- 1Chemical and Biochemical Engineering, Indian Institute of Technology, Patna, India
- 2Department of Rehabilitation, The Second People’s Hospital of Shenzhen, Shenzhen, China
- 3Department of Biotechnology, School of Engineering and Technology, Sharda University, Greater Noida, India
- 4Institute for Biotechnology, St. John’s University, New York City, NY, United States
- 5Department of Biotechnology, School of Applied and Life Sciences, Uttaranchal University, Dehradun, India
- 6Department of Biotechnology Engineering and Food Technology, Chandigarh University, Mohali, India
- 7Department of Pharmaceutical Sciences, College of Pharmacy and Health Sciences, St. John’s University, New York City, NY, United States
Deposition of misfolded proteins and synaptic failure affects the brain in Alzheimer’s disease (AD). Its progression results in amnesia and cognitive impairment. Absence of treatment is due to excessive loss of neurons in the patients and the delayed effects of drugs. The enhanced pluripotency, proliferation, differentiation, and recombination characteristics of stromal cells into nerve cells and glial cells present them as a potential treatment for AD. Successful evidence of action in animal models along with positive results in preclinical studies further encourage its utilization for AD treatment. With regard to humans, cell replacement therapy involving mesenchymal stromal cells, induced-pluripotent stromal cells, human embryonic stromal cells, and neural stems show promising results in clinical trials. However, further research is required prior to its use as stromal cell therapy in AD related disorders. The current review deals with the mechanism of development of anomalies such as Alzheimer’s and the prospective applications of stromal cells for treatment.
Introduction
The epidemiological spread of communicable to non-communicable (not transfer from one living beings to another) diseases has raised the overall burden on healthcare workers worldwide. Neurological illnesses are serious issues that concern both medical professionals and patients when considering non-communicable diseases. Alzheimer’s disease (AD) accounts for 50%–70% of dementia along with it being the top fifth leading cause of death worldwide. By 2050, the worldwide population affected by AD is expected to rise to 152 million (GBD et al., 2019 Dementia Forecasting Collaborators, 2022). The degeneration of neural cells and synaptic connections in certain subcortical regions and cerebral cortex marks the presence of Alzheimer’s disease (AD). Scientists hypothesize that AD is based on cholinergic mechanisms, protein misfolding, and amyloid cascades. The loss of neural cells evident by temporal, parietal, frontal lobe atrophy, along with inflammation, increase in free radicals and the accretion of amyloid peptides and hyperphosphorylated tau protein in the form of plaques and neuro-fibrillary tangles (NFTs), respectively characterize Alzheimer’s disease (Valotassiou et al., 2010). An aggregation of misfolded proteins is the primary cause of this nervous system disorder. Two of these proteins are plaques (β-amyloid) and hyperphosphorylated tau proteins, which are created by the accumulation of Aβ proteins and affect cell-to-cell communication. These proteins also correspond to the formation of intracellular NFTs, which disrupt synaptic networks and reduce neurotransmitter production, respectively. Tangles, which are made of hyperphosphorylated tau protein, obstruct the neuronal internalised transport system that carries vital nutrients to the brain. The remaining 1% of the elderly population has been shown to have presenilin 2 (PS2) and presenilin 1 (PS1) genetic changes, which are responsible for reducing -secretase activity by boosting the synthesis of self-accumulating Aβ42 peptide. The diagnostic criteria for AD is given by the National Institute of Neurological and Communicative Disorders and Stroke (NINCDS) are: 1) the occurrence of disabilities related to cognition (language, orientation, learning and memory, perception behaviour), 2) the neuropsychological testing in case of symptoms concerning dementia syndrome confirmed by neuropsychological testing. There are no standard treatments presented in the literature (Loewenstein et al., 2001). However, the main strategy of treatment in AD reversal relies upon the reduction of Aβ levels. Various researchers across the globe have performed an extensive research to understand the pathogenesis, natural progression, diagnosis, and regenerative principles in the management of AD. However, “disease-modifying” treatments focusing on averting or curing the specific clinical observations and biomarkers related to AD still remain under investigation (Cummings and Fox, 2017). As per the complexities of Alzheimer’s pathophysiology, a multidisciplinary treatment approach is recommended composing of pharmacological therapy, behavioural treatment, and the stimulation of endogenous or exogenous neurogenesis and synaptogenesis (Doody et al., 2008; Liperoti et al., 2008; Radad et al., 2017). The present literature anticipates the current research advancements in stromal cell treatments of AD and discusses the challenges that still need to be addressed.
Cellular therapy in Alzheimer’s disease
In 2004, the World Health Organization (WHO) introduced the idea of medical products of human origin (MPHO), which serve as a platform for the attainment and dispersal of biological therapeutic goods to treat a variety of diseases (Martin, 2017). The use of MPHO to treat diseases has become increasingly important as research in the realms of beneficial and developmental medicine along with pre-clinical examination has progressed. Regenerative medicine aims to rejuvenate at cellular and tissue levels while also achieving restored regulation in the regional milieu (Sharma et al., 2022). To regenerate the tissue of concern, regenerative medicine employs the recombination of the extracellular matrix, growth factors, and most importantly stromal cells.
The notion of “Cellular Therapy” (CT) or “Cytotherapy” is based on the use of paracrine signaling by biomacromolecules to direct stromal cells which are in a state of quiescence for induction or augmentation. CT attempts to induce a curative impact in the concerned area through the transplantation of autologous, allogeneic, or modified cells that are unaccompanied by changes in their biological properties. Recombinant cell-induced pluripotent cells, hematopoietic cells, genetically-modified cells, stromal cells, genetically designed cells, or an amalgamation of all of these cells can be used to create cellular therapeutic products (Kaufman, 2009; Nianias and Themeli 2019; Sharma et al., 2022). The use of human-made medical items to treat neurological problems is still in its early stages. For efficient regeneration of these cells, numerous combinations have to be researched and explored.
Nervous stimulation of stromal cells
The adult stromal cells are stimulated for differentiation via numerous pathways as shown in Figure 1.
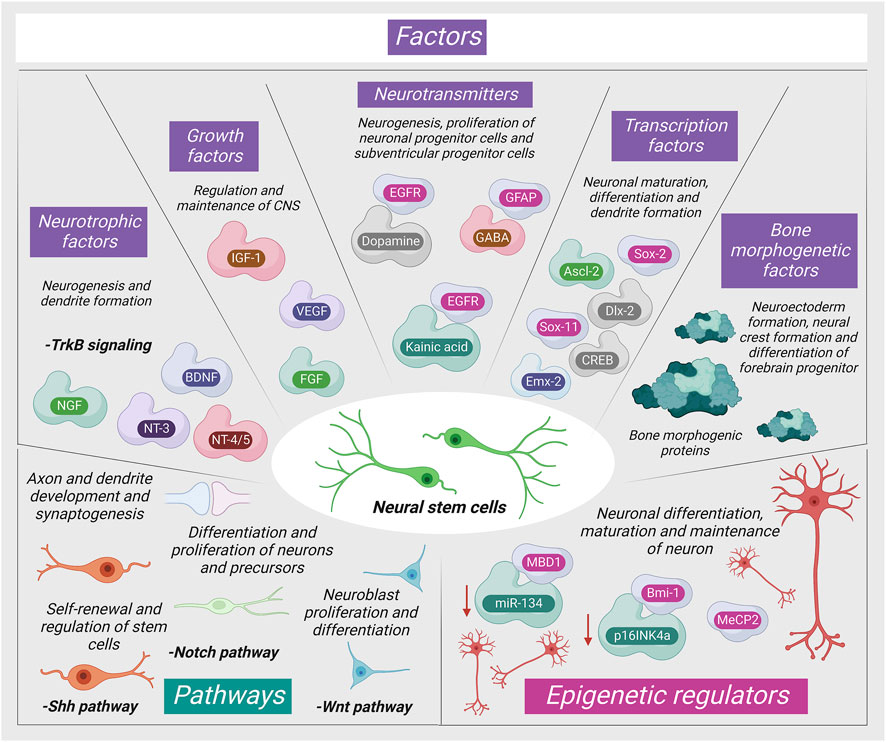
FIGURE 1. Mechanism of neurogenic signaling of stromal cells (MBD1: Methyl-CpG-binding domain protein one; NGF: Nerve Growth Factor; NT-3: Neurotrophin three; MeCP2: Methyl-CpG-binding protein two; BDNF: Brain-derived neurotrophic factor; IGF-1: Insulin-like growth factor; NT-4/5: Neurotrophin 4/5; VEGF: Vascular endothelial growth factor; MeCP2: Methyl-CpG-binding protein two; FGF: Fibroblast growth factor; Mll1: Mixed-lineage leukemia 1).
Significance of stromal cells in Alzheimer’s disease
Currently, the world lacks any proven standard therapy for the treatment of AD. Pharmacological management of AD temporarily improves cognitive symptoms. The targeted research in AD is directed towards 1) anti-oxidation, 2) removal of Aβ accumulation from the brain, and 3) regulation of the tau protein phosphorylation. Due to the advancements in regenerative and translational medicine, the usage of cells for treatment has become the limelight for producing disease-modifying strategies in the treatment of AD. Stromal cells are blank, unspecialized cells that are the result of the replenishment of lost neuronal cells through the differentiation and proliferation of neural cells upon activation via signaling pathways. They essentially restore neuroplasticity and neuromodulation (Ottoboni et al., 2020). In the recent decade, there are evidence to validate the cell-based neuroreplacement treatments for the treatment of AD in pre-clinical and clinical trials. The strategies of stromal cell therapy in AD are depicted in Figure 2. Evidence of the results from successful stromal cell therapy in animal models for AD is tabulated in Table 1.
Neurogenic precursors or neural stromal cells exist in a few restricted areas of the adult brain which shows continued neurogenesis and neuromodulation. Growth and the divergence of the cell into neurons and synapses are regulated by transcription factors and signaling pathways which involve biomolecules that act on neuronal progenitor cells, referred to as neurotransmitters. The genetic modification of neural stromal cells increases migratory efficiency. As a result, it allows to successfully employ them for the enhancement of gene expression and for the delivery of neurotrophic factors which subsequently modify the progression of AD.
Embryonic stromal cells
Embryonic stromal cells (ESCs) are totipotent cells that have a self-renewing ability and differentiation capacity. ESCs are obtained from blastocysts, specifically the inner cell mass. Due to the pluripotent nature of ESCs, tumorigenesis, uncontrolled cellular explosion, and immunogenic rejection may occur. Tang et al. suggested that hippocampal ESC transplantation can be performed without any potential risks to restore cognitive function in Aβ peptide injured rats (J et al., 2008). In this rodent model of AD, ESC-derived neural progenitor cell (NPC) transplantation following commitment to a cholinergic cell phenotype can enhance behavioral and cognitive recovery with the regeneration of cholinergic neurons (Fh et al., 2009). Transplantation in the mouse with ESC-derived NPCs to the meynert basal nucleus resulted in enhanced memory and learning capacity (Liu et al., 2020). Along with that, the enhancement of neurocognitive recovery was also observed when mouse and human ESC-derived basal forebrain cholinergic neurons were transplanted into the transgenic AD mice model (Yue and Jing, 2015). ESCs derived from humans were able to translate into dopaminergic neurons, spinal motor neurons, and astroglial cells (Lee et al., 2007). Neural progenitor cells derived from ESC develop into neuron-like cells and astrocytes which enhances memory and learning performance in AD animal models (J et al., 2008). However, certain ethical concerns are associated with hESCs (Human ESCs) in FDA-approved clinical trials (Liras, 2010; Fouad, 2019). Estimating the effectiveness of transplantation-based therapy is crucial from an ethical standpoint, as is lowering the possibility of therapeutic misunderstanding, minimizing discomfort, and emphasizing the significance of informed permission. Actually, the ethical discussion around stem cell-based therapy demonstrated the challenging balance between the need for prudence and the advancement of clinical studies. For instance, the infinite and unwanted differentiation potential of iPSCs increases the possibility of cancer, human cloning, and the unethical creation of genetically engineered human embryos. Similar safety concerns arise with MSC implantation due to their propensity to promote tumour development and metastasis. As a result, iPSCs are viewed as having higher moral standing than hESCs. However, the greatest safety concern with iPSC-based treatment is the possibility of teratoma development because of the unregulated differentiation. Additionally, the proliferative and differentiation capacity is decreased due to the host cells’ niche differing from that of the in vitro cultivated cells.
Mesenchymal stromal cells
MSCs play a significant role in controlling the symptoms of AD through 1) immunoregulation, 2) neurotrophic, 3) neuroplasticity, and 4) the reduction of Aβ plaque burden which restores the cognitive function (Babaei et al., 2012; Qin et al., 2020). Both in vitro and in vivo, MSCs show a remarkable capacity to trigger the quick clearance of Aβ aggregates. In terms of mechanism, the interaction between microglial cells and MSCs was primarily responsible for the removal of Aβ deposits (Fouad, 2019). Additionally, by expressing CCL5, MSCs can draw more microglial cells from the bone marrow (Fouad, 2019). In this approach, MSCs can inhibit deposition of Aβ plaques and perhaps halt the progression of the illness. Intriguingly, these investigations also showed a decrease in intracellular NFTs. Various preclinical studies have demonstrated the reversal of pathological changes in AD animal models. These studies verified neuroprotection, neuroplasticity, neuromodulation, and the neurogenic effects of MSCs through the neurogenic signaling pathway activation (Fumagalli et al., 2006). These grafted MSCs upregulate the expression of the anti-apoptotic factor, accentuate the AD symptoms, and halt the progression of the AD (Ruzicka et al., 2016). MSC-induced cognition restoration is due to the suppression of microglial activation and reduced levels of Aβ plaques in the brain (Woodruff-Pak, 2008).
The administration of bone marrow-derived MSCs (BM-MSCs) in the intracerebroventricular region ameliorates memory loss in AD model mice by accentuating astrocytic inflammation as well as synaptogenesis. BM-MSCs treated AD model of rodents expressed increased levels of miR-146 exosomes in the hippocampus which ultimately induces synaptogenesis and neurogenesis. Along with that, neuroplasticity was restored and cognitive impairment improved (Nakano et al., 2020). Intravenous injection of BM-MSCs was detected in brain parenchyma within an hour post-injection in the rat AD model which ultimately released growth factors and cytokines to recover neurobehavioral functions and stimulate endogenous regeneration (Harach et al., 2017).
Endovenous introduction of MSCs derived from adipose tissue (AD-MSCs) in rodent-model with AD improved neural functioning. It was accompanied by an increase in the formation of neurons along with differentiation of cells resembling neurons and astrocytes. Advancement in cognitive abilities was evident due to the decrease in accumulation of amyloid plaque and the increase in neural cells. The cells were then observed 12 days after injection (Tian et al., 2012).
Intracerebroventricular transplantation of placenta-derived MSCs (PD-MSCs) demonstrated the inhibitory effect of neuronal apoptosis and memory impairment in Aβ1–42-infused mice (Yun et al., 2013). PD-MSCs decrease the cytokines with pro-inflammatory action and increase the concentration of anti-inflammatory cytokines in the brain. They promote neuronal differentiation and proliferation in the hippocampus of the AD mice model. MSC transplantation in aged AD rats restores motor and cognitive activity (Liu et al., 2020). There are encouraging results in the preclinical trials of MSCs in AD. In one human trial, composed of nine patients, were administered MSCs in AD and have completed phase I which confirms the potential, efficacy, and non-toxicity of MSC injection.
Neural stromal cells
The multipotent nature of neural stromal cells (NSCs) allows them to differentiate into oligodendrocytes, astrocytes, and neurons after transplantation which is why they are an ideal cell-based substitution therapy in degenerative neurological disorders. The sources of NSCs are from primary tissues namely fetus, iPSCs, and ESCs. However, the required NSCs have to be customized for a particular patient to avoid immune rejection. Moreover, the isolation procedure is extremely risky as well. As contrary to other stem cells like ESCs, foetal NSCs, and MSCs, adult neural stem cells (aNSCs) are localised to certain regions of the central nervous system (CNS) and have a low proliferative potential. As a result, the initial isolation and steady in vitro growth of aNSCs are significant technological challenges that must be overcome before aNSCs may be used. Adult CNS surgical samples are often comparatively small (1–2 ml). Since there aren't many resident aNSCs in the tissue, isolation methods have been improved to increase the likelihood that aNSCs will be successfully isolated in the first place. CNS tissues are manually chopped and enzymatically degraded into single cells to get aNSCs. Enzymatic digestion is one among them, and it is important because it directly impacts aNSC survival. Different researchers use different dissociating enzyme compositions and incubation periods. Trypsin, collagenase, and papain have often been utilised, and in some studies, papain dissociation was recommended as the most effective method for the first separation of aNSCs. In the mammalian brain, NSCs exhibit neural homeostasis, repair, and regeneration, and demonstrate neural pleiotropism (Boese et al., 2020).
Administration of both stromal cells and neurotrophic peptides, stimulates differentiation of the neural stromal cells via paracrine signaling. The mentioned peptides increase neural formation in zones of the brain that concern memory such as the subgranular and subventricular zone. These neural stromal cells differentiate into neuronal cells and glial cells (Shohayeb et al., 2018). Genetic engineering of these cells amplifies the release of amyloid plaque degrading enzymes which increases synaptic density by removing Aβ aggregates (Hayashi et al., 2020). The immune protection of MSCs can be provided by the capacity to regulate immune activities thus providing neural protection from inflammation. Aside from that, mi-RNAs and si-RNAs released from these MSCs act to degrade accumulated amyloid plaques.
Transplantation of neural SCs in the lateral ventricle resulted in the formation of neuronal progenitor cells and glial cells. The cells had the capability of migration and regeneration which reduced Aβ deposition and tau phosphorylation and increased neural cell and synapse formation (Hayashi et al., 2020). Findings were proven through the incorporation of fluorescent protein labeled BM-MSCs in the hippocampus of AD diagnosed models where the same results were witnessed (Duncan and Valenzuela, 2017). Human MSCs were able to restore neuromuscular, cognition, and memory function after administration in aged rat models.
Transplanted NSCs in AD animal models possess the ability to replace the destructed neural circuit through neurotrophic factors that counteract the levels of Aβ plaques. NSC transplantation improve the cholinergic neuron numbers and enhance learning and memory abilities (Hayashi et al., 2020). It is unclear whether the observed behavioral rescue, enhanced memory, and learning abilities are due to NSC differentiation or neurotrophic factors in AD models (Hayashi et al., 2020). However, transplanted grafts of Neural SCs increases neurotrophic factor levels that are derived from the brain and enhance the behavioral rescue without altering concentration concerning Aβ or tau in a mutant rodent model of AD with overexpressed hAPP (Marsh and Blurton-Jones, 2017).
In APP/PS1 transgenic mice AD model, intranasal incorporation of human NSCs that differentiate into cholinergic neurons decrease β-amyloid accumulation by increasing neprilysin expression along with enzymes that can degrade β-amyloid. They also downregulate neuroinflammation, synaptic and pericytic loss ultimately rescuing the cognitive function. Intranasal administration of human NSCs possess greatest potential as a noninvasive tool in treating AD (Lu et al., 2021). NSCs ameliorates Aβ levels in AD mouse models by degrading plasmin, cathepsin B, and insulin-degrading enzymes.
Zhang et al. (2019) transplanted human induced neural progenitor cells (iNPCs) into the hippocampal region of brain in wild type immunodeficient AD mice. This study demonstrated the long-term survival, graft-host synaptic connections, neuronal circuits, and regenerated neural network of host hippocampus. AD mice exhibited neuroplasticity, neuromodulation and synaptogenesis with enhanced cognitive and behavioral abilities.
In APP/PS1 murine model of AD, NSC infiltration into fimbria and fornix junction show significant improvement in cognition and memory abilities. NSCs induce microglia activation and phagocytosis of amyloid plaques (McGinley et al., 2018). Without priming the microenvironment with neurotrophic factors, the transplantation of NSCs to the hippocampus would not produce any desired results in AD. In aged animals, the infusion of NGF demonstrated improved cognition. In phase one of the clinical trial, genetically modified fibroblasts coded with NGF gene transplantation into forebrain found to be safe (NGF-gene therapy) for AD (Tuszynski et al., 2005). These evidence provide a right path and new horizon in the management of AD in humans.
Induced pluripotent stromal cells
In 2011, Yahata et al. and Yagi et al. were the first researchers to introduce iPSCs therapy for AD (Yagi et al., 2011; Yahata et al., 2011). Yahata et al. (2011) demonstrated the production of Aβ plaques in neurons through the transplantation of iPSCs from forebrain neurons. Yagi et al. (2011) demonstrated the enhanced Aβ42 secretion by attenuating the dysfunctional condition of familial AD with PS1 (A246E) and PS2 (N141I) mutations.
Induced pluripotent cells can transform into neurospheres, neuronal progenitor cells and, neural cells which is evident in the cortex of rodent. The incorporation of these cells in diseased rodents resulted in increased concentration of Aβ-degrading protease neprilysin (Han et al., 2019). Enhanced synaptic activity and the connection between excitatory and inhibitory neurons have been demonstrated by iPSC-derived neurons (Birey et al., 2017; Xiang et al., 2017). Pure, immature neuronal cells, after incubation, differentiated into mature nerves exhibiting the inhibitory GABAergic and excitatory glutamatergic synapses along with the potentiation of action potentials. Human iPSC derived cortical neuronal cells attenuate the deposition of Aβ plaques and tau protein complexes in the forebrain (Nieweg et al., 2015).
Treatment of AD models by AD-iPSCs derived from neuronal cells consequently suppressed p-tau protein. AD-iPSC derived neuronal cells were able to upregulate AD associated genes which was evident by increased transcription (Hossini et al., 2015). In 5XFAD transgenic AD mouse model, differentiation of protein-iPSCs into glial cells, attenuation of Aβ plaque depositions, and improved cognitive ability were observed. In proteomic analysis, upregulation of oligodendrocyte-related genes in brain when protein-iPSCs are injected were observed (Cha et al., 2017).
Three dimensional spheroids from human iPSCs for AD model were differentiated into neuronal cell lines that led to the reduction of both Aβ40 and Aβ42 thereby potentiating the cell lines to regenerate into neuronal cells (Lee et al., 2016). Three dimensional cerebral organoids recapitulated the human brain architecture including cerebral progenitors in the subventricular and subgranular zone of hippocampus (Machairaki, 2020). Human iPSCs yielded 16 times more extracellular vehicles, exosomes, than MSCs. These exosomes have more potential to attenuate the deposition of Aβ plaques in the brain and demonstrate an increased Aβ42/Aβ40 ratio in AD animal models (Machairaki, 2020).
Clinical trials
Challenges posed by stromal cell treatment in Alzheimer’s disease
The emerging application of clinical therapy by medical experts and researchers in neurological disorders is based on the proof of potential in the preclinical research. The methods discussed have proven to be efficient and safe for the treatment of neural medical conditions. Following preclinical trials, clinical trials (Table 2) have begun to specifically target AD (McGinley et al., 2016). Although certain challenges still obstruct the routine use of cellular therapy in the medical practice, it is important to identify the correct cell types that are used along with understanding the mechanism of action of those cells for a maximum advantage in treatment. For instance, the therapy for treatment of PD requires particular cells such as the dopaminergic substantia nigra neurons (Freed et al., 2001).
Similarly, vascular endothelial cells, neuronal cells, and glial cells are needed for therapy which aims to treat AD and traumatic brain injuries. Considering this, there is a need of specifically differentiated cell lines. Known mechanisms today act through paracrine signalling which involves neurotrophic factors that regulate immune functions. Another potential mechanism could be inducing neural cell formation (Gnecchi et al., 2008). Altogether, it is critical to comprehend these effector processes before using them to treat neurological illnesses.
Results expressing affectivity of stromal cells in animal models are not completely reliable due to numerous reasons. The incomplete aspects and inaccuracy may have more harmful outcomes than beneficial. First being, using rodent models are far from the accurate representation of the physiological state of sickness in humans. Second, the age variation between the two remains at an excessive gap. Third, animal models are still inadequate to show that clinically relevant functional impairments improve. Finally, the animal models may be incapable of indicating the negative consequences of CT (Van der Worp et al., 2010).
The characterization of potential tumour generation remains the next issue in CT. For the treatment of neurological disorders, a healthy average life span and the tumorigenicity potential is important. In this circumstance, even a remote potential of tumour growth through CT would be unbearable. As a result, substantial research into the tumour formation of stromal cells and the derivatives of stromal cells is critical. In order to avoid risk of such terrible incidents and their associated consequences for patients, researchers and healthcare specialists must work together. Furthermore, treatments must be effectively better in terms of safety and effectiveness when compared to conventional therapeutic interventions (Sato et al., 2019).
Stromal cell treatments are linked to moral and ethical concerns along with the possibility of transplant rejection. With these cellular therapies, the cost of customized treatment escalates as a result of poor efficiency in the elicitation of iPSCs from the patient’s diploid cells. In order to increase the effectiveness of these cellular therapies while minimizing cost, technical and methodological improvements are required. The use of SCs in AD treatment raises a lot of unresolved issues. There are no data on which sort of differentiated or undifferentiated pluripotent SCs would be the most efficient for this treatment. Likewise, details on cell concentration, the quantity of effective dosages, and the length of the course of treatment are lacking. It is unclear if a confined (using stereotactic injections) or peripheral transplant should be performed, as well as how cell migration to the afflicted part of the brain happens. There is no proof that SCs treatment works in humans. It is also unknown how SCs can get rid of the inflammatory and toxic environment caused by the Aβ peptide, how healthy cells connect to one another, or how the cell repopulation will influence other metabolic pathways. It is yet unclear if the transplantation of SCs will stop the transmission of pathogenic tau protein between neurons or communication between damaged cells. Finally, since it has been unable to show the safety of this form of therapy for AD, ongoing patient monitoring would be necessary to prevent any negative side effects, both immediate and long-term.
Many SCs treatments are currently in the testing phase. Although SCs products may have a huge potential to cure a wide range of medical conditions, there is not enough scientific proof to guarantee that their usage is safe and has any positive health effects. Despite the fact that SCs have been the subject of several research in animal models of AD, the translation of this approach to the treatment of AD has been hampered by the differences between the molecular patterns of these models and those of people, as well as the conflicting results achieved. Before the advantages of this therapy can be demonstrated in the clinic, further in-depth understanding of the cellular pathways relating to its usage in both animal models and people is required.
Conclusion
In the absence of robust treatment methods for AD, research on SCs seem to be a major prospect. The variety of SCs, iPSCs, MSCs, ESCs and NSCs, can be incorporated invasively and non-invasively. This presents researchers with a wide range of possibilities for the development of customized cellular therapies. The promising results observed in pre-clinical and clinical trials were evident of the decrease in causative agents of AD which encourages its use in humans. Nevertheless, enormous amounts of research are requirement especially in the context of genetic variation due in the occurrence of AD and the inability to conclude negative consequences due to lack of replicable translation models. Issues like accuracy of treatment, mechanistic methodology, and ethical issues also need to be addressed prior to its common medical use.
Furthermore, there are notable changes in the brain’s structure and microenvironment between AD patients and animal models, making it challenging to precisely characterise the positive effects of stem cells in human AD. For the familial form of AD, transgenic animal models have been created, although human AD pathogenesis primarily comprises sporadic instances. This restricts our understanding of how patient-specific stem cell treatment might operate. These issues require future research. Future preclinical studies examining various stem cell sources, kinds, dosages, long-term safety, effectiveness, and exact mechanisms of action are necessary. Using recently developed in vivo biomarkers in clinical research is anticipated to give researchers more control and insight into patient selection and quantity efficacy than was previously feasible using previously known marker kinds. When working with the immune system, neuro factors, enzymes, proteins, and gene therapy, to mention a few examples of applications, among many more, cell extraction and injection enhancements may be tackled from numerous viewpoints. Additionally, as part of the entire development process for the technology, it is crucial to evaluate ethical issues at each stage of building a stem cell-based technology.
Author contributions
SJ has prepared the backbone of the manuscript. RS and AL wrote the original draft of the manuscript with TD and ST. SJ, ST, and Z-SC refined the first draft. Illustrations, NJ. Z-SC and SJ critically revised the manuscript for intellectually correct content. AL and Z-SC supported the project. All authors approved the submitted version.
Conflict of interest
The authors declare that the research was conducted in the absence of any commercial or financial relationships that could be construed as a potential conflict of interest.
Publisher’s note
All claims expressed in this article are solely those of the authors and do not necessarily represent those of their affiliated organizations, or those of the publisher, the editors and the reviewers. Any product that may be evaluated in this article, or claim that may be made by its manufacturer, is not guaranteed or endorsed by the publisher.
References
Babaei, P., Soltani Tehrani, B., and Alizadeh, A. (2012). Transplanted bone marrow mesenchymal stem cells improve memory in rat models of Alzheimer's disease. Stem Cells Int. 2012, e369417. doi:10.1155/2012/369417
Birey, F., Andersen, J., Makinson, C. D., Islam, S., Wei, W., Huber, N., et al. (2017). Assembly of functionally integrated human forebrain spheroids. Nature 545, 54–59. doi:10.1038/nature22330
Boese, A. C., Hamblin, M. H., and Lee, J.-P. (2020). Neural stem cell therapy for neurovascular injury in Alzheimer's disease. Exp. Neurol. 324, 113112. doi:10.1016/j.expneurol.2019.113112
Cha, M.-Y., Kwon, Y.-W., Ahn, H.-S., Jeong, H., Lee, Y. Y., Moon, M., et al. (2017). Protein-induced pluripotent stem cells ameliorate cognitive dysfunction and reduce Aβ deposition in a mouse model of Alzheimer's disease. Stem Cells Transl. Med. 6, 293–305. doi:10.5966/sctm.2016-0081
Cummings, J., and Fox, N. (2017). Defining disease modifying therapy for Alzheimer’s disease. J. Prev. Alzheimers Dis. 4, 109–115. doi:10.14283/jpad.2017.12
Doody, R. S., Gavrilova, S. I., Sano, M., Thomas, R. G., Aisen, P. S., Bachurin, S. O., et al. (2008). Effect of dimebon on cognition, activities of daily living, behaviour, and global function in patients with mild-to-moderate Alzheimer's disease: A randomised, double-blind, placebo-controlled study. Lancet 372, 207–215. doi:10.1016/S0140-6736(08)61074-0
Duncan, T., and Valenzuela, M. (2017). Alzheimer's disease, dementia, and stem cell therapy. Stem Cell Res. Ther. 8, 111. doi:10.1186/s13287-017-0567-5
Fh, M., H, A., K, K., S, T., Mh, N. E., and H, B. (2009). Transplantation of primed or unprimed mouse embryonic stem cell-derived neural precursor cells improves cognitive function in Alzheimerian rats. Differentiation. 78, 59–68. doi:10.1016/j.diff.2009.06.005
Fouad, G. I. (2019). Stem cells as a promising therapeutic approach for Alzheimer’s disease: A review. Bull. Natl. Res. Cent. 43, 52. doi:10.1186/s42269-019-0078-x
Freed, C. R., Greene, P. E., Breeze, R. E., Tsai, W. Y., DuMouchel, W., Kao, R., et al. (2001). Transplantation of embryonic dopamine neurons for severe Parkinson’s disease. N. Engl. J. Med. 344, 710–719. doi:10.1056/NEJM200103083441002
Fumagalli, F., Racagni, G., and Riva, M. A. (2006). The expanding role of BDNF: A therapeutic target for Alzheimer’s disease? Pharmacogenomics J. 6, 8–15. doi:10.1038/sj.tpj.6500337
GBD Steinmetz, J. D., Vollset, S. E., Fukutaki, K., Chalek, J., Abd-Allah, F., et al. (2019). Estimation of the global prevalence of dementia in 2019 and forecasted prevalence in 2050: An analysis for the global burden of disease study 2019. Lancet Public Health 7, e105–e125. doi:10.1016/S2468-2667(21)00249-8
Gnecchi, M., Zhang, Z., Ni, A., and Dzau, V. J. (2008). Paracrine mechanisms in adult stem cell signaling and therapy. Circ. Res. 103, 1204–1219. doi:10.1161/CIRCRESAHA.108.176826
Han, F., Liu, C., Huang, J., Chen, J., Wei, C., Geng, X., et al. (2019). The application of patient-derived induced pluripotent stem cells for modeling and treatment of Alzheimer’s disease. Brain Sci. Adv. 5, 21–40. doi:10.1177/2096595819896178
Harach, T., Jammes, F., Muller, C., Duthilleul, N., Cheatham, V., Zufferey, V., et al. (2017). Administrations of human adult ischemia-tolerant mesenchymal stem cells and factors reduce amyloid beta pathology in a mouse model of Alzheimer's disease. Neurobiol. Aging 51, 83–96. doi:10.1016/j.neurobiolaging.2016.11.009
Hayashi, Y., Lin, H.-T., Lee, C.-C., and Tsai, K.-J. (2020). Effects of neural stem cell transplantation in Alzheimer's disease models. J. Biomed. Sci. 27, 29. doi:10.1186/s12929-020-0622-x
Hossini, A. M., Megges, M., Prigione, A., Lichtner, B., Toliat, M. R., Wruck, W., et al. (2015). Induced pluripotent stem cell-derived neuronal cells from a sporadic Alzheimer's disease donor as a model for investigating AD-associated gene regulatory networks. BMC Genomics 16, 84. doi:10.1186/s12864-015-1262-5
Jun, T., Haiwei, X., Xiaotang, F., Guangji, Z., Zhifang, L., Li, Y., et al. (2008). Embryonic stem cell-derived neural precursor cells improve memory dysfunction in Abeta(1-40) injured rats. Neurosci. Res. 62, 86–96. doi:10.1016/j.neures.2008.06.005
Kaufman, D. S. (2009). Toward clinical therapies using hematopoietic cells derived from human pluripotent stem cells. Blood 114, 3513–3523. doi:10.1182/blood-2009-03-191304
Lee, H.-K., Sanchez, C. V., Chen, M., Morin, P. J., Wells, J. M., Hanlon, E. B., et al. (2016). Three dimensional human neuro-spheroid model of Alzheimer's disease based on differentiated induced pluripotent stem cells. PLOS ONE 11, e0163072. doi:10.1371/journal.pone.0163072
Lee, H., Shamy, G. A., Elkabetz, Y., Schofield, C. M., Harrsion, N. L., Panagiotakos, G., et al. (2007). Directed differentiation and transplantation of human embryonic stem cell-derived motoneurons. Stem Cells 25, 1931–1939. doi:10.1634/stemcells.2007-0097
Liperoti, R., Pedone, C., and Corsonello, A. (2008). Antipsychotics for the treatment of behavioral and psychological symptoms of dementia (BPSD). Curr. Neuropharmacol. 6, 117–124. doi:10.2174/157015908784533860
Liras, A. (2010). Future research and therapeutic applications of human stem cells: General, regulatory, and bioethical aspects. J. Transl. Med. 8, 131. doi:10.1186/1479-5876-8-131
Liu, X.-Y., Yang, L.-P., and Zhao, L. (2020). Stem cell therapy for Alzheimer's disease. World J. Stem Cells 12, 787–802. doi:10.4252/wjsc.v12.i8.787
Loewenstein, D. A., Ownby, R. M., Schram, L., Acevedo, A., Rubert, M., and Argelles, T. (2001). An evaluation of the NINCDS-ADRDA neuropsychological criteria for the assessment of alzheimers disease: A confirmatory factor analysis of single versus multi-factor models. J. Clin. Exp. Neuropsychol. 23, 274–284. doi:10.1076/jcen.23.3.274.1191
Lu, M.-H., Ji, W.-L., Chen, H., Sun, Y.-Y., Zhao, X.-Y., Wang, F., et al. (2021). Intranasal transplantation of human neural stem cells ameliorates Alzheimer's disease-like pathology in a mouse model. Front. Aging Neurosci. 13, 650103. doi:10.3389/fnagi.2021.650103
Machairaki, V. (2020). Human pluripotent stem cells as in vitro models of neurodegenerative diseases. Adv. Exp. Med. Biol. 1195, 93–94. doi:10.1007/978-3-030-32633-3_13
Marsh, S. E., and Blurton-Jones, M. (2017). Neural stem cell therapy for neurodegenerative disorders: The role of neurotrophic support. Neurochem. Int. 106, 94–100. doi:10.1016/j.neuint.2017.02.006
Martin, D. E. (2017). Ethical aspects of medical products of human origin. ISBT Sci. Ser. 12, 281–287. doi:10.1111/voxs.12304
McGinley, L. M., Kashlan, O. N., Bruno, E. S., Chen, K. S., Hayes, J. M., Kashlan, S. R., et al. (2018). Human neural stem cell transplantation improves cognition in a murine model of Alzheimer's disease. Sci. Rep. 8, 14776. doi:10.1038/s41598-018-33017-6
McGinley, L. M., Sims, E., Lunn, J. S., Kashlan, O. N., Chen, K. S., Bruno, E. S., et al. (2016). Human cortical neural stem cells expressing insulin-like growth factor-I: A novel cellular therapy for Alzheimer's disease. Stem Cells Transl. Med. 5, 379–391. doi:10.5966/sctm.2015-0103
Nakano, M., Kubota, K., Kobayashi, E., Chikenji, T. S., Saito, Y., Konari, N., et al. (2020). Bone marrow-derived mesenchymal stem cells improve cognitive impairment in an Alzheimer's disease model by increasing the expression of microRNA-146a in hippocampus. Sci. Rep. 10, 10772. doi:10.1038/s41598-020-67460-1
Nianias, A., and Themeli, M. (2019). Induced pluripotent stem cell (iPSC)–derived lymphocytes for adoptive cell immunotherapy: Recent advances and challenges. Curr. Hematol. Malig. Rep. 14, 261–268. doi:10.1007/s11899-019-00528-6
Nieweg, K., Andreyeva, A., Stegen, B. V., Tanriöver, G., and Gottmann, K. (2015). Alzheimer’s disease-related amyloid-β induces synaptotoxicity in human iPS cell-derived neurons. Cell Death Dis. 6, e1709. doi:10.1038/cddis.2015.72
Ottoboni, L., von Wunster, B., and Martino, G. (2020). Therapeutic plasticity of neural stem cells. Front. Neurol. 11, 148. doi:10.3389/fneur.2020.00148
Qin, C., Lu, Y., Wang, K., Bai, L., Shi, G., Huang, Y., et al. (2020). Transplantation of bone marrow mesenchymal stem cells improves cognitive deficits and alleviates neuropathology in animal models of Alzheimer's disease: A meta-analytic review on potential mechanisms. Transl. Neurodegener. 9, 20. doi:10.1186/s40035-020-00199-x
Radad, K., Moldzio, R., Al-Shraim, M., Kranner, B., Krewenka, C., and Rausch, W. D. (2017). Recent advances on the role of neurogenesis in the adult brain: Therapeutic potential in Parkinson's and Alzheimer's diseases. CNS Neurol. Disord. Drug Targets 16, 740–748. doi:10.2174/1871527316666170623094728
Ruzicka, J., Kulijewicz-Nawrot, M., Rodrigez-Arellano, J. J., Jendelova, P., and Sykova, E. (2016). Mesenchymal stem cells preserve working memory in the 3xTg-AD mouse model of Alzheimer's disease. Int. J. Mol. Sci. 17, E152. doi:10.3390/ijms17020152
Sato, Y., Bando, H., Di Piazza, M., Gowing, G., Herberts, C., Jackman, S., et al. (2019). Tumorigenicity assessment of cell therapy products: The need for global consensus and points to consider. Cytotherapy 21, 1095–1111. doi:10.1016/j.jcyt.2019.10.001
Sharma, S., Jeyaraman, M., and Muthu, S. (2022). Role of stem cell therapy in neurosciences essentials of evidence-based practice of neuroanesthesia and neurocritical care. Massachusetts United States: Academic Press, 163–179. doi:10.1016/B978-0-12-821776-4.00012-3
Shohayeb, B., Diab, M., Ahmed, M., and Ng, D. C. H. (2018). Factors that influence adult neurogenesis as potential therapy. Transl. Neurodegener. 7, 4. doi:10.1186/s40035-018-0109-9
Tian, G., Zhou, J., Wang, J., Xu, B., Li, L., Zhu, F., et al. (2012). Neuronal differentiation of adipose-derived stem cells and their transplantation for cerebral ischemia. Neural Regen. Res. 7, 1992–1999. doi:10.3969/j.issn.1673-5374.2012.25.011
Tuszynski, M. H., Thal, L., Pay, M., Salmon, D. P., U, H. S., Bakay, R., et al. (2005). A phase 1 clinical trial of nerve growth factor gene therapy for Alzheimer disease. Nat. Med. 11, 551–555. doi:10.1038/nm1239
Valotassiou, V., Archimandritis, S., Sifakis, N., Papatriantafyllou, J., and Georgoulias, P. (2010). Alzheimer's disease: Spect and pet tracers for beta-amyloid imaging. Curr. Alzheimer Res. 7, 477–486. doi:10.2174/156720510792231757
Van der Worp, H. B., Howells, D. W., Sena, E. S., Porritt, M. J., Rewell, S., O'Collins, V., et al. (2010). Can animal models of disease reliably inform human studies? PLoS Med. 7, e1000245. doi:10.1371/journal.pmed.1000245
Woodruff-Pak, D. S. (2008). Animal models of Alzheimer’s disease: Therapeutic implications. J. Alzheimers Dis. 15, 507–521. doi:10.3233/jad-2008-15401
Xiang, Y., Tanaka, Y., Patterson, B., Kang, Y.-J., Govindaiah, G., Roselaar, N., et al. (2017). Fusion of regionally specified hPSC-derived organoids models human brain development and interneuron migration. Cell Stem Cell 21, 383–398. doi:10.1016/j.stem.2017.07.007
Yagi, T., Ito, D., Okada, Y., Akamatsu, W., Nihei, Y., Yoshizaki, T., et al. (2011). Modeling familial Alzheimer's disease with induced pluripotent stem cells. Hum. Mol. Genet. 20, 4530–4539. doi:10.1093/hmg/ddr394
Yahata, N., Asai, M., Kitaoka, S., Takahashi, K., Asaka, I., Hioki, H., et al. (2011). Anti-Aβ drug screening platform using human iPS cell-derived neurons for the treatment of Alzheimer’s disease. PLoS One 6, e25788. doi:10.1371/journal.pone.0025788
Yue, C., and Jing, N. (2015). The promise of stem cells in the therapy of Alzheimer's disease. Transl. Neurodegener. 4, 8. doi:10.1186/s40035-015-0029-x
Yun, H.-M., Kim, H. S., Park, K.-R., Shin, J. M., Kang, A. R., il Lee, K., et al. (2013). Placenta-derived mesenchymal stem cells improve memory dysfunction in an Aβ1-42-infused mouse model of Alzheimer's disease. Cell Death Dis. 4, e958. doi:10.1038/cddis.2013.490
Keywords: cellular therapy, mesenchymal stromal cell, Alzheimer’s, clinical trial, management
Citation: Srivastava R, Li A, Datta T, Jha NK, Talukder S, Jha SK and Chen Z-S (2022) Advances in stromal cell therapy for management of Alzheimer’s disease. Front. Pharmacol. 13:955401. doi: 10.3389/fphar.2022.955401
Received: 28 May 2022; Accepted: 08 September 2022;
Published: 04 October 2022.
Edited by:
Mythily Srinivasan, Indiana University, Purdue University Indianapolis, United StatesReviewed by:
Androniki Kretsovali, Foundation for Research and Technology Hellas (FORTH), GreeceVincent Pons, Jackson Laboratory, United States
Longyang Liu, Southern Medical University, China
Copyright © 2022 Srivastava, Li, Datta, Jha, Talukder, Jha and Chen. This is an open-access article distributed under the terms of the Creative Commons Attribution License (CC BY). The use, distribution or reproduction in other forums is permitted, provided the original author(s) and the copyright owner(s) are credited and that the original publication in this journal is cited, in accordance with accepted academic practice. No use, distribution or reproduction is permitted which does not comply with these terms.
*Correspondence: Saurabh Kumar Jha, c2F1cmFiaC5qaGFAc2hhcmRhLmFjLmlu; Zhe-Sheng Chen, Y2hlbnpAc3Rqb2hucy5lZHU=
†These authors have contributed equally to this work and share first authorship