- 1First Clinical Medical School, Shandong University of Traditional Chinese Medicine, Jinan, Shandong, China
- 2Affiliated Hospital of Shandong University of Traditional Chinese Medicine, Shandong Province Hospital of Traditional Chinese Medicine, Jinan, Shandong, China
- 3School of Engineering, The Hong Kong University of Science and Technology, Kowloon, Hong Kong SAR, China
Retinal pigment epithelial cell and neuroretinal damage in age-related macular degeneration (AMD) can lead to serious visual impairments and blindness. Studies have shown that mitophagy, a highly specialized cellular degradation system, is implicated in the pathogenesis of AMD. Mitophagy selectively eliminates impaired or non-functioning mitochondria via several pathways, such as the phosphatase and tensin homolog-induced kinase 1/Parkin, BCL2-interacting protein 3 and NIP3-like protein X, FUN14 domain-containing 1, and AMP-activated protein kinase pathways. This has a major impact on the maintenance of mitochondrial homeostasis. Therefore, the regulation of mitophagy could be a promising therapeutic strategy for AMD. Traditional Chinese medicine (TCM) uses natural products that could potentially prevent and treat various diseases, such as AMD. This review aims to summarize recent findings on mitophagy regulation pathways and the latest progress in AMD treatment targeting mitophagy, emphasizing methods involving TCM.
1 Introduction
Autophagy is a multifunctional degradation system that helps cells maintain homeostasis by encapsulating cytoplasmic proteins, damaged organelles, and pathogens into vesicles (autophagosomes), which subsequently fuse with lysosomes to create autolysosomes, leading to the degradation of the encapsulated cargo and the generation of amino acids, nucleotides, sugars, fatty acids, and adenosine triphosphate (ATP). By assisting in the regulation of protein, nucleic acid, and lipid balances, the modulation of reactive oxidative stress and oxygen species (ROS), and the improvement of mitochondrial function, autophagy contributes to cellular metabolic needs and the renewal of intracellular organelles (Nita and Grzybowski, 2023). Depending on the intracellular lysosomal degradation mechanism, three distinct forms of autophagy—macroautophagy, microautophagy, and chaperone-mediated autophagy—have been distinguished (Mizushima, 2018; Yao and Shen, 2020) (Figure 1). Macroautophagy is the most predominant and conserved type of autophagy and proceeds via five stages—induction, nucleation, extension, fusion, and degradation (Nieto-Torres and Hansen, 2021). During chaperone-mediated autophagy, the chaperone Hsc70 and co-chaperones identify target proteins containing KFERQ-like motifs and then transport these proteins to the lysosomal membrane to bind to the lysosomal receptor lysosomal-associated membrane protein 2A (LAMP2A), triggering receptor multimerization, cargo internalization, and degradation (Gómez-Sintes and Arias, 2021). In contrast, during microautophagy, the target proteins are directly engulfed by the lysosomal membrane through invagination (Fleming et al., 2022).
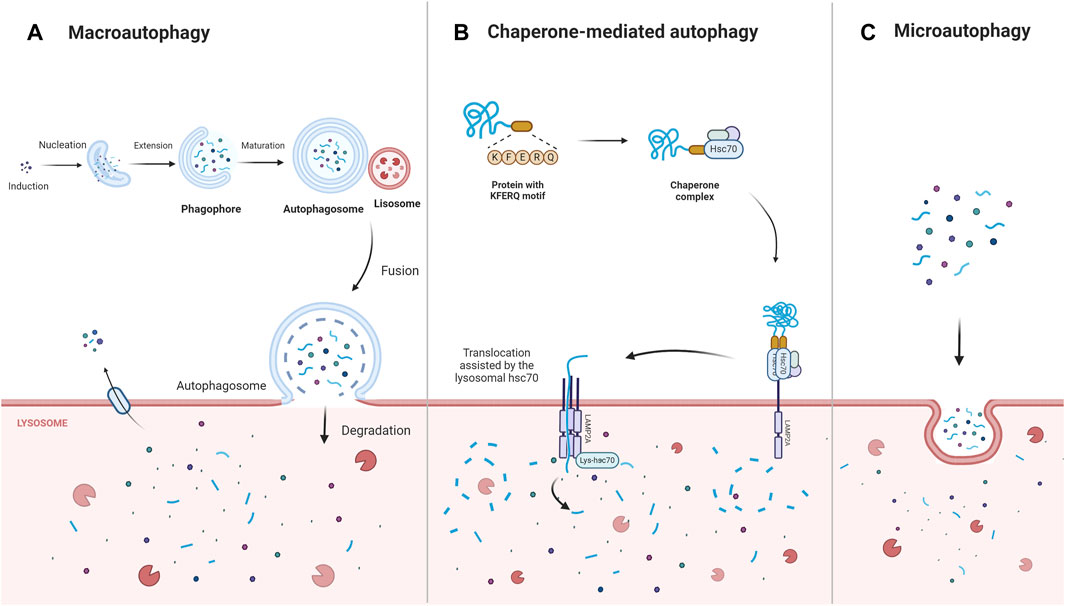
Figure 1. The main types of autophagy: macroautophagy, chaperone- mediated autophagy, microautophagy. Macroautophagy is induced, nucleated, and extended to form a double-membrane structure autophagosome, which wraps around the degraded material and ultimately fuses with the lysosome for degradation. Chaperone-mediated autophagy directly recognizes proteins with KFERQ-related motifs by means of the chaperone protein Hsc70, and the receptor protein LAMP2A on the lysosomal membrane recognizes the KFERQ motifs exposed by the binding protein and guides the target protein into the lysosome for degradation. In contrast, microautophagy directly engulfs the degraded material mainly through the invagination of the lysosomal membrane. Abbreviations: Lysosomal-associated membrane protein 2A (LAMP2A).
Mitophagy, a highly specialized type of autophagy, regulates mitochondrial fission and fusion, thereby eliminating malfunctioning and impaired mitochondria, promoting mitochondrial renewal, preventing ROS overproduction, inhibiting potential cellular oxidative damage, and ensuring mitochondrial quality (Wen et al., 2023). In response to ROS generation, nutrient deficiency, cellular senescence, and other factors, intracellular mitochondria are damaged and depolarized. During mitophagy, the impaired mitochondria are incorporated into autophagosomes, which then fuse with lysosomes, resulting in the breakdown of these organelles (Lu et al., 2023). Mitophagy is also involved in numerous physiological functions, such as delaying the ageing process and cell differentiation. Interference in these processes induces physiological senescence and several age-related diseases (Krantz et al., 2021; Banarase et al., 2023; Sanz et al., 2023). Recent evidence indicates that impaired mitochondrial energy metabolism and age-related diseases share pathological features, such as an increased mutation rate in mitochondrial DNA (mtDNA), impaired electron transport chain function, elevated ROS levels, and the enhanced release of pro-apoptotic factors (Fang et al., 2017). As a result, the dysregulation of mitophagy can induce mitochondrial dysfunction, leading to the progression of age-related diseases.
Age-related macular degeneration (AMD) is a lesion on the macula that primarily affects the retinal pigment epithelium (RPE), photoreceptor cells, Bruch’s membrane, and choroidal multilayered tissue. Gradual degeneration of the outer retina and the formation of new blood vessels between the retina and Bruch’s membrane can advance to geographic atrophic or choroidal neovascular AMD, commonly known as dry and wet AMD, respectively (Figure 2). It is estimated that the worldwide occurrence of AMD stands at 196 million, with projections suggesting an increase to 288 million by 2040 (Xiao et al., 2023). RPE cells are known for high oxygen consumption, continuous photostimulation, and susceptibility to lipid peroxidation product exposure (Kaarniranta et al., 2009). Consequently, oxidative stress and damage play a crucial role in the progression of RPE degeneration in AMD (Handa, 2012; Blasiak et al., 2013). Persistent oxidative stress on RPE cells may result in the accumulation of extracellular deposits and damaged organelles, nucleic acids, lipids, cellular proteins, and lipofuscin granules, thereby increasing the levels of ROS. These excessive levels of oxidized lipoproteins and ROS induce protein misfolding, aggregation, and persistent activation of the innate immune responses, leading to protein accumulation, mitochondrial dysfunction, and inflammasome activation in the RPE cells (Ferrington et al., 2016; Kauppinen et al., 2016; Piippo et al., 2018; Kaarniranta et al., 2019a; Kaarniranta et al., 2020). Mitochondria are the important sites of retinal oxidation and are mainly found within the RPE. Mitochondrial dysfunction reduces oxidative phosphorylation, results in excessive ROS production, increases mtDNA damage and mutations, and enhances the release of pro-inflammatory and pro-apoptotic factors, which induce oxidative stress, inflammatory response, and apoptosis (Murphy, 2009; Kageyama et al., 2012; Kaarniranta et al., 2020). Studies have shown that mitophagy regulates RPE cell damage and apoptosis by maintaining mitochondrial function and protein folding (Dhirachaikulpanich et al., 2022; Hyttinen et al., 2023).
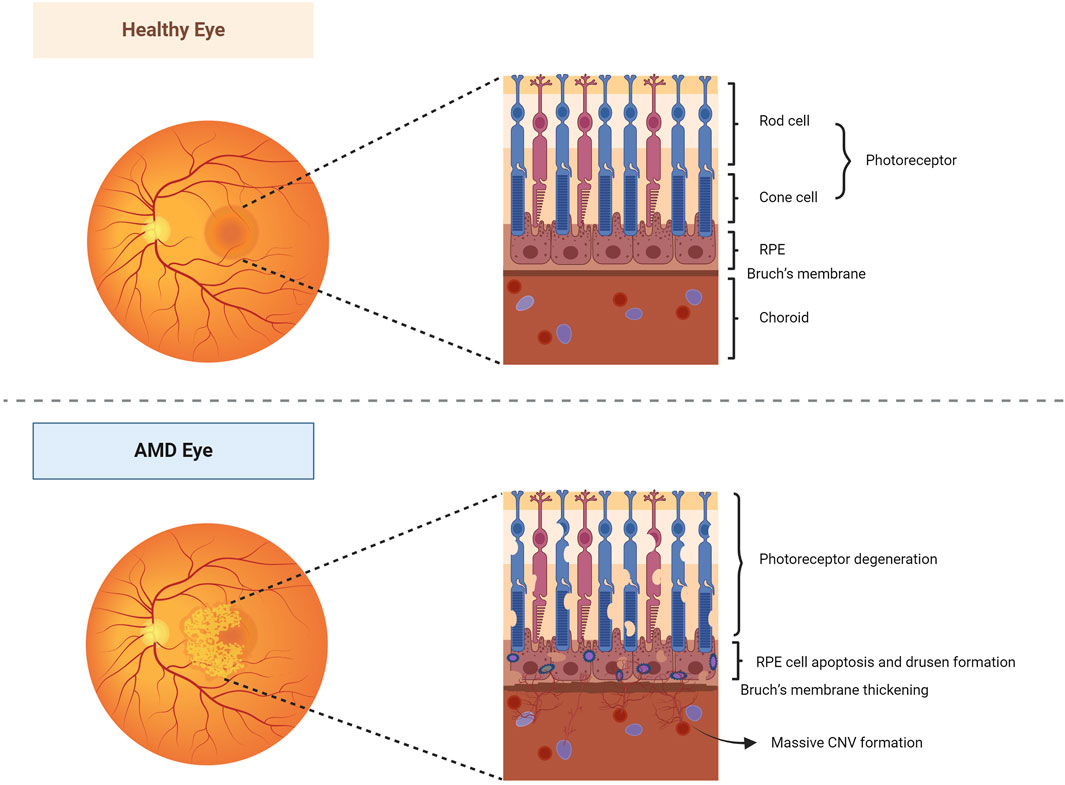
Figure 2. Healthy and AMD-affected eyes. In healthy eyes, the fundus is normal, and the morphology and structure of the photoreceptor cells, RPE layer, Bruch’s membrane, and choroidal layer in the macula are normal. In AMD eyes, the pigmentation of the macular area was disorganized, and a slightly elevated neovascular membrane could be seen locally. Its photoreceptor cells, RPE, Bruch’s membrane, and choroidal multilayer organization changed constantly, resulting in degeneration of photoreceptor cells, apoptosis of RPE cells, pigmentation production, thickening of Bruch’s membrane, and formation of a large number of CNV. Abbreviations: AMD, age-related macular degeneration; RPE, retinal pigment epithelium; CNV, choroidal neovascularization.
Meanwhile, excessive accumulation of extracellular deposits inhibits mitophagy and promotes inflammation in AMD, whereas reduced accumulation restores mitophagy in RPE cells, alleviating AMD (Yu et al., 2023). Damage to mtDNA might be crucial in advancing AMD (Kaarniranta et al., 2019b). Recent reports have indicated that mtDNA damage increases with age; furthermore, elevated mtDNA damage, a higher number of mutations, and reduced DNA repair efficacy have been reported to be associated with the onset and staging of AMD (Piippo et al., 2018). Feher et al. (2006) reported a notable decline in the number of mitochondria and the absence of stromal density and cristae in the RPE of individuals with AMD compared to those in the control group. Zhao et al. (2011) stated that the suppression of oxidative phosphorylation in mouse RPE mitochondria led to the activation of the mechanistic target of the rapamycin (mTOR) pathway in an AMD mouse model. Proteomic analyses performed by Nordgaard et al. (2006) showed the differential expression of mitochondrial refolding and trafficking-related proteins in the RPE of AMD patients compared to that in the non-AMD controls. Subsequent research (Nordgaard et al., 2008) demonstrated that depending on the stage of AMD, the expression of mtHsp70, the mitochondrial translation factor Tu, mitofilin, subunit VIb of the cytochrome c oxidase complex, and α-, β-, and δ-subunits of the catalytic portion of ATP synthase was affected in the RPE. Using an in vitro model of AMD generated by fusing mitochondria-rich platelets from patients with AMD and mitochondrially-depleted retinal pigment epithelial cells (ARPE-19), Dohl et al. (2022) reported increased concentrations of ROS, which could result in mtDNA damage, enhanced antioxidant responses, and increased expression levels of anti-inflammatory proteins. These results suggested that mtDNA damage response was fundamental in preventing AMD and slowing its progression. New findings indicate that boosting autophagy in RPE cells could be an innovative approach to tackle AMD.
Nevertheless, there are few approved mitophagy-modulating medications for AMD treatment. Current clinical AMD therapy includes lifestyle improvements (e.g., caloric restriction and moderate exercise) as well as pharmacological treatments [e.g., antioxidants (D Aloisio et al., 2022), tyrosine kinase inhibitors (Das et al., 2023), antidiabetic drugs (Thee et al., 2021; Mauschitz et al., 2022), anti-vascular endothelial growth factor (anti-VEGF), and other therapies (Francisco and Rowan, 2023; Servillo et al., 2023; Szigiato et al., 2023)]. Lately, traditional Chinese medicine (TCM) has garnered increasing attention for its antioxidant, anti-apoptotic, anti-inflammatory, and lipid-reducing activities, positioning it as a promising treatment option for AMD (Cao et al., 2022a; Yu et al., 2024). This review focuses on the molecular mechanisms governing mitophagy and innovative treatment approaches for employing TCM as a potential AMD therapy.
2 Mitophagy-specific pathways in AMD
2.1 Mitophagy mediated by PINK1/Parkin
Various mechanisms linked to mitophagy, which play a role in the onset of AMD, have been identified. The PTEN-induced putative kinase protein 1 (PINK1)/Parkin-mediated ubiquitination degradation pathway is the most characterized ubiquitin-dependent signaling pathway responsible for the control of mitochondrial structure and function (Bowling et al., 2019). Under normal conditions, PINK1 and Parkin are present at low levels in the mitochondrial outer membrane and cytoplasm, respectively. The cytoplasmic synthesis of PINK1 is followed by its relocation to the mitochondria, which is facilitated by the interaction between translocase of the outer membrane (TOM) and translocase of the inner membrane (TIM) (Kato et al., 2013; Sekine et al., 2019). Subsequently, PINK1 is cleaved by presenilin-associated rhomboid-like (PARL) (Meissner et al., 2015) and mitochondrial-processing protease (MPP) (Bayne and Trempe, 2019), leading to the degradation of PINK1 via the ubiquitin/proteasome pathway (Yamano and Youle, 2013). In response to stress, mitochondria are depolarized, and the level of ROS production increases, thereby inducing oxidative damage. During mitochondrial dysfunction, PINK1 degradation is blocked, causing accumulation at the mitochondrial outer membrane, the recruitment and phosphorylation of Parkin, and the activation of E3 ubiquitin ligase function. Subsequently, PINK1 and Parkin translocate from the cytoplasm to the mitochondrial outer membrane and ubiquitinate mitochondrial component proteins by forming polyubiquitin chains (Sauvé et al., 2022). Autophagy-associated proteins, such as nuclear dot protein 52 (NDP52), TAX1-binding protein-1 (TAX1BP1), optineurin (OPTN), and p62 (Shang et al., 2019; Zachari et al., 2020; Nguyen et al., 2023), recognize ubiquitinated mitochondria through their ubiquitin-binding domains (UBDs) and anchor their cargo to autophagic vesicle membranes via their LC3-interacting region (LIR) motifs, contributing to the formation of mitochondrial autophagosomes. Ultimately, autophagosomes fuse with lysosomes to form mature mitochondrial autolysosomes and trigger mitochondrial degradation (Callegari et al., 2017; Urbina-Varela et al., 2020) (Figure 3). Datta et al. (2023) reported that PINK1 levels were reduced in centro-concave RPE cells obtained from individuals with early AMD. Oxidative stress causes an increase in the concentration of heat shock protein Hsp70 in the lysosomes of RPE cells from patients with AMD, inhibiting the build-up of cytotoxic protein aggregates, alleviating lipofuscin-induced misfolding of intracellular proteins, and activating autophagy-mediated protein hydrolysis, thereby protecting RPE cells from oxidative stress (Kaarniranta et al., 2009; Subrizi et al., 2015). Hsp70 functions as a PINK1 degradation regulator, while Hsp70-interacting proteins, BCL2-associated athanogene 5 (BAG5) and BCL2-associated athanogene 2 (BAG2), play a significant role in regulating PINK1 stability by reducing its ubiquitination. Lowering Hsp70 levels downregulates PINK/Parkin-mediated mitophagy (Zheng et al., 2018a). Accordingly, the mechanism of Hsp70 action that leads to the protection of RPE cells via PINK1-mediated mitophagy could be significant in AMD progression. The regulation of antioxidant production and mitochondrial biosynthesis relies heavily on the involvement of peroxisome proliferator-activated receptor gamma coactivator 1-alpha (PGC-1α) and nuclear factor erythroid 2-related factor 2 (NFE2L2), respectively. These proteins upregulate antioxidant parameters and prevent the mitochondrial injury and apoptosis caused by ROS (Kaarniranta et al., 2020). Within the confines of the retina of NFE2L2/PGC-1α double knockout mice, a dry AMD model, high oxidative stress levels, protein aggregation, the significant upregulation of PINK1/Parkin expression, impaired mitochondrial injury, reduced mitophagy, and aberrant autophagic flux in RPE cells were observed. At the same time, the control group displayed minimal or no PINK1/Parkin activity (Sridevi Gurubaran et al., 2020). These results further demonstrate that controlling PINK1/Parkin-mediated mitophagy could be a viable approach to treating AMD.
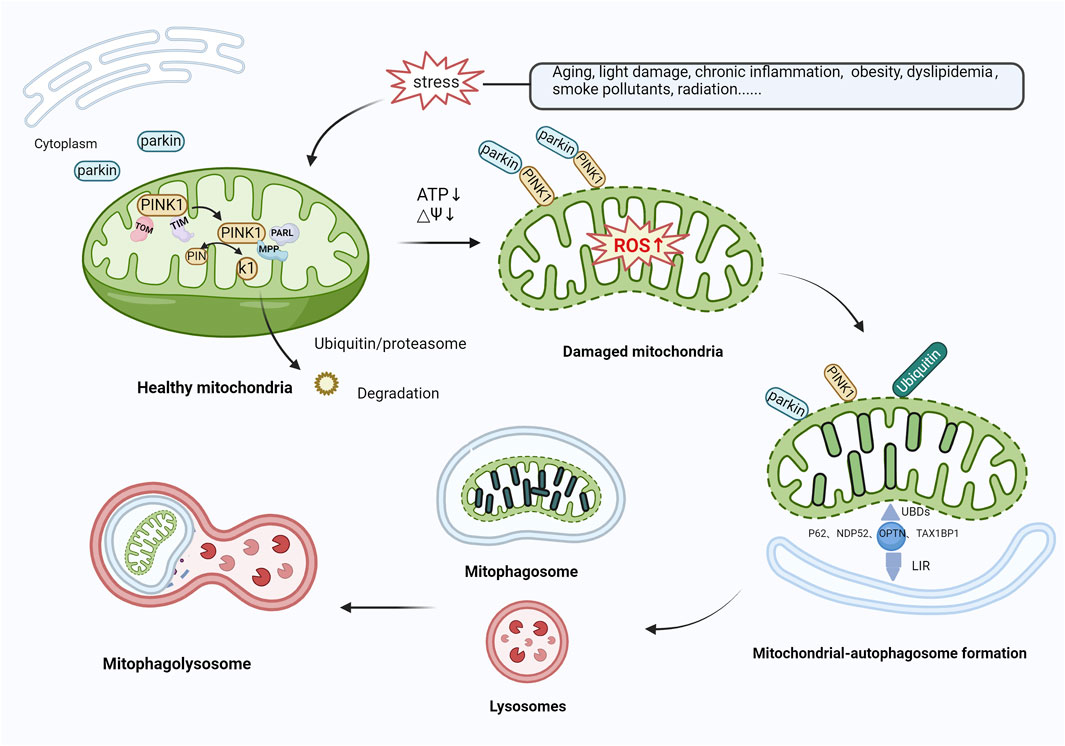
Figure 3. Mitophagy activation mediated by PINK1/Parkin. In healthy mitochondria, PINK1 is transported to mitochondria via TOM and TIM, cleaved by PARL and MPP, and then translocated to the cytoplasm, where it is broken down by the ubiquitin/proteasomal pathway. In response to various stressors, the level of ROS production rises, inducing oxidative damage. PINK1 recognizes damaged mitochondria, accumulates in large quantities in the mitochondrial outer membrane, and recruits and phosphorylates Parkin, activating its E3 ubiquitin ligase function. Both proteins translocate from the cytoplasm to the mitochondrial outer membrane and ubiquitinate mitochondrial proteins by forming polyubiquitin chains. Autophagy-related proteins (e.g., P62, NDP52, OPTN, TAX1BP1) then recognize ubiquitinated mitochondria through UBDs on the one hand, and on the other hand, are anchored to the membrane of autophagic vesicles through the LIR to form mitochondrial autophagosomes, which subsequently merge with lysosomes, leading to mitochondrial degradation. Abbreviations: PINK1, phosphatase and tensin homolog (PTEN)-induced kinase 1; TOM, translocase of outer membrane; TIM, Translocase of inner membrane; PARL, presenilin-associated rhomboid-like; MPP, mitochondrial-processing protease; ROS, reactive oxygen species; NDP52, nuclear dot protein 52; OPTN, optineurin; TAX1BP1, TAX1-binding protein-1; UBDs, Ubiquitin-binding domains; LIR, LC3-interacting region.
2.2 Mitophagy mediated by BNIP3/NIX
Mitochondrial autophagy can also be activated via the BCL2-interacting protein 3 and NIP3-like protein X (BNIP3/NIX) pathway. BNIP3 and NIX, situated in the mitochondrial outer membrane, are hypoxia-inducible factors and are characterized by low expression in physiological states. The latest studies have revealed the role of BNIP3 in controlling apoptosis, alongside its part in mitochondrial quality management through mitophagy (Wang et al., 2013; Lampert et al., 2019). Thomas et al. (2011) reported that, unlike PINK1 and Parkin, BNIP3 and NIX bind directly to autophagosomes, contributing to the activation of mitophagy. BNIP3 and NIX exhibit a 56% protein sequence similarity, and both contain BCL2 homology domain-3 (BH3). Under normal conditions, the interaction between BCL-xl/BCL2 and the BH3 of Beclin-1 results in the formation of Beclin-1/BCL-xl and Beclin-1/BCL2 complexes, effectively inhibiting autophagy. In response to stress, BNIP3/NIX are activated by hypoxia-inducible factor-1 (HIF-1) and interact with BCL-xl/BCL2 to release Beclin-1, subsequently activating mitophagy (Choubey et al., 2021). Furthermore, the control of BNIP3/NIX-mediated mitophagy involves phosphorylation—when BNIP3 Ser17 and Ser24 residues are phosphorylated, BNIP3 attaches to Microtubule-associated protein 1 light chain 3 (LC3) or to LC3 homolog GABA type A receptor-associated protein (GABARAP) through the LIR motif. Nevertheless, when NIX Ser34 and Ser35 residues undergo phosphorylation, NIX forms a bond with LC3, leading to the subsequent binding of LC3 to the γ-aminobutyric acid receptor-associated protein (GABAR) complex, ultimately resulting in the formation of the LC3-GABARAP complex. This complex targets LC3 in the damaged mitochondrial outer membrane and ultimately triggers mitophagy (Zhu et al., 2013; Rogov et al., 2017). BNIP3 contributes to ubiquitin-dependent mitophagy by inducing the mitochondrial movement of Parkin and promoting Parkin-mediated mitophagy. Additionally, BNIP3 inhibits the Ras homolog enriched in the brain (Rheb)/mTOR pathway and initiates autophagy (Figure 4) (Lin et al., 2014). Recent studies demonstrated that RPE in AMD has reduced levels of PINK1 and Parkin, and BNIP3/NIX-mediated mitophagy is the key factor in preserving mitochondrial equilibrium (Fisher et al., 2022; Jiménez-Loygorri et al., 2023). Esteban-Martínez and Boya (2018) reported that in response to metabolic stress, the destabilization of HIF1A/HIF-1 led to the upregulation of mitophagy receptor BNIP3/NIX. Furthermore, BNIP3L-dependent mitophagy-induced metabolic shifts in glycolysis are required for retinal ganglion cell (RGC) neurogenesis and the regulation of pro-inflammatory/M1-type macrophage polarization, which is vital for degenerative diseases such as AMD.
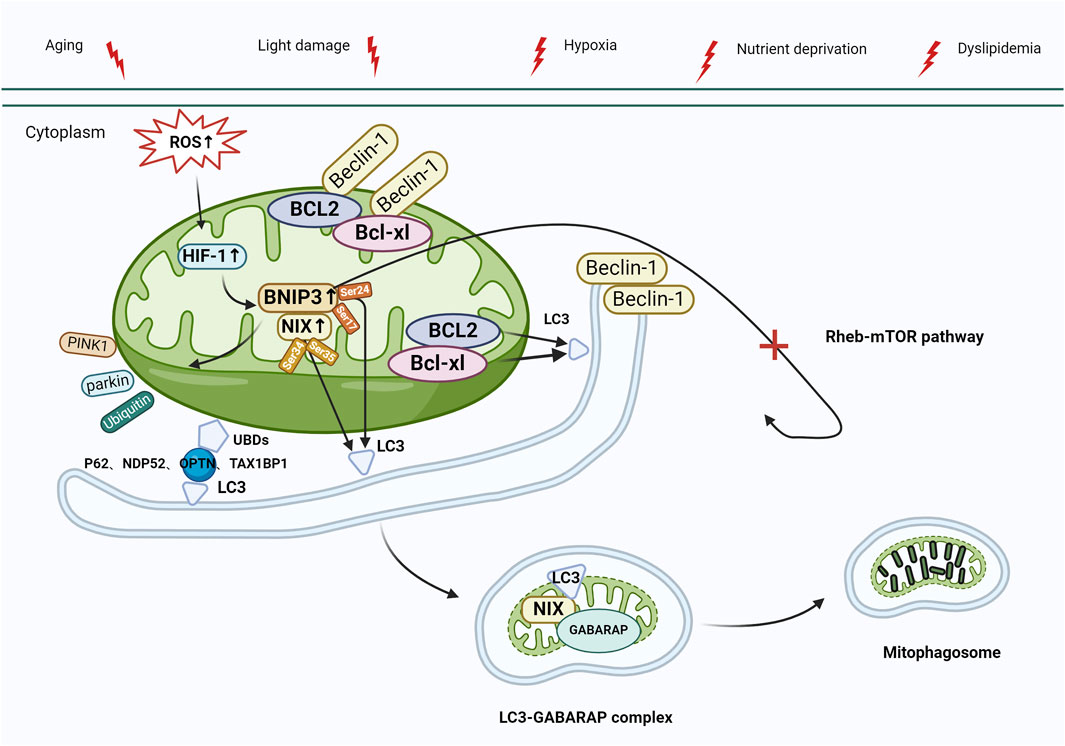
Figure 4. Mitophagy activation mediated by BNIP3/NIX. Under stress (e.g., aging, light damage, hypoxia, nutrient deprivation, and dyslipidemia), elevated levels of ROS activate HIF-1, leading to the upregulation of BNIP3/NIX, which interacts with the Beclin-1/BCL-xl and Beclin-1/BCL2 complexes, releasing Beclin-1, which activates autophagy. BNIP3/NIX-mediated mitophagy can also be regulated by phosphorylation. Ser17 and Ser24 phosphorylated on BNIP3 bind to LC3 via LIR, whereas Ser34 and Ser35 phosphorylated on NIX bind to LC3, and then bind to the GABAR complex to form the LC3-GABARAP complex, which targets LC3 to damaged mitochondrial outer membrane, and eventually initiates mitophagy. In addition, BNIP3 plays a role in the control of ubiquitin-dependent mitophagy by initiating mitochondrial movement of Parkin and facilitating Parkin-mediated mitophagy (process as in Figure 3). Finally, BNIP3 inhibits the Rheb-mTOR pathway, which also leads to autophagy activation. Abbreviations: BNIP3, BCL2-interacting protein 3; NIX, NIP3-like protein X; ROS, reactive oxygen species; HIF-1, Hypoxia-inducible factor-1; LC3, Microtubule-associated protein 1 light chain 3; LIR, LC3-interacting region; GABARAP, GABA type A receptor-associated protein; Rheb, Ras homologue enriched in brain; mTOR, target of the rapamycin machinery.
2.3 Mitophagy mediated by FUNDC1
Mitophagy can also be activated via the FUN14 domain-containing 1 (FUNDC1) pathway. FUNDC1 is a protein located in the outer membrane of the mitochondria, and its increased expression has been reported to trigger mitophagy (Kuang et al., 2016; Wu et al., 2016). The identification of FUNDC1 as a mitophagy receptor has been confirmed by recent studies (Mao et al., 2022). Wu et al. (2016) suggested that the site of FUNDC1 action is the mitochondria-associated endoplasmic reticulum membrane (MAM). FUNDC1, as a MAM-associated protein (Li et al., 2014), has an LIR motif at the amino-terminus that engages with LC3, and the deletion or structural changes to the LIR motif impede the connection between FUNDC1 and LC3, resulting in the downregulation of mitosis. The phosphorylation of FUNDC1, similar to BNIP3/NIX and BNIP3, can have either a positive or negative effect. Under physiological conditions, Src and casein kinase 2 prevent FUNDC1 from binding to LC3 by phosphorylating the Tyr18 residue of LIR, reducing mitophagic activity. When hypoxia or mitochondrial depolarization occurs, phosphoglycerate mutase family member 5 (PGAM5) induces the dephosphorylation of Ser13 (Liu et al., 2012; Chen et al., 2014); the dephosphorylated FUNDC1 then interacts with LC3 to activate mitophagy. Simultaneously, the deubiquitinating enzyme ubiquitin specific peptidase 19 (USP19), which is located in the endoplasmic reticulum, accumulates at the MAM and binds to the mitochondrial outer membrane protein FUNDC1, inducing its deubiquitination, promoting the oligomerization of dynamin-related protein 1 (DRP1), and resulting in mitochondrial fission (Zhang et al., 2022). Additionally, the unc-51-like autophagy-activating kinase 1 (ULK1) complex can translocate into the mitochondria, contributing to the phosphorylation of FUNDC1 Ser17 and the activation of mitophagy (Wu et al., 2014; Mercer et al., 2018). Another mitochondria-associated protein, nucleotide-binding oligomerization domain (NOD)-like receptor X1 (NLRX1), an immune system regulator, is known to diminish inflammatory responses, reduce ROS generation, and regulate autophagy. Increased NLRX1 expression reduces the relative levels of FUNDC1 phosphorylation and NLRP3 inflammasome-associated proteins in ARPE-19 cells, thereby preventing the development of AMD (Wang et al., 2023a). The exact mechanism of FUNDC1 action in mitophagy in AMD is not well characterized; however, studies suggest that the FUNDC1 pathway plays an integral role in obesity and metabolic disorders (Tan et al., 2018; Ren et al., 2020), as the excessive intake of saturated fatty acids affects the stability of mitophagy receptor FUNDC1 and mitochondrial mass, leading to mitochondrial dysfunction, obesity, and metabolic disorders (Chen et al., 2023). Wu et al. (2019) also reported that FUNDC1 deficiency in mice inhibits mitophagy, while a high-fat diet impairs mitochondrial function, resulting in high oxidative stress and heightened inflammatory responses. According to these results, the mitophagy receptor FUNDC1 is essential for maintaining mitochondrial quality, controlling inflammatory responses, and regulating metabolic disorders, possibly via mitogen-activated protein kinase (MAPK) signaling.
2.4 Mitophagy mediated by AMPK
AMP-activated protein kinase (AMPK) is also related to the regulation of mitophagy. AMPK, a ubiquitously expressed serine/threonine kinase, is a highly conserved sensor of cellular energy and nutritional status and a major regulator of cellular metabolism (Rey and Tamargo-Gómez, 2023). AMPK activation plays a role in controlling oxidative stress, inflammation, glycolipid metabolism, mitophagy, and other functions (Cai et al., 2023; Feng et al., 2023; Guo et al., 2023; Zhong et al., 2023; Zhu et al., 2023). AMPK phosphorylates acetyl-CoA carboxylase 1 (ACC1) and mitochondrial fission factor (MFF) on the mitochondrial outer membrane. The interaction among phosphorylated ACC1, MFF, and AMPK in the cytoplasm facilitates the presence of AMPK in or around the mitochondria (Zong et al., 2019). DRP1 is the enzyme that catalyzes mitochondrial fission, while MFF is the primary receptor for DRP1 on the mitochondrial outer membrane. AMPK causes mitochondrial fragmentation by phosphorylating MFF in response to oxidative stress, initiating mitophagy and clearing damaged mitochondrial fragments (Toyama et al., 2016). In another model system, iron overload-induced mesenchymal stem cell (MSC) damage through the AMPK/MFF/DRP1 pathway increased MSC apoptosis, leading to mitochondrial fragmentation and enhanced autophagy (Zheng et al., 2018b). Furthermore, AMPK promotes mitophagy by directly phosphorylating PGC1-α (Figure 5) (Cantó et al., 2009).
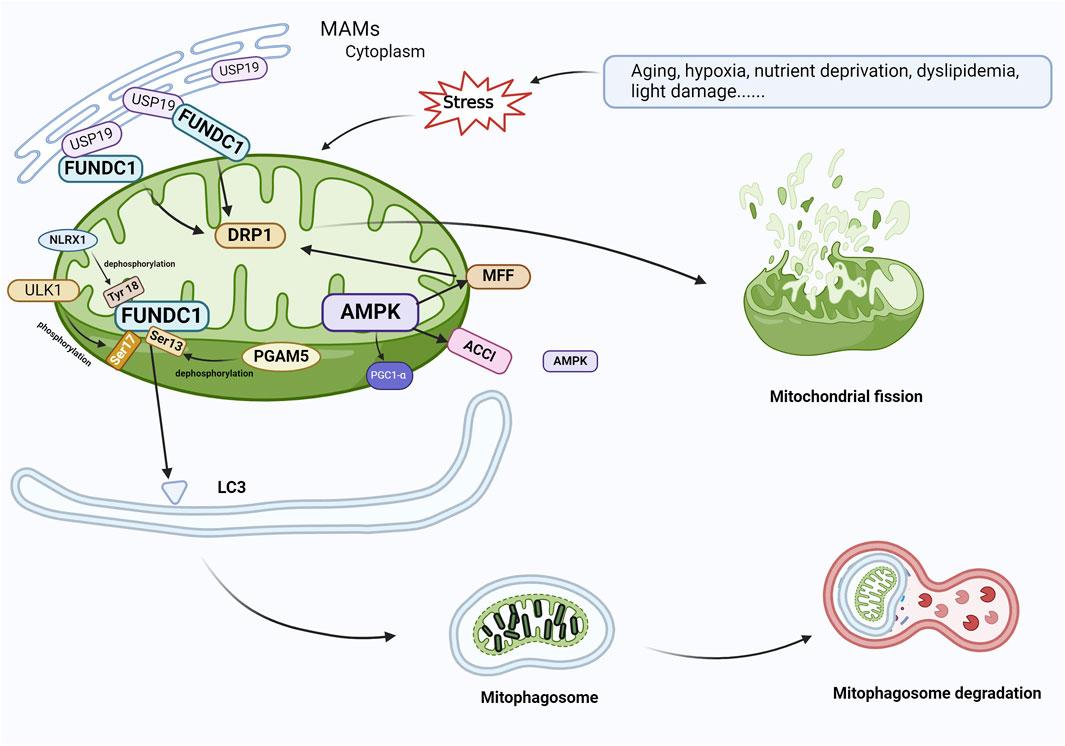
Figure 5. FUNDC1 and AMPK are involved in the regulation of mitophagy. In response to cellular stress, PGAM5 induces the dephosphorylation of Ser13, and FUNDC1 and LC3 interact to induce mitophagy. At the same time, USP19, which accumulates on MAM, attaches to FUNDC1 and triggers deubiquitination of FUNDC1, which promotes oligomerization of DRP1 and leads to mitochondrial fission. ULK1 complex translocates to mitochondria and phosphorylates Ser17 of FUNDC1 to initiate mitophagy, and NLRX1 activates FUNDC1 by dephosphorylating FUNDC1 Tyr 18 and induces mitophagy. During AMPK-mediated mitophagy, ACCl and MFF on the mitochondrial outer membrane, which are substrates for the action of AMPK, contact AMPK in the cytoplasm, resulting in the localization of AMPK within or near mitochondria. AMPK phosphorylates MFF and binds to DRP1 in response to oxidative stress, leading to mitochondrial fission, which initiates mitophagy and elimination of damaged mitochondria. Abbreviations: FUNDC1, FUN14 domain-containing 1; AMPK, AMP-activated protein kinase; PGAM5, phosphoglycerate mutase family member 5; USP19, Ubiquitin Specific Peptidase 19; MAM, mitochondria-associated endoplasmic reticulum membrane; DRP1, dynamin-associated protein 1; ULK1, Unc-51-like autophagy-activated kinase 1; NLRX1, nucleotide-binding oligomerization domain (NOD)-like receptor X1; ACC1, acetyl coenzyme A carboxylase 1; MFF, mitochondrial fission factor.
Zhuang et al. (2022) reported that inhibition of the AMPK pathway induced RPE cell apoptosis, resulting in mitochondrial injury and the suppression of mitophagy. Xu et al. (2018) showed that increasing AMPK activity diminished DNA injury, reduced oxidative stress, and increased the production of mitochondrial energy, thereby protecting photoreceptors and RPE from acute damage, preventing their degeneration, and hindering the development of AMD. These findings confirm that AMPK-mediated mitophagy removes damaged mitochondria and protects mitochondrial function in response to oxidative stress. Furthermore, Salminen et al. (2012) reported that AMPK induced mitochondria generation by upregulating the transcription of PGC-1α target genes and removed impaired mitochondria via ULK1-dependent mitophagy. AMPK agonists also protect the cells by removing misfolded or damaged proteins from RPE cells through mitophagy.
Su et al. (2020) found that TXNIP was induced significantly in human retinal pigment epithelium, Muller glia, and cone photoreceptor cells under high-glucose conditions for five consecutive days, resulting in oxidative stress, ATP reduction, and decreased mitophagic flux. They proposed that TXNIP regulates Parkin/PINK1-mediated mitophagy in dopaminergic neurons under hyperglycemic conditions (Su et al., 2020). In addition, Devi observed that high glucose increased the expression of TXNIP at the mRNA and protein levels significantly in human RPE cell lines and primary human RPE cells. The upregulation of TXNIP was associated with mitochondrial membrane depolarization, fragmentation, and mitophagy access to lysosomes (Devi et al., 2019). Furthermore, Singh proposed that TXNIP leads to mitochondrial dysfunction, oxidative stress, dysfunctional mitophagy phagocytosis, lysosomal instability and inflammation in DR (Singh et al., 2018). The Nrf2/ARE signaling pathway plays an important role in the coordination of mitochondrial biogenesis and mitophagy, and it is involved in regulation of the expression of protective genes against oxidative stress, and regulation of mitochondrial biology and mitophagy. Consequently, it may be an important target for drugs for the treatment of neurodegeneration (Gureev et al., 2020). All the findings above suggest that TXNIP-mitochondria-lysosome and Nrf2-P62 also mediate mitophagy.
3 Mitophagy: a promising therapeutic target for AMD
As summarized in the previous section, mitophagy is strongly associated with the pathogenesis of AMD. Therefore, drugs that activate mitophagy in RPE cells could provide a novel therapeutic strategy for AMD. Recently, numerous mitophagy modulators that either activate or inhibit mitophagy, including AICAR (5-aminoimidazole-4-carboxamide ribonucleotide), an AMP analogue that maintains the optimal function of mitochondria (Ebeling et al., 2022), PGC-1α (an important regulator of mitochondrial biosynthesis) (Hyttinen et al., 2021), human retinal progenitor cells (hRPCs) (Yu et al., 2021), the mitochondria-targeted antioxidant triphenylphosphine (TPP)-nicotinic acid (Kim et al., 2021), melatonin (Mehrzadi et al., 2020), the mitochondrial activator PU-91 (Nashine et al., 2019), the mitochondria-derived peptide variant Humanin G (HNG) (Nashine et al., 2017), and the mitochondria-targeted antioxidant SkQ1 (Telegina et al., 2020; Fisher et al., 2022), have been discovered. mtDNA repair could be another target for improving mitochondrial function. Poly (ADP-ribose) polymerase (PARP1) active base excision repair (BER) and microhomology-mediated end-joining (MMEJ), among others, restore single- and double-strand breaks in mtDNA. Similar to nuclear DNA, nucleic acid complexes of multiple proteins can protect mtDNA from histone binding-induced ROS damage. However, owing to the complexity of mtDNA, its repair needs to be further investigated (Kaarniranta et al., 2020). Furthermore, these studies are still limited to animal and preclinical experiments, and the potential side effects during AMD treatment must be evaluated. A recent study demonstrated that augmenting mitophagic activity in individuals with AMD can impede the progression of the disease (Amini et al., 2023). The complexity of AMD pathogenesis should not be overlooked, as it encompasses a multitude of signaling crosstalks connecting the various layers of retinal structures to the choroid; as a result, positive outcomes are often difficult to achieve using single-agent therapy.
4 Role of TCM in mitophagy regulation in AMD
TCM treats diseases through various active ingredients, which possess antioxidant, anti-ageing, immunomodulatory, and anti-inflammatory properties as well as the ability to regulate mitophagy, and could thus be considered as an alternative therapeutic strategy for AMD. In China, Astragalus mongholicus Bunge, Poria cocos (Schw.) Wolf, Plantago asiatica L., Atractylodes macrocephala Koidz., Angelica sinensis (Oliv.) Diels, Panax ginseng C.A.Mey., Salvia miltiorrhiza Bunge, Curcuma longa L. (Pfahler et al., 2022; Vallée, 2022), Rehmannia glutinosa (Gaertn.) DC., Lycium chinense Mill., and Glycyrrhiza glabra L. (Kozlowski et al., 2015; Lee et al., 2015; Peng et al., 2016; Chen et al., 2021; Li et al., 2022a; Cho et al., 2023; Wei and Tong, 2023) are commonly used for the treatment of AMD. Studies have demonstrated that Fructus lycii can selectively activate and regulate the AMPK and VEGF pathways to enhance mitophagy, thereby preventing the development of retinopathy (Yang et al., 2022c). Another TCM, Mingmu Di Huang Pill, treats AMD by activating the expression of autophagy adaptor-SQSTM1 and AMPK phosphorylation, which can promote the autophagic degradation of Kelch-like ECH-related protein 1 (Keap1), safeguarding RPE cells against oxidative stress damage (Chen et al., 2022) (the main active ingredients, pharmacological actions, and mechanisms of these Chinese medicines are summarized in Table 1). Furthermore, various natural products from TCM, including berberine (BBR), curcumin, artemisinin, paeoniflorin, quercetin, luteolin, naringenin, ivytin (Cao et al., 2022b), urinary apolipid A (UA), ferulic acid, and astaxanthin (Lewis Luján et al., 2022), have been reported to have a mitophagy-regulating effect in numerous diseases by activating several specific pathways, including AMPK, PINK1/Parkin, BNIP3, FUNDC1, as well as LC3 proteins, followed by the induction of autolysosome formation. For example, UA, a novel mitophagy enhancer, initiates mitophagy by decreasing MMP without disrupting the mitochondrial respiratory chain and ROS production. Furthermore, it activates mitophagy by inducing the proper mitochondria function in a dose-dependent manner (Lu et al., 2023) and improves immune function through the PINK1/Parkin-mediated pathway (Denk et al., 2022). A previous study proposed that BBR has anti-inflammatory, antioxidant, antimicrobial, hypotensive, and gastric mucosa protective properties (Wang et al., 2017) as well as the ability to prevent oxidative damage triggered by hydrogen peroxide in human RPE cell line D407 via the activation of AMPK, indicating the potential therapeutic application of BBR for AMD (Li et al., 2018). Wang et al. (2023b) also demonstrated that BBR could act as a potential inducer of mitophagy, inhibiting PINK1 promoter methylation, reversing D-ribose-induced mitochondrial dysfunction, and restoring mitophagy via the PINK1/Parkin pathway, thereby attenuating the ageing process. Lu et al. (2023) reported that curcumin, a diketone extracted from the rhizomes of plants in the Zingiberaceae and Araceae, could potentially enhance Parkin-dependent mitophagy through the AMPK/transcription factor EB (TFEB) signaling pathway to diminish oxidative stress-induced injury to the intestinal barrier and mitochondrial dysfunction (Zhang et al., 2023). By activating AMPK, paeoniflorin, a monoterpene glycoside derived from Paeonia lactiflora, mitigates oxidative stress associated with Nox1/ROS in RPE cells, mitochondrial damage, and endoplasmic reticulum stress while protecting ARPE-19 cells and arresting the advancement of retinal degenerative disorders like AMD (Zhu et al., 2018) (Table 2). The latest evidence suggests that TCM or its active components could regulate mitophagy-related proteins, presenting a promising approach to treating AMD.
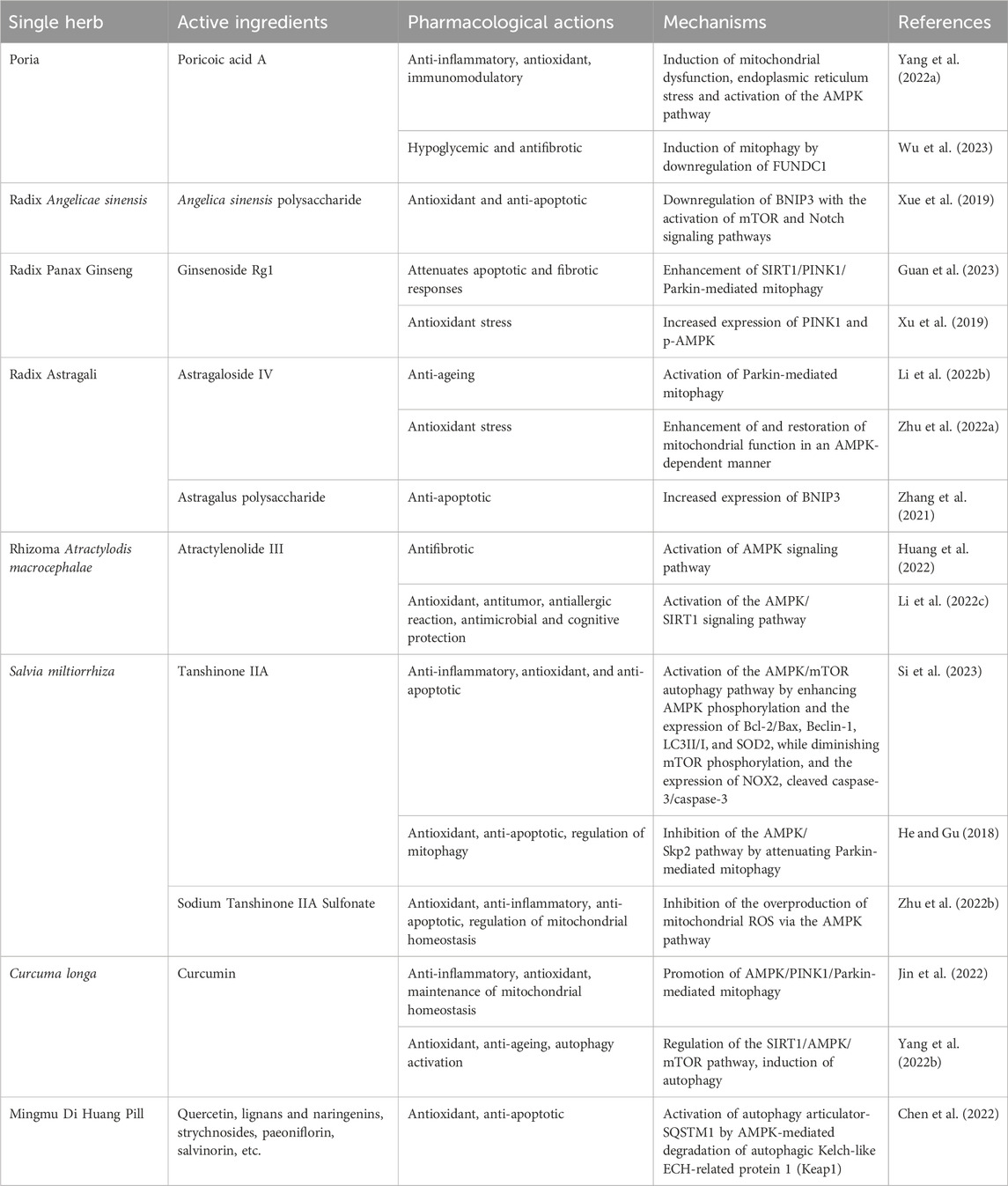
Table 1. Main active ingredients, pharmacological actions, and mechanisms of traditional Chinese medicine herbs and botanical drug decoction frequently used to treat AMD.
5 Conclusion and future directions
Mitophagy is an important mitochondrial quality control mechanism that selectively eliminates dysfunctional mitochondria to maintain cellular homeostasis. The regulation of mitophagy is mediated mainly by PINK1/Parkin, BNIP3/NIX, FUNDC1, and AMPK, and its dysfunction is closely related to AMD development. The use of drugs to increase mitophagy in RPE cells provides new ideas for AMD treatment.
Exploration of the relationship between TCM and mitophagy could offer key insights. The findings of such studies could broaden the scope of research on TCM theories by providing insights into how TCM can prevent and control AMD. In recent years, very few reports on TCM treatment of AMD by modulating mitophagy-related pathways have been published. In the present review, the authors focused on the role of mitophagy-related pathways in AMD pathogenesis, drawing on previous studies. In addition, the authors demonstrated potential ways via which TCM could modulate mitophagy to improve AMD based on three aspects: single botanical drugs, botanical drug decoction, and natural chemical components of TCM. However, although multiple pathways can mediate mitophagy, the correlation between the mediating pathways and the exact mechanism of mitophagy-induced AMD has not been elucidated. In future, more animal experiments and clinical experiments should be conducted under various pathological mechanisms to explore the role of mitophagy in AMD. In addition, studies on the regulation of mitophagy by TCM considering specific pharmacodynamics, target pathway, and mechanism of action should be carried out, to clarify the pathways through which the different active ingredients in single botanical drugs regulate mitophagy, and the mitophagy pathways via which different dosages and combinations of TCM could influence the overall AMD regulation, with a view to provide novel clinical insights and methods for the treatment of AMD with TCM.
Author contributions
YY: Conceptualization, Writing–original draft, Writing–review and editing. GW: Project administration, Writing–review and editing. YL: Supervision, Writing–review and editing. ZM: Writing–review and editing.
Funding
The author(s) declare that financial support was received for the research, authorship, and/or publication of this article. This review was funded by the Traditional Chinese Medicine Science and Technology Development Project of Shandong Province (Grant number M-2022156).
Acknowledgments
The figures in this review were created using the BioRender platform. We extend our gratitude to Editage (www.editage.cn) for their expertise in English language editing.
Conflict of interest
The authors declare that the research was conducted in the absence of any commercial or financial relationships that could be construed as a potential conflict of interest.
Publisher’s note
All claims expressed in this article are solely those of the authors and do not necessarily represent those of their affiliated organizations, or those of the publisher, the editors and the reviewers. Any product that may be evaluated in this article, or claim that may be made by its manufacturer, is not guaranteed or endorsed by the publisher.
References
Amini, M. A., Karbasi, A., Vahabirad, M., Khanaghaei, M., and Alizamir, A. (2023). Mechanistic insight into age-related macular degeneration (AMD): anatomy, epidemiology, genetics, pathogenesis, prevention, implications, and treatment strategies to pace AMD management. Chonnam Med. J. 59, 143–159. doi:10.4068/cmj.2023.59.3.143
Banarase, T. A., Sammeta, S. S., Wankhede, N. L., Mangrulkar, S. V., Rahangdale, S. R., Aglawe, M. M., et al. (2023). Mitophagy regulation in aging and neurodegenerative disease. Biophys. Rev. 15, 239–255. doi:10.1007/s12551-023-01057-6
Bayne, A. N., and Trempe, J.-F. (2019). Mechanisms of PINK1, ubiquitin and Parkin interactions in mitochondrial quality control and beyond. Cell. Mol. Life Sci. 76, 4589–4611. doi:10.1007/s00018-019-03203-4
Blasiak, J., Glowacki, S., Kauppinen, A., and Kaarniranta, K. (2013). Mitochondrial and nuclear DNA damage and repair in age-related macular degeneration. Int. J. Mol. Sci. 14, 2996–3010. doi:10.3390/ijms14022996
Bowling, J. L., Skolfield, M. C., Riley, W. A., Nolin, A. P., Wolf, L. C., and Nelson, D. E. (2019). Temporal integration of mitochondrial stress signals by the PINK1:Parkin pathway. BMC Mol. Cell. Biol. 20, 33. doi:10.1186/s12860-019-0220-5
Cai, J.-L., Zhu, Y.-L., Li, X.-P., Deng, G.-M., Han, Y.-S., Yuan, F.-Y., et al. (2023). Liposomal silybin improves glucose and lipid metabolisms in type 2 diabetes mellitus complicated with non-alcoholic fatty liver disease via AMPK/TGF-β1/Smad signaling. Tohoku J. Exp. Med. 261, 257–265. doi:10.1620/tjem.2023.J050
Callegari, S., Oeljeklaus, S., Warscheid, B., Dennerlein, S., Thumm, M., Rehling, P., et al. (2017). Phospho-ubiquitin-PARK2 complex as a marker for mitophagy defects. Autophagy 13, 201–211. doi:10.1080/15548627.2016.1254852
Cantó, C., Gerhart-Hines, Z., Feige, J. N., Lagouge, M., Noriega, L., Milne, J. C., et al. (2009). AMPK regulates energy expenditure by modulating NAD+ metabolism and SIRT1 activity. Nature 458, 1056–1060. doi:10.1038/nature07813
Cao, Y., Li, X.-Y., Tchivelekete, G. M., Li, X., Zhou, X., He, Z., et al. (2022b). Bioinformatical and biochemical analyses on the protective role of traditional Chinese medicine against age-related macular degeneration. Curr. Eye Res. 47, 1450–1462. doi:10.1080/02713683.2022.2108456
Cao, Y., Li, X. Y., Tchivelekete, G. M., Li, X., Zhou, X., Hou, Z., et al. (2022a). Bioinformatical and biochemical analyses on the protective role of traditional Chinese medicine against age-related macular degeneration. Curr. Eye Res. 47, 1450–1462. doi:10.1080/02713683.2022.2108456
Chen, G., Han, Z., Feng, D., Chen, Y., Chen, L., Wu, H., et al. (2014). A regulatory signaling loop comprising the PGAM5 phosphatase and CK2 controls receptor-mediated mitophagy. Mol. Cell. 54, 362–377. doi:10.1016/j.molcel.2014.02.034
Chen, L., Zhang, Q., Meng, Y., Zhao, T., Mu, C., Fu, C., et al. (2023). Saturated fatty acids increase LPI to reduce FUNDC1 dimerization and stability and mitochondrial function. EMBO Rep. 24, e54731. doi:10.15252/embr.202254731
Chen, X., Zhu, Y., Shi, X., Zuo, J., Hu, T., Wu, H., et al. (2022). Ming-mu-di-huang-pill activates SQSTM1 via AMPK-mediated autophagic KEAP1 degradation and protects RPE cells from oxidative damage. Oxid. Med. Cell. Longev. 2022, 5851315. doi:10.1155/2022/5851315
Chen, X., Zuo, J., Hu, T., Shi, X., Zhu, Y., Wu, H., et al. (2021). Exploration of the effect and mechanism of Fructus lycii, rehmanniae radix praeparata, and Paeonia lactiflora in the treatment of AMD based on network Pharmacology and in vitro experimental verification. Drug Des. Devel Ther. 15, 2831–2842. doi:10.2147/DDDT.S310481
Cho, H. M., Lee, S. J., and Choung, S.-Y. (2023). Protective effects of Panax ginseng berry extract on blue light-induced retinal damage in ARPE-19 cells and mouse retina. J. Ginseng Res. 47, 65–73. doi:10.1016/j.jgr.2022.04.002
Choubey, V., Zeb, A., and Kaasik, A. (2021). Molecular mechanisms and regulation of mammalian mitophagy. Cells 11, 38. doi:10.3390/cells11010038
D Aloisio, R., Di Antonio, L., Toto, L., Rispoli, M., Di Iorio, A., Delvecchio, G., et al. (2022). Choroidal changes in blood flow in patients with intermediate AMD after oral dietary supplement based on astaxanthin, bromelain, vitamin D3, folic acid, lutein, and antioxidants. Med. Kaunas. 58, 1092. doi:10.3390/medicina58081092
Das, N., Chaurasia, S., and Singh, R. P. (2023). A review of emerging tyrosine kinase inhibitors as durable treatment of neovascular age-related macular degeneration. Expert Opin. Emerg. Drugs. 28, 203–211. doi:10.1080/14728214.2023.2259790
Datta, S., Cano, M., Satyanarayana, G., Liu, T., Wang, L., Wang, J., et al. (2023). Mitophagy initiates retrograde mitochondrial-nuclear signaling to guide retinal pigment cell heterogeneity. Autophagy 19, 966–983. doi:10.1080/15548627.2022.2109286
Denk, D., Petrocelli, V., Conche, C., Drachsler, M., Ziegler, P. K., Braun, A., et al. (2022). Expansion of T memory stem cells with superior anti-tumor immunity by Urolithin A-induced mitophagy. Immunity 55, 2059–2073.e8. doi:10.1016/j.immuni.2022.09.014
Devi, T. S., Yuumnamcah, T., Yao, F., Somayajulu, M., Kowluru, R. A., and Singh, L. P. (2019). TXNIP mediates high glucose-induced mitophagic flux and lysosome enlargement in human retinal pigment epithelial cells. Biol. Open 8, bio038521. doi:10.1242/bio.038521
Dhirachaikulpanich, D., de Magalhães, J. P., and Paraoan, L. I. (2022). Investigating the effect of ageing on protein folding chaperones expression in RPE. Investigative Ophthalmol. Vis. Sci. 63, 3904–A0106.
Dohl, J., Schneider, K., Atilano, S., Bao, A., and Kenney, M. C. (2022). Cytoplasmic hybrids of ARPE-19 cells and mitochondria from patients with age-related macular degeneration accurately model reactive oxygen species hallmarks found in vivo. Investigative Ophthalmol. Vis. Sci. 63, 479–A0016.
Ebeling, M. C., Geng, Z., Stahl, M. R., Kapphahn, R. J., Roehrich, H., Montezuma, S. R., et al. (2022). Testing mitochondrial-targeted drugs in iPSC-RPE from patients with age-related macular degeneration. Pharm. (Basel) 15, 62. doi:10.3390/ph15010062
Esteban-Martínez, L., and Boya, P. (2018). BNIP3L/NIX-dependent mitophagy regulates cell differentiation via metabolic reprogramming. Autophagy 14, 915–917. doi:10.1080/15548627.2017.1332567
Fang, E. F., Lautrup, S., Hou, Y., Demarest, T. G., Croteau, D. L., Mattson, M. P., et al. (2017). NAD+ in aging: molecular mechanisms and translational implications. Trends Mol. Med. 23, 899–916. doi:10.1016/j.molmed.2017.08.001
Feher, J., Kovacs, I., Artico, M., Cavallotti, C., Papale, A., and Balacco Gabrieli, C. (2006). Mitochondrial alterations of retinal pigment epithelium in age-related macular degeneration. Neurobiol. Aging 27, 983–993. doi:10.1016/j.neurobiolaging.2005.05.012
Feng, L., Li, B., Cai, M., Zhang, Z., Zhao, Y., Yong, S. S., et al. (2023). Resistance exercise alleviates the prefrontal lobe injury and dysfunction by activating SESN2/AMPK/PGC-1α signaling pathway and inhibiting oxidative stress and inflammation in mice with myocardial infarction. Exp. Neurol. 370, 114559. doi:10.1016/j.expneurol.2023.114559
Ferrington, D. A., Sinha, D., and Kaarniranta, K. (2016). Defects in retinal pigment epithelial cell proteolysis and the pathology associated with age-related macular degeneration. Prog. Retin Eye Res. 51, 69–89. doi:10.1016/j.preteyeres.2015.09.002
Fisher, C. R., Shaaeli, A. A., Ebeling, M. C., Montezuma, S. R., and Ferrington, D. A. (2022). Investigating mitochondrial fission, fusion, and autophagy in retinal pigment epithelium from donors with age-related macular degeneration. Sci. Rep. 12, 21725. doi:10.1038/s41598-022-26012-5
Fleming, A., Bourdenx, M., Fujimaki, M., Karabiyik, C., Krause, G. J., Lopez, A., et al. (2022). The different autophagy degradation pathways and neurodegeneration. Neuron 110, 935–966. doi:10.1016/j.neuron.2022.01.017
Francisco, S. G., and Rowan, S. (2023). Repurposing drugs for treatment of age-related macular degeneration. Adv. Exp. Med. Biol. 1415, 73–77. doi:10.1007/978-3-031-27681-1_12
Gómez-Sintes, R., and Arias, E. (2021). Chaperone-mediated autophagy and disease: implications for cancer and neurodegeneration. Mol. Asp. Med. 82, 101025. doi:10.1016/j.mam.2021.101025
Guan, S., Xin, Y., Ding, Y., Zhang, Q., and Han, W. (2023). Ginsenoside Rg1 protects against cardiac remodeling in heart failure via SIRT1/PINK1/parkin-mediated mitophagy. Chem. Biodivers. 20, e202200730. doi:10.1002/cbdv.202200730
Guo, Y., Jiang, H., Wang, M., Ma, Y., Zhang, J., and Jing, L. (2023). Metformin alleviates cerebral ischemia/reperfusion injury aggravated by hyperglycemia via regulating AMPK/ULK1/PINK1/Parkin pathway-mediated mitophagy and apoptosis. Chem. Biol. Interact. 384, 110723. doi:10.1016/j.cbi.2023.110723
Gureev, A. P., Sadovnikova, I. S., Starkova, N. N., Starkov, A. A., and Popov, V. N. (2020). p62-Nrf2-p62 mitophagy regulatory loop as a target for preventive therapy of neurodegenerative diseases. Brain Sci. 10, 847. doi:10.3390/brainsci10110847
Handa, J. T. (2012). How does the macula protect itself from oxidative stress? Mol. Asp. Med. 33, 418–435. doi:10.1016/j.mam.2012.03.006
He, L., and Gu, K. (2018). Tanshinone IIA regulates colorectal cancer apoptosis via attenuation of Parkin-mediated mitophagy by suppressing AMPK/Skp2 pathways. Mol. Med. Rep. 18, 1692–1703. doi:10.3892/mmr.2018.9087
Huang, M., Jiang, W., Luo, C., Yang, M., and Ren, Y. (2022). Atractylenolide III inhibits epithelial-mesenchymal transition in small intestine epithelial cells by activating the AMPK signaling pathway. Mol. Med. Rep. 25, 98. doi:10.3892/mmr.2022.12614
Hyttinen, J., Blasiak, J., Tavi, P., and Kaarniranta, K. (2021). Therapeutic potential of PGC-1α in age-related macular degeneration (AMD) - the involvement of mitochondrial quality control, autophagy, and antioxidant response. Expert Opin. Ther. Targets 25, 773–785. doi:10.1080/14728222.2021.1991913
Hyttinen, J. M. T., Blasiak, J., and Kaarniranta, K. (2023). Non-coding RNAs regulating mitochondrial functions and the oxidative stress response as putative targets against age-related macular degeneration (AMD). Int. J. Mol. Sci. 24, 2636. doi:10.3390/ijms24032636
Jiménez-Loygorri, J. I., Benítez-Fernández, R., Viedma-Poyatos, Á., Zapata-Muñoz, J., Villarejo-Zori, B., Gómez-Sintes, R., et al. (2023). Mitophagy in the retina: viewing mitochondrial homeostasis through a new lens. Prog. Retin Eye Res. 96, 101205. doi:10.1016/j.preteyeres.2023.101205
Jin, Z., Chang, B., Wei, Y., Yang, Y., Zhang, H., Liu, J., et al. (2022). Curcumin exerts chondroprotective effects against osteoarthritis by promoting AMPK/PINK1/Parkin-mediated mitophagy. Biomed. Pharmacother. 151, 113092. doi:10.1016/j.biopha.2022.113092
Kaarniranta, K., Koskela, A., Felszeghy, S., Kivinen, N., Salminen, A., and Kauppinen, A. (2019a). Fatty acids and oxidized lipoproteins contribute to autophagy and innate immunity responses upon the degeneration of retinal pigment epithelium and development of age-related macular degeneration. Biochimie 159, 49–54. doi:10.1016/j.biochi.2018.07.010
Kaarniranta, K., Pawlowska, E., Szczepanska, J., Jablkowska, A., and Blasiak, J. (2019b). Role of mitochondrial DNA damage in ROS-mediated pathogenesis of age-related macular degeneration (AMD). Int. J. Mol. Sci. 20, 2374. doi:10.3390/ijms20102374
Kaarniranta, K., Salminen, A., Eskelinen, E.-L., and Kopitz, J. (2009). Heat shock proteins as gatekeepers of proteolytic pathways-Implications for age-related macular degeneration (AMD). Ageing Res. Rev. 8, 128–139. doi:10.1016/j.arr.2009.01.001
Kaarniranta, K., Uusitalo, H., Blasiak, J., Felszeghy, S., Kannan, R., Kauppinen, A., et al. (2020). Mechanisms of mitochondrial dysfunction and their impact on age-related macular degeneration. Prog. Retin Eye Res. 79, 100858. doi:10.1016/j.preteyeres.2020.100858
Kageyama, Y., Zhang, Z., Roda, R., Fukaya, M., Wakabayashi, J., Wakabayashi, N., et al. (2012). Mitochondrial division ensures the survival of postmitotic neurons by suppressing oxidative damage. J. Cell. Biol. 197, 535–551. doi:10.1083/jcb.201110034
Kato, H., Lu, Q., Rapaport, D., and Kozjak-Pavlovic, V. (2013). Tom70 is essential for PINK1 import into mitochondria. PLoS One 8, e58435. doi:10.1371/journal.pone.0058435
Kauppinen, A., Paterno, J. J., Blasiak, J., Salminen, A., and Kaarniranta, K. (2016). Inflammation and its role in age-related macular degeneration. Cell. Mol. Life Sci. 73, 1765–1786. doi:10.1007/s00018-016-2147-8
Kim, M. H., Kim, D.-H., Yang, S. G., and Kim, D. Y. (2021). Improved effect of a mitochondria-targeted antioxidant on hydrogen peroxide-induced oxidative stress in human retinal pigment epithelium cells. BMC Pharmacol. Toxicol. 22, 7. doi:10.1186/s40360-020-00471-w
Kozlowski, M. R., Bond, K. E., and Manesh, M. N. (2015). Retina, inhibition of senescence in RPE cells by astragaloside IV: implications for treating AMD. Investigative Ophthalmol. Vis. Sci. 56, 178.
Krantz, S., Kim, Y.-M., Srivastava, S., Leasure, J. W., Toth, P. T., Marsboom, G., et al. (2021). Mitophagy mediates metabolic reprogramming of induced pluripotent stem cells undergoing endothelial differentiation. J. Biol. Chem. 297, 101410. doi:10.1016/j.jbc.2021.101410
Kuang, Y., Ma, K., Zhou, C., Ding, P., Zhu, Y., Chen, Q., et al. (2016). Structural basis for the phosphorylation of FUNDC1 LIR as a molecular switch of mitophagy. Autophagy 12, 2363–2373. doi:10.1080/15548627.2016.1238552
Lampert, M. A., Orogo, A. M., Najor, R. H., Hammerling, B. C., Leon, L. J., Wang, B. J., et al. (2019). BNIP3L/NIX and FUNDC1-mediated mitophagy is required for mitochondrial network remodeling during cardiac progenitor cell differentiation. Autophagy 15, 1182–1198. doi:10.1080/15548627.2019.1580095
Lee, Y., Hussain, A. A., Seok, J.-H., Kim, S.-H., and Marshall, J. (2015). Modulating the transport characteristics of bruch’s membrane with steroidal glycosides and its relevance to age-related macular degeneration (AMD). Investig. Ophthalmol. Vis. Sci. 56, 8403–8418. doi:10.1167/iovs.15-16936
Lewis Luján, L. M., McCarty, M. F., Di Nicolantonio, J. J., Gálvez Ruiz, J. C., Rosas-Burgos, E. C., Plascencia-Jatomea, M., et al. (2022). Nutraceuticals/drugs promoting mitophagy and mitochondrial biogenesis may combat the mitochondrial dysfunction driving progression of dry age-related macular degeneration. Nutrients 14, 1985. doi:10.3390/nu14091985
Li, H., Xu, J., Zhang, Y., Hong, L., He, Z., Zeng, Z., et al. (2022b). Astragaloside IV alleviates senescence of vascular smooth muscle cells through activating Parkin-mediated mitophagy. Hum. Cell. 35, 1684–1696. doi:10.1007/s13577-022-00758-6
Li, Q., Tan, J.-X., He, Y., Bai, F., Li, S.-W., Hou, Y.-W., et al. (2022c). Atractylenolide III ameliorates non-alcoholic fatty liver disease by activating hepatic adiponectin receptor 1-mediated AMPK pathway. Int. J. Biol. Sci. 18, 1594–1611. doi:10.7150/ijbs.68873
Li, S., Gaur, U., Chong, C.-M., Lin, S., Fang, J., Zeng, Z., et al. (2018). Berberine protects human retinal pigment epithelial cells from hydrogen peroxide-induced oxidative damage through activation of AMPK. Int. J. Mol. Sci. 19, 1736. doi:10.3390/ijms19061736
Li, W., Zhang, X., Zhuang, H., Chen, H.-G., Chen, Y., Tian, W., et al. (2014). MicroRNA-137 is a novel hypoxia-responsive microRNA that inhibits mitophagy via regulation of two mitophagy receptors FUNDC1 and NIX. J. Biol. Chem. 289, 10691–10701. doi:10.1074/jbc.M113.537050
Li, Y., Li, X., Li, X., Zeng, Z., Strang, N., Shu, X., et al. (2022a). Non-neglectable therapeutic options for age-related macular degeneration: a promising perspective from traditional Chinese medicine. J. Ethnopharmacol. 282, 114531. doi:10.1016/j.jep.2021.114531
Lin, A., Yao, J., Zhuang, L., Wang, D., Han, J., Lam, E.W.-F., et al. (2014). The FoxO-BNIP3 axis exerts a unique regulation of mTORC1 and cell survival under energy stress. Oncogene 33, 3183–3194. doi:10.1038/onc.2013.273
Liu, L., Feng, D., Chen, G., Chen, M., Zheng, Q., Song, P., et al. (2012). Mitochondrial outer-membrane protein FUNDC1 mediates hypoxia-induced mitophagy in mammalian cells. Nat. Cell. Biol. 14, 177–185. doi:10.1038/ncb2422
Lu, Y., Li, Z., Zhang, S., Zhang, T., Liu, Y., and Zhang, L. (2023). Cellular mitophagy: mechanism, roles in diseases and small molecule pharmacological regulation. Theranostics 13, 736–766. doi:10.7150/thno.79876
Mao, Y., Ren, J., and Yang, L. (2022). FUN14 domain containing 1 (FUNDC1): a promising mitophagy receptor regulating mitochondrial homeostasis in cardiovascular diseases. Front. Pharmacol. 13, 887045. doi:10.3389/fphar.2022.887045
Mauschitz, M. M., Verzijden, T., Schuster, A. K., Elbaz, H., Pfeiffer, N., Khawaja, A., et al. (2022). Association of lipid-lowering drugs and antidiabetic drugs with age-related macular degeneration: a meta-analysis in Europeans. Br. J. Ophthalmol. 107, 1880–1886. bjo-2022-321985. doi:10.1136/bjo-2022-321985
Mehrzadi, S., Hemati, K., Reiter, R. J., and Hosseinzadeh, A. (2020). Mitochondrial dysfunction in age-related macular degeneration: melatonin as a potential treatment. Expert Opin. Ther. Targets 24, 359–378. doi:10.1080/14728222.2020.1737015
Meissner, C., Lorenz, H., Hehn, B., and Lemberg, M. K. (2015). Intramembrane protease PARL defines a negative regulator of PINK1- and PARK2/Parkin-dependent mitophagy. Autophagy 11, 1484–1498. doi:10.1080/15548627.2015.1063763
Mercer, T. J., Gubas, A., and Tooze, S. A. (2018). A molecular perspective of mammalian autophagosome biogenesis. J. Biol. Chem. 293, 5386–5395. doi:10.1074/jbc.R117.810366
Mizushima, N. (2018). A brief history of autophagy from cell biology to physiology and disease. Nat. Cell. Biol. 20, 521–527. doi:10.1038/s41556-018-0092-5
Murphy, M. P. (2009). How mitochondria produce reactive oxygen species. Biochem. J. 417, 1–13. doi:10.1042/BJ20081386
Nashine, S., Cohen, P., Chwa, M., Lu, S., Nesburn, A. B., Kuppermann, B. D., et al. (2017). Humanin G (HNG) protects age-related macular degeneration (AMD) transmitochondrial ARPE-19 cybrids from mitochondrial and cellular damage. Cell. Death Dis. 8, e2951. doi:10.1038/cddis.2017.348
Nashine, S., Subramaniam, S. R., Chwa, M., Nesburn, A., Kuppermann, B. D., Federoff, H., et al. (2019). PU-91 drug rescues human age-related macular degeneration RPE cells; implications for AMD therapeutics. Aging (Albany NY) 11, 6691–6713. doi:10.18632/aging.102179
Nguyen, T. N., Sawa-Makarska, J., Khuu, G., Lam, W. K., Adriaenssens, E., Fracchiolla, D., et al. (2023). Unconventional initiation of PINK1/Parkin mitophagy by Optineurin. Mol. Cell. 83, 1693–1709.e9. doi:10.1016/j.molcel.2023.04.021
Nieto-Torres, J. L., and Hansen, M. (2021). Macroautophagy and aging: the impact of cellular recycling on health and longevity. Mol. Aspects Med. 82, 101020. doi:10.1016/j.mam.2021.101020
Nita, M., and Grzybowski, A. (2023). Antioxidative role of heterophagy, autophagy, and mitophagy in the retina and their association with the age-related macular degeneration (AMD) etiopathogenesis. Antioxidants 12, 1368. doi:10.3390/antiox12071368
Nordgaard, C. L., Berg, K. M., Kapphahn, R. J., Reilly, C., Feng, X., Olsen, T. W., et al. (2006). Proteomics of the retinal pigment epithelium reveals altered protein expression at progressive stages of age-related macular degeneration. Investig. Ophthalmol. Vis. Sci. 47, 815–822. doi:10.1167/iovs.05-0976
Nordgaard, C. L., Karunadharma, P. P., Feng, X., Olsen, T. W., and Ferrington, D. A. (2008). Mitochondrial proteomics of the retinal pigment epithelium at progressive stages of age-related macular degeneration. Investig. Ophthalmol. Vis. Sci. 49, 2848–2855. doi:10.1167/iovs.07-1352
Peng, M.-L., Chiu, H.-F., Chou, H., Liao, H.-J., Chen, S.-T., Wong, Y.-C., et al. (2016). Influence/impact of lutein complex (marigold flower and wolfberry) on visual function with early age-related macular degeneration subjects: a randomized clinical trial. J. Funct. Foods 24, 122–130. doi:10.1016/j.jff.2016.04.006
Pfahler, N., Bielskus, I., Volpe, N. J., Aman, S., Zaparackas, Z., and Knepper, P. A. (2022). Response of drusen volume to curcumin is correlated with risk alleles for age-related macular degeneration. Investigative Ophthalmol. Vis. Sci. 63, 372–F0203.
Piippo, N., Korhonen, E., Hytti, M., Kinnunen, K., Kaarniranta, K., and Kauppinen, A. (2018). Oxidative stress is the principal contributor to inflammasome activation in retinal pigment epithelium cells with defunct proteasomes and autophagy. Cell. Physiol. Biochem. 49, 359–367. doi:10.1159/000492886
Ren, J., Sun, M., Zhou, H., Ajoolabady, A., Zhou, Y., Tao, J., et al. (2020). FUNDC1 interacts with FBXL2 to govern mitochondrial integrity and cardiac function through an IP3R3-dependent manner in obesity. Sci. Adv. 6, eabc8561. doi:10.1126/sciadv.abc8561
Rey, V., and Tamargo-Gómez, I. (2023). From kinases to diseases: investigating the role of AMPK in human pathologies. Kinases Phosphatases 1, 181–205. doi:10.3390/kinasesphosphatases1030012
Rogov, V. V., Suzuki, H., Marinković, M., Lang, V., Kato, R., Kawasaki, M., et al. (2017). Phosphorylation of the mitochondrial autophagy receptor Nix enhances its interaction with LC3 proteins. Sci. Rep. 7, 1131. doi:10.1038/s41598-017-01258-6
Salminen, A., Kaarniranta, K., and Kauppinen, A. (2012). Inflammaging: disturbed interplay between autophagy and inflammasomes. Aging (Albany NY) 4, 166–175. doi:10.18632/aging.100444
Sanz, R. L., Inserra, F., García Menéndez, S., Mazzei, L., Ferder, L., and Manucha, W. (2023). Metabolic syndrome and cardiac remodeling due to mitochondrial oxidative stress involving gliflozins and sirtuins. Curr. Hypertens. Rep. 25, 91–106. doi:10.1007/s11906-023-01240-w
Sauvé, V., Sung, G., MacDougall, E. J., Kozlov, G., Saran, A., Fakih, R., et al. (2022). Structural basis for feedforward control in the PINK1/Parkin pathway. EMBO J. 41, e109460. doi:10.15252/embj.2021109460
Sekine, S., Wang, C., Sideris, D. P., Bunker, E., Zhang, Z., and Youle, R. J. (2019). Reciprocal roles of Tom7 and OMA1 during mitochondrial import and activation of PINK1. Mol. Cell. 73, 1028–1043. doi:10.1016/j.molcel.2019.01.002
Servillo, A., Zucchiatti, I., Sacconi, R., Parravano, M., Querques, L., La Rubia, P., et al. (2023). The state-of-the-art pharmacotherapeutic management of neovascular age-related macular degeneration. Expert Opin. Pharmacother. 24, 197–206. doi:10.1080/14656566.2022.2154145
Shang, H., Xia, Z., Bai, S., Zhang, H. E., Gu, B., and Wang, R. (2019). Downhill running acutely elicits mitophagy in rat soleus muscle. Med. Sci. Sports Exerc 51, 1396–1403. doi:10.1249/MSS.0000000000001906
Si, J., Liu, B., Qi, K., Chen, X., Li, D., Yang, S., et al. (2023). Tanshinone IIA inhibited intermittent hypoxia induced neuronal injury through promoting autophagy via AMPK-mTOR signaling pathway. J. Ethnopharmacol. 315, 116677. doi:10.1016/j.jep.2023.116677
Singh, L. P., Yumnamcha, T., and Devi, T. S. (2018). Mitophagic flux deregulation, lysosomal destabilization and NLRP3 inflammasome activation in diabetic retinopathy: potentials of gene therapy targeting TXNIP and the redox system. Ophthalmol. Res. Rep. 3.
Sridevi Gurubaran, I., Viiri, J., Koskela, A., Hyttinen, J. M. T., Paterno, J. J., Kis, G., et al. (2020). Mitophagy in the retinal pigment epithelium of dry age-related macular degeneration investigated in the nfe2l2/PGC-1α-/- mouse model. Int. J. Mol. Sci. 21, 1976. doi:10.3390/ijms21061976
Su, C. J., Shen, Z., Cui, R. X., Huang, Y., Xu, D. L., Zhao, F. L., et al. (2020). Thioredoxin-interacting protein (TXNIP) regulates Parkin/PINK1-mediated mitophagy in dopaminergic neurons under high-glucose conditions: implications for molecular links between Parkinson’s disease and diabetes. Neurosci. Bull. 36, 346–358. doi:10.1007/s12264-019-00459-5
Subrizi, A., Toropainen, E., Ramsay, E., Airaksinen, A. J., Kaarniranta, K., and Urtti, A. (2015). Oxidative stress protection by exogenous delivery of rhHsp70 chaperone to the retinal pigment epithelium (RPE), a possible therapeutic strategy against RPE degeneration. Pharm. Res. 32, 211–221. doi:10.1007/s11095-014-1456-6
Szigiato, A., Mohan, N., Talcott, K. E., Mammo, D. A., Babiuch, A. S., Kaiser, P. K., et al. (2023). Short-term outcomes of faricimab in patients with neovascular age-related macular degeneration on prior anti-VEGF therapy. Ophthalmol. Retina 8 (23), 10–17. 00437–2. doi:10.1016/j.oret.2023.08.018
Tan, Y., Xu, X., Zhang, Y., and Ren, J. (2018). Abstract 15217: disruption of FUNDC1 binding with SERCA in mitochondria-associated endoplasmic reticulum membrane (MAM) accentuates obesity cardiomyopathy. Circulation 138, A15217. doi:10.1161/circ.138.suppl_1.15217
Telegina, D. V., Kozhevnikova, O. S., Fursova, A. Z., and Kolosova, N. G. (2020). Autophagy as a target for the retinoprotective effects of the mitochondria-targeted antioxidant SkQ1. Biochem. (Mosc). 85, 1640–1649. doi:10.1134/S0006297920120159
Thee, E. F., Vergroesen, J., Ahmadizar, F., Vingerling, J. R., Stricker, B. H. C. H., Kavousi, M., et al. (2021). The effect of antidiabetic drugs in age-related macular degeneration: the Rotterdam study. Investigative Ophthalmol. Vis. Sci. 62, 2940.
Thomas, R. L., Kubli, D. A., and Gustafsson, A. B. (2011). Bnip3-mediated defects in oxidative phosphorylation promote mitophagy. Autophagy 7, 775–777. doi:10.4161/auto.7.7.15536
Toyama, E. Q., Herzig, S., Courchet, J., Lewis, T. L., Losón, O. C., Hellberg, K., et al. (2016). Metabolism. AMP-activated protein kinase mediates mitochondrial fission in response to energy stress. Science 351, 275–281. doi:10.1126/science.aab4138
Urbina-Varela, R., Castillo, N., Videla, L. A., and Del Campo, A. (2020). Impact of mitophagy and mitochondrial unfolded protein response as new adaptive mechanisms underlying old pathologies: sarcopenia and non-alcoholic fatty liver disease. Int. J. Mol. Sci. 21, 7704. doi:10.3390/ijms21207704
Vallée, A. (2022). Curcumin and Wnt/β-catenin signaling in exudative age-related macular degeneration (Review). Int. J. Mol. Med. 49, 79. doi:10.3892/ijmm.2022.5135
Wang, C., Zou, Q., Pu, Y., Cai, Z., and Tang, Y. (2023b). Berberine rescues D-ribose-induced alzheimer’s pathology via promoting mitophagy. Int. J. Mol. Sci. 24, 5896. doi:10.3390/ijms24065896
Wang, E. Y., Gang, H., Aviv, Y., Dhingra, R., Margulets, V., and Kirshenbaum, L. A. (2013). p53 mediates autophagy and cell death by a mechanism contingent on Bnip3. Hypertension 62, 70–77. doi:10.1161/HYPERTENSIONAHA.113.01028
Wang, K., Feng, X., Chai, L., Cao, S., and Qiu, F. (2017). The metabolism of berberine and its contribution to the pharmacological effects. Drug Metab. Rev. 49, 139–157. doi:10.1080/03602532.2017.1306544
Wang, Q., He, F., and Wu, L. (2023a). NLRX1 increases human retinal pigment epithelial autophagy and reduces H2O2-induced oxidative stress and inflammation by suppressing FUNDC1 phosphorylation and NLRP3 activation. Allergol. Immunopathol. Madr. 51, 177–186. doi:10.15586/aei.v51i1.766
Wei, W., and Tong, J. (2023). Research progress in the treatment of age-related macular degeneration with Chinese medicine. J. Contemp. Med. Pract. 8, 103–106.
Wen, X., Tang, L., Zhong, R., Liu, L., Chen, L., and Zhang, H. (2023). Role of mitophagy in regulating intestinal oxidative damage. Antioxidants 12, 480. doi:10.3390/antiox12020480
Wu, H., Wang, Y., Li, W., Chen, H., Du, L., Liu, D., et al. (2019). Deficiency of mitophagy receptor FUNDC1 impairs mitochondrial quality and aggravates dietary-induced obesity and metabolic syndrome. Autophagy 15, 1882–1898. doi:10.1080/15548627.2019.1596482
Wu, W., Li, W., Chen, H., Jiang, L., Zhu, R., and Feng, D. (2016). FUNDC1 is a novel mitochondrial-associated-membrane (MAM) protein required for hypoxia-induced mitochondrial fission and mitophagy. Autophagy 12, 1675–1676. doi:10.1080/15548627.2016.1193656
Wu, W., Tian, W., Hu, Z., Chen, G., Huang, L., Li, W., et al. (2014). ULK1 translocates to mitochondria and phosphorylates FUNDC1 to regulate mitophagy. EMBO Rep. 15, 566–575. doi:10.1002/embr.201438501
Wu, Y., Deng, H., Sun, J., Tang, J., Li, X., and Xu, Y. (2023). Poricoic acid A induces mitophagy to ameliorate podocyte injury in diabetic kidney disease via downregulating FUNDC1. J. Biochem. Mol. Toxicol. 37, e23503. doi:10.1002/jbt.23503
Xiao, J., Zhang, J. Y., Luo, W., He, P. C., and Skondra, D. (2023). The emerging role of gut microbiota in age-related macular degeneration. Am. J. Pathol. 193, 1627–1637. doi:10.1016/j.ajpath.2023.04.006
Xu, L., Kong, L., Wang, J., and Ash, J. D. (2018). Stimulation of AMPK prevents degeneration of photoreceptors and the retinal pigment epithelium. Proc. Natl. Acad. Sci. U. S. A. 115, 10475–10480. doi:10.1073/pnas.1802724115
Xu, Z., Li, C., Liu, Q., Yang, H., and Li, P. (2019). Ginsenoside Rg1 protects H9c2 cells against nutritional stress-induced injury via aldolase/AMPK/PINK1 signalling. J. Cell. Biochem. 120, 18388–18397. doi:10.1002/jcb.29150
Xue, Y., Li, D., Zhang, Y., Gao, H., and Li, H. (2019). Angelica polysaccharide moderates hypoxia-evoked apoptosis and autophagy in rat neural stem cells by downregulation of BNIP3. Artif. Cells Nanomed Biotechnol. 47, 2492–2499. doi:10.1080/21691401.2019.1623228
Yamano, K., and Youle, R. J. (2013). PINK1 is degraded through the N-end rule pathway. Autophagy 9, 1758–1769. doi:10.4161/auto.24633
Yang, C., Zhao, Q., Li, S., Pu, L., Yu, L., Liu, Y., et al. (2022c). Effects of Lycium barbarum L. Polysaccharides on vascular retinopathy: an insight review. Molecules 27, 5628. doi:10.3390/molecules27175628
Yang, L., Shi, J., Wang, X., and Zhang, R. (2022b). Curcumin alleviates D-galactose-induced cardiomyocyte senescence by promoting autophagy via the SIRT1/AMPK/mTOR pathway. Evid. Based Complement. Altern. Med. 2022, 2990843. doi:10.1155/2022/2990843
Yang, T., Tian, S., Wang, Y., Ji, J., and Zhao, J. (2022a). Antitumor activity of pachymic acid in cervical cancer through inducing endoplasmic reticulum stress, mitochondrial dysfunction, and activating the AMPK pathway. Environ. Toxicol. 37, 2121–2132. doi:10.1002/tox.23555
Yao, R., and Shen, J. (2020). Chaperone-mediated autophagy: molecular mechanisms, biological functions, and diseases. MedComm 4, e347. doi:10.1002/mco2.347
Yu, J. J., Azzam, D. B., Chwa, M., Schneider, K., Cho, J.-H., Hsiang, C., et al. (2021). Age-related macular degeneration (AMD) transmitochondrial cybrids protected from cellular damage and death by human retinal progenitor cells (hRPCs). Stem Cells Int. 2021, 6655372. doi:10.1155/2021/6655372
Yu, M., Zhang, M., Fu, P., Wu, M., Yin, X., and Chen, Z. (2023). Research progress of mitophagy in chronic cerebral ischemia. Front. Aging Neurosci. 15, 1224633. doi:10.3389/fnagi.2023.1224633
Yu, Y., Liu, Y., and Meng, Z. (2024). Role of traditional Chinese medicine in age-related macular degeneration: exploring the gut microbiota’s influence. Front. Pharmacol. 15, 1356324. doi:10.3389/fphar.2024.1356324
Zachari, M., Gudmundsson, S. R., Li, Z., Manifava, M., Cugliandolo, F., Shah, R., et al. (2020). Selective autophagy of mitochondria on a ubiquitin-endoplasmic-reticulum platform. Dev. Cell. 55, 251. doi:10.1016/j.devcel.2020.10.002
Zhang, L., Qiu, L., Xu, S., Cheng, X., Wu, J., Wang, Y., et al. (2023). Curcumin induces mitophagy by promoting mitochondrial succinate dehydrogenase activity and sensitizes human papillary thyroid carcinoma BCPAP cells to radioiodine treatment. Toxicol Vitro 93, 105669. doi:10.1016/j.tiv.2023.105669
Zhang, S.-Y., Wang, F., Zeng, X.-J., Huang, Z., and Dong, K.-F. (2021). Astragalus polysaccharide ameliorates steroid-induced osteonecrosis of femoral head through miR-206/HIF-1α/BNIP3 axis. Kaohsiung J. Med. Sci. 37, 1089–1100. doi:10.1002/kjm2.12426
Zhang, Y., Zhuang, H. X., Liu, H., and Feng, D. (2022). Molecular regulations of FUNDC1 at ER-mitochondria contacts under hypoxic stress. Contact (Thousand Oaks) 5, 25152564221092487. doi:10.1177/25152564221092487
Zhao, C., Yasumura, D., Li, X., Matthes, M., Lloyd, M., Nielsen, G., et al. (2011). mTOR-mediated dedifferentiation of the retinal pigment epithelium initiates photoreceptor degeneration in mice. J. Clin. Investigation 121, 369–383. doi:10.1172/JCI44303
Zheng, Q., Huang, C., Guo, J., Tan, J., Wang, C., Tang, B., et al. (2018a). Hsp70 participates in PINK1-mediated mitophagy by regulating the stability of PINK1. Neurosci. Lett. 662, 264–270. doi:10.1016/j.neulet.2017.10.051
Zheng, Q., Zhao, Y., Guo, J., Zhao, S., Fei, C., Xiao, C., et al. (2018b). Iron overload promotes mitochondrial fragmentation in mesenchymal stromal cells from myelodysplastic syndrome patients through activation of the AMPK/MFF/Drp1 pathway. Cell. Death Dis. 9, 515. doi:10.1038/s41419-018-0552-7
Zhong, Y., Shen, Q., Yang, Y., Zhang, R., Zhao, L., and Li, W. (2023). Trilobatin ameliorates HFD/STZ-induced glycolipid metabolism disorders through AMPK-mediated pathways. J. Funct. Foods 103, 105478. doi:10.1016/j.jff.2023.105478
Zhu, J., Chen, H., Guo, J., Zha, C., and Lu, D. (2022b). Sodium tanshinone IIA sulfonate inhibits vascular endothelial cell pyroptosis via the AMPK signaling pathway in atherosclerosis. J. Inflamm. Res. 15, 6293–6306. doi:10.2147/JIR.S386470
Zhu, X., Tao, F., Zhang, L., Yu, Y., Xu, Y., Yang, G., et al. (2022a). Astragaloside IV protects detrusor from partial bladder outlet obstruction-induced oxidative stress by activating mitophagy through AMPK-ULK1 pathway. Oxid. Med. Cell. Longev. 2022, 5757367. doi:10.1155/2022/5757367
Zhu, X., Wang, K., Zhou, F., and Zhu, L. (2018). Paeoniflorin attenuates atRAL-induced oxidative stress, mitochondrial dysfunction and endoplasmic reticulum stress in retinal pigment epithelial cells via triggering Ca2+/CaMKII-dependent activation of AMPK. Arch. Pharm. Res. 41, 1009–1018. doi:10.1007/s12272-018-1059-6
Zhu, Y., He, Y.-J., Yu, Y., Xu, D., Yuan, S.-Y., and Yan, H. (2023). Aldehyde dehydrogenase 2 preserves mitochondrial function in the ischemic heart: a redox-dependent mechanism for AMPK activation by thioredoxin-1. J. Cardiovasc Pharmacol. 83, 93–104. doi:10.1097/FJC.0000000000001499
Zhu, Y., Massen, S., Terenzio, M., Lang, V., Chen-Lindner, S., Eils, R., et al. (2013). Modulation of serines 17 and 24 in the LC3-interacting region of Bnip3 determines pro-survival mitophagy versus apoptosis. J. Biol. Chem. 288, 1099–1113. doi:10.1074/jbc.M112.399345
Zhuang, X., Ma, J., Xu, G., and Sun, Z. (2022). SHP-1 knockdown suppresses mitochondrial biogenesis and aggravates mitochondria-dependent apoptosis induced by all trans retinal through the STING/AMPK pathways. Mol. Med. 28, 125. doi:10.1186/s10020-022-00554-w
Keywords: age-related macular degeneration, mitophagy, oxidative stress, traditional Chinese medicine, mechanism
Citation: Yu Y, Wang G, Liu Y and Meng Z (2024) Potential application of traditional Chinese medicine in age-related macular degeneration—focusing on mitophagy. Front. Pharmacol. 15:1410998. doi: 10.3389/fphar.2024.1410998
Received: 02 April 2024; Accepted: 30 April 2024;
Published: 17 May 2024.
Edited by:
Rajeev K. Singla, Sichuan University, ChinaReviewed by:
Ying Chyi Song, China Medical University, TaiwanLihui Zhu, Shanghai Academy of Agricultural Sciences, China
Tang Biao, Hunan University of Chinese Medicine, China
Dongyue Wang, Sichuan University, China
Copyright © 2024 Yu, Wang, Liu and Meng. This is an open-access article distributed under the terms of the Creative Commons Attribution License (CC BY). The use, distribution or reproduction in other forums is permitted, provided the original author(s) and the copyright owner(s) are credited and that the original publication in this journal is cited, in accordance with accepted academic practice. No use, distribution or reproduction is permitted which does not comply with these terms.
*Correspondence: Gaofeng Wang, NzEwMDE3MzFAc2R1dGNtLmVkdS5jbg==; Yong Liu, NzEwMDA5NTNAc2R1dGNtLmVkdS5jbg==