- 1The First Clinical Medical College, Shanxi Medical University, Taiyuan, Shanxi, China
- 2Department of Plastic and Reconstructive Surgery, First Hospital of Shanxi Medical University, Taiyuan, Shanxi, China
Melanoma is highly invasive and resistant to conventional treatments, accounting for nearly 75% of skin cancer-related deaths globally. Traditional therapies, such as chemotherapy and immunotherapy, often exhibit limited efficacy and are associated with significant side effects due to systemic drug exposure. Microneedles (MNs), as an emerging drug delivery system, offer multiple advantages, including safety, painlessness, minimal invasiveness, and controlled drug release. Among these, hydrogel microneedles (HMNs) stand out due to their extracellular matrix-like structure and swelling-induced continuous hydrogel channels, which enable the direct delivery of therapeutic agents into the tumor microenvironment (TME). This approach enhances drug bioavailability while reducing systemic toxicity, establishing HMNs as a promising platform for melanoma treatment. This review highlights recent advancements in HMNs for melanoma therapy, focusing on their applications in biomarker extraction for early diagnosis and their role in supporting multimodal treatment strategies, such as chemotherapy, immunotherapy, phototherapy, targeted therapy, and combination therapy. Furthermore, the current matrix materials and fabrication techniques for HMNs are discussed. Finally, the limitations of HMNs in melanoma treatment are critically analyzed, and recommendations for future research and development are provided.
1 Introduction
Melanoma, a malignant tumor originating from melanocytes, has shown a globally increasing incidence (1, 2). As one of the deadliest forms of skin cancer, it accounts for over 60,000 deaths annually worldwide (3). Although conventional treatments—including surgical resection, immunotherapy, radiotherapy, chemotherapy, and targeted therapy (4)—have demonstrated some efficacy, their therapeutic potential is often hindered by the highly invasive and drug-resistant nature of the disease (5). Achieving complete remission frequently requires high drug doses, which not only increases treatment complexity but also elevates the risk of severe side effects (6). These limitations underscore the urgent need for innovative approaches to improve therapeutic outcomes through safer and more efficient drug delivery systems.
Transdermal Drug Delivery Systems (TDDS) represent a non-invasive strategy for delivering drugs directly into dermal or subdermal tissues via the skin (7). By bypassing the gastrointestinal tract, TDDS avoids first-pass metabolism, thereby enhancing drug bioavailability at targeted sites. Additionally, TDDS offers a convenient and painless delivery method (8). Among various TDDS techniques, microneedles have emerged as a promising platform due to their ability to penetrate the epidermis and create microchannels while avoiding contact with capillaries and nerves (9). In cancer therapy, microneedles enable localized delivery of anticancer immunogens, adjuvants, and other therapeutic agents, thereby increasing drug concentrations at tumor sites and reducing toxicity to healthy tissues (10). Recent advancements in MN-based systems have highlighted their potential to enhance cancer treatment outcomes. For instance, Song et al. (11) developed a dissolvable microneedle system for delivering nano-photosensitizers. Under near-infrared (NIR) irradiation, this system effectively eliminated residual breast cancer cells, induced immunogenic cell death, reduced tumor recurrence and metastasis, and enhanced systemic immune memory when combined with aPD-L1 therapy. Similarly, Liu et al. (12) designed a microwave-responsive microneedle patch incorporating magnetic biomimetic metal-organic frameworks and platelets. This system activates platelets, releases therapeutic agents, and promotes deeper drug penetration, showing promise for treating deep-seated tumors such as breast cancer and melanoma. Furthermore, D’Amico et al. (13) utilized microneedle patches to deliver cancer vaccines composed of peptide-coated conditionally replicating adenoviruses and tumor-associated antigens, achieving complete tumor rejection in melanoma models.
Microneedles (MNs) can be categorized into five types based on their drug delivery mechanisms: solid, coated, hollow, dissolving, and hydrogel microneedles (Figure 1) (14). Table 1 summarizes the materials, advantages, and disadvantages of these MN types. Among them, hydrogel MNs, made from hydrogel-based materials, mimic the extracellular matrix and exhibit excellent biocompatibility (15). Upon insertion into the skin, their hydrophilic properties allow them to swell and form continuous hydrogel channels, which are particularly advantageous for biomedical applications such as interstitial fluid (ISF) extraction (16). These channels facilitate drug diffusion, ensuring stable and sustained therapeutic effects (17).
HMNs have demonstrated considerable potential in melanoma treatment due to their efficient drug delivery capabilities, non-invasive nature, and high biocompatibility. These properties enable hydrogel microneedles to address the challenge of low bioavailability while minimizing systemic drug exposure (29). This review explores the applications of HMNs in melanoma diagnosis and therapy, including chemotherapy, targeted therapy, immunotherapy, photothermal therapy (PTT), photodynamic therapy (PDT), and combination therapies (Figure 2). Additionally, it provides an overview of the materials and fabrication techniques employed in HMN production, examines their strengths and limitations in the context of melanoma treatment, and outlines future directions for their development. Ultimately, this review aims to establish a foundation for further research on hydrogel microneedle-based drug delivery systems for melanoma, facilitating their clinical translation and contributing to improved patient outcomes.
2 Design of HMNs
HMNs exhibit exceptional water absorption and swelling properties, enabling the gradual release of drugs after skin penetration, thus facilitating sustained therapy (30). Compared to traditional microneedles, HMNs demonstrate superior biocompatibility and allow for tunable drug release rates by adjusting the crosslinking density, which directly influences the hydrogel’s network structure (31).
2.1 Matrix materials
The performance of HMNs is profoundly affected by the selection of matrix materials, which can be broadly categorized into natural and synthetic hydrogels (32). Table 2 summarizes the commonly used materials for HMNs. Natural hydrogels, including silk fibroin (SF) (33), methacrylated hyaluronic acid (MeHA) (34), gelatin methacryloyl (GelMA) (35), sodium alginate (SA) (36), and chitosan (CS) (37), are frequently employed due to their excellent biocompatibility. Synthetic hydrogels, such as poly (vinyl alcohol) (PVA) (38) and poly (methyl vinyl ether-co-maleic acid) (PMVE/MA) (39), provide enhanced mechanical strength and tunable physicochemical properties, making them particularly suited for sophisticated and complex drug delivery systems (40).
Natural hydrogels exhibit low immunogenicity, excellent cellular compatibility, and biodegradability. Their functional groups enable specific responses targeting particular cells or tissues (50, 51). For instance, silk fibroin methacrylate HMNs have been utilized to deliver α-melanocyte-stimulating hormone (α-MSH), effectively improving melanocyte dysfunction in vitiligo patients (41). Yao et al. (42) developed a wound dressing based on a Zn-MOF (metal–organic framework) encapsulated MeHA microneedle array. This dressing demonstrated antibacterial activity, while the hydrolysis of MeHA promoted tissue repair, epithelial regeneration, and angiogenesis. Similarly, GelMA microneedles have been employed as a delivery platform for the alginateoncolytic Newcastle disease virus (NDV), offering a novel approach in cancer immunotherapy (43). Zhou et al. (44) designed calcium ion (Ca²⁺)-crosslinked alginate swellable microneedles, significantly enhancing the transdermal delivery efficiency of acidic drugs, such as 3-O-ethyl ascorbic acid (EAA), while ensuring stable release over a 16-hour period. Additionally, Suriyaamporn et al. (45) developed a chemotherapy patch based on chitosan HMNs loaded with 5-fluorouracil (5-FU) liposomes. This innovative patch not only promotes wound healing and exhibits anti-cancer activity but also demonstrates no significant toxicity to normal cells.
While natural hydrogels are highly biocompatible, they often lack optimal mechanical properties (52). In contrast, synthetic hydrogels can achieve superior mechanical strength and rigidity by modifying polymer structures or adjusting crosslinking degrees (53). For instance, researchers crosslinked PVA and (polyvinylpyrrolidone) PVP with glutaraldehyde at 95°C, incorporating a spiral geometric design to enhance skin contact area, reduce insertion force, and improve stability. Ultraviolet crosslinking further reinforced compressive resistance, resulting in optimized PVA/PVP/HA (hyaluronic acid) microneedles with a fracture force of 0.13 N, far exceeding the 0.058 N required for skin penetration. Additionally, applying micro-vibration at 50 Hz and 100 Hz during insertion reduced skin resistance and minimized fracture risk (54). Similarly, Li et al. (55) developed crosslinked hydrogels using PVA, PVP, and poly(ethylene glycol) diacid (PEGdiacid), optimizing conditions with a PEGdiacid concentration of 2.25% w/w and crosslinking at 150°C for 20 minutes. A 14×14 conical mold was employed to enhance stress distribution, effectively reducing compressive deformation. The optimized microneedles demonstrated a height reduction of only 7.67% under 32 N pressure and achieved insertion depths of 508–522 μm, successfully penetrating the stratum corneum while maintaining structural integrity. These microneedles exhibited excellent mechanical properties and insertion performance, providing robust support for transdermal drug delivery. Furthermore, synthetic hydrogels exhibit enhanced water absorption capabilities compared to their natural counterparts (50). For example, Zhang et al. (46) developed bioinspired adaptive retention microneedles by combining tunable PVA HMNs with mesenchymal stem cell (MSC)-exosomes for transdermal drug delivery in diabetic wound treatment. By employing 3D printing and template replication techniques, the mechanical properties of the PVA hydrogel were modulated using sulfate ions to increase strength, while nitrate ions softened its structure, allowing for tissue adaptation and controlled exosome release. In another study, Al-Badry et al. (47) developed a microneedle array composed of a copolymer of poly(ethylene glycol) (PEG) and PMVE/MA for the transdermal delivery of acyclovir. This microneedle array achieved a 75.56% release of acyclovir within 24 hours, with a transdermal absorption rate 39 times higher than the control. This approach effectively reduces the adverse effects associated with oral administration and enhances patient compliance.
Advancements in medical technology have highlighted the limitations of traditional materials in drug delivery and personalized therapy. To address these challenges and expand their applications, researchers have explored the integration of novel materials into microneedle fabrication. The incorporation of materials such as Laponite, conductive materials, and light-responsive materials has significantly enhanced the overall performance of HMNs. Odinotski et al. (48) developed a HMN biosensor for real-time pH measurement by integrating dopamine(DA)-conjugated HA hydrogel with poly(3,4-ethylenedioxythiophene): polystyrene sulfonate. The HA hydrogel efficiently collected ISF and enabled precise pH measurement, while PEDOT: PSS improved the microneedles’ electrical conductivity and mechanical strength. Experimental results demonstrated that this HMN biosensor achieved 93% accuracy in pH solution testing, showcasing its potential for wearable sensors and health monitoring applications. Zheng et al. (49) designed a composite hydrogel based on photo-crosslinked MeHA and Laponite (LAP). The addition of LAP significantly enhanced the microneedles’ mechanical strength and extended the release duration of antigens, such as ovalbumin (OVA). This improvement promoted better antigen uptake and presentation efficiency, highlighting the composite’s potential in vaccination applications. Therefore, the selection of materials for HMN fabrication is pivotal, as it directly influences drug delivery precision, safety, and therapeutic efficacy.
2.2 Formation mechanism
The gelation of hydrogels occurs through various mechanisms, with the most common being physical cross-linking and chemical cross-linking (56). These mechanisms synergistically form polymer networks with hydrophilic and water-swelling properties (57). The porous structure of these networks facilitates drug encapsulation, minimizing drug loss and enhancing therapeutic efficacy. Additionally, the tunability of the polymers allows for the optimization of microneedles’ physical and chemical properties, enabling controlled drug delivery (58).
2.2.1 Physical cross-linking
Physical cross-linking relies on non-covalent interactions—such as ionic interactions, hydrogen bonding, and hydrophobic interactions—to form hydrogel networks (59). These hydrogels avoid the use of toxic organic solvents, exhibit high biocompatibility, and are reversible since the interactions between polymer chains do not involve covalent bonds (60). Furthermore, physically cross-linked hydrogels can adjust their mechanical properties in response to environmental conditions, such as temperature and pH (61). However, their mechanical performance is generally lower, and the reversible nature of the cross-linking can lead to reduced stability under physiological conditions (62).
2.2.2 Chemical cross-linking
Chemical cross-linking, in contrast, is an irreversible process that involves the formation of covalent bonds between polymer chains. These bonds are typically created through free radical polymerization, functional group reactions, or high-energy radiation-induced reactions, resulting in a stable three-dimensional network (63, 64). Chemically cross-linked hydrogels exhibit superior mechanical strength and elastic modulus, making them ideal for applications requiring high stability and durability (65). However, chemical cross-linking often requires toxic cross-linking agents, and the process may generate harmful byproducts, raising concerns about environmental and human health risks (66). To conclude, by rationally combining or independently utilizing physical and chemical cross-linking methods tailored to specific medical needs, hydrogels with enhanced performance can be designed, achieving an optimal balance of biocompatibility, mechanical strength, and stability.
2.3 Geometric parameters
When designing HMNs, several geometric parameters must be carefully considered, including microneedle shape, length, tip sharpness, and density (67, 68). These factors are critical to the clinical applicability, manufacturing feasibility, and drug-loading capacity of microneedles, directly influencing their performance across various applications (69). Common microneedle shapes include pyramid-shaped (70), cone-shaped (71), claw-inspired (72), shark tooth-shaped (73), and pagoda-shaped bioinspired structures (74). Studies have shown that increasing the number of edges in the cross-sectional shape of the microneedle tip (e.g., square, hexagonal, and octagonal) enhances swelling capacity and drug release rates. However, simpler cross-sectional shapes with fewer edges allow for more thorough drug release (69). Microneedle length is another critical parameter, particularly in relation to pain perception (75). In a double-blind randomized controlled trial, Gill et al. observed that increasing microneedle length from 480 μm to 1450 μm resulted in a 7-fold increase in pain perception, while variations in width, thickness, and tip angles had no significant effect. Additionally, increasing the number of microneedles tenfold (from 5 to 50) caused only a 2.5-fold increase in pain, highlighting microneedle length as the dominant factor influencing pain perception. Tip sharpness also plays a significant role in insertion efficiency and pain reduction. High-resolution imaging studies have revealed that sharper microneedle tips significantly lower insertion force compared to variations in tip angles, thereby minimizing pain (76). Microneedle density has a notable impact on skin recovery. Low-density microneedle arrays (e.g., 121 MNs/cm²) result in less tissue damage and faster recovery, whereas high-density arrays (e.g., 361 MNs/cm²) can cause greater tissue damage and slower recovery. Excessively high-density arrays may compromise the long-term recovery of the skin barrier and induce the “bed of nails” effect, further exacerbating tissue damage and delaying healing (77). In conclusion, optimizing geometric parameters such as shorter microneedle lengths, enhanced tip sharpness, and appropriately spaced microneedle arrays is essential for achieving minimally invasive, painless, and effective drug delivery.
2.4 Preparation methods
The most common preparation methods for HMNs are micromolding technology (78) and 3D printing-based methods (79). Each method offers unique advantages and is suitable for different production requirements.
Micromolding technology is widely used for HMN fabrication due to its reusable micromolds and high-efficiency mass production capabilities (80). Micromolds can be fabricated using a variety of techniques, including thermoforming, injection molding, laser drilling, and polymer casting, to address diverse precision and manufacturing requirements (81, 82). Among these, polydimethylsiloxane (PDMS) is the most commonly used micromold material (75). Its flexibility and hydrophobicity make it an optimal choice for microneedle fabrication (83). PDMS microneedle templates are typically prepared using a replica molding process. In this method, solid MNs—mechanically rigid materials—are used as the master mold, onto which a mixture of PDMS prepolymer and curing agent is cast. After curing, the PDMS template is formed. The polymer solution is then filled into the template, and vacuum or centrifugal forces are applied to ensure uniform distribution. Microneedle formation is completed through drying or photopolymerization, followed by demolding (84, 85). This process is simple, efficient, and cost-effective due to the reusability of PDMS templates, making it ideal for scalable HMN production.
As an additive manufacturing technique, 3D printing constructs three-dimensional structures by depositing materials layer by layer. Its high precision and rapid prototyping capabilities have made it an innovative approach for microneedle fabrication (86). Among the various 3D printing methods, photopolymerization is the most commonly employed technique. Photopolymerization selectively cures photosensitive materials using lasers or light sources to build microneedle structures layer by layer (87). Common photopolymerization methods include Digital Light Processing and Stereo Lithography Apparatus, which enable the high-precision fabrication of HMNs with excellent tensile properties and biocompatibility (88). Compared to traditional micromolding, 3D printing not only allows for the direct fabrication of complex microneedle geometries but also provides precise control over size, shape, and structure, enabling highly customizable designs (89). Additionally, 3D printing can be used to produce microneedle negative molds, which, when combined with replica molding processes, further optimize production workflows. This integration significantly enhances both the flexibility and efficiency of microneedle manufacturing (79).
3 HMNs for melanoma diagnosis
The early clinical manifestations of melanoma closely resemble those of benign pigmented nevi, which limits the diagnostic accuracy of dermoscopy as a non-invasive tool (90). Due to the rapid metastatic progression of melanoma, most patients are diagnosed at advanced stages, leading to poor prognosis (91). This underscores the urgent need for novel biomarkers to improve diagnostic accuracy. Melanoma-associated biomarkers, such as the S100 protein family, miRNA, and exosomes, have been identified in blood (92). While microneedles have been utilized to extract biomarkers like S100B, tyrosinase, and lactate from skin ISF for diagnostic purposes, fluorescence- or colorimetric-based detection methods often lack quantification capabilities, negatively affecting diagnostic specificity (93). Studies have shown that serum S100A1 expression levels are significantly elevated in melanoma patients compared to non-melanoma individuals (94). However, S100A1 overexpression is also associated with other diseases, including breast cancer, cardiovascular diseases, and ovarian cancer, which limits its specificity for melanoma diagnosis (95, 96). To address this challenge, Wang et al. (97) developed a HMN patch based on MeHA for the early diagnosis of melanoma. This microneedle patch extracts S100A1 from skin ISF and utilizes antibody-conjugated magnetic microparticles (MMPs) and polystyrene microparticles (PMPs) for the quantitative measurement of S100A1 concentration, achieving an impressive detection limit of 18.7 ng/mL. Animal experiments demonstrated that this method exhibits higher sensitivity at lower concentrations compared to conventional serum-based detection methods. To enhance usability, the researchers incorporated microfluidic technology, which made the detection results more intuitive and easier to interpret. The integration of microneedles with a microfluidic system streamlined the operational workflow, making it suitable for home use or routine skin examinations (Figure 3). However, despite its high sensitivity, this method is currently limited to detecting S100A1 alone, excluding other potential melanoma biomarkers, which restricts its broader applicability. Additionally, the reliance on a standard curve for quantification introduces the possibility of errors under varying conditions, potentially impacting the accuracy of measurements.
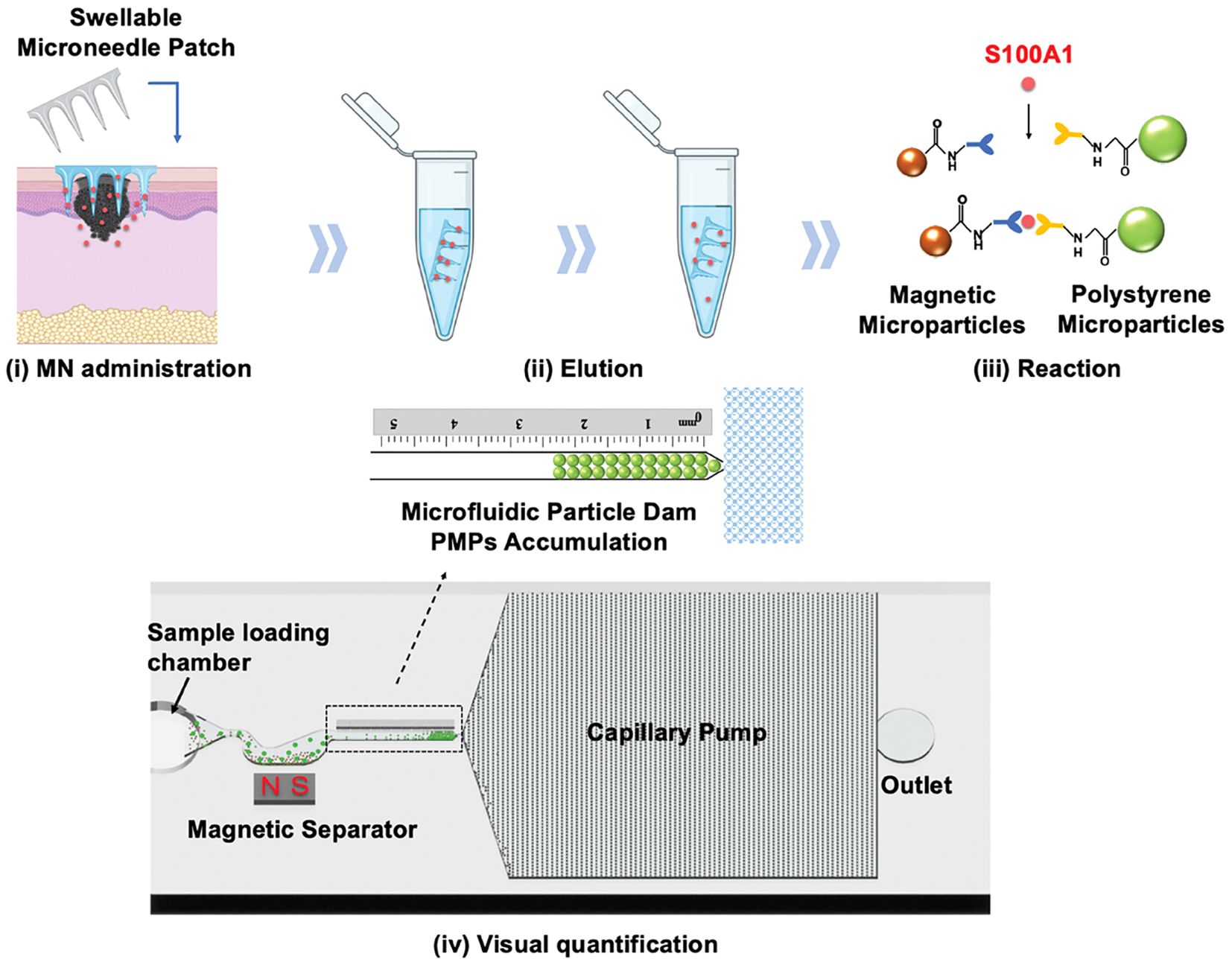
Figure 3. Schematic illustration of the HMN patch for melanoma diagnosis. (i) ISF is extracted using the microneedle patch, (ii) eluted into a centrifuge tube, (iii) where S100A1 binds to MMPs and PMPs, and (iv) its concentration is quantified by measuring PMP accumulation. Copyright permission from Wang et al. (97), Advanced Science, 2024.
4 HMNs for melanoma treatment
HMNs have shown great potential as a drug delivery platform for melanoma treatment. Their unique properties, including excellent drug-controlled release, biocompatibility, and minimally invasive transdermal drug delivery, make them an attractive option for localized melanoma therapy. Compared to conventional treatments, HMNs can significantly reduce systemic toxicity, precisely target tumor sites, enhance therapeutic efficacy, and minimize drug-related side effects. Accordingly, this section provides a comprehensive summary of the applications of HMNs in melanoma treatments, encompassing chemotherapy, immunotherapy, targeted therapy, phototherapy, and synergistic therapy (see Table 3). This overview aims to serve as a valuable reference for developing related therapeutic strategies.
4.1 Microneedles for chemotherapy
Chemotherapy remains a cornerstone in anti-cancer treatments; however, its therapeutic efficacy is significantly hindered by low bioavailability, insufficient drug accumulation in tumor regions, systemic toxicity, and the development of chemoresistance (110, 111). Commonly used chemotherapeutic drugs for melanoma, such as Paclitaxel, Doxorubicin (DOX), and Cisplatin, are associated with systemic toxicity and adverse side effects (112, 113). Delivering chemotherapeutic agents directly to tumor sites via MNs represents a safer and more effective therapeutic strategy.
In drug delivery systems, one of the critical challenges is achieving an optimal drug release rate, as release tends to be either too fast or too slow. To address this, Luo et al. (98) developed a microneedle patch based on GelMA for the sustained and controlled release of the anticancer drug DOX, facilitating effective melanoma treatment. Experimental results indicated that GelMA microneedles loaded with DOX could efficiently penetrate mouse skin. By tuning ultraviolet exposure time and intensity, the crosslinking density of the microneedles could be adjusted, allowing control over their mechanical properties and drug release behavior. Specifically, low crosslinking density resulted in rapid swelling and accelerated drug release, whereas high crosslinking density slowed the release process. Despite its widespread use, the efficacy of DOX is often reduced by P-glycoprotein (P-gp)-mediated multidrug resistance. To overcome this limitation and enhance therapeutic outcomes, Huang et al. (99) developed a novel microneedle system using Dextran Methacrylate (DexMA) hydrogel, which co-delivers DOX and the resistance-reversal drug Trametinib (Tra). This dual-drug system effectively inhibits the efflux of DOX and reverses P-gp-mediated drug resistance. Experimental results demonstrated that the synergistic effect of DOX and Tra significantly improved local anti-melanoma efficacy while minimizing systemic toxicity and side effects. Although microneedles provide sustained drug release, the release rate may be limited by variations in drug solubility, such as the poor water solubility of Tra. Furthermore, the long-term toxicity of DOX to healthy tissues has not been fully evaluated. To address this limitation, Chen and colleagues (100) developed a bioorthogonal catalytic patch based on a PVA matrix to enhance anti-melanoma efficacy while reducing DOX-associated toxicity in healthy tissues. This microneedle array patch was loaded with a composite material consisting of palladium (Pd) nanoparticles and titanium dioxide nanosheets (TiO₂ nanosheets, TNSs), collectively referred to as Pd-TNSs. The palladium nanoparticles act as a catalyst, enabling the activation of the prodrug N-allyloxycarbonyl-doxorubicin (alloc-DOX) via a decaging process, which results in the release of the active drug, DOX (Figure 4). Notably, this reaction occurs exclusively within the specific catalytic environment of the tumor site, thereby minimizing off-target reactions in vivo. In a mouse melanoma model, the combination of alloc-DOX and the microneedle array patch demonstrated superior tumor growth inhibition compared to the group treated with DOX alone. Importantly, alloc-DOX itself exhibited minimal activity in healthy tissues, further reducing the risk of systemic toxicity. Although the methods utilized by the aforementioned research teams have demonstrated outstanding performance in animal studies and in vitro models, they have not yet been fully validated through large-scale clinical trials. Further research is required to assess their clinical applicability and accuracy.
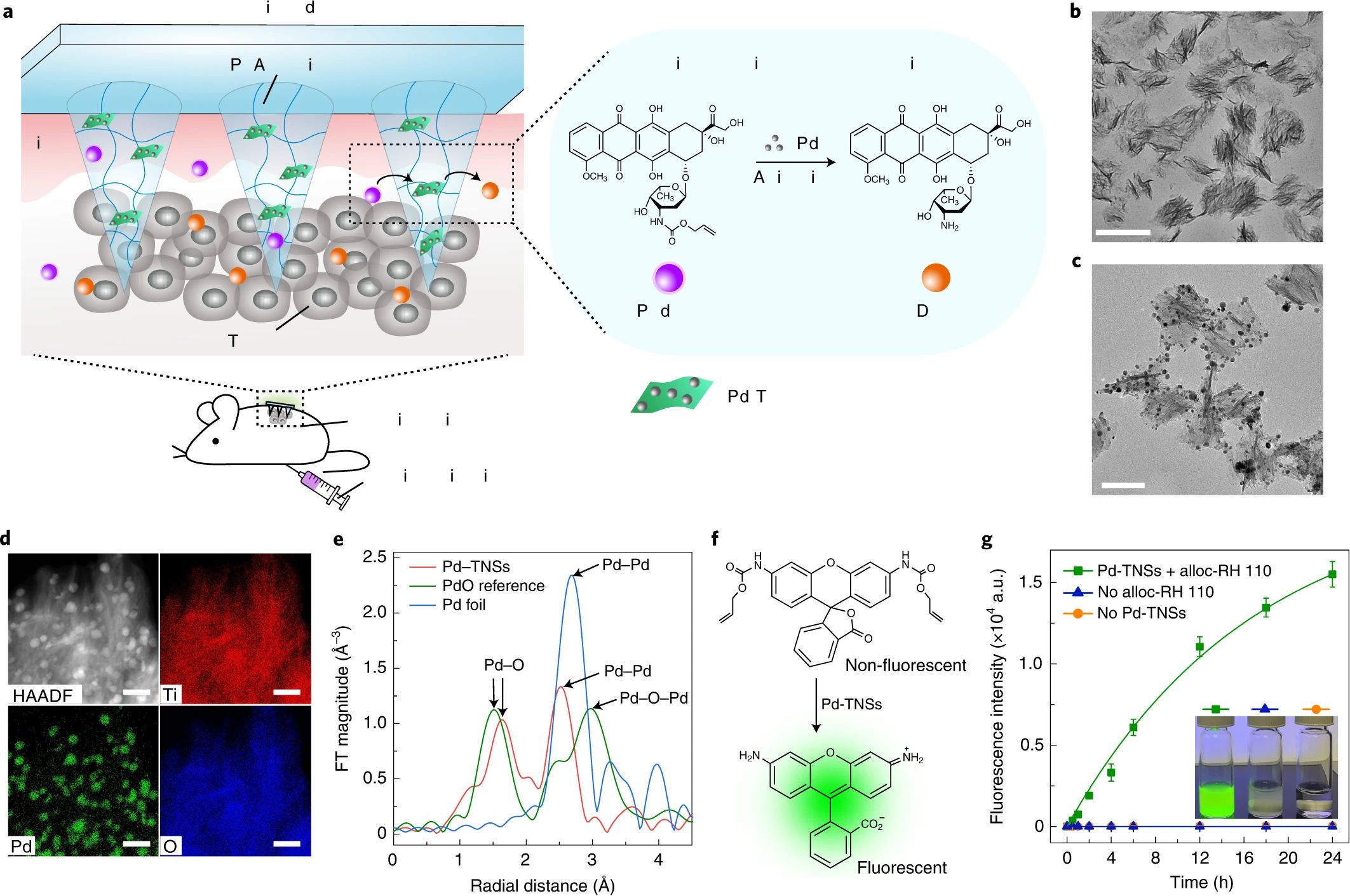
Figure 4. Schematic design of the MN patch for chemotherapy and characterization of Pd-TNSs.(a) Schematic illustration of bioorthogonal catalysis; (b) transmission electron microscope images of TNSs and (c) Pd-TNSs; (d) elemental mapping analysis of Pd-TNSs; (e) structural characterization of palladium in Pd-TNSs through extended X-ray absorption fine structure (EXAFS); (f) schematic representation of the decaging process; (g) fluorescence intensity measurements for monitoring drug activation and release. Copyright permission from Chen et al. (100), Nature Nanotechnology, 2021.
4.2 Microneedles for immunotherapy
Immunotherapy has demonstrated high efficacy in treating melanoma by mobilizing or modulating the body’s immune system to recognize and attack cancer cells, thereby effectively controlling or eliminating the disease (114). Emerging immunotherapy technologies, such as polymer nanoparticles (115) and engineered immune cells (116), have shown great promise in cancer treatment. These technologies primarily facilitate the delivery of immunomodulators or create immunogenic microenvironments at tumor sites to activate immune cells.
Cytosine-phosphate-guanine oligodeoxynucleotides (CpG-ODNs) are innate immune-activating molecules that bind to toll-like receptors on immune cell surfaces, triggering antitumor immune responses (117, 118). However, as highly water-soluble single-stranded DNA molecules, CpG-ODNs are prone to nuclease-mediated degradation, which reduces their therapeutic efficacy (119). Protecting CpG-ODNs from degradation, improving their stability, and addressing the inefficiency and dose variability associated with traditional delivery methods remain major challenges. To overcome these limitations, Dosta et al. (101) encapsulated CpG-ODNs in nanoparticles and developed a crosslinked HA microneedle platform for melanoma treatment. This platform enhances the stability of CpG-ODNs, protects them from enzymatic degradation, and facilitates precise delivery at tumor sites. CpG-ODNs activate bone marrow-derived dendritic cells and bone marrow-derived macrophages by binding to TLR9 receptors on immune cells. This activation induces cytokine release, modulates the TME and stimulates robust antitumor immune responses. Experimental studies have demonstrated that the delivery of CpG-loaded nanoparticles via this microneedle platform significantly slows tumor growth and prolongs survival in melanoma-bearing mice. Moreover, the platform incorporates theranostic capabilities by collecting ISF from the skin to analyze immune markers, enabling precise monitoring of therapeutic efficacy (Figure 5). This dual-function approach integrates treatment and non-invasive biomarker monitoring, improving the overall efficacy of cancer immunotherapy.
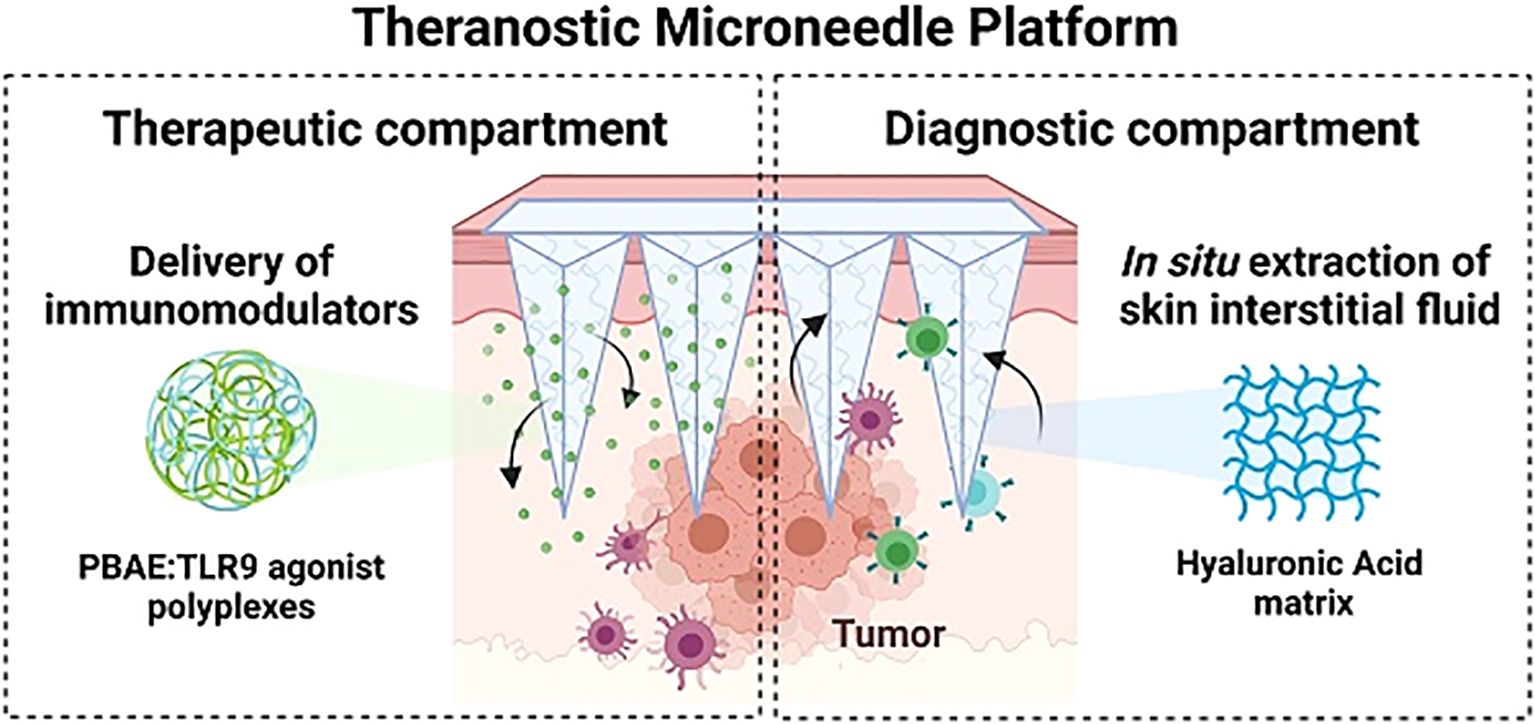
Figure 5. Schematic representation of the microneedle platform designed for CpG-ODN delivery and concurrent extraction of ISF. Copyright permission from Dosta et al. (101), Theranostics, 2023.
Nevertheless, traditional microneedle technology still faces limitations in sensitivity and quantification for biomarker detection after immunotherapy, particularly when monitoring low-concentration cytokines. To address these challenges, Dahis et al. (109) developed a hyaluronic acid HMN technology. This approach allows for the collection of local ISF which contains inflammatory markers closely associated with melanoma immune responses. When combined with ultrasensitive single molecule arrays, it enables precise detection of changes in key cytokine concentrations, such as IFN-β and IL-6. This system was utilized to evaluate immune responses trig-gered by a nanoparticulate stimulator of interferon genes agonist in combi-nation with thermal ablation therapy. This innovative strategy allowed for the dynamic monitoring of inflammation and tumor regression during treatment, under-scoring its potential for non-invasive, real-time monitoring. However, variability in immune system responses among patients poses a significant challenge, as the efficacy of microneedle-delivered drugs may differ between individuals. Customizing treatment strategies based on a patient’s immune status and tumor characteristics remains a critical hurdle. Future advancements in this platform to facilitate tailored treatment approaches could play a pivotal role in the development of precision medicine for cancer therapy.
4.3 Microneedles for targeted therapy
Targeted therapy has significantly improved survival rates in melanoma patients by selectively inhibiting specific genetic mutations or signaling pathways involved in cancer progression (120).
Curcumin, a phytochemical extracted from turmeric, exhibits potent anticancer activity by modulating various cancer-related signaling pathways and enhancing anti-melanoma effects (121). However, curcumin’s extremely limited solubility in aqueous environments severely restricts its absorption and bioavailability in vivo (122). Traditional oral delivery methods fail to overcome these limitations, resulting in insufficient blood concentrations and reduced therapeutic efficacy (123). To address these challenges, Zhou et al. (102) developed a melanoma-targeted microneedle delivery system composed of polymer conjugates of GelMA and β-cyclodextrin (GelMA-β-CD). β-Cyclodextrin plays a key role by forming an inclusion complex with curcumin molecules through its hydrophobic core, thereby improving curcumin’s solubility and stability. This microneedle system demonstrated high anticancer efficacy in B16F10 melanoma cells and 3D tumor models. Compared to traditional delivery methods, microneedle arrays efficiently deliver curcumin directly to melanoma sites, increasing local drug concentrations while minimizing systemic side effects.
Tyrosinase is a key enzyme involved in the formation and progression of melanoma. Studies have demonstrated that tyrosinase inhibitors can suppress melanoma cell growth and induce apoptosis (124). Azelaic acid (AZA), a natural and potent tyrosinase inhibitor, has shown significant potential in inhibiting melanoma progression (125). Similarly, Matrine (MAT), the primary alkaloid extracted from the traditional Chinese herb Sophora flavescens, exhibits antitumor effects by activating the PTEN pathway. In melanoma cell lines, MAT has been shown to effectively inhibit cell proliferation and invasion, as well as induce apoptosis (126, 127). To leverage these therapeutic benefits, Xing et al. (106) developed HMNs using PVA and PVP as primary materials. The HMNs were prepared through infrared radiation heating, followed by annealing. The preparation method involved infrared irradiation at 70°C for 2 minutes, resulting in microneedles with optimal swelling properties, mechanical strength, and biocompatibility. These HMNs were loaded with AZA and MAT for targeted melanoma therapy. In a melanoma mouse model, the drug-loaded HMNs achieved a tumor inhibition rate of 90.53%, significantly outperforming the control group. The microneedles effectively released AZA and MAT simultaneously, maintaining stability during a six-month storage period without significant degradation or the generation of impurities (Figure 6). These findings highlight the potential of HMNs as a promising platform for targeted melanoma therapy, combining high efficacy with excellent stability and biocompatibility.
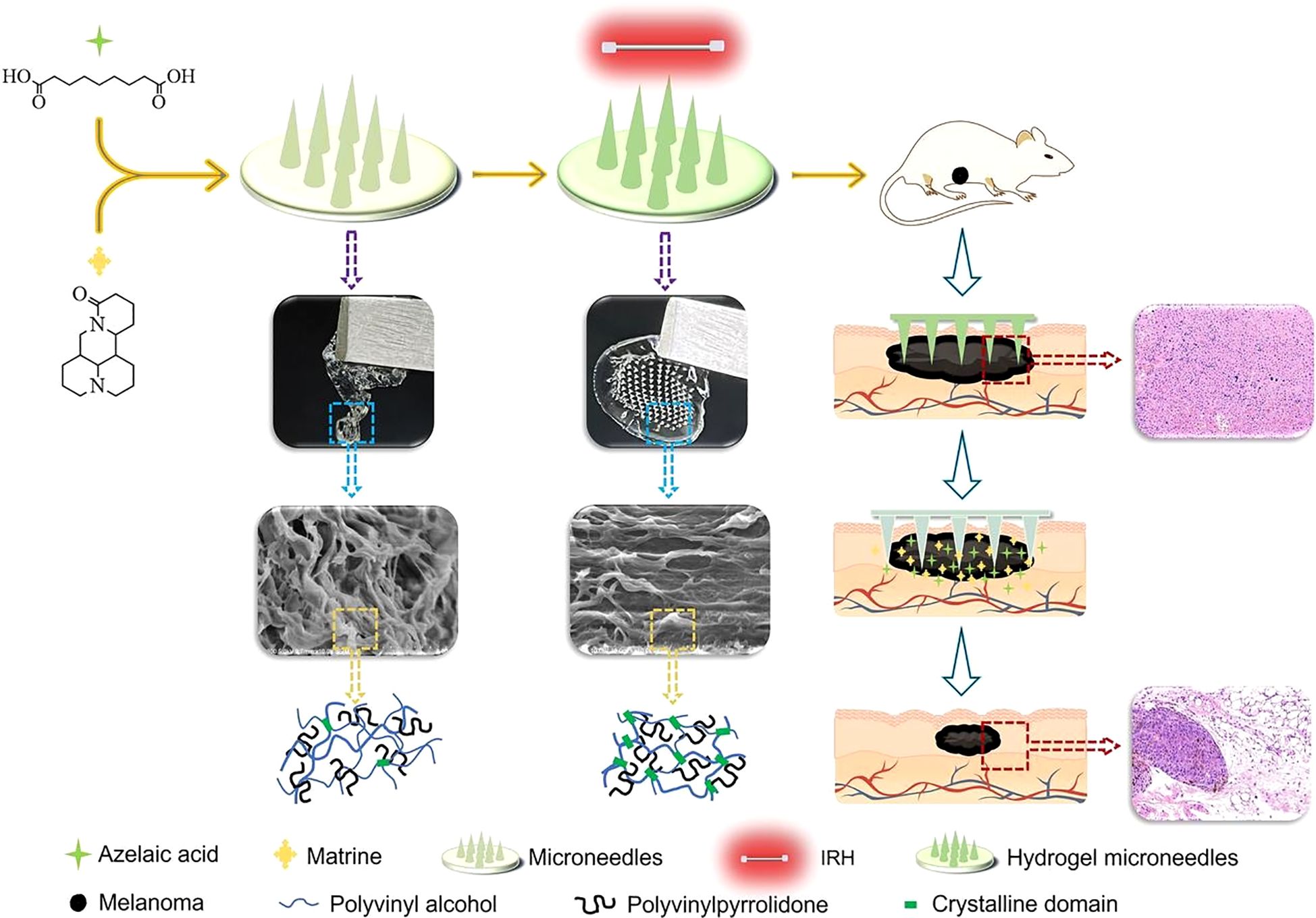
Figure 6. Schematic representation of HMNs for targeted melanoma therapy. Copyright permission from Xing et al. (106), International Journal of Pharmaceutics, 2024.
4.4 Microneedles for phototherapy
Traditional therapies for advanced melanoma are often associated with significant limitations, including severe side effects and inadequate drug concentrations at the tumor site. Although immunotherapy and targeted therapy have shown considerable progress, drug resistance remains a persistent challenge, leading to reduced therapeutic efficacy (128). To overcome these issues, researchers have developed phototherapy approaches such as PTT and PDT. PTT utilizes NIR light to convert light energy into heat, selectively destroying tumor cells while minimizing damage to normal cells (129). Similarly, PDT is a widely recognized non-invasive tumor treatment that involves the activation of photosensitizers to react with oxygen, producing cytotoxic reactive oxygen species (ROS) that selectively destroy tumor cells (130). However, the clinical application of PDT is hindered by several challenges, such as hypoxia in solid tumors (131, 132), skin phototoxicity caused by photosensitizers (133), and the neutralization of ROS by the elevated levels of glutathione (GSH) in cancer cells (134). To address these limitations, Li et al. (104) developed a HMN patch, termed MN-CZCH, as a drug delivery system to enhance the efficacy of PDT (Figure 7). Constructed from hyaluronic acid, the microneedle patch efficiently penetrates the stratum corneum to deliver the photosensitizer 2-(1-hexyloxyethyl)-2-divinylpyropheophorbic-a directly to the tumor site. The patch also incorporates a self-supplying oxygen delivery system consisting of a Cu²⁺-doped porous zeolitic imidazolate framework incorporated with catalase (CZCH). This system facilitates drug delivery while catalyzing the production of oxygen from hydrogen peroxide, thereby alleviating tumor hypoxia. Additionally, copper ions (Cu²⁺) deplete intracellular GSH enhancing the cytotoxic effects of ROS. In a melanoma mouse model, the MN-CZCH group demonstrated superior tumor inhibition under laser irradiation compared to other treatment groups, achieving a tumor growth inhibition rate of 97.7%. This performance was significantly better than that achieved with intravenous or intratumoral injection therapies. Similarly, Chi et al. (108) developed nanocomposite hydrogel microneedles with tunable mechanical strength and controllable transdermal delivery efficiency for PDT in melanoma treatment. This was achieved using an enzyme-mediated strategy. The photosensitizer tetrakis (1-methyl-4-pyridinio) porphyrin (TMPyP) was encapsulated within poly(lactic-co-glycolic acid) nanoparticles, which were then combined with a hyaluronic acid-tyramine (HAT) hydrogel. This combination enhanced the mechanical strength of the microneedles and improved drug release efficiency, providing an effective platform for PDT in melanoma therapy. Phototherapy holds significant promise as an innovative approach for precision tumor treatment.
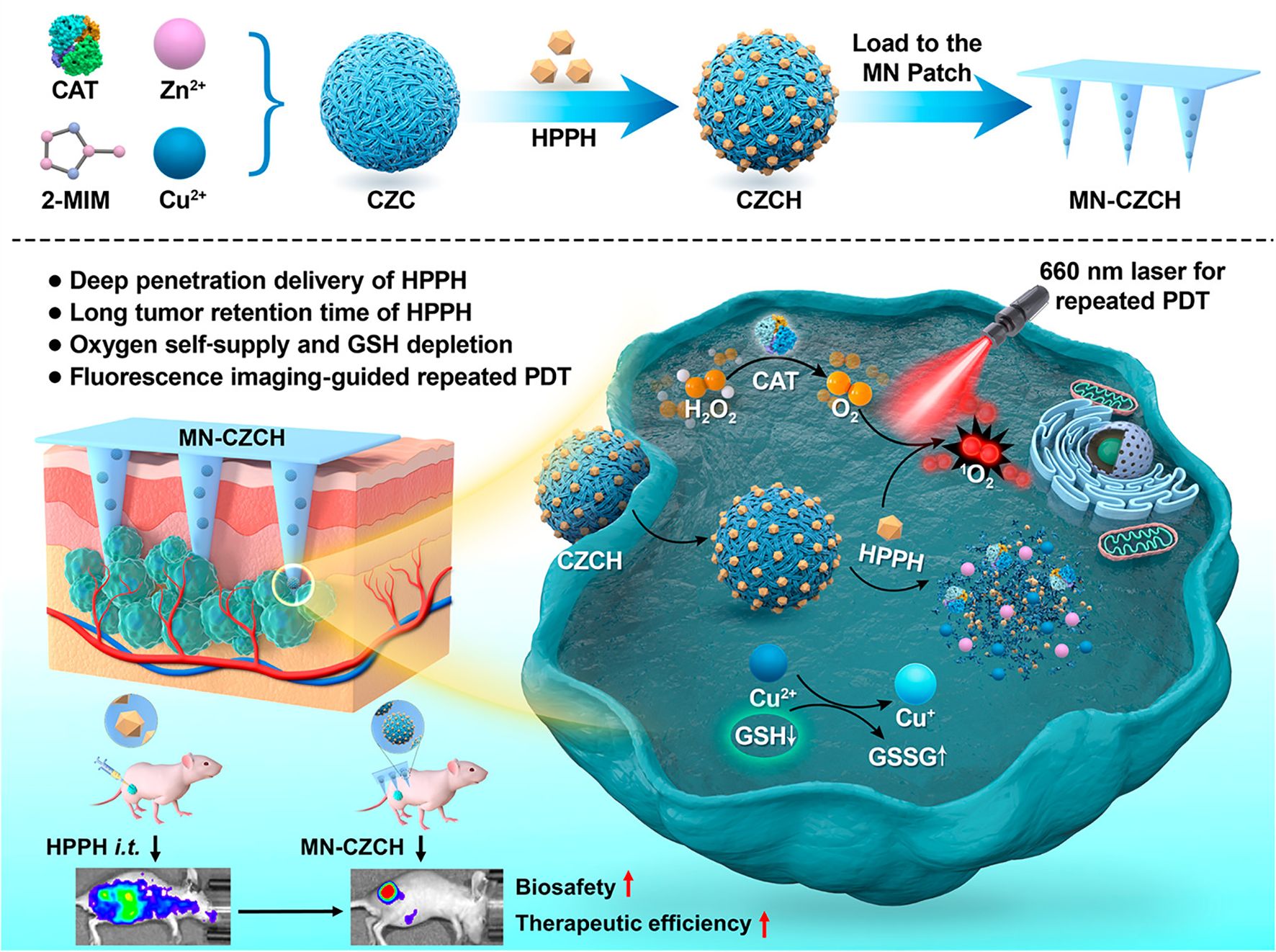
Figure 7. Schematic representation of HMNs for PDT in melanoma treatment. Copyright permission from Li et al. (104), American Chemical Society, 2022.
4.5 Microneedles for synergistic
Therapies Single-treatment approaches for melanoma often fail to achieve optimal outcomes due to limitations such as non-specific drug uptake, uncontrolled release, and systemic side effects (135). In recent years, combination therapy strategies have shown significant promise in addressing these challenges. Compared to monotherapy, microneedles used in combination therapies offer several advantages, including enhanced therapeutic efficacy, reduced drug doses, and decreased dosing frequency (136, 137). Additionally, microneedle-based combination therapies improve treatment convenience and minimize drug-induced damage to normal tissues. These features not only enhance patient compliance but also reduce off-target effects, making them particularly advantageous for melanoma treatment.
4.5.1 Synergistic therapy of chemotherapy and photocatalysis
The standalone application of chemotherapy is often limited by severe side effects and suboptimal efficacy. Combining chemotherapy with photocatalysis offers a promising strategy to address the limitations of single-treatment approaches. Recent studies have highlighted the therapeutic potential of carbon monoxide (CO), an endogenous signaling molecule involved in various physiological and pathological processes (138, 139). In cancer therapy, CO has been shown to enhance tumor cell sensitivity to chemotherapeutic drugs (140), suppress inflammation, and protect normal cells (141, 142). Photocatalytic CO2 reduction technology has emerged as a novel method for controlled CO delivery (143, 144). This approach converts CO2 into CO via a photocatalytic reaction, enabling precise and localized CO generation under light irradiation (145). However, current technologies face challenges, including the low concentration of CO2 at the tumor site and the toxicity of photocatalytic agents, necessitating the development of safer and more efficient delivery platforms. Addressing these challenges, Yu et al. (105) introduced a novel microneedle reactor that integrates photocatalytic CO2 reduction technology for precise, localized CO generation and delivery. This innovative platform enhances tumor responsiveness to chemotherapeutic agents and demonstrates significant inhibitory effects against melanoma (Figure 8). The microneedle reactor is fabricated from a copolymer of hydroxyethyl methacrylate (HEMA) and N-vinyl-2-pyrrolidone (NVP) and incorporates effervescent agents, therapeutic payloads, and photocatalysts. Effervescent agents, such as tartaric acid and sodium bicarbonate, react with body fluids to release CO2, while copper sulfide nanosheets act as the photocatalyst, converting CO2 into CO under 660 nm light irradiation. This process enables the localized and controlled delivery of CO to the tumor site. CO enhances the cytotoxic effects of chemotherapeutic drugs, such as cisplatin, by inhibiting DNA repair mechanisms in tumor cells. In vivo experiments demonstrated that the combination of microneedle-delivered CO and cisplatin treatment achieved superior tumor suppression in a melanoma mouse model. Tumor growth in the combination treatment group was only 1.2 times the initial tumor volume, significantly outperforming other treatment groups. Microneedle systems utilizing photocatalysis-generated CO enhance the sensitivity of chemotherapy by inhibiting DNA repair mechanisms and modulating tumor metabolism. The anti-inflammatory and cytoprotective properties of CO further contribute to mitigating chemotherapy-induced toxicity. Moreover, the microneedle platform enables the integration and controlled release of multiple therapeutic strategies. Despite its potential, this platform still faces challenges, including limited light penetration depth, difficulties in achieving precise control of CO concentrations, and potential risks associated with catalyst residues.
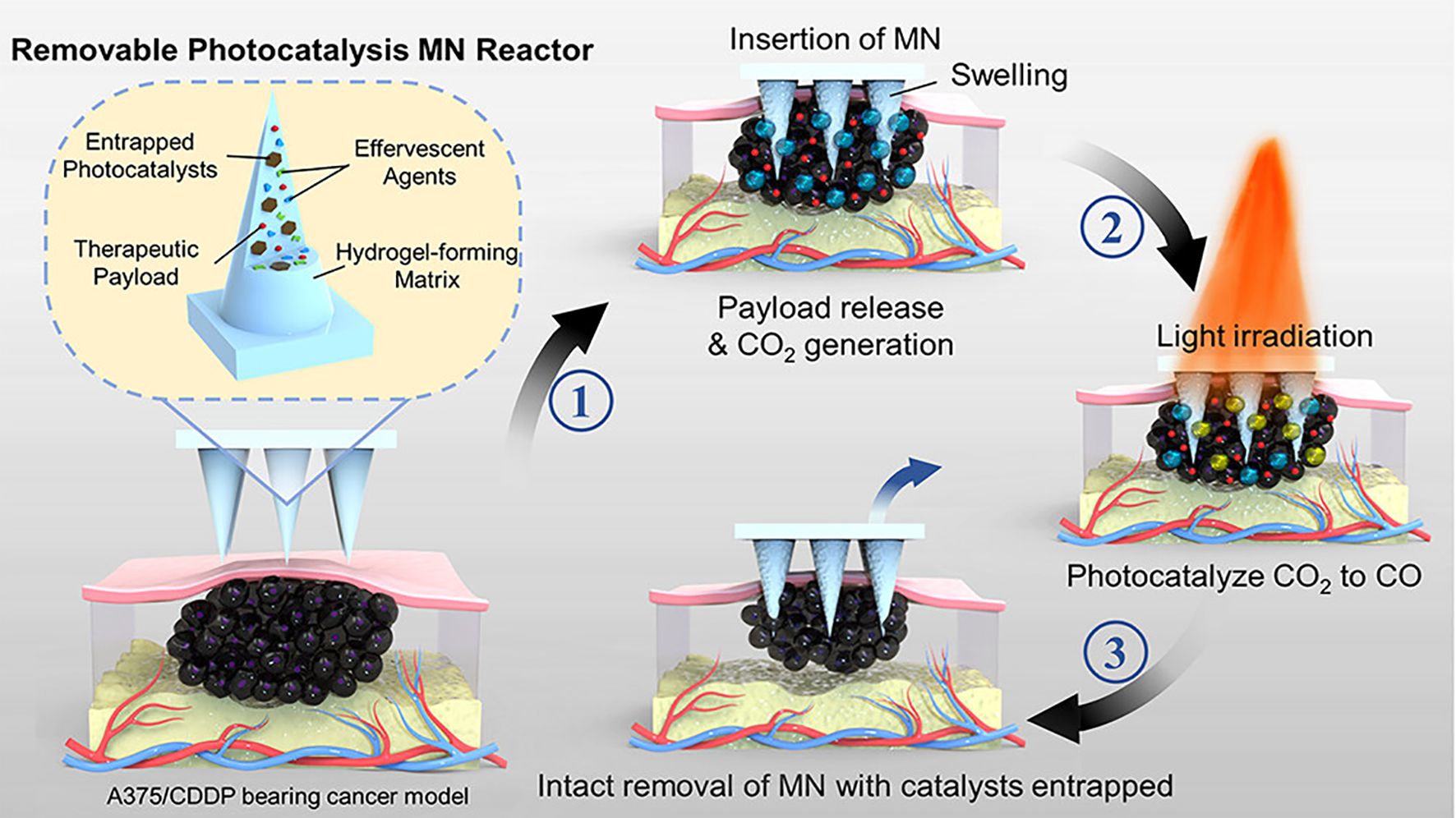
Figure 8. Schematic representation of a HMN platform for synergistic chemotherapy and photocatalytic therapy in the treatment of melanoma. Copyright permission from Yu et al. (105), Nano Letters, 2024.
4.5.2 Synergistic therapies for immunotherapy-related approaches
In melanoma immunotherapy, dendritic cell (DC)-based vaccines have demonstrated the potential to enhance immune responses (146). However, current DC engineering approaches face several limitations, including complex and costly ex vivo operations and poor lymph node homing ability, which reduce their anticancer efficacy (147, 148). To address these issues, Ye et al. (103) developed a melanin-mediated MeHA microneedle patch that integrates PTT with immunotherapy to strengthen antitumor immunity. This microneedle patch incorporates melanin nanoparticles and the immune adjuvant granulocyte-macrophage colony-stimulating factor (GM-CSF). Upon NIR light irradiation, the melanin nanoparticles absorb NIR light and convert it into heat, inducing local hyperthermia in tumor tissues. This process not only directly destroys tumor cells but also activates immune cells. Simultaneously, GM-CSF released from the microneedles promotes the activation of DCs, enabling them to recognize tumor antigens and stimulate T cells, thereby amplifying the antitumor immune response. The experimental results indicated that 87% of treated mice achieved complete tumor regression, and tumor growth was significantly slowed. This dual approach, leveraging both thermal effects and immune activation, effectively treated local tumors while simultaneously stimulating a systemic immune response, offering the potential to inhibit the growth of distant tumors. Microneedle systems for photothermal-immunotherapy offer promising therapeutic advantages. The melanin-mediated photothermal effect induces localized heat shock responses, facilitating the release of inflammatory factors and the recruitment of immune cells. Notably, GM-CSF and whole tumor lysates work synergistically to induce a robust systemic antitumor immune response.
Traditional surgical treatments for melanoma face critical limitations, including the risk of tumor recurrence following excision and inadequate attention to skin regeneration during the treatment process. To address these challenges, Zhang et al. (107) developed a dual-functional microneedle hydrogel system based on hyaluronic acid, enabling synergistic tumor ablation and skin regeneration. This system features a nanomaterial encapsulated in the microneedle tips, comprising Carbon nanotubes@calcium peroxide@tannic acid-Fe/chlorin e6 (CCa@TF/Ce6). Under dual-laser irradiation, these materials generate ROS and heat through PTT and PDT. This process induces immunogenic cell death, promotes DC maturation, and remodels tumor-associated macrophages, ultimately activating autologous immunity to eliminate tumors. In a melanoma mouse model, the dual-laser irradiation group exhibited the strongest anti-melanoma effects, with significantly reduced tumor cell proliferation and a substantial decrease in tumor volume. Following tumor ablation, the microneedle base, composed of 3-aminophenylboronic acid-grafted HA-incorporated PVA hydrogel, facilitated tissue repair by reducing oxidative stress and promoting cell proliferation and migration. These findings underline the development of a therapeutic platform that integrates both antitumor efficacy and tissue regeneration capabilities (Figure 9). Nevertheless, key challenges remain to be addressed, including the validation of long-term efficacy, simplification of treatment protocols, and enhancement of system adaptability for personalized therapies, which are pivotal for advancing clinical translation. This synergistic therapy integrates PTT and PDT to locally generate high temperatures and ROS at the tumor site, effectively killing tumor cells. A notable advantage of this combination is its ability to remain effective under the hypoxic conditions commonly encountered in the tumor microenvironment. Furthermore, the immunogenic cell death induced by PTT and PDT enhances the immune system’s capacity to recognize and eliminate tumor cells. Despite these benefits, the immune-synergistic therapies discussed above also carry potential risks. While immune activation is essential for effective antitumor therapy, excessive immune responses may cause damage to normal tissues or trigger allergic reactions. Healthy skin tissues surrounding the tumor site are particularly vulnerable to immune-mediated inflammatory responses. Additionally, the localized thermal effects of PTT may harm adjacent normal tissues, especially when precise targeting of the treatment area is not achieved. Therefore, optimizing the synergistic ratios of therapeutic components and conducting comprehensive safety evaluations are critical to advancing these therapies toward clinical applications.
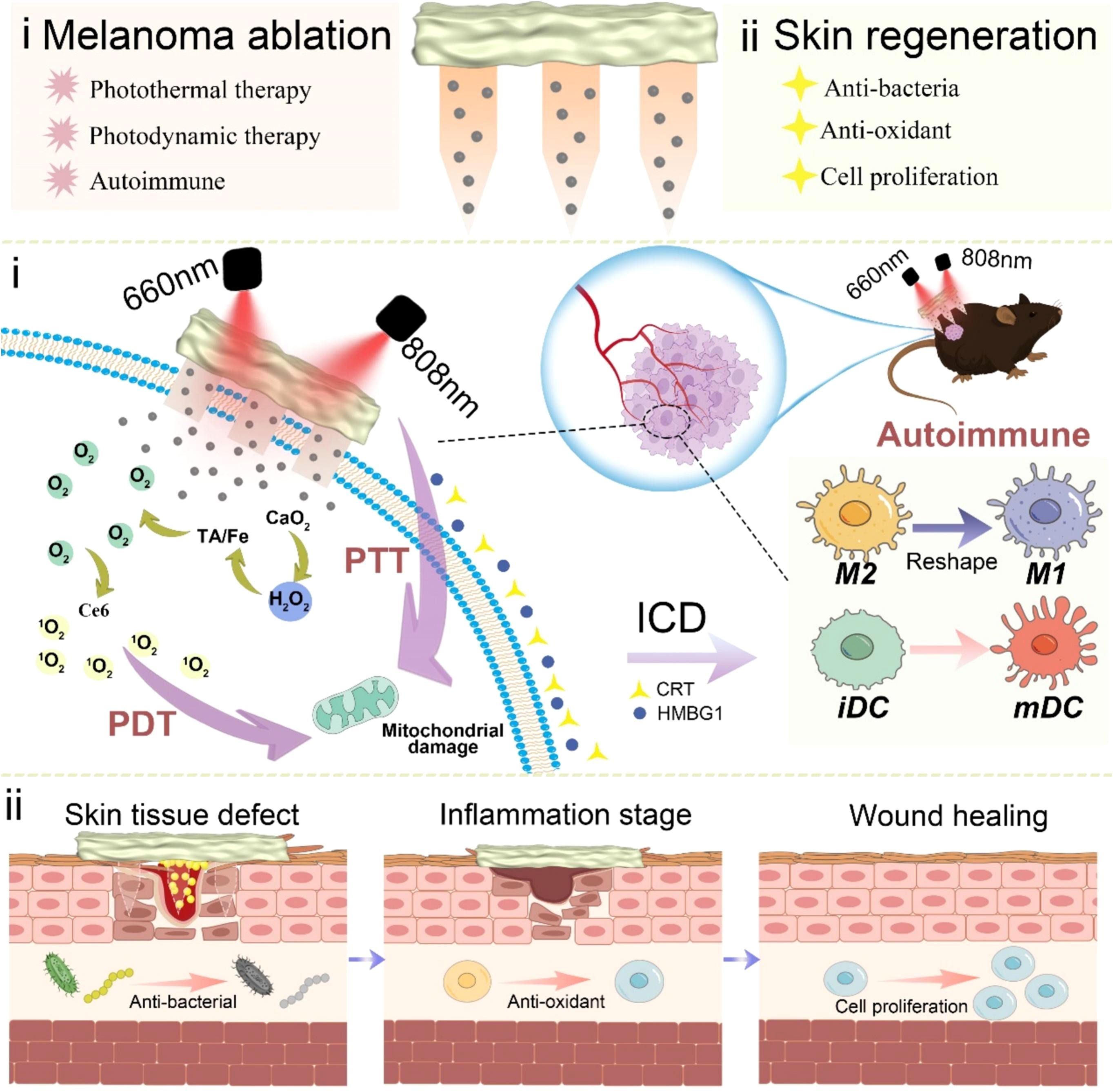
Figure 9. Schematic representation of a HMN platform for immune synergistic therapy in melanoma ablation and subsequent skin regeneration. Copyright permission from Zhang et al. (107), International Journal of Biological Macromolecules, 2024.
5 Conclusion and outlook
Melanoma, known for its high invasiveness, metastatic potential, and resistance to treatment, remains one of the most challenging cancers to manage. In recent years, hydrogel microneedle technology has emerged as a groundbreaking innovation in melanoma care, offering distinct advantages across the entire continuum—from early detection to treatment monitoring. Current research primarily focuses on three key areas: diagnosis, treatment, and materials, with significant progress achieved in each. From a diagnostic perspective, HMN technology has shown great promise in extracting and quantifying melanoma biomarkers, providing innovative, minimally invasive methods for early detection. In terms of therapeutic strategies, chemotherapy, immunotherapy, targeted therapy, phototherapy, and their multimodal synergistic combinations have opened new pathways for improving treatment outcomes. On the materials innovation front, developments range from the creation of foundational materials (e.g., hyaluronic acid, PVA, and GelMA) to intelligent responsive systems and optimized fabrication techniques, such as infrared-assisted manufacturing (149). In practical applications, HMNs enhance tumor sensitivity to therapeutic agents, reduce systemic drug toxicity, and improve drug targeting and bioavailability (150). Their minimally invasive nature enhances patient compliance, while the precise control of drug release opens new possibilities for personalized medicine (151). Furthermore, the integration of therapeutic and monitoring functions into a single platform enables real-time tracking and intervention, paving the way for more comprehensive and effective melanoma treatment strategies.
Despite these promising advancements, several significant challenges persist at both the technical and clinical translation stages. Technically, key challenges include variability in drug release control, insufficient mechanical strength of materials that compromise penetration efficiency, and limitations in drug-loading capacity. Additionally, issues related to the preparation process—such as the scaling up of complex drug delivery systems and achieving uniform dispersion of photocatalysts—need to be addressed to enable broader application. At the clinical translation level, major obstacles remain. Most current research relies heavily on preclinical mouse models, with limited validation in human clinical trials. The primary limitation stems from the inherent differences between animal models and humans, as human and mouse skin structures differ significantly in thickness, density, and cellular composition. Furthermore, the tumor growth cycle in mice is considerably shorter than the progression of melanoma in humans, and mouse models fail to fully replicate the complexity of the human immune microenvironment. Discrepancies in body weight further complicate the conversion of drug dosages between species, creating substantial uncertainty. These fundamental differences intensify the challenges of translating therapeutic effects from preclinical models to human applications. In drug delivery systems, optimizing drug-loading capacity to meet human physiological demands is essential. Additionally, dosing frequency and interval adjustments must be tailored to human physiological characteristics. Safety evaluation also presents significant challenges: the intensity and patterns of immune responses in humans may differ markedly from those in mice, underscoring the urgent need for a more robust adverse reaction monitoring framework. Moreover, variability in individual immune responses adds complexity to tailoring therapies for personalized treatment needs. Long-term safety concerns, including the potential leakage of catalysts and risks associated with nanomaterials, further contribute to uncertainty regarding the clinical applicability of hydrogel microneedle technology.
Looking ahead, research efforts should focus on four key areas. First, in material and technology optimization, there is a need to explore novel intelligent responsive materials and simplify preparation processes. Second, in therapeutic strategy innovation, the focus should be on developing integrated diagnosis and treatment platforms, expanding the range of detectable biomarkers, and optimizing multimodal combination therapies. Third, in clinical translation, it is crucial to strengthen clinical trials, accumulate more in vivo data, and establish standardized safety evaluation systems. Additionally, while most microneedle studies primarily target local primary tumors, metastatic melanoma has been largely overlooked, therefore, future research should focus more on metastatic melanoma. Finally, in interdisciplinary integration, proactive collaboration across multiple disciplines is essential to expedite the translation of research findings into clinical applications. In conclusion, HMN technology holds great potential for melanoma treatment. Future research should focus on overcoming existing technical challenges and accelerating clinical translation to deliver more precise and effective therapies for melanoma patients.
Author contributions
LQZ: Conceptualization, Project administration, Writing – original draft. GQ: Project administration, Writing – original draft. HG: Project administration, Writing – original draft. AJ: Investigation, Writing – original draft. LZ: Investigation, Writing – original draft. XW: Conceptualization, Project administration, Writing – review & editing.
Funding
The author(s) declare that no financial support was received for the research and/or publication of this article.
Conflict of interest
The authors declare that the research was conducted in the absence of any commercial or financial relationships that could be construed as a potential conflict of interest.
Generative AI statement
The author(s) declare that no Generative AI was used in the creation of this manuscript.
Publisher’s note
All claims expressed in this article are solely those of the authors and do not necessarily represent those of their affiliated organizations, or those of the publisher, the editors and the reviewers. Any product that may be evaluated in this article, or claim that may be made by its manufacturer, is not guaranteed or endorsed by the publisher.
Glossary
5-FU: 5-fluorouracil
α-MSH: α-melanocyte-stimulating hormone
alloc-DOX: allyloxycarbonyl-doxorubicin
AZA: azelaic acid
Ca²⁺: calcium ion
CCa@TF/Ce6: Carbon nanotubes@calcium peroxide@tannic acid-Fe/chlorin e6
CO: Carbon monoxide
CpG-ODNs: cytosine-phosphate-guanine oligodeoxynucleotides
CS: chitosan
Cu²⁺: copper ions
CZCH: Cu2+-doped porous zeolitic imidazolate framework incorporated with catalase
DA: dopamine
DexMA: dextran methacrylate
DOX: doxorubicin
EAA: 3-O-ethyl ascorbic acid
GelMA: gelatin methacryloyl
GelMA-β-CD: gelatin methacryloyl and β-cyclodextrin
GM-CSF: granulocyte-macrophage colony-stimulating factor
GSH: glutathione
HA: hyaluronic acid
HAT: hyaluronic acid-tyramine
HEMA: hydroxyethyl methacrylate
HMNs: hydrogel microneedles
ISF: interstitial fluid
LAP: laponite
MAT: matrine
MeHA: methacrylated hyaluronic acid
MMPs: magnetic microparticles
MNs: Microneedles
MOF: metal–organic framework
MSC: mesenchymal stem cell
NDV: Newcastle disease virus
NIR: near-infrared
NVP: N-vinyl-2-pyrrolidone
OVA: ovalbumin
Pd: palladium
PDMS: polydimethylsiloxane
PDT: photodynamic therapy
PEG: poly(ethylene glycol)
PEGdiacid: poly(ethylene glycol) diacid
P-gp: P-glycoprotein
PMPs: polystyrene microparticles
PMVE/MA: poly(methyl vinyl ether-co-maleic acid)
PTT: photothermal therapy
PVA: Poly(vinyl alcohol)
PVP: polyvinylpyrrolidone
ROS: reactive oxygen species
SA: sodium alginate
SF: silk fibroin
TDDS: Transdermal Drug Delivery Systems
TME: tumor microenvironment
TMPyP: tetrakis(1-methyl-4-pyridinio) porphyrin
TNSs: TiO2 nanosheets
Tra: Trametinib
References
1. Siegel RL, Giaquinto AN, Jemal A. Cancer statistics, 2024. CA Cancer J Clin. (2024) 74:12–49. doi: 10.3322/caac.21820
2. Bagheri H, Pourhanifeh MH, Derakhshan M, Mahjoubin-Tehran M, Ghasemi F, Mousavi S, et al. Cxcl-10: A new candidate for melanoma therapy? Cell Oncol (Dordr). (2020) 43:353–65. doi: 10.1007/s13402-020-00501-z
3. SChadendorf D, van Akkooi ACJ, Berking C, Griewank KG, Gutzmer R, Hauschild A, et al. Melanoma. Lancet. (2018) 392:971–84. doi: 10.1016/S0140-6736(18)31559-9
4. Trojaniello C, Luke JJ, Ascierto PA. Therapeutic advancements across clinical stages in melanoma, with a focus on targeted immunotherapy. Front Oncol. (2021) 11:670726. doi: 10.3389/fonc.2021.670726
5. Weiss SA, Wolchok JD, Sznol M. Immunotherapy of melanoma: facts and hopes. Clin Cancer Res. (2019) 25:5191–201. doi: 10.1158/1078-0432.CCR-18-1550
6. Tagliaferri L, Lancellotta V, Fionda B, Mangoni M, Casa C, Di Stefani A, et al. Immunotherapy and radiotherapy in melanoma: A multidisciplinary comprehensive review. Hum Vaccin Immunother. (2022) 18:1903827. doi: 10.1080/21645515.2021.1903827
7. Pan Q, Yu Y, Chen D, Jiao G, Liu X. Enhanced penetration strategies for transdermal delivery. Front Chem Sci Eng. (2020) 14:378–88. doi: 10.1007/s11705-019-1913-1
8. Jeong WY, Kwon M, Choi HE, Kim KS. Recent advances in transdermal drug delivery systems: A review. Biomaterials Res. (2021) 25:24. doi: 10.1186/s40824-021-00226-6
9. Zhang X, Wang Y, Chi J, Zhao Y. Smart microneedles for therapy and diagnosis. Res (Wash D C). (2020) 2020:7462915. doi: 10.34133/2020/7462915
10. Chen Y, Zhu J, Ding J, Zhou W. Recent progress of vaccines administration via microneedles for cancer immunotherapy. Chin Chem Lett. (2024) 35:108706. doi: 10.1016/j.cclet.2023.108706
11. Song C, Wu X, Wang J, Liu R, Zhao Y. Photosensitizer-immunotherapy integrated microneedles for preventing tumor recurrence and metastasis. Nano Today. (2023) 51:101913. doi: 10.1016/j.nantod.2023.101913
12. Liu Z, Liu F, Feng D, Li W, Tan X, Yang N, et al. Microwave-responsive engineered platelet microneedle patch for deep tumor penetration and precision therapy. ACS Appl Materials Interfaces. (2025) 17:10457–69. doi: 10.1021/acsami.4c20896
13. D’Amico C, Fusciello M, Hamdan F, D’Alessio F, Bottega P, Saklauskaite M, et al. Transdermal delivery of pepticrad cancer vaccine using microneedle patches. Bioactive Materials. (2025) 45:115–27. doi: 10.1016/j.bioactmat.2024.11.006
14. Tang H, Cheng X, Liang L, Chen BZ, Liu C, Wang Y. A stimulus responsive microneedle-based drug delivery system for cancer therapy. Biomater Sci. (2024) 12:6274–83. doi: 10.1039/d4bm00741g
15. Lan T, Guo J, Bai X, Huang Z, Wei Z, Du G, et al. Rgd-modified injectable hydrogel maintains islet beta-cell survival and function. J Appl Biomater Funct Mater. (2020) 18:2280800020963473. doi: 10.1177/2280800020963473
16. Chauhan SS, Prasad A, Venuganti VVK. Swellable biopolymer composite microneedle patch for pain-free collection of interstitial fluid to analyze multiple biomolecules. J BioMed Mater Res A. (2025) 113:e37809. doi: 10.1002/jbm.a.37809
17. Aroche AF, Nissan HE, Daniele MA. Hydrogel-forming microneedles and applications in interstitial fluid diagnostic devices. Adv Healthc Mater. (2025) 14:e2401782. doi: 10.1002/adhm.202401782
18. Ahmed Saeed Al-Japairai K, Mahmood S, Hamed Almurisi S, Reddy Venugopal J, Rebhi Hilles A, Azmana M, et al. Current trends in polymer microneedle for transdermal drug delivery. Int J Pharm. (2020) 587:119673. doi: 10.1016/j.ijpharm.2020.119673
19. Tran ML, Nguyen CH, Chu KY, Juang RS. A simplified kinetic modeling of co(2) absorption into water and monoethanolamine solution in hollow-fiber membrane contactors. Membranes (Basel). (2023) 13:494. doi: 10.3390/membranes13050494
20. Kirkby M, Hutton ARJ, Donnelly RF. Microneedle mediated transdermal delivery of protein, peptide and antibody based therapeutics: current status and future considerations. Pharm Res. (2020) 37:117. doi: 10.1007/s11095-020-02844-6
21. Ingrole RSJ, Gill HS. Microneedle coating methods: A review with a perspective. J Pharmacol Exp Ther. (2019) 370:555–69. doi: 10.1124/jpet.119.258707
22. Chen Y, Chen BZ, Wang QL, Jin X, Guo XD. Fabrication of coated polymer microneedles for transdermal drug delivery. J Control Release. (2017) 265:14–21. doi: 10.1016/j.jconrel.2017.03.383
23. Dardano P, De Martino S, Battisti M, Miranda B, Rea I, De Stefano L. One-shot fabrication of polymeric hollow microneedles by standard photolithography. Polymers (Basel). (2021) 13:520. doi: 10.3390/polym13040520
24. Carcamo-Martinez A, Mallon B, Dominguez-Robles J, Vora LK, Anjani QK, Donnelly RF. Hollow microneedles: A perspective in biomedical applications. Int J Pharm. (2021) 599:120455. doi: 10.1016/j.ijpharm.2021.120455
25. Aldawood FK, Andar A, Desai S. A comprehensive review of microneedles: types, materials, processes, characterizations and applications. Polymers (Basel). (2021) 13:2815. doi: 10.3390/polym13162815
26. Yang D, Chen M, Sun Y, Jin Y, Lu C, Pan X, et al. Microneedle-mediated transdermal drug delivery for treating diverse skin diseases. Acta Biomater. (2021) 121:119–33. doi: 10.1016/j.actbio.2020.12.004
27. Wang H, Fu Y, Mao J, Jiang H, Du S, Liu P, et al. Strong and tough supramolecular microneedle patches with ultrafast dissolution and rapid-onset capabilities. Adv Mater. (2022) 34:e2207832. doi: 10.1002/adma.202207832
28. Feng YX, Hu H, Wong YY, Yao X, He ML. Microneedles: an emerging vaccine delivery tool and a prospective solution to the challenges of Sars-Cov-2 mass vaccination. Pharmaceutics. (2023) 15:1349. doi: 10.3390/pharmaceutics15051349
29. Hou X, Li J, Hong Y, Ruan H, Long M, Feng N, et al. Advances and prospects for hydrogel-forming microneedles in transdermal drug delivery. Biomedicines. (2023) 11:2119. doi: 10.3390/biomedicines11082119
30. Ertas YN, Ertas D, Erdem A, Segujja F, Dulchavsky S, Ashammakhi N. Diagnostic, therapeutic, and theranostic multifunctional microneedles. Small. (2024) 20:e2308479. doi: 10.1002/smll.202308479
31. Turner JG, Laabei M, Li S, Estrela P, Leese HS. Antimicrobial releasing hydrogel forming microneedles. Biomater Adv. (2023) 151:213467. doi: 10.1016/j.bioadv.2023.213467
32. Mehta P, Sharma M, Devi M. Hydrogels: an overview of its classifications, properties, and applications. J Mech Behav BioMed Mater. (2023) 147:106145. doi: 10.1016/j.jmbbm.2023.106145
33. Shin D, Hyun J. Silk fibroin microneedles fabricated by digital light processing 3d printing. J Industrial Eng Chem. (2021) 95:126–33. doi: 10.1016/j.jiec.2020.12.011
34. Zhao P, Li Z, Ling Z, Zheng Y, Chang H. Efficient loading and sustained delivery of methotrexate using a tip-swellable microneedle array patch for psoriasis treatment. ACS Biomater Sci Eng. (2024) 10:921–31. doi: 10.1021/acsbiomaterials.3c01810
35. Tian S, Mei J, Zhang L, Wang S, Yuan Y, Li J, et al. Multifunctional hydrogel microneedle patches modulating oxi-inflamm-aging for diabetic wound healing. Small. (2024) 20:e2407340. doi: 10.1002/smll.202407340
36. Wang Y, Yu H, Wang L, Zhang L, Liu J, Chen D, et al. Intelligent microneedle patch based on functionalized alginate and chitosan for long-term self-regulated insulin delivery. Carbohydr Polym. (2025) 348:122885. doi: 10.1016/j.carbpol.2024.122885
37. Bai Y, Liu Z, Niu T, Yi Y, Dou H, Song L, et al. Intelligent microneedles patch with wireless self-sensing and anti-infective actions. Small. (2025) 21:e2411125. doi: 10.1002/smll.202411125
38. Zhao J, Lv J, Ling G, Zhang P. A swellable hydrogel microneedle based on cerium-metal organic frame composite nanozyme for detection of biomarkers. Int J Biol Macromol. (2024) 254:127745. doi: 10.1016/j.ijbiomac.2023.127745
39. Chandran R, Mohd Tohit ER, Stanslas J, Salim N, Tuan Mahmood TM. Investigation and optimization of hydrogel microneedles for transdermal delivery of caffeine. Tissue Eng Part C Methods. (2022) 28:545–56. doi: 10.1089/ten.TEC.2022.0045
40. Li Y, Bi D, Hu Z, Yang Y, Liu Y, Leung WK. Hydrogel-forming microneedles with applications in oral diseases management. Materials (Basel). (2023) 16:4805. doi: 10.3390/ma16134805
41. Chong S, Wei C, Feng L, Guo R. Silk fibroin-based hydrogel microneedles deliver alpha-msh to promote melanosome delivery for vitiligo treatment. ACS Biomater Sci Eng. (2023) 9:3368–78. doi: 10.1021/acsbiomaterials.3c00284
42. Yao S, Chi J, Wang Y, Zhao Y, Luo Y, Wang Y. Zn-mof encapsulated antibacterial and degradable microneedles array for promoting wound healing. Adv Healthc Mater. (2021) 10:e2100056. doi: 10.1002/adhm.202100056
43. Zhang Q, Na J, Liu X, He J. Exploration of the delivery of oncolytic newcastle disease virus by gelatin methacryloyl microneedles. Int J Mol Sci. (2024) 25:2353. doi: 10.3390/ijms25042353
44. Zhou Z, Xing M, Zhang S, Yang G, Gao Y. Process optimization of ca(2+) cross-linked alginate-based swellable microneedles for enhanced transdermal permeability: more applicable to acidic drugs. Int J Pharm. (2022) 618:121669. doi: 10.1016/j.ijpharm.2022.121669
45. Suriyaamporn P, Dechsri K, Charoenying T, Ngawhirunpat T, Rojanarata T, Patrojanasophon P, et al. Multiple strategies approach: A novel crosslinked hydrogel forming chitosan-based microneedles chemowrap patch loaded with 5-fluorouracil liposomes for chronic wound cancer treatment. Int J Biol Macromol. (2024) 279:134973. doi: 10.1016/j.ijbiomac.2024.134973
46. Zhang X, Gan J, Fan L, Luo Z, Zhao Y. Bioinspired adaptable indwelling microneedles for treatment of diabetic ulcers. Adv Mater. (2023) 35:e2210903. doi: 10.1002/adma.202210903
47. Al-Badry AS, Al-Mayahy MH, Scurr DJ. Enhanced transdermal delivery of acyclovir via hydrogel microneedle arrays. J Pharm Sci. (2023) 112:1011–9. doi: 10.1016/j.xphs.2022.11.012
48. Odinotski S, Dhingra K, GhavamiNejad A, Zheng H, GhavamiNejad P, Gaouda H, et al. A conductive hydrogel-based microneedle platform for real-time ph measurement in live animals. Small. (2022) 18:e2200201. doi: 10.1002/smll.202200201
49. Zheng Y, Li Z, Li S, Zhao P, Wang X, Lu S, et al. Separable nanocomposite hydrogel microneedles for intradermal and sustained delivery of antigens to enhance adaptive immune responses. Acta Biomater. (2024) 185:203–14. doi: 10.1016/j.actbio.2024.07.031
50. Ahmad Z, Salman S, Khan SA, Amin A, Rahman ZU, Al-Ghamdi YO, et al. Versatility of hydrogels: from synthetic strategies, classification, and properties to biomedical applications. Gels. (2022) 8:167. doi: 10.3390/gels8030167
51. Shi Z, Gao X, Ullah MW, Li S, Wang Q, Yang G. Electroconductive natural polymer-based hydrogels. Biomaterials. (2016) 111:40–54. doi: 10.1016/j.biomaterials.2016.09.020
52. Zhao L, Zhou Y, Zhang J, Liang H, Chen X, Tan H. Natural polymer-based hydrogels: from polymer to biomedical applications. Pharmaceutics. (2023) 15:2514. doi: 10.3390/pharmaceutics15102514
53. Bashir S, Hina M, Iqbal J, Rajpar AH, Mujtaba MA, Alghamdi NA, et al. Fundamental concepts of hydrogels: synthesis, properties, and their applications. Polymers (Basel). (2020) 12:2702. doi: 10.3390/polym12112702
54. Saifullah KM, Mushtaq A, Azarikhah P, Prewett PD, Davies GJ, Faraji Rad Z. Micro-vibration assisted dual-layer spiral microneedles to rapidly extract dermal interstitial fluid for minimally invasive detection of glucose. Microsystems Nanoengineering. (2025) 11:3. doi: 10.1038/s41378-024-00850-x
55. Li H, Vora LK, Anjani Q, Abraham AM, Cong Y, Moreno-Castellanos N, et al. Quality by design-guided development of hydrogel-forming microneedles for transdermal delivery of enfuvirtide. ACS Appl Materials Interfaces. (2025). doi: 10.1021/acsami.5c00499
56. Ito K. Novel cross-linking concept of polymer network: synthesis, structure, and properties of slide-ring gels with freely movable junctions. Polym J. (2007) 39:489–99. doi: 10.1295/polymj.PJ2006239
57. Bustamante-Torres M, Romero-Fierro D, Arcentales-Vera B, Palomino K, Magana H, Bucio E. Hydrogels classification according to the physical or chemical interactions and as stimuli-sensitive materials. Gels. (2021) 7:182. doi: 10.3390/gels7040182
58. Fu X, Xian H, Xia C, Liu Y, Du S, Wang B, et al. Polymer homologue-mediated formation of hydrogel microneedles for controllable transdermal drug delivery. Int J Pharm. (2024) 666:124768. doi: 10.1016/j.ijpharm.2024.124768
59. Zhang Y, Wang S, Tian Y, Chen L, Du Y, Su G, et al. Multi-physically cross-linked hydrogels for flexible sensors with high strength and self-healing properties. Polymers (Basel). (2023) 15:3748. doi: 10.3390/polym15183748
60. Sun Y, Li D, Yu Y, Zheng Y. Insights into the role of natural polysaccharide-based hydrogel wound dressings in biomedical applications. Gels. (2022) 8:646. doi: 10.3390/gels8100646
61. Mahmoudi C, Tahraoui Douma N, Mahmoudi H, Iurciuc Tincu CE, Popa M. Hydrogels based on proteins cross-linked with carbonyl derivatives of polysaccharides, with biomedical applications. Int J Mol Sci. (2024) 25:7839. doi: 10.3390/ijms25147839
62. Liu J, Tian B, Liu Y, Wan JB. Cyclodextrin-containing hydrogels: A review of preparation method, drug delivery, and degradation behavior. Int J Mol Sci. (2021) 22:13516. doi: 10.3390/ijms222413516
63. Chen X, Yu H, Wang L, Shen D, Li C, Zhou W. Cross-linking-density-changeable microneedle patch prepared from a glucose-responsive hydrogel for insulin delivery. ACS Biomater Sci Eng. (2021) 7:4870–82. doi: 10.1021/acsbiomaterials.1c01073
64. Pan X, Li Y, Pang W, Xue Y, Wang Z, Jiang C, et al. Preparation, characterisation and comparison of glabridin-loaded hydrogel-forming microneedles by chemical and physical cross-linking. Int J Pharm. (2022) 617:121612. doi: 10.1016/j.ijpharm.2022.121612
65. Taaca KLM, Prieto EI, Vasquez MR Jr. Current trends in biomedical hydrogels: from traditional crosslinking to plasma-assisted synthesis. Polymers (Basel). (2022) 14:2560. doi: 10.3390/polym14132560
66. Zhu Y, Zhang Y, Zhou Y. Application progress of modified chitosan and its composite biomaterials for bone tissue engineering. Int J Mol Sci. (2022) 23:6574. doi: 10.3390/ijms23126574
67. Tucak A, Sirbubalo M, Hindija L, Rahic O, Hadziabdic J, Muhamedagic K, et al. Microneedles: characteristics, materials, production methods and commercial development. Micromachines (Basel). (2020) 11:961. doi: 10.3390/mi11110961
68. Fratus M, Alam MA. Performance gain and electro-mechanical design optimization of microneedles for wearable sensor systems. BioMed Microdevices. (2023) 26:4. doi: 10.1007/s10544-023-00683-x
69. Yang P, Song Q, Zhang L, Liu Z, Ma H. Numerical modeling and simulation for microneedles drug delivery: A novel comprehensive swelling-obstruction-mechanics model. Eur J Pharm Biopharm. (2025) 206:114583. doi: 10.1016/j.ejpb.2024.114583
70. Czarczynska-Goslinska B, Goslinski T, Roszak A, Froelich A, Szyk P, Mlynarczyk DT, et al. Microneedle system coated with hydrogels containing protoporphyrin ix for potential application in pharmaceutical technology. Methods Protoc. (2024) 7:73. doi: 10.3390/mps7050073
71. Xu J, Xu D, Xuan X, He H. Advances of microneedles in biomedical applications. Molecules. (2021) 26:5912. doi: 10.3390/molecules26195912
72. Zhang X, Chen G, Sun L, Ye F, Shen X, Zhao Y. Claw-inspired microneedle patches with liquid metal encapsulation for accelerating incisional wound healing. Chem Eng J. (2021) 406:126741. doi: 10.1016/j.cej.2020.126741
73. Guo M, Wang Y, Gao B, He B. Shark tooth-inspired microneedle dressing for intelligent wound management. ACS Nano. (2021) 15:15316–27. doi: 10.1021/acsnano.1c06279
74. Zhang X, Chen G, Cai L, Wang Y, Sun L, Zhao Y. Bioinspired pagoda-like microneedle patches with strong fixation and hemostasis capabilities. Chem Eng J. (2021) 414:128905. doi: 10.1016/j.cej.2021.128905
75. Turner JG, White LR, Estrela P, Leese HS. Hydrogel-forming microneedles: current advancements and future trends. Macromol Biosci. (2021) 21:e2000307. doi: 10.1002/mabi.202000307
76. Gill HS, Denson DD, Burris BA, Prausnitz MR. Effect of microneedle design on pain in human volunteers. Clin J Pain. (2008) 24:585–94. doi: 10.1097/AJP.0b013e31816778f9
77. Gomaa YA, Morrow DI, Garland MJ, Donnelly RF, El-Khordagui LK, Meidan VM. Effects of microneedle length, density, insertion time and multiple applications on human skin barrier function: assessments by transepidermal water loss. Toxicol In Vitro. (2010) 24:1971–8. doi: 10.1016/j.tiv.2010.08.012
78. Wu Y, Tang Z, Ma S, Du L. The promising application of hydrogel microneedles in medical application. J Pharm Pharmacol. (2023) 75:1011–20. doi: 10.1093/jpp/rgad058
79. Loh JM, Lim YJL, Tay JT, Cheng HM, Tey HL, Liang K. Design and fabrication of customizable microneedles enabled by 3d printing for biomedical applications. Bioact Mater. (2024) 32:222–41. doi: 10.1016/j.bioactmat.2023.09.022
80. Ramoller IK, McAlister E, Bogan A, Cordeiro AS, Donnelly RF. Novel design approaches in the fabrication of polymeric microarray patches via micromoulding. Micromachines (Basel). (2020) 11:554. doi: 10.3390/mi11060554
81. Wang M, Hu L, Xu C. Recent advances in the design of polymeric microneedles for transdermal drug delivery and biosensing. Lab Chip. (2017) 17:1373–87. doi: 10.1039/c7lc00016b
82. Shiu P-P, Knopf GK, Ostojic M, Nikumb S. Non-lithographic fabrication of metallic micromold masters by laser machining and welding. Int J Advanced Manufacturing Technol. (2012) 59:157–67. doi: 10.1007/s00170-011-3477-1
83. Kang NW, Kim S, Lee JY, Kim KT, Choi Y, Oh Y, et al. Microneedles for drug delivery: recent advances in materials and geometry for preclinical and clinical studies. Expert Opin Drug Delivery. (2021) 18:929–47. doi: 10.1080/17425247.2021.1828860
84. Wang QL, Zhu DD, Chen Y, Guo XD. A fabrication method of microneedle molds with controlled microstructures. Mater Sci Eng C Mater Biol Appl. (2016) 65:135–42. doi: 10.1016/j.msec.2016.03.097
85. Shahriari MH, Salmani H, Akrami M, Salehi Z. Development of a facile, versatile and scalable fabrication approach of solid, coated, and dissolving microneedle devices for transdermal drug delivery applications. Giant. (2024) 18:100284. doi: 10.1016/j.giant.2024.100284
86. Yang Q, Zhong W, Xu L, Li H, Yan Q, She Y, et al. Recent progress of 3d-printed microneedles for transdermal drug delivery. Int J Pharm. (2021) 593:120106. doi: 10.1016/j.ijpharm.2020.120106
87. Cordeiro AS, Tekko IA, Jomaa MH, Vora L, McAlister E, Volpe-Zanutto F, et al. Two-photon polymerisation 3d printing of microneedle array templates with versatile designs: application in the development of polymeric drug delivery systems. Pharm Res. (2020) 37:174. doi: 10.1007/s11095-020-02887-9
88. Lim SH, Kathuria H, Amir MHB, Zhang X, Duong HTT, Ho PC, et al. High resolution photopolymer for 3d printing of personalised microneedle for transdermal delivery of anti-wrinkle small peptide. J Control Release. (2021) 329:907–18. doi: 10.1016/j.jconrel.2020.10.021
89. Kaliaraj GS, Shanmugam DK, Dasan A, Mosas KKA. Hydrogels-a promising materials for 3d printing technology. Gels. (2023) 9:260. doi: 10.3390/gels9030260
90. Young AT, Vora NB, Cortez J, Tam A, Yeniay Y, Afifi L, et al. The role of technology in melanoma screening and diagnosis. Pigment Cell Melanoma Res. (2021) 34:288–300. doi: 10.1111/pcmr.12907
91. Davis LE, Shalin SC, Tackett AJ. Current state of melanoma diagnosis and treatment. Cancer Biol Ther. (2019) 20:1366–79. doi: 10.1080/15384047.2019.1640032
92. Vaidyanathan R, Soon RH, Zhang P, Jiang K, Lim CT. Cancer diagnosis: from tumor to liquid biopsy and beyond. Lab Chip. (2018) 19:11–34. doi: 10.1039/c8lc00684a
93. Wang J, Lu Z, Cai R, Zheng H, Yu J, Zhang Y, et al. Microneedle-based transdermal detection and sensing devices. Lab Chip. (2023) 23:869–87. doi: 10.1039/d2lc00790h
94. Eisenstein A, Gonzalez EC, Raghunathan R, Xu X, Wu M, McLean EO, et al. Emerging biomarkers in cutaneous melanoma. Mol Diagn Ther. (2018) 22:203–18. doi: 10.1007/s40291-018-0318-z
95. Hoch T, Schulz D, Eling N, Gomez JM, Levesque MP, Bodenmiller B. Multiplexed imaging mass cytometry of the chemokine milieus in melanoma characterizes features of the response to immunotherapy. Sci Immunol. (2022) 7:eabk1692. doi: 10.1126/sciimmunol.abk1692
96. Xiong TF, Pan FQ, Li D. Expression and clinical significance of S100 family genes in patients with melanoma. Melanoma Res. (2019) 29:23–9. doi: 10.1097/CMR.0000000000000512
97. Wang G, Zhang Y, Kwong HK, Zheng M, Wu J, Cui C, et al. On-site melanoma diagnosis utilizing a swellable microneedle-assisted skin interstitial fluid sampling and a microfluidic particle dam for visual quantification of S100a1. Adv Sci (Weinh). (2024) 11:e2306188. doi: 10.1002/advs.202306188
98. Luo Z, Sun W, Fang J, Lee K, Li S, Gu Z, et al. Biodegradable gelatin methacryloyl microneedles for transdermal drug delivery. Adv Healthc Mater. (2019) 8:e1801054. doi: 10.1002/adhm.201801054
99. Huang S, Liu H, Huang S, Fu T, Xue W, Guo R. Dextran methacrylate hydrogel microneedles loaded with doxorubicin and trametinib for continuous transdermal administration of melanoma. Carbohydr Polym. (2020) 246:116650. doi: 10.1016/j.carbpol.2020.116650
100. Chen Z, Li H, Bian Y, Wang Z, Chen G, Zhang X, et al. Bioorthogonal catalytic patch. Nat Nanotechnol. (2021) 16:933–41. doi: 10.1038/s41565-021-00910-7
101. Dosta P, Puigmal N, Cryer AM, Rodriguez AL, Scott E, Weissleder R, et al. Polymeric microneedles enable simultaneous delivery of cancer immunomodulatory drugs and detection of skin biomarkers. Theranostics. (2023) 13:1–15. doi: 10.7150/thno.73966
102. Zhou X, Luo Z, Baidya A, Kim HJ, Wang C, Jiang X, et al. Biodegradable beta-cyclodextrin conjugated gelatin methacryloyl microneedle for delivery of water-insoluble drug. Adv Healthc Mater. (2020) 9:e2000527. doi: 10.1002/adhm.202000527
103. Ye Y, Wang C, Zhang X, Hu Q, Zhang Y, Liu Q, et al. A melanin-mediated cancer immunotherapy patch. Sci Immunol. (2017) 2:5692. doi: 10.1126/sciimmunol.aan5692
104. Li Y, He G, Fu LH, Younis MR, He T, Chen Y, et al. A microneedle patch with self-oxygenation and glutathione depletion for repeatable photodynamic therapy. ACS Nano. (2022) 16:17298–312. doi: 10.1021/acsnano.2c08098
105. Yu W, Fu J, Jia F, Jin Q, Wang Y, Ji J. Removable photocatalysis microneedle reactor for carbon monoxide delivery to enhance chemosensitization. Nano Lett. (2024) 24:10024–31. doi: 10.1021/acs.nanolett.4c01582
106. Xing M, Yang G, Liu H, Zhou Z, Zhang S, Gao Y. Industrializable approach for preparing hydrogel microneedles and their application in melanoma treatment. Int J Pharm. (2024) 653:123883. doi: 10.1016/j.ijpharm.2024.123883
107. Zhang Z, Zhang Z, Zeng W, Li Y, Zhu C. A hyaluronic acid-based dual-functional hydrogel microneedle system for sequential melanoma ablation and skin regeneration. Int J Biol Macromol. (2024) 283:138039. doi: 10.1016/j.ijbiomac.2024.138039
108. Chi Y, Zheng Y, Pan X, Huang Y, Kang Y, Zhong W, et al. Enzyme-mediated fabrication of nanocomposite hydrogel microneedles for tunable mechanical strength and controllable transdermal efficiency. Acta Biomater. (2024) 174:127–40. doi: 10.1016/j.actbio.2023.11.038
109. Dahis D, Dion MZ, Cryer AM, Dosta P, Gilboa T, Alonso M, et al. Monitoring melanoma responses to sting agonism and focused ultrasound thermal ablation using microneedles and ultrasensitive single molecule arrays. Advanced Funct Materials. (2023) 33:2301659. doi: 10.1002/adfm.202301659
110. Luke JJ, Schwartz GK. Chemotherapy in the management of advanced cutaneous Malignant melanoma. Clin Dermatol. (2013) 31:290–7. doi: 10.1016/j.clindermatol.2012.08.016
111. Karpov TE, Rogova A, Akhmetova DR, Tishchenko YA, Chinakova AV, Lipin DV, et al. Encapsulation of a small-molecule drug based on substituted 2-aminothiophenes in calcium carbonate carriers for therapy of melanoma. Biomater Sci. (2024) 12:3431–45. doi: 10.1039/d4bm00390j
112. Li A, Zhao J, Fu J, Cai J, Zhang P. Recent advances of biomimetic nano-systems in the diagnosis and treatment of tumor. Asian J Pharm Sci. (2021) 16:161–74. doi: 10.1016/j.ajps.2019.08.001
113. Gebhardt C, Simon SCS, Weber R, Gries M, Mun DH, Reinhard R, et al. Potential therapeutic effect of low-dose paclitaxel in melanoma patients resistant to immune checkpoint blockade: A pilot study. Cell Immunol. (2021) 360:104274. doi: 10.1016/j.cellimm.2020.104274
114. Zheng DX, Bozym DJ, Tarantino G, Sullivan RJ, Liu D, Jenkins RW. Overcoming resistance mechanisms to melanoma immunotherapy. Am J Clin Dermatol. (2025) 26:77–96. doi: 10.1007/s40257-024-00907-7
115. Sun H, Zhong Z. Bioresponsive polymeric nanoparticles: from design, targeted therapy to cancer immunotherapy. Biomacromolecules. (2025) 26:33–42. doi: 10.1021/acs.biomac.4c01257
116. Irvine DJ, Maus MV, Mooney DJ, Wong WW. The future of engineered immune cell therapies. Science. (2022) 378:853–8. doi: 10.1126/science.abq6990
117. Mellman I, Coukos G, Dranoff G. Cancer immunotherapy comes of age. Nature. (2011) 480:480–9. doi: 10.1038/nature10673
118. Hargadon KM, Johnson CE, Williams CJ. Immune checkpoint blockade therapy for cancer: an overview of fda-approved immune checkpoint inhibitors. Int Immunopharmacol. (2018) 62:29–39. doi: 10.1016/j.intimp.2018.06.001
119. Li M, Yao H, Yi K, Lao YH, Shao D, Tao Y. Emerging nanoparticle platforms for cpg oligonucleotide delivery. Biomater Sci. (2024) 12:2203–28. doi: 10.1039/d3bm01970e
120. Shafi S, Challa B, Parwani AV, Aung TN. New therapies in melanoma: current trends, evolving paradigms, and future perspectives. Cutis. (2023) 112:E32–E9. doi: 10.12788/cutis.0911
121. Younes M, Mardirossian R, Rizk L, Fazlian T, Khairallah JP, Sleiman C, et al. The synergistic effects of curcumin and chemotherapeutic drugs in inhibiting metastatic, invasive and proliferative pathways. Plants (Basel). (2022) 11:2137. doi: 10.3390/plants11162137
122. Mohanty C, Sahoo SK. The in vitro stability and in vivo pharmacokinetics of curcumin prepared as an aqueous nanoparticulate formulation. Biomaterials. (2010) 31:6597–611. doi: 10.1016/j.biomaterials.2010.04.062
123. Gera M, Sharma N, Ghosh M, Huynh DL, Lee SJ, Min T, et al. Nanoformulations of curcumin: an emerging paradigm for improved remedial application. Oncotarget. (2017) 8:66680–98. doi: 10.18632/oncotarget.19164
124. Li J, Feng L, Liu L, Wang F, Ouyang L, Zhang L, et al. Recent advances in the design and discovery of synthetic tyrosinase inhibitors. Eur J Med Chem. (2021) 224:113744. doi: 10.1016/j.ejmech.2021.113744
125. Ismail M, Hassan MHA, Mohamed EIA, Azmy AF, Moawad A, Mohammed R, et al. New insights into the anti-inflammatory and anti-melanoma mechanisms of action of azelaic acid and other fusarium solani metabolites via in vitro and in silico studies. Sci Rep. (2024) 14:14370. doi: 10.1038/s41598-024-63958-0
126. Wei YP, Wang XH, Liu G, Zhang JF, Yang YX, Zhang J, et al. Matrine exerts inhibitory effects in melanoma through the regulation of mir-19b-3p/pten. Int J Oncol. (2018) 53:791–800. doi: 10.3892/ijo.2018.4414
127. Jin H, Sun Y, Wang S, Cheng X. Matrine activates pten to induce growth inhibition and apoptosis in V600ebraf harboring melanoma cells. Int J Mol Sci. (2013) 14:16040–57. doi: 10.3390/ijms140816040
128. Schanknecht E, Bachari A, Nassar N, Piva T, Mantri N. Phytochemical constituents and derivatives of cannabis sativa; bridging the gap in melanoma treatment. Int J Mol Sci. (2023) 24:859. doi: 10.3390/ijms24010859
129. Hasan N, Nadaf A, Imran M, Jiba U, Sheikh A, Almalki WH, et al. Skin cancer: understanding the journey of transformation from conventional to advanced treatment approaches. Mol Cancer. (2023) 22:168. doi: 10.1186/s12943-023-01854-3
130. Correia JH, Rodrigues JA, Pimenta S, Dong T, Yang Z. Photodynamic therapy review: principles, photosensitizers, applications, and future directions. Pharmaceutics. (2021) 13:1332. doi: 10.3390/pharmaceutics13091332
131. D’Aguanno S, Mallone F, Marenco M, Del Bufalo D, Moramarco A. Hypoxia-dependent drivers of melanoma progression. J Exp Clin Cancer Res. (2021) 40:159. doi: 10.1186/s13046-021-01926-6
132. Alvarez N, Sevilla A. Current advances in photodynamic therapy (Pdt) and the future potential of pdt-combinatorial cancer therapies. Int J Mol Sci. (2024) 25:1023. doi: 10.3390/ijms25021023
133. Ibbotson SH, Wong TH, Morton CA, Collier NJ, Haylett A, McKenna KE, et al. Adverse effects of topical photodynamic therapy: A consensus review and approach to management. Br J Dermatol. (2019) 180:715–29. doi: 10.1111/bjd.17131
134. Han HH, Wang HM, Jangili P, Li M, Wu L, Zang Y, et al. The design of small-molecule prodrugs and activatable phototherapeutics for cancer therapy. Chem Soc Rev. (2023) 52:879–920. doi: 10.1039/d2cs00673a
135. Han W, Yu L, Liu Z, Wang C, Zhang Q, Li H, et al. Nir enhanced ph-responsive microneedles for synergetic therapy of melanoma. ChemMedChem. (2025) 20:e202400537. doi: 10.1002/cmdc.202400537
136. Zhao Y, Zhou Y, Yang D, Gao X, Wen T, Fu J, et al. Intelligent and spatiotemporal drug release based on multifunctional nanoparticle-integrated dissolving microneedle system for synergetic chemo-photothermal therapy to eradicate melanoma. Acta Biomater. (2021) 135:164–78. doi: 10.1016/j.actbio.2021.09.009
137. He T, Luo Y, Zhang Q, Men Z, Su T, Fan L, et al. Hyalase-mediated cascade degradation of a matrix barrier and immune cell penetration by a photothermal microneedle for efficient anticancer therapy. ACS Appl Mater Interfaces. (2021) 13:26790–9. doi: 10.1021/acsami.1c06725
138. Roderique JD, Josef CS, Feldman MJ, Spiess BD. A modern literature review of carbon monoxide poisoning theories, therapies, and potential targets for therapy advancement. Toxicology. (2015) 334:45–58. doi: 10.1016/j.tox.2015.05.004
139. Romao CC, Blattler WA, Seixas JD, Bernardes GJ. Developing drug molecules for therapy with carbon monoxide. Chem Soc Rev. (2012) 41:3571–83. doi: 10.1039/c2cs15317c
140. Li Y, Dang J, Liang Q, Yin L. Thermal-responsive carbon monoxide (Co) delivery expedites metabolic exhaustion of cancer cells toward reversal of chemotherapy resistance. ACS Cent Sci. (2019) 5:1044–58. doi: 10.1021/acscentsci.9b00216
141. Chai J, Zhu J, Tian Y, Yang K, Luan J, Wang Y. Carbon monoxide therapy: A promising strategy for cancer. J Mater Chem B. (2023) 11:1849–65. doi: 10.1039/d2tb02599j
142. Ito C, Taguchi K, Yamada T, Hanaya K, Enoki Y, Sugai T, et al. Dual delivery of carbon monoxide and doxorubicin using haemoglobin-albumin cluster: proof of concept for well-tolerated cancer therapy. J Mater Chem B. (2024) 12:5600–8. doi: 10.1039/d4tb00123k
143. Zhu C, Cheng J, Lin H, Yang Z, Huang Y, Lv F, et al. Rational design of conjugated polymers for photocatalytic co(2) reduction: towards localized co production and macrophage polarization. J Am Chem Soc. (2024) 146:24832–41. doi: 10.1021/jacs.4c04980
144. Fu J, Yu W, Qian X, Wang Y, Ji J. A photocatalytic carbon monoxide-generating effervescent microneedle patch for improved transdermal chemotherapy. J Mater Chem B. (2023) 11:5406–15. doi: 10.1039/d2tb02613a
145. Wang SB, Zhang C, Ye JJ, Zou MZ, Liu CJ, Zhang XZ. Near-infrared light responsive nanoreactor for simultaneous tumor photothermal therapy and carbon monoxide-mediated anti-inflammation. ACS Cent Sci. (2020) 6:555–65. doi: 10.1021/acscentsci.9b01342
146. Najafi S, Mortezaee K. Advances in dendritic cell vaccination therapy of cancer. BioMed Pharmacother. (2023) 164:114954. doi: 10.1016/j.biopha.2023.114954
147. Wang J, Zhang Z, Liang R, Chen W, Li Q, Xu J, et al. Targeting lymph nodes for enhanced cancer vaccination: from nanotechnology to tissue engineering. Mater Today Bio. (2024) 26:101068. doi: 10.1016/j.mtbio.2024.101068
148. Aarntzen EH, Srinivas M, Schreibelt G, Heerschap A, Punt CJ, Figdor CG, et al. Reducing cell number improves the homing of dendritic cells to lymph nodes upon intradermal vaccination. Oncoimmunology. (2013) 2:e24661. doi: 10.4161/onci.24661
149. Ji M, Zhan F, Qiu X, Liu H, Liu X, Bu P, et al. Research progress of hydrogel microneedles in wound management. ACS Biomater Sci Eng. (2024) 10:4771–90. doi: 10.1021/acsbiomaterials.4c00972
150. Martins CF, Garcia-Astrain C, Conde J, Liz-Marzan LM. Nanocomposite hydrogel microneedles: A theranostic toolbox for personalized medicine. Drug Delivery Transl Res. (2024) 14:2262–75. doi: 10.1007/s13346-024-01533-w
Keywords: hydrogel microneedles, melanoma, cancer therapy, drug delivery systems, controlled release
Citation: Zhu L, Qiao G, Gao H, Jiang A, Zhang L and Wang X (2025) Enhancing melanoma therapy with hydrogel microneedles. Front. Oncol. 15:1590534. doi: 10.3389/fonc.2025.1590534
Received: 09 March 2025; Accepted: 31 March 2025;
Published: 17 April 2025.
Edited by:
Xiu-Qiong Fu, Hong Kong Baptist University, Hong Kong SAR, ChinaCopyright © 2025 Zhu, Qiao, Gao, Jiang, Zhang and Wang. This is an open-access article distributed under the terms of the Creative Commons Attribution License (CC BY). The use, distribution or reproduction in other forums is permitted, provided the original author(s) and the copyright owner(s) are credited and that the original publication in this journal is cited, in accordance with accepted academic practice. No use, distribution or reproduction is permitted which does not comply with these terms.
*Correspondence: Xiaobing Wang, eGlhb2Jpbmd3MTk3MEAxNjMuY29t