- 1Preclinical R&D, Dr. Willmar Schwabe GmbH & Co. KG, Karlsruhe, Germany
- 2ACARYON GmbH, Berlin, Germany
Background: Disruptions in the gut microbiota metabolism may contribute to the pathophysiology of gut–brain interaction disorders, and correction of intestinal dysbiosis is considered a promising therapeutic approach. Menthacarin, a proprietary fixed combination of Mentha x piperita L. and Carum carvi L. essential oils, is used clinically for the treatment of functional dyspepsia and irritable bowel syndrome. Rodent model data indicate that treatment effects of Menthacarin on visceral hypersensitivity could be mediated via the normalization of gut dysbiosis. However, the impact of Menthacarin on human bacterial gut microbiota has not yet been studied.
Aim: The aim of the present study was to assess whether Menthacarin affects the composition and metabolic activity of human fecal microbiota.
Methods: Fecal slurry samples from 10 healthy volunteers were cultivated for 36 h under anoxic conditions with and without Menthacarin. Relative bacterial abundance at the phylum and genus levels was evaluated using 16S rRNA metagenomic analysis. Short-chain fatty acids (SCFAs) in the supernatants were measured using the LC-MS technology.
Results: Menthacarin induced robust changes in microbial composition at both the phylum and genus levels among the 10 donor microbiomes. The relative abundance of Firmicutes (+13.6 ± 8.6%) and Actinobacteria (+54.9 ± 47.6%) significantly increased, whereas that of Bacteroidetes (−27.7% ± 21.9%) and Proteobacteria (−25.7% ± 12.3%) significantly decreased in the presence of Menthacarin. At the genus level, the most notable changes were significant increases in Bifidobacterium (+105.1 ± 78.4%) and several SCFA-producing genera accompanied by a significant decrease in genera containing members involved in pro-inflammatory processes. In addition, Menthacarin significantly increased the levels of several SCFAs, namely, propionate, butyrate, isobutyrate, valerate, and isovalerate.
Conclusion: Menthacarin alters the microbiota composition and enhances SCFA production in human microbiota samples under in vitro conditions. These effects may contribute to the clinical benefits observed with Menthacarin treatment.
Introduction
Disorders of the gut–brain interaction (DGBIs), such as irritable bowel syndrome (IBS) and functional dyspepsia (FD), are debilitating gastrointestinal disorders. Although the etiology remains unknown, DGBIs are characterized by a combination of gastrointestinal motility disturbance, visceral hypersensitivity, altered mucosal and immune functions, or altered central nervous system processing. In addition, changes in the gut microbiota or dysbiosis have been implicated in the pathogenesis of DGBIs. Studies have shown that patients with IBS and FD often exhibit an altered gut microbiota composition compared to healthy individuals (Brown et al., 2022; Shanahan et al., 2023; Zhang et al., 2024b; Hillestad et al., 2022; Quigley, 2018; Gawey et al., 2024). For instance, a decrease in beneficial bacteria, such as Lactobacillus and Bifidobacterium, and an increase in potentially harmful bacteria have been observed in patients with IBS (Zhang et al., 2024c). However, different studies have produced inconsistent results, and to date, no defined disease-specific dysbiosis profile can be ascribed to patients with IBS (Teige et al., 2024) or FD (Zhou et al., 2022). Despite these limitations in defining a healthy versus disease-associated microbiota profile, treatments targeting microbiota composition and function are currently being studied or have already shown some efficacy in patients with DGBIs. These options include the use of prebiotics, probiotics, antibiotics, fecal transplantation, or fermentable oligo-, di-, and monosaccharides, and polyols diet (FODMAP) (Hillestad et al., 2022). In addition to these approaches specifically designed to address microbiota composition or function, the established pharmacological therapies for treating IBS and FD symptoms could also act by impacting the gut microbiota. This is especially relevant for phytotherapies based on mixtures of natural products, such as essential oils (EOs) from peppermint and caraway that are known to have antibacterial activity. Menthacarin is a proprietary combination of peppermint oil (90 mg WS® 1340) and caraway oil (50 mg WS® 1520) with specified quality. In clinical trials, Menthacarin has shown efficacy in reducing symptoms in patients with functional dyspepsia, including symptoms of IBS (Madisch et al., 2023; Madisch et al., 1999; Madisch et al., 2019; May et al., 2000; Rich et al., 2017; Holtmann et al., 2003). Human pharmacology studies have indicated that Menthacarin reduced gastroduodenal motility (Micklefield et al., 2000). Nonclinical mechanistic studies have confirmed the anti-spasmodic effects of both EOs on small and large human intestinal preparations in organ bath studies, mediated via calcium channel inhibition (Krueger et al., 2020). In addition, both EOs stimulate ion secretion in human intestinal preparations (Krueger et al., 2020). Studies in rodent models showed attenuation of intestinal inflammation by Menthacarin in a colitis model (Alliger et al., 2020) and normalization of visceral hypersensitivity induced by different noxious stimuli (Adam et al., 2006; Botschuijver et al., 2018; Omoloye et al., 2024). In maternally separated rats subjected to water avoidance stress, the induction of visceral hypersensitivity was associated with changes in the composition of the gut microbiome and mycobiome. Interestingly, the reversal of visceral hypersensitivity by Menthacarin was associated with normalization of the mycobiome, suggesting that effects on gut microbial or fungal composition could contribute to treatment effects of Menthacarin. Indeed, antimicrobial activities have been described for EOs, including peppermint oil (Hawrelak et al., 2009; Sharafi et al., 2010; Botschuijver et al., 2018; Neagu et al., 2023; Quintana Soares Lopes et al., 2024), caraway oil (Botschuijver et al., 2018; Hawrelak et al., 2009), and the combination product Menthacarin (Botschuijver et al., 2018), which exerted antibacterial and antimycotic activities in different in vitro assays. These data suggest that modulation of gut microbiota could contribute to the beneficial effects observed with Menthacarin or its individual constituents in nonclinical models and in patients with DGBIs. However, classical plate diffusion assays with isolated pathogens are of limited relevance to predict the effects of Menthacarin on human microbiome composition with a high level of mutual interactions and specific cultivation requirements. In addition, the effects observed in rodent microbiome samples may not be predictive of the human microbiome composition. To overcome these limitations, we employed an optimized anoxic culture system to assess the effect of Menthacarin on human microbiome composition and metabolic function in fecal samples from 10 healthy donors. Sequencing of 16S rRNA and mass spectrometric determination of SCFAs revealed consistent treatment effects of Menthacarin on microbiome composition, resulting in enhancement of SFCA production.
Materials and methods
Test items
Menthacarin® is a proprietary combination of peppermint oil (90 mg WS® 1340) and caraway oil (50 mg WS® 1520) with specified quality. Menthacarin and individual EOs were provided by Dr. Willmar Schwabe GmbH & Co. KG. The EOs fulfilled the specification requirements of the European Pharmacopeia.
Ex vivo cultivation
The present study was conducted using the HUMIPLATE-Platform (ACARYON GmbH, Berlin, Germany), a high-throughput ex vivo gut microbiome simulation model. It was designed to maintain the compositional stability of the microbiome for 48 h while also maintaining the functional capacities of the microbiome intact. Hence, the platform enables close replication of the gut microbiome’s in vivo dynamics in response to the test products.
In this study, feces from 10 healthy donors (five males and five females, Table 1) were used to assess the potential microbiome modulation properties of Menthacarin and its individual constituents, namely, peppermint oil (WS® 1340) and caraway oil (WS® 1520). The microbiomes were provided by ACARYON. The donors provided their samples at the doctor’s offices. Each donor signed a declaration of consent and a transfer of ownership. Strict data protection measures were observed to ensure that the samples could not be traced.
ACA-Medium (ACA-M001; ACARYON GmbH, Berlin, Germany) was inoculated at T0 with donor microbiomes. After 9 h (time for the microbiome to acclimate to the ex vivo conditions), Menthacarin or individual oils were added to each microbiome at a final concentration of 1 mg/mL. Each condition was run in duplicate. All experiments were performed in an anaerobic chamber. After 36 h, microbiomes were harvested. The microbiomes were first assessed for viability before centrifugation to separate the pellets from the cell-free supernatants. The pellets were subjected to 16S rRNA sequencing. The cell-free supernatants were stored at −80°C before being used for quantification of SCFAs (only vehicle and Menthacarin treatments) (Figure 1).
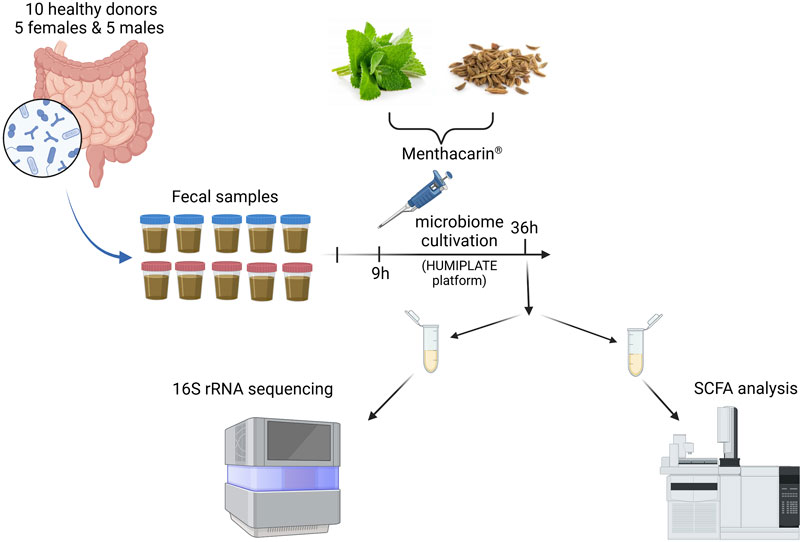
Figure 1. Experimental outline. Created in BioRender. Lehner M. (2025) https://BioRender.com/o98a156.
Microbiome viability
The growth and viability of the microbiomes were controlled by determining the live/dead ratio within the culture using a LIVE/DEAD BacLight Bacterial Viability Kit (Thermo Fisher L7012). The assay was performed according to the manufacturer’s instructions.
Next-generation sequencing
Next-generation sequencing (16S rRNA metagenomic analysis) was performed in a blinded manner.
Sample storage and lysis
The collected samples were stored in a DNA-stabilizing buffer at −20°C until use. For the lysis process, the samples were defrosted and centrifuged at 4,000 g for 15 min. Later, 650 µL of warmed up PW buffer from the QIAamp 96 PowerFecal QIAcube HT Kit (Qiagen, Germany) were added to each sample and vortexed for 20 min.
DNA extraction of stool samples
DNA was extracted with a proprietary in-house process using the QIAamp 96 PowerFecal QIAcube HT Kit on a liquid-handling system (Hamilton StarLine and Tecan EVO) using a vacuum chamber and a high-pressure chamber. The extracted gDNA was stored at −20°C until use.
Library preparation for sequencing with the Illumina MiSeq System
The library preparation was performed following the manual “16S Metagenomic Sequencing Library Preparation—Preparing 16S Ribosomal RNA Gene Amplicons for the Illumina MiSeq System,” using the Master Mix (New England BioLabs) for the target and library amplification and using the 16S V3V4 primer (Eurofins). For the normalization of all samples, a fluorescent dye and the Biotek Synergy HTX plate reader were used to measure DNA concentrations and to calculate the necessary dilution volume per sample. All steps described were nearly fully automated using a liquid-handling system (Hamilton StarLine) that allows the processing of multiple samples simultaneously. Library Denaturing and MiSeq Sample Loading were carried out manually following the Illumina protocol for the MiSeq Reagent Kit v3 (600-cycle). Demultiplexing was performed directly on MiSeq after sequencing, and the resulting FastQ files were generated for subsequent data analysis.
Processing and analysis of sequence data
Paired-end reads from MiSeq (2 × 300 cycles) were merged to reconstruct overlapping sequences with 430–460 base lengths. Chimera and borderline reads were filtered out with the usearch uchime2_ref tool (uchime2_ref command, drive5.com). SILVA 138.1 was used as the database for usearch uchime2_ref.
For taxonomic alignment, Amplicon sequence variants (ASVs) were determined using BLASTn (Nucleotide–Nucleotide BLAST 2.10.1+) against SILVA 138.1 (Release 138.1, arb-silva. de). Alignment identity was required to meet a threshold of at least the following: phylum, 75.0; class, 78.5; order, 82.4; family, 86.5; genus, 94.5; and species, 97.0. RefSeqs/Counts tables were created for all samples using Python package Pandas 1.3.4 (pandas-dev/pandas: Pandas 1.4.3 | Zenodo).
SCFA quantification
Standards and supernatants of microbiome samples were derivatized with aniline, according to the method described by Haidacher et al. (2020). Calibration standards and samples were injected into a CSH Fluoro-Phenyl Column (130 Å, 1.7 µm, 2.1 mm × 150 mm). Acetonitrile (0.1% FA) and ULC-MS water (0.1% FA) were used as the mobile phase. Standards and samples were analyzed using a multiple reaction monitoring (MRM) MS method. This targeted method selects a specific MS2 fragment for quantification, which is generated by defined collision energies for each component. Acquired data of standards and samples were analyzed using Data Analysis (DA) software. Quantification was performed using the generated extracted ion chromatograms (EIC) based on MRM MS2 quantifier signals. The peaks were integrated, and the peak area was used to calculate the amount of SCFAs in each sample on the basis of the calibration curve.
Bioinformatic processing
Copy number normalization
Further analyses were done using Picrust2 (Douglas et al., 2020). The study sequences of the alignment step were placed into a reference tree to determine/predict the copy numbers and the nearest-sequenced taxon index (NSTI index). All study sequences with an NSTI-index > 2 were excluded.
Assessment of alpha diversity
For alpha diversity calculations, ASV counts were rarefied to 10,000 counts per sample. The Shannon index was chosen as the alpha diversity metric, and calculations were performed using the “estimate_richness” diversity function provided by Phyloseq (McMurdie and Holmes, 2013). The R package ggplot (Villanueva and Chen, 2019) was used for visualization.
Assessment of beta diversity
To account for the compositional properties of relative ASV abundance, a total sum-scaling (TSS) transformation was performed for further analysis. This transformation was previously shown to outperform other transformative processes in distance-based analysis.
Bray–Curtis distances between the samples were obtained using “ordination” function in Phyloseq (McMurdie and Holmes, 2013). Compositional differences between the treatment groups were visualized via a principal component analysis, which was conducted using the PCA function of the R package Phyloseq (McMurdie and Holmes, 2013).
Assessment of treatment effects at phylum and genus levels
Variations in genera and phyla were illustrated via the log2 fold change of significantly different taxa. The R package ggplot2 (Villanueva and Chen, 2019) was used for visualization. For quantification of treatment effects, relative changes in abundance levels after test item treatment were expressed as % change vs. control treatment. Heatmaps and graphs containing % change data were generated in GraphPad Prism version 10.1.2 for Windows (GraphPad Software, Boston, Massachusetts, United States, www.graphpad.com).
Statistical analysis
Alpha diversity
An unpaired two-sided t-test was performed for hypothesis testing, and the calculated p-values were adjusted for multiple testing, using the Benjamini and Hochberg correction method (Benjamini and Hochberg, 1995).
Beta diversity
For statistical analysis of beta diversity, Permanova analysis was performed using the adonis2 function from the R package vegan (Oksanen and Blanchet, 2022) and pairedAdonis2 package; the resulting p-values were adjusted for multiple testing using the Benjamini and Hochberg correction method (Benjamini and Hochberg, 1995).
Phyla and genera abundance
To identify differentially abundant taxa between the samples with and without test items, a two-sided paired Wilcoxon signed-rank test was performed with the following:
i) centered-log ratio normalized relative abundances and
ii) relative abundance without test product normalization to 1: the relative abundances in the controls were set to 1, and the relative abundances obtained following cultivation with the product were proportionally normalized.
Statistical significance was set at a p-value <0.05. Analysis was carried out at the genus and phylum levels while only considering samples for statistical comparison with a mean abundance of more than 0.15% in at least one group (with or without Menthacarin).
SCFA quantification
For statistical analysis of treatment effects on SCFA levels, the Wilcoxon matched pairs signed-rank test for comparison of control vs Menthacarin treatment was performed using GraphPad Prism version 10.1.2 for Windows (GraphPad Software, Boston, Massachusetts United States, www.graphpad.com).
Results
Microbiome diversity
Ten microbiomes from 10 healthy donors, 5 males and 5 females of different ages and BMIs, were used in the study (Table 1). The analysis of beta diversity assessed using Bray-Curtis distances and visualized through the principal coordinate analysis plot (PCoA) demonstrated that the 10 microbiomes provided an appropriate diversity for the study (Figure 2).
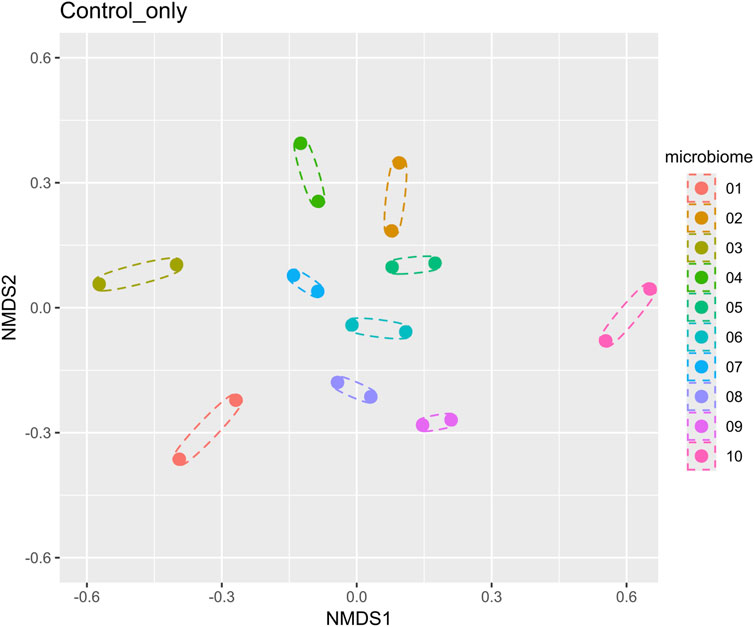
Figure 2. Beta diversity analysis of 10 microbiomes (without Menthacarin) using a nonmetric multidimensional scaling method. Each microbiome has two replicates, which are shown as dotted ellipses.
Effect on alpha diversity
After 36 h of cultivation with Menthacarin, the viability of the microbiomes was above 90% in all individual microbiome samples (Supplementary Table S1), demonstrating that Menthacarin had no toxic effect on the human microbiome. Furthermore, no statistical difference between the microbiomes cultivated with and without Menthacarin could be observed in terms of alpha diversity, demonstrating that Menthacarin did not impact species richness (Figure 3).
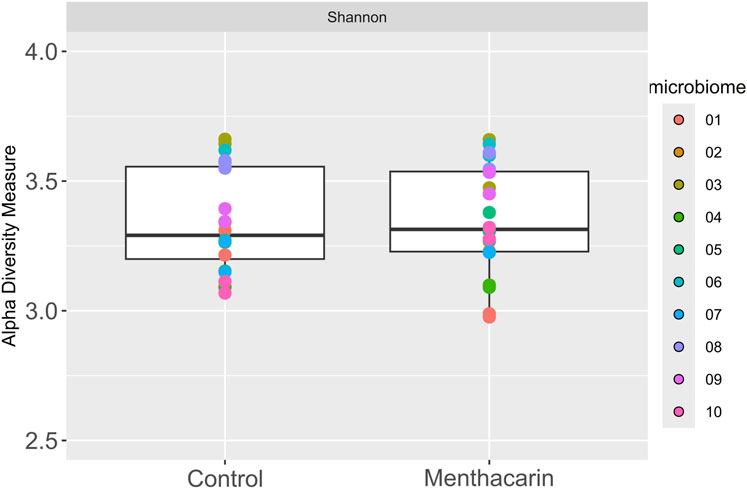
Figure 3. Alpha diversity analysis of 10 microbiomes with and without Menthacarin using Shannon’s index as a metric.
As the study was run with 10 microbiomes, considering the very low abundance and/or low prevalence, taxa may have generated false-positive results. Consequently, to ensure the validity of the results, two successive filters were applied to select the taxa to be considered. First, for each taxon, only microbiomes presenting a relative abundance of at least 0.15% (at the considered taxa level) with or without Menthacarin were considered. Furthermore, only taxa for which at least four of the ten microbiomes fulfilled this condition were considered.
Microbiome modulation at phylum level
At the phylum level, the seven most abundant phyla were found to fit the selection criteria discussed above. In the control samples, Firmicutes was the most abundant phylum (mean: 60.4% and range: 45%–68%), followed by Bacteroidetes (mean: 20.1% and range: 9%–31%), Proteobacteria (mean: 13.8% and range: 7%–20%), and Actinobacteria, the latter being highly variable between different donor samples (mean: 3.1% and range: 0.4%–15.6%). Desulfobacterota (mean: 1.1% and range: 0.1%–5.9%), Verrucobacteria (mean: 1.0% and range: 0.001%–2.7%), and Euryarchaeota (mean: 0.4% and range: 0%–0.9%) were present only in low relative abundance in most samples (Figure 4). The addition of Menthacarin generally maintained the donors’ specific composition while increasing the relative abundance of Firmicutes and Actinobacteria, and decreasing the relative abundance of Bacteroidetes and Proteobacteria (Figure 4; Supplementary Figure S1).
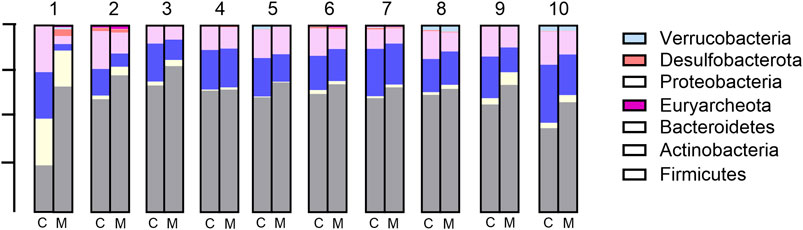
Figure 4. Microbiome composition at the phylum level: relative abundance (%) in control (C) vs Menthacarin (M)-treated microbiomes from 10 donors (1–10).
Menthacarin was found to induce a statistically significant increase in the relative abundance of the phyla Firmicutes (p = 0.0020) and Actinobacteria (p = 0.0059), and a statistically significant decrease in the relative abundance of the phyla Bacteroidetes (p = 0.0039) and Proteobacteria (p = 0.0020) when centered log-ratio transformed relative abundance was used for statistical analysis (Figure 5). Furthermore, although not statistically significant, Menthacarin decreased the relative abundance of the phylum Desulfobacterota in 7 out of 9 considered microbiomes (p = 0.0972; Figure 5).
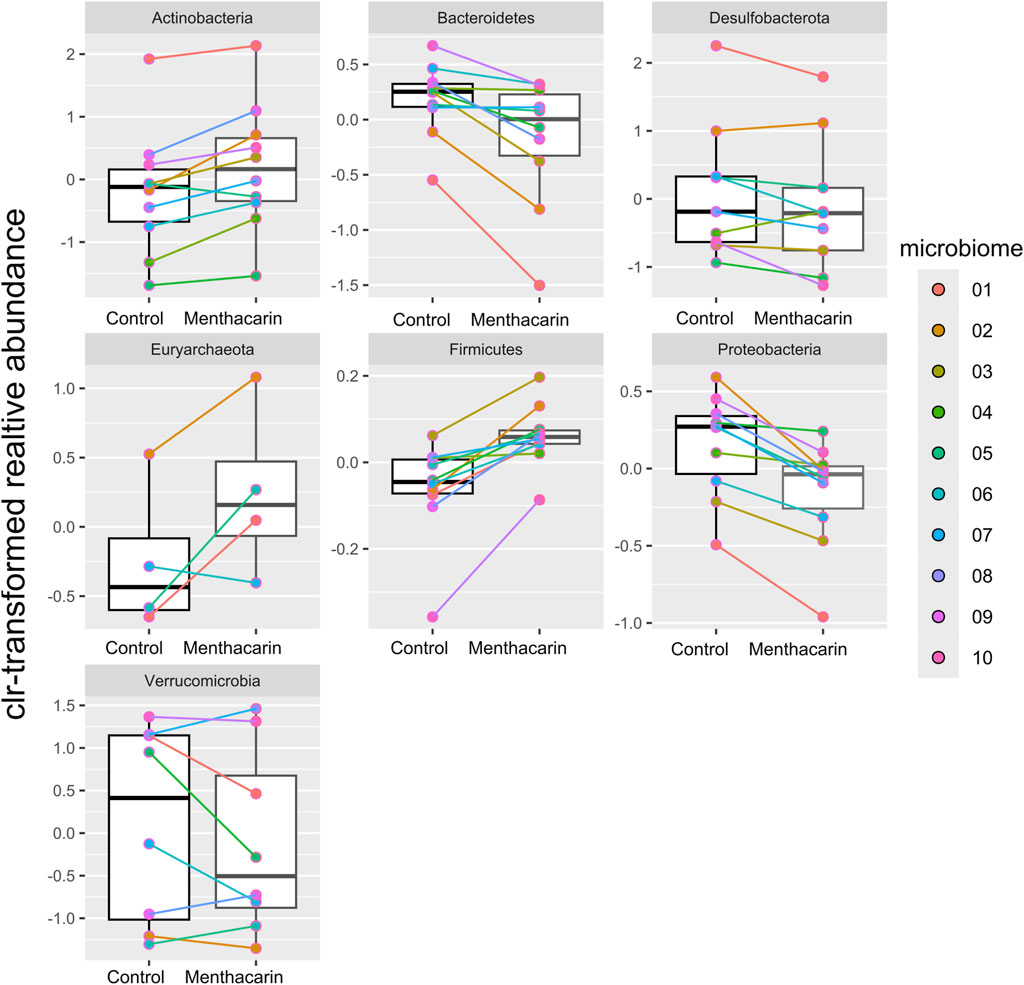
Figure 5. Comparison of centered log-ratio transformed relative abundance between the control and Menthacarin across the considered phyla. Box plots show median, quartile, and min–max with individual microbiome values highlighted in different colors. Individual lines connect corresponding data points for individual microbiomes with and without the addition of Menthacarin. Significant differences between control and Menthacarin treatments were found for Actinobacteria (p = 0.0059), Bacteroidetes (p = 0.0039), Firmicutes (p = 0.0020), and Proteobacteria (p = 0.0020) based on paired two-tailed Wilcoxon’s signed rank-sum test.
For quantification of the effects of Menthacarin on the microbiome composition, we calculated the percentage change induced by Menthacarin in the relative abundance of the four phyla with significant treatment effects. The addition of Menthacarin led to an increase in Firmicutes and Actinobacteria by 13.6% ± 8.6% and 54.9% ± 47.6%, whereas it led to a decrease in Bacteroidetes and Proteobacteria by 27.7% ± 21.9% and 25.7% ± 12.3%, respectively (Figure 6).
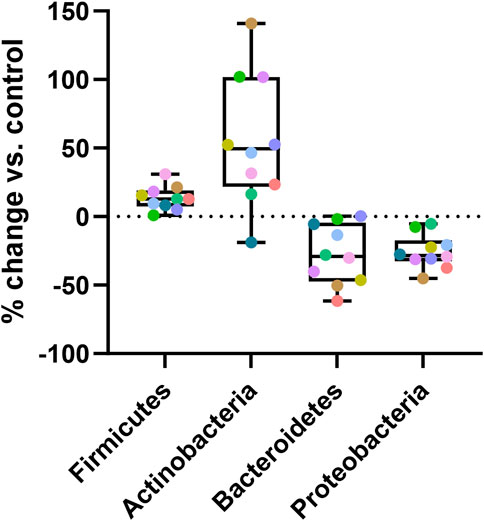
Figure 6. Menthacarin increases Firmicutes and Actinobacteria while decreasing Bacteroidetes and Proteobacteria. Percentage change in the relative abundance at the phylum level induced by Menthacarin (compared to control conditions) is shown. Box and Whiskers plot is presented with quartiles and min–max. Each colored point represents an individual microbiome.
Microbiome modulation at genus level
At the genus level, 173 specific genera (without considering “unclassified”) with relative abundance of at least 0.1% were identified across all 10 microbiomes (Supplementary Figure S2). After filtering, 52 specific genera were considered (Figure 7). For 35 of them, the effects of Menthacarin were qualitatively consistent among all or most of the 10 microbiomes (at least 70% of the considered microbiomes), and 12 presented a statistically significant change in response to Menthacarin (Figure 8).
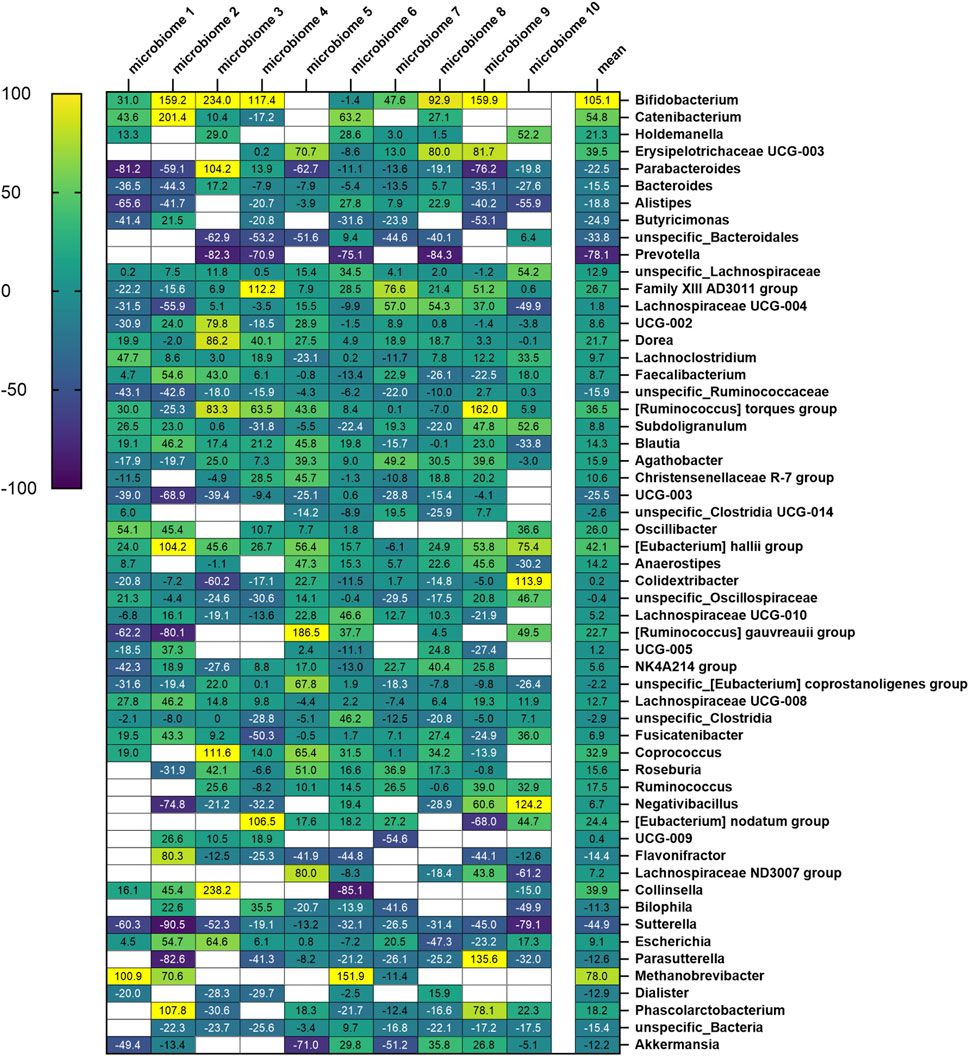
Figure 7. Heatmap of fractional change in relative abundance with Menthacarin (expressed in percentage) across all considered genera. The numbers in each cell denote the fractional change (in %) for each genus across the 10 microbiomes, with the mean values shown in the final column.
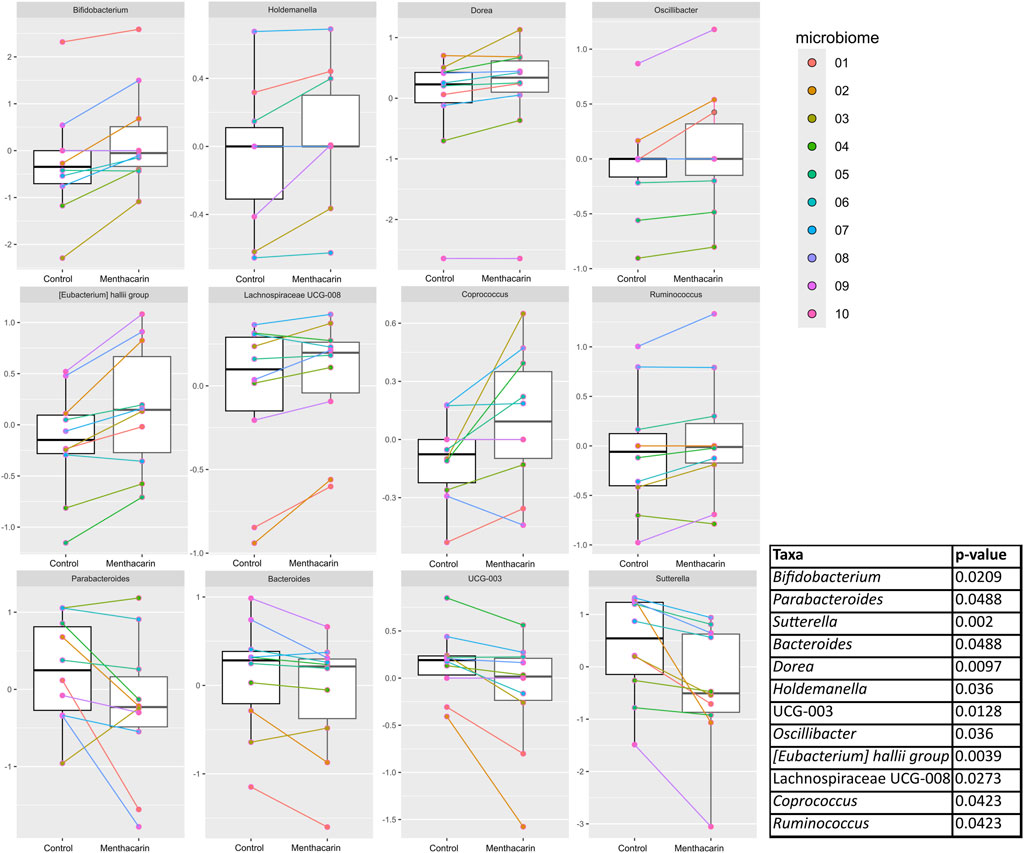
Figure 8. Comparison of centered log-ratio transformed relative abundance between the control and Menthacarin in statistically significantly different genera. Individual lines connect the corresponding data points for individual microbiomes with and without the addition of Menthacarin. A paired two-tailed Wilcoxon’s signed rank-sum test was used for statistical analysis with a p-value <0.05 considered statistically significant.
Menthacarin increases health-promoting and SCFA-producing genera
Menthacarin induced a strong (observed in 7 of 8 considered microbiomes, 105.1% ± 78.4% increase) and statistically significant increase in the relative abundance of the health-promoting genus Bifidobacterium (Figures 7, 8).
In accordance with the statistically significant increase observed in the phylum Firmicutes, Menthacarin was also found to promote several genera belonging to the classes Clostridia and Bacilli. Menthacarin induced a statistically significant increase in the relative abundance of the butyrate-producing genera Eubacterium hallii, Coprococcus, and Ruminococcus (Figures 7, 8). Furthermore, although not statistically significant, Menthacarin induced an increase in the relative abundance of the butyrate-producing genera Blautia, Ruminococcus torque, and Anaerostipes in 7 of 10, in 7 of 10, and in 6 of 8 considered microbiomes, respectively (Figure 7). Altogether, Menthacarin induced an increase in the relative abundance of the main butyrate producers of the class Clostridia (Faecalibacterium, Eubacterium hallii, R. torque, Blautia, Anaerostipes, Coprococcus, Roseburia Ruminococcus, and Subdoligranulum) (Figure 7). Menthacarin also significantly increased the relative abundance of the genera Lachnospiraceae UGC-008, Oscillobacter, and Dorea (Figure 7).
Moreover, Menthacarin promoted members of the class Bacilli. Menthacarin induced a statistically significant increase in the relative abundance of the genus Holdemanella and an increase in the relative abundance of the genera Erysipelotrichaceae UCG-003 and Catenibacterium in 5 of 6 considered microbiomes (Figures 7, 8).
Menthacarin inhibits inflammation-related genera
At the genus level, five specific genera belonging to the phylum Bacteroidetes were considered. The relative abundance of the genera Bacteroides and Parabacteroides was significantly reduced by Menthacarin (Figure 8). Also, the relative abundance of the genera Butyricimonas, Alistipes, and Prevotella mostly decreased; however, the decrease was not statistically significant (Figure 7).
Among the phylum Proteobacteria, two members of the class Gammaproteobacteria were inhibited by Menthacarin. The relative abundance of the genus Sutterella was significantly reduced in the presence of Menthacarin, whereas for the genus Parasutterella, the relative abundance was decreased in 7 of 8 considered microbiomes (Figures 7, 8).
Effects of peppermint and caraway EOs
Treatment of microbiomes with peppermint oil or caraway oil at 1 mg/mL produced qualitatively and quantitatively similar changes in the relative abundance at the phylum level with increases in Firmicutes and Actinobacteria and decreases in Bacteroidetes and Proteobacteria (Supplementary Figure S3). At the genus level, 17 genera were consistently affected (in at least 70% of tested microbiomes) by all three treatments: 9 were affected by Menthacarin and caraway oil, 2 by Menthacarin and peppermint oil, and 6 by peppermint and caraway oil. In addition, 7 genera were only consistently affected by Menthacarin, whereas 2 and 4 genera were uniquely affected by peppermint and caraway oil, respectively (Supplementary Figure S4).
Menthacarin increases SCFA levels
We observed a positive effect of Menthacarin on the relative abundance of several SCFA-producing genera. To assess whether the change in microbial composition translated into altered microbiome metabolite production, we analyzed the concentrations of different SCFAs in the supernatants of the microbiome cultures. As expected from the microbiome changes, Menthacarin significantly increased the production of propionate, butyrate, isobutyrate, valerate, and isovalerate, whereas no effect was observed on acetate levels (Figure 9).
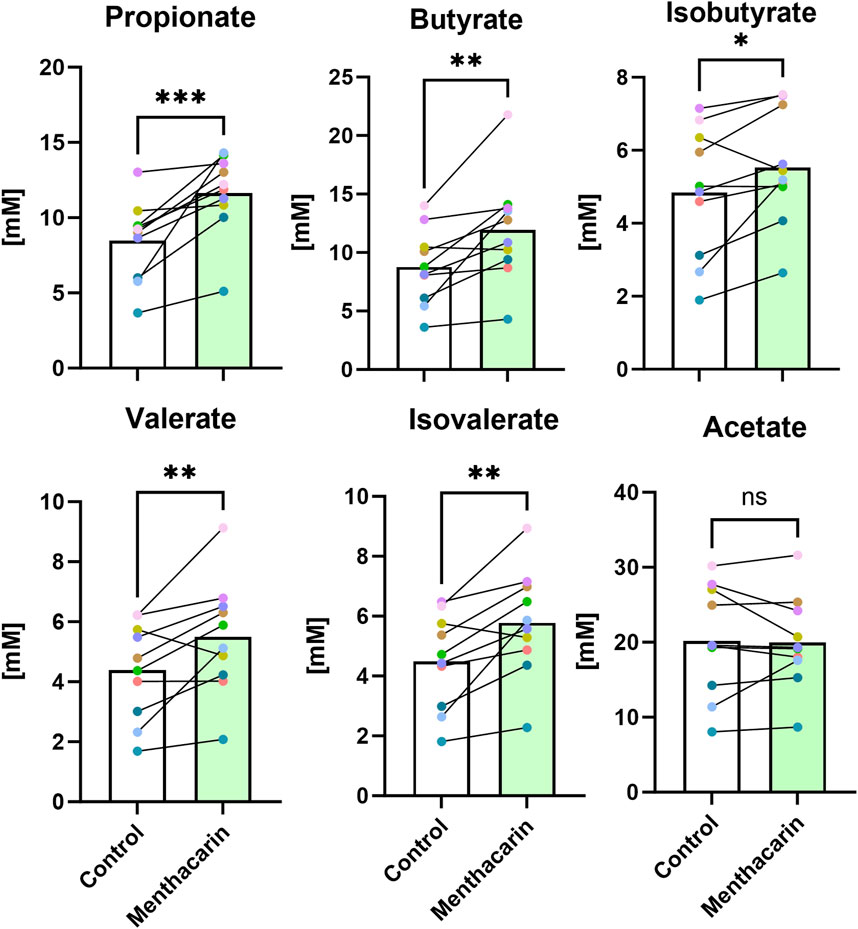
Figure 9. Menthacarin increases the concentration of most SCFAs. Concentrations of short-chained fatty acids in supernatants of control and Menthacarin-treated microbiomes are shown as medians of 10 microbiomes and individual microbiome values. Individual lines connect corresponding data points for individual microbiomes without (control) vs with the addition of Menthacarin. Statistical significance assessed by Wilcoxon matched pairs signed rank test for comparison of control vs. Menthacarin treatment is indicated by asterisks (*p < 0.05, **p < 0.01, and ***p < 0.001).
Discussion
Menthacarin, a proprietary combination of EOs from Mentha x piperita L. and Carum carvi L., has demonstrated clinical efficacy in alleviating symptoms and improving the quality of life in patients with FD (Madisch et al., 2023; Madisch et al., 1999; Rich et al., 2017; Holtmann et al., 2003; Storr and Stracke, 2023) and concomitant IBS symptoms (Madisch et al., 2019). In a rat model of maternal separation and water avoidance stress, Menthacarin normalized the visceral hypersensitivity. This visceral hypersensitivity was associated with changes in the gut microbiome and mycobiome, which were partially corrected by Menthacarin, suggesting that modulation of gut microflora may be a potential mechanism of action (Botschuijver et al., 2018). In the present in vitro study, we assessed the effect of Menthacarin on human microbiome composition and metabolic activity. Using an optimized anoxic incubation protocol of fecal microbiome samples from 10 healthy donors, we observed that Menthacarin induced consistent changes in the microbiome composition among donors. Whereas alpha diversity remained unaffected, Menthacarin significantly increased the relative abundance of the phyla Firmicutes and Actinobacteria, and decreased that of Bacteroidetes and Proteobacteria (Figures 4–6). At the genus level, Menthacarin significantly increased the abundance of health-promoting bacteria such as Bifidobacterium and SCFA-producing genera within the class Clostridia (e.g., Coprococcus, Ruminococcus torques, Dorea, and Eubacterium Hallii) and the class Bacilli (Holdemanella) (Facchin et al., 2024). Conversely, genera from the phyla Bacteroidetes and Proteobacteria, which are considered to be inflammation-related, were significantly (Bacteroides, Parabacteroides, and Sutterella) or numerically decreased (Butyricimonas, Alistipes, Prevotella, and Parasutterella) (Figures 7, 8).
Although the 10 microbiomes displayed different microbiota compositions (Figures 2, 4), the effects of Menthacarin on the four main phyla and most genera mentioned above were qualitatively consistent among all or most microbiomes.
The robustness of the observed microbiota changes was confirmed by testing the single oil constituents of Menthacarin, namely, peppermint and caraway EOs, at the same concentration of 1 mg/mL. Both single EOs produced qualitatively and quantitatively comparable results as Menthacarin on microbiome composition, with increases in Firmicutes and Actinobacteria, as well as corresponding decreases in Bacteroidetes and Proteobacteria (Supplementary Figure S3). Furthermore, among the 35 genera with qualitatively consistent changes in response to Menthacarin, 17 also presented similar changes in response to peppermint oil and caraway oil (Supplementary Figure S4).
One of the most significant recent paradigm shifts in medicine is the recognition of the central role of gut microbiome in human health. The gut microbiome influences almost every aspect of human health and significantly impacts the effectiveness of drugs and treatments (Salvucci, 2019; Hou et al., 2022; Wilkinson et al., 2018; Weersma et al., 2020). Consequently, modulating the composition or functionality of the microbiome has become the main avenue for the prevention and treatment of diseases (Dietert, 2021; Yadav and Chauhan, 2022).
The connection between the gut microbiome and disturbed well-being is particularly relevant for disorders of gut–brain interactions such as IBS and FD (Brown et al., 2022; Gawey et al., 2024; Hillestad et al., 2022; Quigley, 2018; Shanahan et al., 2023; Teige et al., 2024; Zhang et al., 2024b; Zhang et al., 2024c; Zhou et al., 2022; Margolis et al., 2021). Several changes in the microbiome composition have been reported in patients with IBS, including a lower abundance of Firmicutes (Zhuang et al., 2018) and Bifidobacterium (Liu et al., 2017; Zhang et al., 2024c; Kamp et al., 2024) and higher levels of Bacteroides (Zhang et al., 2024c; Kamp et al., 2024; Zhuang et al., 2018) than in healthy controls. In addition, clinical studies have reported beneficial effects of treatments based on live or inactivated Bifidobacterium strains in patients with IBS and FD (Cruchet Muñoz et al., 2024; Srivastava et al., 2024; Zhang et al., 2024a). Ex vivo systems using microbiome samples have demonstrated the ability to reproduce most of the changes identified in vivo. For example, the in vivo effect of a drug like metformin on the murine microbiome has been shown to correlate with effects observed in an ex vivo system (Li et al., 2019). Another example demonstrated that ex vivo systems could simulate the clinical outcomes of repeated prebiotic intake (Van den Abbeele et al., 2023). We employed an ex vivo high-throughput cultivation system (HUMIPLATE, ACARYON GmbH, Germany) that was optimized to maintain the composition and functionality of the microbiome for 48 h. In our experimental setting, Menthacarin produced an increase in Bifidobacterium and reduced the abundance of Bacteroides, suggesting that the microbiome changes induced by Menthacarin have the potential to correct dysbiosis in patients with DGBI.
One limitation of our study design was the use of fecal samples only from healthy donors. Further studies with Menthacarin treatment of microbiomes from patients with DGBI are required to assess the normalization of disease-related dysbiosis. In our study, we tested Menthacarin at a concentration of 1 mg/ml). A single dose of the finished herbal medicinal product contains 140 mg of Menthacarin. Assuming an intestinal volume of 250 mL, a value applied for assessing the intestinal drug–drug-interaction potential (EMA/CHMP/ICH/652460/2022, 2022), a single dose application would result in a luminal concentration of approximately 0.56 mg/mL, suggesting that our test concentration is within a physiologically relevant range.
Extrapolating from microbiome composition data to actual health benefits is challenging due to the complexity of the microbiota and microbiota–host interactions. One approach to reduce this complexity is to analyze the effects on metabolite production, which serves as a common final pathway of microbe–host interactions. Short-chain fatty acids have attracted significant attention as products of microbial metabolism with well-documented beneficial effects on the host cell function and health. Among the activities most relevant for DGBI, SCFAs serve as an energy source for colonocytes and enhance mucus production, which protects the gut lining. In addition, SCFAs, particularly butyrate, have been shown to reduce inflammation and enhance the integrity of the gut barrier (Xiong et al., 2022). In our study, we found significant increases in the levels of all measured SCFAs (propionate, butyrate, isobutyrate, valerate, and isovalerate) after Menthacarin treatment, except for acetate, where no difference was observed (Figure 9). The increase in SCFA production suggests that the observed increases in genera known to be relevant for SCFA production indeed translate into metabolic changes. For patients with DGBI, SCFAs could not only exert beneficial effects by reducing intestinal permeability and inflammation but could also contribute to certain symptoms, especially diarrhea and bloating, depending on the individual situation. Interestingly, the administration of encapsulated butyrate has been shown to reduce symptom severity in patients with IBS and inflammatory bowel diseases (Facchin et al., 2020; Banasiewicz et al., 2013). Additionally, fecal microbiota transplantation in patients with IBS resulted in improvements in symptom scores and quality of life while increasing fecal butyrate, isobutyrate, isovalerate, and valerate levels (El-Salhy et al., 2021). In that study, butyrate levels were inversely correlated with symptom severity in responders, indicating a beneficial effect of (selected) SCFA increase in patients with IBS (El-Salhy et al., 2021).
Our in vitro cultivation system in healthy human microbiomes demonstrates that Menthacarin has the potential to induce changes in the relative microbiome composition, resulting in increased SCFA production. It is tempting to speculate that microbiome modulation could contribute to symptom reductions observed with Menthacarin therapy in animal models of visceral hypersensitivity and patients with FD. However, further nonclinical in vivo studies and patient microbiome data would be needed to verify this hypothesis.
In conclusion, incubation of human fecal samples with Menthacarin produced consistent changes in microbiome composition, characterized by an increase in Firmicutes and Actinobacteria and a decrease in Bacteroidetes and Proteobacteria. In addition, levels of SCFAs were significantly increased by Menthacarin. The data provide initial evidence that microbiome modulation could be a mechanism of action with potential relevance for the observed clinical efficacy of Menthacarin in patients with DGBI.
Data availability statement
The original contributions presented in the study are publicly available. This data can be found here: NCBI BioProject number PRJNA1250407, available at: https://www.ncbi.nlm.nih.gov/sra/PRJNA1250407.
Ethics statement
Ethical approval was not required for the studies involving humans because fecal samples were completely anonymized and could not be traced back to the donors. The studies were conducted in accordance with the local legislation and institutional requirements. The participants provided their written informed consent to participate in this study.
Author contributions
ML: conceptualization, formal analysis, funding acquisition, project administration, supervision, visualization, and writing – original draft. PU: conceptualization, data curation, formal analysis, investigation, methodology, validation, visualization, and writing – review and editing. SC: formal analysis, visualization, and writing – review and editing.
Funding
The author(s) declare that financial support was received for the research and/or publication of this article.
Acknowledgments
We thank Kawe Toutounian and Pabitra Nandy (ACARYON GmbH, Berlin, Germany) for conducting the microbiome studies, performing the bioinformatic analyses, and providing their microbiome expertise.
Conflict of interest
ML and SC are employees of Dr. Willmar Schwabe GmbH & Co. KG, a manufacturer of herbal medicinal products containing the test item Menthacarin®.
The authors declare that this study received funding from Dr. Willmar Schwabe GmbH & Co. KG. The funder had the following involvement in the study: decision to submit the article for publication. PU is an employee of ACARYON GmbH which received payments for conducting the microbiome study.
Generative AI statement
The author(s) declare that no Generative AI was used in the creation of this manuscript.
Publisher’s note
All claims expressed in this article are solely those of the authors and do not necessarily represent those of their affiliated organizations, or those of the publisher, the editors and the reviewers. Any product that may be evaluated in this article, or claim that may be made by its manufacturer, is not guaranteed or endorsed by the publisher.
Supplementary material
The Supplementary Material for this article can be found online at: https://www.frontiersin.org/articles/10.3389/fphar.2025.1569052/full#supplementary-material
References
Adam, B., Liebregts, T., Best, J., Bechmann, L., Lackner, C., Neumann, J., et al. (2006). A combination of peppermint oil and caraway oil attenuates the post-inflammatory visceral hyperalgesia in a rat model. Scand. J. Gastroenterol. 41, 155–160. doi:10.1080/00365520500206442
Alliger, K., Khalil, M., König, B., Weisenburger, S., Koch, E., and Engel, M. (2020). Menthacarin attenuates experimental colitis. Phytomedicine 77, 153212. doi:10.1016/j.phymed.2020.153212
Banasiewicz, T., Krokowicz, Ł., Stojcev, Z., Kaczmarek, B. F., Kaczmarek, E., Maik, J., et al. (2013). Microencapsulated sodium butyrate reduces the frequency of abdominal pain in patients with irritable bowel syndrome. Colorectal Dis. 15, 204–209. doi:10.1111/j.1463-1318.2012.03152.x
Benjamini, Y., and Hochberg, Y. (1995). Controlling the false discovery rate: a practical and powerful approach to multiple testing. J. R. Stat. Soc. Ser. B Methodol. 57, 289–300. doi:10.1111/j.2517-6161.1995.tb02031.x
Botschuijver, S., Welting, O., Levin, E., Maria-Ferreira, D., Koch, E., Montijn, R. C., et al. (2018). Reversal of visceral hypersensitivity in rat by Menthacarin(®), a proprietary combination of essential oils from peppermint and caraway, coincides with mycobiome modulation. Neurogastroenterol. Motil. 30, e13299. doi:10.1111/nmo.13299
Brown, G., Hoedt, E. C., Keely, S., Shah, A., Walker, M. M., Holtmann, G., et al. (2022). Role of the duodenal microbiota in functional dyspepsia. Neurogastroenterol. Motil. 34, e14372. doi:10.1111/nmo.14372
Cruchet Muñoz, S., Verbeke Palma, S., Lera Marqués, L., Espinosa Pizarro, M. N., Malig Mechasqui, J., and Sorensen, K. (2024). Effects of Bifidobacterium longum 35624 in children and adolescents with irritable bowel syndrome. Nutrients 16, 1967. doi:10.3390/nu16121967
Dietert, R. R. (2021). Microbiome first medicine in health and safety. Biomedicines 9, 1099. doi:10.3390/biomedicines9091099
Douglas, G. M., Maffei, V. J., Zaneveld, J., Yurgel, S. N., Brown, J. R., Taylor, C. M., et al. (2020). PICRUSt2: an improved and customizable approach for metagenome inference. bioRxiv, 672295. doi:10.1038/s41587-020-0548-6
El-Salhy, M., Valeur, J., Hausken, T., and Gunnar Hatlebakk, J. (2021). Changes in fecal short-chain fatty acids following fecal microbiota transplantation in patients with irritable bowel syndrome. Neurogastroenterol. Motil. 33, e13983. doi:10.1111/nmo.13983
EMA/CHMP/ICH/652460/2022 (2022). “ICH M12 Guideline on drug interaction studies - step 5,” in EMA/CHMP/ICH/652460/2022.
Facchin, S., Bertin, L., Bonazzi, E., Lorenzon, G., De Barba, C., Barberio, B., et al. (2024). Short-chain fatty acids and human health: from metabolic pathways to current therapeutic implications. Life (Basel) 14, 559. doi:10.3390/life14050559
Facchin, S., Vitulo, N., Calgaro, M., Buda, A., Romualdi, C., Pohl, D., et al. (2020). Microbiota changes induced by microencapsulated sodium butyrate in patients with inflammatory bowel disease. Neurogastroenterol. Motil. 32, e13914. doi:10.1111/nmo.13914
Gawey, B. J., Mars, R. A., and Kashyap, P. C. (2024). The role of the gut microbiome in disorders of gut-brain interaction. Febs J. 292, 1357–1377. doi:10.1111/febs.17200
Haidacher, S., Horvath, T., Hoch, K., Engevik, M., Faith, I., and Haag, A. (2020). Measuring short-chain fatty acids in microbiome-derived samples by LC-MS/MS.
Hawrelak, J. A., Cattley, T., and Myers, S. P. (2009). Essential oils in the treatment of intestinal dysbiosis: a preliminary in vitro study. Altern. Med. Rev. 14, 380–384.
Hillestad, E. M. R., Van Der Meeren, A., Nagaraja, B. H., Bjørsvik, B. R., Haleem, N., Benitez-Paez, A., et al. (2022). Gut bless you: the microbiota-gut-brain axis in irritable bowel syndrome. World J. Gastroenterol. 28, 412–431. doi:10.3748/wjg.v28.i4.412
Holtmann, G., Haag, S., Adam, B., Funk, P., Wieland, V., and Heydenreich, C. J. (2003). Effects of a fixed combination of peppermint oil and caraway oil on symptoms and quality of life in patients suffering from functional dyspepsia. Phytomedicine 10 (Suppl. 4), 56–57. doi:10.1078/1433-187x-00310
Hou, K., Wu, Z. X., Chen, X. Y., Wang, J. Q., Zhang, D., Xiao, C., et al. (2022). Microbiota in health and diseases. Signal Transduct. Target Ther. 7, 135. doi:10.1038/s41392-022-00974-4
Kamp, K. J., Plantinga, A. M., Cain, K. C., Burr, R. L., Tsai, C. S., Wu, Q., et al. (2024). Gut microbiome and symptoms in females with irritable bowel syndrome: a cross-sectional analysis. Benef. Microbes 15, 259–273. doi:10.1163/18762891-bja00015
Krueger, D., Schäuffele, S., Zeller, F., Demir, I. E., Theisen, J., Michel, K., et al. (2020). Peppermint and caraway oils have muscle inhibitory and pro-secretory activity in the human intestine in vitro. Neurogastroenterol. Motil. 32, e13748. doi:10.1111/nmo.13748
Li, L., Abou-Samra, E., Ning, Z., Zhang, X., Mayne, J., Wang, J., et al. (2019). An in vitro model maintaining taxon-specific functional activities of the gut microbiome. Nat. Commun. 10, 4146. doi:10.1038/s41467-019-12087-8
Liu, H. N., Wu, H., Chen, Y. Z., Chen, Y. J., Shen, X. Z., and Liu, T. T. (2017). Altered molecular signature of intestinal microbiota in irritable bowel syndrome patients compared with healthy controls: a systematic review and meta-analysis. Dig. Liver Dis. 49, 331–337. doi:10.1016/j.dld.2017.01.142
Madisch, A., Frieling, T., Zimmermann, A., Hollenz, M., Labenz, J., Stracke, B., et al. (2023). Menthacarin, a proprietary peppermint oil and caraway oil combination, improves multiple complaints in patients with functional gastrointestinal disorders: a systematic review and meta-analysis. Dig. Dis. 41, 522–532. doi:10.1159/000528553
Madisch, A., Heydenreich, C. J., Wieland, V., Hufnagel, R., and Hotz, J. (1999). Treatment of functional dyspepsia with a fixed peppermint oil and caraway oil combination preparation as compared to cisapride. A multicenter, reference-controlled double-blind equivalence study. Arzneimittelforschung 49, 925–932. doi:10.1055/s-0031-1300528
Madisch, A., Miehlke, S., Labenz, J., Stracke, B., and Köhler, S. (2019). Effectiveness of Menthacarin on symptoms of irritable bowel syndrome. Wien Med. Wochenschr 169, 149–155. doi:10.1007/s10354-018-0635-1
Margolis, K. G., Cryan, J. F., and Mayer, E. A. (2021). The microbiota-gut-brain Axis: from motility to mood. Gastroenterology 160, 1486–1501. doi:10.1053/j.gastro.2020.10.066
May, B., Köhler, S., and Schneider, B. (2000). Efficacy and tolerability of a fixed combination of peppermint oil and caraway oil in patients suffering from functional dyspepsia. Aliment. Pharmacol. Ther. 14, 1671–1677. doi:10.1046/j.1365-2036.2000.00873.x
Mcmurdie, P. J., and Holmes, S. (2013). phyloseq: an R package for reproducible interactive analysis and graphics of microbiome census data. PLoS One 8, e61217. doi:10.1371/journal.pone.0061217
Micklefield, G. H., Greving, I., and May, B. (2000). Effects of peppermint oil and caraway oil on gastroduodenal motility. Phytother. Res. 14, 20–23. doi:10.1002/(sici)1099-1573(200002)14:1<20::aid-ptr542>3.0.co;2-z
Neagu, R., Popovici, V., Ionescu, L. E., Ordeanu, V., Popescu, D. M., Ozon, E. A., et al. (2023). Antibacterial and antibiofilm effects of different samples of five commercially available essential oils. Antibiot. (Basel) 12, 1191. doi:10.3390/antibiotics12071191
Oksanen, J. S. G., and Blanchet, F. G. (2022). Vegan: community ecology package. Available online at: https://cran.r-project.org/package=vegan.
Omoloye, A., Weisenburger, S., Lehner, M. D., and Gronier, B. (2024). Menthacarin treatment attenuates nociception in models of visceral hypersensitivity. Neurogastroenterol. Motil. 36, e14760. doi:10.1111/nmo.14760
Quigley, E. M. M. (2018). The gut-brain Axis and the microbiome: clues to pathophysiology and opportunities for novel management strategies in irritable bowel syndrome (IBS). J. Clin. Med. 7, 6. doi:10.3390/jcm7010006
Quintana Soares Lopes, L., Fortes Guerim, P. H., Maldonado, M. E., Wagner, R., Hadlich Xavier, A. C., Gutknecht Da Silva, J. L., et al. (2024). Chemical composition, cytotoxicity, antimicrobial, antibiofilm, and anti-quorum sensing potential of Mentha Piperita essential oil against the oral pathogen Streptococcus mutans. J. Toxicol. Environ. Health A 87, 824–835. doi:10.1080/15287394.2024.2375731
Rich, G., Shah, A., Koloski, N., Funk, P., Stracke, B., Köhler, S., et al. (2017). A randomized placebo-controlled trial on the effects of Menthacarin, a proprietary peppermint- and caraway-oil-preparation, on symptoms and quality of life in patients with functional dyspepsia. Neurogastroenterol. Motil. 29. doi:10.1111/nmo.13132
Salvucci, E. (2019). The human-microbiome superorganism and its modulation to restore health. Int. J. Food Sci. Nutr. 70, 781–795. doi:10.1080/09637486.2019.1580682
Shanahan, E. R., Kang, S., Staudacher, H., Shah, A., Do, A., Burns, G., et al. (2023). Alterations to the duodenal microbiota are linked to gastric emptying and symptoms in functional dyspepsia. Gut 72, 929–938. doi:10.1136/gutjnl-2021-326158
Sharafi, S. M., Rasooli, I., Owlia, P., Taghizadeh, M., and Astaneh, S. D. (2010). Protective effects of bioactive phytochemicals from Mentha piperita with multiple health potentials. Pharmacogn. Mag. 6, 147–153. doi:10.4103/0973-1296.66926
Srivastava, S., Basak, U., Naghibi, M., Vijayakumar, V., Parihar, R., Patel, J., et al. (2024). A randomized double-blind, placebo-controlled trial to evaluate the safety and efficacy of live Bifidobacterium longum CECT 7347 (ES1) and heat-treated Bifidobacterium longum CECT 7347 (HT-ES1) in participants with diarrhea-predominant irritable bowel syndrome. Gut Microbes 16, 2338322. doi:10.1080/19490976.2024.2338322
Storr, M., and Stracke, B. (2023). Menthacarin for long-term treatment of functional dyspepsia - results from a clinical trial follow-up. Z Gastroenterol. 61, 257–267. doi:10.1055/a-1823-1333
Teige, E. S., Sortvik, U., and Lied, G. A. (2024). A systematic review: fecal bacterial profile in patients with irritable bowel syndrome analyzed with the GA-map dysbiosis test based on the 16S rRNA Gene of bacterial species or groups. Clin. Exp. Gastroenterol. 17, 109–120. doi:10.2147/CEG.S451675
Van Den Abbeele, P., Deyaert, S., Thabuis, C., Perreau, C., Bajic, D., Wintergerst, E., et al. (2023). Bridging preclinical and clinical gut microbiota research using the ex vivo SIFR(®) technology. Front. Microbiol. 14, 1131662. doi:10.3389/fmicb.2023.1131662
Villanueva, R. A. M., and Chen, Z. J. (2019). ggplot2: elegant graphics for data analysis (2nd ed.). Meas. Interdiscip. Res. Perspect. 17, 160–167. doi:10.1080/15366367.2019.1565254
Weersma, R. K., Zhernakova, A., and Fu, J. (2020). Interaction between drugs and the gut microbiome. Gut 69, 1510–1519. doi:10.1136/gutjnl-2019-320204
Wilkinson, E. M., Ilhan, Z. E., and Herbst-Kralovetz, M. M. (2018). Microbiota-drug interactions: impact on metabolism and efficacy of therapeutics. Maturitas 112, 53–63. doi:10.1016/j.maturitas.2018.03.012
Xiong, R.-G., Zhou, D.-D., Wu, S.-X., Huang, S.-Y., Saimaiti, A., Yang, Z.-J., et al. (2022). Health benefits and side effects of short-chain fatty acids. Foods 11, 2863. doi:10.3390/foods11182863
Yadav, M., and Chauhan, N. S. (2022). Microbiome therapeutics: exploring the present scenario and challenges. Gastroenterol. Rep. (Oxf) 10, goab046. doi:10.1093/gastro/goab046
Zhang, Q., Li, G., Zhao, W., Wang, X., He, J., Zhou, L., et al. (2024a). Efficacy of Bifidobacterium animalis subsp. lactis BL-99 in the treatment of functional dyspepsia: a randomized placebo-controlled clinical trial. Nat. Commun. 15, 227. doi:10.1038/s41467-023-44292-x
Zhang, X., Chen, L., Zhang, T., Gabo, R., Wang, Q., Zhong, Z., et al. (2024b). Duodenal microbiota dysbiosis in functional dyspepsia and its potential role of the duodenal microbiota in gut-brain axis interaction: a systematic review. Front. Microbiol. 15, 1409280. doi:10.3389/fmicb.2024.1409280
Zhang, X., Jin, W. W., and Wang, H. G. (2024c). Correlation between the neuroendocrine axis, microbial species, inflammatory response, and gastrointestinal symptoms in irritable bowel syndrome. World J. Gastroenterol. 30, 3985–3995. doi:10.3748/wjg.v30.i35.3985
Zhou, L., Zeng, Y., Zhang, H., and Ma, Y. (2022). The role of gastrointestinal microbiota in functional dyspepsia: a review. Front. Physiol. 13, 910568. doi:10.3389/fphys.2022.910568
Keywords: microbiota, Menthacarin, peppermint, caraway, short-chain fatty acids, functional dyspepsia, irritable bowel syndrome, disorders of gut-brain interaction
Citation: Lehner MD, Ulsemer P and Christochowitz S (2025) Menthacarin, a proprietary combination of peppermint and caraway oil, alters cultured human fecal microbiota composition, resulting in increased SCFA production. Front. Pharmacol. 16:1569052. doi: 10.3389/fphar.2025.1569052
Received: 31 January 2025; Accepted: 24 March 2025;
Published: 30 April 2025.
Edited by:
Patricia Rijo, Lusofona University, PortugalReviewed by:
Christian Agyare, Kwame Nkrumah University of Science and Technology, GhanaNidal Jaradat, An-Najah National University, Palestine
Copyright © 2025 Lehner, Ulsemer and Christochowitz. This is an open-access article distributed under the terms of the Creative Commons Attribution License (CC BY). The use, distribution or reproduction in other forums is permitted, provided the original author(s) and the copyright owner(s) are credited and that the original publication in this journal is cited, in accordance with accepted academic practice. No use, distribution or reproduction is permitted which does not comply with these terms.
*Correspondence: Martin D. Lehner, bWFydGluLmxlaG5lckBzY2h3YWJlLmRl