- 1Department of Obstetrics and Gynecology, The First Affiliated Hospital of Zhengzhou University, Zhengzhou, China
- 2School of Basic Medical Sciences, Zhengzhou University, Zhengzhou, China
- 3Cancer Care Centre, St George Hospital, Kogarah, NSW, Australia
- 4St George and Sutherland Clinical Campuses, School of Clinical Medicine, University of New South Wales (UNSW) Sydney, Kensington, NSW, Australia
Radiotherapy is widely used as an indispensable treatment option for cervical cancer patients. However, radioresistance always occurs and has become a big obstacle to treatment efficacy. The reason for radioresistance is mainly attributed to the high repair ability of tumor cells that overcome the DNA damage caused by radiotherapy, and the increased self-healing ability of cancer stem cells (CSCs). Accumulating findings have demonstrated that the tumor microenvironment (TME) is closely related to cervical cancer radioresistance in many aspects, especially in the metabolic processes. In this review, we discuss radiotherapy in cervical cancer radioresistance, and focus on recent research progress of the TME metabolism that affects radioresistance in cervical cancer. Understanding the mechanism of metabolism in cervical cancer radioresistance may help identify useful therapeutic targets for developing novel therapy, overcome radioresistance and improve the efficacy of radiotherapy in clinics and quality of life of patients.
1 Introduction
Cervical cancer is the fourth most common malignant tumor and an important cause of death in women (1). There is a high incidence of cervical cancer in low-income and middle-income countries due to the low popularity of human papillomavirus (HPV) vaccination and cervical cancer screening (1–4). In all cervical cancer, squamous cell carcinoma accounts for 70%, followed by adenocarcinoma accounting for 20% (5). The standard therapies for cervical cancer include surgery, radiotherapy (RT), chemotherapy, and immunotherapy (6). For early-stage cervical cancer, radical surgery is the best option (7). For patients with locally advanced cervical cancer (LACC), concurrent chemoradiotherapy with radical surgery is the standard treatment (8). If patients have recurrent or metastatic cervical cancer, the standard treatment is chemoradiotherapy combined with immunotherapy after surgery, however the prognosis is still very poor (9, 10).
RT is the main treatment choice for LACC (11, 12). It directly causes tumor cell DNA double-strand breaks (DSBs), and the reactive oxygen species (ROS) induced by RT also indirectly causes DNA damage. At the cellular level, radiation-induced DNA damage and DSBs are fatal to cells. However, if the ability of DNA damage repair exceeds DNA damage speed, tumor cells will escape the effect of RT and lead to radioresistance (13–15). Although RT improves the therapeutic effect of cervical cancer patients greatly, the occurrence of radioresistance is still the main challenge for treatment failure (16, 17). Therefore, it is necessary to study the mechanism of radioresistance to overcome this problem and improve the efficacy of RT.
Studies have shown that tumor microenvironment (TME) plays an important role in radioresistance of cervical cancer (12). For instance, hypoxic TME enhanced radioresistance of cervical cancer cells by upregulating hypoxia-inducible factor 1α (HIF-1α) expression (12, 18). HIF-1α knockdown was found to enhance the radiosensitivity of Hela cervical cancer cells (19). In addition, metabolic reprogramming in TME was also reported to affect the efficiency of RT and contribute to cervical cancer radioresistance (20). For example, studies have shown that inhibition of glycolysis and lactic acid production improved the response of cervical cancer cells to single dose RT in ME180 cervical cancer cells (20). Thus, glucose metabolism determines the effect of RT, and metabolic reprogramming provides the key information for clinical cancer treatment. Other studies also suggest that metabolism reprogramming increased radioresistance of cancers (21–23). It was reported that STAT1 upregulated the key glycolytic enzymes lactate dehydrogenase A (LDHA) and pyruvate kinase type M2 (PKM2), promoted the productivity of glycolysis, and then enhanced the resistance to RT in SCC61 human squamous cell carcinoma (23). Serine/threonine kinase AKT mediated enhancement of aerobic glycolysis was reported to promote radioresistance of Hela cervical cancer cells (24). Thus, metabolic reprogramming is a very important factor in cervical cancer radioresistance and is worth of paying more attention.
This review discusses the complicated radioresistant mechanism of cervical cancer and focuses on the recent progress of TME metabolisms on radioresistance. The new research perspectives in this field are able to provide novel ideas and insights to overcome cervical cancer radioresistance with the development of novel targeted therapy.
2 Radiotherapy in cervical cancer treatment and the occurrence of radioresistance
Chemoradiotherapy is the main non-surgical treatment for patients with LACC (25), suggesting that RT is an important aspect in cervical cancer therapy especially in its late stages (25, 26). The main role of RT is demonstrated to destroy cancer cells and shrink tumor sizes, which is applied for postoperative adjuvant therapy. Fractional RT is usually used to treat cancer patients, generally 1.8 ~ 2.0 Gy per day and 5 days per week, for a cycle (26). Different types of cancer RT programs are not the same, and their responses to RT are also different (27).
Various mechanisms related to how RT kills tumor cells have been widely studied. For instance, ionizing radiation can penetrate tissues, destroy chemical bonds, and remove electrons from atoms to treat cancer (28). It also causes chromosomal mutations in cells, leading to cell death (29). Another mechanism that may be the most important one is the DSB of cancer cells after RT (30). In addition, ROS produced by RT indirectly induces cancer cell death (26). Superoxide anion (O2 -), hydrogen peroxide (H2O2) and hydroxyl radical (OH-) are the most common ROS (31, 32), which are effective molecules in RT. These ROS show high reactivity to a variety of cellular macromolecules including DNA, lipids and proteins, and induce biological changes, resulting in tumor cell death (28). Apoptosis, autophagy and necrotic cell death are the common forms of cancer cell death induced by ROS (33). Several clinical treatments, such as photodynamic therapy (PDT), whose mechanism mainly induces ROS to destroy and kill cervical cancer cells (34, 35).
Inhibition of DNA repair has been proved to be a method to improve radiosensitivity of cervical cancer (25). After RT, human DNA repair includes two important pathways homologous recombination (HR) and non-homologous end joining (NHEJ) (30, 36). The HR repair pathway is a precise form of repair that uses undamaged DNA sequences as a template to function in the S and G2 phases of cell cycle (37). Whereas the NHEJ pathway is an error-prone mechanism. It connects broken double-stranded DNA at all stages of the cell cycle, which may cause chromosomal connection errors, such as misalignment or ectopic position (29). DNA repair pathways regulated by HIF-1α, and HR and NHEJ pathways were reported to be inhibited due to hypoxia in breast cancer cells (38). In addition, under hypoxia, the expression of the NHEJ pathway related genes was also found inhibited, resulting in enhanced sensitivity of prostate cancer cells to RT (39). Furthermore, damaged NHEJ was demonstrated to lead to genomic instability, increase the proportion of acquired mutations or translocations, and induce tumorigenesis in leukemia (40, 41). Therefore, several studies have indicated that the key downstream factors in the HR and NHEJ repair pathways may become potential targets for enhancing the sensitivity of RT (42–46). For instance, RAD51 is a highly conserved protein that catalyzes DNA repair through HR repair pathway and modulates the sensitivity of cells to RT (47, 48). Inhibiting RAD51 was found to enhance the radiosensitivity of cervical cancer cells (47, 49, 50). Wang et al. showed that Sulforaphane (SFN) enhanced the radiosensitivity of cervical cancer cells by blocking RAD51 recruitment to the site of injury (49). Other studies also found that Ku70 and Ku80 proteins were involved in the repair of DSBs through NHEJ of DNA strands (51, 52). Cervical cancer cells with the low expression of Ku70 had higher radiosensitivity, and the survival rate of cervical cancer patients with low expression of Ku70 were also higher (53). It was reported that compared with Ku80 positive cervical cancer patients, Ku80 negative patients had a stronger response to RT. Inhibition of Ku80 was found to improve radiosensitivity, which has been confirmed in cervical cancer patients (15, 54). MRE11 is the core of MRN (MRE11-RAD50-NBS1) complex and plays an important role in DNA damage sensing and repairing (15, 55). After DNA damage caused by ionizing radiation, MRE11 recognized and excised DNA DSB ends for further repair (55). RhoC was found to regulate MRE11-mediated DNA repair through Rock2 and regulate radioresistance of cervical cancer (56). NHEJ protein DNA-PKcs was found to be up-regulated in the residual tissues of cervical carcinoma after RT and inhibiting the activity of DNA-PKcs improved the radiosensitivity of cervical cancer (57–60). PARP1 had a high ability to sense DNA damage, and it participated in the repair of single strand broken DNA. PARP1 inhibitor upregulated the sensitivity of cervical cancer to radiation (61, 62). The potential mechanism of radioresistance after RT in cervical cancer is shown in Figure 1.
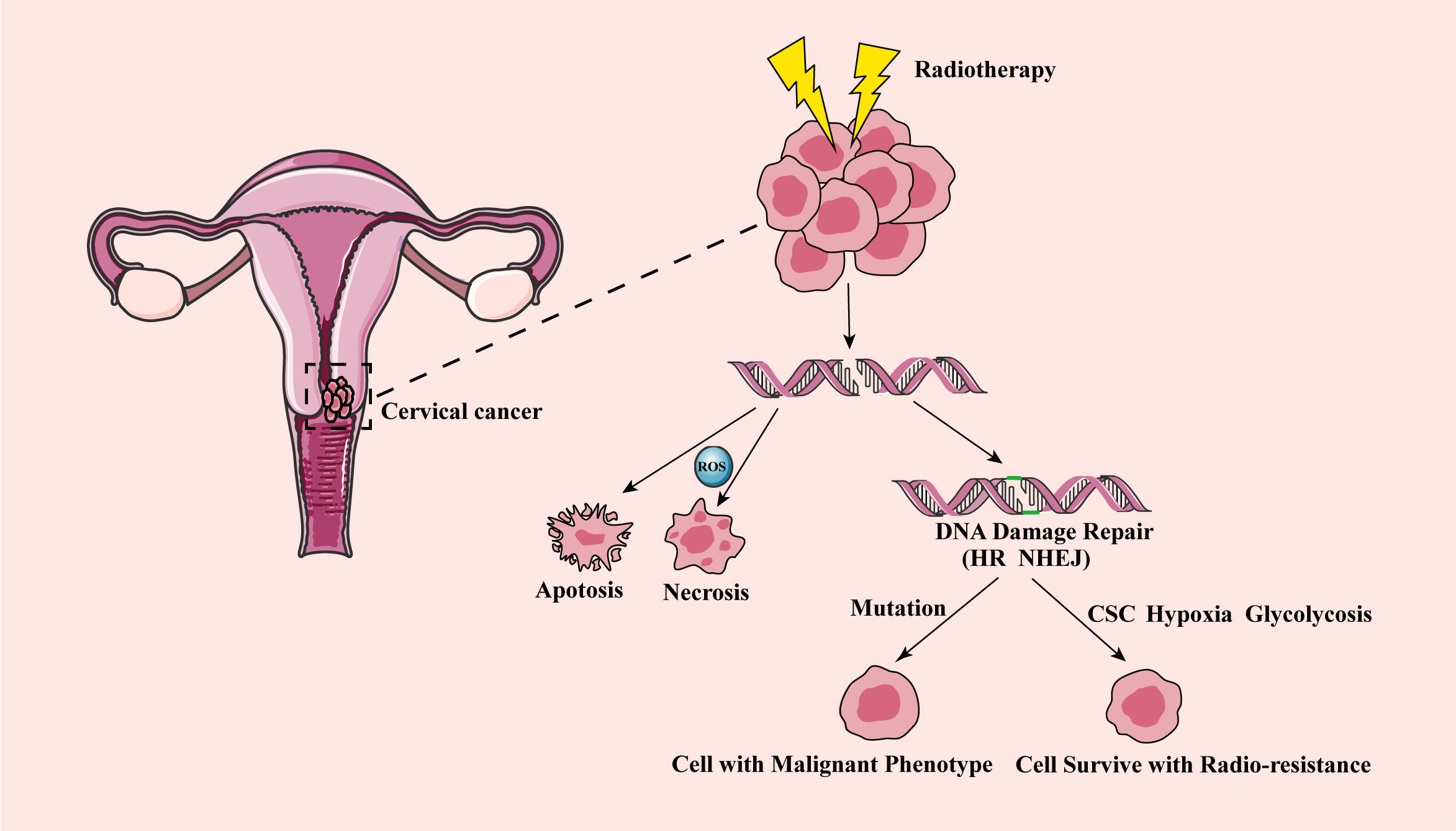
Figure 1 The mechanism of cervical cancer radioresistance after radiotherapy. After RT, DNA double strand breaks in cervical cancer cells, and some cancer cells show apoptosis and necrosis, reaching the effect of RT. Other fraction of cancer cells has DNA damage repair (including HR and NHEJ). The wrong repair path leads to the accumulation of acquired mutations, and tumor aggressivity and recurrence, while the correct repair leads to cell survival and radioresistance. CSC, cancer stem cell; HR, homologous recombination; NHEJ, non-homologous end joining; RT, radiotherapy.
Several oncogenes or tumor suppressor genes were reported to be involved in the regulation of RT. For example, retinoblastoma protein-interacting zinc finger gene (RIZ), located on chromosome 1q36, is a tumor suppressor whose expression is downregulated in a variety of tumors (63, 64). It was found that cervical cancer cells overexpressing RIZ increased the apoptosis rate and DNA damage after RT compared to the control group (65). This study showed that RIZ improved the radiosensitivity of cervical cancer cells and was a potential therapeutic target of RT combined with gene therapy for cervical cancer patients. P53 is an important regulator of cell cycle and DNA repair. After the imbalance of p53 in cervical cancer cells, its regulatory function was confirmed, and the irradiated cells underwent uncontrolled DNA repair, leading to radioresistance (66). P73 has homology with the well-known tumor suppressor gene p53 and is considered as a new tumor suppressor gene. The high expression of p73 was significantly correlated with the radiosensitivity of cervical cancer and played an important role in promoting radiosensitivity of cervical cancer (67). XAV939 is an inhibitor of the Wnt/β-catenin signaling pathway. When combined with RT, XAV939 inhibited proliferation and increased apoptosis of human medulloblastoma cells (68). In cervical cancer, XAV939 promoted apoptosis induced by RT, holding a therapeutic potential in increasing clinical efficacy (69). Inhibiting the repair of DNA damage caused by RT sensitized the radiation response of cervical cancer (70). PXN gene was up regulated in cervical cancer cells, and its overexpression reduced the apoptosis rate after RT, leading to radioresistance (71). The DNA repair molecules and gene regulation cervical cancer are summarized in Table 1.
Inhibitors of DNA repair pathway are crucial to improve the therapeutic efficiency of patients. Some small molecule inhibitors have been used in clinical trials or applied in clinical practice (72). DNA-PK is a key driver of NHEJ pathway, and Pepostertib is an inhibitor of DNA-PK, which destroys DNA repair by inhibiting the activity of DNA-PK to improve the therapeutic effect (73). In one preclinical study, AZD7648 (an effective and specific DNA-PK inhibitor) combined with RT induced tumor regression in mice and improved the therapeutic effect (74). X-ray repair cross complement protein 5 (XRCC5) is an ATP dependent DNA helicase, which provides a start for the DNA repair mechanism of the NHEJ pathway when DNA double strand breaks. Targeting XRCC5 with Myrosin G was found to play a positive therapeutic role (75). CB-5083, as a specific small molecule inhibitor of p97, prevented the decomposition of MRN complex at the DNA damage site during ionizing radiation and damaged DNA repair, thus enhancing the killing function of tumor cells after RT (76). NHEJ inhibitors can be used in combination with standard cancer therapies to reduce therapeutic dose and improve clinical therapeutic effect. Orapanib, a PARP inhibitor, destroyed the localization of base excision repair effector XRCC1 and NHEJ proteins Ku80 and XRCC4, enhanced the sensitivity of cervical cancer cells to cisplatin, demonstrating the potential of PARP inhibitors in the treatment of cervical cancer (77). Santu et al. found that the PARP inhibitor Rucaprib is the most effective radiosensitizer for cervical cancer, which improved the effect of RT for cervical cancer patients (78). The small molecule inhibitors associated with DNA repair are summarized in Table 2. Targeting RT related genes is in a good position to promote DNA damage and apoptosis induced by radiation, which provides a new strategy for cervical cancer RT.
3 Tumor microenvironment contributes to of cervical cancer radioresistance
The presence of immune cells and hypoxia in TME is closely related to cervical cancer radioresistance. For example, the high level of tumor-associated neutrophils was reported to lead to radioresistance in cervical cancer cells and associated with a poor prognosis in patients (18). In addition, many studies have focused on the role of the hypoxic TME in promoting cervical cancer radioresistance (12). The cancer cells in TME usually contain high amount of antioxidants. High levels of glutathione (GSH) were found to boost radioresistance of cervical cancer cells by clearing ROS (82). Therefore, developing radiosensitizers based on reducing antioxidants may be a potential strategy for cervical cancer therapy.
Hypoxia is one of the characteristics of cervical cancer and reduces the effect of RT (83–85). The imbalance between tumor cell growth and neovascularization, as well as the abnormal morphology and function of tumor neovascularization leads to hypoxic microenvironment (86). Hypoxia was demonstrated to enhance the resistance to RT, resulting in a poor prognosis of cervical cancer patients (83, 84, 87).
RT plays an active role in the treatment of cervical cancer; however, this treatment also paradoxically leads to radioresistance through the changes of transcription factors in TME. Epithelial-mesenchymal transition (EMT) is closely related to the effect of RT. Twist is a key transcription factor of EMT, and its expression is positively correlated with hypoxia. Downregulation of Twist reversed the radioresistance induced by hypoxia and enhanced the sensitivity of cervical cancer cells to RT (85). In addition, upregulation of Twist promoted the localization of nuclear epidermal growth factor receptor (EGFR) and the expression of nuclear DNA-PKcs, and enhanced the repair of DNA damage caused by RT (85). Another study showed that Lcn2 interacted with HIF-1α to promote the formation of radioresistant phenotype in nasopharyngeal carcinoma. It was found when Lnc2 was downregulated, the ability of cell colony formation and DNA damage repair were significantly reduced, which indicates that inhibiting Lnc2 improved the effect of RT for nasopharyngeal carcinoma (88). Although RT works for cancer, it paradoxically promotes metastasis and enhances the invasion of cancer through EMT induced by RT (89). Ionizing radiation was reported to activate multiple EMT-induced transcription factors, including HIF-1, ZEB1 and STAT3 (26, 90–92), which activate the corresponding signaling pathways to enhance the EMT ability of tumor cells. In cervical cancer, it has been proved that the EMT induced by RT enhanced the viability and invasiveness of cancer cells (89). In addition, EMT-induced transcription factors endow cells with cancer stem cell (CSC) properties and promote the production of CSCs. CSC is recognized as a radioresistant cell, which plays a role mainly by reducing radiation-induced DNA damage and enhancing DNA repair ability (93).
Immune cells in TME also contribute to the progression of cervical cancer, which makes the immunotherapy an important treatment choice. HPV E6 and E7 proteins were found to promote the expression of programmed cell death protein 1 (PD-1) and programmed death-ligand 1 (PD-L1) in cervical cancer (94). It is widely known that PD-1 and PD-L1 upregulation suppresses the activation of T cells, resulting in immune escape of tumor cells (95). In June 2018, the US Food and Drug Administration (FDA) approved an anti-PD1 antibody pembrolizumab for the treatment of several types of cancer including recurrent or metastatic cervical cancer (96). Another important molecule in suppressing T cell activation is the cytotoxic T-lymphocyte–associated antigen-4 (CTLA-4), and it’s up-regulation leads to immune tolerance of cancer cells (97). PD-L1 blockade and CTLA-4 inhibitor ipilumumab were reported to attenuate tumor induced inhibitory signal transduction, stimulate T cell activation, and play an anti-tumor role (94).
Therefore, targeting TME components is promising for developing novel cervical cancer therapy to reduce radioresistance.
4 Metabolic factors affect cervical cancer radioresistance
The metabolic disorder of cancer cells is closely related to tumorigenesis and therapeutic effects (98). Metabolic reprogramming is regulated by abnormal activation of proto-oncogenes and loss of tumor suppressor genes (99). In addition, TME influences various aspects of the tumor metabolism, including glucose metabolism, amino acid metabolism and lipid metabolism, which may contribute to the modulation of cervical cancer radioresistance (100–102).
4.1 Glucose metabolism and cervical cancer radioresistance
Cervical cancer mainly obtains energy through aerobic glycolysis to maintain the growth and proliferation of cells. Blocking glycolysis inhibits the growth of cancer cells and induces apoptosis, which provides a new approach for the clinical treatment of cervical cancer (103). The process of aerobic glycolysis in cervical cancer cells is mediated by key enzymes such as glucose transporter 1 (GLUT1), LDHA, hexokinase 2 (HK2) and aldolase A (ALDOA), which enhance glucose uptake and lactate production, and promote the progression of cervical cancer (104–108). The Wnt/β-catenin pathway is upregulated in cancer and promotes the activation of Warburg Effect, including the enhancement of enzyme activity in the glycolytic pathway and the acceleration of glutamate decomposition, which in turn promotes the production of lactate. The high lactate microenvironment stimulates the expression of vascular endothelial growth factor (VEGF), promotes tumor angiogenesis and induces cell migration (109). PI3K/mTOR is a key signaling pathway for cell proliferation, and researchers have made great efforts to inhibit this pathway. Li et al. inhibited the glycolysis process of cervical cancer cells through the mTOR inhibitor AZD8055 to fight against the unlimited proliferation of tumor cells (81). Several groups also used NVP-BEZ235 and new 1,3,5-triazine derivatives to double block PI3K/mTOR signaling pathway to inhibit the proliferation of cervical cancer cells, so as to enhance the therapeutic response (79, 80).
Cancer cells choose aerobic glycolysis with less capacity rather than aerobic oxidation with more capacity, which seems to be an uneconomical way. However, cancer cells upregulate GLUT and produce ATP with high efficiency, in order to meet their high energy needs (107). In addition, the metabolic mode of aerobic glycolysis also meets the proliferation needs of cancer cells. Glycolysis produces many metabolic intermediates and precursors, which enter various biosynthetic pathways, such as the pentose phosphate pathway (PPP), to generate amino acids and nucleic acids, and then synthesize biological macromolecules and organelles required for cell proliferation (110, 111). This metabolic mode produces pyruvate, lactic acid, NADPH and hydrogen ions. NADPH converts oxidized glutathione (GSSG) to glutathione (GSH) (Figure 2). These products and glutathione up-regulate the endogenous antioxidant capacity of cells, thereby directly or indirectly working for antagonistic RT (112).
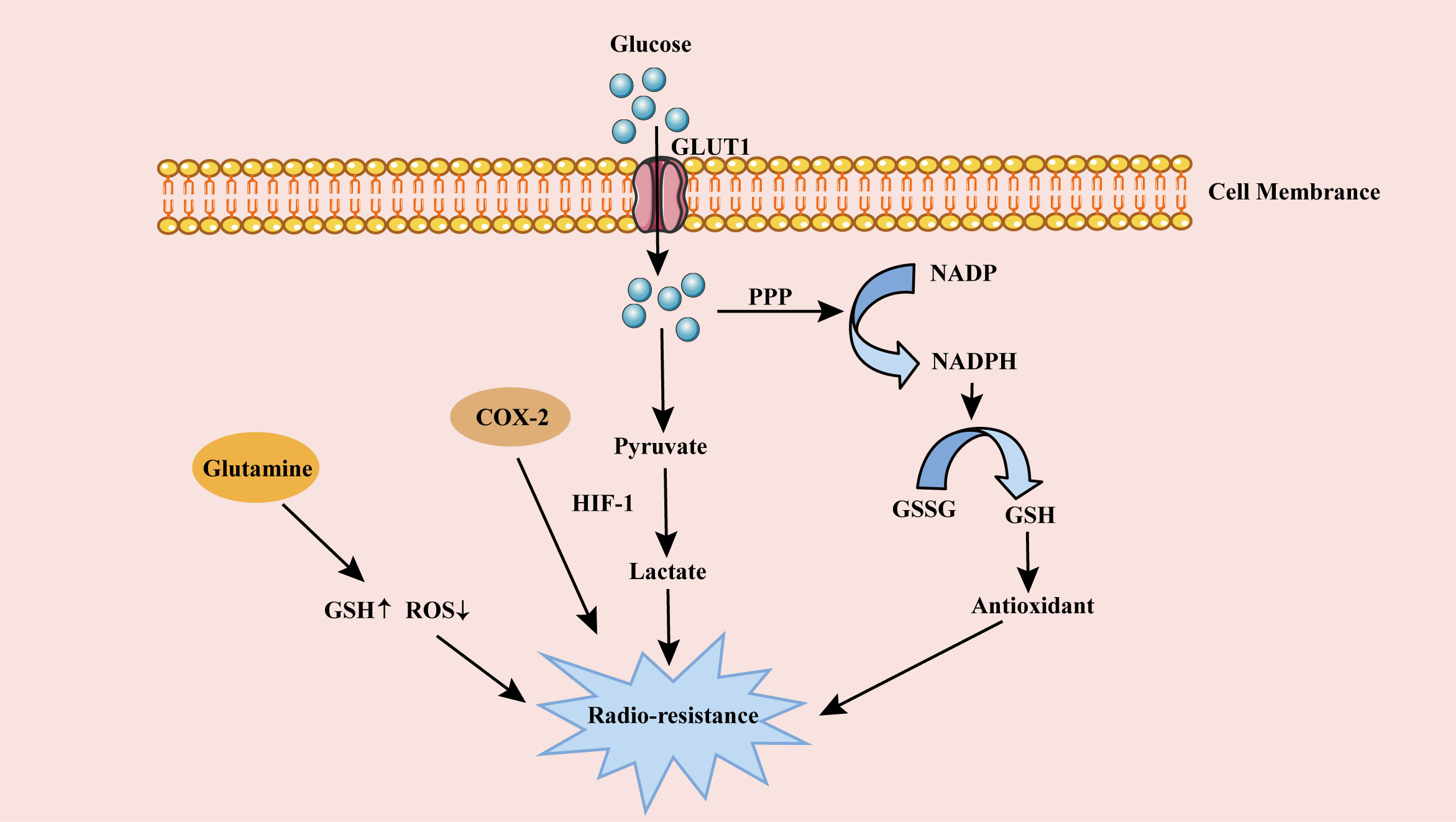
Figure 2 The role of metabolism on cervical cancer radioresistance. Glucose is transported into cells through GLUT1 for glycolysis and produces a large amount of lactic acid, enhancing cervical cancer radioresistance. Glucose in cells also produces GSH through PPP pathway. The antioxidant GSH weakens DSB after RT, resulting in radioresistance of cervical cancer cells. High expression of COX-2 in lipid metabolism and Glutamine in amino acid metabolism also contribute to cervical cancer radioresistance. GLUT1, glucose transporter 1; PPP, pentose phosphate pathway; ROS, reactive oxygen species; HIF-1, hypoxia-inducible factor 1; GSSG, oxidized glutathione; GSH, glutathione; RT, radiotherapy.
4.1.1 GLUT1
GLUT is a family of carrier proteins embedded in the cell membrane to transport glucose, which is widely distributed in various tissues in the body. The distribution of GLUT in vivo and its affinity with glucose molecules are significantly different. Among the 14 glucose transporters, GLUT1 is the most common and widely distributed one (21, 113). It is a human unidirectional protein encoded by SLC2A1 gene that is important for glucose uptake (114). It is up regulated in many types of cancer and metabolic diseases, and involved in the disease progression (115, 116). Inhibition of GLUT1 was found to downregulate glycolysis and inhibited the growth of cervical cancer cells in vitro and in vivo (117). Compared with normal cervical epithelium, the expression of GLUT1 is increased in cervical cancer and is associated with lymphatic metastasis (118).
There are different views on the role of GLUT1 in cervical cancer prognosis. Several lines of evidence suggest that high expression of GLUT1 indicates a poor prognosis of cervical cancer (105, 106, 119–121). However, another study suggested that the expression of GLUT1 was not correlated with the prognosis of cervical cancer (122, 123). This contradict requires more clinical studies to explore the role of GLUT1 in the prognosis of cervical cancer.
It was reported that high expression of GLUT1 in cervical cancer more likely leads to radioresistance (124). In a prospective study, it was found that GLUT1 could be used as a biomarker of cervical cancer radioresistance for individualized treatment (125). Pierre Benoit Ancey et al. found that the loss of GLUT1 in tumor-related neutrophils enhanced the effect of RT in patients with lung cancer. The potential mechanism may be that the lack of GLUT1 weakens the direct killing effect of neutrophils on cancer cells (126). In addition, increased GLUT1 expression was significantly associated with radioresistance in rectal cancer, oral cancer and esophageal cancer (127–130). Therefore, inhibiting the expression of GLUT1 may be used as a new potential therapeutic target to improve RT sensitivity of cancer patients (125).
4.1.2 LDHA
LDHA is a kind of NAD dependent kinase, which is a homologous or heterotetramer molecule mainly located in cytoplasm. LDHA plays a key role in glycolysis to convert pyruvate to lactate and convert NADH to NAD (131). LDHA is up regulated in many cancers, resulting in increased catalytic product lactate and poor prognosis (121, 132–134). High lactic acid forms an acidic TME, promotes tumor to acquire an aggressive phenotype, and increases the risk of tumor metastasis and recurrence (135). It was reported inhibition of LDHA reduced the proliferation and invasion of cervical cancer cells (136, 137).
High lactate level promotes radioresistance of malignant tumor cells. A study on chemoradiotherapy resistance in advanced cervical cancer showed that the expression of LDHA was up-regulated in chemoradiotherapy resistant group by microarray analysis compared to chemoradiotherapy sensitive group (138). Another study also found that a relatively high expression level of LDHA in cervical cancer cells was resistant to RT, which helped cancer cells carry out aerobic glycolysis and promoted radioresistance (139). Therefore, LDHA inhibitors may be a promising strategy to improve the effect of RT and provide a certain foundation for the new drug development (140, 141).
4.1.3 PKM2
PK is another key enzyme in glycolysis, which catalyzes the conversion of phosphoenolpyruvate and adenosine diphosphate to pyruvate that is the last irreversible conversion process of glycolysis (142–144). The M2 subtype PKM2 is highly expressed in many cancers, playing an important role in maintaining the metabolism of cancer cells (143). The high expression level of PKM2 was used as a marker to predict the cervical cancer prognosis (145). One study showed that PKM2 knockdown inhibited EMT via the Wnt/β-catenin pathway, thereby suppressing cervical cancer cell proliferation and invasion (146). Another study reported that knockdown of PKM2 in SiHa and HeLa cervical cancer cells promoted DNA DSBs leading to RT sensitivity (147). In addition, PKM2 knockdown leads to G2/M cell cycle arrest, and the expression of CSC marker NANOG decreases, which significantly enhances the RT effect of cervical cancer cells (147). Zhao et al. found that the high expression of PKM2 was related to the poor prognosis of locally advanced cervical squamous cell carcinoma and contributed to the generation of radioresistance using multivariate COX regression analysis (148). Therefore, targeting PKM2 is a new strategy to improve the RT effect for cervical cancer.
4.1.4 HK2
Glucose can be converted into glucose 6-phosphate under the catalysis of HK2, which is the first step of glycolysis (149). Downregulation of HK2 inhibited glycolysis, reduced glucose consumption and lactate production of cells, and also inhibited cell proliferation and induced apoptosis (137, 150). Whether HK2 regulates the biological behavior and treatment of cancer cells through the glycolytic pathway needs to be further explored. Knockdown of HK2 in cervical cancer cells inhibited proliferation and migration and promoted cell apoptosis (151). Targeting HK2 was found to reduce the glycolysis of cervical cancer cells and enhance the sensitivity of cervical cancer to RT (152). It was showed that increased expression of long non-coding RNA urothelial cancer associated-1 (lncRNA UCA1) by RT enhanced glycolysis by targeting HK2, thereby promoting cervical cancer radioresistance (153). In the established radioresistant cervical cell lines SiHa and HeLa, it was also found that lncRNA UCA1 promoted radioresistance of the cells through enhancement of HK2 and glycolysis (153). Similarly, in the study of locally advanced cervical squamous cell carcinoma, it was found that the high level of HK2 was one of the risk prognostic factors for cervical cancer patients, and associated with the low radiosensitivity (154). Inhibiting HK2 to reduce the dependence of cervical cancer cells on glycolysis is a potential strategy to promote cervical cancer RT.
The glycolysis related proteins in cervical cancer radioresistance are summarized in Table 3.
4.2 Lipid metabolism and cervical cancer radioresistance
Increased lipid uptake and storage in cancer cells contribute to tumor growth and proliferation. More and more evidence shows that lipid metabolism reprogramming plays a very important role in the development of cancer (155–157). The fatty acid is an important component of cell membrane structure, which maintains the fluidity of cell membrane. In case of metabolic emergency, fatty acid decomposition is also the main source of energy (158, 159). Targeting fatty acid metabolism was reported to improve the radiosensitivity of many cancers (160, 161).
In the treatment of cervical cancer patients, reasonable supplementation of high polyunsaturated fatty acids was demonstrated to improve the effect of RT (162). The lipid metabolites in high-resolution magic angle proton magnetic resonance spectroscopy spectrum was used to evaluate the apoptosis of cervical cancer (163). Ferulic acid was effectively combined with RT to increase lipid peroxide of cervical cancer cells and improved radiosensitivity (164). Cyclooxygenase (COX) converted arachidonic acid into prostaglandins, and COX-2 inhibitor phosphorylated p53 in irradiated cervical cancer cells, reducing the cervical cancer radioresistance (102). Currently, the study on lipid metabolism in cervical cancer radioresistance is very limited, but related studies in other types of cancer may give some insights for future investigation. For example, arachidonate 15-lipoxygenase deficiency was found to reduce DNA DSBs induced by RT and induce radioresistance in rectal cancer cells (165). Another study found that targeting fatty acid synthase enhanced the sensitivity of nasopharyngeal carcinoma to RT (161). In addition, targeting carnitine palmitoyl transferase 1 (CPT1A) mediated fatty acid oxidation also improved the effect of RT for nasopharyngeal carcinoma (166).
4.3 Amino acid metabolism and cervical cancer radioresistance
Cancer cells need to obtain enough amino acids for biosynthesis to adapt to the characteristics of rapid proliferation. Amino acid metabolism is closely related to the occurrence and development of cancer, and targeting amino acid metabolism provides a potential therapeutic strategy for cancer treatment (167).
The best-studied amino acid in this metabolic pathway is glutamine, which plays an important role by providing nitrogen and carbon in biosynthesis (168, 169). Studies have shown that glutamine metabolism was closely related to cancer radiosensitivity (170, 171). It has been found that in human cervical cancer samples, the content of phosphate-activated mitochondrial glutaminase2 (GLS2) in the radioresistant group was higher than that in the radiosensitive group. When GLS2 was knocked down, the level of antioxidant glutathione decreased and ROS increased after RT, which promoted the radiosensitivity of cervical cancer cells (172). In another study, the use of glutaminase inhibitors enhanced the radiosensitivity of cervical cancer (101). In addition, glutamine was decomposed by glutaminase, which enhanced the radiosensitivity of prostate cancer (173). Paradoxically, in HeLa cell culture, the addition of supraphysiologic glutamine concentration did not enhance the radioresistance of cervical cancer cells (174). Indepth study is needed about the effect of glutamine on cervical cancer RT. Aminopeptidase N (APN), as a transmembrane exopeptidase, is expressed at a level consistent with the increased malignant behavior of tumors (175). In vitro and in vivo studies showed that APN inhibitor ubenimex enhanced apoptosis and cell damage induced by RT, and improved the radiosensitivity of cervical cancer (176).
Currently, other amino acids regulating metabolism that affects cervical cancer radioresistance have not been reported yet, which requires further study.
5 Conclusions and perspectives
Radioresistance has become a major problem in the management of cervical cancer patients. This review summarizes the mechanism of radioresistance in cervical cancer from different aspects. Most scholars believe that the enhancement of DNA repair ability after RT leads to the reduction of cervical cancer cell death. In addition, EMT and CSCs induced by RT promote radioresistance, leading to cancer recurrence and metastasis.
Here, we focus on TME to investigate how to overcome the radioresistance and promote the efficiency of RT. Hypoxic TME not only directly reduces radiosensitivity due to the reduction of ROS, but also indirectly reduces radiosensitivity by promoting glycolysis. Glucose metabolism is a very important factor in occurrence of radioresistance in cervical cancer. The intermediate products produced by glycolysis are suitable to be used as the precursor of biosynthesis to promote the growth and proliferation of cervical cancer cells. Lactic acid produced by glycolysis promotes radioresistance of cervical cancer cells, and lactic acid inhibitors are expected to become new products to improve the effect of RT. Although the study of lipid metabolism and amino acid metabolism is very limited, we review the important findings related to cervical cancer. Glutamine has a relatively obvious effect on RT and low-level glutamine can increase the content of intracellular ROS to improve sensitivity of cervical cancer cells to RT. In addition, inhibiting the NHEJ pathway to block DNA repair makes tumor cells sensitive to RT, which is also a promising therapeutic strategy (40). In recent years, immunotherapy has become a novel promising treatment, and is used in combination with traditional chemotherapy or RT for advanced and recurrent cervical cancer patients. Anti-PD-1/PD-L1 and anti-CTLA-4 therapies are commonly used in cervical cancer immunotherapy to improve the prognosis of cervical cancer patients. Whether the metabolic programming can give insights for the development of immunotherapy to improve the current cervical cancer treatment is still unknown and worth of putting more efforts for further investigation.
There is no doubt that targeting the key enzymes and intermediates of metabolism is a promising strategy to improve the radiosensitivity of cervical cancer patients. With the in-depth study on the mechanisms related to metabolism with modern omics technologies, it is possible to develop personalized treatment plans to improve the current treatments and prognosis of cervical cancer patients with different metabolic status.
Author contributions
JZ: draft the manuscript and iconography. WT, MC, RG, LQ, and FW: proofread the manuscript and iconography. LC, NL, YL, and JZ: conceptualized, supervised, and finalized the manuscript. All authors contributed to the article and approved the submitted version.
Funding
This work was supported by Henan Provincial Medical Science and Technology Research Plan Joint Provincial and Ministry Youth Project (No.SB201902013), Scientific and Technological Project of Henan Province (No.182102310325 & 192102310069), Henan Province Colleges and Universities Innovative Talent Support Program (No.21HASTIT044), Henan Medical Education Research Project (No.Wjlx2020062), Henan Youth Talent Promotion Project (No.2020HYTP052), Key Research and Development Project of Henan Province (No.222102310539), Henan Province Young and Middle-aged Health Science and Technology Innovative Talent Training Project (No.YXKC2020039), Central Plains Youth Top Talent Project, and High-level Talents Return to China for Research Funding Project.
Conflict of interest
The authors declare that the research was conducted in the absence of any commercial or financial relationships that could be construed as a potential conflict of interest.
Publisher’s note
All claims expressed in this article are solely those of the authors and do not necessarily represent those of their affiliated organizations, or those of the publisher, the editors and the reviewers. Any product that may be evaluated in this article, or claim that may be made by its manufacturer, is not guaranteed or endorsed by the publisher.
References
1. Arbyn M, Weiderpass E, Bruni L, de Sanjosé S, Saraiya M, Ferlay J, et al. Estimates of incidence and mortality of cervical cancer in 2018: A worldwide analysis. Lancet Glob Health (2020) 8(2):e191–203. doi: 10.1016/S2214-109X(19)30482-6
2. Mezei AK, Armstrong HL, Pedersen HN, Campos NG, Mitchell SM, Sekikubo M, et al. Cost-effectiveness of cervical cancer screening methods in low- and middle-income countries: A systematic review. Int J Cancer (2017) 141(3):437–46. doi: 10.1002/ijc.30695
3. Gupta SM, Mania-Pramanik J. Molecular mechanisms in progression of HPV-associated cervical carcinogenesis. J BioMed Sci (2019) 26(1):28. doi: 10.1186/s12929-019-0520-2
4. Ngune I, Kalembo F, Loessl B, Kivuti-Bitok. LW. Biopsychosocial risk factors and knowledge of cervical cancer among young women: A case study from Kenya to inform HPV prevention in Sub-Saharan Africa. PloS One (2020) 15(8):e0237745. doi: 10.1371/journal.pone.0237745
5. Hu K, Wang W, Liu X, Meng Q, Zhang. F. Comparison of treatment outcomes between squamous cell carcinoma and adenocarcinoma of cervix after definitive radiotherapy or concurrent chemoradiotherapy. Radiat Oncol (2018) 13(1):249. doi: 10.1186/s13014-018-1197-5
6. DeBoer RJ, Umutoni V, Bazzett-Matabele L, Katznelson E, Nguyen C, Umwizerwa A, et al. Cervical cancer treatment in Rwanda: Resource-driven adaptations, quality indicators, and patient outcomes. Gynecol Oncol (2022) 164(2):370–8. doi: 10.1016/j.ygyno.2021.12.002
7. Huang H, Feng YL, Wan T, Zhang YN, Cao XP, Huang YW, et al. Effectiveness of sequential chemoradiation vs concurrent chemoradiation or radiation alone in adjuvant treatment after hysterectomy for cervical cancer: The STARS phase 3 randomized clinical trial. JAMA Oncol (2021) 7(3):361–9. doi: 10.1001/jamaoncol.2020.7168
8. Gao S, Du S, Lu Z, Xin J, Gao S, Sun. H. Multiparametric PET/MR (PET and MR-IVIM) for the evaluation of early treatment response and prediction of tumor recurrence in patients with locally advanced cervical cancer. Eur Radiol (2020) 30(2):1191–201. doi: 10.1007/s00330-019-06428-w
9. Cohen PA, Jhingran A, Oaknin A, Denny. L. Cervical cancer. Lancet (2019) 393(10167):169–82. doi: 10.1016/S0140-6736(18)32470-X
10. Feng CH, Mell LK, Sharabi AB, McHale M, Mayadev. JS. Immunotherapy with radiotherapy and chemoradiotherapy for cervical cancer. Semin Radiat Oncol (2020) 30(4):273–80. doi: 10.1016/j.semradonc.2020.05.003
11. Nin DS, Wujanto C, Tan TZ, Lim D, Damen JMA, Wu KY, et al. GAGE mediates radioresistance in cervical cancers via the regulation of chromatin accessibility. Cell Rep (2021) 36(9):109621. doi: 10.1016/j.celrep.2021.109621
12. Zeng X, Wan L, Wang Y, Xue J, Yang H, Zhu Y. Effect of low dose of berberine on the radioresistance of cervical cancer cells via a PI3K/HIF-1 pathway under nutrient-deprived conditions. Int J Radiat Biol (2020) 96(8):1060–7. doi: 10.1080/09553002.2020.1770358
13. Schulz A, Meyer F, Dubrovska A, Borgmann K. Cancer stem cells and radioresistance: DNA repair and beyond. Cancers (Basel) (2019) 11(6):862. doi: 10.3390/cancers11060862
14. Jameel JK, Rao VS, Cawkwell L, Drew PJ. Radioresistance in carcinoma of the breast. Breast (2004) 13(6):452–60. doi: 10.1016/j.breast.2004.08.004
15. Huang RX, Zhou PK. DNA Damage response signaling pathways and targets for radiotherapy sensitization in cancer. Signal Transduct Target Ther (2020) 5(1):60. doi: 10.1038/s41392-020-0150-x
16. Jiao X, Zhang S, Jiao J, Zhang T, Qu W, Muloye GM, et al. Promoter methylation of SEPT9 as a potential biomarker for early detection of cervical cancer and its overexpression predicts radioresistance. Clin Epigenet (2019) 11(1):120. doi: 10.1186/s13148-019-0719-9
17. Feng Y, Wang Z, Yang N, Liu S, Yan J, Song J, et al. Identification of biomarkers for cervical cancer radiotherapy resistance based on RNA sequencing data. Front Cell Dev Biol (2021) 9:724172. doi: 10.3389/fcell.2021.724172
18. Glicksman R, Chaudary N, Pintilie M, Leung E, Clarke B, Sy K, et al. The predictive value of nadir neutrophil count during treatment of cervical cancer: Interactions with tumor hypoxia and interstitial fluid pressure (IFP). Clin Transl Radiat Oncol (2017) 6:15–20. doi: 10.1016/j.ctro.2017.08.002
19. Liu J, Zhang J, Wang X, Li Y, Chen Y, Li K, et al. HIF-1 and NDRG2 contribute to hypoxia-induced radioresistance of cervical cancer hela cells. Exp Cell Res (2010) 316(12):1985–93. doi: 10.1016/j.yexcr.2010.02.028
20. Leung E, Cairns RA, Chaudary N, Vellanki RN, Kalliomaki T, Moriyama EH, et al. Metabolic targeting of HIF-dependent glycolysis reduces lactate, increases oxygen consumption and enhances response to high-dose single-fraction radiotherapy in hypoxic solid tumors. BMC Cancer (2017) 17(1):418. doi: 10.1186/s12885-017-3402-6
21. Tang L, Wei F, Wu Y, He Y, Shi L, Xiong F, et al. Role of metabolism in cancer cell radioresistance and radiosensitization methods. J Exp Clin Cancer Res (2018) 37(1):87. doi: 10.1186/s13046-018-0758-7
22. Lin J, Xia L, Liang J, Han Y, Wang H, Oyang L, et al. The roles of glucose metabolic reprogramming in chemo- and radioresistance. J Exp Clin Cancer Res (2019) 38(1):218. doi: 10.1186/s13046-019-1214-z
23. Pitroda SP, Wakim BT, Sood RF, Beveridge MG, Beckett MA, MacDermed DM, et al. STAT1-dependent expression of energy metabolic pathways links tumour growth and radioresistance to the warburg effect. BMC Med (2009) 7:68. doi: 10.1186/1741-7015-7-68
24. Shimura T, Noma N, Sano Y, Ochiai Y, Oikawa T, Fukumoto M, et al. AKT-mediated enhanced aerobic glycolysis causes acquired radioresistance by human tumor cells. Radiother Oncol (2014) 112(2):302–7. doi: 10.1016/j.radonc.2014.07.015
25. Ni M, Li J, Zhao H, Xu F, Cheng J, Yu M, et al. BRD4 inhibition sensitizes cervical cancer to radiotherapy by attenuating DNA repair. Oncogene (2021) 40(15):2711–24. doi: 10.1038/s41388-021-01735-3
26. Lee SY, Jeong EK, Ju MK, Jeon HM, Kim MY, Kim CH, et al. Induction of metastasis, cancer stem cell phenotype, and oncogenic metabolism in cancer cells by ionizing radiation. Mol Cancer (2017) 16(1):10. doi: 10.1186/s12943-016-0577-4
27. Moding EJ, Kastan MB, Kirsch DG. Strategies for optimizing the response of cancer and normal tissues to radiation. Nat Rev Drug Discovery (2013) 12(7):526–42. doi: 10.1038/nrd4003
28. Kuwahara Y, Tomita K, Roudkenar MH, Roushandeh AM, Urushihara Y, Igarashi K, et al. The effects of hydrogen peroxide and/or radiation on the survival of clinically relevant radioresistant cells. Technol Cancer Res Treat (2020) 19:1533033820980077. doi: 10.1177/1533033820980077
29. Centurione L, Aiello FB. DNA Repair and cytokines: TGF-beta, IL-6, and thrombopoietin as different biomarkers of radioresistance. Front Oncol (2016) 6:175. doi: 10.3389/fonc.2016.00175
30. Zhao Y, Chen S. Targeting DNA double-strand break (DSB) repair to counteract tumor radioresistance. Curr Drug Targets (2019) 20(9):891–902. doi: 10.2174/1389450120666190222181857
31. Kim W, Lee S, Seo D, Kim D, Kim K, Kim E, et al. Cellular stress responses in radiotherapy. Cells (2019) 8(9):1105. doi: 10.3390/cells8091105
32. Fischer N, Seo EJ, Efferth T. Prevention from radiation damage by natural products. Phytomedicine (2018) . 47:192–200. doi: 10.1016/j.phymed.2017.11.005
33. Zou Z, Chang H, Li H, Wang. S. Induction of reactive oxygen species: an emerging approach for cancer therapy. Apoptosis (2017) 22(11):1321–35. doi: 10.1007/s10495-017-1424-9
34. Kim H, Kim MW, Jeong YI, Yang HS. Redox-sensitive and folate-Receptor-Mediated targeting of cervical cancer cells for photodynamic therapy using nanophotosensitizers composed of chlorin e6-conjugated beta-cyclodextrin via diselenide linkage. Cells (2021) 10(9):2190. doi: 10.3390/cells10092190
35. Xin Y, Guo W, Yang C, Huang Q, Zhang P, Zhang L, et al. Photodynamic effects of vitamin K3 on cervical carcinoma cells activating mitochondrial apoptosis pathways. Anticancer Agents Med Chem (2021) 21(1):91–9. doi: 10.2174/1871520620666200814164629
36. Fu S, Jin L, Gong T, Pan S, Zheng S, Zhang X, et al. Effect of sinomenine hydrochloride on radiosensitivity of esophageal squamous cell carcinoma cells. Oncol Rep (2018) 39(4):1601–8. doi: 10.3892/or.2018.6228
37. Wright WD, Shah SS, Heyer. WD. Homologous recombination and the repair of DNA double-strand breaks. J Biol Chem (2018) 293(27):10524–35. doi: 10.1074/jbc.TM118.000372
38. Fanale D, Bazan V, Caruso S, Castiglia M, Bronte G, Rolfo C, et al. Hypoxia and human genome stability: downregulation of BRCA2 expression in breast cancer cell lines. BioMed Res Int (2013) 2013:746858. doi: 10.1155/2013/746858
39. Meng AX, Jalali F, Cuddihy A, Chan N, Bindra RS, Glazer PM, et al. Hypoxia down-regulates DNA double strand break repair gene expression in prostate cancer cells. Radiother Oncol (2005) 76(2):168–76. doi: 10.1016/j.radonc.2005.06.025
40. Valikhani M, Rahimian E, Ahmadi SE, Chegeni R, Safa. M. Involvement of classic and alternative non-homologous end joining pathways in hematologic malignancies: targeting strategies for treatment. Exp Hematol Oncol (2021) 10(1):51. doi: 10.1186/s40164-021-00242-1
41. Lobbardi R, Pinder J, Martinez-Pastor B, Theodorou M, Blackburn JS, Abraham BJ, et al. TOX regulates growth, DNA repair, and genomic instability in T-cell acute lymphoblastic leukemia. Cancer Discovery (2017) 7(11):1336–53. doi: 10.1158/2159-8290.CD-17-0267
42. Zhang J, Chen M, Pang Y, Cheng M, Huang B, Xu S, et al. Flap endonuclease 1 and DNA-PKcs synergistically participate in stabilizing replication fork to encounter replication stress in glioma cells. J Exp Clin Cancer Res (2022) 41(1):140. doi: 10.1186/s13046-022-02334-0
43. Gauthier LR, Granotier C, Hoffschir F, Etienne O, Ayouaz A, Desmaze C, et al. Rad51 and DNA-PKcs are involved in the generation of specific telomere aberrations induced by the quadruplex ligand 360A that impair mitotic cell progression and lead to cell death. Cell Mol Life Sci (2012) 69(4):629–40. doi: 10.1007/s00018-011-0767-6
44. Ma Q, Kai J, Liu Y, Tong Y, Xie S, Zheng H, et al. Targeting Ku86 enhances X-ray-induced radiotherapy sensitivity in serous ovarian cancer cells. Int J Biochem Cell Biol (2020) 121:105705. doi: 10.1016/j.biocel.2020.105705
45. Xu S, Kim J, Tang Q, Chen Q, Liu J, Xu Y, et al. CAS9 is a genome mutator by directly disrupting DNA-PK dependent DNA repair pathway. Protein Cell (2020) 11(5):352–65. doi: 10.1007/s13238-020-00699-6
46. Guo Z, Wang YH, Xu H, Yuan CS, Zhou HH, Huang WH, et al. LncRNA linc00312 suppresses radiotherapy resistance by targeting DNA-PKcs and impairing DNA damage repair in nasopharyngeal carcinoma. Cell Death Dis (2021) 12(1):69. doi: 10.1038/s41419-020-03302-2
47. Sun H, Fan G, Deng C, Wu L. miR-4429 sensitized cervical cancer cells to irradiation by targeting RAD51. J Cell Physiol (2020) 235(1):185–93. doi: 10.1002/jcp.28957
48. Gachechiladze M, Skarda J, Soltermann A, Joerger. M. RAD51 as a potential surrogate marker for DNA repair capacity in solid malignancies. Int J Cancer (2017) 141(7):1286–94. doi: 10.1002/ijc.30764
49. Wang S, Wang Y, Liu X, Yang Y, Wu S, Liu. Y. SFN enhanced the radiosensitivity of cervical cancer cells via activating LATS2 and blocking Rad51/MDC1 recruitment to DNA damage site. Cancers (Basel) (2022) 14(8):1872. doi: 10.3390/cancers14081872
50. Wang X, Gu Y, Liu H, Shi L, Sun. X. Icotinib hydrochloride enhances chemo- and radiosensitivity by inhibiting EGFR signaling and attenuating RAD51 expression and function in hela S3 cells. Onco Targets Ther (2018) 11:1245–58. doi: 10.2147/OTT.S152613
51. Gasinska A, Biesaga B, Widla AJ, Darasz. Z. Positive effect of single nucleotide RAD51 135G>C polymorphism and low Ku70 protein expression on female rectal cancer patients survival after preoperative radiotherapy. Turk J Gastroenterol (2019) 30(1):3–14. doi: 10.5152/tjg.2018.17486
52. Yue X, Bai C, Xie D, Ma T, Zhou. PK. DNA-PKcs: A multi-faceted player in DNA damage response. Front Genet (2020) 11:607428. doi: 10.3389/fgene.2020.607428
53. Wilson CR, Davidson SE, Margison GP, Jackson SP, Hendry JH, West. CM. Expression of Ku70 correlates with survival in carcinoma of the cervix. Br J Cancer (2000) 83(12):1702–6. doi: 10.1054/bjoc.2000.1510
54. Harima Y, Sawada S, Miyazaki Y, Kin K, Ishihara H, Imamura M, et al. Expression of Ku80 in cervical cancer correlates with response to radiotherapy and survival. Am J Clin Oncol (2003) 26(4):e80–5. doi: 10.1097/01.COC.0000077938.48974.59
55. Wang YY, Hung AC, Lo S, Hsieh YC, Yuan. SF. MRE11 as a molecular signature and therapeutic target for cancer treatment with radiotherapy. Cancer Lett (2021) 514:1–11. doi: 10.1016/j.canlet.2021.05.013
56. Pranatharthi A, Thomas P, Udayashankar AH, Bhavani C, Suresh SB, Krishna S, et al. RhoC regulates radioresistance via crosstalk of ROCK2 with the DNA repair machinery in cervical cancer. J Exp Clin Cancer Res (2019) 38(1):392. doi: 10.1186/s13046-019-1385-7
57. Beskow C, Skikuniene J, Holgersson A, Nilsson B, Lewensohn R, Kanter L, et al. Radioresistant cervical cancer shows upregulation of the NHEJ proteins DNA-PKcs, Ku70 and Ku86. Br J Cancer (2009) 101(5):816–21. doi: 10.1038/sj.bjc.6605201
58. Zhuang L, Yu SY, Huang XY, Cao Y, Xiong. HH. [Potentials of DNA-PKcs, Ku80, and ATM in enhancing radiosensitivity of cervical carcinoma cells]. Ai Zheng (2007) 26(7):724–9.
59. van Oorschot B, Granata G, Di Franco S, Ten Cate R, Rodermond HM, Todaro M, et al. Targeting DNA double strand break repair with hyperthermia and DNA-PKcs inhibition to enhance the effect of radiation treatment. Oncotarget (2016) 7(40):65504–13. doi: 10.18632/oncotarget.11798
60. M IJ, van Oorschot B, Oei AL, Krawczyk PM, Rodermond HM, Stalpers LJA, et al. Enhancement of radiation effectiveness in cervical cancer cells by combining ionizing radiation with hyperthermia and molecular targeting agents. Int J Mol Sci (2018) 19(8):2420. doi: 10.3390/ijms19082420
61. Raspaglio G, Buttarelli M, Filippetti F, Battaglia A, Buzzonetti A, Scambia G, et al. Stat1 confers sensitivity to radiation in cervical cancer cells by controlling Parp1 levels: a new perspective for Parp1 inhibition. Cell Death Dis (2021) 12(10):933:2092. doi: 10.1038/s41419-021-04229-y
62. M IJ, van Bochove GGW, Whitton D, Winiarczyk R, Honhoff C, Rodermond H, et al. PARP1-inhibition sensitizes cervical cancer cell lines for chemoradiation and thermoradiation. Cancers (Basel) (2021) 13(9):2092. doi: 10.3390/cancers13092092
63. Zhang C, Zhu Q, He H, Jiang L, Qiang Q, Hu L, et al. RIZ1: a potential tumor suppressor in glioma. BMC Cancer (2015) 15:990. doi: 10.1186/s12885-015-2023-1
64. Liu ZY, Wang JY, Liu HH, Ma XM, Wang CL, Zhang XP, et al. Retinoblastoma protein-interacting zinc-finger gene 1 (RIZ1) dysregulation in human malignant meningiomas. Oncogene (2013) 32(10):1216–22. doi: 10.1038/onc.2012.155
65. Yang S, Xing L, Gu L, Cheng H, Feng Y, Zhang Y. Combination of RIZ1 overexpression and radiotherapy contributes to apoptosis and DNA damage of HeLa and SiHa cervical cancer cells. Basic Clin Pharmacol Toxicol (2018) 123(2):137–46. doi: 10.1111/bcpt.13008
66. Hara T, Omura-Minamisawa M, Kang Y, Cheng C, Inoue T. Flavopiridol potentiates the cytotoxic effects of radiation in radioresistant tumor cells in which p53 is mutated or bcl-2 is overexpressed. Int J Radiat Oncol Biol Phys (2008) 71(5):1485–95. doi: 10.1016/j.ijrobp.2008.03.039
67. Liu SS, Leung RC, Chan KY, Chiu PM, Cheung AN, Tam KF, et al. p73 expression is associated with the cellular radiosensitivity in cervical cancer after radiotherapy. Clin Cancer Res (2004) 10(10):3309–16. doi: 10.1158/1078-0432.CCR-03-0119
68. Renna C, Salaroli R, Cocchi C, Cenacchi. G. XAV939-mediated ARTD activity inhibition in human MB cell lines. PloS One (2015) 10(4):e0124149. doi: 10.1371/journal.pone.0124149
69. Zhang J, Si J, Gan L, Guo M, Yan J, Chen Y, et al. Inhibition of wnt signalling pathway by XAV939 enhances radiosensitivity in human cervical cancer HeLa cells. Artif Cells Nanomed Biotechnol (2020) 48(1):479–87. doi: 10.1080/21691401.2020.1716779
70. Wieringa HW, van der Zee AG, de Vries EG, van Vugt. MA. Breaking the DNA damage response to improve cervical cancer treatment. Cancer Treat Rev (2016) 42:30–40. doi: 10.1016/j.ctrv.2015.11.008
71. Liu Q, Wang J, Tang M, Chen L, Qi X, Li J, et al. The overexpression of PXN promotes tumor progression and leads to radioresistance in cervical cancer. Future Oncol (2018) 14(3):241–53. doi: 10.2217/fon-2017-0474
72. Kopa P, Macieja A, Pastwa E, Majsterek I, Poplawski. T. DNA Double-strand breaks repair inhibitors potentiates the combined effect of VP-16 and CDDP in human colorectal adenocarcinoma (LoVo) cells. Mol Biol Rep (2021) 48(1):709–20. doi: 10.1007/s11033-020-06124-9
73. Patterson-Fortin J, Bose A, Tsai WC, Grochala CJ, Nguyen H, Zhou J, et al. Targeting DNA repair with combined inhibition of NHEJ and MMEJ induces synthetic lethality in TP53-mutant cancers. Cancer Res (2022) CAN-22-1124. doi: 10.1158/0008-5472.CAN-22-1124
74. Nakamura K, Karmokar A, Farrington PM, James NH, Ramos-Montoya A, Bickerton SJ, et al. Inhibition of DNA-PK with AZD7648 sensitizes tumor cells to radiotherapy and induces type I IFN-dependent durable tumor control. Clin Cancer Res (2021) 27(15):4353–66. doi: 10.1158/1078-0432.CCR-20-3701
75. Abegg D, Tomanik M, Qiu N, Pechalrieu D, Shuster A, Commare B, et al. Chemoproteomic profiling by cysteine fluoroalkylation reveals myrocin G as an inhibitor of the nonhomologous end joining DNA repair pathway. J Am Chem Soc (2021) 143(48):20332–42. doi: 10.1021/jacs.1c09724
76. Kilgas S, Singh AN, Paillas S, Then CK, Torrecilla I, Nicholson J, et al. p97/VCP inhibition causes excessive MRE11-dependent DNA end resection promoting cell killing after ionizing radiation. Cell Rep (2021) 35(8):109153. doi: 10.1016/j.celrep.2021.109153
77. Prasad CB, Prasad SB, Yadav SS, Pandey LK, Singh S, Pradhan S, et al. Olaparib modulates DNA repair efficiency, sensitizes cervical cancer cells to cisplatin and exhibits anti-metastatic property. Sci Rep (2017) 7(1):12876. doi: 10.1038/s41598-017-13232-3
78. Saha S, Rundle S, Kotsopoulos IC, Begbie J, Howarth R, Pappworth IY, et al. Determining the potential of DNA damage response (DDR) inhibitors in cervical cancer therapy. Cancers (Basel) (2022) 14(17):4288. doi: 10.3390/cancers14174288
79. Xie G, Wang Z, Chen Y, Zhang S, Feng L, Meng F, et al. Dual blocking of PI3K and mTOR signaling by NVP-BEZ235 inhibits proliferation in cervical carcinoma cells and enhances therapeutic response. Cancer Lett (2017) 388:12–20. doi: 10.1016/j.canlet.2016.11.024
80. Hu J, Zhang Y, Tang N, Lu Y, Guo P, Huang. Z. Discovery of novel 1,3,5-triazine derivatives as potent inhibitor of cervical cancer via dual inhibition of PI3K/mTOR. Bioorg Med Chem (2021) 32:115997. doi: 10.1016/j.bmc.2021.115997
81. Li S, Li Y, Hu R, Li W, Qiu H, Cai H, et al. The mTOR inhibitor AZD8055 inhibits proliferation and glycolysis in cervical cancer cells. Oncol Lett (2013) 5(2):717–21. doi: 10.3892/ol.2012.1058
82. Zhou R, Wang H, Yang Y, Zhang C, Dong X, Du J, et al. Tumor microenvironment-manipulated radiocatalytic sensitizer based on bismuth heteropolytungstate for radiotherapy enhancement. Biomaterials (2019) 189:11–22. doi: 10.1016/j.biomaterials.2018.10.016
83. Yao T, Weng X, Yao Y, Huang C, Li J, Peng Y, et al. ALDH-1-positive cells exhibited a radioresistant phenotype that was enhanced with hypoxia in cervical cancer. BMC Cancer (2020) 20(1):891. doi: 10.1186/s12885-020-07337-8
84. Bachtiary B, Schindl M, Potter R, Dreier B, Knocke TH, Hainfellner JA, et al. Overexpression of hypoxia-inducible factor 1alpha indicates diminished response to radiotherapy and unfavorable prognosis in patients receiving radical radiotherapy for cervical cancer. Clin Cancer Res (2003) 9(6):2234–40.
85. Xiong H, Nie X, Zou Y, Gong C, Li Y, Wu H, et al. Twist1 enhances hypoxia induced radioresistance in cervical cancer cells by promoting nuclear EGFR localization. J Cancer (2017) 8(3):345–53. doi: 10.7150/jca.16607
86. Jing X, Yang F, Shao C, Wei K, Xie M, Shen H, et al. Role of hypoxia in cancer therapy by regulating the tumor microenvironment. Mol Cancer (2019) 18(1):157. doi: 10.1186/s12943-019-1089-9
87. Burri P, Djonov V, Aebersold DM, Lindel K, Studer U, Altermatt HJ, et al. Significant correlation of hypoxia-inducible factor-1alpha with treatment outcome in cervical cancer treated with radical radiotherapy. Int J Radiat Oncol Biol Phys (2003) 56(2):494–501. doi: 10.1016/s0360-3016(02)04579-0
88. Zhang MX, Wang L, Zeng L, Tu. ZW. LCN2 is a potential biomarker for radioresistance and recurrence in nasopharyngeal carcinoma. Front Oncol (2020) 10:605777. doi: 10.3389/fonc.2020.605777
89. Yan S, Wang Y, Yang Q, Li X, Kong X, Zhang N, et al. Low-dose radiation-induced epithelial-mesenchymal transition through NF-kappaB in cervical cancer cells. Int J Oncol (2013) 42(5):1801–6. doi: 10.3892/ijo.2013.1852
90. Dong L, Zhang X, Xiang W, Ni J, Zhou W, Li. H. Post-transcription mediated snail stabilization is involved in radiation exposure induced invasion and migration of hepatocarcinoma cells. BioMed Pharmacother (2018) 103:767–72. doi: 10.1016/j.biopha.2018.04.095
91. Nagaraja SS, Krishnamoorthy V, Raviraj R, Paramasivam A, Nagarajan. D. Effect of trichostatin a on radiation induced epithelial-mesenchymal transition in A549 cells. Biochem Biophys Res Commun (2017) 493(4):1534–41. doi: 10.1016/j.bbrc.2017.10.031
92. Zhang P, Liu H, Xia F, Zhang QW, Zhang YY, Zhao Q, et al. Epithelial-mesenchymal transition is necessary for acquired resistance to cisplatin and increases the metastatic potential of nasopharyngeal carcinoma cells. Int J Mol Med (2014) 33(1):151–9. doi: 10.3892/ijmm.2013.1538
93. Chi HC, Tsai CY, Tsai MM, Yeh CT, Lin. KH. Roles of long noncoding RNAs in recurrence and metastasis of radiotherapy-resistant cancer stem cells. Int J Mol Sci (2017) 18(9):1903. doi: 10.3390/ijms18091903
94. Odiase O, Noah-Vermillion L, Simone BA, Aridgides. PD. The incorporation of immunotherapy and targeted therapy into chemoradiation for cervical cancer: A focused review. Front Oncol (2021) 11:663749. doi: 10.3389/fonc.2021.663749
95. Ferrall L, Lin KY, Roden RBS, Hung CF, Wu. TC. Cervical cancer immunotherapy: Facts and hopes. Clin Cancer Res (2021) 27(18):4953–73. doi: 10.1158/1078-0432.CCR-20-2833
96. Monk BJ, Enomoto T, Kast WM, McCormack M, Tan DSP, Wu X, et al. Integration of immunotherapy into treatment of cervical cancer: Recent data and ongoing trials. Cancer Treat Rev (2022) 106:102385. doi: 10.1016/j.ctrv.2022.102385
97. Minion LE, Tewari KS. Cervical cancer - state of the science: From angiogenesis blockade to checkpoint inhibition. Gynecol Oncol (2018) 148(3):609–21. doi: 10.1016/j.ygyno.2018.01.009
98. Liu Z, Xiao Y, Zhou Z, Mao X, Cai J, Xiong L, et al. Extensive metabolic disorders are present in APC(min) tumorigenesis mice. Mol Cell Endocrinol (2016) 427:57–64. doi: 10.1016/j.mce.2016.03.004
99. Faubert B, Vincent EE, Griss T, Samborska B, Izreig S, Svensson RU, et al. Loss of the tumor suppressor LKB1 promotes metabolic reprogramming of cancer cells via HIF-1alpha. Proc Natl Acad Sci U.S.A. (2014) 111(7):2554–9. doi: 10.1073/pnas.1312570111
100. Rashmi R, Huang X, Floberg JM, Elhammali AE, McCormick ML, Patti GJ, et al. Radioresistant cervical cancers are sensitive to inhibition of glycolysis and redox metabolism. Cancer Res (2018) 78(6):1392–403. doi: 10.1158/0008-5472.CAN-17-2367
101. Rashmi R, Jayachandran K, Zhang J, Menon V, Muhammad N, Zahner M, et al. Glutaminase inhibitors induce thiol-mediated oxidative stress and radiosensitization in treatment-resistant cervical cancers. Mol Cancer Ther (2020) 19(12):2465–75. doi: 10.1158/1535-7163.MCT-20-0271
102. Anoopkumar-Dukie S, Conere T, Houston A, King L, Christie D, McDermott C, et al. The COX-2 inhibitor NS398 selectively sensitizes hypoxic HeLa cells to ionising radiation by mechanisms both dependent and independent of COX-2. Prostaglandins Other Lipid Mediat (2020) 148:106422. doi: 10.1016/j.prostaglandins.2020.106422
103. Liu Z, Zhu W, Kong X, Chen X, Sun X, Zhang W, et al. Tanshinone IIA inhibits glucose metabolism leading to apoptosis in cervical cancer. Oncol Rep (2019) 42(5):1893–903. doi: 10.3892/or.2019.7294
104. Zhang Y, Zhao L, Yang S, Cen Y, Zhu T, Wang L, et al. CircCDKN2B-AS1 interacts with IMP3 to stabilize hexokinase 2 mRNA and facilitate cervical squamous cell carcinoma aerobic glycolysis progression. J Exp Clin Cancer Res (2020) 39(1):281. doi: 10.1186/s13046-020-01793-7
105. Rudlowski C, Becker AJ, Schroder W, Rath W, Buttner R, Moser. M. GLUT1 messenger RNA and protein induction relates to the malignant transformation of cervical cancer. Am J Clin Pathol (2003) 120(5):691–8. doi: 10.1309/4KYN-QM58-62JW-2GD7
106. Gao R, Wu X, Huang Z, Wang B, Li F, Xu H, et al. Anti-tumor effect of aloe-emodin on cervical cancer cells was associated with human papillomavirus E6/E7 and glucose metabolism. Onco Targets Ther (2019) 12:3713–21. doi: 10.2147/OTT.S182405
107. Jiang X, Yuan J, Dou Y, Zeng D, Xiao. S. Lipopolysaccharide affects the proliferation and glucose metabolism of cervical cancer cells through the FRA1/MDM2/p53 pathway. Int J Med Sci (2021) 18(4):1030–8. doi: 10.7150/ijms.47360
108. Ferrer CM, Lynch TP, Sodi VL, Falcone JN, Schwab LP, Peacock DL, et al. O-GlcNAcylation regulates cancer metabolism and survival stress signaling via regulation of the HIF-1 pathway. Mol Cell (2014) 54(5):820–31. doi: 10.1016/j.molcel.2014.04.026
109. Vallee A, Lecarpentier Y, Vallee. JN. The key role of the WNT/beta-catenin pathway in metabolic reprogramming in cancers under normoxic conditions. Cancers (Basel) (2021) 13(21):5557. doi: 10.3390/cancers13215557
110. Zhu Y, Qiu Y, Zhang. X. TKTL1 participated in malignant progression of cervical cancer cells via regulating AKT signal mediated PFKFB3 and thus regulating glycolysis. Cancer Cell Int (2021) 21(1):678. doi: 10.1186/s12935-021-02383-z
111. Ganapathy-Kanniappan S, Geschwind JF. Tumor glycolysis as a target for cancer therapy: progress and prospects. Mol Cancer (2013) 12:152. doi: 10.1186/1476-4598-12-152
112. Hanahan D, Weinberg RA. Hallmarks of cancer: the next generation. Cell (2011) 144(5):646–74. doi: 10.1016/j.cell.2011.02.013
113. Sun L, Zeng X, Yan C, Sun X, Gong X, Rao Y, et al. Crystal structure of a bacterial homologue of glucose transporters GLUT1-4. Nature (2012) 490(7420):361–6. doi: 10.1038/nature11524
114. Mueckler M, Caruso C, Baldwin SA, Panico M, Blench I, Morris HR, et al. Sequence and structure of a human glucose transporter. Science (1985) 229(4717):941–5. doi: 10.1126/science.3839598
115. Dykstra H, LaRose C, Fisk C, Waldhart A, Meng X, Zhao G, et al. TXNIP interaction with GLUT1 depends on PI(4,5)P2. Biochim Biophys Acta Biomembr (2021) 1863(12):183757. doi: 10.1016/j.bbamem.2021.183757
116. Iwasaki K, Yabushita H, Ueno T, Wakatsuki A. Role of hypoxia-inducible factor-1alpha, carbonic anhydrase-IX, glucose transporter-1 and vascular endothelial growth factor associated with lymph node metastasis and recurrence in patients with locally advanced cervical cancer. Oncol Lett (2015) 10(4):1970–8. doi: 10.3892/ol.2015.3524
117. Liu Y, Cao Y, Zhang W, Bergmeier S, Qian Y, Akbar H, et al. A small-molecule inhibitor of glucose transporter 1 downregulates glycolysis, induces cell-cycle arrest, and inhibits cancer cell growth in vitro and in vivo. Mol Cancer Ther (2012) 11(8):1672–82. doi: 10.1158/1535-7163.MCT-12-0131
118. Cheng Y, Chen G, Hong L, Zhou L, Hu M, Li B, et al. How does hypoxia inducible factor-1alpha participate in enhancing the glycolysis activity in cervical cancer? Ann Diagn Pathol (2013) 17(3):305–11. doi: 10.1016/j.anndiagpath.2012.12.002
119. Mayer A, Hockel M, Vaupel. P. Endogenous hypoxia markers in locally advanced cancers of the uterine cervix: reality or wishful thinking? Strahlenther Onkol (2006) 182(9):501–10. doi: 10.1007/s00066-006-1525-9
120. Airley R, Loncaster J, Davidson S, Bromley M, Roberts S, Patterson A, et al. Glucose transporter glut-1 expression correlates with tumor hypoxia and predicts metastasis-free survival in advanced carcinoma of the cervix. Clin Cancer Res (2001) 7(4):928–34.
121. Reyna-Hernandez MA, Alarcon-Romero LDC, Ortiz-Ortiz J, Illades-Aguiar B, Jimenez-Lopez MA, Ocampo-Barcenas A, et al. GLUT1, LDHA, and MCT4 expression is deregulated in cervical cancer and precursor lesions. J Histochem Cytochem (2022) 70(6):437–46. doi: 10.1369/00221554221101662
122. Gaber G, El Achy S, Khedr GA, Parimi V, Helenowksi I, Donnelly ED, et al. Impact of p53, HIF1a, ki-67, CA-9, and GLUT1 expression on treatment outcomes in locally advanced cervical cancer patients treated with definitive chemoradiation therapy. Am J Clin Oncol (2021) 44(2):58–67. doi: 10.1097/COC.0000000000000781
123. Wang J, Ye C, Chen C, Xiong H, Xie B, Zhou J, et al. Glucose transporter GLUT1 expression and clinical outcome in solid tumors: a systematic review and meta-analysis. Oncotarget (2017) 8(10):16875–86. doi: 10.18632/oncotarget.15171
124. Markowska J, Grabowski JP, Tomaszewska K, Kojs Z, Pudelek J, Skrzypczak M, et al. Significance of hypoxia in uterine cervical cancer. multicentre study. Eur J Gynaecol Oncol (2007) 28(5):386–8.
125. Moreno-Acosta P, Vallard A, Carrillo S, Gamboa O, Romero-Rojas A, Molano M, et al. Biomarkers of resistance to radiation therapy: a prospective study in cervical carcinoma. Radiat Oncol (2017) 12(1):120. doi: 10.1186/s13014-017-0856-2
126. Ancey PB, Contat C, Boivin G, Sabatino S, Pascual J, Zangger N, et al. GLUT1 expression in tumor-associated neutrophils promotes lung cancer growth and resistance to radiotherapy. Cancer Res (2021) 81(9):2345–57. doi: 10.1158/0008-5472.CAN-20-2870
127. Kunkel M, Moergel M, Stockinger M, Jeong JH, Fritz G, Lehr HA, et al. Overexpression of GLUT-1 is associated with resistance to radiotherapy and adverse prognosis in squamous cell carcinoma of the oral cavity. Oral Oncol (2007) 43(8):796–803. doi: 10.1016/j.oraloncology.2006.10.009
128. Doki Y, Takachi K, Ishikawa O, Sasaki Y, Miyashiro I, Ohigashi H, et al. Reduced tumor vessel density and high expression of glucose transporter 1 suggest tumor hypoxia of squamous cell carcinoma of the esophagus surviving after radiotherapy. Surgery (2005) 137(5):536–44. doi: 10.1016/j.surg.2005.01.008
129. Saigusa S, Toiyama Y, Tanaka K, Okugawa Y, Fujikawa H, Matsushita K, et al. Prognostic significance of glucose transporter-1 (GLUT1) gene expression in rectal cancer after preoperative chemoradiotherapy. Surg Today (2012) 42(5):460–9. doi: 10.1007/s00595-011-0027-2
130. Korkeila E, Jaakkola PM, Syrjanen K, Pyrhonen S, Sundstrom. J. Pronounced tumour regression after radiotherapy is associated with negative/weak glucose transporter-1 expression in rectal cancer. Anticancer Res (2011) 31(1):311–5.
131. Feng Y, Xiong Y, Qiao T, Li X, Jia L, Han. Y. Lactate dehydrogenase a: A key player in carcinogenesis and potential target in cancer therapy. Cancer Med (2018) 7(12):6124–36. doi: 10.1002/cam4.1820
132. Zhang W, Wang C, Hu X, Lian Y, Ding C, Ming. L. Inhibition of LDHA suppresses cell proliferation and increases mitochondrial apoptosis via the JNK signaling pathway in cervical cancer cells. Oncol Rep (2022) 47(4):77. doi: 10.3892/or.2022.8288
133. Guo Y, Li X, Sun X, Wang J, Yang X, Zhou X, et al. Combined aberrant expression of NDRG2 and LDHA predicts hepatocellular carcinoma prognosis and mediates the anti-tumor effect of gemcitabine. Int J Biol Sci (2019) 15(9):1771–86. doi: 10.7150/ijbs.35094
134. Koukourakis MI, Giatromanolaki A, Panteliadou M, Pouliliou SE, Chondrou PS, Mavropoulou S, et al. Lactate dehydrogenase 5 isoenzyme overexpression defines resistance of prostate cancer to radiotherapy. Br J Cancer (2014) 110(9):2217–23. doi: 10.1038/bjc.2014.158
135. Walenta S, Wetterling M, Lehrke M, Schwickert G, Sundfor K, Rofstad EK, et al. High lactate levels predict likelihood of metastases, tumor recurrence, and restricted patient survival in human cervical cancers. Cancer Res (2000) 60(4):916–21.
136. Luan Y, Zhang W, Xie J, Mao. J. CDKN2A inhibits cell proliferation and invasion in cervical cancer through LDHA-mediated AKT/mTOR pathway. Clin Transl Oncol (2021) 23(2):222–8. doi: 10.1007/s12094-020-02409-4
137. Chen L, Zhong Y, Yang X, Zhang Q, Wu. X. Downregulation of GTSE1 leads to the inhibition of proliferation, migration, and warburg effect in cervical cancer by blocking LHDA expression. J Obstet Gynaecol Res (2021) 47(11):3913–22. doi: 10.1111/jog.15000
138. An JS, Huang MN, Song YM, Li N, Wu LY, Zhan. QM. A preliminary study of genes related to concomitant chemoradiotherapy resistance in advanced uterine cervical squamous cell carcinoma. Chin Med J (Engl) (2013) 126(21):4109–15.
139. Kitahara O, Katagiri T, Tsunoda T, Harima Y, Nakamura Y. Classification of sensitivity or resistance of cervical cancers to ionizing radiation according to expression profiles of 62 genes selected by cDNA microarray analysis. Neoplasia (2002) 4(4):295–303. doi: 10.1038/sj.neo.7900251
140. Schwab M, Thunborg K, Azimzadeh O, von Toerne C, Werner C, Shevtsov M, et al. Targeting cancer metabolism breaks radioresistance by impairing the stress response. Cancers (Basel) (2021) 13(15):3762. doi: 10.3390/cancers13153762
141. Yang Y, Chong Y, Chen M, Dai W, Zhou X, Ji Y, et al. Targeting lactate dehydrogenase a improves radiotherapy efficacy in non-small cell lung cancer: from bedside to bench. J Transl Med (2021) 19(1):170. doi: 10.1186/s12967-021-02825-2
142. Damasceno LEA, Prado DS, Veras FP, Fonseca MM, Toller-Kawahisa JE, Rosa MH, et al. PKM2 promotes Th17 cell differentiation and autoimmune inflammation by fine-tuning STAT3 activation. J Exp Med (2020) 217(10):e20190613. doi: 10.1084/jem.20190613
143. Dayton TL, Jacks T, Vander Heiden. MG. PKM2, cancer metabolism, and the road ahead. EMBO Rep (2016) 17(12):1721–30. doi: 10.15252/embr.201643300
144. Zhang Z, Deng X, Liu Y, Liu Y, Sun L, Chen. F. PKM2, function and expression and regulation. Cell Biosci (2019) 9:52. doi: 10.1186/s13578-019-0317-8
145. Kaura B, Bagga R, Patel. FD. Evaluation of the pyruvate kinase isoenzyme tumor (Tu M2-PK) as a tumor marker for cervical carcinoma. J Obstet Gynaecol Res (2004) 30(3):193–6. doi: 10.1111/j.1447-0756.2004.00187.x
146. Lin Y, Meng F, Lu Z, Chen K, Tao Y, Ouyang Y, et al. Knockdown of PKM2 suppresses tumor progression in human cervical cancer by modulating epithelial-mesenchymal transition via wnt/beta-catenin signaling. Cancer Manag Res (2018) 10:4191–202. doi: 10.2147/CMAR.S178219
147. Lin Y, Zhai H, Ouyang Y, Lu Z, Chu C, He Q, et al. Knockdown of PKM2 enhances radiosensitivity of cervical cancer cells. Cancer Cell Int (2019) 19:129. doi: 10.1186/s12935-019-0845-7
148. Zhao Y, Shen L, Chen X, Qian Y, Zhou Q, Wang Y, et al. High expression of PKM2 as a poor prognosis indicator is associated with radiation resistance in cervical cancer. Histol Histopathol (2015) 30(11):1313–20. doi: 10.14670/HH-11-627
149. Tan VP, Miyamoto S. HK2/hexokinase-II integrates glycolysis and autophagy to confer cellular protection. Autophagy (2015) 11(6):963–4. doi: 10.1080/15548627.2015.1042195
150. Wolf A, Agnihotri S, Micallef J, Mukherjee J, Sabha N, Cairns R, et al. Hexokinase 2 is a key mediator of aerobic glycolysis and promotes tumor growth in human glioblastoma multiforme. J Exp Med (2011) 208(2):313–26. doi: 10.1084/jem.20101470
151. Liu C, Wang X, Zhang. Y. The roles of HK2 on tumorigenesis of cervical cancer. Technol Cancer Res Treat (2019) 18:1533033819871306. doi: 10.1177/1533033819871306
152. Liu Y, Murray-Stewart T, Casero RA Jr., Kagiampakis I, Jin L, Zhang J, et al. Targeting hexokinase 2 inhibition promotes radiosensitization in HPV16 E7-induced cervical cancer and suppresses tumor growth. Int J Oncol (2017) 50(6):2011–23. doi: 10.3892/ijo.2017.3979
153. Fan L, Huang C, Li J, Gao T, Lin Z, Yao. T. Long noncoding RNA urothelial cancer associated 1 regulates radioresistance via the hexokinase 2/glycolytic pathway in cervical cancer. Int J Mol Med (2018) 42(4):2247–59. doi: 10.3892/ijmm.2018.3778
154. Huang X, Liu M, Sun H, Wang F, Xie X, Chen X, et al. HK2 is a radiation resistant and independent negative prognostic factor for patients with locally advanced cervical squamous cell carcinoma. Int J Clin Exp Pathol (2015) 8(4):4054–63.
155. Beloribi-Djefaflia S, Vasseur S, Guillaumon. F. Lipid metabolic reprogramming in cancer cells. Oncogenesis (2016) 5:e189. doi: 10.1038/oncsis.2015.49
156. Baenke F, Peck B, Miess H, Schulze. A. Hooked on fat: the role of lipid synthesis in cancer metabolism and tumour development. Dis Model Mech (2013) 6(6):1353–63. doi: 10.1242/dmm.011338
157. Ackerman D, Simon MC. Hypoxia, lipids, and cancer: surviving the harsh tumor microenvironment. Trends Cell Biol (2014) 24(8):472–8. doi: 10.1016/j.tcb.2014.06.001
158. Currie E, Schulze A, Zechner R, Walther TC, Farese RV Jr. Cellular fatty acid metabolism and cancer. Cell Metab (2013) 18(2):153–61. doi: 10.1016/j.cmet.2013.05.017
159. Koundouros N, Poulogiannis G. Reprogramming of fatty acid metabolism in cancer. Br J Cancer (2020) 122(1):4–22. doi: 10.1038/s41416-019-0650-z
160. Chuang HY, Lee YP, Lin WC, Lin YH, Hwang. JJ. Fatty acid inhibition sensitizes androgen-dependent and -independent prostate cancer to radiotherapy via FASN/NF-kappaB pathway. Sci Rep (2019) 9(1):13284. doi: 10.1038/s41598-019-49486-2
161. Chen J, Zhang F, Ren X, Wang Y, Huang W, Zhang J, et al. Targeting fatty acid synthase sensitizes human nasopharyngeal carcinoma cells to radiation via downregulating frizzled class receptor 10. Cancer Biol Med (2020) 17(3):740–52. doi: 10.20892/j.issn.2095-3941.2020.0219
162. Wuryanti S, Andrijono, Susworo, Witjaksono F. The effect of high poly unsaturated fatty acid (PUFA) dietary supplementation on inflammatory status of patients with advanced cervical cancer on radiation treatment. Acta Med Indones (2015) 47(1):45–9.
163. Lyng H, Sitter B, Bathen TF, Jensen LR, Sundfor K, Kristensen GB, et al. Metabolic mapping by use of high-resolution magic angle spinning 1H MR spectroscopy for assessment of apoptosis in cervical carcinomas. BMC Cancer (2007) 7:11. doi: 10.1186/1471-2407-7-11
164. Sharma A, Rajappa M, Saxena A, Sharma. M. Antioxidant status in advanced cervical cancer patients undergoing neoadjuvant chemoradiation. Br J BioMed Sci (2007) 64(1):23–7. doi: 10.1080/09674845.2007.11732751
165. Na YJ, Kim BR, Kim JL, Kang S, Jeong YA, Park SH, et al. Deficiency of 15-LOX-1 induces radioresistance through downregulation of MacroH2A2 in colorectal cancer. Cancers (Basel) (2019) 11(11):1776. doi: 10.3390/cancers11111776
166. Tan Z, Xiao L, Tang M, Bai F, Li J, Li L, et al. Targeting CPT1A-mediated fatty acid oxidation sensitizes nasopharyngeal carcinoma to radiation therapy. Theranostics (2018) 8(9):2329–47. doi: 10.7150/thno.21451
167. Vettore L, Westbrook RL, D. A. Tennant, new aspects of amino acid metabolism in cancer. Br J Cancer (2020) 122(2):150–6. doi: 10.1038/s41416-019-0620-5
168. Yoo HC, Yu YC, Sung Y, Han. JM. Glutamine reliance in cell metabolism. Exp Mol Med (2020) 52(9):1496–516. doi: 10.1038/s12276-020-00504-8
169. Shanware NP, Mullen AR, DeBerardinis RJ, Abraham. RT. Glutamine: pleiotropic roles in tumor growth and stress resistance. J Mol Med (Berl) (2011) 89(3):229–36. doi: 10.1007/s00109-011-0731-9
170. Blickwedehl J, Olejniczak S, Cummings R, Sarvaiya N, Mantilla A, Chanan-Khan A, et al. The proteasome activator PA200 regulates tumor cell responsiveness to glutamine and resistance to ionizing radiation. Mol Cancer Res (2012) 10(7):937–44. doi: 10.1158/1541-7786.MCR-11-0493-T
171. Souba WW, Klimberg VS, Copeland EM 3rd. Glutamine nutrition in the management of radiation enteritis. JPEN J Parenter Enteral Nutr (1990) 14(4 Suppl):106S–8S. doi: 10.1177/014860719001400414
172. Xiang L, Xie G, Liu C, Zhou J, Chen J, Yu S, et al. Knock-down of glutaminase 2 expression decreases glutathione, NADH, and sensitizes cervical cancer to ionizing radiation. Biochim Biophys Acta (2013) 1833(12):2996–3005. doi: 10.1016/j.bbamcr.2013.08.003
173. Mukha A, Kahya U, Linge A, Chen O, Lock S, Lukiyanchuk V, et al. GLS-driven glutamine catabolism contributes to prostate cancer radiosensitivity by regulating the redox state, stemness and ATG5-mediated autophagy. Theranostics (2021) 11(16):7844–68. doi: 10.7150/thno.58655
174. Santoso JT, Lucci JA 3rd, Coleman RL, Hatch S, Wong P, Miller D, et al. Does glutamine supplementation increase radioresistance in squamous cell carcinoma of the cervix? Gynecol Oncol (1998) 71(3):359–63. doi: 10.1006/gyno.1998.5175
175. Amin SA, Adhikari N, Jha. T. Design of aminopeptidase n inhibitors as anti-cancer agents. J Med Chem (2018) 61(15):6468–90. doi: 10.1021/acs.jmedchem.7b00782
Keywords: radiotherapy, radioresistance, metabolism, tumor microenvironment, cervical cancer
Citation: Zhou J, Lei N, Tian W, Guo R, Chen M, Qiu L, Wu F, Li Y and Chang L (2022) Recent progress of the tumor microenvironmental metabolism in cervical cancer radioresistance. Front. Oncol. 12:999643. doi: 10.3389/fonc.2022.999643
Received: 21 July 2022; Accepted: 27 September 2022;
Published: 12 October 2022.
Edited by:
Maoshan Chen, Army Medical University, ChinaReviewed by:
Elizabeth Ortiz Sánchez, National Institute of Cancerology (INCAN), MexicoKshama Gupta, Mayo Clinic, United States
Copyright © 2022 Zhou, Lei, Tian, Guo, Chen, Qiu, Wu, Li and Chang. This is an open-access article distributed under the terms of the Creative Commons Attribution License (CC BY). The use, distribution or reproduction in other forums is permitted, provided the original author(s) and the copyright owner(s) are credited and that the original publication in this journal is cited, in accordance with accepted academic practice. No use, distribution or reproduction is permitted which does not comply with these terms.
*Correspondence: Lei Chang, ZmNjY2hhbmdsQHp6dS5lZHUuY24=; Yong Li, eS5saUB1bnN3LmVkdS5hdQ==
†These authors have contributed equally to this work