- Division of Neurophysiology and Neuropharmacology, Center for Physiology and Pharmacology, Medical University of Vienna, Vienna, Austria
Introduction: Peripheral nerve endings of dorsal root ganglion (DRG) neurons act as nociceptors and generate action potentials in response to noxious stimuli. Primary cultures of dissociated DRG have been used extensively to study changes neuronal excitability caused by either analgesics or pathological conditions, such as inflammation. The dissociation procedure can be viewed as a form of axotomy, and one might expect a resulting increase in excitability of the neurons during the subsequent culture period. However, changes in firing properties of DRG neurons over time in vitro have not been investigated systematically.
Methods: Thus, the current experiments compared action potential firing in dissociated DRG neurons after one to 7 days in culture and examined Gaussian white noise as novel stimulation paradigm. Primary cultures of DRG neurons were recorded in perforated patch current-clamp. Action potentials were evoked either by a sequence of five rectangular current pulses with increasing amplitudes or by Gaussian white noise of varying RMS amplitudes and frequencies.
Results: Conventional rectangular current injections triggered 19
Discussion: These results demonstrate a decrease in the excitability of DRG neurons from day one to 7 after dissociation and reveal Gaussian white noise as reliable trigger of action potential firing in these neurons.
1 Introduction
The sensation of pain, with the exception of central neuropathic pain, starts at the level of nociceptive neurons. The peripheral nerve endings of these act as nociceptors, i.e., sensors that are able to transduce noxious stimuli into generator potentials which are then transformed into trains of action potentials (Loeser and Treede, 2008). In general, the more action potentials are generated in peripheral nociceptive neurons the more intense the accompanying pain sensation will be, and this holds true for nociceptive as well as inflammatory or neuropathic pain (Middleton et al., 2021). The somata of these neurons are located in trigeminal or dorsal root ganglia (DRG) and are thus easily accessible. Accordingly, numerous publications have investigated action potential firing in neurons of these ganglia in vitro, be it within intact ganglia or in dissociated primary cultures (Körner and Lampert, 2022).
Dissociated primary cultures of sensory ganglia are extremely versatile experimental models, as they do not only offer direct access for patch-clamp investigations of ion channels that control the firing of action potentials and thus neuronal excitability (Körner and Lampert, 2022). They can even be used to mimic pathologic conditions that lead to chronic forms of pain, such as inflammatory or neuropathic pain. With respect to the former, cultures can be exposed to algogenic mediators that are collectively known as inflammatory soup (i.s.; Basbaum et al. (2009). Whether bradykinin (Liu et al., 2010), ATP, or other nucleotides (Yousuf et al., 2011), or serotonin (Salzer et al., 2016) are used, in their presence, DRG neurons fire many more action potentials than in their absence. With respect to neuropathic pain, cultures can be treated, for instance, with the anticancer agent paclitaxel, which causes chemotherapy-induced peripheral neuropathy in human patients and rats (Polomano et al., 2001). This induces a neuropathic phenotype in DRG cultures that is characterized by enhanced neuronal excitability (Li et al., 2021). While such algogenic conditions boost excitability of nociceptive neurons, analgesics lead to the opposite phenomenon. For example, gabapentin, a standard drug in the treatment of neuropathic pain, reduces action potential firing in DRG neurons (Yang et al., 2009). Likewise, N-acetyl-p-benzoquinone imine, an active metabolite of the widely used analgesic paracetamol, has been reported to diminish excitability in such neurons (Ray et al., 2019). Most recently, a high throughput screening platform for the identification of novel analgesics has been devised that is based on optical recordings of action potentials in primary cultures of rat DRG (Liu et al., 2024).
During the preparation of dissociated primary DRG cultures, neuronal cell bodies are separated quite harshly from the axon. Accordingly, the dissociation procedure has been viewed as a model of axotomy, and dissociated DRG cultures were used to investigate consequences of this type of nerve injury. Along this line, muscle lim protein is induced in L4/L5 DRGs after sciatic nerve crush and its expression sharply increases in cultures of DRGs from day 1–5 after dissociation (Levin et al., 2017). Similarly, GTP cyclohydrolase 1 is induced in DRG neurons after nerve injury and in cultures from day 1–4 post dissociation (Cronin et al., 2022). The latter enzyme is rate-limiting in the de novo synthesis of tetrahydrobiopterin which contributes to neuropathic pain. Accordingly, one would expect that the time-dependent rise in protein expression would be paralleled by an increment in membrane excitability when dissociated DRG neurons are kept in culture for increasing periods of time, but this has not been tested (Cronin et al., 2022; Levin et al., 2017).
In principle, action potential firing in DRG neurons is characterized as either phasic or tonic: phasic firing means that only a single or a few action potentials (<4) can be seen at the beginning of a sustained injection (>0.5 s) of depolarizing currents, whereas >5 action potentials are evenly distributed throughout current injection during tonic firing. Using this categorization, several phenotypic features of DRG neurons, such as isolectin B4 positivity (Choi et al., 2007) or the expression of markers of nociceptive function (Viatchenko-Karpinski and Gu, 2016), have been found to correlate with the proportion of neurons that fire in either a phasic or a tonic manner. In addition, the firing properties have been assessed either one or 3–5 days after dissociation, and the percentage of neurons firing tonically was found to be 30 to 40 after one (Ditting et al., 2009; 2016; Lale et al., 2023) vs. <20 after 3–5 days (Sculptoreanu and de Groat, 2007; Sculptoreanu et al., 2009) in culture. Thus, excitability of DRG neurons appears to decrease rather than increase the longer the neurons are kept in culture after dissociation.
To clarify this issue, the current experiments compared firing properties of DRG neurons one, 3 to 4, and 7–10 days after dissociation. After 1 week, virtually none of the neurons investigated was able to fire more than one single action potential during prolonged current injections, and this firing pattern was not altered by inflammatory soup constituents. To reveal whether this apparent limitation of current pulse injections might be overcome by an alternative method of eliciting action potential firing in sensory neurons, Gaussian white noise stimulation was examined. This method has the advantage of delivering wave forms in random order without prejudgment as to their efficacies of triggering action potentials and it has been reported to reliably elicit firing in Aplysia neurons (Bryant and Segundo, 1976) and squid giant axons (Guttman et al., 1974). The results reveal that white noise stimulation is able to generate action potentials at constant frequencies in DRG neurons after 7 days in vitro (DIV) that otherwise produce only a single action potential in response to steady current injections, and this white noise-induced firing is modulated under pro-as well as analgesic conditions.
2 Materials and methods
2.1 Materials
Dimethyl sulfoxide (DMSO), amiloride, retigabine and bulk chemicals for electrophysiological solutions (see 2.3 Electrophysiology) were ordered from Sigma-Aldrich (Vienna, Austria). Retigabine stock solutions of 10 mM in DMSO were prepared and further diluted with external solution to a final concentration of 10
2.2 Primary cultures of rat dorsal root ganglion (DRG) neurons and viral transduction
Primary cultures of DRG neurons were prepared as described before (Ray et al., 2019) in full accordance with the Austrian animal protection law and animal experiment by-laws. Sprague-Dawley rat pups (RRID: MGI:5651135) of both sexes were decapitated 10–15 days after birth. Dissection of dorsal root ganglia was achieved by opening the spine with two longitudinal cuts in the sagittal plane through the vertebral bodies (ventral) and arches (dorsal) and then moving the spinal cord to the side. After isolation, the ganglia were incubated in a collagenase (1.5 mg
2.3 Electrophysiology
Current-clamp recordings were conducted at room temperature (20–24
To test for TRPV1- or ASIC-positive DRG neurons, non-spontaneously firing small- or medium-sized cells (membrane capacitance <30 pF) were clamped to a membrane potential of −70 mV and, every 3 min, superfused with a low pH external solution for 15 s, which elicited an inward current in the majority of cells. If this current was blocked by 10
DRG neuron excitability was first recorded in solvent (DMSO 0.1%) followed by perfusion with an artificial inflammatory soup consisting of ADP (1
2.4 Calcium imaging
Calcium imaging experiments were conducted on a Nikon Eclipse Ti2 microscope with a 40 × 1.25 NA water immersion objective (Nikon, CEE, Vienna, Austria). Neurons expressing jGCamP-8s were excited at 490 nm (pE4000 CoolLed, Andover, United Kingdom) and emission was read out using a GFP filter (excitation filter: 470/40 nm, dichroic: 500 nm, emission filter: 535/50 nm; Semrock, NY, United States). Image acquisition was conducted using an electron-multiplying charge-coupled device (EMCCD) camera (iXon 888 Ultra, Oxford Instruments, Oxford, United Kingdom) in 16-bit mode, at 10-MHz readout speed, EM Gain of 40, and an exposure time of 20 ms, recording one frame every 1 s. Solutions were applied using an Octaflow 2 perfusion system (ALA Scientific Instruments Inc., Clarksburg, NJ, United States), which allows for solution exchange around the cell within 50–150 ms. Cells were perfused using the same external solution as in patch clamp experiments. Calcium transients were triggered during two 20 s periods, and the addition of either 20 mM KCl or 300 nM capsaicin to the external solution was used as stimulation paradigm. Image series were analyzed with ImageJ (Schindelin et al., 2012). First, images were background corrected by subtracting the average intensity of a cell-free region of interest for each image.
2.5 Data analysis and statistics
For current pulse injections, action potentials were counted with the “Threshold Search” function of the Clampfit 10.7 software by setting a threshold value, which was in the range of 30–90 mV more positive to the resting membrane potential. Signals that exceeded that value were regarded as action potentials. The threshold value was chosen individually for each neuron depending on the amplitude of the stimulation pulse. For the analysis of white noise stimulation recordings, traces were first filtered with an 8-pole 600 Hz high-pass Bessel filter to remove the noise. The remaining signals, which corresponded to action potentials, were then detected and counted using the “Template Search” function of the Clampfit 10.7 software (Figure 4A).
Data are shown as arithmetic means
3 Results
3.1 Loss of responsiveness of DRG neurons towards conventional current pulse injections and inflammtory soup constituents during a 7 days culture period
Primary cultures of DRG neurons were recorded in perforated current-clamp mode and subjected to a conventional current-injection pulse protocol. After determination of the rheobases for each individual neuron, action potentials were evoked by injection of five current pulses with increasing amplitudes (1 x, 1.5 x, 2 x, 2.5 x, 3 x), each lasting for 2 s. First, this protocol was applied to neurons (n = 9) within 24 h after preparation (DIV 1): 7 out of 9 neurons displayed tonic action potential firing, 2 showed a phasic firing pattern. On average, DIV 1 neurons fired 19.56
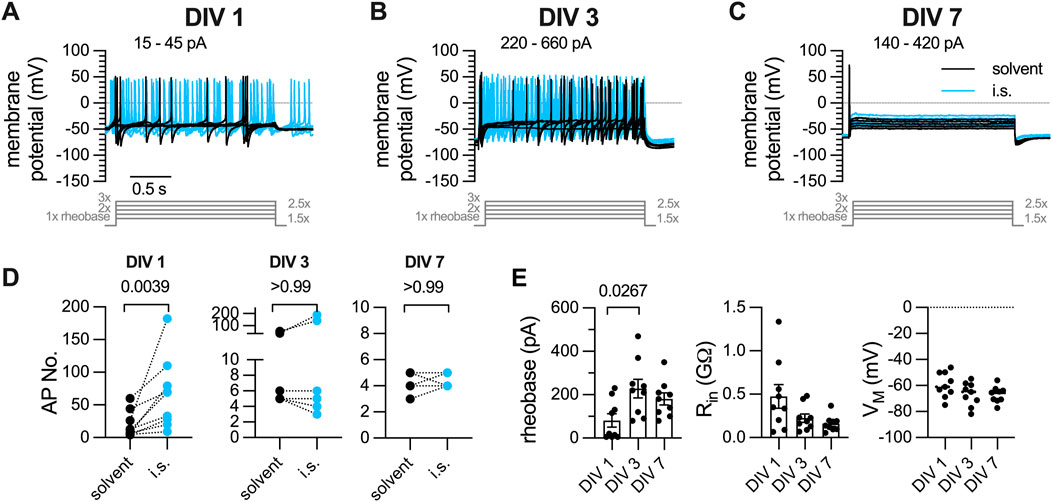
Figure 1. Action potential firing elicited by current pulse injections in DRG neurons after one, three, or 7 days in vitro (DIV). DRG neurons were recorded in perforated current-clamp mode; inflammatory soup (i.s.: ADP 1
A solution mimicking the so-called inflammatory soup (i.s.; Basbaum et al. (2009)) consisting of ADP (1
3.2 responses of DRG neurons are maintained during a 7-day culture period
To reveal whether the apparent decline in membrane excitability might extend to other archetypal responses,
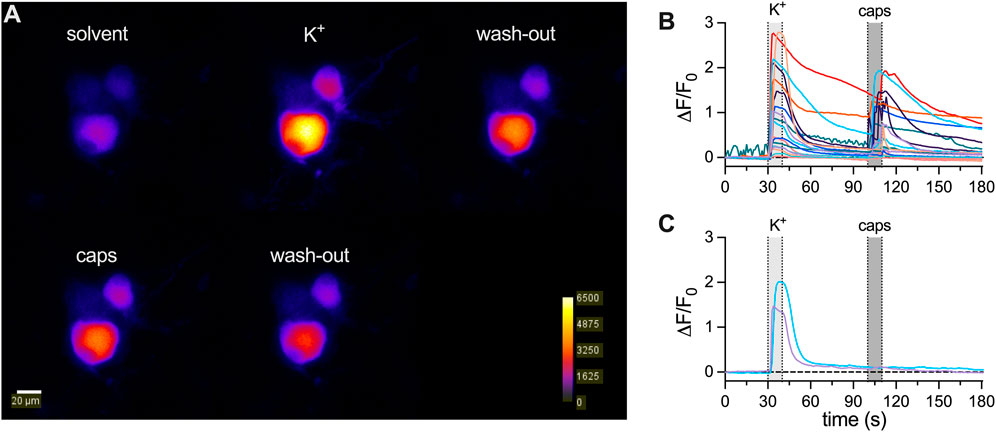
Figure 2.
3.3 Action potential firing of DRG neurons in response to white noise stimulation and inflammatory soup constituents is maintained during a 7-day culture period
The discrepancy between maintained
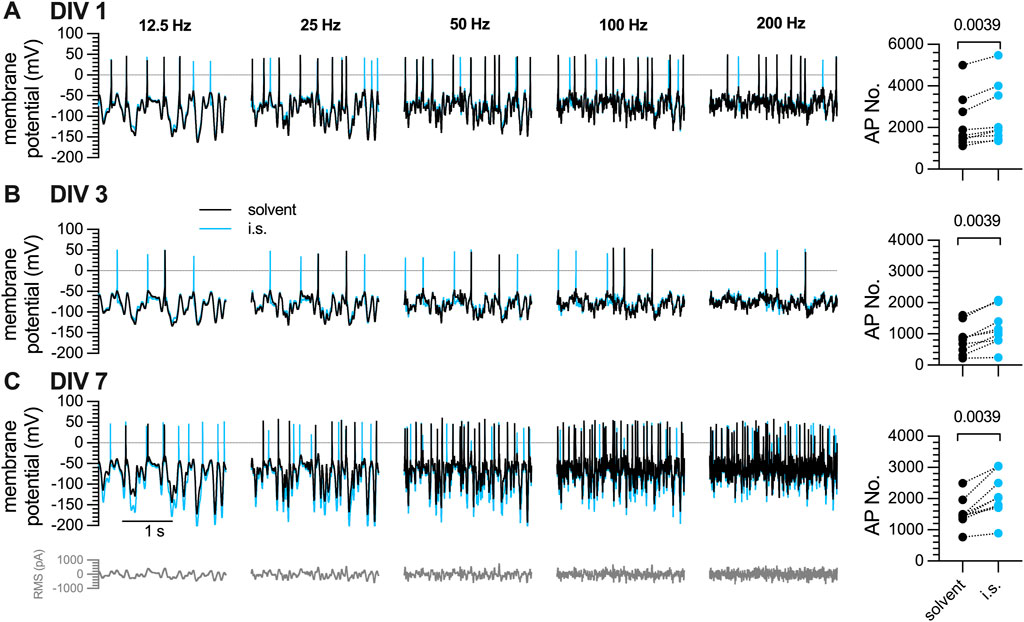
Figure 3. Action potential firing in response to white noise stimuli in DRG neurons after one, three, or 7 days in vitro (DIV). The same set of DRG neurons as in Figure 1 were subjected to white noise currents with RMS amplitudes of 50 pA–350 pA in 50 pA increments and their frequencies ranged from 12.5 Hz, 25 Hz, 50 Hz, 100 Hz, to 200 Hz, as indicated. [(A–C), left panels] The traces shown were obtained with a current amplitude of 200 pA either in solvent (black traces) or in presence of inflammatory soup components (blue traces) (A) after 1 day in vitro (DIV 1), (B) after 3 days in culture (DIV 3), and (C) after 7 days in vitro (DIV 7). [(A–C), right panel] shows the statistical analysis of all action potentials triggered in (A) 1 DIV, (B) DIV 3, and (C) 7 DIV neurons by white noise stimulation in presence of solvent (black circles; n = 9) vs. inflammatory soup (i.s., blue circles; n = 9); the level of statistical significance evaluated by a Wilcoxon matched-pairs signed rank test is indicated above the symbols.
3.4 Semi-automated detection of action potentials triggered by Gaussian white noise stimuli
The Gaussian white noise stimulation protocol triggered an average of 2,285
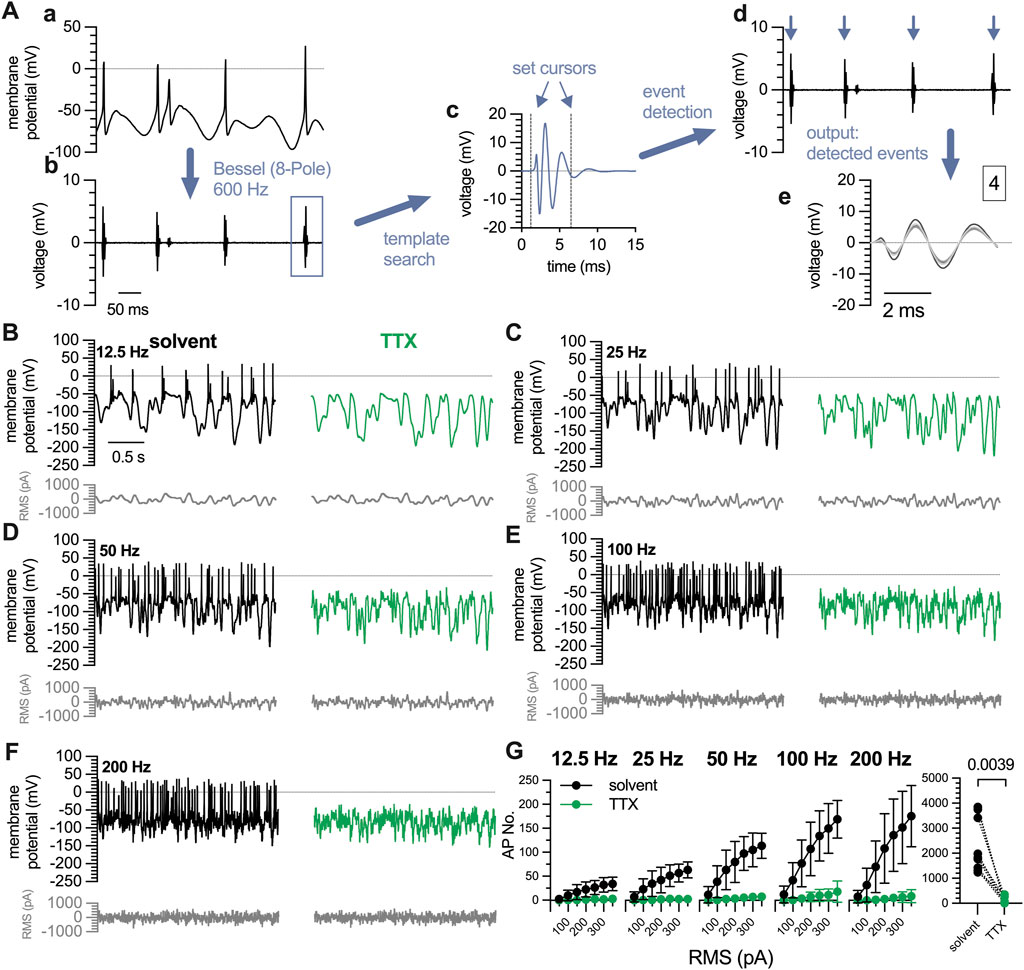
Figure 4. Characterization of action potentials triggered by white noise stimulation in 7 DIV DRG neurons. A complete perforated current-clamp recording consists of 35 traces (10 s each) recorded at filter frequencies of 12.5 Hz, 25 Hz, 50 Hz, 100 Hz, and 200 Hz with RMS amplitudes ranging from 50 pA to 350 pA, in 50 pA increments. (A) Displays the quantification of action potentials triggered by white noise stimulation. (a) For clarity, only 0.5 s of a representative 10 s trace recorded at 12.5 Hz and 200 pA are shown. (b) Traces are filtered using a Bessel (8-pole) high-pass filter at a cut-off frequency of 600 Hz. (c) Then a template search is conducted in Clampfit 10.7 using the template shown in (c). The cursors defining the beginning and the end of the template can be adjusted manually as indicated by the blue arrows. (d) Using this template, events are detected automatically, indicated by blue arrows, and are available for visual inspection. (e) The output window displays all detected events in expanded time scale and indicates the total number of events (here 4). (B–F) show exemplary traces obtained in the very same neuron using a white noise stimulation protocol as indicated in grey below each trace. The white noise currents had RMS amplitudes of 50 pA–350 pA with 50 pA increments and their frequencies ranged from (C) 12.5 Hz, (D) 25 Hz, (E) 50 Hz, (F) 100 Hz, to (G) 200 Hz. The traces shown were obtained with a current amplitude of 200 pA either in solvent (black traces) or in presence of tetrodotoxin (TTX, 1
To probe the validity of the Gaussian white noise trigger and this semi-automated detection method, action potentials were first recorded under standard conditions and thereafter in presence of tetrodotoxin (TTX, 1
3.5 White noise stimulation but not conventional pulse protocols permit the detection of analgesic drug actions
The Gaussian white noise stimulation presented above consisted of 35 individual stimuli lasting for 10 s each, which summed up to a total duration of 350 s. Thus, in time course experiments one could obtain only one value per 6 min. To enable the detection of time-dependent effects of drugs on action potential firing, a modified white noise protocol was generated: this covered stimulation frequencies of 12.5 Hz, 25 Hz, 50 Hz, 100 Hz, and 200 Hz and RMS amplitudes reduced to values from 100 pA to 300 pA in 50 pA increments. This resulted in 25 individual stimuli with a duration of 2.5 s each, summing up to a total stimulation time of 62.5 s. Alternating with this shortened white noise protocol, a conventional pulse protocol was used. This consisted of 5 individual current injections lasting for 3 s each, ranging from 1 x, 1.5 x, 2 x, 2.5 x to 3 x rheobases; this gave a total stimulus time of 15 s (Figures 5A, B). Using this approach, rather stable numbers of action potentials can be triggered over a time period of 10 min in presence of solvent (DMSO 0.1%, Figure 5G). To test the sensitivity of this approach towards inhibitory actions of drugs, the
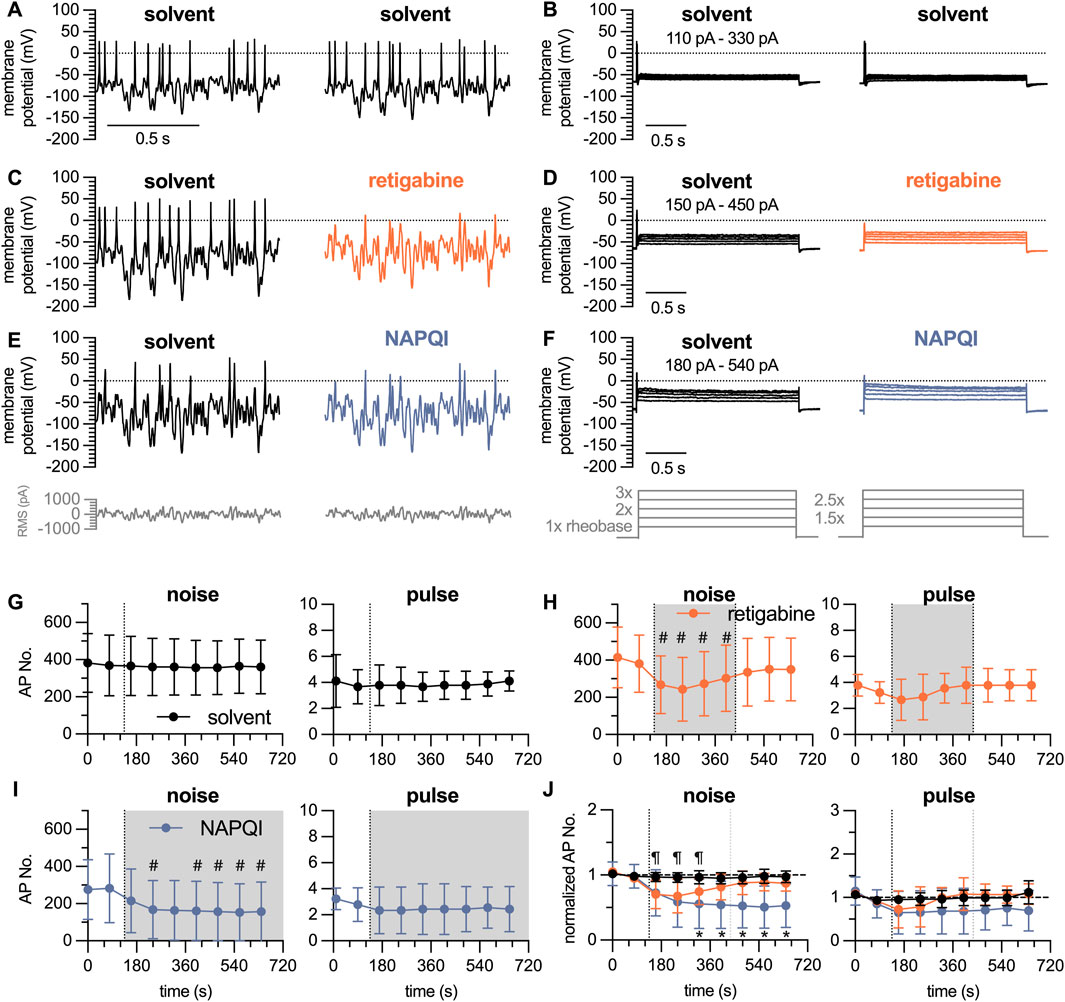
Figure 5. Time course of the effects of analgesics on action potential firing in DIV 7 DRG neurons analyzed by white noise stimulation vs. current pulses. The white noise protocol (noise) consisted of stimulation frequencies ranging from 12.5 Hz, 25 Hz, 50 Hz, 100 Hz and 200 Hz with RMS amplitudes of 100 pA–300 pA in 50 pA increments; each individual stimulus lasted for 2.5 s to give a total white noise stimulation period of approximately 1 minute. This was followed by a 5-step current pulse protocol (pulse) ranging from 1 x to 3 x rheobases with 0.5 x increments, each step lasting for a total of 3 s. After establishing baseline values during a 150 s period (two noise protocols and two pulse protocols) in solvent (DMSO 0.1%), solvent or retigabine were present for 300 s followed by a 300 s wash out period in solvent; alternatively, NAPQI was present for 600 s (A–F) show representative traces in presence of (A, B) solvent only, (C, D) solvent followed by retigabine (10
The paracetamol metabolite N-acetyl-p-benzoquinone imine (NAPQI) has been found to cause a slowly developing decrease in the number of action potentials fired by DIV 1 DRG neurons via a time-dependent irreversible increase in currents through
4 Discussion
Effects of various agents on membrane excitability in neurons can be studied by current clamp recordings. Traditionally, action potential firing is triggered by protocols that inject rectangular current pulses that stay constant over time periods of hundreds of milliseconds or even a few seconds (Hodgkin, 1948; Spindler et al., 1999; Zaika et al., 2006; Dominguez-Rodriguez et al., 2017). This, however, is quite different from the in vivo situation in the nervous system where a single neuron can receive multiple input signals via synaptic connections from other neurons, i.e., excitatory and inhibitory postsynaptic potentials which are then spatially and temporally integrated to generate an output response (Eccles et al., 1966; Chavas and Marty, 2003). Gaussian white noise generates random alternating depolarizing and hyperpolarizing stimuli in the range of a few milliseconds and thereby aims to mimic these physiological conditions. Excitation of nociceptors occurs through stimuli that impinge as mechanical, thermal and/or chemical signals on a group of approximately 30 different transducers. These signals accumulate and dissipate in the vicinity of nociceptors in a random manner and thus lead to a random pattern of generator potentials that are then transformed into action potentials (Reichling and Levine, 1999; Gold and Gebhart, 2010). Again, this random mode of excitation is likely to be mimicked better by Gaussian white noise rather than rectangular current pulses. Additionally, a prolonged depolarizing stimulus leads to voltage-dependent inactivation of voltage-gated sodium channels (Goldman and Schauf, 1972), and their recovery from inactivation is dependent on the extent of a subsequent hyperpolarization (Kuo and Bean, 1994). Therefore, the proportion of inactivated sodium channels must be expected to be larger during injection of current pulses as compared to a white noise stimulus that includes intermittent hyperpolarizations. Based on these considerations, the present experiments employed Gaussian white noise to trigger action potentials in DRG neurons.
Dissociated cultures of DRGs have been used to analyze mechanisms and consequences of axotomy and were found to express injury related proteins in a time-dependent manner, with low levels immediately after dissociation and a rise during the subsequent days in culture (Levin et al., 2017; Cronin et al., 2022). As axonal injury can lead to the development of neuropathic pain (Testa et al., 2024), one would expect that dissociated DRG neurons experience an increase in excitability during extended periods of culturing in parallel with the appearance of axotomy-induced proteins. However, the present data provide evidence to the opposite: DIV 1 neurons fired 19 action potentials during 2 s current injections with amplitudes corresponding to 1 x, 1.5 x, 2 x, 2.5 x, 3 x rheobases and an average rheobases of 81 pA; DIV 3 neurons fired 14 action potentials with an average rheobases of 227 pA, DIV 7 neurons fired 4 action potentials with an average rheobases of 180 pA. Thus, membrane excitability of sensory neurons progressively decreases as they are kept in culture for several days to 1 week.
The tendency of DRG neurons to fire action potentials in response to appropriate stimuli is not fixed, but rather influenced by various parameters such as, for instance, temperature (Jia et al., 2012). More relevant in terms of pathology and therapy is the impact of algogenic and/or analgesic agents on the excitability of DRG neurons. In principle, this excitability is enhanced by toxicants that cause peripheral neuropathy (Li et al., 2021) or mediators of inflammation (Basbaum et al., 2009) and reduced by analgesic drugs (Yang et al., 2009; Ray et al., 2019). Here, numbers of action potentials fired in response to conventional current pulse injections were increased by a mixture of inflammatory mediators in DIV 1, but neither DIV 3 nor DIV 7 neurons. Hence, after 1 day in culture, DRG neurons not only become more resistant towards stimuli that are usually well suited to trigger action potentials, they also lose their capability to react to constituents of the so-called inflammatory soup (Basbaum et al., 2009). Moreover, when neurons can fire only one action potential per current pulse injection, any analgesic drug is rather unlikely to be able to exert inhibitory actions on the firing frequency. Thus, DIV 3 and DIV 7 cultures have lost their potential to reliably display drug-induced changes in excitability when stimulated by injection of conventional current pulses.
As alternative method of triggering action potentials in DRG neurons in primary culture, white noise stimulation was employed. Application of the generated waveforms elicited action potentials in a highly reliable manner over extended periods of time. Filter frequencies and RMS amplitudes of the white noise stimuli were changed to evaluate effects of these parameters on neuronal excitability. Higher frequencies and rising RMS amplitudes led to greater numbers of action potentials. Nevertheless, the general firing pattern remained comparable across different filter frequencies in the same cell (Figure 4). Moreover, numbers of action potentials fired in response to white noise stimulation remained stable over periods of 10 min or even more (Figure 5G), and after normalization, the variability between neurons was limited and by far less pronounced when compared to the results obtained with current pulse injection (Figure 5J). Thus, Gaussian white noise appears to be a very reliable trigger of action potential firing in DRG neurons.
Despite the reliability of white noise stimulation, the resulting firing of action potentials readily responded to the presence of agents that are expected to modulate membrane excitability of DRG neurons. This was not only true for the inflammatory mediators ADP, ATP, bradykinin, histamine, prostaglandin
Taken together, the experiments shown above establish Gaussian white noise as reliable method of eliciting action potential firing in sensory neurons that appears to be even more similar to the physiological situation as current pulse injection. By this stimulation paradigm, the apparent loss of excitability of DRG neurons due to extended culturing periods as observed with current pulse injection can be overcome and at the same time the reactivity of action potential firing towards algogenic and analgesic agents is preserved.
Data availability statement
The raw data supporting the conclusions of this article will be made available by the authors, without undue reservation.
Ethics statement
Ethical approval was not required for the study involving animals in accordance with the local legislation and institutional requirements because research was conducted in primary cultures of dorsal root ganglion neurons of wildtype Sprague-Dawley rats. The animals were humanely sacrificed and the tissue was removed subsequently. According to Austrian Law, no ethics approval by a local animal welfare committee is required.
Author contributions
TL: Formal Analysis, Investigation, Methodology, Visualization, Writing – review and editing. KS: Conceptualization, Data curation, Methodology, Software, Writing – review and editing. KH: Writing – review and editing, Funding acquisition, Formal analysis, Data curation. SB: Data curation, Writing – original draft, Writing – review and editing. IS: Conceptualization, Funding acquisition, Project administration, Resources, Supervision, Validation, Visualization, Writing – original draft, Writing – review and editing.
Funding
The author(s) declare that financial support was received for the research and/or publication of this article. The present work was supported by the Austrian Science Fund (FWF; P32639-B to IS, and P35542-B and P35878-B to KH).
Acknowledgments
The perfect technical assistance of Tanja Wagner, Gabi Gaupmann, and Jarmilla Uhrinova is gratefully acknowledged.
Conflict of interest
The authors declare that the research was conducted in the absence of any commercial or financial relationships that could be construed as a potential conflict of interest.
The author(s) declared that they were an editorial board member of Frontiers, at the time of submission. This had no impact on the peer review process and the final decision.
Generative AI statement
The author(s) declare that no Generative AI was used in the creation of this manuscript.
Publisher’s note
All claims expressed in this article are solely those of the authors and do not necessarily represent those of their affiliated organizations, or those of the publisher, the editors and the reviewers. Any product that may be evaluated in this article, or claim that may be made by its manufacturer, is not guaranteed or endorsed by the publisher.
Supplementary material
The Supplementary Material for this article can be found online at: https://www.frontiersin.org/articles/10.3389/fphar.2025.1561905/full#supplementary-material
Abbreviations
AAV, adeno-associated viral; DIV, days in vitro; DRG, dorsal root ganglion; FBS-hi, fetal bovine serum - heat inactivated; i. s., inflammatory soup; NAPQI, n-acetyl-p-benzoquinone imine; MES, 2-(N-morpholino)ethanesulfonic acid; RMS, root mean square; TTX, tetrodotoxin.
References
Alexander, S. P. H., Mathie, A. A., Peters, J. A., Veale, E. L., Striessnig, J., Kelly, E., et al. (2023). The concise guide to pharmacology 2023/24: ion channels. Br. J. Pharmacol. 180 (Suppl. 2), S145–S222. doi:10.1111/bph.16178
Basbaum, A. I., Bautista, D. M., Scherrer, G., and Julius, D. (2009). Cellular and molecular mechanisms of pain. Cell 139, 267–284. doi:10.1016/j.cell.2009.09.028
Bryant, H. L., and Segundo, J. P. (1976). Spike initiation by transmembrane current: a white-noise analysis. J. Physiol. 260, 279–314. doi:10.1113/jphysiol.1976.sp011516
Chavas, J., and Marty, A. (2003). Coexistence of excitatory and inhibitory gaba synapses in the cerebellar interneuron network. J. Neurosci. 23, 2019–2031. doi:10.1523/JNEUROSCI.23-06-02019.2003
Choi, J.-S., Dib-Hajj, S. D., and Waxman, S. G. (2007). Differential slow inactivation and use-dependent inhibition of nav1.8 channels contribute to distinct firing properties in ib4+ and ib4-drg neurons. J. Neurophysiol. 97, 1258–1265. doi:10.1152/jn.01033.2006
Cronin, S. J. F., Rao, S., Tejada, M. A., Turnes, B. L., Licht-Mayer, S., Omura, T., et al. (2022). Phenotypic drug screen uncovers the metabolic gch1/bh4 pathway as key regulator of egfr/kras-mediated neuropathic pain and lung cancer. Sci. Transl. Med. 14, eabj1531. doi:10.1126/scitranslmed.abj1531
Ditting, T., Freisinger, W., Rodionova, K., Schatz, J., Lale, N., Heinlein, S., et al. (2016). Impaired excitability of renal afferent innervation after exposure to the inflammatory chemokine cxcl1. Am. J. Physiol. Ren. Physiol. 310, F364–F371. doi:10.1152/ajprenal.00189.2015
Ditting, T., Tiegs, G., Rodionova, K., Reeh, P. W., Neuhuber, W., Freisinger, W., et al. (2009). Do distinct populations of dorsal root ganglion neurons account for the sensory peptidergic innervation of the kidney? Am. J. Physiol. Ren. Physiol. 297, F1427–F1434. doi:10.1152/ajprenal.90599.2008
Dominguez-Rodriguez, M., Drobny, H., Boehm, S., and Salzer, I. (2017). Electrophysiological investigation of the subcellular fine tuning of sympathetic neurons by hydrogen sulfide. Front. Pharmacol. 8, 522. doi:10.3389/fphar.2017.00522
Eccles, J. C., Llinás, R., and Sasaki, K. (1966). The excitatory synaptic action of climbing fibres on the purkinje cells of the cerebellum. J. Physiol. 182, 268–296. doi:10.1113/jphysiol.1966.sp007824
Gold, M. S., and Gebhart, G. F. (2010). Nociceptor sensitization in pain pathogenesis. Nat. Med. 16, 1248–1257. doi:10.1038/nm.2235nm.2235
Goldman, L., and Schauf, C. L. (1972). Inactivation of the sodium current in myxicola giant axons. evidence for coupling to the activation process. J. Gen. Physiol. 59, 659–675. doi:10.1085/jgp.59.6.659
Guttman, R., Feldman, L., and Lecar, H. (1974). Squid axon membrane response to white noise stimulation. Biophys. J. 14, 941–955. doi:10.1016/S0006-3495(74)85961-8
Hodgkin, A. L. (1948). The local electric changes associated with repetitive action in a non-medullated axon. J. Physiol. 107, 165–181. doi:10.1113/jphysiol.1948.sp004260
Jia, Z., Ling, J., and Gu, J. G. (2012). Temperature dependence of rapidly adapting mechanically activated currents in rat dorsal root ganglion neurons. Neurosci. Lett. 522, 79–84. doi:10.1016/j.neulet.2012.06.024
Körner, J., and Lampert, A. (2022). Functional subgroups of rat and human sensory neurons: a systematic review of electrophysiological properties. Pflugers Arch. 474, 367–385. doi:10.1007/s00424-021-02656-6
Kuo, C. C., and Bean, B. P. (1994). Na+ channels must deactivate to recover from inactivation. Neuron 12, 819–829. doi:10.1016/0896-6273(94)90335-2
Lale, N., Ditting, T., Hilgers, K. F., Linz, P., Ott, C., Schmieder, R. E., et al. (2023). Afferent neurons of the kidney with impaired firing pattern in inflammation - role of sodium currents? Pflugers Arch. 475, 1329–1342. doi:10.1007/s00424-023-02852-6s00424-023-02852-6
Levin, E., Andreadaki, A., Gobrecht, P., Bosse, F., and Fischer, D. (2017). Nociceptive drg neurons express muscle lim protein upon axonal injury. Sci. Rep. 7, 643. doi:10.1038/s41598-017-00590-1
Li, Y., Marri, T., North, R. Y., Rhodes, H. R., Uhelski, M. L., Tatsui, C. E., et al. (2021). Chemotherapy-induced peripheral neuropathy in a dish: dorsal root ganglion cells treated in vitro with paclitaxel show biochemical and physiological responses parallel to that seen in vivo. Pain 162, 84–96. doi:10.1097/j.pain.0000000000002005
Liu, B., Linley, J. E., Du, X., Zhang, X., Ooi, L., Zhang, H., et al. (2010). The acute nociceptive signals induced by bradykinin in rat sensory neurons are mediated by inhibition of m-type k+ channels and activation of ca2+-activated cl-channels. J. Clin. Invest 120, 1240–1252. doi:10.1172/JCI41084
Liu, P. W., Zhang, H., Werley, C. A., Pichler, M., Ryan, S. J., Lewarch, C. L., et al. (2024). A phenotypic screening platform for chronic pain therapeutics using all-optical electrophysiology. Pain 165, 922–940. doi:10.1097/j.pain.00000000000030900000000000003090
Loeser, J. D., and Treede, R.-D. (2008). The kyoto protocol of iasp basic pain terminology. Pain 137, 473–477. doi:10.1016/j.pain.2008.04.025pain.2008.04.025
Losgott, T., Kudlacek, O., Yang, J.-W., Schicker, K. W., Boehm, S., and Salzer, I. (2025). The paracetamol metabolite n-acetyl-4-benzoquinoneimine (napqi) prevents modulation of kv7 channels via g-protein coupled receptors by interference with pip2 and ca2+ sensitivity. Br. J. Pharmacol. 182, 1341–1357. doi:10.1111/bph.17419
Middleton, S. J., Barry, A. M., Comini, M., Li, Y., Ray, P. R., Shiers, S., et al. (2021). Studying human nociceptors: from fundamentals to clinic. Brain 144, 1312–1335. doi:10.1093/brain/awab048
Passmore, G. M., Selyanko, A. A., Mistry, M., Al-Qatari, M., Marsh, S. J., Matthews, E. A., et al. (2003). Kcnq/m currents in sensory neurons: significance for pain therapy. J. Neurosci. 23, 7227–7236. doi:10.1523/JNEUROSCI.23-18-07227.2003
Polomano, R. C., Mannes, A. J., Clark, U. S., and Bennett, G. J. (2001). A painful peripheral neuropathy in the rat produced by the chemotherapeutic drug, paclitaxel. Pain 94, 293–304. doi:10.1016/S0304-3959(01)00363-3
Ray, S., Salzer, I., Kronschläger, M. T., and Boehm, S. (2019). The paracetamol metabolite n-acetylp-benzoquinone imine reduces excitability in first- and second-order neurons of the pain pathway through actions on kv7 channels. Pain 160, 954–964. doi:10.1097/j.pain.0000000000001474
Reichling, D. B., and Levine, J. D. (1999). The primary afferent nociceptor as pattern generator. Pain Suppl. 6, S103–S109. doi:10.1016/S0304-3959(99)00143-8
Salzer, I., Gantumur, E., Yousuf, A., and Boehm, S. (2016). Control of sensory neuron excitability by serotonin involves 5ht2c receptors and ca(2+)-activated chloride channels. Neuropharmacology 110, 277–286. doi:10.1016/j.neuropharm.2016.08.006
Salzer, I., Ray, S., Schicker, K., and Boehm, S. (2019). Nociceptor signalling through ion channel regulation via gpcrs. Int. J. Mol. Sci. 20, 2488. doi:10.3390/ijms20102488
Schindelin, J., Arganda-Carreras, I., Frise, E., Kaynig, V., Longair, M., Pietzsch, T., et al. (2012). Fiji: an open-source platform for biological-image analysis. Nat. Methods 9, 676–682. doi:10.1038/nmeth.2019
Sculptoreanu, A., Artim, D. E., and de Groat, W. C. (2009). Neurokinins inhibit low threshold inactivating k+ currents in capsaicin responsive drg neurons. Exp. Neurol. 219, 562–573. doi:10.1016/j.expneurol.2009.07.016
Sculptoreanu, A., and de Groat, W. C. (2007). Neurokinins enhance excitability in capsaicin-responsive drg neurons. Exp. Neurol. 205, 92–100. doi:10.1016/j.expneurol.2007.01.038
Spindler, A. J., Noble, S. J., and Noble, D. (1999). Comparison of step and ramp voltage clamp on background currents in Guinea-pig ventricular cells. Exp. Physiol. 84, 865–879. doi:10.1111/j.1469-445x.1999.01790.x
Testa, L., Dotta, S., Vercelli, A., and Marvaldi, L. (2024). Communicating pain: emerging axonal signaling in peripheral neuropathic pain. Front. Neuroanat. 18, 1398400. doi:10.3389/fnana.2024.1398400
Viatchenko-Karpinski, V., and Gu, J. G. (2016). Mechanical sensitivity and electrophysiological properties of acutely dissociated dorsal root ganglion neurons of rats. Neurosci. Lett. 634, 70–75. doi:10.1016/j.neulet.2016.10.011
Yang, R.-H., Wang, W.-T., Chen, J.-Y., Xie, R.-G., and Hu, S.-J. (2009). Gabapentin selectively reduces persistent sodium current in injured type-a dorsal root ganglion neurons. Pain 143, 48–55. doi:10.1016/j.pain.2009.01.020
Yousuf, A., Klinger, F., Schicker, K., and Boehm, S. (2011). Nucleotides control the excitability of sensory neurons via two p2y receptors and a bifurcated signaling cascade. Pain 152, 1899–1908. doi:10.1016/j.pain.2011.04.016
Keywords: Gaussian white noise, neuronal firing, dorsal root ganglion (DRG), dissociated cultures, inflammatory soup, NAPQI, retigabine
Citation: Losgott T, Schicker KW, Hilber K, Boehm S and Salzer I (2025) Gaussian white noise stimulation as an alternative method to excite sensory neurons. Front. Pharmacol. 16:1561905. doi: 10.3389/fphar.2025.1561905
Received: 16 January 2025; Accepted: 31 March 2025;
Published: 22 April 2025.
Edited by:
Mark R. Estacion, Yale University, United StatesReviewed by:
Olga Kopach, St George’s, University of London, United KingdomEnrique Soto, Meritorious Autonomous University of Puebla, Mexico
Copyright © 2025 Losgott, Schicker, Hilber, Boehm and Salzer. This is an open-access article distributed under the terms of the Creative Commons Attribution License (CC BY). The use, distribution or reproduction in other forums is permitted, provided the original author(s) and the copyright owner(s) are credited and that the original publication in this journal is cited, in accordance with accepted academic practice. No use, distribution or reproduction is permitted which does not comply with these terms.
*Correspondence: Isabella Salzer, aXNhYmVsbGEuc2FsemVyQG1lZHVuaXdpZW4uYWMuYXQ=
†These authors have contributed equally to this work