- 1Department of Radiation Oncology, Melvin and Bren Simon Comprehensive Cancer Center, Indiana University School of Medicine, Indianapolis, IN, United States
- 2Department of Biological Sciences, National University of Medical Sciences (NUMS), Rawalpindi, Pakistan
Neutrophils, the most copious leukocytes in human blood, play a critical role in tumorigenesis, cancer progression, and immune suppression. Recently, neutrophils have attracted the attention of researchers, immunologists, and oncologists because of their potential role in orchestrating immune evasion in human diseases including cancer, which has led to a hot debate redefining the contribution of neutrophils in tumor progression and immunity. To make this debate fruitful, this review seeks to provide a recent update about the contribution of neutrophils in immune suppression and tumor progression. Here, we first described the molecular pathways through which neutrophils aid in cancer progression and orchestrate immune suppression/evasion. Later, we summarized the underlying molecular mechanisms of neutrophil-mediated therapy resistance and highlighted various approaches through which neutrophil antagonism may heighten the efficacy of the immune checkpoint blockade therapy. Finally, we have highlighted several unsolved questions and hope that answering these questions will provide a new avenue toward immunotherapy revolution.
1 Introduction
Neutrophils are the most profuse leukocytes, representing 50%–70% of all the circulating leukocytes in human, and are regarded as body’s first responders to injury, infections, and inflammation (1, 2). In response to infection-associated signals, neutrophils initiate multiple effector functions such as the production of neutrophil extracellular traps (NETs), generation of reactive oxygen species (ROS), and production of antibacterial peptides to eradicate pathogens (3, 4). The functional importance of neutrophils had been overlooked previously on the basis of their reported short life span; however, recent studies suggesting that they can survive in the circulation from 19 h to 5 days have ensured renewed attention toward the role of neutrophils under varying biological conditions (5). Since the last two decades, various immunotherapeutic agents have been approved as treatment for multiple human cancers, and most of them mainly focus on the targeting of major immunosuppressive molecules in both tumor and immune cells. Immune checkpoint blockade therapy has been extensively tested and approved as first-line treatment for various cancers (6, 7). Although the development of novel immune checkpoint inhibitors has emerged as a revolutionary milestone in defeating human tumors, and tuning immune system activity for promoting its antitumor activity and overcoming immune suppression, work from multiple groups has shown that immunotherapy treatments have largely failed in most of the patients with solid tumors (8–10). Undoubtedly, several combinatorial treatment approaches have improved these metrics. For instance, anti–programmed death-1 (anti-PD1) monoclonal antibody, pembrolizumab, combined with chemotherapy, has been proven effective in Non-small cell lung cancer (NSCLC) patients whereas treatment with another (anti-PD1) monoclonal antibody, nivolumab, combined with the monoclonal antibody ipilimumab, which enhances the T-cell response by targeting cytotoxic T lymphocyte–associated antigen 4 (CTLA-4), has been fruitful to treat cancer in advanced melanoma patients (11, 12). However, most of the cancer patients are still not getting satisfactory benefits from immune checkpoint blockade therapy due to either a low response rate or higher immune-related toxicities (13). Recently, it has been reported that nearly 44% of the US population of tumor patients is eligible for checkpoint blockade therapy and merely approximately 13% of the patients showed a positive response to it (14). This adverse outlook is associated with multidimensional tumor microenvironment (TME), which endlessly formulates unique resistance mechanisms, thereby leading to a limited response to immunotherapeutic agents (15). Neutrophils are emerging as central effector cells of the innate immune system and are associated with poor outcomes in many types of human cancers, except some specific tumor types (16, 17). Accumulating evidence suggests that neutrophils are key components of TME, drive tumor progression, and limit the efficacy of immunotherapy by establishing immunosuppressive TME (18–20). Furthermore, neutrophils also counteract immunotherapy efficacy by manipulating the adaptive immune system (21, 22). In recent years, boosting the antitumor ability of immune cells, particularly of neutrophils in the tumor niche, has become a major goal in devising new treatment options, owing to aggressive immunosuppressive TME. That is why we have focused on the relationship between neutrophils in tumor progression and immune suppression in this review. We begin with unmasking the molecular pathways by which neutrophils are polarized into the antitumor (N1) or protumor (N2) phenotype and support tumor progression/suppression. Later, we discuss the key concepts related to the critical role of neutrophils in immune suppression/evasion and therapy resistance and highlight the novel strategies for targeting immunosuppressive neutrophils.
2 Neutrophils in cancer progression
Since the last decade, the customary standpoint about neutrophils as a mere bystander in human tumors has been revolutionized and research on the diverse role of neutrophils in cancer progression was established significantly, which can be reflected by numerous recent review papers published in well-reputed journals (23, 24). Increased neutrophil abundance is frequently detected in both cancer patients and tumor-bearing mice (25, 26). A growing body of evidence also suggests that neutrophils function as early responders against inflammatory insult (27, 28). Recently, the neutrophil-to-lymphocyte ratio (NLR) has been used to predict a patient’s tumor or inflammatory status and immunotherapy response in multiple cancer types (29, 30). Neutrophils are capable to infiltrate into tumors and constitute a major portion of the TME (31). Furthermore, association between these tumor-associated neutrophils (TANs) and patient outcomes have also been demonstrated (32). Neutrophils act as a double-edged sword in human cancer, owing to their inimitable potential to either support or inhibit tumor progression. Strong evidence suggests that neutrophils act as a tumor promoter (33–36), while fewer studies have reported that neutrophils may also act as a tumor suppressor (37, 38). Moreover, both the preclinical and clinical trials have shown that TANs contribute in malignant transformation, angiogenesis, and antitumor immunity (39, 40). Based on their role in tumor progression, TANs can be divided into N1 and N2 types. The N1 TANs inhibit tumor growth and increase antitumor immune memory and tumor cell toxicity, while N2 TANs foster tumorigenesis, invasion, metastasis, and immune suppression (41). Tumor cell–driven TME factors often signal for TAN polarization into N1 and N2 types. For instance, transforming growth factor beta (TGF-β), the major immunosuppressive cytokine that is also correlated with poor prognosis in cancer patients, is released by tumor cells in the TME where it polarizes neutrophils to the N2 phenotype and suppresses N1-type neutrophils. On the other hand, interferon beta (IFN-β) in the TME suppresses the N2 neutrophil phenotype and stimulates N1 neutrophils (42, 43) (Figure 1). Changes in the expression of heat-shock proteins (HSPs) have been suggested as danger-associated molecular patterns (DAMPs) because these molecules are highly conserved and their intracellular expression is elevated in response to infection and oxidative stress (44). HSP72 as an endogenous DAMP activates neutrophils via TLR4 signaling (45). A previous study investigated the key role of TLR4 in the programming of N1/N2 neutrophils after stroke. Results showed that the absence of TLR4 increased the amount of N2 neutrophils in ischemic brain (46). Similarly, a recent study has shown that TLR4 regulates neutrophils dynamics in stroke (47). Together, the above findings suggest that HSPs as a danger signal may regulate neutrophil polarization. Here, we have reviewed the tumor- promoting role of neutrophils by focusing on N2-type TANs that exploit diverse mechanisms to promote tumor progression, such as secreting inflammatory cytokines and chemokines (48, 49), releasing NETs (50), and epithelial-to-mesenchymal transition (EMT) (51) (Table 1), which we have discussed in detail in the following subsections.
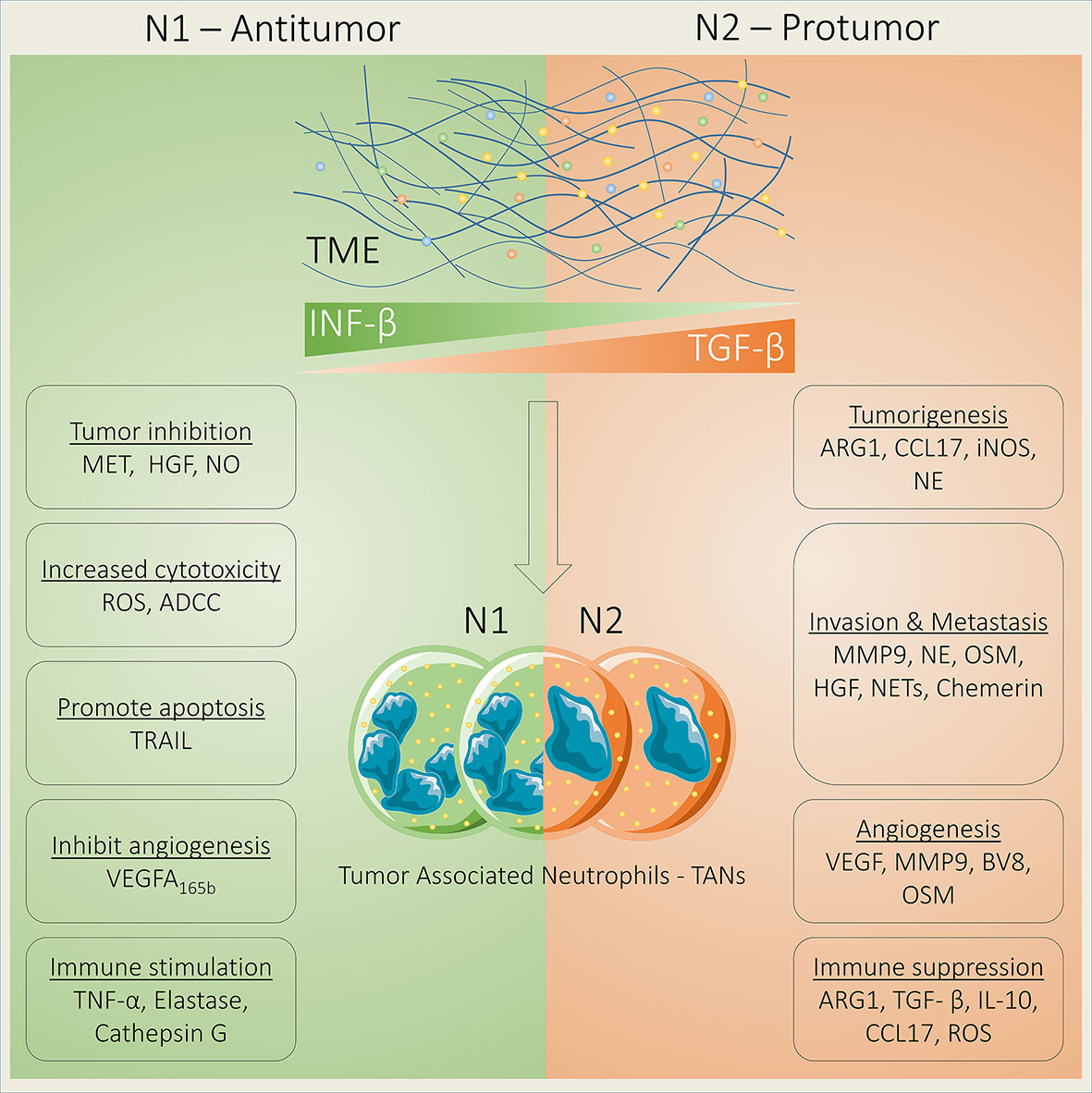
Figure 1 Neutrophils act as tumor suppressor or tumor promoter. Neutrophils act as either a tumor suppressor or promoter depending on their phenotypes that are regulated by transforming growth factor beta (TGF-β) and interferon beta (IFN-β). N1 TANs suppress tumor growth by increasing the expression of MET and HGF binding and NO production. In addition, N1 TANs increase cell toxicity via ADCC and ROS generation and apoptosis by releasing TRAIL; inhibit angiogenesis by releasing antiangiogenic VEGF-A165b; and induce immune stimulation by releasing TNF-α, elastase, and cathepsin G. In contrast, N2 TANs promote tumorigenesis through ARG1, CCL17, iNOS, and NE; foster invasion and metastasis via MMP9, NE, OSM, HGF, NETs, and chemerin; support angiogenesis by releasing VEGF, MMP9, BV8, and OSM; and induce immune suppression through ARG1, TGF-β, IL-10, CCL17, and ROS.
2.1 Inflammatory cytokines and chemokines
Tumor cells induce various inflammatory cytokines, including TNF-α, IL-1β, IL-6, IL-17/18, and IL-23, and growth factors (G-CSF, GM-CSF, and IL-3) to generate neutrophil production and confirm their survival (55–57, 62). Furthermore, various neutrophil-attracting chemokines (CXCL1, CXCL2, CXCL5, CXCL6, and CXCL8) promote the migration of neutrophils to the tumor site through CXCR1 and CXCR2 receptors (54, 63). More recent study has shown that the cancer cell-mediated secretion of CXCL5 drives mature protumorigenic neutrophil infiltration in non-small cell lung cancer and impairs the differentiation of antitumor CD8+ T cells (53). CXCL8 is highly expressed in a wide range of tumor types, and various studies have suggested that the CXCL8 serum level in tumor patients serves as an independent prognostic marker (64, 65). Moreover, it supports tumor progression and promotes resistance to immune checkpoint blockade therapy (65, 66). The function of CXCL8 mainly depends on its binding with two receptors, namely, CXCR1 and CXCR2 (67). Notably, these two receptors are highly expressed on neutrophils (68, 69). The inhibition of CXCR1/2 limits neutrophil infiltration and results in the decreased growth of multiple tumors, including lung adenocarcinoma (70), colorectal cancer (71), and pancreatic ductal adenocarcinoma (72). Furthermore, inflammatory cytokines foster the production of growth factor G-CSF in the TME and further stimulate new neutrophil production in bone marrow (73, 74). In turn, neutrophils stimulate tumor-associated inflammation, thereby leading to tumor progression. In this line, more recently, Shan et al. have investigated interaction among neutrophils, CD4+ T cells, and tumor cells in the gastric TME. Their findings show that CXCL6/CXCL8-CXCR1 chemotaxis arbitrates neutrophil recruitment and accumulation into the gastric TME, an event which upregulates the expression of CD54 and B7-H2 through the activation of the Extracellular signal-regulated kinase (ERK), Nuclear factor kappa B (NF-κB) pathway by tumor-derived TNF-α. Then, neutrophils induce the polarization of the IL-17A-generating Th subsets in a B7-H2-dependent manner, where polarized IL-17A-generating Th cells can be able to wield protumorigenic roles through IL-17A, thereby leading to gastric tumor onset and progression (75). In pancreatic cancer, the expression of neutrophil chemoattractants in tumor cells is increased, following gemcitabine treatment. Subsequently, Gas6-expressing neutrophils infiltrate and accumulate in liver in a CXCR-2-dependent manner. Then, neutrophil-derived Gas6 induces AXL Receptor Tyrosine Kinase (AXL) on metastatic tumor cells and finally contributes to metastatic growth in liver. Furthermore, the pharmacological targeting of the Gas6/AXL axis through warfarin in combination with gemcitabine treatment suppresses metastatic relapse (76). Another latest study has revealed that IL-17-mediated neutrophil infiltration contributes to gastric tumor angiogenesis and maintains tumor persistence (77). These novel findings highlight the crucial role of cytokines and chemokines in neutrophil infiltration to trigger tumor onset and progression and the impact of their blocking to improve efficacy of immunotherapy. Moreover, a deep knowledge about their function in neutrophil maturation and activation can further guide to establish more effective therapeutic strategies.
2.2 Neutrophil extracellular traps
Another important mechanism by which neutrophils promote tumor progression is NETs. NETs are web-like DNA structures and contain the abundance of antimicrobial proteins that are released by neutrophils through a unique program cell death process termed NETosis induced by different pro-inflammatory mediators and microbial stimuli (78, 79). The major NETosis inducers in tumor can vary between different tumor models, but HMGB1 (80) and CXCR1/2 (81, 82) agonists have been found to induce tumor-associated NETosis. Recently, the role of NETs is conspicuously becoming critical in promoting tumor progression (83, 84). Previously, various studies have identified the abundance of NETs in mouse tumor models (59, 85, 86). Recently, the abundance of NETs has been detected and quantified in many types of solid tumors (87) and can be used as tumor biomarker candidates for clinical diagnosis (88, 89). NETs stimulate tumor growth (90) and serve as a scaffold for the inert adhesion or chemotaxis of the cancer cells in different tumor types such as breast, liver, and colon cancer (91, 92). NETs are stimulated by pro-inflammatory cytokines or chemokines during infection (93, 94). And promote tumor progression through diverse mechanisms such as by awakening dormant tumor cells in lungs (95), inducing tumor cell chemotaxis to the liver (84), modulating cancer cell bioenergetics (96), and building a protective coat around cancer for protecting them against drug cytotoxicity (55).
Tumor metastasis is the major reason of huge number of cancer-associated deaths (97). In recent years, emerging studies have shown that NETs foster tumor metastasis in a wide range of cancer types (98–100), whereas NET depletion markedly decreases tumor metastasis (101). In line with these reports, in a more recent study published in Cancer Cell, Xiao et al. have identified a novel mechanism by indicating that NETs promote lung metastasis by degrading TSP-1 protein. In addition, this novel research has revealed a new pathway by which CTSC expression promotes metastatic potential through activating neutrophil membrane–bound PR3 to assist in IL-1β processing and the activation of NF-κB, thereby leading to the upregulation of IL-6 and CCL3 to recruit neutrophils in the metastatic lung niche, while the targeting of CTSC by AZD7986 avoids mouse lung metastasis (92). IL-17 has also been found to promote pancreatic ductal adenocarcinoma progression and immune checkpoint blockade therapy resistance by triggering NET formation, while IL-17 blockade enhances immune checkpoint blockade sensitivity (55). Similarly, another recent study has shown that NETs trap HCC cells and fuel their metastatic potential by activating the TLR4/9-COX2 axis, whereas the inhibition of COX2 by using HCQ and TLR4/9 by Dnase I effectively suppresses HCC metastasis. This combinatorial approach not only efficiently depletes NETs but also abrogates the metastatic ability of the trapped liver cancer cells via undissolved NETs (91).
2.3 Epithelial-to-mesenchymal transition
EMT is a phenotypic switching event where epithelial cells lose their characteristics and undergo mesenchymal transition. The EMT event is orchestrated by the activation of various transcription factors (TFs) such as TWIST1/2, ZEB1/2, and SNAIL1/2 (102), growth factors (TGF-β and Hepatocyte growth factor (HGF), and inflammatory cytokines (TNF-α, CXCL12, IL-6, and IL-8), which, in turn, drive cancer cell invasion (103, 104). Interestingly, same EMT-TFs regulate the expression of various secreted mediators such as growth factors (GM-CSF), cytokine (TNF-α), and chemokines (CXCL6/8/11, CCL2, and GRO) in cancer cells (105–107). In addition, numerous mediators from EMT-induced tumors produce monocytes (CCL2) and neutrophil chemoattractants (GM-CSF, CXCL8, and GRO); the production of these mediators following the induction of EMT regulate the tumor niche immune landscape (108). It has also been reported that neutrophils interact with tumor cells by using the same molecular machinery that induces EMT such as TGF-β, IL-8, CCl2, TNF-α, and IL-17a (61, 100). Consistently, TGF-β pathways stimulate both EMT and the neutrophil pro-tumor phenotype (N2), which is likewise associated with therapy resistance (101). Emerging evidence suggests that neutrophils stimulate tumor progression through inducing EMT (60, 109, 110). It is well documented that both the neutrophils and EMT play a critical role in tumor progression and immunotherapy resistance (105, 111). However, the underlying molecular mechanism of neutrophil-mediated EMT induction remains poorly understood. Here, we provide a recent update about how neutrophils foster tumor through inducing EMT. In gastric cancer stroma, neutrophils promote tumor metastasis by inducing EMT through secreting CXCL5 and IL-17a, whereas antibody-mediated IL-17a blockade suppresses EMT in cancer cells cocultured with TANs (112, 113). In breast tumor, neutrophils induce EMT by producing TIMP-1. TANs-MCF-7 interaction establishes a feedback-loop between MCF-7 cells and TANs with the induction of EMT in cancer cells due to the increased expression of TIMP-1 by CD90. Blocking CD90 decreases tumor metastasis in mice (114). Authors have identified the novel mechanism of the neutrophil-mediated induction of EMT in breast cancer cells by regulating TIMP-1. However, the exact molecular mechanism of how CD90 regulates TIMP-1 expression in neutrophils remains unexplored. In addition, this novel study also provides the foundation to further investigate the therapeutic effect of CD90 blockade in different tumor types.
3 Neutrophils as immune suppressor
Many tumor therapeutic approaches, including radiotherapy, chemotherapy, and immunotherapy, have been widely used to control the level of neutrophil infiltrations or modulate their accumulation and function (25). However, neutrophils are linked with a poor clinical response to these targeted tumor therapies and mediate therapeutic resistance in most of the solid tumors because of their immunosuppressive features (115–117). Accumulating evidence suggests that neutrophils instigate the immunosuppressive TME through instituting brawny crosstalk both in vitro and in vivo with innate immune cells, including natural killer (NK) cells and dendritic cells (DCs), and adaptive immune cells including T cells and B cells (25, 118) (Figure 2). Therefore, it is critical to unmask the involved mechanisms for a better understanding of the crosstalk mediators and signaling pathways that dictate neutrophil infiltration into tumors, which can assist to design new effective therapeutic approaches. In this section, we will discuss the interaction between neutrophils and immune cells and how this crosstalk contributes to neutrophil-mediated immune suppression.
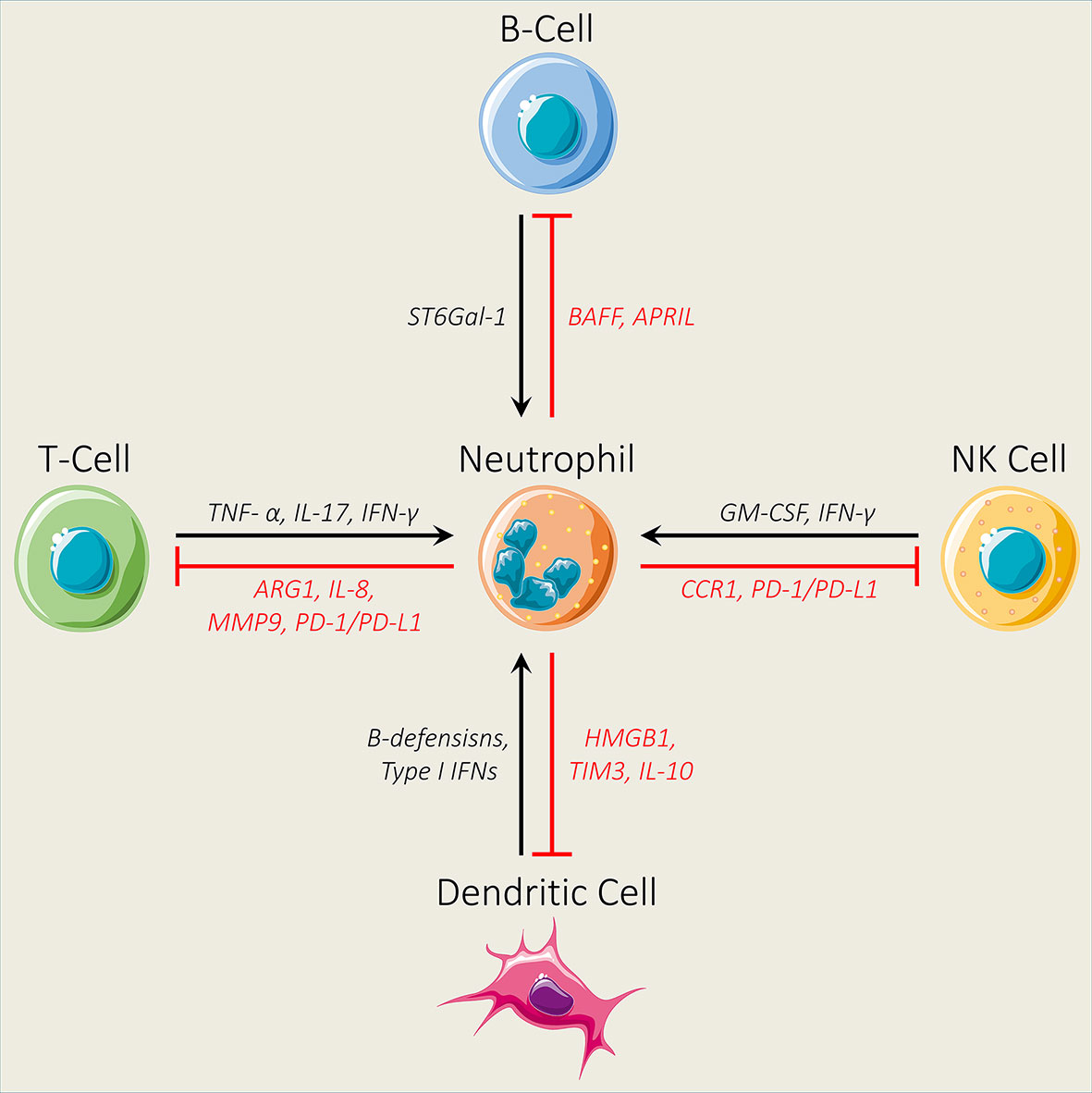
Figure 2 Interaction between neutrophils and different immune cells. Neutrophils impair the infiltration ability of natural killer (NK) cells by decreasing the expression levels of CCR1. In addition, neutrophils inhibit the antitumor activity of NK cells through the PD-1/PD-L1 axis. NK cells regulate neutrophil activation and survival by secreting GM-CSF and IFN-γ. Neutrophils modulate B-cell activation by secreting BAFF and APRIL, while B cells regulate neutrophil production through ST6Gal-1. Furthermore, neutrophils inhibit T-cell activity by increasing the expression levels of ARG1, IL-8, MMP9, and PD-1/PD-L1, while TNF- α, IL-17, and IFN-γ secreted by T cells promote neutrophil activation and recruitment. Finally, neutrophils N2 and dendritic cell interaction via the release of HMGB1 from neutrophils and TIM3 from dendritic cells and the production of IL-10 contribute to dendritic cell inhibition, while dendritic cells regulate neutrophil proliferation and survival by releasing β-defensins and Type 1 IFNs.
3.1 Interaction between neutrophils and T cells
T cells play a major role in effective antitumor immunity and are critical for tumor immunotherapy (119, 120). Recently, various studies have demonstrated the importance of the tumor-specific T-cell priming and activation in the draining lymph node, migration of the T cells toward the tumor site, and the creation of tertiary lymphoid structures inside tumor (112, 121). Primed T cells in the tumor-draining lymph node can respond more efficiently to immune checkpoint blockade compared with those T cells that enter and reside inside tumor (60, 117, 122). However, neutrophils contribute to the immunosuppressive microenvironment by suppressing antitumor T cells (123, 124). Although many scientists have investigated the role of neutrophils in tumor, how neutrophils and T cells communicate with each other to institute immune suppression remains elusive. Emerging evidence has suggested that the suppression of T-cell activity by neutrophils is mediated through the overexpression of ARG1 (125, 126) or the PD-1/PD-L1 axis (127, 128). Recent evidence suggests that TANs are associated with a poor clinical prognosis and also contribute to immune suppression by suppressing the activity of CD8+ T cells. In this event, IL-8 has been identified as a key player that establishes immunosuppressive crosstalk between TANs and CD8+ T cells through recruiting neutrophils into the TME and by inducing JAG2 (129). Another recent study has investigated the underlying molecular mechanism of the neutrophil-mediated inhibition of T-cell activity. Their novel findings demonstrate that neutrophils inhibit antitumor T-cell activity via the MMP-mediated induction of active TGF-β within the colon TME. Importantly, the depletion of neutrophils in mouse adenomas has resulted in decreased tumor burden and a high level of tumor-infiltrating T cells, while T-cell depletion, in turn, enhanced tumor burden and also abolished the valuable effects of neutrophil depletion. Together, these findings demonstrate that neutrophils drive colon tumor progression in mice by repressing the antitumor activity of T cells (130). Interestingly, Minns et al. have recently identified that primed and resting neutrophils contribute to opposite responses in T cells. Their findings show that resting neutrophils inhibit the activation of early-activating CD4+ and CD8+ T cells, while primed neutrophils do not inhibit activation significantly. Furthermore, neutrophils primed with unlike mediators show opposite effects on T cells. Neutrophils primed with LPS and TNF enhance CD4+ T-cell activation, while cytochalasin B/fMLF prime cells repress the activation of the late-stage T cells (131). Accumulating evidence suggests that neutrophils modulate the function of various T-cell subsets Th1, Th2, Th17, γδ T, and Treg cells (132–134). The interaction between TANs and T-cell responses has demonstrated the evidence of direct TAN-mediated inhibition of the Th1 cells and cytotoxic T lymphocytes in tumors. In this perspective, ARG1-expressing human granulocytic cells contribute to the downregulation of the CD3ζ chain on T cells via the depletion of L-arginine and ultimately suppress cytokine secretion and T-cell proliferation. In non-small cell lung cancer patients, ARG1+ neutrophils are increased with the disease stage in treatment-naïve patients and negatively correlated with the CD8+ T-cell population (135). Th17 cells produce different types of cytokines such as GM-CSF, TNF-α, IL-17, and IL-22 and acts as either antitumor or protumor (136, 137). Neutrophils secrete chemokines and cytokines that directly affect Th17 activation and differentiation (138, 139). A more recent study has shown that NETs regulate Th17 cell differentiation and activity through their histone protein components. This modulation of neutrophils, NETs, and histone protein components is mediated through TLR2 in T cells, thereby resulting in STAT3 phosphorylation (3). Coffelt et al. have investigated the critical role of IL-17-secreting cells and cancer-mediated inflammation in a metastasis event. Their findings show that IL-1 β-mediated-IL-17 induction from γδ T cells results in neutrophil expansion and polarization via G-CSF in mammary tumor-bearing mice, while the absence of neutrophils or γδ T cells significantly decreases tumor metastasis (140). These findings demonstrate a novel urbane neutrophil–T-cell interaction and highlight the critical need of exploring a new mediator of this interaction to induce T-cell-mediated adaptive immunity.
3.2 Interaction between neutrophils and B cells
Previously, immune checkpoint blockade therapy mainly focused on the bolstering of effector T lymphocytes; however, work from multiple laboratories have unmasked the fact that B cells are also key players of immunotherapy (141, 142). B cells possess both the protumor and antitumor functions, which mainly depend on their immune-suppressive or immune-stimulatory events and also the tumor type (143, 144). Reflective of both the protumor and antitumor activities of B cells, several clinical trials have been conducted to modulate B-cell functions (145). A recent study has shown that the adoptive transfer of the cancer-specific memory B cells display effective tumor suppression in a murine tumor model, thereby suggesting their clinical worth (146). Furthermore, B cells have also been reported to modulate the functional features of T cells (147, 148). In addition, the existence of PD-1+ and PD-L1+ B cells has been found in several types of human tumors (149–151). The baseline density of B cells can predict a response to immune checkpoint blockade therapy in tumor patients (152). Accumulating evidence suggests that neutrophils directly modulate the response of B cells by regulating cytokine production required for B-cell maturation, differentiation, and survival, such as BAFF and APRIL (153–155). Regarding human cancer, there are supporting evidences about the key role of neutrophils in the differentiation of the B cells. For instance, neutrophils are implicated in the pathogenesis of the B-cell lymphomas by producing APRIL (156). Moreover, it has been found that CXCL8 acts as a mediator to recruit APRIL-expressing neutrophils for diffusing B-cell lymphoma lesions (157). Another important mechanism by which neutrophils promote the development of B-cell chronic lymphocytic leukemia in mice is by elevating the expression level of APRIL and BAFF (158). Furthermore, the critical role of NETs and their crosstalk with CD5+ B cells is another important pathological mechanism driving B-cell chronic lymphocytic leukemia in mice (159). In a recent study in Nature, Petitprez et al. identified that patients with sarcoma immune class highly responded to immune checkpoint inhibitors, while patients from the immune class desert showed no response (160). These results have provided novel insights for changing the care of the patients with soft tissue sarcoma who showed a poor response to immune checkpoint blockade. In melanoma, B-cell signature, and not the T-cell signature, was associated with a response to immune checkpoint blockade. In addition, the density of B cells was enhanced in the tumor of responders compared to non-responding patients (152). B cells have been reported to penetrate into tumor and regulate tumor immunity. However, the profound impact of neutrophils on B cells has been overlooked. A recent study has shown that tumor-associated neutrophils (TANs) drive B-cell recruitment and modulation into plasma cells in the TME. In this event, TNF-α has been found as a key cytokine mediating B-cell chemotaxis by TANs (161). Recently, IL-10+ plasmablasts and IL-10+ B cells have been recovered from tumor-draining lymph nodes and tumor, where tumor patients have demonstrated a higher level of IL-10+ Bregs (162, 163). In addition, the key role of Bregs in regulating CD8+ T-cell responses has been reported in various tumor experimental models (164, 165). Bregs regulate immunity through various anti-inflammatory cytokines, including IL-10 and IL-35 (166). Moreover, Bregs inhibit CD4+ T-cell proliferation and Th1/17 differentiation through IL-10 and IL-35 (167). Accumulating evidence has suggested that neutrophils express IL-10 during inflammatory conditions (168); hence, neutrophils may modulate Breg-mediated immunity regulation by secreting IL-10.
3.3 Interaction between neutrophils and dendritic cells
Dendritic cells (DCs) are the most potent antigen-presenting cells (APCs) and play a critical role in innate and adaptive immune response via arresting, processing and presenting antigens to B and T cells and by activating antitumor T cells (169, 170). The antitumor response mainly depends on APCs to prime naïve T cells (171). Various studies have reported that the cDC1 subset is associated with the induction of tumor-controlling immunity and improved overall survival in many tumor types (172, 173). The activation of neutrophils releases the granule content neutrophils elastase (NE) that induces the polarization of the DC-mediated development of T cells into Th 17 cells, while the blocking of neutrophil activation or NE prevents the production of Th 17 cells (174). Another study has shown that neutrophils promote the development of Th 17 cells from naïve T cells preferentially through their interaction with DCs (175). The neutrophil-derived cathelicidin also induces Th17 and inhibits Th1 differentiation, while cathelicidin-deficient mice inhibit Th17. This study highlights the key role of neutrophils in regulating the T-cell fate through releasing cathelicidin (176). Emerging evidence suggests that DCs play a critical role in triggering immune suppression in response to tumor-associated antigens (177). The DCs migration is crucial for cancer immune surveillance (178). This event includes the migration of DCs into tumor sites, arresting and endocytosing cellular debris or dead tumor cells, and transporting antigens to the tumor- draining lymph nodes to induce the activation of T cells (179). The recruitment of DCs mainly depend on various chemokines including CCL4/5 and XCL1, whereas CCR7 is needed for the migration of DCs to tumor-draining lymph nodes (178). Neutrophils are major producers of CCL4/5; thus, they regulate the recruitment of DCs to the TME (180). DCs as major APCs are associated with the priming of the effector CD4+ and CD8+ T-cell response (181). DCs’ major subsets are monocytes-derived DCs (moDCs), plasmacytoid DCs (pDCs), and conventional CDs (cDCs) (177). DC subset migration plays a critical role in the immune response and tumor onset and progression (182). Migration of cDC trafficking toward lymph node parenchyma for initiating a Th2-cell-dependent immune response is mediated by the chemokines CXCR7 and CXCR8 (182). It has been reported that the subpopulation of the both mouse and human neutrophils has been found to express CXCR7 (183); hence, neutrophils regulate the migration event of cDCs to lymph node parenchyma. Moreover, the recruitment of monocyte-derived DCs (moDCs) to the lymph node is mediated by CCR2 to change their phenotype into CD11c+CD11bhiGr-1+, which induces Th1 responses through IL-12p70 (184). CCR2 is overexpressed in neutrophils and plays a key role in the mobilization of neutrophils from the bone marrow to the liver and as well as the recruitment of neutrophils toward inflammatory sites (185). Thus, neutrophils regulate the migration of moDCs to lymph nodes. pDCs are key producers of type 1 interferons and play an important role in the immune response (186). Recent evidence suggests that neutrophils also regulate the activity of the pDC subset by releasing NETs (187). Together, these findings demonstrate the significance of the interaction between neutrophils and DCs in orchestrating T-cell responses.
3.4 Interaction between neutrophils and natural killer cells
NK cells possess the strong ability of detection and killing malignant or virally infected cells (188–190). In addition, they are also the first lymphocytes that exhibited the natural ability of killing tumor cells and remained unexplored compared with cytotoxic cell therapy toward tumor treatment (191). Clinically, the abundance of NK cells have shown good prognosis in various types of solid tumors (192). Moreover, accumulating evidence has suggested that the increased density of NK cells improves the efficacy of immune checkpoint blockade therapy (193, 194). However, various studies have reported that the function of NK cells is sternly spoiled in patients with cancer and chronic diseases (195, 196). Based on the findings that neutrophils and NK cells are found in the same region of lymph nodes and the spleen, they can make conjugates (197), and neutrophils provide assistance during the intermediate steps of tumor invasion and metastasis through abolishing the activity of NK cells (198), it is believed that neutrophils are key regulators of NK cells. In addition, neutrophils have been found in orchestrating the immune response by attracting NK cells at infection sites and activating them, which, in turn, induce adaptive immune responses through triggering dendritic cell maturation (199). It has been reported that neutrophils cleaved the NK-activating receptor (NKp46) on NK cells by producing serine protease CG and resulted in the loss of the antitumor immunity of NK cells (200). Recently, Sun et al. have investigated the underlying molecular mechanism of neutrophils in the modulation of NK cell immunity. They found that neutrophils reduce the infiltration ability of NK cells in tumor-bearing mice by downregulating CCR1. In addition, their findings show that neutrophils impair the NK cells’ antitumor immunity toward lymphoma and colon cancer cells by impairing NK-activating receptor (NKp46 and NKG2D) responsiveness. The G-CSF led to increased expression of PD-L1 on neutrophils, while IL-8 led to an enhanced expression of PD-1 on NK cells, impelling the inhibition of NK cell immunity via the PD-L1/PD-1 axis (201).
Given the significance of NETs in regulating the tumor immune microenvironment, Teijeira et al. (93) have investigated the interaction between NETs and immune cell population, particularly NK cells. They found that the NET-mediated encapsulation of tumor cells can shield tumor cells from NK cell–mediated cytotoxicity through impeding the interaction between surrounding target cells and immune cells. Tumor cells shielded from NK cell–mediated cytotoxicity trigger tumor metastasis in mice. In addition, authors found that the NET-mediated protective coat around tumor cells against NK cells was lost, following the removal of NETs by using the DNase-I treatment. These novel findings not only unmask the critical role of neutrophils in regulating the antitumor immunity of NK cells but also attract the attention of researchers to explore the NET-mediated shielding of tumor cells and mechanistic link between NETs’ and NK cells’ antitumor immunity. To this end, the development of potent preclinical models to capture how neutrophils physically interact with NK cells in response to immune checkpoint blockade therapy can be a new avenue for designing more effective immunotherapies. In addition, a deep understanding of how neutrophils regulate NK cells’ antitumor activity in different solid tumors can provide a new roadmap for developing immunotherapies to manipulate neutrophil–NK cell communication.
4 Neutrophils in therapy resistance
Accumulating evidence suggests that the aberrant regulation of tumor suppressor genes or oncogenes regulates response to immune checkpoint inhibitors by engaging neutrophils. In lung cancer, the deletion of tumor suppressor STK11/LKB1 enhances the recruitment of tumor-promoting neutrophils and resistance to immune checkpoint blockade therapy (202, 203). Moreover, the activation of c-MET increases the recruitment of reactive neutrophils from the bone marrow to the lymph node and tumor tissues and results in the inhibition of T-cell expansion and function, while the inhibition of c-MET-dependent reactive neutrophil responses facilitates T-cell infiltrations into tumors and increases the efficacy of immunotherapy (204). Previous studies have reported that CXCL5 is engaged in neutrophil recruitment during inflammation (205) and drives neutrophil infiltrations into many types of tumor tissues (206, 207). A more recent study has shown that CXCL5-mediated neutrophil accumulation in lung tumor tissue suppresses the differentiation of CD8+ T cells and promotes resistance to immune checkpoint inhibitors, whereas the blockade of neutrophil infiltration in lung overcomes resistance against immune checkpoint blockade therapy (53). Over the years, tumor treatment has been evolved increasingly, and an overall survival rate of tumor patients has also been improved due to ever-evolving treatment options. Among diverse tumor treatment approaches, immunotherapy has emerged as a promising therapeutic approach (208).. Targeted immunotherapies to inhibit immune checkpoints PD-1/PD-L1 or cytotoxic T lymphocyte antigen 4 for restoring exhausted CD8+ T-cell activity or inducing CD4+ T lymphocyte expansion hold promise in human cancer treatment; however, a limited number of tumor patients have received clinical benefits mainly due to acquired therapeutic resistance (209–212). Therefore, there is a dire need to explore the resistance mechanisms to immune checkpoint inhibitors to improve clinical benefits. Increasing evidence suggests that the existence of the immunosuppressive neutrophils hampers the immune system from effectively killing cancer cells, creating a major barrier for thriving tumor treatment, particularly immunotherapy (22, 213, 214). In colorectal tumor patients, CD177+ neutrophil infiltrations were associated with dismal outcomes in patients who received antiangiogenic bevacizumab treatment (215). In triple- negative breast cancer, neutrophils showed immunosuppressive properties, thereby rendering tumor resistant to immune checkpoint inhibitors (216).
Polymorphonuclear myeloid–derived suppressor cells (PMN-MDSCs) are a type of immature low-density neutrophils (LDNs) and exhibit numerous morphological and phenotypic features of neutrophils (217, 218). It is very difficult to distinguish neutrophils from PMN-MDSCs in the same mouse, owing to the non-existence of appropriate phenotypical markers. Therefore, researchers have compared cells expressing neutrophils/PMN-MDSC-associated markers in tumor-free or tumor-bearing mice. Previously, microarray analysis–based studies have reported that PMN-MDSCs exhibit discrete transcriptomic programs compared with neutrophils. Particularly, neutrophils demonstrated an increased expression of genes associated with NF-κB signaling through IL-1, IL-6, CD-40, TLR, and TNF pathways as well as through lymphotoxin-β receptor signaling, while PMN-MDSCs demonstrated an increased expression of genes linked with the The cAMP-response element bindingprotein (CREB) pathway, G protein signaling, autophagy, and cell cycle regulation. In addition, researchers have also compared naïve neutrophils from the bone marrow with PMN-MDSCs from tumors or the spleen (219). PMN-MDSCs revealed a higher production of pro-inflammatory cytokines as well as the activation of several downstream targets of NF-κB signaling. This may explain the difference between pathologically activated PMN-MDSCs and normal bone marrow neutrophils. In the second study, classically activated neutrophils were compared with PMN-MDSCs, where classically activated neutrophils demonstrated increased levels of TNF, IL-6, and NF-κB signaling compared with PMN-MDSCs (220). Based on cancer patients and healthy donors, Veglia et al. have identified PMN-MDSCs as CD11bhighCD15highCD66bhighCD33highArg1highS100A9highLox1high and classical activated neutrophils as CD11b+CD15+CD16+CD66bhighArg1+/−STAT3-S100A9+LOX− from the peripheral blood of NSCLC patients (221). These findings are consistent with the concept that different stimuli generate both the PMN-MDSCs and classically activated neutrophils. In bladder tumor patients, increased levels of PMN-MDSCs have been detected in tumor tissues and blood mononuclear cells, and this accumulation is associated with the tumor grade and poor prognosis (222). More recently, two studies have investigated the potential role of PMN-MDSCs in immunotherapy response in immune-competent bladder tumor models. In first study, Wang et al. have compared the Bacillus Calmette–Guerin (BCG) intravesical instillation with PA-MSHA in an MB49 orthotopic bladder cancer model. The authors found that PA-MSHA exhibited greater antitumor benefits as compared to BCG, but neither treatment was found to be curative. Their findings showed that high PD-L1 expression and increased levels of PMN-MDSCs hindered the therapeutic efficacy of the treatment (223). This novel study attracts the attention of oncologists for investigating whether increased levels of PMN-MDSCs are correlated with the clinical cases of the non-muscle-invasive bladder tumor that poorly responds to BCG therapy. In the second study, Takeyama et al. have generated cisplatin-resistant bladder tumor cell lines that demonstrated an increased expression of various chemokines, including CXCL1/2 and CCL2. They found that the depletion of PMN-MDSCs decreases the growth of cisplatin-resistant tumor in mice by enhancing the infiltration of the CD8+ T cells; in turn, this depletion increases anti-PD-L1 immunotherapy, suggesting the promising benefit of the combinatorial treatment of anti-PD-1/PD-L1 and PMN-MDSC-incapacitating therapy in urothelial tumor patients.
5 Strategies to target neutrophils
Immune evasion in human tumor has been found as a multifactorial phenomenon. In recent years, this issue has attracted greater attention because resistance mechanisms are found linked with the efficacy of immune checkpoint blockade therapy. Accumulating evidences both in human and mouse models suggests that neutrophils act as immunosuppressive players in the TME. The inflammatory chemokines and cytokines engaged in altering the function of neutrophils constitute new hot topics in the clinical tumor study, both as novel immunotherapy targets and biomarkers (224). Given the stoutness to preclinical studies incriminating neutrophils in immune checkpoint blockade failures, numerous clinical trials are going on to antagonize neutrophil activity combined with immune checkpoint blockade therapy (Table 2). In this section, we will shed light on novel strategies to target neutrophils that include three aspects: limiting neutrophil polarization and recruitment, reducing the immunosuppressive ability of neutrophils, and inhibiting NET production.
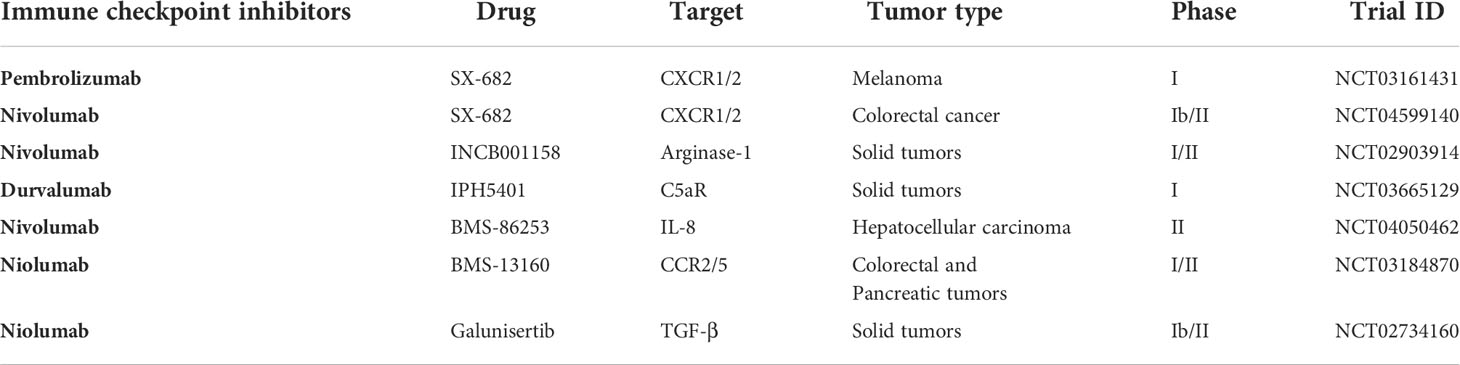
Table 2 List of clinical trials investigating effect of immune checkpoint inhibitors in combination with other drugs to overcome neutrophil-associated resistance in different cancers.
5.1 Limiting neutrophil polarization and recruitment
In this section, we highlight the targeting of various signaling pathways that promote tumor and hinder immunotherapy efficacy through regulating neutrophil polarization and recruitment (Figure 3).
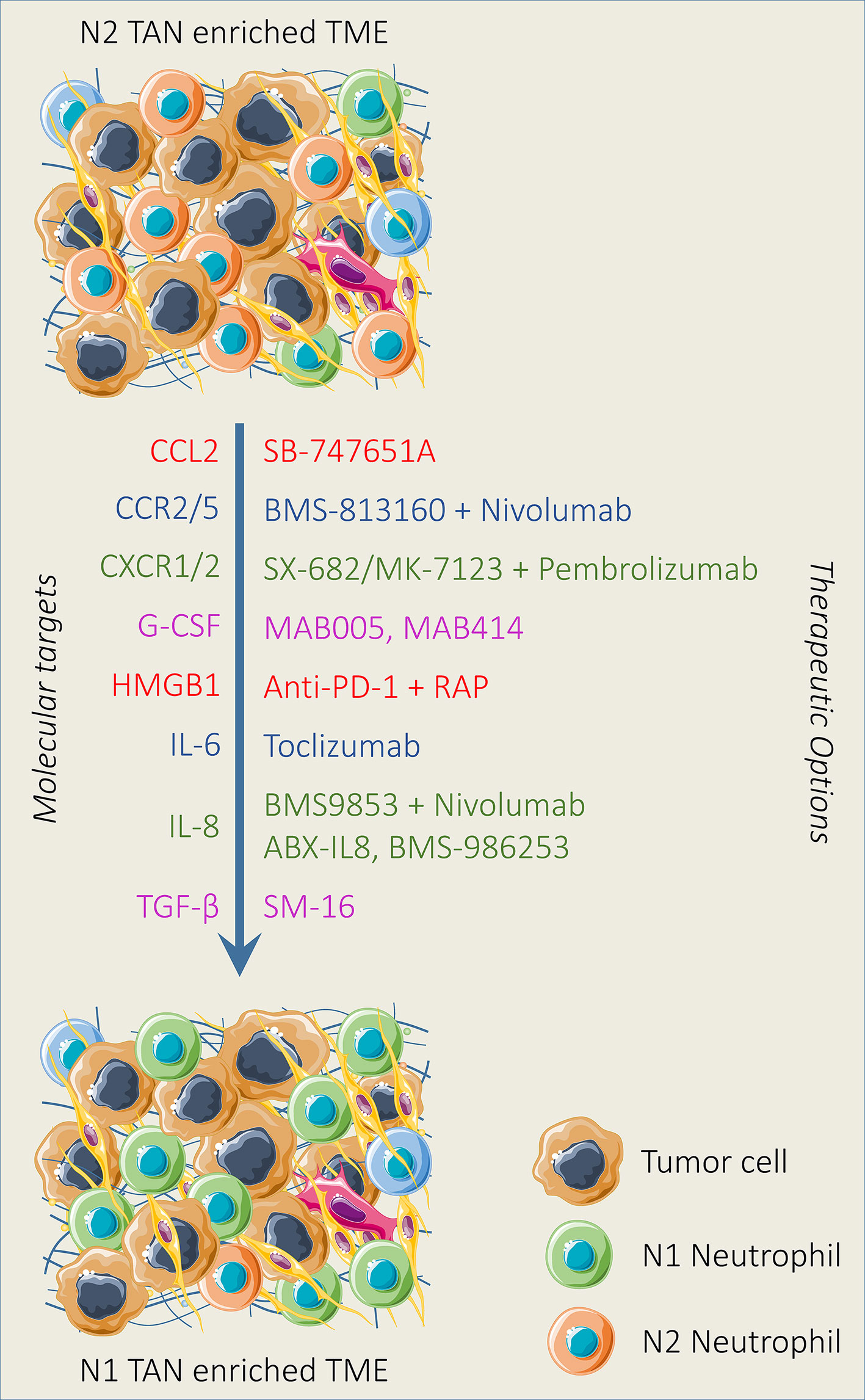
Figure 3 Therapeutic strategies reducing neutrophil recruitment and polarization. Various neutrophil-targeting approaches have been established and demonstrated promising outcomes both in preclinical and clinical settings. Neutrophil recruitment and polarization can be limited by the pharmacological blockade of mediators and downstream pathways alone or in combination with immunotherapy involved in neutrophil recruitment and polarization. In addition, N2 TAN can be reprogrammed into N1 TAN by modulating the activity of TGF-β and IFN-β in the TME.
5.1.1 Transforming growth factor beta blockade
Tumor-associated neutrophils (TANs) are polarized from an antitumor (N1) to a protumor (N2) phenotype after infiltration into the TME (225). As a major immunosuppressive cytokine, TGF-β regulates this phenotypic switch of TANs in the TME, which, in turn, suppresses the antitumor activity of NK cells and T cells (226). It has been suggested that the blockade of the TGF-β pathway suppresses colorectal cancer progression through reversing TAN polarization into the N2 type (227). In NSCLCs, the blockade of TGF-β also inhibits tumor growth through TAN polarization toward antitumor phenotypes (228). Furthermore, the TGF-β pathway plays a critical role in the recruitment of neutrophils to tumor and subsequent resistance of cancer to immune checkpoint inhibitors (52, 210, 229, 230). In recent years, the blockade of TGF-β has emerged as promising approach to induce CD8+ T-cell infiltration and improve immunotherapy efficacy. Martin et al. has recently investigated the potential impact of TGF-β inhibition for modulating immune checkpoint resistance in mice. Results show that the combined treatment of the anti-PD-1 antibody and SRK-181-mIG1 contributes to antitumor responses and survival benefits. In addition, this novel combinatorial strategy has resulted in the reduction of immunosuppressive myeloid cells and induction of intratumoral CD8+ T cells, thereby suggesting that the inhibition of TGF-β can modulate resistance to immune checkpoints (231). Previously, it has been reported that the TGF-β pathway stimulates T-cell exclusion and lymphocyte confiscation at tumor outskirts, thereby leading to tumor metastasis and resistance toward T-cell-mediated immune therapies (232, 233). The repression of CXCR3 activation has been suggested as a new mechanism for T-cell exclusion to tumors mediated by TGF-β signaling. This shows extensive applicability for tuning the efficacy of the CD8+ T -cell-mediated immunotherapies that entailed the infiltrations of the T cells into tumors (234). For instance, evidence suggests that TGF-β is associated with resistance toward immune checkpoint blockade (235); this may be, in part, due to diminished trafficking to tumor via CXCR3 repression. These findings provide a mechanistic rationale for synergy between TGF-β suppression and immune checkpoint blockade (236, 237). Furthermore, clinical trials are also being conducted to evaluate the combined treatment of galunisertib and immune checkpoint inhibitors to target TGF-β in the treatment of solid tumors (NCT02734160). Thus, the combined repression of the TGF-β pathway and immune checkpoints can be a promising avenue to improve the efficacy of forthcoming immunotherapeutic agents.
5.1.2 CXCL8 blockade
CXCL8 (IL-8) has been proposed as a key player of neutrophil recruiter and also an important driver of neutrophil activity. Growing evidence has revealed the crucial role of CXCL8-CXCR1/2 axis in the TME and prognostic significance of the CXCL8 serum level in human cancer, following immune checkpoint blockade therapy (58, 238–240). Advanced NSCLC patients who responded well toward nivolumab, a PD-1 targeted treatment, demonstrated low levels of CXCL8, TNF-α, and IP-10 and high levels of BMP-9 compared to non-responders (241). Recently, two clinical studies published in Nature Medicine have shown that the neutrophil-attractant IL-8 acts as an undesirable predictive factor in tumor patients who received immune checkpoint blockade therapy. Results showed that the elevated IL-8 serum level at baseline was associated with dwindled overall survival and partial response to immune checkpoint inhibitors because of neutrophil-mediated immune suppression (242, 243). Furthermore, the increased CXCL8 serum level is linked with neutrophil expansion and limited clinical outcome from immune checkpoint blockade therapies (244, 245). In ovarian tumor, CXCL8 promotes the recruitment of tumor associated neutrophils in TME and activates JAG2 in tumor-associated neutrophils, which, in turn, suppresses the activity of the CD8+ T cells (129). It has been reported that the anti-CXCL8 monoclonal antibody abolishes the recruitment of neutrophils into tumor and increases the antitumor immunity of triple- negative breast cancer (246). Moreover, various clinical trials of combining the anti-PD1 antibody, nivolumab, and CXCL8 antibodies have also been carried out in both advanced solid tumors (NCT03184870), hepatocellular carcinoma (NCT04050462), and NSCLC (NCT0413379). In triple-negative breast cancer, the direct targeting of CXCL8 by using the monoclonal antibody HuMax-IL8 significantly reduces PMN-MDSC infiltration to the TME and improves immunotherapy efficacy (246). Giving importance to the neutrophil-induced M2-like macrophage phenotype, the blocking of the neutrophil accumulation in the TME represents a promising therapeutic approach to hinder neutrophil-mediated immune suppression. In this line, the latest study has shown that the blocking of CXCL-8 signaling suppresses neutrophil migration and the neutrophil-mediated polarization of anti-inflammatory macrophages in the TME (247). The above discussed findings support that CXCL8 plasma levels can provide a glimpse into the immunosuppressive TME and patients may benefit from immune checkpoint blockade therapy, following the reduction of the CXCL8 level.
5.1.3 Blocking of CXCR1/2 axis
CXCR1 and CXCR2 are major chemokine receptors expressed by neutrophils (248, 249). Both the CXCR1 and CXCR2 chemokine receptors are prognostic biomarkers in many types of human cancers (250–253). The targeting of CXCR1/2 reduces the neutrophil population in several cancer models and also inhibits tumor growth and metastasis (254, 255). In addition, the inhibition of CXCR1/2 by ladarixin decreases neutrophil-dependent airway inflammation in mice (256). SX-682 is another novel potent inhibitor of CXCR1/2 (22, 71) and recently being investigated in many clinical trials in several cancer types such as melanoma, colorectal cancer, and breast cancer (NCT03161431, NCT04574583, and NCT04245397). In lung and breast cancer, the combined inhibition of CXCR1/2 by using the SX-682 inhibitor and anti-PD-1/PD-L1 has effectively controlled tumor growth in a murine model. Grippingly, this combinatorial approach demonstrated enhanced efficacy due to the decreased infiltration of granulocytic MDSC and increased population of activated T cells at the tumor site (257). Moreover, the disruption of CXCR1/2 through SX-682 has shown efficacy in mice (21, 258), and its combinatorial treatment with PD-L1-targeted antibody, pembrolizumab, is also being studied in a phase 1 trial in melanoma (NCT03161421). The mutated Kirsten rat sarcoma virus (KRAS) contributes to resistance against immune checkpoint inhibitors through recruiting PMN-MDSCs in a colorectal cancer mouse model. Mechanistically, oncogenic KRAS mutation inhibits IRF2, thereby leading to the activation of CXCL3, a potent ligand of CXCR2. The blocking of CXCR2 through CXCL3 knockdown or SX-682 treatment in mice reduces PMN-MDSC recruitment and enhances response to anti-PD-1 therapy (71). Therefore, employing an alike rationale in the preclinical models of different cancer types, SX-682 treatment demonstrated promising outcomes in decreasing the infiltration of PMN-MDSCs and synergy with several modes of immunotherapy, including adoptive T-cell transfer, immune checkpoint inhibitors, and NK cell–based therapy (259, 260). In triple-negative breast tumor patients, the phase I clinical trial (NCT02001974) to evaluate the safety of the oral administration of CXCR1/2 inhibitor reparixin has been conducted combined with paclitaxel (261). In addition, the phase II clinical trial (NCT02370238) has also been conducted to investigate the survival of triple-negative breast tumor patients, following the combined treatment of reparixin and paclitaxel.
5.1.4 Blocking IL-17
IL-17 is a prominent cytokine that plays a critical role in tumor progression and immune response (262–265). It is linked with poor prognosis and drives resistance in solid tumors (266, 267) and also acts as a prognostic biomarker in different types of human cancers (268, 269). Recently, Wu et al. have investigated the potential role of IL-17 in regulating breast cancer metastasis and therapy resistance. Results show that IL-17 contributes to breast tumor metastasis and therapy resistance through recruiting neutrophils to the TME (270). Another recent study has shown that IL-17 contributes to immune suppression immunotherapy resistance by increasing the neutrophil population and NET production in the TME. However, the blocking of IL-17 enhances the sensitivity of PD-1 and CTLA4 in pancreatic cancer (55). G-CSF plays a critical role in neutrophil production and recruitment (271, 272). The targeting of the IL-23-IL-17 axis has been found to reduce neutrophil abundance induced by G-CSF (273, 274). Thus, G-CSF inhibition leads to reduce the neutrophil amount and improve antitumor efficacy in several preclinical cancer models (275).
5.2 Reducing immunosuppressive ability of neutrophils
Immunosuppressive neutrophils obstruct the antitumor activity of the immune system and pose a major obstacle in tumor eradication, particularly immunotherapy (276). A deep understanding of various signaling pathways regulating neutrophil activity in tumor progression have enlightened several strategies that exploit antibodies or drugs to block TANs (277, 278). Therefore, the targeting of key pathways that promote immune suppression and regulate neutrophils’ immunosuppressive function can help in reducing the immunosuppressive ability of neutrophils.
5.2.1 ARG1 blockade
ARG1 is an immunosuppressive marker induced almost solely by polymorphonuclear granulocytes (PMNs) in human and regulates both the innate and adaptive immunity (279, 280). ARG1 expression is linked with increased tumor growth and immune suppression (280, 281) and serves as a prognostic biomarker in a wide range of human cancers (282–284). The presence of ARG1 in the TME suppresses both the expression of T-cell receptors and T-cell proliferation, while the inhibition of ARG1 prevents the PMN-mediated suppression of T cells (285). In several xenograft models, ARG1 inhibition has shown delayed tumor growth and enhanced PD-L1 blockade response (286). Based on the above studies, recently, various ARG1 inhibitors, including CB-1158 and OATD-02, have been selected for clinical trials in tumor immunotherapy (287). The CB-1158 potent inhibitor has been found to effectively inhibit human ARG1 (288). Furthermore, clinical trials are also being conducted to evaluate the combined treatment of CB-1158 and immune checkpoint inhibitors in the treatment of solid tumors (NCT02903914, NCT03361228, and NCT03314935). In epithelial ovarian cancer, increased ARG1 expression has been found to contribute in tumor growth and immune suppression, while the blocking of ARG1 mitigated ARG1-mediated tumor progression and immune response (289). OATD-02 inhibitor has been also entered into phase I trials, but this inhibitor has demonstrated low clearance and modest oral bioavailability (290). Importantly, a recent study has evaluated the antitumor effect of the combinatorial treatment of another potent inhibitor of ARG1 (OAT-1746) and anti-PD-1 therapy in a glioma murine model. Results showed that the combined treatment of OAT-1746 and anti-PD-1 antibody reduced tumor growth by decreasing levels of CCL2 and CCL5 in the blood plasma of mouse (291).
5.2.2 Blocking C3A/C5A
The complement system is a major arm of innate immunity (292). C3A and C5A are key components of complement system and regulate immune response and tumor growth in wide range of solid tumors (59, 293–296). In addition, both the C3A and C5A promote resistance to immune checkpoints blockade therapy (283, 284). The C3A and C5A trigger inflammatory response which is crucial step in tumor onset and progression by activating leukocytes, releasing histamine, and stimulating generation of inflammatory mediators such as IL-1, IL-6, IL-1β, IFNγ, and TNF-α (293, 297, 298). A growing body of evidence suggests that C3A and C5A as key components of complement system stimulate neutrophils activation and migration (299–302). Various studies have reported that targeting of C3A and C5A and their receptors is a novel strategy for increasing immunotherapy efficacy (303, 304). Ajona et al. have investigated antitumor synergistic effect of combinatorial inhibition of C5A and PD-1 in the treatment of lung cancer. In this study, they used RMP1-14 antibody to inhibit PD-1 and L-aptamer to block C5A. Authors found that blocking of C5A downregulates immune suppression induced by MDCs as C5A promotes lung cancer onset and progression through inducing the immunosuppressive TME where MDCs are implicated. By using various lung cancer models, the authors found that the combined targeting of C5a and PD-1 significantly suppresses tumor growth and also enhances overall survival rate (305). Moreover, targeting of C5aR increases paclitaxel response in squamous cell carcinoma by reprogramming the immunosuppressive tumor immune microenvironment, thereby leading to improved CD8+ T cell-mediated antitumor immune response (306). In addition to this, the inhibition of complement receptors such as C3aR and C5aR has been proven to be very effective in enhancing the efficacy of immunotherapy (307). Recently, a phase I clinical trial (STELLAR-001) has also been conducted by Innate Pharma to investigate the therapeutic effect of IPH5401 (anti-C5aR) combined with durvalumab (anti-PD-1) in advanced solid tumors (308).
5.3 Targeting of neutrophil extracellular traps
Recently, it has been confirmed that NETs do not only regulate the immune system but are also involved in the pathogenesis of various inflammatory diseases and multiple tumors (309–311). At the initial stage of tumor, NETosis favors EMT induction. The treatment of breast tumor cells with NETs drive the mesenchymal phenotype, leading to tumor progression (312). Moreover, various preclinical studies have also reported that neutrophil accumulation drives metastatic disease through hindering antitumor immune responses, by supporting cancer cell migration and producing neutrophil extracellular traps (NETs) (100, 140, 313, 314). Thus, the targeting of NETs could be a promising strategy for tumor therapy. Various strategies can be adopted for the targeting of NETs such as the blocking of pathways involved in NET production, destroying NET structure, and obstructing NET–tumor interaction (315). CXCL8 has been found to support the production of NETs (316). In addition, Yang et al. have reported that CXCL8 establishes a positive loop between NETs and colorectal cancer liver metastasis (317). Another study has shown that CXCL8 induces the production of NETs through communicating with CXCR2, which, in turn, promotes cancer cell proliferation and migration (318). Therefore, the targeting of the CXCL8-CXCR1/2 axis can be a promising approach for increasing the efficacy of immune checkpoint inhibitors. More recently, Kaiser et al. have investigated the profound impact of CXCL8 blockade in neutrophil activation and NET production. Results show that the targeting of CXCL8-CXCR1/2 by using the anti-IL-8 antibody or a clinically available CXCR1/2 blocker (reparixin) reduces neutrophil activation and NET production in mice (319). Peptidyl arginine deiminase 4 (PAD4) is a critical enzyme associated with the formation of NETs. The blocking of PAD4 reduces NETs protumor effects in various disease models (320, 321). To date, several compounds have been reported for inhibiting PAD activity such as Cl-amidine and BMS-P5. These novel inhibitors effectively abrogate NET formation induced by tumor cells and also set back disease progression (322, 323). In addition, the antitumor drug kaempferol inhibits NET formation by suppressing ROS-PAD4 signaling (324). Another novel PAD4 inhibitor (GSK484) and A2A receptor (CGS21680) effectively block NET formation (325). A more recent study has shown that an Food and Drug Administration (FDA)-approved drug (disulfiram) blocks NET formation and reduces lung injury in rodents (326). Another promising strategy to inhibit NET formation is to demolish the NET structure by using (327). DNase destroys the NET backbone and results in NET degradation (328). In addition, DNase treatment has shown reduced tumor burden in a breast tumor mouse model (329). Coated nanocarriers have demonstrated higher tumor-inhibiting potential due to DNase stability linked with nanocarriers in blood. Dnase nanocarriers effectively digested NETs and suppressed breast tumor lung metastasis configuration (100) (Figure 4). The above findings highlight teamwork between tumor cells, TANs, and the formation of NETs in the TME and the crucial role of NETs in tumor progression and metastasis. In addition, these findings suggest that the combinatorial approach of NET blocking with immunotherapy may move toward clinic.
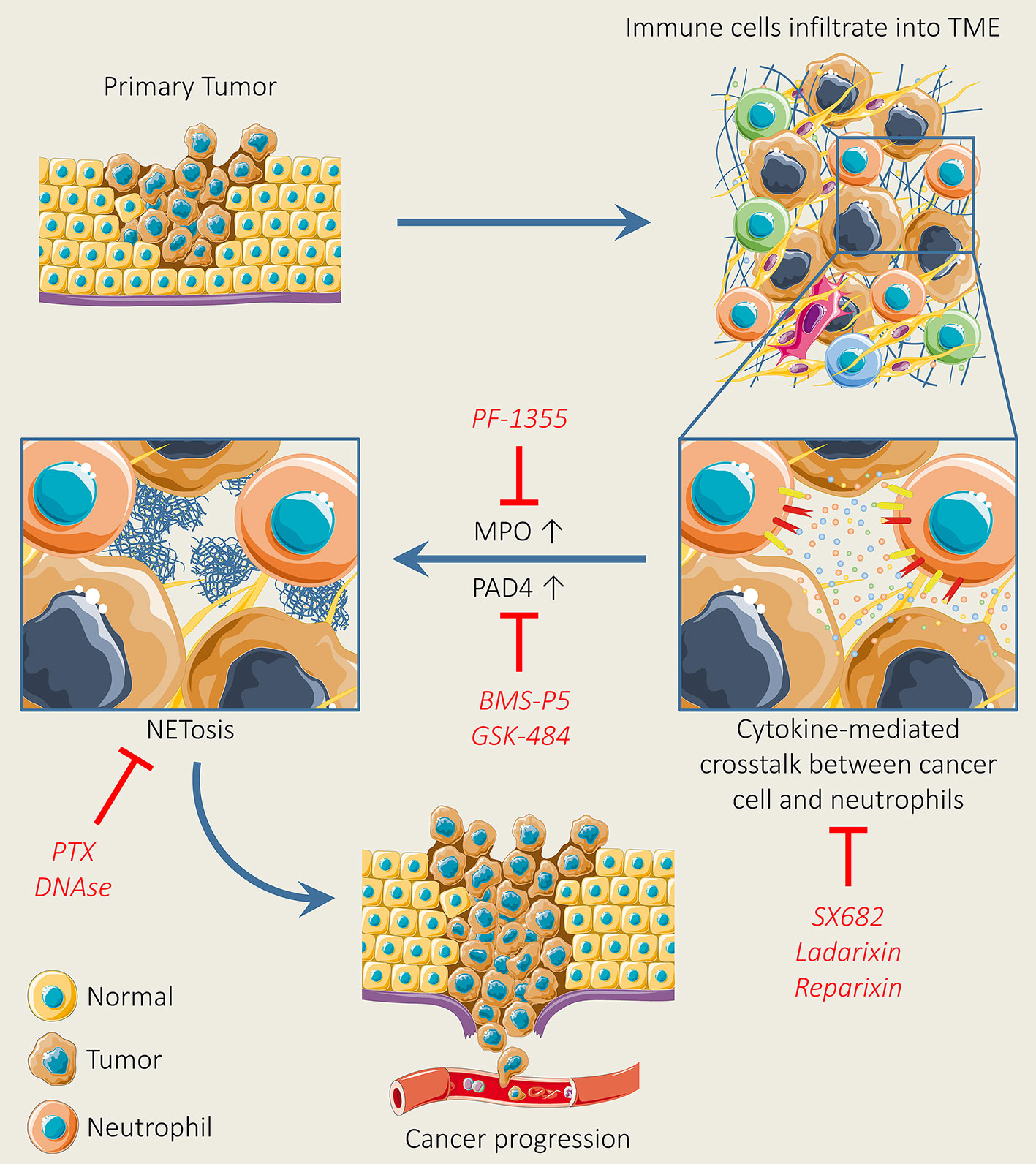
Figure 4 Neutrophil extracellular trap (NET) formation and inhibition for tumor therapy. NET formation is initiated, following the activation of chemokine receptors upon the secretion of various types of chemokines and cytokines secreted by tumor cells. Upon the initiation of NET formation, MPO facilitates cell cytoskeleton degradation, whereas PAD4 triggers histone citrullination, thereby leading to NET release. However, the pharmacological inhibition of chemokines, cytokines, and receptors, MPO, and PAD4 by using selective inhibitors can suppress NET formation. In addition, NET formation can be abolished by using pertussis-toxin and DNase I.
5.4 Potential role of neutrophil-derived extracellular vesicles in tumor
Activated neutrophils have been found to produce a greater amount of extracellular vesicles (EVs) than tumor cells (330). Neutrophils produce EVs either instinctively or in response to different stimuli such as chemokines, cytokines, antibodies, complement component, and bacterial stimulation (331, 332). Neutrophils exploit diverse mechanisms either to promote or suppress tumor. Among them, one key mechanism involves the release of EVs, which induce specific signaling pathways in different target cells and immune cells (333). EVs released from neutrophils exert either a pro-inflammatory or anti-inflammatory effect, mainly depending on environmental conditions (334). Neutrophils hold natural potential to traverse the blood–brain barrier (335) and rapidly penetrate into the glioma tumor site (336). Therefore, in recent years, neutrophil-derived EVs have been studied as drug delivery vehicles in tumor therapy (337). Previously, Wang et al. have shown that neutrophil–exosome-loaded drugs effectively penetrate the blood–brain barrier and migrate into brain. In addition, the intravenous injection of the neutrophil–exosome-loaded PDX efficiently inhibits tumor growth in a glioma mouse model (338). Similarly, neutrophil-carrying PDX have also been found effective in overcoming the blood–brain barrier and suppress glioma recurrence, following tumor surgery (339). More recently, Zhang et al. have engineered neutrophil-derived exosome-like vesicles and investigated the potential of this novel nanocarrier for safe drug delivery into tumor. Results show that this nanocarrier efficiently accumulates at the targeted tumor site under an external magnetic field, suppresses tumor growth, and increases the survival rate in tumor-bearing mice (340).
6 Unsolved mysteries and future perspectives
Over the years, the efficacy of immune checkpoint blockade therapies is obstructed in many cancer settings, which raises new unexplored scientific questions. Meantime, neutrophils have emerged as quintessential warriors of the immune system that play a key role in modulating immunotherapy efficacy; therefore, deep understanding is required for developing effective neutrophil-targeting approaches for tumor therapy. Regarding the mechanistic perspective, we highlight the following unsolved questions that, when answered, may aid in developing effective therapeutic windows to increase immunotherapy efficacy.
(a) What kind of molecular machinery controls the production of the neutrophils recruiting arbitrators in tumor cells?
(b) What kind of trafficking molecules control a precise coordination between chemokines and their receptors in the formation of NETs?
(c) Which trafficking molecules control the transcriptional programming of neutrophils in acquiring their tissue-associated features?
The neutrophil function in tumors is profoundly dictated by a precise TME. The precise TME is regulated by TGF-β and IFN-β. Therefore, modulating the activity of TGF-β and IFN-β in the TME can change the neutrophil phenotype and may unlock the therapeutic potential of the TME in dictating neutrophils as either a tumor promoter or suppressor. Thus, getting control on the desired TME modulation might be a novel approach to improve immunotherapy efficacy. Although this aspiration seems baffling, it can be accomplished by accelerating translational research. Immune checkpoint blockade therapy mainly works by dictating T cells to unleash their potential in killing cancer cells. However, immune-suppressive PMN was found to work as gatekeeper to protect cancer cells from a T-cell attack, which promotes resistance to immune checkpoint blockade therapy (341). Therefore, the identification of novel biomarkers to differentiate immunosuppressive and normal PMN by using high-dimensional mass cytometry and single-cell sequencing technology will be useful for a selective targeting of immunosuppressive PMN. In addition, the identification of novel molecules and pathways involved in the regulation of immunosuppressive PMN will also be helpful for a selective targeting of the immunosuppressive PMN. A better understanding of neutrophil ontogeny is also critical to differentiate different types of neutrophil progenitors. Neutrophils are produced from hematopoietic stem cells (HSCs) in spleen and bone marrow and then progress to common myeloid progenitors (CMP) and committed granulocyte monocyte progenitors (GMP), and then, finally, GMP yields to both neutrophils and monocytes (342). In humans, more recent studies have defined neutrophil-committed progenitors as CD66b–CD64dimCD115– in SSClow CD45dim CD34+ and CD34low/– (343), and early neutrophil progenitors as Lin– CD66b+/low CD15low CD49d+ CD11b– (344). Another more recent study has investigated the neutrophil progenitor commitment event in a human neutrophil deficiency model by using small-molecule alpha-lipoic acid. Authors have identified novel role of alpha-lipoic acid in the regulation of neutrophil lineage specification and also found that the SF3B1-ELK axis controls commitment of the human neutrophil progenitors from CD371+CD115-GMPs (345). Moreover, Zhu et al. identified committed unipotent early-stage neutrophils progenitors from the bone marrow of the human and mouse by using single-cell RNA-sequencing technology and mass cytometry. Results showed that early-stage neutrophil progenitors promote tumor both in humans and mice. Importantly, authors identified human neutrophil progenitors (hNeP) from patients’ blood with melanoma, indicating that hNeP was released from bone marrow in tumor patients and can be detected in human blood (346). As hNeP has been detected as a tumor promotor, therefore, it could be a novel immune-oncology target. Furthermore, a better understanding of the underlying molecular mechanism of the generation of the PMN-MDSCs from their progenitors is also very important in designing novel therapies to target PMN-MDSCs. Accumulating evidence suggests that the developmental joint in granulopoiesis is snuggly choreographed by various growth factors such as G-CSF, GM-CSF, and M-CSF and transcriptional factors. These master regulators play a key role during the development and maturation of the normal granulocytes; however, during tumor burden conditions, this regulatory network is dysregulated and impairs the myeloid differentiation event and drives PMN-MDSC accumulation (347, 348). We hope that this novel immuno-oncology milestone can be a motivational window for preclinical and translational scientists in developing more effective immunotherapies in this space.
Author contributions
KZ, UR, ST, and XH wrote the manuscript. KZ, LJ, WX, and XH reviewed and edited the manuscript. KZ and UR revised the manuscript. XH supervised the project. All authors contributed to the article and approved the submitted version.
Funding
This work was supported by NIH/NCI grants (R01 CA221158-05, R01 CA224493-04, and R01 CA240952-02 to XH). This work was also supported by the IU Simon Comprehensive Cancer Center (Grant P30CA082709).
Conflict of interest
The authors declare that the research was conducted in the absence of any commercial or financial relationships that could be construed as a potential conflict of interest.
Publisher’s note
All claims expressed in this article are solely those of the authors and do not necessarily represent those of their affiliated organizations, or those of the publisher, the editors and the reviewers. Any product that may be evaluated in this article, or claim that may be made by its manufacturer, is not guaranteed or endorsed by the publisher.
References
1. Németh T, Sperandio M, Mócsai A. Neutrophils as emerging therapeutic targets. Nat Rev Drug discovery (2020) 19(4):253–75. doi: 10.1038/s41573-019-0054-z
2. Ng LG, Ostuni R, Hidalgo A. Heterogeneity of neutrophils. Nat Rev Immunol (2019) 19(4):255–65. doi: 10.1038/s41577-019-0141-8
3. Wilson AS, Randall KL, Pettitt JA, Ellyard JI, Blumenthal A, Enders A, et al. Neutrophil extracellular traps and their histones promote Th17 cell differentiation directly via TLR2. Nat Commun (2022) 13(1):1–12. doi: 10.1038/s41467-022-28172-4
4. Dunham-Snary KJ, Surewaard BG, Mewburn JD, Bentley RE, Martin AY, Jones O, et al. Mitochondria in human neutrophils mediate killing of staphylococcus aureus. Redox Biol (2022) 49:102225. doi: 10.1016/j.redox.2021.102225
5. Lahoz-Beneytez J, Elemans M, Zhang Y, Ahmed R, Salam A, Block M, et al. Human neutrophil kinetics: modeling of stable isotope labeling data supports short blood neutrophil half-lives. Blood J Am Soc Hematol (2016) 127(26):3431–8. doi: 10.1182/blood-2016-03-700336
6. Hays E, Bonavida B. YY1 regulates cancer cell immune resistance by modulating PD-L1 expression. Drug Resistance Updates (2019) 43:10–28. doi: 10.1016/j.drup.2019.04.001
7. Kon E, Benhar I. Immune checkpoint inhibitor combinations: current efforts and important aspects for success. Drug Resistance Updates (2019) 45:13–29. doi: 10.1016/j.drup.2019.07.004
8. Fong PC, Retz M, Drakaki A, Massard C, Berry WR, Romano E, et al. Keynote-365 cohort c: Pembrolizumab (pembro) plus enzalutamide (enza) in abiraterone (abi)-pretreated patients (pts) with metastatic castrate resistant prostate cancer (mCRPC). Am Soc Clin Oncol (2019) 37:171–171. doi: 10.1200/JCO.2019.37.7_suppl.171
9. Sharma P, Pachynski RK, Narayan V, Fléchon A, Gravis G, Galsky MD, et al. Nivolumab plus ipilimumab for metastatic castration-resistant prostate cancer: preliminary analysis of patients in the CheckMate 650 trial. Cancer Cell (2020) 38(4):489–99.e3. doi: 10.1016/j.ccell.2020.08.007
10. Pauken KE, Sammons MA, Odorizzi PM, Manne S, Godec J, Khan O, et al. Epigenetic stability of exhausted T cells limits durability of reinvigoration by PD-1 blockade. Science (2016) 354(6316):1160–5. doi: 10.1126/science.aaf2807
11. Gandhi L, Rodríguez-Abreu D, Gadgeel S, Esteban E, Felip E, De Angelis F, et al. Pembrolizumab plus chemotherapy in metastatic non–small-cell lung cancer. N Engl J Med (2018) 378(22):2078–92. doi: 10.1056/NEJMoa1801005
12. Wolchok JD, Chiarion-Sileni V, Gonzalez R, Rutkowski P, Grob J-J, Cowey CL, et al. Overall survival with combined nivolumab and ipilimumab in advanced melanoma. N Engl J Med (2017) 377(14):1345–56. doi: 10.1056/NEJMoa1709684
13. Sun J-Y, Lu X-J. Cancer immunotherapy: current applications and challenges. Cancer Letters (2020) 480:1–3. doi: 10.1016/j.canlet.2020.03.024
14. Haslam A, Prasad V. Estimation of the percentage of US patients with cancer who are eligible for and respond to checkpoint inhibitor immunotherapy drugs. JAMA Network Open (2019) 2(5):e192535–e. doi: 10.1001/jamanetworkopen.2019.2535
15. Bagchi S, Yuan R, Engleman EG. Immune checkpoint inhibitors for the treatment of cancer: clinical impact and mechanisms of response and resistance. Annu Rev Pathol: Mech Dise (2021) 16:223–49. doi: 10.1146/annurev-pathol-042020-042741
16. Giese MA, Hind LE, Huttenlocher A. Neutrophil plasticity in the tumor microenvironment. Blood J Am Soc Hematol (2019) 133(20):2159–67. doi: 10.1182/blood-2018-11-844548
17. Ponzetta A, Carriero R, Carnevale S, Barbagallo M, Molgora M, Perucchini C, et al. Neutrophils driving unconventional T cells mediate resistance against murine sarcomas and selected human tumors. Cell (2019) 178(2):346–60. e24. doi: 10.1016/j.cell.2019.05.047
18. Valero C, Lee M, Hoen D, Weiss K, Kelly DW, Adusumilli PS, et al. Pretreatment neutrophil-to-lymphocyte ratio and mutational burden as biomarkers of tumor response to immune checkpoint inhibitors. Nat Commun (2021) 12(1):1–9. doi: 10.1038/s41467-021-20935-9
19. Geh D, Leslie J, Rumney R, Reeves HL, Bird TG, Mann DA. Neutrophils as potential therapeutic targets in hepatocellular carcinoma. Nat Rev Gastroenterol Hepatol (2022) 19(4):257–73. doi: 10.1038/s41575-021-00568-5
20. Daoud M, Broxtermann PN, Schorn F, Werthenbach JP, Seeger JM, Schiffmann LM, et al. XIAP promotes melanoma growth by inducing tumour neutrophil infiltration. EMBO Rep (2022) 23(6):e53608. doi: 10.15252/embr.202153608
21. Kargl J, Zhu X, Zhang H, Yang GH, Friesen TJ, Shipley M, et al. Neutrophil content predicts lymphocyte depletion and anti-PD1 treatment failure in NSCLC. JCI Insight (2019) 4(24):e130850. doi: 10.1172/jci.insight.130850
22. Sun L, Clavijo PE, Robbins Y, Patel P, Friedman J, Greene S, et al. Inhibiting myeloid-derived suppressor cell trafficking enhances T cell immunotherapy. JCI Insight (2019) 4(7):e126853. doi: 10.1172/jci.insight.126853
23. Veglia F, Tyurin VA, Blasi M, De Leo A, Kossenkov AV, Donthireddy L, et al. Fatty acid transport protein 2 reprograms neutrophils in cancer. Nature (2019) 569(7754):73–8. doi: 10.1038/s41586-019-1118-2
24. Gun SY, Lee SWL, Sieow JL, Wong SC. Targeting immune cells for cancer therapy. Redox Biol (2019) 25:101174. doi: 10.1016/j.redox.2019.101174
25. Shaul ME, Fridlender ZG. Tumour-associated neutrophils in patients with cancer. Nat Rev Clin Oncol (2019) 16(10):601–20. doi: 10.1038/s41571-019-0222-4
26. Uribe-Querol E, Rosales C. Neutrophils in cancer: Two sides of the same coin. J Immunol Res (2015) 2015:983698. doi: 10.1155/2015/983698
27. Tang L, Wang Z, Mu Q, Yu Z, Jacobson O, Li L, et al. Targeting neutrophils for enhanced cancer theranostics. Advanced Materials (2020) 32(33):2002739. doi: 10.1002/adma.202002739
28. Myerson JW, Patel PN, Rubey KM, Zamora ME, Zaleski MH, Habibi N, et al. Supramolecular arrangement of protein in nanoparticle structures predicts nanoparticle tropism for neutrophils in acute lung inflammation. Nat Nanotechnol (2022) 17(1):86–97. doi: 10.1038/s41565-021-00997-y
29. Buonacera A, Stancanelli B, Colaci M, Malatino L. Neutrophil to lymphocyte ratio: An emerging marker of the relationships between the immune system and diseases. Int J Mol Sci (2022) 23(7):3636. doi: 10.3390/ijms23073636
30. Banna G, Di Quattro R, Malatino L, Fornarini G, Addeo A, Maruzzo M, et al. Neutrophil-to-lymphocyte ratio and lactate dehydrogenase as biomarkers for urothelial cancer treated with immunotherapy. Clin Trans Oncol (2020) 22(11):2130–5. doi: 10.1007/s12094-020-02337-3
31. Kargl J, Busch SE, Yang GH, Kim K-H, Hanke ML, Metz HE, et al. Neutrophils dominate the immune cell composition in non-small cell lung cancer. Nat Commun (2017) 8(1):1–11. doi: 10.1038/ncomms14381
32. Wang Y, Zhai J, Zhang T, Han S, Zhang Y, Yao X, et al. Tumor-associated neutrophils can predict lymph node metastasis in early gastric cancer. Front Oncol (2020) 10:570113. doi: 10.3389/fonc.2020.570113
33. Tian S, Chu Y, Hu J, Ding X, Liu Z, Fu D, et al. Tumour-associated neutrophils secrete AGR2 to promote colorectal cancer metastasis via its receptor CD98hc–xCT. Gut (2022) gutjnl-2021-325137. doi: 10.1136/gutjnl-2021-325137
34. Li T-J, Jiang Y-M, Hu Y-F, Huang L, Yu J, Zhao L-Y, et al. Interleukin-17–producing neutrophils link inflammatory stimuli to disease progression by promoting angiogenesis in gastric CancerInterleukin-17–producing neutrophils promoting angiogenesis. Clin Cancer Res (2017) 23(6):1575–85. doi: 10.1158/1078-0432.CCR-16-0617
35. Tyagi A, Wu S-Y, Sharma S, Wu K, Zhao D, Deshpande R, et al. Exosomal miR-4466 from nicotine-activated neutrophils promotes tumor cell stemness and metabolism in lung cancer metastasis. Oncogene (2022) 41(22):3079–92. doi: 10.1038/s41388-022-02322-w
36. Deryugina E, Carré A, Ardi V, Muramatsu T, Schmidt J, Pham C, et al. Neutrophil elastase facilitates tumor cell intravasation and early metastatic events. Iscience. (2020) 23(12):101799. doi: 10.1016/j.isci.2020.101799
37. Matlung HL, Babes L, Zhao XW, van Houdt M, Treffers LW, van Rees DJ, et al. Neutrophils kill antibody-opsonized cancer cells by trogoptosis. Cell Rep (2018) 23(13):3946–59.e6. doi: 10.1016/j.celrep.2018.05.082
38. Brandsma AM, Bondza S, Evers M, Koutstaal R, Nederend M, Jansen J, et al. Potent fc receptor signaling by IgA leads to superior killing of cancer cells by neutrophils compared to IgG. Front Immunol (2019) 10:704. doi: 10.3389/fimmu.2019.00704
39. Cortez-Retamozo V, Etzrodt M, Newton A, Rauch PJ, Chudnovskiy A, Berger C, et al. Origins of tumor-associated macrophages and neutrophils. Proc Natl Acad Sci (2012) 109(7):2491–6. doi: 10.1073/pnas.1113744109
40. Kim J, Bae J-S. Tumor-associated macrophages and neutrophils in tumor microenvironment. Mediators Inflam (2016) 2016:6058147. doi: 10.1155/2016/6058147
41. Piccard H, Muschel R, Opdenakker G. On the dual roles and polarized phenotypes of neutrophils in tumor development and progression. Crit Rev oncol/hematol (2012) 82(3):296–309. doi: 10.1016/j.critrevonc.2011.06.004
42. Flavell RA, Sanjabi S, Wrzesinski SH, Licona-Limón P. The polarization of immune cells in the tumour environment by TGFβ. Nat Rev Immunol (2010) 10(8):554–67. doi: 10.1038/nri2808
43. Pylaeva E, Lang S, Jablonska J. The essential role of type I interferons in differentiation and activation of tumor-associated neutrophils. Front Immunol (2016) 7:629. doi: 10.3389/fimmu.2016.00629
44. Giuliano JS Jr., Lahni PM, Wong HR, Wheeler DS. Pediatric sepsis–part V: extracellular heat shock proteins: alarmins for the host immune system. Open Inflammation J (2011) 4:49. doi: 10.2174/1875041901104010049
45. Wheeler DS, Chase MA, Senft AP, Poynter SE, Wong HR, Page K. Extracellular Hsp72, an endogenous DAMP, is released by virally infected airway epithelial cells and activates neutrophils via toll-like receptor (TLR)-4. Respir Res (2009) 10(1):1–13. doi: 10.1186/1465-9921-10-31
46. Garcia-Culebras A, Duran-Laforet V, Pena-Martinez C, Moraga A, Ballesteros I, Cuartero MI, et al. Role of TLR4 (toll-like receptor 4) in N1/N2 neutrophil programming after stroke. Stroke (2019) 50(10):2922–32. doi: 10.1161/STROKEAHA.119.025085
47. Durán-Laforet V, Peña-Martínez C, García-Culebras A, Cuartero MI, Lo EH, Moro MÁ, et al. Role of TLR4 in neutrophil dynamics and functions: Contribution to stroke pathophysiology. Front Immunol (2021) 4380. doi: 10.3389/fimmu.2021.757872
48. SenGupta S, Hein LE, Xu Y, Zhang J, Konwerski JR, Li Y, et al. Triple-negative breast cancer cells recruit neutrophils by secreting TGF-β and CXCR2 ligands. Front Immunol (2021) 12:659996. doi: 10.3389/fimmu.2021.659996
49. Haider C, Hnat J, Wagner R, Huber H, Timelthaler G, Grubinger M, et al. Transforming growth factor-β and axl induce CXCL5 and neutrophil recruitment in hepatocellular carcinoma. Hepatology (2019) 69(1):222–36. doi: 10.1002/hep.30166
50. Li D, Ji S, Cao B, Shao J, Zhao R, Li H, et al. The significance of neutrophil extracellular traps in colorectal cancer and beyond: From bench to bedside. Front Oncol (2022) 11:12. doi: 10.3389/fonc.2022.848594
51. Qi Y, Liao D, Mei D, Zhang Y, Liu Y. Elevated neutrophil-to-lymphocyte ratio is associated with poor outcomes for melanoma patients treated with PD-1 inhibitor or chemotherapy in a Chinese population. Front Oncol (2020) 10:1752. doi: 10.3389/fonc.2020.01752
52. Jackstadt R, van Hooff SR, Leach JD, Cortes-Lavaud X, Lohuis JO, Ridgway RA, et al. Epithelial NOTCH signaling rewires the tumor microenvironment of colorectal cancer to drive poor-prognosis subtypes and metastasis. Cancer Cell (2019) 36(3):319–36.e7. doi: 10.1016/j.ccell.2019.08.003
53. Simoncello F, Piperno GM, Caronni N, Amadio R, Cappelletto A, Canarutto G, et al. CXCL5-mediated accumulation of mature neutrophils in lung cancer tissues impairs the differentiation program of anticancer CD8 T cells and limits the efficacy of checkpoint inhibitors. Oncoimmunology (2022) 11(1):2059876. doi: 10.1080/2162402X.2022.2059876
54. Safarulla S, Madan A, Xing F, Chandrasekaran A. CXCR2 mediates distinct neutrophil behavior in brain metastatic breast tumor. Cancers. (2022) 14(3):515. doi: 10.3390/cancers14030515
55. Zhang Y, Chandra V, Riquelme Sanchez E, Dutta P, Quesada PR, Rakoski A, et al. Interleukin-17–induced neutrophil extracellular traps mediate resistance to checkpoint blockade in pancreatic cancer. J Exp Med (2020) 217(12):e20190354. doi: 10.1084/jem.20190354
56. Chen Y, Li Y, Guo H, Zhang Z, Zhang J, Dong X, et al. The effects of adoptively transferred IL-23/IL-18-Polarized neutrophils on tumor and collagen-induced arthritis in mice. J Inflammation Res (2021) 14:4669. doi: 10.2147/JIR.S329528
57. Tang D, Zhang D, Heng Y, Zhu X-K, Lin H-Q, Zhou J, et al. Tumor-infiltrating PD-L1+ neutrophils induced by GM-CSF suppress T cell function in laryngeal squamous cell carcinoma and predict unfavorable prognosis. J Inflammation Res (2022) 15:1079. doi: 10.2147/JIR.S347777
58. Xu Q, Ma H, Chang H, Feng Z, Zhang C, Yang X. The interaction of interleukin-8 and PTEN inactivation promotes the malignant progression of head and neck squamous cell carcinoma via the STAT3 pathway. Cell Death Dis (2020) 11(5):1–14. doi: 10.1038/s41419-020-2627-5
59. Guglietta S, Chiavelli A, Zagato E, Krieg C, Gandini S, Ravenda P, et al. Coagulation induced by C3aR-dependent NETosis drives protumorigenic neutrophils during small intestinal tumorigenesis. Nat Commun (2016) 7:11037. doi: 10.1038/ncomms11037
60. Wang Y, Chen J, Yang L, Li J, Wu W, Huang M, et al. Tumor-contacted neutrophils promote metastasis by a CD90-TIMP-1 juxtacrine–paracrine LoopNeutrophils induce EMT by a CD90-TIMP-1 loop. Clin Cancer Res (2019) 25(6):1957–69. doi: 10.1158/1078-0432.CCR-18-2544
61. Qin G, Luo M, Chen J, Dang Y, Chen G, Li L, et al. Reciprocal activation between MMP-8 and TGF-β1 stimulates EMT and malignant progression of hepatocellular carcinoma. Cancer Let (2016) 374(1):85–95. doi: 10.1016/j.canlet.2016.02.001
62. Charles KA, Kulbe H, Soper R, Escorcio-Correia M, Lawrence T, Schultheis A, et al. The tumor-promoting actions of TNF-α involve TNFR1 and IL-17 in ovarian cancer in mice and humans. J Clin Invest (2009) 119(10):3011–23. doi: 10.1172/JCI39065
63. Jablonska J, Wu CF, Andzinski L, Leschner S, Weiss S. CXCR2-mediated tumor-associated neutrophil recruitment is regulated by IFN-β. Int J cancer (2014) 134(6):1346–58. doi: 10.1002/ijc.28551
64. Cheng Y, X-l Ma, Wei Y-Q, Wei X-W. Potential roles and targeted therapy of the CXCLs/CXCR2 axis in cancer and inflammatory diseases. Biochim Biophys Acta (BBA)-Reviews Cancer (2019) 1871(2):289–312. doi: 10.1016/j.bbcan.2019.01.005
65. Fousek K, Horn LA, Palena C. Interleukin-8: A chemokine at the intersection of cancer plasticity, angiogenesis, and immune suppression. Pharmacol Ther (2021) 219:107692. doi: 10.1016/j.pharmthera.2020.107692
66. Alfaro C, Sanmamed MF, Rodríguez-Ruiz ME, Teijeira Á, Oñate C, González Á, et al. Interleukin-8 in cancer pathogenesis, treatment and follow-up. Cancer Treat Rev (2017) 60:24–31. doi: 10.1016/j.ctrv.2017.08.004
67. Liu K, Wu L, Yuan S, Wu M, Xu Y, Sun Q, et al. Structural basis of CXC chemokine receptor 2 activation and signalling. Nature. (2020) 585(7823):135–40. doi: 10.1038/s41586-020-2492-5
68. Capucetti A, Albano F, Bonecchi R. Multiple roles for chemokines in neutrophil biology. Front Immunol (2020) 11:1259. doi: 10.3389/fimmu.2020.01259
69. Steele CW, Karim SA, Leach JD, Bailey P, Upstill-Goddard R, Rishi L, et al. CXCR2 inhibition profoundly suppresses metastases and augments immunotherapy in pancreatic ductal adenocarcinoma. Cancer Cell (2016) 29(6):832–45. doi: 10.1016/j.ccell.2016.04.014
70. Gong L, Cumpian AM, Caetano MS, Ochoa CE, de la Garza MM, Lapid DJ, et al. Promoting effect of neutrophils on lung tumorigenesis is mediated by CXCR2 and neutrophil elastase. Mol Cancer (2013) 12(1):1–14. doi: 10.1186/1476-4598-12-154
71. Liao W, Overman MJ, Boutin AT, Shang X, Zhao D, Dey P, et al. KRAS-IRF2 axis drives immune suppression and immune therapy resistance in colorectal cancer. Cancer Cell (2019) 35(4):559–72.e7. doi: 10.1016/j.ccell.2019.02.008
72. Shang A, Gu C, Zhou C, Yang Y, Chen C, Zeng B, et al. Exosomal KRAS mutation promotes the formation of tumor-associated neutrophil extracellular traps and causes deterioration of colorectal cancer by inducing IL-8 expression. Cell Communication Signaling (2020) 18(1):1–14. doi: 10.1186/s12964-020-0517-1
73. Liu L, Liu Y, Yan X, Zhou C, Xiong X. The role of granulocyte colony−stimulating factor in breast cancer development: a review. Mol Med Rep (2020) 21(5):2019–29. doi: 10.3892/mmr.2020.11017
74. Theyab A, Algahtani M, Alsharif KF, Hawsawi YM, Alghamdi A, Alghamdi A, et al. New insight into the mechanism of granulocyte colony-stimulating factor (G-CSF) that induces the mobilization of neutrophils. Hematology (2021) 26(1):628–36. doi: 10.1080/16078454.2021.1965725
75. Shan ZG, Chen J, Liu JS, Zhang JY, Wang TT, Teng YS, et al. Activated neutrophils polarize protumorigenic interleukin-17A-producing T helper subsets through TNF-α-B7-H2-dependent pathway in human gastric cancer. Clin Trans Med (2021) 11(6):e484. doi: 10.1002/ctm2.484
76. Bellomo G, Rainer C, Quaranta V, Astuti Y, Raymant M, Boyd E, et al. Chemotherapy-induced infiltration of neutrophils promotes pancreatic cancer metastasis via Gas6/AXL signalling axis. Gut (2022) gutjnl-2021-325272. doi: 10.1136/gutjnl-2021-325272
77. Nagaoka K, Shirai M, Taniguchi K, Hosoi A, Sun C, Kobayashi Y, et al. Deep immunophenotyping at the single-cell level identifies a combination of anti-IL-17 and checkpoint blockade as an effective treatment in a preclinical model of data-guided personalized immunotherapy. J immunother Cancer (2020) 8(2):e001358. doi: 10.1136/jitc-2020-001358
78. Thiam HR, Wong SL, Wagner DD, Waterman CM. Cellular mechanisms of NETosis. Annu Rev Cell Dev Biol (2020) 36:191–218. doi: 10.1146/annurev-cellbio-020520-111016
79. Schönrich G, Raftery MJ, Samstag Y. Devilishly radical NETwork in COVID-19: Oxidative stress, neutrophil extracellular traps (NETs), and T cell suppression. Adv Biol regulation (2020) 77:100741. doi: 10.1016/j.jbior.2020.100741
80. Tohme S, Yazdani HO, Al-Khafaji AB, Chidi AP, Loughran P, Mowen K, et al. Neutrophil extracellular traps promote the development and progression of liver metastases after surgical stress. Cancer Res (2016) 76(6):1367–80. doi: 10.1158/0008-5472.CAN-15-1591
81. Nie M, Yang L, Bi X, Wang Y, Sun P, Yang H, et al. Neutrophil extracellular traps induced by IL8 promote diffuse large b-cell lymphoma progression via the TLR9 signaling. Clin Cancer Res (2019) 25(6):1867–79. doi: 10.1158/1078-0432.CCR-18-1226
82. Alfaro C, Teijeira A, Oñate C, Pérez G, Sanmamed MF, Andueza MP, et al. Tumor-produced interleukin-8 attracts human myeloid-derived suppressor cells and elicits extrusion of neutrophil extracellular traps (NETs). Clin Cancer Res (2016) 22(15):3924–36. doi: 10.1158/1078-0432.CCR-15-2463
83. Lee W, Ko SY, Mohamed MS, Kenny HA, Lengyel E, Naora H. Neutrophils facilitate ovarian cancer premetastatic niche formation in the omentum. J Exp Med (2019) 216(1):176–94. doi: 10.1084/jem.20181170
84. Yang L, Liu Q, Zhang X, Liu X, Zhou B, Chen J, et al. DNA Of neutrophil extracellular traps promotes cancer metastasis via CCDC25. Nature. (2020) 583(7814):133–8. doi: 10.1038/s41586-020-2394-6
85. Boone BA, Orlichenko L, Schapiro NE, Loughran P, Gianfrate GC, Ellis JT, et al. The receptor for advanced glycation end products (RAGE) enhances autophagy and neutrophil extracellular traps in pancreatic cancer. Cancer Gene Ther (2015) 22(6):326–34. doi: 10.1038/cgt.2015.21
86. Li M, Lin C, Deng H, Strnad J, Bernabei L, Vogl DT, et al. A novel peptidylarginine deiminase 4 (PAD4) inhibitor BMS-P5 blocks formation of neutrophil extracellular traps and delays progression of multiple myeloma. Mol Cancer Ther (2020) 19(7):1530–8. doi: 10.1158/1535-7163.MCT-19-1020
87. de Andrea CE, Ochoa MC, Villalba-Esparza M, Teijeira Á, Schalper KA, Abengozar-Muela M, et al. Heterogenous presence of neutrophil extracellular traps in human solid tumours is partially dependent on IL-8. J Pathol (2021) 255(2):190–201. doi: 10.1002/path.5753
88. Cedervall J, Zhang Y, Olsson A-K. Tumor-induced NETosis as a risk factor for metastasis and organ failure. Cancer Res (2016) 76(15):4311–5. doi: 10.1158/0008-5472.CAN-15-3051
89. Ravindran M, Khan MA, Palaniyar N. Neutrophil extracellular trap formation: physiology, pathology, and pharmacology. Biomolecules. (2019) 9(8):365. doi: 10.3390/biom9080365
90. Demers M, Wong SL, Martinod K, Gallant M, Cabral JE, Wang Y, et al. Priming of neutrophils toward NETosis promotes tumor growth. Oncoimmunology. (2016) 5(5):e1134073. doi: 10.1080/2162402X.2015.1134073
91. Yang L-Y, Luo Q, Lu L, Zhu W-W, Sun H-T, Wei R, et al. Increased neutrophil extracellular traps promote metastasis potential of hepatocellular carcinoma via provoking tumorous inflammatory response. J Hematol Oncol (2020) 13(1):1–15. doi: 10.1186/s13045-019-0836-0
92. Xiao Y, Cong M, Li J, He D, Wu Q, Tian P, et al. Cathepsin c promotes breast cancer lung metastasis by modulating neutrophil infiltration and neutrophil extracellular trap formation. Cancer Cell (2021) 39(3):423–37. e7. doi: 10.1016/j.ccell.2020.12.012
93. Teijeira Á, Garasa S, Gato M, Alfaro C, Migueliz I, Cirella A, et al. CXCR1 and CXCR2 chemokine receptor agonists produced by tumors induce neutrophil extracellular traps that interfere with immune cytotoxicity. Immunity. (2020) 52(5):856–71. e8. doi: 10.1016/j.immuni.2020.03.001
94. Hedrick CC, Malanchi I. Neutrophils in cancer: heterogeneous and multifaceted. Nat Rev Immunol (2021) 52(5)1–15. doi: 10.1016/j.immuni.2020.03.001
95. Albrengues J, Shields MA, Ng D, Park CG, Ambrico A, Poindexter ME, et al. Neutrophil extracellular traps produced during inflammation awaken dormant cancer cells in mice. Science (2018) 361(6409):eaao4227. doi: 10.1126/science.aao4227
96. Yazdani HO, Roy E, Comerci AJ, van der Windt DJ, Zhang H, Huang H, et al. Neutrophil extracellular traps drive mitochondrial homeostasis in tumors to augment growth. Cancer Res (2019) 79(21):5626–39. doi: 10.1158/0008-5472.CAN-19-0800
97. Fares J, Fares MY, Khachfe HH, Salhab HA, Fares Y. Molecular principles of metastasis: a hallmark of cancer revisited. Signal Transduct Targeted Ther (2020) 5(1):1–17. doi: 10.1038/s41392-020-0134-x
98. Jiang Z-Z, Peng Z-P, Liu X-C, Guo H-F, Zhou M-M, Jiang D, et al. Neutrophil extracellular traps induce tumor metastasis through dual effects on cancer and endothelial cells. Oncoimmunology. (2022) 11(1):2052418. doi: 10.1080/2162402X.2022.2052418
99. Xia X, Zhang Z, Zhu C, Ni B, Wang S, Yang S, et al. Neutrophil extracellular traps promote metastasis in gastric cancer patients with postoperative abdominal infectious complications. Nat Commun (2022) 13(1):1–14. doi: 10.1038/s41467-022-28492-5
100. Park J, Wysocki RW, Amoozgar Z, Maiorino L, Fein MR, Jorns J, et al. Cancer cells induce metastasis-supporting neutrophil extracellular DNA traps. Sci Trans Med (2016) 8(361):361ra138–361ra138. doi: 10.1126/scitranslmed.aag1711
101. Yin H, Lu H, Xiong Y, Ye L, Teng C, Cao X, et al. Tumor-associated neutrophil extracellular traps regulating nanocarrier-enhanced inhibition of malignant tumor growth and distant metastasis. ACS Appl Mater Interfaces (2021) 13(50):59683–94. doi: 10.1021/acsami.1c18660
102. Aiello NM, Maddipati R, Norgard RJ, Balli D, Li J, Yuan S, et al. EMT subtype influences epithelial plasticity and mode of cell migration. Dev Cell (2018) 45(6):681–95.e4. doi: 10.1016/j.devcel.2018.05.027
103. Hedrick CC, Malanchi I. Neutrophils in cancer: Heterogeneous and multifaceted. Nat Rev Immunol (2022) 22(3):173–87. doi: 10.1038/s41577-021-00571-6
104. Yazdani HO, Roy E, Comerci AJ, van der Windt DJ, Zhang H, Huang H, et al. Neutrophil extracellular traps drive mitochondrial homeostasis in tumors to augment GrowthNETs promote tumor growth by altering mitochondrial function. Cancer Res (2019) 79(21):5626–39. doi: 10.1158/0008-5472.CAN-19-0800
105. Koo Y-J, Kim T-J, Min K-J, So K-A, Jung U-S, Hong J-H. CXCL11 mediates TWIST1-induced angiogenesis in epithelial ovarian cancer. Tumor Biol (2017) 39(5):1010428317706226. doi: 10.1177/1010428317706226
106. Low-Marchelli JM, Ardi VC, Vizcarra EA, Van Rooijen N, Quigley JP, Yang J. Twist1 induces CCL2 and recruits macrophages to promote angiogenesis. Cancer Res (2013) 73(2):662–71. doi: 10.1158/0008-5472.CAN-12-0653
107. Hsu DS-S, Wang H-J, Tai S-K, Chou C-H, Hsieh C-H, Chiu P-H, et al. Acetylation of snail modulates the cytokinome of cancer cells to enhance the recruitment of macrophages. Cancer Cell (2014) 26(4):534–48. doi: 10.1016/j.ccell.2014.09.002
108. SenGupta S, Hein LE, Parent CA. The recruitment of neutrophils to the tumor microenvironment is regulated by multiple mediators. Front Immunol (2021) 12. doi: 10.3389/fimmu.2021.734188
109. Hu X, Xiang F, Feng Y, Gao F, Ge S, Wang C, et al. Neutrophils promote tumor progression in oral squamous cell carcinoma by regulating EMT and JAK2/STAT3 signaling through chemerin. Front Oncol (2022) 12. doi: 10.3389/fonc.2022.812044
110. Zhu T, Zou X, Yang C, Li L, Wang B, Li R, et al. Neutrophil extracellular traps promote gastric cancer metastasis by inducing epithelial−mesenchymal transition. Int J Mol Med (2021) 48(1):1–13. doi: 10.1126/scitranslmed.aag1711
111. Ribatti D, Tamma R, Annese T. Epithelial-mesenchymal transition in cancer: a historical overview. Trans Oncol (2020) 13(6):100773. doi: 10.1016/j.tranon.2020.100773
112. Li S, Cong X, Gao H, Lan X, Li Z, Wang W, et al. Tumor-associated neutrophils induce EMT by IL-17a to promote migration and invasion in gastric cancer cells. J Exp Clin Cancer Res (2019) 38(1):1–13. doi: 10.3389/fonc.2022.812044
113. Mao Z, Zhang J, Shi Y, Li W, Shi H, Ji R, et al. CXCL5 promotes gastric cancer metastasis by inducing epithelial-mesenchymal transition and activating neutrophils. Oncogenesis. (2020) 9(7):1–14. doi: 10.1038/s41389-020-00249-z
114. Wang Y, Chen J, Yang L, Li J, Wu W, Huang M, et al. Tumor-contacted neutrophils promote metastasis by a CD90-TIMP-1 juxtacrine–paracrine loop. Clin Cancer Res (2019) 25(6):1957–69. doi: 10.1158/1078-0432.CCR-18-2544
115. Nywening TM, Belt BA, Cullinan DR, Panni RZ, Han BJ, Sanford DE, et al. Targeting both tumour-associated CXCR2+ neutrophils and CCR2+ macrophages disrupts myeloid recruitment and improves chemotherapeutic responses in pancreatic ductal adenocarcinoma. Gut. (2018) 67(6):1112–23. doi: 10.1136/gutjnl-2017-313738
116. Capone M, Giannarelli D, Mallardo D, Madonna G, Festino L, Grimaldi AM, et al. Baseline neutrophil-to-lymphocyte ratio (NLR) and derived NLR could predict overall survival in patients with advanced melanoma treated with nivolumab. J Immunother Cancer (2018) 6(1):1–7. doi: 10.1186/s40425-018-0383-1
117. Russo A, Franchina T, Ricciardi GR, Battaglia A, Scimone A, Berenato R, et al. Baseline neutrophilia, derived neutrophil-to-lymphocyte ratio (dNLR), platelet-to-lymphocyte ratio (PLR), and outcome in non small cell lung cancer (NSCLC) treated with nivolumab or docetaxel. J Cell Physiol (2018) 233(10):6337–43. doi: 10.1002/jcp.26609
118. Wang H, Zhang B, Li R, Chen J, Xu G, Zhu Y, et al. KIAA1199 drives immune suppression to promote colorectal cancer liver metastasis by modulating neutrophil infiltration. Hepatology (2022). doi: 10.1002/hep.32383
119. Liao Y, Huang J, Liu P, Zhang C, Liu J, Xia M, et al. Downregulation of LNMAS orchestrates partial EMT and immune escape from macrophage phagocytosis to promote lymph node metastasis of cervical cancer. Oncogene (2022) 41(13):1931–43. doi: 10.1038/s41388-022-02202-3
120. Gooding AJ, Schiemann WP. Epithelial–mesenchymal transition programs and cancer stem cell phenotypes: Mediators of breast cancer therapy ResistanceEMT programs and CSC chemoresistance. Mol Cancer Res (2020) 18(9):1257–70. doi: 10.1158/1541-7786.MCR-20-0067
121. Guo Y, Lu X, Chen Y, Rendon B, Mitchell RA, Cuatrecasas M, et al. Zeb1 induces immune checkpoints to form an immunosuppressive envelope around invading cancer cells. Sci Adv (2021) 7(21):eabd7455. doi: 10.1126/sciadv.abd7455
122. Meier SL, Satpathy AT, Wells DK. Bystander T cells in cancer immunology and therapy. Nat Cancer (2022) 3(2):143–55. doi: 10.1038/s43018-022-00335-8
123. Han J, Zhao Y, Shirai K, Molodtsov A, Kolling FW, Fisher JL, et al. Resident and circulating memory T cells persist for years in melanoma patients with durable responses to immunotherapy. Nat Cancer (2021) 2(3):300–11. doi: 10.1038/s43018-021-00180-1
124. Topalian SL, Taube JM, Pardoll DM. Neoadjuvant checkpoint blockade for cancer immunotherapy. Science (2020) 367(6477):eaax0182. doi: 10.1126/science.aax0182
125. Moses K, Brandau S. Human neutrophils: their role in cancer and relation to myeloid-derived suppressor cells. Semin Immunol (2016) 28(2):187–196. doi: 10.1016/j.smim.2016.03.018
126. Scapini P, Marini O, Tecchio C, Cassatella MA. Human neutrophils in the saga of cellular heterogeneity: insights and open questions. Immunol Rev (2016) 273(1):48–60. doi: 10.1111/imr.12448
127. Buddhisa S, Rinchai D, Ato M, Bancroft GJ, Lertmemongkolchai G. Programmed death ligand 1 on burkholderia pseudomallei–infected human polymorphonuclear neutrophils impairs T cell functions. J Immunol (2015) 194(9):4413–21. doi: 10.4049/jimmunol.1402417
128. He G, Zhang H, Zhou J, Wang B, Chen Y, Kong Y, et al. Peritumoural neutrophils negatively regulate adaptive immunity via the PD-L1/PD-1 signalling pathway in hepatocellular carcinoma. J Exp Clin Cancer Res (2015) 34(1):1–11. doi: 10.1186/s13046-015-0256-0
129. Yang M, Zhang G, Wang Y, He M, Xu Q, Lu J, et al. Tumour-associated neutrophils orchestrate intratumoural IL-8-driven immune evasion through Jagged2 activation in ovarian cancer. Br J cancer (2020) 123(9):1404–16. doi: 10.1038/s41416-020-1026-0
130. Germann M, Zangger N, Sauvain MO, Sempoux C, Bowler AD, Wirapati P, et al. Neutrophils suppress tumor-infiltrating T cells in colon cancer via matrix metalloproteinase-mediated activation of TGF β. EMBO Mol Med (2020) 12(1):e10681. doi: 10.15252/emmm.201910681
131. Minns D, Smith KJ, Hardisty G, Rossi AG, Gwyer Findlay E. The outcome of neutrophil-T cell contact differs depending on activation status of both cell types. Front Immunol (2021) 12:1049. doi: 10.3389/fimmu.2021.633486
132. Mócsai A. Diverse novel functions of neutrophils in immunity, inflammation, and beyond. J Exp Med (2013) 210(7):1283–99. doi: 10.1084/jem.20122220
133. Scapini P, Cassatella MA. Social networking of human neutrophils within the immune system. Blood J Am Soc Hematol (2014) 124(5):710–9. doi: 10.1182/blood-2014-03-453217
134. Kalyan S, Kabelitz D. When neutrophils meet T cells: beginnings of a tumultuous relationship with underappreciated potential. Eur J Immunol (2014) 44(3):627–33. doi: 10.1002/eji.201344195
135. Liu C-Y, Wang Y-M, Wang C-L, Feng P-H, Ko H-W, Liu Y-H, et al. Population alterations of l-arginase-and inducible nitric oxide synthase-expressed CD11b+/CD14–/CD15+/CD33+ myeloid-derived suppressor cells and CD8+ T lymphocytes in patients with advanced-stage non-small cell lung cancer. J Cancer Res Clin Oncol (2010) 136(1):35–45. doi: 10.1007/s00432-009-0634-0
136. Wilson NJ, Boniface K, Chan JR, McKenzie BS, Blumenschein WM, Mattson JD, et al. Development, cytokine profile and function of human interleukin 17–producing helper T cells. Nat Immunol (2007) 8(9):950–7. doi: 10.1038/ni1497
137. Karpisheh V, Ahmadi M, Abbaszadeh-Goudarzi K, Mohammadpour Saray M, Barshidi A, Mohammadi H, et al. The role of Th17 cells in the pathogenesis and treatment of breast cancer. Cancer Cell Int (2022) 22(1):1–13. doi: 10.1186/s12935-022-02528-8
138. Abi Abdallah DS, Egan CE, Butcher BA, Denkers EY. Mouse neutrophils are professional antigen-presenting cells programmed to instruct Th1 and Th17 T-cell differentiation. Int Immunol (2011) 23(5):317–26. doi: 10.1093/intimm/dxr007
139. Tecchio C, Micheletti A, Cassatella MA. Neutrophil-derived cytokines: facts beyond expression. Front Immunol (2014) 5:508. doi: 10.3389/fimmu.2014.00508
140. Coffelt SB, Kersten K, Doornebal CW, Weiden J, Vrijland K, Hau C-S, et al. IL-17-producing γδ T cells and neutrophils conspire to promote breast cancer metastasis. Nature. (2015) 522(7556):345–8. doi: 10.1038/nature14282
141. Liu X, Hogg GD, DeNardo DG. Rethinking immune checkpoint blockade:’Beyond the T cell’. J ImmunoTher Cancer (2021) 9(1):e001460. doi: 10.1136/jitc-2020-001460
142. Cabrita R, Lauss M, Sanna A, Donia M, Skaarup Larsen M, Mitra S, et al. Tertiary lymphoid structures improve immunotherapy and survival in melanoma. Nature. (2020) 577(7791):561–5. doi: 10.1038/s41586-019-1914-8
143. Kim SS, Sumner WA, Miyauchi S, Cohen EE, Califano JA, Sharabi AB. Role of b cells in responses to checkpoint blockade immunotherapy and overall survival of cancer patients. Clin Cancer Res (2021) 27(22):6075–82. doi: 10.1158/1078-0432.CCR-21-0697
144. Sharonov GV, Serebrovskaya EO, Yuzhakova DV, Britanova OV, Chudakov DM. B cells, plasma cells and antibody repertoires in the tumour microenvironment. Nat Rev Immunol (2020) 20(5):294–307. doi: 10.1038/s41577-019-0257-x
145. Lee DS, Rojas OL, Gommerman JL. B cell depletion therapies in autoimmune disease: advances and mechanistic insights. Nat Rev Drug Discovery (2021) 20(3):179–99. doi: 10.1038/s41573-020-00092-2
146. Yang B, Zhang Z, Chen X, Wang X-Y, Qin S, Du L, et al. An Asian-specific variant in human IgG1 represses colorectal tumorigenesis by shaping the tumor microenvironment. J Clin Invest (2022) 132(6):e153454. doi: 10.1172/JCI153454
147. Akrami M, Menzies R, Chamoto K, Miyajima M, Suzuki R, Sato H, et al. Circulation of gut-preactivated naïve CD8+ T cells enhances antitumor immunity in b cell-defective mice. Proc Natl Acad Sci (2020) 117(38):23674–83. doi: 10.1073/pnas.2010981117
148. Zhang B, Vogelzang A, Miyajima M, Sugiura Y, Wu Y, Chamoto K, et al. B cell-derived GABA elicits IL-10+ macrophages to limit anti-tumour immunity. Nature. (2021) 599(7885):471–6. doi: 10.1038/s41586-021-04082-1
149. Wang X, Wang G, Wang Z, Liu B, Han N, Li J, et al. PD-1-expressing b cells suppress CD4+ and CD8+ T cells via PD-1/PD-L1-dependent pathway. Mol Immunol (2019) 109:20–6. doi: 10.1016/j.molimm.2019.02.009
150. Das R, Bar N, Ferreira M, Newman AM, Zhang L, Bailur JK, et al. Early b cell changes predict autoimmunity following combination immune checkpoint blockade. J Clin Invest (2018) 128(2):715–20. doi: 10.1172/JCI96798
151. Guan H, Lan Y, Wan Y, Wang Q, Wang C, Xu L, et al. PD-L1 mediated the differentiation of tumor-infiltrating CD19+ b lymphocytes and T cells in invasive breast cancer. Oncoimmunology. (2016) 5(2):e1075112. doi: 10.1080/2162402X.2015.1075112
152. Helmink BA, Reddy SM, Gao J, Zhang S, Basar R, Thakur R, et al. B cells and tertiary lymphoid structures promote immunotherapy response. Nature. (2020) 577(7791):549–55. doi: 10.1038/s41586-019-1922-8
153. Scapini P, Nardelli B, Nadali G, Calzetti F, Pizzolo G, Montecucco C, et al. G-CSF–stimulated neutrophils are a prominent source of functional BLyS. J Exp Med (2003) 197(3):297–302. doi: 10.1084/jem.20021343
154. Scapini P, Bazzoni F, Cassatella MA. Regulation of b-cell-activating factor (BAFF)/B lymphocyte stimulator (BLyS) expression in human neutrophils. Immunol let (2008) 116(1):1–6. doi: 10.1016/j.imlet.2007.11.009
155. Huard B, McKee T, Bosshard C, Durual S, Matthes T, Myit S, et al. APRIL secreted by neutrophils binds to heparan sulfate proteoglycans to create plasma cell niches in human mucosa. J Clin Invest (2008) 118(8):2887–95. doi: 10.1172/JCI33760
156. Schwaller J, Schneider P, Mhawech-Fauceglia P, McKee T, Myit S, Matthes T, et al. Neutrophil-derived APRIL concentrated in tumor lesions by proteoglycans correlates with human b-cell lymphoma aggressiveness. Blood. (2007) 109(1):331–8. doi: 10.1182/blood-2006-02-001800
157. Manfroi B, McKee T, Mayol JF, Tabruyn S, Moret S, Villiers C, et al. CXCL-8/IL8 produced by diffuse large b-cell lymphomas recruits neutrophils expressing a proliferation-inducing ligand APRIL. Cancer Res (2017) 77(5):1097–107. doi: 10.1158/0008-5472.CAN-16-0786
158. Gätjen M, Brand F, Grau M, Gerlach K, Kettritz R, Westermann J, et al. Splenic marginal zone granulocytes acquire an accentuated neutrophil b-cell helper phenotype in chronic lymphocytic leukemia. Cancer Res (2016) 76(18):5253–65. doi: 10.1158/0008-5472.CAN-15-3486
159. Sangaletti S, Tripodo C, Portararo P, Dugo M, Vitali C, Botti L, et al. Stromal niche communalities underscore the contribution of the matricellular protein SPARC to b-cell development and lymphoid malignancies. Oncoimmunology. (2014) 3(6):e28989. doi: 10.4161/onci.28989
160. Petitprez F, de Reyniès A, Keung EZ, Chen TW-W, Sun C-M, Calderaro J, et al. B cells are associated with survival and immunotherapy response in sarcoma. Nature (2020) 577(7791):556–60. doi: 10.1038/s41586-019-1906-8
161. Shaul ME, Zlotnik A, Tidhar E, Schwartz A, Arpinati L, Kaisar-Iluz N, et al. Tumor-associated neutrophils drive b-cell recruitment and their differentiation to plasma cells. Cancer Immunol Res (2021) 9(7):811–24. doi: 10.1158/2326-6066.CIR-20-0839
162. Chen Z, Zhu Y, Du R, Pang N, Zhang F, Dong D, et al. Role of regulatory b cells in the progression of cervical cancer. Mediators Inflammation (2019) 2019:6519427. doi: 10.1155/2019/6519427
163. Wang X, Li J, Lu C, Wang G, Wang Z, Liu X, et al. IL-10-producing b cells in differentiated thyroid cancer suppress the effector function of T cells but improve their survival upon activation. Exp Cell Res (2019) 376(2):192–7. doi: 10.1016/j.yexcr.2019.01.021
164. Inoue S, Leitner WW, Golding B, Scott D. Inhibitory effects of b cells on antitumor immunity. Cancer Res (2006) 66(15):7741–7. doi: 10.1158/0008-5472.CAN-05-3766
165. Kim S, Fridlender ZG, Dunn R, Kehry MR, Kapoor V, Blouin A, et al. B-cell depletion using an anti-CD20 antibody augments antitumor immune responses and immunotherapy in nonhematopoetic murine tumor models. J Immunother (2008) 31(5):446–57. doi: 10.1097/CJI.0b013e31816d1d6a
166. Choi JK, Yu C-R, Bing SJ, Jittayasothorn Y, Mattapallil MJ, Kang M, et al. IL-27–producing b-1a cells suppress neuroinflammation and CNS autoimmune diseases. Proc Natl Acad Sci (2021) 118(47):e2109548118. doi: 10.1073/pnas.2109548118
167. Peng B, Ming Y, Yang C. Regulatory b cells: the cutting edge of immune tolerance in kidney transplantation. Cell Death dis (2018) 9(2):1–13. doi: 10.1038/s41419-017-0152-y
168. Silvestre-Roig C, Fridlender ZG, Glogauer M, Scapini P. Neutrophil diversity in health and disease. Trends Immunol (2019) 40(7):565–83. doi: 10.1016/j.it.2019.04.012
169. Hägglöf T, Sedimbi SK, Yates JL, Parsa R, Salas BH, Harris RA, et al. Neutrophils license iNKT cells to regulate self-reactive mouse b cell responses. Nat Immunol (2016) 17(12):1407–14. doi: 10.1038/ni.3583
170. Palanichamy A, Bauer JW, Yalavarthi S, Meednu N, Barnard J, Owen T, et al. Neutrophil-mediated IFN activation in the bone marrow alters b cell development in human and murine systemic lupus erythematosus. J Immunol (2014) 192(3):906–18. doi: 10.4049/jimmunol.1302112
171. Ruhland MK, Roberts EW, Cai E, Mujal AM, Marchuk K, Beppler C, et al. Visualizing synaptic transfer of tumor antigens among dendritic cells. Cancer Cell (2020) 37(6):786–99. e5. doi: 10.1016/j.ccell.2020.05.002
172. Fang H, Shao S, Xue K, Yuan X, Qiao P, Zhang J, et al. Neutrophil extracellular traps contribute to immune dysregulation in bullous pemphigoid via inducing b-cell differentiation and antibody production. FASEB J (2021) 35(7):e21746. doi: 10.1096/fj.202100145R
173. Manfroi B, McKee T, Mayol JF, Tabruyn S, Moret S, Villiers C, et al. CXCL-8/IL8 produced by diffuse Large b-cell lymphomas recruits neutrophils expressing a proliferation-inducing ligand APRILNeutrophils in b-cell lymphoma. Cancer Res (2017) 77(5):1097–107. doi: 10.1158/0008-5472.CAN-16-0786
174. Souwer Y, Kormelink TG, Taanman-Kueter EW, Muller FJ, van Capel TM, Varga DV, et al. Human TH17 cell development requires processing of dendritic cell–derived CXCL8 by neutrophil elastase. J Allergy Clin Immunol (2018) 141(6):2286–9. e5. doi: 10.1016/j.jaci.2018.01.003
175. Tamassia N, Arruda-Silva F, Wright HL, Moots RJ, Gardiman E, Bianchetto-Aguilera F, et al. Human neutrophils activated via TLR8 promote Th17 polarization through IL-23. J Leukoc Biol (2019) 105(6):1155–65. doi: 10.1002/JLB.MA0818-308R
176. Minns D, Smith KJ, Alessandrini V, Hardisty G, Melrose L, Jackson-Jones L, et al. The neutrophil antimicrobial peptide cathelicidin promotes Th17 differentiation. Nat Commun (2021) 12(1):1–16. doi: 10.1038/s41467-021-21533-5
177. Gardner A, Ruffell B. Dendritic cells and cancer immunity. Trends Immunol (2016) 37(12):855–65. doi: 10.1016/j.it.2016.09.006
178. Böttcher JP, e Sousa CR. The role of type 1 conventional dendritic cells in cancer immunity. Trends cancer (2018) 4(11):784–92. doi: 10.1016/j.trecan.2018.09.001
179. Engelhardt JJ, Boldajipour B, Beemiller P, Pandurangi P, Sorensen C, Werb Z, et al. Marginating dendritic cells of the tumor microenvironment cross-present tumor antigens and stably engage tumor-specific T cells. Cancer Cell (2012) 21(3):402–17. doi: 10.1016/j.ccr.2012.01.008
180. Tecchio C, Cassatella MA. Neutrophil-derived chemokines on the road to immunity. Semin Immunol (2016) 28(2):119128. doi: 10.1016/j.smim.2016.04.003
181. Noubade R, Majri-Morrison S, Tarbell KV. Beyond cDC1: Emerging roles of DC crosstalk in cancer immunity. Front Immunol (2019) 10:1014. doi: 10.3389/fimmu.2019.01014
182. Liu J, Zhang X, Cheng Y, Cao X. Dendritic cell migration in inflammation and immunity. Cell Mol Immunol (2021) 18(11):2461–71. doi: 10.1038/s41423-021-00726-4
183. Beauvillain C, Cunin P, Doni A, Scotet M, Jaillon S, Loiry M-L, et al. CCR7 is involved in the migration of neutrophils to lymph nodes. Blood J Am Soc Hematol (2011) 117(4):1196–204. doi: 10.1182/blood-2009-11-254490
184. Nakano H, Lin KL, Yanagita M, Charbonneau C, Cook DN, Kakiuchi T, et al. Blood-derived inflammatory dendritic cells in lymph nodes stimulate acute T helper type 1 immune responses. Nat Immunol (2009) 10(4):394–402. doi: 10.1038/ni.1707
185. Xu P, Zhang J, Wang H, Wang G, Wang C-Y, Zhang J. CCR2 dependent neutrophil activation and mobilization rely on TLR4-p38 axis during liver ischemia-reperfusion injury. Am J Trans Res (2017) 9(6):2878. doi: 10.1038/s41423-021-00726-4
186. Seth S, Oberdörfer L, Hyde R, Hoff K, Thies V, Worbs T, et al. CCR7 essentially contributes to the homing of plasmacytoid dendritic cells to lymph nodes under steady-state as well as inflammatory conditions. J Immunol (2011) 186(6):3364–72. doi: 10.4049/jimmunol.1002598
187. Lande R, Ganguly D, Facchinetti V, Frasca L, Conrad C, Gregorio J, et al. Neutrophils activate plasmacytoid dendritic cells by releasing self-DNA–peptide complexes in systemic lupus erythematosus. Sci Trans Med (2011) 3(73):73ra19–9. doi: 10.1126/scitranslmed.3001180
188. Riggan L, Shah S, O’Sullivan TE. Arrested development: Suppression of NK cell function in the tumor microenvironment. Clin Trans Immunol (2021) 10(1):e1238. doi: 10.1002/cti2.1238
189. Chiossone L, Dumas P-Y, Vienne M, Vivier E. Natural killer cells and other innate lymphoid cells in cancer. Nat Rev Immunol (2018) 18(11):671–88. doi: 10.1038/s41577-018-0061-z
190. Dogra P, Rancan C, Ma W, Toth M, Senda T, Carpenter DJ, et al. Tissue determinants of human NK cell development, function, and residence. Cell. (2020) 180(4):749–63. e13. doi: 10.1016/j.cell.2020.01.022
191. Huntington ND, Cursons J, Rautela J. The cancer–natural killer cell immunity cycle. Nat Rev Cancer (2020) 20(8):437–54. doi: 10.1038/s41568-020-0272-z
192. Nersesian S, Schwartz SL, Grantham SR, MacLean LK, Lee SN, Pugh-Toole M, et al. NK cell infiltration is associated with improved overall survival in solid cancers: A systematic review and meta-analysis. Trans Oncol (2021) 14(1):100930. doi: 10.1016/j.tranon.2020.100930
193. Roberts EW, Broz ML, Binnewies M, Headley MB, Nelson AE, Wolf DM, et al. Critical role for CD103+/CD141+ dendritic cells bearing CCR7 for tumor antigen trafficking and priming of T cell immunity in melanoma. Cancer Cell (2016) 30(2):324–36. doi: 10.1016/j.ccell.2016.06.003
194. Salmon H, Idoyaga J, Rahman A, Leboeuf M, Remark R, Jordan S, et al. Expansion and activation of CD103+ dendritic cell progenitors at the tumor site enhances tumor responses to therapeutic PD-L1 and BRAF inhibition. Immunity. (2016) 44(4):924–38. doi: 10.1016/j.immuni.2016.03.012
195. Spranger S, Bao R, Gajewski TF. Melanoma-intrinsic β-catenin signalling prevents anti-tumour immunity. Nature. (2015) 523(7559):231–5. doi: 10.1038/nature14404
196. Böttcher JP, Bonavita E, Chakravarty P, Blees H, Cabeza-Cabrerizo M, Sammicheli S, et al. NK cells stimulate recruitment of cDC1 into the tumor microenvironment promoting cancer immune control. Cell. (2018) 172(5):1022–37. e14. doi: 10.1016/j.ccell.2016.06.003
197. Costantini C, Calzetti F, Perbellini O, Micheletti A, Scarponi C, Lonardi S, et al. Human neutrophils interact with both 6-sulfo LacNAc+ DC and NK cells to amplify NK-derived IFNγ: role of CD18, ICAM-1, and ICAM-3. Blood J Am Soc Hematol (2011) 117(5):1677–86. doi: 10.1016/j.immuni.2016.03.012
198. Spiegel A, Brooks MW, Houshyar S, Reinhardt F, Ardolino M, Fessler E, et al. Neutrophils suppress intraluminal NK cell–mediated tumor cell clearance and enhance extravasation of disseminated carcinoma cells. Cancer discovery (2016) 6(6):630–49. doi: 10.1158/2159-8290.CD-15-1157
199. Riise RE, Bernson E, Aurelius J, Martner A, Pesce S, Della Chiesa M, et al. TLR-stimulated neutrophils instruct NK cells to trigger dendritic cell maturation and promote adaptive T cell responses. J Immunol (2015) 195(3):1121–8. doi: 10.4049/jimmunol.1500709
200. Valayer A, Brea D, Lajoie L, Avezard L, Combes-Soia L, Labas V, et al. Neutrophils can disarm NK cell response through cleavage of NKp46. J leukoc Biol (2017) 101(1):253–9. doi: 10.1189/jlb.3AB0316-140RR
201. Sun R, Xiong Y, Liu H, Gao C, Su L, Weng J, et al. Tumor-associated neutrophils suppress antitumor immunity of NK cells through the PD-L1/PD-1 axis. Trans Oncol (2020) 13(10):100825. doi: 10.1016/j.tranon.2020.100825
202. Cristescu R, Mogg R, Ayers M, Albright A, Murphy E, Yearley J, et al. Pan-tumor genomic biomarkers for PD-1 checkpoint blockade–based immunotherapy. Science (2018) 362(6411):eaar3593. doi: 10.1126/science.aar3593
203. Koyama S, Akbay EA, Li YY, Aref AR, Skoulidis F, Herter-Sprie GS, et al. STK11/LKB1 deficiency promotes neutrophil recruitment and proinflammatory cytokine production to suppress T-cell activity in the lung tumor MicroenvironmentImmunosuppressive effects of STK11/LKB1 loss in lung cancer. Cancer Res (2016) 76(5):999–1008. doi: 10.1158/0008-5472.CAN-15-1439
204. Glodde N, Bald T, van den Boorn-Konijnenberg D, Nakamura K, O’Donnell JS, Szczepanski S, et al. Reactive neutrophil responses dependent on the receptor tyrosine kinase c-MET limit cancer immunotherapy. Immunity. (2017) 47(4):789–802. e9. doi: 10.1016/j.immuni.2017.09.012
205. Nouailles G, Dorhoi A, Koch M, Zerrahn J, Weiner J, Faé KC, et al. CXCL5-secreting pulmonary epithelial cells drive destructive neutrophilic inflammation in tuberculosis. J Clin Invest (2014) 124(3):1268–82. doi: 10.1172/JCI72030
206. Soler-Cardona A, Forsthuber A, Lipp K, Ebersberger S, Heinz M, Schossleitner K, et al. CXCL5 facilitates melanoma cell–neutrophil interaction and lymph node metastasis. J Invest Dermatol (2018) 138(7):1627–35. doi: 10.1016/j.jid.2018.01.035
207. Zhou SL, Dai Z, Zhou ZJ, Wang XY, Yang GH, Wang Z, et al. Overexpression of CXCL5 mediates neutrophil infiltration and indicates poor prognosis for hepatocellular carcinoma. Hepatology. (2012) 56(6):2242–54. doi: 10.1002/hep.25907
208. Lee JB, Kim HR, Ha S-J. Immune checkpoint inhibitors in 10 years: Contribution of basic research and clinical application in cancer immunotherapy. Immune Network (2022) 22(1):e2. doi: 10.4110/in.2022.22.e2
209. Borghaei H, Paz-Ares L, Horn L, Spigel DR, Steins M, Ready NE, et al. Nivolumab versus docetaxel in advanced nonsquamous non–small-cell lung cancer. New Engl J Med (2015) 373(17):1627–39. doi: 10.1056/NEJMoa1507643
210. Havel JJ, Chowell D, Chan TA. The evolving landscape of biomarkers for checkpoint inhibitor immunotherapy. Nat Rev Cancer (2019) 19(3):133–50. doi: 10.1038/s41568-019-0116-x
211. Gide TN, Quek C, Menzies AM, Tasker AT, Shang P, Holst J, et al. Distinct immune cell populations define response to anti-PD-1 monotherapy and anti-PD-1/anti-CTLA-4 combined therapy. Cancer Cell (2019) 35(2):238–55. e6. doi: 10.1016/j.ccell.2019.01.003
212. Wei SC, Levine JH, Cogdill AP, Zhao Y, Anang N-AA, Andrews MC, et al. Distinct cellular mechanisms underlie anti-CTLA-4 and anti-PD-1 checkpoint blockade. Cell. (2017) 170(6):1120–33. e17. doi: 10.1016/j.cell.2017.07.024
213. Siolas D, Vucic E, Cullis J, Choi M, Bar-Sagi D. Accumulation of neutrophils in the tumor microenvironment promotes resistance to immunotherapy in pancreatic cancer. AACR; (2019) 79:2797. doi: 10.1158/1538-7445.AM2019-2797
214. Faget J, Peters S, Quantin X, Meylan E, Bonnefoy N. Neutrophils in the era of immune checkpoint blockade. J Immunother Cancer (2021) 9(7):e002242. doi: 10.1136/jitc-2020-002242
215. Schiffmann LM, Fritsch M, Gebauer F, Günther SD, Stair NR, Seeger JM, et al. Tumour-infiltrating neutrophils counteract anti-VEGF therapy in metastatic colorectal cancer. Br J cancer (2019) 120(1):69–78. doi: 10.1038/s41416-018-0198-3
216. Kim IS, Gao Y, Welte T, Wang H, Liu J, Janghorban M, et al. Immuno-subtyping of breast cancer reveals distinct myeloid cell profiles and immunotherapy resistance mechanisms. Nat Cell Biol (2019) 21(9):1113–26. doi: 10.1038/s41556-019-0373-7
217. Sanchez-Pino MD, Dean MJ, Ochoa AC. Myeloid-derived suppressor cells (MDSC): When good intentions go awry. Cell Immunol (2021) 362:104302. doi: 10.1016/j.cellimm.2021.104302
218. Zhou J, Nefedova Y, Lei A, Gabrilovich D. Neutrophils and PMN-MDSC: Their biological role and interaction with stromal cells. Semin Immunol (2018) 35:19–28. doi: 10.1016/j.smim.2017.12.004
219. Fridlender ZG, Sun J, Mishalian I, Singhal S, Cheng G, Kapoor V, et al. Transcriptomic analysis comparing tumor-associated neutrophils with granulocytic myeloid-derived suppressor cells and normal neutrophils. PloS One (2012) 7(2):e31524. doi: 10.1371/journal.pone.0031524
220. Youn JI, Collazo M, Shalova IN, Biswas SK, Gabrilovich DI. Characterization of the nature of granulocytic myeloid-derived suppressor cells in tumor-bearing mice. J leukoc Biol (2012) 91(1):167–81. doi: 10.1189/jlb.0311177
221. Veglia F, Hashimoto A, Dweep H, Sanseviero E, De Leo A, Tcyganov E, et al. Analysis of classical neutrophils and polymorphonuclear myeloid-derived suppressor cells in cancer patients and tumor-bearing mice. J Exp Med (2021) 218(4):e20201803. doi: 10.1084/jem.20201803
222. Schneider AK, Chevalier MF, Derré L. The multifaceted immune regulation of bladder cancer. Nat Rev Urol (2019) 16(10):613–30. doi: 10.1038/s41585-019-0226-y
223. Wang B, He Z, Yu H, Ou Z, Chen J, Yang M, et al. Intravesical pseudomonas aeruginosa mannose-sensitive hemagglutinin vaccine triggers a tumor-preventing immune environment in an orthotopic mouse bladder cancer model. Cancer Immunol Immunother (2021) 91(1):1–11. doi: 10.1189/jlb.0311177
224. Teijeira A, Garasa S, Ochoa MC, Villalba M, Olivera I, Cirella A, et al. IL8, neutrophils, and NETs in a collusion against cancer immunity and immunotherapy. Clin Cancer Res (2021) 27(9):2383–93. doi: 10.1158/1078-0432.CCR-20-1319
225. Wang X, Qiu L, Li Z, Wang X-Y, Yi H. Understanding the multifaceted role of neutrophils in cancer and autoimmune diseases. Front Immunol (2018) 2456. doi: 10.3389/fimmu.2018.02456
226. Derynck R, Turley SJ, Akhurst RJ. TGFβ biology in cancer progression and immunotherapy. Nat Rev Clin Oncol (2021) 18(1):9–34. doi: 10.1038/s41571-020-0403-1
227. Qin F, Liu X, Chen J, Huang S, Wei W, Zou Y, et al. Anti-TGF-β attenuates tumor growth via polarization of tumor associated neutrophils towards an anti-tumor phenotype in colorectal cancer. J Cancer (2020) 11(9):2580. doi: 10.7150/jca.38179
228. Fang Y, Li X, Jiang Y, Ge Z. Blocking TGF-β expression attenuates tumor growth in lung cancers, potentially mediated by skewing development of neutrophils. J Oncol (2022) 2022:3447185. doi: 10.1155/2022/3447185
229. Papayannopoulos V. Neutrophil extracellular traps in immunity and disease. Nat Rev Immunol (2018) 18(2):134–47. doi: 10.1038/nri.2017.105
230. Teijeira A, Garasa S, Ochoa MC, Villalba M, Olivera I, Cirella A, et al. IL8, neutrophils, and NETs in a collusion against cancer immunity and ImmunotherapyIL8 and NETs in cancer immunotherapy. Clin Cancer Res (2021) 27(9):2383–93. doi: 10.1158/1078-0432.CCR-20-1319
231. Martin CJ, Datta A, Littlefield C, Kalra A, Chapron C, Wawersik S, et al. Selective inhibition of TGFβ1 activation overcomes primary resistance to checkpoint blockade therapy by altering tumor immune landscape. Sci Trans Med (2020) 12(536):eaay8456. doi: 10.1155/2022/3447185
232. Tauriello DV, Palomo-Ponce S, Stork D, Berenguer-Llergo A, Badia-Ramentol J, Iglesias M, et al. TGFβ drives immune evasion in genetically reconstituted colon cancer metastasis. Nature. (2018) 554(7693):538–43. doi: 10.1038/nature25492
233. Mariathasan S, Turley SJ, Nickles D, Castiglioni A, Yuen K, Wang Y, et al. TGFβ attenuates tumour response to PD-L1 blockade by contributing to exclusion of T cells. Nature. (2018) 554(7693):544–8. doi: 10.1038/nature25501
234. Gunderson AJ, Yamazaki T, McCarty K, Fox N, Phillips M, Alice A, et al. TGFβ suppresses CD8+ T cell expression of CXCR3 and tumor trafficking. Nat Commun (2020) 11(1):1–13. doi: 10.1126/scitranslmed.aay8456
235. Chakravarthy A, Khan L, Bensler NP, Bose P, De Carvalho DD. TGF-β-associated extracellular matrix genes link cancer-associated fibroblasts to immune evasion and immunotherapy failure. Nat Commun (2018) 9(1):1–10. doi: 10.1038/s41467-018-06654-8
236. Lan Y, Zhang D, Xu C, Hance KW, Marelli B, Qi J, et al. Enhanced preclinical antitumor activity of M7824, a bifunctional fusion protein simultaneously targeting PD-L1 and TGF-β. Sci Trans Med (2018) 10(424):eaan5488. doi: 10.1126/scitranslmed.aan5488
237. Rodríguez-Ruiz ME, Rodríguez I, Mayorga L, Labiano T, Barbes B, Etxeberria I, et al. TGFβ blockade enhances radiotherapy abscopal efficacy effects in combination with anti-PD1 and anti-CD137 immunostimulatory monoclonal antibodies. Mol Cancer Ther (2019) 18(3):621–31. doi: 10.1158/1535-7163.MCT-18-0558
238. Han Z-J, Li Y-B, Yang L-X, Cheng H-J, Liu X, Chen H. Roles of the CXCL8-CXCR1/2 axis in the tumor microenvironment and immunotherapy. Molecules. (2021) 27(1):137. doi: 10.3390/molecules27010137
239. Pawluczuk E, Łukaszewicz-Zając M, Gryko M, Kulczyńska-Przybik A, Mroczko B. Serum CXCL8 and its specific receptor (CXCR2) in gastric cancer. Cancers. (2021) 13(20):5186. doi: 10.3390/cancers13205186
240. Ha H, Debnath B, Neamati N. Role of the CXCL8-CXCR1/2 axis in cancer and inflammatory diseases. Theranostics. (2017) 7(6):1543. doi: 10.7150/thno.15625
241. Oyanagi J, Koh Y, Sato K, Mori K, Teraoka S, Akamatsu H, et al. Predictive value of serum protein levels in patients with advanced non-small cell lung cancer treated with nivolumab. Lung Cancer (2019) 132:107–13. doi: 10.1016/j.lungcan.2019.03.020
242. Schalper KA, Carleton M, Zhou M, Chen T, Feng Y, Huang S-P, et al. Elevated serum interleukin-8 is associated with enhanced intratumor neutrophils and reduced clinical benefit of immune-checkpoint inhibitors. Nat Med (2020) 26(5):688–92. doi: 10.1038/s41591-020-0856-x
243. Yuen KC, Liu L-F, Gupta V, Madireddi S, Keerthivasan S, Li C, et al. High systemic and tumor-associated IL-8 correlates with reduced clinical benefit of PD-L1 blockade. Nat Med (2020) 26(5):693–8. doi: 10.1038/s41591-020-0860-1
244. Sanmamed M, Perez-Gracia J, Schalper K, Fusco J, Gonzalez A, Rodriguez-Ruiz M, et al. Changes in serum interleukin-8 (IL-8) levels reflect and predict response to anti-PD-1 treatment in melanoma and non-small-cell lung cancer patients. Ann Oncol (2017) 28(8):1988–95. doi: 10.1093/annonc/mdx190
245. Lei Y, Li X, Huang Q, Zheng X, Liu M. Progress and challenges of predictive biomarkers for immune checkpoint blockade. Front Oncol (2021) 11:609. doi: 10.3389/fonc.2021.617335
246. Dominguez C, McCampbell KK, David JM, Palena C. Neutralization of IL-8 decreases tumor PMN-MDSCs and reduces mesenchymalization of claudin-low triple-negative breast cancer. JCI Insight (2017) 2(21):e94296. doi: 10.1172/jci.insight.94296
247. Schimek V, Strasser K, Beer A, Göber S, Walterskirchen N, Brostjan C, et al. Tumour cell apoptosis modulates the colorectal cancer immune microenvironment via interleukin-8-dependent neutrophil recruitment. Cell Death dis (2022) 13(2):1–13. doi: 10.1038/s41419-022-04585-3
248. Russo RC, Garcia CC, Teixeira MM, Amaral FA. The CXCL8/IL-8 chemokine family and its receptors in inflammatory diseases. Expert Rev Clin Immunol (2014) 10(5):593–619. doi: 10.1586/1744666X.2014.894886
249. Bachelerie F, Ben-Baruch A, Burkhardt AM, Combadiere C, Farber JM, Graham GJ, et al. International union of basic and clinical pharmacology. LXXXIX. update on the extended family of chemokine receptors and introducing a new nomenclature for atypical chemokine receptors. Pharmacol Rev (2014) 66(1):1–79. doi: 10.1172/jci.insight.94296
250. Gallegos-Arreola M, Briseño-Zuno C, Figuera L, Sánchez-López J, Zúñiga-González G, Puebla-Pérez A, et al. The rs1008562, rs2234671, and rs3138060 polymorphisms of the CXCR1 gene are associated with breast cancer risk in a Mexican population. Eur Rev Med Pharmacol Sci (2020) 24(19):9990–10002. doi: 10.1038/s41419-022-04585-3
251. Cheng Y, Mo F, Li Q, Han X, Shi H, Chen S, et al. Targeting CXCR2 inhibits the progression of lung cancer and promotes therapeutic effect of cisplatin. Mol cancer (2021) 20(1):1–21. doi: 10.1186/s12943-021-01355-1
252. Cabrero-de Las Heras S, Martínez-Balibrea E. CXC family of chemokines as prognostic or predictive biomarkers and possible drug targets in colorectal cancer. World J gastroenterol (2018) 24(42):4738. doi: 10.1124/pr.113.007724
253. Taki M, Abiko K, Baba T, Hamanishi J, Yamaguchi K, Murakami R, et al. Snail promotes ovarian cancer progression by recruiting myeloid-derived suppressor cells via CXCR2 ligand upregulation. Nat Commun (2018) 9(1):1–12. doi: 10.1038/s41467-018-03966-7
254. Sano M, Ijichi H, Takahashi R, Miyabayashi K, Fujiwara H, Yamada T, et al. Blocking CXCLs–CXCR2 axis in tumor–stromal interactions contributes to survival in a mouse model of pancreatic ductal adenocarcinoma through reduced cell invasion/migration and a shift of immune-inflammatory microenvironment. Oncogenesis. (2019) 8(2):1–12. doi: 10.1038/s41389-018-0117-8
255. Liu G, An L, Zhang H, Du P, Sheng Y. Activation of CXCL6/CXCR1/2 axis promotes the growth and metastasis of osteosarcoma cells in vitro and in vivo. Front Pharmacol (2019) 10:307. doi: 10.3389/fphar.2019.00307
256. Mattos MS, Ferrero MR, Kraemer L, Lopes GAO, Reis DC, Cassali GD, et al. CXCR1 and CXCR2 inhibition by ladarixin improves neutrophil-dependent airway inflammation in mice. Front Immunol (2020) 2579. doi: 10.3389/fimmu.2020.566953
257. Horn LA, Riskin J, Hempel HA, Fousek K, Lind H, Hamilton DH, et al. Simultaneous inhibition of CXCR1/2, TGF-β, and PD-L1 remodels the tumor and its microenvironment to drive antitumor immunity. J immunother Cancer (2020) 8(1):e000326. doi: 10.1136/jitc-2019-000326
258. Lu X, Horner JW, Paul E, Shang X, Troncoso P, Deng P, et al. Effective combinatorial immunotherapy for castration-resistant prostate cancer. Nature. (2017) 543(7647):728–32. doi: 10.1038/nature21676
259. Greene S, Robbins Y, Mydlarz WK, Huynh AP, Schmitt NC, Friedman J, et al. Inhibition of MDSC trafficking with SX-682, a CXCR1/2 inhibitor, enhances NK-cell immunotherapy in head and neck cancer models. Clin Cancer Res (2020) 26(6):1420–31. doi: 10.1158/1078-0432.CCR-19-2625
260. Yang J, Yan C, Vilgelm AE, Chen S-C, Ayers GD, Johnson CA, et al. Targeted deletion of CXCR2 in myeloid cells alters the tumor immune environment to improve antitumor immunity. Cancer Immunol Res (2020) 9(2):200–13. doi: 10.1158/2326-6066.CIR-20-0312
261. Schott AF, Goldstein LJ, Cristofanilli M, Ruffini PA, McCanna S, Reuben JM, et al. Phase ib pilot study to evaluate reparixin in combination with weekly paclitaxel in patients with HER-2–negative metastatic breast cancer. Clin Cancer Res (2017) 23(18):5358–65. doi: 10.1158/1078-0432.CCR-16-2748
262. Hurtado CG, Wan F, Housseau F, Sears CL. Roles for interleukin 17 and adaptive immunity in pathogenesis of colorectal cancer. Gastroenterology. (2018) 155(6):1706–15. doi: 10.1053/j.gastro.2018.08.056
263. Kersten K, Coffelt SB, Hoogstraat M, Verstegen NJ, Vrijland K, Ciampricotti M, et al. Mammary tumor-derived CCL2 enhances pro-metastatic systemic inflammation through upregulation of IL1β in tumor-associated macrophages. Oncoimmunology. (2017) 6(8):e1334744. doi: 10.1158/2326-6066.CIR-20-0312
264. McGeachy MJ, Cua DJ, Gaffen SL. The IL-17 family of cytokines in health and disease. Immunity. (2019) 50(4):892–906. doi: 10.1016/j.immuni.2019.03.021
265. Chen J, Ye X, Pitmon E, Lu M, Wan J, Jellison ER, et al. IL-17 inhibits CXCL9/10-mediated recruitment of CD8+ cytotoxic T cells and regulatory T cells to colorectal tumors. J immunother cancer (2019) 7(1):1–13. doi: 10.1186/s40425-019-0757-z
266. Chen X, Zhao J, Herjan T, Hong L, Liao Y, Liu C, et al. IL-17–induced HIF1α drives resistance to anti–PD-L1 via fibroblast-mediated immune exclusion. J Exp Med (2022) 219(6):e20210693. doi: 10.1080/2162402X.2017.1334744
267. Liu C, Liu R, Wang B, Lian J, Yao Y, Sun H, et al. Blocking IL-17A enhances tumor response to anti-PD-1 immunotherapy in microsatellite stable colorectal cancer. J immunother Cancer (2021) 9(1):e001895corr1. doi: 10.1136/jitc-2020-001895
268. Song Y, Yang M, Zhang H, Sun Y, Tao Y, Li H, et al. IL-17 affects the progression, metastasis, and recurrence of laryngeal cancer via the inhibition of apoptosis through activation of the PI3K/AKT/FAS/FASL pathways. J Immunol Res (2020) 2020:2953191. doi: 10.1155/2020/2953191
269. Wang J, Li H, Zhang H, Chen Y, Cao Y, Li R, et al. Intratumoral IL17-producing cells infiltration correlate with antitumor immune contexture and improved response to adjuvant chemotherapy in gastric cancer. Ann Oncol (2019) 30(2):266–73. doi: 10.1093/annonc/mdy505
270. Wu L, Awaji M, Saxena S, Varney ML, Sharma B, Singh RK. IL-17–CXC chemokine receptor 2 axis facilitates breast cancer progression by up-regulating neutrophil recruitment. Am J pathol (2020) 190(1):222–33. doi: 10.1016/j.ajpath.2019.09.016
271. Casbon A-J, Reynaud D, Park C, Khuc E, Gan DD, Schepers K, et al. Invasive breast cancer reprograms early myeloid differentiation in the bone marrow to generate immunosuppressive neutrophils. Proc Natl Acad Sci (2015) 112(6):E566–E75. doi: 10.1073/pnas.1424927112
272. Thunström Salzer A, Niemiec MJ, Hosseinzadeh A, Stylianou M, Åström F, Röhm M, et al. Assessment of neutrophil chemotaxis upon G-CSF treatment of healthy stem cell donors and in allogeneic transplant recipients. Front Immunol (2018) 1968. doi: 10.3389/fimmu.2018.01968
273. Gaffen SL, Jain R, Garg AV, Cua DJ. The IL-23–IL-17 immune axis: from mechanisms to therapeutic testing. Nat Rev Immunol (2014) 14(9):585–600. doi: 10.1038/nri3707
274. Jin C, Lagoudas GK, Zhao C, Bullman S, Bhutkar A, Hu B, et al. Commensal microbiota promote lung cancer development via γδ T cells. Cell. (2019) 176(5):998–1013. e16. doi: 10.1073/pnas.1424927112
275. Ocana A, Nieto-Jiménez C, Pandiella A, Templeton AJ. Neutrophils in cancer: prognostic role and therapeutic strategies. Mol cancer (2017) 16(1):1–7. doi: 10.1186/s12943-017-0707-7
276. Lecot P, Sarabi M, Pereira Abrantes M, Mussard J, Koenderman L, Caux C, et al. Neutrophil heterogeneity in cancer: from biology to therapies. Front Immunol (2019) 2155. doi: 10.3389/fimmu.2019.02155
277. Bosiljcic M, Cederberg RA, Hamilton MJ, LePard NE, Harbourne BT, Collier JL, et al. Targeting myeloid-derived suppressor cells in combination with primary mammary tumor resection reduces metastatic growth in the lungs. Breast Cancer Res (2019) 21(1):1–16. doi: 10.1186/s13058-019-1189-x
278. Fleming V, Hu X, Weber R, Nagibin V, Groth C, Altevogt P, et al. Targeting myeloid-derived suppressor cells to bypass tumor-induced immunosuppression. Front Immunol (2018) 16(1):398. doi: 10.3389/fimmu.2018.00398
279. Rotondo R, Barisione G, Mastracci L, Grossi F, Orengo AM, Costa R, et al. IL-8 induces exocytosis of arginase 1 by neutrophil polymorphonuclears in nonsmall cell lung cancer. Int J cancer (2009) 125(4):887–93. doi: 10.1002/ijc.24448
280. Rodriguez PC, Ochoa AC, Al-Khami AA. Arginine metabolism in myeloid cells shapes innate and adaptive immunity. Front Immunol (2017) 8:93. doi: 10.3389/fimmu.2017.00093
281. Caldwell RW, Rodriguez PC, Toque HA, Narayanan SP, Caldwell RB. Arginase: a multifaceted enzyme important in health and disease. Physiol Rev (2018) 98(2):641–65. doi: 10.1152/physrev.00037.2016
282. Akiba J, Nakashima O, Hattori S, Naito Y, Kusano H, Kondo R, et al. The expression of arginase-1, keratin (K) 8 and K18 in combined hepatocellular-cholangiocarcinoma, subtypes with stem-cell features, intermediate-cell type. J Clin pathol (2016) 69(10):846–51. doi: 10.1136/jclinpath-2015-203491
283. Ma Z, Lian J, Yang M, Wuyang J, Zhao C, Chen W, et al. Overexpression of arginase-1 is an indicator of poor prognosis in patients with colorectal cancer. Pathology-Research Practice (2019) 215(6):152383. doi: 10.1016/j.prp.2019.03.012
284. Mussai F, Egan S, Hunter S, Webber H, Fisher J, Wheat R, et al. Neuroblastoma arginase activity creates an immunosuppressive microenvironment that impairs autologous and engineered immunity. Cancer Res (2015) 75(15):3043–53. doi: 10.1158/0008-5472.CAN-14-3443
285. Miret JJ, Kirschmeier P, Koyama S, Zhu M, Li YY, Naito Y, et al. Suppression of myeloid cell arginase activity leads to therapeutic response in a NSCLC mouse model by activating anti-tumor immunity. J immunother cancer (2019) 7(1):1–12. doi: 10.1186/s40425-019-0504-5
286. Steggerda SM, Bennett MK, Chen J, Emberley E, Huang T, Janes JR, et al. Inhibition of arginase by CB-1158 blocks myeloid cell-mediated immune suppression in the tumor microenvironment. J immunother cancer (2017) 5(1):1–18. doi: 10.1186/s40425-017-0308-4
287. Blaszczyk R, Brzezinska J, Dymek B, Stanczak PS, Mazurkiewicz M, Olczak J, et al. Discovery and pharmacokinetics of sulfamides and guanidines as potent human arginase 1 inhibitors. ACS med Chem let (2020) 11(4):433–8. doi: 10.1021/acsmedchemlett.9b00508
288. Pham T-N, Liagre B, Girard-Thernier C, Demougeot C. Research of novel anticancer agents targeting arginase inhibition. Drug Discovery Today (2018) 23(4):871–8. doi: 10.1016/j.drudis.2018.01.046
289. Czystowska-Kuzmicz M, Sosnowska A, Nowis D, Ramji K, Szajnik M, Chlebowska-Tuz J, et al. Small extracellular vesicles containing arginase-1 suppress T-cell responses and promote tumor growth in ovarian carcinoma. Nat Commun (2019) 10(1):1–16. doi: 10.1038/s41467-019-10979-3
290. Borek B, Gajda T, Golebiowski A, Blaszczyk R. Boronic acid-based arginase inhibitors in cancer immunotherapy. Bioorganic Med Chem (2020) 28(18):115658. doi: 10.1016/j.bmc.2020.115658
291. Pilanc P, Wojnicki K, Roura A-J, Cyranowski S, Ellert-Miklaszewska A, Ochocka N, et al. A novel oral arginase 1/2 inhibitor enhances the antitumor effect of PD-1 inhibition in murine experimental gliomas by altering the immunosuppressive environment. Front Oncol (2021) 3259. doi: 10.3389/fonc.2021.703465
292. Walport MJ. Complement: first of two parts. N Engl J Med (2001) 344:1058–66. doi: 10.1056/NEJM200104053441406
293. Afshar-Kharghan V. The role of the complement system in cancer. J Clin Invest (2017) 127(3):780–9. doi: 10.1172/JCI90962
294. Shu C, Zha H, Long H, Wang X, Yang F, Gao J, et al. C3a-C3aR signaling promotes breast cancer lung metastasis via modulating carcinoma associated fibroblasts. J Exp Clin Cancer Res (2020) 39(1):1–14. doi: 10.1186/s13046-019-1515-2
295. Ajona D, Ortiz-Espinosa S, Pio R. Complement anaphylatoxins C3a and C5a: Emerging roles in cancer progression and treatment. Semin Cell Dev Biol (2019) 85:153–63. doi: 10.1016/j.semcdb.2017.11.023
296. Ding P, Li L, Li L, Lv X, Zhou D, Wang Q, et al. C5aR1 is a master regulator in colorectal tumorigenesis via immune modulation. Theranostics. (2020) 10(19):8619. doi: 10.7150/thno.45058
297. Arbore G, West EE, Spolski R, Robertson AA, Klos A, Rheinheimer C, et al. T Helper 1 immunity requires complement-driven NLRP3 inflammasome activity in CD4+ T cells. Science (2016) 352(6292):aad1210. doi: 10.1126/science.aad1210
298. Luo L, Deng S, Tang W, Hu X, Yin F, Ge H, et al. Recruitment of IL-1β-producing intermediate monocytes enhanced by C5a contributes to the development of malignant pleural effusion. Thorac Cancer (2022) 13(6):811–23. doi: 10.1111/1759-7714.14324
299. Ortiz-Espinosa S, Morales X, Senent Y, Alignani D, Tavira B, Macaya I, et al. Complement C5a induces the formation of neutrophil extracellular traps by myeloid-derived suppressor cells to promote metastasis. Cancer let (2022) 529:70–84. doi: 10.1016/j.canlet.2021.12.027
300. Skendros P, Mitsios A, Chrysanthopoulou A, Mastellos DC, Metallidis S, Rafailidis P, et al. Complement and tissue factor–enriched neutrophil extracellular traps are key drivers in COVID-19 immunothrombosis. J Clin Invest (2020) 130(11):6151–7. doi: 10.1172/JCI141374
301. Chen Y, Li X, Lin X, Liang H, Liu X, Zhang X, et al. Complement C5a induces the generation of neutrophil extracellular traps by inhibiting mitochondrial STAT3 to promote the development of arterial thrombosis. Thromb J (2022) 20(1):1–14. doi: 10.1186/s12959-022-00384-0
302. Prat-Luri B, Neal C, Passelli K, Ganga E, Amore J, Firmino-Cruz L, et al. The C5a-C5aR1 complement axis is essential for neutrophil recruitment to draining lymph nodes via high endothelial venules in cutaneous leishmaniasis. Cell Rep (2022) 39(5):110777. doi: 10.1016/j.celrep.2022.110777
303. Nandagopal S, Li CG, Xu Y, Sodji QH, Graves EE, Giaccia AJ. C3aR signaling inhibits NK-cell infiltration into the tumor microenvironment in mouse models. Cancer Immunol Res (2021) 10(2):245–58. doi: 10.1158/2326-6066.CIR-21-0435
304. Weiner LM, Surana R, Wang S. Monoclonal antibodies: versatile platforms for cancer immunotherapy. Nat Rev Immunol (2010) 10(5):317–27. doi: 10.1038/nri2744
305. Ajona D, Ortiz-Espinosa S, Moreno H, Lozano T, Pajares MJ, Agorreta J, et al. A combined PD-1/C5a blockade synergistically protects against lung cancer growth and metastasis. Cancer discovery (2017) 7(7):694–703. doi: 10.1158/2159-8290.CD-16-1184
306. Medler TR, Murugan D, Horton W, Kumar S, Cotechini T, Forsyth AM, et al. Complement C5a fosters squamous carcinogenesis and limits T cell response to chemotherapy. Cancer Cell (2018) 34(4):561–78. e6. doi: 10.1016/j.ccell.2018.09.003
307. Wang Y, Zhang H, He Y-W. The complement receptors C3aR and C5aR are a new class of immune checkpoint receptor in cancer immunotherapy. Front Immunol (2019) 10:1574. doi: 10.3389/fimmu.2019.01574
308. Massard C, Cassier P, Bendell J, Marie D, Blery M, Morehouse C, et al. Preliminary results of STELLAR-001, a dose escalation phase I study of the anti-C5aR, IPH5401, in combination with durvalumab in advanced solid tumours. Ann Oncol (2019) 30:v492. doi: 10.1093/annonc/mdz253.029
309. Barnes BJ, Adrover JM, Baxter-Stoltzfus A, Borczuk A, Cools-Lartigue J, Crawford JM, et al. Targeting potential drivers of COVID-19: Neutrophil extracellular traps. J Exp Med (2020) 217(6):e20200652. doi: 10.1084/jem.20200652
310. Blasco A, Coronado M, Hernández-Terciado F, Martín P, Royuela A, Ramil E, et al. Assessment of neutrophil extracellular traps in coronary thrombus of a case series of patients with COVID-19 and myocardial infarction [published online December 29, 2020]. JAMA Cardiol (2020) 6(4):1–6. doi: 10.1001/jamacardio.2020.7308
311. Cahilog Z, Zhao H, Wu L, Alam A, Eguchi S, Weng H, et al. The role of neutrophil NETosis in organ injury: novel inflammatory cell death mechanisms. Inflammation. (2020) 43(6):2021–32. doi: 10.1007/s10753-020-01294-x
312. Martins-Cardoso K, Almeida VH, Bagri KM, Rossi MID, Mermelstein CS, König S, et al. Neutrophil extracellular traps (NETs) promote pro-metastatic phenotype in human breast cancer cells through epithelial–mesenchymal transition. Cancers. (2020) 12(6):1542. doi: 10.3390/cancers12061542
313. Szczerba BM, Castro-Giner F, Vetter M, Krol I, Gkountela S, Landin J, et al. Neutrophils escort circulating tumour cells to enable cell cycle progression. Nature. (2019) 566(7745):553–7. doi: 10.1038/s41586-019-0915-y
314. Wculek SK, Malanchi I. Neutrophils support lung colonization of metastasis-initiating breast cancer cells. Nature. (2015) 528(7582):413–7. doi: 10.1038/nature16140
315. Yang D, Liu J. Neutrophil extracellular traps: A new player in cancer metastasis and therapeutic target. J Exp Clin Cancer Res (2021) 40(1):1–11. doi: 10.1186/s13046-021-02013-6
316. Schedel F, Mayer-Hain S, Pappelbaum KI, Metze D, Stock M, Goerge T, et al. Evidence and impact of neutrophil extracellular traps in malignant melanoma. Pigment Cell melanoma Res (2020) 33(1):63–73. doi: 10.1111/pcmr.12818
317. Yang L, Liu L, Zhang R, Hong J, Wang Y, Wang J, et al. IL-8 mediates a positive loop connecting increased neutrophil extracellular traps (NETs) and colorectal cancer liver metastasis. J Cancer (2020) 11(15):4384. doi: 10.7150/jca.44215
318. Li T, Rong H-M, Zhang C, Zhai K, Tong Z-H. IL-9 deficiency promotes pulmonary Th17 response in murine model of pneumocystis infection. Front Immunol (2018) 9:1118. doi: 10.3389/fimmu.2018.01118
319. Kaiser R, Leunig A, Pekayvaz K, Popp O, Joppich M, Polewka V, et al. Self-sustaining IL-8 loops drive a prothrombotic neutrophil phenotype in severe COVID-19. JCI Insight (2021) 6(18):e150862. doi: 10.1172/jci.insight.150862
320. Wang R, Zhu Y, Liu Z, Chang L, Bai X, Kang L, et al. Neutrophil extracellular traps promote tPA-induced brain hemorrhage via cGAS in mice with stroke. Blood J Am Soc Hematol (2021) 138(1):91–103. doi: 10.1182/blood.2020008913
321. Wong SL, Wagner DD. Peptidylarginine deiminase 4: a nuclear button triggering neutrophil extracellular traps in inflammatory diseases and aging. FASEB J (2018) 32(12):6258–370. doi: 10.1096/fj.201800691R
322. Knuckley B, Causey CP, Jones JE, Bhatia M, Dreyton CJ, Osborne TC, et al. Substrate specificity and kinetic studies of PADs 1, 3, and 4 identify potent and selective inhibitors of protein arginine deiminase 3. Biochemistry. (2010) 49(23):4852–63. doi: 10.1021/bi100363t
323. Lewis HD, Liddle J, Coote JE, Atkinson SJ, Barker MD, Bax BD, et al. Inhibition of PAD4 activity is sufficient to disrupt mouse and human NET formation. Nat Chem Biol (2015) 11(3):189–91. doi: 10.1038/nchembio.1735
324. Zeng J, Xu H, Pz F, Xie J, He J, Yu J, et al. Kaempferol blocks neutrophil extracellular traps formation and reduces tumour metastasis by inhibiting ROS-PAD4 pathway. J Cell Mol Med (2020) 24(13):7590–9. doi: 10.1111/jcmm.15394
325. Ali RA, Gandhi AA, Meng H, Yalavarthi S, Vreede AP, Estes SK, et al. Adenosine receptor agonism protects against NETosis and thrombosis in antiphospholipid syndrome. Nat Commun (2019) 10(1):1–12. doi: 10.1038/s41467-019-09801-x
326. Adrover JM, Carrau L, Daßler-Plenker J, Bram Y, Chandar V, Houghton S, et al. Disulfiram inhibits neutrophil extracellular trap formation and protects rodents from acute lung injury and SARS-CoV-2 infection. JCI Insight (2022) 7(5):e157342. doi: 10.1172/jci.insight.157342
327. Zhan Y, Ling Y, Deng Q, Qiu Y, Shen J, Lai H, et al. HMGB1-mediated neutrophil extracellular trap formation exacerbates intestinal Ischemia/Reperfusion-induced acute lung injury. J Immunol (2022) 208(4):968–78. doi: 10.4049/jimmunol.2100593
328. Caudrillier A, Kessenbrock K, Gilliss BM, Nguyen JX, Marques MB, Monestier M, et al. Platelets induce neutrophil extracellular traps in transfusion-related acute lung injury. J Clin Invest (2012) 122(7):2661–71. doi: 10.1172/JCI61303
329. Cedervall J, Zhang Y, Huang H, Zhang L, Femel J, Dimberg A, et al. Neutrophil extracellular traps accumulate in peripheral blood vessels and compromise organ function in tumor-bearing animals. Cancer Res (2015) 75(13):2653–62. doi: 10.1158/0008-5472.CAN-14-3299
330. Hong C-W. Extracellular vesicles of neutrophils. Immune network (2018) 18(6):e43. doi: 10.4110/in.2018.18.e43
331. Pliyev BK, Kalintseva MV, Abdulaeva SV, Yarygin KN, Savchenko VG. Neutrophil microparticles modulate cytokine production by natural killer cells. Cytokine. (2014) 65(2):126–9. doi: 10.1016/j.cyto.2013.11.010
332. Headland SE, Jones HR, Norling LV, Kim A, Souza PR, Corsiero E, et al. Neutrophil-derived microvesicles enter cartilage and protect the joint in inflammatory arthritis. Sci Trans Med (2015) 7(315):315ra190–315ra190. doi: 10.1126/scitranslmed.aac5608
333. Pfister H. Neutrophil extracellular traps and neutrophil-derived extracellular vesicles: Common players in neutrophil effector functions. Diagnostics. (2022) 12(7):1715. doi: 10.3390/diagnostics12071715
334. Kolonics F, Kajdácsi E, Farkas VJ, Veres DS, Khamari D, Kittel Á, et al. Neutrophils produce proinflammatory or anti-inflammatory extracellular vesicles depending on the environmental conditions. J leukoc Biol (2021) 109(4):793–806. doi: 10.1002/JLB.3A0320-210R
335. Joice SL, Mydeen F, Couraud P-O, Weksler BB, Romero IA, Fraser PA, et al. Modulation of blood–brain barrier permeability by neutrophils: in vitro and in vivo studies. Brain Res (2009) 1298:13–23. doi: 10.1016/j.brainres.2009.08.076
336. Qin J, Chen D, Hu H, Cui Q, Qiao M, Chen B. Surface modification of RGD-liposomes for selective drug delivery to monocytes/neutrophils in brain. Chem Pharm Bulletin (2007) 55(8):1192–7. doi: 10.1248/cpb.55.1192
337. Wang H, Zang J, Zhao Z, Zhang Q, Chen S. The advances of neutrophil-derived effective drug delivery systems: A key review of managing tumors and inflammation. Int J nanomed (2021) 16:7663. doi: 10.2147/IJN.S328705
338. Wang J, Tang W, Yang M, Yin Y, Li H, Hu F, et al. Inflammatory tumor microenvironment responsive neutrophil exosomes-based drug delivery system for targeted glioma therapy. Biomaterials. (2021) 273:120784. doi: 10.1016/j.biomaterials.2021.120784
339. Xue J, Zhao Z, Zhang L, Xue L, Shen S, Wen Y, et al. Neutrophil-mediated anticancer drug delivery for suppression of postoperative malignant glioma recurrence. Nat nanotechnol (2017) 12(7):692–700. doi: 10.1038/nnano.2017.54
340. Zhang J, Ji C, Zhang H, Shi H, Mao F, Qian H, et al. Engineered neutrophil-derived exosome-like vesicles for targeted cancer therapy. Sci Adv (2022) 8(2):eabj8207. doi: 10.1126/sciadv.abj8207
341. Zhang H, Houghton AM. Good cops turn bad: the contribution of neutrophils to immune-checkpoint inhibitor treatment failures in cancer. Pharmacol Ther (2021) 217:107662. doi: 10.1016/j.pharmthera.2020.107662
342. Grieshaber-Bouyer R, Nigrovic PA. Neutrophil heterogeneity as therapeutic opportunity in immune-mediated disease. Front Immunol (2019) 10:346. doi: 10.3389/fimmu.2019.00346
343. Calzetti F, Finotti G, Tamassia N, Bianchetto-Aguilera F, Castellucci M, Canè S, et al. CD66b– CD64dimCD115– cells in the human bone marrow represent neutrophil-committed progenitors. Nat Immunol (2022) 23(5):679–91. doi: 10.1038/s41590-022-01189-z
344. Kwok I, Becht E, Xia Y, Ng M, Teh YC, Tan L, et al. Combinatorial single-cell analyses of granulocyte-monocyte progenitor heterogeneity reveals an early uni-potent neutrophil progenitor. Immunity. (2020) 53(2):303–18. e5. doi: 10.1016/j.immuni.2020.06.005
345. Dong Y, Zhang Y, Zhang Y, Pan X, Bai J, Chen Y, et al. Dissecting the process of human neutrophil lineage determination by using alpha-lipoic acid inducing neutrophil deficiency model. Redox Biol (2022) 10:102392. doi: 10.1016/j.redox.2022.102392
346. Zhu YP, Padgett L, Dinh HQ, Marcovecchio P, Blatchley A, Wu R, et al. Identification of an early unipotent neutrophil progenitor with pro-tumoral activity in mouse and human bone marrow. Cell Rep (2018) 24(9):2329–41. e8. doi: 10.1016/j.celrep.2018.07.097
347. Kramer ED, Abrams SI. Granulocytic myeloid-derived suppressor cells as negative regulators of anticancer immunity. Front Immunol (2020) 53(2):1963. doi: 10.3389/fimmu.2020.01963
Keywords: neutrophils, immunotherapy, immune checkpoints, NETs, EMT
Citation: Zahid KR, Raza U, Tumbath S, Jiang L, Xu W and Huang X (2022) Neutrophils: Musketeers against immunotherapy. Front. Oncol. 12:975981. doi: 10.3389/fonc.2022.975981
Received: 22 June 2022; Accepted: 01 August 2022;
Published: 25 August 2022.
Edited by:
Ala-Eddin Al Moustafa, Qatar University, QatarReviewed by:
Gabriele Multhoff, Technical University of Munich, GermanyStefano Ugel, University of Verona, Italy
Copyright © 2022 Zahid, Raza, Tumbath, Jiang, Xu and Huang. This is an open-access article distributed under the terms of the Creative Commons Attribution License (CC BY). The use, distribution or reproduction in other forums is permitted, provided the original author(s) and the copyright owner(s) are credited and that the original publication in this journal is cited, in accordance with accepted academic practice. No use, distribution or reproduction is permitted which does not comply with these terms.
*Correspondence: Xiumei Huang, eGl1aHVhbmdAaXUuZWR1