- 1Yunnan Provincial Engineering and Research Center for Sustainable Utilization of Honey Bee Resources, Eastern Bee Research Institute, College of Animal Science and Technology, Yunnan Agricultural University, Kunming, China
- 2First clinical medical College, Yunnan University of Chinese Medicine, Kunming, China
- 3Agricultural and Rural Service Center in Wangjiafan Town, Yichang, Hubei, China
Royal jelly acid (10-HDA), an unsaturated fatty acid unique to royal jelly, exhibits a diverse range of biological activities, including hypoglycemic, anti-inflammatory, and anticancer properties. In recent years, the increasing demand for natural product supplements as health enhancers has led to a significant rise in the consumption of royal jelly products for daily wellness. Consequently, the market for royal jelly supplements has expanded considerably. Understanding the mechanisms underlying the biological activities of royal jelly acid is crucial for optimizing its therapeutic applications and guiding the development of innovative royal jelly-based products. This review consolidates current research on the preparation, metabolism and potential pharmacological roles of royal jelly acid in managing cancer, inflammatory disorders, and glucolipid metabolic diseases and explores the molecular mechanisms driving these effects. Future research should leverage advanced analytical techniques to uncover the intricate mechanisms of royal jelly acid’s actions, paving the way for its broader integration into healthcare and clinical settings.
1 Introduction
Natural products have long captured the interest of scientists due to their potent therapeutic benefits and relatively low risk of side effects (Atanasov, et al., 2021). Among these, royal jelly acid—scientifically known as 10-hydroxy-trans-2-decenoic acid (10-HDA, Figure 1)—stands out as a bioactive unsaturated fatty acid with the chemical structure HO-CH2-(CH2)6CH = CH-COOH (C10H18O3), is a unique unsaturated fatty acid found in royal jelly (Hattori, et al., 2007). It is a primary active compound in royal jelly and plays a pivotal role in regulating genetic pathways associated with its biological effects (Spannhoff, et al., 2011). Numerous studies have highlighted the substantial pharmacological potential of royal jelly acid, including its ability to regulate glycolipid metabolism, exert anti-inflammatory effects, and offer neuroprotection (Yu, et al., 2023). Additionally, with its antimicrobial properties (Melliou and Chinou, 2005; Šamšulová, et al., 2023), royal jelly acid has been shown to inhibit a wide range of bacterial strains, making it a promising candidate for combating microbial infections. These attributes have prompted its exploration as a treatment for various conditions, including diabetes (Hu, et al., 2022), colitis (Huang M. et al., 2022), and cancer (Lin, et al., 2020). This review systematically synthesizes research advancements in the preparation methods, metabolic pathways, and therapeutic applications of royal jelly acid through a comprehensive analysis of data curated from multiple scientific databases. It also examines the underlying mechanisms that mediate its pharmacological effects, offering valuable insights into its potential as a treatment for various diseases. Moreover, it underscores the importance of incorporating royal jelly acid into daily healthcare regimens and calls for further investigation into its biological functions and therapeutic potential.
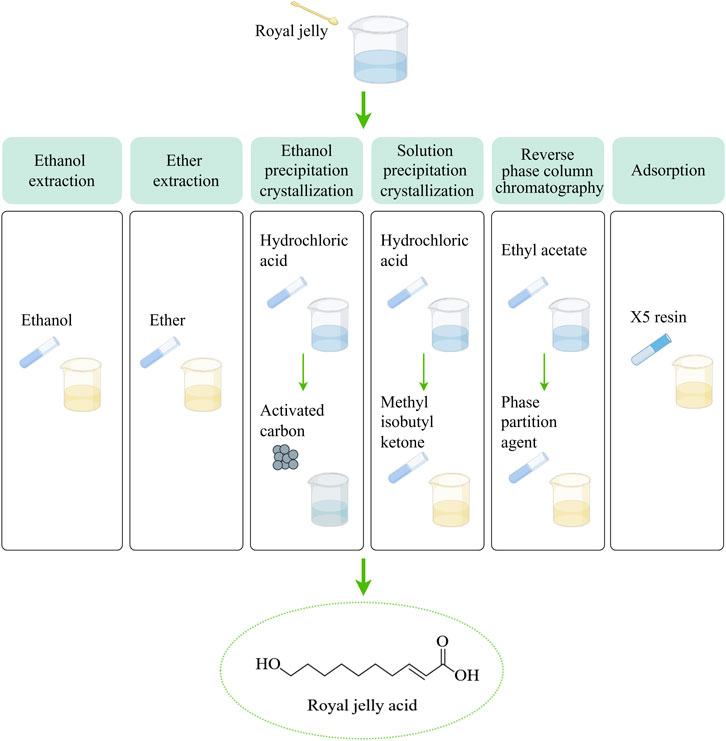
Figure 1. Six methods are used to extract royal jelly acid from royal jelly. In the ethanol extraction method, The lyophilized royal jelly is dissolved in ethanol, and centrifuged, the supernatant is collected and subjected to reverse extraction, crystallization, and drying to isolate royal jelly acid. The ether extraction method builds upon the ethanol extraction method by introducing ether to enhance the extraction process and isolate royal jelly acid more effectively. In the ethanol precipitation crystallization method, first, royal jelly is dissolved in ethanol and centrifuged to obtain the supernatant. The supernatant is acidified with hydrochloric acid, filtered, and dissolved in boiling ethanol. Activated carbon is then added, followed by cooling and crystallization to isolate royal jelly acid. Based on the ethanol precipitation crystallization method, the solution precipitation crystallization method involves melting the solution acidified with hydrochloric acid to form methyl isobutyl ketone. The subsequent steps, including crystallization, follow the ethanol precipitation process. In the reverse phase column chromatography method, first, royal jelly is fractionated using ethyl acetate. ethyl acetate fraction is dissolved in dichloromethane and methanol, the dried and precipitation crystallization in reverse phase column chromatography. In adsorption method, X-5 resin is used to selectively adsorb royal jelly acid from the royal jelly solution. Created by Figdraw.
2 Preparation methods
2.1 Extraction methods
Royal jelly acid can be extracted from royal jelly through several methods, including ethanol extraction (Iegaki, et al., 2020), ether extraction (Antinelli, et al., 2002), ethanol precipitation crystallization (Wang, et al., 2006), solution precipitation crystallization (Wang, et al., 2006), reverse phase column chromatography (Pandeya, et al., 2019) and adsorption method (Zhang and Yang, 2014). The extraction methods are shown in Figure 1. The extraction efficiency of ethanol extraction, ether extraction, ethanol precipitation crystallization and solution precipitation crystallization is generally inefficient, which leads to their gradual phase-out. Reverse phase column chromatography method and adsorption method have garnered attention because of their efficiency and scalability. The X-5 resin demonstrates an adsorption capacity of 9.7 mg/g, with a purity level of approximately 86% (Zhang and Yang, 2014). Its reusability makes this method particularly promising for large-scale industrial applications.
2.2 Synthesis methods
Royal jelly acid can also be synthesized using chemical synthesis and microbial synthesis. Additionally, it is naturally produced in the mandibular glands of worker bees, conclude hydroxylation of stearic acid at the 18th (w) or 17th (o-1) position, then β-oxidation to obtain royal jelly acid (Plettner, et al., 1996). The process thought to be closely linked to the KAT gene (Liu, et al., 2016).
2.2.1 Chemical synthesis method
Six primary chemical synthesis methods are used for royal jelly acid: Wittig reagent alkylene synthesis, ozonolysis, bromination-elimination alkylene synthesis, Knoevenagel condensation, and increased carbon chain elongation synthesis. The principles or processes of these methods are summarized in Table 1. Although these chemical synthesis methods are well-studied, their industrial application remains limited due to several challenges, including generation of multiple by-products, purification complexity, and relatively low yields.
2.2.2 Microbial synthesis method
Microbial synthesis has recently emerged as a promising alternative for producing royal jelly acid, overcoming some of the limitations associated with traditional production methods (Li, et al., 2022). This approach primarily involves the use of Escherichia coli (Fang, et al., 2024; Li, et al., 2022; Wang, et al., 2022) and Pichia pastoris GS115 (Zhang and Yang, 2014) as microbial systems. The first method begins with the use of E. coli to synthesize royal jelly acid from decanoic acid. The mechanism involves the conversion of decanoic acid into decanoyl-CoA by acyl-CoA synthetase. Subsequently, decanoyl-CoA is transformed into trans-dec-2-enoyl-CoA by acyl-CoA dehydrogenase. The intermediate is further converted into trans-2-decenoic acid through the action of acyl-CoA thioesterase. Finally, the hydroxylation of trans-2-decenoic acid at the terminal position, catalyzed by the P450 enzyme, results in the production of 10-hydroxy-2-decenoic acid (royal jelly acid) (Li, et al., 2022). The second method involves the catalytic conversion of 10-hydroxycapric acid into royal jelly acid by using the yeast species P. pastoris GS115 (Zhang and Yang, 2014).
3 Metabolic pathways
Fat metabolism plays a crucial role in maintaining homeostasis within the body (Hansen, et al., 2013). Royal jelly acid undergoes a series of metabolic transformations. Initially, it is metabolized into sebacic acid (SA), which exhibits various physiological activities (Liao, et al., 2024). Subsequently, SA is further broken down into short-chain dicarboxylic acids. These dicarboxylic acids can enter the tricarboxylic acid cycle to release substantial amounts of energy or participate in further metabolic reactions with other bodily substances (Bharathi, et al., 2020). It has been reported that the oxidation of sebacic acid can yield 61 ATP (445.3 kcal/mol), while royal jelly acid may generate over 61 ATP (Liao, et al., 2024). In addition to energy provision, succinate, oxaloacetate, and citrate produced via the tricarboxylic acid cycle play crucial roles in maintaining physiological homeostasis. For instance, citrate synthesis contributes to reduced ketogenesis (Iaconelli, et al., 2010). Furthermore, certain metabolites may continue to release energy through gluconeogenesis (Roden, et al., 2000). Figure 2 presents the metabolic pathways of royal jelly acid. It provides a detailed visualization of royal jelly acid’s metabolic journey.
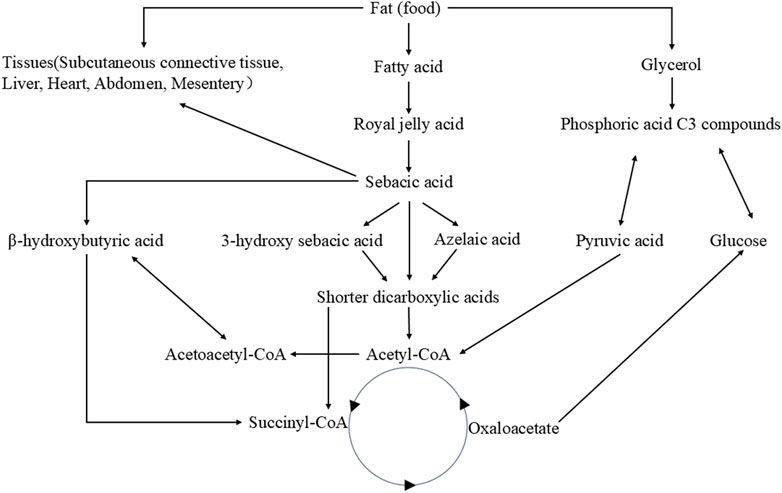
Figure 2. Metabolic pathways of royal jelly acid. The food-derived royal jelly acid is initially oxidized via β-oxidation and ω-oxidation pathways to yield SA. Subsequently, sebacic acid metabolic conversion into shorter-chain dicarboxylic acids. These intermediates are further metabolized through acetyl-CoA to enter the tricarboxylic acid (TCA) cycle, where they undergo complete oxidation to CO2 and H2O, accompanied by substantial energy release. Created by Figdraw.
4 Therapeutic potential
Royal jelly acid has demonstrated a broad spectrum of pharmacological effects, including antibacterial activity (Gao, et al., 2022; Šedivá, et al., 2018), regulation of cancer (Saad Al Shehri, et al., 2023), glucolipid metabolic diseases (Hu, et al., 2022), and inflammatory diseases (Huang S. et al., 2022). Studies have recently begun to unravel the molecular mechanisms that underlie these remarkable bioactivities. This section provides a summary of its pharmacological potential.
4.1 Anti-bacterial potential
In Gram-positive bacteria, Paenibacillus larvae, the causative agent of American foulbrood (AFB), are one of the most devastating bacterial diseases affecting honeybee larvae and pupae (Hansen and and Brødsgaard, 1999). The antibacterial effect of royal jelly acid against P. larvae is strongly pH-dependent, with its activity significantly enhanced under acidic conditions (Šamšulová, et al., 2023; Šedivá, et al., 2018). Staphylococcus aureus is a common human pathogen responsible for various infectious diseases and a major contributor to foodborne illnesses (Bergin, et al., 2017). Royal jelly acid exhibits a minimum inhibitory concentration of 2.25 mg/mL against S. aureus ATCC25923. It inhibits the production of extracellular polymeric substances in S. aureus biofilms, prevents biofilm formation. Moreover, royal jelly acid significantly reduces the hemolytic activity of S. aureus (Gao, et al., 2022). Royal jelly acid has demonstrated antimicrobial activity against a range of Gram-positive bacteria (Melliou and Chinou, 2005; Yang, et al., 2018), including a cause of sepsis and meningitis, Streptococcus alactolyticus (Mylonas, et al., 2020), a cause of skin abscesses, S. intermedius B (Biberstein, et al., 1984), linked to various infections, Staphylococcus xylosus (Battaglia and Garrett-Sinha, 2023), and a major contributor to dental plaque formation, Streptococcus mutans (Forssten, et al., 2010). These findings collectively underscore that royal jelly acid’s potent antibacterial activity, particularly against Gram-positive bacteria, making it a promising candidate for addressing bacterial infections in both humans and animals.
Royal jelly acid demonstrates strong inhibitory effects against several Gram-negative bacteria. Salmonella choleraesuis, a pathogen causing systemic infections and potentially fatal mycotic aneurysms (Chiu, et al., 2004), Vibrio parahaemolyticus, a significant contributor to acute gastroenteritis in humans (Letchumanan, et al., 2014), and Escherichia coli (hemolytic), a strain associated with bacterial pathogenicity (Griffin and Tauxe, 1991), are all strongly inhibited by royal jelly acid (Yang, et al., 2018). Additionally, Salmonella typhimurium induces congestion, edema, and bleeding in the digestive tract (Dar, et al., 2017) and is effectively inhibited by royal jelly acid. Notably, the diHDA-glycerol derivative of royal jelly acid exhibits substantial nonspecific resistance against S. typhimurium (Pollet, et al., 2002). Despite these findings, research on the antibacterial effects of royal jelly acid against a broader range of pathogenic Gram-negative bacteria remains limited. Future studies should focus on exploring its potential against diverse pathogenic bacterial strains and pathogenic microorganisms.
Royal jelly acid also exhibits antifungal properties, effectively inhibiting the growth of various pathogenic fungi. Candida tropicalis is an opportunistic pathogen causing infections under immunocompromised conditions or altered vaginal environments (Chai, et al., 2010). Candida albicans is responsible for infections of the skin, mouth, mucosa, and internal organs (Henriques and Silva, 2021). Candida glabrata causes significant systemic infections (Duggan and Usher, 2023). Royal jelly acid inhibits the growth of these pathogenic fungi (Melliou and Chinou, 2005). By contrast, Saccharomyces cerevisiae, widely used in the production of bread, wine, and other food products (Parapouli, et al., 2020), is unaffected by royal jelly acid, which shows no inhibitory activity against this yeast (Wang, 2008).
4.2 Anti-pest potential
Royal jelly acid demonstrates notable anti-pest properties, targeting a range of vectors and parasites. Aedes aegypti is a primary vector for several significant diseases, such as malaria, dengue fever, chikungunya, and Zika virus (Matthews, 2019). Plasmodium falciparum is the most common and deadly malaria-causing parasite (Maier, et al., 2019). Leishmaniasis is a tropical infection caused by the parasite Leishmania major (Sacks and Noben-Trauth, 2002). Royal jelly acid effectively inhibits Aedes aegypti, P. falciparum K1-strain, and L. major amastigotes. The median lethal concentrations for these three pathogens were 31.4, 2.41, and 3.8 μg/mL, respectively. Royal jelly acid exhibited no toxicity to normal human HEK239T cells, suggesting its safety for human applications (Alkhaibari and Alanazi, 2022).
4.3 Anti-cancer potential
Royal jelly acid has shown promising anticancer effects in liver, lung, colon, and other types of cancer. In liver cancer research, royal jelly acid significantly inhibited ANGPTL8 expression in HepG2 liver cancer cells (Inoue, et al., 2022). HepG2 cells viability were markedly reduced in a dose-dependent matter. Additionally, it promoted apoptotic pathways by increasing the gene expression of Caspase-3, Bax and miR-34a, increasing the protein expression of Caspase-3, PARP, and Bax (Saad Al Shehri, et al., 2023). In another experiment, royal jelly acid markedly reduced Hep3B and HCCLM3 liver cancer cell viability, migration and proliferation, and increased the apoptosis process. Multi-omics analysis determined that royal jelly acid interferes with glycolytic metabolism pathway by reducing lactate production, providing a good antitumor effect in both in vitro and in vivo models (Xu, et al., 2023).
In lung cancer, royal jelly acid inhibited the proliferation of A549, NCI-H460 and NCI-H23 human lung cancer cells, causing nonsignificant toxicity to normal cells. It caused apoptosis in A549 cells through increased the levels of reactive oxygen species. and causes cell cycle arrest at the G0/G1 phase in a time-dependent manner. Furthermore, royal jelly acid modulated key signaling molecules, including increased levels of phosphorylated c-Jun N-terminal kinase (p-JNK), p-p38, and I-κB, whereas reducing the levels of phosphorylated extracellular signal-regulated kinase (p-ERK), p-STAT3, and NF-κB. Moreover, royal jelly acid regulated TGF-β1, SNAI1, GSK-3β, E-cadherin, N-cadherin, and vimentin, inhibiting lung cancer cell migration (Lin, et al., 2020). It suggests that royal jelly acid could potentially be used to treat human lung cancer.
In HCT-116 and SW-480 human colon cancer cells, royal jelly acid has displayed remarkable migratory and invasive effects by inducing significant suppression of promigratory/proinvasive markers, N-cadherin, vimentin and Snail on protein and gene levels (Jovanović, et al., 2022). In WiDr human colon cancer cells, the growth was inhibited by royal jelly acid, and it exhibited anti-inflammatory properties by decreasing NF-κB, IL-8, IL-1β, and TNF-α, and activating IL-1ra (Yang, et al., 2018). Moreover, a combination treatment of royal jelly acid and royal jelly significantly inhibited the growth of CaCo-2 human colon cancer adenocarcinoma cells with decreasing the levels of glutathion and increasing malondialdehyde (Filipič, et al., 2015). While these findings are promising, further in vitro studies are needed to confirm the efficacy of royal jelly acid against colorectal cancer.
In Ehrlich solid tumors, royal jelly acid, either alone or when combined with cyclophosphamide, significantly reduced tumor volume and increased the inhibition rates in mice. The treatment also decreased the expression levels of alpha-fetoprotein and carcinoembryonic antigen tumor, tumor necrosis factor alpha, tumor lipid peroxidation, nitric oxide. Furthermore, it enhanced the activity of antioxidant enzymes, including glutathione peroxidase, catalase enzyme, Superoxide dismutase and the apoptotic genes Caspase-3 and Bax (Albalawi, et al., 2021). Additionally, royal jelly acid may also act as a toll-like receptor four antagonist, which could contribute to its tumor-suppressing effects (Eslami-Kaliji, et al., 2022; Eslami-Kaliji, et al., 2021). Research needs to focus on the combination of royal jelly acid.
Royal jelly acid demonstrated a dose-dependent inhibitory effect on SU-DHL-2 lymphatic cancer cells by modulating key proteins involved in the complement and coagulation cascade pathways, including prothrombin, plasminogen, plasminogen activator, and carboxypeptidyl B2 (Tian, et al., 2024). In HCT116 and MDA-MB-231 breast cancer cells, royal jelly acid reduced histone deacetylase enzyme levels, promoting chromatin lysine residue acetylation and triggering an increase in gene expression levels (Aparecida Dos Santos France, et al., 2024). Moreover, when combined with other royal jelly lipids, royal jelly acid exhibited inhibitory activity on the proliferation of various mammalian cell lines, namely, immortalized C2C12 mouse myoblast cells, PC3 human prostate cancer cells, and SH-SY5Y human neuroblastoma cells. Royal jelly acid also significantly inhibited the proliferation, migration, and tube formation of human umbilical vein endothelial cells (Izuta, et al., 2009), thereby limiting tumor angiogenesis and progression. Ultimately, royal jelly acid has important anti-cancer effects, and more robust in vitro experiments are required. The anti-cancer action of royal jelly acid is represented in Figure 3.
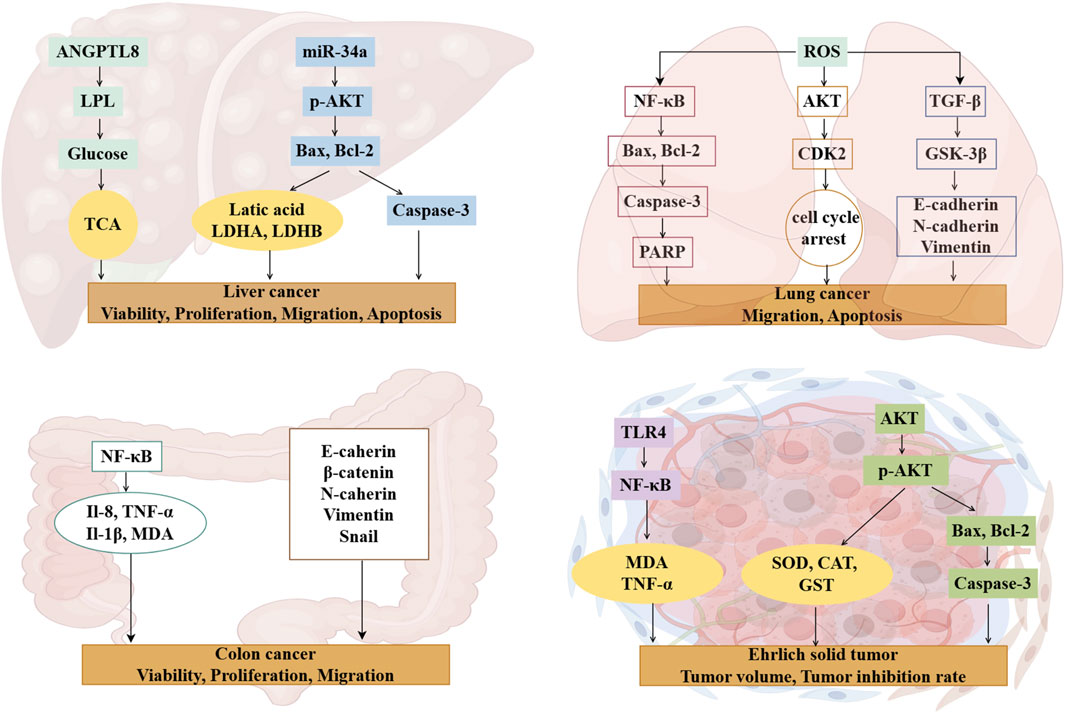
Figure 3. Mechanisms of royal jelly acid on cancer diseases. Royal jelly acid exhibits potential therapeutic benefits against various cancers. In liver cancer, it demonstrates therapeutic potential by modulating key regulators including lactic acid, Caspase-3, and Bax to improve liver cell viability, proliferation, migration, and apoptosis. In lung cancer, royal jelly acid regulates apoptosis-related factors (Bax, Bcl-2, Caspase-3), antioxidant factor (ROS), and anti-inflammatory factor (NF-κB), thereby influencing lung cancer cell migration and apoptosis. For colon cancer, royal jelly acid modulates inflammatory factors (NF-κB, IL-8, IL-1β, TNF-α) and junctional proteins (E-cadherin, β-catenin, N-cadherin), which collectively regulate colorectal cancer cell viability, proliferation, and migration. In Ehrlich solid tumors, royal jelly acid exerts anticancer effects by regulating antioxidant factors (SOD, MDA, CAT) and apoptosis-related factors (Caspase-3, Bax, Bcl-2), leading to reduced tumor volume and enhanced inhibition rate. These findings highlight that royal jelly acid exhibits therapeutic potential against cancer. Created by Figdraw.
4.4 Anti-glucolipid metabolic diseases potential
Royal jelly acid exhibits potent therapeutic effects in the treatment of glycolipid metabolic disorders through its regulatory, anti-inflammatory, and antioxidant properties (Hu, et al., 2022). In hyperlipidemic rats, royal jelly acid significantly reduced the levels of triglycerides, total cholesterol, and β-lipoproteins, whereas increased high-density lipoprotein levels (Xu, et al., 2002). A total of 41 key metabolites played key roles in the exertion of antihyperlipidemic effects, including glutamylaspartic acid, caproic acid and tryptophyl-glutamine (Zhi, et al., 2025). In studies involving type 2 diabetes mice, royal jelly acid displayed strong hypoglycemic effects by modulating inflammatory markers such as IL-6 and TNF-α, and by enhancing antioxidant enzymes, including SOD, CAT, and GPx. The compound improved glucose metabolism through activation of the PI3K/AKT signaling pathway and upregulated the expression of PGC-1α, a key regulator of mitochondrial biogenesis in mice (Hu, et al., 2022). In HepG2 liver cells, royal jelly acid increased phosphorylated AMP-activated protein kinase levels, inhibited aquaporin-9 gene expression, and facilitated glycerol uptake by liver tissues (Watadani, et al., 2017). According to animal studies, royal jelly acid enhances pAMPK phosphorylation and facilitates GLUT4 translocation to the cell membrane, thereby improving glucose uptake in skeletal muscle cells (Usui, et al., 2019). In 3T3-L1 adipocytes, royal jelly acid downregulated oxidative stress markers such as ROS and suppressed the expression of key lipogenic transcription factors, including PPARγ, FABP4, C/EBP-α, SREBP-1c, and leptin (Pandeya, et al., 2019). The compound also scavenged DPPH radicals (Kolayli, et al., 2016) and activated TRPA1 and TRPV1 channels, inducing thermogenesis and augmenting energy expenditure (Terada, et al., 2011). Royal jelly acid evidently has a more beneficial effect on glycolipid metabolic disease improvement. The primary mechanism is depicted in Figure 4.
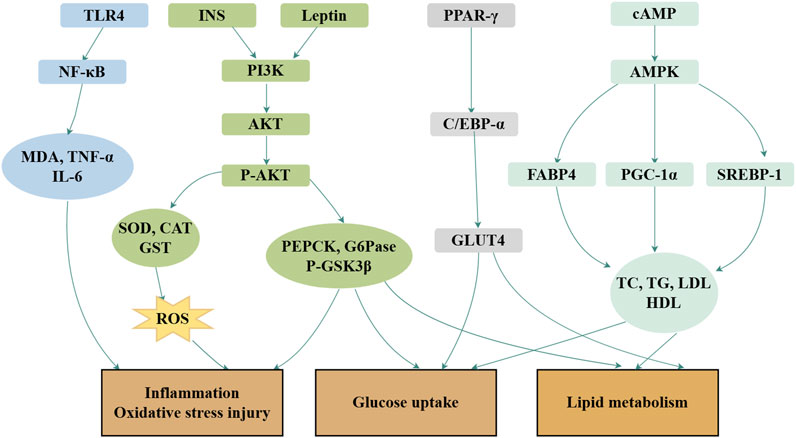
Figure 4. Mechanisms of royal jelly acid on glucolipid metabolic diseases. In glucolipid metabolic diseases, royal jelly acid demonstrates therapeutic potential through multi-target regulatory effects. It modulates lipid factors (TC, TG, LDL, HDL), anti-inflammatory factors (TNF-α, IL-6), and antioxidant factors (SOD, CAT, GST), thereby ameliorating inflammation, oxidative stress injury, glucose uptake, and lipid metabolism dysregulation. Created by Figdraw.
4.5 Anti-inflammatory diseases potential
Inflammation plays a pivotal role in the development and progression of various diseases (Okin and Medzhitov, 2012). Lipopolysaccharide (LPS), commonly used to induce immune responses in mammalian cells, triggers the release of pro-inflammatory cytokines, and serves as a standard model for studying inflammation (Zhang, et al., 2024). In LPS-induced inflammation models generated using RAW264 cells, royal jelly acid exhibits anti-inflammatory effects by inhibiting IκB-ζ and IL-6 expression, although it does not influence TNF-α levels (Spannhoff, et al., 2011). By contrast, in another study, royal jelly acid significantly downregulated TNF-α expression while markedly inhibiting IL-6 and IL-1β production (Huang M. et al., 2022). Further research revealed that royal jelly acid alleviates inflammation by suppressing JNK1/2 and p38 MAPK phosphorylation (Chen, et al., 2016), resulting in reduced IL-6 (Chen, et al., 2016; Huang S. et al., 2022; Sugiyama, et al., 2012) and IL-1β (Huang M. et al., 2022) levels. In BV-2 microglial cells exposed to LPS-induced inflammation, royal jelly acid mitigated inflammation by directly inhibiting the NLRP3 inflammasome or indirectly promoting autophagy (You et al., 2020a). Metabolomic and transcriptomic analyses further highlighted that royal jelly acid modulates pathways involved in amino acid metabolism, antigen processing and presentation, and NOD receptor signaling, which are critical to its anti-inflammatory effects (Huang, et al., 2023). Royal jelly acid has exhibited major protective effects against inflammation both in vitro and in vivo.
Nitric oxide (NO) is a pro-inflammatory mediator that contributes to various inflammatory diseases by generating peroxynitrite anions (Litvinova, et al., 2015). In a model of NO-induced inflammation in RAW264 cells treated with IFN-γ, royal jelly acid significantly reduced NO production and suppressed the activation of the NO synthase promoter. It also inhibited the expression of IRF-8, TNF-α, and NF-κB, while leaving IRF-1 expression unaffected (Takahashi, et al., 2012). Similar findings were observed in another inflammatory RAW264 cell model, where LPS- or IFN-β-mediated NO production was significantly reduced by royal jelly acid, which concurrently increased TNF-α and NF-κB levels (Sugiyama, et al., 2012).
Several in vivo studies have further validated the anti-inflammatory properties of royal jelly acid. In a mouse model of colitis, royal jelly acid significantly reduced the expression of key inflammasome components, including TXNIP, NLRP3, ASC, Caspase-1, GSDMD, N-GSDMD, IL-1β, and IL-18, while in THP1 cells, it inhibited LPS/ATP-induced inflammasome-mediated pyroptosis, thereby alleviating inflammation and associated colitis (Huang S. et al., 2022). Additionally, in chicken models of intestinal mucosal injury, royal jelly acid reduced the levels of pro-inflammatory cytokines (TNF-α, IL-1β, and IL-6), reversed the upregulation of TLR4 and NF-κB, and ameliorated intestinal mucosal injury (Han, et al., 2023). In rheumatoid arthritis synovial fibroblast cells, royal jelly acid modulated the p38 kinase and c-Jun N-terminal kinase (JNK)-AP-1 signaling pathways, thereby alleviating symptoms of rheumatoid arthritis (Yang, et al., 2010). Although royal jelly acid has demonstrated effective anti-inflammatory properties in vitro and in vivo, more studies are necessary to explore its potential mechanisms in different inflammatory and related conditions. Figure 5 illustrates the main mechanism.
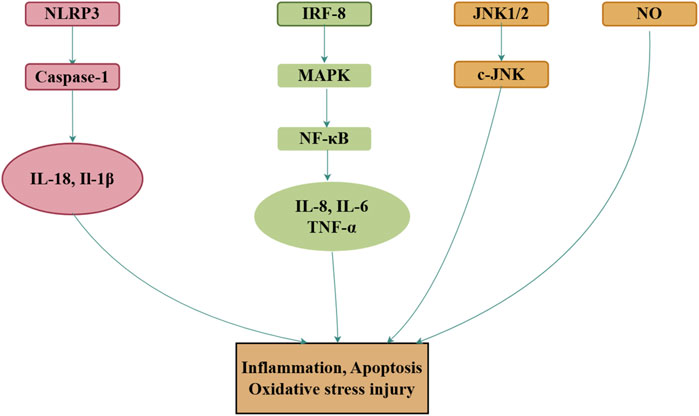
Figure 5. Mechanisms of royal jelly acid on inflammatory diseases. In inflammatory diseases, royal jelly acid demonstrates therapeutic potential by modulating anti-inflammatory factors (TNF-α, IL-17, IL-1β) to regulate inflammation, apoptosis, and oxidative stress injury. Created by Figdraw.
4.6 Anti-neurological diseases potential
Royal jelly acid has demonstrated potential neuroprotective and antidepressant effects across various studies (Dubey, et al., 2024; Gong, et al., 2023; Ito, et al., 2012). It enhances neuronal development, especially when used in conjunction with TAU protein, and displays strong binding affinity for TAU protein kinase and phosphatase (Dubey, et al., 2024). Royal jelly acid activates TRPA1 (Terada, et al., 2011), acetylates histones H3 and H4, and inhibits the expression of active caspase-3 and PARP-1 (Koc, et al., 2025), thus contributing to neuroprotection. In microglial BV2 cells, royal jelly acid alleviates neuroinflammation by promoting the FOXO1-mediated autophagy pathway (You et al., 2020b) It also enhances neurogenesis in cultured neural stem/progenitor cells (Hattori, et al., 2007) and preserves blood–brain barrier integrity by inhibiting the degradation of tight junction proteins via the AMPK/PI3K/AKT signaling pathway (You, et al., 2019). Likely in APP/PS1 mouse models of Alzheimer’s disease, royal jelly significantly improved memory deficits by stimulating the cAMP/PKA/CREB/BDNF pathway and reducing neuronal apoptosis (You, et al., 2018). Moreover, when combined with aspirin to treat memory impairment and neuroinflammation, royal jelly acid synergistically reduced pro-inflammatory mediator levels, inhibited glial cell activation, and alleviated both memory deficits and neuroinflammation (You, et al., 2022). Incorporating royal jelly and royal jelly acid as supplements could be a hopeful therapeutic strategy for neurological ailments.
4.7 Other beneficial potentials
In life extension and immune enhancement, The queen bee, which eats royal jelly for its entire life, lives about ten times longer than the worker bee. Studies have shown that royal jelly acid, a special fatty acid in royal jelly, can also prolong lifespan and boost immunity in various models. Studies on Caenorhabditis elegans revealed that lipid mixtures containing royal jelly acid increased lifespan by activating the FOXO transcription factor DAF-16 and reducing insulin/IGF-1 signaling (IIS) (Honda, et al., 2011). Interestingly, it also extended lifespan through mechanisms IIS-independent mechanisms involving dietary restriction and TOR signaling pathways (Honda, et al., 2015). Additionally, in cyclophosphamide-induced immunocompromised mice, royal jelly acid restored body weight and increased thymus and spleen mass. It enhanced DNA/RNA/protein activity, while stimulating pathways that support B lymphocyte affinity maturation, antigen presentation, and macrophage activity (Fan, et al., 2020). These effects indicate its immune regulatory potential. Furthermore, royal jelly acid inhibited T cell proliferation, downregulated IL-2, CD86, MHC II, and IL-12 production, and upregulated IL-10 production, demonstrating its ability to modulate immune responses effectively (Vucevic, et al., 2007). These properties contribute to its role in improving immunity and addressing immune-related diseases.
Royal jelly acid exhibits protective effects in a range of conditions, including testicular toxicity, osteoporosis, and dry eye disease in mice. In studies on rats exposed to PbAc, royal jelly acid improved testicular function. A combination of royal jelly acid and ZnO nanoparticles reduced inflammatory and apoptotic markers (Caspase-3 and Bax); enhanced semen quality; and improved the levels of pituitary and gonadal hormones, antioxidants, and testicular tissue structure (Maher, et al., 2024). Regarding osteoporosis, royal jelly acid interacts with FFAR4 to inhibit NF-κB signaling, which contributes to its anti-osteoporotic effects in mice (Tsuchiya, et al., 2020). While it did not prevent bone loss following ovariectomy, it improved bone density and reduced femoral bone hardness, suggesting potential benefits in bone health (Hanai, et al., 2023). Moreover, royal jelly acid stabilizes acetylcholine levels, which may help alleviate symptoms of dry eye disease, improving tear production and ocular surface health (Yamaga, et al., 2021). Given the anti-inflammatory, anti-bacterial, and anti-cancer properties of royal jelly acid, more research is needed to understand the specific mechanisms of its effects on various diseases.
5 Conclusion
As a natural product, royal jelly acid has exhibited therapeutic potential against various diseases and pathological conditions. Both in vivo and in vitro studies have demonstrated its pharmacological versatility, but much remains to be explored regarding its efficacy and safety in humans. Key research areas include exploring the pharmacokinetics of royal jelly acid, that is, how royal jelly acid is absorbed, metabolized, and excreted in the human body, and its potential for inducing drug resistance; conducting rigorous studies to evaluate its efficacy and safety in treating specific human diseases, including liver cancer, hyperlipidemia and colitis; elucidating the mechanisms underlying anti-inflammatory, antioxidant, and lipid metabolism-regulating effects of royal jelly acid; exploring age-stratified studies, including adolescents, pregnant individuals, and geriatric cohorts; accelerating the clinical research; assessing the safety profile, including potential side effects and interactions with other drugs or conditions; enhancing bioavailability by developing optimized drug formulations to augment its absorption and therapeutic action; and utilizing advanced technologies such as single-cell sequencing, spatial transcriptomics, and gut microbiome analysis to uncover its molecular pathways. Concomitantly, intensified research efforts should prioritize the investigation of royal jelly acid derivatives, particularly diHDA-glycerol derivative of royal jelly acid. Strengthen the development of royal jelly acid-based functional products, including nutraceutical supplements and bioactive beverages. While royal jelly acid holds significant promise for daily healthcare and therapeutic applications, a comprehensive understanding of its mechanisms, safety, and effectiveness remains a significant challenge for future research.
Author contributions
DZ: Conceptualization, Writing – original draft. XH: Supervision, Writing – review and editing. YX: Writing – review and editing. WZ: Writing – review and editing. XG: Conceptualization, Writing – review and editing. YG: Conceptualization, Investigation, Writing – review and editing. XL: Conceptualization, Writing – review and editing. YT: Investigation, Supervision, Writing – review and editing. KD: Data curation, Funding acquisition, Validation, Visualization, Writing – review and editing.
Funding
The author(s) declare that financial support was received for the research and/or publication of this article. This work was supported by the National Natural Science Foundation of China [grant numbers 32360141, 32060241, 31572339]; the Earmarked Fund for China Agriculture Research System of MOF and MARA [grant number CARS-44-KXJ13]; the Special Project for Basic Research of Young Program [grant number 202201AU070240].
Conflict of interest
The authors declare that the research was conducted in the absence of any commercial or financial relationships that could be construed as a potential conflict of interest.
Generative AI statement
The author(s) declare that no Generative AI was used in the creation of this manuscript.
Publisher’s note
All claims expressed in this article are solely those of the authors and do not necessarily represent those of their affiliated organizations, or those of the publisher, the editors and the reviewers. Any product that may be evaluated in this article, or claim that may be made by its manufacturer, is not guaranteed or endorsed by the publisher.
References
Albalawi, A. E., Althobaiti, N. A., Alrdahe, S. S., Alhasani, R. H., Alaryani, F. S., and BinMowyna, M. N. (2021). Anti-tumor effects of queen bee acid (10-hydroxy-2-decenoic acid) alone and in combination with cyclophosphamide and its cellular mechanisms against ehrlich solid tumor in mice. Molecules 26 (22), 7021. doi:10.3390/molecules26227021
Alkhaibari, A. M., and Alanazi, A. D. (2022). Insecticidal, antimalarial, and antileishmanial effects of royal jelly and its three main fatty acids, trans-10-hydroxy-2-decenoic acid, 10-hydroxydecanoic acid, and sebacic acid. Evidence-based complementary Altern. Med. eCAM. 2022, 7425322. doi:10.1155/2022/7425322
Antinelli, J. F., Davico, R., Rognone, C., Faucon, J. P., and Lizzani-Cuvelier, L. (2002). Application of solid/liquid extraction for the gravimetric determination of lipids in royal jelly. J. Agric. Food Chem. 50 (8), 2227–2230. doi:10.1021/jf0112466
Aparecida Dos Santos France, F., Maeda, D. K., Rodrigues, A. B., Ono, M., Lopes Nogueira Marchetti, F., Marchetti, M. M., et al. (2024). Exploring fatty acids from royal jelly as a source of histone deacetylase inhibitors: from the hive to applications in human well-being and health. Epigenetics 19 (1), 2400423. doi:10.1080/15592294.2024.2400423
Atanasov, A. G., Zotchev, S. B., Dirsch, V. M., and Supuran, C. T. (2021). Natural products in drug discovery: advances and opportunities. Nat. Rev. Drug Discov. 20 (3), 200–216. doi:10.1038/s41573-020-00114-z
Battaglia, M., and Garrett-Sinha, L. A. (2023). Staphylococcus xylosus and Staphylococcus aureus as commensals and pathogens on murine skin. Laboratory animal Res. 39 (1), 18. doi:10.1186/s42826-023-00169-0
Bergin, S. P., Holland, T. L., Fowler, V. G., and Tong, S. Y. C. (2017). Bacteremia, sepsis, and infective endocarditis associated with Staphylococcus aureus. Curr. Top. Microbiol. Immunol. 409, 263–296. doi:10.1007/82_2015_5001
Bharathi, S. S., Zhang, Y., Gong, Z., Muzumdar, R., and Goetzman, E. S. (2020). Role of mitochondrial acyl-CoA dehydrogenases in the metabolism of dicarboxylic fatty acids. BBRC 527 (1), 162–166. doi:10.1016/j.bbrc.2020.04.105
Biberstein, E. L., Jang, S. S., and Hirsh, D. C. (1984). Species distribution of coagulase-positive staphylococci in animals. J. Clin. Microbiol. 19 (5), 610–615. doi:10.1128/jcm.19.5.610-615.1984
Chai, L. Y., Denning, D. W., and Warn, P. (2010). Candida tropicalis in human disease. Crit. Rev. Microbiol. 36 (4), 282–298. doi:10.3109/1040841x.2010.489506
Chen, Y., Wang, K., Zhang, Y., Zheng, Y., and Hu, F. (2016). In vitro anti-inflammatory effects of three fatty acids from royal jelly. Mediat. Inflamm. 2016, 3583684. doi:10.1155/2016/3583684
Chiu, C. H., Su, L. H., and Chu, C. (2004). Salmonella enterica serotype Choleraesuis: epidemiology, pathogenesis, clinical disease, and treatment. Clin. Microbiol. Rev. 17 (2), 311–322. doi:10.1128/cmr.17.2.311-322.2004
Dar, M. A., Ahmad, S. M., Bhat, S. A., Ahmed, R., Urwat, U., Mumtaz, P. T., et al. (2017). Salmonella typhimurium in poultry: a review. Worlds Poult. Sci. J. 73 (2), 345–354. doi:10.1017/S0043933917000204
Dubey, R., Sathiyanarayanan, L., Sankaran, S., and Arulmozhi, S. (2024). Nootropic effect of indian royal jelly against okadaic acid induced rat model of alzheimer's disease: inhibition of neuroinflammation and acetylcholineesterase. J. traditional complementary Med. 14 (3), 300–311. doi:10.1016/j.jtcme.2023.11.005
Duggan, S., and Usher, J. (2023). Candida glabrata: a powerhouse of resistance. PLoS Path 19 (10), e1011651. doi:10.1371/journal.ppat.1011651
Eslami-Kaliji, F., Mirahmadi-Zare, S. Z., Nazem, S., Shafie, N., Ghaedi, R., and Asadian-Esfahani, M. H. (2022). A label-free SPR biosensor for specific detection of TLR4 expression; introducing of 10-HDA as an antagonist. Int. J. Biol. Macromol. 217, 142–149. doi:10.1016/j.ijbiomac.2022.07.035
Eslami-Kaliji, F., Sarafbidabad, M., Kiani-Esfahani, A., Mirahmadi-Zare, S. Z., and Dormiani, K. (2021). 10-hydroxy-2-decenoic acid a bio-immunomodulator in tissue engineering; generates tolerogenic dendritic cells by blocking the toll-like receptor4. J. Biomed. Mater. Res. Part A 109 (9), 1575–1587. doi:10.1002/jbm.a.37152
Fan, P., Han, B., Hu, H., Wei, Q., Zhang, X., Meng, L., et al. (2020). Proteome of thymus and spleen reveals that 10-hydroxydec-2-enoic acid could enhance immunity in mice. Expert Opin. Ther. Targets. 24 (3), 267–279. doi:10.1080/14728222.2020.1733529
Fang, K., Xu, Z., Yang, L., Cui, Q., Du, B., Liu, H., et al. (2024). Biosynthesis of 10-hydroxy-2-decenoic acid through a one-step whole-cell catalysis. J. Agric. Food Chem. 72 (2), 1190–1202. doi:10.1021/acs.jafc.3c08142
Feng, Q., Han, T., He, B., Zhang, X., and Li, Z. (2016). The progress in chemical synthesis of 10-hydroxy-2-decenoic acid. J. Chengdu Normal Univ. (32), 101–107.
Filipič, B., Gradišnik, L., Rihar, K., Šooš, E., Pereyra, A., and Potokar, J. (2015). The influence of royal jelly and human interferon-alpha (HuIFN-αN3) on proliferation, glutathione level and lipid peroxidation in human colorectal adenocarcinoma cells in vitro. Arh. Hig. Rada Toksikol. 66 (4), 269–274. doi:10.1515/aiht-2015-66-2632
Forssten, S. D., Björklund, M., and Ouwehand, A. C. (2010). Streptococcus mutans, caries and simulation models. Nutrients 2 (3), 290–298. doi:10.3390/nu2030290
Fray, G. I., Jaeger, R. H., Morgan, E. D., Robinson, R., and Sloan, A. D. B. (1961a). Synthesis of trans-10-hydroxydec-2-enoic acid and related compounds. Tetrahedron 15 (1), 18–25. doi:10.1016/0040-4020(61)80003-3
Fray, G., Jaeger, R., and Robinson, R. (1961b). Synthesis of royal jelly acid. Bee World 42 (1), 12–13. doi:10.1080/0005772X.1961.11096831
Gao, K., Su, B., Dai, J., Li, P., Wang, R., and Yang, X. (2022). Anti-biofilm and anti-hemolysis activities of 10-hydroxy-2-decenoic acid against Staphylococcus aureus. Molecules 27 (5), 1485. doi:10.3390/molecules27051485
Gong, Y., Luo, H., Li, Z., Feng, Y., Liu, Z., and Chang, J. (2023). Metabolic profile of alzheimer's disease: is 10-hydroxy-2-decenoic acid a pertinent metabolic adjuster? Metabolites 13 (8), 954. doi:10.3390/metabo13080954
Griffin, P. M., and Tauxe, R. V. (1991). The epidemiology of infections caused by Escherichia coli O157:H7, other enterohemorrhagic E. coli, and the associated hemolytic uremic syndrome. Epidemiol. Rev. 13, 60–98. doi:10.1093/oxfordjournals.epirev.a036079
Han, L., Zhang, M., Li, F., Su, J., Wang, R., Li, G., et al. (2023). 10-hydroxy-2-decenoic acid alleviates lipopolysaccharide-induced intestinal mucosal injury through anti-inflammatory, antioxidant, and gut microbiota modulation activities in chickens. Front. Microbiol. 14, 1285299. doi:10.3389/fmicb.2023.1285299
Hanai, R., Matsushita, H., Minami, A., Abe, Y., Tachibana, R., Watanabe, K., et al. (2023). Effects of 10-hydroxy-2-decenoic acid and 10-hydroxydecanoic acid in royal jelly on bone metabolism in ovariectomized rats: a pilot study. J. Clin. Med. 12 (16), 5309. doi:10.3390/jcm12165309
Hansen, H., and Brødsgaard, C. J. (1999). American foulbrood: a review of its biology, diagnosis and control. Bee World 80 (1), 5–23. doi:10.1080/0005772X.1999.11099415
Hansen, M., Flatt, T., and Aguilaniu, H. (2013). Reproduction, fat metabolism, and life span: what is the connection? Cell Metab. 17 (1), 10–19. doi:10.1016/j.cmet.2012.12.003
Hattori, N., Nomoto, H., Fukumitsu, H., Mishima, S., and Furukawa, S. (2007). Royal jelly and its unique fatty acid, 10-hydroxy-trans-2-decenoic acid, promote neurogenesis by neural stem/progenitor cells in vitro. Biomed. Res. 28 (5), 261–266. doi:10.2220/biomedres.28.261
Henriques, M., and Silva, S. (2021). Candida albicans virulence factors and its pathogenicity. Microorganisms 9 (4), 704. doi:10.3390/microorganisms9040704
Honda, Y., Araki, Y., Hata, T., Ichihara, K., Ito, M., Tanaka, M., et al. (2015). 10-hydroxy-2-decenoic acid, the major lipid component of royal jelly, extends the lifespan of Caenorhabditis elegans through dietary restriction and target of rapamycin signaling. J. Aging Res. 2015, 425261. doi:10.1155/2015/425261
Honda, Y., Fujita, Y., Maruyama, H., Araki, Y., Ichihara, K., Sato, A., et al. (2011). Lifespan-extending effects of royal jelly and its related substances on the nematode Caenorhabditis elegans. PLoS ONE 6 (8), e23527. doi:10.1371/journal.pone.0023527
Hu, X., Liu, Z., Lu, Y., Chi, X., Han, K., Wang, H., et al. (2022). Glucose metabolism enhancement by 10-hydroxy-2-decenoic acid via the PI3K/AKT signaling pathway in high-fat-diet/streptozotocin induced type 2 diabetic mice. Food Funct. 13 (19), 9931–9946. doi:10.1039/d1fo03818d
Huang, M., Dong, J., Tan, X., Yang, S., Xiao, M., and Wang, D. (2023). Integration of metabolomic and transcriptomic provides insights into anti-inflammatory response to trans-10-hydroxy-2-decenoic acid on LPS-stimulated RAW 264.7 cells. Int. J. Mol. Sci. 24 (16), 12666. doi:10.3390/ijms241612666
Huang, M., Xiao, M., Dong, J., Huang, Y., Sun, H., and Wang, D. (2022a). Synergistic anti-inflammatory effects of graphene oxide quantum dots and trans-10-hydroxy-2-decenoic acid on LPS-stimulated RAW 264.7 macrophage cells. Biomater. Adv. 136, 212774. doi:10.1016/j.bioadv.2022.212774
Huang, S., Tao, R., Zhou, J., Qian, L., and Wu, J. (2022b). Trans-10-hydroxy-2-decenoic acid alleviates dextran sulfate sodium-induced colitis in mice via regulating the inflammasome-mediated pyroptotic pathway and enhancing colonic barrier function. Mol. Nutr. Food Res. 66 (12), e2100821. doi:10.1002/mnfr.202100821
Iaconelli, A., Gastaldelli, A., Chiellini, C., Gniuli, D., Favuzzi, A., Binnert, C., et al. (2010). Effect of oral sebacic Acid on postprandial glycemia, insulinemia, and glucose rate of appearance in type 2 diabetes. Diabetes Care 33 (11), 2327–2332. doi:10.2337/dc10-0663
Iegaki, N., Narita, Y., Hattori, N., Hirata, Y., and Ichihara, K. (2020). Royal jelly reduces depression-like behavior through possible effects on adrenal steroidogenesis in a murine model of unpredictable chronic mild stress. Biosci. Biotechnol. Biochem. 84 (3), 606–612. doi:10.1080/09168451.2019.1691496
Inoue, Y., Ienaga, M., Kamiya, T., Adachi, T., Ohta, M., and Hara, H. (2022). Royal jelly fatty acids downregulate ANGPTL8 expression through the decrease in HNF4α protein in human hepatoma HepG2 cells. Biosci. Biotechnol. Biochem. 86 (6), 747–754. doi:10.1093/bbb/zbac043
Ishmuratov, G. Y., Kharisov, R. Y., Botsman, O. V., Ishmuratova, N. M., and Tolstikov, G. A. (2002). Synthesis of 9-oxo- and 10-hydroxy-2e-decenoic acids. Chem. Nat. Compd. 38 (1), 1–23. doi:10.1023/A:1015759911900
Ito, S., Nitta, Y., Fukumitsu, H., Soumiya, H., Ikeno, K., Nakamura, T., et al. (2012). Antidepressant-like activity of 10-hydroxy-trans-2-decenoic acid, a unique unsaturated fatty acid of royal jelly, in stress-inducible depression-like mouse model. Evidence-based complementary Altern. Med. eCAM 2012, 139140. doi:10.1155/2012/139140
Izuta, H., Chikaraishi, Y., Shimazawa, M., Mishima, S., and Hara, H. (2009). 10-Hydroxy-2-decenoic acid, a major fatty acid from royal jelly, inhibits VEGF-induced angiogenesis in human umbilical vein endothelial cells. Evidence-based complementary Altern. Med. eCAM. 6 (4), 489–494. doi:10.1093/ecam/nem152
Jovanović, M. M., Šeklić, D. S., Rakobradović, J. D., Planojević, N. S., Vuković, N. L., Vukić, M. D., et al. (2022). Royal jelly and trans-10-hydroxy-2-decenoic acid inhibit migration and invasion of colorectal carcinoma cells. Food Technol. Biotechnol. 60 (2), 213–224. doi:10.17113/ftb.60.02.22.7495
Kenneth, N. C., and Sommers, H. A. (1948). Hexamethylene chlorohydrin. Org. Synth. Hexamethylene Chlorohydrin. Org. Synth. 28 (65). doi:10.15227/orgsyn.028.0065
Kharisov, R. Y., Botsman, O. V., Botsman, L. P., Ishmuratova, N. M., Ishmuratov, G. Y., and Tolstikov, G. A. (2002). Synthesis of 10-hydroxy- and 9-oxo-2e-decenoic acids from oleic acid. Chem. Nat. Compd. 38 (2), 145–148. doi:10.1023/A:1019683830012
Koc, C., Aydemir, C. I., Salman, B., Cakir, A., Akbulut, N. H., Karabarut, P. L., et al. (2025). Comparative neuroprotective effects of royal jelly and its unique compound 10-hydroxy-2-decenoic acid on ischemia-induced inflammatory, apoptotic, epigenetic and genotoxic changes in a rat model of ischemic stroke. Nutr. Neurosci. 28 (1), 37–49. doi:10.1080/1028415x.2024.2344141
Kolayli, S., Sahin, H., Can, Z., Yildiz, O., Malkoc, M., and Asadov, A. (2016). A member of complementary medicinal food: anatolian royal jellies, their chemical compositions, and antioxidant properties. J. Evid. Based Complement. Altern. Med. 21 (4), 43–48. doi:10.1177/2156587215618832
Letchumanan, V., Chan, K. G., and Lee, L. H. (2014). Vibrio parahaemolyticus: a review on the pathogenesis, prevalence, and advance molecular identification techniques. Front. Microbiol. 5, 705. doi:10.3389/fmicb.2014.00705
Li, Q., Gu, K., and Cheng, X., (2007). Synthesis of royal jelly acid. Chem. World. (5), 294–297. doi:10.19500/j.cnki.0367-6358.2007.05.011
Li, Y., Wang, J., Wang, F., Wang, L., Wang, L., Xu, Z., et al. (2022). Production of 10-hydroxy-2-decenoic acid from decanoic acid via whole-cell catalysis in engineered Escherichia Coli. ChemSusChem 15 (9), e202102152. doi:10.1002/cssc.202102152
Liao, Z., Alrosan, M., Alu'datt, M. H., and Tan, T. C. (2024). 10-hydroxy decanoic acid, trans-10-hydroxy-2-decanoic acid, and sebacic acid: source, metabolism, and potential health functionalities and nutraceutical applications. J. Food Sci. 89 (7), 3878–3893. doi:10.1111/1750-3841.17143
Lin, X. M., Liu, S. B., Luo, Y. H., Xu, W. T., Zhang, Y., Zhang, T., et al. (2020). 10-HDA Induces ROS-mediatedapoptosis in A549 human lung cancer cells by regulating the MAPK, STAT3, NF-κB, and TGF-β1 signaling pathways. BioMed Res. Int. 2020, 3042636. doi:10.1155/2020/3042636
Litvinova, L., Atochin, D. N., Fattakhov, N., Vasilenko, M., Zatolokin, P., and Kirienkova, E. (2015). Nitric oxide and mitochondria in metabolic syndrome. Front. Physiol. 6, 20. doi:10.3389/fphys.2015.00020
Liu, L., Yang, X., and Wang, R. (2016). The effect of KAT gene silencing by RNAi on the synthesis of 10-HDA in bees. China Biotechnol. 36 (4), 63–68. doi:10.13523/j.cb.20160410
Maher, A. M., Elsanosy, G. A., Ghareeb, D. A., Elblehi, S. S., and Saleh, S. R., (2024). 10-hydroxy decanoic acid and zinc oxide nanoparticles retrieve Nrf2/HO-1 and Caspase-3/Bax/Bcl-2 signaling in lead-induced testicular toxicity. Biol. Trace Elem. Res. doi:10.1007/s12011-024-04374-3
Maier, A. G., Matuschewski, K., Zhang, M., and Rug, M. (2019). Plasmodium falciparum. Trends Parasitol. 35 (6), 481–482. doi:10.1016/j.pt.2018.11.010
Matthews, B. J. (2019). Aedes aegypti. Trends Genet. TIG. 35 (6), 470–471. doi:10.1016/j.tig.2019.03.005
Melliou, E., and Chinou, I. (2005). Chemistry and bioactivity of royal jelly from Greece. J. Agric. Food Chem. 53 (23), 8987–8992. doi:10.1021/jf051550p
Mylonas, C. C., Gomatou, G., Poulakou, G., Moraitou, E., and Syrigos, K. (2020). Human disease caused by Streptococcus alactolyticus: a case report of native valve infective endocarditis and review of the literature. Monaldi archives chest Dis. = Archivio Monaldi per le malattie del torace 90 (4). doi:10.4081/monaldi.2020.1428
Odinokov, V. N., Ishmuratov, G. Y., and Tolstikov, G. A. (1983). A new route for the synthesis of 10-hydroxydec-2E-enoic and dec-2E-enedioic acids. Chem. Nat. Compd. 19 (6), 658–660. doi:10.1007/BF00575161
Okin, D., and Medzhitov, R. (2012). Evolution of inflammatory diseases. Curr. Biol. CB 22 (17), 733–740. doi:10.1016/j.cub.2012.07.029
Pandeya, P. R., Lamichhane, R., Lee, K. H., Kim, S. G., Lee, D. H., Lee, H. K., et al. (2019). Bioassay-guided isolation of active anti-adipogenic compound from royal jelly and the study of possible mechanisms. BMC Complement. Altern. Med. 19 (1), 33. doi:10.1186/s12906-018-2423-2
Parapouli, M., Vasileiadis, A., Afendra, A. S., and Hatziloukas, E. (2020). Saccharomyces cerevisiae and its industrial applications. AIMS Microbiol. 6 (1), 1–31. doi:10.3934/microbiol.2020001
Plettner, E., Slessor, K. N., Winston, M. L., and Oliver, J. E. (1996). Caste-selective pheromone biosynthesis in honeybees. Sci 271 (5257), 1851–1853. doi:10.1126/science.271.5257.1851
Pollet, S., Bottex-Gauthier, C., Li, M., Potier, P., Favier, A., and Vidal, D. (2002). Insight into some of the signaling pathways triggered by a lipid immunomodulator. Immunopharmacol Immunotoxicol 24 (4), 527–546. doi:10.1081/iph-120016034
Quan, Z., Zhang, L., Piao, H., and Song, Z. (1992). Synthesis of10-hydroxy-2-decenoic acid, 15. Journal of Medical Science Yanbian University. 19–20.
Robert, R. (1960). The biological activity and synthesis of royal jelly acid. Croat. Chem. Acta. 32 (3), 119–122.
Roden, M., Stingl, H., Chandramouli, V., Schumann, W. C., Hofer, A., Landau, B. R., et al. (2000). Effects of free fatty acid elevation on postabsorptive endogenous glucose production and gluconeogenesis in humans. Diabetes 49 (5), 701–707. doi:10.2337/diabetes.49.5.701
Saad Al Shehri, Z., Alanazi, A. D., and Alnomasy, S. F. (2023). Anti-cancer effects of queen bee acid (10-hydroxy-2-decenoic acid) and its cellular mechanisms against human hepatoma cells. Molecules 28 (4), 1972. doi:10.3390/molecules28041972
Sacks, D., and Noben-Trauth, N. (2002). The immunology of susceptibility and resistance to Leishmania major in mice. Nat. Rev. Immunol. 2 (11), 845–858. doi:10.1038/nri933
Šamšulová, V., Šedivá, M., Kóňa, J., Klaudiny, J., and Poláková, M. (2023). A comparison of the antibacterial efficacy of carbohydrate lipid-like (Thio)ether, sulfone, and ester derivatives against Paenibacillus larvae. Molecules 28 (6), 2516. doi:10.3390/molecules28062516
Šedivá, M., Laho, M., Kohútová, L., Mojžišová, A., Majtán, J., and Klaudiny, J. (2018). 10-HDA, a major fatty acid of royal jelly, exhibits ph dependent growth-inhibitory activity against different strains of Paenibacillus larvae. Molecules 23 (12), 3236. doi:10.3390/molecules23123236
Spannhoff, A., Kim, Y. K., Raynal, N. J., Gharibyan, V., Su, M. B., Zhou, Y. Y., et al. (2011). Histone deacetylase inhibitor activity in royal jelly might facilitate caste switching in bees. EMBO Rep. 12 (3), 238–243. doi:10.1038/embor.2011.9
Sugiyama, T., Takahashi, K., Tokoro, S., Gotou, T., Neri, P., and Mori, H. (2012). Inhibitory effect of 10-hydroxy-trans-2-decenoic acid on LPS-induced IL-6 production via reducing IκB-ζ expression. Innate Immun. 18 (3), 429–437. doi:10.1177/1753425911416022
Takahashi, K., Sugiyama, T., Tokoro, S., Neri, P., and Mori, H. (2012). Inhibition of interferon-γ-induced nitric oxide production by 10-hydroxy-trans-2-decenoic acid through inhibition of interferon regulatory factor-8 induction. Cell Immunol. 273 (1), 73–78. doi:10.1016/j.cellimm.2011.11.004
Terada, Y., Narukawa, M., and Watanabe, T. (2011). Specific hydroxy fatty acids in royal jelly activate TRPA1. J. Agric. Food Chem. 59 (6), 2627–2635. doi:10.1021/jf1041646
Tian, Y., Liu, X., Wang, J., Zhang, C., and Yang, W. (2024). Antitumor effects and the potential mechanism of 10-HDA against SU-DHL-2 Cells. Pharm. (Basel) 17 (8), 1088. doi:10.3390/ph17081088
Tsuchiya, Y., Hayashi, M., Nagamatsu, K., Ono, T., Kamakura, M., Iwata, T., et al. (2020). The key royal jelly component 10-hydroxy-2-decenoic acid protects against bone loss by inhibiting NF-κB signaling downstream of FFAR4. J. Biol. Chem. 295 (34), 12224–12232. doi:10.1074/jbc.RA120.013821
Usui, S., Soda, M., Iguchi, K., Abe, N., Oyama, M., Nakayama, T., et al. (2019). Down-regulation of aquaporin 9 gene transcription by 10-hydroxy-2-decenoic acid: a major fatty acid in royal jelly. Food Sci. and Nutr. 7 (11), 3819–3826. doi:10.1002/fsn3.1246
Villiéras, J., Rambaud, M., and Graff, M. (1985). Wittig-horner reaction in heterogenous media vii. A new strategy for the total syntheses of the royal jelly acid and the queen substance of honey-bee. Synth. Commun. 15 (7), 569–580. doi:10.1080/00397918508063842
Vucevic, D., Melliou, E., Vasilijic, S., Gasic, S., Ivanovski, P., Chinou, I., et al. (2007). Fatty acids isolated from royal jelly modulate dendritic cell-mediated immune response in vitro. Int. Immunopharmacol. 7 (9), 1211–1220. doi:10.1016/j.intimp.2007.05.005
Wang, L., Wang, L., Wang, R., Wang, Z., Wang, J., Yuan, H., et al. (2022). Efficient biosynthesis of 10-hydroxy-2-decenoic acid using a NAD(P)H regeneration P450 system and whole-cell catalytic biosynthesis. ACS omega 7 (21), 17774–17783. doi:10.1021/acsomega.2c00972
Wang, T., Wang, H., Liu, H., Wang, L., and Wang, R., (2006). Study on the extraction of 10-HDA from fermenting liquid. Liquor-Making Sci. and Technol. 27–29. doi:10.13746/j.njkj.2006.01.004
Watadani, R., Kotoh, J., Sasaki, D., Someya, A., Matsumoto, K., and Maeda, A. (2017). 10-hydroxy-2-decenoic acid, a natural product, improves hyperglycemia and insulin resistance in obese/diabetic KK-Ay mice, but does not prevent obesity. J. veterinary Med. Sci. 79 (9), 1596–1602. doi:10.1292/jvms.17-0348
Xu, D., Mei, X., and Xu, S. (2002). The research of 10-hydroxy-2-decenoic acid on experiment hyperlipoidemic rat. J. Chin. Med. Mater. 25 (5), 346–347. doi:10.13863/j.issn1001-4454.2002.05.023
Xu, H., Li, L., Wang, S., Wang, Z., Qu, L., Wang, C., et al. (2023). Royal jelly acid suppresses hepatocellular carcinoma tumorigenicity by inhibiting H3 histone lactylation at H3K9la and H3K14la sites. Phytomedicine 118, 154940. doi:10.1016/j.phymed.2023.154940
Yamaga, M., Imada, T., Tani, H., Nakamura, S., Yamaki, A., and Tsubota, K. (2021). Acetylcholine and royal jelly fatty acid combinations as potential dry eye treatment components in mice. Nutrients 13 (8), 2536. doi:10.3390/nu13082536
Yang, X. Y., Yang, D. S., Wei, Z., Wang, J. M., Li, C. Y., Hui, Y., et al. (2010). 10-Hydroxy-2-decenoic acid from Royal jelly: a potential medicine for RA. J. Ethnopharmacol. 128 (2), 314–321. doi:10.1016/j.jep.2010.01.055
Yang, Y. C., Chou, W. M., Widowati, D. A., Lin, I. P., and Peng, C. C. (2018). 10-hydroxy-2-decenoic acid of royal jelly exhibits bactericide and anti-inflammatory activity in human colon cancer cells. BMC Complement. Altern. Med. 18 (1), 202. doi:10.1186/s12906-018-2267-9
You, M., Miao, Z., Pan, Y., and Hu, F. (2019). Trans-10-hydroxy-2-decenoic acid alleviates LPS-induced blood-brain barrier dysfunction by activating the AMPK/PI3K/AKT pathway. Eur. J. Pharmacol. 865, 172736. doi:10.1016/j.ejphar.2019.172736
You, M., Miao, Z., Sienkiewicz, O., Jiang, X., Zhao, X., and Hu, F. (2020a). 10-Hydroxydecanoic acid inhibits LPS-induced inflammation by targeting p53 in microglial cells. Int. Immunopharmacol. 84, 106501. doi:10.1016/j.intimp.2020.106501
You, M., Miao, Z., Tian, J., and Hu, F. (2020b). Trans-10-hydroxy-2-decenoic acid protects against LPS-induced neuroinflammation through FOXO1-mediated activation of autophagy. Eur. J. Nutr. 59 (7), 2875–2892. doi:10.1007/s00394-019-02128-9
You, M., Pan, Y., Liu, Y., Chen, Y., Wu, Y., Si, J., et al. (2018). Royal jelly alleviates cognitive deficits and β-amyloid accumulation in APP/PS1 mouse model via activation of the camp/pka/creb/bdnf pathway and inhibition of neuronal apoptosis. Front. Aging Neurosci. 10, 428. doi:10.3389/fnagi.2018.00428
You, M., Wang, K., Pan, Y., Tao, L., Ma, Q., Zhang, G., et al. (2022). Combined royal jelly 10-hydroxydecanoic acid and aspirin has a synergistic effect against memory deficit and neuroinflammation. Food Funct. 13 (4), 2336–2353. doi:10.1039/d1fo02397g
Yu, X., Tu, X., Tao, L., Daddam, J., Li, S., and Hu, F. (2023). Royal jelly fatty acids: chemical composition, extraction, biological activity, and prospect. J. Funct. Foods. 111, 105868. doi:10.1016/j.jff.2023.105868
Zhang, Q., and Yang, S. (2014). Studies on purification of 10-hydroxy-2-decenoic acid from royal jelly by macroporous resins. Apic. China (65), 47–49.
Zhang, X., Tian, X., Wang, Y., Yan, Y., Wang, Y., Su, M., et al. (2024). Application of lipopolysaccharide in establishing inflammatory models. Int. J. Biol. Macromol. 279 (4), 135371. doi:10.1016/j.ijbiomac.2024.135371
Zhi, D., He, X., Yang, L., Xue, Y., Liu, Y., Yue, D., et al. (2025). Royal jelly acid alleviates diet-induced hyperlipidemia through regulation of oxidative stress and tryptophan metabolism. Eur. J. Pharmacol. 998, 177500. doi:10.1016/j.ejphar.2025.177500
Keywords: royal jelly acid, 10-HDA, fatty acids, function, pharmacological effects
Citation: Zhi D, He X, Xue Y, Zhao W, Gong X, Guo Y, Luo X, Tian Y and Dong K (2025) Royal jelly acid: preparation, metabolism and therapeutic potential. Front. Pharmacol. 16:1561351. doi: 10.3389/fphar.2025.1561351
Received: 15 January 2025; Accepted: 09 May 2025;
Published: 26 May 2025.
Edited by:
Ruyu Yao, Chinese Academy of Sciences (CAS), ChinaReviewed by:
Sharifah Alawieyah Syed Mortadza, Putra Malaysia University, MalaysiaAlisson Mácario de Oliveira, State University of Paraíba, Brazil
Copyright © 2025 Zhi, He, Xue, Zhao, Gong, Guo, Luo, Tian and Dong. This is an open-access article distributed under the terms of the Creative Commons Attribution License (CC BY). The use, distribution or reproduction in other forums is permitted, provided the original author(s) and the copyright owner(s) are credited and that the original publication in this journal is cited, in accordance with accepted academic practice. No use, distribution or reproduction is permitted which does not comply with these terms.
*Correspondence: Yakai Tian, dHlremhvbmdAZm94bWFpbC5jb20=; Kun Dong, ZG9uZ2t1bjE5NzIyMDA0QGFsaXl1bi5jb20=
†These authors have contributed equally to this work