- 1School of Life Sciences and Biopharmaceutics, Guangdong Pharmaceutical University, Guangzhou, Guangdong, China
- 2Guangdong Provincial Key Laboratory for Research and Evaluation of Pharmaceutical Preparations, Guangdong Pharmaceutical University, Guangzhou, China
Background: Schizophyllan (SPG), a bioactive polysaccharide from Schizophyllum commune, possesses significant anti-inflammatory, antioxidant, and immunomodulatory properties. The molecular weight of polysaccharides significantly impacts their structural properties and biological functions. However, the functional characteristics of low molecular weight polysaccharides derived from Schizophyllum commune remain inadequately explored.
Methods: This study developed an ultrasound-assisted hydrogen peroxide (H2O2) degradation method to produce low-molecular-weight SPG with enhanced bioactivity. The process was optimized using response surface methodology, focusing on ultrasound duration, ultrasonic power, and H2O2 concentration. This approach effectively reduced the molecular weight of SPG from 4,409,608 Da to 257,500 Da, yielding three distinct variants: SPG-a (257,500 Da), SPG-b (429,300 Da), and SPG-c (364,800 Da). The bioactivity of these variants was assessed through in vitro cell proliferation and migration assays using BJ and HaCaT cells, as well as an in vivo zebrafish larval caudal fin regeneration model.
Results: In vitro, SPG-b significantly promoted cell proliferation, increasing BJ and HaCaT cells growth by 53.69% and 14.59%, respectively, at a concentration of 300 μg/mL (p < 0.05), compared to undegraded SPG. Additionally, scratch assays revealed that SPG-a enhanced BJ cells migration by 24.13% (p < 0.05), while SPG-b exhibited most pronounced effect on HaCaT cells migration (17.12%, p < 0.05), compared to the undegraded SPG. In vivo, SPG-c (3.125 mg/mL) significantly improved fin regeneration rates by 6.97% (p < 0.05) in zebrafish larvae, compared to the undegraded SPG.
Conclusion: This study demonstrates that ultrasound-assisted H2O2 degradation effectively reduces SPG molecular weight while enhancing its functional properties. These findings provide a foundation for the further development of SPG in pharmaceutical and cosmetic applications, highlighting its potential for broader utilization.
1 Introduction
Schizophyllan (SPG), an active polysaccharide originally extracted from the fruiting bodies or fermentation broth of Schizophyllum commune, is generally recognized as a glucose-based homopolysaccharide (Zhang et al., 2013). However, several studies have demonstrated that SPG from different sources may exhibit heteropolysaccharide characteristics (Chen et al., 2020; Zhong et al., 2013; Du et al., 2017). Recently, our study (Patent Application No.: 202411835493.0, pending) revealed that SPG produced via liquid fermentation using the Schizophyllum commune 5.43 strain (GDMCC No. 5.43), is a heteropolysaccharide consisting of glucose, mannitol, galactose, and fucose in a molar ratio of 86:8:5:1. Notably, SPG obtained through liquid fermentation exhibits potent bioactivities, including anti-inflammatory, antioxidant, and anti-aging effects (Deng et al., 2021; Deng et al., 2022; Tu et al., 2023). Despite these advantages, its high molecular weight and viscosity present significant challenges in pharmaceutical and cosmetic applications. These properties limit its bioavailability by impeding efficient absorption and distribution within the body, while the increased viscosity complicates formulation processes, leading to issues such as poor solubility, instability, and difficulties in product consistency. In contrast, low-molecular-weight polysaccharides offer improved absorption, reduced toxicity, and enhanced bioavailability (Zhao et al., 2016). Several methods have been explored to reduce SPG’s molecular weight. Zhong et al. applied ultrasound-assisted treatment to degrade SPG (Zhong et al., 2015), but this approach is hindered by long processing times, high energy consumption. These findings highlight the critical need to develop efficient and controllable methods for SPG molecular weight reduction to optimize its functionality and broaden its applications.
The biological activity of polysaccharides is closely influenced by their molecular weight, degree of polymerization, and conformation (Wang et al., 2024). Degradation methods for polysaccharides can be broadly categorized into physical, chemical, and biological approaches (Li et al., 2022). While physical methods are environmentally friendly, they are costly and may lead to incomplete degradation (Yu et al., 2020). Chemical approaches are straightforward but risk byproduct generation and environmental hazards (Yuan et al., 2020). Biological methods provide uniform products under mild conditions but are expensive and require stringent controls (Dou et al., 2019). Ultrasonic-assisted degradation has emerged as an eco-friendly, efficient alternative for polysaccharide modification (Hu et al., 2022; Wang et al., 2019; Youssouf et al., 2017). In an ultrasonic-assisted H2O2 system, ultrasound enhances cavitation effects and accelerates H2O2 dissociation, producing hydroxyl radicals that promote efficient degradation (Ayanda et al., 2018). This hybrid method combines the advantages of physical and chemical techniques, providing a novel strategy for polysaccharide degradation. However, limited studies have explored this approach specifically for SPG.
Polysaccharides play a critical role in wound healing, which involves vasoconstriction, inflammation, cell proliferation, and tissue remodeling (Pugliese et al., 2018; de Oliveira and Wilson, 2020; Ridiandries et al., 2018; Rodrigues et al., 2019). Fungal polysaccharides, in particular, demonstrate significant potential in promoting tissue regeneration (Sharifi-Rad et al., 2020). For instance, polysaccharides derived from Trametes versicolor have been shown to enhance cell proliferation and expedite wound healing (Teymoorian et al., 2024), while extracts from Lignosus rhinocerotis exhibit notable wound repair capabilities (Yap et al., 2023). In comparison, SPG has unique properties in promoting wound healing. Compared with other substances, SPG has good biocompatibility and low toxicity, and it can enhance the body’s immune response to promote tissue repair(Ansari et al., 2025). Despite these promising characteristics, the application of SPG in wound healing and tissue regeneration remains underexplored, particularly regarding the influence of molecular weight variations on its bioactivity.
This study employed ultrasonic-assisted H2O2 degradation to produce SPG fractions with varying molecular weights. The process parameters, including ultrasonic duration, ultrasonic power, and H2O2 concentration, were systematically optimized using response surface methodology (RSM). The resulting SPG fractions were evaluated for their structural properties, antioxidant activity, and effects on the proliferation and migration of human skin fibroblasts (BJ) and human keratinocytes (HaCaT). Additionally, their tissue regeneration potential was assessed using a zebrafish caudal fin regeneration model. This research not only elucidates the mechanisms of SPG degradation under ultrasonic-assisted H2O2 treatment but also highlights the impact of molecular weight on its biological activities, providing a theoretical and technical foundation for the development of low-molecular-weight SPG in pharmaceutical and cosmetic applications.
2 Materials and methods
2.1 Reagents, materials, and instruments
Schizophyllum commune 5.43 (GDMCC No. 5.43), was purchased from Guangdong Microbial Culture Collection Center (Guangzhou, China). 2,2′-Azinobis (3-ethylbenzothiazoline-6-sulfonic) acid Ammonium Salt (ABTS) and 1,1-diphenyl-2-picrylhydrazyl (DPPH) were purchased from Shanghai Macklin Biochemical Technology Co., Ltd. (Shanghai, China). Pullulan polysaccharides were purchased from Showa Denko (Tokyo, Japan). Cyclin B and GAPDH antibodies were purchased from Proteintech (Wuhan, China). CCK-8 and BCA kits were purchased from GLPBIO (Montclair, United States). EdU-594 cell proliferation assay kit was purchased from Beyotime (Shanghai, China). All other chemicals and reagents were of analytical grade and purchased from local suppliers.
The AB zebrafish was provided by Guangzhou Rubi Biotechnology Co., Ltd. HaCaT and BJ cell lines were obtained from Guangdong Marubi Biotechnology Co., Ltd. (Guangzhou, China). High-glucose Dulbecco’s Modified Eagle Medium (DMEM) and trypsin were obtained from Gibco (Grand Island, NY, United States). Fetal bovine serum was obtained from Hangzhou Sijiqing Bioengineering Materials Co., Ltd. (Hangzhou, China).
The following equipment was used: ZQPW-70 shaking incubator with full temperature control, Tianjin Laibote Rui Instrument Equipment Co., Ltd. (Tianjin, China). HC-3026R high-speed refrigerated centrifuge, Anhui Zhongke Zhongjia Scientific Instrument Co., Ltd. (Hefei, China). ELX800 microplate reader, Biotek, (Phoenix, AZ, United States). JY92-LLDN Ultrasound cell disruptor, Ningbo Xinzhi Biotechnology Co., Ltd. (Ningbo, China). NDJ-5S Digital rotational viscometer, Shanghai Genggeng Instrument Equipment Co., Ltd. (Shanghai, China). LC-10A High performance liquid chromatograph, Shimadzu Corporation, (Tokyo, Japan). Thermo Nicoletis 50 Fourier transform infrared spectrometer, ThermoFisher Scientific, (Waltham, MA, United States). JSM-7610F PLUS Field emission scanning electron microscopy, Japan Electronics Co., Ltd. (Tokyo, Japan). D8 ADVANCE X-ray diffractometer, Bruker, (Billerica, MA, United States). Tanon 2,500 Gel imaging system, Tanon Science & Technology Co., Ltd. (Shanghai, China). EVOS M5000 Intelligent Cell Imaging System, Thermo Fisher Scientific (Waltham, MA, United States). SZ680 Continuous Zoom Stereo Microscope, Chongqing Ott Optical Instrument Co., Ltd. (Chongqing, China).
2.2 Extraction and preparation of SPG
SPG was extracted from Schizophyllum commune 5.43 following our previously established method (Deng et al., 2021). Specifically, S. commune 5.43 was cultured in seed medium at 28°C for 3 days to prepare fermentation seed liquid. Subsequently, 5% (v/v) of the seed liquid was inoculated into 250 mL sterile Erlenmeyer flasks containing 100 mL of culture medium. The culture was incubated at 160 rpm and 28°C for 6 days. The medium consisted of 3% glucose, 0.01% KH2PO4, 0.05% MgSO4.7H2O, and 0.3% yeast extract, sterilized at 121°C for 20 min. After fermentation, the culture broth was centrifuged at 12,000 g for 20 min to separate the mycelium from the supernatant. The supernatant was collected for further analysis. The total sugar content and reducing sugar contents were determined using the phenol-sulfuric acid method (Yan et al., 2018) and the DNS method (Huang et al., 2020), respectively.
2.3 Optimization of the degradation process
2.3.1 Single-factor experimental design
The SPG extracted as described in Section 2.2 was subjected to degradation using an approach modified from Li et al. (2023). Single-factor experiments were conducted to optimize ultrasound duration, ultrasound power, and H2O2 concentration. For each experiment, the optimized parameter determined from the preceding test was used as a fixed condition. Based on preliminary experiments and insights gained from previous literature (Wang et al., 2024; Li et al., 2020), we have determined the following parameters: Ultrasound durations of 10, 15, 20, 25, and 30 min, ultrasound powers of 50, 100, 150, 200, and 250 W, and H2O2 concentrations of 1.0%, 2.0%, 3.0%, 4.0%, and 5.0% were evaluated. After each experiment, the kinematic viscosity of SPG samples was measured using a rotary viscometer to determine the effect of each variable. Optimal parameters were identified based on minimum kinematic viscosity.
2.3.2 Evaluation of weight-average molecular weight
The weight-average molecular weight (Mw) of SPG was analyzed using the high-performance liquid chromatography (HPLC) equipped with a refractive index detector and a TSK-Gel G3000 PWXL column, following the method of Gu et al. (2020). SPG solutions prepared in Section 2.2 were filtered through 0.22 µm aqueous microporous membranes before injection. The injection volume was 10 μL, with a flow rate of 0.5 mL/min and a column temperature of 40°C. Pullulan polysaccharides (Mw range: 6.9–883,000 Da) were employed as standards to generate a calibration curve for Mw calculation. This analysis provided an accurate assessment of the molecular weight distribution of SPG after degradation.
2.3.3 Response surface optimization experiment
A Box-Behnken design (BBD) was employed to optimize the degradation process of SPG based on the results of single-factor experiments. This experimental design focused on three key factors: ultrasound power (A), ultrasound duration (B), and H2O2 concentration (C). Each factor was evaluated at three levels (low, medium, high) to assess its effect on Mw of SPG, which served as the response variable (Rachpirom et al., 2023). The specific levels for each factor are presented in Table 1.
2.3.4 Design and analysis
The BBD experimental results were fitted to a quadratic model, and analysis of variance (ANOVA) was conducted to identify the significant factors and interactions influencing Mw. The optimal conditions were determined based on the model predictions and experimental validation.
2.4 Structure characterization
2.4.1 Purification of SPG
Activated carbon (1%, w/v) was added to the fermentation broth, followed by incubation in a water bath at 70 °C for 30 min. The mixture was centrifuged at 5,000 g for 30 min to remove pigments. Proteins were removed using the Sevag method (Tang et al., 2024), and polysaccharides were precipitated with ethanol (Wang et al., 2020). The precipitate was dialyzed in pure water for 24 h and lyophilized to obtain purified SPG.
2.4.2 Congo red experiment
The Congo red assay was conducted to examine the interaction of SPG with Congo red as described by Zhang et al. (Zhang et al., 2020). A 0.3 mg/mL SPG solution was mixed with an equal volume of 80 μmol/L Congo red solution. Various final concentrations of NaOH (0–1.0 mol/L) were added to the mixture. A NaOH solution without SPG served as a control. Absorbance spectra between 400 and 600 nm was recorded using a microplate reader, and shifts in the maximum absorption wavelength were analyzed to evaluate conformational changes.
2.4.3 Ultraviolet-visible absorption spectroscopy (UV-Vis) analysis
The UV-Vis spectra of SPG were measured to detect characteristic polysaccharide groups and impurities, following the method of Zhang et al. (2022). SPG solutions (0.3 mg/mL) were scanned within the wavelength range of 200–600 nm using a UV-Vis spectrophotometer, and spectral features indicative of structural integrity and purity were analyzed.
2.4.4 Fourier transform infrared spectroscopy (FT-IR) analysis
Functional groups of SPG were identified using FT-IR spectroscopy. Lyophilized SPG samples were mixed with KBr ground into a fine powder, and compressed into pellets. FT-IR spectra were recorded in the range of 4,000–400 cm−1. Peak positions and intensities were analyzed to determine the functional groups and chemical composition of SPG.
2.4.5 Diffraction of x-rays (XRD) analysis
The crystal structure of SPG was analyzed using a D2 PHASER X-ray diffractometer, following the method of Kazemi et al. (2019). Lyophilized SPG samples (3.0 mg) were mounted onto the sample holder and scanned across a 2θ range 5°–90°, a step size of 0.013° and a scanning speed of 12°/min. The diffraction patterns were analyzed to assess crystallinity and structural organization.
2.4.6 Scanning electron microscope (SEM) analysis
The microstructure, particle morphology, and surface characteristics of SPG were visualized using field emission scanning electron microscopy. Lyophilized SPG samples (3.0 mg) were mounted on copper stubs with double-sided conductive adhesive tape, coated with a thin layer of gold, and imaged under the SEM. Surface topography and microstructural features were documented for further analysis.
2.5 Determination of antioxidant activity in vitro
2.5.1 DPPH· radical scavenging capacity assay
The DPPH radical scavenging activity of SPG was assessed with slight modifications to the method described by Chen et al. (2022). Briefly, 2 mL of the sample was mixed with an equal volume of 0.1 mM DPPH solution and incubated in the dark at room temperature for 30 min. Absolute ethanol was used as the blank control, while ascorbic acid (Vc) served as the positive control. Each sample was analyzed in triplicate. The absorbance of the mixtures was measured at 517 nm using a microplate reader. The DPPH radical scavenging activity was calculated using the following formula:
where A0 is the absorbance of the DPPH solution in absolute ethanol, A1 is the absorbance of the DPPH solution containing the sample, and A2 is the absorbance of the sample mixed with absolute ethanol.
2.5.2 ABTS+· radical scavenging capacity assay
The ABTS+· radical scavenging activity was determined with slight modifications to the method of Zhang et al. (2012). A 7 mM ABTS+ stock solution was mixed with 7.35 mM potassium persulfate in a 2:1 (v/v) ratio and incubated in the dark at room temperature for 16 h to generate ABTS+· radical. Before use, the solution was diluted with 0.01 M PBS buffer to an absorbance of 0.70 ± 0.02 at 743 nm, serving as the ABTS+ working solution. In the assay, 1.9 mL of ABTS+ working solution was mixed with 100 μL of the sample and incubated in the dark at room temperature for 7 min. Absorbance was measured at 743 nm using a microplate reader. PBS was used as the blank control, and Vc served as the positive control. The ABTS+· radical scavenging activity was calculated using the following formula:
where A0 is the absorbance of the blank control, A1 is the absorbance of the ABTS+ solution containing the sample, and A2 is the absorbance of the solution where the sample was replaced by an equal volume of PBS.
2.5.3 Hydroxyl radical scavenging activity assay
The ⋅OH radical scavenging activity of SPG was determined with slight modifications to the method of Yan et al. (2018) Briefly, 200 μL of the sample was mixed with 600 μL of 6 mM ferrous sulfate solution and 600 μL of 6 mM salicylic acid-ethanol solution. After thorough mixing and incubation at room temperature for 10 min, 600 μL of 8.8 mM hydrogen peroxide solution was added, followed by addition mixing and incubation in the dark for 30 min. The absorbance of the reaction mixture was measured at 517 nm using a microplate reader. Double-distilled water (dd H2O) was used as the as the blank control, and Vc served as the positive control. The ⋅OH radical scavenging activity was calculated using the formula:
where A0 is the absorbance of the blank control, A1 is the absorbance of the sample, and A2 is the absorbance of the reaction mixture in which H2O2 was replaced by dd H2O.
2.6 Cell culture
Human immortalized epidermal cells (HaCaT) and human dermal fibroblasts (BJ) were cultured in high-glucose DMEM supplemented with 10% fetal bovine serum (FBS) and 1% penicillin-streptomycin (P/S). Cells were maintained in a humidified incubator at 37°C with 5% CO2.
2.7 CCK-8 assay
The effect of SPG on cell proliferation was evaluated using the CCK-8 assay, performed according to the manufacturer’s instructions. HaCaT and BJ cells were seeded into 96-well plates at a density of 5.0 × 103 cells per well and incubated for 24 h. Subsequently, the culture medium was replaced with fresh medium containing SPG at various concentrations (0, 50, 100, 200, 300, 400, 500 μg/mL). After 24 h of incubation, the medium was replaced with CCK-8 reagent diluted in medium, and cells incubated at 37°C for 1 h. Absorbance was measured at 450 nm using a microplate reader. Cell viability was calculated to evaluate the proliferative effect of SPG.
2.8 Wound healing scratch migration assay
The wound healing assay was conducted to assess the in vitro wound-healing potential of SPG, with modifications based on the method described by Li et al. (2022). HaCaT and BJ cells were seeded into 24-well plates at a density of 2.5 × 105 cells per well and cultured until confluent. A uniform scratch was made across the monolayer using a sterile 200 μL pipette tip. Detached cells were removed by gently washing the wells twice with PBS, and fresh medium containing 300 μg/mL SPG was added. The medium without SPG served as the blank control. After 48 h of incubation, images of the wound areas were captured using an optical microscope. The wound healing process was analyzed using ImageJ software, and the cell migration rate was calculated using the following formula:
where A0 represents the initial wound area (pixels) at 0 h, and A1 represents the wound area (pixels) at 48 h.
2.9 EdU assay
The EdU assay was performed with modifications based on the method described by Zhang et al. (2021) to evaluate cell proliferation. HaCaT cells were seeded into 24-well plates at a density of 1.0 × 105 cells per well and cultured for 24 h. Subsequently, cells were treated with 100 μg/mL SPG for 48 h. To label proliferative cells, an equal volume of 2× EdU working solution was added, followed by incubation at 37°C for 2 h. After incubation, cells were fixed in 4% paraformaldehyde for 15 min and permeabilized with 0.3% Triton X-100 for 5 min. Nuclei were counterstained with DAPI. Fluorescent images of EdU-positive cells were captured using a high-resolution cell imaging system, and the percentage of EdU-positive cells was quantified using ImageJ software.
2.10 Western blot assay
Western blotting was conducted to investigate the molecular mechanisms underlying SPG-induced proliferation of HaCaT cells, focusing on Cyclin B1 expression. The method was adapted from An et al. (2024) with modifications. Total proteins were extracted from HaCaT cells treated with 100 μg/mL SPG for 48 h using RIPA lysis buffer supplemented with PMSF. Protein concentrations were quantified using a BCA protein assay kit. Equal amounts of protein (30.0 μg) were separated by 12% SDS-PAGE and transferred to PVDF membranes. The membranes were blocked with 5% non-fat milk at room temperature for 2 h and then incubated overnight at 4°C with primary antibodies against Cyclin B1 (1:5,000) and GAPDH (1:5,000). Following overnight incubation, the membranes were washed and incubated with HRP-conjugated secondary antibodies (1:5,000) for 1 h at room temperature. Protein bands were visualized using a chemiluminescence detection system, and the relative expression of Cyclin B1 was quantified using ImageJ software, with GAPDH serving as the internal control.
2.11 Maintenance of larvae zebrafish
Wild-type AB zebrafish were obtained from the Guangzhou Rubi Biotechnology Co., Ltd. and maintained under standardized laboratory conditions (Sun et al., 2019): Key environmental parameters included dissolved oxygen levels >6.0 mg/L, un-ionized ammonia <0.02 mg/L, conductivity at 500–600 μS, nitrate levels <30.0 mg/L, nitrite levels <0.01 mg/L, salinity at 0.6 g/L, and neutral pH. The photoperiod was set to 14 h light:10 h dark, and water temperature was consistently maintained at 28°C. Normally developed embryos were collected following natural spawning and incubated in embryo medium (EM) at 28°C for 72 h. Healthy larvae were selected for subsequent experiments.
2.12 Caudal fin regeneration of zebrafish larvae
The caudal fin regeneration assay was performed with slight modifications to the protocol described by Mathew et al. (2006). Zebrafish larvae were anesthetized in 0.05% (w/v) tricaine solution, and caudal fin amputation was carried out under a stereomicroscope. Post-amputation, larvae were immediately transferred to EM containing 3.125 mg/mL SPG and incubated for at 28°C for 72 h. Control groups were maintained in SPG-free EM under identical conditions. At the end of the incubation period, regenerated caudal fins were imaged using a stereomicroscope, and the fin regeneration area was quantified using ImageJ software. The regenerative effects of SPG were assessed by comparing the fin regeneration areas between the SPG-treated and control groups.
2.13 Data analysis and statistics
All experiments were conducted in triplicate, and results are expressed as mean ± standard deviation (Mean ± SD). Statistical analyses were performed using one-way ANOVA in SPSS 27.0, with significance determined at p < 0.05. Response surface methodology (RSM) analysis was conducted using Design-Expert 13.0. to explore parameter interactions. Graphical representations were generated using GraphPad Prism 10.3 and Origin 2024.
3 Results and discussion
3.1 Effects of different factors on the kinematic viscosity of fermentation supernatant
Numerous studies have demonstrated that factors such as ultrasound duration, ultrasound power, and H2O2 concentration significantly influence the molecular weight of polysaccharides (Liu et al., 2024; Li et al., 2019; Cai et al., 2024). Since the kinematic viscosity of polysaccharides correlates closely with their molecular weight (Shao et al., 2020), a single-factor experiment was conducted to evaluate the effects of these variables on the kinematic viscosity of SPG.
As shown in Figure 1A, with the H2O2 concentration fixed at 2.7% and ultrasound duration set to 20 min, the influence of ultrasonic power on the kinematic viscosity of the fermentation supernatant was analyzed. The kinematic viscosity significantly decreased as the ultrasonic power increased from 0 to 150 W (p < 0.05). This decline can be attributed to the intensified cavitation effect at higher ultrasonic power levels (Ogutu and Mu, 2017), which promotes the decomposition of H2O2 into highly oxidative radicals such as ⋅OH (Ayanda et al., 2018), accelerating SPG degradation. However, when the ultrasound power exceeded 150 W, the viscosity plateaued (p > 0.05), likely due to the saturation of the cavitation effect and a dynamic equilibrium in radical concentration, limiting further degradation. Based on these findings, ultrasonic powers of 150 W and 90 W were chosen as the high and low values, respectively, for subsequent response surface analysis.
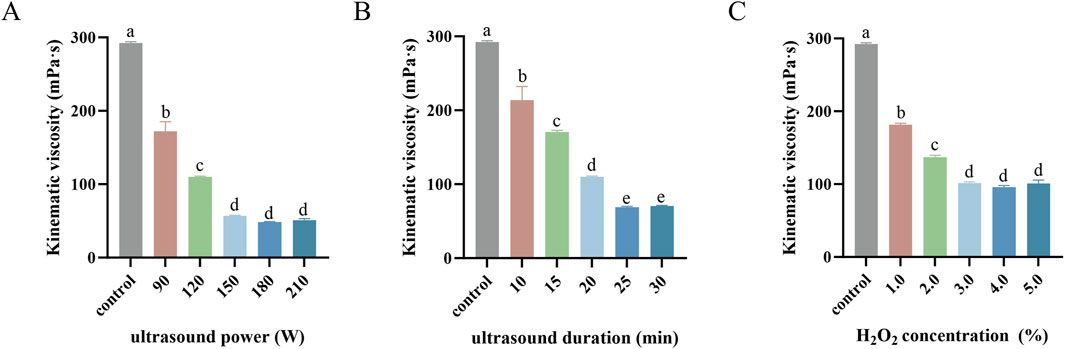
Figure 1. Kinematic viscosity mediated by ultrasound power (A), ultrasound duration (B) and H2O2 concentration (C) in single-factor experiments. Values with different letters indicate a significant difference (p < 0.05). Data are presented as mean ± SD (N = 3). These findings suggest that each factor contributes to viscosity reduction until saturation, beyond which further increases yield diminishing returns.
Figure 1B illustrates the impact of ultrasonic duration on kinematic viscosity with a fixed H2O2 concentration of 2.7% and ultrasonic power of 100 W. Extending the ultrasonic duration from 0 to 25 min resulted in a significant reduction in the kinematic viscosity (p < 0.05), likely due to the cumulative enhancement of the cavitation effect, which increased free radical production and accelerated SPG degradation (Yu et al., 2015; Yan et al., 2016). Beyond 25 min, the viscosity stabilized (p > 0.05), possibly reflecting a steady-state molecular weight of SPG molecules due to maximum shear stress and free radical production capacity being reached (Zhou and Ma, 2006). Therefore, ultrasonic durations of 25 min and 15 min were selected as the high and low values, respectively, for response surface analysis.
Figure 1C shows the effect of H2O2 concentration on kinematic viscosity under fixed conditions of 100 W ultrasonic power and 15 min of ultrasonic duration. Increasing the H2O2 concentration from 1.0% to 3.0% significantly reduced in kinematic viscosity (p < 0.05), likely due to enhanced SPG degradation from increased reactive radical production (Yu et al., 2015). However, beyond 3.0%, no further significant changes were observed (p > 0.05), which may be due to excess H2O2 reacting with ⋅OH radicals to form weaker oxidants such as HO2·, or recombination reactions regenerating H2O2 and reducing free radical availability (Ayanda et al., 2018; Zhou and Ma, 2006). Consequently, H2O2 concentrations of 3.0% and 1.0% were selected as the high and low values, respectively, for response surface analysis.
3.2 Determination of weight-average molecular weight
To further investigate the effects of ultrasonic power, ultrasonic duration, and H2O2 concentration on the Mw of SPG, a RSM model was employed. The Mw of both the undegraded SPG and the 17 experimental samples analyzed through RSM was determined using High-Performance Gel Permeation Chromatography (HPGPC), as shown in Figure 2. Figure 2A presents the HPGPC spectrum of the undegraded SPG, with a molecular weight of 4,409,608 Da. Figures 2B, C display the HPGPC spectra for the 17 experimental samples, revealing Mw values ranging from 257,500 to 434,400 Da (detailed values are listed in Table 2). These findings confirm that the combined application of ultrasound and H2O2 significantly reduced the Mw of SPG, providing valuable experimental data for subsequent investigations.
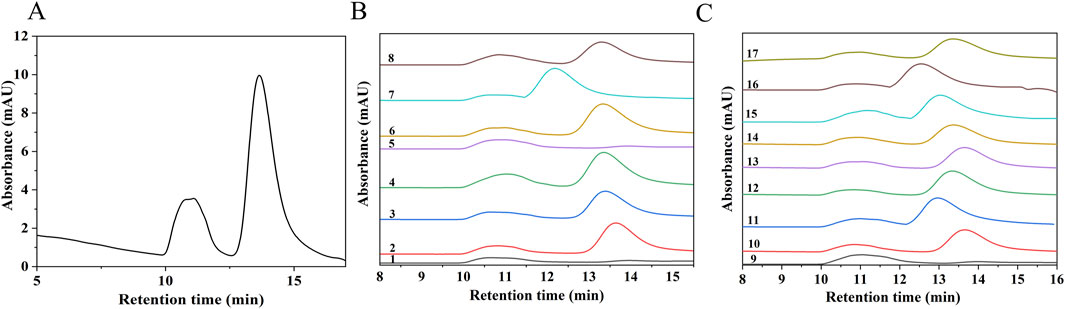
Figure 2. HPGPC chromatograms of SPG before and after degradation. (A) Chromatogram of undegraded SPG. (B, C) Chromatograms of SPG degradation products. Groups 1–17 represent the experimental conditions established through RSM. The retention times of all 17 groups are significantly reduced after degradation, indicating that the combined application of ultrasound and H2O2 effectively reduced the Mw of SPG.
3.3 Model fitting and optimization
3.3.1 Optimization of SPG’s Mw by RSM
This study employed the Box-Behnken Design (BBD) to optimize the effects of ultrasonic power (A), ultrasonic duration (B), and H2O2 concentration (C) on the Mw of SPG. The experimental matrix and corresponding data are presented in Table 2. Regression analysis conducted using Design-Expert 13.0 software provided the following fitted regression equation: Y (Mw) = 2.966 × 105 − 32962.50A − 22700.00B + 10612.50C − 24750.00AB − 14875.00AC + 3800.00BC + 10197.50A2 + 31177.50B2 + 68102.50C2.
The statistical parameters of the regression model are summarized in Table 3. The model yielded an F-value of 10.87 (p < 0.005), confirming its statistical significance. Additionally, the lack-of-fit test resulted in a p-value of 0.063, exceeding the threshold of 0.05, indicating an insignificant lack of fit. These results validate the model’s suitability for accurately representing the experimental data (Fan and Huang, 2023). The coefficient of determination (R2 = 0.9332) and the adjusted R2 (0.847) indicate a strong correlation between predicted and observed values, with minimal error. Additionally, the coefficient of variation (C.V.) is 6.11%, well below the acceptable threshold of 10.0%, reinforcing the model’s reliability and accuracy (Han et al., 2016). Regression analysis reveals significant contributions of both linear terms (A and B) and specific quadratic terms (AB, B2, and C2) to Mw changes (p < 0.05). It is noteworthy that the interaction terms AC and BC, along with higher-order coefficients (A2), were statistically non-significant (p > 0.05). These confirms that ultrasonic power (A) and ultrasonic duration (B) are the primary drivers of SPG degradation (p < 0.05). The non-significance of the interaction terms suggests their effects are independent of H2O2 concentration (C), reinforcing the dominance of ultrasonic parameters.
3.3.2 Analysis of response surfaces
Three-dimensional response surface plots were generated using Design-Expert 13.0 software to visualize the interactions between variables. Circular contour plots indicate weak interactions, while elliptical contours denote significant interactions (Han et al., 2016; Pyun et al., 2015). In the interaction plot between ultrasonic power and ultrasonic duration (Figure 3A), the response surface exhibits a steep gradient, and the elliptical contour plot (Figure 3a) confirms a significant interaction (p = 0.0479 < 0.05). In contrast, the interaction between ultrasonic power and H2O2 concentration (Figure 3B) is characterized by a more gradual response surface and an elliptical contour plot (Figure 3b), suggesting a non-significant interaction (p = 0.1934 > 0.05). Similarly, for ultrasonic duration and H2O2 concentration (Figure 3C), the response surface is steep, but the circular contour plot (Figure 3c) indicates an insignificant interaction (p = 0.7241 > 0.05). These results underscore that ultrasonic power and duration significantly influence Mw during SPG degradation, whereas H2O2 concentration plays a less critical role in these interactions.
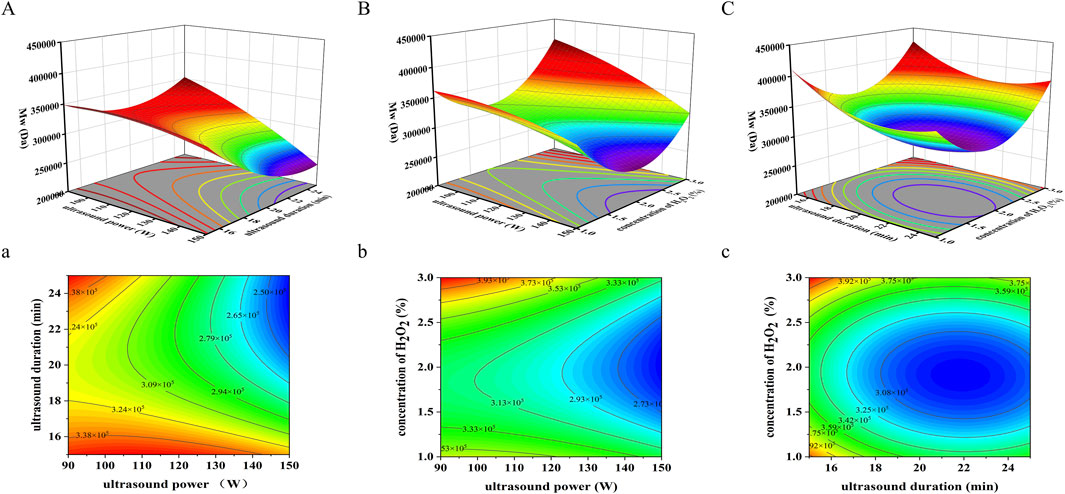
Figure 3. Three-dimensional response surface and contour graphs for the effects on Mw of SPG under the following parameters: (A) 3D response surface plot of ultrasound power (W) and ultrasound duration (min). (a) 2D contour plot of ultrasound power (W) and ultrasound duration (min). (B) 3D response surface plot of ultrasound power (W) and H2O2 concentration (%). (b) 2D contour plot of ultrasound power (W) and H2O2 concentration (%). (C) 3D response surface plot of H2O2 concentration (%) and ultrasound duration (min). (c) 2D contour plot of H2O2 concentration (%) and ultrasound duration (min). These results underscore that ultrasonic power and duration significantly influence Mw during SPG degradation, whereas H2O2 concentration plays a less critical role in these interactions.
Using response surface analysis, the optimal conditions for achieving an Mw of approximately 360,000 Da were predicted: ultrasonic power of 120 W, ultrasonic duration of 19.06 min, and an H2O2 concentration of 1.0%. The experimentally obtained Mw of degraded SPG under these conditions was 489,778 Da, approximately 1.36 times higher than the theoretical prediction. This discrepancy may result from several factors. First, SPG’s high molecular weight and broad molecular weight distribution, which complicate uniform degradation during ultrasonic processing (Zhong et al., 2015). Second, the high viscosity of the SPG aqueous solution, which may impede efficient ultrasound transmission, leading to uneven intensity distribution within the reaction medium (Miano et al., 2019). These factors collectively contribute to deviations from the predicted Mw values.
To assess the biological activity of different molecular weights of SPG, three representative Mw samples were selected from the response surface analysis, SPG-a (257,500 Da, Group 1), SPG-b (429,300 Da, Group 11), and SPG-c (364,800 Da, Group 16). The undegraded SPG (SPG-o) served as the control. All samples underwent purification and structural characterization prior to biological activity evaluations.
3.4 Structure characterization
3.4.1 Ultraviolet-visible spectroscopy analysis
The UV-Vis spectra of SPG, scanned within the range of 200–600 nm (Figure 4A), exhibited no characteristic absorption peaks at 260 nm and 280 nm. This confirms the absence of nucleic acid or protein impurities in the purified SPG samples, indicating a high level of purity suitable for downstream analyses.
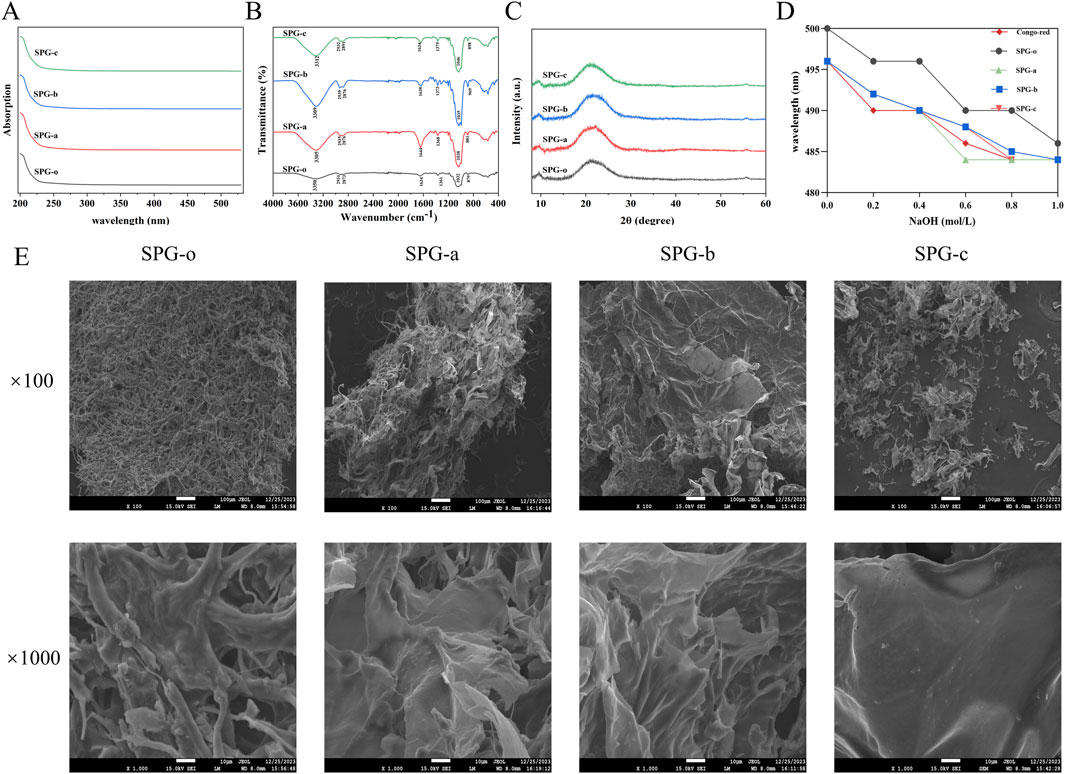
Figure 4. Structural characterization of SPG. (A) UV-Vis spectra. The analysis showed that all SPG samples were absent of nucleic acid and protein impurities. (B) FT-IR spectra. Revealing characteristic polysaccharide absorption peaks and confirming the preservation of pyranose-type polysaccharides and β-glycosidic bonds after degradation. (C) XRD spectra. Indicating SPG’s predominantly amorphous structure, which remains unchanged after ultrasonic-assisted H2O2 degradation. (D) Congo red interaction assay. The triple helical structure of the degraded SPG samples was disrupted. (E) SEM images. Showing changes in surface morphology. SPG-o: Undegraded SPG, Mw = 4,409,608 Da. SPG-a: Degraded SPG, Mw = 257,500 Da. SPG-b: Degraded SPG, Mw = 429,300 Da. SPG-c: Degraded SPG, Mw = 364,800 Da.
3.4.2 Fourier transform infrared spectroscopy analysis
The FT-IR spectra of SPG-o, SPG-a, SPG-b, and SPG-c (Figure 4B), revealed characteristic absorption peaks typical of polysaccharides. The broad and strong absorption peak at 3350 cm−1 corresponds to the O-H stretching vibrations (Li et al., 2023), while the peak near 2,931 cm−1 is attributed to C-H stretching vibrations (Wu et al., 2022). The absorption band around 1,634 cm−1 is associated with the bound water within the SPG matrix (Gao et al., 2022). Additionally, the peaks within the 1,000–1,200 cm−1 range represent the characteristic vibrations of pyranose rings, and the peak near 879 cm−1 indicates the presence of β-glycosidic bonds (Ning et al., 2021). These spectral features confirm that SPG primarily comprises pyranose-type polysaccharides linked by β-glycosidic bonds. Significantly, following ultrasonic-assisted H2O2 degradation, the functional groups in SPG-a, SPG-b, and SPG-c remained unchanged, preserving the pyranose ring structure and glycosidic bond types in the main chain. This indicates that the degradation process did not alter the fundamental chemical composition of SPG.
3.4.3 X-ray diffraction analysis
The crystal structure of SPG was analyzed using X-ray diffraction (XRD) (Figure 4C). The diffraction patterns of SPG-o, SPG-a, SPG-b, and SPG-c exhibited two broad peaks centered at 2θ = 9.7° and 21.7°, characteristic of amorphous materials (Xiao et al., 2022). This confirms that SPG has a predominantly amorphous structure. The ultrasonic-assisted H2O2 degradation process did not induce significant changes in the crystal structure of SPG. The retention of the amorphous nature suggests that the structural integrity of SPG was largely maintained despite molecular weight reduction.
3.4.4 Congo red interaction assay
The binding characteristics of SPG and its structural integrity were evaluated using Congo Red. Polysaccharides with a triple helical structure exhibit a red shift in maximum absorption wavelength upon binding to Congo Red in alkaline conditions. As sodium hydroxide concentration increases, triple helices disassemble into single helices or random coils, resulting in a blue shift in the maximum absorption wavelength (Guo et al., 2021). The undegraded SPG-o displayed a pronounced red shift under alkaline conditions, indicative of its intact triple helical structure (Figure 4D). In contrast, SPG-a, SPG-b, and SPG-c did not exhibit significant red shifts, suggesting disruption of the triple helical conformation. This disruption can be attributed to the cavitation effects, turbulent shear forces, and free radicals (e.g., ·OH, HO2·, H·) generated during the ultrasonic - assisted H2O2 treatment. These forces likely cleave glycosidic bonds and destabilize the hydrogen bonding network, which is essential for maintaining the triple helical structure (Shi et al., 2022). These findings suggest that ultrasonic-assisted H2O2 degradation significantly alters the conformational integrity of SPG, providing a mechanistic basis for its modified physicochemical and biological properties.
3.4.5 Scanning electron microscopy analysis
Scanning electron microscopy (SEM) was used to assess microstructural changes in degraded SPG samples (Figure 4E). The fibrous structures characteristic of SPG-o was replaced by plate-like configurations in SPG-a, SPG-b, and SPG-c. Notably, SPG-c exhibited distinct voids and a more flattened, plate-like morphology. These morphological changes are attributed to ultrasonic treatment, where cavitation effects, shock waves, and turbulent shear forces generate localized high-pressure zones, cleaving glycosidic bonds (Shi et al., 2022). Additionally, free radicals generated by H2O2 decomposition (e.g., ·OH, HO2·, and H·) may interact with glycosidic bonds, promoting cleavage and contributing to the observed microstructural changes (Dai et al., 2017). Similar phenomena were reported by Shi et al. (2022) and Zheng et al. (2022), where ultrasonic treatment significantly altered the surface structures of pectin and chitosan, respectively. These findings highlight the critical role of ultrasonic and oxidative processes in modifying polysaccharide microstructures, which may impact their physicochemical and biological properties.
3.5 In vitro antioxidant activity analysis
The radical scavenging capacity of degraded SPG variants (SPG-a, SPG-b, SPG-c) was evaluated using DPPH·, ABTS+·, and ⋅OH assays, with undegraded SPG-o and Vc as controls (Figure 5). These assays are established methods for assessing the antioxidant activity of polysaccharides. The DPPH radical scavenging assay, valued for its simplicity and reproducibility, showed that SPG-a, SPG-b, and SPG-c exhibited scavenging activities of 83.76% ± 3.68%, 55.43% ± 0.55%, and 60.55% ± 0.77%, respectively, all significantly higher than that of SPG-o (20.37% ± 7.57%, p < 0.05) (Figure 5A). Notably, SPG-a demonstrated the highest scavenging activity among the degraded variants (p < 0.05). These results indicate that ultrasonic-assisted H2O2 degradation significantly enhances SPG’s antioxidant, consistent with the findings from Wu et al. (2022).
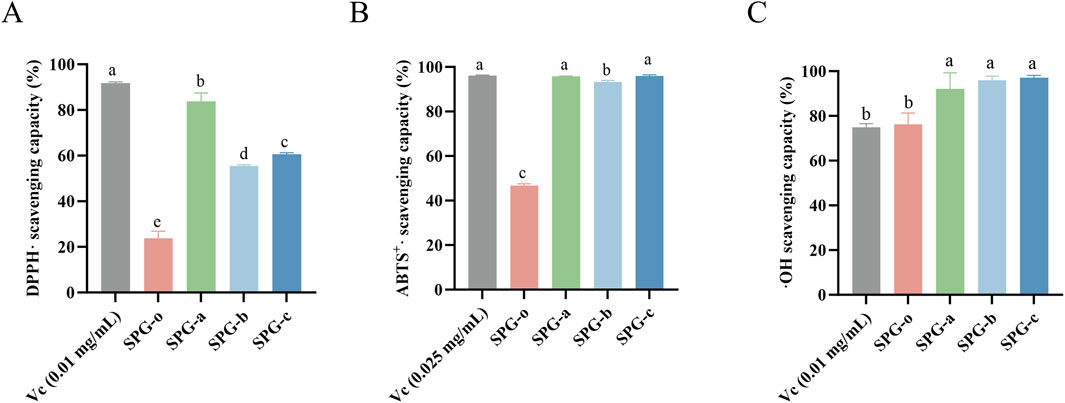
Figure 5. In vitro antioxidant activity among different groups, (A) DPPH scavenging capacity, (B) ABTS+· scavenging capacity, and (C) ⋅OH scavenging capacity. SPG-o: Undegraded SPG, Mw = 4,409,608 Da. SPG-a: Degraded SPG, Mw = 257,500 Da. SPG-b: Degraded SPG, Mw = 429,300 Da. SPG-c: Degraded SPG, Mw = 364,800 Da. Values with different letters indicate a significant difference (p < 0.05). Data are presented as mean ± SD (N = 3). All variants of SPG significantly enhanced the antioxidant capacity against DPPH·, ABTS+·, and ⋅OH.
The ABTS+· radical scavenging, a robust indicator of in vitro antioxidant capacity (Mirzadeh et al., 2020), revealed scavenging activities of 95.79% ± 0.19%, 93.30% ± 0.67%, and 95.91% ± 0.58% for SPG-a, SPG-b, and SPG-c, respectively (Figure 5B). These values significantly higher than that of SPG-o (46.67% ± 0.85%, p < 0.05). Additionally, SPG-a and SPG-c demonstrated significantly greater scavenging activity than SPG-b (p < 0.05).
The ⋅OH radical scavenging assay, a critical measure of antioxidant activity due to the high reactivity and biological damage potential of ⋅OH radicals (Kaźmierczak-Barańska et al., 2020), showed that SPG-a, SPG-b, and SPG-c exhibited scavenging activities of 92.00% ± 7.27%, 95.94% ± 1.83%, and 97.01% ± 1.09%, respectively (Figure 5C). These values significantly exceeded that of SPG-o (76.26% ± 5.00%, p < 0.05).
In summary, the degraded SPG variants, particularly SPG-a and SPG-c, demonstrated robust free radical scavenging capacities. This enhanced activity is attributed to the disruption of the triple helical structure during ultrasonic-assisted H2O2 degradation, which exposes a greater number of reactive hydroxyl groups (Yan et al., 2016). These findings suggest that degraded SPG variants hold significant promise as potent free radical scavengers for applications in antioxidative therapies and functional products.
3.6 Effect of SPG on wound healing in BJ and HaCaT cells
3.6.1 CCK-8 assay
Polysaccharide molecular weight significantly influences their biological activities, including cell proliferation modulation. Studies have shown that lower molecular weight polysaccharides, such as fucoidans, exhibit enhanced cytotoxic and modulatory effects on tumor and immune cells (Ummat et al., 2024; Jiang et al., 2022). The effect of SPG-a, SPG-b, and SPG-c on the proliferation of BJ and HaCaT cells was evaluated using the CCK-8 assay. The results demonstrated a concentration-dependent enhancement in cell proliferation across a range of 50–300 μg/mL (Figures 6A, B). Among the variants, SPG-b exhibited the highest proliferative effect at 300 μg/mL, achieving a proliferation rate of 200.42% ± 11.00% in BJ cells and 177.62% ± 9.16% in HaCaT cells (p < 0.05). The enhanced proliferative effect of SPG-b (Mw = 429,300 Da) can be attributed to its molecular weight, which strikes an optimal balance between bioavailability and cellular interaction. This intermediate molecular weight likely facilitates efficient cellular uptake and better interaction with cell surface receptors (Pan et al., 2024). These results suggest that lower molecular weight SPG variants, particularly SPG-b, significantly enhance cell proliferation, highlighting their potential applications in promoting cellular biological activity and tissue repair.
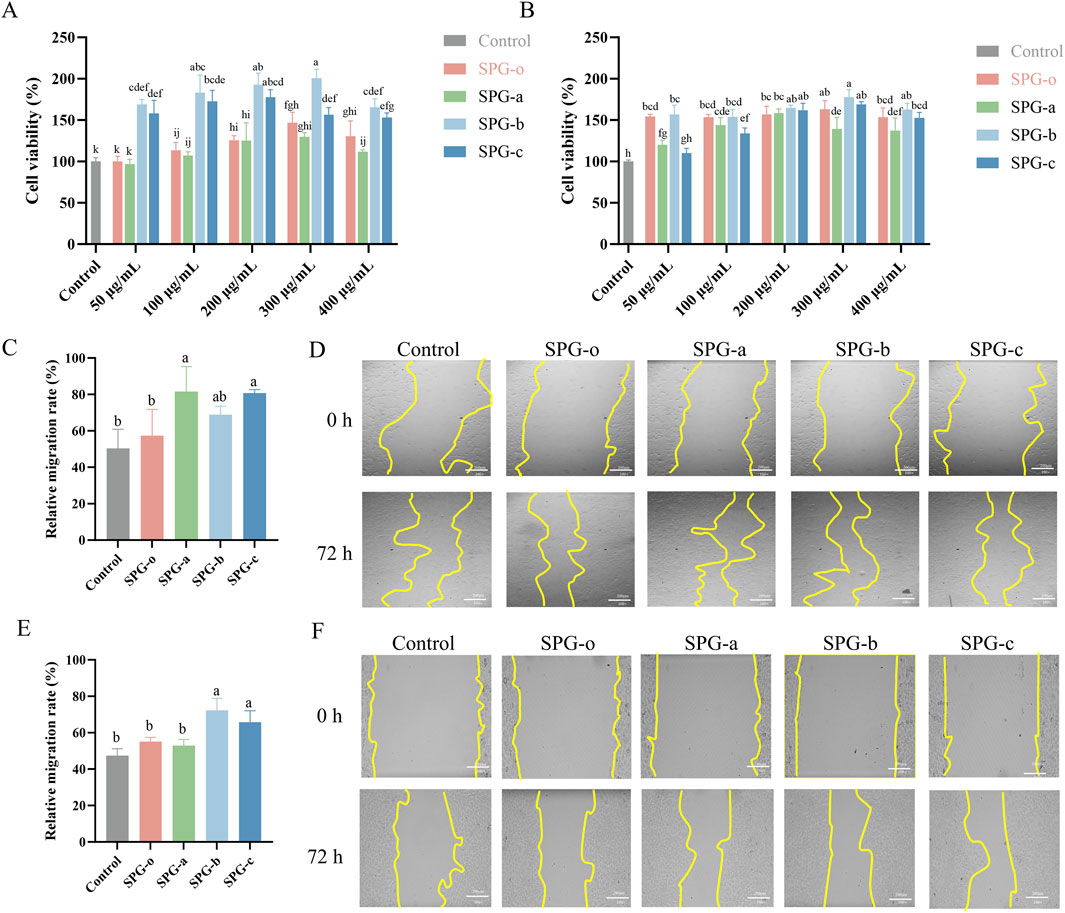
Figure 6. Effects of SPG with different molecular weights on cell viability and migration. Cell viability of BJ (A) and HaCaT (B) cells after 24 h of treatment. Migration rate of BJ cells after 48 h of treatment (C), with representative images (D). Migration rate of HaCaT cells after 48 h of treatment (E), with representative images (F). Scale bar = 200 μm. Untreated cells (Control) were normalized to 100%. SPG-o: Undegraded SPG, Mw = 4,409,608 Da. SPG-a: Degraded SPG, Mw = 257,500 Da. SPG-b: Degraded SPG, Mw = 429,300 Da. SPG-c: Degraded SPG, Mw = 364,800 Da. Values with different letters indicate a significant difference (p < 0.05). Data are presented as mean ± SD (N = 3). SPG-b showed the highest proliferative effect on both BJ and HaCaT cells. Notably, SPG-a most effectively promoted wound healing in fibroblasts, while SPG-b excelled in keratinocyte migration.
3.6.2 Wound healing scratch migration assay
BJ and HaCaT cells play crucial roles in granulation tissue formation and re-epithelialization during wound healing (Zhao et al., 2024; Belvedere et al., 2020). Using a concentration of 300 μg/mL, determined from the CCK-8 assay, the effects of SPG-a, SPG-b, and SPG-c on cellular migration were assessed via a scratch assay. In BJ cells (Figures 6C, D), SPG-o treatment resulted in a wound healing rate of 57.41% ± 14.36%, which did not differ significantly from the control group (50.34% ± 10.51%, p > 0.05). In contrast, SPG-a (81.54% ± 13.78%) and SPG-c (80.70% ± 1.92%) significantly enhanced wound healing rates (p < 0.05). Similarly, in HaCaT cells (Figures 6E, F), SPG-b (72.24% ± 6.67%) and SPG-c (65.81% ± 6.20%) significantly improved wound healing rates compared to the control group (47.46% ± 3.75%, p < 0.05), while SPG-o and SPG-a showed no significant effects (p > 0.05). The results indicate that SPG-a exhibited the highest pro-migratory effect on BJ cells, while SPG-b most effectively promoted HaCaT cell migration. This differences may be attributed to cell type-specific interactions with polysaccharides, including variations in receptor expression, uptake efficiency, and downstream signaling activation (Generalov and Yakovenko, 2023).
For BJ cells, SPG-a, with the lowest molecular weight (Mw = 257,500 Da), may be more readily internalized or interact more effectively with fibroblast-associated receptors, such as CD44, which mediates polysaccharide-induced cell migration and adhesion, as observed with hyaluronic acid (Lin et al., 2023). In contrast, SPG-b, with a molecular weight of 429,300 Da, exhibited the strongest effect on HaCaT cell migration. Larger polysaccharides like SPG-b may engage epidermal growth factor receptors (EGFR) more effectively, activating downstream signaling pathways such as phosphoinositide 3-kinase (PI3K)/Akt and mitogen-activated protein kinase (MAPK), which are crucial for keratinocyte proliferation and migration (Zhou et al., 2025).
These findings suggest that the molecular weight of SPG variants differentially influences fibroblast and keratinocyte migration through distinct receptor-mediated mechanisms, highlighting their potential for tailored wound healing applications. However, further studies are needed to elucidate the precise molecular mechanisms involved.
3.6.3 EdU assay
EdU staining is a highly sensitive method for detecting cell proliferation by labeling cells during the DNA synthesis (S) phase of the cell cycle (Che et al., 2023). As shown in Figures 7A, B, HaCaT cells treated with SPG variants (SPG-o, SPG-a, SPG-b, and SPG-c) for 72 h exhibited significantly higher proportions of EdU-positive cells compared to the control group (32.71% ± 0.31%, p < 0.05). The proportions of EdU-positive cells were 42.42% ± 1.66% for SPG-o, 43.30% ± 0.09% for SPG-a, 50.90% ± 1.26% for SPG-b, and 51.06% ± 0.88% for SPG-c. Notably, the lower molecular weight SPG variants, SPG-b and SPG-c, significantly outperformed SPG-o in promoting EdU incorporation (p < 0.05). These findings are consistent with the results from the CCK-8 and scratch assays, further validating the enhanced proliferative and migratory activities of SPG-b and SPG-c in HaCaT cells. The consistency across multiple experimental approaches underscores the potential of SPG-b and SPG-c as effective agents for promoting cell proliferation and wound healing.
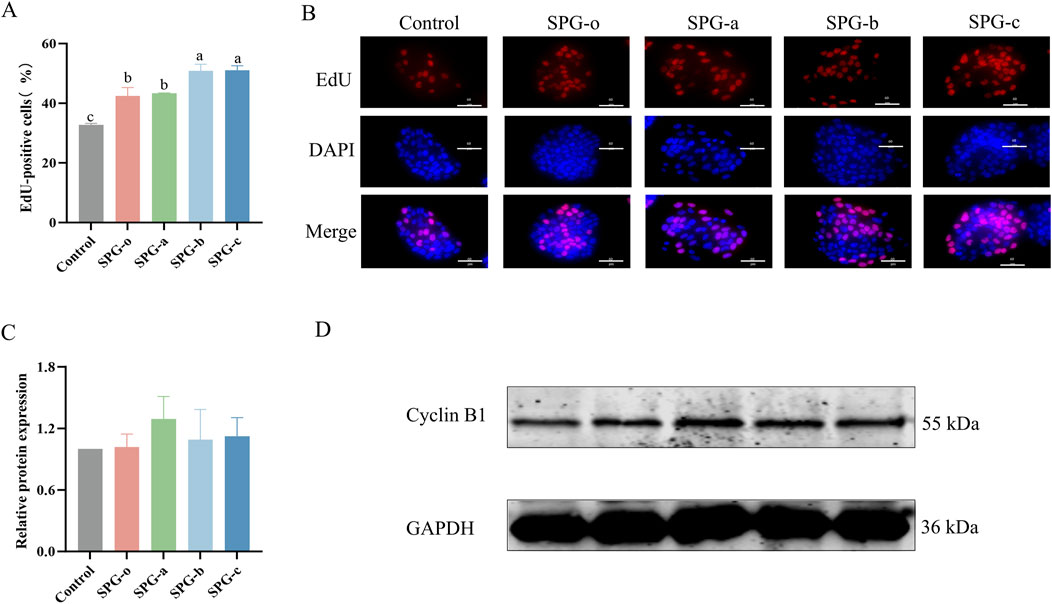
Figure 7. Effects of SPG with different molecular weights on HaCaT cell proliferation and Cyclin B1 expression. Percentage of EdU-positive cells (A). Representative EdU staining images of HaCaT cells (B). EdU - positive are shown in red, DAPI - stained nuclei in blue, and merge is the combination. Scale bar = 60 μm. All SPG variants significantly promoted cell proliferation in HaCaT cells, Quantification of Cyclin B1 expression levels (C) and Western blot analysis using anti-Cyclin B1 antibodies (D) treated with SPG for 48 h. Response of untreated cells (Control) was considered as 1. Western blot results showed that heightened proliferation did not correlate with major changes in Cyclin B1 levels, implying SPG’s proliferative effect might bypass the G2/M cell cycle phase. SPG-o: Undegraded SPG, Mw = 4,409,608 Da. SPG-a: Degraded SPG, Mw = 257,500 Da. SPG-b: Degraded SPG, Mw = 429,300 Da. SPG-c: Degraded SPG, Mw = 364,800 Da. Values with different letters indicate a significant difference (p < 0.05). Data are presented as mean ± SD (N = 3).
3.6.4 Western blot assay
Western blot analysis (Figures 7C, D) revealed that after 48 h of treatment with SPG-a, SPG-b, and SPG-c, Cyclin B1 protein expression in HaCaT cells was slightly elevated compared to the blank control and SPG-o groups. However, these differences were not statistically significant (p > 0.05). Cyclin B1 is crucial during the G2/M phase of the cell cycle, and its expression level reflecting the proportion of cells in this phase (Schmitt et al., 2006). The limited effect of SPG treatment on the G2/M phase of HaCaT cells likely accounts for the lack of significant changes in Cyclin B1 expression. This suggests that the primary mechanism by which SPG variants enhance HaCaT cells proliferation may not involve the G2/M phase. Further investigations are necessary to elucidate the molecular pathways underlying the biological effects of SPG.
3.7 Effect of SPG on fin regeneration in larvae zebrafish
Zebrafish share approximately 87% genomic homology with humans (Cao et al., 2019), and their remarkable ability to regenerate caudal fins (Gemberling et al., 2013) makes them an excellent model for studying the effects of bioactive compounds on wound healing and tissue regeneration. In this study, we employed the zebrafish caudal fin regeneration model to assess the in vivo tissue regenerative potential of SPG variants with different molecular weights. As shown in Figures 8A, B, the fin regeneration rates for SPG-o, SPG-a, SPG-b, and SPG-c were 103.52% ± 7.18%, 109.71% ± 4.81%, 109.04% ± 5.79%, and 110.49% ± 5.94%, respectively, all significantly higher than the control group (98.46% ± 6.91%, p < 0.05). Among these, the lower molecular weight variants, SPG-a and SPG-c, exhibited the most pronounced regenerative effects. These results demonstrate that reducing molecular weight significantly enhances the tissue regenerative activity of SPG, highlighting its potential for applications in tissue repair and regeneration. The zebrafish model not only confirms the bioactivity of SPG variants in a physiologically relevant system but also emphasizes the importance of molecular weight modulation in optimizing the therapeutic potential of bioactive polysaccharides. While zebrafish share genetic similarities with humans, key differences in tissue regeneration mechanisms exist. For instance, prolonged TNF-α and MCP-1 elevation post-injury in zebrafish contrasts with rapid resolution in mammals (Smith, 2024). These disparities highlight the need for validation in advanced mammalian models to fully assess SPG’s therapeutic potential.
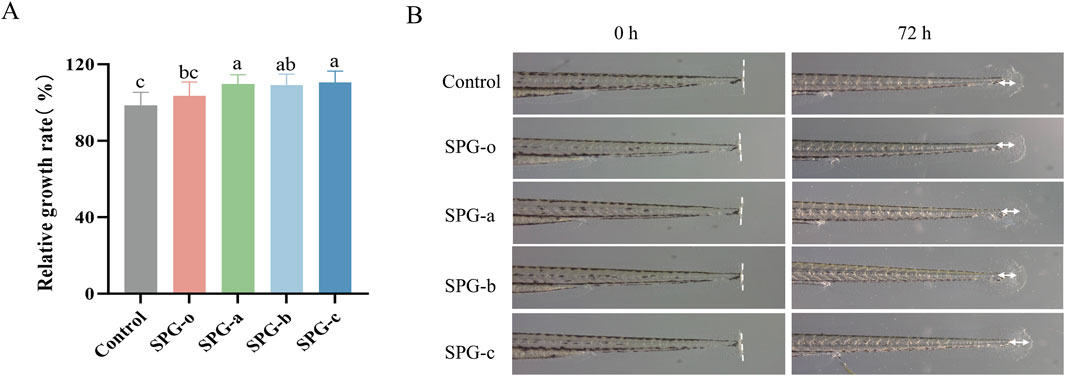
Figure 8. Effect of different molecular weights of SPG exposure on caudal fin regeneration in larval zebrafish. Quantitative analysis of the larval fin regeneration area (A). Representative images showing the larval fin regeneration in group exposed to different molecular weights of SPG (3.125 mg/mL) and the control group (B). SPG-o: Undegraded SPG, Mw = 4,409,608 Da. SPG-a: Degraded SPG, Mw = 257,500 Da. SPG-b: Degraded SPG, Mw = 429,300 Da. SPG-c: Degraded SPG, Mw = 364,800 Da. Values with different letters indicate a significant difference (p < 0.05). Data are presented as mean ± SD (N = 15). All variants of SPG significantly enhanced tissue regeneration in the caudal fins of zebrafish.
These findings underline the crucial role of molecular weight reduction in enhancing the tissue regenerative properties of SPG, establishing it as a promising candidate for tissue repair and regeneration applications. Future research should focus on comprehensive structural analyses of the degraded SPG products, including detailed profiling of monosaccharide composition and potential structural modifications, to clarify their correlation with the observed improvements in bioactivity. While the zebrafish model effectively demonstrates the therapeutic potential of low-molecular-weight SPG, the exact mechanisms underlying its tissue repair and wound healing effects remain unclear. In-depth investigations into the associated signaling pathways and gene expression changes will be essential to unravel its mode of action. Additionally, the use of other experimental models and systems will help further validate the therapeutic potential of SPG and support its development for clinical applications in tissue repair and wound healing.
4 Conclusion
This study successfully synthesized three molecular weight variants of SPG using an optimized ultrasonic-assisted H2O2 degradation process. The structural and functional impacts of this method were systematically analyzed. Spectroscopic analyses, including UV-Vis, FT-IR, and XRD, confirmed that while the functional group composition and crystalline structure of SPG remained intact, the triple-helix conformation was disrupted, significantly altering its microstructure. Biological evaluations demonstrated that the low molecular weight SPG exhibited enhanced bioactivity. In vitro, the low molecular weight SPG showed significantly improved antioxidant capacity and effectively promoted the proliferation and migration of BJ cells and HaCaT cells, both critical for wound healing. In vivo, zebrafish larvae assays confirmed that low molecular weight SPG accelerated caudal fin regeneration, further validating its potential in tissue repair.
In conclusion, molecular weight reduction of SPG significantly enhances its antioxidant and wound healing activities, establishing a solid foundation for its development in cosmetics, pharmaceuticals, and functional foods. This work not only expands the functional scope of SPG but also provides insights into the correlation between molecular weight modulation and the polysaccharide biological. Future research should delve into the specific molecular mechanisms of low molecular weight SPG’s interaction with cellular signaling pathways, as well as its long-term stability and safety for practical application conditions.
Data availability statement
The raw data supporting the conclusions of this article will be made available by the authors without undue reservation.
Ethics statement
The animal study was approved by The Animal Ethics Committee of Guangdong Pharmaceutical University. The study was conducted in accordance with the local legislation and institutional requirements.
Author contributions
HH: Conceptualization, Investigation, Software, Validation, Writing–original draft. YL: Writing–review and editing, Data curation, Methodology. QL: Writing–review and editing, Visualization. FC: Writing–review and editing, Investigation. LZ: Writing–review and editing, Conceptualization, Funding acquisition.
Funding
The author(s) declare that financial support was received for the research and/or publication of this article. This research was funded by Guangdong Pharmaceutical University Research Cultivation Fund, grant number 51309448007.
Conflict of interest
The authors declare that the research was conducted in the absence of any commercial or financial relationships that could be construed as a potential conflict of interest.
Generative AI statement
The author(s) declare that no Generative AI was used in the creation of this manuscript.
Publisher’s note
All claims expressed in this article are solely those of the authors and do not necessarily represent those of their affiliated organizations, or those of the publisher, the editors and the reviewers. Any product that may be evaluated in this article, or claim that may be made by its manufacturer, is not guaranteed or endorsed by the publisher.
References
Ansari, E., Alvandi, H., Kianirad, S., Hatamian-Zarmi, A., and Mokhtari-Hosseini, Z. B. (2025). Research progress on production and biomedical applications of Schizophyllan as a tailor-made polysaccharide: a review. Carbohydr. Polym. 348, 122770. doi:10.1016/j.carbpol.2024.122770
An, Y., Yuan, R., Wang, S., Yang, S., and Zhang, Q. (2024). Effects of silencing NLRP3 gene on proliferation of psoriasis-like HaCaT cells and expressions of cytokines. Cell Mol. Biol. (Noisy-le-grand) 70, 85–89. doi:10.14715/cmb/2024.70.4.13
Ayanda, O. S., Nelana, S. M., and Naidoo, E. B. (2018). Ultrasonic degradation of aqueous phenolsulfonphthalein (PSP) in the presence of nano-Fe/H(2)O(2). Ultrason. Sonochem 47, 29–35. doi:10.1016/j.ultsonch.2018.04.012
Belvedere, R., Pessolano, E., Porta, A., Tosco, A., Parente, L., Petrella, F., et al. (2020). Mesoglycan induces the secretion of microvesicles by keratinocytes able to activate human fibroblasts and endothelial cells: a novel mechanism in skin wound healing. Eur. J. Pharmacol. 869, 172894. doi:10.1016/j.ejphar.2019.172894
Cai, W., Luo, Y., Xue, J., Guo, R., and Huang, Q. (2024). Effect of ultrasound assisted H(2)O(2)/Vc treatment on the hyperbranched Lignosus rhinocerotis polysaccharide: structures, hydrophobic microdomains, and antitumor activity. Food Chem. 450, 139338. doi:10.1016/j.foodchem.2024.139338
Cao, Z., Mao, X., and Luo, L. (2019). Germline Stem cells drive ovary regeneration in zebrafish. Cell Rep. 26, 1709–1717. doi:10.1016/j.celrep.2019.01.061
Che, L., Zhu, C., Huang, L., Xu, H., Ma, X., Luo, X., et al. (2023). Ginsenoside Rg2 promotes the proliferation and Stemness Maintenance of Porcine Mesenchymal Stem cells through Autophagy Induction. Foods 12, 1075. doi:10.3390/foods12051075
Chen, Y., Chen, L., Xiao, Z., and Gao, L. (2022). Effects of enzymolysis and fermentation on the antioxidant activity and functional components of a coarse cereal compound powder based on principal component analysis and microstructure study. J. Food Sci. 87, 3573–3587. doi:10.1111/1750-3841.16217
Chen, Z., Yin, C., Fan, X., Ma, K., Yao, F., Zhou, R., et al. (2020). Characterization of physicochemical and biological properties of Schizophyllum commune polysaccharide extracted with different methods. Int. J. Biol. Macromol. 156, 1425–1434. doi:10.1016/j.ijbiomac.2019.11.183
Dai, Y., Shao, C., Piao, Y., Hu, H., Lu, K., Zhang, T., et al. (2017). The mechanism for cleavage of three typical glucosidic bonds induced by hydroxyl free radical. Carbohydr. Polym. 178, 34–40. doi:10.1016/j.carbpol.2017.09.016
Deng, Y., Huang, Q., Hu, L., Liu, T., Zheng, B., Lu, D., et al. (2021). Enhanced exopolysaccharide yield and antioxidant activities of Schizophyllum commune fermented products by the addition of Radix Puerariae. RSC Adv. 11, 38219–38234. doi:10.1039/d1ra06314f
Deng, Y., Liu, H., Huang, Q., Tu, L., Hu, L., Zheng, B., et al. (2022). Mechanism of Longevity Extension of Caenorhabditis elegans induced by Schizophyllum commune fermented supernatant with added Radix Puerariae. Front. Nutr. 9, 847064. doi:10.3389/fnut.2022.847064
De Oliveira, R. C., and Wilson, S. E. (2020). Fibrocytes, wound healing, and Corneal Fibrosis. Invest. Ophthalmol. Vis. Sci. 61, 28. doi:10.1167/iovs.61.2.28
Dou, Z., Chen, C., and Fu, X. (2019). The effect of ultrasound irradiation on the physicochemical properties and α-glucosidase inhibitory effect of blackberry fruit polysaccharide. Food Hydrocoll. 96, 568–576. doi:10.1016/j.foodhyd.2019.06.002
Du, B., Yang, Y., Bian, Z., and Xu, B. (2017). Characterization and anti-inflammatory potential of an exopolysaccharide from Submerged mycelial culture of Schizophyllum commune. Front. Pharmacol. 8, 252. doi:10.3389/fphar.2017.00252
Fan, Y., and Huang, G. (2023). Preparation and analysis of Pueraria lobata polysaccharides. ACS Biomater. Sci. Eng. 9, 2329–2334. doi:10.1021/acsbiomaterials.2c01479
Gao, T., Hu, W., Zhang, Z., Tang, Z., Chen, Y., Zhang, Z., et al. (2022). An acidic polysaccharide from Oxalis corniculata L. and the preliminary study on its antioxidant activity. J. Food Biochem. 46, e14235. doi:10.1111/jfbc.14235
Gemberling, M., Bailey, T. J., Hyde, D. R., and Poss, K. D. (2013). The zebrafish as a model for complex tissue regeneration. Trends Genet. 29, 611–620. doi:10.1016/j.tig.2013.07.003
Generalov, E., and Yakovenko, L. (2023). Receptor basis of biological activity of polysaccharides. Biophys. Rev. 15, 1209–1222. doi:10.1007/s12551-023-01102-4
Guo, X., Kang, J., Xu, Z., Guo, Q., Zhang, L., Ning, H., et al. (2021). Triple-helix polysaccharides: formation mechanisms and analytical methods. Carbohydr. Polym. 262, 117962. doi:10.1016/j.carbpol.2021.117962
Gu, W., Wang, Y., Zeng, L., Dong, J., Bi, Q., Yang, X., et al. (2020). Polysaccharides from Polygonatum kingianum improve glucose and lipid metabolism in rats fed a high fat diet. Biomed. Pharmacother. 125, 109910. doi:10.1016/j.biopha.2020.109910
Han, Y. L., Gao, J., Yin, Y. Y., Jin, Z. Y., Xu, X. M., and Chen, H. Q. (2016). Extraction optimization by response surface methodology of mucilage polysaccharide from the peel of Opuntia dillenii haw. fruits and their physicochemical properties. Carbohydr. Polym. 151, 381–391. doi:10.1016/j.carbpol.2016.05.085
Huang, F., Hong, R., Yi, Y., Bai, Y., Dong, L., Jia, X., et al. (2020). In vitro digestion and human gut microbiota fermentation of longan pulp polysaccharides as affected by Lactobacillus fermentum fermentation. Int. J. Biol. Macromol. 147, 363–368. doi:10.1016/j.ijbiomac.2020.01.059
Hu, X., Xu, F., Li, J., Li, J., Mo, C., Zhao, M., et al. (2022). Ultrasonic-assisted extraction of polysaccharides from coix seeds: optimization, purification, and in vitro digestibility. Food Chem. 374, 131636. doi:10.1016/j.foodchem.2021.131636
Jiang, F., Ding, Y., Tian, Y., Yang, R., Quan, M., Tong, Z., et al. (2022). Hydrolyzed low-molecular-weight polysaccharide from Enteromorpha prolifera exhibits high anti-inflammatory activity and promotes wound healing. Biomater. Adv. 133, 112637. doi:10.1016/j.msec.2021.112637
Kazemi, M., Khodaiyan, F., Labbafi, M., Saeid Hosseini, S., and Hojjati, M. (2019). Pistachio green hull pectin: optimization of microwave-assisted extraction and evaluation of its physicochemical, structural and functional properties. Food Chem. 271, 663–672. doi:10.1016/j.foodchem.2018.07.212
Kaźmierczak-Barańska, J., Boguszewska, K., Adamus-Grabicka, A., and Karwowski, B. T. (2020). Two Faces of Vitamin C-antioxidative and pro-oxidative agent. Nutrients 12, 1501. doi:10.3390/nu12051501
Li, J., Chen, Z., Shi, H., Yu, J., Huang, G., and Huang, H. (2023). Ultrasound-assisted extraction and properties of polysaccharide from Ginkgo biloba leaves. Ultrason. Sonochem 93, 106295. doi:10.1016/j.ultsonch.2023.106295
Li, J., Li, S., Wu, L., Yang, H., Wei, C., Ding, T., et al. (2019). Ultrasound-assisted fast preparation of low molecular weight fucosylated chondroitin sulfate with antitumor activity. Carbohydr. Polym. 209, 82–91. doi:10.1016/j.carbpol.2018.12.061
Li, M., Ma, F., Li, R., Ren, G., Yan, D., Zhang, H., et al. (2020). Degradation of Tremella fuciformis polysaccharide by a combined ultrasound and hydrogen peroxide treatment: process parameters, structural characteristics, and antioxidant activities. Int. J. Biol. Macromol. 160, 979–990. doi:10.1016/j.ijbiomac.2020.05.216
Lin, C. Y., Basu, K., Ruusala, A., Kozlova, I., Li, Y. S., Skandalis, S. S., et al. (2023). Hyaluronan-induced CD44-iASPP interaction Affects fibroblast migration and Survival. Cancers (Basel) 15, 1082. doi:10.3390/cancers15041082
Liu, Y., Meng, Y., Ji, H., Guo, J., Shi, M., Lai, F., et al. (2024). Structural characteristics and antioxidant activity of a low-molecular-weight jujube polysaccharide by ultrasound assisted metal-free Fenton reaction. Food Chem. X 24, 101908. doi:10.1016/j.fochx.2024.101908
Li, X., Zhang, Z., Wang, L., Zhao, H., Jia, Y., Ma, X., et al. (2022). Three-phase extraction of polysaccharide from Stropharia rugosoannulata: process optimization, structural characterization and bioactivities. Front. Immunol. 13, 994706. doi:10.3389/fimmu.2022.994706
Mathew, L. K., Andreasen, E. A., and Tanguay, R. L. (2006). Aryl hydrocarbon receptor activation inhibits regenerative growth. Mol. Pharmacol. 69, 257–265. doi:10.1124/mol.105.018044
Miano, A. C., Rojas, M. L., and Augusto, P. E. D. (2019). Structural changes caused by ultrasound pretreatment: Direct and indirect demonstration in potato cylinders. Ultrason. Sonochem 52, 176–183. doi:10.1016/j.ultsonch.2018.11.015
Mirzadeh, M., Arianejad, M. R., and Khedmat, L. (2020). Antioxidant, antiradical, and antimicrobial activities of polysaccharides obtained by microwave-assisted extraction method: a review. Carbohydr. Polym. 229, 115421. doi:10.1016/j.carbpol.2019.115421
Ning, X., Liu, Y., Jia, M., Wang, Q., Sun, Z., Ji, L., et al. (2021). Pectic polysaccharides from Radix Sophorae Tonkinensis exhibit significant antioxidant effects. Carbohydr. Polym. 262, 117925. doi:10.1016/j.carbpol.2021.117925
Ogutu, F. O., and Mu, T. H. (2017). Ultrasonic degradation of sweet potato pectin and its antioxidant activity. Ultrason. Sonochem 38, 726–734. doi:10.1016/j.ultsonch.2016.08.014
Pan, Y., Liu, C., Jiang, S., Guan, L., Liu, X., and Wen, L. (2024). Ultrasonic-assisted extraction of a low molecular weight polysaccharide from Nostoc commune Vaucher and its structural characterization and immunomodulatory activity. Ultrason. Sonochem 108, 106961. doi:10.1016/j.ultsonch.2024.106961
Pugliese, E., Coentro, J. Q., Raghunath, M., and Zeugolis, D. I. (2018). Wound healing and scar wars. Adv. Drug Deliv. Rev. 129, 1–3. doi:10.1016/j.addr.2018.05.010
Pyun, D. G., Choi, H. J., Yoon, H. S., Thambi, T., and Lee, D. S. (2015). Polyurethane foam containing rhEGF as a dressing material for healing diabetic wounds: synthesis, characterization, in vitro and in vivo studies. Colloids Surf. B Biointerfaces 135, 699–706. doi:10.1016/j.colsurfb.2015.08.029
Rachpirom, M., Pichayakorn, W., and Puttarak, P. (2023). Box-Behnken design to optimize the cross-linked sodium alginate/mucilage/Aloe vera film: physical and mechanical studies. Int. J. Biol. Macromol. 246, 125568. doi:10.1016/j.ijbiomac.2023.125568
Ridiandries, A., Tan, J. T. M., and Bursill, C. A. (2018). The role of Chemokines in wound healing. Int. J. Mol. Sci. 19, 3217. doi:10.3390/ijms19103217
Rodrigues, M., Kosaric, N., Bonham, C. A., and Gurtner, G. C. (2019). Wound healing: a cellular Perspective. Physiol. Rev. 99, 665–706. doi:10.1152/physrev.00067.2017
Schmitt, E., Boutros, R., Froment, C., Monsarrat, B., Ducommun, B., and Dozier, C. (2006). CHK1 phosphorylates CDC25B during the cell cycle in the absence of DNA damage. J. Cell Sci. 119, 4269–4275. doi:10.1242/jcs.03200
Shao, P., Feng, J., Sun, P., Xiang, N., Lu, B., and Qiu, D. (2020). Recent advances in improving stability of food emulsion by plant polysaccharides. Food Res. Int. 137, 109376. doi:10.1016/j.foodres.2020.109376
Sharifi-Rad, J., Butnariu, M., Ezzat, S. M., Adetunji, C. O., Imran, M., Sobhani, S. R., et al. (2020). Mushrooms-rich Preparations on wound healing: from Nutritional to Medicinal Attributes. Front. Pharmacol. 11, 567518. doi:10.3389/fphar.2020.567518
Shi, Q., Zou, M. Y., Wang, J. H., Song, M. M., Xiong, S. Q., and Liu, Y. (2022). Ultrasonic effects on molecular weight degradation, physicochemical and rheological properties of pectin extracted from Premna microphylla Turcz. Int. J. Biol. Macromol. 221, 1065–1076. doi:10.1016/j.ijbiomac.2022.09.082
Smith, C. (2024). The potential of zebrafish as drug discovery research tool in immune-mediated inflammatory disease. Inflammopharmacology 32, 2219–2233. doi:10.1007/s10787-024-01511-1
Sun, L., Gu, L., Tan, H., Liu, P., Gao, G., Tian, L., et al. (2019). Effects of 17α-ethinylestradiol on caudal fin regeneration in zebrafish larvae. Sci. Total Environ. 653, 10–22. doi:10.1016/j.scitotenv.2018.10.275
Tang, Z., Huang, G., and Huang, H. (2024). Ultrasonic-assisted extraction, analysis and properties of purple mangosteen scarfskin polysaccharide and its acetylated derivative. Ultrason. Sonochem 109, 107010. doi:10.1016/j.ultsonch.2024.107010
Teymoorian, S. K., Nouri, H., and Moghimi, H. (2024). In-vivo and in-vitro wound healing and tissue repair effect of Trametes versicolor polysaccharide extract. Sci. Rep. 14, 3796. doi:10.1038/s41598-024-54565-0
Tu, L., Fan, Y., Deng, Y., Hu, L., Sun, H., Zheng, B., et al. (2023). Production and anti-inflammatory performance of PVA Hydrogels Loaded with Curcumin Encapsulated in Octenyl Succinic Anhydride modified schizophyllan as wound Dressings. Molecules 28, 1321. doi:10.3390/molecules28031321
Ummat, V., Sivagnanam, S. P., Rai, D. K., O'Donnell, C., Conway, G. E., Heffernan, S. M., et al. (2024). Conventional extraction of fucoidan from Irish brown seaweed Fucus vesiculosus followed by ultrasound-assisted depolymerization. Sci. Rep. 14, 6214. doi:10.1038/s41598-024-55225-z
Wang, J., Xu, X., Zou, X., Zhang, R., Jia, X., Dong, L., et al. (2024). Effect of ultrasound assisted H2O2 degradation on longan polysaccharide: degradation kinetics, physicochemical properties and prebiotic activity. Int. J. Biol. Macromol. 282, 136902. doi:10.1016/j.ijbiomac.2024.136902
Wang, L., Li, T., Liu, F., Liu, D., Xu, Y., Yang, Y., et al. (2019). Ultrasonic-assisted enzymatic extraction and characterization of polysaccharides from dandelion (Taraxacum officinale) leaves. Int. J. Biol. Macromol. 126, 846–856. doi:10.1016/j.ijbiomac.2018.12.232
Wang, N., Zhang, X., Wang, S., Guo, Q., Li, Z., Liu, H., et al. (2020). Structural characterisation and immunomodulatory activity of polysaccharides from white asparagus skin. Carbohydr. Polym. 227, 115314. doi:10.1016/j.carbpol.2019.115314
Wu, D.-T., He, Y., Fu, M.-X., Gan, R.-Y., Hu, Y.-C., Peng, L.-X., et al. (2022). Structural characteristics and biological activities of a pectic-polysaccharide from okra affected by ultrasound assisted metal-free Fenton reaction. Food Hydrocoll. 122, 107085. doi:10.1016/j.foodhyd.2021.107085
Xiao, J., Chen, X., Zhan, Q., Zhong, L., Hu, Q., and Zhao, L. (2022). Effects of ultrasound on the degradation kinetics, physicochemical properties and prebiotic activity of Flammulina velutipes polysaccharide. Ultrason. Sonochem 82, 105901. doi:10.1016/j.ultsonch.2021.105901
Yan, J. K., Ding, Z. C., Gao, X., Wang, Y. Y., Yang, Y., Wu, D., et al. (2018). Comparative study of physicochemical properties and bioactivity of Hericium erinaceus polysaccharides at different solvent extractions. Carbohydr. Polym. 193, 373–382. doi:10.1016/j.carbpol.2018.04.019
Yan, J. K., Wang, Y. Y., Ma, H. L., and Wang, Z. B. (2016). Ultrasonic effects on the degradation kinetics, preliminary characterization and antioxidant activities of polysaccharides from Phellinus linteus mycelia. Ultrason. Sonochem 29, 251–257. doi:10.1016/j.ultsonch.2015.10.005
Yap, H. Y., Ariffeen Rosli, M. F., Tan, S. H., Kong, B. H., and Fung, S. Y. (2023). The wound healing potential of Lignosus rhinocerus and other Ethno-myco wound healing agents. Mycobiology 51, 1–15. doi:10.1080/12298093.2022.2164641
Youssouf, L., Lallemand, L., Giraud, P., Soulé, F., Bhaw-Luximon, A., Meilhac, O., et al. (2017). Ultrasound-assisted extraction and structural characterization by NMR of alginates and carrageenans from seaweeds. Carbohydr. Polym. 166, 55–63. doi:10.1016/j.carbpol.2017.01.041
Yuan, D., Li, C., Huang, Q., and Fu, X. (2020). Ultrasonic degradation effects on the physicochemical, rheological and antioxidant properties of polysaccharide from Sargassum pallidum. Carbohydr. Polym. 239, 116230. doi:10.1016/j.carbpol.2020.116230
Yu, G., Chen, Y., Bao, Q., Jiang, Z., Zhu, Y., Ni, H., et al. (2020). A low-molecular-weight ascophyllan prepared from Ascophyllum nodosum: optimization, analysis and biological activities. Int. J. Biol. Macromol. 153, 107–117. doi:10.1016/j.ijbiomac.2020.02.334
Yu, X., Zhou, C., Yang, H., Huang, X., Ma, H., Qin, X., et al. (2015). Effect of ultrasonic treatment on the degradation and inhibition cancer cell lines of polysaccharides from Porphyra yezoensis. Carbohydr. Polym. 117, 650–656. doi:10.1016/j.carbpol.2014.09.086
Zhang, H. R., Wu, S. Y., and Fu, Z. X. (2021). LncRNA-cCSC1 promotes cell proliferation of colorectal cancer through sponging miR-124-3p and upregulating CD44. Biochem. Biophys. Res. Commun. 557, 228–235. doi:10.1016/j.bbrc.2021.04.018
Zhang, S., Liu, B., Yan, G., Wu, H., Han, Y., and Cui, H. (2022). Chemical properties and anti-fatigue effect of polysaccharide from Pholiota nameko. J. Food Biochem. 46, e14015. doi:10.1111/jfbc.14015
Zhang, S., Li, X., Wu, Z., and Kuang, C. (2012). Antioxidant activity of polysaccharide from Camellia Cake against ABTS and DPPH free radicals. Adv. Mat. Res. 550-553, 1545–1549. doi:10.4028/www.scientific.net/amr.550-553.1545
Zhang, W., Xiang, Q., Zhao, J., Mao, G., Feng, W., Chen, Y., et al. (2020). Purification, structural elucidation and physicochemical properties of a polysaccharide from Abelmoschus esculentus L (okra) flowers. Int. J. Biol. Macromol. 155, 740–750. doi:10.1016/j.ijbiomac.2020.03.235
Zhang, Y., Kong, H., Fang, Y., Nishinari, K., and Phillips, G. O. (2013). Schizophyllan: a review on its structure, properties, bioactivities and recent developments. Bioact. Carbohydrates Diet. Fibre 1, 53–71. doi:10.1016/j.bcdf.2013.01.002
Zhao, M., Kang, M., Wang, J., Yang, R., Zhong, X., Xie, Q., et al. (2024). Stem cell-derived Nanovesicles Embedded in Dual-Layered Hydrogel for Programmed ROS Regulation and comprehensive tissue regeneration in Burn wound healing. Adv. Mater 36, e2401369. doi:10.1002/adma.202401369
Zhao, X., Guo, F., Hu, J., Zhang, L., Xue, C., Zhang, Z., et al. (2016). Antithrombotic activity of oral administered low molecular weight fucoidan from Laminaria Japonica. Thromb. Res. 144, 46–52. doi:10.1016/j.thromres.2016.03.008
Zheng, H., Cui, S., Sun, B., Zhang, B., Tao, D., Wang, Z., et al. (2022). Synergistic effect of discrete ultrasonic and H(2)O(2) on physicochemical properties of chitosan. Carbohydr. Polym. 291, 119598. doi:10.1016/j.carbpol.2022.119598
Zhong, K., Liu, L., Tong, L., Zhong, X., Wang, Q., and Zhou, S. (2013). Rheological properties and antitumor activity of schizophyllan produced with solid-state fermentation. Int. J. Biol. Macromol. 62, 13–17. doi:10.1016/j.ijbiomac.2013.08.015
Zhong, K., Zhang, Q., Tong, L., Liu, L., Zhou, X., and Zhou, S. (2015). Molecular weight degradation and rheological properties of schizophyllan under ultrasonic treatment. Ultrason. Sonochem 23, 75–80. doi:10.1016/j.ultsonch.2014.09.008
Zhou, C., and Ma, H. (2006). Ultrasonic degradation of polysaccharide from a red algae (Porphyra yezoensis). J. Agric. Food Chem. 54, 2223–2228. doi:10.1021/jf052763h
Keywords: Schizophyllan, ultrasonic-assisted H2O2, molecular weight, cell proliferation and migration, tissue regeneration
Citation: He H, Liu Y, Li Q, Chen F and Zhou L (2025) Ultrasound-assisted H2O2 degradation enhances the bioactivity of Schizophyllan for wound healing and tissue regeneration. Front. Pharmacol. 16:1562839. doi: 10.3389/fphar.2025.1562839
Received: 18 January 2025; Accepted: 04 March 2025;
Published: 20 March 2025.
Edited by:
Chris A. Bashur, Florida Institute of Technology, United StatesReviewed by:
Kong Xiangdong, Zhejiang Sci-Tech University, ChinaYuhui Liao, Southern Medical University, China
Copyright © 2025 He, Liu, Li, Chen and Zhou. This is an open-access article distributed under the terms of the Creative Commons Attribution License (CC BY). The use, distribution or reproduction in other forums is permitted, provided the original author(s) and the copyright owner(s) are credited and that the original publication in this journal is cited, in accordance with accepted academic practice. No use, distribution or reproduction is permitted which does not comply with these terms.
*Correspondence: Lin Zhou, emhvdWxpbkBnZHB1LmVkdS5jbg==