- 1School of Life Science and Engineering, Southwest Jiaotong University, Chengdu, China
- 2Obesity and Metabolism Medicine-Engineering Integration Laboratory, Department of General Surgery, The Third People’s Hospital of Chengdu, Affiliated Hospital of Southwest Jiaotong University, Chengdu, Sichuan, China
- 3Department of Breast and Thyroid Surgery, The Second Affiliated Hospital of Chongqing Medical University, Chongqing, China
Cancer, the world’s second leading cause of death after cardiovascular diseases, is characterized by hallmarks such as uncontrolled cell growth, metastasis, angiogenesis, hypoxia, and resistance to therapy. Autophagy, a cellular process that can both support and inhibit cancer progression, plays a critical role in cancer development and progression. This process involves the formation of autophagosomes that ultimately fuse with lysosomes to degrade cellular components. A key regulator of this process is Sirtuin 1 (SIRT1), which significantly influences autophagy. This review delves into the role of SIRT1 in modulating autophagy and its broader impacts on carcinogenesis. SIRT1 regulates crucial autophagy mediators, such as AMP-activated protein kinase (AMPK) and mammalian target of rapamycin (mTOR), effectively promoting or suppressing autophagy. Beyond its direct effects on autophagy, SIRT1’s regulatory actions extend to other cell death processes, including apoptosis and ferroptosis, thereby influencing tumor cell proliferation, metastasis, and chemotherapy responses. These insights underscore the complex interplay between SIRT1 and autophagy, with significant implications for cancer therapy. Targeting SIRT1 and its associated pathways presents a promising strategy to manipulate autophagy in cancer treatment. This review underscores the potential of SIRT1 as a therapeutic target, opening new avenues for enhancing cancer treatment efficacy.
Highlights
• As a cell death mechanism, autophagy regulates initiation and progression of carcinogenesis.
• Sirutin family has various cellular functions in which SIRT1 is the most well-known one.
• SIRT1 modulates autophagy and other selective types including mitophagy and lipophagy.
• SIRT-mediated autophagy can regulate apoptosis occurrence in tumor cells.
• SIRT1-mediated autophagy regulation determines the response to cancer chemotherapy.
1 Introduction
Cells utilize autophagy and the ubiquitin-proteasome degradation pathway to dispose of toxic, misfolded, damaged, or unnecessary proteins (Wirawan et al., 2012). Unlike the proteasome, autophagy can degrade a vast array of substrates, including large protein aggregates and entire organelles. Beyond proteins, autophagy also breaks down lipids, DNA, and nuclear RNA, generating new pools of amino acids, fatty acids, and nucleosides for use in anabolic processes. This continual turnover facilitates a cycle of cellular breakdown and renewal (Rabinowitz and White, 2010). Autophagic degradation is carried out by lysosomes, which contain acidic hydrolases such as peptidases, lipases, and nucleases, breaking down large molecules into simpler components. Although all autophagic pathways converge at the lysosomal compartment (or vacuole in yeast), several routes exist to reach these lysosomes. In mammalian cells, three primary autophagy processes are recognized: chaperone-mediated autophagy (CMA), microautophagy, and macroautophagy (Cuervo, 2004). CMA targets proteins with a KFERQ-like motif to the lysosomes, facilitated by heat shock cognate 70 and its co-chaperones, with the lysosomal-associated membrane protein 2 (LAMP-2A) (Kaushik et al., 2011) mediating their subsequent breakdown. Microautophagy involves the lysosomal membrane invaginating to engulf cytoplasmic material, which is then degraded. Macroautophagy, on the other hand, involves the formation of autophagic vacuoles through the creation of autophagic membranes (phagophores) that evolve into double-membraned vesicles called autophagosomes. This form of autophagy is evolutionarily conserved across all eukaryotic cells and has been extensively studied, particularly through mouse models focusing on macroautophagy.
Cancer remains one of the most prevalent diseases globally, irrespective of economic status, with approximately 18.1 million new cases and 9.1 million deaths reported in 2018 (Bray et al., 2018). The extensive research over the past decades into cancer development, progression, detection, and treatment has highlighted the critical nature of early diagnosis and intervention. Without these, cancer often proves fatal. Despite significant advancements, cancer multidrug resistance continues to be a significant obstacle in effective cancer treatment. Chemotherapy remains a cornerstone for treating various malignancies across different stages. Researchers often grapple with understanding the development and potential treatments of cancer, not foreseeing the emergence of drug resistance within their studies. Drug resistance in cancer is complex and broad, making it a challenging phenomenon to elucidate. The understanding of chemotherapeutic resistance mechanisms has expanded greatly, yet the scientific explanations remain limited. The strategies by which tumor cells manage their metabolic pathways and signaling can influence treatment outcomes, such as preventing drug penetration into cancer cells and promoting drug efflux. Numerous studies have also explored whether specific genes are upregulated to foster drug resistance, examining aspects like drug transport through tumor cells, membrane transport protein pathways, target molecule overexpression, direct gene transcription, anti-apoptosis, and enhanced DNA repair, all of which have been implicated in the promotion of drug resistance (Harguindey et al., 2005; Housman et al., 2014; Liang Z. et al., 2014).
Dysregulation of cell death mechanisms is a common feature in carcinogenesis (Figure 1), influencing tumor cell survival, viability, proliferation, metastasis, and response to therapy. Autophagy, a cellular catabolic process, involves the breakdown and recycling of proteins and organelles. It starts with the formation of an autophagosome, a vesicle that fuses with a lysosome containing hydrolytic enzymes. Unlike mitophagy, which specifically targets intracellular organelles, macroautophagy is a non-selective form of autophagy. The complex molecular process of autophagy, which includes nucleation, elongation, and fusion, is facilitated by various proteins, including the autophagy-related (ATG) protein family (Ferro et al., 2020; Ichimura et al., 2000; Mizushima et al., 1998; Zaffagnini and Martens, 2016). Autophagy plays a critical role in balancing environmental substrate availability with cellular metabolic demands. It is activated by nutrient deprivation and oxidative stress through well-regulated pathways linked to energy metabolism, involving key regulators such as mTORC1 and AMPK (Hosokawa et al., 2009; Jung et al., 2009; Egan et al., 2011; Kim et al., 2011). The two primary physiological roles of autophagy are the degradation of defective proteins or organelles for quality control and the recycling of macromolecules under conditions of nutritional stress to meet metabolic needs (Kim and Lee, 2014; Mizushima and Komatsu, 2011).
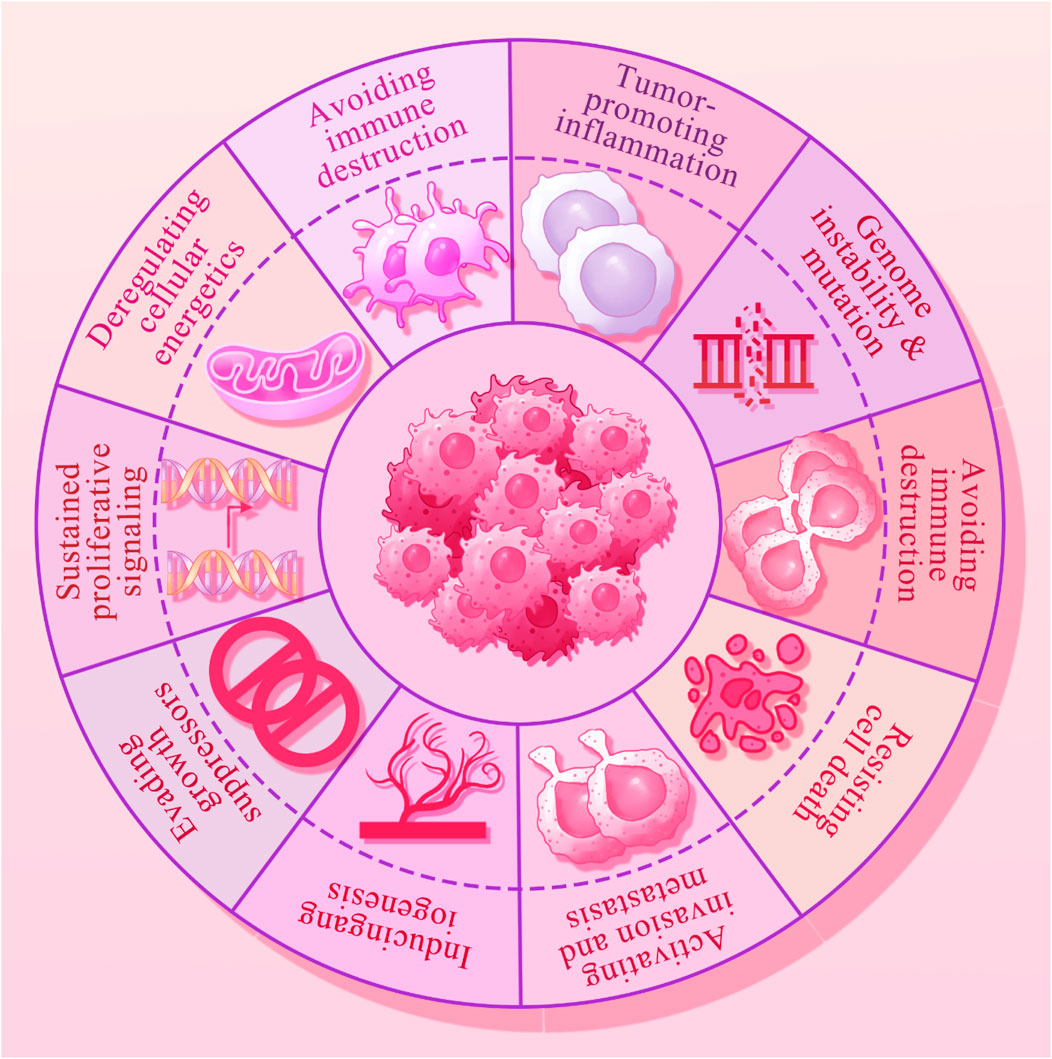
Figure 1. The hallmarks of cancer that include the immune escape, epigenetic alterations, oncogenic inflammation, genomic instability, increased proliferation, angiogenesis, metastasis, cell death resistance and replicative immortality.
In recent years, research has increasingly focused on the role of autophagy in both physiological and pathological contexts. Autophagy serves a dual and crucial role in cancer, where it can either promote or inhibit tumorigenesis. While commonly recognized as a mechanism of cell death, autophagy also influences metastasis and resistance to therapy (Qin et al., 2023; Ashrafizadeh et al., 2022). Consequently, it has become a promising target for pharmacological compounds and nanoparticles in cancer treatment (Ashrafizadeh et al., 2020a; Paskeh et al., 2022). With a better understanding of various autophagy regulators now available, this review concentrates on the role of SIRT1 in autophagy regulation within human cancers. It explores SIRT1’s association with cancer hallmarks and its interactions with apoptosis and ferroptosis.
2 A history of cell death
Cell death is essential for eliminating undesirable or damaged cells, playing a crucial role in animal development, tissue homeostasis, and stress response (Chen et al., 2016). Improper regulation of cell death contributes to various human diseases, including cancer and inflammatory disorders. Oncogenic transformation allows neoplastic cells to develop resistance to cell death, aiding their survival and the accumulation of mutations that promote cancer development (Hanahan and Weinberg, 2011). Many chemotherapeutic drugs work by inducing cell death, making it a fundamental strategy in cancer treatment. Consequently, targeting cell death mechanisms offers a promising approach for developing new anticancer drugs. Cell death can be classified based on morphological and biochemical characteristics into several primary types, such as apoptosis, necrosis, autophagic death, and mitotic catastrophe (Galluzzi et al., 2012). Historically, necrosis was considered a passive and uncontrolled process, while apoptosis was understood as a highly regulated, programmed cell death. However, the past 2 decades of research have revised this view, revealing a regulated form of necrosis. It was discovered that in some cells, inhibiting caspases, which are crucial for apoptosis, did not stop cell death but instead shifted it towards necrotic symptoms (Vercammen et al., 1998). Further studies identified receptor-interacting kinase 1 (RIP1; RIPK1) as a key regulator of this form of necrosis (Holler et al., 2000). Chemical biology research led to the identification of small-molecule inhibitors targeting this cell death pathway (Degterev et al., 2005), specifically inhibiting RIP1 (Degterev et al., 2008). Recent studies have established that RIP3 acts as a downstream mediator of RIP1 (Cho et al., 2009; He et al., 2009; Zhang et al., 2009), with the Mixed Lineage Kinase Domain-Like (MLKL) protein playing a central role in executing cell death (Sun et al., 2012). The physiological and clinical relevance of necrosis has been underscored by various studies in living organisms (Belizário et al., 2015). This regulated form of cell death, now termed necroptosis, involves RIP1, RIP3, and MLKL (Galluzzi et al., 2012) and is essential for its execution. Increasing evidence suggests that necroptosis acts as a protective mechanism by eliminating cancer cells that are resistant to apoptosis, highlighting its significant role in both the biology and therapy of cancer. Table 1 summarizes the dysregulation of cell death mechanisms in human cancers.
3 Different types of autophagy machinery
3.1 Macroautophagy
Macroautophagy, the most extensively examined type of autophagy, plays a crucial role in the breakdown and recycling of cellular components. This process is advantageous in numerous diseases, such as the removal of protein aggregates found in neurodegenerative disorders. Furthermore, macroautophagy has been recognized as a potential therapeutic target in cancer treatment, with its effectiveness depending on the stage of the tumor, its biological characteristics, and the tumor’s microenvironment (Debnath et al., 2023). Autophagosome formation, which involves creating a double-membrane vesicle, is the first step in autophagosomal vesicle generation. These autophagosomes, containing a variety of ATG products, subsequently merge with lysosomes. Lysosomal hydrolases then degrade the autophagosome’s contents. Key protein kinases, ULK1 and ULK2, along with their subunits FIP200, ATG13, and ATG101, initiate autophagosome formation in response to nutritional and energy signals, primarily from mTORC1 signaling. The recruitment of ATG7 and ATG3 is essential when phosphatidylinositol 3-phosphate is activated, facilitating the production of PS3P on autophagic membranes by the specialized Vps34 complex I, which includes Vps34, Beclin-1, ATG14, and Vps15. This complex is vital for cargo recruitment and autophagosome maturation (Zhao et al., 2021; Nakatogawa, 2020). Members of the ATG8 family, divided into two human subfamilies (microtubule-associated protein 1A/1B-light chain 3 (LC3) and GABARAP), are involved in lipid conjugation produced by the Vps34 complex I. Macroautophagy can non-selectively incorporate various materials into autophagosomes, especially under conditions of nutrient scarcity, thereby recycling essential molecules like lipids and amino acids. Consequently, macroautophagy is segmented into four phases: initiation, autophagosome formation, elongation, and fusion of the autophagosome with lysosomes, with each stage meticulously controlled. The final step, fusion, is mediated by SNARE proteins that facilitate the merging with the lysosome.
3.2 Microautophagy
Microautophagy includes two forms: selective and non-selective (Wang L. et al., 2023). Similarly, macroautophagy can also engage in either selective or non-selective absorption and degradation of cargoes. The cellular context influences whether microautophagy targets specific cargoes or functions non-selectively (Mijaljica et al., 2011). Historical studies primarily focused on microautophagy in rat liver before the discovery of ATG genes. In these studies, rat liver lysosomes were observed to invaginate their membranes and engulf various cargoes such as hemoglobin, ovalbumin, lysozyme, ferritin, and Percoll particles, facilitated by their acidic internal pH (Ahlberg et al., 1982; Marzella et al., 1981; Ahlberg and Glaumann, 1985). Certain drugs known as lysosomotropic agents, such as chloroquine, can inhibit the breakdown of these materials within the lysosomes. Findings indicate that the main autophagic response to starvation and refeeding in mice and rat livers is microautophagy (de Waal et al., 1986; Mortimore et al., 1988; Mortimore et al., 1983). However, these studies primarily utilized electron microscopy to observe morphological changes and lacked detailed biochemical evidence of alterations in autophagic activity or the molecular pathways involved. Macroautophagy can selectively target specific cargoes based on environmental conditions. Various selective forms of macroautophagy have been identified, including xenophagy for microorganisms, aggregephagy for protein aggregates, mitophagy for mitochondria, reticulophagy for the endoplasmic reticulum, lysophagy for lysosomes, and ribophagy for ribosomes (Kirkin and Rogov, 2019; Anding and Baehrecke, 2017). Recent research has also highlighted different types of selective microautophagy such as endosomal microautophagy (eMI), micronucleophagy, and micromitophagy, each believed to be regulated by distinct molecular pathways and serving unique functions.
While the direct role of microautophagy in cancer progression modulation has been overlooked, the pathways it influences are better understood (Wang L. et al., 2023). The Wnt signaling pathway regulates various biological processes including development, self-renewal, and immune surveillance (Galluzzi et al., 2019; Nusse and Clevers, 2017). Inhibition of GSK3 triggers the Wnt pathway, and microproteophagy contributes to the degradation of GSK3 and its associated substrate, SMAD4 (Albrecht et al., 2018; Taelman et al., 2010). The degradation of GSK3 by Wnt, facilitated through microproteophagy, depends on the availability of methionine (Albrecht et al., 2019). This establishes a link between microautophagy’s role and the regulation of pathways that influence the proliferation and survival of cancer cells. Furthermore, tumor cells may utilize MDV-induced micromitophagy to enhance their adaptability and survival, underscoring that targeting both macromitophagy and micromitophagy could enhance the efficacy of cancer therapies (Towers et al., 2021).
3.3 CMA
Three types of intracellular lysosomal degradation and autophagy exist, among which CMA is one (Assaye and Gizaw, 2022). CMA specifically targets proteins that are damaged or abnormal for degradation. It distinguishes itself from the other two types of autophagic processes in two keyways. Firstly, it uniquely requires the specific translocation of cargo proteins directly across the lysosomal membrane without enclosing them in a vesicle, allowing these proteins to enter directly into the lysosomal lumen (Auzmendi-Iriarte and Matheu, 2020). Secondly, CMA selectively degrades specific proteins from a larger pool, facilitated by a recognition motif similar to KFERQ found in proteins it targets (Xilouri and Stefanis, 2015). This selectivity enables CMA to degrade only the damaged or abnormal proteins without affecting the normal proteins, even if these are part of a multi-protein complex (Cuervo and Wong, 2014). Furthermore, CMA plays a crucial role in regulating various cellular processes by influencing levels of intracellular enzymes, transcription factors, and cell maintenance proteins. This impacts proteostasis, cellular energetics, and immune system functionality, depending on which proteins are selected for degradation at any given time (Auzmendi-Iriarte and Matheu, 2020; Cuervo and Wong, 2014). Figure 2 illustrates the macroautophagy mechanism.
The role of CMA in cancer therapy has garnered attention. For instance, FDW028 has been found to inhibit lysosomal proteolysis via CMA, which in turn can hinder metastasis in colorectal cancer (Wang M. et al., 2023). Additionally, CMA-mediated degradation of Dicer has been linked to increased metastasis in breast cancer cells (Su CM. et al., 2023). These findings suggest that CMA’s function extends beyond promoting cell death; it also plays a critical role in regulating metastasis and invasion in cancer cells. In prostate cancer, the protein TPD52 has been observed to activate CMA through its interaction with HSPA8/HSC70, leading to enhanced substrate degradation. The upregulation of TPD52 is crucial for promoting growth and stress resistance in prostate cancer cells (Fan et al., 2021). Beyond influencing metastasis, CMA is implicated in regulating growth and drug resistance in various cancers (Ichikawa et al., 2020). Furthermore, CMA is capable of degrading IGF-1Rβ in pancreatic cancer, demonstrating its impact on other significant factors (Xue et al., 2019).
4 Autophagy machinery function in oncology
Studies involving cell cultures and pre-clinical animal models have demonstrated that autophagy, along with genome integrity and anti-inflammatory signaling pathways, plays a crucial role in maintaining tissue homeostasis and preventing pro-oncogenic conditions (Amaravadi et al., 2016; Mathew et al., 2009; Long and McWilliams, 2020). Although there are instances of polymorphisms and altered expression levels of ATG proteins, key autophagy genes are generally unmutated in human cancers (Jiang and Mizushima, 2014). Additionally, autophagy genes are associated with either promoting or inhibiting tumor growth (White, 2015). The discovery of frequent loss of the autophagy regulator Beclin-1 (BECN1) in many cases of human breast, ovarian, and prostate cancers has shed light on the role of autophagy in oncology, suggesting that BECN1 may act as a tumor suppressor gene, particularly in individuals with only one functional copy (Yue et al., 2003; Qu et al., 2003; Karantza-Wadsworth et al., 2007). This theory is supported by findings in heterozygote Becn1 mice, which exhibit an elevated risk of developing hepatic, breast, and lymphoid tumors (Karantza-Wadsworth et al., 2007). While the status of BECN1 as a bona fide tumor suppressor remains under debate, its significant cellular role is undeniable (Li et al., 2017). In recent research, scientists created knock-in mice with a constitutively active Beclin-1 variant (Becn1F121A/F121A) that disrupts the interaction between endogenous Beclin-1 and its inhibitor Bcl-2. This alteration led to increased autophagic activity, improved overall health, extended lifespan, and a lower incidence of age-related spontaneous cancers in these mice (Fernández et al., 2018).
Research has identified patterns of overstimulated, understimulated, and deregulated autophagy (Ozpolat and Benbrook, 2015). The role of autophagy in cancer—whether it is oncogenic or tumor-suppressing—is still a subject of debate (Kroemer and Jäättelä, 2005; Ogier-Denis and Codogno, 2003; Scott et al., 2007; Dalby et al., 2010; Golstein and Kroemer, 2007; Tóth et al., 2002). Autophagy in cancer cells is influenced by various cellular factors, including gene mutations, abnormalities, the activation or inactivation of signaling pathways, and the level of cellular stress. Cancer cells often exhibit a higher rate of autophagy compared to normal cells, which can accelerate their proliferation. For instance, while normal breast epithelial cells typically display high levels of the Beclin-1 protein, these levels are significantly reduced or absent in breast cancer cells (Liang et al., 1999). Beclin-1 is monoallelically deleted in 40%–70% of human breast, prostate, and ovarian cancers (Liang et al., 1999; Qu et al., 2003; Karantza-Wadsworth et al., 2007; Saito et al., 1993), though biallelic mutations in Beclin-1 are rare in human tumors. Instead, other malignancies often show monoallelic deletions. In high-grade malignancies, such as prostate and ovarian cancers, autophagy tends to be downregulated (Liang et al., 2001; Gao et al., 1995). An initial study indicated that inhibiting Beclin-1 accelerated the progression of premalignant lesions caused by agents like the hepatitis B virus, enhanced the emergence of spontaneous cancers in the lung, liver, and lymphomas, and promoted mammary hyperplasia (Liang et al., 1999). This highlights how dysregulation of Beclin-1 and autophagy genes contributes to the development of human cancers. Subsequent research has linked abnormal autophagy to inflammation, DNA damage, genetic instability, insufficient cell turnover, and the production of reactive oxygen species (ROS), all of which are precursors to tumorigenesis and cancer (Table 2) (Karantza-Wadsworth et al., 2007).
5 Chemoresistance regulation by autophagy
Autophagy plays a role in drug resistance in cancer, with chemotherapeutic drugs often limited in their effectiveness due to their induction of protective autophagy, leading to chemoresistance (Hill and Wang, 2020). For instance, cisplatin, commonly used in treating various cancers including ovarian cancer, activates autophagy through the ERK pathway, thereby promoting drug resistance in these cells (Wang and Wu, 2014). Inhibition of autophagy has been shown to sensitize cancer cells to cisplatin (Bao et al., 2015; You et al., 2019), with similar results in lung cancer (Lee et al., 2015). In esophageal cancer, cisplatin-induced autophagy via the class III PI3K pathway enhances treatment efficacy when combined with the autophagy inhibitor 3-Methyladenine (Liu et al., 2011). Similarly, 5-FU, which inhibits DNA synthesis (Park et al., 2013), also induces autophagy leading to chemoresistance (Shuhua et al., 2015). Blocking autophagy has enhanced the effectiveness of 5-FU in colorectal cancer, where ATG genes have been linked to multi-drug resistance (Li et al., 2010). Activation of c-Jun N-terminal kinases (JNK) and phosphorylation of Bcl-2 are key mechanisms in 5-FU-induced autophagy in colon cancer, providing protection to cancer cells (Park et al., 2013). This phenomenon is also observed in gallbladder carcinoma, where inhibiting autophagy with chloroquine enhances the cytotoxic effects of 5-FU (Liang X. et al., 2014). In estrogen receptor-positive breast cancer, suppression of autophagy can resensitize cells to tamoxifen (Samaddar et al., 2008). In prostate cancer, elevated levels of the tumor suppressor candidate gene, nitrogen permease regulator-like 2, increase resistance to Everolimus by enhancing autophagy via the mTOR pathway (Chen et al., 2019). Autophagy also interacts with apoptosis, often protecting cancer cells from drug-induced cell death. In breast cancer, treatment with Epirubicin induces autophagy in MCF-7 cells, shielding them from apoptosis. However, inhibition of autophagy can resensitize these drug-resistant cells to therapy (Sun et al., 2011). In osteosarcoma, common chemotherapeutics induce overexpression of HSP90AA1, regulating autophagy through the PI3K/Akt/mTOR pathway and apoptosis through JNK/p38, highlighting the intricate interactions of these pathways in drug resistance (Xiao et al., 2018). A comprehensive understanding of these mechanisms is vital for developing new treatments. Novel strategies are emerging that target drug resistance by inhibiting autophagy, enhancing the efficacy of chemotherapy (An et al., 2015; O'Donovan et al., 2011; Zhang et al., 2016; Zhang et al., 2010; Fan et al., 2010; Ahn and Lee, 2011; Carew et al., 2007; Ge et al., 2014). Combining anti-cancer drugs with autophagy inhibitors, such as using cisplatin with autophagy suppression, has increased cytotoxicity in cells (Su et al., 2017; Claerhout et al., 2010). Similarly, pairing 5-FU with the autophagy inhibitor hydroxychloroquine has shown increased effects in colon cancer (Sasaki et al., 2010).
Autophagy is thought to play a crucial role in both the development of cancers and their treatment (Pu et al., 2022). Although many patients experience significant benefits from chemotherapy, acquired drug resistance has become a major obstacle to successful treatment. Numerous studies have demonstrated that a variety of chemotherapeutic agents can induce autophagy (Condello et al., 2020; Ashrafizadeh et al., 2020b), which is linked to increased resistance to chemotherapy. Chemotherapy typically triggers apoptosis in cancer cells, but these cells often initiate autophagy as a defense mechanism to avoid apoptosis, thereby reducing the efficacy of the treatment. Liu et al. (2013) used MTT and Hoechst 33342 staining, along with flow cytometry, to detect apoptosis in A549 lung cancer cells post-chemotherapy. They also employed the autophagy inhibitor 3-methyladenine (3-MA) to explore the relationship between autophagy and apoptosis. Their findings indicated that drugs like cisplatin (DDP) and paclitaxel can induce both autophagy and apoptosis in A549 cells. Additionally, studies have revealed that autophagy can render salivary gland adenoid cystic carcinoma cells resistant to DDP, often leading to chemotherapy failure (Tan et al., 2020). Using transmission electron microscopy, the autophagy marker LC3 can be identified, and the presence of minimal levels of p62 also suggests autophagy triggered by DDP. Moreover, downregulating Beclin-1 using 3-MA or RNA interference has been shown to increase apoptosis induced by DDP. As a result, the activation of protective autophagy by chemotherapy contributes to an increase in chemotherapeutic resistance in tumor cells.
6 SIRT1: Cellular functions and oncological importance
6.1 Structure and cellular functions
Sirtuins are characterized by a conserved catalytic domain, NAD + binding domains, and variable NH2- and COOH-terminal sections (Jiao and Gong, 2020; Frye, 1999; Yamamoto et al., 2007). These proteins differ in their functions, catalytic activities, and cellular localizations, influenced by their distinct amino acid sequences. Human sirtuins are classified into four categories: Class I, closely related to yeast Sir2, includes SIRT1, SIRT2, and SIRT3; Class II consists of SIRT4; Class III is represented by SIRT5; and Class IV includes both SIRT6 and SIRT7 (Frye, 2000). SIRT1, which is composed of 747 amino acids, features the longest terminal extensions, including a conserved catalytic core (244–512 residues), a COOH-terminal region (1–180 residues), and an NH2-terminal region (513–747 residues) (Kumar and Chauhan, 2016). The nuclear localization signal (KRKKRK) within the 41–46th residues of SIRT1 explains its presence in the nucleus (Frye, 1999). However, SIRT1 is also found in the cytoplasm in some cell types, indicating dual localization (Jin et al., 2007; Moynihan et al., 2005; Stünkel et al., 2007). SIRT1’s ability to shuttle between the nucleus and cytoplasm (Yanagisawa et al., 2018) is regulated by nuclear import and export sequences within its NH2-terminal region (Tanno et al., 2007). Other sirtuins have distinct subcellular locations: SIRT2 typically resides in the cytoplasm, though it can shuttle to the nucleus (North et al., 2003; Inoue et al., 2007); SIRT3, SIRT4, and SIRT5 are primarily mitochondrial, with SIRT3 being shown to move to the mitochondria from the nucleus post UV exposure or etoposide treatment (Scher et al., 2007). SIRT6 and SIRT7, like SIRT1, are located in the nucleus, with SIRT7 localized specifically to the nucleolus and SIRT6 associated with chromatin (Michishita et al., 2005). SIRT1 plays a significant role in regulating various biological and cellular processes, such as aging, metabolism, and inflammation (Chen et al., 2021). Figure 3 illustrates the functions of SIRT1 in these biological events.
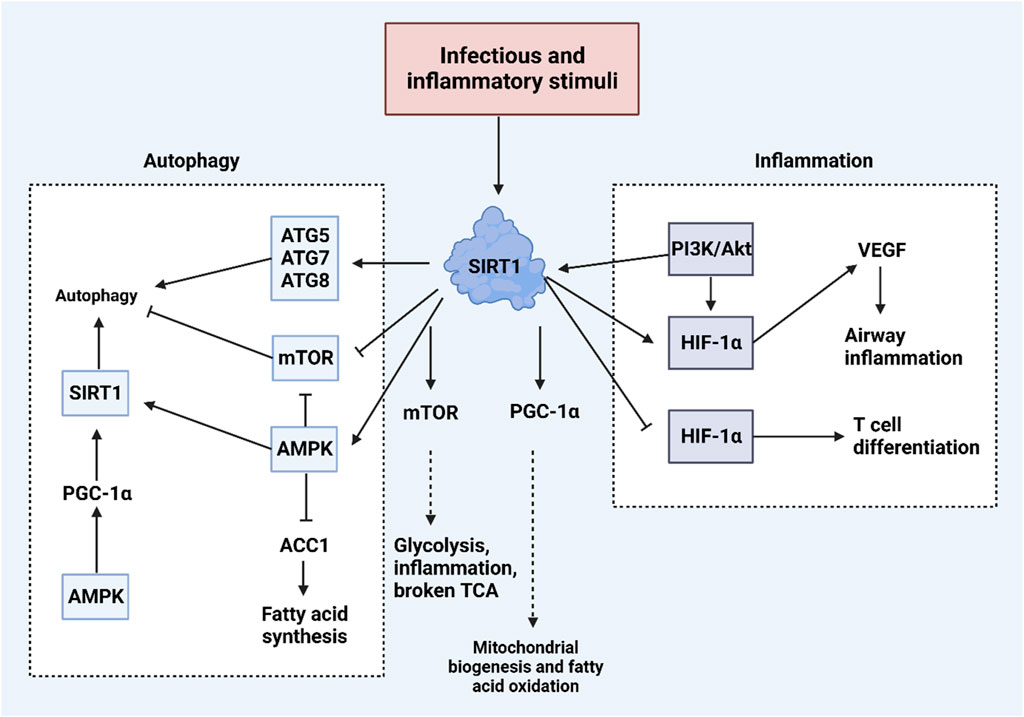
Figure 3. An overview of biological and cellular functions of SIRT1. Exposure to infectious and inflammatory stimuli can lead to an increase in SIRT1 expression, which plays a crucial role in regulating both inflammation and autophagy within cells. SIRT1 enhances autophagy by increasing the levels of ATG proteins such as ATG5, ATG7, and ATG8. Additionally, SIRT1 activates AMPK and suppresses mTOR, further promoting autophagy. In terms of regulating inflammation, SIRT1 interacts with the PI3K/Akt pathway and HIF-1α, illustrating its comprehensive role in cellular response mechanisms. (Kim et al., 2022).
6.2 Role in cancer
Despite the cellular functions of SIRT1, increasing evidence has underscored its role as a potential regulator of tumorigenesis. SIRT1 interacts with various signaling networks to influence the carcinogenesis process. It is upregulated in colorectal cancer and downregulates p53 expression through deacetylation, reducing miR-101 levels, while enhancing KPNA3 expression to promote metastasis and drug resistance (Wang XW. et al., 2023). Additionally, cytoplasmic SIRT1 may contribute to the formation and survival of polypoid giant tumor cells, leading to paclitaxel resistance in ovarian tumors (Xu H. et al., 2023). Conditions such as glucose deprivation and oxidative stress can trigger SIRT1 upregulation, which mediates β-catenin deacetylation, facilitating its transfer from the nucleus to the cytoplasm to decrease glycolysis and enhance fatty acid oxidation (Wei et al., 2023). Importantly, USP14 can increase the stability of SIRT1 by preventing its deubiquitination, promoting fatty acid oxidation in macrophages, which leads to M2 polarization and tumorigenesis (He et al., 2023). In terms of SIRT1’s oncogenic role, inhibiting it can disrupt tumorigenesis; for instance, LITAF increases FOXO1 levels, leading to SIRT1 downregulation, which diminishes the stemness and malignant phenotype of tumor cells (Guan et al., 2023). SIRT1 also regulates fatty acid oxidation in tumor cells. NSD2 boosts SIRT1 expression through interaction with AROS, enhancing fatty acid oxidation and reducing responsiveness to radiotherapy (Luo H. et al., 2023). Propofol’s potential as an anti-cancer agent in reducing tumor metastasis is partly attributed to the downregulation of SIRT1 (Wang R. et al., 2023). The transfer of SIRT1 via extracellular vesicles can activate the CD24/Siglec-10 axis, increasing apoptosis in CD8+ T cells and accelerating carcinogenesis (Zheng Q. et al., 2024). Moreover, SIRT1 regulates cell death mechanisms in cancers, such as inhibiting ferroptosis via p53 downregulation, thereby enhancing the survival of gastric tumor cells (Zhao H. et al., 2023). The following sections will delve deeper into the role of SIRT1 in autophagy regulation and associated molecular pathways (Table 3).
When compared to the non-cancerous tissues that were next to EC tissues, ENST00000534735 in EC tissues was dramatically downregulated (Shan et al., 2024). In addition to facilitating apoptosis and pyroptosis, the ectopic expression of ENST00000534735 significantly stopped the capacity of lung cancer cells to proliferate and migrate. The elevation of OSBPL3 through the APMK/SIRT1/NF-κB pathway was able to counteract the tumor-suppressing effects of ENST00000534735 overexpression. This was accomplished by knocking down ENST00000534735, which resulted in an increase in OSBPL3 expression. An excessive amount of ENST00000534735 expression was shown to inhibit the development of EC in the in vivo tumorigenic experiments that were carried out on nude mice. Another study identifies SIRT1 as a target of ISGylation, a post-translational modification by ISG15, which enhances SIRT1’s deacetylase activity by disrupting its interaction with the inhibitor DBC1 (Kang et al., 2024). SIRT1 ISGylation promotes lung cancer progression and reduces the sensitivity of lung cancer cells to DNA damage-based therapies. Elevated ISG15 and SIRT1 levels in lung cancer tissues correlate with poor patient prognosis, suggesting that these biomarkers could aid in patient stratification and outcome evaluation. SIRT1 downregulation in oral cancer cells leads to mitochondrial hyperfusion and drug resistance, while SIRT1 overexpression or activation by gallic acid reverses this effect, promoting apoptosis and restoring cisplatin sensitivity (Patra et al., 2023a). SPC-180002, a novel dual inhibitor of SIRT1/3, disrupts redox homeostasis and mitochondrial function, leading to cell cycle arrest and strong inhibition of cancer cell growth (Cho et al., 2023). MiR-653–3p promotes genomic instability, proliferation, migration, and chemoresistance in colorectal cancer cells by inhibiting SIRT1 and activating the TWIST1 signaling pathway (Wang H. et al., 2023). Doxorubicin-induced SIRT1 promotes redox imbalance and chemoresistance in breast cancer by enhancing cell proliferation, angiogenesis, and metastasis through NRF2 activation and increased glutathione levels (Sahoo et al., 2024). SIRT1 deacetylates and enhances KRASMut activity in lung cancer, and inhibiting SIRT1 or activating p300, which acetylates KRASMut, sensitizes tumors to cisplatin and erlotinib, offering a potential combination therapy for KRASMut lung cancer (Shin et al., 2023). Resveratrol inhibits neutrophil extracellular trap formation by targeting SIRT1, thereby reducing breast cancer metastasis and promoting CD8+ T cell infiltration in a murine model (Yu W. et al., 2023). Therefore, increasing evidences highlight the function of SIRT1 in the regulation of cancer progression and interaction with various molecular pathways (Wang XW. et al., 2023; Xu H. et al., 2023; Li X. et al., 2023; Zhang X. et al., 2023; Liu S. et al., 2024).
7 General discussion of SIRT1 in autophagy regulation in cancer
The process of mitotic chromosomal condensation is largely dependent on the presence of condensin (Hirano, 2002). Condensin I and condensin II are the names given to the two distinct forms of condensin complexes that may be found in a wide variety of eukaryotic cells (Hirano et al., 1997). The conventional condensin complex is composed of three distinct non-SMC subunits in addition to the same pair of core subunits that are referred to as structural maintenance of chromosomes (SMC) family proteins (Kimura and Hirano, 2000). Within human cells, the non-SMC subunits of condensin I are denoted by the letters NCAPD2, NCAPG, and NCAPH. On the other hand, the comparable subunits in the condensin II complex are denoted by the letters NCAPD3, NCAPG2, and NCAPH2 (Hirano et al., 1997). Condensin I has three non-SMC subunits, and one of them is called NCAPD2. This component may be found on chromosome 12p13.3. Previous research on NCAPD2 has mostly concentrated on its role in mitotic chromosomal condensation and segregation. This is because NCAPD2 is an essential component of the cell cycle. In addition, a number of studies have demonstrated that NCAPD2 is linked to a number of neurodevelopmental diseases, including Alzheimer’s disease, autism, Parkinson’s disease, and others, which suggests that it may have a function in the development of the central nervous system (Lee et al., 2008; Li et al., 2009; Sanders et al., 2012; Zhang et al., 2014). The abnormal expression of NCAPD2 in triple-negative breast cancer has the potential to function as an independent prognostic factor (Zhang et al., 2020). Through its involvement in the Ca2+/CAMKK/AMPK/mTORC1 pathway and the PARP-1/SIRT1 axis, NCAPD2 is able to suppress autophagy and impede autophagic flux. NCAPD2 is a tumor promoter that may be found in both in vitro and in vivo settings. In an AOM/DSS-induced mouse model, suppression of the development of colorectal cancer by NCAPD2 deletion is seen (Jing et al., 2021). 4-dmH targets tNOX and SIRT1, inhibiting their activity and inducing apoptosis (Islam et al., 2024a). SIRT1 in EML4-ALK G1202R and EML4-ALK L1196M mutant drug-resistant cells was downregulated compared with EML4-ALK NSCLC cells (Yang et al., 2024). The high expression of SIRT1 was related to the longer survival time of patients with lung cancer. Activation of SIRT1 induced autophagy and suppressed the invasion and migration of mutant cells. Further experiments indicated that the activation of SIRT1 inhibited the phosphorylation level of mTOR and S6K by upregulating the expression of AMPK, thus activating autophagy. SIRT1 can significantly enhanced the sensitivity of mutant cells to crizotinib, improved its ability to promote apoptosis of mutant cells, and inhibited cell proliferation.
A number of transcription factors, including p53, E2F1, FOXO, NF-θβ, and c-Myc, have been identified as targets for SIRT1 (Mao et al., 2014). These interactions are responsible for the formation of cancer and the spread of disease to other parts of the body in a variety of malignancies (Ayob and Ramasamy, 2018; Wong et al., 2021; Ong and Ramasamy, 2018). Overexpression of SIRT1 in HCC has the potential to contribute to the survival and proliferation of tumor cells (Chen et al., 2011; Jang KY. et al., 2012; Molla et al., 2020), as well as to the promotion of metastasis (Hao et al., 2014). SIRT1 is mostly found in the nucleus, where it plays a function in the development of tumors. However, it has been suggested that cytoplasmic sirtuin 1 may play a role in the suppression of tumors in HCC (Farcas et al., 2019; Song et al., 2014). SIRT1 is also known to influence chemoresistance in a variety of malignancies, including ovarian, breast, and gastric cancers (An et al., 2020; Mvunta et al., 2017; Wang et al., 2019b). However, the involvement of SIRT1 in the chemoresistance of HCC is not well understood. A study investigates the role of SIRT1 in sorafenib-resistant HCC, revealing that increased SIRT1 levels promote autophagy and activate NF-ĸβ signaling in resistant cells (Chan et al., 2024). Silencing SIRT1 downregulates autophagy and restores NF-ĸβ activity by failing to deacetylate key proteins, suggesting that the SIRT1/autophagy/NF-ĸβ axis plays a crucial role in HCC progression and resistance, with potential implications for therapeutic strategies.
There was a significant amount of RACGAP1 found in the cells of stomach cancer. Gastric cancer cell proliferation, migration, and invasion were all enhanced when RACGAP1 was overexpressed (Yan et al., 2024). In addition, the inhibition of RACGAP1 led to the induction of autophagy and death in cells. In addition, the expression of SIRT1 and Mfn2 was also inhibited by RACGAP1. In the tissues of EC tumors, FIRRE and SIRT1 were found to be elevated, whereas miR-199b-5p was shown to be downregulated. By sponging miR-199b-5p and suppressing autophagy, FIRRE knockdown was able to improve the susceptibility of EC cells to radiation doses (Cai et al., 2024). The microRNA known as miR-199b-5p was able to act as a negative regulator of SIRT1. In the absence of this information, SIRT1 has the potential to deacetylate BECN1 protein and take part in FIRRE-mediated autophagy. The activation of FIRRE resulted in an enhancement in the sensitivity of EC radiation in vivo. By inhibiting autophagy and proliferation, as well as inducing apoptosis in HCT116 and HT29 cells, ZMIZ1 knockdown was found to have a substantial therapeutic effect (Huang et al., 2024). Both the mRNA level of SIRT1 and the protein level of the SIRT1-specific substrate, acetylated FOXO3a, were considerably reduced as a result of ZMIZ1 knockdown. However, the mRNA level of SIRT1 was not changed by the knockdown. The relationship between SIRT1 and ZMIZ1 in HCT116 and HT29 cells was brought to light by immunoprecipitation tests. There was an increase in the intracellular ubiquitination of SIRT1 due to ZMIZ1. The effects of ZMIZ knockdown on proliferation, autophagy, and apoptosis in HCT116 and HT29 cells were reduced by targeting SIRT1 by knockdown or pharmacological inhibition. The drug-resistant oesophageal cancer cells exhibit increased autophagy and SIRT1 expression, both of which are linked to enhanced cell migration and the EMT (Zhang et al., 2024b). Inhibiting autophagy or SIRT1 reduced these processes. Additionally, a SIRT1 inhibitor effectively suppressed tumor growth in a mouse xenograft model without significant toxicity, suggesting that SIRT1 plays a key role in autophagy-driven drug resistance in oesophageal cancer. The adipose triglyceride lipase (ATGL) is highly expressed in CRC and is associated with poor prognosis (Su BC. et al., 2023). ATGL promotes CRC cell proliferation by inhibiting the mTOR signaling pathway and activating autophagy. Additionally, ATGL regulates autophagy by increasing SIRT1 expression. These findings suggest that ATGL contributes to CRC growth through the upregulation of autophagy and SIRT1. The electro-acupuncture (EA) can alleviate CRC in mice by reducing inflammation and promoting autophagy through the SIRT1/miR-215/Atg14 axis (Li J. et al., 2023). EA treatment decreased tumor numbers, inflammation, and DAI scores, while increasing body weight and SIRT1 expression. SIRT1 overexpression was shown to suppress miR-215 and enhance Atg14 expression, suggesting that EA exerts its anti-CRC effects by regulating this molecular pathway. The ubiquitin-conjugating enzyme E2C (UBE2C) promotes the malignant progression of endometrial cancer by inhibiting autophagy (Zhao R. et al., 2023). UBE2C suppresses autophagy by inducing ubiquitination and degradation of SIRT1, leading to reduced expression of autophagy-related genes. Knockdown of UBE2C in cancer cells enhanced autophagy and increased apoptosis, while overexpression of UBE2C promoted tumor growth in a mouse model. However, rapamycin, an autophagy activator, reversed the tumor growth and apoptosis inhibition caused by UBE2C overexpression. SIRT1 regulates mitotic catastrophe (MC) through autophagy and BubR1 signaling. Degradation of SIRT1 increased MC, while overexpression of SIRT1 reduced MC by decreasing apoptotic and multinuclear cells and promoting autophagy. Additionally, SIRT1 was shown to bind to the promoter of BubR1, a key component of the spindle assembly checkpoint, increasing its expression and reducing MC (Zhao et al., 2022).
8 SIRT1/AMPK axis in autophagy regulation
AMPK, a crucial metabolic regulator, restores depleted ATP levels and maintains energy balance, especially when cells are stressed. Targeting AMPK has shown promise in treating metabolic syndrome and type 2 diabetes (Steinberg and Kemp, 2009; Yuan et al., 2023). AMPK enhances metabolic processes by inhibiting glucose production in the liver, improving insulin sensitivity, reducing fatty acid synthesis and esterification, increasing glucose uptake in muscles, and reducing proinflammatory changes (Ruderman and Prentki, 2004). Small molecules such as cellular AMP allosterically activate AMPK by binding to the CBS1 domain, while AMP or ADP binding to CBS3 alters AMPK’s phosphorylation status (Xiao et al., 2011). These interactions trigger structural changes in the AMPK complex, enabling phosphorylation at the Thr-172 site on the AMPKα subunit (Hawley et al., 1996; STEIN et al., 2000), and are further enhanced by various upstream kinases that also phosphorylate the Thr-172 site, fully activating AMPK (Liu et al., 2014). AMPK acts as a regulator of autophagy in various cancers, with growing evidence suggesting that SIRT1 serves as an upstream mediator of AMPK in this role. Quercetin, a natural compound, induces apoptosis and toxic autophagy in lung cancer, where increased SIRT1 levels upregulate AMPK, leading to autophagy-mediated apoptosis (Guo et al., 2021). Similarly, ghrelin enhances SIRT1 expression to activate AMPK and induce autophagy, although this SIRT1/AMPK-mediated autophagy does not significantly trigger apoptosis (Heshmati et al., 2020). The SIRT1/AMPK axis has been studied across different tumor types, influencing tumorigenesis progression. For example, diallyl trisulfide induces pro-death autophagy in hepatocellular carcinoma through the AMPK/SIRT1 axis (Sun et al., 2022). Additionally, since mTOR is downstream of AMPK, SIRT1’s regulation of AMPK impacts mTOR, a key autophagy regulator (Ye et al., 2017). Calycosin activates the SIRT1/AMPK axis to inhibit the Akt/mTOR pathway, stimulating autophagy-mediated apoptosis in cancer cells (El-Kott et al., 2019). Nitrosative stress can also induce autophagy in breast cancer by upregulating SIRT1 and its interaction with AMPK (Chakraborty et al., 2019). Thus, SIRT1 is integral in regulating AMPK and downstream targets, influencing autophagy in human cancers.
9 SIRT1/mTOR axis in autophagy regulation
mTOR, a highly conserved serine/threonine kinase, orchestrates cellular metabolism, proliferation, and apoptosis (Xie et al., 2023). It forms two distinct complexes: mTOR complex 1 (mTORC1) and mTOR complex 2 (mTORC2), with mTORC1 being more sensitive to rapamycin and containing the regulatory-associated protein of mTOR (RAPTOR) (Ben-Sahra and Manning, 2017). mTOR responds to three main types of upstream signals: immune activation, environmental stress, and nutrient availability (Chi, 2012). These signals can either upregulate or downregulate mTOR, influencing cell growth, division, and survival, as well as regulating protein synthesis and catabolism. Downstream of mTOR, translational regulation is mediated by factors such as the eIF4E binding protein 1 (4E-BP1) and p70S6 Kinase (S6 Kinase), illustrating another facet of mTOR signaling (Zou et al., 2020; Tan and Miyamoto, 2016; Kennedy and Lamming, 2016). SIRT1 interacts with mTOR to regulate autophagy in human cancers. For instance, ATGL, identified as an oncogenic factor in colorectal tumors, promotes proliferation and correlates with poor prognosis by downregulating mTOR, thus facilitating pro-survival autophagy (Su BC. et al., 2023). SIRT1 regulators have emerged as autophagy modulators in cancer. MHY2245, an inhibitor of SIRT1, suppresses the PKM2/mTOR axis, stimulating autophagy and accelerating apoptosis, which leads to growth reduction in ovarian tumors (Yousafzai et al., 2021). The downregulation of SIRT1/2 can induce protective autophagy in lung cancer by increasing the acetylation of HSPA5, which in turn elevates ATF4 and DDIT4 levels, suppressing mTOR and promoting pro-survival autophagy (Mu et al., 2019). Thus, both AMPK and mTOR play significant roles in the regulation of autophagy in human cancers (Figure 4).
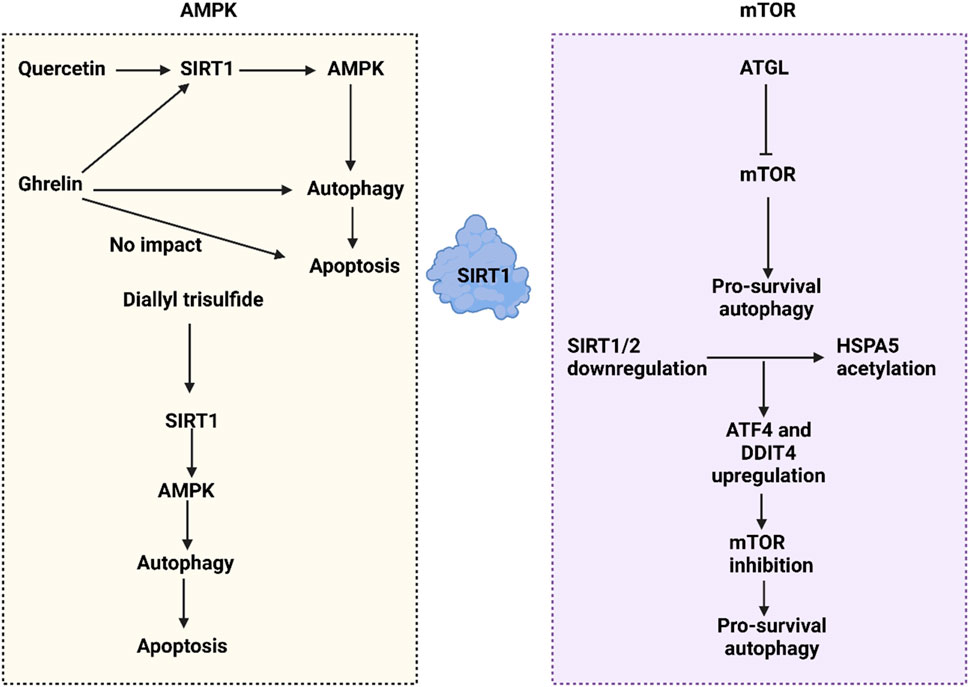
Figure 4. The SIRT1-mediated autophagy regulation in cancer through affecting mTOR and AMPK pathways. The interaction between SIRT1 and AMPK clearly illustrates that SIRT1 upregulates AMPK to promote autophagy. Various compounds influence the SIRT1/AMPK axis; for example, quercetin activates the SIRT1/AMPK/autophagy pathway to stimulate apoptosis. Ghrelin also activates the SIRT1/AMPK/autophagy axis, though it does not lead to cell death. Additionally, the downregulation of SIRT1/2 enhances the acetylation of HSPA5, which in turn increases ATF4 and DDIT4 levels, leading to the downregulation of mTOR and facilitating pro-survival autophagy.
10 SIRT1-mediated autophagy regulation in cancer drug resistance
A major challenge in oncology is the issue of drug resistance, a problem that shares similarities with antimicrobial therapy in terms of rapidly adapting threats, primarily originating from within, such as cancerous cells, and to a lesser extent from external sources like bacteria. Early chemotherapeutics such as nitrogen mustard and aminopterin were initially effective, putting many tumors into remission. However, similar to antimicrobial chemotherapy, they often led to drug resistance and disease relapse. Drawing on strategies from antimicrobial therapy, oncology first attempted to overcome resistance through polychemotherapy, which involves administering a sequence of drugs, each with a different mechanism of action. This approach has been empirically successful in treating certain diseases like some types of lymphoma, breast cancer, and testicular cancer. Consequently, combination chemotherapy became the foundation of systemic cancer treatment, frequently used alongside surgery and tailored radiation therapy. Over time, these combinations grew more complex, and dose intensity strategies were introduced to enhance antitumor efficacy. This involved reducing intervals between chemotherapy cycles or increasing drug dosages, supported by myeloid and other growth factors to manage drug-induced myelotoxicity and sustain ongoing treatment. Despite nearly 5 decades of success, by the early 21st century, it became evident that surgery, radiation, and combination chemotherapy were not curative for many types of tumors (Vasan et al., 2019; Goodman et al., 1946; Farber and Diamond, 1948; Crofton, 1959; DeVita et al., 1980; Bonadonna et al., 1976; Bosl et al., 1986; Hryniuk and Bush, 1984; Citron et al., 2003; Sternberg et al., 2001).
Chemotherapy drugs induce mitochondrial dysfunction to trigger apoptosis in tumor cells. Conversely, CDK9 inhibitors enhance the stability and dephosphorylation of SIRT1. Subsequently, elevated SIRT1 levels lead to the degradation of FOXO3, which in turn suppresses BNIP3-induced stability of PINK1. Additionally, CDK9 inhibitors can inhibit the SIRT1/FOXO9/BNIP3 axis and the PINK1/PRKN pathway, thereby suppressing mitophagy. This inhibition of mitophagy by CDK9 inhibitors contributes to increased mitochondrial dysfunction, ultimately promoting apoptosis in hepatocellular carcinoma (Yao et al., 2022). Despite evidence suggesting that increased stability and upregulation of SIRT1 can suppress mitophagy and enhance apoptosis, some findings overexpressed SIRT1 can facilitate drug resistance in tumor cells. Specifically, SIRT1 can mediate the deacetylation of Beclin-1, which activates protective autophagy and promotes resistance to cisplatin in bladder cancer (Sun et al., 2023).
Autophagy induction during tumorigenesis serves to supply cancer cells with the necessary components for growth by degrading organelles and proteins (White et al., 2015). Modifying autophagy levels has emerged as a promising strategy in cancer treatment (Li et al., 2017). Lipophagy, a selective form of autophagy that degrades lipids, plays a role in modulating lipid metabolism and maintaining intracellular lipid homeostasis (Zhang et al., 2018). Various genes, enzymes, transcription regulators, and other molecules regulate lipophagy (Maan et al., 2018; Madrigal-Matute and Cuervo, 2016). Additionally, de novo lipogenesis is linked to the development of drug resistance in cancer (Zhang et al., 2018; Maan et al., 2018; Beloribi-Djefaflia et al., 2016). For instance, low expression of miR-425 can elevate SIRT1 levels, thereby stimulating pro-survival lipophagy and enhancing resistance to sorafenib in liver cancer (Sun G. et al., 2021). Conversely, the independent regulation of SIRT1 and autophagy can also influence drug resistance. Jaridon 6, for example, inhibits the PI3K/Akt/mTOR axis to induce autophagy and reduces SIRT1 expression, weakening drug resistance in gastric tumors (Fu et al., 2021). A notable aspect of SIRT1-mediated autophagy is its role in various human cancers. The lncRNA H19, which has oncogenic properties in colorectal cancer, mediates 5-fluorouracil resistance by sponging miR-194–5p to elevate SIRT1 expression, thus promoting autophagy-induced resistance to 5-fluorouracil in colorectal tumors (Wang et al., 2018). Thus, the interaction between SIRT1 and autophagy plays a critical role in determining the responsiveness of tumor cells to chemotherapy.
11 SIRT1-mediated autophagy and apoptosis crosstalk
11.1 Basics of apoptosis
During the initiation and intermediate stages of apoptosis, a variety of metabolic activities occur alongside significant morphological changes. These changes include cytoplasmic filament aggregation, nuclear membrane shrinkage, cell fragmentation, the formation of apoptotic bodies, and plasma membrane blebbing (Elmore, 2007; Power et al., 2002). These changes are mainly observed in the nucleus, cell membrane, cytoplasm, and mitochondria, and can be detected through microscopic, light, and fluorescence microscopy methods (Elmore, 2007; Savill and Fadok, 2000). Apoptosis is triggered by environmental signals originating from two primary sources: external signals from other cells and signals from physical contact with adjacent cells. At the onset of apoptosis, cells begin to lose contact with neighboring cells and tightly pack their internal components without releasing them outside, thus preventing inflammation and contamination in the surrounding environment (Rosenblatt et al., 2001; Ferri and Kroemer, 2001). Surrounding cells recognize these apoptotic cells and facilitate their internalization and degradation without triggering an inflammatory response. Apoptosis proceeds via two major pathways: intrinsic and extrinsic. The extrinsic pathway is activated by the interaction of death receptors with their ligands, leading to the activation of caspase 8. This activation can directly induce cell death or further activate caspase 3 or Bid, a process that can be inhibited by cellular FLICE-like inhibitory proteins (cFLIP) (Kiraz et al., 2016). The intrinsic pathway, on the other hand, is initiated by genomic damage and proceeds via the mitochondrial pathway. This involves the activation of Bax, a pro-apoptotic member of the Bcl-2 family. At the mitochondrial membrane, anti-apoptotic proteins such as Bcl-2 and Bcl-XL inhibit Bax’s activity. The release of cytochrome c from mitochondria leads to the formation of apoptosomes, complexes involving cytochrome c, APAF-1, and procaspase 9. The assembly of these complexes triggers the caspase activation cascade, converting procaspase-3 to active caspase 3. Bid, another pro-apoptotic Bcl-2 family member, facilitates communication between the intrinsic and extrinsic pathways. Caspase 8 cleavage of Bid enhances the release of mitochondrial cytochrome C, further driving the apoptotic process (Kiraz et al., 2016).
11.2 SIRT1-mediated autophagy and apoptosis crosstalk
The interactions between autophagy and apoptosis play a crucial role in human cancers, with SIRT1 acting as a key mediator. This section discusses the relationship between SIRT1-induced autophagy and apoptosis. SIRT1 upregulation is essential for initiating autophagy. UBE2C promotes the ubiquitination of SIRT1, leading to its degradation and decreased stability, which in turn reduces H4K16 deacetylation, suppressing autophagy at an epigenetic level. In endometrial cancer, autophagy is critical for inducing apoptosis, hence UBE2C’s regulation of autophagy affects the autophagy-apoptosis interplay (Zhao R. et al., 2023). In some cases, autophagy can inhibit apoptosis in human cancers. For instance, SIRT1 translocation from the nucleus to the cytoplasm increases Beclin-1 expression, thereby promoting autophagy. This protective autophagy then inhibits the release of cytochrome C from mitochondria, suppressing the caspase-3/PARP pathway and preventing apoptosis in bladder cancer (Sun et al., 2023). Additionally, the response of autophagy to cellular stress is pivotal, as evidenced by increased SIRT1 and FoxO1 levels under glucose deprivation in gastric cancer, which boosts Rab7 expression and autophagy, supporting tumor cell survival. Conversely, inhibiting autophagy can enhance apoptosis, underscoring the supportive role of autophagy in this context (Zhu M. et al., 2023). In colorectal cancer, SIRT1 typically stimulates autophagy to inhibit apoptosis. However, using catalpol, a natural product with anticancer and epigenetic properties, leads to miR-34a upregulation, which suppresses the SIRT1/autophagy axis and triggers apoptosis in colorectal tumor cells (Qiao et al., 2020). Although SIRT1 is primarily seen as an upstream autophagy mediator in cancers, autophagy can also influence SIRT1, impacting tumorigenesis regulation. For example, autophagy-induced SIRT1 degradation can enhance radiotherapy-mediated apoptosis in prostate cancer, showing its potential to reduce radio-resistance (Wang et al., 2022). Epigenetic modifications and miRNA dysregulation in tumor cells also affect cancer progression and treatment responses (Ashrafizadeh et al., 2021). In lung cancer, miR-124 and miR-142 downregulation of SIRT1 suppresses supportive autophagy, enhancing cisplatin sensitivity and promoting apoptosis (Song et al., 2019). Furthermore, the anticancer compound elaiophylin decreases SIRT1 and its downstream target Nrf2, inhibiting mitophagy and accelerating apoptosis in lung tumors (Ji et al., 2022). Thus, the interplay between SIRT1-mediated autophagy and apoptosis is integral to the regulation of carcinogenesis (Figure 4).
12 SIRT1-mediated autophagy and ferroptosis crosstalk: New perspectives
Ferroptosis, an iron-dependent form of regulated cell death characterized by the accumulation of lipid peroxides on cellular membranes, was first identified in a proteomics study by the Stockwell laboratory and colleagues in 2012 (Dixon et al., 2012; Lei et al., 2022). This process is distinct from apoptosis and other forms of cell death in several ways, including its unique mechanisms and morphological characteristics. Cells undergoing ferroptosis do not exhibit chromatin condensation or form apoptotic bodies; instead, they typically have smaller mitochondria with fewer mitochondrial cristae compared to normal or apoptosis-resistant cells (Dixon et al., 2012; Stockwell et al., 2017). These cells also accumulate harmful lipid peroxides (Jiang et al., 2021), arising from an imbalance between antioxidant activities that prevent ferroptosis and the pro-ferroptotic processes. When the imbalance exceeds the cell’s capacity to cope, leading to a critical overload of lipid peroxides, ferroptosis is triggered (Yang et al., 2014; Bersuker et al., 2019; Doll et al., 2019; Kraft et al., 2020; Soula et al., 2020; Mao et al., 2021; Ingold et al., 2018). Additionally, ferroptosis differs in its molecular mechanisms from other types of cell death, which involve specific executioner proteins like caspase in apoptosis, gasdermin D in pyroptosis, or MLKL in necroptosis. Furthermore, the oxidized phospholipid profiles are distinctive to ferroptosis, setting it apart from other cell death types (Galluzzi et al., 2018; Wiernicki et al., 2020; Kagan et al., 2017).
Recent research has underscored the interplay between autophagy and ferroptosis in various human cancers, illuminating their roles in tumorigenesis regulation. In lung cancer, inducing ferroptosis has been shown to curb tumor growth, with curcumin enhancing this process by promoting toxic autophagy in lung tumor cells (Tang X. et al., 2021). In ovarian cancer, studies have investigated the expression levels of C-MYC and NCOA4 and their relationship with cancer malignancy. Findings indicate a significant correlation, where C-MYC appears to suppress NCOA4 expression by directly interacting with its mRNA, influencing ferroptosis negatively. This interaction reduces NCOA4 levels, decreases ROS production, and inhibits mitophagy, leading to increased proliferation and invasion of ovarian cancer cells. Furthermore, C-MYC is implicated in reducing NCOA4-mediated ferroptosis, enhancing cancer cell invasion and immune evasion (Jin et al., 2022). In head and neck cancer, the induction of ferritinophagy, a specific form of autophagy, is crucial for promoting ferroptosis (Lee J. et al., 2022). Conversely, in cervical cancer, Cdc25A enhances PKM2 dephosphorylation, which upregulates ErB2 expression and inhibits autophagy-induced ferroptosis (Wang et al., 2021). Additionally, in bladder cancer, although GPX4 acts to inhibit ferroptosis, autophagy facilitates the degradation of GPX4, augmenting the efficacy of Fin56 in stimulating ferroptosis (Sun Y. et al., 2021). These findings highlight the complex interactions and crosstalk between autophagy and ferroptosis in cancer regulation. Given the role of SIRT1 as a regulator of autophagy, further exploration into how SIRT1-mediated autophagy might influence ferroptosis is warranted, offering potential new avenues for cancer therapy.
13 SIRT1 modulators in cancer
There are various types of sirtuins, with SIRT1 being particularly well-studied for its dual role in cancer progression and inhibition. Researchers have explored pathways to activate or inhibit SIRT1, given its critical regulatory impact on tumor promotion and suppression (Carafa et al., 2019). Recent studies have identified several chemotherapeutic agents that target SIRT1, derived from both synthetic and natural bioactive compounds (Patra et al., 2023b). Among these, the polyphenolic antioxidant resveratrol has been highlighted for its anticancer properties, including antioxidant, immunomodulatory, anti-inflammatory, and pro-apoptotic effects. Resveratrol has shown effectiveness against multiple solid tumors and is known to influence autophagy, suggesting that it might trigger autophagic cell death (ACD) as an alternative cell death mechanism when apoptosis is compromised (Patra et al., 2022; Patra et al., 2021). This activation of SIRT1 by resveratrol could be particularly useful in treating drug-resistant cancer cells and eliminating cancer stem cells (Pervaiz and Holme, 2009). Another agent, gallic acid, known for inhibiting autophagy flux, can also activate SIRT1 and induce ATG cell death (Patra et al., 2020a; Patra et al., 2020b; Chang et al., 2021). Additionally, synthetic compound 5 has been shown to induce autophagic and mitophagic cell death in glioblastoma cells through SIRT1 activation (Yao et al., 2018). Indirect evidence also suggests that SRT1720, SRT2183, and SRT1460, as activators of SIRT1, may modulate autophagy to initiate cancer cell death pathways (Pacholec et al., 2010). Abrus agglutinin, another SIRT1 activator, mediates lipophagy leading to apoptotic cell death through ROS production induced by free fatty acids (Panda et al., 2020). Increased SIRT1 expression is associated with the onset of carcinogenesis and malignant transformation, making SIRT1 inhibition a potential therapeutic strategy. The SIRT1 inhibitor EX527, for example, can acetylate p53 in the presence of etoposide (Solomon et al., 2006), potentially triggering apoptotic cell death and inhibiting protective autophagy (Brooks and Gu, 2008). Despite its mixed results in cancer therapy, EX527 has progressed to phase three clinical trials for Huntington’s disease. The combination of chemotherapy with other SIRT1 inhibitors, such as suramins, JGB1741, tenovins, salermide, sirtinol, and other class III HDAC inhibitors, might enhance the efficacy of cancer treatments by regulating autophagy and inducing associated cell death (Lin and Fang, 2013; Heltweg et al., 2006; Lara et al., 2009; Kalle et al., 2010; Lain et al., 2008; Asaka et al., 2015). The latest inhibitor, MHY2245, affects PKM2/mTOR signaling in ovarian cancer cells, promoting autophagy alongside cell cycle arrest in the G2/M phase and potentially initiating autophagy-associated cell death (Table 4) (Tae et al., 2020).
A chemical known as silybin has been shown to inhibit SIRT1 and increase p53 acetylation, in addition to its anticancer properties (Yousafzai et al., 2021). Moreover, silybin and the SIRT1 inhibitor cambinol were produced in mice and employed for in vitro research according to dosage and time dependent parameters. When it comes to lung adenocarcinoma, silybin has been demonstrated to be an efficient inhibitor of adenocarcinoma, and it has the potential to be utilized as a therapeutic intervention (Liang Z. et al., 2014). HDACs inhibitor tenovin-6 induces apoptosis, suppresses cell migration and invasion, and eliminates cancer stem cells (CSCs) in uveal melanoma (Dai et al., 2016). The progression of uveal melanoma (UM) and the diagnosis have remained pitiful. Tenovin-6 has all of these effects. Inducing a senescence-like growth arrest, perhaps having anticancer potential, and causing an impairment in the activation of the Ras/MAPK pathway are all outcomes of sirtinol, which is another inhibitor. Despite this, sirtinol was found to have an influence on the activation of Akt/PKB as well as the tyrosine phosphorylation of receptors for EGF and IGF-I on the receptors (Ota et al., 2006). On the other hand, SIRT1 suppression by EX527 dramatically decreased the tumor growth of HEC1B and HHUA endometrial cancer. This was due to the fact that SIRT1 overexpression caused cisplatin resistance in HHUA cells, which in turn accelerated carcinogenesis in nude mice. In the treatment of cisplatin-resistant cancer, a combination of EX527 and cisplatin has the potential to be an effective targeted therapy (Asaka et al., 2015). According to computational docking studies, EX527 is solely specific for SIRT1 rather than other sirtuin members. However, Sirtinol, Nicotinamide, and Salermide are all direct targets of inhibitors SIRT1 and 2, and they all have the specific inhibitory action for SIRT1. Salermide is also a direct target of SIRT2. EX527 enhanced carcinogenesis in SCID mice in comparison to the control group, regardless of whether it induces apoptosis and DNA damage in vitro (Oon et al., 2015). This suggests that the current method to inhibiting SIRT1 by EX527 in vitro and in vivo both pancreatic tumor models is unexpectedly the opposite of what was seen in vitro. In addition, a study that used short interfering RNA to target SIRT1 found that knocking down SIRT1 can result in the death of cells in the MCF-7 patient line (Peck et al., 2010). MiR-29c overexpression in cisplatin-resistant cancer cells was shown to directly target SIRT1 mRNA and suppress SIRT1 expression. This was demonstrated by Zhang et al. to regulate cell progression and apoptosis, as well as to restore chemosensitivity to cisplatin (Zhang and Luo, 2018). MiR-34a mediated SIRT1 suppression mediates apoptotic activation and chemosensitivity (Herbert et al., 2014). In addition, it is believed that SIRT1 is responsible for accelerating cell growth. In the study of SIRT1’s cellular processes in colorectal cancer, clinical data and patient samples were combined, and a mechanical technique was discovered to regulate p53 and FRA-1 via SIRT1. This approach was verified to be directly related with EMT (Cheng et al., 2016).
14 Function of SIRT1 as biomarker
In terms of genetic and epigenetic background, dietary habits, and environmental influences, it has been demonstrated that there are substantial disparities between the populations of Asians and Caucasians (Hur et al., 2008; Tarabay et al., 2016). Not only are these elements necessary for the beginning and advancement of cancer, but they are also necessary for the spread of cancer to other parts of the body (Chatterjee et al., 2018; Pavlidis and Pavlidis, 2018). Mutations and widespread polymorphisms of SIRT1 were discovered in cancer lines produced by Chinese and Japanese individuals (Shimoyama et al., 2011; Shimoyama et al., 2012; Chen et al., 2015; Lv et al., 2017) as well as 41 cancer lines (Han J. et al., 2013). We suggest that differences in SIRT1 mutations and polymorphisms may be one of the causes for differences in predicting OS and TNM stage and lymphatic metastasis of cancer on the basis of SIRT1 expression. This is despite the fact that the data on SIRT1 mutations and polymorphisms are extremely uncommon. It is important to conduct further research on this (Mei et al., 2016). It is well knowledge that metastasis is a factor that may be used to independently predict a bad prognosis for a variety of cancer types (Tsutsumi et al., 2012; Funazo et al., 2017; Ambe et al., 2018). There was a correlation between the higher expression of SIRT1 and OS, DFS, EFS, and PFS. There is a correlation between SIRT1 overexpression and TNM stage, lymph node metastasis, and distant metastasis (Sun et al., 2019); however, there is no correlation with tumor size, tissue invasion depth, differentiation, gender, or age. The overexpression of SIRT1 was found to be predictive with a worse overall survival, as well as a higher TNM stage and lymphatic metastases, in the Asian population, particularly in China. Consequently, the overexpression of SIRT1 may lead to lymphatic metastasis of malignancies, which in turn results in poor overall survival, disease-free survival, event-free survival, and progression-free survival statistics. One of the possible underlying mechanisms for metastasis is the presence of molecular events and biological processes that are mediated by SIRT1. The results of our meta-analysis are in agreement with the findings of SIRT1 being upregulated more frequently in T3 + T4, lymph node metastases, and TNM stage of colorectal cancer patients (Jiang et al., 2014). SIRT1 expression was not connected with these clinicopathological aspects, but rather a poor predictive biomarker of colorectal cancer patients (Byles et al., 2012). This is despite the fact that SIRT1 over-expression was proven to be associated with distant metastasis and histological grade (Jang S-H. et al., 2012). There was a propensity for a high SIRT1 expression to be related with positive lymph node metastasis, despite the fact that a study did not find any significant differences in lymph node metastasis compared to other studies (Otsuka et al., 2022). A high expression of SIRT1 was shown to be strongly linked with lymph node metastasis, according to the findings of two studies that were included in this comparative analysis. In breast cancer (Wu et al., 2012) and colorectal cancer (Zu et al., 2016), there has been reported to be a connection between SIRT1 expression and lymph node metastasis. Furthermore, it has been revealed that SIRT1 expression is implicated in cell migration in prostate cancer (Byles et al., 2012) and non-small-cell lung cancer (Han L. et al., 2013).
SIRT1, as a key regulator of cellular processes such as DNA repair, apoptosis, autophagy, and metabolism, has become a potential therapeutic target in cancer therapy, where both SIRT1 inducers and inhibitors are being explored for different cancer contexts. SIRT1 inducers are of particular interest in cancers where SIRT1 functions as a tumor suppressor. In many cancers, SIRT1 activation promotes genomic stability and DNA repair by deacetylating important regulators such as p53 and FOXO proteins, thereby reducing the accumulation of DNA damage. This function helps prevent oncogenesis by preserving the integrity of the genome. Inducers of SIRT1, such as resveratrol and other small molecules, have been shown to activate SIRT1’s deacetylase activity, which leads to the suppression of tumor progression through the inhibition of cancer cell proliferation and the promotion of apoptosis. Resveratrol, a naturally occurring polyphenol, has garnered attention for its ability to activate SIRT1 and its subsequent anti-cancer effects, particularly in cancers like breast and prostate cancer, where SIRT1’s tumor-suppressive role has been documented. In addition to promoting apoptosis, SIRT1 activation also stimulates autophagy, a process that allows cancer cells to degrade damaged organelles and proteins, thus reducing oxidative stress and promoting cell survival under stress conditions. This duality makes SIRT1 inducers promising for cancers where oxidative stress plays a significant role, offering a cytoprotective effect in normal tissues while targeting cancerous growth. On the other hand, SIRT1 inhibitors are being explored in cancer types where SIRT1 acts as a tumor promoter, particularly in cases of drug resistance and aggressive cancers. For example, in cancers such as hepatocellular carcinoma, pancreatic cancer, and some forms of leukemia, SIRT1 is often upregulated, which leads to enhanced survival of cancer cells through the suppression of apoptosis and the activation of pro-survival pathways. In such cases, inhibiting SIRT1 can restore the cell’s sensitivity to apoptosis-inducing therapies. SIRT1 inhibitors, such as EX527 and nicotinamide, have been shown to enhance the effectiveness of chemotherapeutic agents like cisplatin by increasing the acetylation and activity of pro-apoptotic factors such as p53. By preventing SIRT1 from deacetylating key regulators of apoptosis and cell death, these inhibitors can sensitize cancer cells to treatment, overcoming resistance and leading to more effective cancer eradication. Additionally, SIRT1 inhibitors may interfere with the autophagic survival pathways, further increasing cancer cell susceptibility to stress and cytotoxicity. However, the use of SIRT1 inhibitors must be approached cautiously, as prolonged inhibition of SIRT1 can disrupt normal cellular homeostasis, potentially leading to adverse effects such as metabolic dysregulation or damage to normal tissues. Therefore, identifying the cancer-specific roles of SIRT1 and tailoring the application of its inducers and inhibitors is critical for developing precise and effective cancer therapies.
15 Conclusion and future perspectives
The sirtuin family, particularly SIRT1, plays a crucial role in regulating cellular and biological processes. While SIRT1 is essential for normal physiological functions, its dysregulation has been linked to the pathogenesis of various diseases, including cancer. Recent studies have shown that SIRT1 is dysregulated in multiple tumor types, including brain, gastrointestinal, gynecological, and reproductive tumors. Given SIRT1’s influence on numerous pathways and its regulation by diverse upstream mediators, it is critical to delineate the specific mechanisms through which SIRT1 modulates tumorigenesis. Additionally, autophagy, a process extending beyond cell death, has been recognized for its role in tumor cell behavior, impacting cell death, growth, viability, metastasis, and therapy resistance. This review focuses on the interaction between autophagy and SIRT1 in regulating tumorigenesis. Notably, while autophagy generally contributes to protein degradation, it can specifically regulate SIRT1 by targeting it for degradation, thereby suppressing its activity. However, most research has concentrated on how SIRT1 regulates autophagy, with findings that SIRT1 can activate autophagy, including specialized forms like mitophagy and lipophagy. Such regulation can contribute to drug resistance in cancer. The impact of SIRT1-mediated autophagy on cancer drug resistance is yet to be thoroughly investigated across different cancer types and with various chemotherapeutic agents, including topoisomerases. Moreover, SIRT1’s regulation of autophagy often involves major autophagy regulators such as AMPK and mTOR. Intriguingly, SIRT1-mediated autophagy can influence apoptosis in cancer cells; for example, SIRT1-induced pro-survival autophagy can decrease apoptosis, whereas toxic autophagy can enhance it. Despite the development of several SIRT1 regulators, their direct effects on autophagy have not been extensively studied. Future research should focus on drug discovery and the development of small molecules that target SIRT1 to modulate autophagy in cancer treatment.
SIRT1 plays a dual role in cancer, acting as both a tumor suppressor and a tumor promoter depending on the cellular context. As a tumor suppressor, SIRT1 deacetylates and activates key regulatory proteins such as p53, FOXO transcription factors, and RB, which are involved in cell cycle arrest, DNA repair, and apoptosis. This promotes cellular homeostasis and reduces the likelihood of oncogenic transformation. Additionally, SIRT1’s role in maintaining genomic stability and preventing oxidative stress further supports its tumor-suppressive functions, particularly in early stages of cancer development. In various cancer types, SIRT1 overexpression has been linked to reduced tumorigenicity and enhanced sensitivity to chemotherapy. Conversely, SIRT1 can also act as a tumor promoter, particularly in advanced cancers, where it aids in tumor progression by promoting cell survival and resistance to stress. SIRT1 has been shown to inhibit apoptosis by deacetylating and inactivating pro-apoptotic factors, such as p53 and E2F1, leading to enhanced tumor cell survival. It also contributes to the activation of oncogenic pathways, including those involving NF-κB and MYC, which drive cancer cell proliferation and metastasis. Moreover, SIRT1 has been implicated in promoting drug resistance by modulating autophagy and DNA repair pathways, making tumors more resilient to conventional therapies. This dual nature of SIRT1 highlights the importance of context in determining its role in cancer progression.
SIRT’s role in autophagy is tightly linked to several key molecular pathways, such as the mTOR (mechanistic target of rapamycin) and AMPK (AMP-activated protein kinase) pathways. SIRT1 influences these pathways in ways that either promote or regulate autophagy, depending on the cellular context. SIRT1 activates autophagy primarily by deacetylating various proteins involved in the autophagic machinery, such as ATG5, ATG7, and ATG8, and also deacetylates the transcription factor FOXO3, promoting the expression of autophagy-related genes, including LC3. In the mTOR pathway, a major negative regulator of autophagy, SIRT1 indirectly inhibits mTOR, promoting autophagy. This inhibition occurs through the activation of TSC1/2, a negative regulator of mTORC1, and by activating AMPK, which enhances the inhibition of mTORC1. Under nutrient-rich conditions, mTOR suppresses autophagy by preventing autophagosome formation, but SIRT1-mediated inhibition of mTOR reverses this effect. On the other hand, SIRT1 activates AMPK by deacetylating liver kinase B1 (LKB1), which leads to the phosphorylation of TSC2 and RAPTOR, thus promoting autophagy. Activated AMPK also directly phosphorylates ULK1, an initiator of autophagy. This interaction between SIRT1 and AMPK is critical in energy-deficient states, allowing cells to initiate autophagy to survive under stress. SIRT1 also affects autophagy through its interaction with p53, a tumor suppressor that inhibits autophagy when acetylated. By deacetylating and inactivating cytoplasmic p53, SIRT1 reduces its inhibitory effects on autophagy. Furthermore, SIRT1 modulates FOXO transcription factors, particularly FOXO1 and FOXO3, which promote the expression of autophagy-related genes when deacetylated by SIRT1. This enhances autophagic processes, especially during stress conditions. Additionally, SIRT1 influences mitochondrial autophagy (mitophagy) by deacetylating and activating PGC-1α, a key regulator of mitochondrial biogenesis and energy metabolism. Another important autophagy regulator influenced by SIRT1 is Beclin-1, a key protein in autophagosome formation. SIRT1 interacts with and enhances the activity of Beclin-1, further promoting autophagy. Moreover, SIRT1 affects multiple molecular pathways, such as its interaction with mTOR and AMPK, highlighting its central role in coordinating cellular energy homeostasis and stress responses. By integrating signals from various pathways, including mTOR, AMPK, FOXO, and p53, SIRT1 balances cell survival and degradation under stress conditions. These mechanisms demonstrate the significant role of SIRT1 in promoting autophagy, making it a crucial factor in cellular health, energy regulation, and potential therapeutic targets for diseases linked to autophagy dysfunction.
As a NAD + -dependent deacetylase, SIRT1 influences cancer cell survival by modulating stress responses, DNA repair, and the tumor microenvironment, contributing to the development of resistance to chemotherapy and targeted therapies. Understanding the relationship between SIRT1 and drug resistance, particularly through autophagy, is essential to developing effective therapeutic strategies. SIRT1 contributes to drug resistance in multiple cancer types by promoting cancer cell survival under stress. It deacetylates and activates various transcription factors, such as p53, FOXO, and NF-ĸB, which are involved in cellular stress responses and apoptosis. Through these interactions, SIRT1 enhances the ability of cancer cells to withstand chemotherapeutic agents and resist apoptosis. For example, in breast cancer, SIRT1 has been shown to deacetylate and inhibit p53, a tumor suppressor, allowing cancer cells to escape apoptosis induced by DNA-damaging agents. Additionally, in hepatocellular carcinoma (HCC), SIRT1-mediated pathways are associated with resistance to sorafenib, a common drug used in HCC treatment. Autophagy is a cellular degradation process that plays a dual role in cancer, acting as a tumor suppressor in early stages and a survival mechanism in advanced cancers. SIRT1 is a key regulator of autophagy, particularly under conditions of stress, such as nutrient deprivation or chemotherapy. By deacetylating autophagy-related proteins (ATGs) and transcription factors like FOXO1/FOXO3, SIRT1 promotes the formation of autophagosomes and enhances the autophagic flux, allowing cancer cells to recycle cellular components and sustain energy production during chemotherapy-induced stress. The SIRT1-autophagy axis has been implicated in drug resistance across various cancer types. For instance, in colorectal cancer, SIRT1 activation enhances autophagy, which protects cancer cells from chemotherapy-induced apoptosis by degrading damaged organelles and proteins. In drug-resistant esophageal cancer cells, SIRT1 upregulation has been linked to increased autophagy, leading to enhanced cell survival and resistance to chemotherapy. In these cases, inhibition of SIRT1 or autophagy sensitizes cancer cells to chemotherapy, indicating the pivotal role of the SIRT1-autophagy pathway in mediating drug resistance. SIRT1 promotes autophagy by deacetylating key regulators of the autophagic process, such as Beclin-1 and LC3. It also regulates autophagy-related miRNAs, including miR-34a and miR-215, which affect the expression of autophagy proteins like Atg14. Furthermore, SIRT1 inhibits mTOR (mechanistic target of rapamycin), a negative regulator of autophagy, through pathways involving AMPK activation, thus promoting autophagy under stress conditions. This activation of autophagy by SIRT1 enables cancer cells to maintain cellular homeostasis and evade the cytotoxic effects of chemotherapy. In drug-resistant cancer cells, increased SIRT1-mediated autophagy allows the cells to clear damaged components and maintain survival despite the presence of chemotherapeutic agents. For example, in ovarian cancer, SIRT1-mediated autophagy has been shown to contribute to resistance to cisplatin, while in gastric cancer, SIRT1 enhances autophagy to protect cancer cells from apoptosis induced by 5-fluorouracil. Blocking SIRT1 or inhibiting autophagy in these models reverses drug resistance, further highlighting the importance of this pathway in maintaining cancer cell survival during treatment.
The research on SIRT1 and its role in autophagy has advanced significantly, but its complexity presents several limitations. One key challenge is the dual role of SIRT1 in cancer, where it can function as both a tumor suppressor and promoter depending on the context. In some cancers, SIRT1 activation supports autophagy and cell survival, while in others, it triggers apoptosis and suppresses tumor growth. The context-specific roles of SIRT1, as well as the dual nature of autophagy, complicate the development of generalized therapeutic strategies. This complexity makes it difficult to predict when SIRT1-mediated autophagy would either aid or hinder treatment, especially given the need to target specific cellular environments in cancer. Another limitation stems from the incomplete understanding of the molecular mechanisms behind SIRT1’s regulation of autophagy. While SIRT1’s interactions with autophagy-related proteins like Beclin-1 and FOXO have been noted, the precise pathways it influences remain unclear. This knowledge gap limits the ability to fully exploit SIRT1 as a therapeutic target. Furthermore, SIRT1 is involved in various cellular pathways, including those regulating metabolism and DNA repair, which complicates its therapeutic targeting. The potential for off-target effects or unwanted consequences from influencing multiple pathways simultaneously represents a significant challenge in developing SIRT1-targeted therapies. Currently available SIRT1 activators and inhibitors lack the specificity needed for effective clinical application. Compounds such as resveratrol and EX527 not only target SIRT1 but also affect other members of the sirtuin family and related pathways, leading to potential side effects. Furthermore, finding the optimal dosage and timing of SIRT1 modulation is challenging because over-activation or inhibition of SIRT1 can either promote survival or induce apoptosis in cancer cells. This delicate balance underscores the need for more selective and precise pharmacological tools to modulate SIRT1 activity in a controlled manner. Translating promising preclinical results into clinical practice has proven difficult, particularly due to differences between animal models and human physiology. Tumor heterogeneity further complicates the development of SIRT1-targeted therapies, as the role of SIRT1 and autophagy can vary not only between different cancer types but also within different regions of the same tumor. Additionally, cancer cells can develop resistance to SIRT1 modulators, limiting the long-term effectiveness of these treatments. Understanding and overcoming these resistance mechanisms will be essential for successful clinical application. Finally, the long-term safety of SIRT1-targeted therapies remains uncertain. SIRT1 is involved in many critical cellular processes, including aging and DNA repair, so long-term inhibition or activation could have adverse effects, such as metabolic disorders or neurodegenerative diseases. Moreover, reliable biomarkers to predict patient response to SIRT1-targeted therapies are lacking, making it difficult to assess which patients would benefit most from these treatments. Addressing these limitations will be crucial to advancing SIRT1-targeted therapies into clinical practice, offering new hope for effective cancer treatments.
Although the function of SIRT1 in the regulation of autophagy was covered in the present review, there are also other members of SIRT family participating in the regulation of autophagy. SIRT2, primarily localized in the cytoplasm, has been shown to regulate autophagy through its deacetylation of key autophagic proteins and its involvement in energy metabolism. SIRT2 can deacetylate FOXO1 and FOXO3, transcription factors that upregulate autophagy-related genes. Additionally, SIRT2 affects autophagy by modulating the acetylation of LC3, a key autophagy marker. By promoting LC3 deacetylation, SIRT2 enhances autophagosome formation and autophagic flux. SIRT2 has also been linked to the regulation of mTOR, a key negative regulator of autophagy. By inhibiting mTOR activity, SIRT2 indirectly promotes autophagy under conditions of nutrient stress. However, SIRT2’s role in autophagy can be complex, as in some contexts, it has been observed to inhibit autophagy and promote cell proliferation, particularly in cancer. SIRT3, SIRT4, and SIRT5 are mitochondrial sirtuins that regulate autophagy through their effects on mitochondrial function and metabolism. SIRT3 is the most well-studied of the three and plays a key role in regulating mitophagy, a specific form of autophagy that targets damaged mitochondria for degradation. SIRT3 deacetylates several mitochondrial proteins, enhancing mitochondrial respiration and reducing oxidative stress, which can influence autophagy activation. In response to cellular stress, SIRT3 can enhance autophagy by deacetylating and activating FOXO3, which upregulates autophagy-related genes such as Beclin-1 and LC3. Additionally, SIRT3 inhibits mTOR signaling by promoting the activation of AMPK, an energy sensor that stimulates autophagy. SIRT4, while less studied, has been shown to inhibit autophagy through its role in regulating mitochondrial glutamine metabolism. By inhibiting glutamate dehydrogenase (GDH), SIRT4 suppresses the production of ATP and thus limits the energy supply needed for autophagy, leading to decreased autophagic activity. SIRT5, a mitochondrial lysine demalonylase and desuccinylase, can also regulate mitochondrial function and oxidative stress, although its direct involvement in autophagy is still under investigation. SIRT6 is primarily a nuclear sirtuin involved in DNA repair and metabolic regulation, but it also influences autophagy. SIRT6 can enhance autophagy by promoting the activation of the AMPK pathway, leading to the inhibition of mTOR, thus stimulating autophagy. Additionally, SIRT6 regulates autophagy by deacetylating histones at the promoters of autophagy-related genes, promoting their transcription. For example, SIRT6-mediated deacetylation of histone H3 at lysine 9 (H3K9) near the promoter region of genes such as ATG5 and ATG12 enhances autophagy induction. SIRT6 also affects the autophagy-lysosomal pathway, which is critical for maintaining cellular homeostasis, particularly during stress. SIRT7, another nuclear sirtuin, has a more indirect role in autophagy regulation. SIRT7 primarily regulates ribosomal RNA (rRNA) transcription and protein synthesis, which affects cellular growth and metabolism. By modulating metabolic pathways, SIRT7 influences the availability of nutrients and energy, which can impact autophagy activation. Interestingly, SIRT7’s suppression of autophagy has been linked to its role in cancer, where it promotes cancer cell survival by limiting autophagic processes. SIRT7 has been observed to deacetylate and inhibit proteins involved in autophagy initiation, thus reducing autophagic flux in certain cancer contexts. More information about SIRT2, SIRT3, SIRT4, SIRT5, SIRT6 and SIRT7 can be found in these reviews (Chen et al., 2020; Wang et al., 2019c; Torrens-Mas et al., 2017; Alhazzazi et al., 2011; Wang et al., 2020; Tomaselli et al., 2020; Bringman-Rodenbarger et al., 2018; Lagunas-Rangel, 2023; Fiorentino et al., 2021; Tang M. et al., 2021).
Author contributions
YT: Conceptualization, Data curation, Investigation, Methodology, Project administration, Resources, Software, Supervision, Validation, Visualization, Writing–original draft, Writing–review and editing. WJ: Conceptualization, Data curation, Formal Analysis, Investigation, Methodology, Project administration, Resources, Software, Supervision, Validation, Visualization, Writing–original draft, Writing–review and editing. YL: Conceptualization, Data curation, Formal Analysis, Investigation, Methodology, Project administration, Resources, Software, Supervision, Validation, Writing–original draft, Writing–review and editing. QD: Conceptualization, Data curation, Investigation, Methodology, Project administration, Resources, Software, Supervision, Validation, Writing–original draft, Writing–review and editing.
Funding
The author(s) declare that no financial support was received for the research, authorship, and/or publication of this article.
Conflict of interest
The authors declare that the research was conducted in the absence of any commercial or financial relationships that could be construed as a potential conflict of interest.
Publisher’s note
All claims expressed in this article are solely those of the authors and do not necessarily represent those of their affiliated organizations, or those of the publisher, the editors and the reviewers. Any product that may be evaluated in this article, or claim that may be made by its manufacturer, is not guaranteed or endorsed by the publisher.
References
Ahlberg, J., and Glaumann, H. (1985). Uptake--microautophagy--and degradation of exogenous proteins by isolated rat liver lysosomes. Effects of pH, ATP, and inhibitors of proteolysis. Exp. Mol. pathology 42 (1), 78–88. doi:10.1016/0014-4800(85)90020-6
Ahlberg, J., Marzella, L., and Glaumann, H. (1982). Uptake and degradation of proteins by isolated rat liver lysosomes. Suggestion of a microautophagic pathway of proteolysis. Laboratory investigation; a J. Tech. methods pathology 47 (6), 523–532.
Ahn, J. H., and Lee, M. (2011). Suppression of autophagy sensitizes multidrug resistant cells towards Src tyrosine kinase specific inhibitor PP2. Cancer Lett. 310 (2), 188–197. doi:10.1016/j.canlet.2011.06.034
Albrecht, L. V., Bui, M. H., and De Robertis, E. M. (2019). Canonical Wnt is inhibited by targeting one-carbon metabolism through methotrexate or methionine deprivation. Proc. Natl. Acad. Sci. U. S. A. 116 (8), 2987–2995. doi:10.1073/pnas.1820161116
Albrecht, L. V., Ploper, D., Tejeda-Muñoz, N., and De Robertis, E. M. (2018). Arginine methylation is required for canonical Wnt signaling and endolysosomal trafficking. Proc. Natl. Acad. Sci. U. S. A. 115 (23), E5317–e5325. doi:10.1073/pnas.1804091115
Alhazzazi, T. Y., Kamarajan, P., Verdin, E., and Kapila, Y. L. (2011). SIRT3 and cancer: tumor promoter or suppressor? Biochimica Biophysica Acta (BBA)-Reviews Cancer 1816 (1), 80–88. doi:10.1016/j.bbcan.2011.04.004
Amaravadi, R., Kimmelman, A. C., and White, E. (2016). Recent insights into the function of autophagy in cancer. Genes and Dev. 30 (17), 1913–1930. doi:10.1101/gad.287524.116
Ambe, P. C., Gödde, D., Stoerkel, S., Zirngibl, H., and Bönicke, L. (2018). Extra nodular metastasis is a poor prognostic factor for overall survival in node-positive patients with colorectal cancer. Int. J. Colorectal Dis. 33, 403–409. doi:10.1007/s00384-018-2991-0
An, Y., Wang, B., Wang, X., Dong, G., Jia, J., and Yang, Q. (2020). SIRT1 inhibits chemoresistance and cancer stemness of gastric cancer by initiating an AMPK/FOXO3 positive feedback loop. Cell death and Dis. 11 (2), 115. doi:10.1038/s41419-020-2308-4
An, Y., Zhang, Z., Shang, Y., Jiang, X., Dong, J., Yu, P., et al. (2015). miR-23b-3p regulates the chemoresistance of gastric cancer cells by targeting ATG12 and HMGB2. Cell death and Dis. 6 (5), e1766. doi:10.1038/cddis.2015.123
Anding, A. L., and Baehrecke, E. H. (2017). Cleaning house: selective autophagy of organelles. Dev. Cell 41 (1), 10–22. doi:10.1016/j.devcel.2017.02.016
Asaka, R., Miyamoto, T., Yamada, Y., Ando, H., Mvunta, D. H., Kobara, H., et al. (2015). Sirtuin 1 promotes the growth and cisplatin resistance of endometrial carcinoma cells: a novel therapeutic target. Laboratory investigation; a J. Tech. methods pathology 95 (12), 1363–1373. doi:10.1038/labinvest.2015.119
Ashrafizadeh, M., Ahmadi, Z., Farkhondeh, T., and Samarghandian, S. (2020a). Autophagy regulation using luteolin: new insight into its anti-tumor activity. Cancer Cell Int. 20 (1), 537. doi:10.1186/s12935-020-01634-9
Ashrafizadeh, M., Paskeh, M. D. A., Mirzaei, S., Gholami, M. H., Zarrabi, A., Hashemi, F., et al. (2022). Targeting autophagy in prostate cancer: preclinical and clinical evidence for therapeutic response. J. Exp. and Clin. Cancer Res. 41 (1), 105. doi:10.1186/s13046-022-02293-6
Ashrafizadeh, M., Tavakol, S., Ahmadi, Z., Roomiani, S., Mohammadinejad, R., and Samarghandian, S. (2020b). Therapeutic effects of kaempferol affecting autophagy and endoplasmic reticulum stress. Phytotherapy Res. PTR 34 (5), 911–923. doi:10.1002/ptr.6577
Ashrafizadeh, M., Zarrabi, A., Hushmandi, K., Hashemi, F., Moghadam, E. R., Owrang, M., et al. (2021). Lung cancer cells and their sensitivity/resistance to cisplatin chemotherapy: role of microRNAs and upstream mediators. Cell. Signal. 78, 109871. doi:10.1016/j.cellsig.2020.109871
Assaye, M. A., and Gizaw, S. T. (2022). Chaperone-mediated autophagy and its implications for neurodegeneration and cancer. Int. J. general Med. 15, 5635–5649. doi:10.2147/IJGM.S368364
Auzmendi-Iriarte, J., and Matheu, A. (2020). Impact of chaperone-mediated autophagy in brain aging: neurodegenerative diseases and glioblastoma. Front. aging Neurosci. 12, 630743. doi:10.3389/fnagi.2020.630743
Ayob, A. Z., and Ramasamy, T. S. (2018). Cancer stem cells as key drivers of tumour progression. J. Biomed. Sci. 25 (1), 20. doi:10.1186/s12929-018-0426-4
Bao, L., Jaramillo, M. C., Zhang, Z., Zheng, Y., Yao, M., Zhang, D. D., et al. (2015). Induction of autophagy contributes to cisplatin resistance in human ovarian cancer cells. Mol. Med. Rep. 11 (1), 91–98. doi:10.3892/mmr.2014.2671
Belizário, J., Vieira-Cordeiro, L., and Enns, S. (2015). Necroptotic cell death signaling and execution pathway: lessons from knockout mice. Mediat. Inflamm. 2015, 128076. doi:10.1155/2015/128076
Beloribi-Djefaflia, S., Vasseur, S., and Guillaumond, F. (2016). Lipid metabolic reprogramming in cancer cells. Oncogenesis 5 (1), e189. doi:10.1038/oncsis.2015.49
Ben-Sahra, I., and Manning, B. D. (2017). mTORC1 signaling and the metabolic control of cell growth. Curr. Opin. Cell Biol. 45, 72–82. doi:10.1016/j.ceb.2017.02.012
Bersuker, K., Hendricks, J. M., Li, Z., Magtanong, L., Ford, B., Tang, P. H., et al. (2019). The CoQ oxidoreductase FSP1 acts parallel to GPX4 to inhibit ferroptosis. Nature 575 (7784), 688–692. doi:10.1038/s41586-019-1705-2
Bonadonna, G., Brusamolino, E., Valagussa, P., Rossi, A., Brugnatelli, L., Brambilla, C., et al. (1976). Combination chemotherapy as an adjuvant treatment in operable breast cancer. N. Engl. J. Med. 294 (8), 405–410. doi:10.1056/NEJM197602192940801
Bosl, G. J., Gluckman, R., Geller, N. L., Golbey, R. B., Whitmore, W. F., Herr, H., et al. (1986). VAB-6: an effective chemotherapy regimen for patients with germ-cell tumors. J. Clin. Oncol. official J. Am. Soc. Clin. Oncol. 4 (10), 1493–1499. doi:10.1200/JCO.1986.4.10.1493
Bray, F., Ferlay, J., Soerjomataram, I., Siegel, R. L., Torre, L. A., and Jemal, A. (2018). Global cancer statistics 2018: GLOBOCAN estimates of incidence and mortality worldwide for 36 cancers in 185 countries. CA a cancer J. Clin. 68 (6), 394–424. doi:10.3322/caac.21492
Bringman-Rodenbarger, L. R., Guo, A. H., Lyssiotis, C. A., and Lombard, D. B. (2018). Emerging roles for SIRT5 in metabolism and cancer. Antioxidants and redox Signal. 28 (8), 677–690. doi:10.1089/ars.2017.7264
Brooks, C. L., and Gu, W. (2008). p53 Activation: a case against Sir. Cancer Cell 13 (5), 377–378. doi:10.1016/j.ccr.2008.04.009
Byles, V., Zhu, L., Lovaas, J. D., Chmilewski, L. K., Wang, J., Faller, D. V., et al. (2012). SIRT1 induces EMT by cooperating with EMT transcription factors and enhances prostate cancer cell migration and metastasis. Oncogene 31 (43), 4619–4629. doi:10.1038/onc.2011.612
Cai, J., Wang, R., Chen, Y., Zhang, C., Fu, L., and Fan, C. (2024). LncRNA FIRRE regulated endometrial cancer radiotherapy sensitivity via the miR-199b-5p/SIRT1/BECN1 axis-mediated autophagy. Genomics 116 (1), 110750. doi:10.1016/j.ygeno.2023.110750
Carafa, V., Altucci, L., and Nebbioso, A. (2019). Dual tumor suppressor and tumor promoter action of sirtuins in determining malignant phenotype. Front. Pharmacol. 10, 38. doi:10.3389/fphar.2019.00038
Carew, J. S., Nawrocki, S. T., Kahue, C. N., Zhang, H., Yang, C., Chung, L., et al. (2007). Targeting autophagy augments the anticancer activity of the histone deacetylase inhibitor SAHA to overcome Bcr-Abl-mediated drug resistance. Blood 110 (1), 313–322. doi:10.1182/blood-2006-10-050260
Chakraborty, S., Datta, S., and Ghosh, S. (2019). Induction of autophagy under nitrosative stress: a complex regulatory interplay between SIRT1 and AMPK in MCF7 cells. Cell. Signal. 64, 109411. doi:10.1016/j.cellsig.2019.109411
Chan, H. Y., Ramasamy, T. S., Chung, F. F., and Teow, S. Y. (2024). Role of sirtuin 1 (SIRT1) in regulation of autophagy and nuclear factor-kappa Beta (NF-ĸβ) pathways in sorafenib-resistant hepatocellular carcinoma (HCC). Cell Biochem. biophysics 82 (2), 959–968. doi:10.1007/s12013-024-01247-3
Chang, W.-T., Huang, S.-C., Cheng, H.-L., Chen, S.-C., and Hsu, C.-L. (2021). Rutin and gallic acid regulates mitochondrial functions via the SIRT1 pathway in C2C12 myotubes. Antioxidants 10 (2), 286. doi:10.3390/antiox10020286
Chatterjee, A., Rodger, E. J., and Eccles, M. R. (2018). “Epigenetic drivers of tumourigenesis and cancer metastasis,” in Seminars in cancer biology (Elsevier), 149–159.
Chen, D., Yu, J., and Zhang, L. (2016). Necroptosis: an alternative cell death program defending against cancer. Biochimica Biophysica Acta (BBA) - Rev. Cancer 1865 (2), 228–236. doi:10.1016/j.bbcan.2016.03.003
Chen, G., Huang, P., and Hu, C. (2020). The role of SIRT2 in cancer: a novel therapeutic target. Int. J. cancer 147 (12), 3297–3304. doi:10.1002/ijc.33118
Chen, J., Chen, H., and Pan, L. (2021). SIRT1 and gynecological malignancies (Review). Oncol. Rep. 45 (4), 43. doi:10.3892/or.2021.7994
Chen, J., Zhang, B., Wong, N., Lo, A. W., To, K. F., Chan, A. W., et al. (2011). Sirtuin 1 is upregulated in a subset of hepatocellular carcinomas where it is essential for telomere maintenance and tumor cell growth. Cancer Res. 71 (12), 4138–4149. doi:10.1158/0008-5472.CAN-10-4274
Chen, Z., Jiang, Q., Zhu, P., Chen, Y., Xie, X., Du, Z., et al. (2019). NPRL2 enhances autophagy and the resistance to Everolimus in castration-resistant prostate cancer. Prostate 79 (1), 44–53. doi:10.1002/pros.23709
Chen, Z., Zhai, Y., Zhang, W., Teng, Y., and Yao, K. (2015). Single nucleotide polymorphisms of the sirtuin 1 (SIRT1) gene are associated with age-related macular degeneration in Chinese han individuals: a case–control pilot study. Medicine 94 (49), e2238. doi:10.1097/MD.0000000000002238
Cheng, F., Su, L., Yao, C., Liu, L., Shen, J., Liu, C., et al. (2016). SIRT1 promotes epithelial–mesenchymal transition and metastasis in colorectal cancer by regulating Fra-1 expression. Cancer Lett. 375 (2), 274–283. doi:10.1016/j.canlet.2016.03.010
Chi, H. (2012). Regulation and function of mTOR signalling in T cell fate decisions. Nat. Rev. Immunol. 12 (5), 325–338. doi:10.1038/nri3198
Cho, Y., Challa, S., Moquin, D., Genga, R., Ray, T. D., Guildford, M., et al. (2009). Phosphorylation-driven assembly of the RIP1-RIP3 complex regulates programmed necrosis and virus-induced inflammation. Cell 137 (6), 1112–1123. doi:10.1016/j.cell.2009.05.037
Cho, Y., Hwang, J. W., Park, N. J., Moon, J., Ali, K. H., Seo, Y. H., et al. (2023). SPC-180002, a SIRT1/3 dual inhibitor, impairs mitochondrial function and redox homeostasis and represents an antitumor activity. Free Radic. Biol. and Med. 208, 73–87. doi:10.1016/j.freeradbiomed.2023.07.033
Citron, M. L., Berry, D. A., Cirrincione, C., Hudis, C., Winer, E. P., Gradishar, W. J., et al. (2003). Randomized trial of dose-dense versus conventionally scheduled and sequential versus concurrent combination chemotherapy as postoperative adjuvant treatment of node-positive primary breast cancer: first report of Intergroup Trial C9741/Cancer and Leukemia Group B Trial 9741. J. Clin. Oncol. official J. Am. Soc. Clin. Oncol. 21 (8), 1431–1439. doi:10.1200/JCO.2003.09.081
Claerhout, S., Verschooten, L., Van Kelst, S., De Vos, R., Proby, C., Agostinis, P., et al. (2010). Concomitant inhibition of AKT and autophagy is required for efficient cisplatin-induced apoptosis of metastatic skin carcinoma. Int. J. cancer 127 (12), 2790–2803. doi:10.1002/ijc.25300
Condello, M., Mancini, G., and Meschini, S. (2020). The exploitation of liposomes in the inhibition of autophagy to defeat drug resistance. Front. Pharmacol. 11, 787. doi:10.3389/fphar.2020.00787
Crofton, J. (1959). Chemotherapy of pulmonary tuberculosis. Br. Med. J. 1 (5138), 1610–1614. doi:10.1136/bmj.1.5138.1610
Cuervo, A. M. (2004). Autophagy: many paths to the same end. Mol. Cell. Biochem. 263 (1-2), 55–72. doi:10.1023/b:mcbi.0000041848.57020.57
Cuervo, A. M., and Wong, E. (2014). Chaperone-mediated autophagy: roles in disease and aging. Cell Res. 24 (1), 92–104. doi:10.1038/cr.2013.153
Cui, Y., Tian, J., Wang, Z., Guo, H., Zhang, H., Wang, Z., et al. (2023). Fructose-induced mTORC1 activation promotes pancreatic cancer progression through inhibition of autophagy. Cancer Res. 83 (24), 4063–4079. doi:10.1158/0008-5472.CAN-23-0464
Dai, W., Zhou, J., Jin, B., and Pan, J. (2016). Class III-specific HDAC inhibitor Tenovin-6 induces apoptosis, suppresses migration and eliminates cancer stem cells in uveal melanoma. Sci. Rep. 6, 22622. doi:10.1038/srep22622
Dalby, K. N., Tekedereli, I., Lopez-Berestein, G., and Ozpolat, B. (2010). Targeting the prodeath and prosurvival functions of autophagy as novel therapeutic strategies in cancer. Autophagy 6 (3), 322–329. doi:10.4161/auto.6.3.11625
Debnath, J., Gammoh, N., and Ryan, K. M. (2023). Autophagy and autophagy-related pathways in cancer. Nat. Rev. Mol. Cell Biol. 24 (8), 560–575. doi:10.1038/s41580-023-00585-z
Degterev, A., Hitomi, J., Germscheid, M., Ch'en, I. L., Korkina, O., Teng, X., et al. (2008). Identification of RIP1 kinase as a specific cellular target of necrostatins. Nat. Chem. Biol. 4 (5), 313–321. doi:10.1038/nchembio.83
Degterev, A., Huang, Z., Boyce, M., Li, Y., Jagtap, P., Mizushima, N., et al. (2005). Chemical inhibitor of nonapoptotic cell death with therapeutic potential for ischemic brain injury. Nat. Chem. Biol. 1 (2), 112–119. doi:10.1038/nchembio711
DeVita, V. T., Simon, R. M., Hubbard, S. M., Young, R. C., Berard, C. W., Moxley, J. H., et al. (1980). Curability of advanced Hodgkin's disease with chemotherapy. Long-term follow-up of MOPP-treated patients at the National Cancer Institute. Ann. Intern. Med. 92 (5), 587–595. doi:10.7326/0003-4819-92-5-587
de Waal, E. J., Vreeling-Sindelárová, H., Schellens, J. P., Houtkooper, J. M., and James, J. (1986). Quantitative changes in the lysosomal vacuolar system of rat hepatocytes during short-term starvation. A morphometric analysis with special reference to macro- and microautophagy. Cell tissue Res. 243 (3), 641–648. doi:10.1007/BF00218073
Dixon, S. J., Lemberg, K. M., Lamprecht, M. R., Skouta, R., Zaitsev, E. M., Gleason, C. E., et al. (2012). Ferroptosis: an iron-dependent form of nonapoptotic cell death. Cell 149 (5), 1060–1072. doi:10.1016/j.cell.2012.03.042
Doll, S., Freitas, F. P., Shah, R., Aldrovandi, M., da Silva, M. C., Ingold, I., et al. (2019). FSP1 is a glutathione-independent ferroptosis suppressor. Nature 575 (7784), 693–698. doi:10.1038/s41586-019-1707-0
Du, R., Zhao, P., Wu, S., Gao, Y., Wu, R., Yang, M., et al. (2021). Sendeng-4 suppressed melanoma growth by induction of autophagy and apoptosis. Evidence-based complementary Altern. Med. eCAM 2021, 5519973. doi:10.1155/2021/5519973
Egan, D. F., Shackelford, D. B., Mihaylova, M. M., Gelino, S., Kohnz, R. A., Mair, W., et al. (2011). Phosphorylation of ULK1 (hATG1) by AMP-activated protein kinase connects energy sensing to mitophagy. Sci. (New York, NY) 331 (6016), 456–461. doi:10.1126/science.1196371
El-Kott, A. F., Al-Kahtani, M. A., and Shati, A. A. (2019). Calycosin induces apoptosis in adenocarcinoma HT29 cells by inducing cytotoxic autophagy mediated by SIRT1/AMPK-induced inhibition of Akt/mTOR. Clin. Exp. Pharmacol. and physiology 46 (10), 944–954. doi:10.1111/1440-1681.13133
Elmore, S. (2007). Apoptosis: a review of programmed cell death. Toxicol. Pathol. 35 (4), 495–516. doi:10.1080/01926230701320337
Fan, Q. W., Cheng, C., Hackett, C., Feldman, M., Houseman, B. T., Nicolaides, T., et al. (2010). Akt and autophagy cooperate to promote survival of drug-resistant glioma. Sci. Signal. 3 (147), ra81. doi:10.1126/scisignal.2001017
Fan, Y., Hou, T., Gao, Y., Dan, W., Liu, T., Liu, B., et al. (2021). Acetylation-dependent regulation of TPD52 isoform 1 modulates chaperone-mediated autophagy in prostate cancer. Autophagy 17 (12), 4386–4400. doi:10.1080/15548627.2021.1917130
Farber, S., and Diamond, L. K. (1948). Temporary remissions in acute leukemia in children produced by folic acid antagonist, 4-aminopteroyl-glutamic acid. N. Engl. J. Med. 238 (23), 787–793. doi:10.1056/NEJM194806032382301
Farcas, M., Gavrea, A. A., Gulei, D., Ionescu, C., Irimie, A., Catana, C. S., et al. (2019). SIRT1 in the development and treatment of hepatocellular carcinoma. Front. Nutr. 6, 148. doi:10.3389/fnut.2019.00148
Fernández, Á. F., Sebti, S., Wei, Y., Zou, Z., Shi, M., McMillan, K. L., et al. (2018). Disruption of the beclin 1–BCL2 autophagy regulatory complex promotes longevity in mice. Nature 558 (7708), 136–140. doi:10.1038/s41586-018-0162-7
Ferri, K. F., and Kroemer, G. (2001). Organelle-specific initiation of cell death pathways. Nat. Cell Biol. 3 (11), E255–E263. doi:10.1038/ncb1101-e255
Ferro, F., Servais, S., Besson, P., Roger, S., Dumas, J.-F., and Brisson, L. (2020). Autophagy and mitophagy in cancer metabolic remodelling. Seminars Cell and Dev. Biol. 98, 129–138. doi:10.1016/j.semcdb.2019.05.029
Fiorentino, F., Carafa, V., Favale, G., Altucci, L., Mai, A., and Rotili, D. (2021). The two-faced role of SIRT6 in cancer. Cancers 13 (5), 1156. doi:10.3390/cancers13051156
Frye, R. A. (1999). Characterization of five human cDNAs with homology to the yeast SIR2 gene: Sir2-like proteins (sirtuins) metabolize NAD and may have protein ADP-ribosyltransferase activity. Biochem. biophysical Res. Commun. 260 (1), 273–279. doi:10.1006/bbrc.1999.0897
Frye, R. A. (2000). Phylogenetic classification of prokaryotic and eukaryotic Sir2-like proteins. Biochem. biophysical Res. Commun. 273 (2), 793–798. doi:10.1006/bbrc.2000.3000
Fu, L., Han, B. K., Meng, F. F., Wang, J. W., Wang, T. Y., Li, H. J., et al. (2021). Jaridon 6, a new diterpene from Rabdosia rubescens (Hemsl.) Hara, can display anti-gastric cancer resistance by inhibiting SIRT1 and inducing autophagy. Phytotherapy Res. PTR 35 (10), 5720–5733. doi:10.1002/ptr.7231
Funazo, T., Nomizo, T., and Kim, Y. H. (2017). Liver metastasis is associated with poor progression-free survival in patients with non–small cell lung cancer treated with nivolumab. J. Thorac. Oncol. 12 (9), e140–e141. doi:10.1016/j.jtho.2017.04.027
Galluzzi, L., Spranger, S., Fuchs, E., and López-Soto, A. (2019). WNT signaling in cancer immunosurveillance. Trends Cell Biol. 29 (1), 44–65. doi:10.1016/j.tcb.2018.08.005
Galluzzi, L., Vitale, I., Aaronson, S. A., Abrams, J. M., Adam, D., Agostinis, P., et al. (2018). Molecular mechanisms of cell death: recommendations of the nomenclature committee on cell death 2018. Cell death Differ. 25 (3), 486–541. doi:10.1038/s41418-017-0012-4
Galluzzi, L., Vitale, I., Abrams, J., Alnemri, E., Baehrecke, E., Blagosklonny, M., et al. (2012). Molecular definitions of cell death subroutines: recommendations of the Nomenclature Committee on Cell Death 2012. Cell Death and Differ. 19 (1), 107–120. doi:10.1038/cdd.2011.96
Gao, X., Zacharek, A., Salkowski, A., Grignon, D. J., Sakr, W., Porter, A. T., et al. (1995). Loss of heterozygosity of the BRCA1 and other loci on chromosome 17q in human prostate cancer. Cancer Res. 55 (5), 1002–1005.
Ge, J., Chen, Z., Huang, J., Chen, J., Yuan, W., Deng, Z., et al. (2014). Upregulation of autophagy-related gene-5 (ATG-5) is associated with chemoresistance in human gastric cancer. PloS one 9 (10), e110293. doi:10.1371/journal.pone.0110293
Golstein, P., and Kroemer, G. (2007). Cell death by necrosis: towards a molecular definition. Trends Biochem. Sci. 32 (1), 37–43. doi:10.1016/j.tibs.2006.11.001
Goodman, L. S., Wintrobe, M. M., Dameshek, W., Goodman, M. J., Gilman, A., and McLennan, M. T. (1946). Nitrogen mustard therapy; use of methyl-bis (beta-chloroethyl) amine hydrochloride and tris (beta-chloroethyl) amine hydrochloride for Hodgkin's disease, lymphosarcoma, leukemia and certain allied and miscellaneous disorders. J. Am. Med. Assoc. 132, 126–132. doi:10.1001/jama.1946.02870380008004
Guan, J., Zhang, Z. Y., Sun, J. H., Wang, X. P., Zhou, Z. Q., and Qin, L. (2023). LITAF inhibits colorectal cancer stemness and metastatic behavior by regulating FOXO1-mediated SIRT1 expression. Clin. and Exp. metastasis 40 (4), 309–320. doi:10.1007/s10585-023-10213-x
Guo, H., Ding, H., Tang, X., Liang, M., Li, S., Zhang, J., et al. (2021). Quercetin induces pro-apoptotic autophagy via SIRT1/AMPK signaling pathway in human lung cancer cell lines A549 and H1299 in vitro. Thorac. cancer 12 (9), 1415–1422. doi:10.1111/1759-7714.13925
Han, J., Hubbard, B. P., Lee, J., Montagna, C., Lee, H.-W., Sinclair, D. A., et al. (2013a). Analysis of 41 cancer cell lines reveals excessive allelic loss and novel mutations in the SIRT1 gene. Cell Cycle 12 (2), 263–270. doi:10.4161/cc.23056
Han, L., Liang, X. H., Chen, L. X., Bao, S. M., and Yan, Z. Q. (2013b). SIRT1 is highly expressed in brain metastasis tissues of non-small cell lung cancer (NSCLC) and in positive regulation of NSCLC cell migration. Int. J. Clin. Exp. pathology 6 (11), 2357–2365.
Hanahan, D., and Weinberg, R. A. (2011). Hallmarks of cancer: the next generation. Cell 144 (5), 646–674. doi:10.1016/j.cell.2011.02.013
Hao, C., Zhu, P. X., Yang, X., Han, Z. P., Jiang, J. H., Zong, C., et al. (2014). Overexpression of SIRT1 promotes metastasis through epithelial-mesenchymal transition in hepatocellular carcinoma. BMC cancer 14, 978. doi:10.1186/1471-2407-14-978
Harguindey, S., Orive, G., Luis Pedraz, J., Paradiso, A., and Reshkin, S. J. (2005). The role of pH dynamics and the Na+/H+ antiporter in the etiopathogenesis and treatment of cancer. Two faces of the same coin--one single nature. Biochimica biophysica acta 1756 (1), 1–24. doi:10.1016/j.bbcan.2005.06.004
Hawley, S. A., Davison, M., Woods, A., Davies, S. P., Beri, R. K., Carling, D., et al. (1996). Characterization of the AMP-activated protein kinase kinase from rat liver and identification of threonine 172 as the major site at which it phosphorylates AMP-activated protein kinase. J. Biol. Chem. 271 (44), 27879–27887. doi:10.1074/jbc.271.44.27879
He, F., Chen, Y., He, D., and He, S. (2023). USP14-mediated deubiquitination of SIRT1 in macrophage promotes fatty acid oxidation amplification and M2 phenotype polarization. Biochem. biophysical Res. Commun. 646, 19–29. doi:10.1016/j.bbrc.2022.12.076
He, S., Wang, L., Miao, L., Wang, T., Du, F., Zhao, L., et al. (2009). Receptor interacting protein kinase-3 determines cellular necrotic response to TNF-alpha. Cell 137 (6), 1100–1111. doi:10.1016/j.cell.2009.05.021
Heltweg, B., Gatbonton, T., Schuler, A. D., Posakony, J., Li, H., Goehle, S., et al. (2006). Antitumor activity of a small-molecule inhibitor of human silent information regulator 2 enzymes. Cancer Res. 66 (8), 4368–4377. doi:10.1158/0008-5472.CAN-05-3617
Herbert, K. J., Cook, A. L., and Snow, E. T. (2014). SIRT1 inhibition restores apoptotic sensitivity in p53-mutated human keratinocytes. Toxicol. Appl. Pharmacol. 277 (3), 288–297. doi:10.1016/j.taap.2014.04.001
Heshmati, M., Soltani, A., Sanaei, M. J., Nahid-Samiei, M., Shirzad, H., Jami, M. S., et al. (2020). Ghrelin induces autophagy and CXCR4 expression via the SIRT1/AMPK axis in lymphoblastic leukemia cell lines. Cell. Signal. 66, 109492. doi:10.1016/j.cellsig.2019.109492
Hill, C., and Wang, Y. (2020). The importance of epithelial-mesenchymal transition and autophagy in cancer drug resistance. Cancer drug Resist. (Alhambra, Calif.) 3 (1), 38–47. doi:10.20517/cdr.2019.75
Hirano, T. (2002). The ABCs of SMC proteins: two-armed ATPases for chromosome condensation, cohesion, and repair. Genes and Dev. 16 (4), 399–414. doi:10.1101/gad.955102
Hirano, T., Kobayashi, R., and Hirano, M. (1997). Condensins, chromosome condensation protein complexes containing XCAP-C, XCAP-E and a Xenopus homolog of the Drosophila Barren protein. Cell 89 (4), 511–521. doi:10.1016/s0092-8674(00)80233-0
Holler, N., Zaru, R., Micheau, O., Thome, M., Attinger, A., Valitutti, S., et al. (2000). Fas triggers an alternative, caspase-8–independent cell death pathway using the kinase RIP as effector molecule. Nat. Immunol. 1 (6), 489–495. doi:10.1038/82732
Hosokawa, N., Hara, T., Kaizuka, T., Kishi, C., Takamura, A., Miura, Y., et al. (2009). Nutrient-dependent mTORC1 association with the ULK1–Atg13–FIP200 complex required for autophagy. Mol. Biol. Cell 20 (7), 1981–1991. doi:10.1091/mbc.e08-12-1248
Housman, G., Byler, S., Heerboth, S., Lapinska, K., Longacre, M., Snyder, N., et al. (2014). Drug resistance in cancer: an overview. Cancers 6 (3), 1769–1792. doi:10.3390/cancers6031769
Hryniuk, W., and Bush, H. (1984). The importance of dose intensity in chemotherapy of metastatic breast cancer. J. Clin. Oncol. official J. Am. Soc. Clin. Oncol. 2 (11), 1281–1288. doi:10.1200/JCO.1984.2.11.1281
Hu, J., Dong, S. W., Pei, Y., Wang, J., Zhang, J., and Wei, X. P. (2021). LncRNA MITA1 promotes gefitinib resistance by inducing autophagy in lung cancer cells. Biochem. biophysical Res. Commun. 551, 21–26. doi:10.1016/j.bbrc.2021.02.130
Huang, M., Wang, J., Zhang, Z., and Zuo, X. (2024). ZMIZ1 regulates proliferation, autophagy and apoptosis of colon cancer cells by mediating ubiquitin-proteasome degradation of SIRT1. Biochem. Genet. 62 (4), 3245–3259. doi:10.1007/s10528-023-10573-9
Hur, Y., Kaprio, J., Iacono, W., Boomsma, D., McGue, M., Silventoinen, K., et al. (2008). Genetic influences on the difference in variability of height, weight and body mass index between Caucasian and East Asian adolescent twins. Int. J. Obes. 32 (10), 1455–1467. doi:10.1038/ijo.2008.144
Ichikawa, A., Fujita, Y., Hosaka, Y., Kadota, T., Ito, A., Yagishita, S., et al. (2020). Chaperone-mediated autophagy receptor modulates tumor growth and chemoresistance in non-small cell lung cancer. Cancer Sci. 111 (11), 4154–4165. doi:10.1111/cas.14629
Ichimura, Y., Kirisako, T., Takao, T., Satomi, Y., Shimonishi, Y., Ishihara, N., et al. (2000). A ubiquitin-like system mediates protein lipidation. Nature 408 (6811), 488–492. doi:10.1038/35044114
Ingold, I., Berndt, C., Schmitt, S., Doll, S., Poschmann, G., Buday, K., et al. (2018). Selenium utilization by GPX4 is required to prevent hydroperoxide-induced ferroptosis. Cell 172 (3), 409–422. doi:10.1016/j.cell.2017.11.048
Inoue, T., Hiratsuka, M., Osaki, M., Yamada, H., Kishimoto, I., Yamaguchi, S., et al. (2007). SIRT2, a tubulin deacetylase, acts to block the entry to chromosome condensation in response to mitotic stress. Oncogene 26 (7), 945–957. doi:10.1038/sj.onc.1209857
Islam, A., Chang, Y. C., Chen, X. C., Weng, C. W., Chen, C. Y., Wang, C. W., et al. (2024a). Water-soluble 4-(dimethylaminomethyl)heliomycin exerts greater antitumor effects than parental heliomycin by targeting the tNOX-SIRT1 axis and apoptosis in oral cancer cells. eLife 12. doi:10.7554/eLife.87873
Islam, A., Chang, Y. C., Tsao, N. W., Wang, S. Y., and Chueh, P. J. (2024b). Calocedrus formosana essential oils induce ROS-mediated autophagy and apoptosis by targeting SIRT1 in colon cancer cells. Antioxidants (Basel, Switz.) 13 (3), 284. doi:10.3390/antiox13030284
Jang, K. Y., Noh, S. J., Lehwald, N., Tao, G. Z., Bellovin, D. I., Park, H. S., et al. (2012a). SIRT1 and c-Myc promote liver tumor cell survival and predict poor survival of human hepatocellular carcinomas. PloS one 7 (9), e45119. doi:10.1371/journal.pone.0045119
Jang, S.-H., Min, K.-W., Paik, S. S., and Jang, K.-S. (2012b). Loss of SIRT1 histone deacetylase expression associates with tumour progression in colorectal adenocarcinoma. J. Clin. pathology 65 (8), 735–739. doi:10.1136/jclinpath-2012-200685
Ji, J., Wang, K., Meng, X., Zhong, H., Li, X., Zhao, H., et al. (2022). Elaiophylin inhibits tumorigenesis of human lung adenocarcinoma by inhibiting mitophagy via suppression of SIRT1/nrf2 signaling. Cancers 14 (23), 5812. doi:10.3390/cancers14235812
Jiang, K., Lyu, L., Shen, Z., Zhang, J., Zhang, H., Dong, J., et al. (2014). Overexpression of SIRT1 is a poor prognostic factor for advanced colorectal cancer. Chin. Med. J. 127 (11), 2021–2024. doi:10.3760/cma.j.issn.0366-6999.20140044
Jiang, P., and Mizushima, N. (2014). Autophagy and human diseases. Cell Res. 24 (1), 69–79. doi:10.1038/cr.2013.161
Jiang, X., Stockwell, B. R., and Conrad, M. (2021). Ferroptosis: mechanisms, biology and role in disease. Nat. Rev. Mol. Cell Biol. 22 (4), 266–282. doi:10.1038/s41580-020-00324-8
Jiao, F., and Gong, Z. (2020). The beneficial roles of SIRT1 in neuroinflammation-related diseases. Oxidative Med. Cell. Longev. 2020, 6782872. doi:10.1155/2020/6782872
Jin, J., Fan, Z., Long, Y., Li, Y., He, Q., Yang, Y., et al. (2024). Matrine induces ferroptosis in cervical cancer through activation of piezo1 channel. Phytomedicine Int. J. phytotherapy Phytopharm. 122, 155165. doi:10.1016/j.phymed.2023.155165
Jin, Q., Yan, T., Ge, X., Sun, C., Shi, X., and Zhai, Q. (2007). Cytoplasm-localized SIRT1 enhances apoptosis. J. Cell. physiology 213 (1), 88–97. doi:10.1002/jcp.21091
Jin, Y., Qiu, J., Lu, X., and Li, G. (2022). C-MYC inhibited ferroptosis and promoted immune evasion in ovarian cancer cells through NCOA4 mediated ferritin autophagy. Cells 11 (24), 4127. doi:10.3390/cells11244127
Jing, Z., He, X., Jia, Z., Sa, Y., Yang, B., and Liu, P. (2021). NCAPD2 inhibits autophagy by regulating Ca(2+)/CAMKK2/AMPK/mTORC1 pathway and PARP-1/SIRT1 axis to promote colorectal cancer. Cancer Lett. 520, 26–37. doi:10.1016/j.canlet.2021.06.029
Jung, C. H., Jun, C. B., Ro, S.-H., Kim, Y.-M., Otto, N. M., Cao, J., et al. (2009). ULK-Atg13-FIP200 complexes mediate mTOR signaling to the autophagy machinery. Mol. Biol. Cell 20 (7), 1992–2003. doi:10.1091/mbc.e08-12-1249
Kagan, V. E., Mao, G., Qu, F., Angeli, J. P., Doll, S., Croix, C. S., et al. (2017). Oxidized arachidonic and adrenic PEs navigate cells to ferroptosis. Nat. Chem. Biol. 13 (1), 81–90. doi:10.1038/nchembio.2238
Kalle, A. M., Mallika, A., Badiger, J., Talukdar, P., and Sachchidanand, (2010). Inhibition of SIRT1 by a small molecule induces apoptosis in breast cancer cells. Biochem. biophysical Res. Commun. 401 (1), 13–19. doi:10.1016/j.bbrc.2010.08.118
Kang, J. A., Kim, Y. J., Jang, K. Y., Moon, H. W., Lee, H., Lee, S., et al. (2024). SIRT1 ISGylation accelerates tumor progression by unleashing SIRT1 from the inactive state to promote its deacetylase activity. Exp. and Mol. Med. 56 (3), 656–673. doi:10.1038/s12276-024-01194-2
Karantza-Wadsworth, V., Patel, S., Kravchuk, O., Chen, G., Mathew, R., Jin, S., et al. (2007). Autophagy mitigates metabolic stress and genome damage in mammary tumorigenesis. Genes Dev. 21 (13), 1621–1635. doi:10.1101/gad.1565707
Kaushik, S., Bandyopadhyay, U., Sridhar, S., Kiffin, R., Martinez-Vicente, M., Kon, M., et al. (2011). Chaperone-mediated autophagy at a glance. J. Cell Sci. 124 (Pt 4), 495–499. doi:10.1242/jcs.073874
Kennedy, B. K., and Lamming, D. W. (2016). The mechanistic target of rapamycin: the grand conducTOR of metabolism and aging. Cell metab. 23 (6), 990–1003. doi:10.1016/j.cmet.2016.05.009
Kim, J., Kundu, M., Viollet, B., and Guan, K.-L. (2011). AMPK and mTOR regulate autophagy through direct phosphorylation of Ulk1. Nat. Cell Biol. 13 (2), 132–141. doi:10.1038/ncb2152
Kim, J. K., Silwal, P., and Jo, E. K. (2022). Sirtuin 1 in host defense during infection. Cells 11 (18), 2921. doi:10.3390/cells11182921
Kim, K. H., and Lee, M.-S. (2014). Autophagy—a key player in cellular and body metabolism. Nat. Rev. Endocrinol. 10 (6), 322–337. doi:10.1038/nrendo.2014.35
Kimura, K., and Hirano, T. (2000). Dual roles of the 11S regulatory subcomplex in condensin functions. Proc. Natl. Acad. Sci. 97 (22), 11972–11977. doi:10.1073/pnas.220326097
Kiraz, Y., Adan, A., Kartal Yandim, M., and Baran, Y. (2016). Major apoptotic mechanisms and genes involved in apoptosis. Tumor Biol. 37 (7), 8471–8486. doi:10.1007/s13277-016-5035-9
Kirkin, V., and Rogov, V. V. (2019). A diversity of selective autophagy receptors determines the specificity of the autophagy pathway. Mol. Cell 76 (2), 268–285. doi:10.1016/j.molcel.2019.09.005
Kraft, V. A. N., Bezjian, C. T., Pfeiffer, S., Ringelstetter, L., Müller, C., Zandkarimi, F., et al. (2020). GTP cyclohydrolase 1/tetrahydrobiopterin counteract ferroptosis through lipid remodeling. ACS central Sci. 6 (1), 41–53. doi:10.1021/acscentsci.9b01063
Kroemer, G., and Jäättelä, M. (2005). Lysosomes and autophagy in cell death control. Nat. Rev. Cancer 5 (11), 886–897. doi:10.1038/nrc1738
Kumar, A., and Chauhan, S. (2016). How much successful are the medicinal chemists in modulation of SIRT1: a critical review. Eur. J. Med. Chem. 119, 45–69. doi:10.1016/j.ejmech.2016.04.063
Lagunas-Rangel, F. A. (2023). Role of SIRT5 in cancer. Friend or foe? Biochimie 209, 131–141. doi:10.1016/j.biochi.2023.02.008
Lain, S., Hollick, J. J., Campbell, J., Staples, O. D., Higgins, M., Aoubala, M., et al. (2008). Discovery, in vivo activity, and mechanism of action of a small-molecule p53 activator. Cancer Cell 13 (5), 454–463. doi:10.1016/j.ccr.2008.03.004
Lara, E., Mai, A., Calvanese, V., Altucci, L., Lopez-Nieva, P., Martinez-Chantar, M., et al. (2009). Salermide, a Sirtuin inhibitor with a strong cancer-specific proapoptotic effect. Oncogene 28 (6), 781–791. doi:10.1038/onc.2008.436
Lee, J., You, J. H., and Roh, J. L. (2022b). Poly(rC)-binding protein 1 represses ferritinophagy-mediated ferroptosis in head and neck cancer. Redox Biol. 51, 102276. doi:10.1016/j.redox.2022.102276
Lee, J. G., Shin, J. H., Shim, H. S., Lee, C. Y., Kim, D. J., Kim, Y. S., et al. (2015). Autophagy contributes to the chemo-resistance of non-small cell lung cancer in hypoxic conditions. Respir. Res. 16, 138. doi:10.1186/s12931-015-0285-4
Lee, J. H., Cheng, R., Rogaeva, E., Meng, Y., Stern, Y., Santana, V., et al. (2008). Further examination of the candidate genes in chromosome 12p13 locus for late-onset alzheimer disease. Neurogenetics 9, 127–138. doi:10.1007/s10048-008-0122-8
Lee, S. J., Choi, Y. J., Kim, H. I., Moon, H. E., Paek, S. H., Kim, T. Y., et al. (2022a). Platycodin D inhibits autophagy and increases glioblastoma cell death via LDLR upregulation. Mol. Oncol. 16 (1), 250–268. doi:10.1002/1878-0261.12966
Lei, G., Zhuang, L., and Gan, B. (2022). Targeting ferroptosis as a vulnerability in cancer. Nat. Rev. Cancer 22 (7), 381–396. doi:10.1038/s41568-022-00459-0
Li, H., Sun, Y., Yao, Y., Ke, S., Zhang, N., Xiong, W., et al. (2024b). USP8-governed GPX4 homeostasis orchestrates ferroptosis and cancer immunotherapy. Proc. Natl. Acad. Sci. U. S. A. 121 (16), e2315541121. doi:10.1073/pnas.2315541121
Li, H., Yu, K., Hu, H., Zhang, X., Zeng, S., Li, J., et al. (2024a). METTL17 coordinates ferroptosis and tumorigenesis by regulating mitochondrial translation in colorectal cancer. Redox Biol. 71, 103087. doi:10.1016/j.redox.2024.103087
Li, J., Han, Y., Zhou, M., Liu, N., Li, H., Huang, G., et al. (2023b). Electroacupuncture ameliorates AOM/DSS-induced mice colorectal cancer by inhibiting inflammation and promoting autophagy via the SIRT1/miR-215/Atg14 axis. Aging 15 (22), 13194–13212. doi:10.18632/aging.205236
Li, J., Hou, N., Faried, A., Tsutsumi, S., and Kuwano, H. (2010). Inhibition of autophagy augments 5-fluorouracil chemotherapy in human colon cancer in vitro and in vivo model. Eur. J. cancer (Oxford, Engl. 1990) 46 (10), 1900–1909. doi:10.1016/j.ejca.2010.02.021
Li, L., Liu, W., Sun, Q., Zhu, H., Hong, M., and Qian, S. (2021). Decitabine downregulates TIGAR to induce apoptosis and autophagy in myeloid leukemia cells. Oxidative Med. Cell. Longev. 2021, 8877460. doi:10.1155/2021/8877460
Li, X., Song, D., Chen, Y., Huang, C., Liu, A., Wu, Q., et al. (2023a). NSD2 methylates AROS to promote SIRT1 activation and regulates fatty acid metabolism-mediated cancer radiotherapy. Cell Rep. 42 (10), 113126. doi:10.1016/j.celrep.2023.113126
Li, Y., Chu, L. W., Li, Z., Yik, P.-Y., and Song, Y.-Q. (2009). A study on the association of the chromosome 12p13 locus with sporadic late-onset alzheimer’s disease in Chinese. Dementia geriatric cognitive Disord. 27 (6), 508–512. doi:10.1159/000218740
Li, Y. J., Lei, Y. H., Yao, N., Wang, C. R., Hu, N., Ye, W. C., et al. (2017). Autophagy and multidrug resistance in cancer. Chin. J. cancer 36 (1), 52. doi:10.1186/s40880-017-0219-2
Liang, X., Tang, J., Liang, Y., Jin, R., and Cai, X. (2014a). Suppression of autophagy by chloroquine sensitizes 5-fluorouracil-mediated cell death in gallbladder carcinoma cells. Cell and Biosci. 4 (1), 10. doi:10.1186/2045-3701-4-10
Liang, X. H., Jackson, S., Seaman, M., Brown, K., Kempkes, B., Hibshoosh, H., et al. (1999). Induction of autophagy and inhibition of tumorigenesis by beclin 1. Nature 402 (6762), 672–676. doi:10.1038/45257
Liang, X. H., Yu, J., Brown, K., and Levine, B. (2001). Beclin 1 contains a leucine-rich nuclear export signal that is required for its autophagy and tumor suppressor function. Cancer Res. 61 (8), 3443–3449.
Liang, Z., Yang, Y., Wang, H., Yi, W., Yan, X., Yan, J., et al. (2014b). Inhibition of SIRT1 signaling sensitizes the antitumor activity of silybin against human lung adenocarcinoma cells in vitro and in vivo. Mol. cancer Ther. 13 (7), 1860–1872. doi:10.1158/1535-7163.MCT-13-0942
Lin, Z., and Fang, D. (2013). The roles of SIRT1 in cancer. Genes and cancer 4 (3-4), 97–104. doi:10.1177/1947601912475079
Liu, C., Zhou, L., Chen, J., Yang, Z., Chen, S., Wang, X., et al. (2023). Galectin-7 promotes cisplatin efficacy by facilitating apoptosis and G3BP1 degradation in cervical cancer. Biochem. Pharmacol. 217, 115834. doi:10.1016/j.bcp.2023.115834
Liu, D., Yang, Y., Liu, Q., and Wang, J. (2011). Inhibition of autophagy by 3-MA potentiates cisplatin-induced apoptosis in esophageal squamous cell carcinoma cells. Med. Oncol. N. Lond. Engl. 28 (1), 105–111. doi:10.1007/s12032-009-9397-3
Liu, F., Liu, D., Yang, Y., and Zhao, S. (2013). Effect of autophagy inhibition on chemotherapy-induced apoptosis in A549 lung cancer cells. Oncol. Lett. 5 (4), 1261–1265. doi:10.3892/ol.2013.1154
Liu, J., Lu, X., Zeng, S., Fu, R., Wang, X., Luo, L., et al. (2024a). ATF3-CBS signaling axis coordinates ferroptosis and tumorigenesis in colorectal cancer. Redox Biol. 71, 103118. doi:10.1016/j.redox.2024.103118
Liu, S., Wang, Z., Hu, L., Ye, C., Zhang, X., Zhu, Z., et al. (2024b). Pan-cancer analysis of super-enhancer-induced LINC00862 and validation as a SIRT1-promoting factor in cervical cancer and gastric cancer. Transl. Oncol. 45, 101982. doi:10.1016/j.tranon.2024.101982
Liu, X., Chhipa, R. R., Nakano, I., and Dasgupta, B. (2014). The AMPK inhibitor compound C is a potent AMPK-independent antiglioma agent. Mol. cancer Ther. 13 (3), 596–605. doi:10.1158/1535-7163.MCT-13-0579
Long, M., and McWilliams, T. G. (2020). Monitoring autophagy in cancer: from bench to bedside. Seminars Cancer Biol. 66, 12–21. doi:10.1016/j.semcancer.2019.05.016
Luo, H., Zhang, H., Mao, J., Cao, H., Tao, Y., Zhao, G., et al. (2023b). Exosome-based nanoimmunotherapy targeting TAMs, a promising strategy for glioma. Cell death and Dis. 14 (4), 235. doi:10.1038/s41419-023-05753-9
Luo, P., Zhang, Q., Shen, S., An, Y., Yuan, L., Wong, Y. K., et al. (2023a). Mechanistic engineering of celastrol liposomes induces ferroptosis and apoptosis by directly targeting VDAC2 in hepatocellular carcinoma. Asian J. Pharm. Sci. 18 (6), 100874. doi:10.1016/j.ajps.2023.100874
Lv, Y., Lin, S., and Peng, F. (2017). SIRT1 gene polymorphisms and risk of lung cancer. Cancer management and research 2017 9, 381–386.
Maan, M., Peters, J. M., Dutta, M., and Patterson, A. D. (2018). Lipid metabolism and lipophagy in cancer. Biochem. biophysical Res. Commun. 504 (3), 582–589. doi:10.1016/j.bbrc.2018.02.097
Madrigal-Matute, J., and Cuervo, A. M. (2016). Regulation of liver metabolism by autophagy. Gastroenterology 150 (2), 328–339. doi:10.1053/j.gastro.2015.09.042
Mao, B., Hu, F., Cheng, J., Wang, P., Xu, M., Yuan, F., et al. (2014). SIRT1 regulates YAP2-mediated cell proliferation and chemoresistance in hepatocellular carcinoma. Oncogene 33 (11), 1468–1474. doi:10.1038/onc.2013.88
Mao, C., Liu, X., Zhang, Y., Lei, G., Yan, Y., Lee, H., et al. (2021). DHODH-mediated ferroptosis defence is a targetable vulnerability in cancer. Nature 593 (7860), 586–590. doi:10.1038/s41586-021-03539-7
Marzella, L., Ahlberg, J., and Glaumann, H. (1981). Autophagy, heterophagy, microautophagy and crinophagy as the means for intracellular degradation. Virchows Arch. B, Cell Pathol. Incl. Mol. Pathol. 36 (2-3), 219–234. doi:10.1007/BF02912068
Mathew, R., Karp, C. M., Beaudoin, B., Vuong, N., Chen, G., Chen, H.-Y., et al. (2009). Autophagy suppresses tumorigenesis through elimination of p62. Cell 137 (6), 1062–1075. doi:10.1016/j.cell.2009.03.048
Mei, Z., Duan, C., Li, C., Cui, L., and Ogino, S. (2016). Prognostic role of tumor PIK3CA mutation in colorectal cancer: a systematic review and meta-analysis. Ann. Oncol. 27 (10), 1836–1848. doi:10.1093/annonc/mdw264
Michishita, E., Park, J. Y., Burneskis, J. M., Barrett, J. C., and Horikawa, I. (2005). Evolutionarily conserved and nonconserved cellular localizations and functions of human SIRT proteins. Mol. Biol. Cell 16 (10), 4623–4635. doi:10.1091/mbc.e05-01-0033
Mijaljica, D., Prescott, M., and Devenish, R. J. (2011). Microautophagy in mammalian cells: revisiting a 40-year-old conundrum. Autophagy 7 (7), 673–682. doi:10.4161/auto.7.7.14733
Mizushima, N., and Komatsu, M. (2011). Autophagy: renovation of cells and tissues. Cell 147 (4), 728–741. doi:10.1016/j.cell.2011.10.026
Mizushima, N., Noda, T., Yoshimori, T., Tanaka, Y., Ishii, T., George, M. D., et al. (1998). A protein conjugation system essential for autophagy. Nature 395 (6700), 395–398. doi:10.1038/26506
Molla, M. D., Dessie, G., Akalu, Y., and Ayelign, B. (2020). Hepatocellular expression of SIRT1 and its effect on hepatocellular carcinoma progression: a future therapeutic perspective. Int. J. hepatology 2020, 2374615. doi:10.1155/2020/2374615
Mortimore, G. E., Hutson, N. J., and Surmacz, C. A. (1983). Quantitative correlation between proteolysis and macro- and microautophagy in mouse hepatocytes during starvation and refeeding. Proc. Natl. Acad. Sci. U. S. A. 80 (8), 2179–2183. doi:10.1073/pnas.80.8.2179
Mortimore, G. E., Lardeux, B. R., and Adams, C. E. (1988). Regulation of microautophagy and basal protein turnover in rat liver. Effects of short-term starvation. J. Biol. Chem. 263 (5), 2506–2512. doi:10.1016/s0021-9258(18)69235-x
Moynihan, K. A., Grimm, A. A., Plueger, M. M., Bernal-Mizrachi, E., Ford, E., Cras-Méneur, C., et al. (2005). Increased dosage of mammalian Sir2 in pancreatic beta cells enhances glucose-stimulated insulin secretion in mice. Cell metab. 2 (2), 105–117. doi:10.1016/j.cmet.2005.07.001
Mu, N., Lei, Y., Wang, Y., Wang, Y., Duan, Q., Ma, G., et al. (2019). Inhibition of SIRT1/2 upregulates HSPA5 acetylation and induces pro-survival autophagy via ATF4-DDIT4-mTORC1 axis in human lung cancer cells. Apoptosis Int. J. Program. Cell death 24 (9-10), 798–811. doi:10.1007/s10495-019-01559-3
Mvunta, D. H., Miyamoto, T., Asaka, R., Yamada, Y., Ando, H., Higuchi, S., et al. (2017). SIRT1 regulates the chemoresistance and invasiveness of ovarian carcinoma cells. Transl. Oncol. 10 (4), 621–631. doi:10.1016/j.tranon.2017.05.005
Nakatogawa, H. (2020). Mechanisms governing autophagosome biogenesis. Nat. Rev. Mol. Cell Biol. 21 (8), 439–458. doi:10.1038/s41580-020-0241-0
North, B. J., Marshall, B. L., Borra, M. T., Denu, J. M., and Verdin, E. (2003). The human Sir2 ortholog, SIRT2, is an NAD+-dependent tubulin deacetylase. Mol. Cell 11 (2), 437–444. doi:10.1016/s1097-2765(03)00038-8
Nusse, R., and Clevers, H. (2017). Wnt/β-Catenin signaling, disease, and emerging therapeutic modalities. Cell 169 (6), 985–999. doi:10.1016/j.cell.2017.05.016
O'Donovan, T. R., O'Sullivan, G. C., and McKenna, S. L. (2011). Induction of autophagy by drug-resistant esophageal cancer cells promotes their survival and recovery following treatment with chemotherapeutics. Autophagy 7 (5), 509–524. doi:10.4161/auto.7.6.15066
Ogier-Denis, E., and Codogno, P. (2003). Autophagy: a barrier or an adaptive response to cancer. Biochimica biophysica acta 1603 (2), 113–128. doi:10.1016/s0304-419x(03)00004-0
Ong, A. L. C., and Ramasamy, T. S. (2018). Role of Sirtuin1-p53 regulatory axis in aging, cancer and cellular reprogramming. Ageing Res. Rev. 43, 64–80. doi:10.1016/j.arr.2018.02.004
Oon, C. E., Strell, C., Yeong, K. Y., Östman, A., and Prakash, J. (2015). SIRT1 inhibition in pancreatic cancer models: contrasting effects in vitro and in vivo. Eur. J. Pharmacol. 757, 59–67. doi:10.1016/j.ejphar.2015.03.064
Ota, H., Tokunaga, E., Chang, K., Hikasa, M., Iijima, K., Eto, M., et al. (2006). Sirt1 inhibitor, Sirtinol, induces senescence-like growth arrest with attenuated Ras-MAPK signaling in human cancer cells. Oncogene 25 (2), 176–185. doi:10.1038/sj.onc.1209049
Otsuka, R., Sakata, H., Murakami, K., Kano, M., Endo, S., Toyozumi, T., et al. (2022). SIRT1 expression is a promising prognostic biomarker in esophageal squamous cell carcinoma: a systematic review and meta-analysis. Cancer Diagnosis and Prognosis 2 (2), 126–133. doi:10.21873/cdp.10086
Ozpolat, B., and Benbrook, D. M. (2015). Targeting autophagy in cancer management - strategies and developments. Cancer Manag. Res. 7, 291–299. doi:10.2147/CMAR.S34859
Pacholec, M., Bleasdale, J. E., Chrunyk, B., Cunningham, D., Flynn, D., Garofalo, R. S., et al. (2010). SRT1720, SRT2183, SRT1460, and resveratrol are not direct activators of SIRT1♦. J. Biol. Chem. 285 (11), 8340–8351. doi:10.1074/jbc.M109.088682
Panda, P. K., Patra, S., Naik, P. P., Praharaj, P. P., Mukhopadhyay, S., Meher, B. R., et al. (2020). Deacetylation of LAMP1 drives lipophagy-dependent generation of free fatty acids by Abrus agglutinin to promote senescence in prostate cancer. J. Cell. physiology 235 (3), 2776–2791. doi:10.1002/jcp.29182
Park, J. M., Huang, S., Wu, T. T., Foster, N. R., and Sinicrope, F. A. (2013). Prognostic impact of Beclin 1, p62/sequestosome 1 and LC3 protein expression in colon carcinomas from patients receiving 5-fluorouracil as adjuvant chemotherapy. Cancer Biol. and Ther. 14 (2), 100–107. doi:10.4161/cbt.22954
Paskeh, M. D. A., Entezari, M., Clark, C., Zabolian, A., Ranjbar, E., Farahani, M. V., et al. (2022). Targeted regulation of autophagy using nanoparticles: new insight into cancer therapy. Biochimica Biophysica Acta (BBA) - Mol. Basis Dis. 1868 (3), 166326. doi:10.1016/j.bbadis.2021.166326
Patra, S., Bhol, C. S., Panigrahi, D. P., Praharaj, P. P., Pradhan, B., Jena, M., et al. (2020b). Gamma irradiation promotes chemo-sensitization potential of gallic acid through attenuation of autophagic flux to trigger apoptosis in an NRF2 inactivation signalling pathway. Free Radic. Biol. Med. 160, 111–124. doi:10.1016/j.freeradbiomed.2020.06.022
Patra, S., Mishra, S. R., Behera, B. P., Mahapatra, K. K., Panigrahi, D. P., Bhol, C. S., et al. (2022). “Autophagy-modulating phytochemicals in cancer therapeutics: current evidences and future perspectives,” in Seminars in cancer biology (Elsevier), 205–217.
Patra, S., Panda, P. K., Naik, P. P., Panigrahi, D. P., Praharaj, P. P., Bhol, C. S., et al. (2020a). Terminalia bellirica extract induces anticancer activity through modulation of apoptosis and autophagy in oral squamous cell carcinoma. Food Chem. Toxicol. 136, 111073. doi:10.1016/j.fct.2019.111073
Patra, S., Pradhan, B., Nayak, R., Behera, C., Rout, L., Jena, M., et al. (2021). “Chemotherapeutic efficacy of curcumin and resveratrol against cancer: chemoprevention, chemoprotection, drug synergism and clinical pharmacokinetics,” in Seminars in cancer biology (Elsevier), 310–320.
Patra, S., Praharaj, P. P., Singh, A., and Bhutia, S. K. (2023b). Targeting SIRT1-regulated autophagic cell death as a novel therapeutic avenue for cancer prevention. Drug Discov. Today 28 (9), 103692. doi:10.1016/j.drudis.2023.103692
Patra, S., Singh, A., Praharaj, P. P., Mohanta, N. K., Jena, M., Patro, B. S., et al. (2023a). SIRT1 inhibits mitochondrial hyperfusion associated mito-bulb formation to sensitize oral cancer cells for apoptosis in a mtROS-dependent signalling pathway. Cell death and Dis. 14 (11), 732. doi:10.1038/s41419-023-06232-x
Pavlidis, E. T., and Pavlidis, T. E. (2018). Current molecular and genetic aspects of pancreatic cancer, the role of metastasis associated proteins (MTA): a review. J. Investigative Surg. 31 (1), 54–66. doi:10.1080/08941939.2016.1269854
Peck, B., Chen, C. Y., Ho, K. K., Di Fruscia, P., Myatt, S. S., Coombes, R. C., et al. (2010). SIRT inhibitors induce cell death and p53 acetylation through targeting both SIRT1 and SIRT2. Mol. cancer Ther. 9 (4), 844–855. doi:10.1158/1535-7163.MCT-09-0971
Pervaiz, S., and Holme, A. L. (2009). Resveratrol: its biologic targets and functional activity. Antioxidants and redox Signal. 11 (11), 2851–2897. doi:10.1089/ars.2008.2412
Power, C., Fanning, N., and Redmond, H. P. (2002). Cellular apoptosis and organ injury in sepsis: a review. Shock 18 (3), 197–211. doi:10.1097/00024382-200209000-00001
Pu, Y., Wang, J., and Wang, S. (2022). Role of autophagy in drug resistance and regulation of osteosarcoma. Mol. Clin. Oncol. 16 (3), 1–6.
Qi, Y., Qi, W., Liu, S., Sun, L., Ding, A., Yu, G., et al. (2020). TSPAN9 suppresses the chemosensitivity of gastric cancer to 5-fluorouracil by promoting autophagy. Cancer Cell Int. 20, 4. doi:10.1186/s12935-019-1089-2
Qiao, P. F., Yao, L., and Zeng, Z. L. (2020). Catalpol-mediated microRNA-34a suppresses autophagy and malignancy by regulating SIRT1 in colorectal cancer. Oncol. Rep. 43 (4), 1053–1066. doi:10.3892/or.2020.7494
Qin, Y., Ashrafizadeh, M., Mongiardini, V., Grimaldi, B., Crea, F., Rietdorf, K., et al. (2023). Autophagy and cancer drug resistance in dialogue: pre-clinical and clinical evidence. Cancer Lett. 570, 216307. doi:10.1016/j.canlet.2023.216307
Qu, X., Yu, J., Bhagat, G., Furuya, N., Hibshoosh, H., Troxel, A., et al. (2003). Promotion of tumorigenesis by heterozygous disruption of the beclin 1 autophagy gene. J. Clin. Invest. 112 (12), 1809–1820. doi:10.1172/JCI20039
Rabinowitz, J. D., and White, E. (2010). Autophagy and metabolism. Sci. (New York, NY) 330 (6009), 1344–1348. doi:10.1126/science.1193497
Rosenblatt, J., Raff, M. C., and Cramer, L. P. (2001). An epithelial cell destined for apoptosis signals its neighbors to extrude it by an actin-and myosin-dependent mechanism. Curr. Biol. 11 (23), 1847–1857. doi:10.1016/s0960-9822(01)00587-5
Ruderman, N., and Prentki, M. (2004). AMP kinase and malonyl-CoA: targets for therapy of the metabolic syndrome. Nat. Rev. Drug Discov. 3 (4), 340–351. doi:10.1038/nrd1344
Sahoo, S., Kumari, S., Pulipaka, S., Chandra, Y., and Kotamraju, S. (2024). SIRT1 promotes doxorubicin-induced breast cancer drug resistance and tumor angiogenesis via regulating GSH-mediated redox homeostasis. Mol. Carcinog. doi:10.1002/mc.23809
Saito, H., Inazawa, J., Saito, S., Kasumi, F., Koi, S., Sagae, S., et al. (1993). Detailed deletion mapping of chromosome 17q in ovarian and breast cancers: 2-cM region on 17q21.3 often and commonly deleted in tumors. Cancer Res. 53 (14), 3382–3385.
Samaddar, J. S., Gaddy, V. T., Duplantier, J., Thandavan, S. P., Shah, M., Smith, M. J., et al. (2008). A role for macroautophagy in protection against 4-hydroxytamoxifen-induced cell death and the development of antiestrogen resistance. Mol. cancer Ther. 7 (9), 2977–2987. doi:10.1158/1535-7163.MCT-08-0447
Sanders, S. J., Murtha, M. T., Gupta, A. R., Murdoch, J. D., Raubeson, M. J., Willsey, A. J., et al. (2012). De novo mutations revealed by whole-exome sequencing are strongly associated with autism. Nature 485 (7397), 237–241. doi:10.1038/nature10945
Sasaki, K., Tsuno, N. H., Sunami, E., Tsurita, G., Kawai, K., Okaji, Y., et al. (2010). Chloroquine potentiates the anti-cancer effect of 5-fluorouracil on colon cancer cells. BMC cancer 10, 370. doi:10.1186/1471-2407-10-370
Savill, J., and Fadok, V. (2000). Corpse clearance defines the meaning of cell death. Nature 407 (6805), 784–788. doi:10.1038/35037722
Scher, M. B., Vaquero, A., and Reinberg, D. (2007). SirT3 is a nuclear NAD+-dependent histone deacetylase that translocates to the mitochondria upon cellular stress. Genes Dev. 21 (8), 920–928. doi:10.1101/gad.1527307
Scott, R. C., Juhász, G., and Neufeld, T. P. (2007). Direct induction of autophagy by Atg1 inhibits cell growth and induces apoptotic cell death. Curr. Biol. CB 17 (1), 1–11. doi:10.1016/j.cub.2006.10.053
Shan, S., Wang, X., Qian, L., Wang, C., and Zhao, S. (2024). ENST00000534735 inhibits proliferation and migration, promotes apoptosis and pyroptosis of endometrial cancer via OSBPL3 through APMK/SIRT1/NF-κB pathway. Heliyon 10 (4), e25281. doi:10.1016/j.heliyon.2024.e25281
Shen, J., Yang, H., Qiao, X., Chen, Y., Zheng, L., Lin, J., et al. (2023). The E3 ubiquitin ligase TRIM17 promotes gastric cancer survival and progression via controlling BAX stability and antagonizing apoptosis. Cell death Differ. 30 (10), 2322–2335. doi:10.1038/s41418-023-01221-1
Shen, T., Cai, L. D., Liu, Y. H., Li, S., Gan, W. J., Li, X. M., et al. (2018). Ube2v1-mediated ubiquitination and degradation of Sirt1 promotes metastasis of colorectal cancer by epigenetically suppressing autophagy. J. Hematol. and Oncol. 11 (1), 95. doi:10.1186/s13045-018-0638-9
Shimoyama, Y., Mitsuda, Y., Tsuruta, Y., Suzuki, K., Hamajima, N., and Niwa, T. (2012). SIRTUIN 1 gene polymorphisms are associated with cholesterol metabolism and coronary artery calcification in Japanese hemodialysis patients. J. Ren. Nutr. 22 (1), 114–119. doi:10.1053/j.jrn.2011.10.025
Shimoyama, Y., Suzuki, K., Hamajima, N., and Niwa, T. (2011). Sirtuin 1 gene polymorphisms are associated with body fat and blood pressure in Japanese. Transl. Res. 157 (6), 339–347. doi:10.1016/j.trsl.2011.02.004
Shin, D. H., Jo, J. Y., Choi, M., Kim, K. H., Bae, Y. K., and Kim, S. S. (2023). Oncogenic KRAS mutation confers chemoresistance by upregulating SIRT1 in non-small cell lung cancer. Exp. and Mol. Med. 55 (10), 2220–2237. doi:10.1038/s12276-023-01091-0
Shuhua, W., Chenbo, S., Yangyang, L., Xiangqian, G., Shuang, H., Tangyue, L., et al. (2015). Autophagy-related genes Raptor, Rictor, and Beclin1 expression and relationship with multidrug resistance in colorectal carcinoma. Hum. Pathol. 46 (11), 1752–1759. doi:10.1016/j.humpath.2015.07.016
Solomon, J. M., Pasupuleti, R., Xu, L., McDonagh, T., Curtis, R., DiStefano, P. S., et al. (2006). Inhibition of SIRT1 catalytic activity increases p53 acetylation but does not alter cell survival following DNA damage. Mol. Cell. Biol. 26 (1), 28–38. doi:10.1128/MCB.26.1.28-38.2006
Song, S., Luo, M., Song, Y., Liu, T., Zhang, H., and Xie, Z. (2014). Prognostic role of SIRT1 in hepatocellular carcinoma. J. Coll. Physicians Surg. Pak 24 (11), 849–854.
Song, X., Kong, F., Zong, Z., Ren, M., Meng, Q., Li, Y., et al. (2019). miR-124 and miR-142 enhance cisplatin sensitivity of non-small cell lung cancer cells through repressing autophagy via directly targeting SIRT1. RSC Adv. 9 (9), 5234–5243. doi:10.1039/c8ra09914f
Soula, M., Weber, R. A., Zilka, O., Alwaseem, H., La, K., Yen, F., et al. (2020). Metabolic determinants of cancer cell sensitivity to canonical ferroptosis inducers. Nat. Chem. Biol. 16 (12), 1351–1360. doi:10.1038/s41589-020-0613-y
Stein, S. C., Woods, A., Jones, N. A., Davison, M. D., and Carling, D. (2000). The regulation of AMP-activated protein kinase by phosphorylation. Biochem. J. 345 (3), 437–443. doi:10.1042/0264-6021:3450437
Steinberg, G. R., and Kemp, B. E. (2009). AMPK in health and disease. Physiol. Rev. 89 (3), 1025–1078. doi:10.1152/physrev.00011.2008
Sternberg, C. N., de Mulder, P. H., Schornagel, J. H., Théodore, C., Fossa, S. D., van Oosterom, A. T., et al. (2001). Randomized phase III trial of high-dose-intensity methotrexate, vinblastine, doxorubicin, and cisplatin (MVAC) chemotherapy and recombinant human granulocyte colony-stimulating factor versus classic MVAC in advanced urothelial tract tumors: European Organization for Research and Treatment of Cancer Protocol no. 30924. J. Clin. Oncol. official J. Am. Soc. Clin. Oncol. 19 (10), 2638–2646. doi:10.1200/JCO.2001.19.10.2638
Stockwell, B. R., Friedmann Angeli, J. P., Bayir, H., Bush, A. I., Conrad, M., Dixon, S. J., et al. (2017). Ferroptosis: a regulated cell death nexus linking metabolism, redox biology, and disease. Cell 171 (2), 273–285. doi:10.1016/j.cell.2017.09.021
Stünkel, W., Peh, B. K., Tan, Y. C., Nayagam, V. M., Wang, X., Salto-Tellez, M., et al. (2007). Function of the SIRT1 protein deacetylase in cancer. Biotechnol. J. 2 (11), 1360–1368. doi:10.1002/biot.200700087
Su, B. C., Xiao, K. M., Wang, K. L., Yang, S. F., Huang, Z. X., and Luo, J. W. (2023b). ATGL promotes colorectal cancer growth by regulating autophagy process and SIRT1 expression. Med. Oncol. N. Lond. Engl. 40 (12), 350. doi:10.1007/s12032-023-02148-w
Su, C. M., Hsu, T. W., Chen, H. A., Wang, W. Y., Huang, C. Y., Hung, C. C., et al. (2023a). Chaperone-mediated autophagy degrade Dicer to promote breast cancer metastasis. J. Cell. physiology 238 (4), 829–841. doi:10.1002/jcp.30979
Su, Z., Li, G., Liu, C., Ren, S., Deng, T., Zhang, S., et al. (2017). Autophagy inhibition impairs the epithelial-mesenchymal transition and enhances cisplatin sensitivity in nasopharyngeal carcinoma. Oncol. Lett. 13 (6), 4147–4154. doi:10.3892/ol.2017.5963
Sun, G., Yang, L., Wei, S., Jin, H., Li, B., and Li, H. (2021b). miR-425 regulates lipophagy via SIRT1 to promote sorafenib resistance in liver cancer. Oncol. Lett. 22 (4), 695. doi:10.3892/ol.2021.12956
Sun, L., Wang, H., Wang, Z., He, S., Chen, S., Liao, D., et al. (2012). Mixed lineage kinase domain-like protein mediates necrosis signaling downstream of RIP3 kinase. Cell 148 (1), 213–227. doi:10.1016/j.cell.2011.11.031
Sun, M., Du, M., Zhang, W., Xiong, S., Gong, X., Lei, P., et al. (2019). Survival and clinicopathological significance of SIRT1 expression in cancers: a meta-analysis. Front. Endocrinol. 10, 121. doi:10.3389/fendo.2019.00121
Sun, S., Liu, X., Wei, X., Zhang, S., and Wang, W. (2022). Diallyl trisulfide induces pro-apoptotic autophagy via the AMPK/SIRT1 signalling pathway in human hepatocellular carcinoma HepG2 cell line. Food and Nutr. Res. 66. doi:10.29219/fnr.v66.8981
Sun, T., Peng, H., Mao, W., Ma, L., Liu, H., Mai, J., et al. (2021a). Autophagy-mediated negative feedback attenuates the oncogenic activity of YAP in pancreatic cancer. Int. J. Biol. Sci. 17 (13), 3634–3645. doi:10.7150/ijbs.61795
Sun, W. L., Chen, J., Wang, Y. P., and Zheng, H. (2011). Autophagy protects breast cancer cells from epirubicin-induced apoptosis and facilitates epirubicin-resistance development. Autophagy 7 (9), 1035–1044. doi:10.4161/auto.7.9.16521
Sun, Y., Berleth, N., Wu, W., Schlütermann, D., Deitersen, J., Stuhldreier, F., et al. (2021c). Fin56-induced ferroptosis is supported by autophagy-mediated GPX4 degradation and functions synergistically with mTOR inhibition to kill bladder cancer cells. Cell death and Dis. 12 (11), 1028. doi:10.1038/s41419-021-04306-2
Sun, Y., Liu, X., Tong, H., Yin, H., Li, T., Zhu, J., et al. (2023). SIRT1 promotes cisplatin resistance in bladder cancer via Beclin1 deacetylation-mediated autophagy. Cancers 16 (1), 125. doi:10.3390/cancers16010125
Tae, I. H., Son, J. Y., Lee, S. H., Ahn, M.-Y., Yoon, K., Yoon, S., et al. (2020). A new SIRT1 inhibitor, MHY2245, induces autophagy and inhibits energy metabolism via PKM2/mTOR pathway in human ovarian cancer cells. Int. J. Biol. Sci. 16 (11), 1901–1916. doi:10.7150/ijbs.44343
Taelman, V. F., Dobrowolski, R., Plouhinec, J. L., Fuentealba, L. C., Vorwald, P. P., Gumper, I., et al. (2010). Wnt signaling requires sequestration of glycogen synthase kinase 3 inside multivesicular endosomes. Cell 143 (7), 1136–1148. doi:10.1016/j.cell.2010.11.034
Tan, Q., Liu, Y., Deng, X., Chen, J., Tsai, P. J., Chen, P. H., et al. (2020). Autophagy: a promising process for the treatment of acetaminophen-induced liver injury. Archives Toxicol. 94 (9), 2925–2938. doi:10.1007/s00204-020-02780-9
Tan, V. P., and Miyamoto, S. (2016). Nutrient-sensing mTORC1: integration of metabolic and autophagic signals. J. Mol. Cell. Cardiol. 95, 31–41. doi:10.1016/j.yjmcc.2016.01.005
Tang, L., Zhang, H., Zhou, F., Wei, Q., Du, M., Wu, J., et al. (2024). Targeting autophagy overcomes cancer-intrinsic resistance to CAR-T immunotherapy in B-cell malignancies. Cancer Commun. Lond. Engl. 44 (3), 408–432. doi:10.1002/cac2.12525
Tang, M., Tang, H., Tu, B., and Zhu, W.-G. (2021b). SIRT7: a sentinel of genome stability. Open Biol. 11 (6), 210047. doi:10.1098/rsob.210047
Tang, X., Ding, H., Liang, M., Chen, X., Yan, Y., Wan, N., et al. (2021a). Curcumin induces ferroptosis in non-small-cell lung cancer via activating autophagy. Thorac. cancer 12 (8), 1219–1230. doi:10.1111/1759-7714.13904
Tanno, M., Sakamoto, J., Miura, T., Shimamoto, K., and Horio, Y. (2007). Nucleocytoplasmic shuttling of the NAD+-dependent histone deacetylase SIRT1. J. Biol. Chem. 282 (9), 6823–6832. doi:10.1074/jbc.M609554200
Tarabay, M., Elshazli, R., and Settin, A. (2016). African vs. Caucasian and Asian difference for the association of interleukin-10 promotor polymorphisms with type 2 diabetes mellitus (a meta-analysis study). Meta gene 9, 10–17. doi:10.1016/j.mgene.2016.02.006
Tian, Q., Zhang, P., Wang, Y., Si, Y., Yin, D., Weber, C. R., et al. (2023). A novel triptolide analog downregulates NF-κB and induces mitochondrial apoptosis pathways in human pancreatic cancer. eLife 12, e85862. doi:10.7554/eLife.85862
Tomaselli, D., Steegborn, C., Mai, A., and Rotili, D. (2020). Sirt4: a multifaceted enzyme at the crossroads of mitochondrial metabolism and cancer. Front. Oncol. 10, 474. doi:10.3389/fonc.2020.00474
Torrens-Mas, M., Oliver, J., Roca, P., and Sastre-Serra, J. (2017). SIRT3: oncogene and tumor suppressor in cancer. Cancers 9 (7), 90. doi:10.3390/cancers9070090
Tóth, S., Nagy, K., Pálfia, Z., and Réz, G. (2002). Cellular autophagic capacity changes during azaserine-induced tumour progression in the rat pancreas. Up-regulation in all premalignant stages and down-regulation with loss of cycloheximide sensitivity of segregation along with malignant transformation. Cell tissue Res. 309 (3), 409–416. doi:10.1007/s00441-001-0506-7
Towers, C. G., Wodetzki, D. K., Thorburn, J., Smith, K. R., Caino, M. C., and Thorburn, A. (2021). Mitochondrial-derived vesicles compensate for loss of LC3-mediated mitophagy. Dev. Cell 56 (14), 2029–2042.e5. doi:10.1016/j.devcel.2021.06.003
Tsutsumi, S., Watanabe, R., Tabe, Y., Fujii, T., Morita, H., Kigure, W., et al. (2012). Extranodal metastasis predicts poor survival in advanced colorectal cancer. Hepato-gastroenterology 59 (114), 372–374. doi:10.5754/hge10139
Varghese, B., Chianese, U., Capasso, L., Sian, V., Bontempo, P., Conte, M., et al. (2023). SIRT1 activation promotes energy homeostasis and reprograms liver cancer metabolism. J. Transl. Med. 21 (1), 627. doi:10.1186/s12967-023-04440-9
Vasan, N., Baselga, J., and Hyman, D. M. (2019). A view on drug resistance in cancer. Nature 575 (7782), 299–309. doi:10.1038/s41586-019-1730-1
Vercammen, D., Beyaert, R., Denecker, G., Goossens, V., Van Loo, G., Declercq, W., et al. (1998). Inhibition of caspases increases the sensitivity of L929 cells to necrosis mediated by tumor necrosis factor. J. Exp. Med. 187 (9), 1477–1485. doi:10.1084/jem.187.9.1477
Wang, C., Liu, Y., Zhu, Y., and Kong, C. (2020). Functions of mammalian SIRT4 in cellular metabolism and research progress in human cancer. Oncol. Lett. 20 (4), 11–1. doi:10.3892/ol.2020.11872
Wang, C., Zeng, J., Li, L. J., Xue, M., and He, S. L. (2021). Cdc25A inhibits autophagy-mediated ferroptosis by upregulating ErbB2 through PKM2 dephosphorylation in cervical cancer cells. Cell death and Dis. 12 (11), 1055. doi:10.1038/s41419-021-04342-y
Wang, H., Liang, Y., Zhao, L., Deng, J., Li, Y., Zhao, H., et al. (2023f). miR-653-3p promotes genomic instability of colorectal cancer cells via targeting SIRT1/TWIST1 signaling pathway. Biochimica biophysica acta Mol. basis Dis. 1869 (8), 166821. doi:10.1016/j.bbadis.2023.166821
Wang, J., and Wu, G. S. (2014). Role of autophagy in cisplatin resistance in ovarian cancer cells. J. Biol. Chem. 289 (24), 17163–17173. doi:10.1074/jbc.M114.558288
Wang, K. X., Yan, C., Yang, X., Zhu, P. Y., Cui, W. W., Ye, C., et al. (2022). Enhanced autophagy promotes radiosensitivity by mediating Sirt1 downregulation in RM-1 prostate cancer cells. Biochem. biophysical Res. Commun. 609, 84–92. doi:10.1016/j.bbrc.2022.03.142
Wang, L., Klionsky, D. J., and Shen, H.-M. (2023b). The emerging mechanisms and functions of microautophagy. Nat. Rev. Mol. Cell Biol. 24 (3), 186–203. doi:10.1038/s41580-022-00529-z
Wang, M., Han, D., Yuan, Z., Hu, H., Zhao, Z., Yang, R., et al. (2018). Long non-coding RNA H19 confers 5-Fu resistance in colorectal cancer by promoting SIRT1-mediated autophagy. Cell death and Dis. 9 (12), 1149. doi:10.1038/s41419-018-1187-4
Wang, M., Zhang, Z., Chen, M., Lv, Y., Tian, S., Meng, F., et al. (2023c). FDW028, a novel FUT8 inhibitor, impels lysosomal proteolysis of B7-H3 via chaperone-mediated autophagy pathway and exhibits potent efficacy against metastatic colorectal cancer. Cell death and Dis. 14 (8), 495. doi:10.1038/s41419-023-06027-0
Wang, R., Li, S., Hou, Q., Zhang, B., Chu, H., Hou, Y., et al. (2023e). Propofol inhibits colon cancer cell stemness and epithelial-mesenchymal transition by regulating SIRT1, Wnt/β-catenin and PI3K/AKT/mTOR signaling pathways. Discov. Oncol. 14 (1), 137. doi:10.1007/s12672-023-00734-y
Wang, S., Nie, J., Xu, K., Liu, Y., Tong, W., Li, A., et al. (2023a). YY1 is regulated by ALKBH5-mediated m6A modification and promotes autophagy and cancer progression through targeting ATG4B. Aging 15 (18), 9590–9613. doi:10.18632/aging.205037
Wang, X. W., Jiang, Y. H., Ye, W., Shao, C. F., Xie, J. J., and Li, X. (2023d). SIRT1 promotes the progression and chemoresistance of colorectal cancer through the p53/miR-101/KPNA3 axis. Cancer Biol. and Ther. 24 (1), 2235770. doi:10.1080/15384047.2023.2235770
Wang, Y., Chen, Y., Chen, X., Liang, Y., Yang, D., Dong, J., et al. (2019a). Angelicin inhibits the malignant behaviours of human cervical cancer potentially via inhibiting autophagy. Exp. Ther. Med. 18 (5), 3365–3374. doi:10.3892/etm.2019.7985
Wang, Y., Yang, J., Hong, T., Chen, X., and Cui, L. (2019c). SIRT2: controversy and multiple roles in disease and physiology. Ageing Res. Rev. 55, 100961. doi:10.1016/j.arr.2019.100961
Wang, Y., Zeng, J., Wu, W., Xie, S., Yu, H., Li, G., et al. (2019b). Nicotinamide N-methyltransferase enhances chemoresistance in breast cancer through SIRT1 protein stabilization. Breast cancer Res. BCR 21 (1), 64. doi:10.1186/s13058-019-1150-z
Wei, Z., Xia, J., Li, J., Cai, J., Shan, J., Zhang, C., et al. (2023). SIRT1 promotes glucolipid metabolic conversion to facilitate tumor development in colorectal carcinoma. Int. J. Biol. Sci. 19 (6), 1925–1940. doi:10.7150/ijbs.76704
White, E. (2015). The role for autophagy in cancer. J. Clin. investigation 125 (1), 42–46. doi:10.1172/JCI73941
White, E., Mehnert, J. M., and Chan, C. S. (2015). Autophagy, metabolism, and cancer. Clin. cancer Res. official J. Am. Assoc. Cancer Res. 21 (22), 5037–5046. doi:10.1158/1078-0432.CCR-15-0490
Wiernicki, B., Dubois, H., Tyurina, Y. Y., Hassannia, B., Bayir, H., Kagan, V. E., et al. (2020). Excessive phospholipid peroxidation distinguishes ferroptosis from other cell death modes including pyroptosis. Cell death and Dis. 11 (10), 922. doi:10.1038/s41419-020-03118-0
Wirawan, E., Berghe, T. V., Lippens, S., Agostinis, P., and Vandenabeele, P. (2012). Autophagy: for better or for worse. Cell Res. 22 (1), 43–61. doi:10.1038/cr.2011.152
Wong, M. M., Chan, H. Y., Aziz, N. A., Ramasamy, T. S., Bong, J. J., Ch'ng, E. S., et al. (2021). Interplay of autophagy and cancer stem cells in hepatocellular carcinoma. Mol. Biol. Rep. 48 (4), 3695–3717. doi:10.1007/s11033-021-06334-9
Wu, J., Guo, Q., Li, J., Yuan, H., Xiao, C., Qiu, J., et al. (2023). Loperamide induces protective autophagy and apoptosis through the ROS/JNK signaling pathway in bladder cancer. Biochem. Pharmacol. 218, 115870. doi:10.1016/j.bcp.2023.115870
Wu, M., Wei, W., Xiao, X., Guo, J., Xie, X., Li, L., et al. (2012). Expression of SIRT1 is associated with lymph node metastasis and poor prognosis in both operable triple-negative and non-triple-negative breast cancer. Med. Oncol. N. Lond. Engl. 29 (5), 3240–3249. doi:10.1007/s12032-012-0260-6
Xiao, B., Sanders, M. J., Underwood, E., Heath, R., Mayer, F. V., Carmena, D., et al. (2011). Structure of mammalian AMPK and its regulation by ADP. Nature 472 (7342), 230–233. doi:10.1038/nature09932
Xiao, L., Zhang, Y., Luo, Q., Guo, C., Chen, Z., and Lai, C. (2023). DHRS4-AS1 regulate gastric cancer apoptosis and cell proliferation by destabilizing DHX9 and inhibited the association between DHX9 and ILF3. Cancer Cell Int. 23 (1), 304. doi:10.1186/s12935-023-03151-x
Xiao, X., Wang, W., Li, Y., Yang, D., Li, X., Shen, C., et al. (2018). HSP90AA1-mediated autophagy promotes drug resistance in osteosarcoma. J. Exp. and Clin. cancer Res. CR 37 (1), 201. doi:10.1186/s13046-018-0880-6
Xie, Y., Lei, X., Zhao, G., Guo, R., and Cui, N. (2023). mTOR in programmed cell death and its therapeutic implications. Cytokine and Growth Factor Rev. 71-72, 66–81. doi:10.1016/j.cytogfr.2023.06.002
Xilouri, M., and Stefanis, L. (2015). Chaperone mediated autophagy to the rescue: a new-fangled target for the treatment of neurodegenerative diseases. Mol. Cell. Neurosci. 66 (Pt A), 29–36. doi:10.1016/j.mcn.2015.01.003
Xu, H., Zeng, S., Wang, Y., Yang, T., Wang, M., Li, X., et al. (2023b). Cytoplasmic SIRT1 promotes paclitaxel resistance in ovarian carcinoma through increased formation and survival of polyploid giant cancer cells. J. pathology 261 (2), 210–226. doi:10.1002/path.6167
Xu, Z., Wang, X., Sun, W., Xu, F., Kou, H., Hu, W., et al. (2023a). RelB-activated GPX4 inhibits ferroptosis and confers tamoxifen resistance in breast cancer. Redox Biol. 68, 102952. doi:10.1016/j.redox.2023.102952
Xue, N., Lai, F., Du, T., Ji, M., Liu, D., Yan, C., et al. (2019). Chaperone-mediated autophagy degradation of IGF-1Rβ induced by NVP-AUY922 in pancreatic cancer. Cell. Mol. life Sci. CMLS 76 (17), 3433–3447. doi:10.1007/s00018-019-03080-x
Yamamoto, H., Schoonjans, K., and Auwerx, J. (2007). Sirtuin functions in health and disease. Mol. Endocrinol. (Baltim. Md) 21 (8), 1745–1755. doi:10.1210/me.2007-0079
Yan, T., Lu, G., Shang, R., Hu, J., Zhu, C., and Jin, L. (2024). RACGAP1 drives proliferation, migration and invasion and suppresses autophagy of gastric cancer cells via inhibiting SIRT1/Mfn2. Physiol. Int. 111 (1), 35–46. doi:10.1556/2060.2023.00235
Yanagisawa, S., Baker, J. R., Vuppusetty, C., Koga, T., Colley, T., Fenwick, P., et al. (2018). The dynamic shuttling of SIRT1 between cytoplasm and nuclei in bronchial epithelial cells by single and repeated cigarette smoke exposure. PloS one 13 (3), e0193921. doi:10.1371/journal.pone.0193921
Yang, Q., Sun, K., Gao, T., Gao, Y., Yang, Y., Li, Z., et al. (2024). SIRT1 silencing promotes EMT and Crizotinib resistance by regulating autophagy through AMPK/mTOR/S6K signaling pathway in EML4-ALK L1196M and EML4-ALK G1202R mutant non-small cell lung cancer cells. Mol. Carcinog. doi:10.1002/mc.23799
Yang, W. S., SriRamaratnam, R., Welsch, M. E., Shimada, K., Skouta, R., Viswanathan, V. S., et al. (2014). Regulation of ferroptotic cancer cell death by GPX4. Cell 156 (1-2), 317–331. doi:10.1016/j.cell.2013.12.010
Yao, J., Wang, J., Xu, Y., Guo, Q., Sun, Y., Liu, J., et al. (2022). CDK9 inhibition blocks the initiation of PINK1-PRKN-mediated mitophagy by regulating the SIRT1-FOXO3-BNIP3 axis and enhances the therapeutic effects involving mitochondrial dysfunction in hepatocellular carcinoma. Autophagy 18 (8), 1879–1897. doi:10.1080/15548627.2021.2007027
Yao, Z.-q., Zhang, X., Zhen, Y., He, X.-Y., Zhao, S., Li, X.-F., et al. (2018). A novel small-molecule activator of Sirtuin-1 induces autophagic cell death/mitophagy as a potential therapeutic strategy in glioblastoma. Cell death and Dis. 9 (7), 767. doi:10.1038/s41419-018-0799-z
Ye, Z., Fang, B., Pan, J., Zhang, N., Huang, J., Xie, C., et al. (2017). miR-138 suppresses the proliferation, metastasis and autophagy of non-small cell lung cancer by targeting Sirt1. Oncol. Rep. 37 (6), 3244–3252. doi:10.3892/or.2017.5619
You, Y., Bi, F. F., Jiang, Y., Xu, Y. T., An, Y. Y., Li, D., et al. (2019). BRCA1 affects the resistance and stemness of SKOV3-derived ovarian cancer stem cells by regulating autophagy. Cancer Med. 8 (2), 656–668. doi:10.1002/cam4.1975
Yousafzai, N. A., Jin, H., Ullah, M., and Wang, X. (2021). Recent advances of SIRT1 and implications in chemotherapeutics resistance in cancer. Am. J. cancer Res. 11 (11), 5233–5248.
Yu, H. Y., Yang, L., Liu, Y. C., and Yu, A. J. (2023a). Sulforaphene suppressed cell proliferation and promoted apoptosis of COV362 cells in endometrioid ovarian cancer. PeerJ 11, e16308. doi:10.7717/peerj.16308
Yu, W., Wang, Z., Dai, P., Sun, J., Li, J., Han, W., et al. (2023b). The activation of SIRT1 by resveratrol reduces breast cancer metastasis to lung through inhibiting neutrophil extracellular traps. J. drug Target. 31 (9), 962–975. doi:10.1080/1061186X.2023.2265585
Yuan, W., Fang, W., Zhang, R., Lyu, H., Xiao, S., Guo, D., et al. (2023). Therapeutic strategies targeting AMPK-dependent autophagy in cancer cells. Biochimica Biophysica Acta (BBA) - Mol. Cell Res. 1870 (7), 119537. doi:10.1016/j.bbamcr.2023.119537
Yue, Z., Jin, S., Yang, C., Levine, A. J., and Heintz, N. (2003). Beclin 1, an autophagy gene essential for early embryonic development, is a haploinsufficient tumor suppressor. Proc. Natl. Acad. Sci. 100 (25), 15077–15082. doi:10.1073/pnas.2436255100
Zaffagnini, G., and Martens, S. (2016). Mechanisms of selective autophagy. J. Mol. Biol. 428 (9), 1714–1724. doi:10.1016/j.jmb.2016.02.004
Zhang, B., Shen, P., Yin, X., Dai, Y., Ding, M., and Cui, L. (2020). Expression and functions of cellular prion proteins in immunocytes. Scand. J. Immunol. 91 (3), e12854. doi:10.1111/sji.12854
Zhang, D.-W., Shao, J., Lin, J., Zhang, N., Lu, B.-J., Lin, S.-C., et al. (2009). RIP3, an energy metabolism regulator that switches TNF-induced cell death from apoptosis to necrosis. Sci. (New York, NY) 325 (5938), 332–336. doi:10.1126/science.1172308
Zhang, L. H., Yang, A. J., Wang, M., Liu, W., Wang, C. Y., Xie, X. F., et al. (2016). Enhanced autophagy reveals vulnerability of P-gp mediated epirubicin resistance in triple negative breast cancer cells. Apoptosis Int. J. Program. Cell death 21 (4), 473–488. doi:10.1007/s10495-016-1214-9
Zhang, N., Qi, Y., Wadham, C., Wang, L., Warren, A., Di, W., et al. (2010). FTY720 induces necrotic cell death and autophagy in ovarian cancer cells: a protective role of autophagy. Autophagy 6 (8), 1157–1167. doi:10.4161/auto.6.8.13614
Zhang, P., Liu, L., Huang, J., Shao, L., Wang, H., Xiong, N., et al. (2014). Non-SMC condensin I complex, subunit D2 gene polymorphisms are associated with Parkinson’s disease: a Han Chinese study. Genome 57 (5), 253–257. doi:10.1139/gen-2014-0032
Zhang, S., Yang, Y., Huang, S., Deng, C., Zhou, S., Yang, J., et al. (2019). SIRT1 inhibits gastric cancer proliferation and metastasis via STAT3/MMP-13 signaling. J. Cell. physiology 234 (9), 15395–15406. doi:10.1002/jcp.28186
Zhang, W., Dai, J., Hou, G., Liu, H., Zheng, S., Wang, X., et al. (2023a). SMURF2 predisposes cancer cell toward ferroptosis in GPX4-independent manners by promoting GSTP1 degradation. Mol. Cell 83 (23), 4352–4369.e8. doi:10.1016/j.molcel.2023.10.042
Zhang, W., and Luo, P. (2018). MicroRNA-29c restores cisplatin sensitivity in liver cancer through direct inhibition of sirtuin 1 expression. Oncol. Lett. 16 (2), 1543–1550. doi:10.3892/ol.2018.8801
Zhang, X., Dong, Y., Li, W., He, M., Shi, Y., Han, S., et al. (2024b). The mechanism by which SIRT1 regulates autophagy and EMT in drug-resistant oesophageal cancer cells. Life Sci. 343, 122530. doi:10.1016/j.lfs.2024.122530
Zhang, X., Lao, M., Yang, H., Sun, K., Dong, Y., He, L., et al. (2024a). Targeting cancer-associated fibroblast autophagy renders pancreatic cancer eradicable with immunochemotherapy by inhibiting adaptive immune resistance. Autophagy 20 (6), 1314–1334. doi:10.1080/15548627.2023.2300913
Zhang, X., Wu, X., Yao, W., and Wang, Y. H. (2023b). A tumor-suppressing role of TSPYL2 in thyroid cancer: through interacting with SIRT1 and repressing SIRT1/AKT pathway. Exp. Cell Res. 432 (1), 113777. doi:10.1016/j.yexcr.2023.113777
Zhang, Y., Kong, Y., Ma, Y., Ni, S., Wikerholmen, T., Xi, K., et al. (2021). Loss of COPZ1 induces NCOA4 mediated autophagy and ferroptosis in glioblastoma cell lines. Oncogene 40 (8), 1425–1439. doi:10.1038/s41388-020-01622-3
Zhang, Z., Yao, Z., Chen, Y., Qian, L., Jiang, S., Zhou, J., et al. (2018). Lipophagy and liver disease: new perspectives to better understanding and therapy. Biomed. and Pharmacother. = Biomedecine and Pharmacother. 97, 339–348. doi:10.1016/j.biopha.2017.07.168
Zhao, H., Ding, Y., and Zhang, L. (2023d). SIRT1/APE1 promotes the viability of gastric cancer cells by inhibiting p53 to suppress ferroptosis. Open Med. Wars. Pol. 18 (1), 20220620. doi:10.1515/med-2022-0620
Zhao, R., Liu, Y., Wang, Z., Zhang, J., Zhang, W., Zhou, X., et al. (2023e). UBE2C-mediated autophagy inhibition via ubiquitination of SIRT1 contributes to endometrial cancer progression. Mol. cancer Res. MCR 21 (6), 564–577. doi:10.1158/1541-7786.MCR-22-0825
Zhao, T. L., Qi, Y., Wang, Y. F., Wang, Y., Liang, H., and Pu, Y. B. (2023b). 5-methoxytryptophan induced apoptosis and PI3K/Akt/FoxO3a phosphorylation in colorectal cancer. World J. gastroenterology 29 (47), 6148–6160. doi:10.3748/wjg.v29.i47.6148
Zhao, W., Wang, Q., Li, L., Xie, C., Wu, Y., Gautam, M., et al. (2022). SIRT1 regulates mitotic catastrophe via autophagy and BubR1 signaling. Mol. Cell. Biochem. 477 (12), 2787–2799. doi:10.1007/s11010-022-04470-9
Zhao, Y., Liu, Z., Liu, G., Zhang, Y., Liu, S., Gan, D., et al. (2023a). Neutrophils resist ferroptosis and promote breast cancer metastasis through aconitate decarboxylase 1. Cell metab. 35 (10), 1688–1703.e10. doi:10.1016/j.cmet.2023.09.004
Zhao, Y., Ma, R., Wang, C., Hu, R., Wu, W., Sun, X., et al. (2023c). CAPG interference induces apoptosis and ferroptosis in colorectal cancer cells through the P53 pathway. Mol. Cell. probes 71, 101919. doi:10.1016/j.mcp.2023.101919
Zhao, Y. G., Codogno, P., and Zhang, H. (2021). Machinery, regulation and pathophysiological implications of autophagosome maturation. Nat. Rev. Mol. Cell Biol. 22 (11), 733–750. doi:10.1038/s41580-021-00392-4
Zheng, H., Liu, J., Cheng, Q., Zhang, Q., Zhang, Y., Jiang, L., et al. (2024a). Targeted activation of ferroptosis in colorectal cancer via LGR4 targeting overcomes acquired drug resistance. Nat. cancer 5 (4), 572–589. doi:10.1038/s43018-023-00715-8
Zheng, Q., Du, X., Zhang, J., Liu, Y., Dong, W., Dai, X., et al. (2024b). Delivery of SIRT1 by cancer-associated adipocyte-derived extracellular vesicles regulates immune response and tumorigenesis of ovarian cancer cells. Clin. and Transl. Oncol. 26 (1), 190–203. doi:10.1007/s12094-023-03240-3
Zheng, Y., Wu, J., Chen, H., Lin, D., Chen, H., Zheng, J., et al. (2023). KLF4 targets RAB26 and decreases 5-FU resistance through inhibiting autophagy in colon cancer. Cancer Biol. and Ther. 24 (1), 2226353. doi:10.1080/15384047.2023.2226353
Zhou, N., Wei, Z. X., and Qi, Z. X. (2019). Inhibition of autophagy triggers melatonin-induced apoptosis in glioblastoma cells. BMC Neurosci. 20 (1), 63. doi:10.1186/s12868-019-0545-1
Zhu, M., Wei, C., Wang, H., Han, S., Cai, L., Li, X., et al. (2023b). SIRT1 mediated gastric cancer progression under glucose deprivation through the FoxO1-Rab7-autophagy axis. Front. Oncol. 13, 1175151. doi:10.3389/fonc.2023.1175151
Zhu, T., Cen, Y., Chen, Z., Zhang, Y., Zhao, L., Wang, J., et al. (2022). Oncogenic circTICRR suppresses autophagy via binding to HuR protein and stabilizing GLUD1 mRNA in cervical cancer. Cell death and Dis. 13 (5), 479. doi:10.1038/s41419-022-04943-1
Zhu, X., Su, Q., Xie, H., Song, L., Yang, F., Zhang, D., et al. (2023a). SIRT1 deacetylates WEE1 and sensitizes cancer cells to WEE1 inhibition. Nat. Chem. Biol. 19 (5), 585–595. doi:10.1038/s41589-022-01240-y
Zou, Z., Tao, T., Li, H., and Zhu, X. (2020). mTOR signaling pathway and mTOR inhibitors in cancer: progress and challenges. Cell and Biosci. 10 (1), 31–11. doi:10.1186/s13578-020-00396-1
Keywords: autophagy, apoptosis, ferroptosis, drug resistance, sirtuin family, SIRT1
Citation: Tang Y, Ju W, Liu Y and Deng Q (2024) The role of SIRT1 in autophagy and drug resistance: unveiling new targets and potential biomarkers in cancer therapy. Front. Pharmacol. 15:1469830. doi: 10.3389/fphar.2024.1469830
Received: 24 July 2024; Accepted: 13 September 2024;
Published: 30 September 2024.
Edited by:
Hailin Tang, Sun Yat-sen University Cancer Center (SYSUCC), ChinaReviewed by:
Cheng Hu, Third Affiliated Hospital of Sun Yat-sen University, ChinaRenjun Gu, Nanjing University of Chinese Medicine, China
Jin Zhang, University of Mississippi Medical Center, United States
Copyright © 2024 Tang, Ju, Liu and Deng. This is an open-access article distributed under the terms of the Creative Commons Attribution License (CC BY). The use, distribution or reproduction in other forums is permitted, provided the original author(s) and the copyright owner(s) are credited and that the original publication in this journal is cited, in accordance with accepted academic practice. No use, distribution or reproduction is permitted which does not comply with these terms.
*Correspondence: Qin Deng, MzAwMzUyQGhvc3BpdGFsLmNxbXUuZWR1LmNu; Yanjun Liu, bGl1eWFuanVuXzAwMUAxNjMuY29t
†These authors have contributed equally to this work