- 1State Key Laboratory of Environmental Criteria and Risk Assessment, Chinese Research Academy of Environmental Sciences, Beijing, China
- 2Center for Global Health, School of Public Health, Nanjing Medical University, Nanjing, China
- 3Institute of Biological and Chemical Systems – Biological Information Processing, Karlsruhe Institute of Technology (KIT), Karlsruhe, Germany
Kolmer–Agduhr (KA) cells are a subgroup of interneurons positioned adjacent to the neurocoele with cilia on the apical surface protruding into the central canal of the spinal cord. Although KA cells were identified almost a century ago, their development and functions are only beginning to be unfolded. Recent studies have revealed the characteristics of KA cells in greater detail, including their spatial distribution, the timing of their differentiation, and their specification via extrinsic signaling and a unique combination of transcription factors in zebrafish and mouse. Cell lineage-tracing experiments have demonstrated that two subsets of KA cells, named KA’ and KA” cells, differentiate from motoneuronal progenitors and floor-plate precursors, respectively, in both zebrafish and mouse. Although KA’ and KA” cells originate from different progenitors/precursors, they each share a common set of transcription factors. Intriguingly, the combination of transcription factors that promote the acquisition of KA’ cell characteristics differs from those that promote a KA” cell identity. In addition, KA’ and KA” cells exhibit separable neuronal targets and differential responses to bending of the spinal cord. In this review, we summarize what is currently known about the genetic programs defining the identities of KA’ and KA” cell identities. We then discuss how these two subgroups of KA cells are genetically specified.
Introduction
Kolmer–Agduhr (KA) cells are a group of cerebrospinal fluid (CSF)-contacting neurons (CSF-cNs). The term KA cell was first proposed by N. Dale et al. in 1987 (Dale et al., 1987b) to name a class of neurons that lie in the ventrolateral spinal cord and contact the cerebrospinal fluid in frog embryos (Roberts and Clarke, 1982); even earlier observations of cells with KA cell morphologies were made by Kolmer and Agduhr, who observed and described them in the spinal cords of most classes of vertebrates (Kolmer, 1921, 1925, 1931; Agduhr, 1922; Vigh-Teichmann and Vigh, 1983). Using antibodies against the neurotransmitter γ-aminobutyric acid (GABA) and an enzyme glutamic acid decarboxylase (GAD), numerous studies have reported the anatomy of KA cells in greater detail, including their axonal projection patterns, their appearance during development, and their distribution and organization in frogs (Dale et al., 1987a, b; Binor and Heathcote, 2001) and zebrafish (Bernhardt et al., 1992). For example, in frog (Xenopus laevis) embryos, KA cell have a pear-shaped soma (Roberts and Clarke, 1982). These GABA-positive KA cells distribute in the ventral part of the spinal cord in two orderly rows adjacent to the neurocoele (Dale et al., 1987b). There are numerous microvilli and one or two cilia on the apical surface of KA cells that project into the central canal of the spinal cord (Roberts and Clarke, 1982; Binor and Heathcote, 2001). Differentiated KA cells first appear at stage 25, and then one cell is continuously generated every 12 min on each side of the spinal cord (Dale et al., 1987b).
According to the location and origin of KA cells in zebrafish, two subsets of KA cells termed KA’ and KA” have been distinguished. KA” cells are distributed in the lateral floor plate (LFP), while the relatively dorsal KA’ cells localize in the motoneuron progenitor (pMN) domain (Park, 2004; Shin et al., 2007; Yang et al., 2010). Cell fate-mapping experiments showed that all KA’ cells are derived from Olig2+ precursors in the pMN domain (Park, 2004), while KA” cells differentiate from nkx2.2a+/nkx2.2b+/nkx2.9+ progenitors in the lateral floor plate (LFP). Most KA cells are born around 16.5 h postfertilization (hpf) in zebrafish (Schäfer et al., 2007; Yang et al., 2010; Huang et al., 2012).
Similar subsets of KA cells are observed in the mouse spinal cord, where these cells are named CSF-cN’ and CSF-cN” (Petracca et al., 2016). CSF-cN’ cells are derived from Nkx6+/Pax6+ progenitors positioned in the p2 neural progenitor domain and in the dorsal part of the oligodendrogenic (pOL) domain. In contrast, CSF-cN” cells originate from Nkx2.2+/Foxa2+ precursors in the boundary between the p3 neural progenitor domain and the floor plate. Most CSF-cN cells are born around embryonic days 13–14 (E13–E14) (Petracca et al., 2016).
Neurons with somata that have similar characteristics to those of KA cells in terms of shape, position, and/or expression of GABA have been reported in the lancelet (Vígh et al., 2004), lamprey (Meléndez-Ferro et al., 2003; Jalalvand et al., 2014), dogfish (Sueiro et al., 2004), eel and trout (Roberts et al., 1995), newt (Harper and Roberts, 1993), and macaque (Macaca fascicularis) (Djenoune et al., 2014). Based on these comparative histological data, vertebrate KA cells are thought to be derived from an ancient epithelial neuron-like ectodermal cell (Vígh et al., 2004). This notion was further supported by a recent discovery of KA cells in the marine annelid (Platynereis dumerilii) (Vergara et al., 2017). Notably, compared with KA cells in the lamprey (Jalalvand et al., 2014) and zebrafish (Djenoune et al., 2017), mouse KA cells do not produce somatostatin (Petracca et al., 2016). There are thus important differences in the molecular identities of KA cells that have evolved over time.
The functions of KA cells have puzzled researchers for almost a century. According to the location and morphology of KA cells; the suggested physiological roles of these cells are mechanosensory or chemosensory (Kolmer, 1921; Agduhr, 1922; Vigh-Teichmann and Vigh, 1983). One recent in vivo experiment has demonstrated that KA cells have a direct mechanosensory function to sense CSF flow via polycystic kidney disease 2-like 1 (Pkd2l1) channels in the zebrafish spinal cord (Sternberg et al., 2018). In addition, there is evidence that KA cells may play a role as mechanoreceptors and chemoreceptors due to their expression of an acid-sensing ion channel (ASIC3) in lampreys (Jalalvand et al., 2016).
Knowledge of the shared expression of transcription factors and GABA neurotransmitter in KA’/CSF-cN’ and KA”/CSF-cN” cells allows one to ask how their common identities are genetically programmed. In this review, we will describe the gene expression patterns of KA/CSF-cN cells and summarize progress in the quest to understand how KA cell fates are specified. Finally, we will discuss possible future directions to provide additional details of the genetic programs that define a KA/CSF-cN cell fate.
KA/CSF-cN Cells Are Gabaergic Interneurons
Several characteristics of KA cells are provided in Figure 1 and Table 1. Cells with similar characteristics to those of KA cells, such as expressing the genes encoding Gad2 (formerly Gad65)/Gad1 (formerly Gad67) enzymes for the synthesis of GABA from glutamate, as well as releasing GABA have been identified in the lamprey (Jalalvand et al., 2014, 2016), dogfish (Sueiro et al., 2004), zebrafish (Bernhardt et al., 1992; Yang et al., 2010), frog (Dale et al., 1987b), mouse (Djenoune et al., 2014; Orts-Del’Immagine et al., 2014; Petracca et al., 2016), rat (Kútna et al., 2014), and macaque (Macaca fascicularis) spinal cords (Djenoune et al., 2014). Collectively, these findings demonstrate that KA cells are GABAergic interneurons that exhibit a long ascending ipsilateral axon. Of note, expressions of genes encoding somatostatin (sst), urotensin II-related peptides 1 (urp1) and 2 (urp2), and serotonin (5-hydroxytryptamine, 5-TH) are observed in lamprey (Jalalvand et al., 2014), dogfish (Sueiro et al., 2004) and zebrafish KA cells (Quan et al., 2015) (Djenoune et al., 2017), suggesting that KA/CSF-cN cells may play a role in exerting neuroendocrine activities.
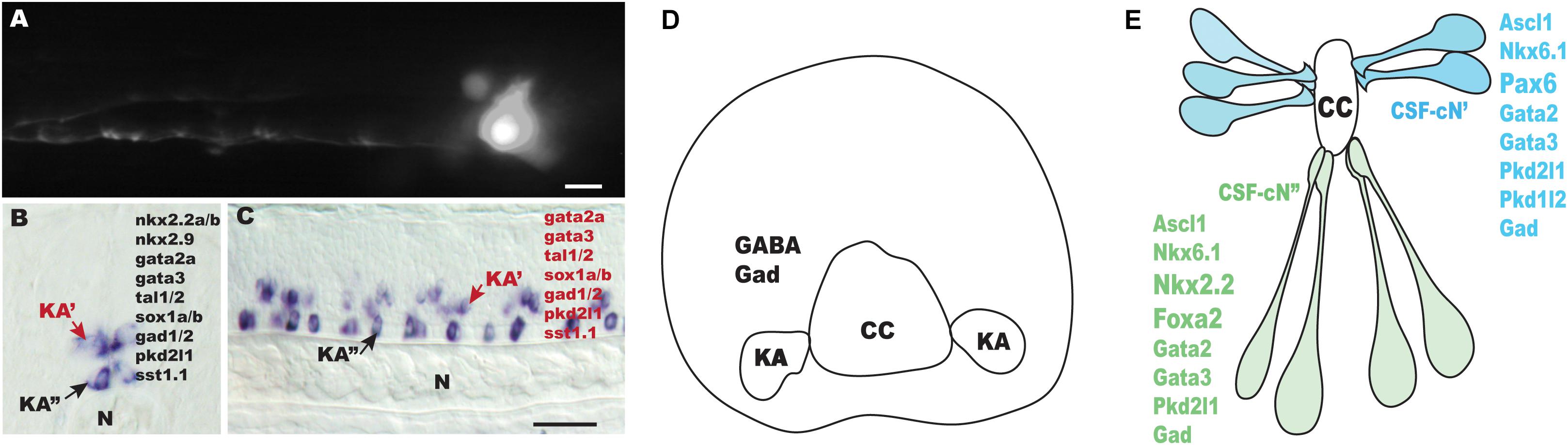
Figure 1. Outline of Kolmer–Agduhr (KA)/cerebrospinal fluid (CSF)-contacting neuron (cN) cells in different species. (A) sox1a+ KA” cell at 24-hpf-labeled transiently by injection of a GFP reporter cassette [TgBAC (sox1a:eGFP)] in zebrafish embryos at one-to-two cell stage. (B,C) Lateral view (B) and cross-section (C) of a 24-hpf zebrafish embryo hybridized with a tal2 probe. (D) A scheme of a transverse section through the spinal cord of a frog embryo (stages 37–38). (E) A scheme of mouse CSF-cN cells (E14.5). N, notochord; CC, central canal. Scale bars: 25 μm in (A); 50 μm in (B,C).
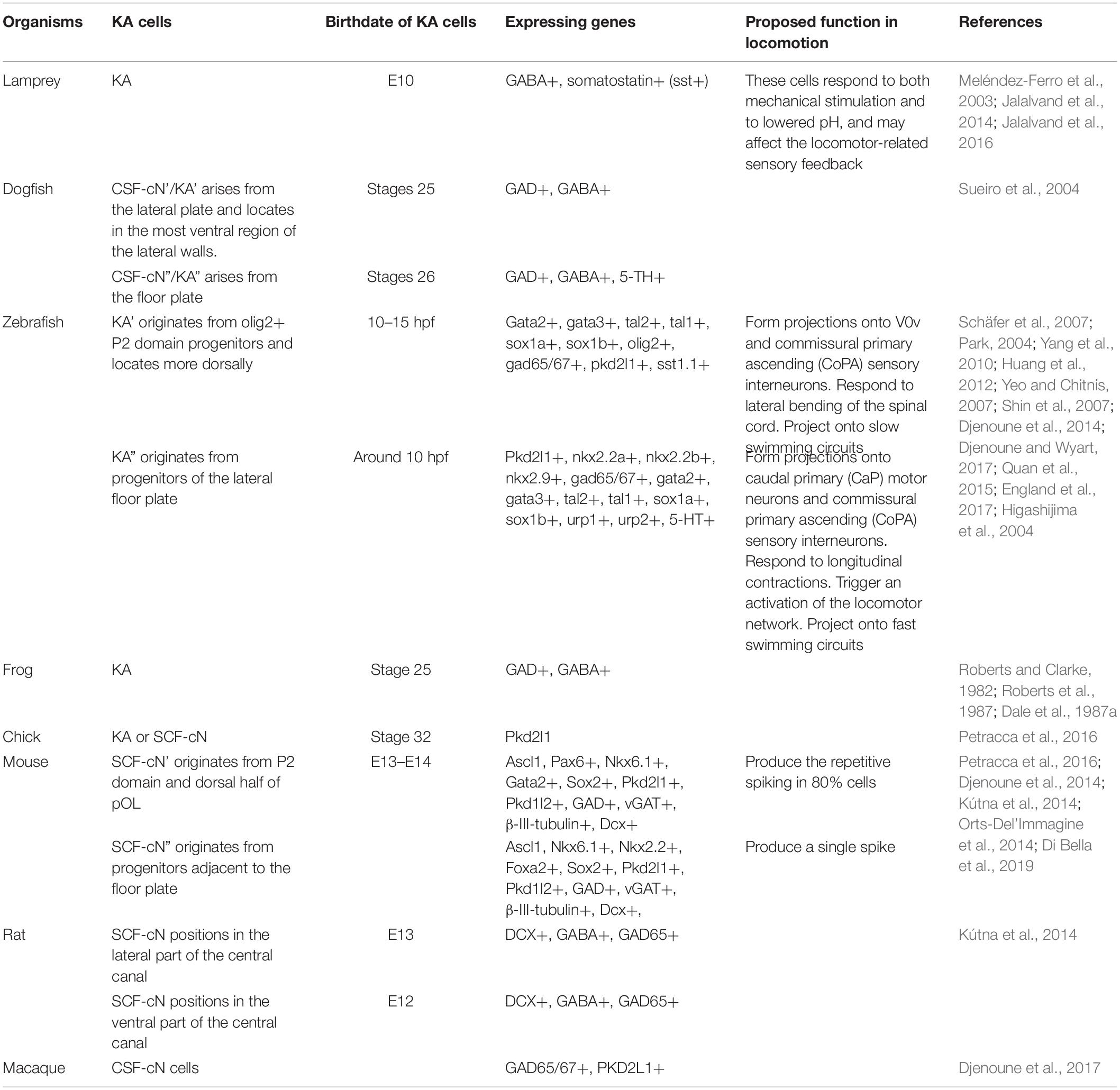
Table 1. The characteristics of Kolmer–Agduhr (KA)/cerebrospinal fluid (CSF)-contacting neuron (cN) cells.
Subsets of KA/CSF-cN Cells Are Differentiated From Different Developmental Origins
KA cells are subdivided into the KA”/CSF-cNs” ventral subgroup and KA’/CSF-cNs’ dorsal subgroup in zebrafish (Yang et al., 2010), dogfish (Sueiro et al., 2004), mouse (Petracca et al., 2016), and rat (Kútna et al., 2014). Considering the distinct locations of each of these KA cells subtypes, it is hypothesized that KA’ and KA” cells are generated from different developmental origins. Based on cell fate mapping and clonal analysis, indeed, KA’ cells are distinguished as a subgroup of interneurons expressing olig2:EGFP+/GABA+ in zebrafish embryos, whereas KA” cells are found to be generated from LFP nkx2.2+/nkx2.9+ progenitors. Evidence supports that KA’ cells are differentiated from olig2+ progenitors. First, cell fate-mapping experiments have shown that in zebrafish, all KA’ cells are derived from the olig2+ precursors in the pMN domain, which also produces motoneurons (Park, 2004). Second, morpholino knockdown of olig2 abolishes cells expressing KA’ markers including tal2 and gad65/67 (Yang et al., 2010). In contrast, current evidence supports that KA” cells are differentiated from LFP nkx2.2+/nkx2.9+ progenitors. Specifically, nkx2.2+/nkx2.9+ progenitors divide both symmetrically and asymmetrically and form KA” cells in zebrafish embryos (Huang et al., 2012). In addition, morpholino knockdown of nkx2.2a, nkx2.2b, and nkx2.9 completely eliminates KA” cells expressing the markers gata2a, gata3, sox1a, sox1b, tal2, and gad65/67 in the LFP (Yang et al., 2010; Gerber et al., 2019). Furthermore, a subset of KA” cells expressing tal2+/nkx2.2b+ differentiates into sim1+/huC/D+ V3 interneurons, and thus, tal2+/nkx2.2b+ cells are postulated to be p3 neural progenitor cells (Schäfer et al., 2007). This notion has been further supported by a recent report that shows that in gata2a mutants, KA” cells lose their identities, and that there is a concomitant increase in the number of cells expressing the V3-specific gene, single-minded homolog 1a (sim1a), which encodes a leucine zipper/PAS transcription factor gene single-minded homolog 1a (Andrzejczuk et al., 2018).
To determine the developmental origins of KA cells in mouse, newly born Pkd2l1-expressing CSF-cN cells have been mapped in relation to the domains marked by transcription factors including Nkx6.1, Pax6, Nkx2.2, and Olig2. These experiments have shown that 70% of CSF-cN’s arise from the Nkx6.1+/Pax6+ progenitors located dorsal to Olig2+ ventricular cells, (which marks the p2 neural progenitor domain), whereas the other 30% of these cells are differentiated from the dorsal half of the Olig2+ pOL domain; in contrast, CSF-cN”s were found to originate from the Nkx2.2+/Foxa2+ cells positioned in the floor plate (Petracca et al., 2016). Taken together, the current evidence supports that at least two subgroups of KA/CSF-cN cells develop from distinct progenitors in zebrafish and mouse. Of note, regardless of the different origins of CSF-cN’ and CSF-cN” cells in Ascl1-deficient mice, both of these CSF-cN subtypes fail to differentiate, and CSF-cN precursors are instead converted into non-neuronal ependymocytes (Di Bella et al., 2019), suggesting that Ascl1 may play a role as a selector for controlling the fate of CSF-cN cells and ependymocytes in mouse.
Transcription Factors Driving the Identities of KA/CSF-cN Cells
To better understand how KA/CSF-cN cells are generated, several studies have made progress by investigating the genetic programs that regulate KA cell development. Currently, at least 10 transcription factors have been identified to be involved in specifying KA/CSF-cN cells in zebrafish and/or mouse.
Nkx2.2 and nkx2.9 each contain highly conserved homeobox and NK2-specific domains and belong to the family of class II transcription factors. Zebrafish have two nkx2.2 genes, namely, nkx2.2a and nkx2.2b (Schäfer et al., 2005). The spatial expressions of nkx2.2a, nkx2.2b, and nkx2.9 are restricted to the LFP (Schäfer et al., 2005) (Yang et al., 2010). In the zebrafish LFP, there are at least three different cell groups positioned along the anteroposterior axis. One of these subgroups has been identified as KA” cells and expresses nkx2.2a, nkx2.2b, nkx2.9, and tal2. The functions of Nkx2.2a, Nkx2.2b, and Nkx2.9 are necessary for guiding the identity of gad65/67 expressing KA” cells in a functionally redundant manner (Yang et al., 2010). The second subgroup of cells expressing nkx2.2a and nkx2.9 are thought to be undifferentiated LFP progenitor cells. Differentiated KA” cells downregulate the expressions of nkx2.2a and nkx2.9 (Huang et al., 2012). The third subgroup of tal2+/nkx2.2b+ cells differentiates into sim1+ V3 postmitotic interneurons (Schäfer et al., 2007). Morpholino knockdown experiments have revealed that nkx2.2a and nkx2.2b are required for the formation of LFP cells, but are not essential for defining tal2+/nkx2.2b+ cells. Furthermore, cells expressing foxa2 and nkx2.2b represent the non-neuronal floor plate cells and proliferate during early neurogenesis (Schäfer et al., 2007).
In mouse, CSF-cN” cells express Nkx2.2 and Foxa2; however, they do not express Lmx1b, a marker of the non-neurogenic floor plate, or Pax6, suggesting that Pkd2l1+ CSF-cN” neurons developed from the boundary between the p3 ventricular zone and the floor plate (Petracca et al., 2016). Nkx2.2 is expressed in CSF-cN” cells, but it is not essential for the differentiation of Pkd2l1+ CSF-cN” cells because no difference is observed in the number of Pkd2l1+ KA” cells in Nkx2.2 mutants compared to that in controls (Petracca et al., 2016). One possible explanation for this result is that there is functional redundancy of Nkx2.2 and Nkx2.9 for specification of Pkd2l1+ CSF-cN” cells, as found in zebrafish. However, whether these different cell types exist in the mouse LFP remains unclear.
There are two nkx6 homologs in zebrafish, named nkx6.1 and nkx6.2. They are each expressed in the ventral spinal cord, including within the floor plate and pMN domain. In the absence of Nkx6.1 and Nkx6.2 proteins, middle primary motoneurons (MiPs) develop a hybrid phenotype consisting of morphological characteristics of both motoneurons and interneurons; however, the number of GABA-positive cells produced from the pMN domain and LFP do not change (Cheesman, 2004; Hutchinson et al., 2007). In mouse, Nkx6.1 is expressed by both CSF-cN’ and CSF-cN” cell progenitors. CSF-cN’ and CSF-cN” cells are derived from Nkx6.1+/Pax6+ and Nkx6.1+/Nkx2.2+/Foxa2+ progenitors, respectively, but the functions of Nkx6.1 in the specification of CSF-cN subtypes have not yet been reported.
Gata2a and gata3 belong to the C4 zinc-finger family and are expressed by the V2b, V2s, KA”, and KA’ cells in zebrafish (Batista et al., 2008; Gerber et al., 2019; Yang et al., 2010). Morpholino knockdown of gata3 eliminates KA’ cell formation (Yang et al., 2010). Consistent with this finding, several KA’ markers, including tal2, gad65/67, pkd2l1, and sst1.1 are completely abolished in gata3 mutants (Andrzejczuk et al., 2018), suggesting that Gata3 is required for specifying KA’ cells. While knockdown of gata2a dramatically reduces gad65/67-expressing KA” cells, the expressions of several KA” markers, including gata3, tal1, sox1a, gad65/67, pkd2l1, and urp1 are eliminated in gata2a mutants (Yang et al., 2010) (Andrzejczuk et al., 2018). These data suggest that gata2a and gata3 denote distinct regulatory networks for specifying KA” and KA’ cells, respectively, despite gata2a and gata3 being expressed in both KA” and KA’ cells. In mouse, CSF-cN cells are identified as late born neurons appearing at E14.5 and express Gata2, Gata3, Pkd2l1, and Pkd1l2; however, the functions of Gata3 and Gata2 in CSF-cN cells have not yet been reported (Petracca et al., 2016).
Olig2, a basic helix–loop–helix (bHLH) transcription factor, plays a pivotal role in oligodendrocytic and motoneuronal differentiation. Olig2 is expressed in proliferative ventral neuronal precursors, primary motoneurons, and oligodendrocytic progenitors in zebrafish (Park, 2004). Cell tracking experiments have suggested that all KA’ cells are differentiated from the Olig2+ progenitors in zebrafish and that the function of Olig2 is required for the production of KA’ cells from progenitors in the pMN domain (Park, 2004; Yang et al., 2010). In mouse, nearly 70% of CSF-cN’ cells are produced from progenitors with a p2 identity, whereas only 30% originate from the Olig2+ cells. One possible explanation is that Olig2 may be transiently expressed by p2 progenitors, but that CSF-cN’ cells differentiate several days later. Hence, it remains to be determined whether Olig2 plays a role in the development of mouse CSF-cN’ cells.
Tal1 and tal2 belong to the family of bHLH transcription factors. Both of tal1 and tal2 share 50% identical amino acids and are expressed by KA,” KA,’ and V2b cells in zebrafish (Andrzejczuk et al., 2018; Pinheiro et al., 2004; Yang et al., 2010). Genetic inhibition of tal1 in homozygous tal1 mutants abolishes the expressions of gata3, gata2a, tal2, sox1a, sox1b, gad65/67, pkd2l1, and sst1.1 in KA’ cells, whereas knockdown of tal2 causes a reduction in the KA” markers, gad65/67 expression (Andrzejczuk et al., 2018; Yang et al., 2010), even though tal1 and tal2 are expressed in both KA” and KA’ cells. This suggests that tal1 and tal2 may combine with different transcription factors and form a distinct regulatory network to differentially specify KA” and KA’ cells.
Sox1a and sox1b belong to group B of the Sox gene family and share 86% amino acid sequence identity. Sox1a and sox1b are expressed by KA,” KA,’ V2b, and V2s interneurons in zebrafish (Andrzejczuk et al., 2018) (Gerber et al., 2019). Knockdown of sox1a and sox1b results in a significant increase in the expression levels of V2b markers, including tal2, gata2a, gata3, and gad65/67 in the V2 domain, whereas markers for KA cells are unaffected. In agreement with this finding, sox1a and sox1b mutants only affect the expression levels of V2b markers (Gerber et al., 2019), indicating that sox1a and sox1b are expressed by KA cells, but that they are dispensable for KA cell specification. In mouse, Sox1, the ortholog of zebrafish sox1a and sox1b, is expressed in the ventricular progenitor zone in the spinal cord and in V2c interneurons. In the absence of Sox1, V2c interneurons become reprogrammed toward the V2b cell fate, suggesting that Sox1 is essential for the specification of the V2c interneuronal fate (Panayi et al., 2010). However, it remains to be determined whether the function of Sox1 plays a role in specifying CSF-cN cells in mouse.
Ascl1, a bHLH transcription factor, is expressed by the CSF-cN lineage and plays an important role in CSF-cN development (Di Bella et al., 2019). In mice lacking Ascl1, expressions of Gata2, Gata3, Pkd2l1, and Pkd1l2 in CSF-cN cells are abolished, and prospective CSF-cN progenitors instead adopt the morphology of central canal ependymocytes. Remarkably, simultaneous knockdown of ascl1a and ascl1b in zebrafish results in a reduction (∼40%) of pkd2l1+ KA cells without eliminating either KA’ or KA” cells, suggesting that the activity of Ascl1 in defining the identities of KA/CSF-cN cell identity in zebrafish differs from that in mouse, the latter of which is fully dependent on Ascl1.
Pax6 is a member of transcription factors containing a paired box. In mouse, Pax6 is expressed by most dorsal subgroups of Pkd2l1+ CSF-cN’ cells, and the expression of Pax6 is sharply downregulated during CSF-cN’ neurogenesis (Petracca et al., 2016). In the absence of Pax6, the number of Pkd2l1+ CSF-cN’ cells is almost entirely diminished, whereas the number of CSF-cN” cells positive for Pkd2l1, Nkx2.2, and Foxa2 remain unchanged, suggesting that Pax6 is only required for specifying Pkd2l1-expressing CSF-cN’ cells (Petracca et al., 2016). Despite these findings in mouse, it remains unclear whether Pax6 plays a similar role in specifying KA’ cells in zebrafish.
Transcription Factors That Are Not Expressed in KA Cells but Are Involved in Their Specifying
Islet1 is a member of the subfamily of LIM homeobox genes, a class of genes that control cell-fate programs in vertebrates. Zebrafish islet1 is expressed by motoneurons and plays a prominent role in motoneuronal development (Hutchinson, 2006). Dorsally projecting MiPs express islet1. KA’ cells do not express islet1; however, knockdown of islet1 significantly increases the number of GABA-expressing ventrolateral descending (VeLD) interneurons and KA’ cells, without disrupting the number of GABA-expressing cells at the location in which KA” cells are normally located (Hutchinson, 2006). Consistent with this finding, misexpression of Islet1 significantly reduces the number of GABA-expressing VeLD (V2b) interneurons and KA’ cells, whereas the number of cells in the KA” position is not changed compared with that in the control (Hutchinson, 2006). A possible explanation for this phenomenon is that zebrafish Iselt1 may function to promote the formation of primary motoneuron formation and mediate a switch between motoneuronal and interneuronal fates in the pMN domain. Although this study only determined the number of GABA-expressing KA and VeLD cells in the absence or misexpression of Iselt1, several other lines of evidence support the idea that KA’ cells, but not the VeLD interneurons, may be the target of iselt1-mediated patterning. First, KA’ cells are derived from Olig2+ progenitors positioned in the pMN domain, and the activity of Olig2 is required for KA’ cell specification (Park, 2004; Yang et al., 2010). Second, the effects of misexpression of islet1 is limited to a subset of interneurons produced from the pMN domain (Hutchinson, 2006). Third, VeLD/V2b interneurons express lhx3 but not islet1 (Appel, 1995). Fourth, the number of V2b is unchanged in the absence of Olig2, whereas a lack of Olig2 abolishes nearly all primary motoneurons expressing islet2, as well as nearly all KA’ cells (Park, 2004; Yang et al., 2010).
Lhx3 and lhx4 genes belong to the family of LIM homeodomain transcription factor and play pivotal roles in motoneuronal and interneuronal differentiation. In the absence of lhx3 and lhx4, primary motoneurons develop a hybrid identity in which islet-expressing neurons coexpress GABA and gad, and form ipsilateral ascending axons, a characteristic property of the KA’ cells (Seredick et al., 2014). Evidence supports the idea that Lhx3 and Lhx4 may regulate Notch signaling, which in turn promotes the expression of gad in primary motoneurons. Forced-expression experiments have demonstrated that Lhx3 promotes the specification of circumferential descending (CiD) interneurons, (also known as V2a interneurons) at the expense of KA’ cells. Although lhx3 and lhx4 are not expressed in KA’ cells, Lhx proteins can regulate the expression levels of gad and GABA in primary motoneurons and influence axonal projections to acquire the phenotype of ipsilaterally ascending axons (Seredick et al., 2014).
Potential Markers of KA/CSF-cN Cells
Pkd1l2a and Pkd2l1
The polycystic kidney disease (PKD) gene family encodes transmembrane proteins that share a conserved polycystin-cation-channel domain. Several lines of evidence support that genes encoding PKD 1-like 2a (pkd1l2a) and pkd2l1 are expressed by all KA” and KA’ cells in zebrafish embryos (Djenoune et al., 2014; England et al., 2017), while Pkd2l1 is also expressed in mouse and macaque KA cells (Djenoune et al., 2014). Approximately 15% of PKD2L1+ KA cells are GABA/GAD67 negative in the adult mouse spinal cord. PKD2L1+ KA cells are not serotonergic (5-HT) or catecholaminergic [marked by tyrosine hydroxylase (TH) expression] (Djenoune et al., 2014). A potential explanation for this discrepancy may be due to differences in embryonic and adult tissues. In vivo experiments suggest that pkd2l1 is required for KA cells to detect CSF flow in zebrafish embryos; however, Pkd2l1 is not required for KA cell differentiation (Sternberg et al., 2018).
KA’/CSF-cN’ and KA”/CSF-cN” Cells Share Common Transcription Factors but Differ in Terms of Their Regulatory Networks
We and others have shown that KA’ and KA” cells share a group of transcription factors including gata2a, gata3, tal1, tal2, sox1a, and sox1b in zebrafish embryos (Yang et al., 2010; Andrzejczuk et al., 2018; Gerber et al., 2019). However, the genetic programs regulating KA’ and KA” development are distinct from one another. Morpholino knockdown analyses have indicated that gata3 is required for KA,’ but not KA” cell specification, whereas gata2a and tal2 are indispensable for specification of KA” but not KA’ cells (Yang et al., 2010). Consistent with these results, analyses of tal1, gata2a, and gata3 mutant have demonstrated that Gata2a is required for specifying KA” cell identity, and that Gata3 and Tal1 are required for defining KA’ cell fate (Andrzejczuk et al., 2018). Deficiency of gata2a results in a loss of cells in the LFP (where KA” cells are generated) that expresses gata3, tal2, tal1, sox1a, sox1b, gad65/67, urp1, and pkd2l1, but not a loss of such cells in the dorsal spinal cord where KA’ cells normally form (Yang et al., 2010; Andrzejczuk et al., 2018; Gerber et al., 2019) (Yang et al., unpublished observations). In addition, a significant increase in the number of slc17a6a/b and sim1a-expressing cells is observed in gata2a mutant (Andrzejczuk et al., 2018), suggesting that at least some KA” cells shift to become V3 interneurons or adopt a hybrid V3/KA” fate in the absence of gata2a. Further investigation has revealed that knockdown of tal2 eliminates the expression of gad65/67 in KA” cells, whereas the expressions of gata2a and gata3 in KA” cells are unchanged. Taken together, current evidence suggests that gata2a acts upstream of tal2 and sox1a in KA” cells, which in turn drive the expressions of gad65/67, urp1, and pkd2l1 in KA” cells.
In the absence of Gata3 protein, KA’ cells that express gata2a, tal1, tal2, sox1a, sox1b, gad65/67, sst1.1, and pkd2l1 are abolished, whereas there is no change in the number of KA” cells expressing gata2a, tal1, tal2, sox1a, sox1b, gad65/67, sst1.1, and pkd2l1 (Yang et al., 2010; Andrzejczuk et al., 2018; Gerber et al., 2019) (Yang et al. unpublished observations). Similarly, in tal1 mutants, expressions of gata2a, tal2, sox1b, gad65/67, sst1.1, and pkd2l1 in KA’ cells are completely abolished, and gata3 and sox1a-expressing KA’ cells are dramatically reduced. In contrast, there is no effect on the number of KA” cells (Andrzejczuk et al., 2018). Furthermore, an increase in the number of phosphor-histone H3-positive/olig2-positive cells positioned in the pMN domain (where KA’ cells are generated) is observed in both gata3 and tal1 mutants, suggesting that loss of the function of Gata3 and/or Tal1 may promote cells to become mitotically active precursors, which in turn block/delay KA’ cell differentiation. Similarly, Gata2/3 are expressed in mouse CSF-cN’ and CSF-cN” cells, although expressions of Tal1 and Tal2 were not examined in this study (Petracca et al., 2016). Gene function analysis demonstrates that Pax6 is exclusively required for the production of CSF-cN’ cells from progenitors in the p2-pOL domain. In contrast, Nkx2.2 is dispensable for the production of CSF-cN” cells despite CSF-cN” cells expressing Nkx2.2. Despite these recent findings, further studies are needed to elucidate the functions of Gata2, Gata3, Tal1, and Tal2 in regulating the CSF-cN cell differentiation in mouse.
Specifications of KA’/CSF-cN’ and KA”/CSF-cN” Cells Are Differently Regulated by Hedgehog and Delta-Notch Signaling
Hedgehog signaling plays a pivotal role in defining the KA” cell fate in a concentration- and duration-dependent manner (Strähle et al., 2004; Schäfer et al., 2007; Huang et al., 2012). Loss of sonic hedgehog (Shh) signaling in homozygous mutants of the ligand Shh (sonic-you, syu), the signal transducer smoothened (slow-muscle-omitted, smo), and the transcription factors Gli1 (detour, dtr) and Gli2 (you-too, yot) completely eliminates expressions of several markers, namely, nkx2.2a, nkx2.2b, nkx2.9, and tal2 in the LFP and in KA” cells (Yang et al., 2010) and Yang et al., unpublished observations) (Schäfer et al., 2007). In contrast, the expression of tal2 in KA” cells is normal in heterozygous dtr and yot mutants (Schäfer et al., 2007), suggesting that compared with those in nkx2.2b+/foxa2+ LFP cells, relatively lower levels of hedgehog activity are required for forming KA” cells (Nkx2.2b+/Tal2+) and Sim1-positive V3 interneurons in zebrafish (Schäfer et al., 2007). In agreement with this, the LFP progenitors remain responsive to hedgehog, whereas differentiated KA” cells lose their responses (Huang et al., 2012). Further evidence indicates that forced expression of Gli1 reduces the number of KA” cells and increases in nkx2.9-expressing LFP cells, suggesting that termination of hedgehog signaling is essential for KA” cell differentiation (Huang et al., 2012). In addition, activation of hedgehog signaling by ectopic expression of Shh or the dominant-negative form of PKA mRNA induces numerous tal2-expressing KA” cells, as well as dorsally located KA’ cells (Huang et al., 2012). Intriguingly, expression of tal2 in more dorsally located cells, which might represent KA’ cells and V2b interneurons, is unaffected in the absence of Gli2 (Schäfer et al., 2007). This phenomenon appears to hold true in embryos incubated in cyclopamine from the shield stage to the 22 somite stage, in which tal2-positive KA” cells are completely eliminated, whereas the tal2-positive KA’ cells are not, and V2b interneurons also likely exist (Schäfer et al., 2007). These results suggest that hedgehog signaling may play differential roles in specifying KA” and KA’ cells.
Comparative studies suggest that the functions of hedgehog signaling in mouse differ from those in zebrafish (England et al., 2011). Hedgehog signaling is required to induce both V3 interneurons in the p3 domain and some motoneurons in the pMN domain. Loss of Shh signaling in mouse results in severely decreased numbers of V1 and V0v cells, in which case only a few V2 interneurons form, and there is a complete elimination of motoneurons. Additionally, a lack of hedgehog signaling in zebrafish embryos results in most V3 domain cells not forming and motoneurons being dramatically reduced (England et al., 2011). However, it is unclear whether hedgehog signaling plays a role in defining the CSF-cN identities in mouse.
Notch signaling has been implicated in KA cell development (Schäfer et al., 2007; Shin et al., 2007; Yeo and Chitnis, 2007; Huang et al., 2012). Absence of Notch signaling in the zebrafish mutant, mindbomb (mib), which encodes an E3 ubiquitin ligase and is necessary for efficient Notch signaling (Itoh et al., 2003), results in loss of both LFP and KA” cells (Schäfer et al., 2007; Yeo and Chitnis, 2007). In addition, early blocking of Notch signaling by expressing a dominant-negative form of Xenopus suppressor of Hairless [Su(H)] or inhibitors at 7 hpf leads to a reduction in the number of KA” cells, as that observed in the mib mutant (Schäfer et al., 2007; Yeo and Chitnis, 2007; Huang et al., 2012). Inhibition of Notch signaling from 10 to 25 hpf results in a significant increase in the number of tal2-expressing KA” cells at the expense of nkx2.9-expressing FLP cells (Huang et al., 2012). Conversely, activation of Notch signaling by the induced Notch intracellular domain (NICD) at 10 hpf almost completely eliminates tal2-expressing KA” cells, but increases the LFP cells expressing nkx2.9 (Huang et al., 2012). In contrast, blocking Notch signaling at 17 hpf does not affect the number of KA” cells (Yeo and Chitnis, 2007). Furthermore, knockdown of Jagged2, a ligand of Notch receptors, causes a significant increase in the number of KA” cells and secondary motor neurons (SMNs), as well as a significant decrease in the rate of cell division. These data suggest that Jagged2-mediated signaling is not only required to maintain a group of dividing precursors, but that it also plays a role in regulating the number of KA” cells. Notch signaling also plays a pivotal role in specifying KA’ cells. In the absence of Notch signaling, primary motoneurons are formed at the expense of KA’ cells. In contrast, an excess of Notch signaling induces KA’ cell formation at the expense of PMNs in zebrafish, suggesting that Notch signaling promotes KA’ cell identity and inhibits primary motoneuronal fate (Shin et al., 2007). These lines of evidence support that Notch signaling plays an essential role in KA cell differentiation. Hence, specification of KA” cells initially depends on the activation and then the attenuation of both Notch and hedgehog signaling (Huang et al., 2012).
The Transcriptional Regulatory Logic That Drives KA/CSF-cN Identity
Based on findings by our lab and other research groups (Park, 2004; Yeo and Chitnis, 2007; Yang et al., 2010; Petracca et al., 2016; Andrzejczuk et al., 2018; Di Bella et al., 2019; Gerber et al., 2019), here, we summarize the regulatory network guiding the KA/CSF-cN differentiation and identity (see Figure 2). Considering that KA/CSF-cN cells are GABAergic neurons, we summarize the transcriptional regulatory network guiding GABAergic neuronal identity in the mouse telencephalon, midbrain, hindbrain, and dorsal spinal cord (Figure 2). A line of evidence supports that the genetic program guiding GABAergic fate is likely dependent on multiple transcription factors in different regions, rather than by universal regulators that govern differentiation of all GABAergic neurons (Achim et al., 2014; Hobert and Kratsios, 2019). Furthermore, there is conceivable evidence supporting that differences in the transcription regulatory networks controlling generation of the diversity of GABAergic neurons may depend on the respective selector gene being either selectively antagonized by a repressor and/or assisted by region-specific cofactors (Hobert and Kratsios, 2019). Nevertheless, transcription factors including proneural genes (e.g., Ascl1, Helt) and postmitotic subtype selector genes (e.g., Gata2, Gata3, Tal1, and Tal2) appear to be repeatedly employed for driving GABAergic identity in mouse. In particular, functions of PTF1A and GATA2/TAL1 have been demonstrated to play a role as a GABAergic, rather than glutamatergic selectors in the dorsal and ventral spinal cord, respectively. In addition, Dlx1/2, Gata2, and Gata2/Tal2 have been suggested to play roles as selectors for GABAergic neuronal identity in the mouse telencephalon, diencephalon, and midbrain, respectively (Achim et al., 2014; Figure 2).
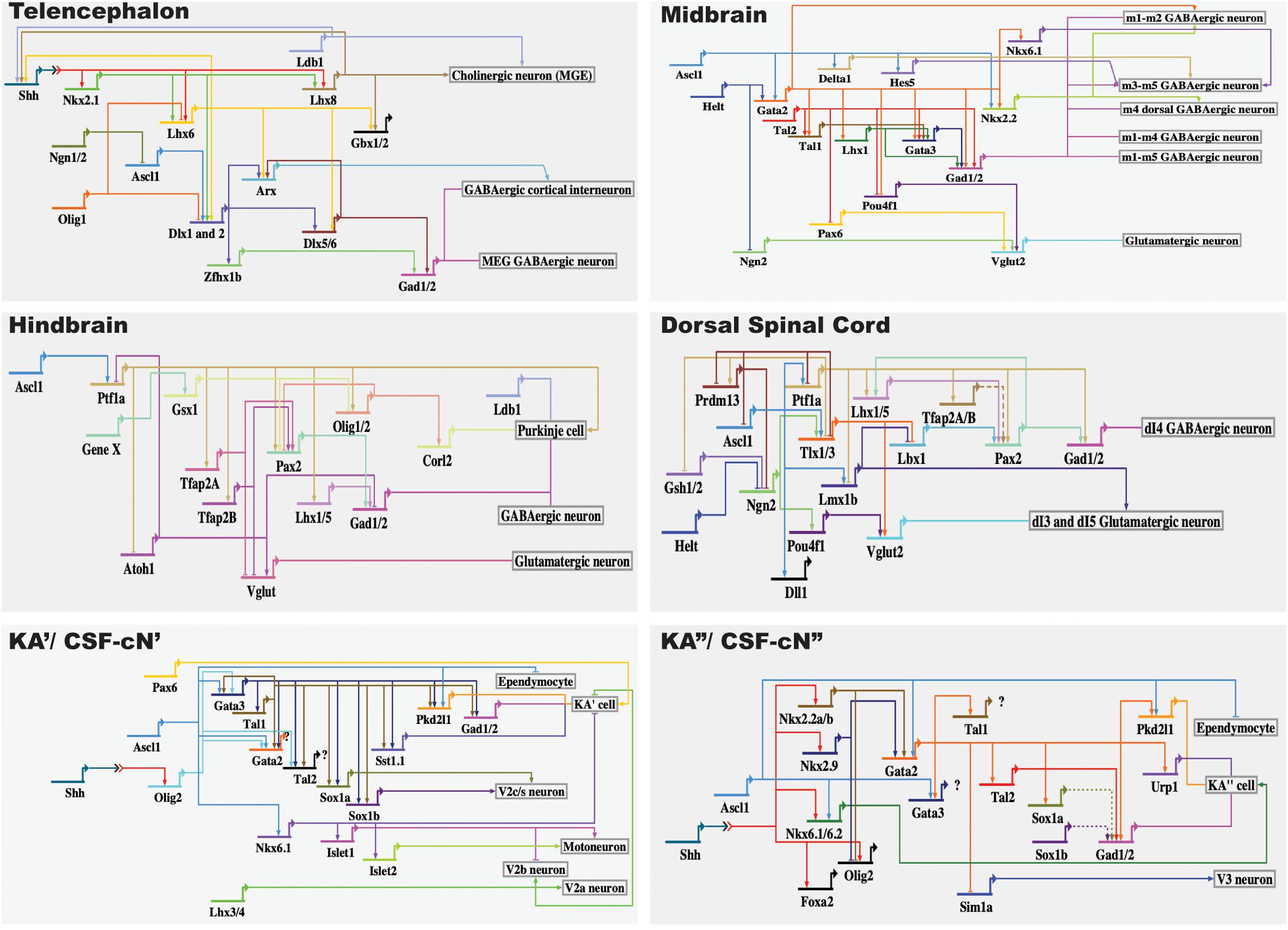
Figure 2. Gene regulatory network leading to differentiation of GABAergic interneurons. Of note, arrows do not necessarily reflect direct interactions between genes or proteins. The BioTapestry was used for building the GRN (http://www.biotapestry.org/).
KA’ and KA” cells share a class of the transcription factors, including gata2, gata3, tal1, tal2, sox1a, and sox1b, despite these cellular subtypes having different developmental origins. This is in agreement with observations that similar neurons, such as dopaminergic and GABAergic neuronal classes with distinct lineages, appear to be specified by the same terminal selector type transcription factors in C. elegans (Gendrel et al., 2016). We surmise here, as suggested via terminal selectors elucidated previously (Hobert, 2016) that gata3/tal1 and gata2/tal2 may serve as terminal selectors controlling KA’ and KA” differentiation, respectively, by combining cis-regulatory motifs associated with gad1/2 and/or pkd2l1/pkd1l2 genes in zebrafish. Gata3 and tal1 are expressed in KA’ and KA” cells; however, genetic removal of gata3 and tal1 only leads to a failure of KA’ to be differentiated from precursors. Similarly, gata2/tal2 are expressed in both KA’ and KA” cells, but genetic removal of gata2 and tal2 only leads to a failure of KA” cells to acquire a GABAergic identity (Yang et al., 2010; Andrzejczuk et al., 2018). This is consistent with the function of Gata2 in specifying GABAergic identity in the mouse midbrain and in rhombomere 1. Gata2 is required for GABAergic neuronal differentiation in the midbrain. However, expressions of the GABAergic marker genes, Gata3 and Gad1, in GABAergic precursors of rhombomere 1 are not altered in the Gata2 mutants (Kala et al., 2009). The most likely explanation for these observations is that the differences in the cooperation of gata3/tal1 and gata2/tal2 for specifying the KA’ and KA” cell identity may be related to the different lineages of KA’ and KA” cells. Gata3 may cooperate with the cofactor tal1 to define KA’ identity. In line with this hypothesis, deficiency of tal1 phenocopies the characteristics of gata3 mutants, including the elimination of KA’ cells expressing gata2a, gata3, tal2, and gad1/2 (Andrzejczuk et al., 2018). Similar to findings in tal1 mutants, in the absence of gata3, expressions of gata2a, tal1, tal2, and gad1/2 are abolished in KA’ cells. In addition, deletion of tal1 phenocopies loss of expression of Tg(-8.1gata1-EGFP) in the V2b region observed in gata2a/gata3 double mutants, suggesting that gata2a and gata3 may cooperate with their cofactor, tal1, as a functional complex for specifying V2b interneurons in zebrafish (Andrzejczuk et al., 2018).
In mouse, both CSF-cN’ cells and V2b interneurons share the expressions of Gata2 and Gata3. However, evidence supports that CSF-cN’ cells are different from early born GATA2 and GATA3-expressing V2b interneurons. In contrast with the finding that Foxn4 is essential for V2b interneuronal specification, differentiation of CSF-cN’ cells is unchanged in the Foxn4 mutants. Moreover, the activity of PAX6 is indispensable for CSF-cN’ specification, but V2b interneurons are not affected in Pax6 mutant mice (Petracca et al., 2016).
Differences Between KA/CSF-cN Differentiation in Mouse and Zebrafish
Since the underlying mechanisms and signaling controlling the formations of the medial floor plate and LFP are different in mouse and zebrafish (Strähle et al., 2004), the genetic programs defining the identity of KA/CSF-cN cells may differ in these two vertebrate species. Indeed, in Ascl1-deficient mouse, CSF-cN cells fail to initiate differentiation, and the precursors are converted into ependymal cells. In contrast, in the knockdown of ascl1a and ascl1b, KA cells are still formed, despite a decrease in the numbers of KAs observed in zebrafish (Di Bella et al., 2019). Whether Ascl1 plays a similar role in differentiation of early born KA/CSF-cN cells in Xenopus and lamprey as that does in zebrafish remains to be elucidated. In addition, observations have shown that CSF-cN cells are differentiated only after a neurogenic-to-gliogenic switch of spinal precursors in mice, rats, and chicks (Petracca et al., 2016) (Kútna et al., 2014) (Di Bella et al., 2019). In contrast to findings in mouse, in zebrafish and Xenopus, KA cells are produced simultaneously with primary motoneurons and other interneurons.
Discussion
Although it is currently known that gata3/tal1 and gata2/tal2 drive KA’ and KA” identities, respectively, in the zebrafish spinal cord, it remains unclear how gata3/tal1 and gata2/tal2 genes are selected and functionally define these two groups of KA cells despite all of these genes being expressed in both KA’ and KA” cells. In addition, at least some KA” cells change from a GABAergic identity to a glutamatergic V3 interneuronal identity or acquire a hybrid V3/KA” identity in gata2a mutant zebrafish. Furthermore, in the absence of both gata3 and tal1, an increase in the numbers of phosphor-histone H3-labeled precursors and olig2-positive cells is observed in the pMN domain, from which KA’ cells are produced, suggesting a failure of KA’ cells in terminal differentiation. Although gata2/3 and tal1/2 encode highly related proteins and act via both the distinct and redundant functions in the central nervous system and during hematopoietic development, it is not known how these genes are functional as selector genes and/or activators for exiting the cell cycle.
Perspectives
We currently know that gata2/3 and tal1/2 are critical for specifying KA’ and KA” cells, respectively, but the crucial details remain unknown as to how these two subgroups of KA cells that originated from two different progenitor domains are encoded at the genomic, epigenomic, and transcriptomic levels via transcription factors, particularly in terms of KA/CSF-cN cells that are present in all vertebrate species that have been studied. Based on a mechanistic understanding of this regulatory network, transient expression of ASCL1 and DLX2 is sufficient to convert human pluripotent stem cells exclusively into GABAergic neurons with characteristics of forebrain GABAergic neurons. Remarkably, a combination of Ascl1 and Dlx2 with other transcription factors, including Arx, Brn4, Ebf1, Gata2, Gbx1, Gsx2, Ikaros, Islet1, Lhx6, Lmo2, Lmo3, Meis1, Meis2, Oct6, Otp, Pbx1, and Ptf1a does not drive the cells to generate the different subtypes of GABAergic neurons (Yang et al., 2017), suggesting that much remains unknown regarding how these GABAergic cells are differentiated and specified. It has been indicated that regulatory elements as binding hubs are critical for regulating spatiotemporal gene expression patterns and cell lineage specifications. Although cis-regulatory control of gene expression is a complex process, dependent on distal sequences, spatial organization of the chromosome, and chromatin or epigenetic states and advances in genetics, genomics, and developmental neurobiology have helped to gain further insight into the genetically encoded wiring diagram that ultimately gives rise to KA/CSF-cN cells. In particular, single-cell RNA-sequencing methods have been demonstrated in characterizing cellular diversity and transcriptional regulation of the brain and spinal cord, shedding the new light on revealing the regulatory networks that specify KA/CSF-cN identities.
Author Contributions
LY wrote the manuscript. FW prepared the figures. US read and commented on drafts of this manuscript. All authors contributed to the article and approved the submitted version.
Funding
This work was supported by the Ministry of Human Resources and Social Security of China and the State Key Laboratory of Environmental Criteria and Risk Assessment, Chinese Research Academy of Environmental Sciences.
Conflict of Interest
The authors declare that the research was conducted in the absence of any commercial or financial relationships that could be construed as a potential conflict of interest.
References
Achim, K., Salminen, M., and Partanen, J. (2014). Mechanisms regulating GABAergic neuron development. Cell. Mol. Life Sci. 71, 1395–1415. doi: 10.1007/s00018-013-1501-3
Agduhr, E. (1922). Über ein zentrales sinnesorgan (?) bei den vertebraten. Z. Anat. Entwicklungsgesch. 66, 223–360. doi: 10.1007/bf02593586
Andrzejczuk, L. A., Banerjee, S., England, S. J., Voufo, C., Kamara, K., and Lewis, K. E. (2018). Tal1, Gata2a, and Gata3 have distinct functions in the development of V2b and cerebrospinal fluid-contacting KA spinal neurons. Front. Neurosci. 12:170. doi: 10.3389/fnins.2018.00170
Appel, B. (1995). Motoneuron fate specification revealed by patterned LIM homebox gene expression in embryonic zebrafish. Development 121, 4117–4125.
Batista, M. F., Jacobstein, J., and Lewis, K. E. (2008). Zebrafish V2 cells develop into excitatory CiD and Notch signalling dependent inhibitory VeLD interneurons. Dev. Biol. 322, 263–275. doi: 10.1016/j.ydbio.2008.07.015
Bernhardt, R. R., Patel, C. K., Wilson, S. W., and Kuwada, J. Y. (1992). Axonal trajectories and distribution of GABAergic spinal neurons in wildtype and mutant zebrafish lacking floor plate cells. J. Comp. Neurol. 326, 263–272. doi: 10.1002/cne.903260208
Binor, E., and Heathcote, R. D. (2001). Development of GABA-immunoreactive neuron patterning in the spinal cord. J. Comp. Neurol. 438, 1–11. doi: 10.1002/cne.1298
Cheesman, S. E. (2004). Zebrafish and fly Nkx6 proteins have similar CNS expression patterns and regulate motoneuron formation. Development 131, 5221–5232. doi: 10.1242/dev.01397
Dale, N., Roberts, A., Ottersen, O. P., and Storm-Mathisen, J. (1987a). The development of a population of spinal cord neurons and their axonal projections revealed by GABA immunocytochemistry in frog embryos. Proc. R. Soc. Lond. B. Biol. Sci. 232, 205–215. doi: 10.1098/rspb.1987.0069
Dale, N., Roberts, A., Ottersen, O. P., and Storm-Mathisen, J. (1987b). The morphology and distribution of “Kolmer-Agduhr cells”, a class of cerebrospinal-fluid-contacting neurons revealed in the frog embryo spinal cord by GABA immunocytochemistry. Proc. R. Soc. Lond. B. Biol. Sci. 232, 193–203. doi: 10.1098/rspb.1987.0068
Di Bella, D. J., Carcagno, A. L., Bartolomeu, M. L., Pardi, M. B., Löhr, H., Siegel, N., et al. (2019). Ascl1 balances neuronal versus ependymal fate in the spinal cord central canal. Cell Rep. 28, 2264–2274. doi: 10.1016/j.celrep.2019.07.087
Djenoune, L., Desban, L., Gomez, J., Sternberg, J. R., Prendergast, A., Langui, D., et al. (2017). The dual developmental origin of spinal cerebrospinal fluid-contacting neurons gives rise to distinct functional subtypes. Sci. Rep. 7, 1–14.
Djenoune, L., Khabou, H., Joubert, F., Quan, F. B., Figueiredo, S. N., Bodineau, L., et al. (2014). Investigation of spinal cerebrospinal fluid-contacting neurons expressing PKD2L1: evidence for a conserved system from fish to primates. Front. Neuroanat. 8:26. doi: 10.3389/fnana.2014.00026
Djenoune, L., and Wyart, C. (2017). Light on a sensory interface linking the cerebrospinal fluid to motor circuits in vertebrates. J. Neurogenet. 31, 113–127. doi: 10.1080/01677063.2017.1359833
England, S., Batista, M. F., Mich, J. K., Chen, J. K., and Lewis, K. E. (2011). Roles of hedgehog pathway components and retinoic acid signalling in specifying zebrafish ventral spinal cord neurons. Development 138, 5121–5134. doi: 10.1242/dev.066159
England, S. J., Campbell, P. C., Banerjee, S., Swanson, A. J., and Lewis, K. E. (2017). Identification and expression analysis of the complete family of Zebrafish pkd genes. Front. Cell Dev. Biol. 5:5. doi: 10.3389/fcell.2017.00005
Gendrel, M., Atlas, E. G., and Hobert, O. (2016). A cellular and regulatory map of the GABAergic nervous system of C. elegans. eLife 5:e17686.
Gerber, V., Yang, L., Takamiya, M., Ribes, V., Gourain, V., Peravali, R., et al. (2019). The HMG box transcription factors Sox1a and Sox1b specify a new class of glycinergic interneuron in the spinal cord of zebrafish embryos. Development 146, 1–13.
Harper, C. E., and Roberts, A. (1993). Spinal cord neuron classes in embryos of the smooth newt Triturus vulgaris: a horseradish peroxidase and immunocytochemical study. Philos. Trans. R. Soc. Lond. B. Biol. Sci. 340, 141–160. doi: 10.1098/rstb.1993.0053
Higashijima, S. I., Schaefer, M., and Fetcho, J. R. (2004). Neurotransmitter properties of spinal interneurons in embryonic and larval zebrafish. J. Comp. Neurol. 480, 19–37. doi: 10.1002/cne.20279
Hobert, O. (2016). Terminal selectors of neuronal identity. Curr. Top. Dev. Biol. 116, 455–475. doi: 10.1016/bs.ctdb.2015.12.007
Hobert, O., and Kratsios, P. (2019). Neuronal identity control by terminal selectors in worms, flies, and chordates. Curr. Opin. Neurobiol. 56, 97–105. doi: 10.1016/j.conb.2018.12.006
Huang, P., Xiong, F., Megason, S. G., and Schier, A. F. (2012). Attenuation of notch and hedgehog signaling is required for fate specification in the spinal cord. PLoS Genet. 8:e1002762. doi: 10.1371/journal.pone.1002762
Hutchinson, S. A. (2006). Islet1 and Islet2 have equivalent abilities to promote motoneuron formation and to specify motoneuron subtype identity. Development 133, 2137–2147. doi: 10.1242/dev.02355
Hutchinson, S. A., Cheesman, S. E., Hale, L. A., Boone, J. Q., and Eisen, J. S. (2007). Nkx6 proteins specify one zebrafish primary motoneuron subtype by regulating late islet1 expression. Development 134, 1671–1677. doi: 10.1242/dev.02826
Itoh, M., Kim, C.-H., Palardy, G., Oda, T., Jiang, Y.-J., Maust, D., et al. (2003). Mind bomb is a ubiquitin ligase that is essential for efficient activation of Notch signaling by Delta. Dev. Cell 4, 67–82. doi: 10.1016/s1534-5807(02)00409-4
Jalalvand, E., Robertson, B., Wallén, P., and Grillner, S. (2016). Ciliated neurons lining the central canal sense both fluid movement and pH through ASIC3. Nat. Commun. 7:10002.
Jalalvand, E., Robertson, B., Wallén, P., Hill, R. H., and Grillner, S. (2014). Laterally projecting cerebrospinal fluid-contacting cells in the lamprey spinal cord are of two distinct types. J. Comp. Neurol. 522, 1753–1768. doi: 10.1002/cne.23542
Kala, K., Haugas, M., Lillevali, K., Guimera, J., Wurst, W., Salminen, M., et al. (2009). Gata2 is a tissue-specific post-mitotic selector gene for midbrain GABAergic neurons. Development 136, 253–262. doi: 10.1242/dev.029900
Kolmer, W. (1921). Das “sagitallorgan” der wirbeltiere. Z. Anat. Entwicklungsgesch. 60, 652–717. doi: 10.1007/978-3-642-65104-5_12
Kolmer, W. (1925). Weitere beitrage zur kenntnis des sagittalorgans der wirbeltiere. Verh. Anat. Ges. JENA 60, 252–257.
Kolmer, W. (1931). Uber das sagittalorgan ein zentrales sinnesorgan der wirbeltiere, in besondere bein Affen. Z. Zellforsch. Mikrosk. Anat. 13, 236–248. doi: 10.1007/bf00406356
Kútna, V., Ševc, J., Gombalová, Z., Matiašová, A., and Daxnerová, Z. (2014). Enigmatic cerebrospinal fluid-contacting neurons arise even after the termination of neurogenesis in the rat spinal cord during embryonic development and retain their immature-like characteristics until adulthood. Acta Histochem. 116, 278–285. doi: 10.1016/j.acthis.2013.08.004
Meléndez-Ferro, M., Pérez-Costas, E., Villar-Cheda, B., Rodríguez-Muñoz, R., Anadón, R., and Rodicio, M. C. (2003). Ontogeny of γ-aminobutyric acid-immunoreactive neurons in the rhombencephalon and spinal cord of the sea lamprey. J. Comp. Neurol. 464, 17–35. doi: 10.1002/cne.10773
Orts-Del’Immagine, A., Kastner, A., Tillement, V., Tardivel, C., Trouslard, J., and Wanaverbecq, N. (2014). Morphology, distribution and phenotype of polycystin kidney disease 2-like 1-positive cerebrospinal fluid contacting neurons in the brainstem of adult mice. PLoS One 9:e87748. doi: 10.1371/journal.pone.0087748
Panayi, H., Panayiotou, E., Orford, M., Genethliou, N., Mean, R., Lapathitis, G., et al. (2010). Sox1 is required for the specification of a novel p2-derived interneuron subtype in the mouse ventral spinal cord. J. Neurosci. 30, 12274–12280. doi: 10.1523/jneurosci.2402-10.2010
Park, H.-C. (2004). Spatial and temporal regulation of ventral spinal cord precursor specification by Hedgehog signaling. Development 131, 5959–5969. doi: 10.1242/dev.01456
Petracca, Y. L., Sartoretti, M. M., Di Bella, D. J., Marin-Burgin, A., Carcagno, A. L., Schinder, A. F., et al. (2016). The late and dual origin of cerebrospinal fluid-contacting neurons in the mouse spinal cord. Development 143, 880–891. doi: 10.1242/dev.129254
Pinheiro, P., Gering, M., and Patient, R. (2004). The basic helix-loop-helix transcription factor, Tal2, marks the lateral floor plate of the spinal cord in zebrafish. Gene Expr. Patterns 4, 85–92. doi: 10.1016/s1567-133x(03)00145-5
Quan, F. B., Dubessy, C., Galant, S., Kenigfest, N. B., Djenoune, L., Leprince, J., et al. (2015). Comparative distribution and in vitro activities of the urotensin II-related peptides URP1 and URP2 in zebrafish: Evidence for their colocalization in spinal cerebrospinal fluid-contacting neurons. PLoS One 10:e0119290. doi: 10.1371/journal.pone.0119290
Roberts, A., and Clarke, J. D. W. (1982). The neuroanatomy of an amphibian embryo spinal cord. Philos. Trans. R. Soc. B Biol. Sci. 296, 195–212. doi: 10.1098/rstb.1982.0002
Roberts, A., Dale, N., Ottersen, O. P., and Storm-Mathisen, J. (1987). The early development of neurons with GABA immunoreactivity in the CNS of Xenopus laevis embryos. J. Comp. Neurol. 261, 435–449. doi: 10.1002/cne.902610308
Roberts, B. L., Maslam, S., Scholten, G., and Smit, W. (1995). Dopaminergic and GABAergic cerebrospinal fluid-contacting neurons along the central canal of the spinal cord of the eel and trout. J. Comp. Neurol. 354, 423–437. doi: 10.1002/cne.903540310
Schäfer, M., Kinzel, D., Neuner, C., Schartl, M., Volff, J. N., and Winkler, C. (2005). Hedgehog and retinoid signalling confines nkx2.2b expression to the lateral floor plate of the zebrafish trunk. Mech. Dev. 122, 43–56. doi: 10.1016/j.mod.2004.09.002
Schäfer, M., Kinzel, D., and Winkler, C. (2007). Discontinuous organization and specification of the lateral floor plate in zebrafish. Dev. Biol. 301, 117–129. doi: 10.1016/j.ydbio.2006.09.018
Seredick, S., Hutchinson, S. A., Van Ryswyk, L., Talbot, J. C., and Eisen, J. S. (2014). Lhx3 and Lhx4 suppress Kolmer-Agduhr interneuron characteristics within zebrafish axial motoneurons. Development 141, 3900–3909. doi: 10.1242/dev.105718
Shin, J., Poling, J., Park, H. C., and Appel, B. (2007). Notch signaling regulates neural precursor allocation and binary neuronal fate decisions in zebrafish. Development 134, 1911–1920. doi: 10.1242/dev.001602
Sternberg, J. R., Prendergast, A. E., Brosse, L., Cantaut-Belarif, Y., Thouvenin, O., Orts-Del’Immagine, A., et al. (2018). Pkd2l1 is required for mechanoception in cerebrospinal fluid-contacting neurons and maintenance of spine curvature. Nat. Commun. 9, 1–10.
Strähle, U., Lam, C. S., Ertzer, R., and Rastegar, S. (2004). Vertebrate floor-plate specification: variations on common themes. Trends Genet. 20, 155–162. doi: 10.1016/j.tig.2004.01.002
Sueiro, C., Carrera, I., Molist, P., Rodríguez-Moldes, I., and Anadón, R. (2004). Distribution and development of glutamic acid decarboxylase immunoreactivity in the spinal cord of the dogfish Scyliorhinus canicula (elasmobranchs). J. Comp. Neurol. 478, 189–206. doi: 10.1002/cne.20285
Vergara, H. M., Bertucci, P. Y., Hantz, P., Tosches, M. A., Achim, K., Vopalensky, P., et al. (2017). Whole-organism cellular gene-expression atlas reveals conserved cell types in the ventral nerve cord of Platynereis dumerilii. Proc. Natl. Acad. Sci. U.S.A. 114, 5878–5885. doi: 10.1073/pnas.1610602114
Vígh, B., Manzano e Silva, M. J., Frank, C. L., Vincze, C., Czirok, S. J., and Szabó, A. (2004). The system of cerebrospinal fluid-contacting neurons. Its supposed role in the nonsynaptic signal transmission of the brain. Histol. Histopathol. 19, 607–628.
Vigh-Teichmann, I., and Vigh, B. (1983). The system of cerebrospinal fluid-contacting neurons. Arch. Histol. Jpn. 46, 427–468.
Yang, L., Rastegar, S., and Strahle, U. (2010). Regulatory interactions specifying Kolmer-Agduhr interneurons. Development 137, 2713–2722. doi: 10.1242/dev.048470
Yang, N., Chanda, S., Marro, S., Ng, Y.-H., Janas, J. A., Haag, D., et al. (2017). Generation of pure GABAergic neurons by transcription factor programming. Nat. Methods 14, 621–628. doi: 10.1038/nmeth.4291
Keywords: Kolmer–Agduhr cells, cerebrospinal fluid-contacting neurons, transcription factors, transcriptional regulatory network, GABAergic interneuron
Citation: Yang L, Wang F and Strähle U (2020) The Genetic Programs Specifying Kolmer–Agduhr Interneurons. Front. Neurosci. 14:577879. doi: 10.3389/fnins.2020.577879
Received: 30 June 2020; Accepted: 15 September 2020;
Published: 09 October 2020.
Edited by:
Michael Piper, The University of Queensland, AustraliaReviewed by:
Nathalie Dehorter, Australian National University, AustraliaCarol Schuurmans, Sunnybrook Health Sciences Centre, Canada
Copyright © 2020 Yang, Wang and Strähle. This is an open-access article distributed under the terms of the Creative Commons Attribution License (CC BY). The use, distribution or reproduction in other forums is permitted, provided the original author(s) and the copyright owner(s) are credited and that the original publication in this journal is cited, in accordance with accepted academic practice. No use, distribution or reproduction is permitted which does not comply with these terms.
*Correspondence: Lixin Yang, eWFuZ2x4QGNyYWVzLm9yZy5jbg==