- Department of Pharmaceutical Sciences, School of Pharmacy, College of Health and Human Sciences, North Dakota State University, Fargo, ND, United States
Brain-targeted gene delivery across the blood-brain barrier (BBB) is a significant challenge in the 21st century for the healthcare sector, particularly in developing an effective treatment strategy against Alzheimer’s disease (AD). The Internal architecture of the brain capillary endothelium restricts bio-actives entry into the brain. Additionally, therapy with nucleic acids faces challenges like vulnerability to degradation by nucleases and potential immune responses. Functionalized nanocarrier-based gene delivery approaches have resulted in safe and effective platforms. These nanoparticles (NPs) have demonstrated efficacy in protecting nucleic acids from degradation, enhancing transport across the BBB, increasing bioavailability, prolonging circulation time, and regulating gene expression of key proteins involved in AD pathology. We provided a detailed review of several nanocarriers and targeting ligands such as cell-penetrating peptides (CPPs), endogenous proteins, and antibodies. The utilization of functionalized NPs extends beyond a singular system, serving as a versatile platform for customization in related neurodegenerative diseases. Only a few numbers of bioactive regimens can go through the BBB. Thus, exploring functionalized NPs for brain-targeted gene delivery is of utmost necessity. Currently, genes are considered high therapeutic potential molecules for altering any disease-causing gene. Through surface modification, nanoparticulate systems can be tailored to address various diseases by replacing the target-specific molecule on their surface. This review article presents several nanoparticulate delivery systems, such as lipid NPs, polymeric micelles, exosomes, and polymeric NPs, for nucleic acids delivery to the brain and the functionalization strategies explored in AD research.
1 Introduction
Alzheimer’s Disease (AD) is recognized as the leading cause of dementia among people aged 65 and older. It is characterized by the loss of neuronal cells due to the buildup of extracellular amyloid plaques and intraneuronal neurofibrillary tangles in the brain (Bloom, 2014; Singh et al., 2022). This sequence of events eventually leads to reduced cognition, memory loss, and trouble with performing everyday tasks (Burns and Iliffe, 2009). Consequently, it poses a significant burden on global health, affecting millions of individuals and their families. The World Health Organization (WHO) projects an increase in dementia cases globally from about 55 million in 2019 to over 139 million by the year 2050, with AD accounting for 60%–70% of cases (WHO, 2023).1 As the population continues to age, the prevalence of AD will be on the rise, making it a major public health concern.
1.1 Treatment options for AD
Currently, treatment strategies for AD mainly focus on addressing symptoms rather than treating the underlying cause. Until recently, the Food and Drug Administration (FDA) has approved various treatments, encompassing cholinesterase inhibitors such as donepezil, rivastigmine, and galantamine, along with glutamate regulators like memantine and anti-amyloid monoclonal antibodies such as lecanemab and aducanumab.
1.1.1 Anti-Amyloid Monoclonal Antibodies
Aducanumab, marketed as Aduhelm, marks the first disease-modifying medication approved for individuals with AD. Aducanumab received FDA approval in June 2021 (Dunn et al., 2021). Nonetheless, the European Medicine Agency (EMA) refused marketing authorization for aducanumab in December 2021 because results from clinical studies did not convincingly show the effectiveness of the drug in AD treatment, and brain scan images showed brain swelling or bleeding, questioning the safety of the drug (Villain et al., 2022). As an IgG1 monoclonal antibody, it targets extracellular β-amyloid (Aβ) plaques in the brain, binding to and assisting in removing them. Aducanumab is administered intravenously for an hour every 4 weeks (Dunn et al., 2021; Walsh et al., 2021). Despite its conditional approval, clinical studies on Aducanumab show a decline in Aβ plaque burden, but this reduction does not correlate with any beneficial effects on cognition among patients. The aducanumab FDA approval notice has lifted the spirits of patients living with AD and advocacy organizations. Apart from being the initial treatment aimed at modifying the disease’s pathology, it is anticipated to pave the way for developing comparable therapies in the near future. However, despite receiving accelerated approval from FDA for AD treatment in 2021, Aducanumab is scheduled to be discontinued by its manufacturer, Biogen, in 2024. This decision is part of a strategic reallocation of resources towards other treatments, including Lecanemab, to further advance the development of novel therapeutic approaches. Notably, the discontinuation is not predicated on concerns about safety or efficacy (Biogen, 2024).2 The company announced that individuals who are currently participating in clinical trials for Aducanumab will be able to continue doing so until 24 May 2024. In addition, those getting prescriptions will have continued availability until 1 November 2024 (Alzheimer’s Association, 2024).3
In January 2023, the FDA approved Lecanemab (Leqembi), a novel disease-modifying human monoclonal antibody targeting amyloid-beta (Aβ), for the treatment of AD. Lecanemab is given intravenously at a 10 mg/kg dose every 2 weeks (Swanson et al., 2021).
Lecanemab demonstrated effectiveness in reducing beta-amyloid accumulation, thereby slowing cognitive decline. It has also exhibited favorable tolerability (Chowdhury and Chowdhury, 2023). As of March 2024, Lacanemab is still under review by EMA (Eisai, 2024).4
1.1.2 Cholinesterase inhibitors
At present, cholinesterase inhibitors stand as the primary pharmacological intervention for AD. Clinically, the three principal cholinesterase inhibitors employed are donepezil, rivastigmine, and galantamine. AD is characterized by a concurrent decline in cholinergic neurons and a reduced acetylcholine levels within the brain cortex. Numerous studies have demonstrated that augmenting acetylcholine levels in individuals with dementia contributes to the reduction of cognitive decline. The mechanism of action of cholinesterase inhibitors involves hindering the degradation of acetylcholine, leading to enhanced cholinergic activity, thereby benefiting the patient (Hampel et al., 2019). The FDA approved donepezil in 1996. Its associated side effects include sleep disturbances, irregular heart rhythms, seizures, and nausea (Shintani and Uchida, 1997). Subsequently, in 1997 and 2001, FDA approved rivastigmine and galantamine, respectively, for AD pharmacotherapy. Donepezil and galantamine received approval through the European mutual recognition procedures in 1997 (2011 in the Netherlands) and 2000 respectively, while rivastigmine was approved through EMA in 1998 (Dekker et al., 2019). Similar to donepezil, both rivastigmine and galantamine operate by reversibly inhibiting acetylcholinesterase (AChE), thereby enhancing the intrinsic activity of acetylcholine on cholinergic receptors (McShane et al., 2019). Donepezil is given as an oral tablet at a dose of 5 or 10 mg/day, but for moderate to severe cases of AD, a dose of 23 mg/day oral tablet is administered. Rivastigmine is administered as a transdermal patch at a dose of 13.3 mg every 24 h. Galantamine is used for mild to moderate AD cases, and it is administered orally at 16–24 mg/day (Deardorff et al., 2015). Although cholinesterase inhibitors have been utilized in treating AD, they specifically target symptom relief in patients and do not hinder the progression of the condition. The use of donepezil results in slight improvements in cognitive function but does not improve the treatment costs, caregiver duties, or length of hospital stays (Knowles, 2006).
1.1.3 Glutamatergic modulators
The brain relies on glutamate as its primary neurotransmitter for excitatory signaling. Overstimulation, especially in N-methyl-D-aspartate (NMDA) receptors, can lead to neurodegeneration. However, completely blocking NMDA receptors has led to notable adverse effects. Therefore, the development of memantine, an uncompetitive NMDA receptor antagonist, provides therapeutic advantages by regulating NMDA receptor activation. This strategy aims to safeguard patients from inhibitory effects resulting from excessive activation (Johnson and Kotermanski, 2006). Memantine is employed to manage moderate-to-severe AD in various regions, including the United States, China, Canada, and Europe. It obtained approval from FDA in 2003 and EMA in 2002 (Dekker et al., 2019). In the central nervous system, memantine functions as an antagonist to N-methyl-D-aspartate glutamate receptors (NMDAR), offering a therapeutic approach for AD (Popik et al., 2003). It is administered orally at 5–20 mg/day (Reisberg et al., 2003).
Despite memantine being used to treat AD, its therapeutic benefits mainly target symptom management in patients rather than halting the advancement of the condition. Studies suggest that memantine is proven to be a safe and effective means of improving cognitive function in individuals with advanced AD. However, there is insufficient evidence documenting clinical advantages for those in the early stages of AD (Tampi and van Dyck, 2007).
2 Nucleic acids as a therapeutic target for AD
Addressing the genetic foundations of numerous diseases is swiftly transitioning from a theoretical concept to reality. This is exemplified by the recent endorsements from FDA and EMA for several nucleic-acid-based therapeutics (Kulkarni et al., 2021), and this can be attributed primarily to the distinctive benefits offered by nucleic acid drugs. Unlike traditional pharmaceuticals, which predominantly focus on proteins, genetic medications regulate gene expression to elicit therapeutic outcomes. Introducing exogenous nucleic acids into cells to address malfunctioning genes presents an appealing approach for achieving highly targeted, long-lasting, and potentially curative therapeutic outcomes in inherited and acquired disorders. MicroRNAs (miRNAs), small interfering RNAs (siRNAs), and antisense oligonucleotides (ASOs) represent conventional nucleic acid drugs designed to inhibit target genes through complementary binding to the target RNA.
In contrast, plasmid DNAs (pDNAs) and messenger RNAs (mRNAs) are frequently employed to enhance the expression of specific genes. In addition, clustered regularly interspaced short palindromic repeats (CRISPR)/Cas systems exhibit greater versatility, enabling modulation of target gene expression by amplifying, suppressing, and correcting it (Lu et al., 2023). The approval of the nucleic acid aptamer Macugen by FDA and EMA for the treatment of age-related macular degeneration highlights the potential of nucleic-acid based technology for neurological diseases, including AD (Cunningham et al., 2005). The active substance of Macugen, pegaptanib, was designed to block the action of VEGF, a peptide associated with the clinical manifestation of AD. However, Macugen was discontinued by its manufacturer, Eyetech, mainly due to the superiority of other VEGF inhibitors on the market (Louie, 2022). This progress underscores the promise of leveraging nucleic acid-based approaches in developing innovative treatments for AD. Nonetheless, utilizing nucleic acids as therapeutic agents poses challenges due to their vulnerability to nuclease degradation, potential to trigger immune responses, and unfavorable physicochemical properties that hinder their easy entry into cells. The heightened negative charge density of the nucleic acids further impedes cellular uptake by target cells. In contrast to conventional drugs, the distinct characteristics of nucleic acids for therapeutic purposes necessitate delivery through vectors. Overall, the effectiveness of nucleic acid therapy heavily depends on the delivery vectors guiding the nucleic acid to the intended target sites (Lin and Qi, 2023).
2.1 Nucleic acids and their mechanism of action
Nucleic acids are derived from monomers called nucleotides. Each nucleotide consists of a five-carbon sugar, a nitrogenous base, and at least one phosphate group. Naturally occurring nucleic acids such as ribonucleic acid (RNA) and deoxyribonucleic acid (DNA), play key roles in gene regulation and transmission of genetic information (Minchin and Lodge, 2019). These endogenous genes encode proteins by specifying the amino acid sequence required for protein synthesis. Factors such as gene mutations and chromosomal damage could lead to the development of genetic disorders (Kapeli et al., 2017). In clinical settings, exogenous nucleic acids have been used to treat genetic disorders by supplementing deficient proteins or inhibiting the expression of malfunctioning genes. In this section, we review the mechanisms of action of nucleic acids-based molecules while highlighting their therapeutic potential in AD therapy (Figure 1).
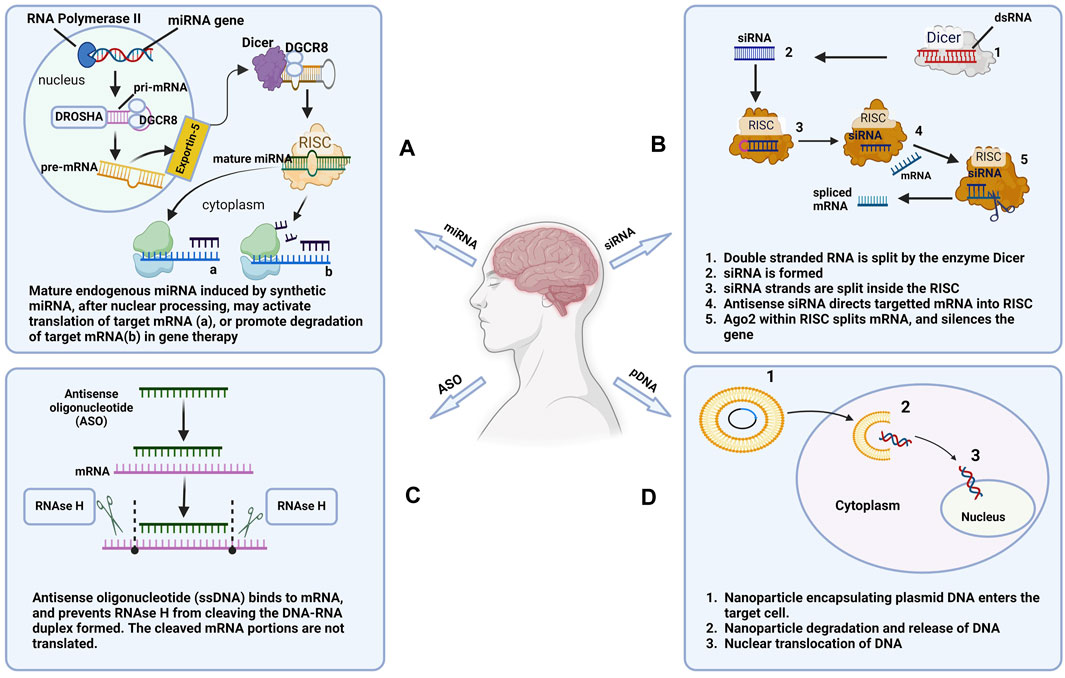
Figure 1. Mechanisms of action of commonly used nucleic acids. (A) microRNA (miRNA), (B) Small interfering RNA (siRNA), (C) Antisense oligonucleotide (ASO), and (D) Plasmid DNA (pDNA) used in AD therapy (Created in Biorender.com).
2.1.1 Plasmid DNA (pDNA)
pDNA usually consists of circular DNA, ranging from 2,000 to 20,000 base pairs. Gene therapy with pDNA involves cloning therapeutically functioning target genes into pDNA (Han et al., 2009). pDNA is frequently derived from recombinant Escherichia coli and utilized after purification to remove endotoxins and RNA. Plasmids are efficiently used in gene therapy due to their stability. Following the cell’s absorption of DNA, it must travel to the nucleus. Under certain conditions, pDNA is integrated into the genetic material of the host chromosome and transmitted to the next-generation through chromosome replication and cell division. The genetic information encoded by the pDNA is translated into a protein (Prather et al., 2003). The basic components of pDNA are the elements necessary for maintenance and reproduction in bacteria, as well as the elements necessary for expression in mammals. The components within bacteria encompass replication origins, genes conferring antibiotic resistance, and additional markers for plasmid amplification. Mammalian expression elements comprise of enhancer/promoter sequences derived from mammals or viruses to drive gene expression. These elements typically include the 5′ UTR, containing reporter transgenes, polyadenylation sequences, and introns (Gill et al., 2009). Naturally occurring apolipoprotein E (APOE) gene plays a role in AD by mediating processes such as cholesterol transport, neuronal signaling, amyloid-beta clearance, and synaptic plasticity. As a result, targeting malfunctioning APOE genes in AD patients with APOE pDNA could serve as a viable therapeutic option (Hunsberger et al., 2019).
Currently, novel therapeutic approaches using pDNA are being explored for neurological conditions, including AD. Researchers have explored stimulating neurotrophins such as Brain-derived neurotrophic factor (BDNF) and Vascular endothelial growth factor (VEGF) in the brain. The neurotrophin BDNF induces the expression of Neurosecretory protein VGF (non-acronymic), which has been shown to decline in AD patients. A study has demonstrated that BDNF and VGF play a significant role in cognitive function. Thus, plasmid forms of BDNF and VGF can potentially alleviate AD symptoms (Lin et al., 2015). In a study conducted in animal models of AD, immunization with a plasmid encoding an Aβ1-42 trimeric fusion protein together with Gal4 responsive promoter elements increased the expression of antigens and the generation of anti-Aβ42 antibodies. The results of the study highlight the potential of pDNA in AD therapy (Qu et al., 2010).
2.1.2 Messenger RNA (mRNA)
mRNA is a lengthy, unbranched polynucleotide consisting of a single strand, ranging from 500 to 5,000 nucleotides. The structure consists of a 3′ poly(A) tail 5′ cap, a 3′ untranslated region, a 5′untranslated region, and an open reading frame (ORF). Upon entering cells, the open reading frame section expresses the encoded protein. At the same time, the remaining regions of the mRNA safeguard it and control its translation (Cobb, 2015). Generally, mRNA translation does not require entry into the cell nucleus, as the process can occur inside the cytoplasm.
Furthermore, mRNA does not integrate into the host’s DNA, ensuring efficient expression of proteins in slowly dividing cells, like neuronal cells. In addition, the risk of serious adverse effects such as carcinogenesis is reduced with mRNA therapy (Sahin et al., 2014). The strength of mRNA in disease management encompasses safety, efficiency, and flexibility. By adjusting regulations and optimizing sequences, a notable enhancement in mRNA stability and translation efficiency can be achieved. Messenger RNA has been shown to be an important therapeutic marker in the diagnosis and treatment of AD. For instance, late stages of AD development are characterized by high levels of beta-site amyloid precursor protein cleaving enzyme 1 (BACE1) mRNA. As such, silencing BACE1 mRNA is a viable therapeutic approach (Holsinger et al., 2002). Attenuated levels of BDNF mRNA were found in Alzheimer patients, further highlighting the potential of mRNA gene therapy in the management of AD (Phillips et al., 1991). The therapeutic potential of mRNAs in AD therapy has been emphasized in research studies using animal models. A study demonstrated that mRNA can be utilized to induce the expression of Neprilysin in the brain of mice. This transmembrane protein can break down individual amyloid beta (Aβ) molecules and small clusters, leading to a decrease in the accumulation of Aβ (Lin et al., 2016).
2.1.3 Small interfering RNA (siRNA)
siRNAs are non-coding RNAs with 20–24 nucleotides that can cause post-transcriptional silencing of the desired gene. The first stage of ribonucleic acid interference entails the processing and cleaving of large double-stranded RNA by the enzyme Dicer into small siRNAs (McNamara et al., 2006). Once formed, the siRNA strands are split up inside the RNA-induced silencing complex (RISC). The more stable 5ʹ end of the siRNA strand is usually added to the active RISC complex. Subsequently, the specific mRNA being targeted is directed towards the RISC by the single-stranded antisense siRNA. After alignment, a protein within the RISC complex, Ago2, catalyzes the cleavage of mRNA (Hammond et al., 2000; Song et al., 2004). While siRNA shows promise in drug development, various barriers hinder its widespread clinical application. Naked siRNA exhibits drawbacks such as poor pharmacokinetic behavior, adverse reactions, and stability concerns.
Regarding stability, siRNA’s phosphodiester bond is susceptible to degradation by ribonucleases and phosphatases. In addition, siRNA is rapidly degraded into fragments by endonucleases and exonucleases following systemic administration, reducing the bioavailability of siRNA at the target sites of action. Although siRNA typically operates effectively when its antisense strand perfectly matches the target mRNA, the RNA-induced silencing complex (RISC) can tolerate some mismatches, which may inadvertently result in unintended gene silencing (Hu et al., 2020). Researchers have investigated siRNA effects on gene silencing, specifically targeting genes associated with AD. Genes encoding Presenilin-1 (PSEN 1), amyloid precursor protein (APP), and APOE have been targeted explicitly with siRNA to induce silencing (Taylor, 2023). A study showed that siRNA can be used to knock down the GSK3β gene, reducing amyloid beta levels in the in vitro AD models (Gupta et al., 2022).
2.1.4 Antagomirs (antimiRs) and microRNAs (miRNAs) mimics
miRNAs, short in nucleotide length and highly conserved in nature, alter gene expression by imitating endogenous miRNAs. The inhibition of gene expression by miRNA involves the repressing of translation or mRNA degradation. In contrast, antimiRs act by blocking the action of endogenous miRNAs (Jonas and Izaurralde, 2015). The transcription of miRNA by RNA polymerases II or III creates a lengthy primary miRNA with a poly(A) tail and a 5′cap. The microprocessor complex processes primary miRNAs in the nucleus into precursor miRNAs, which are small 70-nucleotide hairpin structures. Exportin 5 facilitates the transportation of precursor miRNAs to the cytoplasm. Consequently, the enzyme Dicer converts them into double-stranded miRNA duplexes of around 22 nucleotides (Ha and Kim, 2014). The therapeutic potential of antimiRs and miRNA mimics has been highlighted in several studies. First, the administration of anti-miR candidate AM-206 significantly increased the brain levels of BDNF. In addition, improvements in neurogenesis and memory were observed in AD animal models treated with AM-206 (Lee et al., 2012). In another study, artificial miRNA targeting acetyl-CoA acyl transferase decreased the accumulation of amyloid beta plaque and improved cognitive performance in an AD mouse model (Murphy et al., 2013). Multiple studies have indicated that distinct miRNAs can control various stages of tau processing, such as splicing and post-translational modifications. The hyperphosphorylation of endogenous tau in the adult forebrain when Dicer is depleted suggests that miRNA plays a direct role in tau-related neuropathies. Subsequently, it was demonstrated that the decrease in the miR-15/107 family in the brains of AD patients is directly associated with this phenomenon. Several miRNAs family have been shown to decrease the expression of mitogen activated protein kinases (MAPK 1/3) (Moncini et al., 2017; Safa et al., 2020). MAPK1/3 phosphorylate tau, in a process linked to the existence of NFTs and senile plaques. A study showed that miR-26a regulated the activity of glycogen synthase kinase 3 beta, which is involved in hyperphosphorylation of tau protein. In addition, glycogen synthase kinase 3 beta is associated with the creation of amyloid beta in the brains of AD patients (Maqbool et al., 2016).
2.1.5 Short hairpin RNA (shRNA)
shRNA molecules can be classified into two primary groups: microRNA-adapted shRNA and simple stem-loop shRNA. The longer microRNA-adapted shRNA design, typically exceeding 250 nucleotides, closely mimics native pri-microRNA molecules found in cells. This design features a shRNA stem structure with microRNA-like mismatches, connected by a loop and bordered by 5′ and 3′endogenous microRNA sequences. This configuration enhances the stability and processing of the shRNA by cellular machinery, leading to more efficient gene silencing through RNA interference (Silva et al., 2005). The simple stem-loop shRNA, 50–70 nucleotides long, is transcribed within the nucleus with the guidance of an RNA Polymerase III promoter. The RNA polymerase II promoter produces a primary transcript with a stem-loop structure resembling a hairpin. In the next step, a complex of RNA-binding domain protein DGCR8 and the RNase III enzyme Drosha processes stem-loop structure inside the cell nucleus (Lee et al., 2003). Exportin 5 facilitates the transportation of the pre-shRNA molecule to the cytoplasm. In the cytoplasm, the pre-shRNA is attached to a complex consisting of the RNase III enzyme Dicer and TRBP/PACT. This compound eliminates the hairpin loop, forming a double-stranded siRNA with 2 nucleotide 3′overhangs. Subsequently, the Dicer complex loads RNA molecules onto the RISC complex’s Ago2 protein and degrades complementing mRNA (Cullen, 2004). ShRNA-induced gene silencing shows potential as a therapeutic strategy for treating AD. Recent studies have shown that Transient Receptor Potential Canonical (TRPC) genes play a crucial role in important cellular processes such as neuronal growth, synapse development, and neuronal differentiation (Tai et al., 2009). Following the injection of a vector carrying TRPC6-specific shRNA into the hippocampal dentate gyrus to knockout TRPC6, there were observed impairments in spatial learning and social recognition memory in the mice that received the treatment after a 4-week duration compared to the control group (Xie et al., 2021).
2.1.6 Antisense oligonucleotides (ASOs)
ASOs are synthetic, short, single-stranded oligonucleotides designed to target mRNA transcripts specifically. They regulate protein production by interfering with the mRNA’s function. Antisense mechanisms encompass various strategies such as recruiting RNase H to cleave mRNA, altering splicing in pre-mRNA, and physically blocking pre-mRNAs. As an illustration, RNase H-mediated cleavage requires the construction of a brief DNA oligonucleotide that forms an RNA-DNA duplex by binding to the target mRNA. Subsequently, endogenous RNase H recognizes this duplex and proceeds to cleave it. Generally, antisense oligonucleotides that regulate pre-mRNA splicing can rectify defective RNA and eradicate splice variants associated with diseases (Bennett and Swayze, 2010).
3 Route of administration of nanotherapeutics to the central nervous system (CNS)
There are several ways in which nanoparticles (NPs) encapsulating nucleic acids can be delivered to the CNS for the treatment of neurological diseases. Delivery routes such as intravenous, intranasal, intracerebroventricular, intrathecal, and intraparenchymal are the most common routes of administration being explored in research and clinical settings (Figure 2).
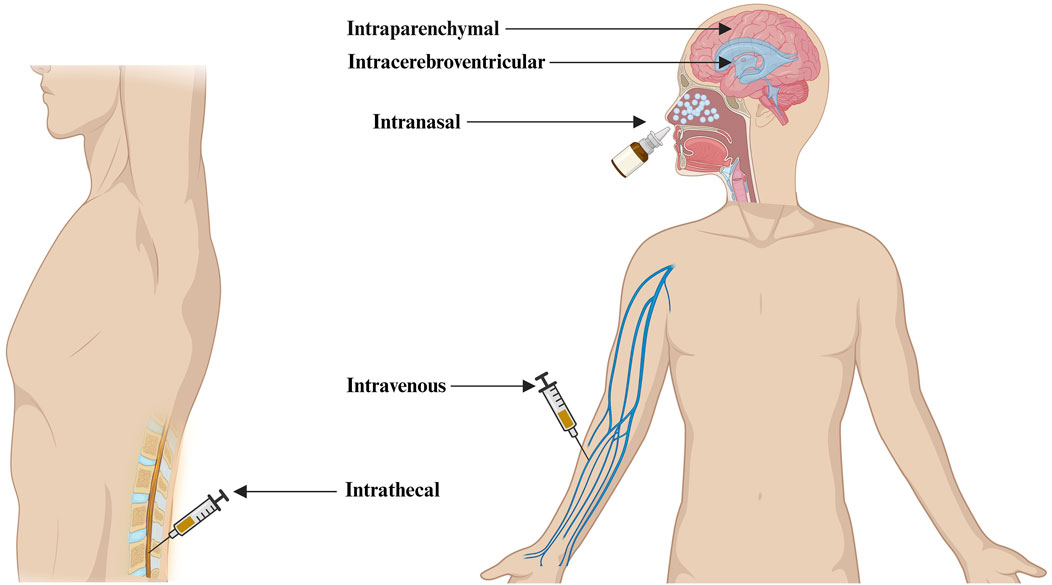
Figure 2. Different routes of administration of nanotherapeutics to the CNS for the treatment of Alzheimer’s disease (Created in Biorender.com).
Direct CNS delivery using intracerebroventricular, Intrathecal, and Intraparenchymal administration routes has the advantage of delivering a much higher concentration of neurotherapeutics into the cerebrospinal fluid or parenchyma space, improving neurotherapeutics retention in the CNS. The intranasal delivery route holds significant potential for administering nanotherapeutics directly to the brain. Nonetheless, its effectiveness is still being explored due to uncertainties surrounding bioavailability and the challenges of ensuring appropriate dosing (D'Souza et al., 2023). Nanotherapeutics administered through an intravenous route goes directly into the bloodstream and may trigger the activation of complement. This activation can result in the release of anaphylatoxins and proinflammatory mediators. One mechanism is the opsonization of NPs with C3b, which interacts with phagocytes (Moghimi et al., 2019). PEGylation of NPs has emerged as a widely employed strategy to mitigate complement activation. A group of scientists conducted preliminary experiments utilizing PEGylated liposomes, affirming the superiority of carboxy-mediated PEGs over their methoxy-terminated counterparts in suppressing complement activation (Yang et al., 2010). Another study has demonstrated that the incorporation of methoxypoly (ethylene glycol) m-PEG-phospholipid into the liposomal bilayer effectively eliminates the risk of rapid vesicle clearance by mononuclear phagocytic cells, achieved through the suppression of protein adsorption and blood opsonization (Pannuzzo et al., 2020). Despite these polymers’ protective effect on NPs against immune system recognition, available data shows the potential of PEG-specific antibodies to form following intravenous administration of PEG-coated liposomes. As a result, these antibodies lead to quicker PEG-liposome clearance from the bloodstream, altering the pharmacokinetic profile of subsequently administered doses. Hence, it is essential to acknowledge that generating particle-specific antibodies may influence both the safety and efficacy of nanoparticle-based therapeutics (Zolnik et al., 2010). Surface modification of NPs with CPPs and brain-specific targeting ligands have been shown to enhance the intravenous administration of bio-actives to the brain (Arora et al., 2021). We have presented the advantages and limitations of different routes of administration of nanotherapeutics to the CNS (Table 1).
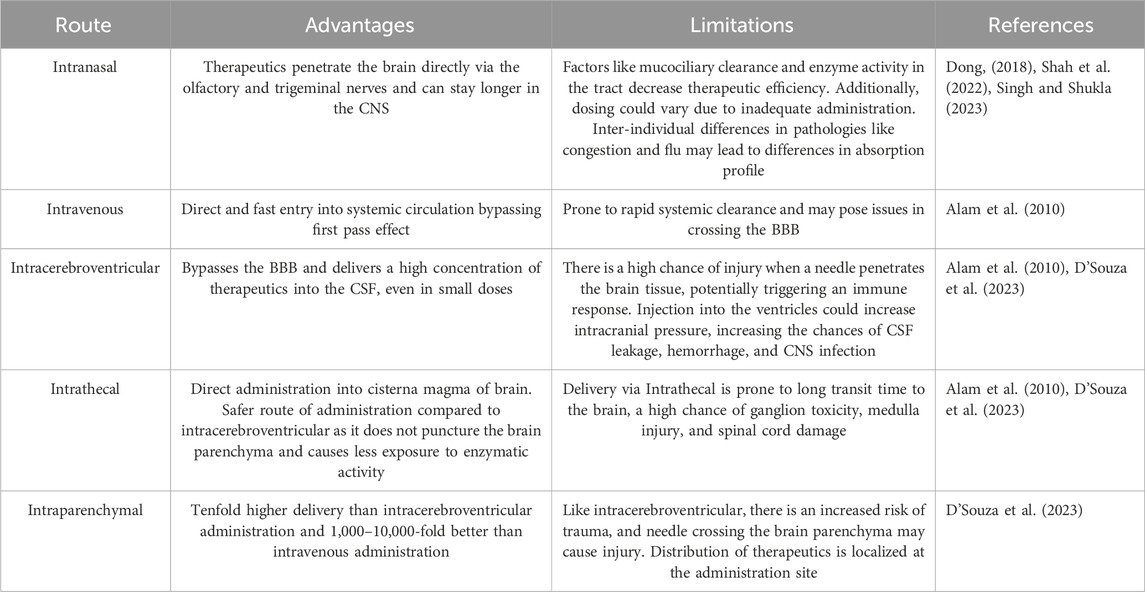
Table 1. Advantages and limitations of different routes of administration of nanotherapeutics to the CNS.
4 Functionalized NPs for delivering nucleic acids to the brain for the treatment of AD
Functionalized NPs assist in the delivery of nucleic acids to the brain for neurodegenerative disorders, providing a chance to improve transportation across the blood-brain barrier (BBB) and contributing positively to therapeutic payload to specific sites within the brain due to their small size and distinctive features. Recent literature has demonstrated that NPs ranging from 12 to 340 nm in size can cross the BBB. Studies have established that endocytosis, a process influenced by size, allows smaller NPs, particularly those less than 100 nm, to be easily engulfed by cells. Additionally, NPs with a positively charged zeta potential enhance BBB penetration through adsorptive-mediated transcytosis (Lombardo et al., 2020). Functionalization of NPs involves surface modifications utilizing targeting ligands such as CPPs, endogenous proteins, or antibodies. This modification strategy serves to mitigate off-target effects, overcome receptor saturation, and augment internalization via receptor-mediated transport across the BBB. Consequently, this increases the uptake of nanosized particles by neuronal cells, thereby optimizing therapeutic efficacy. Functionalized NPs can be customized to carry various nucleic acid payloads like genes, siRNAs, and ASOs without the concerns of viral replication or immune reactions. Additionally, the ability to scale up production and the flexibility in synthesizing NPs make them more suitable for meeting the requirements of nucleic acid therapies (Figure 3).
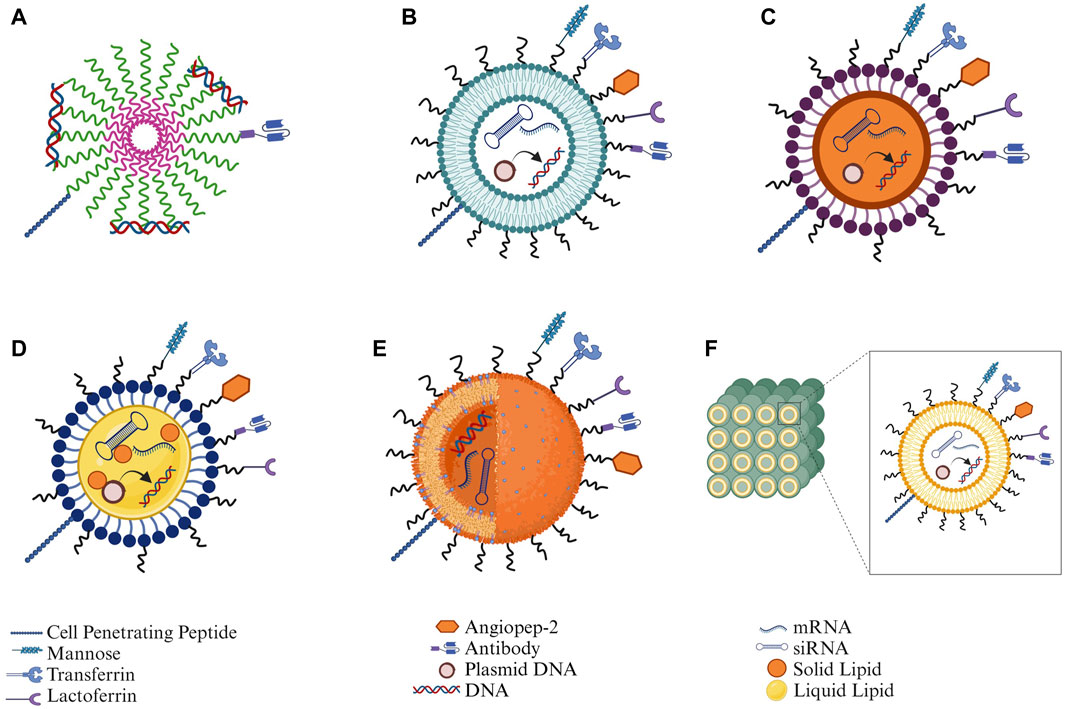
Figure 3. Structural depiction of different types of nanoparticles along with functionalization strategies for nucleic acid delivery: (A) Polymeric micelles, (B) Liposomes, (C) Solid lipid nanoparticles (SLNs), (D) Nanostructured lipid carriers (NLCs), (E) Polymeric nanoparticles, and (F) Cubosomes (Created in Biorender.com).
4.1 Lipid nanoparticles (LNPs)
LNPs are a blend of helper and functional lipids. The main component of functional lipids is an ionizable cationic lipid, and helper lipids such as cholesterol, polyethylene glycol-lipid (PEG-lipid), distearoyl phosphatidylcholine (DSPC), 1,2-distearoyl-sn-glycero-3-phosphorylcholine (DSPC), 1,2-dioleoyl-sn-glycero-3-phosphocholine (DOPC), 1,2-dioleoyl-sn-glycero-3-phosphorylethanolamine (DOPE), 1-stearoyl-2-oleoyl-sn-glycero-3-phosphocholine (SOPC). The ionizable cationic lipids allow for efficient encapsulation of nucleic acids, resulting in a neutral LNP surface charge in circulation, therefore promoting the escape of nucleic acids to cytosol following endocytosis (Kulkarni et al., 2017; Kulkarni et al., 2018). Understanding the many forms of LNPs and their unique properties is essential for the effective delivery of nucleic acid to the brain for the treatment of neurological disorders. Lipid-based carriers can be classified into several categories based on their physicochemical characteristics and how they are being formulated.
4.1.1 Solid Lipid Nanoparticles (SLNs)
SLNs represent lipid-based nanocarrier systems in solid form, primarily comprising lipids such as fatty acids, triglycerides, or modified lipid nanostructures with diameters ranging from 10 to 1,000 nm. These biocompatible vehicles facilitate the distribution of lipophilic and hydrophilic drugs across their solid hydrophobic lipid core (Kaur et al., 2008). Recently, there has been growing interest in SLNs as potential drug delivery systems for brain targeting. SLNs are increasingly recognized for their potential as safe and most cost-effective drug carriers for treating neurodegenerative (Shivananjegowda et al., 2023). Erythropoietin-loaded SLNs showed improved memory in animal models of AD (Dara et al., 2019). In vitro and in vivo experiments show the multifaceted characteristics of SLNs, such as improved bioavailability of active pharmaceuticals, specificity to target sites, regulated drug release profile, minimal immune response with stealth properties, substantial drug loading capacity, and adaptability to various pharmaceutical as well as biopharmaceutical formulations (Martins et al., 2013). Chitosan-coated SLNs provided total encapsulation of BACE1 siRNA, which aided transportation and delivery to olfactory nerves and protected the nucleic acid against enzymatic degradation, hence preserving its therapeutic effect (Rassu et al., 2017). These distinct properties collectively position SLNs as an ideal and versatile drug delivery system with great potential for advancing nucleic acid delivery to the brain.
4.1.2 Nanostructured lipid carriers (NLCs)
NLCs are colloidal systems characterized by binary lipid mixtures featuring a liquid lipid phase embedded within a solid lipid matrix. Unlike SLNs, NLCs circumvent issues of recrystallization and phase separation of encapsulated pharmacological compounds. They offer greater encapsulation efficiency due to their regulated release and increased stability. Additionally, they minimize drug expulsion during storage, ensuring sustained drug levels in the brain over prolonged periods (Fang et al., 2013; Pardeshi and Belgamwar, 2020; Pinheiro et al., 2020).
4.1.3 Liposomes
Artificial lipid-based bilayered vesicles have become one of the most widely used and effective lipid nanocarriers for neurological disorders. Liposomal nanocarriers offer a significant advantage due to their lipophilic properties, primarily in protecting drugs from degradation, thus enhancing their pharmacokinetic profile (Sonali et al., 2016). A study by a group of scientists proved that the concentrations often employed in animal studies showed no toxicity in the blood while in vitro studies showed no interference with the generation of nitric oxide. However, because liposomes lack a targeting molecule, conjugation with peptides, aptamers, or antibodies is crucial to induce targeting effects (Fu et al., 2018; Marcos-Contreras et al., 2020; Ye et al., 2022). Another study showed that functionalized liposomes protect high molecular weight anionic nucleic acids from enzymatic degradation supporting their efficient neuronal targeting and delivery to the brain (Dos Santos Rodrigues et al., 2019b).
4.1.4 Cubosomes
Cubosomes are NPs, which are self-assembling liquid crystalline particles of a certain surfactant with a proper water ratio, as opposed to solid particles. The microstructure of cubosomes gives them unique qualities that are desirable for drug delivery (Garg and Saraf, 2007; Wu et al., 2012). Cubosomes have received much attention recently due to their high drug payload and encapsulation efficiency as well as their ability to stabilize peptides (Landau and Rosenbusch, 1996; Sadhale and Shah, 1999; Barauskas et al., 2005; Azhari et al., 2021), making them probable non-viral vector for nucleic acid delivery to the brain.
4.1.5 Functionalization strategies used in LNPs
LNPs are functionalized via different strategies like surface modification with target ligands such as cell-penetrating peptides, exogenous proteins, and antibodies. CPPs like rabies virus glycoprotein (RVG) peptide, penetratin (PEN) peptide, and exogenous proteins like transferrin (Tf) and lactoferrin (Lf) are some of the common targeting ligands widely used for targeted nucleic acid delivery.
4.1.5.1 Functionalization with cell-penetrating peptides (CPPs)
Researchers have introduced more than 100 CPPs so far which includes cationic and anionic CPPs. CPPs can elevate the cell penetration of a loaded cargo (Sharma et al., 2012). These CPPs have a specific protein sequence known as the protein transduction domain which is responsible for cell penetration (Schmidt et al., 2010). The underlying mechanism of cell penetration of CPPs is still unclear (Reissmann, 2014). The cationic-amphiphilic character of CPPs, such as PEN, helps NPs interact with the lipid components of the cellular membrane and then internalize the cargo into the cell (Zhang et al., 2016). CPPs and brain-specific targeting ligands are being used alone or in combination to facilitate active and efficient gene delivery. A recent study emphasized that formulations incorporating CPPs exhibited brain accumulation levels ranging from 8 to 10 times higher than formulations without the incorporation of CPPs. This observed improvement signifies the efficacy of the K16ApoE CPP in facilitating enhanced delivery of the construct to the brain, emphasizing its potential as a crucial component for optimizing drug transport across biological barriers and elevating therapeutic impact (Ahlschwede et al., 2019).
Transactivating transcriptional activator (TAT), discovered in 1988, is derived from human immunodeficiency virus 1(HIV-1) (Frankel and Pabo, 1988). TAT is considered a powerful transactivator that cells can take up as a viral growth factor. It is composed of 86 amino acids, and since then, it has been used to elevate the transfection potential of LNPs for brain delivery. Transferrin (Tf) is a ligand that harnesses the specificity of Tf-mediated binding to Tf-receptors in brain endothelial cells. An in vivo study of liposomes conjugated with TAT and Tf, aiming to improve the penetrability of pharmaceutical formulations through the BBB, shows that TAT-Tf liposomes are efficacious in transporting therapeutic DNA into the brains of mice. This study signifies a promising advancement in targeted drug delivery, highlighting the potential of surface-modified liposomes to facilitate the transport of therapeutic agents across biological barriers for enhanced therapeutic outcomes (Dos Santos Rodrigues et al., 2019a). Nevertheless, TAT presents a potential challenge in AD therapy, as it can trigger Aβ deposition, tau phosphorylation, and subsequent neuronal demise. These considerations are paramount in developing formulations to cross the BBB for AD treatment (Giunta et al., 2009; Di et al., 2016; Hategan et al., 2017). Recent behavioral studies have revealed promising outcomes with a novel fusion peptide combining BDNF with TAT. This fusion peptide effectively targets multiple biochemical pathways in the brain, significantly enhancing spatial memory, restoring memory-associated proteins, and improving cognitive functions in AD-like animal models (Wu et al., 2015).
RVG peptide is a 29-amino acid peptide derived from Rabies virus glycoprotein, a 505 amino acid type 1 membrane glycoprotein, that can bind precisely to neuronal cells’ acetylcholine receptor (nAchR). This allows for nucleic acid entry into neuronal cells via receptor-mediated nucleic acid transport, resulting in efficient gene delivery to the brain (Kumar et al., 2007; Son et al., 2011; Huey et al., 2017; Dos Santos Rodrigues et al., 2019b).
Angiopep-2, a 19 amino acid peptide from the Kunitz domain, binds preferentially to Low-density lipoprotein receptor-related protein-1 (LRP1) and has enhanced penetrability through the BBB (Bertrand et al., 2011; Xin et al., 2012). In a comparative study, a group of scientists reported that SLNs loaded with Angiopep-2 led to prolonged survival of the animal due to increased drug delivery level to the glioma (Kadari et al., 2018). Covalent surface modification of SLN with synthetic decapeptide derived from the receptor binding region of human Apolipoprotein E (mApoE) enhances penetration of NPs when delivered intra-nasally (Dal Magro et al., 2017).
4.1.5.2 Functionalization with exogenous proteins
Tf-liposomal formulation improved the transport of poor brain penetrability agents to the brain in both in vitro and in vivo studies (Chen et al., 2016). Lactoferrin (Lf) is a cationic iron-binding protein belonging to the transferrin family, characterized by multifaceted functionalities such as anticarcinogenic and anti-inflammatory properties. The Lf exhibits diverse interactions, including engagement with the LDL receptor-related protein, facilitating its transportation to the brain through receptor-mediated endocytosis (Huang et al., 2007). The Lf expression has been observed to be significantly upregulated in both neurons and glia in AD, indicating potential for brain targeting (Kawamata et al., 1993). A study formulated Lf-liposomes, which acts as a brain-specific targeting ligand. The formulation exhibited an improved absorption profile in primary brain capillary endothelial cells, a more remarkable ability to penetrate the BBB in vitro, increased accumulation of the formulation in the brain, and decreased cellular toxicity compared to conventionally formulated liposomes (Chen et al., 2010). Surface Lf has also been shown to efficiently transport Nerve Growth Factor (NGF) as Lf/NGF-liposomes across the BBB, inhibiting the degeneration of SK-N-MC cells with Aβ-induced neurotoxicity (Kuo and Wang, 2014).
In another study with plasmid NGF and plasmid BDNF using CPPs and ligands such as Tf and mannose (MAN), the formulation facilitated enhanced cell penetration and effective receptor targeting to deliver nucleic acids to the brain, showing positive therapeutic responses in vivo (Rodrigues et al., 2020).
4.1.5.3 Functionalization with antibodies
Recently, it was revealed that a new collection of antibodies targeting receptor-mediated transcytosis (RMT) was shown to bind both the human and mouse BBB in brain tissue sections. To improve brain-specific delivery, a study created an efficient RMT antibody-targeted liposomal system employing antibody (scFv46.1). scFv46.1-modified liposomes were shown to be absorbed by cells in vitro. In vivo evaluations showed that antibody-liposome loaded with pralidoxime accumulated in the brain ten times higher than the liposomal control group. These results point to the antibody-liposomal formulation’s potential as a tailored synthetic vehicle for CNS medication delivery (Ye et al., 2022).
Furthermore, using Tf as a targeting ligand has limitations, including the potential to saturate Tf-receptors in the body, thereby interfering with iron uptake. To address this issue, antibodies binding to Tf-receptors through non-Tf sites are being explored as an alternative. OX26, an anti-Tf-receptor monoclonal antibody, interacts with the Tf-receptor’s extracellular domain without hindering Tf-binding in rats. Like Tf, OX26 can be used to conjugate drug carriers for BBB penetration. Human basic fibroblast growth factor (bFGF), a potent neuroprotective agent, was conjugated with Streptavidin and OX26, and brain uptake of neurotrophin bFGF was seen to increase (Wu et al., 2002). For neuroimaging in AD, radio-iodinated Aβ (125I-Aβ40) can be linked to OX26 using a streptavidin-biotin linker after intravenous injection (Wong et al., 2019).
4.1.6 Functionalized LNPs for nucleic acid delivery in AD
The transport and transfection of ApoE2 gene in the in vitro BBB model were markedly improved by dual functionalized liposomes with CPPs such as RVG, PEN, and the brain targeting ligand Mannose (MAN). Despite the inherent challenges in gene delivery to the brain, dual-functionalized liposomes successfully targeted the brain. They expressed the genetic cargo in brain cells, resulting in considerably elevated ApoE expression in the brains of C57BL/6 mice (Arora et al., 2021). This emphasizes how bi-functionalized liposomes could be an efficient gene delivery method in the treatment of AD. In the brains of three-month-old APP/PS1 mice, liposomes dual modified with PEN and Tf encasing chitosan-pNGF complexes boosted NGF levels, stimulated the formation of new cells, and decreased the levels of harmful soluble and insoluble Aβ peptides (Rodrigues et al., 2020). BDNF gene therapy utilizing bi-functionalized liposomes increased pre- and post-synaptic protein levels above baseline levels in age-matched wild-type animals. Furthermore, compared to untreated age-matched transgenic AD mice controls, a significant decrease in the plaque load was seen in treated age-matched AD groups. These findings imply that amyloid pathology may be prevented or attenuated with BDNF restoration via neurotrophin therapy (Arora et al., 2022).
Additionally, the delivery of BACE1 siRNA through functionalized SLNs containing a short peptide of RVG (RVG-19) and coated with chitosan altered the system’s positive Zeta Potential, mucoadhesiveness, and the ability for the siRNA to penetrate epithelial cells, improving intracellular transport (Rassu et al., 2017). In vivo and in vitro data of PEGylated anionic liposomes demonstrated more efficient packaging of siRNA, leading to BACE1 gene silencing; roughly a 60% reduction in BACE1 mRNA compared to the untreated group and 30% protein silencing (Tagalakis et al., 2014).
4.2 Polymeric micelles
Polymeric micelles are composed of amphiphilic block copolymers which aggregate to form nano scale assemblies (1–200 nm). There are two functional sections in polymeric micelles, the inner part is known as the core, and the outer as the corona. Commonly, the core and corona are composed of hydrophobic and hydrophilic polymers respectively. The corona is responsible for in vivo pharmacokinetics whereas the core controls the drug entrapment, drug release, and stability properties. The exclusiveness of polymeric micelles is associated with their structural construction; the inner core can encapsulate the hydrophobic drugs, whereas the corona can be tailored to achieve the specific desired characteristics (Gothwal et al., 2016). As discussed in this review, nucleic acid delivery to the brain is challenging. Therefore, polymeric micelles have been extensively used in brain-targeted nucleic acid deliveries to curtail AD progression.
4.2.1 Functionalization strategies used in polymeric micelles
Polymeric micelles have been functionalized with different CPPs to achieve higher cell penetration. Available literature shows different CPPs such as Tat, RGD, and PEN were used. Recently, these CPPs have been extensively used to improve the cell-penetrating potential of polymeric micelles. For example, study showed that TAT functionalized poly-(N-3-carbobenzyloxy-lysine) (CPCL) polymeric micelles improved the gene transfection (Wang GH. et al., 2018). Similarly, PEG-PCL polymeric micelles functionalized with TAT improved in vitro cell penetration (Ming et al., 2019). The TAT CPPs have been explored for intranasal brain delivery of siRNA (Kanazawa et al., 2013). Even multi-CPPs functionalization has improved the transfection potential of polymeric micelles. For instance, RGD/TAT functionalization on poly (ethylene oxide)-block-poly(3-caprolactone)(PEO-b-PCL) polymeric micelles also improved in vitro co-delivery of bio-actives (Xiong et al., 2010). In another study, multi functionalized chitosan polymeric micelles showed effective transport across the BBB (Lamptey et al., 2022).
Besides TAT, PEN has been documented as a widely used CPP in elevating nanocarrier internalization across the BBB. It is composed of 16 amino acid sequences (Khafagy El et al., 2010; Gartziandia et al., 2016). The available literature confirms that PEN peptide can enhance the BBB permeability without exerting any cytotoxicity to the tissues (Liu et al., 2014; Nielsen et al., 2014). Multi-functionalized PEN tagged with chitosan polymeric micelles have shown higher in vitro transfection without causing any significant toxicity (Layek and Singh, 2013). Higher gene transfection was also achieved in the brain when multi-functionalized chitosan polymeric micelles were tagged with PEN (Gothwal et al., 2023a; Gothwal et al., 2023b). Similarly, Angiopep CPP was explored with PEG-PCL polymeric micelles for effective drug delivery across the BBB (Xin et al., 2011). RVG decorated polymeric micelles demonstrated its promising siRNA delivering potential to the brain (Huo et al., 2015). Limited literature availability on applications of CPP functionalized polymeric micelles in AD shows that this area can be explored for developing effective treatment strategies against AD.
4.2.2 Functionalized polymeric micelles for nucleic acid delivery in AD
Functionalized polymeric micelles have proved their potential in gene delivery against AD. A study investigated the transfection potential of polymeric micelles to deliver siRNA targeting BACE1 and APP (therapeutic targets of AD). Linear polyethyleneimine (LPEI) was grafted with polyethylene glycol (PEG) and loaded with siRNA. The in vitro outcomes showed selective knockdown in the mouse neuroblastoma (N2a) cells, and in vivo imaging proved the accumulation of polymeric micelles in the brain of mice. Additionally, in vivo results demonstrated the efficient knockdown of BACE1 in the brain (Shyam et al., 2015). The CPP functionalization improved gene transfection significantly.
Another group of scientists reported TAT functionalized polyethylene glycol (PEG)-polycaprolactone (PCL) polymeric micelles to deliver siRNA/dextran (10 kDa) to the brain via intranasal administration. The rats were sacrificed after 15 min and 1 h of intranasal and intravenous administration. The in vivo research findings provided higher transfer of siRNA to the brain through intranasal administration over intravenous after 1 h of administration (Kanazawa et al., 2013). Similarly, our laboratory has also explored the multi-functionalized chitosan polymeric micelles for comparative analysis of intranasal and intravenous administration. The chitosan polymeric micelles were functionalized with oleic acid (OA) mannose followed by CPPs- TAT and MAN. The multi-functionalized chitosan polymeric micelles were polyplexed with pVGF and investigated for transfection in C57BL/6J mice through intranasal and intravenous administration. Our findings showed higher transfection of pVGF in the multi-functionalized chitosan polymeric micelles in intranasal and intravenous groups over un-functionalized polymeric micelles. Overall higher transfection was observed in the intranasal administered animal groups over intravenous treated groups (Lamptey et al., 2022). We further explored PEN-CPP functionalized chitosan polymeric micelles for intranasal administration of pVGF into the brain. The chitosan was multi-functionalized with oleic acid (OA), followed by PEN and MAN, and finally polyplexed with pVGF. After intranasal administration, the multi-functionalized polymeric micelles showed significantly higher transfection in the mice brains (Gothwal et al., 2023a). In a different study, pApoE2 was used with PEN-conjugated multi-functionalized polymeric micelles and investigated for brain transfection after intranasal administration. The in vitro findings showed higher transfection of pApoE2 in primary astrocytes and primary neurons by dual-functionalized micelles. The in vivo results were also in the notion of in vitro findings, multi-functionalized polymeric micelles-treated mice showed higher ApoE2 expression in the brain over un-functionalized polymeric micelles (Gothwal et al., 2023b).
Polymeric micelle is a promising nanocarrier for gene delivery but limited available literature shows that polymeric micelles can be explored for gene delivery to the brain in AD. Functionalization of polymeric micelles with CPPs can serve as a promising nanocarrier platform in gene delivery to develop effective treatment approaches against AD progression. Polymeric micelles can also be explored with other ligands, including antibodies, amino acid sequences, or in combination to improve the targeted delivery across the BBB. This approach could be effective in targeting different receptors abundantly present on the BBB.
4.3 Exosomes
Exosomes are vesicular structures of endo-lysosomal origin ranging from 30 nm to 150 nm in size secreted by cells into extracellular fluids such as blood, cerebrospinal fluid (CSF), urine, saliva, etc. They consist of double-layered lipid membranes interspersed with transmembrane proteins encapsulating nucleic acids, proteins, or bioactive molecules in their aqueous core (Kalluri and LeBleu, 2020). The composition of exosomes is a function of the cell source and its pathological state. The common proteins in exosomes are either of endosomal origin, i.e., annexins, flotillin, tetraspins, or cell surface proteins collagen, integrin, and galectin. The lipid components include cholesterol, ceramides, phosphoglycerides, and short and long chain fatty-acyl side chains. The composition of the exosomes has its effect on physiochemical properties but is not limited to blood opsonization, blood circulation, tissue penetration, or renal clearance (Conlan et al., 2017). Although the functions of exosomes are yet to be established, it is suggested that they are involved in the removal of unnecessary constituents from the cell, like proteins, lipids, or nucleic acids (Kowal et al., 2014) to maintain cellular homeostasis and combat stress (Han et al., 2022), facilitating cellular communication between cells and maintaining the extracellular matrix.
The formation of exosomes starts with the invagination of the plasma membrane into the cytosol to form an intraluminal vesicle (ILV) (Edgar, 2016). Various proteins of ILVs are recycled back into the plasma membrane, and additional cargos are introduced into them to form multivesicular bodies (MVBs). MVBs either fuse with lysosomes to be degraded or fuse with the plasma membrane to release exosomes into the cytosol (Han et al., 2022) (Figure 4).
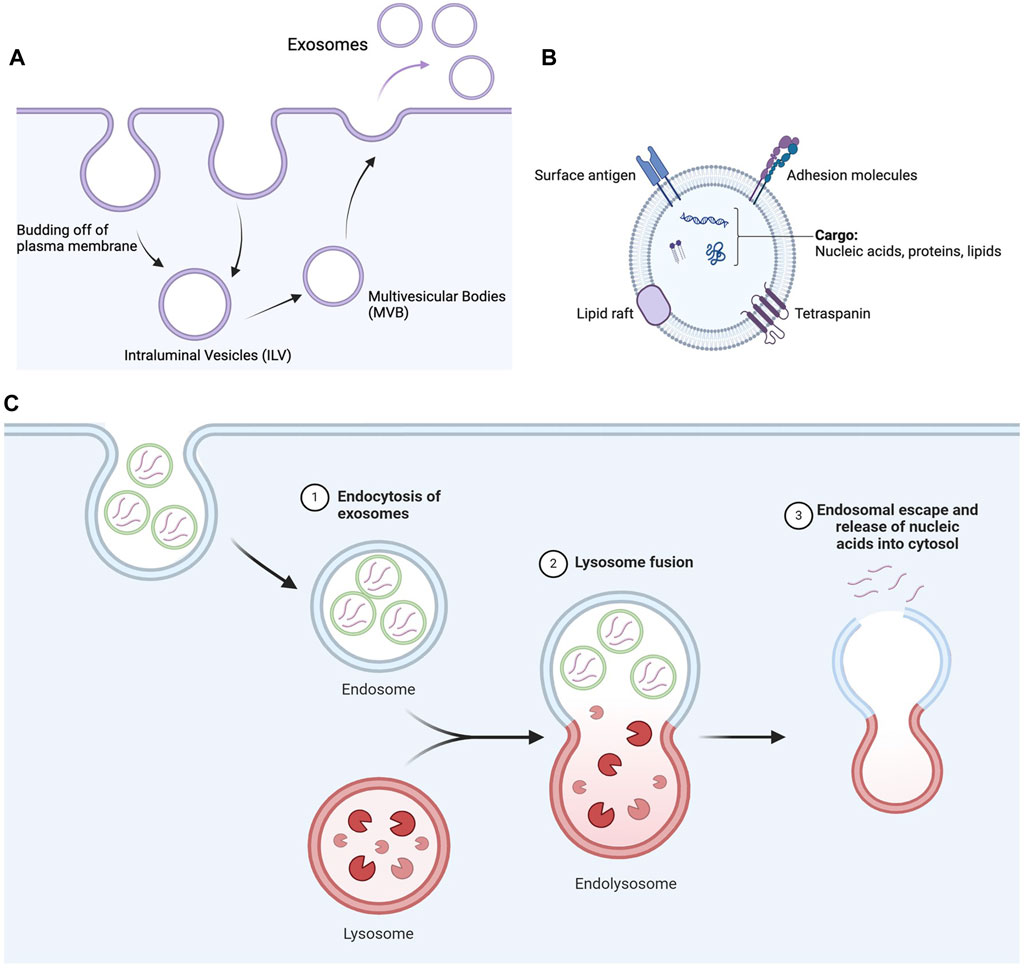
Figure 4. Secretion of exosomes and nucleic acid delivery mediated by exosomes. (A) Schematic flow of formation of exosomes inside cells. The invagination of the plasma membrane forms ILV, which matures into MVB. MVB then finally releases exosomes from the plasma membrane. (B) Schematic diagram of exosomes representing their lipid architecture, protein markers, and encapsulated contents. (C) Exosomes are internalized by cells through endocytosis, such as clathrin-mediated endocytosis and micropinocytosis, depending on the nature of the exosomes and cell type. The internalized exosomes then fuse with lysosomes to form endolysosomes, eventually escaping the endolysosomal pathway to release nucleic acids into the cytosol (Created in Biorender.com).
The most used method for isolating exosomes includes ultracentrifugation in combination with density gradient centrifugation. This method is labor intensive and time-consuming and requires large amount of samples for isolation. As a result, advanced methods like ultrafiltration or affinity-based capture of exosomes are used that are quick and yield a high amount of exosomes (Zeringer et al., 2015).
4.3.1 Functionalization strategies used in exosomes
Due to their biological origin, exosomes are non-immunogenic, biocompatible, and physiologically stable (Salunkhe et al., 2020). As a result, they are getting increased attention from the drug delivery community towards delivering cargo ranging from small molecules, nucleic acids, and proteins for therapeutic and diagnostic purposes (Donoso-Quezada et al., 2020). The tropism of exosomes is governed by the cell type during isolation, the membrane composition, and the pathological state of the host. Biodistribution studies in mice reported that administration of these exosomes in vivo results in their distribution mostly in the liver, spleen, kidney, lung, and gastrointestinal tract while being cleared rapidly from the blood (Choi et al., 2021). Localization towards the brain was minimal (Tian et al., 2018). Engineering exosomes, thus, is an innovative approach to enhance the utility of exosomes in CNS disorders, including AD. Here are some engineering strategies adopted to functionalize exosomes and enhance their delivery to the brain (Table 2).
4.3.1.1 Chemical functionalization of exosomes
The surface of exosomes is enriched with amine/carboxylic acid-terminated phospholipids and transmembrane proteins, which can be functionalized by targeting moiety through a direct chemical reaction (Tian et al., 2018). Click chemistry is the process of choice for chemical functionalization strategy compared to conventional chemical reactions, which involve noxious solvents and reaction conditions that are detrimental to the integrity of exosomes (Salunkhe et al., 2020). Click chemistry reactions are quick, executed in aqueous solvent conditions, generating high yield, and have less rigorous separation procedures. Some click chemistry reactions employed in exosomes are thiol-maleimide coupling, EDC/NHS coupling, and azide-alkyne cycloaddition coupling (Rayamajhi and Aryal, 2020).
4.3.1.2 Physical functionalization of exosomes
The fluid nature of the exosome membrane has the property to reassemble into its original vesicular organization after brief disruption by physical forces. This property is the core principle of the functionalization achieved through physical forces. The physical forces used to dissemble the membrane integrity are sonication, extrusion, and freeze-thaw cycles (Haney et al., 2015). Additionally, a hydrophobic insertion strategy using functionalized liposomes is also used. In this, after the application of physical forces, the disrupted exosomes are exposed to functionalized liposomes, which ultimately results in a hybridized liposome-exosome system with targeting ligands (Rayamajhi and Aryal, 2020). One fundamental flaw with this method is the release of internal constituents during the process.
4.3.1.3 Biological functionalization of exosomes
The protein machinery of exosome-producing cells can be exploited to engineer transmembrane protein fused with targeting ligands as a biological functionalization method. Lamp, GPI, CD63, CD9, CD81, etc., are the transmembrane protein cohort in exosomes that can be used as a functionalization base (Rayamajhi and Aryal, 2020). Essentially, exosome-producing cells are loaded with expression vectors (plasmids/viruses) containing chimeric genes where the targeting ligand genetic code is fused with the transmembrane protein genetic code. The exosome-producing cells process these chimeric genes to produce transmembrane protein expressing targeting ligand on its surface.
4.3.2 Functionalized exosomes for nucleic acid delivery in AD
A group of scientists tested biologically functionalized exosomes to downregulate BACE1 protein via siRNA for the management of AD. The researchers isolated exosomes from the culture of primary dendritic cells of C57Bl/6 mice bone marrow. The biological functionalization was achieved through plasmid constructs encoding CNS-specific peptide RVG on the genetic backbone of transmembrane exosome protein Lamp2b. siRNA was loaded by electroporation into the exosomes and injected intravenously. The functionalized exosomes demonstrated significant knockdown of BACE1 protein (66% ± 15%, p < 0.001) and β amyloid 1-42 (55%, p < 0.05), a critical pathological marker of AD, suggesting its application in the management of AD (Alvarez-Erviti et al., 2011). A recent study investigated the therapeutic efficacy of nucleic acid microRNA (miRNA) exosomes against AD. MiR-29a and miR-29b, the family of miR-29 family, are downregulated in AD and play a critical role in regulating BACE1 and neuronal apoptosis. As a result, the researchers used exosomes isolated from bone marrow mesenchymal stem cells (r-BMSCs) for miRNA delivery. The exosomes were biologically modified with vectors consisting of miRNA constructs such that released exosomes were rich in miR-29. The isolated exosomes were then injected intrahippocampally into the Wistar rat model of AD. A significant increase in miR-29 expression was observed in the hippocampus of the rats (p < 0.05), which resulted in a reversal of cognitive impairment observed in the Barnes maze (Jahangard et al., 2020).
4.4 Polymeric NPs
Polymeric NPs are colloidal NPs used as nanocarrier platforms for drugs and nucleic acid deliveries against different ailments (Reis et al., 2006). Polymeric NPs have shown greater advantages over other nanocarriers, i.e., being biodegradable, compatibility with therapeutic agents, improved shelf-life, and improved release kinetics (Reis et al., 2006; Davis, 2009). The application of functionalized polymeric NPs for nucleic acid delivery in CNS disorders is a promising approach in medicine. Herein, we discuss various functionalization strategies of polymeric NPs for transporting therapeutic genes across the BBB for the treatment of AD.
4.4.1 Functionalized polymeric NPs for nucleic acid delivery in AD
Current strategies for nucleic acid delivery across the BBB have some limitations for clinical translation, such as the risk of inflammation and invasive administration techniques (Singer et al., 2005; Shyam et al., 2015). The functionalization of polymeric NPs offers excellent targeting potential for delivering nucleic acid into the brain without triggering any adverse response. Recently, functionalized PEGylated Poly (2-(N,N-dimethylamino)ethylmethacrylate) (PEG-PDMAEMA) NPs were used to deliver siRNA to the amyloid plaque precisely in AD mice. The NPs were functionalized with QSH and CGN. The d-peptides QSH and CGN also play an important role in delivering the siRNA. CGN is a d- CGNHPHLAKYNGT, and a phase displayed l-peptide that has the affinity to bind with brain capillary endothelial cells whereas, QSH, a d-QSHYRHISPAQV specifically binds to Aβ1−42 and selectively targets the amyloid plaques. An intravenous administration of the functionalized NPs containing siRNA led to higher accumulation around the Aβ plaques in transgenic AD mice brain and inhibited BACE1 production, thereby decreasing Aβ formation (Zheng et al., 2017).
Similarly, a group of researchers designed PEGylated poly (2-(N,N-dimethylamino) ethyl methacrylate) (PEGPDMAEMA) polymeric NPs for delivering siRNA across the BBB specifically to the neurons. The nanocarrier was modified with CGN and Tet1 targeting ligands. After caudal vein administration in APP/PS1 transgenic mice, a notable decrease in BACE1 mRNA level and Aβ plaques were observed. Additionally, significant hippocampal neurogenesis was also observed (Wang P. et al., 2018).
The delivery of BACE1 siRNA to neuronal cells helps to improve cognitive dysfunction in transgenic AD mice. Glycosylated polymeric siRNA (Gal-NP@siRNA) nanomedicine has been developed to target BACE1 (Zhou et al., 2020). A salt bridge of guanidinium-phosphate (Gu+/PO34−) was used to stabilize the Gal-NP@siRNA through hydrogen bonding. The glycosylated nano delivery system enhanced the BBB penetration through glucose transporter-1 receptors. Administration of Gal-NP@siRNA in APP/PS1 transgenic AD mouse model specifically targets BACE1 and suppresses the expression of BACE1, thereby decreasing amyloid-β formation (Zhou et al., 2020).
Another study developed a functionalized polymeric nanoparticle based on PEGylated dendrigraft poly-L-lysines (DGLs) for co-delivering BACE 1-AS shRNA and peptides. The aim was to reduce Aβ plaques and inhibit p-tau associated fibrils for the treatment of AD. The DGLs were modified with brain-targeted RVG29 and D-peptide through a bi-functional PEG linker, resulting in DGLs-PEG-RVG29-D-peptide NPs. Multiple dosing treatments in transgenic AD mice revealed that the therapeutic gene downregulated the expression of BACE1, resulting in the reduction of Aβ plaques. Additionally, the formation of intracellular tau-fibrils decreased due to the inhibition of tau-fibril formation by D-peptides (Liu et al., 2016).
BDNF has been reported as a key element in neurodegenerative diseases. However, this therapy has some drawbacks, such as exogenous protein safety, including other side effects like neuropathic pain and seizures. Recently, poly (β amino esters) polymers (PBAE) NPs-based delivery of BDNF mRNA was reported for AD. Neuron-specific miRNA targeting modification was done on mRNA to prevent BDNF protein expression in neurons. The astrocytes released BDNF to support the neuronal function, significantly increasing memory improvement in the double transgenic AD mice model (APP/PS1) (Li et al., 2023).
The Rho/ROCK pathway is believed to control neuronal loss and inhibition of axonal growth associated with AD. Blocking the Rho/ROCK pathway could be a possible treatment to promote neuritic extension and regenerate the axon. Therefore, ROCK II-siRNA was delivered to the brain using polyethyleneimine (PEI) NPs modified by PEGylation (PEG). Administration of this formulation by intracranial injection in a senescence-accelerated mouse (SAM) model of AD was found to improve significant cognitive impairment by enhancing Choline acetyltransferase (ChAT) activity in the hippocampus region (Wen et al., 2014).
In addition, RVG29-modified PEGylated PLGA NPs were used to deliver BACE1-AS shRNA-encoded plasmid and epigallocatechin-3-gallate (EGCG) to the brain. Therapeutic (shRNA) gene and EGCG delivery in double transgenic (APP/PS1) mice showed the downregulation of BACE1 enzyme as well as amyloid-beta because of synergistic therapeutic effect (Lv et al., 2020) (Table 3).
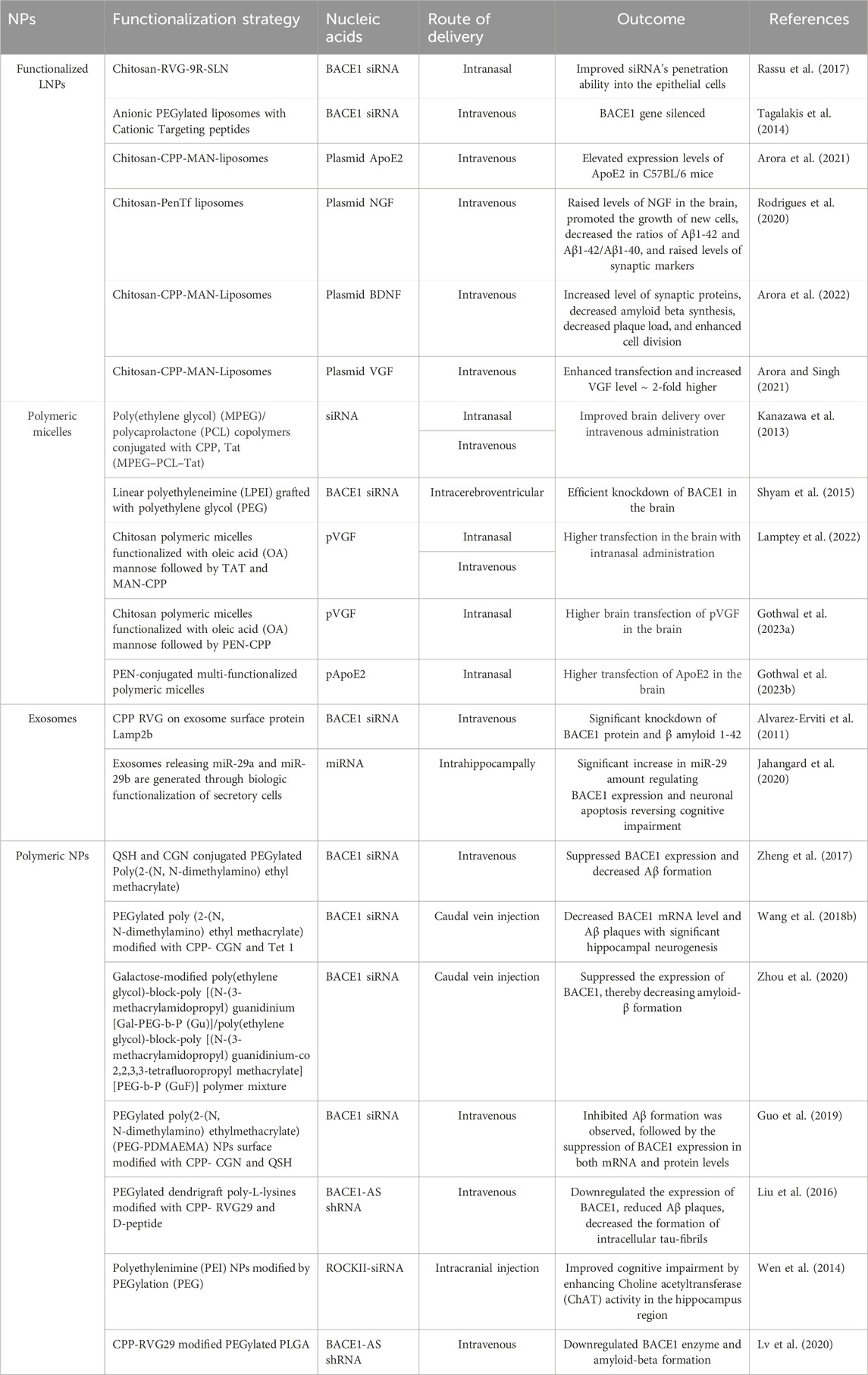
Table 3. Summarized preclinical studies semonstrating the efficacy of functionalized NPs used for nucleic acids delivery to the brain for the treatment of AD.
5 Advancements in nucleic acid delivery for AD therapy
Currently, there are no evidence of clinical studies utilizing NPs for nucleic acid delivery to the brain against AD. However, there are ongoing open-label phase 1 clinical trial using adeno-associated virus serotype 2 (AAV2) for BDNF and APOE2 delivery for AD. ASOs approved for clinical use are efficiently distributed within the brain and spinal cord, highlighting their potential in treating neurodegenerative disorders. The stability of ASO formulations is conferred through chemical modifications (Smith et al., 2006). The antisense oligonucleotide, MAPTRx, has been shown to inhibit the formation of tau protein through endogenous ribonuclease H-1 mediated degradation of MAPT mRNA. In preclinical studies, administration of mRNA-targeting ASOs resulted in a significant reduction of intracellular tau in a mouse model of tauopathy. Furthermore, ASO effectively reduced neuronal and cognitive impairments and significantly inhibited the cell-to-cell spread of oligomerized tau in the study (Takeda et al., 2015). The findings of the study, coupled with the safety profile of MAPTRx, provided the basis for a phase Ib clinical trial to assess its therapeutic potential in human subjects with AD. The study primarily aimed to evaluate safety, with a secondary focus on the pharmacokinetics of MAPTRx in cerebrospinal fluid (Mummery et al., 2023). An additional exploratory objective was to analyze the concentration of CSF total-tau protein. Mild or moderate adverse events were documented in 94% of patients treated with MAPTRx and 75% of those who received the placebo. Notably, there were no reports of serious adverse events among patients treated with MAPTRx. In addition, there was a significant decline in CSF total-tau exceeding 50% from baseline at the 24-week mark after the final dose in cohorts receiving either four doses of 60 mg or two doses of 115 mg of MAPTRx (Mummery et al., 2023). Based on the positive outcome of the Phase Ib trial, Biogen began a Phase II trial in August 2022 to compare two dosages and two injection schedules to placebo in 735 AD patients with moderate cognitive impairment or mild dementia (Alzforum, 2023).5 Participants will be given high or low dosage BIIB080 (IONIS-MAPTRx) every 24 weeks, a high dose every 12 weeks, or a placebo for 72 weeks. The primary outcome is the dosage response in changes in Clinical Dementia Rating Scale Sum of Boxes (CDR-SB) from baseline after 76 weeks. Secondary indicators include Activities of Daily Living for Mild Cognitive Impairment (ADCS-ADL-MCI), Alzheimer’s Disease Assessment Scale–Cognitive Subscale (ADAS-Cog 13), Mini Mental State Examination (MMSE), Integrated Alzheimer’s Disease Rating Scale (iADRS), Alzheimer’s disease composite score (ADCOMS), and adverse events. The experiment is planned to end in 2030 (Trial Registration: NCT05399888) (Biogen, 2022).6 Several drug products for AD are already available in the market, with the most recently approved drugs by FDA listed in this article. Additionally, there are still ongoing clinical trials exploring various drug options for AD (Tables 4–6).
6 Conclusion and future perspectives
This review summarized nucleic acid delivery to the brain using different functionalized nanocarrier platforms to bypass biological barriers and improve target specificity. The existing therapies for AD, including amyloid removal antibodies, provide only symptomatic relief to patients without any interference in halting the disease progression. In such scenario, nucleic acid therapy with pDNA, mRNA, siRNA, miRNA, shRNA, and ASOs holds great promise as a novel therapeutic target. However, standalone delivery of nucleic acid poses significant challenges like susceptibility to degradation during circulation or within the endolysosomal pathway of cells, initiation of immune response, and minimal cellular uptake due to its high negative charge density. Encapsulating these nucleic acids in vectors such as functionalized NPs helps to overcome biological barriers and enhance the targeted nucleic acid delivery to the brain. Developing suitable vectors requires multidisciplinary integration due to several factors such as physiological barriers, vector performance after administration, and undesired interaction with tissue and drugs/genes. The presence of the BBB restricts the transportation of exogenous molecules to the brain; therefore, it must be emphasized in vector development.
Furthermore, gene delivery with NPs holds great promise in targeting associated proteins contributing to AD, which are typically considered non-druggable. This technology also has the potential to extend to associated neurological disorders like Parkinson’s Disease and Amyotrophic Lateral Sclerosis, among others, in a similar fashion to viral vectors. For instance, UniQure Biopharma B.V. used viral vectors for phase 1 and 2 clinical trials (NCT04120493 and NCT05243017) for Huntington’s disease treatment. Similarly, Neurologix Inc. used a viral vector for glutamic acid decarboxylase (GAD) gene delivery in Parkinson’s disease patients (NCT00643890). The complexity in the synthesis of these NPs, their optimization catered to clinical application, and the poorly understood fate of the NPs inside the body present a drawback in the success of this therapy in the future. Studies concerning the undesired genetic effects of nucleic acids, like gene silencing and nonspecific gene expression, warrant further investigation. Typically, preclinical investigations are conducted within relatively constrained experimental conditions and by a limited number of animal models. Hence, research should incorporate diverse statistical, mathematical, and biological models to enhance the likelihood of regulatory approvals for further clinical trials. Researchers must also strive to consider the final dosage form for human use while conducting preclinical investigations, ensuring that formulations have the potential to be translated into clinically applicable therapeutics. The recent advancements highlighted in this review article provide a structured comprehension that can inform the utilization of functionalized NPs in gene therapy for AD. We anticipate that the careful selection of scientifically validated genes, specific promoters, and promoter control mechanisms, coupled with the development of functionalized nanocarriers, will bring gene therapy approaches for AD closer to practical implementation. Additionally, harnessing progress in genomics and precision medicine allows for tailoring NP-based therapies to meet the specific needs of AD patients, considering factors such as their genetic predisposition, disease stage, and other relevant variables. As research in this field advances and nanotechnologies evolve, gene therapy is poised to play an increasingly important role in therapeutics against AD, offering new hope for patients and healthcare providers alike.
Author contributions
CM: Writing–original draft, Writing–review and editing. BC: Writing–original draft, Writing–review and editing. AG: Writing–original draft, Writing–review and editing. AM: Writing–original draft, Writing–review and editing. BT: Writing–original draft, Writing–review and editing. BL: Writing–original draft, Writing–review and editing. JS: Conceptualization, Formal Analysis, Funding acquisition, Resources, Supervision, Validation, Writing–original draft, Writing–review and editing.
Funding
The author(s) declare that financial support was received for the research, authorship, and/or publication of this article. This work was supported by the National Institutes of Health grants RF1 AG068034, RF1 AG068034-01A1S1 and R01 AG08398.
Conflict of interest
The authors declare that the research was conducted in the absence of any commercial or financial relationships that could be construed as a potential conflict of interest.
Publisher’s note
All claims expressed in this article are solely those of the authors and do not necessarily represent those of their affiliated organizations, or those of the publisher, the editors and the reviewers. Any product that may be evaluated in this article, or claim that may be made by its manufacturer, is not guaranteed or endorsed by the publisher.
Footnotes
1www.who.int/news-room/fact-sheets/detail/dementia
2www.biogen.com/medicines.html
3www.alz.org/alzheimers-dementia/treatments/medications-for-memory
4www.eisai.com/news/2024/news202404.html
5https://www.alzforum.org/therapeutics/biib080/
6https://clinicaltrials.gov/study/NCT05399888/
References
Ahlschwede, K. M., Curran, G. L., Rosenberg, J. T., Grant, S. C., Sarkar, G., Jenkins, R. B., et al. (2019). Cationic carrier peptide enhances cerebrovascular targeting of nanoparticles in Alzheimer's disease brain. Nanomedicine 16, 258–266. doi:10.1016/j.nano.2018.09.010
Alam, M. I., Beg, S., Samad, A., Baboota, S., Kohli, K., Ali, J., et al. (2010). Strategy for effective brain drug delivery. Eur. J. Pharm. Sci. 40 (5), 385–403. doi:10.1016/j.ejps.2010.05.003
Alvarez-Erviti, L., Seow, Y., Yin, H., Betts, C., Lakhal, S., and Wood, M. J. (2011). Delivery of siRNA to the mouse brain by systemic injection of targeted exosomes. Nat. Biotechnol. 29 (4), 341–345. doi:10.1038/nbt.1807
Alzforum (2023). Therapeutics. BIIB080. Available at: https://www.alzforum.org/therapeutics/biib080/ (Accessed April 18, 2024).
Alzheimer’s Association (2024). Medications for memory, cognition and dementia-related behaviors. Available at: www.alz.org/alzheimers-dementia/treatments/medications-for-memory/ (Accessed April 17, 2024).
Arora, S., Kanekiyo, T., and Singh, J. (2022). Functionalized nanoparticles for brain targeted BDNF gene therapy to rescue Alzheimer's disease pathology in transgenic mouse model. Int. J. Biol. Macromol. 208, 901–911. doi:10.1016/j.ijbiomac.2022.03.203
Arora, S., Layek, B., and Singh, J. (2021). Design and validation of liposomal ApoE2 gene delivery system to evade blood-brain barrier for effective treatment of Alzheimer's disease. Mol. Pharm. 18 (2), 714–725. doi:10.1021/acs.molpharmaceut.0c00461
Arora, S., and Singh, J. (2021). In vitro and in vivo optimization of liposomal nanoparticles based brain targeted vgf gene therapy. Int. J. Pharm. 608, 121095. doi:10.1016/j.ijpharm.2021.121095
Azhari, H., Younus, M., Hook, S. M., Boyd, B. J., and Rizwan, S. B. (2021). Cubosomes enhance drug permeability across the blood-brain barrier in zebrafish. Int. J. Pharm. 600, 120411. doi:10.1016/j.ijpharm.2021.120411
Barauskas, J., Johnsson, M., and Tiberg, F. (2005). Self-assembled lipid superstructures: beyond vesicles and liposomes. Nano Lett. 5 (8), 1615–1619. doi:10.1021/nl050678i
Bennett, C. F., and Swayze, E. E. (2010). RNA targeting therapeutics: molecular mechanisms of antisense oligonucleotides as a therapeutic platform. Annu. Rev. Pharmacol. Toxicol. 50, 259–293. doi:10.1146/annurev.pharmtox.010909.105654
Bertrand, Y., Currie, J. C., Poirier, J., Demeule, M., Abulrob, A., Fatehi, D., et al. (2011). Influence of glioma tumour microenvironment on the transport of ANG1005 via low-density lipoprotein receptor-related protein 1. Br. J. Cancer 105 (11), 1697–1707. doi:10.1038/bjc.2011.427
Biogen (2022). A study to learn about the safety of BIIB080 and whether it can improve symptoms of participants with mild cognitive impairment due to Alzheimer's disease (AD) or mild AD dementia between 50 to 80 Years of age (CELIA). ClinicalTrials.gov identifier: NCT05399888. Available at: https://clinicaltrials.gov/study/NCT05399888/ (Accessed April 17, 2024).
Biogen (2024). Medicines. Available at: www.biogen.com/medicines.html/ (Accessed April 17, 2024).
Bloom, G. S. (2014). Amyloid-β and tau: the trigger and bullet in Alzheimer disease pathogenesis. JAMA Neurol. 71 (4), 505–508. doi:10.1001/jamaneurol.2013.5847
Budd Haeberlein, S., Aisen, P. S., Barkhof, F., Chalkias, S., Chen, T., Cohen, S., et al. (2022). Two randomized phase 3 studies of aducanumab in early Alzheimer's disease. J. Prev. Alzheimers Dis. 9 (2), 197–210. doi:10.14283/jpad.2022.30
Chen, H., Tang, L., Qin, Y., Yin, Y., Tang, J., Tang, W., et al. (2010). Lactoferrin-modified procationic liposomes as a novel drug carrier for brain delivery. Eur. J. Pharm. Sci. 40 (2), 94–102. doi:10.1016/j.ejps.2010.03.007
Chen, Z. L., Huang, M., Wang, X. R., Fu, J., Han, M., Shen, Y. Q., et al. (2016). Transferrin-modified liposome promotes α-mangostin to penetrate the blood-brain barrier. Nanomedicine 12 (2), 421–430. doi:10.1016/j.nano.2015.10.021
Choi, H., Choi, Y., Yim, H. Y., Mirzaaghasi, A., Yoo, J.-K., and Choi, C. (2021). Biodistribution of exosomes and engineering strategies for targeted delivery of therapeutic exosomes. Tissue Eng. Regen. Med. 18 (4), 499–511. doi:10.1007/s13770-021-00361-0
Chowdhury, S., and Chowdhury, N. S. (2023). Novel anti-amyloid-beta (Aβ) monoclonal antibody lecanemab for Alzheimer's disease: a systematic review. Int. J. Immunopathol. Pharmacol. 37, 3946320231209839. doi:10.1177/03946320231209839
Cobb, M. (2015). Who discovered messenger RNA? Curr. Biol. 25 (13), R526–R532. doi:10.1016/j.cub.2015.05.032
Conlan, R. S., Pisano, S., Oliveira, M. I., Ferrari, M., and Pinto, I. M. (2017). Exosomes as reconfigurable therapeutic systems. Trends Mol. Med. 23 (7), 636–650. doi:10.1016/j.molmed.2017.05.003
Cui, G.-H., Guo, H.-D., Li, H., Zhai, Y., Gong, Z.-B., Wu, J., et al. (2019). RVG-modified exosomes derived from mesenchymal stem cells rescue memory deficits by regulating inflammatory responses in a mouse model of Alzheimer’s disease. Immun. Ageing 16 (1), 10–12. doi:10.1186/s12979-019-0150-2
Cullen, B. R. (2004). Transcription and processing of human microRNA precursors. Mol. Cell 16 (6), 861–865. doi:10.1016/j.molcel.2004.12.002
Cunningham, E. T., Adamis a, P., Altaweel, M., Aiello, L. P., Bressler, N. M., D'amico, D. J., et al. (2005). A phase II randomized double-masked trial of pegaptanib, an anti-vascular endothelial growth factor aptamer, for diabetic macular edema. Ophthalmology 112 (10), 1747–1757. doi:10.1016/j.ophtha.2005.06.007
Dal Magro, R., Ornaghi, F., Cambianica, I., Beretta, S., Re, F., Musicanti, C., et al. (2017). ApoE-modified solid lipid nanoparticles: a feasible strategy to cross the blood-brain barrier. J. Control Release 249, 103–110. doi:10.1016/j.jconrel.2017.01.039
Dara, T., Vatanara, A., Sharifzadeh, M., Khani, S., Vakilinezhad, M. A., Vakhshiteh, F., et al. (2019). Improvement of memory deficits in the rat model of Alzheimer's disease by erythropoietin-loaded solid lipid nanoparticles. Neurobiol. Learn Mem. 166, 107082. doi:10.1016/j.nlm.2019.107082
Davis, M. E. (2009). The first targeted delivery of siRNA in humans via a self-assembling, cyclodextrin polymer-based nanoparticle: from concept to clinic. Mol. Pharm. 6 (3), 659–668. doi:10.1021/mp900015y
Deardorff, W. J., Feen, E., and Grossberg, G. T. (2015). The use of cholinesterase inhibitors across all stages of Alzheimer's disease. Drugs Aging 32 (7), 537–547. doi:10.1007/s40266-015-0273-x
Dekker, M., Bouvy, J. C., O'rourke, D., Thompson, R., Makady, A., Jonsson, P., et al. (2019). Alignment of European regulatory and health technology assessments: a review of licensed products for Alzheimer's disease. Front. Med. (Lausanne) 6, 73. doi:10.3389/fmed.2019.00073
Di, F. G., Catania, M., Maderna, E., Morbin, M., Moda, F., Colombo, L., et al. (2016). Tackling amyloidogenesis in Alzheimer's disease with A2V variants of Amyloid-β. Sci. Rep. 6, 20949. doi:10.1038/srep20949
Dong, X. (2018). Current strategies for brain drug delivery. Theranostics 8 (6), 1481–1493. doi:10.7150/thno.21254
Donoso-Quezada, J., Ayala-Mar, S., and González-Valdez, J. (2020). State-of-the-art exosome loading and functionalization techniques for enhanced therapeutics: a review. Crit. Rev. Biotechnol. 40 (6), 804–820. doi:10.1080/07388551.2020.1785385
Dos Santos Rodrigues, B., Banerjee, A., Kanekiyo, T., and Singh, J. (2019a). Functionalized liposomal nanoparticles for efficient gene delivery system to neuronal cell transfection. Int. J. Pharm. 566, 717–730. doi:10.1016/j.ijpharm.2019.06.026
Dos Santos Rodrigues, B., Lakkadwala, S., Kanekiyo, T., and Singh, J. (2019b). Development and screening of brain-targeted lipid-based nanoparticles with enhanced cell penetration and gene delivery properties. Int. J. Nanomedicine 14, 6497–6517. doi:10.2147/IJN.S215941
D'souza, A., Nozohouri, S., Bleier, B. S., and Amiji, M. M. (2023). CNS delivery of nucleic acid therapeutics: beyond the blood-brain barrier and towards specific cellular targeting. Pharm. Res. 40 (1), 77–105. doi:10.1007/s11095-022-03433-5
Dunn, B., Stein, P., and Cavazzoni, P. (2021). Approval of aducanumab for alzheimer disease-the FDA's perspective. JAMA Intern Med. 181 (10), 1276–1278. doi:10.1001/jamainternmed.2021.4607
Edgar, J. R. (2016). Q&A: what are exosomes, exactly? BMC Biol. 14 (1), 46–47. doi:10.1186/s12915-016-0268-z
Eisai (2024). The scientific advisory group (SAG) to convene to discuss the marketing authorization application for lecanemab in the EU. 2024 release. Available at: www.eisai.com/news/2024/news202404.html/ (Accessed April 17, 2024).
Fang, C. L., Al-Suwayeh, S. A., and Fang, J. Y. (2013). Nanostructured lipid carriers (NLCs) for drug delivery and targeting. Recent Pat. Nanotechnol. 7 (1), 41–55. doi:10.2174/18722105130105
Frankel, a D., and Pabo, C. O. (1988). Cellular uptake of the tat protein from human immunodeficiency virus. Cell 55 (6), 1189–1193. doi:10.1016/0092-8674(88)90263-2
Fu, C., Xiang, Y., Li, X., and Fu, A. (2018). Targeted transport of nanocarriers into brain for theranosis with rabies virus glycoprotein-derived peptide. Mater Sci. Eng. C Mater Biol. Appl. 87, 155–166. doi:10.1016/j.msec.2017.12.029
Fuhrmann, G., Serio, A., Mazo, M., Nair, R., and Stevens, M. M. (2015). Active loading into extracellular vesicles significantly improves the cellular uptake and photodynamic effect of porphyrins. J. Control. release 205, 35–44. doi:10.1016/j.jconrel.2014.11.029
Garg, G., and Saraf, S. (2007). Cubosomes: an overview. Biol. Pharm. Bull. 30 (2), 350–353. doi:10.1248/bpb.30.350
Gartziandia, O., Egusquiaguirre, S. P., Bianco, J., Pedraz, J. L., Igartua, M., Hernandez, R. M., et al. (2016). Nanoparticle transport across in vitro olfactory cell monolayers. Int. J. Pharm. 499 (1-2), 81–89. doi:10.1016/j.ijpharm.2015.12.046
Gill, D. R., Pringle, I. A., and Hyde, S. C. (2009). Progress and prospects: the design and production of plasmid vectors. Gene Ther. 16 (2), 165–171. doi:10.1038/gt.2008.183
Giunta, B., Hou, H., Zhu, Y., Rrapo, E., Tian, J., Takashi, M., et al. (2009). HIV-1 Tat contributes to Alzheimer's disease-like pathology in PSAPP mice. Int. J. Clin. Exp. Pathol. 2 (5), 433–443.
Gothwal, A., Khan, I., and Gupta, U. (2016). Polymeric micelles: recent advancements in the delivery of anticancer drugs. Pharm. Res. 33 (1), 18–39. doi:10.1007/s11095-015-1784-1
Gothwal, A., Lamptey, R. N. L., and Singh, J. (2023a). Multifunctionalized cationic chitosan polymeric micelles polyplexed with pVGF for noninvasive delivery to the mouse brain through the intranasal route for developing therapeutics for Alzheimer's disease. Mol. Pharm. 20 (6), 3009–3019. doi:10.1021/acs.molpharmaceut.3c00031
Gothwal, A., Lamptey, R. N. L., Trivedi, R., Chaulagain, B., and Singh, J. (2023b). Non-invasive intranasal delivery of pApoE2: effect of multiple dosing on the ApoE2 expression in mice brain. Int. J. Mol. Sci. 24 (16), 13019. doi:10.3390/ijms241613019
Guo, Q., Zheng, X., Yang, P., Pang, X., Qian, K., Wang, P., et al. (2019). Small interfering RNA delivery to the neurons near the amyloid plaques for improved treatment of Alzheimer׳s disease. Acta Pharm. Sin. B 9 (3), 590–603. doi:10.1016/j.apsb.2018.12.010
Gupta, S., Singh, V., Ganesh, S., Singhal, N. K., and Sandhir, R. (2022). siRNA mediated GSK3β knockdown targets insulin signaling pathway and rescues Alzheimer’s disease pathology: evidence from in vitro and in vivo studies. ACS Appl. Mater. Interfaces 14 (1), 69–93. doi:10.1021/acsami.1c15305
Ha, M., and Kim, V. N. (2014). Regulation of microRNA biogenesis. Nat. Rev. Mol. Cell Biol. 15 (8), 509–524. doi:10.1038/nrm3838
Hammond, S. M., Bernstein, E., Beach, D., and Hannon, G. J. (2000). An RNA-directed nuclease mediates post-transcriptional gene silencing in Drosophila cells. Nature 404 (6775), 293–296. doi:10.1038/35005107
Hampel, H., Mesulam, M. M., Cuello, a C., Khachaturian, a S., Vergallo, A., Farlow, M. R., et al. (2019). Revisiting the cholinergic hypothesis in Alzheimer's disease: emerging evidence from translational and clinical research. J. Prev. Alzheimers Dis. 6 (1), 2–15. doi:10.14283/jpad.2018.43
Han, Q.-F., Li, W.-J., Hu, K.-S., Gao, J., Zhai, W.-L., Yang, J.-H., et al. (2022). Exosome biogenesis: machinery, regulation, and therapeutic implications in cancer. Mol. Cancer 21 (1), 207–226. doi:10.1186/s12943-022-01671-0
Han, Y., Liu, S., Ho, J., Danquah, M. K., and Forde, G. M. (2009). Using DNA as a drug—bioprocessing and delivery strategies. Chem. Eng. Res. Des. 87 (3), 343–348. doi:10.1016/j.cherd.2008.09.010
Haney, M. J., Klyachko, N. L., Zhao, Y., Gupta, R., Plotnikova, E. G., He, Z., et al. (2015). Exosomes as drug delivery vehicles for Parkinson's disease therapy. J. Control. release 207, 18–30. doi:10.1016/j.jconrel.2015.03.033
Haroon, K., Zheng, H., Wu, S., Liu, Z., Tang, Y., Yang, G.-Y., et al. (2024). Engineered exosomes mediated targeted delivery of neuroprotective peptide NR2B9c for the treatment of traumatic brain injury. Int. J. Pharm. 649, 123656. doi:10.1016/j.ijpharm.2023.123656
Hategan, A., Bianchet, M. A., Steiner, J., Karnaukhova, E., Masliah, E., Fields, A., et al. (2017). HIV Tat protein and amyloid-β peptide form multifibrillar structures that cause neurotoxicity. Nat. Struct. Mol. Biol. 24 (4), 379–386. doi:10.1038/nsmb.3379
Holsinger, R. M., Mclean, C. A., Beyreuther, K., Masters, C. L., and Evin, G. (2002). Increased expression of the amyloid precursor beta-secretase in Alzheimer's disease. Ann. Neurol. 51 (6), 783–786. doi:10.1002/ana.10208
Hu, B., Zhong, L., Weng, Y., Peng, L., Huang, Y., Zhao, Y., et al. (2020). Therapeutic siRNA: state of the art. Signal Transduct. Target Ther. 5 (1), 101. doi:10.1038/s41392-020-0207-x
Huang, L. K., Kuan, Y. C., Lin, H. W., and Hu, C. J. (2023). Clinical trials of new drugs for Alzheimer disease: a 2020-2023 update. J. Biomed. Sci. 30 (1), 83. doi:10.1186/s12929-023-00976-6
Huang, R. Q., Ke, W. L., Qu, Y. H., Zhu, J. H., Pei, Y. Y., and Jiang, C. (2007). Characterization of lactoferrin receptor in brain endothelial capillary cells and mouse brain. J. Biomed. Sci. 14 (1), 121–128. doi:10.1007/s11373-006-9121-7
Huey, R., Hawthorne, S., and Mccarron, P. (2017). The potential use of rabies virus glycoprotein-derived peptides to facilitate drug delivery into the central nervous system: a mini review. J. Drug Target 25 (5), 379–385. doi:10.1080/1061186X.2016.1223676
Hunsberger, H. C., Pinky, P. D., Smith, W., Suppiramaniam, V., and Reed, M. N. (2019). The role of APOE4 in Alzheimer's disease: strategies for future therapeutic interventions. Neuronal Signal 3 (2), Ns20180203. doi:10.1042/NS20180203
Huo, H., Gao, Y., Wang, Y., Zhang, J., Wang, Z. Y., Jiang, T., et al. (2015). Polyion complex micelles composed of pegylated polyasparthydrazide derivatives for siRNA delivery to the brain. J. Colloid Interface Sci. 447, 8–15. doi:10.1016/j.jcis.2015.01.043
Jahangard, Y., Monfared, H., Moradi, A., Zare, M., Mirnajafi-Zadeh, J., and Mowla, S. J. (2020). Therapeutic effects of transplanted exosomes containing miR-29b to a rat model of Alzheimer’s disease. Front. Neurosci. 14, 564. doi:10.3389/fnins.2020.00564
Johnson, J. W., and Kotermanski, S. E. (2006). Mechanism of action of memantine. Curr. Opin. Pharmacol. 6 (1), 61–67. doi:10.1016/j.coph.2005.09.007
Jonas, S., and Izaurralde, E. (2015). Towards a molecular understanding of microRNA-mediated gene silencing. Nat. Rev. Genet. 16 (7), 421–433. doi:10.1038/nrg3965
Kadari, A., Pooja, D., Gora, R. H., Gudem, S., Kolapalli, V. R. M., Kulhari, H., et al. (2018). Design of multifunctional peptide collaborated and docetaxel loaded lipid nanoparticles for antiglioma therapy. Eur. J. Pharm. Biopharm. 132, 168–179. doi:10.1016/j.ejpb.2018.09.012
Kalluri, R., and Lebleu, V. S. (2020). The biology, function, and biomedical applications of exosomes. Science 367 (6478), eaau6977. doi:10.1126/science.aau6977
Kanazawa, T., Akiyama, F., Kakizaki, S., Takashima, Y., and Seta, Y. (2013). Delivery of siRNA to the brain using a combination of nose-to-brain delivery and cell-penetrating peptide-modified nano-micelles. Biomaterials 34 (36), 9220–9226. doi:10.1016/j.biomaterials.2013.08.036
Kapeli, K., Martinez, F. J., and Yeo, G. W. (2017). Genetic mutations in RNA-binding proteins and their roles in ALS. Hum. Genet. 136 (9), 1193–1214. doi:10.1007/s00439-017-1830-7
Kaur, I. P., Bhandari, R., Bhandari, S., and Kakkar, V. (2008). Potential of solid lipid nanoparticles in brain targeting. J. Control Release 127 (2), 97–109. doi:10.1016/j.jconrel.2007.12.018
Kawamata, T., Tooyama, I., Yamada, T., Walker, D. G., and Mcgeer, P. L. (1993). Lactotransferrin immunocytochemistry in Alzheimer and normal human brain. Am. J. Pathol. 142 (5), 1574–1585.
Khafagy El, S., Morishita, M., Ida, N., Nishio, R., Isowa, K., and Takayama, K. (2010). Structural requirements of penetratin absorption enhancement efficiency for insulin delivery. J. Control Release 143 (3), 302–310. doi:10.1016/j.jconrel.2010.01.019
Kim, G., Kim, M., Lee, Y., Byun, J. W., and Lee, M. (2020). Systemic delivery of microRNA-21 antisense oligonucleotides to the brain using T7-peptide decorated exosomes. J. Control. release 317, 273–281. doi:10.1016/j.jconrel.2019.11.009
Knowles, J. (2006). Donepezil in Alzheimer's disease: an evidence-based review of its impact on clinical and economic outcomes. Core Evid. 1 (3), 195–219.
Kowal, J., Tkach, M., and Théry, C. (2014). Biogenesis and secretion of exosomes. Curr. Opin. Cell Biol. 29, 116–125. doi:10.1016/j.ceb.2014.05.004
Kulkarni, J. A., Cullis, P. R., and Van Der Meel, R. (2018). Lipid nanoparticles enabling gene therapies: from concepts to clinical utility. Nucleic Acid. Ther. 28 (3), 146–157. doi:10.1089/nat.2018.0721
Kulkarni, J. A., Myhre, J. L., Chen, S., Tam, Y. Y. C., Danescu, A., Richman, J. M., et al. (2017). Design of lipid nanoparticles for in vitro and in vivo delivery of plasmid DNA. Nanomedicine Nanotechnol. Biol. Med. 13 (4), 1377–1387. doi:10.1016/j.nano.2016.12.014
Kulkarni, J. A., Witzigmann, D., Thomson, S. B., Chen, S., Leavitt, B. R., Cullis, P. R., et al. (2021). The current landscape of nucleic acid therapeutics. Nat. Nanotechnol. 16 (6), 630–643. doi:10.1038/s41565-021-00898-0
Kumar, P., Wu, H., Mcbride, J. L., Jung, K. E., Kim, M. H., Davidson, B. L., et al. (2007). Transvascular delivery of small interfering RNA to the central nervous system. Nature 448 (7149), 39–43. doi:10.1038/nature05901
Kuo, Y. C., and Wang, C. T. (2014). Protection of SK-N-MC cells against β-amyloid peptide-induced degeneration using neuron growth factor-loaded liposomes with surface lactoferrin. Biomaterials 35 (22), 5954–5964. doi:10.1016/j.biomaterials.2014.03.082
Lamptey, R. N. L., Gothwal, A., Trivedi, R., Arora, S., and Singh, J. (2022). Synthesis and characterization of fatty acid grafted chitosan polymeric micelles for improved gene delivery of VGF to the brain through intranasal route. Biomedicines 10 (2), 493. doi:10.3390/biomedicines10020493
Landau, E. M., and Rosenbusch, J. P. (1996). Lipidic cubic phases: a novel concept for the crystallization of membrane proteins. Proc. Natl. Acad. Sci. U. S. A. 93 (25), 14532–14535. doi:10.1073/pnas.93.25.14532
Layek, B., and Singh, J. (2013). Cell penetrating peptide conjugated polymeric micelles as a high performance versatile nonviral gene carrier. Biomacromolecules 14 (11), 4071–4081. doi:10.1021/bm401204n
Lee, D., Slomkowski, M., Hefting, N., Chen, D., Larsen, K. G., Kohegyi, E., et al. (2023). Brexpiprazole for the treatment of agitation in alzheimer dementia: a randomized clinical trial. JAMA Neurol. 80 (12), 1307–1316. doi:10.1001/jamaneurol.2023.3810
Lee, S. T., Chu, K., Jung, K. H., Kim, J. H., Huh, J. Y., Yoon, H., et al. (2012). miR-206 regulates brain-derived neurotrophic factor in Alzheimer disease model. Ann. Neurol. 72 (2), 269–277. doi:10.1002/ana.23588
Lee, Y., Ahn, C., Han, J., Choi, H., Kim, J., Yim, J., et al. (2003). The nuclear RNase III Drosha initiates microRNA processing. Nature 425 (6956), 415–419. doi:10.1038/nature01957
Lexeo, T. (2019). Gene therapy for APOE4 homozygote of Alzheimer's disease. ClinicalTrials.gov identifier: NCT03634007. Available at: https://clinicaltrials.gov/study/NCT03634007/ (Accessed April 17, 2024).
Li, H., Cao, Y., Ye, J., Yang, Z., Chen, Q., Liu, X., et al. (2023). Engineering brain-derived neurotrophic factor mRNA delivery for the treatment of Alzheimer’s disease. Chem. Eng. J. 466, 143152. doi:10.1016/j.cej.2023.143152
Lin, C. Y., Perche, F., Ikegami, M., Uchida, S., Kataoka, K., and Itaka, K. (2016). Messenger RNA-based therapeutics for brain diseases: an animal study for augmenting clearance of beta-amyloid by intracerebral administration of neprilysin mRNA loaded in polyplex nanomicelles. J. Control Release 235, 268–275. doi:10.1016/j.jconrel.2016.06.001
Lin, M., and Qi, X. (2023). Advances and challenges of stimuli-responsive nucleic acids delivery system in gene therapy. Pharmaceutics 15 (5), 1450. doi:10.3390/pharmaceutics15051450
Lin, W. J., Jiang, C., Sadahiro, M., Bozdagi, O., Vulchanova, L., Alberini, C. M., et al. (2015). VGF and its C-terminal peptide TLQP-62 regulate memory formation in Hippocampus via a BDNF-TrkB-dependent mechanism. J. Neurosci. 35 (28), 10343–10356. doi:10.1523/JNEUROSCI.0584-15.2015
Liu, D., Lin, B., Shao, W., Zhu, Z., Ji, T., and Yang, C. (2014). In vitro and in vivo studies on the transport of PEGylated silica nanoparticles across the blood-brain barrier. ACS Appl. Mater Interfaces 6 (3), 2131–2136. doi:10.1021/am405219u
Liu, Y., An, S., Li, J., Kuang, Y., He, X., Guo, Y., et al. (2016). Brain-targeted co-delivery of therapeutic gene and peptide by multifunctional nanoparticles in Alzheimer's disease mice. Biomaterials 80, 33–45. doi:10.1016/j.biomaterials.2015.11.060
Lombardo, S. M., Schneider, M., Türeli, a E., and Günday Türeli, N. (2020). Key for crossing the BBB with nanoparticles: the rational design. Beilstein J. Nanotechnol. 11, 866–883. doi:10.3762/bjnano.11.72
Lu, Z. G., Shen, J., Yang, J., Wang, J. W., Zhao, R. C., Zhang, T. L., et al. (2023). Nucleic acid drug vectors for diagnosis and treatment of brain diseases. Signal Transduct. Target Ther. 8 (1), 39. doi:10.1038/s41392-022-01298-z
Lv, L., Yang, F., Li, H., and Yuan, J. (2020). Brain-targeted co-delivery of β-amyloid converting enzyme 1 shRNA and epigallocatechin-3-gallate by multifunctional nanocarriers for Alzheimer's disease treatment. IUBMB Life 72 (8), 1819–1829. doi:10.1002/iub.2330
Maqbool, M., Mobashir, M., and Hoda, N. (2016). Pivotal role of glycogen synthase kinase-3: a therapeutic target for Alzheimer's disease. Eur. J. Med. Chem. 107, 63–81. doi:10.1016/j.ejmech.2015.10.018
Marcos-Contreras, O. A., Greineder, C. F., Kiseleva, R. Y., Parhiz, H., Walsh, L. R., Zuluaga-Ramirez, V., et al. (2020). Selective targeting of nanomedicine to inflamed cerebral vasculature to enhance the blood-brain barrier. Proc. Natl. Acad. Sci. U. S. A. 117 (7), 3405–3414. doi:10.1073/pnas.1912012117
Martins, S. M., Sarmento, B., Nunes, C., Lúcio, M., Reis, S., and Ferreira, D. C. (2013). Brain targeting effect of camptothecin-loaded solid lipid nanoparticles in rat after intravenous administration. Eur. J. Pharm. Biopharm. 85 (3 Pt A), 488–502. doi:10.1016/j.ejpb.2013.08.011
Mcnamara, J. O., Andrechek, E. R., Wang, Y., Viles, K. D., Rempel, R. E., Gilboa, E., et al. (2006). Cell type-specific delivery of siRNAs with aptamer-siRNA chimeras. Nat. Biotechnol. 24 (8), 1005–1015. doi:10.1038/nbt1223
Mcshane, R., Westby, M. J., Roberts, E., Minakaran, N., Schneider, L., Farrimond, L. E., et al. (2019). Memantine for dementia. Cochrane Database Syst. Rev. 3 (3), Cd003154. doi:10.1002/14651858.CD003154.pub6
Minchin, S., and Lodge, J. (2019). Understanding biochemistry: structure and function of nucleic acids. Essays Biochem. 63 (4), 433–456. doi:10.1042/EBC20180038
Ming, Y., Xiao, Y., Tian, Y., and Zhou, S. (2019). Cell-membrane penetration of tat-conjugated polymeric micelles: effect of tat coating density. Macromol. Biosci. 19 (4), e1800364. doi:10.1002/mabi.201800364
Moghimi, S. M., Simberg, D., Skotland, T., Yaghmur, A., and Hunter, a C. (2019). The interplay between blood proteins, complement, and macrophages on nanomedicine performance and responses. J. Pharmacol. Exp. Ther. 370 (3), 581–592. doi:10.1124/jpet.119.258012
Moncini, S., Lunghi, M., Valmadre, A., Grasso, M., Del Vescovo, V., Riva, P., et al. (2017). The miR-15/107 family of microRNA genes regulates cdk5r1/p35 with implications for Alzheimer's disease pathogenesis. Mol. Neurobiol. 54 (6), 4329–4342. doi:10.1007/s12035-016-0002-4
Mummery, C. J., Börjesson-Hanson, A., Blackburn, D. J., Vijverberg, E. G. B., De Deyn, P. P., Ducharme, S., et al. (2023). Tau-targeting antisense oligonucleotide MAPT(Rx) in mild Alzheimer's disease: a phase 1b, randomized, placebo-controlled trial. Nat. Med. 29 (6), 1437–1447. doi:10.1038/s41591-023-02326-3
Murphy, S. R., Chang, C. C., Dogbevia, G., Bryleva, E. Y., Bowen, Z., Hasan, M. T., et al. (2013). Acat1 knockdown gene therapy decreases amyloid-β in a mouse model of Alzheimer's disease. Mol. Ther. 21 (8), 1497–1506. doi:10.1038/mt.2013.118
Nielsen, E. J., Yoshida, S., Kamei, N., Iwamae, R., Khafagy El, S., Olsen, J., et al. (2014). In vivo proof of concept of oral insulin delivery based on a co-administration strategy with the cell-penetrating peptide penetratin. J. Control Release 189, 19–24. doi:10.1016/j.jconrel.2014.06.022
Pannuzzo, M., Esposito, S., Wu, L. P., Key, J., Aryal, S., Celia, C., et al. (2020). Overcoming nanoparticle-mediated complement activation by surface PEG pairing. Nano Lett. 20 (6), 4312–4321. doi:10.1021/acs.nanolett.0c01011
Pardeshi, C. V., and Belgamwar, V. S. (2020). Improved brain pharmacokinetics following intranasal administration of N,N,N-trimethyl chitosan tailored mucoadhesive NLCs. Mater. Technol. 35 (5), 249–266. doi:10.1080/10667857.2019.1674522
Phillips, H. S., Hains, J. M., Armanini, M., Laramee, G. R., Johnson, S. A., and Winslow, J. W. (1991). BDNF mRNA is decreased in the hippocampus of individuals with Alzheimer's disease. Neuron 7 (5), 695–702. doi:10.1016/0896-6273(91)90273-3
Pinheiro, R. G. R., Granja, A., Loureiro, J. A., Pereira, M. C., Pinheiro, M., Neves, a R., et al. (2020). Quercetin lipid nanoparticles functionalized with transferrin for Alzheimer's disease. Eur. J. Pharm. Sci. 148, 105314. doi:10.1016/j.ejps.2020.105314
Popik, P., Wrobel, M., Rygula, R., Bisaga, A., and Bespalov, a Y. (2003). Effects of memantine, an NMDA receptor antagonist, on place preference conditioned with drug and nondrug reinforcers in mice. Behav. Pharmacol. 14 (3), 237–244. doi:10.1097/00008877-200305000-00008
Practical Neurology (2023). Neurology drug and device approvals. Pract. Neurol. (US) 23. Available at: https://practicalneurology.com/articles/2024-jan-feb/special-report-2023-neurology-drug-device-approvals/ (Accessed April 17, 2024).
Prather, K. J., Sagar, S., Murphy, J., and Chartrain, M. (2003). Industrial scale production of plasmid DNA for vaccine and gene therapy: plasmid design, production, and purification. Enzyme Microb. Technol. 33 (7), 865–883. doi:10.1016/s0141-0229(03)00205-9
Qu, B.-X., Lambracht-Washington, D., Fu, M., Eagar, T. N., Stüve, O., and Rosenberg, R. N. (2010). Analysis of three plasmid systems for use in DNA A beta 42 immunization as therapy for Alzheimer's disease. Vaccine 28 (32), 5280–5287. doi:10.1016/j.vaccine.2010.05.054
Rassu, G., Soddu, E., Posadino, a M., Pintus, G., Sarmento, B., Giunchedi, P., et al. (2017). Nose-to-brain delivery of BACE1 siRNA loaded in solid lipid nanoparticles for Alzheimer's therapy. Colloids Surf. B Biointerfaces 152, 296–301. doi:10.1016/j.colsurfb.2017.01.031
Rayamajhi, S., and Aryal, S. (2020). Surface functionalization strategies of extracellular vesicles. J. Mater. Chem. B 8 (21), 4552–4569. doi:10.1039/d0tb00744g
Reis, C. P., Neufeld, R. J., Ribeiro, a J., and Veiga, F. (2006). Nanoencapsulation I. Methods for preparation of drug-loaded polymeric nanoparticles. Nanomedicine 2 (1), 8–21. doi:10.1016/j.nano.2005.12.003
Reisberg, B., Doody, R., Stöffler, A., Schmitt, F., Ferris, S., Möbius, H. J., et al. (2003). Memantine in moderate-to-severe Alzheimer's disease. N. Engl. J. Med. 348 (14), 1333–1341. doi:10.1056/NEJMoa013128
Reissmann, S. (2014). Cell penetration: scope and limitations by the application of cell-penetrating peptides. J. Pept. Sci. 20 (10), 760–784. doi:10.1002/psc.2672
Rodrigues, B. D. S., Kanekiyo, T., and Singh, J. (2020). Nerve growth factor gene delivery across the blood-brain barrier to reduce beta amyloid accumulation in AD mice. Mol. Pharm. 17 (6), 2054–2063. doi:10.1021/acs.molpharmaceut.0c00218
Sadhale, Y., and Shah, J. C. (1999). Stabilization of insulin against agitation-induced aggregation by the GMO cubic phase gel. Int. J. Pharm. 191 (1), 51–64. doi:10.1016/s0378-5173(99)00288-4
Safa, A., Abak, A., Shoorei, H., Taheri, M., and Ghafouri-Fard, S. (2020). MicroRNAs as regulators of ERK/MAPK pathway: a comprehensive review. Biomed. Pharmacother. 132, 110853. doi:10.1016/j.biopha.2020.110853
Sahin, U., Karikó, K., and Türeci, Ö. (2014). mRNA-based therapeutics--developing a new class of drugs. Nat. Rev. Drug Discov. 13 (10), 759–780. doi:10.1038/nrd4278
Salunkhe, S., Basak, M., Chitkara, D., and Mittal, A. (2020). Surface functionalization of exosomes for target-specific delivery and in vivo imaging & tracking: strategies and significance. J. Control. release 326, 599–614. doi:10.1016/j.jconrel.2020.07.042
Schmidt, N., Mishra, A., Lai, G. H., and Wong, G. C. (2010). Arginine-rich cell-penetrating peptides. FEBS Lett. 584 (9), 1806–1813. doi:10.1016/j.febslet.2009.11.046
Shah, P., Lalan, M., and Barve, K. (2022). Intranasal delivery: an attractive route for the administration of nucleic acid based therapeutics for CNS disorders. Front. Pharmacol. 13, 974666. doi:10.3389/fphar.2022.974666
Sharma, G., Modgil, A., Sun, C., and Singh, J. (2012). Grafting of cell-penetrating peptide to receptor-targeted liposomes improves their transfection efficiency and transport across blood-brain barrier model. J. Pharm. Sci. 101 (7), 2468–2478. doi:10.1002/jps.23152
Shintani, E. Y., and Uchida, K. M. (1997). Donepezil: an anticholinesterase inhibitor for Alzheimer's disease. Am. J. Health Syst. Pharm. 54 (24), 2805–2810. doi:10.1093/ajhp/54.24.2805
Shivananjegowda, M. G., Hani, U., Osmani, R. a. M., Alamri, a H., Ghazwani, M., Alhamhoom, Y., et al. (2023). Development and evaluation of solid lipid nanoparticles for the clearance of Aβ in Alzheimer's disease. Pharmaceutics 15 (1), 221. doi:10.3390/pharmaceutics15010221
Shyam, R., Ren, Y., Lee, J., Braunstein, K. E., Mao, H.-Q., and Wong, P. C. (2015). Intraventricular delivery of siRNA nanoparticles to the central nervous system. Mol. Ther. - Nucleic Acids 4, e242. doi:10.1038/mtna.2015.15
Silva, J. M., Li, M. Z., Chang, K., Ge, W., Golding, M. C., Rickles, R. J., et al. (2005). Second-generation shRNA libraries covering the mouse and human genomes. Nat. Genet. 37 (11), 1281–1288. doi:10.1038/ng1650
Singer, O., Marr, R. A., Rockenstein, E., Crews, L., Coufal, N. G., Gage, F. H., et al. (2005). Targeting BACE1 with siRNAs ameliorates Alzheimer disease neuropathology in a transgenic model. Nat. Neurosci. 8 (10), 1343–1349. doi:10.1038/nn1531
Singh, A., Ujjwal, R. R., Naqvi, S., Verma, R. K., Tiwari, S., Kesharwani, P., et al. (2022). Formulation development of tocopherol polyethylene glycol nanoengineered polyamidoamine dendrimer for neuroprotection and treatment of Alzheimer disease. J. Drug Target 30 (7), 777–791. doi:10.1080/1061186X.2022.2063297
Singh, S., and Shukla, R. (2023). Nanovesicular-mediated intranasal drug therapy for neurodegenerative disease. AAPS PharmSciTech 24 (7), 179. doi:10.1208/s12249-023-02625-5
Smith, R. A., Miller, T. M., Yamanaka, K., Monia, B. P., Condon, T. P., Hung, G., et al. (2006). Antisense oligonucleotide therapy for neurodegenerative disease. J. Clin. Invest. 116 (8), 2290–2296. doi:10.1172/JCI25424
Son, S., Hwang, D. W., Singha, K., Jeong, J. H., Park, T. G., Lee, D. S., et al. (2011). RVG peptide tethered bioreducible polyethylenimine for gene delivery to brain. J. Control Release 155 (1), 18–25. doi:10.1016/j.jconrel.2010.08.011
Sonali, S. R. P., Singh, N., Sharma, G., Vijayakumar, M. R., Koch, B., Singh, S., et al. (2016). Transferrin liposomes of docetaxel for brain-targeted cancer applications: formulation and brain theranostics. Drug Deliv. 23 (4), 1261–1271. doi:10.3109/10717544.2016.1162878
Song, J. J., Smith, S. K., Hannon, G. J., and Joshua-Tor, L. (2004). Crystal structure of Argonaute and its implications for RISC slicer activity. Science 305 (5689), 1434–1437. doi:10.1126/science.1102514
Swanson, C. J., Zhang, Y., Dhadda, S., Wang, J., Kaplow, J., Lai, R. Y. K., et al. (2021). A randomized, double-blind, phase 2b proof-of-concept clinical trial in early Alzheimer's disease with lecanemab, an anti-Aβ protofibril antibody. Alzheimers Res. Ther. 13 (1), 80. doi:10.1186/s13195-021-00813-8
Tagalakis, a D., Lee, D. H., Bienemann, a S., Zhou, H., Munye, M. M., Saraiva, L., et al. (2014). Multifunctional, self-assembling anionic peptide-lipid nanocomplexes for targeted siRNA delivery. Biomaterials 35 (29), 8406–8415. doi:10.1016/j.biomaterials.2014.06.003
Tai, Y., Feng, S., Du, W., and Wang, Y. (2009). Functional roles of TRPC channels in the developing brain. Pflugers Arch. 458 (2), 283–289. doi:10.1007/s00424-008-0618-y
Takeda, S., Wegmann, S., Cho, H., Devos, S. L., Commins, C., Roe, a D., et al. (2015). Neuronal uptake and propagation of a rare phosphorylated high-molecular-weight tau derived from Alzheimer's disease brain. Nat. Commun. 6, 8490. doi:10.1038/ncomms9490
Tampi, R. R., and Van Dyck, C. H. (2007). Memantine: efficacy and safety in mild-to-severe Alzheimer's disease. Neuropsychiatr. Dis. Treat. 3 (2), 245–258. doi:10.2147/nedt.2007.3.2.245
Taylor, C. (2023). siRNA drug delivery across the blood brain barrier in Alzheimer's. Eur. Pharm. Rev.
Tian, T., Zhang, H.-X., He, C.-P., Fan, S., Zhu, Y.-L., Qi, C., et al. (2018). Surface functionalized exosomes as targeted drug delivery vehicles for cerebral ischemia therapy. Biomaterials 150, 137–149. doi:10.1016/j.biomaterials.2017.10.012
Tuszynski, M. (2022). A clinical trial of AAV2-BDNF gene therapy in early Alzheimer's disease and mild cognitive impairment. ClinicalTrials.gov identifier: NCT05040217. Available at: https://clinicaltrials.gov/study/NCT05040217/ (Accessed April 17, 2024).
Villain, N., Planche, V., and Levy, R. (2022). High-clearance anti-amyloid immunotherapies in Alzheimer's disease. Part 1: meta-analysis and review of efficacy and safety data, and medico-economical aspects. Rev. Neurol. 178 (10), 1011–1030. doi:10.1016/j.neurol.2022.06.012
Walsh, S., Merrick, R., Milne, R., and Brayne, C. (2021). Aducanumab for Alzheimer's disease? Bmj 374, n1682. doi:10.1136/bmj.n1682
Wang, G. H., Cai, Y. Y., Du, J. K., Li, L., Li, Q., Yang, H. K., et al. (2018a). TAT-conjugated chitosan cationic micelle for nuclear-targeted drug and gene co-delivery. Colloids Surf. B Biointerfaces 162, 326–334. doi:10.1016/j.colsurfb.2017.11.066
Wang, P., Zheng, X., Guo, Q., Yang, P., Pang, X., Qian, K., et al. (2018b). Systemic delivery of BACE1 siRNA through neuron-targeted nanocomplexes for treatment of Alzheimer's disease. J. Control Release 279, 220–233. doi:10.1016/j.jconrel.2018.04.034
Wen, X., Wang, L., Liu, Z., Liu, Y., and Hu, J. (2014). Intracranial injection of PEG-PEI/ROCK II-siRNA improves cognitive impairment in a mouse model of Alzheimer's disease. Int. J. Neurosci. 124 (9), 697–703. doi:10.3109/00207454.2013.877014
WHO (2023). Dementia. Available at: www.who.int/news-room/fact-sheets/detail/dementia/ (Accessed April 17, 2024).
Wong, E., Liao, G. P., Chang, J. C., Xu, P., Li, Y. M., and Greengard, P. (2019). GSAP modulates γ-secretase specificity by inducing conformational change in PS1. Proc. Natl. Acad. Sci. U. S. A. 116 (13), 6385–6390. doi:10.1073/pnas.1820160116
Wu, D., Song, B. W., Vinters, H. V., and Pardridge, W. M. (2002). Pharmacokinetics and brain uptake of biotinylated basic fibroblast growth factor conjugated to a blood-brain barrier drug delivery system. J. Drug Target 10 (3), 239–245. doi:10.1080/10611860290022679
Wu, H., Li, J., Zhang, Q., Yan, X., Guo, L., Gao, X., et al. (2012). A novel small Odorranalectin-bearing cubosomes: preparation, brain delivery and pharmacodynamic study on amyloid-β₂₅₋₃₅-treated rats following intranasal administration. Eur. J. Pharm. Biopharm. 80 (2), 368–378. doi:10.1016/j.ejpb.2011.10.012
Wu, J.-Y., Li, Y.-J., Hu, X.-B., Huang, S., Luo, S., Tang, T., et al. (2021). Exosomes and biomimetic nanovesicles-mediated anti-glioblastoma therapy: a head-to-head comparison. J. Control. release 336, 510–521. doi:10.1016/j.jconrel.2021.07.004
Wu, Y., Luo, X., Liu, X., Liu, D., Wang, X., Guo, Z., et al. (2015). Intraperitoneal administration of a novel TAT-BDNF peptide ameliorates cognitive impairments via modulating multiple pathways in two Alzheimer's rodent models. Sci. Rep. 5, 15032. doi:10.1038/srep15032
Xie, R., Wang, Z., Liu, T., Xiao, R., Lv, K., Wu, C., et al. (2021). AAV delivery of shRNA against TRPC6 in mouse Hippocampus impairs cognitive function. Front. Cell Dev. Biol. 9, 688655. doi:10.3389/fcell.2021.688655
Xin, H., Jiang, X., Gu, J., Sha, X., Chen, L., Law, K., et al. (2011). Angiopep-conjugated poly(ethylene glycol)-co-poly(ε-caprolactone) nanoparticles as dual-targeting drug delivery system for brain glioma. Biomaterials 32 (18), 4293–4305. doi:10.1016/j.biomaterials.2011.02.044
Xin, H., Sha, X., Jiang, X., Zhang, W., Chen, L., and Fang, X. (2012). Anti-glioblastoma efficacy and safety of paclitaxel-loading Angiopep-conjugated dual targeting PEG-PCL nanoparticles. Biomaterials 33 (32), 8167–8176. doi:10.1016/j.biomaterials.2012.07.046
Xiong, X. B., Uludağ, H., and Lavasanifar, A. (2010). Virus-mimetic polymeric micelles for targeted siRNA delivery. Biomaterials 31 (22), 5886–5893. doi:10.1016/j.biomaterials.2010.03.075
Yang, A., Liu, W., Li, Z., Jiang, L., Xu, H., and Yang, X. (2010). Influence of polyethyleneglycol modification on phagocytic uptake of polymeric nanoparticles mediated by immunoglobulin G and complement activation. J. Nanosci. Nanotechnol. 10 (1), 622–628. doi:10.1166/jnn.2010.1738
Ye, Z., Gastfriend, B. D., Umlauf, B. J., Lynn, D. M., and Shusta, E. V. (2022). Antibody-targeted liposomes for enhanced targeting of the blood-brain barrier. Pharm. Res. 39 (7), 1523–1534. doi:10.1007/s11095-022-03186-1
Yu, Y., Li, W., Mao, L., Peng, W., Long, D., Li, D., et al. (2021). Genetically engineered exosomes display RVG peptide and selectively enrich a neprilysin variant: a potential formulation for the treatment of Alzheimer’s disease. J. drug Target. 29 (10), 1128–1138. doi:10.1080/1061186X.2021.1929257
Zeringer, E., Barta, T., Li, M., and Vlassov, a V. (2015). Strategies for isolation of exosomes. Cold Spring Harb. Protoc. 2015 (4), 319–323. doi:10.1101/pdb.top074476
Zhang, D., Wang, J., and Xu, D. (2016). Cell-penetrating peptides as noninvasive transmembrane vectors for the development of novel multifunctional drug-delivery systems. J. Control Release 229, 130–139. doi:10.1016/j.jconrel.2016.03.020
Zheng, X., Pang, X., Yang, P., Wan, X., Wei, Y., Guo, Q., et al. (2017). A hybrid siRNA delivery complex for enhanced brain penetration and precise amyloid plaque targeting in Alzheimer's disease mice. Acta Biomater. 49, 388–401. doi:10.1016/j.actbio.2016.11.029
Zhou, Y., Zhu, F., Liu, Y., Zheng, M., Wang, Y., Zhang, D., et al. (2020). Blood-brain barrier-penetrating siRNA nanomedicine for Alzheimer's disease therapy. Sci. Adv. 6 (41), eabc7031. doi:10.1126/sciadv.abc7031
Keywords: Alzheimer’s disease, functionalized nanoparticles, gene delivery, nucleic acids, blood-brain barrier, cell-penetrating peptides, targeting ligands
Citation: Muolokwu CE, Chaulagain B, Gothwal A, Mahanta AK, Tagoe B, Lamsal B and Singh J (2024) Functionalized nanoparticles to deliver nucleic acids to the brain for the treatment of Alzheimer’s disease. Front. Pharmacol. 15:1405423. doi: 10.3389/fphar.2024.1405423
Received: 22 March 2024; Accepted: 03 May 2024;
Published: 24 May 2024.
Edited by:
Panoraia Siafaka, European University Cyprus, CyprusReviewed by:
Yiannis Sarigiannis, University of Nicosia, CyprusRahul Shukla, National Institute of Pharmaceutical Education and Research, India
Seetha Harilal, Kerala University of Health Sciences, India
Copyright © 2024 Muolokwu, Chaulagain, Gothwal, Mahanta, Tagoe, Lamsal and Singh. This is an open-access article distributed under the terms of the Creative Commons Attribution License (CC BY). The use, distribution or reproduction in other forums is permitted, provided the original author(s) and the copyright owner(s) are credited and that the original publication in this journal is cited, in accordance with accepted academic practice. No use, distribution or reproduction is permitted which does not comply with these terms.
*Correspondence: Jagdish Singh, amFnZGlzaC5zaW5naEBuZHN1LmVkdQ==