- 1Department of Respiratory and Critical Care Medicine, Affiliated Hospital of Yangzhou University, Yangzhou University, Yangzhou, China
- 2Department of Microbiology, Medical College, Yangzhou University, Yangzhou, China
Introduction: Acinetobacter baumannii has been recognized as a major public health concern, and carbapenems have been considered the first-line treatment for Acinetobacter baumannii infections. However, their widespread and prolonged use has led to the emergence of carbapenem-resistant Acinetobacter baumannii (CRAB), which has become a significant nosocomial pathogen. The control and treatment of CRAB infections have become a global challenge.
Methods: Logistic regression was applied to investigate the clinical characteristics and prognostic risk factors of CRAB infections. Multilocus sequence typing (MLST) of three clinical CRAB isolates was carried out to determine their genotype. The antibacterial efficacy of meropenem combined with ciprofloxacin was evaluated using checkerboard and growth curve assays. Transcriptomics analysis was futher used to analysis the molecular mechanism related to the combination treatment.
Results: Logistic regression analysis identified the duration of antibiotic use, glucocorticoid application, C-reactive protein levels, and creatine kinase-MB levels as independent risk factors for poor prognosis in CRAB patients. Multilocus sequence typing of three CRAB isolates revealed that all isolates were ST208 genotype. Checkerboard and Growth Curve assays demonstrated that the combination of ciprofloxacin and meropenem had significant bactericidal effects on CRAB, outer membrane permeability, membrane potential, and reactive oxygen species generation. Transcriptomic analysis revealed that the combination inhibited efflux pump function, reduced iron uptake, and impacted metabolic pathways, membrane protein synthesis, and stress responses, thereby enhancing bacterial killing.
Discussion: The findings from this study underscore the synergistic effect of ciprofloxacin and meropenem not only offers a potential alternative treatment strategy but also highlights the importance of combination therapy in overcoming antibiotic resistance, which pose a significant threat to public health.
Introduction
Acinetobacter baumannii (AB) is a Gram-negative, aerobic, non-fermentative bacterium primarily associated with hospital-related infections (Maragakis and Perl, 2008). It can cause respiratory tract infections, bloodstream infections, and more (Sievert et al., 2013). When immune function is compromised or invasive procedures such as intubation, catheter insertion, or surgery are performed, AB can breach mucosal barriers, enter the bloodstream, or spread to other tissues, leading to infection (Iovleva et al., 2022). AB has strong antibiotic resistance and clonal spread capabilities (Garnacho-Montero and Amaya-Villar, 2010). In fact, multidrug-resistant (MDR), extensively drug-resistant (XDR), and pan-drug-resistant (PDR) AB strains have been reported globally (Weinberg et al., 2020). In China, AB has become one of the most important pathogens in nosocomial infections (Dijkshoorn et al., 2007). Multidrug-resistant Acinetobacter baumannii (MDRAB) refers to AB strains resistant to multiple classes of antibiotics, which are more likely to infect immunocompromised or underlying disease patients (Paterson and Doi, 2007).
In the past, infections caused by MDRAB have significantly increased (Munoz-Price et al., 2013). Although carbapenems were once considered the first choice for treating MDRAB infections, the rapid rise of carbapenem resistance globally in recent years has raised widespread concern (Dinc et al., 2013). In Asia, Eastern Europe, and Latin America, carbapenem resistance rates have exceeded 30%–90% (O’Donnell et al., 2021; Shields et al., 2023). Additionally, carbapenems are considered one of the main risk factors for the emergence and spread of MDRAB (Garnacho-Montero et al., 2015). The natural and acquired resistance of AB limits the treatment options for CRAB infections (Shields et al., 2023). CRAB strains often exhibit extensive drug resistance (XDR), meaning resistance to all antibiotics except polymyxins and tigecycline. However, CRAB strains resistant to polymyxins and/or tigecycline have been increasingly reported, significantly increasing the difficulty of clinical treatment (Bell and Park, 2023; Zhao et al., 2023). The high incidence of pan-drug resistance in many clinical isolates is attributed to the complex resistance mechanisms of this pathogen, including biofilm formation, active efflux pumps, and more (Antimicrobial Resistance et al., 2022). As a result, CRAB has become one of the most challenging nosocomial pathogens (Tiku et al., 2021). The main mechanism of carbapenem resistance in AB is the production of carbapenemases (classes D, A, and B) (Bartal et al., 2022). In CRAB, the most common are OXA-type β-lactamases, which are divided into multiple subgroups, with over 400 OXA-type enzymes identified (Evans et al., 2013). Previous studies have shown that CRAB can form biofilms on various material surfaces, including medical devices, rubber, and endotracheal tubes (Greene et al., 2016). Biofilm formation increases the risk of device-associated infections (Pour et al., 2011). Outer membrane proteins (OMPs) are a class of integral membrane proteins embedded in the outer membrane, with OmpA being one of the most abundant porins in the CRAB outer membrane (Uppalapati et al., 2020). Studies have shown that OmpA not only participates in the process of expelling antibiotics from the periplasmic space through the outer membrane but also works in conjunction with the inner membrane efflux system (Confer and Ayalew, 2013). Additionally, OmpA promotes biofilm formation, further enhancing the survival and persistence of AB, and its overexpression is closely associated with a significant increase in pneumonia, bacteremia, and mortality in patients (Sanchez-Encinales et al., 2017). Similarly, the AdeABC efflux pump is highly expressed in CRAB, which is closely related to its resistance to carbapenem antibiotics. Studies have shown that the AdeABC effluxau pump significantly reduces the effective concentration of antibiotics within the cell by expelling them from the cell, thereby enhancing CRAB resistance (Paul et al., 2022; Tamma et al., 2022). In response to these phenomena, researchers have focused on developing various combination therapy regimens, including minocycline/tigecycline and colistin/tigecycline (Castanheira et al., 2014; Menegucci et al., 2019). Although these alternative regimens have shown some efficacy in treating nosocomial infections, their effectiveness remains limited.
In this study, data were collected from 137 patients with carbapenem-resistant A. baumannii (CRAB) infections and 131 patients with non-carbapenem-resistant A. baumannii infections admitted to Yangzhou University Affiliated Hospital from July 2021 to September 2022. A series of analyses were conducted on clinical resistance patterns, biochemical characteristics, and prognostic risk factors. The aim is to provide theoretical insights for clinical antimicrobial strategies and improve the poor prognosis of CRAB patients. Due to the different resistance mechanisms of AB to carbapenems and fluoroquinolones, it is unclear whether the combined use of these two drugs can effectively kill CRAB and its specific mechanisms. Thus, three CRAB strains with strong biofilm formation were selected, and the in vitro antibacterial activity of meropenem combined with ciprofloxacin against CRAB was studied.
Materials and methods
Selection of study subjects
This study selected clinical data from 137 CRAB patients and 131 AB patients hospitalized at the Affiliated Hospital of Yangzhou University from July 2021 to September 2022. The age range of all patients was 16–94 years, with an average age of 69 years; 174 were male (64.9%) and 94 were female (35.1%); 196 patients (73.1%) were over 60 years old. All strains were isolated from sputum specimens of hospitalized patients.
Inclusion criteria
New or progressive radiographic pulmonary infiltrates appearing 48 h after admission, accompanied by at least two clinical infection symptoms (temperature >38°C or <35.5°C, leukocytosis or leukopenia, purulent secretions). For elderly patients, clinical manifestations may be atypical, such as altered mental status, anorexia, fatigue, or lack of fever. Inclusion criteria also included bacteriological test results.
Exclusion criteria
Patients were excluded if they met any of the following conditions: (i) AB detected within 48 h of admission; (ii) age under 16 years; (iii) incomplete clinical data; (iv) duplicate specimens from the same or different sites of the same patient; (v) bacterial colonization without clinical symptoms, radiographic, or laboratory abnormalities.
Detection of β-lactamase genes in A baumannii isolates
We screened for common β-lactamase genes (blaPER, blaSHV, blaIMP, blaVIM, blaSIM, blaNDM-1, blaAmpC, blaOXA-23, blaOXA-24, blaOXA-51, blaOXA-143, blaOXA-58), and sent the primers to Nanjing GenScript Biotechnology Co., Ltd. for synthesis. PCR detection was performed on CRAB 27 and CRAB 49. The final reaction volume for PCR amplification was 20 μL, containing 1 μL of template, 10 μL of Mix, 1 μL each of forward and reverse primers, and 7 μL of ddH2O. The amplification conditions were as follows: pre-denaturation at 95°C for 5 min, denaturation at 95°C for 30 s, annealing for 30 s, extension at 72°C for 1 min, and 35 cycles. The PCR products were subjected to agarose gel electrophoresis and photographed for documentation. The primer sequences are shown in Table 1.
Bacteria culture and biofilm screening
Bacteria culture and isolation were performed according to the standards of the “National Clinical Laboratory Procedures” (fourth edition). For the same site of the same patient, only the first isolated strain was analyzed. Strain identification was conducted using the Vitek 2 Compact automated microbiology analyzer and Vitek MS automated mass spectrometer from bioMérieux, France. Five strains isolated from clinical samples were inoculated onto LB agar plates and isolated using the four-quadrant streaking method. The inoculated plates were incubated overnight at 37°C, and uniform single colonies were selected using an inoculating loop. The selected single colonies were inoculated into 2 mL of LB medium and cultured overnight at 37°C and 200 rpm in a shaker. The cultured bacterial solution was mixed with 40% glycerol at a 1:1 ratio and stored at −80°C for subsequent experiments. Next, the stored bacterial solution was inoculated into 1 mL of MH broth at a 1:100 ratio and cultured until the OD600 nm value reached 0.5. Then, 10 µL of the bacterial solution with an OD600 nm of 0.5 was added to 990 µL of MH broth for a 1:100 dilution. The diluted bacterial solution (200 µL) was added to a 96-well microplate, with three replicates for each strain and 200 µL of MH medium as a blank control. The microplate was incubated statically at 37°C for 18 h. After incubation, the supernatant was discarded, and the wells were washed twice with PBS, stained with 0.4% crystal violet for 30 min, and washed twice again with PBS. Finally, the dye was eluted with 95% ethanol, and the absorbance was measured at OD590 nm using a microplate reader.
Antibiotic susceptibility testing
Single colonies were inoculated into 10 mL of MH broth and cultured at 37°C and 200 rpm until the OD600 nm reached 0.5. The bacterial solution was then diluted 100-fold to a final concentration of 1 × 106 CFU/mL. Different concentrations of antibiotic solutions (0.5, 1, 2, 4, 8, 16, 32, 64 μg/mL) were prepared, and 100 µL of the corresponding concentration of antibiotic was added to each test well. Another 100 µL of the diluted bacterial solution was added to each well, with a negative control well containing 200 µL of MH medium. After thorough mixing, the plates were incubated at 37°C for 18 h. After incubation, the OD600 nm value was measured using a microplate reader. The minimum inhibitory concentration (MIC) of the antibiotic was defined as the lowest concentration that inhibited bacterial growth. Antibiotic susceptibility results were determined according to the 2021 Clinical and Laboratory Standards Institute (CLSI) guidelines and classified as resistant, intermediate, or sensitive. Quality control strains included Escherichia coli ATCC 25922 and Pseudomonas aeruginosa ATCC 27853. All experimental operations strictly followed standard operating procedures. All experiments were repeated three times to ensure reproducibility and reliability of the results.
Data collection
Complete medical records of CRAB patients meeting the inclusion criteria were collected, including the following: (i) Basic patient information: gender, age, history of diabetes, history of invasive procedures, glucocorticoid use, length of hospital stay, length of ICU stay, duration of mechanical ventilation, antibiotic use; (ii) Clinical indicators and antibiotic susceptibility results; (iii) Hospital outcomes: outcomes were classified as improvement (including cure and clinical and/or laboratory and radiographic improvement) and poor prognosis (including death and non-recovery).
Multilocus sequence typing (MLST)
PCR amplification of seven housekeeping genes (gltA, gyrB, gdhB, recA, cpn60, gpi, rpoD) was performed using internationally recognized primers. The amplification products were sequenced by Nanjing GenScript Biotechnology Co., Ltd. According to the Oxford MLST scheme, MLST typing analysis was performed on strains AB1, AB2, and AB3. The obtained gene sequences were uploaded to the Acinetobacter baumannii PubMLST database (http://pubmlst.org/abaumannii/) to determine their sequence type (ST) by comparison with existing sequences in the database. The total volume of the PCR reaction system was 25 μL, including 1 µL of primer, 1 µL of template, 12.5 µL of PCR Mix, and 9.5 µL of ddH2O. The PCR amplification conditions were set as follows: pre-denaturation at 94°C for 5 min; denaturation at 94°C for 30 s, annealing for 30 s, extension at 72°C for 1 min, for a total of 30 cycles; final extension at 72°C for 10 min. Primer sequences and annealing temperatures are detailed in Supplementary Table S1. All experiments were performed with three technical replicates to ensure the reproducibility and reliability of the results.
Checkerboard broth microdilution and the growth curve assays
To evaluate the in vitro antibacterial activity of antibiotic combinations against CRAB, a checkerboard broth microdilution assay was first performed. Based on the results of the previous antibiotic susceptibility tests, the MICs of the two antibiotics were determined, and their concentrations were further diluted to one to two times the MIC. According to the two-fold dilution method, the two antibiotics were diluted along the vertical and horizontal coordinates of the 96-well plate, with 50 µL of the corresponding concentration of antibiotic MH broth added to each well. After overnight culture at 37°C, single colonies were transferred to 5 mL of fresh MH broth and cultured until the OD590 nm reached 0.5 (1 × 108 CFU/mL), then diluted 100-fold to a final concentration of 1 × 106 CFU/mL. Each well was inoculated with 100 µL of the diluted bacterial solution and mixed with 100 µL of MH broth containing antibiotics, with negative and blank controls set. After mixing, the plates were incubated at 37°C for 18 h, and the optical density (OD) at 600 nm was measured using a microplate reader. The bacterial synergistic inhibition rate was calculated using the following formula: Bacterial synergistic inhibition rate (%) = [(OD positive control group − OD negative control group) − (OD sample - OD negative control group)]/(OD positive control group − OD negative control group) × 100%. The fractional inhibitory concentration index (FICI) of the combined drugs was calculated using the following formula: FICI = MIC meropenem (combination)/MIC meropenem (single) + MIC ciprofloxacin (combination)/MIC ciprofloxacin (single). FICI ≤0.5, synergy; 0.5≤ FICI ≤4, no interaction; FICI >4, antagonism (Vidaillac et al., 2012). Additionally, the growth curve assay was performed on the CRAB2 strain. After streaking single colonies using the quadrant method, they were cultured overnight at 37°C and transferred to 5 mL of fresh MH broth at a 1:100 ratio, cultured until the OD590 nm was 0.5 (1 × 108 CFU/mL), and then diluted to 1 × 106 CFU/mL. Subsequently, the two antibiotics were added to the bacterial solution alone or in combination, with no antibiotics added as the control group. The cultures were incubated at 37°C and 200 rpm, and 200 µL samples were taken every 2 h, and the OD600 nm was measured using a microplate reader to monitor bacterial growth (YOURASSOWSKY et al., 1985).
Data analysis
Analysis of CRAB resistance, intermediate resistance, and sensitivity was performed using SPSS 26.0 software for statistical analysis. For quantitative data that follow a normal distribution or an approximate normal distribution, they are presented as mean ± standard deviation. Independent-samples t-test or one-way ANOVA (depending on the number of groups) is used for analysis. If the data do not meet the requirements of normal distribution or homogeneity of variance, they are described by median/interquartile range. The Mann-Whitney U test for two independent samples or the Kruskal–Wallis test for multiple independent samples is used for result analysis. Categorical variables or ranked data are described using percentages. The chi-square test or Fisher’s exact probability method is used for between-group difference analysis.
Transcriptomic analysis
The AB-27 strain of CRAB was selected for transcriptomic analysis. After culturing AB-27 in Mueller-Hinton broth (MHB) to the early logarithmic growth phase, the strain was treated with a combination of ciprofloxacin and meropenem. Following RNA quality assessment, cDNA synthesis, fragmentation, and sequencing were performed. The cDNA library was then amplified and sequenced using the Illumina HiSeq™ platform. Technical services and subsequent bioinformatics analysis were provided by Guangzhou Gene Denovo Biotechnology Co., Ltd. Differentially expressed genes (DEGs) were identified through comparative analysis of RNA-Seq data. Differential expression analysis was conducted using the DESeq2 package, and genes with significant differences were selected based on the predefined thresholds (P < 0.05, |log2FoldChange| > 1). For the selected DEGs, Gene Ontology (GO) enrichment analysis was performed using the R package clusterProfiler. The GO annotations were categorized into three main terms: Biological Process (BP), Molecular Function (MF), and Cellular Component (CC). The significance of each GO term was calculated using Fisher’s exact test, followed by multiple testing correction using the Benjamini–Hochberg method. GO terms with a P-value <0.05 were considered significantly enriched. KEGG pathway enrichment analysis was performed on the DEGs using the KEGG database. KEGG enrichment analysis was conducted using the R package clusterProfiler, which calculates the enrichment of DEGs across various KEGG pathways using Fisher’s exact test, followed by False Discovery Rate (FDR) correction for P-values. Pathways with a P-value <0.05 were considered significantly enriched.
Results
Distribution of CRAB strains
In this study, 137 carbapenem-resistant A. baumannii (CRAB) strains were isolated from sputum samples. These strains were primarily obtained from the Intensive Care Unit (ICU) (103 cases), the Department of Respiratory Medicine (12 cases), and the Department of Neurosurgery (11 cases), accounting for 75.2%, 8.8%, and 8.0% of the total, respectively (Supplementary Table S2).
Antibiotic resistance of CRAB strains
In this study, carbapenem resistant Acinetobacter baumannii strains were selected for research. Clinical antimicrobial susceptibility tests on 137 CRAB strains against 24 antimicrobial agents were conducted. Results showed that among β-lactam antibiotics, resistance rate to carbapenem antibiotics was the highest, reaching 99.27%. This was followed by third-generation cephalosporins, with a resistance rate exceeding 96%. The resistance rates to broad-spectrum penicillins (such as piperacillin and ticarcillin) were also high, exceeding 95%.CRAB exhibited moderate resistance to tigecycline, with a resistance rate of 42.33%, while minocycline still showed good antibacterial activity against CRAB, with a resistance rate of 25.54% (Supplementary Table S3).
Comparison of clinical characteristics between CRAB and AB
To compare the clinical characteristics between the CRAB group and the AB group, we conducted a comparative analysis of multiple parameters in both groups of patients. These parameters included the number of invasive procedures, length of hospital stay, length of ICU stay, duration of mechanical ventilation, types and duration of antibiotic use, conjugated bilirubin levels, alanine aminotransferase (ALT) levels, aspartate aminotransferase (AST) levels, urea levels, and the number of unresolved cases. We found that the CRAB group had higher values in all these indicators compared to the AB group, with statistically significant differences between the two groups (P < 0.05). The number of patients undergoing invasive procedures was 129 in the CRAB group and 111 in the AB group, accounting for 94.16% and 84.73%, respectively. The length of hospital stay was 21 ± 11 days for the CRAB group and 17 ± 9 days for the AB group. The length of ICU stay was 13 ± 9 days for the CRAB group and 5 ± 7 days for the AB group. The duration of mechanical ventilation was 9 ± 8 days for the CRAB group and 3 ± 6 days for the AB group. The number of types of antibiotics used was 4 ± 7 in the CRAB group and 2 ± 1 in the AB group. The duration of antibiotic use was 18 ± 9 days in the CRAB group and 13 ± 7 days in the AB group. The conjugated bilirubin level was 6.32 ± 22.40 μmol/L in the CRAB group and 3.69 ± 14.68 μmol/L in the AB group. The average alanine aminotransferase (ALT) level was 36.00 U/L in the CRAB group and 23.00 U/L in the AB group. The average aspartate aminotransferase (AST) level was 28.00 U/L in the CRAB group and 41.00 U/L in the AB group. The urea level was 11.19 ± 7.00 mmol/L in the CRAB group and 8.41 ± 5.67 mmol/L in the AB group. The number of unresolved cases was 53 in the CRAB group and 25 in the AB group, accounting for 38.69% and 19.08%, respectively (Supplementary Table S4).
Clinical characteristics of poor prognosis in CRAB patients
To elucidate the prognosis of patients with CRAB infection, we compared multiple parameters between the non-improvement group and the improvement group. The results showed that the non-improvement group had higher values in ICU stay days, mechanical ventilation days, white blood cell count, neutrophil count, C-reactive protein level, procalcitonin level, conjugated bilirubin, unconjugated bilirubin, aspartate aminotransferase, urea, creatinine, and creatine kinase-MB compared to the improvement group, with statistically significant differences between the two groups (P < 0.05). Specifically, the ICU stay days were 15 ± 11 days in the non-improvement group and 11 ± 8 days in the improvement group; mechanical ventilation days were 11 ± 9 days in the non-improvement group and 8 ± 8 days in the improvement group; white blood cell count was 12.17 ± 5.19 × 109/L in the non-improvement group and 9.82 ± 3.53 × 109/L in the improvement group; neutrophil count was 11.37 ± 7.00 × 109/L in the non-improvement group and 8.05 ± 3.20 × 109/L in the improvement group; C-reactive protein level was 102.19 ± 77.72 mg/L in the non-improvement group and 50.09 ± 39.31 mg/L in the improvement group; procalcitonin level was 4.49 ± 8.57 ng/L in the non-improvement group and 0.68 ± 1.64 ng/L in the improvement group; conjugated bilirubin was 14.60 ± 34.45 μmol/L in the non-improvement group and 1.10 ± 2.67 μmol/L in the improvement group; unconjugated bilirubin was 16.06 ± 12.83 μmol/L in the non-improvement group and 10.24 ± 6.61 μmol/L in the improvement group; aspartate aminotransferase average level was 53.00 U/L in the non-improvement group and 36.00 U/L in the improvement group; urea was 13.84 ± 8.40 mmol/L in the non-improvement group and 9.52 ± 5.36 mmol/L in the improvement group; creatinine was 126.59 ± 94.72 μmol/L in the non-improvement group and 83.21 ± 75.63 μmol/L in the improvement group; creatine kinase-MB was 19.45 ± 26.03 U/L in the non-improvement group and 7.64 ± 6.40 U/L in the improvement group. These data indicate that the clinical manifestations and laboratory indicators of patients in the non-improvement group were significantly worse than those in the improvement group (Supplementary Table S5).
Risk factors for prognosis of CRAB infection
The results of the logistic multivariate regression analysis showed that the duration of antibiotic use, application of glucocorticoids, C-reactive protein levels, and creatine kinase isoenzyme levels were independent risk factors for poor prognosis in CRAB patients (P < 0.05) (Table 2). Additionally, C-reactive protein and creatine kinase isoenzyme levels have certain predictive value in forecasting poor prognosis in CRAB patients, with an AUC of 0.69 for C-reactive protein and an AUC of 0.64 for creatine kinase isoenzyme (Figure 1).
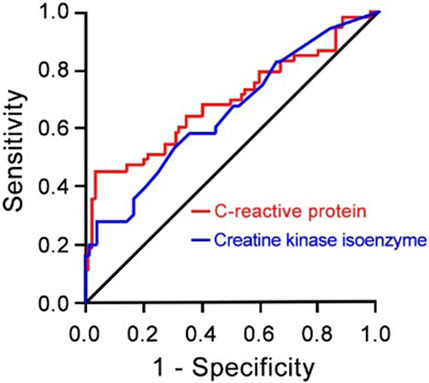
Figure 1. Receiver Operating Characteristic (ROC) Curve for C-reactive Protein (red lines) and Creatine Kinase Isoenzyme (blue lines) as Risk Factors for Prognosis of CRAB Infection (N = 137) compared to AB patients (N = 131). CRAB, carbapenem-resistant Acinetobacter baumannii; AB, Acinetobacter baumannii.
Biofilm strength and antibiotic susceptibility testing
We tested the biofilm formation ability of five CRAB strains, with results reflected by OD590 nm values. The results indicated that strain 2 had the strongest biofilm formation ability, followed by strains 1 and 3 (Figure 2). Therefore, we selected these three strong biofilm-forming CRAB strains for further antibiotic susceptibility testing. We found that all strains exhibited high levels of resistance (MIC ≥64 μg/mL) to meropenem (MEM), imipenem (IPM), gentamicin (GEN), ticarcillin (TIC), ceftriaxone (CRO), tetracycline (TET), and vancomycin (VCA). Notably, CRAB1 had minimum inhibitory concentrations (MICs) of 4 μg/mL for ciprofloxacin (CIP), while CRAB2 and CRAB3 had MICs of 8 μg/mL for this drugs, respectively. These results indicate that these strains exhibit intermediate sensitivity to levofloxacin and ciprofloxacin. Additionally, all strains were highly sensitive to tigecycline (TGC) (MIC <1 μg/mL) (Table 3).
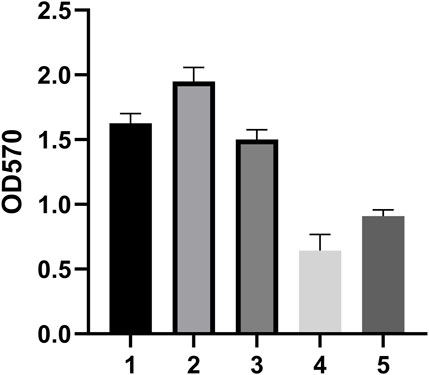
Figure 2. Biofilm Formation Ability of 5 Clinically Isolated CRAB Strains. The data are expressed as mean ± SD derived from three biological replicates. The Kruskal–Wallis test was utilized to assess differences in fluorescence alterations among the four groups, followed by the Mann-Whitney U test for pairwise comparisons between groups. (A–D) Bars sharing the same annotation indicate no statistically significant differences between groups, while bars with different annotations denote statistically significant differences between groups.
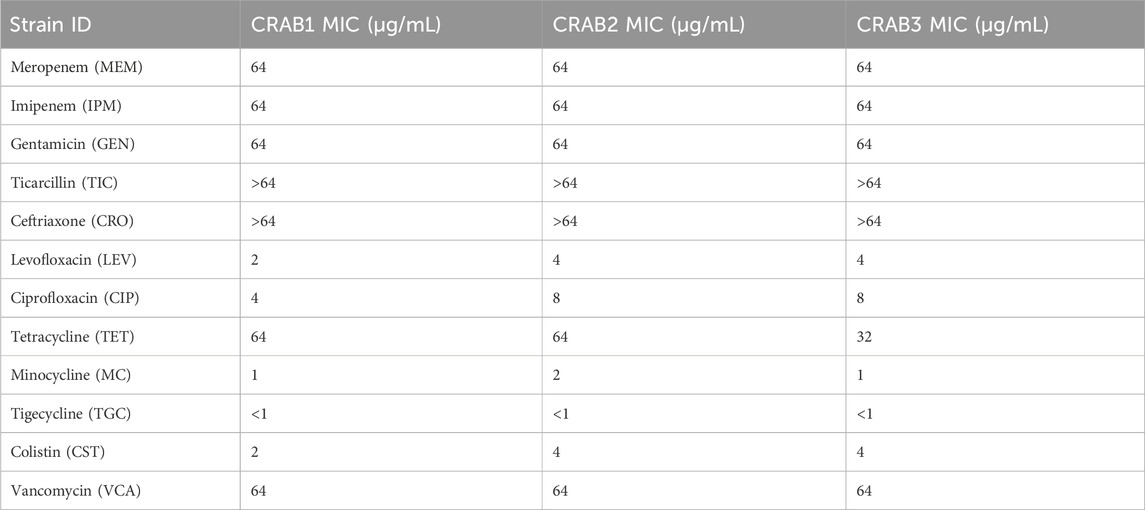
Table 3. Antibiotic Susceptibility test results (All experiments were conducted with three technical replicates).
The β-lactamase gene carriage status of the two CRAB strains
Two CRAB strains (CRAB27 and CRAB49) were collected for analyzing the synergistic effects of carbapenem antibiotics and ciprofloxacin in bactericidal experiments. Prior to the experiments, the β-lactamase gene carriage status of both CRAB strains was analyzed, with the results shown in Figure 3. PCR detection revealed that both CRAB strains carried the blaVIM, blaOXA-51, blaAmpC, and blaOXA-23 genes. The blaVIM gene encodes a Class B metallo-β-lactamase (MBL), while blaOXA-51 and blaOXA-23 encode Class D β-lactamases (OXA enzymes), and blaAmpC encodes a Class C β-lactamase (AmpC enzyme). There were no significant differences in the β-lactamase genotypes between the two CRAB strains, indicating that they likely share similar resistance mechanisms. By confirming the absence of significant genotypic differences between the strains, potential interference from genotypic variations in subsequent resistance mechanism studies can be ruled out. This facilitates a more accurate assessment of the impact of combination therapy on CRAB resistance.
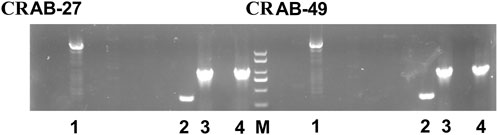
Figure 3. The β-lactamase gene carriage status of CRAB-27 and CRAB-49. (M: Marker; 1: blaVIM; 2: blaOXA-51; 3: blaAmpC; 4: blaOXA-23).
Checkerboard assay and growth curve
The checkerboard assay results indicated that the combination of carbapenem antibiotics and ciprofloxacin exhibited significant synergistic effects against AB27 and AB49. In contrast, the combination of carbapenem antibiotics and colistin showed no interaction against AB27 and AB49. We further used the growth curve of bacteria to describe the bactericidal effects of different antibiotics on CRAB. According to the curve analysis, meropenem alone (blue line) had poor inhibitory effects on the strains, with the OD600 nm value gradually increasing over 24 h, indicating continuous bacterial growth. Ciprofloxacin alone (green line) showed some inhibitory effects compared to meropenem but still failed to completely inhibit bacterial growth. When meropenem was combined with ciprofloxacin (orange line), the OD600 nm value was significantly lower than in other groups within 24 h, indicating a significant bactericidal effect of this combination. In summary, The FICI of MEM combined with CIP is 0.375, indicating that the combination of the two has a synergistic antibacterial effect against CRAB. The FICI of MEM combined with COL is greater than 0.5, indicating that the combination of the two does not have a synergistic effect (Table 4). This demonstrates the potential advantage of the combination therapy of MEM and CIP against CRAB strains (Figure 4).
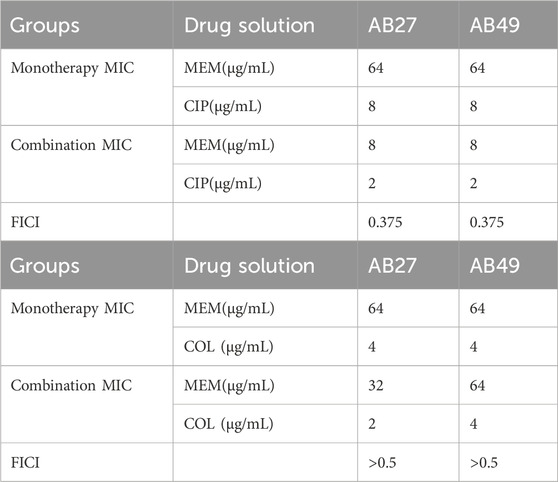
Table 4. The FICI values of MEM in combination with two antimicrobial agents (Replications: three times).
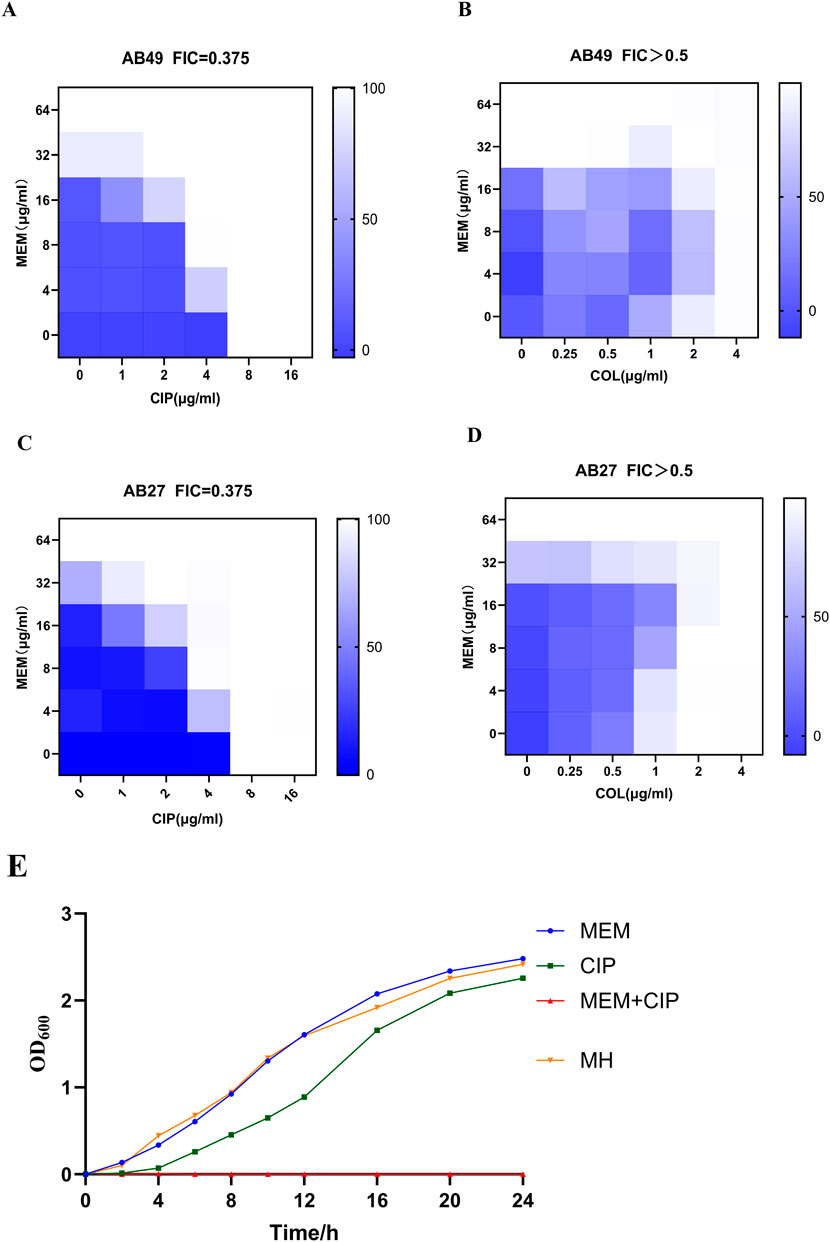
Figure 4. Inhibitory Effects of Different Antibiotics on CRAB. (A) Bactericidal effects of MEM with CIP on AB49; (B) Bactericidal effects of MEM with COL on AB49; (C) Bactericidal effects of MEM with CIP on AB27; (D) Bactericidal effects of MEM with COL on AB27; (E) Representative growth curves of AB27 (MEM:Meropenem; CIP:Ciprofloxacin; COL:Colistin). Data were presented as mean ± SD from three biological replicates.
The synergy mechanism of meropenem with ciprofloxacin
To further elucidate the effects of various antibiotics on CRAB, we examined the impact of different antibiotic treatments on the outer membrane permeability, membrane integrity, membrane potential, and reactive oxygen species (ROS) production of CRAB. The results showed that the combination of meropenem and ciprofloxacin significantly increased the outer membrane permeability of CRAB (P < 0.001), with the combined use of meropenem and ciprofloxacin being more effective than either drug alone. Membrane integrity analysis indicated that the meropenem group had significantly higher membrane integrity than other groups (P < 0.01). In contrast, the ciprofloxacin alone and combination groups did not show significant differences in membrane integrity but were both lower than the meropenem group. Membrane potential measurements showed that the membrane potential of the meropenem and ciprofloxacin combination group was significantly higher than that of the control group (P < 0.05), indicating a significant synergistic effect of this combination in altering membrane potential. In terms of ROS production, both the ciprofloxacin alone and combination groups significantly increased ROS levels, although the combination group showed higher ROS production, the difference was not statistically significant compared to the control group (Figure 5).
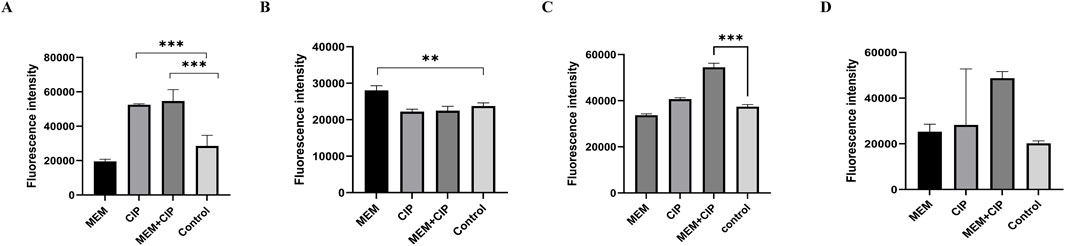
Figure 5. Effects of Different Antibiotics on CRAB. (A) Effects of different antibiotic treatments on the outer membrane of CRAB; (B) Effects of different antibiotic treatments on the membrane integrity of CRAB; (C) Effects of different antibiotic treatments on the membrane potential of CRAB; (D) Different antibiotic treatments altered ROS production levels in CRAB (MEM, Meropenem; CIP, Ciprofloxacin). The data are expressed as mean ± SD derived from three biological replicates. The Kruskal–Wallis test was utilized to assess differences in fluorescence alterations among the four groups, followed by the Mann-Whitney U test for pairwise comparisons between groups.
ST typing results of CRAB
In this study, multilocus sequence typing (MLST) was used to analyze the sequence types of carbapenem-resistant A. baumannii strains. The results revealed that strains 8, 27, and 49 al l belonged to Sequence Type 208 (ST208). These strains shared identical allele numbers across seven loci: gltA, cnp60, gdhB, gpi, gyrB, rpoD, and recA. This finding suggests that the strains have a common genetic background and likely originated from the same clonal group (Table 5).
Inhibition of CRAB energy metabolism and efflux activity by meropenem combined with ciprofloxacin
To investigate the synergistic bactericidal mechanism of ciprofloxacin combined with meropenem against CRAB, the transcriptome of the AB-27 strain was analyzed after ciprofloxacin-meropenem combination treatment. The results showed that, compared to the control group, 480 genes were upregulated and 112 genes were downregulated in CRAB following the combined treatment (Figure 6A). GO enrichment analysis revealed that the differentially expressed genes (DEGs) under meropenem combined with ciprofloxacin treatment (Figure 6B) are primarily associated with the following biological functional categories: Biological Processes: The DEGs are significantly enriched in cellular processes, metabolic processes, localization, and response to stimuli, indicating that the drug treatment has a substantial impact on the basic biological activities of the bacteria. Molecular Functions: The DEGs are predominantly enriched in catalytic activity, binding activity, and transport activity, which are closely related to the bacteria’s metabolic and adaptive responses. Cellular Components: The DEGs are mainly concentrated in cellular components and protein complexes, suggesting that the treatment may induce alterations in the structure and function of the bacterial cell. KEGG pathway analysis further revealed the biological pathways associated with these differentially expressed genes (DEGs) (Figure 6C). The most significantly enriched pathways include: Metabolic Pathways, with the Global and Overview Maps (132 genes) and Amino Acid Metabolism (48 genes) being the most prominently enriched. Other important pathways include Energy Metabolism, Carbohydrate Metabolism, and Cofactor and Vitamin Metabolism, suggesting that the drug treatment may affect bacterial growth and antibiotic resistance by altering metabolic pathways. Genetic Information Processing pathways, such as Folding, Sorting, and Degradation (12 genes) and Transcription (11 genes), indicating that the combined treatment may impact gene expression and protein synthesis in the bacteria. Cellular Processes and Environmental Information Processing pathways were also enriched, highlighting the bacterial adaptive response to environmental changes.
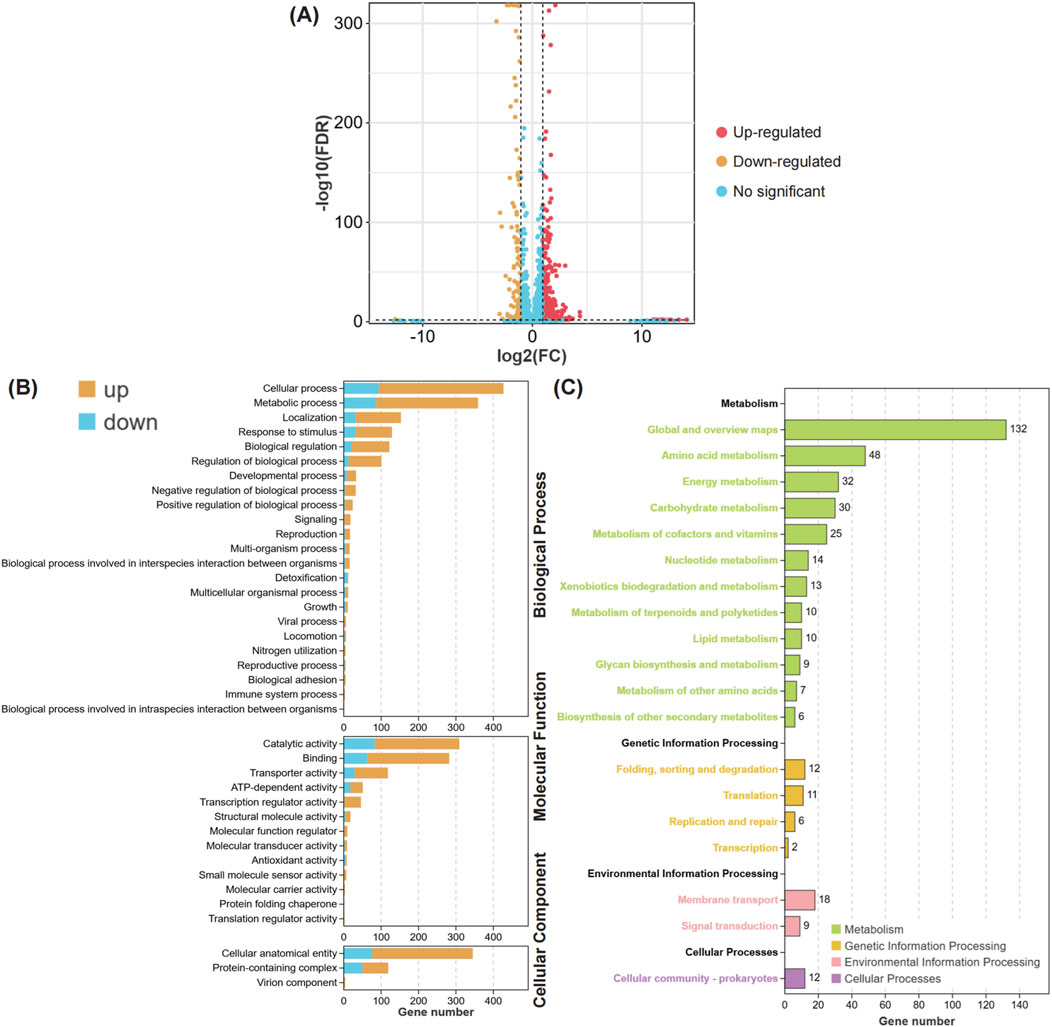
Figure 6. The biological functions of differential expression genes in MIPCEM compared to MH. (A) The Volcano plot of differentially expressed genes. The points with different colors represent different alteration trends, Red, Upregulated; Yellow, Downregulated; Blue, No-significant trend. (B) The Bar plot of GO enrichment for differentially expressed genes. The figure is divided into three panels based on different types of GO Terms, including biological process, molecular function, and cellular component. The bars in different colors represent the pathway enrichment results for upregulated (Yellow) and downregulated (Blue) genes, respectively. (C) The Bar plot of KEGG enrichment for differentially expressed genes. The bar with different colors represent the different types biological pathways, including metabolism (green), genetic information processing (yellow), environmental information processing (fresh pink), and cellular processes (pink).
Discussion
In recent years, A. baumannii has become one of the main pathogens of hospital-acquired infections (HAIs). With the widespread use of antibiotics in clinical settings, the resistance rate of Acinetobacter baumannii to carbapenem antibiotics has been increasing annually. By 2017, the World Health Organization (WHO) had listed it as a pathogen that poses a significant threat to human health (Boral et al., 2019; Xie et al., 2018). The results of this study indicated that the detection rate of CRAB in our hospital’s ICU, respiratory department, and neurosurgery department is relatively high, at 75.2%, 8.8%, and 8.0%, respectively, which is consistent with previous reports on the distribution of CRAB in clinical departments (Qian et al., 2015). This phenomenon may be related to several factors: first, patients hospitalized in these departments are usually critically ill and have weakened immune systems; second, the routine use of various invasive procedures and long-term broad-spectrum antibiotic treatments. Therefore, medical staff in relevant departments should be vigilant, strengthen environmental hygiene monitoring and disinfection, and strictly follow aseptic procedures to reduce the risk of iatrogenic cross-infection. Additionally, this study shows that CRAB specimens are mainly derived from sputum, which is consistent with previous clinical studies (Lin et al., 2020). This indicates that CRAB primarily causes respiratory infections in our hospital. Therefore, it is particularly important to focus on and strengthen infection prevention and control measures in key departments.
This study shows that the resistance rates of common clinical antibiotics, including β-lactams (excluding fourth-generation cephalosporins), aminoglycosides (excluding tobramycin), and quinolones, all exceed 90%. Additionally, the resistance rate of tetracycline antibiotics is also rising, with the resistance rate of doxycycline exceeding 94%. Kristina et al. (Cerniauskiene et al., 2023) studied the antibiotic resistance of Acinetobacter baumannii from 2016 to 2017 and from 2021 to 2022, finding that the proportion of doxycycline-resistant Acinetobacter baumannii nearly doubled, which is consistent with the tetracycline resistance rate observed in our study. Furthermore, the resistance rate of sulfonamides is also higher than 50%. Currently, only polymyxin shows 100% intermediate sensitivity to Acinetobacter baumannii. However, there have been reports worldwide of the emergence of colistin-resistant Acinetobacter baumannii, and its resistance rate is gradually increasing with the widespread use of colistin (CAI et al., 2012). In this study, the resistance rate of Acinetobacter baumannii to polymyxin in our hospital is 0%, which differs from the results of Trebosc et al. (2019), possibly due to different antibiotic usage habits in different hospitals. Although Acinetobacter baumannii remains sensitive to polymyxin, the emergence of resistance to polymyxin poses a significant challenge to clinical anti-infection treatment. Therefore, developing new treatment strategies remains urgent.
This study found that the proportion of CRAB patients undergoing invasive procedures was significantly higher than that of the AB group. Additionally, CRAB patients had longer hospital stays, ICU stays, mechanical ventilation days, and more types and longer durations of antibiotic use compared to the AB group. CRAB patients also had higher levels of conjugated bilirubin, alanine aminotransferase (ALT), aspartate aminotransferase (AST), and urea, indicating that CRAB patients generally have more severe conditions involving multiple organ dysfunction, leading to a significantly higher incidence of poor prognosis compared to the AB group. Among CRAB patients, those with poor prognosis had higher rates of glucocorticoid use, longer ICU stays, and longer mechanical ventilation days than those with improved conditions. Additionally, patients with poor prognosis had higher white blood cell counts, neutrophil ratios, C-reactive protein (CRP), procalcitonin (PCT), conjugated bilirubin, unconjugated bilirubin, aspartate aminotransferase (AST), urea, creatinine, and creatine kinase isoenzyme (CK-MB) levels than those with improved conditions, which is consistent with previous studies (Borges Duarte and Goncalves Rodrigues, 2022). Therefore, exploring the risk factors and resistance of hospital-acquired infections caused by Acinetobacter baumannii and reducing the chances of infection are crucial for early treatment of patients. This study shows that the duration of antibiotic use, application of glucocorticoids, C-reactive protein, and creatine kinase isoenzyme are independent risk factors for poor prognosis in CRAB infection patients. ROC curve analysis shows that C-reactive protein and creatine kinase isoenzyme have certain predictive value for poor prognosis in CRAB patients (AUC = 0.69, AUC = 0.64), which is consistent with previous reports (Zhou et al., 2019). However, the clinical biochemical indicators collected in this study are relatively limited, which is related to the lack of clinical examination data of patients and is one of the limitations of this study. Future studies need to collect a large amount of basic information and clinical biochemical indicators of hospitalized patients infected with Acinetobacter baumannii over a long period to further enrich the research results.
Acinetobacter baumannii can form complex biofilm structures, allowing it to colonize biological and non-biological surfaces for extended periods. Once a biofilm is formed, the difficulty of treating Acinetobacter infections increases significantly, and the risk of transmission between patients also increases, potentially leading to uncontrollable infection outbreaks. Therefore, this study tested the biofilm formation ability of five CRAB strains and selected three strong biofilm-forming CRAB strains for antibiotic susceptibility testing. The results showed that these strains were resistant to all antibiotics except tigecycline, which is consistent with previous reports (Maleki et al., 2022). We performed MLST typing analysis on three CRAB strains, and the results showed that all three strains were ST208. The allele sequences of seven housekeeping genes, gltA, gyrB, gdhB, cpn60, gpi, and rpoD, were identical, while the similarity of the recA gene was 98.92%. This result indicates that ST208 strains are highly conserved, widely distributed, and strongly resistant to carbapenem antibiotics, making them prone to global spread, consistent with the report by Gao et al. (2022) We further conducted antibiotic combination experiments on CRAB strains, and the results showed that the combined use of meropenem and ciprofloxacin could reduce each other’s MIC, indicating a synergistic effect between these two drugs. This result is consistent with the study by Bilal et al. on the combined use of meropenem and ciprofloxacin against P. aeruginosa (another Gram-negative bacillus) (BILAL et al., 2020). Specifically, the combination of 8 μg/mL meropenem and 4 μg/mL ciprofloxacin had a significant bactericidal effect on carbapenem-resistant Acinetobacter baumannii, while the same effect was not achieved when these two drugs were used alone. To our knowledge, this is the first study to report the combined use of meropenem and ciprofloxacin in experiments on carbapenem-resistant Acinetobacter baumannii and confirm its significant bactericidal effect.
The resistance of Acinetobacter baumannii to carbapenem antibiotics is mainly mediated by the production of β-lactamases, which can catalyze the hydrolysis of β-lactam antibiotics. Based on differences in sequence motifs and hydrolysis mechanisms, β-lactamases can be divided into four classes: A, B, C, and D. Among them, class D β-lactamases, also known as oxacillinases (OXAs) or carbapenem-hydrolyzing class D β-lactamases (CHDLs), can inactivate a broad spectrum of β-lactam antibiotics, especially antibiotics of the OXA-10 family, and have become the main mechanism of carbapenem resistance (Antunes and Fisher, 2014). Bacterial biofilms are the primary means by which Acinetobacter baumannii establishes and survives long-term in patients. Additionally, Acinetobacter baumannii can persist in hospital environments by forming biofilms. Biofilms create a physical barrier that impedes the penetration of antimicrobial agents; on the other hand, the metabolic activity within biofilms slows down, reducing the bacteria’s response rate to antimicrobial agents, leading to multidrug resistance in Acinetobacter baumannii (Qin and Wang, 2019). Efflux pumps are one of the key mechanisms by which bacteria resist the action of antibiotics, actively expelling antibiotics out of bacterial cells to reduce intracellular drug concentrations and prevent them from reaching their intended targets (Ding et al., 2023). The AdeJ gene, belonging to the resistance-nodulation-division (RND) family of efflux pumps, plays a crucial role in this process by expelling multiple antibiotics from bacterial cells, leading to multidrug resistance (MDR) (Zhang et al., 2021). In carbapenem-resistant Acinetobacter baumannii (CRAB) strains, the overexpression of AdeJ, along with other efflux pumps such as AdeB and AbeM, is closely associated with high levels of resistance, particularly to carbapenem antibiotics, which are often used as a last resort in clinical treatment (Rafiei et al., 2022). As a significant member of the RND family, AdeJ can expel various antibiotics, including β-lactams, ciprofloxacin, and tetracyclines. Studies have shown that the overexpression of AdeJ in CRAB strains is significantly correlated with their resistance characteristics (Zack et al., 2024). Like most organisms, AB requires iron as an essential element for survival and proliferation during infection (Miethke and Marahiel, 2007). AB secretes siderophores, such as aerobactin, enterobactin, salmochelin, and yersiniabactin, which tightly bind extracellular iron and transport it back into the bacteria (Gonzalez-Ferrer et al., 2021). In this process, ATP-binding cassette (ABC) transporter substrate-binding proteins play a key role by utilizing the energy generated from ATP hydrolysis to transport iron-bound siderophores across the membrane into the cell, a mechanism closely related to AB’s survival in iron-deficient environments (Abdallah et al., 2023). The cysteine ABC transporter substrate-binding protein is part of the ABC transport system, specifically responsible for recognizing and transporting cysteine from the extracellular environment into the cell, which is crucial for bacterial nutrient uptake, metabolic regulation, and adaptation to adverse environmental conditions (Ohtsu et al., 2015). Additionally, it plays an important role in the pathogenicity of pathogens and their ability to colonize the host, making it a potential target for antibacterial drugs (Davies et al., 2021). Our study found that the combined use of ciprofloxacin and meropenem significantly disrupted the integrity of CRAB biofilms, increased membrane permeability, and caused significant membrane depolarization. Ultimately, these changes led to elevated ROS levels within CRAB, triggering oxidative stress responses. Furthermore, our transcriptomic analysis revealed that the combination of ciprofloxacin and meropenem significantly reduced the expression of adeJ and ABC transporter permease in CRAB, effectively inhibiting the function of CRAB efflux pumps and their Fe2+ uptake. GO and KEGG enrichment analysis results indicated that the combined treatment of meropenem and ciprofloxacin significantly affected bacterial metabolic pathways, membrane protein synthesis, and stress responses, particularly by regulating metabolism-related genes and pathways, altering the bacteria’s ability to adapt to environmental changes, thereby effectively killing CRAB.
In summary, this study preliminarily explored the bactericidal effect of meropenem combined with ciprofloxacin on CRAB. The results showed that these two drugs have a significant synergistic effect, reducing their respective MICs and enhancing their bactericidal effect. Meropenem is primarily metabolized by the kidneys, so dose adjustments are necessary in patients with renal impairment; ciprofloxacin is metabolized through the blood, kidneys, and bile. However, patients with CRAB infections are often critically ill and frequently suffer from multiple organ failure. In such patients, high-dose medication may further impair organ function. Therefore, a reasonable combination therapy and dose reduction to protect organ function are crucial. The limitation of this study is the small number of strains used, and the specific mechanism of the synergistic bactericidal effect of meropenem and ciprofloxacin was not thoroughly investigated. Future studies will expand the number of strains and delve into the synergistic bactericidal mechanism to provide more reliable theoretical evidence for clinical medication.
Conclusion
Our findings demonstrate that CRAB patients had more severe clinical outcomes compared to the non-resistant AB group, with significantly longer hospital stays, ICU admissions, mechanical ventilation duration, and antibiotic use. Multi-organ dysfunction and higher rates of adverse outcomes were also more prevalent in the CRAB group. Logistic regression identified prolonged antibiotic use, corticosteroid use, elevated C-reactive protein, and creatine kinase isoenzyme levels as independent predictors of poor prognosis. CRAB displayed broad resistance to common antibiotics but remained partially sensitive to tigecycline. The combination of meropenem and ciprofloxacin exhibited synergistic antibacterial effects by lowering the MIC and enhancing bactericidal activity. Certain CRAB strains showed strong biofilm formation, complicating treatment. MLST analysis identified these strains as ST208, suggesting clonal origins. Mechanistically, meropenem and ciprofloxacin disrupted the CRAB outer membrane, increasing permeability, depolarizing membrane potential, and inducing oxidative stress. Transcriptomic analysis revealed inhibition of efflux pump function, reduced iron uptake, and significant disruption of metabolic pathways, membrane protein synthesis, and stress responses, leading to enhanced bacterial clearance. This study underscores the potential of combination therapy in overcoming CRAB resistance and improving treatment outcomes.
Data availability statement
The data obtained have been approved by the Ethics Committee of the Affiliated Hospital of Yangzhou University, and the ethical approval number is 2021-YKL4-28-004. All data are legal and compliant. Due to ethical requirements, the original data cannot be uploaded to public datasets. However, if readers wish to access the data, they may request the information from the corresponding author atMDkwOTA0QHl6dS5lZHUuY24=and the raw data supporting the conclusion of this article will be made available by the authors, without undue reservation.
Ethics statement
All participants signed informed consent forms, and the ethical review was completed under the supervision of the Ethics Committee of the Affiliated Hospital of Yangzhou University (protocol code: 2021-YKL4-28-004, date of approval: 2021-04-28). No potentially identifiable human images or data are presented in this study.
Author contributions
YF: Data curation, Writing – review and editing. XC: Writing – original draft, Writing – review and editing. YS: Writing – review and editing. TG: Formal Analysis, Writing – review and editing. FW: Project administration, Writing – original draft. FJ: Data curation, Writing – review and editing. JZ: Writing – original draft, Writing – review and editing.
Funding
The author(s) declare that financial support was received for the research and/or publication of this article. This research was funded by the Clinical Translational Research Project of the Medical Innovation and Translation Special Fund of Yangzhou University grant (AHYZUZHXM 202106), the Open Project Program of Jiangsu Key Laboratory of Zoonosis (No. R2202) and 2023 Yangzhou Social Development Project (No. YZ2023098) and Yangzhou Key Discipline Cultivation Project (YZYXZDXK-010).
Acknowledgments
The A. baumannii strains included in this study was kindly given by the department of clinical laboratory in the Affiliated Hospital of Yangzhou University. The study was conducted in accordance with the Declaration of Helsinki, and approved by the Affiliated Hospital of Yangzhou University (protocol code: 2021-YKL4-28-004, date of approval: 2021-04-28). The authors affirm that human research participants provided informed consent for publication. This research was funded by the Clinical Translational Research Project of the Medical Innovation and Translation Special Fund of Yangzhou University grant (AHYZUZHXM 202106), the Open Project Program of Jiangsu Key Laboratory of Zoonosis (No. R2202) and 2023 Yangzhou Social Development Project (No. YZ2023098) and Yangzhou Key Discipline Cultivation Project (YZYXZDXK-010). All authors made a significant contribution to the work reported, whether that is in the conception, study design, execution, acquisition of data, analysis and interpretation, or in all these areas. All authors have drafted or written, or substantially revised or critically reviewed the article. All authors have agreed to the published version of the manuscript and be accountable for the contents of the article and no conflicts of interest in this work.
Conflict of interest
The authors declare that the research was conducted in the absence of any commercial or financial relationships that could be construed as a potential conflict of interest.
Generative AI statement
The author(s) declare that no Generative AI was used in the creation of this manuscript.
Publisher’s note
All claims expressed in this article are solely those of the authors and do not necessarily represent those of their affiliated organizations, or those of the publisher, the editors and the reviewers. Any product that may be evaluated in this article, or claim that may be made by its manufacturer, is not guaranteed or endorsed by the publisher.
Supplementary material
The Supplementary Material for this article can be found online at: https://www.frontiersin.org/articles/10.3389/fphar.2025.1534155/full#supplementary-material
References
Abdallah, S., Kassem, M., Mohamed, N., and Bahey-El-Din, M. (2023). Exploration of the potential of the ATP-binding cassette (ABC) transporter antigen as a vaccine candidate against Acinetobacter baumannii infections. J. Adv. Pharm. Res. 7 (2), 111–119. doi:10.21608/aprh.2023.177396.1202
Antimicrobial Resistance Collaborators Ikuta, K. S., Sharara, F., Swetschinski, L., Robles Aguilar, G., Gray, A., et al. (2022). Global burden of bacterial antimicrobial resistance in 2019: a systematic analysis. Lancet 399 (10325), 629–655. doi:10.1016/s0140-6736(21)02724-0
Antunes, N. T., and Fisher, J. F. (2014). Acquired class D beta-lactamases. Antibiot. (Basel) 3 (3), 398–434. doi:10.3390/antibiotics3030398
Bartal, C., Rolston, K. V. I., and Nesher, L. (2022). Carbapenem-resistant Acinetobacter baumannii: colonization, infection and current treatment options. Infect. Dis. Ther. 11 (2), 683–694. doi:10.1007/s40121-022-00597-w
Bell, T. D., and Park, S. C. (2023). Colistin - that was fun, but now we’re done. NEJM Evid. 2 (1), EVIDe2200298. doi:10.1056/EVIDe2200298
Bilal, H., Bergen, P. J., Tait, J. R., Wallis, S. C., Peleg, A. Y., Roberts, J. A., et al. (2020). Clinically relevant epithelial lining fluid concentrations of meropenem with ciprofloxacin provide synergistic killing and resistance suppression of hypermutable Pseudomonas aeruginosa in a dynamic biofilm model. Antimicrob. Agents Chemother. 64 (7), 004699-e520. doi:10.1128/AAC.00469-20
Boral, B., Unaldi, O., Ergin, A., Durmaz, R., and Eser, Ö. K.Acinetobacter Study Group (2019). A prospective multicenter study on the evaluation of antimicrobial resistance and molecular epidemiology of multidrug-resistant Acinetobacter baumannii infections in intensive care units with clinical and environmental features. Ann. Clin. Microbiol. Antimicrob. 18 (1), 19. doi:10.1186/s12941-019-0319-8
Borges Duarte, D. F., and Goncalves Rodrigues, A. (2022). Acinetobacter baumannii: insights towards a comprehensive approach for the prevention of outbreaks in health-care facilities. APMIS 130 (6), 330–337. doi:10.1111/apm.13227
Cai, Y., Chai, D., Wang, R., Liang, B., and Bai, N. (2012). Colistin resistance of Acinetobacter baumannii: clinical reports, mechanisms and antimicrobial strategies. J. Antimicrob. Chemother. 67 (7), 1607–1615. doi:10.1093/jac/dks084
Castanheira, M., Mendes, R. E., and Jones, R. N. (2014). Update on Acinetobacter species: mechanisms of antimicrobial resistance and contemporary in vitro activity of minocycline and other treatment options. Clin. Infect. Dis. 59 (Suppl. 6), S367–S373. doi:10.1093/cid/ciu706
Cerniauskiene, K., Dambrauskiene, A., and Vitkauskiene, A. (2023). Associations between beta-lactamase types of Acinetobacter baumannii and antimicrobial resistance. Med. Kaunas. 59 (8), 1386. doi:10.3390/medicina59081386
Confer, A. W., and Ayalew, S. (2013). The OmpA family of proteins: roles in bacterial pathogenesis and immunity. Vet. Microbiol. 163 (3-4), 207–222. doi:10.1016/j.vetmic.2012.08.019
Davies, J. S., Currie, M. J., Wright, J. D., Newton-Vesty, M. C., North, R. A., Mace, P. D., et al. (2021). Selective nutrient transport in bacteria: multicomponent transporter systems reign supreme. Front. Mol. Biosci. 8, 699222. doi:10.3389/fmolb.2021.699222
Dijkshoorn, L., Nemec, A., and Seifert, H. (2007). An increasing threat in hospitals: multidrug-resistant Acinetobacter baumannii. Nat. Rev. Microbiol. 5 (12), 939–951. doi:10.1038/nrmicro1789
Dinc, G., Demiraslan, H., Elmali, F., Ahmed, S. S., Metan, G., Alp, E., et al. (2013). Efficacy of sulbactam and its combination with imipenem, colistin and tigecycline in an experimental model of carbapenem-resistant Acinetobacter baumannii sepsis. Chemotherapy 59 (5), 325–329. doi:10.1159/000356755
Ding, Y., Hao, J., Xiao, W., Ye, C., Xiao, X., Jian, C., et al. (2023). Role of efflux pumps, their inhibitors, and regulators in colistin resistance. Front. Microbiol. 14, 1207441. doi:10.3389/fmicb.2023.1207441
Evans, B. A., Hamouda, A., and Amyes, S. G. (2013). The rise of carbapenem-resistant Acinetobacter baumannii. Curr. Pharm. Des. 19 (2), 223–238. doi:10.2174/138161213804070285
Gao, Y., Li, H., Chen, H., Zhang, J., Wang, R., Wang, Z., et al. (2022). Origin, phylogeny, and transmission of the epidemic clone ST208 of carbapenem-resistant Acinetobacter baumannii on a global scale. Microbiol. Spectr. 10 (3), e0260421. doi:10.1128/spectrum.02604-21
Garnacho-Montero, J., and Amaya-Villar, R. (2010). Multiresistant Acinetobacter baumannii infections: epidemiology and management. Curr. Opin. Infect. Dis. 23 (4), 332–339. doi:10.1097/QCO.0b013e32833ae38b
Garnacho-Montero, J., Amaya-Villar, R., Ferrandiz-Millon, C., Díaz-Martín, A., López-Sánchez, J. M., and Gutiérrez-Pizarraya, A. (2015). Optimum treatment strategies for carbapenem-resistant Acinetobacter baumannii bacteremia. Expert Rev. Anti Infect. Ther. 13 (6), 769–777. doi:10.1586/14787210.2015.1032254
Gonzalez-Ferrer, S., Penaloza, H. F., Budnick, J. A., Bain, W. G., Nordstrom, H. R., Lee, J. S., et al. (2021). Finding order in the chaos: outstanding questions in Klebsiella pneumoniae pathogenesis. Infect. Immun. 89 (4), 006933-e720. doi:10.1128/IAI.00693-20
Greene, C., Vadlamudi, G., Newton, D., Foxman, B., and Xi, C. (2016). The influence of biofilm formation and multidrug resistance on environmental survival of clinical and environmental isolates of Acinetobacter baumannii. Am. J. Infect. Control 44 (5), e65–e71. doi:10.1016/j.ajic.2015.12.012
Iovleva, A., Mustapha, M. M., Griffith, M. P., Komarow, L., Luterbach, C., Evans, D. R., et al. (2022). Carbapenem-resistant Acinetobacter baumannii in U.S. Hospitals: diversification of circulating lineages and antimicrobial resistance. mBio 13 (2), e0275921. doi:10.1128/mbio.02759-21
Lin, J., Gao, X., Cui, Y., Sun, W., Shen, Y., Shi, Q., et al. (2020). Increased multidrug resistant isolates: new clinical burdens for 66 hospitals in Shanghai, 2015 to 2017. Ann. Transl. Med. 8 (4), 112. doi:10.21037/atm.2019.12.91
Maleki, A., Kaviar, V. H., Koupaei, M., Haddadi, M. H., Kalani, B. S., Valadbeigi, H., et al. (2022). Molecular typing and antibiotic resistance patterns among clinical isolates of Acinetobacter baumannii recovered from burn patients in Tehran, Iran. Front. Microbiol. 13, 994303. doi:10.3389/fmicb.2022.994303
Maragakis, L. L., and Perl, T. M. (2008). Acinetobacter baumannii: epidemiology, antimicrobial resistance, and treatment options. Clin. Infect. Dis. 46 (8), 1254–1263. doi:10.1086/529198
Menegucci, T. C., Fedrigo, N. H., Lodi, F. G., Albiero, J., Nishiyama, S. A. B., Mazucheli, J., et al. (2019). Pharmacodynamic effects of sulbactam/meropenem/polymyxin-B combination against extremely drug resistant Acinetobacter baumannii using checkerboard information. Microb. Drug Resist 25 (9), 1266–1274. doi:10.1089/mdr.2018.0283
Miethke, M., and Marahiel, M. A. (2007). Siderophore-based iron acquisition and pathogen control. Microbiol. Mol. Biol. Rev. 71 (3), 413–451. doi:10.1128/MMBR.00012-07
Munoz-Price, L. S., Arheart, K., Nordmann, P., Boulanger, A. E., Cleary, T., Alvarez, R., et al. (2013). Eighteen years of experience with Acinetobacter baumannii in a tertiary care hospital. Crit. Care Med. 41 (12), 2733–2742. doi:10.1097/CCM.0b013e318298a541
O’Donnell, J. N., Putra, V., and Lodise, T. P. (2021). Treatment of patients with serious infections due to carbapenem-resistant Acinetobacter baumannii: how viable are the current options? Pharmacotherapy 41 (9), 762–780. doi:10.1002/phar.2607
Ohtsu, I., Kawano, Y., Suzuki, M., Morigasaki, S., Saiki, K., Yamazaki, S., et al. (2015). Uptake of L-cystine via an ABC transporter contributes defense of oxidative stress in the L-cystine export-dependent manner in Escherichia coli. PLoS One 10 (3), e0120619. doi:10.1371/journal.pone.0120619
Paterson, D. L., and Doi, Y. (2007). A step closer to extreme drug resistance (XDR) in gram-negative bacilli. Clin. Infect. Dis. 45 (9), 1179–1181. doi:10.1086/522287
Paul, M., Carrara, E., Retamar, P., Tängdén, T., Bitterman, R., Bonomo, R. A., et al. (2022). European Society of Clinical Microbiology and Infectious Diseases (ESCMID) guidelines for the treatment of infections caused by multidrug-resistant Gram-negative bacilli (endorsed by European society of intensive care medicine). Clin. Microbiol. Infect. 28 (4), 521–547. doi:10.1016/j.cmi.2021.11.025
Pour, N. K., Dusane, D. H., Dhakephalkar, P. K., Zamin, F. R., Zinjarde, S. S., and Chopade, B. A. (2011). Biofilm formation by Acinetobacter baumannii strains isolated from urinary tract infection and urinary catheters. FEMS Immunol. Med. Microbiol. 62 (3), 328–338. doi:10.1111/j.1574-695X.2011.00818.x
Qian, Y., Dong, X., Wang, Z., Yang, G., and Liu, Q. (2015). Distributions and types of multidrug-resistant Acinetobacter baumannii in different departments of a general hospital. Jundishapur J. Microbiol. 8 (9), e22935. doi:10.5812/jjm.22935
Qin, L.-J., and Wang, X. (2019). A review on Acinetobacter baumannii. J. Acute Dis. 8 (1), 16. doi:10.4103/2221-6189.250373
Rafiei, E., Shahini Shams Abadi, M., Zamanzad, B., and Gholipour, A. (2022). The frequency of efflux pump genes expression in Acinetobacter baumannii isolates from pulmonary secretions. Amb. Express 12 (1), 103. doi:10.1186/s13568-022-01444-4
Sanchez-Encinales, V., Alvarez-Marin, R., Pachon-Ibanez, M. E., Fernández-Cuenca, F., Pascual, A., Garnacho-Montero, J., et al. (2017). Overproduction of outer membrane protein A by Acinetobacter baumannii as a risk factor for nosocomial pneumonia, bacteremia, and mortality rate increase. J. Infect. Dis. 215 (6), 966–974. doi:10.1093/infdis/jix010
Shields, R. K., Paterson, D. L., and Tamma, P. D. (2023). Navigating available treatment options for carbapenem-resistant Acinetobacter baumannii-calcoaceticus complex infections. Clin. Infect. Dis. 76 (Suppl. 2), S179–S193. doi:10.1093/cid/ciad094
Sievert, D. M., Ricks, P., Edwards, J. R., Schneider, A., Patel, J., Srinivasan, A., et al. (2013). Antimicrobial-resistant pathogens associated with healthcare-associated infections: summary of data reported to the national healthcare safety network at the centers for disease control and prevention, 2009–2010. Infect. Control Hosp. Epidemiol. 34 (1), 1–14. doi:10.1086/668770
Tamma, P. D., Aitken, S. L., Bonomo, R. A., Mathers, A. J., van Duin, D., and Clancy, C. J. (2022). Infectious diseases society of America 2022 guidance on the treatment of extended-spectrum beta-lactamase producing enterobacterales (ESBL-E), carbapenem-resistant enterobacterales (CRE), and Pseudomonas aeruginosa with difficult-to-treat resistance (DTR-P. aeruginosa). Clin. Infect. Dis. 75 (2), 187–212. doi:10.1093/cid/ciac268
Tiku, V., Kofoed, E. M., Yan, D., Kang, J., Xu, M., Reichelt, M., et al. (2021). Outer membrane vesicles containing OmpA induce mitochondrial fragmentation to promote pathogenesis of Acinetobacter baumannii. Sci. Rep. 11 (1), 618. doi:10.1038/s41598-020-79966-9
Trebosc, V., Gartenmann, S., Totzl, M., Lucchini, V., Schellhorn, B., Pieren, M., et al. (2019). Dissecting colistin resistance mechanisms in extensively drug-resistant Acinetobacter baumannii clinical isolates. mBio 10 (4), 010833-e1119. doi:10.1128/mBio.01083-19
Uppalapati, S. R., Sett, A., and Pathania, R. (2020). The outer membrane proteins OmpA, CarO, and OprD of Acinetobacter baumannii confer a two-pronged defense in facilitating its success as a potent human pathogen. Front. Microbiol. 11, 589234. doi:10.3389/fmicb.2020.589234
Vidaillac, C., Benichou, L., and Duval, R. E. (2012). In vitro synergy of colistin combinations against colistin-resistant Acinetobacter baumannii, Pseudomonas aeruginosa, and Klebsiella pneumoniae isolates. Antimicrob. agents Chemother. 56 (9), 4856–4861. doi:10.1128/AAC.05996-11
Weinberg, S. E., Villedieu, A., Bagdasarian, N., Karah, N., Teare, L., and Elamin, W. F. (2020). Control and management of multidrug resistant Acinetobacter baumannii: a review of the evidence and proposal of novel approaches. Infect. Prev. Pract. 2 (3), 100077. doi:10.1016/j.infpip.2020.100077
Xie, R., Zhang, X. D., Zhao, Q., Peng, B., and Zheng, J. (2018). Analysis of global prevalence of antibiotic resistance in Acinetobacter baumannii infections disclosed a faster increase in OECD countries. Emerg. Microbes Infect. 7 (1), 31. doi:10.1038/s41426-018-0038-9
Yourassowsky, E., VAN DER Linden, M., Lismont, M., Crokaert, F., and Glupczynski, Y. (1985). Effect on growth curve patterns of brief exposure of bacteria to different concentrations of β-lactam antibiotics. J. Antimicrob. Chemother. 15 (Suppl. l_A), 27–36. doi:10.1093/jac/15.suppl_a.27
Zack, K. M., Sorenson, T., and Joshi, S. G. (2024). Types and mechanisms of efflux pump systems and the potential of efflux pump inhibitors in the restoration of antimicrobial susceptibility, with a special reference to Acinetobacter baumannii. Pathogens 13 (3), 197. doi:10.3390/pathogens13030197
Zhang, Z., Morgan, C. E., Bonomo, R. A., and Yu, E. W. (2021). Cryo-EM determination of eravacycline-bound structures of the ribosome and the multidrug efflux pump AdeJ of Acinetobacter baumannii. mBio 12 (3), e0103121. doi:10.1128/mBio.01031-21
Zhao, Y., Qian, C., Ye, J., Li, Q., Zhao, R., Qin, L., et al. (2023). Convergence of plasmid-mediated colistin and tigecycline resistance in Klebsiella pneumoniae. Front. Microbiol. 14, 1221428. doi:10.3389/fmicb.2023.1221428
Keywords: carbapenem-resistant Acinetobacter baumannii (CRAB), meropenem, ciprofloxacin, biofilm formation, transcriptomics
Citation: Feng Y, Chen X, Sun Y, Guo T, Wu F, Jin F and Zhou J (2025) Synergistic effect and mechanism of meropenem with ciprofloxacin against carbapenem-resistant Acinetobacter baumannii. Front. Pharmacol. 16:1534155. doi: 10.3389/fphar.2025.1534155
Received: 25 November 2024; Accepted: 01 May 2025;
Published: 15 May 2025.
Edited by:
Xiangji Liu, Frontage Laboratories, Inc., United StatesReviewed by:
Aimé Gabriel Fankam, University of Dschang, CameroonArijit Bhattacharya, Adamas University, India
Copyright © 2025 Feng, Chen, Sun, Guo, Wu, Jin and Zhou. This is an open-access article distributed under the terms of the Creative Commons Attribution License (CC BY). The use, distribution or reproduction in other forums is permitted, provided the original author(s) and the copyright owner(s) are credited and that the original publication in this journal is cited, in accordance with accepted academic practice. No use, distribution or reproduction is permitted which does not comply with these terms.
*Correspondence: Jun Zhou, MDkwOTA0QHl6dS5lZHUuY24=
†These authors have contributed equally to this work and share first authorship