- 1Student Research Committee, Kermanshah University of Medical Sciences, Kermanshah, Iran
- 2Pharmaceutical Sciences Research Center, Health Institute, Kermanshah University of Medical Sciences, Kermanshah, Iran
- 3Student Research Committee, USERN Office, Lorestan University of Medical Sciences, Khorramabad, Iran
- 4Student Research Committee, Qom University of Medical Sciences, Qom, Iran
- 5Department of Tissue Engineering and Applied Cell Sciences, School of Medicine, Qom University of Medical Sciences, Qom, Iran
- 6Cellular and Molecular Research Center, Qom University of Medical Sciences, Qom, Iran
- 7Department of Immunology, School of Medicine, Iran University of Medical Sciences, Tehran, Iran
- 8Iran University of Medical Sciences, Deputy of Health, Tehran, Iran
- 9Nervous System Stem Cells Research Center, Semnan University of Medical Sciences, Semnan, Iran
- 10Department of Medical Microbiology, Faculty of Medicine, Shahed University, Tehran, Iran
Cancer is a complex and heterogeneous disease that often requires multifaceted treatment strategies to achieve optimal therapeutic outcomes. Given the limitations of single-agent therapies, particularly in the face of intricate biological signaling networks and treatment resistance, there is a growing need for combinatory approaches. This article presents a novel hypothesis: the simultaneous use of ribosome-inactivating proteins (RIPs) and mesenchymal stem cells (MSCs) or MSC-derived extracellular vesicles (EVs) in cancer treatment. RIPs, with their potent cytotoxic properties, can target tumor cells effectively, while MSCs, known for their tumor-homing abilities and regenerative potential, can serve as delivery vehicles, potentially enhancing the targeting precision and reducing the systemic toxicity of RIPs. This hypothesis explores the synergistic potential of combining these two therapeutic modalities, leveraging the advantages of both techniques to create a more effective cancer treatment strategy. By combining RIPs’ ability to inhibit protein synthesis with MSCs or MSC-derived EVs’ capability to modulate the tumor microenvironment and deliver therapeutic agents. This approach offers a promising avenue for overcoming cancer’s inherent complexity. However, challenges remain, such as optimizing dosing protocols, addressing safety concerns, and ensuring efficient drug delivery. Future research and clinical trials are necessary to validate this combination as a viable cancer therapy.
1 Introduction
Cancer has emerged as a significant public health issue, with more than 52,900 new diagnoses and over 27,000 deaths each day. Projections suggest that by 2040, the number of new cancer cases will reach 28 million annually, with 16.2 million deaths globally. To effectively decrease cancer mortality rates worldwide, it is essential to focus on the broad adoption of personalized and targeted treatments, coupled with increased investment in the development of advanced cancer therapies (1). These alarming statistics surrounding cancer have intensified efforts to either prevent the disease or detect it at an early stage, when treatments tend to be less invasive, more costeffective, and have higher chances of success. However, the precise mechanisms by which tissues undergo malignant transformation remain a topic of debate and controversy, complicating efforts toward cancer prevention and early intervention (2, 3). Cancer, a serious and often life-threatening condition, is marked by the uncontrolled proliferation and spread of abnormal cells within the body. The disease poses major challenges for both patients and their families, impacting them not only physically but also emotionally and financially (4–6). The growing incidence of cancer has also increased the economic burden on healthcare systems worldwide. Projections indicate that global cancer drug sales will soar from $193 billion USD in 2022 to $377 billion USD by 2027. This increase is driven by earlier cancer detection, improved survival rates that extend treatment durations, broader access to innovative cancer therapies, and the ongoing introduction of new drugs into the market (7). Conventional cancer treatments face significant limitations due to their associated radiotoxicity (such as xerostomia, hepatotoxicity, or pneumonia) and adverse effects, including genetic mutations, cytotoxicity, and the development of drug resistance (8). Advanced anticancer therapies require the development of targeted biological strategies in order to maximize efficacy while reducing toxicity (9).
While various cancer therapies have shown success in clinical applications, significant challenges such as drug resistance and low response rates remain unresolved. To address these issues, ongoing research focuses on discovering new therapeutic agents. Among these, ribosomeinactivating proteins (RIPs) have gained remarkable attention due to their potent anticancer properties. RIPs can inhibit protein synthesis and induce tumor cell death at extremely low concentrations, making them promising candidates for cancer treatment, provided they can effectively target cytosolic ribosomes (10–12). Recent developments in genetic recombination technology have facilitated the large-scale production of recombinant RIPs, simplifying the creation of various fusion proteins. Building on these technological advances, RIPs with enhanced druggability have been designed. Despite these improvements, the clinical application of RIPs for cancer treatment continues to face major hurdles. The main challenge is effective drug delivery, hindered by biological barriers within the body, including vascular, intratumoral, and intracellular barriers. To overcome these obstacles, innovative drug delivery systems (DDS) based on prodrug strategies have been developed (10).
Mesenchymal stem cells (MSCs) have become a promising tool for delivering therapeutic agents directly to tumor sites due to their unique properties, making them an effective approach for drug delivery strategies. They exhibit unique biological properties, such as differentiation capabilities, easy isolation, large-scale expansion, immune system modulation, and tumortargeting abilities. However, conflicting reports on their tumor-suppressive and -supportive effects pose challenges for their clinical application in cancer therapy (13–15).
Recent studies have indicated that MSCs may also exhibit additional beneficial effects through paracrine pathways, involving the generation and release of soluble factors and extracellular vesicles (EVs) mediating immune-modulatory and trophic functions (16–18). Emerging research highlights the potential of exosomes derived from MSCs as a promising cell-free alternative to traditional MSC-based therapies. These exosomes have garnered attention for their ability to mediate the therapeutic effects of MSCs while offering distinct advantages, including improved safety profiles, ease of storage, simplified transportation, and more convenient administration compared to MSC transplantation methods. As nanoscale vesicles, MSC-derived exosomes can encapsulate and deliver diverse biomolecules such as nanomedicines, functional proteins, mRNAs, and microRNAs (miRNAs) (14, 19, 20).
Cancer, with its diverse nature, rapid development of treatment resistance, and complex cellular pathways, poses significant challenges for single-agent therapies. It has become increasingly clear that the future of effective cancer treatment lies in combinatorial therapies (7, 21, 22). In the present scenario, the prime focus is on exploring potential of RIPs and MSCs/MSC-EVs to treat cancer. It reviews strategies aimed at minimizing the systemic toxicity of RIPs and addresses the current limitations of MSC therapy, while also highlighting the promise of combinatorial approaches. The review emphasizes how RIPs and MSCs/MSC-EVs can complement each other through synergistic mechanisms to offer a more effective therapeutic strategy. However, it is crucial to acknowledge that, while these approaches show considerable promise in preclinical studies, their success in clinical settings may vary.
2 Components of the tumor microenvironment
Cancers are complex ecosystems composed of tumor cells along with a variety of noncancerous cells, all embedded within a modified extracellular matrix (23). In addition to the tumors itself, it’s essential to study the dynamic tumors microenvironment (TME). The TME is primarily made up of malignant cells, endothelial cells, immune cells, diverse stromal cells, and extracellular matrix (ECM) components. Paget introduced the “seed and soil” hypothesis a decade ago, likening tumor cells to seeds and the tumor microenvironment to soil, underscoring the strong interdependence between tumor cell growth and their surrounding environment (24). Once thought to be mere bystanders in tumorigenesis, these host cells are now recognized as key contributors to cancer pathogenesis. The cellular makeup and functional state of the TME can vary widely based on the organ of origin, the inherent properties of the cancer cells, tumor stage, and patient-specific factors (23). Consequently, the TME is crucial in influencing tumor development, invasion, and the effectiveness of anti-cancer therapies (24). Before delving into the therapeutic strategies discussed in this review, we will first provide an overview of the essential components of the TME. Targeted anticancer agents and chemotherapies exploit inherent vulnerabilities in cancer cells. However, evidence suggests that the local TME can influence tumor behavior, either promoting or inhibiting its growth. Drugs that target cancer cell weaknesses have also been shown to affect the TME. Understanding these effects can help develop drug combinations that not only directly inhibit cancer cells but also exert indirect anticancer effects on the TME (25).
Recent studies have revealed that the TME’s cellular interactions play a role in cancer’s start and development. Malignant TME cells fail to effectively stimulate or target the immune system during the early stages of tumor development. Over time, these cells acquire the ability to evade the natural immune response and start inhibiting the adaptive immune response (26–28).
3 RIPs in cancer therapy
RIPs are a family of cytotoxic enzymes known for their ability to inhibit protein synthesis in eukaryotic cells. They achieve this by cleaving a specific adenine residue from the 28S rRNA within the 60S ribosomal subunit through N-glycosidase activity, thereby blocking the function of the ribosome. Initially discovered in the castor oil plant, Ricinus communis, RIPs have since been identified in a wide range of higher plants, and to a lesser extent, in fungi and bacteria (29). RIPs have been identified across a broad spectrum of plant species, spanning approximately 17 families, as well as in bacteria, fungi, and algae. Moreover, certain animal tissues have also been reported to exhibit RIP-like activity. A significant concentration of RIPs has been discovered within specific plant families, such as Caryophyllaceae, Sambucaceae, Euphorbiaceae, Cucurbitaceae, Poaceae, Phytolaccaceae, and Rosaceae. RIPs have also been localized in various plant tissues, including leaves, seeds, roots, and tubers. Bacterial species are known to produce type II RIPs, such as Shiga toxins. For example, specific strains of Escherichia coli synthesize Shiga toxin type 1 (Stx1) and Shiga toxin type 2 (Stx2), exhibiting enzymatic activity analogous to their plant counterparts. Similarly, several fungal species, including Volvariella volvacea, Flammulina velutipes, Hypsizigus marmoreus, and Lyophyllum shimeji, have been shown to produce RIPs.
Additionally, a RIP has been identified in algae, specifically Laminaria japonica A. Notably, RIPrelated genes have also been detected in the genomes of two mosquito species (30).
Various techniques have been employed for the purification of RIPs, tailored to specific sources and applications. Tobacco RIP (TRIP), isolated from Nicotiana tabacum leaves, was purified using ion exchange and gel filtration chromatography in combination with yeast ribosome depurination assays (31). Moschatin, a novel RIP from pumpkin Cucurbita moschata seeds, was purified through a series of steps, including ammonium sulfate precipitation, CM-cellulose 52 column chromatography, Blue Sepharose CL-6B affinity chromatography, and FPLC sizeexclusion chromatography (32). Similarly, an antiviral 25 kDa RIP from Celosia plumosa leaves were purified using a combination of 60% ammonium sulfate precipitation, FPLC-based anion and cation exchange chromatography with 10 and 50 mM NaCl, size-exclusion chromatography in 50 mM NaCl, and SDS-PAGE (10%) (33). MAP30, a type-I RIP from Momordica charantia was cloned, expressed in Escherichia coli, and purified to over 95% purity using Ni–NTA affinity chromatography (34).
RIPs are classified into three types based on their structure, mature proteins, isoelectric point (pI) value, molecular weight, and function. Type I RIPs are single-chain proteins, approximately 30 kDa in size, that possess N-glycosidase activity, such as trichosanthin (TCS) and cucurmosin. TCS, also known as monorcharin, is an example of a type 1 RIP with catalytic activity due to its solitary polypeptide chain. Type II RIPs, like ricin and abrin, are more complex, consisting of an enzymatic A-chain similar to Type I RIPs, linked to a B-chain with lectin properties that target specific sugar molecules. Type III RIPs, like JIP60 and maize ribosome-inactivating protein, a less common group, have been identified only in maize and barley, and their additional domains are not yet fully understood (35–37).
In recent years, RIPs have attracted significant attention for their potential use in cancer therapy. Some RIPs have demonstrated strong cytotoxicity towards cancer cells while exhibiting lower toxicity towards normal cells. They primarily induce apoptosis in cancer cells, though the precise mechanisms are still under investigation (36).
The toxic effects of certain type 2 RIPs, such as the toxicity of castor and jequirity beans to humans and animals, as well as the abortifacient properties of some Cucurbitaceae species, have been recognized since ancient times, long before the underlying proteins were identified. Until the early 1970s, the primary focus of scientific interest in plant RIPs was their toxicity. Subsequently, numerous RIPs were isolated and classified based on their structural and toxicological characteristics, with type 2 RIPs often demonstrating greater toxicity compared to type 1 RIPs. Notably, the cellular toxicity of type 2 RIPs generally aligns with their toxicity to animals and humans, albeit with some exceptions (38).
Type 2 RIPs are composed of two functional components: a toxic A-chain (N-β-glycosylase) and a cell-binding B-chain, which are connected by an interchain disulfide bond and hydrophobic interactions. The B-chain, which possesses lectin activity, binds to galactose residues on the surface of mammalian cells, facilitating the entry of the A-chain into the cells to exert its cytotoxic effects. In contrast, type 1 RIPs lack the lectin-containing B-chain, which significantly limits their ability to penetrate cells and results in lower cytotoxicity. Additionally, noncanonical type 3 RIPs have been identified in members of the Poaceae family. Examples include barley JIP-60, which contains a C-terminal domain of unknown function, and maize b-32, which requires proteolytic activation to achieve enzymatic functionality (39).
Despite their potential as innovative anti-tumor agents, RIPs also exhibit serious toxic side effects, including systemic anaphylaxis, immunogenicity, and general toxicity (29). A major challenge in developing RIPs for therapeutic use is their nonspecific toxicity, which poses a significant risk to healthy cells during treatment. Furthermore, RIPs require effective cellular uptake to exert their toxic effects, but they often lack the ability to independently cross the plasma membrane. This limitation highlights the need for advanced delivery systems capable of targeting specific cells while sparing healthy tissues. The upcoming sections will explore the mechanism of action, discuss the challenges posed by various barriers, and propose potential solutions to address these issues.
3.1 RIPs mechanism of action
RIPs with RNA N-glycosidase activity have attracted significant attention in cancer research. Since the identification of RIPs as potent toxins derived from Ricinus communis in the 1990s, numerous RIPs have been isolated and characterized from various plant and fungal species. These proteins, including ricin, sarcin, and trichosanthin, primarily function through depurination. RIPs target ribosomal subunit binding domains, where they cleave the sarcin-ricin loop in rRNA, a region essential for ribosome assembly and GTP hydrolysis. By impairing GTP hydrolysis, RIPs disrupt the energy supply required for the ribosome’s unidirectional movement during the elongation stage, thereby inhibiting elongation factors, halting protein synthesis, and ultimately inducing cell death (40).
RIPs play a critical role in inhibiting protein synthesis by targeting the eukaryotic ribosome, a process that requires their entry into the cell. The entry mechanism of RIPs varies depending on the type. Type II RIPs are known to bind to glycoproteins and glycolipids on the cell membrane, facilitating their entry via endocytosis. Once inside, they are transported from the Golgi apparatus to the endoplasmic reticulum through retrograde transport (41). The enzymatic components of these RIPs are released into the cytosol after exploiting the ER-associated degradation pathway, allowing them to reach the ribosomes and exert their inhibitory function (35).
In contrast, Type I RIPs, which lack sugar-binding activity, face more challenges in entering cells. They may enter to some extent by interacting with phospholipids in the cell membrane, but this process is not well understood. To enhance their entry, Type I RIPs are often conjugated with carriers like monoclonal antibodies, enabling them to specifically target and kill cells, particularly in cancer therapies. This has led to the development and study of various immunotoxins that leverage Type I RIPs for targeted cancer treatment. Once inside the cell, RIPs can induce apoptosis through multiple signaling pathways. The primary pathways include mitochondrial-mediated apoptosis, death receptor-mediated apoptosis, and ER stress-mediated apoptosis (29).
3.2 Cellular Mechanism of RIPs
3.2.1 Mitochondrial-mediated apoptosis
This pathway involves the generation of reactive oxygen species (ROS) and the disruption of intracellular Ca2+ balance, leading to changes in mitochondrial membrane potential and permeability. These changes trigger the release of proapoptotic factors such as cytochrome c, apoptosis-inducing factor (AIF), and second mitochondriaderived activator of caspases (Smac), which activate caspase-9 and downstream caspase-3, leading to DNA fragmentation and cell death (29, 42).
3.2.2 Death receptor-mediated apoptosis
This pathway is initiated when death receptors on the cell surface, such as Fas, bind to their respective ligands. This binding activates procaspase-8, which then activates caspase-3, driving the cell into apoptosis. In certain cells, RIPs can trigger this pathway, amplifying the apoptotic signal and leading to mitochondrial collapse (43).
3.2.3 ER stress-mediated apoptosis
When cells are exposed to stressors such as toxins or hypoxia, misfolded proteins accumulate in the ER, leading to ER stress. RIPs can exacerbate this stress, resulting in the activation of ER stress-related proteins like Bip and CHOP, which subsequently activate caspase-4 and caspase-12. This pathway is another route through which RIPs can induce apoptosis, particularly in cancer cells (44).
Overall, RIPs utilize these cellular mechanisms to induce apoptosis in target cells, making them potent candidates for cancer therapy. However, understanding these mechanisms in greater detail is essential for improving the effectiveness and specificity of RIP-based treatments in clinical settings. The entry pathways of type I RIPs, type II RIPs, and immunotoxins are shown in Figure 1. cellular mechanisms of RIPs are presented in Table 1.
3.2.3.1 Biological barriers in RIPs delivery
RIPs face significant challenges from the body’s bio-barriers when attempting to reach tumor sites. These problems include endothelial and epithelial cell membranes, the reticuloendothelial system, complex blood vessel networks, abnormal blood flow, and the high interstitial pressure within tumors. Additionally, the tumor cell membranes themselves pose a final barrier, hindering the transfection of RIPs into the cells. Overcoming these barriers is crucial for the effective delivery of RIPs and other anticancer therapies. Therefore, developing strategies to bypass these bio-barriers is essential for advancing protein-based cancer treatments (10). During the journey from the administration site to the target location, drugs face numerous biological barriers. Below, we provide a brief overview of these obstacles (Figure 2).
3.2.4 Vascular bio-barriers
The body’s vascular system, including endothelial and epithelial cell membranes, acts as a barrier that prevents RIPs from reaching tumor sites. The selective permeability of these membranes recognizes RIPs as foreign agents, thus blocking their passage (48). The blood-brain barrier (BBB) is a particularly challenging obstacle, controlling the transport of essential nutrients and oxygen into the brain. For RIPs to cross the BBB, they must either exploit specific receptors or navigate through tight junctions between endothelial cells (49). Additionally, factors such as slow blood flow and the presence of immune cells can further impede the delivery of RIPs by deactivating the drug molecules, leading to reduced therapeutic efficacy (50).
3.2.5 Intratumoral bio-barriers
Once RIPs reach the tumor vicinity, they encounter the dense extracellular matrix and high interstitial fluid pressure within the tumor, which further obstructs their penetration. Tumors often have disorganized and leaky vascular networks, leading to abnormal blood flow and pressure gradients that complicate drug delivery. These conditions create a physical barrier that prevents RIPs from effectively reaching and accumulating within the tumor (51). The extracellular matrix, which becomes denser and stiffer as the tumor grows, also plays a critical role in limiting drug penetration, thereby increasing the risk of metastasis and reducing the overall effectiveness of the therapy (52).
3.2.6 Intracellular bio-barriers
Even after navigating through vascular and tumoral barriers, RIPs must overcome cellular barriers to exert their therapeutic effects. The cellular membrane, with its negative charge, can resist the entry of positively charged nanoparticles and large molecules like RIPs. Endocytosis, the primary pathway for cellular uptake, is size-dependent and may involve different mechanisms, such as clathrin-mediated or caveolae-mediated endocytosis. However, once inside the cell, RIPs may face degradation within the harsh lysosomal environment, which can prevent them from reaching their target in the cytosol and thereby reduce their ability to kill tumor cells (53).
In summary, RIPs face multiple bio-barriers at various stages of delivery, from vascular transport to cellular entry. Overcoming these barriers is critical for improving the delivery efficiency and therapeutic outcomes of RIP-based cancer treatments. Developing innovative strategies to bypass or mitigate these obstacles remains a key focus in advancing protein-based cancer therapies (10).
4 Delivery strategies of RIPs for improving anti-cancer activity
The delivery of drugs from their administration site to their target location is hindered by numerous biological barriers. Among these, intracellular barriers pose a significant challenge in the effective delivery of cytotoxic proteins. To address this, researchers have explored various approaches and proposed innovative systems to overcome this limitation. One such advancement is the use of the TAT peptide, a widely utilized cell-penetrating peptide (CPP) in drug delivery, which demonstrates the ability to transport a range of cargoes, including small molecules, proteins, genes, and nanoparticles, into virtually all cell types. Despite its versatility, the nonselective nature of TAT-mediated cell internalization presents a challenge in ensuring that the drug remains inactive and safe during circulation, while selectively activating it at the tumor site (10). To address these limitations, several advanced strategies have been developed, including: the Antibody Targeted Triggered Electrically Modified Prodrug Type Strategy (ATTEMPTS), enzymetriggered systems, pH-sensitive and charge-sensitive systems, nanotechnology-based approaches, light-activated internalization systems and Voltage-Gated Channel Targeting. Table 2 summarizes the mechanism of each method, highlighting its advantages and disadvantages.
5 Role of RIPs as tumor suppressors
5.1 Effects of RIPs on breast cancer
Several RIPs have been reported for their anti-cancer and anti-tumor activities. By activating caspase-8 and caspase in breast tumor cells, RIPs induce cell cycle arrest, decrease tumor growth and volume, and restrict cell survival. An immunotoxin is designed to kill various cells and solid tumors based on the RIP model. Superior structural and functional characteristics are found in Saporin-S6 immunoconjugates. Saporin-S6 is immune to proteolysis and denaturation, is highly catalytic, and causes less damage to healthy cells. Type I RIPs have been found to inhibit breast tumor cell proliferation in vitro and in vivo (61–63). Human epidermal growth factor receptor 2 (HER2) and fibroblast growth factor-inducible 14-kDa protein (Fn14) are frequently co-expressed in human breast tumors, and HER2 directly induces an increase in Fn14 expression, making tumor cells sensitive to an immunotoxin made by fusing Fn14 antibodies to recombinant gelonin (designated hSGZ) (64). Recombinant gelonin (rGel) can be enzymatically blocked from producing proteins in tumor cells thanks to hSGZ’s ability to rapidly internalize and distribute it to the cytoplasm. Since Fn14 promotes the migration and invasion of breast cancer cells, one may wonder if there is a way to harm tumor cells while simultaneously decreasing Fn14 expression. Since MAP30 can reduce HER2 expression, leading to decreased Fn14 expression, and hSGZ can target Fn14-positive cells and exert its function without increasing the invasive capacity of tumor cells, we hypothesize that using them together might achieve a better outcome, and breast tumor cells can be sequentially treated with MAP30 and hSGZ. However, further testing is needed to confirm this hypothesis. Many receptors on cell membranes are expressed at low levels by healthy cells but at high levels by cancerous ones. In contrast to normal breast tissues, tumor tissues express estrogen receptors (ER) at significantly greater levels in around 75% of breast cancer cases (P = 0.001) (29). The proliferation-inhibiting effects of marmorin in ER-positive breast cancer cells include the ER-mediated signaling system, which is the target of numerous medicines. It has been hypothesized that marmorin might starve tumors to death by reducing the number of blood vessels in vivo (65), due to its capacity to inhibit angiogenesis by reducing the survival of human umbilical vein endothelial cells in vitro. In mice with a xenograft of the MDA-MB-231 tumor, marmorin causes DNA damage and endoplasmic reticulum stress, leading to the activation of apoptosis (65). Although RIPs may one day be used as novel anti-tumor agents, they are not without serious side effects, such as anaphylaxis, immunogenicity, and toxicity that can affect the entire body. Deng et al. (66) investigated the anti-tumor efficacy of polyethylene glycol (PEG)-modified -MMC in breast carcinoma and found that PEGylation of -MMC increases the half-life of -MMC and reduces non-specific toxicity. There was a marked improvement in anti-tumor activity with a manageable level of adverse responses with -MMC-PEG.
A novel functional gelonin fusion protein, denoted as Trx-PVGLIG-pHLIP-gelonin (TPpG), was genetically engineered and characterized for the first time. TPpG is a multi-functional construct composed of four distinct components, each designed to fulfill a specific role in tumor targeting and therapy. The thioredoxin (Trx) tag enhances the solubility and stability of the fusion protein, while the MMP-2/9-cleavable peptide (PVGLIG) ensures responsiveness to the tumor microenvironment by being cleaved in the presence of overexpressed MMP-2/9 enzymes. The pHLIP (pH Low Insertion Peptide), with a specific sequence (AAEQNPIYWARYADWLFTTPLLLLDLALLVDADEGT), confers tumor acidity-targeting capability, and gelonin, a plant-derived ribosome-inactivating protein, serves as the cytotoxic agent (67).
Asim Pervaiz et al. (2023) investigated the deregulation of anticancer genes (NOXA, PAR-4, TRAIL) in breast cancer and their induction by riproximin, a ribosome-inactivating plant protein. Expression analysis in 45 clinical samples and two breast cancer cell lines showed NOXA deregulation, PAR-4 downregulation, and TRAIL upregulation, correlating with disease severity. Riproximin significantly induced these genes at transcriptomic and protein levels, highlighting its potential therapeutic role. Bioinformatics analysis confirmed their involvement in key cellular pathways. This study suggests riproximin as a promising agent for restoring anticancer gene expression in breast cancer (68).
Shen et al. developed a TME-responsive nanoplatform using a pH-sensitive polymer and a cationic lipid-like compound for cytotoxic saporin delivery in breast cancer therapy. This approach enhances intracellular uptake, promotes endosomal escape, and effectively inhibits tumor growth, highlighting its potential for targeted protein-based treatments (69).
5.2 Effects of RIPs on leukemia and lymphoma
TCS inhibits the proliferation of B-lymphocyte cell lines by arresting them in the S-phase of the cell cycle, and it causes death in T-lymphocyte cell lines (70). Cucurmosin (type I RIP) is said to be more effective than TCS at killing chronic myelogenous leukaemia K562 cells, despite the fact that both drugs suppress cell growth by downregulating P210Bcr-Ab1 and tyrosine kinase (71). Lymphoma and leukaemia may be inhibited by TCS’s anti-proliferative properties. Tlymphocyte cell death is induced by blocking the cell cycle in the S phase, while B-lymphocyte growth is stifled (72). Type 2 RIP ripoximin inhibited cell proliferation, apoptosis resistance, and motility by downregulating Rho GTPases in MDA-MB-231 and MCF-7 human cells. IL24/MDA7, an anti-cancer cytokine, and the ER stress-related GADD genes were both elevated by ripoximin (73). Curcin 1 of the ribosomal inhibitory protein family reduces tumor cell growth. T-cell leukaemia is one type of cancer that may be inhibited by viscumarticulatum RIP. Apoptosis is triggered alongside the elevation of mitochondrial potential via early articulating D signaling. Compared to RTA alone, RTA combined with anti-HER2 scFv 4D5 and the endoplasmic reticulum-targeting peptide KDEL was 440 times more effective against ovarian cancer cells (74). RIPs ITs have potent anti-cancer effects, especially against haematological diseases, which are more easily treatable than solid tumors. Improved ITs should be directed at the differentiation clusters that can be identified on the surface of haematological cells. Human lymphoma and leukaemia surface proteins CD7, CD2, CD19, and CD22 were used to create Sap-So6 (75–78).
The growth of many different types of leukemia and lymphoma cell lines is considerably slowed by trichosanthin. Cucurmosin and TCS inhibit tyrosine kinase, which in turn suppresses cell proliferation by downregulating P210Bcr-Ab1 (79).
Several lines of leukemia and lymphoma cells are sensitive to TCS’s anti-proliferative effects (80). Importantly, TCS can cause damage to leukemia and lymphoma cells in distinct ways. TCS suppresses the proliferation of B-lymphocyte cell lines by causing them to enter an S-phase cell cycle arrest, while it promotes apoptosis in T-lymphocyte cell lines (80). It has been hypothesized that cucurmosin is more effective than TCS at killing chronic myelogenous leukemia K562 cells, although both compounds decrease cell proliferation by downregulating P210Bcr-Ab1 and tyrosine kinase (71). It has been found that the combination of cucurmosin plus trans-retinoic acid or arsenic trioxide synergistically increases these effects on the human acute promyelocytic leukemia NB4 cell line (81).
The cytotoxicity of Articulatin-D, the first cytotoxic RIP with a B-chain missing sugar-binding function, was demonstrated in vitro against a variety of leukemia and lymphoma cell lines, with Jurkat cells showing the highest sensitivity, followed by Molt-4, U-937, HL-60, and Raji cells (82). Articulatin-D’s unique physical features make it a promising starting point for the development of immunotoxins that can target and kill tumor cells with pinpoint accuracy. Emerging immunotoxins combine a toxin fragment with an antibody or cytokine to create a specific therapeutic agent. Several studies (83–85) and clinical evaluations (86–88) have reported the efficacy of immunotoxins made from saporin and rGel in the treatment of cancer. Cancer cells may be located by their membrane proteins CD22, CD7, CD19, and CD38, and the immunotoxins built against them use the corresponding antibodies HB22.7, HB2, BU12, and OKT10. Cytotoxic activity of HB22.7-saporin against a panel of non-Hodgkin’s lymphoma (NHL) cell lines and considerable inhibition of tumor growth in a xenograft model of NHL was demonstrated (88). Selective cytotoxicity against human acute lymphoblastic leukemia has been demonstrated in vitro and in vivo (89) using HB2-saporin, BU12-saporin, and OKT10-saporin. Treatment with rGelBLyS, rGel fused to a B-lymphocyte stimulator, significantly increased survival in a mouse model of disseminated lymphoma or leukemia (xenograft model) and significantly decreased tumor burden; in this context, cell death was not induced by caspase activation but was partially mediated by the ribotoxic stress response, as reported by Luster et al. (90) Nuclear factor kappa B (NF-B) activity, essential for cellular proliferation and survival, was inhibited by the combination of the rGel-BLyS fusion toxin and the proteasome inhibitor bortezomib (91).
5.3 Effects of RIPs on other cancers
Immunotoxins, made from substances like saporin and rGel, are effective against cancer. In a xenograft model of NHL, HB22.7-saporin was demonstrated to dramatically inhibit tumor growth. It was also cytotoxic against a panel of non-Hodgkin’s lymphoma (NHL) cell lines (92). RIP’s Impact on Other Forms of Cancer Recent studies in cell culture and mice have shown that MAP30 has anticancer action. For instance, MAP30 decreased cell viability and S Phase arrest in a dose- and time-dependent manner in HepG2 cells. Tumor volume was also reduced in HepG2-bearing mice as a result of MAP30-induced apoptosis and necrosis. In HepG2 cells, cucurmosin triggered G0/G1 cell cycle arrest and death, which translated to potent anti-tumor action in vivo. Abrus agglutinin induces cascade activation and inhibits act phosphorylation and NF-B expression in HepG2 cells (93). Furthermore, Luffa cylindrica recombinant luffin exhibits cytotoxicity in vitro against several tumor cell lines. In a dose- and time-dependent way, recombinant luffin suppressed the growth of JEG-3 (human placental choriocarcinoma), HepG2 (human hepatoma), and MCF-7 (human breast cancer) cell lines (94). Trichosanthes kirilowii’s tianhua (TH-R) has been shown to suppress the growth of the human lung cancer A549 cell line by dose- and time-dependently interrupting the G0/G1 phase of the cell cycle and inducing apoptosis (95). Cell survival, cell proliferation, angiogenesis, and inflammation are just some of the cellular processes that have been demonstrated to be regulated by fibroblast growth factor-inducible 14 (Fn14), a member of the tumor necrosis factor (TNF) receptor superfamily. Fn14 is expressed locally at significantly higher levels in wounded and diseased tissue (96), despite being expressed at relatively low levels in normal tissues. Highly cytotoxic to an FN14-expressing tumor cell line was recombinant gelonin (rGel), a type I RIP conjugated to an anti-Fn14 monoclonal antibody (ITEM-4). Nude mice carrying a xenograft of T-24 human bladder cancer cells showed improved long-term tumor growth inhibition after receiving an immunoconjugate with ITEM-4-rGel (97). Hepatoma and other malignancies in relation to RIPs. Anti-tumor effect of MAP30 was demonstrated in cell culture and mice. Time- and dose-dependent MAP30 inhibition of cell viability and S-phase arrest were observed in HepG2 cells; furthermore, MAP30-induced apoptosis and necrosis resulted in tumor volume decrease in HepG2-bearing mice (98). These effects of cucurmosin translated into strong anti-tumor properties in vivo (99), and G0/G1 arrest and death were triggered in HepG2 cells. Abrus agglutinin inhibits Akt phosphorylation and NF-B expression in HepG2 cells (100), and it also triggers the caspase cascade.
Morrison and colleagues developed a bioinspired delivery platform to enhance the therapeutic potential of saporin, a ribosome-inactivating plant toxin with poor membrane permeability. Using a pH-responsive pseudopeptide, poly (L-lysine isophthalamide) grafted with 50% L-phenylalanine (PP50), they significantly improved saporin’s cytotoxicity in A549 lung cancer cells and 3D spheroids under mildly acidic conditions. PP50/saporin inhibited protein synthesis, activated caspases 3/7, 8, and 9, and induced apoptosis via micropinocytosis and caveolae-mediated endocytosis. Fluorescent labeling confirmed saporin delivery to the cytoplasm and nucleus. These findings establish PP50 as a promising platform for intracellular delivery of protein therapeutics, enhancing cancer treatment efficacy (101).
In the study in 2023 analyzed the expression of anticancer genes (NOXA, PAR-4, TRAIL) in colorectal cancer (CRC) and their induction by riproximin, a ribosomal-inactivating plant protein. Their study revealed a consistent downregulation of anticancer genes in CRC, with NOXA and PAR-4 significantly suppressed during liver metastasis, while TRAIL showed early induction. Riproximin effectively upregulated these genes in CRC cell lines, suggesting its potential as a therapeutic agent (102).
In 2024, the research team investigated a novel antitumor strategy by exploring the synergistic effects of QS-21, a cationic amphiphilic saponin, and MAP30, a RIP, in inducing lysosomedependent cell death (LDCD). Their study highlights that while QS-21 alone induces lysosomal membrane permeabilization (LMP) without cytotoxicity, its combination with MAP30 significantly enhances apoptosis in tumor cells at low concentrations. Mechanistically, QS-21 facilitates the escape of MAP30 from lysosomes into the endoplasmic reticulum, where MAP30 suppresses lysophagy by downregulating LC3 proteins. This inhibition prevents the clearance of damaged lysosomes, leading to the accumulation of lysosomal contents such as cathepsins in the cytoplasm, ultimately triggering LDCD. The findings suggest that the coadministration of QS-21 and MAP30 could serve as a promising synergistic antitumor approach by amplifying lysosomal disruption (103).
Ren et al. (2025) developed optimized lipid nanoparticles (LNPs) to enhance protein delivery for cancer immunotherapy.These LNPs efficiently delivered various therapeutic proteins, including saporin and IL-10, which inhibited tumor growth and enhanced T cell responses in melanoma models. This study highlights the potential of LNPs for advancing protein-based therapies in cancer treatment (104).
5.4 Challenges and future directions
The therapeutic potential of RIPs, particularly in anti-HIV and anti-cancer applications, has gained attention over the past decades (105). Despite this, RIPs have been used in clinical trials as part of immunotoxins to treat malignant tumors (106). RIPs face several challenges in therapeutic applications. Despite their immunosuppressive activity, plant-derived RIPs often trigger immune responses, potentially leading to allergic reactions (35). Additionally, their short plasma half-life limits exposure to target cells, necessitating repeated administration, which in turn increases immune reactions. Furthermore, neurotoxicity has been observed in HIV-infected patients treated with RIPs like TCS, and accessing solid tumor masses presents a significant obstacle to effectiveness (107). Advances such as PEGylation have been explored to improve RIPs’ pharmacological properties by increasing their half-life and reducing antigenicity, with the potential to enhance tumor targeting (69). Although PEGylation has extended plasma half-life and reduced antigenicity, challenges remain, such as non-selective cytotoxicity. Immunotoxins combined with chemotherapy or other ITs offer promising strategies to enhance efficacy while minimizing side effects. Studies have shown that combining RIP-based ITs can improve outcomes, as seen in clinical trials involving treatments for acute graft-versus-host disease and lung cancer (105). However, severe side effects have led to the failure of some RIP-based drugs in clinical trials. New techniques like photochemical internalization and conjugation with albumin-binding peptides provide promising avenues for future research and development (105).
Current cancer therapeutics often lack precise tumor specificity, resulting in limited efficacy and off-target effects that can compromise treatment outcomes. Targeted therapies, such as immunotoxins with bispecific antibodies or tumor-specific expression of RIPs, offer potential solutions. These strategies must undergo extensive preclinical studies to evaluate their safety and effectiveness. Factors like immunogenicity, toxicity, and dosage must be considered when translating preclinical findings to clinical applications. While saporin-containing immunotoxins have shown promise in clinical trials, more research is needed to refine tumor-specific expression strategies and enhance RIP-based therapies (106).
As mentioned in the first section, cancer presents formidable challenges to treatment due to its diverse nature, rapid development of therapeutic resistance, and the complexity of its cellular pathways. In the treatment of cancer, the diverse nature of the disease and its rapid resistance to therapies present significant obstacles for single-agent approaches like RIPs. While RIPs exhibit potent anti-cancer effects, their toxicity and lack of specificity pose challenges to their clinical application. To overcome these limitations, combining RIPs with MSCs offers a promising therapeutic strategy. MSCs possess unique tumor-targeting properties and can serve as carriers for RIPs, allowing for more precise delivery of the cytotoxic proteins directly to the tumor site, minimizing off-target effects. Furthermore, MSCs have immunomodulatory capabilities, which can help reduce the immune responses triggered by RIPs, improving the safety profile of this combination therapy. Unlike MSCs, which have the potential to differentiate into undesired cell types or contribute to tumor progression under certain conditions, EVs lack the ability to proliferate or transform, reducing safety concerns. MSCs and their EVs offer a complementary mechanism by leveraging their intrinsic tumor-tropism. MSCs can migrate to tumor sites, delivering therapeutic agents directly to the microenvironment. However, their use raises concerns about their potential to promote tumor growth or metastasis under certain conditions. In contrast, MSCderived EVs circumvent many of these risks while retaining the therapeutic benefits (108).
By leveraging the tumor-homing ability of MSCs and the potent anti-cancer activity of RIPs, this combination could significantly enhance therapeutic efficacy while reducing systemic toxicity. As a potential hypothesis, this synergistic approach could be tested to evaluate how well it addresses the challenges of cancer treatment, including drug resistance and therapeutic targeting.
In the next section, we will explore the advantages, disadvantages, and challenges of MSCs to develop strategy for cancer therapy and advantages of MSC-derived EVs over MCSs. Next, we explore the possibility of combining these two treatment strategies.
6 MSCs and their dual role in cancer therapy
MSCs are multipotent stem cells known for their high differentiation potential and self-renewal capabilities. Initially identified in adult bone marrow in the late 1960s, MSCs were later named for their ability to differentiate into various cell types (109–112). So far, MSCs have been isolated from various tissues including bone marrow and adipose tissue, umbilical cord, peripheral blood, dermis, liver, epidermis, and skeletal muscle, etc. (14, 18, 109, 113).
The reciprocal interactions between cancer cells and the non-cancerous stromal and immune cells within the TME are essential in the pathophysiology of cancer. Factors secreted by tumor cells influence the tumor-tropism, reprogramming, and fate of MSCs, while MSCs, in turn, can impact the fate and progression of cancer cells through the secretion of chemokines and cytokines. MSCs secrete various effector molecules that mediate their dual roles in either supporting or suppressing tumor growth. Regardless of their origin, MSCs play a critical role in the TME, contributing to either tumor-suppressive or tumor-supportive conditions (114).
Over the past two decades, MSCs have been widely used in regenerative medicine and cancer treatment due to their ability to influence tumor behavior and their tumor-targeting properties. However, despite these promising traits, MSCs have also been found to support tumor growth in some cases. These conflicting findings highlight the need for careful consideration of MSCs’ complex biological characteristics in therapeutic applications to prevent unintended consequences (13).
These dual and often contradictory functions must be carefully considered when therapeutic MSCs are used, as they may adopt tumor-supportive roles within the TME, similar to tumorassociated MSCs (TA-MSCs). The subsequent sections will explore the different pathways through which MSCs can exert either tumor-suppressive or tumor-supportive effects (13) Various sources of MSCs and their dual roles in either supporting or suppressing tumor growth are schematically shown in Figure 3.
6.1 Tumor-supportive properties of MSCs
Cancer progression is affected by the TME, which consists of stroma, non-cancerous cells, and malignant cells. Multiple cell groups might be present in the extracellular matrix and tumor stroma. Fibroblasts, endothelial cells, and myofibroblasts are some of the many kinds of immune cells. After reaching tumor sites, MSCs join the stroma (115). Tumor growth is stimulated when these cells engage with cancer cells. Evidence from studies using co-implanted cancer cells (116) and mouse models of breast cancer (117) showed that MSCs may increase tumor growth and metastasis. MSCs are drawn to tumor where they can aid in the growth and spread of cancer (118, 119). Receptors and chemokines that encourage the migration of other support cells to tumors are likely mediators of the processes that allow MSCs to home (120). These include angiogenic factors (FGF, HIF1, and VEGF), chemokines (CCL5, CCL2, CXCL12, and CCL22), and inflammation factors (TGF, TNF, IL-8, and IL-1) (121, 122). Recruiting MSCs to different malignancies may have a significant effect on the course of the tumor -Metastasis and tumor -promoting MSCs were demonstrated in a rodent model of breast cancer (123). Here, we investigate the impact of MSCs on the growth of tumor. The supportive mechanisms of MSCs on tumor growth are presented in Table 3.
6.2 Tumor-suppressive properties of MSCs
While many studies have shown that MSCs promote tumor growth, others have shown that they inhibit tumor growth (Figure 3). As a result, mesenchymal stem cells (MSCs) are thought to inhibit tumor growth by increasing inflammatory cell infiltration (142), stopping angiogenesis (143), decreasing Wnt and AKT signaling (144, 145), causing cell cycle arrest and death, and preventing angiogenesis (146, 147). MSCs isolated from adipose tissue secreted interferon-, which inhibited the growth of MCF-7 cells, according to recent studies by Ryu et al. (148). In addition, MSCs primed with IFN- or cultured in 3D systems can secrete TRAIL, causing tumor-cell-specific apoptosis (149). The primary mechanisms by which MSCs exert their tumor-suppressive effects are summarized in this section (Table 3).
6.3 Signaling pathways regulated by MSCs
Cancer progression is intricately linked to various signaling pathways, which are modulated by MSCs in ways that can influence tumor growth. Key pathways affected by MSCs include PI3K/AKT, JAK/STAT, Wnt, Hippo, MYC, and NF-κB (Figure 4).
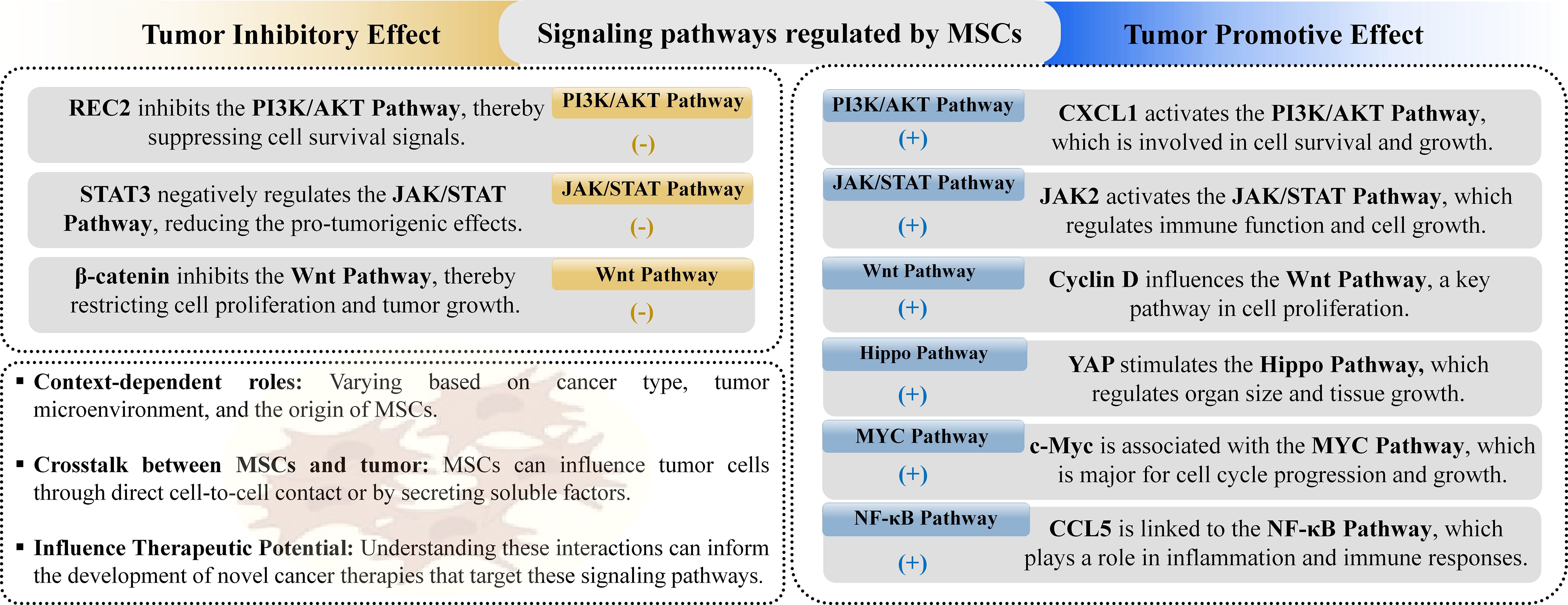
Figure 4. MSCs influence cancer-related signaling pathways, playing both tumor-promoting and tumorsuppressing roles. They achieve this by either upregulating or downregulating specific pathways involved in tumor growth and inhibition.
Among these pathways, the PI3K/AKT pathway is notably significant, as its aberrant activation or inhibition is frequently observed in various cancers, contributing to increased cell proliferation, drug resistance, and stem-cell-like phenotypes (150). PI3K, activated by numerous upstream factors, and AKT, a key downstream effector, are involved in multiple signaling cascades that influence cancer development (151). Evidence suggests that MSCs can affect tumor growth and metastasis through the PI3K/AKT pathway by promoting or inhibiting various cancer processes. For instance, MSCs associated with breast cancer can enhance mammosphere formation and create a tumor-friendly environment via this pathway, while MSC-conditioned media has been shown to boost the progression of head and neck cancer (152, 153). The JAK/STAT signaling pathway, another evolutionarily conserved cascade, plays a important role in regulating growth factors and cytokines, impacting various tissue development trajectories and disease progression, including cancer. Dysregulation of this pathway has been linked to tumorigenesis, with specific STAT proteins contributing to cancer cell malignancy (154). Studies on MSC interactions with tumors via JAK/STAT pathways reveal mixed roles. For example, IL-6 secreted by MSCs in colorectal cancer can activate JAK2/STAT3 signaling (155), promoting cancer progression (156), whereas MSC-conditioned media has been reported to inhibit STAT3 levels in breast cancer, suggesting potential anti-tumor effects (157). The Wnt signaling pathway, crucial for tissue development and cancer regulation, exhibits complex interactions with MSCs, with some studies showing MSCs modulating Wnt signaling to enhance cancer cell proliferation and metastasis, while others indicate that MSCs secrete Wnt antagonists to inhibit cancer growth (158). Similarly, the Hippo signaling pathway, involved in regulating cell growth and organ development, has been shown to influence cancer progression when modulated by MSCs, although evidence is limited and further investigation is needed (159). The MYC signaling pathway, associated with oncogenesis and poor prognosis in various cancers, is influenced by MSCs that can either promote tumor growth or enhance drug resistance (158). Lastly, the NF-κB pathway, a critical regulator in cancer progression, is implicated in MSC-mediated pro-tumor effects through its involvement in stemness and chemoresistance. Overall, these findings underscore the intricate role of MSCs in cancer signaling pathways, highlighting the need for targeted research to elucidate their therapeutic potential and impact on tumor biology (160).
6.4 MSC-derived EVs and their role in cancer therapy
A major challenge facing potential therapeutic applications of MSCs lies in their safety profile. MSCs are known to play a dual role in immune regulation; while they exhibit immunosuppressive properties in certain contexts, they can also act as antigen-presenting cells, thereby triggering immune responses. Additionally, MSCs carry a risk of tumorigenicity, either through their direct malignant transformation or by indirectly promoting the growth of existing tumor cells. Evidence indicates that MSCs are present in several tumor types, such as gastric adenocarcinoma, lipoma, and osteosarcoma, underscoring their potential role in tumor development. Consequently, it is crucial to carefully evaluate the risks and benefits of MSC-based therapies for each patient (161).
In recent years, there has been growing interest in the use of EVs derived from MSCs (MSCEVs). These EVs act as carriers of bioactive molecules, such as RNAs and proteins, transferring their cargo from parent cells to target cells and mimicking the biological functions of their origin cells. Emerging evidence suggests that MSC-EVs retain the therapeutic properties of MSCs while mitigating the safety concerns associated with live cell therapies. As a result, MSC-EVs are increasingly being considered as a promising alternative to MSCs, paving the way for cell-free therapies in future clinical applications (162).
Efficient and scalable isolation of pure EVs is critical for advancing biological research and clinical applications. Various EVs isolation methods have been developed, including centrifugation, polymer precipitation, size-dependent separation, immunoaffinity capture, and microfluidic technologies. Centrifugation, the gold standard, is effective but time-intensive and yields low purity. Polymer precipitation, often using PEG, enhances EVs recovery but introduces polymer impurities. Size-dependent methods, such as ultrafiltration and size exclusion chromatography (SEC), offer better purity but lack specificity for EVs subtypes. Immunocapture selectively isolates EVs based on surface markers (e.g., CD9, CD63, CD81) but is limited by sample size and tumor heterogeneity. Emerging microfluidic technologies leverage EVs properties such as size, density, and immunoreactivity, offering advantages like high sensitivity, rapid processing, and cost-effectiveness while being compatible with conventional methods (163).
Accurate identification and quality control of EVs are essential for their use in biological research and clinical applications. Established methods such as electron microscopy, capillary electrophoresis, nano-plasmonic sensing dynamic light scattering, and nanoparticle tracking analysis enable characterization of EVs physical properties like size and morphology, although each has limitations related to sample preparation or measurement consistency (164). Omics analyses further reveal the molecular composition of EVs, highlighting their enrichment in noncoding RNAs (e.g., miRNAs, circRNAs, lncRNAs) and functional proteins. EVs proteins are categorized into membrane-associated proteins, cytoplasmic proteins, and contaminants from the purification process, with markers like CD90 and CD29 aiding MSC-EVs identification. Techniques like western blotting, ELISA, and flow cytometry further support EVs characterization. Despite advances, improved methodologies for EVs isolation and identification are critical to provide their therapeutic potential (165).
6.5 MSC-EVs and cancer therapy resistance
Therapy resistance represents one of the most significant challenges in clinical cancer treatment, contributing to higher recurrence rates and increased mortality. Understanding the underlying mechanisms of therapeutic resistance is essential for devising effective strategies to eradicate cancer and prevent relapse. Cancer therapy resistance is often attributed to several mechanisms, including rare pre-existing drug-resistant clones that drive recurrence and treatment failure; the adaptive responses of cancer cells, which involve phenotypic plasticity (e.g., transdifferentiation, dedifferentiation and metabolic alterations); and changes within the TME, such as the activation of cancer stem cells (166). Additionally, drug-specific mechanisms, such as enhanced drug efflux, inactivation, or modifications to drug targets, also play a role. To address these challenges, new approaches are needed to uncover the fundamental drivers of resistance and implement strategies to counteract them, thereby improving treatment efficacy. MSC-EVs have emerged as key players in cancer development, progression, and therapy resistance. Their potential as an anticancer modality for clinical use has been widely recognized. Table 4 summarizes recent progress in understanding how MSC-EVs contribute to therapy resistance, with a particular focus on their roles in radiotherapy, chemotherapy, targeted therapy, immunotherapy, and endocrine therapy (165).
6.6 MSC-EVs as drug-delivery tools for anti-cancer drugs
EVs offer several advantages as drug delivery systems due to their ability to carry diverse bioactive molecules, such as nucleic acids, proteins, and other therapeutic compounds. Their natural biocompatibility, low immunogenicity, and capacity to bypass biological barriers make them highly suitable for targeted drug delivery applications. Compared to traditional drug delivery methods, MSC-derived EVs provide unique advantages, including improved cellular uptake by target cells, extended circulation time, and enhanced stability and protection of therapeutic cargo. Moreover, the specific properties of MSCs, such as their immunomodulatory and tissue repair capabilities, are reflected in the composition and functionality of their EVs. These attributes not only enhance the therapeutic potential of MSC-derived EVs as drug carriers but also contribute to broader therapeutic effects, such as modulating immune responses, promoting angiogenesis, and facilitating tissue regeneration (178, 179).
MSC-derived EVs have a promising future as drug delivery vehicles, but there are still some obstacles to overcome before they can be successfully applied in clinical settings. Key hurdles include improving targeting specificity, ensuring consistent and scalable production, and refining isolation and purification techniques to maximize therapeutic efficacy. Addressing these obstacles is essential to fully realize the potential of MSC-derived EVs in clinical practice (180).
Extensive studies highlight the potential of miRNAs as therapeutic agents in cancer, emphasizing their dual inhibitory and restorative roles. Dysregulated miRNAs, resulting from cancer progression, play critical roles in tumor initiation and development, functioning either as onco-miRs or tumor-suppressor miRNAs (TS-miRNAs). Restoring regulatory miRNAs in cancer cells has shown promising therapeutic effects, such as inhibiting proliferation, migration, invasion, angiogenesis, and metastasis while enhancing chemosensitivity and inducing apoptosis via target gene regulation (19). The success of miRNA-based cancer therapy largely depends on the efficacy of the delivery system. Notably, MSC-derived EVs have been validated as effective carriers for therapeutic miRNAs across various malignancies, presenting a novel, highly promising approach to cancer treatment. However, despite the anticancer potential of MSCs, their role in tumor progression remains controversial and warrants consideration. EVs -based therapies offer a safer alternative to live cell injections, addressing safety concerns. However, advancing the clinical application of MSC-derived EVs requires further research to understand MSC biology, clarify tumor-site homing mechanisms, develop efficacy and safety standards, and refine methods for delivering therapeutic molecules like miRNAs (19). Table 5 provides a concise overview of the miRNAs used for restoration therapy in various cancers.
6.7 Challenges and future directions
Despite extensive global efforts in academic and pharmaceutical research, current anticancer therapies are effective in treating only a limited number of neoplasms. Terms such as big killers, which refer to tumors with persistently high mortality rates, along with concepts like undruggable cancer targets and chemoresistance, underscore the significant challenges faced in cancer treatment. Moreover, critical factors such as metastasis, tumor microenvironment, tumor heterogeneity, metabolic reprogramming, and resistance to immunotherapy play central roles in modulating tumor responses to therapy. However, these factors continue to lack robust therapeutic interventions or modulators (209).
MSCs are widely used for treating various diseases due to their ability to target damaged tissues, differentiate into multiple cell types, and offer diverse therapeutic effects. However, their use in cancer therapy has shown mixed results, with both anti-tumor and pro-tumor effects observed in preclinical studies. Despite these challenges, recent MSC-based therapies offer hope for personalized, effective cancer treatments. One key advancement is the use of MSCs as “Trojan horses” to deliver therapeutic agents directly to tumors. Understanding the interaction between MSCs and cancer cells is critical for improving the safety of these therapies. Additionally, MSCderived EVs present a promising alternative to live-cell therapy, potentially addressing safety concerns (210, 211). EVs, secretomes, and exosomes are key mediators of cell-to-cell communication, helping maintain physiological balance and influencing disease development. Due to their low immunogenicity, biodegradability, minimal toxicity, and ability to transport bioactive molecules across biological barriers, they are seen as promising alternatives to stem cell therapy. MSC-derived EVs, exosomes, and secretomes have demonstrated regenerative, antiinflammatory, and immunomodulatory properties in treating various human diseases (212).
A comprehensive understanding of the biological roles of MSC-derived extracellular vesicles (MSC-EVs) could pave the way for more effective strategies to overcome or reverse cancer therapy resistance, thereby enhancing treatment outcomes. Additionally, MSC-EVs have emerged as promising carriers for drug and biomolecular delivery, with ongoing research exploring their potential to mitigate the adverse effects of cancer therapies (165).
Several challenges must be addressed before MSC-EVs can be effectively utilized in experimental and clinical settings. First, the complexity and heterogeneity of MSC-EVs necessitate the development of standardized protocols for their nomenclature, classification, isolation, and characterization. Second, safety concerns pose significant barriers to their clinical application, as MSC-EVs have demonstrated both tumor-promoting and tumor-suppressing properties depending on the cancer type and MSC source (158). Studies have reported that MSC-EVs may stimulate cancer cell proliferation, inhibit apoptosis, and promote angiogenesis through the transfer of miRNAs, limiting their clinical potential (213). Third, improving the bioavailability of MSC-EVs is critical, as their tumor-homing properties are compromised by off-target uptake and rapid clearance by macrophages in the mononuclear phagocyte system (MPS), leading to accumulation in organs like the liver, spleen, and lungs. Lastly, the low yield and limited efficiency of natural MSC-EVs in drug delivery hinder their large-scale application (214). While MSCs from sources such as bone marrow, umbilical cord, and adipose tissue are commonly used for EVs production, the difficulty in obtaining adequate samples further restricts their availability for clinical use.
To enhance the therapeutic efficacy of RIPs and MSC-derived EVs in cancer treatment and address their limitations, combining RIPs with MSC-derived EVs offers a promising strategy. MSC-derived EVs, known for their regenerative, anti-inflammatory, and immunomodulatory properties, provide a biologically compatible and low-toxicity delivery system that can improve the bioavailability and targeted delivery of RIPs to tumor cells. The use of EVs can improve the immunogenicity and short half-life of RIPs, while their ability to traverse biological barriers ensures more effective delivery to tumor sites. Previous studies have highlighted the potential synergistic effects of combining EVs with RIPs. Large bioactive molecules, such as dextran and the RIP (saporin), have been loaded into HeLa cell exosome (215, 216).
As a result, the combination of RIPs with MSC-derived EVs represents a novel and innovative method for cancer treatment, with the potential to maximize the strengths of both techniques. This approach may increase therapeutic efficiency, reduce off-target toxicity, and offer a more personalized and effective cancer treatment. Further investigation into this combination therapy is warranted, and it could pave the way for a new class of cancer treatments that harness the benefits of both RIPs and MSC-derived EVs. The combination of MSC-derived EVs and RIPs could enhance the therapeutic efficacy against cancer. MSC-derived EVs can serve as delivery vehicles for RIPs, thereby concentrating the therapeutic effects at the tumor site while minimizing systemic toxicity.
For example, in 2021, the research team developed a novel strategy for intracellular delivery using macropinocytosis-inducible EVs modified with antimicrobial protein CAP18-derived cellpenetrating peptides. They identified that dimerized (sC18)2 peptides, derived from the CAP18 antimicrobial protein, can be easily incorporated into EV membranes and significantly enhance their cellular uptake. By inducing macropinocytosis via glycosaminoglycan-dependent mechanisms, these modified EVs exhibited increased internalization into targeted cells. The technique demonstrated high efficacy in delivering the cytotoxic protein saporin, encapsulated in EVs by electroporation, highlighting its potential for targeted intracellular delivery of biofunctional molecules and advancing EV-based drug delivery systems (217).
The Nakase Research Group also developed saporin-encapsulated exosomes designed for specific receptor targeting and enhanced cytosolic delivery. This was achieved by modifying functional peptides on exosomal membranes. They introduced innovative techniques for exosomebased saporin delivery, providing a detailed explanation of exosomal properties and peptide-based methods (215, 218, 219).
Zuppone et al. (2024) developed a new EV loading protocol that enhances the encapsulation efficiency of therapeutic toxins while preserving EV properties. Unlike conventional methods, their approach utilizes temporary pH alteration with alkaline sodium carbonate, achieving superior cargo incorporation without altering vesicle size, morphology, or uptake. This method outperformed electroporation, particularly for encapsulating the ribosome-inactivating toxin saporin, which remained protected from degradation and exhibited enhanced cytotoxicity against cancer cells. Their findings demonstrate a promising EV-based drug delivery strategy that maintains vesicle integrity while improving therapeutic efficacy (220).
7 Rationale for combination therapy in cancer treatment
Advancements in our understanding of cancer biology, including inter- and intra-tumoral heterogeneity and the intricate interactions between tumors and their microenvironment, have highlighted the growing significance of combination therapies in targeting multiple pathways concurrently. Cancer is characterized by a wide range of genetic, epigenetic, proteomic, and metabolomic alterations, which collectively contribute to the diverse outcomes associated with the disease. This diversity often involves the dysregulation of several signaling pathways, even within a single tumor (221). In addition to tumor-specific factors, the dynamic TME plays a crucial role in cancer progression. The TME comprises various cellular and non-cellular components connected through complex pathways that mediate communication among cancer cells, CSCs, and their surroundings. Consequently, a multi-target approach may offer a more effective cancer treatment strategy compared to the traditional “silver bullet” model of targeting a single pathway (222). The rationale for combination therapy stems from key hallmarks of oncogenesis, including the polygenic mutational basis of most malignancies, as well as challenges such as tumor recurrence, metastasis, and the development of resistance to single-agent therapies, including targeted treatments. Monotherapy approaches aimed at specific signaling pathways have demonstrated limited success in addressing these issues. This underscores the urgent need to develop innovative combinatorial strategies to replace or enhance conventional treatment regimens (7).
In the era of -omics technologies and big data, computational methods are emerging as increasingly favorable therapeutic tools. Through signature matching screens, cancer cells’ proteomic, metabolomic, and genomic profiles can be compared with those of treated cells. This allows researchers to predict which treatments are most effective by identifying those that reverse dysregulation and restore a normal -omics profile (7). The widespread adoption of -omics approaches, combined with advancements in data storage, machine learning algorithms, and computational modeling, has significantly enhanced our understanding of cancer biology and the mechanisms of drug action. These innovations enable the generation of disease-related and drugrelated datasets that facilitate the development of computational drug networks. These networks, using in-silico methods, can efficiently predict the efficacy of existing therapies against specific cancer targets and aid in identifying optimal patient responders and relevant disease biomarkers (209). Artificial intelligence (AI), machine learning (ML), and deep learning (DL) also play critical roles in discovering potential therapeutic strategies. For instance, text mining techniques can uncover novel associations between drugs and diseases. A recent study employed text mining of PubMed data to identify metastasis-related genes in cancer and suggest repurposed drugs that may effectively target these genes (223).
Multimodal therapy incorporates combinations of treatment methods to address the multifaceted nature of complex diseases such as cancer. This approach recognizes that a single treatment modality may not adequately tackle all aspects of a disease. By leveraging complementary mechanisms of action, multimodal therapies aim to enhance treatment efficacy, overcome resistance mechanisms, and improve patient outcomes. This strategy is particularly valuable in targeting both cancer cells and their microenvironment, offering a more comprehensive approach to cancer management (7, 224).
8 Challenges of combination therapy
The polygenic mutational basis of cancer often renders single-agent therapies inadequate in controlling tumor growth and preventing recurrence, largely due to the development of resistance mechanisms. Consequently, combination therapies have become a preferred strategy (225).
Regulatory approvals for combination treatments typically rely on randomized phase II or III clinical trials demonstrating improved survival outcomes compared to the standard of care. However, designing and implementing combination therapy trials is inherently more complex than monotherapy trials. These trials must carefully account for the intricacies of drug interactions, efficacy, and overall therapeutic benefit (226). The substantial financial, resources, and time demands of clinical trials present a significant barrier to the progression of therapies into phase III studies, particularly when single-agent efficacy is a prerequisite for further evaluation. Singleagent activity is often assessed in randomized trials rather than uncontrolled phase II studies, which are more common in cancer. However, randomized trials require large sample sizes to detect small effects, imposing additional financial burdens (227, 228). This challenge is exacerbated by low patient enrollment rates in clinical trials, with approximately 40% of cancer trials failing due to insufficient patient accrual (229, 230). These factors highlight the inefficiencies in the current clinical trial framework for evaluating combination therapies (227). Importantly, drugs that show limited efficacy as single agents in phase II trials may still exhibit significant therapeutic potential when used in combination. Consequently, these agents are often prematurely excluded from further evaluation, underscoring the need for revised strategies in clinical trial design to better accommodate and assess combination therapies (231).
9 Conclusion
Combining RIPs with MSCs/MSC- derived EVs offers a novel approach to cancer therapy by maximizing the benefits of both therapeutic modalities. By targeting tumor cells more precisely, reducing toxicity, and enhancing the pharmacokinetics of RIPs, this method presents a promising avenue for the future of cancer treatment. Further investigation into this combination therapy is warranted, and it could pave the way for a new class of cancer treatments that harness the benefits of both RIPs and MSC- EVs. The combination of MSC- EVs and RIPs could enhance the therapeutic efficacy against cancer. Despite their potential, there are several challenges and limitations associated with using RIPs and MSC- EVs in cancer treatment. One challenge is the need for further research and clinical trials to establish the optimal dosing, administration route, and treatment protocols for these therapies. Additionally, the cost of producing and purifying RIPs can be a limiting factor for their widespread use. For MSCs/MSC-EVs, there are challenges related to their isolation, expansion, and quality control, as well as concerns about their potential to promote tumor growth. These challenges need to be addressed in order to fully realize the potential of RIPs and MSCs/MSC-EVs in cancer treatment. As combination therapies become more central to cancer treatment strategies, the MSCs/MSC- EVs -RIP approach could serve as a pivotal advancement in the fight against cancer. However, further research and rigorous validation are essential to fully understand the mechanisms involved and to optimize the clinical application of this combined strategy.
Author contributions
SF: Conceptualization, Data curation, Investigation, Software, Writing – original draft. MM: Conceptualization, Investigation, Software, Writing – original draft. MY: Conceptualization, Software, Writing – review & editing. AF: Data curation, Software, Writing – review & editing. AS: Writing – review & editing. MA: Project administration, Supervision, Validation, Writing – review & editing. FF: Project administration, Supervision, Validation, Writing – review & editing. HA: Methodology, Project administration, Supervision, Validation, Writing – original draft, Writing – review & editing
Funding
The author(s) declare that no financial support was received for the research and/or publication of this article.
Conflict of interest
The authors declare that the research was conducted in the absence of any commercial or financial relationships that could be construed as a potential conflict of interest.
Generative AI statement
The author(s) declare that no Generative AI was used in the creation of this manuscript.
Publisher’s note
All claims expressed in this article are solely those of the authors and do not necessarily represent those of their affiliated organizations, or those of the publisher, the editors and the reviewers. Any product that may be evaluated in this article, or claim that may be made by its manufacturer, is not guaranteed or endorsed by the publisher.
References
1. Yue W, Xu Z, Tayyab M, Wang L, Ye Z, Zhang J. Schottky junction enhanced H2 evolution for graphitic carbon nitride-NiS composite photocatalysts. J Colloid Interface Sci. (2024) 657:133–41. doi: 10.1016/j.jcis.2023.11.092
2. Jassim A, Rahrmann EP, Simons BD, Gilbertson RJ. Cancers make their own luck: theories of cancer origins. Nat Rev cancer. (2023) 23:710–24. doi: 10.1038/s41568-023-00602-5
3. Kalaki NS, Ahmadzadeh M, Dehghan M, Rabori VS, Davoudi S, Afkhami H. Investigation of CST7 and hsa-miR-4793-5p gene expression in breast cancer. Biochem Biophysics Rep. (2024) 40:101863. doi: 10.1016/j.bbrep.2024.101863
4. Gupta D, Roy P, Sharma R, Kasana R, Rathore P, Gupta TK. Recent nanotheranostic approaches in cancer research. Clin Exp Med. (2024) 24:8. doi: 10.1007/s10238-023-01262-3
5. Moslemi M, Moradi Y, Dehghanbanadaki H, Afkhami H, Khaledi M, Sedighimehr N, et al. The association between ATM variants and risk of breast cancer: a systematic review and meta-analysis. BMC cancer. (2021) 21:1–12. doi: 10.1186/s12885-020-07749-6
6. Sohrabi E, Moslemi M, Rezaie E, Nafissi N, Khaledi M, Afkhami H, et al. The tissue expression of MCT3, MCT8, and MCT9 genes in women with breast cancer. Genes Genomics. (2021) 43:1065–77. doi: 10.1007/s13258-021-01116-w
7. Weth FR, Hoggarth GB, Weth AF, Paterson E, White MP, Tan ST, et al. Unlocking hidden potential: advancements, approaches, and obstacles in repurposing drugs for cancer therapy. Br J Cancer. (2024) 130:703–15. doi: 10.1038/s41416-023-02502-9
8. Zhang Y, Zhang M, Song H, Dai Q, Liu C. Tumor microenvironment-responsive polymer-based RNA delivery systems for cancer treatment. Small Methods. (2024) 2024:2400278. doi: 10.1002/smtd.202400278
9. Piña MJ, Girotti A, Santos M, Rodríguez-Cabello JC, Arias FJ. Biocompatible ELR-based polyplexes coated with MUC1 specific aptamers and targeted for breast cancer gene therapy. Mol pharmaceutics. (2016) 13:795–808. doi: 10.1021/acs.molpharmaceut.5b00712
10. Asrorov AM, Gu Z, Min KA, Shin MC, Huang Y. Advances on tumor-targeting delivery of cytotoxic proteins. ACS Pharmacol Trans sci. (2019) 3:107–18. doi: 10.1021/acsptsci.9b00087
11. Shapira A, Benhar I. Toxin-based therapeutic approaches. Toxins. (2010) 2:2519–83. doi: 10.3390/toxins2112519
12. Yousefy MH, Afkhami H, Salehi S, Honari H. Expression, purification, characterization, and cytotoxic evaluation of the ML1-STxB fusion protein in E. coli. World Journal of Stem Cells (2023) 8:73. doi: 10.21203/rs.3.rs-2527517/v1
13. Taheri M, Tehrani HA, Dehghani S, Rajabzadeh A, Alibolandi M, Zamani N, et al. Signaling crosstalk between mesenchymal stem cells and tumor cells: Implications for tumor suppression or progression. Cytokine Growth Factor Rev. (2024). doi: 10.1016/j.cytogfr.2024.01.004
14. Andalib E, Kashfi M, Mahmoudvand G, Rezaei E, Mahjoor M, Torki A, et al. Application of hypoxiamesenchymal stem cells in treatment of anaerobic bacterial wound infection: wound healing and infection recovery. Front Microbiol. (2023) 14:1251956. doi: 10.3389/fmicb.2023.1251956
15. Farokhi S, Razavi Z-S, Mavaei M, Shadab A, Afkhami H, Sardarabadi H. New perspectives on arteriosclerosis treatment using nanoparticles and mesenchymal stem cells. Discover Appl Sci. (2024) 6:1–40. doi: 10.1007/s42452-024-06113-8
16. Ma CY, Zhai Y, Li CT, Liu J, Xu X, Chen H, et al. Translating mesenchymal stem cell and their exosome research into GMP compliant advanced therapy products: Promises, problems and prospects. Medicinal Res Rev. (2024) 44:919–38. doi: 10.1002/med.22002
17. Teymouri S, Yousefi MH, Heidari S, Farokhi S, Afkhami H, Kashfi M. Beyond antibiotics: mesenchymal stem cells and bacteriophages-new approaches to combat bacterial resistance in wound infections. Mol Biol Rep. (2024) 52:64. doi: 10.1007/s11033-024-10163-x
18. Aliniay-Sharafshadehi S, Yousefi MH, Kashfi M, Afkhami H, Ghoreyshiamiri SM. Exploring the Therapeutic Potential of Different Sources of Mesenchymal stem cells: Novel Approach to Combat Burn Wound Infections. Front Microbiol. (2024) 15:1495011. doi: 10.3389/fmicb.2024.1495011
19. Sohrabi B, Dayeri B, Zahedi E, Khoshbakht S, Nezamabadi Pour N, Ranjbar H, et al. Mesenchymal stem cell (MSC)-derived exosomes as novel vehicles for delivery of miRNAs in cancer therapy. Cancer Gene Ther. (2022) 29:1105–16. doi: 10.1038/s41417-022-00427-8
20. Fakouri A, Razavi Z-S, Mohammed AT, Hussein AHA, Afkhami H, Hooshiar MH. Applications of mesenchymal stem cell-exosome components in wound infection healing: new insights. Burns Trauma. (2024) 12:tkae021. doi: 10.1093/burnst/tkae021
21. Yarahmadi A, Afkhami H. The role of microbiomes in gastrointestinal cancers: new insights. Front Oncol. (2024) 13:1344328. doi: 10.3389/fonc.2023.1344328
22. Shadab A, Mahjoor M, Abbasi-Kolli M, Afkhami H, Moeinian P, Safdarian A-R. Divergent functions of NLRP3 inflammasomes in cancer: a review. Cell Communication Signaling. (2023) 21:232. doi: 10.1186/s12964-023-01235-9
23. De Visser KE, Joyce JA. The evolving tumor microenvironment: From cancer initiation to metastatic outgrowth. Cancer Cell. (2023) 41:374–403. doi: 10.1016/j.ccell.2023.02.016
24. Liu J, Zhang J, Gao Y, Jiang Y, Guan Z, Xie Y, et al. Barrier permeation and improved nanomedicine delivery in tumor microenvironments. Cancer Letters. (2023) 562:216166. doi: 10.1016/j.canlet.2023.216166
25. Tatarova Z. Using the tumour microenvironment to improve therapy efficacy. Nat Rev Cancer. (2024) 2024:1–. doi: 10.1038/s41568-024-00693-8
26. Angell H, Galon J. From the immune contexture to the Immunoscore: the role of prognostic and predictive immune markers in cancer. Curr Opin Immunol. (2013) 25:261–7. doi: 10.1016/j.coi.2013.03.004
27. Dong H, Strome SE, Salomao DR, Tamura H, Hirano F, Flies DB, et al. Tumor-associated B7-H1 promotes T-cell apoptosis: a potential mechanism of immune evasion. Nat Med. (2002) 8:793800. doi: 10.1038/nm730
28. Wang T, Niu G, Kortylewski M, Burdelya L, Shain K, Zhang S, et al. Regulation of the innate and adaptive immune responses by Stat-3 signaling in tumor cells. Nat Med. (2004) 10:48–54. doi: 10.1038/nm976
29. Zeng M, Zheng M, Lu D, Wang J, Jiang W, Sha O. Anti-tumor activities and apoptotic mechanism of ribosome-inactivating proteins. Chin J cancer. (2015) 34:1–10. doi: 10.1186/s40880-015-0030-x
30. Zhu F, Zhou Y-K, Ji Z-L, Chen X-R. The plant ribosome-inactivating proteins play important roles in defense against pathogens and insect pest attacks. Front Plant Sci. (2018) 9:146. doi: 10.3389/fpls.2018.00146
31. Sharma N, Park S-W, Vepachedu R, Barbieri L, Ciani M, Stirpe F, et al. Isolation and characterization of an RIP (ribosome-inactivating protein)-like protein from tobacco with dual enzymatic activity. Plant Physiol. (2004) 134:171–81. doi: 10.1104/pp.103.030205
32. Xia HC, Li F, Li Z, Zhang ZC. Purification and characterization of Moschatin, a novel type I ribosomeinactivating protein from the mature seeds of pumpkin (Cucurbita moschata), and preparation of its immunotoxin against human melanoma cells. Cell Res. (2003) 13:369–74. doi: 10.1038/sj.cr.7290182
33. Gholizadeh A. Purification of a ribosome-inactivating protein with antioxidation and root developer potencies from Celosia plumosa. Physiol Mol Biol Plants. (2019) 25:243–51. doi: 10.1007/s12298-018-0577-5
34. Lv QY. Recombinant expression and purification of a MAP30-cell penetrating peptide fusion protein with higher anti-tumor bioactivity. Protein Expression Purification. (2015) 111:17. doi: 10.1016/j.pep.2015.03.008
35. Puri M, Kaur I, Perugini MA, Gupta RC. Ribosome-inactivating proteins: current status and biomedical applications. Drug Discovery Today. (2012) 17:774–83. doi: 10.1016/j.drudis.2012.03.007
36. Sharma A, Gupta S, Sharma NR, Paul K. Expanding role of ribosome-inactivating proteins: From toxins to therapeutics. IUBMB Life. (2023) 75:82–96. doi: 10.1002/iub.v75.2
37. Barbier J, Gillet D. Ribosome inactivating proteins: from plant defense to treatments against human misuse or diseases. Plant Physiology. MDPI (2018). p. 160.
38. Stirpe F, Battelli MG. Ribosome-inactivating proteins: progress and problems. Cell Mol Life Sci CMLS. (2006) 63:1850–66. doi: 10.1007/s00018-006-6078-7
39. Ragucci S, Russo V, Clemente A, Campanile MG, Oliva MA, Landi N, et al. Hortensins, type 1 ribosome-inactivating proteins from seeds of red mountain spinach: isolation, characterization, and their effect on glioblastoma cells. Toxins. (2024) 16:135. doi: 10.3390/toxins16030135
40. Jia W, Yuan J, Li S, Cheng B. The role of dysregulated mRNA translation machinery in cancer pathogenesis and therapeutic value of ribosome-inactivating proteins. Biochim Biophys Acta (BBA)-Reviews Cancer. (2023) 40:189018. doi: 10.1016/j.bbcan.2023.189018
41. Stirpe F. Ribosome-inactivating proteins. Toxicon. (2004) 44:371–83. doi: 10.1016/j.toxicon.2004.05.004
42. Webster KA. Mitochondrial membrane permeabilization and cell death during myocardial infarction: roles of calcium and reactive oxygen species. Future Cardiol. (2012) 8:863–84. doi: 10.2217/fca.12.58
43. Schmitz I, Kirchhoff S, Krammer PH. Regulation of death receptor-mediated apoptosis pathways. Int J Biochem Cell Biol. (2000) 32:1123–36. doi: 10.1016/S1357-2725(00)00048-0
44. Citores La, Ferreras JM, Muñoz R, Benıtez J, Jiménez P, Girbés T. Targeting cancer cells with ́ transferrin conjugates containing the non-toxic type 2 ribosome-inactivating proteins nigrin b or ebulin l. Cancer Letters. (2002) 184:29–35. doi: 10.1016/S0304-3835(02)00169-6
45. Shi Y. A structural view of mitochondria-mediated apoptosis. Nat Struct Biol. (2001) 8:394–401. doi: 10.1038/87548
46. Yi X, Yin X-M, Dong Z. Inhibition of Bid-induced apoptosis by Bcl-2: tBid insertion, Bax translocation, and Bax/Bak oligomerization suppressed. J Biol Chem. (2003) 278:16992–9. doi: 10.1074/jbc.M300039200
47. Pan WL, Wong JH, Fang EF, Chan YS, Ng TB, Cheung RCF. Preferential cytotoxicity of the type I ribosome inactivating protein alpha-momorcharin on human nasopharyngeal carcinoma cells under normoxia and hypoxia. Biochem Pharmacol. (2014) 89:329–39. doi: 10.1016/j.bcp.2014.03.004
48. Su C, Liu Y, Li R, Wu W, Fawcett JP, Gu J. Absorption, distribution, metabolism and excretion of the biomaterials used in Nanocarrier drug delivery systems. Advanced Drug delivery Rev. (2019) 143:97114. doi: 10.1016/j.addr.2019.06.008
49. Upadhyay RK. Drug delivery systems, CNS protection, and the blood brain barrier. BioMed Res Int. (2014) 2014:869269. doi: 10.1155/2014/869269
51. Azzi S, Hebda JK, Gavard J. Vascular permeability and drug delivery in cancers. Front Oncol. (2013) 3:211. doi: 10.3389/fonc.2013.00211
52. Eble JA, Niland S. The extracellular matrix in tumor progression and metastasis. Clin Exp metastasis. (2019) 36:171–98. doi: 10.1007/s10585-019-09966-1
53. Setayesh-Mehr Z, Poorsargol M. Toxic proteins application in cancer therapy. Mol Biol Rep. (2021) 48:3827–40. doi: 10.1007/s11033-021-06363-4
54. Shin MC, Zhang J, Min KA, He H, David AE, Huang Y, et al. PTD-modified ATTEMPTS for enhanced toxin-based cancer therapy: an in vivo proof-of-concept study. Pharm Res. (2015) 32:2690703. doi: 10.1007/s11095-015-1653-y
55. Chen Y, Zhang M, Jin H, Tang Y, Wang H, Xu Q, et al. Intein-mediated site-specific synthesis of tumor-targeting protein delivery system: Turning PEG dilemma into prodrug-like feature. Biomaterials. (2017) 116:57–68. doi: 10.1016/j.biomaterials.2016.11.033
56. Vila-Caballer M, Codolo G, Munari F, Malfanti A, Fassan M, Rugge M, et al. A pH-sensitive stearoylPEG-poly (methacryloyl sulfadimethoxine)-decorated liposome system for protein delivery: an application for bladder cancer treatment. J Controlled Release. (2016) 238:31–42. doi: 10.1016/j.jconrel.2016.07.024
57. Provoda CJ, Stier EM, Lee K-D. Tumor cell killing enabled by listeriolysin O-liposome-mediated delivery of the protein toxin gelonin. J Biol Chem. (2003) 278:35102–8. doi: 10.1074/jbc.M305411200
58. Tang Y, Liang J, Wu A, Chen Y, Zhao P, Lin T, et al. Co-delivery of trichosanthin and albendazole by nano-self-assembly for overcoming tumor multidrug-resistance and metastasis. ACS Appl materials interfaces. (2017) 9:26648–64. doi: 10.1021/acsami.7b05292
59. Weyergang A, Fremstedal AS, Skarpen E, Peng Q, Mohamedali KA, Eng MS, et al. Light-enhanced VEGF121/rGel: A tumor targeted modality with vascular and immune-mediated efficacy. J Controlled release. (2018) 288:161–72. doi: 10.1016/j.jconrel.2018.09.005
60. Pessentheiner AR, Pelzmann HJ, Walenta E, Schweiger M, Groschner LN, Graier WF, et al. NAT8L (N-acetyltransferase 8-like) accelerates lipid turnover and increases energy expenditure in brown adipocytes. J Biol Chem. (2013) 288:36040–51. doi: 10.1074/jbc.M113.491324
61. Fang EF, Zhang CZY, Zhang L, Wong JH, Chan YS, Pan WL, et al. Trichosanthin inhibits breast cancer cell proliferation in both cell lines and nude mice by promotion of apoptosis. (2012). doi: 10.1371/journal.pone.0041592
62. Lee-Huang S, Huang P, Sun Y, Chen H, Kung H, Murphy W. Inhibition of MDA-MB-231 human breast tumor xenografts and HER2 expression by anti-tumor agents GAP31 and MAP30. Anticancer Res. (2000) 20:653–9.
63. Roh H, Pippin JA, Green DW, Boswell CB, Hirose CT, Mokadam N, et al. HER2/neu antisense targeting of human breast carcinoma. Oncogene. (2000) 19:6138–43. doi: 10.1038/sj.onc.1204001
64. Zhou H, Hittelman WN, Yagita H, Cheung LH, Martin SS, Winkles JA, et al. Antitumor activity of a humanized, bivalent immunotoxin targeting fn14-positive solid tumors. Cancer Res. (2013) 73:4439–50. doi: 10.1158/0008-5472.CAN-13-0187
65. Pan WL, Wong JH, Fang EF, Chan YS, Ye XJ, Ng TB. Differential inhibitory potencies and mechanisms of the type I ribosome inactivating protein marmorin on estrogen receptor (ER)-positive and ER-negative breast cancer cells. Biochim Biophys Acta (BBA)-Molecular Cell Res. (2013) 1833:987–96. doi: 10.1016/j.bbamcr.2012.12.013
66. Deng N-h, Wang L, He Q-c, Zheng J-c, Meng Y, Meng Y-F, et al. PEGylation alleviates the nonspecific toxicities of Alpha-Momorcharin and preserves its antitumor efficacy in vivo. Drug Delivery. (2016) 23:95–100. doi: 10.3109/10717544.2014.905652
67. Ding G-B, Cao H, Zhu C, Chen F, Ye J, Li B-C, et al. Biosynthesized tumor acidity and MMP dualresponsive plant toxin gelonin for robust cancer therapy. Biomaterials Sci. (2024) 12:346–60. doi: 10.1039/D3BM01779F
68. Pervaiz A, Naseem N, Saleem T, Raza SM, Shaukat I, Kanwal K, et al. Anticancer genes (NOXA, PAR4, TRAIL) are de-regulated in breast cancer patients and can be targeted by using a ribosomal inactivating plant protein (riproximin). Mol Biol Rep. (2023) 50:5209–21. doi: 10.1007/s11033-023-08477-3
69. Shen Q, Xu L, Li R, Wu G, Li S, Saw PE, et al. A tumor microenvironment (TME)-responsive nanoplatform for systemic saporin delivery and effective breast cancer therapy. Chem Commun. (2021) 57:2563–6. doi: 10.1039/D0CC07808E
70. Maqsood Q, Sumrin A, Mahnoor M, Waseem M, Ameen E, Tabassum N. Potential use of ribosome inactivating proteins (RIPS) as cancer therapeutic and its indigenous sources. Ann Med Health Sci Res. (2021) 11.
71. Hou X, Meehan EJ, Xie J, Huang M, Chen M, Chen L. Atomic resolution structure of cucurmosin, a novel type 1 ribosome-inactivating protein from the sarcocarp of Cucurbita moschata. J Struct Biol. (2008) 164:81–7. doi: 10.1016/j.jsb.2008.06.011
72. Landi N, Ruocco MR, Ragucci S, Aliotta F, Nasso R, Pedone PV, et al. Quinoa as source of type 1 ribosome inactivating proteins: A novel knowledge for a revision of its consumption. Food Chem. (2021) 342:128337. doi: 10.1016/j.foodchem.2020.128337
73. Lestariana WLW. Identification and test of active protein resemble Ribosomr-inactivating proteins (RIPs) on Kaemferia rotunda Linn. J Med Sci (Berkala Ilmu Kedokteran). (2003) 35.
74. Lin Y, Xu L, Li Y, Wu X, Liu Y, Zhu H, et al. Ribosome-inactivating proteins of Bougainvillea glabra uncovered polymorphism and active site divergence. Toxins. (2021) 13:331. doi: 10.3390/toxins13050331
75. Weng A. A novel adenine-releasing assay for ribosome-inactivating proteins. J Chromatogr B. (2018) 1072:300–4. doi: 10.1016/j.jchromb.2017.11.038
76. Wong JH, Bao H, Ng TB, Chan HHL, Ng CCW, Man GCW, et al. New ribosome-inactivating proteins and other proteins with protein synthesis–inhibiting activities. Appl Microbiol Biotechnol. (2020) 104:4211–26. doi: 10.1007/s00253-020-10457-7
77. Yaqoob R, Noureen A, Ujjan JA, Achakzai WM, Saddozai S, Saleem N, et al. Functional importance and anti-tumor activities of ribosome inactivation protein (RIPs). Ann Romanian Soc Cell Biol. (2021) 2021:18747–63.
78. De Zaeytijd J, Van Damme EJ. Extensive evolution of cereal ribosome-inactivating proteins translates into unique structural features, activation mechanisms, and physiological roles. Toxins. (2017) 9:123. doi: 10.3390/toxins9040123
79. Zhou Y, Li X-P, Kahn JN, Tumer NE. Functional assays for measuring the catalytic activity of ribosome inactivating proteins. Toxins. (2018) 10:240. doi: 10.3390/toxins10060240
80. Wang Y-Y, Ouyang D-Y, Zheng Y-T. Mechanism of trichosanthin against human leukemia/lymphoma cells in vitro. Zhongguo Shi Yan Xue Ye Xue Za Zhi. (2007) 15:729–32.
81. Xle J-M, Liu M, Liu T-B, Chen M-H, Yang A-Q, Yang P. Effects of cucurmosin combined with common chemotherapeutics on proliferation and apoptosis of NB4 cells. Zhongguo shi yan xue ye xue za zhi. (2012) 20:1327–31.
82. Das MK, Sharma RS, Mishra V. A cytotoxic type-2 ribosome inactivating protein (from leafless mistletoe) lacking sugar binding activity. Int J Biol macromolecules. (2011) 49:1096–103. doi: 10.1016/j.ijbiomac.2011.09.006
83. Cao Y, Marks JD, Marks JW, Cheung LH, Kim S, Rosenblum MG. Construction and characterization of novel, recombinant immunotoxins targeting the Her2/neu oncogene product: in vitro and in vivo studies. Cancer Res. (2009) 69:8987–95. doi: 10.1158/0008-5472.CAN-09-2693
84. Polito L, Bortolotti M, Mercatelli D, Battelli MG, Bolognesi A. Saporin-S6: a useful tool in cancer therapy. Toxins. (2013) 5:1698–722. doi: 10.3390/toxins5101698
85. Zhou H, Ekmekcioglu S, Marks JW, Mohamedali KA, Asrani K, Phillips KK, et al. The TWEAK receptor Fn14 is a therapeutic target in melanoma: immunotoxins targeting Fn14 receptor for Malignant melanoma treatment. J Invest Dermatol. (2013) 133:1052–62. doi: 10.1038/jid.2012.402
86. Borthakur G, Rosenblum MG, Talpaz M, Daver N, Ravandi F, Faderl S, et al. Phase 1 study of an anti-CD33 immunotoxin, humanized monoclonal antibody M195 conjugated to recombinant gelonin (HUM-195/rGEL), in patients with advanced myeloid Malignancies. Haematologica. (2013) 98:217. doi: 10.3324/haematol.2012.071092
87. Polito L, Bortolotti M, Pedrazzi M, Bolognesi A. Immunotoxins and other conjugates containing saporin-s6 for cancer therapy. Toxins. (2011) 3:697–720. doi: 10.3390/toxins3060697
88. Kato J, O’Donnell RT, Abuhay M, Tuscano JM. Efficacy and toxicity of a CD22-targeted antibodysaporin conjugate in a xenograft model of non-Hodgkin’s lymphoma. Oncoimmunology. (2012) 1:146975. doi: 10.4161/onci.21815
89. Flavell D, Boehm D, Noss A, Warnes S, Flavell S. Therapy of human T-cell acute lymphoblastic leukaemia with a combination of anti-CD7 and anti-CD38-SAPORIN immunotoxins is significantly better than therapy with each individual immunotoxin. Br J cancer. (2001) 84:571–8. doi: 10.1054/bjoc.2000.1633
90. Luster TA, Mukherjee I, Carrell JA, Cho YH, Gill J, Kelly L, et al. Fusion toxin BLyS-gelonin inhibits growth of Malignant human B cell lines in vitro and in vivo. (2012). doi: 10.1371/journal.pone.0047361
91. Lyu M-A, Pham LV, Sung B, Tamayo AT, Ahn KS, Hittelman WN, et al. The therapeutic effects of rGel/BLyS fusion toxin in in vitro and in vivo models of mantle cell lymphoma. Biochem Pharmacol. (2012) 84:451–8. doi: 10.1016/j.bcp.2012.05.019
92. Wani SS, Dar PA, Zargar SM, Dar TA. Therapeutic potential of medicinal plant proteins: present status and future perspectives. Curr Protein Pept Sci. (2020) 21:443–87. doi: 10.2174/1389203720666191119095624
93. Weise C, Schrot A, Wuerger LT, Adolf J, Gilabert-Oriol R, Sama S, et al. An unusual type I ribosomeinactivating protein from Agrostemma githago L. Sci Rep. (2020) 10:15377. doi: 10.1038/s41598-020-72282-2
94. Liu L, Wang R, He W, He F, Huang G. Cloning and soluble expression of mature α-luffin from Luffa cylindrica and its antitumor activities in vitro. Acta Biochim Biophys Sin. (2010) 42:585–92. doi: 10.1093/abbs/gmq056
95. Li C-T, Lin C-H, Kao T-Y, Wu M-F, Yeh C-S, Yeh K-T, et al. The mechanisms of action of Tianhua™ on antitumor activity in lung cancer cells. Pharm Biol. (2010) 48:1302–9. doi: 10.3109/13880201003789432
96. Han ES, Mekasha S, Ingalls RR. Fibroblast growth factor-inducible 14 (Fn14) is expressed in the lower genital tract and may play a role in amplifying inflammation during infection. J Reprod Immunol. (2010) 84:16–23. doi: 10.1016/j.jri.2009.09.009
97. Zhou H, Marks JW, Hittelman WN, Yagita H, Cheung LH, Rosenblum MG, et al. Development and characterization of a potent immunoconjugate targeting the Fn14 receptor on solid tumor cells. Mol Cancer Ther. (2011) 10:1276–88. doi: 10.1158/1535-7163.MCT-11-0161
98. Fang EF, Zhang CZY, Wong JH, Shen JY, Li CH, Ng TB. The MAP30 protein from bitter gourd (Momordica charantia) seeds promotes apoptosis in liver cancer cells in vitro and in vivo. Cancer Lett. (2012) 324:66–74. doi: 10.1016/j.canlet.2012.05.005
99. Xie J, Que W, Liu H, Liu M, Yang A, Chen M. Anti-proliferative effects of cucurmosin on human hepatoma HepG2 cells. Mol Med Rep. (2012) 5:196–201. doi: 10.3892/mmr.2011.605
100. Mukhopadhyay S, Panda PK, Das DN, Sinha N, Behera B, Maiti TK, et al. Abrus agglutinin suppresses human hepatocellular carcinoma in vitro and in vivo by inducing caspase-mediated cell death. Acta Pharmacologica Sinica. (2014) 35:814–24. doi: 10.1038/aps.2014.15
101. Morrison G, Henry N, Kopytynski M, Chen R. A bioinspired pseudopeptide-based intracellular delivery platform enhances the cytotoxicity of a ribosome-inactivating protein through multiple death pathways. Biomaterials Sci. (2024) 12:5010–22. doi: 10.1039/D4BM00600C
102. Pervaiz A, Saleem T, Kanwal K, Raza SM, Iqbal S, Zepp M, et al. Expression profiling of anticancer genes in colorectal cancer patients and their in vitro induction by riproximin, a ribosomal inactivating plant protein. J Cancer Res Clin Oncol. (2023) 149:4825–37. doi: 10.1007/s00432-022-04410-6
103. Chen P, Cao X-W, Dong J-W, Zhao J, Wang F-J. Saponin and ribosome-inactivating protein synergistically trigger lysosome-dependent apoptosis by inhibiting lysophagy: potential to become a new antitumor strategy. Mol Pharmaceutics. (2024) 21:2993–3005. doi: 10.1021/acs.molpharmaceut.4c00140
104. Ren L, Zhao Z, Chao Y, Yu P, Mei Z, Du B, et al. Optimization of lipid nanoparticles with robust efficiency for the delivery of protein therapeutics to augment cancer immunotherapy. Advanced Sci. (2025) 2025:2500844. doi: 10.1002/advs.202500844
105. Lu J-Q, Zhu Z-N, Zheng Y-T, Shaw P-C. Engineering of ribosome-inactivating proteins for improving pharmacological properties. Toxins. (2020) 12:167. doi: 10.3390/toxins12030167
106. Yang Y-x, Wang X-y, Lin T, Sun Y, Yu Y-c, Zhu Z-h. Opportunities and challenges for ribosomeinactivating proteins in traditional Chinese medicine plants. Toxicon. (2023), 107278. doi: 10.1016/j.toxicon.2023.107278
107. Pulliam L, McGrath M. GLQ223® exacerbation of indirect HIV-associated neurotoxicity in vitro. Antiviral Res. (1991) 15:82. doi: 10.1016/0166-3542(91)90159-O
108. Cortes-Dericks L, Galetta D. The therapeutic potential of mesenchymal stem cells in lung cancer: benefits, risks and challenges. Cell Oncol. (2019) 42:727–38. doi: 10.1007/s13402-019-00459-7
109. Timaner M, Tsai KK, Shaked Y eds. The multifaceted role of mesenchymal stem cells in cancer. In: Seminars in Cancer Biology. Elsevier.
110. Mirshekar M, Afkhami H, Razavi S, Jazi FM, Darban-Sarokhalil D, Ohadi E, et al. Potential antibacterial activity and healing effect of topical administration of bone marrow and adipose mesenchymal stem cells encapsulated in collagen-fibrin hydrogel scaffold on full-thickness burn wound infection caused by Pseudomonas aeruginosa. Burns. (2023) 49:1944–57. doi: 10.1016/j.burns.2023.01.005
111. Sheikhi K, Ghaderi S, Firouzi H, Afkhami H, Yarahmadi A. Recent advances in mesenchymal stem cell therapy for multiple sclerosis: clinical applications and challenges. Front Cell Dev Biol. (2025) 13:1517369. doi: 10.3389/fcell.2025.1517369
112. Kamrani S, Naseramini R, Khani P, Razavi ZS, Afkhami H, Atashzar MR, et al. Mesenchymal stromal cells in bone marrow niche of patients with multiple myeloma: a double-edged sword. Cancer Cell Int. (2025) 25:117. doi: 10.1186/s12935-025-03741-x
113. Yarahmadi A, Hosseininejad R, Rezaie A, Mohammadi N, Afkhami H. Mesenchymal stem cell and their extracellular vesicle therapy for neurological disorders: traumatic brain injury and beyond. Front Neurol. 16:1472679. doi: 10.3389/fneur.2025.1472679
114. Berger L, Shamai Y, Skorecki KL, Tzukerman M. Tumor specific recruitment and reprogramming of mesenchymal stem cells in tumorigenesis. Stem Cells. (2016) 34:1011–26. doi: 10.1002/stem.2269
115. Zhao Y, Shen M, Wu L, Yang H, Yao Y, Yang Q, et al. Stromal cells in the tumor microenvironment: accomplices of tumor progression? Cell Death Dis. (2023) 14:587. doi: 1038/s41419-023-06110-6
116. Jahangiri B, Khalaj-Kondori M, Asadollahi E, Kian Saei A, Sadeghizadeh M. Dual impacts of mesenchymal stem cell-derived exosomes on cancer cells: unravelling complex interactions. J Cell Communication Signaling. (2023) 17:1229–47. doi: 10.1007/s12079-023-00794-3
117. Xu W-t, Bian Z-y, Fan Q-m, Li G, Tang T-t. Human mesenchymal stem cells (hMSCs) target osteosarcoma and promote its growth and pulmonary metastasis. Cancer letters. (2009) 281:32–41. doi: 10.1016/j.canlet.2009.02.022
118. Hill BS, Pelagalli A, Passaro N, Zannetti A. Tumor-educated mesenchymal stem cells promote prometastatic phenotype. Oncotarget. (2017) 8:73296. doi: 10.18632/oncotarget.20265
119. Afkhami H, Yarahmadi A, Bostani S, Yarian N, Haddad MS, Lesani SS, et al. Converging frontiers in cancer treatment: the role of nanomaterials, mesenchymal stem cells, and microbial agents—challenges and limitations. Discover Oncol. (2024) 15:1–35. doi: 10.1007/s12672-024-01590-0
120. De Becker A, Van Riet I. Homing and migration of mesenchymal stromal cells: how to improve the efficacy of cell therapy? World J Stem Cells. (2016) 8:73. doi: 10.4252/wjsc.v8.i3.73
121. Bissell MJ, Radisky D. Putting tumours in context. Nat Rev Cancer. (2001) 1:46–54. doi: 10.1038/35094059
122. Coffelt SB, Hughes R, Lewis CE. Tumor-associated macrophages: effectors of angiogenesis and tumor progression. Biochim Biophys Acta (BBA)-Reviews Cancer. (2009) 1796:11–8. doi: 10.1016/j.bbcan.2009.02.004
123. Karnoub AE, Dash AB, Vo AP, Sullivan A, Brooks MW, Bell GW, et al. Mesenchymal stem cells within tumour stroma promote breast cancer metastasis. Nature. (2007) 449:557–63. doi: 10.1038/nature06188
124. Krueger TE, Thorek DL, Meeker AK, Isaacs JT, Brennen WN. Tumor-infiltrating mesenchymal stem cells: Drivers of the immunosuppressive tumor microenvironment in prostate cancer? Prostate. (2019) 79:320–30. doi: 10.1002/pros.23738
125. Ungerer C, Quade-Lyssy P, Radeke HH, Henschler R, Königs C, Köhl U, et al. Galectin-9 is a suppressor of T and B cells and predicts the immune modulatory potential of mesenchymal stromal cell preparations. Stem Cells Dev. (2014) 23:755–66. doi: 10.1089/scd.2013.0335
126. Lopatina T, Bruno S, Tetta C, Kalinina N, Porta M, Camussi G. Platelet-derived growth factor regulates the secretion of extracellular vesicles by adipose mesenchymal stem cells and enhances their angiogenic potential. Cell Communication Signaling. (2014) 12:1–12. doi: 10.1186/1478-811X-12-26
127. Akimoto K, Kimura K, Nagano M, Takano S, To’a Salazar G, Yamashita T, et al. Umbilical cord bloodderived mesenchymal stem cells inhibit, but adipose tissue-derived mesenchymal stem cells promote, glioblastoma multiforme proliferation. Stem Cells Dev. (2013) 22:1370–86. doi: 10.1089/scd.2012.0486
128. Kalluri R. The biology and function of fibroblasts in cancer. Nat Rev Cancer. (2016) 16:582–98. doi: 10.1038/nrc.2016.73
129. Paino F, La Noce M, Di Nucci D, Nicoletti GF, Salzillo R, De Rosa A, et al. Human adipose stem cell differentiation is highly affected by cancer cells both in vitro and in vivo: implication for autologous fat grafting. Cell Death disease. (2018) 8:e2568–e. doi: 10.1038/cddis.2016.308
130. Chen B, Cai T, Huang C, Zang X, Sun L, Guo S, et al. G6PD-NF-κB-HGF signal in gastric cancerassociated mesenchymal stem cells promotes the proliferation and metastasis of gastric cancer cells by upregulating the expression of HK2. Front Oncol. (2021) 11:648706. doi: 10.3389/fonc.2021.648706
131. McLean K, Gong Y, Choi Y, Deng N, Yang K, Bai S, et al. Human ovarian carcinoma–associated mesenchymal stem cells regulate cancer stem cells and tumorigenesis via altered BMP production. J Clin Invest. (2011) 121:3206–19. doi: 10.1172/JCI45273
132. Mi Z, Bhattacharya SD, Kim VM, Guo H, Talbot LJ, Kuo PC. Osteopontin promotes CCL5mesenchymal stromal cell-mediated breast cancer metastasis. Carcinogenesis. (2011) 32:477–87. doi: 10.1093/carcin/bgr009
133. Thomas C, Karnoub AE. Lysyl oxidase at the crossroads of mesenchymal stem cells and epithelialmesenchymal transition. Oncotarget. (2013) 4:376. doi: 10.18632/oncotarget.v4i3
134. Maxwell PJ, Neisen J, Messenger J, Waugh DJ. Tumor-derived CXCL8 signaling augments stromaderived CCL2-promoted proliferation and CXCL12-mediated invasion of PTEN-deficient prostate cancer cells. Oncotarget. (2014) 5:4895. doi: 10.18632/oncotarget.v5i13
135. Vianello F, Villanova F, Tisato V, Lymperi S, Ho K-K, Gomes AR, et al. Bone marrow mesenchymal stromal cells non-selectively protect chronic myeloid leukemia cells from imatinib-induced apoptosis via the CXCR4/CXCL12 axis. Haematologica. (2010) 95:1081. doi: 10.3324/haematol.2009.017178
136. Li H-J, Reinhardt F, Herschman HR, Weinberg RA. Cancer-stimulated mesenchymal stem cells create a carcinoma stem cell niche via prostaglandin E2 signaling. Cancer discov. (2012) 2:840–55. doi: 10.1158/2159-8290.CD-12-0101
137. Ho IA, Toh HC, Ng WH, Teo YL, Guo CM, Hui KM, et al. Human bone marrow-derived mesenchymal stem cells suppress human glioma growth through inhibition of angiogenesis. Stem Cells. (2013) 31:14655. doi: 10.1002/stem.1247
138. Du L, Tao X, Shen X. Human umbilical cord mesenchymal stem cell-derived exosomes inhibit migration and invasion of breast cancer cells via miR-21-5p/ZNF367 pathway. Breast Cancer. (2021) 28:82937. doi: 10.1007/s12282-021-01218-z
139. Serhal R, Saliba N, Hilal G, Moussa M, Hassan GS, El Atat O, et al. Effect of adipose-derived mesenchymal stem cells on hepatocellular carcinoma: In vitro inhibition of carcinogenesis. World J gastroenterol. (2019) 25:567. doi: 10.3748/wjg.v25.i5.567
140. Fathi E, Vandghanooni S, Montazersaheb S, Farahzadi R. Mesenchymal stem cells promote caspase-3 expression of SH-SY5Y neuroblastoma cells via reducing telomerase activity and telomere length. Iranian J basic Med Sci. (2021) 24:1583. doi: 10.22038/IJBMS.2021.59400.13187
141. Tang R-j, Shen S-n, Zhao X-y, Nie Y-z, Xu Y-j, Ren J, et al. Mesenchymal stem cells-regulated Treg cells suppress colitis-associated colorectal cancer. Stem Cell Res Ther. (2015) 6:1–11. doi: 10.1186/s13287-015-0055-8
142. Ryu H, Oh J-E, Rhee K-J, Baik SK, Kim J, Kang SJ, et al. Adipose tissue-derived mesenchymal stem cells cultured at high density express IFN-β and suppress the growth of MCF-7 human breast cancer cells. Cancer letters. (2014) 352:220–7. doi: 10.1016/j.canlet.2014.06.018
143. Zhang F, Guo J, Zhang Z, Qian Y, Wang G, Duan M, et al. Mesenchymal stem cell-derived exosome: A tumor regulator and carrier for targeted tumor therapy. Cancer letters. (2022) 526:29–40. doi: 10.1016/j.canlet.2021.11.015
144. Qiao L, Xu Z, Zhao T, Zhao Z, Shi M, Zhao RC, et al. Suppression of tumorigenesis by human mesenchymal stem cells in a hepatoma model. Cell Res. (2008) 18:500–7. doi: 10.1038/cr.2008.40
145. Zhu Y, Sun Z, Han Q, Liao L, Wang J, Bian C, et al. Human mesenchymal stem cells inhibit cancer cell proliferation by secreting DKK-1. Leukemia. (2009) 23:925–33. doi: 10.1038/leu.2008.384
146. Xuan X, Tian C, Zhao M, Sun Y, Huang C. Mesenchymal stem cells in cancer progression and anticancer therapeutic resistance. Cancer Cell Int. (2021) 21:595. doi: 10.1186/s12935-021-02300-4
147. Afkhami H, Mahmoudvand G, Fakouri A, Shadab A, Mahjoor M, Komeili Movahhed T. New insights in application of mesenchymal stem cells therapy in tumor microenvironment: Pros and cons. Front Cell Dev Biol. (2023) 11:1255697. doi: 10.3389/fcell.2023.1255697
148. Madrigal M, Rao KS, Riordan NH. A review of therapeutic effects of mesenchymal stem cell secretions and induction of secretory modification by different culture methods. J Trans Med. (2014) 12:1–14. doi: 10.1186/s12967-014-0260-8
149. Du J, Zhou L, Chen X, Yan S, Ke M, Lu X, et al. IFN-γ-primed human bone marrow mesenchymal stem cells induce tumor cell apoptosis in vitro via tumor necrosis factor-related apoptosis-inducing ligand. Int J Biochem Cell Biol. (2012) 44:1305–14. doi: 10.1016/j.biocel.2012.04.015
150. Jiang N, Dai Q, Su X, Fu J, Feng X, Peng J. Role of PI3K/AKT pathway in cancer: the framework of Malignant behavior. Mol Biol Rep. (2020) 47:4587–629. doi: 10.1007/s11033-020-05435-1
151. Faes S, Dormond O. PI3K and AKT: unfaithful partners in cancer. Int J Mol Sci. (2015) 16:21138–52. doi: 10.3390/ijms160921138
152. Yan X-l, Fu C-j, Chen L, Qin J-h, Zeng Q, Yuan H-f, et al. Mesenchymal stem cells from primary breast cancer tissue promote cancer proliferation and enhance mammosphere formation partially via EGF/EGFR/Akt pathway. Breast Cancer Res Treat. (2012) 132:153–64. doi: 10.1007/s10549-011-1577-0
153. Starska-Kowarska K. The role of mesenchymal stem/stromal cells in head and neck cancer– regulatory mechanisms of tumorigenic and immune activity, chemotherapy resistance, and therapeutic benefits of the use of stromal cell-based pharmacological strategies. Breast Cancer Research and Treatment. (2024) 132:153–64. doi: 10.20944/preprints202406.0771.v1
154. Groner B, von Manstein V. Jak Stat signaling and cancer: Opportunities, benefits and side effects of targeted inhibition. Mol Cell endocrinol. (2017) 451:1–14. doi: 10.1016/j.mce.2017.05.033
155. Mahjoor M, Mahmoudvand G, Farokhi S, Shadab A, Kashfi M, Afkhami H. Double-edged sword of JAK/STAT signaling pathway in viral infections: novel insights into virotherapy. Cell Communication Signaling. (2023) 21:272. doi: 10.1186/s12964-023-01240-y
156. Zhang X, Hu F, Li G, Li G, Yang X, Liu L, et al. Human colorectal cancer-derived mesenchymal stem cells promote colorectal cancer progression through IL-6/JAK2/STAT3 signaling. Cell Death disease. (2018) 9:25. doi: 10.1038/s41419-017-0176-3
157. He N, Kong Y, Lei X, Liu Y, Wang J, Xu C, et al. MSCs inhibit tumor progression and enhance radiosensitivity of breast cancer cells by down-regulating Stat3 signaling pathway. Cell Death disease. (2018) 9:1026. doi: 10.1038/s41419-018-0949-3
158. Lan T, Luo M, Wei X. Mesenchymal stem/stromal cells in cancer therapy. J Hematol Oncol. (2021) 14:1–16. doi: 10.1186/s13045-021-01208-w
159. Zhu C, Li L, Zhao B. The regulation and function of YAP transcription co-activator. Acta Biochim Biophys Sin. (2015) 47:16–28. doi: 10.1093/abbs/gmu110
160. Hoesel B, Schmid JA. The complexity of NF-κB signaling in inflammation and cancer. Mol cancer. (2013) 12:1–15. doi: 10.1186/1476-4598-12-86
161. Cheng Y, Cao X, Qin L. Mesenchymal stem cell-derived extracellular vesicles: a novel cell-free therapy for sepsis. Front Immunol. (2020) 11:647. doi: 10.3389/fimmu.2020.00647
162. Kou M, Huang L, Yang J, Chiang Z, Chen S, Liu J, et al. Mesenchymal stem cell-derived extracellular vesicles for immunomodulation and regeneration: a next generation therapeutic tool? Cell Death Dis. (2022) 13:580. doi: 10.1038/s41419-022-05034-x
163. De Sousa KP, Rossi I, Abdullahi M, Ramirez MI, Stratton D, Inal JM. Isolation and characterization of extracellular vesicles and future directions in diagnosis and therapy. Wiley Interdiscip Reviews: Nanomed Nanobiotechnol. (2023) 15:e1835. doi: 10.1002/wnan.v15.1
164. Konoshenko MY, Lekchnov EA, Vlassov AV, Laktionov PP. Isolation of extracellular vesicles: general methodologies and latest trends. BioMed Res Int. (2018) 2018:8545347. doi: 10.1155/2018/8545347
165. Shan C, Liang Y, Wang K, Li P. Mesenchymal stem cell-derived extracellular vesicles in Cancer Therapy Resistance: from Biology to Clinical Opportunity. Int J Biol Sci. (2024) 20:347. doi: 10.7150/ijbs.88500
166. Bukowski K, Kciuk M, Kontek R. Mechanisms of multidrug resistance in cancer chemotherapy. Int J Mol Sci. (2020) 21:3233. doi: 10.3390/ijms21093233
167. Wan F-Z, Chen K-H, Sun Y-C, Chen X-C, Liang R-B, Chen L, et al. Exosomes overexpressing miR-34c inhibit Malignant behavior and reverse the radioresistance of nasopharyngeal carcinoma. J Trans Med. (2020) 18:1–19. doi: 10.1186/s12967-019-02203-z
168. de Araujo Farias V, O’Valle F, Serrano-Saenz S, Anderson P, Andrés E, López-Peñalver J, et al. Exosomes derived from mesenchymal stem cells enhance radiotherapy-induced cell death in tumor and metastatic tumor foci. Mol Cancer. (2018) 17:1–12. doi: 10.1186/s12943-018-0867-0
169. Ji R, Zhang B, Zhang X, Xue J, Yuan X, Yan Y, et al. Exosomes derived from human mesenchymal stem cells confer drug resistance in gastric cancer. Cell Cycle. (2015) 14:2473–83. doi: 10.1080/15384101.2015.1005530
170. Jia Z, Zhu H, Sun H, Hua Y, Zhang G, Jiang J, et al. Adipose mesenchymal stem cell-derived exosomal microRNA-1236 reduces resistance of breast cancer cells to cisplatin by suppressing SLC9A1 and the Wnt/β-catenin signaling. Cancer Manage Res. (2020) 18:8733–44. doi: 10.2147/CMAR.S270200
171. Munoz JL, Bliss SA, Greco SJ, Ramkissoon SH, Ligon KL, Rameshwar P. Delivery of functional antimiR-9 by mesenchymal stem cell–derived exosomes to glioblastoma multiforme cells conferred chemosensitivity. Mol Therapy-Nucleic Acids. (2013) 2. doi: 10.1038/mtna.2013.60
172. Li H, Yang C, Shi Y, Zhao L. Exosomes derived from siRNA against GRP78 modified bone-marrowderived mesenchymal stem cells suppress Sorafenib resistance in hepatocellular carcinoma. J nanobiotechnol. (2018) 16:1–13. doi: 10.1186/s12951-018-0429-z
173. Zhang X, Yang Y, Yang Y, Chen H, Tu H, Li J. Exosomes from bone marrow microenvironmentderived mesenchymal stem cells affect CML cells growth and promote drug resistance to tyrosine kinase inhibitors. Stem Cells Int. (2020) 2020:8890201. doi: 10.1155/2020/8890201
174. Crompot E, Van Damme M, Pieters K, Vermeersch M, Perez-Morga D, Mineur P, et al. Extracellular vesicles of bone marrow stromal cells rescue chronic lymphocytic leukemia B cells from apoptosis, enhance their migration and induce gene expression modifications. Haematologica. (2017) 102:1594. doi: 10.3324/haematol.2016.163337
175. Biswas S, Mandal G, Roy Chowdhury S, Purohit S, Payne KK, Anadon C, et al. Exosomes produced by mesenchymal stem cells drive differentiation of myeloid cells into immunosuppressive M2-polarized macrophages in breast cancer. J Immunol. (2019) 203:3447–60. doi: 10.4049/jimmunol.1900692
176. Wei Y, Lai X, Yu S, Chen S, Ma Y, Zhang Y, et al. Exosomal miR-221/222 enhances tamoxifen resistance in recipient ER-positive breast cancer cells. Breast Cancer Res Treat. (2014) 147:423–31. doi: 10.1007/s10549-014-3037-0
177. Gan J, Liu S, Zhang Y, He L, Bai L, Liao R, et al. MicroRNA-375 is a therapeutic target for castrationresistant prostate cancer through the PTPN4/STAT3 axis. Exp Mol Med. (2022) 54:1290–305. doi: 10.1038/s12276-022-00837-6
178. Mendt M, Kamerkar S, Sugimoto H, McAndrews K, Wu C, Gagea M, et al. Generation and testing of clinical-grade exosomes for pancreatic cancer. JCI Insight. (2018). doi: 10.1172/jci.insight.99263
179. Rani S, Ryan AE, Griffin MD, Ritter T. Mesenchymal stem cell-derived extracellular vesicles: toward cell-free therapeutic applications. Mol Ther. (2015) 23:812–23. doi: 10.1038/mt.2015.44
180. Klimova D, Pastorakova A, Tomka M, Altaner C, Repiska V. Small extracellular vesicles derived from mesenchymal stem/stromal cells as drug-delivery tools for anti-cancer drugs. J Drug Delivery Sci Technol. (2024) 23:105999. doi: 10.1016/j.jddst.2024.105999
181. O’brien K, Khan S, Gilligan K, Zafar H, Lalor P, Glynn C, et al. Employing mesenchymal stem cells to support tumor-targeted delivery of extracellular vesicle (EV)-encapsulated microRNA-379. Oncogene. (2018) 37:2137–49. doi: 10.1038/s41388-017-0116-9
182. Shojaei S, Hashemi SM, Ghanbarian H, Sharifi K, Salehi M, Mohammadi-Yeganeh S. Delivery of miR-381-3p mimic by mesenchymal stem cell-derived exosomes inhibits triple negative breast cancer aggressiveness; an in vitro study. Stem Cell Rev Rep. (2021) 17:1027–38. doi: 10.1007/s12015-020-10089-4
183. Vakhshiteh F, Rahmani S, Ostad SN, Madjd Z, Dinarvand R, Atyabi F. Exosomes derived from miR34a-overexpressing mesenchymal stem cells inhibit in vitro tumor growth: A new approach for drug delivery. Life Sci. (2021) 266:118871. doi: 10.1016/j.lfs.2020.118871
184. Naseri Z, Oskuee RK, Jaafari MR, Forouzandeh Moghadam M. Exosome-mediated delivery of functionally active miRNA-142-3p inhibitor reduces tumorigenicity of breast cancer in vitro and in vivo. Int J nanomedicine. (2018), 7727–47. doi: 10.2147/IJN.S182384
185. Naseri Z, Oskuee RK, Forouzandeh-Moghadam M, Jaafari MR. Delivery of LNA-antimiR-142-3p by mesenchymal stem cells-derived exosomes to breast cancer stem cells reduces tumorigenicity. Stem Cell Rev Rep. (2020) 16:541–56. doi: 10.1007/s12015-019-09944-w
186. Yuan L, Liu Y, Qu Y, Liu L, Li H. Exosomes derived from MicroRNA-148b-3p-overexpressing human umbilical cord mesenchymal stem cells restrain breast cancer progression. Front Oncol. (2019) 9:1076. doi: 10.3389/fonc.2019.01076
187. Xu H, Zhao G, Zhang Y, Jiang H, Wang W, Zhao D, et al. Mesenchymal stem cell-derived exosomal microRNA-133b suppresses glioma progression via Wnt/β-catenin signaling pathway by targeting EZH2. Stem Cell Res Ther. (2019) 10:1–14. doi: 10.1186/s13287-019-1446-z
188. Kim R, Lee S, Lee J, Kim M, Kim WJ, Lee HW, et al. Exosomes derived from microRNA-584 transfected mesenchymal stem cells: novel alternative therapeutic vehicles for cancer therapy. BMB Rep. (2018) 51:406. doi: 10.5483/BMBRep.2018.51.8.105
189. Katakowski M, Buller B, Zheng X, Lu Y, Rogers T, Osobamiro O, et al. Exosomes from marrow stromal cells expressing miR-146b inhibit glioma growth. Cancer letters. (2013) 335:201–4. doi: 10.1016/j.canlet.2013.02.019
190. Lee HK, Finniss S, Cazacu S, Bucris E, Ziv-Av A, Xiang C, et al. Mesenchymal stem cells deliver synthetic microRNA mimics to glioma cells and glioma stem cells and inhibit their cell migration and selfrenewal. Oncotarget. (2013) 4:346. doi: 10.18632/oncotarget.v4i2
191. Lang FM, Hossain A, Gumin J, Momin EN, Shimizu Y, Ledbetter D, et al. Mesenchymal stem cells as natural biofactories for exosomes carrying miR-124a in the treatment of gliomas. Neuro-oncology. (2018) 20:380–90. doi: 10.1093/neuonc/nox152
192. Zhang Z, Guo X, Guo X, Yu R, Qian M, Wang S, et al. MicroRNA-29a-3p delivery via exosomes derived from engineered human mesenchymal stem cells exerts tumour suppressive effects by inhibiting migration and vasculogenic mimicry in glioma. Aging (Albany NY). (2021) 13:5055. doi: 10.18632/aging.202424
193. Yu L, Gui S, Liu Y, Qiu X, Zhang G, Zhang Xa, et al. Exosomes derived from microRNA-199aoverexpressing mesenchymal stem cells inhibit glioma progression by down-regulating AGAP2. Aging (Albany NY). (2019) 11:5300. doi: 10.18632/aging.102092
194. Ding X, Xu L, Sun X, Zhao X, Gao B, Cheng Y, et al. Human bone marrow-derived mesenchymal stem cell-secreted exosomes overexpressing microrna-124-3p inhibit DLBCL progression by downregulating NFATc1. Aging (Albany NY). (2020) 13:5055. doi: 10.21203/rs.3.rs-117708/v1
195. Chen H-L, Li J-J, Jiang F, Shi W-J, Chang G-Y. MicroRNA-4461 derived from bone marrow mesenchymal stem cell exosomes inhibits tumorigenesis by downregulating COPB2 expression in colorectal cancer. Bioscience biotechnol Biochem. (2020) 84:338–46. doi: 10.1080/09168451.2019.1677452
196. Xu Y, Shen L, Li F, Yang J, Wan X, Ouyang M. microRNA-16-5p-containing exosomes derived from bone marrow-derived mesenchymal stem cells inhibit proliferation, migration, and invasion, while promoting apoptosis of colorectal cancer cells by downregulating ITGA2. J Cell Physiol. (2019) 234:21380–94. doi: 10.1002/jcp.v234.11
197. Li T, Wan Y, Su Z, Li J, Han M, Zhou C. Mesenchymal stem cell-derived exosomal microRNA-39405p inhibits colorectal cancer metastasis by targeting integrin α6. Digestive Dis Sci. (2021) 66:1916–27. doi: 10.1007/s10620-020-06458-1
198. Lou G, Chen L, Xia C, Wang W, Qi J, Li A, et al. MiR-199a-modified exosomes from adipose tissuederived mesenchymal stem cells improve hepatocellular carcinoma chemosensitivity through mTOR pathway. J Exp Clin Cancer Res. (2020) 39:1–9. doi: 10.1186/s13046-019-1512-5
199. Lou G, Song X, Yang F, Wu S, Wang J, Chen Z, et al. Exosomes derived from miR-122-modified adipose tissue-derived MSCs increase chemosensitivity of hepatocellular carcinoma. J Hematol Oncol. (2015) 8:1–11. doi: 10.1186/s13045-015-0220-7
200. Xu Y, Lai Y, Cao L, Li Y, Chen G, Chen L, et al. Human umbilical cord mesenchymal stem cells-derived exosomal microRNA-451a represses epithelial–mesenchymal transition of hepatocellular carcinoma cells by inhibiting ADAM10. RNA Biol. (2021) 18:1408–23. doi: 10.1080/15476286.2020.1851540
201. Ding Y, Cao F, Sun H, Wang Y, Liu S, Wu Y, et al. Exosomes derived from human umbilical cord mesenchymal stromal cells deliver exogenous miR-145-5p to inhibit pancreatic ductal adenocarcinoma progression. Cancer letters. (2019) 442:351–61. doi: 10.1016/j.canlet.2018.10.039
202. Xu Q, Liu X, Cai Y, Yu Y, Chen W. RNAi-mediated ADAM9 gene silencing inhibits metastasis of adenoid cystic carcinoma cells. Tumor Biol. (2010) 31:217–24. doi: 10.1007/s13277-010-0034-8
203. Shang S, Wang J, Chen S, Tian R, Zeng H, Wang L, et al. Exosomal miRNA-1231 derived from bone marrow mesenchymal stem cells inhibits the activity of pancreatic cancer. Cancer Med. (2019) 8:7728–40. doi: 10.1002/cam4.v8.18
204. Xie C, Du L-Y, Guo F, Li X, Cheng B. Exosomes derived from microRNA-101-3p-overexpressing human bone marrow mesenchymal stem cells suppress oral cancer cell proliferation, invasion, and migration. Mol Cell Biochem. (2019) 458:11–26. doi: 10.1007/s11010-019-03526-7
205. Wang L, Yin P, Wang J, Wang Y, Sun Z, Zhou Y, et al. Delivery of mesenchymal stem cells-derived extracellular vesicles with enriched miR-185 inhibits progression of OPMD. Artif cells nanomedicine Biotechnol. (2019) 47:2481–91. doi: 10.1080/21691401.2019.1623232
206. He Z, Li W, Zheng T, Liu D, Zhao S. Human umbilical cord mesenchymal stem cells-derived exosomes deliver microRNA-375 to downregulate ENAH and thus retard esophageal squamous cell carcinoma progression. J Exp Clin Cancer Res. (2020) 39:1–18. doi: 10.1186/s13046-020-01631-w
207. Jia Y, Ding X, Zhou L, Zhang L, Yang X. Mesenchymal stem cells-derived exosomal microRNA-1395p restrains tumorigenesis in bladder cancer by targeting PRC1 Vol. 40. . 4 CRINAN ST, LONDON, N1 9XW, ENGLAND: SPRINGERNATURE CAMPUS (2021). p. 246 2023.
208. Cai H, Yang X, Gao Y, Xu Z, Yu B, Xu T, et al. Exosomal microRNA-9-3p secreted from BMSCs downregulates ESM1 to suppress the development of bladder cancer. Mol Therapy-Nucleic Acids. (2019) 18:787–800. doi: 10.1016/j.omtn.2019.09.023
209. Computer-aided drug repurposing for cancer therapy: approaches and opportunities to challenge anticancer targets. Semin Cancer Biol. (2021). doi: 10.1016/j.semcancer.2019.09.023
210. Hmadcha A, Martin-Montalvo A, Gauthier BR, Soria B, Capilla-Gonzalez V. Therapeutic potential of mesenchymal stem cells for cancer therapy. Front bioengineering Biotechnol. (2020) 8:43. doi: 10.3389/fbioe.2020.00043
211. Mahmoudvand G, Karimi Rouzbahani A, Razavi ZS, Mahjoor M, Afkhami H. Mesenchymal stem cell therapy for non-healing diabetic foot ulcer infection: new insight. Front bioengineering Biotechnol. (2023) 11:1158484. doi: 10.3389/fbioe.2023.1158484
212. Gemayel J, Chaker D, El Hachem G, Mhanna M, Salemeh R, Hanna C, et al. Mesenchymal stem cells-derived secretome and extracellular vesicles: perspective and challenges in cancer therapy and clinical applications. Clin Trans Oncol. (2023) 25:2056–68. doi: 10.1007/s12094-023-03115-7
213. Dong L, Pu Y, Zhang L, Qi Q, Xu L, Li W, et al. Human umbilical cord mesenchymal stem cell-derived extracellular vesicles promote lung adenocarcinoma growth by transferring miR-410. Cell Death disease. (2018) 9:218. doi: 10.1038/s41419-018-0323-5
214. Wiklander OP, Nordin JZ, O’Loughlin A, Gustafsson Y, Corso G, Mäger I, et al. Extracellular vesicle in vivo biodistribution is determined by cell source, route of administration and targeting. J extracellular vesicles. (2015) 4:26316. doi: 10.3402/jev.v4.26316
215. Nakase I, Futaki S. Combined treatment with a pH-sensitive fusogenic peptide and cationic lipids achieves enhanced cytosolic delivery of exosomes. Sci Rep. (2015) 5:10112. doi: 10.1038/srep10112
216. Sun Y, Ren D, Yang C, Yang W, Zhao J, Zhou Y, et al. TRIM15 promotes the invasion and metastasis of pancreatic cancer cells by mediating APOA1 ubiquitination and degradation. Biochim Biophys Acta (BBA)-Molecular Basis Disease. (2021) 1867:166213. doi: 10.1016/j.bbadis.2021.166213
217. Noguchi K, Obuki M, Sumi H, Klussmann M, Morimoto K, Nakai S, et al. Macropinocytosisinducible extracellular vesicles modified with antimicrobial protein CAP18-derived cell-penetrating peptides for efficient intracellular delivery. Mol Pharmaceutics. (2021) 18:3290–301. doi: 10.1021/acs.molpharmaceut.1c00244
218. Nakase I, Noguchi K, Aoki A, Takatani-Nakase T, Fujii I, Futaki S. Arginine-rich cell-penetrating peptide-modified extracellular vesicles for active macropinocytosis induction and efficient intracellular delivery. Sci Rep. (2017) 7:1991. doi: 10.1038/s41598-017-02014-6
219. Nakase I, Kobayashi NB, Takatani-Nakase T, Yoshida T. Active macropinocytosis induction by stimulation of epidermal growth factor receptor and oncogenic Ras expression potentiates cellular uptake efficacy of exosomes. Sci Rep. (2015) 5:10300. doi: 10.1038/srep10300
220. Zuppone S, Zarovni N, Noguchi K, Loria F, Morasso C, Lõhmus A, et al. Novel loading protocol combines highly efficient encapsulation of exogenous therapeutic toxin with preservation of extracellular vesicles properties, uptake and cargo activity. Discover Nano. (2024) 19:76. doi: 10.1186/s11671-024-04022-8
221. Nouri Z, Fakhri S, Nouri K, Wallace CE, Farzaei MH, Bishayee A. Targeting multiple signaling pathways in cancer: The rutin therapeutic approach. Cancers. (2020) 12:2276. doi: 10.3390/cancers12082276
222. Roma-Rodrigues C, Mendes R, Baptista PV, Fernandes AR. Targeting tumor microenvironment for cancer therapy. Int J Mol Sci. (2019) 20:840. doi: 10.3390/ijms20040840
223. Detroja TS, Gil-Henn H, Samson AO. Text-mining approach to identify hub genes of cancer metastasis and potential drug repurposing to target them. J Clin Med. (2022) 11:2130. doi: 10.3390/jcm11082130
224. Mokhtari RB, Homayouni TS, Baluch N, Morgatskaya E, Kumar S, Das B, et al. Combination therapy in combating cancer. Oncotarget. (2017) 8:38022. doi: 10.18632/oncotarget.16723
225. Devita Jr.VT, Young RC, Canellos GP. Combination versus single agent chemotherapy: a review of the basis for selection of drug treatment of cancer. Cancer. (1975) 35:98–110. doi: 10.1002/1097-0142(197501)35:1<98::AID-CNCR2820350115>3.0.CO;2-B
226. Michel MC, Staskin D. Study designs for evaluation of combination treatment: focus on individual patient benefit. Biomedicines. (2022) 10:270. doi: 10.3390/biomedicines10020270
227. Day D, Siu LL. Approaches to modernize the combination drug development paradigm. Genome Med. (2016) 8:1–14. doi: 10.1186/s13073-016-0369-x
228. Gaber T, Schönbeck K, Hoff H, Tran CL, Strehl C, Lang A, et al. CTLA-4 mediates inhibitory function of mesenchymal stem/stromal cells. Int J Mol Sci. (2018) 19:2312. doi: 10.3390/ijms19082312
229. Lee SJC, Murphy CC, Geiger AM, Gerber DE, Cox JV, Nair R, et al. Conceptual model for accrual to cancer clinical trials. J Clin Oncol. (2019) 37:1993. doi: 10.1200/JCO.19.00101
230. Sharif S, Ghahremani M, Soleimani M. Delivery of exogenous miR-124 to glioblastoma multiform cells by Wharton’s jelly mesenchymal stem cells decreases cell proliferation and migration, and confers chemosensitivity. Stem Cell Rev Rep. (2018) 14:236–46. doi: 10.1007/s12015-017-9788-3
Keywords: ribosome-inactivating proteins (RIP), mesenchymal stem Cell (MSC), MSC-derived extracellular vesicles (EVs), cancer, cancer treatment
Citation: Mavaei M, Farokhi S, Yousefi MH, Fakouri A, Shadab A, Abdolmohammadi MH, Fallahian F and Afkhami H (2025) Exploring two tumor treatment strategies: effectiveness of ribosome inactivating proteins and mesenchymal stem cells/MSC derived extracellular vesicles in cancer treatment. Front. Oncol. 15:1533065. doi: 10.3389/fonc.2025.1533065
Received: 23 November 2024; Accepted: 15 April 2025;
Published: 13 May 2025.
Edited by:
Bekir Cinar, Clark Atlanta University, United StatesReviewed by:
Fatemeh Talebian, The Ohio State University, United StatesNarendra Sankpal, Saint Joseph Hospital Medical Center Phoenix, United States
Copyright © 2025 Mavaei, Farokhi, Yousefi, Fakouri, Shadab, Abdolmohammadi, Fallahian and Afkhami. This is an open-access article distributed under the terms of the Creative Commons Attribution License (CC BY). The use, distribution or reproduction in other forums is permitted, provided the original author(s) and the copyright owner(s) are credited and that the original publication in this journal is cited, in accordance with accepted academic practice. No use, distribution or reproduction is permitted which does not comply with these terms.
*Correspondence: Mohammad Hossein Abdolmohammadi, bWguYWJkb2xtb2hhbW1hZGlAZ21haWwuY29t; Faranak Fallahian, ZmFyYW5hay5mYWxsYWhpYW5AZ21haWwuY29t; Hamed Afkhami, aGFtZWRhZmtoYW1pNzBAZ21haWwuY29t
†These authors share first authorship