- Department of Biology, Division of Microbiology, Universität Erlangen-Nürnberg, Erlangen, Germany
Campylobacter jejuni is a typical zoonotic bacterium, colonizing the gut of many bird species as commensal. In humans, C. jejuni is a major foodborne pathogen. Infection of humans causes campylobacteriosis in the small intestine, constituting a main source of bacteria-dependent gastroenteritis cases worldwide. In particular, the ingestion of under-cooked rooster meat, raw milk and contaminated water, as well as cross-contamination of ready-to-eat food after handling raw chicken meat, are responsible for the majority of C. jejuni infections. As a consequence, infected individuals may acquire watery and/or bloody diarrhea associated with abdominal pain, and eventually post-infection illnesses of the neural system and joints, including the Guillain-Barré, Miller Fisher and Reiter syndromes. One therapeutic strategy is to reduce C. jejuni colonization in chicken farms using vaccination, bacteriocins and phage therapy protocols. Prevention approaches during poultry meat processing comprise the compliance to high hygiene standards. Furthermore, substantial progress has been also made in recent years to combat campylobacteriosis using established mouse and in vitro cell model systems. In this regard, specific C. jejuni colonization- and pathogenicity-associated components were considered as favored treatment structures, targeting bacterial movement, host cell interaction, intracellular survival, propagation and spread of the bacteria. This has been complemented by a number of pharmaceutical compounds to reduce C. jejuni-induced epithelial cell damage, inflammation and apoptosis in infected mice. Here we review these novel treatment and prevention as well as “One World - One Health” approaches that aim to diminish the consequences of acute campylobacteriosis and post-infection sequelae in humans.
1 Introduction
Zoonoses represent major infectious diseases, which are transmitted among animals and humans by bacteria, fungi, viruses, parasites and prions (Plowright et al., 2017; Hasso-Agopsowicz et al., 2021). Around 75% of all infectious diseases are originally transferred from animals to humans, often via contaminated food (Sharan et al., 2023). Annual statistics from the World Health Organization document that approximately 550 million cases of illness are foodborne, including 220 million very young children up to 4 years of age (WHO, 2020). Bacteria-dependent zoonotic diseases in the human intestine are dominated by pathogens of two genera, Campylobacter and Salmonella. The European Food Safety Authority (EFSA) and the European Centre for Disease Prevention and Control (2024) reported for 16 investigated European countries that campylobacteriosis and salmonellosis are the first and second most common zoonoses of humans, respectively. Although the overall number of Salmonella cases reported worldwide has somewhat fallen in recent years, the numbers of Campylobacter infections remain constantly high, which causes enormous health problems. For the European Union, the costs of campylobacteriosis were assessed to comprise around 2.4 billion euros annually (EFSA, 2014), while the costs in the U.S. are up to 11.3 billion dollars per year (Hoffmann et al., 2024). Thus, Campylobacter is a typical zoonotic and foodborne pathogen that generates an important economic burden in the societies. Species of the genus Campylobacter can be regularly found in several natural ecosystems, including surface water and in the gut of birds and various agriculturally important animals (Huang et al., 2015; Mulder et al., 2020; Mughini-Gras et al., 2021). In addition, two predominant Campylobacter subspecies (C. jejuni and less frequently C. coli) can colonize chicken and other farm animals as commensal microbes. Thus, bacterial contamination commonly occurs during slaughter, which always leads to the transfer of C. jejuni to meat (Kingsbury et al., 2023). Consequently, the main transmission to humans occurs via the consumption of C. jejuni-positive rooster meat products. In addition, unpasteurized milk (Kenyon et al., 2020) and contaminated water (Gilpin et al., 2020; Jansen et al., 2024) were also reported as sources of the infection.
Upon intake by the fecal-oral route, C. jejuni passes the upper gastrointestinal tract, followed by colonizing the lower small and large intestines (Bereswill et al., 2011a; Kaakoush et al., 2015). The infectious dose is quite low at a few hundred bacteria. C. jejuni then penetrates the intestinal epithelium and triggers acute inflammatory responses by activation of the human innate immune machinery (Lobo de Sá et al., 2021a). Therefore, the course of campylobacteriosis depends on the immune status of the infected person and varies from mild, mildly inflammatory, self-limiting symptoms to severe, vastly inflammatory and blood-containing diarrhoea (Burnham and Hendrixson, 2018; Heimesaat M. M. et al., 2021). These symptoms can be associated with harsh abdominal pain that commonly last for a week or longer. Occasionally, C. jejuni infections can also trigger bacteremia and immunologically-mediated post-infectious diseases with an autoimmune component (Backert et al., 2017). These include neural diseases such as the Guillain-Barré and Miller-Fisher syndromes (GBS and MFS), as well as joint inflammation associated with Reiter’s syndrome (RS) or reactive arthritis, which can occur several weeks after C. jejuni clearance (Diggikar et al., 2024; Hughes, 2024; Leonhard et al., 2024). In addition, C. jejuni infections can also lead to the development of post-infectious irritable bowel syndrome (PI-IBS) and may trigger episodes of chronic inflammatory bowel disease such as ulcerative colitis and Crohn’s disease (Burnham and Hendrixson, 2018; Lobo de Sá et al., 2021a; Heimesaat M. M. et al., 2021). However, the molecular basis of these diseases is still not fully elucidated. Consequently, there is an urgent need for a broader knowledge about the health and disease mechanisms of humans associated with domestic animals and wildlife. To this end, the “One World-One Health” approach is one promising strategy for ensuring better control of zoonotic infectious diseases (One World One Health, 2021; Backert, 2021; Pitt and Gunn, 2024). Furthermore, new drug therapeutics, bacteriophages and vaccines against campylobacteriosis have been under development in recent years. Current strategies also aim to inhibit the inflammatory and cell damage responses by C. jejuni, which may be protective against secondary diseases such as GBS, MFS and various chronic inflammatory processes. In this review article, we discuss the current status of anti-Campylobacter treatments and recent developments in this important field of human health protection.
1.1 Campylobacter jejuni virulence factors and interactions with host cells
The molecular mechanisms of C. jejuni pathogenesis have been investigated using infection of cultured cell lines, various animal model systems and in clinical studies. Campylobacteriosis is characterised by disruption of the intestinal epithelial barrier and inflammation triggered by infiltrating immune cells (model in Figure 1). The invading bacteria move very efficiently with the support of their propelling flagella under the guidance of chemotactic receptors (Korolik, 2019; Cayrou et al., 2021). Afterwards, C. jejuni colonizes the mucus layer and attaches to intestinal epithelial cells that is mediated by specific bacterial adhesion factors (Tegtmeyer et al., 2021). Most notably, the adhesins FlpA and CadF have been characterized thoroughly as binding factors of the extracellular matrix protein fibronectin and associated integrin receptors (Konkel et al., 2020). It was reported that intimate attachment of bacteria to the epithelium is ultimately connected with the capability of C. jejuni to invade these cells (O Cróinín and Backert, 2012; Konkel et al., 2020; Tegtmeyer et al., 2021). Host cell entry by C. jejuni is supported by effector proteins, the Campylobacter invasion antigens (such as CiaB and CiaD), which are secreted via a type-III secretion system (T3SS), also encoded by the flagellum (Young G. M. et al., 1999; Konkel et al., 2004; Song et al., 2004; Negretti et al., 2021). Altogether, it was reported that CadF, FlpA and CiaD can cooperatively stimulate host guanine exchange factors (GEFs such as Vav2, Tiam-1, Dock180 and IQGAP1), which activate members of the small Rho GTPases (Rac1 and Cdc42) to trigger actin polymerization and C. jejuni invasion (Krause-Gruszczynska et al., 2007; Krause-Gruszczynska et al., 2011; Boehm et al., 2011; Larson et al., 2013; Eucker and Konkel, 2012; Negretti et al., 2021). Remarkably, C. jejuni was shown to survive in vacuoles that are formed inside epithelial cells (Watson and Galán, 2008). It appears that C. jejuni-containing vacuoles deviate from the canonical endocytosis route that dampens its transport to lysosomes, while uptake of C. jejuni by macrophages leads to lysosome formation and subsequently killing of the pathogen (Watson and Galán, 2008).
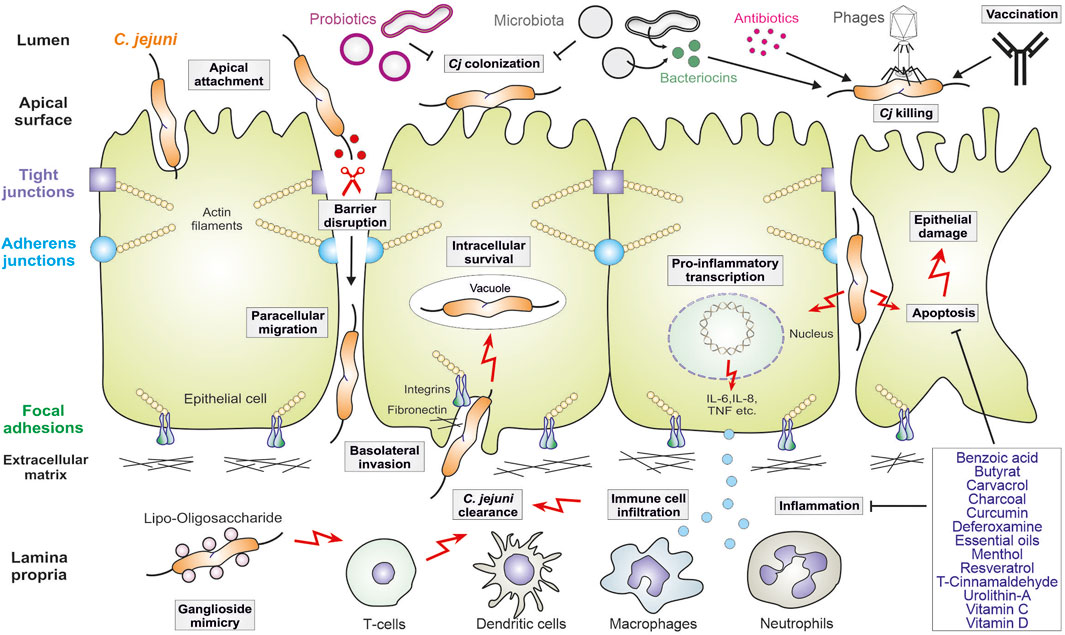
Figure 1. Model for C. jejuni virulence mechanisms in human gut infection and therapeutic intervention approaches. The pathogen adheres to polarized intestinal epithelial cells, followed by opening of tight and adherens junctions and basolateral invasion. C. jejuni also triggers pro-inflammatory signaling leading to the secretion of cytokines that attract the indicated immune cells, which infiltrate to the site of infection. In this scenario, apoptosis is also induced leading to additional epithelial cell damage. The crosstalk of C. jejuni with the intestinal microbiota is indicated. Intervention procedures include the use of probiotics, some of which inhibit C. jejuni colonization, as well as bacteriocins, vaccination and phage application. Some antibiotics can be applied, but antibiotic resistances are common in C. jejuni. Thus, various indicated drug compounds are new promising therapeutic candidates to inhibit inflammation and apoptosis in the infected intestine. For more details, see the text.
During these interactions with host cells, C. jejuni expose their endotoxin lipooligosaccharide (LOS) to the human immune system, which triggers highly potent inflammatory responses (Figure 1). LOS is a glycolipid consisting of an oligosaccharide moiety and a lipid A core that is recognised by toll-like receptor 4 (TLR4), which activates a cascade of kinases and the pro-inflammatory transcription factor NF-κB (Kuijf et al., 2010; Kløve et al., 2020; Heimesaat M. M. et al., 2021). In response to LOS, dendritic cells and macrophages attract neutrophil granulocytes, which produce reactive oxygen species (ROS) and inflammatory mediators that cause epithelial destruction through the induction of apoptotic cell death (Figure 1). These processes can be intensified by the cytolethal distending toxin, called CDT, which is expressed and secreted by numerous, but not all C. jejuni strains (Lara-Tejero and Galán, 2000). Apoptosis results in ulcerative tissue destruction, and the release of pro-inflammatory cytokines and chemokines (including IL-1β, IL-6, IL-8, IL-12, IL-17, IL-22, IL-23, IFN-γ and TNF) intensifies the inflammatory reactions (Heimesaat M. M. et al., 2021; Lobo de Sá et al., 2021a). In addition, activated T cells can produce the cytokines IL-4 and IL-10, which serve as anti-inflammatory factors that counteract severe courses of campylobacteriosis by dampening the immune responses (Heimesaat M. M. et al., 2021). Interestingly, some C. jejuni isolates can even increase the inflammatory host response by sialylating their LOS (Kuijf et al., 2010). This could explain the variability of the LOS associated with different disease outcomes upon infection with individual bacterial strains. For example, sialylation of the oligosaccharide portion of LOS enhances binding to and activation of TLR4, thus driving acute inflammation (Kuijf et al., 2010; Stephenson et al., 2013). Finally, sialylated oligosaccharide chains in C. jejuni LOS also resemble gangliosides in the human brain. Thus, individual LOS structural variants can induce the production of anti-ganglioside antibodies in the human host, which can subsequently lead to axonal destruction associated with GBS and MFS (Diggikar et al., 2024; Hughes, 2024; Leonhard et al., 2024).
1.2 Epithelial barrier disruption by Campylobacter jejuni protease HtrA
The cellular lining in every epithelium is a highly organized polarized structure that protects inner tissues from the potentially harmful impact of intruding microbes (Buckley and St Johnston, 2022). Adjacent epithelial cells are connected at their apical sides by proteins in the tight junction (TJ) followed by adherens junction (AJ) that maintain a barrier function and enable selective paracellular diffusion (Zihni et al., 2016). These pivotal cellular junctions that regulate cell motility and molecular signaling via the associated host cytoskeleton were found to be a key target for many bacterial pathogens (Sharafutdinov et al., 2022a). A major C. jejuni virulence factor targeting the host TJ and AJ proteins is serine protease HtrA, the structurally active form of which are four trimers assembled into a dodecamer (Zarzecka et al., 2020; Tegtmeyer et al., 2021). C. jejuni HtrA, similar to Helicobacter pylori HtrA, possesses a hydrophobic amino acid at position 171, which was found to be crucial for protease trimer stability (Zarzecka et al., 2023). To date, the identified host cell substrates for C. jejuni HtrA are the tight junction proteins occludin and claudin-8, and the adherens junction protein E-cadherin (Linz et al., 2023), the cleavage sites of which were mapped to the extracellular loop 2 of occludin, to the extracellular loop 1 of claudin-8, and to the extracellular domain 5 of E−cadherin (Boehm et al., 2012; Boehm et al., 2015; Harrer et al., 2019; Sharafutdinov et al., 2020). Cleavage leads to barrier disruption that allows the influx of bacterial antigens and toxins, as well as transmigration of C. jejuni to the serosal side (Lobo de Sá et al., 2021a; Heimesaat et al., 2023a). In vitro experiments showed that protein cleavage was time-dependent and positively correlated with bacterial transmigration (Sharafutdinov et al., 2024), but opening of the cell junctions also enabled transmigration of commensals such as the intestinal Escherichia coli or Lactococcus lactis (Sharafutdinov et al., 2022b). Therefore, the HtrA-dependent mechanism can partially explain how microbiota can be translocated across the intestinal epithelia, interact with host immune cells and activate the release of various cytokines upon infection, which can exacerbate disease symptoms. HtrA is released from C. jejuni within shed outer membrane vesicles (OMVs) (Elmi et al., 2016). However, recent results showed that the cell junction-damaging proteolytic activity is mediated via HtrA exposed on the bacterial outer membrane upon direct bacterial-host contact (Sharafutdinov et al., 2024).
1.3 Animal models of Campylobacter jejuni infection
For decades, research on C. jejuni-caused campylobacteriosis has been hampered by the lack of suitable animal models. A variety of different animal species have been tested, and some are used to investigate the interaction of humans with the invading C. jejuni. However, all of the currently used animal models are associated with their individual problems in that they do not fully reproduce all aspects of C. jejuni infection in humans (Mousavi et al., 2021a). In this context, we’d like to quote a truism attributed to George Box: “All models are wrong; some models are useful.” (Box, 1976). Generally, all models represent approximations, that mimic one or several aspects of the pathogen infection and of the associated symptoms observed in humans. Thus, while no model is ever completely accurate, the question is whether it can provide useful information that advances our understanding of the pathogen-host interaction. Several useful animal models for C. jejuni infection are discussed below.
1.3.1 Chickens
Chickens and also various wild birds such as ducks, geese and seagulls are natural hosts and frequently colonized by C. jejuni. Natural colonization usually follows initial infection with very few bacteria. In broiler chicken farms, stable natural colonization usually occurs after the second or third week of life (Sahin et al., 2003; Cawthraw and Newell, 2010; Hermans et al., 2011), but stable colonization has also been demonstrated in 6-day-old chickens (Connerton et al., 2018). Experimental infection is usually performed by direct administration of a suspension of mid-log growing C. jejuni into the crop of chicken chicks. Infection with 105 CFU achieved 100% colonization of the chicken intestine within 48 h (Szott et al., 2020) and resulted in C. jejuni loads as high as 106–108 bacterial cells per g intestinal content (Ridley et al., 2008; Szott et al., 2020). Then, high pathogen loads are maintained for weeks, and the birds spread the pathogen with their feces. Possible transmission to poultry breeding and raising facilities is of high concern due to the potential risk for human health. However, despite the high pathogen loads, the immune system of the birds is largely non-responsive to C. jejuni colonization, and the avian hosts do not develop overt disease symptoms. The observed absence of disease symptoms has been attributed to an overall low inflammatory responses. Even spread of the bacteria to extra-intestinal organs, including spleen and liver, was reported to not trigger clinical signs of inflammation (Young C. R. et al., 1999; Young et al., 2007; Hermans et al., 2012b). However, recent studies on chickens experimentally infected with C. jejuni detected a Th17 inflammatory response that was mediated by IFNγ and IL-17 signaling (Connerton et al., 2018; Chick et al., 2025). The poor avian immune response to C. jejuni is connected to the reduced recognition of bacterial LOS. While the C. jejuni LOS is a potent activator of TLR4 in humans (Kuijf et al., 2010) as discussed above, it fails to stimulate the avian TLR4 counterpart. Therefore, in contrast to strong acute inflammatory responses seen in humans, the inability of the avian immune system to detect C. jejuni causes a benign colonization, which makes C. jejuni a commensal in the avian host (Young et al., 2007).
Which genes are necessary for C. jejuni colonization? Transposon mutagenesis identified 22 C. jejuni genes involved in colonization of the gastrointestinal tract of chickens, several of which were associated with chemotaxis and motility (Hendrixson and DiRita, 2004). The flagellar T3SS was reported to affect pathogenesis in humans via secretion of virulence factors such as CiaA. The flagellar regulatory system also controls expression of FlaA and other flagellar filament proteins, including the so-called flagellar co-expressed determinants (Feds) that were shown to be important for commensal colonization of chickens (Barrero-Tobon and Hendrixson, 2012). Subsequent research revealed that the abundance of short-chain fatty acids (SCFAs) produced by the resident microbiota as well as organic acids modulate the expression of genes involved in C. jejuni commensalism in the chicken host (Luethy et al., 2017). Interestingly, C. jejuni can discriminate between different intestinal regions based on the concentration of SCFAs and lactate, which allows C. jejuni to colonize its preferred location in the intestinal tract. Here, the C. jejuni two-component system (TCS) BumSR senses the concentration of the microbiota-generated intestinal metabolite butyrate by its predicted cytoplasmic sensor kinase BumS and regulates transcription of target genes via its transcription activator BumR. Thus, the BumSR TCS is important for the commensal colonization of the avian host (Goodman et al., 2020).
1.3.2 Mammalian hosts
The chicken model is suitable for unraveling and investigating the bacterial factors that are necessary to establish natural colonization of the avian host or to test the ability of vaccines or antimicrobial compounds to prevent colonization with C. jejuni. However, the chicken model cannot be used for the analysis of the complex interactions during human C. jejuni-caused campylobacteriosis. Other animal models using newborn pigs, weanling ferrets, gnotobiotic canine pups, and primates have several shortcomings as reviewed previously (Fox et al., 1987; Vitovec et al., 1989; Babakhani et al., 1993). Apart from ethical considerations concerning the use of primates, those animal models are associated with high costs and difficulties that require special equipment and trained staff because of handling issues. Due to the limited availability of genetically standardized animals those models do not allow the use of a large number of animals necessary to investigate the pathogen-host interaction in detail (Fox et al., 1987; Vitovec et al., 1989; Babakhani et al., 1993; Backert, 2021). Below we discuss two established mouse models for C. jejuni infection that have recently yielded many new and important results.
1.3.3 Mice and the gut microbiota
Mice are highly convenient for the study of bacterial pathogenicity, and they are indeed used in infection models of many different pathogens. The use of mice has several advantages, including ease of handling due to their size, low-cost housing, and their high fertility. Reaching the reproductive age within 2 months and a short gestation period of only 3 weeks allow for three to four generations a year. In addition, mouse lineages are highly inbred so that all offspring of the same sex can be considered genetically identical, which greatly increases reproducibility in animal experiments over the time course of infections and between laboratories. Moreover, the availability of many different genetically modified mouse lineages with altered immune components allows to investigate the roles of each in control and/or clearance of the bacterial infection and disease.
Unfortunately, the mouse model of C. jejuni infection is associated with a number of problems. A major drawback is that mice are generally about 500 times more resistant to TLR4 ligands such as LPS and LOS than humans (Vaure and Liu, 2014). Thus, mouse TLR4 is only poorly stimulated by C. jejuni LOS, and mice do not react with a robust pro-inflammatory immune response to C. jejuni infection (Stahl et al., 2014). In addition, C. jejuni only poorly, if at all, colonizes conventional wild-type mice, which led to the term “colonization resistance” in regard to the inability to establish efficient colonization (Jesudason et al., 1989; Dorrell and Wren, 2007; Bereswill et al., 2011b; Masanta et al., 2013). Due to these major complications, work with the mouse model has been neglected for years. Interestingly, in contrast to wild-type mice, stable C. jejuni colonization was successfully achieved in germ-free adult mice (Yrios and Balish, 1986a; 1986b; Chang and Miller, 2006). However, germfree mice are known to have an hampered innate immune system (Haag and Siegmund, 2015), which raises the possibility that either the immune answer or the intestinal microbiota or both may be involved in preventing stable C. jejuni infection in mice. A major breakthrough in mouse model-based work on campylobacteriosis was achieved after the observation that pre-treatment of mice by oral administration of broad-spectrum antibiotics, which essentially killed the entire intestinal microbiota, enabled stable experimental colonization with C. jejuni (Bereswill et al., 2011a), suggesting that the microbiota prevented C. jejuni from colonizing mice. Additional evidence came from experiments in which those secondary abiotic mice were orally re-inoculated either with mouse intestinal microbiota or with human-derived intestinal microbiota. These animals, which then permanently carried the human or murine microbiota, were subsequently infected with C. jejuni and assessed for bacterial loads of the pathogen. In contrast to secondary abiotic mice and mice carrying human intestinal microbiota, in which C. jejuni infection was maintained for 6 weeks, mice re-inoculated with mouse intestinal microbiota cleared C. jejuni within 2 days. Thus, the re-introduction of the mouse microbiota re-established the previous colonization resistance, clearly pointing to the microbiota as the cause of the colonization resistance (Bereswill et al., 2011b).
While the possibility to infect those secondary abiotic mice with C. jejuni after oral inoculation represented a major step as it overcame the colonization resistance, the problem remained that the infected mice did not display the symptoms known from human campylobacteriosis (Bereswill et al., 2011b; Heimesaat and Bereswill, 2015). Here, the availability of multiple different mouse lineages with altered immune components came to the rescue in the form of mice deficient in IL-10, because IL-10−/− mice developed intestinal immunopathology after oral infection with C. jejuni (Mansfield et al., 2007; Mansfield et al., 2008; Bell et al., 2009). Microbiota-depleted IL-10−/− mice could be stably infected with C. jejuni and developed an acute enterocolitis with bloody inflammatory diarrhea within 1 week post infection and showed symptoms typical for severe campylobacteriosis in immunocompromised human patients (Haag et al., 2012c; Heimesaat et al., 2014a; Heimesaat et al., 2017; Mousavi et al., 2020a). Likewise, C. jejuni infection of secondary abiotic mice lacking single Ig IL-1 receptor-related molecule (SIGIRR) reproduced a typical intestinal pathology. The infected Sigirr−/− mice showed strongly elevated expression of pro-inflammatory cytokines such as TNF, IFN-γ, and IL-17 compared to wild-type mice (Stahl et al., 2014). However, the overall clinical disease symptoms were moderate, and the C. jejuni infection was self-limiting (Stahl et al., 2014; Stahl and Vallance, 2015).
The infant mouse model that utilizes conventional 3-weeks-old mice carrying their natural intestinal microbiota avoids some of the above problems and dependencies on altered immune components. When only 3 weeks old, which is immediately after weaning, young mice were found to be more susceptible to C. jejuni infection than adult mice (Haag et al., 2012b). Young mice developed self-limiting acute enteritis within 1 week post infection, which was accompanied by inflammation of the colon mucosa and diarrhea with bloody discharge (Haag et al., 2012b; Heimesaat et al., 2014b; Heimesaat et al., 2016), and C. jejuni was also detected in extra-intestinal organs. Thus, the infant mouse model of C. jejuni infection mimics key features of human campylobacteriosis. The murine gut microbiota are known to change after weaning and to stabilize in their composition in the weeks after (Schloss et al., 2012), and young mice were found to carry more E. coli and less Lactobacillus in their intestines compared to adult mice (Haag et al., 2012b; Haag et al., 2012a). Subsequent research showed that addition of E. coli to the intestinal microbiota of adult mice increased C. jejuni loads during infection, effectively mitigating the colonization resistance against C. jejuni and suggesting that the proportion of enterobacteria may affect the susceptibility (Haag et al., 2012c). Along those lines, it is interesting to speculate that the relative amount of enterobacteria in the large intestine may also affect the severity of campylobacteriosis in humans, from (almost) asymptomatic carriage via mild diarrhea to severe bloody diarrhea with abdominal cramps.
In general, the development and advancement of novel and modified mouse models of C. jejuni infection in the last 2 decades greatly contributed to our understanding of C. jejuni interactions with the human host and the associated pathogenicity. However, despite the exciting progress that has been made, the mouse models of C. jejuni infection are still far from ideal. In order to enable C. jejuni infection, infant mice are used or adult mice are subjected to a 10-week course of broad-spectrum antibiotic treatment administered via the drinking water (Bereswill et al., 2011a). Those secondary abiotic mice are then orally inoculated twice with 109 CFU of mid-log-phase growing bacteria, usually on two consecutive days, to establish stable infection (Schmidt et al., 2019a; Schmidt et al., 2019b). In contrast, infection of the human host only requires oral uptake of a very limited number of bacteria, likely only a few hundred, to establish initial colonization of the intestinal mucus layer (Heimesaat M. M. et al., 2020).
1.4 Therapeutics and prevention strategies
The most common transmission route of C. jejuni to humans occurs through contaminated food, with the poultry being a major source followed by cattle (Ahmed and Gulhan, 2022). Complex biocontrol of the food processing at all stages, from harvesting and slaughter to the storage and transport to consumers, might help to reduce the burdens of C. jejuni contamination (Alter and Reich, 2021; Taha-Abdelaziz et al., 2023). Additionally, Campylobacter emission into environment that is responsible for the bacterial persistence, can be significantly reduced by simultaneous application of various intervention strategies (Szott and Friese, 2021). C. jejuni infections usually do not require medical treatment, however, in severe cases antibiotic treatment is recommended (Zainol et al., 2024). As a result of improper antibiotic use both in humans and animals, the global growth of antibiotic resistance imposes high risk of common antibiotics to become ineffective against Campylobacter infections (Barata et al., 2024). Substantial research has been performed to identify alternative prevention and treatment strategies, as summarized in Table 1 and discussed below. Most of the therapeutic compounds that we summarized below are natural substances produced by plants or bacteria. The majority of these compounds was only recently tested in their ability to inhibit growth of C. jejuni, mostly in mouse experiments, some in cell lines in vitro. As the majority of those papers were published very recently, there are almost no data yet concerning their application in humans. Therefore, we listed those potential therapeutic compounds as promising.
1.4.1 Antibiotics
While in most infected individuals the C. jejuni infection is self-limiting, usually no specific treatment is needed for mild and even moderate symptoms, but is recommended, however, for severe manifestations (Zainol et al., 2024). The European Centre for Disease Prevention and Control (ECDC) reported 137,107 confirmed cases of campylobacteriosis in 30 European countries in 2022. Of the 44,876 cases with a recorded hospitalization status (hospitalized or not), 10,551 (23.5%) were treated at hospitals (European Centre for Disease Prevention and Control, 2024). Likewise, in 2023, 148,181 campylobacteriosis cases were reported in the European Union with 12,194 cases (23.9%) requiring hospitalizations (European Food Safety Authority European Centre for Disease Prevention and Control, 2024). Based on a large combined case-control and source attribution study, a substantial proportion (31%) of human Campylobacter infections were treated with antibiotics (Rosner et al., 2017). However, the use of antibiotics may be difficult in controlling C. jejuni-caused diarrhea, because C. jejuni developed resistance against several antibiotics that have been widely used in veterinary medicine since the 1990s, including tetracycline (Wozniak-Biel et al., 2017), fluoroquinolones (Nachamkin et al., 2002), macrolides (Gibreel et al., 2005), and others (Veronese and Dodi, 2024). Inappropriate use of antibiotics promotes the growing spread of antibiotic resistance, which makes available treatments increasingly ineffective (Barata et al., 2024). As such, selection pressure needs to be removed from the source, so that antibiotic resistance does not confer a competitive advantage to resistant isolates. However, as a recent study from the Netherlands suggests, “reducing antimicrobial use in livestock alone may not be sufficient to reduce antimicrobial resistance among human Campylobacter infections” (Deng et al., 2024). An alarmingly increasing number of isolates already exhibits multidrug resistance, including C. jejuni from Portugal (Santos-Ferreira et al., 2022) and Brazil (Dias et al., 2021), but most dramatically in Asia, where the proportion of multidrug resistant isolates is close to 100% (Shin et al., 2012; Thomrongsuwannakij et al., 2017). Azithromycin is usually the first-line treatment for C. jejuni infections, particularly in cases where symptoms are severe or the patient is immunocompromised. Depending on the geographic area and guidelines, antibiotics of second choice might include fluoroquinolones, rifaximin and trimethoprim-sulfamethoxazole (Veronese and Dodi, 2024). However, due to high (33.1%) to extremely high (100%) resistance levels in Europe, fluoroquinolones cannot be recommended for the treatment of Campylobacter infections in humans (European Food Safety Authority & European Centre for Disease Prevention and Control, 2024). Interestingly, experimental depletion of the microbiome in mice by the combination of cefoperazone/sulbactam, as discussed above, resulted in long-term colonization of C. jejuni in the intestine (Chen et al., 2024). Another study showed that 8-week long pretreatment by either combination of ampicillin/sulbactam or the antibiotic cocktail (ampicillin, ciprofloxacin, imipenem, metronidazole, and vancomycin) effectively depleted the microbiota in IL-10−/− mice enabling sufficient C. jejuni colonization (Heimesaat et al., 2022). These data suggest, that in case of resistance antibiotics therapy may backfire and exacerbate C. jejuni colonization. Also, the campylobacteriosis-specific nature of disease associated with dehydration of the organism complicates the control of antibiotic concentrations at the infection site (Heimesaat M. M. et al., 2021). Therefore, alternative treatment strategies especially in resistant C. jejuni infections are urgently needed.
1.4.2 Benzoic acid
Naturally present in plants and animals, benzoic acid and its derivatives are known as food supplements for preservation and/or flavoring purposes. For example, salts of benzoic acid (especially sodium benzoate) can exhibit antimicrobial properties via bacterial membrane disruption (del Olmo et al., 2017). Supplementing broiler chickens with benzoic acid led to a decrease in gut pH, which might be beneficial for a healthy microflora, and upregulated gene expression of the junction proteins E-cadherin and claudin-1, which contributes to restoring the integrity of the epithelial barrier (Yu et al., 2024). Antibacterial susceptibility testing on C. jejuni isolates in vitro showed antimicrobial activity of benzoic acid alone and exhibited synergistic effects in combination with other organic acids, e.g., sorbic acid, propionic acid or acetic acid (Peh et al., 2020). However, in vivo infection of IL-10−/− mice showed no antimicrobial activity of benzoic acid alone, and only in combination with butyric, caprylic and sorbic acids C. jejuni loads were reduced in the duodenum, but not in the stomach, ileum or colon (Du et al., 2023). Interestingly, treatment with these organic acids improved overall clinical outcome with reduced diarrheal symptoms in C. jejuni infected IL-10−/− mice, reduced inflammatory immune responses and dampened secretion of pro-inflammatory cytokines in the colon and mesenteric lymph nodes and the serum (Du et al., 2023). Treatment with benzoic acid alone, similarly to the combination of organic acids, reduced the diarrheal symptoms, alleviated apoptotic cell responses and reduced pro-inflammatory immune reactions (Du et al., 2024). Therefore, benzoic acid appears a promising non-antibiotic immune-modulatory treatment against C. jejuni infections.
1.4.3 Butyrate
Butyrate is a salt of butyric acid, which is physiologically produced by intestinal bacteria, is important for the function of immune system, energy metabolism and even the nervous system (Du et al., 2021). In vitro studies revealed antimicrobial activity of butyric acid against Gram-negative pathogens Staphylococcus pseudintermedius and Acinetobacter baumannii through affecting the bacterial membrane integrity (Kennedy et al., 2019). Treatment of C. jejuni-infected IL-10−/− mice with butyrate led to reduced diarrheal severity and frequency (Du et al., 2022). Furthermore, butyrate treatment of infected mice significantly reduced intestinal pro-inflammatory immune responses as shown by lower numbers of apoptotic cells and neutrophils, less distinct TNF secretion in mesenteric lymph nodes and lower IL-6 and MCP-1 concentrations in the ileum (Du et al., 2022). Therefore, the above studies indicate that butyrate is a promising molecule for the development of new strategies to combat acute campylobacteriosis.
1.4.4 Activated charcoal
Activated charcoal is an adsorbent with exceptionally few side effects and widely used in management of diarrhea to clear the intoxicating particles from the body (Senderovich and Vierhout, 2018). Treatment of C. jejuni-infected IL-10−/− mice with activated charcoal resulted in lower intestinal pathogen loads as well as alleviated clinical signs of diarrhea and diminished pro-inflammatory immune responses in the intestinal tract of mice (Bereswill et al., 2021b). When activated charcoal was used for the treatment of the C. jejuni infected human gut microbiota associated (hma) IL-10−/− mice, the campylobacteriosis symptoms and pro-inflammatory signaling were significantly reduced (Heimesaat et al., 2024d). Interestingly, activated charcoal treatment did not affect the fecal microbiota composition that was shifted towards higher enterobacterial numbers and lower loads of obligate anaerobic species in hma IL-10−/− mice (Heimesaat et al., 2024d). Therefore, the group proposed that activated charcoal might be a promising antibiotics-independent option for the treatment of human campylobacteriosis.
1.4.5 Deferoxamine
Deferoxamine is an iron chelator and siderophore that is naturally present in the bacterium Streptomyces pilosus, and as a medication used to treat patients with the acute or chronic iron overload (Rahimzadeh et al., 2023). In turn, iron acquisition plays an important role not only for Campylobacter survival, but also for effective host colonization (Miller et al., 2009). Therefore, limiting free iron using additional iron chelators such as deferoxamine might be an effective strategy against bacterial colonization. Deferoxamine prophylactically given to microbiota-depleted IL-10−/− mice 7 days prior C. jejuni infection did not affect bacterial loads in the mice intestine, but significantly improved the clinical outcome of infected mice at day six post-infection (Bereswill et al., 2023). The pre-treatment with deferoxamine resulted in less epithelial cell apoptosis and reduced intestinal and systemic immune responses in IL-10−/− mice (Bereswill et al., 2023). Furthermore, application of deferoxamine in C. jejuni-infected hma IL-10−/− mice at 2 days post infection, diminished recruitment of colonic neutrophils and T lymphocytes and inhibited ex-vivo IFN-γ secretion in the colon, the kidneys and in the serum (Mousavi et al., 2024). Interestingly, non-covalent fusion of deferoxamine with chitosan and iron (III) sharply potentiated its anti-bacterial activity against Staphylococcus aureus and E. coli as shown in vitro and in vivo using rat peritonitis infection model (Khubiev et al., 2023). Therefore, it would be interesting to test such promising deferoxamine derivatives in campylobacteriosis animal models, which might offer a new non-antibiotic therapeutic strategy.
1.4.6 Phytochemicals
Phytochemicals, which comprise plant-derived antimicrobial agents including extracts, essential oils and purified compounds, have been extensively studied for their antimicrobial potential (Alvarez-Martínez et al., 2021; Balta et al., 2021c). They have been reported to exhibit different antimicrobial mechanisms, most of which are based on disruption of the bacterial membrane. Because the Campylobacter infection site is normally limited to the gastrointestinal tract, the application of natural plans-based treatments, that could be orally administered, represents a promising treatment strategy (Alvarez-Martínez et al., 2021).
1.4.6.1 Carvacrol
Carvacrol is a phenolic monoterpene naturally found in essential oils obtained from various plants such as oregano, bergamot and thyme, and which shows promising antimicrobial and antioxidant properties (Maczka et al., 2023). In vitro treatment of Caco-2 cells with carvacrol significantly reduced attachment, invasion and translocation of C. jejuni (Upadhyay et al., 2017). Four-day carvacrol diet in secondary abiotic IL-10−/− mice prior C. jejuni infection reduced pathogen loads in their intestines at 6 days post-infection, and furthermore significantly alleviated acute enterocolitis symptoms (Mousavi et al., 2020b). In addition, carvacrol-fed mice showed decreased intestinal apoptosis rate and diminished pro-inflammatory signaling as evidenced by lower IFN-γ, TNF, MCP-1 and IL-6 concentrations in the intestine (Mousavi et al., 2020b). Importantly, carvacrol treatment restricted C. jejuni translocation from the intestine to extra-intestinal compartments of mice, suggesting positive effect of carvacrol on the intestinal barrier. Carvacrol in combination with butyrate, ellagic acid and 20-fucosyl-lactose showed even more pronounced anti-inflammatory effects in C. jejuni infected mice by diminishing IFN-γ, TNF, and IL-6 secretion in colon, liver, kidneys, lungs, and serum samples (Mousavi et al., 2023a). In order to simulate the human gut microbiota, secondary abiotic IL-10−/− mice were subjected to oral transplantation of fecal microbiota from human donors (Foote et al., 2023). The hma IL-10−/− mice were further infected with C. jejuni and treated with carvacrol, which eventually dampened intestinal and extra-intestinal inflammatory responses during acute campylobacteriosis (Foote et al., 2023). Interestingly, prophylactical pretreatment of hma IL-10−/− mice with carvacrol a week before C. jejuni infection significantly mitigated acute campylobacteriosis without affecting the fecal gut microbiota composition (Heimesaat et al., 2024a). Treatment of hma IL-10−/− mice after C. jejuni infection with carvacrol alone or in combination with deferoxamine, deoxycholic acid and 2-fucosyl-lactose showed comparable results with diminished immune response in the colon, kidneys and serum, establishing carvacrol as effective anti-C. jejuni compound (Mousavi et al., 2024). In chickens, a diet supplemented with carvacrol resulted in changes in the gut microbiome, with an increasing relative abundance of Lactobacillus and reduction of Campylobacter spp. (Kelly et al., 2017).
1.4.6.2 Trans-cinnamaldehyde
Trans-cinnamaldehyde is an aromatic aldehyde naturally found in the bark of cinnamon tree and has demonstrated significant antimicrobial activity via disruption of the bacterial cell membrane (Jimenez et al., 2024). In vitro studies showed that trans-cinnamaldehyde can effectively reduce C. jejuni attachment, invasion and translocation in Caco-2 cells (Upadhyay et al., 2017). Prophylactic treatment of IL-10−/− mice with trans-cinnamaldehyde starting 7 days prior C. jejuni infection did not affect the bacterial colonization but alleviated colonic epithelial cell apoptosis and resulted in less pronounced pro-inflammatory responses (Heimesaat et al., 2023b). The immune-modulatory effects upon trans-cinnamaldehyde treatment were not restricted to the intestinal tract of IL-10−/− mice and were also observed in the liver and kidneys (Heimesaat et al., 2023b). The above studies identify trans-cinnamaldehyde as a promising prophylactic option for future studies to combat C. jejuni infections.
1.4.6.3 Curcumin
Curcumin is a polyphenol produced by plants of the Curcuma longa species and exhibits a wide variety of biological activities including antimicrobial, antioxidant, anti-inflammatory, anticancer and many others (Sharifi-Rad et al., 2020). Food supplementation of secondary abiotic IL-10−/− mice with curcumin 1 week prior C. jejuni infection resulted in less pronounced histopathological changes and epithelial cell apoptosis in the colon (Heimesaat et al., 2024c). Furthermore, curcumin pretreatment reduced pro-inflammatory innate and adaptive immune responses in C. jejuni-infected mice (Heimesaat et al., 2024c). Given its food safety and effectiveness, curcumin warrants further studies for potential application of this polyphenolic compound for the prevention of campylobacteriosis and related post-infectious complications.
1.4.6.4 Menthol
Menthol is a cyclic monoterpene found within essential oils obtained from plants of the Mentha genus, with pronounced anti-bacterial and anti-inflammatory activities (Kamatou et al., 2013). Treatment of C. jejuni-infected microbiota-depleted IL-10−/− mice with menthol from day two until day six post-infection did not affect the pathogen loads, but reduced the number of colonic T cells (Bandick et al., 2023). Furthermore, application of menthol in combination with the plant-derived extracts used in herbal medicine, alleviated C. jejuni-induced diarrheal symptoms in infected mice (Bandick et al., 2023). Interestingly, when synthetic menthol was given to hma IL-10−/− mice prophylactically starting 1 week before C. jejuni infection, bacterial loads and immune cell recruitment in the colon were reduced with minimal effects on the human fecal gut microbiota (Heimesaat et al., 2024b). The discussed preclinical placebo-controlled intervention studies indicate on a promising potential of the menthol application against acute campylobacteriosis as an alternative non-antibiotic treatment strategy.
1.4.6.5 Resveratrol
Resveratrol is a polyphenolic molecule naturally found in grapes and therefore present in wines, and in some other plants. Resveratrol plays a protective role against various microbial pathogens (Keylor et al., 2015). Resveratrol treatment of C. jejuni-infected secondary abiotic IL-10−/− mice improved the clinical conditions, reduced colonic epithelial apoptosis and dampened innate and adaptive immune cell responses (Heimesaat M. et al., 2020). Importantly, resveratrol treatment could effectively rescue colonic epithelial barrier function in C. jejuni infected mice as shown by the transmural electrical resistance assay (Heimesaat M. et al., 2020). Mechanistically, C. jejuni-infection disrupted the epithelial barrier in human colonic HT-29/B6 cell monolayers via mis-localization of the TJ proteins occludin and claudin-5, which was restored after resveratrol treatment (Lobo de Sá et al., 2021b). Prophylactic administration of resveratrol to hma IL-10−/− mice starting 1 week before C. jejuni infection diminished the pathogen-induced colonic T and B cell responses and reduced intestinal secretion of pro-inflammatory nitric oxide, IL-6, TNF, and IFN-γ, which altogether alleviated clinical signs of acute campylobacteriosis (Heimesaat et al., 2023c).
1.4.6.6 Essential oils
Essential oils are secondary metabolites of plants that can normally be extracted from various parts of the plant via hydrodistillation (Alvarez-Martínez et al., 2021). Various essential oils including those of cardamom, clove, garlic, cumin, lemon and coriander were studied for their potential use in the treatment of campylobacteriosis. Thus, treatment of microbiota-depleted and C. jejuni-infected IL-10−/− mice with essential oils of either cardamom, clove, garlic or cumin alleviated clinical signs of acute campylobacteriosis in all cases (Bereswill et al., 2021a; Heimesaat et al., 2021b; Heimesaat et al., 2021c; Mousavi et al., 2021b). Additionally, treatment with essential oils dampened pro-inflammatory signaling in intestinal and extra-intestinal sites of infected IL-10−/− mice. Interestingly, infected IL-10−/− mice treated with essential oils of either cardamom, clove, garlic or cumin, resulted in significantly reduced bacterial loads in the ileum (Bereswill et al., 2021a; Heimesaat et al., 2021b; Heimesaat et al., 2021c; Mousavi et al., 2021b). Prophylactic supplementation of abiotic IL-10−/− mice with essential oils of either lemon or coriander improved the clinical outcome of acute campylobacteriosis that was associated with reduced secretion of distinct pro-inflammatory molecules in mesenteric lymph nodes, kidneys and serum of infected mice (Mousavi et al., 2023b).
1.4.7 Probiotics
Probiotics can play an important role in anti-Campylobacter strategies by their application both in farm animals by reducing bacterial loads in poultry and in humans by attenuating the infection severity (Balta et al., 2022). Probiotic bacteria, such as Lactobacillus and Bifidobacterium, show a promising potential in inhibiting C. jejuni colonization and infection via different mechanisms including competition for attachment sites, co-aggregation with the pathogen and production of antimicrobial compounds, such as hydrogen peroxide and/or lactic acid (Nishiyama et al., 2014). Administration of a mixture of eight different probiotic strains of Streptococcus, Bifidobacterium and Lactobacillus, known as VSL#3, to secondary abiotic, C. jejuni-infected mice, reduced pro-inflammatory (IL-6 and MCP-1) and induced anti-inflammatory (IL-10) immune responses (Ekmekciu et al., 2017). Treatment of microbiota-depleted and C. jejuni-infected IL-10−/− mice with a commercially available probiotic Aviguard® improved clinical outcome and resulted in less distinct pro-inflammatory immune responses in the intestinal and extra-intestinal sites of infected mice (Heimesaat et al., 2021d). Reassociation of secondary abiotic mice with commensal murine Lactobacillus johnsonii as a prophylactic regimen 14 days before C. jejuni infection ameliorated pathogen-induced colonic apoptosis (Bereswill et al., 2017). When the probiotic was given as a therapeutic regimen 7 days after C. jejuni-infection, the numbers of colonic B lymphocyte decreased, which was not the case with the prophylactic regimen. Both prophylactic and therapeutic L. johnsonii administration reduced ileal TNF and nitric oxide, and serum IL-6 levels, resulting in ameliorated pro-inflammatory cytokine responses in C. jejuni-infected mice (Bereswill et al., 2017). Interestingly, recent studies using Caenorhabditis elegans for infection proposed a new reliable model for a large scale screening for lactic acid bacteria antagonistic to C. jejuni, which can easily be translated to mouse or chicken models (Jin et al., 2021).
1.4.8 Urolithin-A
Urolithins are metabolites with bioactive properties processed by gut microbiota from ellagitannins found in fruits, berries and nuts (Tow et al., 2022). Urolithin-A treatment of C. jejuni-infected microbiota-depleted IL-10−/− mice improved clinical outcome of infected mice and slightly reduced the pathogen loads in their ileum, but not in the colon (Mousavi et al., 2021c). Additionally, urolithin-A treatment reduced intestinal and extra-intestinal pro-inflammatory immune responses in C. jejuni-infected IL-10−/− mice, which was associated with less pronounced macroscopic and microscopic inflammatory sequelae (Mousavi et al., 2021c). The above discussed preclinical murine intervention study proposes urolithin-A as a promising treatment option for alleviating acute human campylobacteriosis.
1.4.9 Vitamin C
Ascorbic acid, also known as vitamin C, is an essential micronutrient that cannot be synthesized by humans. Due to its antioxidant and immune-modulatory properties, vitamin C has a beneficial influence both on innate and adaptive immune responses against pathogens (Carr and Maggini, 2017). Several in vitro studies demonstrated inhibitory and even bactericidal effects of vitamin C on C. jejuni growth and viability (Mousavi et al., 2019a). Vitamin C administration to the secondary abiotic IL-10−/− mice starting 4 days prior C. jejuni infection alleviated clinical symptoms of campylobacteriosis and reduced pathogen-induced apoptosis rates in colonic epithelia (Mousavi et al., 2020c). Additionally, vitamin C administration dampened pro-inflammatory immune cell responses in infected mice, as shown by lower numbers of macrophages/monocytes as well as T and B cells in their intestines (Mousavi et al., 2020c). Therefore, the preclinical intervention study using secondary abiotic IL-10−/− mice as a C. jejuni infection model provides a new promising approach for prophylaxis and treatment of acute campylobacteriosis by vitamin C administration.
1.4.10 Vitamin D
Vitamin D, which is in fact a secosteroid hormone, is known for the regulation of calcium homeostasis and gene expression associated with immunological responses against bacterial, fungal and viral pathogens (Kroner et al., 2015). RNA-Seq analysis of colonic samples obtained from C. jejuni-infected patients indicated that the vitamin D biosynthesis pathway is inhibited, as a putative target for the pathogen (Bücker et al., 2018). Cytotoxicity assay conducted on the intestinal HT-29/B6 cells showed that vitamin D supplementation could abolish the cytotoxic effect induced by the pathogen (Bücker et al., 2018). Treatment of secondary abiotic IL-10−/− mice with synthetic 25-OH-cholecalciferol starting 4 days before infection resulted in less frequent C. jejuni-induced diarrhea and counteracted intestinal cell damage (Mousavi et al., 2019b). Importantly, 25-OH-cholecalciferol treatment reduced the numbers of colonic innate and adaptive immune cells along with decreased intestinal concentrations of pro-inflammatory mediators including IL-6, MCP1, and IFN-γ (Mousavi et al., 2019b). Mechanistically, it seems that C. jejuni targets the vitamin D receptor (VDR) pathway via VDR/retinoid X receptor (RXR) interaction, as was shown by immunofluorescence microscopy analysis (Lobo de Sá et al., 2021c). Interestingly, C. jejuni-induced paracellular leakiness due to mislocalization of the TJ proteins claudin-4 and occludin to cytoplasm could be inhibited by vitamin D treatment, which restored epithelial barrier integrity (Lobo de Sá et al., 2021c). Therefore, in vitro studies along with preclinical intervention studies provide evidence that vitamin D might constitute an effective approach to treat acute campylobacteriosis and prevent the following sequelae.
1.4.11 Amalgamation of antimicrobials
Another approach assessed mixtures of several compounds in their effect on C. jejuni. For example, a novel phenolic antimicrobial named Auranta 3001 that mainly consisted of citrus extract, oregano extract, and grape seed extract, was found to greatly inhibit adhesion to and invasion of human epithelial cells by C. jejuni and C. coli, to reduce motility of the bacterial pathogens, and to decrease expression of genes known to be important for the function of the type VI secretion system (Sima et al., 2018). Importantly, this preparation reduced C. jejuni and C. coli loads in the cecum of infected chickens from around 8-log10 in the control groups to 2-log10 in the groups treated with Auranta 3001 after oral inoculation (Sima et al., 2018). In addition, the antimicrobial greatly reduced adherence of C. coli to chicken skin during slaughter by 3-log10 CFU (Balta et al., 2021a). In another study the authors combined maltodextrin, citric acid, sodium citrate, malic acid, citrus extract and olive extract and assessed the effect of the antimicrobial mixture on MDCK cells infected either with C. jejuni, Salmonella enterica or Clostridium perfringens (Balta et al., 2021b). This concoction significantly reduced the virulence of all three pathogens against the cell line. Specifically, as shown by measuring the transepithelial resistance (TEER), the antimicrobial restored the integrity of tight junctions, in part through increased expression of TJ proteins zonula occludens 1 (ZO-1) and occludin (Balta et al., 2021b).
1.5 Bacteriophages
Bacteriophages (phages) are viruses that can selectively infect specific bacterial species or strains, and therefore attract an increasing interest as they can target pathogenic bacteria without affecting beneficial microbiota and without exhibiting cytotoxicity to the host compared to some antibiotics (Baral, 2023). So far, phage treatment proved to be effective against bacterial pathogens including multidrug resistant S. aureus, Pseudomonas aeruginosa and A. baumannii, thus opening new strategies to overcome the rising problem of antibiotic resistance (Yang et al., 2021). Early studies by Wagenaar et al. demonstrated effectiveness of phage administration both to groups of young and pre-harvesting elder chickens, with the reduction of C. jejuni loads by 1–3 logs (Wagenaar et al., 2005). Later, multiple studies have shown the effectiveness of phage therapy by reducing Campylobacter loads in both settings in vitro when meat was experimentally infected and then treated, and in vivo when phages were administered directly to chickens (Kittler et al., 2021). While bacteriophages are considered as drugs and medicinal products in the U.S. and Europe, respectively, the lack of a legal basis for the use of phages slows down the development of phage applications (Jorda et al., 2023). Analysis of Campylobacter population dynamics during phage application on a commercial broiler farm demonstrated higher colonization capacity of isolates susceptible to phages in in vitro tests (Kittler et al., 2014). Surprisingly, bacteria susceptible to phages exhibited increased motility and gamma-glutamyl transferase activity promoting bacterial colonization (Kittler et al., 2014). Therefore, it is highly important to understand how the correlation between C. jejuni colonization potential and phage susceptibility can influence phage therapy in commercial broiler houses.
1.6 Bacteriocins
Bacteriocins (BCN) are bacteria-produced, small antimicrobial peptides that are already widely used as food preservatives, and have a promising potential in developing new treatment approaches against various microbial infections (Sugrue et al., 2024). In terms of campylobacteriosis, research has focused on preventing C. jejuni colonization in poultry, predominantly chickens. The first bacteriocins effective against C. jejuni were isolated from Bacillus circulans and Paenibacillus polymyxa and were identified as class IIa bacteriocins (Svetoch et al., 2005). Of those, BCN B 602 produced by P. polymyxa was tested for its ability to prevent C. jejuni infection in broiler chicks. While the untreated control birds showed typical colonization levels of 107–108 C. jejuni per Gram cecal content, C. jejuni was not detected in any of the chicks fed with B 602-treated feed prior to infection (Svetoch et al., 2005). BCN OR7 isolated from Lactobacillus salivarus also significantly reduced carriage of C. jejuni from 108 CFU to approximately 20 CFU C jejuni per Gram of cecal material (Stern et al., 2006). Similarly, assessment of BCN B 602 and BCN OR7 in turkey chicks resulted in effective colonization with Campylobacter coli in the control group, but no detectable levels of C. coli in the BCN-treated turkey poults (Cole et al., 2006). Another bacteriocin isolated from Lactobacillus salivarius, BCN SMXD51, which was only analyzed in vitro, demonstrated antimicrobial activity against C. jejuni through growth reduction by two logs (Messaoudi et al., 2012). A further interesting bacteriocin, BCN E 50-52, was produced from an Enterococcus faecium isolate originally isolated from cecal content of commercial broilers (Svetoch et al., 2008). Initial in vitro experiments showed inhibition of a broad range of different pathogens, including C. jejuni, Yersinia, Salmonella, Shigella, Morganella, Staphylococcus, and Listeria. Treatment of C. jejuni-colonized broiler chicks with BCN E 50-52 reduced C. jejuni loads by 6-log10, but did not result in complete elimination of the pathogen. The treated broilers still carried 102–103 CFU C jejuni per Gram cecal content (Svetoch et al., 2008). Another highly active bacteriocin, enterocin E−760, was isolated from another Enterococcus isolate characterized as E. durum/faecium/hirae. BCN E−760 exhibited a broad-spectrum antimicrobial activity by inhibiting the growth of several important pathogens, including C. jejuni, S. enterica, E. coli, Shigella dysenteriae and others (Line et al., 2008). When administered as food supplement, BCN E−760 dramatically reduced the bacterial loads in young chicken by 8-log10 CFU/g cecal material, with no C. jejuni detected in any of the treated groups (Line et al., 2008). Further, this study showed that BCN E−760 treatment of mature broilers naturally colonized with Campylobacter starting 3 days before slaughter effectively reduced the bacterial loads by 5-to-6-log10 to below the detection limit of 102 CFU/g (Line et al., 2008). Of all these analyzed BCNs, BCN E−760 was estimated to be the most effective. Regardless of whether the birds were treated with BCN E−760 before the experimental infection or the birds were already colonized by high C. jejuni numbers before BCN E−760 was administered, treatment resulted in the complete eradication of C. jejuni in 90% of the experiments or reduced the pathogen numbers by at least 7-log10 (Svetoch and Stern, 2010). Interestingly, when chickens colonized with C. jejuni were fed with BCN-supplemented chicken feed, C. jejuni numbers were effectively reduced. In contrast, treatment with viable bacterial cultures of the BCN-producing strain as a probiotic were ineffective in reducing C. jejuni in the treated birds (Stern et al., 2008).
1.7 Vaccine development
The development of vaccines for the prevention of Campylobacter-caused intestinal disease has been going on for well over 2 decades. Given that contaminated poultry meat is considered the main source of infection (Hermans et al., 2012a), large efforts have been made to prevent primary infection in broilers, on the rationale that elimination of C. jejuni from poultry would greatly reduce the disease burden in humans (de Zoete et al., 2007; Nothaft et al., 2016; Nothaft et al., 2021). There are several requirements that have to be met. The vaccine has to (i) be safe, (ii) elicit an efficient immune response, (iii) reliably protect against C. jejuni colonization, not just one strain or lineage, but the entire variability of the species, (iv) ideally, show cross-protection against C. coli, (v) be very easy to administer, ideally orally, and (vi) be very cheap to produce large-scale.
Similar to many other vaccines, killed entire C. jejuni cells were tested as vaccine candidate. Unfortunately, vaccination with this formalin-killed whole-cell vaccine failed to reduce the C. jejuni burden (Okamura et al., 2012). Likewise, crude cell lysates were administered, but only reduced C. jejuni colonization levels by about 10-fold (1-log10), and thus showed very limited effects (Annamalai et al., 2013). In addition, several protein-based, DNA-based and carbohydrate-based vaccine candidates have been tested in their ability to prevent C. jejuni colonization in chickens (Pumtang-On et al., 2021). Protein-based preparations included flagellin subunit FlaA (Wassenaar et al., 1993; Khoury and Meinersmann, 1995; Huang et al., 2010; Neal-McKinney et al., 2014), the multidrug efflux pump component CmeC (Jin et al., 2001; Zeng et al., 2010), the lipoproteins CjaA and CjaC (Layton et al., 2011; Laniewski et al., 2014; Wang et al., 2020), the adhesin CadF (Konkel et al., 1999b; Neal-McKinney et al., 2014), effector protein CiaB (Konkel et al., 1999a), the iron binding protein Dps (Theoret et al., 2012), and nanoparticle-encapsulated outer membrane proteins (OMPs) (Annamalai et al., 2013). Despite the induction of promising target-specific antibody production in most cases, the elicited immune response was associated with problems. The vaccination either provided only limited protection, lacked cross-protection or targeted epitopes with high genetic variability, or resulted in varying protection levels that depended on how the vaccine was administered, or did not sufficiently prevent C. jejuni colonization (Khoury and Meinersmann, 1995; Zeng et al., 2010; Layton et al., 2011; Theoret et al., 2012; Annamalai et al., 2013; Neal-McKinney et al., 2014). Another approach identified several C. jejuni OMPs as possible vaccine candidates in silico (Meunier et al., 2016) and used DNA-based preparations of hemolysin activation/secretion protein or flagellin protein FlgL to vaccinate chickens, but those showed either no effect or provided limited reduction of C. jejuni numbers (Meunier et al., 2017; Meunier et al., 2018). A recent review of completed vaccine trials summarized the data from several studies and listed the vaccine efficacy in terms of reduction of C. jejuni colonization (Pumtang-On et al., 2021). This overview concluded that a vaccine consisting of 125 µg crude cell lysate supplemented with OMPs covered in nanoparticles showed the highest efficacy against C. jejuni colonization. C. jejuni loads were reduced by 5.7-log10 CFU per Gram of fecal content, and thus showed 90% efficacy (Annamalai et al., 2013). However, while the OMP vaccine reduced C. jejuni cecal loads below the detection limit of 10 CFU per ml cecal content in all vaccinated chickens, the vaccine was only effective when administered subcutaneously. After oral vaccination, 63% of the tested chickens were still positive for C. jejuni (Annamalai et al., 2013). Given, that only subcutaneous vaccine administration provided significant protection against C. jejuni infection, this vaccine may not be suitable for large-scale use in broiler farms. Another vaccine strategy that also resulted in efficient protection of chickens against C. jejuni consisted of oral immunization of chickens with a live, avirulent S. enterica sv. Typhimurium strain expressing C. jejuni gene cjaA (cj0982c). The vaccinated birds not only developed serum IgG and mucosal IgA antibody responses against the C. jejuni CjaA membrane protein (and also Salmonella OMPs), but importantly greatly reduced the ability of C. jejuni to colonize the chicken intestinal tract, which was estimated to 89% efficacy (Wyszynska et al., 2004; Laniewski et al., 2014).
Other vaccine development efforts focused on C. jejuni carbohydrates (Keo et al., 2011; Hodgins et al., 2015; Nothaft et al., 2016). Vaccination of chickens with a capsular polysaccharide - diphtheria toxoid conjugated vaccine, which was administered subcutaneously, only resulted in a 0.6-log10 reduction in C. jejuni numbers compared to the non-vaccinated control birds (Hodgins et al., 2015). Based on research showing that C. jejuni express multiple different surface carbohydrates including O-linked and N-linked glycans (Szymanski et al., 2003) and that N-glycosylation of surface proteins promotes C. jejuni fitness (Szymanski et al., 2002; Alemka et al., 2013), N-glycan was evaluated as a potential vaccine candidate to control C. jejuni in chickens (Nothaft et al., 2016; Nothaft et al., 2021). N-glycan, that is common to all C. jejuni and C. coli strains, consists of a heptasaccharide composed of five units of N-acetylgalactosamine, one unit glucose and one unit diacetamido-trideoxy-D-glucopyranose (Young et al., 2002). Chickens vaccinated by oral administration of 108 live E. coli cells expressing C. jejuni N-glycan instead of E. coli O-antigen showed up to 8-log10 reduction in C. jejuni in cecal content (Nothaft et al., 2016). Unfortunately, not all birds responded to vaccination, which was associated with differences in the intestinal microbiota (Nothaft et al., 2017), a problem that had also affected other vaccine trials. Increased levels of Clostridia, specifically OTUs classified as Clostridium spp., Ruminococcaceae and Lachnospiraceae, were found to be beneficial for vaccine efficacy (Nothaft et al., 2021), and co-administration of the live N-glycan-based vaccine with the Clostridiales bacterium Anaerosporobacter mobilis that was identified in good vaccine responder chickens or with the probiotic bacterium Lactobacillus reuteri significantly improved vaccine performance (Nothaft et al., 2017). Thus, vaccine development and improvement will very likely continue.
1.8 The “One World–One Health” strategy
The “One World-One Health” (or short “One Health”) concept originated from the “One Medicine” approach, which suggested the establishment of an interdisciplinary cooperative research alliance of human and veterinary medicine to better control specific diseases, as originally designed by Calvin W. Schwabe about 40 years ago (Schwabe, 1984). The rationale of this initiative is to obtain a more comprehensive understanding of human and animal health and disease. In this context, the Wildlife Conservation Society has published the “Manhattan principles”, synopsizing practical methodologies and common standards in this concept (One World One Health, 2020). In addition, the so-called “Tripartite Concept Note” has been published, which represents a strategic paper for a partnership between the World Health Organization (WHO), the World Organization for Animal Health (WOAH), and the Food and Agriculture Organization of the United States (2025), focusing on the health risks during interactions of humans with domestic animals, wildlife and natural ecosystems, including the fight against antimicrobial resistances and specific microbial diseases. These ideas have been further optimized in recent years as a promising strategy for better control of zoonotic infectious diseases, additionally involving environmental health (One World One Health, 2021; Pitt and Gunn, 2024; Zhang et al., 2024). As a result, this concept was recently expanded to the “Quadripartite collaboration on One Health” that was initiated by the WHO, the WOAH, the FAO, and the United Nations Environment Programme (UNEP) to sustainably balance and optimize the health of humans, animals, plants and the environment (FAO, UNEP, WHO and WOAH, 2022). The One Health concept is particularly important for Campylobacter as a major zoonotic pathogen in the bacterial kingdom (Heimesaat M. M. et al., 2021). An example for its implementation is the PAC-Campy consortium that was launched under the direction of the Federal Ministry of Education and Research (Pac-Campy, 2023). This association is part of the National Research Network for “Zoonotic Infectious Diseases” and investigated Campylobacter infections in Germany from 2017–2023. The increasing number of reported cases of campylobacteriosis in humans emphasized the need to better understand the Campylobacter population genetics, the molecular basis of the infection, and to develop new strategies for the prevention and control of the pathogen and treatment of the disease (Lobo de Sá et al., 2021a; Golz and Stingl, 2021; Püning et al., 2021; Szott and Friese, 2021; Tegtmeyer et al., 2021; Linz et al., 2023). The overall objective of the PAC-Campy consortium was to reduce the contamination of food with Campylobacter and the incidence of these infections in humans. Poultry for fattening is a major natural reservoir for the pathogen, and there is a clear link between the presence of Campylobacter in broilers and Campylobacter infections in humans that can be traced back to the consumption of contaminated chicken meat (Ben Romdhane and Merle, 2021; Epping et al., 2021). Therefore, the particular aim of the network was to reduce the contamination of poultry meat with Campylobacter, and thus the number of infections in humans through intensive co-operation between all partners. To this end, possible intervention strategies along the production chain for poultry food products are being analyzed in terms of their efficiency and practical feasibility (McKenna et al., 2020; Pangga et al., 2025). Based on the data obtained, the research network has developed management strategies and intervention models such as bacteriophages and tested them under field conditions (Alter and Reich, 2021; Kittler et al., 2021). In addition, PAC-Campy provided the public health service and industry with efficient intervention measures (BMBF, 2021; Backert, 2021; Pac-Campy, 2023). In complementary work, the research network developed infection models in mice tested with numerous therapeutic compounds discussed above in order to establish innovative approaches for combating infections in humans and investigating the course of outbreaks (Mousavi et al., 2021a; Foote et al., 2023; Heimesaat et al., 2023b; Omarova et al., 2023).
2 Conclusion and perspectives
C. jejuni is a major globally distributed zoonotic pathogen with a high infectivity in humans. The pathogen cannot yet be effectively eliminated from the poultry food chain and represents one of the four key sources of diarrheal disease worldwide (WHO, 2020; WHO, 2024). This high incidence of the disease and the potential of late complications brands this pathogen as a serious threat for mankind both from medical and socio-economic viewpoints. Therefore, “One World-One Health”-based control processes and intervention strategies have been recently developed in order to minimize the occurrence of the bacteria in livestock and reduce contamination during food production. For example, new technological decontamination measures should help to improve hygiene at the fattening, slaughter and processing levels. At the same time, general awareness of foodborne pathogens such as C. jejuni must be further promoted among the human population through public information campaigns. The focus here should be on kitchen hygiene during processing of raw poultry meat products, which can even distribute the pathogen to other food. In addition, much progress has been made in recent years to unravel in detail the molecular mechanisms of C. jejuni pathogenicity, which are now also utilized to develop new therapeutics, phage protocols and vaccines against campylobacteriosis. Future studies should therefore intensify the search for and the investigation of novel anti-bacterial and anti-inflammatory compounds to reduce the C. jejuni load in the gut, and to impede associated inflammation and apoptosis, which in turn may not only diminish diarrhea, but also help to protect against secondary diseases caused by the pathogen such as GBS, MFS, RS or various chronic inflammatory processes in humans.
Author contributions
IS: Writing – original draft, Writing – review and editing. BL: Writing – original draft, Writing – review and editing. NT: Writing – original draft, Writing – review and editing. SB: Writing – original draft, Writing – review and editing.
Funding
The author(s) declare that financial support was received for the research and/or publication of this article. This work was supported by the German Federal Ministry of Education and Research (BMBF) through project IP9/01KI1725E in the PAC-Campylobacter Research Consortium and by the Deutsche Forschungsgemeinschaft (DFG, German Science Fundation) - [project 533962379].
Conflict of interest
The authors declare that the research was conducted in the absence of any commercial or financial relationships that could be construed as a potential conflict of interest.
Generative AI statement
The author(s) declare that no Generative AI was used in the creation of this manuscript.
Publisher’s note
All claims expressed in this article are solely those of the authors and do not necessarily represent those of their affiliated organizations, or those of the publisher, the editors and the reviewers. Any product that may be evaluated in this article, or claim that may be made by its manufacturer, is not guaranteed or endorsed by the publisher.
References
Ahmed, N. A., and Gulhan, T. (2022). Campylobacter in wild birds: is it an animal and public health concern? Front. Microbiol. 12, 812591. doi:10.3389/fmicb.2021.812591
Alemka, A., Nothaft, H., Zheng, J., and Szymanski, C. M. (2013). N-glycosylation of Campylobacter jejuni surface proteins promotes bacterial fitness. Infect. Immun. 81, 1674–1682. doi:10.1128/IAI.01370-12
Alter, T., and Reich, F. (2021). Management strategies for prevention of Campylobacter infections through the poultry food chain: a European perspective. Curr. Top. Microbiol. Immunol. 431, 79–102. doi:10.1007/978-3-030-65481-8_4
Alvarez-Martínez, F., Barrajón-Catalán, E., Herranz-López, M., and Micol, V. (2021). Antibacterial plant compounds, extracts and essential oils: an updated review on their effects and putative mechanisms of action. Phytomedicine 90, 153626. doi:10.1016/j.phymed.2021.153626
Annamalai, T., Pina-Mimbela, R., Kumar, A., Binjawadagi, B., Liu, Z., Renukaradhya, G. J., et al. (2013). Evaluation of nanoparticle-encapsulated outer membrane proteins for the control of Campylobacter jejuni colonization in chickens. Poult. Sci. 92, 2201–2211. doi:10.3382/ps.2012-03004
Babakhani, F. K., Bradley, G. A., and Joens, L. A. (1993). Newborn piglet model for campylobacteriosis. Infect. Immun. 61, 3466–3475. doi:10.1128/Iai.61.8.3466-3475.1993
S. Backert (2021). Fighting Campylobacter infections-towards a one health approach (Nature Switzerland: Springer). 978-3-030-65480-1. doi:10.1007/978-3-030-65481-8
Backert, S., Tegtmeyer, N., Ó’Cróinín, T., Boehm, M., and Heimesaat, M. M. (2017). “Human campylobacteriosis,” in Features, detection and prevention of foodborne disease: Campylobacter (Klein, G: Elsevier), 1–25.
Balta, I., Butucel, E., Stef, L., Pet, I., Gradisteanu-Pircalabioru, G., Chifiriuc, C., et al. (2022). Anti-Campylobacter probiotics: latest mechanistic insights. Foodborne Pathog. Dis. 19, 693–703. doi:10.1089/fpd.2022.0039
Balta, I., Linton, M., Pinkerton, L., Kelly, C., Stef, L., Pet, I., et al. (2021c). The effect of natural antimicrobials against Campylobacter spp. and its similarities to Salmonella spp., Listeria spp., Escherichia coli, Vibrio spp., Clostridium spp. and Staphylococcus spp. Food control. 121, 107745. doi:10.1016/j.foodcont.2020.107745
Balta, I., Linton, M., Pinkerton, L., Kelly, C., Ward, P., Stef, L., et al. (2021a). The effect of natural antimicrobials on the Campylobacter coli T6SS+/- during in vitro infection assays and on their ability to adhere to chicken skin and carcasses. Int. J. Food Microbiol. 338, 108998. doi:10.1016/j.ijfoodmicro.2020.108998
Balta, I., Marcu, A., Linton, M., Kelly, C., Gundogdu, O., Stef, L., et al. (2021b). Mixtures of natural antimicrobials can reduce Campylobacter jejuni, Salmonella enterica and Clostridium perfringens infections and cellular inflammatory response in MDCK cells. Gut Pathog. 13, 37. doi:10.1186/s13099-021-00433-5
Bandick, R., Busmann, L., Mousavi, S., Shayya, N., Piwowarski, J., Granica, S., et al. (2023). Therapeutic effects of oral application of menthol and extracts from tormentil (Potentilla erecta), raspberry leaves (Rubus idaeus), and loosestrife (Lythrum salicaria) during acute murine campylobacteriosis. Pharmaceutics 15 (10), 2410. doi:10.3390/pharmaceutics15102410
Baral, B. (2023). Phages against killer superbugs: an enticing strategy against antibiotics-resistant pathogens. Front. Pharmacol. 14, 1036051. doi:10.3389/fphar.2023.1036051
Barata, R., Saavedra, M., and Almeida, G. (2024). A decade of antimicrobial resistance in human and animal Campylobacter spp. isolates. Antibiotics 13 (9), 904. doi:10.3390/antibiotics13090904
Barrero-Tobon, A. M., and Hendrixson, D. R. (2012). Identification and analysis of flagellar coexpressed determinants (Feds) of Campylobacter jejuni involved in colonization. Mol. Microbiol. 84 (2), 352–369. doi:10.1111/j.1365-2958.2012.08027.x
Bell, J. A., St Charles, J. L., Murphy, A. J., Rathinam, V. A., Plovanich-Jones, A. E., Stanley, E. L., et al. (2009). Multiple factors interact to produce responses resembling spectrum of human disease in Campylobacter jejuni infected C57BL/6 IL-10-/- mice. BMC Microbiol. 9, 57. doi:10.1186/1471-2180-9-57
Ben Romdhane, R., and Merle, R. (2021). The data behind risk analysis of Campylobacter jejuni and Campylobacter coli infections. Curr. Top. Microbiol. Immunol. 431, 25–58. doi:10.1007/978-3-030-65481-8_2
Bereswill, S., Ekmekciu, I., Escher, U., Fiebiger, U., Stingl, K., and Heimesaat, M. (2017). Lactobacillus johnsonii ameliorates intestinal, extra-intestinal and systemic pro-inflammatory immune responses following murine Campylobacter jejuni infection. Sci. Rep. 7 (1), 2138. doi:10.1038/s41598-017-02436-2
Bereswill, S., Fischer, A., Plickert, R., Haag, L. M., Otto, B., Kuhl, A. A., et al. (2011a). Novel murine infection models provide deep insights into the “menage a trois” of Campylobacter jejuni, microbiota and host innate immunity. PLoS One 6, e20953. doi:10.1371/journal.pone.0020953
Bereswill, S., Mousavi, S., Weschka, D., Buczkowski, A., Schmidt, S., and Heimesaat, M. (2021a). Peroral clove essential oil treatment ameliorates acute campylobacteriosis-results from a preclinical murine intervention study. Microorganisms 9 (4), 735. doi:10.3390/microorganisms9040735
Bereswill, S., Mousavi, S., Weschka, D., Buczkowski, A., Schmidt, S., and Heimesaat, M. (2023). Iron deprivation by oral deferoxamine application alleviates acute campylobacteriosis in a clinical murine Campylobacter jejuni infection model. Biomolecules 13 (1), 71. doi:10.3390/biom13010071
Bereswill, S., Mousavi, S., Weschka, D., and Heimesaat, M. (2021b). Disease-alleviating effects of peroral activated charcoal treatment in acute murine campylobacteriosis. Microorganisms 9 (7), 1424. doi:10.3390/microorganisms9071424
Bereswill, S., Plickert, R., Fischer, A., Kühl, A. A., Loddenkemper, C., Batra, A., et al. (2011b). What you eat is what you get: novel Campylobacter models in the quadrangle relationship between nutrition, obesity, microbiota and susceptibility to infection. Eur. J. Microbiol. Immunol. Bp. 1 (3), 237–248. doi:10.1556/EuJMI.1.2011.3.8
BMBF (2021). Available online at: https://www.gesundheitsforschung-bmbf.de/de/pac-campy-pravention-und-bekampfung-von-campylobacter-infektionen-ein-one-health-ansatz-7199.php (Accessed February 07, 2025).
Boehm, M., Hoy, B., Rohde, M., Tegtmeyer, N., Bæk, K. T., Oyarzabal, O. A., et al. (2012). Rapid paracellular transmigration of Campylobacter jejuni across polarized epithelial cells without affecting TER: role of proteolytic-active HtrA cleaving E-cadherin but not fibronectin. Gut Pathog. 4 (1), 3. doi:10.1186/1757-4749-4-3
Boehm, M., Krause-Gruszczynska, M., Rohde, M., Tegtmeyer, N., Takahashi, S., Oyarzabal, O. A., et al. (2011). Major host factors involved in epithelial cell invasion of Campylobacter jejuni: role of fibronectin, integrin beta1, FAK, Tiam-1, and DOCK180 in activating Rho GTPase Rac1. Front. Cell Infect. Microbiol. 1, 17. eCollection 2011. doi:10.3389/fcimb.2011.00017
Boehm, M., Lind, J., Backert, S., and Tegtmeyer, N. (2015). Campylobacter jejuni serine protease HtrA plays an important role in heat tolerance, oxygen resistance, host cell adhesion, invasion, and transmigration. Eur. J. Microbiol. Immunol. (Bp) 5 (1), 68–80. doi:10.1556/EUJMI-D-15-00003
Bücker, R., Krug, S., Moos, V., Bojarski, C., Schweiger, M., Kerick, M., et al. (2018). Campylobacter jejuni impairs sodium transport and epithelial barrier function via cytokine release in human colon. Mucosal Immunol. 11 (2), 474–485. doi:10.1038/mi.2017.66
Buckley, C. E., and St Johnston, D. (2022). Apical-basal polarity and the control of epithelial form and function. Nat. Rev. Mol. Cell Biol. 23 (8), 559–577. doi:10.1038/s41580-022-00465-y
Burnham, P. M., and Hendrixson, D. R. (2018). Campylobacter jejuni: collective components promoting a successful enteric lifestyle. Nat. Rev. Microbiol. 16 (9), 551–565. doi:10.1038/s41579-018-0037-9
Carr, A., and Maggini, S. (2017). Vitamin C and immune function. Nutrients 9 (11), 1211. doi:10.3390/nu9111211
Cawthraw, S. A., and Newell, D. G. (2010). Investigation of the presence and protective effects of maternal antibodies against Campylobacter jejuni in chickens. Avian. Dis. 54, 86–93. doi:10.1637/9004-072709-Reg.1
Cayrou, C., Barratt, N. A., Ketley, J. M., and Bayliss, C. D. (2021). Phase variation during host colonization and invasion by Campylobacter jejuni and other Campylobacter species. Front. Microbiol. 12, 705139. doi:10.3389/fmicb.2021.705139
Chang, C., and Miller, J. F. (2006). Campylobacter jejuni colonization of mice with limited enteric flora. Infect. Immun. 74, 5261–5271. doi:10.1128/IAI.01094-05
Chen, H., Zhang, Y., Pan, Y., Wu, L., Wang, W., Zhang, H., et al. (2024). Antibiotic-induced microbiome depletion promotes intestinal colonization by Campylobacter jejuni in mice. BMC Microbiol. 24 (1), 156. doi:10.1186/s12866-024-03313-5
Chick, H. M., Williams, L. K., Sparks, N., Khattak, F., Vermeij, P., Frantzen, I., et al. (2025). Campylobacter jejuni ST353 and ST464 cause localized gut inflammation, crypt damage, and extraintestinal spread during large- and small-scale infection in broiler chickens. Appl. Environ. Microbiol. e0161424, e0161424. doi:10.1128/aem.01614-24
Cole, K., Farnell, M. B., Donoghue, A. M., Stern, N. J., Svetoch, E. A., Eruslanov, B. N., et al. (2006). Bacteriocins reduce Campylobacter colonization and alter gut morphology in Turkey poults. Poult. Sci. 85, 1570–1575. doi:10.1093/ps/85.9.1570
Connerton, P. L., Richards, P. J., Lafontaine, G. M., O'Kane, P. M., Ghaffar, N., Cummings, N. J., et al. (2018). The effect of the timing of exposure to Campylobacter jejuni on the gut microbiome and inflammatory responses of broiler chickens. Microbiome 6, 88. doi:10.1186/s40168-018-0477-5
Del Olmo, A., Calzada, J., and Nuñez, M. (2017). Benzoic acid and its derivatives as naturally occurring compounds in foods and as additives: uses, exposure, and controversy. Crit. Rev. Food Sci. Nutr. 57 (14), 3084–3103. doi:10.1080/10408398.2015.1087964
Deng, H., Chaname Pinedo, L. E., Meijs, A. P., Sanders, P., Veldman, K. T., Brouwer, M. S. M., et al. (2024). Reducing antimicrobial use in livestock alone may be not sufficient to reduce antimicrobial resistance among human Campylobacter infections: an ecological study in The Netherlands. Epidemiol. Infect. 152, e148. doi:10.1017/S0950268824001511
de Zoete, M. R., van Putten, J. P., and Wagenaar, J. A. (2007). Vaccination of chickens against Campylobacter. Vaccine 25, 5548–5557. doi:10.1016/j.vaccine.2006.12.002
Dias, T. S., Nascimento, R. J., Machado, L. S., Abreu, D. L. C., do Nascimento, E. R., Pereira, V. L. A., et al. (2021). Comparison of antimicrobial resistance in thermophilic Campylobacter strains isolated from conventional production and backyard poultry flocks. Br. Poult. Sci. 62, 188–192. doi:10.1080/00071668.2020.1833302
Diggikar, P. M., Pancholi, T., Yammanuru, B., and Mundada, M. R. J. (2024). Exploring treatments for a rare guillain-barré variant: a case report of miller-Fisher syndrome. Cureus 16 (7), e65561. doi:10.7759/cureus.65561
Dorrell, N., and Wren, B. W. (2007). The second century of Campylobacter research: recent advances, new opportunities and old problems. Curr. Opin. Infect. Dis. 20, 514–518. doi:10.1097/QCO.0b013e3282a56b15
Du, K., Bereswill, S., and Heimesaat, M. (2021). A literature survey on antimicrobial and immune-modulatory effects of butyrate revealing non-antibiotic approaches to tackle bacterial infections. Eur. J. Microbiol. Immunol. (Bp) 11 (1), 1–9. doi:10.1556/1886.2021.00001
Du, K., Foote, M., Mousavi, S., Buczkowski, A., Schmidt, S., Bereswill, S., et al. (2022). Less pronounced immunopathological responses following oral butyrate treatment of Campylobacter jejuni-infected mice. Microorganisms 10 (10), 1953. doi:10.3390/microorganisms10101953
Du, K., Foote, M., Mousavi, S., Buczkowski, A., Schmidt, S., Peh, E., et al. (2023). Combination of organic acids benzoate, butyrate, caprylate, and sorbate provides a novel antibiotics-independent treatment option in the combat of acute campylobacteriosis. Front. Microbiol. 14, 1128500. eCollection 2023. doi:10.3389/fmicb.2023.1128500
Du, K., Mousavi, S., Foote, M., Bereswill, S., and Heimesaat, M. (2024). Therapeutic effects of oral benzoic acid application during acute murine campylobacteriosis. Eur. J. Microbiol. Immunol. (Bp) 14 (3), 243–260. doi:10.1556/1886.2024.00059
Ekmekciu, I., Fiebiger, U., Stingl, K., Bereswill, S., and Heimesaat, M. (2017). Amelioration of intestinal and systemic sequelae of murine Campylobacter jejuni infection by probiotic VSL#3 treatment. Gut Pathog. 9, 17. eCollection 2017. doi:10.1186/s13099-017-0168-y
Elmi, A., Nasher, F., Jagatia, H., Gundogdu, O., Bajaj-Elliott, M., Wren, B., et al. (2016). Campylobacter jejuni outer membrane vesicle-associated proteolytic activity promotes bacterial invasion by mediating cleavage of intestinal epithelial cell E-cadherin and occludin. Cell. Microbiol. 18, 561–572. doi:10.1111/cmi.12534
Epping, L., Antão, E. M., and Semmler, T. (2021). Population biology and comparative genomics of Campylobacter species. Curr. Top. Microbiol. Immunol. 431, 59–78. doi:10.1007/978-3-030-65481-8_3
Eucker, T. P., and Konkel, M. E. (2012). The cooperative action of bacterial fibronectin-binding proteins and secreted proteins promote maximal Campylobacterjejuni invasion of host cells by stimulating membrane ruffling. Cell Microbiol. 14 (2), 226–238. doi:10.1111/j.1462-5822.2011.01714.x
European Centre for Disease Prevention and Control (ECDC) (2024). The European union one health 2023 zoonoses report. EFSA J. 22, e9106. doi:10.2903/j.efsa.2024.9106
European Centre for Disease Prevention and Control (2024). “Campylobacteriosis” in ECDC. Annual epidemiological report for 2022 (Stockholm: ECDC). Available online at: https://www.ecdc.europa.eu/en/publications-data/campylobacteriosis-annual-epidemiological-report-2022.
European Food Safety Authority (2014). EFSA explains zoonotic diseases: Campylobacter. Eur. Food Saf. Auth. Available online at: https://data.europa.eu/doi/10.2805/59450. doi:10.2805/59450
European Centre for Disease Prevention and Control (ECDC). (2024). The European Union summary report on antimicrobial resistance in zoonotic and indicator bacteria from humans, animals and food in 2021-2022. EFSA J. 22, e8583. doi:10.2903/j.efsa.2024.8583
FAO, UNEP, WHO, and WOAH (2022). “One health joint plan of action (2022-2026),” in Working together for the health of humans, animals, plants and the environment (Rome). doi:10.4060/cc2289en
Food and Agriculture Organization of the United States (2025). World organisation for animal health, WHO (2010) the FAO–OIE–WHO collaboration: a tripartite concept note. Available online at: https://www.who.int/publications/m/item/the-fao-oie-who-collaboration (Accessed February 07, 2025).
Foote, M., Du, K., Mousavi, S., Bereswill, S., and Heimesaat, M. (2023). Therapeutic oral application of carvacrol alleviates acute campylobacteriosis in mice harboring a human gut microbiota. Biomolecules 13 (2), 320. doi:10.3390/biom13020320
Fox, J. G., Ackerman, J. I., Taylor, N., Claps, M., and Murphy, J. C. (1987). Campylobacter jejuni infection in the ferret - an animal-model of human campylobacteriosis. Am. J. Vet. Res. 48, 85–90. doi:10.2460/ajvr.1987.48.01.85
Gibreel, A., Kos, V. N., Keelan, M., Trieber, C. A., Levesque, S., Michaud, S., et al. (2005). Macrolide resistance in Campylobacter jejuni and Campylobacter coli: molecular mechanism and stability of the resistance phenotype. Antimicrob. Agents Chemother. 49, 2753–2759. doi:10.1128/AAC.49.7.2753-2759.2005
Gilpin, B. J., Walker, T., Paine, S., Sherwood, J., Mackereth, G., Wood, T., et al. (2020). A large scale waterborne Campylobacteriosis outbreak, Havelock North, New Zealand. J. Infect. 81, 390–395. doi:10.1016/j.jinf.2020.06.065
Golz, J. C., and Stingl, K. (2021). Natural competence and horizontal gene transfer in Campylobacter. Curr. Top. Microbiol. Immunol. 431, 265–292. doi:10.1007/978-3-030-65481-8_10
Goodman, K. N., Powers, M. J., Crofts, A. A., Trent, M. S., and Hendrixson, D. R. (2020). Campylobacter jejuni BumSR directs a response to butyrate via sensor phosphatase activity to impact transcription and colonization. Proc. Natl. Acad. Sci. U. S. A. 117 (21), 11715–11726. doi:10.1073/pnas.1922719117
Haag, L. M., Fischer, A., Otto, B., Grundmann, U., Kuhl, A. A., Gobel, U. B., et al. (2012b). Campylobacter jejuni infection of infant mice: acute enterocolitis is followed by asymptomatic intestinal and extra-intestinal immune responses. Eur. J. Microbiol. Immunol. (Bp) 2, 2–11. doi:10.1556/EuJMI.2.2012.1.2
Haag, L. M., Fischer, A., Otto, B., Plickert, R., Kuhl, A. A., Gobel, U. B., et al. (2012a). Campylobacter jejuni induces acute enterocolitis in gnotobiotic IL-10-/- mice via Toll-like-receptor-2 and -4 signaling. PLoS One 7, e40761. doi:10.1371/journal.pone.0040761
Haag, L. M., Fischer, A., Otto, B., Plickert, R., Kuhl, A. A., Gobel, U. B., et al. (2012c). Intestinal microbiota shifts towards elevated commensal Escherichia coli loads abrogate colonization resistance against Campylobacter jejuni in mice. Plos One 7 (5), e35988. doi:10.1371/journal.pone.0035988
Haag, L. M., and Siegmund, B. (2015). Intestinal microbiota and the innate immune system - a crosstalk in Crohn's disease pathogenesis. Front. Immunol. 6, 489. doi:10.3389/fimmu.2015.00489
Harrer, A., Bucker, R., Boehm, M., Zarzecka, U., Tegtmeyer, N., Sticht, H., et al. (2019). Campylobacter jejuni enters gut epithelial cells and impairs intestinal barrier function through cleavage of occludin by serine protease HtrA. Gut Pathog. 11, 4. eCollection 2019. doi:10.1186/s13099-019-0283-z
Hasso-Agopsowicz, M., Lopman, B. A., Lanata, C. F., Rogawski McQuade, E. T., Kang, G., Prudden, H. J., et al. (2021). World Health Organization Expert Working Group: recommendations for assessing morbidity associated with enteric pathogens. Vaccine 39 (52), 7521–7525. doi:10.1016/j.vaccine.2021.11.033
Heimesaat, M., Backert, S., Alter, T., and Bereswill, S. (2023a). Molecular targets in Campylobacter infections. Biomolecules 13 (3), 409. doi:10.3390/biom13030409
Heimesaat, M., Langfeld, L., Schabbel, N., Mousavi, S., and Bereswill, S. (2024a). Carvacrol prophylaxis improves clinical outcome and dampens apoptotic and pro-inflammatory immune responses upon Campylobacter jejuni infection of human microbiota-associated IL-10-/- mice. Eur. J. Microbiol. Immunol. (Bp) 14 (2), 166–179. doi:10.1556/1886.2024.00009
Heimesaat, M., Langfeld, L., Schabbel, N., Shayya, N., Mousavi, S., and Bereswill, S. (2024b). Menthol pretreatment alleviates Campylobacter jejuni-induced enterocolitis in human gut microbiota-associated IL-10-/- mice. Biomolecules 14 (3), 290. doi:10.3390/biom14030290
Heimesaat, M., Mousavi, S., Bandick, R., and Bereswill, S. (2022). Campylobacter jejuni infection induces acute enterocolitis in IL-10-/- mice pretreated with ampicillin plus sulbactam. Eur. J. Microbiol. Immunol. (Bp) 12 (3), 73–83. doi:10.1556/1886.2022.00014
Heimesaat, M., Mousavi, S., De Sá, F., Peh, E., Schulzke, J., Bücker, R., et al. (2024c). Oral curcumin ameliorates acute murine campylobacteriosis. Front. Immunol. 15, 1363457. eCollection 2024. doi:10.3389/fimmu.2024.1363457
Heimesaat, M., Mousavi, S., Escher, U., De Sa, F., Peh, E., Schulzke, J., et al. (2020b). Resveratrol alleviates acute Campylobacter jejuni induced enterocolitis in a preclinical murine intervention study. Microorganisms 8 (12), 1858. doi:10.3390/microorganisms8121858
Heimesaat, M., Mousavi, S., Weschka, D., and Bereswill, S. (2021b). Anti-pathogenic and immune-modulatory effects of peroral treatment with cardamom essential oil in acute murine campylobacteriosis. Microorganisms 9 (1), 169. doi:10.3390/microorganisms9010169
Heimesaat, M., Mousavi, S., Weschka, D., and Bereswill, S. (2021c). Garlic essential oil as promising option for the treatment of acute campylobacteriosis-results from a preclinical placebo-controlled intervention study. Microorganisms 9 (6), 1140. doi:10.3390/microorganisms9061140
Heimesaat, M., Mousavi, S., Weschka, D., and Bereswill, S. (2023b). Not only for Christmas: prophylactic oral application of trans-cinnamaldehyde alleviates acute murine campylobacteriosis. Eur. J. Microbiol. Immunol. (Bp) 13 (2), 45–56. doi:10.1556/1886.2023.00024
Heimesaat, M., Schabbel, N., Langfeld, L., Shayya, N., Mousavi, S., and Bereswill, S. (2023c). Prophylactic oral application of resveratrol to alleviate acute campylobacteriosis in human gut microbiota associated IL-10-/- mice. Eur. J. Microbiol. Immunol. (Bp) 13 (4), 135–149. doi:10.1556/1886.2023.00042
Heimesaat, M., Schabbel, N., Langfeld, L., Shayya, N., Mousavi, S., and Bereswill, S. (2024d). Prophylactic oral application of activated charcoal mitigates acute campylobacteriosis in human gut microbiota-associated IL-10-/- mice. Biomolecules 14 (2), 141. doi:10.3390/biom14020141
Heimesaat, M., Weschka, D., Mousavi, S., and Bereswill, S. (2021d). Treatment with the probiotic product Aviguard® alleviates inflammatory responses during Campylobacter jejuni-induced acute enterocolitis in mice. Int. J. Mol. Sci. 22 (13), 6683. doi:10.3390/ijms22136683
Heimesaat, M. M., Alutis, M., Grundmann, U., Fischer, A., Tegtmeyer, N., Böhm, M., et al. (2014a). The role of serine protease HtrA in acute ulcerative enterocolitis and extra-intestinal immune responses during Campylobacter jejuni infection of gnotobiotic IL-10 deficient mice. Front. Cell. Infect. Microbiol. 4, 77. doi:10.3389/fcimb.2014.00077
Heimesaat, M. M., Backert, S., Alter, T., and Bereswill, S. (2021a). Human campylobacteriosis-A serious infectious threat in a one health perspective. Curr. Top. Microbiol. Immunol. 431, 1–23. doi:10.1007/978-3-030-65481-8_1
Heimesaat, M. M., and Bereswill, S. (2015). Murine infection models for the investigation of Campylobacter jejuni-host interactions and pathogenicity. Berl. Munch. Tierarztl. Wochenschr. 128, 98–103.
Heimesaat, M. M., Fischer, A., Alutis, M., Grundmann, U., Boehm, M., Tegtmeyer, N., et al. (2014b). The impact of serine protease HtrA in apoptosis, intestinal immune responses and extra-intestinal histopathology during Campylobacter jejuni infection of infant mice. Gut Pathog. 6, 16. eCollection 2014. doi:10.1186/1757-4749-6-16
Heimesaat, M. M., Grundmann, U., Alutis, M. E., Fischer, A., and Bereswill, S. (2017). Absence of nucleotide-oligomerization- domain-2 is associated with less distinct disease in Campylobacter jejuni infected secondary abiotic IL-10 deficient mice. Front. Cell Infect. Microbiol. 7, 322. eCollection 2017. doi:10.3389/fcimb.2017.00322
Heimesaat, M. M., Grundmann, U., Alutis, M. E., Fischer, A., Göbel, U. B., and Bereswill, S. (2016). Colonic expression of genes encoding inflammatory mediators and gelatinases during Campylobacter jejuni infection of conventional infant mice. Eur. J. Microbiol. Immunol. (Bp) 6 (2), 137–146. eCollection 2016 Jun 24. doi:10.1556/1886.2016.00009
Heimesaat, M. M., Schmidt, A. M., Mousavi, S., Escher, U., Tegtmeyer, N., Wessler, S., et al. (2020a). Peptidase PepP is a novel virulence factor of Campylobacter jejuni contributing to murine campylobacteriosis. Gut Microbes 12, 1770017. doi:10.1080/19490976.2020.1770017
Hendrixson, D. R., and DiRita, V. J. (2004). Identification of Campylobacter jejuni genes involved in commensal colonization of the chick gastrointestinal tract. Mol. Microbiol. 52 (2), 471–484. doi:10.1111/j.1365-2958.2004.03988.x
Hermans, D., Pasmans, F., Heyndrickx, M., Van Immerseel, F., Martel, A., Deun, K., et al. (2012b). A tolerogenic mucosal immune response leads to persistent Campylobacter jejuni colonization in the chicken gut. Crit. Rev. Microbiol. 38, 17–29. doi:10.3109/1040841x.2011.615298
Hermans, D., Pasmans, F., Messens, W., Martel, A., Van Immerseel, F., Rasschaert, G., et al. (2012a). Poultry as a host for the zoonotic pathogen Campylobacter jejuni. Vector Borne Zoonotic Dis. 12, 89–98. doi:10.1089/vbz.2011.0676
Hermans, D., Van Deun, K., Martel, A., Van Immerseel, F., Messens, W., Heyndrickx, M., et al. (2011). Colonization factors of Campylobacter jejuni in the chicken gut. Vet. Res. 42 (1), 82. doi:10.1186/1297-9716-42-82
Hodgins, D. C., Barjesteh, N., St Paul, M., Ma, Z., Monteiro, M. A., and Sharif, S. (2015). Evaluation of a polysaccharide conjugate vaccine to reduce colonization by Campylobacter jejuni in broiler chickens. BMC Res. Notes 8, 204. doi:10.1186/s13104-015-1203-z
Hoffmann, S., White, A. E., McQueen, R. B., Ahn, J. W., Gunn-Sandell, L. B., and Scallan Walter, E. J. (2024). Economic burden of foodborne illnesses acquired in the United States. Foodborne pathogens Dis. doi:10.1089/fpd.2023.0157
Huang, H., Brooks, B. W., Lowman, R., and Carrillo, C. D. (2015). Campylobacter species in animal, food, and environmental sources, and relevant testing programs in Canada. Can. J. Microbiol. 61 (10), 701–721. doi:10.1139/cjm-2014-0770
Huang, J. L., Yin, Y. X., Pan, Z. M., Zhang, G., Zhu, A. P., Liu, X. F., et al. (2010). Intranasal immunization with chitosan/pCAGGS-flaA nanoparticles inhibits Campylobacter jejuni in a White Leghorn model. J. Biomed. Biotechnol. 2010, 589476. doi:10.1155/2010/589476
Hughes, R. A. C. (2024). Guillain-Barré syndrome: history, pathogenesis, treatment, and future directions. Eur. J. Neurol. 31 (11), e16346. doi:10.1111/ene.16346
Jansen, L., Birn, R., Koirala, S., Oppegard, S., Loeck, B., Hamik, J., et al. (2024). Campylobacteriosis outbreak linked to municipal water, Nebraska, USA, 20211. Emerg. Infect. Dis. 30, 1998–2005. doi:10.3201/eid3010.231509
Jesudason, M. V., Hentges, D. J., and Pongpech, P. (1989). Colonization of mice by Campylobacter jejuni. Infect. Immun. 57, 2279–2282. doi:10.1128/iai.57.8.2279-2282.1989
Jimenez, B., Awwad, F., and Desgagné-Penix, I. (2024). Cinnamaldehyde in focus: antimicrobial properties, biosynthetic pathway, and industrial applications. Antibiotics 13 (11), 1095. doi:10.3390/antibiotics13111095
Jin, S. M., Joe, A., Lynett, J., Hani, E. K., Sherman, P., and Chan, V. L. (2001). JlpA, a novel surface-exposed lipoprotein specific to Campylobacter jejuni, mediates adherence to host epithelial cells. Mol. Microbiol. 39, 1225–1236. doi:10.1111/j.1365-2958.2001.02294.x
Jin, X., He, Y., Zhou, Y., Chen, X., Lee, Y., Zhao, J., et al. (2021). Lactic acid bacteria that activate immune gene expression in Caenorhabditis elegans can antagonise Campylobacter jejuni infection in nematodes, chickens and mice. BMC Microbiol. 21 (1), 169. doi:10.1186/s12866-021-02226-x
Jorda, J., Lorenzo-Rebenaque, L., Montoro-Dasi, L., Marco-Fuertes, A., Vega, S., Marin, C., et al. (2023). Phage-based biosanitation strategies for minimizing persistent Salmonella and Campylobacter bacteria in poultry. Animals 13 (24), 3826. doi:10.3390/ani13243826
Kaakoush, N. O., Castaño-Rodríguez, N., Mitchell, H. M., and Man, S. M. (2015). Global epidemiology of Campylobacter infection. Clin. Microbiol. Rev. 28 (3), 687–720. doi:10.1128/CMR.00006-15
Kamatou, G. P. P., Vermaak, I., Viljoen, A. M., and Lawrence, B. M. (2013). Menthol: a simple monoterpene with remarkable biological properties. Phytochemistry 96, 15–25. doi:10.1016/j.phytochem.2013.08.005
Kelly, C., Gundogdu, O., Pircalabioru, G., Cean, A., Scates, P., Linton, M., et al. (2017). The in vitro and in vivo effect of carvacrol in preventing Campylobacter infection, colonization and in improving productivity of chicken broilers. Foodborne pathogens Dis. 14 (6), 341–349. doi:10.1089/fpd.2016.2265
Kennedy, G., Min, M., Fitzgerald, J., Nguyen, M., Schultz, S., Crum, M., et al. (2019). Inactivation of the bacterial pathogens Staphylococcus pseudintermedius and Acinetobacter baumannii by butanoic acid. J. Appl. Microbiol. 126, 752–763. doi:10.1111/jam.14180
Kenyon, J., Inns, T., Aird, H., Swift, C., Astbury, J., Forester, E., et al. (2020). Campylobacter outbreak associated with raw drinking milk, North West England, 2016. Epidemiol. Infect. 148, e13. doi:10.1017/S0950268820000096
Keo, T., Collins, J., Kunwar, P., Blaser, M. J., and Iovine, N. M. (2011). Campylobacter capsule and lipooligosaccharide confer resistance to serum and cationic antimicrobials. Virulence 2, 30–40. doi:10.4161/viru.2.1.14752
Keylor, M., Matsuura, B., and Stephenson, C. (2015). Chemistry and biology of resveratrol-derived natural products. Chem. Rev. 115, 8976–9027. doi:10.1021/cr500689b
Khoury, C. A., and Meinersmann, R. J. (1995). A genetic hybrid of the Campylobacter jejuni flaA gene with LT-B of Escherichia coli and assessment of the efficacy of the hybrid protein as an oral chicken vaccine. Avian Dis. 39, 812–820. doi:10.2307/1592418
Khubiev, O., Esakova, V., Egorov, A., Bely, A., Golubev, R., Tachaev, M., et al. (2023). Novel non-Toxic highly antibacterial chitosan/Fe(III)-Based nanoparticles that contain a deferoxamine-trojan horse ligands: combined synthetic and biological studies. Processes 11 (3), 870. doi:10.3390/pr11030870
Kingsbury, J. M., Horn, B., Armstrong, B., Midwinter, A., Biggs, P., Callander, M., et al. (2023). The impact of primary and secondary processing steps on Campylobacter concentrations on chicken carcasses and portions. Food Microbiol. 110, 104168. doi:10.1016/j.fm.2022.104168
Kittler, S., Fischer, S., Abdulmawjood, A., Glünder, G., and Klein, G. (2014). Colonisation of a phage susceptible Campylobacter jejuni population in two phage positive broiler flocks. PLOS One 9 (4), e94782. doi:10.1371/journal.pone.0094782
Kittler, S., Steffan, S., Peh, E., and Plötz, M. (2021). Phage biocontrol of Campylobacter: a one health approach. Curr. Top. Microbiol. Immunol. 431, 127–168. doi:10.1007/978-3-030-65481-8_6
Kløve, S., Genger, C., Weschka, D., Mousavi, S., Bereswill, S., and Heimesaat, M. M. (2020). Toll-like receptor-4 is involved in mediating intestinal and extra-intestinal inflammation in Campylobacter coli-infected secondary abiotic IL-10-/- mice. Microorganisms 8 (12), 1882. doi:10.3390/microorganisms8121882
Konkel, M. E., Gray, S. A., Kim, B. J., Garvis, S. G., and Yoon, J. (1999b). Identification of the enteropathogens Campylobacter jejuni and Campylobacter coli based on the cadF virulence gene and its product. J. Clin. Microbiol. 37, 510–517. doi:10.1128/Jcm.37.3.510-517.1999
Konkel, M. E., Kim, B. J., Rivera-Amill, V., and Garvis, S. G. (1999a). Bacterial secreted proteins are required for the internalization of Campylobacter jejuni into cultured mammalian cells. Mol. Microbiol. 32, 691–701. doi:10.1046/j.1365-2958.1999.01376.x
Konkel, M. E., Klena, J. D., Rivera-Amill, V., Monteville, M. R., Biswas, D., Raphael, B., et al. (2004). Secretion of virulence proteins from Campylobacter jejuni is dependent on a functional flagellar export apparatus. J. Bacteriol. 186, 3296–3303. doi:10.1128/JB.186.11.3296-3303.2004
Konkel, M. E., Talukdar, P. K., Negretti, N. M., and Klappenbach, C. M. (2020). Taking control: Campylobacter jejuni binding to fibronectin sets the stage for cellular adherence and invasion. Front. Microbiol. 11, 564. doi:10.3389/fmicb.2020.00564
Korolik, V. (2019). The role of chemotaxis during Campylobacter jejuni colonisation and pathogenesis. Curr. Opin. Microbiol. 47, 32–37. doi:10.1016/j.mib.2018.11.001
Krause-Gruszczynska, M., Boehm, M., Rohde, M., Tegtmeyer, N., Takahashi, S., Buday, L., et al. (2011). The signaling pathway of Campylobacter jejuni-induced Cdc42 activation: role of fibronectin, integrin beta1, tyrosine kinases and guanine exchange factor Vav2. Cell Commun. Signal. 9, 32. doi:10.1186/1478-811X-9-32
Krause-Gruszczynska, M., Rohde, M., Hartig, R., Genth, H., Schmidt, G., Keo, T., et al. (2007). Role of the small Rho GTPases Rac1 and Cdc42 in host cell invasion of Campylobacter jejuni. Cell Microbiol. 9, 2431–2444. doi:10.1111/j.1462-5822.2007.00971.x
Kroner, J., Sommer, A., and Fabri, M. (2015). Vitamin D every day to keep the infection away? Nutrients 7, 4170–4188. doi:10.3390/nu7064170
Kuijf, M. L., Samsom, J. N., van Rijs, W., Bax, M., Huizinga, R., Heikema, A. P., et al. (2010). TLR4-mediated sensing of Campylobacter jejuni by dendritic cells is determined by sialylation. J. Immunol. 185, 748–755. doi:10.4049/jimmunol.0903014
Laniewski, P., Kuczkowski, M., Chrzastek, K., Wozniak, A., Wyszynska, A., Wieliczko, A., et al. (2014). Evaluation of the immunogenicity of Campylobacter jejuni CjaA protein delivered by Salmonella enterica sv. Typhimurium strain with regulated delayed attenuation in chickens. World J. Microbiol. Biotechnol. 30, 281–292. doi:10.1007/s11274-013-1447-5
Lara-Tejero, M., and Galán, J. E. (2000). A bacterial toxin that controls cell cycle progression as a deoxyribonuclease I-like protein. Science 290, 354–357. doi:10.1126/science.290.5490.354
Larson, C. L., Samuelson, D. R., Eucker, T. P., O'Loughlin, J. L., and Konkel, M. E. (2013). The fibronectin-binding motif within FlpA facilitates Campylobacter jejuni adherence to host cell and activation of host cell signaling. Emerg. Microbes Infect. 2 (10), e65. doi:10.1038/emi.2013.65
Layton, S. L., Morgan, M. J., Cole, K., Kwon, Y. M., Donoghue, D. J., Hargis, B. M., et al. (2011). Evaluation of Salmonella-vectored Campylobacter peptide epitopes for reduction of Campylobacter jejuni in broiler chickens. Clin. Vaccine Immunol. 18, 449–454. doi:10.1128/CVI.00379-10
Leonhard, S. E., Papri, N., Querol, L., Rinaldi, S., Shahrizaila, N., and Jacobs, B. C. (2024). Guillain-Barré syndrome. Nat. Rev. Dis. Prim. 10 (1), 97. doi:10.1038/s41572-024-00580-4
Line, J., Svetoch, E., Eruslanov, B., Perelygin, V., Mitsevich, E., Mitsevich, I., et al. (2008). Isolation and purification of enterocin E-760 with broad antimicrobial activity against gram-positive and gram-negative bacteria. Antimicrob. Agents. Chemother. 52, 1094–1100. doi:10.1128/AAC.01569-06
Linz, B., Sharafutdinov, I., Tegtmeyer, N., and Backert, S. (2023). Evolution and role of proteases in Campylobacter jejuni lifestyle and pathogenesis. Biomolecules 13 (2), 323. doi:10.3390/biom13020323
Lobo De Sá, F. D., Backert, S., Nattramilarasu, P., Mousavi, S., Sandle, G., Bereswill, S., et al. (2021c). Vitamin D reverses disruption of gut epithelial barrier function caused by Campylobacter jejuni. Int. J. Mol. Sci. 22 (16), 8872. doi:10.3390/ijms22168872
Lobo De Sá, F. D., Heimesaat, M. M., Bereswill, S., Nattramilarasu, P. K., Schulzke, J. D., and Bucker, R. (2021b). Resveratrol prevents Campylobacter jejuni-induced leaky gut by restoring occludin and claudin-5 in the paracellular leak pathway. Front. Pharmacol. 12, 640572. doi:10.3389/fphar.2021.640572
Lobo De Sá, F. D., Schulzke, J. D., and Bucker, R. (2021a). Diarrheal mechanisms and the role of intestinal barrier dysfunction in Campylobacter infections. Curr. Top. Microbiol. Immunol. 431, 203–231. doi:10.1007/978-3-030-65481-8_8
Luethy, P. M., Huynh, S., Ribardo, D. A., Winter, S. E., Parker, C. T., and Hendrixson, D. R. (2017). Microbiota-derived short-chain fatty acids modulate expression of Campylobacter jejuni determinants required for commensalism and virulence. mBio 8 (3), e00407-17–e00417. doi:10.1128/mBio.00407-17
Maczka, W., Twardawska, M., Grabarczyk, M., and Winska, K. (2023). Carvacrol-A natural phenolic compound with antimicrobial properties. Antibiotics 12 (5), 824. doi:10.3390/antibiotics12050824
Mansfield, L. S., Bell, J. A., Wilson, D. L., Murphy, A. J., Elsheikha, H. M., Rathinam, V. A., et al. (2007). C57BL/6 and congenic interleukin-10-deficient mice can serve as models of Campylobacter jejuni colonization and enteritis. Infect. Immun. 75, 1099–1115. doi:10.1128/IAI.00833-06
Mansfield, L. S., Patterson, J. S., Fierro, B. R., Murphy, A. J., Rathinam, V. A., Kopper, J. J., et al. (2008). Genetic background of IL-10(-/-) mice alters host-pathogen interactions with Campylobacter jejuni and influences disease phenotype. Microb. Pathog. 45, 241–257. doi:10.1016/j.micpath.2008.05.010
Masanta, W. O., Heimesaat, M. M., Bereswill, S., Tareen, A. M., Lugert, R., Gross, U., et al. (2013). Modification of intestinal microbiota and its consequences for innate immune response in the pathogenesis of campylobacteriosis. Clin. Dev. Immunol. 2013, 526860. doi:10.1155/2013/526860
McKenna, A., Ijaz, U. Z., Kelly, C., Linton, M., Sloan, W. T., Green, B. D., et al. (2020). Impact of industrial production system parameters on chicken microbiomes: mechanisms to improve performance and reduce Campylobacter. Microbiome 8, 128. doi:10.1186/s40168-020-00908-8
Messaoudi, S., Kergourlay, G., Dalgalarrondo, M., Choiset, Y., Ferchichi, M., Prevost, H., et al. (2012). Purification and characterization of a new bacteriocin active against Campylobacter produced by Lactobacillus salivarius SMXD51. Food Microbiol. 32, 129–134. doi:10.1016/j.fm.2012.05.002
Meunier, M., Guyard-Nicodeme, M., Hirchaud, E., Parra, A., Chemaly, M., and Dory, D. (2016). Identification of novel vaccine candidates against Campylobacter through reverse vaccinology. J. Immunol. Res. 2016, 5715790. doi:10.1155/2016/5715790
Meunier, M., Guyard-Nicodeme, M., Vigouroux, E., Poezevara, T., Beven, V., Quesne, S., et al. (2017). Promising new vaccine candidates against Campylobacter in broilers. PLoS One 12, e0188472. doi:10.1371/journal.pone.0188472
Meunier, M., Guyard-Nicodeme, M., Vigouroux, E., Poezevara, T., Beven, V., Quesne, S., et al. (2018). A DNA prime/protein boost vaccine protocol developed against Campylobacter jejuni for poultry. Vaccine 36, 2119–2125. doi:10.1016/j.vaccine.2018.03.004
Miller, C., Williams, P., and Ketley, J. (2009). Pumping iron: mechanisms for iron uptake by Campylobacter. Microbiology-SGM 155, 3157–3165. doi:10.1099/mic.0.032425-0
Mousavi, S., Bereswill, S., and Heimesaat, M. (2019a). Immunomodulatory and antimicrobial effects of vitamin C. Eur. J. Microbiol. Immunol. (Bp) 9 (3), 73–79. doi:10.1556/1886.2019.00016
Mousavi, S., Bereswill, S., and Heimesaat, M. M. (2020a). Novel clinical Campylobacter jejuni infection models based on sensitization of mice to lipooligosaccharide, a major bacterial factor triggering innate immune responses in human campylobacteriosis. Microorganisms 8 (4), 482. doi:10.3390/microorganisms8040482
Mousavi, S., Bereswill, S., and Heimesaat, M. M. (2021a). Murine models for the investigation of colonization resistance and innate immune responses in Campylobacter jejuni infections. Curr. Top. Microbiol. Immunol. 431, 233–263. doi:10.1007/978-3-030-65481-8_9
Mousavi, S., Busmann, L., Bandick, R., Shayya, N., Bereswill, S., and Heimesaat, M. (2023a). Oral application of carvacrol, butyrate, ellagic acid, and 2'-fucosyl-lactose to mice suffering from acute campylobacteriosis - results from a preclinical placebo-controlled intervention study. Eur. J. Microbiol. Immunol. (Bp) 13 (3), 88–105. doi:10.1556/1886.2023.00037
Mousavi, S., De Sá, F., Schulzke, J., Bücker, R., Bereswill, S., and Heimesaat, M. (2019b). Vitamin D in acute campylobacteriosis-results from an intervention study applying a clinical Campylobacter jejuni induced enterocolitis model. Front. Immunol. 10, 2094. doi:10.3389/fimmu.2019.02094
Mousavi, S., Escher, U., Thunhorst, E., Kittler, S., Kehrenberg, C., Bereswill, S., et al. (2020c). Vitamin C alleviates acute enterocolitis in Campylobacter jejuni infected mice. Sci. Rep. 10 (1), 2921. doi:10.1038/s41598-020-59890-8
Mousavi, S., Foote, M., Du, K., Bandick, R., Bereswill, S., and Heimesaat, M. (2024). Oral treatment of human gut microbiota associated IL-10-/- mice suffering from acute campylobacteriosis with carvacrol, deferoxamine, deoxycholic acid, and 2-fucosyl-lactose. Front. Microbiol. 15, 1290490. doi:10.3389/fmicb.2024.1290490
Mousavi, S., Schmidt, A., Escher, U., Kittler, S., Kehrenberg, C., Thunhorst, E., et al. (2020b). Carvacrol ameliorates acute campylobacteriosis in a clinical murine infection model. Gut Pathog. 12, 2. doi:10.1186/s13099-019-0343-4
Mousavi, S., Weschka, D., Bereswill, S., and Heimesaat, M. (2021b). Immune-modulatory effects upon oral application of cumin-essential-oil to mice suffering from acute campylobacteriosis. Pathogens 10 (7), 818. doi:10.3390/pathogens10070818
Mousavi, S., Weschka, D., Bereswill, S., and Heimesaat, M. (2021c). Preclinical evaluation of oral urolithin-A for the treatment of acute campylobacteriosis in Campylobacter jejuni infected microbiota-depleted IL-10-/- mice. Pathogens 10 (1), 7. doi:10.3390/pathogens10010007
Mousavi, S., Weschka, D., Bereswill, S., and Heimesaat, M. (2023b). Disease alleviating effects following prophylactic lemon and coriander essential oil treatment in mice with acute campylobacteriosis. Front. Microbiol. 14, 1154407. doi:10.3389/fmicb.2023.1154407
Mughini-Gras, L., Pijnacker, R., Coipan, C., Mulder, A. C., Fernandes Veludo, A., de Rijk, S., et al. (2021). Sources and transmission routes of campylobacteriosis: a combined analysis of genome and exposure data. J. Infect. 82, 216–226. doi:10.1016/j.jinf.2020.09.039
Mulder, A. C., Franz, E., de Rijk, S., Versluis, M. A. J., Coipan, C., Buij, R., et al. (2020). Tracing the animal sources of surface water contamination with Campylobacter jejuni and Campylobacter coli. Water Res. 187, 116421. doi:10.1016/j.watres.2020.116421
Nachamkin, I., Ung, H., and Li, M. (2002). Increasing fluoroquinolone resistance in Campylobacter jejuni, Pennsylvania, USA, 1982–2001. Emerg. Infect. Dis. 8, 1501–1503. doi:10.3201/eid0812.020115
Neal-McKinney, J. M., Samuelson, D. R., Eucker, T. P., Nissen, M. S., Crespo, R., and Konkel, M. E. (2014). Reducing Campylobacter jejuni colonization of poultry via vaccination. PLoS One 9, e114254. doi:10.1371/journal.pone.0114254
Negretti, N. M., Gourley, C. R., Talukdar, P. K., Clair, G., Klappenbach, C. M., Lauritsen, C. J., et al. (2021). The Campylobacter jejuni CiaD effector co-opts the host cell protein IQGAP1 to promote cell entry. Nat. Commun. 12 (1), 1339. doi:10.1038/s41467-021-21579-5
Nishiyama, K., Seto, Y., Yoshioka, K., Kakuda, T., Takai, S., Yamamoto, Y., et al. (2014). Lactobacillus gasseri SBT2055 reduces infection by and colonization of Campylobacter jejuni. PLOS One 9 (9), e108827. eCollection 2014. doi:10.1371/journal.pone.0108827
Northcutt, J., Smith, D., Ingram, K. D., Hinton, A.Jr., and Musgrove, M. (2007). Recovery of bacteria from broiler carcasses after spray washing with acidified electrolyzed water or sodium hypochlorite solutions. Poult. Sci. 86 (10), 2239–2244. doi:10.1093/ps/86.10.2239
Northcutt, J. K., Smith, D. P., Musgrove, M. T., Ingram, K. D., and Hinton, A. (2005). Microbiological impact of spray washing broiler carcasses using different chlorine concentrations and water temperatures. Poult. Sci. 84 (10), 1648–1652. doi:10.1093/ps/84.10.1648
Nothaft, H., Davis, B., Lock, Y. Y., Perez-Munoz, M. E., Vinogradov, E., Walter, J., et al. (2016). Engineering the Campylobacter jejuni N-glycan to create an effective chicken vaccine. Sci. Rep. 6, 26511. doi:10.1038/srep26511
Nothaft, H., Perez-Munoz, M. E., Gouveia, G. J., Duar, R. M., Wanford, J. J., Lango-Scholey, L., et al. (2017). Coadministration of the Campylobacter jejuni N-Glycan-Based vaccine with probiotics improves vaccine performance in broiler chickens. Appl. Environ. Microbiol. 83 (23), 015233–e1617. doi:10.1128/AEM.01523-17
Nothaft, H., Perez-Munoz, M. E., Yang, T., Murugan, A. V. M., Miller, M., Kolarich, D., et al. (2021). Improving chicken responses to glycoconjugate vaccination against Campylobacter jejuni. Front. Microbiol. 12, 734526. doi:10.3389/fmicb.2021.734526
O Cróinín, T., and Backert, S. (2012). Host epithelial cell invasion by Campylobacter jejuni: trigger or zipper mechanism? Front. Cell Infect. Microbiol. 2, 25. doi:10.3389/fcimb.2012.00025
Okamura, M., Tominaga, A., Ueda, M., Ohshima, R., Kobayashi, M., Tsukada, M., et al. (2012). Irrelevance between the induction of anti-Campylobacter humoral response by a bacterin and the lack of protection against homologous challenge in Japanese jidori chickens. J. Vet. Med. Sci. 74 (1), 75–78. doi:10.1292/jvms.11-0286
Omarova, S., Awad, K., Moos, V., Püning, C., Gölz, G., Schulzke, J., et al. (2023). Intestinal barrier in post-Campylobacter jejuni irritable bowel syndrome. Biomolecules 13 (3), 449. doi:10.3390/biom13030449
One World One Health (2020). The Manhattan principles. Available online at: https://oneworldonehealth.wcs.org/About-Us/Mission/The-Manhattan-Principles.aspx (Accessed February 07, 2025).
One World One Health (2021). One World - one Health™ - MISSION statement. Available online at: https://oneworldonehealth.wcs.org/About-Us/Mission.aspx (Accessed February 07, 2025).
Pac-Campy (2023). Available online at: https://www.zoonosen.net/en/forschungsnetz/verbunde-nachwuchsgruppen/pac-campy (Accessed February 07, 2025).
Pangga, G. M., Star-Shirko, B., Psifidi, A., Xia, D., Corcionivoschi, N., Kelly, C., et al. (2025). Impact of commercial gut health interventions on caecal metagenome and broiler performance. Microbiome 13, 30. doi:10.1186/s40168-024-02012-7
Peh, E., Kittler, S., Reich, F., and Kehrenberg, C. (2020). Antimicrobial activity of organic acids against Campylobacter spp. and development of combinations - a synergistic effect? PLOS One 15 (9), e0239312. doi:10.1371/journal.pone.0239312
Pitt, S. J., and Gunn, A. (2024). The one health concept. Br. J. Biomed. Sci. 81, 12366. doi:10.3389/bjbs.2024.12366
Plowright, R. K., Parrish, C. R., McCallum, H., Hudson, P. J., Ko, A. I., Graham, A. L., et al. (2017). Pathways to zoonotic spillover. Nat. Rev. Microbiol. 15 (8), 502–510. doi:10.1038/nrmicro.2017.45
Pumtang-On, P., Mahony, T. J., Hill, R. A., and Vanniasinkam, T. (2021). A systematic review of Campylobacter jejuni vaccine candidates for chickens. Microorganisms 9 (2), 397. doi:10.3390/microorganisms9020397
Püning, C., Su, Y., Lu, X., and Gölz, G. (2021). Molecular mechanisms of Campylobacter biofilm formation and quorum sensing. Curr. Top. Microbiol. Immunol. 43, 293–319. doi:10.1007/978-3-030-65481-8_11
Rahimzadeh, M., Rahimzadeh, M., Kazemi, S., Moghadamnia, A., Amiri, M., and Moghadamnia, A. (2023). Iron; Benefits or threatens (with emphasis on mechanism and treatment of its poisoning). Hum. Exp. Toxicol. 42, 9603271231192361. doi:10.1177/09603271231192361
Ridley, A. M., Toszeghy, M. J., Cawthraw, S. A., Wassenaar, T. M., and Newell, D. G. (2008). Genetic instability is associated with changes in the colonization potential of Campylobacter jejuni in the avian intestine. J. Appl. Microbiol. 105, 95–104. doi:10.1111/j.1365-2672.2008.03759.x
Rosner, B. M., Schielke, A., Didelot, X., Kops, F., Breidenbach, J., Willrich, N., et al. (2017). A combined case-control and molecular source attribution study of human Campylobacter infections in Germany, 2011–2014. Sci. Rep. 7, 5139. doi:10.1038/s41598-017-05227-x
Sahin, O., Luo, N., Huang, S., and Zhang, Q. (2003). Effect of Campylobacter-specific maternal antibodies on Campylobacter jejuni colonization in young chickens. Appl. Environ. Microbiol. 69, 5372–5379. doi:10.1128/AEM.69.9.5372-5379.2003
Santos-Ferreira, N., Ferreira, V., and Teixeira, P. (2022). Occurrence and multidrug resistance of Campylobacter in chicken meat from different production systems. Foods 11, 1827. doi:10.3390/foods11131827
Schloss, P. D., Schubert, A. M., Zackular, J. P., Iverson, K. D., Young, V. B., and Petrosino, J. F. (2012). Stabilization of the murine gut microbiome following weaning. Gut Microbes 3 (4), 383–393. doi:10.4161/gmic.21008
Schmidt, A. M., Escher, U., Mousavi, S., Boehm, M., Backert, S., Bereswill, S., et al. (2019b). Protease activity of Campylobacter jejuni HtrA modulates distinct intestinal and systemic immune responses in infected secondary abiotic IL-10 deficient mice. Front. Cell. Infect. Microbiol. 9, 79. doi:10.3389/fcimb.2019.00079
Schmidt, A. M., Escher, U., Mousavi, S., Tegtmeyer, N., Boehm, M., Backert, S., et al. (2019a). Immunopathological properties of the Campylobacter jejuni flagellins and the adhesin CadF as assessed in a clinical murine infection model. Gut Pathog. 11, 24. doi:10.1186/s13099-019-0306-9
Senderovich, H., and Vierhout, M. (2018). Is there a role for charcoal in palliative diarrhea management? Curr. Med. Res. Opin. 34 (7), 1253–1259. doi:10.1080/03007995.2017.1416345
Sharafutdinov, I., Esmaeili, D. S., Harrer, A., Tegtmeyer, N., Sticht, H., and Backert, S. (2020). Campylobacter jejuni serine protease HtrA cleaves the tight junction component claudin-8. Front. Cell. Infect. Microbiol. 10, 590186. doi:10.3389/fcimb.2020.590186
Sharafutdinov, I., Knorr, J., Rottner, K., Backert, S., and Tegtmeyer, N. (2022a). Cortactin: a universal host cytoskeletal target of Gram-negative and Gram-positive bacterial pathogens. Mol. Microbiol. 118, 623–636. doi:10.1111/mmi.15002
Sharafutdinov, I., Tegtmeyer, N., Musken, M., and Backert, S. (2022b). Campylobacter jejuni serine protease HtrA induces paracellular transmigration of microbiota across polarized intestinal epithelial cells. Biomolecules 12 (4), 521. doi:10.3390/biom12040521
Sharafutdinov, I., Tegtmeyer, N., Rohde, M., Olofsson, A., Rehman, Z., Arnqvist, A., et al. (2024). Campylobacter jejuni surface-bound protease HtrA, but not the secreted protease nor protease in shed membrane vesicles, disrupts epithelial cell-to-cell junctions. Cells 13 (3), 224. doi:10.3390/cells13030224
Sharan, M., Vijay, D., Yadav, J. P., Bedi, J. S., and Dhaka, P. (2023). Surveillance and response strategies for zoonotic diseases: a comprehensive review. Sci. One Health 2, 100050. doi:10.1016/j.soh.2023.100050
Sharifi-Rad, J., El Rayess, Y., Rizk, A., Sadaka, C., Zgheib, R., Zam, W., et al. (2020). Turmeric and its major compound curcumin on health: bioactive effects and safety profiles for food, pharmaceutical, biotechnological and medicinal applications. Front. Pharmacol. 11, 01021. doi:10.3389/fphar.2020.01021
Shin, E., Oh, Y., Kim, M., Jung, J., and Lee, Y. (2012). Antimicrobial resistance patterns and corresponding multilocus sequence types of the Campylobacter jejuni isolates from human diarrheal samples. Microb. Drug Resist. 19, 110–116. doi:10.1089/mdr.2012.0099
Sima, F., Stratakos, A. C., Ward, P., Linton, M., Kelly, C., Pinkerton, L., et al. (2018). A novel natural antimicrobial can reduce the in vitro and in vivo pathogenicity of T6SS positive Campylobacter jejuni and Campylobacter coli chicken isolates. Front. Microbiol. 9, 2139. doi:10.3389/fmicb.2018.02139
Song, Y. C., Jin, S., Louie, H., Ng, D., Lau, R., Zhang, Y., et al. (2004). FlaC, a protein of Campylobacter jejuni TGH9011 (ATCC43431) secreted through the flagellar apparatus, binds epithelial cells and influences cell invasion. Mol. Microbiol. 53, 541–553. doi:10.1111/j.1365-2958.2004.04175.x
Stahl, M., Ries, J., Vermeulen, J., Yang, H., Sham, H. P., Crowley, S. M., et al. (2014). A novel mouse model of Campylobacter jejuni gastroenteritis reveals key pro-inflammatory and tissue protective roles for Toll-like receptor signaling during infection. PLoS Pathog. 10, e1004264. doi:10.1371/journal.ppat.1004264
Stahl, M., and Vallance, B. A. (2015). Insights into Campylobacter jejuni colonization of the mammalian intestinal tract using a novel mouse model of infection. Gut Microbes 6, 143–148. doi:10.1080/19490976.2015.1016691
Stephenson, H. N., John, C. M., Naz, N., Gundogdu, O., Dorrell, N., Wren, B. W., et al. (2013). Campylobacter jejuni lipooligosaccharide sialylation, phosphorylation, and amide/ester linkage modifications fine-tune human Toll-like receptor 4 activation. J. Biol. Chem. 288, 19661–19672. doi:10.1074/jbc.M113.468298
Stern, N. J., Eruslanov, B. V., Pokhilenko, V. D., Kovalev, Y. N., Volodina, L. L., Perelygin, V. V., et al. (2008). Bacteriocins reduce Campylobacter jejuni colonization while bacteria producing bacteriocins are ineffective. Microb. Ecol. Health Dis. 20, 74–79. doi:10.1080/08910600802030196
Stern, N. J., Svetoch, E. A., Eruslanov, B. V., Perelygin, V. V., Mitsevich, E. V., Mitsevich, I. P., et al. (2006). Isolation of a Lactobacillus salivarius strain and purification of its bacteriocin, which is inhibitory to Campylobacter jejuni in the chicken gastrointestinal system. Antimicrob. Agents Chemother. 50, 3111–3116. doi:10.1128/AAC.00259-06
Sugrue, I., Ross, R., and Hill, C. (2024). Bacteriocin diversity, function, discovery and application as antimicrobials. Nat. Rev. Microbiol. 22 (9), 556–571. doi:10.1038/s41579-024-01045-x
Svetoch, E., and Stern, N. (2010). Bacteriocins to control Campylobacter spp. in poultry - a review. Poult. Sci. 89 (8), 1763–1768. doi:10.3382/ps.2010-00659
Svetoch, E., Stern, N., Eruslanov, B., Kovalev, Y., Volodina, L., Perelygin, V., et al. (2005). Isolation of Bacillus circulans and Paenibacillus polymyxa strains inhibitory to Campylobacter jejuni and characterization of associated bacteriocins. J. Food Prot. 68 (1), 11–17. doi:10.4315/0362-028x-68.1.11
Svetoch, E. A., Eruslanov, B. V., Perelygin, V. V., Mitsevich, E. V., Mitsevich, I. P., Borzenkov, V. N., et al. (2008). Diverse antimicrobial killing by Enterococcus faecium E 50-52 bacteriocin. J. Agric. Food Chem. 56, 1942–1948. doi:10.1021/jf073284g
Szott, V., and Friese, A. (2021). Emission sources of Campylobacter from agricultural farms, impact on environmental contamination and intervention strategies. Curr. Top. Microbiol. Immunol. 431, 103–125. doi:10.1007/978-3-030-65481-8_5
Szott, V., Reichelt, B., Alter, T., Friese, A., and Roesler, U. (2020). In vivo efficacy of carvacrol on Campylobacter jejuni prevalence in broiler chickens during an entire fattening period. Eur. J. Microbiol. Immunol. (Bp) 10, 131–138. doi:10.1556/1886.2020.00011
Szymanski, C. M., Burr, D. H., and Guerry, P. (2002). Campylobacter protein glycosylation affects host cell interactions. Infect. Immun. 70, 2242–2244. doi:10.1128/IAI.70.4.2242-2244.2002
Szymanski, C. M., Logan, S. M., Linton, D., and Wren, B. W. (2003). Campylobacter - a tale of two protein glycosylation systems. Trends Microbiol. 11, 233–238. doi:10.1016/s0966-842x(03)00079-9
Taha-Abdelaziz, K., Singh, M., Sharif, S., Sharma, S., Kulkarni, R., Alizadeh, M., et al. (2023). Intervention strategies to control Campylobacter at different stages of the food chain. Microorganisms 11 (1), 113. doi:10.3390/microorganisms11010113
Tegtmeyer, N., Sharafutdinov, I., Harrer, A., Esmaeili, D. S., Linz, B., and Backert, S. (2021). Campylobacter virulence factors and molecular host-pathogen interactions. Curr. Top. Microbiol. Immunol. 431, 169–202. doi:10.1007/978-3-030-65481-8_7
Theoret, J. R., Cooper, K. K., Zekarias, B., Roland, K. L., Law, B. F., Curtiss, R., et al. (2012). The Campylobacter jejuni Dps homologue is important for in vitro biofilm formation and cecal colonization of poultry and may serve as a protective antigen for vaccination. Clin. Vaccine Immunol. 19, 1426–1431. doi:10.1128/CVI.00151-12
Thomrongsuwannakij, T., Blackall, P. J., and Chansiripornchai, N. (2017). A study on Campylobacter jejuni and Campylobacter coli through commercial broiler production chains in Thailand: antimicrobial resistance, the characterization of dna gyrase subunit A mutation, and genetic diversity by flagellin A gene restriction fragmen. Avian Dis. 61, 186–197. doi:10.1637/11546-120116-Reg.1
Tow, W., Chee, P., Sundralingam, U., and Palanisamy, U. (2022). The therapeutic relevance of urolithins, intestinal metabolites of ellagitannin-rich food: a systematic review of in vivo studies. Nutrients 14 (17), 3494. doi:10.3390/nu14173494
Upadhyay, A., Arsi, K., Wagle, B. R., Upadhyaya, I., Shrestha, S., Donoghue, A. M., et al. (2017). Trans-cinnamaldehyde, carvacrol, and eugenol reduce Campylobacter jejuni colonization factors and expression of virulence genes in vitro. Front. Microbiol. 8, 713. doi:10.3389/fmicb.2017.00713
Vaure, C., and Liu, Y. (2014). A comparative review of toll-like receptor 4 expression and functionality in different animal species. Front. Immunol. 5, 316. doi:10.3389/fimmu.2014.00316
Veronese, P., and Dodi, I. (2024). Campylobacter jejuni/coli infection: is it still a concern? Microorganisms 12 (12), 2669. doi:10.3390/microorganisms12122669
Vitovec, J., Koudela, B., Sterba, J., Tomancova, I., Matyas, Z., and Vladik, P. (1989). The gnotobiotic piglet as a model for the pathogenesis of Campylobacter jejuni infection. Zentralbl. Bakteriol. 271 (1), 91–103. doi:10.1016/s0934-8840(89)80058-1
Wagenaar, J. A., Van Bergen, M. A., Mueller, M. A., Wassenaar, T. M., and Carlton, R. M. (2005). Phage therapy reduces Campylobacter jejuni colonization in broilers. Vet. Microbiol. 109 (3-4), 275–283. doi:10.1016/j.vetmic.2005.06.002
Wang, C. W., Zhou, H. Z., Guo, F. F., Yang, B., Su, X., Lin, J., et al. (2020). Oral immunization of chickens with Lactococcus lactis expressing cjaA temporarily reduces Campylobacter jejuni colonization. Foodborne Pathog. Dis. 17 (6), 366–372. doi:10.1089/fpd.2019.2727
Wassenaar, T. M., Vanderzeijst, B. A. M., Ayling, R., and Newell, D. G. (1993). Colonization of chicks by motility mutants of Campylobacter jejuni demonstrates the importance of flagellin-a expression. J. Gen. Microbiol. 139 (Pt 6), 1171–1175. doi:10.1099/00221287-139-6-1171
Watson, R. O., and Galán, J. E. (2008). Campylobacter jejuni survives within epithelial cells by avoiding delivery to lysosomes. PLoS Pathog. 4 (1), e14. doi:10.1371/journal.ppat.0040014
WHO (2020). World Health Organisation. Campylobacter. Available online at: https://www.who.int/news-room/fact-sheets/detail/campylobacter (Accessed February 07, 2025).
WHO (2024). World Health Organisation. Available online at: https://www.who.int/news-room/fact-sheets/detail/diarrhoeal-disease (Accessed February 07, 2025).
Wozniak-Biel, A., Bugla-Płoskonska, G., Kielsznia, A., Korzekwa, K., Tobiasz, A., Korzeniowska-Kowal, A., et al. (2017). High prevalence of resistance to fluoroquinolones and tetracycline Campylobacter spp. isolated from poultry in Poland. Microb. Drug Resist. 24, 314–322. doi:10.1089/mdr.2016.0249
Wyszynska, A., Raczko, A., Lis, M., and Jagusztyn-Krynicka, E. K. (2004). Oral immunization of chickens with avirulent Salmonella vaccine strain carrying C. jejuni 72Dz/92 cjaA gene elicits specific humoral immune response associated with protection against challenge with wild-type Campylobacter. Vaccine 22, 1379–1389. doi:10.1016/j.vaccine.2003.11.001
Yang, B., Fang, D., Lv, Q., Wang, Z., and Liu, Y. (2021). Targeted therapeutic strategies in the battle against pathogenic bacteria. Front. Pharmacol. 12, 673239. doi:10.3389/fphar.2021.673239
Young, C. R., Ziprin, R. L., Hume, M. E., and Stanker, L. H. (1999b). Dose response and organ invasion of day-of-hatch leghorn chicks by different isolates of Campylobacter jejuni. Avian Dis. 43, 763–767. doi:10.2307/1592745
Young, G. M., Schmiel, D. H., and Miller, V. L. (1999a). A new pathway for the secretion of virulence factors by bacteria: the flagellar export apparatus functions as a protein-secretion system. Proc. Natl. Acad. Sci. U.S.A. 96, 6456–6461. doi:10.1073/pnas.96.11.6456
Young, K. T., Davis, L. M., and DiRita, V. J. (2007). Campylobacter jejuni: molecular biology and pathogenesis. Nat. Rev. Microbiol. 5 (9), 665–679. doi:10.1038/nrmicro1718
Young, N. M., Brisson, J. R., Kelly, J., Watson, D. C., Tessier, L., Lanthier, P. H., et al. (2002). Structure of the N-linked glycan present on multiple glycoproteins in the Gram-negative bacterium, Campylobacter jejuni. J. Biol. Chem. 277, 42530–42539. doi:10.1074/jbc.M206114200
Yrios, J. W., and Balish, E. (1986a). Colonization and infection of athymic and euthymic germfree mice by Campylobacter jejuni and Campylobacter fetus subsp. Fetus. Infect. Immun. 53, 378–383. doi:10.1128/iai.53.2.378-383.1986
Yrios, J. W., and Balish, E. (1986b). Pathogenesis of Campylobacter spp. in athymic and euthymic germfree mice. Infect. Immun. 53, 384–392. doi:10.1128/iai.53.2.384-392.1986
Yu, C., Xu, H., Jiang, J., Tan, Z., Peng, X., Liu, S., et al. (2024). Effects of benzoic acid or benzocal-50 supplementation on growth performance and intestinal health in mixed-sex Ross 308 chickens under a high stocking density. J. Appl. Poult. Res. 33 (3), 100454. doi:10.1016/j.japr.2024.100454
Zainol, M., Safiyanu, M., Abd Aziz, S., Omar, A., Chuang, K., and Mariatulqabtiah, A. (2024). Campylobacteriosis and control strategies against campylobacters in poultry farms. J. Microbiol. Biotechnol. 34 (5), 987–993. doi:10.4014/jmb.2311.11045
Zarzecka, U., Grinzato, A., Kandiah, E., Cysewski, D., Berto, P., SkóRko-Glonek, J., et al. (2020). Functional analysis and cryo-electron microscopy of Campylobacter jejuni serine protease HtrA. Gut Microbes 12 (1), 1–16. doi:10.1080/19490976.2020.1810532
Zarzecka, U., Tegtmeyer, N., Sticht, H., and Backert, S. (2023). Trimer stability of Helicobacter pylori HtrA is regulated by a natural mutation in the protease domain. Med. Microbiol. Immunol. 212 (3), 241–252. doi:10.1007/s00430-023-00766-9
Zeng, X., Xu, F., and Lin, J. (2010). Development and evaluation of CmeC subunit vaccine against Campylobacter jejuni. J. Vaccines Vaccin. 1, 112. doi:10.4172/2157-7560.1000112
Zhang, Q., Liu, J., Han, L., Li, X., Zhang, C., Guo, Z., et al. (2024). How far has the globe gone in achieving One Health? Current evidence and policy implications based on global One Health index. Sci. One Health 3, 100064. doi:10.1016/j.soh.2024.100064
Keywords: Campylobacterjejuni, gut microbiota, epithelial barrier, One Health, phytochemicals, probiotics, bacteriophage, vaccine
Citation: Sharafutdinov I, Linz B, Tegtmeyer N and Backert S (2025) Therapeutic and protective approaches to combat Campylobacter jejuni infections. Front. Pharmacol. 16:1572616. doi: 10.3389/fphar.2025.1572616
Received: 07 February 2025; Accepted: 16 April 2025;
Published: 06 May 2025.
Edited by:
Roland Bücker, Charité University Medicine Berlin, GermanyReviewed by:
Ozan Gundogdu, University of London, United KingdomKerstin Stingl, NRL for Campylobacter, United Kingdom
Copyright © 2025 Sharafutdinov, Linz, Tegtmeyer and Backert. This is an open-access article distributed under the terms of the Creative Commons Attribution License (CC BY). The use, distribution or reproduction in other forums is permitted, provided the original author(s) and the copyright owner(s) are credited and that the original publication in this journal is cited, in accordance with accepted academic practice. No use, distribution or reproduction is permitted which does not comply with these terms.
*Correspondence: Steffen Backert, c3RlZmZlbi5iYWNrZXJ0QGZhdS5kZQ==
†Present address: Irshad Sharafutdinov, Department of Microbiology and Immunology, University of California, San Francisco, CA, United States