- 1Key Laboratory of Active Substances and Biological Mechanisms of Ginseng Efficacy, Jilin Provincial Key Laboratory of Bio-Macromolecules of Chinese Medicine, Ministry of Education, Northeast Asia Research Institute of Traditional Chinese Medicine, Changchun University of Chinese Medicine, Changchun, Jilin, China
- 2GCP Department, Affiliated Hospital of Changchun University of Chinese Medicine, Changchun, China
- 3College of Integrated Traditional Chinese and Western Medicine, Changchun University of Chinese Medicine, Changchun, China
- 4College of Traditional Chinese Medicine, Changchun University of Chinese Medicine, Changchun, China
Background: Respiratory diseases are common and frequent diseases. Due to the high pathogenicity and side effects of respiratory diseases, the discovery of new strategies for drug treatment is a hot area of research. Scutellaria baicalensis Georgi (SBG) has been used as a medicinal herb in China for over 2000 years. Baicalin (BA) is a flavonoid active ingredient extracted from SBG that BA has been found to exert various pharmacological effects against respiratory diseases. However, there is no comprehensive review of the mechanism of the effects of BA in treating respiratory diseases. This review aims to summarize the current pharmacokinetics of BA, baicalin-loaded nano-delivery system, and its molecular mechanisms and therapeutical effects for treating respiratory diseases.
Method: This review reviewed databases such as PubMed, NCBI, and Web of Science from their inception to 13 December 2022, in which literature was related to “baicalin”, “Scutellaria baicalensis Georgi”, “COVID-19”, “acute lung injury”, “pulmonary arterial hypertension”, “asthma”, “chronic obstructive pulmonary disease”, “pulmonary fibrosis”, “lung cancer”, “pharmacokinetics”, “liposomes”, “nano-emulsions”, “micelles”, “phospholipid complexes”, “solid dispersions”, “inclusion complexes”, and other terms.
Result: The pharmacokinetics of BA involves mainly gastrointestinal hydrolysis, the enteroglycoside cycle, multiple metabolic pathways, and excretion in bile and urine. Due to the poor bioavailability and solubility of BA, liposomes, nano-emulsions, micelles, phospholipid complexes, solid dispersions, and inclusion complexes of BA have been developed to improve its bioavailability, lung targeting, and solubility. BA exerts potent effects mainly by mediating upstream oxidative stress, inflammation, apoptosis, and immune response pathways. It regulates are the NF-κB, PI3K/AKT, TGF-β/Smad, Nrf2/HO-1, and ERK/GSK3β pathways.
Conclusion: This review presents comprehensive information on BA about pharmacokinetics, baicalin-loaded nano-delivery system, and its therapeutic effects and potential pharmacological mechanisms in respiratory diseases. The available studies suggest that BA has excellent possible treatment of respiratory diseases and is worthy of further investigation and development.
1 Introduction
Respiratory diseases are a significant worldwide health problem with high morbidity and mortality rates. Smoking, radiation, dust, bacteria, viruses, mycoplasma, and obesity can all cause pulmonary diseases, which are coronary viral disease-2019 (COVID-19), acute lung injury (ALI), asthma, chronic obstructive pulmonary disease (COPD), pulmonary arterial hypertension (PAH), pulmonary fibrosis (PF), and lung cancer (LC) (Venkatesan et al., 2007; Kumar et al., 2018). Due to its susceptibility and poor prognosis, drug development in this field has been of great interest.
Chinese herbal medicine, as one of the natural products, has been a rich source of discovery compounds (Hu et al., 2021). Scutellaria baicalensis Georgi (SBG) is a Lamiaceae family herb widely used in East Asian countries to treat diseases (Shang et al., 2010). Baicalin (BA), baicalein (BE), wogonin, and wogonoside are the main flavonoid compounds found in SBG (Pan et al., 2021). As the main effective constituent, BA has been found to have therapeutic effects on cardiovascular, hepatobiliary, brain, nervous system, and intestinal diseases (Jin et al., 2019; Song et al., 2020; Xin et al., 2020; Ganguly et al., 2022). BA has been extensively reported to treat respiratory diseases (Deng et al., 2022; Ju et al., 2022; Li et al., 2022). However, the widespread use of BA is still a challenge due to its low solubility and poor bioavailability (Haider et al., 2018; Pramual et al., 2022). And the molecular mechanism of BA in treating respiratory diseases has yet to be reported based on a summary of reliable evidence.
Herein, we discuss a comprehensive report of BA on pharmacokinetics and baicalin-loaded nano-delivery system. In addition, we have summarized studies related to BA for the treatment of COVID-19, ALI, asthma, PAH, COPD, PF, and LC. And the main therapeutic targets and the critical signal pathways of BA against respiratory diseases were summarized. This review could provide a theoretical basis for further pharmacological studies and clinical applications of BA for the prevention and treatment of respiratory diseases.
2 Literature survey databases
This review reviewed databases such as PubMed, NCBI, and Web of Science from their inception to 13 December 2022, using terms including “baicalin”, “Scutellaria baicalensis Georgi”, “COVID-19”, “acute lung injury”, “pulmonary arterial hypertension”, “asthma”, “chronic obstructive pulmonary disease”, “pulmonary fibrosis”, “lung cancer”, “pharmacokinetics”, “liposomes”, “nano-emulsions”, “micelles”, “phospholipid complexes”, “solid dispersions”, “inclusion complexes”, and other terms. The pharmacokinetics of BA, strategies to improve bioavailability, therapeutic effects, and potential pharmacological mechanisms were summarized. Further research in this area is informed and recommended.
3 Pharmacokinetics of BA
As a glycoside flavonoid, BA has low aqueous solubility and poor membrane permeability, reducing its oral bioavailability and limiting its clinical application (Taiming and Xuehua, 2006; Ju et al., 2022). In vivo, BA is rapidly hydrolyzed to its glycosidic ligand BE, which shows excellent permeability and lipophilicity (Wang et al., 2020a). Liu et al. investigated the absorption mechanisms of BA and BE in rats and discovered that BA was moderately absorbed in the stomach and poorly absorbed in the small intestine and colon. While BE was well absorbed in the stomach and small intestine and relatively little in the colon (Taiming and Xuehua, 2006). After intravenous BA administration, the drug concentration decreases from high to low in the major organ tissues in the following order: kidney, lung, liver, and spleen (Wei et al., 2016). Notably, BA is the main metabolite in the blood, whether an oral BA or BE (Akao et al., 2013).
The metabolism of BA is a crucial factor in determining its efficacy and toxicity. The first step in the metabolism of BA is the hydrolysis of the glycoside into its ligand BE by the enzyme β-glucuronidase produced by intestinal microbiota (Xing et al., 2005). The type and abundance of intestinal microbes can impact the metabolic processes of BA in the intestine, making the intestinal microbiota an important factor in the metabolic processes of BA (Xie et al., 2020). This was confirmed by conducting studies in a rat model of antibiotic-pretreated rats, which showed that the maximum serum concentration (Cmax), terminal half-life, elimination rate constant, and plasma drug concentration-time curve (AUC) values were significantly altered compared to normal rats. Moreover, gut microbiota, such as Escherichia coli and Streptococcus spp., can produce β-glucuronidase which enhances the metabolism of BA (Ishihara et al., 2002).
After metabolism in the intestine, BA undergoes glucuronidation (Zeng et al., 2022). Extensive hepatic and intestinal first-pass glucuronidation of BA has been found in both humans and rats (Akao et al., 2000; Zhang et al., 2007). BA and its glycosidic ligand exhibit inhibitory effects in the liver on UDP-glucuronosyltransferase (UGT) isoforms, including UGT1A1, 1A6, 1A9, and 2B7 (Teng et al., 2013). As one of the essential phase II mechanisms, those UGT isoforms might affect the bioavailability of BA.
Following glucuronidation, the hepatic-intestinal circulation of BA is comprised of several key steps, including uptake by the liver from the blood, excretion from the liver into the bile, transport of the bile to the duodenum for reabsorption, and finally return to the liver via the portal circulation (Xing et al., 2005). During this process, BA has two potential two sites of absorption. The first site is the upper intestine, which may directly absorb BA, and the second site is located in the colon in the form of glycosylated ligands (Lu et al., 2007).
As with the intestine, extensive metabolism of BA occurs in the liver. After oral administration of BA to rats, 32 metabolites were detected in blood and urine. During this process, various reactions were found in rats, including methylation, hydrolysis, hydroxylation, methoxylation, glucuronide conjugation, sulfate conjugation, and complex reactions. The liver and kidney are the most important organs for metabolite distribution (Zhang et al., 2015). Finally, BA is excreted mainly as gluconate in the bile, accompanied by a small amount of urine (Xing et al., 2005).
In the intestine, β-glucuronidase, which is produced by the intestinal flora, hydrolyzes BA to BE. Glucuronidation is a significant metabolic pathway for BA, which undergoes various reactions in the liver and kidneys, the main metabolic organs. The elimination of metabolites occurs primarily through bile and urine. The biotransformation of BA requires the metabolic enzymes UGT and β-glucuronidase. The pharmacokinetics of BA can also be affected by various factors, including the administration route. The study by (Xing et al., 2005) found that the AUC values for BA administered intravenously (37 μmol/kg) and orally (224 μmol/kg) were 33.57 ± 1.8 (nmol·h/mL) and 4.43 ± 0.4 (nmol·h/mL), respectively. This indicates that the intravenous administration of BA was 6.05 times lower in dose but 7.57 times higher in AUC compared to the oral administration. However, BA’s low bioavailability limits its clinical application, leading to the development of various formulations to enhance its solubility, bioavailability, and lung targeting potential.
4 Baicalin-loaded nano-delivery system
In recent years, the combination of nanoscience and biologically active natural compounds has been frequently investigated to create safe, biodegradable, and biocompatible drug delivery systems. Poor bioavailability is the main problem limiting BA in treating respiratory diseases (Xu et al., 2022a). In recent years, the development of new formulations for BA has been of increasing interest to the pharmaceutical field. Various nano-sized delivery systems have been explored, including liposomes, nano-emulsions, micelles, phospholipid complexes, solid dispersions, and inclusion complexes (Li J. et al., 2009; Li et al., 2013; Yue et al., 2013; Zhao et al., 2013; Wu et al., 2014; Zhang et al., 2014) (Tables 1, 2).
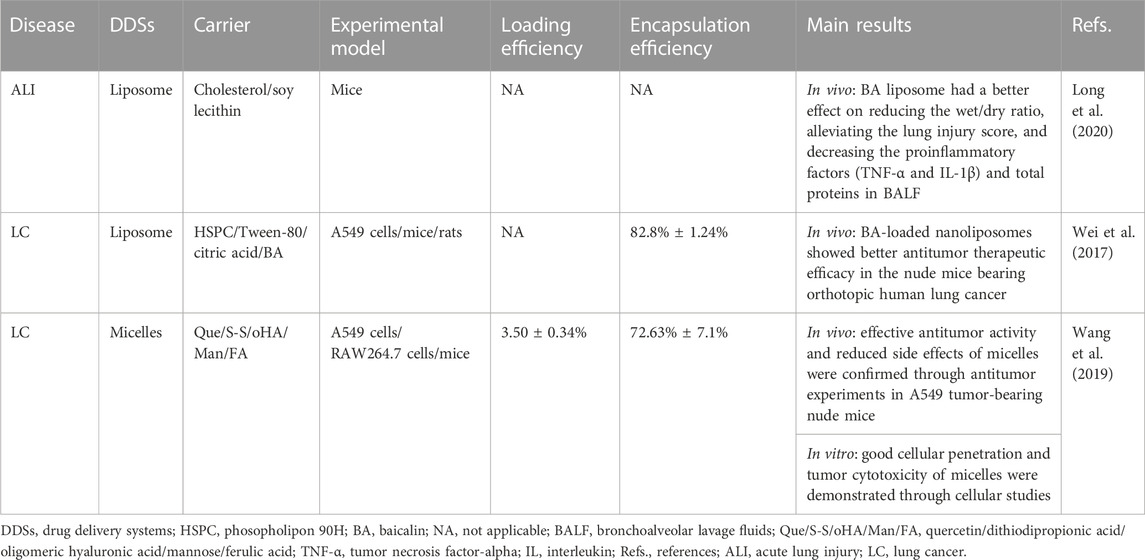
TABLE 2. Drug delivery systems for baicalin studied in preclinical acute lung injury and lung cancer models.
4.1 Liposomes
Liposomes are the most widely studied nano drug delivery system due to their synergistic delivery system that increases drug solubility (Huang et al., 2022; Ramedani et al., 2022). It has been demonstrated that the advantage of BA-loaded nanoliposomes for treating respiratory diseases is lung targeting (Wei et al., 2016; Wei et al., 2017). Research has shown that the BA liposome (L-BA) improves oral availability and tissue distribution (Wei et al., 2014). Compared to the same dose of BA, L-BA (100 mg/kg, tail-vein injection) more significantly reduced the W/D ratio, lung injury score, pro-inflammatory factors, and protein in total bronchoalveolar lavage fluid in a mouse model of lipopolysaccharide (LPS)-induced ALI (Long et al., 2020). In particular, the daily increase in tumor weight of BALB/c nude mice injected with A549 human lung cancer tumor cells was significantly reduced by L-BA (100 mg/kg) after intravenous injection of the drug, resulting in increased the survival rate of mice (Wei et al., 2017). L-BA was modified with folic acid and polyethylene glycol to improve further tumor targeting, which exhibited higher cellular uptake than non-targeted liposomes (Chen et al., 2016).
4.2 Nano-emulsions
Nano-emulsions offer unique advantages and properties that increase water solubility and dynamic stability, improve therapeutic efficacy, and reduce adverse effects and toxic reactions (Pangeni et al., 2018). Due to the sustained-release characteristics of the nano-emulsions containing BA, it is very effective in increasing oral availability. A novel nano-emulsion has been proven to improve systemic exposure to BA by promoting intestinal absorption and lymphatic transport (Wu et al., 2018). Zhao et al. (2013) prepared BA-loaded nano-emulsions by internal and external drug addition. And they found that both were superior to BA suspensions in terms of Cmax and AUC0-∞.
4.3 Micelles
Micelles are synthesized from amphiphilic surfactants in the aqueous phase, which provides hydrophobic cores as reservoirs to increase the solubility of water-insoluble drugs (Gaucher et al., 2005). The carrier material containing a mixture of Pluronic P123 copolymer and sodium taurocholate in bundle gum increased the Cmax and AUC in rats by 1.77 and 1.54 times more than the BA suspension, respectively (Zhang et al., 2016). The therapeutic effects of BA in micelles have been investigated in preclinical LC models. Novel carrier materials have been screened for structures targeting nano-micelles (named “nano-dandelion”) for the simultaneous delivery of curcumin and BA. The nano-dandelion improved cellular uptake and cytotoxicity in the A549 cells, exhibiting better anti-cancer effects. In addition, by aggregating more readily at the tumor site, tail vein injection nano-dandelion exerts a more effective tumor suppression effect than free drug (Wang et al., 2019).
4.4 Phospholipid complexes
As with liposomes, phospholipid complexes can improve the affinity of BA to solubility and cell membranes (Qin et al., 2018). It has been shown that orally administered SBG extract-phospholipid complex improves solubility and bioavailability in vivo (Chen S. et al., 2022). Although phospholipid complexes can improve bioavailability, the increased lipophilicity leads to poor solubility and dispersion of the drug in the aqueous phase (Huang et al., 2019; Wang et al., 2020b). Self-emulsifying drug delivery systems (SEDDS) are isotropic mixtures of drugs, lipids, and surfactants, which are well-suited for lipophilic drugs with limited solubility (Salawi, 2022). A preparation for improving the oral availability of BA has been developed by combining a phospholipid complex with a SEDDS, which has been shown to enhance BA transport and relative bioavailability (Wu et al., 2014).
4.5 Solid dispersions
Solid dispersions improve the bioavailability of drugs by reducing the particle size to an absolute minimum, thereby increasing the wettability of the drug (Vasconcelos et al., 2007). Li et al. (2013) have developed that the BA-polyvinylpyrrolidone coprecipitate was prepared by the solid phase dispersion technique solvent method. Compared to the BA raw material capsules, the relative bioavailability of the coprecipitate capsules was 338.2% ± 93.2%. BA mesoporous carbon nanopowder (MCN) solid dispersions offer more advantages over pure BA as an oral delivery system, including shorter time to peak concentration and higher Cmax and AUC. Significantly, it improves solubility and oral bioavailability without gastrointestinal and renal toxicity (Cui et al., 2016).
4.6 Inclusion complexes
Cyclodextrins have been widely used as functional excipients in inclusion formulations (Gandhi et al., 2020). Li et al. (2017) synthesized β-cyclodextrin (β-CD) and BA host-guest inclusion complexes to exert better therapeutic effects. And the inclusion complex found that the AUC0-t, AUC0-∞, and the relative oral bioavailability increased by 2.65, 2.53, and 2 times compared with free BA. To further improve the solubility and stability of the complexation of BA with β-CD, researchers structurally modified the BA complex to synthesize hydroxypropyl-β-CD to enhance antioxidant capacity, which is the most reactive form (Li et al., 2009a).
Due to the low bioavailability of BA, researchers have synthesized various novel formulations of BA to increase its solubility, bioavailability, targeting, cellular uptake, and retention time in vivo, increasing the therapeutic effect of BA. However, studies of these novel formulation forms are still in the pre-clinical stage, and further evaluation is needed to determine whether they can be used in the clinic.
5 The protection of BA against respiratory diseases
5.1 COVID-19 prevention
In 2019, the unprecedented COVID-19 was caused by the new coronavirus severe acute respiratory syndrome coronavirus 2 (SARS-CoV-2), which has become a global public health concern (Kciuk et al., 2022; Murali et al., 2022). BA has been shown to inhibit SARS-CoV-2 RNA-dependent RNA polymerase activity and exhibited significant antiviral activity against SARS-CoV-2 in vitro (Zandi et al., 2021). Evidence shows that cytokine storm (CS) was a pivotal stage in the advancement of COVID-19 (Hach et al., 2022; Jing et al., 2022; Shevtsova et al., 2022). You et al. (2022) used the method of network pharmacology to analyze the possible pathway of BA inhibiting CS. The enrichment analysis results showed that the tumor necrosis factor-α (TNF-α) pathway and IL (interleukin)-17 pathway might be potentially significant pathways for BA to inhibit CS. Furthermore, BA (200 mg/kg, gavage) exerts an anti-inflammatory effect by decreasing the expression of TNF-α, which alleviates CS against lung injury in vivo. As is well known, SARS-CoV-2 uses the ACE2 to enter cells, and ACE2 cleaves angiotensin (Ang) II into Ang 1–7, which is thought to exert a cellular response primarily through the receptor Mas to counteract RAS activation in several organs (Letko et al., 2020; South et al., 2020). A study by Wei reported that BA inhibits Ang II-induced oxidative damage and dysfunction in endothelial cells through activation of the ACE2/Ang-(1–7)/Mas axis and upregulation of the PI3K/AKT/eNOS pathway, thereby exerting a protective effect on endothelial cells (Wei et al., 2015).
From the above summary, we found that BA prevented COVID-19 through antiviral activity, inhibiting CS and protecting against endothelial cell dysfunction and oxidative damage (Figure 1; Table 3). In the future, clinical evaluation of BA for the treatment of COVID-19 needs to be performed.
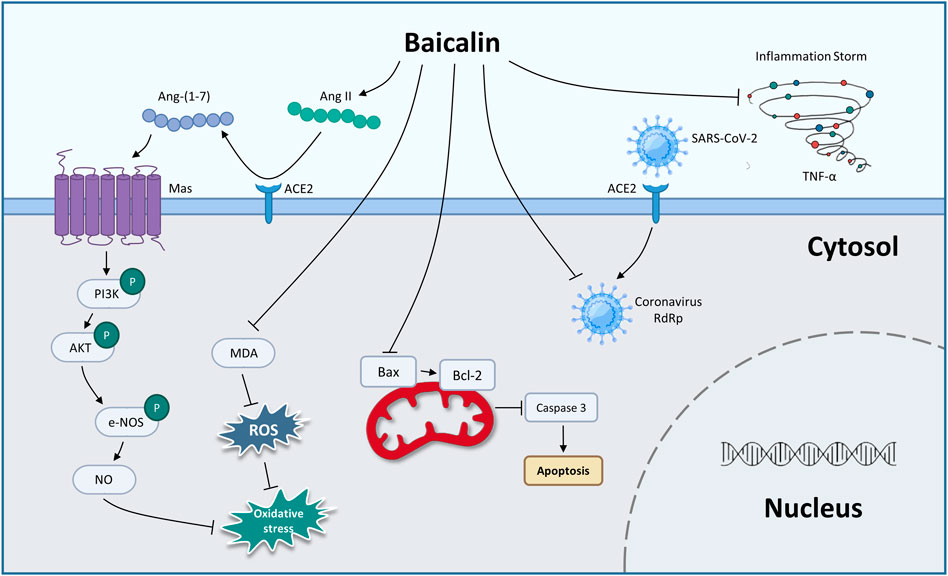
FIGURE 1. The therapeutic mechanism of baicalin on coronary viral disease-2019 (graphics courtesy of freepik.com).
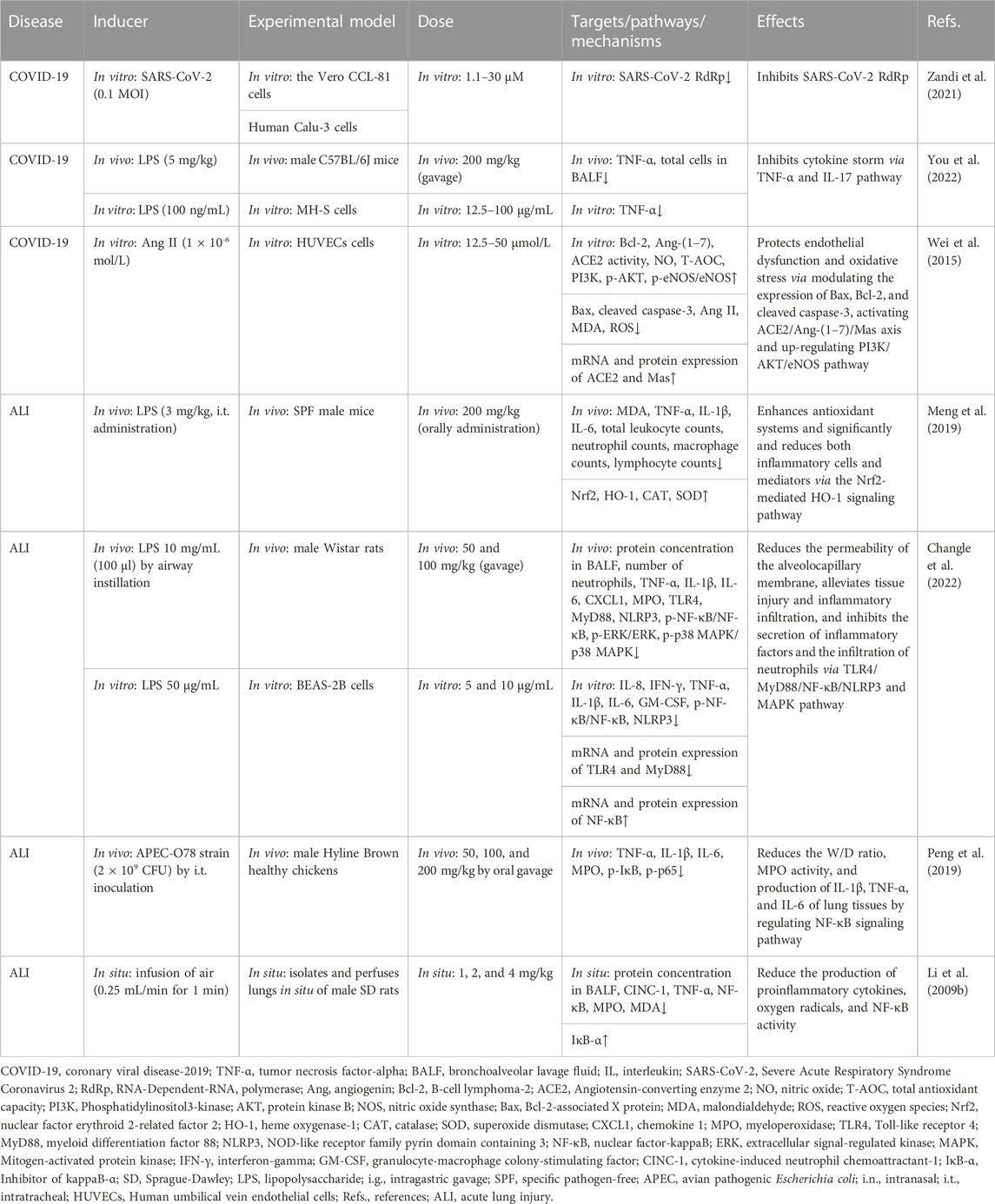
TABLE 3. Summary of the targets/pathways/mechanisms and effects of baicalin on coronary viral disease 2019 and acute lung injury.
5.2 Acute lung injury
ALI is a life-threatening disease with a high fatality rate in a clinical setting (Guo et al., 2022). Severe lung infection, pulmonary contusion, and pulmonary embolism are the direct causes of ALI (He et al., 2021). The main pathological features are acute inflammation and apoptosis of alveolar epithelial cells (Allen and Kurdowska, 2014; Liu et al., 2020; Gao et al., 2021).
Using the LPS-induced ALI model, BA might be a novel strategy for lung protection, mainly due to BA (200 mg/kg, orally administration) inhibited the expression of TNF-α, IL-1β, IL-6, and malondialdehyde (MDA) and restored antioxidative enzyme activities, including superoxide dismutase (SOD) and catalase (CAT). The suppression of inflammatory and oxidant responses was mediated by activation of the nuclear erythroid factor 2 (Nrf2)-mediated heme oxygenase-1 (HO-1) pathway (Meng et al., 2019). In addition, another similar model study conducted by Zhu et al. demonstrated that 50 mg/kg and 100 mg/kg of BA (gavage) significantly alleviated the permeability of the alveolocapillary membrane and tissue injury through the toll-like receptor 4 (TLR4)/myeloid differentiation factor 88 (MyD88)/nuclear factor-kappa B (NF-κB)/nod-like receptor pyrin containing 3 (NLRP3) pathway and the mitogen-activated protein kinase (MAPK) pathway (Changle et al., 2022). The BA-induced attenuation of lung injury via the NF-κB anti-inflammatory pathway was confirmed in another lung injury model. Peng showed that BA (80 mg/kg, oral gavage) might alleviate lung injury in an avian pathogenic E. coli-induced model by inhibiting the phosphorylation of NF-κB (Peng et al., 2019). The findings demonstrated that BA modulated the NF-κB anti-inflammatory pathway, suggesting that it may contribute to reversing pulmonary injury. In a study of the air embolism-induced ALI model, BA (1, 2, and 4 mg/kg) was added into the lung perfusate, alleviating lung injury in isolated lungs by suppressing the proinflammatory cytokines, oxygen radicals, and NF-κB activity (Li et al., 2009b).
From the above summary, BA exerts anti-oxidative stress and anti-inflammatory effects through the Nrf2-mediated HO-1 pathway. CAT antioxidant enzyme, transforming growth factor-β (TGF-β), and granulocyte-macrophage colony-stimulating factor (GM-CSF) are involved in relieving ALI regulated by BA. And the inhibition of lung inflammation via the NF-κB pathway might play a pivotal role in attenuating pulmonary damage by BA (Table 3).
5.3 Asthma
Bronchial asthma is a chronic airway disease predominantly characterized by chronic inflammation and hyperresponsiveness, leading to cough, wheezing, shortness of breath, and chest tightness (Olin and Wechsler, 2014; Banno et al., 2020; Yasuda et al., 2020). It is widely believed that cytokines and inflammatory cells such as eosinophils, neutrophils, lymphocytes, and mast cells are involved in asthma (Yasuda et al., 2020; Zhang et al., 2020).
Ma et al. (2014) confirmed that BA (10–40 mg/kg, gavage) alleviated ovalbumin (OVA)-induced allergic asthma by inhibiting airway resistance, eosinophil count, and IL-4 level, recovering lung compliance, and increasing the expression of IFN-γ. And the study of molecular mechanisms might be associated with the decrease of IL-17A. Another similar model also confirmed the importance of immune regulation in asthma. Xu et al. (2017a) demonstrated that intragastric gavage (i.g.) administration of BA at a dose of 10, 25, and 65 mg/kg protected OVA and LPS-induced allergic asthma in mice models by maintaining Th17/Treg balance. In the study of Sun, researchers administered BA (gavage) dosages ranging from 25 to 100 mg/kg/day to mice with OVA-induced allergic asthma. The result demonstrated that BA reduced the expression of TGF-β1, IL-13, and vascular endothelial growth factor in tissue remodeling by suppressing the activation of the extracellular signal-regulated kinase (ERK) pathway and RAS expressions (Sun et al., 2013). Liu et al. (2016) found BA (10, 25, and 50 mg/kg, i.g. administration) could reduce the expression of immunoglobulin E (IgE), IL-6, TNF-α, CC-chemokine receptor (CCR) 7, C-C chemokine ligand (CCL)19/CCL21 in the OVA-induced mice model, inhibiting airway inflammation via the NF-κB pathway.
According to above research, immune modulation and inhibition of inflammatory responses appear to be important mechanisms for BA to treat asthma. It is critical for BA to relieve asthma by inhibiting the ERK pathway and inhibiting CCR7, CCL19/CCL21, and NF-κB pathway (Table 4).
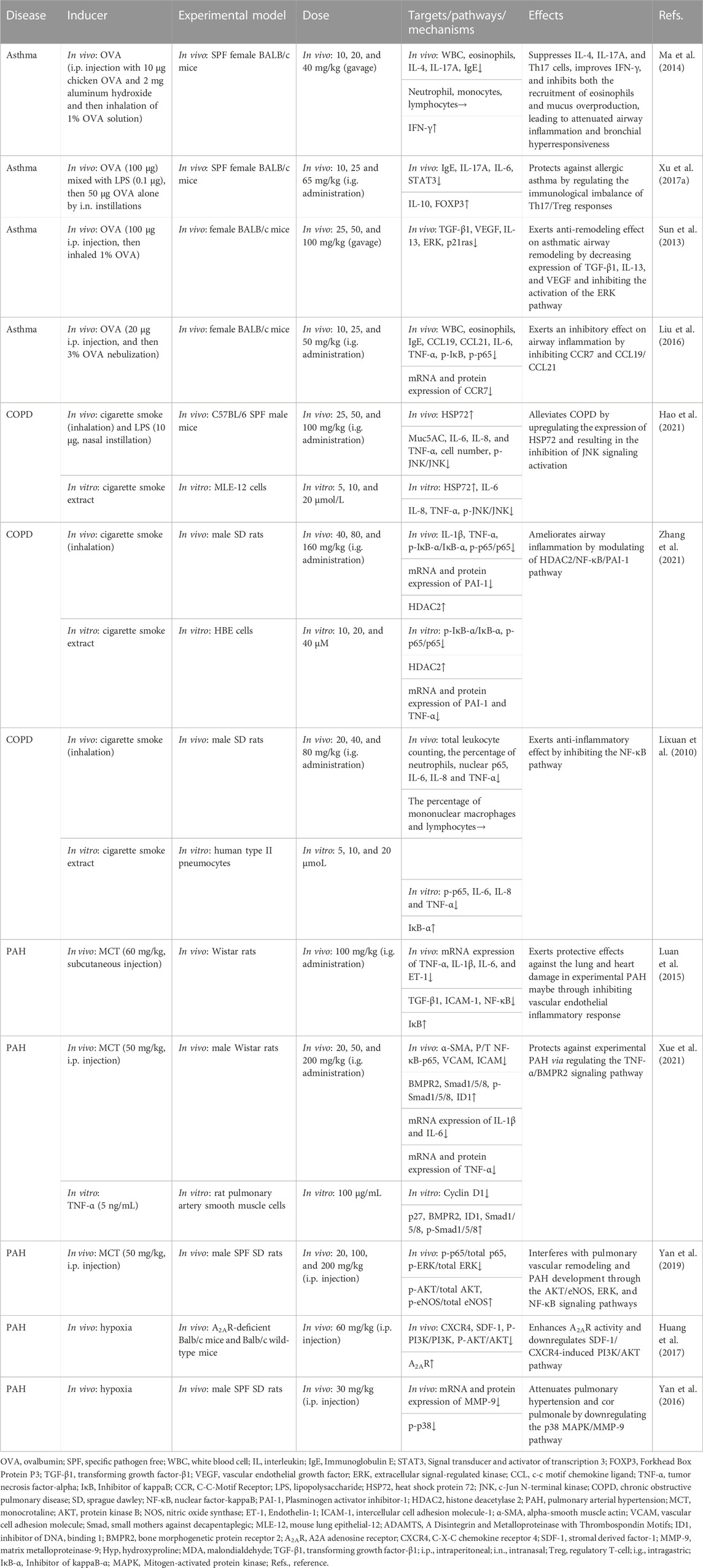
TABLE 4. Summary of the targets/pathways/mechanisms and effects of baicalin on asthma, chronic obstructive pulmonary disease, and pulmonary arterial hypertension.
5.4 Chronic obstructive pulmonary disease
COPD is characterized by airway remodeling and progressive lung inflammation, which are the main features of an increase in the number of alveolar macrophages, neutrophils, and T lymphocytes (Barnes, 2014; Chang et al., 2014). Cigarette smoking is one of the primary causes of COPD, resulting in 5 million deaths annually (Jones et al., 2017; Labaki and Rosenberg, 2020).
Hao et al. (2021) utilized cigarette smoke extract-induced mouse lung epithelial cell model and cigarette smoke and LPS-induced mouse model to investigate the anti-inflammatory effect of BA in COPD. They found that BA (25, 50, and 100 mg/kg, i.g. administration) inhibited inflammatory cell infiltration and the secretion of pro-inflammatory factors by regulating the heat shock protein 72 (HSP72)-mediated c-Jun N-terminal kinases (JNK) pathway. In addition, Zhang et al. showed that BA (i.g. administration) dosages ranging from 40 to 160 mg/kg suppressed the expression of inflammatory factors in cigarette smoke (extract)-induced human bronchial epithelial cells and airway tissues through suppressing the inflammatory response, enhancing histone deacetylase 2 protein expression, and inhibiting the activation of NF-κB and its downstream target of PAI-1 (Zhang et al., 2021). Using the same model, a study by Zeng et al. indicated that BA (20, 40, and 80 mg/kg, i.g. administration) reduced cigarette smoke-induced inflammation in human type II pneumocytes and rats by suppressing IL-6, IL-8, and TNF-α via the inhibition of NF-κB activation (Lixuan et al., 2010).
According to recent advances, the mechanism of COPD mitigation by BA revolves around inhibition of the inflammatory response, mainly through tight regulation of the HSP72-mediated JNK pathway, HDAC2/NF-κB/PAI-1 pathway, and NF-κB pathway (Table 4).
5.5 Pulmonary arterial hypertension
PAH is a progressive and fatal disease with rapid onset and mortality in 7 people for every 100 person-years in Asian countries (Chung et al., 2015). Pulmonary endothelial cell dysfunction, pulmonary artery smooth muscle proliferation, and right ventricular hypertrophy are all involved in the development and formation of PAH (Xu et al., 2022b).
In a study by Luan et al. (2015), the rats with monocrotaline (MCT)-induced PAH were administered BA (i.g. administration) at a daily dose of 100 mg/kg. The results showed that BA downregulated the mRNA levels of TNF-α, IL-13, IL-6, and ET-1 in pulmonary tissues via blocking the activation of the NF-κB signaling pathway to attenuate PAH. Utilizing the same model, Xue et al. (2021) found that BA (20, 50, and 200 mg/kg, i.g. administration) profoundly reduced the mRNA and protein levels of inflammatory factors, excessive proliferation, migration of pulmonary artery smooth muscle cells, and vascular remodeling via the TNF-α/bone morphogenetic protein receptor 2 (BMPR2) signaling pathway. In an MCT-induced rat model, BA (20, 100, and 200 mg/kg, i.p. injection) protected the rats from the severity of pulmonary vascular remodeling and cardiorespiratory injury via AKT/ERK/NF-κB signaling pathways and p-eNOS protein (Yan et al., 2019). In another PAH model, the relief of chronic hypoxia-induced PAH by BA (60 mg/kg, i.p. injection) through the AKT pathway was confirmed. In addition, the results of in vivo studies suggested that BA performed a therapeutic role via the attenuation of the adenosine A2A receptor-induced SDF-1/CXCR4/PI3K/AKT pathway (Huang et al., 2017). Yan et al. (2016) demonstrated that 30 mg/kg of BA (i.p. injection) relieved the hypoxia-induced PAH model by mitigating pulmonary hypertension, pulmonary arteriole, and right ventricular remodeling and ameliorating hypoxic cor pulmonale by suppressing the p38 MAPK pathway and the expression of matrix metalloprotein (MMP)-9.
In conclusion, the suppression of inflammatory factors, the TNF-α/BMPR2 pathway, the SDF-1/CXCR4/PI3K/AKT pathway, and the p38 MAPK pathway seem to be essential to the regulation of PAH by BA (Table 4).
5.6 Pulmonary fibrosis
PF is a chronic, progressive, and deadly lung disease primarily affecting middle-aged and older people (Sharif, 2017). It is generally accepted that the occurrence of PF is abnormal wound healing after repeated alveolar injury in genetically susceptible individuals, leading to the destruction of the lung parenchyma, and deposition of extracellular matrix in fibroblasts and alveolar epithelium. In addition, improperly activated alveolar epithelial cells (AECs) and fibroblasts cause the fibrotic response (King et al., 2011; Ptasinski et al., 2021).
A recent study indicated that 50 mg/kg BA (i.p. administration) effectively suppresses bleomycin (BLM)-induced PF in rats by decreasing the expression of hydroxyproline, TGF-β, collagen I, collagen III, and TNF-α, enhancing the function of anti-oxidative stress, and inhibiting apoptotic protein expression. Moreover, this study also showed that 80 μg/mL BA reverses BLM-induced fibroblast proliferation, regulating the expression of phosphoinositide 3-kinase (PI3K)/AKT and calcium/calmodulin-dependent kinase II in vitro (Zhao et al., 2020). Another in vivo study revealed that 120 mg/kg BA (i.p. administration) was found to block the TGF-β1-induced ERK1/2 signaling pathway via the adenosine A2A receptor, leading to the alleviation of fibrosis caused by BLM (Huang et al., 2016). In recent years, the targeted omics approach for biomarkers discovery has been an effective approach for evaluating the effectiveness and mechanisms of BA (Ullah et al., 2022). In the study of Chang et al. (2021), the researchers administered 25–100 mg/kg BA to rat models with PF caused by BLM. The results demonstrated that BA ameliorated the levels of MDA and SOD to relieve oxidative stress. Furthermore, BA was identified to potentially alleviate PF by regulating four vital metabolic markers, including taurine, hypotaurine, glutathione, and glycerophospholipid metabolism.
TGF-β acts as a potent driver of PF progression by induction of epithelial-mesenchymal transition (EMT) (Qian et al., 2018). At doses of 2–50 μM, BA appears to be implicated in the radiation-induced EMT of AECs by inhibiting TGF-β and ERK/GSK signaling pathways mediated fibrosis (Lu et al., 2017). In terms of immunoregulatory mechanisms, i.p. injection of 100 mg/kg BA decreased PF in mouse models caused by silica by altering the Th17/Treg responses by lowering Th17 cells, activating Treg cells, and inhibiting IL-6 and IL-23 (Liu et al., 2015).
In summary, BA alleviates PF through a variety of mechanisms, including inhibition of the inflammatory response, oxidative stress, EMT, apoptosis and immune modulation (Table 5).
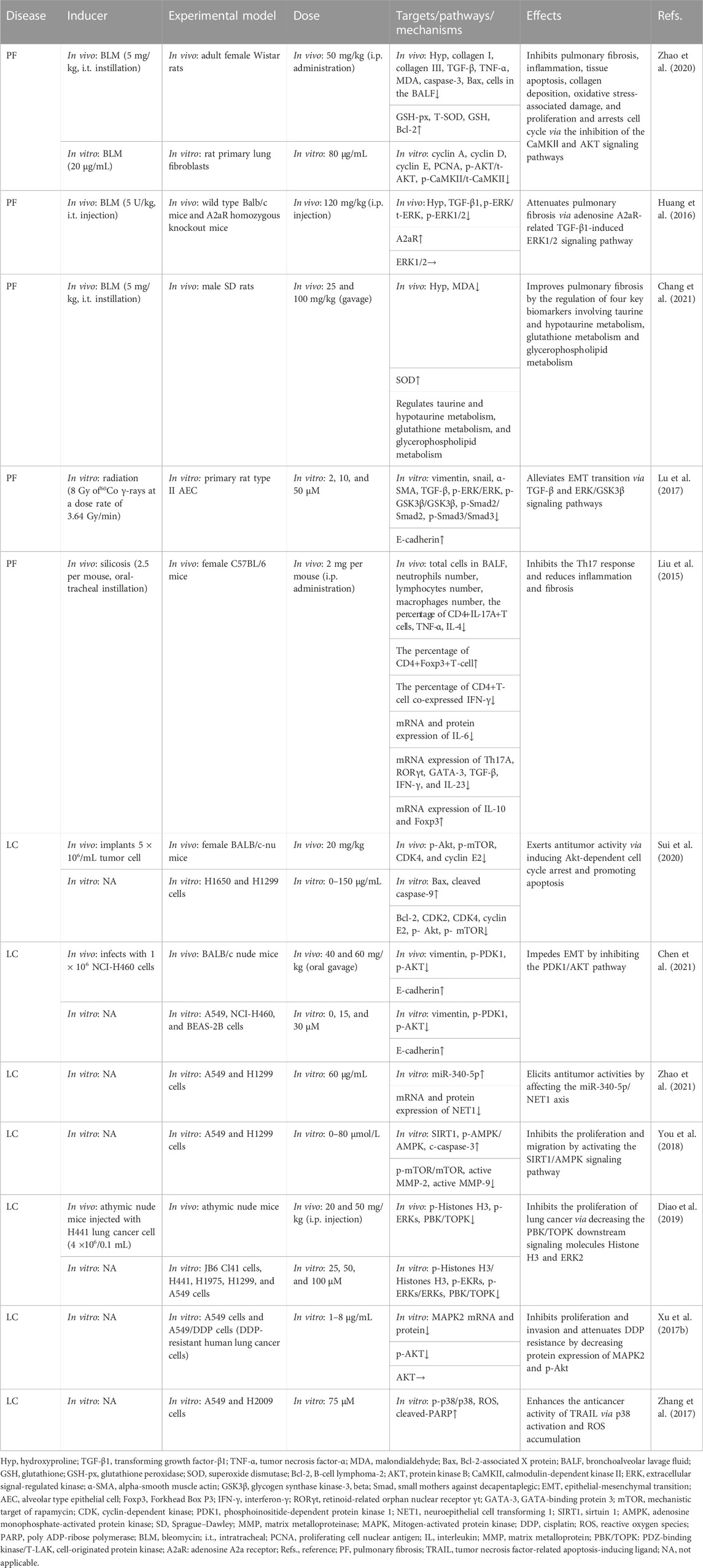
TABLE 5. Summary of the targets/pathways/mechanisms and effects of baicalin on pulmonary fibrosis and lung cancer.
5.7 Lung cancer
LC remains the primary cause of cancer deaths in the world (Wei et al., 2015; Saman et al., 2022). As the most common subtype of LC, non-small cell lung cancer (NSCLC) accounts for around 85% of LC cases (Fiero et al., 2019). Currently, surgery, chemotherapy, radiation, and targeted molecular treatment are the main treatment options for LC patients (South et al., 2020). However, the side effects and toxicity of these techniques have become limiting factors in the clinical treatment of lung cancer (Luan et al., 2015; Yamamoto et al., 2022).
Sui et al. (2020) found that 20 mg/kg of BA can trigger apoptosis and block cell cycle G1/S transition in carcinoma of the lung cells via the AKT/mechanistic target of rapamycin (mTOR) pathway. NSCLC is highly prone to invasion and metastasis, which are closely associated with the occurrence of EMT (Xia et al., 2014). In this regard, Chen reported that BA inhibited the EMT of NSCLC through the PDK1/AKT pathway (Chen et al., 2021). Recently, the importance of miRNAs in cancer progression has been reported (He et al., 2005; Lee and Dutta, 2007). Zhao et al. (2021) discovered that BA (60 μg/mL) restrained cell proliferation and invasion, promoted apoptosis, and arrested the G1/S phase via the miR-340-5p/NET1 axis, exerting anti-lung cancer effects. In an in vitro study, BA (20–80 μmol/L) inhibited cell viability, triggered apoptosis, and decreased cell migration and invasion by inhibiting the activation of the sirtuin 1 (SIRT1)/adenosine monophosphate-activated protein kinase (AMPK) signaling pathway (You et al., 2018). In several studies, overexpression of PDZ-binding kinase/T-LAK cell-originated protein kinase (PBK/TOPK) has been related to a poor prognosis and is a crucial target for anticancer drugs (Lei et al., 2013; Han et al., 2021). Diao et al. (2019) found that BA inhibited PBK/TOPK activities by directly binding with PBK/TOPK in vitro and in vivo.
Despite the successful efficacy of chemotherapy, there is growing concerned about chemotherapeutic agents developing resistance (Siddik, 2003; Apps et al., 2015; Pan et al., 2019). Considering this, researchers found that combining BA and DDP (cisplatin) inhibited tumor cells significantly more than DDP or BA alone in A549 and A549/DDP cells (DDP-resistant cells). The attenuation of the resistance of DDP was connected with downregulating MARK2 and p-Akt proteins (Xu et al., 2017b). In addition, tumor necrosis factor-related apoptosis-inducing ligand (TRAIL) is a promising target for overcoming cancer cell resistance (von Karstedt et al., 2017). BA (75 μM) can increase TRAIL-induced apoptosis and intracellular reactive oxygen species generation in cancer cells via activating p38 MAPK, enhancing the antitumor effectiveness of TRAIL (Zhang et al., 2017). The findings of the current investigations suggested that targeting MAPK is a significant pathway to reduce drug resistance in cancer. The AKT/mTOR, the PDK1/AKT, the SIRT1/AMPK pathways, and the regulation of active MMP-2, active MMP-9, vimentin, and E-cadherin are connected with the relief of LC (Table 5).
6 Discussion and outlook
Through this review, we have attempted to summarize a comprehensive review of research advances for BA in pharmacokinetics, strategies to improve bioavailability, and the treatment of disorders related to the respiratory system. Despite the poor bioavailability and solubility of BA, the development of DDSs for BA has improved the bioavailability, pulmonary targeting, and solubility, thereby improving the efficacy. The multiple pharmacological activities of BA suggest a broad prospect for the prevention and treatment of ALI, PF, COVID-19, LC, PAH, COPD, and asthma (Figure 2). At present, studies on BA are still in the preclinical stage. This review provides a valuable reference for subsequent pharmacological studies and clinical applications of BA.
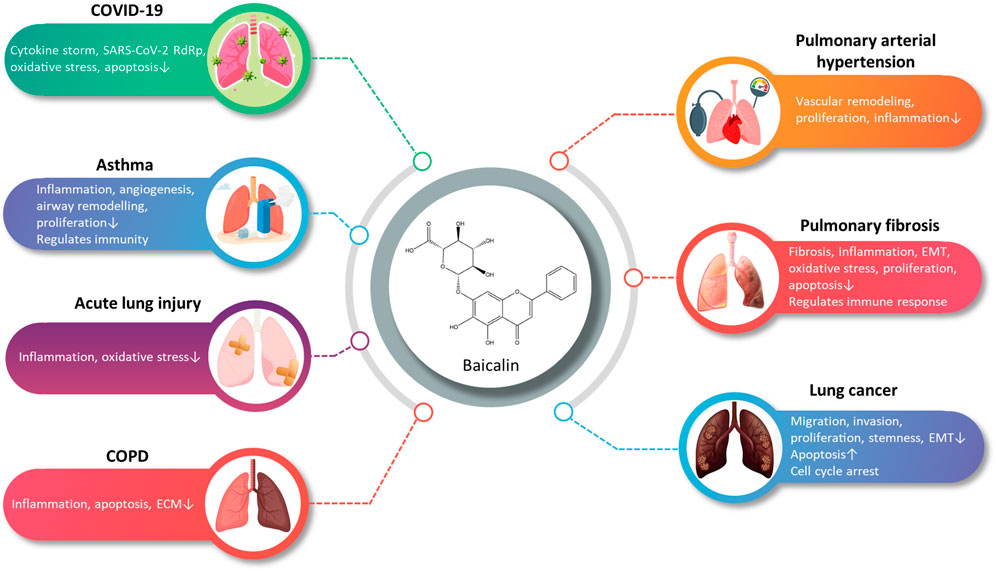
FIGURE 2. A summary of the main pharmacological effects of baicalin and its mechanisms involved in respiratory diseases (graphics courtesy of freepik.com).
Considerable preclinical studies have shown that BA has excellent therapeutic potential to treat respiratory diseases in both in vivo and in vitro settings. BA exerts therapeutic effects mainly through mediating upstream oxidative stress, inflammation, apoptosis, and immune response pathways. In the regulation of oxidative stress, the PI3K/AKT/eNOS pathway and the Nrf2-mediated HO-1 pathway are critical factors associated with the protective effects of BA on COVID-19 and ALI. Regarding the inflammatory response, TNF-α, IL-1β, IL-6, GM-CSF, IL-8, IL-4, and IL-10 are involved in BA to alleviate COVID-19, ALI, PF, COPD, PAH, and asthma. Regarding the regulation of apoptosis molecules, caspase-3, caspase-9, Bcl-2, and Bax are essential factors in alleviating LC, PF, and COVID-19. Immune modulation therapy has sparked the interest of researchers. The underlying mechanisms of BA in the treatment of immune modulation on PF and asthma manifest as the regulation of Th17/Treg responses by promoting Treg cell differentiation, inhibiting the expression of Th17A, and reducing the levels of IL-6, IFN-γ, IL-4, and IL-23 (Figure 3). Although the target of BA for the treatment of respiratory diseases is still inconclusive. Phosphodiesterase 4 (PDE4) might be a potential therapeutic target for treating respiratory diseases. And PDE4 already has targeted inhibitors approved for treating severe COPD, such as roflumilast (Crocetti et al., 2022; Herrmann et al., 2022). In addition, GSK-256066, a highly selective inhaled PDE4 inhibitor, has been in phase II clinical trials for asthma and COPD (Pagès et al., 2009). BA was found to selectively inhibit the enzymatic activity of PDE4A and 4B to relieve allergic asthma (Park et al., 2016). Thus, it indicates that PDE4 may be a candidate target for BA to target respiratory diseases, which requires further experimental proof. Importantly, we discovered that BA exhibits anti-cancer effects via inducing apoptosis and oxidative stress, which is the opposite of BA for the treatment of PF. It suggests that BA exerts a bidirectional regulatory effect to protect the lung. The multiple pharmacological activities suggest that BA is a promising compound for the prevention and treatment of ALI, PF, COVID-19, LC, PAH, COPD, and asthma (Figure 4).
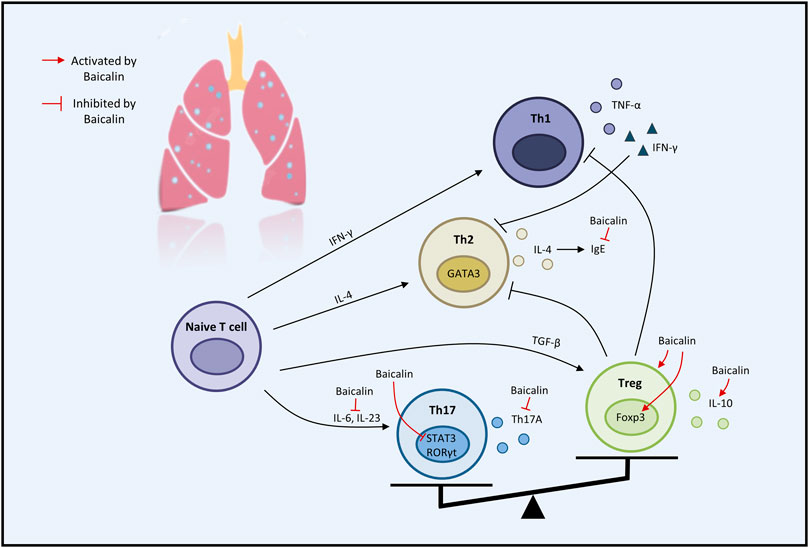
FIGURE 3. The therapeutic effects of baicalin on immune modulation on respiratory disease (graphics courtesy of freepik.com).
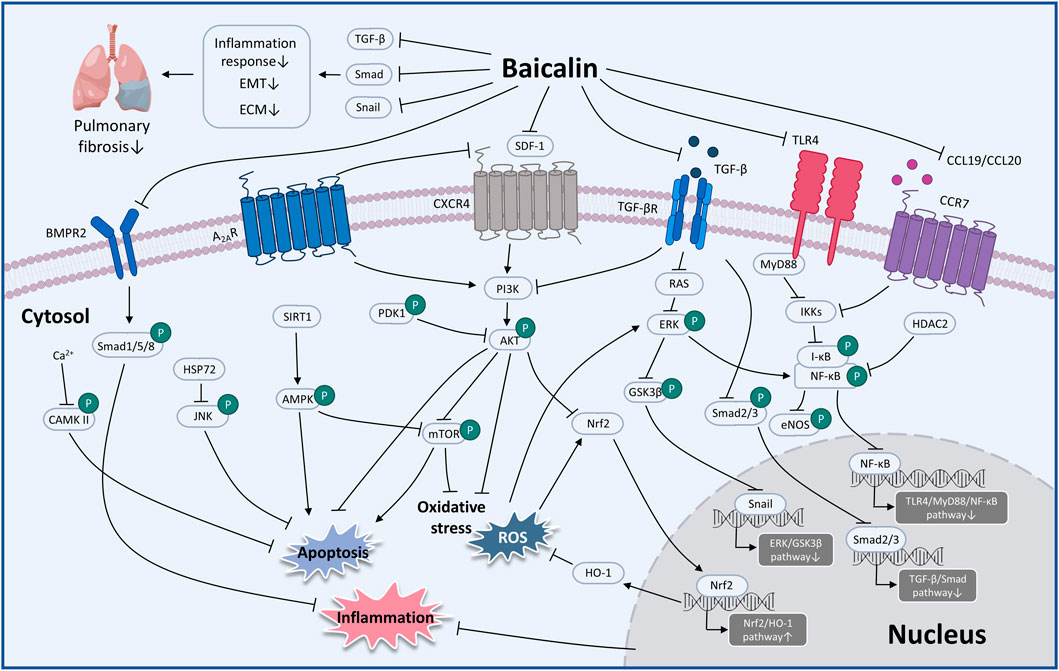
FIGURE 4. The therapeutic mechanism and critical pathways of baicalin against respiratory diseases (graphics courtesy of figdraw.com/static/index.html#/ and freepik.com).
This review identifies a plausible mechanism for the therapeutic efficacy of BA in treating respiratory diseases. However, the experiments have almost always verified the conclusion in vivo and in vitro. More clinical evidence is needed to verify efficacy. Several aspects of BA need to be investigated before its clinical application. Firstly, the poor water solubility of BA leads to low oral bioavailability. Researchers have developed strategies to improve the bioavailability of BA, including liposomes, nano-emulsions, micelles, phospholipid complexes, solid dispersions, and inclusion complexes (Li J. et al., 2009; Li et al., 2013; Yue et al., 2013; Zhao et al., 2013; Wu et al., 2014; Zhang et al., 2014). Modified versions of these different formulations of BA would be carried out in animal experiments to find their optimal effective dose. And there is also the issue of acute and long-term toxicity of BA in animal models of different respiratory diseases. Secondly, therapeutic doses of BA varied widely across studies. This may be due to disease type, disease state, route of administration, time point of administration (prophylactic or therapeutic administration), number of days of treatment, age, weight, sex of the animals, and environmental factors, etc. Thus, when BA is used as a treatment for respiratory diseases, it needs to be tailored to the specific situation. In addition, as a common dosage form for the treatment of respiratory diseases, inhalation of BA has not been studied. BA is reported to be insoluble in water that may be the main reason for limiting its candidate for the development of inhalation preparation. A number of new formulations have been developed to improve water solubility, including inclusion complexes and phospholipid complexes, etc. BA has shown improved properties as an inhalant component of chinese herbal compound. Transnasal aerosol inhalation of Tanreqing (containing BA) increased the drug concentration of BA in the lungs and showed better anti-inflammatory effects on LPS-induced ALI in mice, compared to intravenous administration (Chen T. F. et al., 2022). This indicates the potential of BA as an inhalation. Finally, with the advancement of multi-omics studies, those approaches can be used to explore the mechanism of the effects of BA in the treatment of respiratory diseases and to gain a clearer understanding of the multi-target regulatory network of BA. In general, this review presents novel perspectives on the pharmacological effects of BA on respiratory diseases. It must be acknowledged that this review also has some limitations, including the source of BA, the experimental method, and the choice of dose.
Author contributions
SS and LD collected, analyzed, and reviewed the literature, and wrote the main manuscript; GL, TC, MZ, XuL, ML, HQ, and JC assembled figures/tables; ZiW, JM, YW, and QW added and checked references; ZeW and XiL designed and supervised the manuscript; ZeW and XiL revised the final version of the manuscript. All authors have read and agreed to the published version of the manuscript.
Funding
This work was supported by the Key Research and Development Project of Jilin Province (No. 20200404057YY), Jilin Administration of Traditional Chinese Medicine (2022221), and the Science and Technology Development Plan Project of Jilin Province (YDZJ202301ZYTS136, YDZJ202202CXJD049).
Acknowledgments
We thank FIGDRAW (figdraw.com/static/index.html) and freepik (freepik.com) for their graphics courtesy assistance during the preparation of this manuscript.
Conflicts of interest
The authors declare that the research was conducted in the absence of any commercial or financial relationships that could be construed as a potential conflict of interest.
Publisher’s note
All claims expressed in this article are solely those of the authors and do not necessarily represent those of their affiliated organizations, or those of the publisher, the editors and the reviewers. Any product that may be evaluated in this article, or claim that may be made by its manufacturer, is not guaranteed or endorsed by the publisher.
References
Akao, T., Kawabata, K., Yanagisawa, E., Ishihara, K., Mizuhara, Y., Wakui, Y., et al. (2000). Baicalin, the predominant flavone glucuronide of scutellariae radix, is absorbed from the rat gastrointestinal tract as the aglycone and restored to its original form. J. Pharm. Pharmacol. 52 (12), 1563–1568. doi:10.1211/0022357001777621
Akao, T., Sato, K., He, J. X., Ma, C. M., and Hattori, M. (2013). Baicalein 6-O-β-D-glucopyranuronoside is a main metabolite in the plasma after oral administration of baicalin, a flavone glucuronide of scutellariae radix, to rats. Biol. Pharm. Bull. 36 (5), 748–753. doi:10.1248/bpb.b12-00850
Allen, T. C., and Kurdowska, A. (2014). Interleukin 8 and acute lung injury. Arch. Pathol. Lab. Med. 138 (2), 266–269. doi:10.5858/arpa.2013-0182-RA
Apps, M. G., Choi, E. H., and Wheate, N. J. (2015). The state-of-play and future of platinum drugs. Endocr. Relat. Cancer 22 (4), R219–R233. doi:10.1530/ERC-15-0237
Banno, A., Reddy, A. T., Lakshmi, S. P., and Reddy, R. C. (2020). Bidirectional interaction of airway epithelial remodeling and inflammation in asthma. Clin. Sci. (Lond) 134 (9), 1063–1079. doi:10.1042/CS20191309
Barnes, P. J. (2014). Cellular and molecular mechanisms of chronic obstructive pulmonary disease. Clin. Chest Med. 35 (1), 71–86. doi:10.1016/j.ccm.2013.10.004
Chang, H., Meng, H. Y., Bai, W. F., and Meng, Q. G. (2021). A metabolomic approach to elucidate the inhibitory effects of baicalin in pulmonary fibrosis. Pharm. Biol. 59 (1), 1016–1025. doi:10.1080/13880209.2021.1950192
Chang, Y., Al-Alwan, L., Alshakfa, S., Audusseau, S., Mogas, A. K., Chouiali, F., et al. (2014). Upregulation of IL-17a/F from human lung tissue explants with cigarette smoke exposure: Implications for COPD. Respir. Res. 15 (1), 145. doi:10.1186/s12931-014-0145-7
Changle, Z., Cuiling, F., Feng, F., Xiaoqin, Y., Guishu, W., Liangtian, S., et al. (2022). Baicalin inhibits inflammation of lipopolysaccharide-induced acute lung injury toll like receptor-4/myeloid differentiation primary response 88/nuclear factor-kappa B signaling pathway. J. Tradit. Chin. Med. 42 (2), 200–212. doi:10.19852/j.cnki.jtcm.20211214.004
Chen, J., Yuan, C. B., Yang, B., and Zhou, X. (2021). Baicalin inhibits EMT through PDK1/AKT signaling in human nonsmall cell lung cancer. J. Oncol. 2021, 4391581. doi:10.1155/2021/4391581
Chen, S., Xie, Q., Yang, M., Shi, Y., Shi, J., and Zeng, X. (2022). Scutellaria baicalensis extract-phospholipid complex: Preparation and initial pharmacodynamics research in rats. Curr. Pharm. Biotechnol. 23 (6), 847–860. doi:10.2174/1389201022666210729142257
Chen, T. F., Song, L., Gao, Y. H., Li, H., Li, J. L., Hou, H. P., et al. (2022). Pharmacokinetics of baicalin and oroxyloside in plasma and different tissues of rats after transnasal aerosol inhalation and intravenous injection of Tanreqing. Front. Pharmacol. 13, 951613. doi:10.3389/fphar.2022.951613
Chen, Y., Minh, L. V., Liu, J., Angelov, B., Drechsler, M., Garamus, V. M., et al. (2016). Baicalin loaded in folate-PEG modified liposomes for enhanced stability and tumor targeting. Colloids Surf. B Biointerfaces 140, 74–82. doi:10.1016/j.colsurfb.2015.11.018
Chung, W. J., Park, Y. B., Jeon, C. H., Jung, J. W., Ko, K. P., Choi, S. J., et al. (2015). Baseline characteristics of the Korean registry of pulmonary arterial hypertension. J. Korean Med. Sci. 30 (10), 1429–1438. doi:10.3346/jkms.2015.30.10.1429
Crocetti, L., Floresta, G., Cilibrizzi, A., and Giovannoni, M. P. (2022). An overview of PDE4 inhibitors in clinical trials: 2010 to early 2022. Molecules 27 (15), 4964. doi:10.3390/molecules27154964
Cui, L., Sune, E., Song, J., Wang, J., Jia, X. B., and Zhang, Z. H. (2016). Characterization and bioavailability study of baicalin-mesoporous carbon nanopowder solid dispersion. Pharmacogn. Mag. 12 (48), 326–332. doi:10.4103/0973-1296.192199
Deng, L., Li, S., Zhou, L., Ye, S., and Wang, J., (2022). Protective effect and mechanism of baicalin on lung inflammatory injury in BALB/cJ mice induced by PM2.5. Ecotoxicol. Environ. Saf. 248, 114329. doi:10.1016/j.ecoenv.2022.114329
Diao, X., Yang, D., Chen, Y., and Liu, W. (2019). Baicalin suppresses lung cancer growth by targeting PDZ-binding kinase/T-LAK cell-originated protein kinase. Biosci. Rep. 39 (4). doi:10.1042/BSR20181692
Fiero, M. H., Roydhouse, J. K., Vallejo, J., King-Kallimanis, B. L., Kluetz, P. G., and Sridhara, R. (2019). US Food and Drug Administration review of statistical analysis of patient-reported outcomes in lung cancer clinical trials approved between January, 2008, and December, 2017. Lancet Oncol. 20 (10), e582–e589. doi:10.1016/S1470-2045(19)30335-3
Gandhi, S. R., Quintans, J. D. S. S., Gandhi, G. R., Araujo, A. A. D. S., and Quintans Junior, L. J. (2020). The use of cyclodextrin inclusion complexes to improve anticancer drug profiles: A systematic review. Expert Opin. Drug Deliv. 17 (8), 1069–1080. doi:10.1080/17425247.2020.1776261
Ganguly, R., Gupta, A., and Pandey, A. K. (2022). Role of baicalin as a potential therapeutic agent in hepatobiliary and gastrointestinal disorders: A review. World J. Gastroenterol. 28 (26), 3047–3062. doi:10.3748/wjg.v28.i26.3047
Gao, J., Teng, L., Yang, S., Huang, S., Li, L., Zhou, L., et al. (2021). MNK as a potential pharmacological target for suppressing LPS-induced acute lung injury in mice. Biochem. Pharmacol. 186, 114499. doi:10.1016/j.bcp.2021.114499
Gaucher, G., Dufresne, M. H., Sant, V. P., Kang, N., Maysinger, D., and Leroux, J. C. (2005). Block copolymer micelles: Preparation, characterization and application in drug delivery. J. Control Release 109 (1-3), 169–188. doi:10.1016/j.jconrel.2005.09.034
Guo, B., Zuo, Z., Di, X., Huang, Y., Gong, G., Xu, B., et al. (2022). Salidroside attenuates HALI via IL-17A-mediated ferroptosis of alveolar epithelial cells by regulating Act1-TRAF6-p38 MAPK pathway. Cell Commun. Signal 20 (1), 183. doi:10.1186/s12964-022-00994-1
Hach, T., Shakeri-Nejad, K., Bigaud, M., Dahlke, F., de Micco, M., Petricoul, O., et al. (2022). Rationale for use of sphingosine-1-phosphate receptor modulators in COVID-19 patients: Overview of scientific evidence. J. Interferon Cytokine Res. 2022, 0078. doi:10.1089/jir.2022.0078
Haider, M., Hassan, M. A., Ahmed, I. S., and Shamma, R. (2018). Thermogelling platform for baicalin delivery for versatile biomedical applications. Mol. Pharm. 15 (8), 3478–3488. doi:10.1021/acs.molpharmaceut.8b00480
Han, Z., Li, L., Huang, Y., Zhao, H., and Luo, Y. (2021). PBK/TOPK: A therapeutic target worthy of attention. Cells 10 (2), 371. doi:10.3390/cells10020371
Hao, D., Li, Y., Shi, J., and Jiang, J. (2021). Baicalin alleviates chronic obstructive pulmonary disease through regulation of HSP72-mediated JNK pathway. Mol. Med. 27 (1), 53. doi:10.1186/s10020-021-00309-z
He, L., Thomson, J. M., Hemann, M. T., Hernando-Monge, E., Mu, D., Goodson, S., et al. (2005). A microRNA polycistron as a potential human oncogene. Nature 435 (7043), 828–833. doi:10.1038/nature03552
He, Y. Q., Zhou, C. C., Yu, L. Y., Wang, L., Deng, J. L., Tao, Y. L., et al. (2021). Natural product derived phytochemicals in managing acute lung injury by multiple mechanisms. Pharmacol. Res. 163, 105224. doi:10.1016/j.phrs.2020.105224
Herrmann, F. E., Wollin, L., and Nickolaus, P. (2022). BI 1015550 is a PDE4B inhibitor and a clinical drug candidate for the oral treatment of idiopathic pulmonary fibrosis. Front. Pharmacol. 13, 838449. doi:10.3389/fphar.2022.838449
Hu, Q., Zhang, W., Wu, Z., Tian, X., Xiang, J., Li, L., et al. (2021). Baicalin and the liver-gut system: Pharmacological bases explaining its therapeutic effects. Pharmacol. Res. 165, 105444. doi:10.1016/j.phrs.2021.105444
Huang, J., Chen, P. X., Rogers, M. A., and Wettig, S. D. (2019). Investigating the phospholipid effect on the bioaccessibility of rosmarinic acid-phospholipid complex through a dynamic gastrointestinal in vitro model. Pharmaceutics 11 (4), 156. doi:10.3390/pharmaceutics11040156
Huang, S., Zhai, B., Fan, Y., Sun, J., Cheng, J., Zou, J., et al. (2022). Development of paeonol liposomes: Design, optimization, in vitro and in vivo evaluation. Int. J. Nanomedicine 17, 5027–5046. doi:10.2147/IJN.S363135
Huang, X., He, Y., Chen, Y., Wu, P., Gui, D., Cai, H., et al. (2016). Baicalin attenuates bleomycin-induced pulmonary fibrosis via adenosine A2a receptor related TGF-β1-induced ERK1/2 signaling pathway. BMC Pulm. Med. 16 (1), 132. doi:10.1186/s12890-016-0294-1
Huang, X., Wu, P., Huang, F., Xu, M., Chen, M., Huang, K., et al. (2017). Baicalin attenuates chronic hypoxia-induced pulmonary hypertension via adenosine A(2A) receptor-induced SDF-1/CXCR4/PI3K/AKT signaling. J. Biomed. Sci. 24 (1), 52. doi:10.1186/s12929-017-0359-3
Ishihara, M., Homma, M., Kuno, E., Watanabe, M., and Kohda, Y. (2002). [Combination use of kampo-medicines and drugs affecting intestinal bacterial flora]. Yakugaku Zasshi 122 (9), 695–701. doi:10.1248/yakushi.122.695
Jin, X., Liu, M. Y., Zhang, D. F., Zhong, X., Du, K., Qian, P., et al. (2019). Baicalin mitigates cognitive impairment and protects neurons from microglia-mediated neuroinflammation via suppressing NLRP3 inflammasomes and TLR4/NF-κB signaling pathway. CNS Neurosci. Ther. 25 (5), 575–590. doi:10.1111/cns.13086
Jing, H., Wu, X., Xiang, M., Liu, L., Novakovic, V. A., and Shi, J. (2022). Pathophysiological mechanisms of thrombosis in acute and long COVID-19. Front. Immunol. 13, 992384. doi:10.3389/fimmu.2022.992384
Jones, B., Donovan, C., Liu, G., Gomez, H. M., Chimankar, V., Harrison, C. L., et al. (2017). Animal models of COPD: What do they tell us? Respirology 22 (1), 21–32. doi:10.1111/resp.12908
Ju, J., Li, Z., and Shi, Q. (2022). Baicalin inhibits inflammation in rats with chronic obstructive pulmonary disease by the TLR2/MYD88/NF-κBp65 signaling pathway. Evid. Based Complement. Altern. Med. 2022, 7273387. doi:10.1155/2022/7273387
Kciuk, M., Mujwar, S., Rani, I., Munjal, K., Gielecińska, A., Kontek, R., et al. (2022). Computational bioprospecting guggulsterone against ADP ribose phosphatase of SARS-CoV-2. Molecules 27 (23), 8287. doi:10.3390/molecules27238287
King, T. E., Pardo, A., and Selman, M. (2011). Idiopathic pulmonary fibrosis. Lancet 378 (9807), 1949–1961. doi:10.1016/S0140-6736(11)60052-4
Kumar, A., Cherian, S. V., Vassallo, R., Yi, E. S., and Ryu, J. H. (2018). Current concepts in pathogenesis, diagnosis, and management of smoking-related interstitial lung diseases. Chest 154 (2), 394–408. doi:10.1016/j.chest.2017.11.023
Labaki, W. W., and Rosenberg, S. R. (2020). Chronic obstructive pulmonary disease. Ann. Intern Med. 173 (3), Itc17–itc32. doi:10.7326/AITC202008040
Lee, Y. S., and Dutta, A. (2007). The tumor suppressor microRNA let-7 represses the HMGA2 oncogene. Genes Dev. 21 (9), 1025–1030. doi:10.1101/gad.1540407
Lei, B., Liu, S., Qi, W., Zhao, Y., Li, Y., Lin, N., et al. (2013). PBK/TOPK expression in non-small-cell lung cancer: Its correlation and prognostic significance with Ki67 and p53 expression. Histopathology 63 (5), 696–703. doi:10.1111/his.12215
Letko, M., Marzi, A., and Munster, V. (2020). Functional assessment of cell entry and receptor usage for SARS-CoV-2 and other lineage B betacoronaviruses. Nat. Microbiol. 5 (4), 562–569. doi:10.1038/s41564-020-0688-y
Li, B., He, M., Li, W., Luo, Z., Guo, Y., Li, Y., et al. (2013). Dissolution and pharmacokinetics of baicalin-polyvinylpyrrolidone coprecipitate. J. Pharm. Pharmacol. 65 (11), 1670–1678. doi:10.1111/jphp.12146
Li, J., Jiang, Q., Deng, P., Chen, Q., Yu, M., Shang, J., et al. (2017). The formation of a host-guest inclusion complex system between β-cyclodextrin and baicalin and its dissolution characteristics. J. Pharm. Pharmacol. 69 (6), 663–674. doi:10.1111/jphp.12708
Li, J., Zhang, M., Chao, J., and Shuang, S. (2009). Preparation and characterization of the inclusion complex of baicalin (BG) with beta-CD and HP-beta-CD in solution: An antioxidant ability study. Spectrochim. Acta A Mol. Biomol. Spectrosc. 73 (4), 752–756. doi:10.1016/j.saa.2009.03.025
Li, L., Liu, Q., Shi, L., Zhou, X., Wu, W., Wang, X., et al. (2022). Baicalin prevents fibrosis of human trabecular meshwork cells via inhibiting the MyD88/NF-κB pathway. Eur. J. Pharmacol. 938, 175425. doi:10.1016/j.ejphar.2022.175425
Li, M. H., Huang, K. L., Wu, S. Y., Chen, C. W., Yan, H. C., Hsu, K., et al. (2009). Baicalin attenuates air embolism-induced acute lung injury in rat isolated lungs. Br. J. Pharmacol. 157 (2), 244–251. doi:10.1111/j.1476-5381.2009.00139.x
Liu, J., Wei, Y., Luo, Q., Xu, F., Zhao, Z., Zhang, H., et al. (2016). Baicalin attenuates inflammation in mice with OVA-induced asthma by inhibiting NF-κB and suppressing CCR7/CCL19/CCL21. Int. J. Mol. Med. 38 (5), 1541–1548. doi:10.3892/ijmm.2016.2743
Liu, T., Dai, W., Liu, F., Chen, Y., and Weng, D., (2015). Baicalin alleviates silica-induced lung inflammation and fibrosis by inhibiting the Th17 response in C57bl/6 mice. J. Nat. Prod. 78 (12), 3049–3057. doi:10.1021/acs.jnatprod.5b00868
Liu, Y., Xiang, D., Zhang, H., Yao, H., and Wang, Y. (2020). Hypoxia-inducible factor-1: A potential target to treat acute lung injury. Oxid. Med. Cell Longev. 2020, 8871476. doi:10.1155/2020/8871476
Lixuan, Z., Jingcheng, D., Wenqin, Y., Jianhua, H., Baojun, L., and Xiaotao, F. (2010). Baicalin attenuates inflammation by inhibiting NF-kappaB activation in cigarette smoke induced inflammatory models. Pulm. Pharmacol. Ther. 23 (5), 411–419. doi:10.1016/j.pupt.2010.05.004
Long, Y., Xiang, Y., Liu, S., Zhang, Y., Wan, J., Yang, Q., et al. (2020). Baicalin liposome alleviates lipopolysaccharide-induced acute lung injury in mice via inhibiting TLR4/JNK/ERK/NF-κB pathway. Mediat. Inflamm. 2020, 8414062. doi:10.1155/2020/8414062
Lu, J., Zhong, Y., Lin, Z., Lin, X., Chen, Z., Wu, X., et al. (2017). Baicalin alleviates radiation-induced epithelial-mesenchymal transition of primary type II alveolar epithelial cells via TGF-β and ERK/GSK3β signaling pathways. Biomed. Pharmacother. 95, 1219–1224. doi:10.1016/j.biopha.2017.09.037
Lu, T., Song, J., Huang, F., Deng, Y., Xie, L., Wang, G., et al. (2007). Comparative pharmacokinetics of baicalin after oral administration of pure baicalin, Radix scutellariae extract and Huang-Lian-Jie-Du-Tang to rats. J. Ethnopharmacol. 110 (3), 412–418. doi:10.1016/j.jep.2006.09.036
Luan, Y., Chao, S., Ju, Z. Y., Wang, J., Xue, X., Qi, T. G., et al. (2015). Therapeutic effects of baicalin on monocrotaline-induced pulmonary arterial hypertension by inhibiting inflammatory response. Int. Immunopharmacol. 26 (1), 188–193. doi:10.1016/j.intimp.2015.01.009
Ma, C., Ma, Z., and Fu, Q. (2014). Anti-asthmatic effects of baicalin in a mouse model of allergic asthma. Phytother. Res. 28 (2), 231–237. doi:10.1002/ptr.4983
Meng, X., Hu, L., and Li, W. (2019). Baicalin ameliorates lipopolysaccharide-induced acute lung injury in mice by suppressing oxidative stress and inflammation via the activation of the Nrf2-mediated HO-1 signaling pathway. Naunyn Schmiedeb. Arch. Pharmacol. 392 (11), 1421–1433. doi:10.1007/s00210-019-01680-9
Murali, M., Gowtham, H. G., Shilpa, N., Krishnappa, H. K. N., Ledesma, A. E., Jain, A. S., et al. (2022). Exploration of anti-HIV phytocompounds against SARS-CoV-2 main protease: Structure-based screening, molecular simulation, ADME analysis and conceptual DFT studies. Molecules 27 (23), 8288. doi:10.3390/molecules27238288
Olin, J. T., and Wechsler, M. E. (2014). Asthma: Pathogenesis and novel drugs for treatment. Bmj 349, g5517. doi:10.1136/bmj.g5517
Pagès, L., Gavaldà, A., and Lehner, M. D. (2009). PDE4 inhibitors: A review of current developments (2005 - 2009). Expert Opin. Ther. Pat. 19 (11), 1501–1519. doi:10.1517/13543770903313753
Pan, L., Cho, K. S., Yi, I., To, C. H., Chen, D. F., and Do, C. W. (2021). Baicalein, baicalin, and wogonin: Protective effects against ischemia-induced neurodegeneration in the brain and retina. Oxid. Med. Cell Longev. 2021, 8377362. doi:10.1155/2021/8377362
Pan, X., Chen, Y., Shen, Y., and Tantai, J. (2019). Knockdown of TRIM65 inhibits autophagy and cisplatin resistance in A549/DDP cells by regulating miR-138-5p/ATG7. Cell Death Dis. 10 (6), 429. doi:10.1038/s41419-019-1660-8
Pangeni, R., Panthi, V. K., Yoon, I. S., and Park, J. W. (2018). Preparation, characterization, and in vivo evaluation of an oral multiple nanoemulsive system for Co-delivery of pemetrexed and quercetin. Pharmaceutics 10 (3), 158. doi:10.3390/pharmaceutics10030158
Park, K., Lee, J. S., Choi, J. S., Nam, Y. J., Han, J. H., Byun, H. D., et al. (2016). Identification and characterization of baicalin as a phosphodiesterase 4 inhibitor. Phytother. Res. 30 (1), 144–151. doi:10.1002/ptr.5515
Peng, L. Y., Yuan, M., Song, K., Yu, J. L., Li, J. H., Huang, J. N., et al. (2019). Baicalin alleviated APEC-induced acute lung injury in chicken by inhibiting NF-κB pathway activation. Int. Immunopharmacol. 72, 467–472. doi:10.1016/j.intimp.2019.04.046
Pramual, S., Lirdprapamongkol, K., Atjanasuppat, K., Chaisuriya, P., Niamsiri, N., and Svasti, J. (2022). PLGA-lipid hybrid nanoparticles for overcoming paclitaxel tolerance in anoikis-resistant lung cancer cells. Molecules 27 (23), 8295. doi:10.3390/molecules27238295
Ptasinski, V. A., Stegmayr, J., Belvisi, M. G., Wagner, D. E., and Murray, L. A. (2021). Targeting alveolar repair in idiopathic pulmonary fibrosis. Am. J. Respir. Cell Mol. Biol. 65 (4), 347–365. doi:10.1165/rcmb.2020-0476TR
Qian, W., Cai, X., Qian, Q., Zhang, W., and Wang, D. (2018). Astragaloside IV modulates TGF-β1-dependent epithelial-mesenchymal transition in bleomycin-induced pulmonary fibrosis. J. Cell Mol. Med. 22 (9), 4354–4365. doi:10.1111/jcmm.13725
Qin, L., Niu, Y., Wang, Y., and Chen, X. (2018). Combination of phospholipid complex and submicron emulsion techniques for improving oral bioavailability and therapeutic efficacy of water-insoluble drug. Mol. Pharm. 15 (3), 1238–1247. doi:10.1021/acs.molpharmaceut.7b01061
Ramedani, A., Sabzevari, O., and Simchi, A. (2022). Hybrid ultrasound-activated nanoparticles based on graphene quantum dots for cancer treatment. Int. J. Pharm. 629, 122373. doi:10.1016/j.ijpharm.2022.122373
Salawi, A. (2022). Self-emulsifying drug delivery systems: A novel approach to deliver drugs. Drug Deliv. 29 (1), 1811–1823. doi:10.1080/10717544.2022.2083724
Saman, H., Raza, A., Patil, K., Uddin, S., and Crnogorac-Jurcevic, T. (2022). Non-invasive biomarkers for early lung cancer detection. Cancers (Basel) 14 (23), 5782. doi:10.3390/cancers14235782
Shang, X., He, X., He, X., Li, M., Zhang, R., Fan, P., et al. (2010). The genus Scutellaria an ethnopharmacological and phytochemical review. J. Ethnopharmacol. 128 (2), 279–313. doi:10.1016/j.jep.2010.01.006
Sharif, R. (2017). Overview of idiopathic pulmonary fibrosis (IPF) and evidence-based guidelines. Am. J. Manag. Care 23, S176–s182.
Shevtsova, Y. A., Goryunov, K. V., Babenko, V. A., Pevzner, I. B., Vtorushina, V. V., Inviyaeva, E. V., et al. (2022). Development of an in vitro model of SARS-CoV-induced acute lung injury for studying new therapeutic approaches. Antioxidants (Basel) 11 (10), 1910. doi:10.3390/antiox11101910
Siddik, Z. H. (2003). Cisplatin: Mode of cytotoxic action and molecular basis of resistance. Oncogene 22 (47), 7265–7279. doi:10.1038/sj.onc.1206933
Song, X., Gong, Z., Liu, K., Kou, J., Liu, B., and Liu, K. (2020). Baicalin combats glutamate excitotoxicity via protecting glutamine synthetase from ROS-induced 20S proteasomal degradation. Redox Biol. 34, 101559. doi:10.1016/j.redox.2020.101559
South, A. M., Brady, T. M., and Flynn, J. T. (2020). ACE2 (Angiotensin-Converting enzyme 2), COVID-19, and ACE inhibitor and Ang II (angiotensin II) receptor blocker use during the pandemic: The pediatric perspective. Hypertension 76 (1), 16–22. doi:10.1161/HYPERTENSIONAHA.120.15291
Sui, X., Han, X., Chen, P., Wu, Q., Feng, J., Duan, T., et al. (2020). Baicalin induces apoptosis and suppresses the cell cycle progression of lung cancer cells through downregulating akt/mTOR signaling pathway. Front. Mol. Biosci. 7, 602282. doi:10.3389/fmolb.2020.602282
Sun, J., Li, L., Wu, J., Liu, B., Gong, W., Lv, Y., et al. (2013). Effects of baicalin on airway remodeling in asthmatic mice. Planta Med. 79 (3-4), 199–206. doi:10.1055/s-0032-1328197
Taiming, L., and Xuehua, J. (2006). Investigation of the absorption mechanisms of baicalin and baicalein in rats. J. Pharm. Sci. 95 (6), 1326–1333. doi:10.1002/jps.20593
Teng, Y., Nian, H., Zhao, H., Chen, P., and Wang, G. (2013). Biotransformation of baicalin to baicalein significantly strengthens the inhibition potential towards UDP-glucuronosyltransferases (UGTs) isoforms. Pharmazie 68 (9), 763–767.
Ullah, I., Yang, L., Yin, F. T., Sun, Y., Li, X. H., Li, J., et al. (2022). Multi-omics approaches in colorectal cancer screening and diagnosis, recent updates and future perspectives. Cancers (Basel) 14 (22), 5545. doi:10.3390/cancers14225545
Vasconcelos, T., Sarmento, B., and Costa, P. (2007). Solid dispersions as strategy to improve oral bioavailability of poor water soluble drugs. Drug Discov. Today 12 (23-24), 1068–1075. doi:10.1016/j.drudis.2007.09.005
Venkatesan, N., Punithavathi, D., and Babu, M. (2007). Protection from acute and chronic lung diseases by curcumin. Adv. Exp. Med. Biol. 595, 379–405. doi:10.1007/978-0-387-46401-5_17
von Karstedt, S., Montinaro, A., and Walczak, H. (2017). Exploring the TRAILs less travelled: TRAIL in cancer biology and therapy. Nat. Rev. Cancer 17 (6), 352–366. doi:10.1038/nrc.2017.28
Wang, B., Zhang, W., Zhou, X., Liu, M., Hou, X., Cheng, Z., et al. (2019). Development of dual-targeted nano-dandelion based on an oligomeric hyaluronic acid polymer targeting tumor-associated macrophages for combination therapy of non-small cell lung cancer. Drug Deliv. 26 (1), 1265–1279. doi:10.1080/10717544.2019.1693707
Wang, C. Z., Zhang, C. F., Luo, Y., Yao, H., Yu, C., Chen, L., et al. (2020). Baicalein, an enteric microbial metabolite, suppresses gut inflammation and cancer progression in Apc(Min/+) mice. Clin. Transl. Oncol. 22 (7), 1013–1022. doi:10.1007/s12094-019-02225-5
Wang, M., You, S. K., Lee, H. K., Han, M. G., Lee, H. M., Pham, T. M. A., et al. (2020). Development and evaluation of docetaxel-phospholipid complex loaded self-microemulsifying drug delivery system: Optimization and in vitro/ex vivo studies. Pharmaceutics 12 (6), 544. doi:10.3390/pharmaceutics12060544
Wei, X., Zhu, X., Hu, N., Zhang, X., Sun, T., Xu, J., et al. (2015). Baicalin attenuates angiotensin II-induced endothelial dysfunction. Biochem. Biophys. Res. Commun. 465 (1), 101–107. doi:10.1016/j.bbrc.2015.07.138
Wei, Y., Guo, J., Zheng, X., Wu, J., Zhou, Y., Yu, Y., et al. (2014). Preparation, pharmacokinetics and biodistribution of baicalin-loaded liposomes. Int. J. Nanomedicine 9, 3623–3630. doi:10.2147/IJN.S66312
Wei, Y., Liang, J., Zheng, X., Liu, H., and Yang, H., (2017). Lung-targeting drug delivery system of baicalin-loaded nanoliposomes: Development, biodistribution in rabbits, and pharmacodynamics in nude mice bearing orthotopic human lung cancer. Int. J. Nanomedicine 12, 251–261. doi:10.2147/IJN.S119895
Wei, Y., Pi, C., Yang, G., Xiong, X., Lan, Y., Yang, H., et al. (2016). LC-UV determination of baicalin in rabbit plasma and tissues for application in pharmacokinetics and tissue distribution studies of baicalin after intravenous administration of liposomal and injectable formulations. Molecules 21 (4), 444. doi:10.3390/molecules21040444
Wu, H., Long, X., Yuan, F., Chen, L., Pan, S., Liu, Y., et al. (2014). Combined use of phospholipid complexes and self-emulsifying microemulsions for improving the oral absorption of a BCS class IV compound, baicalin. Acta Pharm. Sin. B 4 (3), 217–226. doi:10.1016/j.apsb.2014.03.002
Wu, L., Bi, Y., and Wu, H. (2018). Formulation optimization and the absorption mechanisms of nanoemulsion in improving baicalin oral exposure. Drug Dev. Ind. Pharm. 44 (2), 266–275. doi:10.1080/03639045.2017.1391831
Xia, Y., Wu, Y., Liu, B., Wang, P., and Chen, Y. (2014). Downregulation of miR-638 promotes invasion and proliferation by regulating SOX2 and induces EMT in NSCLC. FEBS Lett. 588 (14), 2238–2245. doi:10.1016/j.febslet.2014.05.002
Xie, Y., Hu, F., Xiang, D., Lu, H., Li, W., Zhao, A., et al. (2020). The metabolic effect of gut microbiota on drugs. Drug Metab. Rev. 52 (1), 139–156. doi:10.1080/03602532.2020.1718691
Xin, L., Gao, J., Lin, H., Qu, Y., Shang, C., Wang, Y., et al. (2020). Regulatory mechanisms of baicalin in cardiovascular diseases: A review. Front. Pharmacol. 11, 583200. doi:10.3389/fphar.2020.583200
Xing, J., Chen, X., and Zhong, D. (2005). Absorption and enterohepatic circulation of baicalin in rats. Life Sci. 78 (2), 140–146. doi:10.1016/j.lfs.2005.04.072
Xu, D., Hu, Y. H., Gou, X., Li, F. Y., Yang, X. Y. C., Li, Y. M., et al. (2022). Oxidative stress and antioxidative therapy in pulmonary arterial hypertension. Molecules 27 (12), 3724. doi:10.3390/molecules27123724
Xu, L., Li, J., Zhang, Y., Zhao, P., and Zhang, X. (2017). Regulatory effect of baicalin on the imbalance of Th17/Treg responses in mice with allergic asthma. J. Ethnopharmacol. 208, 199–206. doi:10.1016/j.jep.2017.07.013
Xu, W., Niu, Y., Ai, X., Xia, C., Geng, P., Zhu, H., et al. (2022). Liver-targeted nanoparticles facilitate the bioavailability and anti-HBV efficacy of baicalin in vitro and in vivo. Biomedicines 10 (4), 900. doi:10.3390/biomedicines10040900
Xu, Z., Mei, J., and Tan, Y. (2017). Baicalin attenuates DDP (cisplatin) resistance in lung cancer by downregulating MARK2 and p-Akt. Int. J. Oncol. 50 (1), 93–100. doi:10.3892/ijo.2016.3768
Xue, X., Zhang, S., Jiang, W., Wang, J., Xin, Q., Sun, C., et al. (2021). Protective effect of baicalin against pulmonary arterial hypertension vascular remodeling through regulation of TNF-α signaling pathway. Pharmacol. Res. Perspect. 9 (1), e00703. doi:10.1002/prp2.703
Yamamoto, T., Katsuta, Y., Sato, K., Tsukita, Y., Umezawa, R., Takahashi, N., et al. (2022). Longitudinal analyses and predictive factors of radiation-induced lung toxicity-related parameters after stereotactic radiotherapy for lung cancer. PLoS One 17 (12), e0278707. doi:10.1371/journal.pone.0278707
Yan, G., Wang, J., Yi, T., Cheng, J., Guo, H., He, Y., et al. (2019). Baicalin prevents pulmonary arterial remodeling in vivo via the AKT/ERK/NF-κB signaling pathways. Pulm. Circ. 9 (4), 2045894019878599. doi:10.1177/2045894019878599
Yan, S., Wang, Y., Liu, P., Chen, A., Chen, M., Yao, D., et al. (2016). Baicalin attenuates hypoxia-induced pulmonary arterial hypertension to improve hypoxic cor pulmonale by reducing the activity of the p38 MAPK signaling pathway and MMP-9. Evid. Based Complement. Altern. Med. 2016, 2546402. doi:10.1155/2016/2546402
Yasuda, Y., Nagano, T., Kobayashi, K., and Nishimura, Y. (2020). Group 2 innate lymphoid cells and the house dust mite-induced asthma mouse model. Cells 9 (5), 1178. doi:10.3390/cells9051178
You, J., Cheng, J., Yu, B., Duan, C., and Peng, J. (2018). Baicalin, a Chinese herbal medicine, inhibits the proliferation and migration of human non-small cell lung carcinoma (NSCLC) cells, A549 and H1299, by activating the SIRT1/AMPK signaling pathway. Med. Sci. Monit. 24, 2126–2133. doi:10.12659/msm.909627
You, J., Li, H., Fan, P., Yang, X., Wei, Y., Zheng, L., et al. (2022). Inspiration for COVID-19 treatment: Network analysis and experimental validation of baicalin for cytokine storm. Front. Pharmacol. 13, 853496. doi:10.3389/fphar.2022.853496
Yue, P. F., Li, Y., Wan, J., Wang, Y., Yang, M., Zhu, W. F., et al. (2013). Process optimization and evaluation of novel baicalin solid nanocrystals. Int. J. Nanomedicine 8, 2961–2973. doi:10.2147/IJN.S44924
Zandi, K., Musall, K., Oo, A., Cao, D., Liang, B., Hassandarvish, P., et al. (2021). Baicalein and baicalin inhibit SARS-CoV-2 RNA-dependent-RNA polymerase. Microorganisms 9 (5), 893. doi:10.3390/microorganisms9050893
Zeng, W., Liu, X., Wu, Y., Cai, Y., Li, Z., Ye, F., et al. (2022). Dysregulated hepatic UDP-glucuronosyltransferases and flavonoids glucuronidation in experimental colitis. Front. Pharmacol. 13, 1053610. doi:10.3389/fphar.2022.1053610
Zhang, H., Liu, B., Jiang, S., Wu, J. F., Qi, C. H., Mohammadtursun, N., et al. (2021). Baicalin ameliorates cigarette smoke-induced airway inflammation in rats by modulating HDAC2/NF-κB/PAI-1 signalling. Pulm. Pharmacol. Ther. 70, 102061. doi:10.1016/j.pupt.2021.102061
Zhang, H., Yang, X., Zhao, L., Jiao, Y., Liu, J., and Zhai, G. (2016). In vitro and in vivo study of Baicalin-loaded mixed micelles for oral delivery. Drug Deliv. 23 (6), 1933–1939. doi:10.3109/10717544.2015.100870
Zhang, H., Zhao, L., Chu, L., Han, X., and Zhai, G. (2014). Preparation, optimization, characterization and cytotoxicity in vitro of Baicalin-loaded mixed micelles. J. Colloid Interface Sci. 434, 40–47. doi:10.1016/j.jcis.2014.07.045
Zhang, J., Cai, W., Zhou, Y., Liu, Y., Wu, X., Li, Y., et al. (2015). Profiling and identification of the metabolites of baicalin and study on their tissue distribution in rats by ultra-high-performance liquid chromatography with linear ion trap-Orbitrap mass spectrometer. J. Chromatogr. B Anal. Technol. Biomed. Life Sci. 985, 91–102. doi:10.1016/j.jchromb.2015.01.018
Zhang, J., Zhou, Y., Gu, H., Tang, H., and Rong, Q., (2020). LncRNA-AK149641 associated with airway inflammation in an OVA-induced asthma mouse model. J. Bioenerg. Biomembr. 52 (5), 355–365. doi:10.1007/s10863-020-09844-6
Zhang, L., Lin, G., and Zuo, Z. (2007). Involvement of UDP-glucuronosyltransferases in the extensive liver and intestinal first-pass metabolism of flavonoid baicalein. Pharm. Res. 24 (1), 81–89. doi:10.1007/s11095-006-9126-y
Zhang, L., Wang, X., Wang, R., Zheng, X., and Li, H., (2017). Baicalin potentiates TRAIL-induced apoptosis through p38 MAPK activation and intracellular reactive oxygen species production. Mol. Med. Rep. 16 (6), 8549–8555. doi:10.3892/mmr.2017.7633
Zhao, F., Zhao, Z., Han, Y., Li, S., Liu, C., and Jia, K. (2021). Baicalin suppresses lung cancer growth phenotypes via miR-340-5p/NET1 axis. Bioengineered 12 (1), 1699–1707. doi:10.1080/21655979.2021.1922052
Zhao, H., Li, L., Liu, J., Gao, Y., and Mu, K., (2020). Baicalin alleviates bleomycin-induced pulmonary fibrosis and fibroblast proliferation in rats via the PI3K/AKT signaling pathway. Mol. Med. Rep. 21 (6), 2321–2334. doi:10.3892/mmr.2020.11046
Zhao, L., Wei, Y., Huang, Y., He, B., Zhou, Y., and Fu, J. (2013). Nanoemulsion improves the oral bioavailability of baicalin in rats: In vitro and in vivo evaluation. Int. J. Nanomedicine 8, 3769–3779. doi:10.2147/IJN.S51578
Glossary
ACE2 angiotensin-converting enzyme-2
AECs alveolar epithelial cells
ALI acute lung injury
AMPK adenosine monophosphate-activated protein kinase
Ang II angiotensin II
AUC plasma drug concentration-time curve
BA Baicalin
BE Baicalein
BLM bleomycin
BMPR2 bone morphogenetic protein receptor 2
CAT catalase
CCR CC-chemokine receptor
CCL C-C chemokine ligand
Cmax maximum serum concentration
COPD chronic obstructive pulmonary disease
COVID-19 coronary viral disease-2019
CS cytokine storm
DDP cisplatin
DDSs drug delivery systems
EMT Epithelial-mesenchymal transition
ERK extracellular signal-regulated kinase
GM-CSF Granulocyte-macrophage colony stimulating factor
HO-1 heme oxygenase-1
HSP72 heat shock protein 72
i.g. intragastric gavage
i.p. intraperitoneal
IgE immunoglobulin E
IL interleukin
JNK c-Jun N-terminal kinases
L-BA BA liposome
LC lung cancer
LPS lipopolysaccharide
MAPK mitogen-activated protein kinase
MCN mesoporous carbon nanopowder
MCT monocrotaline
MDA malondialdehyde
MMP matrix metalloprotein
mTOR mechanistic target of rapamycin
MyD88 myeloid differentiation factor 88
NF-κB nuclear factor-kappa B
NLRP3 nod-like receptor pyrin containing 3
Nrf2 nuclear erythroid factor 2
NSCLC Non-small cell lung cancer
OVA ovalbumin
PAH pulmonary arterial hypertension
PAI-1 plasminogen activator inhibitor-1
PBK/TOPK PDZ-binding kinase/T-LAK cell-originated protein kinase
PDE4 phosphodiesterase 4
PF pulmonary fibrosis
PI3K Phosphoinositide 3-kinase
SARS-CoV-2 severe acute respiratory syndrome coronavirus 2
SBG Scutellaria baicalensis Georgi
SEDDS self-emulsifying drug delivery systems
SIRT1 sirtuin 1
SOD superoxide dismutase
TCM traditional Chinese medicine
TGF-β transforming growth factor-β
TLR4 Toll Like Receptor 4
TNF-α tumor necrosis factor-α
TRAIL Tumor necrosis factor-related apoptosis-inducing ligand
UGT UDP-glucuronosyltransferase
VEGF vascular endothelial growth factor
β-CD β-cyclodextrin
Keywords: baicalin, respiratory diseases, molecular mechanisms, pharmacological action, pharmacokinetics, baicalin-loaded nano-delivery system
Citation: Song S, Ding L, Liu G, Chen T, Zhao M, Li X, Li M, Qi H, Chen J, Wang Z, Wang Y, Ma J, Wang Q, Li X and Wang Z (2023) The protective effects of baicalin for respiratory diseases: an update and future perspectives. Front. Pharmacol. 14:1129817. doi: 10.3389/fphar.2023.1129817
Received: 22 December 2022; Accepted: 13 February 2023;
Published: 16 March 2023.
Edited by:
Poonam Arora, Shree Guru Gobind Singh Tricentenary University, IndiaReviewed by:
Zhenhua Jia, Hebei Yiling Hospital, ChinaAn Kang, Nanjing University of Chinese Medicine, China
Copyright © 2023 Song, Ding, Liu, Chen, Zhao, Li, Li, Qi, Chen, Wang, Wang, Ma, Wang, Li and Wang. This is an open-access article distributed under the terms of the Creative Commons Attribution License (CC BY). The use, distribution or reproduction in other forums is permitted, provided the original author(s) and the copyright owner(s) are credited and that the original publication in this journal is cited, in accordance with accepted academic practice. No use, distribution or reproduction is permitted which does not comply with these terms.
*Correspondence: Xiangyan Li, eGlhbmd5YW5fbGkxOTgxQDE2My5jb20=; Zeyu Wang, emV5dTc4MTAyMkAxNjMuY29t
†These authors have contributed equally to this work