- Department of Emergency, Suining Central Hospital in Sichuan Province, Suining, Sichuan, China
Diquat is a widely used bipyridyl herbicide that is extensively applied in agricultural production and water management due to its high efficacy in weed control. However, its environmental persistence and the toxic effects it induces have raised widespread concern. Studies show that Diquat primarily enters the body through the digestive tract, leading to poisoning. The core mechanism of its toxicity involves reactive oxygen species (ROS)-induced oxidative stress, which not only directly damages the intestinal barrier function but also exacerbates inflammation and systemic toxicity by disrupting the balance of the gut microbiota and the normal production of metabolic products. This review systematically summarizes the physicochemical properties of Diquat, with a focus on analyzing the mechanisms by which it damages the gut tissue structure, barrier function, and microbiota after digestive tract exposure. The aim is to provide theoretical support for a deeper understanding of Diquat’s toxic mechanisms and its digestive tract-centered toxic characteristics, laying a scientific foundation for the development of effective interventions and protective strategies against its toxicity.
1 Introduction
Diquat (1,1′-ethylene-2,2′-bipyridyl) is a bipyridyl herbicide widely used in agriculture and non-cultivated land weed control due to its rapid action and broad-spectrum efficacy (Wilkinson, 1969). Its high water solubility and stability in acidic and neutral environments contribute to its persistent environmental residues, although it can degrade into simple organic compounds and inorganic salts under alkaline conditions (Jones and Vale, 2000). Although Diquat exhibits lower acute toxicity compared to the related herbicide paraquat, it still poses a significant threat to ecosystems due to its potential for bioaccumulation and long-term persistence in soil and water bodies (Wilkinson, 1969; Akhavein and Linscott, 1968).
The toxicity of Diquat arises from its redox cycling capability, which generates reactive oxygen species (ROS) such as superoxide anions (O2−·) and hydroxyl radicals (OH·) (Ren et al., 2024; Fussell et al., 2011; Aloise et al., 2022). These ROS cause oxidative damage to lipids, proteins, and DNA, ultimately leading to multi-organ dysfunction. The kidney is the primary target organ, where Diquat can induce acute tubular necrosis, mitochondrial dysfunction, and apoptosis (Xing et al., 2020; Yu et al., 2022; Chen et al., 2024; Lai et al., 2024; Wu et al., 2022a). In addition, lung and nervous system injuries have also been reported, which in severe cases can progress to multiple organ failure (Ren et al., 2024; Sun et al., 2020; Zhang et al., 2022). However, existing studies have largely focused on systemic toxicity following absorption, overlooking the gastrointestinal tract as both an exposure route and a direct target organ.
The intestine is a critical interface for nutrient absorption, immune regulation, and microbial symbiosis (Takiishi et al., 2017). The integrity of the intestinal epithelial barrier is maintained by tight junction proteins such as ZO-1 and occludin, which prevent pathogen invasion and systemic inflammation (Gieryńska et al., 2022). The gut microbiota further modulates host metabolism, immune responses, and resistance to oxidative stress (Ma et al., 2025). Disruption of barrier function, dysbiosis, or chronic inflammation can destabilize this balance and is closely associated with systemic diseases such as metabolic disorders and neurodegenerative conditions (Hrncir, 2022; Zhao et al., 2023). Therefore, intestinal health serves as a central line of defense against damage from exogenous toxicants.
Recent studies have identified the gut as a key target of Diquat toxicity (Cao et al., 2020; Clark and Hurst, 1970; Rawlings et al., 1994). Oral ingestion, the primary route of exposure, allows Diquat to directly damage intestinal epithelial cells, disrupt tight junctions, and trigger ROS-mediated inflammatory responses (Huang et al., 2025; Jin et al., 2021; Xu et al., 2023). Diquat also alters gut microbiota composition, reducing the abundance of Lactobacillus while enriching pathogenic bacteria, thereby exacerbating both intestinal and systemic toxicity (Fu et al., 2021; Wen et al., 2024a). Moreover, Diquat promotes a vicious cycle of oxidative stress and immune dysregulation by inhibiting antioxidant defenses and activating pro-inflammatory pathways such as NF-κB and MAPK (Xu et al., 2023; Xu Q. et al., 2022). Nevertheless, the molecular mechanisms linking gut-specific injury to systemic organ failure remain unclear.
Current research on Diquat toxicity has been overly focused on liver and kidney dysfunction, while the role of intestinal mechanisms in systemic pathology remains insufficiently integrated. Key unresolved questions include: (1) How does Diquat-induced disruption of the intestinal barrier facilitate toxin translocation and injury to distal organs? (2) Do gut microbiota-derived metabolites modulate Diquat toxicity? (3) Can targeting intestinal integrity or microbial balance alleviate multiple organ dysfunction? Moreover, although antioxidants (e.g., resveratrol) and probiotics have shown promise in preclinical models, the mechanisms by which they restore gut-immune interactions remain to be fully elucidated. This review synthesizes existing evidence on Diquat-induced structural damage to the gut, microbial dysbiosis, and immune activation, highlighting how these processes amplify systemic toxicity. We propose a gut-centered intervention strategy to bridge the knowledge gap between local intestinal injury and systemic effects, offering new perspectives for detoxification and clinical management.
2 Physicochemical properties and metabolic process of diquat
2.1 Basic chemical structure and properties
Diquat has the full chemical name of 1,1′-ethylene-2,2′-bipyridinium. It is a bipyridyl compound with a molecular formula of C12H12N2 (ionic form) or C12H12Br2N2 (dibromide form), with molecular weights of 184.24 and 344.05, respectively. It exists as odorless yellow crystals, while the commercial products are often dark green or reddish-brown, exhibiting high water solubility (solubility 712 g/L, pH 5.2). Under acidic and neutral conditions, Diquat demonstrates high stability, but it is prone to hydrolysis in alkaline solutions. Its half-life in aquatic environments ranges from 2 to 10 days, primarily degrading into simple organic molecules and inorganic salts (Wilkinson, 1969; Kim et al., 2024; Summers and Black, 1968). These properties confer strong environmental persistence and potential ecological toxicity to Diquat.
2.2 Forms of diquat in the environment and its metabolism
In the environment, the form of Diquat primarily depends on the medium in which it is found. In soil, Diquat is easily adsorbed onto soil particles, resulting in low mobility and slow degradation, primarily relying on microbial and chemical processes for breakdown (Kim et al., 2024). In water, Diquat can be removed through photodegradation and microbial activity after binding with suspended particles. Although its degradation rate is relatively fast, the degradation products may pose secondary toxicity to aquatic organisms. Due to Diquat’s accumulation behavior, it may pose long-term threats to organisms and ecosystems in the environment (Summers and Black, 1968).
2.3 Absorption, distribution, metabolism, excretion, and organ damage in animals
The primary route of absorption of Diquat is through the digestive tract, with a low absorption rate (<10%) (Duan et al., 2024). Once inside the body, Diquat enters tissues via diffusion or active transport through ion pumps and rapidly distributes throughout the system (Magalhães et al., 2018). Autopsy findings from patients who died from DQ poisoning have shown that tissue (and fluid) concentrations of DQ vary depending on the time of death. For instance, in a patient who died 14 h after ingestion, the concentration of DQ from highest to lowest was: urine > vitreous humor > lungs > liver > brain tissue > kidneys (McCarthy and Speth, 1983). During metabolism, Diquat undergoes a redox cycle to generate highly reactive DQ+, which further binds with oxygen molecules to form superoxide anion (O2−) and other ROSs. These ROSs significantly induce cellular damage, primarily manifested as lipid peroxidation, protein oxidation, and DNA damage, which in turn triggers oxidative stress, leading to disruption of cell membrane structures, mitochondrial dysfunction, and cell apoptosis (Fussell et al., 2011; Awad et al., 1994; Wolfgang et al., 1991; Zhang et al., 2006; Blakeman et al., 1998; Wen et al., 2024b; Gupta et al., 2000). The excretion of Diquat primarily occurs through urine and feces, with most of it being excreted in its unmetabolized parent form (Jones and Vale, 2000; Basilicata et al., 2022; Guo et al., 2023; Magalhães et al., 2018). However, Diquat that is not effectively eliminated continues to induce toxic reactions in the body. The main renal toxicity of Diquat is acute kidney injury (AKI), with ROS being a key pathological inducer in its molecular mechanism. ROS attacks the polyunsaturated fatty acids in the renal tubular cell membranes, leading to lipid peroxidation and membrane structural damage (Chen et al., 2024; Wu et al., 2024). Studies have also found that Diquat induces gasdermin E (GSDME) cleavage through mitochondrial dysfunction, triggering pyroptosis and leading to damage of renal tubular cells (Chen et al., 2024). Additionally, Diquat activates the HMGB1/IκBα/NF-κB signaling axis, inducing the massive release of pro-inflammatory cytokines, which exacerbates renal interstitial inflammation and fibrosis (Huang et al., 2023). Diquat that is not eliminated causes irreversible structural and functional damage to the kidneys through these mechanisms (Yu et al., 2022). In the liver, the toxicity of Diquat is dominated by mitochondrial dysfunction and signaling pathway disorders. Diquat downregulates the expression of SIRT1, and inhibits the activity of mitochondrial complexes (I, II, III, and V), leading to a significant reduction in ATP synthesis, which further induces energy crisis and apoptosis in hepatocytes (Jia et al., 2020; Zhang et al., 2020). At the same time, Diquat promotes hepatocyte apoptosis by inhibiting the expression of anti-apoptotic proteins and enhancing the activity of pro-apoptotic factors (Fu et al., 2001; Hong et al., 2009; Luo et al., 2024). Moreover, Diquat increases the expression of autophagy-related proteins (such as Beclin-1 and LC3-II), but the abnormal accumulation of p62 indicates that autophagy function is not effectively completed, further aggravating the stress response and damage in hepatocytes (Chen et al., 2021). Diquat-induced ROSs also stimulate hepatocytes to release pro-inflammatory cytokines (such as IL-1β and IL-18), enhancing liver inflammation and promoting liver damage (Luo et al., 2024). Although the respiratory system is not the primary target of Diquat, its toxicity still causes damage through ROS and inflammatory signaling pathways. Diquat significantly activates the Nrf2 signaling axis in lung tissue, which, on the one hand, induces antioxidant defense, but on the other hand, exacerbates oxidative stress and damages lung tissue due to the excessive generation of ROS (Fussell et al., 2011; Sun et al., 2020; Wu et al., 2012). Diquat induces systemic oxidative stress and inflammatory responses throughout the body, leading to multiple organ dysfunction syndrome (MODS) (Huang et al., 2021; Yoshioka et al., 1992).
3 The relationship between diquat and gut dysfunction
The toxic mechanism of Diquat focuses on multi-organ damage induced by ROS (Ren et al., 2024; Chen et al., 2024; Jin et al., 2021; Luo et al., 2024; Qu et al., 2024). However, it is worth noting that existing literature reports indicate that the main route of Diquat poisoning is through ingestion, while other routes of exposure are relatively rare. Therefore, the gut, as the main exposure target for Diquat, plays a crucial role in the poisoning process. Diquat not only directly damages the tissue structure of the gut but also exacerbates systemic toxicity by disrupting the gut microbiota and impairing gut immune homeostasis. The specific mechanisms of these effects include oxidative stress, abnormal activation of inflammatory signaling pathways, autophagy dysregulation, and barrier function disruption (Cao et al., 2020; Xu et al., 2023; Xu Q. et al., 2022; Wen et al., 2024b; Cao et al., 2018; Feng et al., 2023; Hao et al., 2021; Liang et al., 2023; Wu et al., 2019). The following will focus on the latest research progress regarding the impact of Diquat on gut tissue structure, microbiota, and immune homeostasis, as well as its potential molecular mechanisms, providing theoretical support for further exploration of its toxicity mechanisms and intervention strategies (Figure 1).
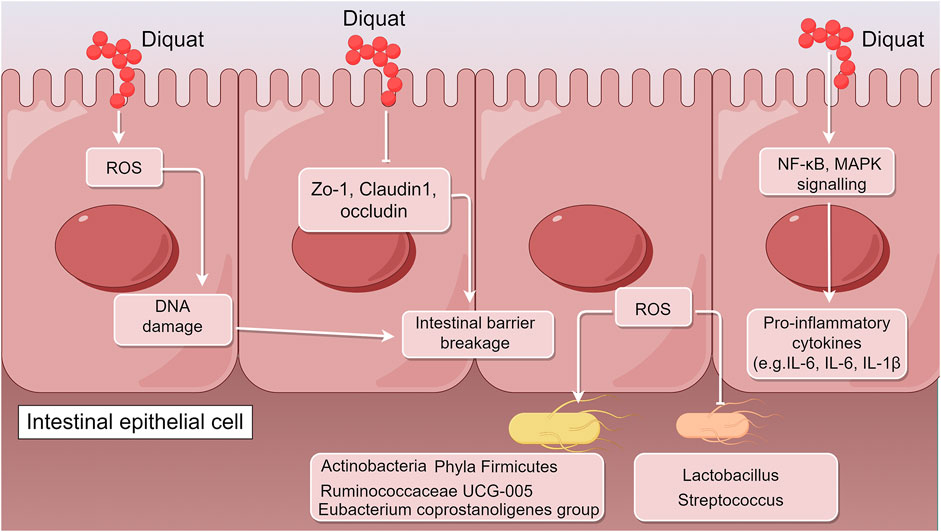
Figure 1. Schematic illustration of the molecular mechanisms underlying Diquat-induced intestinal dysfunction (by Figdraw). After entering the body primarily through the digestive tract, Diquat first acts on intestinal tissues, triggering a range of pathological responses including oxidative stress, barrier disruption, microbial dysbiosis, and immune imbalance. Its toxic effects are manifested through several pathways: Diquat induces the generation of ROS, significantly downregulates the expression of tight junction proteins (ZO-1, occludin, claudin-1), and increases intestinal permeability. ROS also activate inflammatory pathways such as NF-κB and MAPK, leading to the excessive release of pro-inflammatory cytokines (TNF-α, IL-6, IL-1β), thereby forming a vicious cycle of inflammation and barrier damage. Additionally, beneficial bacteria (e.g., Lactobacillus) are reduced, while pathogenic bacteria increase in abundance.
3.1 Specific effects of diquat on gut tissue structure
The damage to the intestinal epithelial barrier caused by Diquat is one of its core toxic effects, primarily manifested as the disruption of gut barrier function and the loss of mechanical barrier integrity. At the molecular level, Diquat significantly downregulates the expression of tight junction proteins (such as ZO-1, claudin-1, and occludin) by inducing oxidative stress. These tight junction proteins are key components in maintaining the barrier function between intestinal epithelial cells. A reduction in their expression leads to the loosening of cell-cell connections, significantly increasing intestinal permeability. This damage to the mechanical barrier allows toxins and pathogens in the intestinal lumen to more easily penetrate the barrier and invade tissues, further exacerbating intestinal inflammation (Jin et al., 2021; Cao et al., 2018; Liang et al., 2023; Cao et al., 2019; Wen et al., 2020). Additionally, Diquat activates the NF-κB signaling pathway, leading to the massive release of pro-inflammatory cytokines (such as TNF-α, IL-6, and IL-1β). These pro-inflammatory cytokines not only trigger inflammatory responses but also further suppress the expression of tight junction proteins, thereby creating a vicious cycle of inflammation and barrier damage (Xu Q. et al., 2022; Xu et al., 2023).
Oxidative stress is another key mechanism underlying the toxic effects of Diquat (Cao et al., 2020; Magalhães et al., 2018; Cao et al., 2018; Feng et al., 2023; Liang et al., 2023). The molecular action of Diquat generates a large amount of ROS through its enzymatic reactions, including superoxide anion (O2−), hydrogen peroxide (H2O2), and hydroxyl radicals (OH·). These ROSs are powerful oxidants that can directly cause oxidative damage to the cell membranes, proteins, and DNA of intestinal cells (Afzal et al., 2023). Diquat significantly increases the levels of malondialdehyde (MDA) in intestinal tissues, a marker of lipid peroxidation, while inhibiting the activity of antioxidant enzymes such as superoxide dismutase (SOD) and glutathione peroxidase (GSH-Px), further weakening the cell’s defense against oxidative stress (Zhang et al., 2021). Moreover, excess ROSs also promote the expression of pro-inflammatory factors by activating the NF-κB pathway, enhancing the inflammatory response, and thereby exacerbating intestinal damage (Zhang et al., 2021).
In terms of mitochondrial function, Diquat induces excessive ROS production, which directly damages mitochondrial structure and function (Choi et al., 2018; Gou et al., 2024; Nisar et al., 2015). A significant decrease in mitochondrial membrane potential (MMP) is an early sign of mitochondrial dysfunction, indicating impaired mitochondrial oxidative phosphorylation and energy metabolism (Khan et al., 2022; Gottlieb et al., 2003). Diquat also induces pathological changes such as mitochondrial swelling, cristae rupture, and vacuolization, further affecting mitochondrial metabolic function. To cope with this damage, cells typically activate mitochondrial autophagy through the PINK1/Parkin signaling pathway, marking and degrading damaged mitochondria to maintain mitochondrial homeostasis. However, excessive autophagy induced by Diquat may lead to further deterioration of mitochondrial function (Cao et al., 2020). Furthermore, Diquat interferes with the function of the endoplasmic reticulum-mitochondria-associated membranes (MAM), disrupting the transport of Ca2+ between the endoplasmic reticulum and mitochondria, further weakening mitochondrial activity and intestinal cell metabolic function (Li et al., 2022).
It is worth emphasizing that mitochondria, as key organelles regulating cellular metabolism, signal transduction, and cell fate, play an indispensable role in maintaining the integrity of the intestinal epithelial barrier (Rath and Haller, 2022). Recent studies have shown that mitochondria in intestinal epithelial cells not only contribute to energy supply—particularly through oxidative phosphorylation to produce ATP—but also directly participate in the structural and functional regulation of the mucosal barrier (Wang et al., 2023; Ho and Theiss, 2022). Mitochondrial dysfunction can impair intestinal structure through multiple mechanisms. Firstly, impaired oxidative phosphorylation leads to reduced ATP production, which in turn suppresses the expression and stability of tight junction proteins, disrupts intercellular connections in the intestinal epithelium, and increases intestinal permeability (Song et al., 2023). Secondly, damaged mitochondria release large amounts of ROS and mitochondrial DNA (mtDNA), which act as danger-associated molecular patterns (DAMPs) that activate the NLRP3 inflammasome and NF-κB pathway, amplifying the inflammatory response (Zong et al., 2024). Thirdly, disruption of local oxygen homeostasis compromises the anaerobic environment, resulting in the depletion of beneficial anaerobic bacteria and further weakening the mucosal barrier’s anti-inflammatory and reparative functions (Yan et al., 2023). More critically, in diseases such as inflammatory bowel disease and colorectal cancer, mitochondrial dysfunction is not only a consequence of tissue damage but may also serve as an initiating factor in pathogenesis. By regulating apoptosis, autophagy, and cell phenotype transformation, it contributes to disease progression (Kłos and Dabravolski, 2021).
In terms of intestinal morphology, the toxic effects of Diquat manifest as a significant reduction in villus height and an increase in crypt depth (Chen et al., 2023). These morphological changes directly impair the absorption function of the intestine, affecting the effective absorption of nutrients (Groschwitz and Hogan, 2009; Salvo Romero et al., 2015; Turner, 2009). In summary, Diquat induces mitochondrial dysfunction, leading to marked damage to the intestinal epithelial barrier and tissue structure through multiple pathways, including disrupted energy metabolism, amplified inflammation, and microbial dysbiosis. These findings provide theoretical support for the intestinal toxicity mechanisms of oxidative stress-inducing toxicants.
3.2 Specific impact of diquat on the gut microbiota
Diquat’s impact on the gut microbiota manifests as significant dysbiosis, primarily characterized by a reduction in beneficial bacteria and an increase in harmful bacteria. Studies have shown that Diquat markedly reduces the abundance of Lactobacillus and Streptococcus, both of which, despite potentially playing different roles in regulating intestinal barrier function and immune homeostasis, are important components of a healthy gut microbiota (Wen et al., 2024c; Wu et al., 2020; Wu et al., 2022b; Dominguez et al., 2024; Wang et al., 2022). Meanwhile, Diquat exposure leads to an increased relative abundance of members from the Phylum Firmicutes and Actinobacteria, Ruminococcaceae UCG-005 and Eubacterium coprostanoligenes (Wen et al., 2024a). This imbalance weakens the protective role of beneficial bacteria, while harmful bacteria further promote inflammation and barrier damage (Fu et al., 2021). At the molecular level, Diquat exacerbates dysbiosis through oxidative stress mechanisms. Elevated ROS levels directly inhibit the survival of Lactobacillus, while Actinobacteria and Ruminococcaceae exhibit stronger tolerance to the oxidative stress environment, allowing them to proliferate (Fu et al., 2021). In addition, the expression of tight junction proteins (such as occludin, claudin-1, and ZO-1) is significantly reduced, further compromising the intestinal barrier and creating conditions favorable for the overgrowth of harmful bacteria (Chelakkot et al., 2018; He et al., 2023; Ulluwishewa et al., 2011). This dysbiosis also affects the production of intestinal metabolites, such as a significant decrease in indole-3-methanol and butyrate levels, which not only weakens the intestinal anti-inflammatory and antioxidant capabilities but also impacts the repair functions of the intestinal barrier (Fu et al., 2021). Through the disruption of the microbiota-metabolite axis, Diquat not only alters the microbiota structure but also disturbs gut signaling and metabolic regulation by influencing the production of metabolites (such as uridine and indole) (Fu et al., 2021; Saito, 1986). The reduction of Lactobacillus and associated metabolites is directly related, and the absence of these metabolic products further impairs the microbiota’s antioxidant and anti-inflammatory abilities (Fu et al., 2021). Overall, Diquat shapes an ecological environment favorable to harmful bacteria through oxidative stress and the disruption of the microbiota-metabolite axis, thereby exacerbating the dysbiosis of the gut microbiota.
3.3 Specific effects of diquat on gut immune homeostasis
Diquat significantly increases the secretion of pro-inflammatory cytokines (such as TNF-α, IL-6, and IL-1β), exacerbating chronic inflammatory responses in the gut (Xu Q. et al., 2022; Xu et al., 2023; Wen et al., 2024c; Wang et al., 2019; Nong et al., 2023). Oxidative stress not only directly activates intestinal macrophages but also further amplifies the intensity of the pro-inflammatory response (Zhang et al., 2021). Molecular mechanism studies show that Diquat-induced ROSs promote the phosphorylation levels of IκB, NF-κB, and ERK1/2, thereby upregulating the gene expression of pro-inflammatory cytokines (Xu et al., 2023). In addition, Diquat enhances the phosphorylation levels of key molecules in the MAPK signaling pathway, including p38, ERK1/2, and JNK, further amplifying the cascade effect of inflammation. These mechanisms collectively drive the persistent inflammatory state in the gut (Wu et al., 2019; Xu Q. et al., 2022; Xu et al., 2023; Liu et al., 2023). Notably, anti-inflammatory therapy studies have shown that antioxidants such as vitamin D3 and resveratrol can significantly alleviate Diquat-induced intestinal inflammation, offering potential therapeutic strategies for intervention (Zhang et al., 2021; Xun et al., 2021; Prakash et al., 2024).
In addition, Diquat significantly weakens the immune barrier function of the intestinal mucosa (Cao et al., 2018; Cao et al., 2019; Gou et al., 2024; Chen et al., 2023; Xun et al., 2021; Chen et al., 2022). This is specifically manifested by reduced secretion of immunoglobulins (such as IgA and IgG) and the downregulation of tight junction proteins in the intestine (such as ZO-1, claudin-1, and occludin), leading to increased intestinal permeability and impaired mucosal barrier function (Wen et al., 2020; Wang et al., 2021). This indicates that Diquat-induced oxidative stress not only triggers chronic inflammation but also causes multiple damages to the intestinal barrier through immune regulatory dysfunction, exacerbating the systemic risks to gut health.
4 The mechanisms of antioxidants and probiotics in protecting the intestinal barrier
Resveratrol has shown significant effects in antioxidant activity and intestinal barrier protection by activating the Nrf2 signaling pathway. Its mechanisms of action include enhancing the activity of antioxidant enzymes (such as SOD, GSH-Px, and HO-1), thereby significantly reducing the levels of ROS induced by oxidative stress (Xun et al., 2021; Zhou et al., 2022). Additionally, resveratrol regulates the AhR/Nrf2 pathway, inhibiting the expression of pro-inflammatory factors (such as TNF-α and IL-6), while increasing the abundance of tight junction proteins (such as occludin, claudin-1, and ZO-1), thereby repairing the damaged intestinal barrier function (Xun et al., 2021; Zhou et al., 2022). Further studies have shown that resveratrol also induces mitophagy, alleviating mitochondrial damage and providing multiple layers of protection to mitigate inflammation and support gut health (Cao et al., 2019).
Taurine plays a crucial role in protecting the intestinal barrier, primarily by significantly enhancing the expression of tight junction proteins (such as claudin-1, ZO-1, and occludin), thereby improving intestinal barrier function (Wen et al., 2020). Additionally, taurine effectively alleviates oxidative stress-induced damage to the intestines by regulating immune responses, demonstrating its unique advantage in maintaining intestinal homeostasis (Wen et al., 2020).
Stevioside, a natural antioxidant, protects the intestinal barrier by inhibiting the NF-κB and MAPK signaling pathways. Studies have shown that stevioside significantly increases the activity of antioxidant enzymes (such as T-SOD, CAT, and GSH-Px) and downregulates the expression of pro-inflammatory factors (such as IL-6 and TNF-α), thereby reducing inflammatory responses (Xu et al., 2023). Furthermore, stevioside maintains the integrity of the intestinal barrier by enhancing the expression of tight junction proteins and reducing the permeability of intestinal cells (Xu et al., 2023).
Lactobacillus and their extracellular vesicles (EVs) have demonstrated significant antioxidant effects and gut barrier protection by regulating the Nrf2 signaling pathway (Feng et al., 2023; Hao et al., 2021; Qiao et al., 2020; Wang et al., 2013; Xu et al., 2018). For example, Pediococcus pentosaceus ZJUAF-4 and other Lactobacillus can improve the composition of gut microbiota while activating Nrf2, thereby significantly reducing ROS produced by oxidative stress (Hao et al., 2021). Additionally, these probiotics and their EVs can maintain the expression of tight junction proteins, effectively repairing gut barrier function and reducing oxidative stress-induced gut damage (Feng et al., 2023). These findings provide new strategies and approaches for the prevention and treatment of gut-related diseases.
The above studies suggest that different types of antioxidants and probiotics, through regulating oxidative stress, inflammatory responses, and the expression of tight junction proteins, work together to protect and repair the gut barrier. This provides scientific evidence for improving gut health and treating related diseases.
5 Discussion
As a bipyridyl herbicide, Diquat has traditionally been studied for its toxic mechanisms primarily in relation to oxidative stress-induced damage in organs such as the liver and kidneys. However, recent studies have increasingly revealed that the intestine is not only the primary route of exposure but also a central target of its toxic effects (Cao et al., 2020; Jin et al., 2021; Liang et al., 2023; Xu X. et al., 2022). This review summarizes the mechanisms by which Diquat disrupts intestinal structure, microbial balance, and immune homeostasis, and explores how these local injuries may amplify systemic toxicity through the “gut–organ axis”. Notably, although Diquat has been banned in regions such as the European Union and the United Kingdom due to its ecological toxicity and health risks (EC Regulation No 1107/2009), it is still used for weed control in agriculture and non-cultivated land in certain developing countries. Moreover, residual Diquat in the environment may continue to pose long-term threats to ecosystems and human health via the food chain. Therefore, in-depth investigation of its toxicological mechanisms remains essential for ecological restoration in contaminated areas, clinical management of poisoning cases, and toxicity assessment of structurally related herbicides.
Following oral intake, Diquat directly targets intestinal epithelial cells, where it generates excessive ROS through redox cycling. This leads to the downregulation of tight junction proteins such as ZO-1, occludin, and claudin-1, thereby significantly increasing intestinal permeability (Jin et al., 2021; Cao et al., 2019). The resulting barrier dysfunction facilitates the translocation of endotoxins and unmetabolized Diquat molecules from the gut lumen into the systemic circulation, subsequently triggering a systemic inflammatory response via activation of the TLR4/NF-κB signaling pathway (Cui et al., 2023; Huang et al., 2024; Liu et al., 2021; Zhan et al., 2025). In this process, the gut microbiota plays a pivotal “amplifier” role: Diquat inhibits the proliferation of beneficial bacteria such as Lactobacillus, while promoting the enrichment of potentially pathogenic taxa such as Actinobacteria and Ruminococcaceae, leading to a reduction in key microbial metabolites including indole-3-methanol, 5-hydroxyindole-3-acetic acid, and uridine (Fu et al., 2021). These small molecules are normally involved in maintaining intestinal barrier integrity and immune tolerance through activation of the aryl hydrocarbon receptor (AhR). Their depletion exacerbates mucosal damage and inflammatory cascades (Kahalehili et al., 2020; Dang et al., 2023). This resulting cycle of “oxidative stress–microbial dysbiosis–inflammatory amplification” may serve as a key driving force in the development of MODS.
Growing evidence suggests that Diquat-induced intestinal injury is closely associated with dysfunction in distal organs through multiple molecular pathways. On one hand, microbial dysbiosis leads to reduced levels of key metabolites such as tryptophan and uridine, thereby compromising antioxidant defenses in peripheral organs (Christen et al., 1990). On the other hand, mitochondrial damage in intestinal epithelial cells can result in the release of mtDNA, which activates the cGAS–STING signaling pathway and triggers a systemic inflammatory storm (Newman and Shadel, 2023; Cai et al., 2024). Additionally, Diquat may interfere with autophagic flux—indicated by the abnormal accumulation of p62—facilitating the transport of misfolded proteins via exosomes and potentially inducing an unfolded protein response (UPR) in distal organs, although this hypothesis remains to be experimentally validated (Chen et al., 2021).
Given the central role of the intestine in Diquat toxicity, targeted intervention strategies show considerable potential. For example, resveratrol can enhance antioxidant capacity by activating the Nrf2 pathway and regulate AhR signaling to restore microbial metabolite homeostasis (Prakash et al., 2024; Meng et al., 2020). However, existing research has several limitations: first, most studies use acute high-dose exposure models, which fail to simulate the real-world scenario of low-dose chronic exposure in the environment; second, the spatiotemporal dynamics of microbiota-host metabolic interactions remain unclear; third, the strain-specific mechanisms of probiotics lack systematic comparative studies. Therefore, future research should incorporate new technologies such as organoid co-cultures and multi-omics analysis to elucidate the precise roles of key strains and metabolites (e.g., butyrate) in the detoxification process of Diquat.
The main strength of this study lies in its novel approach, systematically integrating the mechanisms of Diquat-induced intestinal toxicity from three dimensions: structural damage, microbial imbalance, and immune disruption. It introduces new hypotheses, such as “gut-derived ROS diffusion” and “microbiota-metabolite axis imbalance”, offering fresh perspectives that challenge the traditional liver and kidney-centered toxicological framework. This not only deepens our understanding of the inherent toxicity of bipyridyl herbicides but also provides a theoretical foundation for toxicity assessment and detoxification strategies.
Nevertheless, it is important to acknowledge the limitations of existing research: for example, human-related data is lacking, and most evidence comes from rodent models; the experimental models are relatively simplistic and fail to represent real-world scenarios of long-term low-dose exposure; the complexity of the gut microbiome is high, influenced by confounding factors such as diet and genetics, which complicates the interpretation of Diquat-specific effects; additionally, the selective mechanisms by which Diquat affects different microbiota remain unclear and may be related to differences in its structure’s ability to scavenge ROS or its sensitivity to metabolic pathways.
Future research should focus on the following key areas: first, the mechanisms by which Diquat selectively suppresses specific microbiota should be elucidated, exploring whether it causes dysbiosis through ROS tolerance, metabolic dependence, or membrane protein affinity; second, more realistic long-term low-dose exposure models should be developed to clarify the compensatory mechanisms of the intestinal barrier and the critical points of dysregulation; third, epidemiological studies on populations exposed to Diquat should be conducted to identify potential biomarkers, such as serum zonulin and microbiota profiles; finally, there is a need to develop responsive nanoparticle delivery systems to facilitate the targeted supplementation of key detoxification metabolites (e.g., indole-3-methanol), enhancing the precision and translational potential of intervention strategies.
In conclusion, the systemic toxicity induced by Diquat is essentially a reflection of the gradual progression of localized intestinal injury into a systemic pathological process. Adopting the “intestinal barrier-microbiota-immune” framework for research not only helps reinterpret its toxicological mechanisms but also provides a theoretical foundation for developing targeted detoxification strategies centered on the intestine. Even though Diquat has been banned in many regions, the “gut-organ axis” mechanism revealed by this “model toxin” still holds significant theoretical and practical value for environmental pollution management and ecological toxicology research.
Author contributions
CH: Conceptualization, Formal Analysis, Writing – original draft, Writing – review and editing. GC: Conceptualization, Investigation, Writing – original draft, Writing – review and editing. YJ: Resources, Software, Writing – review and editing. RJ: Data curation, Writing – review and editing. XW: Writing – review and editing. NT: Writing – review and editing.
Funding
The author(s) declare that no financial support was received for the research and/or publication of this article.
Conflict of interest
The authors declare that the research was conducted in the absence of any commercial or financial relationships that could be construed as a potential conflict of interest.
Generative AI statement
The author(s) declare that no Generative AI was used in the creation of this manuscript.
Publisher’s note
All claims expressed in this article are solely those of the authors and do not necessarily represent those of their affiliated organizations, or those of the publisher, the editors and the reviewers. Any product that may be evaluated in this article, or claim that may be made by its manufacturer, is not guaranteed or endorsed by the publisher.
Abbreviations
AKI, acute kidney injury; EVs, extracellular vesicles; GSDME, gasdermin E; GSH-Px, glutathione peroxidase; MAM, mitochondria-associated membranes; MDA, malondialdehyde; MMP, mitochondrial membrane potential; MODS, multiple organ dysfunction syndrome; ROS, reactive oxygen species; SOD, superoxide dismutase.
References
Afzal, S., Abdul Manap, A. S., Attiq, A., Albokhadaim, I., Kandeel, M., and Alhojaily, S. M. (2023). From imbalance to impairment: the central role of reactive oxygen species in oxidative stress-induced disorders and therapeutic exploration. Front. Pharmacol. 14, 1269581. doi:10.3389/fphar.2023.1269581
Akhavein, A. A., and Linscott, D. L. (1968). The dipyridylium herbicides, paraquat and diquat. Residue Rev. 23, 97–145. doi:10.1007/978-1-4615-8437-7_6
Aloise, D. M., Memon, A., and Zaldivar, A. (2022). Diquat herbicide organophosphate poisoning and multi-organ failure: a case report. Cureus 14 (7), e27241. doi:10.7759/cureus.27241
Awad, J. A., Burk, R. F., and Roberts, L. J. (1994). 2nd: effect of selenium deficiency and glutathione-modulating agents on diquat toxicity and lipid peroxidation in rats. J. Pharmacol. Exp. Ther. 270 (3), 858–864.
Basilicata, P., Pieri, M., Simonelli, A., Capasso, E., Casella, C., Noto, T., et al. (2022). Diquat poisoning: care management and medico-legal implications. Toxics 10 (4), 166. doi:10.3390/toxics10040166
Blakeman, D. P., Ryan, T. P., Jolly, R. A., and Petry, T. W. (1998). Protein oxidation: examination of potential lipid-independent mechanisms for protein carbonyl formation. J. Biochem. Mol. Toxicol. 12 (3), 185–190. doi:10.1002/(sici)1099-0461(1998)12:3<185::aid-jbt7>3.0.co;2-h
Cai, Y., Li, S., Yang, Y., Duan, S., Fan, G., Bai, J., et al. (2024). Intestinal epithelial damage-derived mtDNA activates STING-IL12 axis in dendritic cells to promote colitis. Theranostics 14 (11), 4393–4410. doi:10.7150/thno.96184
Cao, S., Shen, Z., Wang, C., Zhang, Q., Hong, Q., He, Y., et al. (2019). Resveratrol improves intestinal barrier function, alleviates mitochondrial dysfunction and induces mitophagy in diquat challenged piglets(1). Food Funct. 10 (1), 344–354. doi:10.1039/c8fo02091d
Cao, S., Wang, C., Yan, J., Li, X., Wen, J., and Hu, C. (2020). Curcumin ameliorates oxidative stress-induced intestinal barrier injury and mitochondrial damage by promoting Parkin dependent mitophagy through AMPK-TFEB signal pathway. Free Radic. Biol. Med. 147, 8–22. doi:10.1016/j.freeradbiomed.2019.12.004
Cao, S., Wu, H., Wang, C., Zhang, Q., Jiao, L., Lin, F., et al. (2018). Diquat-induced oxidative stress increases intestinal permeability, impairs mitochondrial function, and triggers mitophagy in piglets. J. Anim. Sci. 96 (5), 1795–1805. doi:10.1093/jas/sky104
Chelakkot, C., Ghim, J., and Ryu, S. H. (2018). Mechanisms regulating intestinal barrier integrity and its pathological implications. Exp. Mol. Med. 50 (8), 103–109. doi:10.1038/s12276-018-0126-x
Chen, J., Chen, D., Yu, B., Luo, Y., Zheng, P., Mao, X., et al. (2022). Chlorogenic acid attenuates oxidative stress-induced intestinal mucosa disruption in weaned pigs. Front. Vet. Sci. 9, 806253. doi:10.3389/fvets.2022.806253
Chen, J., Su, Y., Lin, R., Lin, F., Shang, P., Hussain, R., et al. (2021). Effects of acute diquat poisoning on liver mitochondrial apoptosis and autophagy in ducks. Front. Vet. Sci. 8, 727766. doi:10.3389/fvets.2021.727766
Chen, K., Tang, Y., Lan, L., Li, M., and Lu, Z. (2024). Autophagy mediated FTH1 degradation activates gasdermin E dependent pyroptosis contributing to diquat induced kidney injury. Food Chem. Toxicol. 184, 114411. doi:10.1016/j.fct.2023.114411
Chen, Y., Zhang, H., Li, Y., and Wang, T. (2023). Pterostilbene confers protection against diquat-induced intestinal damage with potential regulation of redox status and ferroptosis in broiler chickens. Oxid. Med. Cell Longev., 8258354. doi:10.1155/2023/8258354
Choi, S. E., Park, Y. S., and Koh, H. C. (2018). NF-κB/p53-activated inflammatory response involves in diquat-induced mitochondrial dysfunction and apoptosis. Environ. Toxicol. 33 (10), 1005–1018. doi:10.1002/tox.22552
Christen, S., Peterhans, E., and Stocker, R. (1990). Antioxidant activities of some tryptophan metabolites: possible implication for inflammatory diseases. Proc. Natl. Acad. Sci. U. S. A. 87 (7), 2506–2510. doi:10.1073/pnas.87.7.2506
Clark, D. G., and Hurst, E. W. (1970). The toxicity of diquat. Br. J. Ind. Med. 27 (1), 51–55. doi:10.1136/oem.27.1.51
Cui, S., Zhang, X., Wang, C., Sun, C., Shi, L., Kan, B., et al. (2023). Study on the therapeutic effect of glucocorticoids on acute kidney injury in rats exposed to diquat. Biomed. Pharmacother. 166, 115310. doi:10.1016/j.biopha.2023.115310
Dang, G., Wen, X., Zhong, R., Wu, W., Tang, S., Li, C., et al. (2023). Pectin modulates intestinal immunity in a pig model via regulating the gut microbiota-derived tryptophan metabolite-AhR-IL22 pathway. J. Anim. Sci. Biotechnol. 14 (1), 38. doi:10.1186/s40104-023-00838-z
Dominguez, K., Pearah, A. N., Lindon, A. K., Worthington, L. A., Carter, R. R., John-Lewis Edwards, N., et al. (2024). The impact of butyrate on group B Streptococcus-induced intestinal barrier disruption. Infect. Immun. 92 (10), e0020024. doi:10.1128/iai.00200-24
Duan, M., Yang, B., Cheng, X., Shen, F., Lu, X., and Wang, F. (2024). Two cases of diquat poisoning in adolescent children. Italian J. Pediatr. 50 (1), 80. doi:10.1186/s13052-024-01640-x
Feng, S., Liu, Y., Xu, J., Fan, J., Li, J., Wu, Z., et al. (2023). Three strains of Lactobacillus derived from piglets alleviated intestinal oxidative stress induced by diquat through extracellular vesicles. Nutrients 15 (19), 4198. doi:10.3390/nu15194198
Fu, Q., Tan, Z., Shi, L., and Xun, W. (2021). Resveratrol attenuates diquat-induced oxidative stress by regulating gut microbiota and metabolome characteristics in piglets. Front. Microbiol. 12, 695155. doi:10.3389/fmicb.2021.695155
Fu, Y., Sies, H., and Lei, X. G. (2001). Opposite roles of selenium-dependent glutathione peroxidase-1 in superoxide generator diquat- and peroxynitrite-induced apoptosis and signaling. J. Biol. Chem. 276 (46), 43004–43009. doi:10.1074/jbc.M106946200
Fussell, K. C., Udasin, R. G., Gray, J. P., Mishin, V., Smith, P. J., Heck, D. E., et al. (2011). Redox cycling and increased oxygen utilization contribute to diquat-induced oxidative stress and cytotoxicity in Chinese hamster ovary cells overexpressing NADPH-cytochrome P450 reductase. Free Radic. Biol. Med. 50 (7), 874–882. doi:10.1016/j.freeradbiomed.2010.12.035
Gieryńska, M., Szulc-Dąbrowska, L., Struzik, J., Mielcarska, M. B., and Gregorczyk-Zboroch, K. P. (2022). Integrity of the intestinal barrier: the involvement of epithelial cells and microbiota-A mutual relationship. Anim. (Basel) 12 (2), 145. doi:10.3390/ani12020145
Gottlieb, E., Armour, S. M., Harris, M. H., and Thompson, C. B. (2003). Mitochondrial membrane potential regulates matrix configuration and cytochrome c release during apoptosis. Cell Death Differ. 10 (6), 709–717. doi:10.1038/sj.cdd.4401231
Gou, F., Cai, F., Li, X., Lin, Q., Zhu, J., Yu, M., et al. (2024). Mitochondria-associated endoplasmic reticulum membranes involve in oxidative stress-induced intestinal barrier injury and mitochondrial dysfunction under diquat exposing. Environ. Toxicol. 39 (7), 3906–3919. doi:10.1002/tox.24232
Groschwitz, K. R., and Hogan, S. P. (2009). Intestinal barrier function: molecular regulation and disease pathogenesis. J. Allergy Clin. Immunol. 124 (1), 3–20. doi:10.1016/j.jaci.2009.05.038
Guo, H., Li, L., and Gao, L. (2023). Paraquat and diquat: recent updates on their pretreatment and analysis methods since 2010 in biological samples. Molecules 28 (2), 684. doi:10.3390/molecules28020684
Gupta, S., Husser, R. C., Geske, R. S., Welty, S. E., and Smith, C. V. (2000). Sex differences in diquat-induced hepatic necrosis and DNA fragmentation in Fischer 344 rats. Toxicol. Sci. 54 (1), 203–211. doi:10.1093/toxsci/54.1.203
Hao, L., Cheng, Y., Su, W., Wang, C., Lu, Z., Jin, M., et al. (2021). Pediococcus pentosaceus ZJUAF-4 relieves oxidative stress and restores the gut microbiota in diquat-induced intestinal injury. Appl. Microbiol. Biotechnol. 105 (4), 1657–1668. doi:10.1007/s00253-021-11111-6
He, Z., Deng, N., Zheng, B., Gu, Y., Chen, J., Li, T., et al. (2023). Apple peel polyphenol alleviates antibiotic-induced intestinal dysbiosis by modulating tight junction proteins, the TLR4/NF-κB pathway and intestinal flora. Food Funct. 14 (14), 6678–6689. doi:10.1039/d3fo01358h
Ho, G. T., and Theiss, A. L. (2022). Mitochondria and inflammatory bowel diseases: toward a stratified therapeutic intervention. Annu. Rev. Physiol. 84, 435–459. doi:10.1146/annurev-physiol-060821-083306
Hong, J. Y., Lebofsky, M., Farhood, A., and Jaeschke, H. (2009). Oxidant stress-induced liver injury in vivo: role of apoptosis, oncotic necrosis, and c-Jun NH2-terminal kinase activation. Am. J. Physiol. Gastrointest. Liver Physiol. 296 (3), G572–G581. doi:10.1152/ajpgi.90435.2008
Hrncir, T. (2022). Gut microbiota dysbiosis: triggers, consequences, diagnostic and therapeutic options. Microorganisms 10 (3), 578. doi:10.3390/microorganisms10030578
Huang, K., Li, Y., Zhang, Y., and Zhu, M. (2025). Alleviation effect of taxifolin on diquat-induced damage to porcine intestinal epithelial cells. Biochem. Biophys. Res. Commun. 748, 151318. doi:10.1016/j.bbrc.2025.151318
Huang, S., Lin, S., Zhou, S., Huang, Z., Li, Y., Liu, S., et al. (2023). Soluble thrombomodulin alleviates Diquat-induced acute kidney injury by inhibiting the HMGB1/IκBα/NF-κB signalling pathway. Food Chem. Toxicol. 178, 113871. doi:10.1016/j.fct.2023.113871
Huang, T., Rao, G., Zhao, Z., Xu, N., Zhou, M., and Ou, R. (2024). Protective effect of tumor necrosis factor receptor-associated factor 6 inhibitor C25-140 on acute kidney injury induced by diquat poisoning in mice. Zhonghua Wei Zhong Bing Ji Jiu Yi Xue 36 (12), 1273–1278. doi:10.3760/cma.j.cn121430-20230906-00751
Huang, Y., Zhang, R., Meng, M., Chen, D., and Deng, Y. (2021). High-dose diquat poisoning: a case report. J. Int. Med. Res. 49 (6), 3000605211026117. doi:10.1177/03000605211026117
Jia, P., Ji, S., Zhang, H., Chen, Y., and Wang, T. (2020). Piceatannol ameliorates hepatic oxidative damage and mitochondrial dysfunction of weaned piglets challenged with diquat. Anim. (Basel) 10 (7), 1239. doi:10.3390/ani10071239
Jin, Y., Zhai, Z., Jia, H., Lai, J., Si, X., and Wu, Z. (2021). Kaempferol attenuates diquat-induced oxidative damage and apoptosis in intestinal porcine epithelial cells. Food Funct. 12 (15), 6889–6899. doi:10.1039/d1fo00402f
Jones, G. M., and Vale, J. A. (2000). Mechanisms of toxicity, clinical features, and management of diquat poisoning: a review. J. Toxicol. Clin. Toxicol. 38 (2), 123–128. doi:10.1081/clt-100100926
Kahalehili, H. M., Newman, N. K., Pennington, J. M., Kolluri, S. K., Kerkvliet, N. I., Shulzhenko, N., et al. (2020). Dietary indole-3-carbinol activates AhR in the gut, alters Th17-microbe interactions, and exacerbates insulitis in NOD mice. Front. Immunol. 11, 606441. doi:10.3389/fimmu.2020.606441
Khan, T., Waseem, R., Zehra, Z., Aiman, A., Bhardwaj, P., Ansari, J., et al. (2022). Mitochondrial dysfunction: pathophysiology and mitochondria-targeted drug delivery approaches. Pharmaceutics 14 (12), 2657. doi:10.3390/pharmaceutics14122657
Kim, S., Chen, J., Cheng, T., Gindulyte, A., He, J., He, S., et al. (2024). PubChem 2025 update. Nucleic Acids Res. 53 (D1), D1516–D1525. doi:10.1093/nar/gkae1059
Kłos, P., and Dabravolski, S. A. (2021). The role of mitochondria dysfunction in inflammatory bowel diseases and colorectal cancer. Int. J. Mol. Sci. 22 (21), 11673. doi:10.3390/ijms222111673
Lai, K., Wang, J., Lin, S., Chen, Z., Lin, G., Ye, K., et al. (2024). Sensing of mitochondrial DNA by ZBP1 promotes RIPK3-mediated necroptosis and ferroptosis in response to diquat poisoning. Cell Death Differ. 31 (5), 635–650. doi:10.1038/s41418-024-01279-5
Li, X., Zhu, J., Lin, Q., Yu, M., Lu, J., Feng, J., et al. (2022). Effects of curcumin on mitochondrial function, endoplasmic reticulum stress, and mitochondria-associated endoplasmic reticulum membranes in the jejunum of oxidative stress piglets. J. Agric. Food Chem. 70 (29), 8974–8985. doi:10.1021/acs.jafc.2c02824
Liang, C., Ren, Y., Tian, G., He, J., Zheng, P., Mao, X., et al. (2023). Dietary glutathione supplementation attenuates oxidative stress and improves intestinal barrier in diquat-treated weaned piglets. Arch. Anim. Nutr. 77 (2), 141–154. doi:10.1080/1745039x.2023.2199806
Liu, L., Chen, D., Yu, B., Luo, Y., Huang, Z., Zheng, P., et al. (2021). Influences of selenium-enriched yeast on growth performance, immune function, and antioxidant capacity in weaned pigs exposure to oxidative stress. Biomed. Res. Int., 5533210. doi:10.1155/2021/5533210
Liu, Z., Li, Z., Zheng, Z., Li, N., Mu, S., Ma, Y., et al. (2023). Effects of L-theanine on intestinal morphology, barrier function, and MAPK signaling pathways in diquat-challenged piglets. Anim. Biotechnol. 34 (4), 1112–1119. doi:10.1080/10495398.2021.2013857
Luo, J., Wu, X., Chen, D., Yu, B., and He, J. (2024). Dietary ferulic acid supplementation enhances antioxidant capacity and alleviates hepatocyte pyroptosis in diquat challenged piglets. J. Anim. Sci. Biotechnol. 15 (1), 134. doi:10.1186/s40104-024-01086-5
Ma, B., Barathan, M., Ng, M. H., and Law, J. X. (2025). Oxidative stress, gut microbiota, and extracellular vesicles: interconnected pathways and therapeutic potentials. Int. J. Mol. Sci. 26 (7), 3148. doi:10.3390/ijms26073148
Magalhães, N., Carvalho, F., and Dinis-Oliveira, R. J. (2018). Human and experimental toxicology of diquat poisoning: toxicokinetics, mechanisms of toxicity, clinical features, and treatment. Hum. Exp. Toxicol. 37 (11), 1131–1160. doi:10.1177/0960327118765330
McCarthy, L. G., and Speth, C. P. (1983). Diquat intoxication. Ann. Emerg. Med. 12 (6), 394–396. doi:10.1016/s0196-0644(83)80474-0
Meng, X., Zhou, J., Zhao, C. N., Gan, R. Y., and Li, H. B. (2020). Health benefits and molecular mechanisms of resveratrol: a narrative review. Foods 9 (3), 340. doi:10.3390/foods9030340
Newman, L. E., and Shadel, G. S. (2023). Mitochondrial DNA release in innate immune signaling. Annu. Rev. Biochem. 92, 299–332. doi:10.1146/annurev-biochem-032620-104401
Nisar, R., Hanson, P. S., He, L., Taylor, R. W., Blain, P. G., and Morris, C. M. (2015). Diquat causes caspase-independent cell death in SH-SY5Y cells by production of ROS independently of mitochondria. Arch. Toxicol. 89 (10), 1811–1825. doi:10.1007/s00204-015-1453-5
Nong, K., Liu, Y., Fang, X., Qin, X., Liu, Z., and Zhang, H. (2023). Effects of the vitamin D3 on alleviating the oxidative stress induced by diquat in wenchang chickens. Anim. (Basel) 13 (4), 711. doi:10.3390/ani13040711
Prakash, V., Bose, C., Sunilkumar, D., Cherian, R. M., Thomas, S. S., and Nair, B. G. (2024). Resveratrol as a promising nutraceutical: implications in gut microbiota modulation, inflammatory disorders, and colorectal cancer. Int. J. Mol. Sci. 25 (6), 3370. doi:10.3390/ijms25063370
Qiao, L., Dou, X., Yan, S., Zhang, B., and Xu, C. (2020). Biogenic selenium nanoparticles synthesized by Lactobacillus casei ATCC 393 alleviate diquat-induced intestinal barrier dysfunction in C57BL/6 mice through their antioxidant activity. Food Funct. 11 (4), 3020–3031. doi:10.1039/d0fo00132e
Qu, J., Pei, H., Li, X. Z., Li, Y., Chen, J. M., Zhang, M., et al. (2024). Erythrocyte membrane biomimetic EGCG nanoparticles attenuate renal injury induced by diquat through the NF-κB/NLRP3 inflammasome pathway. Front. Pharmacol. 15, 1414918. doi:10.3389/fphar.2024.1414918
Rath, E., and Haller, D. (2022). Intestinal epithelial cell metabolism at the interface of microbial dysbiosis and tissue injury. Mucosal Immunol. 15 (4), 595–604. doi:10.1038/s41385-022-00514-x
Rawlings, J. M., Wyatt, I., and Heylings, J. R. (1994). Evidence for redox cycling of diquat in rat small intestine. Biochem. Pharmacol. 47 (7), 1271–1274. doi:10.1016/0006-2952(94)90401-4
Ren, Y., Guo, F., and Wang, L. (2024). Imaging findings and toxicological mechanisms of nervous system injury caused by diquat. Mol. Neurobiol. 61 (11), 9272–9283. doi:10.1007/s12035-024-04172-x
Saito, K. (1986). Effect of diquat on cell growth and macromolecule synthesis in cultured pneumocytes. Tohoku J. Exp. Med. 148 (1), 35–39. doi:10.1620/tjem.148.35
Salvo Romero, E., Alonso Cotoner, C., Pardo Camacho, C., Casado Bedmar, M., and Vicario, M. (2015). The intestinal barrier function and its involvement in digestive disease. Rev. Esp. Enferm. Dig. 107 (11), 686–696. doi:10.17235/reed.2015.3846/2015
Song, C., Chai, Z., Chen, S., Zhang, H., Zhang, X., and Zhou, Y. (2023). Intestinal mucus components and secretion mechanisms: what we do and do not know. Exp. and Mol. Med. 55 (4), 681–691. doi:10.1038/s12276-023-00960-y
Summers, L. A., and Black, A. L. (1968). Herbicidal activity of an aromatic analogue of diquat. Nature 218 (5146), 1067–1068. doi:10.1038/2181067a0
Sun, Y. Q., Xian, X. H., Gao, H. B., Yuan, L., Xiao, H., Lv, B. P., et al. (2020). The expression of Nrf2 in the lung tissue of rats with acute diquat poisoning and the distribution of diquat in lungs. Zhonghua Lao Dong Wei Sheng Zhi Ye Bing Za Zhi 38 (5), 327–331. doi:10.3760/cma.j.cn121094-20191109-00536
Takiishi, T., Fenero, C. I. M., and Câmara, N. O. S. (2017). Intestinal barrier and gut microbiota: shaping our immune responses throughout life. Tissue Barriers 5 (4), e1373208. doi:10.1080/21688370.2017.1373208
Turner, J. R. (2009). Intestinal mucosal barrier function in health and disease. Nat. Rev. Immunol. 9 (11), 799–809. doi:10.1038/nri2653
Ulluwishewa, D., Anderson, R. C., McNabb, W. C., Moughan, P. J., Wells, J. M., and Roy, N. C. (2011). Regulation of tight junction permeability by intestinal bacteria and dietary components. J. Nutr. 141 (5), 769–776. doi:10.3945/jn.110.135657
Wang, A. N., Cai, C. J., Zeng, X. F., Zhang, F. R., Zhang, G. L., Thacker, P. A., et al. (2013). Dietary supplementation with Lactobacillus fermentum I5007 improves the anti-oxidative activity of weanling piglets challenged with diquat. J. Appl. Microbiol. 114 (6), 1582–1591. doi:10.1111/jam.12188
Wang, C., Cao, S., Zhang, Q., Shen, Z., Feng, J., Hong, Q., et al. (2019). Dietary tributyrin attenuates intestinal inflammation, enhances mitochondrial function, and induces mitophagy in piglets challenged with diquat. J. Agric. Food Chem. 67 (5), 1409–1417. doi:10.1021/acs.jafc.8b06208
Wang, J. F., Shi, C. Y., and Ying, H. Z. (2022). Cephalosporins-induced intestinal dysbiosis exacerbated pulmonary endothelial barrier disruption in streptococcus pneumoniae-infected mice. Front. Cell Infect. Microbiol. 12, 997368. doi:10.3389/fcimb.2022.997368
Wang, S., Bai, M., Xu, K., Shao, Y., Yang, Z., Xiong, X., et al. (2021). Effects of coated cysteamine on oxidative stress and inflammation in weaned pigs. Anim. (Basel) 11 (8), 2217. doi:10.3390/ani11082217
Wang, Y., Lai, H., Zhang, T., Wu, J., Tang, H., Liang, X., et al. (2023). Mitochondria of intestinal epithelial cells in depression: are they at a crossroads of gut-brain communication? Neurosci. Biobehav Rev. 153, 105403. doi:10.1016/j.neubiorev.2023.105403
Wen, C., Guo, Q., Wang, W., Duan, Y., Zhang, L., Li, J., et al. (2020). Taurine alleviates intestinal injury by mediating tight junction barriers in diquat-challenged piglet models. Front. Physiol. 11, 449. doi:10.3389/fphys.2020.00449
Wen, X., Tang, S., Wan, F., Zhong, R., Chen, L., and Zhang, H. (2024b). The PI3K/Akt-Nrf2 signaling pathway and mitophagy synergistically mediate hydroxytyrosol to alleviate intestinal oxidative damage. Int. J. Biol. Sci. 20 (11), 4258–4276. doi:10.7150/ijbs.97263
Wen, X., Wan, F., Zhong, R., Chen, L., and Zhang, H. (2024a). Hydroxytyrosol alleviates intestinal oxidative stress by regulating bile acid metabolism in a piglet model. Int. J. Mol. Sci. 25 (11), 5590.
Wen, X., Wan, F., Zhong, R., Chen, L., and Zhang, H. (2024c). Hydroxytyrosol alleviates intestinal oxidative stress by regulating bile acid metabolism in a piglet model. Int. J. Mol. Sci. 25 (11), 5590. doi:10.3390/ijms25115590
Wilkinson, V. (1969). Ecological effects of diquat. Nature 224 (5219), 618–619. doi:10.1038/224618a0
Wolfgang, G. H., Jolly, R. A., Donarski, W. J., and Petry, T. W. (1991). Inhibition of diquat-induced lipid peroxidation and toxicity in precision-cut rat liver slices by novel antioxidants. Toxicol. Appl. Pharmacol. 108 (2), 321–329. doi:10.1016/0041-008x(91)90121-t
Wu, H., Xie, S., Miao, J., Li, Y., Wang, Z., Wang, M., et al. (2020). Lactobacillus reuteri maintains intestinal epithelial regeneration and repairs damaged intestinal mucosa. Gut Microbes 11 (4), 997–1014. doi:10.1080/19490976.2020.1734423
Wu, J., Jia, Y., Liao, Y., Yang, D., Ren, H., Xie, Z., et al. (2024). Protective effect and mechanism of CoQ10 in mitochondrial dysfunction in diquat-induced renal proximal tubular injury. J. Biochem. Mol. Toxicol. 38 (11), e70023. doi:10.1002/jbt.70023
Wu, K. C., Zhang, Y., and Klaassen, C. D. (2012). Nrf2 protects against diquat-induced liver and lung injury. Free Radic. Res. 46 (10), 1220–1229. doi:10.3109/10715762.2012.700709
Wu, Y., Cui, S., Wang, W., Jian, T., Kan, B., and Jian, X. (2022a). Kidney and lung injury in rats following acute diquat exposure. Exp. Ther. Med. 23 (4), 275. doi:10.3892/etm.2022.11201
Wu, Y., Jha, R., Li, A., Liu, H., Zhang, Z., Zhang, C., et al. (2022b). Probiotics (Lactobacillus plantarum HNU082) supplementation relieves ulcerative colitis by affecting intestinal barrier functions, immunity-related gene expression, gut microbiota, and metabolic pathways in mice. Microbiol. Spectr. 10 (6), e0165122. doi:10.1128/spectrum.01651-22
Wu, Y., Wang, B., Xu, H., Tang, L., Li, Y., Gong, L., et al. (2019). Probiotic Bacillus attenuates oxidative stress- induced intestinal injury via p38-mediated autophagy. Front. Microbiol. 10, 2185. doi:10.3389/fmicb.2019.02185
Xing, J., Chu, Z., Han, D., Jiang, X., Zang, X., Liu, Y., et al. (2020). Lethal diquat poisoning manifesting as central pontine myelinolysis and acute kidney injury: a case report and literature review. J. Int. Med. Res. 48 (7), 300060520943824. doi:10.1177/0300060520943824
Xu, C., Qiao, L., Guo, Y., Ma, L., and Cheng, Y. (2018). Preparation, characteristics and antioxidant activity of polysaccharides and proteins-capped selenium nanoparticles synthesized by Lactobacillus casei ATCC 393. Carbohydr. Polym. 195, 576–585. doi:10.1016/j.carbpol.2018.04.110
Xu, Q., Liu, M., Chao, X., Zhang, C., Yang, H., Chen, J., et al. (2022). Acidifiers attenuate diquat-induced oxidative stress and inflammatory responses by regulating NF-κB/MAPK/COX-2 pathways in IPEC-J2 cells. Antioxidants (Basel) 11 (10). doi:10.3390/antiox11102002
Xu, Q., Liu, M., Chao, X., Zhang, C., Yang, H., Chen, J., et al. (2023). Stevioside improves antioxidant capacity and intestinal barrier function while attenuating inflammation and apoptosis by regulating the NF-κB/MAPK pathways in diquat-induced oxidative stress of IPEC-J2 cells. Antioxidants (Basel) 12 (5), 1070. doi:10.3390/antiox12051070
Xu, X., Wei, Y., Hua, H., Zhu, H., Xiao, K., Zhao, J., et al. (2022). Glycine alleviated intestinal injury by inhibiting ferroptosis in piglets challenged with diquat. Anim. (Basel) 12 (22), 3071. doi:10.3390/ani12223071
Xun, W., Fu, Q., Shi, L., Cao, T., Jiang, H., and Ma, Z. (2021). Resveratrol protects intestinal integrity, alleviates intestinal inflammation and oxidative stress by modulating AhR/Nrf2 pathways in weaned piglets challenged with diquat. Int. Immunopharmacol. 99, 107989. doi:10.1016/j.intimp.2021.107989
Yan, Q., Jia, S., Li, D., and Yang, J. (2023). The role and mechanism of action of microbiota-derived short-chain fatty acids in neutrophils: from the activation to becoming potential biomarkers. Biomed. Pharmacother. 169, 115821. doi:10.1016/j.biopha.2023.115821
Yoshioka, T., Sugimoto, T., Kinoshita, N., Shimazu, T., Hiraide, A., and Kuwagata, Y. (1992). Effects of concentration reduction and partial replacement of paraquat by diquat on human toxicity: a clinical survey. Hum. Exp. Toxicol. 11 (4), 241–245. doi:10.1177/096032719201100401
Yu, G., Wang, J., Jian, T., Shi, L., Zhao, L., Li, Y., et al. (2022). Case series: diquat poisoning with acute kidney failure, myocardial damage, and rhabdomyolysis. Front. Public Health 10, 991587. doi:10.3389/fpubh.2022.991587
Zhan, S., Wu, L., Lv, Y., Huang, W., Ge, C., Hu, Z., et al. (2025). Lactobacillus reuteri alleviates diquat induced hepatic impairment and mitochondrial dysfunction via activation of the Nrf2 antioxidant system and suppression of NF-κB inflammatory response. Poult. Sci. 104 (5), 104997. doi:10.1016/j.psj.2025.104997
Zhang, H., Chen, Y., Chen, Y., Jia, P., Ji, S., Xu, J., et al. (2020). Comparison of the effects of resveratrol and its derivative pterostilbene on hepatic oxidative stress and mitochondrial dysfunction in piglets challenged with diquat. Food Funct. 11 (5), 4202–4215. doi:10.1039/d0fo00732c
Zhang, H., Liu, Y., Fang, X., Gu, L., Luo, C., Chen, L., et al. (2021). Vitamin D3 protects mice from diquat-induced oxidative stress through the NF-κB/Nrf2/HO-1 signaling pathway. Oxid. Med. Cell Longev. 2021, 6776956. doi:10.1155/2021/6776956
Zhang, L., Wei, W., Xu, J., Min, F., Wang, L., Wang, X., et al. (2006). Inhibitory effect of melatonin on diquat-induced lipid peroxidation in vivo as assessed by the measurement of F2-isoprostanes. J. Pineal Res. 40 (4), 326–331. doi:10.1111/j.1600-079X.2005.00311.x
Zhang, X. Y., Li, G. Q., and Liu, B. (2022). The research progress of nervous system damage caused by diquat poisoning. Zhonghua Lao Dong Wei Sheng Zhi Ye Bing Za Zhi 40 (8), 636–640. doi:10.3760/cma.j.cn121094-20210713-00350
Zhao, M., Chu, J., Feng, S., Guo, C., Xue, B., He, K., et al. (2023). Immunological mechanisms of inflammatory diseases caused by gut microbiota dysbiosis: a review. Biomed. Pharmacother. 164, 114985. doi:10.1016/j.biopha.2023.114985
Zhou, N., Tian, Y., Liu, W., Tu, B., Xu, W., Gu, T., et al. (2022). Protective effects of resveratrol and apigenin dietary supplementation on serum antioxidative parameters and mRNAs expression in the small intestines of diquat-challenged pullets. Front. Vet. Sci. 9, 850769. doi:10.3389/fvets.2022.850769
Keywords: Diquat, gut health, molecular mechanisms, toxic effects, protective strategies
Citation: He C, Cai G, Jia Y, Jiang R, Wei X and Tao N (2025) Effect of Diquat on gut health: molecular mechanisms, toxic effects, and protective strategies. Front. Pharmacol. 16:1562182. doi: 10.3389/fphar.2025.1562182
Received: 17 January 2025; Accepted: 28 April 2025;
Published: 12 May 2025.
Edited by:
Rosa Serio, University of Palermo, ItalyReviewed by:
Akinleye Stephen Akinrinde, University of Ibadan, NigeriaNajm Alsadat Madani, State University of New York, United States
Copyright © 2025 He, Cai, Jia, Jiang, Wei and Tao. This is an open-access article distributed under the terms of the Creative Commons Attribution License (CC BY). The use, distribution or reproduction in other forums is permitted, provided the original author(s) and the copyright owner(s) are credited and that the original publication in this journal is cited, in accordance with accepted academic practice. No use, distribution or reproduction is permitted which does not comply with these terms.
*Correspondence: Xiaolan Wei, MTM5ODI1MjA5MDNAMTYzLmNvbQ==; Ning Tao, dG4xODE3NTkzOThAMTYzLmNvbQ==
†These authors have contributed equally to this work