- 1Affiliated Yongkang First People’s Hospital, School of Pharmacy, Hangzhou Medical College, Hangzhou, Zhejiang, China
- 2School of Pharmaceutical Sciences, Hangzhou Medical College, Hangzhou, Zhejiang, China
- 3Zhejiang Provincial Key Laboratory of Laboratory Animals and Safety Research, Hangzhou Medical College, Hangzhou, Zhejiang, China
- 4School of Laboratory Medicine and Bioengineering, Hangzhou Medical College, Hangzhou, Zhejiang, China
- 5Chemical Biology Research Center, School of Pharmaceutical Sciences, Wenzhou Medical University, Wenzhou, Zhejiang, China
Liver fibrosis, a compensatory repair response to chronic liver injury, is caused by various pathogenic factors, and hepatic stellate cell (HSC) activation and phenotypic transformation are regarded as key events in its progression. Ferroptosis, a novel form of programmed cell death, is also closely related to different pathological processes, including those associated with liver diseases. Here, we investigated the effect of doxofylline (DOX), a xanthine derivative with potent anti-inflammatory activity, on liver fibrosis as well as the associated mechanism. Our results indicated that in mice with CCl4-induced liver fibrosis, DOX attenuated hepatocellular injury and the levels of liver fibrosis indicators, inhibited the TGF-β/Smad signaling pathway, and significantly downregulated the expression of HSC activation markers, both in vitro and in vivo. Furthermore, inducing ferroptosis in activated HSCs was found to be critical for its anti-liver fibrosis effect. More importantly, ferroptosis inhibition using the specific inhibitor, deferoxamine (DFO) not only abolished DOX-induced ferroptosis, but also led to resistance to the anti-liver fibrosis effect of DOX in HSCs. In summary, our results showed an association between the protective effect of DOX against liver fibrosis and HSC ferroptosis. Thus, DOX may be a promising anti-hepatic fibrosis agent.
Introduction
Liver fibrosis, which is characterized by a disease course that is long and reversible, is a repair response to chronic liver injury caused by various factors. Without active and effective intervention, it can progress to liver cirrhosis or even liver cancer (Lee et al., 2015). Therefore, developing effective liver fibrosis prevention and reversal strategies has become a major challenge worldwide. Current mainstream studies suggest that hepatic stellate cell (HSC) activation is a key event in the development of liver fibrosis. With increasing interest in developing antifibrotic therapies, there is a need for cell lines that preserve the in vivo phenotype of human HSCs to elucidate pathways of human hepatic fibrosis. Therefore, LX-2 was established which characterised human HSC cell lines. LX-2 was generated by spontaneous immortalisation in low serum conditions. LX-2 express α smooth muscle actin, vimentin, and glial fibrillary acid protein, as visualised by immunocytochemistry. Similar to primary HSCs, LX-2 express key receptors regulating liver fibrosis, including platelet derived growth factor receptor β (PDGF-R), obese receptor long form (Ob-RL), and discoidin domain receptor 2 (DDR2), and also proteins involved in matrix remodelling; matrix metalloproteinase (MMP)-2, tissue inhibitor of matrix metalloproteinase (TIMP)-2, and MT1-MMP, as determined by western analyses. LX-2 had a retinoid phenotype typical of stellate cells. Microarray analyses showed strong similarity in gene expression between primary HSCs and either LX-2 (98.7%), with expression of multiple neuronal genes (Xu et al., 2005). Activited HSC affects the normal functioning of hepatocytes and Kupffer cells, further aggravating the pathological state of the liver (Tacke and Trautwein, 2015). Therefore, inhibiting the activation and proliferation of HSCs and inducing their death is a plausible treatment strategy for liver fibrosis.
Ferroptosis is a form of programmed cell death caused by iron-dependent oxidative damage. Unlike other forms of programmed cell death, ferroptosis is characterized by the excessive accumulation of lipid reactive oxygen species (ROS), mitochondrial wrinkling, and increased mitochondrial membrane density (Dixon et al., 2012; Li et al., 2020). Intracellular iron level is mainly regulated by transferrin receptor which accounts for transporting extracellular iron-transferrin complex into cells via clathrin-mediated endocytosis, ferritin composed of light chain and heavy chain and responsible for storing iron, and ferroportin in charge of iron exportation. The increased iron disrupts membrane integrity by peroxidizing polyunsaturated fatty acid (PUFA) chains of membrane phospholipids via two pathways. One is to serve as a cofactor for nonheme iron-containing lipoxygenase to enzymatically catalyze PUFA peroxidation. The other is to react with H2O2 via Fenton reaction to generate toxic hydroxyl radicals, which have potent capacity to peroxidize PUFA (Ooko et al., 2015; Chen et al., 2021). In recent years, the role of ferroptosis in the development of liver fibrosis has attracted increased attention and has become a research hotspot in the field of liver fibrosis. Notably, several studies have shown that large amounts of Fe2+ are stored in HSCs and that ferroptosis can affect the development of liver fibrosis by regulating the Fe2+ content of HSCs as well as the degree of lipid peroxidation. Therefore, targeting HSC ferroptosis could be a novel strategy to treat liver fibrosis (Anderson and Shah, 2013).
Additionally, doxofylline (DOX, Figure 1A), a xanthine derivative with a long-acting bronchodilator effect (Page, 2010), is regarded as a substitute for theophylline owing to its higher efficacy and fewer side effects (Matera et al., 2017). DOX has also been reported to be a non-selective phosphodiesterase (PDE) inhibitor with good anti-inflammatory activity. However, its effects on liver fibrosis remain unclear. Therefore, in this study, we attempted to clarify its effect on ferroptosis and further elucidate the mechanism underlying its action in liver fibrosis.
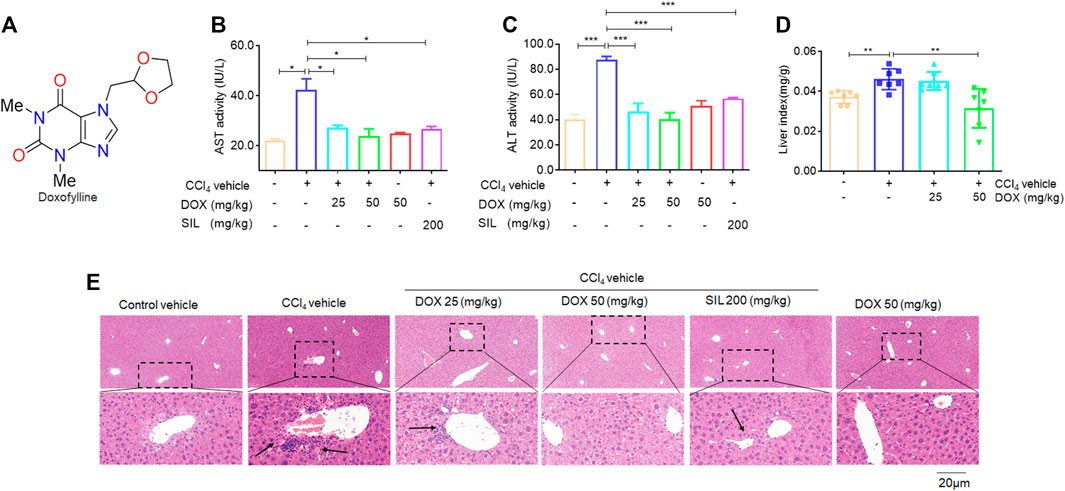
FIGURE 1. Effects of DOX serum ALT/AST activity and liver H&E staining. (A) The chemical structures of DOX. (B) AST activity. (C) ALT activity. Data were expressed as means ± SEM (n = 3). (D) Liver index (n = 7). (E) Liver H&E staining. Typical images were chosen from each experimental group. (original magnification ×400, upper images; partial enlarged pictures, down images). *p < 0.05; ***p < 0.001.
Materials and methods
Chemical compounds and reagents
Kits for detecting serum alanine (ALT)/aspartate aminotransferase (AST) activity, liver hydroxyproline content, and glutathione (GSH) from LX-2 cells were purchased from Jiancheng Bioengineering Institute (Nanjing, China). The Phen Green SK diacetate (PGSK) kit was purchased from Glpbio (Montclair, CA, United States), cell counting Kit-8 (CCK-8) was purchased from APE×BIO Technology LLC (Houston, TX, United States), the 2′,7′-dichlorodihydrofluorescein diacetate (DCFH-DA) assay kit was purchased from Solarbio (Beijing, China) and MitoLite™ Red FX600 Kit was purchased from AAT Bioquest, Inc. (Sunnyvale, CA, United States). 2.5% glutaraldehyde solution was purchased from Solarbio (Beijing, China). Further, antibodies against α-smooth muscle actin (α-SMA), vimentin (VIM), desmin (DES), SMAD family member 2/3 (Smad 2/3), p-Smad 2/3, transforming growth factor-beta (TGF-β), glutathione peroxidase 4 (GPX4), solute carrier family-7 member-11 (SLC7A11), solute carrier family 40 member 1 (SLC40A1), transferrin, transferrin receptor and glyceraldehyde-3-phosphate dehydrogenase (GAPDH) were purchased from Cell Signaling Technology (Danvers, MA, United States). Furthermore, the FDA staining kit was purchased from AAT Bioquest (Sunnyvale, CA, United States), FerroOrange was purchased from Goryo Chemical Inc. (Hokkaido, Japan), and deferoxamine (DFO) was purchased from Sigma-Aldrich (St. Louis, MO, United States). Peroxidase-conjugated or fluorescein isothiocyanate (FITC)-conjugated antibodies were purchased from Jackson ImmunoResearch (West Grove, PA, United States), TRIzol reagent was purchased from Invitrogen Life Technology (Carlsbad, CA, United States), the PrimeScript Master Mix and SYBR Premix Ex Taq were purchased from Yeasen Biotechnology Co., Ltd. (Shanghai, China). Doxofylline (DOX) and silymarin (SIL) were purchased from Yuanye Bio-Technology Co., Ltd. (Shanghai, China).
Experimental animals
C57BL/6 mice (20 ± 2 g) were purchased from the Experimental Animal Center of Hangzhou Medical College (Hangzhou, China). The animals were maintained under controlled temperature (22°C ± 1°C), humidity (50%), and light (12-h light/12-h dark cycle) conditions. All the animals received humane care according to the institutional animal care guidelines of the Experimental Animal Ethical Committee of Hangzhou Medical College. The mice were randomly divided into six groups, namely, the: 1) vehicle control (n = 7), 2) CCl4 model (n = 7), 3) CCl4+DOX (25 mg/kg) (n = 7), 4) CCl4+DOX (50 mg/kg) (n = 7), 5) DOX (50 mg/kg) (n = 7), and 6) CCl4+silymarin (SIL) (0.2 g/kg) (n = 5) groups. Thereafter, that mice were treated for 2 weeks with CCl4 (intraperitoneal injection, mixed with olive oil in the ratio 1:3, 2 mL/kg twice per week) and then for additional 4 weeks with both CCl4 (twice per week), DOX or SIL (intragastric administration, every day). After the treatment period, the mice were sacrificed, and their plasma and liver tissue samples were collected.
Analysis of serum ALT/AST activities
Serum ALT and AST (Nanjing Jiancheng Bioengineering Institute) activities were assessed according to the manufacturer’s instructions.
Liver histological evaluation
Liver tissue samples were fixed in 4% paraformaldehyde for 24 h, sectioned (4 μm) and stained with haematoxylin-eosin (H&E) for the histological observation of liver injury, Staining with masson’s trichrome and sirius red were also performed to observe collagen deposition in the liver of the mice.
Measurement of liver hydroxyproline content
Liver hydroxyproline content was determined using the alkaline hydrolysis method in accordance with the manufacturer’s instruction (Nanjing Jiancheng Bioengineering Institute, Nanjing, China).
Immunofluorescence staining with α-SMA
The liver sections were incubated overnight with α-SMA antibody in a humidified chamber at 4°C. Thereafter, the sections were incubated again with FITC-conjugated secondary antibody for 1 h. Finally, images were captured using an inverted IX81 microscope (Olympus, Tokyo, Japan).
Immunohistochemical staining
Liver sections were blocked with 3% hydrogen peroxide and 3% bovine serum albumin (BSA) for 1 h. After rinsing once with PBS (phosphate buffer saline), diluted vimentin antibody was added to the sections followed by incubation overnight at 4°C. Next, goat anti-rabbit secondary antibody was added dropwise and the mixture was incubated at room temperature for 1 h. After washing with PBS, the tissue sections were developed with 3,3-Diaminobenzidine tetrahydrochloride (DAB) chromogenic solution and the nuclei were counterstained with hematoxylin. This was followed by thorough washing, after which the sections were dehydrated using 75% and 85% alcohol for 6 min and then washed with absolute ethanol and n-butanol, respectively, until they became transparent. Next, the tissue slices were then mounted on slides and their pathological characteristics were observed under a light microscope.
Cell culture
LX-2 cells were cultured in dulbecco’s modified eagle medium (DMEM) supplemented with 10% [v/v] fetal bovine serum (FBS), 2 mM glutamine, 100 U/mL penicillin, and 100 mg/mL streptomycin. Heparg cells were cultured in RPMI1640 supplemented with 10% [v/v] fetal bovine serum, 2 mM glutamine, 100 U/mL penicillin and 100 mg/mL streptomycin.
Cell viability assay
For assay of viability, LX-2 cells and heparg cells was seeded onto 96-well plates with a density of 5 × 103 cells per well and treated by DOX (25, 50, 100, and 250 μM) for 48 h. Viability of cells in the 96-well plates were assayed using a CCK-8 kit (APE×BIO Technology, United States). The CCK-8 was incubated with cells at 37°C for 1 h. Then the 96-well plates was measured using a multifunctional enzyme labeler (Molecular devices, CA) at 405 nm.
Cell death
Cell death was assessed using a FDA staining kit (AAT Bioquest Sunnyvale, CA). LX-2 cells were seeded onto 6-well plates with a density of 1 × 105 cells per well and treated by DOX (10, 25 μM) for 48 h. Briefly, cells were stained in 10 μM FDA (Beyotime, China) in PBS for 1 h at 37°C. Finally, the samples were washed with PBS and imaged immediately. The samples were observed with a fluorescence inverted microscope (EVOS M7000, United States).
Transmission electron microscopy
LX-2 cells were seeded onto 6-well plates with a density of 1 × 105 cells per well and treated by DOX (25 μM) for 48 h. The cells were harvested by centrifugation at 3,000 rpm for 5 min. Then the cells were fixed by adding 2.5% glutaraldehyde solution. The samples were observed by the bio-TEM (Hitachi H-7000, Japan).
GSH measurement
To measure total GSH levels, LX-2 cells was seeded onto 6-well plates with a density of 1 × 105 cells per well and treated by DOX (10, 25 μM) for 48 h. The samples were determined using a glutathione assay kit (Nanjing Jiancheng Bioengineering Institute, China) following a standard protocol.
ROS measurements
To measure ROS levels, LX-2 cells was seeded onto 6-well plates with a density of 1 × 105 cells per well and treated by DOX (10, 25 μM) for 48 h. The cells were stained in 10 μM 2,7-Dichlorodi-hydrofluorescein diacetate, DCFH-DA (Solarbio, China) in DMEM for 1 h at 37°C. Next the samples were washed with PBS and imaged. The samples were observed with a fluorescence inverted microscope (EVOS M7000, United States).
Iron level determination
Iron levels were measured as described below. LX-2 cells were seeded onto 6-well plates with a density of 1 × 105 cells per well and treated by DOX (10, 25 μM) for 48 h. The cells were stained in 10 μM Phen Green SK (Glpbio, United States) in PBS for 1 h at 37°C. Finally, the samples were imaged immediately. LX-2 cells were seeded onto 6-well plates with a density of 1 × 105 cells per well and treated by DOX (10, 25 μM) for 48 h. The cells were stained in 1 mM Ferro orange (Dojingo, Japan) in PBS for 1 h at 37°C. Next nuclei of the LX-2 cells were incubated with DAPI (Biosharp, China) for 30 min. Finally, the constructs were imaged immediately. All samples as described above were observed with a fluorescence inverted microscope (EVOS M7000, United States).
Mitochondrion detection
Mitochondria were stained using MitoLite™ Red FX600 Kit (AAT Bioquest, United States). LX-2 cells were seeded onto 6-well plates with a density of 1 × 105 cells per well and treated by DOX (10, 25 μM) for 48 h. Next cells were stained in the buffer containing a 1:500 dilution of Mitolite™ Red FX600 for 1 h at 37°C. Then nuclei of the LX-2 cells were incubated with DAPI (Biosharp, China) for 30 min. Finally, the samples were washed with PBS three times again. The samples were observed with a fluorescence inverted microscope (EVOS M7000, United States).
Real-time PCR analysis
Total ribonucleic acid (RNA) was extracted from liver tissue samples and LX-2 cells and heparg cells using TRIzol reagent. Next, complementary deoxyribonucleic acid (cDNA) was synthesized using the PrimeScript RT Master Mix kit, and real-time PCR was performed using the SYBR green premix according to the manufacturer’s instructions. The relative expression levels of target genes were then normalized to actin, analyzed using the delta-delta-CT method, and expressed as ratios relative to the vehicle control group. The primers used are listed in Supplementary Table S1.
Western blot analysis
Liver and cell protein samples from mice in the different groups were isolated using a lysis buffer. This was followed by centrifugation at 3,000 g for 20 min at 4°C, after which supernatant samples were collected. Next, the protein concentration in each sample was then determined and normalized. Thereafter, the protein samples were separated via sodium dodecyl sulfate-polyacrylamide gel electrophoresis and transferred onto polyvinylidene fluoride membranes, which were then incubated with primary and secondary antibodies followed by the visualization of the proteins in the cell membranes using a chemiluminescence kit.
Statistical analysis
Data are expressed as mean ± standard error of the mean (SEM). Further, significant differences were determined by performing one-way analysis of variance (ANOVA) with least significant difference (LSD) post hoc tests, and statistical significance was set at p < 0.05.
Results
DOX attenuated CCl4-induced liver injury in mice
In this study, we investigated whether therapeutic DOX administration could improve fibrotic liver damage. A classic CCl4-induced hepatic fibrosis mouse model was established, followed by DOX treatment. The therapeutic effect of DOX was then investigated by detecting changes in liver injury indices. From Figures 1B, C, it is evident that DOX treatment (25 and 50 mg/kg) significantly reduced serum AST/ALT activity, compared with the CCl4 model group. Reportedly, the liver index (liver weight/body weight ratio) has been found to be positively correlated with the degree of liver damage (Wu et al., 2015). Similarly, our results indicated that mice in the model group demonstrated significantly higher liver weight/body weight ratios than their counterparts in the normal group, and DOX treatment significantly lowered this index relative to its value for the model group (Figure 1D). Moreover, H&E staining of pathological liver sections showed that DOX ameliorated CCl4-induced liver injury in mice, reversing inflammatory cell infiltration and hepatocyte necrosis (Figure 1E). SIL is a well-known hepato-protective drug (Abd Elmaaboud et al., 2021). The results of serum ALT/AST activity and liver histological evaluation also indicated that it attenuates CCl4-induced liver injury (Figures 1B, C, E). Additionally, the DOX-only treatment (50 mg/kg) exert any significant effect on serum ALT/AST level and liver histomorphology compared with these characteristics for mice in the control group (Figures 1B, C, E). Therefore, DOX significantly improved the pharmacological effects of CCl4-induced liver injury in mice.
DOX attenuated CCl4-induced liver fibrosis in mice
Next, we determined how DOX alleviated pathological changes in CCl4-induced hepatic fibrosis in mice. First, we assessed the level of hydroxyproline, which is considered as a gold standard marker for liver fibrosis (Wu et al., 2015). The results thus obtained showed that hydroxyproline was significantly elevated in liver tissue samples from mice in the CCl4 model group, while the administration of DOX (25 and 50 mg/kg) significantly alleviated this phenomenon (Figure 2A). Additionally, DOX administration resulted in a significant decrease in the upregulated liver Col1a1 and Col3a1 mRNA expression levels induced by CCl4 (Figures 2B, C). Our results also indicated that the pathogenesis of liver fibrosis involved the excessive accumulation of ECM, which has as main component, collagen. In this study, we used two staining methods (Masson’s trichrome and Sirius red staining) to investigate the effect of DOX on collagen deposition. The two staining results showed increased collagen deposition in samples from mice in the CCl4-induced liver fibrosis group, while this effect was completely normalized by DOX treatment (Figures 2D, E). In contrast, the DOX-only treatment of mice without liver fibrosis had no significant effect on collagen deposition in mouse liver tissue (Figures 2D, E).
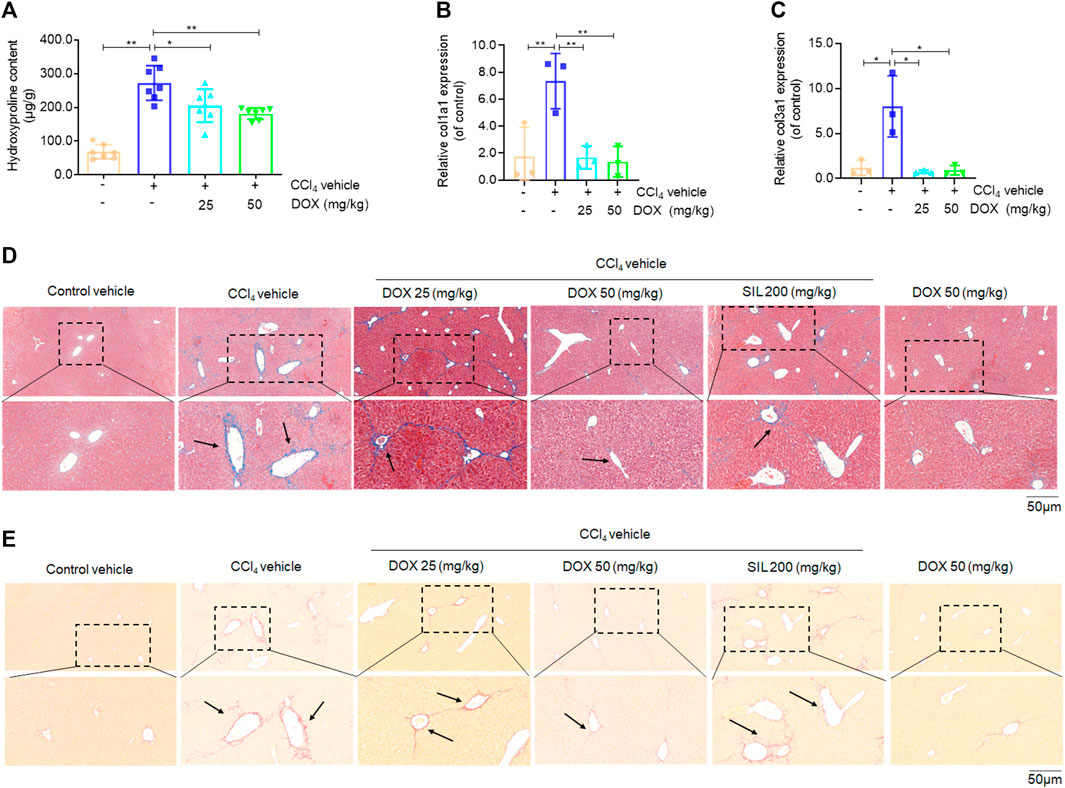
FIGURE 2. Effects of DOX on liver hydroxyproline content, serum markers of fibrosis and liver collagen expression. (A) Liver hydroxyproline content (n = 7). (B,C) Liver Col1a1 (Collagen, type I, α1) and Col3a1 (Collagen, type III, α1) (n = 3). (D) Liver masson’s trichrome staining. Typical images were chosen from each experimental group. Black arrows indicate collagen deposition. (original magnification ×200, upper images; partial enlarged pictures, down images). (E) Liver sirius red staining. Typical images were chosen from each experimental group. Black arrows indicate collagen deposition. (original magnification ×200, upper images; partial enlarged pictures, down images). Data were expressed as means ± SEM. *p < 0.05; **p < 0.01.
DOX inhibited HSCs activation in CCl4-treated mice
Activated HSCs constitute the main source of ECM, hence play a major role in hepatic fibrosis. In this study, we investigated the inhibitory effects of DOX on HSC activation in vivo, based the mRNA levels of α-SMA, vimentin, and desmin, which are markers of activated HSCs (Puche et al., 2013; Greenhalgh et al., 2015). Our results in this regard showed that DOX treatment (25 and 50 mg/kg) significantly downregulated the mRNA and protein expression levels of hepatic α-SMA, vimentin, and desmin, compared with their levels for the CCl4 group (Figures 3A–E). Moreover, hepatic α-SMA immunofluorescence staining showed that DOX treatment resulted in an obvious decrease in the number of α-SMA-positive cells in CCl4-treated mice (Figure 3F). Similarly, hepatic vimentin immunohistochemical staining showed that DOX treatment resulted in a significantly reduced number of vimentin-positive cells in CCl4-treated mice (Figure 3G).
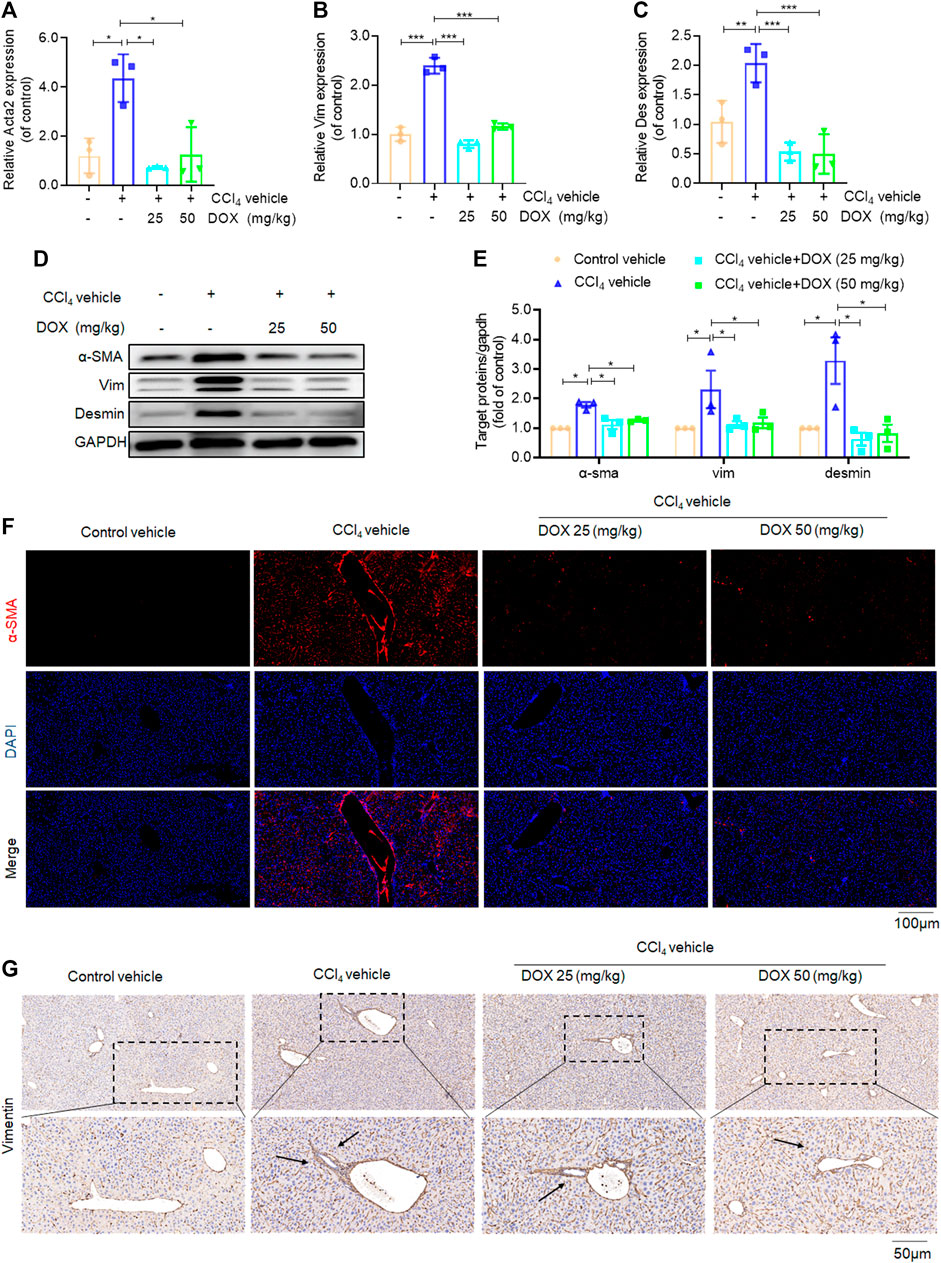
FIGURE 3. Effects of DOX on HSCs activation in vivo. (A–C) Liver Acta2 (α-SMA), Vim (Vimentin), Des (Desmin) mRNA expression (n = 3). (D,B) The expression of α-SMA, vim and desmin proteins in CCl4-induced mice liver tissues treated with DOX (25, 50 mg/kg) was detected by Western blot, and GAPDH was used as a loading control. (E) The quantitative result of α-SMA, vim and desmin. The results represent three independent experiments. (F) Liver α-SMA immunofluorescence staining (original magnification×100). (G) Liver Vim immunohistochemical staining (original magnification×100). Typical images are chosen from each experimental group. Black arrows indicate positive staining. Data were expressed as means ± SEM. *p < 0.05; **p < 0.01; ***p < 0.001.
DOX suppressed HSCs activation by inhibiting TGF-β/smad signaling in vivo
TGF-β, which shows association with all key links in the pathogenesis of liver fibrosis, is one of the most potent pro-fibrotic cytokines. This implies that the TGF-β/Smad signaling pathway plays an extremely important role in the activation of HSCs (Yang et al., 2003). In this study, we further used real-time PCR and western blot analysis to investigate the effect of DOX on the key proteins of this signaling pathway. The results of real-time PCR showed that DOX treatment decreased TGF-β mRNA expression in mice (25 and 50 mg/kg) (Figure 4A). Further, as shown in Figures 4B–D, Western blot analysis revealed that the CCl4-induced p-Smad2/3 upregulation at the protein level was reversed in mice with CCl4-induced liver fibrosis following treatment with DOX (25 and 50 mg/kg).
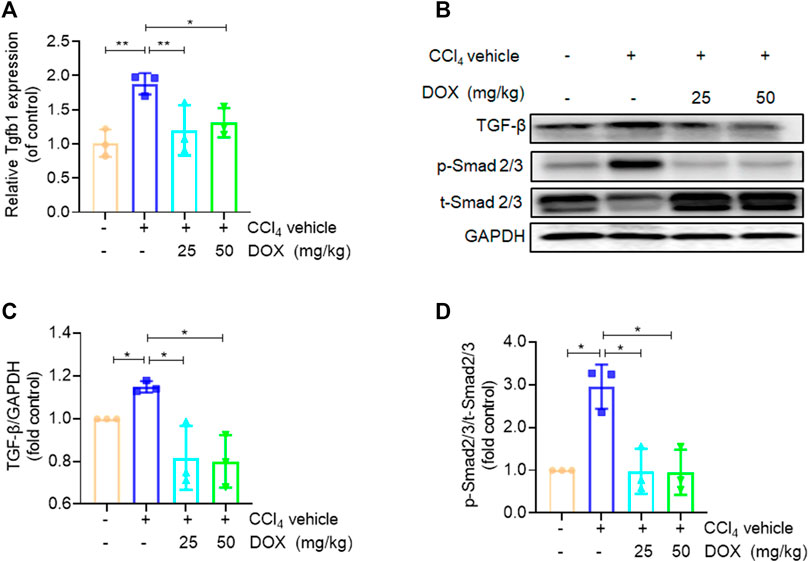
FIGURE 4. Effects of DOX TGF-β/Smad signalling in vivo. (A) Liver Tgfb1 (TGF-β), mRNA expression (n = 3). (B) The expression of TGF-β, p-Smad2/3 and t-Smad 2/3 proteins in CCl4-induced mice liver tissues treated with DOX (25 mg/kg, 50 mg/kg) was detected by Western blot, and GAPDH was used as a loading control. The results represent three independent experiments. (C) The quantitative result of TGF-β. The results represent three independent experiments. (D) The quantitative result of p-Smad2/3. The results represent three independent experiments. Data were expressed as means ± SEM. *p < 0.05; **p < 0.01.
DOX inhibited HSC activation in vitro
Our findings indicated that DOX ameliorated liver injury and fibrosis in vivo. Thus, we performed in vitro experiments to further corroborate these in vivo experimental results. Our findings, based on real-time PCR analysis, revealed that HSC activation markers, α-SMA, COL1A1, FN1, VIM, and DES were significantly downregulated by DOX in a dose-dependent manner (10 and 25 μM) following treatment for 48 h (Figures 5A–E). Moreover, western blot analysis revealed that DOX (10 and 25 μM) remarkably downregulated α-SMA, VIM, and desmin expression in activated HSCs (Figures 5F, G).
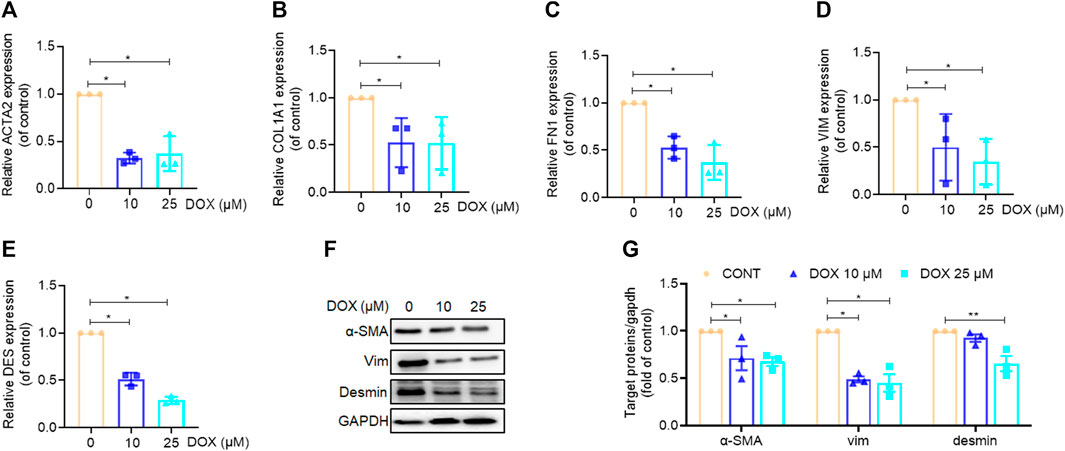
FIGURE 5. Effects of DOX inhibited HSC activation in vitro. LX-2 cells treated with DOX (10, 25 μM) for 48 h (A–E) LX-2 cell ACTA2 (α-SMA), COL1A1, FN1, VIM (Vimentin), DES (Desmin), mRNA expression (n = 3). (F) The expression of LX-2 cell α-SMA, vimentin and desmin proteins was detected by Western blot, and GAPDH was used as a loading control. (G) The quantitative result of α-SMA, vimentin and desmin. The results represent three independent experiments. Data were expressed as means ± SEM. *p < 0.05; **p < 0.01.
DOX triggered activated HSC ferroptosis in vitro
Previous in vivo and in vitro experiments have demonstrated that DOX significantly inhibits HSC activation; however, it is still unclear whether DOX treatment drives the ferroptosis of activated HSCs. Our findings in this study revealed that DOX induced HSC death at 48 h after treatment with different doses. Furthermore, FDA staining demonstrated reduced green fluorescence expression with increasing drug dosage, indicating that DOX significantly induced the death of activated HSCs (Figure 6A). A cell counting Kit-8 (CCK-8) analysis also showed that DOX remarkably suppressed HSC viability (Figure 6B). Reportedly, transferrin, GPX4, SLC7A11, and SLC40A1 are the key regulators of ferroptosis. Transferrin (TRF, Tf) is responsible for carrying iron absorbed by the digestive tract and released by the degradation of red blood cells (Gomme et al., 2005). The solute carrier family-7 member-11 (SLC7A11) is the main member of Systerm x c-, responsible for the uptake of extracellular cystine into the cytoplasm and the output of glutamic acid, which is then reduced to cysteine for the biosynthesis of GSH. GSH is synthesized from glutamic acid, cysteine and glycine, which directly affects the activity of GPX4 (Koppula et al., 2018). GPX4 is an anti-lipid peroxidase, responsible for catalyzing toxic unsaturated lipid hydroperoxides into non-toxic fatty alcohols, inhibiting ROS production, protecting cells from the effects of lipid peroxides, and playing an important role in inhibiting ferroptosis (Seibt et al., 2019). SLC40A1 (Solute Carrier Family 40 Member 1) is currently the only member of the SLC40 transporter family, and is currently the only transfer protein found to promote the transfer of iron from the cell to the outside of cell (Pietrangelo, 2004). We found that DOX (25, 50, 100, 250 μM) has no significant effect not only on the viability of liver parenchyma cells, but also on the expression of ferroptosis related proteins (Transferrin, GPX4, SLX7A11, SLC40A1, Supplementary Figure S1). Next, we investigated whether DOX mediated the activation of the ferroptotic mode in vitro by measuring ferroptosis-associated biomarkers (Hirschhorn and Stockwell, 2019). PGSK and FerroOrange were used for intracellular Fe2+ detection. As shown in Figures 6C–F, DOX treatment markedly triggered HSC ferroptosis, which was characterized by a decrease in GSH content, elimination of ROS scavenging enzymes, and increased iron levels, Since, we found differences which are statistically significant but slight, the biological meaning of these findings should be confirmed in further studies. Furthermore, electron microscopy images of mitochondria samples confirmed the occurrence of ferroptosis. We also observed that DOX-treated LX-2 cells showed smaller, ruptured, and broken mitochondria, which represent the morphological changes associated with ferroptotic cells, when compared with un-treated LX-2 cells (Cao and Dixon, 2016) (Figure 6G). Furthermore, the mitochondrial red fluorescent probe is a cationic dye that can selectively accumulate in the mitochondria through mitochondrial membrane potential gradients. The fluorescence intensity is proportional to the mitochondrial potential, and stronger fluorescence indicates higher mitochondrial potential, that is, healthier mitochondria, which proves that mitochondria are less affected by ferroptosis. In our results, mitochondrial staining revealed that DOX (10, 25 μM) significantly reduced the number of mitochondrion-positive HSCs (Figure 6H). Similarly, Our results show that DOX (10 and 25 μM) can increase the protein expression of transferrin and transferrin receptor in a dose-dependent manner; At the same time, in our study show that GPX4, SLC7A11, and SLC40A1 were reduced by DOX (10 and 25 μM) at both the mRNA and protein levels in LX-2 cells compared with the control (Figures 6I–K). Overall, these findings indicated that the inhibition of HSC activation is associated with DOX-induced HSC ferroptosis in vitro.
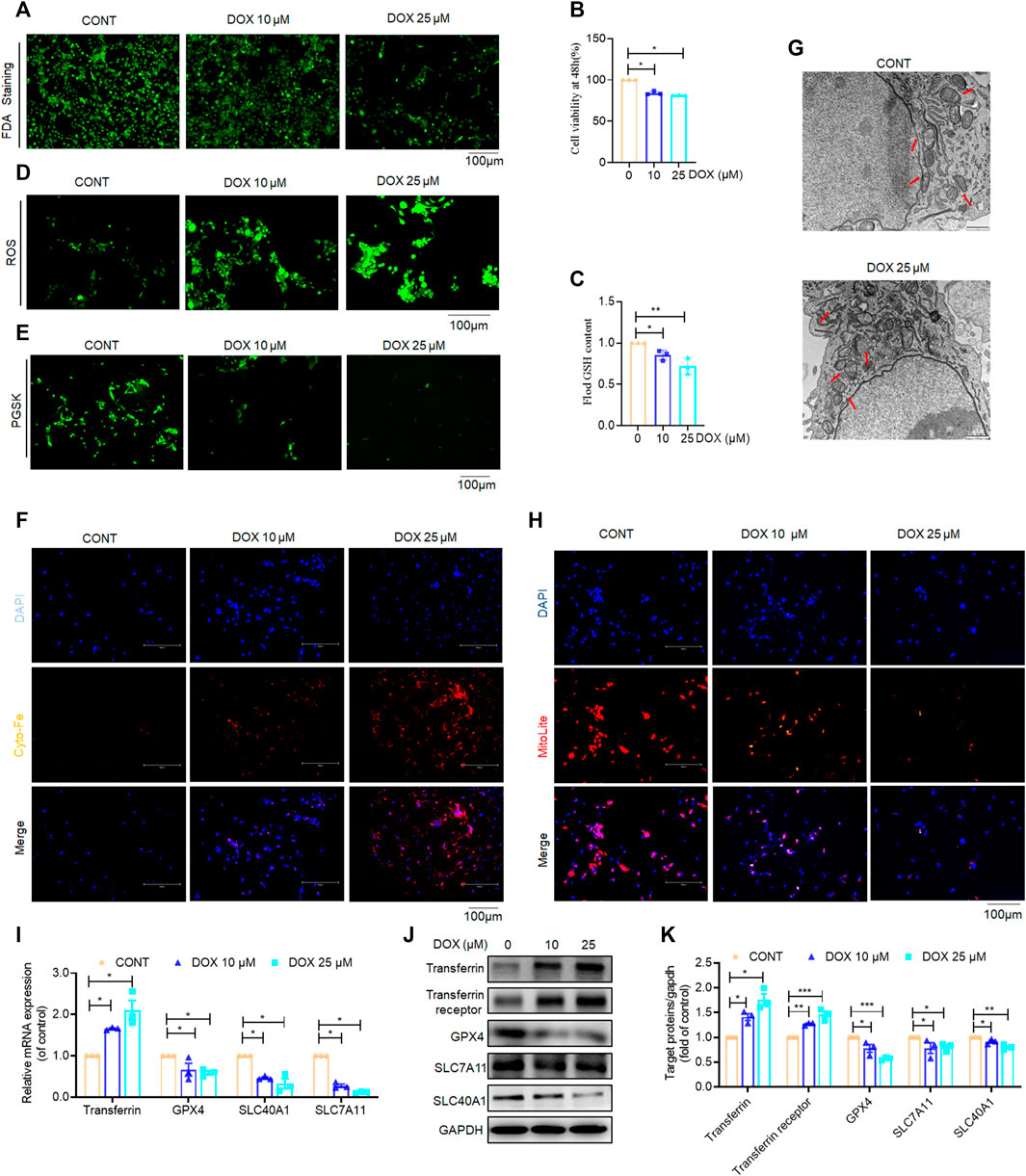
FIGURE 6. Effects of DOX triggers activated HSC ferroptosis in vitro. LX-2 was treated with DOX (10, 25 μM) for 48 h (A) FDA staining for evaluating cell death. LX-2 was stained in 10 μM FDA and the samples were observed with a fluorescence inverted microscope. (B) Cell Count Kit-8 analysis of cell viability in LX-2. CCK-8 was incubated with LX-2 at 37°C for 1 h and the samples was measured using a multifunctional enzyme labeler at 405 nm. (C) Cellular GSH amount (n = 3). (D) The ROS level in LX-2. LX-2 was stained in 10 μM DCFH-DA in DMEM for 1 h at 37°C and the samples were observed with a fluorescence inverted microscope. (E) Free iron levels in LX-2 were measured by Phen Green SK probe. LX-2 cells were stained in 10 μM Phen Green SK in PBS for 1 h at 37°C and the samples were imaged immediately. (F) Representative fluorescent images of intracellular iron level were identified by using FerroOrange (orange). Nuclei are stained by DAPI (blue). LX-2 was stained in 1 mM Ferro orange in PBS for 1 h at 37°C and constructs were imaged immediately. All samples as described (E,F) were observed with a fluorescence inverted microscope. (G) The mitochondria morphology was observed by transmission electron microscopy and mitochondrial length was summarized. (H) Mitochondrion staining detection. LX-2 cells were stained in the buffer containing a 1:500 dilution of MitoliteTM Red FX600 for 1 h at 37°C and the samples were observed with a fluorescence inverted microscope. (I) LX-2 cell transferrin, GPX-4, SLC7A11, SLC40A1 mRNA expression (n = 3). (J) The expression of LX-2 cell transferrin, transferrin receptor, GPX-4, SLC7A11, SLC40A1 proteins was detected by Western blot, and GAPDH was used as a loading control. (K) The quantitative result of transferrin, transferrin receptor, GPX-4, SLC7A11, SLC40A1. The results represent three independent experiments. For the statistics of each panel in this figure, data were expressed as means ± SEM. *p < 0.05; **p < 0.01; ***p < 0.001.
Ferroptosis inhibition abolished DOX-induced anti-fibrosis effects in vitro
To investigate whether ferroptosis plays a role in the anti-fibrotic effect of DOX, the ferroptosis-specific inhibitor, deferoxamine (DFO) was used to inhibit ferroptosis in activated HSCs (Wang et al., 2017). Additionally, activated LX-2 cells were exposed to DOX as well as DFO. The results of FDA staining as well as CCK-8 assay showed that LX-2 cells treated with DOX showed suppressed HSC viability, whereas DFO rescued this growth inhibition effect of DOX (Figures 7A, B). Additionally, DOX-treated LX-2 cells demonstrated remarkably increased ROS and iron levels compared with their untreated counterparts. Conversely, this effect on ferroptosis induction was weakened by co-treatment with DFO (Figures 7C–E). Mitochondrial staining further indicated that DOX treatment (25 μM) significantly reduced the number of mitochondria-positive cells, and DFO treatment significantly blocked the ferroptosis-promoting effect of DOX (Figure 7F). Real-time PCR analysis, performed to explore the expression of HSC activation- and ferroptosis-associated biomarkers showed that DOX treatment significantly downregulated the gene expression levels of the HSC activation- and ferroptosis-associated markers, whereas this function was inhibited by DFO (Figures 7G, H). Overall, these findings suggested that DFO inhibition abolished DOX-induced anti-fibrotic effects in vitro.
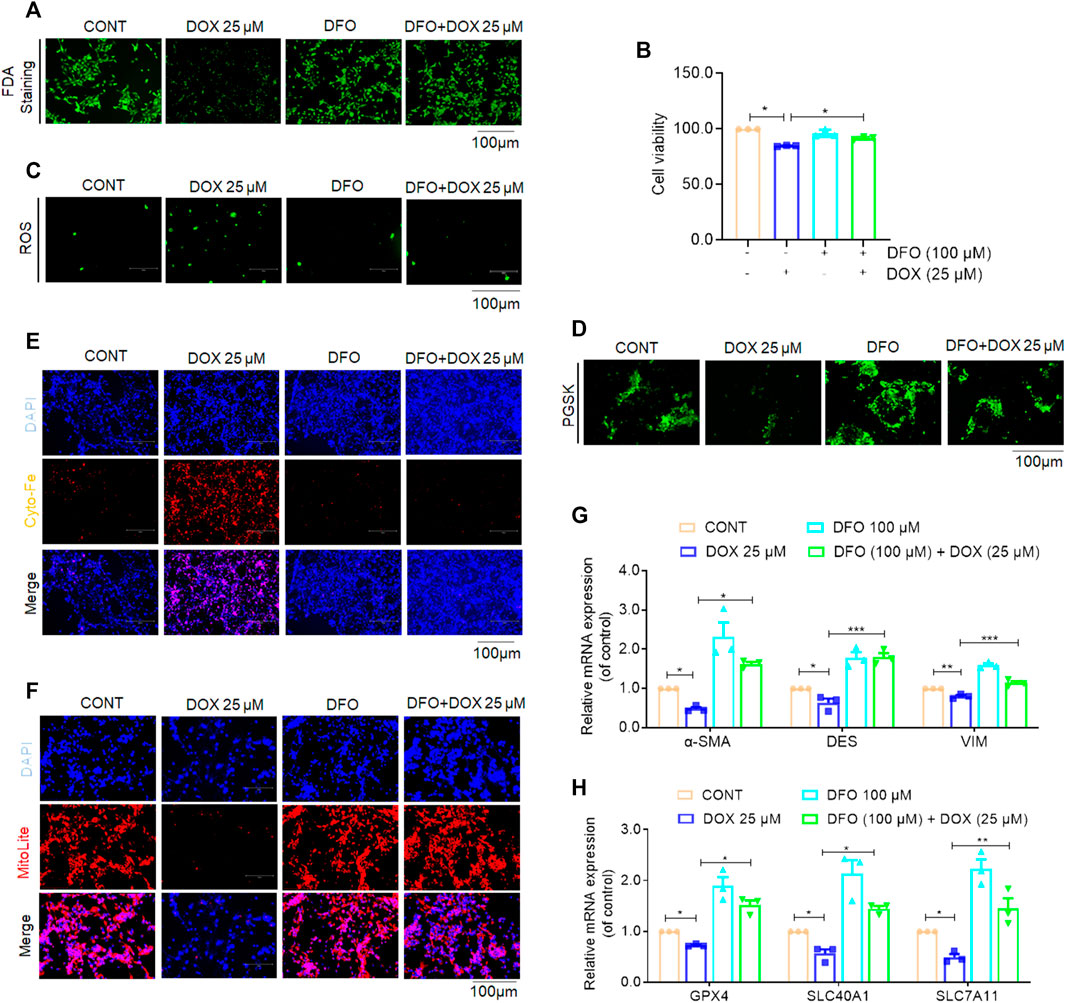
FIGURE 7. Inhibition of ferroptosis impaired DOX-induced anti-fibrosis effects in vitro. Activated LX-2 cells were exposed for 24 h to 100 μM DFO followed by 25 μM DOX treatment. (A) FDA staining for evaluating cell death. LX-2 was stained in 10 μM FDA and the samples were observed with a fluorescence inverted microscope. (B) Cell Count Kit-8 analysis of cell viability in LX-2. CCK-8 was incubated with LX-2 at 37°C for 1 h and the samples was measured using a multifunctional enzyme labeler at 405 nm. (C) The ROS level in LX-2. LX-2 was stained in 10 μM DCFH-DA in DMEM for 1 h at 37°C and the samples were observed with a fluorescence inverted microscope. (D) Free iron levels in LX-2 were measured by Phen Green SK probe. LX-2 cells were stained in 10 μM Phen Green SK in PBS for 1 h at 37°C and the samples were imaged immediately. (E) Representative fluorescent images of intracellular iron level were identified by using FerroOrange (orange). Nuclei are stained by DAPI (blue). LX-2 was stained in 1 mM Ferro orange in PBS for 1 h at 37°C and samples were imaged immediately. All samples as described (D,E) were observed with a fluorescence inverted microscope. (F) Mitochondrion staining detection. LX-2 cells were stained in the buffer containing a 1:500 dilution of MitoliteTM Red FX600 for 1 h at 37°C and the samples were observed with a fluorescence inverted microscope. (G) The mRNA expression of α-SMA, DES and VIM (n = 3). (H) The mRNA expression of GPX-4, SLC40A1 and SLC7A11 (n = 3). For the statistics of each panel in this figure, data were expressed as means ± SEM. *p < 0.05; **p < 0.01; ***p < 0.001.
Discussion
In this study, several key findings were made. First, we observed that DOX significantly attenuated hepatocellular injury and liver fibrosis in mice in the CCl4 group. Second, treatment with DOX significantly decreased the expression levels of HSC activation markers both in vitro and in vivo. Further, our results also indicated that DOX alleviated liver fibrosis by inducing ferroptosis in HSC and reducing ECM production. Thus, DOX may be useful in clinical practice as a treatment for liver fibrosis.
Liver fibrosis is a complex process, and its pathogenesis is based on increased synthesis or decreased degradation of the ECM, resulting in ECM deposition (Lee et al., 2015). HSCs normally store lipids in the liver. When the liver is damaged, they can be activated to become myofibroblasts, producing large amounts of ECM; therefore, their activation represents the pathogenesis of liver fibrosis (Tacke and Trautwein, 2015). This also implies that inducing activated HSC death is an important strategy for the treatment of liver fibrosis. Ferroptosis, a newly discovered form of programmed cell death, is significantly different from other types of programmed death processes, such as apoptosis, necrosis, and autophagy, at the morphological, biochemical, and genetic levels (Dixon et al., 2012). Several recent studies indicate that a variety of cytokines, signaling pathways (Zhang et al., 2020a; Zhang et al., 2020b; Z; Zhang et al., 2018; Zhu et al., 2021) affect the occurrence of ferroptosis and participate in liver fibrosis development. Tripartite motif-containing protein 26 (TRIM26) induces HSC ferroptosis through the regulation of solute carrier family-7 member-11 (SLC7A11) ubiquitination, thereby ameliorating liver fibrosis (Zhu et al., 2021). In addition to the above-mentioned targets for regulating ferroptosis, it has also been reported that several drugs can treat liver fibrosis by inducing ferroptosis in HSCs. Specifically, sorafenib and erastin can induce ferroptosis in HSCs to alleviate liver fibrosis (Z. Zhang et al., 2018). Magnesium isoglycyrrhizinate (MgIG) can upregulate heme oxygenase 1 (HO-1) expression, leading to intracellular iron deposition, lipid peroxide accumulation, the induction of ferroptosis in HSC, and the inhibition of liver fibrosis (Sui et al., 2018). Further, artesunate ultimately induces ferroptosis in HSCs and ameliorates liver fibrosis development by depleting glutathione (GSH), reducing glutathione peroxidase 4 (GPX4) activity, and favoring ROS accumulation (Kong et al., 2019). Interestingly, in this current study we also identified that a drug with high efficacy and low toxicity, DOX, alleviated liver fibrosis and induced ferroptosis in HSCs.
DOX has been used for patients with asthma and chronic obstructive pulmonary disease (Page, 2010). DOX increases intracellular cyclic adenosine monophosphate concentrations by inhibiting intracellular phosphodiesterase activity, and this in turn reduces the incidence of airway pressure and barometric injury in patients (Y. Zhang et al., 2016), and reportedly, DOX, via nucleotide-binding oligomerization domain, leucine-rich repeat and pyrin domain-containing 3-thioredoxin-interacting protein (NLRP3-TXNIP) inflammasome activation, mitigates epithelial inflammation (Jiao et al., 2020). Moreover, compared with aminophylline, DOX has fewer cardiovascular adverse effects, a higher safety profile, and better user experience (Rao et al., 2013). Based on these previous studies, we investigated whether DOX exerts a therapeutic effect on liver fibrosis as well as the possible mechanisms.
Studies have shown that hepatocyte injury is one of the key factors to promote the formation of liver fibrous scar (Kisseleva and Brenner, 2021). In our study showed that DOX also reduced the elevated serum ALT/AST activities in CCl4-treated mice, and the liver histological analysis showed that DOX significant reduce hepatic infiltration of immune cells, swelling and necrosis of hepatocytes in CCl4-treated mice. These evidences show that DOX protection against hepatocellular injury induced by CCl4. We observed that DOX ameliorated liver fibrosis and inhibited HSC activation both in vivo and in vitro. Additionally, our findings indicated that DOX treatment significantly impaired HSC viability via inducing ferroptosis. Primarily, Electron microscopy and mitochondrial staining provided direct evidence that DOX induced ferroptosis in HSCs. Furthermore, the ferroptosis markers iron and ROS level in HSCs increasing significantly induced by DOX, while GSH content was decreased. Meanwhile, DOX can increase the protein expression of transferrin and transferrin receptor in a dose-dependent manner; GPX4, SLC7A11, and SLC40A1, which have been recognized as critical upstream factors of ferroptosis (Pietrangelo, 2004; Gomme et al., 2005; Koppula et al., 2018; Seibt et al., 2019), was inhibited significantly by DOX. Then the inhibition of ferroptosis using the specific inhibitor, DFO not only abolished the ferroptosis-inducing effect of DOX, but also resisted its anti-fibrosis effect. These findings above indicated that the anti-fibrotic effect of DOX is closely related to the induction of ferroptosis in HSCs.
In conclusion, our findings overall indicated for the first time that DOX elicits its anti-fibrotic effects through the regulation of HSC ferroptosis (Figure 8). Additionally, DOX-only treatment of mice without liver fibrosis exhibited no significant effect on serum ALT/AST levels or liver histomorphology. This observation suggests that DOX may be useful in clinical settings as treatment of liver fibrosis. However, in this study, we did not explore the in vivo distribution of DOX; this was a limitation of this study. Therefore, the further evaluation of the in vivo metabolic profile of DOX should be realized in future studies.
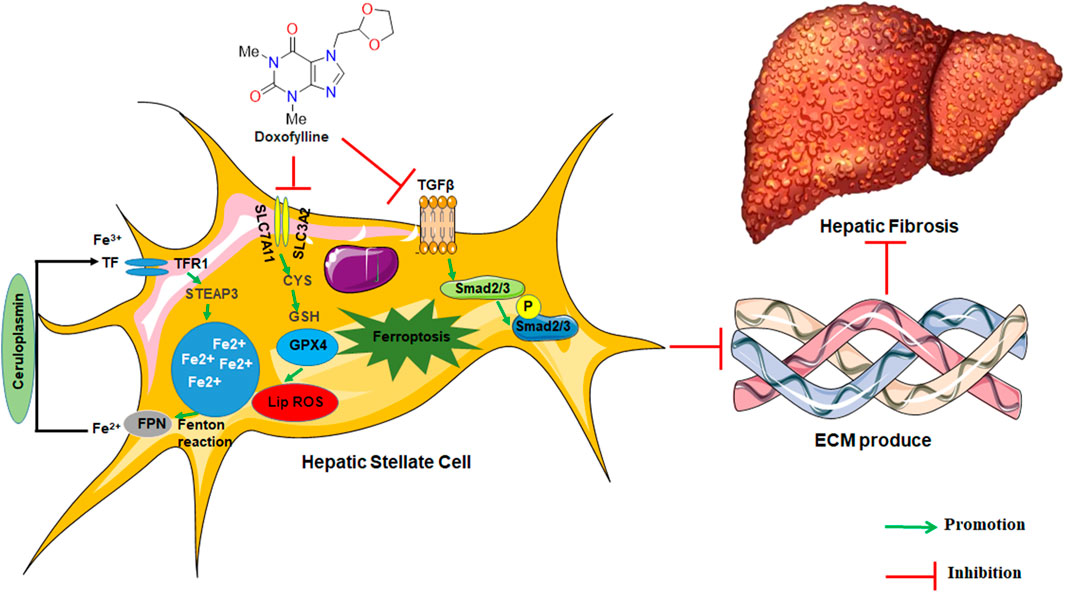
FIGURE 8. The graphic illustration of the mechanism of doxofylline protecting against liver fibrosis.
Data availability statement
The original contributions presented in the study are included in the article/Supplementary Material, further inquiries can be directed to the corresponding authors.
Ethics statement
The animal study was reviewed and approved by the Experimental Animal Ethical Committee of Hangzhou Medical College.
Author contributions
YiZ and GL contributed to the literature search and study design. LX, JP, and XX participated in the drafting of the article. LX, MZ, YWZ, XH, LY, LC, JR, JY, and YMZ carried out the experiments. GL revised the manuscript. YiZ contributed to data collection and analysis.
Funding
This study was supported by Zhejiang Provincial Natural Science Foundation of China under Grant No. LQ23H280021, the General Scientific Research Project of Department of Education of Zhejiang Province (Y202045353 to YiZ), Basic Scientific Research Project of Hangzhou Medical College (KYYB202106 to YiZ).
Conflicts of interest
The authors declare that the research was conducted in the absence of any commercial or financial relationships that could be construed as a potential conflict of interest.
Publisher’s note
All claims expressed in this article are solely those of the authors and do not necessarily represent those of their affiliated organizations, or those of the publisher, the editors and the reviewers. Any product that may be evaluated in this article, or claim that may be made by its manufacturer, is not guaranteed or endorsed by the publisher.
Supplementary material
The Supplementary Material for this article can be found online at: https://www.frontiersin.org/articles/10.3389/fphar.2023.1135366/full#supplementary-material
References
Abd Elmaaboud, M., Khattab, H., and Shalaby, S. (2021). Hepatoprotective effect of linagliptin against liver fibrosis induced by carbon tetrachloride in mice. Can. J. Physiol. Pharmacol. 99 (3), 294–302. doi:10.1139/cjpp-2020-0049
Anderson, E. R., and Shah, Y. M. (2013). Iron homeostasis in the liver. Compr. Physiol. 3 (1), 315–330. doi:10.1002/cphy.c120016
Cao, J. Y., and Dixon, S. J. (2016). Mechanisms of ferroptosis. Cell. Mol. Life. Sci. 73 (11-12), 2195–2209. doi:10.1007/s00018-016-2194-1
Chen, X., Li, J., Kang, R., Klionsky, D. J., and Tang, D. (2021). Ferroptosis: Machinery and regulation. Autophagy 17 (9), 2054–2081. doi:10.1080/15548627.2020.1810918
Dixon, S. J., Lemberg, K. M., Lamprecht, M. R., Skouta, R., Zaitsev, E. M., Gleason, C. E., et al. (2012). Ferroptosis: An iron-dependent form of nonapoptotic cell death. Cell 149 (5), 1060–1072. doi:10.1016/j.cell.2012.03.042
Gomme, P. T., McCann, K. B., and Bertolini, J. (2005). Transferrin: Structure, function and potential therapeutic actions. Drug. Discov. Today. 10 (4), 267–273. doi:10.1016/s1359-6446(04)03333-1
Greenhalgh, S. N., Conroy, K. P., and Henderson, N. C. (2015). Cre-ativity in the liver: Transgenic approaches to targeting hepatic nonparenchymal cells. Hepatology 61 (6), 2091–2099. doi:10.1002/hep.27606
Hirschhorn, T., and Stockwell, B. R. (2019). The development of the concept of ferroptosis. Free. Radic. Biol. Med. 133, 130–143. doi:10.1016/j.freeradbiomed.2018.09.043
Jiao, P., Li, W., Shen, L., Li, Y., Yu, L., and Liu, Z. (2020). The protective effect of doxofylline against lipopolysaccharides (LPS)-induced activation of NLRP3 inflammasome is mediated by SIRT1 in human pulmonary bronchial epithelial cells. Artif. Cells. Nanomed. Biotechnol. 48 (1), 687–694. doi:10.1080/21691401.2020.1730391
Kisseleva, T., and Brenner, D. (2021). Molecular and cellular mechanisms of liver fibrosis and its regression. Nat. Rev. Gastroenterol. Hepatol. 18 (3), 151–166. doi:10.1038/s41575-020-00372-7
Kong, Z., Liu, R., and Cheng, Y. (2019). Artesunate alleviates liver fibrosis by regulating ferroptosis signaling pathway. Biomed. Pharmacother. 109, 2043–2053. doi:10.1016/j.biopha.2018.11.030
Koppula, P., Zhang, Y., Zhuang, L., and Gan, B. (2018). Amino acid transporter SLC7A11/xCT at the crossroads of regulating redox homeostasis and nutrient dependency of cancer. Cancer. Commun. (Lond) 38 (1), 12. doi:10.1186/s40880-018-0288-x
Lee, Y. A., Wallace, M. C., and Friedman, S. L. (2015). Pathobiology of liver fibrosis: A translational success story. Gut 64 (5), 830–841. doi:10.1136/gutjnl-2014-306842
Li, J., Cao, F., Yin, H. L., Huang, Z. J., Lin, Z. T., Mao, N., et al. (2020). Ferroptosis: Past, present and future. Cell. death. Dis. 11 (2), 88. doi:10.1038/s41419-020-2298-2
Matera, M. G., Page, C., and Cazzola, M. (2017). Doxofylline is not just another theophylline. Int. J. Chron. Obstruct. Pulmon. Dis. 12, 3487–3493. doi:10.2147/copd.S150887
Ooko, E., Saeed, M. E., Kadioglu, O., Sarvi, S., Colak, M., Elmasaoudi, K., et al. (2015). Artemisinin derivatives induce iron-dependent cell death (ferroptosis) in tumor cells. Phytomedicine 22 (11), 1045–1054. doi:10.1016/j.phymed.2015.08.002
Page, C. P. (2010). Doxofylline: A "novofylline. Pulm. Pharmacol. Ther. 23 (4), 231–234. doi:10.1016/j.pupt.2010.04.002
Pietrangelo, A. (2004). The ferroportin disease. Blood. Cells. Mol. Dis. 32 (1), 131–138. doi:10.1016/j.bcmd.2003.08.003
Puche, J. E., Saiman, Y., and Friedman, S. L. (2013). Hepatic stellate cells and liver fibrosis. Compr. Physiol. 3 (4), 1473–1492. doi:10.1002/cphy.c120035
Rao, R. N., Prasad, K. G., Naidu Ch, G., Saida, S., and Agwane, S. B. (2013). Development of a validated LC-MS/MS method for determination of doxofylline on rat dried blood spots and urine: Application to pharmacokinetics. J. Pharm. Biomed. Anal. 78-79, 211–216. doi:10.1016/j.jpba.2013.02.017
Seibt, T. M., Proneth, B., and Conrad, M. (2019). Role of GPX4 in ferroptosis and its pharmacological implication. Free. Radic. Biol. Med. 133, 144–152. doi:10.1016/j.freeradbiomed.2018.09.014
Sui, M., Jiang, X., Chen, J., Yang, H., and Zhu, Y. (2018). Magnesium isoglycyrrhizinate ameliorates liver fibrosis and hepatic stellate cell activation by regulating ferroptosis signaling pathway. Biomed. Pharmacother. 106, 125–133. doi:10.1016/j.biopha.2018.06.060
Tacke, F., and Trautwein, C. (2015). Mechanisms of liver fibrosis resolution. J. Hepatol. 63 (4), 1038–1039. doi:10.1016/j.jhep.2015.03.039
Wang, H., An, P., Xie, E., Wu, Q., Fang, X., Gao, H., et al. (2017). Characterization of ferroptosis in murine models of hemochromatosis. Hepatology 66 (2), 449–465. doi:10.1002/hep.29117
Wu, X., Zhang, F., Xiong, X., Lu, C., Lian, N., Lu, Y., et al. (2015). Tetramethylpyrazine reduces inflammation in liver fibrosis and inhibits inflammatory cytokine expression in hepatic stellate cells by modulating NLRP3 inflammasome pathway. IUBMB. Life. 67 (4), 312–321. doi:10.1002/iub.1348
Xu, L., Hui, A. Y., Albanis, E., Arthur, M. J., O'Byrne, S. M., Blaner, W. S., et al. (2005). Human hepatic stellate cell lines, LX-1 and LX-2: New tools for analysis of hepatic fibrosis. Gut 54 (1), 142–151. doi:10.1136/gut.2004.042127
Yang, C., Zeisberg, M., Mosterman, B., Sudhakar, A., Yerramalla, U., Holthaus, K., et al. (2003). Liver fibrosis: Insights into migration of hepatic stellate cells in response to extracellular matrix and growth factors. Gastroenterology 124 (1), 147–159. doi:10.1053/gast.2003.50012
Zhang, Y., Zeng, K., Wang, J., Gao, H., Nan, Y., and Zheng, X. (2016). Identifying the antiasthmatic target of doxofylline using immobilized β2 -adrenoceptor based high-performance affinity chromatography and site-directed molecular docking. J. Mol. Recognit. 29 (10), 492–498. doi:10.1002/jmr.2549
Zhang, Z., Guo, M., Li, Y., Shen, M., Kong, D., Shao, J., et al. (2020a). RNA-binding protein ZFP36/TTP protects against ferroptosis by regulating autophagy signaling pathway in hepatic stellate cells. Autophagy 16 (8), 1482–1505. doi:10.1080/15548627.2019.1687985
Zhang, Z., Guo, M., Shen, M., Kong, D., Zhang, F., Shao, J., et al. (2020b). The BRD7-P53-SLC25A28 axis regulates ferroptosis in hepatic stellate cells. Redox. Biol. 36, 101619. doi:10.1016/j.redox.2020.101619
Zhang, Z., Yao, Z., Wang, L., Ding, H., Shao, J., Chen, A., et al. (2018). Activation of ferritinophagy is required for the RNA-binding protein ELAVL1/HuR to regulate ferroptosis in hepatic stellate cells. Autophagy 14 (12), 2083–2103. doi:10.1080/15548627.2018.1503146
Zhu, Y., Zhang, C., Huang, M., Lin, J., Fan, X., and Ni, T. (2021). TRIM26 induces ferroptosis to inhibit hepatic stellate cell activation and mitigate liver fibrosis through mediating SLC7A11 ubiquitination. Front. Cell. Dev. Biol. 9, 644901. doi:10.3389/fcell.2021.644901
Glossary
ALT/AST Alanine/Aspartate aminotransferase
ANOVA Analysis of variance
α-SMA α-Smooth muscle actin
BSA Bovine serum albumin
CCK-8 Cell counting Kit-8
CCl4 Carbon tetrachloride
cDNA Complementary deoxyribonucleic acid
DDR2 Discoidin domain receptor 2
DAB,3 3-Diaminobenzidine tetrahydrochloride
DAPI 4′, 6-diamidino-2-phenylindole
DCFH-DA Dichlorodihydrofluorescein diacetate
DES Desmin
DFO deferoxamine
DMEM Dulbecco’s modified eagle medium
DOX Doxorubicin
ECM Extracellular matrix
FBS Fetal bovine serum
FDA Fluorescein diacetate
FITC Fluorescein isothiocyanate
GAPDH Glyceraldehyde-3-phosphate dehydrogenase
GPX4 Glutathione peroxidase 4
GSH glutathione
H&E Hematoxylin-eosin
HO-1 Heme oxygenase 1
NLRP3-TXNIP Nucleotide-binding oligomerization domain, leucine—rich repeat and pyrin domain-containing 3—thioredoxin-interacting protein
HSC Hepatic stellate cell
LSD Least significant difference
MMP-2 Matrix metalloproteinase
MgIG Magnesium isoglycyrrhizinate
Ob-RL Obese receptor long form
PDGF-R Platelet derived growth factor receptor β
PUFA Peroxidizing polyunsaturated fatty acid
PBS Phosphate buffer saline
PDE Phosphodiesterase
PGSK Phen Green SK diacetate
RNA ribonucleic acid
ROS Reactive oxygen species
SEM Standard error of the mean
SIL Silymarin
SLC7A11 Solute carrier family-7 member-11
SLC40A1 Solute carrier family 40 member 1
Smad 2/3 SMAD family member 2/3
TIMP-2 Matrix metalloproteinase-2
TEM Transmission electron microscope
TGF-β Transforming growth factor-beta
VIM Vimentin
Keywords: liver fibrosis, doxofylline, hepatic stellate cells, ferroptosis, deferoxamine (DFO)
Citation: Xu L, Zhang M, Pan J, Xu X, Zhang Y, Han X, Yin L, Chen L, Ren J, Yu J, Zhang Y, Liang G and Zhang Y (2023) Doxofylline ameliorates liver fibrosis by regulating the ferroptosis signaling pathway. Front. Pharmacol. 14:1135366. doi: 10.3389/fphar.2023.1135366
Received: 31 December 2022; Accepted: 08 March 2023;
Published: 17 March 2023.
Edited by:
Xiaoying Yang, Xuzhou Medical University, ChinaReviewed by:
Prasanna K. Santhekadur, JSS Academy of Higher Education and Research, IndiaGaetano Cairo, University of Milan, Italy
Copyright © 2023 Xu, Zhang, Pan, Xu, Zhang, Han, Yin, Chen, Ren, Yu, Zhang, Liang and Zhang. This is an open-access article distributed under the terms of the Creative Commons Attribution License (CC BY). The use, distribution or reproduction in other forums is permitted, provided the original author(s) and the copyright owner(s) are credited and that the original publication in this journal is cited, in accordance with accepted academic practice. No use, distribution or reproduction is permitted which does not comply with these terms.
*Correspondence: Guang Liang, wzmcliangguang@163.com; Yi Zhang, 1020437493@qq.com