- 1Department of Radiotherapy, The People’s Hospital of Liaoning Province, Shenyang, Liaoning, China
- 2Department of Thoracic Surgery, Shenyang Chest Hospital and Tenth People’s Hospital, Shenyang, Liaoning, China
Tertiary lymphoid structures (TLS) are ectopic lymphoid structures that form in non-lymphoid tissues in response to chronic inflammatory stimulation. Structurally and functionally resembling lymph nodes, TLS are primarily composed of B cells, T cells, dendritic cells, and other immune cell populations. Critically, TLS serve as direct sites for initiating anti-tumor immune responses. Within tumors, TLS facilitate the accumulation of immune cells—particularly effector subsets such as cytotoxic T cells and antibody-producing B cells—in the tumor microenvironment, thereby establishing a localized hub for both cellular and humoral immunity. This localized immune activation correlates with improved patient prognosis and enhanced responses to immunotherapy. In this review, we summarize the organization, formation drivers, detection markers, and the interplay between TLS and tumor-associated genes. Furthermore, we discuss the potential of TLS as biomarkers for immunotherapy efficacy and their translational clinical applications.
1 Components of TLS
Tertiary lymphoid structures (TLS) comprise diverse immune and stromal components, including B cells, T cells, follicular dendritic cells (FDCs), fibroblastic reticular cells (FRCs), stromal cells, dendritic cells (DCs), macrophages, and endothelial cells (1, 2). Within this organized architecture, FDCs predominantly localize to B cell zones, where they express an array of surface receptors to efficiently present antigens and orchestrate B cell differentiation into antibody-producing cells (3). Meanwhile, endothelial cells occupy TLS peripheries and T cell-rich regions, collaborating with fibroblasts to modulate T cell activity. Together, these spatially and functionally distinct cellular interactions not only establish the structural foundation of TLS but also sustain immune responses within the tumor microenvironment (TME) (4, 5)(Figure 1).
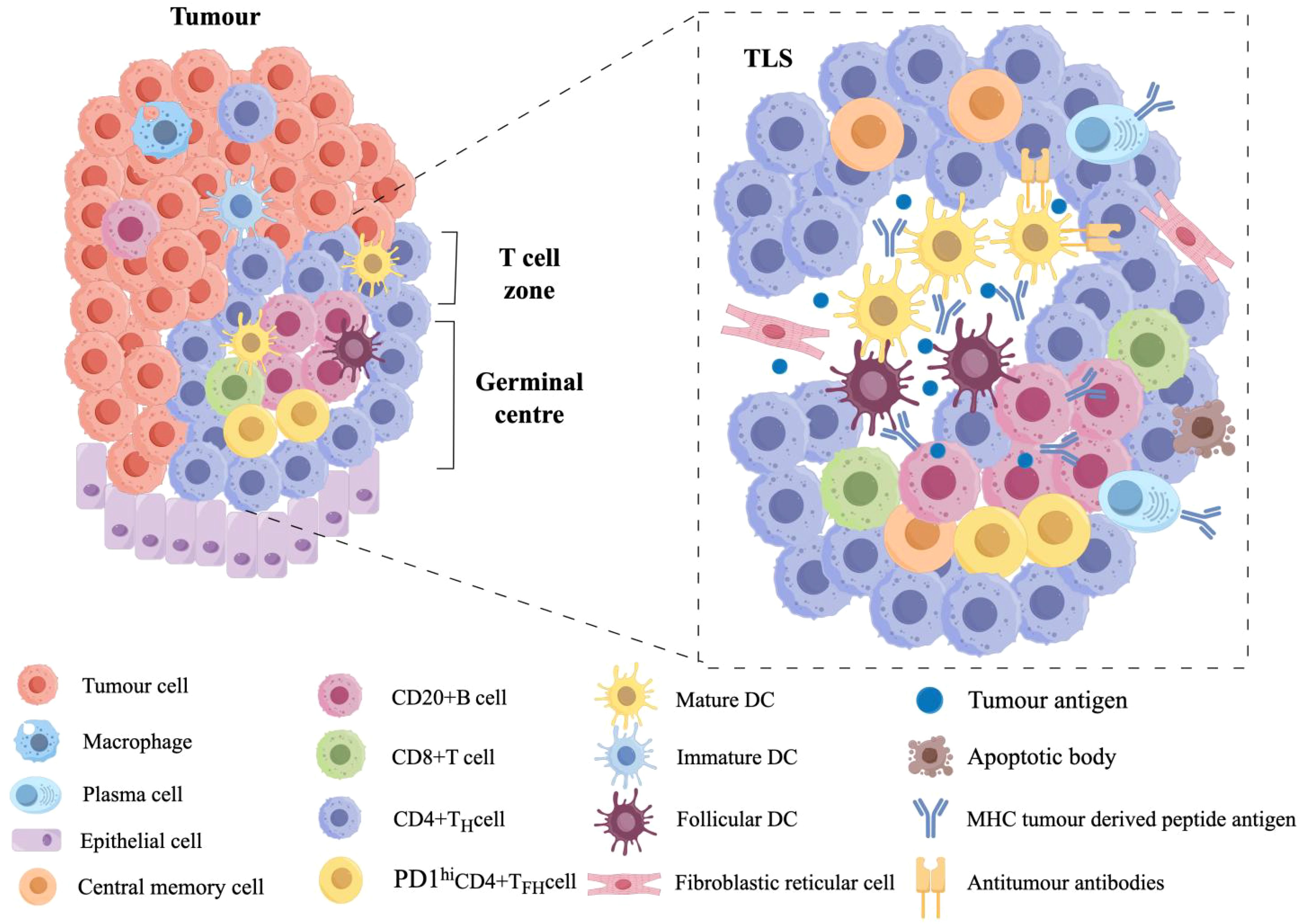
Figure 1. The structural composition of TLS (Tertiary Lymphoid Structures) in the tumor microenvironment. The TLS primarily contains B cells, follicular dendritic cells (FDCs), T cells, fibroblastic reticular cells (FRCs), stromal cells, dendritic cells, neutrophils, macrophages, and endothelial cells. Among these, FDCs are typically located in the B-cell zone, expressing various receptors to facilitate antigen presentation to B cells, thereby promoting the production of highly active antibodies. Endothelial cells are situated at the periphery of the TLS. Additionally, within the T-cell zone, there are some FRCs that interact with the local microenvironment to regulate T-cell function.
1.1 B cells in TLS
In antitumor humoral immunity, the orchestrated activation, proliferation, and differentiation of B cells constitute a critical effector mechanism. Within mature TLS, class switch recombination (CSR) and somatic hypermutation (SHM) are the main events of B cell activation. The resulting high-affinity IgG/IgA antibodies specifically bind to tumor cell antigens, triggering antibody-dependent cellular cytotoxicity (ADCC) and thereby enhancing anti-tumor immunity (6–13). For instance, in renal cell carcinoma, Meylan et al. demonstrated that tumors with high TLS signature gene expression exhibited markedly elevated clonal indices for immunoglobulin heavy (IgH) and light chains (IgL), with increased IgH clonality showing particular prominence compared to TLS-low tumors (14). These findings reveal antigen-driven B cell selection within TLS, accompanied by robust SHM and CSR activity that culminates in plasma cell differentiation. Taken together, these data position TLS as pivotal hubs for B cell maturation into either memory B cells or antibody-secreting plasma cells, thereby establishing tumor-targeted humoral immunity through IgG/IgA production (Figure 2).
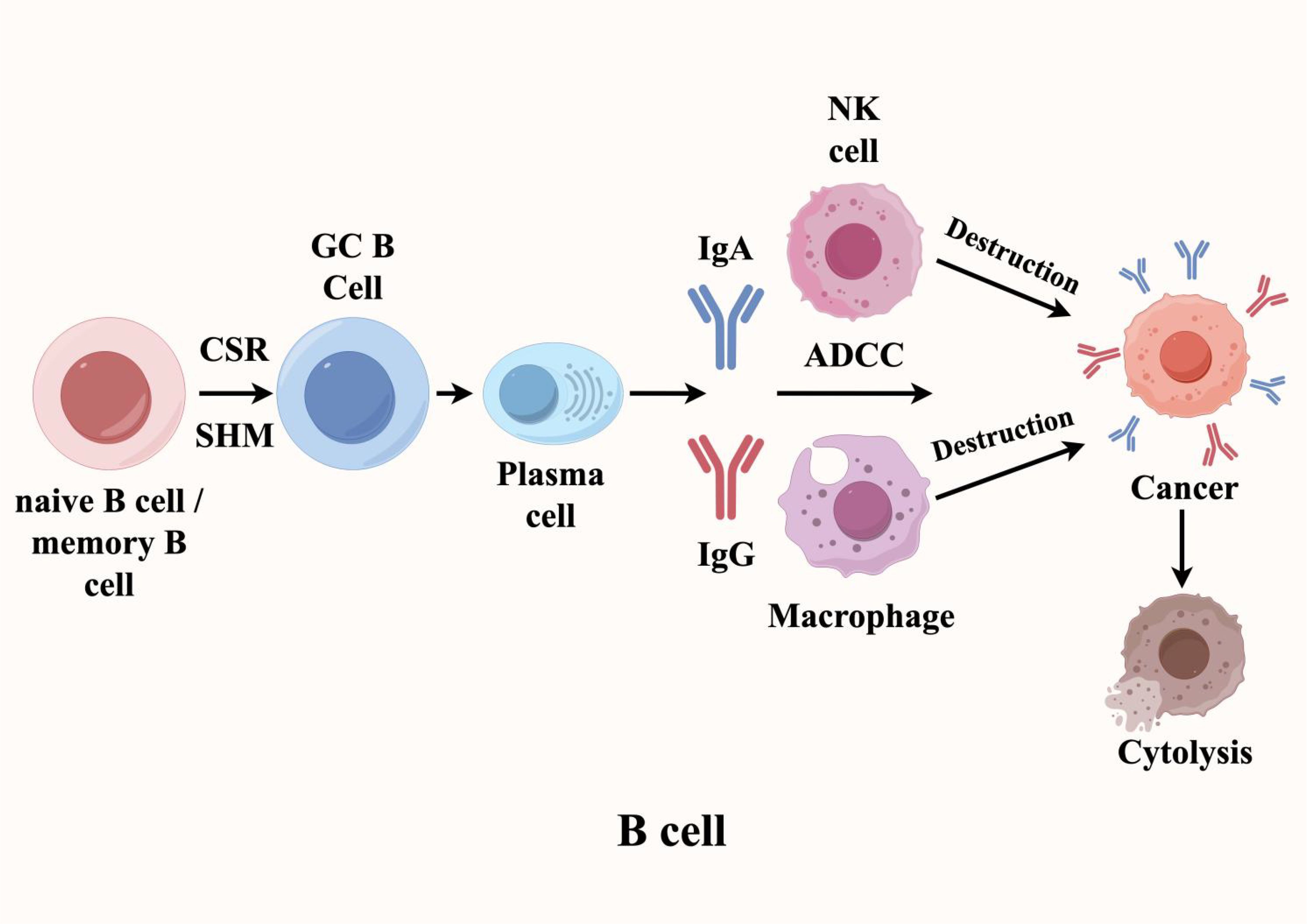
Figure 2. B cells play a dual role in TLS (Tertiary Lymphoid Structures), exhibiting both anti-tumor and tumor-promoting effects. In the tumor microenvironment, when B cells within TLS encounter tumor-associated antigens, they differentiate into memory B cells and plasma cells through the synergistic actions of somatic hypermutation (SHM) and class-switch recombination (CSR). The plasma cells then secrete high-affinity IgG and IgA antibodies targeting specific antigens on the surface of tumor cells, thereby inducing antibody-dependent cellular cytotoxicity (ADCC).
Clinical evidence further underscores the prognostic significance of TLS-associated humoral immunity. In head and neck squamous cell carcinoma (HNSCC), the presence of IgA- and IgG1-secreting plasma cells within TLS correlates strongly with improved patient outcomes (15). Mechanistically, studies in ovarian cancer mouse models reveal that T follicular helper (Tfh) cells orchestrate antibody class switching in TLS-resident B cells, thereby amplifying their antitumor potency. Moreover, across diverse malignancies—including cutaneous melanoma, omental ovarian cancer metastases, and gastroesophageal adenocarcinoma—B cells within tumor-associated TLS exhibit substantially more pronounced immunoglobulin gene rearrangement and clonal expansion than their peripheral counterparts (16–20). Together, these consistent observations highlight that antigen-driven B cell activation and proliferation within TLS represent a hallmark feature of productive antitumor immunity.
However, the TME harbors immature TLS that may foster B cell differentiation into regulatory B cells (Bregs). These immunosuppressive Bregs secrete cytokines like TGF-β, actively remodeling the immune landscape to promote tumor immune evasion (21–23). Notably, the functional impact of TLS exhibits striking cancer-type specificity—while in prostate cancer, a unique plasma cell subset suppresses CD8+ T cell activity, other tumor types may experience polyclonal B cell activation that propels macrophage polarization toward an immunosuppressive phenotype (24). Through these multifaceted mechanisms, TLS-resident B cells dynamically modulate the TME, ultimately dictating immunotherapy responses (25–27). This context-dependent functional plasticity underscores the dual roles of B cells in tumor immunity, ranging from effector responses to immunosuppression.
1.2 T cells in TLS
The TME hosts immune cells with multiple functional capacities, where T lymphocytes serve as the central mediators of anti-tumor immunity. As such, their activity within the local microenvironment fundamentally determines immunotherapy outcomes (28, 29). Within TLS, a specialized immune niche, antigen-presenting cells (APCs) - particularly DCs - prime T cell responses by presenting tumor-specific antigens, driving their activation, clonal expansion, and effector differentiation. This cascade culminates in potent cellular immunity against tumors. Specifically, CD8+ cytotoxic T lymphocytes (CTLs) directly eliminate tumor cells through dual mechanisms: (1) release of cytotoxic granules (e.g., granzyme B) and (2) secretion of pro-apoptotic cytokines (e.g., TNF-α) (30). Meanwhile, CD4+ T helper cells amplify immune responses by producing IFN-γ, which enhances CTL function (31). Importantly, a subset of activated T cells differentiates into memory T cells, establishing durable immunological memory that enables rapid tumor antigen recall responses (Figure 3).
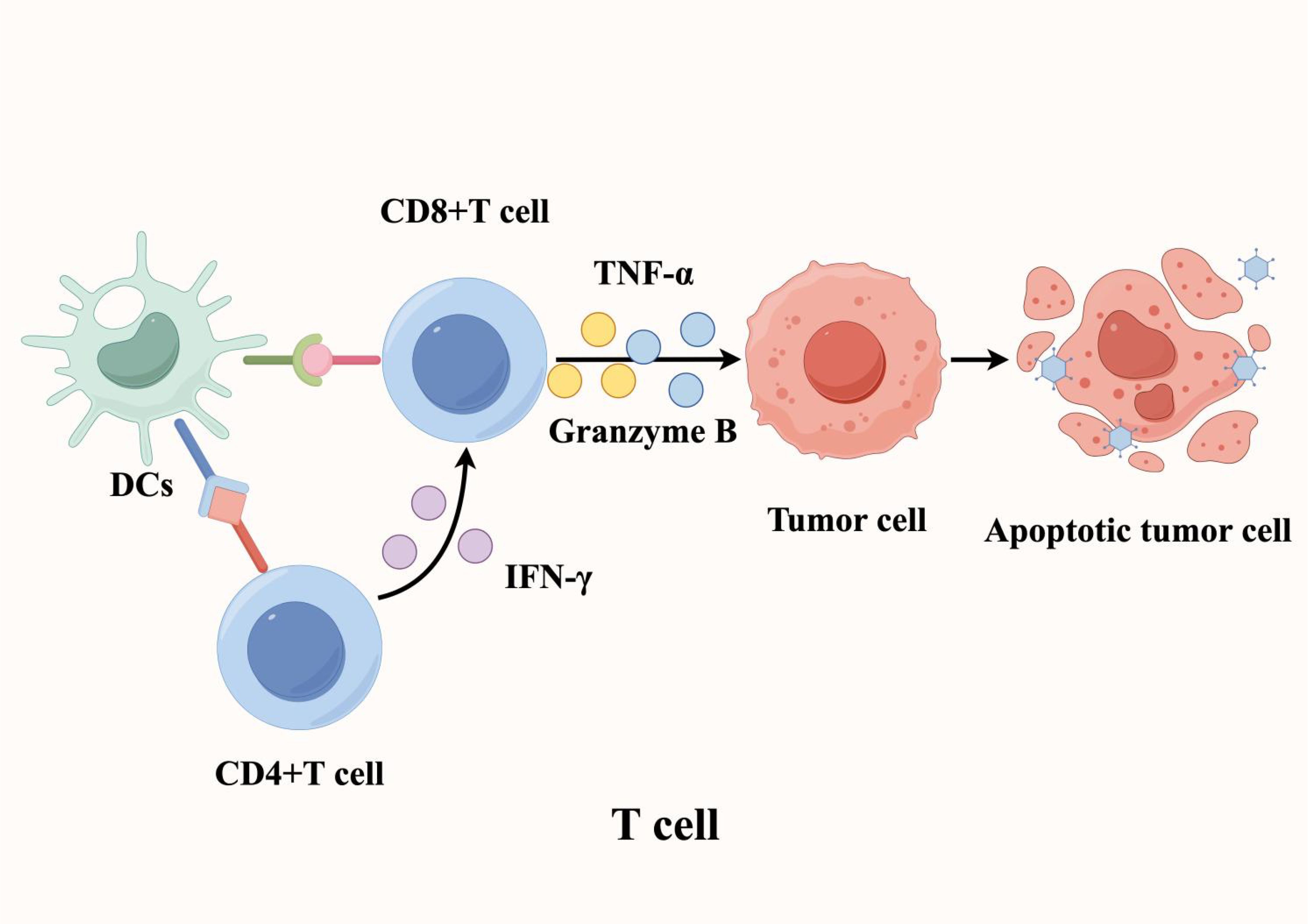
Figure 3. T cells play a crucial role in mediating anti-tumor immunity within tertiary lymphoid structures. Antigen-presenting cells (APCs), such as dendritic cells (DCs), process and present tumor-specific antigens to T cells, triggering their activation, proliferation, and differentiation. This process ultimately enhances tumor-targeted cellular immune responses and promotes tumor cell death.
Research indicates that the vast majority of T cells within TLS in lung cancer tissues are effector memory T cells, with only a small number being central memory T cells and other T cell subsets (32–34). Notably, researchers also observed that CD8+ T cells within TLS in the TME exhibit significant cytotoxic characteristics. This observation aligns with findings across multiple cancer types—such as lung cancer, colorectal cancer (CRC), and pancreatic cancer—where the density distribution of TLS in tumor tissues is positively correlated with T cell infiltration (35–37). Additionally, in HNSCC, the Tfh cell signature gene set is associated with a favorable prognosis, further underscoring the functional importance of TLS (38). Beyond cytotoxic CD8+ T cells, TLS is enriched with CD4+ T cells skewed towards the Th1 phenotype and regulatory T cells (Tregs) with immune regulatory functions (39). These findings reveal a complex and dynamic immune cell network within TLS, where the infiltration of cytotoxic CD8+ T cells suggests their key role in combating tumors. In parallel, the presence of Th1-skewed CD4+ T cells further enhances this immune response by promoting the effector mechanisms of the anti-tumor immune reaction. Crucially, as an organized structure, TLS allows T cells to directly interact with tumor edge cells to exert antitumor effects. In contrast, in patients with diffuse immune cell distribution, macrophages infiltrating the tumor tissue increase the enrichment of Ki-67+ Tregs, which in turn inhibit the immune function of T cells. This contrast confirms that TLS plays a more important antitumor role compared to diffusely distributed immune cells. In summary, within the TME, TLS functions as a structure similar to secondary lymphoid organs (SLOs), capable of regulating the proliferation and differentiation of T cells, thereby exerting potent antitumor immune responses.
1.3 FDCs in TLS
In the TME, tumor-associated mature TLSs are similar to SLOs, with the FDCs within them serving as a key subpopulation of cells that regulate humoral immunity. Specifically, FDCs in the TME are a special type of DC, mainly distributed in the B cell area and germinal center (GC) regions of TLSs, where they play a crucial role in B-cell mediated/humoral responses (40) (Figure 4). Within TLSs, FDCs interact with B cells through specific surface molecules such as CD21, CD23, and CD35, thereby promoting B cell activation, proliferation, and differentiation. Beyond direct cell–cell contact, FDCs can also present antigens to B cells, assisting them in recognizing tumor-specific antigens and facilitating B cell class switching and high-frequency mutation (somatic hypermutation) (41). Additionally, FDCs in TLSs can store and present antigens, a process that helps induce the formation of immunological memory. This memory function ensures that upon re-exposure to the same tumor antigen, memory B cells are rapidly reactivated to mount an effective and specific antibody response.
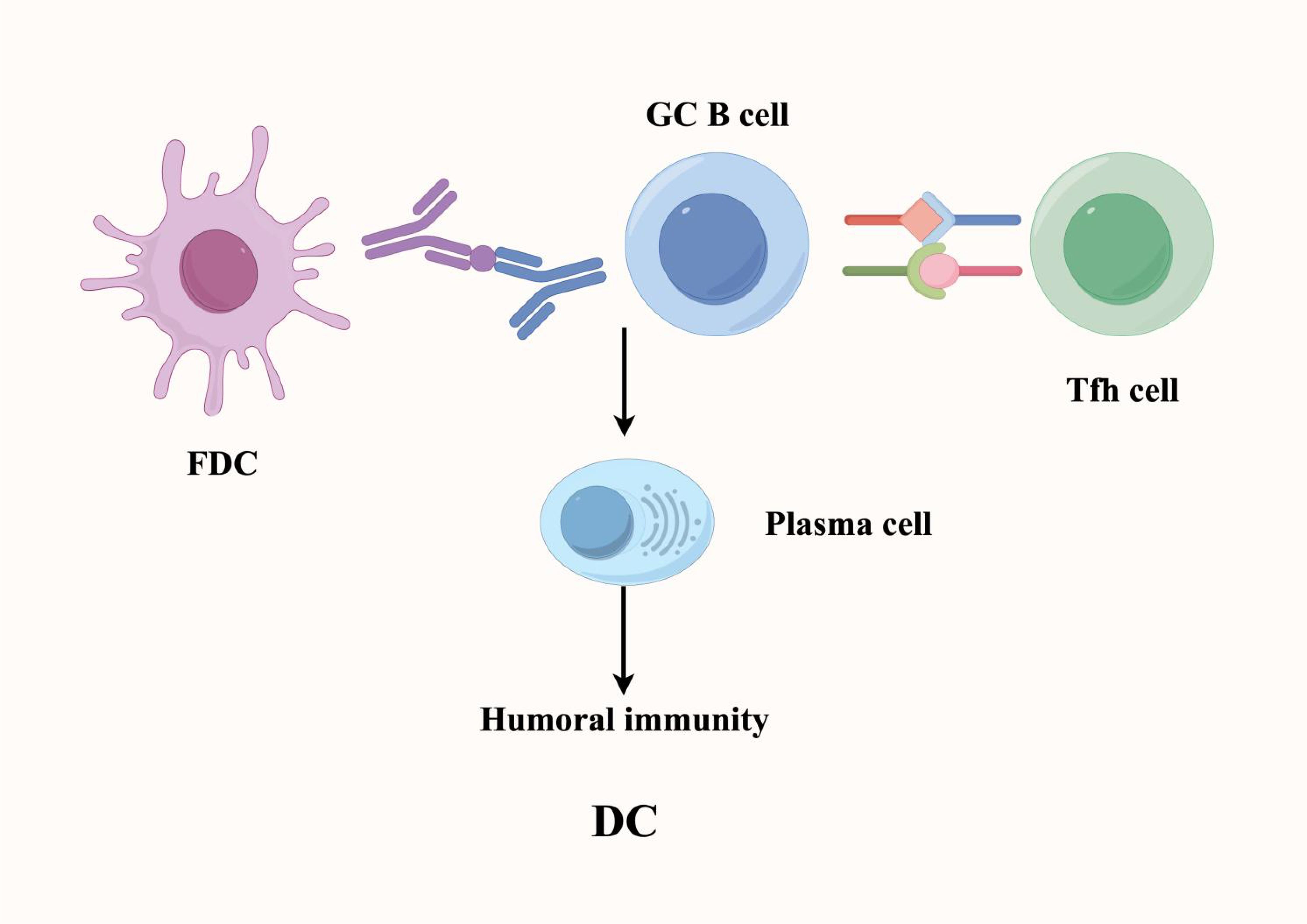
Figure 4. Within tertiary lymphoid structures, follicular dendritic cells (FDCs) sustain B cell-mediated humoral immune responses and maintain immunological memory. FDCs provide critical signaling cues and cellular support to promote B cell differentiation into antibody-secreting plasma cells. These plasma cells produce high-affinity antibodies targeting tumor-associated antigens, thereby enhancing tumor-specific humoral immune responses.
Moreover, in TLSs, the signals and cytokines provided by FDCs promote the differentiation of B cells into plasma cells, which then produce high-affinity antibodies against tumor antigens, ultimately enhancing the humoral immune response against tumors. Supporting their clinical relevance, in small cell renal cancer, increased infiltration of DCs in TLSs is closely related to lower recurrence and mortality risks (42). Furthermore, the density of DC infiltration in TLSs is negatively correlated with the infiltration of exhausted T cells in the tumor, suggesting a protective role of FDC-mediated immunity. In addition to their role in humoral regulation, FDCs recruit immune cells such as T cells, B cells, and macrophages into TLSs by secreting cytokines and chemokines, thereby helping maintain the structure and function of TLSs. This coordinated recruitment enhances local immune surveillance and immune response, further solidifying TLSs as critical regulators of antitumor immunity (43).
1.4 High endothelial venule and TLS
In the TME, high endothelial venules (HEVs) are key structures for the formation, maturation, and functional maintenance of TLS, and also serve as structural markers for TLS identification (44–46). Functionally, HEVs provide a rapid conduit for immune cells, enabling efficient recruitment of T cells, B cells, and other immune cells into the TLS compartment. This organized migration allows these immune cells to engage in direct interactions with tumor cells or other immune subsets, thereby enhancing immune surveillance and potentiating antitumor immune responses. Through this mechanism, HEVs facilitate the dynamic accumulation of effector lymphocytes at the tumor site, significantly enhancing tumor recognition and elimination by the immune system.
Clinically, the presence of HEVs in TLS shows a strong positive correlation with the degree of immune cell infiltration in the tumor, particularly for effector populations such as T cells, B cells, and macrophages (47). This association explains why HEV development is indicative of a favorable prognosis for cancer patients (48). Importantly, research has demonstrated that across multiple malignancies—including melanoma, head and neck squamous cell carcinoma, and breast cancer—HEV formation in TLS correlates with the activation and proliferation of immune cells within TLS microenvironments.
In summary, through their dual roles in immune cell recruitment and activation, HEVs not only contribute to the formation and structural integrity of TLS but also establish an immunologically active antitumor microenvironment, thereby amplifying the immune system’s surveillance and cytotoxic capacity against tumor cells (49).
2 The driving mechanism of TLS
SLOs are distributed throughout the body, including major sites such as lymph nodes, spleen, tonsils, Peyer’s patches, and mucosa-associated lymphoid tissue (MALT). These organized structures enable the immune system to sample antigens from various tissues and facilitate coordinated interactions between different immune cells, thereby promoting the induction of adaptive immune responses. Interestingly, under chronic inflammatory conditions, lymphoid tissue can undergo extranodal seeding and form TLSs at the site of inflammation (50). To better understand the development of these ectopic lymphoid aggregates, they can be structurally and functionally compared with the formation of SLOs during embryogenesis.
The seeding and organization of SLOs, particularly lymph nodes and Peyer’s patches, are initiated by a series of highly orchestrated events involving interactions between hematopoietic cells and non-lymphoid stromal cells. These interactions are mediated by cytokines, chemokines, adhesion molecules, and survival factors, which collectively regulate the spatial and temporal progression of SLOs development. The formation of SLOs begins early in embryonic development when hematopoietic lymphoid tissue inducer (LTi) cells, characterized by the expression of transcription factors RORγt and Id2, colonize the lymph node primordium. These LTi cells, which differentiate from fetal liver precursors, express lymphotoxin α1β2 (LTα1β2), which binds to the lymphotoxin β receptor (LTβR) on mesenchymal lymphoid tissue organizer (LTo) cells. This interaction drives the initial steps of SLOs formation, inducing the expression of adhesion molecules such as vascular cell adhesion molecule 1 (VCAM1), intercellular adhesion molecule 1 (ICAM1), mucosal addressin cell adhesion molecule 1 (MAdCAM1), and peripheral node addressin (PNAd), as well as the production of lymphoid chemokines including CCL19, CCL21, and CXCL13. These molecules collectively regulate the recruitment of immune cells to the lymphoid niche and the development of HEVs. The subsequent compartmentalization of newly formed lymphoid follicles is achieved through the segregated expression of homeostatic chemokines, such as CCL19+ and/or CCL21+ FRCs and CXCL13+ FDCs, which guide the distribution of lymphocytes expressing corresponding CCR7 and CXCR5 receptors, thereby enabling the formation of distinct T cell and B cell zones. Notably, the positive feedback loop induced by lymphoid chemokine secretion is essential for maintaining the lymphoid niche, as signaling through CXCR5 expressed on B cells and LTi cells can induce LTα1β2 expression (51, 52).
TLS exhibit significant anatomical similarities to SLOs, although most TLS in tissues lack a surrounding capsule. This structural distinction may allow their cellular components to directly interact with the surrounding tissue but also potentially exposes resident immune cells in TLS to large molecules in the inflammatory microenvironment. While the formation of TLS and SLOs was initially thought to be induced by the same molecular factors, such as LTα1β2-LTβR signaling and local expression of adhesion molecules and lymphoid chemokines, recent studies suggest that the cell types involved are not identical, and the precise stimuli driving TLS formation remain unclear. Additionally, several TLS molecular inducers independent of lymphotoxin signaling have been reported. Importantly, much of our understanding of the cellular and molecular processes driving TLS formation has been derived from autoimmune disease and chronic infection models, highlighting the need for caution when extrapolating these findings to other physiological or pathological contexts.
Regarding the upstream initiation mechanisms of TLS, it is currently unclear whether classical LTi cells are required to initiate local mesenchymal accumulation, or whether locally aggregated immune cells can substitute for LTi cells. Several immune cell subsets that may fulfill this role mainly include Th17 cells and Innate Lymphoid Cell 3 (ILC3), which have been observed in contexts such as allograft rejection, autoimmunity, chronic inflammation, or cancer in both mice and humans. Notably, unlike the formation of SLOs, the induction of TLS may not always depend on lymphotoxins. For example, Interleukin 17 (IL-17) produced by T cells can induce the expression of CXCL13 and CCL19 in mouse stromal cells, thereby promoting the formation of inducible Bronchus-Associated Lymphoid Tissue (iBALT), a type of TLS that forms in lung tissue. Consistent with this lymphotoxin-independent pathway, lymphoid aggregates do form in LTα-/- mice, although these structures exhibit impaired organization, lacking clear separation of T and B cell zones and functional HEVs, and thus may not be considered typical TLS. To further elucidate the role of the local tissue environment in determining the composition of TLS, studies have demonstrated that transgenic expression of different cytokines and chemokines in mouse models can induce TLS with distinct characteristics. For example, tissue-specific expression of CXCL13 induces B cell aggregates that are lacking FDC networks, while expression of TNF and CXCL12 induces small lymphocyte infiltrates, mainly composed of B cells and scattered T cells. Moreover, although CCL19 and CCL21, the ligands of CCR7, induce aggregates of similar composition, the structures induced by CCL21 expression are larger and more organized, suggesting a dose- or context-dependent effect on lymphoid neogenesis (53).
Huge differences in TLS components have been detected across various human cancers. For example, DC-LAMP+ DCs are frequently observed in the TLS of NSCLC but are rare in other cancer types. Similarly, Tfh cells have primarily been documented in the TLSs of breast cancer (54). However, these findings must be interpreted cautiously, as most available data derive from studies employing inconsistent TLS markers. Consequently, there is still a lack of standardized parameters for rigorous assessment and large-scale analysis of TLS heterogeneity among cancers. Emerging evidence suggests that TLS heterogeneity correlates with the degree of TLS maturation. For instance, based on structural similarity with SLOs, three maturation stages have been identified in NSCLC, hepatocellular carcinoma (HCC), and CRC (55). The least organized stage comprises dense lymphocyte aggregates without FDCs or distinct T/B cell zones. Primary follicle-like TLSs contain FDCs but lack GC reactions, whereas fully mature secondary follicle-like TLSs exhibit active GCs, indicating functional maturity (56). Although numerous factors influencing TLS formation have been characterized, the precise molecular determinants of the TME that promote or suppress TLS formation remain incompletely understood. To clarify this, we propose a framework categorizing TMEs where TLSs are absent: (1) a “restrictive” environment, where TLS formation is actively inhibited, and (2) an “insufficient” environment, lacking essential drivers (e.g., antigens). In summary, TLS formation and development involve a multi-step, dynamic process. Pro-inflammatory signals from immune cells initiate TLS induction, while activated fibroblasts serve as organizers, establishing lymphocyte structures and secreting chemokines to recruit cells. Through these interactions, TLSs differentiate, mature, and ultimately exert immune effects.
3 TLS markers and detection
Currently, TLS have been identified in various tumor types, including lung cancer, pancreatic cancer, CRC, and melanoma. However, due to the lack of standardized marker strategies, significant differences exist in TLS detection across cancer types, which complicates direct cross-cancer comparisons. To assess TLS presence and composition, commonly used methods include: hematoxylin and eosin staining (H&E), immunohistochemistry (IHC), multiplex immunohistochemistry (mIHC)/multiplex immunofluorescence (mIF), and sequencing-based assays.
4 Tumor-associated genes and TLS
4.1 EGFR mutations and TLS
In 2016, Mansuet-Lupo et al. first reported that EGFR mutations were more frequent in lung adenocarcinoma (LUAD) patients with high-density TLS (57). Subsequent studies confirmed this finding, demonstrating that EGFR mutations were enriched in TLS containing mature dendritic DCs and served as a key determinant of the tumor immune microenvironment (TIME) (58). These mutations may influence immune responses by modulating cytokine and chemokine secretion, thereby promoting T cell-mediated protective immunity.
However, the relationship between EGFR mutations and TLS remains controversial (59, 60). While some studies support this association, others found no significant difference in EGFR mutation rates between LUAD patients with or without TLS. Notably, Feng et al. observed that regardless of EGFR mutation status, patients with high TLS density had significantly better overall survival (OS) and progression-free interval (PFI) than those with low TLS density, suggesting TLS density may be an independent prognostic factor.
Furthermore, Gu et al. investigated ethnic differences in the relationship between tumor mutational burden (TMB) and genetic mutations in LUAD (61). Their findings revealed that EGFR-mutant LUAD patients of Chinese descent had better prognosis compared to Caucasian patients, highlighting the importance of ethnic considerations in LUAD prognosis. Although TMB has emerged as a potential biomarker for LUAD, its predictive value requires further validation due to inconsistent findings across studies.
4.2 HER-2 and TLS
Liu et al. conducted a retrospective analysis of 248 invasive breast cancer cases, revealing that HER2-positive tumors were significantly more likely to exhibit TLS expression (62). Their findings demonstrated that the HER2-positive cohort displayed: (1) increased infiltration of mature FDCs, and (2) elevated expression of lymphoid chemokines (CCL19, CCL21, and CXCL13) known to be critical for TLS formation and maintenance. A subsequent meta-analysis reinforced these observations, confirming a positive correlation between TLS presence and HER2 mutation status while additionally associating TLS with improved clinical outcomes, including both disease-free survival (DFS) and overall survival (OS) (63).
Further investigations in HER2-positive breast cancer patients undergoing surgical treatment revealed significant correlations between TLS characteristics (both density and maturity) and HER2-related parameters (immune scores and gene copy numbers) (64). Intriguingly, comparative analysis showed that the high-TLS group exhibited marked upregulation of key immune checkpoint genes relative to the low-TLS group. Based on these findings, the researchers proposed two potential mechanistic explanations: First, HER2 protein overexpression or related mutations may serve as immunogenic factors that actively recruit lymphocytes and facilitate TLS formation. Second, HER2+ ductal carcinoma in situ (DCIS) might promote macrophage infiltration, thereby enhancing antigen presentation and immune activation processes essential for TLS development. Notably, this association appears to be tumor type-specific, as no similar relationship between TLS and HER2 mutations has been observed in LUAD studies. This distinction underscores the importance of considering cancer-specific microenvironments when evaluating TLS formation mechanisms.
4.3 TP53 and TLS
In lung adenocarcinoma (LUAD), tumors with TP53 mutations exhibit higher CD8+ T cell density, mature DC infiltration, and enhanced immunogenicity. Despite these immunogenic features, no direct correlation has been found between TP53 mutations and TLS expression in LUAD. This contrasts with other cancers: for example, CRC, gastric cancer, and oral squamous cell carcinoma (OSCC) show a negative correlation between TLS presence and TP53 mutations, whereas breast cancer and low-grade gliomas with higher TLS scores tend to have a higher frequency of TP53 mutations. These divergent observations suggest a context-dependent role of TP53 in TLS regulation (65, 66). A possible explanation is that TP53-mutant tumors upregulate chemokines (CXCL9, CXCL10, CXCL11), which promote T cell effector functions and memory B cell phenotypes, thereby driving TLS formation in certain tumor types but not others.
4.4 BRAF and TLS
In lung cancer, BRAF mutations do not significantly influence the composition of the TIME. In contrast, in non-metastatic colorectal cancer (nmCRC), BRAF mutation status is associated with TLS formation, which follows a sequential maturation process that ultimately leads to GC development. Notably, BRAF mutations positively correlate with TLS density and maturity, particularly in BRAF V600E-mutant tumors, which exhibit higher TLS numbers (67). Meanwhile, in melanoma, approximately 66% of cases harbor BRAF mutations, yet the relationship between BRAF and TLS remains poorly characterized (68). Here, the BRAF V600E mutation regulates IL-1α/β transcription in melanocytes, leading to upregulation of immunosuppressive genes (e.g., PD-1 ligands, COX2) in cancer-associated fibroblasts. Preclinically, BRAF inhibition suppresses IL-1α production in melanoma cell lines, implying a potential indirect role in TLS modulation. However, a study of 177 melanoma cases found no correlation between B-cell transcriptional signatures (a TLS marker) and BRAF mutation status, further underscoring the context-dependent nature of BRAF-TLS interactions (69, 70).
4.5 BRCA and TLS
The genes encoding BRCA1/2 are involved in the detection and repair of DNA alterations and are frequently mutated in tumors such as breast or ovarian cancer. Consistent with this role, LIN et al. found that in most tumors, including breast, prostate, and endometrial cancers, many mutations (such as BRCA mutations) are positively correlated with TLS scores (71). Specifically, in ovarian cancer, HER-2/neu+ tumors, and triple-negative breast cancer (TNBC), BRCA-mutated tumors exhibit strong CD8+ T-cell infiltration (72). Supporting this observation, Solinas et al. detected an average of 4.5 TLS per square centimeter of tumor tissue in 75% of TNBC cases (73). While in BRCA-mutated tumors, tumor-infiltrating lymphocytes (TILs) were observed, with some studies suggesting that TILs are the strongest independent predictor of TLS, the study showed no difference in the density, location, or composition of TLS between BRCA-mutated and non-mutated TNBC cohorts. This discrepancy may be because mutations arising from DNA repair defects are largely independent of immune status, while TLS quantity is indirectly influenced by this process and occurs stochastically (74).
Furthermore, other studies have found that in BRCA1/2 protein-positive breast cancer tissues, the proportion of Treg infiltration in the local microenvironment decreases. Since Tregs suppress T-cell and DC-cell responses, reducing HEV formation and CCL21 expression, their reduction could diminish the inhibitory effect on TLS formation (75, 76). However, contrasting these findings, in high-grade serous ovarian cancer, no correlation was found between TLS and mutation load, BRCA1/2 status, or differentiation antigens.
4.6 DNA methylation and TLS
By conducting DNA methylation analysis on ccRCC samples with different TLS statuses, significant differences in methylation profiles were observed (77). Notably, in CRC, the TLS-positive group exhibited the CpG island methylator phenotype (CIMP)-high subtype. Further supporting this observation, GO enrichment analysis revealed that TLS-positive tumors were associated with positive regulation of biological processes such as RNA polymerase II promoter transcription and intercellular adhesion. These findings suggest that the epigenetic background can predict whether TLS-positive ccRCC tumors exhibit invasive behavior. Moreover, certain adhesion factors may recruit lymphocytes through a multi-step adhesion cascade mechanism, a critical step in TLS formation.
Beyond ccRCC, DNA methylation changes were also observed between TLS subgroups in EBV-negative gastric cancer patients (78). Specifically, the low-density TLS subgroup exhibited epigenetic silencing, primarily affecting immune-related transcription factors such as GFI1 and IRF4. As previously established, Tfh cells are localized at the center of TLS, marking the initial stage of TLS formation before GC appearance. Additionally, fibroblasts play a pivotal role in TLS development (79, 80).
Epigenetic regulation further influences TLS formation through SATB1, a nuclear matrix-binding protein that organizes chromatin loops and recruits epigenetic modifiers in immune cells, driving their phenotypes and inflammatory responses. Interestingly, Tfh cells express low levels of SATB1 (81). Supporting this, CHAURIO et al. demonstrated SATB1’s role as a “genomic organizer” in Tfh differentiation using a mouse model (82). Mechanistically, SATB1 induces the expression of ICOS, a key costimulatory factor for Tfh, by binding ~60 bp upstream of the ICOS transcription start site (83). Consequently, SATB1 deficiency leads to elevated ICOS levels, enhancing Tfh differentiation and increasing IL-21, CXCL13, and IgG1 antibody production by B cells. Simultaneously, SATB1 loss impairs naive T cell differentiation into Foxp3+ Treg cells, reducing their inhibitory effect on TLS formation. In summary, SATB1 silencing in CD4+ T cells suppresses the ICOS promoter, thereby promoting their differentiation into functional Tfh cells while inhibiting Treg generation. This creates a permissive microenvironment for B cell recruitment, chemokine activation, and TLS development.
5 TLS and tumor prognosis
TLS was first discovered in solid tumors of NSCLC patients, where the high density of these structures was associated with long-term survival (84). Subsequent studies revealed that the favorable prognostic value of TLS extends to a wide range of cancers, including CRC, lung cancer, breast cancer, melanoma, and pancreatic cancer, where TLS density consistently correlates with improved clinical outcomes (85–89). Notably, this prognostic significance is often independent of TNM staging.
Recently, ZHANG et al. identified TLS in cervical cancer for the first time, demonstrating that their abundance is influenced by tumor invasion depth, preoperative chemotherapy, HPV infection status, and PD-1 expression levels (90). Critically, TLS presence in these patients was linked to better prognosis. Beyond prognosis, TLS is increasingly recognized as a predictive biomarker for treatment response across multiple therapies, including chemotherapy, targeted therapy, and immunotherapy (91). For example, in TNBC, TLS density correlates with pathological complete response (pCR) after neoadjuvant chemotherapy and predicts superior outcomes for patients receiving targeted therapy. Similarly, in HER-2/neu+ tumors treated with trastuzumab, high TLS density is associated with improved DFS. In GIST patients, high TLS levels are linked to reduced imatinib resistance, lower recurrence rates, and prolonged OS (92). Furthermore, TLS shows promise in predicting response to immune checkpoint blockade (ICB). In melanoma, pre-treatment of CD20+ B cells within TLS coordinate tumor inflammation and enhance PD-1+ T cell activation upon anti-PD-1 therapy, thereby predicting ICB response and survival (93). This association is corroborated in NSCLC, soft tissue sarcoma, and urothelial carcinoma, where high TLS density correlates with better ICB outcomes (94–96). Collectively, these findings position TLS as a robust biomarker for patient stratification across cancer types and therapies.
The induction of TLS may represent an attractive therapeutic strategy for cancer treatment. Supporting this concept, in hepatoblastoma (HB) with APC mutations, TLS formation was significantly increased in paired tumor biopsies following cisplatin-based chemotherapy (97). This chemotherapy-induced TLS formation may promote immunogenic cell death (ICD), resulting in neoantigen release that is captured by DCs to initiate anti-tumor immune responses. Consistent with this immunostimulatory role, NSCLC patients treated with neoadjuvant anti-PD-1 therapy showed TLS enrichment that positively correlated with improved treatment response rates (98). However, the therapeutic impact appears context-dependent, as demonstrated by contrasting findings in squamous lung cancer where neoadjuvant chemotherapy impaired TLS maturation, caused GC loss, and abolished their prognostic value (99).
While numerous studies have demonstrated the association between high TLS density and improved long-term survival in various tumors, emerging evidence reveals a more complex relationship. In breast cancer, for instance, TLS presence correlated with prolonged survival in metastatic lesions (lung, liver, brain, and ovary), yet paradoxically, high TLS density was associated with worse outcomes in primary tumors (100). This dichotomy extends to other malignancies: Finkin et al. reported poorer prognosis in HCV-negative, non-alcoholic HCC patients with TLS, while Giraldo et al. identified a distinct TLS subtype (NTLS-DC) in clear cell renal cell carcinoma (ccRCC) that predicted shorter DFS and OS (101). Notably, these NTLS-DCs, located in tumor cores, exhibited low MHC II expression and contained immature DCs. Similarly, in advanced classical Hodgkin lymphoma, high TLS density unexpectedly increased mortality risk (102).
The dual role of TLS may stem from their functional heterogeneity. In aggressive cancers, TLS may foster immunosuppression through: (1) cytokine-mediated inhibitory microenvironments; (2) TLS-resident Tregs that dampen anti-tumor T cell responses; and (3) IL-10-secreting B cells that impair tumor-specific immunity. Moreover, tumor-specific antibodies generated in TLS might paradoxically suppress immunity via inhibitory Fc receptor signaling. However, whether these observations represent cancer-type-specific phenomena or broader patterns requires further investigation.
Spatial distribution adds another layer of complexity. In HCC, TLS within adjacent normal liver tissue correlated with higher recurrence risk or lacked prognostic value, in stark contrast to tumor-core TLS which predicted better outcomes (103). This spatial dichotomy was replicated in intrahepatic cholangiocarcinoma, where intratumoral TLS conferred survival benefits while peritumoral TLS associated with worse prognosis. Emerging evidence suggests this discrepancy may reflect compositional differences: intratumoral TLS harbor elevated Tfh and Treg populations compared to their peritumoral counterparts. To resolve these controversies, future studies should employ advanced animal models to elucidate TLS dynamics and microenvironmental interactions across tumor stages and locations.
6 TLS and immune therapy
The classical paradigm held that tumor-specific immune responses required DC migration from primary tumors to SLOs, where naïve CD4+ T cell activation occurred following tumor antigen presentation. However, emerging evidence challenges this view, demonstrating that efficient antigen presentation to T cells can occur directly within TLS in the TME, bypassing the need for DC trafficking to distant lymph nodes (104). This localized process enables more rapid and efficient antitumor immunity, with important implications for cancer immunotherapy. These findings have catalyzed significant interest in understanding TLS functions during immune checkpoint inhibitor (ICI) therapy. Unlike conventional cytotoxic therapies, ICB exerts antitumor effects by harnessing endogenous immunity a mechanism that highlights the critical role of TLS as specialized immune niches within the TIME (105). The TIME represents an intricate ecosystem of diverse immune cell populations that collectively influence tumor progression, treatment response, and clinical outcomes. As ectopic lymphoid organs, TLS positioned within or adjacent to tumors dramatically increase lymphocyte-tumor cell interactions, potentially amplifying local immune responses. This spatial advantage may explain their association with enhanced immunotherapeutic efficacy observed in multiple malignancies.
Mounting clinical evidence has established a bidirectional correlation between TLS presence and ICI efficacy: not only can TLS density predict ICI treatment outcomes, but conversely, ICI responsiveness may reflect TLS status. Compelling data demonstrate that ICI responders exhibit significantly higher intratumoral TLS densities compared to non-responders, suggesting that TLS-mediated T cell/B cell crosstalk potentiates antitumor immunity (106). Capitalizing on these prognostic associations, TLS induction has emerged as a promising immunotherapeutic strategy. Preclinical studies have validated the feasibility of generating ectopic TLS through localized delivery of lymphoid-organizing factors, including lymphotoxin, TNFα, LIGHT, CXCL13, CCL19, CCL21, and CXCL12 in murine models - providing a mechanistic foundation for clinical translation (107–111). Anatomically, TLS predominantly localize to stromal and peritumoral regions across multiple malignancies, including melanoma, NSCLC, HCC, and CSCC (112, 113). Notably, TLS occur with particularly high frequency in CSCC and CRCs, a phenomenon potentially attributable to the skin and gut’s specialized immune surveillance systems as barrier organs (114, 115). This anatomical predisposition suggests that TLS-rich microenvironments may amplify antitumor immunity through enhanced immune cell priming and activation.
While TLS can enhance tumor suppression, their presence may also trigger autoimmune reactions in normal tissues. As TLS form and mature within or around tumors, a large number of tumor-associated antigens are released. These antigens further activate immune cells, which can then migrate via the bloodstream and infiltrate normal tissues. Upon encountering self-antigens resembling tumor-associated antigens, these activated immune cells may mistakenly attack healthy tissues, leading to autoimmune pathology (116). Although data on such events are scarce, the potential for immune-related adverse outcomes cannot be ignored. Therefore, further research is needed to better harness TLS for antitumor immunity while minimizing autoimmune risks.
7 Standardization of TLS evaluation
Standardizing the qualitative and quantitative assessment of TLS is a crucial yet challenging task. Current evaluation methods primarily rely on histopathological approaches, utilizing immunohistochemistry and multiplex immunofluorescence techniques to identify the composition and spatial distribution of immune cell types within TLS. While this assessment approach is effective and intuitive to some extent, several major issues remain: First is subjectivity. Histopathological evaluation largely depends on the expertise of pathologists, leading to discrepancies in TLS interpretation among experts across different laboratories and research centers. Second, there is a lack of standardized assessment criteria for TLS. Currently, methods for evaluating TLS vary across research institutions, including inconsistencies in TLS quantification and the labeling of immune cells within TLS. These two major shortcomings limit the comparability and applicability of TLS research findings, hindering the clinical adoption of TLS as a predictive biomarker. Without unified standards, different evaluation results cannot be directly compared, making it difficult to translate research outcomes into practical clinical guidelines.
To address these issues, the following steps should be taken: First, it is essential to establish unified criteria for TLS evaluation, including standardization of immunohistochemical staining protocols, selection of lymphocyte markers and quantification methods for positivity, as well as clear criteria for assessing TLS maturity. Second, artificial intelligence (AI)-assisted analysis of digital pathology images should be employed to standardize the evaluation of TLS distribution density, maturity, and internal immune cell composition. This approach can reduce pathologist subjectivity, improve accuracy and reproducibility, and facilitate global adoption, thereby enhancing the translation of research findings into clinical practice. Finally, multicenter and multi-cohort validation is needed to refine TLS assessment standards. Testing and validating TLS evaluation methods across diverse populations and tumor types will improve the generalizability and reliability of the approach.
8 Conclusion
Recent studies highlighting the prognostic and predictive value of TLS in cancer have renewed interest in these ectopic immune aggregates as potential mediators of antitumor immunity. While direct evidence demonstrating the unique characteristics of immune responses originating within TLS remains scarce, cross-cancer analyses have consistently affirmed their clinical significance. However, the absence of standardized markers to define and characterize TLS represents a major translational hurdle that must be overcome to establish them as reliable biomarkers. To advance the field, we propose that a comprehensive definition of “TLS status” should integrate multidimensional characteristics, including cellular composition, spatial organization, maturation stage, and functional output. Such a framework would not only clarify their role in cancer immunity but also facilitate their clinical implementation as biomarkers. Importantly, systematic elucidation of TLS-associated molecular signatures through multi-omics approaches could further refine their prognostic and predictive value. Looking forward, a deeper mechanistic understanding of TLS functions—particularly their context-dependent roles in both antitumor immunity and autoimmune toxicity—will be essential to harness their full potential as therapeutic targets across cancer and chronic inflammatory diseases.
Author contributions
CL: Writing – original draft. JC: Writing – review & editing.
Funding
The author(s) declare that no financial support was received for the research and/or publication of this article.
Acknowledgments
We sincerely thank the free drawing support provided by the Figdraw platform (www.fgdraw.com). Figures in the paper were drawn using Figdraw.
Conflict of interest
The authors declare that the research was conducted in the absence of any commercial or financial relationships that could be construed as a potential conflict of interest.
Generative AI statement
The author(s) declare that no Generative AI was used in the creation of this manuscript.
Publisher’s note
All claims expressed in this article are solely those of the authors and do not necessarily represent those of their affiliated organizations, or those of the publisher, the editors and the reviewers. Any product that may be evaluated in this article, or claim that may be made by its manufacturer, is not guaranteed or endorsed by the publisher.
References
1. Zou J, Zhang Y, Zeng Y, Peng Y, Liu J, Xiao C, et al. Tertiary lymphoid structures: A potential biomarker for anti-cancer therapy. Cancers (Basel). (2022) 14:5968. doi: 10.3390/cancers14235968
2. Zhang E, Ding C, Li S, Zhou X, Aikemu B, Fan X, et al. Roles and mechanisms of tumour-infiltrating B cells in human cancer: a new force in immunotherapy. Biomark Res. (2023) 11:28. doi: 10.1186/s40364-023-00460-1
3. Laumont CM and Nelson BH. B cells in the tumor microenvironment: Multi-faceted organizers, regulators, and effectors of anti-tumor immunity. Cancer Cell. (2023) 41:466–89. doi: 10.1016/j.ccell.2023.02.017
4. Mulligan JK, Rosenzweig SA, and Young MR. Tumor secretion of VEGF induces endothelial cells to suppress T cell functions through the production of PGE2. J Immunother. (2010) 33:126–35. doi: 10.1097/CJI.0b013e3181b91c9c
5. Dieu-Nosjean MC, Goc J, Giraldo NA, Sautès-Fridman C, and Fridman WH. Tertiary lymphoid structures in cancer and beyond. Trends Immunol. (2014) 35:571–80. doi: 10.1016/j.it.2014.09.006
6. Bod L and Shalapour S. B cells spatial organization defines their phenotype and function in cancer "Tell me with whom you consort, and I will tell you who you are" - Goethe. Curr Opin Immunol. (2024) 91:102504. doi: 10.1016/j.coi.2024.102504
7. Verstegen NJM, Pollastro S, Unger PA, Marsman C, Elias G, Jorritsma T, et al. Single-cell analysis reveals dynamics of human B cell differentiation and identifies novel B and antibody-secreting cell intermediates. Elife. (2023) 12:e83578. doi: 10.7554/eLife.83578
8. Chi X, Li Y, and Qiu X. V(D)J recombination, somatic hypermutation and class switch recombination of immunoglobulins: mechanism and regulation. Immunology. (2020) 160:233–47. doi: 10.1111/imm.13176
9. Kroeger DR, Milne K, and Nelson BH. Tumor-infiltrating plasma cells are associated with tertiary lymphoid structures, cytolytic T-cell responses, and superior prognosis in ovarian cancer. Clin Cancer Res. (2016) 22:3005–15. doi: 10.1158/1078-0432.CCR-15-2762
10. Germain C, Gnjatic S, Tamzalit F, Knockaert S, Remark R, Goc J, et al. Presence of B cells in tertiary lymphoid structures is associated with a protective immunity in patients with lung cancer. Am J Respir Crit Care Med. (2014) 189:832–44. doi: 10.1164/rccm.201309-1611OC
11. Montfort A, Pearce O, Maniati E, Vincent BG, Bixby L, Böhm S, et al. A strong B-cell response is part of the immune landscape in human high-grade serous ovarian metastases. Clin Cancer Res. (2017) 23:250–62. doi: 10.1158/1078-0432.CCR-16-0081
12. Yaeger R, Chatila WK, Lipsyc MD, Hechtman JF, Cercek A, Sanchez-Vega F, et al. Clinical sequencing defines the genomic landscape of metastatic colorectal cancer. Cancer Cell. (2018) 33:125–136.e3. doi: 10.1016/j.ccell.2017.12.004
13. Biswas S, Mandal G, Payne KK, Anadon CM, Gatenbee CD, Chaurio RA, et al. IgA transcytosis and antigen recognition govern ovarian cancer immunity. Nature. (2021) 591:464–70. doi: 10.1038/s41586-020-03144-0
14. Meylan M, Petitprez F, Becht E, Bougoüin A, Pupier G, Calvez A, et al. Tertiary lymphoid structures generate and propagate anti-tumor antibody-producing plasma cells in renal cell cancer. Immunity. (2022) 55:527–541.e5. doi: 10.1016/j.immuni.2022.02.001
15. Wieland A, Patel MR, Cardenas MA, Eberhardt CS, Hudson WH, Obeng RC, et al. Defining HPV-specific B cell responses in patients with head and neck cancer. Nature. (2021) 597:274–8. doi: 10.1038/s41586-020-2931-3
16. Cipponi A, Mercier M, Seremet T, Baurain JF, Théate I, van den Oord J, et al. Neogenesis of lymphoid structures and antibody responses occur in human melanoma metastases. Cancer Res. (2012) 72:3997–4007. doi: 10.1158/0008-5472.CAN-12-1377
17. Nielsen JS, Sahota RA, Milne K, Kost SE, Nesslinger NJ, Watson PH, et al. CD20+ tumor-infiltrating lymphocytes have an atypical CD27- memory phenotype and together with CD8+ T cells promote favorable prognosis in ovarian cancer. Clin Cancer Res. (2012) 18:3281–92. doi: 10.1158/1078-0432.CCR-12-0234
18. Schlößer HA, Thelen M, Lechner A, Wennhold K, Garcia-Marquez MA, Rothschild SI, et al. B cells in esophago-gastric adenocarcinoma are highly differentiated, organize in tertiary lymphoid structures and produce tumor-specific antibodies. Oncoimmunology. (2018) 8:e1512458. doi: 10.1080/2162402X.2018.1512458
19. Coronella JA, Spier C, Welch M, Trevor KT, Stopeck AT, Villar H, et al. Antigen-driven oligoclonal expansion of tumor-infiltrating B cells in infiltrating ductal carcinoma of the breast. J Immunol. (2002) 169:1829–36. doi: 10.4049/jimmunol.169.4.1829
20. Nzula S, Going JJ, and Stott DI. Antigen-driven clonal proliferation, somatic hypermutation, and selection of B lymphocytes infiltrating human ductal breast carcinomas. Cancer Res. (2003) 63:3275–80.
21. Zirakzadeh AA, Sherif A, Rosenblatt R, Ahlén Bergman E, Winerdal M, Yang D, et al. Tumour-associated B cells in urothelial urinary bladder cancer. Scand J Immunol. (2020) 91:e12830. doi: 10.1111/sji.12830
22. Murakami Y, Saito H, Shimizu S, Kono Y, Shishido Y, Miyatani K, et al. Increased regulatory B cells are involved in immune evasion in patients with gastric cancer. Sci Rep. (2019) 9:13083. doi: 10.1038/s41598-019-49581-4
23. Zhou X, Su YX, Lao XM, Liang YJ, and Liao GQ. CD19(+)IL-10(+) regulatory B cells affect survival of tongue squamous cell carcinoma patients and induce resting CD4(+) T cells to CD4(+)Foxp3(+) regulatory T cells. Oncol. (2016) 53:27–35. doi: 10.1016/j.oraloncology.2015.11.003
24. Shalapour S, Lin XJ, Bastian IN, Brain J, Burt AD, Aksenov AA, et al. Inflammation-induced IgA+ cells dismantle anti-liver cancer immunity [published correction appears in Nature. 2017 Dec 21;552(7685):430. doi: 10.1038/nature25028.] [published correction appears in Nature. 2018 Sep;561(7721):E1. doi: 10.1038/s41586-018-0304-y. Nature. (2017) 551:340–5. doi: 10.1038/nature24302
25. Mantovani A, Sozzani S, Locati M, Allavena P, and Sica A. Macrophage polarization: tumor-associated macrophages as a paradigm for polarized M2 mononuclear phagocytes. Trends Immunol. (2002) 23:549–55. doi: 10.1016/s1471-4906(02)02302-5
26. Kinker GS, Vitiello GAF, Ferreira WAS, Chaves AS, Cordeiro de Lima VC, and Medina TDS. B cell orchestration of anti-tumor immune responses: A matter of cell localization and communication. Front Cell Dev Biol. (2021) 9:678127. doi: 10.3389/fcell.2021.678127
27. Xiao X, Lao XM, Chen MM, Liu RX, Wei Y, Ouyang FZ, et al. PD-1hi identifies a novel regulatory B-cell population in human hepatoma that promotes disease progression. Cancer Discov. (2016) 6:546–59. doi: 10.1158/2159-8290.CD-15-1408
28. Balta E, Wabnitz GH, and Samstag Y. Hijacked immune cells in the tumor microenvironment: molecular mechanisms of immunosuppression and cues to improve T cell-based immunotherapy of solid tumors. Int J Mol Sci. (2021) 22:5736. doi: 10.3390/ijms22115736
29. Xing J, Zhang J, and Wang J. The immune regulatory role of adenosine in the tumor microenvironment. Int J Mol Sci. (2023) 24:14928. doi: 10.3390/ijms241914928
30. Oliveira G and Wu CJ. Dynamics and specificities of T cells in cancer immunotherapy. Nat Rev Cancer. (2023) 23:295–316. doi: 10.1038/s41568-023-00560-y
31. Śledzińska A, Vila de Mucha M, Bergerhoff K, Hotblack A, Demane DF, Ghorani E, et al. Regulatory T cells restrain interleukin-2- and blimp-1-dependent acquisition of cytotoxic function by CD4+ T cells. Immunity. (2020) 52:151–166.e6. doi: 10.1016/j.immuni.2019.12.007
32. Goc J, Germain C, Vo-Bourgais TK, Lupo A, Klein C, Knockaert S, et al. Dendritic cells in tumor-associated tertiary lymphoid structures signal a Th1 cytotoxic immune contexture and license the positive prognostic value of infiltrating CD8+ T cells. Cancer Res. (2014) 74:705–15. doi: 10.1158/0008-5472.CAN-13-1342
33. de Chaisemartin L, Goc J, Damotte D, Validire P, Magdeleinat P, Alifano M, et al. Characterization of chemokines and adhesion molecules associated with T cell presence in tertiary lymphoid structures in human lung cancer. Cancer Res. (2011) 71:6391–9. doi: 10.1158/0008-5472.CAN-11-0952
34. Engelhard VH, Rodriguez AB, Mauldin IS, Woods AN, Peske JD, and Slingluff CL Jr. Immune cell infiltration and tertiary lymphoid structures as determinants of antitumor immunity. J Immunol. (2018) 200:432–42. doi: 10.4049/jimmunol.1701269
35. Di Caro G, Bergomas F, Grizzi F, Doni A, Bianchi P, Malesci A, et al. Occurrence of tertiary lymphoid tissue is associated with T-cell infiltration and predicts better prognosis in early-stage colorectal cancers. Clin Cancer Res. (2014) 20:2147–58. doi: 10.1158/1078-0432.CCR-13-2590
36. Goc J, Fridman WH, Hammond SA, Sautès-Fridman C, and Dieu-Nosjean MC. Tertiary lymphoid structures in human lung cancers, a new driver of antitumor immune responses. Oncoimmunology. (2014) 3:e28976. doi: 10.4161/onci.28976
37. Alsughayyir J, Pettigrew GJ, and Motallebzadeh R. Spoiling for a fight: B lymphocytes as initiator and effector populations within tertiary lymphoid organs in autoimmunity and transplantation. Front Immunol. (2017) 8:1639. doi: 10.3389/fimmu.2017.01639
38. Cillo AR, Kürten CHL, Tabib T, Qi Z, Onkar S, Wang T, et al. Immune landscape of viral- and carcinogen-driven head and neck cancer. Immunity. (2020) 52:183–199.e9. doi: 10.1016/j.immuni.2019.11.014
39. Schürch CM, Bhate SS, Barlow GL, Phillips DJ, Noti L, Zlobec I, et al. Coordinated Cellular Neighborhoods Orchestrate Antitumoral Immunity at the Colorectal Cancer Invasive Front [published correction appears in Cell. 2020 Oct 29;183(3):838. doi: 10.1016/j.cell.2020.10.021. Cell. (2020) 182:1341–1359.e19. doi: 10.1016/j.cell.2020.07.005
40. Sautès-Fridman C, Petitprez F, Calderaro J, and Fridman WH. Tertiary lymphoid structures in the era of cancer immunotherapy. Nat Rev Cancer. (2019) 19:307–25. doi: 10.1038/s41568-019-0144-6
41. Rodriguez AB and Engelhard VH. Insights into tumor-associated tertiary lymphoid structures: novel targets for antitumor immunity and cancer immunotherapy. Cancer Immunol Res. (2020) 8:1338–45. doi: 10.1158/2326-6066.CIR-20-0432
42. Giraldo NA, Becht E, Pagès F, Skliris G, Verkarre V, Vano Y, et al. Orchestration and prognostic significance of immune checkpoints in the microenvironment of primary and metastatic renal cell cancer. Clin Cancer Res. (2015) 21:3031–40. doi: 10.1158/1078-0432.CCR-14-2926
43. Munoz-Erazo L, Rhodes JL, Marion VC, and Kemp RA. Tertiary lymphoid structures in cancer - considerations for patient prognosis. Cell Mol Immunol. (2020) 17:570–5. doi: 10.1038/s41423-020-0457-0
44. Jones E, Gallimore A, and Ager A. Defining high endothelial venules and tertiary lymphoid structures in cancer. Methods Mol Biol. (2018) 1845:99–118. doi: 10.1007/978-1-4939-8709-2_7
45. Jiang Q, Tian C, Wu H, Min L, Chen H, Chen L, et al. Tertiary lymphoid structure patterns predicted anti-PD1 therapeutic responses in gastric cancer. Chin J Cancer Res. (2022) 34:365–82. doi: 10.21147/j.issn.1000-9604.2022.04.05
46. Vella G, Hua Y, and Bergers G. High endothelial venules in cancer: Regulation, function, and therapeutic implication. Cancer Cell. (2023) 41:527–45. doi: 10.1016/j.ccell.2023.02.002
47. Vella G, Guelfi S, and Bergers G. High endothelial venules: A vascular perspective on tertiary lymphoid structures in cancer. Front Immunol. (2021) 12:736670. doi: 10.3389/fimmu.2021.736670
48. Zhan Z, Shi-Jin L, Yi-Ran Z, Zhi-Long L, Xiao-Xu Z, Hui D, et al. High endothelial venules proportion in tertiary lymphoid structure is a prognostic marker and correlated with anti-tumor immune microenvironment in colorectal cancer. Ann Med. (2023) 55:114–26. doi: 10.1080/07853890.2022.2153911
49. Fridman WH, Zitvogel L, Sautès-Fridman C, and Kroemer G. The immune contexture in cancer prognosis and treatment. Nat Rev Clin Oncol. (2017) 14:717–34. doi: 10.1038/nrclinonc.2017.101
50. Aloisi F and Pujol-Borrell R. Lymphoid neogenesis in chronic inflammatory diseases. Nat Rev Immunol. (2006) 6:205–17. doi: 10.1038/nri1786
51. Arroz-Madeira S, Bekkhus T, Ulvmar MH, and Petrova TV. Lessons of vascular specialization from secondary lymphoid organ lymphatic endothelial cells. Circ Res. (2023) 132:1203–25. doi: 10.1161/CIRCRESAHA.123.322136
52. Gago da Graça C, van Baarsen LGM, and Mebius RE. Tertiary lymphoid structures: diversity in their development, composition, and role. J Immunol. (2021) 206:273–81. doi: 10.4049/jimmunol.2000873
53. Sato Y, Silina K, van den Broek M, Hirahara K, and Yanagita M. The roles of tertiary lymphoid structures in chronic diseases. Nat Rev Nephrol. (2023) 19:525–37. doi: 10.1038/s41581-023-00706-z
54. Gu-Trantien C, Loi S, Garaud S, Equeter C, Libin M, de Wind A, et al. CD4+ follicular helper T cell infiltration predicts breast cancer survival. J Clin Invest. (2013) 123:2873–92. doi: 10.1172/JCI67428
55. Calderaro J, Petitprez F, Becht E, Laurent A, Hirsch TZ, Rousseau B, et al. Intra-tumoral tertiary lymphoid structures are associated with a low risk of early recurrence of hepatocellular carcinoma. J Hepatol. (2019) 70:58–65. doi: 10.1016/j.jhep.2018.09.003
56. Schumacher TN and Thommen DS. Tertiary lymphoid structures in cancer. Science. (2022) 375:eabf9419. doi: 10.1126/science.abf9419
57. Mansuet-Lupo A, Alifano M, Pécuchet N, Biton J, Becht E, Goc J, et al. Intratumoral immune cell densities are associated with lung adenocarcinoma gene alterations. Am J Respir Crit Care Med. (2016) 194:1403–12. doi: 10.1164/rccm.201510-2031OC
58. Biton J, Mansuet-Lupo A, Pécuchet N, Alifano M, Ouakrim H, Arrondeau J, et al. TP53, STK11, and EGFR mutations predict tumor immune profile and the response to anti-PD-1 in lung adenocarcinoma. Clin Cancer Res. (2018) 24:5710–23. doi: 10.1158/1078-0432.CCR-18-0163
59. Feng H, Yang F, Qiao L, Zhou K, Wang J, Zhang J, et al. Prognostic significance of gene signature of tertiary lymphoid structures in patients with lung adenocarcinoma. Front Oncol. (2021) 11:693234. doi: 10.3389/fonc.2021.693234
60. Tamiya Y, Nakai T, Suzuki A, Mimaki S, Tsuchihara K, Sato K, et al. The impact of tertiary lymphoid structures on clinicopathological, genetic and gene expression characteristics in lung adenocarcinoma. Lung Cancer. (2022) 174:125–32. doi: 10.1016/j.lungcan.2022.11.001
61. Gu W, Wang N, Gu W, Qiu Y, Zhang H, Liang J, et al. Molecular gene mutation profiles, TMB and the impact of prognosis in Caucasians and east Asian patients with lung adenocarcinoma. Transl Lung Cancer Res. (2020) 9:629–38. doi: 10.21037/tlcr-20-457
62. Liu X, Tsang JYS, Hlaing T, Hu J, Ni YB, Chan SK, et al. Distinct tertiary lymphoid structure associations and their prognostic relevance in HER2 positive and negative breast cancers. Oncologist. (2017) 22:1316–24. doi: 10.1634/theoncologist.2017-0029
63. Wang B, Liu J, Han Y, Deng Y, Li J, and Jiang Y. The presence of tertiary lymphoid structures provides new insight into the clinicopathological features and prognosis of patients with breast cancer. Front Immunol. (2022) 13:868155. doi: 10.3389/fimmu.2022.868155
64. Lee HJ, Kim JY, Park IA, Song IH, Yu JH, Ahn JH, et al. Prognostic significance of tumor-infiltrating lymphocytes and the tertiary lymphoid structures in HER2-positive breast cancer treated with adjuvant trastuzumab. Am J Clin Pathol. (2015) 144:278–88. doi: 10.1309/AJCPIXUYDVZ0RZ3G
65. Tokunaga R, Nakagawa S, Sakamoto Y, Nakamura K, Naseem M, Izumi D, et al. 12-Chemokine signature, a predictor of tumor recurrence in colorectal cancer. Int J Cancer. (2020) 147:532–41. doi: 10.1002/ijc.32982
66. Li Q, Liu X, Wang D, Wang Y, Lu H, Wen S, et al. Prognostic value of tertiary lymphoid structure and tumour infiltrating lymphocytes in oral squamous cell carcinoma. Int J Sci. (2020) 12:24. doi: 10.1038/s41368-020-00092-3
67. Posch F, Silina K, Leibl S, Mündlein A, Moch H, Siebenhüner A, et al. Maturation of tertiary lymphoid structures and recurrence of stage II and III colorectal cancer. Oncoimmunology. (2017) 7:e1378844. doi: 10.1080/2162402X.2017.1378844
68. Davies H, Bignell GR, Cox C, Stephens P, Edkins S, Clegg S, et al. Mutations of the BRAF gene in human cancer. Nature. (2002) 417:949–54. doi: 10.1038/nature00766
69. Salem D, Chelvanambi M, Storkus WJ, and Fecek RJ. Cutaneous melanoma: mutational status and potential links to tertiary lymphoid structure formation. Front Immunol. (2021) 12:629519. doi: 10.3389/fimmu.2021.629519
70. Cabrita R, Lauss M, Sanna A, Donia M, Skaarup Larsen M, Mitra S, et al. Tertiary lymphoid structures improve immunotherapy and survival in melanoma [published correction appears in Nature. 2020 Apr;580(7801):E1. doi: 10.1038/s41586-020-2155-6. Nature. (2020) 577:561–5. doi: 10.1038/s41586-019-1914-8
71. Lin Z, Huang L, Li S, Gu J, Cui X, and Zhou Y. Pan-cancer analysis of genomic properties and clinical outcome associated with tumor tertiary lymphoid structure. Sci Rep. (2020) 10:21530. doi: 10.1038/s41598-020-78560-3
72. Solinas C, Carbognin L, De Silva P, Criscitiello C, and Lambertini M. Tumor-infiltrating lymphocytes in breast cancer according to tumor subtype: Current state of the art. Breast. (2017) 35:142–50. doi: 10.1016/j.breast.2017.07.005
73. Solinas C, Marcoux D, Garaud S, Vitória JR, Van den Eynden G, de Wind A, et al. BRCA gene mutations do not shape the extent and organization of tumor infiltrating lymphocytes in triple negative breast cancer. Cancer Lett. (2019) 450:88–97. doi: 10.1016/j.canlet.2019.02.027
74. Paijens ST, Vledder A, de Bruyn M, and Nijman HW. Tumor-infiltrating lymphocytes in the immunotherapy era. Cell Mol Immunol. (2021) 18:842–59. doi: 10.1038/s41423-020-00565-9
75. Joshi NS, Akama-Garren EH, Lu Y, Lee DY, Chang GP, Li A, et al. Regulatory T cells in tumor-associated tertiary lymphoid structures suppress anti-tumor T cell responses. Immunity. (2015) 43:579–90. doi: 10.1016/j.immuni.2015.08.006
76. Colbeck EJ, Jones E, Hindley JP, Smart K, Schulz R, Browne M, et al. Treg depletion licenses T cell-driven HEV neogenesis and promotes tumor destruction. Cancer Immunol Res. (2017) 5:1005–15. doi: 10.1158/2326-6066.CIR-17-0131
77. Masuda T, Tanaka N, Takamatsu K, Hakozaki K, Takahashi R, Anno T, et al. Unique characteristics of tertiary lymphoid structures in kidney clear cell carcinoma: prognostic outcome and comparison with bladder cancer. J Immunother Cancer. (2022) 10:e003883. doi: 10.1136/jitc-2021-003883
78. Ager A and May MJ. Understanding high endothelial venules: Lessons for cancer immunology. Oncoimmunology. (2015) 4:e1008791. doi: 10.1080/2162402X.2015.1008791
79. Nayar S, Campos J, Smith CG, Iannizzotto V, Gardner DH, Mourcin F, et al. Immunofibroblasts are pivotal drivers of tertiary lymphoid structure formation and local pathology. Proc Natl Acad Sci U S A. (2019) 116:13490–7. doi: 10.1073/pnas.1905301116
80. Rodriguez AB, Peske JD, Woods AN, Leick KM, Mauldin IS, Meneveau MO, et al. Immune mechanisms orchestrate tertiary lymphoid structures in tumors via cancer-associated fibroblasts. Cell Rep. (2021) 36:109422. doi: 10.1016/j.celrep.2021.109422
81. Georgiev H, Ravens I, Papadogianni G, Halle S, Malissen B, Loots GG, et al. Shared and unique features distinguishing follicular T helper and regulatory cells of peripheral lymph node and peyer's patches. Front Immunol. (2018) 9:714. doi: 10.3389/fimmu.2018.00714
82. Chaurio RA, Anadon CM, Lee Costich T, Payne KK, Biswas S, Harro CM, et al. TGF-β-mediated silencing of genomic organizer SATB1 promotes Tfh cell differentiation and formation of intra-tumoral tertiary lymphoid structures. Immunity. (2022) 55:115–128.e9. doi: 10.1016/j.immuni.2021.12.007
83. Crotty S. T follicular helper cell differentiation, function, and roles in disease. Immunity. (2014) 41:529–42. doi: 10.1016/j.immuni.2014.10.004
84. Dieu-Nosjean MC, Antoine M, Danel C, Heudes D, Wislez M, Poulot V, et al. Long-term survival for patients with non-small-cell lung cancer with intratumoral lymphoid structures. J Clin Oncol. (2008) 26:4410–7. doi: 10.1200/JCO.2007.15.0284
85. Trajkovski G, Ognjenovic L, Karadzov Z, Jota G, Hadzi-Manchev D, Kostovski O, et al. Tertiary lymphoid structures in colorectal cancers and their prognostic value. Open Access Maced J Med Sci. (2018) 6:1824–8. doi: 10.3889/oamjms.2018.341
86. Ahmed A and Halama N. Tertiary lymphoid structures in colorectal cancer liver metastases: association with immunological and clinical parameters and chemotherapy response. Anticancer Res. (2020) 40:6367–73. doi: 10.21873/anticanres.14657
87. Li R, Berglund A, Zemp L, Dhillon J, Putney R, Kim Y, et al. The 12-CK score: global measurement of tertiary lymphoid structures. Front Immunol. (2021) 12:694079. doi: 10.3389/fimmu.2021.694079
88. Fukuhara M, Muto S, Inomata S, Yamaguchi H, Mine H, Takagi H, et al. The clinical significance of tertiary lymphoid structure and its relationship with peripheral blood characteristics in patients with surgically resected non-small cell lung cancer: a single-center, retrospective study. Cancer Immunol Immunother. (2022) 71:1129–37. doi: 10.1007/s00262-021-03067-3
89. Hiraoka N, Ino Y, Yamazaki-Itoh R, Kanai Y, Kosuge T, and Shimada K. Intratumoral tertiary lymphoid organ is a favourable prognosticator in patients with pancreatic cancer. Br J Cancer. (2015) 112:1782–90. doi: 10.1038/bjc.2015.145
90. Zhang Y, Li J, Yang F, Zhang X, Ren X, and Wei F. Relationship and prognostic significance of IL-33, PD-1/PD-L1, and tertiary lymphoid structures in cervical cancer. J Leukoc Biol. (2022) 112:1591–603. doi: 10.1002/JLB.5MA0322-746R
91. Girard JP, Moussion C, and Förster R. HEVs, lymphatics and homeostatic immune cell trafficking in lymph nodes. Nat Rev Immunol. (2012) 12:762–73. doi: 10.1038/nri3298
92. Lin Q, Tao P, Wang J, Ma L, Jiang Q, Li J, et al. Tumor-associated tertiary lymphoid structure predicts postoperative outcomes in patients with primary gastrointestinal stromal tumors. Oncoimmunology. (2020) 9:1747339. doi: 10.1080/2162402X.2020.1747339
93. Griss J, Bauer W, Wagner C, Simon M, Chen M, Grabmeier-Pfistershammer K, et al. B cells sustain inflammation and predict response to immune checkpoint blockade in human melanoma. Nat Commun. (2019) 10:4186. doi: 10.1038/s41467-019-12160-2
94. Cottrell TR, Thompson ED, Forde PM, Stein JE, Duffield AS, Anagnostou V, et al. Pathologic features of response to neoadjuvant anti-PD-1 in resected non-small-cell lung carcinoma: a proposal for quantitative immune-related pathologic response criteria (irPRC). Ann Oncol. (2018) 29:1853–60. doi: 10.1093/annonc/mdy218
95. Petitprez F, de Reyniès A, Keung EZ, Chen TW, Sun CM, Calderaro J, et al. B cells are associated with survival and immunotherapy response in sarcoma. Nature. (2020) 577:556–60. doi: 10.1038/s41586-019-1906-8
96. Gao J, Navai N, Alhalabi O, Siefker-Radtke A, Campbell MT, Tidwell RS, et al. Neoadjuvant PD-L1 plus CTLA-4 blockade in patients with cisplatin-ineligible operable high-risk urothelial carcinoma. Nat Med. (2020) 26:1845–51. doi: 10.1038/s41591-020-1086-y
97. Morcrette G, Hirsch TZ, Badour E, Pilet J, Caruso S, Calderaro J, et al. APC germline hepatoblastomas demonstrate cisplatin-induced intratumor tertiary lymphoid structures. Oncoimmunology. (2019) 8:e1583547. doi: 10.1080/2162402X.2019.1583547
98. Sánchez-Alonso S, Setti-Jerez G, Arroyo M, Hernández T, Martos MI, Sánchez-Torres JM, et al. A new role for circulating T follicular helper cells in humoral response to anti-PD-1 therapy. J Immunother Cancer. (2020) 8:e001187. doi: 10.1136/jitc-2020-001187
99. Siliņa K, Soltermann A, Attar FM, Casanova R, Uckeley ZM, Thut H, et al. Germinal centers determine the prognostic relevance of tertiary lymphoid structures and are impaired by corticosteroids in lung squamous cell carcinoma. Cancer Res. (2018) 78:1308–20. doi: 10.1158/0008-5472.CAN-17-1987
100. Lee M, Heo SH, Song IH, Rajayi H, Park HS, Park IA, et al. Presence of tertiary lymphoid structures determines the level of tumor-infiltrating lymphocytes in primary breast cancer and metastasis. Mod Pathol. (2019) 32:70–80. doi: 10.1038/s41379-018-0113-8
101. Finkin S, Yuan D, Stein I, Taniguchi K, Weber A, Unger K, et al. Ectopic lymphoid structures function as microniches for tumor progenitor cells in hepatocellular carcinoma. Nat Immunol. (2015) 16:1235–44. doi: 10.1038/ni.3290
102. Scott DW, Chan FC, Hong F, Rogic S, Tan KL, Meissner B, et al. Gene expression-based model using formalin-fixed paraffin-embedded biopsies predicts overall survival in advanced-stage classical Hodgkin lymphoma. J Clin Oncol. (2013) 31:692–700. doi: 10.1200/JCO.2012.43.4589
103. Ding GY, Ma JQ, Yun JP, Chen X, Ling Y, Zhang S, et al. Distribution and density of tertiary lymphoid structures predict clinical outcome in intrahepatic cholangiocarcinoma. J Hepatol. (2022) 76:608–18. doi: 10.1016/j.jhep.2021.10.030
104. Fridman WH, Petitprez F, Meylan M, Chen TW, Sun CM, Roumenina LT, et al. B cells and cancer: To B or not to B? J Exp Med. (2021) 218:e20200851. doi: 10.1084/jem.20200851
105. Ribas A and Wolchok JD. Cancer immunotherapy using checkpoint blockade. Science. (2018) 359:1350–5. doi: 10.1126/science.aar4060
106. Helmink BA, Reddy SM, Gao J, Zhang S, Basar R, Thakur R, et al. B cells and tertiary lymphoid structures promote immunotherapy response. Nature. (2020) 577:549–55. doi: 10.1038/s41586-019-1922-8
107. Mounzer RH, Svendsen OS, Baluk P, Bergman CM, Padera TP, Wiig H, et al. Lymphotoxin-alpha contributes to lymphangiogenesis. Blood. (2010) 116:2173–82. doi: 10.1182/blood-2009-12-256065
108. Zhang Q, Lu Y, Proulx ST, Guo R, Yao Z, Schwarz EM, et al. Increased lymphangiogenesis in joints of mice with inflammatory arthritis. Arthritis Res Ther. (2007) 9:R118. doi: 10.1186/ar2326
109. Lee Y, Chin RK, Christiansen P, Sun Y, Tumanov AV, Wang J, et al. Recruitment and activation of naive T cells in the islets by lymphotoxin beta receptor-dependent tertiary lymphoid structure. Immunity. (2006) 25:499–509. doi: 10.1016/j.immuni.2006.06.016
110. Luther SA, Lopez T, Bai W, Hanahan D, and Cyster JG. BLC expression in pancreatic islets causes B cell recruitment and lymphotoxin-dependent lymphoid neogenesis. Immunity. (2000) 12:471–81. doi: 10.1016/s1074-7613(00)80199-5
111. Luther SA, Bidgol A, Hargreaves DC, Schmidt A, Xu Y, Paniyadi J, et al. Differing activities of homeostatic chemokines CCL19, CCL21, and CXCL12 in lymphocyte and dendritic cell recruitment and lymphoid neogenesis. J Immunol. (2002) 169:424–33. doi: 10.4049/jimmunol.169.1.424
112. Stowman AM, Hickman AW, Mauldin IS, Mahmutovic A, Gru AA, and Slingluff CL Jr. Lymphoid aggregates in desmoplastic melanoma have features of tertiary lymphoid structures. Melanoma Res. (2018) 28:237–45. doi: 10.1097/CMR.0000000000000439
113. Wu YH, Wu F, Yan GR, Zeng QY, Jia N, Zheng Z, et al. Features and clinical significance of tertiary lymphoid structure in cutaneous squamous cell carcinoma. J Eur Acad Dermatol Venereol. (2022) 36:2043–50. doi: 10.1111/jdv.18464
114. Kabashima K, Honda T, Ginhoux F, and Egawa G. The immunological anatomy of the skin. Nat Rev Immunol. (2019) 19:19–30. doi: 10.1038/s41577-018-0084-5
115. Jacobson A, Yang D, Vella M, and Chiu IM. The intestinal neuro-immune axis: crosstalk between neurons, immune cells, and microbes. Mucosal Immunol. (2021) 14:555–65. doi: 10.1038/s41385-020-00368-1
Keywords: tertiary lymphoid structure, TLS, immunotherapy, tumor immune microenvironment, TME
Citation: Liu C and Cao J (2025) The pivotal role of tertiary lymphoid structures in the tumor immune microenvironment. Front. Oncol. 15:1616904. doi: 10.3389/fonc.2025.1616904
Received: 23 April 2025; Accepted: 05 May 2025;
Published: 22 May 2025.
Edited by:
Zong Sheng Guo, University at Buffalo, United StatesReviewed by:
Michihisa Kono, Dana–Farber Cancer Institute, United StatesAlessandra Rossi, Sapienza University of Rome, Italy
Copyright © 2025 Liu and Cao. This is an open-access article distributed under the terms of the Creative Commons Attribution License (CC BY). The use, distribution or reproduction in other forums is permitted, provided the original author(s) and the copyright owner(s) are credited and that the original publication in this journal is cited, in accordance with accepted academic practice. No use, distribution or reproduction is permitted which does not comply with these terms.
*Correspondence: Jiandong Cao, Y2pkODU3MTM4NTk4QDE2My5jb20=