- 1Pharmaceutical Sciences Research Center, Health Institute, Kermanshah University of Medical Sciences, Kermanshah, Iran
- 2Student Research Committee, Kermanshah University of Medical Sciences, Kermanshah, Iran
- 3Departamento de Ciencias del Ambiente, Facultad de Química y Biología, Universidad de Santiago de Chile, Santiago, Chile
The novel coronavirus 2019 (COVID-19) caused by severe acute respiratory syndrome coronavirus 2 (SARS-CoV-2) has made a wide range of manifestations. In this regard, growing evidence is focusing on COVID-19 neurological associations; however, there is a lack of established pathophysiological mechanisms and related treatments. Accordingly, a comprehensive review was conducted, using electronic databases, including PubMed, Scopus, Web of Science, and Cochrane, along with the author’s expertize in COVID-19 associated neuronal signaling pathways. Besides, potential phytochemicals have been provided against neurological signs of COVID-19. Considering a high homology among SARS-CoV, Middle East Respiratory Syndrome and SARS-CoV-2, revealing their precise pathophysiological mechanisms seems to pave the road for the treatment of COVID-19 neural manifestations. There is a complex pathophysiological mechanism behind central manifestations of COVID-19, including pain, hypo/anosmia, delirium, impaired consciousness, pyramidal signs, and ischemic stroke. Among those dysregulated neuronal mechanisms, neuroinflammation, angiotensin-converting enzyme 2 (ACE2)/spike proteins, RNA-dependent RNA polymerase and protease are of special attention. So, employing multi-target therapeutic agents with considerable safety and efficacy seems to show a bright future in fighting COVID-19 neurological manifestations. Nowadays, natural secondary metabolites are highlighted as potential multi-target phytochemicals in combating several complications of COVID-19. In this review, central pathophysiological mechanisms and therapeutic targets of SARS-CoV-2 has been provided. Besides, in terms of pharmacological mechanisms, phytochemicals have been introduced as potential multi-target agents in combating COVID-19 central nervous system complications.
Introduction
Phylogenetic studies on the genomic structure, introduced various types of coronaviruses (CoVs), including NL63, 229E, OC43, HKU1, middle east respiratory syndrome (MERS)-CoV, severe acute respiratory syndrome coronavirus (SARS-CoV), and severe acute respiratory syndrome coronavirus 2 (SARS-CoV-2) (Gurung et al., 2020a; Vellingiri et al., 2020), divided into four groups of alpha (229E and NL63), beta (OC43 and HKU1), gamma and delta coronaviruses. Among coronaviruses, alpha and beta groups cause respiratory manifestations in human (Gurung et al., 2020b; Gurung et al., 2020c; Rasool et al., 2020). Recently, a new strain of coronaviruses, namely SARS-CoV-2 has been found, belonging to a distinct class of beta coronaviruses (Divani et al., 2020). SARS-CoV-2 made a deadly disease, termed coronavirus disease 2019 (COVID-19) with devastating manifestation all over the world (Fitriani et al., 2020; Nemoto et al., 2020). The large and positive sense RNA genome with a size of 27–32 kb, as well as an envelope with spike (S1 and S2)/conjugated proteins (Holmes and Lai, 1996; Davidson et al., 2020) are associated with COVID-19 symptoms over a period of 2–14 days. Studies have revealed that when viruses enter to the lung tissue cells and proliferate, cause alveolar and interstitial inflammatory secretion and edema that leads to alveolar gas exchange impairment and hypoxia in the central nervous system (CNS), thereby increases anaerobic metabolism in the mitochondria of brain cells (Wu et al., 2020c). Besides, SARS-CoV enters the nasal passage and triggers neural inflammatory responses through dysregulation of the immune system. The entry factors for SARS-CoV-2 are highly expressed in nasal epithelial cells (Sungnak et al., 2020). As a consequence, CoVs enters the brain via the olfactory tract in the early stages of nasal vaccination or infection (Mori, 2015; Wu et al., 2020c; Desforges et al., 2020). Accordingly, this virus is not limited to the respiratory system but invades peripheral nerves and enters the CNS then causes/aggravates neurodegenerative disorders (Matsuda et al., 2004; Vellingiri et al., 2020). Research has shown the presence of SARS-CoV in cortex, hippocampus, spinal cord, brain stem, cerebellum, striatum, colliculus superior, and hypothalamus (Jacomy and Talbot, 2003). Consequently, COVID-19 patients have shown neurological symptoms, including headache, dizziness, hypogeusia, nausea, vomiting, and anosmia (Ahmad and Rathore, 2020; Vellingiri et al., 2020).
From the pathophysiological point of view, the spike protein in the morphology of COVID-19 bind to angiotensin-converting enzyme (ACE)-receptors on alveolar epithelial cell type 2 (AT2), primed by transmembrane protease serine 2 (TMPRSS2) to allow coronavirus entry (Marchetti et al., 2018; Li et al., 2019b; Wang et al., 2020b; Vallamkondu et al., 2020). Experimental evidence indicated that COVID-19 enters the lung via the respiratory tract and invades AT2 cells to generate a surfactant regarding declining related tension within alveoli to alleviate collapsing pressure. Also, ACE2 is presented in kidney, heart, enterocytes, pancreas, endothelial cells and widely distributed in brain to facilitate the SARS-CoV-2 entry into the cells (Li et al., 2003; Diao et al., 2020). The neural distribution of ACE2 was controversial at first. While a quantitative real-time RT-PCR study showed low levels of ACE2 mRNA in the human brain (Harmer et al., 2002), immunohistochemistry results indicated that ACE2 protein expression was restricted to arterial and endothelial smooth muscle cells (Hamming et al., 2004). Additionally, the predominant expression of ACE2 in glial cells was shown in brain primary cell cultures (Gallagher et al., 2006). Complementary evidence showed the widespread presence of ACE2 mRNA and protein throughout the brain (Doobay et al., 2007) or brainstem (Lin et al., 2008). Finding SARS-CoV in brains of infected patients also confirmed related distribution of ACE2 (Ding et al., 2004; Gu et al., 2005; Xu et al., 2005; Xia and Lazartigues, 2008).
As a critical sign of COVID-19, neuroinflammation occurs through elevated levels of neuronal interleukin (IL)-1β, IL-2, IL-4, IL-6, IL-8, IL-10, IL-12, tumor necrosis factor-α (TNF-α), interferon-gamma (IFN-γ), granulocyte colony-stimulating factor (GMCSF), IFN-γ-induced protein 10 (IP-10), monocyte chemoattractant protein-1 (MCP1), macrophage inflammatory protein 1α (MIP1α), and T cell expression (Xu et al., 2005; Yarmohammadi et al., 2020). The coronaviruses release inflammatory mediators to stimulate macrophages. These macrophages activate IL-1, IL-6, TNF-α, C-X-C motif chemokine ligand 10 (CXCL10) and chemokine ligand 2 (CCL2). Prevailing evidence is showing that CoVs reach the neurons, astrocytes, and/or microglia in CNS. Consequently, microglia and astrocytes play major roles in neuroinflammation and released inflammatory mediators (Murta et al., 2020). These cytokines and chemokines causes vasodilation and also increased capillary penetrance that causes declined surfactant stage in AP-2 cells which in turn lead to alveolar collapse and perturbation in gaseous exchange (Zaki et al., 2012; Wu et al., 2020a; Guan et al., 2020; Yang et al., 2020). In the other level of disease there is an increased level of inflammatory mediators via CD4+ and also increased generation of neutrophils and macrophages using IL-17, IL-21, and IL-22 that causes difficulties in breathing, hypoxemia, and cough (de Wit et al., 2016; Gao et al., 2020; Wan et al., 2020). In addition to the elevation of neuronal inflammatory mediators in CNS and related neuronal associations, ACE2/spike proteins and downstream mediators, RNA-dependent RNA polymerase (RdRP)/proteases, seem to be golden targets in stopping related neuronal signs.
Unfortunately, up to now there is no antiviral drug or vaccine for the treatment of coronaviruses infection, although candidate phytochemicals can be promising factors with antiviral potentials for the treatment of infection (Liu and Du, 2012; Gurung et al., 2020b). Some previous research has indicated neurological manifestations in coronaviruses (Ahmed et al., 2020; Yavarpour-Bali and Ghasemi-Kasman, 2020). Besides, limited studies suggested natural products and candidate phytochemicals as helpful agents for the prevention and treatment of coronaviruses (Hasan et al., 2020; Majnooni et al., 2020; Zhou and Huang, 2020). In this study, an extensive review was performed on neurological manifestations of coronaviruses, as well as the effects of candidate phytochemicals on the aforementioned signaling pathways. Additionally, this is the first review on highlighting phytochemicals with antiviral and neuroprotective effects, which targets the neural pathogenic pathways of CoVs (termed candidate phytochemicals) regarding the prevention and treatment of COVID-19 neuronal signs.
Study Design
We used electronic databases (e.g., Scopus, PubMed, Medline, and Web of Science) and related articles in other sources, to conduct a comprehensive review on the neurological manifests of coronaviruses, as well as the phytochemicals effects. The keywords (“Severe Acute Respiratory Syndrome” OR “SARS” OR “Middle East Respiratory Syndrome” OR “MERS” OR “Coronavirus disease 2019” OR “COVID-19” OR “SARS-CoV” OR “SARS-CoV-2”) AND (“neurological sign” OR “neurological manifestation” OR “neuron” OR “nerve” OR “central nervous system” OR “CNS” OR “brain” OR “neurology” OR “neuropathy” OR “stroke” OR “multiple sclerosis” OR “encephalitis” OR “encephalopathy”) [title/abstract/keywords] were used. All the phytochemicals possessing both the antiviral and neuroprotective activities with the keywords (“phytochemical” OR “secondary metabolite” OR “plant” OR “polyphenol” OR “phenolic compound” OR “flavonoid” OR “alkaloid” OR “terpen” OR “terpenoid” OR “quinone”) were also searched in the whole text. Overall, the phytochemicals with reported antiviral and neuroprotective effects possessing the potential of modulating coronaviruses pathophysiological mechanisms were included. Data were collected without language and date restrictions until October 2020. The screening of retrieved articles was also done. on the reference lists/citation. Regarding completing review on the electronic databases, hand searching also was done relying on the authors' expertize on the SARS-CoVs pathophysiological mechanisms in CNS and candidate phytochemicals.
Neuronal Manifestations of Coronaviruses
Experimental evidence showed two types of neurological manifestations referring to the CNS and peripheral nervous system (PNS). Of the PNS, there are various neurological associations such as hypogeusia, hyposmia, impaired eye movement, trigeminal neuropathy, Miller-Fisher syndrome, polyneuritis cranialis, rhabdomyolysis, Guillain-Barré Syndrome, and olfactory dysfunction (Ahmad and Rathore, 2020; de Freitas Ferreira et al., 2020; Mochan and Modi, 2020; Nordvig et al., 2020; Pascual-Goñi et al., 2020; Yavarpour-Bali and Ghasemi-Kasman, 2020). COVID-19 also causes CNS impairment such as cerebrovascular disorders, acute ischemic stroke (1–3%), intracranial haemorrhage (0.5%), encephalitis (brain inflammation), demyelination, meningitis, polyneuritis cranialis, vasculitis, and skeletal muscular damage (Li et al., 2016; Dorche et al., 2020; Filatov et al., 2020; Mao et al., 2020; Moriguchi et al., 2020; Zhou et al., 2020). It has been shown that 229E and OC43 coronavirus strains invade to neuroblastoma, neuroglioma, astrocytoma, microglial, and oligodendrocytic cell cultures (Cheng et al., 2020b) toward revealing neuronal complications. Werner and co-workers have indicated additional symptoms of several cases, such as acute necrotizing encephalopathy, neck stiffness, bilateral ankle clonus, positive Brudzinski, left Babinski, and right Chaddock signs (Werner et al., 2020). Other neuronal symptoms of COVID-19 are ataxia, refractory status epilepticus (Xu et al., 2005), neuron denaturation/necrosis, broad gliocytes hyperplasia with gliosome formation (Yassin et al., 2020), myalgia, dyspnea (Prakash et al., 2020), taste and smell dysfunctions, acute cerebrovascular and oculomotor nerve palsy (Nepal et al., 2020). Mao et al. indicated that elevated creatine phosphokinase (CPK), C-reactive protein (CRP), D-dimer, necrotizing myopathy, thick filament myopathy, critical illness myopathy (nonspecific), and acute quadriplegic myopathy are other neural manifestation of COVID-19 (Mao et al., 2020; Suri et al., 2020; Warner, 2020). Reports have also shown other neurological manifestations such as Bickerstaff’s encephalitis, critical illness myopathy, severe lymphopenia, thrombocytopenia and uremia, facial diplegia, and toxin associated myopathy and neuropathy (Wu et al., 2017; Gulati et al., 2020; Zheng et al., 2020). Of the clinical behavioral signs, headache, syncope, agitation, delirium, dysgeusia, fatigue, dizziness, acute confusion, sleep disorders, changed the level of consciousness, and altered mental status, have been observed in COVID-19 patients (Stewart et al., 1992; Dessau et al., 2001; Lau et al., 2004; Wang et al., 2020a; Wang et al., 2020c; Wu et al., 2020c; Dorche et al., 2020; Helms et al., 2020; Mochan and Modi, 2020; Nalleballe et al., 2020). The aforementioned neurological signs are being manifested in 84% of patients with COVID-19 (Wang et al., 2020c; Helms et al., 2020).
Severe respiratory syndrome as one of the critical impairment of COVID-19 may result in systemic hypoxia, hypercarbia, and anaerobic metabolism resulting in neuronal swelling and brain edema/damage (Suri et al., 2020). SARS-CoV-2 also invades to the spinal cord and causes acute inflammation of gray and white matter in the spinal cord (myelitis), which was recognized by the acute flaccid myelitis of lower limbs, urinary and bowel incontinence (Zhao et al., 2020). Evidence has shown a close relationship between COVID-19 and Parkinson disease, increased motor symptoms (e.g., tremor), freezing of gait or dyskinesias, and declined the efficacy of dopaminergic medication (Macht et al., 2007; Zach et al., 2017; Ehgoetz Martens et al., 2018). Interestingly, it seems to be a near linkage between dopamine synthesis pathway and COVID-19 pathophysiology. In this line, dopa decarboxylase, as a regulatory enzyme in dopamine pathway is meaningfully co-expressed with ACE2 receptor. On the other hand, SARS-COV virus downregulates ACE2 in consistent with dopamine synthesis alteration (Kuba et al., 2005). Besides, as dopamine is expressed in the alveolar epithelial cells, it also contributes in lung immunity, as well as what ACE2 does (Bone et al., 2017). Accordingly, considering the critical role of dopamine deficiency in Parkinson’s disease, the SARS-CoV-2 virus may cause such sporadic signs COVID-19 patients (Rietdijk et al., 2017).
Additionally, evidence indicated that CoVs may play an essential function in the pathogenesis of multiple sclerosis (Saleki et al., 2020). The CoVs isolated from multiple sclerosis patients were neutralized using the patients’ serum. This revealed the destructive role of CoVs in the pathogenesis of multiple sclerosis (Burks et al., 1980). Growing studies are evaluating the use of immunomodulatory/disease-modifying agents in multiple sclerosis patients with COVID-19. Results declared an increased risk of COVID-19 complications in those treated patients (Baysal-Kirac and Uysal, 2020; Ramanathan et al., 2020). Decision on continuing/stopping the immunotherapy in these patients is closely dependent on disease severity and activity (Giovannoni et al., 2020).
Orsucci and co-others have revealed that there are olfactory and gustatory function impairments as common neural disorders in patients of COVID-19 (Orsucci et al., 2020). It has shown in CNS-CoV disease, there is a lower level of lymphocytes, eosinophils and a higher level of neutrophils as well as monocyte (Saleki et al., 2020). Also, Toscano et al. observed Guillain–Barré syndrome, lower limb weakness and paresthesia, facial diplegia followed by ataxia and paresthesia, flaccid tetraparesis or tetraplegia in COVID-19 (Toscano et al., 2020). Researchers in several cases observed tonic-clonic seizure, anxiety, psychotic symptoms, meningeal irritation signs, extensor plantar response, encephalitis, dysphagia, dysarthria, bulbar impairment and massive hemorrhagic conversion (Wang et al., 2020a).
THE Pathophysiological Mechanistic Pathways of Coronaviruses in Central Nervous System
Experimental evidence has indicated that coronaviruses invade to neurons and glial cells to induce an unfolded protein response (UPR) regarding necroptosis in neuronal cells (Meessen-Pinard et al., 2017). As previously mentioned, coronaviruses caused neuronal damages and death along with related neuroinflammatory responses (Morfopoulou et al., 2016). There are multiple mechanisms by which SARS-CoV-2 enters the CNS and causes associated complications. Those mechanisms are blood-mediated contamination (hematogenous), neuronal-mediated infection (neurogenic), immunodeficient related damage, direct respiratory infection, and hypoxic injury (Ahmed et al., 2020) which are described as following. During the hematogenous manner, CoVs crossed the blood-brain barrier (BBB) and entered the brain. This occurs via two mechanisms, by direct penetration of the virus particle crossing the BBB or by hijacking peripheral blood cells (Bohmwald et al., 2018). In the latter way of invasion, Human coronavirus OC43 (HCoV-OC43) accesses the CNS via the neurogenic way to be appeared in the cell bodies and dendrites of olfactory neurons, then spread in hippocampus, cortex and spinal cord (Niu et al., 2020a). During the viremia phase of illness, BBB disruption causes a direct virus entrance to the brain. Spreading/disseminating of SARS-CoV-2 from the cribriform bone in nearby proximity to the olfactory bulb, and brain causes in seven days (Baig et al., 2020). Besides, peripheral invasion of nerve terminals by CoVs through the connected synapse leads to the virus entry to the CNS (Ahmad and Rathore, 2020). Additionally, systemic hypoxia resulted from severe pneumonia causes vasodilatation, anaerobic metabolism, hypoxia and accumulation of toxic compounds lead to brain damage (Tu et al., 2020).
One of the most widely accepted neuropathological mechanisms of SARS-CoV-2 is hyper-inflammatory state (Yavarpour-Bali and Ghasemi-Kasman, 2020). Accordingly, the immune-mediated damage is resulted from cytokine storms, as well as the activation of T lymphocytes, endothelial cells, and macrophages which leads into vascular leakage, coagulation, and end-organ damage (Mehta et al., 2020; Tveito, 2020). It was shown that coronavirus triggers innate immunity associated with the release of microglial-induced INF-α/β (Savarin et al., 2018). In this regard, several cytokines and chemokines are released by microglia and astrocytes such as IL-1α, IL-1β, IL-6, IFN-γ, TNF-α, and CXCL10 (Joseph et al., 1993). Li et al. indicated the increased levels of many inflammatory mediators in the cerebrospinal fluid, including IL-6, IL-8, MCP-1, and granulocyte-macrophage colony-stimulating factor (GM-CSF) in COVID-19 patients (Li et al., 2016). During early stage of CoV neuroinfection, CXCL10 and CXCL9 are present in the peripheral blood of patient affected by IFN-γ (Jiang et al., 2005). Experiment has shown that CoVs play a destructive role in acute disseminated encephalomyelitis (ADEM) correlated with increased inflammatory mediators such as IL-6, IFN-γ, TNF-α, CXCL9, and CXCL10 (Kothur et al., 2016). It has been demonstrated that there is a direct correlation between the levels of IL-1β, IL-6, IL-8, TNF-α, IL-10 and COVID-19 central inflammatory complications such as neuromyelitis optica (also known as Devic's disease), transverse myelitis, acute disseminated encephalomyelitis, amyotrophic lateral sclerosis, herpes simplex encephalitis, Parkinson’s disease, traumatic brain injury, epilepsy, and stroke (Vezzani et al., 2002; Rodney et al., 2018; Vezzani et al., 2019; West et al., 2019). In this line, it has shown that IL-2 and IL-2 receptors (IL-2R) have important signals for T cell activation via Janus kinase/signal transducer and activator of transcription (JAK/STAT) signaling pathway (Fu et al., 2018; Shi et al., 2020). The transcription factor nuclear factor-κB (NF-κB) is another essential regulator in immune system, which is activated in lung inflammatory immunopathology-induced by SARS-CoV (DeDiego et al., 2014; Catanzaro et al., 2020).
As previously mentioned, studies have suggested several mechanisms for entering the SARS-CoV-2 to the nervous system, although the exact mechanism is not clear (Yavarpour-Bali and Ghasemi-Kasman, 2020). Scientists have suggested that coronaviruses enter the olfactory bulb/epithelium, then penetrates to CNS. So, make the anosmia or hyposmia as the neural manifestation of COVID-19. Recently additional studies have suggested different mechanisms for anosmia in COVID-19, such as olfactory cleft syndrome, mucosal obstruction, direct damage of olfactory sensory neurons, impairment of the olfactory perception center and cytokine storm in the brain (Yazdanpanah et al., 2020). Released inflammatory factors altered the penetrance of the BBB and increased inflammatory cascade (Singhi, 2011). Studies have also shown that deficiency in neuronal endoplasmic reticulum (ER) leads to the activation of UPR-induced by SARS-CoV (Chan et al., 2006; Ron and Walter, 2007). Until now, some related signaling pathways have shown functional roles in the UPR processing, such as ATF6, phospho-extracellular signal-regulated kinase (p-ERK)/eIF2-alpha and IRE1/XBP1 (Ron and Walter, 2007). Favreau et al. indicated that HCoV-OC43 induced UPR and causes neuronal death by caspase-3 activation and nuclear fragmentation (Favreau et al., 2009). From another mechanistic point, studies suggested that SARS-CoV-2 induces severe inflammation that leads to thrombosis. SARS-CoV-2 also binds to toll-like receptors (TLR) and causes the synthesis and liberation of IL-1. As a matter of fact, TLRs activate biochemical cascade by inflammasome activation as well as type I interferon (IFN) which is released as an important player against viral infection (Marchetti et al., 2018; Conti et al., 2020; Vaninov, 2020).
Role of RENIN-ANGIOTENSIN System in the Neuronal Manifestations of Coronaviruses
It has been shown that SARS-CoV-2 mainly enters the CNS via the ACE2 or TMPRSS2 receptors. These receptors are expressed in the glial cells of brain/spinal cord and thereby facilitates the invasion of coronavirus to the spinal cord, which is essential for the host cell entry of SARS-CoV-2 and also plasma membrane fusion (El Tabaa and El Tabaa, 2020; Nemoto et al., 2020). Also, it has been indicated that when coronavirus enters the cells, ACE2 will break and shed by ADAM Metallopeptidase Domain 17 (ADAM17) into the membrane space (Li and De Clercq, 2020). Studies suggested that phosphorylation of ACE2 at Ser680 inhibits ubiquitination of ACE2 and also increase related membrane expression (Amraei and Rahimi, 2020). It has been indicated that renin-angiotensin system (RAS), including angiotensin II (Ang II), ACE, ACE2, angiotensin type-1 receptor (AT1R), angiotensin type-2 receptor (AT2R), and Mas receptor (MAS), plays critical physiological functions. Research suggested that Ang II prevents COVID-19 infection through binding to ATR1 and activating ACE2 internalization, then declining ERK1/2 and p38 mitogen-activated protein kinase (MAPK) pathway (Koka et al., 2008; Fernandes et al., 2011; Divani et al., 2020). Recent reports indicated that Ang II act via two G protein-coupled receptors (GPCR) such as AT1R, angiotensin type-2 receptor (AT2R) which expressed in human lung tissue. Besides, the activations of Ang II can be mediated by AT1R through enhancing several signaling pathways such as MAPK/ERK, IP3/diacylglycerol, tyrosine kinases, and NF-κB (Balakumar and Jagadeesh, 2014; El Tabaa and El Tabaa, 2020). In a parallel way, AT1 stimulates monocytes, macrophages and vascular smooth muscle cells to generate TNF-α and IL-6 (Balakumar and Jagadeesh, 2014). Additionally, Ang II promotes vasoconstriction, released pro-inflammatory cytokine, vascular endothelial dysfunction, and platelet aggregation (Nakashima et al., 2006; Shatanawi et al., 2011). There is also a relationship between Ang II and endothelin-1 (ET-1). Indeed ET-1 has an important function in Ang II-induced endothelial dysfunction and platelet activation through inducing IL-6 release (Touyz and Schiffrin, 1993; Browatzki et al., 2000). In order to reduce SARS-CoV-2 entry and related side effects, ACE2 activity should be declined. It has been found that ACE2 is a critical enzyme in the RAS, which has a critical function role in the human body. In this pathway, renin generated in the kidneys cleaves angiotensinogen from the liver, producing Ang I and then is cleaved by ACE into Ang-II (the substrate of ACE2). Ang I binds to the AT1R and AT2R as well as the RAS system has an important function in SARS-CoV-2 infection (Battagello et al., 2020).
In addition to the critical role of blood, hypoxia, ACE2, neuroinflammation in the neuronal pathogenesis of COVID-19, modulating RdRP/3-chymotrypsin-like protease (3CLpro) and papain-like protease (PLpro), as critical enzymes involved in the replication of SARS-CoV-2, is of great importance. There are also several receptors, namely CD209L (L-SIGN), CD209 (DC-SIGN), neuropilin receptors (NRPs), and CD147/Basigin, which facilitate SARS-CoV-2 entry (Amraei and Rahimi, 2020). As described, there is a close interconnection between the aforementioned dysregulated signaling pathways. In this line, providing multi-target agents capable of a simultaneous modulation of the aforementioned targets could pave the road against COVID-19 neurological manifestations.
Importance of Natural Products in Combating COVID-19 General Manifestations
The widespread pandemic of COVID-19 disease, by infecting millions of people, and thousands of killing around the world, has triggered researchers to make a diligent effort regarding finding potential drugs or vaccines against SARS-CoV-2. However, these efforts have not yet reached credible drugs due to the inherent complexity of the SARS-CoV-2 pathogenicity/complications (Sharma et al., 2020). Due to their simultaneous effects on multi-therapeutic targets and low side effects, phytochemicals including alkaloids, flavonoids, polyphenols, quinones, and terpenoids are of the most promising options for finding effective treatment against SARS-CoV-2 (Efferth and Koch, 2011; Mani et al., 2020).
Recent studies showed that three main targets, including main proteases, as well as S protein interaction with ACE2, have attracted the most attention of researchers to discover effective drugs against SARS-CoV-2 from phytochemicals. Additionally, phytochemicals potentially target neuroinflammation to combat related neuronal signs in COVID-19.
Potential of Phytochemicals Against COVID-19 Neurological Associations
Recently, no drug or vaccine has been developed for the treatment/prevention of SARS-CoV-2. Phytochemicals have shown to play critical antiviral biological activities and health benefits in CNS (Kähkönen et al., 1999). As previously mentioned, there are several major targets for phytochemicals against coronavirus such as ACE2, spike protein, TMPRSS2, 3CLpro, RdRp and PLpro, which among them ACE2 plays an important role regarding the initial stage of SARS-CoV-2 invasion into the cells/neurons (Huang et al., 2020b). Also, 3CLpro and PLpro play vital roles in SARS-CoV-2 maturation and replication (Xue et al., 2008; Ryu et al., 2010a).
The potential of phytochemicals in suppressing neuroinflammation induced by SARS-CoV-2 has also made them promising agents in combating neuronal signs of COVID-19.
Phytochemicals Inhibit Neuroinflammation and Neural Manifestations in COVID-19
As previously mentioned, hyper-inflammation is one of the critical neuropathological mechanisms of SARS-CoV-2 in line with the release of IL-2, IL-6, IL-7, IL-10 and TNF-α (Yang et al., 2020). Studies also suggested elevated levels of IL-8, MCP-1, IFN-γ, CXCL9, CXCL10 and GM-CSF in COVID-19 patients (Li et al., 2016; Marchetti et al., 2018; Conti et al., 2020; Vaninov, 2020) regarding triggering the neuronal manifestations. Systemic inflammation following the leukocyte activation prior to its BBB migrating is another major mechanism toward viral neurological complications (Campbell et al., 2014). The released inflammatory agents change the BBB permeability, triggers the neuroinflammatory flows and drive neuronal hyper-excitability through the activation of glutamate receptors, leading to acute seizure (Libbey et al., 2011; Yavarpour-Bali and Ghasemi-Kasman, 2020). Considering the crucial role of inflammation in the neuropathogenesis of COVID-19, phytochemicals with neuronal anti-inflammatory effects could pave the road in combating related neuronal manifestations. Recent reports also have declared the critical role of phytochemicals in health care through their antiviral (Fitriani et al., 2020) and the inhibition of neuroinflammatory-interconnected pathways (Abbaszadeh et al., 2020). Phytochemicals with potential antioxidant and anti-inflammatory effects (e.g., carotenoids and polyphenols) interact with major transcription factors such as Nrf2 and NF-κB (Iddir et al., 2020).
Naringin (Figure 1) is a phenolic phytochemical belonging to the flavonoid class, possessing anti-neuroinflammatory (Chen et al., 2016; Chtourou et al., 2016; Ngwa et al., 2020), and antiviral (Ng et al., 1996) effects with the potential of being used in the prevention/treatment of COVID-19 (Dabaghian et al., 2020). Naringin also inhibits the expression level of cyclooxygenase (COX)-2, inducible nitric oxide synthase (iNOS), IL-1β and IL-6 via suppressing high mobility group box 1 (HMGB1) in COVID-19 (Park et al., 2003; Huang et al., 2020a). It also declined the expression level of p38MAPK to inhibit HMGB1 generation of inflammatory mediators and associated lung injury (Gil et al., 2016; Kim et al., 2019b). According to the critical destructive role of inflammatory mediators in the neurological signs of COVID-19, naringin seems to be a hopeful anti-inflammatory/antiviral candidate in combating related neuronal manifestations. As an aglycone form of naringin, naringenin has similarly shown anti-neuroinflammatory (Nouri et al., 2019; Alberca et al., 2020), and antiviral effects with the potential of being used against COVID-19 (Tutunchi et al., 2020). We have previously shown the neuroprotective potential of naringenin through modulation of inflammatory mediators (NF-κB, TNF-α, IL-1β, etc) and microglia activation in the CNS (Nouri et al., 2019), thereby it could mitigate the neuronal signs of COVID-19 mediated by the inflammatory mediators. As another phenolic compound, resveratrol has shown promising beneficial effects against COVID-19, through the activation of ERK1/2 and SIR1 signaling pathways related to survival, DNA protection (Levy et al., 2020; Ma and Li, 2020), and anti-neuroinflammatory responses (Bastianetto et al., 2015). It also inhibits neuroapoptosis by reducing FGF-2 and suppressing NF-κB (Xu et al., 2018). Considering the critical role of the aforementioned inflammatory mediators in COVID-19 (Yarmohammadi et al., 2020), resveratrol could potentially decline neuroinflammatory signs of COVID-19 patients (Chen et al., 2005). As a major natural derivative of resveratrol, polydatin potentially decline the neural levels of NF-κB, TNF-α, IL-1β, IL-6, IL-8, prostaglandin E2 (PGE2), NO, COX-2, iNOS, matrix metalloproteinase (MMP)-3 and MMP-9, thereby could be a novel agents in combating neuronal inflammatory manifestation in COVID-19 (Lo Muzio et al., 2020). A recent study by Bonucci et al., has also introduced polydatin as a protective phytochemical against COVID-19 (Bonucci et al., 2020). So, focusing on their ameliorating effects against neuroinflammation, as well as related antiviral properties, resveratrol and plydatin derivative could be of candidate phytochemicals in combating neuronal signs of COVID-19.
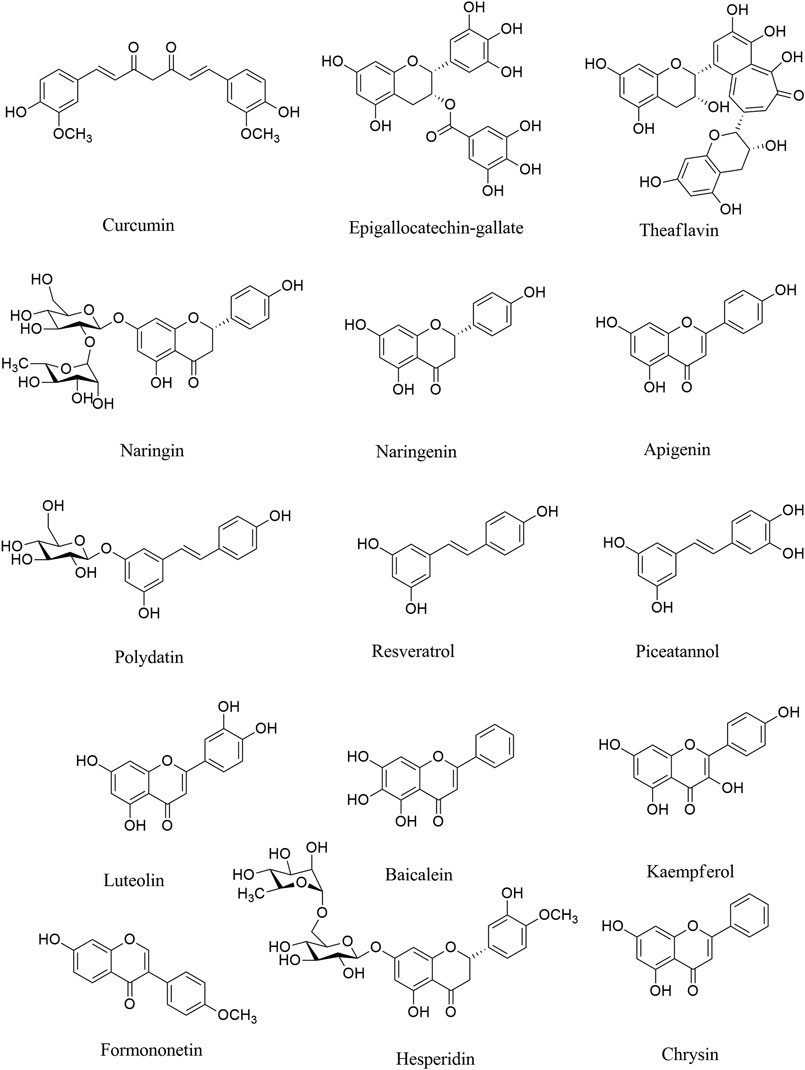
FIGURE 1. Chemical structures of selected polyphenols/flavonoids with the potential of being used against COVID-19 neurological manifestations.
Consistently, evidence has shown that epigallocatechin gallate (EGCG), as a natural polyphenolic compound, plays important functions such as antitumorigenic, anti-inflammatory, antibacterial, antioxidative, and antiproliferative effects (Chacko et al., 2010; Ge et al., 2018; Mhatre et al., 2020). The anti-neuroinflammatory effects through inhibiting microglia activation, and suppressing inflammatory mediators (Abbaszadeh et al., 2020), as well as antiviral effects of EGCG (Steinmann et al., 2013) make it a potential polyphenol for the treatment of neurological symptom in COVID-19. Green tea with the prominent phytochemicals of such polyphenols, including EGCG, epicatechin gallate, epicatechin and catechin plays both the antiviral (Chojnacka et al., 2020), anti-SARS-CoV-2 (Ghosh et al., 2020) and anti-neuroinflammatory activities (Calis et al., 2020), thereby could play promising role in combating COVID-19 neural complications. EGCG has employed several other mechanisms to suppress SARS-CoV-2 in different steps of virus life cycle (Jang et al., 2020).
As another polyphenol, formononetin declined neuroinflammation by decreasing the levels of TNF-α, IL-6, IL-1β, PGE2, iNOS, and COX-2. Evidence indicated that formononetin inhibited neuroinflammation through suppressing NF-κB signaling pathway, thereby could be a novel drug for the neurological manifestation of COVID-19 (El-Bakoush and Olajide, 2018; An et al., 2020). Formononetin was shown to modulate MAPK, ERK, p38, JNK pathway and downstream mediators to play antiviral effects and inhibit infection-induced inflammation (Wang et al., 2015; Lalani and Poh, 2020). Recent reports also have considered the formononetin as one of major plant-derived secondary metabolites with acceptable effectiveness against COVID-19 (Mirzaie et al., 2020). Consistently, theaflavins are other phenolic compounds with antiviral, anti-inflammatory, antioxidative, and antibacterial effects (Higdon and Frei, 2003; Lambert and Yang, 2003). Theaflavins also suppressed the levels of inflammatory mediators such as COX-2, TNF-α, intercellular adhesion molecule 1 (ICAM-1), and NF-κB mRNA (Mhatre et al., 2020). The aforementioned effects of theaflavins, as well as its antiviral potentials (Zu et al., 2012), could introduce it as a useful treatment against the neurological sign of COVID-19, via modulation of neuronal IL-1β, IL-6, TNF-α, IL-10, glial fibrillary acidic protein and Bax. As well as related interaction with ACE2/spike proteins, and main proteases. Based on molecular dynamic analysis Kumar et al. indicated that some other phenolic compounds play important roles in the inhibition of SARS-CoV-2 such as rosmarinic acid, ferulic acid, ursonic acid, piperine, gingerol, curcumin, and silymarin (Kumar et al., 2020). Previously the neuroprotective effects of such plant-derived secondary metabolites have been reported through inhibiting the inflammatory-interconnected mediators (Abbaszadeh et al., 2020; Fakhri et al., 2020b). Among the aforementioned phytochemicals, ferulic acid, silymarin and curcumin possess particular anti-neuroinflammatory effects, in addition to related antiviral effects (Dutta et al., 2009; Borah et al., 2013; Ghosh et al., 2017). The anti-neuroinflammatory effects of curcumin is applied through suppressing microglia cells (Ghasemi et al., 2019). Other flavonoids like luteoloside and baicalein also possess potential modulatory effects against neuroinflammation, toward antiviral effects (Nagai et al., 1995; Cao et al., 2016; Li et al., 2019a; Welcome, 2020).
Among other classes of phytochemical compounds, phytosterols also have shown potential anti-inflammatory effects (Dash et al., 2020). Of those compounds, stigmasterol and β-sitosterol reduced the expression of COX-2, TNF-α, iNOS, IL-6, IL-1β, PGE2 and NF-κB (Philip et al., 2018). Consequently, Krupanidhi et al. indicated the antiviral effects of stigmasterol and β-sitosterol against the SARS-CoV-2 by computational studies. So, considering the antiviral and anti-inflammatory potential of stigmasterol and β-sitosterol, they could be potential agents in combating COVID-19 neurological signs (Krupanidhi et al., 2020).
Additionally, several lines of evidence indicated that asiaticoside (a saponin), borneol (a terpene), catalpol (an iridoid) as other phytochemicals declined the neuronal levels of TNF-α, IL-6, TLR4, NF-κB, IL-β and IL-8, thus may be hopeful agents against neurological symptoms in COVID-19 (Welcome, 2020). In fact, since inflammation triggers several cascades of CNS pathogenesis in COVID-19, suppressing related mediators could potentially ameliorate related symptoms. Among other phytochemicals, some alkaloids also show promising anti-inflammatory and antiviral effects (Chen et al., 2015; Powers and Setzer, 2016), with the potential of being used against COVID-19 (Bleasel and Peterson, 2020). This effect of alkaloids was also confirmed by a recent in silico study by Garg and Roy. In their study, two alkaloids of sophaline D and thalimonine indicated potential antiviral activities by suppressing main viral proteases (Garg and Roy, 2020) and inflammatory pathways (Varadinova et al., 1996; Pour et al., 2019).
Several other phytochemicals play important roles in the inhibition of SARS-CoV-2 such as sarsasapogenin (a steroidal sapogenin), novobiocin (a coumarin), and alpha terpinyl acetate (a terpenoid) (Kumar et al., 2020). Previously the neuroprotective effects of such plant-derived secondary metabolites have been reported through inhibiting the inflammatory-interconnected mediators (Abbaszadeh et al., 2020; Fakhri et al., 2020b).
Cannabinoids also possess critical anti-inflammatory roles in viral diseases (Walter and Stella, 2004; Rizzo et al., 2020). These compounds are major constituents of the cannabis plant. The physiological roles of cannabinoids and cannabis are primarily mediated by the cannabinoid receptors (CB1R and CB2R), endocannabinoids, and related metabolic enzymes which are widely distributed throughout the body, especially CNS. The mediators of cannabinoid receptors are being considered as potential targets for numerous disorders, including those correlated with inflammation and autoimmune dysregulation (Rizzo et al., 2020). Prevailing evidence are indicating the pivotal anti-inflammatory and immunoregulatory effects of cannabis-derived cannabinoids, through suppressing cytokines, inhibition of immune cell migration/proliferation (Almogi-Hazan and Or, 2020). Besides, selective cannabinoid agonists present a novel way regarding the treatment of virus-associated neuroinflammation. Considering their growing global acceptance for medicinal uses (Onaivi et al., 2020), cannabinoids seem to be of potential agents against inflammatory cytokine and related mortality in COVID-19 (Onaivi and Sharma, 2020).
Overall, phytochemicals with the potential of modulating the immune system and attributed neuronal cytokine storm could pave the road in combating COVID-19 neuronal complications.
Phytochemicals Inhibit ACE2, and Spike Protein Thereby Neural Manifestations in COVID-19
As previously mentioned, SARS-CoV-2 enters the CNS via the ACE2 or TMPRSS2 receptors (El Tabaa and El Tabaa, 2020; Nemoto et al., 2020). In order to decline SARS-CoV-2 entry to neural cells, ACE2 activity should be declined (Battagello et al., 2020). Spike (S) glycoprotein as the main SARS-CoV-2 structural protein with a critical role in binding to the host cell and protecting the virus against some of the host species antibodies, is another target of phytochemicals (Schoeman and Fielding, 2019).
ACE2 is an enzyme found in the outer membrane of the human cell that acts as a binding site for the S protein. Several studies have shown that there is a strong interaction between ACE2 and S protein. So, blocking ACE2 is also another phytochemical strategy to fight SARS-CoV-2 (Li et al., 2005).
Flavonoids reduce the ACE2 expression through activating Nrf2, thereby combat SARS-CoV-2 (Mendonca and Soliman, 2020; Muchtaridi et al., 2020). Based on the molecular docking mutagenesis study and experimental verification results, hesperidin, chrysin and emodin can be used for COVID-19 treatment (Basu et al., 2020). An in silico study indicated that kaempferol, quercetin, and fisetin bind to the hACE2-S-protein complex, near the interface of hACE2 and S protein binding (Pandey et al., 2020). In a recent study by Rebas et al., 2020 the neuroprotective effects of the aforementioned compounds have been shown. So, kaempferol, quercetin and fisetin are of promising flavonoids against COVID-19 neurological signs. Two in silico studies showed that quercetin, quercetin 3-glucuronide-7-glucoside, quercetin 3-vicianoside, absinthin, glabridin, and gallic acid gave better binding energy (BE) with ACE2 (Joshi et al., 2020) toward inhibiting COVID-19 (Joshi et al., 2020).
Through the same molecular docking analysis piceatannol also has shown neuroprotective responses (Zhang et al., 2018; Talebi et al., 2020) with the potential of binding to ACE2, thereby playing a critical role in the prevention and treatment of COVID-19 (Wahedi et al., 2020; Ahmad et al., 2020). The phytochemicals, baicalin, scutellarin, and hesperetin, also bind to ACE2, regarding reducing neurological symptoms in COVID-19 (Cheng et al., 2020a). Several in silico studies showed that the binding energy of hesperidin with the SARS-CoV-2 spike protein, and main proteases are lower than that of ritonavir, lopinavir, and indinavir. It could introduce hesperidin as an effective antiviral agent. Hesperidin also has shown to counteract the cell damaging induced by virus infection, inflammation and free radicals (Bellavite and Donzelli, 2020). Many of other phenolic compounds, including naringenin, hesperetin, hesperidin, and baicalin, showed potential inhibitory effects on ACE2 activity, thereby showed potential effects on COVID-19 and related neural manifestations (Muchtaridi et al., 2020). In another study, stilbene-based compounds especially resveratrol, are promising candidate phytochemicals acting via disrupting spike protein and human ACE2 receptor complex (Wahedi et al., 2020).
EGCG and theaflavin gallate seem to be of promising phytochemicals in targeting spike-protein central channel of SARS-CoV-2 (Maiti and Banerjee, 2020). In a recent study by Kulkarni et al., 2020 some terpenoids such as carvacrol, geraniol, anethole, l-4-terpineol,cinnamyl acetate, thymol and pulegone, and other phenolic as cinnamaldehyde were effective antiviral agents with potential inhibitory effects on viral spike protein. In this line, nimbin (a triterpenoid) and curcumin (polyphenol) showed high binding affinity regarding interacting with ACE2 and the S protein (Maurya et al., 2020). Consistently, Chen and Due estimated the BE of ACE2 interaction with the flavonoid glycoside scutellarin and the triterpenoid glycyrrhizin as a -14.9 and -9 kcal/mol, respectively, that were more strong than other studied phytochemicals including baicalin, hesperetin, and nicotianamine (Chen and Du, 2020). A study by Vardhan et al., showed that one hundred fifty-four analogous of limonoids and triterpenoids showed potential inhibitory effects on ACE2, 3CLpro, PLpro, spike protein, and RdRp. Another in silico study also showed that limonin, obacunone, ursolic acid, glycyrrhizic acid, 7-deacetyl-7-benzoylgedunin, maslinic acid, and corosolic acid effectively target SARS-CoV-2 proteins (Vardhan and Sahoo, 2020).
Evaluated by molecular docking analysis, dithymoquinone (a quinone, Figure 2) showed neuroprotective responses (Zhang et al., 2018; Talebi et al., 2020) through binding to ACE2, to show key roles in the prevention and treatment of COVID-19 (Wahedi et al., 2020; Ahmad et al., 2020). As a potential phytochemical of Nigella sativa L. (Ranunculaceae), dithymoquinone, with binding affinity of -8.6 kcal/mol, showed a higher potential of binding at SARS-CoV-2 ACE2 (Ahmad et al., 2020). According to the molecular modeling results on SARS-CoV-2, a new indazole alkaloid from the seeds of N. sativa, nigellidine meaningfully bind to active sites of SARS-CoV-2 (Maiti et al., 2020).
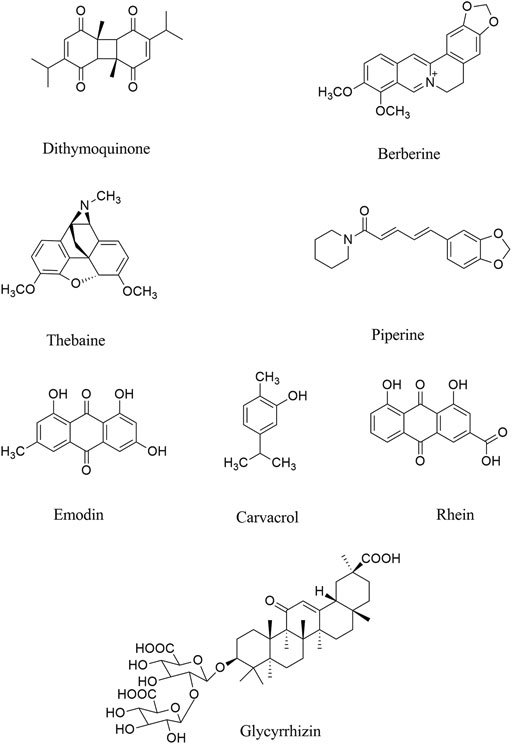
FIGURE 2. Chemical structures of selected alkaloids/terpenes/quinones with the potential of being used against COVID-19 neurological manifestations.
Parvez and co-workers, in an in silico study, showed that two chalcones azobechalcone (binding energy [BE], −14.4 kcal/mol) and isolophirachalcone (BE, −12.8 kcal/mol) as well as two alkaloids fangchinoline (BE, −12.5 kcal/mol) and tetrandrine (BE, −12.6 kcal/mol) have shown high binding affinity to S protein of SARS-CoV-2 (Parvez et al., 2020). Also, three alkaloids, including cepharanthine, fangchinoline, and tetrandrine inhibited the S protein of Human-CoV-OC43 expression at 5 µM (Kim et al., 2019a), as previously showed anti-inflammatory roles in viral diseases. In another study, Ho and co-workers showed that anthraquinone emodin (IC50, 200 µM) blocked the interaction between ACE2 and S protein (Okamoto et al., 2001; Ho et al., 2007).
In a survey by Niu et al. glabridin, genistein, chrysoeriol, and tectorigenin have been introduced as phytochemicals affecting miRNAs of ACE2 (Niu et al., 2020b). In vitro investigation showed that rhoifolin, δ-viniferin, myritilin, homoflavone A, lactucopicrin15-oxalate, nympholide A, afzelin, biorobin, phyllaemblicin B, cyanidin, baicalin, scutellarin, glycyrrhizin, tangeretin, pro-cyanidin, nobiletin, brazilein, galangin, acetoxychavicol acetate (ACA) and delphinidin are among other phytochemicals which inhibit ACE to suppress COVID-19 (Maroli et al., 2020; Muchtaridi et al., 2020).
Recent reports confirmed that there are several other phytochemicals, which inhibited ACE2 activity, including neohesperidin, nobiletin, scutellarin, nicotinamin, and glycyrinodin (Muchtaridi et al., 2020). As another natural product with antiviral properties, glycyrrhizic acid binds to ACE2, thereby could be used for treatment of COVID-19 neurological signs (Pilcher, 2003). Luteolin also inhibited furin proteins which breakdown the S protein in SARS-CoV. Similarly, herbacetin inhibited the interaction between S protein and ACE2. Accordingly, these phytochemicals can be useful for treating/managing neurological manifestation of COVID-19 by targeting the ACE2/spike proteins to suppress the penetration/attachment of SARS-CoV-2 to the CNS cells, what triggers the neurological signs (Wu et al., 2020b). Overall, evidence has shown berberine, thebaine, piperine (as alkaloids), withaferin A (steroidal lactone), nimbin, embelin, cafestol, murrayanine, murrayaquinone-A and andrographolide are phytochemicals with the potential antiviral effects for example through binding to spike protein in SARS-CoV-2, as well as ACE2 receptor (Grover et al., 2011; Boukhatem and Setzer, 2020; Gupta et al., 2020; Parida et al., 2020). Consistent docking results showed the same acceptable inhibitory effects against SARS-CoV-2.
The main phytochemicals with reported inhibitory effects on ACE2 and spike proteins are presented in Table 1.
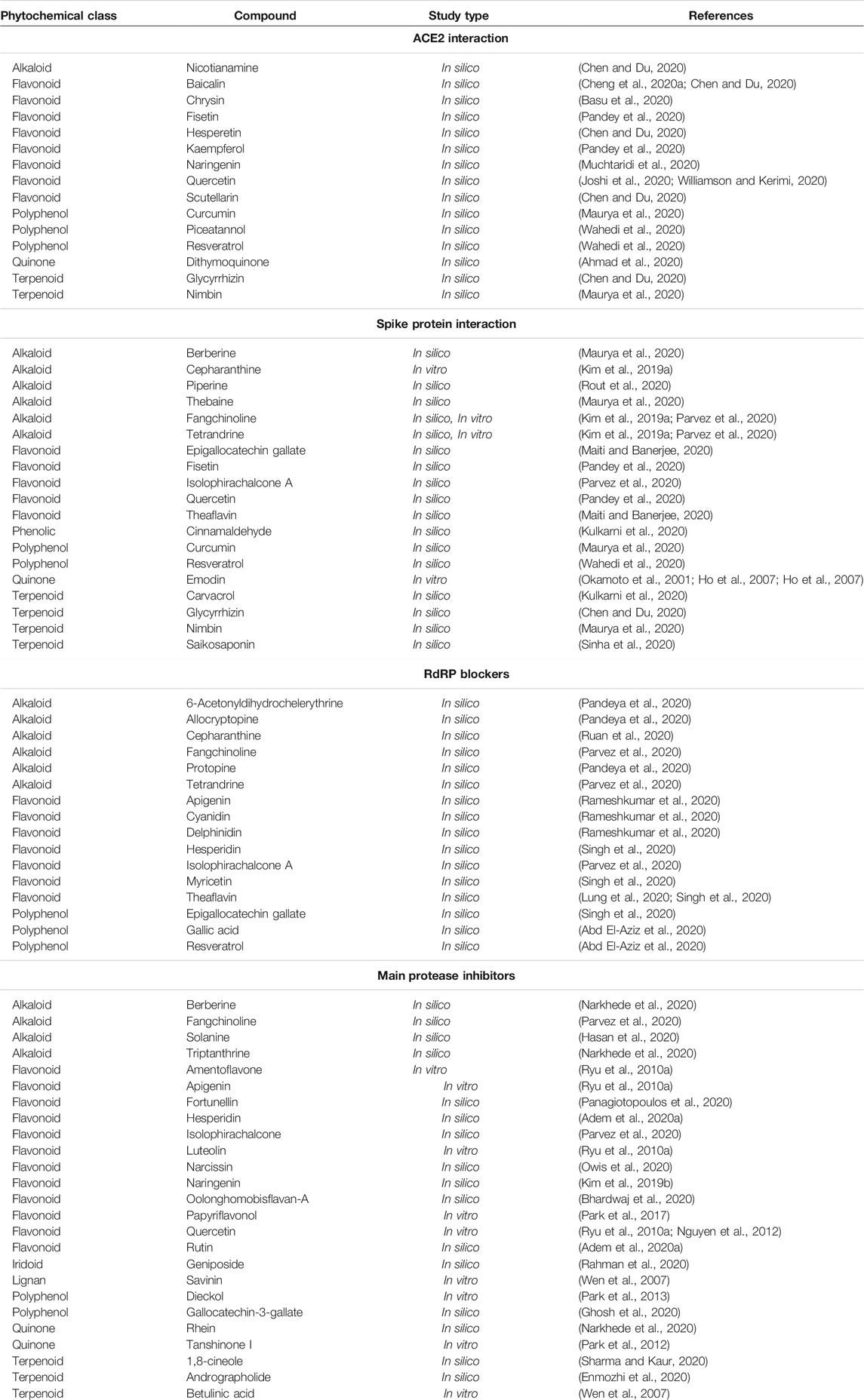
TABLE 1. Selected/candidate phytochemicals with inhibitory effects on ACE2, spike proteins, protease, and RdRP in combating COVID-19 neurological signs.
Phytochemicals Inhibit RdRp, 3CLpro and PLpro, Thereby Neural Manifestations in COVID-19
Ongoing studies are consisting on the key role of RdRp, 3CLpro and PLpro, in the neuropathogenesis of SARS-CoV-2. Proteases especially 3CLpro and PLpro, play critical roles in SARS-CoV-2 maturation and replication, and are of the main targets of anti-SARS-CoV-2 phytochemicals (Xue et al., 2008; Ryu et al., 2010a; Shamsi et al., 2016). Polyphenols, especially flavonoids, are among the phytochemicals with anti-SARS effects through inhibiting proteases (Senthilvel et al., 2013; Shamsi et al., 2016; Annunziata et al., 2020). Adem and co-workers, in a molecular docking study on 80 flavonoids showed that 24 of them had suitable interaction with the main protease of SARS-CoV-2, of which hesperidin and rutin had the highest interaction (Adem et al., 2020a). In another in silico report, four hundred fifty-eight flavonoids were screened, which among them apigenin 7-(6″-malonylglucoside), cyanidin-3-(p-coumaroyl)-rutinoside-5-glucoside, delphinidin 3-O-beta-D-glucoside 5-O-(6-coumaroyl-beta-D-glucoside), albireodelphin, and (-)-Maackiain-3-O-glucosyl-6″-O-malonate possessed the most potential in inhibiting SARS-CoV-2. The aforementioned flavonoids showed the highest binding energy values against RdRP, and S proteins of SARS-CoV-2 (Rameshkumar et al., 2020). Another study on twenty-three flavonoids and twenty-five chalcones compounds, showed that the compounds were capable of blocking main proteases. In their study, cyanidin inhibited RNA polymerase and, quercetin blocked the viral spike. As previously mentioned, RdRp catalyzes SARS-CoV-2 RNA replication and thereby is considered an important target for antiviral drug design. Molecular docking investigation revealed that EGCG, theaflavin, theaflavin-3′-O-gallate, theaflavin-3′-gallate, theaflavin 3,3′-digallate, hesperidin, quercetagetin, and myricetin bind to the active site of RdRp (Singh et al., 2020). Overall, flavonoids and indole chalcones could combat SARS-CoV-2 (Vijayakumar et al., 2020). Additional evidence confirmed that quercetin and kaempferol possess beneficial anti-inflammatory, antioxidant, antiviral, antiallergic effects which potentially inhibits SARS-CoV 3CLpro, PLpro, and S protein (Di Pierro et al., 2020). Accordingly, docking evidence indicated quercetin and kaempferol as promising compounds against SARS-CoV-2. So, these phytochemicals could decline neurological manifestations in COVID-19 patients (Ryu et al., 2010b). In a recent in silico report by Gorla et al. (2020) silymarin, and biochanin A were proposed as bioflavonoids possessing the most acceptable interaction with ACE2/spike protein of SARS-CoV-2. Also, an in silico study indicated that naringenin inhibited 3CLpro chains, thereby may be a promising phytochemical for alleviating neurological symptoms in COVID-19 patients (Kim et al., 2019b). Papyriflavonol A as a prenylated flavone inhibited the PLpro and 3CLpro of SARS-CoV at 3.7 and 103.6 µM, respectively (Park et al., 2017). Also, Ryu and co-workers showed that a biflavonoid, amentoflavone, blocked the 3CLpro at 8.3 µM while apigenin, luteolin, and quercetin inhibited the enzyme at 280.8, 20.2, and 23.8 µM, respectively (Ryu et al., 2010a; Yao et al., 2018; Istifli et al., 2020). Oolonghomobisflavan-A (Bhardwaj et al., 2020), narcissin (Owis et al., 2020), isolophirachalcone (Parvez et al., 2020), fortunellin (Panagiotopoulos et al., 2020), dieckol (Park et al., 2013), gallocatechin-3-gallate (Ghosh et al., 2020) are other polyphenols with inhibitory effects on SARS-CoV-2 proteases.
Theaflavins, a group of polyphenols formed after the fermentation of green tea, have a very strong affinity to bind to RdRp (Lung et al., 2020; Singh et al., 2020). Lung and co-workers reported that theaflavin had a high affinity for RdRp of SARS-CoV2, SARS-CoV, and MERS-CoV (Lung et al., 2020). Also, Singh et al. (2020) showed that theaflavin-3,3′-digallate, theaflavin-3′-gallate, theaflavin-3′-O-gallate, and theaflavin had the highest affinity for RdRp with -9.9, -9.6, -9.6, and -9.3 kcal/mol bonding energy, respectively. EGCG and hesperidin (Singh et al., 2020), isolophirachalcone A (Parvez et al., 2020), gallic acid and resveratrol (Abd El-Aziz et al., 2020) are other polyphenols with anti-SARS-CoV-2 activities through the high binding affinity to RdRp.
Of other classes of phytochemicals, solanine is a steroidal alkaloid that interacts with two clusters of amino acids of the C3-like protease. The first cluster consists His163, His164, Met165, and Pro168 and the latter contains Asp187, Gln189, and Ala191 (Hasan et al., 2020). There are several other alkaloid that interact with C3-like protease such as solasurine, omatidenol, cycloartanol, diosgenin, lupeol and purpurin (Hasan et al., 2020). Besides, the alkaloids including cepharanthine (Ruan et al., 2020), fangchinoline and tetrandrine (Parvez et al., 2020), protopine, 6-Acetonyldihydrochelerythrine, and allocryptopine (Pandeya et al., 2020) showed strong binding to SARS-CoV-2 RdRp in docking studies.
Nsp15 is responsible for protein interference with the innate immune response, which is essential in the function of coronavirus. Studies indicated that sarsasapogenin, ursonic acid, apigenin, curcumin, ajmalicine, novobiocin, silymarin, alpha amyrin, pomolic acid, carnosol, asiatic acid, reserpine, betulinic acid, platanic acid, taspine, alphitolic acid, taxifolin, wogonin, chlorogenic acid, afromosin, gliotoxin, psoralen, carinatine rhinacanthin, caffeic acid, coriandrin, scopoletin, cordycepin, ricinoleic acid, alpha asarone, allicin and aranotin as other phytochemicals, can bind to Nsp15 protein, thereby could be useful factors for inhibitors of COVID-19 (Kumar et al., 2020; Umesh et al., 2020). In a research by Adem et al., showed the beneficial effects of caffeic acid derivatives were shown as inhibitors of SARS-CoV-2, via inhibition of COVID-19 Nsp15, main proteases, and spike protein (Adem et al., 2020b).
In addition to alkaloids and flavonoids, terpenoids and quinones are other phytochemicals with inhibitory effects on main proteases of SARS-CoV-2. In an in silico study, some natural products against SARS-CoV-2 anthraquinones such as rhein and crysophanic acid as well as the alkaloids such as indican, indigo, berberine, tryptanthrine and terpenes (e.g., bicylogermecrene and glycyrrhizin) showed a strong interaction with SARS-CoV-2 main protease. In their study based on the lowest binding energy, rhein (BE, −8.9 kcal/mol) and tryptanthrine (BE, −8.2 kcal/mol) were introduced as suitable candidates against SARS-CoV-2 (Narkhede et al., 2020). Andrographolide (Enmozhi et al., 2020), 1,8-cineole (Sharma and Kaur, 2020), betulinic acid and savinin (Wen et al., 2007), geniposide (Rahman et al., 2020), and tanshinone I (Park et al., 2012) are other phytochemicals with anti-SARS-CoV-2 activities via the blocking the SARS-CoV-2 proteases. In a similar study, silibinin, dihydrorobinetin, peonidin, robinetin, 5-deoxygalangin, scutellarein, purpurin, isorhamnetin, tricetin, gossypetin, norathyriol, coumestrol, isosakuranetin, pectolinarigenin, tangeritin, nobiletin, pratensein, hispidulin, baicalein, morin, urolithin A, acacetin, pelargonidin, irilone, pinocembrin, malvidin, dalbergin, butein, biochanin A, fustin, 5-hydroxyflavone, pinostrobin, pinobanksin, datiscetin, galangin, cyanidin, daidzein, glycitein, wogonin, phloretin, urolithin B, angolensin, pinosylvin, formononetin, liquiritigenin, prunetin, alpinetin, biochanin A, rhapontigenin, equol, piceatannol, isorhapontigenin, danshensu, eugenin, sinapic acid, pterostilbene, pyrogallol, resacetophenone, syringic acid, p-coumaric acid, paeonol, protocatechuic acid, tyrosol, catechol, 4-ethylphenol and cinnamic acid as natural product binding to SARS-CoV-2 RdRp (Kurokawa et al., 2001; Bosch-Barrera et al., 2020; Singh et al., 2020). Consistently, based on an study of Umesh et al. (2020) carnosol, rosmanol, and arjunglucoside-I, as natural phytochemicals have shown potential inhibitory effects on SARS-CoV main protease using molecular docking approach. In a recent study by Chojnacka et al., some biologically active phytochemicals like quercetin, betulinic acid, luteolin, indigo, aloeemodine, and quinomethyl triterpenoids, or gallates were of potential key antiviral agents in blocking viral proteases (Chojnacka et al., 2020). Additional studies have shown several phytochemicals such as 18-hydroxy-3-epi-alphayohimbine, vincapusine, alloyohimbine, and gummadiol, toward the inhibition of SARS-CoV 3CLpro, SARS-CoV-2 3CLpro, and MERS-CoV 3CLpro toward the treatment of COVID-19 neuronal associations (Bhardwaj et al., 2020). Phytochemicals with the potential of inhibiting RdRP and proteases are also presented in Table 1.
Pharmacokinetic Interaction and BBB Permeability of Phytochemicals: AN Approach to Novel Delivery Systems
However, the neuroprotective effects of such phytochemicals have been provided in several studies, estimations of the permeability through the BBB of the phytochemicals were assessed by the SwissADME program (Daina et al., 2017). Information on the estimations of permeability through the BBB, as well as predict absorption, distribution, metabolism, and excretion (ADME) parameters, pharmacokinetic properties, druglike nature and medicinal chemistry friendliness are shown in Supplementary Table S1. Among the fifty-five phytochemicals, the screening of BBB permeability gave fifteen compounds with a positive effect. Among these are the monoterpenoids 1,8-cineole and carvacrol; the alkaloids 6-acetonyldihydrochelerythrine, allocryptopine, berberine, piperine, protopine, thebaine, and triptanthin; the flavonoid chrysin; the quinones dithymoquinone and tanshinone I; the phenolic compounds resveratrol and cinnamaldehyde; and the lignan savinin. To overcome the aforementioned pharmacokinetic drawbacks of some phytochemicals, novel delivery systems are being applied regarding increasing their penetration to BBB. Accordingly, nano-formulations, polymeric micelles, nano-/micro-emulsions, nano-gels, solid lipid nano-particles, polymer composites, and liposome/phospholipid have been studied so far (Abbaszadeh et al., 2020; Fakhri et al., 2020a).
As previously mentioned, inflammatory conditions play critical roles during the pathogenesis of COVID-19 disease. It is worth noting that inflammation could increase the BBB penetration of phytochemicals to facilitate their central permeation. This pathophysiological condition simplifies the CNS penetration of those phytochemicals with limitations in their penetration.
Discussion
COVID-19 pandemic is an important threat to human life. Up to now, no effective drug or vaccination has been provided to combat various complications in COVID-19. So, finding therapeutic agents to combat related manifestations in COVID-19, is of great importance. Among different complications of COVID-19 the neurological manifestations have attracted particular attention. Growing evidence is highlighting the involvement of multiple dysregulated mechanisms behind the pathophysiology of COVID-19 neurological manifestations, including hypoxia, neuroinflammation, ACE2/spike proteins, and related enzymes in virus proliferation (e.g., RdRP, 3CLpro, and PLpro). So, providing multi-target agents could pave the road in combating associated neuronal manifestations in COVID-19. For many years, the plant kingdom has shown promising antiviral, and anti-neuroinflammatory results. Accordingly, the hope regarding identifying new applications for the candidate phytochemicals has a successful history in complementary/alternative medicine. We previously showed the antiviral approaches and therapeutic targets of plant-derived secondary metabolites in various steps of viruses life cycle, including penetration, uncoating, replication, and release (Pour et al., 2019). In the present study, potential phytochemicals with antiviral effects and modulatory potentials against neuroinflammation, ACE2/spike protein, and related main proteases in the virus life cycle have been highlighted regarding inhibiting the penetration/attachment and replication phases of coronaviruses (Figure 3). Among the aforementioned phytochemicals, in silico/in vitro results introduced polyphenols (mainly flavonoids), alkaloids, and terpenes/terpenoids as potential candidates in counteracting the neurological signs of COVID-19. Although the BBB limits the CNS penetration of some phytochemicals, the disease-related inflammatory conditions as well as novel delivery systems could potentially overcome the BBB dynamic and drawback the limitation. As the results, flavonoids like naringin and it aglycone (naringenin), theaflavins, silymarin, curcumin, EGCG, polyphenol resveratrol and its derivative (polydatin), as well as some phytosterols and cannabinoids showed the most simultaneous anti-neuroinflammatory and antiviral potentials in combating SARS-CoV-2 neural complications. To suppress the viral penetration/attachment the flavonoids hesperidin, chrysin, kaempferol, quercetin, fisetin, baicalin, naringenin, EGCG, and theaflavin as well as some terpenes chalcones, glycyrrhizin, nimbin and alkaloids like berberin, thebaine, piperine as well as terpenoids have shown a more potential future in targeting ACE2/spike proteins. Consequently, regarding targeting the main proteases of coronaviruses flavonoids apigenin, cyaniding, delphinidin, EGCG, theaflavin, naringenin, hesperidin, quercetin and kaempferol, as well as some chalcones, steroidal alkaloid, terpenoids, and quinones are of potential candidates in inhibiting the main proteases of coronaviruses. Overall, the aforementioned phytochemicals have shown growing evidence to be of potential agents in combating neurological signs of COVID-19 through attenuation of neuroinflammation, ACE2/spike proteins, and main proteases.
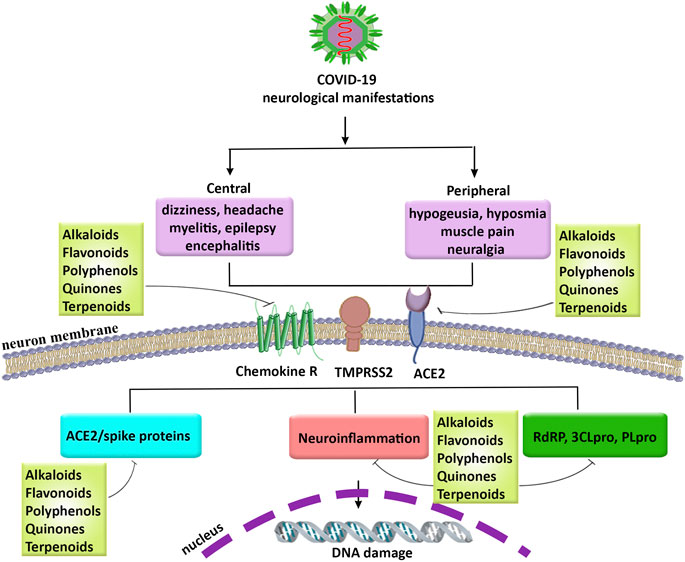
FIGURE 3. The neurological manifestations in COVID-19, related pathophysiological mechanisms, and promising role of phytochemicals. COVID-19: coronavirus 2019, PLpro: papain-like protease, RdRP: RNA-dependent RNA polymerase, SARS-CoV-2: severe acute respiratory syndrome coronavirus 2, TMPRSS2: transmembrane protease, serine 2, 3CLpro: 3-chymotrypsin-like cysteine protease.
Such studies could pave the road regarding finding novel therapeutic agents in combating neurological manifestations in COVID-19. Further reports are required to reveal the precise dysregulated pathways responsible for COVID-19 neurological signs, as well as potential therapeutic phytochemicals.
Author Contributions
Conceptualization, SF, MF, and JE; drafting the manuscript, SF, SP, MM; Software: SF, review and editing the paper: SF, MF, and JE; All authors have read, revised and agreed to the published version of the manuscript.
Conflict of Interest
The authors declare that the research was conducted in the absence of any commercial or financial relationships that could be construed as a potential conflict of interest.
Acknowledgments
JE gratefully acknowledges funding from CONICYT (PAI/ACADEMIA N°79160109).
Supplementary Material
The Supplementary Material for this article can be found online at: https://www.frontiersin.org/articles/10.3389/fphar.2020.621099/full#supplementary-material.
References
Abbaszadeh, F., Fakhri, S., and Khan, H. (2020). Targeting apoptosis and autophagy following spinal cord injury: therapeutic approaches to polyphenols and candidate phytochemicals. Pharmacol. Res. 160, 105069. doi:10.1016/j.phrs.2020.105069
Abd El-Aziz, N. M., Shehata, M. G., Awad, O. M. E., and El-Sohaimy, S. A. (2020). Inhibition of COVID-19 RNA-dependent RNA polymerase by natural bioactive compounds: molecular docking analysis. Research Square [Pre-print]. doi:10.21203/rs.3.rs-25850/v1
Adem, S., Eyupoglu, V., Sarfraz, I., Rasul, A., and Ali, M. (2020a). Identification of potent COVID-19 main protease (Mpro) inhibitors from natural polyphenols: an in silico strategy unveils a hope against CORONA. Preprints. doi:10.20944/preprints202003.0333.v1
Adem, Ş., Eyupoglu, V., Sarfraz, I., Rasul, A., Zahoor, A. F., Ali, M., et al. (2020b). Caffeic acid derivatives (CAFDs) as inhibitors of SARS-CoV-2: CAFDs-based functional foods as a potential alternative approach to combat COVID-19. Phytomedicine 33, 153310. doi:10.1016/j.phymed.2020.153310
Ahmad, I., and Rathore, F. A. (2020). Neurological manifestations and complications of COVID-19: a literature review. J. Clin. Neurosci. 77, 8–12. doi:10.1016/j.jocn.2020.05.017
Ahmad, S., Abbasi, H. W., Shahid, S., Gul, S., and Abbasi, S. W. (2020). Molecular docking, simulation and MM-PBSA studies of Nigella sativa compounds: a computational quest to identify potential natural antiviral for COVID-19 treatment. J. Biomol. Struct. Dyn. 21, 1–9. doi:1080/07391102.2020.1775129
Ahmed, M. U., Hanif, M., Ali, M. J., Haider, M. A., Kherani, D., Memon, G. M., et al. (2020). Neurological manifestations of COVID-19 (SARS-CoV-2): a review. Front. Neurol. 11, 518–519. doi:10.3389/fneur.2020.00518
Alberca, R. W., Teixeira, F. M. E., Beserra, D. R., de Oliveira, E. A., de Souza Andrade, M. M., and Pietrobon, A. J. (2020). Perspective: the potential effects of naringenin in COVID-19. Front. Immunol. 11, 570919. doi:10.3389/fimmu.2020.570919
Almogi-Hazan, O., and Or, R. (2020). Cannabis, the endocannabinoid system and immunity—the Journey from the Bedside to the Bench and Back. Int. J. Mol. Sci. 21 (12), 4448. doi:10.3390/ijms21124448
Amraei, R., and Rahimi, N. (2020). COVID-19, renin-angiotensin system and endothelial dysfunction. Cells 9 (7), 1652. doi:10.3390/cells9071652
An, J., Chen, B., Kang, X., Zhang, R., Guo, Y., and Zhao, J. (2020). Neuroprotective effects of natural compounds on LPS-induced inflammatory responses in microglia. Am J Transl Res. 12 (6), 2353–2378. doi:10.1101/2019.12.25.888529
Annunziata, G., Zamparelli, M. S., Santoro, C., Ciampaglia, R., Stornaiuolo, M., and Tenore, G. C. (2020). May polyphenols have a role against coronavirus infection? An overview of in vitro evidence. Front. Med. 7, 240. doi:10.3389/fmed.2020.00240
Baig, A. M., Khaleeq, A., Ali, U., and Syeda, H. (2020). Evidence of the COVID-19 virus targeting the CNS: tissue distribution, host-virus interaction, and proposed neurotropic mechanisms. ACS Chem. Neurosci. 11 (7), 995–998. doi:10.1021/acschemneuro.0c00122
Balakumar, P., and Jagadeesh, G. (2014). A century old renin-angiotensin system still grows with endless possibilities: AT1 receptor signaling cascades in cardiovascular physiopathology. Cell. Signal. 26 (10), 2147–2160. doi:10.1016/j.cellsig.2014.06.011
Bastianetto, S., Ménard, C., and Quirion, R. (2015). Neuroprotective action of resveratrol. Biochim. Biophys. Acta 1852 (6), 1195–1201. doi:10.1016/j.bbadis.2014.09.011
Basu, A., Sarkar, A., and Maulik, U. (2020). Molecular docking study of potential phytochemicals and their effects on the complex of SARS-CoV2 spike protein and human ACE2. Sci. Rep. 10, 17699. doi:10.1038/s41598-020-74715-4
Battagello, D. S., Dragunas, G., Klein, M. O., Ayub, A. L. P., Velloso, F. J., and Correa, R. G. (2020). Unpuzzling COVID-19: tissue-related signaling pathways associated with SARS-CoV-2 infection and transmission. Clin. Sci. (Lond.) 134 (16), 2137–2160. doi:10.1042/CS20200904
Baysal-Kirac, L., and Uysal, H. (2020). COVID-19 associate neurological complications. Neurol. Sci Neurophysiol. 37 (1), 1–3. doi:10.4103/2636-865X.283930
Bellavite, P., and Donzelli, A. (2020). Hesperidin and SARS-CoV-2: new light on the healthy function of citrus fruits. Antioxidants 9 (8), 742. doi:10.3390/antiox9080742
Bhardwaj, V. K., Singh, R., Sharma, J., Rajendran, V., Purohit, R., and Kumar, S. (2020). Identification of bioactive molecules from Tea plant as SARS-CoV-2 main protease inhibitors. J. Biomol. Struct. Dyn. 2020, 1–10. doi:10.1080/07391102.2020.1766572
Bleasel, M. D., and Peterson, G. M. (2020). Emetine, ipecac, ipecac alkaloids and analogues as potential antiviral agents for coronaviruses. Pharmaceuticals 13 (3), 51. doi:10.3390/ph13030051
Bohmwald, K., Gálvez, N. M. S., Ríos, M., and Kalergis, A. M. (2018). Neurologic alterations due to respiratory virus infections. Front. Cell. Neurosci. 12, 386. doi:10.3389/fncel.2018.00386
Bone, N. B., Liu, Z., Pittet, J. F., and Zmijewski, J. W. (2017). Frontline Science: D1 dopaminergic receptor signaling activates the AMPK-bioenergetic pathway in macrophages and alveolar epithelial cells and reduces endotoxin-induced ALI. J. Leukoc. Biol. 101 (2), 357–365. doi:10.1189/jlb.3HI0216-068RR
Bonucci, M., Raggi, R., and Vacca, R. A. (2020). Polydatin and its potential protective effect on COVID-19. Clin. Nutr. 12, 111–157. doi:10.1016/j.clnu.2020.09.052
Borah, A., Paul, R., Choudhury, S., Choudhury, A., Bhuyan, B., Das Talukdar, A., et al. (2013). Neuroprotective potential of silymarin against CNS disorders: insight into the pathways and molecular mechanisms of action. CNS Neurosci. Ther. 19 (11), 847–853. doi:10.1111/cns.12175
Bosch-Barrera, J., Martin-Castillo, B., Buxó, M., Brunet, J., Encinar, J. A., and Menendez, J. A. (2020). Silibinin and SARS-CoV-2: dual targeting of host cytokine storm and virus replication machinery for clinical management of COVID-19 patients. J. Clin. Med. 9 (6), 1770. doi:10.3390/jcm9061770
Boukhatem, M. N., and Setzer, W. N. (2020). Aromatic herbs, medicinal plant-derived essential oils, and phytochemical extracts as potential therapies for coronaviruses: future perspectives. Plants 9 (6), 800. doi:10.3390/plants9060800
Browatzki, M., Schmidt, J., Kübler, W., and Kranzhöfer, R. (2000). Endothelin-1 induces interleukin-6 release via activation of the transcription factor NF-kappaB in human vascular smooth muscle cells. Basic Res. Cardiol. 95 (2), 98–105. doi:10.1007/s003950050170
Burks, J. S., DeVald, B., Jankovsky, L. D., and Gerdes, J. C. (1980). Two coronaviruses isolated from central nervous system tissue of two multiple sclerosis patients. Science 209 (4459), 933–934. doi:10.1126/science.7403860
Calis, Z., Mogulkoc, R., and Baltaci, A. K. (2020). The roles of flavonols/flavonoids in neurodegeneration and neuroinflammation. Mini Rev. Med. Chem. 20 (15), 1475–1488. doi:10.2174/1389557519666190617150051
Campbell, J. H., Ratai, E. M., Autissier, P., Nolan, D. J., Tse, S., and Miller, A. D. (2014). Anti-α4 antibody treatment blocks virus traffic to the brain and gut early, and stabilizes CNS injury late in infection. PLoS Pathog. 10 (12), e1004533. doi:10.1371/journal.ppat.1004533
Cao, Z., Ding, Y., Ke, Z., Cao, L., Li, N., Ding, G., et al. (2016). Luteoloside acts as 3C protease inhibitor of enterovirus 71 in vitro. PloS One 11 (2), e0148693. doi:10.1371/journal.pone.0148693
Catanzaro, M., Fagiani, F., Racchi, M., Corsini, E., Govoni, S., and Lanni, C. (2020). Immune response in COVID-19: addressing a pharmacological challenge by targeting pathways triggered by SARS-CoV-2. Signal Transduct. Target Ther. 5 (1), 84. doi:10.1038/s41392-020-0191-1
Chacko, S. M., Thambi, P. T., Kuttan, R., and Nishigaki, I. (2010). Beneficial effects of green tea: a literature review. Chin. Med. 5, 13. doi:10.1186/1749-8546-5-13
Chan, C. P., Siu, K. L., Chin, K. T., Yuen, K. Y., Zheng, B., and Jin, D. Y. (2006). Modulation of the unfolded protein response by the severe acute respiratory syndrome coronavirus spike protein. J. Virol. 80 (18), 9279–9287. doi:10.1128/JVI.00659-06
Chen, C. N., Lin, C. P., Huang, K. K., Chen, W. C., Hsieh, H. P., Liang, P. H., et al. (2005). Inhibition of SARS-CoV 3C-like protease activity by theaflavin-3,3′-digallate (TF3). Evid. Based Compl. Alternat. Med. 2 (2), 209–215. doi:10.1093/ecam/neh081
Chen, D., Su, A., Fu, Y., Wang, X., Lv, X., and Xu, W. (2015). Harmine blocks herpes simplex virus infection through downregulating cellular NF-κB and MAPK pathways induced by oxidative stress. Antivir. Res. 123, 27–38. doi:10.1016/j.antiviral.2015.09.003
Chen, H., and Du, Q. (2020). Potential natural compounds for preventing SARS-CoV-2 (2019-nCoV) infection. Preprints doi:10.20944/preprints202001.0358.v3
Chen, R., Qi, Q. L., Wang, M. T., and Li, Q. Y. (2016). Therapeutic potential of naringin: an overview. Pharm. Biol. 54 (12), 3203–3210. doi:10.1080/13880209.2016.1216131
Cheng, L., Zheng, W., Li, M., Huang, J., Bao, S., Xu, Q., et al. (2020a). Citrus fruits are rich in flavonoids for immunoregulation and potential targeting ACE2. Fresh Citrus Fruits 32, 479–507. doi:10.1007/978-1-4684-8792-3_20
Cheng, Q., Yang, Y., and Gao, J. (2020b). Infectivity of human coronavirus in the brain. EBioMedicine 56, 102799. doi:10.1016/j.ebiom.2020.102799
Chojnacka, K., Witek-Krowiak, A., Skrzypczak, D., Mikula, K., and Młynarz, P. (2020). Phytochemicals containing biologically active polyphenols as an effective agent against Covid-19-inducing coronavirus. J. Funct. Foods 73, 104146. doi:10.1016/j.jff.2020.104146
Chtourou, Y., Aouey, B., Aroui, S., Kebieche, M., and Fetoui, H. (2016). Anti-apoptotic and anti-inflammatory effects of naringin on cisplatin-induced renal injury in the rat. Chem. Biol. Interact. 243, 1–9. doi:10.1016/j.cbi.2015.11.019
Conti, P., Ronconi, G., Caraffa, A., Gallenga, C., Ross, R., Frydas, I., et al. (2020). Induction of pro-inflammatory cytokines (IL-1 and IL-6) and lung inflammation by Coronavirus-19 (COVI-19 or SARS-CoV-2): anti-inflammatory strategies. J. Biol. Regul. Homeost. Agents 34 (2), 327–331. doi:10.23812/CONTI-E
Dabaghian, F., Khanavi, M., and Zarshenas, M. M. (2020). Bioactive compounds with possible inhibitory activity of Angiotensin-Converting Enzyme-II; a gate to manage and prevent COVID-19. Med. Hypotheses 143, 109841. doi:10.1016/j.mehy.2020.109841
Daina, A., Michielin, O., and Zoete, V. (2017). SwissADME: a free web tool to evaluate pharmacokinetics, drug-likeness and medicinal chemistry friendliness of small molecules. Sci. Rep. 7, 42717. doi:10.1038/srep42717
Dash, R., Mitra, S., Ali, M., Oktaviani, D. F., Hannan, M., Choi, S. M., et al. (2020). Phytosterols: targeting neuroinflammation in neurodegeneration. Curr. Pharmaceut. Des. 12, 124–127. doi:10.2174/1381612826666200628022812
Davidson, A. M., Wysocki, J., and Batlle, D. (2020). Interaction of SARS-CoV-2 and other coronavirus with ACE (Angiotensin-Converting enzyme)-2 as their main receptor: therapeutic implications. Hypertension 76 (5), 1339–1349. doi:10.1161/HYPERTENSIONAHA.120.15256
de Freitas Ferreira, A. C. A., Romão, T. T., SIlva Macedo, Y., Pupe, C., and Nascimento, O. J. (2020). COVID‐19 and herpes zoster co‐infection presenting with trigeminal neuropathy. Eur. J. Neurol. 24, 39–44. doi:10.1111/ene.14361
de Wit, E., van Doremalen, N., Falzarano, D., and Munster, V. J. (2016). SARS and MERS: recent insights into emerging coronaviruses. Nat. Rev. Microbiol. 14 (8), 523–534. doi:10.1038/nrmicro.2016.81
DeDiego, M. L., Nieto-Torres, J. L., Regla-Nava, J. A., Jimenez-Guardeño, J. M., Fernandez-Delgado, R., and Fett, C. (2014). Inhibition of NF-κB-mediated inflammation in severe acute respiratory syndrome coronavirus-infected mice increases survival. J. Virol. 88 (2), 913–924. doi:10.1128/JVI.02576-13
Desforges, M., Le Coupanec, A., Dubeau, P., Bourgouin, A., Lajoie, L., Dubé, M., et al. (2020). Human coronaviruses and other respiratory viruses: underestimated opportunistic pathogens of the central nervous system?. Viruses 12 (1), 14. doi:10.3390/v12010014
Dessau, R. B., Lisby, G., and Frederiksen, J. L. (2001). Coronaviruses in brain tissue from patients with multiple sclerosis. Acta Neuropathol. 101 (6), 601–604. doi:10.1007/s004010000331
Di Pierro, F., Khan, A., Bertuccioli, A., Maffioli, P., Derosa, G., Khan, S., et al. (2020). Quercetin Phytosome® as a potential drug for covid-19. Minerva Gastroenterol. Dietol. 14, 173. doi:10.23736/s1121-421x.20.02771-3
Diao, B., Feng, Z., Wang, C., Wang, H., Liu, L., Wang, C., et al. (2020). Human kidney is a target for novel severe acute respiratory syndrome coronavirus 2 (SARS-CoV-2) infection. MedRxiv. doi:10.1101/2020.03.04.20031120
Ding, Y., He, L., Zhang, Q., Huang, Z., Che, X., and Hou, J. (2004). Organ distribution of severe acute respiratory syndrome (SARS) associated coronavirus (SARS-CoV) in SARS patients: implications for pathogenesis and virus transmission pathways. J. Pathol. 203 (2), 622–630. doi:10.1002/path.1560
Divani, A. A., Andalib, S., Di Napoli, M., Lattanzi, S., Hussain, M. S., and Biller, J. (2020). Coronavirus disease 2019 and stroke: clinical manifestations and pathophysiological insights. J. Stroke Cerebrovasc. Dis. 29 (8), 104941. doi:10.1016/j.jstrokecerebrovasdis.2020.104941
Doobay, M. F., Talman, L. S., Obr, T. D., Tian, X., Davisson, R. L., and Lazartigues, E. (2007). Differential expression of neuronal ACE2 in transgenic mice with overexpression of the brain renin-angiotensin system. Am. J. Physiol. Regul. Integr. Comp. Physiol. 292 (1), R373–R381. doi:10.1152/ajpregu.00292.2006
Dutta, K., Ghosh, D., and Basu, A. (2009). Curcumin protects neuronal cells from Japanese encephalitis virus-mediated cell death and also inhibits infective viral particle formation by dysregulation of ubiquitin-proteasome system. J. Neuroimmune Pharmacol. 4 (3), 328–337. doi:10.1007/s11481-009-9158-2
Efferth, T., and Koch, E. (2011). Complex interactions between phytochemicals. The multi-target therapeutic concept of phytotherapy. Curr. Drug Targets 12 (1), 122–132. doi:10.2174/138945011793591626
Ehgoetz Martens, K. A., Hall, J. M., Georgiades, M. J., Gilat, M., Walton, C. C., Matar, E., et al. (2018). The functional network signature of heterogeneity in freezing of gait. Brain 141 (4), 1145–1160. doi:10.1093/brain/awy019
El Tabaa, M. M., and El Tabaa, M. M. (2020). Targeting Neprilysin (NEP) pathways: a potential new hope to defeat COVID-19 ghost. Biochem. Pharmacol. 178, 114057. doi:10.1016/j.bcp.2020.114057
El-Bakoush, A., and Olajide, O. A. (2018). Formononetin inhibits neuroinflammation and increases estrogen receptor beta (ERβ) protein expression in BV2 microglia. Int. Immunopharm. 61, 325–337. doi:10.1016/j.intimp.2018.06.016
Enmozhi, S. K., Raja, K., Sebastine, I., and Joseph, J. (2020). Andrographolide as a potential inhibitor of SARS-CoV-2 main protease: an in silico approach. J. Biomol. Struct. Dyn. 1-7. doi:10.1080/07391102.2020.1760136
Fakhri, S., Khodamorady, M., Naseri, M., Farzaei, M. H., and Khan, H. (2020a). The ameliorating effects of anthocyanins on the cross-linked signaling pathways of cancer dysregulated metabolism. Pharmacol. Res. 159, 104895. doi:10.1016/j.phrs.2020.104895
Fakhri, S., Pesce, M., Patruno, A., Moradi, S. Z., Iranpanah, A., Farzaei, M. H., et al. (2020b). Attenuation of Nrf2/Keap1/ARE in Alzheimer’s disease by plant secondary metabolites: a mechanistic review. Molecules 25 (21), 4926. doi:10.3390/molecules25214926
Favreau, D. J., Desforges, M., St-Jean, J. R., and Talbot, P. J. (2009). A human coronavirus OC43 variant harboring persistence-associated mutations in the S glycoprotein differentially induces the unfolded protein response in human neurons as compared to wild-type virus. Virology 395 (2), 255–267. doi:10.1016/j.virol.2009.09.026
Fernandes, T., Hashimoto, N. Y., Magalhães, F. C., Fernandes, F. B., Casarini, D. E., and Carmona, A. K. (2011). Aerobic exercise training-induced left ventricular hypertrophy involves regulatory MicroRNAs, decreased angiotensin-converting enzyme-angiotensin ii, and synergistic regulation of angiotensin-converting enzyme 2-angiotensin (1-7). Hypertension 58 (2), 182–189. doi:10.1161/hypertensionaha.110.168252
Filatov, A., Sharma, P., Hindi, F., and Espinosa, P. S. (2020). Neurological complications of coronavirus disease (COVID-19): encephalopathy. Cureus 12 (3), e7352. doi:10.7759/cureus.7352
Fitriani, I. N., Utami, W., Zikri, A. T., and Santoso, P. (2020). Silico approach of potential phytochemical inhibitor from Moringa oleifera, Cocos nucifera, Allium cepa, Psidium guajava, and Eucalyptus globulus for the treatment of COVID-19 by Molecular Docking. Research Square [Preprint PPR191112]. doi:10.21203/rs.3.rs-42747/v1
Fu, X., Xu, M., Song, Y., Li, Y., Zhang, H., Zhang, J., et al. (2018). Enhanced interaction between SEC2 mutant and TCR Vβ induces MHC II-independent activation of T cells via PKCθ/NF-κB and IL-2R/STAT5 signaling pathways. J. Biol. Chem. 293 (51), 19771–19784. doi:10.1074/jbc.RA118.003668
Gallagher, P. E., Chappell, M. C., Ferrario, C. M., and Tallant, E. A. (2006). Distinct roles for ANG II and ANG-(1-7) in the regulation of angiotensin-converting enzyme 2 in rat astrocytes. Am. J. Physiol. Cell Physiol. 290 (2), C420–C426. doi:10.1152/ajpcell.00409.2004
Gao, Y., Li, T., Han, M., Li, X., Wu, D., and Xu, Y. (2020). Diagnostic utility of clinical laboratory data determinations for patients with the severe COVID-19. J. Med. Virol. 92 (7), 791–796. doi:10.1002/jmv.25770
Garg, S., and Roy, A. (2020). In silico analysis of selected alkaloids against main protease (Mpro) of COVID-19. Chem. Biol. Interact. 21, 109309. doi:10.1016/j.cbi.2020.109309
Ge, M., Xiao, Y., Chen, H., Luo, F., Du, G., and Zeng, F. (2018). Multiple antiviral approaches of (-)-epigallocatechin-3-gallate (EGCG) against porcine reproductive and respiratory syndrome virus infection in vitro. Antivir. Res. 158, 52–62. doi:10.1016/j.antiviral.2018.07.012
Ghasemi, F., Bagheri, H., Barreto, G. E., Read, M. I., and Sahebkar, A. (2019). Effects of curcumin on microglial cells. Neurotox. Res. 36 (1), 12–26. doi:10.1007/s12640-019-00030-0
Ghosh, R., Chakraborty, A., Biswas, A., and Chowdhuri, S. (2020). Evaluation of green tea polyphenols as novel corona virus (SARS CoV-2) main protease (Mpro) inhibitors–an in silico docking and molecular dynamics simulation study. J. Biomol. Struct. Dyn. 22, 1–13. doi:10.1080/07391102.2020.1779818
Ghosh, S., Basak, P., Dutta, S., Chowdhury, S., and Sil, P. C. (2017). New insights into the ameliorative effects of ferulic acid in pathophysiological conditions. Food Chem. Toxicol. 103, 41–55. doi:10.1016/j.fct.2017.02.028
Gil, M., Kim, Y. K., Hong, S. B., and Lee, K. J. (2016). Naringin decreases TNF-α and HMGB1 release from LPS-stimulated macrophages and improves survival in a CLP-induced sepsis mice. PloS One 11 (10), e0164186. doi:10.1371/journal.pone.0164186
Giovannoni, G., Hawkes, C., Lechner-Scott, J., Levy, M., Waubant, E., and Gold, J. (2020). The COVID-19 pandemic and the use of MS disease-modifying therapies. Mult Scler Relat Disord 39, 102073. doi:10.1016/j.msard.2020.102073
Gorla, U. S., Rao, G. K., Kulandaivelu, U. S., Alavala, R. R., and Panda, S. P. (2020). Lead finding from selected flavonoids with antiviral (SARS-CoV-2) potentials against COVID-19: an in-silico evaluation. Comb. Chem. High Throughput Screen. 11, 27. doi:10.2174/1386207323999200818162706
Grover, A., Agrawal, V., Shandilya, A., Bisaria, V. S., and Sundar, D. (2011). Non-nucleosidic inhibition of Herpes simplex virus DNA polymerase: mechanistic insights into the anti-herpetic mode of action of herbal drug withaferin A. BMC Bioinform. 12 Suppl 13 (Suppl. 13), S22. doi:10.1186/1471-2105-12-S13-S22
Gu, J., Gong, E., Zhang, B., Zheng, J., Gao, Z., and Zhong, Y. (2005). Multiple organ infection and the pathogenesis of SARS. J. Exp. Med. 202 (3), 415–424. doi:10.1084/jem.20050828
Guan, W.-j., Ni, Z. Y., Hu, Y., Liang, W. H., Ou, C. Q., and He, J. X. (2020). Clinical characteristics of coronavirus disease 2019 in China. N. Engl. J. Med. 382 (18), 1708–1720. doi:10.1056/NEJMoa2002032
Gulati, A., Pomeranz, C., Qamar, Z., Thomas, S., Frisch, D., George, G., et al. (2020). A comprehensive review of manifestations of novel coronaviruses in the context of deadly COVID-19 global pandemic. Am. J. Med. Sci. 360 (1), 5–34. doi:10.1016/j.amjms.2020.05.006
Gupta, S., Singh, V., Varadwaj, P. K., Chakravartty, N., Katta, A. K. M., Lekkala, S. P., et al. (2020). Secondary metabolites from spice and herbs as potential multitarget inhibitors of SARS-CoV-2 proteins. J. Biomol. Struct. Dyn. 27, 1–20. doi:10.1080/07391102.2020.1837679
Gurung, A. B., Ali, M. A., Lee, J., Abul Farah, M., and Al-Anazi, K. M. (2020a). In silico screening of FDA approved drugs reveals ergotamine and dihydroergotamine as potential coronavirus main protease enzyme inhibitors. Saudi J. Biol. Sci. 27 (10), 2674–2682. doi:10.1016/j.sjbs.2020.06.005
Gurung, A. B., Ali, M. A., Lee, J., Farah, M. A., and Al-Anazi, K. M. (2020c). Unravelling lead antiviral phytochemicals for the inhibition of SARS-CoV-2 Mpro enzyme through in silico approach. Life Sci. 255, 117831. doi:10.1016/j.lfs.2020.117831
Gurung, A. B., Ali, M. A., Lee, J., Farah, M. A., and Al-Anazi, K. M. (2020b). Structure-based virtual screening of phytochemicals and repurposing of FDA approved antiviral drugs unravels lead molecules as potential inhibitors of coronavirus 3C-like protease enzyme. J. King Saud Univ. Sci. 32 (6), 2845–2853. doi:10.1016/j.jksus.2020.07.007
Hamming, I., Timens, W., Bulthuis, M., Lely, A., Navis, G., and van Goor, H. (2004). Tissue distribution of ACE2 protein, the functional receptor for SARS coronavirus. A first step in understanding SARS pathogenesis. J. Pathol. 203 (2), 631–637. doi:10.1002/path.1570
Harmer, D., Gilbert, M., Borman, R., and Clark, K. L. (2002). Quantitative mRNA expression profiling of ACE 2, a novel homologue of angiotensin converting enzyme. FEBS Lett. 532 (1–2), 107–110. doi:10.1016/s0014-5793(02)03640-2
Hasan, A., Al Mahamud, R., Jannat, K., Afroze, T., Bondhon, B.-n. F., Fariba, M. H., et al. (2020). Phytochemicals from Solanum surattense Burm. f. have high binding affinities for C-3 like main protease of COVID-19 (SARS-CoV-2). J Med Plants Stud 8 (4), 20–26. doi:10.26434/chemrxiv.12115359.v2
Helms, J., Kremer, S., Merdji, H., Clere-Jehl, R., Schenck, M., and Kummerlen, C. (2020). Neurologic features in severe SARS-CoV-2 infection. N. Engl. J. Med. 382 (23), 2268–2270. doi:10.1056/NEJMc2008597
Higdon, J. V., and Frei, B. (2003). Tea catechins and polyphenols: health effects, metabolism, and antioxidant functions. Crit. Rev. Food Sci. Nutr. 43 (1), 89–143. doi:10.1080/10408690390826464
Ho, T. Y., Wu, S. L., Chen, J. C., Li, C. C., and Hsiang, C. Y. (2007). Emodin blocks the SARS coronavirus spike protein and angiotensin-converting enzyme 2 interaction. Antivir. Res. 74 (2), 92–101. doi:10.1016/j.antiviral.2006.04.014
Holmes, K. V., and Lai, M. (1996). Coronaviridae: the viruses and their replication. Fields Virol. 1, 1075–1093
Huang, C., Wang, Y., Li, X., Ren, L., Zhao, J., and Hu, Y. (2020a). Clinical features of patients infected with 2019 novel coronavirus in Wuhan, China. Lancet 395 (10223), 497–506. doi:10.1016/S0140-6736(20)30183-5
Huang, F., Li, Y., Leung, E. L.-H., Liu, X., Liu, K., Wang, Q., et al. (2020b). A review of therapeutic agents and Chinese herbal medicines against SARS-COV-2 (COVID-19). Pharmacol. Res. 7, 104929. doi:10.1016/j.phrs.2020.104929
Iddir, M., Brito, A., Dingeo, G., Fernandez Del Campo, S. S., Samouda, H., La Frano, M. R., et al. (2020). Strengthening the immune system and reducing inflammation and oxidative stress through diet and Nutrition: considerations during the COVID-19 crisis. Nutrients 12 (6), 1562. doi:10.3390/nu12061562
Istifli, E. S., Netz, P. A., Husunet, M. T., Sarikurkcu, C., and Tepe, B. (2020). In silico analysis of the interactions of certain flavonoids with the receptor-binding domain of 2019 novel coronavirus and cellular proteases and their pharmacokinetic properties. J. Biomol. Struct. Dyn. 14, 1–15. doi:10.1080/07391102.2020.1840444
Jacomy, H., and Talbot, P. J. (2003). Vacuolating encephalitis in mice infected by human coronavirus OC43. Virology 315 (1), 20–33. doi:10.1016/s0042-6822(03)00323-4
Jang, M., Park, Y. I., Cha, Y. E., Park, R., Namkoong, S., Lee, J. I., et al. (2020). Tea polyphenols EGCG and theaflavin inhibit the activity of SARS-CoV-2 3CL-protease in vitro. Evid Based Compl. Alternat. Med. 20, 5630838. doi:10.1155/2020/5630838
Jiang, Y., Xu, J., Zhou, C., Wu, Z., Zhong, S., Liu, J., et al. (2005). Characterization of cytokine/chemokine profiles of severe acute respiratory syndrome. Am. J. Respir. Crit. Care Med. 171 (8), 850–857. doi:10.1164/rccm.200407-857OC
Joseph, J., Grun, J. L., Lublin, F. D., and Knobler, R. L. (1993). Interleukin-6 induction in vitro in mouse brain endothelial cells and astrocytes by exposure to mouse hepatitis virus (MHV-4, JHM). J. Neuroimmunol. 42 (1), 47–52. doi:10.1016/0165-5728(93)90211-g
Joshi, T., Joshi, T., Sharma, P., Mathpal, S., Pundir, H., Bhatt, V., et al. (2020). In silico screening of natural compounds against COVID-19 by targeting Mpro and ACE2 using molecular docking. Eur. Rev. Med. Pharmacol. Sci. 24 (8), 4529–4536. doi:10.26355/eurrev_202004_21036
Kähkönen, M. P., Hopia, A. I., Vuorela, H. J., Rauha, J. P., Pihlaja, K., Kujala, T. S., et al. (1999). Antioxidant activity of plant extracts containing phenolic compounds. J. Agric. Food Chem. 47 (10), 3954–3962. doi:10.1021/jf990146l
Kim, D. E., Min, J. S., Jang, M. S., Lee, J. Y., Shin, Y. S., Park, C. M., et al. (2019a). Natural bis-benzylisoquinoline alkaloids-tetrandrine, fangchinoline, and cepharanthine, inhibit human coronavirus OC43 infection of MRC-5 human lung cells. Biomolecules 9 (11), 696. doi:10.3390/biom9110696
Kim, V. Y., Batty, A., Li, J., Kirk, S. G., Crowell, S. A., and Jin, Y. (2019b). Glutathione Reductase promotes fungal clearance and suppresses inflammation during systemic Candida albicans infection in mice. J. Immunol. 203 (8), 2239–2251. doi:10.4049/jimmunol.1701686
Koka, V., Huang, X. R., Chung, A. C., Wang, W., Truong, L. D., and Lan, H. Y. (2008). Angiotensin II up-regulates angiotensin I-converting enzyme (ACE), but down-regulates ACE2 via the AT1-ERK/p38 MAP kinase pathway. Am. J. Pathol. 172 (5), 1174–1183. doi:10.2353/ajpath.2008.070762
Kothur, K., Wienholt, L., Mohammad, S. S., Tantsis, E. M., Pillai, S., and Britton, P. N. (2016). Utility of CSF cytokine/chemokines as markers of active intrathecal inflammation: comparison of demyelinating, anti-NMDAR and enteroviral encephalitis. PloS One 11 (8), e0161656. doi:10.1371/journal.pone.0161656
Krupanidhi, S., Abraham Peele, K., Venkateswarulu, T. C., Ayyagari, V. S., Nazneen Bobby, M., John Babu, D., et al. (2020). Screening of phytochemical compounds of Tinospora cordifolia for their inhibitory activity on SARS-CoV-2: an in silico study. J. Biomol. Struct. Dyn. 15, 19 doi:10.1080/07391102.2020.1787226
Kuba, K., Imai, Y., Rao, S., Gao, H., Guo, F., and Guan, B. (2005). A crucial role of angiotensin converting enzyme 2 (ACE2) in SARS coronavirus-induced lung injury. Nat. Med. 11 (8), 875–879. doi:10.1038/nm1267
Kulkarni, S. A., Nagarajan, S. K., Ramesh, V., Palaniyandi, V., Selvam, S. P., and Madhavan, T. (2020). Computational evaluation of major components from plant essential oils as potent inhibitors of SARS-CoV-2 spike protein. J. Mol. Struct. 1221, 128823. doi:10.1016/j.molstruc.2020.128823
Kumar, S., Kashyap, P., Chowdhury, S., Kumar, S., Panwar, A., and Kumar, A. (2020). Identification of phytochemicals as potential therapeutic agents that binds to Nsp15 protein target of coronavirus (SARS-CoV-2) that are capable of inhibiting virus replication. Phytomedicine 153317, 153317. doi:10.1016/j.phymed.2020.153317
Kurokawa, M., Hozumi, T., Tsurita, M., Kadota, S., Namba, T., and Shiraki, K. (2001). Biological characterization of eugeniin as an anti-herpes simplex virus type 1 compound in vitro and in vivo. J. Pharmacol. Exp. Therapeut. 297 (1), 372–379. doi:10.1349/ddlp.3008
Lalani, S., and Poh, C. L. (2020). Flavonoids as antiviral agents for Enterovirus A71 (EV-A71). Viruses 12 (2), 184. doi:10.3390/v12020184
Lambert, J. D., and Yang, C. S. (2003). Mechanisms of cancer prevention by tea constituents. J. Nutr. 133 (10), 3262S–3267S. doi:10.1093/jn/133.10.3262S
Lau, K. K., Yu, W. C., Chu, C. M., Lau, S. T., Sheng, B., and Yuen, K. Y. (2004). Possible central nervous system infection by SARS coronavirus. Emerg. Infect. Dis. 10 (2), 342. doi:10.3201/eid1002.030638
Levy, E., Delvin, E., Marcil, V., and Spahis, S. (2020). Can phytotherapy with polyphenols serve as a powerful approach for the prevention and therapy tool of novel coronavirus disease 2019 (COVID-19)?. Am. J. Physiol. Endocrinol. Metab. 319 (4), E689–E708. doi:10.1152/ajpendo.00298.2020
Li, F., Li, W., Farzan, M., and Harrison, S. C. (2005). Structure of SARS coronavirus spike receptor-binding domain complexed with receptor. Science 309 (5742), 1864–1868. doi:10.1126/science.1116480
Li, G., and De Clercq, E. (2020). Therapeutic options for the 2019 novel coronavirus (2019-nCoV). Nat. Rev. Drug Discov. 19 (3), 149–150. doi:10.1038/d41573-020-00016-0
Li, Q., Tian, Z., Wang, M., Kou, J., Wang, C., Rong, X., et al. (2019a). Luteoloside attenuates neuroinflammation in focal cerebral ischemia in rats via regulation of the PPARγ/Nrf2/NF-κB signaling pathway. Int. Immunopharm. 66, 309–316. doi:10.1016/j.intimp.2018.11.044
Li, W., Moore, M. J., Vasilieva, N., Sui, J., Wong, S. K., and Berne, M. A. (2003). Angiotensin-converting enzyme 2 is a functional receptor for the SARS coronavirus. Nature 426 (6965), 450–454. doi:10.1038/nature02145
Li, Y., Li, H., Fan, R., Wen, B., Zhang, J., and Cao, X. (2016). Coronavirus infections in the central nervous system and respiratory tract show distinct features in hospitalized children. Intervirology 59 (3), 163–169. doi:10.1159/000453066
Li, Z., Tomlinson, A. C., Wong, A. H., Zhou, D., Desforges, M., Talbot, P. J., et al. (2019b). The human coronavirus HCoV-229E S-protein structure and receptor binding. Elife 8, e51230. doi:10.7554/eLife.51230
Libbey, J. E., Kennett, N. J., Wilcox, K. S., White, H. S., and Fujinami, R. S. (2011). Interleukin-6, produced by resident cells of the central nervous system and infiltrating cells, contributes to the development of seizures following viral infection. J. Virol. 85 (14), 6913–6922. doi:10.1128/JVI.00458-11
Lin, Z., Chen, Y., Zhang, W., Chen, A. F., Lin, S., and Morris, M. (2008). RNA interference shows interactions between mouse brainstem angiotensin AT1 receptors and angiotensin-converting enzyme 2. Exp. Physiol. 93 (5), 676–684. doi:10.1113/expphysiol.2007.041657
Liu, A.-L., and Du, G.-H. (2012). “Antiviral properties of phytochemicals,” in Dietary phytochemicals and microbes. Editor A. Patra (Dordrecht: Springer), 93–126. doi:10.1007/978-94-007-3926-0_3
Lo Muzio, L., Bizzoca, M. E., and Ravagnan, G. (2020). New intriguing possibility for prevention of coronavirus pneumonitis: natural purified polyphenols. Oral Dis. 14, 77. doi:10.1111/odi.13518
Lung, J., Lin, Y. S., Yang, Y. H., Chou, Y. L., Shu, L. H., Cheng, Y. C., et al. (2020). The potential chemical structure of anti-SARS-CoV-2 RNA-dependent RNA polymerase. J. Med. Virol. 92 (6), 693–697. doi:10.1002/jmv.25761
Ma, B.-n., and Li, X.-j. (2020). Resveratrol extracted from Chinese herbal medicines: a novel therapeutic strategy for lung diseases. Chin Herb Med. 13, 33. doi:10.1016/j.chmed.2020.07.003[Epub ahead of print]
Macht, M., Kaussner, Y., Möller, J. C., Stiasny-Kolster, K., Eggert, K. M., Krüger, H. P., et al. (2007). Predictors of freezing in Parkinson's disease: a survey of 6,620 patients. Mov. Disord. 22 (7), 953–956. doi:10.1002/mds.21458
Maiti, S., Banerjee, A., Nazmeen, A., Kanwar, M., and Das, S. (2020). Active-site Molecular docking of Nigellidine with nucleocapsid- NSP2-MPro of COVID-19 and to human IL1R-IL6R and strong antioxidant role of Nigella-sativa in experimental rats. J. Drug Target. 12, 1–23. doi:10.1080/1061186X.2020.1817040
Maiti, S., and Banerjee, A. (2020). Epigallocatechin-gallate and theaflavin-gallate interaction in SARS CoV-2 spike-protein central-channel with reference to the hydroxychloroquine interaction: Bioinformatics and molecular docking study. Drug Dev. Res. 26, 121–223. doi:10.1002/ddr.21730[Epub ahead of print]
Majnooni, M. B., Fakhri, S., Shokoohinia, Y., Kiyani, N., Stage, K., Mohammadi, P., et al. (2020). Phytochemicals: potential therapeutic interventions against coronaviruses-associated lung injury. Front. Pharmacol. 11, 1744. doi:10.3389/fphar.2020.588467
Mani, J. S., Johnson, J. B., Steel, J. C., Broszczak, D. A., Neilsen, P. M., Walsh, K. B., et al. (2020). Natural product-derived phytochemicals as potential agents against coronaviruses: a review. Virus Res. 284, 197989. doi:10.1016/j.virusres.2020.197989
Mao, L., Jin, H., Wang, M., Hu, Y., Chen, S., and He, Q. (2020). Neurologic manifestations of hospitalized patients with coronavirus disease 2019 in Wuhan, China. JAMA Neurol. 77 (6), 683–690. doi:10.1001/jamaneurol.2020.1127
Marchetti, C., Swartzwelter, B., Koenders, M. I., Azam, T., Tengesdal, I. W., and Powers, N. (2018). NLRP3 inflammasome inhibitor OLT1177 suppresses joint inflammation in murine models of acute arthritis. Arthritis Res. Ther. 20 (1), 169. doi:10.1186/s13075-018-1664-2
Maroli, N., Bhasuran, B., Natarajan, J., and Kolandaivel, P. (2020). The potential role of procyanidin as a therapeutic agent against SARS-CoV-2: a text mining, molecular docking and molecular dynamics simulation approach. J. Biomol. Struct. Dyn. 1, 1–16. doi:10.1080/07391102.2020.1823887
Matsuda, K., Park, C., Sunden, Y., Kimura, T., Ochiai, K., Kida, H., et al. (2004). The vagus nerve is one route of transneural invasion for intranasally inoculated influenza a virus in mice. Vet. Pathol. 41 (2), 101–107. doi:10.1354/vp.41-2-101
Maurya, V. K., Kumar, S., Prasad, A. K., Bhatt, M. L. B., and Saxena, S. K. (2020). Structure-based drug designing for potential antiviral activity of selected natural products from Ayurveda against SARS-CoV-2 spike glycoprotein and its cellular receptor. VirusDisease 31 (2), 179–193. doi:10.1007/s13337-020-00598-8
Meessen-Pinard, M., Le Coupanec, A., Desforges, M., and Talbot, P. J. (2017). Pivotal role of receptor-interacting protein kinase 1 and mixed lineage kinase domain-like in neuronal cell death induced by the human neuroinvasive coronavirus OC43. J. Virol. 91 (1), e01513–16. doi:10.1128/JVI.01513-16
Mehta, P., McAuley, D. F., Brown, M., Sanchez, E., Tattersall, R. S., Manson, J. J., et al. (2020). COVID-19: consider cytokine storm syndromes and immunosuppression. Lancet 395 (10229), 1033–1034. doi:10.1016/S0140-6736(20)30628-0
Mendonca, P., and Soliman, K. F. (2020). Flavonoids activation of the transcription factor Nrf2 as a hypothesis approach for the prevention and modulation of SARS-CoV-2 infection severity. Antioxidants 9 (8), 659. doi:10.3390/antiox9080659
Mhatre, S., Srivastava, T., Naik, S., and Patravale, V. (2020). Antiviral activity of green tea and black tea polyphenols in prophylaxis and treatment of COVID-19: a review. Phytomedicine 15, 153286. doi:10.1016/j.phymed.2020.153286
Mirzaie, A., Halaji, M., Dehkordi, F. S., Ranjbar, R., and Noorbazargan, H. (2020). A narrative literature review on traditional medicine options for treatment of corona virus disease 2019 (COVID-19). Compl. Ther. Clin. Pract. 40, 101214. doi:10.1016/j.ctcp.2020.101214
Mochan, A., and Modi, G. (2020). Neurological involvement with COVID-19 review. Wits J Clin Med. 2 (2), 135–140. doi:10.18772/26180197.2020.v2n2a5
Morfopoulou, S., Brown, J. R., Davies, E. G., Anderson, G., Virasami, A., and Qasim, W. (2016). Human coronavirus OC43 associated with fatal encephalitis. N. Engl. J. Med. 375 (5), 497–498. doi:10.1056/NEJMc1509458
Mori, I. (2015). Transolfactory neuroinvasion by viruses threatens the human brain. Acta Virol. 59 (4), 338–349. doi:10.4149/av_2015_04_338
Moriguchi, T., Harii, N., Goto, J., Harada, D., Sugawara, H., and Takamino, J. (2020). A first case of meningitis/encephalitis associated with SARS-Coronavirus-2. Int. J. Infect. Dis. 94, 55–58. doi:10.1016/j.ijid.2020.03.062
Muchtaridi, M., Fauzi, M., Khairul Ikram, N. K., Mohd Gazzali, A., and Wahab, H. A. (2020). Natural flavonoids as potential angiotensin-converting enzyme 2 inhibitors for anti-SARS-CoV-2. Molecules 25 (17), 3980. doi:10.3390/molecules25173980
Murta, V., Villarreal, A., and Ramos, A. J. (2020). Severe acute respiratory syndrome coronavirus 2 impact on the central nervous system: are astrocytes and microglia main players or merely Bystanders?. ASN neuro 12, 1759091420954960. doi:10.1177/1759091420954960
Nagai, T., Moriguchi, R., Suzuki, Y., Tomimori, T., and Yamada, H. (1995). Mode of action of the anti-influenza virus activity of plant flavonoid, 5,7,4′-trihydroxy-8-methoxyflavone, from the roots of Scutellaria baicalensis. Antivir. Res. 26 (1), 11–25. doi:10.1016/0166-3542(94)00062-d
Nakashima, H., Suzuki, H., Ohtsu, H., Chao, J. Y., Utsunomiya, H., Frank, G. D., et al. (2006). Angiotensin II regulates vascular and endothelial dysfunction: recent topics of Angiotensin II type-1 receptor signaling in the vasculature. Curr. Vasc. Pharmacol. 4 (1), 67–78. doi:10.2174/157016106775203126
Nalleballe, K., Onteddu, S. R., Sharma, R., Dandu, V., Brown, A., and Jasti, M. (2020). Spectrum of neuropsychiatric manifestations in COVID-19. Brain Behav. Immun. 88, 71–74. doi:10.1016/j.bbi.2020.06.020
Narkhede, R. R., Pise, A. V., Cheke, R. S., and Shinde, S. D. (2020). Recognition of natural products as potential inhibitors of COVID-19 main protease (Mpro): in-silico evidences. Nat Prod Bioprospect 10 (5), 297–306. doi:10.1007/s13659-020-00253-1
Nemoto, W., Yamagata, R., Nakagawasai, O., Nakagawa, K., Hung, W. Y., and Fujita, M. (2020). Effect of spinal angiotensin-converting enzyme 2 activation on the formalin-induced nociceptive response in mice. Eur. J. Pharmacol. 872, 172950. doi:10.1016/j.ejphar.2020.172950
Nepal, G., Rehrig, J. H., Shrestha, G. S., Shing, Y. K., Yadav, J. K., and Ojha, R. (2020). Neurological manifestations of COVID-19: a systematic review. Crit. Care 24 (1), 421. doi:10.1186/s13054-020-03121-z10.1186/s13054-020-03121-z
Ng, T., Ling, J. M., Wang, Z. T., Cai, J. N., and Xu, G. J. (1996). Examination of coumarins, flavonoids and polysaccharopeptide for antibacterial activity. Gen. Pharmacol. 27 (7), 1237–1240. doi:10.1016/0306-3623(95)02143-4
Nguyen, T. T., Woo, H. J., Kang, H. K., Nguyen, V. D., Kim, Y. M., and Kim, D. W. (2012). Flavonoid-mediated inhibition of SARS coronavirus 3C-like protease expressed in Pichia pastoris. Biotechnol. Lett. 34 (5), 831–838. doi:10.1007/s10529-011-0845-8
Ngwa, W., Kumar, R., Thompson, D., Lyerly, W., Moore, R., Reid, T.-E., et al. (2020). Potential of flavonoid-inspired phytomedicines against COVID-19. Molecules 25 (11), 2707. doi:10.3390/molecules25112707
Niu, J., Shen, L., Huang, B., Ye, F., Zhao, L., Wang, H., et al. (2020a). Non-invasive bioluminescence imaging of HCoV-OC43 infection and therapy in the central nervous system of live mice. Antivir. Res. 173, 104646. doi:10.1016/j.antiviral.2019.104646
Niu, W., Wu, F., Cui, H., Cao, W., Chao, Y., Wu, Z., et al. (2020b). Network Pharmacology analysis to identify phytochemicals in traditional Chinese medicines that may regulate ACE2 for the treatment of COVID-19. Evid Based Compl. Alternat. Med. 23, 7493281. doi:10.1155/2020/7493281
Nordvig, A. S., Rimmer, K. T., Willey, J. Z., Thakur, K. T., Boehme, A. K., Vargas, W. S., et al. (2020). Potential neurological manifestations of COVID-19. Neurol. Clin. Pract. 14, 119. doi:10.1212/CPJ.0000000000000897
Nouri, Z., Fakhri, S., El-Senduny, F. F., Sanadgol, N., Abd-ElGhani, G. E., Farzaei, M. H., et al. (2019). On the neuroprotective effects of naringenin: pharmacological targets, signaling pathways, molecular mechanisms, and clinical perspective. Biomolecules 9 (11), 690. doi:10.3390/biom9110690
Okamoto, M., Ono, M., and Baba, M. (2001). Suppression of cytokine production and neural cell death by the anti-inflammatory alkaloid cepharanthine: a potential agent against HIV-1 encephalopathy. Biochem. Pharmacol. 62 (6), 747–753. doi:10.1016/s0006-2952(01)00692-x
Onaivi, E. S., and Sharma, V. (2020). Cannabis for COVID-19: can cannabinoids quell the cytokine storm?. Future Sci OA 6 (8), FSO625. doi:10.2144/fsoa-2020-0124
Onaivi, E. S., Singh Chauhan, B. P., and Sharma, V. (2020). Challenges of cannabinoid delivery: how can nanomedicine help?. Nanomedicine 15 (21), 2023–2028. doi:10.2217/nnm-2020-0221
Orsucci, D., Ienco, E. C., Nocita, G., Napolitano, A., and Vista, M. (2020). Neurological features of COVID-19 and their treatment: a review. Drugs Context 9, 2020–2025. doi:10.7573/dic.2020-5-12021
Owis, A. I., El-Hawary, M. S., El Amir, D., Aly, O. M., Abdelmohsen, U. R., and Kamel, M. S. (2020). Molecular docking reveals the potential of Salvadora persica flavonoids to inhibit COVID-19 virus main protease. RSC Adv. 10 (33), 19570–19575. doi:10.1039/D0RA03582C
Panagiotopoulos, A. A., Kotzampasi, D.-M., Sourvinos, G., Kampa, M., Pirintsos, S., Castanas, E., et al. (2020). The natural polyphenol fortunellin is a dimerization inhibitor of the SARS-CoV-2 3C-like proteinase, revealed by molecular simulations. arXiv preprint arXiv:2007.
Pandey, P., Rane, J. S., Chatterjee, A., Kumar, A., Khan, R., Prakash, A., et al. (2020). Targeting SARS-CoV-2 spike protein of COVID-19 with naturally occurring phytochemicals: an in silico study for drug development. J. Biomol. Struct. Dyn. 34, 1–11. doi:10.1080/07391102.2020.1796811
Pandeya, K., Ganeshpurkar, A., and Mishra, M. K. (2020). Natural RNA dependent RNA polymerase inhibitors: molecular docking studies of some biologically active alkaloids of Argemone mexicana. Med. Hypoth. 4, 23–27. doi:10.1016/j.mehy.2020.109905
Parida, P. K., Paul, D., and Chakravorty, D. (2020). The natural way forward: molecular dynamics simulation analysis of phytochemicals from Indian medicinal plants as potential inhibitors of SARS‐CoV‐2 targets. Phytother Res. 43, 145–171. doi:10.1002/ptr.6868
Park, J.-Y., Kim, J. H., Kwon, J. M., Kwon, H. J., Jeong, H. J., Kim, Y. M., et al. (2013). Dieckol, a SARS-CoV 3CL(pro) inhibitor, isolated from the edible brown algae Ecklonia cava. Bioorg. Med. Chem. 21 (13), 3730. doi:10.1016/j.bmc.2013.04.026
Park, J.-Y., Yuk, H. J., Ryu, H. W., Lim, S. H., Kim, K. S., Park, K. H., et al. (2017). Evaluation of polyphenols from Broussonetia papyrifera as coronavirus protease inhibitors. J. Enzym. Inhib. Med. Chem. 32 (1), 504–515. doi:10.1080/14756366.2016.1265519
Park, J. S., Arcaroli, J., Yum, H. K., Yang, H., Wang, H., and Yang, K. Y. (2003). Activation of gene expression in human neutrophils by high mobility group box 1 protein. Am. J. Physiol. Cell Physiol. 284 (4), C870–C879. doi:10.1152/ajpcell.00322.2002
Park, J. Y., Kim, J. H., Kim, Y. M., Jeong, H. J., Kim, D. W., Park, K. H., et al. (2012). Tanshinones as selective and slow-binding inhibitors for SARS-CoV cysteine proteases. Bioorg. Med. Chem. 20 (19), 5928–5935. doi:10.1016/j.bmc.2012.07.038
Parvez, M. S. A., Azim, K. F., Imran, A. S., Raihan, T., Begum, A., Shammi, T. S., et al. (2020). Virtual screening of plant metabolites against main protease, RNA-dependent RNA polymerase and spike protein of SARS-CoV-2: Therapeutics option of COVID-19. arXiv preprint arXiv:2005.11254
Pascual-Goñi, E., Fortea, J., Martínez-Domeño, A., Rabella, N., Tecame, M., Gómez-Oliva, C., et al. (2020). COVID-19-associated ophthalmoparesis and hypothalamic involvement. Neurol Neuroimmunol Neuroinflamm 7 (5), e823. doi:10.1212/NXI.0000000000000823
Philip, S., Tom, G., and Vasumathi, A. V. (2018). Evaluation of the anti-inflammatory activity of Tinospora cordifolia (Willd.) Miers chloroform extract—a preclinical study. J. Pharm. Pharmacol. 70 (8), 1113–1125. doi:10.1111/jphp.12932
Pour, P. M., Fakhri, S., Asgary, S., Farzaei, M. H., and Echeverría, J. (2019). The signaling pathways, and therapeutic targets of antiviral agents: focusing on the antiviral approaches and clinical perspectives of anthocyanins in the management of viral diseases. Front. Pharmacol. 10, 1207. doi:10.3389/fphar.2019.01207
Powers, C. N., and Setzer, W. (2016). An in-silico investigation of phytochemicals as antiviral agents against dengue fever. Comb. Chem. High Throughput Screen. 19 (7), 516–536. doi:10.2174/1386207319666160506123715
Prakash, A., Singh, H., Sarma, P., Bhattacharyya, A., Dhibar, D. P., Balaini, N., et al. (2020). nCoV-2019 infection induces neurological outcome and manifestation, linking its historical ancestor SARS-CoV & MERS-CoV: a systematic review and meta-analysis. Resaerch Square [Pre-print]. doi:10.21203/rs.3.rs-35790/v1
Rahman, N., Basharat, Z., Yousuf, M., Castaldo, G., Rastrelli, L., and Khan, H. (2020). Virtual screening of natural products against type II transmembrane serine protease (TMPRSS2), the priming agent of coronavirus 2 (SARS-CoV-2). Molecules 25 (10), 2271. doi:10.3390/molecules25102271
Ramanathan, K., Antognini, D., Combes, A., Paden, M., Zakhary, B., Ogino, M., et al. (2020). Planning and provision of ECMO services for severe ARDS during the COVID-19 pandemic and other outbreaks of emerging infectious diseases. Lancet Respir Med. 8 (5), 518–526. doi:10.1016/S2213-2600(20)30121-1
Rameshkumar, M. R., Indu, P., Arunagirinathan, N., Venkatadri, B., El-Serehy, H. A., and Ahmad, A. (2020). Computational selection of flavonoid compounds as inhibitors against SARS-CoV-2 main protease, RNA-dependent RNA polymerase and spike proteins: a molecular docking study. Saudi J. Biol. Sci. 14, 33–39. doi:10.1016/j.sjbs.2020.10.028
Rasool, N., Akhtar, A., and Hussain, W. (2020). Insights into the inhibitory potential of selective phytochemicals against Mpro of 2019-nCoV: a computer-aided study. Struct. Chem. 1, 1–7. doi:10.1007/s11224-020-01536-6
Rebas, E., Rzajew, J., Radzik, T., and Zylinska, L. (2020). Neuroprotective polyphenols: a modulatory action on Neurotransmitter pathways. Curr. Neuropharmacol. 18 (5), 431–445. doi:10.2174/1570159X18666200106155127
Rietdijk, C. D., Perez-Pardo, P., Garssen, J., van Wezel, R. J., and Kraneveld, A. D. (2017). Exploring Braak's hypothesis of Parkinson's disease. Front. Neurol. 8, 37. doi:10.3389/fneur.2017.00037
Rizzo, M. D., Henriquez, J. E., Blevins, L. K., Bach, A., Crawford, R. B., and Kaminski, N. E. (2020). Targeting cannabinoid receptor 2 on peripheral leukocytes to attenuate inflammatory mechanisms implicated in HIV-associated Neurocognitive disorder. J. Neuroimmune Pharmacol. 19, 55–63. doi:10.1007/s11481-020-09918-7
Rodney, T., Osier, N., and Gill, J. (2018). Pro- and anti-inflammatory biomarkers and traumatic brain injury outcomes: a review. Cytokine 110, 248–256. doi:10.1016/j.cyto.2018.01.012
Ron, D., and Walter, P. (2007). Signal integration in the endoplasmic reticulum unfolded protein response. Nat. Rev. Mol. Cell Biol. 8 (7), 519–529. doi:10.1038/nrm2199
Rout, J., Swain, B. C., and Tripathy, U. (2020). Silico investigation of spice molecules as potent inhibitor of SARS-CoV-2. ChemRxiv [Preprint]. doi:10.26434/chemrxiv.12323615.v1
Ruan, Z., Liu, C., Guo, Y., He, Z., Huang, X., Jia, X., et al. (2020). SARS‐CoV‐2 and SARS‐CoV: virtual screening of potential inhibitors targeting RNA‐dependent RNA polymerase activity (NSP12). J. Med. Virol. 19, 321–355. doi:10.1002/jmv.26222
Ryu, Y. B., Jeong, H. J., Kim, J. H., Kim, Y. M., Park, J. Y., and Kim, D. (2010a). Biflavonoids from Torreya nucifera displaying SARS-CoV 3CL(pro) inhibition. Bioorg. Med. Chem. 18 (22), 7940–7947. doi:10.1016/j.bmc.2010.09.035
Ryu, Y. B., Jeong, H. J., Kim, J. H., Kim, Y. M., Park, J. Y., and Kim, D. (2010b). Biflavonoids from Torreya nucifera displaying SARS-CoV 3CL(pro) inhibition. Bioorg. Med. Chem. 18 (22), 7940–7947. doi:10.1016/j.bmc.2010.09.035
Saleki, K., Banazadeh, M., Saghazadeh, A., and Rezaei, N. (2020). The involvement of the central nervous system in patients with COVID-19. Rev. Neurosci. 31 (4), 453–456. doi:10.1515/revneuro-2020-0026
Savarin, C., Dutta, R., and Bergmann, C. C. (2018). Distinct gene profiles of bone marrow-derived macrophages and microglia during neurotropic coronavirus-induced demyelination. Front. Immunol. 9, 1325. doi:10.3389/fimmu.2018.01325
Schoeman, D., and Fielding, B. C. (2019). Coronavirus envelope protein: current knowledge. Virol. J. 16 (1), 69–22. doi:10.1186/s12985-019-1182-0
Senthilvel, P., Lavanya, P., Kumar, K. M., Swetha, R., Anitha, P., and Bag, S. (2013). Flavonoid from Carica papaya inhibits NS2B-NS3 protease and prevents Dengue 2 viral assembly. Bioinformation 9 (18), 889–895. doi:10.6026/97320630009889
Shamsi, T. N., Parveen, R., and Fatima, S. (2016). Characterization, biomedical and agricultural applications of protease inhibitors: a review. Int. J. Biol. Macromol. 91, 1120–1133. doi:10.1016/j.ijbiomac.2016.02.069
Sharifian-Dorche, M., Huot, P., Osherov, M., Wen, D., Saveriano, A., Giacomini, P., et al. (2020). Neurological complications of coronavirus infection; a comparative review and lessons learned during the COVID-19 pandemic. J. Neurol. Sci. 417, 117085. doi:10.1016/j.jns.2020.117085
Sharma, A. D., and Kaur, I. (2020). Eucalyptol (1,8-cineole) from eucalyptus essential Oil a potential inhibitor of COVID 19 corona virus infection by molecular docking studies. Notulae Scientia Biologicae 12, 536–545. doi:10.20944/preprints202003.0455.v1
Sharma, A., Tiwari, S., Deb, M. K., and Marty, J. L. (2020). Severe acute respiratory syndrome coronavirus-2 (SARS-CoV-2): a global pandemic and treatment strategies. Int. J. Antimicrob. Agents 56 (2), 106054. doi:10.1016/j.ijantimicag.2020.106054
Shatanawi, A., Romero, M. J., Iddings, J. A., Chandra, S., Umapathy, N. S., Verin, A. D., et al. (2011). Angiotensin II-induced vascular endothelial dysfunction through RhoA/Rho kinase/p38 mitogen-activated protein kinase/arginase pathway. Am. J. Physiol. Cell Physiol. 300 (5), C1181–C1192. doi:10.1152/ajpcell.00328.2010
Shi, H., Wang, W., Yin, J., Ouyang, Y., Pang, L., Feng, Y., et al. (2020). The inhibition of IL-2/IL-2R gives rise to CD8+ T cell and lymphocyte decrease through JAK1-STAT5 in critical patients with COVID-19 pneumonia. Cell Death Dis. 11 (6), 429–438. doi:10.1038/s41419-020-2636-4
Singh, S., Sk, M. F., Sonawane, A., Kar, P., and Sadhukhan, S. (2020). Plant-derived natural polyphenols as potential antiviral drugs against SARS-CoV-2 via RNA‐dependent RNA polymerase (RdRp) inhibition: an in-silico analysis. J. Biomol. Struct. Dyn. 1–16. doi:10.1080/07391102.2020.1796810
Singhi, P. (2011). Infectious causes of seizures and epilepsy in the developing world. Dev. Med. Child Neurol. 53 (7), 600–609. doi:10.1111/j.1469-8749.2011.03928.x
Sinha, S. K., Shakya, A., Prasad, S. K., Singh, S., Gurav, N. S., Prasad, R. S., et al. (2020). An in-silico evaluation of different Saikosaponins for their potency against SARS-CoV-2 using NSP15 and fusion spike glycoprotein as targets. J. Biomol. Struct. Dyn. 12. 144–149. doi:10.1080/07391102.2020.1762741
Steinmann, J., Buer, J., Pietschmann, T., and Steinmann, E. (2013). Anti-infective properties of epigallocatechin-3-gallate (EGCG), a component of green tea. Br. J. Pharmacol. 168 (5), 1059–1073. doi:10.1111/bph.12009
Stewart, J. N., Mounir, S., and Talbot, P. J. (1992). Human coronavirus gene expression in the brains of multiple sclerosis patients. Virology 191 (1), 502–505. doi:10.1016/0042-6822(92)90220-j
Sungnak, W., Huang, N., Bécavin, C., Berg, M., Queen, R., and Litvinukova, M. (2020). SARS-CoV-2 entry factors are highly expressed in nasal epithelial cells together with innate immune genes. Nat. Med. 26 (5), 681–687. doi:10.1038/s41591-020-0868-6
Suri, V., Suri, K., Jain, S., and Suri, K. (2020). Neurological manifestations of COVID-19 (severe acute respiratory syndrome coronavirus 2). Apollo Med. 17 (3), 157–160. doi:10.4103/am.am_63_20
Talebi, M., Talebi, M., Farkhondeh, T., and Samarghandian, S. (2020). Biological and therapeutic activities of thymoquinone: focus on the Nrf2 signaling pathway. Phytother Res. 15, 190–242. doi:10.1002/ptr.6905
Toscano, G., Palmerini, F., Ravaglia, S., Ruiz, L., Invernizzi, P., Cuzzoni, M. G., et al. (2020). Guillain–Barré syndrome associated with SARS-CoV-2. N. Engl. J. Med. 5, 51–56. doi:10.1056/NEJMc200919
Touyz, R. M., and Schiffrin, E. L. (1993). Effects of angiotensin II and endothelin-1 on platelet aggregation and cytosolic pH and free Ca2+ concentrations in essential hypertension. Hypertension 22 (6), 853–862. doi:10.1161/01.HYP.22.6.853
Tu, H., Tu, S., Gao, S., Shao, A., and Sheng, J. (2020). The epidemiological and clinical features of COVID-19 and lessons from this global infectious public health event. J. Infect. 81 (1), 1–9. doi:10.1016/j.jinf.2020.04.011
Tutunchi, H., Naeini, F., Ostadrahimi, A., and Hosseinzadeh‐Attar, M. J. (2020). Naringenin, a flavanone with antiviral and anti‐inflammatory effects: a promising treatment strategy against COVID‐19. Phytother Res. 9, 313–339. doi:10.1002/ptr.6781
Tveito, K. (2020). Cytokinstormer ved covid-19?. Tidsskrift for Den norske legeforening 24, 1402020. doi:10.4045/tidsskr.20.0239
Umesh, D., Selvaraj, C., Singh, S. K., and Dubey, V. K. (2020). Identification of new anti-nCoV drug chemical compounds from Indian spices exploiting SARS-CoV-2 main protease as target. J. Biomol. Struct. Dyn. 14, 1–9. doi:10.1080/07391102.2020.1763202
Vallamkondu, J., John, A., Wani, W. Y., Ramadevi, S., Jella, K. K., Reddy, P. H., et al. (2020). SARS-CoV-2 pathophysiology and assessment of coronaviruses in CNS diseases with a focus on therapeutic targets. Biochim. Biophys. Acta (BBA) - Mol. Basis Dis. 1866 (10), 165889. doi:10.1016/j.bbadis.2020.165889
Vaninov, N. (2020). In the eye of the COVID-19 cytokine storm. Nat. Rev. Immunol. 20 (5), 277. doi:10.1038/s41577-020-0305-6
Varadinova, T., Shishkov, S, , Ivanovska, N., , Velcheva, M., , Danghaaghin, S., , Samadanghiin, Z., , et al. (1996). Antiviral and immunological activity of a new pavine alkaloid (‐)‐Thalimonine isolated from Thalictrum simplex. Phytother Res. 10 (5), 414–417. doi:10.1002/(SICI)1099-1573(199608)10:5<414::AID-PTR876>3.0.CO;2-5
Vardhan, S., and Sahoo, S. K. (2020). In silico ADMET and molecular docking study on searching potential inhibitors from limonoids and triterpenoids for COVID-19. arXiv preprint arXiv:2005.07955.
Vellingiri, B., Jayaramayya, K., Iyer, M., Narayanasamy, A., Govindasamy, V., and Giridharan, B. (2020). COVID-19: a promising cure for the global panic. Sci. Total Environ. 725, 138277. doi:138277.10.1016/j.scitotenv.2020.138277
Vezzani, A., Moneta, D., Richichi, C., Aliprandi, M., Burrows, S. J., Ravizza, T., et al. (2002). Functional role of inflammatory cytokines and antiinflammatory molecules in seizures and epileptogenesis. Epilepsia 43 Suppl 5, 30–35. doi:10.1046/j.1528-1157.43.s.5.14.x
Vezzani, A., Balosso, S., and Ravizza, T. (2019). Neuroinflammatory pathways as treatment targets and biomarkers in epilepsy. Nat. Rev. Neurol. 15 (8), 459–472. doi:10.1038/s41582-019-0217-x
Vijayakumar, B. G., Ramesh, D., Joji, A., and Kannan, T. (2020). In silico pharmacokinetic and molecular docking studies of natural flavonoids and synthetic indole chalcones against essential proteins of SARS-CoV-2. Eur. J. Pharmacol. 886, 173448. doi:10.1016/j.ejphar.2020.173448
Wahedi, H. M., Ahmad, S., and Abbasi, S. W. (2020). Stilbene-based natural compounds as promising drug candidates against COVID-19. J. Biomol. Struct. Dyn. 1, 1–10. doi:10.1080/07391102.2020.1762743
Walter, L., and Stella, N. (2004). Cannabinoids and neuroinflammation. Br. J. Pharmacol. 141 (5), 775–785. doi:10.1038/sj.bjp.0705667
Wan, S., Yi, Q., Fan, S., Lv, J., Zhang, X., Guo, L., et al. (2020). Characteristics of lymphocyte subsets and cytokines in peripheral blood of 123 hospitalized patients with 2019 novel coronavirus pneumonia (NCP). MedRxiv. doi:10.1101/2020.02.10.20021832
Wang, H., Zhang, D., Ge, M., Li, Z., Jiang, J., and Li, Y. (2015). Formononetin inhibits enterovirus 71 replication by regulating COX- 2/PGE₂ expression. Virol. J. 12 (1), 35. doi:10.1186/s12985-015-0264-x
Wang, L., Shen, Y., Li, M., Chuang, H., Ye, Y., Zhao, H., et al. (2020a). Clinical manifestations and evidence of neurological involvement in 2019 novel coronavirus SARS-CoV-2: a systematic review and meta-analysis. J. Neurol. 1, 13. doi:10.1007/s00415-020-09974-2
Wang, Q., Zhang, Y., Wu, L., Niu, S., Song, C., Zhang, Z., et al. (2020b). Structural and functional basis of SARS-CoV-2 entry by using human ACE2. Cell 181 (4), 894–899. doi:10.1016/j.cell.2020.03.045
Wang, Y., Wang, Y., Chen, Y., and Qin, Q. (2020c). Unique epidemiological and clinical features of the emerging 2019 novel coronavirus pneumonia (COVID-19) implicate special control measures. J. Med. Virol. 92 (6), 568–576. doi:10.1002/jmv.25748
Warner, R. (2020). Neurological and neuromuscular manifestations in SARS-CoV-2: review of literature and case series. Research Square [Preprint]. doi:10.21203/rs.3.rs-37519/v1]
Welcome, M. O. (2020). Blood brain barrier inflammation and potential therapeutic role of phytochemicals. PharmaNutrition 11, 100177. doi:10.1016/j.phanu.2020.100177
Wen, C. C., Kuo, Y. H., Jan, J. T., Liang, P. H., Wang, S. Y., Liu, H. G., et al. (2007). Specific plant terpenoids and lignoids possess potent antiviral activities against severe acute respiratory syndrome coronavirus. J. Med. Chem. 50 (17), 4087–4095. doi:10.1021/jm070295s
Werner, C., Scullen, T., Mathkour, M., Zeoli, T., Beighley, A., Kilgore, M. D., et al. (2020). Neurological impact of coronavirus disease of 2019: practical considerations for the Neuroscience community. World Neurosurg 139, 344–354. doi:10.1016/j.wneu.2020.04.222
West, P. K., Viengkhou, B., Campbell, I. L., and Hofer, M. J. (2019). Microglia responses to interleukin-6 and type I interferons in neuroinflammatory disease. Glia 67 (10), 1821–1841. doi:10.1002/glia.23634
Williamson, G., and Kerimi, A. (2020). Testing of natural products in clinical trials targeting the SARS-CoV-2 (Covid-19) viral spike protein-angiotensin converting enzyme-2 (ACE2) interaction. Biochem. Pharmacol. 178, 114123. doi:10.1016/j.bcp.2020.114123
Wu, C., Chen, X., Cai, Y., Zhou, X., Xu, S., Huang, H., et al. (2020a). Risk factors associated with acute respiratory distress syndrome and death in patients with coronavirus disease 2019 pneumonia in Wuhan, China. JAMA Intern. Med 180 (7), 934–943. doi:10.1001/jamainternmed.2020.0994
Wu, C., Liu, Y., Yang, Y., Zhang, P., Zhong, W., Wang, Y., et al. (2020b). Analysis of therapeutic targets for SARS-CoV-2 and discovery of potential drugs by computational methods. Acta Pharm. Sin. B 10 (5), 766–788. doi:10.1016/j.apsb.2020.02.008
Wu, Q., Zhou, L., Sun, X., Yan, Z., Hu, C., Wu, J., et al. (2017). Altered lipid metabolism in recovered SARS patients twelve years after infection. Sci. Rep. 7, 9110. doi:10.1038/s41598-017-09536-z
Wu, Y., Xu, X., Chen, Z., Duan, J., Hashimoto, K., Yang, L., et al. (2020c). Nervous system involvement after infection with COVID-19 and other coronaviruses. Brain Behav. Immun. 87, 18–22. doi:10.1016/j.bbi.2020.03.031
Xia, H., and Lazartigues, E. (2008). Angiotensin-converting enzyme 2 in the brain: properties and future directions. J. Neurochem. 107 (6), 1482–1494. doi:10.1111/j.1471-4159.2008.05723.x
Xu, J., Zhong, S., Liu, J., Li, L., Li, Y., Wu, X., et al. (2005). Detection of severe acute respiratory syndrome coronavirus in the brain: potential role of the chemokine mig in pathogenesis. Clin. Infect. Dis. 41 (8), 1089–1096. doi:10.1086/444461
Xu, L., Botchway, B. O. A., Zhang, S., Zhou, J., and Liu, X. (2018). Inhibition of NF-κB signaling pathway by resveratrol improves spinal cord injury. Front. Neurosci. 12, 690. doi:10.3389/fnins.2018.00690
Xue, X., Yu, H., Yang, H., Xue, F., Wu, Z., Shen, W., et al. (2008). Structures of two coronavirus main proteases: implications for substrate binding and antiviral drug design. J. Virol. 82 (5), 2515–2527. doi:10.1128/JVI.02114-07
Yang, X., Yu, Y., Xu, J., Shu, H., Xia, J., Liu, H., et al. (2020). Clinical course and outcomes of critically ill patients with SARS-CoV-2 pneumonia in Wuhan, China: a single-centered, retrospective, observational study. Lancet Respir. Med. 8 (5), 475–481. doi:10.1016/S2213-2600(20)30079-5
Yao, C., Xi, C., Hu, K., Gao, W., Cai, X., Qin, J., et al. (2018). Inhibition of enterovirus 71 replication and viral 3C protease by quercetin. Virol. J. 15 (1), 116. doi:10.1186/s12985-018-1023-6
Yarmohammadi, A., Yarmohammadi, M., Fakhri, S., and Khan, H. (2021). Targeting pivotal inflammatory pathways in COVID-19: a mechanistic review. Eur. J. Pharmacol., 890, 173620. doi:10.1016/j.ejphar.2020.173620
Yassin, A., Nawaiseh, M., Alsherbini, K., El-Salem, K., Soudah, O., and Abu-Rub, M. (2020). Neurological manifestations and complications of coronavirus disease 2019 (COVID-19): a systematic review and Meta-analysis. Research Square [Pre-print]. doi:10.21203/rs.3.rs-39952/v1
Yavarpour-Bali, H., and Ghasemi-Kasman, M. (2020). Update on neurological manifestations of COVID-19. Life Sci. 257, 118063. doi:10.1016/j.lfs.2020.118063
Yazdanpanah, N., Saghazadeh, A., and Rezaei, N. (2020). Anosmia: a missing link in the neuroimmunology of coronavirus disease 2019 (COVID-19). Rev. Neurosci. 31 (7), 691–701. doi:10.1515/revneuro-2020-0039
Zach, H., Dirkx, M., Pasman, J. W., Bloem, B. R., and Helmich, R. C. (2017). The patient's perspective: the effect of levodopa on Parkinson symptoms. Park. Relat. Disord. 35, 48–54. doi:10.1016/j.parkreldis.2016.11.015
Zaki, A. M., Van Boheemen, S., Bestebroer, T. M., Osterhaus, A. D., and Fouchier, R. A. (2012). Isolation of a novel coronavirus from a man with pneumonia in Saudi Arabia. N. Engl. J. Med. 367 (19), 1814–1820. doi:10.1056/NEJMoa1211721
Zhang, Y., Zhang, L. H., Chen, X., Zhang, N., and Li, G. (2018). Piceatannol attenuates behavioral disorder and neurological deficits in aging mice via activating the Nrf2 pathway. Food Funct 9 (1), 371–378. doi:10.1039/c7fo01511a
Zhao, K., Huang, J., Dai, D., Feng, Y., Liu, L., and Nie, S. (2020). Acute myelitis after SARS-CoV-2 infection: a case report. MedRxiv. doi:10.1101/2020.03.16.20035105
Zheng, Y. Y., Ma, Y. T., Zhang, J. Y., and Xie, X. (2020). COVID-19 and the cardiovascular system. Nat. Rev. Cardiol. 17 (5), 259–260. doi:10.1038/s41569-020-0360-5
Zhou, J., and Huang, J. (2020). Current findings regarding natural components with potential anti-2019-nCoV activity. Front Cell Dev Biol. 8, 589. doi:10.3389/fcell.2020.00589
Zhou, L., Zhang, M., Wang, J., and Gao, J. (2020). Sars-Cov-2: underestimated damage to nervous system. Trav. Med. Infect. Dis. 47, 121–139. doi:10.1016/j.tmaid.2020.101642
Keywords: coronaviruses, COVID-19, SARS-CoV-2, neurology, nervous system, phytochemicals, pharmacology, signaling pathways
Citation: Fakhri S, Piri S, Majnooni MB, Farzaei MH and Echeverría J (2021) Targeting Neurological Manifestations of Coronaviruses by Candidate Phytochemicals: A Mechanistic Approach. Front. Pharmacol. 11:621099. doi: 10.3389/fphar.2020.621099
Received: 25 October 2020; Accepted: 08 December 2020;
Published: 20 January 2021.
Edited by:
Vincent Kam Wai Wong, Macau University of Science and Technology, MacauReviewed by:
Dezső Csupor, University of Szeged, HungarySubhash Chandra Mandal, Government of West Bengal, India
Sigrun Lange, University of Westminster, United Kingdom
Copyright © 2021 Fakhri, Piri, Majnooni, Farzaei and Echeverría. This is an open-access article distributed under the terms of the Creative Commons Attribution License (CC BY). The use, distribution or reproduction in other forums is permitted, provided the original author(s) and the copyright owner(s) are credited and that the original publication in this journal is cited, in accordance with accepted academic practice. No use, distribution or reproduction is permitted which does not comply with these terms.
*Correspondence: Mohammad Hosein Farzaei, bWguZmFyemFlaUBnbWFpbC5jb20=; Javier Echeverría, amF2aWVyLmVjaGV2ZXJyaWFtQHVzYWNoLmNs