- 1VA Palo Alto Health Care System, Palo Alto, CA, United States
- 2Stanford University School of Medicine, Stanford, CA, United States
- 3Departments of Pediatrics, Medicine, Oncology, Radiation Oncology, and Biological Chemistry, and McKusick-Nathans Institute of Genetic Medicine, Vascular Program, Institute for Cell Engineering, Johns Hopkins University School of Medicine, Baltimore, MD, United States
Lymphedema is a chronic inflammatory disorder characterized by edema, fat deposition, and fibrotic tissue remodeling. Despite significant advances in lymphatic biology research, our knowledge of lymphedema pathology is incomplete. Currently, there is no approved pharmacological therapy for this debilitating disease. Hypoxia is a recognized feature of inflammation, obesity, and fibrosis. Understanding hypoxia-regulated pathways in lymphedema may provide new insights into the pathobiology of this chronic disorder and help develop new medicinal treatments.
Introduction
Lymphedema manifests as an initial accumulation of extracellular fluid and heightened inflammation with progressive chronic tissue remodeling characterized by fat deposition and fibrosis (Jiang et al., 2018; Rockson et al., 2019; Li et al., 2020). In the United States, lymphedema afflicts more than five million patients, most of them being cancer survivors who have undergone lymph node removal or radiotherapy (Rockson, 2018; Kataru et al., 2019). Current treatments for lymphedema are physiotherapy and compression garments, but these interventions only transiently reduce edema being ineffective in preventing or reversing disease progression (Rockson, 2008). There is an urgent need to improve the understanding of lymphedema pathogenesis and develop pharmacological therapies.
Prior research demonstrated that hypoxia regulates tumor lymphangiogenesis by promoting lymphatic endothelial expression of Prox-1 and pro-growth molecules such as VEGFC/VEGFR3 and CXCL12/CXCR4 (Irigoyen et al., 2007; Zhou et al., 2013; Ji, 2014; Morfoisse et al., 2015). Emerging precinlinal research indicates that lymphedema tissues are hypoxic with metabolic abnormalities (Poeze, 2006; Tabibiazar et al., 2006; Zampell et al., 2012a; Jiang et al., 2020). These studies suggest that hypoxia-regulated signaling may play a crucial role in lymphedema progression through modulating lymphangiogenesis.
Research over the last 15 years implicates inflammation as a crucial factor promoting lymphedema (Tabibiazar et al., 2006; Ly et al., 2017; Azhar et al., 2020). Clinical studies demonstrated lymphedema tissues are inflamed (Lin et al., 2012). SNP analysis revealed inflammatory gene variants that increase the risk of lymphedema in patients following breast cancer surgery (Leung et al., 2014). Immune cell profiling has revealed that lymphedematous tissues exhibit increased CD4+ T cells, including Th1, Th2, Th17, and regulatory T cells (Tregs) (Avraham et al., 2013; Gousopoulos et al., 2016; Ogata et al., 2016). Th2 cells aggravate lymphedema by augmenting tissue fibrosis (Avraham et al., 2013; Ly et al., 2019), whereas Th1 and Th17 cells promote non-productive lymphangiogenesis and exacerbate lymphedema by enhancing macrophage VEGFC production (Ogata et al., 2016). Tregs are beneficial but insufficient in suppressing effector cells in lymphedema (Gousopoulos et al., 2016). Innate immunity also contributes to lymphedema pathogenesis (Ly et al., 2017; Jiang et al., 2018; Azhar et al., 2020). For instance, dendritic cells (DCs) activate T cells and promote lymphedema (Li et al., 2020); macrophages exert context-dependent effects on lymphedema pathophysiology (Zampell et al., 2012b; Ghanta et al., 2015); and the myeloid cell-derived lipid mediator leukotriene B4 (LTB4) promotes lymphedema and blocking LTB4 production reverses the disease (Tian et al., 2017). Prolonged inflammation and edema ultimately induce chronic tissue pathology including excessive fat accumulation and fibrosis that are irresponsive to physiotherapy and compression garments (Jiang et al., 2018; Jiang et al., 2019a; Azhar et al., 2020; Li et al., 2020).
Anti-inflammatory therapies, including ketoprofen (NSAID) and Th2 cell inhibition, improve skin pathology but are ineffective in reducing limb volume and fat deposition (Rockson et al., 2018; Mehrara et al., 2021). Findings from these pilot clinical trials suggest that anti-inflammatory therapy alone may not be sufficient to reverse lymphedema. A better understanding of the mechanisms involved in inflammation, tissue fibrosis, and adipose expansion will provide a fuller view of lymphedema pathogenesis. As tissue hypoxia and cellular responses to hypoxic stress regulate immune function, adipose pathology, and fibrosis in various chronic diseases (Semenza, 2003; Trayhurn et al., 2008; Eltzschig and Carmeliet, 2011; Trayhurn, 2013; Manresa et al., 2014; Taylor and Colgan, 2017; Lee et al., 2019; Semenza, 2019; Colgan et al., 2020; Lee et al., 2020; Chen and Gaber, 2021), knowledge of hypoxia-regulated pathways in lymphedema will deepen our understanding of this disease. Here we review how hypoxic stress may influence key lymphedema pathology.
Hypoxia and Hypoxia-Inducible Factors
Oxygen homeostasis is an organizing principle for understanding human physiology and pathobiology (Semenza, 2012). Hypoxia reflects conditions in which oxygen demand exceeds supply (Cavadas et al., 2013). The most common causes of tissue hypoxia include vascular insufficiency, edema, and inflammation (Lee et al., 2020). Mammals have evolved systemic adaptive mechanisms to cope with hypoxic stress by increasing ventilation, cardiac output, new vessel growth, and circulating red blood cell numbers (Lee et al., 2020). In contrast, edema and inflammation-associated hypoxia discretely influence the bioenergetics of various cell types within affected tissues and regulate disease pathogenesis (Poeze, 2006; Eltzschig and Carmeliet, 2011; Palazon et al., 2014; Colgan et al., 2020). The best-studied mechanism of responses to hypoxia involves hypoxia-inducible factors (HIFs). HIFs are heterodimers comprised of two subunits: the constitutively expressed β subunit (HIF-1β) and one of the α subunits (HIF-1α or HIF-2α) (Prabhakar and Semenza, 2015; Lee et al., 2020). Under normoxic conditions, the α subunits are subject to proteasome-mediated degradation involving enzymatic activities of dioxygenases termed prolyl hydroxylases (PHDs) and the von Hippel-Lindau tumor suppressor protein (pVHL) in the presence of ferrous iron (Fe2+) and α-ketoglutarate (Semenza, 2012; Lee et al., 2020). Reduced oxygen availability leads to HIF-α stabilization through mechanisms involving paradoxically increased production of reactive oxygen species (ROS) by complex III of the electron transport chain in mitochondria, as well as increased production of L-2-hydroxyglutarate (L-2HG), succinate, and fumarate (Nathan and Cunningham-Bussel, 2013; Lee et al., 2020). Activated HIF-αs govern a variety of biological processes through transcriptional regulation of several thousand downstream targets that are responsible for cell differentiation, survival, and metabolic adaptation (Keith et al., 2011; Lee et al., 2020). HIF-1α and HIF-2α share about 48% amino acid identity and regulate both overlapping and distinct downstream genes (Tian et al., 1997; Majmundar et al., 2010). Notably, HIF-1α is more active in conditions with intense hypoxia, while HIF-2α functions more prominently under mild or physiological hypoxia (Koh and Powis, 2012) or at homeostasis (Jiang et al., 2019b; Pasupneti et al., 2020).
HIF and Lymphangiogenesis in Lymphedema
The evolution of secondary lymphedema involves early regenerative lymphangiogenesis following lymphatic injury and late lymphatic remodeling when the disease progresses (Rutkowski et al., 2006; Mihara et al., 2012). Evidence from both preclinical and clinical studies indicates that lymphedema tissues are hypoxic with metabolic derangement (Tabibiazar et al., 2006; Zampell et al., 2012a; Jiang et al., 2020). Lymph stasis and inflammation stabilize HIF-1α in mouse lymphedema, and this high tissue HIF-1α expression is required for reparative lymphangiogenesis and wound healing (Zampell et al., 2012a). Mechanistically, HIF-1α induces LEC (lymphatic endothelial cell) VEGFC and VEGFR3 expression and promotes lymphangiogenesis (Min et al., 2011; Zampell et al., 2012a; Han et al., 2019). LEC HIF-1α deletion transiently aggravates edema in a mouse-tail lymphedema model, corroborating a critical role of LEC HIF-1α in lymphatic regeneration immediately post-surgery (Jiang et al., 2020). By contrast, lymphatic HIF-2α plays a crucial role in embryonic lymphatic development, adult lymphatic maintenance, and lymphatic regeneration in lymphedema by activating TIE2 signaling, which is required for lymphatic vascular maturation and maintenance (Dellinger et al., 2008; Kajiya et al., 2012; Kim et al., 2017; Jiang et al., 2020). Interestingly, lymphatic HIF-2α decreases in lymphedematous tissues, a phenomenon likely attributable to heightened IFN-γ and HIF-1α expression (Takeda et al., 2010; Meneses and Wielockx, 2016; Jiang et al., 2020; Becker et al., 2021). In blood vasculature, HIF-2α is an endothelial survival factor (Jiang et al., 2019b; Pasupneti et al., 2020). HIF-2α also regulates vascular maturation and stabilization (Skuli et al., 2012), whereas HIF-1α tends to promote vascular sprouting (Koh and Powis, 2012). Further, HIF-2α regulates the expression of lymphatic lineage marker Prox-1 (Zhou et al., 2013). Therefore, increased HIF-1α expression with concomitant HIF-2α reduction in LECs may exacerbate lymphatic malfunctioning by promoting non-productive lymphangiogenesis and LEC phenotypic transition in chronic stage lymphedema.
HIF and Inflammation in Lymphedema
Hypoxia and inflammation often coexist in pathological conditions (Bartels et al., 2013; Taylor and Colgan, 2017; Colgan et al., 2020). For example, increased immune cell infiltration in inflammatory loci increases oxygen consumption, disrupting the balance between demand and supply of O2, consequently promoting hypoxia (Eltzschig and Carmeliet, 2011). Conversely, hypoxia and HIFs promote immune dysregulation, which drives tissue dysfunction and disease progression; the degree of hypoxia determines the extent to which HIF activation in immune cells and subsequent downstream effects (Taylor and Colgan, 2017). Hypoxia modulates both innate and adaptive immunity (Taylor and Colgan, 2017). The coevolving of hypoxia and inflammation is a feature of various diseases such as inflammatory bowel disease, rheumatoid arthritis, and acute lung injury (Watts and Walmsley, 2019). Hypoxia and inflammation also appear to influence lymphedema pathogenesis (Jiang et al., 2020).
HIFs control macrophage differentiation. Specifically, HIF-1α promotes macrophage differentiation into an M1 lineage, while HIF-2α fosters M2 polarization (Imtiyaz et al., 2010; Takeda et al., 2010; Domblides et al., 2018). Macrophages may differentiate primarily into the M2 phenotype in lymphedema (Ghanta et al., 2015). However, macrophages localized in fat tissue within lymphedematous skin display the M1 phenotype (Tashiro et al., 2017). Divergent macrophage differentiation in different tissue compartments may explain why macrophages play protective or harmful roles in lymphedema in a context-dependent manner (Kataru et al., 2009; Ginhoux and Jung, 2014; Ghanta et al., 2015). We showed that myeloid cell-specific HIF-2α deficiency aggravates tissue swelling following lymphedema surgery (Jiang et al., 2020), suggesting M2 macrophages may promote lymphatic repair and alleviate edema in subacute mouse-tail lymphedema. More in-depth characterization of HIF isoform expression in macrophages during the acute and chronic phases of lymphedema will enhance our understanding of how HIFs modulate macrophage differentiation and regulate lymphedema pathology. Future investigation of macrophage biology in lymphedema should also go beyond the overly simplified M1, M2 dichotomy (Ginhoux and Jung, 2014).
HIF-1α controls the balance of Th17/Treg cell differentiation (Dang et al., 2011; Hsiao et al., 2015; Tao et al., 2015; Clever et al., 2016). Specifically, HIF-1α induces the expression of the transcriptional factor RORγT and the Th17 signature gene IL-17A that requires STAT3 activation; concomitantly, HIF-1α promotes proteasome-mediated degradation of FOXP3, the key factor for Treg differentiation (Dang et al., 2011; Darce et al., 2012). HIF-1α also favors Th17 over Treg differentiation by skewing T cell glycolytic metabolism (Shi et al., 2011). HIF-2α, by contrast, is crucial for Treg stability and function, and this regulation may partly depend on suppressing HIF-1α gene transcription and protein accumulation (Hsu et al., 2020). It is worth noting that HIF-1α may also promote Treg differentiation and play a role in suppressing mucosal inflammation (Clambey et al., 2012). While hypoxia results in a shift toward Th2 response (Lederer et al., 1999), it is not entirely clear how HIF isoforms may differentially regulate Th1 and Th2 differentiation. CD4+ T cell expansion is a prominent pathology in lymphedema (Ly et al., 2017; Jiang et al., 2018; Azhar et al., 2020). HIF isoforms, therefore, could conceivably modulate the differentiation and function of different immune compartments in lymphedema.
HIF and Adipose Expansion in Lymphedema
Hypoxia plays a pathogenic role in obesity (Norouzirad et al., 2017). Hypoxia induces adipocyte death and consequent macrophage recruitment (Cinti et al., 2005; Yin et al., 2009); these infiltrating cells, regulated primarily by HIF-1α, play a crucial role in initiating obesity-related disorders, including metabolic reprogramming, insulin resistance, and inflammation (Mazzatti et al., 2012). Accordingly, adipocyte-specific HIF-1α deletion improves insulin sensitivity and diminishes adipose mass in high-fat diet-fed mice (Jiang et al., 2011). These studies demonstrated that adipocyte expression of HIF-1α promotes obesity and related pathologies. By contrast, adipocyte HIF-2α protects against metabolic maladaptation, insulin resistance, and inflammation in diet-induced obesity (García-Martín et al., 2016). Aberrant fat accumulation and metabolism in lymphedema resemble that of obesity (Mehrara and Greene, 2014). For instance, lymphedema patients exhibit increased serum levels of adipokines such as adiponectin and leptin (Zaleska and Olszewski, 2017). Lymphedema tissues also express increased proinflammatory cytokines such as TNF-α, IL-6, and IL-1β (de Ferranti and Mozaffarian, 2008; Jiang et al., 2018). In particular, high IL-6 expression appears to associate with fat deposition in both lymphedema and obesity (Olszewski et al., 1992; Mohamed-Ali et al., 1997; Fried et al., 1998; Cuzzone et al., 2014). Hypoxia also influences adipose pathology through modulating the differentiation and function of infiltrating macrophages (Engin, 2017). Adipose tissue hypoxia promotes proinflammatory M1 macropahge differentiation and strengthens the production of IL-6, IL-1β, and iNOS (Fujisaka et al., 2013). In addition, hypoxic macropahges in an environment with increased saturated free fatty acids also enhance adipocyte IL-6 and MCP-1 production (Snodgrass et al., 2016). Whether HIF-α isoforms similarly regulate fat accumulation in lymphedema as observed in the context of obesity is an open question. Interestingly, in visceral adipose tissue (VAT), estrogen signaling modulates stromal cell expression of the HIF-1α target gene CD73 (Synnestvedt et al., 2002), which leads to a divergent Treg population in female adipose tissue compared to males (Vasanthakumar et al., 2020). These studies support the notion that hypoxia may regulate adipose tissue inflammation in a sexually dimorphic manner. This concept can be used to explain why lymphedema preferentially develops in females (Trincot and Caron, 2019).
HIF and Fibrosis in Lymphedema
Fibrosis, characterized by excessive deposition of extracellular matrix (ECM) components such as collagen and fibronectin, is a dysregulated tissue repair response following various types of tissue injury with chronic inflammation (Henderson et al., 2020). Fibroblasts are the critical source of excessively produced ECM in remodeled fibrotic tissue (Henderson et al., 2020). A recent single-cell RNA-Seq analysis of keloid and scleroderma samples identified four subpopulations of skin fibroblasts: secretory-papillary, secretory-reticular, mesenchymal, and proinflammatory; among which the percentage of mesenchymal fibroblast is the primary fibroblast subtype involved in skin fibrosis (Deng et al., 2021). Fibrotic tissues often display features of chronic hypoxia, and hypoxia-regulated signaling regulates collagen production, accumulation, and crosslinking (Gilkes et al., 2013; Gilkes et al., 2014; Xiong and Liu, 2017). Hypoxia promotes fibroblast transition to a myofibroblast-like phenotype and skin fibrosis through HIF-1α-mediated activation of the TGF-β and NF-κB signaling pathways (Distler et al., 2007; Zhao et al., 2017; Lei et al., 2019). Intriguingly, HIF-1α-mediated fibroblast to myofibroblast differentiation depends on augmented glycolysis and increased succinate production (Xie et al., 2015). Specifically, elevated lactate levels resulting from augmented fibroblast glycolytic metabolism stimulate TGF-β signaling by reducing extracellular pH and activating latent TGF-β (Kottmann et al., 2012). HIF-1α may also enhance myofibroblast collagen production by inducing glutaminase 1 (GLS1) expression and promoting glutaminolysis (Ge et al., 2018; Xiang et al., 2019; Bouthelier and Aragonés, 2020). Collectively, HIF-1α-dependent hypoxic responses foster myofibroblast differentiation and ECM protein production by reprogramming metabolism and activating the TGF-β pathway (Gibb et al., 2020). Studies have also demonstrated that Th2 and Th17 cells and macrophages are involved in tissue fibrosis (Barron and Wynn, 2011; Wynn and Vannella, 2016; Vannella and Wynn, 2017; Henderson et al., 2020). Therefore, HIF isoforms may regulate fibrotic remodeling by controlling the differentiation of CD4+ T helper cells and macrophages.
Fibrosis in lymphedema affects the dermis and subcutaneous tissue, representing a crucial factor promoting tissue hardening and non-pitting edema (Rutkowski et al., 2010; Zampell et al., 2012c; Gardenier et al., 2016; Tashiro et al., 2017). Additionally, diffuse tissue fibrosis worsens lymphatic dysfunction by directly impairing lymphatic vascular regeneration (Lynch et al., 2015). Collecting lymphatic vessels also undergo fibrotic remodeling, including excessive collagen deposition and disorganized expansion of the smooth muscle cell layer; these structural changes decrease lymphatic vessel contractility, aggravate drainage deficiency, and increase lymph leakage (Olszewski, 2002; Mihara et al., 2012; Gardenier et al., 2016). Currently, studies investigating hypoxia in promoting fibrosis in lymphedema are limited. Knowledge on how hypoxia may influence the phenotype of lymphatic vascular cells and enhance fibrotic tissue remodeling will improve our understanding of molecular pathways involved in lymphedema progression.
Concluding Remarks
Chronic lymphedema poses a significant health burden on afflicted patients and requires effective pharmacological treatments. Hypoxia and HIF-mediated responses appear to regulate lymphedema pathogenesis (Figure 1). Available data concerning the roles of HIF isoforms in lymphangiogenesis, inflammation, fatty expansion, and fibrotic tissue remodeling suggested a possible opposing role of HIF isoforms, with HIF-2α possibly playing beneficial effects in lymphedema. A fuller exploration of hypoxia-regulated molecular pathways in lymphatic endothelial, immune, and stromal compartments will enhance our understanding of lymphedema pathogenesis and likely provide novel therapeutic targets to improve lymphatic repair, adipose expansion, and fibrotic remodeling in lymphedema.
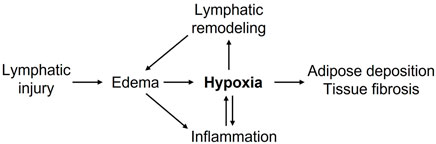
FIGURE 1. Putative roles of hypoxia in promoting lymphedema pathogenesis. Lymphatic injury-caused edema promotes inflammation and tissue hypoxia. Decreased tissue oxygenation further enhances inflammatory responses, lymphatic vascular remodeling, adipose deposition, tissue fibrosis, and collectively exacerbates lymphedema.
Author Contributions
WT, DK, AM, RV, SR, GS, MN, and XJ contributed to the design, writing, and review of this mini-review. All authors contributed to the article and approved the submitted version.
Funding
This work was supported by NIH grants R01 HL141105 (to MN), HL150583 (to XJ), and Stanford Endowed Chair Funds (to MN and SG).
Conflict of Interest
The authors declare that the research was conducted in the absence of any commercial or financial relationships that could be construed as a potential conflict of interest.
Publisher’s Note
All claims expressed in this article are solely those of the authors and do not necessarily represent those of their affiliated organizations or those of the publisher, the editors, and the reviewers. Any product that may be evaluated in this article, or claim that may be made by its manufacturer, is not guaranteed or endorsed by the publisher.
References
Avraham, T., Zampell, J. C., Yan, A., Elhadad, S., Weitman, E. S., Rockson, S. G., et al. (2013). Th2 Differentiation Is Necessary for Soft Tissue Fibrosis and Lymphatic Dysfunction Resulting from Lymphedema. FASEB J. 27, 1114–1126. doi:10.1096/fj.12-222695
Azhar, S. H., Lim, H. Y., Tan, B. K., and Angeli, V. (2020). The Unresolved Pathophysiology of Lymphedema. Front. Physiol. 11, 137. doi:10.3389/fphys.2020.00137
Barron, L., and Wynn, T. A. (2011). Fibrosis Is Regulated by Th2 and Th17 Responses and by Dynamic Interactions between Fibroblasts and Macrophages. Am. J. Physiol. Gastrointest. Liver Physiol. 300, G723–G728. doi:10.1152/ajpgi.00414.2010
Bartels, K., Grenz, A., and Eltzschig, H. K. (2013). Hypoxia and Inflammation Are Two Sides of the Same coin. Proc. Natl. Acad. Sci. U S A. 110, 18351–18352. doi:10.1073/pnas.1318345110
Becker, J., Schwoch, S., Zelent, C., Sitte, M., Salinas, G., and Wilting, J. (2021). Transcriptome Analysis of Hypoxic Lymphatic Endothelial Cells Indicates Their Potential to Contribute to Extracellular Matrix Rearrangement. Cells 10, 8. doi:10.3390/cells10051008
Bouthelier, A., and Aragonés, J. (2020). Role of the HIF Oxygen Sensing Pathway in Cell Defense and Proliferation through the Control of Amino Acid Metabolism. Biochim. Biophys. Acta Mol. Cel Res 1867, 118733. doi:10.1016/j.bbamcr.2020.118733
Cavadas, M. A., Nguyen, L. K., and Cheong, A. (2013). Hypoxia-inducible Factor (HIF) Network: Insights from Mathematical Models. Cell Commun Signal 11, 42. doi:10.1186/1478-811X-11-42
Chen, Y., and Gaber, T. (2021). Hypoxia/HIF Modulates Immune Responses. Biomedicines 9, 260. doi:10.3390/biomedicines9030260
Cinti, S., Mitchell, G., Barbatelli, G., Murano, I., Ceresi, E., Faloia, E., et al. (2005). Adipocyte Death Defines Macrophage Localization and Function in Adipose Tissue of Obese Mice and Humans. J. Lipid Res. 46, 2347–2355. doi:10.1194/jlr.M500294-JLR200
Clambey, E. T., McNamee, E. N., Westrich, J. A., Glover, L. E., Campbell, E. L., Jedlicka, P., et al. (2012). Hypoxia-inducible Factor-1 Alpha-dependent Induction of FoxP3 Drives Regulatory T-Cell Abundance and Function during Inflammatory Hypoxia of the Mucosa. Proc. Natl. Acad. Sci. U S A. 109, E2784–E2793. doi:10.1073/pnas.1202366109
Clever, D., Roychoudhuri, R., Constantinides, M. G., Askenase, M. H., Sukumar, M., Klebanoff, C. A., et al. (2016). Oxygen Sensing by T Cells Establishes an Immunologically Tolerant Metastatic Niche. Cell 166, 1117–e14. e14. doi:10.1016/j.cell.2016.07.032
Colgan, S. P., Furuta, G. T., and Taylor, C. T. (2020). Hypoxia and Innate Immunity: Keeping up with the HIFsters. Annu. Rev. Immunol. 38, 341–363. doi:10.1146/annurev-immunol-100819-121537
Cuzzone, D. A., Weitman, E. S., Albano, N. J., Ghanta, S., Savetsky, I. L., Gardenier, J. C., et al. (2014). IL-6 Regulates Adipose Deposition and Homeostasis in Lymphedema. Am. J. Physiol. Heart Circ. Physiol. 306, H1426–H1434. doi:10.1152/ajpheart.01019.2013
Dang, E. V., Barbi, J., Yang, H. Y., Jinasena, D., Yu, H., Zheng, Y., et al. (2011). Control of T(H)17/T(reg) Balance by Hypoxia-Inducible Factor 1. Cell 146, 772–784. doi:10.1016/j.cell.2011.07.033
Darce, J., Rudra, D., Li, L., Nishio, J., Cipolletta, D., Rudensky, A. Y., et al. (2012). An N-Terminal Mutation of the Foxp3 Transcription Factor Alleviates Arthritis but Exacerbates Diabetes. Immunity 36, 731–741. doi:10.1016/j.immuni.2012.04.007
de Ferranti, S., and Mozaffarian, D. (2008). The Perfect Storm: Obesity, Adipocyte Dysfunction, and Metabolic Consequences. Clin. Chem. 54, 945–955. doi:10.1373/clinchem.2007.100156
Dellinger, M., Hunter, R., Bernas, M., Gale, N., Yancopoulos, G., Erickson, R., et al. (2008). Defective Remodeling and Maturation of the Lymphatic Vasculature in Angiopoietin-2 Deficient Mice. Dev. Biol. 319, 309–320. doi:10.1016/j.ydbio.2008.04.024
Deng, C. C., Hu, Y. F., Zhu, D. H., Cheng, Q., Gu, J. J., Feng, Q. L., et al. (2021). Single-cell RNA-Seq Reveals Fibroblast Heterogeneity and Increased Mesenchymal Fibroblasts in Human Fibrotic Skin Diseases. Nat. Commun. 12, 3709. doi:10.1038/s41467-021-24110-y
Distler, J. H., Jüngel, A., Pileckyte, M., Zwerina, J., Michel, B. A., Gay, R. E., et al. (2007). Hypoxia-induced Increase in the Production of Extracellular Matrix Proteins in Systemic Sclerosis. Arthritis Rheum. 56, 4203–4215. doi:10.1002/art.23074
Domblides, C., Lartigue, L., and Faustin, B. (2018). Metabolic Stress in the Immune Function of T Cells, Macrophages and Dendritic Cells. Cells 7, 68. doi:10.3390/cells7070068
Eltzschig, H. K., and Carmeliet, P. (2011). Hypoxia and Inflammation. N. Engl. J. Med. 364, 656–665. doi:10.1056/NEJMra0910283
Engin, A. (2017). Adipose Tissue Hypoxia in Obesity and its Impact on Preadipocytes and Macrophages: Hypoxia Hypothesis. Adv. Exp. Med. Biol. 960, 305–326. doi:10.1007/978-3-319-48382-5_13
Fried, S. K., Bunkin, D. A., and Greenberg, A. S. (1998). Omental and Subcutaneous Adipose Tissues of Obese Subjects Release Interleukin-6: Depot Difference and Regulation by Glucocorticoid. J. Clin. Endocrinol. Metab. 83, 847–850. doi:10.1210/jcem.83.3.4660
Fujisaka, S., Usui, I., Ikutani, M., Aminuddin, A., Takikawa, A., Tsuneyama, K., et al. (2013). Adipose Tissue Hypoxia Induces Inflammatory M1 Polarity of Macrophages in an HIF-1α-dependent and HIF-1α-independent Manner in Obese Mice. Diabetologia 56, 1403–1412. doi:10.1007/s00125-013-2885-1
García-Martín, R., Alexaki, V. I., Qin, N., Rubín de Celis, M. F., Economopoulou, M., Ziogas, A., et al. (2016). Adipocyte-Specific Hypoxia-Inducible Factor 2α Deficiency Exacerbates Obesity-Induced Brown Adipose Tissue Dysfunction and Metabolic Dysregulation. Mol. Cell. Biol. 36, 376–393. doi:10.1128/MCB.00430-15
Gardenier, J. C., Hespe, G. E., Kataru, R. P., Savetsky, I. L., Torrisi, J. S., Nores, G. D. G., et al. (2016). Diphtheria Toxin-Mediated Ablation of Lymphatic Endothelial Cells Results in Progressive Lymphedema. JCI Insight 1, e84095. doi:10.1172/jci.insight.84095
Ge, J., Cui, H., Xie, N., Banerjee, S., Guo, S., Dubey, S., et al. (2018). Glutaminolysis Promotes Collagen Translation and Stability via α-Ketoglutarate-mediated mTOR Activation and Proline Hydroxylation. Am. J. Respir. Cel Mol Biol 58, 378–390. doi:10.1165/rcmb.2017-0238OC
Ghanta, S., Cuzzone, D. A., Torrisi, J. S., Albano, N. J., Joseph, W. J., Savetsky, I. L., et al. (2015). Regulation of Inflammation and Fibrosis by Macrophages in Lymphedema. Am. J. Physiol. Heart Circ. Physiol. 308, H1065–H1077. doi:10.1152/ajpheart.00598.2014
Gibb, A. A., Lazaropoulos, M. P., and Elrod, J. W. (2020). Myofibroblasts and Fibrosis: Mitochondrial and Metabolic Control of Cellular Differentiation. Circ. Res. 127, 427–447. doi:10.1161/CIRCRESAHA.120.316958
Gilkes, D. M., Bajpai, S., Chaturvedi, P., Wirtz, D., and Semenza, G. L. (2013). Hypoxia-inducible Factor 1 (HIF-1) Promotes Extracellular Matrix Remodeling under Hypoxic Conditions by Inducing P4HA1, P4HA2, and PLOD2 Expression in Fibroblasts. J. Biol. Chem. 288, 10819–10829. doi:10.1074/jbc.M112.442939
Gilkes, D. M., Semenza, G. L., and Wirtz, D. (2014). Hypoxia and the Extracellular Matrix: Drivers of Tumour Metastasis. Nat. Rev. Cancer 14, 430–439. doi:10.1038/nrc3726
Ginhoux, F., and Jung, S. (2014). Monocytes and Macrophages: Developmental Pathways and Tissue Homeostasis. Nat. Rev. Immunol. 14, 392–404. doi:10.1038/nri3671
Gousopoulos, E., Proulx, S. T., Bachmann, S. B., Scholl, J., Dionyssiou, D., Demiri, E., et al. (2016). Regulatory T Cell Transfer Ameliorates Lymphedema and Promotes Lymphatic Vessel Function. JCI Insight 1, e89081. doi:10.1172/jci.insight.89081
Han, T., Yan, J., Chen, H., Ji, Y., Chen, J., Cui, J., et al. (2019). HIF-1α Contributes to Tube Malformation of Human Lymphatic Endothelial Cells by Upregulating VEGFR-3. Int. J. Oncol. 54, 139–151. doi:10.3892/ijo.2018.4623
Henderson, N. C., Rieder, F., and Wynn, T. A. (2020). Fibrosis: from Mechanisms to Medicines. Nature 587, 555–566. doi:10.1038/s41586-020-2938-9
Hsiao, H. W., Hsu, T. S., Liu, W. H., Hsieh, W. C., Chou, T. F., Wu, Y. J., et al. (2015). Deltex1 Antagonizes HIF-1α and Sustains the Stability of Regulatory T Cells In Vivo. Nat. Commun. 6, 6353. doi:10.1038/ncomms7353
Hsu, T. S., Lin, Y. L., Wang, Y. A., Mo, S. T., Chi, P. Y., Lai, A. C., et al. (2020). HIF-2α Is Indispensable for Regulatory T Cell Function. Nat. Commun. 11, 5005. doi:10.1038/s41467-020-18731-y
Imtiyaz, H. Z., Williams, E. P., Hickey, M. M., Patel, S. A., Durham, A. C., Yuan, L. J., et al. (2010). Hypoxia-inducible Factor 2alpha Regulates Macrophage Function in Mouse Models of Acute and Tumor Inflammation. J. Clin. Invest. 120, 2699–2714. doi:10.1172/JCI39506
Irigoyen, M., Ansó, E., Martínez, E., Garayoa, M., Martínez-Irujo, J. J., and Rouzaut, A. (2007). Hypoxia Alters the Adhesive Properties of Lymphatic Endothelial Cells. A Transcriptional and Functional Study. Biochim. Biophys. Acta 1773, 880–890. doi:10.1016/j.bbamcr.2007.03.001
Ji, R. C. (2014). Hypoxia and Lymphangiogenesis in Tumor Microenvironment and Metastasis. Cancer Lett. 346, 6–16. doi:10.1016/j.canlet.2013.12.001
Jiang, C., Qu, A., Matsubara, T., Chanturiya, T., Jou, W., Gavrilova, O., et al. (2011). Disruption of Hypoxia-Inducible Factor 1 in Adipocytes Improves Insulin Sensitivity and Decreases Adiposity in High-Fat Diet-Fed Mice. Diabetes 60, 2484–2495. doi:10.2337/db11-0174
Jiang, X., Nicolls, M. R., Tian, W., and Rockson, S. G. (2018). Lymphatic Dysfunction, Leukotrienes, and Lymphedema. Annu. Rev. Physiol. 80, 49–70. doi:10.1146/annurev-physiol-022516-034008
Jiang, X., Tian, W., Granucci, E. J., Tu, A. B., Kim, D., Dahms, P., et al. (2020). Decreased Lymphatic HIF-2α Accentuates Lymphatic Remodeling in Lymphedema. J. Clin. Invest. 130, 5562–5575. doi:10.1172/JCI136164
Jiang, X., Tian, W., Nicolls, M. R., and Rockson, S. G. (2019). The Lymphatic System in Obesity, Insulin Resistance, and Cardiovascular Diseases. Front. Physiol. 10, 1402. doi:10.3389/fphys.2019.01402
Jiang, X., Tian, W., Tu, A. B., Pasupneti, S., Shuffle, E., Dahms, P., et al. (2019). Endothelial Hypoxia-Inducible Factor-2α Is Required for the Maintenance of Airway Microvasculature. Circulation 139, 502–517. doi:10.1161/CIRCULATIONAHA.118.036157
Kajiya, K., Kidoya, H., Sawane, M., Matsumoto-Okazaki, Y., Yamanishi, H., Furuse, M., et al. (2012). Promotion of Lymphatic Integrity by angiopoietin-1/Tie2 Signaling during Inflammation. Am. J. Pathol. 180, 1273–1282. doi:10.1016/j.ajpath.2011.11.008
Kataru, R. P., Jung, K., Jang, C., Yang, H., Schwendener, R. A., Baik, J. E., et al. (2009). Critical Role of CD11b+ Macrophages and VEGF in Inflammatory Lymphangiogenesis, Antigen Clearance, and Inflammation Resolution. Blood 113, 5650–5659. doi:10.1182/blood-2008-09-176776
Kataru, R. P., Wiser, I., Baik, J. E., Park, H. J., Rehal, S., Shin, J. Y., et al. (2019). Fibrosis and Secondary Lymphedema: Chicken or Egg? Transl Res. 209, 68–76. doi:10.1016/j.trsl.2019.04.001
Keith, B., Johnson, R. S., and Simon, M. C. (2011). HIF1α and HIF2α: Sibling Rivalry in Hypoxic Tumour Growth and Progression. Nat. Rev. Cancer 12, 9–22. doi:10.1038/nrc3183
Kim, J., Park, D. Y., Bae, H., Park, D. Y., Kim, D., Lee, C. K., et al. (2017). Impaired angiopoietin/Tie2 Signaling Compromises Schlemm's Canal Integrity and Induces Glaucoma. J. Clin. Invest. 127, 3877–3896. doi:10.1172/JCI94668
Koh, M. Y., and Powis, G. (2012). Passing the Baton: the HIF Switch. Trends Biochem. Sci. 37, 364–372. doi:10.1016/j.tibs.2012.06.004
Kottmann, R. M., Kulkarni, A. A., Smolnycki, K. A., Lyda, E., Dahanayake, T., Salibi, R., et al. (2012). Lactic Acid Is Elevated in Idiopathic Pulmonary Fibrosis and Induces Myofibroblast Differentiation via pH-dependent Activation of Transforming Growth Factor-β. Am. J. Respir. Crit. Care Med. 186, 740–751. doi:10.1164/rccm.201201-0084OC
Lederer, J. A., Rodrick, M. L., and Mannick, J. A. (1999). The Effects of Injury on the Adaptive Immune Response. Shock 11, 153–159. doi:10.1097/00024382-199903000-00001
Lee, J. W., Ko, J., Ju, C., and Eltzschig, H. K. (2019). Hypoxia Signaling in Human Diseases and Therapeutic Targets. Exp. Mol. Med. 51, 1–13. doi:10.1038/s12276-019-0235-1
Lee, P., Chandel, N. S., and Simon, M. C. (2020). Cellular Adaptation to Hypoxia through Hypoxia Inducible Factors and beyond. Nat. Rev. Mol. Cel Biol 21, 268–283. doi:10.1038/s41580-020-0227-y
Lei, R., Li, J., Liu, F., Li, W., Zhang, S., Wang, Y., et al. (2019). HIF-1α Promotes the Keloid Development through the Activation of TGF-β/Smad and TLR4/MyD88/NF-Κb Pathways. Cell Cycle 18, 3239–3250. doi:10.1080/15384101.2019.1670508
Leung, G., Baggott, C., West, C., Elboim, C., Paul, S. M., Cooper, B. A., et al. (2014). Cytokine Candidate Genes Predict the Development of Secondary Lymphedema Following Breast Cancer Surgery. Lymphat Res. Biol. 12, 10–22. doi:10.1089/lrb.2013.0024
Li, C. Y., Kataru, R. P., and Mehrara, B. J. (2020). Histopathologic Features of Lymphedema: A Molecular Review. Int. J. Mol. Sci. 21, 2546. doi:10.3390/ijms21072546
Lin, S., Kim, J., Lee, M. J., Roche, L., Yang, N. L., Tsao, P. S., et al. (2012). Prospective Transcriptomic Pathway Analysis of Human Lymphatic Vascular Insufficiency: Identification and Validation of a Circulating Biomarker Panel. PLoS One 7, e52021. doi:10.1371/journal.pone.0052021
Ly, C. L., Kataru, R. P., and Mehrara, B. J. (2017). Inflammatory Manifestations of Lymphedema. Int. J. Mol. Sci. 18, 171. doi:10.3390/ijms18010171
Ly, C. L., Nores, G. D. G., Kataru, R. P., and Mehrara, B. J. (2019). T Helper 2 Differentiation Is Necessary for Development of Lymphedema. Transl Res. 206, 57–70. doi:10.1016/j.trsl.2018.12.003
Lynch, L. L., Mendez, U., Waller, A. B., Gillette, A. A., Guillory, R. J., and Goldman, J. (2015). Fibrosis Worsens Chronic Lymphedema in Rodent Tissues. Am. J. Physiol. Heart Circ. Physiol. 308, H1229–H1236. doi:10.1152/ajpheart.00527.2013
Majmundar, A. J., Wong, W. J., and Simon, M. C. (2010). Hypoxia-inducible Factors and the Response to Hypoxic Stress. Mol. Cel 40, 294–309. doi:10.1016/j.molcel.2010.09.022
Manresa, M. C., Godson, C., and Taylor, C. T. (2014). Hypoxia-sensitive Pathways in Inflammation-Driven Fibrosis. Am. J. Physiol. Regul. Integr. Comp. Physiol. 307, R1369–R1380. doi:10.1152/ajpregu.00349.2014
Mazzatti, D., Lim, F. L., O'Hara, A., Wood, I. S., and Trayhurn, P. (2012). A Microarray Analysis of the Hypoxia-Induced Modulation of Gene Expression in Human Adipocytes. Arch. Physiol. Biochem. 118, 112–120. doi:10.3109/13813455.2012.654611
Mehrara, B. J., and Greene, A. K. (2014). Lymphedema and Obesity: Is There a Link? Plast. Reconstr. Surg. 134, 154e–160e. doi:10.1097/PRS.0000000000000268
Mehrara, B. J., Park, H. J., Kataru, R. P., Bromberg, J., Coriddi, M., Baik, J. E., et al. (2021). Pilot Study of Anti-th2 Immunotherapy for the Treatment of Breast Cancer-Related Upper Extremity Lymphedema. Biology (Basel) 10, 934. doi:10.3390/biology10090934
Meneses, A. M., and Wielockx, B. (2016). PHD2: from Hypoxia Regulation to Disease Progression. Hypoxia (Auckl) 4, 53–67. doi:10.2147/HP.S53576
Mihara, M., Hara, H., Hayashi, Y., Narushima, M., Yamamoto, T., Todokoro, T., et al. (2012). Pathological Steps of Cancer-Related Lymphedema: Histological Changes in the Collecting Lymphatic Vessels after Lymphadenectomy. PLoS One 7, e41126. doi:10.1371/journal.pone.0041126
Min, Y., Ghose, S., Boelte, K., Li, J., Yang, L., and Lin, P. C. (2011). C/EBP-δ Regulates VEGF-C Autocrine Signaling in Lymphangiogenesis and Metastasis of Lung Cancer through HIF-1α. Oncogene 30, 4901–4909. doi:10.1038/onc.2011.187
Mohamed-Ali, V., Goodrick, S., Rawesh, A., Katz, D. R., Miles, J. M., Yudkin, J. S., et al. (1997). Subcutaneous Adipose Tissue Releases Interleukin-6, but Not Tumor Necrosis Factor-Alpha, In Vivo. J. Clin. Endocrinol. Metab. 82, 4196–4200. doi:10.1210/jcem.82.12.4450
Morfoisse, F., Renaud, E., Hantelys, F., Prats, A. C., and Garmy-Susini, B. (2015). Role of Hypoxia and Vascular Endothelial Growth Factors in Lymphangiogenesis. Mol. Cel Oncol 2, e1024821. doi:10.1080/23723556.2015.1024821
Nathan, C., and Cunningham-Bussel, A. (2013). Beyond Oxidative Stress: an Immunologist's Guide to Reactive Oxygen Species. Nat. Rev. Immunol. 13, 349–361. doi:10.1038/nri3423
Norouzirad, R., González-Muniesa, P., and Ghasemi, A. (2017). Hypoxia in Obesity and Diabetes: Potential Therapeutic Effects of Hyperoxia and Nitrate. Oxid Med. Cel Longev 2017, 5350267. doi:10.1155/2017/5350267
Ogata, F., Fujiu, K., Matsumoto, S., Nakayama, Y., Shibata, M., Oike, Y., et al. (2016). Excess Lymphangiogenesis Cooperatively Induced by Macrophages and CD4(+) T Cells Drives the Pathogenesis of Lymphedema. J. Invest. Dermatol. 136, 706–714. doi:10.1016/j.jid.2015.12.001
Olszewski, W. L. (2002). Contractility Patterns of normal and Pathologically Changed Human Lymphatics. Ann. N. Y Acad. Sci. 979, 52–59. discussion 76-9. doi:10.1111/j.1749-6632.2002.tb04867.x
Olszewski, W. L., Jamal, S., Lukomska, B., Manokaran, G., and Grzelak, I. (1992). Immune Proteins in Peripheral Tissue Fluid-Lymph in Patients with Filarial Lymphedema of the Lower Limbs. Lymphology 25, 166–171.
Palazon, A., Goldrath, A. W., Nizet, V., and Johnson, R. S. (2014). HIF Transcription Factors, Inflammation, and Immunity. Immunity 41, 518–528. doi:10.1016/j.immuni.2014.09.008
Pasupneti, S., Tian, W., Tu, A. B., Dahms, P., Granucci, E., Gandjeva, A., et al. (2020). Endothelial HIF-2α as a Key Endogenous Mediator Preventing Emphysema. Am. J. Respir. Crit. Care Med. 202, 983–995. doi:10.1164/rccm.202001-0078OC
Poeze, M. (2006). Tissue-oxygenation Assessment Using Near-Infrared Spectroscopy during Severe Sepsis: Confounding Effects of Tissue Edema on StO2 Values. Intensive Care Med. 32, 788–789. doi:10.1007/s00134-006-0121-x
Prabhakar, N. R., and Semenza, G. L. (2015). Oxygen Sensing and Homeostasis. Physiology (Bethesda) 30, 340–348. doi:10.1152/physiol.00022.2015
Rockson, S. G. (2008). Diagnosis and Management of Lymphatic Vascular Disease. J. Am. Coll. Cardiol. 52, 799–806. doi:10.1016/j.jacc.2008.06.005
Rockson, S. G., Keeley, V., Kilbreath, S., Szuba, A., and Towers, A. (2019). Cancer-associated Secondary Lymphoedema. Nat. Rev. Dis. Primers 5, 22. doi:10.1038/s41572-019-0072-5
Rockson, S. G. (2018). Lymphedema after Breast Cancer Treatment. N. Engl. J. Med. 379, 1937–1944. doi:10.1056/NEJMcp1803290
Rockson, S. G., Tian, W., Jiang, X., Kuznetsova, T., Haddad, F., Zampell, J., et al. (2018). Pilot Studies Demonstrate the Potential Benefits of Antiinflammatory Therapy in Human Lymphedema. JCI Insight 3, e123775. doi:10.1172/jci.insight.123775
Rutkowski, J. M., Markhus, C. E., Gyenge, C. C., Alitalo, K., Wiig, H., and Swartz, M. A. (2010). Dermal Collagen and Lipid Deposition Correlate with Tissue Swelling and Hydraulic Conductivity in Murine Primary Lymphedema. Am. J. Pathol. 176, 1122–1129. doi:10.2353/ajpath.2010.090733
Rutkowski, J. M., Moya, M., Johannes, J., Goldman, J., and Swartz, M. A. (2006). Secondary Lymphedema in the Mouse Tail: Lymphatic Hyperplasia, VEGF-C Upregulation, and the Protective Role of MMP-9. Microvasc. Res. 72, 161–171. doi:10.1016/j.mvr.2006.05.009
Semenza, G. L. (2012). Hypoxia-inducible Factors in Physiology and Medicine. Cell 148, 399–408. doi:10.1016/j.cell.2012.01.021
Semenza, G. L. (2019). Pharmacologic Targeting of Hypoxia-Inducible Factors. Annu. Rev. Pharmacol. Toxicol. 59, 379–403. doi:10.1146/annurev-pharmtox-010818-021637
Semenza, G. L. (2003). Targeting HIF-1 for Cancer Therapy. Nat. Rev. Cancer 3, 721–732. doi:10.1038/nrc1187
Shi, L. Z., Wang, R., Huang, G., Vogel, P., Neale, G., Green, D. R., et al. (2011). HIF1alpha-dependent Glycolytic Pathway Orchestrates a Metabolic Checkpoint for the Differentiation of TH17 and Treg Cells. J. Exp. Med. 208, 1367–1376. doi:10.1084/jem.20110278
Skuli, N., Majmundar, A. J., Krock, B. L., Mesquita, R. C., Mathew, L. K., Quinn, Z. L., et al. (2012). Endothelial HIF-2α Regulates Murine Pathological Angiogenesis and Revascularization Processes. J. Clin. Invest. 122, 1427–1443. doi:10.1172/JCI57322
Snodgrass, R. G., Boß, M., Zezina, E., Weigert, A., Dehne, N., Fleming, I., et al. (2016). Hypoxia Potentiates Palmitate-Induced Pro-inflammatory Activation of Primary Human Macrophages. J. Biol. Chem. 291, 413–424. doi:10.1074/jbc.M115.686709
Synnestvedt, K., Furuta, G. T., Comerford, K. M., Louis, N., Karhausen, J., Eltzschig, H. K., et al. (2002). Ecto-5'-nucleotidase (CD73) Regulation by Hypoxia-Inducible Factor-1 Mediates Permeability Changes in Intestinal Epithelia. J. Clin. Invest. 110, 993–1002. doi:10.1172/JCI15337
Tabibiazar, R., Cheung, L., Han, J., Swanson, J., Beilhack, A., An, A., et al. (2006). Inflammatory Manifestations of Experimental Lymphatic Insufficiency. Plos Med. 3, e254. doi:10.1371/journal.pmed.0030254
Takeda, N., O'Dea, E. L., Doedens, A., Kim, J. W., Weidemann, A., Stockmann, C., et al. (2010). Differential Activation and Antagonistic Function of HIF-{alpha} Isoforms in Macrophages Are Essential for NO Homeostasis. Genes Dev. 24, 491–501. doi:10.1101/gad.1881410
Tao, J. H., Barbi, J., and Pan, F. (2015). Hypoxia-inducible Factors in T Lymphocyte Differentiation and Function. A Review in the Theme: Cellular Responses to Hypoxia. Am. J. Physiol. Cel Physiol 309, C580–C589. doi:10.1152/ajpcell.00204.2015
Tashiro, K., Feng, J., Wu, S. H., Mashiko, T., Kanayama, K., Narushima, M., et al. (2017). Pathological Changes of Adipose Tissue in Secondary Lymphoedema. Br. J. Dermatol. 177, 158–167. doi:10.1111/bjd.15238
Taylor, C. T., and Colgan, S. P. (2017). Regulation of Immunity and Inflammation by Hypoxia in Immunological Niches. Nat. Rev. Immunol. 17, 774–785. doi:10.1038/nri.2017.103
Tian, H., McKnight, S. L., and Russell, D. W. (1997). Endothelial PAS Domain Protein 1 (EPAS1), a Transcription Factor Selectively Expressed in Endothelial Cells. Genes Dev. 11, 72–82. doi:10.1101/gad.11.1.72
Tian, W., Rockson, S. G., Jiang, X., Kim, J., Begaye, A., Shuffle, E. M., et al. (2017). Leukotriene B4 Antagonism Ameliorates Experimental Lymphedema. Sci. Transl Med. 9, eaal3820. doi:10.1126/scitranslmed.aal3920
Trayhurn, P. (2013). Hypoxia and Adipose Tissue Function and Dysfunction in Obesity. Physiol. Rev. 93, 1–21. doi:10.1152/physrev.00017.2012
Trayhurn, P., Wang, B., and Wood, I. S. (2008). Hypoxia in Adipose Tissue: a Basis for the Dysregulation of Tissue Function in Obesity? Br. J. Nutr. 100, 227–235. doi:10.1017/S0007114508971282
Trincot, C. E., and Caron, K. M. (2019). Lymphatic Function and Dysfunction in the Context of Sex Differences. ACS Pharmacol. Transl Sci. 2, 311–324. doi:10.1021/acsptsci.9b00051
Vannella, K. M., and Wynn, T. A. (2017). Mechanisms of Organ Injury and Repair by Macrophages. Annu. Rev. Physiol. 79, 593–617. doi:10.1146/annurev-physiol-022516-034356
Vasanthakumar, A., Chisanga, D., Blume, J., Gloury, R., Britt, K., Henstridge, D. C., et al. (2020). Sex-specific Adipose Tissue Imprinting of Regulatory T Cells. Nature 579, 581–585. doi:10.1038/s41586-020-2040-3
Watts, E. R., and Walmsley, S. R. (2019). Inflammation and Hypoxia: HIF and PHD Isoform Selectivity. Trends Mol. Med. 25, 33–46. doi:10.1016/j.molmed.2018.10.006
Wynn, T. A., and Vannella, K. M. (2016). Macrophages in Tissue Repair, Regeneration, and Fibrosis. Immunity 44, 450–462. doi:10.1016/j.immuni.2016.02.015
Xiang, L., Mou, J., Shao, B., Wei, Y., Liang, H., Takano, N., et al. (2019). Glutaminase 1 Expression in Colorectal Cancer Cells Is Induced by Hypoxia and Required for Tumor Growth, Invasion, and Metastatic Colonization. Cell Death Dis 10, 40. doi:10.1038/s41419-018-1291-5
Xie, N., Tan, Z., Banerjee, S., Cui, H., Ge, J., Liu, R. M., et al. (2015). Glycolytic Reprogramming in Myofibroblast Differentiation and Lung Fibrosis. Am. J. Respir. Crit. Care Med. 192, 1462–1474. doi:10.1164/rccm.201504-0780OC
Xiong, A., and Liu, Y. (2017). Targeting Hypoxia Inducible Factors-1α as a Novel Therapy in Fibrosis. Front. Pharmacol. 8, 326. doi:10.3389/fphar.2017.00326
Yin, J., Gao, Z., He, Q., Zhou, D., Guo, Z., and Ye, J. (2009). Role of Hypoxia in Obesity-Induced Disorders of Glucose and Lipid Metabolism in Adipose Tissue. Am. J. Physiol. Endocrinol. Metab. 296, E333–E342. doi:10.1152/ajpendo.90760.2008
Zaleska, M. T., and Olszewski, W. L. (2017). Serum Immune Proteins in Limb Lymphedema Reflecting Tissue Processes Caused by Lymph Stasis and Chronic Dermato-Lymphangio-Adenitis (Cellulitis). Lymphat Res. Biol. 15, 246–251. doi:10.1089/lrb.2017.0003
Zampell, J. C., Elhadad, S., Avraham, T., Weitman, E., Aschen, S., Yan, A., et al. (2012). Toll-like Receptor Deficiency Worsens Inflammation and Lymphedema after Lymphatic Injury. Am. J. Physiol. Cel Physiol 302, C709–C719. doi:10.1152/ajpcell.00284.2011
Zampell, J. C., Yan, A., Avraham, T., Daluvoy, S., Weitman, E. S., and Mehrara, B. J. (2012). HIF-1α Coordinates Lymphangiogenesis during Wound Healing and in Response to Inflammation. FASEB J. 26, 1027–1039. doi:10.1096/fj.11-195321
Zampell, J. C., Yan, A., Elhadad, S., Avraham, T., Weitman, E., and Mehrara, B. J. (2012). CD4(+) Cells Regulate Fibrosis and Lymphangiogenesis in Response to Lymphatic Fluid Stasis. PLoS One 7, e49940. doi:10.1371/journal.pone.0049940
Zhao, B., Guan, H., Liu, J. Q., Zheng, Z., Zhou, Q., Zhang, J., et al. (2017). Hypoxia Drives the Transition of Human Dermal Fibroblasts to a Myofibroblast-like Phenotype via the TGF-β1/Smad3 Pathway. Int. J. Mol. Med. 39, 153–159. doi:10.3892/ijmm.2016.2816
Keywords: HIF, lymphedema, inflammation, lymphangiogensis, hypoxia
Citation: Jiang X, Tian W, Kim D, McQuiston AS, Vinh R, Rockson SG, Semenza GL and Nicolls MR (2022) Hypoxia and Hypoxia-Inducible Factors in Lymphedema. Front. Pharmacol. 13:851057. doi: 10.3389/fphar.2022.851057
Received: 08 January 2022; Accepted: 14 March 2022;
Published: 28 March 2022.
Edited by:
Gerard Bannenberg, Global Organization for EPA and DHA Omega-3s (GOED), United StatesReviewed by:
Raghu P. Kataru, Memorial Sloan Kettering Cancer Center, United StatesFrancesca Bianchini, University of Florence, Italy
Copyright © 2022 Jiang, Tian, Kim, McQuiston, Vinh, Rockson, Semenza and Nicolls. This is an open-access article distributed under the terms of the Creative Commons Attribution License (CC BY). The use, distribution or reproduction in other forums is permitted, provided the original author(s) and the copyright owner(s) are credited and that the original publication in this journal is cited, in accordance with accepted academic practice. No use, distribution or reproduction is permitted which does not comply with these terms.
*Correspondence: Xinguo Jiang, eGluZ3VvakBzdGFuZm9yZC5lZHU=; Wen Tian, YW15dGlhbkBzdGFuZm9yZC5lZHU=; Mark R. Nicolls, bW5pY29sbHNAc3RhbmZvcmQuZWR1
†These authors have contributed equally to this work