- 1 Department of Tourism and Leisure, Hsing Wu University, Taipei, Taiwan
- 2 Research Center of Natural Cosmeceuticals Engineering, Xiamen Medical College, Xiamen, China
- 3 Department of Biological Science and Technology, China University of Science and Technology, Taipei, Taiwan
- 4 Chill Beauty Corporation, Taipei, Taiwan
- 5 Cancer Genome Research Center, Chang Gung Memorial Hospital at Linkou, Taoyuan, Taiwan
Buddleja davidii Franch. [Scrophulariaceae] commonly found in the mountainous regions of Taiwan, is used as herbal medicine around the world. In spite of this, no research has been conducted on the physiological activities of B. davidii extracts from different parts of the plant and from plants grown at different altitudes. In this study, B. davidii flower, stem, and leaf extracts were prepared using distilled water, methanol, and 60% ethanol as extraction solvents. The total phenolic content of the extracts served as an indicator of their activities. Our results indicated high bioactivity in the water extract of B. davidii flowers grown at 1,500 m, the 60% ethanol extract of B. davidii stems grown at 1,000 m, and the methanol extract of B. davidii leaves grown at 1,500 m. The freeze-dried leaf extract exhibited the highest antioxidant activity, which may be attributed to its abundance of phenylethanoid glycosides and flavonoids. The major bioactive components of the flower extract were crocin, crocetin, quercetin, and rutin. Those in the stem extract were luteolin, naringenin, quercetin, acacetin, and apigenin; and in the leaf extract were verbascoside, isoverbascoside and oleanolic acid. These compounds were potentially responsible for the antiaging and anti-inflammatory activity of the flower extract (IC50: 28.6–125.1 mg/L), the antibacterial activity of the stem extract (minimum inhibitory concentration: 60–100 mg/L), and the antityrosinase activity of the leaf extract (IC50: 38.17 mg/L). For example, the antiaging activity of B. davidii flower extract was found to be superior to or comparable with that of the positive controls, which include EGCG (IC50: 67.2–162.8 mg/L), 1,10-phenanthroline (IC50: 46.7 mg/L), gallic acid (IC50: 132.6 mg/L), and tannic acid (IC50: 140.3 mg/L). Moreover, these extracts can be deemed safe, as they demonstrated no toxic effects on CCD-966SK, HEMn, and RAW264.7 cells at a concentration of 200 mg/L. To our knowledge, this is the first report revealing differences in activities of B. davidii extracts based on plant part and altitudes. The findings provide insights for potential applications of the identified bioactive compounds in health foods, herbal medicines, and cosmetics.
1 Introduction
Medicinal plants have long been cultivated as sources of therapeutically active compounds. Some herbal medicines even have lower side effects than conventional medicines (Nguyen and Kim, 2020). The butterfly bush (Buddleja davidii Franch. [Scrophulariaceae]) is a medicinal plant from the Scrophulariaceae family and is cultivated for commercial use. B. davidii generally grows on mountain slopes at altitudes of 800–3,000 m (Ebeling et al., 2008). The genus Buddleja is found in the drier subtropical regions of Asia, western Europe, southern and eastern Africa, and parts of America that lie south of the southwestern states of the United States of America. Several species have been introduced as ornamental plants because their flowers can attract butterflies. Furthermore, B. davidii grows widely due to its potential invasive power and adaptability (Houghton et al., 2003; Ahmad et al., 2009; Chen et al., 2020).
Previous studies have analyzed extracts from various parts of Buddleja and detected phenylpropanoids, flavonoids, saponins, terpenoids, sterols, and lignin (Ying and Wan, 2012). Chemical profiles of the essential oils from Buddleja perfoliata have been reported, and the main components included cubenol, germacrene D-4-ol, β-eudesmol, and cis-verbenol (Cuevas-Cianca et al., 2022). These bioactive compounds possess activities that are anticarcinogenic, health-promoting, wound-healing, antioxidant, antiaging, anti-inflammatory, and antimicrobial, while exhibiting low toxicity and eliciting few side effects (Wu et al., 2012; Khan et al., 2019; Nguyen and Kim, 2020; Getahun et al., 2021). The metabolites of B. davidii are known for their antioxidant, anti-inflammatory, and photoprotective activities (Chen et al., 2020). The flowers, stems, and leaves of B. davidii contain different types and concentrations of bioactive substances, including flavonoids, carotenoids, phenols, and vitamins (Ying and Wan, 2012; Diretto et al., 2021). Thus, the different parts of B. davidii likely contribute differently to the pharmacological and cosmeceutical activities of the plant, but these differences have not been clearly delineated yet.
The accumulation of melanin in the epidermal layer leads to melanogenesis, which is related to skin aging. Tyrosinase is the key enzyme responsible for melanin production (Mapunya et al., 2012). Furthermore, aging and skin wrinkles are often caused by the proteolytic degradation of the extracellular matrix, a process that is significantly associated with increased dermal activities of collagenase, elastase, hyaluronidase, matrix metalloproteinase-1 (MMP-1), and MMP-2 (Maity et al., 2011; Buhren et al., 2020). Some compounds derived from B. davidii may serve as nutritional ingredients and whitening agents in the cosmetic sector. However, the lack of a pharmacopeia of the chemical constituents of B. davidii extracts limits the in-depth study of the pharmaceutical and cosmeceutical potential of various parts of B. davidii plants grown at different altitudes. Buddleja plants are also cultivated in the mountainous regions of Taiwan (Chen et al., 2010). However, there have been no investigations into the impact of diverse growth environments on the pharmaceutical and cosmetic constituents found in different parts of B. davidii.
In this study, bioactive compounds were extracted from various parts of native B. davidii plants cultivated at different altitudes by using distilled water, 100% methanol, and 60% ethanol and evaluated their antioxidant, antityrosinase, antimicrobial, anti-inflammatory, and antiwrinkle properties of these extracts. The novelty of this research lies in the absence of prior literature addressing the effects of different altitudes on the biological activities of extracts. The mechanisms underlying the strong physiological effects of the extracts were elucidated by determining the compositions and amounts of phenolic acid, flavonoids, triterpenes, phenylethanoid glycosides, and other possible bioactive compounds in the extracts. Furthermore, the cytotoxicity levels of the extracts with high physiological activity were evaluated to illustrate their safety. Finally, the binding between the chemical components of B. davidii extracts and core enzymatic targets was evaluated through molecular docking, which is an essential method in structural molecular biology and computer-aided drug development (Jin et al., 2021).
2 Materials and methods
2.1 Plant material and extraction procedure
Buddleja davidii plants were collected during the autumn of 2022 from three different mountainous regions (altitudes of 1,000 m, 1,500 m, and 2,000 m) in Ren’ai Township, Nantou County, Taiwan. B. davidii was identify by a plant doctor named Bau-Yuan Hu, and voucher specimens (20221101-20221103) were submitted to the herbarium at China University of Science and Technology in Taiwan. The flowers, stems and leaves of B. davidii were collected, respectively.
The gathered samples were rinsed with distilled water, air-dried naturally for 2 days, pulverized to powders, sifted through a 0.5-mm mesh, stored in a drying box, and extracted with distilled water, 100% methanol, or 60% ethanol at a liquid-to-solid ratio of 10:1 (10 g sample per 100 mL solvent) in a 300-mL Erlenmeyer flask at 250 rpm for 1 h at 26oC. That is, each different solvent was used to extract different plant samples. The crude extracts were passed through a Whatman filter (0.45 μm), and the resulting filtrates were analyzed for total phenolic content (TPC). The extracts with high TPC were subsequently concentrated at 60°C for the aqueous extract, 50°C for the ethanolic extract, and 45°C for the methanolic extract, utilizing a rotary vacuum evaporator (Panchum Scientific Corp., Kaohsiung City, Taiwan), and the resulting residues were then subjected to lyophilization for further analysis, employing a shelf freeze dryer (Uniss Corp., Taipei City, Taiwan).
2.2 Microbial strains, cells, and regents
Abiding by the regulatory guidelines in the United States Pharmacopeia 51 (antimicrobial effectiveness testing) and considering the microbes associated with skin diseases, we assessed the antimicrobial effectiveness of the freeze-dried extracts from B. davidii flowers, stems, and leaves against various microorganisms, including Pseudomonas aeruginosa ATCC 9027, Staphylococcus aureus ATCC 6538, Escherichia coli ATCC 8739, Candida albicans ATCC 10231, Aspergillus brasiliensis ATCC 16404, Cutibacterium acnes ATCC 6919, and Epidermophyton floccosum ATCC 18397. The strains that were subjected to testing were acquired from the Bioresource Collection and Research Center (BCRC) located in Hsinchu, Taiwan. Normal HEMn cells (C-102-5C) were obtained from Cascade Biologics (Portland, OR, United States). The CCD-966SK (BCRC 60153, human skin fibroblast cell line) and RAW264.7 cells (BCRC 60001, mouse macrophagic cell line) were purchased from the BCRC. Mushroom tyrosinase (≥1,000 units/mg) along with chemicals (purity ≥99%) were purchased from Sigma-Aldrich Chemistry, located in St. Louis, United States. Lipopolysaccharide (LPS) was isolated from E. coli O111:B4. Proinflammatory cytokines were obtained from Invitrogen (Austria).
2.3 Analysis of total phenolic content and total flavonoid content
Earlier research has highlighted a favorable link between TPC, TFC, and antioxidant activity (Li et al., 2009; Muflihah et al., 2021); therefore, TPC and TFC were used as rapidly measurable indicators to screen B. davidii extracts. The TPC of B. davidii extracts was quantified in terms of gallic acid equivalents (GAE) utilizing the methodology established by Kujala et al. (2000) with slight modifications implemented. The extracts (0.5 mL) underwent oxidation using 1 mL of a 10-fold diluted Folin–Ciocalteu phenol reagent for 10 min. Subsequently, the reaction was neutralized with 1 mL of a 7.5% Na2CO3 solution and allowed to proceed for 3 h. Following this, the mixture was subjected to centrifugation at 5,000 rpm for 10 min. The absorbance of the supernatant was recorded at a wavelength of 760 nm using an ultraviolet–visible spectrophotometer (UV-2600i, Shimadzu, Japan). Gallic acid, serving as our benchmark solution, was also processed as previously outlined. The TPC was quantified either by measuring OD760 or by estimating it in the mg-GAE/g-dried extract, utilizing the calibration curve derived from standard solutions of gallic acid.
The total flavonoid content (TFC) was quantified utilizing the aluminum chloride colorimetric technique, and the results were reported as mg-rutin equivalents (RE)/g-dry weight (Pourmorad et al., 2006). Briefly, 100 μL of B. davidii extracts were mixed with 30 μL of a 5% NaNO2 solution for 6 min at 26oC. Subsequently, 30 μL of a 10% Al(NO3)3 solution was introduced to the mixture and incubated for an additional 6 min, followed by 0.4 mL of a 1 M NaOH solution and enough methanol to adjust the total volume to 1 mL. Half an hour later, we took a peek at the reaction mixture’s absorbance, capturing its essence at 500 nm with the help of an ultraviolet–visible spectrophotometer. Rutin, used as a standard solution, was also processed per the aforementioned method. The TFC was calculated from the calibration curve derived from standard solutions of rutin.
2.4 Evaluation of antityrosinase activity and cellular melanin levels
The antityrosinase activities of B. davidii extracts were evaluated utilizing the methodology established by Wu et al. (2018). The extracts derived from flower, stem, and leaf of B. davidii were diluted to 0–200 mg/L in dimethyl sulfoxide (DMSO) solution. Subsequently, a 30-μL sample was combined with 970 μL of phosphate-buffered saline (0.05 mM). The mixture was incorporated into a solution comprising 1 mL of L-tyrosine at a concentration of 100 mg/L and 1 mL of mushroom tyrosinase solution at an activity level of 350 U/mL and mixed homogeneously in the dark. The absorbance of the reaction mixture was assessed at a wavelength of 490 nm following a 20-min incubation period. α-Arbutin and kojic acid served as the positive control agents in the study. The half-maximal inhibitory concentration (IC50) is defined as the concentration of the extract that results in a 50% reduction of the initial tyrosinase activity. The antityrosinase activity of an extract is defined as the percentage of inhibition of tyrosinase, calculated using the formula provided below (Equation 1) (Wang et al., 2017).
where A is OD490 in the absence of the extract (control), B is OD490 without both the extract and tyrosinase (blank of A), C is OD490 in the presence of the extract and tyrosinase (experimental group), and D is OD490 in the absence of the enzyme (blank of C).
Cellular tyrosinase activity and melanin content in HEMn cells were conducted utilizing the methodology described by Wu et al. (2018). HEMn cells (2 × 105 cells/well) were cultured in 24-well plates in Medium 254 supplemented with HMGS at a temperature of 37°C for a duration of 24 h in an atmosphere containing 5% CO2. The cells were subjected to treatment with extracts ranging from 0 to 200 mg/L for 24 h. Following this treatment, the cells were washed with phosphate-buffered saline, subsequently lysed using a cell lysis solution. The lysate was then sonicated at a cool 4°C for 10 min utilizing a Qsonica ultrasonic sonicator from Newtown, CT (United States) and centrifuged at 6,500 g for 15 min employing a micro-ultracentrifuge from Thermo Fisher Scientific in Waltham, MA (United States). To measure the tyrosinase activity within HEMn cells, the lysate supernatants were reacted with 2.5 mM L-3,4-dihydroxyphenylalanine for 1 h, and the OD475 of the solution was measured on the Epoch ELISA reader (BioTek Instruments, Santa Clara, CA, United States). In order to assess the melanin concentration in HEMn cells, the cell pellets were heated and dissolved in a solution of 1 N NaOH with 10% DMSO at a temperature of 80°C for 1 h. The OD405 of the solution was assessed utilizing an ELISA reader, and the melanin concentration was estimated from the OD405 measurement by employing a standard curve derived from synthetic melanin.
2.5 Cell viability assay
The viability of HEMn, CCD-966SK, and RAW264.7 cells lines was determined utilizing the 3-(4,5-dimethylthiazol-2-yl)-2,5-diphenyltetrazolium bromide (MTT) colorimetric assay, in accordance with the methodology outlined by Sittisart and Chitsomboon (2014). Briefly, 5 × 105 cells were incubated overnight in each well of a 96-well plate at 37°C under 5% CO2. Subsequently, 0–500 mg/L of B. davidii extracts were incubated with the cells for 24 h. The media were removed, and 0.1 mL of 0.5 g/L MTT solution was incubated with the cells for 1 h (HEMn, CCD-966SK) or 4 h (RAW264.7). The MTT solution was removed via aspiration, and 0.1 mL of dimethyl sulfoxide was introduced to each well to facilitate the solubilization of the formazan crystals. The optical density at 570 nm (OD570) of the solution was assessed utilizing an ELISA reader. Cell viability was quantified as the OD570 value of the sample as a percentage of the OD570 value of the blank.
2.6 Analysis of scavenging activity of DPPH and ABTS
The levels of the 2,2-diphenyl-1-picrylhydrazyl (DPPH) free radical and the 2,2′-azino-bis(3-ethylbenzothiazoline-6-sulfonic acid) (ABTS) free radical are widely used indexes of the antioxidant activity exhibited by natural substances. They were conducted in accordance with the methodologies outlined by Wu et al. (2018) and Merchán-Arenas et al. (2011), respectively. Briefly, 1 mL of 0–200 mg/L B davidii extract in the dark. The absorbance of the mixture was assessed at a wavelength of 517 nm utilizing an ultraviolet–visible spectrophotometer, and subsequent measurements were taken following a 1-h incubation period. The DPPH scavenging activity of B. davidii extracts was determined using the following formula (Equation 2).
where A0 represents OD517 measured without the extract, while A denotes OD517 measured in the presence of the extract.
The ABTS radical cation was produced by generated by combining a 2.45 mM K2S2O8 solution with a 7 mM solution of ABTS in a 1:1 ratio and allowing the mixture to react for 16 h in a dark. The ABTS solution that was prepared underwent dilution to achieve an optical density of 0.7 at a wavelength of 734 nm, and 20 μL of 0–200 mg/L B davidii extracts were mixed evenly with 20 μL of this solution. After an incubation of 3 min, the absorbance of this mixture was assessed at a wavelength of 734 nm utilizing an ELISA reader. Butylated hydroxytoluene (BHT) was served as a positive control for DPPH and ABTS assay. The ABTS scavenging activity of the B. davidii extracts was determined using the following equation (Equation 3).
where A0 is OD734 without the extract and A is OD734 with the extract.
The IC50 values for the DPPH and ABTS scavenging activities of B. davidii extracts represented 50% scavenging activity (Merchán-Arenas et al., 2011).
2.7 Analysis of antiwrinkle activity
The activity associated with anti-wrinkle or anti-aging effects is significantly linked to the inhibition of enzymes such as collagenase, elastase, hyaluronidase, MMP-2, and MMP-1 (Wu et al., 2018). Thus, we assayed the activities of these enzymes to estimate antiwrinkle activity of the extracts. Collagenase activity was assessed utilizing a modified fluorogenic dye-quenched (DQ)-gelatin assay, as outlined by Li et al. (2020). Briefly, 10 μL of 0–300 mg/L B davidii extract was mixed with 10 μL of 1 U/mL collagenase and 80 μL of buffer solution in each well of a 96-well plate and reacted for 15 min. Following this, 100 μL of 15 μg/mL DQ-gelatin solution was incorporated into the mixture. The absorbance of the resulting solution was subsequently measured at an excitation wavelength of 485 nm and an emission wavelength of 528 nm using a Synergy 2 microplate reader (BioTek Instruments, Santa Clara, CA, United States) to estimate the proteolysis rate of gelatin. Elastase activity assay was conducted in accordance with the methodology outlined by Wu et al. (2018). In summary, B. davidii extracts were diluted with buffer solution composed of 500 mM NaCl, 100 mM HEPES, and 0.05% Tween 20 in DMSO, and this mixture was applied to each well of a 96-well plate. Subsequently, 20 μL of the diluted extracts was reacted with 50 μL of 0.171 U/mL porcine pancreatic elastase and incubated at 37°C for a duration of 10 min. Finally, 5 μL of methoxysuccinyl-Ala-Ala-Pro-Val-p-nitroanilide was introduced into each well, and the absorbance of the mixture was quantified at 420 nm utilizing an ELISA reader. The activity of hyaluronidase was evaluated utilizing a spectrophotometric technique (Sumantran et al., 2007). Briefly, hyaluronidase (800 U/mL), hyaluronic acid (HA) substrate (0.40 mg/mL), and B. davidii extracts (0–300 mg/L) were reacted at 37°C for 60 min. Hyaluronidase activity was estimated by monitoring the percentage of undigested HA substrate, which was determined by measuring the absorbance of the reaction mixture at a wavelength of 415 nm utilizing an ELISA reader. The activities of MMP-1 and MMP-2 were determined in CCD-966SK cells utilizing human MMP-1 and MMP-2 ELISA kits from RayBiotech (Norcross, GA, United States), following the methodology outlined by Chen et al. (2022). Epigallocatechin (EGCG), 1,10-phenanthroline, gallic acid, ursolic acid or tannic acid were used as positive controls in the antiwrinkle assay, if required.
2.8 Analysis of anti-inflammatory activity
Anti-inflammatory activity was evaluated in RAW264.7 cells. The cells were inoculated at a density of 5 × 105 cells per well in a 96-well plate and subsequently incubated for 24 h. Subsequent to the renewal of the culture medium, the cells in each well were subjected to incubation with 20 μL of 0–300 mg/L B davidii extracts for 1 h. This was followed by the introduction of 1 μg/mL LPS to induce inflammatory responses for 24 h. The concentration of reactive oxygen species (ROS) was conducted utilizing the dichloro-dihydro-fluorescein diacetate assay, with absorbance being taken at excitation and emission wavelengths of 485 nm and 535 nm, respectively, using a Synergy 2 microplate reader (Sittisart and Chitsomboon, 2014). The concentration of nitric oxide (NO) was quantified utilizing a commercially available Griess reagent kit, with absorbance being conducted at a wavelength of 530 nm with the help of an ELISA reader and the concentration being extrapolated from a standard curve of nitrite solution (Intayoung et al., 2016). The levels of tumor necrosis factor-α (TNF-α) and interleukin-6 (IL-6) were quantified utilizing commercially available ELISA kits (R&D systems Inc., United States) in accordance with the manufacturer’s guidelines and with the help of standard curves for each cytokine (Liu et al., 2012). In anti-inflammatory study, indomethacin was served as the positive control.
2.9 Determination of minimum inhibitory concentration and minimum fungicidal concentration
The minimum inhibitory concentration (MIC) of B. davidii extracts against E. coli, S. aureus, P. aeruginosa, and C. acnes was assessed utilizing a tube dilution methodology (Rahman et al., 2013). Briefly, we mixed 2 mL of B. davidii extracts at various concentrations with 2 mL of tryptic soy broth and added a splash of 1 mL of inoculum (5 × 106 cfu/mL) in a test tube. This delightful concoction was then set to incubate at 35°C. C. acnes was cultured for 48 h under anaerobic conditions, whereas the other bacterial species were cultured for 24 h under aerobic conditions. The MIC was estimated by monitoring the change in OD600 of the bacterial suspensions using an ultraviolet–visible spectrophotometer. The lowest concentration of B. davidii extracts that inhibited the observable growth of the tested bacteria was considered as the MIC.
The antifungal activity of B. davidii extracts was evaluated using a conventional plate count method (Chen et al., 2022). Briefly, 1 mL of B. davidii extracts of different concentrations were incubated with 1 mL of inoculum (5 × 107 cfu/mL or spores/mL) and 100 mL of broth in a conical flask, which was then incubated at 24°C for 3 days (C. albicans) and a full week (A. brasiliensis and E. floccosum). The minimum fungicidal concentration (MFC) is defined as the lowest concentration of extracts that caused the mortality of 99.9% of the inoculated microorganisms.
2.10 Quantification of chemical compositions
The primary chemical compositions of B. davidii extracts were examined utilizing high-performance liquid chromatography (HPLC) (Hitachi, Tokyo, Japan) on a reversed-phase Econosil column (5 μm, 4.6 × 250 mm), at a flow rate of 1.0 mL/min with an injection volume of 20 µL. The chromatographic method was modified from that of Chen et al. (2022). The gradient elution protocol, utilizing solution A (deionized water) and solution B (acetonitrile) was implemented as detailed below: 0 min, 85% A; 25 min, 40% A; 50 min, 15% A; 75 min, 40% A; and 100 min, 85% A. The detection wavelength was in the range of 200–500 nm. Certain triterpenes (e.g., oleanic acid and ursolic acid) were analyzed at 210 nm (Yang et al., 2012); picrocrocin at 250 nm (Koulakiotis et al., 2015); isoflavone, alcohol, and phenolic compounds at 280 nm; phenylethanoid glycosides at 334 nm (Zhang et al., 2019); flavonoids at 350 nm (Crozier et al., 1997); and certain carotenoids (e.g., crocin and crocetin) at 440 nm. The identification of individual compounds was accomplished by comparing their retention times with those of the corresponding standards, utilizing identical experimental conditions. The concentrations of all identified compounds were quantified using calibration curves that correlate the concentrations of standard samples with their corresponding peak area values, resulting in linearity coefficients (R2 > 0.99).
2.11 Molecular docking analysis
Molecular docking calculations can provide more molecular biological information, such as the hydrogen bond force provider and the number of providers of small molecules or proteins, the polar and non-polar interaction relationships between small molecules and protein activation sites, and the configurational performance of small molecules at protein activation sites (Nwakulite et al., 2021). Molecular docking simulations were conducted on the main identified components of B. davidii flower, stem, and leaf extracts. The three-dimensional structures of selected chemical compounds sourced from the Human Metabolome Database, while structures of enzymes were acquired from the Protein Data Bank database. Molecular docking was conducted utilizing the iGEMDOCK 2.1 software, employing the following parameters: a population size of 300, a total of 80 generations, and a solution number of 100. The optimal docking conformation was identified as the one exhibiting the lowest energy value.
2.12 Statistical analysis
Data were analyzed utilizing a one-way analysis of variance, subsequently followed by Duncan’s test and expressed as the means ± standard deviations derived from at least three independent experiments. Statistically significant differences were identified with a p-value of less than 0.05. We utilized IBM SPSS, version 26 (SPSS, Chicago, IL, United States) to dive into the data.
3 Results and discussion
3.1 Effects of extraction solvent and cultivation altitudes on TPC
We used different solvents (distilled water, 100% methanol, 60% ethanol) to obtain extracts from the flowers, stems, and leaves of B. davidii pants cultivated at different altitudes (1,000 m, 1,500 m, 2,000 m). TPC was considered an index of the biological potency of the crude extract of B. davidii. The flower extract with the highest TPC (OD760 = 1.62 ± 0.02) was obtained using distilled water from B. davidii plants grown at an altitude of 1,500 m (Figure 1a). The stem extract with the highest TPC (OD760 = 1.51 ± 0.01) was obtained using 60% ethanol from B. davidii plants grown at an altitude of 1,000 m (Figure 1b). The leaf extract with the highest TPC (OD760 = 1.81 ± 0.02) was obtained using 100% methanol from B. davidii plants grown at an altitude of 1,500 m (Figure 1c). The TPC might be affected by the altitude, as indicated by the fact that herbs grown at higher altitudes tended to have higher phenolic content (Pandey et al., 2018). The increased ultraviolet radiation and lower temperatures at higher altitudes may stimulate the production of polyphenols and related bioactive compounds, which played an active role in stress response (Gülsoy et al., 2023). B. davidii extracts with a higher TPC likely contain more bioactive components. Zengin et al. (2015) found a positive correlation between the antioxidant activity of plant extracts and their TPC. For subsequent experiments, we used the freeze-dried water extract of B. davidii flowers (grown at 1,500 m), the freeze-dried ethanol extract of B. davidii stems (grown at 1,000 m), and the freeze-dried methanol extract of B. davidii leaves (grown at 1,500 m). In addition to latitude, the growth of plants at different phenological stages also influenced their physiological activity (Sharifi-Rad et al., 2020; 2022a; 2022b). By assessing the physiological traits of B. davidii growing at different latitudes, we chose various parts of B. davidii from different altitudes for potential future uses.
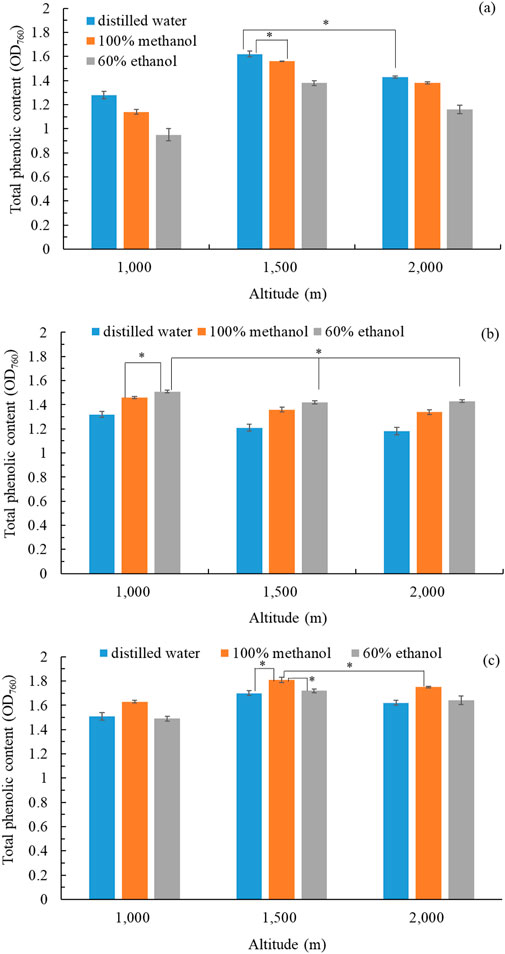
Figure 1. Total phenolic content in extracts: (a) flower extract; (b) stem extract; (c) leaf extracts of B. davidii plants collected from different altitudes and extracted using different solvents. Data are expressed as the means ± standard deviations of 3 independent experiments. Significant difference was expressed by *p < 0.05.
3.2 Cytotoxicity and antityrosinase activity
The prioritization of safety over pharmacological efficacy is of paramount importance. Therefore, we evaluated the cytotoxicity of B. davidii flower, stem, and leaf extracts on HEMn, CCD-966SK, and RAW264.7 cell lines utilizing the MTT assay. Cytotoxicity was assessed by comparing cell viability to the control group. HEMn cell viability was significantly reduced when treated with B. davidii leaf extracts at concentrations exceeding 250 mg/L (Figure 2a). The viability of CCD-966SK cells was not significantly affected by treatment with flower, stem, and leaf extracts with concentrations of 500 mg/L (Figure 2b). The viability of RAW264.7 cells was significantly affected by treatment with leaf and flower extracts with concentrations higher than 250 mg/L (Figure 2c). These findings suggest that HEMn and RAW264.7 cells were more vulnerable to B. davidii extracts than CCD-966SK cells. Moreover, B. davidii extracts from all three parts having a concentration up to 200 mg/L were safe for cells. Antityrosinase activity was assayed using extracts having concentrations less than 200 mg/L. Nguyen and Kim (2020) reported that B. davidii aqueous extracts exerted no significant cytotoxic effects on human HaCaT cells at concentrations reaching 100 mg/L.
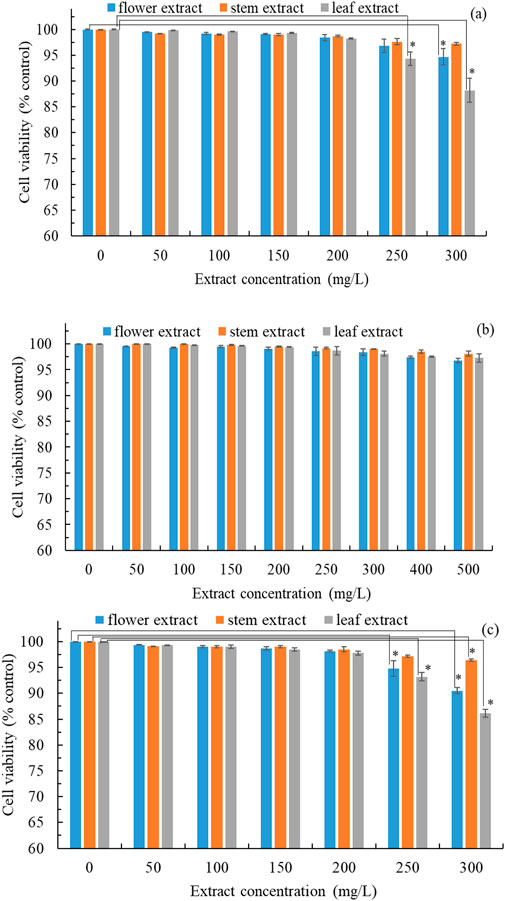
Figure 2. Cytotoxic effects of B. davidii extracts (flower, stem, and leaf) on (a) HEMn cells; (b) CCD-966SK cells; (c) RAW 264.7 cells. Data are expressed as the means ± standard deviations of 3 independent experiments. (*p < 0.05 vs blank control).
Tyrosinase is a crucial enzyme involved in the biosynthesis of melanin; therefore, the inhibition of tyrosinase activity can decrease melanin production and enhance skin whitening (Ho et al., 2021). The extracellular antityrosinase activity of extracts from B. davidii exhibited an increase in correlation with concentration (Figure 3a). A nonlinear regression analysis indicated that the IC50 values for the antityrosinase activity of flower, stem, and leaf extracts were 50.6 ± 2.4, 78.2 ± 2.6, and 41.4 ± 1.3 mg/L, respectively, implying that B. davidii leaf extracts exhibited the highest skin-whitening activity among the three extracts. The antityrosinase activity by the leaf extracts was found to be more effective than that of the positive control α-arbutin (IC50: 67.6 ± 3.5 mg/L) but slightly inferior to that of kojic acid (IC50: 32.6 mg/L). Subsequently, we evaluated the impact of different concentrations of B. davidii extracts on the melanin levels in HEMn cells. The melanin content exhibited a decline in correlation with the increase in extract concentration and antityrosinase activity (Figures 3b–d). A nonlinear regression analysis indicated that the IC50 values for the intracellular antityrosinase activity of flower, stem, and leaf extracts were 53.6 ± 2.5 mg/L, 91.8 ± 3.2 mg/L, and 38.2 ± 1.9 mg/L, respectively, which is consistent with the results obtained from the extracellular experiment.
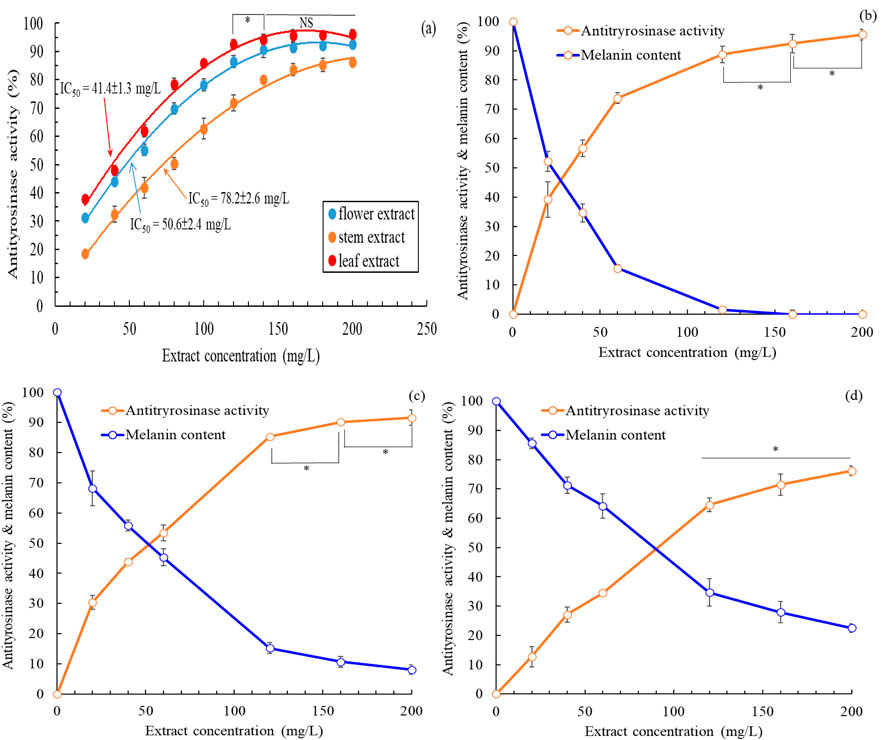
Figure 3. (a) Effects of B. davidii extracts (flower, stem, and leaf) on antityrosinase activity; (b) Effects on the melanin content and antityrosinase activity of HEMn cells of the (b) leaf extract, (c) flower extract, (d) and stem extract. Data are expressed as the means ± standard deviations of 3 independent experiments. (NS = not significant p > 0.05, *p < 0.05).
The intracellular antityrosinase activity of B. davidii leaf extract was higher than its extracellular antityrosinase activity, whereas the opposite trend was observed for flower and stem extracts. The flower and stem extracts were simultaneously associated with increasing antityrosinase activity and decreasing melanin content within HEMn cells. Therefore, inhibition of tyrosinase activity was the primary mechanism underlying the inhibition of melanin production by B. davidii flower and stem extracts (Lim et al., 2021). However, B. davidii leaf extract did not display similar behavior, hinting that the underlying mechanism was more complex (e.g., modulation of the regulators of signaling pathways involving PKA/CREB, ERK, AKT, and GSK3β/β-catenin) (Kim and Hyun, 2022). Mukherjee et al. (2018) suggested that a compound may inhibit extracellular and intracellular tyrosinase to different extents because the enzyme differs in its active site oxidative state, substrate specificity, and amino acid sequence in the two locations.
3.3 Analysis of antioxidant and antiaging activity
The DPPH and ABTS scavenging activity assays are the preferred methods for evaluating the antioxidant activity of compounds. Table 1 presents the TPC, TFC, and the scavenging activity against DPPH and ABTS of freeze-dried B. davidii extracts (flowers, stems, and leaves). The DPPH scavenging activity (IC50: 18.3 ± 0.6 mg/L) of the leaf extract was the highest among the three extracts and was comparable to that (IC50: 16.3 mg/L) of the methanol extract of Buddleja asiatica (El-Sayed et al., 2008). The ABTS scavenging activity of the flower (IC50: 22.6 ± 0.9 mg/L) and leaf (IC50: 23.7 ± 0.2 mg/L) extracts was found to be superior to that of the stem extract. B. davidii leaf extracts exhibited the highest TPC and TFC among the three extracts. Higher antioxidant activities in plant extracts may suggest an abundance of phenolic compounds or carotene derivatives (Mahlke et al., 2009), with additional evidence provided in the results of Section 3.6. The DPPH and ABTS radical scavenging activities exhibited by all three B. davidii extracts were found to be more effective than those of the positive control BHT (IC50: 58.1 ± 1.2 mg/L and 50.6 ± 0.37 mg/L, respectively).
To maintain vibrant and radiant skin, the inhibition of collagenase, elastase, hyaluronidase, MMP-1, and MMP-2 within the skin is crucial. Table 2 presents the antiaging activity of freeze-dried extracts from different parts of B. davidii. B. davidii flower extract exhibited the best antiaging activity, with IC50 values for inhibiting aging-related enzymes in the range of 62.7–125.1 mg/L, followed by the leaf and stem extracts. B. davidii flower extract presented better antiaging activity than some positive controls, such as EGCG (IC50: 90.6 ± 3.7 mg/L, 67.2 ± 1.4 mg/L, and 162.8 ± 7.1 mg/L for anti-MMP-1, anti-MMP-2, and antielastase activities, respectively), 1,10-phenanthroline (IC50: 46.7 ± 0.5 mg/L for anticollagenase activity), gallic acid (IC50: 132.6 ± 10.3 mg/L for anticollagenase activity), ursolic acid (IC50: 32.8 ± 4.2 mg/L for antielastase activity), and tannic acid (IC50: 140.3 ± 11.6 mg/L for antihyaluronidase activity). Buddleja officinalis (a close relative of B. davidii) has been shown to inhibit the aging-related enzymes elastase and MMPs (Kucukguven and Khalil, 2013; Chen et al., 2023).

Table 2. Antiaging activity (IC50, mg/L) of freeze-dried extracts from different parts of Buddleja davidii.
3.4 Examination of anti-inflammatory activity
The overproduction of proinflammatory mediator by macrophages can result in various inflammatory diseases. Therefore, one effective therapeutic strategy involves lowering the levels of inflammatory mediators and cytokines. Figure 4 shows the anti-inflammatory activities of the 3 B. davidii extracts, expressed as IC50 values for the inhibition of various inflammatory mediators and cytokines. The flower extract had the lowest IC50 value among all three extracts for the inhibition of ROS, NO, and TNF-α, implying that it possessed the strongest anti-inflammatory activity, with the leaf and stem extracts showing comparatively lesser effects. Specifically, the anti-inflammatory activity of the flower extract was 2.4–3.7 times more effective than that of the stem extract and 1.8 to 2.4 times more effective than that of the leaf extract. The IC50 of B. davidii flower extract for inhibiting TNF-α production (54.2 ± 0.8 mg/L) was inferior to those of cyclocitralosides A and B, pure compounds isolated from B. officinalis flower extract (IC50: 6.83–7.71 mg/L; Huang et al., 2021), and the positive control indomethacin (a potent nonsteroidal anti-inflammatory drug) (IC50: 51.4 ± 1.2 mg/L). Furthermore, Nguyen and Kim (2020) reported that B. davidii aqueous extract exerted the strongest inhibitory effect on the inflammatory processes associated with C. acnes. A higher concentration of flavonoids in the leaf extract of Buddleja scordioides was associated with high anti-inflammatory activity (Macías-Cortés et al., 2022). These results clearly illustrate the utility of using B. davidii extracts, especially the flower extract, as a natural anti-inflammatory ingredient for skin care.
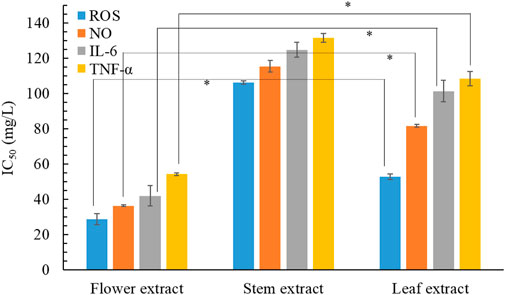
Figure 4. Effects of B. davidii extracts (flower, stem, and leaf) on the production of NO, ROS, IL-6, and TNF-α in LPS-stimulated RAW264.7 cells. Significant difference was expressed by *p < 0.05.
3.5 Analysis of antimicrobial activity
Wu et al. (2018) reported that antimicrobial activity is positively correlated with antioxidant activity. Table 3 lists the antimicrobial characteristics of freeze-dried extracts from different parts of B. davidii. The stem extract of B. davidii demonstrated the most significant antimicrobial activity, with the leaf and flower extracts exhibiting comparatively lower levels of efficacy. Pendota et al. (2013) discovered that Buddleja salviifolia leaf extract possessed antimicrobial properties that resulted from additive effects or synergistic interactions between the active compounds in the extract. In the present investigation, the MIC of B. davidii stem extract against S. aureus, E. coli, P. aeruginosa, and C. acnes was determined to be between 60 and 100 mg/L. This efficacy is notably higher than that observed for a hexane extract of Buddleja perfoliate, which exhibited an MIC of 200 mg/L (Cuevas-Cianca et al., 2022). The MFC of B. davidii stem extract against C. albicans, A. brasiliensis, and E. flocosum (tinea pedis related pathogen) was in the range of 30–50 mg/L, which was superior to that of chloroform extracts of Buddleja globosa (MFC: 100 mg/L) and B. davidii stembark (MFC: 62.5 mg/L) (Houghton et al., 2003). Given that it is safe to apply to the skin at a dose between 30 and 100 mg/L (Figure 2), B. davidii stem extract can potentially be used in cosmetic products whose purpose is to clean, sterilize, and preserve the skin.
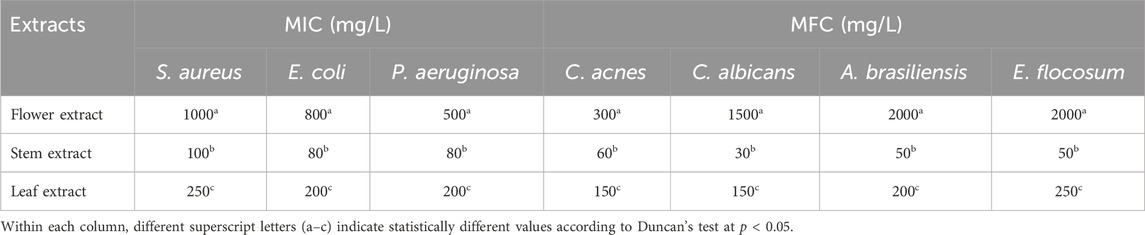
Table 3. Minimum inhibitory concentration (MIC) and minimum fungicidal concentration (MFC) of freeze-dried extracts from different parts of Buddleja davidii.
3.6 Identification of bioactive compounds
Table 4 lists the major chemical components (relative concentrations >0.5%) detected in the three B. davidii extracts. A total of 21, 16, and 21 major active components were detected using HPLC in the flower, stem, and leaf extracts, respectively (Table 4). In order of decreasing abundance, the flower extract primarily contained crocin, crocetin, quercetin, sinapic acid, rutin, and picrocrocin. Crocin, crocetin, and picrocrocin are carotenoids that have anti-inflammatory and antiaging activities (Roniawati et al., 2021; Roshanravan and Ghaffari, 2022). Moreover, the flower extract contained sinapic acid, a bioactive phenolic acid with anti-inflammatory and antiaging activities (Chen, 2016), and rutin, a flavonoid with antiaging activity (Choi et al., 2016). The presence of these components might explain why B. davidii flower extract exhibited better anti-inflammatory and antiaging activities than the other two extracts. The major components of the stem extract were luteolin, oleanolic acid, naringenin, quercetin, acacetin, and apigenin. Luteolin, quercetin, acacetin, naringenin, and apigenin are flavonoids that possess antimicrobial activities (Zhou et al., 2014; Ayeleso et al., 2017; Aghababaei and Hadidi, 2023), which can explain why B. davidii stem extract exerted higher antimicrobial activities than the other two extracts. The primary constituents identified in the leaf extract were oleanolic acid, verbascoside, luteolin, isoverbascoside, luteolin-7-O-β-D-glucoside, ursolic acid, apigenin, and martynoside, similar to the composition of an 80% methanol extract of B. polystachya leaves (Getahun et al., 2021). Verbascoside, isoverbascoside, and martynoside are phenylethanoid glycosides; oleanolic acid and ursolic acid are triterpenes; and luteolin, luteolin-7-O-β-D-glucoside, and apigenin are flavonoids, all of which were detected in B. davidii leaf extract. Burger et al. (2017) proposed that luteolin and apigenin can serve as skin whitening agents. Moreover, martynoside has been shown to exert considerable skin whitening activity (Muñoz et al., 2013), and verbascoside, isoverbascoside, oleanolic acid, and ursolic acid offer photoprotection against ultraviolet radiation to prevent melanin production (Díaz-Barradas et al., 2016; Gómez-Hernández et al., 2021), which can explain why B. davidii leaf extract exhibited better skin whitening activity than the other two extracts. Even so, different compounds within the extracts may have potential synergistic effects on their physiological activity, which will be investigated in future studies.
3.7 Molecular docking analysis
Molecular ducking study could help understand the binding mode and binding interactions between the inhibitory compounds and the target site. We performed molecular docking to explore the binding interactions between the predominant bioactive compounds and the active sites of target enzymes selected based on the physiological activities of these compounds. We especially focused on research on antiaging and antityrosinase activities. B. davidii flower extract possessed the best antiaging activity among the three extracts (Table 2), so we subjected the major chemical components of B. davidii flower extract: crocin, crocetin, quercetin, sinapic acid, picrocrocin, and rutin to molecular docking with aging-related enzymes (Table 5). The findings indicated that the binding affinities of each of the different compounds for any given enzyme differed from each other. A reduced binding energy signifies a higher affinity of the small molecule ligand for the catalytic site (Xu et al., 2024). Crocin exhibited the best binding energies against MMP-2 (PDB code: 8H78) and hyaluronidase (PDB code: 2PE4) (−133.34 and −167.41 kcal/mol, respectively). Crocetin exhibited the best binding energy against elastase (PDB code: 1FLE) (−140.17 kcal/mol). Quercetin had the best binding energy against MMP-1 (PDB code: 3SHI) (−148.21 kcal/mol), whereas rutin had the best binding energy against collagenase (PDB code: 2CLT) (−135.37 kcal/mol). Bastani et al. (2022) proposed that the potent antioxidant activity of crocin may inhibit the activities of MMP-2 and hyaluronidase. Bari et al. (2023) reported that crocetin could confer protection from oxidative stress-induced damage through its antielastase activity. In addition, quercetin could downregulate MMP-1 expression (Beken et al., 2020), and rutin exhibited good antiaging activity (Phumat et al., 2023).
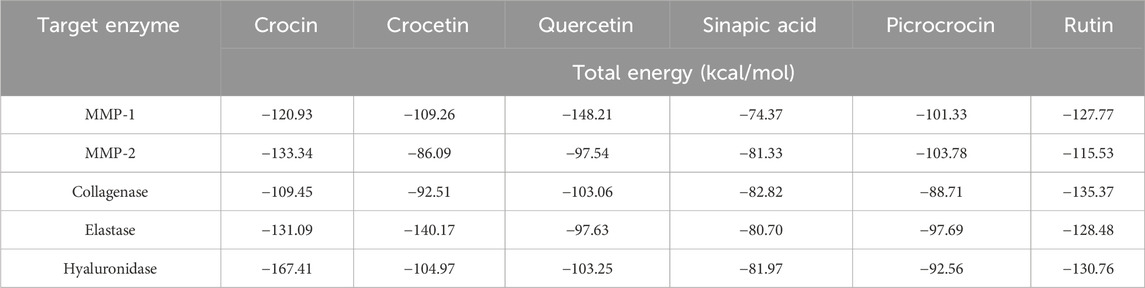
Table 5. Results of molecular docking analysis of crocin, crocetin, quercetin, sinapic acid, picrocrocin, and rutin.
B. davidii leaf extract exhibited the best antityrosinase activity among the three extracts (Figure 3), so its major ingredients were docked against tyrosinase. The binding energies of oleanolic acid, verbascoside, luteolin, isoverbascoside, ursolic acid, and apigenin to tyrosinase (PDB code: 5M8L) were −120.88, −136.86, −101.54, −112.07, −86.29, and −95.93 kcal/mol, respectively. Verbascoside exhibited the best binding energy to tyrosinase. Matos et al. (2022) reported that verbascoside showed good tyrosinase inhibition activity.
Among the various compounds examined, quercetin exhibited the most favorable docking interactions with MMP-1, facilitated by the formation of a π–alkyl bond with Pro177, a π–sigma bond with Pro146, and H-bonds with Arg165, Arg202, Asp124, and Asn143 (Figure 5a). Crocin exhibited the best docking interactions with MMP-2, facilitated by the formation of an alkyl bond with Val41, C−H bonds with Pro166, Asn147, and Asp33, and H-bonds with Arg49, Ser165, Tyr144, Thr145, and Leu106 (Figure 5b). Rutin demonstrated the most favorable docking interactions with collagenase, facilitated by the establishment of an alkyl bond with Lys437, C−H bonds with Pro341 and Ser295, and H-bonds with Asp390, Glu389, Glu436, Glu339, and Asn438 (Figure 5c). Crocetin demonstrated the most favorable docking interactions with elastase, which were attributed to the establishment of an ionic bond with Arg31, π–alkyl bonds with Ile19 and Phe54, and H-bonds with Leu20 and Thr175 (Figure 5d). Crocin demonstrated optimal docking interactions with hyaluronidase, facilitated by the formation of C−H bonds with residues Glu131 and Gly203, as well as H-bonds with Trp130, Arg134, Asp206, Tyr202, Tyr208, Tyr210, Arg265, Asp292, Glu325, Thr293, and Asn295 (Figure 5e). Verbascoside demonstrated the most favorable docking interactions with tyrosinase, facilitated by the formation of a π–carbon bond with Arg374, an unfavorable bump with Gln390, C−H interactions with Arg321 and Gly389, π–alkyl interactions with Leu382 and Val319, as well as H-bonds with Tyr362, Asn385, and Gly386 (Figure 5f). Subbaiyan et al. (2020) indicated that these H-bonds played a central role in maintaining the structural stability of the protein–ligand complex. Conversely, unfavorable bump interactions revealed poor binding between amino acids and ligands (Zainab et al., 2020). Based on the experimental and molecular docking results, we conclude that the main ingredients of B. davidii flower and leaf extracts are responsible for their observed antityrosinase and antiaging properties.
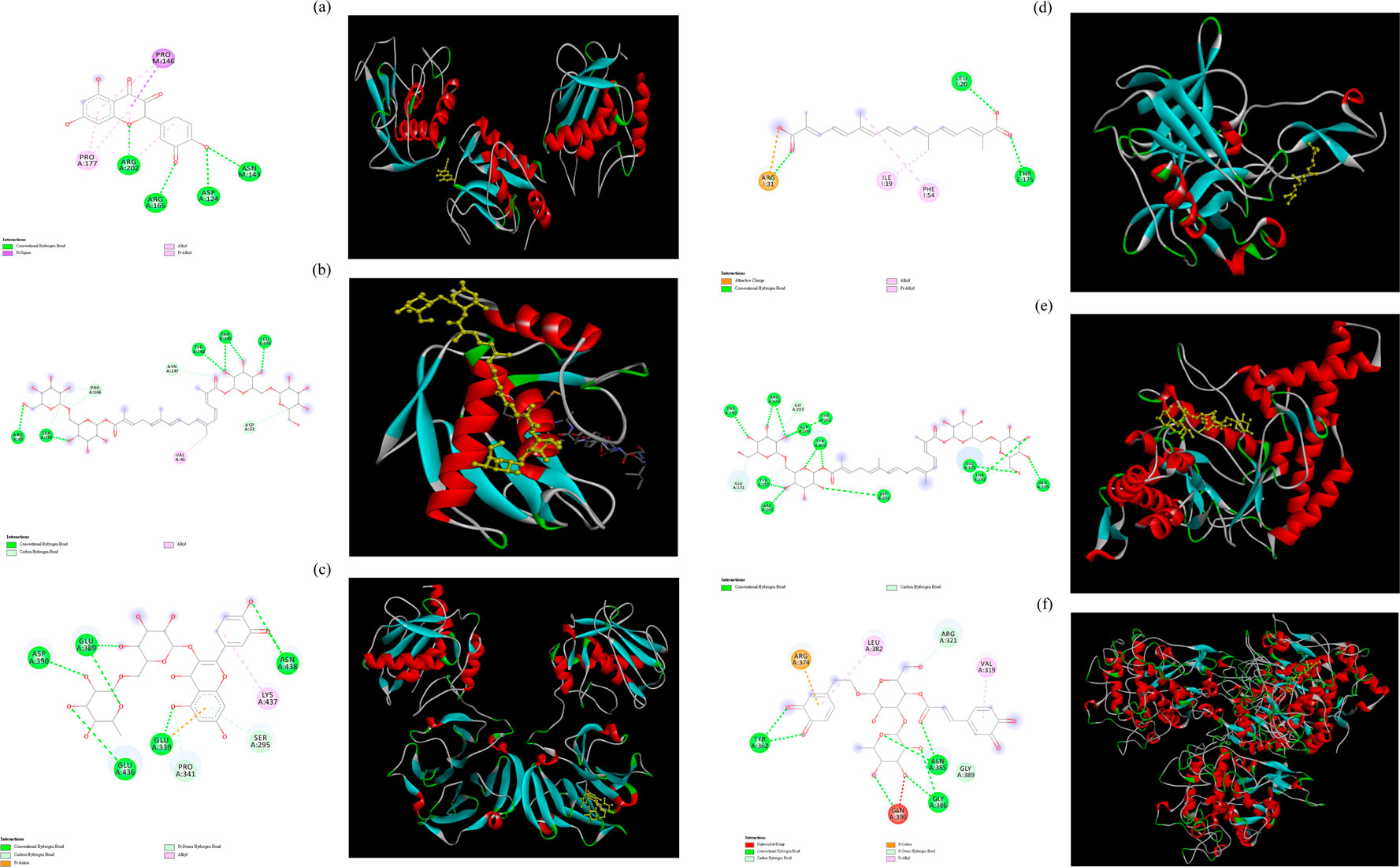
Figure 5. Molecular docking interactions and docking complex of the optimal phytochemical compounds against tested enzymes. (a) molecular docking of the interactions between quercetin and MMP-1; (b) molecular docking of the interactions between crocin and MMP-2; (c) molecular docking of the interactions between rutin and collagenase; (d) molecular docking of the interactions between crocetin and elastase; (e) molecular docking of the interactions between crocin and hyaluronidase. (f) molecular docking of the interactions between verbascoside and tyrosinase.
4 Conclusion
In the present study, we demonstrated that B. davidii extracts contained phenylethanoid glycosides, flavonoids, phenolic acids, carotenoids, and triterpenes. The extracts from all three plant parts possessed potent antioxidant, antiaging, antimicrobial, or/and anti-inflammatory activities. Antiaging/anti-inflammatory activity was high in the flower extract, antimicrobial activity was high in the stem extract, and skin-whitening activity was high in the leaf extract. Molecular docking analysis further suggested that crocin, crocetin, quercetin, and rutin in the flower extract were responsible for its antiaging activity, whereas verbascoside in the leaf extract was responsible for its antityrosinase activity. In conclusion, this serves as a foundation for future studies exploring the physiological properties of B. davidii extracts and their bioactive compounds. Products containing B. davidii extracts can be developed for use in cosmetics, health products, medicine, and clinical studies. Thus, we select various parts of B. davidii from different altitudes for utilization. B. davidii cultivated in Taiwan shows promise for several practical applications. The underlying mechanisms of action will need to be investigated further in the future.
Data availability statement
The original contributions presented in the study are included in the article/supplementary material, further inquiries can be directed to the corresponding author.
Ethics statement
Ethical approval was not required for the studies on humans in accordance with the local legislation and institutional requirements because only commercially available established cell lines were used.
Author contributions
C-YC: Investigation, Resources, Writing – original draft. G-HW: Investigation, Resources, Writing – original draft. Yu-CC: Data curation, Investigation, Validation, Writing – original draft. SL: Data curation, Investigation, Validation, Writing – review and editing. Y-TL: Data curation, Formal Analysis, Methodology, Writing – review and editing. Y-LL: Investigation, Validation, Writing – original draft. Yi-CC: Conceptualization, Funding acquisition, Supervision, Writing – review and editing.
Funding
The author(s) declare that financial support was received for the research and/or publication of this article. This research was funded by National Science and Technology Council, grant number NSTC 111-2622-E-157-002 and NSTC 112-2313-B-157-001-MY3.
Acknowledgments
The authors would like to thank Tzu-Ling Huang for help with partially analytical measurements.
Conflict of interest
Author SL was employed by Chill Beauty Corporation.
The remaining authors declare that the research was conducted in the absence of any commercial or financial relationships that could be construed as a potential conflict of interest.
The author(s) declared that they were an editorial board member of Frontiers, at the time of submission. This had no impact on the peer review process and the final decision.
Generative AI statement
The author(s) declare that no Generative AI was used in the creation of this manuscript.
Publisher’s note
All claims expressed in this article are solely those of the authors and do not necessarily represent those of their affiliated organizations, or those of the publisher, the editors and the reviewers. Any product that may be evaluated in this article, or claim that may be made by its manufacturer, is not guaranteed or endorsed by the publisher.
References
Aghababaei, F., and Hadidi, M. (2023). Recent advances in potential health benefits of quercetin. Pharmaceuticals 16, 1020. doi:10.3390/ph16071020
Ahmad, I., Ahmad, N., and Wang, F. (2009). Antioxidant phenylpropanoid glycosides from Buddleja davidii. J. Enzyme. Inhib. Med. Chem. 24, 993–997. doi:10.1080/14756360802565072
Ayeleso, T. B., Matumba, M. G., and Mukwevho, E. (2017). Oleanolic acid and its derivatives: biological activities and therapeutic potential in chronic diseases. Molecules 22, 1915. doi:10.3390/molecules22111915
Bari, E., Perteghella, S., Rassu, G., Gavini, E., Petretto, G. L., Bonferoni, M. C., et al. (2023). Sericin/crocetin micro/nanoparticles for nucleus pulposus cells regeneration: an “active” drug delivery system. Front. Pharmacol. 14, 1129882. doi:10.3389/fphar.2023.1129882
Bastani, S., Vahedian, V., Rashidi, M., Mir, A., Mirzaei, S., Alipourfard, I., et al. (2022). An evaluation on potential anti-oxidant and anti-inflammatory effects of crocin. Biomedi. Pharmacother. 153, 113297. doi:10.1016/j.biopha.2022.113297
Beken, B., Serttas, R., Yazicioglu, M., Turkekul, K., and Erdogan, S. (2020). Quercetin improves inflammation, oxidative stress, and impaired wound healing in atopic dermatitis model of human keratinocytes. Pediatr. Allergy Immunol. Pulmonol. 33, 69–79. doi:10.1089/ped.2019.1137
Buhren, B. A., Schrumpf, H., Gorges, K., Reiners, O., Bölke, E., Fischer, J. W., et al. (2020). Dose-and time-dependent effects of hyaluronidase on structural cells and the extracellular matrix of the skin. Eur. J. Med. Res. 25, 60. doi:10.1186/s40001-020-00460-z
Burger, P., Monchot, A., Bagarri, O., Chiffolleau, P., Azoulay, S., Fernandez, X., et al. (2017). Whitening agents from Reseda luteola L. and their chemical characterization using combination of CPC, UPLC-HRMS and NMR. Cosmetics 4, 51. doi:10.3390/cosmetics4040051
Chen, C. (2016). Sinapic acid and its derivatives as medicine in oxidative stress-induced diseases and aging. Oxid. Med. Cell. Longev. 2016, 3571614. doi:10.1155/2016/3571614
Chen, C. Y., Hu, C. Y., Chen, Y. H., Li, Y. T., and Chung, Y. C. (2022). Submerged fermentation with Lactobacillus brevis significantly improved the physiological activities of Citrus aurantium flower extract. Heliyon 8, e10498. doi:10.1016/j.heliyon.2022.e10498
Chen, G., Sun, W., and Sun, H. (2010). Leaf epidermal characteristics of Asiatic Buddleja L. under scanning electron microscope: insights into chromosomal and taxonomic significance. Flora Morphol. Distrib. Funct. Ecol. 205, 777–785. doi:10.1016/j.flora.2010.04.002
Chen, R., Zhang, Y., Patel, N., Wu, K., Li, M., Mo, X., et al. (2023). CARD9 mediated MAPK/NF-κB signal pathway participates in the pathophysiological process of septic hepatitis: the role of tiliroside. Int. Immunopharmacol. 124, 110275. doi:10.1016/j.intimp.2023.110275
Chen, Y., Montero, L., Luo, J., Li, J., and Schmitz, O. J. (2020). Application of the new at-column dilution (ACD) modulator for the two-dimensional RP×HILIC analysis of Buddleja davidii. Anal. Bioanal. Chem. 412, 1483–1495. doi:10.1007/s00216-020-02392-3
Choi, S. J., Lee, S. N., Kim, K., Joo, D. H., Shin, S., Lee, J., et al. (2016). Biological effects of rutin on skin aging. Int. J. Mol. Med. 38, 357–363. doi:10.3892/ijmm.2016.2604
Crozier, A., Jensen, E., Lean, M. E., and McDonald, M. S. (1997). Quantitative analysis of flavonoids by reversed-phase high-performance liquid chromatography. J. Chromatogr. A 761, 315–321. doi:10.1016/S0021-9673(96)00826-6
Cuevas-Cianca, S. I., Leal, A. C. L., Hernández, L. R., Arreola, E. S., and Bach, H. (2022). Antimicrobial, toxicity, and anti-inflammatory activities of Buddleja perfoliata Kunth. Phytomed. Plus 2, 100357. doi:10.1016/j.phyplu.2022.100357
Díaz-Barradas, M. C., Costa, C., Correia, O., León-González, A. J., Navarro-Zafra, I., Zunzunegui, M., et al. (2016). Pentacyclic triterpenes responsible for photoprotection of Corema album (L.) D. Don white berries. Biochem. Syst. Ecol. 67, 103–109. doi:10.1016/j.bse.2016.05.009
Diretto, G., López-Jiménez, A. J., Ahrazem, O., Frusciante, S., Song, J., Rubio-Moraga, Á., et al. (2021). Identification and characterization of apocarotenoid modifiers and carotenogenic enzymes for biosynthesis of crocins in Buddleja davidii flowers. J. Exp. Bot. 72, 3200–3218. doi:10.1093/jxb/erab053
Ebeling, S. K., Hensen, I., and Auge, H. (2008). The invasive shrub Buddleja davidii performs better in its introduced range. Divers. Distrib. 14, 225–233. doi:10.1111/j.1472-4642.2007.00422.x
El-Sayed, M. M., Abdel-Hameed, E. S. S., Ahmed, W. S., and El-Wakil, E. A. (2008). Non-phenolic antioxidant compounds from Buddleja asiatica. Z. Naturforsch. C 63, 483–491. doi:10.1515/znc-2008-7-803
Getahun, A., Kifle, Z. D., Ambikar, D., and Atnafie, S. A. (2021). In vivo evaluation of 80% methanolic leaves crude extract and solvent fractions of Buddleja polystachya fresen (Buddlejaceae) for wound healing activity in normal and diabetic mice. Metab. Open 11, 100110. doi:10.1016/j.metop.2021.100110
Gómez-Hernández, M. A., Flores-Merino, M. V., Sánchez-Flores, J. E., Burrola-Aguilar, C., Zepeda-Gómez, C., Nieto-Trujillo, A., et al. (2021). Photoprotective activity of Buddleja cordata cell culture methanolic extract on UVB-irradiated 3T3-swiss albino fibroblasts. Plants 10, 266. doi:10.3390/plants10020266
Gülsoy, E., Kaya, E. D., Türkhan, A., Bulut, M., Koyuncu, M., Güler, E., et al. (2023). The effect of altitude on phenolic, antioxidant and fatty acid compositions of some Turkish hazelnut (Coryllus avellana L.) Cultivars. Molecules 28, 5067. doi:10.3390/molecules28135067
Ho, C. C., Ng, S. C., Chuang, H. L., Chen, J. Y., Wen, S. Y., Kuo, C. H., et al. (2021). Seven traditional Chinese herbal extracts fermented by Lactobacillus rhamnosus provide anti-pigmentation effects by regulating the CREB/MITF/tyrosinase pathway. Environ. Toxicol. 36, 654–664. doi:10.1002/tox.23069
Houghton, P. J., Mensah, A. Y., Iessa, N., and Hong, L. Y. (2003). Terpenoids in Buddleja: relevance to chemosystematics, chemical ecology and biological activity. Phytochem 64, 385–393. doi:10.1016/s0031-9422(03)00264-4
Huang, F. B., Liang, N., Hussain, N., Zhou, X. D., Ismail, M., Xie, Q. L., et al. (2021). Anti-inflammatory and antioxidant activities of chemical constituents from the flower buds of Buddleja officinalis. Nat. Prod. Res. 36, 3031–3042. doi:10.1080/14786419.2021.1952577
Intayoung, P., Limtrakul, P., and Yodkeeree, S. (2016). Antiinflammatory activities of crebanine by inhibition of NF-κB and AP-1 activation through suppressing MAPKs and Akt signaling in LPS-induced RAW264.7 macrophages. Biol. Pharm. Bull. 39, 54–61. doi:10.1248/bpb.b15-00479
Jin, D., An, X., Zhang, Y., Zhao, S., Duan, L., Duan, Y., et al. (2021). Potential mechanism prediction of herbal medicine for pulmonary fibrosis associated with SARS-CoV-2 infection based on network analysis and molecular docking. Front. Pharmacol. 12, 602218. doi:10.3389/fphar.2021.602218
Khan, S., Ullah, H., and Zhang, L. (2019). Bioactive constituents form Buddleja species. Pak. J. Pharm. Sci. 32, 721–741.
Kim, T., and Hyun, C. G. (2022). Imperatorin positively regulates melanogenesis through signaling pathways involving PKA/CREB, ERK, AKT, and GSK3β/β-catenin. Molecules 27, 6512. doi:10.3390/molecules27196512
Koulakiotis, N., Gikas, E., Iatrou, G., Lamari, F., and Tsarbopoulos, A. (2015). Quantitation of crocins and picrocrocin in saffron by HPLC: application to quality control and phytochemical differentiation from other crocus taxa. Planta Med. 81, 606–612. doi:10.1055/s-0035-1545873
Kucukguven, A., and Khalil, R. A. (2013). Matrix metalloproteinases as potential targets in the venous dilation associated with varicose veins. Curr. Drug Targets 14, 287–324. doi:10.2174/138945013804998972
Kujala, T. S., Loponen, J. M., Klika, K. D., and Pihlaja, K. (2000). Phenolics and betacyanins in red beetroot (Beta vulgaris) root: distribution and effect of cold storage on the content of total phenolics and three individual compounds. J. Agric. Food Chem. 48, 5338–5342. doi:10.1021/jf000523q
Li, H., Dasilva, N. A., Liu, W., Xu, J., Dombi, G. W., Dain, J. A., et al. (2020). Thymocid®, a standardized black cumin (Nigella sativa) seed extract, modulates collagen cross-linking, collagenase and elastase activities, and melanogenesis in murine B16F10 melanoma cells. Nutrients 12, 2146. doi:10.3390/nu12072146
Li, X., Wu, X., and Huang, L. (2009). Correlation between antioxidant activities and phenolic contents of radix Angelicae sinensis (Danggui). Molecules 14, 5349–5361. doi:10.3390/molecules14125349
Lim, W. Y., Chan, E. W. C., Phan, C. W., and Wong, C. W. (2021). Tyrosinase inhibiting extracts from coastal plants as potential additives in skin whitening formulations. Curr. Appl. Sci. Technol. 21, 481–494.
Liu, T., Li, J., Liu, Y., Xiao, N., Suo, H., Xie, K., et al. (2012). Short-chain fatty acids suppress lipopolysaccharide-induced production of nitric oxide and proinflammatory cytokines through inhibition of NF-κB pathway in RAW264. 7 cells. Inflammation 35, 1676–1684. doi:10.1007/s10753-012-9484-z
Macías-Cortés, E., Gallegos-Infante, J. A., Rocha-Guzmán, N. E., Moreno-Jiménez, M. R., Cervantes-Cardoza, V., Castillo-Herrera, G. A., et al. (2022). Antioxidant and anti-inflammatory polyphenols in ultrasound-assisted extracts from salvilla (Buddleja scordioides Kunth). Ultrason. Sonochem. 83, 105917. doi:10.1016/j.ultsonch.2022.105917
Mahlke, J. D., Boligon, A. A., Machado, M. M., Spader, T. B., Alves, S. H., Canto-Dorow, T. D., et al. (2009). In vitro antimicrobial and antioxidant activities of a crude extract and fractions from Buddleja thyrsoides Lam. leaves. Quím. Nova 32, 277–281. doi:10.1590/s0100-40422009000200002
Maity, N., Nema, N. K., Abedy, M. K., Sarkar, B. K., and Mukherjee, P. K. (2011). Exploring Tagetes erecta Linn flower for the elastase, hyaluronidase and MMP-1 inhibitory activity. J. Ethnopharmacol. 137, 1300–1305. doi:10.1016/j.jep.2011.07.064
Mapunya, M. B., Nikolova, R. V., and Lall, N. (2012). Melanogenesis and antityrosinase activity of selected South African plants. Evid. Based. Complement. Altern. Med. 2012, 374017. doi:10.1155/2012/374017
Matos, P., Paranhos, A., Batista, M. T., and Figueirinha, A. (2022). Synergistic effect of DIBOA and verbascoside from Acanthus mollis leaf on tyrosinase inhibition. Int. J. Mol. Sci. 23, 13536. doi:10.3390/ijms232113536
Merchán Arenas, D. R., Muñoz Acevedo, A., Vargas Méndez, L. Y., and Kouznetsov, V. V. (2011). Scavenger activity evaluation of the clove bud essential oil (Eugenia caryophyllus) and eugenol derivatives employing ABTS+· decolorization. Sci. Pharm. 79, 779–792. doi:10.3797/scipharm.1109-11
Muflihah, Y. M., Gollavelli, G., and Ling, Y. C. (2021). Correlation study of antioxidant activity with phenolic and flavonoid compounds in 12 Indonesian indigenous herbs. Antioxidants 10, 1530. doi:10.3390/antiox10101530
Mukherjee, P. K., Biswas, R., Sharma, A., Banerjee, S., Biswas, S., and Katiyar, C. K. (2018). Validation of medicinal herbs for anti-tyrosinase potential. J. Herb. Med. 14, 1–16. doi:10.1016/j.hermed.2018.09.002
Muñoz, E., Avila, J. G., Alarcón, J., Kubo, I., Werner, E., and Céspedes, C. L. (2013). Tyrosinase inhibitors from Calceolaria integrifolia sl: Calceolaria talcana aerial parts. J. Agric. Food Chem. 61, 4336–4343. doi:10.1021/jf400531h
Nguyen, A. T., and Kim, K. Y. (2020). Inhibition of proinflammatory cytokines in Cutibacterium acnes-induced inflammation in HaCaT cells by using Buddleja davidii aqueous extract. Int. J. Inflamm. 2020, 8063289. doi:10.1155/2020/8063289
Nwakulite, A., Obeagu, E. I., Nwanjo, H. U., Nwosu, D. C., Nnatuanya, I. N., Eze, R., et al. (2021). Studies on molecular docking of moringa oleifera leaf phytochemical constituents on alpha glucosidase, alpha amylase and dipeptidyl peptidase. J. Pharm. Res. Int. 33, 239–245. doi:10.9734/jpri/2021/v33i28A31527
Pandey, G., Khatoon, S., Pandey, M. M., and Rawat, A. K. S. (2018). Altitudinal variation of berberine, total phenolics and flavonoid content in Thalictrum foliolosum and their correlation with antimicrobial and antioxidant activities. J. Ayurveda. Integr. Med. 9, 169–176. doi:10.1016/j.jaim.2017.02.010
Pendota, S. C., Aderogba, M. A., Ndhlala, A. R., and Van Staden, J. (2013). Antimicrobial and acetylcholinesterase inhibitory activities of Buddleja salviifolia (L.) Lam. leaf extracts and isolated compounds. J. Ethnopharmacol. 148, 515–520. doi:10.1016/j.jep.2013.04.047
Phumat, P., Chaichit, S., Potprommanee, S., Preedalikit, W., Sainakham, M., Poomanee, W., et al. (2023). Influence of Benincasa hispida peel extracts on antioxidant and anti-aging activities, including molecular docking simulation. Foods 12, 3555. doi:10.3390/foods12193555
Pourmorad, F., Hosseinimehr, S. J., and Shahabimajd, N. (2006). Antioxidant activity, phenol and flavonoid contents of some selected Iranian medicinal plants. Afr. J. Biotechnol. 5, 1142–1145.
Rahman, M. A., Imran, T. B., and Islam, S. (2013). Antioxidative, antimicrobial and cytotoxic effects of the phenolics of Leea indica leaf extract. Saudi J. Biol. Sci. 20, 213–225. doi:10.1016/j.sjbs.2012.11.007
Roniawati, I., Putriana, N. A., Putri, A. N., and Nur’aini, Y. A. (2021). Review: saffron’s activity as an active ingredient in cosmetics. Indones. J. Pharm. 3, 74–81. doi:10.24198/idjp.v3i2.34876
Roshanravan, N., and Ghaffari, S. (2022). The therapeutic potential of Crocus sativus Linn.: a comprehensive narrative review of clinical trials. Phytother. Res. 36, 98–111. doi:10.1002/ptr.7286
Sharifi-Rad, M., Epifano, F., Fiorito, S., and Álvarez-Suarez, J. M. (2020). Phytochemical analysis and biological investigation of Nepeta juncea Benth. different extracts. Plants 9, 646. doi:10.3390/plants9050646
Sharifi-Rad, M., Mohanta, Y. K., Pohl, P., Jaradat, N., Aboul-Soud, M. A., and Zengin, G. (2022a). Variation of phytochemical constituents, antioxidant, antibacterial, antifungal, and anti-inflammatory properties of Grantia aucheri (Boiss.) at different growth stages. Microb. Pathog. 172, 105805. doi:10.1016/j.micpath.2022.105805
Sharifi-Rad, M., Pohl, P., Epifano, F., Zengin, G., Jaradat, N., and Messaoudi, M. (2022b). Teucrium polium (L.): phytochemical screening and biological activities at different phenological stages. Molecules 27, 1561. doi:10.3390/molecules27051561
Sittisart, P., and Chitsomboon, B. (2014). Intracellular ROS scavenging activity and downregulation of inflammatory mediators in RAW264.7 macrophage by fresh leaf extracts of Pseuderanthemum palatiferum. Evid. Based Complement. Altern. Med. 2014, 309095. doi:10.1155/2014/309095
Subbaiyan, A., Ravichandran, K., Singh, S. V., Sankar, M., Thomas, P., Dhama, K., et al. (2020). In silico molecular docking analysis targeting SARSCoV-2 spike protein and selected herbal constituents. J. Pure Appl. Microbiol. 14, 989–998. doi:10.22207/JPAM.14.SPL1.37
Sumantran, V. N., Kulkarni, A. A., Harsulkar, A., Wele, A., Koppikar, S. J., Chandwaskar, R., et al. (2007). Hyaluronidase and collagenase inhibitory activities of the herbal formulation Triphala guggulu. J. Biosci. 32, 755–761. doi:10.1007/s12038-007-0075-3
Wang, G. H., Chen, C. Y., Tsai, T. H., Chen, C. K., Cheng, C. Y., Huang, Y. H., et al. (2017). Evaluation of tyrosinase inhibitory and antioxidant activities of Angelica dahurica root extracts for four different probiotic bacteria fermentations. J. Biosci. Bioeng. 123, 679–684. doi:10.1016/j.jbiosc.2017.01.003
Wu, J., Yi, W., Jin, L., Hu, D., and Song, B. (2012). Antiproliferative and cell apoptosis inducing activities of compounds from Buddleja davidii in Mgc-803 cells. Cell Div. 7, 20. doi:10.1186/1747-1028-7-20
Wu, L., Chen, C. Y., Cheng, C. Y., Dai, H., Ai, Y., Lin, C. H., et al. (2018). Evaluation of tyrosinase inhibitory, antioxidant, antimicrobial, and antiaging activities of Magnolia officinalis extracts after Aspergillus niger fermentation. Biomed. Res. Int. 2018, 5201786. doi:10.1155/2018/5201786
Xu, Z., Zhu, X., Lian, J., Guan, Y., and Zhao, Z. (2024). Anti-melanin and tyrosinase inhibition mechanism of polydatin and 6″-O-lauryl polydatin synthesized by microwave-assisted enzymatic catalysis. Food Biosci. 57, 103605. doi:10.1016/j.fbio.2024.103605
Yang, Y. C., Wei, M. C., and Huang, T. C. (2012). Optimisation of an ultrasound-assisted extraction followed by RP-HPLC separation for the simultaneous determination of oleanolic acid, ursolic acid and oridonin content in Rabdosia rubescens. Phytochem. Anal. 23, 627–636. doi:10.1002/pca.2365
Ying, C., and Wan, D. (2012). Quantitative determination of total and individual flavonoids in stems and leaves of Buddleja davidii and Buddleja albiflora. Pharmacogn. Mag. 8, 273–279. doi:10.4103/0973-1296.103651
Zainab, B., Ayaz, Z., Alwahibi, M. S., Khan, S., Rizwana, H., Soliman, D. W., et al. (2020). In-silico elucidation of Moringa oleifera phytochemicals against diabetes mellitus. Saudi J. Biol. Sci. 27, 2299–2307. doi:10.1016/j.sjbs.2020.04.002
Zengin, G., Uysal, S., Ceylan, R., and Aktumsek, A. (2015). Phenolic constituent, antioxidative and tyrosinase inhibitory activity of Ornithogalum narbonense L. from Turkey: a phytochemical study. Ind. Crops Prod. 70, 1–6. doi:10.1016/j.indcrop.2015.03.012
Zhang, Y., Zou, X. L., Wang, Y. L., Gao, L., and Chou, G. X. (2019). Determining the levels of four phenylethanoid glycosides and five triterpene acids in Liuwei Dihuang capsule using solid phase extraction with HPLC-UV. J. Anal. Methods Chem. 209, 7609438. doi:10.1155/2019/7609438
Keywords: antioxidant, antiaging, anti-inflammatory, Buddleja davidii Franch. [Scrophulariaceae], bioactive, cytotoxicity
Citation: Chen C-Y, Wang G-H, Chang Y-C, Liu S, Lin Y-T, Lai Y-L and Chung Y-C (2025) Cosmeceutical application of extracts from the flowers, stems, and leaves of Buddleja davidii grown at different altitudes. Front. Pharmacol. 16:1551134. doi: 10.3389/fphar.2025.1551134
Received: 06 January 2025; Accepted: 24 April 2025;
Published: 09 May 2025.
Edited by:
Claudio Ferrante, University of Studies G. d’Annunzio Chieti and Pescara, ItalyReviewed by:
Majid Sharifi-Rad, Zabol University, IranWahyu Widowati, Maranatha Christian University, Indonesia
Chee Chin Chu, Taylor’s University, Malaysia
Copyright © 2025 Chen, Wang, Chang, Liu, Lin, Lai and Chung. This is an open-access article distributed under the terms of the Creative Commons Attribution License (CC BY). The use, distribution or reproduction in other forums is permitted, provided the original author(s) and the copyright owner(s) are credited and that the original publication in this journal is cited, in accordance with accepted academic practice. No use, distribution or reproduction is permitted which does not comply with these terms.
*Correspondence: Ying-Chien Chung, ycchung@cc.cust.edu.tw
† These authors have contributed equally to this work