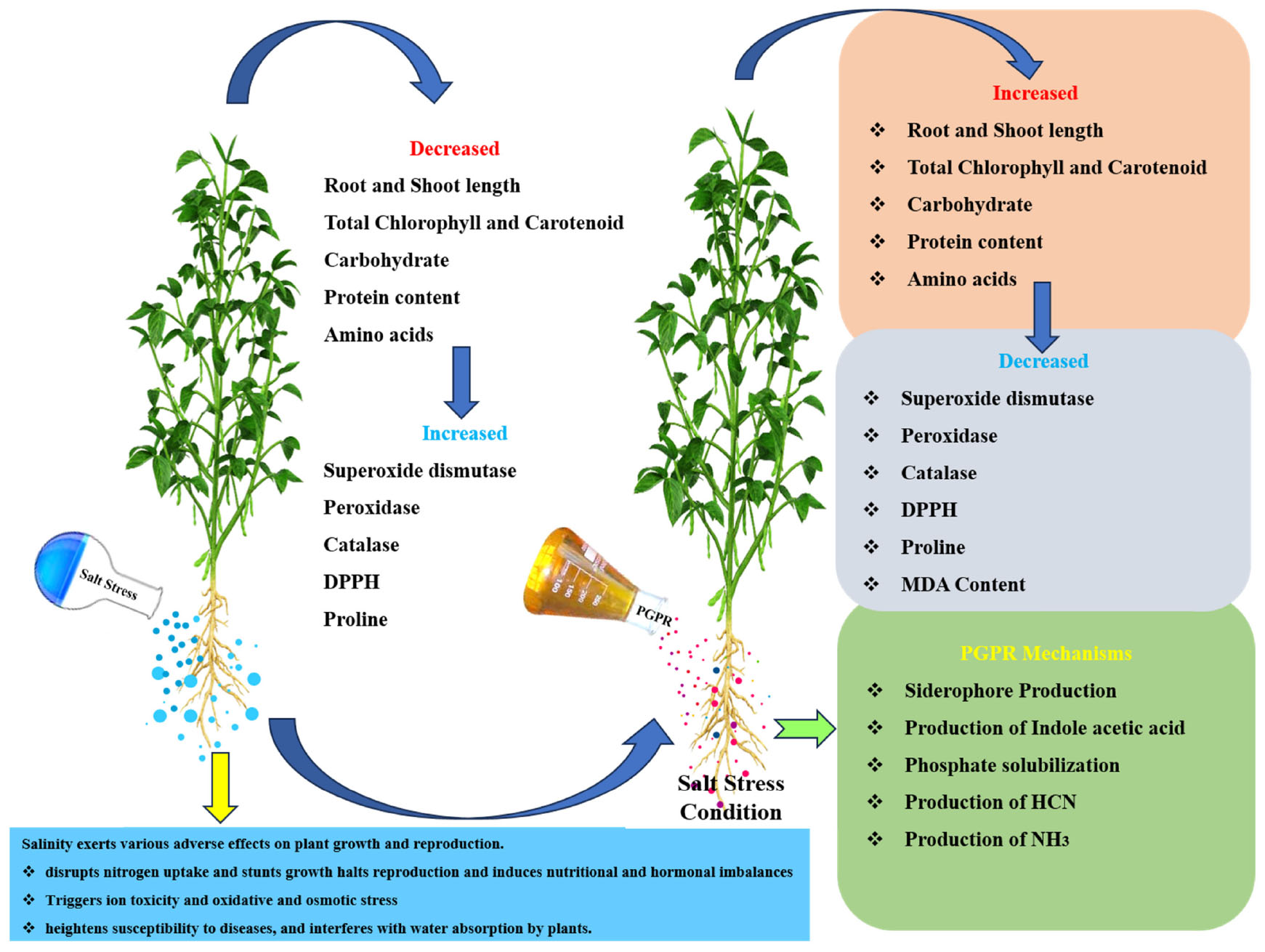
Graphical Abstract. Role of Bacillus flexus in enhancing sesame growth under salt stress through PGPR mechanisms and improved physiological responses.
Highlights
• Salt-tolerant PGPR strain protects inoculated sesame plants against salinity stress.
• The effect of PAS1 treatment on sesame plant showed restoration of physiological traits from salinity stress condition to normal condition.
• Bacillus flexus inoculation increased antioxidant defense mechanisms under salt conditions.
• The findings of this study strongly support future efforts to manage plant salinity stress with the development of effective bioinoculants for agricultural use.
Introduction
Climate change is the most pressing challenge of our era, impacting the earth in diverse ways. Rising sea levels, driven by climate change, are causing saltwater intrusion into agricultural lands, increasing soil salinity. This salinization ultimately contributes to global food insecurity and diminished agricultural productivity (Khan et al., 2021; Mukhopadhyay et al., 2021; Gulzar et al., 2025). Globally, 20% of irrigated lands are severely damaged by salinity (Singh, 2022; Patel et al., 2023), and this land degradation is projected to reach 50% by 2050 (Dias et al., 2021). Approximately 70% of yield loss in cereal crops, including wheat, rice, sorghum, sesame, and barley, is attributed to soil contamination from salinity and sodicity (Sagar et al., 2022a; Awaad, 2023).
Salinity, an abiotic chemical stress, is characterized by the accumulation of soluble salts in the rhizosphere, adversely affecting plant metabolism in two primary ways (Kalam et al., 2020; Khan et al., 2021). First, high salt concentrations create hyper-osmotic and hypertonic environments that damage the root system, impairing water and nutrient uptake (Seleiman et al., 2022; Zhang et al., 2024). This leads to secondary stresses, such as oxidative stress, which induces membrane instability through DNA and protein denaturation and lipid peroxidation. Ultimately, these effects trigger programmed cell death (apoptosis) and cause the deterioration of the entire plant (Debnath et al., 2021; Paes de Melo et al., 2022).
Remediation of saline soils traditionally involves inorganic amendments (e.g., gypsum, lime, sulfuric acid derivatives, and synthetic fertilizers) and organic amendments (e.g., green manure, farmyard manure, and industrial wastes such as press mud) (Kapadia et al., 2021; Kour et al., 2021). However, these methods have shown limited success in improving stress tolerance in economically important crops under field conditions due to several factors (Soto-Gómez and Pérez-Rodríguez, 2022). Plant growth-promoting rhizobacteria (PGPR) has recently gained attention as a sustainable and eco-friendly approach to enhance crop production in salt-affected lands (Nasab and Sayyed, 2019; Sagar et al., 2022b; Kapadia et al., 2022; Jabborova et al., 2025). These beneficial bacteria colonize plant roots, thrive in hyper-saline environments, and improve plant growth and yield (Choudhary et al., 2022; Kumawat et al., 2022). Rhizobacteria have been reported to promote plant growth effectively, even under saline conditions (Ayuso-Calles et al., 2021; Basu et al., 2021). Halophilic and halo-tolerant PGPR employ several mechanisms to withstand severe environmental stresses and minimize yield loss (Hernández-Canseco et al., 2022). These include synthesizing osmolytes to maintain cellular osmotic balance, regulating ion transporters to reduce the toxic effects of Na+ and Cl– ions, and activating plant defense systems to scavenge reactive oxygen species (ROS) and alleviate oxidative stress (Kusale et al., 2021; Chattha et al., 2022). Furthermore, these microorganisms produce phytohormones, fix nitrogen, solubilize phosphate, generate volatile compounds, and produce antifungal or antibacterial metabolites (Hamid et al., 2021; Koza et al., 2022; Dave et al., 2024; Praveen et al., 2024; Anbalagan et al., 2025; Bright et al., 2025). They also aid in mobilizing mineral ions, enhancing photosynthesis, and regulating osmotic pressure through the heterodimerization of acids (Burnap, 2023; Sethi et al., 2025). Additionally, PGPR contribute to plant defense by producing antioxidants, betaines, and other compounds that mitigate the harmful effects of ROS and toxins (Sagar et al., 2020; Hasanuzzaman et al., 2021).
Sesamum indicum L. is a valuable oilseed crop cultivated widely in India, China, Thailand, Mexico, Guatemala, El Salvador, Afghanistan, Pakistan, Bangladesh, Indonesia, Sri Lanka, Saudi Arabia, and Turkey (Morris et al., 2023). Sesame seeds are highly valued for their rich oil, protein, and antioxidant content, making them essential in food, nutraceuticals, pharmaceuticals, and various industries (Wei et al., 2022). They offer health benefits such as reducing cancer risk, mitigating mucosal, colon, and liver damage, lowering serum cholesterol levels, and improving vitamin E activity and α-tocopherol availability (Zainal et al., 2022). Sesame seeds also help reduce thiobarbituric acid reactive substances (TBARS) (Mitsiopoulou et al., 2021). Lignan in sesame seeds acts as a phytoestrogens and is converted to enterolactone, which is crucial in preventing hormone-dependent cancers (e.g., breast and prostate) and cardiovascular diseases (Jang et al., 2022).
The present study aimed to evaluate the role of halo-tolerant plant growth-promoting (PGP) microorganisms in sesame. Plant parameters for evaluation include the enhancement of growth and yield and morpho-physiological and biochemical traits. The findings will help explore the potential of salt-tolerant PGPR strains as biofertilizers to mitigate yield loss caused by salt stress in sesame crops. Ultimately, this research may contribute to developing effective biomass solutions for managing complex, saline soils.
Materials and methods
Isolation of potent PGPR
Plant growth promoting rhizobacteria cultures were isolated from the saline soil of Kanniyakumari, Tamil Nadu, India (8°09′′20′′N, 77°27′27′′E). Using the serial dilution method, 100 μL aliquots were spread onto nutrient agar (NA) plates, the plates were incubated at 30 ± 2°C for 24 h. Morphologically distinct bacterial colonies were selected, isolated, and purified. The purified isolate designated PAS1 was used for further studies.
Gram-staining and biochemical properties of the isolate
Purified culture of each isolate was stained using Gram’s staining and observed under an oil immersion microscope at 100 × magnification (Coico, 2006). The catalase activity of the bacterial isolates was tested according to Whittenbury (1964) method. A small amount of bacterial colony was mixed in a drop of 3% hydrogen peroxide and observed for the evolution of gas bubbles as an indication of a positive catalase test. The ability of the isolate to utilize citrate as the sole carbon source was assessed using Simmons citrate agar medium, as described by MacWilliams (2009). The inoculated plates were incubated at 30°C for 7 days and observed for color change from green to blue as an indication of a positive citrate test.
Evaluation of plant growth-promoting traits
Siderophore production
The Chrome Azurol Sulfonate (CAS) agar plate method was used to assess siderophore production (Alexander and Zuberer, 1991; Patel et al., 2018). Bacterial isolates were grown on CAS at 30°C for 24–48 h and observed for the development of an orange halo around the colonies. For quantitative estimation, bacterial culture was grown in succinate medium at 30°C for 24–48 h, and the amount of siderophore was estimated according to Himpsl and Mobley (2019) and expressed as siderophore units (SU), using the following formula.
where As is the absorbance of the sample (CAS reagent mixed with the bacterial supernatant), and Ar is the absorbance of the reference (CAS reagent mixed with uninoculated medium).
Indole acetic acid production
Production of IAA by isolates was determined using the method of Gordon and Weber (1951). The bacterial isolates were grown in Luria and Bertani (LB) broth enriched with tryptophan (100 μg ml–1) at 30 ± 2°C for 72 h. These culture supernatants from the isolate were combined with Salkowski’s reagent in a 1:2 ratio and observed for the development of a pink color as an indication of IAA production. The amount of IAA produced was measured spectrophotometrically at 530 nm, using an IAA calibration curve (10–100 μg ml–1).
Ammonia production
For this, 24 h old bacterial cultures were individually grown in 10 ml of peptone at 30 ± 2°C for 48 h. Following incubation, 0.5 ml of Nessler’s reagent was added, and observed for color change from brown to yellow as a signal of the presence of ammonia (Hills, 1940).
Hydrogen cyanide production
Hydrogen cyanide production was assessed using the procedure described by Lorck (1948). Bacterial isolates were inoculated into LB broth containing glycine, and Whatman No. 1 filter paper soaked in a solution of 0.5% C6H3N3O7 and 2% Na2CO3 was placed on top of the inside of the tubes. A color change of the filter paper from orange to red indicated HCN production.
Phosphate solubilization
Phosphate (P) solubilization was determined using Pikovskaya’s agar medium (Nautiyal, 1999). Bacterial colonies were spot-inoculated at the center of the plates and incubated for 7 days at 30°C and observed for the formation of clear halo zones of P solubilization around the colonies. The P solubilization ability was quantified by calculating the Solubilization Index (SI) using the following formula:
Identification of isolate through 16s rRNA sequencing
Genomic DNA was extracted from the screened multifunctional PGPB strain using a commercial bacterial genomic DNA extraction kit, following the manufacturer’s instructions. The extracted DNA was used as a template for polymerase chain reaction (PCR) amplification of the 16s rRNA gene, using universal bacterial primers 27F (5′-AGAGTTTGATCCTGGCTCAG-3′) and 1492R (5′-GGTTACCTTGTTACGACTT-3′). The PCR reaction mixture (25 μL) contained 1 μL of DNA template, 0.5 μL of primer 27F, 0.5 μL of primer 1492R, 12.5 μL of 2x Taq PCR Master Mix, and 10.5 μL of nuclease-free water. PCR conditions were as follows: initial denaturation at 93°C for 3 min, followed by 32 cycles of denaturation at 93°C for 30 s, annealing at 56°C for 30 s, and extension at 72°C for 2 min with a final extension at 72°C for 7 min. The amplified PCR products were subjected to bidirectional sequencing by BGI. The obtained sequences were assembled and analyzed using BLAST against the NCBI GenBank database for species-level identification. A phylogenetic tree was constructed using the neighbor-joining method in MEGA version 11.0, and the reliability of the tree was assessed through bootstrap analysis with 1,000 replicates (Sanschagrin and Yergeau, 2014).
Pot experiment design
Various physicochemical properties of the soil were assessed according to the methods described by Jackson (1985). The selected bacterial isolate B. flexus (PAS1) was grown in LB broth at 28 ± 1°C for 24 h under a shaking at 100 rpm. After incubation, the bacterial culture was applied to the soil in pots.
Treatment 1: Control
Treatment 2: Control+PAS1
Treatment 3: NaCl 100 mM
Treatment 4: NaCl 100 mM+PAS1
Treatment 5: NaCl 200 mM
Treatment 6: NaCl 200 mM+PAS1
The experiment included treatments with B. flexus (PAS1) in control soil and soils supplemented with NaCl at concentrations of 0 mM, 100 mM, and 200 mM, with each treatment replicated three times. Sesame seeds (TMV7) were soaked in the PAS1 inoculum for 1 h before being sown in the treated pots. The physicochemical properties of the test soil were pH 8.02, EC 3.17 dS/m, K+ 62 kg/acre, Na+ 10.12 me/L, SAR 5.72 mmolc/L, and ESP 8.12%. Based on these properties, the soil was classified as saline. Plants were harvested after 45 days, and root and shoot length measurements, metabolites, and antioxidant enzymes were recorded.
Seed dormancy, germination, and morphological traits
Sesame seeds were surface sterilized and plated on 0.6% agar (pH 5.7) for 1 h. The seeds were then exposed to light conditions for 4 days, and germination frequency was recorded. For light-mediated germination assays, seeds were stored dry at 25°C for 2–5 months before being surface sterilized, plated on 0.6% agar with 0.01% (v/v) ethanol (mock) for 1 h, and treated with salt stress (Bentsink and Koornneef, 2008). The growth of the radical defines germination. After 45 days, the growth of sesame seedlings was examined, and growth parameters such as root length and shoot length were measured.
Determination of chlorophyll content and carotenoids
The total chlorophyll content and carotenoids in the fresh leaves of plants were determined using the method outlined by Arnon (1949).
Primary metabolites
Estimation of carbohydrate
The total carbohydrate content of the leaf was determined using the protocol Hedge and Hofreiter (1962). 0.5 g of dried leaves were ground with 10 mL of 1 N sulfuric acid, then transferred to a test tube and heated at 100°C for 24 h. Then, 1 mL of sugar solution was mixed with 1 mL of 5% phenol solution, after which 5.0 mL of sulfuric acid was added. Incubation was carried out for 20 min at 23–30°C. The total carbohydrate content was measured at 490 nm.
Estimation of protein
The total protein content in the leaves was measured following the method of Bradford (1976). Plant leaves were first powdered in liquid nitrogen and then homogenized with a 50 mM Na-phosphate buffer (pH 7.6). The homogenate was centrifuged at 6,000 × g for 20 min at 4°C, and the absorbance of the supernatant was recorded at 595 nm. The protein content was estimated and the standard curve was created using the Bovine Serum Albumin (BSA) standards.
Estimation of amino acids
The leaf extract was blended with 3 mL of 80% (v/v) methanol and subjected to incubation in hot water at 70°C for 30 min. Following this, an equal volume of 5% phenol and 1.5 ml of concentrated H2SO4 was introduced to the mixture, which was subsequently incubated in the dark for an additional 30 min. The absorbance of the resulting reaction mixture was measured at 490 nm (Roland and Gross, 1954).
Estimation of proline
The proline content in fresh leaves was determined as per Bates (1973). Fresh leaves (0.1 g) were homogenized in 3 mL of 3% sulfosalicylic acid and heated at 95°C for 15 min. The homogenate was then centrifuged at 8,000 × g for 10 min. The supernatant was mixed with equal glacial acetic acid, 2% acid ninhydrin, and 6 M orthophosphoric acid. This mixture was heated in a boiling water bath for 30 min and then cooled to room temperature for 30 min. After adding 1 mL of toluene and shaking vigorously for 30 s, the absorbance of the upper toluene phase was measured at 520 nm using toluene as a blank. Free proline content was determined by comparing the absorbance to a standard curve of pure L-proline and was calculated based on fresh weight, expressed as mg per 100 g FW.
Antioxidant enzyme activities
Scavenging of the DPPH radical analysis
2,2-diphenyl-1-picrylhydrazyl (DPPH) radical scavenging activity was assessed according to the method described by Brand-Williams et al. (1995). A total of 1 g of plant powder was mixed with 2 mL of 80% (v/v) methanol and shaken for 24 h at 25°C. The mixture was then centrifuged at 12,000 × g for 20 min, and the supernatant was collected. This supernatant was combined with an equal volume of DPPH solution (0.1 M), and the reaction mixtures were incubated in the dark for 40 min. The absorbance was then measured at 515 nm.
SOD, CAT, and POD enzyme activities
Superoxide dismutase (SOD) activity was assessed following the method outlined by Flohé and Ötting (1984). One unit of the enzyme is defined as the amount of SOD that inhibits 50% of nitro blue tetrazolium at 25°C. The reaction mixture consisted of 50 mM sodium phosphate buffer (pH 7.6), 13 mM methionine, 75 μM NBT, 2 μM riboflavin, 0.1 mM EDTA, and 0–50 μL of enzyme extract, with riboflavin being added last. The tube was then shaken and exposed to a 40-watt fluorescent lamp for 15 min, and absorbance was subsequently measured at 560 nm.
Catalase (CAT) activity was measured by converting H2O2 to water and oxygen, following the method described by Lück (1965a). For the peroxidase (POD) activity assay, the reaction mixture consisted of 750 μL of 50 mM phosphate buffer, 100 μL of 20 mM guaiacol, 100 μL of 40 mM H2O2, and 100 μL of enzyme extract. The absorbance of the mixture was recorded every 20 s at 470 nm for 3 min (Lück, 1965b).
Estimation of lipid peroxidation
Two grams of fresh leaf tissue was homogenized in 600 μL of 0.1% trichloroacetic acid (TCA). The homogenate was centrifuged at 15,000 × g for 20 min at 4°C. A total of 1.5 mL of 20% TCA containing 0.5% Thio barbituric acid (TBA) was added to the supernatant, and the mixture was heated at 95°C for 25 min. After heating, the mixture was cooled and centrifuged at 15,000 × g for 5 min at 4°C. The absorbance of the supernatant was then measured at 532 nm. The results were expressed as μmol MDA per gram of fresh weight (FW) (Moore and Roberts, 1998).
Data analysis
The experiments were conducted in triplicate (n = 3). Graphs were generated using Prism software version 5.01 (Graph Pad Software Inc., United States), and data were analyzed using SPSS 16.0 employing One-way ANOVA. Statistical significance was determined using Tukey’s multiple comparison tests (P < 0.05).
Results
Isolation of salt-tolerant PGPR strains and identification of PGPR characteristics
Bacterial isolate obtained from high-salinity areas of the Kanyakumari district, India, produced siderophore production, IAA, ammonia, HCN, and solubilized phosphate. It was Gram-positive, motile, rod and exhibited catalase activity and utilized citrate (Supplementary Table 1).
Analysis of 16s rRNA sequencing followed by a phylogenetic tree
PCR amplification of the 16s rRNA gene was carried out for the identification of the selected bacterial isolate. The amplified sequence was analyzed through the NCBI BLAST search and has been submitted to the GenBank (NCBI accession number: PP275111). The isolate was identified as Bacillus flexus. A phylogenetic tree was constructed based on the 16s rRNA gene sequence using the neighbor-joining method in MEGA version 11.0. The tree indicated that the isolate belongs to the genus Bacillus and clustered closely with B. flexus, thereby confirming its identity (Figure 1).
Effect of B. flexus on salt stress on S. indicum growth parameters
The plant was grown in different concentrations of NaCl (Control, 100 mM, and 200 mM). The shoot lengths of the inoculated were 21.7, 19.3, and 17.1, and the shoot lengths of the non-inoculated were 20.5, 18.6, and 16.2. This inoculated strain gave good results when grown under NaCl compared to the control (Figure 2a). Similar to shoot length, the root length of the inoculated was 7.3, 5.4, 3.9, and the root length of the non-inoculated was 6.5, 4.7, and 3.1. As mentioned above, root length in inoculation showed better results than the control (Figure 2b).
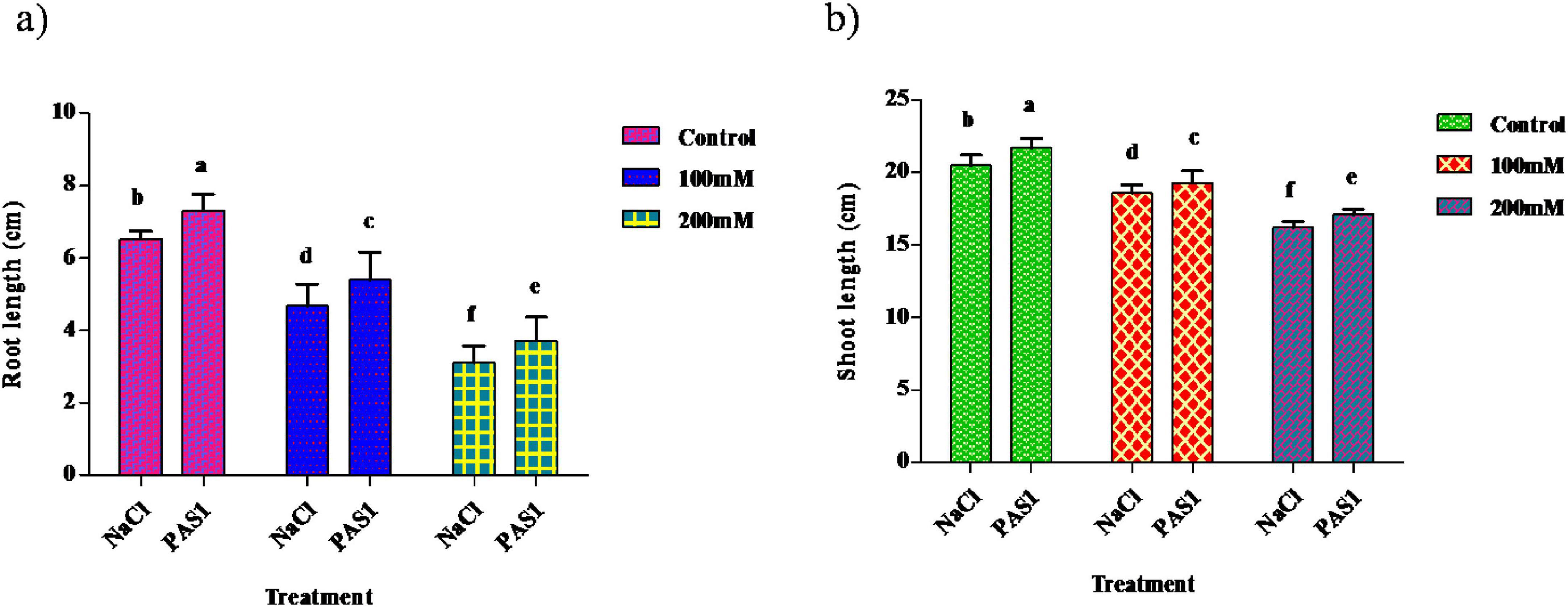
Figure 2. The effect of plant growth-promoting rhizobacteria (PGPR) B. flexus (PAS1) on plant growth parameters was evaluated in pots subjected to salt stress. The parameters measured were root (a) and shoot (b) length. Error bars represent the standard deviation (n = 3). Small alphabetical letters above the error bars indicate significant differences between treatments according to Tukey’s post-hoc test (p = 0.05).
Determination of total chlorophyll (TC) and carotenoids in S. indicum with B. flexus under salt stress
Chlorophyll is essential in photosynthesis and is plants’ light energy source. As soil salinity increases, chlorophyll decreases. The chlorophyll content in the plant was higher in the inoculated samples (4.4, 3.1, and 2.6 mg g–1) compared to the control (4.0, 2.8, and 2.1 mg g–1) (Figure 3a). Similarly, a substantial increase in carotenoid content was (0.97, 0.69, and 0.57 mg g–1) was evident over the control (0.29, 0.62, and 0.48 mg g–1) (Figure 3b). However, the amount of chlorophyll pigments and carotenoid content decreased with increasing salinity.
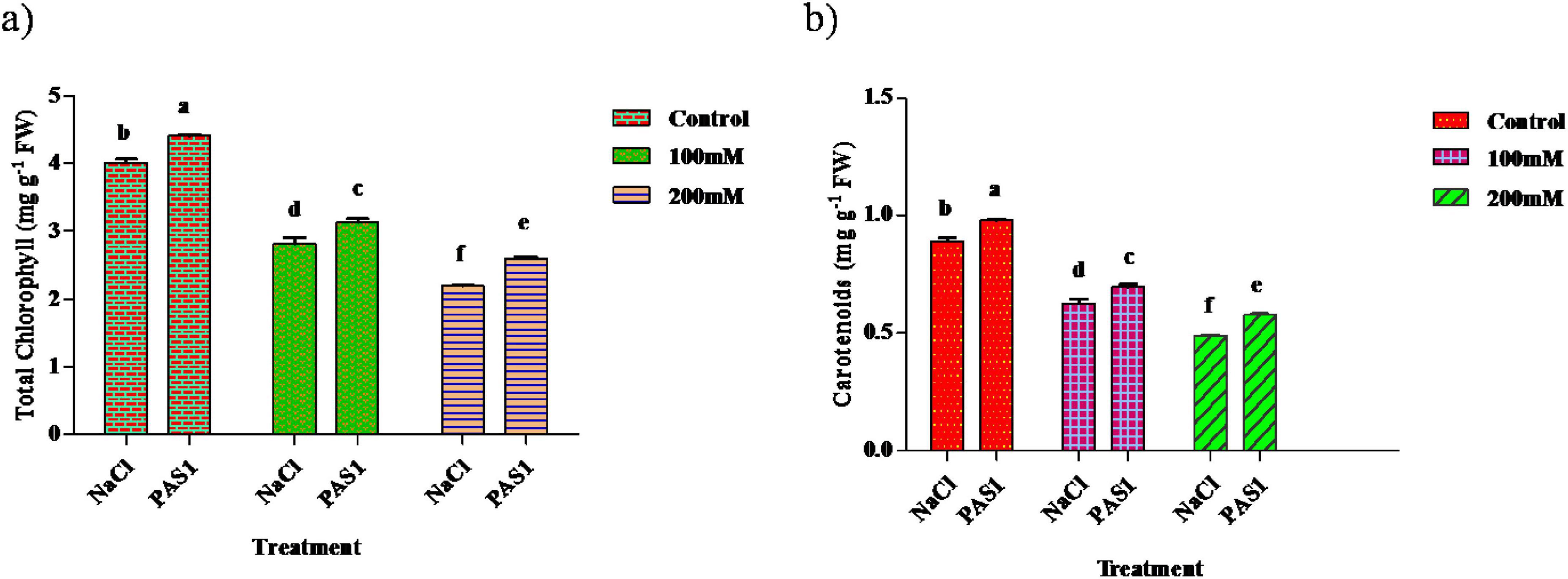
Figure 3. The effect of plant growth-promoting rhizobacteria (PGPR) B. flexus (PAS1) on photosynthetic pigments, total chlorophyll (a), and carotenoid (b) levels under NaCl stress in sesame leaves was investigated. Error bars represent the standard deviation (n = 3). Small alphabetical letters above the error bars indicate significant differences between treatments according to Tukey’s post-hoc test (p = 0.05).
Determination of primary metabolites in S. indicum with B. flexus under salt stress
Regarding carbohydrate content, a significant increase was observed in inoculated plants compared to control. These are 61.34, 59.83, 57.53,60.27, 57.06, and 55.03 mg g–1 inoculated and non-inoculated, respectively (Figure 4a). Also, the protein level was higher in inoculated plants (3.31, 3.22, and 3.10 mg g–1), than in untreated (control) plants 3.20, 3.13, and 3.08 mg g–1 (Figure 4b). Amino acid concentration also increased in plants grown under salt stress. A higher amino acid content was recorded in PGPR treated plants (1.15, 1.33, and 1.57 mg g–1) than control plants (1.15, 1.26, and 1.48 mg g–1) (Figure 4c). Higher levels of proline contents were observed in PGPR treated plant grown under salinity to be 0.0176, 0.0137, 0.0017 mg g–1 higher in untreated plants. Also, treated plants exhibited lower proline levels with values of 0.0153, 0.0115, and 0.0022 mg g–1 compared to the control (Figure 4d).
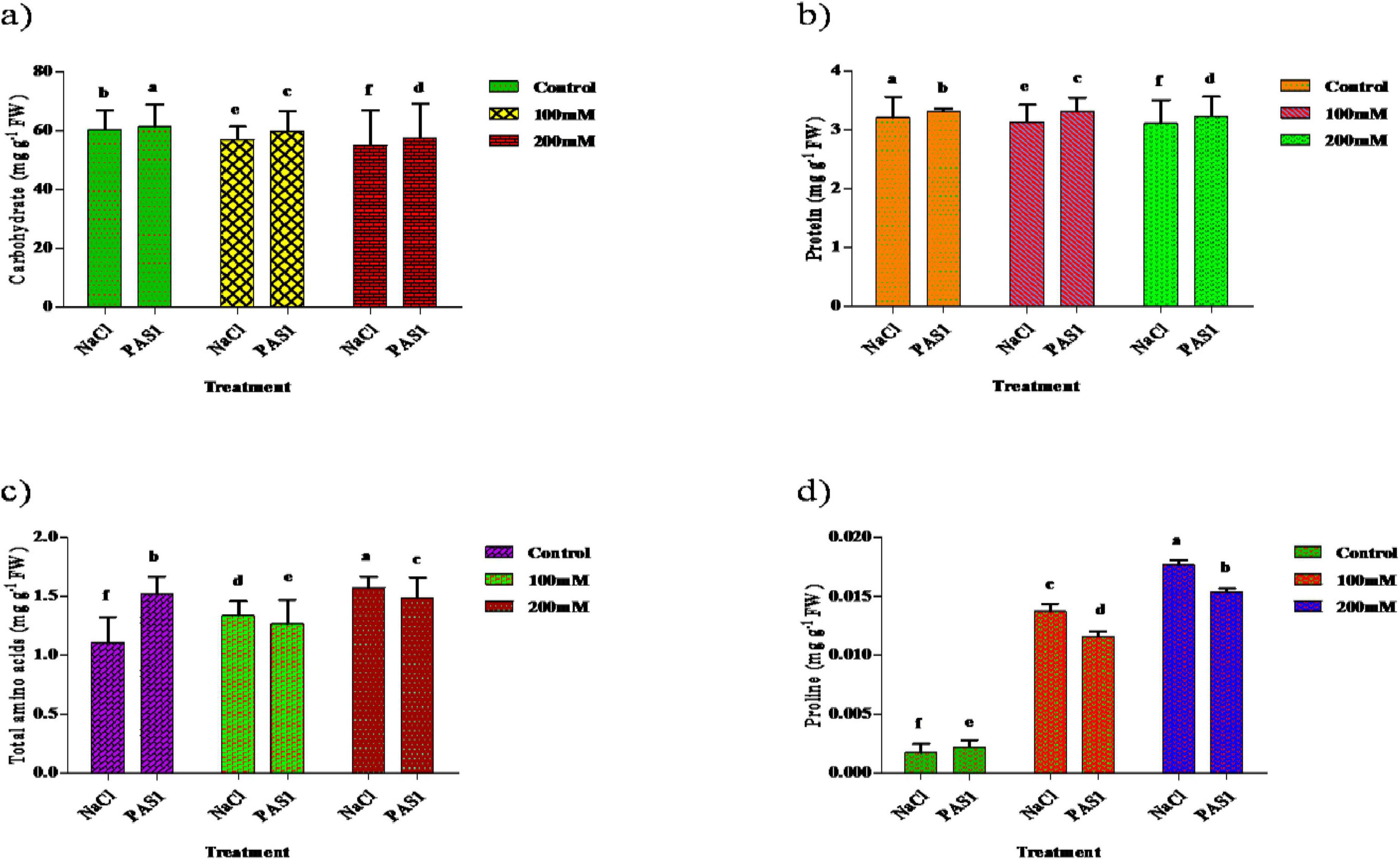
Figure 4. The effect of plant growth-promoting rhizobacteria (PGPR) B. flexus (PAS1) on total carbohydrate (a), protein (b), amino acids (c), and proline (d) levels under NaCl stress in sesame leaves was investigated. Error bars represent the standard deviation (n = 3). Small alphabetical letters above the error bars indicate significant differences between treatments according to Tukey’s post-hoc test (p = 0.05).
Determination of antioxidant enzyme activity in S. indicum with B. flexus under salt stress
2,2-diphenyl-1-picrylhydrazyl activity increased significantly in both control and PGPR treatments. Specifically, the activity increased by 23.8% at 100 mM NaCl and 59.5% at 200 mM NaCl, while the PAS1-treated showed increases of 13.6% and 45.5%. SOD, POD, and CAT activities were also higher in NaCl-treated plants compared to control. Significant increases were observed for POD, SOD, and CAT with 5.44%, 13.64%, and 31.14% increases in NaCl treatment of 0, 100, and 200 mM, respectively. The lowest MDA levels were observed in control plants, with 25.6 μmol g-1 FW for NaCl and 24.1 μM g–1 FW for PGPR treatments. At 100 mM NaCl, MDA increased to 34.7 μmol g–1 FW in NaCl-treated plants and 32.5 1 μmol g–1 FW in PGPR-treated plants. At 200 mM NaCl, MDA levels increased to 42.3 μmol g–1 FW and 39.6 μmol g–1 FW, respectively. The increase in MDA was lower in PGPR-treated plants compared to untreated plants. Co-application of B. flexus with NaCl stress further enhanced the antioxidant enzyme activity observed with NaCl stress. The activity of antioxidant enzymes, including SOD, POD, CAT, and MDA content under NaCl stress, significantly increased during the experiment in cultivars compared to the control (Figure 5).
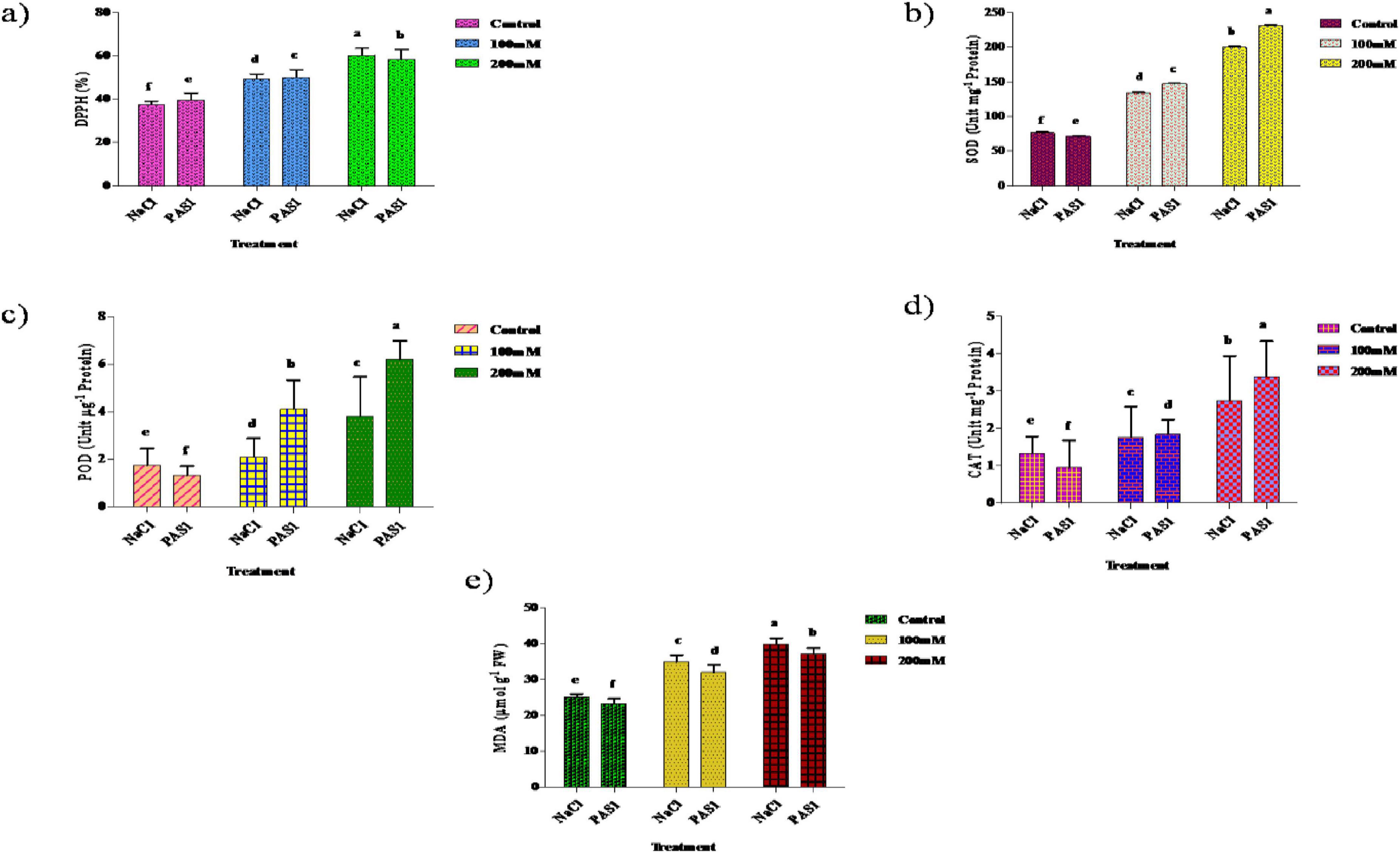
Figure 5. The effect of plant growth-promoting rhizobacteria (PGPR) B. flexus (PAS1) on DPPH % (a), SOD (b), POD (c), CAT (d), and MDA (e) content levels under NaCl stress in sesame leaves was investigated. Error bars represent the standard deviation (n = 3). Small alphabetical letters above the error bars indicate significant differences between treatments according to Tukey’s post-hoc test (p = 0.05).
Pearson’s correlation coefficient analysis
A Pearson’s correlation analysis was performed to determine the relationship between morphological, physiological, biochemical, and antioxidant traits under salt stress (Figure 6). The analysis revealed a strong, significant positive correlation (p < 0.01) among shoot length (SL), root length (RL), total chlorophyll (TCH), carotenoids, carbohydrate content (CHO), and protein content. This indicates that these parameters are positively associated with each other and play a major role in plant growth promotion. Similarly, a significant positive correlation (p < 0.01) was observed among amino acid content, proline accumulation, DPPH activity, SOD, POD, CAT, and MDA. In contrast, a significant negative correlation (p < 0.05) was found between growth parameters (SL and RL) and stress such as proline, DPPH, SOD, CAT, and MDA. TCH and carotenoids also negatively correlated with proline, DPPH, SOD, and MDA, while CHO was negatively correlated with MDA. Additionally, negative correlations were noted between SL, RL and amino acids, POD; TCH and carotenoids with amino acids, POD, CAT; CHO with amino acids, proline, DPPH, SOD, POD, CAT; and protein with all stress including amino acids, proline, DPPH, SOD, POD, CAT, and MDA.
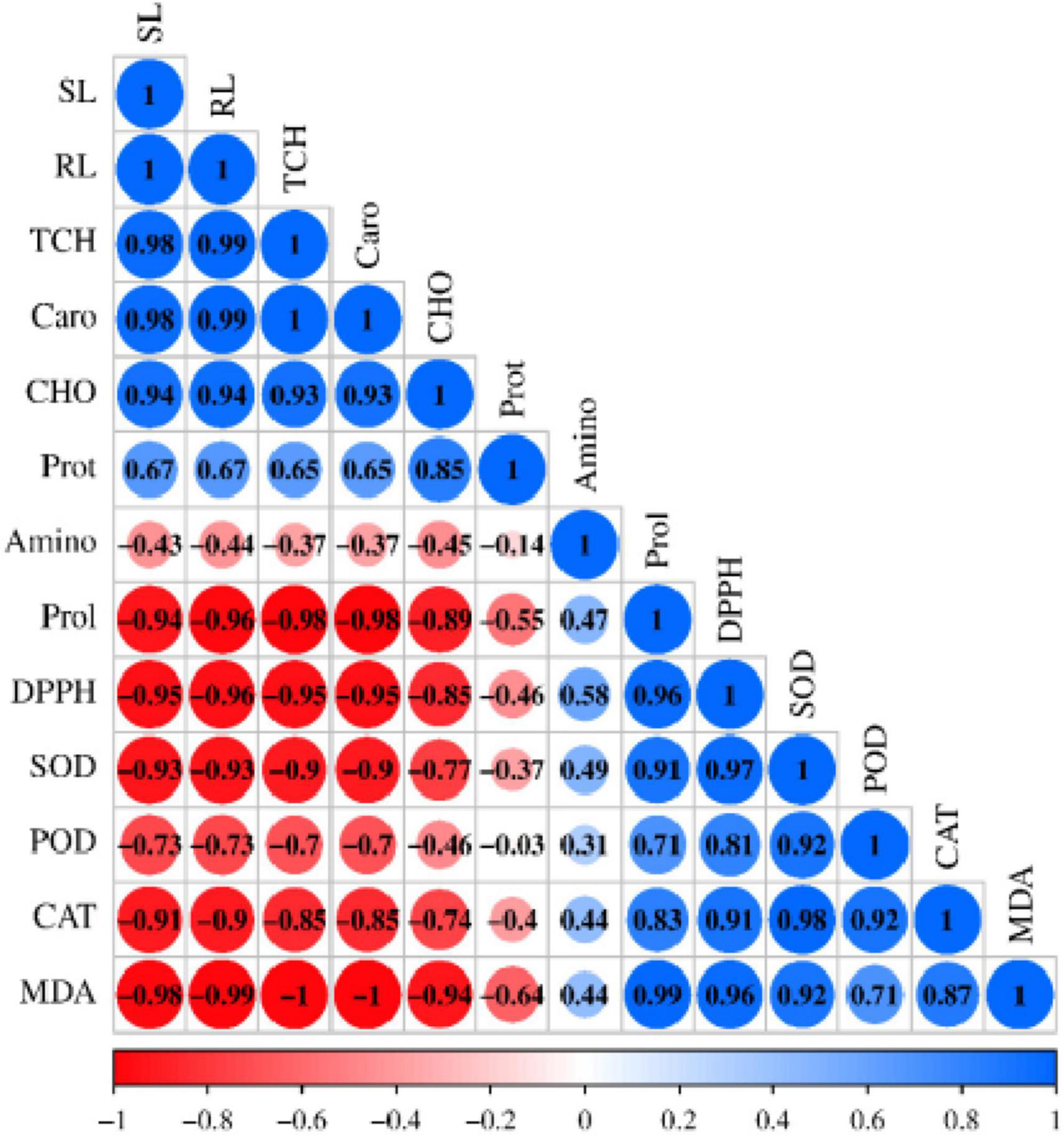
Figure 6. Pearson’s correlation analysis was performed on the morphological, physiological, and biochemical attributes of sesame treated with plant growth-promoting rhizobacteria (PGPR) under salt stress. The attributes analyzed include SL (shoot length), RL (root length), TCH (total chlorophyll), Caro (carotenoids), CHO (carbohydrate), Prot (protein), Amino (amino acid), Prol (proline), DPPH, SOD, POD, CAT, and MDA (lipid peroxidation).
Discussion
Various crop plants thrive in diverse regions worldwide, often overcoming challenges from varying climatic conditions, soil types, and pests. Among these challenges, salt stress is a significant abiotic stress that significantly impacts agriculture (Ashraf and Munns, 2022; Al-Turki et al., 2023). This study analyzed soil samples collected from the rhizosphere of plants growing in saline-affected soil. PGPR are soil-dwelling microorganisms that enhance plant growth through direct or indirect mechanisms during root colonization and contribute to increased crop yields (Rai et al., 2023; Saini et al., 2023). Several studies have identified beneficial PGPR based on their growth-promoting properties (Passari et al., 2016; Singh et al., 2019; Bright et al., 2025; Sethi et al., 2025). For example, various Pseudomonas and Bacillus strains promote plant growth by producing indole-3-acetic acid (IAA), stimulating root tip growth, and enhancing phototropism (Paravar et al., 2023; Sagar et al., 2024). PGPR can also mitigate salinity stress and improve nitrogen uptake by plants (Kapadia et al., 2021; Rana et al., 2022). Phosphate solubilization, mediated by the release of inorganic and organic acids, converts insoluble phosphate in the soil into orthophosphate, making it available for plant uptake (Parray et al., 2023; Yu et al., 2024). Siderophore, produced by PGPR, facilitates iron acquisition by plants through the solubilization of organic and inorganic minerals in the soil (Pattnaik et al., 2021; Omar et al., 2022), thereby promoting root and shoot elongation (Meena et al., 2020). Furthermore, PGPR can control soil-borne pathogens by producing hydrogen cyanide (HCN), protecting plants from diseases, particularly root damage, and indirectly promoting plant growth (Sehrawat et al., 2022). Our study demonstrated that the isolated PGPR produced IAA, solubilized phosphate, and generated ammonia, siderophores, and HCN, consistent with the findings of (Mazumdar et al., 2020). The bacterial isolate was identified as B. flexus based on 16s rRNA gene sequencing, and a phylogenetic tree was constructed using the neighbor-joining method (Kapadia et al., 2022; Zhou et al., 2022). Previous studies have reported significant reductions in root and shoot length in various crop plants under salt stress (Kumar et al., 2021; Vafa et al., 2024). PGPR induce physiological changes in plant tissues, promoting growth and improving various plant parameters (Chauhan et al., 2022; Bright et al., 2025). For example, a study on wheat treated with P. fluorescens and B. licheniformis demonstrated improved growth under saline conditions (Orozco-Mosqueda et al., 2020). Similarly, Azospirillum lipoferum, Azospirillum brasilense, and Bacillus spp. have been shown to enhance plant growth under different salt stress concentrations (0, 50 mM) (Rabiei et al., 2020; Sagar et al., 2022b). Our results are consistent with those of Jalil and Ansari (2020), who demonstrated that plants protect themselves against salt stress by producing compatible solutes such as carbohydrates, proteins, and amino acids. These solutes are responsible for intracellular osmotic adjustment, production of antioxidant enzymes, excess ROS reduction, and membrane stability maintenance (Parveen et al., 2021; Hamidian et al., 2023). Plants employ various conservation strategies to enhance growth and counteract the detrimental effects of salt stress (Nasab and Sayyed, 2019; Ayub et al., 2020). In our study, proline concentration was higher under salt stress, similar to the findings of Patriarca et al. (2021). Proline has been shown to reduce enzymatic degradation induced by NaCl and other stresses in plants, thereby reducing the activity of antioxidant enzymes (Shahid et al., 2022). Proline acts as an osmoprotectants and a scavenger of hydroxyl radicals (Hosseinifard et al., 2022), ensuring membrane and sub-cellular structural integrity (Maslennikova et al., 2023). It also protects cellular functions by scavenging ROS (Feng et al., 2024). The DPPH scavenging activity of plant extracts indicated the presence of antioxidant activity. In our study, both plant and leaf extracts exhibited DPPH scavenging activity under salt stress, similar to the observations of Azeem et al. (2023). One of the primary plant defense strategies against reactive oxygen species (ROS) involves buffering ROS levels. Plants employ a coordinated defense mechanism involving antioxidant enzymes such as SOD, POD, and CAT (Kesawat et al., 2023). The activities of CAT, SOD, and POD in salt-stressed sage plants in our study were consistent with those reported by Hussain et al. (2023). Furthermore, the application of NaCl enhanced the activities of CAT, SOD, and POD, indicating oxidative damage in salt-stressed sage, similar to observations in other crops (Sheikhalipour et al., 2024). Lipid peroxidation, indicated by MDA levels, signifies membrane damage and leakage under salt stress (Syeed et al., 2021). Consistent with our findings, low MDA levels have been associated with salt tolerance in various studies. For example, salt-tolerant tomato cultivars (Khan et al., 2019; Raja et al., 2022) and salt-resistant tobacco plants (Wang et al., 2022) exhibited reduced lipid peroxidation, reflecting their ability to minimize oxidative damage under salinity. Salt stress can induce the biosynthesis of endogenous nitric oxide (NO), which acts as a direct ROS scavenger or a signaling molecule, thus reducing ROS levels and oxidative damage in stressed plants (Hasanuzzaman et al., 2021; Gao et al., 2022). PGPR improves the characteristics of crop plants and oilseeds and enhances yield (Kusale et al., 2021; Nagrale et al., 2023). Each PGPR strain uniquely supports plant growth in various polluted environments, contributing significantly to plant growth and product quality. The findings of this study strongly suggest that rhizobacteria, which produce PGPR traits and possess plant growth-promoting properties, play a key role in enhancing the ability of oil-yielding plants like sesame to adapt to saline environments.
Conclusion
The study revealed that B. flexus from the contaminated area was resistant to NaCl. It enhances sesame growth under salt stress, which improves photosynthesis, transpiration, and photosynthetic pigments. In addition, B. flexus facilitates the availability of essential nutrients such as nitrogen, calcium, and iron in the soil. B. flexus halo-tolerant PGPR strains act as biofertilizers when cultivated in saline soils under salt-stressed conditions. This highlights the potential of improving these PGPR strains to mitigate the adverse effects of salt stress on plant growth and promote sustainable agriculture in salt-affected areas. In conclusion, this study suggests the critical role of B. flexus as a halo-tolerant PGPR in mitigating salt stress and promoting plant growth. These findings have considerable potential for practical applications in agricultural systems such as sustainable agriculture.
Data availability statement
The original contributions presented in this study are included in this article/Supplementary material, further inquiries can be directed to the corresponding authors.
Author contributions
DS: Investigation, Methodology, Writing – original draft. SA: Formal Analysis, Funding acquisition, Writing – review and editing. JB: Formal Analysis, Validation, Writing – review and editing. HE: Formal Analysis, Validation, Writing – review and editing. SL: Conceptualization, Formal Analysis, Project administration, Supervision, Writing – original draft, Writing – review and editing. SM: Formal Analysis, Validation, Writing – review and editing. SN: Investigation, Methodology, Writing – original draft. RS: Formal Analysis, Validation, Writing – review and editing, Writing – original draft.
Funding
The author(s) declare that financial support was received for the research and/or publication of this article. This work research was funded by Deanship of Graduate, Studies and Scientific Research at Qassim University (QU-APC-2025), Saudi Arabia and industrial fund R.J130000.7609.4C646, Universiti Teknologi Malaysia, Malaysia.
Acknowledgments
The Researchers would like to thank the Deanship of Graduate Studies and Scientific Research at Qassim University, Saudi Arabia for financial support (QU-APC-2025). The authors recognize and thank the Department of Botany, School of Life Sciences, Periyar University, Salem, 636 011, India, for providing Infrastructural Facilities.
Conflict of interest
The authors declare that the research was conducted in the absence of any commercial or financial relationships that could be construed as a potential conflict of interest.
Generative AI statement
The authors declare that no Generative AI was used in the creation of this manuscript.
Publisher’s note
All claims expressed in this article are solely those of the authors and do not necessarily represent those of their affiliated organizations, or those of the publisher, the editors and the reviewers. Any product that may be evaluated in this article, or claim that may be made by its manufacturer, is not guaranteed or endorsed by the publisher.
Supplementary material
The Supplementary Material for this article can be found online at: https://www.frontiersin.org/articles/10.3389/fmicb.2025.1590854/full#supplementary-material
References
Alexander, D. B., and Zuberer, D. A. (1991). Use of chrome azurol S reagents to evaluate siderophore production by rhizosphere bacteria. Biol. Fertil. Soils 12, 39–45. doi: 10.1007/BF00369386
Al-Turki, A., Murali, M., Omar, A. F., Rehan, M., and Sayyed, R. Z. (2023). Recent advances in PGPR-mediated resilience toward interactive effects of drought and salt stress in plants. Front. Microbiol. 14:1214845. doi: 10.3389/fmicb.2023.1214845
Anbalagan, S. A., Appusamy, S., Kumaresan, P. V., Chellappan, G., Narayanan, S., Rangasamy, A., et al. (2025). Deciphering the biocontrol potential of Trichoderma asperellum (Tv1) against fusarium -nematode wilt complex in tomato. J. Basic Microbiol. 65:e2400595. doi: 10.1002/jobm.202400595
Arnon, D. I. (1949). Copper enzymes in isolated chloroplasts. Polyphenoloxidase in Beta vulgaris. Plant Physiol. 24, 1–15. doi: 10.1104/pp.24.1.1
Ashraf, M., and Munns, R. (2022). Evolution of approaches to increase the salt tolerance of crops. CRC. Crit. Rev. Plant Sci. 41, 128–160. doi: 10.1080/07352689.2022.2065136
Awaad, H. A. (2023). Salinity Resilience and Sustainable Crop Production Under Climate Change. Cham: Springer, doi: 10.1007/978-3-031-48542-8
Ayub, M. A., Ahmad, H. R., Ali, M., Rizwan, M., Ali, S., Zia ur Rehman, M., et al. (2020). “Salinity and its tolerance strategies in plants,” in Plant Life Under Changing Environment, eds S. Prasad, N. Ramawat, and K. Dubey (Amsterdam: Elsevier), 47–76. doi: 10.1016/B978-0-12-818204-8.00003-5
Ayuso-Calles, M., Flores-Félix, J. D., and Rivas, R. (2021). Overview of the role of rhizobacteria in plant salt stress tolerance. Agronomy 11:1759. doi: 10.3390/agronomy11091759
Azeem, M., Pirjan, K., Qasim, M., Mahmood, A., Javed, T., Muhammad, H., et al. (2023). Salinity stress improves antioxidant potential by modulating physio-biochemical responses in Moringa oleifera Lam. Sci. Rep. 13:2895. doi: 10.1038/s41598-023-29954-6
Basu, A., Prasad, P., Das, S. N., Kalam, S., Sayyed, R. Z., Reddy, M. S., et al. (2021). Plant growth promoting rhizobacteria (PGPR) as green bioinoculants: Recent developments, constraints, and prospects. Sustainability 13:1140. doi: 10.3390/su13031140
Bentsink, L., and Koornneef, M. (2008). Seed dormancy and germination. Arab. B. 6:e0119. doi: 10.1199/tab.0119
Bradford, M. M. (1976). A rapid and sensitive method for the quantitation of microgram quantities of protein utilizing the principle of protein-dye binding. Anal. Biochem. 72, 248–254. doi: 10.1016/0003-2697(76)90527-3
Brand-Williams, W., Cuvelier, M. E., and Berset, C. (1995). Use of a free radical method to evaluate antioxidant activity. LWT Food Sci. Technol. 28, 25–30. doi: 10.1016/S0023-6438(95)80008-5
Bright, J. P., Maheshwari, H. S., Thangappan, S., Perveen, K., Bukhari, N. A., Mitra, D., et al. (2025). Biofilmed-PGPR: Next-generation bioinoculant for plant growth promotion in rice under changing climate. Rice Sci. 32, 94–106. doi: 10.1016/j.rsci.2024.08.008
Burnap, R. L. (2023). Cyanobacterial bioenergetics in relation to cellular growth and productivity. Adv. Biochem. Eng. Biotechnol. 183, 25–64. doi: 10.1007/10_2022_215
Chattha, M. U., Hassan, M. U. U., Khan, I., Nawaz, M., Shah, A. N., Sattar, A., et al. (2022). Hydrogen peroxide priming alleviates salinity induced toxic effect in maize by improving antioxidant defense system, ionic homeostasis, photosynthetic efficiency and hormonal crosstalk. Mol. Biol. Rep. 49, 5611–5624. doi: 10.1007/s11033-022-07535-6
Chauhan, A., Saini, R., and Sharma, J. C. (2022). Plant growth promoting rhizobacteria and their biological properties for soil enrichment and growth promotion. J. Plant Nutr. 45, 273–299. doi: 10.1080/01904167.2021.1952221
Choudhary, M., Chandra, P., Dixit, B., Nehra, V., Choudhary, U., and Choudhary, S. (2022). Plant growth-promoting microbes: Role and prospective in amelioration of salt stress. Commun. Soil Sci. Plant Anal. 53, 1692–1711. doi: 10.1080/00103624.2022.2063316
Coico, R. (2006). Gram staining. Curr. Protoc. Microbiol. A.3C.1–A.3C.2. doi: 10.1002/9780471729259.mca03cs00
Dave, A., Ingle, S., Perveen, K., Bukhari, N. A., Sayyed, R., and Mastinu, A. (2024). Harnessing plant growth–promoting and wilt-controlling biopotential of a consortium of actinomycetes and mycorrhizae in pigeon pea. J. Phytopathol. 172:13399. doi: 10.1111/jph.13399
Debnath, S., Chandel, R. K., Devi, K., and Khan, Z. (2021). “Mechanism and molecular response of induced genotoxicity and oxidative stress in plants,” in Induced Genotoxicity and Oxidative Stress in Plants, eds Z. Khan, M. Ansari, and D. Shahwar (Singapore: Springer Singapore), 213–227. doi: 10.1007/978-981-16-2074-4_8
Dias, T. A. C., Lora, E. E. S., Maya, D. M. Y., and Olmo, O. A. (2021). Global potential assessment of available land for bioenergy projects in 2050 within food security limits. Land Policy 105:105346. doi: 10.1016/j.landusepol.2021.105346
Feng, L., Li, Q., Zhou, D., Jia, M., Liu, Z., Hou, Z., et al. (2024). B. subtilis CNBG-PGPR –1 induces methionine to regulate ethylene pathway and ROS scavenging for improving salt tolerance of tomato. Plant J. 117, 193–211. doi: 10.1111/tpj.16489
Flohé, L., and Ötting, F. (1984). Superoxide dismutase assays. Methods Enzymol. 105, 93–104. doi: 10.1016/S0076-6879(84)05013-8
Gao, Z., Zhang, J., Zhang, J., Zhang, W., Zheng, L., Borjigin, T., et al. (2022). Nitric oxide alleviates salt-induced stress damage by regulating the ascorbate–glutathione cycle and Na+/K+ homeostasis in Nitraria tangutorum Bobr. Plant Physiol. Biochem. 173, 46–58. doi: 10.1016/j.plaphy.2022.01.017
Gordon, S. A., and Weber, R. P. (1951). Colorimetric estimation of indoleacetic acid. Plant Physiol. 26, 192–195. doi: 10.1104/pp.26.1.192
Gulzar, Y., Reegu, F. A., Soomro, A. B., Mir, M. S., Zahir, A., and Onn, C. W. (2025). Exploring the economic implications of IoT adoption in agriculture: A cost-benefit study in Jazan, Saudi Arabia. Sarhad J. Agric. 41: 51–65. doi: 10.17582/journal.sja/2025/41.1.51.65
Hamid, B., Zaman, M., Farooq, S., Fatima, S., Sayyed, R. Z., Baba, Z. A., et al. (2021). Bacterial plant biostimulants: A sustainable way towards improving growth, productivity, and health of crops. Sustainability 13:2856. doi: 10.3390/su13052856
Hamidian, M., Movahhedi-Dehnavi, M., Sayyed, R. Z., Almalki, W. H., Gafur, A., and Fazeli-Nasab, B. (2023). Co-application of Mycorrhiza and methyl jasmonate regulates morpho-physiological and antioxidant responses of Crocus sativus (Saffron) under salinity stress conditions. Sci. Rep. 13:7378. doi: 10.1038/s41598-023-34359-6
Hasanuzzaman, M., Raihan, M. R. H., Masud, A. A. C., Rahman, K., Nowroz, F., Rahman, M., et al. (2021). Regulation of reactive oxygen species and antioxidant defense in plants under salinity. Int. J. Mol. Sci. 22:9326. doi: 10.3390/ijms22179326
Hedge, J. E., and Hofreiter, B. T. (1962). Estimation of Carbohydrate: Methods in Carbohydrate Chemistry. New York, NY: Academic Press, 17–22.
Hernández-Canseco, J., Bautista-Cruz, A., Sánchez-Mendoza, S., Aquino-Bolaños, T., and Sánchez-Medina, P. S. (2022). Plant growth-promoting halobacteria and their ability to protect crops from abiotic stress: An eco-friendly alternative for saline soils. Agronomy 12:804. doi: 10.3390/agronomy12040804
Hills, G. M. (1940). Ammonia production by pathogenic bacteria. Biochem. J. 34, 1057–1069. doi: 10.1042/bj0341057
Himpsl, S. D., and Mobley, H. L. T. (2019). Siderophore detection using chrome azurol S and cross-feeding assays. Methods Mol. Biol. 2021, 97–108. doi: 10.1007/978-1-4939-9601-8_10
Hosseinifard, M., Stefaniak, S., Ghorbani Javid, M., Soltani, E., Wojtyla, Ł, and Garnczarska, M. (2022). Contribution of exogenous proline to abiotic stresses tolerance in plants: A review. Int. J. Mol. Sci. 23:5186. doi: 10.3390/ijms23095186
Hussain, S., Ahmed, S., Akram, W., Sardar, R., Abbas, M., and Yasin, N. A. (2023). Selenium-Priming mediated growth and yield improvement of turnip under saline conditions. Int. J. Phytoremediation 26, 1–17. doi: 10.1080/15226514.2023.2261548
Jabborova, D., Jabbarov, Z., Abdrakhmanov, T., Fayzullaev, O., Saharan, B. S., Perveen, K., et al. (2025). Assessing the synergistic effects of biochar, hydrogel, and biofertilizer on growth and physiological traits of wheat in saline environments. Funct. Plant. Biol. 52:FP24277 doi: 10.1071/FP24277
Jalil, S. U., and Ansari, M. I. (2020). Physiological Role of Gamma-Aminobutyric Acid in Salt Stress Tolerance. Berlin: Springer, 337–350. doi: 10.1007/978-3-030-40277-8_13
Jang, W. Y., Kim, M.-Y., and Cho, J. Y. (2022). Antioxidant, anti-inflammatory, anti-menopausal, and anti-cancer effects of lignans and their metabolites. Int. J. Mol. Sci. 23:15482. doi: 10.3390/ijms232415482
Kalam, S., Basu, A., Ahmad, I., Sayyed, R. Z., El-Enshasy, H. A., Dailin, D. J., et al. (2020). Recent understanding of soil acidobacteria and their ecological significance: A critical review. Front. Microbiol. 11:580024. doi: 10.3389/fmicb.2020.580024
Kapadia, C., Patel, N., Rana, A., Vaidya, H., Alfarraj, S., Ansari, M. J., et al. (2022). Evaluation of plant growth-promoting and salinity ameliorating potential of halophilic bacteria isolated from saline soil. Front. Plant Sci. 13:946217. doi: 10.3389/fpls.2022.946217
Kapadia, C., Sayyed, R. Z., El Enshasy, H. A., Vaidya, H., Sharma, D., Patel, N., et al. (2021). Halotolerant microbial consortia for sustainable mitigation of salinity stress, growth promotion, and mineral uptake in tomato plants and soil nutrient enrichment. Sustainability 13:8369. doi: 10.3390/su13158369
Kesawat, M. S., Satheesh, N., Kherawat, B. S., Kumar, A., Kim, H.-U., Chung, S.-M., et al. (2023). Regulation of reactive oxygen species during salt stress in plants and their crosstalk with other signaling molecules—current perspectives and future directions. Plants 12:864. doi: 10.3390/plants12040864
Khan, A., Sayyed, R. Z., and Seifi, S. (2019). Rhizobacteria: Legendary Soil Guards in Abiotic Stress Management. Berlin: Springer, 327–343. doi: 10.1007/978-981-13-6536-2_15
Khan, N., Ali, S., Shahid, M. A., Mustafa, A., Sayyed, R. Z., and Curá, J. A. (2021). Insights into the interactions among roots, rhizosphere, and rhizobacteria for improving plant growth and tolerance to abiotic stresses: A review. Cells 10:1551. doi: 10.3390/cells10061551
Kour, D., Kaur, T., Devi, R., Yadav, A., Singh, M., Joshi, D., et al. (2021). Beneficial microbiomes for bioremediation of diverse contaminated environments for environmental sustainability: Present status and future challenges. Environ. Sci. Pollut. Res. 28, 24917–24939. doi: 10.1007/s11356-021-13252-7
Koza, N., Adedayo, A., Babalola, O., and Kappo, A. (2022). Microorganisms in plant growth and development: Roles in abiotic stress tolerance and secondary metabolites secretion. Microorganisms 10:1528. doi: 10.3390/microorganisms10081528
Kumar, S., Li, G., Yang, J., Huang, X., Ji, Q., Liu, Z., et al. (2021). Effect of salt stress on growth, physiological parameters, and ionic concentration of water dropwort (Oenanthe javanica) cultivars. Front. Plant Sci. 12:660409. doi: 10.3389/fpls.2021.660409
Kumawat, K. C., Nagpal, S., and Sharma, P. (2022). Potential of plant growth-promoting rhizobacteria-plant interactions in mitigating salt stress for sustainable agriculture: A review. Pedosphere 32, 223–245. doi: 10.1016/S1002-0160(21)60070-X
Kusale, S. P., Attar, Y. C., Sayyed, R. Z., El Enshasy, H., Hanapi, S. Z., Ilyas, N., et al. (2021). Inoculation of Klebsiella variicola Alleviated salt stress and improved growth and nutrients in wheat and maize. Agronomy 11:927. doi: 10.3390/agronomy11050927
Lorck, H. (1948). Production of hydrocyanic acid by bacteria. Physiol. Plant. 1, 142–146. doi: 10.1111/j.1399-3054.1948.tb07118.x
Lück, H. (1965a). “Catalase,” in Methods of Enzymatic Analysis, ed. Hans-Ulrich Bergmeyer (Amsterdam: Elsevier), 885–894. doi: 10.1016/B978-0-12-395630-9.50158-4
Lück, H. (1965b). “Peroxidase,” in Methods of Enzymatic Analysis, ed. Hans-Ulrich Bergmeyer (Amsterdam: Elsevier), 895–897. doi: 10.1016/B978-0-12-395630-9.50159-6
Maslennikova, D., Knyazeva, I., Vershinina, O., Titenkov, A., and Lastochkina, O. (2023). Seed treatment with sodium nitroprusside ensures a long-term physiological and protective effect on wheat under salinity. Life 13:1499. doi: 10.3390/life13071499
Mazumdar, D., Saha, S. P., and Ghosh, S. (2020). Isolation, screening and application of a potent PGPR for enhancing growth of Chickpea as affected by nitrogen level. Int. J. Veg. Sci. 26, 333–350. doi: 10.1080/19315260.2019.1632401
Meena, M., Swapnil, P., Divyanshu, K., Kumar, S., Harish, Tripathi, Y. N., et al. (2020). PGPR-mediated induction of systemic resistance and physiochemical alterations in plants against the pathogens: Current perspectives. J. Basic Microbiol. 60, 828–861. doi: 10.1002/jobm.202000370
Mitsiopoulou, C., Sotirakoglou, K., Labrou, N. E., and Tsiplakou, E. (2021). The effect of whole sesame seeds on milk chemical composition, fatty acid profile and antioxidant status in goats. Livest. Sci. 245:104452. doi: 10.1016/j.livsci.2021.104452
Moore, K., and Roberts, L. J. (1998). Measurement of lipid peroxidation. Free Radic. Res. 28, 659–671. doi: 10.3109/10715769809065821
Morris, J. B., Dierig, D., Heinitz, C., Hellier, B., Bradley, V., and Marek, L. (2023). Vulnerability of U.S. new and industrial crop genetic resources. Ind. Crops Prod. 206:117364. doi: 10.1016/j.indcrop.2023.117364
Mukhopadhyay, R., Sarkar, B., Jat, H. S., Sharma, P. C., and Bolan, N. S. (2021). Soil salinity under climate change: Challenges for sustainable agriculture and food security. J. Environ. Manage. 280:111736. doi: 10.1016/j.jenvman.2020.111736
Nagrale, D. T., Chaurasia, A., Kumar, S., Gawande, S. P., Hiremani, N. S., Shankar, R., et al. (2023). PGPR: The treasure of multifarious beneficial microorganisms for nutrient mobilization, pest biocontrol and plant growth promotion in field crops. World J. Microbiol. Biotechnol. 39:100. doi: 10.1007/s11274-023-03536-0
Nasab, B. F., and Sayyed, R. (2019). “Plant growth promoting rhizobacteria and salinity stress: A journey into the soil,” in Plant Growth Promoting Rhizobacteria for sustainable stress Management Vol 1 Abiotic Stress Management, Vol. 2019, eds R. Sayyed, N. Arora, and M. S. Reddy (Singapore: Springer), 21–34. doi: 10.1007/978-981-13-6536-2_2
Nautiyal, C. S. (1999). An efficient microbiological growth medium for screening phosphate solubilizing microorganisms. FEMS Microbiol. Lett. 170, 265–270. doi: 10.1111/j.1574-6968.1999.tb13383.x
Omar, A. F., Abdelmageed, A. H. A., Al-Turki, A., Abdelhameid, N. M., Sayyed, R. Z., and Rehan, M. (2022). Exploring the plant growth-promotion of four streptomyces strains from rhizosphere soil to enhance cucumber growth and yield. Plants 11:3316. doi: 10.3390/plants11233316
Orozco-Mosqueda, M. C., Glick, B. R., and Santoyo, G. (2020). ACC deaminase in plant growth-promoting bacteria (PGPB): An efficient mechanism to counter salt stress in crops. Microbiol. Res. 235:126439. doi: 10.1016/j.micres.2020.126439
Paes de Melo, B., Carpinetti, P., de, A., Fraga, O. T., Rodrigues-Silva, P. L., et al. (2022). Abiotic stresses in plants and their markers: A practice view of plant stress responses and programmed cell death mechanisms. Plants 11:1100. doi: 10.3390/plants11091100
Paravar, A., Piri, R., Balouchi, H., and Ma, Y. (2023). Microbial seed coating: An attractive tool for sustainable agriculture. Biotechnol. Rep. 37:e00781. doi: 10.1016/j.btre.2023.e00781
Parray, J. A., Egamberdieva, D., Abd_Allah, E. F., and Sayyed, R. Z. (2023). Editorial: soil microbiome metabolomics: A way forward to sustainable intensification. Front. Sustain. Food Syst. 7:1251054. doi: 10.3389/fsufs.2023.1251054
Parveen, A., Ahmar, S., Kamran, M., Malik, Z., Ali, A., Riaz, M., et al. (2021). Abscisic acid signaling reduced transpiration flow, regulated Na ion homeostasis and antioxidant enzyme activities to induce salinity toleranc. Environ. Technol. Innov. 24:101808. doi: 10.1016/j.eti.2021.101808
Passari, A. K., Mishra, V. K., Leo, V. V., Gupta, V. K., and Singh, B. P. (2016). Phytohormone production endowed with antagonistic potential and plant growth promoting abilities of culturable endophytic bacteria isolated from Clerodendrum colebrookianum Walp. Microbiol. Res. 193, 57–73. doi: 10.1016/j.micres.2016.09.006
Patel, P. R., Shaikh, S. S., and Sayyed, R. Z. (2018). Modified chrome azurol S method for detection and estimation of siderophores having affinity for metal ions other than iron. Environ. Sustain. 1, 81–87. doi: 10.1007/s42398-018-0005-3
Patel, P., Sayyed, R. Z., and Patel, H. (2023). “PGPR: A sustainable agricultural mitigator for stressed agro-environments,” in Plant Growth Promoting Microorganisms of Arid Region, eds R. Mawar, R. Sayyed, and S. Sharma (Singapore: Springer Nature Singapore), 303–318. doi: 10.1007/978-981-19-4124-5_14
Patriarca, E. J., Cermola, F., D’Aniello, C., Fico, A., Guardiola, O., De Cesare, D., et al. (2021). The multifaceted roles of proline in cell behavior. Front. Cell Dev. Biol. 9:728576. doi: 10.3389/fcell.2021.728576
Pattnaik, S., Mohapatra, B., and Gupta, A. (2021). Plant growth-promoting microbe mediated uptake of essential nutrients (Fe, P, K) for crop stress management: Microbe–soil–plant continuum. Front. Agron. 3:689972. doi: 10.3389/fagro.2021.689972
Praveen, V., Sudha Appusami, A., Selva Amala, A., Ramalakshmi, R., Ramjegathesh, A. S., Fanish, S., et al. (2024). Plant Growth-Promotion and Tri-Trophic Interaction of Trichoderma asperellum Against Root Rot of Black Gram. 851:85175978. doi: 10.22541/au.172114768.85175978/v1
Rabiei, Z., Hosseini, S. J., Pirdashti, H., and Hazrati, S. (2020). Physiological and biochemical traits in coriander affected by plant growth-promoting rhizobacteria under salt stress. Heliyon 6: e05321. doi: 10.1016/j.heliyon.2020.e05321
Rai, S., Omar, A. F., Rehan, M., Al-Turki, A., Sagar, A., Ilyas, N., et al. (2023). Crop microbiome: Their role and advances in molecular and omic techniques for the sustenance of agriculture. Planta 257:27. doi: 10.1007/s00425-022-04052-5
Raja, V., Wani, U. M., Wani, Z. A., Jan, N., Kottakota, C., Reddy, M. K., et al. (2022). Pyramiding ascorbate–glutathione pathway in Lycopersicum esculentum confers tolerance to drought and salinity stress. Plant Cell Rep. 41, 619–637. doi: 10.1007/s00299-021-02764-8
Rana, K. L., Kour, D., Kaur, T., Negi, R., Devi, R., Yadav, N., et al. (2022). Endophytic nitrogen-fixing bacteria: Untapped treasurer for agricultural sustainability. J. Appl. Biol. Biotechnol. 11, 75–93. doi: 10.7324/JABB.2023.110207
Roland, J. F., and Gross, A. M. (1954). Quantitative determination of amino acids. Anal. Chem. 26, 502–505. doi: 10.1021/ac60087a022
Sagar, A., Rai, S., Ilyas, N., Sayyed, R. Z., Al-Turki, A. I., El Enshasy, H. A., et al. (2022a). Halotolerant rhizobacteria for salinity-stress mitigation: Diversity, mechanisms and molecular approaches. Sustainability 14:490. doi: 10.3390/su14010490
Sagar, A., Rai, S., Sharma, S., Perveen, K., Bukhari, N. A., Sayyed, R. Z., et al. (2024). Molecular characterization reveals biodiversity and biopotential of rhizobacterial isolates of Bacillus Spp. Microb. Ecol. 87:83. doi: 10.1007/s00248-024-02397-w
Sagar, A., Sayyed, R. Z., Ramteke, P. W., Sharma, S., Marraiki, N., Elgorban, A. M., et al. (2020). ACC deaminase and antioxidant enzymes producing halophilic Enterobacter sp. PR14 promotes the growth of rice and millets under salinity stress. Physiol. Mol. Biol. Plants 26, 1847–1854. doi: 10.1007/s12298-020-00852-9
Sagar, A., Yadav, S. S., Sayyed, R. Z., Sharma, S., and Ramteke, P. W. (2022b). Bacillus subtilis: A Multifarious Plant Growth Promoter, Biocontrol Agent, and Bioalleviator of Abiotic Stress. Berlin: Springer, 561–580. doi: 10.1007/978-3-030-85465-2_24
Saini, S., Lohani, S., Khati, P., and Rani, V. (2023). “PGPR-mediated mitigation of biotic and abiotic stress in plants,” in Advanced Microbial Technology for Sustainable Agriculture and Environment, eds S. Gangola, S. Kumar, and S. Joshi (Amsterdam: Elsevier), 199–227. doi: 10.1016/B978-0-323-95090-9.00013-3
Sanschagrin, S., and Yergeau, E. (2014). Next-generation sequencing of 16S ribosomal RNA gene amplicons. J. Vis. Exp. 90:51709. doi: 10.3791/51709
Sehrawat, A., Sindhu, S. S., and Glick, B. R. (2022). Hydrogen cyanide production by soil bacteria: Biological control of pests and promotion of plant growth in sustainable agriculture. Pedosphere 32, 15–38. doi: 10.1016/S1002-0160(21)60058-9
Seleiman, M., Talha Aslam, M., Ahmed Alhammad, B., Umair Hassan, M., Maqbool, R., Umer Chattha, M., et al. (2022). Salinity stress in wheat: Effects, mechanisms and management strategies. Phyton (B. Aires). 91, 667–694. doi: 10.32604/phyton.2022.017365
Sethi, G., Behera, K. K., Sayyed, R., Adarsh, V., Sipra, B. S., Singh, L., et al. (2025). Enhancing soil health and crop productivity: The role of zinc-solubilizing bacteria in sustainable agriculture. Plant Growth Regul. 1–17. doi: 10.1007/s10725-025-01294-7
Shahid, S., Shahbaz, M., Maqsood, M. F., Farhat, F., Zulfiqar, U., Javed, T., et al. (2022). Proline-induced modifications in morpho-physiological, biochemical and yield attributes of Pea (Pisum sativum L.) cultivars under salt stress. Sustainability 14:13579. doi: 10.3390/su142013579
Sheikhalipour, M., Kulak, M., Mohammadi, S. A., Esmaielpour, B., Nouraein, M., Kocak, M. Z., et al. (2024). Foliar application of either melatonin or sodium nitpoprusside regulates the antioxidant status, and the morpho-physiological attributes and essential oil production in sage (Salvia officinalis L.) under salinity stress. Sci. Hortic. (Amsterdam). 323:112526. doi: 10.1016/j.scienta.2023.112526
Singh, A. (2022). Soil salinity: A global threat to sustainable development. Soil Use Manag. 38, 39–67. doi: 10.1111/sum.12772
Singh, M. P., Singh, P., Singh, R. K., Sayyed, R. Z., and Sharma, A. (2019). “Plant small RNAs: Big players in biotic stress responses,” in Plant Growth Promoting Rhizobacteria for Sustainable Stress Management. Microorganisms for Sustainability, vol 13, ed. R. Sayyed (Singapore: Springer), 217–239. doi: 10.1007/978-981-13-6986-5_8
Soto-Gómez, D., and Pérez-Rodríguez, P. (2022). Sustainable agriculture through perennial grains: Wheat, rice, maize, and other species. A review. Agric. Ecosyst. Environ. 325:107747. doi: 10.1016/j.agee.2021.107747
Syeed, S., Sehar, Z., Masood, A., Anjum, N. A., and Khan, N. A. (2021). Control of elevated ion accumulation, oxidative stress, and lipid peroxidation with salicylic acid-induced accumulation of glycine betaine in salinity-exposed Vigna radiata L. Appl. Biochem. Biotechnol. 193, 3301–3320. doi: 10.1007/s12010-021-03595-9
Vafa, Z. N., Sohrabi, Y., Mirzaghaderi, G., Heidari, G., Rizwan, M., and Sayyed, R. Z. (2024). Effect of bio-fertilizers and seaweed extract on growth and yield of wheat (Triticum aestivum L.) under different irrigation regimes: Two-year field study. Chemosphere 364:143068. doi: 10.1016/j.chemosphere.2024.143068
Wang, Y., Cui, Y., Liu, B., Wang, Y., Sun, S., Wang, J., et al. (2022). Lilium pumilum stress-responsive NAC transcription factor LpNAC17 enhances salt stress tolerance in tobacco. Front. Plant Sci. 13:993841. doi: 10.3389/fpls.2022.993841
Wei, P., Zhao, F., Wang, Z., Wang, Q., Chai, X., Hou, G., et al. (2022). Sesame (Sesamum indicum L.): A comprehensive review of nutritional value, phytochemical composition, health benefits, development of food, and industrial applications. Nutrients 14:4079. doi: 10.3390/nu14194079
Whittenbury, R. (1964). Hydrogen peroxide formation and catalase activity in the lactic acid bacteria. J. Gen. Microbiol. 35, 13–26. doi: 10.1099/00221287-35-1-13
Yu, X., Xiong, F., Zhou, C., Luo, Z., Zhou, Z., Chen, J., et al. (2024). Uranium bioprecipitation mediated by a phosphate-solubilizing Enterobacter sp. N1-10 and remediation of uranium-contaminated soil. Sci. Total Environ. 906:167688. doi: 10.1016/j.scitotenv.2023.167688
Zainal, Z., Khaza’ai, H., Kutty Radhakrishnan, A., and Chang, S. K. (2022). Therapeutic potential of palm oil vitamin E-derived tocotrienols in inflammation and chronic diseases: Evidence from preclinical and clinical studies. Food Res. Int. 156:111175. doi: 10.1016/j.foodres.2022.111175
Zhang, D., Tian, C., and Mai, W. (2024). Exogenous sodium and calcium alleviate drought stress by promoting the succulence of Suaeda salsa. Plants 13:721. doi: 10.3390/plants13050721
Keywords: PGPR traits, B. flexus, metabolites, antioxidant, salt stress
Citation: Sridhar D, Alheswairini SS, Barasarathi J, Enshasy HAE, Lalitha S, Mir SH, Nithyapriya S and Sayyed R (2025) Halophilic rhizobacteria promote growth, physiology and salinity tolerance in Sesamum indicum L. grown under salt stress. Front. Microbiol. 16:1590854. doi: 10.3389/fmicb.2025.1590854
Received: 10 March 2025; Accepted: 24 April 2025;
Published: 14 May 2025.
Edited by:
Abhinav Aeron, Chonbuk National University, Republic of KoreaReviewed by:
Ajit Kumar Passari, Scotland’s Rural College, United KingdomAngelica Rodriguez Dorantes, National Polytechnic Institute (IPN), Mexico
Copyright © 2025 Sridhar, Alheswairini, Barasarathi, Enshasy, Lalitha, Mir, Nithyapriya and Sayyed. This is an open-access article distributed under the terms of the Creative Commons Attribution License (CC BY). The use, distribution or reproduction in other forums is permitted, provided the original author(s) and the copyright owner(s) are credited and that the original publication in this journal is cited, in accordance with accepted academic practice. No use, distribution or reproduction is permitted which does not comply with these terms.
*Correspondence: Sundaram Lalitha, bGFsaXRoYWJvdEBwZXJpeWFydW5pdmVyc2l0eS5hYy5pbg==; Riyaz Sayyed, c2F5eWVkcnpAZ21haWwuY29t