- 1Wenzhou Key Laboratory of Sanitary Microbiology, Department of Microbiology and Immunology, School of Laboratory Medicine, Wenzhou Medical University, Wenzhou, China
- 2Central Laboratory, The First Affiliated Hospital of Wenzhou Medical University, Wenzhou, China
The first-line treatment for colorectal cancer (CRC) is 5-fluorouracil (5-FU). However, the efficacy of this treatment is sometimes limited owing to chemoresistance as well as treatment-associated intestinal mucositis and other adverse events. Growing evidence suggests that certain phytochemicals have therapeutic and cancer-preventing properties. Further, the synergistic interactions between many such plant-derived products and chemotherapeutic drugs have been linked to improved therapeutic efficacy. Polysaccharides extracted from Albuca bracteata (Thunb.) J.C.Manning and Goldblatt (ABP) have been reported to exhibit anti-oxidant, anti-inflammatory, and anti-tumor properties. In this study, murine CRC cells (CT26) and a murine model of CRC were used to examine the anti-tumor properties of ABP and explore the mechanism underlying the synergistic interactions between ABP and 5-FU. Our results revealed that ABP could inhibit tumor cell proliferation, invasion, and migratory activity in vitro and inhibited tumor progression in vivo by suppressing β-catenin signaling. Additionally, treatment with a combination of ABP and 5-FU resulted in better outcomes than treatment with either agent alone. Moreover, this combination therapy resulted in the specific enrichment of Ruminococcus, Anaerostipes, and Oscillospira in the intestinal microbiota and increased fecal short-chain fatty acid (SCFA) levels (acetic acid, propionic acid, and butyric acid). The improvement in the intestinal microbiota and the increase in beneficial SCFAs contributed to enhanced therapeutic outcomes and reduced the adverse effects of 5-FU. Together, these data suggest that ABP exhibits anti-neoplastic activity and can effectively enhance the efficacy of 5-FU in CRC treatment. Therefore, further research on the application of ABP in the development of novel anti-tumor drugs and adjuvant compounds is warranted and could improve the outcomes of CRC patients.
Introduction
Colorectal cancer (CRC) is the third most common cause of tumor-related death worldwide (Siegel et al., 2020; Siegel et al., 2021). Currently, the standard treatment for CRC consists of a combination of surgery and chemotherapy, with 5-fluorouracil (5-FU) serving as the first-line drug of choice in most cases (Shionome et al., 2013). Treatment with 5-FU restricts tumor cell growth and causes apoptosis via extensive DNA damage. However, many CRC patients eventually develop chemoresistance to 5-FU. In addition, 5-FU does not specifically target tumor cells and thus also causes damage to normal cells, resulting in potentially severe adverse effects. Moreover, 5-FU treatment can also alter the gut microbiota (Wardill et al., 2014; Hamouda et al., 2017), which influences intestinal barrier function and drives colon inflammation (Cai et al., 2021).
The gut microbiota plays a vital role in maintaining intestinal homeostasis, mucus barrier integrity, host immune responses, drug toxicity (Liu et al., 2020), and response to anti-cancer drugs (Karin et al., 2014). Polysaccharides, considered potential prebiotics, have beneficial effects on host intestinal health and may attenuate chemotherapy-induced intestinal mucositis (Shang et al., 2018; Gori et al., 2019). The anti-tumor effects of polysaccharides can be partially attributed to the regulation of the gut microbiota (Liu et al., 2019) and increases in fecal short-chain fatty acids (SCFAs) (Wang et al., 2017). Natural anti-tumor compounds with few or no adverse effects are appealing alternatives to adjuvant drugs in cancer treatment (Haq et al., 2019). Liu et al. found that tea polysaccharides can inhibit CT26 tumor cell proliferation and metastasis via the IL-6/STAT3 signaling pathway (Liu et al., 2018). Liang et al. found that Ganoderma lucidum polysaccharides can upregulate JNK expression via the MAPK pathway and thereby induce apoptosis in HCT-116 cells (Liang et al., 2014). Feng et al. found that Atractylodes macrocephala polysaccharides enhance anti-tumor immune responses by driving lymphocyte proliferation and elevating the production of IL-6, IFN-λ, and TNF-α (Feng et al., 2019). As such, these biocompatible phytochemicals represent promising adjuvant chemotherapeutic drugs for the treatment of various cancers (Liu, 2004).
Albuca bracteata (Thunb.) J.C.Manning and Goldblatt (AB), formerly known as Ornithogalum caudatum Aiton, is an evergreen perennial herb from the Asparagaceae family. It is native to southern Africa but has long been cultivated in China. AB is a herbal remedy for hepatitis, diabetes, parotitis, and several other diseases in both traditional Chinese medicine (TCM) and African folk medicine (Zhang et al., 2017). Molecular analyses have ascribed the bioactive properties of AB to a variety of saponins, polysaccharides, and flavonoids, which exhibit anti-neoplastic, anti-oxidant, anti-diabetic, and anti-inflammatory effects (Zhou et al., 2005). Chen et al. reported that AB has a relatively high content of polysaccharides, which can be effectively extracted using the ultrasonic extraction technique (Chen et al., 2012). AB polysaccharides (ABP) may be good candidates for anti-oxidant and immunostimulating agents in medicine and functional foods. However, few studies, with the exception of one demonstrated that ABP treatment suppresses murine S180 sarcoma growth, have focused on the specific anti-tumor properties of ABP. Moreover, to our knowledge, no study has explored the effect of ABP treatment in the context of gastrointestinal tumors.
β-catenin is a multifunctional oncogenic protein that plays an essential role in developmental processes (Clevers and Nusse, 2012). Abnormal activation of the Wnt/β-catenin pathway in the context of CRC results in excess accumulation of nuclear β-catenin, further driving tumor cell proliferation and malignancy and ultimately facilitating CRC development and progression (Reya and Clevers, 2005). Apart from its role in cell growth and adhesion, the activated canonical Wnt/β-catenin signaling pathway also promotes tumor bulking, recurrence, and resistance to chemotherapy in cancer stem cells (Reya and Clevers, 2005; Malanchi et al., 2008). High levels of β-catenin induce chemoresistance in CRC cells (Jiang et al., 2018; Uppada et al., 2018), and increased expression of β-catenin has been observed in drug-resistant CRC cells (He et al., 2018). Saito et al. found that β-catenin silencing is sufficient to sensitize CRC cells to chemotherapeutic treatment (Saifo et al., 2010). Accordingly, agents capable of inhibiting β-catenin could improve the clinical outcomes of cancer patients when used in combination with standard therapies.
Herein, we explored the potential therapeutic utility of ABP treatment and mechanisms underlying the potential anti-neoplastic synergy between 5-FU and ABP in vivo and in vitro, hoping to find a new strategy for CRC treatment. We determined that ABP can suppress the migratory, proliferative, and invasive activity of CRC cells by inhibiting the Wnt/β-catenin signaling pathway. Further, we found that ABP can enhance the ability of 5-FU to suppress CRC, likely by downregulating β-catenin, improving the overall composition of the intestinal microbiome, and augmenting SCFA levels. As such, our data suggest that ABP may be a promising anti-tumor agent and could serve as valuable adjuvant agents in chemotherapy for CRC.
Materials and Methods
ABP Preparation
ABP were extracted from AB bulbs and purified using a previously published protocol (Chen et al., 2012). The total carbohydrate, uronic acid, and protein content of the resultant extract was 92.58, 1.63, and 1.70%, respectively, and the average molecular weight was 18.3 kDa.
Cell Culture
The CT26 murine cell line was purchased from the Type Culture Collection of the Chinese Academy of Sciences (Shanghai, China). Cells were cultured in RPMI-1640 medium containing 10% fetal bovine serum (Thermo Fisher Scientific, United States) at 37°C in a humidified incubator containing 5% CO2.
CCK-8 Assay
A Cell Counting Kit-8 (CCK-8, Dojindo, Japan) assay was performed using the manufacturer’s instructions to assess cell viability (Wang et al., 2020). Briefly, at the end of the treatment period, 10 μL of CCK-8 solution was added to each well. After a 3-h incubation at 37°C, the absorbance at 450 nm was measured.
EdU Uptake Assay
A BeyoClick™ EdU Cell Proliferation Kit with Alexa Fluor 488 (BeyoTime) was used according to the manufacturer’s instructions to monitor cell proliferation (Wang et al., 2019). Briefly, at the end of the treatment period, EdU (10 μM) was added to each well, and cells were incubated for 2 h at 37°C. The cells were then fixed with 4% paraformaldehyde, stained with an Apollo Dye Solution, and subjected to Hoechst 33,342 nuclear counterstaining. EdU-stained cells were visualized by using a fluorescence microscopy (Leica, Wetzlar, Germany), and images were processed using ImageJ software.
Wound Healing and Transwell Assays
Wound healing assay was performed as previously described (Shen et al., 2020). CT26 cells were seeded into 6-well plates and cultured to confluence. The cell monolayer was scratched with a 100-μL pipette tip to generate a scratch wound. Recovery was allowed to occur for 24 h in serum-free RPMI-1640 containing the appropriate ABP concentrations, following which the cells were observed using an optical microscope. The migration area was analyzed under an inverted microscope using ImageJ software.
The transwell assay was also performed as described previously (Wang et al., 2020). For this assay, 24-well Boyden tissue culture plates (Corning, MA, United States) with an 8-μm-pore polycarbonate membrane were used. CT26 cells were seeded into the upper chambers, and 200 μL of serum-free RPMI-1640 was added (migration, 2×105 cells, 24 h; invasion, 4×105 cells, 48 h). The numbers of migrating or invading cells were counted in randomly chosen fields from duplicate chambers using an optical microscope. There were three replicates for each treatment, and each experiment was repeated thrice.
Western Blot
Western blots were performed as described previously (Luo et al., 2021). RIPA buffer (Thermo Fisher, Waltham, MA, United States) was used to extract the total protein from samples. ECL Western Blotting Substrate (Thermo Fisher, Waltham, MA, United States) was used to detect protein bands on a Bio-Rad Gel Doc XR + system (Bio-Rad, United States). The primary antibodies used were as follows: anti-β-catenin (#ab68183, Abcam, United Kingdom), anti-Cyclin D1 (#ab40754, Abcam, United Kingdom), anti-c-Myc (#ab32072, Abcam, United Kingdom), anti-E-Cadherin (#3195, Cell Signaling Technology, United States), anti-Vimentin (#5741, Abcam, United Kingdom), anti-PCNA (#ab29, Abcam, United Kingdom), anti-COX-2 (#AF7003, Affinity Biosciences, United States), and anti-GAPDH (#ab181602, Abcam, United Kingdom).
Animal Studies
Animal experiments were performed using protocols approved by the Institutional Animal Care and Use Committee of Wenzhou Medical University (wydw 2021-0224). CT26 cells are homologous tumors of BALB/c mice. According to reports in the literature (Li et al., 2019; Wan et al., 2020), the success rate of modeling of CT26 on BALB/c female mice was satisfied. BALB/c mice (female, 4–5 weeks old, Laboratory Animal Center, Wenzhou Medical University) were kept in a climate-controlled room of 20–22°C with a 12 h/12 h light-dark cycle. Each of the 24 mice received a subcutaneous injection of 5×105 tumor cells in the right thigh. On day 5 after injection, they were randomized into four groups (n = 6/group): control, 5-FU, ABP, and 5-FU + ABP based on tumor size at the start of the treatment. ABP were administered to the latter two groups via oral gavage (0.5 mg/ml; 0.2 ml/mouse) once per day for 16 days, whereas the other two groups received distilled water. Further, groups 2 and 4 received 5-FU via intraperitoneal injection for 10 days (20 mg/kg/mouse on each day), whereas the other two groups received sterilized distilled water. The body weight and tumor growth (volume = 0.5 × length × [width]2) in all animals were measured every alternate day. At the end of interval period, tumors were immediately removed after the animal was sacrificed, and weighed, fixed, or kept at −80°C.
16S rRNA Gene Sequencing and Microbiome Analysis
After euthanasia at the end of treatment, mouse fecal samples were collected and preserved at −80°C. Part of samples were used for microbiome composition analysis. 16S rRNA gene amplicon sequencing was performed using Illumina sequencing technology as previously described (Peng et al., 2019). Briefly, bacterial DNA was extracted from mouse feces using an E. Z.N.A.® soil DNA Kit (Omega Bio-Tek, Norcross, GA, United States) according to the manufacturer’s instructions. The V3–V4 hypervariable region of the 16 sRNA gene was amplified using the primers 338F (5ʹ-ACTCCTACGGGAGGCAGCA-3ʹ) and 806R (5ʹ-GGACTACHVGGGTWTCTAAT-3ʹ). Amplified fragments were mixed in equimolar amounts and subjected to paired 2 × 300 bp sequencing on an Illumina MiSeq system. The Atacama soil microbiome tutorial from Qiime2docs was used for bioinformatics analyses along with custom scripts (https://docs.qiime2.org/2019.1/).
Analysis of SCFAs
Fecal SCFA levels at the end of the intervention period were measured using a Trace GC Ultra Gas Chromatograph coupled with an ISQ Mass Spectrometer (TRACE 1310-ISQ 7000, Thermo, MA, United States). Final data were normalized based on fecal weight.
Immunohistochemical Staining
Immunohistochemistry (IHC) analyses were conducted as described earlier (Li et al., 2018). Briefly, tumor tissue was fixed in 4% paraformaldehyde and embedded in paraffin using standard techniques. Sections (5 μm) were obtained and stained with anti-Ki67 (#ab152976, Abcam, United Kingdom) antibodies. Images were acquired using a fluorescence microscope (DP80, Olympus, Japan).
Statistical Analysis
Data are presented as means ± standard deviation (SD) or median (interquartile range). Normally distributed data showing variance homogeneity were analyzed by student’s t-tests, other data were analyzed via Kruskal–Wallis tests using SPSS 23.0 (SPSS Company, Inc., United States). p < 0.05 was the threshold for significance (n.s., not significant, *p < 0.05, **p < 0.01, and ***p < 0.001).
Results
ABP Treatment Suppresses CRC Cell Proliferation
To explore the effect of ABP treatment on CRC cells, we treated CT26 cells with a range of ABP concentrations and then explored their proliferation and viability through CCK-8 and EdU uptake assays. ABP treatment significantly impaired CT26 cell growth in a time- and dose-dependent manner (Figure 1A), and the EdU uptake assay yielded similar findings (Figures 1B,C).
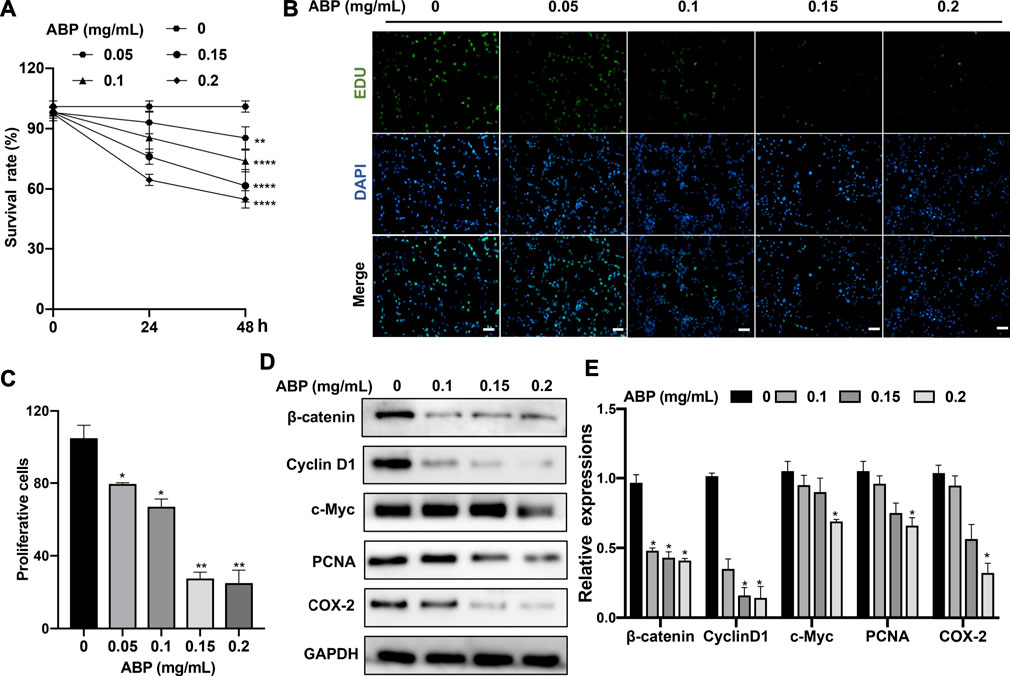
FIGURE 1. ABP treatment suppresses the proliferation of CRC cells. (A) CCK-8 assay examining the proliferation-suppressing effect of ABP on CT26 cells. (B–C) EdU uptake assay examining the proliferation-suppressing effect of ABP on CT26 cells. Scale bar = 50 μm (×200 magnification). (D–E) Western blot examining the effect of ABP on the protein levels of β-catenin, Cyclin D1, c-Myc, PCNA, COX-2, and GAPDH in CT26 cells. Compared with the untreated group, *p < 0.05, **p < 0.01.
To further elucidate the mechanisms underlying the observed results, western blots were performed. They revealed that ABP treatment inhibited the expression of β-catenin, c-Myc, Cyclin D1, COX-2, and PCNA (Figures 1D,E). There is a corresponding change in mRNA levels (Supplementary Figure S1). The results thus suggested that ABP inhibit the growth of CRC cells in vitro by suppressing the Wnt/β-catenin pathway.
ABP Treatment Suppresses the Migratory and Invasive Activity of CRC Cells
The effects of ABP treatment on CRC cell migration and invasion were assessed using a transwell assay. Treatment with ABP at doses of 0.1 and 0.15 mg/ml resulted in a 38.3 ± 4.81% (p < 0.01) and 46.7 ± 6.24% (p < 0.01) decrease in the percentage of migratory cells, respectively. This was accompanied by a 42.6 ± 5.7% (p < 0.01) and 50.2 ± 4.1% (p < 0.01) decrease in the frequency of invasive cells (Figures 2A,B). Consistent with these results, wound healing assays showed that ABP treatment reduced the wound closure rate from 34 ± 3.4% in control samples to 19 ± 3.6% (0.1 mg/ml, p < 0.01) and 15 ± 3.1% (0.15 mg/ml, p < 0.01) in ABP treated ones (Figures 2C,D). Western blot analyses for epithelial–mesenchymal transition (EMT)-related proteins showed that ABP treatment suppressed Vimentin and enhanced E-cadherin expression in CT26 cells (Figures 2E,F).
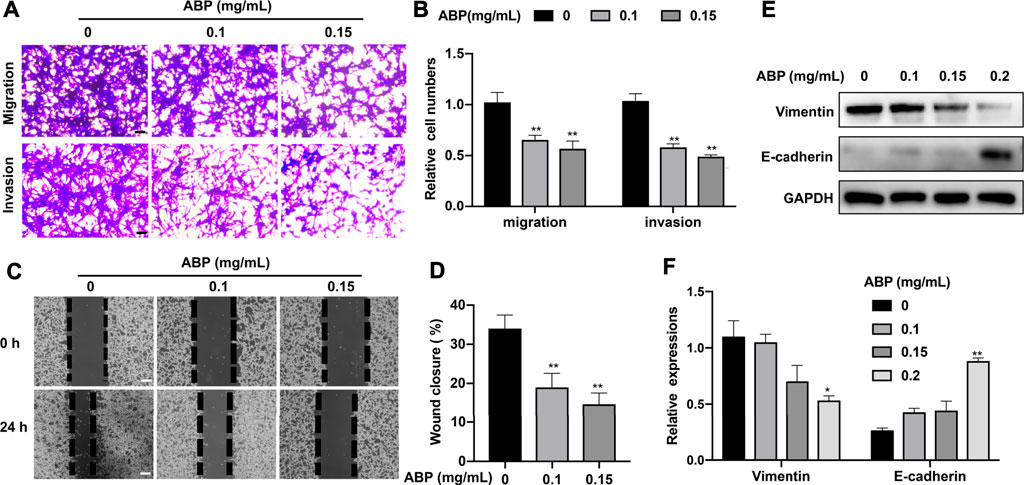
FIGURE 2. ABP treatment suppresses the migratory and invasive activity of CRC cells. (A-B) Transwell assay examining the negative effect of ABP on the migration and invasion of CT26 cells. Scale bar = 50 μm. (C-D) Wound healing assay examining the negative effect of ABP on the migration and invasion of CT26 cells. Scale bar = 250 μm. (E-F) Western blot examining the effect of ABP on the protein levels of Vimentin, E-cadherin, and GAPDH in CT26 cells. Compared with the untreated group, *p < 0.05, **p < 0.01.
ABP Treatment Suppresses the Growth and Metastasis of CRC Cells by Downregulating β-Catenin
Given our aforementioned results, which suggested that ABP suppress the expression of β-catenin and its related proteins, we speculated that β-catenin was an essential anti-tumor target of ABP in CT26 cells. To verify this hypothesis, we used an agonist of β-catenin, SKL 2001 (MCE, China), to manipulate β-catenin activity. We examined the effects of ABP treatment on CT26 cells with or without SKL2001 in vitro and analyzed subsequently. Results indicated that SKL2001 reversed the ABP-induced β-catenin downregulation (Figures 3A,B) and partially attenuated the ABP-induced inhibition of CRC cell proliferation (Figure 3C, p < 0.01), SKL2001 also evidently reversed the inhibitory effect of ABP on tumor cell migration (p < 0.01) and invasion (p < 0.05) (Figures 3D,E). These data demonstrated that ABP act as β-catenin inhibitors and inhibit the migration, invasion, and growth of CRC cells, at least in part, by downregulating β-catenin.
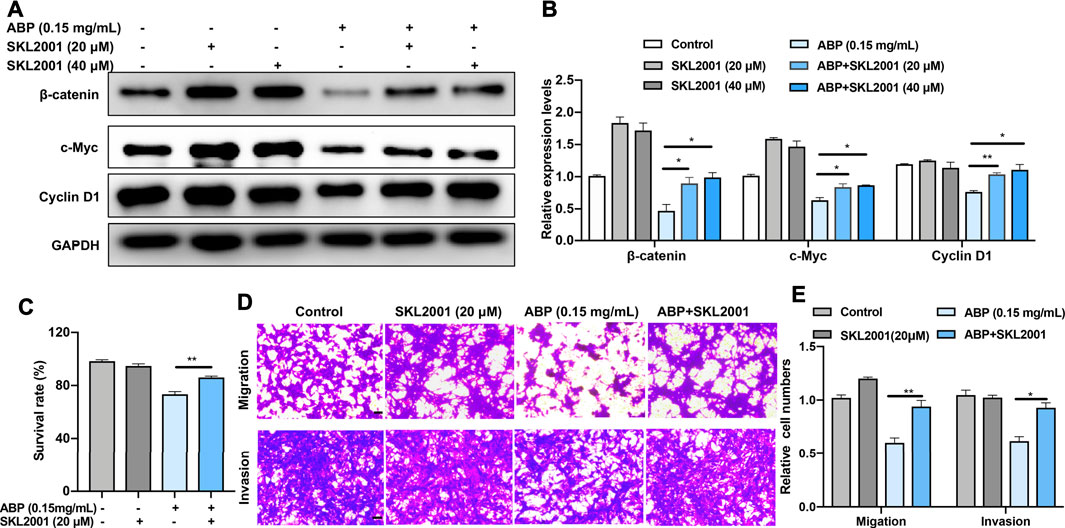
FIGURE 3. ABP treatment suppresses the growth and metastasis of CRC cells by downregulating β-catenin. (A–B) Western blot examining the effects of SKL 2001 (β-catenin activator) on β-catenin, c-Myc, and Cyclin D1 levels in CT26 cells treated with ABP. (C) CCK-8 assay examining the rescue effects of SKL2001 treatment on CT26 cells treated with ABP. (D–E) Transwell assay examining the rescue effects of SKL2001 treatment on CT26 cells treated with ABP. Scale bar = 50 μm. Compared with the ABP group, *p < 0.05, **p < 0.01.
ABP and 5-FU Exhibit Synergistic Anti-Tumor Efficacy Against CT26 Cells
A β-catenin inhibitor, XAV939, was used to assess the role of β-catenin in the cellular response to 5-FU. Subsequent analysis indicated that at doses of 1 μg/ml (p < 0.01) and 2 μg/ml (Figure 4A, p < 0.01), XAV939 enhances the cytotoxic effects of 5-FU on CT26 cells. We also used 2 siRNAs specific for β-catenin to knock down β-catenin expression in CT26 cells (Supplementry Figure S2). This result indicated that decreased β-catenin expression might enhance the chemotherapeutic efficacy of 5-FU. We then explored the combined effects of ABP (0.15 mg/ml) and 5-FU (0–10 μg/ml) on CT26 cells. CCK-8 assays showed that the survival rate in the combination treatment group was significantly lower than those in the ABP or 5-FU treatment group alone (Figures 4B,C). The effects of drug combinations were checked by using online software SynergyFinder (https://synergyfinder.org/) (Ianevski et al., 2020) and Compusyn (http://www.combosyn.com/) (Chou, 2010). The HSA synergy score (16.05), the Loewe synergy score (10.67), and the combination index (CI) (0.7 to 1.0) indicated that ABP and 5-FU had a slight synergistic effect in vitro (Supplementary Figure S3).
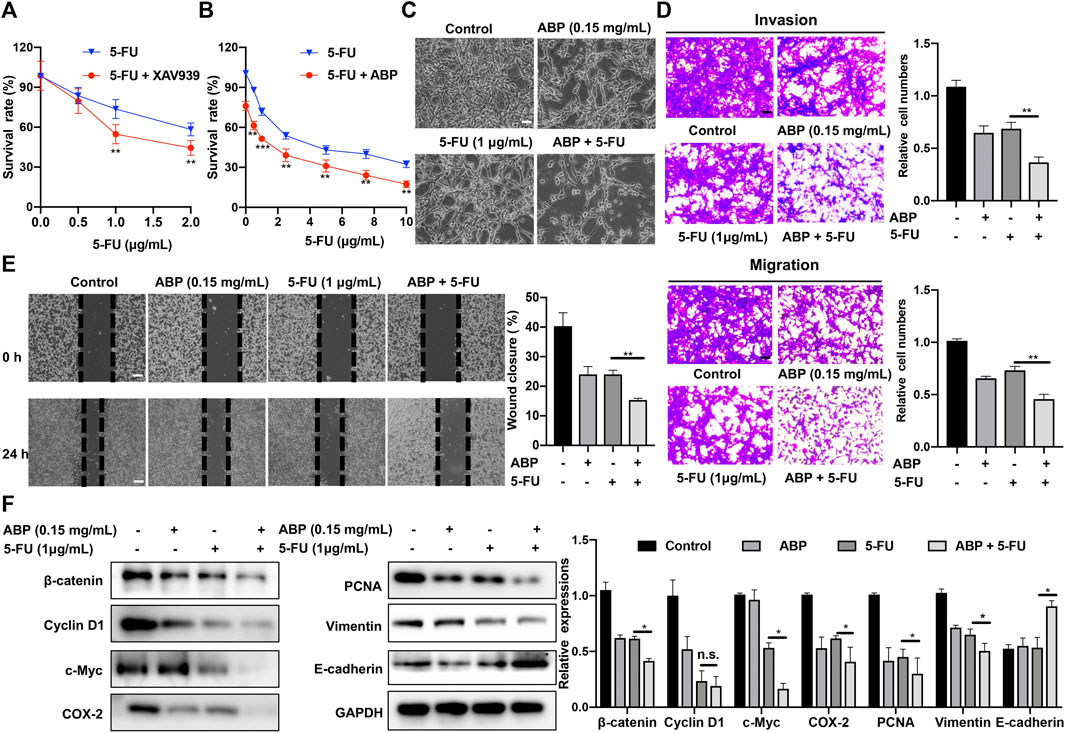
FIGURE 4. ABP enhances the anti-tumor efficacy of 5-FU against CT26 cells. (A-B) CCK-8 assay examining the effects of XAV939 (β-catenin inhibitor) and ABP on the anti-tumor efficacy of 5-FU against CT26 cells. (C) Cellular morphology of CT26 cells treated with ABP, 5-FU, and ABP + 5-FU for 24 h. Scale bar = 50 μm. (D) Transwell assays examining the enhancing effect of ABP on the anti-tumor efficacy of 5-FU against CT26 cells. Scale bar = 50 μm. (E) Wound healing assay examining the enhancing effect of ABP on the anti-tumor efficacy of 5-FU against CT26 cells. Scale bar = 250 μm. (F) Western blot examining the protein levels of β-catenin, Cyclin D1, c-Myc, COX-2, PCNA, Vimentin, E-cadherin, an GAPDH in CT26 cells treated with ABP and 5-FU. Compared with the 5-FU group, *p < 0.05, **p < 0.01, n. s., no significance.
The effects of combination treatment on metastasis ability of CT26 cells were also evaluated by Transwell assays. Compared with 5-FU alone, the combination treatment significantly decreased migratory and invasive abilities by 38.3 ± 0.8% (p < 0.01) and 47.3 ± 5.3% (p < 0.01), respectively (Figure 4D). Furthermore, the combination treatment reduced the wound closure rate from 24 ± 2.5% of 5-FU alone to 14 ± 1.6% (Figure 4E, p < 0.01). Western blots showed that the expression levels of β-catenin, Cyclin D1, c-Myc, COX-2, PCNA, and Vimentin in the combination treatment cells were all lower than those in 5-FU alone, while the expression of E-cadherin was higher (Figure 4F). These data suggested that ABP had a synergistic effect with 5-FU on CRC tumor cell growth, migration, and invasion.
ABP and 5-FU Exhibit Synergistic Anti-Tumor Efficacy in Tumor-Bearing Mice
The anti-tumor efficacy of ABP in vivo was assessed in a BALB/c mouse model of subcutaneous CRC. ABP treatment significantly impaired tumor growth (Figures 5A–C); the average tumor weight in the ABP group (1.440 ± 0.401 g) was significantly lower than that in the control group (2.389 ± 0.228 g, p < 0.001) (Figures 5B,C). Further, the average tumor weight in the combination treatment group (0.449 ± 0.119 g) was also much lower than that in the 5-FU treatment group (0.781 ± 0.151 g, p < 0.01) (Figures 5B,C).
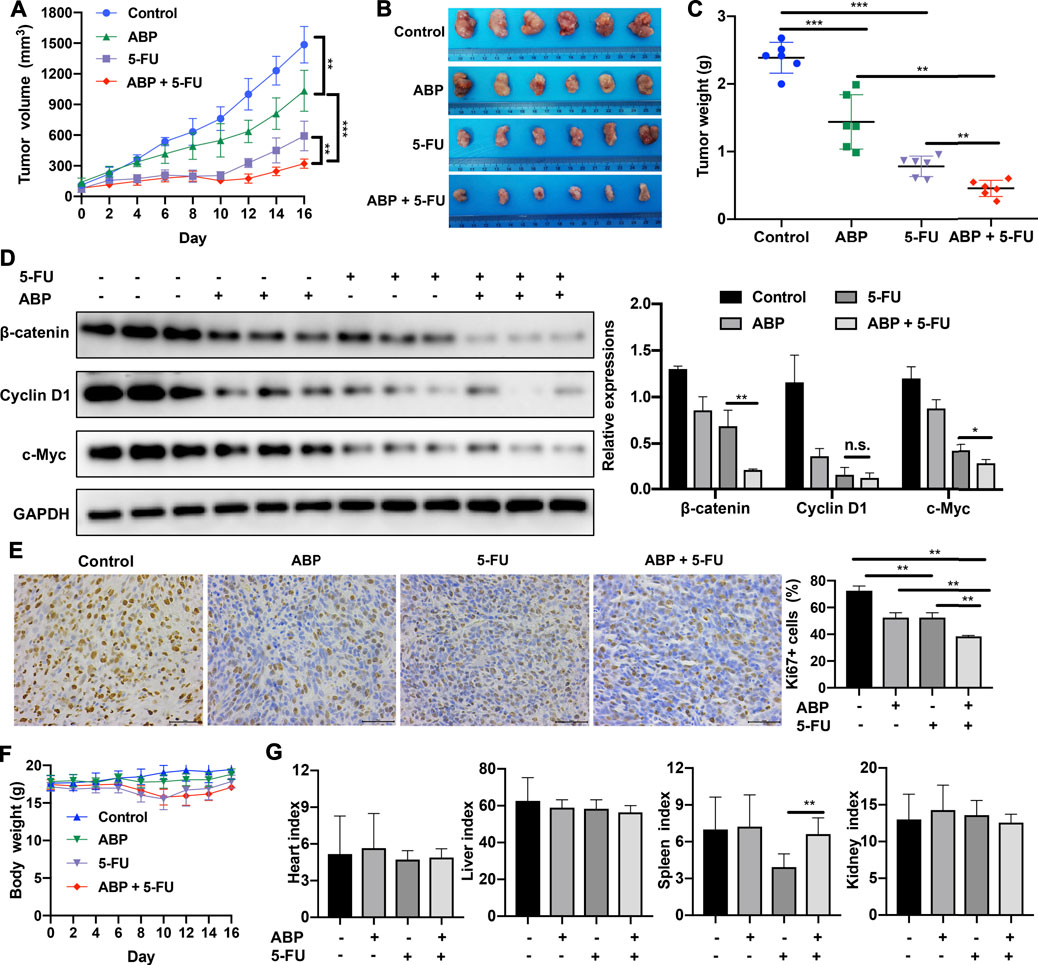
FIGURE 5. ABP exhibits synergistic anti-tumor effects when administered in combination with 5-FU in mice bearing CT26 tumors. (A) Tumor volumes measured at the indicated time points. Data are shown as mean ± SD (n = 6 mice at each time point). (B) Image of tumors resected from sacrificed mice at the end of the treatment period. (C) Tumor weights measured after surgical tumor removal, represented as mean ± SD. (D) Western blot examining the levels of β-catenin, Cyclin D1, and c-Myc in subcutaneous tumors, with GAPDH used as the internal control. (E) Images of Ki67 immunohistochemical staining (× 400) in tumor cells and quantification of Ki67-positive tumor cells. Scale bar = 50 μm. (F) Body weights measured every 2 days. (G) Organ indexes calculated as the ratio of organ weight (mg) to body weight (g) at the end of the treatment period. Data are means ± SD (n = 6). *p < 0.05, **p < 0.01, ***p < 0.001.
Western blots showed that ABP treatment reduced the expression levels of β-catenin, Cyclin D1, and c-Myc in tumor tissue samples (Figure 5D). IHC staining showed that ABP treatment reduced Ki-67 expression in tumor cells (Figure 5E). Significant body weight losses were observed in groups treated by 5-FU (Figure 5F, p < 0.01), but there was no statistical difference between the 5-FU and the combination group. The 5-FU group had the lowest spleen-to-body weight ratio (3.936 ± 1.071) and the combination treatment group had a significantly higher one (6.626 ± 1.311) (Figure 5G, p < 0.01). These data suggested that ABP exerted anti-tumor effects on CT26 tumor-bearing mice when used alone and provided synergistic anti-tumor effects when combined with 5-FU.
ABP and 5-FU Treatment Improves the Composition of the Intestinal Flora in Mouse Models of CRC
To explore whether the anti-tumor effects of ABP were associated with changes in intestinal flora, 16S rRNA gene sequencing of fecal flora was performed after treatment, and the species diversity was evaluated at the operational taxonomic unit (OUT) level. The combination treatment group had a higher alpha diversity than the 5-FU alone group (faith_pd index [p = 0.032], chao1 index [p = 0.095]) (Figures 6A,B, Supplementary Table S1), suggesting that the combination treatment improved the richness of intestinal flora. Principal component analysis (Figures 6C,D) and principal coordinates analysis (Figures 6E,F) showed clear differences between the control and ABP groups (R = 0.204, p = 0.021) and between the combination and 5-FU groups (R = 0.231, p = 0.011), indicating that ABP treatment significantly affected microbial composition. The composition of intestinal flora at the phylum and family level were shown in Figure 7A (Supplementary Table S2). To clarify the specific changes in the microbial taxa, we further analyzed the relative abundance of 22 predominant genera in the four groups. The results showed that at the genus level, the combination treatment group had significantly higher relative abundance of Roseburia, Clostridium, Anaerotruncus, Bacteroides, Dehalobacterium, Corprococcus, Prevotella and Oscillospira (Figure 7B), while the 5-FU treatment group had a highest level of Enterococcus and Lactobaccilus.
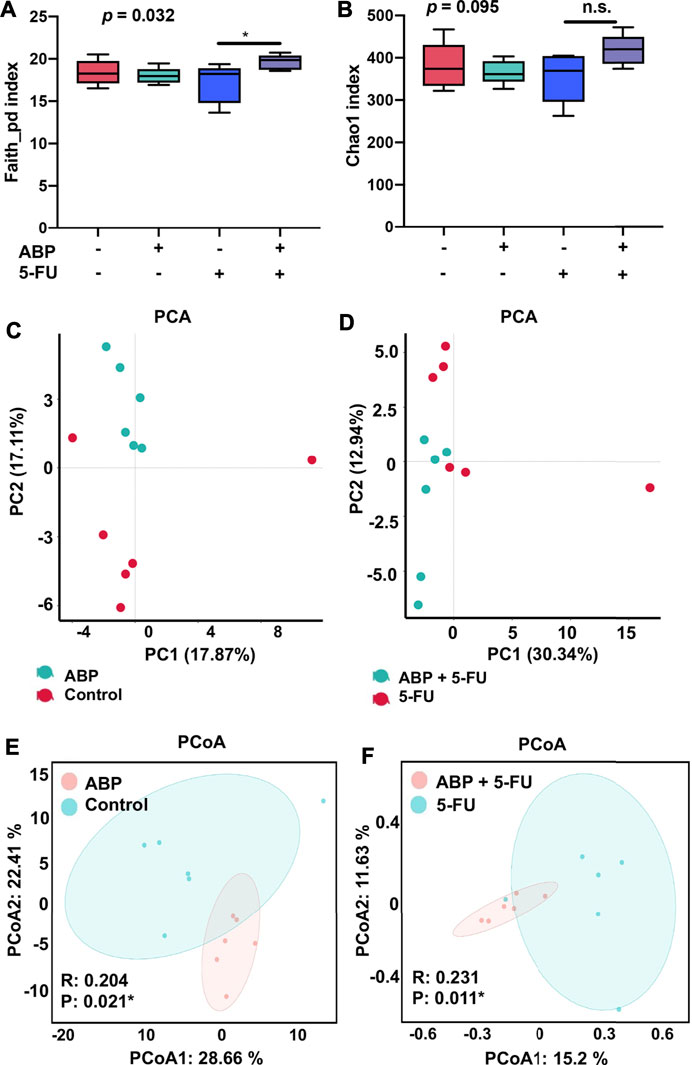
FIGURE 6. ABP treatment alters the abundance and diversity of gut microbes in mice bearing CT26 tumors. (A-B) Analysis of alpha diversity; (A) Faith_pd index. (B) chao1 index. (C-F) Analysis of beta diversity. (C-D) Principal components analysis (PCA). (E-F) principal coordination analysis (PCoA) (n = 6 mice per group).
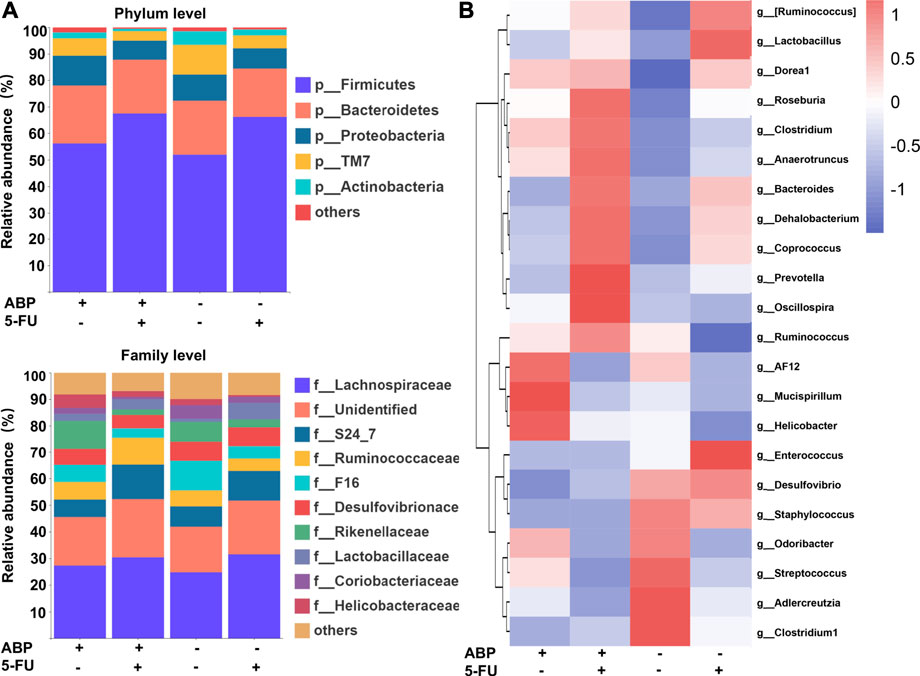
FIGURE 7. ABP treatment improves the composition of the intestinal microflora. (A) Bar plots of bacterial taxa present in the feces at the phylum and family levels based on relative abundance. (B) Heatmap of bacterial taxa present in the feces at the genus level based on relative abundance.
To further identify microbial taxa that serve as biomarkers for different groups, we performed liner discriminate analysis (LDA) and effect size measurements (LEfSe). Biomarkers for each group ranked by effect size (p = 0.05, LDA score > 2) were shown in Figures 8A,B. To further clarify the taxa modulated by ABP, the Kruskal–Wallis test was applied to analyze the difference among the four groups. Compared with the 5-FU group, the combination treatment group harbored a higher relative abundance of some SCFA-producing bacteria (f_Ruminococcaceae, g_Ruminococcus, g_Anaerostipes, g_Oscillospira, g_Parabacteroides) and a lower relative abundance of certain potentially pathogenic, inflammation-promoting bacteria (f_Staphylococcus, g_Staphylococcus, s_Staphylococcus sciuri, s_ Ruminoccocus gnavus). Moreover, compared with the control group, the ABP treatment groups had a higher relative abundance of some SCFA-producing bacteria (g_Alistipes, g_Roseburia) and a lower abundance of potentially pathogenic bacteria (g_Ralstonia, g_Staphylococcus, s_Staphylococcus sciuri) (Figure 8C, Supplementary Table S3).
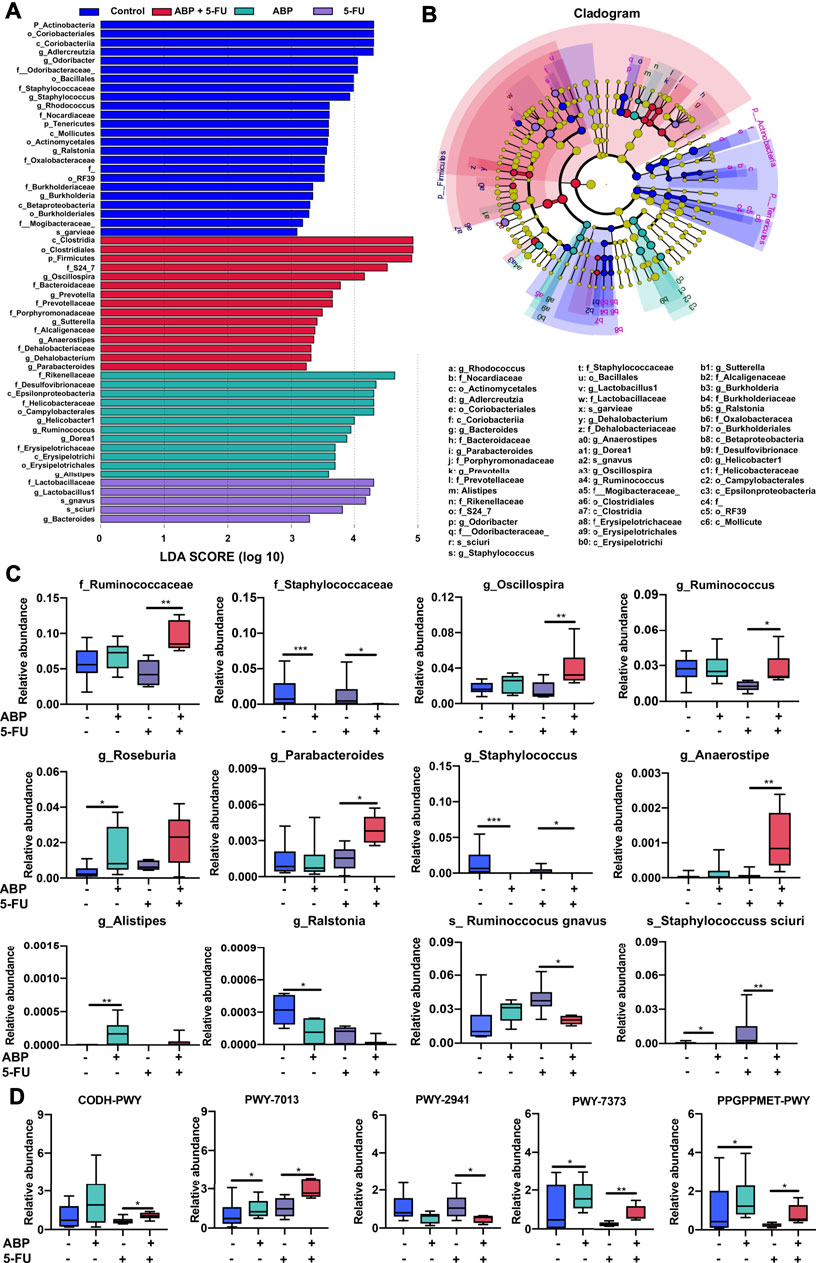
FIGURE 8. ABP treatment leads to the enrichment of beneficial bacteria and influences metabolic pathways. (A-B) Bacterial taxa differences among the four groups, observed using LEfSe analysis. (C) Box plots demonstrating the characteristic bacteria at the family, genus, and species levels. (D) Difference in functional metabolic pathways among the groups. *p < 0.05, **p < 0.01, ***p < 0.001.
Compared to the 5-FU group, the relative abundance of bacteria involved in the PWY-7373 (superpathway of demethylmenaquinol-6 biosynthesis II), PWY-7013 (L-1,2-propanediol degradation), PPGPPMET-PWY (ppGpp biosynthesis), and CODH-PWY (reductive acetyl coenzyme A pathway) pathways was higher. In contrast, the PWY-2941 (L-lysine biosynthesis II) pathway was lower in the combination treatment groups than in the 5-FU group (Figure 8D, Supplementary Table S4). These data suggested that ABP treatment may ameliorate the dysbiosis induced by 5-FU, and combined treatment could enhance the therapeutic efficacy of 5-FU in part by increasing the levels of beneficial bacteria and improving metabolite composition.
ABP Treatment Improves the Fecal SCFA Levels in Mouse Models of CRC
To elucidate the positive effects of ABP treatment on the metabolic activity of the intestinal flora, the concentrations of fecal SCFAs were measured using gas chromatography–mass spectrometry (GC–MS). PCoA and PLSDA analysis indicated that 5-FU treatment resulted in a distinct fecal SCFA composition profile (Figures 9A,B). A heat map was generated to visualize the data more intuitively. As shown in Figure 9C, the concentration of each beneficial SCFA in the 5-FU treatment group was lower than that of the other two groups. From the standpoint of positive impact, the combination treatment elevated all beneficial SCFA, especially the butyric acid (Figure 9C). The concentration of acetic acid (p < 0.01), propionic acid (p < 0.05), butyric acid (p < 0.05), valeric acid (p < 0.01), and caproic acid (p = 0.13) were higher in the combination treatment group than that in the 5-FU treatment group. In contrast, concentration of isovaleric acid (p < 0.01) and isobutyric acid (p = 0.23) were higher in the 5-FU treatment group (Figures 9C,D, Supplementary Table S5). These data suggested that 5-FU depleted most beneficial SCFAs, and ABP treatment could elevate the levels of beneficial SCFAs in the feces of CT26 tumor-bearing mice.
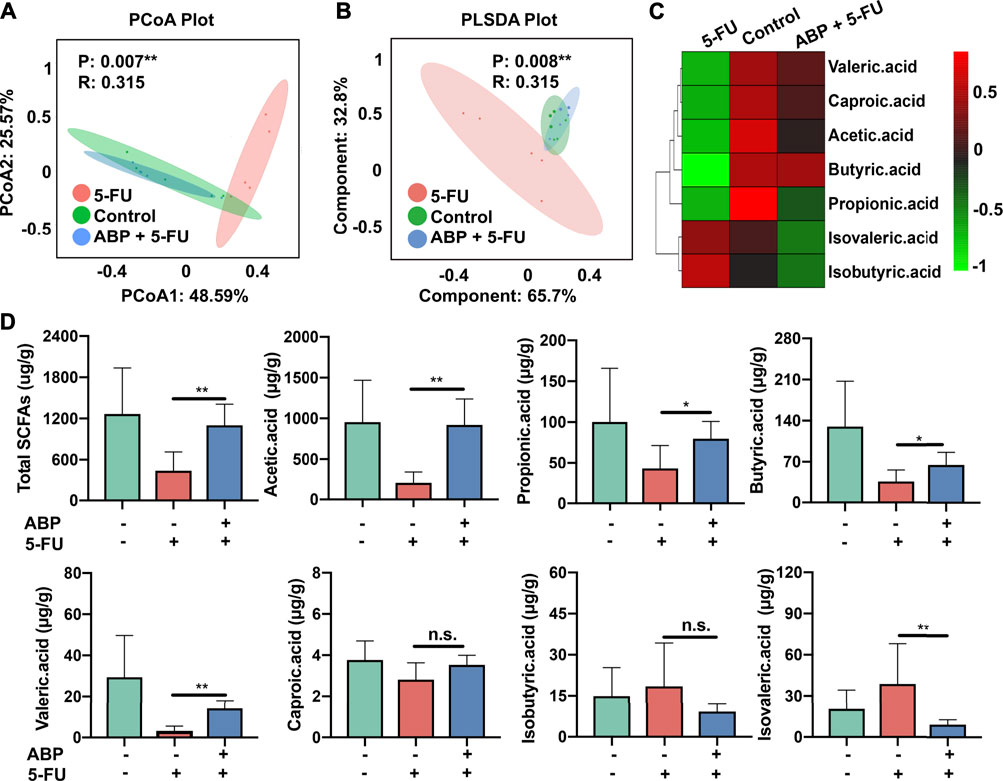
FIGURE 9. ABP treatment improves the concentrations of fecal short-chain fatty acids in CRC model mice. (A-B) PCoA and PLS-DA analysis. (C-D) Heatmap and Bar plots for fecal SCFA content. *p < 0.05, ** represents p < 0.01.
Discussion
CRC is the second leading cause of cancer-related death in the United States and the third most common cancer worldwide. The use of 5-FU has been a standard approach for CRC treatment since the 1950s (Cho et al., 2020), and 5-FU-based chemotherapy remains the predominant approach for CRC, especially metastatic CRC (Van Cutsem et al., 2016). However, in addition to severe immunosuppressive effects and drug toxicity, 5-FU treatment also leads to primary and secondary chemoresistance (Xie N. et al., 2012). Given the enormous damage caused by cancer and the continuous emergence of chemoresistance, novel drugs and anti-tumor treatments are required. Further, the use of conventional chemotherapeutic agents in combination with natural compounds to provide synergistic anti-tumor effects could also be vastly helpful.
Natural products have served as important anti-tumor agents and have been developed for clinical use (Zi et al., 2019). Moreover, anti-tumor compounds with few or no adverse effects hold promise as drug alternatives or adjuvant agents in cancer treatment (Haq et al., 2019). Many plant-derived compounds such as tanshinones, curcumin, and Ganoderma lucidum polysaccharides have drawn great attention because of their broad-spectrum anti-tumor effects and high efficacy (Liang et al., 2014; Kaya et al., 2019; Fu et al., 2020).
Owing to their broad array of anti-inflammatory, anti-neoplastic, anti-oxidant, anti-bacterial, and anti-diabetic properties, plant polysaccharides have been the focus of intensive research (Ji et al., 2018). AB, a herbal remedy for hepatitis, diabetes, and many types of cancers (Zhang et al., 2017), has been used in TCM in some regions of China for several decades. Phytochemical examinations have confirmed that AB has broad bioactive properties owing to the various saponins, polysaccharides, flavonoids, and cholestane glycosides it contains (Zhou et al., 2005; Iguchi et al., 2017). Considering that TCM preparations of AB are generally decoctions and are administered orally, and given the high levels of potentially prebiotic polysaccharides AB contains (Chen et al., 2012), we speculated that ABP could contribute to the anti-tumor effects of AB. On testing this hypothesis, we found that ABP treatment effectively inhibits the growth, migration, and invasion of CRC cells in vitro and suppresses subcutaneous tumor growth in vivo.
CRC development is a complex, multi-step process, involving abnormalities in the Wnt/β-catenin, Hedgehog, Notch, TGF-β, Jak-STAT, and Ras-Raf-MAPK pathways (Calvert and Frucht, 2002). Aberrant Wnt/β-catenin pathway activation is evident in an estimated 90% of CRC tumors, and this pathway is therefore thought to play a central role in the development and malignant growth of such tumors (Kimelman and Xu, 2006). Abnormal activation of the classical Wnt signaling pathway results in high levels of β-catenin, driving EMT induction and cancer stem cell development, thereby enhancing the overall malignancy of CRC tumors (Ghahhari and Babashah, 2015). Cyclin D1 and c-Myc are downstream members of the β-catenin signaling pathway, and their overexpression results in enhanced proliferation and malignant transformation (Hsieh et al., 2012; Lin et al., 2015). Further, high c-Myc expression is also associated with poorer long-term outcomes in CRC patients (Matsushita et al., 2006). The EMT process is tightly linked to tumor cell invasion and metastasis, and it is characterized by E-cadherin downregulation and elevated N-cadherin and Vimentin expression (Vu and Datta, 2017).
We found that ABP treatment suppresses the expression of β-Catenin and related proteins, including c-Myc, Cyclin D1, PCNA, COX-2, and Vimentin, and elevates E-cadherin levels in a dose-dependent manner. β-catenin is crucial for Wnt/β-catenin signaling. Given that SKL2001 reverses ABP-induced β-catenin downregulation and cell proliferation, we concluded that β-Catenin was a crucial target of ABP in CT26 cells and suppressed the proliferative ability and malignancy of these cells at least partially by downregulating β-catenin and altering the expression of downstream genes.
Chemoresistance to 5-FU remains a major obstacle in CRC treatment. Drebber et al. reported that CRC patients with high levels of β-catenin show low sensitivity to 5-FU treatment (Drebber et al., 2011). In line with prior reports (Xie J. et al., 2012; Regmi et al., 2015), in our study, treatment with the β-catenin inhibitor XAV939 confirmed that the suppression of β-catenin signaling was sufficient to enhance the sensitivity of CRC cells to 5-FU. We further explored the effect of combined treatment with ABP and 5-FU on CT26 cells. The in vitro results showed that combined treatment provided better anti-tumor efficacy than ABP or 5-FU treatment alone. Further analysis indicated that ABP and 5-FU showed minor synergistic effects (Supplementary Figure S3).
Given the prebiotic potential of polysaccharides, we speculated that the efficacy of combination treatment would be better in vivo. We found that in a murine model of CRC, the anti-tumor efficacy of combination treatment was much better than that of single-agent treatment with 5-FU or ABP. Further, the spleen index was higher in the combination treatment group than in 5-FU-treated mice (Figures 5B,C,G). Moreover, a fascinating prolongation of the suppression period (4 days) was observed in the combination group (Figure 5A), while tumor proliferation resumed soon after the cessation of chemotherapy in the 5-FU-treated group.
To reveal the multiple mechanisms underlying the effects of ABP on CRC in vivo, western blot analyses were performed. We examined changes in proteins involved in the Wnt/β-catenin signaling pathway, and the results corroborated our in vitro findings.
Various factors contribute to CRC oncogenesis, including diet, drug intake, and environmental conditions, and all of these can shape the composition, function, and metabolite production capabilities of the intestinal microbiota (Wang et al., 2018). Billions of bacteria reside within the human colon, where they serve as essential bridges between the host and specific drug responses and functional nutrients, contributing to both normal homeostasis and dysbiosis-associated pathological conditions such as inflammatory bowel disease (Backhed et al., 2005; Raman et al., 2013).
Polysaccharides improve immune responses and chemosensitivity in cancer patients (Yin et al., 2015), and the anti-tumor effects of polysaccharides are partially due to their regulation of gut microbiota and promotion of fecal SCFA levels (Wang et al., 2017). In the colon, SCFAs serve as an essential energy source for epithelial cells and can regulate key inflammatory processes within the colon to maintain normal host physiology (Kim et al., 2014; Schulz et al., 2014; Yang et al., 2020). Hence, we analyzed the fecal microbiome composition and fecal SCFA concentration to clarify the anti-tumor functions of ABP. 16S rRNA sequencing revealed that the genera Ruminococcus, Anaerostipes, Oscillospira, Alistipes, and Roseburia were enriched in fecal samples from the ABP treatment group. These bacteria are potential producers of SCFAs (Gophna et al., 2017; Kasahara et al., 2018; La Reau and Suen, 2018; Markowiak-Kopec and Slizewska, 2020; Parker et al., 2020). Data also indicated that ABP inhibited the growth of certain pathogenic inflammation-promoting bacteria (g_Staphylococcus, s_Staphylococcus sciuri, s_-Ruminoccocus gnavus). It was clear that ABP treatment ameliorated the dysbiosis induced by 5-FU and improved the intestinal flora composition in CRC model mice to a certain extent.
Butyric acid is a crucial SCFA in the intestinal tract, and it can kill CRC cells and inhibit their migration (Wang et al., 2020). GC–MS results revealed that ABP could increase the levels of some beneficial SCFAs (acetic acid, propionic acid, and butyric acid) in mice treated with 5-FU. The changes in fecal SCFA levels in this study were consistent with shifts in the intestinal microbiome composition. We thus speculated that in addition to promoting β-catenin downregulation, ABP also promotes anti-tumor effects in vivo by increasing the abundance of SCFA-producing bacteria and the levels of beneficial SCFAs. Such improvement in the intestinal microbiome and associated metabolite production may, in turn, reverse the 5-FU-induced immunosuppression and dysbiosis.
Together, our data indicate that ABP treatment alone or in combination with 5-FU could reduce the expression of β-catenin and related proteins in CRC cells. ABP treatment synergistically enhances the anti-tumor efficacy of 5-FU by promoting β-Catenin downregulation, improving the composition of intestinal flora, and elevating fecal SCFA levels (Figure 10). The results imply that ABP may be a favorable anti-cancer agent for enhancing the efficacy of chemotherapy.
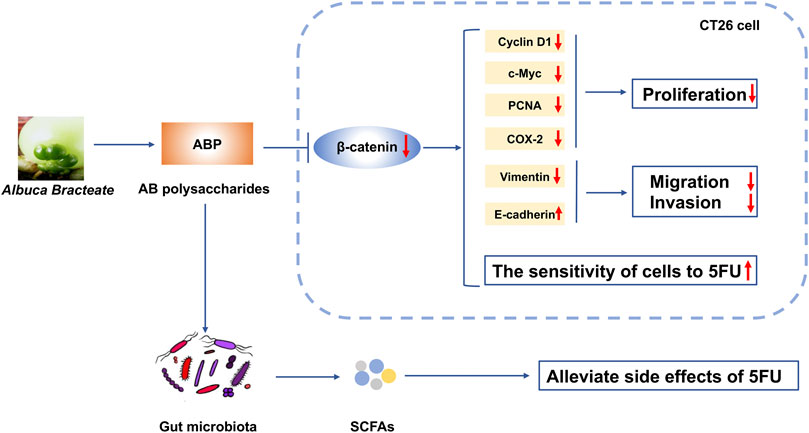
FIGURE 10. Schematic diagram depicting the mechanism of action underlying the effect of ABP as a regulator of CRC progression. ABP suppresses CRC cell proliferation, migration, and invasion by influencing the Wnt/β-catenin signaling pathway. ABP treatment further increases the sensitivity of CRC cells to 5-FU and thereby decreases the incidence of 5-FU-related adverse effects. ABP, Albuca Bracteate polysaccharides; CRC, colorectal cancer.
Our study suggests that ABP is a promising anti-cancer drug with the potential to serve as a valuable chemotherapy adjuvant agent for the clinical treatment of CRC. However, there are some limitations of the study. First, further preclinical research is required to investigate the pharmacologic action of ABP, although our data showed that β-catenin signaling is involved in the anti-tumor effect of ABP. Second, the anti-tumor effects of ABP were only performed in CT26, a murine colon carcinoma cell line. Effects and underlying mechanisms of ABP on human CRC cell lines in vitro and in vivo models will require intensive research.
Data Availability Statement
The datasets presented in this study can be found in online repositories. The names of the repository/repositories and accession number(s) can be found in the article/Supplementary Material. The 16S rRNA gene sequence data (accession number: PRJNA733597) have been deposited in the NCBI’s Sequence Read Archive database.
Ethics Statement
The animal study was reviewed and approved by the Animal Ethics Committee of Wenzhou Medical University, China (wydw 2021-0224). Written informed consent was obtained from the owners for the participation of their animals in this study.
Author Contributions
YL, LJ, and JD conceived and supervised the project and designed the experiments. JD and XY contributed to the design of the experiments, XY performed most of the experiments, and JD and XY analyzed the results and wrote the manuscript. JX, ZQ, ZJ, and YW performed a part of the experiments. XY, YT, XB, and LP analyzed the data and created the figures. SL, QY, and ZQ performed the literature review. LJ and JD revised the manuscript. YL, LJ, and JD are the corresponding authors. All authors provided their final approval for the publication of this manuscript.
Funding
This research was supported by the Fundamental Public Welfare Research Program of Zhejiang Province (No. LGF18H180012).
Conflict of Interest
The authors declare that the research was conducted in the absence of any commercial or financial relationships that could be construed as a potential conflict of interest.
Publisher’s Note
All claims expressed in this article are solely those of the authors and do not necessarily represent those of their affiliated organizations, or those of the publisher, the editors and the reviewers. Any product that may be evaluated in this article, or claim that may be made by its manufacturer, is not guaranteed or endorsed by the publisher.
Acknowledgments
The authors thank Mr. Guohua Deng for his kind help with cultivating the AB on his farm. We also express our gratefulness to Professor Pan Li of Zhejiang University and Professor Haibin Tong of Wenzhou University for helping us with the identification of AB and ABP. We also extend our appreciation to Microeco Tech Co. Ltd., Shenzhen, China, for their sequencing and metabolomic profiling services.
Supplementary Material
The Supplementary Material for this article can be found online at: https://www.frontiersin.org/articles/10.3389/fphar.2021.736627/full#supplementary-material
References
Bäckhed, F., Ley, R. E., Sonnenburg, J. L., Peterson, D. A., and Gordon, J. I. (2005). Host-bacterial Mutualism in the Human Intestine. Science 307 (5717), 1915–1920. doi:10.1126/science.1104816
Cai, B., Pan, J., Chen, H., Chen, X., Ye, Z., Yuan, H., et al. (2021). Oyster Polysaccharides Ameliorate Intestinal Mucositis and Improve Metabolism in 5-Fluorouracil-Treated S180 Tumour-Bearing Mice. Carbohydr. Polym. 256, 117545. doi:10.1016/j.carbpol.2020.117545
Calvert, P. M., and Frucht, H. (2002). The Genetics of Colorectal Cancer. Ann. Intern. Med. 137 (7), 603–612. doi:10.7326/0003-4819-137-7-200210010-00012
Chen, R., Li, Y., Dong, H., Liu, Z., Li, S., Yang, S., et al. (2012). Optimization of Ultrasonic Extraction Process of Polysaccharides from Ornithogalum Caudatum Ait and Evaluation of its Biological Activities. Ultrason. Sonochem. 19 (6), 1160–1168. doi:10.1016/j.ultsonch.2012.03.008
Cho, Y. H., Ro, E. J., Yoon, J. S., Mizutani, T., Kang, D. W., Park, J. C., et al. (2020). 5-FU Promotes Stemness of Colorectal Cancer via P53-Mediated WNT/β-catenin Pathway Activation. Nat. Commun. 11 (1), 5321. doi:10.1038/s41467-020-19173-2
Chou, T. C. (2010). Drug Combination Studies and Their Synergy Quantification Using the Chou-Talalay Method. Cancer Res. 70 (2), 440–446. doi:10.1158/0008-5472.CAN-09-1947
Clevers, H., and Nusse, R. (2012). Wnt/β-catenin Signaling and Disease. Cell 149 (6), 1192–1205. doi:10.1016/j.cell.2012.05.012
Drebber, U., Madeja, M., Odenthal, M., Wedemeyer, I., Mönig, S. P., Brabender, J., et al. (2011). β-Catenin and Her2/neu Expression in Rectal Cancer: Association with Histomorphological Response to Neoadjuvant Therapy and Prognosis. Int. J. Colorectal Dis. 26 (9), 1127–1134. doi:10.1007/s00384-011-1213-9
Feng, Z., Yang, R., Wu, L., Tang, S., Wei, B., Guo, L., et al. (2019). Atractylodes Macrocephala Polysaccharides Regulate the Innate Immunity of Colorectal Cancer Cells by Modulating the TLR4 Signaling Pathway. Onco Targets Ther. 12, 7111–7121. doi:10.2147/OTT.S219623
Fu, L., Han, B., Zhou, Y., Ren, J., Cao, W., Patel, G., et al. (2020). The Anticancer Properties of Tanshinones and the Pharmacological Effects of Their Active Ingredients. Front. Pharmacol. 11, 193. doi:10.3389/fphar.2020.00193
Ghahhari, N. M., and Babashah, S. (2015). Interplay between microRNAs and WNT/β-catenin Signalling Pathway Regulates Epithelial-Mesenchymal Transition in Cancer. Eur. J. Cancer 51 (12), 1638–1649. doi:10.1016/j.ejca.2015.04.021
Gophna, U., Konikoff, T., and Nielsen, H. B. (2017). Oscillospira and Related Bacteria - from Metagenomic Species to Metabolic Features. Environ. Microbiol. 19 (3), 835–841. doi:10.1111/1462-2920.13658
Gori, S., Inno, A., Belluomini, L., Bocus, P., Bisoffi, Z., Russo, A., et al. (2019). Gut Microbiota and Cancer: How Gut Microbiota Modulates Activity, Efficacy and Toxicity of Antitumoral Therapy. Crit. Rev. Oncol. Hematol. 143, 139–147. doi:10.1016/j.critrevonc.2019.09.003
Hamouda, N., Sano, T., Oikawa, Y., Ozaki, T., Shimakawa, M., Matsumoto, K., et al. (2017). Apoptosis, Dysbiosis and Expression of Inflammatory Cytokines Are Sequential Events in the Development of 5-Fluorouracil-Induced Intestinal Mucositis in Mice. Basic Clin. Pharmacol. Toxicol. 121 (3), 159–168. doi:10.1111/bcpt.12793
Haq, S. H., Al-Ruwaished, G., Al-Mutlaq, M. A., Naji, S. A., Al-Mogren, M., Al-Rashed, S., et al. (2019). Antioxidant, Anticancer Activity and Phytochemical Analysis of Green Algae, Chaetomorpha Collected from the Arabian Gulf. Sci. Rep. 9 (1), 18906. doi:10.1038/s41598-019-55309-1
He, L., Zhu, H., Zhou, S., Wu, T., Wu, H., Yang, H., et al. (2018). Wnt Pathway Is Involved in 5-FU Drug Resistance of Colorectal Cancer Cells. Exp. Mol. Med. 50 (8), 101–112. doi:10.1038/s12276-018-0128-8
Hsieh, T. C., Yang, C. J., Lin, C. Y., Lee, Y. S., and Wu, J. M. (2012). Control of Stability of Cyclin D1 by Quinone Reductase 2 in CWR22Rv1 Prostate Cancer Cells. Carcinogenesis 33 (3), 670–677. doi:10.1093/carcin/bgs016
Ianevski, A., Giri, A. K., and Aittokallio, T. (2020). SynergyFinder 2.0: Visual Analytics of Multi-Drug Combination Synergies. Nucleic Acids Res. 48 (W1), W488–W493. doi:10.1093/nar/gkaa216
Iguchi, T., Kuroda, M., Naito, R., Watanabe, T., Matsuo, Y., Yokosuka, A., et al. (2017). Structural Characterization of Cholestane Rhamnosides from Ornithogalum Saundersiae Bulbs and Their Cytotoxic Activity against Cultured Tumor Cells. Molecules 22 (8). doi:10.3390/molecules22081243
Ji, X., Peng, Q., and Wang, M. (2018). Anti-colon-cancer Effects of Polysaccharides: A Mini-Review of the Mechanisms. Int. J. Biol. Macromol 114, 1127–1133. doi:10.1016/j.ijbiomac.2018.03.186
Jiang, S., Song, C., Gu, X., Wang, M., Miao, D., Lv, J., et al. (2018). Ubiquitin-Specific Peptidase 22 Contributes to Colorectal Cancer Stemness and Chemoresistance via Wnt/β-Catenin Pathway. Cell Physiol Biochem 46 (4), 1412–1422. doi:10.1159/000489156
Karin, M., Jobin, C., and Balkwill, F. (2014). Chemotherapy, Immunity and Microbiota-Aa New Triumvirate? Nat. Med. 20 (2), 126–127. doi:10.1038/nm.3473
Kasahara, K., Krautkramer, K. A., Org, E., Romano, K. A., Kerby, R. L., Vivas, E. I., et al. (2018). Interactions between Roseburia Intestinalis and Diet Modulate Atherogenesis in a Murine Model. Nat. Microbiol. 3 (12), 1461–1471. doi:10.1038/s41564-018-0272-x
Kaya, P., Lee, S. R., Lee, Y. H., Kwon, S. W., Yang, H., Lee, H. W., et al. (2019). Curcumae Radix Extract Decreases Mammary Tumor-Derived Lung Metastasis via Suppression of C-C Chemokine Receptor Type 7 Expression. Nutrients 11 (2). doi:10.3390/nu11020410
Kim, C. H., Park, J., and Kim, M. (2014). Gut Microbiota-Derived Short-Chain Fatty Acids, T Cells, and Inflammation. Immune Netw. 14 (6), 277–288. doi:10.4110/in.2014.14.6.277
Kimelman, D., and Xu, W. (2006). Beta-Catenin Destruction Complex: Insights and Questions from a Structural Perspective. Oncogene 25 (57), 7482–7491. doi:10.1038/sj.onc.1210055
La Reau, A. J., and Suen, G. (2018). The Ruminococci: Key Symbionts of the Gut Ecosystem. J. Microbiol. 56 (3), 199–208. doi:10.1007/s12275-018-8024-4
Li, C., Li, Z., Zhang, T., Wei, P., Li, N., Zhang, W., et al. (2019). 1H NMR-Based Metabolomics Reveals the Antitumor Mechanisms of Triptolide in BALB/c Mice Bearing CT26 Tumors. Front. Pharmacol. 10, 1175. doi:10.3389/fphar.2019.01175
Li, Y., Li, H., Liu, S., Pan, P., Su, X., Tan, H., et al. (2018). Pirfenidone Ameliorates Lipopolysaccharide-Induced Pulmonary Inflammation and Fibrosis by Blocking NLRP3 Inflammasome Activation. Mol. Immunol. 99, 134–144. doi:10.1016/j.molimm.2018.05.003
Liang, Z., Yi, Y., Guo, Y., Wang, R., Hu, Q., and Xiong, X. (2014). Chemical Characterization and Antitumor Activities of Polysaccharide Extracted from Ganoderma Lucidum. Int. J. Mol. Sci. 15 (5), 9103–9116. doi:10.3390/ijms15059103
Lin, J. T., Li, H. Y., Chang, N. S., Lin, C. H., Chen, Y. C., and Lu, P. J. (2015). WWOX Suppresses Prostate Cancer Cell Progression through Cyclin D1-Mediated Cell Cycle Arrest in the G1 Phase. Cell Cycle 14 (3), 408–416. doi:10.4161/15384101.2014.977103
Liu, L., Li, M., Yu, M., Shen, M., Wang, Q., Yu, Y., et al. (2019). Natural Polysaccharides Exhibit Anti-tumor Activity by Targeting Gut Microbiota. Int. J. Biol. Macromol 121, 743–751. doi:10.1016/j.ijbiomac.2018.10.083
Liu, L., Liu, Z., Li, H., Cao, Z., Li, W., Song, Z., et al. (2020). Naturally Occurring TPE-CA Maintains Gut Microbiota and Bile Acids Homeostasis via FXR Signaling Modulation of the Liver-Gut Axis. Front. Pharmacol. 11, 12. doi:10.3389/fphar.2020.00012
Liu, L. Q., Nie, S. P., Shen, M. Y., Hu, J. L., Yu, Q., Gong, D., et al. (2018). Tea Polysaccharides Inhibit Colitis-Associated Colorectal Cancer via Interleukin-6/STAT3 Pathway. J. Agric. Food Chem. 66 (17), 4384–4393. doi:10.1021/acs.jafc.8b00710
Liu, R. H. (2004). Potential Synergy of Phytochemicals in Cancer Prevention: Mechanism of Action. J. Nutr. 134 (12 Suppl. l), 3479S–3485S. doi:10.1093/jn/134.12.3479S
Luo, L., Sun, W., Zhu, W., Li, S., Zhang, W., Xu, X., et al. (2021). BCAT1 Decreases the Sensitivity of Cancer Cells to Cisplatin by Regulating mTOR-Mediated Autophagy via Branched-Chain Amino Acid Metabolism. Cell Death Dis 12 (2), 169. doi:10.1038/s41419-021-03456-7
Malanchi, I., Peinado, H., Kassen, D., Hussenet, T., Metzger, D., Chambon, P., et al. (2008). Cutaneous Cancer Stem Cell Maintenance Is Dependent on Beta-Catenin Signalling. Nature 452 (7187), 650–653. doi:10.1038/nature06835
Markowiak-Kopeć, P., and Śliżewska, K. (2020). The Effect of Probiotics on the Production of Short-Chain Fatty Acids by Human Intestinal Microbiome. Nutrients 12 (4), 1107. doi:10.3390/nu12041107
Matsushita, K., Takenouchi, T., Shimada, H., Tomonaga, T., Hayashi, H., Shioya, A., et al. (2006). Strong HLA-DR Antigen Expression on Cancer Cells Relates to Better Prognosis of Colorectal Cancer Patients: Possible Involvement of C-Myc Suppression by Interferon-Gamma In Situ. Cancer Sci. 97 (1), 57–63. doi:10.1111/j.1349-7006.2006.00137.x
Parker, B. J., Wearsch, P. A., Veloo, A. C. M., and Rodriguez-Palacios, A. (2020). The Genus Alistipes: Gut Bacteria with Emerging Implications to Inflammation, Cancer, and Mental Health. Front. Immunol. 11, 906. doi:10.3389/fimmu.2020.00906
Peng, J., Lu, X., Xie, K., Xu, Y., He, R., Guo, L., et al. (2019). Dynamic Alterations in the Gut Microbiota of Collagen-Induced Arthritis Rats Following the Prolonged Administration of Total Glucosides of Paeony. Front Cel Infect Microbiol 9, 204. doi:10.3389/fcimb.2019.00204
Raman, M., Ambalam, P., Kondepudi, K. K., Pithva, S., Kothari, C., Patel, A. T., et al. (2013). Potential of Probiotics, Prebiotics and Synbiotics for Management of Colorectal Cancer. Gut Microbes 4 (3), 181–192. doi:10.4161/gmic.23919
Regmi, S. C., Park, S. Y., Kim, S. J., Banskota, S., Shah, S., Kim, D. H., et al. (2015). The Anti-tumor Activity of Succinyl Macrolactin A Is Mediated through the β-Catenin Destruction Complex via the Suppression of Tankyrase and PI3K/Akt. PLoS One 10 (11), e0141753. doi:10.1371/journal.pone.0141753
Reya, T., and Clevers, H. (2005). Wnt Signalling in Stem Cells and Cancer. Nature 434 (7035), 843–850. doi:10.1038/nature03319
Saifo, M. S., Rempinski, D. R., Rustum, Y. M., and Azrak, R. G. (2010). Targeting the Oncogenic Protein Beta-Catenin to Enhance Chemotherapy Outcome against Solid Human Cancers. Mol. Cancer 9, 310. doi:10.1186/1476-4598-9-310
Schulz, M. D., Atay, C., Heringer, J., Romrig, F. K., Schwitalla, S., Aydin, B., et al. (2014). High-fat-diet-mediated Dysbiosis Promotes Intestinal Carcinogenesis Independently of Obesity. Nature 514 (7523), 508–512. doi:10.1038/nature13398
Shang, Q., Jiang, H., Cai, C., Hao, J., Li, G., and Yu, G. (2018). Gut Microbiota Fermentation of marine Polysaccharides and its Effects on Intestinal Ecology: An Overview. Carbohydr. Polym. 179, 173–185. doi:10.1016/j.carbpol.2017.09.059
Shen, L., Lei, S., Zhang, B., Li, S., Huang, L., Czachor, A., et al. (2020). Skipping of Exon 10 in Axl Pre-mRNA Regulated by PTBP1 Mediates Invasion and Metastasis Process of Liver Cancer Cells. Theranostics 10 (13), 5719–5735. doi:10.7150/thno.42010
Shionome, Y., Lin, W. H., Shiao, H. Y., Hsieh, H. P., Hsu, J. T., and Ouchi, T. (2013). A Novel aurora-A Inhibitor, BPR1K0609S1, Sensitizes Colorectal Tumor Cells to 5-fluorofracil (5-FU) Treatment. Int. J. Biol. Sci. 9 (4), 403–411. doi:10.7150/ijbs.5806
Siegel, R. L., Miller, K. D., Goding Sauer, A., Fedewa, S. A., Butterly, L. F., Anderson, J. C., et al. (2020). Colorectal Cancer Statistics, 2020. CA Cancer J. Clin. 70 (3), 145–164. doi:10.3322/caac.21601
Siegel, R. L., Miller, K. D., Fuchs, H. E., and Jemal, A. (2021). Cancer Statistics, 2021. CA A. Cancer J. Clin. 71 (1), 7–33. doi:10.3322/caac.21654
Uppada, S. B., Gowrikumar, S., Ahmad, R., Kumar, B., Szeglin, B., Chen, X., et al. (2018). MASTL Induces Colon Cancer Progression and Chemoresistance by Promoting Wnt/β-Catenin Signaling. Mol. Cancer 17 (1), 111. doi:10.1186/s12943-018-0848-3
Van Cutsem, E., Cervantes, A., Adam, R., Sobrero, A., Van Krieken, J. H., Aderka, D., et al. (2016). ESMO Consensus Guidelines for the Management of Patients with Metastatic Colorectal Cancer. Ann. Oncol. 27 (8), 1386–1422. doi:10.1093/annonc/mdw235
Vu, T., and Datta, P. K. (2017). Regulation of EMT in Colorectal Cancer: A Culprit in Metastasis. Cancers (Basel) 9 (12). doi:10.3390/cancers9120171
Wan, Y., Zhang, Y., Wang, G., Mwangi, P. M., Cai, H., and Li, R. (2020). Recombinant KRAS G12D Protein Vaccines Elicit Significant Anti-tumor Effects in Mouse CT26 Tumor Models. Front. Oncol. 10, 1326. doi:10.3389/fonc.2020.01326
Wang, C. Z., Huang, W. H., Zhang, C. F., Wan, J. Y., Wang, Y., Yu, C., et al. (2018). Role of Intestinal Microbiome in American Ginseng-Mediated colon Cancer Prevention in High Fat Diet-Fed AOM/DSS Mice [corrected]. Clin. Transl Oncol. 20 (3), 302–312. doi:10.1007/s12094-017-1717-z
Wang, R., Sun, Y., Yu, W., Yan, Y., Qiao, M., Jiang, R., et al. (2019). Downregulation of miRNA-214 in Cancer-Associated Fibroblasts Contributes to Migration and Invasion of Gastric Cancer Cells through Targeting FGF9 and Inducing EMT. J. Exp. Clin. Cancer Res. 38 (1), 20. doi:10.1186/s13046-018-0995-9
Wang, S., Li, Q., Zang, Y., Zhao, Y., Liu, N., Wang, Y., et al. (2017). Apple Polysaccharide Inhibits Microbial Dysbiosis and Chronic Inflammation and Modulates Gut Permeability in HFD-Fed Rats. Int. J. Biol. Macromol 99, 282–292. doi:10.1016/j.ijbiomac.2017.02.074
Wang, W., Fang, D., Zhang, H., Xue, J., Wangchuk, D., Du, J., et al. (2020). Sodium Butyrate Selectively Kills Cancer Cells and Inhibits Migration in Colorectal Cancer by Targeting Thioredoxin-1. Onco Targets Ther. 13, 4691–4704. doi:10.2147/OTT.S235575
Wardill, H. R., Bowen, J. M., and Gibson, R. J. (2014). New Pharmacotherapy Options for Chemotherapy-Induced Alimentary Mucositis. Expert Opin. Biol. Ther. 14 (3), 347–354. doi:10.1517/14712598.2014.874412
Xie, J., Xiang, D. B., Wang, H., Zhao, C., Chen, J., Xiong, F., et al. (2012a). Inhibition of Tcf-4 Induces Apoptosis and Enhances Chemosensitivity of colon Cancer Cells. PLoS One 7 (9), e45617. doi:10.1371/journal.pone.0045617
Xie, N., Wang, Y., Wang, Q., Li, F. R., and Guo, B. (2012b). Lipoteichoic Acid of Bifidobacterium in Combination with 5-fluorouracil Inhibit Tumor Growth and Relieve the Immunosuppression. Bull. Cancer 99 (5), E55–E63. doi:10.1684/bdc.2012.1571
Yang, W., Yu, T., Huang, X., Bilotta, A. J., Xu, L., Lu, Y., et al. (2020). Intestinal Microbiota-Derived Short-Chain Fatty Acids Regulation of Immune Cell IL-22 Production and Gut Immunity. Nat. Commun. 11 (1), 4457. doi:10.1038/s41467-020-18262-6
Yin, X., Ying, J., Li, L., Zhang, H., and Wang, H. (2015). A Meta-Analysis of Lentinan Injection Combined with Chemotherapy in the Treatment of Nonsmall Cell Lung Cancer. Indian J. Cancer 52 Suppl 1 (Suppl. 1), e29–31. doi:10.4103/0019-509X.168953
Zhang, Y., Fang, F., Fan, K., Zhang, Y., Zhang, J., Guo, H., et al. (2017). Effective Cytotoxic Activity of OSW-1 on colon Cancer by Inducing Apoptosis In Vitro and In Vivo. Oncol. Rep. 37 (6), 3509–3519. doi:10.3892/or.2017.5582
Zhou, Y., Garcia-Prieto, C., Carney, D. A., Xu, R. H., Pelicano, H., Kang, Y., et al. (2005). OSW-1: a Natural Compound with Potent Anticancer Activity and a Novel Mechanism of Action. J. Natl. Cancer Inst. 97 (23), 1781–1785. doi:10.1093/jnci/dji404
Keywords: polysaccharide, colorectal cancer, 5-fluorouracil, β-catenin, gut microbiota, short-chain fatty acids
Citation: Yuan X, Xue J, Tan Y, Yang Q, Qin Z, Bao X, Li S, Pan L, Jiang Z, Wang Y, Lou Y, Jiang L and Du J (2021) Albuca Bracteate Polysaccharides Synergistically Enhance the Anti-Tumor Efficacy of 5-Fluorouracil Against Colorectal Cancer by Modulating β-Catenin Signaling and Intestinal Flora. Front. Pharmacol. 12:736627. doi: 10.3389/fphar.2021.736627
Received: 05 July 2021; Accepted: 12 August 2021;
Published: 03 September 2021.
Edited by:
Zhe-Sheng Chen, St. John's University, United StatesReviewed by:
Wenjun Wu, Fox chase Cancer Center, United StatesRishil Kathawala, St. John’s University, United States
Hadiar Rahman, National Institutes of Health (NIH), United States
Copyright © 2021 Yuan, Xue, Tan, Yang, Qin, Bao, Li, Pan, Jiang, Wang, Lou, Jiang and Du. This is an open-access article distributed under the terms of the Creative Commons Attribution License (CC BY). The use, distribution or reproduction in other forums is permitted, provided the original author(s) and the copyright owner(s) are credited and that the original publication in this journal is cited, in accordance with accepted academic practice. No use, distribution or reproduction is permitted which does not comply with these terms.
*Correspondence: Yongliang Lou, lyl@wmu.edu.cn; Lei Jiang, jiangleistone79@163.com; Jimei Du, djm@wmu.edu.cn