- 1Department of Respiratory and Critical Care Medicine, Aerospace Center Hospital, Peking University Aerospace School of Clinical Medicine, Beijing, China
- 2Department of Pathogen Biology, School of Basic Medical Sciences, Tianjin Medical University, Tianjin, China
- 3Department of Pharmaceutical Sciences, College of Pharmacy and Health Sciences, St. John’s University, Queens, NY, United States
- 4Department of Pharmacy, Aerospace Center Hospital, Peking University Aerospace School of Clinical Medicine, Beijing, China
- 5Beijing Key Laboratory of Drug Target and Screening Research, Institute of Materia Medica, Chinese Academy of Medical Sciences and Peking Union Medical College, Beijing, China
- 6National Clinical Research Center for Cancer, Tianjin’s Clinical Research Center for Cancer, Key Laboratory of Cancer Prevention and Therapy, Tianjin Medical University Cancer Institute and Hospital, Tianjin, China
Lung cancer is the leading cause of global cancer-related deaths. Platinum-based chemotherapy is the first-line treatment for the most common type of lung cancer, i.e., non-small-cell lung cancer (NSCLC), but its therapeutic efficiency is limited by chemotherapeutic resistance. Therefore, it is vital to develop effective therapeutic modalities that bypass the common molecular mechanisms associated with chemotherapeutic resistance. Ferroptosis is a form of non-apoptotic regulated cell death characterized by iron-dependent lipid peroxidation (LPO). Ferroptosis is crucial for the proper therapeutic efficacy of lung cancer-associated chemotherapies. If targeted as a novel therapeutic mechanism, ferroptosis modulators present new opportunities for increasing the therapeutic efficacy of lung cancer chemotherapy. Emerging studies have revealed that the pharmacological induction of ferroptosis using natural compounds boosts the efficacy of chemotherapy in lung cancer or drug-resistant cancer. In this review, we first discuss chemotherapeutic resistance (or chemoresistance) in lung cancer and introduce the core mechanisms behind ferroptosis. Then, we comprehensively summarize the small-molecule compounds sourced from traditional medicines that may boost the anti-tumor activity of current chemotherapeutic agents and overcome chemotherapeutic resistance in NSCLC. Cumulatively, we suggest that traditional medicines with ferroptosis-related anticancer activity could serve as a starting point to overcome chemotherapeutic resistance in NSCLC by inducing ferroptosis, highlighting new potential therapeutic regimens used to overcome chemoresistance in NSCLC.
1 Introduction
Lung cancer is broadly classified into two types: small-cell lung cancer (SCLC) and non-small-cell lung cancer (NSCLC). SCLC and NSCLC comprise >85% of all cases, are highly prevalent, and are very aggressive, with an estimated 2.2 million new cases and 1.8 million deaths in 2020 (Leiter et al., 2023). Globally, lung cancer is the second leading cause of cancer death after breast cancer in women and is the leading cause of cancer mortality in men (Sung et al., 2021).
Although multiple approaches including surgery, immunotherapy, targeted therapy, and radiotherapy are recommended for NSCLC patients, systemic chemotherapy is still the mainstay regimen for NSCLC, especially for advanced-stage patients. Platinum-based chemotherapy is recommended as the standard first-line regimen for patients with advanced NSCLC and is also prescribed for patients at earlier stages (Nagasaka and Gadgeel, 2018). Platinum-based chemotherapy is frequently combined with gemcitabine, pemetrexed, and vinorelbine or taxanes as first-line therapeutic regimens for NSCLC. However, the therapeutic efficacy of this regimen varies remarkably among individuals and is limited by chemoresistance (Yin et al., 2016). Therefore, understanding the novel molecular mechanism behind chemoresistance in lung cancer will be vital to develop effective therapies (Herbst et al., 2018; Lim and Ma, 2019).
Ferroptosis, a new form of non-apoptotic regulated cell death (RCD) characterized by iron-dependent lipid peroxidation (LPO), is suggested to play a vital role in anti-tumor activity (Dixon et al., 2012; Lei et al., 2021; Wang et al., 2023; Wang et al., 2023). Emerging evidence has revealed that the induction of ferroptosis by ferroptosis-related small-molecule compounds suppresses tumor growth (Yin et al., 2022; Xing et al., 2023). Ferroptosis-inducing bioactive compounds could exert anti-tumor activity by inducing ferroptosis, boosting the intrinsic anti-tumor activity of chemotherapeutic agents, or altogether surmounting existing chemoresistance in lung cancer (Tabnak et al., 2021; Wu et al., 2021; Yin et al., 2022; Yin et al., 2022; Koeberle et al., 2023; Xing et al., 2023).
Recent publications have discovered that using traditional medicines to pharmacologically induce ferroptosis holds great therapeutic potential by either boosting the efficacy of chemotherapy or overcoming chemoresistance in NSCLC. In this review, we first introduce the role of chemoresistance in lung cancer and then discuss the core mechanisms of ferroptosis. We then comprehensively summarize small-molecule compounds from traditional medicines that may boost the anti-tumor activity of chemotherapeutic agents or overcome chemotherapy drug resistance in NSCLC. Cumulatively, we suggest that the pharmacological induction of ferroptosis by traditional medicines with ferroptosis-related anticancer activity could overcome chemotherapy resistance in NSCLC, potentially producing therapeutic regimens that may overcome chemoresistance in NSCLC.
2 Chemoresistance in lung cancer
Chemotherapy remains a dominant treatment cornerstone for many types of cancers at different stages (El-Hussein et al., 2021). Conventional chemotherapy remains a cornerstone in the treatment of patients with NSCLC, especially those with advanced-stage disease (Min and Lee, 2021). Platinum-based chemotherapy is still the standard treatment option and mainstay regimen for patients with SCLC (Herzog et al., 2021). However, the development of chemoresistance, i.e., resistance to chemotherapeutic agents, poses a significant challenge and obstacle to the treatment efficiency of patients with NSCLC (Min and Lee, 2021). Although most patients with SCLC initially have a good response to platinum-based chemotherapy, most patients develop chemoresistance within 1 year (Jin et al., 2023), making chemoresistance almost a universal driving factor behind patient mortality (Herzog et al., 2021). Therefore, it is necessary to understand the mechanisms underlying chemoresistance to develop efficacious chemotherapeutic approaches for lung cancer.
3 Core mechanisms of ferroptosis
Ferroptosis is a new form of RCD characterized by the iron-dependent oxidative modification of phospholipid membranes (Dixon et al., 2012; Stockwell, 2022; Yin et al., 2022; Gu et al., 2023; Huo et al., 2023) (Figure 1). Ferroptosis reflects an imbalance between ferroptosis defense systems and promoting factors (Lei et al., 2022). When the latter overrides the former, lethal lipid peroxides accumulate on cellular membranes, leading to membrane rupture and cell death (Hadian and Stockwell, 2020; Chen et al., 2021; Lei et al., 2022).
3.1 Ferroptosis prerequisites
3.1.1 Iron homeostasis
Iron functions as a cofactor for iron-dependent enzymes, i.e., arachidonate lipoxygenases (ALOXs) and cytochrome P450 oxidoreductase (POR), or catalyzes the Fenton reaction to promote LPO during the process of ferroptosis (Lei et al., 2022). The overproduction of ROS and reactive nitrogen species (RNS) can directly damage lipid membranes. In an iron-catalyzed process, ROS (such as LO• or HO•) can react with polyunsaturated fatty acid (PUFA)-containing phospholipids (PUFA-PLs) to produce lipid hydroperoxides through the Fenton reaction. The Fenton reaction is an Fe2+-catalyzed reaction that converts hydrogen peroxide (H2O2) to toxic HO•, triggering LPO (Ryter et al., 2007; Dos Santos et al., 2023). In the enzymatic LPO pathway, Fe2+ promotes the activity of iron-dependent peroxidases, in which LOXs initiate the dioxygenation of the membrane PUFA-PLs (Chen et al., 2020; David et al., 2022).
3.1.2 Lipid peroxidation
PUFA-PLs are the substrates for LPO during ferroptosis (Hadian and Stockwell, 2020). There are two pathways for LPO, the non-enzymatic and enzymatic LPO pathways (Hassannia et al., 2019; Chen et al., 2021; Liang et al., 2022). The non-enzymatic LPO pathway is a radical-driven chain reaction-dependent auto-oxidation of lipids, in which ROS initiate PUFA oxidation. The hydroxyl radical (OH·), a highly mobile water-soluble form of ROS produced from Fenton reactions, is involved in initiating LPO (Ayala et al., 2014). One OH· first abstracts a hydrogen radical from a PUFA to produce a lipid radical (L•), which rapidly reacts with molecular oxygen (O2) to yield a lipid peroxyl radical (LOO•). LOO• subsequently abstracts a hydrogen radical from an adjacent PUFA, producing lipid hydroperoxide (LOOH). In the presence of ferrous iron, LOOH is converted to an alkoxyl radical (LO•), which subsequently reacts with an adjacent PUFA to initiate another lipid radical chain reaction. When the ferroptosis defense systems that keep LPO in check fail, this iron- and oxygen-catalyzed oxidation process can lead to membrane destruction and cell death (Hassannia et al., 2019).
Enzymatic LPO is mediated by the activity of ALOXs and POR in a controlled manner. Iron initiates the Fenton reaction by functioning as an essential cofactor for ALOXs and POR. In enzymatic processes, acyl-coenzyme A synthetase long-chain family member 4 (ACSL4) catalyzes the generation of PUFA-CoAs by ligating free PUFAs with CoA to form phospholipids (Dixon et al., 2015; Doll et al., 2017). Then, acyl groups are inserted into lysophospholipids by lysophosphatidylcholine acyltransferase 3 (LPCAT3), which incorporates free PUFAs into phospholipids (PLs) to generate PUFA-PLs (Dixon et al., 2015; Kagan et al., 2017). The incorporated PUFA-PLs are then peroxidated by PORs and ALOXs by labile iron and O2 to generate PUFA-PL hydroperoxides (PUFA-PL-OOH) or peroxidated PUFA-PLs (Hadian and Stockwell, 2020; Zou et al., 2020). Malondialdehyde (MDA) and 4-hydroxynonenal (4-HNE) are the two secondary products of LPO activity, leading to the formation of membrane pores and, from such cell death, ferroptosis (Tang and Kroemer, 2020).
3.2 Ferroptosis defense mechanisms
Cellular antioxidant systems constitute the ferroptosis defense systems, which directly neutralize lipid peroxides (Gu et al., 2023). Five major ferroptosis defense systems exist with specific subcellular localizations.
3.2.1 SLC7A11-GSH-GPX4 axis
The solute carrier family 7 member 11-reduced glutathione (GSH)–glutathione–glutathione peroxidase 4 (SLC7A11-GSH-GPX4) axis is the first well-defined ferroptosis defense system discovered (Lei et al., 2022; Sun et al., 2022). As such, GPX4 has been identified as a key inhibitor of ferroptosis (Dixon et al., 2012; Friedmann Angeli et al., 2014; Yang et al., 2014; Ingold et al., 2018; Forcina and Dixon, 2019). GPX4 is a lipid repair enzyme (Brigelius-Flohé and Maiorino, 2013; Brigelius-Flohé and Flohé, 2020), which converts LOOH to non-toxic PL alcohols, concomitantly oxidizing two reduced GSHs into an oxidized glutathione (GSSG) (Ursini et al., 1982; Seibt et al., 2019). Solute carrier family 3 member 2 (SLC3A2), also known as system Xc−(Sato et al., 1999; Koppula et al., 2021), and SLC7A11, also known as xCT, mediate antiporter activity by which intracellular glutamate is exported and extracellular cystine is imported (Sato et al., 1999; Koppula et al., 2018). Cytosolic NADPH is then used to reduce cystine into cysteine, which functions as the precursor for GSH, the cofactor required for the GPX4-induced detoxification of LPO (Koppula et al., 2021).
3.2.2 FSP1-CoQH2 system
Ubiquinone (coenzyme Q10 or CoQ10), a component of mitochondria and diverse membranes, works as a second endogenous mechanism to inhibit LPO and ferroptosis. Ferroptosis suppressor protein 1 (FSP1) localizes to the plasma membrane and was first discovered to operate independently of GPX4 to halt ferroptosis (Bersuker et al., 2019; Doll et al., 2019), which reduces ubiquinone CoQ10 to regenerate CoQ10-H2(CoQ10 ubiquinol), acting as a NAD(P)H-dependent oxidoreductase. This traps LOO•, thereby suppressing ferroptosis by inhibiting LPO. FSP1 halts ferroptosis by repairing damage to the plasma membrane and by activating the endosomal sorting complex required for transport III (ESCRT-III) complex (Dai et al., 2020; Pedrera et al., 2021).
3.2.3 GCH1-BH4 system
The GTP cyclohydrolase 1 (GCH1)–tetrahydrobiopterin (BH4) system is identified as the second suppressor of ferroptosis independent of GPX4 (Kraft et al., 2020; Soula et al., 2020). GCH1 mediates the production of the radical-trapping antioxidant BH4, which functions as a cofactor for aromatic amino acid hydroxylases (Kraft et al., 2020; Soula et al., 2020).
3.2.4 DHODH-CoQH2 system
The dihydroorotate dehydrogenase (DHODH)–dihydroubiquione (CoQH2) system is the third ferroptosis defense system independent of GPX4, which detoxifies mitochondrial lipid peroxides compensating for GPX4 loss (Mao et al., 2021). In the inner mitochondrial membrane, DHODH, originally discovered to be involved in pyrimidine synthesis, reduces CoQ10 to CoQH2, thereby reducing mitochondrial CoQ10, analogous to the function of FSP1 in the extramitochondrial membranes (Mao et al., 2021). Once GPX4 is acutely inactivated, DHODH-mediated flux is significantly increased to promote the generation of CoQH2, which neutralizes LPO and halts ferroptosis that originates from the mitochondria (Mao et al., 2021).
3.2.5 MBOAT1/2-MUFA system
The MBOAT1/2-PE-MUFA system is a newly identified ferroptosis defense system independent of GPX4 and FSP1, discovered by Jiang et al. In the MBOAT1/2-PE-MUFA system, new phospholipid-modifying enzymes O-acyltransferase domain-containing 1 (MBOAT1) and O-acyltransferase domain-containing 2 (MBOAT2) work as ferroptosis suppressors (Liang et al., 2023). PE-PUFA is the preferred substrate for PL peroxidation, dictating ferroptosis sensitivity (Doll et al., 2017; Kagan et al., 2017). As a lyso-PL acyltransferase (LPLAT), the membrane-bound MBOAT2 selectively transfers monounsaturated fatty acids (MUFAs) into lyso-phosphatidylethanolamine (lyso-PE), thereby decreasing cellular PE-PUFA and increasing cellular PE-MUFA, eventually inhibiting ferroptosis. The estrogen receptor (ER) and androgen receptor (AR) directly transcriptionally upregulate MBOAT1 and MBOAT2, respectively. Meanwhile, the ER or AR antagonist boosts the anti-tumor activity of ferroptosis inducers in AR+ prostate cancer and ER+ breast cancer, even in tumors with drug resistance.
3.2.6 SC5D-7-DHC axis
The lathosterol oxidase (SC5D)–7-dehydrocholesterol (7-DHC) axis is a newly identified inhibitor of ferroptosis, discovered by Freitas et al. (2024), Li et al. (2024), Freitas et al. (2024), Li et al. (2024), and Li et al. (2024), who both reported a previously unknown natural inhibitor of ferroptosis, i.e., 7-DHC. Synthesized in the endoplasmic reticulum, 7-DHC is found on the cell membrane and mitochondria. It is generated in the cholesterol synthesis pathway, which includes the intermediates of zymosterol/lathosterol and the enzymes EBP, SC5D, and DHCR7. When radicals attack phospholipids, the lipid is oxidized, and it fragments. Here, 7-DHC absorbs radicals and inhibits lipid peroxidation in both the plasma membrane and mitochondria by diverting the peroxidation pathway from phospholipids, thus mitigating ferroptosis.
4 Reversing chemotherapy resistance by inducing ferroptosis in NSCLC
New reports suggest that small-molecule drugs may function as ferroptosis-inducing bioactive compounds, enhancing chemotoxicity toward cancers (Yin et al., 2022; Xing et al., 2023; Li et al., 2024). Small-molecule drugs are organic compounds that impact cellular activity, which, due to their low molecular weight, can provide high cellular permeability. Small-molecule drugs are generally derived from two major practices: isolation from natural products or rational design to target proteins with a known function (Ibarrola-Villava et al., 2018; Niu et al., 2023). Emerging ferroptosis-inducing bioactive compounds (Figure 2) could boost the anti-tumor activity of ferroptosis induced by chemotherapeutic agents, overcoming chemotherapeutic drug resistance in NSCLC (Figure 3). Table 1 lists some natural compounds that induce ferroptosis to overcome chemoresistance in NSCLC.
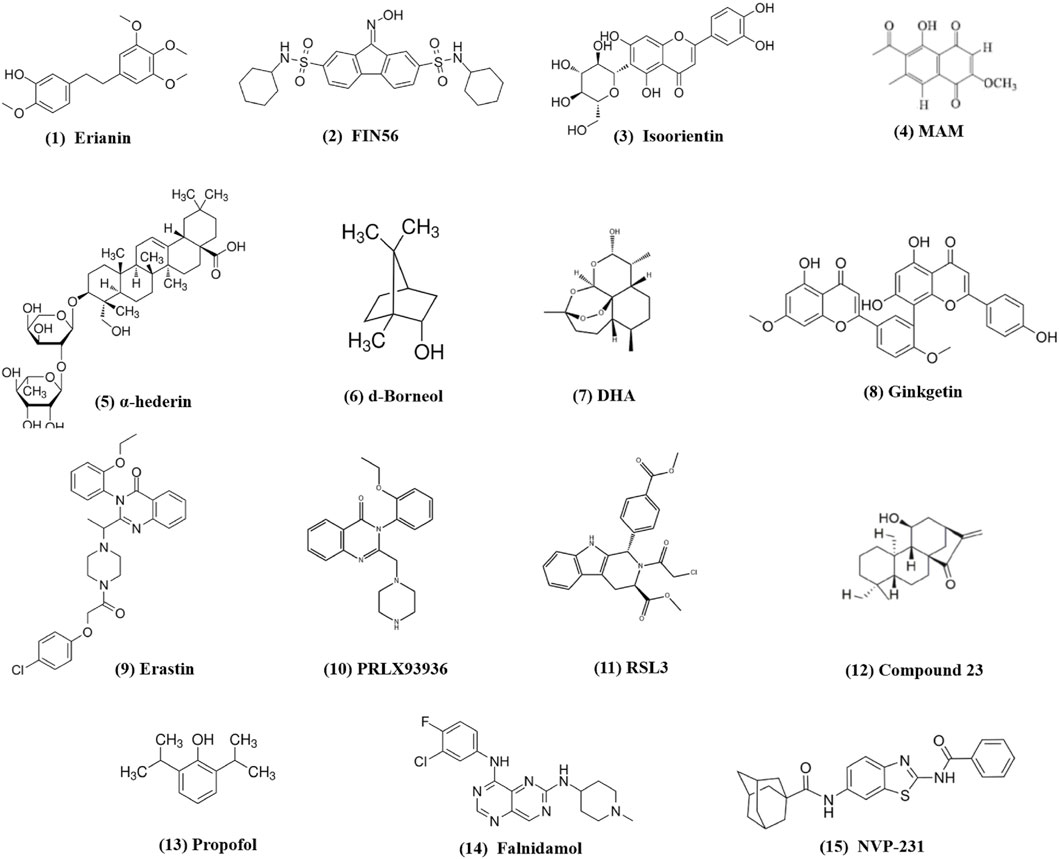
Figure 2. Chemical structures of small-molecule compounds from traditional medicines inducing ferroptosis in lung cancer.
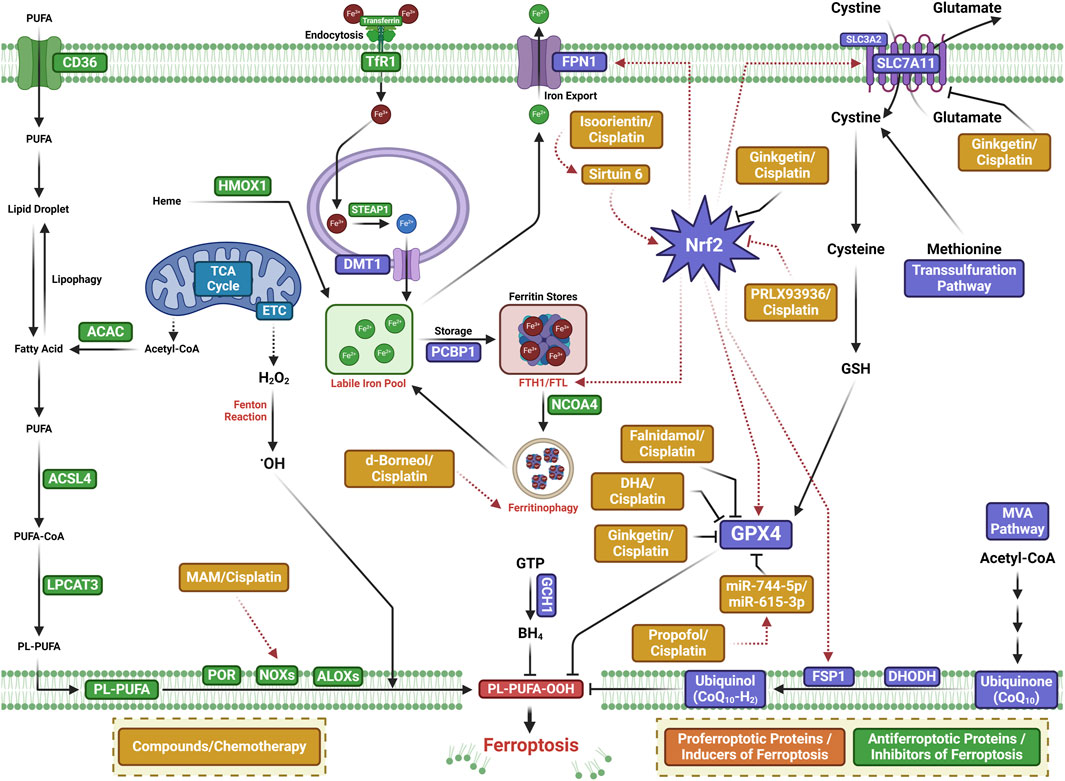
Figure 3. Mechanisms of ferroptosis-inducing small-molecule compounds that may boost the anti-tumor activity of chemotherapeutic agents or overcome chemotherapy drug resistance in lung cancer.
4.1 Reversing chemotherapeutic resistance in lung cancer—inducing ferroptosis with natural products
One of the major active components of Dendrobii caulis and phytoestrogen is erianin, which has anti-tumor, anti-diabetic retinopathy, anti-inflammatory, antibacterial, and anti-psoriasis effects (Li et al., 2023). Erianin significantly attenuates lung cancer stemness and enhances sensitivity of lung cancer cells to 5-FU (Lin et al., 2020). The ferroptosis inhibitor Fer-1 attenuates the erianin-mediated inhibition of sphere formation in lung cancer cells, suggesting that erianin inhibits lung cancer stemness by facilitating ferroptosis (Lin et al., 2020).
Isoorientin is a natural C-glucosyl flavone that has multiple pharmacological activities, including anti-inflammatory, robust antioxidant, and anti-tumor activities (Li et al., 2020; Xu et al., 2020; Ziqubu et al., 2020; Liu et al., 2021; Cui et al., 2023). Previous experiments have shown that it promotes apoptosis by the ROS-mediated MAPK/STAT3/NF-κB signaling pathway in A549 lung cancer cells (Xu et al., 2020). Further study has revealed that isoorientin overcomes drug resistance by inducing ferroptosis via the sirtuin 6 (SIRT6)/nuclear factor-erythroid factor 2-related factor 2 (Nrf2)/GPX4 signaling pathway in lung cancer (Feng et al., 2023). Isoorientin boosts the anti-tumor activity of cisplatin, as evidenced by significantly decreasing the viability of drug-resistant cells, a notable decrease in glutathione concentration, and a substantial increase in intracellular iron, MDA, and ROS production in vitro and in vivo. Mechanistically, isoorientin overcomes drug resistance by downregulating SIRT6/Nrf2/GPX4 in lung cancer cells (Feng et al., 2023).
As a natural bioactive juglone derivative, 2-methoxy-6-acetyl-7-methyljuglone (2-methoxystypandrone, MAM) has anticancer, anti-inflammatory, antimicrobial, antioxidant, and anti-HIV properties (Khalil et al., 2022). MAM inhibits cancer progression by promoting apoptosis, necroptosis, and deregulation signaling pathways in colon cancer cells, glioblastoma, lung cancer, and breast cancer (Sun et al., 2016; Sun et al., 2017; Sun et al., 2019; Yu et al., 2020). Anticancer activity against lung cancer was corroborated by other studies, which reported that MAM induces significant cell death in cisplatin- and AZD9291-resistant lung cancer cells, being completely reversed by NQO1 siRNA, NQO1 inhibitors, or iron chelators (Yu et al., 2023). Mechanistically, MAM triggers ROS generation by binding to activate NQO1, increasing LIP and LPO. MAM suppresses tumor growth in a tumor xenograft zebrafish model. These studies suggest that MAM induces ferroptosis by activating NQO1 in drug-resistant NSCLC cells, highlighting a novel therapeutic regimen to overcome drug resistance via inducing NQO1-mediated ferroptosis in NSCLC (Yu et al., 2023).
As a natural bioactive molecule very abundant in aromatic and medicinal plants (AMPs), α-hederin has various pharmacological activities, particularly anticancer activity in several cancers including colorectal, lung, esophageal, breast, hepatic, colon, ovarian, and gastric cancers (Belmehdi et al., 2023). Recent experiments substantiate previous indications that α-hederin has anticancer activity against lung cancer (Wu et al., 2022). α-Hederin inhibited cancer cell proliferation, invasion, and migration in NSCLC in vitro and in vivo. α-Hederin increases the sensitivity of NSCLC cells to cisplatin by promoting ferroptosis and apoptosis (Wu et al., 2022).
The natural borneol obtained from the fresh branches and leaves of Cinnamomum camphora (L.), J. Presl d-borneol has refreshing and awakening effects and is usually used for treating cerebrovascular and cardiovascular diseases. Borneol has anti-inflammatory, penetration-promoting, and sedative, analgesic, and antibacterial properties. Borneol can also boost the anti-tumor effects of chemotherapeutic drugs in NSCLC, human esophageal squamous cell carcinoma, gliomas, and hepatocellular carcinoma (Chen et al., 2015; Meng et al., 2018; Cao et al., 2019; Li et al., 2022). Further study has revealed that d-borneol exerts anticancer activity in cisplatin-resistant NSCLC cells by inducing ferroptosis (Li et al., 2022). d-Borneol enhances tumor-inhibiting effects of cisplatin by promoting ferroptosis, as evidenced by the increased production of ROS and MDA and decreased expression of GSH, Trx, SOD, and heme oxygenase-1. Mechanistically, the combination of d-borneol and cisplatin induces ferroptosis by facilitating nuclear receptor coactivator 4 (NCOA4)-mediated ferritinophagy and modulating intracellular iron ion transport via decreasing PCBP2 and increasing PRNP (Li et al., 2022).
Dihydroartemisinin (DHA), an active derivative of artemisinin originally developed in China, is the first-line treatment for malaria (Dai et al., 2021). DHA has anticancer activity by boosting the efficacy of chemotherapy, targeted therapy, and even radiotherapy in a wide range of cancer types (Li et al., 2021). Recent studies have suggested that DHA boosts the efficacy of targeted therapy and immunotherapy by inducing ferroptosis in lung cancer cells (Li et al., 2022; Han et al., 2023; Lai et al., 2023). DHA facilitates chlorin e6-induced photodynamic therapy by inducing ferroptosis, inhibiting GPX4, and enhancing ROS in lung cancer cells (Han et al., 2022).
Ginkgetin (GK) is a natural biflavone with anticancer, anti-inflammatory, antimicrobial, anti-adipogenic, and neuroprotective activities (Adnan et al., 2020). GK has anticancer activities in a wide range of cancer types including lung cancer (Lou et al., 2017; Ho et al., 2018; Liu et al., 2022; Wu et al., 2023). In EGFR wild-type NSCLC, GK facilitates the therapeutic effect of cisplatin by inducing ferroptosis and downregulating Nrf2/HO-1 (Lou et al., 2021).
Compound 23, i.e., 11β-Hydroxy-ent-16-kaurene-15-one, is one of the ent-kaurane diterpenoids from Chinese liverworts Jungermannia tetragona Lindenb and has strong anti-tumor activity in several cancer cell lines. Compound 23 induces both apoptosis and ferroptosis by increasing cellular ROS levels in HepG2 cells. Compound 23 increases the sensitivity of cisplatin-resistant A549/CDDP cancer cells by inducing ferroptosis and apoptosis, suggesting that ent-kaurane derivatives overcome chemoresistance to cisplatin by inducing ferroptosis (Sun et al., 2021).
4.2 Reversing chemotherapy resistance through inducing ferroptosis by small-molecular drugs in lung cancer
The utilization of ferroptosis-modulating small molecules or compounds is a new novel strategy to enhance chemotherapy outcomes (Yin et al., 2022), potentially acting as a vector to treat chemotherapeutic resistance (Koeberle et al., 2023). Functioning as a type 3 ferroptosis inducer, the ferroptosis-inducing agent 56 (FIN56) promotes ferroptosis by facilitating the autophagy-dependent protein degradation of GPX4 (Shimada et al., 2016; Sun et al., 2021). FIN56 combined with cisplatin increases cellular ROS levels, decreases antioxidant gene expression, and boosts the cisplatin cytotoxic effect in the A549 cell line, indicating that inducing ferroptosis is a promising strategy in cisplatin-resistant cancer cells (Golbashirzadeh et al., 2023).
Initially identified as a small-molecule compound that selectively kills tumor cells, erastin is an inducer of ferroptosis by modulating system XC−, p53, and the voltage-dependent anion channel (VDAC). Erastin can increase tumor sensitivity to chemotherapy and radiotherapy, highlighting a promising potential in cancer therapy (Zhao et al., 2020). Erastin and sorafenib induce ferroptosis in CDDP-resistant N5CP NSCLC cells, as evidenced by the accumulation of intracellular lipid ROS. Erastin and sorafenib, alone or in combination with CDDP, inhibit the growth of N5CP cells in vivo (Li et al., 2020).
An analog of erastin, PRLX93936, has demonstrated synergistic effects against NSCLC cells. The combination of PRLX93936 and cisplatin induces ferroptosis, as evidenced by the increased production of ROS, LPO, and Fe2+. Mechanistically, the cotreatment of PRLX93936 with cisplatin induces ferroptosis by inhibiting Nrf2-dependant GPX4 (Liang et al., 2021).
RAS-selective lethal 3 (RSL3) induces ferroptosis by inhibiting GPX4. RSL3 facilitates the anticancer effect of cisplatin in vitro and in vivo (Zhang et al., 2020). Recent studies have shown that propofol, an intravenous anesthetic agent traditionally and widely used for sedation and general anesthesia, exhibits anti-tumor activity against cancer progression in vitro and in vivo (Wang et al., 2018; Gu et al., 2022). Propofol can boost the anti-tumor activity of cisplatin in lung cancer (Huang et al., 2020; Ling et al., 2022; Quan et al., 2022). Mechanistically, propofol decreases cisplatin resistance in NSCLC by inducing ferroptosis, accomplished by upregulating the miR-744-5p/miR-615-3p axis and inhibiting GPX4 (Han et al., 2023).
NVP-231, a ceramide kinase (CERK) inhibitor, induces ferroptosis in mutant KRAS NSCLC cells by increasing the VDAC-regulated mitochondrial membrane potential and the generation of ROS. NVP-231 synergized NSCLC to cisplatin through the upregulation of VDAC1 (Vu et al., 2022).
5 Conclusion and future perspectives
In conclusion, this review summarized the novel role of ferroptosis in lung cancer and provides an overview on how pro-ferroptotic molecules may be used to overcome chemotherapeutic resistance. During the past decade, ferroptosis has attracted considerable interest in lung cancer research for its anti-tumor activity, which is thought to boost the efficacy of chemotherapy. In this review, we comprehensively summarized the small-molecule compounds from traditional medicines that may boost the anti-tumor activity of chemotherapeutic agents or overcome chemotherapy drug resistance in NSCLC, both of which serve as starting points to develop ferroptosis-related anticancer drugs for NSCLC. Small-molecule compounds that induce ferroptosis have specific targets. Erastin targets system Xc− to prevent cysteine import, which causes GSH depletion. RSL3 is a covalent inhibitor of GPX4 that causes the accumulation of lipid peroxides. In contrast to classical small molecules, traditional medicines have the advantage of polypharmacology. For example, ginkgetin regulates Nrf2, SLC7A11, and GPX4 at the same time.
The FDA has approved some ferroptosis-targeting small-molecule compounds for the evaluation of clinical trials with NSCLC patients (Li et al., 2024). However, research on ferroptosis is an emerging field still in its infancy. Significanr research is needed to bridge the gap from where we are to where we need to be in order to provide satisfactory biological outcomes. First, research on the role of ferroptosis in NSCLC is still ongoing, and the specific functions of ferroptosis remain ambiguous, hence warranting further investigation. As such, inevitable challenges still remain before the practical application of these treatment modalities. Second, the epigenetic modification of ferroptosis in cancer is an emerging field. The epigenetic modification of ferroptosis is identified in NSCLC, which, when dysregulated, can be feasibly targeted by small-molecule compounds. However, the practical application of these treatment modalities in NSCLC still has a long way to go. Third, many key components of the ferroptosis pathway, i.e., the principal proteins and enzymes engaged in the induction and inhibition of ferroptosis are transcriptionally controlled by Nrf249−53. More research is needed to discover new mechanisms that regulate ferroptosis and the role of Nrf2 in inhibiting ferroptosis, which will repurpose old drugs, i.e., Nrf2 inhibitors, as ferroptosis inducers to kill NSCLC. Nrf2 inhibitors may then be an optimal approach to treat NSCLC, but this requires further investigation. Fourth, the identification of biomarkers for ferroptosis sensitivity or resistance is urgently needed for accurately predicting the efficiency of inducing ferroptosis. Fifth, an assessment of the safety and potential toxicity of the small-molecule compounds that induce ferroptosis should be considered as this is a crucial consideration for potential therapeutic agents.
In conclusion, ferroptosis has been identified as a critical RCD triggered by ferroptosis-inducing bioactive compounds in NSCLC. Thus, the small-molecule compounds from traditional medicines hold great potential in NSCLC therapy, especially when combined with conventional chemotherapy by boosting the anti-tumor activity of chemotherapeutic agents or overcoming chemotherapy drug resistance in NSCLC. Natural-product ferroptosis-inducing small molecules may serve as an excellent starting point for the further development of ferroptosis-related anticancer drugs to overcome NSCLC chemotherapeutic resistance.
Author contributions
YW: conceptualization, writing–original draft, funding acquisition, and writing–review and editing. JH: writing–review and editing and data curation. JSF: writing–review and editing and visualization. YL: data curation and writing–review and editing. ZR: writing–review and editing. JW: writing–review and editing and conceptualization. YF: writing–review and editing. JC: writing–review and editing and funding acquisition. HW: conceptualization, investigation, and writing–original draft.
Funding
The author(s) declare that financial support was received for the research, authorship, and/or publication of this article. This work was supported in part by the Science Foundation of AMHT (2022YK01), the National Natural Science Foundation of China (82271895, 82072752, and 81172498), and Key Programs of the Science Foundation of Heilongjiang Province (ZD 2019H009).
Conflict of interest
The authors declare that the research was conducted in the absence of any commercial or financial relationships that could be construed as a potential conflict of interest.
Publisher’s note
All claims expressed in this article are solely those of the authors and do not necessarily represent those of their affiliated organizations, or those of the publisher, the editors, and the reviewers. Any product that may be evaluated in this article, or claim that may be made by its manufacturer, is not guaranteed or endorsed by the publisher.
References
Adnan, M., Rasul, A., Hussain, G., Shah, M. A., Zahoor, M. K., Anwar, H., et al. (2020). Ginkgetin: a natural biflavone with versatile pharmacological activities. Food Chem. Toxicol. 145, 111642. doi:10.1016/j.fct.2020.111642
Ayala, A., Muñoz, M. F., and Argüelles, S. (2014). Lipid peroxidation: production, metabolism, and signaling mechanisms of malondialdehyde and 4-hydroxy-2-nonenal. Oxid. Med. Cell Longev. 2014, 360438. doi:10.1155/2014/360438
Belmehdi, O., Taha, D., Abrini, J., Ming, L. C., Khalid, A., Abdalla, A. N., et al. (2023). Anticancer properties and mechanism insights of α-hederin. Biomed. Pharmacother. 165, 115205. doi:10.1016/j.biopha.2023.115205
Bersuker, K., Hendricks, J. M., Li, Z., Magtanong, L., Ford, B., Tang, P. H., et al. (2019). The CoQ oxidoreductase FSP1 acts parallel to GPX4 to inhibit ferroptosis. Nature 575 (7784), 688–692. doi:10.1038/s41586-019-1705-2
Brigelius-Flohé, R., and Flohé, L. (2020). Regulatory phenomena in the glutathione peroxidase superfamily. Antioxid. Redox Signal 33 (7), 498–516. doi:10.1089/ars.2019.7905
Brigelius-Flohé, R., and Maiorino, M. (2013). Glutathione peroxidases. Biochim. Biophys. Acta 1830 (5), 3289–3303. doi:10.1016/j.bbagen.2012.11.020
Cao, W. Q., Li, Y., Hou, Y. J., Yang, M. X., Fu, X. Q., Zhao, B. S., et al. (2019). Enhanced anticancer efficiency of doxorubicin against human glioma by natural borneol through triggering ROS-mediated signal. Biomed. Pharmacother. 118, 109261. doi:10.1016/j.biopha.2019.109261
Chen, J., Li, L., Su, J., Li, B., Zhang, X., and Chen, T. (2015). Proteomic analysis of G2/M arrest triggered by natural borneol/curcumin in HepG2 cells, the importance of the reactive oxygen species-p53 pathway. J. Agric. Food Chem. 63, 6440–6449. doi:10.1021/acs.jafc.5b01773
Chen, X., Kang, R., Kroemer, G., and Tang, D. (2021). Ferroptosis in infection, inflammation, and immunity. J. Exp. Med. 218, e20210518. doi:10.1084/jem.20210518
Chen, X., Li, J., Kang, R., Klionsky, D. J., and Tang, D. (2021). Ferroptosis: machinery and regulation. Autophagy 17 (9), 2054–2081. doi:10.1080/15548627.2020.1810918
Chen, X., Yu, C., Kang, R., and Tang, D. (2020). Iron metabolism in ferroptosis. Front. Cell Dev. Biol. 8, 590226. doi:10.3389/fcell.2020.590226
Cui, T., Lan, Y., Lu, Y., Yu, F., Lin, S., Fu, Y., et al. (2023). Isoorientin ameliorates H(2)O(2)-induced apoptosis and oxidative stress in chondrocytes by regulating MAPK and PI3K/Akt pathways. Aging 15, 4861–4874. doi:10.18632/aging.204768
Cui, Z., Li, D., Zhao, J., and Chen, K. (2022). Falnidamol and cisplatin combinational treatment inhibits non-small cell lung cancer (NSCLC) by targeting DUSP26-mediated signal pathways. Free Radic. Biol. Med. 183, 106–124. doi:10.1016/j.freeradbiomed.2022.03.003
Dai, E., Zhang, W., Cong, D., Kang, R., Wang, J., and Tang, D. (2020). AIFM2 blocks ferroptosis independent of ubiquinol metabolism. Biochem. Biophys. Res. Commun. 523 (4), 966–971. doi:10.1016/j.bbrc.2020.01.066
Dai, X., Zhang, X., Chen, W., Chen, Y., Zhang, Q., Mo, S., et al. (2021). Dihydroartemisinin: a potential natural anticancer drug. Int. J. Biol. Sci. 17, 603–622. doi:10.7150/ijbs.50364
David, S., Jhelum, P., Ryan, F., Jeong, S. Y., and Kroner, A. (2022). Dysregulation of iron homeostasis in the central nervous system and the role of ferroptosis in neurodegenerative disorders. Antioxid. Redox Signal 37 (1-3), 150–170. doi:10.1089/ars.2021.0218
Dixon, S. J., Lemberg, K. M., Lamprecht, M. R., Skouta, R., Zaitsev, E. M., Gleason, C. E., et al. (2012). Ferroptosis: an iron-dependent form of nonapoptotic cell death. Cell 149 (5), 1060–1072. doi:10.1016/j.cell.2012.03.042
Dixon, S. J., Winter, G. E., Musavi, L. S., Lee, E. D., Snijder, B., Rebsamen, M., et al. (2015). Human haploid cell genetics reveals roles for lipid metabolism genes in nonapoptotic cell death. ACS Chem. Biol. 10 (7), 1604–1609. doi:10.1021/acschembio.5b00245
Doll, S., Freitas, F. P., Shah, R., Aldrovandi, M., da Silva, M. C., Ingold, I., et al. (2019). FSP1 is a glutathione-independent ferroptosis suppressor. Nature 575 (7784), 693–698. doi:10.1038/s41586-019-1707-0
Doll, S., Proneth, B., Tyurina, Y. Y., Panzilius, E., Kobayashi, S., Ingold, I., et al. (2017). ACSL4 dictates ferroptosis sensitivity by shaping cellular lipid composition. Nat. Chem. Biol. 13 (1), 91–98. doi:10.1038/nchembio.2239
Dos Santos, A. F., Fazeli, G., Xavier da Silva, T. N., and Friedmann Angeli, J. P. (2023). Ferroptosis: mechanisms and implications for cancer development and therapy response. Trends Cell Biol. 33, 1062–1076. doi:10.1016/j.tcb.2023.04.005
Duvigneau, J. C., Trovato, A., Müllebner, A., Miller, I., Krewenka, C., Krenn, K., et al. (2020). Cannabidiol protects dopaminergic neurons in mesencephalic cultures against the complex I inhibitor rotenone via modulation of heme oxygenase activity and bilirubin. Antioxidants (Basel) 9, 135. doi:10.3390/antiox9020135
El-Hussein, A., Manoto, S. L., Ombinda-Lemboumba, S., Alrowaili, Z. A., and Mthunzi-Kufa, P. (2021). A review of chemotherapy and photodynamic therapy for lung cancer treatment. Anticancer Agents Med. Chem. 21, 149–161. doi:10.2174/1871520620666200403144945
Feng, S., Li, Y., Huang, H., Huang, H., Duan, Y., Yuan, Z., et al. (2023). Isoorientin reverses lung cancer drug resistance by promoting ferroptosis via the SIRT6/Nrf2/GPX4 signaling pathway. Eur. J. Pharmacol. 954, 175853. doi:10.1016/j.ejphar.2023.175853
Forcina, G. C., and Dixon, S. J. (2019). GPX4 at the crossroads of lipid homeostasis and ferroptosis. Proteomics 19 (18), e1800311. doi:10.1002/pmic.201800311
Freitas, F. P., Alborzinia, H., Dos Santos, A. F., Nepachalovich, P., Pedrera, L., Zilka, O., et al. (2024). 7-Dehydrocholesterol is an endogenous suppressor of ferroptosis. Nature 626, 401–410. doi:10.1038/s41586-023-06878-9
Friedmann Angeli, J. P., Schneider, M., Proneth, B., Tyurina, Y. Y., Tyurin, V. A., Hammond, V. J., et al. (2014). Inactivation of the ferroptosis regulator Gpx4 triggers acute renal failure in mice. Nat. Cell Biol. 16 (12), 1180–1191. doi:10.1038/ncb3064
Golbashirzadeh, M., Heidari, H. R., Talebi, M., and Yari Khosroushahi, A. (2023). Ferroptosis as a potential cell death mechanism against cisplatin-resistant lung cancer cell line. Adv. Pharm. Bull. 13, 176–187. doi:10.34172/apb.2023.019
Gu, L., Pan, X., Wang, C., and Wang, L. (2022). The benefits of propofol on cancer treatment: decipher its modulation code to immunocytes. Front. Pharmacol. 13, 919636. doi:10.3389/fphar.2022.919636
Gu, Y., Li, Y., Wang, J., Zhang, L., Zhang, J., and Wang, Y. (2023). Targeting ferroptosis: paving new roads for drug design and discovery. Eur. J. Med. Chem. 247, 115015. doi:10.1016/j.ejmech.2022.115015
Hadian, K., and Stockwell, B. R. (2020). SnapShot: ferroptosis. Cell 181 (5), 1188–1188.e1. doi:10.1016/j.cell.2020.04.039
Han, B., Liu, Y., Zhang, Q., and Liang, L. (2023). Propofol decreases cisplatin resistance of non-small cell lung cancer by inducing GPX4-mediated ferroptosis through the miR-744-5p/miR-615-3p axis. J. Proteomics 274, 104777. doi:10.1016/j.jprot.2022.104777
Han, N., Li, L. G., Peng, X. C., Ma, Q. L., Yang, Z. Y., Wang, X. Y., et al. (2022). Ferroptosis triggered by dihydroartemisinin facilitates chlorin e6 induced photodynamic therapy against lung cancerthrough inhibiting GPX4 and enhancing ROS. Eur. J. Pharmacol. 919, 174797. doi:10.1016/j.ejphar.2022.174797
Han, N., Yang, Z. Y., Xie, Z. X., Xu, H. Z., Yu, T. T., Li, Q. R., et al. (2023). Dihydroartemisinin elicits immunogenic death through ferroptosis-triggered ER stress and DNA damage for lung cancer immunotherapy. Phytomedicine 112, 154682. doi:10.1016/j.phymed.2023.154682
Hassannia, B., Vandenabeele, P., and Vanden Berghe, T. (2019). Targeting ferroptosis to iron out cancer. Cancer Cell 35 (6), 830–849. doi:10.1016/j.ccell.2019.04.002
Herbst, R. S., Morgensztern, D., and Boshoff, C. (2018). The biology and management of non-small cell lung cancer. Nature 553, 446–454. doi:10.1038/nature25183
Herzog, B. H., Devarakonda, S., and Govindan, R. (2021). Overcoming chemotherapy resistance in SCLC. J. Thorac. Oncol. 16, 2002–2015. doi:10.1016/j.jtho.2021.07.018
Ho, L., Cheng, H., Wang, J., Simon, J. E., Wu, Q., Zhao, D., et al. (2018). A comprehensive database and analysis framework to incorporate multiscale data types and enable integrated analysis of bioactive polyphenols. Mol. Pharm. 15, 840–850. doi:10.1021/acs.molpharmaceut.7b00412
Huang, Y., Lei, L., and Liu, Y. (2020). Propofol improves sensitivity of lung cancer cells to cisplatin and its mechanism. Med. Sci. Monit. 26, e919786. doi:10.12659/MSM.919786
Huo, L., Liu, C., Yuan, Y., Liu, X., and Cao, Q. (2023). Pharmacological inhibition of ferroptosis as a therapeutic target for sepsis-associated organ damage. Eur. J. Med. Chem. 257, 115438. doi:10.1016/j.ejmech.2023.115438
Ibarrola-Villava, M., Cervantes, A., and Bardelli, A. (2018). Preclinical models for precision oncology. Biochim. Biophys. Acta Rev. Cancer 1870, 239–246. doi:10.1016/j.bbcan.2018.06.004
Ingold, I., Berndt, C., Schmitt, S., Doll, S., Poschmann, G., Buday, K., et al. (2018). Selenium utilization by GPX4 is required to prevent hydroperoxide-induced ferroptosis. Cell 172 (3), 409–422. doi:10.1016/j.cell.2017.11.048
Jin, Y., Chen, Y., Qin, Z., Hu, L., Guo, C., and Ji, H. (2023). Understanding SCLC heterogeneity and plasticity in cancer metastasis and chemotherapy resistance. Acta Biochim. Biophys. Sin. (Shanghai) 55, 948–955. doi:10.3724/abbs.2023080
Kagan, V. E., Mao, G., Qu, F., Angeli, J. P. F., Doll, S., Croix, C. S., et al. (2017). Oxidized arachidonic and adrenic PEs navigate cells to ferroptosis. Nat. Chem. Biol. 13 (1), 81–90. doi:10.1038/nchembio.2238
Khalil, A., Qazi, A. S., Nasir, A., Ahn, M. J., Shah, M. A., Ahmad, M. S., et al. (2022). 2-Methoxy-6-Acetyl-7-Methyljuglone: a bioactive phytochemical with potential pharmacological activities. Anticancer Agents Med. Chem. 22, 687–693. doi:10.2174/1871520621666210623095636
Koeberle, S. C., Kipp, A. P., Stuppner, H., and Koeberle, A. (2023). Ferroptosis-modulating small molecules for targeting drug-resistant cancer: challenges and opportunities in manipulating redox signaling. Med. Res. Rev. 43, 614–682. doi:10.1002/med.21933
Koppula, P., Zhang, Y., Zhuang, L., and Gan, B. (2018). Amino acid transporter SLC7A11/xCT at the crossroads of regulating redox homeostasis and nutrient dependency of cancer. Cancer Commun. (Lond). 38 (1), 12. doi:10.1186/s40880-018-0288-x
Koppula, P., Zhuang, L., and Gan, B. (2021). Cystine transporter SLC7A11/xCT in cancer: ferroptosis, nutrient dependency, and cancer therapy. Protein Cell 12 (8), 599–620. doi:10.1007/s13238-020-00789-5
Kraft, V., Bezjian, C. T., Pfeiffer, S., Ringelstetter, L., Müller, C., Zandkarimi, F., et al. (2020). GTP cyclohydrolase 1/tetrahydrobiopterin counteract ferroptosis through lipid remodeling. ACS Cent. Sci. 6 (1), 41–53. doi:10.1021/acscentsci.9b01063
Lai, X. Y., Shi, Y. M., and Zhou, M. M. (2023). Dihydroartemisinin enhances gefitinib cytotoxicity against lung adenocarcinoma cells by inducing ROS-dependent apoptosis and ferroptosis. Kaohsiung J. Med. Sci. 39, 699–709. doi:10.1002/kjm2.12684
Lei, G., Mao, C., Yan, Y., Zhuang, L., and Gan, B. (2021). Ferroptosis, radiotherapy, and combination therapeutic strategies. Protein Cell 12, 836–857. doi:10.1007/s13238-021-00841-y
Lei, G., Zhuang, L., and Gan, B. (2022). Targeting ferroptosis as a vulnerability in cancer. Nat. Rev. Cancer 22 (7), 381–396. doi:10.1038/s41568-022-00459-0
Leiter, A., Veluswamy, R. R., and Wisnivesky, J. P. (2023). The global burden of lung cancer: current status and future trends. Nat. Rev. Clin. Oncol. 20, 624–639. doi:10.1038/s41571-023-00798-3
Li, G., Zhang, H., Lai, H., Liang, G., Huang, J., Zhao, F., et al. (2023). Erianin: a phytoestrogen with therapeutic potential. Front. Pharmacol. 14, 1197056. doi:10.3389/fphar.2023.1197056
Li, J., Yuan, J., Li, Y., Wang, J., Gong, D., Xie, Q., et al. (2022). d-Borneol enhances cisplatin sensitivity via p21/p27-mediated S-phase arrest and cell apoptosis in non-small cell lung cancer cells and a murine xenograft model. Cell. Mol. Biol. Lett. 27, 61. doi:10.1186/s11658-022-00362-4
Li, J., Yuan, J., Li, Y., Wang, J., Xie, Q., Ma, R., et al. (2022). d-Borneol enhances cisplatin sensitivity via autophagy dependent EMT signaling and NCOA4-mediated ferritinophagy. Phytomedicine 106, 154411. doi:10.1016/j.phymed.2022.154411
Li, L. G., Peng, X. C., Yu, T. T., Xu, H. Z., Han, N., Yang, X. X., et al. (2022). Dihydroartemisinin remodels macrophage into an M1 phenotype via ferroptosis-mediated DNA damage. Front. Pharmacol. 13, 949835. doi:10.3389/fphar.2022.949835
Li, Q., Ma, Q., Cheng, J., Zhou, X., Pu, W., Zhong, X., et al. (2021). Dihydroartemisinin as a sensitizing agent in cancer therapies. Onco Targets Ther. 14, 2563–2573. doi:10.2147/OTT.S297785
Li, S., Wang, A., Wu, Y., He, S., Shuai, W., Zhao, M., et al. (2024a). Targeted therapy for non-small-cell lung cancer: new insights into regulated cell death combined with immunotherapy. Immunol. Rev. 321 (1), 300–334. doi:10.1111/imr.13274
Li, Y., Ran, Q., Duan, Q., Jin, J., Wang, Y., Yu, L., et al. (2024b). 7-Dehydrocholesterol dictates ferroptosis sensitivity. Nature 626, 411–418. doi:10.1038/s41586-023-06983-9
Li, Y., Yan, H., Xu, X., Liu, H., Wu, C., and Zhao, L. (2020). Erastin/sorafenib induces cisplatin-resistant non-small cell lung cancer cell ferroptosis through inhibition of the Nrf2/xCT pathway. Oncol. Lett. 19, 323–333. doi:10.3892/ol.2019.11066
Li, Y., Zhao, Y., Tan, X., Liu, J., Zhi, Y., Yi, L., et al. (2020). Isoorientin inhibits inflammation in macrophages and endotoxemia mice by regulating glycogen synthase kinase 3β. Mediat. Inflamm. 2020, 8704146. doi:10.1155/2020/8704146
Liang, D., Feng, Y., Zandkarimi, F., Wang, H., Zhang, Z., Kim, J., et al. (2023). Ferroptosis surveillance independent of GPX4 and differentially regulated by sex hormones. Cell 186, 2748–2764.e22. doi:10.1016/j.cell.2023.05.003
Liang, D., Minikes, A. M., and Jiang, X. (2022). Ferroptosis at the intersection of lipid metabolism and cellular signaling. Mol. Cell 82 (12), 2215–2227. doi:10.1016/j.molcel.2022.03.022
Liang, Z., Zhao, W., Li, X., Wang, L., Meng, L., and Yu, R. (2021). Cisplatin synergizes with PRLX93936 to induce ferroptosis in non-small cell lung cancer cells. Biochem. Biophys. Res. Commun. 569, 79–85. doi:10.1016/j.bbrc.2021.06.088
Lim, Z. F., and Ma, P. C. (2019). Emerging insights of tumor heterogeneity and drug resistance mechanisms in lung cancer targeted therapy. J. Hematol. Oncol. 12, 134. doi:10.1186/s13045-019-0818-2
Lin, Q., Hou, S., Dai, Y., Jiang, N., and Lin, Y. (2020). Monascin exhibits neuroprotective effects in rotenone model of Parkinson's disease via antioxidation and anti-neuroinflammation. Neuroreport 31, 637–643. doi:10.1097/WNR.0000000000001467
Ling, Q., Wu, S., Liao, X., Liu, C., and Chen, Y. (2022). Anesthetic propofol enhances cisplatin-sensitivity of non-small cell lung cancer cells through N6-methyladenosine-dependently regulating the miR-486-5p/RAP1-NF-κB axis. BMC Cancer 22, 765. doi:10.1186/s12885-022-09848-y
Liu, Q., Chen, L., Yin, W., Nie, Y., Zeng, P., and Yang, X. (2022). Anti-tumor effect of ginkgetin on human hepatocellular carcinoma cell lines by inducing cell cycle arrest and promoting cell apoptosis. Cell Cycle 21, 74–85. doi:10.1080/15384101.2021.1995684
Liu, S. C., Huang, C. S., Huang, C. M., Hsieh, M. S., Huang, M. S., Fong, I. H., et al. (2021). Isoorientin inhibits epithelial-to-mesenchymal properties and cancer stem-cell-like features in oral squamous cell carcinoma by blocking Wnt/β-catenin/STAT3 axis. Toxicol. Appl. Pharmacol. 424, 115581. doi:10.1016/j.taap.2021.115581
Lou, J. S., Bi, W. C., Chan, G., Jin, Y., Wong, C. W., Zhou, Z. Y., et al. (2017). Ginkgetin induces autophagic cell death through p62/SQSTM1-mediated autolysosome formation and redox setting in non-small cell lung cancer. Oncotarget 8, 93131–93148. doi:10.18632/oncotarget.21862
Lou, J. S., Zhao, L. P., Huang, Z. H., Chen, X. Y., Xu, J. T., Tai, W. C., et al. (2021). Ginkgetin derived from Ginkgo biloba leaves enhances the therapeutic effect of cisplatin via ferroptosis-mediated disruption of the Nrf2/HO-1 axis in EGFR wild-type non-small-cell lung cancer. Phytomedicine 80, 153370. doi:10.1016/j.phymed.2020.153370
Lv, J., Wang, Z., and Liu, H. (2023). Erianin suppressed lung cancer stemness and chemotherapeutic sensitivity via triggering ferroptosis. Environ. Toxicol. 39, 479–486. doi:10.1002/tox.23832
Mao, C., Liu, X., Zhang, Y., Lei, G., Yan, Y., Lee, H., et al. (2021). DHODH-mediated ferroptosis defence is a targetable vulnerability in cancer. Nature 593 (7860), 586–590. doi:10.1038/s41586-021-03539-7
Meng, X., Dong, X., Wang, W., Yang, L., Zhang, X., Li, Y., et al. (2018). Natural borneol enhances paclitaxel-induced apoptosis of ESCC cells by inactivation of the PI3K/AKT. J. Food Sci. 83, 1436–1443. doi:10.1111/1750-3841.14143
Min, H. Y., and Lee, H. Y. (2021). Mechanisms of resistance to chemotherapy in non-small cell lung cancer. Arch. Pharm. Res. 44, 146–164. doi:10.1007/s12272-021-01312-y
Nagasaka, M., and Gadgeel, S. M. (2018). Role of chemotherapy and targeted therapy in early-stage non-small cell lung cancer. Expert Rev. Anticancer Ther. 18, 63–70. doi:10.1080/14737140.2018.1409624
Niu, Z. X., Wang, Y. T., Lu, N., Sun, J. F., Nie, P., and Herdewijn, P. (2023). Advances of clinically approved small-molecule drugs for the treatment of non-small cell lung cancer. Eur. J. Med. Chem. 261, 115868. doi:10.1016/j.ejmech.2023.115868
Pedrera, L., Espiritu, R. A., Ros, U., Weber, J., Schmitt, A., Stroh, J., et al. (2021). Ferroptotic pores induce Ca(2+) fluxes and ESCRT-III activation to modulate cell death kinetics. Cell Death Differ. 28 (5), 1644–1657. doi:10.1038/s41418-020-00691-x
Quan, Y., Li, S., Wang, Y., Liu, G., Lv, Z., and Wang, Z. (2022). Propofol and sevoflurane alleviate malignant biological behavior and cisplatin resistance of xuanwei lung adenocarcinoma by modulating the wnt/β-catenin pathway and PI3K/AKT pathway. Anticancer Agents Med. Chem. 22, 2098–2108. doi:10.2174/1871520621666211026092405
Ryter, S. W., Kim, H. P., Hoetzel, A., Park, J. W., Nakahira, K., Wang, X., et al. (2007). Mechanisms of cell death in oxidative stress. Antioxid. Redox Signal 9 (1), 49–89. doi:10.1089/ars.2007.9.49
Sato, H., Tamba, M., Ishii, T., and Bannai, S. (1999). Cloning and expression of a plasma membrane cystine/glutamate exchange transporter composed of two distinct proteins. J. Biol. Chem. 274 (17), 11455–11458. doi:10.1074/jbc.274.17.11455
Seibt, T. M., Proneth, B., and Conrad, M. (2019). Role of GPX4 in ferroptosis and its pharmacological implication. Free Radic. Biol. Med. 133, 144–152. doi:10.1016/j.freeradbiomed.2018.09.014
Shimada, K., Skouta, R., Kaplan, A., Yang, W. S., Hayano, M., Dixon, S. J., et al. (2016). Global survey of cell death mechanisms reveals metabolic regulation of ferroptosis. Nat. Chem. Biol. 12, 497–503. doi:10.1038/nchembio.2079
Soula, M., Weber, R. A., Zilka, O., Alwaseem, H., La, K., Yen, F., et al. (2020). Metabolic determinants of cancer cell sensitivity to canonical ferroptosis inducers. Nat. Chem. Biol. 16 (12), 1351–1360. doi:10.1038/s41589-020-0613-y
Stockwell, B. R. (2022). Ferroptosis turns 10: emerging mechanisms, physiological functions, and therapeutic applications. Cell. 185 (14), 2401–2421. doi:10.1016/j.cell.2022.06.003
Sun, W., Bao, J., Lin, W., Gao, H., Zhao, W., Zhang, Q., et al. (2016). 2-Methoxy-6-acetyl-7-methyljuglone (MAM), a natural naphthoquinone, induces NO-dependent apoptosis and necroptosis by H2O2-dependent JNK activation in cancer cells. Free Radic. Biol. Med. 92, 61–77. doi:10.1016/j.freeradbiomed.2016.01.014
Sun, W., Wu, X., Gao, H., Yu, J., Zhao, W., Lu, J. J., et al. (2017). Cytosolic calcium mediates RIP1/RIP3 complex-dependent necroptosis through JNK activation and mitochondrial ROS production in human colon cancer cells. Free Radic. Biol. Med. 108, 433–444. doi:10.1016/j.freeradbiomed.2017.04.010
Sun, W., Yu, J., Gao, H., Wu, X., Wang, S., Hou, Y., et al. (2019). Inhibition of lung cancer by 2-methoxy-6-acetyl-7-methyljuglone through induction of necroptosis by targeting receptor-interacting protein 1. Antioxid. Redox Signal. 31, 93–108. doi:10.1089/ars.2017.7376
Sun, Y., Berleth, N., Wu, W., Schlütermann, D., Deitersen, J., Stuhldreier, F., et al. (2021). Fin56-induced ferroptosis is supported by autophagy-mediated GPX4 degradation and functions synergistically with mTOR inhibition to kill bladder cancer cells. Cell Death Dis. 12, 1028. doi:10.1038/s41419-021-04306-2
Sun, Y., Qiao, Y., Liu, Y., Zhou, J., Wang, X., Zheng, H., et al. (2021). ent-Kaurane diterpenoids induce apoptosis and ferroptosis through targeting redox resetting to overcome cisplatin resistance. Redox Biol. 43, 101977. doi:10.1016/j.redox.2021.101977
Sun, Y., Xia, X., Basnet, D., Zheng, J. C., Huang, J., and Liu, J. (2022). Mechanisms of ferroptosis and emerging links to the pathology of neurodegenerative diseases. Front. Aging Neurosci. 14, 904152. doi:10.3389/fnagi.2022.904152
Sung, H., Ferlay, J., Siegel, R. L., Laversanne, M., Soerjomataram, I., Jemal, A., et al. (2021). Global cancer statistics 2020: GLOBOCAN estimates of incidence and mortality worldwide for 36 cancers in 185 countries. CA Cancer J. Clin. 71, 209–249. doi:10.3322/caac.21660
Tabnak, P., HajiEsmailPoor, Z., and Soraneh, S. (2021). Ferroptosis in lung cancer: from molecular mechanisms to prognostic and therapeutic opportunities. Front. Oncol. 11, 792827. doi:10.3389/fonc.2021.792827
Tang, D., and Kroemer, G. (2020). Ferroptosis. Curr. Biol. 30, R1292–R1297. doi:10.1016/j.cub.2020.09.068
Ursini, F., Maiorino, M., Valente, M., Ferri, L., and Gregolin, C. (1982). Purification from pig liver of a protein which protects liposomes and biomembranes from peroxidative degradation and exhibits glutathione peroxidase activity on phosphatidylcholine hydroperoxides. Biochim. Biophys. Acta 710 (2), 197–211. doi:10.1016/0005-2760(82)90150-3
Vu, N. T., Kim, M., Stephenson, D. J., MacKnight, H. P., and Chalfant, C. E. (2022). Ceramide kinase inhibition drives ferroptosis and sensitivity to cisplatin in mutant KRAS lung cancer by dysregulating VDAC-mediated mitochondria function. Mol. Cancer Res. 20, 1429–1442. doi:10.1158/1541-7786.MCR-22-0085
Wang, J., Cheng, C. S., Lu, Y., Ding, X., Zhu, M., Miao, C., et al. (2018). Novel findings of anti-cancer property of propofol. Anticancer Agents Med. Chem. 18, 156–165. doi:10.2174/1871520617666170912120327
Wang, Y., Hu, J., Wu, S., Fleishman, J. S., Li, Y., Xu, Y., et al. (2023). Targeting epigenetic and posttranslational modifications regulating ferroptosis for the treatment of diseases. Signal Transduct. Target Ther. 8, 449. doi:10.1038/s41392-023-01720-0
Wang, Y., Wu, X., Ren, Z., Li, Y., Zou, W., Chen, J., et al. (2023). Overcoming cancer chemotherapy resistance by the induction of ferroptosis. Drug resist. updat. 66, 100916. doi:10.1016/j.drup.2022.100916
Wu, L., Qian, C., Zhang, W., Shi, M., Chen, X., Wang, Y., et al. (2023). Ginkgetin suppresses ovarian cancer growth through inhibition of JAK2/STAT3 and MAPKs signaling pathways. Phytomedicine 116, 154846. doi:10.1016/j.phymed.2023.154846
Wu, S., Zhu, C., Tang, D., Dou, Q. P., Shen, J., and Chen, X. (2021). The role of ferroptosis in lung cancer. Biomark. Res. 9, 82. doi:10.1186/s40364-021-00338-0
Wu, Y., Wang, D., Lou, Y., Liu, X., Huang, P., Jin, M., et al. (2022). Regulatory mechanism of α-hederin upon cisplatin sensibility in NSCLC at safe dose by destroying GSS/GSH/GPX2 axis-mediated glutathione oxidation-reduction system. Biomed. Pharmacother. 150, 112927. doi:10.1016/j.biopha.2022.112927
Xing, N., Du, Q., Guo, S., Xiang, G., Zhang, Y., Meng, X., et al. (2023). Ferroptosis in lung cancer: a novel pathway regulating cell death and a promising target for drug therapy. Cell Death Discov. 9, 110. doi:10.1038/s41420-023-01407-z
Xu, W. T., Shen, G. N., Li, T. Z., Zhang, Y., Zhang, T., Xue, H., et al. (2020). Isoorientin induces the apoptosis and cell cycle arrest of A549 human lung cancer cells via the ROS-regulated MAPK, STAT3 and NF-κB signaling pathways. Int. J. Oncol. 57, 550–561. doi:10.3892/ijo.2020.5079
Yang, W. S., SriRamaratnam, R., Welsch, M. E., Shimada, K., Skouta, R., Viswanathan, V. S., et al. (2014). Regulation of ferroptotic cancer cell death by GPX4. Cell 156 (1-2), 317–331. doi:10.1016/j.cell.2013.12.010
Yin, J. Y., Li, X., Zhou, H. H., and Liu, Z. Q. (2016). Pharmacogenomics of platinum-based chemotherapy sensitivity in NSCLC: toward precision medicine. Pharmacogenomics 17, 1365–1378. doi:10.2217/pgs-2016-0074
Yin, L., Liu, P., Jin, Y., Ning, Z., Yang, Y., and Gao, H. (2022). Ferroptosis-related small-molecule compounds in cancer therapy: strategies and applications. Eur. J. Med. Chem. 244, 114861. doi:10.1016/j.ejmech.2022.114861
Yu, J., Zhong, B., Jin, L., Hou, Y., Ai, N., Ge, W., et al. (2020). 2-Methoxy-6-acetyl-7-methyljuglone (MAM) induced programmed necrosis in glioblastoma by targeting NAD(P)H: quinone oxidoreductase 1 (NQO1). Free Radic. Biol. Med. 152, 336–347. doi:10.1016/j.freeradbiomed.2020.03.026
Yu, J., Zhong, B., Zhao, L., Hou, Y., Ai, N., Lu, J. J., et al. (2023). Fighting drug-resistant lung cancer by induction of NAD(P)H:quinone oxidoreductase 1 (NQO1)-mediated ferroptosis. Drug resist. updat. 70, 100977. doi:10.1016/j.drup.2023.100977
Zhang, X., Sui, S., Wang, L., Li, H., Zhang, L., Xu, S., et al. (2020). Inhibition of tumor propellant glutathione peroxidase 4 induces ferroptosis in cancer cells and enhances anticancer effect of cisplatin. J. Cell. Physiol. 235, 3425–3437. doi:10.1002/jcp.29232
Zhao, Y., Li, Y., Zhang, R., Wang, F., Wang, T., and Jiao, Y. (2020). The role of erastin in ferroptosis and its prospects in cancer therapy. Onco Targets Ther. 13, 5429–5441. doi:10.2147/OTT.S254995
Ziqubu, K., Dludla, P. V., Joubert, E., Muller, C., Louw, J., Tiano, L., et al. (2020). Isoorientin: a dietary flavone with the potential to ameliorate diverse metabolic complications. Pharmacol. Res. 158, 104867. doi:10.1016/j.phrs.2020.104867
Keywords: lung cancer, non-small-cell lung cancer, ferroptosis, chemotherapy, chemoresistance, traditional medicines
Citation: Wang Y, Hu J, Fleishman JS, Li Y, Ren Z, Wang J, Feng Y, Chen J and Wang H (2024) Inducing ferroptosis by traditional medicines: a novel approach to reverse chemoresistance in lung cancer. Front. Pharmacol. 15:1290183. doi: 10.3389/fphar.2024.1290183
Received: 07 September 2023; Accepted: 22 April 2024;
Published: 23 May 2024.
Edited by:
Xiao Zhang, Shanghai Jiao Tong University, ChinaReviewed by:
Junjian Wang, Sun Yat-sen University, ChinaRong Cai, Shanghai Jiao Tong University, China
Shaohui Wang, Chengdu University of Traditional Chinese Medicine, China
Copyright © 2024 Wang, Hu, Fleishman, Li, Ren, Wang, Feng, Chen and Wang. This is an open-access article distributed under the terms of the Creative Commons Attribution License (CC BY). The use, distribution or reproduction in other forums is permitted, provided the original author(s) and the copyright owner(s) are credited and that the original publication in this journal is cited, in accordance with accepted academic practice. No use, distribution or reproduction is permitted which does not comply with these terms.
*Correspondence: Yukuan Feng, ZmVuZ3l1a3VhbkB0am11Y2guY29t; Jichao Chen, Y2hlbl9odHp4eXlAc2luYS5jb20=; Hongquan Wang, d2hvbmdxdWFuQGFsdS5mdWRhbi5lZHUuY24=
†These authors have contributed equally to this work