- 1School of Pharmacy, Changchun University of Chinese Medicine, Changchun, China
- 2Affiliated Hospital of Changchun University of Chinese Medicine, Changchun, China
Dysregulation of the epigenetic enzyme-mediated transcription of oncogenes or tumor suppressor genes is closely associated with the occurrence, progression, and prognosis of tumors. Based on the reversibility of epigenetic mechanisms, small-molecule compounds that target epigenetic regulation have become promising therapeutics. These compounds target epigenetic regulatory enzymes, including DNA methylases, histone modifiers (methylation and acetylation), enzymes that specifically recognize post-translational modifications, chromatin-remodeling enzymes, and post-transcriptional regulators. Few compounds have been used in clinical trials and exhibit certain therapeutic effects. Herein, we summarize the classification and therapeutic roles of compounds that target epigenetic regulatory enzymes in cancer treatment. Finally, we highlight how the natural compounds berberine and ginsenosides can target epigenetic regulatory enzymes to treat cancer.
1 Introduction
The concept of epigenetics was first introduced in 1942 by Waddington, a British scientist who defined “epigenetics” as changes in the phenotype without underlying genotypic changes to explain altered growth and development (1). Epigenetics is now widely recognized as the regulatory mechanisms by which a heritable phenotype is changed without altering the DNA sequence. Epigenetic changes, including DNA/RNA methylation, histone modifications, nucleosome localization, non-coding RNA (ncRNA) expression, and chromatin 3D structure, are involved in cellular growth, development, and function (2). These epigenetic modifications constitute the specific epigenome of an individual organism and provide a regulatory mechanism for cellular diversity. Recently, epigenetics has gained attention in fields such as medicine, exerting a profound impact on the research and treatment of diseases such as cancer.
Epigenetic modifications catalyzed by epigenetic regulatory enzymes are important for regulating chromatin structure and gene expression. Imbalanced gene expression can be one of the main mechanisms underlying diseases such as cancer. In particular, thee aberrant expression of oncogenes, tumor suppressor genes, or cancer-related genes by dysregulated epigenetic regulatory enzymes can trigger tumorigenesis by modulating basic processes, such as DNA repair, cell proliferation, and mortality (3, 4). Therefore, epigenetic marks such as DNA methylation, histone modifications, and ncRNA expression have been identified as potential biomarkers for the early diagnosis and prognosis of cancers (5, 6). In recent years, many small-molecule compounds targeting epigenetic regulatory enzymes have been discovered, some of which are promising anticancer drugs.
The discovery and development of inhibitors targeting epigenetic regulatory enzymes are extensively described in this review. Further, we summarize the functions of berberine (BBR) and ginsenosides, natural compounds capable of targeting epigenetic enzymes in cancer. Additionally, we discuss promises and challenges that lie ahead of us.
2 DNA Methylation and Its Role in Cancer Treatment
2.1 DNA Methylation
DNA methylation is a stable epigenetic event in intracellular processes, such as cell differentiation, and is involved in the lineage classification and quality control of stem cells (7). In humans, DNA methylation occurs almost exclusively at cytosine residues in CpG sequences. These dinucleotides are dispersed unevenly across the genome, and most are heavily methylated. In contrast, CpG-rich regions known as CpG islands (CpGIs) remain largely unmethylated, especially in promoter regions (8). However, altered CpGI methylation patterns during cancer progression result in both genome-wide hypomethylation and site-specific CpGI hypermethylation (9). Therefore, DNA methylation provides a useful molecular marker for cancer diagnosis and therapeutics (10). In mammals, DNA methyltransferases (DNMTs) are responsible for transferring methyl donor S-adenosyl-L-methionine (SAM) to the 5′-residue of cytosine (5′-C) in DNA (Figure 1A). The DNMT family includes DNMT1, DNMT2, DNMT3A, DNMT3B, and DNMT3L, which differ based on their structural characteristics and functional domains (Figure 1B). For example, the Pro-Trp-Trp-Pro (PWWP) domain of DNMT3A/3B recognizes the di- or tri-methylation of histone H3 lysine 36 (H3K36) to activate gene expression (11, 12), whereas the ATRX-like domain of DNMT3A and XXC-BAH1 domain of DNMT1 interact with deacetylase HDAC1 to repress gene expression (13, 14). Further, the C-terminal catalytic methyltransferase (MTase) domain of DNMT3A mediates homo- and heterodimerization to regulate progressive DNA methylation (15, 16). Cleavage between the N- and C-terminal domains reportedly affects the relative preference of DNMTs for unmethylated and hemi-methylated DNA (17). DNMTs preferentially bind to hemi-methylated CpG sites (18).
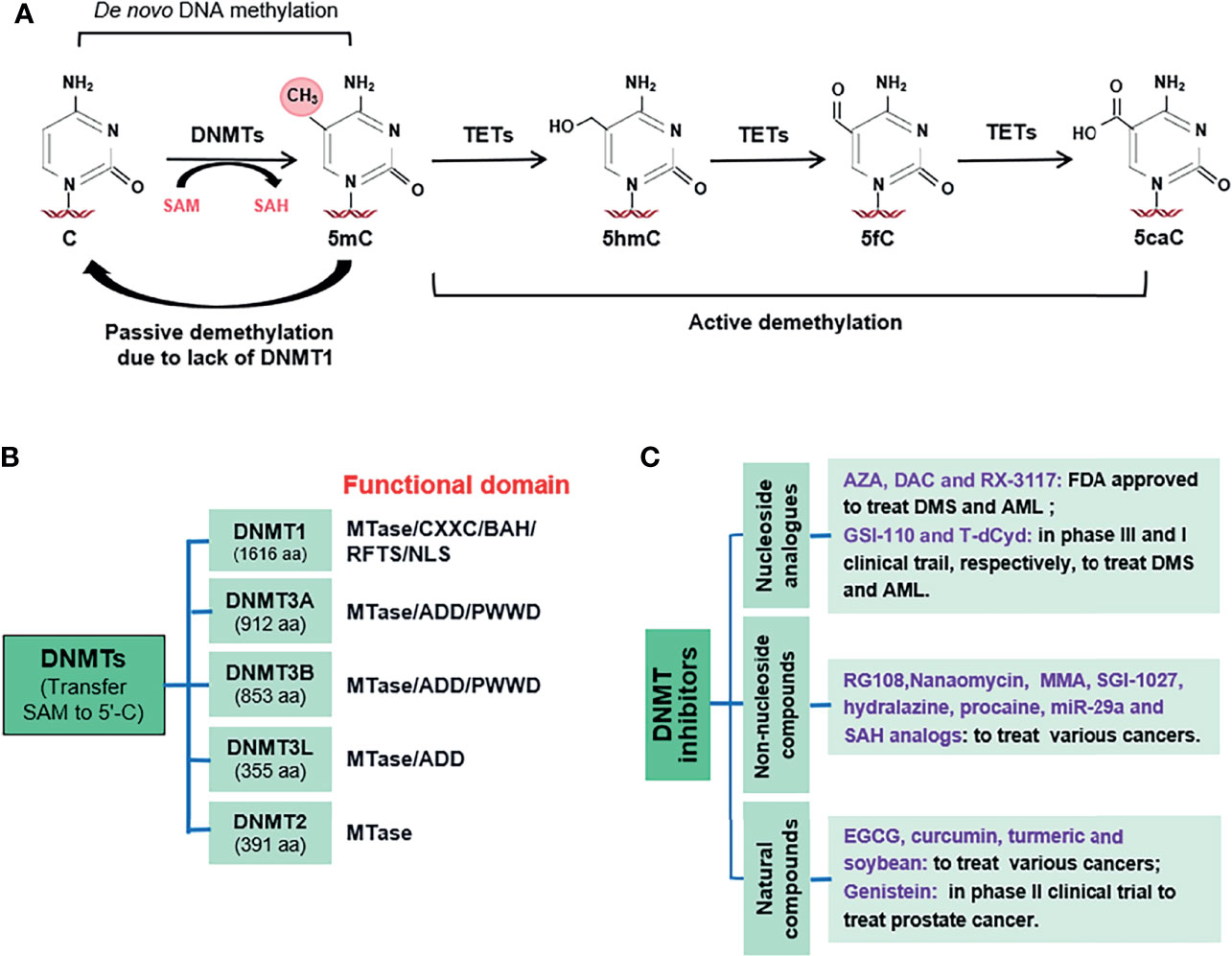
Figure 1 Types of DNA methyltransferases (DNMTs) and antitumor activity of its inhibitors: (A). Schematic of DNA methyltransferases (DNMTs) transferring methyl donor S-adenosyl-L-methionine (SAM) to cytosine (5 ‘C) 5’- residues in DNA or removing SAM from DNA. (B). Functional domains of DNMTs. (DNMT1, DNMT2, DNMT3A, DNMT3B and DNMT3L) (C). Three types of DNMTs inhibitors.
DNA demethylation can occur either passively or actively. DNA demethylation or “erasing DNA methylation” can occur passively when DNA is replicated and the modification is not re-established. One example of passive DNA demethylation is the absence of methylation owing to a lack of DNMT1, whereas another is the removal of methyl groups from cytosine (5-mC) by ten-eleven translocation proteins (TETs) in a replication-independent manner. TETs mainly oxidize 5-mC to form 5-hydroxymethylcytosine (5hmC), 5-formylcytosine (5fC), and 5-carboxycytosine (5caC) (19, 20). The catalytic domain of TETs consists of a double-stranded β-helix domain and cysteine-rich domain at the carboxyl end (21). In addition, TET1 and TET3 contain CXXC domains at their N-terminus, which are composed of two Cys4-type zinc finger motifs that promote DNA binding (22). Importantly, DNA methylation can be recognized by methyl-CpG-binding proteins (MBPs), which bind and interpret methylated DNA to initiate gene silencing by recruiting other factors (23). MBPs can be classified as methyl-CpG-binding domain (MBD) proteins as follows: su(var) 3-9, enhancer of zeste, and trithorax (SET), RING-associated, and zinc finger (24). To date, 11 proteins in the MBD family have been identified, including methyl-CpG-binding protein 2, MBDs 1–6, SETB1/2, and BAZ2A/B (25). In addition to the MBD domain, SETB1/2 and BAZ2A/B also contain other domains, such as SET, DNA-binding homeobox and different transcription factors, plant homeodomain (PHD), and bromodomain (BRD). Although they cannot interact with 5mC residues, MBPs can bind to methylated or acetylated histones to participate in heterochromatin formation and transcriptional inhibition by coordinating H3K9 demethylation, histone H4 deacetylation, and DNA methylation, which are essential for the epigenetic silencing of ribosomal DNA (26, 27).
2.2 Inhibitors Targeting DNMTs (DNMTis)
In view of the hypermethylation of CpGIs in the promoter region of most cancers, DNMT inhibitors (DNMTis) have been developed for tumor treatment. DNMTis are mainly divided into three types, nucleoside analogs (NAs), non-nucleoside compounds, and natural compounds (Figure 1C) (28). Compounds that inhibit DNMTs lead to hypomethylation across cell divisions, subsequently inducing the expression of tumor suppressors. Using methylation-specific PCR, Chan et al. demonstrated substantial demethylation of all latent and lytic Epstein-Barr virus promoters in nasopharyngeal cancer patients after treatment with 5-azacytidine (a DNMTi) (29). DNMTis such as azacitidine, decitabine, guadecitabine, and 4-thio-2-deoxycytidine have been examined in clinical anti-tumor trials (30–32) (Table 1). Non-nucleoside compounds with various chemical scaffolds have also been studied (63). Compounds such as RG108, nanaomycin A, mithramycin A, SGI-1027, hydralazine, procaine, S-adenosyl-L-homocysteine analogs, and miR-29a have been shown to suppress the activity of DNMTs (33–40). Among these, hydralazine has been shown to be an effective demethylation agent and tumor suppressor gene transcriptional reactivator (36). In a phase II clinical study, hydralazine in combination with standard cytotoxic chemotherapy reactivated tumor suppressor genes silenced by DNA methylation and increased chemotherapy efficacy in prostate cancer (33). Interestingly, some natural compounds, such as (-)-epigallocatechin-3-gallate, curcumin, and genistein from green tea/soybean, also reportedly block the activity of DNMTs (36, 37). Genistein and related soy isoflavones reportedly reactivate methylation-silenced genes to delay the progression of breast or prostate cancer by directly blocking DNMT. Although many DNMTis have been identified, few have been applied clinically as current DNMTis are nonselective cytosine analogs that induce cytotoxic side effects (64).
3 Histone Methylation as an Anticancer Target
3.1 Histone Methylation
Histone methylation, a unique post-translational modification catalyzed by histone MTases (HMTs), occurs at both lysine (K) and arginine (R) residues. Abnormal histone methyl modification plays an important role in the proliferation, apoptosis, differentiation, and invasion of tumor cells. Thus, blocking these abnormal modifications has become a new direction in tumor therapeutics (65, 66). The key steps of the histone methylation process, including HMT inhibitors (HMTis) and histone lysine demethylases (KDMs), are shown in Figures 2A, B. Lysine methylation occurs in mono-, di-, and tri-states, whereas arginine methylation only occurs in mono-and di-states. These methyl marks contribute to transcriptional regulation and serve as platforms for the recruitment of effector proteins. Most HMTs contain the SET domain. Methylation occurs at lysine residues K4, K9, K27, K36, and K79 of histone H3 and K20 of histone H4 (Figure 2B). In general, methylation at H3K9, H3K27, and H4K20 correlates with transcriptional repression, whereas methylation at H3K4, H3K36, and H3K79 corresponds with gene transcription (67). H3K9me2/me3, H3K27me2/me3, and H4K20me3 often appear on heterochromatin where genes remain silent (68).
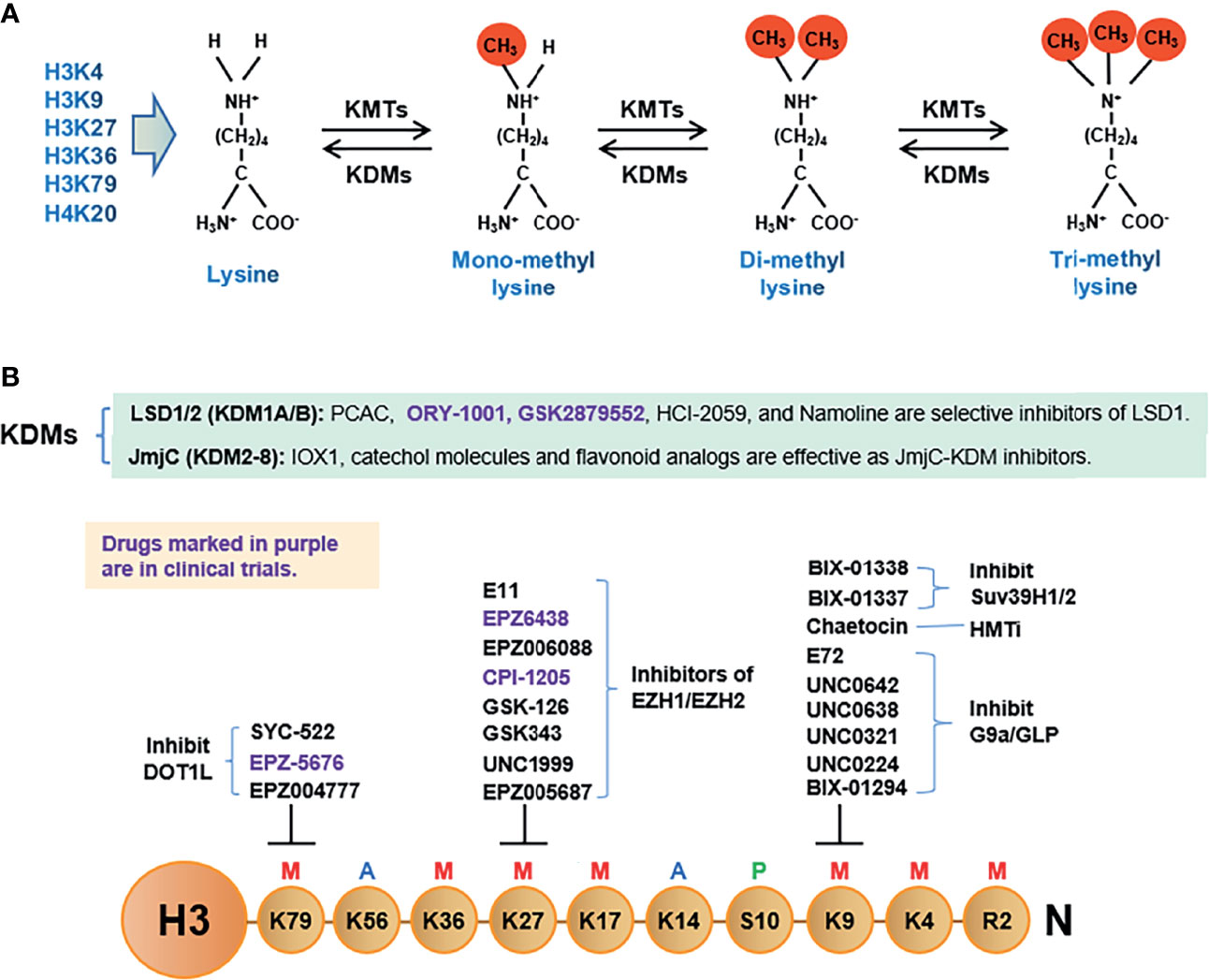
Figure 2 The histone methylation process, inhibitors of histone lysine methyltransferases (HMTs) and histone lysine demethylases (KDMs). (A). Schematic diagram of three states of lysine methylation: mono, di, and tri states. (B). A summary of the histone methylation process and inhibitors of HMTs and KDMs.
There are two families of histone demethylases, lysine-specific demethylases (LSDs) and Jumonji C (JmjC) domain-containing lysine demethylases (JmjC-KDMs). The LSD family includes LSD1/KDM1A and LSD2/KDM1B proteins, which contain the N-terminal Swi3p, Rsc8p, and Moira (SWIRM) domains, a flavin adenine dinucleotide-binding motif (FAD), and a C-terminal amine oxidase domain that is responsible for LSD activity in an FAD-dependent manner (69). Both LSD1 and LSD2 function as corepressors through the demethylation of mono- or di-methyl marks on H3K4 (70). However, LSD1 can also act as a coactivator of the androgen receptor via the demethylation of H3K9me1/me2 (71). The JmjC-KDM family includes iron- and α-ketoglutarate-dependent dioxygenases, which can be divided into KDM 2–8 subfamilies. Members of the JmjC-KDM family are responsible for the demethylation of all statuses of H3K4, H3K9, H3K27, H3K36, H3K79, and H4K20 through the co-substrate 2-oxoglutarate, dioxygen, and Fe (II) as a cofactor (42, 72). The lysine residues mentioned previously herein are prone to methylation and play critical roles in tumorigenesis (73, 74).
3.2 Histone Methyltransferase Inhibitors (HMTis)
Histone methylation is a hot topic in tumor epigenetic modification. This modification is associated with the biological behavior of tumor cells and plays a role in the development of tumors. In this section, we focus on a subclass of epigenetic regulators, namely histone methyltransferases. To date, hundreds of HMTs have been identified, including lysine and arginine MTases (47). Several HMTs have been linked to different types of cancer. However, in most cases, we only have limited knowledge regarding the molecular mechanisms by which the HMTs contribute to disease development. HMTis can be classified according to their specificity for different types of methyltransferases. Here, we summarize the current knowledge regarding some of the best validated examples of HMTis inhibiting tumorigenesis and discuss their potential mechanisms of action.
3.2.1 Inhibitors of H3K9 HMTs
Most HMTs are present as closely homologous pairs. For example, the catalytic SET domains of G9a and GLP share 77% sequence identity and are present as a stoichiometric heterometric complex (75). In cells, they are responsible for H3K9 methylation and G9a/GLP-mediated H3K9me2, which are highly associated with transcriptional repression (76). A recent study reported high expression of G9a in various cancers, such as prostate/colon/lung cancers, multiple myeloma, and lymphocytic leukemia, indicating that G9a inhibitors might suppress cancer proliferation (41, 77). BIX-01294 was first found as a G9a/GLP-specific inhibitor that can modulate global H3K9me2 levels in cells (78). Although specific G9a/GLP inhibitors, such as UNC0224, UNC0321, E72, UNC0638, and UNC0642, have been developed, they have not been used in clinical trials because of their cell toxicity or poor bioavailability.
3.2.2 Inhibitors of H3K27 HMTs
In mammals, polycomb repressive complex 2 exhibits HMT activity on H3K27 via catalytic subunits enhancer of zeste homologs 1/2 (EZH1/2) (79). In Drosophila, EZH1 and 2 are mainly responsible for maintaining the spatial expression pattern of homeobox (HOX) genes (80). Aberrant EZH2 expression has been associated with various human cancers. For example, the overexpression of EZH2 has been detected in prostate, breast, and other cancers, suggesting that it might serve as a prognostic marker for cancers (43, 81). Further, wild-type and mutant EZH2 cooperatively regulate and maintain the hypertrimethylation of H3K27, which inhibits the proliferation of lymphoma cells by abnormally silencing PCR2-target genes (47).
Inhibitors of EZH1/2 can be classified into three groups according to their basic skeleton structure as follows: those with the pyridone-indazole scaffold, which includes EPZ005687, UNC1999, and GSK343 (48); those with the pyridone-indole scaffold, which includes GSK-126, CPI-1205, and E11 (41, 78); and those with the pyridone-phenyl scaffold, which includes EPZ006088 and EPZ6438 (tazemetostat) (45). More recently, several non-SAM-derived inhibitors of the catalytic activity of EZH2 have been discovered. Among them are GSK126 and EPZ005687, inhibitors effective against EZH2 mutant lymphomas, and EI1, a low MW inhibitor that blocks diffuse large B-cell lymphoma proliferation (46). Tazemetostat has been recently approved for relapsed/refractory after two or more lines of therapy in the presence of an EZH2 mutation or independent of an EZH2 mutation in the absence of other options (82). Combined tazemetostat and MAPKis enhances the differentiation of papillary thyroid cancer cells harboring BRAFV600E by synergistically decreasing the global trimethylation of H3K27me (44). UNC1999, a modified inhibitor, improves the specificity of EZH2 and achieves better oral bioavailability (83). As a second-generation compound, EPZ6438 shows improved potency, pharmacokinetic properties, and selectivity for EZH1 than EPZ005687 (81). Both EPZ6438 and CPI-1205 are currently undergoing clinical trials for solid tumors or B-cell lymphoma (84) (Table 1).
3.2.3 Inhibitors of H3K79 HMTs
DOT1-like protein (DOT1L), an enzyme responsible for H3K79 methylation, does not contain the SET catalytic domain and displays a class I SAM-dependent MTase fold (85). In cells, DOT1/DOT1L-mediated H3K79 methylation is involved in various biological processes, including gene transcription, the cell cycle, and DNA damage repair (86). DOT1L interacts with mixed lineage leukemia (MLL) translocation fusion proteins, such as AF10, ENL, AF9, and AF4, resulting in the DOT1L-mediated H3K79 methylation of target genes. Therefore, DOT1L has become a potential target for developing therapeutic drugs to treat leukemia.
To date, more than 20 DOT1L inhibitors have been reported. Among them, EPZ004777 was first found to selectively kill leukemic cells by repressing DOT1L-mediated H3K79 methylation (87). EPZ-5676, an optimized version of EPZ004777, forms hydrogen bonds with residues Asp222, Glu186, Gly163, and Asp161 of DOT1L to prevent cellular H3K79 methylation. EPZ-5676 has been used against leukemia in phase I clinical trials (88). Another DOT1L inhibitor, SYC-522, effectively delayed the progression of MLL in the preclinical phase by suppressing H3K79 methylation and reducing the expression of two important leukemia-related genes, HOXA9 and MEIS1. Additionally, SYC-522 significantly reduces the expression of CCND1 and BCL2L1, which are important regulators of the cell cycle and anti-apoptotic signaling pathways (49) (Table 1).
3.3 Inhibitors Targeting Protein Arginine Methyltransferases (PRMTis)
The protein arginine MTase (PRMT) family includes nine enzymes divided into three types, type I PRMT, CARM1, and PRMT5. In cells, PRMTs catalyze the methylation of arginine residues on histones. PRMT dysfunction is associated with the occurrence of several cancers.
PRMTis are also classified into three types based on their corresponding PRMT type and have been investigated in the early preclinical stage (Table 1). Type I PRMTis include AMI-1, AMI-6, DB75, GSK3368715, and MS023 (50, 89). MS049, TP-064, and EZM2302 exhibit the highly selective inhibition of CARM1 (PRMT4) (51), and the latter two compounds can be effectively used to treat multiple myeloma (MM) by selectively blocking CARM1 (90, 91). Interestingly, PRMT5 inhibitors, such as EPZ015666 (GSK3235025), EPZ015938 (GSK3326595), and LLY-283, possess high anti-tumor activities. EPZ015666 was used against NHL in clinical trials by blocking SmD3 methylation (52–54).
3.4 Inhibitors Targeting Histone Lysine Demethyltransferases (KDMis)
Many small-molecule compounds have emerged as lysine demethyltransferase inhibitors (KDMis), some of which have entered different clinical stages as anti-tumor drug candidates (Table 1). Inhibitors of both the LSD/KDM and JmjC-KDM family proteins have been shown to block the catalytic domain to reduce catalytic activity. One of the most potent LSD1 inhibitors, tranylcypromine (PCPA), causes the irreversible inhibition of LSD1 by forming a covalent adduct with the FAD cofactor of LSD1 (92). This process destroys the catalytic group of the histone lysine demethyltransferase, which inhibits the activity of the enzyme and inactivates it. Based on the chemo-type scaffold, a series of PCPA derivatives have been designed and shown to exert anti-tumor effects (55). Two recently developed PCPA derivatives, ORY-1001 and GSK2879552, promoted the differentiation of acute myeloid leukemia (AML) and limited the growth of small cell lung cancer (SCLC) in a phase 1 clinical trial aimed to assess their roles against AML and SCLC (60, 61). These two PCPA derivatives exhibit higher selectivity for LSD1 than for PCPA (93). Therefore, PCPA derivatives have the potential to become new epigenetic anticancer drugs. In addition, HCI-2509, a potent small-molecule inhibitor of LSD1, hinders the growth of and exerts the cytotoxic effects on neuroblastoma (NB) cells via p53 (62). Of the thieno[3,2-b]pyrrole-5-carboxamides, novel reversible inhibitors of KDM1A, that showed a remarkable anti-clonogenic cell growth effect on MLL-AF9 human leukemia cells (59) (Table 1).
Various structural scaffolds, including hydroxamic acid, hydroxyquinoline analogs, and cyclic peptides, reportedly function as effective JmjC-KDM inhibitors (25). For example, the 8-hydroxyquinoline derivative IOX1 can block many KDM isoforms (94). Several catechol molecules and flavonoid analogs have also been identified as JmjC-KDM inhibitors (25). However, the aforementioned compounds are still in the developmental phase.
3.5 Inhibitors Targeting Specific Functional Domains of Methyl-Lysine Readers
The methylation of lysine residues in N-terminal tails of histones H3 and H4 widely mediates biological processes in cells. In recent decades, various proteins containing specific functional domains that recognize methyl-lysine on histones have been identified, such as methyl-lysine reader proteins. Methyl-lysine readers are approximately categorized into chromodomain, PHD finger domain, Tudor domain, PWWP domain, WD40 repeat (WDR) domain, and malignant brain tumor (MBT) domain families (5). These proteins exhibit different abilities to recognize methylated lysine residues according to their different functional domains.
Chromodomain proteins are further classified into heterochromatin protein 1 (HP1)/polycomb (Pc), chromo-ATPase/helicase-DNA-binding (CHD), chromobarrel domain, and chromodomain Y (CDY) families (5). Both HP1/Pc and CDY proteins show strong preference for trimethylated H3K9 and H3K27 (68). Moreover, CHD proteins recognize methyl-lysine residues on H3K4 (95), whereas chromobarrel domain proteins interact with methylated H3K36 and H4K20 (96). In addition, both PHD and MBT domain proteins recognize methylated H3K4 (97).
Methyl-lysine reader proteins play important roles in regulating many cellular processes, such as development, the cell cycle, stress responses, and oncogenesis, and have increasingly become the focus of epigenetic research. Inhibitors of methyl-lysine reader proteins, such as MS37452A, SW2_110A, and UCN3866, have been found to inhibit the growth of cancer cells as selective inhibitors of Pc chromobox (CBX) and CDY proteins (98, 99). Additionally, several compounds have been identified as PHD inhibitors (100). For example, macrocyclic calixarenes can disrupt the binding of ING2 PHD to H3K4me, disulfiram, amiodarone, and tegaserod to prevent interactions between JARID1A PHD3 and H3K4me3 (2, 6). Moreover, benzimidazole can be selectively docked in methylated H3K4, preventing the binding of the Pygo-BCL9 chromatin reader to H3K4me PHD (101). Thus, many proteins targeting methyl-lysine readers have been shown to exert anticancer effects (102).
4 Histone Acetylation as a Target for Anti-Tumor Drug Development
4.1 Histone Acetylation
Acetylation of the ε-amino group of a lysine residue was first discovered with histones in 1968, but the responsible enzymes, histone acetyltransferases and deacetylases, were not identified until the mid-1990s (103). Histone acetylation is a reversible process that occurs via the addition of an acetyl group to the ε-amino of the lysine residue at the midamino end and tail of the histone. This process is dynamically controlled by histone acetyltransferases (HATs), lysine acetyltransferases, and histone deacetylases (HDACs) (Table 2). Lysine residues on histones are prone to acetylation, resulting in a decrease in the positive charge and weakening of the interaction between histones and DNA (104).
There are three major families of HATs, general control non-derepressible 5 (Gcn5)-related N-acetyltransferases (GNATs), p300/CBP, and MYST proteins. p300 (adenoviral E1A-associated protein of 300 kDa) and CBP (CREB-binding protein) form a pair of paralogous transcriptional co-activators. Members of the GNAT family include HAT1, yeast Gcn5, and its metazoan orthologs GCN5 and PCAF (p300/CBP-associated factor) (103). HATs are classified into types A and B based on their cellular location. Type A is responsible for acetylating histones associated with chromatin, whereas type B acetylates newly translated histones in the cytoplasm. Nuclear HATs can be divided into two categories based on their sequence homology and shared structural features. The GCN5-related N-acetyltransferase (GNAT) family, which includes GCN5 and p300/CBP-associating factor (PCAF), can acetylate lysine residues on histones H2B, H3, and H4. Meanwhile, the MOZ, YBF2/SAS3, SAS2, and TIP60 (MYST) families of proteins are characterized by a highly conserved MYST domain (105).
Acetyl groups on lysine residues must be removed by HDACs. Dependent on sequence similarity and cofactor dependency, HDACs are grouped into four classes and two families, the classical and silent information regulator 2 (Sir2)-related protein (sirtuin) families. In humans, members of the classical family include HDAC1, 2, 3, and 8 (class I); HDAC4, 5, 6, 7, 9, and 10 (class II); and HDAC11 (class IV). They share sequence similarity and require Zn2+ for deacetylase activity. The sirtuin family contains seven members (SIRT1–7, class III), which show no sequence resemblance to members of the classical family and require NAD+ as the cofactor (106, 107).
4.2 Inhibitors Targeting Histone Acetyltransferases (HATis)
Imbalanced HAT expression and acetylation levels in tumorigenesis make HATs suitable targets for drug development. In preclinical experiments, many small-molecule compounds have been screened as potential HATis to regulate histone acetylation and reduce tumor growth (Table 3). These compounds include isothiazolone-based chemical compounds, the natural compounds garcinol and embelin (108, 109), the pyrazolone-containing small molecule C646 (124), and the pyridoisothiazole derivatives PU139 and PU141, which block PCAF and/or p300 (110). For example, PU139 retards the growth of NB by blocking Gcn5, PCAF, CBP, and p300 (110).
4.3 Inhibitors Targeting Histone Lysine Deacetylases (HDACis)
Global histone acetylation levels are frequently decreased in cancer cells. Correcting imbalanced acetylation in tumor cells can be achieved by reducing the activity of HDACs using HDACis (Table 3). The first HDACi discovered was trichostatin A, a dienohydroxamic acid obtained from Streptomyces that effectively suppressed zinc-dependent HDACs in the preclinical stage (111). Notably, numerous pan-HDAC inhibitors (P-HDACi) such as vorinostat (also known as suberoylanilide hydroxamic acid, SAHA) (112), belinostat (PXD-101) (113), panobinostat (LBH589) (114), pracinostat (MEI Pharma) (115), romidepsin (FK228) (116), and chidamide (CS055, HBI-8000) (117), have been approved by the FDA to treat different cancers, including primary cutaneous T-cell lymphoma, peripheral T-cell lymphoma, MM, and AML. Moreover, several P-HDACis, including resminostat (4SC-201) (118), givinostat (ITF2357) (119), quisinostat (JNJ-26481585) (120), entinostat (121), and mocetinostat (122), have been evaluated clinically for Hodgkin’s lymphoma, polycythemia, ovarian cancer, and other carcinomas. Furthermore, both sirtinol and nicotinamide have exhibited activity against breast cancer as SIRT inhibitors (123, 125) (Table 3). In addition to the compounds mentioned, novel HDACis are constantly being developed (25).
4.4 Inhibitors Targeting Specific Functional Domains of Acetyl-Lysine Readers
Acetyl-lysine on histones can also be recognized by readers with specific functional domains, such as PHD finger, Yaf9, ENL, AF9, Taf14, and Sas5 (YEATS), and BRD. PHD finger proteins recognize acetylated, un-acetylated, or methylated histones, with the PHD finger domains in MLL4 (KMT2D) and MLL3 (KMT2C) targeting H4K16 acetylation and involved in the interaction between MLL4/3 and males absent on the first (MOF) (1278). YEATS proteins interact with acetylated histones H3K9, H3K14, and H3K27 (126).
Many BRD proteins are involved in chromatin-remodeling or chromatin-modifying enzymes. BRDs in HATs act as protein–protein interaction modules that specifically recognize acetylated histones to regulate gene transcription, including H4K5, H4K8, H4K12, H4K16, H4K20, H3K14, and H3K36 (5). BRD proteins are the most widely studied acetyl-lysine readers and have been found in many nuclear proteins, including HATs, HMTs, chromatin-remodeling enzymes, and transcriptional co-activators (127). At present, several inhibitors that target the acetyl-binding pocket of BRDs or BRD extraterminal proteins (BETs) have been discovered (25) (Table 4). Among them, BET inhibitors such as RVX-208 (RVX00022), I-BET762 (GSK525762), FT-1101, CPI-0610, BAY1238094, INCB054329, PLX51107, GSK2820151, ZEN003694, BMS-986158, BI 894999, ABBV-075, GS-5829 (128, 129), and OTX015 (MK-8628) have been tested for their anti-tumor effects against numerous types of cancers in clinical trials (130, 131) (Table 4). Moreover, several novel BRD inhibitors, including I-BRD9, BI-7273, and BI-9564, can specifically target BRD9 and possess anti-tumor activity (132, 133).
5 Epigenetic Enzymes as Anticancer Targets of Natural Compounds and Their Active Components
Natural compounds and their active components have been widely used in traditional medicine in China, Japan, South Korea, and other countries for their various pharmacological effects. Increasing natural compounds have demonstrated high anticancer activity, providing potential candidates for developing multifunctional tumor-targeted drugs. However, their precise mechanisms of action remain unclear. Here, we focus on BBR (C20H18NO4) and ginsenosides, natural compounds that have undergone extensive preclinical investigation and play anti-tumor roles by targeting epigenetic enzymes and ncRNAs (Figure 3).
5.1 Berberine, a Natural Compound With Epigenetic Regulatory Activity
BBR, the main alkaloid in the herbal medicine Coptis, and its derivatives exhibit effective anti-tumor activity (Table 5). The functional mechanism of BBR is closely related to its regulation of epigenetic chromatin-modifying enzymes, as the activities of multiple enzymes involved in histone acetylation and methylation, such as CBP/p300, SIRT3, KDM6A, SETD7, and HDAC8, are altered when myeloma U266 cells are treated with BBR (138). Furthermore, BBR treatment leads to the increased acetylation of histones H3 and H4 and suppresses total HDAC activity, further retarding the growth of human lung cancer A549 cells (139). Chen et al. demonstrated that BBR reduces the expression of both EZH2 and H3K27me3 in esophageal carcinoma (134). Further, pseudodehydrocorydaline (a protoberberine alkaloid) selectively suppresses the activity of HMT G9a and decreases the expression of H3K9me2 in MCF-7 breast cancer cells via CT13 occupation of the binding site of histone H3, suggesting that CT13 might provide a novel scaffold for synthetic G9a inhibitors (135). In addition to modifying histones, BBR also regulates DNMTs. BBR reportedly accesses chromatin in hepatoma HepG2 cells, resulting in increased global genome methylation and reduced methylation in promoter region CpG sites of cytochrome P450 2B6 (CYP2B6), cytochrome P450 3A4 (CYP3A4), and glucose regulated protein 78 (GRP78) (136). In addition, BBR effectively reduces the expression of DNMT1/3B and promotes p53-hypomethylation, thus further altering the p53-dependent signaling pathway to hinder the growth of myeloma U266 cells (137, 140).
5.2 Anti-Tumor Epigenetic Regulatory Effects of Ginsenosides
Ginsenosides, derived from saponins of ginseng, have a steroid-like hydrophobic backbone connected to one or more sugar moieties and are generally believed to be the major bioactive constituents of ginseng (141). Ginsenosides are divided into two groups based on their chemical structures, panaxatriol (Re, Rf, Rg1, Rg2, and Rh1) and panaxadiol (Rb1, Rb2, Rb3, Rc, Rj, Rg3, and Rh2) (142) (Table 6). Although ginsenosides possess various pharmacological activities, including anti-inflammatory, anti-allergic, anti-fatigue, anti-stress, and anti-cancer properties (152), their basic biological characteristics have not been fully studied. Recent studies have demonstrated that epigenetic mechanisms might be involved in pharmacological effects of ginsenosides (153).
Genome-wide DNA methylation analysis revealed that ginsenoside Rh2 inhibits the growth of breast cancer MCF-7 cells by reducing long interspersed nucleotide element methylation and the expression of hypermethylated genes involved in tumorigenesis (143). Similarly, ginsenoside Rg3 treatment downregulates hypermethylated tRNA methyltransferase 1-like (TRMT1L), proteasome 26S subunit, ATPase 6 (PSMC6), and NADPH oxidase 4 (NOX4), while upregulating hypomethylated ST3 beta-galactoside alpha-2, 3-sialyltransferase 4 (ST3GAL4), RNLS, and KDM5A in breast cancer MCF-7 cells to block tumor growth (154). Ginsenosides also block DNMTs by modulating their target genes. Compound K (the main metabolite of ginseng saponin) suppresses DNMT1 expression to reduce the proliferation of colorectal cancer (CRC) cells by reactivating the epigenetically silenced RUNX3 gene (144). Ginsenoside Rg3 treatment decreases the expression of DNMT1/3A/3B and increases the acetylation of histones H3K9/K14 and H4K5/K12/K16 to inhibit the growth of ovarian cancer cells (145). Treatment with 20(s)-ginsenoside Rh2 suppresses the proliferation of K562 and KG1-α leukemia cells by reducing the expression and activity of HDACs, including HDAC1/C2/C6, suggesting that 20(s)-ginsenoside Rh2 acts as an HDACi (155). Interestingly, treatment with ginsenoside Rh2 also suppresses PDZ-binding kinase/T-LAK cell-originated protein kinase (PBK/TOPK), which retards the proliferation of tumor cells through the ERK1/2 signaling pathway (156).
A recent study reported the ability of ginsenosides to suppress cancer by regulating miRNAs (150, 157). The activity of ginsenoside Rh2 against different types of cancer cells is mediated by upregulating miR-146a-5p, miR-21, miR-491, and miR128 (158–160) or by downregulating miR-4295, miR-31, and miR-638 (146, 161–163). In addition, treatment with the ginsenoside Rh2 reduces anti-tumor drug resistance in breast cancer cells by reducing the expression of miR-222, miR-34a, and miR-29a (147). Further, treatment with 20(S)-ginsenoside Rg3 reverses epithelial–mesenchymal transition in ovarian cancer cells by downregulating DNMT3A-mediated miR-145 (148). Similarly, ginsenoside Rg3 treatment downregulates miR-221 to reduce epithelial–mesenchymal transition in human oral squamous carcinoma cells (149). 20(S)-ginsenoside Rg3-mediated miR-532-3p/miR-324-5p also represses the expression of pyruvate kinase M2 (PMK2), resulting in an anti-tumor effect (164, 165).
Ginsenosides also modulate lncRNAs to hamper the growth of cancer cells (150, 166). Treatment with ginsenoside Rh2 suppresses the lncRNA C3orf67 in breast cancer MCF-7 cells (151). Moreover, ginsenoside Rg3 binds to the promoters of two lncRNAs, regulatory factor X-antisense 1 (RFX-AS1) and syntaxin-binding protein 5-antisense 1 (STXBP5-AS1), to alter DNA methylation, thus inhibiting the growth of breast cancer MCF-7 cells (56) (Table 6). Thus, ginsenosides mediate the expression of DNMTs and lncRNAs in tumor growth. In summary, natural compounds and their active components, targeting epigenetic enzymes, have therapeutic potential for cancer treatment (57).
6 Conclusions and Perspectives
Epigenomic alterations mediated by epigenetic regulatory enzymes have a profound effect on many hallmarks of cancer, including malignant self-renewal, differentiation blockade, evasion of cell death, and tissue invasiveness (167). The anticancer roles of inhibitors targeting epigenetic regulatory enzymes provide attractive targets for novel drugs, even if enzymes that selectively regulate the target genes are not well known (168). Some HATis, HDACis, and DNMTis have been approved as anticancer epidrugs. However, the use of most epigenetic regulatory enzyme inhibitors is limited by their poor bioavailability, cytotoxicity, and specificity. Therefore, developing effective drugs that target epigenetic enzymes remains challenging. An increasing number of studies has demonstrated that many natural compounds and their active components target epigenetic enzymes to successfully delay cancer progression, suggesting attractive alternatives for anticancer treatments.
Author Contributions
YJ, TL, HL, YL, and DL participated in writing, editing, and creating figures. All authors have read and approved the final manuscript.
Funding
This research was funded by the National Natural Science Foundation of China (Grant Nos. 82003985 and 81973712). The Science and Technology Research Project of the Jilin Provincial Department of Education (Grant No. JJKH20210995KJ, JJKH20210995KJ), and Jilin Province Science and Technology Development Project in China (Grant No. 20210204013YY).
Conflict of Interest
The authors declare that the research was conducted in the absence of any commercial or financial relationships that could be construed as a potential conflict of interest.
Publisher’s Note
All claims expressed in this article are solely those of the authors and do not necessarily represent those of their affiliated organizations, or those of the publisher, the editors and the reviewers. Any product that may be evaluated in this article, or claim that may be made by its manufacturer, is not guaranteed or endorsed by the publisher.
Abbreviations
AML, acute myeloid leukemia; BBR, berberine; BET, BRD-extra terminal protein; BRD, bromodomain; CHD, chromo-ATPase/helicase-DNA-binding; CDY, chromodomain Y; CpGI, CpG island; CRC, colorectal cancer; DNMT, DNA methylase; DNMTi, DNMT inhibitor; DOT1, disruptor of telomeric silencing 1; DOT1L, DOT1-like; EZH1/2, enhancer of zeste homologs 1/2; FAD, flavin adenine dinucleotide; GNAT, GCN5-related N-acetyltransferase; HAT, histone acetyltransferase; HDAC, histone deacetylase; HMT, histone methyltransferase; HMTi, HMT inhibitor; HP1, heterochromatin protein 1; JmjC, Jumonji C; KDM, lysine demethylase; KMT, lysine methyltransferase; LSD, lysine-specific demethylase; MBD, methyl-CpG-binding domain; MBP, methyl-CpG-binding protein; MBT, malignant brain tumor; mC; miRNA, microRNA; MLL, mixed lineage leukemia; MM, multiple myeloma; MTase, methyltransferase; MYST, MOZ, YBF2/SAS3, SAS2, and TIP60; NA, nucleoside analog; NB, neuroblastoma; ncRNA, noncoding RNA; NHL, non-Hodgkin’s lymphoma; PHD, plant homeodomain; PRMT, protein arginine methyltransferase; PRMTi, PRMT inhibitor; PWWP, Pro-Trp-Trp-Pro; SAM, S-adenosylmethionine; SCLC, small cell lung cancer; SET, su(var) 3-9, enhancer of zeste, and trithorax; SIRT, sirtuin; TET, ten-eleven translocation.
References
2. Allis CD, Jenuwein T. The Molecular Hallmarks of Epigenetic Control. Nat Rev Genet (2016) 17(8):487–500. doi: 10.1038/nrg.2016.59
3. Miranda Furtado CL, Dos Santos Luciano MC, Silva Santos RD, Furtado GP, Moraes MO, Pessoa C. Epidrugs: Targeting Epigenetic Marks in Cancer Treatment. Epigenetics (2019) 14(12):1164–76. doi: 10.1080/15592294.2019.1640546
4. Park JW, Han JW. Targeting Epigenetics for Cancer Therapy. Arch Pharm Res (2019) 42(2):159–70. doi: 10.1007/s12272-019-01126-z
5. Cai SF, Levine RL. Genetic and Epigenetic Determinants of AML Pathogenesis. Semin Hematol (2019) 56(2):84–9. doi: 10.1053/j.seminhematol.2018.08.001
6. Zhu H, Wei T, Cai Y, Jin J. Small Molecules Targeting the Specific Domains of Histone-Mark Readers in Cancer Therapy. Molecules (2020) 25(3):578. doi: 10.3390/molecules25030578
7. Wagner W, Frobel J, Goetzke R. Epigenetic Quality Check - How Good are Your Mesenchymal Stromal Cells? Epigenomics (2016) 8(7):889–94. doi: 10.2217/epi-2016-0054
8. Lin CC, Chen YP, Yang WZ, Shen JCK, Yuan HS. Structural Insights Into Cpg-Specific DNA Methylation by Human DNA Methyltransferase 3B. Nucleic Acids Res (2020) 48(7):3949–61. doi: 10.1093/nar/gkaa111
9. Xie W, Baylin SB, Easwaran H. DNA Methylation in Senescence, Aging and Cancer. Oncoscience (2019) 6(1-2):291–3. doi: 10.18632/oncoscience.476
10. Porcellini E, Laprovitera N, Riefolo M, Ravaioli M, Garajova I, Ferracin M. Epigenetic and Epitranscriptomic Changes in Colorectal Cancer: Diagnostic, Prognostic, and Treatment Implications. Cancer Lett (2018) 419:84–95. doi: 10.1016/j.canlet.2018.01.049
11. Baubec T, Colombo DF, Wirbelauer C, Schmidt J, Burger L, Krebs AR, et al. Genomic Profiling of DNA Methyltransferases Reveals a Role for DNMT3B in Genic Methylation. Nature (2015) 520(7546):243–7. doi: 10.1038/nature14176
12. Weinberg DN, Papillon-Cavanagh S, Chen H, Yue Y, Chen X, Rajagopalan KN, et al. The Histone Mark H3k36me2 Recruits DNMT3A and Shapes the Intergenic DNA Methylation Landscape. Nature (2019) 573(7773):281–6. doi: 10.1038/s41586-019-1534-3
13. Chen X, Pan X, Zhang W, Guo H, Cheng S, He Q, et al. Epigenetic Strategies Synergize With PD-L1/PD-1 Targeted Cancer Immunotherapies to Enhance Antitumor Responses. Acta Pharm Sin B (2020) 10(5):723–33. doi: 10.1016/j.apsb.2019.09.006
14. Fuks F, Burgers WA, Godin N, Kasai M, Kouzarides T. Dnmt3a Binds Deacetylases and is Recruited by a Sequence-Specific Repressor to Silence Transcription. EMBO J (2001) 20(10):2536–44. doi: 10.1093/emboj/20.10.2536
15. Jurkowska RZ, Rajavelu A, Anspach N, Urbanke C, Jankevicius G, Ragozin S, et al. Oligomerization and Binding of the Dnmt3a DNA Methyltransferase to Parallel DNA Molecules: Heterochromatic Localization and Role of Dnmt3L. J Biol Chem (2011) 286(27):24200–7. doi: 10.1074/jbc.M111.254987
16. Holz-Schietinger C, Matje DM, Reich NO. Mutations in DNA Methyltransferase (DNMT3A) Observed in Acute Myeloid Leukemia Patients Disrupt Processive Methylation. J Biol Chem (2012) 287(37):30941–51. doi: 10.1074/jbc.M112.366625
17. Ren W, Gao L, Song J. Structural Basis of DNMT1 and DNMT3A-Mediated DNA Methylation. Genes (Basel) (2018) 9(12):620. doi: 10.3390/genes9120620
18. Bestor TH. Activation of Mammalian DNA Methyltransferase by Cleavage of a Zn Binding Regulatory Domain. EMBO J (1992) 11(7):2611–7. doi: 10.1002/j.1460-2075.1992.tb05326.x
19. Parry A, Rulands S, Reik W. Active Turnover of DNA Methylation During Cell Fate Decisions. Nat Rev Genet (2021) 22(1):59–66. doi: 10.1038/s41576-020-00287-8
20. Ito S, Shen L, Dai Q, Wu SC, Collins LB, Swenberg JA, et al. Tet Proteins can Convert 5-Methylcytosine to 5-Formylcytosine and 5-Carboxylcytosine. Science (2011) 333(6047):1300–3. doi: 10.1126/science.1210597
21. Tahiliani M, Koh KP, Shen Y, Pastor WA, Bandukwala H, Brudno Y, et al. Conversion of 5-Methylcytosine to 5-Hydroxymethylcytosine in Mammalian DNA by MLL Partner TET1. Science (2009) 324(5929):930–5. doi: 10.1126/science.1170116
22. Long HK, Blackledge NP, Klose RJ. ZF-Cxxc Domain-Containing Proteins, Cpg Islands and the Chromatin Connection. Biochem Soc Trans (2013) 41(3):727–40. doi: 10.1042/bst20130028
23. Hodges AJ, Hudson NO, Buck-Koehntop BA. Cys2His2 Zinc Finger Methyl-Cpg Binding Proteins: Getting a Handle on Methylated DNA. J Mol Biol (2019) 16:S0022–2836(19)30567-4. doi: 10.1016/j.jmb.2019.09.012
24. Moore LD, Le T, Fan G. DNA Methylation and its Basic Function. Neuropsychopharmacology (2013) 38(1):23–38. doi: 10.1038/npp.2012.112
25. Biswas S, Rao CM. Epigenetic Tools (the Writers, the Readers and the Erasers) and Their Implications in Cancer Therapy. Eur J Pharmacol (2018) 837:8–24. doi: 10.1016/j.ejphar.2018.08.021
26. Du Q, Luu PL, Stirzaker C, Clark SJ. Methyl-Cpg-Binding Domain Proteins: Readers of the Epigenome. Epigenomics (2015) 7(6):1051–73. doi: 10.2217/epi.15.39
27. Hendrich B, Tweedie S. The Methyl-Cpg Binding Domain and the Evolving Role of DNA Methylation in Animals. Trends Genet (2003) 19(5):269–77. doi: 10.1016/s0168-9525(03)00080-5
28. Xu P, Hu G, Luo C, Liang Z. DNA Methyltransferase Inhibitors: An Updated Patent Review (2012-2015). Expert Opin Ther Pat (2016) 26(9):1017–30. doi: 10.1080/13543776.2016.1209488
29. Chan AT, Tao Q, Robertson KD, Flinn IW, Mann RB, Klencke B, et al. Azacitidine Induces Demethylation of the Epstein-Barr Virus Genome in Tumors. J Clin Oncol (2004) 22(8):1373–81. doi: 10.1200/jco.2004.04.185
30. Brien GL, Valerio DG, Armstrong SA. Exploiting the Epigenome to Control Cancer-Promoting Gene-Expression Programs. Cancer Cell (2016) 29(4):464–76. doi: 10.1016/j.ccell.2016.03.007
31. Thottassery JV, Sambandam V, Allan PW, Maddry JA, Maxuitenko YY, Tiwari K, et al. Novel DNA Methyltransferase-1 (DNMT1) Depleting Anticancer Nucleosides, 4’-Thio-2’-Deoxycytidine and 5-Aza-4’-Thio-2’-Deoxycytidine. Cancer Chemother Pharmacol (2014) 74(2):291–302. doi: 10.1007/s00280-014-2503-z
32. Christman JK. 5-Azacytidine and 5-Aza-2’-Deoxycytidine as Inhibitors of DNA Methylation: Mechanistic Studies and Their Implications for Cancer Therapy. Oncogene (2002) 21(35):5483–95. doi: 10.1038/sj.onc.1205699
33. Lin RK, Hsu CH, Wang YC. Mithramycin a Inhibits DNA Methyltransferase and Metastasis Potential of Lung Cancer Cells. Anticancer Drugs (2007) 18(10):1157–64. doi: 10.1097/CAD.0b013e3282a215e9
34. Datta J, Ghoshal K, Denny WA, Gamage SA, Brooke DG, Phiasivongsa P, et al. A New Class of Quinoline-Based DNA Hypomethylating Agents Reactivates Tumor Suppressor Genes by Blocking DNA Methyltransferase 1 Activity and Inducing its Degradation. Cancer Res (2009) 69(10):4277–85. doi: 10.1158/0008-5472.Can-08-3669
35. Villar-Garea A, Fraga MF, Espada J, Esteller M. Procaine is a DNA-Demethylating Agent With Growth-Inhibitory Effects in Human Cancer Cells. Cancer Res (2003) 63(16):4984–9.
36. Zambrano P, Segura-Pacheco B, Perez-Cardenas E, Cetina L, Revilla-Vazquez A, Taja-Chayeb L, et al. A Phase I Study of Hydralazine to Demethylate and Reactivate the Expression of Tumor Suppressor Genes. BMC Cancer (2005) 5:44. doi: 10.1186/1471-2407-5-44
37. Isakovic L, Saavedra OM, Llewellyn DB, Claridge S, Zhan L, Bernstein N, et al. Constrained (L-)-s-Adenosyl-L-Homocysteine (SAH) Analogues as DNA Methyltransferase Inhibitors. Bioorg Med Chem Lett (2009) 19(10):2742–6. doi: 10.1016/j.bmcl.2009.03.132
38. Liu B, Ma H, Liu Q, Xiao Y, Pan S, Zhou H, et al. Mir-29b/Sp1/FUT4 Axis Modulates the Malignancy of Leukemia Stem Cells by Regulating Fucosylation via Wnt/β-Catenin Pathway in Acute Myeloid Leukemia. J Exp Clin Cancer Res (2019) 38(1):200. doi: 10.1186/s13046-019-1179-y
39. Fang MZ, Chen D, Sun Y, Jin Z, Christman JK, Yang CS. Reversal of Hypermethylation and Reactivation of P16ink4a, Rarbeta, and MGMT Genes by Genistein and Other Isoflavones From Soy. Clin Cancer Res (2005) 11(19 Pt 1):7033–41. doi: 10.1158/1078-0432.Ccr-05-0406
40. Medina-Franco JL, López-Vallejo F, Kuck D, Lyko F. Natural Products as DNA Methyltransferase Inhibitors: A Computer-Aided Discovery Approach. Mol Divers (2011) 15(2):293–304. doi: 10.1007/s11030-010-9262-5
41. Ishiguro K, Kitajima H, Niinuma T, Maruyama R, Nishiyama N, Ohtani H, et al. Dual EZH2 and G9a Inhibition Suppresses Multiple Myeloma Cell Proliferation by Regulating the Interferon Signal and IRF4-MYC Axis. Cell Death Discov (2021) 7(1):7. doi: 10.1038/s41420-020-00400-0
42. Cerami E, Gao J, Dogrusoz U, Gross BE, Sumer SO, Aksoy BA, et al. The Cbio Cancer Genomics Portal: An Open Platform for Exploring Multidimensional Cancer Genomics Data. Cancer Discov (2012) 2(5):401–4. doi: 10.1158/2159-8290.Cd-12-0095
43. Fernández-Serrano M, Winkler R, Santos JC, Le Pannérer MM, Buschbeck M, Roué G. Histone Modifications and Their Targeting in Lymphoid Malignancies. Int J Mol Sci (2021) 23(1):253. doi: 10.3390/ijms23010253
44. Zhong H, Zhou J, An XH, Hua YR, Lai YF, Zhang R, et al. Natural Product-Based Design, Synthesis and Biological Evaluation of 2’,3,4,4’-Tetrahydrochalcone Analogues as Antivitiligo Agents. Bioorg Chem (2019) 87:523–33. doi: 10.1016/j.bioorg.2019.03.054
45. Morschhauser F, Tilly H, Chaidos A, McKay P, Phillips T, Assouline S, et al. Tazemetostat for Patients With Relapsed or Refractory Follicular Lymphoma: An Open-Label, Single-Arm, Multicentre, Phase 2 Trial. Lancet Oncol (2020 ) 21(11):1433–42. doi: 10.1016/S1470-2045(20)30441-1
46. Fu H, Cheng L, Sa R, Jin Y, Chen L. Combined Tazemetostat and Mapki Enhances Differentiation of Papillary Thyroid Cancer Cells Harbouring BRAFV600E by Synergistically Decreasing Global Trimethylation of H3K27. J Cell Mol Med (2020) 24(6):3336–45. doi: 10.1111/jcmm.15007
47. Liu Q, Wang MW. Histone Lysine Methyltransferases as Anti-Cancer Targets for Drug Discovery. Acta Pharmacol Sin (2016) 37(10):1273–80. doi: 10.1038/aps.2016.64
48. Dilworth D, Barsyte-Lovejoy D. Targeting Protein Methylation: From Chemical Tools to Precision Medicines. Cell Mol Life Sci (2019) 76(15):2967–85. doi: 10.1007/s00018-019-03147-9
49. Liu W, Deng L, Song Y, Redell M. DOT1L Inhibition Sensitizes MLL-Rearranged AML to Chemotherapy. PloS One (2014) 9(5):e98270. doi: 10.1371/journal.pone.0098270
50. Fedoriw A, Rajapurkar SR, O’Brien S, Gerhart SV, Mitchell LH, Adams ND, et al. Anti-Tumor Activity of the Type I PRMT Inhibitor, GSK3368715, Synergizes With PRMT5 Inhibition Through MTAP Loss. Cancer Cell (2019) 36(1):100–14.e25. doi: 10.1016/j.ccell.2019.05.014
51. Shen Y, Szewczyk MM, Eram MS, Smil D, Kaniskan H, de Freitas RF, et al. Discovery of a Potent, Selective, and Cell-Active Dual Inhibitor of Protein Arginine Methyltransferase 4 and Protein Arginine Methyltransferase 6. J Med Chem (2016) 59(19):9124–39. doi: 10.1021/acs.jmedchem.6b01033
52. Chan-Penebre E, Kuplast KG, Majer CR, Boriack-Sjodin PA, Wigle TJ, Johnston LD, et al. A Selective Inhibitor of PRMT5 With In Vivo and In Vitro Potency in MCL Models. Nat Chem Biol (2015) 11(6):432–7. doi: 10.1038/nchembio.1810
53. Bonday ZQ, Cortez GS, Grogan MJ, Antonysamy S, Weichert K, Bocchinfuso WP, et al. LLY-283, a Potent and Selective Inhibitor of Arginine Methyltransferase 5, PRMT5, With Antitumor Activity. ACS Med Chem Lett (2018) 9(7):612–7. doi: 10.1021/acsmedchemlett.8b00014
54. Gerhart SV, Kellner WA, Thompson C, Pappalardi MB, Zhang XP, Montes de Oca R, et al. Activation of the P53-MDM4 Regulatory Axis Defines the Anti-Tumour Response to PRMT5 Inhibition Through Its Role in Regulating Cellular Splicing. Sci Rep (2018) 8(1):9711. doi: 10.1038/s41598-018-28002-y
55. Liu K, Liu Y, Lau JL, Min J. Epigenetic Targets and Drug Discovery Part 2: Histone Demethylation and DNA Methylation. Pharmacol Ther (2015) 151:121–40. doi: 10.1016/j.pharmthera.2015.04.001
56. Ham J, Jeong D, Park S, Kim HW, Kim H, Kim SJ. Ginsenoside Rg3 and Korean Red Ginseng Extract Epigenetically Regulate the Tumor-Related Long Noncoding RNAs RFX3-AS1 and STXBP5-AS1. J Ginseng Res (2019) 43(4):625–34. doi: 10.1016/j.jgr.2019.02.004
57. Song H, Liu D, Dong S, Zeng L, Wu Z, Zhao P, et al. Epitranscriptomics and Epiproteomics in Cancer Drug Resistance: Therapeutic Implications. Signal Transduct Target Ther (2020) 5(1):193. doi: 10.1038/s41392-020-00300-w
58. Ohnston G, Ramsey HE, Liu Q, Wang J, Stengel KR, Sampathi S, et al. Nascent Transcript and Single-Cell RNA-Seq Analysis Defines the Mechanism of Action of the LSD1 Inhibitor INCB059872 in Myeloid Leukemia. Gene (2020) 752:144758. doi: 10.1016/j.gene.2020.144758
59. Vianello P, Sartori L, Amigoni F. Thieno [3, 2-B] Pyrrole-5-Carboxamides as New Reversible Inhibitors of Histone Lysine Demethylase KDM1A/LSD1. Part 2: Structure-Based Drug Design and Structure–Activity Relationship[J]. J Med Chem (2017) 60(5):1693–715. doi: 10.1021/acs.jmedchem.6b01019
60. Bauer TM, Besse B, Martinez-Marti A, Trigo JM, Moreno V, Garrido P, et al. Phase I, Open-Label, Dose-Escalation Study of the Safety, Pharmacokinetics, Pharmacodynamics, and Efficacy of GSK2879552 in Relapsed/Refractory SCLC. J Thorac Oncol (2019) 14(10):1828–38. doi: 10.1016/j.jtho.2019.06.021
61. Helin K, Dhanak D. Chromatin Proteins and Modifications as Drug Targets. Nature (2013) 502(7472):480–8. doi: 10.1038/nature12751
62. Gupta S, Doyle K, Mosbruger TL, Butterfield A, Weston A, Ast A, et al. Reversible LSD1 Inhibition With HCI-2509 Induces the P53 Gene Expression Signature and Disrupts the MYCN Signature in High-Risk Neuroblastoma Cells. Oncotarget (2018) 9(11):9907–24. doi: 10.18632/oncotarget.24035
63. Ciechomska M, Roszkowski L, Maslinski W. DNA Methylation as a Future Therapeutic and Diagnostic Target in Rheumatoid Arthritis. Cells (2019) 8(9):953. doi: 10.3390/cells8090953
64. Singh M, Kumar V, Sehrawat N, Yadav M, Chaudhary M, Upadhyay SK, et al. Current Paradigms in Epigenetic Anticancer Therapeutics and Future Challenges. Semin Cancer Biol (2021) 22:S1044–579X(21)00063-8. doi: 10.1016/j.semcancer
65. Yin X, Yang S, Zhang M, Yue Y. The Role and Prospect of JMJD3 in Stem Cells and Cancer. BioMed Pharmacother (2019) 118:109384. doi: 10.1016/j.biopha.2019.109384
66. Cao H, Li L, Yang D, Zeng L, Yewei X, Yu B, et al. Recent Progress in Histone Methyltransferase (G9a) Inhibitors as Anticancer Agents. Eur J Med Chem (2019) 179:537–46. doi: 10.1016/j.ejmech.2019.06.072
67. Hon GC, Hawkins RD, Ren B. Predictive Chromatin Signatures in the Mammalian Genome. Hum Mol Genet (2009) 18(R2):R195–201. doi: 10.1093/hmg/ddp409
68. Vermeulen M, Eberl HC, Matarese F, Marks H, Denissov S, Butter F, et al. Quantitative Interaction Proteomics and Genome-Wide Profiling of Epigenetic Histone Marks and Their Readers. Cell (2010) 142(6):967–80. doi: 10.1016/j.cell.2010.08.020
69. Maiques-Diaz A, Somervaille TC. LSD1: Biologic Roles and Therapeutic Targeting. Epigenomics (2016) 8(8):1103–16. doi: 10.2217/epi-2016-0009
70. Shi Y, Whetstine JR. Dynamic Regulation of Histone Lysine Methylation by Demethylases. Mol Cell (2007) 25(1):1–14. doi: 10.1016/j.molcel.2006.12.010
71. Metzger E, Wissmann M, Yin N, Müller JM, Schneider R, Peters AH, et al. LSD1 Demethylates Repressive Histone Marks to Promote Androgen-Receptor-Dependent Transcription. Nature (2005) 437(7057):436–9. doi: 10.1038/nature04020
72. Labbé RM, Holowatyj A, Yang ZQ. Histone Lysine Demethylase (KDM) Subfamily 4: Structures, Functions and Therapeutic Potential. Am J Transl Res (2013) 6(1):1–15.
73. Dimitrova E, Turberfield AH, Klose RJ. Histone Demethylases in Chromatin Biology and Beyond. EMBO Rep (2015) 16(12):1620–39. doi: 10.15252/embr.201541113
74. Kang JY, Kim JY, Kim KB, Park JW, Cho H, Hahm JY, et al. KDM2B Is a Histone H3K79 Demethylase and Induces Transcriptional Repression via Sirtuin-1-Mediated Chromatin Silencing. FASEB J (2018) 32(10):5737–50. doi: 10.1096/fj.201800242R
75. Murn J, Shi Y. The Winding Path of Protein Methylation Research: Milestones and New Frontiers. Nat Rev Mol Cell Biol (2017) 18(8):517–27. doi: 10.1038/nrm.2017.35
76. Salzberg AC, Harris-Becker A, Popova EY, Keasey N, Loughran TP, Claxton DF, et al. Genome-Wide Mapping of Histone H3k9me2 in Acute Myeloid Leukemia Reveals Large Chromosomal Domains Associated With Massive Gene Silencing and Sites of Genome Instability. PloS One (2017) 12(3):e0173723. doi: 10.1371/journal.pone.0173723
77. Chae YC, Kim JY, Park JW, Kim KB, Oh H, Lee KH, et al. FOXO1 Degradation via G9a-Mediated Methylation Promotes Cell Proliferation in Colon Cancer. Nucleic Acids Res (2019) 47(4):1692–705. doi: 10.1093/nar/gky1230
78. Lee CH, Yu JR, Granat J, Saldaña-Meyer R, Andrade J, LeRoy G, et al. Automethylation of PRC2 Promotes H3K27 Methylation and is Impaired in H3K27M Pediatric Glioma. Genes Dev (2019) 33(19-20):1428–40. doi: 10.1101/gad.328773.119
79. Żylicz JJ, Heard E. Molecular Mechanisms of Facultative Heterochromatin Formation: An X-Chromosome Perspective. Annu Rev Biochem (2020) 89:255–82. doi: 10.1146/annurev-biochem-062917-012655
80. Zhao Y, Hu Z, Li J, Hu T. EZH2 Exacerbates Breast Cancer by Methylating and Activating STAT3 Directly. J Cancer (2021) 12(17):5220–30. doi: 10.7150/jca.50675
81. Park SH, Fong KW, Mong E, Martin MC, Schiltz GE, Yu J. Going Beyond Polycomb: EZH2 Functions in Prostate Cancer. Oncogene (2021) 40(39):5788–98. doi: 10.1038/s41388-021-01982-4
82. Li C, Wang Y, Gong Y, Zhang T, Huang J, Tan Z, et al. Finding an Easy Way to Harmonize: A Review of Advances in Clinical Research and Combination Strategies of EZH2 Inhibitors. Clin Epigenet (2021) 13(1):62. doi: 10.1186/s13148-021-01045-1
83. Gulati N, Béguelin W, Giulino-Roth L. Enhancer of Zeste Homolog 2 (EZH2) Inhibitors. Leuk Lymphoma (2018) 59(7):1574–85. doi: 10.1080/10428194.2018.1430795
84. Chen Y, Liu X, Li Y, Quan C, Zheng L, Huang K. Lung Cancer Therapy Targeting Histone Methylation: Opportunities and Challenges. Comput Struct Biotechnol J (2018) 16:211–23. doi: 10.1016/j.csbj.2018.06.001
85. Gsell C, Richly H, Coin F, Naegeli H. A Chromatin Scaffold for DNA Damage Recognition: How Histone Methyltransferases Prime Nucleosomes for Repair of Ultraviolet Light-Induced Lesions. Nucleic Acids Res (2020) 48(4):1652–68. doi: 10.1093/nar/gkz1229
86. Nguyen AT, Zhang Y. The Diverse Functions of Dot1 and H3K79 Methylation. Genes Dev (2011) 25(13):1345–58. doi: 10.1101/gad.2057811
87. Sarno F, Nebbioso A, Altucci L. DOT1L: A Key Target in Normal Chromatin Remodelling and in Mixed-Lineage Leukaemia Treatment. Epigenetics (2020) 15(5):439–53. doi: 10.1080/15592294.2019.1699991
88. Raj U, Kumar H, Gupta S, Varadwaj PK. Novel DOT1L Receptornatural Inhibitors Involved in Mixed Lineage Leukemia: A Virtual Screening, Molecular Docking and Dynamics Simulation Study. Asian Pac J Cancer Prev (2015) 16(9):3817–25. doi: 10.7314/apjcp.2015.16.9.3817
89. Dominici C, Sgarioto N, Yu Z, Sesma-Sanz L, Masson JY, Richard S, et al. Synergistic Effects of Type I PRMT and PARP Inhibitors Against Non-Small Cell Lung Cancer Cells. Clin Epigenet (2021) 13(1):54. doi: 10.1186/s13148-021-01037-1
90. Drew AE, Moradei O, Jacques SL, Rioux N, Boriack-Sjodin AP, Allain C, et al. Identification of a CARM1 Inhibitor With Potent In Vitro and In Vivo Activity in Preclinical Models of Multiple Myeloma. Sci Rep (2017) 7(1):17993. doi: 10.1038/s41598-017-18446-z
91. Nakayama K, Szewczyk MM, Dela Sena C, Wu H, Dong A, Zeng H, et al. TP-064, a Potent and Selective Small Molecule Inhibitor of PRMT4 for Multiple Myeloma. Oncotarget (2018) 9(26):18480–93. doi: 10.18632/oncotarget.24883
92. Schmidt DM, McCafferty DG. Trans-2-Phenylcyclopropylamine Is a Mechanism-Based Inactivator of the Histone Demethylase LSD1. Biochemistry (2007) 46(14):4408–16. doi: 10.1021/bi0618621
93. Finley A, Copeland RA. Small Molecule Control of Chromatin Remodeling. Chem Biol (2014) 21(9):1196–210. doi: 10.1016/j.chembiol.2014.07.024
94. Yoo J, Jeon YH, Cho HY, Lee SW, Kim GW, Lee DH, et al. Advances in Histone Demethylase KDM3A as a Cancer Therapeutic Target. Cancers (Basel) (2020) 12(5):1098. doi: 10.3390/cancers12051098
95. Schnetz MP, Bartels CF, Shastri K, Balasubramanian D, Zentner GE, Balaji R, et al. Genomic Distribution of CHD7 on Chromatin Tracks H3K4 Methylation Patterns. Genome Res (2009) 19(4):590–601. doi: 10.1101/gr.086983.108
96. Weaver TM, Morrison EA, Musselman CA. Reading More Than Histones: The Prevalence of Nucleic Acid Binding Among Reader Domains. Molecules (2018) 23(10):2614. doi: 10.3390/molecules23102614
97. Ali M, Daze KD, Strongin DE, Rothbart SB, Rincon-Arano H, Allen HF, et al. Molecular Insights Into Inhibition of the Methylated Histone-Plant Homeodomain Complexes by Calixarenes. J Biol Chem (2015) 290(38):22919–30. doi: 10.1074/jbc.M115.669333
98. Ren C, Morohashi K, Plotnikov AN, Jakoncic J, Smith SG, Li J, et al. Small-Molecule Modulators of Methyl-Lysine Binding for the CBX7 Chromodomain. Chem Biol (2015) 22(2):161–8. doi: 10.1016/j.chembiol.2014.11.021
99. Wang S, Denton KE, Hobbs KF, Weaver T, McFarlane JMB, Connelly KE, et al. Optimization of Ligands Using Focused DNA-Encoded Libraries to Develop a Selective, Cell-Permeable CBX8 Chromodomain Inhibitor. ACS Chem Biol (2020) 15(1):112–31. doi: 10.1021/acschembio.9b00654
100. Stuckey JI, Dickson BM, Cheng N, Liu Y, Norris JL, Cholensky SH, et al. A Cellular Chemical Probe Targeting the Chromodomains of Polycomb Repressive Complex 1. Nat Chem Biol (2016) 12(3):180–7. doi: 10.1038/nchembio.2007
101. Miller TC, Rutherford TJ, Birchall K, Chugh J, Fiedler M, Bienz M. Competitive Binding of a Benzimidazole to the Histone-Binding Pocket of the Pygo PHD Finger. ACS Chem Biol (2014) 9(12):2864–74. doi: 10.1021/cb500585s
102. Milosevich N, Hof F. Chemical Inhibitors of Epigenetic Methyllysine Reader Proteins. Biochemistry (2016) 55(11):1570–83. doi: 10.1021/acs.biochem.5b01073
103. Yang XJ, Seto E. Hats and Hdacs: From Structure, Function and Regulation to Novel Strategies for Therapy and Prevention. Oncogene (2007) 26(37):5310–8. doi: 10.1038/sj.onc.1210599
104. Ikebe J, Sakuraba S, Kono H. H3 Histone Tail Conformation Within the Nucleosome and the Impact of K14 Acetylation Studied Using Enhanced Sampling Simulation. PloS Comput Biol (2016) 12(3):e1004788. doi: 10.1371/journal.pcbi.1004788
105. Su J, Wang F, Cai Y, Jin J. The Functional Analysis of Histone Acetyltransferase MOF in Tumorigenesis. Int J Mol Sci (2016) 17(1):99. doi: 10.3390/ijms17010099
106. Gregoretti IV, Lee YM, Goodson HV. Molecular Evolution of the Histone Deacetylase Family: Functional Implications of Phylogenetic Analysis. J Mol Biol (2004) 338(1):17–31. doi: 10.1016/j.jmb.2004.02.006
107. Whittle JR, Powell MJ, Popov VM, Shirley LA, Wang C, Pestell RG. Sirtuins, Nuclear Hormone Receptor Acetylation and Transcriptional Regulation. Trends Endocrinol Metab (2007) 18(9):356–64. doi: 10.1016/j.tem.2007.07.007
108. Yao Z, Chen Y, Cao W, Shyh-Chang N. Chromatin-Modifying Drugs and Metabolites in Cell Fate Control. Cell Prolif (2020) 53(11):e12898. doi: 10.1111/cpr.12898
109. Richters A, Koehler AN. Epigenetic Modulation Using Small Molecules - Targeting Histone Acetyltransferases in Disease. Curr Med Chem (2017) 24(37):4121–50. doi: 10.2174/0929867324666170223153115
110. Gajer JM, Furdas SD, Gründer A, Gothwal M, Heinicke U, Keller K, et al. Histone Acetyltransferase Inhibitors Block Neuroblastoma Cell Growth In Vivo. Oncogenesis (2015) 4(2):e137. doi: 10.1038/oncsis.2014.51
111. Li Y, Wang F, Chen X, Wang J, Zhao Y, Li Y, et al. Zinc-Dependent Deacetylase (HDAC) Inhibitors With Different Zinc Binding Groups. Curr Top Med Chem (2019) 19(3):223–41. doi: 10.2174/1568026619666190122144949
112. Banerjee NS, Moore DW, Broker TR, Chow LT. Vorinostat, a Pan-HDAC Inhibitor, Abrogates Productive HPV-18 DNA Amplification. Proc Natl Acad Sci USA (2018) 115(47):E11138–47. doi: 10.1073/pnas.1801156115
113. McDermott J, Jimeno A. Belinostat for the Treatment of Peripheral T-Cell Lymphomas. Drugs Today (Barc) (2014) 50(5):337–45. doi: 10.1358/dot.2014.50.5.2138703
114. Eleutherakis-Papaiakovou E, Kanellias N, Kastritis E, Gavriatopoulou M, Terpos E, Dimopoulos MA. Efficacy of Panobinostat for the Treatment of Multiple Myeloma. J Oncol (2020) 2020:7131802. doi: 10.1155/2020/7131802
115. Morschhauser F, Flinn IW, Advani R, Sehn LH, Diefenbach C, Kolibaba K, et al. Polatuzumab Vedotin or Pinatuzumab Vedotin Plus Rituximab in Patients With Relapsed or Refractory non-Hodgkin Lymphoma: Final Results From a Phase 2 Randomised Study (ROMULUS). Lancet Haematol (2019) 6(5):e254–e65. doi: 10.1016/s2352-3026(19)30026-2
116. Shimony S, Horowitz N, Ribakovsky E, Rozovski U, Avigdor A, Zloto K, et al. Romidepsin Treatment for Relapsed or Refractory Peripheral and Cutaneous T-Cell Lymphoma: Real-Life Data From a National Multicenter Observational Study. Hematol Oncol (2019) 37(5):569–77. doi: 10.1002/hon.2691
117. Xu Y, Zhang P, Liu Y. Chidamide Tablets: HDAC Inhibition to Treat Lymphoma. Drugs Today (Barc) (2017) 53(3):167–76. doi: 10.1358/dot.2017.53.3.2595452
118. Soukupova J, Bertran E, Peñuelas-Haro I, Urdiroz-Urricelqui U, Borgman M, Kohlhof H, et al. Resminostat Induces Changes in Epithelial Plasticity of Hepatocellular Carcinoma Cells and Sensitizes Them to Sorafenib-Induced Apoptosis. Oncotarget (2017) 8(66):110367–79. doi: 10.18632/oncotarget.22775
119. Chifotides HT, Bose P, Verstovsek S. Givinostat: An Emerging Treatment for Polycythemia Vera. Expert Opin Investig Drugs (2020) 29(6):525–36. doi: 10.1080/13543784.2020.1761323
120. Child F, Ortiz-Romero PL, Alvarez R, Bagot M, Stadler R, Weichenthal M, et al. Phase II Multicentre Trial of Oral Quisinostat, a Histone Deacetylase Inhibitor, in Patients With Previously Treated Stage IB-IVA Mycosis Fungoides/Sézary Syndrome. Br J Dermatol (2016) 175(1):80–8. doi: 10.1111/bjd.14427
121. Trapani D, Esposito A, Criscitiello C, Mazzarella L, Locatelli M, Minchella I, et al. Entinostat for the Treatment of Breast Cancer. Expert Opin Investig Drugs (2017) 26(8):965–71. doi: 10.1080/13543784.2017.1353077
122. Batlevi CL, Crump M, Andreadis C, Rizzieri D, Assouline SE, Fox S, et al. A Phase 2 Study of Mocetinostat, a Histone Deacetylase Inhibitor, in Relapsed or Refractory Lymphoma. Br J Haematol (2017) 178(3):434–41. doi: 10.1111/bjh.14698
123. Wang J, Kim TH, Ahn MY, Lee J, Jung JH, Choi WS, et al. Sirtinol, a Class III HDAC Inhibitor, Induces Apoptotic and Autophagic Cell Death in MCF-7 Human Breast Cancer Cells. Int J Oncol (2012) 41(3):1101–9. doi: 10.3892/ijo.2012.1534
124. Wang YM, Gu ML, Meng FS, Jiao WR, Zhou XX, Yao HP, et al. Histone Acetyltransferase P300/CBP Inhibitor C646 Blocks the Survival and Invasion Pathways of Gastric Cancer Cell Lines. Int J Oncol (2017) 51(6):1860–8. doi: 10.3892/ijo.2017.4176
125. Wang T, Cui H, Ma N, Jiang Y. Nicotinamide-Mediated Inhibition of SIRT1 Deacetylase Is Associated With the Viability of Cancer Cells Exposed to Antitumor Agents and Apoptosis. Oncol Lett (2013) 6(2):600–4. doi: 10.1038/s41467-019-10324-8
126. Shvedunova M, Akhtar A. Modulation of Cellular Processes by Histone and Non-Histone Protein Acetylation. Nat Rev Mol Cell Biol (2022) 18:29168. doi: 10.1038/s41580-021-00441-y
127. Ferri E, Petosa C, McKenna CE. Bromodomains: Structure, Function and Pharmacology of Inhibition. Biochem Pharmacol (2016) 106:1–18. doi: 10.1016/j.bcp.2015.12.005
128. Lu P, Shen Y, Yang H, Wang Y, Jiang Z, Yang X, et al. BET Inhibitors RVX-208 and PFI-1 Reactivate HIV-1 From Latency. Sci Rep (2017) 7(1):16646. doi: 10.1038/s41598-017-16816-1
129. Leal AS, Williams CR, Royce DB, Pioli PA, Sporn MB, Liby KT. Bromodomain Inhibitors, JQ1 and I-BET 762, as Potential Therapies for Pancreatic Cancer. Cancer Lett (2017) 394:76–87. doi: 10.1016/j.canlet.2017.02.021
130. Zhu X, Park S, Lee WK, Cheng SY. Potentiated Anti-Tumor Effects of Beti by Meki in Anaplastic Thyroid Cancer. Endocr Relat Cancer (2019) 26(9):739–50. doi: 10.1530/erc-19-0107
131. Gerlach D, Tontsch-Grunt U, Baum A, Popow J, Scharn D, Hofmann MH, et al. The Novel BET Bromodomain Inhibitor BI 894999 Represses Super-Enhancer-Associated Transcription and Synergizes With CDK9 Inhibition in AML. Oncogene (2018) 37(20):2687–701. doi: 10.1038/s41388-018-0150-2
132. Theodoulou NH, Bamborough P, Bannister AJ, Becher I, Bit RA, Che KH, et al. Discovery of I-BRD9, a Selective Cell Active Chemical Probe for Bromodomain Containing Protein 9 Inhibition. J Med Chem (2016) 59(4):1425–39. doi: 10.1021/acs.jmedchem.5b00256
133. Martin LJ, Koegl M, Bader G, Cockcroft XL, Fedorov O, Fiegen D, et al. Structure-Based Design of an In Vivo Active Selective Brd9 Inhibitor. J Med Chem (2016) 59(10):4462–75. doi: 10.1021/acs.jmedchem.5b01865
134. Shahabipour F, Caraglia M, Majeed M, Derosa G, Maffioli P, Sahebkar A. Naturally Occurring Anti-Cancer Agents Targeting EZH2. Cancer Lett (2017) 400:325–35. doi: 10.1016/j.canlet.2017.03.020
135. Chen J, Lin X, Park KJ, Lee KR, Park HJ. Identification of Protoberberine Alkaloids as Novel Histone Methyltransferase G9a Inhibitors by Structure-Based Virtual Screening. J Comput Aided Mol Des (2018) 32(9):917–28. doi: 10.1007/s10822-018-0156-4
136. Zhang L, Miao XJ, Wang X, Pan HH, Li P, Ren H, et al. Antiproliferation of Berberine Is Mediated by Epigenetic Modification of Constitutive Androstane Receptor (CAR) Metabolic Pathway in Hepatoma Cells. Sci Rep (2016) 6:28116. doi: 10.1038/srep28116
137. Qing Y, Hu H, Liu Y, Feng T, Meng W, Jiang L, et al. Berberine Induces Apoptosis in Human Multiple Myeloma Cell Line U266 Through Hypomethylation of P53 Promoter. Cell Biol Int (2014) 38(5):563–70. doi: 10.1002/cbin.10206
138. Wang Z, Liu Y, Xue Y, Hu H, Ye J, Li X, et al. Berberine Acts as a Putative Epigenetic Modulator by Affecting the Histone Code. Toxicol In Vitro (2016) 36:10–7. doi: 10.1016/j.tiv.2016.06.004
139. Kalaiarasi A, Anusha C, Sankar R, Rajasekaran S, John Marshal J, Muthusamy K, et al. Plant Isoquinoline Alkaloid Berberine Exhibits Chromatin Remodeling by Modulation of Histone Deacetylase to Induce Growth Arrest and Apoptosis in the A549 Cell Line. J Agric Food Chem (2016) 64(50):9542–50. doi: 10.1021/acs.jafc.6b04453
140. Liu D, Meng X, Wu D, Qiu Z, Luo H. A Natural Isoquinoline Alkaloid With Antitumor Activity: Studies of the Biological Activities of Berberine. Front Pharmacol (2019) 10:9. doi: 10.3389/fphar.2019.00009
141. Shi ZY, Zeng JZ, Wong AST. Chemical Structures and Pharmacological Profiles of Ginseng Saponins. Molecules (2019) 24(13):2443. doi: 10.3390/molecules24132443
142. Hu HY, Li KP, Wang XJ, Liu Y, Lu ZG, Dong RH, et al. Set9, NF-κb, and MicroRNA-21 Mediate Berberine-Induced Apoptosis of Human Multiple Myeloma Cells. Acta Pharmacol Sin (2013) 34(1):157–66. doi: 10.1038/aps.2012.161
143. Lee H, Lee S, Jeong D, Kim SJ. Ginsenoside Rh2 Epigenetically Regulates Cell-Mediated Immune Pathway to Inhibit Proliferation of MCF-7 Breast Cancer Cells. J Ginseng Res (2018) 42(4):455–62. doi: 10.1016/j.jgr.2017.05.003
144. Kang KA, Kim HS, Kim DH, Hyun JW. The Role of a Ginseng Saponin Metabolite as a DNA Methyltransferase Inhibitor in Colorectal Cancer Cells. Int J Oncol (2013) 43(1):228–36. doi: 10.3892/ijo.2013.1931
145. Zhao L, Shou H, Chen L, Gao W, Fang C, Zhang P. Effects of Ginsenoside Rg3 on Epigenetic Modification in Ovarian Cancer Cells. Oncol Rep (2019) 41(6):3209–18. doi: 10.3892/or.2019.7115
146. Chen Y, Shang H, Zhang S, Zhang X. Ginsenoside Rh2 Inhibits Proliferation and Migration of Medulloblastoma Daoy by Down-Regulation of MicroRNA-31. J Cell Biochem (2018) 119(8):6527–34. doi: 10.1002/jcb.26716
147. Jin Y, Huynh DTN, Nguyen TLL, Jeon H, Heo KS. Therapeutic Effects of Ginsenosides on Breast Cancer Growth and Metastasis. Arch Pharm Res (2020) 43(8):773–87. doi: 10.1007/s12272-020-01265-8
148. Li J, Lu J, Ye Z, Han X, Zheng X, Hou H, et al. 20(s)-Rg3 Blocked Epithelial-Mesenchymal Transition Through DNMT3A/Mir-145/FSCN1 in Ovarian Cancer. Oncotarget (2017) 8(32):53375–86. doi: 10.18632/oncotarget.18482
149. Zheng X, Zhou Y, Chen W, Chen L, Lu J, He F, et al. Ginsenoside 20(s)-Rg3 Prevents PKM2-Targeting Mir-324-5p From H19 Sponging to Antagonize the Warburg Effect in Ovarian Cancer Cells. Cell Physiol Biochem (2018) 51(3):1340–53. doi: 10.1159/000495552
150. Liu TJ, Hu S, Qiu ZD, Liu D. Anti-Tumor Mechanisms Associated With Regulation of Non-Coding RNA by Active Ingredients of Chinese Medicine: A Review. Front Oncol (2020) 10:634936. doi: 10.3389/fonc.2020.634936
151. Jeong D, Ham J, Park S, Kim HW, Kim H, Ji HW, et al. Ginsenoside Rh2 Suppresses Breast Cancer Cell Proliferation by Epigenetically Regulating the Long Noncoding RNA C3orf67-AS1. Am J Chin Med (2019) 47(7):1643–58. doi: 10.1142/s0192415x19500848
152. Christensen LP. Ginsenosides Chemistry, Biosynthesis, Analysis, and Potential Health Effects. Adv Food Nutr Res (2009) 55:1–99. doi: 10.1016/s1043-4526(08)00401-4
153. Wan Q, Ma X, Zhang ZJ, Sun T, Xia F, Zhao G, et al. Ginsenoside Reduces Cognitive Impairment During Chronic Cerebral Hypoperfusion Through Brain-Derived Neurotrophic Factor Regulated by Epigenetic Modulation. Mol Neurobiol (2017) 54(4):2889–900. doi: 10.1007/s12035-016-9868-4
154. Ham J, Lee S, Lee H, Jeong D, Park S, Kim SJ. Genome-Wide Methylation Analysis Identifies NOX4 and KDM5A as Key Regulators in Inhibiting Breast Cancer Cell Proliferation by Ginsenoside Rg3. Am J Chin Med (2018) 46(6):1333–55. doi: 10.1142/s0192415x18500702
155. Liu ZH, Li J, Xia J, Jiang R, Zuo GW, Li XP, et al. Ginsenoside 20(s)-Rh2 as Potent Natural Histone Deacetylase Inhibitors Suppressing the Growth of Human Leukemia Cells. Chem Biol Interact (2015) 242:227–34. doi: 10.1016/j.cbi.2015.10.014
156. Yang J, Yuan D, Xing T, Su H, Zhang S, Wen J, et al. Ginsenoside Rh2 Inhibiting HCT116 Colon Cancer Cell Proliferation Through Blocking PDZ-Binding Kinase/T-LAK Cell-Originated Protein Kinase. J Ginseng Res (2016) 40(4):400–8. doi: 10.1016/j.jgr.2016.03.007
157. Ashrafizadeh M, Ahmadi Z, Mohammadinejad R, Farkhondeh T, Samarghandian S. MicroRNAs Mediate the Anti-Tumor and Protective Effects of Ginsenosides. Nutr Cancer (2020) 72(8):1264–75. doi: 10.1080/01635581.2019.1675722
158. Chen W, Chu S, Li H, Qiu Y. MicroRNA-146a-5p Enhances Ginsenoside Rh2-Induced Anti-Proliferation and the Apoptosis of the Human Liver Cancer Cell Line Hepg2. Oncol Lett (2018) 16(4):5367–74. doi: 10.3892/ol.2018.9235
159. Wang X, Wang Y. Ginsenoside Rh2 Mitigates Pediatric Leukemia Through Suppression of Bcl-2 in Leukemia Cells. Cell Physiol Biochem (2015) 37(2):641–50. doi: 10.1159/000430383
160. Chen Y, Zhang Y, Song W, Zhang Y, Dong X, Tan M. Ginsenoside Rh2 Inhibits Migration of Lung Cancer Cells Under Hypoxia via Mir-491. Anticancer Agents Med Chem (2019) 19(13):1633–41. doi: 10.2174/1871520619666190704165205
161. Wu N, Wu GC, Hu R, Li M, Feng H. Ginsenoside Rh2 Inhibits Glioma Cell Proliferation by Targeting MicroRNA-128. Acta Pharmacol Sin (2011) 32(3):345–53. doi: 10.1038/aps.2010.220
162. Gao Q, Zheng J. Ginsenoside Rh2 Inhibits Prostate Cancer Cell Growth Through Suppression of MicroRNA-4295 That Activates CDKN1A. Cell Prolif (2018) 51(3):e12438. doi: 10.1111/cpr.12438
163. Li M, Zhang D, Cheng J, Liang J, Yu F. Ginsenoside Rh2 Inhibits Proliferation But Promotes Apoptosis and Autophagy by Down-Regulating MicroRNA-638 in Human Retinoblastoma Cells. Exp Mol Pathol (2019) 108:17–23. doi: 10.1016/j.yexmp.2019.03.004
164. Zhou Y, Zheng X, Lu J, Chen W, Li X, Zhao L. Ginsenoside 20(s)-Rg3 Inhibits the Warburg Effect via Modulating DNMT3A/Mir-532-3p/HK2 Pathway in Ovarian Cancer Cells. Cell Physiol Biochem (2018) 45(6):2548–59. doi: 10.1159/000488273
165. Cheng Z, Xing D. Ginsenoside Rg3 Inhibits Growth and Epithelial-Mesenchymal Transition of Human Oral Squamous Carcinoma Cells by Down-Regulating Mir-221. Eur J Pharmacol (2019) 853:353–63. doi: 10.1016/j.ejphar.2019.03.040
166. Xiang Y, Guo Z, Zhu P, Chen J, Huang Y. Traditional Chinese Medicine as a Cancer Treatment: Modern Perspectives of Ancient But Advanced Science. Cancer Med (2019) 8(5):1958–75. doi: 10.1002/cam4.2108
167. Ahuja N, Sharma AR, Baylin SB. Epigenetic Therapeutics: A New Weapon in the War Against Cancer. Annu Rev Med (2016) 67:73–89. doi: 10.1146/annurev-med-111314-035900
Keywords: epigenetic regulatory enzymes, cancer therapeutics, inhibitors, small molecule, epidrug development
Citation: Jin Y, Liu T, Luo H, Liu Y and Liu D (2022) Targeting Epigenetic Regulatory Enzymes for Cancer Therapeutics: Novel Small-Molecule Epidrug Development. Front. Oncol. 12:848221. doi: 10.3389/fonc.2022.848221
Received: 10 January 2022; Accepted: 04 March 2022;
Published: 28 March 2022.
Edited by:
Zhe-Sheng Chen, St. John’s University, United StatesReviewed by:
Oronza Antonietta Botrugno, San Raffaele Scientific Institute (IRCCS), ItalyZhihui Liu, National Cancer Institute (NIH), United States
Copyright © 2022 Jin, Liu, Luo, Liu and Liu. This is an open-access article distributed under the terms of the Creative Commons Attribution License (CC BY). The use, distribution or reproduction in other forums is permitted, provided the original author(s) and the copyright owner(s) are credited and that the original publication in this journal is cited, in accordance with accepted academic practice. No use, distribution or reproduction is permitted which does not comply with these terms.
*Correspondence: Yangyang Liu, bHl5Y2N1Y21AMTYzLmNvbQ==; Da Liu, bGl1ZGFfMTk4NkAxNjMuY29t
†These authors have contributed equally to this work