- 1Department of Neurosurgery, Wuxi No. 2 People’s Hospital (Jiangnan University Medical Center), Wuxi, China
- 2Wuxi School of Medicine, Jiangnan University, Wuxi, China
- 3Wuxi Neurosurgical Institute, Wuxi, China
- 4Neurological Medicine Research Centre, Jiangnan University, Wuxi, China
Objectives: This investigation sought to explore the inhibitory impact of wogonin on prolactinoma and elucidate its underlying mechanisms through network pharmacology, molecular docking (MD), and molecular biology experiments.
Methods: Target identification for wogonin and prolactinoma was conducted using relevant databases, followed by protein-protein interaction (PPI) analysis of intersecting targets via the STRING database. Functional and pathway enrichment analyses were executed utilizing Gene Ontology (GO) and Kyoto Encyclopedia of Genes and Genomes (KEGG) methodologies. Hub genes were identified from the PPI network, and MD was utilized to assess the binding patterns and interaction strength between wogonin and hub targets. Network pharmacological findings were further validated through in vivo and in vitro experiments.
Results: A sum of 137 drug targets for wogonin and 3,942 disease targets for prolactinoma were identified, with 37 overlapping targets. Nine hub genes were screened, including KDR, EGFR, BCL2, IL6, ESR1, MYC, CCL2, PTGS2, and ESR2. GO and KEGG analyses revealed that wogonin was closely associated with several critical signaling cascades. MD analysis confirmed robust binding interactions between wogonin and the identified hub targets. Cellular experiments suggested that wogonin suppressed cell proliferation and triggered apoptosis in prolactinoma cells in a time- and concentration-dependent manner, primarily via inhibition of the PI3K/AKT signaling cascades. Animal studies further revealed that wogonin markedly suppressed tumor growth and enhanced prolactinoma sensitivity to bromocriptine.
Conclusion: These findings suggest that wogonin exerts its anti-prolactinoma effects via multiple targets and signaling cascades, establishing a robust scientific basis for the development and screening of novel anti-prolactinoma therapeutics.
1 Introduction
Prolactinoma, the most prevalent type of pituitary adenoma, accounts for approximately 40%–66% of functional pituitary adenomas (Tritos and Miller, 2023; Petersenn and Giustina, 2020). Although benign and not life-threatening, prolactinomas markedly impair patients’ quality of life due to clinical manifestations such as amenorrhea, galactorrhea, infertility, and visual disturbances (Castle-Kirszbaum et al., 2024; Auriemma et al., 2023). The primary treatment strategy involves dopamine agonists (Auriemma et al., 2023; Inder and Jang, 2022), however, many patients experience adverse effects or develop resistance (Maiter, 2019; Cheng et al., 2024; Vermeulen et al., 2020), undermining therapeutic efficacy and increasing recurrence risk (Fukuhara et al., 2022). Consequently, identifying novel therapeutic targets and drugs is critical to enhancing treatment outcomes and improving patient quality of life. Traditional Chinese medicines (TCM) serve as a valuable resource for new drug development due to their multi-component, multi-target characteristics, and low toxicity profiles (Wang et al., 2020; Liu Y. et al., 2020). Notably, approximately 52% of antineoplastic agents approved by the U.S. FDA are derived from natural products (Newman and Cragg, 2016). Wogonin (Wog), a flavonoid isolated from the Chinese herb Scutellaria baicalensis Georgi, demonstrates multiple biological functions, encompassing anti-inflammatory, antioxidant, anti-allergic, and antibacterial properties (Liao et al., 2021; Guo et al., 2022; Wang et al., 2021). Increasing evidence supports its antitumor efficacy across various malignancies (Banik et al., 2022; Zhang et al., 2022; Yang et al., 2020). For instance, wog promotes apoptosis in tumor cells by activating BAX proteins, with this effect in human prostate cancer cells linked to increased intracellular P53 expression (Lee et al., 2008). Furthermore, wog triggers apoptosis in lymphoma and breast cancer cells by suppressing anti-apoptotic protein levels, specifically Mcl-1, which belongs to the Bcl-2 family, and Survivin (Huang et al., 2012; Polier et al., 2015). Although its antitumor potential in prolactinoma has yet to be elucidated, wog’s established bioactivity underscores its value as a promising candidate for anticancer drug development.
Network pharmacology (NP) has emerged as an innovative discipline tailored to the multi-level and multi-target nature of TCM formulas (Zhao et al., 2023; Shang et al., 2023; Lin et al., 2023). It enables comprehensive analysis of drug-disease interactions from multiple perspectives, elucidating the mechanisms of action through gene functions and signaling pathways (Wu et al., 2024). This approach has gained recognition for its accuracy and reliability in exploring complex biological systems (Wang Y. X. et al., 2022; Liu Q. et al., 2023). In this study, the key molecular targets, biological processes, and signaling pathways underlying the effects of wog on prolactinoma were systematically identified and experimentally validated using a NP framework. These findings provide a robust theoretical and experimental foundation for future scientific investigations into the medicinal applications of wog.
2 Methods and materials
2.1 Chemicals and antibodies
Wogonin (W820521-50 mg, purity ≥99%) and bromocriptine (BRC) (B860726-50 mg, purity >99%) were acquired from Shanghai Macklin Biochemical Technology Co., Ltd. (Shanghai, China). Horse serum, fetal bovine serum, penicillin-streptomycin, and Ham’s F12K medium were supplied by Wuhan Procell Life Science and Technology Co., Ltd. (Wuhan, China). Primary antibodies, including anti-GAPDH (T0004), anti-PI3K (AF6242), anti-AKT (AF6261), and anti-Bax (AF0120), were obtained from Affinity Biosciences (Jiangsu, China). The anti-phospho-PI3K antibody (YP0224) was procured from ImmunoWay Biotechnology (Beijing, China), while anti-phospho-AKT (4060T), anti-Cleaved Caspase-3 (9661S), and anti-Cleaved Caspase-9 (9507S) were sourced from Cell Signaling Technology (Danvers, MA). A CCK-8 kit (MAO218) was obtained from Melone Pharmaceutical Co., Ltd. (Dalian, China), and an ELISA kit (RA20563) was supplied by Bioswamp (Wuhan, China). All reagents and antibodies were used in accordance with the manufacturers protocols.
2.2 Screening of wogonin drug targets
The Traditional Chinese Medicine Systems Pharmacology Database and Analysis Platform (TCMSP) was queried using the keyword “wogonin” to retrieve related targets, which were then matched to corresponding gene names via the UniProt database (https://www.uniprot.org). Unmatched genes were excluded. Additional drug targets were identified through searches of SwissTargetPrediction (http://swisstargetprediction.ch) and STITCH (http://stitch.embl.de) (Zhang et al., 2021; Li et al., 2023). After combining data from these sources and removing duplicates, the drug targets of wog were finalized.
2.3 Screening for prolactinoma disease targets
The GSE119063 dataset was procured through the Gene Expression Omnibus (GEO) database (https://www.ncbi.nlm.nih.gov/geo) (Wang Y. et al., 2022), and GEO2R was utilized to ascertain differentially expressed genes (DEGs) between prolactinoma tissues and normal pituitary tissues. DEGs were selected grounded in |logFC| > 1 and P < 0.05 thresholds (Zhang et al., 2023). Bioinformatics website (https://www.bioinformatics.com.cn) was utilized to generate volcano plots and heatmap (He et al., 2019). Prolactinoma-related disease targets were retrieved from the GeneCards (https://www.genecards.org) and PharmGKB (https://www.pharmgkb.org) databases utilizing the keyword “prolactinoma” with GeneCards results filtered by a relevance score >5 (Weng et al., 2023). Therapeutic targets for prolactinoma were compiled by merging these datasets and removing duplicates.
2.4 Identification of drug and disease common targets
Potential therapeutic targets for wog in prolactinoma were identified by intersecting drug targets and disease targets utilizing Venny 2.1.0 (https://bioinfogp.cnb.csic.es/tools/venny) (He et al., 2023).
2.5 Development of protein-protein interaction (PPI) network and identification of hub targets
The intersecting targets were analyzed in the STRING database (https://cn.string-db.org) to construct PPI networks (Li et al., 2022), which were subsequently visualized using Cytoscape software. Node parameters, encompassing betweenness unDir (BU), closeness unDir (CU), and degree unDir (DU), were computed utilizing the CentiScape plug-in (Liu H. et al., 2020). Targets with values exceeding threshold parameters for BU, CU, and DU were designated as hub targets for wog in prolactinoma therapy.
2.6 GO and KEGG enrichment analyses
The shared targets identified were analyzed using the DAVID database (https://david.ncifcrf.gov) for Gene Ontology (GO) enrichment and Kyoto Encyclopedia of Genes and Genomes (KEGG) pathway enrichment (Wang Y. et al., 2022; Luo et al., 2023). GO enrichment analysis categorized the targets into biological processes (BP), cellular components (CC), and molecular functions (MF), utilizing a significance threshold of P < 0.05. The top 10 enriched categories were visualized using bioinformatics website and presented as bubble charts (Madikyzy et al., 2022).
2.7 Molecular docking (MD) analysis
MD analysis of wog with hub targets was conducted using AutoDockTools-1.5.7 (He et al., 2023; Ji et al., 2023). The mol2 file of wog was retrieved from TCMSP, followed by hydrogen atom addition, ligand definition, and torsion bond selection. The processed ligand was saved in PDBQT format. Protein structures were procured from the PDB database (https://www.rcsb.org), preprocessed, and exported to PDBQT format. Docking parameters were configured, and docking simulations were performed using Autogrid and AutoDock. The structure exhibiting minimal binding energy was selected as the optimal result and rendered utilizing PyMOL (Ji et al., 2023; Lu et al., 2023).
2.8 Cell culture
Rat pituitary tumor cell lines MMQ and GH3 were procured from Wuhan Procell Life Science and Technology Co., Ltd. (Wuhan, China). The cells underwent cultivation in Ham’s F12K medium enriched with 15% horse serum, 2.5% fetal bovine serum, and 1% penicillin-streptomycin, sustained at 37°C within a 5% CO2 incubator (Selek et al., 2023).
2.9 Cell viability assay
The effects of wog on cell viability were assessed utilizing a CCK-8 kit. MMQ and GH3 cells were placed in 96-well plates at 1 × 104 cells/well. Experimental groups included blank controls, untreated controls, and drug-treated groups, with five replicate wells per group. Outliers (maximum and minimum values) were excluded to minimize errors. After 24 h of initial culture, cells underwent exposure to multiple drug concentrations across 24, 48, and 72-hour periods. A volume of 10 µL CCK-8 solution was introduced into wells containing 100 µL medium, succeeded by incubation at 37°C for 3–4 h. The optical density measurements were procured at 450 nm utilizing a Multiskan FC microplate reader (Thermo Scientific, United States).
2.10 Colony formation assay
For colony formation assay, GH3 cells in the logarithmic growth phase were seeded in six-well plates at 1,000–2,000 cells/well, utilizing three replicates per group. After cell attachment (24 h), a fresh complete medium containing varying drug concentrations was introduced. Cells were kept at 37°C in a 5% CO2 atmosphere for 14–21 days, with medium replacement every 4–5 days. The resulting colonies were fixed, visualized with 0.1% crystal violet staining, imaged, and enumerated.
2.11 Enzyme-linked immunosorbent assay
To measure prolactin (PRL) secretion, MMQ and GH3 cells were placed in six-well plates at 1 × 105 cells/well, utilizing three replicates per group. Cell supernatants were harvested after 72 h of drug treatment, and PRL levels were quantified utilizing a rat PRL ELISA kit per the supplier’s protocols. OD values were ascertained at 450 nm utilizing a Multiskan FC microplate reader (Thermo Scientific, United States). PRL concentrations in cell supernatants were calculated in ng/mL based on a standard curve generated with PRL standards provided in the kit.
2.12 Western blot analysis
GH3 and MMQ cells in the logarithmic growth phase were kept in Petri dishes and subjected to drug treatments for 72 h. Protein extraction was conducted utilizing RIPA lysis buffer comprising 1 mM enzyme and phosphatase blockers, with subsequent centrifugation executed at 12,000 rpm for 15 min under 4°C to isolate the supernatant. Protein levels were quantified utilizing a BCA assay kit, after which 5 × SDS-PAGE Loading Buffer was introduced, and the samples were denatured at 100°C for 10 min. The protein samples underwent SDS-PAGE separation, membrane transfer to PVDF, and BSA blocking for 1 h. Primary antibody incubation occurred overnight at 4°C, succeeded by secondary antibody application for 2 h at ambient temperature. An automated chemiluminescence imaging platform (Tanon-4600, Shanghai, China) enabled protein detection, while ImageJ software facilitated band intensity measurement. The experimental procedure was replicated independently five times.
2.13 Tumor cell xenograft nude mouse models
A xenograft model involving subcutaneous tumors was implemented using BALB/c nude mice to assess wog’s in vivo efficacy against prolactinoma. Female BALB/c nude mice (n = 20), aged 4–6 weeks, were obtained from SPF (Suzhou) Biotechnology Co., Ltd. and maintained in specific pathogen-free environments. All experimental procedures were conducted in compliance with the Regulations on the Management of Laboratory Animals and sanctioned by the Animal Ethics Committee of Jiangnan University (Ethics No. JN.No20240630b0240912 [371]). To develop the prolactinoma model, GH3 cells (roughly 1.0 × 107) were introduced via subcutaneous injection into the mice’s lateral abdomen. Tumor dimensions were recorded every alternate day, with volume calculations following the formula: volume = 1/2 × length × width2. Upon tumors reaching 2–4 mm, the mice underwent random distribution into four groups: Control group, Wog group, BRC group and Wog + BRC group (n = 5). Mice in the Wog group received daily intragastric administration of wog (120 mg/kg/day i.g.); mice in the BRC group received daily intragastric administration of BRC (1 mg/kg/day i.g.); and the combined treatment group received daily administration of both BRC and wog. After 14 days of treatment, tumors were excised for measurement, weighing, and immunohistochemical analysis (Tang C. et al., 2021). To evaluate potential drug toxicity, internal organs (heart, liver, spleen, lungs, and kidneys) and blood samples were procured for histopathological examination via HE staining and serum biochemical analysis.
2.14 Immunohistochemistry
Immunohistochemical staining was executed on paraffin-embedded tumor tissue sections to evaluate target protein expression. Following dewaxing and hydration, the slides were treated with citric acid antigen retrieval solution and antigen restoration process. Non-specific binding was prevented by treating the slides with 10% BSA for 30 min at ambient temperature, and the excess solution was gently removed. The slides were then exposed to the primary antibody overnight at 4°C, succeeded by exposed to horseradish peroxidase-conjugated secondary antibody for 20 min at ambient temperature. Nuclear staining was conducted with hematoxylin to visualize nuclei. Images of stained sections were captured using a microscope.
2.15 Hematoxylin-eosin (HE)
For HE staining, tissue samples were paraffin-embedded and sectioned following dehydration and clearing. Deparaffinization was performed with xylene and ethanol, after which the specimens were stained with hematoxylin for 5 min. Following differentiation, the specimens were counterstained with 0.5% eosin for 1 min, rinsed with water, and exposed to ethanol dehydration and xylene clearing. The specimens were sealed with neutral gum and examined microscopically, with representative images captured.
2.16 Statistical analysis
Experimental data underwent statistical analysis utilizing GraphPad Prism version 9.0. Each experiment was performed in triplicate or more, with outcomes presented as mean ± standard deviation (SD). Group comparisons were made utilizing one-way ANOVA, with statistical significance established at p < 0.05 (*p < 0.05, **p < 0.01, ***p < 0.005, ****p < 0.0001; ns means no significance).
3 Results
3.1 Identification of gene targets for wogonin in prolactinoma
A total of 35, 103, and 10 pharmacological targets of wog were identified through the TCMSP, SwissTargetPrediction, and STITCH databases, respectively. After eliminating duplicates and erroneous entries, 137 unique pharmacological targets were finalized. Differential expression analysis of the GSE119063 dataset from the GEO database identified 3,909 DEGs associated with prolactinoma (Figures 1A,B). Additionally, 46 disease-related genes were procured from the GeneCards database and 8 from PharmGKB. Integrating these datasets and removing redundancies resulted in 3,942 prolactinoma-associated targets. By intersecting the pharmacological and disease target datasets, 37 overlapping genes were identified (Figure 1C).
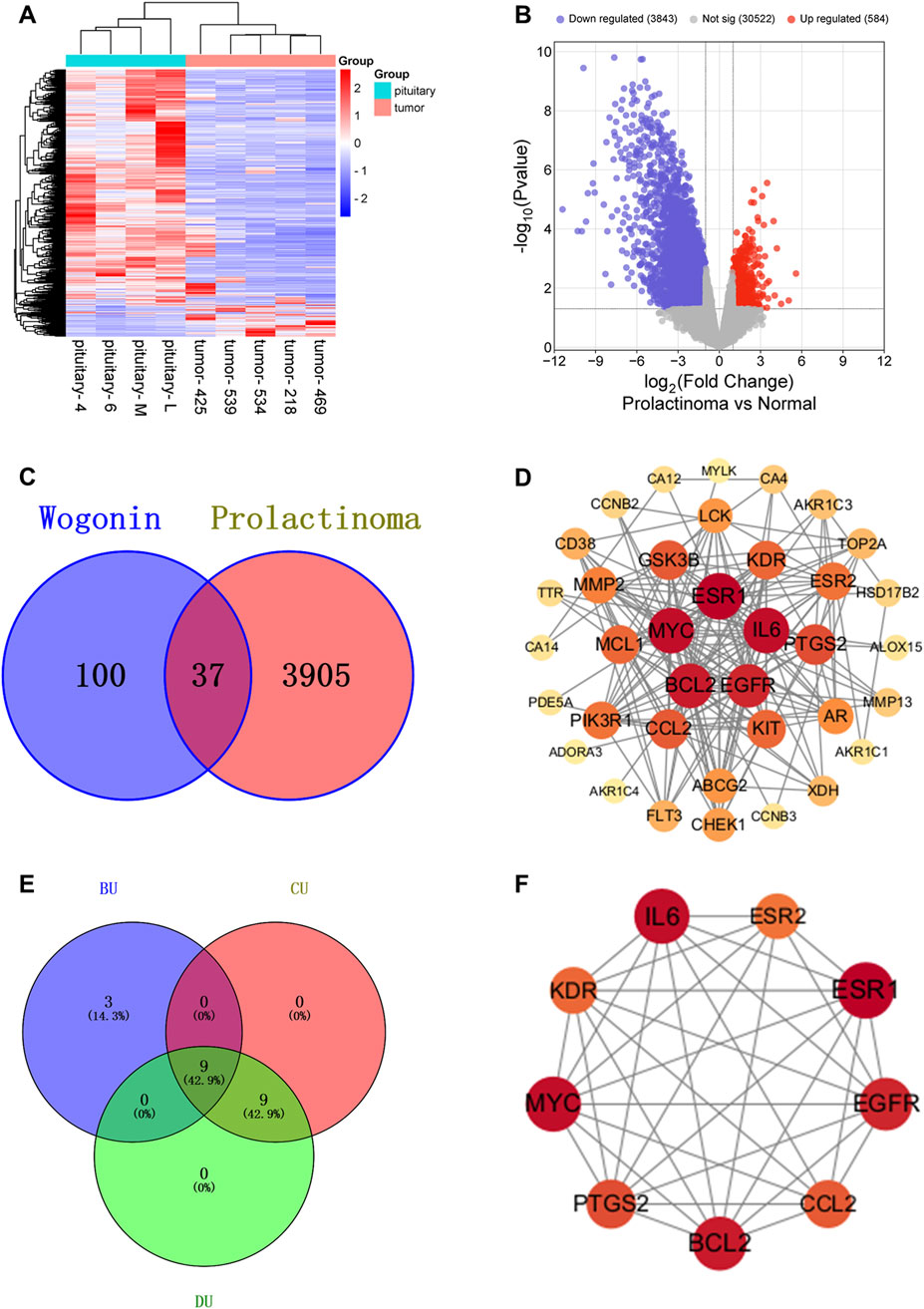
Figure 1. Relevant targets, PPI network and hub targets: (A). Volcano plot of prolactinoma DEGs in the GEO database (B). Heatmap of prolactinoma DEGs in the GEO database (C). Venn diagram showing the common part of wogonin and prolactinoma (D). PPI network of potential targets for wogonin therapy of prolactinoma (E,F). Hub targets for wogonin therapy of prolactinoma.
3.2 Development of PPI network and detection of hub targets
The intersecting genes were analyzed for PPI relationships utilizing the STRING database, and the generated PPI network was rendered via the Cytoscape tool. The network comprised 37 nodes and 180 edges (Figure 1D), with node size and color intensity proportional to their degree values. Using the CentiScape plugin, hub targets were ascertained grounded in criteria for BU > 37.29729729729731, CU > 0.0142901334866273, and DU > 9.72972972972973 (Figures 1E,F). This analysis highlighted 9 hub targets: KDR, EGFR, BCL2, IL6, ESR1, MYC, CCL2, PTGS2, and ESR2 (Table 1).
3.3 GO and KEGG pathway enrichment analyses
Functional enrichment analysis identified 142 BP, 14 CC, 52 MF terms, and 69 signaling pathways markedly associated with P < 0.05. The top 10 terms from GO and KEGG pathway enrichment were depicted in bubble plots. Key BP terms included negative regulation of apoptotic processes, phosphorylation, cellular response to jasmonic acid, and the transmembrane receptor protein tyrosine kinase signaling pathway. CC terms were primarily related to macromolecular complexes, membranes, apical plasma membranes, membrane rafts, and receptor complexes. MF terms were enriched in activities such as estradiol 17-beta-dehydrogenase, dihydrotestosterone 17-beta-dehydrogenase, and transmembrane receptor protein tyrosine kinase (Figure 2A). KEGG pathway examination suggested substantial enrichment in pathways encompassing pathways in cancer, PI3K/Akt signaling, EGFR tyrosine kinase inhibitor resistance, endocrine resistance, and central carbon metabolism in cancer (Figure 2B). Among these, the PI3K/Akt signaling cascade was notably associated with cancer progression, highlighting its critical function in tumor cell proliferation, apoptosis, and invasion (He et al., 2021; Dworakowska et al., 2009). These observations suggest that wog may exert therapeutic effects in prolactinoma through modulation of the PI3K/Akt signaling cascade.
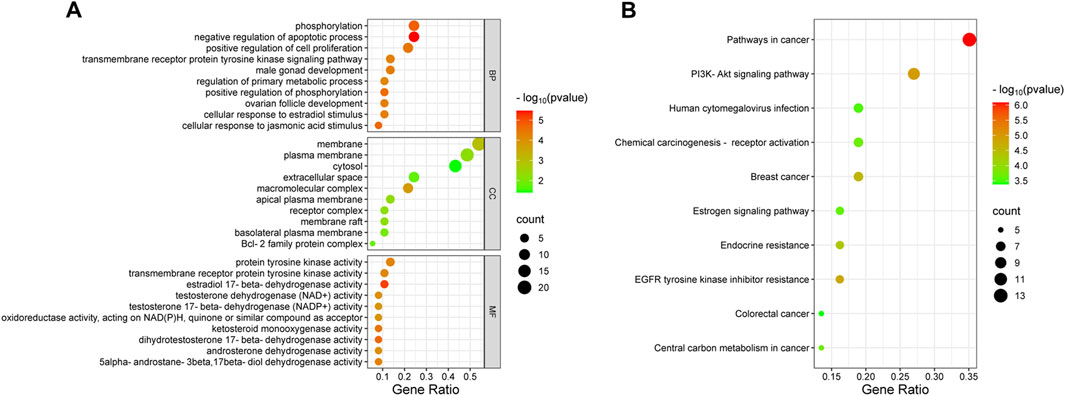
Figure 2. Bubble plot of enrichment analysis: (A). GO functional enrichment analysis of wogonin in prolactinoma (B). KEGG pathway enrichment analysis of wogonin in prolactinoma.
3.4 Molecular docking
MD analysis was conducted to assess the interactions between wog and the identified hub targets, thereby verifying the reliability of these interactions. A lower binding energy between a small molecule ligand and a protein receptor indicates stronger and more stable affinity. The MD results showed that the binding energies between wog and the target proteins ranged from −3.83 to −6.67 kcal/mol (Table 2). Wog is tightly linked to amino acid residues through hydrogen bonds (Figure 3). The analysis demonstrated robust binding affinities between wog and the hub targets, supporting its potential therapeutic efficacy in prolactinoma treatment.
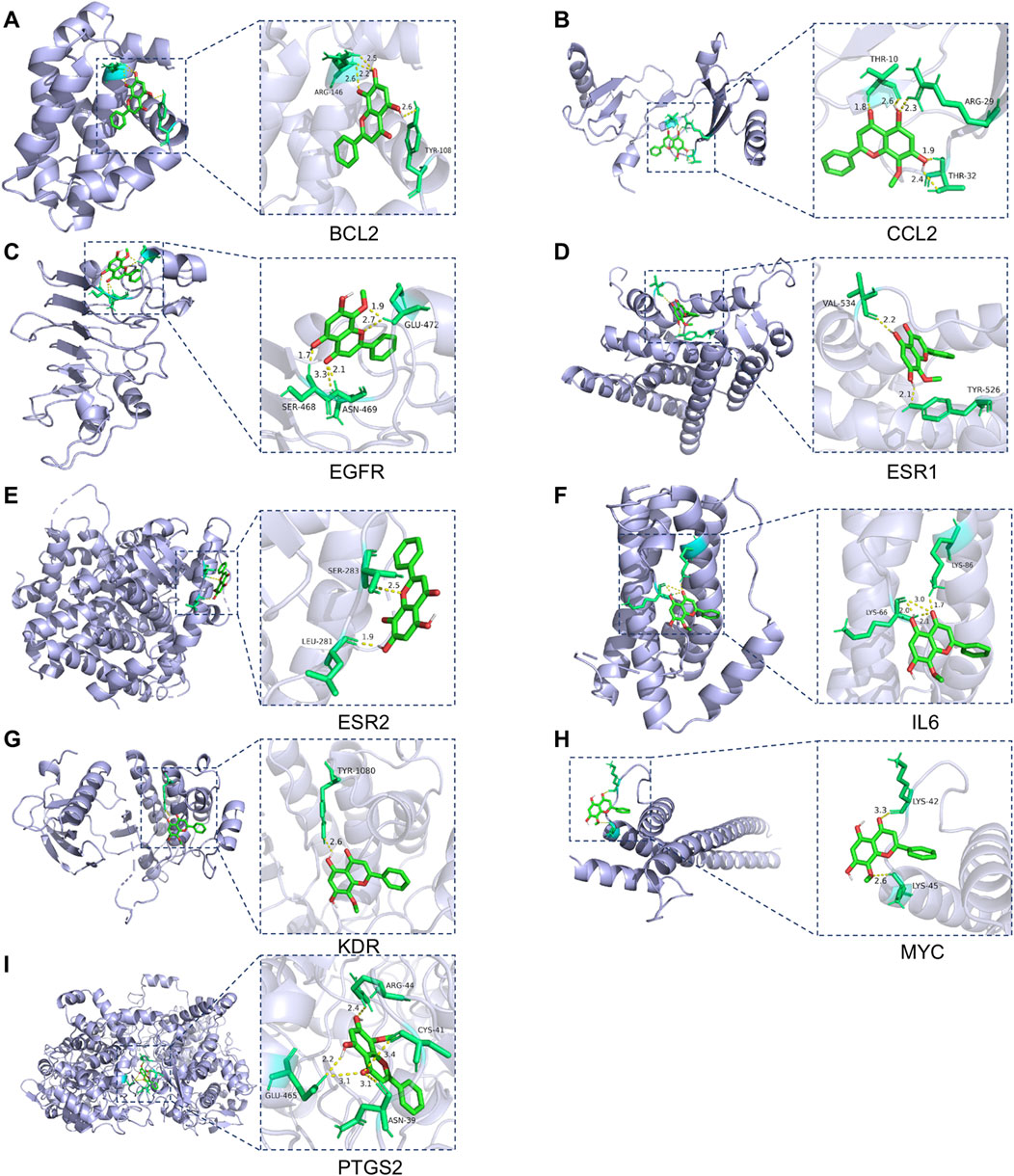
Figure 3. Molecular docking pattern of wogonin and hub target proteins: (A). Wogonin-BCL2, (B). Wogonin-CCL2, (C). Wogonin-EGFR, (D). Wogonin-ESR1, (E). Wogonin-ESR2, (F). Wogonin-IL6, (G). Wogonin-KDR, (H). Wogonin-MYC, (I). Wogonin-PTGS2.
3.5 Wogonin inhibits the prolactinoma cell proliferation
To examine how wog influences prolactinoma cell growth, GH3 and MMQ cells underwent treatment with diverse wog doses (0, 25, 37.5, 50, 75, 100, 150 and 200 μM) for 24, 48, and 72 h. Wog suppressed prolactinoma cell proliferation in a time- and concentration-dependent manner (Figures 4A,B). The calculated IC50 values for GH3 and MMQ cells were 61.99 μM and 107 μM, respectively, after 72 h of treatment.
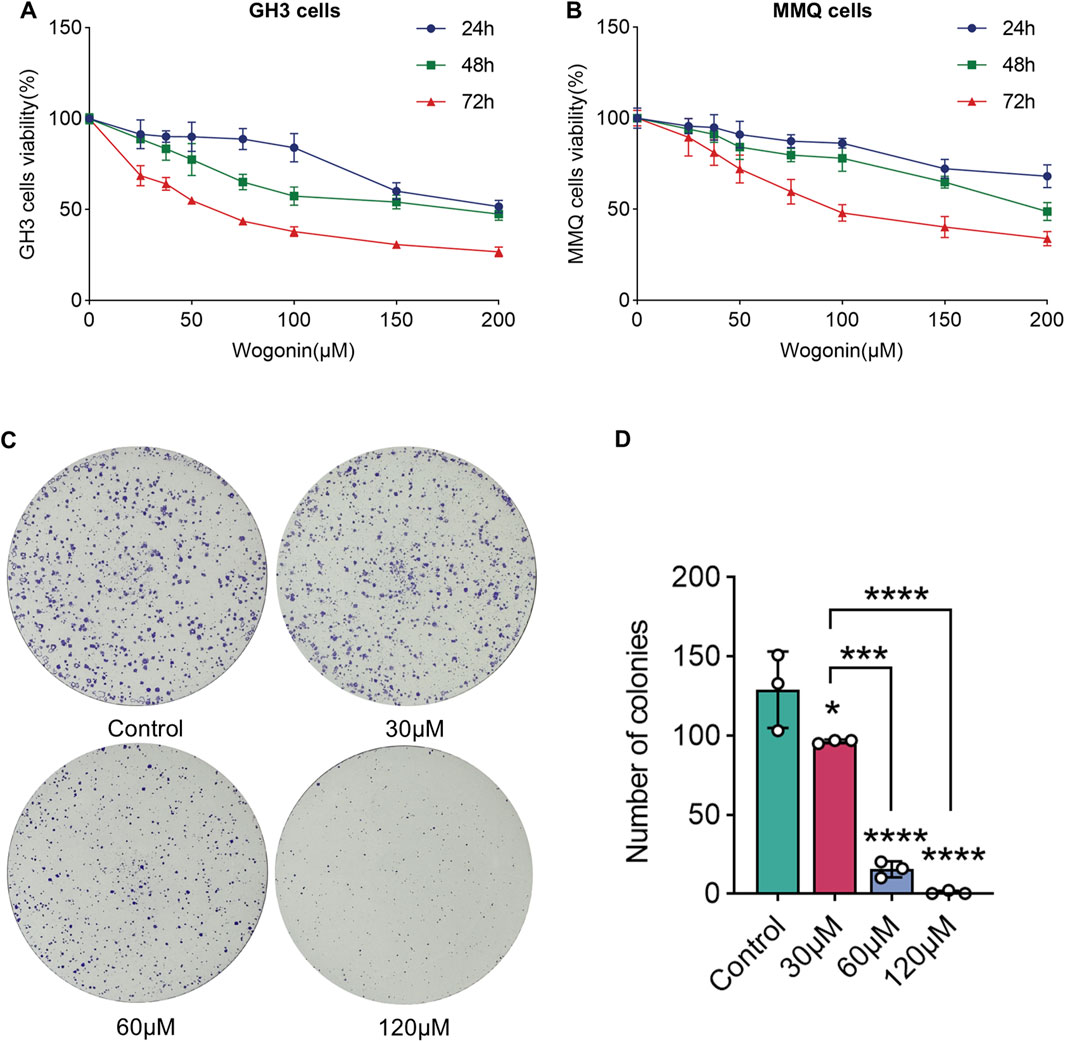
Figure 4. Effects of wogonin on cell viability and colony formation: GH3 cell (A) and MMQ cell (B) survival was determined by the CCK-8 assay (C). GH3 cells were treated with wogonin at concentrations of 0, 30, 60 and 120 μM (D). The statistical graph of the result of the colony formation. (****p < 0.0001, ***p < 0.005, **p < 0.01, *p < 0.05, Except for * on the n-zig-zag line, all other * indicate comparisons with the control group).
3.6 Wogonin inhibits the colony formation ability of prolactinoma cell
Further experiments were executed to evaluate wog’s influence on colony formation. GH3 cells were exposed to 30 μM, 60 μM, and 120 μM wog, respectively. A significant reduction in colony formation was observed when treated with wog at concentrations of 30 μM and 60 μM. When the concentration of wog was 120 μM, colony formation was nearly completely inhibited (Figures 4C,D). These results indicate that wog effectively suppresses the colony formation ability of prolactinoma cells in vitro.
3.7 Wogonin inhibits PI3K/AKT pathway phosphorylation and induces apoptosis in prolactinoma cells
NP analysis indicated that wog’s tumor-suppressing activity potentially operates through PI3K/AKT pathway modulation. To test this hypothesis, GH3 and MMQ cells were exposed to diverse concentrations of wog for 72 h, and the PI3K/AKT pathway protein level was examined by Western blotting. Compared to the control group, wog markedly reduced phosphorylated PI3K (p-PI3K) and phosphorylated AKT (p-AKT) levels in GH3 cell (Figures 5A,B) and MMQ cell (Figures 5C,D). Given prior evidence that wog induces apoptosis in various tumor cells, its apoptotic effect on prolactinoma cells was also examined. Western blot analysis suggested that treatment with wog notably elevated pro-apoptotic protein levels in GH3 cell (Figures 6A,B) and MMQ cell (Figures 6C,D), encompassing Bax, cleaved caspase-9, and cleaved caspase-3. These results collectively indicate that wog inhibits the PI3K/AKT signaling pathway and promotes apoptosis in prolactinoma cells.
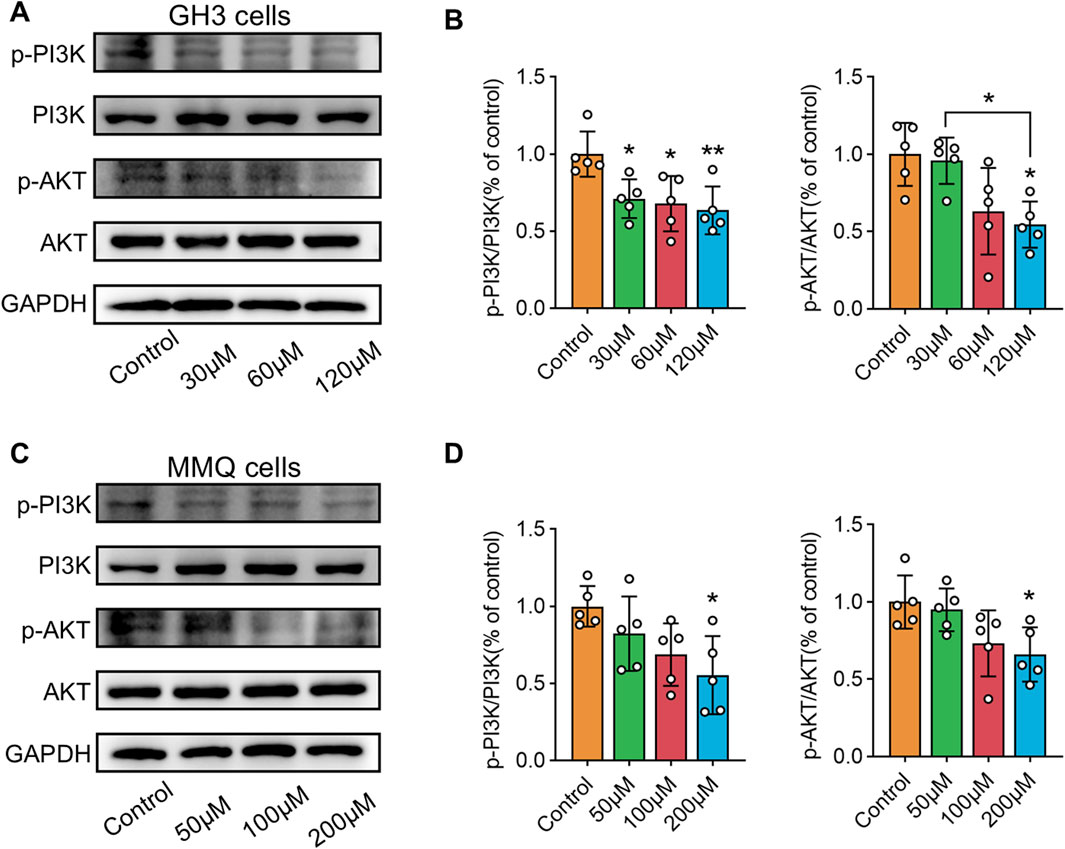
Figure 5. Protein expression levels of p-PI3K and p-AKT expression in prolactinoma cells were detected by Western blot. GAPDH was used as an internal control. n = 5/group: (A). GH3 cells were treated with wogonin at concentrations of 0, 30, 60 and 120 μM for 72 h (B). Statistical graph of Western blot results of GH3 cells (C). MMQ cells were treated with wogonin at concentrations of 0, 50, 100 and 200 μM for 72 h (D). Statistical graph of Western blot results of MMQ cells. (**p < 0.01, *p < 0.05. Except for * on the n-zig-zag line, all other * indicate comparisons with the control group).
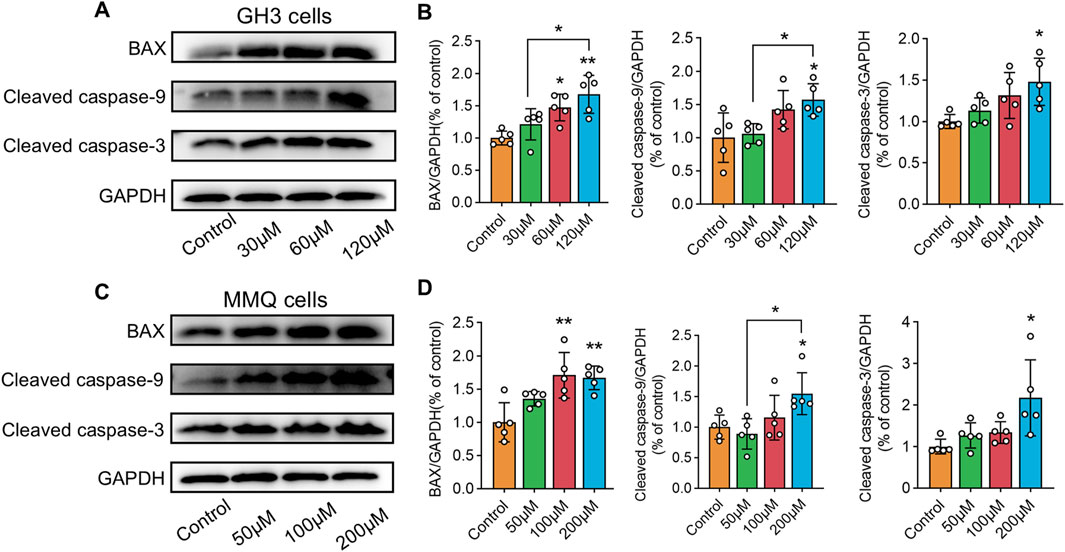
Figure 6. Protein expression levels of Bax, cleaved caspase-9 and cleaved caspase-3 expression in prolactinoma cells were detected by Western blot. GAPDH was used as an internal control. n = 5/group: (A). GH3 cells were treated with wogonin at concentrations of 0, 30, 60 and 120 μM for 72 h (B). Statistical graph of Western blot results of GH3 cells (C). MMQ cells were treated with wogonin at concentrations of 0, 50, 100 and 200 μM for 72 h (D). Statistical graph of Western blot results of MMQ cells. (**p < 0.01, *p < 0.05, Except for * on the n-zig-zag line, all other * indicate comparisons with the control group).
3.8 Wogonin inhibits PRL secretion in prolactinoma cells
PRL secretion levels in cell supernatants were measured using an ELISA kit following 72 h of wog treatment at various concentrations. In GH3 cells, wog treatment did not affect PRL secretion (Figure 7A). However, in MMQ cells, a marked decline in PRL levels was evident at 200 μM relative to the control group (Figure 7B). At lower concentrations (50 μM and 100 μM), no significant changes in PRL secretion were detected.
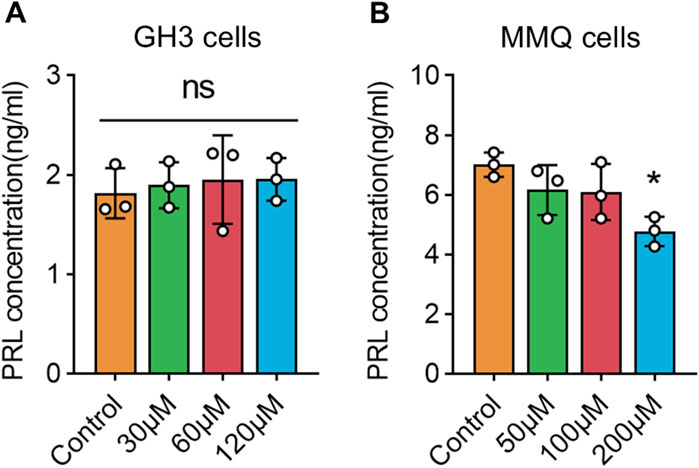
Figure 7. Levels of PRL secretion under wogonin treatment in prolactinoma cells: (A). GH3 cells were treated with wogonin at concentrations of 0, 30, 60 and 120 μM for 72 h (B). MMQ cells were treated with wogonin at concentrations of 0, 50, 100 and 200 μM for 72 h (*p < 0.05, all * indicate comparisons with the control group, ns means no significance).
3.9 Wogonin inhibits prolactinoma cell growth in vivo
To examine how wog impacts prolactinoma development in vivo, a subcutaneous tumor xenograft model was developed using nude mice implanted with rat pituitary tumor cells. Following tumor formation, the mice were separated into four equal groups (n = 5 per group). Wog treatment markedly suppressed tumor growth (Figure 8A), with tumor size and weight in the Wog + BRC group markedly diminished relative to the control group or the single-drug treatment groups (Figures 8B,C). Immunohistochemical analysis revealed markedly reduced Ki-67, p-PI3K, and p-AKT levels in tumors treated with wog and BRC (Figure 8D). The combination therapy further enhanced the suppression of these proteins compared to individual treatments. These results demonstrate that wog inhibits prolactinoma cell proliferation and has a synergistic therapeutic effect with BRC, primarily through inhibition of the PI3K/AKT signaling pathway. Drug toxicity assessments, including HE staining of major organs, revealed no significant pathological changes in the treated groups (Supplementary Figure S2). Serum biochemical analyses confirmed that wog did not cause notable damage to liver or kidney function or to the myocardium (Supplementary Figure S1), suggesting a favorable safety profile for wog at the administered doses. Taken together, these results suggest that wog can induce apoptosis in prolactinoma cells by inhibiting phosphorylation of the PI3K/AKT pathway (Figure 9).
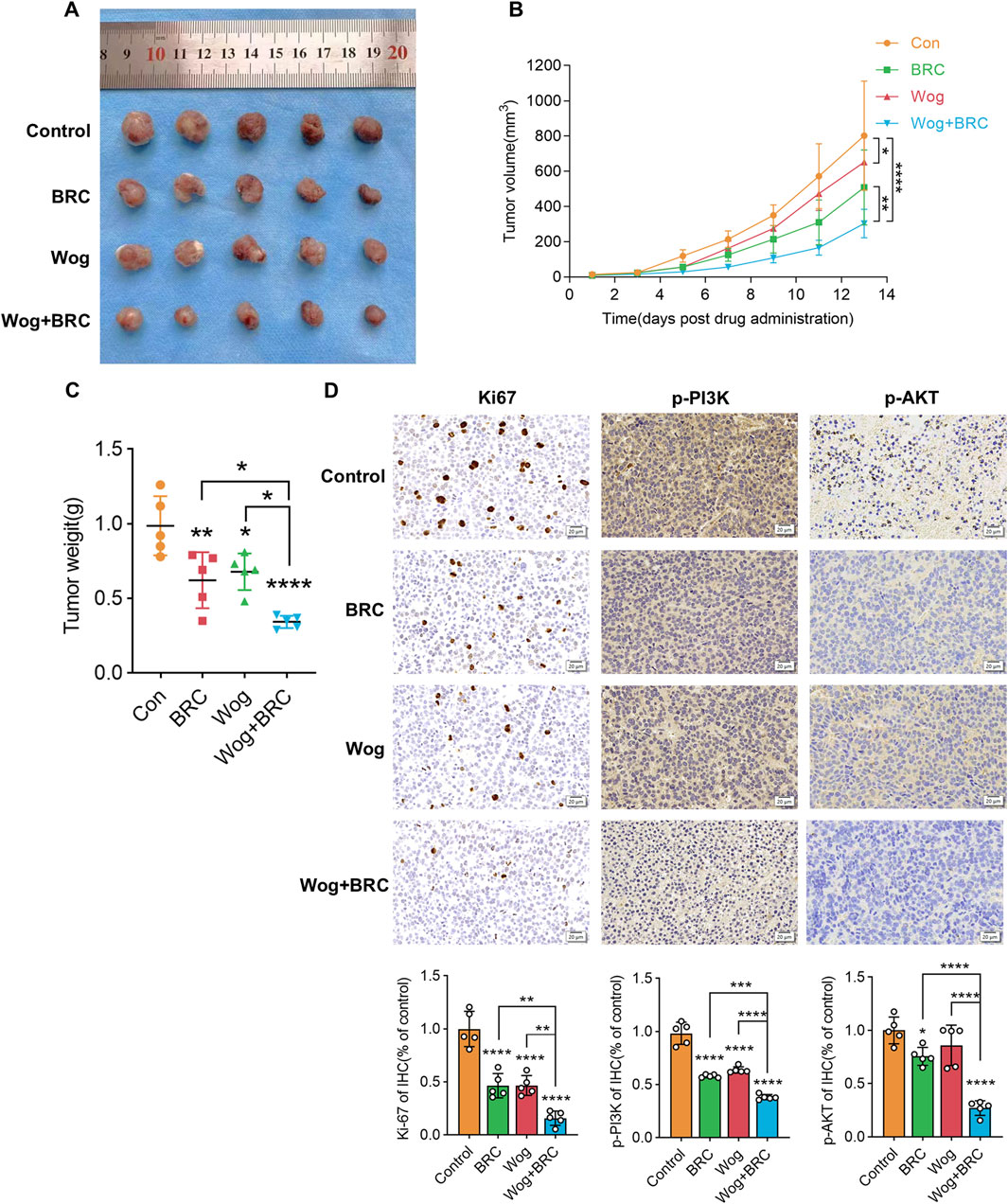
Figure 8. Subcutaneous xenograft model and drug treatment in vivo: (A) Representative images of xenograft tumors in nude mice (B,C). After tumor formation, the tumor volume (B) and weight (C) of each group were assessed (D). The immunohistochemical analyses of Ki-67, p-PI3K and p-AKT in tumor samples of each group. (****p < 0.0001, ***p < 0.005, **p < 0.01, *p < 0.05. Except for * on the n-zig-zag line, all other * indicate comparisons with the control group).
4 Discussion
BRC, the first-line clinical treatment for prolactinoma (Inder and Jang, 2022), suppresses prolactin secretion through negative feedback by activating dopamine receptors in the anterior pituitary. While this treatment effectively reduces prolactin levels and tumor size in the majority of patients, approximately 20% exhibit resistance to BRC (Maiter, 2019; Molitch, 2005). For these cases, temozolomide has demonstrated some efficacy as an adjunct to surgical and radiotherapeutic interventions (Tang H. et al., 2021). However, no definitive consensus exists regarding the optimal therapeutic approach, underscoring the urgent need for alternative strategies to manage dopamine-resistant prolactinomas.
The use of TCM in oncology has a longstanding history, with Scutellaria baicalensis Georgi being a prominent example. One of its primary bioactive constituents, wog, exhibits potent antitumor properties through various mechanisms (Huynh et al., 2017; Liu et al., 2024; Liu X. et al., 2023), encompassing apoptosis induction, angiogenesis inhibition, cell cycle arrest, suppression of tumor invasion and metastasis, and regulation of telomerase activity. This study employed a systematic approach to identify the potential molecular targets of wog for prolactinoma treatment, incorporating multiple analytical perspectives. Nine hub targets were subsequently identified, and MD analysis confirmed strong binding affinities between wog and these targets. These findings highlight wog’s potential as a promising therapeutic agent against prolactinoma, offering mechanistic insights into its antitumor efficacy.
GO and KEGG enrichment analyses revealed that wog exerts therapeutic effects on prolactinomas through various biological mechanisms. Key signaling pathways enriched in the analysis include Pathways in cancer, PI3K/Akt signaling, EGFR tyrosine kinase inhibitor resistance, endocrine resistance, and central carbon metabolism in cancer. Among these, the PI3K/AKT pathway emerges as a critical intracellular signaling cascade intricately linked to cellular growth, proliferation, metabolism, and tumorigenesis (He et al., 2021; Fresno et al., 2004). This pathway is regulated by upstream tyrosine kinase receptors and modulated by PI3K. Aberrant stimulation of the PI3K/AKT signaling cascade has been extensively reported as a driver of tumor progression, poor prognosis, and the emergence of therapeutic resistance (Wei et al., 2019; Song et al., 2020; Tufail et al., 2024). In prolactinoma, activation of the PI3K/Akt pathway is closely associated with cell proliferation (Ferraris, 2022). As a result, this pathway has become a prominent target in cancer therapy, with several small-molecule inhibitors such as Alpelisib, a PI3K inhibitor, and Capivasertib, a selective AKT inhibitor, showing promising anti-tumor efficacy in clinical studies, particularly for cancers harboring PI3K or AKT mutations (Savas et al., 2022; Turner et al., 2023).
The results of NP analyses were further validated through both in vitro and in vivo experiments. The results of cellular experiments showed that inhibited the phosphorylation of the PI3K/AKT pathway and induced apoptosis in prolactinoma cells. The results showed that GH3 cells and MMQ cells had different sensitivities to wog, the reason for which may be related to the different receptors on the surface of the 2 cells and the degree of activation of intracellular signaling pathways. In in vivo experiments, wog inhibited proliferation of prolactinoma. When combined with BRC, it demonstrated synergistic therapeutic effects on prolactinoma. However, the study has several limitations. First, the drug and disease targets were sourced from public databases such as TCMSP and GEO, which may contain incomplete or outdated data due to inconsistent update frequencies. Second, the experimental focus was limited to the molecular biology of the PI3K/AKT pathway, without exploring other potential mechanisms in depth. Third, the study relied on rat prolactinoma cells, which may not fully reflect the physiological and pathological processes in humans. Subsequent investigations ought to broaden the experimental parameters to encompass human-derived cells and explore additional pathways to improve the reliability, clinical relevance, and translational potential of the findings.
5 Conclusion
In summary, wog effectively inhibits the proliferation of prolactinoma cells and promotes tumor cell apoptosis in a time- and concentration-dependent manner. Furthermore, its antitumor efficacy is markedly enhanced when used in combination with BRC. These results underscore wog’s potential as a promising, safe, and effective therapeutic agent for prolactinoma, offering a novel avenue for future drug development and clinical treatment strategies.
Data availability statement
The datasets presented in this study can be found in online repositories. The names of the repository/repositories and accession number(s) can be found below: Du, zhiyong (2025), “Wogonin”, Mendeley Data, v2, doi: 10.17632/jtyrvxgwwv.2, https://data.mendeley.com/datasets/jtyrvxgwwv/2.
Ethics statement
The animal study was approved by Animal Ethics Committee of Jiangnan University. The study was conducted in accordance with the local legislation and institutional requirements.
Author contributions
ZD: Conceptualization, Formal Analysis, Investigation, Methodology, Validation, Writing – original draft, Writing – review and editing. CS: Formal Analysis, Investigation, Methodology, Writing – original draft. JwW: Investigation, Methodology, Writing – original draft. HG: Methodology, Writing – original draft. JlW: Methodology, Writing – original draft. YZ: Methodology, Writing – original draft. XW: Methodology, Writing – original draft. LS: Funding acquisition, Project administration, Resources, Supervision, Writing – original draft, Writing – review and editing. QW: Funding acquisition, Project administration, Resources, Supervision, Writing – original draft, Writing – review and editing.
Funding
The author(s) declare that financial support was received for the research and/or publication of this article. This research was supported by the “Taihu Talent Plan” Healthcare High-end Talent (THRC0009), National Natural Science Foundation of China (32400811), Jiangsu Provincial Key Research and Development Program for Social Development (BE2022695), The Fund of “the Fourteenth Five-Year Plan” of Jiangsu Provincial Key Medical Discipline (ZDXK202227) and Key Medical Discipline of Wuxi (ZDXK2021004).
Conflict of interest
The authors declare that the research was conducted in the absence of any commercial or financial relationships that could be construed as a potential conflict of interest.
Generative AI statement
The author(s) declare that no Generative AI was used in the creation of this manuscript.
Publisher’s note
All claims expressed in this article are solely those of the authors and do not necessarily represent those of their affiliated organizations, or those of the publisher, the editors and the reviewers. Any product that may be evaluated in this article, or claim that may be made by its manufacturer, is not guaranteed or endorsed by the publisher.
Supplementary material
The Supplementary Material for this article can be found online at: https://www.frontiersin.org/articles/10.3389/fphar.2025.1546285/full#supplementary-material
References
Auriemma, R. S., Pirchio, R., Pivonello, C., Garifalos, F., and Colao, A. (2023). Approach to the patient with prolactinoma. J. Clin. Endocrinol. Metab. 108 (9), 2400–2423. doi:10.1210/clinem/dgad174
Banik, K., Khatoon, E., Harsha, C., Rana, V., Parama, D., Thakur, K. K., et al. (2022). Wogonin and its analogs for the prevention and treatment of cancer: a systematic review. Phytother. Res. 36 (5), 1854–1883. doi:10.1002/ptr.7386
Castle-Kirszbaum, M., Biermasz, N., Kam, J., and Goldschlager, T. (2024). Quality of life in Prolactinoma: a systematic review. Pituitary 27 (3), 239–247. doi:10.1007/s11102-024-01392-1
Cheng, J., Xie, W., Chen, Y., Sun, Y., Gong, L., Wang, H., et al. (2024). Drug resistance mechanisms in dopamine agonist-resistant prolactin pituitary neuroendocrine tumors and exploration for new drugs. Drug Resist Updat 73, 101056. doi:10.1016/j.drup.2024.101056
Dworakowska, D., Wlodek, E., Leontiou, C. A., Igreja, S., Cakir, M., Teng, M., et al. (2009). Activation of RAF/MEK/ERK and PI3K/AKT/mTOR pathways in pituitary adenomas and their effects on downstream effectors. Endocr. Relat. Cancer 16 (4), 1329–1338. doi:10.1677/ERC-09-0101
Ferraris, J. (2022). Is prolactin receptor signaling a target in dopamine-resistant prolactinomas? Front. Endocrinol. (Lausanne) 13, 1057749. doi:10.3389/fendo.2022.1057749
Fresno, V. J. A., Casado, E., de Castro, J., Cejas, P., Belda-Iniesta, C., and González-Barón, M. (2004). PI3K/Akt signalling pathway and cancer. Cancer Treat. Rev. 30 (2), 193–204. doi:10.1016/j.ctrv.2003.07.007
Fukuhara, N., Nishiyama, M., and Iwasaki, Y. (2022). Update in pathogenesis, diagnosis, and therapy of prolactinoma. Cancers (Basel) 14 (15), 3604. doi:10.3390/cancers14153604
Guo, B., Zhao, C., Zhang, C., Xiao, Y., Yan, G., Liu, L., et al. (2022). Elucidation of the anti-inflammatory mechanism of Er Miao San by integrative approach of network pharmacology and experimental verification. Pharmacol. Res. 175, 106000. doi:10.1016/j.phrs.2021.106000
He, J. P., Zhao, M., Zhang, W. Q., Huang, M. Y., Zhu, C., Cheng, H. Z., et al. (2019). Identification of gene expression changes associated with uterine receptivity in mice. Front. Physiol. 10, 125. doi:10.3389/fphys.2019.00125
He, Q., Liu, C., Wang, X., Rong, K., Zhu, M., Duan, L., et al. (2023). Exploring the mechanism of curcumin in the treatment of colon cancer based on network pharmacology and molecular docking. Front. Pharmacol. 14, 1102581. doi:10.3389/fphar.2023.1102581
He, Y., Sun, M. M., Zhang, G. G., Yang, J., Chen, K. S., Xu, W. W., et al. (2021). Targeting PI3K/Akt signal transduction for cancer therapy. Signal Transduct. Target Ther. 6 (1), 425. doi:10.1038/s41392-021-00828-5
Huang, K. F., Zhang, G. D., Huang, Y. Q., and Diao, Y. (2012). Wogonin induces apoptosis and down-regulates survivin in human breast cancer MCF-7 cells by modulating PI3K-AKT pathway. Int. Immunopharmacol. 12 (2), 334–341. doi:10.1016/j.intimp.2011.12.004
Huynh, D. L., Sharma, N., Kumar Singh, A., Singh Sodhi, S., Zhang, J. J., Mongre, R. K., et al. (2017). Anti-tumor activity of wogonin, an extract from Scutellaria baicalensis, through regulating different signaling pathways. Chin. J. Nat. Med. 15 (1), 15–40. doi:10.1016/S1875-5364(17)30005-5
Inder, W. J., and Jang, C. (2022). Treatment of prolactinoma. Med. (Kaunas) 58 (8), 1095. doi:10.3390/medicina58081095
Ji, L., Song, T., Ge, C., Wu, Q., Ma, L., Chen, X., et al. (2023). Identification of bioactive compounds and potential mechanisms of scutellariae radix-coptidis rhizoma in the treatment of atherosclerosis by integrating network pharmacology and experimental validation. Biomed. Pharmacother. 165, 115210. doi:10.1016/j.biopha.2023.115210
Lee, D. H., Kim, C., Zhang, L., and Lee, Y. J. (2008). Role of p53, PUMA, and Bax in wogonin-induced apoptosis in human cancer cells. Biochem. Pharmacol. 75 (10), 2020–2033. doi:10.1016/j.bcp.2008.02.023
Li, T., Li, W., Guo, X., Tan, T., Xiang, C., and Ouyang, Z. (2023). Unraveling the potential mechanisms of the anti-osteoporotic effects of the Achyranthes bidentata-Dipsacus asper herb pair: a network pharmacology and experimental study. Front. Pharmacol. 14, 1242194. doi:10.3389/fphar.2023.1242194
Li, X., Wei, S., Niu, S., Ma, X., Li, H., Jing, M., et al. (2022). Network pharmacology prediction and molecular docking-based strategy to explore the potential mechanism of Huanglian Jiedu Decoction against sepsis. Comput. Biol. Med. 144, 105389. doi:10.1016/j.compbiomed.2022.105389
Liao, H., Ye, J., Gao, L., and Liu, Y. (2021). The main bioactive compounds of Scutellaria baicalensis Georgi. for alleviation of inflammatory cytokines: a comprehensive review. Biomed. Pharmacother. 133, 110917. doi:10.1016/j.biopha.2020.110917
Lin, F., Zhang, G., Yang, X., Wang, M., Wang, R., Wan, M., et al. (2023). A network pharmacology approach and experimental validation to investigate the anticancer mechanism and potential active targets of ethanol extract of Wei-Tong-Xin against colorectal cancer through induction of apoptosis via PI3K/AKT signaling pathway. J. Ethnopharmacol. 303, 115933. doi:10.1016/j.jep.2022.115933
Liu, H., Zhou, Q., Wei, W., Qi, B., Zeng, F., Bao, N., et al. (2020b). The potential drug for treatment in pancreatic adenocarcinoma: a bioinformatical study based on distinct drug databases. Chin. Med. 15, 26. doi:10.1186/s13020-020-00309-x
Liu, Q., Li, L., Zheng, D., Jin, S., Guan, X., Fu, Z., et al. (2023a). Mechanism of ShuiJingDan in treating acute gouty arthritis flares based on network pharmacology and molecular docking. Drug Des. Devel Ther. 17, 3493–3505. doi:10.2147/DDDT.S436360
Liu, X., Peng, X., Cen, S., Yang, C., Ma, Z., and Shi, X. (2023b). Wogonin induces ferroptosis in pancreatic cancer cells by inhibiting the Nrf2/GPX4 axis. Front. Pharmacol. 14, 1129662. doi:10.3389/fphar.2023.1129662
Liu, Y., Lu, L., Cheng, P., Zhang, S., Xu, Y., Hu, D., et al. (2024). Wogonin inhibits colorectal cancer proliferation and epithelial mesenchymal transformation by suppressing phosphorylation in the AKT pathway. Am. J. Chin. Med. 52 (4), 1155–1172. doi:10.1142/S0192415X24500460
Liu, Y., Yang, S., Wang, K., Lu, J., Bao, X., Wang, R., et al. (2020a). Cellular senescence and cancer: focusing on traditional Chinese medicine and natural products. Cell Prolif. 53 (10), e12894. doi:10.1111/cpr.12894
Lu, S., Sun, X., Zhou, Z., Tang, H., Xiao, R., Lv, Q., et al. (2023). Mechanism of Bazhen decoction in the treatment of colorectal cancer based on network pharmacology, molecular docking, and experimental validation. Front. Immunol. 14, 1235575. doi:10.3389/fimmu.2023.1235575
Luo, J. J., Chen, X. H., Liang, P. Y., Zhao, Z., Wu, T., Li, Z. H., et al. (2023). Mechanism of anti-hyperuricemia of isobavachin based on network pharmacology and molecular docking. Comput. Biol. Med. 155, 106637. doi:10.1016/j.compbiomed.2023.106637
Madikyzy, M., Tilegen, M., Nazarbek, G., Mu, C., Kutzhanova, A., Li, X., et al. (2022). Honghua extract mediated potent inhibition of COVID-19 host cell pathways. Sci. Rep. 12 (1), 14296. doi:10.1038/s41598-022-15338-9
Maiter, D. (2019). Management of dopamine agonist-resistant prolactinoma. Neuroendocrinology 109 (1), 42–50. doi:10.1159/000495775
Molitch, M. E. (2005). Pharmacologic resistance in prolactinoma patients. Pituitary 8 (1), 43–52. doi:10.1007/s11102-005-5085-2
Newman, D. J., and Cragg, G. M. (2016). Natural products as sources of new drugs from 1981 to 2014. J. Nat. Prod. 79 (3), 629–661. doi:10.1021/acs.jnatprod.5b01055
Petersenn, S., and Giustina, A. (2020). Diagnosis and management of prolactinomas: current challenges. Pituitary 23 (1), 1–2. doi:10.1007/s11102-019-01025-y
Polier, G., Giaisi, M., Kohler, R., Müller, W. W., Lutz, C., Buss, E. C., et al. (2015). Targeting CDK9 by wogonin and related natural flavones potentiates the anti-cancer efficacy of the Bcl-2 family inhibitor ABT-263. Int. J. Cancer 136 (3), 688–698. doi:10.1002/ijc.29009
Savas, P., Lo, L. L., Luen, S. J., Blackley, E. F., Callahan, J., Moodie, K., et al. (2022). Alpelisib monotherapy for PI3K-altered, pretreated advanced breast cancer: a phase II study. Cancer Discov. 12 (9), 2058–2073. doi:10.1158/2159-8290.CD-21-1696
Selek, A., Halbutogullari, Z. S. U., Aydemir, C. I., Cetinarslan, B., Canturk, Z., Tarkun, I., et al. (2023). Letrozole decreased testosterone-induced cell proliferation and prolactin secretion also increased apoptosis in MMQ and GH3 rat prolactinoma cell lines. Mol. Neurobiol. 60 (5), 2442–2454. doi:10.1007/s12035-023-03220-2
Shang, L., Wang, Y., Li, J., Zhou, F., Xiao, K., Liu, Y., et al. (2023). Mechanism of Sijunzi Decoction in the treatment of colorectal cancer based on network pharmacology and experimental validation. J. Ethnopharmacol. 302 (Pt A), 115876. doi:10.1016/j.jep.2022.115876
Song, F., Hu, B., Cheng, J. W., Sun, Y. F., Zhou, K. Q., Wang, P. X., et al. (2020). Anlotinib suppresses tumor progression via blocking the VEGFR2/PI3K/AKT cascade in intrahepatic cholangiocarcinoma. Cell Death Dis. 11 (7), 573. doi:10.1038/s41419-020-02749-7
Tang, C., Zhu, J., Yuan, F., Yang, J., and Cai, X. (2021a). Curcumin sensitizes prolactinoma cells to bromocriptine by activating the ERK/EGR1 and inhibiting the AKT/GSK-3β signaling pathway in vitro and in vivo. Mol. Neurobiol. 58 (12), 6170–6185. doi:10.1007/s12035-021-02541-4
Tang, H., Cheng, Y., Huang, J., Li, J., Zhang, B., and Wu, Z. B. (2021b). Case report: temozolomide treatment of refractory prolactinoma resistant to dopamine agonists. Front. Endocrinol. (Lausanne) 12, 616339. doi:10.3389/fendo.2021.616339
Tritos, N. A., and Miller, K. K. (2023). Diagnosis and management of pituitary adenomas: a review. JAMA 329 (16), 1386–1398. doi:10.1001/jama.2023.5444
Tufail, M., Wan, W. D., Jiang, C., and Li, N. (2024). Targeting PI3K/AKT/mTOR signaling to overcome drug resistance in cancer. Chem. Biol. Interact. 396, 111055. doi:10.1016/j.cbi.2024.111055
Turner, N. C., Oliveira, M., Howell, S. J., Dalenc, F., Cortes, J., Gomez Moreno, H. L., et al. (2023). Capivasertib in hormone receptor-positive advanced breast cancer. N. Engl. J. Med. 388 (22), 2058–2070. doi:10.1056/NEJMoa2214131
Vermeulen, E., D'Haens, J., Stadnik, T., Unuane, D., Barbe, K., Van Velthoven, V., et al. (2020). Predictors of dopamine agonist resistance in prolactinoma patients. BMC Endocr. Disord. 20 (1), 68. doi:10.1186/s12902-020-0543-4
Wang, S., Feng, Y., Han, X., Cai, X., Yang, L., Liu, C., et al. (2021). Inhibition of virulence factors and biofilm formation by wogonin attenuates pathogenicity of Pseudomonas aeruginosa PAO1 via targeting pqs -Sensing system. Int. J. Mol. Sci. 22 (23), 12699. doi:10.3390/ijms222312699
Wang, Y., Yuan, Y., Wang, W., He, Y., Zhong, H., Zhou, X., et al. (2022b). Mechanisms underlying the therapeutic effects of Qingfeiyin in treating acute lung injury based on GEO datasets, network pharmacology and molecular docking. Comput. Biol. Med. 145, 105454. doi:10.1016/j.compbiomed.2022.105454
Wang, Y., Zhang, Q., Chen, Y., Liang, C. L., Liu, H., Qiu, F., et al. (2020). Antitumor effects of immunity-enhancing traditional Chinese medicine. Biomed. Pharmacother. 121, 109570. doi:10.1016/j.biopha.2019.109570
Wang, Y. X., Yang, Z., Wang, W. X., Huang, Y. X., Zhang, Q., Li, J. J., et al. (2022a). Methodology of network pharmacology for research on Chinese herbal medicine against COVID-19: a review. J. Integr. Med. 20 (6), 477–487. doi:10.1016/j.joim.2022.09.004
Wei, C., Dong, X., Lu, H., Tong, F., Chen, L., Zhang, R., et al. (2019). LPCAT1 promotes brain metastasis of lung adenocarcinoma by up-regulating PI3K/AKT/MYC pathway. J. Exp. Clin. Cancer Res. 38 (1), 95. doi:10.1186/s13046-019-1092-4
Weng, J., Wu, X. F., Shao, P., Liu, X. P., and Wang, C. X. (2023). Medicine for chronic atrophic gastritis: a systematic review, meta- and network pharmacology analysis. Ann. Med. 55 (2), 2299352. doi:10.1080/07853890.2023.2299352
Wu, J. W., Gao, W., Shen, L. P., Chen, Y. L., Du, S. Q., Du, Z. Y., et al. (2024). Leonurus japonicus Houtt. modulates neuronal apoptosis in intracerebral hemorrhage: insights from network pharmacology and molecular docking. J. Ethnopharmacol. 330, 118223. doi:10.1016/j.jep.2024.118223
Yang, D., Guo, Q., Liang, Y., Zhao, Y., Tian, X., Ye, Y., et al. (2020). Wogonin induces cellular senescence in breast cancer via suppressing TXNRD2 expression. Arch. Toxicol. 94 (10), 3433–3447. doi:10.1007/s00204-020-02842-y
Zhang, L., Han, L., Wang, X., Wei, Y., Zheng, J., Zhao, L., et al. (2021). Exploring the mechanisms underlying the therapeutic effect of Salvia miltiorrhiza in diabetic nephropathy using network pharmacology and molecular docking. Biosci. Rep. 41 (6). doi:10.1042/BSR20203520
Zhang, L., Yang, Z., Li, X., Hua, Y., Fan, G., and He, F. (2023). Anti-atherosclerotic effects of naringenin and quercetin from Folium Artemisiae argyi by attenuating Interleukin-1 beta (IL-1β)/matrix metalloproteinase 9 (MMP9): network pharmacology-based analysis and validation. BMC Complement. Med. Ther. 23 (1), 378. doi:10.1186/s12906-023-04223-1
Zhang, T., Liu, M., Liu, Q., and Xiao, G. G. (2022). Wogonin increases gemcitabine sensitivity in pancreatic cancer by inhibiting Akt pathway. Front. Pharmacol. 13, 1068855. doi:10.3389/fphar.2022.1068855
Keywords: network pharmacology, molecular docking, prolactinoma, wogonin, PI3K/Akt signaling pathway, apoptosis
Citation: Du Z, Sun C, Wu J, Gao H, Wu J, Zhou Y, Wu X, Shen L and Wang Q (2025) Wogonin inhibits the proliferation of prolactinoma through the PI3K/AKT signaling pathway. Front. Pharmacol. 16:1546285. doi: 10.3389/fphar.2025.1546285
Received: 16 December 2024; Accepted: 23 April 2025;
Published: 15 May 2025.
Edited by:
Yan Zhang, Shenyang Pharmaceutical University, ChinaReviewed by:
Xu Weidong, Jiangsu University, ChinaYuwei He, The Affiliated Hospital of Qingdao University, China
Copyright © 2025 Du, Sun, Wu, Gao, Wu, Zhou, Wu, Shen and Wang. This is an open-access article distributed under the terms of the Creative Commons Attribution License (CC BY). The use, distribution or reproduction in other forums is permitted, provided the original author(s) and the copyright owner(s) are credited and that the original publication in this journal is cited, in accordance with accepted academic practice. No use, distribution or reproduction is permitted which does not comply with these terms.
*Correspondence: Qing Wang, d3hleXdxbmpAMTYzLmNvbQ==; Liping Shen, ZGcxOTMwMDM0QHNtYWlsLm5qdS5lZHUuY24=