- 1School of Medical College, Taizhou University, Taizhou, Jiaojiang, Zhejiang, China
- 2School of Pharmaceutical Sciences, Wenzhou Medical University, Wenzhou, China
- 3Department of Pharmacy, Municipal Hospital Affiliated to Taizhou University, Taizhou, China
Non-small cell lung cancer (NSCLC) has become one of the most common malignant tumors. Emerging evidence has shown that tumor resistance to apoptosis by damaging or bypassing apoptotic cell death is a major contributor to poor responses to therapy in patients with NSCLC. Pyroptosis is a new type of cytolytic and inflammatory programmed death distinct from apoptosis. Currently, pyroptosis has been reported to cause a strong inflammatory response and significant tumor suppression. It is considered a promising therapeutic strategy and prognosis for NSCLC. In this review, we summarized the characteristics of pyroptosis from its underlying basis and role in NSCLC, thereby providing the potential of pyroptosis as a therapeutic strategy and highlighting the challenges of activating pyroptosis in NSCLC treatment.
1. Introduction
Globally, non-small cell lung cancer (NSCLC) accounts for 85% of common lung cancer types and is mainly divided into lung adenocarcinoma (LUAD) and lung squamous cell carcinoma (LUSC) (1). The efficacy of NSCLC treatment largely depends on the stage of the disease in which the patient is diagnosed. Adjuvant therapy can prolong postoperative survival, but only a small number of patients with NSCLC have resectable tumors. Over the past two decades have been witnessed that molecular targeted therapies and immunotherapies for NSCLC with markedly improved outcomes (1). However, the majority of advanced NSCLC patients develop resistance to apoptosis under these treatments and eventually progress and death (2). Thus, for patients with locally advanced or metastatic disease, the prognosis and 5-year survival rates remain extraordinarily poor (3). Through bypassing apoptosis or activating non-apoptotic programmed cell death in NSCLC has attracted great attention and is crucial for the development of new therapeutic approaches.
Pyroptosis, also known as inflammatory necrosis, is a new non-apoptotic programmed cell death mediated by inflammatory responses, and is related to many diseases, such as infectious, spontaneous inflammation, and autoimmune conditions (4). Since the discovery in 1986 that anthrax lethal toxin (LT) caused cell death and rapid release of cellular contents in primary mouse macrophages (5), researchers have discovered caspase families and characterized and explained their function preliminarily over the next 15 years (6–8), while pyroptosis was discovered in 1992 and the term pyroptosis was coined in 2001 (9, 10). It was first hypothesized in 2002 that inflammasomes activate inflammatory caspases and process pro-IL-1β (11), and mouse caspase-11 can trigger non-classical inflammasome activation. It was later established in 2012 that gram-negative bacteria can utilize lipopolysaccharide (LPS) to activate caspase-11 intracellularly. This activation is independent of the traditional LPS extracellular receptor toll-like receptor 4 (TLR4) (12). It was also been reported that caspase-1 or caspase-11/4/5 in the activated state can cleave gasdermin D (GSDMD) to form GSDMD-N-terminal (GSDMD-NT) and GSDMD-C-terminal (GSDMD-CT). Moreover, the GSDMD-CT can oligomerize to form pores and induce pyroptosis (13). In 2017, the results of Wang et al. and Rogers et al. discovered that conventional chemotherapeutic drugs are able to cause cell death via pyroptosis when tumor-expressing gasdermin E (GSDME) is cleaved by activated caspase-3 to form GSDME-N-terminal (GSDME-NT) and GSDME-C-terminal (GSDME-CT) in various cancers. The membrane perforates and releases interleukin-1β (IL-1β), interleukin-18 (IL-18), and cellular contents into the extracellular space and activates inflammatory response (14, 15). In 2020, it was reported that granzyme B (GzmB) can activate pyroptosis by cleaving GSDME, which stimulates antitumor immune responses and further inhibits tumor growth (16). Additionally, it was found that granzyme A (GzmA) is released from cytotoxic lymphocytes and then enters target cells through perforin, where it causes cell pyroptosis by hydrolyzing GSDMB in the same year (17). Quite recently, streptococcal pyrogenic exotoxin B (SpeB) was found to trigger keratinocyte pyroptosis by cleaving GSDMA after Gln246, resulting in the release of an active N-terminal fragment that induces pyroptosis. This renewed our understanding of pyroptosis (18) (Figure 1). The discovery that pyroptosis in cancers eliminates apoptosis- or necroptosis-resistant cancer cells has also advanced our understanding of cancer and provided new ideas for its management. Significant progress has also been made in identifying the underlying mechanisms and prognosis of pyroptosis in NSCLC (Figures 2 and 3). Moreover, lung cancer cells tend to trigger an autoimmune response, which sets off a series of inflammatory cascades. Therefore, targeting pyroptosis can provide a new and promising approach to treating lung cancer. To provide potential perspectives for the treatment of NSCLC, we for the first time primarily summarized the between cardinal mechanisms and role of pyroptosis and NSCLC in this review.
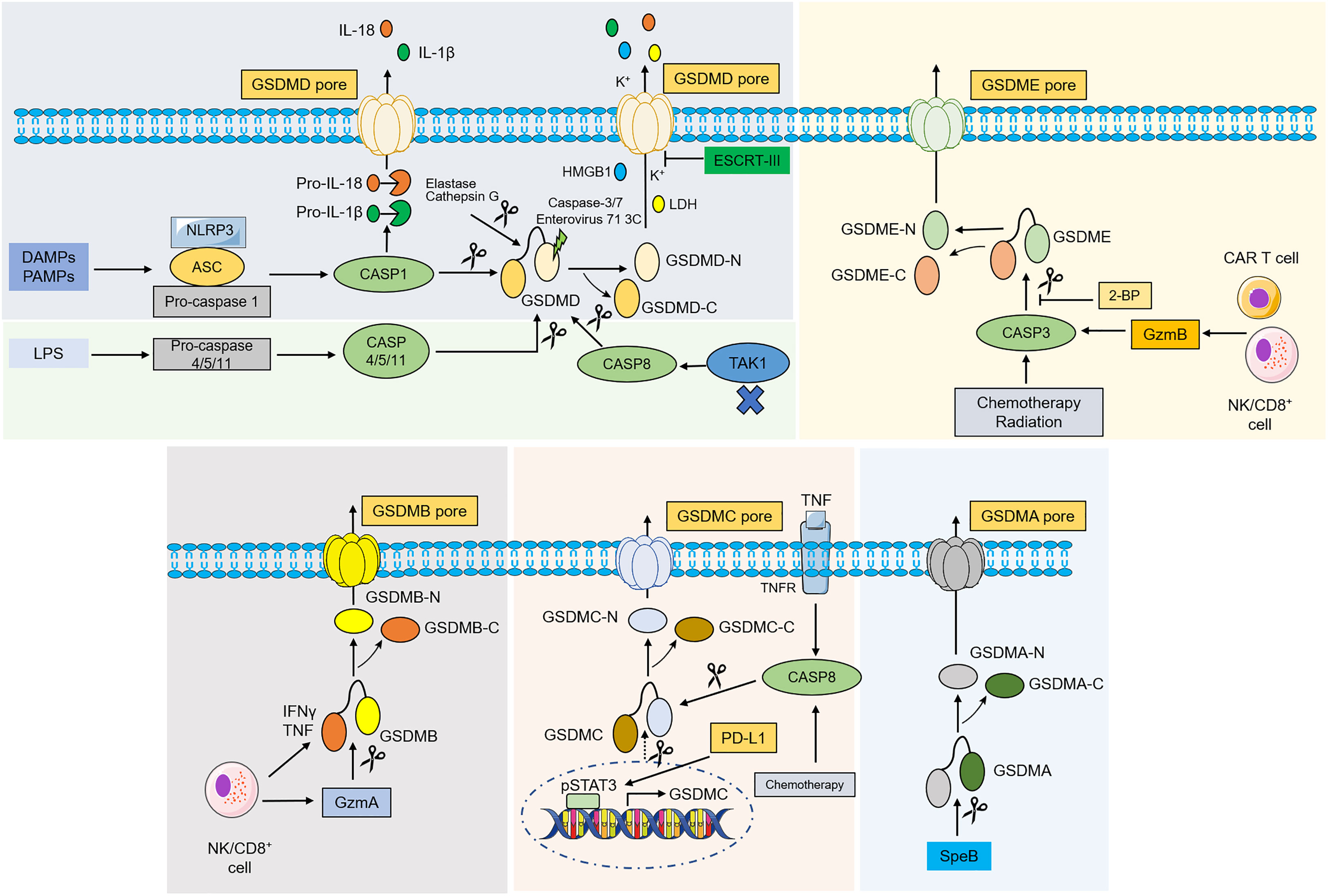
Figure 2 Molecular mechanism of gasdermins-mediated pyroptosis. In classical pathway, PAMPs and DAMPs are recognized by various stimuli and then activate conformational change of the NLRP3 inflammasome, and NLRP3 recruits ASC and binds to pro-caspase-1 to form NLRP3- ASC-pro-caspase-1 protein complex, which then cleaves pro-caspase-1 into caspase-1. Activated caspase-1 destroys the intramolecular auto-inhibitory structure of GSDMD by cleaving GSDMD, which promotes GSDMD-NT generation and induces pyroptosis. Caspase-1 can also recognize and catalyze pro-IL-1β, pro-IL-18, leading to maturation of IL-1β, IL-18. In the noncanonical pathway, caspase-4/5/11 are recognized by LPS from Gram-negative bacteria in the cytoplasm, which then lead to pyroptosis by cleaving GSDMD. Inhibiting TAK1 can stimulate the activation of caspase-8, which cleaves GSDMD and causes pyroptosis. An elastase and cathepsin G can also cleave GSDMD and activates pyroptosis. ESCRT-III complex repairs the membrane by removing the GSDMD pore through exocytosis. Caspase-3, caspase-7 and enterovirus 71 3C cleave the GSDMD and generate a nonfunctional N-terminus. Under the action of chemotherapy, radiation and GzmB, caspase-3 was activated and cleaves GSDME to liberate the cytotoxic N-GSDME, inducing pyroptosis. 2-BP can reverse caspase-3/GSDME-mediated pyroptosis. In addition, GSDMB is cleaved by GzmA, while under hypoxia conditions, GSDMC is cleaved by TNFα-activated-caspase-8 and transcriptionally upregulated through pSTAT3 interaction with PD-L1. SpeB directly activates GSDMA and induce pyroptosis. Abbreviations: DAMPs, danger-associated molecular patterns; PAMPs, pathogen associated molecular patterns; ASC, apoptosis-associated speck-like protein contain a CARD; LPS, lipopolysaccharides; NLRP3, NLR family pyrin domain-containing 3; TAK1, transforming growth factor beta-activated kinase 1; LDH, lactate dehydrogenase; HMGB1, high-mobility group box 1; IL, interleukin; TAK1, transforming growth factor beta-activated kinase 1. ESCRT, endosomal sorting complex required for transport; GzmA/B, granzyme A/B; 2-BP, 2-bromopalmitate; SpeB, streptococcal pyrogenic exotoxin B; pSTAT3, phospho-signal transducer and activator of transcription 3; PD-L1, programmed death-ligand 1.
2. The molecular mechanism of pyroptosis
2.1. The canonical pathway
The canonical pyroptotic death is dependent on caspase-1 and inflammasome assembly and is accompanied by GSDMD cleavage and release of IL-1β and IL-18 (13, 19–21). Inflammasomes are multi-protein complexes comprised of sensor proteins, apoptosis-associated speck-like protein containing a C-terminal caspase recruitment domain (ASC), and pro-caspase-1 that can assemble in response to microbial infections, pathogen-associated molecular patterns (PAMPs) (e.g., lipoteichoicacid, peptidoglycan, glycans, and LPS), and damage-associated molecular patterns (DAMPs) (e.g., high mobility group box 1, heat shock proteins, and DNA) (22). Upon intracellular encounter with various risk factors, a range of intracellular sensor proteins, such as the nucleotide-binding oligomerization domain (NOD)-like receptor (NLR) family (e.g., NLRP3, NLRP1, NLRC4), absent in melanoma 2 (AIM2), or pyrin proteins, homooligomerize and recruit the ASC, bridging inflammasome sensors with pro-caspase-1 and leading to mature caspase-1 activation through self-cleavage (22). The GSDMD was discovered as a potent pyroptosis executioner for caspase-1 and mouse caspase-11 or human caspase-4 and 5 (19–22). The pro-inflammatory cytokines IL-1β and IL-18 are matured under the activation of caspase-1 and cleave GSDMD after a conserved Asp275 residue (Asp276 residue for mouse GSDMD) in the flexible peptide linker to form GSDMD-NT and GSDMD-CT, and further form GSDMD pore on plasma membrane through the liberated GSDMD-NT (23, 24). The IL-1β and IL-18 are released into the extracellular milieu through the GSDMD pore. This facilitates immune cell infiltration and establishes an inflammatory microenvironment (25). In addition, excessive GSDMD pore formation enables the release of other pro-inflammatory contents, including high-mobility group box 1 (HMGB1) and lactate dehydrogenase (LDH), thereby exacerbating inflammatory responses and recruiting immune cells (26, 27). It is worth noting that cells may release IL-1β and IL-18 after inflammasome activation without cell death. However, the exact mechanism is unclear (28).
2.2. The non-canonical pathway
Unlike the canonical inflammasome pathway, the non-canonical pathway relies on caspase-4/5/11. These pro-caspases can be activated by direct binding of the caspase activation and recruitment domain (CARD) to intracellular LPS, which contributes to stimulate caspase-mediated cleavage of GSDMD and ultimately causes pyroptosis (29, 30). However, caspases-4/5/11 are unable to cleave pro-IL-1β/pro-IL-18 but can mediate the efflux of potassium ions and the maturation and secretion of IL-1β/IL-18 via the NLRP3/caspase-1 pathway (31). Recent studies have shown that a p10 product generated from specific autocleavage at Asp289/Asp285/Asp316 in caspase-4/11/1, respectively, is necessary for GSDMD cleavage and pyroptosis induction (32). In addition to caspase-1/4/5/11, GSDMD can be cleaved by caspase-8 during Yersinia infection which can inhibit the transforming growth factor-β (TGF-β)-activated kinase 1 (TAK1) in mouse macrophages (33, 34). Moreover, an elastase released from cytoplasmic granules and cathepsin G can directly induce pyroptosis of neutrophils and monocytes, respectively, in a GSDMD-dependent manner (35).
Several other negative regulations of pyroptosis pathways have also been reported. In monocytes and macrophages, active apoptotic caspases-3 and 7 were found to inactivate GSDMD by cleaving GSDMD at the Asp87 residue, which in turn induces apoptosis, but not pyroptosis (36). Enterovirus A71 (EV-A71) is one of the major pathogens causing hand-foot-and-mouth disease (HFMD) in infants and young children (37). It was found that EV-A71 can cleave GSDMD at the Gln193-Gly194 residue using utilize a 3C protease, resulting in a truncated GSDMD-NT lacking pore-forming capabilities, residues 1-193, and inhibition of pyroptosis (38). The generation of GSDMD pores is the main cellular mechanism for the release of inflammatory molecules and cell pyroptosis. Preventing or prolonging the process of pyroptosis through repairing GSDMD pores may be another promising approach to inhibiting pyroptosis. The endosomal sorting complex required for transport (ESCRT), a peripheral membrane protein complex, that plays an important role in repairing cell membrane damage (39). In the ESCRT system, ESCRT-III was found to preserve the integrity of the cytoplasmic membrane to restrain pyroptosis (40). Mechanistically, calcium ions enter the cells through GSDMD pores as signaling molecules and launch membrane repair by enrolling the ESCRT-III machinery into damaged membrane regions (40).
2.3. The caspase-3-mediated pathways
The cytosol contains a group of structurally related cysteine proteases known as caspases, all of which can specifically break peptide bonds after aspartic acid residues (41, 42). Caspase-8 and caspase-3 are originally defined as the promoter and effector of apoptotic caspases in the apoptosis process, respectively (43, 44). However, it has been discovered recently that the activation of caspase-3 or caspase-8 is not specific to apoptosis. Caspase-3 after chemotherapeutic drug activation cleaves human GSDME at position 270 amino acid residue to produce GSDME-NT in tumor cells with high GSDME expression, which causes pyroptosis instead of apoptosis. However, apoptosis occurs in cancer cells with low GSDME levels (14, 15). Hence, the high level of GSDME has the function of converting apoptosis to pyroptosis upon internal and external death stimuli. A commonly used inhibitor of protein palmitoylation, 2-bromopalmitate (2-BP), can affect the localization and function of proteins on the membrane structures (45). A recent study found that 2-BP can inhibit the GSDME-CT palmitoylation and cause a switch from pyroptosis to apoptosis upon treatment with chemotherapeutic agents, but the detailed mechanism needs to be further studied (46).
2.4. The granzyme-mediated pathway
Granzyme is an exogenous serine protease derived from cytoplasm particles released by cytotoxic lymphocytes (CTLs) and natural killer cells (NK) and has pro-apoptotic functions (47, 48). Recently, studies have found that granzyme proteases, such as GzmA and GzmB, can induce pyroptosis of cancer cells by cleaving GSDM family members (16, 17). For example, chimeric antigen receptor (CAR) T cells from NK cells and CD8+ T lymphocytes released GzmB into tumor cells in a perforin (PFN)-dependent manner, and then GzmB directly processed GSDME after D270 residue, a site also cleaved by caspase-3, releasing cytotoxic GSDME-NT to form pores in plasmalemma (16). Additionally, it has been demonstrated that NK and CD8+ T cells can induce pyroptosis in cancer cells that express GSDMB to promote tumor clearance via the GzmA-GSDMB axis, moreover, this process is enhanced by interferon-γ (IFN-γ) and tumor necrosis factor (TNF). This study demonstrated the idea that the pyroptosis process does not involve caspase activation for the first time (17). More importantly, it was found that cancer cell pyroptosis under the action of Gmzs may amplify inflammation in tumor microenvironments (TMEs), thus recruiting more immune cells and further promoting antitumor immunity and suppressing tumor growth (49). However, cytokine release syndrome (CRS) can be developed in this process, which is a serious and common side effect observed during CAR T-cell therapy (49, 50).
2.5. Alternative pyroptosis pathways
Up to now, several alternative pathways have also been elucidated in addition to the above-mentioned pyroptotic pathways. For example, under hypoxic conditions, TNF-α binds to the tumor necrosis factor receptor (TNFR) and subsequently activates caspase-8 expression. When the expression of GSDMC is upregulated in cells through the programmed death ligand 1 (PD-L1)/phospho-signaling transducer and activator of transcription (pSTAT3) pathway transcriptionally, then caspase-8 specifically cleaves GSDMC at Asp240 to form GSDMC-CT and eventually stimulates pyroptosis (51). More recently, Liu Xing et al. reported that streptococcal pyrogenic exotoxin B (SpeB) triggers keratinocyte pyroptosis and further suppresses systemic infection by cleaving GSDMA after Gln246, releasing an active N-terminal fragment to form a GSDMA pore and ultimately triggering pyroptosis (18).
3. Pyroptosis in NSCLC-associated signaling pathways
3.1. GSDMs
Currently, the human GSDMs superfamily consists of GSDMA, GSDMB, GSDMC, GSDMD, GSDME, and DFNB59 (52). The activating enzymes and biological functions of GSDMD, GSDME, and GSDMC in NSCLC have only recently been made known. GSDMD was first identified as having the function of inducing pyroptosis. Gao et al. for the first time demonstrated the biological effects of GSDMD in NSCLC, and their clinical data showed LUAD and LUSC specimens had significantly higher GSDMD protein levels than matched neighboring tumor specimens (53). In addition, NSCLC patients with larger tumor size and more advanced tumor lymph node metastasis (TNM) stage occurred in patients with GSDMD overexpression. It is found that GSDMD overexpression is an independent poor prognostic biomarker in LUAD, but not in LUSC. Silencing GSDMD expression induced apoptosis, instead of pyroptosis by activating both NLRP3/caspase−1 and caspase−3−mediated mitochondrial pathways in vitro and in vivo. Meanwhile, knockdown of GSDMD in NSCLC cells were found to undergo apoptosis in the absence of exogenous stimuli, whereas GSDMD-deficient immune cells suffered from apoptosis in the presence of pyroptotic stimuli such as LPS and adenosine triphosphate (ATP). In addition, the epidermal growth factor receptor (EGFR)/protein kinase B (Akt) signal transduction was modulated by GSDMD in NSCLC cell lines. A weakened inflammatory response in GSDMD−deficient NSCLC cells may interrupt the negative feedback regulation that activates the phosphoinositide-3-kinase (PI3K)/Akt pathway. Full-length GSDMD may also play a role in regulating this pathway, but the more nuanced crosstalk between the GSDMD and EGFR/Akt in NSCLC requires further study (53). Xi et al. found that among the GSDM family, only GSDMD gene expression was positively correlated with CD8+ T cell marker genes in cytotoxic T lymphocytes (CTLs) across LUAD, LUSC, and melanoma tumor samples in The Cancer Genome Atlas (TCGA) cohorts, suggesting that the function of GSDMD gene could be related with CD8+ T cells. Furthermore, GSDMD was found to colocalize with GzmB near immune synapses and was essential for an optimal response of CTL to lung cancer cells (54). In addition to GSDMD, the mechanism of GSDME-mediated NSCLC pyroptosis was reported. Compared to paired normal lung tissues, the expressions of GSDME, caspase-3, and caspase-8 were significantly were increased in most lung cancer tissues, and GSDME was mainly found in the cytoplasm and a small amount in the cell membrane in lung cancer tissues. However, the level of GSDME was positively correlated with the postoperative survival rate of patients with lung cancer (55). Similarly, GSDME was modestly upregulated in EGFR-mutant, and downregulation in serine/threonine kinase 11 (STK11)- or Kelch-like ECH-associated protein 1 (KEAP1)/Nuclear factor (erythroid-derived 2)-like 2 (NFE2L2)-mutant in LUAD patients based on the TCGA dataset. Loss of GSDME or caspase-3 significantly attenuated GSDME-dependent pyroptosis in A549, PC9, or NCI-H3122 cancer cells (56). Although the correlation between decreased GSDME levels and tumor size, clinical stage, age, or tumor recurrence rate in NSCLC patients was no significance, however, NSCLC patients with lower GSDME expression had shorter survival times, higher mortality rate, and lower degree of T-cell infiltration after platinum treatment (57). Additionally, Zhang et al. found that both chemotherapeutics paclitaxel and cisplatin (DDP) could induce pyroptosis in A549 cells, the pyroptosis induction by DDP was more severe than that caused by paclitaxel, suggesting that DDP may be more preferable than paclitaxel for the treatment of NSCLC with high GSDME expression (58). It has also been demonstrated that targeted antitumor agents can induce pyroptosis of NSCLC cells by activating the mitochondrial apoptosis-GSDME signaling pathway. GSDME overexpression tended to promote targeted antitumor agent and chemotherapy drug sensitivity to regress NSCLC (56). Dasatinib at low concentrations (0.2 µM) increased GSDME and GSDMD protein levels in A549 cells and further induced pyroptosis, nonetheless, 10 µM dasatinib failed to induce cleavage of GSDME in A549 cells (59). The expression of GSDMC is associated with various cancer (60). It was found that GSDMC is up-regulated in both LUAD tissues and cell lines, especially in radio-resistant LUAD cells. A high level of GSDMC suggested a poor prognosis in LUAD (61). Mechanistically, the expression of GSDMC in LUAD cells may be regulated by DNA hypomethylation (61).
3.2. Toll-like receptor 4/NOD-like receptor protein 3
Toll-like receptor (TLRs), a type of pattern recognition receptor (PRRs), plays an important role in multiple diseases (62). It is known that TLR4 can activate the NLRP3 inflammasome and further increase the release of IL-1β and IL-18 (63, 64). Recent investigations have identified that compared with matched normal tissues and human immortalized lung epithelial cell line, the levels of NLRP3, caspase-1, IL-1β, and IL-18 in NSCLC tissues and cells are lower. Additionally, knockdown or reduction of NLRP3 expression significantly promoted NSCLC cell viability and decreased the IL-1β and IL-18 expression, suggesting that NLRP3-caspase-1-IL-1β and IL-18 inflammation pathways play an important antitumor role in NSCLC (65, 66). Meanwhile, Yuan et al. demonstrated that silencing or decreasing TLR4 expression inhibits NLRP3 inflammasome activation and reduces reactive oxygen species (ROS) and calcium ion levels, thus suppressing pyroptosis in NSCLC cells. It was also observed that inhibition of TLR4 by cucurbitacin B treatment suppressed NSCLC growth via pyroptosis in a dose-independent manner (67). As a result, the TLR4-mediated NLRP3 signaling pathway is a potential target for NSCLC therapy.
3.3. ROS/Nuclear factor-κB
Oxidative stress is characterized by a biological state of imbalance between the production and elimination of ROS, which is mainly produced by damaged mitochondria (68, 69). The excessive level of ROS can activate NF-κB, leading to a persistent and strong inflammatory response (70). ROS has also been considered to be one of the exciters that activate NLRP3 inflammasome (71). NF-κB is abnormally activated in NSCLC tissues and cells, and an increase of in NF-κB can promote NSCLC cells pyroptosis (72, 73). Polyphyllin V was recently found to induce pyroptosis in NSCLC cells through the stimulation of the ROS/NF-κB/NLRP3/GSDMD axis. N-acetyl-L-cysteine (NAC) has the potential to inhibit NF-κB and NLRP3 expression and pyroptosis (73). Conversely, ROS has been shown to induce pyroptosis by inhibiting NF‐κB signaling (74). Li et al. found that increasing ROS levels in NSCLC cells with a small molecule ROS inducer can induce apoptosis and pyroptosis, and ROS-mediated NF-κB inhibition may be one of the mechanisms of pyroptosis (75). Meanwhile, Chen et al. also reported that NF-κB system suppression exhibited good antitumor activity in NSCLC cells by switching apoptosis to pyroptosis in vitro and in vivo (76, 77).
3.4. Long noncoding RNAs and microRNAs
The development of numerous cancers, including NSCLC, and the emergence of drug resistance was strongly correlated with lncRNAs and miRNAs (78, 79). According to recent studies, lncRNA X inactive-specific transcript (XIST) was aberrantly overexpressed in NSCLC tissues and cell lines and was especially in NSCLC tumors treated with (DDP) (80, 81). Knock-down of lncRNA-XIST promoted NSCLC cell apoptosis and pyroptosis (80, 81). Liu et al. found that lncRNA-XIST knockdown induced NLRP3 inflammasome activation and affected ROS-induced pyroptotic cell death by regulating miRNA-335/superoxide dismutase 2 (SOD2) in A549 cells (80). Moreover, the sensitivity of NSCLC cells to DDP increased after lncrNA-XIST knockout. Additionally, the carcinogenesis roles and abilities to promote DDP resistance of lncRNA-XIST were largely associated with the reduction of nuclear translocation of TGF-β effector mothers against decapentaplegic homolog 2 (SMAD2), which further prevented the transcription of p53 and NLRP3 (81). Song et al. also demonstrated several lncRNAs are associated with pyroptosis, and generated a new risk signal for predicting LUAD prognosis, providing new prospects for promoting personalized treatment for LUAD patients (82). Shi et al. showed miRNA-556-5p, a newly discovered pyroptosis-related gene that regulates DDP-chemoresistance in NSCLC. They found that miRNA-556-5p level was obviously up-regulated in the DDP-chemoresistance NSCLC tissues and cells. Further experiments confirmed that knocking out miRNA-556-5p suppresses NSCLC cell viability by up-regulating NLRP-mediated pyroptotic cell death. Interestingly, the pyroptotic effects of miRNA-556-5p silencing were reversed by necrosulfonamide (NSA) and NLRP3 reduction, suggesting that the miRNA-556-5p/NLRP3 pathway can regulate DDP-chemoresistance in NSCLC (83).
3.5. Protein 53
The tumor suppressor gene p53 is a major transcription factor, and activated p53 can inhibit tumorigenesis by regulating the transcription of a series of genes. Mutant p53 has no tumor-suppressive activity, but instead acquires a dominant-negative effect and novel tumorigenic functions (84). NSCLC occurrence and progression are closely correlated with p53 (85). Mounting evidence suggests that p53 also inhibits NSLCLC tumors by promoting pyroptosis. Zhang et al. for the first time revealed that p53 positively correlates with pyroptosis in NSCLC tumor tissues (86). According to their findings, overexpression of p53 induced pyroptosis and the mRNA and protein levels of NLRP3, ASC, and cleaved caspase-1 were all increased. Based on chromatin immunoprecipitation (ChIP) analysis, they found that P53 can interact directly with NLRP3. Furthermore, high expression of p53 markedly inhibited NSCLC growth by inducing pyroptosis, suggesting that p53 can be considered a potent regulator capable of mediating pyroptosis in NSCLC effectively (86).
3.6. Maternal embryonic leucine zipper kinase
MELK is a fascinating cell cycle-dependent protein kinase belonging to the sucrose non-fermenting 1 (Snf1)/adenosine monophosphate-activated kinase (AMPK) family and is highly expressed in most malignant tumors (87). MELK overexpression is related to poor prognosis of cancer. MELK is implicated in tumor cell cycle regulation, proliferation, apoptosis, invasion, and metastasis (87). Lai et al. found that high MELK expression was negatively correlated with LUAD survival. The migration and invasion activities of LUAD cells was inhibited after knockdown of MELK expression by decreasing the levels of twist family BHLH transcription factor 1 (Twist1), snail family transcriptional repressor 2 (Snai2), matrix metalloproteinase 7 (MMP7), and N-catenin. In terms of the molecular mechanism of MELK in LUAD, inhibition of MELK by the specific inhibitor of MELK OTSSP167 arrested the G2/M phase of LUAD cells via the polo-like kinase 1 (PLK-1)/cell division cycle (CDC25C)/cyclin-dependent kinase 1 (CDK1) pathway and triggered the caspase-3/GSDME-mediated pyroptosis. Therefore, MELK may be a promising biological target for LUAD in clinic in the future (88).
3.7. Apurinic/apyrimidinic endonuclease 1
APE1, a biological enzyme with multiple functions, mainly primarily stimulates several transcription factors in DNA repair and redox. APE1 also plays a part in genomic DNA oxidation and alkylation base repair by recognizing and cutting nucleotide chains at the 5’-apurinic (AP) site during the DNA base excision repair (BER) process (89, 90). Recent studies have found that APE1 is highly expressed in a variety of cancers, and knockdown of MELK expression decreases cancer cell proliferation and induces apoptosis (91). Long et al. concluded that APE1 is a poor prognosis marker in NSCLC, and the suppression of APE1 with the APE1 inhibitor NO.0449-0145 could promote the accumulation of unrepaired DNA damage in NSCLC cells continuously and induce DNA damage, apoptosis, pyroptosis, and necroptosis in vitro and in vivo, as well as overcome both DDP- and erlotinib-resistance in NSCLC. In terms of the pyroptosis mechanism, APE1 downregulation induced by NO.0449-0145 can upregulate the expression of caspase-4, GSDMD-NT, and IL-1β (92).
3.8. Secretoglobin family 3A member 2
SCGB3A2 (also named uteroglobin-related protein 1, UGRP1 and high in normal-2, HIN-2), the first member of the SCGB gene superfamily, that is highly expressed in airway epithelial Club cells with various biological functions, including anti-lung cancer activity (93–95). Yokoyama et al. found that SCGB3A2 chaperones LPS to the cytosol through the heparan sulfate (HS) of the cell surface receptor syndecan-1 (SDC1) and activates the caspase-11/NLRP3/GSDMD pathway to lead to pyroptosis in murine Lewis lung carcinoma (LLC) cells (96). Recent studies further show that SCGB3A2 exhibits marked anticancer activity against 5 out of 11 human NSCLC cell lines, while no effect on small cell lung cancer cell lines. Interestingly, better survival outcomes were observed in patients with LUAD having high SCGB3A2 expression. Moreover, it was found that SCGB3A2 and LPS together induce growth inhibition of NCI-H596 cancer cells through caspase-4/GSDMD-mediated pyroptosis (97). Therefore, SCGB3A2 uses the machinery of pyroptosis for the elimination of human NSCLC via the non-canonical inflammasome pathway.
4. The role of pyroptosis in NSCLC therapy
4.1. Pyroptosis is a potent aide in chemotherapy, targeted therapy, and immunotherapy
4.1.1. Chemotherapy
Paclitaxel and DDP are first-line chemotherapy drugs used for a variety of other malignancies including advanced NSCLC (98, 99). Mechanicall, paclitaxel and DDP lead to tumor cell apoptosis mainly by targeting microtubules and DNA, respectively (100). Recent studies have shown that in addition to apoptosis, paclitaxel and DDP can trigger A549 cells pyroptosis via the caspase-3/GSDME pathway, with DDP triggering more pronounced pyroptosis as well as higher levels of caspase-3 and GSDME-NT than paclitaxel (58). Nevertheless, chemotherapy regimens cannot effectively improve the overall survival (OS) of NSCLC because apparent apoptotic resistance is common in these patients (2). Therefore, other types of non-apoptotic programmed cell death, such as pyroptosis, should be explored to enhance chemotherapy sensitivity. A recent investigation has suggested that GSDME-induced pyroptosis can increase the chemotherapy sensitivity of NSCLC cells to DDP. The study further suggested that GSDME silencing can reverse DDP-induced NSCLC growth inhibition rather than tumor regression in vivo (57). Natural products, repurposed drugs, small molecule compounds, and conventional chemotherapeutic agents have all been found to induce pyroptosis in NSCLC. For example, doxycycline and simvastatin are originally broad-spectrum tetracycline-class antibiotics and antilipemic agents, respectively (65, 101). As studies progressed, it became clear that both repurposed drugs had strong anticancer effects on NSCLC via the NLRP3-caspase-1-mediated pyroptosis pathway. Wu et al. reported three synthetic small molecule NF-κB inhibitors that exhibit good antitumor activity by switching apoptosis to pyroptosis (75–77) A small molecule compound NO.0449-0145 was also discovered as an APE1 inhibitor through high-throughput virtual screening of small molecule libraries by Long et al. Furthermore, the level of caspase-4 and GSDMD in vitro and in vivo was upregulated following NO.0449-0145 treatment (92). Due to excellent anticancer and safety properties, natural products are considered another promising alternative for first-line cancer prevention and treatment (102). Polyphyllin VI is a natural product that induces pyroptosis through the ROS/NF-κB/NLRP3/GSDMD signaling axis in NSCLC, suggesting that polyphyllin VI is a potential therapeutic option for NSCLC patients (73). Cucurbitacin B (CuB) is a natural tetracyclic triterpenoid derived from cucurbitaceae and cruciferous plants. Recent study showed that CuB is an effective pyroptosis inducer in NSCLC in vivo and in vitro by stimulating the TLR4/NLRP3/GSDMD-dependent signal pathway (67). Cordyceps militaris extract (CME) was also found to induce apoptosis and pyroptosis via the caspase-3/poly (ADP-ribose) (PARP)/GSDME pathway in the A549 cells, providing fundamental insights into the clinical application of CME in patients with NSCLC (103).
4.1.2. Targeted therapy
Molecularly targeted therapies are one of the main effective strategies for stimulating apoptosis machinery in the treatment of NSCLC (104). Compared with traditional chemotherapy, molecularly targeted therapy drugs can kill tumor cells in a targeted manner with mild side effects and high safety (104). However, resistance to molecularly targeted therapies often leads to poor anticancer outcomes and even therapeutic failure (2). Lu et al. concluded that GSDME-dependent cell pyroptosis is another widespread cancer death mode, and GSDME overexpression promotes small molecular targeted inhibitor sensitivity. This result suggest that pyroptosis may serve as an ally to improve the antitumor efficacy of targeted drugs in NSCLC. Additionally, they found that several kirsten rat sarcoma virus (KRAS), the epidermal growth factor receptor (EGFR), or anaplastic lymphoma kinase (ALK) small molecule targeted inhibitors uniformly activate the mitochondrial intrinsic apoptotic pathway to facilitate caspase-3 cleavage of GSDME to induce NSCLC cell pyroptosis, in addition, to concurrently inducing cellular apoptosis. The results of this study change the traditional viewpoint that that molecular targeted therapy can only induce cell death through the apoptotic pathway, established the clinical biological correlation between GSDME expression and pyroptosis, and propose that pyroptosis can improve the sensitivity and antitumor efficacy of targeted drugs, although these effects are decreased when apoptotic feature is unabridged (56).
4.1.3. Immunotherapy
Unlike non-inflammatory apoptosis, pyrotopia is a type of cell death that induces a strong inflammatory response and in some cases is considered an immunogenic cell death (30). Although many researches are needed to clarify the relationship between pyroptosis and anticancer immunity currently, several studies suggest that pyroptosis exerts antitumor effects by enhancing immune activation and function in NSCLC. For example, CTLs are able to release the contents of potentially anti-immune cytotoxic particles into the immune synapse formed with target cancer cells (105). Moreover, CTLs can release perforin and Gzms onto target cells and then promote pore formation and cell lysis. These morphological changes are similar to pyroptosis (47). Xi et al. showed that CTL with higher expression of GSDMD had stronger inhibition effects on lung cancer cells. CTL-mediated pyroptosis is partly via activation of caspase-4. They observed that silencing caspase-4 reversed CTL activation and GSDMD-mediated pyroptosis in NSCLC cell line H1299. Furthermore, CTL cytotoxicity towards H1299 cells and human CTLs was diminished following GSDMD knockdown, indicating that GSDMD-induced pyroptosis was required for CD8+ T cell cytotoxicity (54). Peng et al. also reported that the cell survival rate of GSDME-silenced LLC tumor was elevated after DDP treatment in nude mice, while a prominent reduction in tumor volume was observed in GSDME-overexpressing LLC xenograft after DDP treatment in C57 mice. Because nude mice lack a thymus and C57 mice have a normal immune function, the results suggested that GSDME is involved in the antitumor therapy via immune regulation. Further study found that the number of tumor-infiltrating CD3+ T cells was enhanced when increased GSDME expression and the levels of chemokines that recruit T-cells after DDP treatment, indicating that GSDME may induce T-cell activation in mediating T-cell infiltration into tumor tissue (57). Although anticancer immunotherapeutic strategies have demonstrated efficacy against lymphoid tumors, they are less effective against solid tumors owing to the presence of an immunosuppressive microenvironment induced through programmed death-1 (PD-1) (106). To interfere with PD-1 signaling to enhance immunotherapy for solid tumors, NK92 cells inspired a chimeric costimulatory converting receptor (CCCR) (CCCR-NK92) was established by Lu et al., and these CCCR-NK92 cells can reversed the immune suppressive effects of PD-1 by switching the negative PD-1 signal to an activating signal, and hence effectively enhanced the antitumor activity against H1299 cancer cells by triggering GSDME-induced pyroptosis (107). In addition, CCCR-NK92 cells significantly inhibited tumor growth in vivo, suggesting this CCCR-NK92 cells may provide an effective option for promoting NSCLC immunotherapy (107).
4.2. The prognostic role of pyroptosis-related biomarkers in NSCLC
4.2.1. The prognostic value and mechanism of GSDMs in NSCLC
The GSDMD family, especially GSDMC, GSDMD, and GSDME can be consider as potential markers for the diagnosis and prognosis of lung cancer patients, including NSCLC. Dysregulated GSDMC is associated with a variety of cancers and its functions are tissue-specific (60). GSDMC is found to be significantly overexpressed in LUAD tissues and cell lines compared to adjacent normal tissues, suggesting that GSDMC is a promising promoting cancer biomarker in LUAD []61. Moreover, LUAD patients with lower GSDMC levels showed preferable first progression and OS, and GSDMC expression in LUAD cells may be regulated by DNA hypomethylation (61). DNA hypomethylation plays an important role in regulating the transcription of important oncogenes in various cancers including LUAD (108). The preferentially expressed phosphoribosylaminoimidazole carboxylase (PAICS) is the common tumor promoter in LUAD and can be activated by promoter DNA hypomethylation. Tumor suppression caused by overexpression of adenosine deaminase RNA-specific B1 (ADARB1) is the result of DNA demethylation signal transduction, and inactivation of DNA methylation using a DNA methyltransferase inhibitor significantly enhances ADARB1-mediated metastatic inhibition of LUAD cells (109). In a study by Wei et al., GSDMC was hypomethylated in the lung tissue of patients with LUAD compared with normal lung tissue, and in all cases, GSDMC expression was significantly negatively correlated with its methylation status (61). The expression of GSDMC was negatively correlated with the methylation degree of two CpG sites (cg05316065 and cg26073844) in its promoter region (61). The longer the OS of patients with LUAD, the higher the degree of GSDMC methylation. These suggest that DNA hypomethylation plays an important role in the overexpression of GSDMC, and up-regulated GSDMC may serve as an independent predictor of poor prognosis in patients with LUAD (61). The level of GSDMD protein in lung cancer was significantly upregulated, and the expression of GSDMD was not correlated with age, gender, and lymph node metastasis in patients with LUAD; high expression of GSDMD was significantly correlated with tumor size and later TNM staging in LUAD patients. However, the expression of GSDMD was only significantly correlated with the patient’s TNM stage in LUSC, and the inconsistent results may be due to differences in histological subtypes of the tumor (53). The above results indicated that GSDMD can be regard as an independent prognostic biomarker in LUAD patients. Meanwhile, the expression level of GSDME in lung cancer tissue is significantly higher than that in adjacent tissue, patients with lung cancer having a high GSDME expression have less lymph node metastasis and longer postoperative survival, although the decrease in GSDME level was not significantly correlated with tumor size, clinical stage, age, or tumor recurrence rate (55, 110). In addition, the expression level of GSDME was positively correlated with that of caspase-3, and hence caspase-3 is also an important prognostic biomarker in lung cancer patients (55).
4.2.2. The prognostic value and mechanism of other pyroptosis-related genes in NSCLC
Mounting evidence indicates that abnormally expressed lncRNA has prognostic value in a variety of biological and pathological processes. Song et al. discovered that five pyroptosis-related genes, including lncRNAs-GSEC, FAM83A-AS1, AL606489.1, AL034397.3, and AC010980.2, were significantly associated with OS in LUAD patients. Among these genes, GSEC, FAM83A-AS1, AL606489.1, and AC010980.2 are considered as potentially dangerous lncRNAs, while AL034397.3 is a defensive lncRNA (111). Moreover, an immune-related risk model was established using AL034397.3 showed that AL034397.3 is a reliable predictive marker of LUAD prognosis. Other studies have also confirmed that immunity, autophagy, and ferroptosis are closely related to AC010980.2 in LUAD. AC010980.2 could be a carcinogene to predict the prognosis of LUAD patients (112, 113). The study by Lin et al. found novel pyroptosis-related prognostic genes in patients with LUAD including NLRP7, NLRP1, NLRP2, NOD1, and caspase-6 (114). Prognostic analysis showed patients with low expressions of NLRP7, NLRP1, NLRP2, and NOD1 and high expression of caspase-6 always have poor survival, respectively. Moreover, the above five pyroptosis-related prognostic biomarkers are closely related to immune infiltration, indicating that pyroptosis can exert antitumor efficacy by mediating LUAD in the tumor immune microenvironment. Also, miRNA-335-5p and lncRNA KCNQ1OT1 are related to the prognosis of LUAD patients, and further study showed that the lncRNA KCNQ1OT1/miRNA-335-5p/NLRP1/NLRP7 regulatory axis may play a crucial role in the progression of LUAD (114). Additionally, IL-6, NOD1, and caspase-4 are pivot genes and could be used as potential prognostic factors for early-stage LUSC. Among these, IL-6 has been established as a potent independent risk factor for OS in early-stage LUSC patients, and patients with higher levels of IL-6 expression tend to have advanced LUSC and large cell lung cancer (115). NOD1 may be an antioncogene of lung cancer, and high expression of caspase-4 is related to poorer survival outcomes, possibly due to the negative regulation of pyroptosis by caspase-4.
The lack of comprehensive and personalized treatment is one of the reasons for the high mortality rate associated with NSCLC. Therefore, identifying new genes for early prognostic detection may be of great significance for discovering new therapeutic targets for NSCLC. The above studies have shown that the expression of pyroptosis-related genes can affect the prognosis of patients with NSCLC. Compared with the TNM staging system, pyroptosis-related genes may be used as markers of lung cancer prognosis in the future.
5. Future perspective and conclusion
In this review, we described for the first time the relationship between the cellular physiology and molecular mechanisms of pyroptosis and NSCLC (Figures 2 and 3). Pyroptosis is a defense mechanism against pathogenic insults that has recently been linked to GSDMs-dependent programmed death, which is distinct from apoptosis, necrosis, autophagy, and ferroptosis and has a unique mode of occurrence. Although the underlying mechanism that regulates pyroptosis has not been fully revealed, it has been implicated in the regulation of multiple signaling pathways and intracellular biological processes in NSCLC progression. Numerous studies have shown that NSCLC cell lines exhibit severe chemoresistance due to resistance to apoptosis, and therefore the induction of pyroptosis appears promising for NSCLC treatment. It has also been indicated that the pyroptosis-based therapeutic strategies, including chemotherapy, targeted therapy, and immunotherapy may be able to inhibit the progression of NSCLC in vitro or in vivo and target pyroptosis, enhancing anti-NSCLC cancer efficacy (Table 1). Meanwhile, various pyroptosis-related genes with great clinical significance were identified, and they play important roles in tumor immunity and can be used to predict the prognosis of LUAD and LUSC, but the scope of bioinformatics analysis should be expanded, and relevant biological experiments at the cell, animal, and tissue level should be performed to further confirm the scientificity of these genes.
However, there are still several pressing issues that require further investigation. As an inflammatory cell death mode, the fatal cytokine release syndrome (CRS) could be occurred during pyroptosis therapy, which damages adjacent or other normal tissues and further leads to a series of adverse effects. In other words, the improper control of pyroptosis cannot provide benefits to patients. How to regulate the pyroptosis of tumor cells to achieve the desired therapeutic effect while avoiding the necrosis of normal cells remains to be explored. Perhaps targeting the pyroptosis of tumor cells could become a promising anticancer therapy approach in the future. Targeted inhibitors were found to induce NSCLC cell death by inducing pyroptosis, indicating the therapeutic possibility of targeting pyroptosis. Moreover, pyroptosis induction is strongly associated with carcinogenesis effects and a poor prognosis. Meanwhile, the activation of both GSDMD and GSDME can trigger pyroptosis in NSCLC. Whether the activation of GSDMD or GSDME is more beneficial is currently unclear and further research is needed.
Author contributions
Study concept, design and drafting of the manuscript: JBW and JZW. Write the manuscript: XC. All authors have read and agreed to the published version of the manuscript. All authors contributed to the article and approved the submitted version.
Funding
The work was supported by the National Natural Science Foundation of China (No. 81903074), the Medical Health Science and Technology Project of Zhejiang Provincial Health Commission (No. 2020KY366 and 2021KY399).
Conflict of interest
The authors declare that the research was conducted in the absence of any commercial or financial relationships that could be construed as a potential conflict of interest.
Publisher’s note
All claims expressed in this article are solely those of the authors and do not necessarily represent those of their affiliated organizations, or those of the publisher, the editors and the reviewers. Any product that may be evaluated in this article, or claim that may be made by its manufacturer, is not guaranteed or endorsed by the publisher.
References
1. Thai AA, Solomon BJ, Sequist LV, Gainor JF, Heist RS. Lung cancer. Lancet (2021) 398(10299):535–54. doi: 10.1016/S0140-6736(21)00312-3
2. Denisenko TV, Budkevich IN, Zhivotovsky B. Cell death-based treatment of lung adenocarcinoma. Cell Death Dis (2018) 9(2):117. doi: 10.1038/s41419-017-0063-y
3. Hirsch FR, Scagliotti GV, Mulshine JL, Kwon R, Curran WJ Jr, Wu YL, et al. Lung cancer: current therapies and new targeted treatments. Lancet (2017) 389(10066):299–311. doi: 10.1016/S0140-6736(16)30958-8
4. Broz P, Pelegrin P, Shao F. The gasdermins, a protein family executing cell death and inflammation. Nat Rev Immunol (2020) 20(3):143–57. doi: 10.1038/s41577-019-0228-2
5. Friedlander AM. Macrophages are sensitive to anthrax lethal toxin through an acid-dependent process. J Biol Chem (1986) 261(16):7123–6. doi: 10.1016/S0021-9258(17)38364-3
6. Black RA, Kronheim SR, Merriam JE, March CJ, Hopp TP. A pre-aspartate-specific protease from human leukocytes that cleaves pro-interleukin-1 beta. J Biol Chem (1989) 264(10):5323–6. doi: 10.1016/S0021-9258(18)83546-3
7. Chen Y, Smith MR, Thirumalai K, Zychlinsky A. A bacterial invasin induces macrophage apoptosis by binding directly to ICE. EMBO J (1996) 15(15):3853–60. doi: 10.1002/j.1460-2075.1996.tb00759.x
8. lnemri ES, Livingston DJ, Nicholson DW, Salvesen G, Thornberry NA, Wong WW, et al. Human ICE/CED-3 protease nomenclature. Cell (1996) 87(2):171. doi: 10.1016/s0092-8674(00)81334-3
9. Zychlinsky A, Prevost MC, Sansonetti PJ. Shigella flexneri induces apoptosis in infected macrophages. Nature (1992) 358(6382):167–9. doi: 10.1038/358167a0
10. Cookson BT, Brennan MA. Pro-inflammatory programmed cell death. Trends Microbiol (2001) 9(3):113–4. doi: 10.1016/S0966-842X(00)01936-3
11. Martinon F, Burns K, Tschopp J. The inflammasome: a molecular platform triggering activation of inflammatory caspases and processing of proIL-beta. Mol Cell (2002) 10(2):417–26. doi: 10.1016/S1097-2765(02)00599-3
12. Broz P, Ruby T, Belhocine K, Bouley DM, Kayagaki N, Dixit VM, et al. Caspase-11 increases susceptibility to salmonella infection in the absence of caspase-1. Nature (2012) 490(7419):288–91. doi: 10.1038/nature11419
13. Liu X, Zhang Z, Ruan J, Pan Y, Magupalli VG, Wu H, et al. Inflammasome-activated gasdermin d causes pyroptosis by forming membrane pores. Nature (2016) 535(7610):153–8. doi: 10.1038/nature18629
14. Wang Y, Gao W, Shi X, Ding J, Liu W, He H, et al. Chemotherapy drugs induce pyroptosis through caspase-3 cleavage of a gasdermin. Nature (2017) 547(7661):99–103. doi: 10.1038/nature22393
15. Rogers C, Fernandes-Alnemri T, Mayes L, Alnemri D, Cingolani G, Alnemri ES. Cleavage of DFNA5 by caspase-3 during apoptosis mediates progression to secondary necrotic/pyroptotic cell death. Nat Commun (2017) 8:14128. doi: 10.1038/ncomms14128
16. Zhang Z, Zhang Y, Xia S, Kong Q, Li S, Liu X, et al. Gasdermin e suppresses tumour growth by activating anti-tumour immunity. Nature (2020) 579(7799):415–20. doi: 10.1038/s41586-020-2071-9
17. Zhou Z, He H, Wang K, Shi X, Wang Y, Su Y, et al. Granzyme a from cytotoxic lymphocytes cleaves GSDMB to trigger pyroptosis in target cells. Science (2020) 368(6494):eaaz7548. doi: 10.1126/science.aaz7548
18. Deng W, Bai Y, Deng F, Pan Y, Mei S, Zheng Z, et al. Streptococcal pyrogenic exotoxin b cleaves GSDMA and triggers pyroptosis. Nature (2022) 602(7897):496–502. doi: 10.1038/s41586-021-04384-4
19. He WT, Wan H, Hu L, Chen P, Wang X, Huang Z, et al. Gasdermin d is an executor of pyroptosis and required for interleukin-1beta secretion. Cell Res (2015) 25(12):1285–98. doi: 10.1038/cr.2015.139
20. Van Gorp H, Lamkanfi M. The emerging roles of inflammasome-dependent cytokines in cancer development. EMBO Rep (2019) 20(6):e47575. doi: 10.15252/embr.201847575
21. Xia X, Wang X, Cheng Z, Qin W, Lei L, Jiang J, et al. The role of pyroptosis in cancer: pro-cancer or pro-"host"? Cell Death Dis (2019) 10(9):650. doi: 10.1038/s41419-019-1883-8
22. Lamkanfi M, Dixit VM. In retrospect: The inflammasome turns 15. Nature (2017) 548(7669):534–5. doi: 10.1038/548534a
23. Chen X, He WT, Hu L, Li J, Fang Y, Wang X, et al. Pyroptosis is driven by non-selective gasdermin-d pore and its morphology is different from MLKL channel-mediated necroptosis. Cell Res (2016) 26(9):1007–20. doi: 10.1038/cr.2016.100
24. Sborgi L, Ruhl S, Mulvihill E, Pipercevic J, Heilig R, Stahlberg H, et al. GSDMD membrane pore formation constitutes the mechanism of pyroptotic cell death. EMBO J (2016) 35(16):1766–78. doi: 10.15252/embj.201694696
25. Aglietti RA, Dueber EC. Recent insights into the molecular mechanisms underlying pyroptosis and gasdermin family functions. Trends Immunol (2017) 38(4):261–71. doi: 10.1016/j.it.2017.01.003
26. Hou L, Yang Z, Wang Z, Zhang X, Zhao Y, Yang H, et al. NLRP3/ASC-mediated alveolar macrophage pyroptosis enhances HMGB1 secretion in acute lung injury induced by cardiopulmonary bypass. Lab Invest (2018) 98(8):1052–64.
27. Lamkanfi M, Sarkar A, Vande Walle L, Vitari AC, Amer AO, Wewers MD, et al. Inflammasome-dependent release of the alarmin HMGB1 in endotoxemia. J Immunol (2010) 185(7):4385–92.
28. Yu P, Zhang X, Liu N, Tang L, Peng C, Chen X. Pyroptosis: mechanisms and diseases. Signal Transduct Target Ther (2021) 6(1):128. doi: 10.1038/s41392-021-00507-5
29. Shi J, Zhao Y, Wang Y, Gao W, Ding J, Li P, et al. Inflammatory caspases are innate immune receptors for intracellular LPS. Nature (2014) 514(7521):187–92. doi: 10.1038/nature13683
30. Tan Y, Chen Q, Li X, Zeng Z, Xiong W, Li G, et al. Pyroptosis: a new paradigm of cell death for fighting against cancer. J Exp Clin Cancer Res (2021) 40(1):153. doi: 10.1186/s13046-021-01959-x
31. Shi J, Gao W, Shao F. Pyroptosis: Gasdermin-mediated programmed necrotic cell death. Trends Biochem Sci (2017) 42(4):245–54. doi: 10.1016/j.tibs.2016.10.004
32. Wang K, Sun Q, Zhong X, Zeng M, Zeng H, Shi X, et al. Structural mechanism for GSDMD targeting by autoprocessed caspases in pyroptosis. Cell (2020) 180(5):941–55.e920. doi: 10.1016/j.cell.2020.02.002
33. Orning P, Weng D, Starheim K, Ratner D, Best Z, Lee B, et al. Pathogen blockade of TAK1 triggers caspase-8-dependent cleavage of gasdermin d and cell death. Science (2018) 362(6418):1064–9. doi: 10.1126/science.aau2818
34. Sarhan J, Liu BC, Muendlein HI, Li P, Nilson R, Tang AY, et al. Caspase-8 induces cleavage of gasdermin d to elicit pyroptosis during yersinia infection. Proc Natl Acad Sci USA (2018) 115(46):E10888–97. doi: 10.1073/pnas.1809548115
35. Burgener SS, Leborgne NGF, Snipas SJ, Salvesen GS, Bird PI, Benarafa C. Cathepsin G inhibition by Serpinb1 and Serpinb6 prevents programmed necrosis in neutrophils and monocytes and reduces GSDMD-driven inflammation. Cell Rep (2019) 27(12):3646–56.e3645. doi: 10.1016/j.celrep.2019.05.065
36. Taabazuing CY, Okondo MC, Bachovchin DA. Pyroptosis and apoptosis pathways engage in bidirectional crosstalk in monocytes and macrophages. Cell Chem Biol (2017) 24(4):507–514.e504. doi: 10.1016/j.chembiol.2017.03.009
37. Nguyen-Tran H, Messacar K. Preventing enterovirus A71 disease: another promising vaccine for children. Lancet (2022) 399(10336):1671–3. doi: 10.1016/S0140-6736(22)00380-4
38. Lei X, Zhang Z, Xiao X, Qi J, He B, Wang J. Enterovirus 71 inhibits pyroptosis through cleavage of gasdermin d. J Virol (2017) 91(18):e01069–17. doi: 10.1128/JVI.01069-17
39. Ruhl S, Shkarina K, Demarco B, Heilig R, Santos JC, Broz P. ESCRT-dependent membrane repair negatively regulates pyroptosis downstream of GSDMD activation. Science (2018) 362(6417):956–60. doi: 10.1126/science.aar7607
40. Gong YN, Guy C, Olauson H, Becker JU, Yang M, Fitzgerald P, et al. ESCRT-III acts downstream of MLKL to regulate necroptotic cell death and its consequences. Cell (2017) 169(2):286–300.e216. doi: 10.1016/j.cell.2017.03.020
41. Lamkanfi M, Declercq W, Kalai M, Saelens X, Vandenabeele P. Alice In caspase land. a phylogenetic analysis of caspases from worm to man. Cell Death Differ (2002) 9(4):358–61. doi: 10.1038/sj.cdd.4400989
42. Miller DK. The role of the caspase family of cysteine proteases in apoptosis. Semin Immunol (1997) 9(1):35–49. doi: 10.1006/smim.1996.0058
43. Van Opdenbosch N, Lamkanfi M. Caspases in cell death, inflammation, and disease. Immunity (2019) 50(6):1352–64. doi: 10.1016/j.immuni.2019.05.020
44. Kesavardhana S, Malireddi RKS, Kanneganti TD. Caspases in cell death, inflammation, and pyroptosis. Annu Rev Immunol (2020) 38:567–95. doi: 10.1146/annurev-immunol-073119-095439
45. Zheng B, DeRan M, Li X, Liao X, Fukata M, Wu X. 2-bromopalmitate analogues as activity-based probes to explore palmitoyl acyltransferases. J Am Chem Soc (2013) 135(19):7082–5. doi: 10.1021/ja311416v
46. Hu L, Chen M, Chen X, Zhao C, Fang Z, Wang H, et al. Chemotherapy-induced pyroptosis is mediated by BAK/BAX-caspase-3-GSDME pathway and inhibited by 2-bromopalmitate. Cell Death Dis (2020) 11(4):281. doi: 10.1038/s41419-020-2476-2
47. Voskoboinik I, Whisstock JC, Trapani JA. Perforin and granzymes: function, dysfunction and human pathology. Nat Rev Immunol (2015) 15(6):388–400. doi: 10.1038/nri3839
48. Golstein P, Griffiths GM. An early history of T cell-mediated cytotoxicity. Nat Rev Immunol (2018) 18(8):527–35. doi: 10.1038/s41577-018-0009-3
49. Liu Y, Fang Y, Chen X, Wang Z, Liang X, Zhang T, et al. Gasdermin e-mediated target cell pyroptosis by CAR T cells triggers cytokine release syndrome. Sci Immunol (2020) 5(43):eaax7969. doi: 10.1126/sciimmunol.aax7969
50. Miao L, Zhang Z, Ren Z, Li Y. Reactions related to CAR-T cell therapy. Front Immunol (2021) 12:663201. doi: 10.3389/fimmu.2021.663201
51. Hou J, Zhao R, Xia W, Chang CW, You Y, Hsu JM, et al. PD-L1-mediated gasdermin c expression switches apoptosis to pyroptosis in cancer cells and facilitates tumour necrosis. Nat Cell Biol (2020) 22(10):1264–75. doi: 10.1038/s41556-020-0575-z
52. Liu X, Xia S, Zhang Z, Wu H, Lieberman J. Channelling inflammation: gasdermins in physiology and disease. Nat Rev Drug Discovery (2021) 20(5):384–405. doi: 10.1038/s41573-021-00154-z
53. Gao J, Qiu X, Xi G, Liu H, Zhang F, Lv T, et al. Downregulation of GSDMD attenuates tumor proliferation via the intrinsic mitochondrial apoptotic pathway and inhibition of EGFR/Akt signaling and predicts a good prognosis in nonsmall cell lung cancer. Oncol Rep (2018) 40(4):1971–84. doi: 10.3892/or.2018.6634
54. Xi G, Gao J, Wan B, Zhan P, Xu W, Lv T, et al. GSDMD is required for effector CD8(+) T cell responses to lung cancer cells. Int Immunopharmacol (2019) 74:105713. doi: 10.1016/j.intimp.2019.105713
55. Huang YL, Zhang GH, Zhu Q, Wu X, Wu LG. Expression levels of caspase-3 and gasdermin e and their involvement in the occurrence and prognosis of lung cancer. Cancer Rep (Hoboken) (2021):e1561. doi: 10.21203/rs.3.rs-189686/v1
56. Lu H, Zhang S, Wu J, Chen M, Cai MC, Fu Y, et al. Molecular targeted therapies elicit concurrent apoptotic and GSDME-dependent pyroptotic tumor cell death. Clin Cancer Res (2018) 24(23):6066–77. doi: 10.1158/1078-0432.CCR-18-1478
57. Peng Z, Wang P, Song W, Yao Q, Li Y, Liu L, et al. GSDME enhances cisplatin sensitivity to regress non-small cell lung carcinoma by mediating pyroptosis to trigger antitumor immunocyte infiltration. Signal Transduct Target Ther (2020) 5(1):159. doi: 10.1038/s41392-020-00274-9
58. Zhang CC, Li CG, Wang YF, Xu LH, He XH, Zeng QZ, et al. Chemotherapeutic paclitaxel and cisplatin differentially induce pyroptosis in A549 lung cancer cells via caspase-3/GSDME activation. Apoptosis (2019) 24(3-4):312–25. doi: 10.1007/s10495-019-01515-1
59. Zhang J, Chen Y, He Q. Distinct characteristics of dasatinib-induced pyroptosis in gasdermin e-expressing human lung cancer A549 cells and neuroblastoma SH-SY5Y cells. Oncol Lett (2020) 20(1):145–54. doi: 10.3892/ol.2020.12406
60. Cui YQ, Meng F, Zhan WL, Dai ZT, Liao X. High expression of GSDMC is associated with poor survival in kidney clear cell cancer. BioMed Res Int (2021) 2021:5282894. doi: 10.1155/2021/5282894
61. Wei J, Xu Z, Chen X, Wang X, Zeng S, Qian L, et al. Overexpression of GSDMC is a prognostic factor for predicting a poor outcome in lung adenocarcinoma. Mol Med Rep (2020) 21(1):360–70. doi: 10.3892/mmr.2019.10837
62. Fitzgerald KA, Kagan JC. Toll-like receptors and the control of immunity. Cell (2020) 180(6):1044–66. doi: 10.1016/j.cell.2020.02.041
63. Yang J, Wise L, Fukuchi KI. TLR4 cross-talk with NLRP3 inflammasome and complement signaling pathways in alzheimer's disease. Front Immunol (2020) 11:724. doi: 10.3389/fimmu.2020.00724
64. Liu T, Zhang L, Joo D, Sun SC. NF-kappaB signaling in inflammation. Signal Transduct Target Ther (2017) 2:17023. doi: 10.1038/sigtrans.2017.23
65. Wang F, Liu W, Ning J, Wang J, Lang Y, Jin X, et al. Simvastatin suppresses proliferation and migration in non-small cell lung cancer via pyroptosis. Int J Biol Sci (2018) 14(4):406–17. doi: 10.7150/ijbs.23542
66. Xie J, Zhuan B, Wang H, Wang Y, Wang X, Yuan Q, et al. Huaier extract suppresses non-small cell lung cancer progression through activating NLRP3-dependent pyroptosis. Anat Rec (Hoboken) (2021) 304(2):291–301. doi: 10.1002/ar.24307
67. Yuan R, Zhao W, Wang Q-Q, He J, Han S, Gao H, et al. Cucurbitacin b inhibits non-small cell lung cancer in vivo and in vitro by triggering TLR4/NLRP3/GSDMD-dependent pyroptosis. Pharmacol Res (2021) 170:105748. doi: 10.1016/j.phrs.2021.105748
68. Wang J, Sun D, Huang L, Wang S, Jin Y. Targeting reactive oxygen species capacity of tumor cells with repurposed drug as an anticancer therapy. Oxid Med Cell Longev (2021) 2021:8532940. doi: 10.1155/2021/8532940
69. Taucher E, Mykoliuk I, Fediuk M, Smolle-Juettner FM. Autophagy, oxidative stress and cancer development. Cancers (Basel) (2022) 14(7):1637. doi: 10.3390/cancers14071637
70. Alharbi KS, Fuloria NK, Fuloria S, Rahman SB, Al-Malki WH, Javed Shaikh MA, et al. Nuclear factor-kappa b and its role in inflammatory lung disease. Chem Biol Interact (2021) 345:109568. doi: 10.1016/j.cbi.2021.109568
71. Zhou R, Yazdi AS, Menu P, Tschopp J. A role for mitochondria in NLRP3 inflammasome activation. Nature (2011) 469(7329):221–5. doi: 10.1038/nature09663
72. Dimitrakopoulos FD, Kottorou AE, Kalofonou M, Kalofonos HP. The fire within: NF-kappaB involvement in non-small cell lung cancer. Cancer Res (2020) 80(19):4025–36. doi: 10.1158/0008-5472
73. Teng JF, Mei QB, Zhou XG, Tang Y, Xiong R, Qiu WQ, et al. Polyphyllin VI induces caspase-1-Mediated pyroptosis via the induction of ROS/NF-kappaB/NLRP3/GSDMD signal axis in non-small cell lung cancer. Cancers (Basel) (2020) 12(1):193. doi: 10.3390/cancers12010193
74. Fouani L, Kovacevic Z, Richardson DR. Targeting oncogenic nuclear factor kappa b signaling with redox-active agents for cancer treatment. Antioxid Redox Signal (2019) 30(8):1096–123. doi: 10.1089/ars.2017.7387
75. Li Q, Chen L, Dong Z, Zhao Y, Deng H, Wu J, et al. Piperlongumine analogue L50377 induces pyroptosis via ROS mediated NF-kappaB suppression in non-small-cell lung cancer. Chem Biol Interact (2019) 313:108820. doi: 10.1016/j.cbi.2019.108820
76. Chen L, Li Q, Zheng Z, Xie J, Lin X, Jiang C, et al. Design and optimize n-substituted EF24 as effective and low toxicity NF-kappaB inhibitor for lung cancer therapy via apoptosis-to-pyroptosis switch. Chem Biol Drug Des (2019) 94(1):1368–77. doi: 10.1111/cbdd.13514
77. Chen L, Weng B, Li H, Wang H, Li Q, Wei X, et al. A thiopyran derivative with low murine toxicity with therapeutic potential on lung cancer acting through a NF-kappaB mediated apoptosis-to-pyroptosis switch. Apoptosis (2019) 24(1-2):74–82. doi: 10.1007/s10495-018-1499-y
78. Winkle M, El-Daly SM, Fabbri M, Calin GA. Noncoding RNA therapeutics - challenges and potential solutions. Nat Rev Drug Discovery (2021) 20(8):629–51. doi: 10.1038/s41573-021-00219-z
79. Goodall GJ, Wickramasinghe VO. RNA In cancer. Nat Rev Cancer (2021) 21(1):22–36. doi: 10.1038/s41568-020-00306-0
80. Liu J, Yao L, Zhang M, Jiang J, Yang M, Wang Y. Downregulation of LncRNA-XIST inhibited development of non-small cell lung cancer by activating miR-335/SOD2/ROS signal pathway mediated pyroptotic cell death. Aging (Albany NY) (2019) 11(18):7830–46. doi: 10.18632/aging.102291
81. Xu X, Zhou X, Chen Z, Gao C, Zhao L, Cui Y. Silencing of lncRNA XIST inhibits non-small cell lung cancer growth and promotes chemosensitivity to cisplatin. Aging (Albany NY) (2020) 12(6):4711–26. doi: 10.18632/aging.102673
82. Song J, Sun Y, Cao H, Liu Z, Xi L, Dong C, et al. A novel pyroptosis-related lncRNA signature for prognostic prediction in patients with lung adenocarcinoma. Bioengineered (2021) 12(1):5932–49. doi: 10.1080/21655979.2021.1972078
83. Shi F, Zhang L, Liu X, Wang Y. Knock-down of microRNA miR-556-5p increases cisplatin-sensitivity in non-small cell lung cancer (NSCLC) via activating NLR family pyrin domain containing 3 (NLRP3)-mediated pyroptotic cell death. Bioengineered (2021) 12(1):6332–42. doi: 10.1080/21655979.2021.1971502
84. Levine AJ. p53: 800 million years of evolution and 40 years of discovery. Nat Rev Cancer (2020) 20(8):471–80. doi: 10.1038/s41568-020-0262-1
85. Stewart DJ. Tumor and host factors that may limit efficacy of chemotherapy in non-small cell and small cell lung cancer. Crit Rev Oncol Hematol (2010) 75(3):173–234. doi: 10.1016/j.critrevonc.2009.11.006
86. Zhang T, Li Y, Zhu R, Song P, Wei Y, Liang T, et al. Transcription factor p53 suppresses tumor growth by prompting pyroptosis in non-Small-Cell lung cancer. Oxid Med Cell Longev (2019) 2019:8746895. doi: 10.1155/2019/8746895
87. McDonald IM, Graves LM. Enigmatic MELK: The controversy surrounding its complex role in cancer. J Biol Chem (2020) 295(24):8195–203. doi: 10.1074/jbc.REV120.013433
88. Tang Q, Li W, Zheng X, Ren L, Liu J, Li S, et al. MELK is an oncogenic kinase essential for metastasis, mitotic progression, and programmed death in lung carcinoma. Signal Transduct Target Ther (2020) 5(1):279. doi: 10.1038/s41392-020-00288-3
89. Bhakat KK, Mantha AK, Mitra S. Transcriptional regulatory functions of mammalian AP-endonuclease (APE1/Ref-1), an essential multifunctional protein. Antioxid Redox Signal (2009) 11(3):621–38. doi: 10.1089/ars.2008.2198
90. Whitaker AM, Freudenthal BD. APE1: A skilled nucleic acid surgeon. DNA Repair (Amst) (2018) 71:93–100. doi: 10.1016/j.dnarep.2018.08.012
91. Poletto M, Malfatti MC, Dorjsuren D, Scognamiglio PL, Marasco D, Vascotto C, et al. Inhibitors of the apurinic/apyrimidinic endonuclease 1 (APE1)/nucleophosmin (NPM1) interaction that display anti-tumor properties. Mol Carcinog (2016) 55(5):688–704. doi: 10.1002/mc.22313
92. Long K, Gu L, Li L, Zhang Z, Li E, Zhang Y, et al. Small-molecule inhibition of APE1 induces apoptosis, pyroptosis, and necroptosis in non-small cell lung cancer. Cell Death Dis (2021) 12(6):503. doi: 10.1038/s41419-021-03804-7
93. Chiba Y, Kurotani R, Kusakabe T, Miura T, Link BW, Misawa M, et al. Uteroglobin-related protein 1 expression suppresses allergic airway inflammation in mice. Am J Respir Crit Care Med (2006) 173(9):958–64. doi: 10.1164/rccm.200503-456OC
94. Kurotani R, Tomita T, Yang Q, Carlson BA, Chen C, Kimura S. Role of secretoglobin 3A2 in lung development. Am J Respir Crit Care Med (2008) 178(4):389–98. doi: 10.1164/rccm.200707-1104OC
95. Kurotani R, Okumura S, Matsubara T, Yokoyama U, Buckley JR, Tomita T, et al. Secretoglobin 3A2 suppresses bleomycin-induced pulmonary fibrosis by transforming growth factor beta signaling down-regulation. J Biol Chem (2011) 286(22):19682–92. doi: 10.1074/jbc.M111.239046
96. Yokoyama S, Cai Y, Murata M, Tomita T, Yoneda M, Xu L, et al. A novel pathway of LPS uptake through syndecan-1 leading to pyroptotic cell death. Elife (2018) 7:e37854. doi: 10.7554/eLife.37854
97. Yokoyama S, Nakayama S, Xu L, Pilon AL, Kimura S. Secretoglobin 3A2 eliminates human cancer cells through pyroptosis. Cell Death Discovery (2021) 7(1):12. doi: 10.1038/s41420-020-00385-w
98. Chang WJ, Sun JM, Lee JY, Ahn JS, Ahn MJ, Park K. A retrospective comparison of adjuvant chemotherapeutic regimens for non-small cell lung cancer (NSCLC): paclitaxel plus carboplatin versus vinorelbine plus cisplatin. Lung Cancer (2014) 84(1):51–5. doi: 10.1016/j.lungcan.2014.01.017
99. Fennell DA, Summers Y, Cadranel J, Benepal T, Christoph DC, Lal R, et al. Cisplatin in the modern era: The backbone of first-line chemotherapy for non-small cell lung cancer. Cancer Treat Rev (2016) 44:42–50. doi: 10.1016/j.ctrv.2016.01.003
100. Arnal I, Wade RH. How does taxol stabilize microtubules? Curr Biol (1995) 5(8):900–8. doi: 10.1016/s0960-9822(95)00180-1
101. Alsaadi M, Tezcan G, Garanina EE, Hamza S, McIntyre A, Rizvanov AA, et al. Doxycycline attenuates cancer cell growth by suppressing NLRP3-mediated inflammation. Pharm (Basel) (2021) 14(9):852. doi: 10.3390/ph14090852
102. Sparreboom A, Cox MC, Acharya MR, Figg WD. Herbal remedies in the united states: potential adverse interactions with anticancer agents. J Clin Oncol (2004) 22(12):2489–503. doi: 10.1200/JCO.2004.08.182
103. Hu Z, Lai Y, Ma C, Zuo L, Xiao G, Gao H, et al. Cordyceps militaris extract induces apoptosis and pyroptosis via caspase-3/PARP/GSDME pathways in A549 cell line. Food Sci Nutr (2022) 10(1):21–38. doi: 10.1002/fsn3.2636
104. Wang M, Herbst RS, Boshoff C. Toward personalized treatment approaches for non-small-cell lung cancer. Nat Med (2021) 27(8):1345–56. doi: 10.1038/s41591-021-01450-2
105. Chavez-Galan L, Arenas-Del Angel MC, Zenteno E, Chávez R, Lascurain R. Cell death mechanisms induced by cytotoxic lymphocytes. Cell Mol Immunol (2009) 6(1):15–25. doi: 10.1038/cmi.2009.3
106. McGowan E, Lin Q, Ma G, Yin H, Chen S, Lin Y. PD-1 disrupted CAR-T cells in the treatment of solid tumors: Promises and challenges. BioMed Pharmacother (2020) 121:109625. doi: 10.1016/j.biopha.2019.109625
107. Lu C, Guo C, Chen H, Zhang H, Zhi L, Lv T, et al. A novel chimeric PD1-NKG2D-41BB receptor enhances antitumor activity of NK92 cells against human lung cancer H1299 cells by triggering pyroptosis. Mol Immunol (2020) 122:200–6. doi: 10.1016/j.molimm.2020.04.016
108. Zhou S, Yan Y, Chen X, Wang X, Zeng S, Qian L, et al. Roles of highly expressed PAICS in lung adenocarcinoma. Gene (2019) 692:1–8. doi: 10.1016/j.gene.2018.12.064
109. Wang X, Xu Z, Ren X, Chen X, Wei J, Lin W, et al. Function of low ADARB1 expression in lung adenocarcinoma. PloS One (2019) 14(9):e0222298. doi: 10.1371/journal.pone.0222298
110. Huang Y, Zhang G, Zhu Q, Wu X, Wu L. Role of cytokines released during pyroptosis in non-small cell lung cancer. Cancer Manag Res (2021) 13:7399–409. doi: 10.2147/CMAR.S330232
111. Li X, He J. A novel pyroptosis-related gene signature for early-stage lung squamous cell carcinoma. Int J Gen Med (2021) 14:6439–53. doi: 10.2147/IJGM.S331975
112. Wu L, Wen Z, Song Y, Wang L. A novel autophagy-related lncRNA survival model for lung adenocarcinoma. J Cell Mol Med (2021) 25(12):5681–90. doi: 10.1111/jcmm.16582
113. Guo Y, Qu Z, Li D, Bai F, Xing J, Ding Q, et al. Identification of a prognostic ferroptosis-related lncRNA signature in the tumor microenvironment of lung adenocarcinoma. Cell Death Discovery (2021) 7(1):190. doi: 10.1038/s41420-021-00576-z
114. Lin W, Chen Y, Wu B, Chen Y, Li Z. Identification of the pyroptosis related prognostic gene signature and the associated regulation axis in lung adenocarcinoma. Cell Death Discovery (2021) 7(1):161. doi: 10.1038/s41420-021-00557-2
Keywords: pyroptosis, NSCLC, programmed cell death, prognosis, therapy
Citation: Chen X, Wu J and Wang J (2022) Pyroptosis: A new insight of non-small-cell lung cancer treatment. Front. Oncol. 12:1013544. doi: 10.3389/fonc.2022.1013544
Received: 07 August 2022; Accepted: 09 November 2022;
Published: 29 November 2022.
Edited by:
Tao Liu, University of New South Wales, AustraliaReviewed by:
Zhang Jianglin, Jinan University, ChinaLuisa Lanfrancone, European Institute of Oncology (IEO), Italy
Copyright © 2022 Chen, Wu and Wang. This is an open-access article distributed under the terms of the Creative Commons Attribution License (CC BY). The use, distribution or reproduction in other forums is permitted, provided the original author(s) and the copyright owner(s) are credited and that the original publication in this journal is cited, in accordance with accepted academic practice. No use, distribution or reproduction is permitted which does not comply with these terms.
*Correspondence: Jiabing Wang, wangjiabing@tzc.edu.cn
†These authors have contributed equally to this work