- 1Division of Medical Oncology, The Ohio State University Comprehensive Cancer Center, Columbus, OH, United States
- 2Division of Pharmaceutics and Pharmacology, The Ohio State University College of Pharmacy, Columbus, OH, United States
- 3Drug Development Institute, The Ohio State University Comprehensive Cancer Center, Columbus, OH, United States
Objective: Seventy percent of newly diagnosed breast cancers are estrogen receptor-α positive and HER2/neu negative. First-line treatments incorporate endocrine therapy and cyclin-dependent kinase 4/6 inhibitors. However, therapy resistance occurs in most patients. Hence, there is an urgent need for effective second-line treatments. We previously showed that the potent estrogen receptor-β agonists, OSU-ERβ-12 and LY500307, synergized with the selective estrogen receptor modulator, tamoxifen, in vitro. Furthermore, we showed that these compounds inhibited endocrine-resistant and cyclin-dependent kinase 4/6-inhibitor-resistant estrogen receptor α-positive cell lines in vitro. Here, we used fulvestrant- and abemaciclib-resistant T47D-derived cell line xenografts to determine the efficacy of the combination of OSU-ERβ-12 and LY500307 with tamoxifen in vivo.
Results: Despite efficacy in vitro, treatments failed to reduce xenograft tumor volumes. Hence, we conclude that this treatment strategy lacks direct cancer cell-intrinsic cytotoxic efficacy in vivo.
Introduction
Breast cancer is the most frequently diagnosed cancer in the world among women; estrogen receptor-α (ERα)-positive HER2-negative (ERα+/HER2-) breast cancer is the most common subtype (1–3). There are two nuclear estrogen receptors: ERα, which evidence has shown to be oncogenic in breast cancer, and estrogen receptor-β (ERβ), which is believed to be a tumor suppressor (4–9). First-line treatments incorporate endocrine therapies, which suppress estrogen production or modulate ERα (10, 11). However, breast cancer cells inevitably develop resistance to endocrine therapy through the emergence of activating mutations of the ESR1 gene from which ERα is expressed or increased signaling by multiple different receptor tyrosine kinases and c-Myc (12–15).
The development of cyclin-dependent kinase 4/6 (CDK4/6) inhibitors such as ribociclib, abemaciclib, and palbociclib, revolutionized the management of metastatic ERα+ breast cancer, doubling progression-free survival on first-line therapy (16–18). However, these agents were introduced more than seven years ago, and many patients have since progressed (19–21). Resistance to CDK4/6 inhibitors occurs through multiple mechanisms, including the amplification of cell cycle genes such as cyclin E and CDK6 and loss of tumor suppressors such as RB1 and the Hippo pathway component FAT1 (22). As a result of the heterogeneity of these resistance mechanisms, it has been challenging to develop therapies that overcome CDK4/6 inhibitor resistance. Moreover, these cancers are concurrently resistant to endocrine agents and responses to second-line endocrine therapy are often brief (23). Hence, most of the ongoing ERα+/HER2- breast cancer research is focused on developing improved second-line therapies (11). Most current strategies focus on new selective estrogen receptor modulators (23–25).
Our previous research indicated that highly specific agonists of ERβ inhibit the growth of ERα+ breast cancer cells in vitro, including endocrine-resistant and CDK4/6 inhibitor-resistant breast cancer cell lines. Moreover, our previous data suggested that selective ER modulators (SERM), such as tamoxifen, synergize with ERβ agonists against ERα+ breast cancer cell lines (27). In this report, we examined the potential efficacy of this novel strategy in vivo using a cell line xenograft model of endocrine and CDK4/6 inhibitor-resistant breast cancer.
Materials and methods
Drug formulation and drug administration
The Drug Development Institute (DDI) at The Ohio State University (OSU) synthesized OSU-ERβ-12, as previously described (26). OSU-ERβ-12 was administered as a solution in 20% hydroxypropyl-β-cyclodextrin (HPBCD) (Sigma H107). Tamoxifen (Sigma T5648). LY500307 was synthesized and provided by the OSU DDI. Due to the hydrophobic nature of LY500307, extra light olive oil (Bertolli) was used as vehicle. Treatments included combination treatment with 100 mg/kg of OSU-ERβ-12 + 20 mg/kg of tamoxifen in 100 µl of 20% HPBCD, given via oral gavage daily; 100 mg/kg of OSU-ERβ-12 in 100 µl of 20% HPBCD, given via oral gavage daily; 20 mg/kg of tamoxifen in 100 µl of 20% HPBCD, given via oral gavage daily; 100 µl 20% of HPBCD, given via oral gavage daily; 10 mg/kg of OSU-ERβ-12 + 20 mg/kg tamoxifen in 100 µl of 20% HPBCD, given via subcutaneous injection daily; 50 mg/kg of OSU-ERβ-12 + 10 mg/kg of tamoxifen in 100 µl of 20% HPBCD, given via 2 subcutaneous injections in 2 separate locations on the body (for a total dose of 100 mg/kg of OSU-ERβ-12 + 20 mg/kg of tamoxifen) daily; and 30 mg/kg of LY500307 + 20 mg/kg of tamoxifen in 100 µl of extra light olive oil given via oral gavage daily. Dose levels were chosen based on the pharmacokinetic properties of OSU-ERβ-12 and were designed to provide peak plasma concentrations that exceeded the half maximal inhibitory concentration (IC50) of the drugs by at least 2-fold and to fully activate ERβ without activation of ERα (26).
Cell culture
T47D cells, (ATCC HTB-133; NCI-DTP Cat# T-47D, RRID: CVCL_0553), concurrently resistant to fulvestrant and abemaciclib, were selected by continuous culture in incremental concentrations over six months. Viability assays confirmed IC50 levels of more than 3 times that of the parental cell line, as described previously, thus verifying resistance to each agent (27). Cells were diluted to a concentration of 5 million cells per 100 µl in phosphate-buffered saline (PBS) in individual syringes for mammary fat pad injection.
Cell pellets were prepared by allowing the fulvestrant- and abemaciclib-resistant T47D cell line to grow overnight, followed by treatment with 5 µM, which was the estimated IC50, of OSU-ERβ-12 or LY500307 for 72 hours. Control cells were treated with dimethyl sulfoxide (DMSO) vehicle. Fresh media and drugs were replaced every alternate day. After 72 hours of treatment, the cells were collected and centrifuged to obtain a cell pellet.
Mouse maintenance
Animals were maintained on a 12-hour light/12-hour dark schedule and had free access to standard food and water. Ovariectomized NCG mice (strain 572, NOD-SCID-γ-/-) that are known to lack T cells, B cells, and NK cells (Charles River Laboratory) were used for cell-line xenografts of T47D cells. Mice were euthanized by carbon dioxide inhalation and confirmed with cervical dislocation.
Subcutaneous implantation of estradiol pellet
From 3 days before the mammary fat pad injection, to 5 days after, the mice received 0.2 mg of ibuprofen/ml of drinking water. 2 days before the mammary fat pad injection, the mice were anesthetized and received a 0.1 mg/kg subcutaneous injection of buprenorphine. Hair was shaved from the upper back between the shoulder blades. The exposed skin was cleaned with alternating rounds of betadine and 70% ethanol and repeated three times each. Press’n Seal (GLAD) was used as a surgical drape over the surgery area. A 10G trocar (Innovative Research of America, MP-182) was used to implant the 60-day release 0.72 mg 17β estradiol pellet (Innovative Research of America, SE-121) under the skin. The wound was closed with a drop of Vetbond Tissue Adhesive. Mice recovered under a heat lamp until ready to return to their home cages.
Mouse mammary fat pad injection
On the day of mammary fat pad injection, the mice were anesthetized and received a 1 mg/kg subcutaneous injection of buprenorphine. The mice continued to receive 0.2 mg of ibuprofen/ml of drinking water. The mice were shaved in the inguinal region to access the lower mammary fat pad. The skin was cleaned with betadine and 70% ethanol, and a drape was used as described above. A 1 cm-long longitudinal incision was made, and the mammary fat pad was identified. Cells were injected directly into the fat pad, which was then tucked back inside the incision. The wound was closed with a drop of tissue adhesive.
Data collection
Mice were weighed before drug treatments began and twice weekly during the experiment. After tumor cell injection, the length and width of the tumor were measured twice weekly using calipers. The tumor volume was calculated using the following formula: (4/3) x π*L x W x W, where width was the smaller of the two length measurements. Once the tumor reached a volume of 500 mm3, treatment began. Treatment groups were randomly assigned to mice via a random group generator. Each treatment group included 8-9 mice. Treatments were administered 5 days a week for 6 weeks for a total of 30 doses. Twice weekly tumor measurements continued during treatment administration. Study termination criteria included a tumor volume measuring 1.6 cm in diameter, tumor ulceration, or morbid condition. Mice were euthanized after 6 weeks of treatment, and tumors were removed, flash-frozen in dry ice, and stored at -80°C.
Blood collection and drug concentration analysis
Blood was collected via submandibular vein sampling 2 hours and 24 hours after drug administration. The drug concentrations of OSU-ERβ-12 and LY500307 in cell pellets, plasma, and tumors were measured by Charles River Laboratories (Wilmington, MA) by high-performance liquid chromatography/mass spectrometry (HPLC-MS). Drug concentrations were expressed in micromoles/L.
Statistical analysis
Group differences in tumor volume changes over time were analyzed with a linear mixed model using R 4.10 (Vienna, Austria). In the linear mixed model, time points, treatment groups (with vehicle treatment as the reference), and interactions between time and treatment groups served as predictors. Group differences in tumor weight were assessed with a one-way analysis of variance (ANOVA) model, and partial η2 was used to indicate effect size. Group differences in mouse survival status (Y/N) were evaluated with a logistic regression model. Group differences in LY500307 drug concentration were assessed using Wilcoxon’s rank sum test. SPSS version 28.0 (IBM Corp., Armonk, NY) was used for these calculations. Group differences in mouse weight changes over time were analyzed by linear mixed model using R 4.10. For this model, time and treatment groups (vehicle as the reference) and interactions between treatment and time served as predictors. Pairwise comparisons of OSU-ERβ-12 drug concentration were assessed using Dunn’s test with concentration in the cell pellet serving as the reference group. GraphPad Prism version 10.0.2 software (Boston, MA) was used to calculate this test, and a two-sided alpha level of 0.05 was adopted.
Results
Tumor volume and weight
Tumor volume, measured twice weekly, increased over time across all treatments (p ≤ 0.001) (Figure 1A). However, there was no statistically significant difference between any treatment group and the vehicle-treated group over 6 weeks of drug administration. Tumors were harvested after the completion of 6 weeks of drug administration. There was no significant difference between tumor weight of any treatment group and vehicle treated group (p=0.969) (Figure 1B). All treatment groups demonstrated a small magnitude of effect on tumor weight as indicated by partial ƞ2 = 0.029.
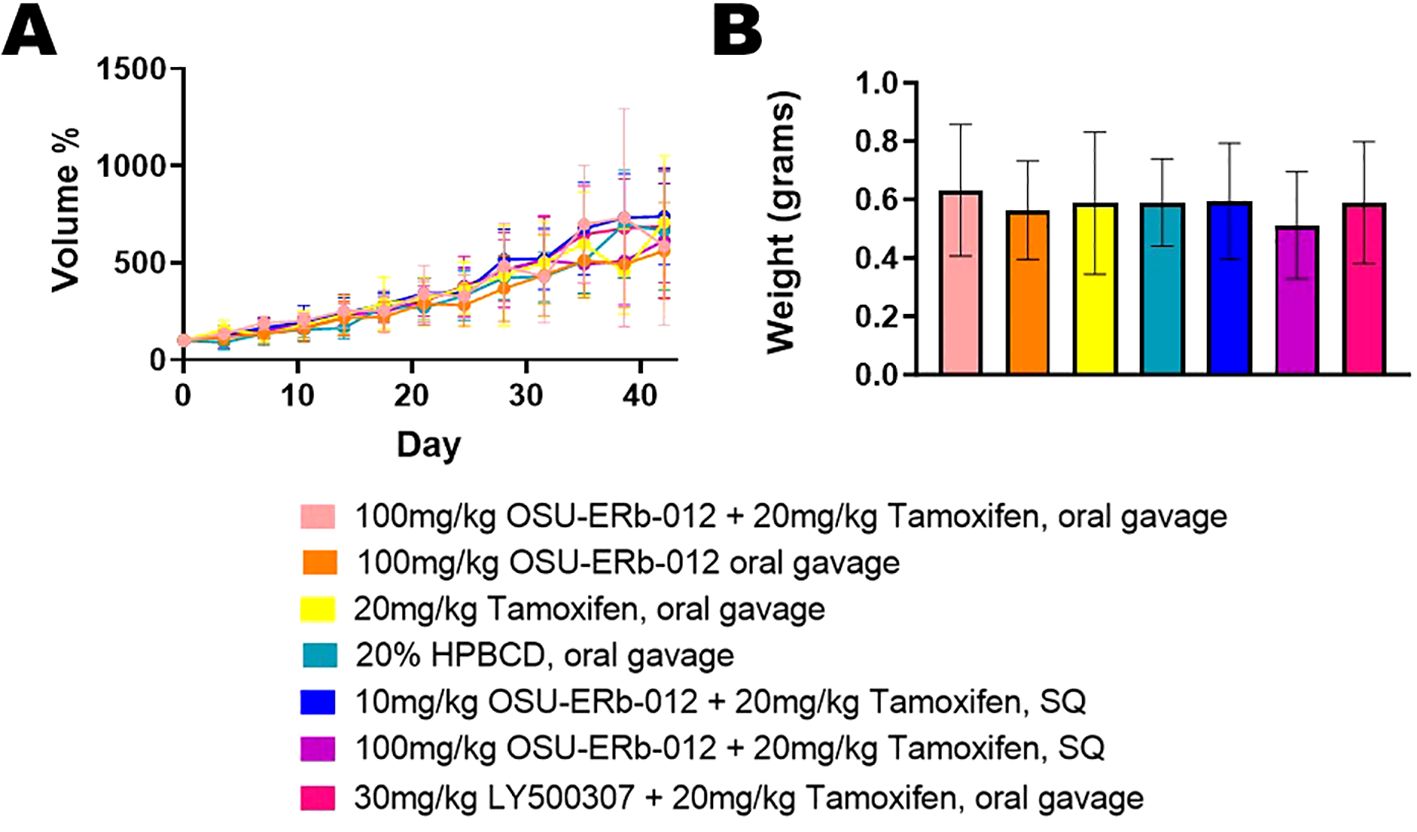
Figure 1. Tumor Volume and Tumor Weight. (A) Depicted tumor volumes were normalized to their pretreatment volumes; (pretreatment volumes set at 100% on Day 0). The average tumor volume per treatment group is shown by the day of treatment. Group differences in tumor volume change over time were analyzed with a linear mixed model. Time points, treatment groups (with vehicle treatment as the reference), and interactions between time and treatment groups served as predictors. Beta coefficient = 324.096, standard error = 19.743, p ≤ 0.001. None of the treatments were significantly different compared to vehicle control. (B) The average tumor weight per treatment group after completion of 6 weeks of drug administration is shown. Group differences in tumor weight were assessed with a one-way analysis of variance (ANOVA) model, and partial η2 was used to indicate effect size. There was no significant difference in tumor weight between each treatment and vehicle treatment. p=0.969, partial ƞ2 = 0.029, indicating a small magnitude of effect size.
Mouse survival and weight
Mouse weight decreased over time, indicating a possible adverse or toxic effect of the treatments or tumor burden (Figure 2A). Compared to vehicle treatment, the OSU-ERβ-12 oral gavage (p=0.001) and 100 mg OSU-ERβ-12 + tamoxifen subcutaneous (SQ) (p<0.001) groups lost significantly more weight over time, while the group treated with tamoxifen alone (p<0.001) lost significantly less weight over time.
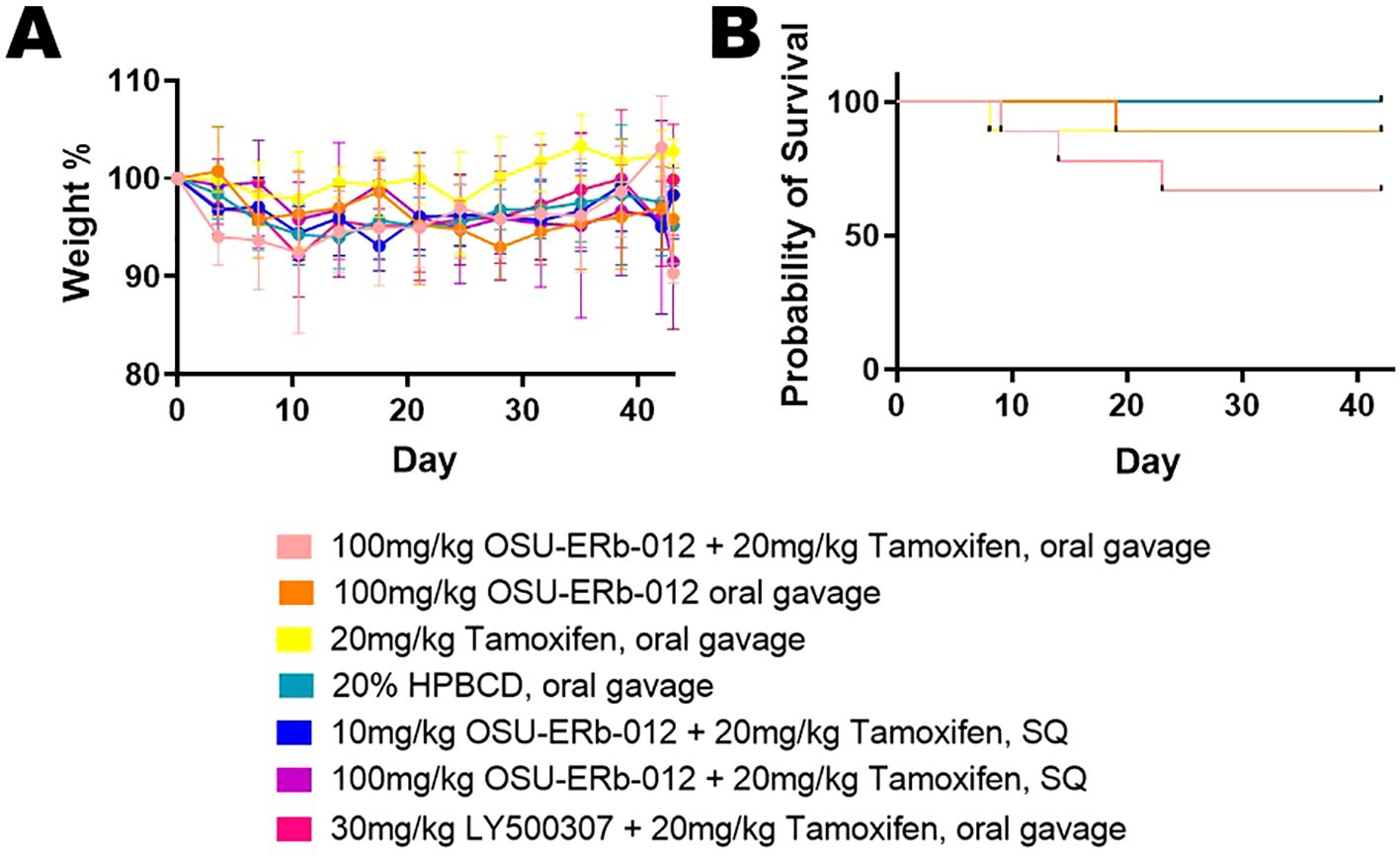
Figure 2. Mouse Weight and Mouse Survival. (A) Mouse weights were normalized to their pretreatment weights; (pretreatment weights set at 100% on Day 0). Group differences in mouse weight changes over time, analyzed by linear mixed model, showed that overall mouse weight decreased significantly over time. For this model, time and treatment groups, and interactions between treatment and time served as predictors. With the 20% HPBCD vehicle treatment being the reference group, the OSU-ERβ-12 oral gavage treatment (p=0.001) and 100 mg OSU-ERβ-12 + tamoxifen SQ treatment (p<0.001) significantly enhanced the decreasing trend, while the tamoxifen alone group (p<0.001) significantly weakened the decreasing trend. (B) Mouse Survival: The probability of mouse survival is shown by the day of treatment. At the beginning of the experiment n = 9 mice for treatments: OSU-ERβ-12 + tamoxifen oral gavage, OSU-ERβ-12 oral gavage, tamoxifen oral gavage, LY500307 + tamoxifen oral gavage; and n = 8 mice for treatments: 10 mg OSU-ERβ-12 + tamoxifen SQ, 100 mg OSU-ERβ-12 + tamoxifen SQ, and 20% HPBCD vehicle. Over the course of the experiment, two mice on OSU-ERβ-12 + tamoxifen oral gavage treatment expired during treatment; a third was euthanized due to veterinary concerns. One mouse on OSU-ERβ-12 oral gavage treatment expired during treatment. One mouse each from the tamoxifen alone and LY500307 + tamoxifen treatment groups was euthanized due to veterinary concerns. Group differences in mouse survival status (Y/N) were evaluated with a logistic regression model. None of the treatment groups were significantly different from vehicle treatment in terms of mortality.
Two mice on OSU-ERβ-12 + tamoxifen oral gavage treatment expired during treatment; a third was euthanized due to veterinary concern. One mouse on OSU-ERβ-12 oral gavage treatment expired during treatment. One mouse each from the tamoxifen alone and LY500307 + tamoxifen treatment groups was euthanized due to veterinary concerns. None of the treatment groups were significantly different from the vehicle treatment group in terms of survival (Figure 2B).
Drug level concentrations in plasma and tumors
The concentration of OSU-ERβ-12 in the cell pellet was 290-fold higher (p=0.002, pellet mean rank = 22.50, tumor mean rank = 10.50) compared to the tumors (Figure 3A). The peak concentration (Cmax) of LY500307 in the plasma was a little more than half of the IC50 that was established in vitro for endocrine-resistant and CDK4/6 inhibitor-resistant T47D cells (27) (Figure 3B). The large error bar for Cmax is attributed to a single mouse whose measured plasma concentration was 100-fold higher than the other three mice measured. We hypothesize this is an error in measurement. The concentration of LY50037 in the cell pellet was 1,076-fold higher than in the tumors (p=0.021, pellet mean rank = 6.50, tumor mean rank = 2.50) (Figure 3C).
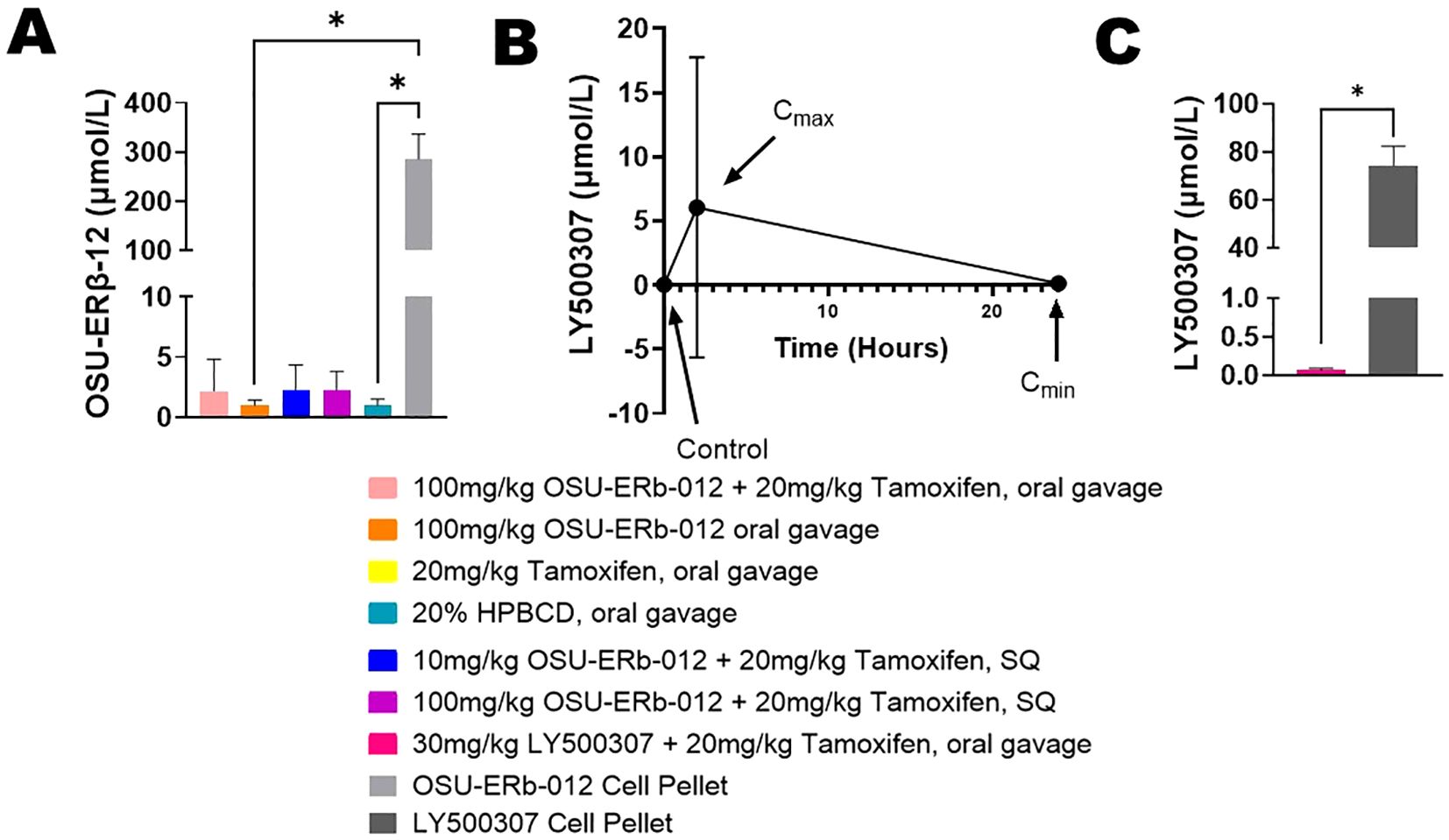
Figure 3. Drug Level Concentrations. (A) OSU-ERβ-12 concentration in tumors compared to cell pellets. Briefly, fulvestrant- and abemaciclib-resistant T47D cells were treated with OSU-ERβ-12 and pelleted after 72 hours to determine cellular concentrations OSU-ERβ-12 in the absence of a complex systemic environment; This is compared to the measured OSU-ERβ-12 concentrations achieved in tumors in vivo. Measurements were obtained by HPLC-MS. Pairwise comparisons of OSU-ERβ-12 drug concentration were assessed using Dunn’s test with concentration in the cell pellet serving as the reference group, and a two-sided alpha level of 0.05 was adopted. The concentration of OSU-ERβ-12 in the OSU-ERβ-12 treated cell pellets was significantly higher than OSU-ERβ-12 oral gavage treated tumors (p=0.0320) and 20% HPBCD vehicle-treated tumors (p=0.0465). Drug concentrations in other groups were not statistically significantly different from those in OSU-ERβ-12 -treated cell pellets, despite a 100-fold difference, due to multiple comparisons. (B) LY500307 concentration in plasma of mice treated with LY500307. Briefly, blood was collected before, 2 hours after, and 24 hours after LY500307 administration, to determine the peak serum concentration (Cmax) and minimum serum concentration (Cmin) achieved. Measurements were obtained by HPLC-MS. (C) LY500307 concentration in tumors compared to cell pellets treated with LY500307. Briefly, fulvestrant- and abemaciclib-resistant T47D cells were treated with LY500307 and pelleted after 72 hours to determine cellular concentrations LY500307 in the absence of a complex systemic environment; This is compared to the measured LY500307 concentrations achieved in tumors in vivo. Measurements were obtained by HPLC-MS. Group differences in LY500307 drug concentration, assessed using Wilcoxon’s rank sum test, showed that the concentration of LY500307 in cell pellets was significantly higher than that in LY500307 treated tumors (p=0.021). (*indicates p<0.05).
Discussion
The 100 mg/kg treatment dose of OSU-ERβ-12 was 10-fold higher than pharmacokinetic testing suggested was necessary to achieve peak plasma concentrations (28). This suggests that peak concentrations greater than the IC50 could be achieved with this dosing schedule. However, our peak plasma concentrations were lower than anticipated which may be due to a change in vehicle the drug was dissolved into (5% DMSO/5% Tween 20/water vs HPBCD). Despite being dosed aggressively, the drug combinations lacked efficacy in vivo, which was unexpected based on our in vitro results (27). We believe that the lack of efficacy may be due to the low intratumoral drug concentrations, at which concentration had no effect in vitro.
As a comparison, in vitro fulvestrant- and abemaciclib-resistant cells were also treated with the ERβ agonists to determine what cellular concentration were possible, in the absence of the complex systemic environment. Intratumoral drug concentrations were significantly less than those achieved in pellets of cells treated at the IC50 concentration. The concentration of OSU-ERβ-12 in cell pellets was 290-fold higher compared to intratumoral concentrations, and the concentration of LY500307 in cell pellets was 1076-fold higher than intratumoral concentrations. The concentration of LY500307 in plasma was similar to that in tumors.
Hence, although we utilized a dose that was 10-fold higher than pharmacokinetic testing suggested was necessary, we failed to achieve intratumoral concentrations that were similar to those in the cell pellets, which were established to be cytotoxic in vitro. One explanation is that as ERβ agonists are nuclear receptor agonists and hydrophobic, plasma protein binding may have resulted in low “free” concentrations. As free (or unbound) drug exercises its biological activity, low levels would result in low physiological response. Another explanation is that ERβ agonists may concentrate in lipid-rich cell organelles. This would explain our observed cell pellet concentrations that far exceeded the concentration in the medium. Our experiments had 80% power to detect 2-fold differences assuming a coefficient of variance of 50%. As treatment groups were sufficiently large, we do not believe that higher numbers would improve the data. We are currently exploring OSU-ERβ-12 in combination with immune checkpoint inhibitors, which is based on similar work published with other ERβ agonists (29, 30).
Limitations
A limitation of this study is the lack of a pharmacodynamic marker for ERβ activation. However, unique markers for ERβ-specific activation are not well established (31).
Data availability statement
The raw data supporting the conclusions of this article will be made available by the authors, without undue reservation.
Ethics statement
Ethical approval was not required for the studies on humans in accordance with the local legislation and institutional requirements because only commercially available established cell lines were used. The animal study was approved by Ohio State University’s Institutional Animal Care and Use Committee. The study was conducted in accordance with the local legislation and institutional requirements.
Author contributions
LM: Formal Analysis, Investigation, Validation, Writing – original draft, Writing – review & editing, Methodology. CC: Conceptualization, Investigation, Writing – review & editing. MX: Formal Analysis, Writing – review & editing. JD: Investigation, Writing – review & editing. JM: Writing – review & editing. MC: Conceptualization, Investigation, Project administration, Writing – review & editing.
Funding
The author(s) declare that financial support was received for the research and/or publication of this article. This study is supported by the National Comprehensive Cancer Network (NCCN) through a grant provided by Lilly. Neither Lilly nor NCCN are the sponsors of this study. The research reported in this publication was also supported by The Ohio State University Comprehensive Cancer Center and by a National Cancer Institute (NCI)/National Institutes of Health (NIH) grant (P30CA016058). This publication was also supported, in part, by a grant from the National Center for Advancing Translational Sciences of the NIH (KL2TR002734). Institutions that provided funding support had no role in the design or conduct of this study or the preparation of the manuscript. The content is solely the responsibility of the authors and does not necessarily represent the official views of the NIH.
Acknowledgments
The authors would like to thank scientific editor Angela Dahlberg, Division of Medical Oncology, The Ohio State University Comprehensive Cancer Center, for editing this manuscript. This work was supported by the Drug Discovery Shared Resource and the Drug Development Institute within the Comprehensive Cancer Center at The Ohio State University.
Conflict of interest
The authors declare that the research was conducted in the absence of any commercial or financial relationships that could be construed as a potential conflict of interest.
Publisher’s note
All claims expressed in this article are solely those of the authors and do not necessarily represent those of their affiliated organizations, or those of the publisher, the editors and the reviewers. Any product that may be evaluated in this article, or claim that may be made by its manufacturer, is not guaranteed or endorsed by the publisher.
Abbreviations
ANOVA, analysis of variance; CDK4/6, cyclin-dependent kinase 4/6; DMSO, dimethyl sulfoxide; EC50, half maximal effective concentration; ERα, estrogen receptor-α; ERβ, estrogen receptor-β; HPBCD, hydroxypropyl β cyclodextrin; HPLC-MS, high-performance liquid chromatography/mass spectrometry; IC50, half maximal inhibitory concentration; PBS, phosphate buffered saline; SERM, selective ER modulators; SQ, subcutaneous; µl, microliter; µM, micromolar; mg, milligram; kg, kilogram.
References
1. Ferlay J, Colombet M, Soerjomataram I, Mathers C, Parkin DM, Piñeros M, et al. Estimating the global cancer incidence and mortality in 2018: GLOBOCAN sources and methods. Int J Cancer. (2019) 144:1941–53. doi: 10.1002/ijc.v144.8
2. Hanker AB, Sudhan DR, Arteaga CL. Overcoming endocrine resistance in breast cancer. Cancer Cell. (2020) 37:496–513. doi: 10.1016/j.ccell.2020.03.009
3. Howlader N, Altekruse SF, Li CI, Chen VW, Clarke CA, Ries LA, et al. US incidence of breast cancer subtypes defined by joint hormone receptor and HER2 status. J Natl Cancer Inst. (2014) 106(5):dju055. doi: 10.1093/jnci/dju055
4. Mal R, Magner A, David J, Datta J, Vallabhaneni M, Kassem M, et al. Estrogen receptor beta (ERβ): A ligand activated tumor suppressor. Front Oncol. (2020) 10:587386. doi: 10.3389/fonc.2020.587386
5. Duan R, Ginsburg E, Vonderhaar BK. Estrogen stimulates transcription from the human prolactin distal promoter through AP1 and estrogen responsive elements in T47D human breast cancer cells. Mol Cell Endocrinol. (2008) 281:9–18. doi: 10.1016/j.mce.2007.10.004
6. Jia M, Dahlman-Wright K, Gustafsson J. Estrogen receptor alpha and beta in health and disease. Best Pract Res Clin Endocrinol Metab. (2015) 29:557–68. doi: 10.1016/j.beem.2015.04.008
7. Paruthiyil S, Parmar H, Kerekatte V, Cunha GR, Firestone GL, Leitman DC. Estrogen receptor beta inhibits human breast cancer cell proliferation and tumor formation by causing a G2 cell cycle arrest. Cancer Res. (2004) 64:423–8. doi: 10.1158/0008-5472.CAN-03-2446
8. Bardin A, Boulle N, Lazennec G, Vignon F, Pujol P. Loss of ERbeta expression as a common step in estrogen-dependent tumor progression. Endocr Relat Cancer. (2004) 11:537–51. doi: 10.1677/erc.1.00800
9. Palmieri C, Cheng GJ, Saji S, Zelada-Hedman M, Wärri A, Weihua Z, et al. Estrogen receptor beta in breast cancer. Endocr Relat Cancer. (2002) 9:1–13. doi: 10.1677/erc.0.0090001
10. Reinbolt RE, Mangini N, Hill JL, Levine LB, Dempsey JL, Singaravelu J, et al. Endocrine therapy in breast cancer: the neoadjuvant, adjuvant, and metastatic approach. Semin Oncol Nurs. (2015) 31:146–55. doi: 10.1016/j.soncn.2015.02.002
11. Basile D, Gerratana L, Corvaja C, Pelizzari G, Franceschin G, Bertoli E, et al. First- and second-line treatment strategies for hormone-receptor (HR)-positive HER2-negative metastatic breast cancer: A real-world study. Breast. (2021) 57:104–12. doi: 10.1016/j.breast.2021.02.015
12. Rasha F, Sharma M, Pruitt K. Mechanisms of endocrine therapy resistance in breast cancer. Mol Cell Endocrinol. (2021) 532:111322. doi: 10.1016/j.mce.2021.111322
13. Ali S, Coombes RC. Endocrine-responsive breast cancer and strategies for combating resistance. Nat Rev Cancer. (2002) 2:101–12. doi: 10.1038/nrc721
14. Clarke R, Tyson JJ, Dixon JM. Endocrine resistance in breast cancer–An overview and update. Mol Cell Endocrinol. (2015) 418 Pt 3:220–34. doi: 10.1016/j.mce.2015.09.035
15. Lei JT, Anurag M, Haricharan S, Gou X, Ellis MJ. Endocrine therapy resistance: new insights. Breast. (2019) 48 Suppl 1:S26–s30. doi: 10.1016/S0960-9776(19)31118-X
16. Finn RS, Martin M, Rugo HS, Jones S, Im SA, Gelmon K, et al. Palbociclib and letrozole in advanced breast cancer. N Engl J Med. (2016) 375:1925–36. doi: 10.1056/NEJMoa1607303
17. Goetz MP, Toi M, Campone M, Sohn J, Paluch-Shimon S, Huober J, et al. MONARCH 3: abemaciclib as initial therapy for advanced breast cancer. J Clin Oncol. (2017) 35:3638–46. doi: 10.1200/JCO.2017.75.6155
18. Hortobagyi GN, Stemmer SM, Burris HA, Yap YS, Sonke GS, Paluch-Shimon S, et al. Ribociclib as first-line therapy for HR-positive, advanced breast cancer. N Engl J Med. (2016) 375:1738–48. doi: 10.1056/NEJMoa1609709
19. Gnant M, Turner NC, Hernando C. Managing a long and winding road: estrogen receptor-positive breast cancer. Am Soc Clin Oncol Educ Book. (2023) 43:e390922. doi: 10.1200/EDBK_390922
20. Scheidemann ER, Shajahan-Haq AN. Resistance to CDK4/6 inhibitors in estrogen receptor-positive breast cancer. Int J Mol Sci. (2021) 22(22):12292. doi: 10.3390/ijms222212292
21. Zhou FH, Downton T, Freelander A, Hurwitz J, Caldon CE, Lim E. CDK4/6 inhibitor resistance in estrogen receptor positive breast cancer, a 2023 perspective. Front Cell Dev Biol. (2023) 11:1148792. doi: 10.3389/fcell.2023.1148792
22. Li Z, Razavi P, Li Q, Toy W, Liu B, Ping C, et al. Loss of the FAT1 tumor suppressor promotes resistance to CDK4/6 inhibitors via the hippo pathway. Cancer Cell. (2018) 34:893–905.e898. doi: 10.1016/j.ccell.2018.11.006
23. Bidard FC, Kaklamani VG, Neven P, Streich G, Montero AJ, Forget F, et al. Elacestrant (oral selective estrogen receptor degrader) Versus Standard Endocrine Therapy for Estrogen Receptor-Positive, Human Epidermal Growth Factor Receptor 2-Negative Advanced Breast Cancer: Results From the Randomized Phase III EMERALD Trial. J Clin Oncol. (2022) 40:3246–56. doi: 10.1200/JCO.22.00338
24. Jhaveri KL, Neven P, Casalnuovo ML, Kim S-B, Tokunaga E, Aftimos P, et al. Imlunestrant with or without abemaciclib in advanced breast cancer. N Engl J Med. doi: 10.1056/NEJMoa2410858
25. Damodaran S, O’Sullivan CC, Elkhanany A, Anderson IC, Barve M, Blau S, et al. Open-label, phase II, multicenter study of lasofoxifene plus abemaciclib for treating women with metastatic ER+/HER2- breast cancer and an ESR1 mutation after disease progression on prior therapies: ELAINE 2. Ann Oncol. (2023) 34:1131–40. doi: 10.1016/j.annonc.2023.09.3103
26. Sedlák D, Wilson TA, Tjarks W, Radomska HS, Wang H, Kolla JN, et al. Structure-activity relationship of para-carborane selective estrogen receptor β Agonists. J Med Chem. (2021) 64:9330–53. doi: 10.1021/acs.jmedchem.1c00555
27. Datta J, Willingham N, Manouchehri JM, Schnell P, Sheth M, David JJ, et al. Activity of estrogen receptor β Agonists in therapy-resistant estrogen receptor-positive breast cancer. Front Oncol. (2022) 12:857590. doi: 10.3389/fonc.2022.857590
28. Adeluola AA, Radomska HS, Wilson TA, Kulp SK, Kabat A, Helms TH, et al. The elucidation of species-specific receptor pharmacology: A case study using subtype-selective para- and meta-carborane estrogen receptor agonists. J Pharmacol Exp Ther. (2025) 392:100001. doi: 10.1124/jpet.123.001874
29. Yuan B, Clark CA, Wu B, Yang J, Drerup JM, Li T, et al. Estrogen receptor beta signaling in CD8(+) T cells boosts T cell receptor activation and antitumor immunity through a phosphotyrosine switch. J Immunother Cancer. (2021) 9(1):e001932. doi: 10.1136/jitc-2020-001932
30. Huang S, Zhou N, Zhao L, Gimple RC, Ahn YH, Zhang P, et al. Pharmacological activation of estrogen receptor beta overcomes tumor resistance to immune checkpoint blockade therapy. iScience. (2020) 23:101458. doi: 10.1016/j.isci.2020.101458
Keywords: ER+ breast cancer, ERβ, CDK4/6 inhibitor-resistant, endocrine-resistant, breast cancer
Citation: Marcho LM, Coss CC, Xu M, Datta J, Manouchehri JM and Cherian MA (2025) Potent estrogen receptor β agonists with inhibitory activity in vitro, fail to suppress xenografts of endocrine-resistant cyclin-dependent kinase 4/6 inhibitor-resistant breast cancer cells. Front. Oncol. 15:1441896. doi: 10.3389/fonc.2025.1441896
Received: 31 May 2024; Accepted: 07 March 2025;
Published: 26 March 2025.
Edited by:
Zheng Wang, Shanghai Jiao Tong University, ChinaReviewed by:
Shrikanth Gadad, Texas Tech University Health Sciences Center El Paso, United StatesNorbert Nass, Brandenburg Medical School Theodor Fontane, Germany
Copyright © 2025 Marcho, Coss, Xu, Datta, Manouchehri and Cherian. This is an open-access article distributed under the terms of the Creative Commons Attribution License (CC BY). The use, distribution or reproduction in other forums is permitted, provided the original author(s) and the copyright owner(s) are credited and that the original publication in this journal is cited, in accordance with accepted academic practice. No use, distribution or reproduction is permitted which does not comply with these terms.
*Correspondence: Mathew A. Cherian, bWF0aGV3LmNoZXJpYW5Ab3N1bWMuZWR1