- 1Cardiovascular Department, Xiyuan Hospital, China Academy of Chinese Medical Sciences, Beijing, China
- 2Graduate School, Beijing University of Chinese Medicine, Beijing, China
- 3Department of Geratology, Xiyuan Hospital, China Academy of Chinese Medical Sciences, Beijing, China
- 4State Key Laboratory of Traditional Chinese Medicine Syndrome, Xiyuan Hospital, China Academy of Chinese Medical Sciences, Beijing, China
Cardiovascular diseases (CVDs) are currently the leading cause of death worldwide. In 2022, the CVDs contributed to 19.8 million deaths globally, accounting for one-third of all global deaths. With an aging population and changing lifestyles, CVDs pose a major threat to human health. Mitochondria-associated endoplasmic reticulum membranes (MAMs) are communication platforms between cellular organelles and regulate cellular physiological functions, including apoptosis, autophagy, and programmed necrosis. Further research has shown that MAMs play a critical role in the pathogenesis of CVDs, including myocardial ischemia and reperfusion injury, heart failure, pulmonary hypertension, and coronary atherosclerosis. This suggests that MAMs could be an important therapeutic target for managing CVDs. The goal of this study is to summarize the protein complex of MAMs, discuss its role in the pathological mechanisms of CVDs in terms of its functions such as Ca2+ transport, apoptotic signaling, and lipid metabolism, and suggest the possibility of MAMs as a potential therapeutic approach.
1 Introduction
Cardiovascular diseases (CVDs), such as cardiomyopathy, heart failure (HF), hypertension, and atherosclerosis, are a major cause of mortality worldwide (Amini et al., 2021; Ren et al., 2021). In 2022 alone, it caused 19.8 million deaths worldwide, accounting for 33% of global deaths. It is noteworthy that 34% of these deaths occur before the age of 70 (Mensah et al., 2023). Given the aging population and lifestyle changes, this disease poses a significant challenge to human health. Due to the severe lethality of the disease, the study of its pathogenesis has been the focus of pharmacological attention.
Mitochondria are considered to be the primary site for aerobic respiration and energy production. Additionally, they are key regulators of reactive oxygen species (ROS) production, inflammation, metabolism, and cell death (He et al., 2022). It has been demonstrated through numerous studies that mitochondria play a crucial role in CVDs. For example, recent research has shown that mitochondrial energy metabolism and translation are crucial factors in cardiac regeneration (Li et al., 2023; Gao et al., 2023). A recent study found that the promotion of MCM8 and E3 ubiquitin ligase tripartite motif containing 21 (TRIM21) by nitric oxide can mediate mitochondrial autophagy, which in turn helps to maintain normal coronary artery function (Lin et al., 2023). The endoplasmic reticulum (ER) is a crucial site for protein synthesis and folding. It is an interconnected network of different morphologies that extend throughout the cytoplasm, forming abundant contacts with other organelles. Additionally, the ER plays a role in regulating Ca2+ and lipid metabolism (Parkkinen et al., 2023; Zheng et al., 2023). Maintaining ER protein homeostasis is crucial for preserving cellular secretory function. It is important to note that long-term ER stress can result in cellular defects and CVDs (Ren et al., 2021). Additionally, interactions between organelles are essential for organelle function and overall cellular homeostasis (Gatta and Levine, 2017). Mitochondria-associated endoplasmic reticulum membranes (MAMs) are considered to be communication platforms that regulate cellular physiological functions between mitochondria and endoplasmic reticulum organelles in both directions, which may be believed to influence cell fate (Wang et al., 2021; Zhang et al., 2023; Yang and Luan, 2023). According to the report, dysfunctions in the structure and function of MAMs can lead to the development of various pathological conditions and diseases in the body, including CVDs (Missiroli et al., 2018).
Recently, there has been an increasing number of studies investigating the role of MAM proteins in CVDs. For instance, one study discovered that FUN14 domain-containing 1 (FUNDC1) facilitates the formation of MAMs, leading to elevated expression of vascular endothelial growth factor R2 and ultimately promoting angiogenesis (Wang et al., 2021). The transmembrane protein 215 (TMEM215) is a transmembrane protein located on the endoplasmic reticulum that inhibits BIK (BCL-2 interacting killer)-regulated ER-to-mitochondria Ca2+ inward flow, thereby preventing endothelial cell apoptosis during vascular recovery (Zhang et al., 2023). Lon protease 1 (LonP1), a novel protein localized to MAMs, is involved in regulating cardiomyocyte function and maintaining normal cardiac function (Li et al., 2023). The purpose of this study is to describe the structure and function of MAMs, elaborate on their mechanism of action in CVDs, and discuss interventions related to the prevention or treatment of CVDs.
2 The components of mitochondria-associated endoplasmic reticulum membranes
MAMs are regions where the outer mitochondrial membrane (OMM) and certain areas of the ER membrane overlap without membrane fusion. It was first isolated from rat liver in the 1990s (Vance, 1990). Under electron microscopy, the distance between the OMM and the ER was maintained at 10–25 nm (Csordás et al., 2006). Proteomic analysis revealed that MAMs contain over 1000 enriched proteins across various species and tissues (Poston et al., 2013; Wang et al., 2018). Studies have shown that proteins in MAMs exhibit dynamic behavior, with some being transient while others are persistent (Janikiewicz et al., 2018). The biological functions of MAMs are carried out through mitochondria-endoplasmic reticulum contacts (MERCs) (Barazzuol et al., 2021), which are protein complexes that perform a variety of functions such as Ca2+ signaling, lipid metabolism, oxidative stress, autophagy, apoptosis, etc (Zhang et al., 2023; Yang and Luan, 2023). Loss of MERCs homeostasis is manifested by Ca2+ overload, accumulation of unfolded or misfolded proteins, etc., which in turn leads to cell degeneration and pathology.
The proteins of MERCs were classified into three categories based on mass spectrometry analysis. These categories include 1) MAMs-resident proteins, which are present only on MAMs; 2) MAMs-enriched proteins, which are also present in other regions of the cell; and 3) MAMs-associated proteins, which are temporarily located on MAMs (Poston et al., 2013). Among the major proteins associated with the cardiovascular system are the inositol 1,4,5-trisphosphate receptor (IP3R), glucose-regulated protein 75 (GRP75), voltage-dependent anion channel 1 (VDAC1), sigma 1 receptor (Sig1R), mitofusin 1 and 2 (MFN1/2), vesicle-associated membrane protein-associated protein B (VAPB), protein tyrosine phosphatase interacting protein 51 (PTPIP51), fission protein 1 (Fis1), B-cell receptor-associated protein 31 (BAP31), phosphofurin acidic cluster sorting protein 2 (PACS2), etc. Further, the proteins can be classified according to their functions, including Ca2+ regulatory proteins, redox regulatory proteins, lipid synthesis and transport proteins, autophagy-related proteins, etc (Li et al., 2022). The focus of this study is on the mechanism of these proteins in cardiovascular homeostasis and metabolic mechanisms associated with CVDs.
2.1 IP3R1-GRP75-VDAC1 complex
The IP3R1 protein is located on the surface of the ER and facilitates the transfer of Ca2+ from the ER to the cytoplasm. The VDAC1 protein is an ion channel located on the OMM that regulates metabolites and ions within the mitochondria. Additionally, it is capable of promoting Ca2+ into the mitochondria. The GRP75 protein acts as a bridge between IP3R and VDAC, forming the intact protein complex and facilitating the interaction between IP3R and VDAC (Basso et al., 2020). The IP3R1-GRP75-VDAC1 complex has been found to play a significant role in regulating the cardiovascular system. Specifically, IP3R1 has been identified as a central player in the development of cardiac hypertrophy (Nakayama et al., 2010; Garcia et al., 2017), while IP3R1 in vascular smooth muscle cells (VSMCs) has been shown to contribute to peripheral vasoconstriction during HF (Dridi et al., 2022). Additionally, one study reported that a reduction in VDAC1 during myocardial ischemic injury resulted in a decrease in the area of myocardial infarction (Feng et al., 2019).
2.2 BAP31-Fis1 complex
BAP31 is a protein located in the ER, while Fis1 is in the OMM. In the process of apoptosis, procaspase-8 is recruited to the BAP31-Fis1 platform, which leads to the processing of BAP31 and the formation of p20BAP31. This signaling pathway activates mitochondria for apoptosis by releasing Ca2+ from the ER (Iwasawa et al., 2011). Furthermore, it is noted that PACS2 proteins play a critical role in regulating MAMs. If PACS2 protein is depleted, BAP31 is cleaved into p20, which leads to the release of Ca2+ from the ER to the mitochondria. This, in turn, triggers the recruitment of dynamin-related protein 1 (Drp1) to the mitochondria to activate mitochondrial fission, ultimately resulting in the fragmentation of the mitochondrial network (Li et al., 2020). During prolonged hypobaric hypoxia, it has been observed that the proteins PACS2 related to MAMs and mitochondrial autophagy are downregulated. Studies have shown that knockdown and knock-in of PACS2 can cause cardiomyocyte injury, exacerbation, and recovery of right heart dysfunction (Yang et al., 2023). Additionally, research has demonstrated that stabilizing BAP31 in septic cardiomyopathy can help protect cardiac function (Zhang et al., 2020). Moreover, Zhou and colleagues have found that the desumoylation of endothelial cell Fis1 may help maintain mitochondria, which could potentially prevent hypoxic pulmonary hypertension (Zhou et al., 2023).
2.3 VAPB-PTPIP51 complex
VAPB is a protein found in the ER that is responsible for vesicle trafficking and the unfolded protein response. PTPIP51 is located in the OMM and regulates cell development and tumorigenesis (Vinay Kumar et al., 2014; Gómez-Suaga et al., 2019). It has been suggested that VAPB forms a complex with PTPIP51 to maintain the structure of MAMs and regulate the transport of Ca2+ (Gómez-Suaga et al., 2019). Research has indicated that the VAPBP56S mutation results in a heightened attraction to PTPIP51, which facilitates the transfer of Ca2+ from the ER to the mitochondria. The reduction of Ca2+ transport occurs when a gene is suppressed (De Vos et al., 2012). Disruption of the structure of MAMs affects Ca2+ conductance and ATP synthesis (Paillusson et al., 2017). Furthermore, the VAPB-PTPIP51 complex’s function is influenced by α-synuclein (Gómez-Suaga et al., 2019) and TAR DNA-binding domain protein 43 (Stoica et al., 2014). The transfer of phosphatidylserine (PS) from the ER to the mitochondria is promoted by the interaction between ORP5/8 on the ER and PTPIP51 on the OMM. It has been observed that depletion of ORP5/8 leads to defects in mitochondrial morphology and respiratory function (Galmes et al., 2016). One study discovered that PTPIP51 was significantly upregulated in myocardial ischemia-reperfusion (I/R) (Qiao et al., 2017). Additionally, they found that specific knockdown of PTPIP51 reduced myocardial infarction size. According to a study, it was found that the expression of the VAPB-PTPIP51 complex was significantly reduced in a hypertensive mouse model (Liu T. et al., 2023).
2.4 MFN2-MFN1/2 complex
The protein MFN2, which is responsible for mitochondrial fusion, is present in both the OMM and the ER (de Brito and Scorrano, 2008). It forms hetero- or homodimers with MFN1/2 on the OMM to regulate the distance between organelles and coordinate the ER and mitochondrial dynamics (Naon et al., 2016; Filadi et al., 2018). However, there are conflicting findings in current studies. One study showed that when MFN2 was removed or silenced, the distance between the ER and mitochondria decreased, resulting in an increased transfer of inositol trisphosphate (IP3)-induced Ca2+ from the ER to mitochondria (Filadi et al., 2015). However, another study found that the acute removal of MFN2 resulted in a decrease in mitochondrial uptake of Ca2+ released from the ER (Naon et al., 2016). Moreover, it has been observed that the knockdown of MFN2 leads to mitochondrial swelling, degeneration, and increased oxidative damage, which ultimately results in apoptosis (Han et al., 2020).
The MFN2-MFN1/2 complex is involved in the regulation of the cardiovascular system. One study discovered that MFN2 expression was downregulated in myocardium hypertrophied compared to normal myocardium (Sun et al., 2019). Furthermore, in an animal study, the overexpression of MFN2 inhibited the formation of atherosclerosis (Guo et al., 2007). In a study conducted on mice, the researchers found that the absence of MFN1/2 in hearts prevented acute myocardial infarction (Hall et al., 2016).
3 The functions of mitochondria-associated endoplasmic reticulum membranes
MAMs are recognized as important functional regions within cells where multiple biological events occur, such as Ca2+ signaling, lipid metabolism, mitochondrial dynamics, apoptosis, etc (Giamogante et al., 2021; Li et al., 2022).
3.1 Ca2+ signaling
Ca2+ is known to play a crucial role in cell proliferation, growth, and death (Giorgi et al., 2018). The transduction of Ca2+ between the ER and mitochondria is a complex process that involves various protein complexes. The IP3R1-GRP75-VDAC1 complex is considered one of the most prominent regulatory pathways (Basso et al., 2020). The ER releases Ca2+ through IP3R1, resulting in the formation of a region of high Ca2+ concentration near the ER. Additionally, VDAC1, a Ca2+ uptake channel located on the OMM, connects to GRP75 through the cytoplasm. It is worth noting that neither overexpression nor deficiency of GRP75 has been observed to alter the contact distance between the ER and mitochondria. However, it has been found that deficiency of GRP75 reduces the Ca2+ uptake of mitochondria (Szabadkai et al., 2006; Honrath et al., 2017). This protein complex also serves as a molecular scaffold for other Ca2+ regulators. Studies have shown that pyruvate dehydrogenase kinases 4 (Thoudam et al., 2019), DJ-1 (Liu et al., 2019; Basso et al., 2020), and Sig1R (Su et al., 2016) interact with the ER protein chaperone binding immunoglobulin protein (BiP) to maintain the stability of the IP3R-GRP75-VDAC complex, thereby enhancing its Ca2+ regulatory function. Additionally, transient receptor potential melastatine 8 and ryanodine receptor (RyR) are also involved in Ca2+ regulation (Bidaux et al., 2018).
The function of the cardiovascular system is highly dependent on Ca2+ signaling. According to a study, the depletion of the protein kinase RNA-like endoplasmic reticulum kinase (PERK) in cells with diabetic cardiomyopathy resulted in decreased activity of calcineurin and RyR2 channels, which impaired intracellular Ca2+ accumulation (Liu et al., 2014). This may be responsible for ventricular arrhythmias in diabetic cardiomyopathy. Additionally, in cardiomyocytes, FUNDC1, located in the OMM, directly binds to IP3R2 and regulates Ca2+ release. Abnormal Ca2+ metabolism is associated with mitochondrial fission, which may lead to cardiac dysfunction and HF (Wu et al., 2017). Additionally, Ca2+ is known to play a crucial role in vasoconstriction and resistance in VSMCs, and transient receptor potential vanilloid (TRPV) 4 channels have been shown to mediate Ca2+ signaling, thereby regulating blood pressure bidirectionally (Chen et al., 2022).
3.2 Synthesis and transfer of lipids
It is worth noting that while the majority of enzymes involved in lipid synthesis are located in the membranes of the ER, there are also some present in mitochondrial membranes (Rowland and Voeltz, 2012; Petrungaro and Kornmann, 2019; Dong et al., 2024). MAMs are enriched with proteins related to lipid metabolisms, such as phosphatidylserine synthase 1/2 (PSS1/2), phosphatidylethanolamine N-methyltransferase (PEMT) 2, fatty acid CoA ligase 4, phosphatidylserine decarboxylase (PSD), caveolin-1 (CAV1), diacylglycerol O-acyltransferase, and Acyl-coenzyme A: cholesterol acyltransferase/sterol O-acyltransferase (ACAT/SOAT) (Luan et al., 2022; Wang et al., 2021; Zhang et al., 2023). The regulation of common cellular phospholipids, including phosphatidylcholine (PC), phosphatidylethanolamine (PE), and PS, is co-regulated by multiple proteins on MAMs. The PSS1/2 enzyme synthesizes PS on the ER, which is then transferred to the OMM through the ORP5/8-PTPIP51 complex. Subsequently, the PSD on the inner mitochondrial membrane (IMM) converts it to PE (Kimura and Kimura, 2021). PE is transferred from the mitochondria to the ER, where it undergoes PEMT2 methylation to generate PC (Rowland and Voeltz, 2012).
CAV1 is considered a crucial element of MAMs and is believed to play a role in regulating intracellular steroid and lipoprotein metabolism (Sala-Vila et al., 2016). ACAT is responsible for esterifying free cholesterol and storing cholesteryl esters in lipid droplets. It is worth noting that defects in ACAT function have been associated with atherosclerosis (Dove et al., 2006). Phosphatidic acid is synthesized in the ER and then translocated to the mitochondria for modification, ultimately resulting in the production of cardiolipin. This molecule has been shown to possess cardioprotective properties (Zhao and Wang, 2020). There is a strong association between PE and triacylglycerols with CVDs (Stegemann et al., 2014). The presence of excess lipids has been observed to create an intracellular environment that promotes Drp1 acetylation. As a result, there is an increase in its activity and mitochondrial translocation, which has been linked to cardiomyocyte dysfunction and death (Hu et al., 2020).
3.3 Mitochondrial dynamics
Mitochondria are organelles that undergo constant division and fusion, which is crucial for cellular function (Youle and van der Bliek, 2012). MFN1/2 and optic atrophy 1 (OPA1) are proteins involved in the regulation of mitochondrial fusion on MAMs, where MFN1/2 regulates the fusion of the OMM and OPA1 regulates the fusion of the IMM (Mishra and Chan, 2016). According to a study, a significant proportion of mitochondrial fission (84%) and fusion (59%) events appear to occur on MAMs, as observed through microscopy (Guo et al., 2018). Mitochondrial fission-mediated contraction takes place at the location of ER tubule-mitochondria contact (Friedman et al., 2011). Several proteins located in MAMs are involved in mitochondrial fission, including Drp1, Fis1, mitochondrial fission factor, and mitochondrial dynamics protein of 49 and 51 kDa, which form a protein complex that tightens mitochondria and initiates fission. Additionally, FUNDC1, inverted formin 2, syntaxin 17 (STX17), and ras analog in brain 32 are also involved (Ji et al., 2017; Luan et al., 2022).
Mitochondrial fusion prevents the loss of mitochondrial DNA and maintains mitochondrial protein synthesis, which is essential for proper mitochondrial function. Studies have shown that imbalances in protein activity may lead to mitochondrial disruption and increased damage to the cardiovascular system. For instance, excessive activity of Drp1 can lead to cardiac dysfunction due to excessive mitochondrial fragmentation (Hu et al., 2020). Metastasis-associated lung adenocarcinoma transcript 1 has been shown to inhibit mitochondrial dynamics and apoptosis through the miR-26b-5p/MFN1 pathway to improve cardiac microcirculation after myocardial infarction (Chen et al., 2021).
3.4 Autophagy
Autophagy is a biological process that is conserved in eukaryotic cells. It is regulated by autophagy-related genes and corresponding proteins (Feng et al., 2015). Studies have shown that autophagosomal membranes and many proteins related to autophagy are associated with MAMs, such as autophagosome markers autophagy-related (ATG) 5/14 (Hamasaki et al., 2013), and mechanistic target of rapamycin (mTOR) complex 2 (Colombi et al., 2011; Gomez-Suaga et al., 2017), a key inducer of autophagy. Furthermore, the VAPB-PTPIP51 complex on MAMs has been found to regulate autophagy (Wu et al., 2023). The depletion of MFN2 has been shown to significantly impair the generation of autophagy induced by starvation (Hailey et al., 2010). Autophagy is a process that selectively removes damaged mitochondria. PTEN-induced kinase 1, located in the damaged OMM, promotes Parkin translocation from the cytoplasm to the OMM, ubiquitinates the OMM protein MFN2 and the ion channel protein (VDAC), and thus promotes mitochondrial degradation (Gelmetti et al., 2017; Barazzuol et al., 2020). Additionally, hypoxia-induced FUNDC1, located in the OMM, has been reported to function as a mitochondrial receptor. It recruits autophagosomes and triggers mitochondrial degradation in response to hypoxia. Furthermore, FUNDC1 has been found to recruit Drp1 in MAMs, thereby promoting mitochondrial fission and autophagy (Wu et al., 2016).
Autophagic is considered to be crucial for maintaining the cardiovascular system. It has been suggested that excessive or insufficient autophagy may contribute to the development of CVDs (Gatica et al., 2015). The knockdown of essential autophagy genes, such as ATG5/7, can result in defective cardiac morphogenesis, particularly valve development and ventricular septum (Lee et al., 2014). In a sepsis model, it was observed that the heart increased autophagy by overexpressing Beclin-1. The inhibition of the mTOR signaling pathway was found to ameliorate septic cardiac dysfunction and alleviate inflammation and fibrosis. Conversely, knocking down Beclin-1 resulted in the opposite effect (Sun et al., 2018). Recent findings suggest that nitric oxide promotes mitochondrial autophagy, which is mediated by MCM8 and the E3 ubiquitin ligase TRIM21, and helps maintain normal coronary artery function (Lin et al., 2023).
3.5 Apoptosis
Apoptosis is a process of active cell death that is genetically controlled, also referred to as programmed cell death. Ca2+ transfer from the ER to the mitochondria plays a crucial role in apoptosis (Carpio et al., 2021). Ca2+ can easily pass through the OMM with the aid of ion channels, while the IMM is impermeable and Ca2+ can only enter through the mitochondrial Ca2+ uniporter, which has a relatively weak affinity for Ca2+ (Xu et al., 2020). Mitochondrial permeability transition pores (mPTP) can be formed when Ca2+ interacts with cyclophilin D and adenine nucleotide translocator. Excessive Ca2+ uptake by mitochondria can cause mPTP opening, which may lead to mitochondrial swelling and rupture of the OMM. This rupture can promote the release of apoptotic factors, including cytochrome C (Carraro et al., 2020). Several factors can impact Ca2+ levels in mitochondria, either directly or indirectly, by affecting the IP3R-GRP75-VDAC complex. For example, it has been observed that Akt, a serine-threonine protein kinase located in MAMs, phosphorylates IP3R. This leads to a reduction in Ca2+ release from the ER and a decrease in cellular sensitivity to Ca2+-dependent apoptosis (Marchi et al., 2017). Additionally, Bcl-2, a member of the Bcl-2 family located on MAMs, can bind to the central regulatory domain of IP3R to inhibit Ca2+ release. It can also indirectly inhibit IP3Rs by regulating their phosphorylation. In contrast, it has been observed that the proapoptotic proteins Bax and Bak of the Bcl-2 family regulate Ca2+ in the ER by binding to IP3R1 and replacing Bcl-2 (Rowland and Voeltz, 2012).
It is worth noting that apoptosis is closely related to the onset, progression, and regression of CVDs. A study reported that oxidative stress promotes PM2.5-induced cardiac injury in hyperlipidemic mice by activating apoptosis (Meng et al., 2022). Another study reported that inhibiting endothelial cell apoptosis can improve vascular dysfunction in vascular complications of type 2 diabetes (Su et al., 2018). Furthermore, a recent study has discovered that TMEM215 regulates Ca2+-mediated apoptosis by inhibiting MAMs, thereby modulating vascular pruning (Zhang et al., 2023).
3.6 Oxidative stress
MAMs are known to play a crucial role in regulating intracellular ROS and Ca2+. It has been reported that ER stress triggers Ca2+ release from the ER to mitochondria through MAMs. Conversely, ROS produced by mitochondria affects the ER, which can worsen ER stress and promote increased Ca2+ release. This, in turn, can lead to mitochondrial dysfunction and apoptosis or necrosis. Endoplasmic Reticulum oxidoreductase 1-alpha (Ero1α) is a critical regulator of protein folding and ER redox homeostasis. Its localization on MAMs is over 75% in oxygen-rich conditions (Gilady et al., 2010; Anelli et al., 2012). One study reported that homocysteine promotes Ero1α expression to produce H2O2 and further triggers ER oxidative stress (Wu et al., 2019). P66Shc, an oxidoreductase located on MAMs, interacts with cytochrome C to produce ROS (H2O2) as a signaling molecule for apoptosis (Giorgio et al., 2005). Another study found that Ca2+ induces the formation of H2O2 nanostructural domains at the MAMs, which modulate Ca2+ signaling and mitochondrial activity (Booth et al., 2016).
It is increasingly evident that oxidative stress contributes to the pathogenesis of CVDs (Senoner and Dichtl, 2019). For instance, one study reported that oxidative stress is associated with endothelial dysfunction in CVDs (Shaito et al., 2022). Another study reported that the promotion of PM2.5-induced cardiac injury in hyperlipidemic mice was associated with the activation of RyR2-regulated Ca2+ channels and apoptosis due to oxidative stress (Meng et al., 2022). Additionally, there are also studies showing that oxidative stress plays a crucial role in the development of HF and that its progression can be inhibited by anti-oxidative stress regulation of activating transcription factor 4 (Wang et al., 2022).
4 Mitochondria-associated endoplasmic reticulum membranes contribute to the mechanisms of several cardiovascular diseases
4.1 Myocardial infarction/Myocardial I/R injury
Acute myocardial infarction (AMI) is a significant cause of mortality globally. Hemodialysis is a vital tool to save the lives of AMI patients, but the resulting myocardial I/R injury is unavoidable. Studies have shown that the pathophysiologic mechanisms of I/R injury are associated with Ca2+ overload, ER stress, oxidative stress, mitochondrial autophagy, and apoptosis (He et al., 2022). Myocardial I/R injury is characterized by mPTP-mediated cell death. Mitochondrial Ca2+ overload triggers the opening of mPTP, resulting in mitochondrial swelling and the release of pro-apoptotic factors (Gao et al., 2020). One study suggested that mitochondrial autophagy could be a potential therapeutic target for treating I/R injury (Yang et al., 2019). After I/R injury, researchers found that liproxstatin-1 protected the heart by decreasing VDAC1 levels and mitochondrial ROS while inducing an increase in the antioxidant glutathione peroxidase 4 (Feng et al., 2019). Moreover, mitochondrial dynamics play an important role in myocardial I/R injury. As ischemia causes mitochondrial fragmentation, which is largely dependent on Drp1 and is associated with increased ROS and Ca2+ overload. Promoting mitochondrial fusion or inhibiting mitochondrial fragmentation may help protect the heart from I/R injury (Chen et al., 2023).
4.2 Diabetic cardiomyopathy
Diabetic cardiomyopathy (DCM) is a cardiomyopathy that is not related to hypertension or coronary artery disease. It is closely associated with a high incidence of HF and mortality in diabetic patients (Liu et al., 2024). The pathology of DCM is characterized by hypertrophy, necrosis, and apoptosis of cardiomyocytes, as well as myocardial interstitial fibrosis. The pathogenesis of DCM is a complex issue that involves multiple factors, such as insulin resistance, myocardial energy metabolism disorder, oxidative stress, inflammatory response, Ca2+ imbalance, autophagy, etc (Ritchie and Abel, 2020). Recent studies have suggested that there is a close association between MAMs and the development of DCM. Specifically, high glucose-induced aberrations of MAMs and mitochondrial dysfunction have been implicated in the pathogenesis of cardiomyopathy (Salin Raj et al., 2023). One study showed that elevated glucose levels were associated with increased levels of FUNDC1, IP3R2, and MAMs, resulting in mitochondrial dysfunction and increased Ca2+ transfer (Wu et al., 2019). Additionally, an in vitro study found that cardiomyocytes cultured in high-glucose and high-fat media displayed excessive mitochondrial fission and low MFN2 expression. The restoration of MFN2 was found to have a positive impact on mitochondrial membrane potential, reducing mitochondrial oxidative stress and alleviating mitochondrial dysfunction in cardiomyocytes (Hu et al., 2019). Furthermore, it was observed that PERK deficiency exhibited a protective effect against high glucose-induced cardiomyocyte apoptosis by reducing ROS-mediated activation of the PERK signaling pathway, which causes ER stress-induced apoptosis (Liu et al., 2013).
4.3 Cardiac hypertrophy/HF
Cardiac hypertrophy is a significant paleopathology of HF. According to studies, TRPV1 has been found to promote the formation of MAMs and stabilize mitochondrial function through the AMP-activated protein kinase/MFN2 pathway in cardiomyocytes. This has been shown to effectively prevent stress-induced cardiac hypertrophy (Wang et al., 2022). HF is a condition that can occur as a result of various CVDs. It is characterized by structural and/or functional abnormalities of the heart that can lead to increased intracardiac pressure and/or insufficient cardiac output, both at rest and during exercise (McDonagh et al., 2021). Previous studies have shown that Ca2+ homeostasis and mitochondrial function play a key role in cardiac remodeling and HF (Chaanine, 2021). FUNDC1 is localized to MAMs and regulates Ca2+ release from the ER by binding to the ER-resident protein IP3R2. Disruption of these interactions can lead to reduced mitochondrial and cytoplasmic Ca2+, which may result in aberrant mitochondrial fission and dysfunction. Ultimately, this may contribute to cardiac dysfunction and HF (Wu et al., 2017). LonP1 is a protease that is known to be localized in the MAMs. Studies have shown that LonP1 deficiency can damage the integrity of MAMs and mitochondrial fusion, which may lead to the activation of the unfolded protein response within the ER. This could potentially result in remodeling of the heart and eventual progression to HF (Li et al., 2023).
4.4 Myocardial injury
Recently, MAMs have been shown to have an important role in myocardial injury. Specifically, studies have demonstrated that dibutyl phthalate can induce ER stress in cardiomyocytes, leading to an increase in MAMs and subsequent mitochondrial damage caused by abnormal Ca2+ transfer. Furthermore, the production of mitochondrial ROS can activate the NLRP3 inflammasome and pyroptosis in cardiomyocytes, which can ultimately result in cardiac injury (Li et al., 2023). In a study, sheep were used as research subjects and were administered Mo and Cd through continuous gavage for 50 days (Peng et al., 2023). The study found that this led to cardiac autophagy and damage to myocardial morphology in sheep, which was attributed to ER stress, mitochondrial dysfunction, and structural disruption of MAMs. According to a recent study, the formation of the MAMs protein FUNDC1 and intracellular Ca2+ levels are regulated by sepsis through the IL-6/STAT3 pathway, which can result in mitochondrial disruption, expression of mitochondrial autophagy proteins, and ROS production, ultimately leading to myocardial dysfunction (Jiang et al., 2022). Moreover, ATG, a scaffolding molecule located on MAMs, may play a significant role in obesity-induced cardiomyopathy. Recently study suggested that STX17 may contribute to cardiac damage associated with obesity through the formation of MAMs, which can lead to mitochondrial Ca2+ overload, O2- accumulation, and lipid peroxidation (Xu et al., 2023). Additionally, long-term consumption of diets high in fat and sucrose may cause abnormal lipid accumulation in both endothelial and myocardial cells in individuals with metabolic syndrome due to induced CAV1 expression. Lipid accumulation and lipotoxicity may have an impact on the destruction of MAMs and mitochondrial remodeling in cardiomyocytes, which could potentially lead to cardiomyocyte apoptosis, cardiac dysfunction, and remodeling (Liu et al., 2023).
4.5 Other CVDs
Recently, it has been found that MAMs have a crucial role in the proliferation of VSMCs. Under hypoxic conditions, the downregulation of the Nogo-B receptor leads to the destruction of MAMs. This, in turn, enhances the phosphorylation of IP3R3 via pAkt and promotes VSMCs proliferation (Yang D. et al., 2019). Furthermore, it has been noted that PEMT located in MAMs plays a significant role in the regulation of phospholipid metabolism. Another study reported that diet-induced atherosclerosis can be prevented by PEMT knockdown (Li et al., 2023). MAMs are significantly involved in age-related CVDs. Specifically, during cardiac aging, the integrity of MAM contact sites was disrupted and the dynamic balance of Ca2+ was dysregulated. Additionally, the expression level of Cisd2, a longevity gene located on MAMs, was observed to decrease with cardiac aging. It is worth noting that high levels of Cisd2 may have a positive effect on delaying cardiac aging and improving age-related cardiac dysfunction (Yeh et al., 2020). Recent studies have suggested that TMEM215 may protect endothelial cells from apoptosis by inhibiting BIK-mediated Ca2+ flow in MAMs by facilitating BiP/BIK complex interactions. The reduction of TMEM215 resulted in notable changes, including an increase in the number of MAMs, a decrease in the distance between the OMM and the ER membrane, and an elevation in mitochondrial Ca2+ and Cyto C levels. These changes are known to help with the regulation of vascular pruning (Zhang et al., 2023).
5 Mitochondria-associated endoplasmic reticulum membranes as potential targets for cardiovascular diseases
Scholars have proposed and explored the possibility of MAMs as potential new targets for the prevention or treatment of CVDs (Table 1). One reported that hyperglycemia was found to increase interactions between mitochondria and ER, as well as mitochondrial apoptosis (Yang et al., 2017). However, exogenous H2S was observed to reduce mitochondrial apoptotic proteins, cytochrome c, mPTP opening, and MFN2 expression, which had a cardioprotective effect. Additionally, researchers discovered that the herbal formula Yiqi Huoxue reduced the Sigma-1 receptor (a chaperone protein on MAMs) and increased IP3R2 expression in infarcted rats, which helped prevent cardiomyocyte hypertrophy (Lou et al., 2021). Another study reported that FeA was found to attenuate MAM alterations induced by high glucose and ameliorate cardiomyopathy in diabetic rats (Salin Raj et al., 2023). This was achieved by activating the pro-apoptotic protein PACS2/IP3R2/FUNDC1/VDAC1 pathway. A recent study found that the herbal extract Luteolin may attenuate palmitic acid-induced lipotoxic myocardial injury via the ER stress-mitochondrial apoptosis pathway (Xiang et al., 2023). However, these pieces of evidence have only been attempted before clinical trials, and further validation and clinical translation are needed in the future.
6 Summary and outlook
MAMs, physical connections between the ER and mitochondria, have been found to play a crucial role in regulating cellular metabolism in both physiological and pathological states. This study provides an overview of the structure and function of MAMs, as well as their mechanism of action in CVDs. Additionally, the clinical significance of MAM-based interventions is discussed. A comprehensive understanding of the regulatory mechanisms of the MAMs in CVDs is important for identifying new therapeutic targets for the prevention or treatment of CVDs (Figure 1). For instance, while ketogenic diets are popular among diabetic patients, it is important to consider their safety. A study found that the ketogenic diet improved metabolic markers and reduced MAMs in patients (Tao et al., 2021). However, the study also points out that the ketogenic diet promoted cardiac fibrosis by inhibiting mitochondrial and T-regulatory cell function. Similarly, Sor has toxic side effects on the heart during tumor treatment and may affect the continuation of treatment. Excitingly, researchers found Sor appears to mediate the downregulation of MFN2 in a toxic-dependent manner, which leads to over-formation of MAMs and Ca2+ overload (Song et al., 2022). This, in turn, causes necrotic apoptosis in cardiomyocytes. Furthermore, overexpression of MFN2 inhibits cardiomyocyte necrotic apoptosis without interfering with the antitumor effect. This approach enables the potential for extended treatment of tumors. In addition, it should be noted that exercise preconditioning has the potential to regulate MAMs, which may contribute to a protective effect on the heart (Lv et al., 2022).
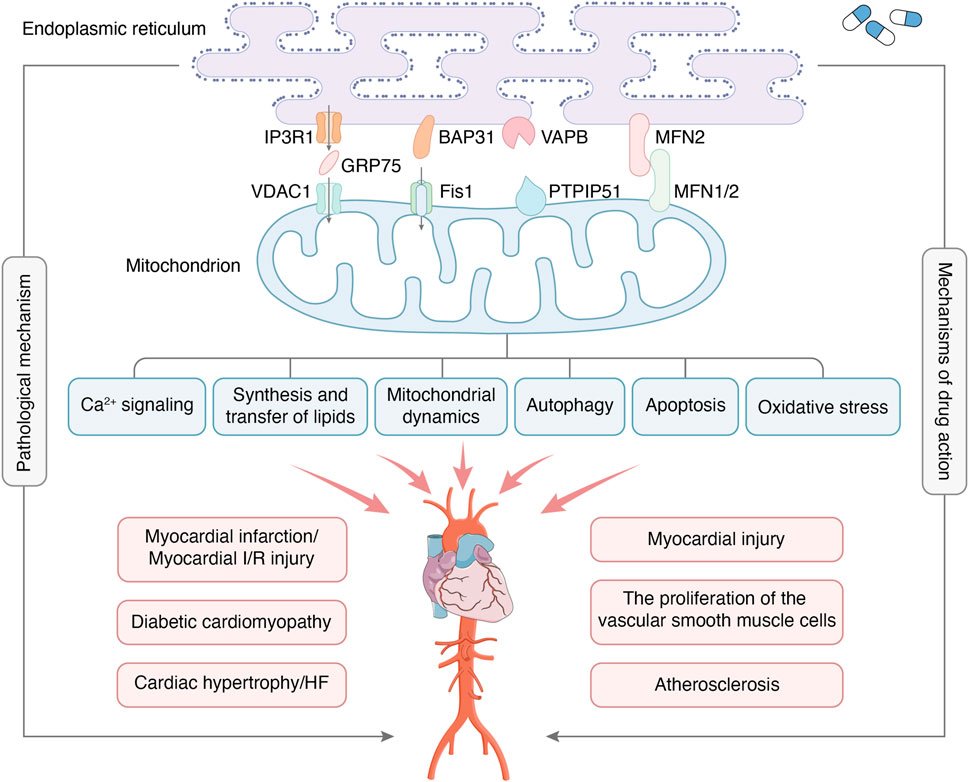
Figure 1. MAMs are regions where the OMM and certain areas of the ER membrane overlap without membrane fusion, which enables communication between organelles and bi-directionally regulates cellular functions through connectivity proteins. The major protein complexes on MAMs include IP3R1-GRP75-VDAC1, BAP31-Fis1, VAPB-PTPIP51, MFN2-MFN1/2, etc. The main functions of MAMs include Ca2+ signaling, synthesis and transfer of lipids, mitochondrial dynamics, autophagy, apoptosis, oxidative stress, etc. MAMs are crucial in developing CVDs, including myocardial infarction, myocardial I/R injury, diabetic cardiomyopathy, etc. At the same time, MAMs have also become the target of drug therapy for CVDs.
Although the structure and function of MAMs and their relationship to CVDs have been recognized, there are still some unknown areas waiting to be explored by humans. Firstly, it is important to investigate whether MAMs have the same structure and function in different tissues of the cardiovascular system. If not, what are the key mechanisms by which they function? Secondly, it is necessary to examine the effects of age, gender, and behavioral factors such as late nights, alcohol consumption, and lack of exercise on MAMs. Do these factors contribute to CVDs through MAMs? Finally, as an important signaling platform, can MAMs play a preventive role as early markers of CVDs? In conclusion, a deeper understanding of the MAMs platform is needed to propose optimal strategies for the prevention and treatment of CVDs.
Author contributions
YD: Conceptualization, Writing–review and editing. NL: Conceptualization, Writing–original draft. DZ: Writing–review and editing. LG: Writing–review and editing. QS: Visualization, Writing–review and editing. YL: Visualization, Writing–review and editing. GR: Supervision, Writing–review and editing. XM: Supervision, Writing–review and editing.
Funding
The author(s) declare that financial support was received for the research, authorship, and/or publication of this article. National Natural Science Foundation of China Project (82374280); National Natural Science Foundation of China Youth Science Fund Project (82104677).
Acknowledgments
I would like to show my deepest gratitude to my supervisor XM, who has walked me through all the stages of the writing of this thesis.
Conflict of interest
The authors declare that the research was conducted in the absence of any commercial or financial relationships that could be construed as a potential conflict of interest.
Publisher’s note
All claims expressed in this article are solely those of the authors and do not necessarily represent those of their affiliated organizations, or those of the publisher, the editors and the reviewers. Any product that may be evaluated in this article, or claim that may be made by its manufacturer, is not guaranteed or endorsed by the publisher.
References
Amini, M., Zayeri, F., and Salehi, M. (2021). Trend analysis of cardiovascular disease mortality, incidence, and mortality-to-incidence ratio: results from global burden of disease study 2017. BMC Public Health 21 (1), 401. doi:10.1186/s12889-021-10429-0
Anelli, T., Bergamelli, L., Margittai, E., Rimessi, A., Fagioli, C., Malgaroli, A., et al. (2012). Ero1α regulates Ca(2+) fluxes at the endoplasmic reticulum-mitochondria interface (MAM). Antioxid. Redox Signal. 16 (10), 1077–1087. doi:10.1089/ars.2011.4004
Barazzuol, L., Giamogante, F., Brini, M., and Calì, T. (2020). PINK1/Parkin mediated mitophagy, Ca2+ signalling, and ER-mitochondria contacts in Parkinson's disease. Int. J. Mol. Sci. 21 (5), 1772. doi:10.3390/ijms21051772
Barazzuol, L., Giamogante, F., and Calì, T. (2021). Mitochondria associated membranes (MAMs): architecture and physiopathological role. Cell Calcium 94, 102343. doi:10.1016/j.ceca.2020.102343
Basso, V., Marchesan, E., and Ziviani, E. (2020). A trio has turned into a quartet: DJ-1 interacts with the IP3R-Grp75-VDAC complex to control ER-mitochondria interaction. Cell Calcium 87, 102186. doi:10.1016/j.ceca.2020.102186
Bidaux, G., Gordienko, D., Shapovalov, G., Farfariello, V., Borowiec, A. S., Iamshanova, O., et al. (2018). 4TM-TRPM8 channels are new gatekeepers of the ER-mitochondria Ca2+ transfer. Biochim. Biophys. Acta. Mol. Cell Res. 1865 (7), 981–994. doi:10.1016/j.bbamcr.2018.04.007
Booth, D. M., Enyedi, B., Geiszt, M., Várnai, P., and Hajnóczky, G. (2016). Redox nanodomains are induced by and control calcium signaling at the ER-mitochondrial interface. Mol. Cell 63 (2), 240–248. doi:10.1016/j.molcel.2016.05.040
Carpio, M. A., Means, R. E., Brill, A. L., Sainz, A., Ehrlich, B. E., and Katz, S. G. (2021). BOK controls apoptosis by Ca2+ transfer through ER-mitochondrial contact sites. Cell Rep. 34 (10), 108827. doi:10.1016/j.celrep.2021.108827
Carraro, M., Jones, K., Sartori, G., Schiavone, M., Antonucci, S., Kucharczyk, R., et al. (2020). The unique cysteine of F-ATP synthase OSCP subunit participates in modulation of the permeability transition pore. Cell Rep. 32 (9), 108095. doi:10.1016/j.celrep.2020.108095
Chaanine, A. H. (2021). Metabolic remodeling and implicated calcium and signal transduction pathways in the pathogenesis of heart failure. Int. J. Mol. Sci. 22 (19), 10579. doi:10.3390/ijms221910579
Chen, W., Zhao, H., and Li, Y. (2023). Mitochondrial dynamics in health and disease: mechanisms and potential targets. Signal Transduct. Target. Ther. 8 (1), 333. doi:10.1038/s41392-023-01547-9
Chen, Y., Li, S., Zhang, Y., Wang, M., Li, X., Liu, S., et al. (2021). The lncRNA Malat1 regulates microvascular function after myocardial infarction in mice via miR-26b-5p/Mfn1 axis-mediated mitochondrial dynamics. Redox Biol. 41, 101910. doi:10.1016/j.redox.2021.101910
Chen, Y. L., Daneva, Z., Kuppusamy, M., Ottolini, M., Baker, T. M., Klimentova, E., et al. (2022). Novel smooth muscle Ca2+-signaling nanodomains in blood pressure regulation. Circulation 146 (7), 548–564. doi:10.1161/CIRCULATIONAHA.121.058607
Colombi, M., Molle, K. D., Benjamin, D., Rattenbacher-Kiser, K., Schaefer, C., Betz, C., et al. (2011). Genome-wide shRNA screen reveals increased mitochondrial dependence upon mTORC2 addiction. Oncogene 30 (13), 1551–1565. doi:10.1038/onc.2010.539
Csordás, G., Renken, C., Várnai, P., Walter, L., Weaver, D., Buttle, K. F., et al. (2006). Structural and functional features and significance of the physical linkage between ER and mitochondria. J. Cell Biol. 174 (7), 915–921. doi:10.1083/jcb.200604016
de Brito, O. M., and Scorrano, L. (2008). Mitofusin 2 tethers endoplasmic reticulum to mitochondria. Nature 456 (7222), 605–610. doi:10.1038/nature07534
De Vos, K. J., Mórotz, G. M., Stoica, R., Tudor, E. L., Lau, K. F., Ackerley, S., et al. (2012). VAPB interacts with the mitochondrial protein PTPIP51 to regulate calcium homeostasis. Hum. Mol. Genet. 21 (6), 1299–1311. doi:10.1093/hmg/ddr559
Dong, J., Chen, L., Ye, F., Tang, J., Liu, B., Lin, J., et al. (2024). Mic19 depletion impairs endoplasmic reticulum-mitochondrial contacts and mitochondrial lipid metabolism and triggers liver disease. Nat. Commun. 15 (1), 168. doi:10.1038/s41467-023-44057-6
Dove, D. E., Su, Y. R., Swift, L. L., Linton, M. F., and Fazio, S. (2006). ACAT1 deficiency increases cholesterol synthesis in mouse peritoneal macrophages. Atherosclerosis 186 (2), 267–274. doi:10.1016/j.atherosclerosis.2005.08.005
Dridi, H., Santulli, G., Gambardella, J., Jankauskas, S. S., Yuan, Q., Yang, J., et al. (2022). IP3 receptor orchestrates maladaptive vascular responses in heart failure. J. Clin. Invest. 132 (4), e152859. doi:10.1172/JCI152859
Feng, Y., Madungwe, N. B., Imam Aliagan, A. D., Tombo, N., and Bopassa, J. C. (2019). Liproxstatin-1 protects the mouse myocardium against ischemia/reperfusion injury by decreasing VDAC1 levels and restoring GPX4 levels. Biochem. Biophys. Res. Commun. 520 (3), 606–611. doi:10.1016/j.bbrc.2019.10.006
Feng, Y., Yao, Z., and Klionsky, D. J. (2015). How to control self-digestion: transcriptional, post-transcriptional, and post-translational regulation of autophagy. Trends Cell Biol. 25 (6), 354–363. doi:10.1016/j.tcb.2015.02.002
Filadi, R., Greotti, E., and Pizzo, P. (2018). Highlighting the endoplasmic reticulum-mitochondria connection: focus on Mitofusin 2. Pharmacol. Res. 128, 42–51. doi:10.1016/j.phrs.2018.01.003
Filadi, R., Greotti, E., Turacchio, G., Luini, A., Pozzan, T., and Pizzo, P. (2015). Mitofusin 2 ablation increases endoplasmic reticulum-mitochondria coupling. Proc. Natl. Acad. Sci. U. S. A. 112 (17), E2174–E2181. doi:10.1073/pnas.1504880112
Friedman, J. R., Lackner, L. L., West, M., DiBenedetto, J. R., Nunnari, J., and Voeltz, G. K. (2011). ER tubules mark sites of mitochondrial division. Science 334 (6054), 358–362. doi:10.1126/science.1207385
Galmes, R., Houcine, A., van Vliet, A. R., Agostinis, P., Jackson, C. L., and Giordano, F. (2016). ORP5/ORP8 localize to endoplasmic reticulum-mitochondria contacts and are involved in mitochondrial function. EMBO Rep. 17 (6), 800–810. doi:10.15252/embr.201541108
Gao, F., Liang, T., Lu, Y. W., Pu, L., Fu, X., Dong, X., et al. (2023). Reduced mitochondrial protein translation promotes cardiomyocyte proliferation and heart regeneration. Circulation 148 (23), 1887–1906. doi:10.1161/CIRCULATIONAHA.122.061192
Gao, P., Yan, Z., and Zhu, Z. (2020). Mitochondria-associated endoplasmic reticulum membranes in cardiovascular diseases. Front. Cell Dev. Biol. 8, 604240. doi:10.3389/fcell.2020.604240
Garcia, M. I., Karlstaedt, A., Chen, J. J., Amione-Guerra, J., Youker, K. A., Taegtmeyer, H., et al. (2017). Functionally redundant control of cardiac hypertrophic signaling by inositol 1,4,5-trisphosphate receptors. J. Mol. Cell. Cardiol. 112, 95–103. doi:10.1016/j.yjmcc.2017.09.006
Gatica, D., Chiong, M., Lavandero, S., and Klionsky, D. J. (2015). Molecular mechanisms of autophagy in the cardiovascular system. Circ. Res. 116 (3), 456–467. doi:10.1161/CIRCRESAHA.114.303788
Gatta, A. T., and Levine, T. P. (2017). Piecing together the patchwork of contact sites. Trends Cell Biol. 27 (3), 214–229. doi:10.1016/j.tcb.2016.08.010
Gelmetti, V., De Rosa, P., Torosantucci, L., Marini, E. S., Romagnoli, A., Di, R. M., et al. (2017). PINK1 and BECN1 relocalize at mitochondria-associated membranes during mitophagy and promote ER-mitochondria tethering and autophagosome formation. Autophagy 13 (4), 654–669. doi:10.1080/15548627.2016.1277309
Giamogante, F., Poggio, E., Barazzuol, L., Covallero, A., and Calì, T. (2021). Apoptotic signals at the endoplasmic reticulum-mitochondria interface. Adv. Protein Chem. Struct. Biol. 126, 307–343. doi:10.1016/bs.apcsb.2021.02.007
Gilady, S. Y., Bui, M., Lynes, E. M., Benson, M. D., Watts, R., Vance, J. E., et al. (2010). Ero1alpha requires oxidizing and normoxic conditions to localize to the mitochondria-associated membrane (MAM). Cell Stress Chaperones 15 (5), 619–629. doi:10.1007/s12192-010-0174-1
Giorgi, C., Danese, A., Missiroli, S., Patergnani, S., and Pinton, P. (2018). Calcium dynamics as a machine for decoding signals. Trends Cell Biol. 28 (4), 258–273. doi:10.1016/j.tcb.2018.01.002
Giorgio, M., Migliaccio, E., Orsini, F., Paolucci, D., Moroni, M., Contursi, C., et al. (2005). Electron transfer between cytochrome c and p66Shc generates reactive oxygen species that trigger mitochondrial apoptosis. Cell 122 (2), 221–233. doi:10.1016/j.cell.2005.05.011
Gomez-Suaga, P., Paillusson, S., and Miller, C. C. J. (2017). ER-mitochondria signaling regulates autophagy. Autophagy 13 (7), 1250–1251. doi:10.1080/15548627.2017.1317913
Gómez-Suaga, P., Pérez-Nievas, B. G., Glennon, E. B., Lau, D. H. W., Paillusson, S., Mórotz, G. M., et al. (2019). The VAPB-PTPIP51 endoplasmic reticulum-mitochondria tethering proteins are present in neuronal synapses and regulate synaptic activity. Acta Neuropathol. Commun. 7 (1), 35. doi:10.1186/s40478-019-0688-4
Guo, Y., Li, D., Zhang, S., Yang, Y., Liu, J. J., Wang, X., et al. (2018). Visualizing intracellular organelle and cytoskeletal interactions at nanoscale resolution on millisecond timescales. Cell 175 (5), 1430–1442. doi:10.1016/j.cell.2018.09.057
Guo, Y. H., Chen, K., Gao, W., Li, Q., Chen, L., Wang, G. S., et al. (2007). Overexpression of Mitofusin 2 inhibited oxidized low-density lipoprotein induced vascular smooth muscle cell proliferation and reduced atherosclerotic lesion formation in rabbit. Biochem. Biophys. Res. Commun. 363 (2), 411–417. doi:10.1016/j.bbrc.2007.08.191
Hailey, D. W., Rambold, A. S., Satpute-Krishnan, P., Mitra, K., Sougrat, R., Kim, P. K., et al. (2010). Mitochondria supply membranes for autophagosome biogenesis during starvation. Cell 141 (4), 656–667. doi:10.1016/j.cell.2010.04.009
Hall, A. R., Burke, N., Dongworth, R. K., Kalkhoran, S. B., Dyson, A., Vicencio, J. M., et al. (2016). Hearts deficient in both Mfn1 and Mfn2 are protected against acute myocardial infarction. Cell Death Dis. 7 (5), e2238. doi:10.1038/cddis.2016.139
Hamasaki, M., Furuta, N., Matsuda, A., Nezu, A., Yamamoto, A., Fujita, N., et al. (2013). Autophagosomes form at ER-mitochondria contact sites. Nature 495 (7441), 389–393. doi:10.1038/nature11910
Han, S., Nandy, P., Austria, Q., Siedlak, S. L., Torres, S., Fujioka, H., et al. (2020). Mfn2 ablation in the adult mouse Hippocampus and cortex causes neuronal death. Cells 9 (1), 116. doi:10.3390/cells9010116
He, J., Liu, D., Zhao, L., Zhou, D., Rong, J., Zhang, L., et al. (2022b). Myocardial ischemia/reperfusion injury: mechanisms of injury and implications for management (Review). Exp. Ther. Med. 23 (6), 430. doi:10.3892/etm.2022.11357
He, X., Liu, J., and Zang, W. J. (2022a). Mitochondrial homeostasis and redox status in cardiovascular diseases: protective role of the vagal system. Free Radic. Biol. Med. 178, 369–379. doi:10.1016/j.freeradbiomed.2021.12.255
Honrath, B., Metz, I., Bendridi, N., Rieusset, J., Culmsee, C., and Dolga, A. M. (2017). Glucose-regulated protein 75 determines ER-mitochondrial coupling and sensitivity to oxidative stress in neuronal cells. Cell Death Discov. 3, 17076. doi:10.1038/cddiscovery.2017.76
Hu, L., Ding, M., Tang, D., Gao, E., Li, C., Wang, K., et al. (2019). Targeting mitochondrial dynamics by regulating Mfn2 for therapeutic intervention in diabetic cardiomyopathy. Theranostics 9 (13), 3687–3706. doi:10.7150/thno.33684
Hu, Q., Zhang, H., Gutiérrez Cortés, N., Wu, D., Wang, P., Zhang, J., et al. (2020). Increased Drp1 acetylation by lipid overload induces cardiomyocyte death and heart dysfunction. Circ. Res. 126 (4), 456–470. doi:10.1161/CIRCRESAHA.119.315252
Iwasawa, R., Mahul-Mellier, A. L., Datler, C., Pazarentzos, E., and Grimm, S. (2011). Fis1 and Bap31 bridge the mitochondria-ER interface to establish a platform for apoptosis induction. EMBO J. 30 (3), 556–568. doi:10.1038/emboj.2010.346
Janikiewicz, J., Szymański, J., Malinska, D., Patalas-Krawczyk, P., Michalska, B., Duszyński, J., et al. (2018). Mitochondria-associated membranes in aging and senescence: structure, function, and dynamics. Cell Death Dis. 9 (3), 332. doi:10.1038/s41419-017-0105-5
Ji, W. K., Chakrabarti, R., Fan, X., Schoenfeld, L., Strack, S., and Higgs, H. N. (2017). Receptor-mediated Drp1 oligomerization on endoplasmic reticulum. J. Cell Biol. 216 (12), 4123–4139. doi:10.1083/jcb.201610057
Jiang, T., Peng, D., Shi, W., Guo, J., Huo, S., Men, L., et al. (2022). IL-6/STAT3 signaling promotes cardiac dysfunction by upregulating FUNDC1-dependent mitochondria-associated endoplasmic reticulum membranes formation in sepsis mice. Front. Cardiovasc. Med. 8, 790612. doi:10.3389/fcvm.2021.790612
Kimura, A. K., and Kimura, T. (2021). Phosphatidylserine biosynthesis pathways in lipid homeostasis: toward resolution of the pending central issue for decades. FASEB J. 35 (1), e21177. doi:10.1096/fj.202001802R
Lee, E., Koo, Y., Ng, A., Wei, Y., Luby-Phelps, K., Juraszek, A., et al. (2014). Autophagy is essential for cardiac morphogenesis during vertebrate development. Autophagy 10 (4), 572–587. doi:10.4161/auto.27649
Li, B., Huo, S., Du, J., Zhang, X., Zhang, J., Wang, Q., et al. (2023c). Dibutyl phthalate causes heart damage by disrupting Ca2+ transfer from endoplasmic reticulum to mitochondria and triggering subsequent pyroptosis. Sci. Total Environ. 892, 164620. doi:10.1016/j.scitotenv.2023.164620
Li, C., Li, L., Yang, M., Zeng, L., and Sun, L. (2020). PACS-2: a key regulator of mitochondria-associated membranes (MAMs). Pharmacol. Res. 160, 105080. doi:10.1016/j.phrs.2020.105080
Li, J., Xin, Y., Li, J., Chen, H., and Li, H. (2023d). Phosphatidylethanolamine N-methyltransferase: from functions to diseases. Aging Dis. 14 (3), 879–891. doi:10.14336/AD.2022.1025
Li, X., Wu, F., Günther, S., Looso, M., Kuenne, C., Zhang, T., et al. (2023a). Inhibition of fatty acid oxidation enables heart regeneration in adult mice. Nature 622 (7983), 619–626. doi:10.1038/s41586-023-06585-5
Li, Y., Huang, D., Jia, L., Shangguan, F., Gong, S., Lan, L., et al. (2023b). LonP1 links mitochondria-ER interaction to regulate heart function. Res. (Wash. D. C.) 6, 0175. doi:10.34133/research.0175
Li, Y. E., Sowers, J. R., Hetz, C., and Ren, J. (2022). Cell death regulation by MAMs: from molecular mechanisms to therapeutic implications in cardiovascular diseases. Cell Death Dis. 13 (5), 504. doi:10.1038/s41419-022-04942-2
Lin, M., Xian, H., Chen, Z., Wang, S., Liu, M., Liang, W., et al. (2023). MCM8-mediated mitophagy protects vascular health in response to nitric oxide signaling in a mouse model of Kawasaki disease. Nat. Cardiovasc. Res. 2, 778–792. doi:10.1038/s44161-023-00314-x
Liu, I. F., Lin, T. C., Wang, S. C., Yen, C. H., Li, C. Y., Kuo, H. F., et al. (2023b). Long-term administration of Western diet induced metabolic syndrome in mice and causes cardiac microvascular dysfunction, cardiomyocyte mitochondrial damage, and cardiac remodeling involving caveolae and caveolin-1 expression. Biol. Direct 18 (1), 9. doi:10.1186/s13062-023-00363-z
Liu, P., Zhang, Z., Chen, H., and Chen, Q. (2024). Pyroptosis: mechanisms and links with diabetic cardiomyopathy. Ageing Res. Rev. 94, 102182. doi:10.1016/j.arr.2023.102182
Liu, T., Wang, L., Chen, G., Tong, L., Ye, X., Yang, H., et al. (2023a). PDZD8-mediated endoplasmic reticulum-mitochondria associations regulate sympathetic drive and blood pressure through the intervention of neuronal mitochondrial homeostasis in stress-induced hypertension. Neurobiol. Dis. 183, 106173. doi:10.1016/j.nbd.2023.106173
Liu, Y., Ma, X., Fujioka, H., Liu, J., Chen, S., and Zhu, X. (2019). DJ-1 regulates the integrity and function of ER-mitochondria association through interaction with IP3R3-Grp75-VDAC1. Proc. Natl. Acad. Sci. U. S. A. 116 (50), 25322–25328. doi:10.1073/pnas.1906565116
Liu, Z., Cai, H., Zhu, H., Toque, H., Zhao, N., Qiu, C., et al. (2014). Protein kinase RNA-like endoplasmic reticulum kinase (PERK)/calcineurin signaling is a novel pathway regulating intracellular calcium accumulation which might be involved in ventricular arrhythmias in diabetic cardiomyopathy. Cell. Signal. 26 (12), 2591–2600. doi:10.1016/j.cellsig.2014.08.015
Liu, Z. W., Zhu, H. T., Chen, K. L., Dong, X., Wei, J., Qiu, C., et al. (2013). Protein kinase RNA-like endoplasmic reticulum kinase (PERK) signaling pathway plays a major role in reactive oxygen species (ROS)-mediated endoplasmic reticulum stress-induced apoptosis in diabetic cardiomyopathy. Cardiovasc. Diabetol. 12, 158. doi:10.1186/1475-2840-12-158
Lou, L., Li, C., Wang, J., Wu, A., Zhang, T., Ma, Z., et al. (2021). Yiqi Huoxue preserves heart function by upregulating the Sigma-1 receptor in rats with myocardial infarction. Exp. Ther. Med. 22 (5), 1308. doi:10.3892/etm.2021.10743
Luan, Y., Jin, Y., Zhang, P., Li, H., and Yang, Y. (2022). Mitochondria-associated endoplasmic reticulum membranes and cardiac hypertrophy: molecular mechanisms and therapeutic targets. Front. Cardiovasc. Med. 9, 1015722. doi:10.3389/fcvm.2022.1015722
Lv, Y., Cheng, L., and Peng, F. (2022). Compositions and functions of mitochondria-associated endoplasmic reticulum membranes and their contribution to cardioprotection by exercise preconditioning. Front. Physiol. 13, 910452. doi:10.3389/fphys.2022.910452
Marchi, S., Bittremieux, M., Missiroli, S., Morganti, C., Patergnani, S., Sbano, L., et al. (2017). Endoplasmic reticulum-mitochondria communication through Ca2+ signaling: the importance of mitochondria-associated membranes (MAMs). Adv. Exp. Med. Biol. 997, 49–67. doi:10.1007/978-981-10-4567-7_4
McDonagh, T. A., Metra, M., Adamo, M., Gardner, R. S., Baumbach, A., Böhm, M., et al. (2021). 2021 ESC Guidelines for the diagnosis and treatment of acute and chronic heart failure. Eur. Heart J. 42 (36), 3599–3726. doi:10.1093/eurheartj/ehab368
Meng, M., Jia, R., Wei, M., Meng, X., Zhang, X., Du, R., et al. (2022). Oxidative stress activates Ryr2-Ca2+ and apoptosis to promote PM2.5-induced heart injury of hyperlipidemia mice. Environ. Saf. 232, 113228. doi:10.1016/j.ecoenv.2022.113228
Mensah, G. A., Fuster, V., and Roth, G. A. (2023). A heart-healthy and stroke-free world: using data to inform global action. J. Am. Coll. Cardiol. 82 (25), 2343–2349. doi:10.1016/j.jacc.2023.11.003
Mishra, P., and Chan, D. C. (2016). Metabolic regulation of mitochondrial dynamics. J. Cell Biol. 212 (4), 379–387. doi:10.1083/jcb.201511036
Missiroli, S., Patergnani, S., Caroccia, N., Pedriali, G., Perrone, M., Previati, M., et al. (2018). Mitochondria-associated membranes (MAMs) and inflammation. Cell Death Dis. 9 (3), 329. doi:10.1038/s41419-017-0027-2
Nakayama, H., Bodi, I., Maillet, M., DeSantiago, J., Domeier, T. L., Mikoshiba, K., et al. (2010). The IP3 receptor regulates cardiac hypertrophy in response to select stimuli. Circ. Res. 107 (5), 659–666. doi:10.1161/CIRCRESAHA.110.220038
Naon, D., Zaninello, M., Giacomello, M., Varanita, T., Grespi, F., Lakshminaranayan, S., et al. (2016). Critical reappraisal confirms that Mitofusin 2 is an endoplasmic reticulum-mitochondria tether. Proc. Natl. Acad. Sci. U. S. A. 113 (40), 11249–11254. doi:10.1073/pnas.1606786113
Paillusson, S., Gomez-Suaga, P., Stoica, R., Little, D., Gissen, P., Devine, M. J., et al. (2017). α-Synuclein binds to the ER-mitochondria tethering protein VAPB to disrupt Ca2+ homeostasis and mitochondrial ATP production. Acta Neuropathol. 134 (1), 129–149. doi:10.1007/s00401-017-1704-z
Parkkinen, I., Their, A., Asghar, M. Y., Sree, S., Jokitalo, E., and Airavaara, M. (2023). Pharmacological regulation of endoplasmic reticulum structure and calcium dynamics: importance for neurodegenerative diseases. Pharmacol. Rev. 75 (5), 959–978. doi:10.1124/pharmrev.122.000701
Peng, C., Yang, S., Yang, F., Xiong, Z., Liu, Q., Liao, S., et al. (2023). Crosstalk between Mfn2-mediated mitochondria associated membranes disorder and autophagy induced by molybdenum and cadmium in sheep heart. Food Chem. Toxicol. 174, 113660. doi:10.1016/j.fct.2023.113660
Petrungaro, C., and Kornmann, B. (2019). Lipid exchange at ER-mitochondria contact sites: a puzzle falling into place with quite a few pieces missing. Curr. Opin. Cell Biol. 57, 71–76. doi:10.1016/j.ceb.2018.11.005
Poston, C. N., Krishnan, S. C., and Bazemore-Walker, C. R. (2013). In-depth proteomic analysis of mammalian mitochondria-associated membranes (MAM). J. Proteomics 79, 219–230. doi:10.1016/j.jprot.2012.12.018
Qiao, X., Jia, S., Ye, J., Fang, X., Zhang, C., Cao, Y., et al. (2017). PTPIP51 regulates mouse cardiac ischemia/reperfusion through mediating the mitochondria-SR junction. Sci. Rep. 7, 45379. doi:10.1038/srep45379
Ren, J., Bi, Y., Sowers, J. R., Hetz, C., and Zhang, Y. (2021). Endoplasmic reticulum stress and unfolded protein response in cardiovascular diseases. Nat. Rev. Cardiol. 18 (7), 499–521. doi:10.1038/s41569-021-00511-w
Ritchie, R. H., and Abel, E. D. (2020). Basic mechanisms of diabetic heart disease. Circ. Res. 126 (11), 1501–1525. doi:10.1161/CIRCRESAHA.120.315913
Rowland, A. A., and Voeltz, G. K. (2012). Endoplasmic reticulum-mitochondria contacts: function of the junction. Nat. Rev. Mol. Cell. Biol. 13 (10), 607–625. doi:10.1038/nrm3440
Sala-Vila, A., Navarro-Lérida, I., Sánchez-Alvarez, M., Bosch, M., Calvo, C., López, J. A., et al. (2016). Interplay between hepatic mitochondria-associated membranes, lipid metabolism and caveolin-1 in mice. Sci. Rep. 6, 27351. doi:10.1038/srep27351
Salin Raj, P., Nair, A., Preetha Rani, M. R., Rajankutty, K., Ranjith, S., and Raghu, K. G. (2023). Ferulic acid attenuates high glucose-induced MAM alterations via PACS2/IP3R2/FUNDC1/VDAC1 pathway activating proapoptotic proteins and ameliorates cardiomyopathy in diabetic rats. Int. J. Cardiol. 372, 101–109. doi:10.1016/j.ijcard.2022.12.003
Senoner, T., and Dichtl, W. (2019). Oxidative stress in cardiovascular diseases: still a therapeutic target? Nutrients 11 (9), 2090. doi:10.3390/nu11092090
Shaito, A., Aramouni, K., Assaf, R., Parenti, A., Orekhov, A., Yazbi, A. E., et al. (2022). Oxidative stress-induced endothelial dysfunction in cardiovascular diseases. Front. Biosci. (Landmark Ed.) 27 (3), 105. doi:10.31083/j.fbl2703105
Song, Z., Song, H., Liu, D., Yan, B., Wang, D., Zhang, Y., et al. (2022). Overexpression of MFN2 alleviates sorafenib-induced cardiomyocyte necroptosis via the MAM-CaMKIIδ pathway in vitro and in vivo. Theranostics 12 (3), 1267–1285. doi:10.7150/thno.65716
Stegemann, C., Pechlaner, R., Willeit, P., Langley, S. R., Mangino, M., Mayr, U., et al. (2014). Lipidomics profiling and risk of cardiovascular disease in the prospective population-based Bruneck study. Circulation 129 (18), 1821–1831. doi:10.1161/CIRCULATIONAHA.113.002500
Stoica, R., De Vos, K. J., Paillusson, S., Mueller, S., Sancho, R. M., Lau, K. F., et al. (2014). ER-mitochondria associations are regulated by the VAPB-PTPIP51 interaction and are disrupted by ALS/FTD-associated TDP-43. Nat. Commun. 5, 3996. doi:10.1038/ncomms4996
Su, J., An, X. R., Li, Q., Li, X. X., Cong, X. D., and Xu, M. (2018). Improvement of vascular dysfunction by argirein through inhibiting endothelial cell apoptosis associated with ET-1/Nox4 signal pathway in diabetic rats. Sci. Rep. 8 (1), 12620. doi:10.1038/s41598-018-30386-w
Su, T. P., Su, T. C., Nakamura, Y., and Tsai, S. Y. (2016). The sigma-1 receptor as a pluripotent modulator in living systems. Trends Pharmacol. Sci. 37 (4), 262–278. doi:10.1016/j.tips.2016.01.003
Sun, D., Li, C., Liu, J., Wang, Z., Liu, Y., Luo, C., et al. (2019). Expression profile of microRNAs in hypertrophic cardiomyopathy and effects of microRNA-20 in inducing cardiomyocyte hypertrophy through regulating gene MFN2. DNA Cell Biol. 38 (8), 796–807. doi:10.1089/dna.2019.4731
Sun, Y., Yao, X., Zhang, Q. J., Zhu, M., Liu, Z. P., Ci, B., et al. (2018). Beclin-1-Dependent autophagy protects the heart during sepsis. Circulation 138 (20), 2247–2262. doi:10.1161/CIRCULATIONAHA.117.032821
Szabadkai, G., Bianchi, K., Várnai, P., De Stefani, D., Wieckowski, M. R., Cavagna, D., et al. (2006). Chaperone-mediated coupling of endoplasmic reticulum and mitochondrial Ca2+ channels. J. Cell Biol. 175 (6), 901–911. doi:10.1083/jcb.200608073
Tao, J., Chen, H., Wang, Y. J., Qiu, J. X., Meng, Q. Q., Zou, R. J., et al. (2021). Ketogenic diet suppressed T-regulatory cells and promoted cardiac fibrosis via reducing mitochondria-associated membranes and inhibiting mitochondrial function. Oxid. Med. Cell. Longev. 2021, 5512322. doi:10.1155/2021/5512322
Thoudam, T., Ha, C. M., Leem, J., Chanda, D., Park, J. S., Kim, H. J., et al. (2019). PDK4 augments ER-mitochondria contact to dampen skeletal muscle insulin signaling during obesity. Diabetes 68 (3), 571–586. doi:10.2337/db18-0363
Vance, J. E. (1990). Phospholipid synthesis in a membrane fraction associated with mitochondria. J. Biol. Chem. 265 (13), 7248–7256. doi:10.1016/s0021-9258(19)39106-9
Vinay Kumar, C., Kumar, K. M., Swetha, R., Ramaiah, S., and Anbarasu, A. (2014). Protein aggregation due to nsSNP resulting in P56S VABP protein is associated with amyotrophic lateral sclerosis. J. Theor. Biol. 354, 72–80. doi:10.1016/j.jtbi.2014.03.027
Wang, C., Dai, X., Wu, S., Xu, W., Song, P., and Huang, K. (2021b). FUNDC1-dependent mitochondria-associated endoplasmic reticulum membranes are involved in angiogenesis and neoangiogenesis. Nat. Commun. 12 (1), 2616. doi:10.1038/s41467-021-22771-3
Wang, N., Wang, C., Zhao, H., He, Y., Lan, B., Sun, L., et al. (2021a). The MAMs structure and its role in cell death. Cells 10 (3), 657. doi:10.3390/cells10030657
Wang, X., Wen, Y., Dong, J., Cao, C., and Yuan, S. (2018). Systematic in-depth proteomic analysis of mitochondria-associated endoplasmic reticulum membranes in mouse and human testes. Proteomics 18 (14), e1700478. doi:10.1002/pmic.201700478
Wang, X., Zhang, G., Dasgupta, S., Niewold, E. L., Li, C., Li, Q., et al. (2022a). ATF4 protects the heart from failure by antagonizing oxidative stress. Circ. Res. 131 (1), 91–105. doi:10.1161/CIRCRESAHA.122.321050
Wang, Y., Li, X., Xu, X., Qu, X., and Yang, Y. (2022b). Transient receptor potential vanilloid type 1 protects against pressure overload-induced cardiac hypertrophy by promoting mitochondria-associated endoplasmic reticulum membranes. J. Cardiovasc. Pharmacol. 80 (3), 430–441. doi:10.1097/FJC.0000000000001301
Wang, Y., Zhang, X., Wen, Y., Li, S., Lu, X., Xu, R., et al. (2021c). Endoplasmic reticulum-mitochondria contacts: a potential therapy target for cardiovascular remodeling-associated diseases. Front. Cell Dev. Biol. 9, 774989. doi:10.3389/fcell.2021.774989
Wu, H., Chen, W., Chen, Z., Li, X., and Wang, M. (2023). Novel tumor therapy strategies targeting endoplasmic reticulum-mitochondria signal pathways. Ageing Res. Rev. 88, 101951. doi:10.1016/j.arr.2023.101951
Wu, S., Lu, Q., Ding, Y., Wu, Y., Qiu, Y., Wang, P., et al. (2019b). Hyperglycemia-driven inhibition of AMP-activated protein kinase α2 induces diabetic cardiomyopathy by promoting mitochondria-associated endoplasmic reticulum membranes in vivo. Circulation 139 (16), 1913–1936. doi:10.1161/CIRCULATIONAHA.118.033552
Wu, S., Lu, Q., Wang, Q., Ding, Y., Ma, Z., Mao, X., et al. (2017). Binding of FUN14 domain containing 1 with inositol 1,4,5-trisphosphate receptor in mitochondria-associated endoplasmic reticulum membranes maintains mitochondrial dynamics and function in hearts in vivo. Circulation 136 (23), 2248–2266. doi:10.1161/CIRCULATIONAHA.117.030235
Wu, W., Lin, C., Wu, K., Jiang, L., Wang, X., Li, W., et al. (2016). FUNDC1 regulates mitochondrial dynamics at the ER-mitochondrial contact site under hypoxic conditions. EMBO J. 35 (13), 1368–1384. doi:10.15252/embj.201593102
Wu, X., Zhang, L., Miao, Y., Yang, J., Wang, X., Wang, C. C., et al. (2019a). Homocysteine causes vascular endothelial dysfunction by disrupting endoplasmic reticulum redox homeostasis. Redox Biol. 20, 46–59. doi:10.1016/j.redox.2018.09.021
Xiang, Y., Liang, X., Bao, C. Y., and liu, T. (2023). The role of Luteolin in regulating the endoplasmic reticulum stress-mitochondrial apoptosis pathway in lipotoxic myocardial injury. J. Hubei Univ. Sci. Technol. Med. Sci. 37 (03), 185–189+195+180. doi:10.16751/j.cnki.2095-4646.2023.03.0185
Xu, H., Yu, W., Sun, M., Bi, Y., Wu, N. N., Zhou, Y., et al. (2023). Syntaxin17 contributes to obesity cardiomyopathy through promoting mitochondrial Ca2+ overload in a Parkin-MCUb-dependent manner. Metabolism 143, 155551. doi:10.1016/j.metabol.2023.155551
Xu, H. X., Cui, S. M., Zhang, Y. M., and Ren, J. (2020). Mitochondrial Ca2+ regulation in the etiology of heart failure: physiological and pathophysiological implications. Acta Pharmacol. Sin. 41 (10), 1301–1309. doi:10.1038/s41401-020-0476-5
Yang, F., Yu, X., Li, T., Wu, J., Zhao, Y., Liu, J., et al. (2017). Exogenous H2S regulates endoplasmic reticulum-mitochondria cross-talk to inhibit apoptotic pathways in STZ-induced type I diabetes. Am. J. Physiol. Endocrinol. Metab. 312 (3), E190–E203. doi:10.1152/ajpendo.00196.2016
Yang, J., Sun, M., Chen, R., Ye, X., Wu, B., Liu, Z., et al. (2023). Mitochondria-associated membrane protein PACS2 maintains right cardiac function in hypobaric hypoxia. iScience 26 (4), 106328. doi:10.1016/j.isci.2023.106328
Yang, M., Linn, B. S., Zhang, Y., and Ren, J. (2019a). Mitophagy and mitochondrial integrity in cardiac ischemia-reperfusion injury. Acta. Mol. Basis Dis. 1865 (9), 2293–2302. doi:10.1016/j.bbadis.2019.05.007
Yang, Y., and Luan, Y. (2023). Editorial: recent advances in mitochondria-associated endoplasmic reticulum membranes (MAMs) in heart-related diseases. Front. Cardiovasc. Med. 10, 1168152. doi:10.3389/fcvm.2023.1168152
Yang, Y. D., Li, M. M., Xu, G., Feng, L., Zhang, E. L., Chen, J., et al. (2019b). Nogo-B receptor directs mitochondria-associated membranes to regulate vascular smooth muscle cell proliferation. Int. J. Mol. Sci. 20 (9), 2319. doi:10.3390/ijms20092319
Yeh, C. H., Chou, Y. J., Kao, C. H., and Tsai, T. F. (2020). Mitochondria and calcium homeostasis: Cisd2 as a big player in cardiac ageing. Int. J. Mol. Sci. 21 (23), 9238. doi:10.3390/ijms21239238
Youle, R. J., and van der Bliek, A. M. (2012). Mitochondrial fission, fusion, and stress. Science 337 (6098), 1062–1065. doi:10.1126/science.1219855
Zhang, J., Wang, L., Xie, W., Hu, S., Zhou, H., Zhu, P., et al. (2020). Melatonin attenuates ER stress and mitochondrial damage in septic cardiomyopathy: a new mechanism involving BAP31 upregulation and MAPK-ERK pathway. J. Cell. Physiol. 235 (3), 2847–2856. doi:10.1002/jcp.29190
Zhang, P., Yan, X., Zhang, X., Liu, Y., Feng, X., Yang, Z., et al. (2023b). TMEM215 prevents endothelial cell apoptosis in vessel regression by blunting BIK-regulated ER-to-mitochondrial Ca influx. Circ. Res. 133 (9), 739–757. doi:10.1161/CIRCRESAHA.123.322686
Zhang, Y., Yao, J., Zhang, M., Wang, Y., and Shi, X. (2023a). Mitochondria-associated endoplasmic reticulum membranes (MAMs): possible therapeutic targets in heart failure. Cardiovasc. Med. 10, 1083935. doi:10.3389/fcvm.2023.1083935
Zhao, H., and Wang, T. (2020). PE homeostasis rebalanced through mitochondria-ER lipid exchange prevents retinal degeneration in Drosophila. PLoS Genet. 16 (10), e1009070. doi:10.1371/journal.pgen.1009070
Zheng, S., Wang, X., Zhao, D., Liu, H., and Hu, Y. (2023). Calcium homeostasis and cancer: insights from endoplasmic reticulum-centered organelle communications. Trends Cell Biol. 33 (4), 312–323. doi:10.1016/j.tcb.2022.07.004
Zhou, X., Jiang, Y., Wang, Y., Fan, L., Zhu, Y., Chen, Y., et al. (2023). Endothelial FIS1 DeSUMOylation protects against hypoxic pulmonary hypertension. Circ. Res. 133 (6), 508–531. doi:10.1161/CIRCRESAHA.122.321200
Glossary
Keywords: cardiovascular diseases, mitochondria-associated endoplasmic reticulum membrane, therapeutic target, coronary atherosclerosis, myocardial ischemia
Citation: Ding Y, Liu N, Zhang D, Guo L, Shang Q, Liu Y, Ren G and Ma X (2024) Mitochondria-associated endoplasmic reticulum membranes as a therapeutic target for cardiovascular diseases. Front. Pharmacol. 15:1398381. doi: 10.3389/fphar.2024.1398381
Received: 11 March 2024; Accepted: 05 April 2024;
Published: 17 April 2024.
Edited by:
Yuxiang Dong, University of Nebraska Medical Center, United StatesReviewed by:
Derek Leas, University of Florida, United StatesWangbin Wu, University of Nebraska Medical Center, United States
Copyright © 2024 Ding, Liu, Zhang, Guo, Shang, Liu, Ren and Ma. This is an open-access article distributed under the terms of the Creative Commons Attribution License (CC BY). The use, distribution or reproduction in other forums is permitted, provided the original author(s) and the copyright owner(s) are credited and that the original publication in this journal is cited, in accordance with accepted academic practice. No use, distribution or reproduction is permitted which does not comply with these terms.
*Correspondence: Xiaochang Ma, bWF4aWFvY2hhbmcxMTE4QDE2My5jb20=
†These authors have contributed equally to this work