- 1School of Medicine, Yan’an University, Yan’an, China
- 2College of Physical Education, Yan’an University, Yan’an, China
Ferroptosis is a non-apoptotic mode of programmed cell death characterized by iron dependence and lipid peroxidation. Since the ferroptosis was proposed, researchers have revealed the mechanisms of its formation and continue to explore effective inhibitors of ferroptosis in disease. Recent studies have shown a correlation between ferroptosis and the pathological mechanisms of neurodegenerative diseases, as well as diseases involving tissue or organ damage. Acting on ferroptosis-related targets may provide new strategies for the treatment of ferroptosis-mediated diseases. This article specifically describes the metabolic pathways of ferroptosis and summarizes the reported mechanisms of action of natural and synthetic small molecule inhibitors of ferroptosis and their efficacy in disease. The paper also describes ferroptosis treatments such as gene therapy, cell therapy, and nanotechnology, and summarises the challenges encountered in the clinical translation of ferroptosis inhibitors. Finally, the relationship between ferroptosis and other modes of cell death is discussed, hopefully paving the way for future drug design and discovery.
1 Introduction
Ferroptosis was first observed in 2003 and officially named by Dixon et al., in 2012. Ferroptosis is a programmed cell death characterized by iron dependence and lipid peroxidation (Chen et al., 2021). Ferroptosis is morphologically and biochemically distinct from traditional modes of cell death such as necrosis, apoptosis, and autophagy. Morphologically, ferroptosis is mostly characterized by smaller mitochondria, increased membrane density, reduced or absent mitochondrial ridges, and normal cell size but a lack of chromatin cohesion (Sun et al., 2023). Biochemically, large amounts of unsaturated fatty acids in the cell membrane undergo lipid peroxidation in response to Fe2+ or lipoxygenase (LOX), triggering ferroptosis (Tang et al., 2021). Ferroptosis mainly involves three pathways: iron metabolism, lipid metabolism, and antioxidants system. Disturbed iron metabolism triggers the Fenton reaction and induces the accumulation of ROS, accumulation of lipid peroxides, and insufficient Glutathione Peroxidase 4 (GPX4) leading to the difficult conversion of lipid hydroperoxides are at the core of the ferroptosis induced by iron metabolism disorders.
With the proposal of ferroptosis, ferroptosis has been found to mediate the pathogenesis of many diseases. In recent years, it has been found that ferroptosis inhibitors also play an important role in neurodegenerative diseases (e.g., stroke (Millán et al., 2021), Alzheimer’s disease (AD) (Jakaria et al., 2021), spinal cord injury (SCI) (Yu et al., 2023)), Acute kidney injury (AKI) (Guerrero-Mauvecin et al., 2023). Intervening in ferroptosis may therefore lead to new therapeutic strategies for the disease. Several drugs have been reported to play an inhibitory role in iron-death-mediated diseases. This review systematically describes the metabolic pathways of ferroptosis and summarizes the mechanisms of action of natural and synthetic small-molecule inhibitors of ferroptosis as well as their applications in disease. Non-traditional therapeutic approaches such as gene therapy, cell therapy, drug combinations and nano-delivery in ferroptosis-mediated diseases are also presented. In addition, the challenges encountered in the preclinical experimental stage and clinical translation of ferroptosis inhibitors are summarised. Finally, the relationship between ferroptosis and other modes of cell death is discussed in the hope of providing new ideas for future drug design and development and clinical translational applications.
2 Small molecule ferroptosis inhibitors
2.1 Inhibition of ferroptosis via the iron metabolism pathway
Iron is one of the important trace elements in the human body and is a key causative factor in ROS accumulation and ferroptosis. Under normal conditions, Fe3+ is found in serum transferrin (TF), The membrane protein Transferrin Receptor1 (TFR1), Six-Transmembrane Epithelial Antigen of Prostate 3 (STEAP3) enzyme, Divalent Metal Transporter 1 (DMT1), Nuclear Receptor Co-Activator 4 (NRC4), and the serum transferrin (TF) enzyme, and in the serum transferrin (TF) and TFR1 enzymes. Epithelial Antigen of Prostate 3 (STEAP3) enzyme, Divalent Metal Transporter 1 (DMT1), and Nuclear receptor coactivator 4 (NCOA4) (Gryzik et al., 2021), Membrane iron transport protein 1 (Ferroportin1, FPN1), Ferritin, Promimin 2, Ceruloplasmin (CP), etc. are involved in the transport to cells and participate in the reaction in vivo (Scarpellini et al., 2023; Zhang et al., 2024a). However, the excess of Fe2+ in the organism under certain pathological conditions, on the one hand, induces the accumulation of ROS in the body through the Fenton reaction; on the other hand, iron participates in the catalytic process of metabolic enzymes, such as LOX, as a cofactor of various phospholipid peroxidases, which in turn accelerates lipid peroxidation and induces ferroptosis (Du and Guo, 2022; Sun et al., 2023) (e.g., Figure 1). Therefore, regulating Fe2+ levels in the body could potentially inhibit ferroptosis and aid in disease treatment.
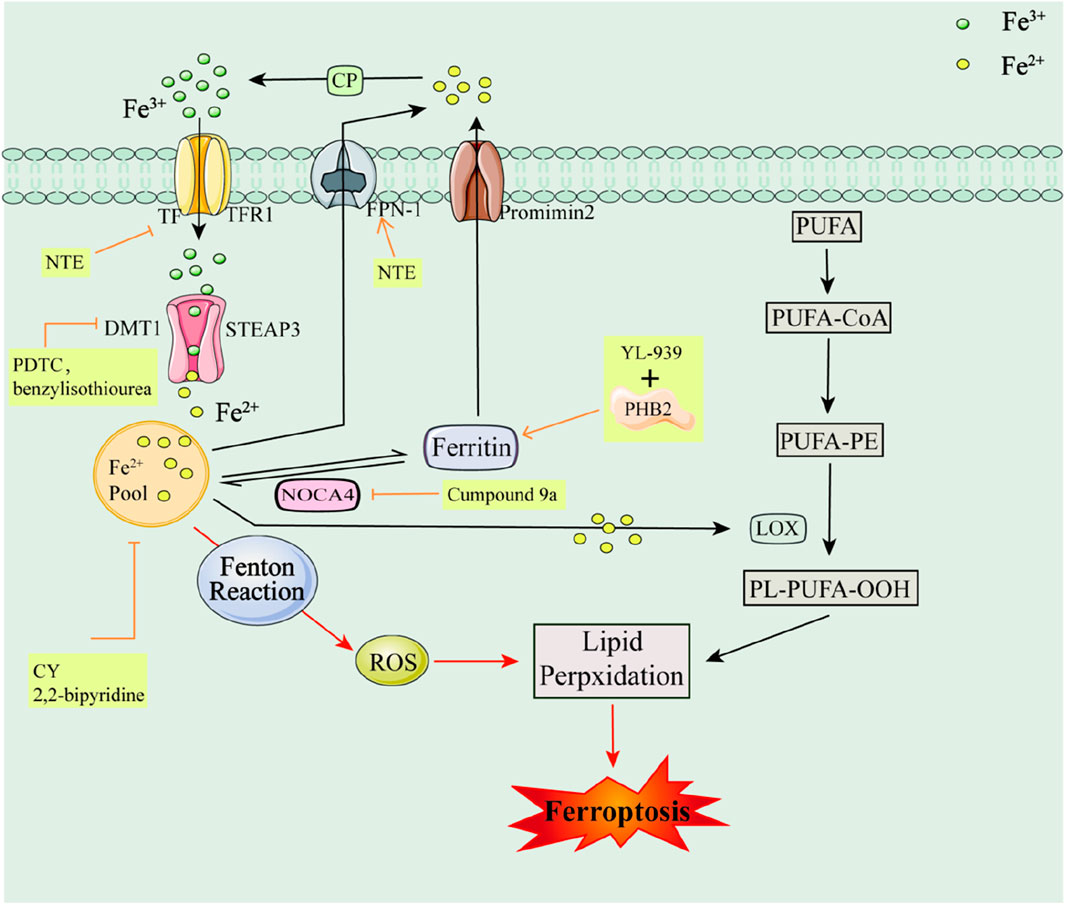
Figure 1. Iron metabolism pathways and their associated inhibitors of ferroptosis Serum Transferrin (TF); The membrane protein Transferrin Receptor1 (TFR1); Six-Transmembrane Epithelial Antigen of Prostate 3 (STEAP3); Ceruloplasmin (CP); Divalent Metal Transporter 1 (DMT1); Polyunsaturated fatty acids (PUFAs); Phosphatidyl Ethanolamine-Polyunsaturated Fatty Acids (PE-PUFA); Lipoxygenase (LOX); Ferritin; Membrane iron transport protein 1 (Ferroportin1, FPN1); Nuclear receptor coactivator 4 (NCOA4); Lipid hydroperoxide (PL-PUFA-OOH); Promimin 2.
2.1.1 Iron chelators
Deferoxamine (DFO) has been approved by the Food and Drug Administration (FDA) for subcutaneous injection to mitigate elastin-induced ferroptosis in an in vitro ferroptosis model (Abdul et al., 2021). DFO inhibits ferroptosis by chelating free intracellular Fe3+, down-regulating ROS, and up-regulating intracellular levels of GPX4, the ferritin heavy chain (FTH1), and Cystine/glutamic acid reverse transporter (System Xc-) (Zhang et al., 2020; Zeng et al., 2021). Moreover, DFO has been shown to inhibit ferroptosis in SCI (Yao et al., 2019), but whether it directly protects neurons from ferroptosis is unclear. At the same time, DFO also plays a role in ischemic stroke (IS), which significantly reduces the area of cerebral infarction (Millán et al., 2021), and plays a certain neuroprotective role against the damage of neurological function after cerebral ischemia (Jones et al., 2022). However, a short DFO half-life was found during treatment. At the same time, two oral drugs, Deferiprone (DFP) and Deferasirox (DFX), have been developed to address the short half-life of DFO, but side effects such as granulocyte deficiency (Lecornec et al., 2022)and renal failure (Kattamis, 2019)are still present during treatment. To address the limitations of DFO, DFP, and DFX such as low oral activity, low efficacy, and side effects, Chen et al. introduced a sacrificial site for glucuronidation and designed and synthesized a novel oral iron chelator, CN128, and found that CN128 was more effective and efficacious orally (Chen et al., 2020), and it has been used in clinical trials in β-thalassemia patients after regular blood transfusions.
Deferric Amine Compounds DFA1 bind iron through two molecules of oxygen in the phenolic hydroxyl group and one molecule of nitrogen in the amine. Research has shown that DFA1 efficiently chelates iron in vivo and ex vivo, outperforming DFO. It has also been observed to alleviate iron overload-induced ferroptosis and reduce cytotoxicity in a mouse model of iron overload when administered orally and intravenously (Feng et al., 2022). Furthermore, DFA1 shows promise as a lead compound due to its high oral (Feng et al., 2022).
DFP was shown to have nephroprotective effects in a glycerol-induced AKI mouse model. Zhang et al. synthesized 25 novel iron chelator cinnamamide-hydroxypyridone derivatives by combining the iron chelating properties of DFP with the free radical scavenging capabilities of phenolic acids. Their assessment included assays for ABTS radical scavenging, Fe3+ affinity, oxygen radical absorbance capacity (ORAC), and the inhibition of Erastin-induced ferroptosis in HT22 cells. It was shown that compound 9c has both chelating iron ions and antioxidant properties. Compound 9c exhibited the strongest inhibition of ferroptosis, almost 10 times more potent than DFP (EC50 = 14.89 μM). Additionally, the researchers observed that compound 9c significantly alleviated cisplatin (CP)-induced AKI in the HEK293T cell model (Rayatpour et al., 2022).
Dexrazoxane (DXZ) is the only drug approved by the FDA that can be used to prevent doxorubicin (DOX)-induced cardiotoxicity. DXZ has been reported to reverse DOX-induced ferroptosis mainly by chelating mitochondrial iron, and its co-administration with Ferrostatin-1 (Fer-1) increased survival in cardiomyopathic rats. Moreover, DOX upregulated GPX4 as well as FTH1 in H9c2 cells (Zhang et al., 2021; Huang et al., 2024). Meanwhile, studies have reported that 2,2′-Bipyridine exerts ferroptosis inhibition by chelating intra-mitochondrial iron (Chen et al., 2020). 1,10-Phenanthroline resembles and downregulates mitochondrial ROS accumulation and inhibits ferroptosis induced by zero-valent iron nanoparticles in vitro with 2,2′-Bipyridine (Huang et al., 2019; Chen et al., 2020). Furthermore, the N, N-dimethylaniline structure of the novel iron chelator GIF-2197-r is crucial for ferrous ion coordination and ferritin resistance (Hirata et al., 2023). In addition, Yael Avramovich-Tirosh et al. reported that the synthetic iron chelator [5-(N-methyl-N-propargylaminomethyl)-8-hydroxyquinoline] (M-30), which permeates the BBB and reduces cellular APP and Aβ production levels, was shown to alleviate the intellectual disability in mice after AD in an in vivo animal model (Avramovich-Tirosh et al., 2007; Zhang et al., 2022).
In addition, studies have shown that natural compounds also can chelate iron. The flavonoid baicalein significantly reverses elastin-induced downregulation of iron accumulation, GSH, and GPX4 in cells (Xie et al., 2016). Experimental screening yielded Hinokitiol, a natural molecule carrying an α-hydroxy ketone skeleton with strong iron chelating properties, which can activate nuclear factor red factor 2-related factor 2 (Nrf2), providing a basis for further neuroprotection (Sridharan and Sivaramakrishnan, 2018). Tannins (TA), which complex with iron without binding to endogenous iron-containing molecules, are also an effective measure for the treatment of diseases associated with iron overload (Phiwchai et al., 2018). BMS536924 a dual inhibitor of insulin-like growth and insulin receptor protects against the induction of iron-dead cells and can act as an iron chelator to block ferroptosis (Kuganesan et al., 2021). Thymus β4 is an endogenous iron chelator that regulates ferroptosis by affecting free iron ions and ROS (Lachowicz et al., 2022). Ciclopirox (CPX) (Eberhard et al., 2009; Lin J. et al., 2021; Lu et al., 2022) has been approved by the FDA for antifungal therapy, and in recent years it has been found that intraperitoneal injection can significantly inhibit the growth of non-small cell lung cancer (NSCLC). Therefore, the use of iron chelation therapy in clinical practice still holds great promise.
2.1.2 Non-iron chelators
In addition to iron chelators, several compounds have been found to inhibit ferroptosis by modulating iron metabolism and are used in disease treatment.
Yang et al. screened a non-classical inhibitor of ferroptosis, YL-939, from chemical libraries. They found that YL-939 binds to its target inhibitor 2PHB2 and promotes ferritin expression to reduce iron levels to inhibit ferroptosis and alleviate ferroptosis-mediated liver injury (Yang et al., 2022). Compound YL-939 provides a new intervention strategy for diseases associated with ferroptosis.
Phenotypic screening and structural modification identification of benzimidazole derivatives and discovery of a novel ferroptosis inhibitor compound 9a by Fang et al. They found that compound 9a inhibited ferroptosis mainly by stabilizing intracellular Fe2+. In the middle cerebral artery occlusion (MCAO) model, compound 9a was found to significantly alleviate neurological impairment after IS. In addition, compound 9a can bind to NCOA4 and break the NCOA4-FTH1 interaction (Fang et al., 2021).
A National Cancer Institute screen found that the polycyclic aromatic compound NSC306711 blocked iron uptake by the Tf-TfR pathway and inhibited ferroptosis. At the same, unlike classical lattice protein-mediated endocytosis of Tf receptors, this drug is also known as ferritin due to its different previous endocytosis pathway that induces internalization and degradation of unoccupied Tf receptors (Horonchik and Wessling-Resnick, 2008).
Study demonstrates that DMT1 inhibitors reduce DMT1-mediated non-transferrin bound iron (NTBI) and play a role in ferroptosis-mediated disease (Sun et al., 2023). These include inhibitors such as pyrrolidine dithiobarbamate (PDTC) (Wetli et al., 2006) and benzylisothiourea (Zhang et al., 2012).
The complex Chinese herb Naotai formula extract (NTE) has been reported to modulate FPN-1, downregulate TFR1 and DMT1 levels, and reduce ROS and MDA accumulation (Yang T. et al., 2021). NTE was found to upregulate rat Recombinant Solute Carrier Family 7, Member 11 (SLC7A11), GPX4, and GSH levels in the MCAO model (Lan et al., 2020; Qiu et al., 2022). Meanwhile, the flavonoid compound Carthamin yellow (CY) has been shown in ex vivo and in vivo experiments to be useful in myocardial ischemia-reperfusion (MIRI) injury and to reduce ROS. In recent years, Guo et al. found downregulation of Fe2+, ROS, reverse transcription of Acyl-CoA Synthetase Long-Chain Family Member 4 (ACSL4), Fe2+, TFR1, Glutathione (GSH), SOD, and MDA levels in the brain and improvement of infarct size in the rat brain after administration of the drug in MCAO models (Guo et al., 2021; Chen G. et al., 2022). In addition, the natural flavonoid Farrerol (FA) may alleviate ferroptosis by inhibiting iron accumulation and lipid peroxidation (Wu et al., 2022).
2.2 Inhibition of ferroptosis via the lipid metabolism pathway
Lipid peroxidation is central to triggering ferroptosis. It was shown that Polyunsaturated fatty acids (PUFA) of arachidonic acid (AA)/adrenaline (AdA) were acylated and esterified to AdA-Phosphatidyl Ethanolamine (AA-PE) and AA-PE with the participation of ACSL4, coenzyme A, and lysophosphatidylcholine acyltransferase-3 (LPCAT3). Ultimately, PE-PUFA leads to lipid peroxidation and induces ferroptosis through both non-enzymatic and enzymatic reactions (Naowarojna et al., 2023; Pope and Dixon, 2023). The non-enzymatic reaction that requires the participation of ROS generated by Fe2+ mediated Fenton reaction. In contrast, enzymatic reactions may be more complex. The enzymatic reaction generally catalyzes the oxidation of PUFAs with the involvement of LOX to produce PE-PUFA-OOH and its derivatives and reactive aldehydes, including Malondialdehyde (MDA) and 4-hydroxynonenal (4HNE), which in turn induce ferroptosis, but these aldehydes, in turn, lead to impaired nucleic acid and protein functions (Pope and Dixon, 2023). Cytochrome P450 oxidoreductase (POR) also promotes lipid peroxidation and induces ferroptosis with the involvement of two cofactors, Flavin mononucleotide (FMN) and Flavin adenine dinucleotide (FAD) (Koppula et al., 2021). In addition, iron can also catalyze the metabolic activity of two enzymes, LOX and POR (Zou et al., 2020) (e.g., Figure 2).
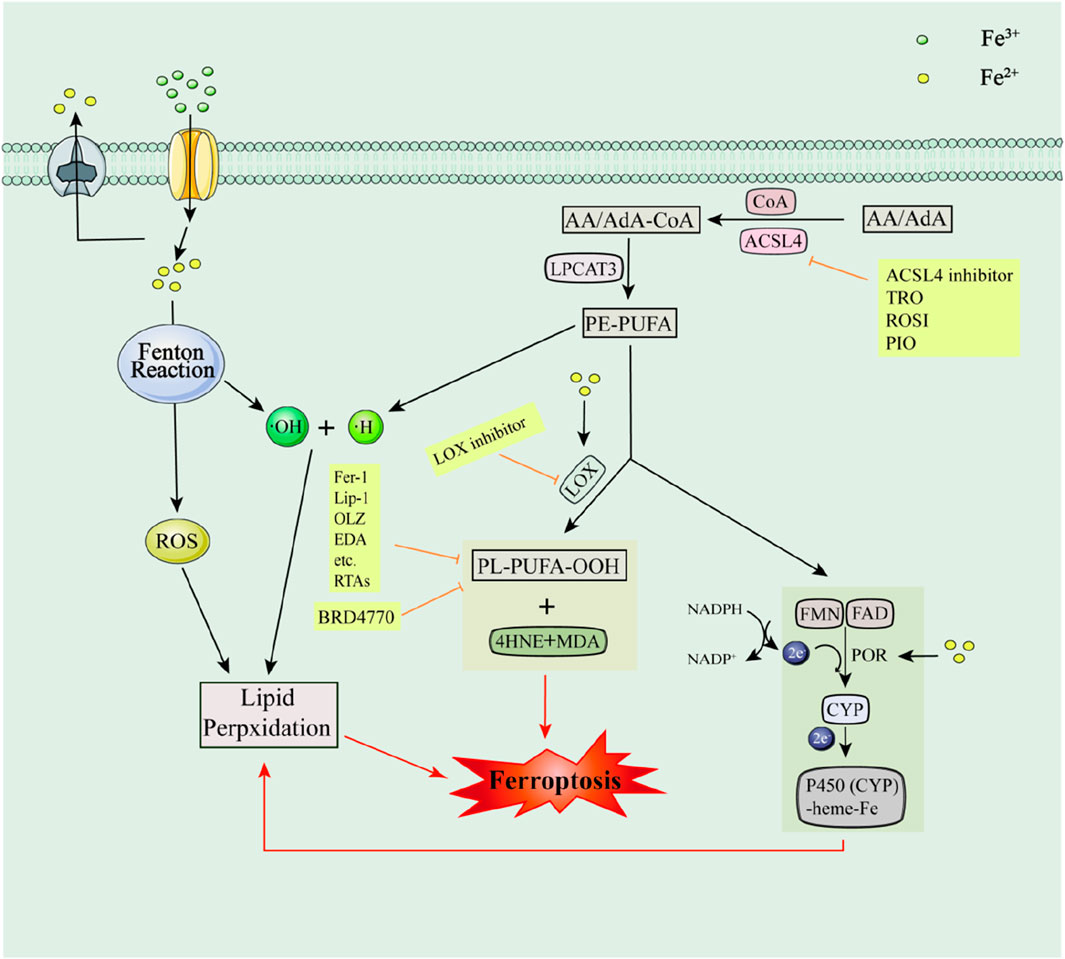
Figure 2. Lipid metabolic pathways and their associated inhibitors of ferroptosis. Acyl-CoA Synthetase Long-Chain Family Member 4 (ACSL4); Recombinant Solute Carrier Family 3, Member 2 (SLC3A2); Malondialdehyde (MDA); 4-hydroxynonenal (4HNE); Cytochrome P450 oxidoreductase (POR); Flavin mononucleotide (FMN); Flavin adenine dinucleotide (FAD).
2.2.1 Free radical trapping antioxidants
Free radical trapping antioxidants (RTAs) inhibit ferroptosis and protect hydrocarbon systems by trapping lipid peroxyl radicals. Fer-1 and Liproxstatin-1 (Lip-1) (Zilka et al., 2017), both obtained by high-throughput screening of small molecule libraries, rapidly transfer H atoms from their arylamine portions to lipid radicals to inhibit lipid peroxidation and prevent free radical chain reactions in membrane PUFA lipids (Bayır et al., 2020; Scarpellini et al., 2023). Current researchers have identified quite a few other exogenous RTAs and many endogenous RTAs that play a role in iron-death-mediated diseases (e.g., Table 1).
2.2.1.1 Endogenous free radical trapping antioxidants
Vitamin E and the trace mineral selenium (Se) form a complementary antioxidant system (Saito, 2021). Vitamin E inhibits lipid peroxide production by reducing Fe3+ in LOX-15 (Tavakol and Seifalian, 2022), but it acts less potently than Fer-1 and Lip-1. Furthermore, it was found that neurological damage caused by vitamin E deficiency in COVID-19 patients was strongly associated with ferroptosis. (Tavakol and Seifalian, 2022).
Melatonin (MLT) is different from vitamin E (Zhang et al., 2023). It can regulate ferritin, lipid peroxidation, and antioxidant capacity (Kajarabille and Latunde-Dada, 2019). MLT was found to inhibit ferroptosis in subarachnoid haemorrhage-mediated neuronal injury by activating genes such as Nrf2 and Heme oxygenase-1 (HO-1) (Ma et al., 2023). Furthermore, MLT alleviates retinal damage and retinal ganglion cells (RGC) death by inhibiting p53-mediated ferroptosis (Zhang et al., 2023). Thus MTL is expected to be a potential therapeutic agent for the treatment of ferroptosis-mediated diseases.
Vitamin K (VK) is a redox-active naphthoquinone, including chlorophyll quinone, menaquinone-4 (MK-4), and menaquinone in three forms (Hirschhorn and Stockwell, 2022). VK is converted to hydroquinone (VKH2) by VK epoxide reductase (VKOR) (Shearer and Okano, 2018). And in a mouse model with a genetic deletion of GPX4, MK-4 showed a protective effect on tissues (Mishima et al., 2022). At the same time, VK reductase Ferroptosis Inhibitory Protein 1 (FSP1) inhibits ferroptosis by reducing VK to VKH2 and prevents lipid peroxidation by depleting NAD(P)H (Li et al., 2023). In addition, recently Kolbrink et al. reported that VK1 may act as a potent endogenous antioxidant to ameliorate AKI (Kolbrink et al., 2022).
2.2.1.2 Exogenous free radical trapping antioxidants
Fer-1, the first synthetic inhibitor of ferroptosis, stabilises free radicals, reduces ROS, and has been strongly implicated in a variety of diseases (Skouta et al., 2014; Wang et al., 2023), including acute lung injury (ALI) (Liu et al., 2020). In structure-activity relationship (SAR) analysis (Saito, 2021), it was found that N-cyclohexyl acts as a lipophilic anchor playing an important role in maintaining Fer-1 activity (Scarpellini et al., 2023). Moreover, both amine groups and lipophilic anchors are essential for maintaining Fer-1 activity. Studies have reported that Fer-1 inhibits ferroptosis both in vivo and in vitro with significant in vitro inhibition. Structural analysis of Fer-1 by researchers yielded the structurally stable compound SRS11-92, but it is less active and less stable in plasma metabolism (Linkermann et al., 2014). Further researchers used elastin-induced HT-1080 cells as a model of ferroptosis, using SRS11-92 as a starting point for optimization. They first introduced a sulfonamide instead of the unstable ester and introduced a benzyl ring 2 on the NH to obtain the sulfonamide analogue compound 38 (UAMC-2418). Compound 38 has high stability but low solubility. Thus, the researchers introduced solubility-enhancing groups into compound 38 to obtain compound 39 (UAMC-3203). Compound 39 showed stronger solubility, stability, and pharmacokinetic values than 38, was protective against multiple organ damage in mice showed no toxicity, and was overall superior to Fer-1 (Scarpellini et al., 2023). Meanwhile, the nanomaterial poly(2-oxazoline)-Fer-1 significantly improved the potency of Fer-1 (Morrow et al., 2022), providing a new idea for the treatment of ferroptosis.
Lip-1 is a newly discovered ferroptosis inhibitor that contains a spiroquinoxaline amine scaffold, functioning similarly to Fer-1 without affecting other cell death pathways. It is both active and soluble, and was introduced around the same time as the Fer-1 derivative UAMC-3203. Various research studies have highlighted the significance of Lip-1 in treating different neurological disorders and cancers. A recent study revealed that a Lip-1 analog, Lip-2, successfully prevented ferroptosis induced by serum in human proximal tubular epithelial cells from patients with lupus nephritis (Class IV), while also exhibiting improved pharmacokinetics (Alli et al., 2023).
To obtain more potent inhibitors, Yang et al. screened the phenothiazine derivatives isoprozine as well as phenothiazine from the Selleck (USA) library of biologically active compounds and they further explored the structure–activity of phenothiazine derivatives (Shah et al., 2017). By substituting different functional groups, they found that compound 51 with methylcytosine had better activity and exerted its antioxidant capacity mainly by trapping free radicals, thus protecting against elastin-induced cellular ferroptosis. It is worth noting that the compound has good pharmacokinetics as well as good BBB penetration, which is essential for the treatment of CNS diseases. Compound 51 was further evaluated in the MCAO model, which showed a significant reduction in lesion volume (Yang et al., 2021). However, it has a high hERG activity, so they further optimized the structure based on the antioxidant and ferroptosis inhibition properties of compounds with phenothiazine scaffolds to obtain 2-vinyl-10H-phenothiazine derivatives. Compound 7J was found to have the best ferroptosis inhibitory activity by SAR study, 7J showed good ROS scavenging ability and could alleviate DOX-induced cardiotoxicity with a good pharmacokinetic profile and no significant toxicity in vitro and ex vivo (You et al., 2022).
Edaravone (EDA), a free radical scavenger approved for treating ischemic stroke (IS) and amyotrophic lateral sclerosis (ALS) (Rothstein, 2017). Recent studies have reported that EDA predominantly activates the Nrf2-FPN pathway, upregulates Nrf2, FPN, and GPX4, and downregulates inflammatory factors to inhibit ferroptosis and alleviate cerebral ischemia-reperfusion injury (CIRI). In addition, it was found that EDA upregulated GPX4 and xCT, downregulated ACSL4 and LOX-5, and inhibited neuronal cell ferroptosis during the acute phase of SCI (Pang et al., 2022).
A novel ferroptosis inhibitor, olanzapine (OLZ), was found to trap free radicals and inhibit ferroptosis in RSL3-induced hippocampal neuronal cells of HT22 mice (EC50 = 1.18 μM) (Jiang et al., 2023; Drabczyk et al., 2023). To improve its inhibitory effect, the researchers optimized its structure. Forty-two thiophene benzodiazepine derivatives (compounds 4–45) were first designed and synthesized and seven compounds (compound 21 and compounds 31–36) were selected by analyzing their tectonic relationships for better ferroptosis inhibition activity (Jiang et al., 2023). After HT22 cytotoxicity evaluation compound 36 was found to have low cytotoxicity (CC50 = 18.8 μM) and its inhibitory activity was 16 times higher than that of OLZ (EC50 = 0.074 μM). Thus, the discovery of OLZ derivatives solves the problem of easy metabolism and low efficacy of Fer-1 and offers hope for ferroptosis-mediated diseases (Jiang et al., 2023).
Recent studies have reported that mitochondria-targeted nitrogen oxide RTA has an effective inhibitory effect on ferroptosis. Nitrogen oxides catalyze the cross-disproportionation of alkyl peroxyl and hydroperoxyl radicals, allowing them to form two substances in unsaturated hydrocarbons as uniquely effective RTAs. Ability of Targeted Nitrogen Oxides XJB-5-131 and JP4-039 to prevent the Occurrence of Cytosolic ferroptosis in HT-1080 Cells as Olefinic Peptide Isoforms (Krainz et al., 2016). Optimisation of JP4-039 by Manwika Charaschanya et al. found compound (S)-6c to be the most potent inhibitor of ferroptosis in HT-1080 cells (approximately 30 times more active than JP4-039) (Charaschanya et al., 2022a; Charaschanya et al., 2022b).
In recent years, copper complex diacetylbis (N(4)-methylthio- semicarbazonato) copper(II) (CuATSM) (Kuo et al., 2019) has been an efficient RTA. The study reports that CuATSM reduces the area of murine cerebral infarction and oxidative stress and exerts antioxidant and neuroprotective effects in acute IS in a transient MCAO (tMCAO) model (Shi et al., 2021). CuATSM can be used in combination with EDA to upregulate this effect (Shi et al., 2021). In addition, CuATSM also exerts a neuroprotective effect in ALS, but the pathological mechanism is currently unknown (Kuo et al., 2019; Yang et al., 2023).
The investigators phenotypically analyzed a new 4-hydroxyl pyrazole scaffold for ferroptosis inhibitor 4-hydroxyl pyrazole derivatives (HW-3) (EC50 = 120.1 ± 3.5 nM) and synthesized a series of 4-hydroxyl pyrazole derivatives based on the backbone structure of HW-3. And it was found that compound 25 exhibited the strongest inhibition of ferroptosis (EC50 = 8.6 ± 2.2 nM). In addition, cellular-level studies have found that compound 25 exhibits more potent inhibition of ferroptosis than Fer-1 (Ying et al., 2024), offering hope for ferroptosis-mediated diseases.
2.2.2 Lipoxygenase inhibitors
Lipoxygenase (LOX) is thought to be a central player in ferroptosis. It induces lipid peroxidation by reacting with ROS and catalysing PUFA, which in turn induces ferroptosis. It has been found that humans have six LOX isoforms, ALOX5, ALOX12, ALOX12B, ALOX15, ALOX15B, and ALOXE3, and there are already some cells that can be rescued by LOX inhibitors. LOX inhibitors inhibit LOX primarily by trapping free radicals (Shah et al., 2018a), which in turn inhibits lipid peroxidation. The most relevant inhibitors reported in the article are LOX-5 inhibitors, including zileuton (Liu et al., 2015; Lee et al., 2022), MK-886 (Shi et al., 2023), BWA4C (Franchi-Miller and Saffar, 1995), PD146176 (Walters et al., 2018) and others. Furthermore, it was found that the LOX-5 inhibitor Zileuton is involved in oxidative stress in retinal pigment epithelium (RPE) cells and regulates retinal ROS. It provides an effective solution for the treatment of retinal diseases (Liu et al., 2015; Lee et al., 2022). LOX-5 expressed during inflammation uptakes apoptotic cells, activates resident macrophage populations, and thus maintains apoptotic cell tolerance (Kapralov et al., 2020). Also, ML351 specifically inhibits ALOX-15 and alleviates erastin-induced cardiac ischemia/reperfusion (I/R) injury (Cai et al., 2023; Horinouchi et al., 2024). In addition, the LOX-5/12 inhibitor docebenone (AA-861), and the LOX-12/15 inhibitor baicalein have all been found to inhibit lipid peroxidation (Scarpellini et al., 2023) and play a role in ferroptosis-mediated diseases.
2.2.3 Acyl-CoA Synthetase Long-Chain Family Member 4 inhibitors
The insulin sensitising drugs thiazolidinediones (TZD) are a class of peroxisome proliferator activatedreceptor γ (PPARγ) activators. Troglitazone (TRO), rosiglitazone (ROSI) and pioglitazone (PIO) were found to effectively and specifically inhibit ACSL4 (Kim et al., 2001), and TRO being TZD-protective due to its 6-chromophoranol structure (Doll et al., 2017). Pharmacological evaluation of ACSL4 revealed that GPX4KO mice treated with ROSI showed better inhibition of ferroptosis (Doll et al., 2017). In addition, thrombin inhibitors also inhibit ACLS4 and ferroptosis (Zhang et al., 2024a).
2.2.4 Other inhibitors
Histone methyltransferase inhibitors (BRD4770) is comparable to Fer-1 inhibition at optimal concentrations (Chen et al., 2022b; Chen et al., 2022c) and may be useful in the treatment of stenosis. The researchers screened and found that BRD4770 exhibited significant ferroptosis inhibition. They further found that BRD4770 inhibited lipid peroxidation by down-regulating PLO and MDA levels and increasing 4-HNE expression. And BRD4770 upregulated the mRNA levels of ferroptosis regulators SLC7A11, Recombinant Solute Carrier Family 3, Member 2 (SLC3A2), GPX4, and FSP1. Furthermore, it was found that BRD4770 alleviated cognitive impairment and downregulated aortic lipid peroxidation in a mouse model of AD (Chen et al., 2022b). Thus, BRD4770 is expected to be an effective molecular drug for the treatment of AD.
Recently Florencio Porto Freitas et al. identified 7-dehydrocholesterol (7-DHC), an endogenous ferroptosis inhibitor, as one of the lipid components susceptible to autotrophication in vivo. They prepared soybean phosphatidylcholine (PC) monolayers loaded with 7-DHC and found that 7-DHC is preferentially oxidised in vitro and that the oxidation of 7-DHC in vitro is critical for the inhibition of (phospho)lipid peroxidation. Subsequently, using the iron/ascorbate couple as a model for oxidative sources, they found that 7-DHC accumulation protects (phosphoric acid) lipids from autoxidation and subsequent rupture and is different from previous lipid peroxidation, mainly due to its better reactivity to peroxyl radicals. In addition, 7-DHC accumulation significantly reduces metabolic stress in the body (Angeli et al., 2021; Freitas et al., 2024). Thus, 7-DHC regulates ferroptosis in an easily overlooked manner by inhibiting lipid peroxidation and bringing new therapeutic ideas to ferroptosis-mediated diseases.
In recent years, researchers have isolated seven abolane-type sesquiterpenoids (PBSs) from the deep sea fungus Aspergillus floridus YPH1 (Li et al., 2023b), with compound 7 showing a selective inhibitory effect on Erastin/RSL3-induced ferroptosis similar to Fer-1. However, unlike Fer-1, compound 7 exhibited negligible radical scavenging activity in 2,2-diphenyl-1-picrylhydrazyl (DPPH) assays (Li et al., 2023b). Additionally, PBSs25 gossypol acetic acid (GAA) was found to protect cardiomyocytes from ferroptosis in vitro by reducing chelated iron and lipid peroxidation. In vivo studies showed that GAA significantly upregulated GPX4 (Lin et al., 2021).
2.3 Inhibition of ferroptosis via antioxidant action
The antioxidant system is central to impeding ferroptosis by stabilizing or extinguishing free radicals and thereby inhibiting lipid peroxidation (Tan et al., 2023) (e.g., Figure 3.). System Xc-is an important antioxidant system involved in the regulation of ferroptosis. System Xc-passes through a heterodimer of the light-chain subunit SLC7A11 and the heavy-chain subunit SLC3A2, and glutamate-cysteine ligase (GCL) and glutathione synthase (GSS) transfer cystine to the cell and synthesise glutathione (GSH). Then, GSH is converted to Oxidized glutathione (GSSG) with the participation of glutathione peroxidation GPX4 to eliminate toxic lipid peroxides (Dar et al., 2024). Moreover, in the presence of GSH GPX4 converts toxic lipid peroxides into non-toxic lipocalciferol, which protects cells from lipid peroxidation and thus inhibits ferroptosis (Dar et al., 2024).
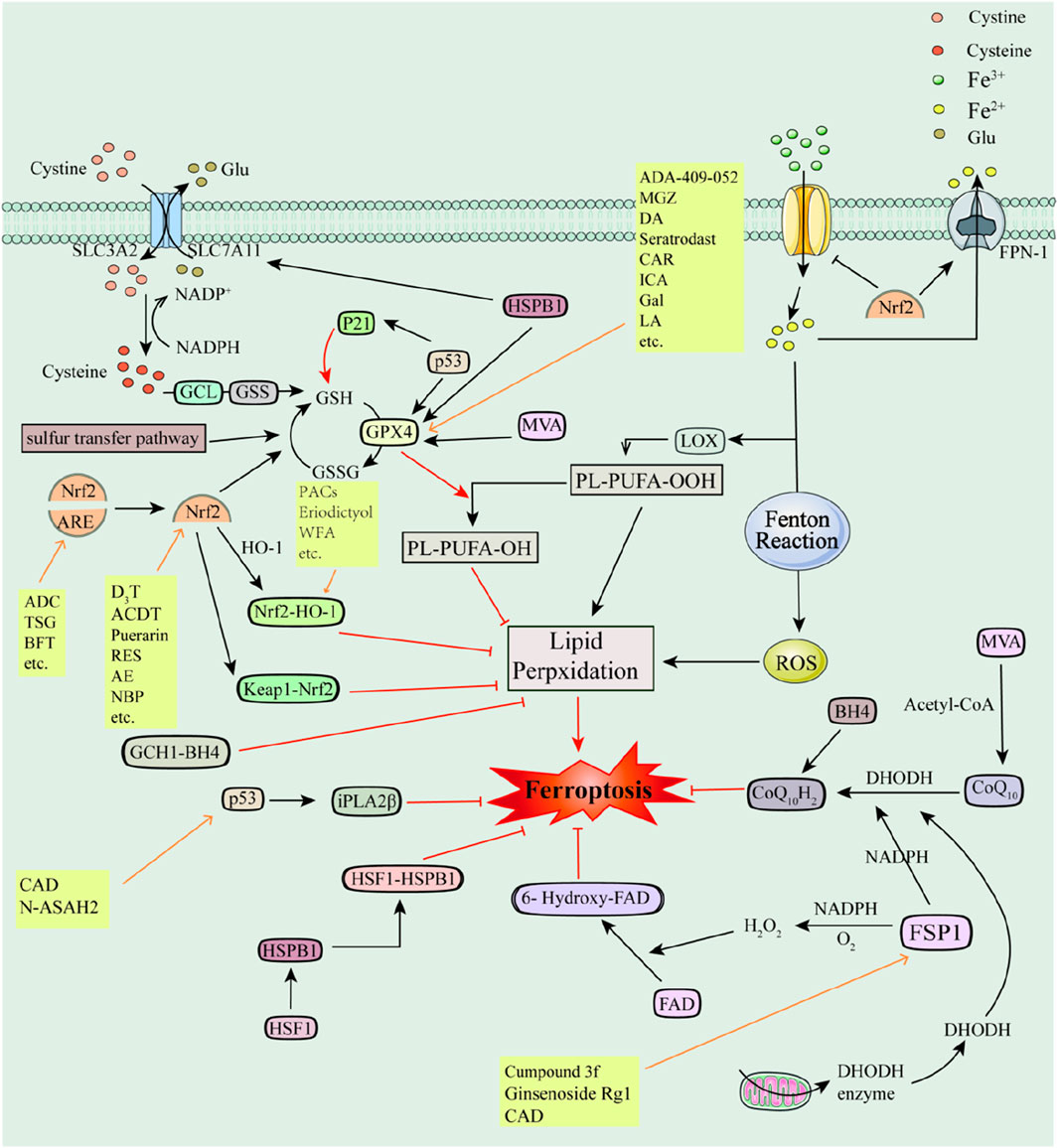
Figure 3. Antioxidant pathways and their associated inhibitors of ferroptosis Recombinant Solute Carrier Family 7, Member 11 (SLC7A11); Recombinant Solute Carrier Family 3, Member 2 (SLC3A2); Glutamate-cysteine ligase (GCL); Glutathione Synthase (GSS); Glutathione (GSH); Glutathione Peroxidase 4 (GPX4); Oxidized glutathione, GSSG; Glutamic acid (Glu); Cystine (Cys); Nuclear factor E2-related factor 2 (Nrf2); p53 (tumor suppressor); Recombinant Kelch-like ECH Associated Protein 1 (Keap1); Antioxidant response element (ARE); Heme oxygenase-1 (HO-1); Ferroptosis Inhibitory Protein 1 (FSP1); Coenzyme Q10 (CoQ10); Panthenol (CoQ10H2); Nicotinamide Adenine Dinucleotide Phosphate (NADPH); Dihydroorotate dehydrogenase (DHODH); GTP Cyclohydrolase-1 (GCH1); Tetrahydrobiopterin (BH4); GTP Cyclohydrolase-1-Tetrahydrobiopterin (GCH1-BH4); Mevalonate (MVA); Heat Shock Protein Beta-1 (HSPB1); Heat shock factor (HSF1); 6-hydroxy-FAD; p53-iPLA2β axis; p21; Acetyl-coenzyme A (Acetyl-CoA).
The anti-oxidative stress transcription factor Nrf2 and the tumor protein p53 are the two most studied transcription factors in ferroptosis. Nrf2 regulates a variety of antioxidant enzymes and proteins to exert antioxidant effects and inhibit ferroptosis, including Superoxide Dismutases (SODs), HO-1, NAD(P)H-associated enzymes, GPX4, catalase (CAT), FPN, TFR, TF, System Xc-, ferrochelatase (FECH), etc. (Punziano et al., 2024). p53, as an effective oncogene, promotes ferroptosis mainly through the canonical and non-canonical pathways (Dixon et al., 2012). However, recent studies have found that the p53-GPX4 axis eliminates lipid peroxidation, the p53-iPLA2β axis eliminates free radicals, and p53 transactivates iPLA2β and inhibits ferroptosis when cells are low in damage from lipid peroxidation (Gnanapradeepan et al., 2022). In addition, p53 induces GSH production by P21 (also known as cell cycle protein-dependent kinase inhibitor 1A, CDKN1A), which in turn upregulates GPX4 thereby inhibiting ferroptosis (Tarangelo and Dixon, 2018; Tarangelo et al., 2018; Tarangelo et al., 2022) (e.g., Figure 3).
FSP1 can inhibit ferroptosis by generating H2O2 in the presence of O2 and NADPH and then converting FAD to 6-hydroxy-FAD (Lv et al., 2023; Nakamura et al., 2023). Meanwhile, FSP1 can reduce Coenzyme Q10 (CoQ10) to panthenol (CoQ10H2) with the involvement of NADPH and thus inhibit ferroptosis (Li et al., 2023; Zhang et al., 2023). CoQ10 is a lipophilic RTA (Li et al., 2023; Zhang et al., 2023). Furthermore, the NADPH-FSP1-CoQ10 pathway is independent of the Xc-GSH-GPX4 pathway and cooperates with it to inhibit lipid peroxidation and ferroptosis (Bersuker et al., 2019). Dihydroorotate dehydrogenase (DHODH) can also independently reduce CoQ10 to CoQ10H2, thereby protecting cells from lipid peroxidation (Amos et al., 2023a; Amos et al., 2023b) (e.g., Figure 3).
Other antioxidant pathways involved in ferroptosis include GTP Cyclohydrolase-1-Tetrahydrobiopterin (GCH1-BH4), Heat shock factor-Heat Shock Protein Beta-1 (HSF1-HSPB1), the mevalonate (MVA) pathway, Sulfur transfer pathway, the glutaminolysis pathway, and so on. Overexpression of the ferroptosis regulator GCH1 inhibits lipid peroxidation and promotes BH4 synthesis (Xu et al., 2023). BH4 is a potent endogenous RTA and participates in CoQ10H2 formation. The GCH1-BH4 pathway has endogenous antioxidant effects and is independent of the Xc-GSH-GPX4 axis and the NADPH-FSP1-CoQ10 axis (Akiyama et al., 2023). Transcription factor HSF1 promotes HSPB1 transcription and forms the HSF1-HSPB1 pathway (Sun et al., 2015). HSPB1 is a negative regulator of ferroptosis that upregulates GPX4, SLC7A11, and G6PD and HSPB1 overexpression attenuates ischemic-hypoxic brain damage in neonatal rats (Doshi et al., 2010; Liang et al., 2023). MVA plays a regulatory role in ferroptosis. On the one hand, by regulating selenocysteine tRNA which in turn promotes GPX4 synthesis. And on the other hand, MVA can synthesize CoQ10 in the presence of acetyl coenzyme A, which in turn participates in the Xc-GSH-GPX4 axis and the NADPH-FSP1-CoQ10 pathway and is involved in the regulation of ferroptosis (Xing et al., 2023). The Sulfur transfer pathway plays a role in the maintenance of redox homeostasis and oxidative stress, mainly due to the upregulation of intracellular Cys, GSH, and GSSG and the inhibition of ROS as a result of Cys-tRNA synthetase (CARS) deficiency (Floros et al., 2022; Sun et al., 2023). Glutamine catabolism provides sufficient GSH and ATP for ferroptosis and also plays a supporting role in ferroptosis-mediated diseases (Durante, 2019; Shin et al., 2020; Gagliardi et al., 2023; Xiao et al., 2023) (e.g., Figure 3). Several ferroptosis inhibitors have been reported to inhibit ferroptosis by modulating the antioxidant pathway.
2.3.1 The System Xc-GSH-GPX4 axis
The heat shock protein 90 (HSP90) plays an important role in protein maturation, stabilisation, and activation (Wickramaratne et al., 2023). A triterpenoid compound 2-amino-5-chloro-N, 3-dimethylbenzamide (CDDO) was identified by Wu et al. It inhibits HSP90, which in turn inhibits GPX4 degradation and lipid peroxidation, and downregulates ROS, protecting cells from damage caused byferroptosis (Wu et al., 2019). Furthermore, in 2022 Liu et al. found that a series of disulfide compounds, such as disulfiram (DSF) and fursultiamine, could disrupt the interaction of GPX4 with HSC70, which in turn inhibited GPX4 degradation and protected cells from ferroptosis (Liu et al., 2022).
In 2021 Meike Hedwig Keuters et al. found that the novel ferroptosis inhibitor arylthiazyne derivative small molecule (ADA-409-052) inhibited tert-butyl hydroperoxide (TBHP)-induced lipid peroxidation and prevented ferroptosis induced by GSH, as well as GPX4 deficiency. It is rapidly absorbed after oral administration in a mouse model. In addition, administration of the drug in a thrombotic mouse model revealed a significant decrease in the area of cerebral edema, the area of cerebral infarction, and the expression of pro-inflammatory factors in mice (Keuters et al., 2021). Thus, small molecule inhibitors such as ADA-409-052 offer new therapeutic strategies for neurological disorders as well as acute brain injury.
In 2023, Qi et al., 2023 reported that the antidiabetic drug mitoglitazone (MGZ) upregulated GPX4 and reduced lipid peroxidation, thereby significantly alleviating renal injury in mice after I/R, providing a new therapeutic strategy to ameliorate renal I/R injury. In addition, MGZ can also exert a nephroprotective effect by maintaining the normal morphology of mitochondria.
The catecholamine neurotransmitter Dopamine (DA) plays a role in human cognitive functions. It was shown that DA enhances the stability of GPX4 protein (Costa and Schoenbaum, 2022). Increased survival of DA ergic neurons and reversal of ROS, GPX4, and FTH1 levels in SNpc after moxibustion application in a Parkinson’s disease (PD) model and improvement of motor deficits in it. And DA agonists reduce the risk of side effects during recovery (Ding et al., 2023). In addition, levodopa is approved for use in patients with early or late stroke and can be used in combination with physiotherapy (Obi et al., 2018). DA-mediated ferroptosis has been mentioned in other diseases and is expected to lead to new research directions in ferroptosis-mediated diseases.
The study reports that Se inhibits ferroptosis and protects neurons by enhancing GPX4 expression. Se acts in the antioxidant pathway mainly by containing selenoproteins (Ramakrishnan et al., 2022; Choi et al., 2023). The study reports that Se inhibits ferroptosis and protects neurons by enhancing GPX4 expression (Alim et al., 2019). Inadequate levels of organismal Se downregulate GPX4 and antioxidant enzymes and upregulate ROS, MDA, and LPO (Xu et al., 2023). Se alleviates cerebral ischemia-induced neurological damage by activating GPX4 in IS (Alim et al., 2019). Iron, MDA and 4-HNE were found to be downregulated and promote the FSP1/GPX4 pathway after sodium selenite injection in a rat model of SCI, which in turn improved motor function in rats (Chen et al., 2022). In addition, injection of double selenium nanospheres (CLNDSe) in an AD mouse model revealed abnormal microglia protofibrils or tau protein-targeted aggregation outside of Aβ42, and its crossing of the BBB into the brain significantly downregulated ROS, ACSL4, and COX-2, and upregulated FTH1, GPX1, and GPX4, ultimately inhibiting ferroptosis and ameliorating cognitive deficits in APP/PS1 mice (Solovyev et al., 2018). Overall, the trace element Se has been found to play a role in a variety of diseases by mediating ferroptosis.
Recently Li et al., 2019 identified a potent GPX4 metastable site and obtained eight GPX4 metastable activators by structural analysis and computational design (Scarpellini et al., 2023). They inhibit ferroptosis by activating GPX4 enzyme activity (IC50 > 100 μM). Compound PKUMDL-LC-101 and its analogue PKUMDL-LC-101-D04 most efficiently activated and increased GPX4 activity in intact cells while inhibiting ferroptosis in a cellular model (Scarpellini et al., 2023). These compounds provide an effective strategy for the future development of activators for other protein targets and also lead to new therapeutic strategies for lipid peroxidation-related diseases such as neurodegenerative diseases (Li et al., 2018; Li et al., 2019).
Upregulation of GPX4 levels and downregulation of lipid ROS in neurons after treatment with the thromboxane A2 receptor antagonist Seratrodast and consequent inhibition of neuronal cell ferroptosis in erastin-induced hippocampal HT22 cells. And Seratrodast increased GPX4 expression and shortened seizure duration and prolonged seizure latency in a mouse model of epilepsy (Noack et al., 2017). Thus Seratrodast could play a role in several diseases by inhibiting ferroptosis.
Using APP/PS1 mice as subjects, researchers found that the monoterpene glycoside paeoniflorin (PF) improved cognitive performance and downregulated the levels of Fe2+, MAD, and ROS in brain tissue, which reduced oxidative damage and thus alleviated the neurological damage in AD mice (Zhang and Wei, 2020; Zhai et al., 2023). And PF can reverse AKI by inhibiting SLC7A11-mediated ferroptosis (Ma et al., 2023). In 2019, Guan et al. found that the monoterpene oenol carvacrol (CAR) (Abdul Ghani et al., 2023) reduced PLO levels in ischemic gerbil brain tissue and inhibited ferroptosis by up-regulating GPX4, which exerted neuroprotective effects in both in vivo and ex vivo models of IS. Thus, CAR has the potential to be an effective therapeutic agent for IS. In 2021, Li et al. found that injection of Ginkgolide B (GB), the active ingredient of terpene lactones (Wang et al., 2020), in an animal model of AD reversed the levels of TFR1 and NOCA4 and upregulated the expression of Nrf2 and GPX4 in the brains of SAMP8 mice, which exhibited neuroprotective effects in AD mice (Hébert et al., 2022).
The lanolin-type triterpenoid Pachymic Acid (PA) has a variety of pharmacological properties. Liu et al. established a cellular myocardial infarction (MI) model after administration of 20 μg/mL and found that the inhibitory effect of oxygen-glucose deprivation/reperfusion (OGD/R) on cell viability was reversed and the levels of MDA, ROS, and Fe2+ were downregulated, and the expression of GSH, SLC7A11, and GPX4 was increased. Meanwhile, PA inhibits cardiomyocyte ferroptosis in a dose-dependent manner and attenuates MIRI injury in mice (Liu et al., 2024).
Flavonoids are an important class of phenolic metabolites in plants with good antioxidant effects. The bioflavonoid Kaempferol (KF) has shown neuroprotective effects in neurological disorders such as IS and AD. Recently, it was found that KF activated the Nrf2/SLC7A11/GPX4 signalling pathway and enhanced antioxidant capacity, which in turn reversed OGDR-induced ferroptosis (Holland et al., 2020; Kim et al., 2023) and alleviated neuronal cell damage. The natural flavonoid compound Icaritin (ICA) plays a role in a variety of diseases such as IS, AD, depression, and others. It directly binds to Nrf2 and promotes GPX4 transcription, which in turn inhibits ferroptosis after IRI and ameliorates brain damage (Choi et al., 2023). In recent years, ICA compounds significantly enhanced GSH-Ps, SOD activities and alleviated oxidative stress injury in mouse brain tissue after gastric administration of ICA compounds in APP/PS1 double transgenic mice. In addition, ICA was found to alleviate cognitive deficits in AD mice in a dose-dependent manner in the Morris water maze (VWM) (Angeloni et al., 2019; Zheng et al., 2023).
Total flavonoids from Aspergillus membranaceus (TFA) play a role in neurodegenerative diseases. Gao et al., 2024 found that TFA prevented SH-SY5Y cell neurotoxicity by increasing GSH and GSH/GSSG ratios and decreasing ROS, and showed significant neuroprotection in MPTP/MPP-induced in vitro and in vivo PD mouse models. Simultaneous injection of the flavonoid galangin (Gal) after I/R injury in VWM revealed a significant reduction in lipid peroxidation levels and an upregulation of SLC7A11 and GPX4 expression in the gerbil brain, which resulted in the inhibition of ferroptosis and the protection of hippocampal neurons in the gerbil brain after I/R (Guan et al., 2021; Hassanein et al., 2023). At the same time, gerbils showed significant improvement in the area of learning memory. Recently, Yang et al. found that Gal prevented iron overload and lipid peroxidation in a MIRI model and significantly attenuated myocardial fiber damage, reduced cerebral infarct size, and improved cardiac function by targeting the GPX4/Nrf2 signalling pathway in mice after MIRI (Salama and Elshafey, 2021). Thus flavonoids are a promising inhibitor of ferroptosis.
Α-lipoic acid (LA) reverses folic acid (FA)-induced AKI. In 2021, Xue et al. found that LA upregulated GSH, GPX4, and downregulated ROS as well as lipid peroxidation, and that supplementation with LA reversed the low expression of SLC7A11 (Li et al., 2021). In addition, LA also inhibits ferroptosis and attenuates fluoride-induced liver injury by modulating the Xc-GSH-GPX4 axis and chelating iron (Cho et al., 2022).
In addition to the above-mentioned small molecule drugs, many herbal medicines have also been found to play a role in herbal medicines have also been found to play a role in ferroptosis-mediated diseases. For example, Lv et al. established a diabetic nephropathy (DN) mouse model and found that Fe2+ was downregulated and SLC7A11, GPX4 content, and GSH/GSSG content, and GSH/GSSG were upregulated in the renal tissues of mice after San-Huang-Yi-Shen capsule (SHYS) administration and that it alleviated renal injury (Su et al., 2021; Lv et al., 2023). At the same time, Modified Shoutai Pill, also known as Jianwei Shoutai Pill (JSP), upregulated GSH, GPX4, downregulated MDA levels, and ACSL4 protein expression in the placenta of Recurrent Pregnancy Loss (RPL) mice, and was shown to protect against RSL3-induced lipid metabolism (Zhang et al., 2023; Lai et al., 2024). Futhermore, Angong Niuhuang Wan (AGNHW) has recently been found to exert neuroprotective effects by modulating GPX4-related signaling pathways and inhibiting ferroptosis (Bai et al., 2024).
2.3.2 Nrf2
Uridine, composed of uracil and ribose, exhibits anti-inflammatory (Jeengar et al., 2017), antioxidant (Adant et al., 2022), and anti-aging (Krylova et al., 2021) properties. Kai Lai et al. (2023) discovered an increase in uridine phosphorylase 1 (UPP1) in an ALI model induced by lipopolysaccharide (LPS), leading to elevated uridine levels and the upregulation of SLC7A11, GPX4, and HO-1 expression. Moreover, Uridine was found to inhibit macrophage ferroptosis by activating the Nrf2 signaling pathway, with the protective effect enhanced by Fer-1. Therefore, supplementing with Uridine may offer potential therapeutic benefits for ALI (Lai et al., 2023).
Dithiolethiones, lipophilic organosulfur compounds (Ansari et al., 2018), such as 3H-1,2-dithiole-3-thione (D3T) and 5-amino-3-thioxo-3H-(1,2)dithiole -4-carboxylic acid ethyl ester (ACDT), activate Nrf2 and increase system Xc- and GSH levels in the erastin-induced ferroptosis model, demonstrating antioxidant effects and significant inhibition of ferroptosis akin to Fer-1 (Kulkarni et al., 2023). Treatment with D3T and ACDT upregulated Nrf2-mediated expression of ferritin and FPN1, while also protecting U-87MG cells from iron overload-induced cytotoxicity (Kulkarni et al., 2023). Additionally, D3T upregulates silent information regulator 1 (SIRT1), Nrf2, and HO-1, ameliorating cognitive deficits in the Tg2576 AD mouse model (Cui et al., 2018). Notably, D3T exhibits neuroprotective effects in neuroinflammation and IS, suggesting its potential as a treatment option for ferroptosis-related diseases.
In 2022, Fan et al., 2023 discovered that the anaesthetic Propofol (Islam et al., 2020) could have a strong antioxidant effect by modulating Nrf2/GPX4 through protein blotting, transmission electron microscopy, and glutathione assays. Furthermore, in a mouse model of CIRI, Propofol was shown to effectively prevent ferroptosis and protect against neuronal damage in the brain after cerebral ischemia/reperfusion.
Metformin (Met), a biguanide derivative commonly used in the first-line treatment of type 2 diabetes, has also shown promise in various other conditions such as cancer, cardiovascular disease, and neurological disorders (Ala and Ala, 2021). Recent research has unveiled Met’s potential role in spinal cord injury (SCI) by inhibiting oxidative stress through the Nrf2/ARE pathway (Wang et al., 2020) and promoting neural regeneration post-SCI, offering new therapeutic possibilities. Additionally, studies have indicated that Met upregulates GPX4 (Ma et al., 2021), reduces MDA levels, and provides protection against neurological impairments following cerebral ischemia (Cai Z. et al., 2023).
The novel chlorane diterpenoid analogue Ajudecunoid C (ADC) is more effective in ferroptosis inhibition. It has been shown that ADC mainly acts on the Nrf2-AREs pathway and scavenges free radicals to exert an ferroptosis inhibitory effect, and ADC may interfere with the Keap1-Nrf2 pathway and activate the Nrf2-AREs pathway (Tan et al., 2021). In addition, the diterpenoid Dehydroabietic Acid activates the Keap1/Nrf2-ARE signalling pathway and attenuates non-alcoholic fatty liver disease (NAFLD). Therefore, researchers have proposed that diterpenoids may be effective compounds for inhibiting ferroptosis (Tan et al., 2021).
Biopolyphenolics Proanthocyanidins (PACs) are effective free radical scavengers. PACs have been reported to also have the ability to regulate LOX (Zhou et al., 2020). PACs have been found to inhibit ferroptosis and play a role in a variety of diseases by altering the expression of iron-related factors. For example, PACs upregulate the expression of GSH, GPX4, SLC7A11, Nrf2, and HO-1 and downregulate the expression of TFR1, ACSL4 and regulate SCI, thereby promoting ferroptosis-mediated functional recovery in SCI mice (Zhou et al., 2020). In addition, a large number of studies have shown that PACs activate the Nrf2-HO-1 pathway and ameliorate CIRI, bringing a new direction for CIRI treatment (Chumboatong et al., 2020). In 2022, Yuan et al. found downregulation of oxidative stress and neuronal death after injection of the phenolic substance geraniin by the middle cerebral artery occlusion-reperfusion (MCAO/R), and OGD/R models, as well as by neurological scoring assays, CCK8 assessment, TUNEL staining, detection of cells by flow cytometry, and Western blotting assessment (Chen et al., 2021). Meanwhile, they found that the infarcted portion of brain tissue upregulated ex vivo and in vivo Nrf2, HO-1 protein expression in a concentration-dependent manner in the tMCAO model (Chumboatong et al., 2020). Thus, the neuroprotective effect of geraniin may be closely related to the Nrf2/HO-1 signalling pathway, but further experimental elucidation is needed in the future.
Flavonoids have been implicated in ferroptosis-related diseases through their interaction with Nrf2. For example, quercetin (QCT), a natural flavonoid, has been shown to alleviate renal injury by activating the Nrf2-HO-1 signaling pathway (Kato et al., 2023). Studies using an AKI-I/R model demonstrated that QCT administration led to increased cell viability, upregulation of ALC7A11, SLC3A2, and GSH expression, as well as a reduction in MDA and lipid ROS content in mice (Cruz-Gregorio and Aranda-Rivera, 2023). QCT also shows promise in the treatment of neurological disorders such as Parkinson’s disease (PD) (Jiang et al., 2023). Eriodictyol, another flavonoid, was found to upregulate Nrf2/HO-1 and reduce ROS levels in a renal injury model, providing protection against CP-induced AKI (Badi et al., 2024). Furthermore, Eriodictyol has been shown to inhibit ferroptosis and improve cognitive deficits in APP/PS1 mice through activation of the Nrf2/HO-1 pathway (Li et al., 2022; Zhang et al., 2022). Naringenin, a flavonoid derivative from citrus extract, has demonstrated efficacy in alleviating myocardial injury by modulating the Nrf2/system Xc-/GPX4 axis (Shamsi et al., 2021). It also shows potential in mitigating ferroptosis induced by silver nanoparticles in human bronchial epithelial BEAS-2B cells through the Nrf2/HO-1 axis (Zhang et al., 2022b). Hesperidin and naringin have both been found to inhibit ferroptosis by upregulating the Nrf2 pathway, offering protection to human myeloid cells (Tang et al., 2022; Zhu et al., 2023).
The isoflavone tectorigenin protects against unilateral ureteral obstruction and renal injury in rats by affecting NADPH oxidase 4 (NOX4) expression (Li et al., 2022). Biochanin A, an active isoflavone of Astragalus membranaceus, inhibits ferroptosis by modulating the Nrf2/system Xc-/GPX4 signalling pathway, decreasing TFR1 and ferritin levels, and relieves knee osteoarthritis (KOA) (He et al., 2023). Licorice extract chalcone isoiquiritin apioside inhibits ferroptosis mediated ALI through upregulation of HIF-α and HO-1 proteins (Zhongyin et al., 2022). Puerarin, a natural isoflavone extracted from Pueraria Mirifica, was found to be protective against neuronal cell damage in mice by modulating Nrf2 in the OGD/R model (Li and Liu, 2024). It was also demonstrated to downregulate Fe2+, cyclooxygenase 2 (COX2), and upregulate Nrf2 and its downstream related ferritin (including SLC7A11, GPX4, HO-1) expression to reduce lipid peroxidation and inhibit retinal ferroptosis (Song et al., 2024). In addition, Puerarin was found to downregulate p53 and effectively improve neurological impairments in patients with ischemic brain injury in combination with conventional treatment in the clinic (Hou et al., 2024).
The non-flavonoid polyphenolic compound Resveratrol (RES) was found to promote ferroptosis-mediated recovery of motor function in SCI mice by modulating the Nrf2/GPX4 pathway in an SCI model (Ni et al., 2023). Meanwhile, RES protects human bronchial epithelial cells (BEAS-2B) from ferroptosis by acting on the ferroptosis pathway and inhibits diabetic periodontitis-induced ferroptosis in alveolar osteoblasts (Li et al., 2023c), which offers the possibility of disease treatment. Furthermore, Kosuke Kato et al. showed that quercetin and RES inhibit ferroptosis by inhibiting iron-catalyzed hydroxyl radicals and are independent of the Nrf2-ARE pathway (Kato et al., 2023).
The natural molecule Hinokitiol has a stronger iron chelating ability than DFO. Platycodone activates Nrf2, upregulates SLC7A11, GPX4, heme oxygenase-1 (HO-1) and exerts antioxidant effects (Abeydeera et al., 2022; Xi et al., 2022). The study demonstrated that the injection of flatulin in the rat MCAO model significantly inhibited ferroptosis and reduced the size of cerebral infarcts and alleviated the neurological damage after cerebral ischemia in rats, providing new insights into the IS (Jayakumar et al., 2013). Meanwhile, Hinokitiol alleviates neurological aspects of behavioural disorders caused by the neurotoxin 6-hydroxy DA (6-OHDA), providing a new treatment option for PD (Xi et al., 2022). Thus Hinokitiol holds promise for more diseases.
The natural anthraquinone derivative aloe-emodin (AE) attenuates adriamycin (the doxorubicin, DOX)-induced cardiomyocyte toxicity in H9c2 rats (He Y. et al., 2023). The researchers assessed the molecular mechanism of action of Nrf2 by Western blot, luciferase reporter gene assay, and qRT-PCR analysis, and detected the changes of intracellular ROS, and lipids by fluorescence assay. The results showed that AE could activate Nrf2, upregulate the expression of SLC7A11 and GPX4, and exhibit significant antioxidant capacity, thus reducing oxidative stress (He et al., 2023).
The bioactive steroid ester Withaferin A (WFA) modulates endothelial cell apoptosis after traumatic brain injury (Şeker Karatoprak et al., 2022). In recent years, WFA has been experimentally found to have the ability to inhibit ferroptosis and to play a role in many diseases associated with ferroptosis. It has been shown that WFA can activate the Nrf2/HO-1 pathway and reduce oxidative stress to inhibit neuronal cell injury after ICH, thus exerting neuroprotective effects. Meanwhile, the combination of WFA with Fer-1 increased this neuroprotective effect (Zhou et al., 2023), bringing new hope for ICH treatment.
Arbutin (ARB) was found to reduce ROS, and MAD accumulation, upregulate GSH, and exert a mitigating effect on alcoholic fatty liver disease (ALD) and liver injury by modulating the Nrf2/HO-1 pathway and oxidative stress. In addition, ARB can promote m6A methylation of SLC7A11 by inhibiting FTO, which in turn inhibits ferroptosis and exerts a mitigating effect on NAFLD in vitro and in vivo (Jiang et al., 2023).
Dl-3-n-butylphthalide (NBP), an extract of celery, has been widely used in stroke, dementia, and ischemic diseases. In 2022 Ye et al. suggested that injection of NBP inhibited erastin-induced accumulation of iron and ROS, downregulated TFH, and upregulated Nrf2, which in turn inhibited ferroptosis and reversed the damage to DAergic neurons (MES23.5 cells) (Ye et al., 2023).
Tetrahydroxy stilbene glycoside (TSG), an active ingredient of Polygonum tigrinum, enhances memory and locomotor activity in aged rats mainly by restoring mitochondrial function and plays a role in AD (Gao et al., 2023). In an AD rat model, TSG administration modulates the Nrf2-HO-1 pathway and protects against hippocampal neuronal damage in mice. Also TSG enhanced antioxidant capacity by activating GSH/GPX4/ROS and Keap1/Nrf2/ARE signalling pathways (Zhao et al., 2023). Recent studies have shown that BFT, a fat-soluble derivative of vitamin B1 synthesis, can also rescue cognitive deficits in mice in the APP/PS1 mouse model through activation of the Nrf2/ARE pathway (Pan et al., 2016).
In addition to the above drugs, small molecule drugs such as Dihydromyricetin (DHM) (Liang et al., 2014; Xu et al., 2023), β-Caryophyllene(BCP) (Hu et al., 2022), Forsythoside A (FA) (Zhang et al., 2024b), 15, 16-Dihydrotanshinone I (DHT) (Roth et al., 2023) (Table 1) have been demonstrated to play a role in neurological impairment-related disorders by modulating the signalling factors associated with the Nrf2 pathway. However, their specific mechanisms still need to be verified more further.
2.3.3 Other antioxidant pathways
Phenotypic analysis of the HT22 cell model revealed that the diphenylbutene derivative DPT could inhibit ferroptosis (EC50 = 12.0 mM) and was non-toxic. Researchers have designed and synthesised 14 DPT analogues (Fang et al., 2022) using DPT as a backbone and chemically to enhance the inhibitory activity. By analysis, it was found that 3f, 3m, and 3n exhibited stronger inhibition of ferroptosis. Next, scaffolding analysis was carried out and it was found that R1 of compound 3f was substituted with 3-OCH3 and 4-OH on the A ring, and R1 of compounds 3m and 3n was substituted with 3-OCH3 and 4-OH on both the A and B rings. A comparison of activities revealed that compound 3f was significantly more active than the latter two mentioned above. Also, the stronger ferroptosis inhibition ability and superior biological activity of compound 3f were demonstrated by morphological analysis. Further DPPH assay DPT was found to be different from RTAs such as Fer-1 and DFO but inhibited ferroptosis by up-regulating FSP1 protein levels (Fang et al., 2022). In addition, compound 3f was found to alleviate the impairment of neurological function after cerebral ischemia to a certain extent in a rat MCAO model (Fang et al., 2022), which is expected to be a new prospect for the treatment of neurological disorders.
Ginsenoside Rg1, an active component of ginseng, has been shown to protect the kidney from damage by reducing oxidative stress. Following Rg1 treatment, iron content, FTL, FTH, and MDA levels in renal tissues were significantly reduced, while GPX4, FSP1, and GSH levels were elevated (Guo et al., 2022). Recent studies have demonstrated that knocking down FSP1 eliminates the inhibitory effect of ginsenosides on ferroptosis, and the administration of ginsenosides effectively alleviates Sepsis-induced Acute Kidney Injury (SI-AKI) (Guo et al., 2023).
In vivo experiments have shown that the natural chalcone cardamonin (CAD) inhibits ferroptosis and improves cartilage damage in rats by modulating the p53/SLC7A11/GPX4 signaling pathway. Additionally, CAD has been found to have a similar effect as DFO in osteoarthritis (OA), with CAD being more effective in improving cartilage damage in OA (Gong et al., 2023).
3 Alternative approaches to targeting ferroptosis
In addition to the small molecule ferroptosis inhibitors traditionally used to inhibit ferroptosis that can play a role in ferroptosis-mediated diseases, gene regulatory approaches, cellular therapies, and nano-targeted delivery can also exhibit ferroptosis inhibition by all along the correlates of ferroptosis (Table 2), and there are some differences in treatment efficiency between them (Table 3).
3.1 Gene regulation method
MicroRNAs (miRNAs) are small non-coding RNAs that negatively regulate gene expression and are implicated in various diseases. In a study by Huang et al., in 2022, miR-134-5p was found to be upregulated in bronchopulmonary dysplasia (BPD) in preterm infants, leading to ROS and Fe2+ accumulation and GPX4 downregulation in these patients. Conversely, inhibiting miR-134-5p reversed these effects and significantly improved BPD (Lan et al., 2023). Another key miRNA, miR-3587, regulates HO-1 and is involved in renal ischemia-reperfusion injury. Tao et al. demonstrated in 2022 that injecting a miR-3587 inhibitor post-establishment of an ex vivo IR model enhanced HO-1 and GPX4 expression and cellular activity, thus safeguarding renal tissues from IR injury (Tao et al., 2021).
lncRNAs are long-stranded non-coding RNAs, more than 200 nt in length, which can affect downstream miRNAs but cannot be converted into proteins. In 2017, Wu et al. found that lncRNA-N1LR was neuroprotective in IS-I/R by inhibiting p53 phosphorylation on serine 15 and inactivating p53 (Wu et al., 2017). lncRNA-SNHG14 was significantly upregulated in the nutlin3a-resistant osteosarcoma (OS) cell line NR-SJSA1, which resulted in downregulation of miR-206, upregulation of SLC7A11 expression and inhibition of ferroptosis (Li et al., 2023). lncRNA-MEG3 overexpression modulates miR-885-5p-SLC7A11 signalling pathway, upregulates GPX4, and thereby alleviates erastin-induced ferroptosis in chondrocytes (Zhu et al., 2024).
Prominin2, a pentameric transmembrane protein, plays a crucial role in mediating iron efflux. It facilitates the formation of multivesicular bodies (MVBs) and exosomes containing iron-rich proteins, allowing the removal of excess intracellular iron via these exosomes, thus preventing cellular ferroptosis. Additionally, Fer-1 has been shown to mitigate the decline in cellular function resulting from prominin2 depletion (Brown et al., 2019). In a study conducted in 2017, Yan et al., 2022 discovered that the small molecule protein-protein interaction (PPI) inhibitor K-181 could suppress p53 transcription and ameliorate neurological impairments post-ischemic stroke (IS) by increasing the expression of the p53 repressor Mdmx, consequently inhibiting p53 transcription in the brains of mice following IS. Embryonic lethal-abnormal vision like protein 1 (ELAVL1) is an RNA-binding protein that enhances mRNA stability and regulates translation, thereby influencing gene expression (Du et al., 2022). ELAVL1 is notably upregulated in the I/R model. Research has shown that silencing ELAVL1 leads to an increase in GSH, GPX4, and SLC7A11 expression, while decreasing Fe2+, ROS, and MAD levels in rat brain tissues, ultimately preventing ferroptosis and reducing ischemic brain damage in rats (Du et al., 2022).
In addition, several other genes have been discovered to influence ferroptosis-related diseases by regulating factors associated with ferroptosis. For instance, in 2022, Sean K. Ryan et al. identified the ferroptosis susceptibility gene SEC24B through a comprehensive gene screening process. This gene inhibits ferroptosis and is implicated in neurological disorders by modulating iron-regulated proteins such as transferrin, thus maintaining iron homeostasis within cellular iron pools (Ryan et al., 2023). In a separate study in 2023, Hu et al., 2023 demonstrated that CDGSH iron-sulfur domain 2 (CISD2) was upregulated and activated the Nrf2/HO-1 signaling pathway in both the MCAO mouse model and the OGD/R HT22 cell model, mimicking in vivo and ex vivo conditions of cerebral ischemia and reperfusion. This activation led to increased survival rates of HT22 cells, while also reducing Fe2+ content and exhibiting antioxidant properties. Furthermore, CISD2 was found to be effective in conditions such as IS-I/R and ICH (Yeh et al., 2022).
3.2 Cellular therapy
3.2.1 Neuregulin1β
With the development of stem cell research, the use of neural stem cells (NSCs) in the treatment of diseases is now of great interest. It has been found that exogenous NSCs can repair damaged tissues and play a role in neurological diseases such as IS (Calabrese et al., 2022). In 2022, Zhai et al. introduced human umbilical cord mesenchymal stem cells (hUC-MSCs) obtained by growth factor induction into NSCs and added NSCs by adding growth factor and neuregulin1β (NRG1β) to obtain NSCs-NRG1β. They found improved neurological function and reduced area of cerebral infarction in the rats injected with NSCs-10 nM NRG1β group in the rat MCAO/R model, and they also found an increase in the levels of GPX4 and SLC7A11 and a downregulation of p53 expression (Zhai et al., 2022). 2023, they obtained the same results with NSCs-10 nM NRG1β intervention in oxygen OGD/R-injured PC12 cells (Zhai et al., 2023). Thus NSCs provide a new direction for the future treatment of neurological diseases. However, many issues including ethical issues, therapeutic efficacy, and safety of exogenous NSC transplantation remain to be resolved.
3.2.2 Neutrophils therapy
To address the problems of antioxidant enzymes in IS therapy, in 2022, Wang et al. designed an albumin-conditioned nanoparticle based on co-encapsulation with antioxidases catalase (CAT) and superoxide dismutase 1 (SOD1) in a “neutrophil piggybacking” strategy. In the MCAO model, Neutrophil therapy delivered SOD1/CAT and Se to the site of brain injury in mice and significantly alleviated the area of cerebral infarction in mice after IS (Wang et al., 2022). In addition, the delivery of Se successfully upregulated GPX4 expression. At the same time, in addition to Neutrophil therapy, macrophages and monocytes also have this delivery capacity (Wang et al., 2022). Therefore, this neutrophil piggybacking strategy holds the promise of facilitating the application of nanomedicines in the central nervous system.
3.3 Relationship between nanoparticles-mediated ferroptosis and disease
The nanodelivery system is a sub-particulate carrier drug delivery system that modulates drug release rate and increases biofilm permeability. Numerous studies have reported that targeted ferroptosis nanomedicines can improve the low targeting and low solubility of conventional ferroptosis inhibitors (Żur and Farajpour, 2022). Also, several ferroptosis nanocarrier drugs have been used for ferroptosis-mediated diseases such as AKI, stroke, etc.
3.3.1 Individual use of nanoparticles in ferroptosis-mediated disease
The injection of DFO in a model of Idiopathic pulmonary fibrosis (IPF) was found to increase survival (from 50% to 90%) and reverse the IPF phenotype in mice. However, low solubility and low targeting during drug delivery remain an insurmountable gap in DFO therapy. To solve this problem, the researchers prepared DFO nanomedicines and used a pulmonary drug-delivery system (PDDS) instead of oral administration or injection, and the biological effect of the drugs was significantly improved (Devkota et al., 2021).
The miR-134-5p inhibitor proposed by the investigators for the treatment of bronchopulmonary dysplasia (BPD) achieves a certain therapeutic effect, but it still suffers from the problems of conventional drugs such as drop targeting and low solubility. To better improve drug utilisation, the investigators further designed and synthesised the targeted ROS-responsive nanocarrier PCC-R8-ROS@miR-134-5p inhibitor (Lan et al., 2023), which more efficiently delivered the miR-134-5p inhibitor to the alveolar epithelial cells, providing a more efficient therapeutic strategy for BPD. However, in the future, improving encapsulation efficiency may remain a major challenge for delivering miRNAs.
AKI, a severe syndrome of renal insufficiency, was the focus of a study by Wang et al., 2021. They developed ultrasmall KCa(H2O)2 [FeIII(CN)6]-H2O nanoparticles, known as CaPB nano-enzymes, to function as a multienzymatic mimic. These nano-enzymes were effective in inhibiting ferroptosis by scavenging reactive oxygen/nitrogen species (RO/NSs) and treating AKI. The study found that CaPB nano-enzymes upregulated GPX4 both in vivo and ex vivo post intravenous administration, leading to kidney protection from oxidative damage and improved drug efficiency and targeting. Furthermore, the potential clinical application of CaPB nano-enzymes in AKI and other RO/NSs-related kidney diseases was highlighted. Xie et al., 2022 also contributed to the field by introducing gallic acid-gallium polyvinyl pyrrolidone nanoparticles (GGP NPs) as iron removers that could reduce intracellular free iron and mitochondrial dysfunction. These nanoparticles were able to downregulate iron-death-related substances like NADPH, GSH, GPX4, and ferritin, effectively suppressing ferroptosis-mediated AKI. Additionally, GGP NPs showed promise in ameliorating renal tubular injury and mitochondrial damage.
ICH remains an important cause of morbidity and mortality worldwide. Wang et al. presented a magnetically targeted nanocarrier loaded with the PPARγ agonist 15d-PGJ2-MNPs, which, when administered intravenously, activated PPARγ receptors on macrophages around haematomas, attenuated brain damage, and improved sensory and motor functions in mice after ICH (Wang et al., 2023). In addition, Yang et al. found that curcumin nanoparticles (Curcumin-NPs, Cur-NPs) could be effective in Cur delivery and provide ideas for the treatment of neurological impairment after ICH (Yang et al., 2021).
IS, a prevalent clinical neurological disorder, constitutes 85% of all strokes globally. Li et al., 2022 demonstrated that poly (lactic acid)-glycolic acid copolymerization-nanoparticles (SOD-PLGA-NPs) possess potent free radical scavenging capabilities, reducing cerebral infarct size in mice post-IS and ameliorating neurological deficits resulting from cerebral ischemia in the MCAO mouse model. Moreover, the nano delivery system enhances drug penetration through the blood-brain barrier.
Acute Liver Injury, a rare and life-threatening condition, was addressed by Shan et al. through the synthesis of ultrasmall poly (acrylic) acid coated Mn3O4 nanoparticles (PAA@Mn3O4-NPs, PMO), which effectively scavenge ROS, inhibit lipid peroxidation and ferroptosis, and mitigate Acute Liver Injury induced by acetaminophen and ischemia/reperfusion in mice. Furthermore, intravenous administration of PMO exhibits superior biocompatibility (Shan et al., 2023).
Myocardial Injury is a common complication of sepsis. Liu et al. designed and synthesised small biocompatible and MRI-visible melanin nanoparticles (MMPP) that could attenuate myocardial Injury. In ex vivo experiments, the investigators found that MMPP scavenges ROS and inhibits ferroptosis-mediated cardiomyocyte injury. In addition, MMPP downregulates oxidative stress induced by inflammatory factors (Alcalá-Alcalá et al., 2023).
Retinal pigment epithelial cells (RPE) are essential for maintaining the normal function and survival of photoreceptor cells. Oxidative stress and ferrous ion accumulation play a role in retinal degenerative diseases. Tang et al. found that a potent iron-conjugated nanoscale Prussian blue analogue, KCa [FeIII(CN)6] (CaPB), rescued retinal structure and visual function by preventing RPE degradation and was effective in preventing RPE lesions in mouse models (Tang et al., 2021).
3.3.2 Targeted nanotechnology in combination with other drugs
The current use of targeted nanotechnology has to some extent improved the problems of traditional drug targeting, solubility, and time window. Recent studies have reported that targeted nanotechnology in combination with conventional drugs has improved drug targeting even further. In a rat model of cerebral ischemia-induced neurological impairment, the co-administration of tissue plasminogen activator (tPA) with nanoliposomal Fasudil-Lip showed better neuroprotection, and prolonged therapeutic time window (TTW) and improved drug targeting (Fukuta et al., 2017). Therefore, we can speculate whether ferroptosis inhibitors could also better address issues such as drug targeting by combining them with nanoliposome-encapsulated drugs.
4 Clinical diseases mediated by ferroptosis
Ferroptosis, a newly discovered form of cell death, has been found to have strong links with pathophysiological processes in neurological disorders, organ damage, and cardiovascular diseases.
AD is the most prevalent type of dementia, characterized by amyloid-β (Aβ) deposition in senile plaques (SPs) and intracellular neurofibrillary tangles (NFTs) formed due to hyperphosphorylation of tau proteins (Aggleton et al., 2016). Clinical research indicates that iron accumulation and oxidative stress are the primary pathological changes in the brains of AD patients. Aβ has been reported to convert intracellular iron to ferrous iron, leading to increased Aβ plaque formation, elevated ferritin expression, and worsening oxidative damage and cognitive impairments (Lane et al., 2023; Singh et al., 2024). Moreover, high levels of iron in the brain trigger the production of free radicals and induce oxidative stress. Studies have shown increased levels of HNE and acrolein, as well as upregulation of lipid peroxidation-related enzymes in the brains of individuals with AD. Additionally, reduced levels of GSH and inactivation of GPX4 have been observed in both animal models of AD and postmortem brain specimens (Yoo et al., 2010). Therefore, targeting ferroptosis inhibition could serve as a promising therapeutic approach for AD.
PD is characterized by the loss of dopaminergic neurons in the Substantia Nigra (SN) (Bae et al., 2021). The pathogenic mechanisms are multifaceted and involve α-synuclein accumulation, lipid peroxidation damage, iron deposition, oxidative stress, and inflammation. Among these, the production of lipid peroxides by α-synuclein within the cell membrane to induce ferroptosis plays a crucial role in the development of PD. Both iron accumulation and oxidative stress have long been linked to the progressive loss of dopaminergic neurons in PD patients, with dopaminergic neuron loss potentially further triggering ferroptosis (Thapa et al., 2022). Studies have shown that following the onset of PD, levels of DMT1 were elevated, leading to increased intracellular iron input, subsequently promoting dopaminergic neuronal death and α-syn accumulation. Additionally, a decrease in GSH levels post-PD activated 12-LOX, resulting in LOOH accumulation in the brain and worsening PD symptoms (Shibu et al., 2021). Furthermore, the use of ferroptosis inhibitors like DFP has shown promise in mitigating PD-related damage (Lin et al., 2022; Ding et al., 2023). Overall, exploring the relationship between ferroptosis and PD pathomechanisms may provide effective treatment options for patients with PD.
IS is associated with various pathological changes including ROS accumulation, ion metabolism disorders, and oxidative stress. Following cerebral ischemia, lipid peroxidation and iron accumulation are common characteristics of ferroptosis. Research has demonstrated that altered blood-brain barrier permeability post cerebral ischemia exacerbates ischemic injury, leading to disruptions in iron metabolism within brain tissues (Yang et al., 2022). This disruption induces the production of ROS through the Fenton reaction, along with an increase in ferritin, TFR1, and DMT1 expression in the brain. The accumulation of iron in the brain triggers ROS production via the Fenton reaction, worsening brain damage (DeGregorio-Rocasolano et al., 2019). Moreover, the brain contains high levels of unsaturated lipids that generate excess ROS, activating Nrf2 and p53, which further exacerbates oxidative stress and brain damage. Studies in rat MCAO models have shown elevated lipid peroxidation levels and reduced GSH levels (Millán et al., 2021). Thus, amelioration of neurologic impairment after IS may be facilitated by inhibiting relevant targets of ferroptosis.
SCI is a severe traumatic neurological disorder characterized by high levels of polyunsaturated fatty acids in the spinal cord, leading to oxidative stress. Studies have shown that rats with SCI exhibit iron overload in the motor cortex, resulting in the accumulation of Reactive Oxygen Species (ROS) and the induction of ferroptosis. Pathological changes such as iron deposition, lipid peroxide accumulation, and downregulation of GPX4 are commonly observed in both SCI patients and animal models (Wei et al., 2021). The activation of neuroglia after SCI results in the secretion of inflammatory factors, contributing to iron overload in the motor cortex (Li et al., 2023). Furthermore, the use of ferroptosis inhibitors like DFO and Fer-1 has been found to enhance motor function recovery following SCI (Li and Jia, 2023).
AKI is a common renal disease in clinical practice, and recent research suggests that ferroptosis may play a crucial role in IRI-AKI. During ischemia, AKI is exacerbated by elevated levels of free iron in the kidney, downregulation of GPX4 and SLC7A11, and depletion of blood GSH, leading to the activation of ferroptosis and subsequent tubular necrosis (Borawski and Malyszko, 2020). Zhao et al., 2020 demonstrated that iron deficiency worsened rhabdomyolysis (RM)-induced AKI. Additionally, ferroptosis inhibitors like Fer-1 and pioglitazone have been shown to reverse AKI damage in a mouse model by targeting ferroptosis, offering promising avenues for AKI treatment (Hosohata et al., 2022). Acute liver injury is another condition with high clinical mortality rates, and recent studies have highlighted the potential of Nrf2 activation in mitigating liver injury (Zhu et al., 2024). Furthermore, research by Yamada et al., 2020 indicated that upregulation of ferroptosis markers (iron ions, lipid peroxide levels) exacerbated liver injury in hepatic IRI models, but treatment with ferroptosis inhibitors like Fer-1 significantly reduced injury severity.
Studies have shown that ferroptosis is associated with MIRI, heart failure, atherosclerosis, myocardial infarction, and other cardiovascular diseases. Iron metabolism and lipid metabolism play important roles in the regulation of cardiovascular disease. Of these, iron ion disorders are the most common. TFR1 expression was found to be upregulated during MIRI (Miyamoto et al., 2022). In contrast, TFR1 inhibitors such as DMT1i are protective against ferroptosis-mediated heart disease. In cardiovascular disease, LOX expression is upregulated, which in turn promotes lipid peroxidation and ferroptosis (Fratta Pasini et al., 2023). Meanwhile, inhibition of ACSL4 improves cardiac function and prevents heart failure (Ito et al., 2021). Furthermore, Upregulation of SLC7A11 and GPX4 Downregulates the Mouse Lipid Peroxidation and Iron Levels in the Endothelium of Mouse Aorta and Attenuates Atherosclerotic Injury (Bai et al., 2020).
5 Challenges encountered in the clinical translation of ferroptosis inhibitors
There have always been several problems in the development of drugs. In general, new drugs tend to face many difficulties and challenges before they are launched on the market. Since ferroptosis was proposed, the development of ferroptosis inhibitors as well as their clinical application has also become a topic of increasing interest for researchers. Although many small-molecule ferroptosis inhibitors have been developed and continuously optimised, most of them are still difficult to use successfully in the clinic.
5.1 Challenges encountered in preclinical trials
Several drugs have been shown to inhibit ferroptosis in animal experiments, but they mostly suffer from low stability, low solubility, low targeting, low safety, toxicity, poor pharmacokinetics, and low activity (Guo et al., 2023), so very few of them have entered clinical studies. For example, Fer-1, the first synthetic inhibitor of ferroptosis, has been shown to play a role in many diseases in animal models (Skouta et al., 2014; Wang et al., 2023), but is still in the experimental stage, making it difficult to cross over to clinical trials. Mainly due to its low stability and low solubility, the researchers designed and synthesised compound 39 based on Fer-1, compound 39 can significantly improve its ferroptosis inhibition potency (Linkermann et al., 2014; Scarpellini et al., 2023). Meanwhile, phenothiazine-based derivatives compound 51, although it has shown neuroprotective effects in IS animal models, still has high hERG inhibitory activity (Yang et al., 2021; You et al., 2022). Se has long been shown to alleviate diseases such as iron-death-mediated stroke by enhancing adaptive transcription, but Ishraq Alim et al. found a parabolic dose curve after Se supplementation in vitro and in vivo experiments, raising concerns about the mode of Se delivery as well as its safety. Moreover, Se-mediated GPX4 regulation and neuronal protection are extremely complex and therefore difficult to successfully translate into the clinic (Alim et al., 2019). Although DFO reversed the survival of IPF mice, it still suffers from low solubility and targeting during delivery (Guo et al., 2023). In addition, the natural substance RES is still in the preclinical experimental stage and is difficult to translate because of its problems such as low water solubility, easy degradation, low activity, and poor pharmacokinetics (Pharmacokinetics, PK) (Chung et al., 2020; Ni et al., 2023). Therefore, there is a need for further improvement of the drug thus better marketing of the drug to clinical studies.
5.2 Challenges encountered in clinical studies
After a drug has gone through the preclinical phase of experimentation, the drug needs to be tested in clinical trials (Moujalled et al., 2021; Iasonos and OQuigley, 2021). Several ferroptosis inhibitors have also made it to the clinical translational stage in recent years, but they still present challenges in clinical studies.
Vitamin E was found to significantly reduce cognitive performance in patients with mild to moderate AD in a multicentre, randomised, double-blind, placebo-controlled trial of AD from 2007 to 2012. However, some studies claim that vitamin E supplementation does not reduce the risk of AD and slows down its pathogenesis (Dysken et al., 2014; Kryscio et al., 2017; Ashraf and So, 2020).
In 2013, DFP significantly improved motor function in patients with PD in a 12-month phase II clinical trial of PD, but patients who continued to take DFP showed diminished improvement in motor function as well as reduced Neutrophils and granulocyte deficiencies (Devos et al., 2014; Martin-Bastida et al., 2017). In addition, Mohsen Saleh Elalfy et al. conducted a clinical trial on DFP for the treatment of transfusion-dependent thalassemia children in 2016. They found that the safety and efficacy of the drug was improved when DFP was administered to thalassemia patients, but adverse effects such as diarrhea, vomiting, colic, neutropenia, and elevated liver enzymes were still present (Elalfy et al., 2018).
In 2019, an injection of Edaravone dexborneol in phase II multicentre, randomised, double-blind, multi-dose, active-controlled clinical trial in patients with IS found that patients experienced a reduction in acute brain injury, but continued to have itching and AKI side effects (Xu et al., 2019).
Copper compound CuII(ATSM) exhibits neuroprotective effects in phase I clinical trials in ALS and PD (Southon et al., 2020). Meanwhile, CuII (ATSM) has completed phase I trials in ALS patients and entered phase II clinical trials, but it has been found to exhibit spinal cord immunocompromise in patients in the clinical trial phase (Liddell et al., 2023).
In recent years, it has been found that ferroptosis-mediated neurological impairment can be significantly alleviated by thrombin inhibition. Meanwhile, the thrombin inhibitor Dabigatran is currently in Phase III clinical trial in IS (NCT03961334) (Tuo et al., 2022). In April 2023, the thrombin inhibitor Argatroban concluded a Phase IV clinical trial in IS (NCT03740958) and was found to significantly reduce adverse prognosis when used in combination with Aspirin (Peng, 2023).
Therefore, there is a need for researchers to further optimise drugs to improve drug utilisation, reduce side effects and treat ferroptosis-mediated diseases.
6 Ferroptosis in relation to other modes of cell death
Ferroptosis is a type of programmed cell death that is distinguished from apoptosis, necrotic apoptosis, autophagy, pyroptosis and cuproptosis in both morphological changes and biochemical functions. However, a growing body of research suggests that there is a link between ferroptosis and all of the above modes of cell death.
6.1 Ferroptosis and apoptosis
Apoptosis, the first identified regulatory cell death modality, is governed by apoptosis-related genes. The initiation of apoptosis is often characterized by morphological alterations such as cell shrinkage, chromatin fragmentation, and membrane blistering and disintegration. Apoptosis encompasses caspase-dependent intrinsic apoptosis, death receptor-mediated extrinsic apoptosis, and receptor-dependent extrinsic apoptosis. Research indicates that Tumor Necrosis Factor-α (TNF-α) plays a crucial role as an inflammatory factor that triggers ferroptosis. Additionally, Kai et al. (2020) reported the induction of chondrocyte apoptosis through the upregulation of TNF-α (Yang et al., 2021). Furthermore, Ma et al., 2022 demonstrated that Fer-1 mitigates noise-induced hearing loss by targeting TFR1-mediated ferroptosis and the p53-AIFM2 apoptosis pathway.
6.2 Ferroptosis and necroptosis
Necroptosis is a type of regulatory necrosis that is often caused by a variety of physicochemical stimuli, such as inflammatory factors and ATP depletion and is not dependent on caspases. Necrotic apoptosis is followed by morphological changes such as rupture of cell membranes, translucent cytoplasm, swollen organelles, increased cell volume, and chromatin condensation. Iron overload was found to affect cellular redox homeostasis and activate necrotic apoptosis and ferroptosis. In recent years, the ferroptosis inhibitor Lip-1 was found to be effective in inhibiting necrotic apoptosis during cerebral ischemia-reperfusion. Meanwhile, the necrotic apoptosis inhibitor Necrostatin-1 effectively inhibited the activation of proteins associated with ferroptosis (Yuk et al., 2021). In addition, Nigratine (also known as 6E11) was found to inhibit both necrotic apoptosis and ferroptosis in human bronchial-like organs (Delehouzé et al., 2022). Thus, ferroptosis and apoptosis have complex interactions and are closely related.
6.3 Ferroptosis and autophagy
Autophagy is the process of removing and degrading intracellular material through lysosomes, which includes macroautophagy, microautophagy, and chaperone-mediated autophagy. This process plays a vital role in maintaining cellular homeostasis and preventing cell overgrowth. Studies have shown a close relationship between ferroptosis and ferritin autophagy, adipose autophagy, and chaperone-mediated autophagy (Lee et al., 2023). Moreover, ROS accumulation, p53 activation, and Nrf2 signaling pathway activation all induced autophagy and ferroptosis. Autophagy-dependent ferroptosis has been implicated in kidney-related diseases and cardiovascular diseases. Additionally, research by Du et al., 2023 demonstrated that rapamycin injection enhanced autophagy, leading to the downregulation of TFR1, which inhibited ferroptosis and reduced cognitive deficits in a Sepsis-associated encephalopathy (SAE) model in mice.
6.4 Ferroptosis and pyroptosis
Pyroptosis, also known as cellular inflammatory necrosis, is programmed cell death induced by activation of inflammatory vesicles and is mainly regulated by cysteinyl asparagine-1, -4, -5, and -11-dependent signaling pathways (Teng et al., 2023). Pyroptosis was accompanied by morphological changes such as cell swelling, membrane rupture, and leakage of cytoplasmic components. It has been shown that high intracellular ROS accumulation promotes pyroptosis and that severe oxidative stress induces upregulation of pyroptosis. In recent years, it has been found that a mixed form of cell death, “PAN apoptosis”, consisting of TNF-α and interferon gamma (IFNγ) or Proadrenomedullin N-terminal 20 peptide (PAMP), can activate pyroptosis and apoptosis at the same time. IFNγ can form an important anti-ferroptosis system with SLC3A2 (Ai et al., 2024).
6.5 Ferroptosis and cuproptosis
Cuproptosis is defined as the aggregation of proteins, proteotoxic stress, and eventual cell death triggered by copper binding to the lipolytic enzymes of the tricarboxylic acid (TCA) cycle. This form of regulated cell death, dependent on copper, was termed “copper tumor” in 2022, and has been linked to the metabolic pathways of ferroptosis. Similar to ferroptosis, cuproptosis leads to notable alterations in mitochondrial structure, such as shrinkage and membrane rupture. The mitochondrial TCA cycle serves as a crucial nexus between ferroptosis and copper tumors, playing a pivotal role in cuproptosis (Jhelum and David, 2022). Furthermore, GSH has been identified as a key player in both ferroptosis and cuproptosis. GSH binds copper, reducing protein aggregation in cuproptosis. Moreover, iron-sulfur cluster proteins, essential cofactors for maintaining redox balance and iron levels, are produced as auxiliary enzymes in cuproptosis, with their levels significantly decreasing following cuproptosis initiation. Notably, protein aggregates can interfere with the function of iron-sulfur clusters (Prasad Panda and Kesharwani, 2023).
7 Discussion and conclusion
Ferroptosis inhibitors have displayed some efficacy in ferroptosis-related diseases, yet face significant challenges in making a substantial impact. These obstacles stem from the limitations of current small molecule drugs, characterized by poor selectivity, targeting, solubility, pharmacokinetic properties, adverse reactions, efficacy, in vivo toxicity, and side effects. On the other hand, some of the ferroptosis mechanisms themselves are unclear, and some of the mechanisms have been proposed but are still unknown (e.g., GCH1-BH4 pathway, HSF1-HSPB1 pathway, sulfur-transfer pathway, MVA pathway, etc.), which has become an important obstacle in the development of small molecule drugs. In addition, the complexity of the pathological mechanisms of some diseases is unclear, and the controversial relationship between ferroptosis and other modes of cell death is also an issue that needs to be addressed for the application of ferroptosis inhibitors to disease.
Overall, this review specifically describes the metabolic pathways of ferroptosis. A comprehensive summary of natural and synthetic small molecule drugs that treat ferroptosis-mediated diseases by acting on targets of ferroptosis and their therapeutic efficacy in disease. Meanwhile, this paper describes the mechanism of action of therapeutic approaches such as gene regulatory approaches, cell therapy, and nanodelivery in ferroptosis-mediated diseases. And it summarizes the problems encountered during the clinical translation of ferroptosis inhibitors and drugs that are currently in clinical translation. Finally, the relationship between ferroptosis and other forms of cell death modalities such as apoptosis is discussed. It is hoped that this will provide new ideas for the development of future ferroptosis inhibitors and provide effective therapeutic options for future ferroptosis-mediated diseases.
Author contributions
LZ: Methodology, Investigation, Data curation, Writing–original draft. YL: Writing–original draft, Investigation, Data curation. YX: Writing–original draft, Data curation, Conceptualization. XB: Writing–original draft, Supervision, Investigation, Conceptualization. RQ: Writing–original draft, Project administration, Methodology, Investigation, Conceptualization. XZ: Writing–original draft, Supervision, Software, Investigation, Conceptualization. YY: Writing–original draft, Methodology, Investigation, Conceptualization. XL: Writing–review and editing.
Funding
The author(s) declare that financial support was received for the research, authorship, and/or publication of this article. This review was supported by the grants from Shaanxi Provincial Health Research (2022E010), Shaanxi Provincial Education Department Scientific Research Program (22JK0612 to XL and 23JK0731 to YX), and Yan’an University Program (2023JBZR-017 to YX).
Conflict of interest
The authors declare that the research was conducted in the absence of any commercial or financial relationships that could be construed as a potential conflict of interest.
Publisher’s note
All claims expressed in this article are solely those of the authors and do not necessarily represent those of their affiliated organizations, or those of the publisher, the editors and the reviewers. Any product that may be evaluated in this article, or claim that may be made by its manufacturer, is not guaranteed or endorsed by the publisher.
References
Abdul, Y., Li, W., Ward, R., Abdelsaid, M., Hafez, S., Dong, G., et al. (2021). Deferoxamine treatment prevents post-stroke vasoregression and neurovascular unit remodeling leading to improved functional outcomes in type 2 male diabetic rats: role of endothelial ferroptosis. Transl. Stroke Res. 12, 615–630. doi:10.1007/s12975-020-00844-7
Abdul Ghani, M. A., Ugusman, A., Latip, J., and Zainalabidin, S. (2023). Role of terpenophenolics in modulating inflammation and apoptosis in cardiovascular diseases: a review. Int. J. Mol. Sci. 24, 5339. doi:10.3390/ijms24065339
Abeydeera, N., Yu, B., Pant, B. D., Kim, M. H., and Huang, S. D. (2022). Harnessing the toxicity of dysregulated iron uptake for killing Staphylococcus aureus: reality or mirage? Biomater. Sci. 10, 474–484. doi:10.1039/d1bm01743h
Adant, I., Bird, M., Decru, B., Windmolders, P., Wallays, M., de Witte, P., et al. (2022). Pyruvate and uridine rescue the metabolic profile of OXPHOS dysfunction. Mol. Metab. 63, 101537. doi:10.1016/j.molmet.2022.101537
Aggleton, J. P., Pralus, A., Nelson, A. J., and Hornberger, M. (2016). Thalamic pathology and memory loss in early Alzheimer's disease: moving the focus from the medial temporal lobe to Papez circuit. Brain 139, 1877–1890. doi:10.1093/brain/aww083
Ai, Y., Meng, Y., Yan, B., Zhou, Q., and Wang, X. (2024). The biochemical pathways of apoptotic, necroptotic, pyroptotic, and ferroptotic cell death. Mol. Cell 84, 170–179. doi:10.1016/j.molcel.2023.11.040
Akbari Moqadam, F., Pieters, R., and den Boer, M. L. (2013). The hunting of targets: challenge in miRNA research. Leukemia 27, 16–23. doi:10.1038/leu.2012.179
Akiyama, H., Carter, B. Z., Andreeff, M., and Ishizawa, J. (2023). Molecular mechanisms of ferroptosis and updates of ferroptosis studies in cancers and leukemia. Cells 12, 1128. doi:10.3390/cells12081128
Ala, M., and Ala, M. (2021). Metformin for cardiovascular protection, inflammatory bowel disease, osteoporosis, periodontitis, polycystic ovarian syndrome, neurodegeneration, cancer, inflammation and senescence: what is next? ACS Pharmacol. Transl. Sci. 4, 1747–1770. doi:10.1021/acsptsci.1c00167
Alcalá-Alcalá, S., Casarrubias-Anacleto, J. E., Mondragón-Guillén, M., Tavira-Montalvan, C. A., Bonilla-Hernández, M., Gómez-Galicia, D. L., et al. (2023). Melanin nanoparticles obtained from preformed recombinant melanin by bottom-up and top-down approaches. Polym. (Basel) 15, 2381. doi:10.3390/polym15102381
Alim, I., Caulfield, J. T., Chen, Y., Swarup, V., Geschwind, D. H., Ivanova, E., et al. (2019). Selenium drives a transcriptional adaptive Program to block ferroptosis and treat stroke. Cell 177, 1262–1279. doi:10.1016/j.cell.2019.03.032
Alli, A. A., Desai, D., Elshika, A., Conrad, M., Proneth, B., Clapp, W., et al. (2023). Kidney tubular epithelial cell ferroptosis links glomerular injury to tubulointerstitial pathology in lupus nephritis. Clin. Immunol. 248, 109213. doi:10.1016/j.clim.2022.109213
Amos, A., Amos, A., Wu, L., and Xia, H. (2023a). The Warburg effect modulates DHODH role in ferroptosis: a review. Cell Commun. Signal 21, 100. doi:10.1186/s12964-022-01025-9
Amos, A., Jiang, N., Zong, D., Gu, J., Zhou, J., Yin, L., et al. (2023b). Depletion of SOD2 enhances nasopharyngeal carcinoma cell radiosensitivity via ferroptosis induction modulated by DHODH inhibition. BMC Cancer 23, 117. doi:10.1186/s12885-022-10465-y
Angeli, J. P. F., Freitas, F. P., Nepachalovich, P., Puentes, L., Zilka, O., Inague, A., et al. (2021). 7-Dehydrocholesterol is an endogenous suppressor of ferroptosis. Res. Square. doi:10.21203/rs.3.rs-943221/v1
Angeloni, C., Barbalace, M. C., and Hrelia, S. (2019). Icariin and its metabolites as potential protective phytochemicals against alzheimer's disease. Front. Pharmacol. 10, 271. doi:10.3389/fphar.2019.00271
Ansari, M. I., Khan, M. M., Saquib, M., Khatoon, S., and Hussain, M. K. (2018). Dithiolethiones: a privileged pharmacophore for anticancer therapy and chemoprevention. Future Med. Chem. 10, 1241–1260. doi:10.4155/fmc-2017-0281
Ashraf, A., and So, P. W. (2020). Spotlight on ferroptosis: iron-dependent cell death in alzheimer's disease. Front. Aging Neurosci. 12, 196. doi:10.3389/fnagi.2020.00196
Avramovich-Tirosh, Y., Amit, T., Bar-Am, O., Zheng, H., Fridkin, M., and Youdim, M. B. (2007). Therapeutic targets and potential of the novel brain-permeable multifunctional iron chelator-monoamine oxidase inhibitor drug, M-30, for the treatment of Alzheimer's disease. J. Neurochem. 100, 490–502. doi:10.1111/j.1471-4159.2006.04258.x
Badi, R. M., Khaleel, E. F., Satti, H. H., and Monir, R. (2024). Eriodictyol attenuates doxorubicin-induced nephropathy by activating the AMPK/Nrf2 signalling pathway. J. Tradit. Complement. Med. 14, 203–214. doi:10.1016/j.jtcme.2023.11.003
Bae, Y. J., Kim, J. M., Sohn, C. H., Choi, J. H., Choi, B. S., Song, Y. S., et al. (2021). Imaging the Substantia Nigra in Parkinson disease and other parkinsonian syndromes. Radiology 300, 260–278. doi:10.1148/radiol.2021203341
Bai, T., Li, M., Liu, Y., Qiao, Z., and Wang, Z. (2020). Inhibition of ferroptosis alleviates atherosclerosis through attenuating lipid peroxidation and endothelial dysfunction in mouse aortic endothelial cell. Free Radic. Biol. Med. 160, 92–102. doi:10.1016/j.freeradbiomed.2020.07.026
Bai, X., Zheng, E., Tong, L., Liu, Y., Li, X., Yang, H., et al. (2024). Angong Niuhuang Wan inhibit ferroptosis on ischemic and hemorrhagic stroke by activating PPARγ/AKT/GPX4 pathway. J. Ethnopharmacol. 321, 117438. doi:10.1016/j.jep.2023.117438
Bayır, H., Anthonymuthu, T. S., Tyurina, Y. Y., Patel, S. J., Amoscato, A. A., Lamade, A. M., et al. (2020). Achieving life through death: redox biology of lipid peroxidation in ferroptosis. Cell Chem. Biol. 27, 387–408. doi:10.1016/j.chembiol.2020.03.014
Berezutsky, M. A., Durnova, N. A., and Romanteeva, Y. V. (2022). Neurobiological effects of gastrodin and its possible use in neurology and psychiatry. Zh Nevrol. Psikhiatr Im. S S Korsakova 122, 27–34. doi:10.17116/jnevro202212208127
Bersuker, K., Hendricks, J. M., Li, Z., Magtanong, L., Ford, B., Tang, P. H., et al. (2019). The CoQ oxidoreductase FSP1 acts parallel to GPX4 to inhibit ferroptosis. Nature 575, 688–692. doi:10.1038/s41586-019-1705-2
Borawski, B., and Malyszko, J. (2020). Iron, ferroptosis, and new insights for prevention in acute kidney injury. Adv. Med. Sci. 65, 361–370. doi:10.1016/j.advms.2020.06.004
Brown, C. W., Amante, J. J., Chhoy, P., Elaimy, A. L., Liu, H., Zhu, L. J., et al. (2019). Prominin2 drives ferroptosis resistance by stimulating iron export. Dev. Cell 51, 575–586. doi:10.1016/j.devcel.2019.10.007
Cai, W., Liu, L., Shi, X., Liu, Y., Wang, J., Fang, X., et al. (2023a). Alox15/15-HpETE aggravates myocardial ischemia-reperfusion injury by promoting cardiomyocyte ferroptosis. Circulation 147, 1444–1460. doi:10.1161/CIRCULATIONAHA.122.060257
Cai, Z., Wu, X., Song, Z., Sun, S., Su, Y., Wang, T., et al. (2023b). Metformin potentiates nephrotoxicity by promoting NETosis in response to renal ferroptosis. Cell Discov. 9, 104. doi:10.1038/s41421-023-00595-3
Calabrese, E. J., Calabrese, V., Dhawan, G., Kapoor, R., and Giordano, J. (2022). Hormesis and neural stem cells. Free Radic. Biol. Med. 178, 314–329. doi:10.1016/j.freeradbiomed.2021.12.003
Charaschanya, M., Maskrey, T. S., LaPorte, M. G., Janjic, J. M., and Wipf, P. (2022a). Synthesis and optimization of nitroxide-based inhibitors of ferroptotic cell death in cancer cells and macrophages. Acs Med. Chem. Lett. 13, 403–408. doi:10.1021/acsmedchemlett.1c00561
Charaschanya, M., Maskrey, T. S., LaPorte, M. G., Janjic, J. M., and Wipf, P. (2022b). Synthesis and optimization of nitroxide-based inhibitors of ferroptotic cell death in cancer cells and macrophages. ACS Med. Chem. Lett. 13, 403–408. doi:10.1021/acsmedchemlett.1c00561
Chen, B., Li, X., Liu, J., Li, Y., Dai, W., Chen, Y., et al. (2021b). Ferroptosis-inhibitory effect and possible mechanisms of ellagitannin geraniin. ChemistryOpen 10, 737–739. doi:10.1002/open.202000255
Chen, G., Li, C., Zhang, L., Yang, J., Meng, H., Wan, H., et al. (2022a). Hydroxysafflor yellow A and anhydrosafflor yellow B alleviate ferroptosis and parthanatos in PC12 cells injured by OGD/R. Free Radic. Biol. Med. 179, 1–10. doi:10.1016/j.freeradbiomed.2021.12.262
Chen, L., Huang, J., Yao, Z. M., Sun, X. R., Tong, X. H., Hu, M., et al. (2023). Procyanidins alleviated cerebral ischemia/reperfusion injury by inhibiting ferroptosis via the Nrf2/HO-1 signaling pathway. Molecules 28, 3582. doi:10.3390/molecules28083582
Chen, Q., Li, L., and Xie, H. (2021c). Research progress of different types of stem cells in treatment of ischemic stroke. Zhongguo Xiu Fu Chong Jian Wai Ke Za Zhi 35, 111–117. doi:10.7507/1002-1892.202004160
Chen, W., Yuan, X., Li, Z., Lu, Z., Kong, S., Jiang, H., et al. (2020a). CN128: a new orally active hydroxypyridinone iron chelator. J. Med. Chem. 63, 4215–4226. doi:10.1021/acs.jmedchem.0c00137
Chen, X., Kang, R., Kroemer, G., and Tang, D. (2021a). Broadening horizons: the role of ferroptosis in cancer. Nat. Rev. Clin. Oncol. 18, 280–296. doi:10.1038/s41571-020-00462-0
Chen, X., Yu, C., Kang, R., and Tang, D. (2020b). Iron metabolism in ferroptosis. Front. Cell Dev. Biol. 8, 590226. doi:10.3389/fcell.2020.590226
Chen, Y., He, Y., Wei, X., and Jiang, D. S. (2022c). Targeting regulated cell death in aortic aneurysm and dissection therapy. Pharmacol. Res. 176, 106048. doi:10.1016/j.phrs.2021.106048
Chen, Y., Yi, X., Huo, B., He, Y., Guo, X., Zhang, Z., et al. (2022b). BRD4770 functions as a novel ferroptosis inhibitor to protect against aortic dissection. Pharmacol. Res. 177, 106122. doi:10.1016/j.phrs.2022.106122
Chen, Y. X., Zuliyaer, T., Liu, B., Guo, S., Yang, D. G., Gao, F., et al. (2022d). Sodium selenite promotes neurological function recovery after spinal cord injury by inhibiting ferroptosis. Neural Regen. Res. 17, 2702–2709. doi:10.4103/1673-5374.339491
Cho, S., Hong, S. J., Kang, S. H., Park, Y., and Kim, S. K. (2022). Alpha-lipoic acid attenuates apoptosis and ferroptosis in cisplatin-induced ototoxicity via the reduction of intracellular lipid droplets. Int. J. Mol. Sci. 23, 10981. doi:10.3390/ijms231810981
Choi, J., Choi, H., and Chung, J. (2023b). Icariin supplementation suppresses the markers of ferroptosis and attenuates the progression of nonalcoholic steatohepatitis in mice fed a methionine choline-deficient diet. Int. J. Mol. Sci. 24, 12510. doi:10.3390/ijms241512510
Choi, J. A., Lee, E. H., Cho, H., and Kim, J. H. (2023a). High-dose selenium induces ferroptotic cell death in ovarian cancer. Int. J. Mol. Sci. 24, 1918. doi:10.3390/ijms24031918
Chowdhury, M. R., Chatterjee, C., Ghosh, D., Mukherjee, J., Shaw, S., and Basak, J. (2024). Deciphering miRNA-lncRNA-mRNA interaction through experimental validation of miRNAs, lncRNAs, and miRNA targets on mRNAs in Cajanus cajan. Plant Biol. (Stuttg). doi:10.1111/plb.13639
Chumboatong, W., Khamchai, S., Tocharus, C., Govitrapong, P., and Tocharus, J. (2020). Agomelatine protects against permanent cerebral ischaemia via the Nrf2-HO-1 pathway. Eur. J. Pharmacol. 874, 173028. doi:10.1016/j.ejphar.2020.173028
Chung, I.-M., Subramanian, U., Thirupathi, P., Venkidasamy, B., Samynathan, R., Gangadhar, B. H., et al. (2020). Resveratrol nanoparticles: a promising therapeutic advancement over native resveratrol. Processes 8, 458. doi:10.3390/pr8040458
Conlon, M., Poltorack, C. D., Forcina, G. C., Armenta, D. A., Mallais, M., Perez, M. A., et al. (2021). A compendium of kinetic modulatory profiles identifies ferroptosis regulators. Nat. Chem. Biol. 17, 665–674. doi:10.1038/s41589-021-00751-4
Costa, K. M., and Schoenbaum, G. (2022). Dopamine. Curr. Biol. 32, R817–r824. doi:10.1016/j.cub.2022.06.060
Cruz-Gregorio, A., and Aranda-Rivera, A. K. (2023). Quercetin and ferroptosis. Life (Basel) 13, 1730. doi:10.3390/life13081730
Cui, Y., Ma, S., Zhang, C., Li, D., Yang, B., Lv, P., et al. (2018). Pharmacological activation of the Nrf2 pathway by 3H-1, 2-dithiole-3-thione is neuroprotective in a mouse model of Alzheimer disease. Behav. Brain Res. 336, 219–226. doi:10.1016/j.bbr.2017.09.011
Dar, N. J., John, U., Bano, N., Khan, S., and Bhat, S. A. (2024). Oxytosis/ferroptosis in neurodegeneration: the underlying role of master regulator glutathione peroxidase 4 (GPX4). Mol. Neurobiol. 61, 1507–1526. doi:10.1007/s12035-023-03646-8
DeGregorio-Rocasolano, N., Martí-Sistac, O., and Gasull, T. (2019). Deciphering the iron side of stroke: neurodegeneration at the crossroads between iron dyshomeostasis, excitotoxicity, and ferroptosis. Front. Neurosci. 13, 85. doi:10.3389/fnins.2019.00085
Delehouzé, C., Comte, A., Leon-Icaza, S. A., Cougoule, C., Hauteville, M., Goekjian, P., et al. (2022). Nigratine as dual inhibitor of necroptosis and ferroptosis regulated cell death. Sci. Rep. 12, 5118. doi:10.1038/s41598-022-09019-w
Devkota, H. P., Paudel, K. R., Jha, N. K., Gupta, P. K., Singh, S. K., Chellappan, D. K., et al. (2021). Applications of drug-delivery systems targeting inflammasomes in pulmonary diseases. Nanomedicine (Lond) 16, 2407–2410. doi:10.2217/nnm-2021-0275
Devos, D., Moreau, C., Devedjian, J. C., Kluza, J., Petrault, M., Laloux, C., et al. (2014). Targeting chelatable iron as a therapeutic modality in Parkinson's disease. Antioxid. Redox Signal 21, 195–210. doi:10.1089/ars.2013.5593
Ding, S., Jiang, J., and Li, Y. (2024). Quercetin alleviates PM(2.5)-induced chronic lung injury in mice by targeting ferroptosis. PeerJ 12, e16703. doi:10.7717/peerj.16703
Ding, X. S., Gao, L., Han, Z., Eleuteri, S., Shi, W., Shen, Y., et al. (2023). Ferroptosis in Parkinson's disease: molecular mechanisms and therapeutic potential. Ageing Res. Rev. 91, 102077. doi:10.1016/j.arr.2023.102077
Dixon, S. J., Lemberg, K. M., Lamprecht, M. R., Skouta, R., Zaitsev, E. M., Gleason, C. E., et al. (2012). Ferroptosis: an iron-dependent form of nonapoptotic cell death. Cell 149, 1060–1072. doi:10.1016/j.cell.2012.03.042
Doll, S., Proneth, B., Tyurina, Y. Y., Panzilius, E., Kobayashi, S., Ingold, I., et al. (2017). ACSL4 dictates ferroptosis sensitivity by shaping cellular lipid composition. Nat. Chem. Biol. 13, 91–98. doi:10.1038/nchembio.2239
Doshi, B. M., Hightower, L. E., and Lee, J. (2010). HSPB1, actin filament dynamics, and aging cells. Ann. N. Y. Acad. Sci. 1197, 76–84. doi:10.1111/j.1749-6632.2010.05191.x
Drabczyk, A. K., Kułaga, D., Zaręba, P., Tylińska, W., Bachowski, W., Archała, A., et al. (2023). Eco-friendly synthesis of new olanzapine derivatives and evaluation of their anticancer potential. RSC Adv. 13, 20467–20476. doi:10.1039/d3ra03926a
Du, L., Wu, Y., Jia, Q., Li, J., Li, Y., Ma, H., et al. (2023). Autophagy suppresses ferroptosis by degrading TFR1 to alleviate cognitive dysfunction in mice with SAE. Cell Mol. Neurobiol. 43, 3605–3622. doi:10.1007/s10571-023-01370-4
Du, Y., and Guo, Z. (2022). Recent progress in ferroptosis: inducers and inhibitors. Cell Death Discov. 8, 501. doi:10.1038/s41420-022-01297-7
Du, Y., Zhang, R., Zhang, G., Wu, H., Zhan, S., and Bu, N. (2022). Downregulation of ELAVL1 attenuates ferroptosis-induced neuronal impairment in rats with cerebral ischemia/reperfusion via reducing DNMT3B-dependent PINK1 methylation. Metab. Brain Dis. 37, 2763–2775. doi:10.1007/s11011-022-01080-8
Durante, W. (2019). The emerging role of l-glutamine in cardiovascular health and disease. Nutrients 11, 2092. doi:10.3390/nu11092092
Dysken, M. W., Guarino, P. D., Vertrees, J. E., Asthana, S., Sano, M., Llorente, M., et al. (2014). Vitamin E and memantine in Alzheimer's disease: clinical trial methods and baseline data. Alzheimers Dement. 10, 36–44. doi:10.1016/j.jalz.2013.01.014
Eberhard, Y., McDermott, S. P., Wang, X., Gronda, M., Venugopal, A., Wood, T. E., et al. (2009). Chelation of intracellular iron with the antifungal agent ciclopirox olamine induces cell death in leukemia and myeloma cells. Blood 114, 3064–3073. doi:10.1182/blood-2009-03-209965
Elalfy, M. S., Adly, A., Awad, H., Tarif Salam, M., Berdoukas, V., and Tricta, F. (2018). Safety and efficacy of early start of iron chelation therapy with deferiprone in young children newly diagnosed with transfusion-dependent thalassemia: a randomized controlled trial. Am. J. Hematol. 93, 262–268. doi:10.1002/ajh.24966
Fan, G. B., Li, Y., Xu, G. S., Zhao, A. Y., Jin, H. J., Sun, S. Q., et al. (2023). Propofol inhibits ferroptotic cell death through the nrf2/gpx4 signaling pathway in the mouse model of cerebral ischemia-reperfusion injury. Neurochem. Res. 48, 956–966. doi:10.1007/s11064-022-03822-7
Fang, Y., Chen, X., Tan, Q., Zhou, H., Xu, J., and Gu, Q. (2021). Inhibiting ferroptosis through disrupting the NCOA4-FTH1 interaction: a new mechanism of action. ACS Cent. Sci. 7, 980–989. doi:10.1021/acscentsci.0c01592
Fang, Y., Tan, Q., Zhou, H., Gu, Q., and Xu, J. (2022). Discovery of novel diphenylbutene derivative ferroptosis inhibitors as neuroprotective agents. Eur. J. Med. Chem. 231, 114151. doi:10.1016/j.ejmech.2022.114151
Farmer, L. A., Wu, Z., Poon, J. F., Zilka, O., Lorenz, S. M., Huehn, S., et al. (2022). Intrinsic and extrinsic limitations to the design and optimization of inhibitors of lipid peroxidation and associated cell death. J. Am. Chem. Soc. 144, 14706–14721. doi:10.1021/jacs.2c05252
Feng, Q., Yang, Y., Qiao, Y., Zheng, Y., Yu, X., Liu, F., et al. (2023). Quercetin ameliorates diabetic kidney injury by inhibiting ferroptosis via activating Nrf2/HO-1 signaling pathway. Am. J. Chin. Med. 51, 997–1018. doi:10.1142/S0192415X23500465
Feng, W., Xiao, Y., Zhao, C., Zhang, Z., Liu, W., Ma, J., et al. (2022). New deferric amine compounds efficiently chelate excess iron to treat iron overload disorders and to prevent ferroptosis. Adv. Sci. (Weinh) 9, e2202679. doi:10.1002/advs.202202679
Floros, K. V., Chawla, A. T., Johnson-Berro, M. O., Khatri, R., Stamatouli, A. M., Boikos, S. A., et al. (2022). MYCN upregulates the transsulfuration pathway to suppress the ferroptotic vulnerability in MYCN-amplified neuroblastoma. Cell Stress 6, 21–29. doi:10.15698/cst2022.02.264
Franchi-Miller, C., and Saffar, J. L. (1995). The 5-lipoxygenase inhibitor BWA4C impairs osteoclastic resorption in a synchronized model of bone remodeling. Bone 17, 185–191. doi:10.1016/s8756-3282(95)00173-5
Fratta Pasini, A. M., Stranieri, C., Busti, F., Di Leo, E. G., Girelli, D., and Cominacini, L. (2023). New insights into the role of ferroptosis in cardiovascular diseases. Cells 12, 867. doi:10.3390/cells12060867
Freitas, F. P., Alborzinia, H., Dos Santos, A. F., Nepachalovich, P., Pedrera, L., Zilka, O., et al. (2024). 7-Dehydrocholesterol is an endogenous suppressor of ferroptosis. Nature 626, 401–410. doi:10.1038/s41586-023-06878-9
Fu, F., Lai, Q., Hu, J., Zhang, L., Zhu, X., Kou, J., et al. (2022). Ruscogenin alleviates myocardial ischemia-induced ferroptosis through the activation of BCAT1/BCAT2. Antioxidants (Basel) 11, 583. doi:10.3390/antiox11030583
Fukuta, T., Asai, T., Yanagida, Y., Namba, M., Koide, H., Shimizu, K., et al. (2017). Combination therapy with liposomal neuroprotectants and tissue plasminogen activator for treatment of ischemic stroke. Faseb J. 31, 1879–1890. doi:10.1096/fj.201601209R
Gagliardi, M., Saverio, V., Rossin, F., D'Eletto, M., and Corazzari, M. (2023). Transglutaminase 2 and Ferroptosis: a new liaison? Cell Death Discov. 9, 88. doi:10.1038/s41420-023-01394-1
Gao, D., Hao, J. P., Li, B. Y., Zheng, C. C., Miao, B. B., Zhang, L., et al. (2023). Tetrahydroxy stilbene glycoside ameliorates neuroinflammation for Alzheimer's disease via cGAS-STING. Eur. J. Pharmacol. 953, 175809. doi:10.1016/j.ejphar.2023.175809
Gao, Z., Wang, G., Chen, Y., Yuan, W., Cai, J., Feng, A., et al. (2024). Total flavonoids of Astragalus membranaceus protect against 1-methyl-4-phenyl-1,2,3,6-tetrahydropyridine-induced neurotoxicity in mice by inhibiting ferroptosis through SLC7A11/GPX-4 signaling pathway. Food Sci. Hum. Wellness 13, 414–420. doi:10.26599/fshw.2022.9250035
Ghadi, R., Pandey, P. K., Gabhale, A., Wadikar, A., Dharshini, M., Kuche, K., et al. (2023). Genipin-crosslinked albumin nanoparticles containing neratinib and silibinin: a dual-death therapy for triple negative breast cancer. Int. J. Pharm. 648, 123570. doi:10.1016/j.ijpharm.2023.123570
Gnanapradeepan, K., Indeglia, A., Stieg, D. C., Clarke, N., Shao, C., Dougherty, J. F., et al. (2022). PLTP is a p53 target gene with roles in cancer growth suppression and ferroptosis. J. Biol. Chem. 298, 102637. doi:10.1016/j.jbc.2022.102637
Gonçalves, M. D., Bortoleti, B. T. S., Tomiotto-Pellissier, F., Miranda-Sapla, M. M., Assolini, J. P., Carloto, A. C. M., et al. (2018). Dehydroabietic acid isolated from Pinus elliottii exerts in vitro antileishmanial action by pro-oxidant effect, inducing ROS production in promastigote and downregulating Nrf2/ferritin expression in amastigote forms of Leishmania amazonensis. Fitoterapia 128, 224–232. doi:10.1016/j.fitote.2018.05.027
Gong, Z., Wang, Y., Li, L., Li, X., Qiu, B., and Hu, Y. (2023). Cardamonin alleviates chondrocytes inflammation and cartilage degradation of osteoarthritis by inhibiting ferroptosis via p53 pathway. Food Chem. Toxicol. 174, 113644. doi:10.1016/j.fct.2023.113644
Goujon, M., Liang, Z., Soriano-Castell, D., Currais, A., and Maher, P. (2024). The neuroprotective flavonoids sterubin and fisetin maintain mitochondrial health under oxytotic/ferroptotic stress and improve bioenergetic efficiency in HT22 neuronal cells. Antioxidants (Basel) 13, 460. doi:10.3390/antiox13040460
Gryzik, M., Asperti, M., Denardo, A., Arosio, P., and Poli, M. (2021). NCOA4-mediated ferritinophagy promotes ferroptosis induced by erastin, but not by RSL3 in HeLa cells. Biochim. Biophys. Acta Mol. Cell Res. 1868, 118913. doi:10.1016/j.bbamcr.2020.118913
Guan, X., Li, Z., Zhu, S., Cheng, M., Ju, Y., Ren, L., et al. (2021). Galangin attenuated cerebral ischemia-reperfusion injury by inhibition of ferroptosis through activating the SLC7A11/GPX4 axis in gerbils. Life Sci. 264, 118660. doi:10.1016/j.lfs.2020.118660
Guerrero-Mauvecin, J., Villar-Gómez, N., Rayego-Mateos, S., Ramos, A. M., Ruiz-Ortega, M., Ortiz, A., et al. (2023). Regulated necrosis role in inflammation and repair in acute kidney injury. Front. Immunol. 14, 1324996. doi:10.3389/fimmu.2023.1324996
Guo, H., Zhu, L., Tang, P., Chen, D., Li, Y., Li, J., et al. (2021). Carthamin yellow improves cerebral ischemia-reperfusion injury by attenuating inflammation and ferroptosis in rats. Int. J. Mol. Med. 47, 52. doi:10.3892/ijmm.2021.4885
Guo, J., Chen, L., and Ma, M. (2023a). Ginsenoside Rg1 suppresses ferroptosis of renal tubular epithelial cells in sepsis-induced acute kidney injury via the FSP1-CoQ10-NAD(P)H pathway. Curr. Med. Chem. doi:10.2174/0929867330666230607125054
Guo, J., Wang, R., and Min, F. (2022a). Ginsenoside Rg1 ameliorates sepsis-induced acute kidney injury by inhibiting ferroptosis in renal tubular epithelial cells. J. Leukoc. Biol. 112, 1065–1077. doi:10.1002/JLB.1A0422-211R
Guo, M., Peng, T., Wu, C., Pan, X., and Huang, Z. (2023b). Engineering ferroptosis inhibitors as inhalable nanomedicines for the highly efficient treatment of idiopathic pulmonary fibrosis. Bioeng. (Basel) 10, 727. doi:10.3390/bioengineering10060727
Guo, X., Jin, X., Han, K., Kang, S., Tian, S., Lv, X., et al. (2022b). Iron promotes neurological function recovery in mice with ischemic stroke through endogenous repair mechanisms. Free Radic. Biol. Med. 182, 59–72. doi:10.1016/j.freeradbiomed.2022.02.017
Hassanein, E. H. M., Abd El-Maksoud, M. S., Ibrahim, I. M., Abd-Alhameed, E. K., Althagafy, H. S., Mohamed, N. M., et al. (2023). The molecular mechanisms underlying anti-inflammatory effects of galangin in different diseases. Phytother. Res. 37, 3161–3181. doi:10.1002/ptr.7874
He, Q., Yang, J., Pan, Z., Zhang, G., Chen, B., Li, S., et al. (2023a). Biochanin A protects against iron overload associated knee osteoarthritis via regulating iron levels and NRF2/System xc-/GPX4 axis. Biomed. Pharmacother. 157, 113915. doi:10.1016/j.biopha.2022.113915
He, Y., Xi, J., Fang, J., Zhang, B., and Cai, W. (2023b). Aloe-emodin alleviates doxorubicin-induced cardiotoxicity via inhibition of ferroptosis. Free Radic. Biol. Med. 206, 13–21. doi:10.1016/j.freeradbiomed.2023.06.025
Hébert, M., Bellavance, G., and Barriault, L. (2022). Total synthesis of Ginkgolide C and formal syntheses of ginkgolides A and B. J. Am. Chem. Soc. 144, 17792–17796. doi:10.1021/jacs.2c08351
Hirata, Y., Hashimoto, T., Ando, K., Kamatari, Y. O., Takemori, H., and Furuta, K. (2023). Structural features localizing the ferroptosis inhibitor GIF-2197-r to lysosomes. RSC Adv. 13, 32276–32281. doi:10.1039/d3ra06611h
Hirschhorn, T., and Stockwell, B. R. (2022). Vitamin K: a new guardian against ferroptosis. Mol. Cell 82, 3760–3762. doi:10.1016/j.molcel.2022.10.001
Holland, T. M., Agarwal, P., Wang, Y., Leurgans, S. E., Bennett, D. A., Booth, S. L., et al. (2020). Dietary flavonols and risk of Alzheimer dementia. Neurology 94, e1749–e1756. doi:10.1212/WNL.0000000000008981
Horinouchi, T., Mazaki, Y., and Miwa, S. (2024). Mechanism of cytotoxicity induced by the cigarette smoke extract (CSE) of heated tobacco products in vascular smooth muscle cells: a comparative study of the cytotoxic effects of CSE and the ferroptosis inducer, erastin. J. Pharmacol. Sci. 154, 86–96. doi:10.1016/j.jphs.2023.12.010
Horonchik, L., and Wessling-Resnick, M. (2008). The small-molecule iron transport inhibitor ferristatin/NSC306711 promotes degradation of the transferrin receptor. Chem. Biol. 15, 647–653. doi:10.1016/j.chembiol.2008.05.011
Hosohata, K., Harnsirikarn, T., and Chokesuwattanaskul, S. (2022). Ferroptosis: a potential therapeutic target in acute kidney injury. Int. J. Mol. Sci. 23, 6583. doi:10.3390/ijms23126583
Hou, B., Ma, P., Yang, X., Zhao, X., Zhang, L., Zhao, Y., et al. (2024). In silico prediction and experimental validation to reveal the protective mechanism of Puerarin against excessive extracellular matrix accumulation through inhibiting ferroptosis in diabetic nephropathy. J. Ethnopharmacol. 319, 117281. doi:10.1016/j.jep.2023.117281
Hu, J., Gu, W., Ma, N., Fan, X., and Ci, X. (2022b). Leonurine alleviates ferroptosis in cisplatin-induced acute kidney injury by activating the Nrf2 signalling pathway. Br. J. Pharmacol. 179, 3991–4009. doi:10.1111/bph.15834
Hu, M., Huang, J., Chen, L., Sun, X. R., Yao, Z. M., Tong, X. H., et al. (2023). Upregulation of CDGSH iron sulfur domain 2 attenuates cerebral ischemia/reperfusion injury. Neural Regen. Res. 18, 1512–1520. doi:10.4103/1673-5374.355766
Hu, Q., Zuo, T., Deng, L., Chen, S., Yu, W., Liu, S., et al. (2022a). β-Caryophyllene suppresses ferroptosis induced by cerebral ischemia reperfusion via activation of the NRF2/HO-1 signaling pathway in MCAO/R rats. Phytomedicine 102, 154112. doi:10.1016/j.phymed.2022.154112
Huang, C., Guo, Y., Li, T., Sun, G., Yang, J., Wang, Y., et al. (2024). Pharmacological activation of GPX4 ameliorates doxorubicin-induced cardiomyopathy. Redox Biol. 70, 103024. doi:10.1016/j.redox.2023.103024
Huang, D., Shen, P., Wang, C., Gao, J., Ye, C., and Wu, F. (2022). Calycosin plays a protective role in diabetic kidney disease through the regulation of ferroptosis. Pharm. Biol. 60, 990–996. doi:10.1080/13880209.2022.2067572
Huang, K. J., Wei, Y. H., Chiu, Y. C., Wu, S. R., and Shieh, D. B. (2019). Assessment of zero-valent iron-based nanotherapeutics for ferroptosis induction and resensitization strategy in cancer cells. Biomater. Sci. 7, 1311–1322. doi:10.1039/c8bm01525b
Huang, W., Yu, L., Cai, W., and Ma, C. (2023). Resveratrol protects BEAS-2B cells against erastin-induced ferroptosis through the nrf2/keap1 pathway. Planta Med. 89, 408–415. doi:10.1055/a-1923-4399
Iasonos, A., and OQuigley, J. (2021). Randomised Phase 1 clinical trials in oncology. Br. J. Cancer 125, 920–926. doi:10.1038/s41416-021-01412-y
Islam, A., Islam, M. S., Rahman, M. K., Uddin, M. N., and Akanda, M. R. (2020). The pharmacological and biological roles of eriodictyol. Arch. Pharm. Res. 43, 582–592. doi:10.1007/s12272-020-01243-0
Ito, J., Omiya, S., Rusu, M. C., Ueda, H., Murakawa, T., Tanada, Y., et al. (2021). Iron derived from autophagy-mediated ferritin degradation induces cardiomyocyte death and heart failure in mice. Elife 10, e62174. doi:10.7554/eLife.62174
Jakaria, M., Belaidi, A. A., Bush, A. I., and Ayton, S. (2021). Ferroptosis as a mechanism of neurodegeneration in Alzheimer's disease. J. Neurochem. 159, 804–825. doi:10.1111/jnc.15519
Jayakumar, T., Hsu, W. H., Yen, T. L., Luo, J. Y., Kuo, Y. C., Fong, T. H., et al. (2013). Hinokitiol, a natural tropolone derivative, offers neuroprotection from thromboembolic stroke in vivo. Evid. Based Complement. Altern. Med. 2013, 840487. doi:10.1155/2013/840487
Jeengar, M. K., Thummuri, D., Magnusson, M., Naidu, V. G. M., and Uppugunduri, S. (2017). Uridine ameliorates dextran sulfate sodium (DSS)-Induced colitis in mice. Sci. Rep. 7, 3924. doi:10.1038/s41598-017-04041-9
Jhelum, P., and David, S. (2022). Ferroptosis: copper-iron connection in cuprizone-induced demyelination. Neural Regen. Res. 17, 89–90. doi:10.4103/1673-5374.314300
Jiang, T., Xiao, Y., Zhou, J., Luo, Z., Yu, L., Liao, Q., et al. (2023c). Arbutin alleviates fatty liver by inhibiting ferroptosis via FTO/SLC7A11 pathway. Redox Biol. 68, 102963. doi:10.1016/j.redox.2023.102963
Jiang, X., Teng, X., Shi, H., Cao, L., He, L., and Gu, Q. (2023a). Discovery and optimization of olanzapine derivatives as new ferroptosis inhibitors. Bioorg Chem. 133, 106393. doi:10.1016/j.bioorg.2023.106393
Jiang, Y., Xie, G., Alimujiang, A., Xie, H., Yang, W., Yin, F., et al. (2023b). Protective effects of querectin against MPP(+)-Induced dopaminergic neurons injury via the Nrf2 signaling pathway. Front. Biosci. (Landmark Ed.) 28, 42. doi:10.31083/j.fbl2803042
Jitrangsri, K., Kamata, K., Akiba, M., Yajiri, Y., Ishibashi, M., Tatsuzaki, J., et al. (2022). Is 18α-Glycyrrhizin a real natural product? Improved preparation of 18α-Glycyrrhizin from 18β-Glycyrrhizin as a positive standard for HPLC analysis of licorice extracts. J. Nat. Med. 76, 367–378. doi:10.1007/s11418-021-01589-9
Jones, G., Zeng, L., Stiles, W. R., Park, S. H., Kang, H., Choi, H. S., et al. (2022). Pharmacokinetics and tissue distribution of deferoxamine-based nanochelator in rats. Nanomedicine (Lond) 17, 1649–1662. doi:10.2217/nnm-2022-0159
Kajarabille, N., and Latunde-Dada, G. O. (2019). Programmed cell-death by ferroptosis: antioxidants as mitigators. Int. J. Mol. Sci. 20, 4968. doi:10.3390/ijms20194968
Kapralov, A. A., Yang, Q., Dar, H. H., Tyurina, Y. Y., Anthonymuthu, T. S., Kim, R., et al. (2020). Redox lipid reprogramming commands susceptibility of macrophages and microglia to ferroptotic death. Nat. Chem. Biol. 16, 278–290. doi:10.1038/s41589-019-0462-8
Karami, A., Fakhri, S., Kooshki, L., and Khan, H. (2022). Polydatin: pharmacological mechanisms, therapeutic targets, biological activities, and health benefits. Molecules 27, 6474. doi:10.3390/molecules27196474
Kato, K., Takahashi, M., Oh-Hashi, K., Ando, K., and Hirata, Y. (2023). Quercetin and resveratrol inhibit ferroptosis independently of Nrf2-ARE activation in mouse hippocampal HT22 cells. Food Chem. Toxicol. 172, 113586. doi:10.1016/j.fct.2022.113586
Kattamis, A. (2019). Renal function abnormalities and deferasirox. Lancet Child. Adolesc. Health 3, 2–3. doi:10.1016/S2352-4642(18)30350-X
Keuters, M. H., Keksa-Goldsteine, V., Dhungana, H., Huuskonen, M. T., Pomeshchik, Y., Savchenko, E., et al. (2021). An arylthiazyne derivative is a potent inhibitor of lipid peroxidation and ferroptosis providing neuroprotection in vitro and in vivo. Sci. Rep. 11, 3518. doi:10.1038/s41598-021-81741-3
Kim, J. H., Lewin, T. M., and Coleman, R. A. (2001). Expression and characterization of recombinant rat acyl-CoA synthetases 1, 4, and 5 - selective inhibition by triacsin C and thiazolidinediones. J. Biol. Chem. 276, 24667–24673. doi:10.1074/jbc.M010793200
Kim, M. J., Song, Y. R., Kim, Y. E., Bae, S. J., Lee, W. Y., Bak, S. B., et al. (2023). Kaempferol stimulation of autophagy regulates the ferroptosis under the oxidative stress as mediated with AMP-activated protein kinase. Free Radic. Biol. Med. 208, 630–642. doi:10.1016/j.freeradbiomed.2023.09.008
Kolbrink, B., von Samson-Himmelstjerna, F. A., Messtorff, M. L., Riebeling, T., Nische, R., Schmitz, J., et al. (2022). Vitamin K1 inhibits ferroptosis and counteracts a detrimental effect of phenprocoumon in experimental acute kidney injury. Cell Mol. Life Sci. 79, 387. doi:10.1007/s00018-022-04416-w
Koppula, P., Zhuang, L., and Gan, B. (2021). Cystine transporter SLC7A11/xCT in cancer: ferroptosis, nutrient dependency, and cancer therapy. Protein Cell 12, 599–620. doi:10.1007/s13238-020-00789-5
Krainz, T., Gaschler, M. M., Lim, C., Sacher, J. R., Stockwell, B. R., and Wipf, P. (2016). A mitochondrial-targeted nitroxide is a potent inhibitor of ferroptosis. Acs Central Sci. 2, 653–659. doi:10.1021/acscentsci.6b00199
Krylova, I. B., Selina, E. N., Bulion, V. V., Rodionova, O. M., Evdokimova, N. R., Belosludtseva, N. V., et al. (2021). Uridine treatment prevents myocardial injury in rat models of acute ischemia and ischemia/reperfusion by activating the mitochondrial ATP-dependent potassium channel. Sci. Rep. 11, 16999. doi:10.1038/s41598-021-96562-7
Kryscio, R. J., Abner, E. L., Caban-Holt, A., Lovell, M., Goodman, P., Darke, A. K., et al. (2017). Association of antioxidant supplement use and dementia in the prevention of alzheimer's disease by vitamin E and selenium trial (PREADViSE). JAMA Neurol. 74, 567–573. doi:10.1001/jamaneurol.2016.5778
Kuganesan, N., Dlamini, S., McDaniel, J., Tillekeratne, V. L. M., and Taylor, W. R. (2021). Identification and initial characterization of a potent inhibitor of ferroptosis. J. Cell. Biochem. 122, 413–424. doi:10.1002/jcb.29870
Kulkarni, N., Gadde, R., and Betharia, S. (2023). Dithiolethiones D3T and ACDT protect against iron overload-induced cytotoxicity and serve as ferroptosis inhibitors in U-87 MG cells. Neurochem. Res. 48, 2542–2551. doi:10.1007/s11064-023-03927-7
Kuo, M. T. H., Beckman, J. S., and Shaw, C. A. (2019). Neuroprotective effect of CuATSM on neurotoxin-induced motor neuron loss in an ALS mouse model. Neurobiol. Dis. 130, 104495. doi:10.1016/j.nbd.2019.104495
Lachowicz, J. I., Pichiri, G., Piludu, M., Fais, S., Orrù, G., Congiu, T., et al. (2022). Thymosin β4 is an endogenous iron chelator and molecular switcher of ferroptosis. Int. J. Mol. Sci. 23, 551. doi:10.3390/ijms23010551
Lai, K., Song, C., Gao, M., Deng, Y., Lu, Z., Li, N., et al. (2023). Uridine alleviates sepsis-induced acute lung injury by inhibiting ferroptosis of macrophage. Int. J. Mol. Sci. 24, 5093. doi:10.3390/ijms24065093
Lai, Y., Zhang, Y., Zhang, H., Chen, Z., Zeng, L., Deng, G., et al. (2024). Modified Shoutai Pill inhibited ferroptosis to alleviate recurrent pregnancy loss. J. Ethnopharmacol. 319, 117028. doi:10.1016/j.jep.2023.117028
Lan, B., Ge, J. W., Cheng, S. W., Zheng, X. L., Liao, J., He, C., et al. (2020). Extract of Naotaifang, a compound Chinese herbal medicine, protects neuron ferroptosis induced by acute cerebral ischemia in rats. J. Integr. Med. 18, 344–350. doi:10.1016/j.joim.2020.01.008
Lan, J., Chen, X., Xu, F., Tao, F., Liu, L., Cheng, R., et al. (2023). Self-assembled miR-134-5p inhibitor nanoparticles ameliorate experimental bronchopulmonary dysplasia (BPD) via suppressing ferroptosis. Mikrochim. Acta 190, 491. doi:10.1007/s00604-023-06069-3
Lane, D. J. R., Alves, F., Ayton, S. J., and Bush, A. I. (2023). Striking a NRF2: the rusty and rancid vulnerabilities toward ferroptosis in alzheimer's disease. Antioxid. Redox Signal 39, 141–161. doi:10.1089/ars.2023.0318
Lecornec, N., Castex, M. P., Réguerre, Y., Moreau, P., Marie, I., Garçon, L., et al. (2022). Agranulocytosis in patients with Diamond-Blackfan anaemia (DBA) treated with deferiprone for post-transfusion iron overload: a retrospective study of the French DBA cohort. Br. J. Haematol. 199, 285–288. doi:10.1111/bjh.18366
Lee, J. J., Chang-Chien, G. P., Lin, S., Hsiao, Y. T., Ke, M. C., Chen, A., et al. (2022). 5-Lipoxygenase inhibition protects retinal pigment epithelium from sodium iodate-induced ferroptosis and prevents retinal degeneration. Oxid. Med. Cell Longev. 2022, 1792894. doi:10.1155/2022/1792894
Lee, S., Hwang, N., Seok, B. G., Lee, S., Lee, S. J., and Chung, S. W. (2023). Autophagy mediates an amplification loop during ferroptosis. Cell Death Dis. 14, 464. doi:10.1038/s41419-023-05978-8
Li, C., Deng, X., Xie, X., Liu, Y., Friedmann Angeli, J. P., and Lai, L. (2018). Activation of glutathione peroxidase 4 as a novel anti-inflammatory strategy. Front. Pharmacol. 9, 1120. doi:10.3389/fphar.2018.01120
Li, C., Deng, X., Zhang, W., Xie, X., Conrad, M., Liu, Y., et al. (2019). Novel allosteric activators for ferroptosis regulator glutathione peroxidase 4. J. Med. Chem. 62, 266–275. doi:10.1021/acs.jmedchem.8b00315
Li, C., and Liu, Y. (2024). Puerarin reduces cell damage from cerebral ischemia-reperfusion by inhibiting ferroptosis. Biochem. Biophys. Res. Commun. 693, 149324. doi:10.1016/j.bbrc.2023.149324
Li, J., Yang, J., Zhu, B., Fan, J., Hu, Q., and Wang, L. (2022b). Tectorigenin protects against unilateral ureteral obstruction by inhibiting Smad3-mediated ferroptosis and fibrosis. Phytother. Res. 36, 475–487. doi:10.1002/ptr.7353
Li, J. Z., Fan, B. Y., Sun, T., Wang, X. X., Li, J. J., Zhang, J. P., et al. (2023e). Bioinformatics analysis of ferroptosis in spinal cord injury. Neural Regen. Res. 18, 626–633. doi:10.4103/1673-5374.350209
Li, L., Li, W. J., Zheng, X. R., Liu, Q. L., Du, Q., Lai, Y. J., et al. (2022a). Eriodictyol ameliorates cognitive dysfunction in APP/PS1 mice by inhibiting ferroptosis via vitamin D receptor-mediated Nrf2 activation. Mol. Med. 28, 11. doi:10.1186/s10020-022-00442-3
Li, L., Zhang, Y., Gao, Y., Hu, Y., Wang, R., Wang, S., et al. (2023d). LncSNHG14 promotes nutlin3a resistance by inhibiting ferroptosis via the miR-206/SLC7A11 axis in osteosarcoma cells. Cancer Gene Ther. 30, 704–715. doi:10.1038/s41417-022-00581-z
Li, Q. S., and Jia, Y. J. (2023). Ferroptosis: a critical player and potential therapeutic target in traumatic brain injury and spinal cord injury. Neural Regen. Res. 18, 506–512. doi:10.4103/1673-5374.350187
Li, W., Liang, L., Liu, S., Yi, H., and Zhou, Y. (2023a). FSP1: a key regulator of ferroptosis. Trends Mol. Med. 29, 753–764. doi:10.1016/j.molmed.2023.05.013
Li, X., Zou, Y., Fu, Y. Y., Xing, J., Wang, K. Y., Wan, P. Z., et al. (2021). A-lipoic acid alleviates folic acid-induced renal damage through inhibition of ferroptosis. Front. Physiol. 12, 680544. doi:10.3389/fphys.2021.680544
Li, Y., Huang, Z., Pan, S., Feng, Y., He, H., Cheng, S., et al. (2023c). Resveratrol alleviates diabetic periodontitis-induced alveolar osteocyte ferroptosis possibly via regulation of slc7a11/GPX4. Nutrients 15, 2115. doi:10.3390/nu15092115
Li, Y., Shi, J., Liu, R., Liu, Y., Liu, R., Wu, Z., et al. (2023b). Structure revisions of phenolic bisabolane sesquiterpenes and a ferroptosis inhibitor from the marine-derived fungus Aspergillus versicolor YPH93. J. Nat. Prod. 86, 830–841. doi:10.1021/acs.jnatprod.2c01022
Li, Y. X., Wang, H. B., Jin, J. B., Yang, C. L., Hu, J. B., and Li, J. (2022c). Advances in the research of nano delivery systems in ischemic stroke. Front. Bioeng. Biotechnol. 10, 984424. doi:10.3389/fbioe.2022.984424
Liang, J., López-Valdés, H. E., Martínez-Coria, H., Lindemeyer, A. K., Shen, Y., Shao, X. M., et al. (2014). Dihydromyricetin ameliorates behavioral deficits and reverses neuropathology of transgenic mouse models of Alzheimer's disease. Neurochem. Res. 39, 1171–1181. doi:10.1007/s11064-014-1304-4
Liang, Y., Wang, Y., Zhang, Y., Ye, F., Luo, D., Li, Y., et al. (2023). HSPB1 facilitates chemoresistance through inhibiting ferroptotic cancer cell death and regulating NF-κB signaling pathway in breast cancer. Cell Death Dis. 14, 434. doi:10.1038/s41419-023-05972-0
Liddell, J. R., Hilton, J. B. W., and Crouch, P. J. (2023). Cu(II)(atsm) significantly decreases microglial reactivity in patients with sporadic amyotrophic lateral sclerosis. Neuropathol. Appl. Neurobiol. 49, e12938. doi:10.1111/nan.12938
Lin, J., Zangi, M., Kumar, T., Shakar Reddy, M., Reddy, L. V. R., Sadhukhan, S. K., et al. (2021a). Synthetic derivatives of ciclopirox are effective inhibitors of cryptococcus neoformans. ACS Omega 6, 8477–8487. doi:10.1021/acsomega.1c00273
Lin, J. H., Yang, K. T., Ting, P. C., Luo, Y. P., Lin, D. J., Wang, Y. S., et al. (2021b). Gossypol acetic acid attenuates cardiac ischemia/reperfusion injury in rats via an antiferroptotic mechanism. Biomolecules 11, 1667. doi:10.3390/biom11111667
Lin, K. J., Chen, S. D., Lin, K. L., Liou, C. W., Lan, M. Y., Chuang, Y. C., et al. (2022). Iron brain menace: the involvement of ferroptosis in Parkinson disease. Cells 11, 3829. doi:10.3390/cells11233829
Linkermann, A., Skouta, R., Himmerkus, N., Mulay, S. R., Dewitz, C., De Zen, F., et al. (2014). Synchronized renal tubular cell death involves ferroptosis. Proc. Natl. Acad. Sci. U. S. A. 111, 16836–16841. doi:10.1073/pnas.1415518111
Liu, D., Ding, J., Li, Z., and Lu, Y. (2024). Pachymic acid (PA) inhibits ferroptosis of cardiomyocytes via activation of the AMPK in mice with ischemia/reperfusion-induced myocardial injury. Cell Biol. Int. 48, 46–59. doi:10.1002/cbin.12090
Liu, J. P., Cen, S. Y., Xue, Z., Wang, T. X., Gao, Y., Zheng, J., et al. (2022). A class of disulfide compounds suppresses ferroptosis by stabilizing GPX4. ACS Chem. Biol. 17, 3389–3406. doi:10.1021/acschembio.2c00445
Liu, P., Feng, Y., Li, H., Chen, X., Wang, G., Xu, S., et al. (2020). Ferrostatin-1 alleviates lipopolysaccharide-induced acute lung injury via inhibiting ferroptosis. Cell Mol. Biol. Lett. 25, 10. doi:10.1186/s11658-020-00205-0
Liu, Y., Wang, W., Li, Y., Xiao, Y., Cheng, J., and Jia, J. (2015). The 5-lipoxygenase inhibitor zileuton confers neuroprotection against glutamate oxidative damage by inhibiting ferroptosis. Biol. Pharm. Bull. 38, 1234–1239. doi:10.1248/bpb.b15-00048
Lu, J., Li, Y., Gong, S., Wang, J., Lu, X., Jin, Q., et al. (2022). Ciclopirox targets cellular bioenergetics and activates ER stress to induce apoptosis in non-small cell lung cancer cells. Cell Commun. Signal 20, 37. doi:10.1186/s12964-022-00847-x
Lv, S., Li, H., Zhang, T., Su, X., Sun, W., Wang, Q., et al. (2023b). San-Huang-Yi-Shen capsule ameliorates diabetic nephropathy in mice through inhibiting ferroptosis. Biomed. Pharmacother. 165, 115086. doi:10.1016/j.biopha.2023.115086
Lv, Y., Liang, C., Sun, Q., Zhu, J., Xu, H., Li, X., et al. (2023a). Structural insights into FSP1 catalysis and ferroptosis inhibition. Nat. Commun. 14, 5933. doi:10.1038/s41467-023-41626-7
Ma, L., Liu, X., Zhang, M., Zhou, L., Jiang, L., Gao, L., et al. (2023b). Paeoniflorin alleviates ischemia/reperfusion induced acute kidney injury by inhibiting Slc7a11-mediated ferroptosis. Int. Immunopharmacol. 116, 109754. doi:10.1016/j.intimp.2023.109754
Ma, P. W., Wang, W. L., Chen, J. W., Yuan, H., Lu, P. H., Gao, W., et al. (2022). Treatment with the ferroptosis inhibitor ferrostatin-1 attenuates noise-induced hearing loss by suppressing ferroptosis and apoptosis. Oxid. Med. Cell Longev. 2022, 3373828. doi:10.1155/2022/3373828
Ma, S. J., Li, C., Yan, C., Liu, N., Jiang, G. Y., Yang, H. R., et al. (2023a). Melatonin alleviates early brain injury by inhibiting the NRF2-mediated ferroptosis pathway after subarachnoid hemorrhage. Free Radic. Biol. Med. 208, 555–570. doi:10.1016/j.freeradbiomed.2023.09.012
Ma, W. Q., Sun, X. J., Zhu, Y., and Liu, N. F. (2021). Metformin attenuates hyperlipidaemia-associated vascular calcification through anti-ferroptotic effects. Free Radic. Biol. Med. 165, 229–242. doi:10.1016/j.freeradbiomed.2021.01.033
Mallais, M., Hanson, C. S., Giray, M., and Pratt, D. A. (2023). General approach to identify, assess, and characterize inhibitors of lipid peroxidation and associated cell death. ACS Chem. Biol. 18, 561–571. doi:10.1021/acschembio.2c00897
Manogaran, P., Beeraka, N. M., Paulraj, R. S., Sathiyachandran, P., and Thammaiappa, M. (2023). Impediment of cancer by dietary plant-derived alkaloids through oxidative stress: implications of PI3K/AKT pathway in apoptosis, autophagy, and ferroptosis. Curr. Top. Med. Chem. 23, 860–877. doi:10.2174/1568026623666230111154537
Martin-Bastida, A., Ward, R. J., Newbould, R., Piccini, P., Sharp, D., Kabba, C., et al. (2017). Brain iron chelation by deferiprone in a phase 2 randomised double-blinded placebo controlled clinical trial in Parkinson's disease. Sci. Rep. 7, 1398. doi:10.1038/s41598-017-01402-2
Millán, M., DeGregorio-Rocasolano, N., Pérez de la Ossa, N., Reverté, S., Costa, J., Giner, P., et al. (2021). Targeting pro-oxidant iron with deferoxamine as a treatment for ischemic stroke: safety and optimal dose selection in a randomized clinical trial. Antioxidants (Basel) 10, 1270. doi:10.3390/antiox10081270
Mishima, E., Ito, J., Wu, Z., Nakamura, T., Wahida, A., Doll, S., et al. (2022). A non-canonical vitamin K cycle is a potent ferroptosis suppressor. Nature 608, 778–783. doi:10.1038/s41586-022-05022-3
Miyamoto, H. D., Ikeda, M., Ide, T., Tadokoro, T., Furusawa, S., Abe, K., et al. (2022). Iron overload via heme degradation in the endoplasmic reticulum triggers ferroptosis in myocardial ischemia-reperfusion injury. JACC Basic Transl. Sci. 7, 800–819. doi:10.1016/j.jacbts.2022.03.012
Morrow, J. P., Mazrad, Z. A. I., Bush, A. I., and Kempe, K. (2022). Poly(2-oxazoline) - ferrostatin-1 drug conjugates inhibit ferroptotic cell death. J. Control Release 350, 193–203. doi:10.1016/j.jconrel.2022.08.004
Moujalled, D., Strasser, A., and Liddell, J. R. (2021). Molecular mechanisms of cell death in neurological diseases. Cell Death Differ. 28, 2029–2044. doi:10.1038/s41418-021-00814-y
Nakamura, T., Mishima, E., Yamada, N., Mourão, A. S. D., Trümbach, D., Doll, S., et al. (2023). Integrated chemical and genetic screens unveil FSP1 mechanisms of ferroptosis regulation. Nat. Struct. Mol. Biol. 30, 1806–1815. doi:10.1038/s41594-023-01136-y
Naowarojna, N., Wu, T. W., Pan, Z., Li, M., Han, J. R., and Zou, Y. (2023). Dynamic regulation of ferroptosis by lipid metabolism. Antioxid. Redox Signal 39, 59–78. doi:10.1089/ars.2023.0278
Naskar, A., and Kim, K. S. (2019). Nanomaterials as delivery vehicles and components of new strategies to combat bacterial infections: advantages and limitations. Microorganisms 7, 356. doi:10.3390/microorganisms7090356
Ni, C., Ye, Q., Mi, X., Jiao, D., Zhang, S., Cheng, R., et al. (2023). Resveratrol inhibits ferroptosis via activating NRF2/GPX4 pathway in mice with spinal cord injury. Microsc. Res. Tech. 86, 1378–1390. doi:10.1002/jemt.24335
Noack, V., Pak, K., Jalota, R., Kurabi, A., and Ryan, A. F. (2017). An antioxidant screen identifies candidates for protection of cochlear hair cells from gentamicin toxicity. Front. Cell Neurosci. 11, 242. doi:10.3389/fncel.2017.00242
Obi, K., Amano, I., and Takatsuru, Y. (2018). Role of dopamine on functional recovery in the contralateral hemisphere after focal stroke in the somatosensory cortex. Brain Res. 1678, 146–152. doi:10.1016/j.brainres.2017.10.022
Olivieri, N. F., Sabouhanian, A., and Gallie, B. L. (2019). Single-center retrospective study of the effectiveness and toxicity of the oral iron chelating drugs deferiprone and deferasirox. PLoS One 14, e0211942. doi:10.1371/journal.pone.0211942
Pan, X., Chen, Z., Fei, G., Pan, S., Bao, W., Ren, S., et al. (2016). Long-term cognitive improvement after benfotiamine administration in patients with alzheimer's disease. Neurosci. Bull. 32, 591–596. doi:10.1007/s12264-016-0067-0
Pang, Y., Liu, X., Wang, X., Shi, X., Ma, L., Zhang, Y., et al. (2022). Edaravone modulates neuronal GPX4/ACSL4/5-LOX to promote recovery after spinal cord injury. Front. Cell Dev. Biol. 10, 849854. doi:10.3389/fcell.2022.849854
Park, G., Rim, Y. A., Sohn, Y., Nam, Y., and Ju, J. H. (2024). Replacing animal testing with stem cell-organoids: advantages and limitations. Stem Cell Rev. Rep. doi:10.1007/s12015-024-10723-5
Peng, Z. (2023). Efficacy and safety of Agatroban in improving the prognosis of ischemic stroke patients. Am. J. Transl. Res. 15, 5699–5706.
Phiwchai, I., Yuensook, W., Sawaengsiriphon, N., Krungchanuchat, S., and Pilapong, C. (2018). Tannic acid (TA): a molecular tool for chelating and imaging labile iron. Eur. J. Pharm. Sci. 114, 64–73. doi:10.1016/j.ejps.2017.12.004
Pope, L. E., and Dixon, S. J. (2023). Regulation of ferroptosis by lipid metabolism. Trends Cell Biol. 33, 1077–1087. doi:10.1016/j.tcb.2023.05.003
Popelová, O., Sterba, M., Hasková, P., Simůnek, T., Hroch, M., Guncová, I., et al. (2009). Dexrazoxane-afforded protection against chronic anthracycline cardiotoxicity in vivo: effective rescue of cardiomyocytes from apoptotic cell death. Br. J. Cancer 101, 792–802. doi:10.1038/sj.bjc.6605192
Prasad Panda, S., and Kesharwani, A. (2023). Micronutrients/miRs/ATP networking in mitochondria: clinical intervention with ferroptosis, cuproptosis, and calcium burden. Mitochondrion 71, 1–16. doi:10.1016/j.mito.2023.05.003
Punziano, C., Trombetti, S., Cesaro, E., Grosso, M., and Faraonio, R. (2024). Antioxidant systems as modulators of ferroptosis: focus on transcription factors. Antioxidants (Basel) 13, 298. doi:10.3390/antiox13030298
Qi, Y., Hu, M., Qiu, Y., Zhang, L., Yan, Y., Feng, Y., et al. (2023). Mitoglitazone ameliorates renal ischemia/reperfusion injury by inhibiting ferroptosis via targeting mitoNEET. Toxicol. Appl. Pharmacol. 465, 116440. doi:10.1016/j.taap.2023.116440
Qiu, W., An, S., Wang, T., Li, J., Yu, B., Zeng, Z., et al. (2022). Melatonin suppresses ferroptosis via activation of the Nrf2/HO-1 signaling pathway in the mouse model of sepsis-induced acute kidney injury. Int. Immunopharmacol. 112, 109162. doi:10.1016/j.intimp.2022.109162
Ramakrishnan, M., Arivalagan, J., Satish, L., Mohan, M., Samuel Selvan Christyraj, J. R., Chandran, S. A., et al. (2022). Selenium: a potent regulator of ferroptosis and biomass production. Chemosphere 306, 135531. doi:10.1016/j.chemosphere.2022.135531
Rayatpour, A., Foolad, F., Heibatollahi, M., Khajeh, K., and Javan, M. (2022). Ferroptosis inhibition by deferiprone, attenuates myelin damage and promotes neuroprotection in demyelinated optic nerve. Sci. Rep. 12, 19630. doi:10.1038/s41598-022-24152-2
Rizzardi, N., Pezzolesi, L., Samorì, C., Senese, F., Zalambani, C., Pitacco, W., et al. (2022). Natural astaxanthin is a green antioxidant able to counteract lipid peroxidation and ferroptotic cell death. Int. J. Mol. Sci. 23, 15137. doi:10.3390/ijms232315137
Roth, A., Zhao, P., Soukup, S. T., Guigas, C., Stärke, J., Kulling, S. E., et al. (2023). Chemical Stability and Bioactivity of tanshinone I, tanshinone IIA, cryptotanshinone and dihydrotanshinone in in vitro test systems. Toxicol. Lett. 375, 21–28. doi:10.1016/j.toxlet.2022.12.002
Rothstein, J. D. (2017). Edaravone: a new drug approved for ALS. Cell 171, 725. doi:10.1016/j.cell.2017.10.011
Ryan, S. K., Zelic, M., Han, Y., Teeple, E., Chen, L., Sadeghi, M., et al. (2023). Microglia ferroptosis is regulated by SEC24B and contributes to neurodegeneration. Nat. Neurosci. 26, 12–26. doi:10.1038/s41593-022-01221-3
Saito, Y. (2021). Diverse cytoprotective actions of vitamin E isoforms-role as peroxyl radical scavengers and complementary functions with selenoproteins. Free Radic. Biol. Med. 175, 121–129. doi:10.1016/j.freeradbiomed.2021.08.234
Salama, S. A., Abdel-Bakky, M. S., and Mohamed, A. A. (2022). Upregulation of Nrf2 signaling and suppression of ferroptosis and NF-κB pathway by leonurine attenuate iron overload-induced hepatotoxicity. Chem. Biol. Interact. 356, 109875. doi:10.1016/j.cbi.2022.109875
Salama, S. A., and Elshafey, M. M. (2021). Galangin mitigates iron overload-triggered liver injury: up-regulation of PPARγ and Nrf2 signaling, and abrogation of the inflammatory responses. Life Sci. 283, 119856. doi:10.1016/j.lfs.2021.119856
Scarpellini, C., Klejborowska, G., Lanthier, C., Hassannia, B., Vanden Berghe, T., and Augustyns, K. (2023). Beyond ferrostatin-1: a comprehensive review of ferroptosis inhibitors. Trends Pharmacol. Sci. 44, 902–916. doi:10.1016/j.tips.2023.08.012
Şeker Karatoprak, G., Küpeli Akkol, E., Yücel, Ç., Bahadır Acıkara, Ö., and Sobarzo-Sánchez, E. (2022). Advances in understanding the role of aloe emodin and targeted drug delivery systems in cancer. Oxid. Med. Cell Longev. 2022, 7928200. doi:10.1155/2022/7928200
Shah, R., Margison, K., and Pratt, D. A. (2017). The potency of diarylamine radical-trapping antioxidants as inhibitors of ferroptosis underscores the role of autoxidation in the mechanism of cell death. ACS Chem. Biol. 12, 2538–2545. doi:10.1021/acschembio.7b00730
Shah, R., Shchepinov, M. S., and Pratt, D. A. (2018a). Resolving the role of lipoxygenases in the initiation and execution of ferroptosis. Acs Central Sci. 4, 387–396. doi:10.1021/acscentsci.7b00589
Shah, R., Shchepinov, M. S., and Pratt, D. A. (2018b). Resolving the role of lipoxygenases in the initiation and execution of ferroptosis. ACS Cent. Sci. 4, 387–396. doi:10.1021/acscentsci.7b00589
Shamsi, A., Shahwan, M., Khan, M. S., Husain, F. M., Alhumaydhi, F. A., Aljohani, A. S. M., et al. (2021). Elucidating the interaction of human ferritin with quercetin and naringenin: implication of natural products in neurodegenerative diseases: molecular docking and dynamics simulation insight. ACS Omega 6, 7922–7930. doi:10.1021/acsomega.1c00527
Shan, X., Li, J., Liu, J., Feng, B., Zhang, T., Liu, Q., et al. (2023). Targeting ferroptosis by poly(acrylic) acid coated Mn(3)O(4) nanoparticles alleviates acute liver injury. Nat. Commun. 14, 7598. doi:10.1038/s41467-023-43308-w
Shearer, M. J., and Okano, T. (2018). Key pathways and regulators of vitamin K function and intermediary metabolism. Annu. Rev. Nutr. 38, 127–151. doi:10.1146/annurev-nutr-082117-051741
Shi, K. N., Li, P. B., Su, H. X., Gao, J., and Li, H. H. (2023). MK-886 protects against cardiac ischaemia/reperfusion injury by activating proteasome-Keap1-NRF2 signalling. Redox Biol. 62, 102706. doi:10.1016/j.redox.2023.102706
Shi, X., Ohta, Y., Nakano, Y., Liu, X., Tadokoro, K., Feng, T., et al. (2021). Neuroprotective effect of CuATSM in mice stroke model by ameliorating oxidative stress. Neurosci. Res. 166, 55–61. doi:10.1016/j.neures.2020.05.009
Shibu, M. A., Bharath, M., and Velmurugan, B. K. (2021). Regulating inflammation associated ferroptosis - a treatment strategy for Parkinson disease. Curr. Med. Chem. 28, 6895–6914. doi:10.2174/0929867328666210419125032
Shin, D., Lee, J., You, J. H., Kim, D., and Roh, J. L. (2020). Dihydrolipoamide dehydrogenase regulates cystine deprivation-induced ferroptosis in head and neck cancer. Redox Biol. 30, 101418. doi:10.1016/j.redox.2019.101418
Singh, G., Kesharwani, P., Kumar Singh, G., Kumar, S., Putta, A., and Modi, G. (2024). Ferroptosis and its modulators: a raising target for cancer and Alzheimer's disease. Bioorg Med. Chem. 98, 117564. doi:10.1016/j.bmc.2023.117564
Skouta, R., Dixon, S. J., Wang, J., Dunn, D. E., Orman, M., Shimada, K., et al. (2014). Ferrostatins inhibit oxidative lipid damage and cell death in diverse disease models. J. Am. Chem. Soc. 136, 4551–4556. doi:10.1021/ja411006a
Solovyev, N., Drobyshev, E., Bjørklund, G., Dubrovskii, Y., Lysiuk, R., and Rayman, M. P. (2018). Selenium, selenoprotein P, and Alzheimer's disease: is there a link? Free Radic. Biol. Med. 127, 124–133. doi:10.1016/j.freeradbiomed.2018.02.030
Song, Q., Jian, W., Zhang, Y., Li, Q., Zhao, Y., Liu, R., et al. (2024). Puerarin attenuates iron overload-induced ferroptosis in retina through a nrf2-mediated mechanism. Mol. Nutr. Food Res. 68, e2300123. doi:10.1002/mnfr.202300123
Southon, A., Szostak, K., Acevedo, K. M., Dent, K. A., Volitakis, I., Belaidi, A. A., et al. (2020). Cu(II) (atsm) inhibits ferroptosis: implications for treatment of neurodegenerative disease. Br. J. Pharmacol. 177, 656–667. doi:10.1111/bph.14881
Sridharan, K., and Sivaramakrishnan, G. (2018). Efficacy and safety of iron chelators in thalassemia and sickle cell disease: a multiple treatment comparison network meta-analysis and trial sequential analysis. Expert Rev. Clin. Pharmacol. 11, 641–650. doi:10.1080/17512433.2018.1473760
Su, X., Yu, W., Liu, A., Wang, C., Li, X., Gao, J., et al. (2021). San-huang-yi-shen capsule ameliorates diabetic nephropathy in rats through modulating the gut microbiota and overall metabolism. Front. Pharmacol. 12, 808867. doi:10.3389/fphar.2021.808867
Sun, S., Shen, J., Jiang, J., Wang, F., and Min, J. (2023). Targeting ferroptosis opens new avenues for the development of novel therapeutics. Signal Transduct. Target Ther. 8, 372. doi:10.1038/s41392-023-01606-1
Sun, X., Ou, Z., Xie, M., Kang, R., Fan, Y., Niu, X., et al. (2015). HSPB1 as a novel regulator of ferroptotic cancer cell death. Oncogene 34, 5617–5625. doi:10.1038/onc.2015.32
Tan, M., Yin, Y., Ma, X., Zhang, J., Pan, W., Tan, M., et al. (2023). Glutathione system enhancement for cardiac protection: pharmacological options against oxidative stress and ferroptosis. Cell Death Dis. 14, 131. doi:10.1038/s41419-023-05645-y
Tan, Q., Fang, Y., Peng, X., Zhou, H., Xu, J., and Gu, Q. (2021). A new ferroptosis inhibitor, isolated from Ajuga nipponensis, protects neuronal cells via activating NRF2-antioxidant response elements (AREs) pathway. Bioorg Chem. 115, 105177. doi:10.1016/j.bioorg.2021.105177
Tang, D., Chen, X., Kang, R., and Kroemer, G. (2021a). Ferroptosis: molecular mechanisms and health implications. Cell Res. 31, 107–125. doi:10.1038/s41422-020-00441-1
Tang, G., Pi, L., Guo, H., Hu, Z., Zhou, C., Hu, Q., et al. (2022). Naringin relieves diabetic cardiac autonomic neuropathy mediated by P2Y(14) receptor in superior cervical ganglion. Front. Pharmacol. 13, 873090. doi:10.3389/fphar.2022.873090
Tang, Z., Huo, M., Ju, Y., Dai, X., Ni, N., Liu, Y., et al. (2021b). Nanoprotection against retinal pigment epithelium degeneration via ferroptosis inhibition. Small Methods 5, e2100848. doi:10.1002/smtd.202100848
Tao, W., Liu, F., Zhang, J., Fu, S., Zhan, H., and Qian, K. (2021). miR-3587 inhibitor attenuates ferroptosis following renal ischemia-reperfusion through HO-1. Front. Mol. Biosci. 8, 789927. doi:10.3389/fmolb.2021.789927
Tarangelo, A., and Dixon, S. (2018). The p53-p21 pathway inhibits ferroptosis during metabolic stress. Oncotarget 9, 24572–24573. doi:10.18632/oncotarget.25362
Tarangelo, A., Magtanong, L., Bieging-Rolett, K. T., Li, Y., Ye, J., Attardi, L. D., et al. (2018). p53 suppresses metabolic stress-induced ferroptosis in cancer cells. Cell Rep. 22, 569–575. doi:10.1016/j.celrep.2017.12.077
Tarangelo, A., Rodencal, J., Kim, J. T., Magtanong, L., Long, J. Z., and Dixon, S. J. (2022). Nucleotide biosynthesis links glutathione metabolism to ferroptosis sensitivity. Life Sci. Alliance 5, e202101157. doi:10.26508/lsa.202101157
Tartaglione, I., Origa, R., Kattamis, A., Pfeilstöcker, M., Gunes, S., Crowe, S., et al. (2020). Two-year long safety and efficacy of deferasirox film-coated tablets in patients with thalassemia or lower/intermediate risk MDS: phase 3 results from a subset of patients previously treated with deferasirox in the ECLIPSE study. Exp. Hematol. Oncol. 9, 20. doi:10.1186/s40164-020-00174-2
Tavakol, S., and Seifalian, A. M. (2022). Vitamin E at a high dose as an anti-ferroptosis drug and not just a supplement for COVID-19 treatment. Biotechnol. Appl. Biochem. 69, 1058–1060. doi:10.1002/bab.2176
Teng, Y., Xu, D., Yang, X., Tang, H., Tao, X., Fan, Y., et al. (2023). The emerging roles of pyroptosis, necroptosis, and ferroptosis in non-malignant dermatoses: a review. J. Inflamm. Res. 16, 1967–1977. doi:10.2147/JIR.S409699
Thapa, K., Khan, H., Kanojia, N., Singh, T. G., Kaur, A., and Kaur, G. (2022). Therapeutic insights on ferroptosis in Parkinson's disease. Eur. J. Pharmacol. 930, 175133. doi:10.1016/j.ejphar.2022.175133
Tuo, Q. Z., Liu, Y., Xiang, Z., Yan, H. F., Zou, T., Shu, Y., et al. (2022). Thrombin induces ACSL4-dependent ferroptosis during cerebral ischemia/reperfusion. Signal Transduct. Target Ther. 7, 59. doi:10.1038/s41392-022-00917-z
Walters, J. L. H., De Iuliis, G. N., Dun, M. D., Aitken, R. J., McLaughlin, E. A., Nixon, B., et al. (2018). Pharmacological inhibition of arachidonate 15-lipoxygenase protects human spermatozoa against oxidative stress. Biol. Reprod. 98, 784–794. doi:10.1093/biolre/ioy058
Wang, H., Zheng, Z., Han, W., Yuan, Y., Li, Y., Zhou, K., et al. (2020b). Metformin promotes axon regeneration after spinal cord injury through inhibiting oxidative stress and stabilizing microtubule. Oxid. Med. Cell Longev. 2020, 9741369. doi:10.1155/2020/9741369
Wang, K., Zhang, Y., Mao, W., Feng, W., Lu, S., Wan, J., et al. (2021). Engineering ultrasmall ferroptosis-targeting and reactive oxygen/nitrogen species-scavenging nanozyme for alleviating acute kidney injury. Adv. Funct. Mater. 32. doi:10.1002/adfm.202109221
Wang, L., Lei, Q., Zhao, S., Xu, W., Dong, W., Ran, J., et al. (2020a). Ginkgolide B maintains calcium homeostasis in hypoxic hippocampal neurons by inhibiting calcium influx and intracellular calcium release. Front. Cell Neurosci. 14, 627846. doi:10.3389/fncel.2020.627846
Wang, M., Liu, C. Y., Wang, T., Yu, H. M., Ouyang, S. H., Wu, Y. P., et al. (2020c). (+)-Clausenamide protects against drug-induced liver injury by inhibiting hepatocyte ferroptosis. Cell Death Dis. 11, 781. doi:10.1038/s41419-020-02961-5
Wang, T., Lei, H., Li, X., Yang, N., Ma, C., Li, G., et al. (2023b). Magnetic targeting nanocarriers combined with focusing ultrasound for enhanced intracerebral hemorrhage therapy. Small 19, e2206982. doi:10.1002/smll.202206982
Wang, W., Zhang, Z., Liu, Y., Kong, L., Li, W., Hu, W., et al. (2022a). Nano-integrated cascade antioxidases opsonized by albumin bypass the blood-brain barrier for treatment of ischemia-reperfusion injury. Biomater. Sci. 10, 7103–7116. doi:10.1039/d2bm01401g
Wang, Y., Dong, Z., Zhang, Z., Wang, Y., Yang, K., and Li, X. (2022b). Postconditioning with irisin attenuates lung ischemia/reperfusion injury by suppressing ferroptosis via induction of the Nrf2/HO-1 signal Axis. Oxid. Med. Cell Longev. 2022, 9911167. doi:10.1155/2022/9911167
Wang, Y., Lv, M. N., and Zhao, W. J. (2023a). Research on ferroptosis as a therapeutic target for the treatment of neurodegenerative diseases. Ageing Res. Rev. 91, 102035. doi:10.1016/j.arr.2023.102035
Wei, N., Lu, T., Yang, L., Dong, Y., and Liu, X. (2021). Lipoxin A4 protects primary spinal cord neurons from Erastin-induced ferroptosis by activating the Akt/Nrf2/HO-1 signaling pathway. FEBS Open Bio 11, 2118–2126. doi:10.1002/2211-5463.13203
Wetli, H. A., Buckett, P. D., and Wessling-Resnick, M. (2006). Small-molecule screening identifies the selanazal drug ebselen as a potent inhibitor of DMT1-mediated iron uptake. Chem. Biol. 13, 965–972. doi:10.1016/j.chembiol.2006.08.005
Wickramaratne, A. C., Liao, J. Y., Doyle, S. M., Hoskins, J. R., Puller, G., Scott, M. L., et al. (2023). J-Domain proteins form binary complexes with Hsp90 and ternary complexes with Hsp90 and Hsp70. J. Mol. Biol. 435, 168184. doi:10.1016/j.jmb.2023.168184
Wu, Y., Qian, J., Li, K., Li, W., Yin, W., and Jiang, H. (2022). Farrerol alleviates collagenase-induced tendinopathy by inhibiting ferroptosis in rats. J. Cell Mol. Med. 26, 3483–3494. doi:10.1111/jcmm.17388
Wu, Y., Ran, L., Yang, Y., Gao, X., Peng, M., Liu, S., et al. (2023). Deferasirox alleviates DSS-induced ulcerative colitis in mice by inhibiting ferroptosis and improving intestinal microbiota. Life Sci. 314, 121312. doi:10.1016/j.lfs.2022.121312
Wu, Z., Geng, Y., Lu, X., Shi, Y., Wu, G., Zhang, M., et al. (2019). Chaperone-mediated autophagy is involved in the execution of ferroptosis. Proc. Natl. Acad. Sci. U. S. A. 116, 2996–3005. doi:10.1073/pnas.1819728116
Wu, Z., Wu, P., Zuo, X., Yu, N., Qin, Y., Xu, Q., et al. (2017). LncRNA-N1LR enhances neuroprotection against ischemic stroke probably by inhibiting p53 phosphorylation. Mol. Neurobiol. 54, 7670–7685. doi:10.1007/s12035-016-0246-z
Xi, J., Zhang, Z., Wang, Z., Wu, Q., He, Y., Xu, Y., et al. (2022). Hinokitiol functions as a ferroptosis inhibitor to confer neuroprotection. Free Radic. Biol. Med. 190, 202–215. doi:10.1016/j.freeradbiomed.2022.08.011
Xiao, F.-J., Zhang, D., Wu, Y., Jia, Q.-H., Zhang, L., Li, Y.-X., et al. (2019). miRNA-17-92 protects endothelial cells from erastin-induced ferroptosis through targeting the A20-ACSL4 axis. Biochem. Biophysical Res. Commun. 515, 448–454. doi:10.1016/j.bbrc.2019.05.147
Xiao, Z., Deng, S., Liu, H., Wang, R., Liu, Y., Dai, Z., et al. (2023). Glutamine deprivation induces ferroptosis in pancreatic cancer cells. Acta Biochim. Biophys. Sin. (Shanghai) 55, 1288–1300. doi:10.3724/abbs.2023029
Xie, X., Zhang, Y., Su, X., Wang, J., Yao, X., Lv, D., et al. (2022). Targeting iron metabolism using gallium nanoparticles to suppress ferroptosis and effectively mitigate acute kidney injury. Nano Res. 15, 6315–6327. doi:10.1007/s12274-022-4257-y
Xie, Y., Song, X., Sun, X., Huang, J., Zhong, M., Lotze, M. T., et al. (2016). Identification of baicalein as a ferroptosis inhibitor by natural product library screening. Biochem. Biophys. Res. Commun. 473, 775–780. doi:10.1016/j.bbrc.2016.03.052
Xing, K., Bian, X., Shi, D., Dong, S., Zhou, H., Xiao, S., et al. (2023). miR-612 enhances RSL3-induced ferroptosis of hepatocellular carcinoma cells via mevalonate pathway. J. Hepatocell. Carcinoma 10, 2173–2185. doi:10.2147/JHC.S433332
Xu, J., Wang, Y., Wang, A., Gao, Z., Gao, X., Chen, H., et al. (2019). Safety and efficacy of Edaravone Dexborneol versus edaravone for patients with acute ischaemic stroke: a phase II, multicentre, randomised, double-blind, multiple-dose, active-controlled clinical trial. Stroke Vasc. Neurol. 4, 109–114. doi:10.1136/svn-2018-000221
Xu, L., Liu, Y., Chen, X., Zhong, H., and Wang, Y. (2023a). Ferroptosis in life: to be or not to be. Biomed. Pharmacother. 159, 114241. doi:10.1016/j.biopha.2023.114241
Xu, S., Kang, Z., Li, K., Li, X., Zhang, Y., and Gao, X. J. (2023b). Selenium deficiency causes iron death and inflammatory injury through oxidative stress in the mice gastric mucosa. Biol. Trace Elem. Res. 202, 1150–1163. doi:10.1007/s12011-023-03754-5
Xu, S., Kang, Z., Li, K., Li, X., Zhang, Y., and Gao, X. J. (2024). Selenium deficiency causes iron death and inflammatory injury through oxidative stress in the mice gastric mucosa. Biol. Trace Elem. Res. 202, 1150–1163. doi:10.1007/s12011-023-03754-5
Xu, Z., Zhang, M., Wang, W., Zhou, S., Yu, M., Qiu, X., et al. (2023c). Dihydromyricetin attenuates cisplatin-induced acute kidney injury by reducing oxidative stress, inflammation and ferroptosis. Toxicol. Appl. Pharmacol. 473, 116595. doi:10.1016/j.taap.2023.116595
Yamada, N., Karasawa, T., Wakiya, T., Sadatomo, A., Ito, H., Kamata, R., et al. (2020). Iron overload as a risk factor for hepatic ischemia-reperfusion injury in liver transplantation: potential role of ferroptosis. Am. J. Transpl. 20, 1606–1618. doi:10.1111/ajt.15773
Yan, H., Sasaki, T., Kanki, H., Hirata, Y., Nishiyama, K., Hisada, S., et al. (2022). MDMX elevation by a novel Mdmx-p53 interaction inhibitor mitigates neuronal damage after ischemic stroke. Sci. Rep. 12, 21110. doi:10.1038/s41598-022-25427-4
Yang, C., Han, M., Li, R., Zhou, L., Zhang, Y., Duan, L., et al. (2021c). Curcumin nanoparticles inhibiting ferroptosis for the enhanced treatment of intracerebral hemorrhage. Int. J. Nanomedicine 16, 8049–8065. doi:10.2147/IJN.S334965
Yang, J., Hu, S., Bian, Y., Yao, J., Wang, D., Liu, X., et al. (2021d). Targeting cell death: pyroptosis, ferroptosis, apoptosis and necroptosis in osteoarthritis. Front. Cell Dev. Biol. 9, 789948. doi:10.3389/fcell.2021.789948
Yang, K., Zeng, L., Yuan, X., Wang, S., Ge, A., Xu, H., et al. (2022b). The mechanism of ferroptosis regulating oxidative stress in ischemic stroke and the regulation mechanism of natural pharmacological active components. Biomed. Pharmacother. 154, 113611. doi:10.1016/j.biopha.2022.113611
Yang, T., Chen, X., Mei, Z., Liu, X., Feng, Z., Liao, J., et al. (2021a). An integrated analysis of network Pharmacology and experimental validation to reveal the mechanism of Chinese medicine formula naotaifang in treating cerebral ischemia-reperfusion injury. Drug Des. Devel Ther. 15, 3783–3808. doi:10.2147/DDDT.S328837
Yang, W., Liu, X., Song, C., Ji, S., Yang, J., Liu, Y., et al. (2021b). Structure-activity relationship studies of phenothiazine derivatives as a new class of ferroptosis inhibitors together with the therapeutic effect in an ischemic stroke model. Eur. J. Med. Chem. 209, 112842. doi:10.1016/j.ejmech.2020.112842
Yang, W., Mu, B., You, J., Tian, C., Bin, H., Xu, Z., et al. (2022a). Non-classical ferroptosis inhibition by a small molecule targeting PHB2. Nat. Commun. 13, 7473. doi:10.1038/s41467-022-35294-2
Yang, Y., Rowe, D., McCann, H., Shepherd, C. E., Kril, J. J., Kiernan, M. C., et al. (2023). Treatment with the copper compound CuATSM has no significant effect on motor neuronal pathology in patients with ALS. Neuropathol. Appl. Neurobiol. 49, e12919. doi:10.1111/nan.12919
Yao, X., Zhang, Y., Hao, J., Duan, H. Q., Zhao, C. X., Sun, C., et al. (2019). Deferoxamine promotes recovery of traumatic spinal cord injury by inhibiting ferroptosis. Neural Regen. Res. 14, 532–541. doi:10.4103/1673-5374.245480
Ye, Z., Li, C., Liu, S., Liang, H., Feng, J., Lin, D., et al. (2023). Dl-3-n-butylphthalide activates Nrf2, inhibits ferritinophagy, and protects MES23.5 dopaminergic neurons from ferroptosis. Chem. Biol. Interact. 382, 110604. doi:10.1016/j.cbi.2023.110604
Yeh, C. H., Shen, Z. Q., Lin, C. C., Lu, C. K., and Tsai, T. F. (2022). Rejuvenation: turning back time by enhancing CISD2. Int. J. Mol. Sci. 23, 14014. doi:10.3390/ijms232214014
Ying, D., Shen, X., Wang, S., Chen, J., Wu, Z., Chen, W., et al. (2024). Discovery of 4-hydroxyl pyrazole derivatives as potent ferroptosis inhibitors. Eur. J. Med. Chem. 263, 115913. doi:10.1016/j.ejmech.2023.115913
Yoo, M. H., Gu, X., Xu, X. M., Kim, J. Y., Carlson, B. A., Patterson, A. D., et al. (2010). Delineating the role of glutathione peroxidase 4 in protecting cells against lipid hydroperoxide damage and in Alzheimer's disease. Antioxid. Redox Signal 12, 819–827. doi:10.1089/ars.2009.2891
You, J., Yang, W., Ma, R., Xia, A., Zhang, G., Fang, Z., et al. (2022). Discovery of 2-vinyl-10H-phenothiazine derivatives as a class of ferroptosis inhibitors with minimal human Ether-a-go-go related gene (hERG) activity for the treatment of DOX-induced cardiomyopathy. Bioorg Med. Chem. Lett. 74, 128911. doi:10.1016/j.bmcl.2022.128911
Yu, Z., Cheng, X., Pan, W., Yu, C., and Duan, Y. (2023). The ferroptosis activity is associated with neurological recovery following chronic compressive spinal cord injury. Neural Regen. Res. 18, 2482–2488. doi:10.4103/1673-5374.371378
Yuan, M., Liu, G., Zheng, X., Li, P., Liu, J., Wang, S., et al. (2017). Effects of puerarin combined with conventional therapy on ischemic stroke. Exp. Ther. Med. 14, 2943–2946. doi:10.3892/etm.2017.4922
Yuk, H., Abdullah, M., Kim, D. H., Lee, H., and Lee, S. J. (2021). Necrostatin-1 prevents ferroptosis in a RIPK1- and Ido-independent manner in hepatocellular carcinoma. Antioxidants (Basel) 10, 1347. doi:10.3390/antiox10091347
Zeng, X., An, H., Yu, F., Wang, K., Zheng, L., Zhou, W., et al. (2021). Benefits of iron chelators in the treatment of Parkinson's disease. Neurochem. Res. 46, 1239–1251. doi:10.1007/s11064-021-03262-9
Zhai, L., Pei, H., Shen, H., Yang, Y., Han, C., and Guan, Q. (2023a). Paeoniflorin suppresses neuronal ferroptosis to improve the cognitive behaviors in Alzheimer's disease mice. Phytother. Res. 37, 4791–4800. doi:10.1002/ptr.7946
Zhai, Q. Y., Ren, Y. Q., Ni, Q. S., Song, Z. H., Ge, K. L., and Guo, Y. L. (2022). Transplantation of human umbilical cord mesenchymal stem cells-derived neural stem cells pretreated with Neuregulin1β ameliorate cerebral ischemic reperfusion injury in rats. Biomolecules 12, 428. doi:10.3390/biom12030428
Zhai, Q. Y., Ye, Y. H., Ren, Y. Q., Song, Z. H., Ge, K. L., Cheng, B. H., et al. (2023b). Neuroprotective effects of neural stem cells pretreated with neuregulin1β on PC12 cells exposed to oxygen-glucose deprivation/reoxygenation. Neural Regen. Res. 18, 618–625. doi:10.4103/1673-5374.350207
Zhang, D., Jia, X., Lin, D., and Ma, J. (2023a). Melatonin and ferroptosis: mechanisms and therapeutic implications. Biochem. Pharmacol. 218, 115909. doi:10.1016/j.bcp.2023.115909
Zhang, F., Lin, B., Huang, S., Wu, P., Zhou, M., Zhao, J., et al. (2023b). Melatonin alleviates retinal ischemia-reperfusion injury by inhibiting p53-mediated ferroptosis. Antioxidants (Basel) 12, 1173. doi:10.3390/antiox12061173
Zhang, H., Wang, Z., Liu, Z., Du, K., and Lu, X. (2021). Protective effects of dexazoxane on rat ferroptosis in doxorubicin-induced cardiomyopathy through regulating HMGB1. Front. Cardiovasc Med. 8, 685434. doi:10.3389/fcvm.2021.685434
Zhang, L., Bai, X. Y., Sun, K. Y., Li, X., Zhang, Z. Q., Liu, Y. D., et al. (2024a). A new perspective in the treatment of ischemic stroke: ferroptosis. Neurochem. Res. 49, 815–833. doi:10.1007/s11064-023-04096-3
Zhang, L., Lang, F., Feng, J., and Wang, J. (2024b). Review of the therapeutic potential of Forsythiae Fructus on the central nervous system: active ingredients and mechanisms of action. J. Ethnopharmacol. 319, 117275. doi:10.1016/j.jep.2023.117275
Zhang, L., Wang, S., Ma, Y., Song, Y., Li, D., Liang, X., et al. (2023d). Shoutai Wan regulates glycolysis imbalance at the maternal-fetal interface in threatened abortion mice. J. Ethnopharmacol. 312, 116502. doi:10.1016/j.jep.2023.116502
Zhang, L., and Wei, W. (2020). Anti-inflammatory and immunoregulatory effects of paeoniflorin and total glucosides of paeony. Pharmacol. Ther. 207, 107452. doi:10.1016/j.pharmthera.2019.107452
Zhang, S., Gou, S., Zhang, Q., Yong, X., Gan, B., and Jia, D. (2023c). FSP1 oxidizes NADPH to suppress ferroptosis. Cell Res. 33, 967–970. doi:10.1038/s41422-023-00879-z
Zhang, X., Li, M., Wu, H., Fan, W., Zhang, J., Su, W., et al. (2022b). Naringenin attenuates inflammation, apoptosis, and ferroptosis in silver nanoparticle-induced lung injury through a mechanism associated with Nrf2/HO-1 axis: in vitro and in vivo studies. Life Sci. 311, 121127. doi:10.1016/j.lfs.2022.121127
Zhang, X., Wu, S., Guo, C., Guo, K., Hu, Z., Peng, J., et al. (2022c). Vitamin E exerts neuroprotective effects in pentylenetetrazole kindling epilepsy via suppression of ferroptosis. Neurochem. Res. 47, 739–747. doi:10.1007/s11064-021-03483-y
Zhang, Y., Fan, B. Y., Pang, Y. L., Shen, W. Y., Wang, X., Zhao, C. X., et al. (2020). Neuroprotective effect of deferoxamine on erastininduced ferroptosis in primary cortical neurons. Neural Regen. Res. 15, 1539–1545. doi:10.4103/1673-5374.274344
Zhang, Y., Wang, M., and Chang, W. (2022a). Iron dyshomeostasis and ferroptosis in Alzheimer's disease: molecular mechanisms of cell death and novel therapeutic drugs and targets for AD. Front. Pharmacol. 13, 983623. doi:10.3389/fphar.2022.983623
Zhang, Z., Kodumuru, V., Sviridov, S., Liu, S., Chafeev, M., Chowdhury, S., et al. (2012). Discovery of benzylisothioureas as potent divalent metal transporter 1 (DMT1) inhibitors. Bioorg Med. Chem. Lett. 22, 5108–5113. doi:10.1016/j.bmcl.2012.05.129
Zhao, D., Yang, K., Guo, H., Zeng, J., Wang, S., Xu, H., et al. (2023). Mechanisms of ferroptosis in Alzheimer's disease and therapeutic effects of natural plant products: a review. Biomed. Pharmacother. 164, 114312. doi:10.1016/j.biopha.2023.114312
Zhao, Z., Wu, J., Xu, H., Zhou, C., Han, B., Zhu, H., et al. (2020). XJB-5-131 inhibited ferroptosis in tubular epithelial cells after ischemia-reperfusion injury. Cell Death Dis. 11, 629. doi:10.1038/s41419-020-02871-6
Zheng, L., Wu, S., Jin, H., Wu, J., Wang, X., Cao, Y., et al. (2023). Molecular mechanisms and therapeutic potential of icariin in the treatment of Alzheimer's disease. Phytomedicine 116, 154890. doi:10.1016/j.phymed.2023.154890
Zhongyin, Z., Wei, W., Juan, X., and Guohua, F. (2022). Isoliquiritin apioside relieves intestinal ischemia/reperfusion-induced acute lung injury by blocking Hif-1α-mediated ferroptosis. Int. Immunopharmacol. 108, 108852. doi:10.1016/j.intimp.2022.108852
Zhou, H., Yin, C., Zhang, Z., Tang, H., Shen, W., Zha, X., et al. (2020). Proanthocyanidin promotes functional recovery of spinal cord injury via inhibiting ferroptosis. J. Chem. Neuroanat. 107, 101807. doi:10.1016/j.jchemneu.2020.101807
Zhou, Z. X., Cui, Q., Zhang, Y. M., Yang, J. X., Xiang, W. J., Tian, N., et al. (2023). Withaferin A inhibits ferroptosis and protects against intracerebral hemorrhage. Neural Regen. Res. 18, 1308–1315. doi:10.4103/1673-5374.355822
Zhu, C., Chen, B., He, X., Li, W., Wang, S., Zhu, X., et al. (2024a). LncRNA MEG3 suppresses erastin-induced ferroptosis of chondrocytes via regulating miR-885-5p/SLC7A11 axis. Mol. Biol. Rep. 51, 139. doi:10.1007/s11033-023-09095-9
Zhu, H., Cen, J., Hong, C., Wang, H., Wen, Y., He, Q., et al. (2023b). Targeting labile iron-mediated ferroptosis provides a potential therapeutic strategy for rhabdomyolysis-induced acute kidney injury. ACS Chem. Biol. 18, 1294–1304. doi:10.1021/acschembio.2c00914
Zhu, J., Sun, R., Yan, C., Sun, K., Gao, L., Zheng, B., et al. (2023a). Hesperidin mitigates oxidative stress-induced ferroptosis in nucleus pulposus cells via Nrf2/NF-κB axis to protect intervertebral disc from degeneration. Cell Cycle 22, 1196–1214. doi:10.1080/15384101.2023.2200291
Zhu, K., Zhu, X., Liu, S., Yu, J., Wu, S., and Hei, M. (2022). Glycyrrhizin attenuates hypoxic-ischemic brain damage by inhibiting ferroptosis and neuroinflammation in neonatal rats via the HMGB1/GPX4 pathway. Oxid. Med. Cell Longev. 2022, 8438528. doi:10.1155/2022/8438528
Zhu, Z., Chambers, S., and Bhatia, M. (2024b). Suppressing the substance P-NK1R signalling protects mice against sepsis-associated acute inflammatory injury and ferroptosis in the liver and lungs. Antioxidants (Basel) 13, 300. doi:10.3390/antiox13030300
Zilka, O., Poon, J. F., and Pratt, D. A. (2021). Radical-trapping antioxidant activity of copper and nickel bis(thiosemicarbazone) complexes underlies their potency as inhibitors of ferroptotic cell death. J. Am. Chem. Soc. 143, 19043–19057. doi:10.1021/jacs.1c08254
Zilka, O., Shah, R., Li, B., Friedmann Angeli, J. P., Griesser, M., Conrad, M., et al. (2017). On the mechanism of cytoprotection by ferrostatin-1 and liproxstatin-1 and the role of lipid peroxidation in ferroptotic cell death. ACS Cent. Sci. 3, 232–243. doi:10.1021/acscentsci.7b00028
Zou, Y., Li, H., Graham, E. T., Deik, A. A., Eaton, J. K., Wang, W., et al. (2020). Cytochrome P450 oxidoreductase contributes to phospholipid peroxidation in ferroptosis. Nat. Chem. Biol. 16, 302–309. doi:10.1038/s41589-020-0472-6
Keywords: ferroptosis, inhibitors, iron metabolism, lipid metabolism, antioxidants system, clinical translation
Citation: Zhang L, Luo YL, Xiang Y, Bai XY, Qiang RR, Zhang X, Yang YL and Liu XL (2024) Ferroptosis inhibitors: past, present and future. Front. Pharmacol. 15:1407335. doi: 10.3389/fphar.2024.1407335
Received: 26 March 2024; Accepted: 06 May 2024;
Published: 23 May 2024.
Edited by:
Hua Li, Air Force Medical University, ChinaReviewed by:
Feng Zhang, Nanjing University of Chinese Medicine, ChinaAhmed A. Elmarakby, Augusta University, United States
Yassine Kaddouri, Mohamed Premier University, Morocco
Copyright © 2024 Zhang, Luo, Xiang, Bai, Qiang, Zhang, Yang and Liu. This is an open-access article distributed under the terms of the Creative Commons Attribution License (CC BY). The use, distribution or reproduction in other forums is permitted, provided the original author(s) and the copyright owner(s) are credited and that the original publication in this journal is cited, in accordance with accepted academic practice. No use, distribution or reproduction is permitted which does not comply with these terms.
*Correspondence: Xiao Long Liu, bHhsMzI4MUAxNjMuY29t
†These authors have contributed equally to this work and share first authorship