- 1Key Laboratory of Neuroregeneration of Jiangsu and Ministry of Education, Co-Innovation Center of Neuroregeneration, NMPA Key Laboratory for Research and Evaluation of Tissue Engineering Technology Products, Nantong University, Nantong, China
- 2Department of Surgery, Nantong Tumor Hospital, Affiliated Tumor Hospital of Nantong University, Medical School of Nantong University, Nantong, China
Cancer remains the second leading cause of death worldwide. Tumor invasion and metastasis pose significant challenges for clinical management. In addition to the traditional pathways of metastasis such as hematologic or lymphatic transmission, perineural invasion (PNI) has become a unique mechanism of metastasis, which is closely associated with neuropathic pain, motor deficits, and poor prognosis. PNI is often observed in malignant tumors of the pancreas, head and neck, gastrointestinal tract, and lungs, and it reflects a unique neurotropic transfer behavior utilizing neural networks. Despite its clinical significance, targeted therapies for PNI are still lacking. This review synthesizes current evidences regarding PNI, elucidates the clinical significance of PNI in tumor metastasis, prognosis, and neurological dysfunction. By integrating the latest advances in multi-omics, we analyzed the potential key molecular pathways and tumor microenvironment drivers of PNI, and proposed future research directions for developing PNI-specific therapies to improve patient outcomes.
1 Introduction
According to data from the World Health Organization, cancer is now the second leading cause of death worldwide (1). The uncontrolled growth of tumors, invasion into surrounding tissues, and their ability to metastasize to distant organs are not only key indicators of malignancy but also pose significant challenges in cancer prevention and treatment (2). In addition to common metastatic routes, such as direct extension, hematogenous spread, lymphatic dissemination, and implantation metastasis (3), perineural invasion (PNI) has emerged in recent years as a distinct and noteworthy mode of metastasis, also known as neurotropic or perineural cancer dissemination. PNI is defined as the involvement of at least 33% of the nerve circumference or the presence of cancer cells in any of the three layers of the nerve sheath (4), and this invasion can cause symptoms such as pain, numbness, muscle weakness, and other neurological dysfunction, all of which can negatively impact treatment outcomes and the patients’ quality of life. PNI is commonly observed in tumors located in areas with rich nerve networks, such as the head and neck, lungs, pancreas, stomach, colon, and rectum (5), and has been recognized as a significant pathological feature of various malignant tumors (6). For example, postoperative pathological examination of pancreatic cancer often shows significant PNI (Figure 1).
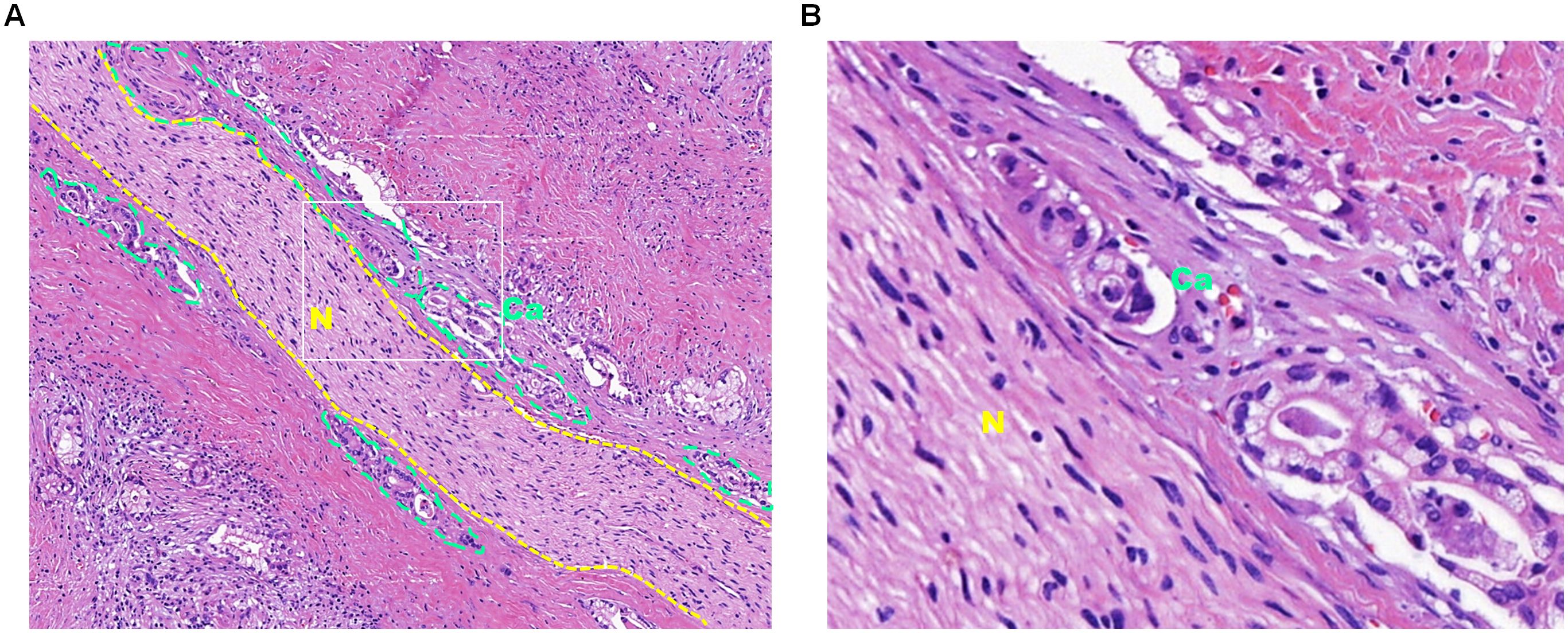
Figure 1. Hematoxylin and eosin staining of pancreatic cancer tissue subjected to perineural invasion. (A) Hematoxylin and eosin staining of pancreatic cancer tissue at 10× magnification. The dotted yellow lines outline the nerve tissue, and the dotted green lines outline the cancer cells that invade the nerve. (B) Enlarged view of the white box in panel A at 40× magnification, with “Ca” indicating cancer tissue and “N” indicating nerve tissue.
Despite increasing awareness of PNI, targeted therapies addressing this pathological phenomenon are unavailable. This review aims to clarify the significance of PNI in malignant tumors, including the relationship between PNI and tumor metastasis, prognosis, and neurological dysfunction, summarize the key signaling pathways involved in PNI and the influence of tumor microenvironment on PNI, and propose future research directions.
2 Significance of PNI
2.1 Impact on tumor metastasis
2.1.1 Differences between PNI and lymphatic, hematogenous metastasis
Initially, PNI was thought to be an extension of lymphatic or hematogenous metastasis. However, the occurrence of PNI in the absence of lymphatic or vascular infiltration indicates that PNI is a distinct pathological entity (7). The differences between PNI and lymphatic, hematogenous metastasis were described in detail in Table 1. They differ mechanically, anatomically, and clinically (8–13). PNI places more emphasis on local neural aggression, while lymphatic and hematogenous routes represent regional and systemic dissemination, respectively. Understanding these distinctions can help develop personalized treatment strategies and improving patient outcomes.
2.1.2 Main molecular mechanisms of PNI promoting metastasis
PNI is not limited to local invasion; it can also act as a source for distant metastasis. In certain tumor types, PNI may be the sole mechanism of metastatic spread (7). PNI promotes the metastasis of malignant tumors through multidimensional molecular interactions.
Tumor cells that invade nerves tend to have enhanced interactions with the perineuronal blood vessels and lymphatic networks. For example, PNI-positive prostate cancer cells overexpress vascular endothelial growth factor C (VEGFC), promoting lymphatic metastasis of prostate cancer (14). During the PNI process of prostate cancer, cancer cells can induce the formation of abnormal neovascularization networks within the nerve and simultaneously promote vascular invasion (15).
PNI establishes a specialized neuro-tumor interactive microenvironment (16). Within this milieu, neurons and Schwann cells (SCs) release a spectrum of regulatory mediators such as neurotrophic factors (e.g. nerve growth factor (NGF), brain derived neurotrophic factor), chemokines (e.g. C-X3-C motif chemokine ligand 1, ephrin type-A receptor 2, C-C motif chemokine ligand (CCL) 2), axon guidance molecules (e.g. Semaphorin 3D) and neurotransmitters (e.g. glutamate, acetylcholine, dopamine) (17–19). These neural-derived signaling molecules activate corresponding receptors on cancer cells through paracrine signaling, triggering downstream cascades that drive the activation of metastasis-associated signaling pathways (20). Table 2 summarizes the main molecular mechanisms of PNI promoting tumor metastasis (21–24).
MMP, matrix metalloproteinase; VEGF-C, vascular endothelial growth factor C; cAMP/PKA, cyclic adenosine monophosphate/protein kinase A; CCL21, C-C motif chemokine ligand 21; NGF, nerve growth factor; BDNF, brain derived neurotrophic factor; CX3CL1, C-X3-C motif chemokine ligand 1; EPHA2, ephrin type-A receptor 2; SEMA3D, semaphorin 3D; TrkA, tyrosine receptor kinase A; TrkB, tyrosine kinase receptor B; RAS/MAPK, RAS-mitogen-activated protein kinase; PI3K/AKT, phosphatidylinositol 3-kinase/protein kinase B; JAK2/STAT3, Janus kinase 2/signal transducers and activators of transcription 3; NK1R, neurokinin-1 receptor; NF-κB, nuclear factor-kappaB; TNF-α, tumor necrosis factor alpha; IL-6, interleukin 6
2.2 Prognostic implications
PNI is closely associated with the prognosis of patients with malignant tumors (25, 26). In periocular skin squamous cell carcinoma, the higher the degree of PNI, the greater the risk of tumor recurrence, regional metastasis, and high mortality (27). In oral squamous cell carcinoma, PNI predicts the risk of lymph nodes occult metastasis (28). In pancreatic carcinoma, PNI-positive patients had an increased risk of liver metastasis (29). In patients with resectable pancreatic cancer undergoing preoperative chemotherapy with gemcitabine, PNI can serve as an important indicator for evaluating surgical outcomes and recurrence, and it is a significant independent factor related to postoperative disease-free survival (30). The incidence of PNI is high among patients with head and neck cancer, particularly those receiving radiotherapy, where an elevated pretreatment PNI is significantly correlated with poorer overall survival, distant metastasis-free survival, and progression-free survival (31). Approximately 20%-31% of patients with intrahepatic cholangiocarcinoma (ICC) exhibit PNI, which is associated with reduced overall and recurrence-free survival, as well as an increased tendency for immunosuppressive metastasis. After receiving adjuvant therapy with TEGIO, GEMOX, or capecitabine, patients with ICC complicated by PNI have improved survival, indicating a favorable response to adjuvant chemotherapy (32). In patients who have undergone potentially curative surgery for ICC, PNI is a strong independent predictor of tumor recurrence and long-term survival; therefore, it should be routinely assessed in clinical practice (33).
2.3 Neurological complications
2.3.1 Cancer-related neuropathic pain mechanism
PNI is a key factor in the development of cancer pain, and pain itself can also serve as a predictor of PNI (34, 35). The mechanisms by which PNI contributes to cancer pain are complex and involve various factors, such as nerve compression, neuroinflammation, and neuronal damage (36). Neuropathies resulting from PNI, along with the upregulation of cancer-associated fibroblasts (CAFs), tumor-associated macrophages (TAMs), neurotrophic factors, and substance in the tumor microenvironment, can exacerbate neuropathic pain (34, 37). Additionally, PNI can lead to abnormalities in nerve conduction, resulting in the amplification or abnormal transmission of pain signals (38). Targeting signaling pathways associated with PNI, such as NGF/tyrosine receptor kinase A (TrkA), may effectively alleviate cancer pain and improve treatment outcomes for tumors (39).
2.3.2 Neurological dysfunction syndromes in PNI-associated cancers
PNI disrupts nerve structure and impairs nerve conductive function, leading to muscle weakness or paralysis in the areas innervated by the affected nerves. In patients with advanced prostate cancer, tumors may directly invade the lumbosacral plexus, resulting in radiculopathy of the lower limbs, characterized by weakness, numbness, and loss of control over bowel or bladder function (40, 41). Head and neck cancers, particularly squamous cell carcinoma and adenoid cystic carcinoma, can directly invade the facial nerve, resulting in slowly progressive facial paralysis (42, 43). PNI may also lead to autonomic nerve dysfunction (44). PNI of ICC primarily affects the sympathetic nerve (32), and inhibiting sympathetic nerve activity can improve prognosis and prolong survival in these patients (45). Additionally, suppressing sympathetic signaling in patients with pancreatic ductal adenocarcinoma (PDAC) may increase the efficacy of anticancer drugs, reduce the tumor burden, and prolong survival (46).
3 Molecular pathways involved in PNI
PNI is a bidirectional process that involves interactions between cancer cells and nerves rather than being triggered solely by the unidirectional invasion of nerves by cancer cells (47). This bidirectional cellular interplay is governed by evolutionarily conserved molecular pathways that orchestrate both neurotropic attraction and tumor advancement. Systematic investigation of these signaling cascades serves dual purposes: elucidating the mechanistic basis of PNI while simultaneously identifying therapeutically actionable targets for clinical intervention.
3.1 NGF/TrKA signaling pathway
NGF binds to the high-affinity receptor TrkA and the low-affinity receptor p75NTR, playing crucial roles not only in neurodevelopment but also in pain perception, immune regulation, and the occurrence and progression of tumors in adults (48–50). Increased expression of NGF and its receptors in tumor cells is associated with a greater frequency and severity of PNI (51, 52). For example, in cholangiocarcinoma, activation of the NGF/TrkA pathway promotes the proliferation and invasion of cancer cells. Elevated levels of NGF bind to TrkA in nerve sheaths, facilitating the development of PNI (50, 53). In the extracellular matrix (ECM) of ICCs, NGF expression is upregulated, which activates TrkA receptors on tumor cells through a paracrine mechanism, thereby promoting PNI (54). In pancreatic cancer, the NGF/TrkA pathway enhances PNI by promoting the Warburg effect and upregulating the expression of miR-21-5p (52). Pancreatic cancer cells typically exhibit high levels of NGF, and knocking down TrkA can inhibit PNI in these cells (55). Additionally, NGF acts as a key driver of neuroinflammation in the tumor microenvironment of pancreatic cancer, and its inhibition can significantly reduce tumor-associated PNI and alleviate cancer-related pain (56, 57). Furthermore, the low-affinity NGF receptor p75NTR can act as a chemotactic agent, attracting cancer cells toward nerve tissues and thereby promoting PNI (51). Additionally, NGF released by SCs may facilitate the migration of glial cells during the process of PNI (58, 59).
3.2 Notch signaling pathway
The Notch signaling pathway is a highly conserved mechanism that plays a critical role in various physiological processes (60). Aberrant activation of this pathway is closely associated with PNI. In oral squamous cell carcinoma, strong expression of Notch1 and Notch4 is linked to PNI (61, 62). Similarly, elevated expression of Notch4 is closely related to PNI in salivary gland adenoid cystic carcinoma (63, 64). Additionally, the expression of the Notch ligand DLL4 and the γ-secretase complex component nicastrin is also associated with PNI (65, 66). Moreover, the Notch signaling pathway is a key driver of epithelial–mesenchymal transition (EMT) induced by transforming growth factor-beta, which promotes tumor invasion and metastasis by regulating molecules such as Twist, Snail, and E-cadherin (67–69). Components of the Notch pathway, particularly Notch4, are highly expressed in various tumors complicated by PNI, making them potential biomarkers for predicting PNI and poor prognosis. Targeting the Notch pathway, such as by silencing Notch4 with siRNA, can effectively inhibit the PNI capabilities of tumor cells, indicating its potential as a therapeutic target.
3.3 c-Jun N-terminal kinase signaling pathway
JNK, a member of the mitogen-activated protein kinase (MAPK) family, can be activated by various extracellular signals, such as growth factors and inflammatory mediators (70, 71). The JNK signaling pathway plays a crucial role in tumor-associated PNI. In PDAC, glial cell line-derived neurotrophic factor (GDNF) secreted by SCs can promote PNI and metastasis by activating the JNK/ZEB1/EMT axis (17). GDNF expression is significantly elevated in oral cancer tissues, and GDNF treatment enhances the phosphorylation of extracellular signal-regulated kinases (ERK), p38, and JNK, which increases the DNA-binding activity of AP-1 and increases the expression of matrix metalloproteinases (MMPs). This, in turn, increases the migratory capacity of tumor cells. Notably, the inhibition of MAPK or AP-1 effectively reduces GDNF-induced PNI (72). In salivary gland adenoid cystic carcinoma, the phosphorylation levels of JNK and p38 are closely associated with PNI. Elevated levels of phosphorylated p38 are considered an independent prognostic factor for poor prognosis, whereas JNK activation is correlated with tumor invasiveness (73). In rectal cancer, serine protease inhibitor Kazal type 1 (SPINK1) enhances the proliferation and migration of cancer cells by activating epidermal growth factor receptor-downstream ERK, p38, and JNK signaling pathways. Elevated SPINK1 expression is obviously associated with the PNI and is a crucial prognostic indicator for overall survival and disease-free survival (74). BAP1 is a tumor suppressor, and its downregulation is linked to the invasiveness of ICC. BAP1 exerts its tumor-suppressive effects by inhibiting the JNK/c-Jun pathway, suggesting that the JNK signaling pathway could serve as a potential therapeutic target in ICC (75, 76).
Notably, JNK1 and JNK2 play distinct functional roles in various cancers. Specific inhibition of JNK2 increases the oncogenic potential of pancreatic cancer cells, whereas inhibition of JNK1 has the opposite effect (77). These findings suggest that targeted regulation of JNK isoforms may provide new strategies for cancer therapy.
3.4 Wnt signaling pathway
The Wnt signaling pathway is a crucial regulator of tissue homeostasis and is closely associated with cancer, playing a significant role in PNI. This pathway can be categorized into two types: β-catenin-dependent (canonical) signaling and β-catenin-independent (noncanonical) signaling. The canonical Wnt signaling pathway stabilizes and promotes the accumulation of β-catenin when Wnt ligands bind to Frizzled and low-density lipoprotein receptor-related protein, allowing β-catenin to enter the nucleus and initiate the transcription of target genes. In contrast, the noncanonical Wnt signaling pathway regulates cell polarity and movement through different mechanisms (78).
Both the canonical and noncanonical Wnt signaling pathways promote tumor PNI in a highly tissue-specific manner. In head and neck squamous cell carcinoma, a secreted inhibitor of the Wnt signaling pathway DKK1 is upregulated, which can promote PNI by activating the phosphatidylinositol 3-kinase/protein kinase B (PI3K/AKT) signaling pathway (79). In gastric cancer, increased expression of USP15 triggers the nuclear translocation of β-catenin, increasing cell proliferation and promoting PNI (80). In pancreatic cancer, the miRNA tRF-19-PNR8YPJZ activates the Wnt pathway, increasing cell proliferation and metastasis and promoting PNI (81). In advanced metastatic bladder cancer, the upregulation of SFRP2 promotes EMT and promote PNI (81). In prostate cancer, dysfunction of primary cilia leads to enhanced Wnt signaling, promoting the occurrence of PNI (82). Furthermore, both canonical and noncanonical Wnt signaling can increase tumor invasiveness and promote PNI by regulating EMT-related transcription factors such as Snail2 and ASPP2 (83, 84).
3.5 Emerging mechanisms unveiled by multi-omics profiling
Recent advances in single-cell RNA sequencing (scRNA-seq), spatial transcriptomics, and proteomics have significantly advanced our understanding of PNI. These technologies collectively unravel the molecular complexity of PNI by dissecting cellular heterogeneity, spatial dynamics, and functional interactions.
scRNA-seq has identified tumor subpopulations with distinct neurotropic properties. In prostate cancer, basal/intermediate epithelial cells expressing polymeric immunoglobulin receptor, MMP-7, and anterior gradient 2 were associated with PNI progression, while trajectory analysis suggested luminal cells as potential precursors (85). Similarly, in distal cholangiocarcinoma, hypoxia-prone NEAT1+ tumor cells and hypermetabolic GFAP+ dedifferentiated SCs formed a lactate-high mobility group box-1 axis driving invasion (86). SC plasticity, characterized by upregulated secretory proteins (SCG2, VGF), was also observed in pancreatic cancer, mirroring nerve injury responses (87). scRNA-seq further revealed immune microenvironment shifts, such as CD161+CD8+ T cells in PDAC, which exhibited dual cytotoxic and immunosuppressive roles correlated with survival (88).
Spatial transcriptomics has mapped molecular gradients and immune landscapes at nerve-tumor interfaces. In oral squamous cell carcinoma, nerves near tumors showed enriched stress response (HSPA1A) and growth signaling (EGFR), fading with increasing distance (89). PDAC studies highlighted axon guidance pathways and endocannabinoid metabolism in PNI, alongside immune “cold” niches marked by PD-L1 upregulation (90). Spatial profiling also enabled practical innovations, such as MHC-II-targeted peptide probes in adenoid cystic carcinoma (91), which dynamically labeled SCs in PNI regions for intraoperative guidance.
Proteomics validated pathway activities and post-translational modifications underlying PNI. In PDAC, VGF-mediated neurite extension was confirmed in vitro (87), while cholinergic signaling in PNI suppressed CD8+ T-cell recruitment via CCL5 downregulation, promoting Th2 polarization (92). Metabolic reprogramming, such as lactate-induced SC dedifferentiation in distal cholangiocarcinoma (86), further underscored microenvironmental crosstalk. Epigenetic proteomics in head and neck cancer linked DNA hypermethylation (CDH1, TIMP3) and histone modifications (H3K27me3) to EMT and PNI progression (93).
PNI process is regulated by chemokines, growth factors, cell adhesion and epigenetics (6, 18, 94–116). A thorough understanding of the molecular mechanisms involved is essential for developing targeted interventions against PNI (Table 3).
4 Tumor microenvironment constituents orchestrating PNI
The tumor microenvironment (TME) comprises heterogeneous components, including vascular/lymphatic networks, ECM, stromal/immune cells, secretory factors, noncoding RNAs, and extracellular vesicles, that collectively establish a pro-metastatic niche (117). Within this ecosystem, SCs, the principal myelinating glia of peripheral nerves, emerges as pivotal orchestrators of PNI. Beyond SCs, CAFs, TAMs, and infiltrating T cells further potentiate PNI via autocrine-paracrine signaling axes, highlighting the multicellular cooperativity with TME (118). The following sections describe how they drive PNI from the perspective of non-tumor cell components of TME.
4.1 SCs
Within the tumor microenvironment, SCs act as bridges at the tumor–nerve interface, playing a role in both the nerve supply to tumors and the invasion of tumors into adjacent nerves (119, 120). In tumors with high rates of PNI, such as ICC, the expression of SC markers such as S100B and glial fibrillary acidic protein (GFAP) is obviously elevated (Figure 2).
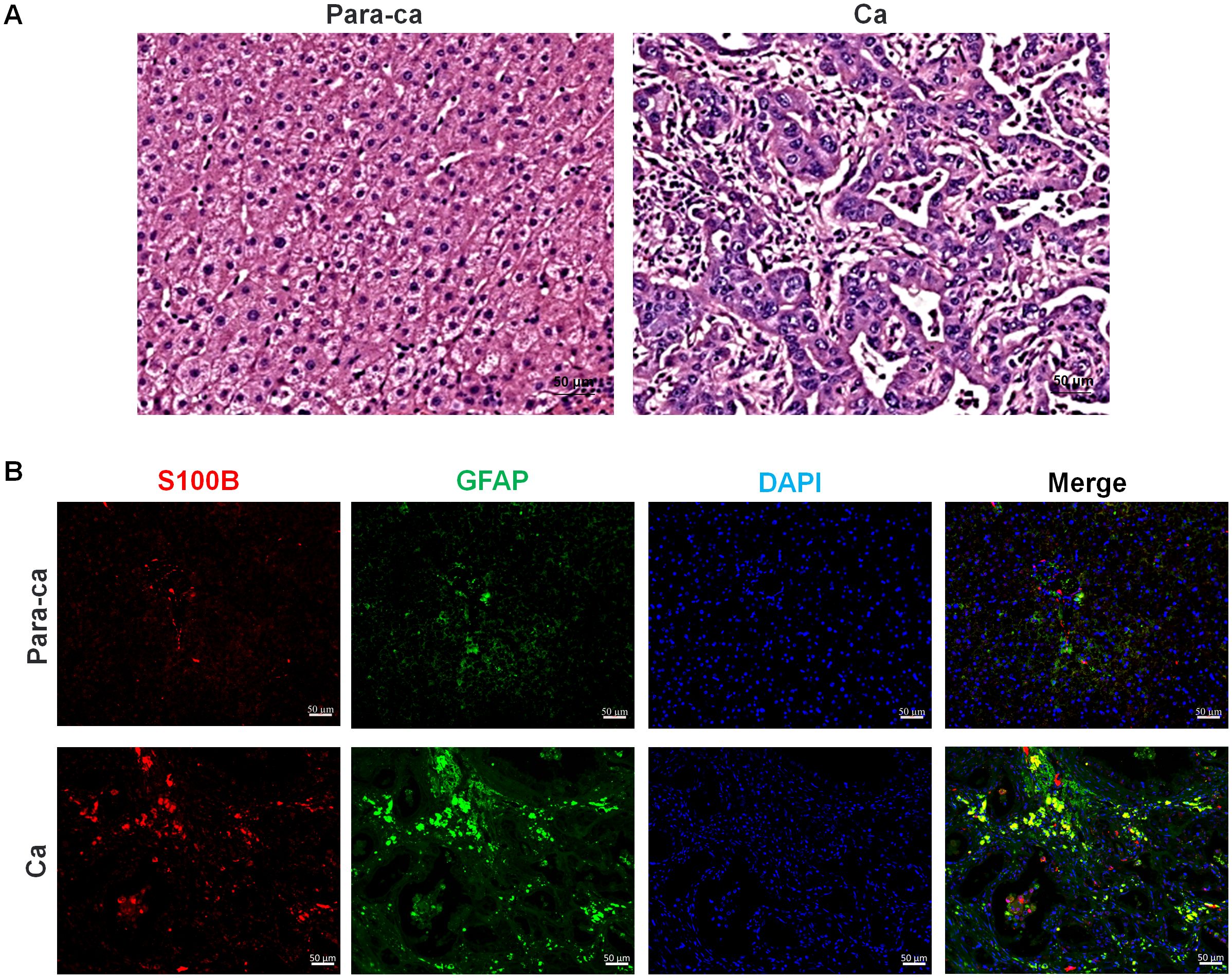
Figure 2. Pathological section staining of intrahepatic cholangiocarcinoma (ICC) with para-carcinoma and carcinoma tissues. (A) H&E staining of para-carcinoma and carcinoma tissues. (B) S100B and GFAP immunofluorescence staining of para-carcinoma and carcinoma tissues. Red, S100B; Green, GFAP; Blue, DAPI, and Merge is their overlap. Bar=50 μm.
SCs derived from neural crest precursors differentiate postnatally into two functionally distinct subtypes: myelinating SCs, which insulate large axons via myelin proteins (MBP, MPZ), and non-myelinating SCs (including Remak SCs) that ensheath small-caliber axons while expressing markers such as p75NTR and GFAP (121, 122). This developmental plasticity is recapitulated during nerve injury, where mature SCs dedifferentiate into a repair phenotype characterized by c-Jun/Sox2 upregulation and myelin gene suppression, promoting nerve regeneration (123). However, this process is hijacked by cancers to facilitate PNI (124). SCs guide cancer migration via neural cell adhesion molecule 1-dependent protrusion formation, enabling cancer dispersion along nerves. This mechanism is similar to the repair phenotype of SCs after nerve injury, while in cancer, it directly leads to the invasion of nerve tissue by cancer cells (125). In PDAC, c-Jun-activated SCs form tumor-activated Schwann cell tracks that enhance cancer cell motility and promote PNI. However, the use of a c-Jun N-terminal kinase inhibitor (e.g., SP600125) for SCs can effectively reduce the activation of pro-invasive SC (126). PDAC-derived extracellular vesicles upregulate p75NTR in SCs, which is a biomarker closely related to PNI (127). Similarly, colon cancer-derived exosomal miR-21-5p suppresses Von Hippel-Lindau expression in SCs, stabilizing hypoxia-inducible factor-1α to elevate NGF secretion, which reciprocally activates ERK signaling and promotes PNI in cancer cells (128).
However, not all reprogrammed SCs exhibit tumor-promoting functions; reprogrammed SCs may also exert tumor-suppressive effects. Emerging evidence indicates that pancreatic SCs undergo adaptive reprogramming during early carcinogenesis to support protective anti-tumor neuronal responses. Using sparse genetic labeling, the study demonstrated that SCs in early PDAC overexpress neurotrophic factors, particularly GDNF. This altered SCs phenotype drives enhanced sympathetic neuronal projections, thereby establishing an inhibitory microenvironment that suppresses cancer progression (129).
ScRNA-seq has unveiled the dynamic heterogeneity of cellular subpopulations within the TME. SCs exhibit functional heterogeneity in the TME and regulate tumor progression through interactions with immune and stromal cells. In PNI-positive distal cholangiocarcinoma, five SCs subpopulations have been identified, with GFAP+ dedifferentiated SCs displaying hypermetabolic characteristics. Lactate in hypoxic TME induces GFAP-dedifferentiation in SCs, which promotes cancer cell invasion and progression via upregulation of HMGB1 (86). In vestibular schwannomas, tumor-associated SCs comprise “repair-like” and MHC-II antigen-presenting subpopulations that actively recruit macrophages through CSF1 signaling, mimicking nerve injury repair processes to drive tumor growth. Tumors exhibiting this “injury-like” phenotype demonstrate larger volumes and activation of nerve repair-associated transcription factors (130). In plexiform neurofibromas, NF1 deficiency drives SCs differentiation into five subpopulations, including a Schwann cell progenitor-like population characterized by elevated expression of Postn and CD74. These SCs aberrantly interact with immune/stromal cells through PROS1-AXL, FGF-FGFR, and MIF-CD74/NF-κB pathways, establishing a TME dominated by immune and stromal components, with conserved mechanisms across murine and human models (131). Spatial mapping of neuroblastomas reveals that a subset of malignant cells adopts mesenchymal states sharing molecular features with SCs precursors, while spatial compartmentalization segregates malignant cells from immune infiltrates. Epigenetic regulation, particularly DNA methylation patterns, correlates with clinical outcomes (132).
Mechanistically, the functional heterogeneity of SCs within the TME manifests through: i) Differentiation into distinct subpopulations, including repair-like, antigen-presenting, and progenitor-like phenotypes; ii) Recruitment of immune cells or augmentation of sympathetic neuronal activity via secretion of factors such as CSF1, GDNF, and MIF; iii) Formation of a pro-tumorigenic microenvironment through evolutionarily conserved signaling pathways (e.g., CD74-NF-κB) that mediate crosstalk with immune and stromal cells. Therapeutically, potential strategies include targeting SC-driven immune recruitment (e.g., CSF1 blockade), dysregulated signaling pathways (e.g., CD74 and AXL inhibition), or epigenetic modulation. Furthermore, the association between SC precursor-like cells and tumor spatial heterogeneity highlights novel opportunities for modulating the TME.
4.2 CAFs
In the tumor microenvironment, CAFs play a central role and exhibit high heterogeneity. They can originate from fibroblasts in adjacent tissues, transdifferentiated cells within the tumor, bone marrow-derived mesenchymal cells, or precursor cells derived from adipocytes, endothelial cells, mesothelial cells, or pericytes (133–135). Single-cell sequencing has categorized CAFs into several subgroups, including myofibroblastic CAFs, inflammatory CAFs, immune-regulatory CAFs, antigen-presenting CAFs, vascular CAFs, and metabolic CAFs. These subgroups are conserved across different organs, cancer subtypes, and species but also present some organ-specific characteristics (136).
The heterogeneity of CAFs leads to diverse functions, including the promotion of cancer cell growth, angiogenesis, ECM remodeling, and the recruitment of suppressive immune cells. In some cases, they may even exert tumor-suppressive effects (137). CAFs can alter the tumor microenvironment through the secretion of growth factors, cytokines, and matrix metalloproteinases, thereby facilitating tumorigenesis and metastasis. A previous study revealed that CAFs are significantly associated with PNI in patients with breast cancer and indicate a poor prognosis (138). Coculturing prostate cancer cells with CAFs enhances the expression of critical component of the Hippo signaling pathway, Yes-associated protein 1, which in turn elevates the secretion of NGF and promote the occurrence of PNI in cancer cells (139). Additionally, CAFs drive PNI in pancreatic cancer cells through the SOX4/MMP11 signaling axis (140). Aberrant activation of SOX4 facilitates the transition of inflammatory CAFs to myofibroblastic CAFs, further increasing the expression of MMP11. Myofibroblastic CAFs also contribute to EMT, thereby enhancing the invasive capacity of pancreatic cancer cells and promoting PNI (140).
4.3 TAMs
TAMs are critical components of the tumor microenvironment, which originate from circulating monocytes and resident tissue macrophages. Tumor-derived cytokines actively recruit TAMs to the tumor site. TAMs are important sources of epithelial growth factor within tumor tissue (141) and can also produce a variety of other cytokines, including platelet-derived growth factor, transforming growth factor beta-β, hepatocyte growth factor, and basic fibroblast growth factor (bFGF), thus promoting tumor cell proliferation and survival, acting as “signal transducers” in the interactions between tumors and neurons.
TAMs are closely associated with the PNI of tumors, primarily influencing cancer progression by promoting EMT (142). Prior to PNI, TAMs accumulate near tumor cells and assist in the polarization and migration of these cells through the GDNF/RET pathway. The number of TAMs surrounding the tumor-invading nerves is greater than that surrounding normal nerves (34). In patients with pancreatic cancer accompanied by PNI, the infiltration of TAMs in tumor tissue is significantly greater than that in patients without PNI (143).
TAMs can also collaborate with other cells in the tumor microenvironment to promote PNI. They can stimulate CAFs to produce leukemia inhibitory factor and SLIT2, inducing the plasticity of dorsal root ganglia (DRGs) and facilitating cancer cell PNI through the JAK/STAT3/AKT and N-cadherin/β-catenin pathways (135). Additionally, TAMs can activate pancreatic stellate cells (PSCs) and promote PNI via cholinergic signaling (144). In PDAC, TAMs can activate PSCs through the bFGF/PI3K/Akt/c-myc/GFAP pathway. PSCs can secrete IL-33, which recruits macrophages into the perineural environment, promoting the M2 polarization of macrophages and, consequently, tumor growth. The positive feedback loop between SCs and TAMs involving bFGF and IL-33 is essential in the process of PNI in PDAC (145). Furthermore, CCR2-expressing inflammatory monocytes are preferentially recruited to PNI sites by CCL2 released from SCs, where they differentiate into macrophages that promote PNI through the release of cathepsin B (115).
4.4 T cells
T cells, as important immune cells, play crucial roles in the tumor microenvironment, which can not only exert antitumor effects, but also be suppressed by tumor cells and their microenvironment. The main types of T cells include (i) effector T cells (CD8+ T cells), which induce apoptosis in tumor cells by recognizing tumor-specific antigens and releasing cytotoxic molecules such as perforin and granzyme; (ii) helper T cells (CD4+ T cells), which enhance the immune response by activating B cells, macrophages, and dendritic cells through the secretion of cytokines such as IL-2 and IFN-γ; and (iii) regulatory T cells, which play important roles in maintaining immune tolerance and suppressing excessive immune responses. In the tumor microenvironment, regulatory T cells typically inhibit the activity of CD8+ T cells, aiding tumors in evading immune surveillance.
PNI is associated with T-cell infiltration. In patients with serous ovarian cancer, the severity of PNI is negatively correlated with the infiltration of CD4+ T cells in tumor tissue (146). Severe PNI can lead to an immunosuppressive microenvironment in PDAC, which is associated with elevated levels of acetylcholine (Ach) during severe PNI. Increased Ach levels inhibit the expression of CCL5 through HDAC1, impairing the ability of pancreatic cancer cells to recruit CD8+ T cells. Ach also directly suppresses the production of IFN-γ by CD8+ T cells in a dose-dependent manner and promotes the differentiation of Th2 cells (92). In primary cutaneous squamous cell carcinoma with PNI, infiltrating lymphocytes are predominantly CD8+ T cells, which exhibit a relatively high frequency of immune checkpoints such as PD-1, CTLA-4, and Tim-3. These immune checkpoints inhibit T-cell activity through various mechanisms, allowing tumor cells to evade the surveillance of the immune system (147). Additionally, in head and neck squamous cell carcinoma with PNI, sensory nerves can directly interact with the adaptive immune system by releasing calcitonin gene-related peptides, which reduces the number of CD4+ T cells in the tumor microenvironment while activating CD8+ T cells, thereby accelerating tumor growth (148).
5 Conclusion and future perspectives
Although significant progress has been made in understanding the role of neuro-tumor crosstalk in tumor progression, many uncertainties remain regarding the specific mechanisms by which cancer regulates nerves. These include neurogenesis and nerve remodeling in the tumor microenvironment, as well as the development of PNI. Future research should focus on the following areas.
5.1 Bidirectional mechanism of PNI
PNI is both the process by which tumors invade nerves and the mechanism through which nerves promote tumor cell behavior. This interaction relies on a range of molecular mediators, including neurotrophic factors, chemokines, cell adhesion molecules, axon guidance cues, neurotransmitters, neuropeptides, and matrix metalloproteinases. A more detailed understanding of the specific roles of these mediators in PNI could offer valuable insights for developing targeted therapies. Future research should prioritize multi-omics integration to map spatiotemporal PNI dynamics, leveraging AI for predictive modeling and therapeutic optimization.
5.2 Effect of the tumor microenvironment on PNI
The tumor microenvironment plays a crucial role in PNI. The complex interactions among SCs, immune cells, mesenchymal stem cells, and fibroblasts create the necessary conditions for the initiation and progression of PNI. Targeting neurotrophic axes (GDNF/RET, NGF/TrkA) and metabolic pathways (e.g., lactate transport) may disrupt nerve-tumor crosstalk, while reversing cholinergic immunosuppression or enhancing CD161+CD8+ T-cell cytotoxicity could reshape immune responses. Studying the interactions between different cell types in the tumor microenvironment and exploring approaches to modulate the tumor microenvironment to inhibit PNI could provide new strategies for cancer treatment.
5.3 Discovery of new therapeutic targets
In-depth investigations of the molecules and signaling pathways involved in PNI are crucial for identifying new therapeutic targets. The reliance of cancer cells on nerves for their oncogenic phenotype provides a foundation for targeting these mechanisms. Approaches such as surgery or drugs offer significant translational potential in cancer neuroscience. For example, chemical pancreatic denervation in patients with nonresectable pancreatic cancer has demonstrated potential for pain relief and improved survival (120). However, these denervation strategies may be accompanied by severe side effects, including persistent diarrhea and sexual dysfunction. Future research should focus on optimizing efficacy while minimizing these adverse effects. Clinically, spatial omics-based classifiers (e.g., nerve-tumor distance) and 3D models will refine diagnostics and drug testing. These efforts promise to translate mechanistic insights into therapies for PNI-prone malignancies, ultimately improving patient outcomes.
5.4 Development of specific in vivo and in vitro models of PNI
In-depth research on PNI depends on improving existing experimental models and developing specialized models to help researchers investigate the roles of different cell types in PNI, the tumor microenvironment factors that influence PNI, and phenomena such as immune evasion and inflammatory responses in the tumor microenvironment. Particular attention should be given to the pathological changes and molecular mechanisms occurring in the very early stages of PNI, as well as during the phase of harmful hypersensitivity reactions. The development of specialized PNI models can also aid in identifying potential biomarkers and, when combined with cutting-edge biotechnologies (such as multi-omics and super-resolution imaging), can facilitate the development of new diagnostic tools, ultimately, enabling early intervention in PNI and increasing the effectiveness of cancer therapies.
In conclusion, a deeper understanding of the biological characteristics of PNI not only helps reveal the mechanisms of cancer progression but also opens new avenues for developing novel treatment strategies. Ongoing research in this field will provide a crucial foundation for the early diagnosis and treatment of cancer.
Author contributions
MW: Formal Analysis, Investigation, Methodology, Writing – original draft. NP: Formal Analysis, Investigation, Methodology, Writing – original draft. XB: Investigation, Methodology, Writing – review & editing. FC: Investigation, Methodology, Writing – review & editing. YZ: Conceptualization, Supervision, Validation, Writing – review & editing. QC: Conceptualization, Methodology, Supervision, Validation, Writing – review & editing.
Funding
The author(s) declare that financial support was received for the research and/or publication of this article. This study received funding from the National Natural Science Foundation of China (Grant No. 82071355 to Q.C.), Nantong University Clinical Medicine Special Research Fund (Grant No. 2024JY051 to Y.L.Z.). We thank the Priority Academic Program Development of Jiangsu Higher Education Institutions (PAPD) for the research platform.
Acknowledgments
We thank Dr. Xiaoxia Jin for providing the relevant pathological sections and technical support.
Conflict of interest
The authors declare that the research was conducted in the absence of any commercial or financial relationships that could be construed as a potential conflict of interest.
Generative AI statement
The author(s) declare that no Generative AI was used in the creation of this manuscript.
Publisher’s note
All claims expressed in this article are solely those of the authors and do not necessarily represent those of their affiliated organizations, or those of the publisher, the editors and the reviewers. Any product that may be evaluated in this article, or claim that may be made by its manufacturer, is not guaranteed or endorsed by the publisher.
Abbreviations
PNI, perineural invasion; ICC, intrahepatic cholangiocarcinoma; CAFs, cancer-associated fibroblasts; TAMs, tumor-associated macrophages; NGF, nerve growth factor; TrkA, tyrosine receptor kinase A; ECM, extracellular matrix; SCs, Schwann cells; EMT, epithelial–mesenchymal transition; JNK, c-Jun N-terminal kinase; MAPK, mitogen-activated protein kinase; AP-1, activator protein 1; GDNF, glial cell line-derived neurotrophic factor; ERK, extracellular signal-regulated kinases; bFGF, basic fibroblast growth factor; DRGs, dorsal root ganglia; PSCs, pancreatic stellate cells; CAM, chorioallantoic membrane; SPINK1, serine protease inhibitor Kazal type 1; CCL, C-C motif chemokine ligand; MMPs, Matrix metalloproteinases; PDAC, pancreatic ductal adenocarcinoma; scRNA-seq, single-cell RNA sequencing;
References
1. Cao W, Chen HD, Yu YW, Li N, Chen WQ. Changing profiles of cancer burden worldwide and in China: a secondary analysis of the global cancer statistics 2020. Chin Med J (Engl). (2021) 134:783–91. doi: 10.1097/CM9.0000000000001474
3. de Visser KE, Joyce JA. The evolving tumor microenvironment: From cancer initiation to metastatic outgrowth. Cancer Cell. (2023) 41:374–403. doi: 10.1016/j.ccell.2023.02.016
4. Nagakawa T, Kayahara M, Ueno K, Ohta T, Konishi I, Ueda N, et al. A clinicopathologic study on neural invasion in cancer of the pancreatic head. Cancer. (1992) 69:930–5. doi: 10.1002/1097-0142(19920215)69:4<930::aid-cncr2820690416>3.0.co;2-r
5. Liu Q, Ma Z, Cao Q, Zhao H, Guo Y, Liu T, et al. Perineural invasion-associated biomarkers for tumor development. BioMed Pharmacother. (2022) 155:113691. doi: 10.1016/j.biopha.2022.113691
6. He S, Chen CH, Chernichenko N, Bakst RL, Barajas F, Deborde S, et al. GFRα1 released by nerves enhances cancer cell perineural invasion through GDNF-RET signaling. Proc Natl Acad Sci U S A. (2014) 111:E2008–17. doi: 10.1073/pnas.1402944111
7. Liebig C, Ayala G, Wilks JA, Berger DH, Albo D. Perineural invasion in cancer: a review of the literature. Cancer. (2009) 115:3379–91. doi: 10.1002/cncr.24396
8. Sekiguchi S, Ishida Y, Tomino M, Ohseto K. Two cases of splanchnic nerve block with epidural anesthesia in patients unable to maintain prone position due to pancreatic pain. Palliat Med Rep. (2023) 4:274–7. doi: 10.1089/pmr.2023.0031
9. Hauerslev KR, Madsen AH, Overgaard J, Damsgaard TE, Christiansen P. Long-term follow-up on shoulder and arm morbidity in patients treated for early breast cancer. Acta Oncol. (2020) 59:851–8. doi: 10.1080/0284186X.2020.1745269
10. Okuma Y, Kashima J, Watanabe K, Homma S. Survival analysis and pathological features of advanced non-small cell lung cancer with miliary pulmonary metastases in patients harboring epidermal growth factor receptor mutations. J Cancer Res Clin Oncol. (2018) 144:1601–11. doi: 10.1007/s00432-018-2681-x
11. Masuda C, Onishi S, Yorozu K, Kurasawa M, Morinaga M, Wakita D, et al. PD-L1 and VEGF dual blockade enhances anti-tumor effect on brain metastasis in hematogenous metastasis model. Clin Exp Metastasis. (2024) 41:909–24. doi: 10.1007/s10585-024-10309-y
12. Yuan H, Zhang Y, Liu F, Wu Y, Huang X, Liu X, et al. Exploring the biological mechanism and clinical value of perineural invasion in pancreatic cancer. Cancer Lett. (2025) 613:217515. doi: 10.1016/j.canlet.2025.217515
13. Mou A, Li H, Chen XL, Fan YH, Pu H. Tumor size measured by multidetector CT in resectable colon cancer: correlation with regional lymph node metastasis and N stage. World J Surg Oncol. (2021) 19:179. doi: 10.1186/s12957-021-02292-5
14. Zeng XZ, Huang ZS, Fang HP, Wu JY, Huang QX, Zhuang CB, et al. Coexpression of TLR9 and VEGF-C is associated with lymphatic metastasis in prostate cancer. Asian J Androl. (2022) 24:380–5. doi: 10.4103/aja202167
15. Ghinea N, Robin B, Pichon C, Leclere R, Nicolas A, Chnecker C, et al. Vasa nervorum angiogenesis in prostate cancer with perineural invasion. Prostate. (2019) 79:640–6. doi: 10.1002/pros.23771
16. Zhang Z, Lv ZG, Lu M, Li H, Zhou J. Nerve-tumor crosstalk in tumor microenvironment: From tumor initiation and progression to clinical implications. Biochim Biophys Acta Rev Cancer. (2024) 1879:189121. doi: 10.1016/j.bbcan.2024.189121
17. Chen Y, Zhang W, Zeng Y, Yang P, Li Y, Liang X, et al. GDNF-induced phosphorylation of MUC21 promotes pancreatic cancer perineural invasion and metastasis by activating RAC2 GTPase. Oncogene. (2024) 43:2564–77. doi: 10.1038/s41388-024-03102-4
18. Jurcak NR, Rucki AA, Muth S, Thompson E, Sharma R, Ding D, et al. Axon guidance molecules promote perineural invasion and metastasis of orthotopic pancreatic tumors in mice. Gastroenterology. (2019) 157:838–50.e6. doi: 10.1053/j.gastro.2019.05.065
19. Tian Z, Ou G, Su M, Li R, Pan L, Lin X, et al. TIMP1 derived from pancreatic cancer cells stimulates Schwann cells and promotes the occurrence of perineural invasion. Cancer Lett. (2022) 546:215863. doi: 10.1016/j.canlet.2022.215863
20. Fan Y, Zhang B, Du X, Wang B, Yan Q, Guo L, et al. Regulating tumorigenicity and cancer metastasis through TRKA signaling. Curr Cancer Drug Targets. (2024) 24:271–87. doi: 10.2174/1568009623666230904150957
21. Hsieh KJ, Addae-Mensah K, Alrohaibani Y, Goad A, Learned K. Perineural spread of tumor in the skull base and head and neck. Maxillofac Surg Clin North Am. (2023) 35:399–412. doi: 10.1016/j.coms.2023.02.004
22. Whitehead MT, Choudhri AF, Grimm J, Nelson MD. Rhombencephalosynapsis as a cause of aqueductal stenosis: an under-recognized association in hydrocephalic children. Pediatr Radiol. (2014) 44:849–56. doi: 10.1007/s00247-014-2877-4
23. Ji T, Ma K, Wu H, Cao TA, Substance P. (SP)/neurokinin-1 receptor axis promotes perineural invasion of pancreatic cancer and is affected by lncRNA LOC389641. J Immunol Res. (2022) 2022:5582811. doi: 10.1155/2022/5582811
24. Li X, Ma G, Ma Q, Li W, Liu J, Han L, et al. Neurotransmitter substance P mediates pancreatic cancer perineural invasion via NK-1R in cancer cells. Mol Cancer Res. (2013) 11:294–302. doi: 10.1158/1541-7786.MCR-12-0609
25. Duraker N, Sişman S, Can G. The significance of perineural invasion as a prognostic factor in patients with gastric carcinoma. Surg Today. (2003) 33:95–100. doi: 10.1007/s005950300020
26. Jiang P, Li X, Wang S, Liu Y. Prognostic significance of PNI in patients with pancreatic head cancer undergoing laparoscopic pancreaticoduodenectomy. Front Surg. (2022) 9:897033. doi: 10.3389/fsurg.2022.897033
27. Tong JY, Huilgol SC, James C, Rajak S, Selva D. Perineural invasion and perineural spread in periocular squamous cell carcinoma. Eye (Lond). (2023) 37:875–84. doi: 10.1038/s41433-022-02306-w
28. Jin W, Zhu M, Zheng Y, Wu Y, Ding X, Wu H, et al. Perineural invasion, lactate dehydrogenase, globulin, and serum sodium predicting occult metastasis in oral cancer. Dis. (2022) 28:132–41. doi: 10.1111/odi.13750
29. Lu M, Xiu DR, Guo LM, Yuan CH, Zhang LF, Tao LY. Extrapancreatic neuropathy correlates with early liver metastasis in pancreatic head adenocarcinoma. Onco Targets Ther. (2019) 12:11083–95. doi: 10.2147/OTT.S221844
30. Takahashi H, Ohigashi H, Ishikawa O, Gotoh K, Yamada T, Nagata S, et al. Perineural invasion and lymph node involvement as indicators of surgical outcome and pattern of recurrence in the setting of preoperative gemcitabine-based chemoradiation therapy for resectable pancreatic cancer. Ann Surg. (2012) 255:95–102. doi: 10.1097/SLA.0b013e31823d813c
31. Schmitd LB, Scanlon CS, D’Silva NJ. Perineural invasion in head and neck cancer. J Dent Res. (2018) 97:742–50. doi: 10.1177/0022034518756297
32. Meng XL, Lu JC, Zeng HY, Chen Z, Guo XJ, Gao C, et al. The clinical implications and molecular features of intrahepatic cholangiocarcinoma with perineural invasion. Hepatol Int. (2023) 17:63–76. doi: 10.1007/s12072-022-10445-1
33. Wei T, Zhang XF, He J, Popescu I, Marques HP, Aldrighetti L, et al. Prognostic impact of perineural invasion in intrahepatic cholangiocarcinoma: multicenter study. Br J Surg. (2022) 109:610–6. doi: 10.1093/bjs/znac098
34. Wang J, Chen Y, Li X, Zou X. Perineural invasion and associated pain transmission in pancreatic cancer. Cancers (Basel). (2021) 13(14):4594. doi: 10.3390/cancers13184594
35. Salvo E, Campana WM, Scheff NN, Nguyen TH, Jeong SH, Wall I, et al. Peripheral nerve injury and sensitization underlie pain associated with oral cancer perineural invasion. Pain. (2020) 161:2592–602. doi: 10.1097/j.pain.0000000000001986
36. Demir IE, Friess H, Ceyhan GO. Neural plasticity in pancreatitis and pancreatic cancer. Nat Rev Gastroenterol Hepatol. (2015) 12:649–59. doi: 10.1038/nrgastro.2015.166
37. Bahar ME, Kim HJ, Kim DR. Targeting the RAS/RAF/MAPK pathway for cancer therapy: from mechanism to clinical studies. Signal Transduct Target Ther. (2023) 8:455. doi: 10.1038/s41392-023-01705-z
38. Shi RJ, Ke BW, Tang YL, Liang XH. Perineural invasion: A potential driver of cancer-induced pain. Biochem Pharmacol. (2023) 215:115692. doi: 10.1016/j.bcp.2023.115692
39. Zhu Y, Colak T, Shenoy M, Liu L, Pai R, Li C, et al. Nerve growth factor modulates TRPV1 expression and function and mediates pain in chronic pancreatitis. Gastroenterology. (2011) 141:370–7. doi: 10.1053/j.gastro.2011.03.046
40. Capek S, Howe BM, Tracy JA, García JJ, Amrami KK, Spinner RJ. Prostate cancer with perineural spread and dural extension causing bilateral lumbosacral plexopathy: case report. J Neurosurg. (2015) 122:778–83. doi: 10.3171/2014.12.JNS141339
41. Markowski MC, Wobker SE, Strowd RE, Antonarakis ES. Lumbosacral plexus involvement as the first site of metastatic recurrence in a patient with CTNNB1-mutant prostate cancer. Clin Genitourin Cancer. (2016) 14:e417–22. doi: 10.1016/j.clgc.2016.02.001
42. Lesnik DJ, Boey HP. Perineural invasion of the facial nerve by a cutaneous squamous cell cancer: a case report. Ear Nose Throat J. (2004) 83:824, 6–7. doi: 10.1177/014556130408301213
43. Caldemeyer KS, Mathews VP, Righi PD, Smith RR. Imaging features and clinical significance of perineural spread or extension of head and neck tumors. Radiographics. (1998) 18:97–110. doi: 10.1148/radiographics.18.1.9460111
44. Xu W, Liu J, Zhang J, Lu J, Guo J. Tumor microenvironment crosstalk between tumors and the nervous system in pancreatic cancer: Molecular mechanisms and clinical perspectives. Biochim Biophys Acta Rev Cancer. (2024) 1879:189032. doi: 10.1016/j.bbcan.2023.189032
45. Saloman JL, Albers KM, Rhim AD, Davis BM. Can stopping nerves, stop cancer? Trends Neurosci. (2016) 39:880–9. doi: 10.1016/j.tins.2016.10.002
46. Renz BW, Takahashi R, Tanaka T, Macchini M, Hayakawa Y, Dantes Z, et al. beta2 adrenergic-neurotrophin feedforward loop promotes pancreatic cancer. Cancer Cell. (2018) 33:75–90.e7. doi: 10.1016/j.ccell.2017.11.007
47. Bakst RL, Wong RJ. Mechanisms of perineural invasion. J Neurol Surg B Skull Base. (2016) 77:96–106. doi: 10.1055/s-0036-1571835
48. Ahmadi N, Kelly G, Low TH, Clark J, Gupta R. Molecular factors governing perineural invasion in Malignancy. Surg Oncol. (2022) 42:101770. doi: 10.1016/j.suronc.2022.101770
49. Hirose M, Kuroda Y, Murata E. NGF/trkA signaling as a therapeutic target for pain. Pain Pract. (2016) 16:175–82. doi: 10.1111/papr.12342
50. Shen FZ, Zhang BY, Feng YJ, Jia ZX, An B, Liu CC, et al. Current research in perineural invasion of cholangiocarcinoma. J Exp Clin Cancer Res. (2010) 29:24. doi: 10.1186/1756-9966-29-24
51. Bahmad HF, Gogola S, Rejzer M, Stoyanov K, Gomez AS, Valencia AK, et al. Unraveling the mysteries of perineural invasion in benign and Malignant conditions. Curr Oncol. (2023) 30:8948–72. doi: 10.3390/curroncol30100647
52. Peng T, Guo Y, Gan Z, Ling Y, Xiong J, Liang X, et al. Nerve growth factor (NGF) encourages the neuroinvasive potential of pancreatic cancer cells by activating the warburg effect and promoting tumor derived exosomal miRNA-21 expression. Oxid Med Cell Longev. (2022) 2022:8445093. doi: 10.1155/2022/8445093
53. Yue XJ, Xu LB, Zhu MS, Zhang R, Liu C. Over-expression of nerve growth factor-beta in human cholangiocarcinoma QBC939 cells promote tumor progression. PloS One. (2013) 8:e62024. doi: 10.1371/journal.pone.0062024
54. Yang XQ, Xu YF, Guo S, Liu Y, Ning SL, Lu XF, et al. Clinical significance of nerve growth factor and tropomyosin-receptor-kinase signaling pathway in intrahepatic cholangiocarcinoma. World J Gastroenterol. (2014) 20:4076–84. doi: 10.3748/wjg.v20.i14.4076
55. Peng Y, Wang Y, Zhou C, Mei W, Zeng C. PI3K/akt/mTOR pathway and its role in cancer therapeutics: are we making headway? Front Oncol. (2022) 12:819128. doi: 10.3389/fonc.2022.819128
56. Li J, Kang R, Tang D. Cellular and molecular mechanisms of perineural invasion of pancreatic ductal adenocarcinoma. Cancer Commun (Lond). (2021) 41:642–60. doi: 10.1002/cac2.12188
57. Selvaggi F, Melchiorre E, Casari I, Cinalli S, Cinalli M, Aceto GM, et al. Perineural invasion in pancreatic ductal adenocarcinoma: from molecules towards drugs of clinical relevance. Cancers (Basel). (2022) 14(23):5793. doi: 10.3390/cancers14235793
58. Deborde S, Wong RJ. How Schwann cells facilitate cancer progression in nerves. Cell Mol Life Sci. (2017) 74:4405–20. doi: 10.1007/s00018-017-2578-x
59. Wang K, Demir IE, D’Haese JG, Tieftrunk E, Kujundzic K, Schorn S, et al. The neurotrophic factor neurturin contributes toward an aggressive cancer cell phenotype, neuropathic pain and neuronal plasticity in pancreatic cancer. Carcinogenesis. (2014) 35:103–13. doi: 10.1093/carcin/bgt312
60. Hori K, Sen A, Artavanis-Tsakonas S. Notch signaling at a glance. J Cell Sci. (2013) 126:2135–40. doi: 10.1242/jcs.127308
61. de Freitas Filho SAJ, Coutinho-Camillo CM, Oliveira KK, Bettim BB, Pinto CAL, Kowalski LP, et al. Prognostic implications of ALDH1 and notch1 in different subtypes of oral cancer. J Oncol. (2021) 2021:6663720. doi: 10.1155/2021/6663720
62. Mk H, Prince S, Mohan AM, Krishnan KV, Devi A. Association of Notch4 with metastasis in human oral squamous cell carcinoma. Life Sci. (2016) 156:38–46. doi: 10.1016/j.lfs.2016.05.019
63. Ding LC, She L, Zheng DL, Huang QL, Wang JF, Zheng FF, et al. Notch-4 contributes to the metastasis of salivary adenoid cystic carcinoma. Oncol Rep. (2010) 24:363–8. doi: 10.3892/or_00000868
64. Chen W, Zhang H, Wang J, Cao G, Dong Z, Su H, et al. Lentiviral-mediated gene silencing of Notch-4 inhibits in vitro proliferation and perineural invasion of ACC-M cells. Oncol Rep. (2013) 29:1797–804. doi: 10.3892/or.2013.2317
65. Boustan A, Jahangiri R, Ghalehno AD, Khorsandi M, Mosaffa F, Jamialahmadi K. Expression analysis elucidates the roles of Nicastrin, Notch4, and Hes1 in prognosis and endocrine-therapy resistance in ER-positive breast cancer patients. Res Pharm Sci. (2023) 18:78–88. doi: 10.4103/1735-5362.363598
66. Kim G, Jung J, Kim JW, Kim JY. Low HES-1 and positive DLL4 expression predicts poor prognosis of colorectal cancers. Pathology. (2023) 55:52–7. doi: 10.1016/j.pathol.2022.07.008
67. Deshmukh AP, Vasaikar SV, Tomczak K, Tripathi S, den Hollander P, Arslan E, et al. Identification of EMT signaling cross-talk and gene regulatory networks by single-cell RNA sequencing. Proc Natl Acad Sci U S A. (2021) 118(19):e2102050118. doi: 10.1073/pnas.2102050118
68. Li Y, Ma J, Qian X, Wu Q, Xia J, Miele L, et al. Regulation of EMT by Notch signaling pathway in tumor progression. Curr Cancer Drug Targets. (2013) 13:957–62. doi: 10.2174/15680096113136660101
69. Dimova I, Popivanov G, Djonov V. Angiogenesis in cancer - general pathways and their therapeutic implications. J BUON. (2014) 19:15–21.
70. Bode AM, Dong Z. The functional contrariety of JNK. Mol Carcinog. (2007) 46:591–8. doi: 10.1002/mc.20348
71. Liu J, Lin A. Role of JNK activation in apoptosis: a double-edged sword. Cell Res. (2005) 15:36–42. doi: 10.1038/sj.cr.7290262
72. Chuang JY, Tsai CF, Chang SW, Chiang IP, Huang SM, Lin HY, et al. Glial cell line-derived neurotrophic factor induces cell migration in human oral squamous cell carcinoma. Oncol. (2013) 49:1103–12. doi: 10.1016/j.oraloncology.2013.08.009
73. Handra-Luca A, Mauguen A, Ménard P, Fouret P. Coordinated expression of activated mitogen-activated protein kinases in salivary gland adenoid cystic carcinoma. Hum Pathol. (2008) 39:1590–6. doi: 10.1016/j.humpath.2008.04.008
74. Chen YT, Tseng TT, Tsai HP, Kuo SH, Huang MY, Wang JY, et al. Serine protease inhibitor Kazal type 1 (SPINK1) promotes proliferation, migration, invasion and radiation resistance in rectal cancer patients receiving concurrent chemoradiotherapy: a potential target for precision medicine. Hum Cell. (2022) 35:1912–27. doi: 10.1007/s13577-022-00776-4
75. Yuan D, Huang S, Berger E, Liu L, Gross N, Heinzmann F, et al. Kupffer Cell-Derived Tnf Triggers Cholangiocellular Tumorigenesis through JNK due to Chronic Mitochondrial Dysfunction and ROS. Cancer Cell. (2017) 31:771–89.e6. doi: 10.1016/j.ccell.2017.05.006
76. Chen XX, Yin Y, Cheng JW, Huang A, Hu B, Zhang X, et al. BAP1 acts as a tumor suppressor in intrahepatic cholangiocarcinoma by modulating the ERK1/2 and JNK/c-Jun pathways. Cell Death Dis. (2018) 9:1036. doi: 10.1038/s41419-018-1087-7
77. Tian X, Traub B, Shi J, Huber N, Schreiner S, Chen G, et al. c-Jun N-terminal kinase 2 suppresses pancreatic cancer growth and invasion and is opposed by c-Jun N-terminal kinase 1. Cancer Gene Ther. (2022) 29:73–86. doi: 10.1038/s41417-020-00290-5
78. Jung YS, Park JI. Wnt signaling in cancer: therapeutic targeting of Wnt signaling beyond beta-catenin and the destruction complex. Exp Mol Med. (2020) 52:183–91. doi: 10.1038/s12276-020-0380-6
79. Wang J, Li Q, Liang F, Du X, Song P, Wu T, et al. Dickkopf-1 drives perineural invasion via PI3K-AKT signaling pathway in head and neck squamous cancer. MedComm (2020). (2024) 5:e518. doi: 10.1002/mco2.518
80. Zhong M, Zhou L, Fang Z, Yao YY, Zou JP, Xiong JP, et al. Ubiquitin-specific protease 15 contributes to gastric cancer progression by regulating the Wnt/β-catenin signaling pathway. World J Gastroenterol. (2021) 27:4221–35. doi: 10.3748/wjg.v27.i26.4221
81. Lai HY, Chiu CC, Kuo YH, Tsai HH, Wu LC, Tseng WH, et al. High stromal SFRP2 expression in urothelial carcinoma confers an unfavorable prognosis. Front Oncol. (2022) 12:834249. doi: 10.3389/fonc.2022.834249
82. Hassounah NB, Nagle R, Saboda K, Roe DJ, Dalkin BL, McDermott KM. Primary cilia are lost in preinvasive and invasive prostate cancer. PloS One. (2013) 8:e68521. doi: 10.1371/journal.pone.0068521
83. Wu ZQ, Li XY, Hu CY, Ford M, Kleer CG, Weiss SJ. Canonical Wnt signaling regulates Slug activity and links epithelial-mesenchymal transition with epigenetic Breast Cancer 1, Early Onset (BRCA1) repression. Proc Natl Acad Sci U S A. (2012) 109:16654–9. doi: 10.1073/pnas.1205822109
84. Gujral TS, Chan M, Peshkin L, Sorger PK, Kirschner MW, MacBeath G. A noncanonical Frizzled2 pathway regulates epithelial-mesenchymal transition and metastasis. Cell. (2014) 159:844–56. doi: 10.1016/j.cell.2014.10.032
85. Zhang B, Wang S, Fu Z, Gao Q, Yang L, Lei Z, et al. Single-cell RNA sequencing reveals intratumoral heterogeneity and potential mechanisms of Malignant progression in prostate cancer with perineural invasion. Front Genet. (2022) 13:1073232. doi: 10.3389/fgene.2022.1073232
86. Zu Z, Zhang C, Shi J, Chen K, Tang H, Hu K, et al. Single-cell analysis reveals that GFAP. Cell Death Dis. (2025) 16:215. doi: 10.1038/s41419-025-07543-x
87. Alrawashdeh W, Jones R, Dumartin L, Radon TP, Cutillas PR, Feakins RM, et al. Perineural invasion in pancreatic cancer: proteomic analysis and in vitro modelling. Mol Oncol. (2019) 13:1075–91. doi: 10.1002/1878-0261.12463
88. Chen Q, Yin H, Jiang Z, He T, Xie Y, Mao W, et al. Poor clinical outcomes and immunoevasive contexture in CD161. J Immunother Cancer. (2024) 12(3):e008694. doi: 10.1136/jitc-2023-008694
89. Schmitd LB, Perez-Pacheco C, Bellile EL, Wu W, Casper K, Mierzwa M, et al. Spatial and transcriptomic analysis of perineural invasion in oral cancer. Clin Cancer Res. (2022) 28:3557–72. doi: 10.1158/1078-0432.CCR-21-4543
90. Lakis V, Chan NL, Lyons R, Blackburn N, Nguyen TH, Chang C, et al. Spatial transcriptomics reveals novel mechanisms involved in perineural invasion in pancreatic ductal adenocarcinomas. Cancers (Basel). (2025) 17(5):852. doi: 10.3390/cancers17050852
91. Yuan X, Dong Z, Zhang B, Li Q, Jiang W. Combining single-cell spatial transcriptomics and molecular simulation to develop in vivo probes targeting the perineural invasion region of adenoid cystic carcinoma. Heliyon. (2024) 10:e34628. doi: 10.1016/j.heliyon.2024.e34628
92. Yang MW, Tao LY, Jiang YS, Yang JY, Huo YM, Liu DJ, et al. Perineural invasion reprograms the immune microenvironment through cholinergic signaling in pancreatic ductal adenocarcinoma. Cancer Res. (2020) 80:1991–2003. doi: 10.1158/0008-5472.CAN-19-2689
93. Hurník P, Chyra Z, Ševčíková T, Štembírek J, Trtková KS, Gaykalova DA, et al. Epigenetic regulations of perineural invasion in head and neck squamous cell carcinoma. Front Genet. (2022) 13:848557. doi: 10.3389/fgene.2022.848557
94. Shang J, Sheng L, Wang K, Shui Y, Wei Q. Expression of neural cell adhesion molecule in salivary adenoid cystic carcinoma and its correlation with perineural invasion. Oncol Rep. (2007) 18:1413–6. doi: 10.3892/or.18.6.1413
95. Na’ara S, Amit M, Gil Z. L1CAM induces perineural invasion of pancreas cancer cells by upregulation of metalloproteinase expression. Oncogene. (2019) 38:596–608. doi: 10.1038/s41388-018-0458-y
96. Sroka IC, Chopra H, Das L, Gard JM, Nagle RB, Cress AE. Schwann cells increase prostate and pancreatic tumor cell invasion using laminin binding A6 integrin. J Cell Biochem. (2016) 117:491–9. doi: 10.1002/jcb.25300
97. Lin C, Ren Z, Yang X, Yang R, Chen Y, Liu Z, et al. Nerve growth factor (NGF)-TrkA axis in head and neck squamous cell carcinoma triggers EMT and confers resistance to the EGFR inhibitor erlotinib. Cancer Lett. (2020) 472:81–96. doi: 10.1016/j.canlet.2019.12.015
98. Lv CY, Zhou T, Chen W, Yin XD, Yao JH, Zhang YF. Preliminary study correlating CX3CL1/CX3CR1 expression with gastric carcinoma and gastric carcinoma perineural invasion. World J Gastroenterol. (2014) 20:4428–32. doi: 10.3748/wjg.v20.i15.4428
99. Yin L, Li J, Wang J, Pu T, Wei J, Li Q, et al. MAOA promotes prostate cancer cell perineural invasion through SEMA3C/PlexinA2/NRP1-cMET signaling. Oncogene. (2021) 40:1362–74. doi: 10.1038/s41388-020-01615-2
100. Binmadi NO, Yang YH, Zhou H, Proia P, Lin YL, Batista De Paula AM, et al. Plexin-B1 and semaphorin 4D cooperate to promote perineural invasion in a RhoA/ROK-dependent manner. Am J Pathol. (2012) 180:1232–42. doi: 10.1016/j.ajpath.2011.12.009
101. de Franchis V, Petrungaro S, Pizzichini E, Camerini S, Casella M, Somma F, et al. Cholangiocarcinoma Malignant traits are promoted by schwann cells through TGFβ Signaling in a model of perineural invasion. Cells. (2024) 13(5):366. doi: 10.3390/cells13050366
102. Roger E, Martel S, Bertrand-Chapel A, Depollier A, Chuvin N, Pommier RM, et al. Schwann cells support oncogenic potential of pancreatic cancer cells through TGFβ signaling. Cell Death Dis. (2019) 10:886. doi: 10.1038/s41419-019-2116-x
103. Zhang L, Wang H, Li C, Zhao Y, Wu L, Du X, et al. VEGF-A/neuropilin 1 pathway confers cancer stemness via activating wnt/β-catenin axis in breast cancer cells. Cell Physiol Biochem. (2017) 44:1251–62. doi: 10.1159/000485455
104. Huang C, Li Y, Guo Y, Zhang Z, Lian G, Chen Y, et al. MMP1/PAR1/SP/NK1R paracrine loop modulates early perineural invasion of pancreatic cancer cells. Theranostics. (2018) 8:3074–86. doi: 10.7150/thno.24281
105. Feng Y, Hu X, Liu G, Lu L, Zhao W, Shen F, et al. M3 muscarinic acetylcholine receptors regulate epithelial-mesenchymal transition, perineural invasion, and migration/metastasis in cholangiocarcinoma through the AKT pathway. Cancer Cell Int. (2018) 18:173. doi: 10.1186/s12935-018-0667-z
106. Guo K, Ma Q, Li J, Wang Z, Shan T, Li W, et al. Interaction of the sympathetic nerve with pancreatic cancer cells promotes perineural invasion through the activation of STAT3 signaling. Mol Cancer Ther. (2013) 12:264–73. doi: 10.1158/1535-7163.MCT-12-0809
107. Dezong G, Zhongbing M, Qinye F, Zhigang Y. Carvedilol suppresses migration and invasion of Malignant breast cells by inactivating Src involving cAMP/PKA and PKCδ signaling pathway. J Cancer Res Ther. (2014) 10:998–1003. doi: 10.4103/0973-1482.137664
108. Su D, Guo X, Huang L, Ye H, Li Z, Lin L, et al. Tumor-neuroglia interaction promotes pancreatic cancer metastasis. Theranostics. (2020) 10:5029–47. doi: 10.7150/thno.42440
109. Islam F, Gopalan V, Pillai S, Lu CT, Kasem K, Lam AK. Promoter hypermethylation inactivate tumor suppressor FAM134B and is associated with poor prognosis in colorectal cancer. Genes Chromosomes Cancer. (2018) 57:240–51. doi: 10.1002/gcc.22525
110. Bidar N, Rezaei T, Amini M, Jebelli A, Mokhtarzadeh A, Baradaran B. ZNF677 downregulation by promoter hypermethylation as a driver event through gastric tumorigenesis. Exp Mol Pathol. (2021) 121:104663. doi: 10.1016/j.yexmp.2021.104663
111. Lukosiute-Urboniene A, Mazeike A, Kazokaite M, Silkuniene G, Silkunas M, Barauskas V, et al. Epigenetic regulation of APAF-1 through DNA methylation in pancreatic cancer. Anticancer Res. (2020) 40:3765–79. doi: 10.21873/anticanres.14366
112. Zhang M, Xian HC, Dai L, Tang YL, Liang XH. MicroRNAs: emerging driver of cancer perineural invasion. Cell Biosci. (2021) 11:117. doi: 10.1186/s13578-021-00630-4
113. Yu EH, Tu HF, Wu CH, Yang CC, Chang KW. MicroRNA-21 promotes perineural invasion and impacts survival in patients with oral carcinoma. J Chin Med Assoc. (2017) 80:383–8. doi: 10.1016/j.jcma.2017.01.003
114. Cheng K, Pan J, Liu Q, Ji Y, Liu L, Guo X, et al. Exosomal lncRNA XIST promotes perineural invasion of pancreatic cancer cells via miR-211-5p/GDNF. Oncogene. (2024) 43:1341–52. doi: 10.1038/s41388-024-02994-6
115. Bakst RL, Xiong H, Chen CH, Deborde S, Lyubchik A, Zhou Y, et al. Inflammatory monocytes promote perineural invasion via CCL2-mediated recruitment and cathepsin B expression. Cancer Res. (2017) 77:6400–14. doi: 10.1158/0008-5472.CAN-17-1612
116. Li X, Wang Y, Zhai Z, Mao Q, Chen D, Xiao L, et al. Predicting response to immunotherapy in gastric cancer via assessing perineural invasion-mediated inflammation in tumor microenvironment. J Exp Clin Cancer Res. (2023) 42:206. doi: 10.1186/s13046-023-02730-0
117. Guo S, Deng CX. Effect of stromal cells in tumor microenvironment on metastasis initiation. Int J Biol Sci. (2018) 14:2083–93. doi: 10.7150/ijbs.25720
118. Qian BZ, Pollard JW. Macrophage diversity enhances tumor progression and metastasis. Cell. (2010) 141:39–51. doi: 10.1016/j.cell.2010.03.014
119. Cai Z, Yao H, Chen J, Ahmed AA, Li C, Hu X, et al. Schwann cells in pancreatic cancer: Unraveling their multifaceted roles in tumorigenesis and neural interactions. Cancer Lett. (2024) 587:216689. doi: 10.1016/j.canlet.2024.216689
120. Demir IE, Boldis A, Pfitzinger PL, Brunner E, Klose N, Kehl T, et al. Investigation of Schwann cells at neoplastic cell sites before the onset of cancer invasion. J Natl Cancer Inst. (2014) 106(8):dju184. doi: 10.1093/jnci/dju184
121. Jessen KR, Mirsky R. The origin and development of glial cells in peripheral nerves. Nat Rev Neurosci. (2005) 6:671–82. doi: 10.1038/nrn1746
122. Wolbert J, Li X, Heming M, Mausberg AK, Akkermann D, Frydrychowicz C, et al. Redefining the heterogeneity of peripheral nerve cells in health and autoimmunity. Proc Natl Acad Sci U S A. (2020) 117:9466–76. doi: 10.1073/pnas.1912139117
123. Zhang Y, Zhao Q, Chen Q, Xu L, Yi S. Transcriptional control of peripheral nerve regeneration. Mol Neurobiol. (2023) 60:329–41. doi: 10.1007/s12035-022-03090-0
124. Jessen KR, Mirsky R. The repair Schwann cell and its function in regenerating nerves. J Physiol. (2016) 594:3521–31. doi: 10.1113/JP270874
125. Deborde S, Omelchenko T, Lyubchik A, Zhou Y, He S, McNamara WF, et al. Schwann cells induce cancer cell dispersion and invasion. J Clin Invest. (2016) 126:1538–54. doi: 10.1172/JCI82658
126. Deborde S, Gusain L, Powers A, Marcadis A, Yu Y, Chen CH, et al. Reprogrammed schwann cells organize into dynamic tracks that promote pancreatic cancer invasion. Cancer Discov. (2022) 12:2454–73. doi: 10.1158/2159-8290.CD-21-1690
127. Wong FC, Merker SR, Bauer L, Han Y, Le VMH, Wenzel C, et al. Extracellular vesicles from pancreatic cancer and its tumor microenvironment promote increased Schwann cell migration. Br J Cancer. (2025) 132:326–39. doi: 10.1038/s41416-024-02915-0
128. Han S, Wang D, Huang Y, Zeng Z, Xu P, Xiong H, et al. A reciprocal feedback between colon cancer cells and Schwann cells promotes the proliferation and metastasis of colon cancer. J Exp Clin Cancer Res. (2022) 41:348. doi: 10.1186/s13046-022-02556-2
129. Rangel-Sosa MM, Mann F, Chauvet S. Pancreatic Schwann cell reprogramming supports cancer-associated neuronal remodeling. Glia. (2024) 72:1840–61. doi: 10.1002/glia.24586
130. Barrett TF, Patel B, Khan SM, Mullins RDZ, Yim AKY, Pugazenthi S, et al. Single-cell multi-omic analysis of the vestibular schwannoma ecosystem uncovers a nerve injury-like state. Nat Commun. (2024) 15:478. doi: 10.1038/s41467-023-42762-w
131. Kershner LJ, Choi K, Wu J, Zhang X, Perrino M, Salomonis N, et al. Multiple Nf1 Schwann cell populations reprogram the plexiform neurofibroma tumor microenvironment. JCI Insight. (2022) 7(18):e154513. doi: 10.1172/jci.insight.154513
132. Patel AG, Ashenberg O, Collins NB, Segerstolpe Å, Jiang S, Slyper M, et al. A spatial cell atlas of neuroblastoma reveals developmental, epigenetic and spatial axis of tumor heterogeneity. bioRxiv. (2024). doi: 10.1101/2024.01.07.574538
133. Sahai E, Astsaturov I, Cukierman E, DeNardo DG, Egeblad M, Evans RM, et al. A framework for advancing our understanding of cancer-associated fibroblasts. Nat Rev Cancer. (2020) 20:174–86. doi: 10.1038/s41568-019-0238-1
134. Song M, He J, Pan QZ, Yang J, Zhao J, Zhang YJ, et al. Cancer-associated fibroblast-mediated cellular crosstalk supports hepatocellular carcinoma progression. Hepatology. (2021) 73:1717–35. doi: 10.1002/hep.31792
135. Zhao Y, Shen M, Wu L, Yang H, Yao Y, Yang Q, et al. Stromal cells in the tumor microenvironment: accomplices of tumor progression? Cell Death Dis. (2023) 14:587. doi: 10.1038/s41419-023-06110-6
136. Ishii G, Ochiai A, Neri S. Phenotypic and functional heterogeneity of cancer-associated fibroblast within the tumor microenvironment. Adv Drug Delivery Rev. (2016) 99:186–96. doi: 10.1016/j.addr.2015.07.007
137. Özdemir BC, Pentcheva-Hoang T, Carstens JL, Zheng X, Wu CC, Simpson TR, et al. Depletion of carcinoma-associated fibroblasts and fibrosis induces immunosuppression and accelerates pancreas cancer with reduced survival. Cancer Cell. (2014) 25:719–34. doi: 10.1016/j.ccr.2014.04.005
138. Kim HS, Noh YK, Min KW, Kim DH, Kwon MJ, Pyo JS, et al. Cancer-associated fibroblasts together with a decline in CD8+ T cells predict a worse prognosis for breast cancer patients. Ann Surg Oncol. (2024) 31:2114–26. doi: 10.1245/s10434-023-14715-6
139. Shen T, Li Y, Wang D, Su Y, Li G, Shang Z, et al. YAP1-TEAD1 mediates the perineural invasion of prostate cancer cells induced by cancer-associated fibroblasts. Biochim Biophys Acta Mol Basis Dis. (2022) 1868:166540. doi: 10.1016/j.bbadis.2022.166540
140. Ge F, Zeng C, Wang J, Liu X, Zheng C, Zhang H, et al. Cancer-associated fibroblasts drive early pancreatic cancer cell invasion via the SOX4/MMP11 signaling axis. Biochim Biophys Acta Mol Basis Dis. (2024) 1870:166852. doi: 10.1016/j.bbadis.2023.166852
141. Yin M, Li X, Tan S, Zhou HJ, Ji W, Bellone S, et al. Tumor-associated macrophages drive spheroid formation during early transcoelomic metastasis of ovarian cancer. J Clin Invest. (2016) 126:4157–73. doi: 10.1172/JCI87252
142. Zhang Y, Ding X, Zhang X, Li Y, Xu R, Li HJ, et al. Unveiling the contribution of tumor-associated macrophages in driving epithelial-mesenchymal transition: a review of mechanisms and therapeutic Strategies. Front Pharmacol. (2024) 15:1404687. doi: 10.3389/fphar.2024.1404687
143. Zeng L, Guo Y, Liang J, Chen S, Peng P, Zhang Q, et al. Perineural invasion and TAMs in pancreatic ductal adenocarcinomas: review of the original pathology reports using immunohistochemical enhancement and relationships with clinicopathological features. J Cancer. (2014) 5:754–60. doi: 10.7150/jca.10238
144. Mu W, Wang Z, Zöller M. Ping-pong-tumor and host in pancreatic cancer progression. Front Oncol. (2019) 9:1359. doi: 10.3389/fonc.2019.01359
145. Zhang B, Guo X, Huang L, Zhang Y, Li Z, Su D, et al. Tumor-associated macrophages and Schwann cells promote perineural invasion via paracrine loop in pancreatic ductal adenocarcinoma. Br J Cancer. (2024) 130:542–54. doi: 10.1038/s41416-023-02539-w
146. Zheng Z, Li X, Chen G, Chen J, Zhu X, Teng Y. Transcriptome analyses reveal new insights on key determinants of perineural invasion in high-grade serous ovarian cancer. Front Cell Dev Biol. (2023) 11:1109710. doi: 10.3389/fcell.2023.1109710
147. Linedale R, Schmidt C, King BT, Ganko AG, Simpson F, Panizza BJ, et al. Elevated frequencies of CD8 T cells expressing PD-1, CTLA-4 and Tim-3 within tumor from perineural squamous cell carcinoma patients. PloS One. (2017) 12:e0175755. doi: 10.1371/journal.pone.0175755
Keywords: malignant tumors, perineural invasion, matastasis, molecular mechanisms, tumor microenvironment, neuro-tumor crosstalk
Citation: Wang M, Pu N, Bo X, Chen F, Zhou Y and Cheng Q (2025) Significance and mechanisms of perineural invasion in malignant tumors. Front. Oncol. 15:1572396. doi: 10.3389/fonc.2025.1572396
Received: 07 February 2025; Accepted: 18 April 2025;
Published: 12 May 2025.
Edited by:
Lei Liang, Zhejiang Provincial People’s Hospital, ChinaReviewed by:
Xiuzhi Jia, Lishui University, ChinaYan Qi, Central People’s Hospital of Zhanjiang, China
Copyright © 2025 Wang, Pu, Bo, Chen, Zhou and Cheng. This is an open-access article distributed under the terms of the Creative Commons Attribution License (CC BY). The use, distribution or reproduction in other forums is permitted, provided the original author(s) and the copyright owner(s) are credited and that the original publication in this journal is cited, in accordance with accepted academic practice. No use, distribution or reproduction is permitted which does not comply with these terms.
*Correspondence: Qiong Cheng, Y3ExOTgxQG50dS5lZHUuY24=; Yilong Zhou, MTM2NDg5MDAwM0BxcS5jb20=
†These authors have contributed equally to this work