- 1Department of Endocrinology, Hospital of Chengdu University of Traditional Chinese Medicine, Chengdu, Sichuan, China
- 2Department of Emergency, Hospital of Chengdu University of Traditional Chinese Medicine, Chengdu, Sichuan, China
- 3Affiliated Hospital of Jiangxi University of Chinese Medicine, Nanchang, Jiangxi, China
- 4TCM Regulating Metabolic Diseases Key Laboratory of Sichuan Province, Hospital of Chengdu University of Traditional Chinese Medicine, Chengdu, Sichuan, China
Salvia miltiorrhiza Bunge [Lamiaceae; Salviae miltiorrhizae radix et rhizoma] is a traditional Chinese medication used extensively as a therapeutic agent against atherosclerosis (AS) because of its substantial cardiovascular protective properties as well as ability to regulate the signaling cascades and molecular targets involved in AS. Preclinical research has shown that the hydrophilic metabolites of S. miltiorrhiza Bunge represented by danshensu (DSS), salvianolic acid A (SAA), and salvianolic acid B (SAB) can reduce endothelial dysfunction, inhibit smooth muscle cell migration and proliferation, block platelet aggregation, have antithrombotic properties, and modulate vascular tone. Furthermore, studies have shown that salvianolic acid is clinically beneficial, while some evidence also supports its safety and effectiveness in diseases linked to AS. The present study is a review of the anti-atherosclerotic pharmacological activities, pharmacokinetic characteristics, drug interactions, and safety evaluations of salvianolic acid over the last 20 years. Herein, we focus on the cellular targets linked to AS; clarify the molecular mechanisms of the anti-atherosclerotic activities of DSS, SAA, and SAB; and discuss the future needs and priorities in light of the limitations of the existing studies. This review is intended to establish the groundwork and offer a thorough perspective for deeper investigations of the studies, clinical uses, and product development efforts of salvianolic acid as a natural modulator of AS.
1 Introduction
Atherosclerosis (AS) constitutes a multifaceted pathological process characterized by chronic non-specific inflammation within the arterial walls (Wolf and Ley, 2019). The core pathogenesis of AS entails a complex interplay of lipid metabolism dysregulation, vascular endothelial dysfunction, inflammatory cell infiltration, and subsequent foam cell formation, ultimately culminating in atherosclerotic plaque development and vascular remodeling (Figure 1) (Mauersberger et al., 2022; Orecchioni et al., 2022; Wang et al., 2015a; Xu et al., 2021; Zheng et al., 2017). Epidemiological evidence has consistently demonstrated that AS-related cardiovascular diseases, including coronary heart disease, myocardial infarction, and stroke, remain the primary causes of mortality globally (Souilhol et al., 2018). Notably, the incidence of these AS-associated diseases continues to escalate in developing countries, posing significant public health challenges (Cainzos-Achirica et al., 2020; Poller et al., 2020). Current anti-AS therapies like statins, proprotein convertase subtilisin/kexin type 9 (PCSK-9) inhibitors, and antiplatelet agents has certain limitations: statins are known to cause myotoxicity (1.9%–30% incidence) and hepatotoxicity; aspirin increases the risk of gastrointestinal bleeding (9.4%); clopidogrel shows resistance due to CYP2C19 polymorphism; PCSK-9 inhibitors are costly, and their interventional procedures have high restenosis rates (34.8%–73.1%) and expenses (Abd and Jacobson, 2011; Ariesen et al., 2006; Kashani et al., 2006; Montastruc, 2023; Pradhan et al., 2024; Turner and Pirmohamed, 2019; Ward et al., 2019; Wu et al., 2017). These challenges highlight the urgent need for safer multitarget anti-AS drugs.
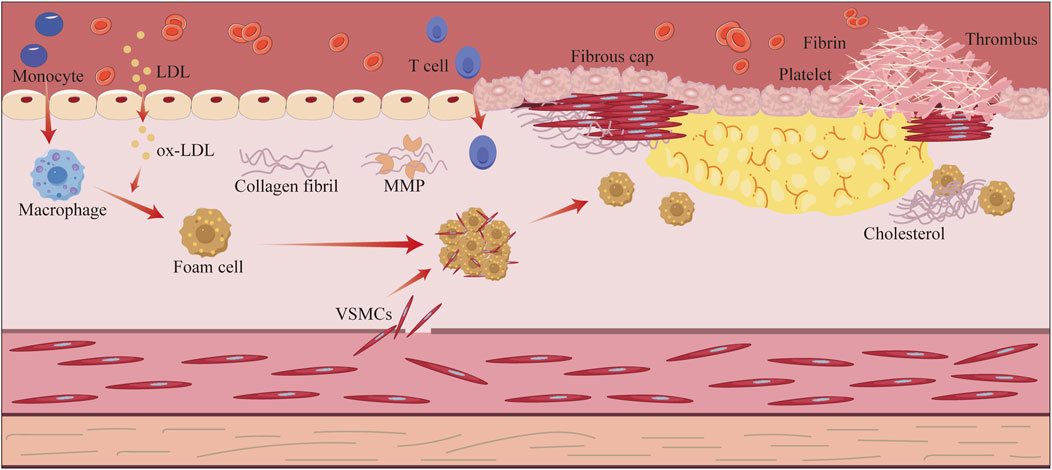
Figure 1. Schematic overview of the pathological processes related to atherosclerosis. Atherosclerosis begins with endothelial damage triggered by risk factors, such as hypertension, hyperglycemia, and hyperlipidemia. The increased permeability of the damaged endothelium encourages infiltration of low-density lipoprotein (LDL) into the endothelium, which is converted to oxidized LDL. Monocytes migrate to the endothelium and differentiate into macrophages, which phagocytose the oxidized LDL and transform into foam cells, forming lipid streaks. Subsequently, smooth muscle cells migrate to the intima and secrete collagen, which wraps around the lipid core to form a fibrous plaque. The gradual increase in plaque size leads to narrowing of the arterial lumen; when inflammation disrupts the fibrous cap, the plaque ruptures and exposes the lipid core, ultimately triggering platelet aggregation and thrombus formation.
The water-soluble metabolites of Salvia miltiorrhiza Bunge [Lamiaceae; Salviae miltiorrhizae radix et rhizoma], particularly the salvianolic acid analogs (including danshensu (DSS), salvianolic acid A (SAA), salvianolic acid B (SAB), rosmarinic acid (RA), and protocatechuic acid (PCA)) (Figure 2), have garnered significant attention in the prevention and treatment of AS owing to their well-defined pharmacophoric properties and comprehensive mechanisms (Duan et al., 2021; Li et al., 2018; Qin et al., 2015; Yang et al., 2019; Zhang et al., 2021a; Zhang et al., 2021b; Zhou et al., 2005a). Research has demonstrated that salvianolic acid exerts multifaceted protective effects on endothelial cells through multiple pathways, including the suppression of yes-associated protein (YAP)/transcriptional coactivator with PDZ-binding motif (TAZ)/c-Jun N-terminal kinase (JNK)-mediated endothelial inflammation, inhibition of NLR family pyrin domain containing 3 (NLRP3)-associated endothelial pyroptosis, modulation of silent mating type information regulation 2 homolog-1 (SIRT1)-mediated oxidative stress and autophagy, and regulation of mitogen-activated protein kinase (MEK)/extracellular signal-regulated kinase (ERK)-associated endothelial apoptosis. Notably, salvianolic acid exhibits targeted therapeutic effects on the key pathological processes of AS, including vascular smooth muscle cell (VSMC) proliferation and migration, macrophage lipid metabolism regulation, endothelial progenitor cell differentiation, and platelet activation inhibition (Jia et al., 2019; Li et al., 2018; Xu et al., 2018b). These multicellular multitarget synergistic mechanisms present distinct advantages over conventional single-target synthetic drugs. Furthermore, salvianolic acid demonstrates significant pharmacokinetic interactions, reducing statin clearance by 43% (to 57% of the original levels) and enhancing irbesartan plasma concentration by 49.9%, thereby highlighting its potential in combination therapy.
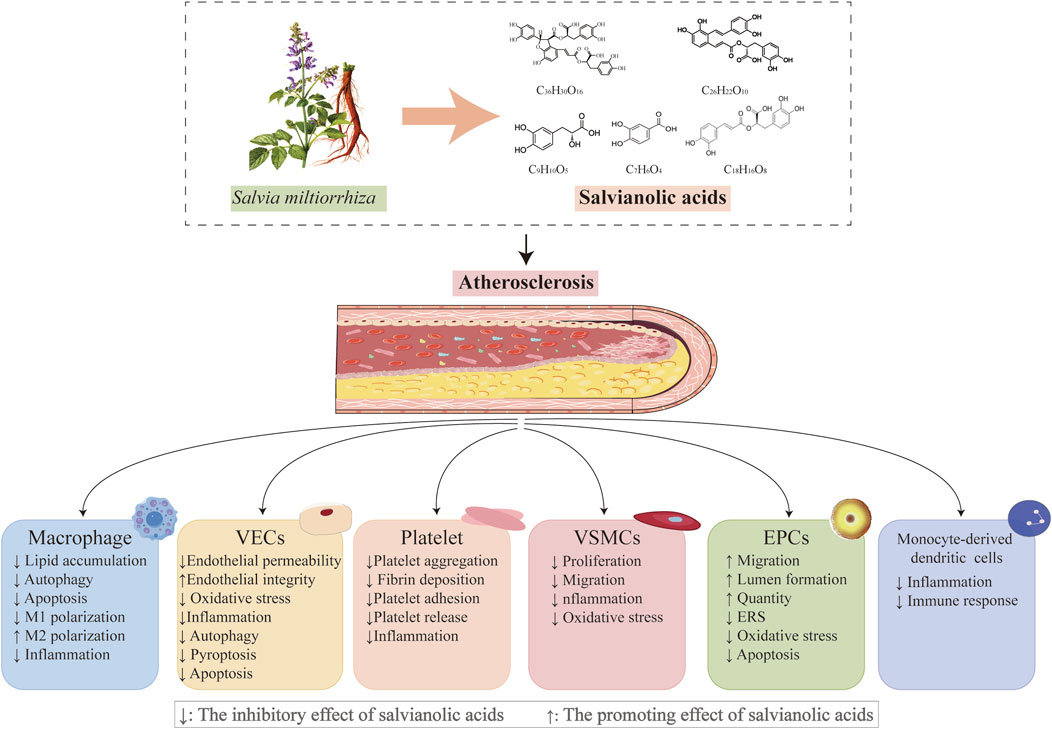
Figure 2. Salvianolic acids regulate important cellular processes in atherosclerosis; plant morphology of Salvia miltiorrhiza Bunge, whose major water-soluble active metabolites and their chemical structures mediate vasoprotective actions (chemical structure of danshensu (PubChem CID: 11600642), molecular formula: C9H10O5; chemical structure of salvianolic acid A (PubChem CID: 5281793), molecular formula: C26H22O10; chemical structure of salvianolic acid B (PubChem CID: 6451084), molecular formula: C36H30O16; chemical structure of rosmarinic acid (PubChem CID: 5281792), molecular formula: C18H16O8; chemical structure of protocatechuic acid (PubChem CID: 72), and molecular formula: C7H6O4).
Hence, the present work focuses on the multidimensional anti-AS mechanisms of salvianolic-acid-based components, revealing their integrated regulatory properties that distinguish them from existing single-target drugs by systematically resolving their regulatory networks on vascular endothelial cells (VECs), macrophage polarization, and platelet activation. By combining these with clinical evidence, toxicity evaluations, pharmacokinetic data, and drug–drug interactions, we comprehensively evaluate the academic and application prospects of salvianolic acid in the prevention and treatment of AS in the current era of precision medicine. In addition to highlighting the therapeutic potential and safety of salvianolic acid in AS, we address the limitations of the current review and discuss some priorities for future research with the aim of providing a comprehensive perspective for further studies.
2 Research methodology
We conducted a literature search of three scientific databases, namely, Web of Science, PubMed, and Embase, without using filters and applied the following keywords in appropriate combinations to obtain the relevant search formula: “salvianolic acid,” “salvianolic acid A,” “salvianolic acid B,” “danshensu,” “rosmarinic acid,” “lithospermic acid,” “protocatechualdehyde,” “protocatechuic acid,” “salvianolic acid C,” “atherosclerosis,” “anti-atherosclerotic effect,” “vascular endothelial cell,” “vascular smooth muscle cell,” “leukocyte,” “monocytes,” “macrophages,” “endothelial progenitor cell,” “mast cell,” “neutrophils,” “platelet.” The therapeutic roles and mechanism investigations of salvianolic acid in AS are the main emphasis of this review. To eliminate articles unrelated to our study subject, we reviewed the titles, abstracts, and full text of works published over the previous 24 years. Parallelly, we conducted a relevancy search to find references to pertinent research and literature reviews. A total of 90 references pertaining to the review topic were ultimately included after eliminating duplicates using the automated and manual duplicate identification technique of Endnote 20 (Figure 3).
3 Salvianolic acids and atherosclerotic vascular disorders: emerging action mechanisms and cellular targets
Salvianolic acid is the water-soluble active ingredient of S. miltiorrhiza Bunge and shows increasing evidence of vasoprotective properties (Ma et al., 2020; Xu et al., 2018a; Zhao et al., 2023). Salvianolic acid is notable for its anti-atherosclerotic actions in a range of animal and cellular models linked with AS, such as VECs, VSMCs, platelets, macrophages, and endothelial progenitor cells (EPCs) (Ma et al., 2022; Tang et al., 2022; Xie et al., 2023; Zeng et al., 2023; Zhang et al., 2024a) (Table 1). By altering several of these biological processes, salvianolic acid slows the progression of AS (Figure 4). Furthermore, salvianolic acid reduces changes in vascular tone caused by mechanical stimulation via altering calcium ion transport and blood flow shear, indicating that it may have additional benefits in treating AS (Pan et al., 2022). Crucially, salvianolic acid protects against AS at various stages of its development. During the initial phases of AS, salvianolic acid protects by reducing endothelial damage; as the disease advances, crucial elements of AS development, including platelet activation, macrophage polarization, and VSMC migration and proliferation, are also inhibited by salvianolic acid (Sun et al., 2012; Zhao et al., 2020; Zhou et al., 2020). To better demonstrate the anti-atherosclerotic properties of salvianolic acid, we explore the cellular pathways linked with AS and the precise action mechanisms of salvianolic acid in these pathways. Understanding these processes will help us better appreciate and utilize the potential of salvianolic acid in treating AS.
3.1 Restoration of endothelial function
VECs are essential for preserving both the vascular function and integrity of the internal environment (Xu et al., 2021). Several studies have demonstrated that endothelial dysfunction and injury occur frequently before vascular wall structural lesions and are the causes of AS with strong links to its pathological development (Libby et al., 2011). It has been proposed that endothelial dysfunction typified by hyperpermeability, increased oxidative stress, and chronic inflammation is a hallmark of several human vascular illnesses, including diabetes mellitus, hypertension, and AS, that affect the development and progression of AS (Davignon and Ganz, 2004; Tamargo et al., 2023; Xu et al., 2021). The atherosclerotic process is initiated by endothelial barrier dysfunction, which then facilitates monocyte infiltration and triggers localized inflammation that are subsequently amplified through nuclear factor kappa-B (NF-κB) pathway activation to exacerbate oxidative stress. This pathological cascade not only induces direct mitochondrial structural damage but also establishes a self-perpetuating “inflammation and oxidative stress” cycle mediated by NLRP3 inflammasome activation (Tanase et al., 2023). Progressive mitochondrial dysfunction disrupts cellular metabolism and energy homeostasis, initially stimulating protective autophagy as a compensatory mechanism (Li et al., 2021a). However, persistent damage ultimately results in autophagic flux impairment and subsequent accumulation of dysfunctional mitochondria, which concurrently activate both the apoptotic and pyroptotic cell death pathways. Consequently, AS progresses from the initial lipid-driven phase (characterized by autophagy-mediated protection) to the advanced vulnerable plaque stage (marked by pyroptosis-dominated inflammatory destruction), establishing a pathogenic cascade of endothelial dysfunction, metabolic dysregulation, and cell death activation. Thus, we explain the various aspects of endothelial dysfunction as well as the action mechanisms and intervention targets of salvianolic acids to improve endothelial dysfunction.
3.1.1 Improvement of endothelial permeability and integrity
The structural integrity of the vascular endothelium is vital for sustaining vascular function and providing a non-thrombogenic lining for the cardiovascular system (Abdelsalam et al., 2019). When the cellular connection is compromised, cell permeability increases and numerous substances like low-density lipoproteins (LDLs) can flow through the endothelial barrier. Cytoskeletal changes result in cell shrinkage, which increases the cell gap. Cellular tight junction composition, cytoskeleton development, and epithelial cell polarity are all significantly influenced by zonula occludens-1 (ZO-1) (Beutel et al., 2019). Liu et al. (2021a) investigated the effects of various concentrations of SAA (1, 3, and 10 μmol/L) on oxygen-glucose deprivation (OGD)-induced human brain microvascular endothelial cells (HBMECs; their findings demonstrated that SAA at a concentration of 10 μmol/L exerted protective effects on VECs by modulating the vascular endothelial growth factor A (VEGFA)-proto-oncogene tyrosine-protein kinase Src-guanine nucleotide exchange factor VAV2-ras related C3 botulinum toxin substrate 1 (Rac1)-p21 activated kinase (PAK) signaling pathway. Specifically, this protective mechanism involved the reversal of matrix metalloprotein (MMP) production elevation, suppression of ZO-1 protein degradation, and restoration of cell viability diminished by OGD induction (Liu et al., 2021a). MMP-9 is known to promote VEC permeability by dissolving the extracellular matrix and disrupting the cellular junctions to enhance cell movement and the intercellular gap (Li et al., 2021b). SAA can prevent vascular remodeling, which not only preserves the integrity of the endothelial monolayer but also reduces endothelial activity in rats with endothelial dysfunction. This is directly linked to the suppression of MMP-9 production (Teng et al., 2016). The permeability barrier of endothelial cells is maintained in part by CD31 (Lertkiatmongkol et al., 2016); here, SAA retains the intensity of CD31 by mediating the hypoxia-inducible factor 1 alpha (HIF1α)/heat shock factor 1 (HSF1)/CD31 signaling pathway, which has a protective effect on the endothelial permeability barrier (Li et al., 2022). Tyrosine phosphorylation of connexins, which directly affects the structure of VECs, is another method by which tumor necrosis factor-α (TNF-α) promotes endothelial gap junction development and connexin redistribution that increase endothelial permeability (He et al., 2024; Takei et al., 2024). In contrast, DSS and SAB attenuate VE-cadherin phosphorylation and signaling chaperone β-catenin through the tyrosine phosphorylation signaling pathway (Ding and Yuan, 2007). Research has shown that DSS and SAB prevent TNF-α damage to the VEC barrier by suppressing the activation of extracellular regulated protein kinases (ERKs) and lowering the production of vascular endothelial growth factor (VEGF) (Ding et al., 2005). Additionally, SAB (20 µM) was found to change the endothelial permeability via the cyclic guanosine monophosphate (cGMP)/protein kinase G (PKG)/NF-κB signaling cascade, which involves the paracellular pathway (downregulation of occludin and claudin-5) and transcellular pathway (upregulation of caveolin-1 and caveolin-2). Furthermore, SAB mediates the reactive oxygen species (ROS)/HIF-1α/VEGF and miR-200b/VEGF signaling pathways to ameliorate high glucose (HG)-induced endothelial dysfunction by inducing miR-200 (Ding et al., 2005). SAB also directly inhibits VEGF-induced elevation of vascular endothelial permeability (Qui et al., 2001). RA demonstrates dose-dependent inhibitory effects (1, 10, 50, and 100 µM) on both the expression of adhesion molecules and THP-1 monocyte adhesion to endothelial cells (ECs). These protective mechanisms were achieved through effective modulation of the ox-LDL-mediated ROS/p38 mitogen-activated protein kinase (p38 MAPK)/forkhead box protein O (FOXO)1/thioredoxin-interacting protein (TXNIP)/NLRP3 signaling pathway while simultaneously suppressing endothelial monolayer permeability (Nyandwi et al., 2022). Thus, salvianolic acids may help treat endothelial dysfunction by preserving the integrity of the cytoskeleton and endothelium while enhancing the cell junctions and permeability.
3.1.2 Inhibition of endothelial inflammation
Inflammation is one of the leading causes of lipid deposition and plaque development in AS (Weber et al., 2023). Several investigations have revealed that patients with AS have proinflammatory markers like C-reactive protein (CRP) and interleukin (IL)-6 and that high levels of these mediators may cause damage and dysfunction of the VECs (He et al., 2019). SAA (20 and 40 µM) reduces AS by preventing inflammation via insulin-inducible gene (Insig)1, renal cancer chromosome 8 (Trc8), and Insig2-mediated ubiquitylation and recombinant 3-hydroxy-3-methylglutaryl coenzyme A reductase (HMGCR) degradation in a dose-dependent manner (Xie et al., 2023). This was primarily shown through the stimulation of anti-inflammatory IL-10 expression and suppression of the synthesis of proinflammatory proteins TNF-α, IL-1β, and IL-6. These findings imply that SAA may have anti-inflammatory effects and slow the progression of AS, highlighting the significance of Trc8-mediated HMGCR degradation in anti-atherosclerotic cases. After being stimulated by several inflammatory cytokines, the VECs create plasminogen activator inhibitor type 1 (PAI-1), which is crucial for advancing AS (Gimenez-Bastida et al., 2016; Khoukaz et al., 2020). SAB (0.05 and 0.15 µM) decreases endothelial damage and dysfunction caused by endothelial inflammation via controlling the NF-κB and ERK-activator protein 1 (AP-1) pathways, which in turn suppress TNF-α-induced plasminogen activator inhibitor-1 (PAI-1) mRNA synthesis and protein release in HUVECs after 12 h and 18 h of intervention (90.5% and 74.6% as well as 75.1% and 64.2%), respectively (Zhou et al., 2005b). SAB also increases the endothelial fibrinolytic activity, decreases PAI-1 expression, and upregulates t-PA and TM expressions while reducing leukocyte adherence to blood arteries as well as reducing vascular inflammation by blocking the TNF-α-induced upregulation of vascular cell adhesion molecule 1 (VCAM-1) and intercellular cell adhesion molecule-1 (ICAM-1) expressions through NF-κB (1, 2.5, 5, 10, and 20 μg/mL of SAB); VCAM-1 notably attenuates the dose-dependent lowering of ICAM-1 expression by 84.5% ± 1.9%, 78.8% ± 1.2%, 58.9% ± 0.4%, 58.7% ± 0.9%, and 57.4% ± 0.3% correspondingly (Chen et al., 2001). By suppressing the expressions of YAP/TAZ and inflammation-related proteins (NF-κB, TNF-α, and JNK) in the VECs, SAB shields them from inflammatory damage and delays the development of AS (Yang et al., 2020b). This process is strongly linked to the control of the YAP/TAZ/JNK pathway over oxidized LDL (ox-LDL) formation and its anti-inflammatory properties. The suppression of NF-κB-regulated proinflammatory mediators, which can dramatically and dose-dependently downregulate the expressions of ICAM-1, IL-1 β, IL-6, IL-8, and monocyte chemoattractant protein-1 (MCP-1), is also linked to the anti-inflammatory actions of SAB (Xu et al., 2015). Since interferon gamma (IFN-γ) is a major cytokine that causes AS, blocking its effects on the inflammatory responses could be a valuable strategy for preventing AS (Li et al., 2023b). SAB suppresses the Janus kinase (JAK)-signal transducer and activator of transcription (STAT) signaling pathway triggered by IFN-γ, in addition to JAK2 and STAT1 phosphorylation and IP-10 promoter activity. These lower the release of CXC chemokines, lowering T-cell recruitment and slowing the progression of AS (Chen et al., 2011). PCA demonstrates significant anti-inflammatory properties in an IL-1β-induced endothelial cell model of diabetic endothelial dysfunction, primarily through enhancement of the phosphorylation of both endothelial nitric oxide synthase (eNOS) and aktin protein kinase B (Akt) (treatment is dose-dependent with statistical significance at 100 nM). Notably, pharmacological inhibition of Akt phosphorylation resulted in sustained reduction of p-eNOS/eNOS levels and abrogated PCA’s suppressive effects on proinflammatory cytokine production, suggesting that the anti-inflammatory actions of PCA are mediated by the Akt/eNOS signaling pathway (Chook et al., 2023). The above studies suggest that SAA and SAB are potential candidates for anti-inflammatory actions against VECs.
3.1.3 Inhibition of endothelial pyroptosis
The development of AS is tightly linked to VEC pyroptosis, which is a novel form of inflammatory cell programmed death (He et al., 2021; Zhaolin et al., 2019). Furthermore, the role of NLRP3 inflammatory vesicles in VEC pyroptosis has been demonstrated (Lv et al., 2024). Plaque vulnerability and overexpression of NLRP3 inflammasome-related components were closely correlated, indicating that NLRP3 inflammasome activation may encourage plaque instability (Paramel Varghese et al., 2016). DSS mainly prevents VEC juxtaposition at the initial stage of NLRP3 activation. DSS binds to the recombinant chloride intracellular channel protein 4 (CLIC4) and blocks its membrane localization to suppress the release of IL-1β and IL-18, cleavage of pro-caspase-1, and activation of NLRP3 inflammatory vesicles (Zhang et al., 2024b). In contrast, SAA (5, 10, and 25 µM) dose-dependently interacts with pyruvate kinase isozyme typeM2 (PKM2) to prevent Y105 from being phosphorylated and to prevent PKM2 from moving to the nucleus to cause SAA regulation of cellular focal death dependent on PKM2 (Zhu et al., 2022). Salvianolic acids can thus prevent AS-induced VEC pyroptosis.
3.1.4 Inhibition of endothelial oxidative stress
Oxidative stress of the vascular endothelium is a significant precursor to AS (Suschek et al., 2003). One of the major causes of endothelial dysfunction is increase in ROS buildup in the vessel walls (Ray and Shah, 2005). By modulating the SIRT1-eNOS pathway, SAA (100, 200, and 400 mg/L) dramatically enhances cell viability and inhibits the formation of excess nitric oxide (NO) and ROS in response to HG circumstances in a dose-dependent manner (Zhai et al., 2019). SAA also exerts endothelial protective effects by directly reducing malondialdehyde (MDA), advanced glycation end products (AGEs), NOS activity, and endothelial-type NOS protein expression in rats to inhibit oxidative stress (Yang et al., 2011). SAB (25 mg/kg/d) decreases the increased expression of angiotensin I (AT1) receptor, nicotinamide adenine dinucleotide phosphate hydrogen (NADPH) oxidase, nitrotyrosine, NADPH oxidase (NOX)-2, and NOX-4 proteins as well as excess generation of ROS in the vascular walls (Ling et al., 2017). This was shown to restore damaged endothelial function and dramatically reduce AT1 receptor-dependent vascular oxidative stress. The primary sources of ROS in the vascular system are NOX-2 and NOX-4, which are the two main isoforms of NADPH oxidase (Harel et al., 2017). SAB (80 and 160 mg/kg) was shown to lower the oxidative stress levels in a rat model of vascular endothelial dysfunction caused by blood glucose oscillations, as observed through decreased levels of NOX-2 and NOX-4 proteins as well as MDA (5.367 ± 0.89 and 4.467 ± 0.83, respectively) in the aorta (Ren et al., 2016). The bone morphogenetic protein 4 (BMP4)-ROS cycle in db/db mice causes oxidative stress through BMP4 overexpression, contributing to endothelial dysfunction. SAB improves endothelial function by interfering with the BMP4-ROS cycle to suppress p38 MAPK/JNK/caspase-3 activation (Liu et al., 2021b). Consequently, SAA and SAB may be useful natural substances for treating endothelial dysfunction caused by oxidative stress injury in vascular disorders.
3.1.5 Inhibition of endothelial apoptosis
AS is triggered and promoted by endothelial cell apoptosis (Paone et al., 2019). Thus, one of the most successful preventative and therapeutic approaches for AS involves the use of substances with antiapoptotic properties (Duan et al., 2021). As previously stated, SAB has antioxidant and anti-inflammatory properties that guard against damage to VECs caused by inflammation, oxidative stress, and others. In apoptosis caused by these harmful conditions, SAB has also shown potent antiapoptotic actions. Using the phosphatidylinositol 3-kinase (PI3K)/Akt/rapidly accelerated fibrosarcoma kinase (Raf)/MEK/ERK pathway, hydrogen peroxide (H2O2) causes VEC death while SAB (20 µM) shields rCMECs from the corresponding processes (Liu et al., 2007). SAB also inhibits oxidative stress, p53, and caspase-3 production, which results in antiapoptotic actions in ox-LDL-induced cellular damage (Chen et al., 2017). SAB (60 μg/mL) promotes antiapoptotic effects by increasing antioxidant enzyme activity, inhibiting inflammatory factor secretion, and restoring balanced expressions of proapoptotic and antiapoptotic proteins via the p38MAPK/NF-κB and PI3K/Akt/NF-κB pathways mediated by lectin-like ox-LDL receptor 1 (LOX-1), NOX-4, and ERα receptors (Yang et al., 2018). Here, ox-LDL triggers the intrinsic apoptotic pathway of VECs implicated in mitochondrial autophagy and apoptosis when the glu levels are elevated. Conversely, SAB (1, 10, 50, and 100 µM) dose-dependently protects VECs harmed by ox-LDL in HG circumstances and suppresses the rho-associated coiled-coil containing protein kinase 1 (ROCK1)-mediated apoptotic pathway to prevent apoptotic cell death and mitochondrial breakage (Ko et al., 2020). SAA (100, 200, and 400 mg/L) also prevents apoptosis through elevated glu by upregulating B-cell lymphoma-2 (Bcl-2), downregulating Bcl-2 associated X (Bax), and blocking caspase-3 and caspase-9 activities in a dose-dependent manner (Zhai et al., 2019). In addition to the cellular milieu of elevated glucose, variations in blood glucose levels can induce apoptosis and vascular endothelial dysfunction. In rats with blood glucose fluctuations, SAB was shown to significantly enhance endothelial function; this was achieved by inhibiting oxidative stress, upregulating Bcl-2 proteins, and considerably downregulating Bax proteins, thereby preventing apoptosis of VECs (Ren et al., 2016; Xiang et al., 2022). In conclusion, salvianolic acids prevent apoptosis of VECs and endothelial dysfunction caused by several sources.
3.1.6 Inhibition of endothelial autophagy
The development and instability of AS plaques are facilitated by impaired autophagy that contributes to inflammation and apoptosis (Martinet and De Meyer, 2009; Sergin and Razani, 2014). The creation and rupture of AS plaques is facilitated by oxidatively damaged lipoproteins, inflammatory agents, and metabolites that trigger cellular autophagy, particularly in VECs (Siddiqui et al., 2015). SAB inhibits autophagy in HUVECs by controlling the expressions of microtubule-associated protein light chain 3 (LC3), Beclin1, and P62 via miR-19a/SIRT1 (Guo et al., 2021). SAB at 40 and 80 µM dramatically elevated the levels of Bcl-2, LC3, autophagy-related protein 5 (Atg5), and Beclin1 in response to glucose restriction, while 80 µM of SAB decreased the expressions of Bax, lactate dehydrogenase (LDH), MDA, and superoxide dismutase (SOD) (Chen et al., 2023). However, these protective effects of SAB were lost when Atg5 expression was suppressed, implying that SAB may protect the vasculature by controlling the autophagy of VECs.
3.1.7 Improvement of endothelial mitochondrial dysfunction
Mitochondria are the prominent organelles of energy production whose dysfunction is an essential pathological basis for endothelial dysfunction, which is closely related to the occurrence and development of cardiovascular diseases (Karnewar et al., 2022). Mitochondrial autophagy plays a crucial role in the development of AS; it directly eliminates damaged and defective mitochondria to preserve regular mitochondrial quantity and quality, which are necessary for organisms to maintain healthy mitochondrial homeostasis (Chen et al., 2022d; Pickles et al., 2018; Yang et al., 2020a). Under oxidative stress or inflammatory stimulation conditions, VECs undergo mitochondrial dysfunction characterized by decreased membrane potential and impaired electron transport chain activity to trigger protective autophagy, which eliminates the damaged mitochondria and maintains cellular homeostasis (Zong et al., 2015). However, persistent pathological stimuli can lead to autophagic flux impairment owing to lysosomal system overload or defective lysosomal acidification, resulting in failed degradation of dysfunctional mitochondria. This accumulation of abnormal mitochondria ultimately drives the transition from cytoprotective autophagy to apoptotic cell death in VECs. SAA stimulates mitochondrial production in VECs by activating the adenosine 5′-monophosphate (AMP)-activated protein kinase (AMPK)-mediated peroxisome proliferator-activated receptor gamma coactivator 1-alpha (PGC-1α)/transcription factor a mitochondrial (TFAM) signaling pathway (Wang et al., 2022), which markedly increases AMPK and ACC phosphorylation. SAB dramatically reduces Parkin and Pink1 expressions, downregulates mitochondrial autophagy, and increases mitochondrial activity in HG-induced HUVECs (Xiang et al., 2022); therefore, salvianolic acids may be beneficial natural substances for treating vascular diseases by addressing mitochondrial dysfunction.
3.2 Effects on EPCs
Proliferation, migration, and differentiation of EPCs into mature VECs in the peripheral blood circulation is primarily responsible for the repair process following VEC injury and limited repair induced by the extension of nearby mature VECs (Endtmann et al., 2011). The quantity, migration, proliferation, and cytokine production of circulating EPCs influence the extent to which well-damaged arteries undergo endothelial repair. This information can be used to evaluate endothelial function and forecast the progression of AS. Therefore, there is a critical necessity to determine methods to increase the quantity and functionality of EPCs for preventing and treating AS. DSS (60 mg/kg/d) increases the survivability of EPCs, prevents apoptosis, and improves EPC capacity to migrate and create lumens by encouraging stromal cell-derived factor 1 alpha (SDF-1α) and CXC motif chemokine receptor 4 (CXCR4) expressions as well as AKT phosphorylation. AMD3100 (a CXCR4 antagonist) and LY294002 (a PI3K antagonist) have been reported to partially inhibit this ability (Yin et al., 2017). However, SAA (2.5, 5.0, and 10 mg/kg/d) can enhance neovascularization by upregulating VEGF, VEGFR-2, and MMP-9 expressions while preserving the quantity and functionality of EPCs (Li et al., 2014). SAB triggers the mechanistic target of the rapamycin (mTOR)/p70 ribosomal protein S6 kinase (p70S6K)/eukaryotic translation initiation factor 4E-binding protein 1 (4EBP1) pathway, thereby inhibiting the expressions of mitogen-activated protein kinase kinase (MKK) 3/6-p38MAPK-activating transcription factor 2 (ATF2) and ERK1/2 while increasing EPC migration, improving tube formation, and preventing cellular damage caused by H2O2 (Tang et al., 2014). The present study reveals the molecular processes in the oxidative signaling cascade involved in SAB; we identify potential targets for intervention to prevent endothelial-damage-mediated vascular injury along with applications concerning endothelial-damage-mediated vascular disease and downregulated ROS levels (162.0% ± 9.9% and 136.7% ± 13.9, respectively). However, endoplasmic reticulum stress (ERS) causes BM-EPCs to produce NLRP3 and release mitochondrial ROS. Meanwhile, SAB prevents endothelial damage linked to ERS by controlling the adhesion proteoglycan-4/Rac1/ATF2 and AMPK/FOXO4/Krüppel-like factor (KLF)2 pathways, thereby preventing NLRP3 inflammasome-mediated cellular pyroptosis (Tang et al., 2022). Thus, salvianolic acids mitigate vascular endothelial damage, improve EPC mobilization, and restore EPC functions by efficiently inhibiting ERS, oxidative stress, and apoptosis while restoring their migratory capacity (Figure 5).
3.3 Inhibition of VSMC proliferation and migration
VSMC proliferation and migration are essential factors in the pathogenesis of AS (Basatemur et al., 2019). VSMCs multiply, move to the subendothelial layer of the vasculature, and contribute to the formation of plaques when the endothelial cells are injured (Wang et al., 2015a). The progression of AS is significantly influenced by this proliferation and migration, which occur early in the process. Sun et al. (2012) discovered that SAA (0.01–0.1 µM) blocks the platelet-derived growth factor receptor (PDGFR) β-ERK1/2 cascade to prevent PDGF-BB from causing VSMC migration and proliferation in a concentration-dependent manner. However, the influence of SAA on VSMC proliferation is not limited to stopping the cell cycle in the G0/G1 phase; it is also intimately linked to the cyclic adenosine monophosphate (cAMP)/protein kinase a (PKA)/camp response element-binding protein (CREB) signaling cascade, which inhibits endothelial proliferation and increases p21/Cip1 (Sun et al., 2012; Sun et al., 2016b). However, SAB (5 μmol/L) suppresses the growth of VSMCs produced by Ang II and endothelial hyperplasia caused by carotid artery ligation in vitro by downregulating miR-146a and the positive cell cycle regulators KLF5 and cyclin D1 (Zhao et al., 2019). In addition, SAB (10 µM) significantly decreases the intima/media area ratio (0.010% ± 0.009%) by blocking lipopolysaccharide (LPS)-induced expression of cyclooxygenase-2 (COX-2) from 3.8 ± 0.3 to 1.5 ± 0.2, inhibiting ERK and JNK phosphorylation, attenuating prostaglandin E2 production, and increasing NADPH oxidase activity (Chen et al., 2006). SAB also successfully improves the suppression of FOXO1 activity and increases the expression of miR-486a-5p to reduce inflammation caused by HG in VSMCs (Zhang et al., 2024a). SDF-1α has an important regulatory role in VSMC migration and proliferation during neointimal development (Li et al., 2012b). SAB successfully suppresses chemokine (CXC motif) receptor 4 (CXCR4) expression and prevents SDF-1α/CXCR4-induced cell migration and proliferation (Pan et al., 2012). This implies that SAB might prevent the growth of neoplastic endothelial hyperplasia by acting as a CXCR4 receptor antagonist. According to numerous studies, MMPs, specifically MMP-2 and MMP-9, facilitate VSMC migration from the mid-membrane to the endothelium, resulting in neointimal hyperplasia (Brauninger et al., 2023; Dai et al., 2019; Sun et al., 2016a). SAB (5, 10, and 20 µM) inhibits MMP-2 and MMP-9 activities as well as downregulates JNK and ERK phosphorylation in a concentration-dependent manner to decrease LPS-induced migration of VSMCs (Lin et al., 2007). The antioxidant protein heme oxygenase-1 (HO-1) prevents the formation of AS and shields vascular cells against neointimal hyperplasia after vascular injury (Juan et al., 2001; Tulis et al., 2001). SAB prevents PDGF-induced VSMC migration and proliferation by scavenging ROS and upregulating HO-1 expression (Lee et al., 2014). Research has demonstrated that RA exerts multiple protective effects on VSMCs through distinct molecular mechanisms, such as suppression of PDGF-BB-induced VSMC proliferation, migration, and phenotypic switching via activation of the nuclear factor erythroid 2-related factor 2 (Nrf2)/antioxidant response element (ARE) pathway (Chen et al., 2022a); RA mitigates nicotine-induced vascular inflammation and retards AS progression by inhibiting the ROS-NLRP3-CRP axis as well as attenuates LPS-induced inflammatory responses in VSMCs through blockade of the MAPK/NF-κB pathway (Chen et al., 2022b). Furthermore, PCA has been shown to inhibit A7r5 cell proliferation through AMPK pathway activation mediated by downregulation of fatty acid synthase (FAS) expression, suppression of AKT phosphorylation and S-phase kinase-associated protein 2 (Skp2) protein synthesis, and induction of cell cycle arrest at the G0/G1 phase via coordinated activation of both the p53/p21Cip1 and Skp2 pathways (Lin et al., 2015). Subsequent research has shown that HO-1 knockdown reduces the capacity of SAB to control atherogenic processes in VSMCs induced by PDGF. These works demonstrate the pivotal functions of HO-1 and the contributions of its activation to the anti-atherosclerotic actions of SAB. Salvianolic acids may thus work against AS through various mechanisms, including antiproliferative and antimigratory actions on VSMCs (Figure 6).
3.4 Regulation of macrophages in AS
The presence of a mass infiltration of macrophages is one of the pathological underpinnings for the development of AS (Wilson, 2022). One of the factors contributing to plaque development in AS is macrophages, which are engaged in intraplaque neovascularization, fibrous cap, lipid necrotic core, and plaque inflammation (Martinet and De Meyer, 2009). The uptake of ox-LDL by macrophages initiates a pathological cascade characterized by cholesterol crystal (CHC) deposition, which triggers inflammasome activation and subsequent release of proinflammatory cytokines, including IL-1β, thereby promoting macrophage polarization toward the proinflammatory M1 phenotype (Luo et al., 2025). This M1 polarization establishes a self-amplifying inflammatory loop through ROS generation to exacerbate cellular apoptosis. When the apoptotic macrophages fail to undergo timely efferocytosis by the neighboring cells, they progress to secondary necrosis and release proinflammatory contents that contribute to necrotic core formation. In advanced stages of AS, persistent lipid accumulation and the sustained inflammatory microenvironment suppress anti-inflammatory M2 polarization, impairing both efferocytosis and cholesterol efflux mechanisms. These pathological alterations lead to progressive lipid accumulation, necrotic core expansion, and fibrous cap thinning to ultimately promote plaque destabilization and accelerate atherosclerotic progression (Farahi et al., 2021).
3.4.1 Suppression of macrophage inflammation
Inflammation is a hallmark of the pathophysiological processes involved in AS, and macrophages are crucial in these events. The production of foam cells from macrophages in the subendothelial region, which coincides with macrophage proliferation during AS plaque formation, is a characteristic of early AS (Emanuel et al., 2014; Lv et al., 2014; Shen et al., 2023). Furthermore, compared to stable plaques, susceptible plaques in AS exhibit more significant macrophage infiltration (Seneviratne et al., 2013). Therefore, inflammatory reactions mediated by macrophages play essential roles in the formation of AS. Reduction of the inflammatory responses, prevention of plaque rupture, and improvement of AS progression depend on inhibiting macrophage infiltration and multiplication (Tang et al., 2015). AS development is tightly linked to the classical NF-κB signaling pathway, which is mediated by the inhibitor of nuclear factor kappa-B kinase (IKKβ) subunit (Gwon et al., 2020). DSS demonstrates strong anti-inflammatory effects in macrophages through the IKKβ/NF-κB pathway. These effects have been primarily observed in the inhibition of Ltf, Ccr7, IFN-γ, and Cxcl9 expressions; reductions of arterial plaque development and areas in mice; and improvement of the stability of susceptible plaques (Ye et al., 2020; Zeng et al., 2023). LPS stimulates the release of proinflammatory factors involved in inflammatory reactions, translocation of NF-κB to the nucleus, and phenotypic shift of macrophages to the M1 phenotype (Bosca et al., 2005; Herrera-Velit et al., 1997; Jeong et al., 2005; Moller et al., 2012; Ni Gabhann et al., 2014; Shapira et al., 1996). By blocking IKKα/β activation via the p38-HO-1 pathway through blocking of IKKα/β phosphorylation and NF-κB translocation to the nucleus, SAA might decrease the transcriptional activation of NF-κB; this suggests that SAA has anti-inflammatory effects on LPS-stimulated RAW 264.7 cells. The toll-like receptor 4 (TLR4) is an essential receptor of LPS that promotes inflammation (Baeuerle, 1998). Induction of THP-1 cells with LPS (1 μg/mL) for 24 h was shown to significantly upregulate proinflammatory cytokines, with the expression levels of IL-1β, IL-6, and TNF-α reaching 60.4 ± 5.5, 109.1 ± 19.5, and 1,297.29 ± 94.09, respectively, demonstrating successful induction of M1 macrophage polarization. SAB (0.72, 3.60, and 17.95 μg/mL) exerts anti-inflammatory effects through the TLR4/NF-κB signaling pathway, significantly reducing the levels of LPS-induced TLR4, p-IκBα, and p-p65 proteins as well as preventing nuclear translocation of NF-κB and degradation of IκBα (Liu et al., 2018a). Furthermore, SAB inhibition of LPS-induced macrophage inflammation has been linked to increased arginase activity, decreased NO generation, and increased expression of the cytoprotective protein HO-1 (Joe et al., 2012). RAW264.7 cells induced with ox-LDL (100 μg/mL) or LPS (100 μg/mL) for 24 h showed significantly increased secretion of proinflammatory cytokines, including IL-1β, IL-6, TNF-α, and iNOS, confirming successful polarization toward the M1 macrophage phenotype. By preventing activation of the MAPKs/NF-κB pathway, reducing lipid buildup, and reducing the expressions of inflammatory components in the aorta and RAW264.7 cells, SAB (25 mg/kg) has been shown to exert anti-atherosclerotic effects in high-fat-diet-induced LDLR−/−mice, ox-LDL, or LPS-induced RAW264.7 cells (1.25, 2.5, and 5 μg/mL) (Zhang et al., 2022). Therefore, one of the key biological targets of salvianolic acids for anti-atherosclerotic effects is the inhibitory action on macrophage inflammation caused by various stressors.
3.4.2 Inhibition of macrophage polarization
The quantity and polarization state of the invading macrophages determine the size and stability of the plaques (Xia et al., 2020). By lowering the amount of the membrane receptor protein Mincle and phosphorylating the downstream effector molecule Syk, SAB prevents macrophage M1 phenotype (proinflammatory) polarization, highlighting that Mincle is a target protein for the anti-inflammatory actions of SAB (Li et al., 2021d). One of the methods by which SAB controls macrophage polarization to provide anti-inflammatory effects is promoting the growth of anti-inflammatory (M2) phenotype macrophages while simultaneously suppressing the number of M1 phenotype macrophages. Niu et al. (2022) found that SAB (15 mg/kg/d) supports blood perfusion by increasing the quantity of M2 phenotype macrophages via the SIRT1/PI3K/AKT pathway. Following 24 h of stimulation with LPS (100 ng/mL) and IFN-γ (2.5 ng/mL), RAW264.7 cells exhibited morphological changes from round to oval or spindle shapes and increased expressions of M1 markers (iNOS, TNF-α, and IL-6), thereby confirming successful M1 polarization. Treatment with SAB (100, 150, and 200 µM) significantly downregulated M1-associated proinflammatory factors while upregulating M2 markers (Arg-1 and IL-10), indicating SAB-mediated M1-to-M2 phenotype switching. This phenotypic transformation was found to be autophagy-dependent, as evidenced by the reversal of LC3-II, Beclin1, and p62 alterations upon 24 h of treatment with the autophagy inhibitor 3-MA (5 mM). Further mechanistic studies using the protein kinase C (PKC) agonist PMA (100 nM/mL) revealed the involvement of NF-κB pathway activation and AKT/mTOR inhibition in SAB-induced autophagy. These findings collectively demonstrate that SAB promotes macrophage phenotype switching through autophagy modulation via NF-κB activation and AKT/mTOR pathway inhibition (Zou et al., 2022). Zhao et al. (2020) demonstrated that LPS (100 ng/mL) stimulation of BMDMs for 24 h significantly upregulated mRNA expressions of the M1 polarization markers (TNF-α, IL-6, iNOS, and CCL2), confirming successful M1 polarization. Conversely, SAB treatment (5, 10, and 20 µM) exerted concentration-dependent effects, including inhibition of mTORC1-mediated glycolysis, downregulation of M1 marker expressions (IL-6, iNOS, CCL2, and TNF-α), and upregulation of M2-associated genes (Arg1, Clec10a, and Mrc); these coordinated effects promote macrophage switching from proinflammatory M1 to anti-inflammatory M2 phenotype polarization (Zhao et al., 2020). PCA exerts dual regulatory effects on macrophage polarization by suppressing M1 polarization through inhibition of the PI3K-AKT/NF-κB signaling pathway and promoting M2 polarization by activating both STAT6 and peroxisome proliferator-activated receptor (PPAR)γ pathways. This coordinated modulation of the M1–M2 phenotypic transition in both J774 cells and mouse bone-marrow-derived macrophages contributes to its therapeutic efficacy in alleviating AS progression (Liu et al., 2019). These findings provide a better theoretical basis for further research and development of salvianolic acids as therapeutic agents for restoring macrophage polarization and treating AS.
3.4.3 Inhibition of macrophage apoptosis
One of the treatment strategies for advanced AS is decreasing macrophage apoptosis while preserving phagocytosis as this process exacerbates plaque development (Simion et al., 2020). SAA has similar effects to PI3K inhibitors in that it counteracts Ang-II-induced macrophage apoptosis and oxidative stress (Li et al., 2016). These effects are demonstrated by the increase in TUNEL-positive cells, upregulation of SOD and Bax expressions, and decreases in Bcl-2, MDA, p-NF-κB, p-AKT 473, and p-AKT 308 expressions. Here, autophagy is controlled by the inhibitory AKT/mTOR signaling pathway (Chang et al., 2013). According to research, the buildup of CHCs and subsequent inflammatory responses are intimately linked to the instability of AS plaques (Duewell et al., 2010; Whiting and Watts, 1983). Sun et al. (2021) showed that SAB reduces apoptosis in RAW264.7 cells and improves autophagy dysfunction caused by CHC by modulating the AKT/mTOR signaling pathway. PCA (25 µM) promotes nuclear translocation of Nrf2 and suppresses ROS overproduction during the initial phase of oxidative stress via JNK pathway activation; it also mediates JNK/Nrf2 signaling-dependent regulation of the endogenous antioxidant defense system. This coordinated action plays a crucial role in the cytoprotective properties of PCA against oxidative-stress-induced apoptosis in macrophages (Vari et al., 2015) (Figure 7).
3.4.4 Improvement of macrophage lipid metabolism
One of the leading causes of AS lesion progression is disturbed macrophage lipid metabolism along with foam cell production (Li et al., 2021c). AS development can be slowed by boosting cholesterol export because the accumulated cholesterol encourages macrophages to convert into foam cells (Moore et al., 2013). SAB stimulates the ox-LDL-activated THP-1 cells to increase apoA-1 and HDL-mediated cholesterol efflux through the ABCA1/PPAR-g/LXRα pathway (Yue et al., 2015). Although CD36 overexpression has been shown to prevent ischemia–reperfusion injury, CD36 deficiency can reduces the progression of AS (Shu et al., 2022). Wang et al. (2010b) suggested SAB (1, 10, and 100 µM) as one of the potential natural compounds for CD36 antagonism (where CD36 levels were reduced by 11.2%, 48.9%, and 78.3%, respectively); here, SAB had a higher binding affinity, indicating that it was a more potent CD36 antagonist than hexarelin (kinetics by association: 264.3 M−1s−1 vs. 240.1 M−1s−1; dissociation constant: 9.89 × 10−4 s−1 vs. 5.48 × 10−3 s−1; affinity: 3.74 μM vs. 22.8 μM) that binds directly to CD36 to reduce lipid uptake (17.65%). SAB was found to exert this effect in a CD36-dependent manner after knockdown or overexpression of CD36 in macrophages (Bao et al., 2012; Wang et al., 2010b); this implies that SAB has a strong and targeted inhibitory effect on the CD36 pathway. One of the chemokines essential for the development of AS that accumulates in the plaques is CC chemokine ligand-20 (CCL-20)/macrophage inflammatory protein-3α (Schutyser et al., 2003). Reduced lipid accumulation and decreased AS plaque extension were the outcomes of considerable inhibition of CCL-20 production and CCR6 expression by SAA in TNF-α-stimulated RAW264.7 cells (Zhang et al., 2014). Additionally, SAA was shown to suppress p-p38 through the MAPK pathway. RA enhances the cholesterol efflux capacity in macrophages by upregulating ATP-binding cassette transporter (ABC)A1 and ABCG1 expressions. Mechanistic studies reveal that RA differentially regulates ABCG1 expression via multiple signaling pathways, including JAK2/STAT3 activation, JNK modulation, and PKC-mediated regulation of the ERK1/2/p38 MAPK cascade (Nyandwi et al., 2021). According to the above reports, salvianolic acids are beneficial for enhancing lipid metabolism in macrophages and controlling AS thereof.
3.4.5 Modulation of macrophage cytostasis
Macrophages play a crucial role in tissue homeostasis through efferocytosis, which is the process of apoptotic cell clearance. However, this essential function is significantly impaired in advanced atherosclerotic plaques, contributing to sustained inflammation and necrotic core expansion (Doran et al., 2020; Morioka et al., 2019; Yurdagul et al., 2017). Mechanistic studies have shown that PCA enhances macrophage efferocytic capacity through a novel miR-10b-mediated pathway and that PCA promotes microRNA-10b secretion into the extracellular vesicles, thereby reducing intracellular miR-10b levels; this reduction derepresses KLF4, which is a key transcriptional regulator, and subsequently upregulates MerTK receptor expression to enhance efferocytic efficiency (Li et al., 2023a). Oral PCA administration has been reported to significantly improve macrophage efferocytosis in atherosclerotic plaques via the miR-10b-KLF4-MerTK axis, resulting in reduced apoptotic cell accumulation and attenuated inflammatory responses in vivo (Li et al., 2023a). These findings not only establish the therapeutic potential of PCA through activation of the KLF4-MerTK pathway but also provide a novel strategy for AS management by targeting the efferocytic mechanisms, thereby highlighting the therapeutic potential of modulating apoptotic cell clearance in cardiovascular pathologies.
3.5 Effects on monocyte-derived dendritic cells
Foam cell formation is crucial for the development of AS and is mediated by monocyte-derived dendritic cells using exophagic breakdown of aggregated LDLs (Haka et al., 2015). By inhibiting the TLR4/MyD88-mediated p38-MAPK pathway as well as upregulating PPARγ nuclear translocation and transcription through the PPARγ signaling cascade, SAB efficiently prevents ox-LDL-induced maturation of h-monDC to consequently reduce the immunological responses and cellular inflammation (Sun et al., 2011). PCA also demonstrates significant anti-atherogenic effects by suppressing monocyte adhesion to TNF-R-activated endothelial cells, inhibiting NF-κB pathway activation, and attenuating early atherosclerotic lesion formation in vitro (Wang et al., 2010a).
3.6 Inhibition of platelet activation and antithrombosis
AS develops as a result of alterations in the vascular structure and functions caused by various mechanical and chemical factors that damage the VECs. This allows infiltration of the inflammatory cells, adhesion and aggregation of platelets, and deposition of fibrin to form thrombi (Massberg et al., 2010; Pan et al., 2009). Therefore, prevention of platelet aggregation is crucial for the management of cerebrovascular and cardiovascular disorders (Davi and Patrono, 2007).
DSS exerts potent antiplatelet effects through SIRT1/ROS/mtDNA pathway modulation, demonstrating a concentration-dependent inhibition (1–500 µM) of platelet aggregation induced by multiple agonists, including U46619 (1 µM), TRAP-6 (2 µM), and adenosine diphosphate (ADP; 10 µM). This unique mechanism provides effective antithrombotic protection without compromising hemostatic balance, thereby minimizing bleeding risk; however, the SIRT1 inhibitor EX-527 (10 µM) reverses this effect, indicating that DSS suppresses ROS generation and prevents platelet activation by upregulating SIRT1 expression (Xue et al., 2022). The capacity of DSS to alter blood rheology without compromising the coagulation system is linked to its antithrombotic action. In a comprehensive preclinical evaluation, male SD rats and ICR mice received oral administration of DSS (15, 30, and 60 mg/kg) or aspirin (20 mg/kg) for 7 d. Pharmacological assessments were then conducted using both in vitro platelet aggregation assays and an in vivo arteriovenous shunt thrombosis model complemented by a venous thrombosis model. The results showed that DSS treatment significantly improved the hemorheological parameters, as evidenced by the lower blood and plasma viscosities, while inhibiting platelet aggregation caused by arachidonic acid (AA) and ADP. This can be explained by the ability of DSS to precisely suppress COX-2 while restoring the equilibrium between prostacyclin (PGI2) and thromboxane A2 (TXA2). Additionally, SAA has good antithrombotic activity, as evidenced by its dose-dependent inhibition of thrombus growth in the arteriovenous shunt model, activation of adenylate cyclase to increase cAMP levels for inhibiting platelet aggregation and release, and improvement of erythrocyte pressure and whole blood viscosity as two indicators of blood rheology (Fan et al., 2010; Yu et al., 2014). Huang et al. (2010) discovered that SAA primarily inhibits the PI3K pathway to influence platelet activation and exert antithrombotic effects. SAA specifically decreases platelet–fibrinogen binding ability and ADP-induced P-selectin expression on the platelet surface, further preventing ADP-mediated platelet–leukocyte aggregation (Yuan et al., 2017). The antithrombotic effectiveness of SAA is further enhanced by its ability to prevent platelet spreading on the fibrinogen matrix efficiently. SAB inhibits platelet adhesion to collagen in a dose-dependent manner by blocking α2β1-collagen interactions, as demonstrated by reduced platelet deposition under physiological shear rates, decreased α2β1 antibody binding in flow cytometry, and inhibited soluble α2β1-collagen binding in solid-phase assays (Wu et al., 2008). SAB interacts with P2Y1 and P2Y12 platelets to modestly reduce adhesion and aggregation (Liu et al., 2014; Liu et al., 2018b).
One of the key targets in the development of antithrombotic medications is the platelet surface protease-activated receptor, which is triggered by thrombin and is a potent agonist of platelet activation. These receptors are essential for platelet activation, aggregation, and blood coagulation (Coppens et al., 2012; Coughlin, 2000; Han et al., 2021). Neves et al. (2024) employed a comprehensive approach combining platelet aggregation assays, coagulation tests, perfusion chamber experiments, and in vivo microscopy; using intrinsic fluorescence spectrophotometry and isothermal titration calorimetry, they characterized the thrombin inhibition kinetics and binding affinity of SAB. Their groundbreaking work identified SAB as the first direct plant-derived inhibitor of thrombin, demonstrating dual antithrombotic mechanisms through both thrombin-dependent (active site blockade) and thrombin-independent pathways (Neves et al., 2024). Salvianolic acids have shown great promise in antiplatelet and antithrombotic therapies. Inflammation is an essential factor in thrombosis that is closely related to the coagulation and fibrinolytic systems (Lopes-Bezerra and Filler, 2003; Wei et al., 2022). For example, TNF-α can accumulate in the relevant thrombosis and coagulation pathways, and its overexpression can increase the risk of thrombosis. SAB inhibits TNF-α-induced increases in PAI-1 and TF secretion levels as well as the production of FⅩa and FIIa through the NF-κB/JNK/P38MAPK pathway (Zheng et al., 2021). Platelet apoptosis regulation has emerged as a promising therapeutic strategy for preventing atherosclerotic thrombosis. PCA exerts protective effects by scavenging ROS and preventing H2O2-induced downregulation of the PI3K/AKT/glycogen synthase kinase 3β (GSK3β) signaling axis, thereby mitigating oxidative-stress-induced platelet apoptosis (Ya et al., 2021). Furthermore, PCA demonstrates significant antiplatelet activity against shear-stress-induced platelet aggregation (SIPA), which is a critical factor in arterial disease progression, by inhibiting the binding of von Willebrand factor to glycoprotein Ib as the primary event initiating SIPA (Kim et al., 2012). Additionally, RA exhibits platelet aggregation inhibition via modulation of ERp57, a protein disulfide isomerase family member, suggesting an alternative mechanism for platelet function regulation (Zou et al., 2018). The above studies demonstrated that salvianolic acids are promising candidates in antiplatelet and antithrombotic therapies (Figure 5).
3.7 Regulation of vascular tone
Vasodilatory dysfunction increases the risk of AS while accelerating the breakdown of smooth muscle and endothelial cells (Chen et al., 2024). Rat coronary arteries were shown to be dilated by DSS, with an IC50 value of 71.5 ± 11.0 μg/mL and effective dose ranging from 0.02 to 0.35 mg/mL (Lam et al., 2007). Inhibiting the L-type voltage-dependent Ca2+ channels in VSMCs and, to a lesser extent, activating K+ channels are the primary mechanisms by which vasodilatory effects are produced (Lam et al., 2007). SAA has a protective effect on impaired vascular reactivity; SAA treatment was shown to restore impaired endothelium-dependent diastolic function in rats by reversing the oxidative stress caused by hyperglycemia as well as improving the aortic ring contractility (Wang et al., 2009). Furthermore, by preventing Ang-II and ET-1 from overactivating the vascular smooth muscle L-type calcium channels, SAA preserves the relaxation function of vascular smooth muscle (Lin et al., 2022). SAA also stimulates F-actin bundling, controls the rearrangement of the actin cytoskeleton, and stabilizes the translation-actin complex, all of which improve coronary artery contractility and blood flow (Zhong et al., 2018). SAB reduces aortic ring relaxation and HUVEC alignment in the direction of shear stress by efficiently blocking mechanical-stimulus-induced Ca2+ currents through the Yoda1-induced Piezo1 channels and antagonizing ion flow through these channels (Pan et al., 2022). However, the effective dose of SAB differed between studies. For instance, in a 5-hydroxytryptamine (5-HT)-preconditioned preparation, approximately 0.72 mg/mL of SAB eliminated CaCl2-induced vasoconstriction; however, in a KCl-preconditioned preparation, 1.44 mg/mL of SAB resulted in 90% inhibition (Lam et al., 2006). Briefly, salvianolic acids can restore the endothelial-dependent stretch and smooth muscle relaxation functions of blood vessels by modulating vascular mechanotransduction.
4 Safety evaluations and clinical evidence for salvianolic acids
Salvianolic acids are effective against several AS-related cellular components and molecular targets, but their safety remains to be assessed. Acute toxicity studies revealed a median lethal dose (LD50) of 2,356.33 mg/kg for DSS in mice, while rat studies demonstrated an excellent safety profile with no mortality or toxicity signs at 1,500 mg/kg (Gao et al., 2009). Subchronic toxicity assessments were used to further confirm the safety of DSS, which showed no mortality or histopathological changes in rats following 90 d of administration at doses up to 450 mg/kg (Gao et al., 2009). Injection-site sclerosis was seen only in the high-dose group after 2–3 weeks of continuous administration (normalized after 3–4 d of stopping the drug), according to a toxicity evaluation of DSS in Beagle dogs after 3 months of continuous infusion at doses of 17, 50, and 150 mg/kg/d (Li et al., 2009). Acute toxicity studies showed an LD50 of 1,161.2 mg/kg for SAA in mice without pathological abnormalities, while studies on Beagle dogs revealed an minimum lethal dose (MLD) of 682 mg/kg and maximum non-lethal dose (MNLD) of 455 mg/kg accompanied by hepatic and renal pathologies. A 4-week repeated-dose study identified dose-dependent toxicity at 300 mg/kg, which manifested as reduced hemoglobin level, decreased hematocrit, and hepatorenal impairment (Yang et al., 2022). Genotoxicity assessments through bacterial reverse mutation and mouse bone marrow micronucleus tests demonstrated no mutagenic effects or hematopoietic function alterations (Yang et al., 2022). Nephrotoxicity is a significant side-effect of the clinical use of cisplatin. Interestingly, DSS (60 mg/kg) protects against cisplatin-induced nephrotoxicity by activating Nrf2/HO-1 and blocking the NF-κB pathway (Yu et al., 2021). Magnesium lithospermate B as a magnesium salt derivative of SAB has shown nephroprotective effects by mitigating cisplatin-induced acute kidney injury through preservation of mitochondrial function (Shen et al., 2022). Furthermore, SAB (2 mg/kg) demonstrates cardioprotective synergy with arsenic trioxide (ATO) by alleviating ATO-induced cardiac dysfunction and myocardial damage (Wang et al., 2013).
Clinical evaluation in a phase I randomized double-blind placebo-controlled trial confirmed the excellent safety profile of SAB injection in healthy Chinese volunteers for single doses up to 300 mg and repeated doses of 250 mg for 5 d (Cheng et al., 2023). A single intravenous infusion of 100 or 200 mg of salvia polyphenols was tolerated well by the participants in a randomized open single-dose study of the compound in a healthy population. Salvianolic acid salt (85% SAB concentration) was evaluated for its impacts on platelets in patients with acute coronary syndrome (ACS); the results showed that it did not affect hemostasis in ACS patients and improved the antiplatelet effects of conventional antiplatelet medication (Liu et al., 2014). According to a different study, SAA also prevented platelet aggregation and activation in diabetic individuals but their diabetes-related comorbidities did not lower this inhibitory impact (Zhou et al., 2020).
The above information indicates that although the safety, tolerability, and effects of salvianolic acids on platelet augmentation have all been investigated partially, high-quality evidence is still required to support clinical usage of the drug as a novel antiplatelet treatment. A comprehensive literature review across PubMed, Web of Science, and Embase revealed that there were no existing studies on the reproductive toxicity of salvianolic acid, including fertility, embryofetal development, and perinatal effects. This gap likely stems from two factors: (1) historical research emphasis on pharmacodynamic mechanisms rather than systematic toxicity assessments; (2) the substantial time and financial investments required for ICH S5(R3)-compliant multigenerational reproductive toxicity studies. To address these, future research should implement a tiered approach of initial developmental toxicity screening using zebrafish embryo models, followed by extended one-generation reproductive toxicity studies in SD rats with detailed monitoring of F1 generation reproductive organ histopathology and sex hormone dynamics. Ultimately, large-scale multicenter clinical trials (such as phase IV pharmacovigilance studies) should establish human risk prediction models, thereby completing the safety profile of salvianolic acids and supporting its evidence-based clinical applications.
5 Drug interactions
Several studies have demonstrated the potential therapeutic roles of salvianolic acids in AS-related diseases. Compared to controls who received rosuvastatin alone, concurrent treatment with DSS (46 mg/kg) increased systemic exposure to rosuvastatin by more than twice and lowered plasma clearance to more than 57%. According to the current findings, CYPs and/or organic anion-transporting peptides may mediate these interactions (Wen and Xiong, 2011). The Cmax of DSS increased from 0.05 mg/mL to 0.11 mg/mL, while the area under the curve (AUC) value increased from 5.20 mg/mL·min to 18.47 mg/mL·min when combined with warfarin (Zhou et al., 2012). However, aspirin had no discernible impacts on the pharmacokinetic characteristics of DSS (Zhou et al., 2012). According to another investigation, DSS considerably increased the plasma levels of aspirin (Zhang et al., 2020). Thus, when consuming warfarin or aspirin along with herbal remedies and preparations containing DSS, it is necessary to monitor their plasma concentrations. Coadministration of DSS and irbesartan changed the metabolism of irbesartan by increasing its AUC(0-t) (9,573 ± 441 vs. 16,157 ± 559 μg/L·h) and Cmax (821 ± 24 vs. 1,231 ± 44 μg/L), thereby prolonging t1/2 (13.39 ± 0.98 vs. 16.04 ± 1.21 h), decreasing clearance, improving the stability of in vitro metabolism, and lowering endogenous clearance (from 38.14 ± 10.24 μL/min/mg protein to 28.51 ± 9.06 μL/min/mg protein) (Li and Liu, 2024).
Regarding the drug interactions with DSS as a natural medication, strong cardioprotective impacts of DSS were observed when used in conjunction with salvinorin, which may be linked to the regulation of Bax, Bcl-2, and calpain I to exert antiapoptotic effects as well as activation of Nrf2 to boost the antioxidant effects (Li et al., 2012a). According to reports, the combination of SAB and astragaloside IV (AsIV) can effectively prevent and treat AS; this combination can positively affect lipid metabolism, antioxidative stress, and protection of the vascular endothelium. However, compared to the administration of single agents, the combination of SAB and AsIV had a more substantial antioxidant impact and an inhibitory effect on lipid deposition in the artery walls (Kong et al., 2022). Ligustrazine and SAB were observed to decrease the late apoptotic rate of rCMECs under high-shear flow conditions; however, their combination lowered the persistence of apoptosis rather than shielding cells from early death, inhibiting apoptosis synergistically (Li et al., 2008). By restoring the balanced production of proapoptotic and antiapoptotic proteins as well as the balanced redox state of cells through p38MAPK/NF-κB and PI3K/AKT/NF-κB, SAB and ginsenosides have been shown to produce concerted effects to counteract apoptosis (Yang et al., 2018). In the zebrafish vasculature, cotreatment with SAB and ferulic acid increased the expressions of specific VEGF receptors and ligands while promoting the proliferation and cell cycle progression of endothelial cells. The AUC and Cmax of SAB increased by 51.7% and 27.7%, respectively, when coupled with metoprolol (a common medication used to treat hypertension); when mixed with metoprolol acid, the AUC and Cmax of SAB decreased by 26.5% and 19.6%, respectively (Wan et al., 2010). Briefly, DSS and SAB produce synergistic effects with certain drugs and herbal monomers in treating AS, lipid-lowering, antiplatelet, anticoagulation, and antihypertensive disorders. Notably, the drug interactions of SAA with associated medications for AS therapy are rarely reported. Hence, further research efforts are necessary to determine whether the addition of salvianolic acids to traditional anti-atherosclerotic medications may lower or enhance the likelihood of their side-effects, such as hepatic impairment and elevated risk of bleeding.
6 Pharmacokinetic characteristics of salvianolic acids and their preparations
Salvianolic acids and their preparations have been proven to have potential therapeutic effects in AS-related disorders via numerous studies. Table 2 lists the pharmacokinetic properties of these preparations in various people and animals. Comparative pharmacokinetic analysis across species (rats, rabbits, beagles, and humans) have revealed significant interspecies variations in the absorption kinetics, metabolic pathways, and elimination profiles of salvianolic acids (Table 2). Beagles emerged as the most relevant preclinical model for oral formulation development, given that their gastrointestinal physiology (including pH environment and transit time) closely resembles that of humans. Conversely, rat-derived pharmacokinetic data require cautious clinical extrapolation owing to species-specific differences, such as significantly enhanced hepatic CYP450 metabolic activity and pronounced first-pass effects that may substantially underestimate systemic drug exposure. However, distribution metabolism studies have revealed that the primary metabolic reaction of DSS in rats is the formation of methylated DSS, with 4-hydroxy-3-methoxyphenyllactic acid as the main metabolite (Wang et al., 2015b). SAA has two metabolic pathways in rats, including methylation and glucuronidation, which produce five metabolites (SAA-monoglucuronide, monomethyl-SAA-monoglucuronide, monomethyl-SAA, dimethyl-SAA, and dimethyl-SAA-monoglucuronide) (Shen et al., 2009). Similarly, the primary metabolic pathway of SAB in rats is methylation that produces nine metabolites, including methylated metabolites of SAB and lithospermic acid (LSA), as well as decarboxylation and methylation metabolites of LSA, salvianolic acid S (SAS), and dehydrated SAS (Qi et al., 2013).
Salvianolic acids have limited oral bioavailability, similar to many natural compounds. Among these, DSS has 13.72% absolute bioavailability and brief duration of body circulation (Meng et al., 2019). According to preclinical research, the absolute oral bioavailabilities of SAA are roughly 0.6% and 1.84% in rats and beagles, respectively (Sun et al., 2013). This is mainly because the substance is found in an ionic state in the digestive system and has low bioavailability owing to its difficulty passing through the intestinal biomembrane (Chen et al., 2022c). Furthermore, because SAB has poor permeability and stability, its oral bioavailability in rats is only around 2.3% (Wu et al., 2006). The above issues can be successfully resolved with the advancement of pharmaceutical research and creation of novel formulations. Tanshinol borneol ester was loaded into nanostructured lipid carriers modified with polyethylene glycol and administered intravenously; the results of this effort were a significant increase in the area under the blood concentration vs. time curve, longer plasma retention time, and lower clearance. Hence, this formulation is the recommended option for the sustained release of DSS in vivo and may be among the most promising therapies for managing cardiovascular disorders (Yuan et al., 2018). To increase oral bioavailability, a solid self-microemulsifying drug delivery system was developed in another study and loaded with many active metabolites of S. miltiorrhiza Bunge as a novel approach to oral medication delivery (Bi et al., 2016).
Because of their structural characteristics, salvianolic acids have low absorption, hampering their clinical applicability even as promising natural substances in AS-related diseases. Novel advancements in materials sciences and related pharmaceutical sciences have made it feasible to load drug using a range of cutting-edge materials, significantly boosting the bioavailability and precisely delivering to the drug targets via various pathways. However, several critical aspects of salvianolic acids require further investigations and optimization, including development of pharmaceutical formulations to enhance stability and bioavailability, comprehensive evaluations of efficacy and safety in AS-related models, and validation of stability-enhancing technologies to facilitate clinical translation. These advancements are essential for promoting the clinical application and therapeutic potential of salvianolic acids.
7 Conclusion and future directions
Numerous research studies have demonstrated the positive pharmacological and therapeutic effects of salvianolic acids on AS. Salvianolic acids can influence the actions of several cellular components, such as endothelial cells, smooth muscle cells, macrophages, EPCs, and other immune cells, which are linked to the development of AS and can slow its progression. The therapeutic benefits of SAB, SAA, and DSS for AS include multitarget and multipathway effects that are strongly linked to the regulation of oxidative stress, inflammation, autophagy, apoptosis, platelet aggregation, thrombosis, vascular tone, and other processes. However, the current study entails specific practical issues that prevent salvianolic acids from being used in clinical settings in the future.
7.1 Clarifying direct action targets
Published studies do not thoroughly examine the deeper mechanisms and particular action connections of the anti-atherosclerotic properties of salvianolic acids. Salvianolic acids have tremendous potential in the treatment of AS, as demonstrated by their ability to influence multilayer signaling cascades. In light of the available literature, we analyzed the anti-atherosclerotic cellular components and related mechanisms of SAA, SAB, and DSS. However, previous studies have not examined the precise active metabolic components; instead, these studies concentrated on a single cellular element or method of inhibiting autophagy, apoptosis, and inflammation. For instance, the degree of lipid metabolism in macrophages plays a significant role in controlling AS. To date, most studies have only examined whether salvianolic acids can help control the levels of lipid metabolism rather than the upstream mechanisms of this effect. To systematically explore the direct targets of salvianolic acids, the following integrated strategies are proposed. First, multiomics integration can be employed to construct a multitarget regulatory network complemented by molecular docking and molecular dynamics simulations to predict the binding modes between salvianolic acids and their targets, thereby prioritizing candidates for experimental validation. Second, two distinct types of bioprobes can be developed: (1) bioorthogonal probes derived from the core structures of salvianolic acids to enable target visualization and enrichment via click chemistry; (2) activity-based probes of salvianolic acid analogs that can covalently label target proteins for precise identification through mass spectrometry. Additionally, cellular thermal shift analysis may be used to assess changes in the thermal stability of the target protein upon salvianolic acid binding to provide orthogonal validation. This synergistic approach combining multiomics analysis, computational modeling, and chemical biology techniques offers a reliable experimental basis for comprehensively elucidating the direct molecular targets of salvianolic acids.
7.2 Strengthening clinical evidence
The clinical evidence base for salvianolic acids in AS management remains limited and has several methodological constraints: (1) current AS-related trials predominantly consist of single-center small-scale exploratory studies lacking rigorous randomization, blinding, or placebo controls; (2) unoptimized dosing regimens due to insufficient dose-response studies have led to inconsistent efficacy evaluation criteria; (3) while randomized controlled trials (RCTs) of salvianolic acid injection have demonstrated efficacy in cerebral perfusion disorders, these neurological-focused findings cannot be directly extrapolated to AS management; (4) existing safety data are primarily derived from short-term assessments in healthy populations, with inadequate representation of high-risk subgroups (such as diabetic patients or those with vulnerable plaques), thereby limiting the clinical generalizability of the current findings.
Future research efforts should therefore adopt comprehensive strategies to strengthen the evidence chain: (1) conduct multicenter large-scale randomized double-blind placebo-controlled phase II/III trials to evaluate the efficacies of salvianolic acids, both as monotherapy and in combination with standard care (such as statins), on the hard endpoints of AS (such as major adverse cardiovascular events and plaque regression); (2) implement population pharmacokinetic and pharmacodynamic (PPK-PD) modeling to optimize personalized dosing strategies; (3) incorporate real-world evidence (such as electronic health records) to assess the efficacy and safety in complex patient populations, thereby addressing the external validity limitations of RCT findings.
7.3 Clarifying the effectiveness and safety of drug interactions
Emerging evidence indicates the synergistic potential between salvianolic acids and conventional anti-atherosclerotic therapies, although mechanistic elucidation and clinical translation demand rigorous validation. While the current investigations predominantly address pharmacokinetic parameters, three critical knowledge gaps require systematic exploration. The first gap is statin synergism, where the proposed complementary mechanisms between salvianolic-acid-mediated ABCA1/PPAR-γ/LXRα activation and statin-induced HMG-CoA reductase inhibition necessitate quantitative validation. Here, the essential research efforts should prioritize (a) systematic characterization of CYP3A4 inhibitory potency of salvianolic acids and the dose-dependent effects on statin metabolism as well as (b) multicenter RCTs evaluating the efficacy of combination therapy in plaque stabilization and cardiovascular mortality reduction, with standardized monitoring of the hepatic transaminases and creatinine kinase levels. The second gap involves anticoagulant interactions, where the potential pharmacodynamic interactions require rigorous investigation through (a) warfarin metabolism inhibition and plasma protein binding competition, which may elevate the AUC and INR values as well as (b) synergistic COX-1 inhibition with aspirin to potentially enhance the antiplatelet effects while increasing the risk of gastrointestinal bleeding. Development of pharmacodynamic models and patient-specific monitoring protocols (INR, fecal occult blood) based on individual risk factors (age, ulcer history) is also essential. The third gap entails multicomponent synergism, where systematic analyses of the effects of different isomers of salvianolic acids are needed on CYP450 isoforms, transporters, and signaling pathways through in vitro screening and AS animal models to elucidate the multicomponent synergistic mechanisms and optimize clinical combination strategies.
7.4 Pharmacological mechanisms and comparative advantages
Comparative analyses of some natural anti-atherosclerotic agents reveal distinct yet complementary mechanisms. (1) Resveratrol (RSV): This agent primarily targets endothelial regulation through SIRT1/AMPK/eNOS activation and TGF/ERK pathway inhibition to improve vasodilation and suppress smooth muscle proliferation (Guo et al., 2022; Parsamanesh et al., 2021). (2) Curcumin: This agent focuses on NLRP3 inflammasome-mediated inflammation to modulate macrophage polarization (inhibiting iron-induced M1 phenotype) and platelet activation (reducing P-selectin expression), albeit with limited endothelial repair capacity (Benameur et al., 2023; Hussain et al., 2022; Momtazi-Borojeni et al., 2019). (3) Quercetin: This is a dietary flavonoid requiring intestinal microbial metabolism for activation that exerts antioxidant (LDL reduction) and anti-inflammatory effects while indirectly improving lipid metabolism through gut microbiota modulation. However, the clinical outcomes remain inconsistent owing to bioavailability limitations and individual metabolic variations (Papakyriakopoulou et al., 2022; Terao, 2023). (4) Salvianolic acids demonstrate superior multitarget efficacy through YAP/TAZ/JNK-NLRP3 axis inhibition to block endothelial inflammation and pyroptosis; SIRT1-mediated autophagy activation for oxidative stress clearance; MEK/ERK pathway modulation to inhibit endothelial apoptosis and promote progenitor cell differentiation; and comprehensive regulation of smooth muscle migration, macrophage lipid metabolism, and platelet activation. Unlike the endothelial focus of RSV, anti-inflammatory specialization of curcumin, or microbiota-dependent effects of quercetin, salvianolic acids offer a systematic therapeutic approach encompassing endothelial homeostasis, plaque stabilization, and vascular regeneration. Future research efforts could thus employ systems pharmacology procedures to explore natural product synergies (such as salvianolic acid and curcumin nanoformulations) and utilize advanced models (organoid arrays, patient-derived plaque systems) to quantitatively assess target engagement and pathway modulation efficiency to optimize clinical applications.
In conclusion, the primary water-soluble metabolites of S. miltiorrhiza Bunge show sound vasoprotective effects in the treatment of AS and can be used as candidate drugs for the prevention and treatment of AS owing to its intricate pathological mechanisms and complex interactions between different cellular components. However, there remain certain disadvantages and shortcomings in the practical applications of salvianolic acids because of their low stability, significant individual variances, lack of clinical trial data, and numerous constraints. Future advancements in the clinical applications and development of salvianolic acids for the treatment of AS-related diseases will require more investigations of the interactions, high-quality clinical trials, optimization of drug formulations and dosing regimens, as well as integration of research and development of novel formulations, modern technological tools like artificial intelligence and computer science, and in-depth studies in molecular biology and pharmacology. These efforts are expected to yield valuable drug templates for advanced clinical applications.
Author contributions
QL: writing – original draft, and writing – review and editing. XL: visualization and writing – review and editing. XX: writing – original draft and writing – review and editing. ZC: supervision and writing – review and editing. TL: supervision, writing – review and editing. YS: writing – review and editing. HX: supervision and writing – review and editing. HG: writing – review and editing. CX: supervision and writing – review and editing.
Funding
The author(s) declare that financial support was received for the research and/or publication of this article. This work was supported by the innovation team and talents cultivation program of the National Administration of Traditional Chinese Medicine (no. ZYYCXTD-C-202209), Study on the effects of Shenqi Compound series on cardiovascular benefit in diabetes mellitus based on macrovascular protective effects, Sichuan Provincial Department of Science and Technology (no. 2022ZDZX0022), and Chinese Medicine Prevention and Treatment of Endocrine Metabolic Diseases Science and Industry Innovation Team (no. 2022C012).
Conflict of interest
The authors declare that the research was conducted in the absence of any commercial or financial relationships that could be construed as a potential conflict of interest.
Generative AI statement
The authors declare that no Generative AI was used in the creation of this manuscript.
Publisher’s note
All claims expressed in this article are solely those of the authors and do not necessarily represent those of their affiliated organizations, or those of the publisher, the editors and the reviewers. Any product that may be evaluated in this article, or claim that may be made by its manufacturer, is not guaranteed or endorsed by the publisher.
References
Abd, T. T., and Jacobson, T. A. (2011). Statin-induced myopathy: a review and update. Expert Opin. Drug Saf. 10 (3), 373–387. doi:10.1517/14740338.2011.540568
Abdelsalam, S. S., Korashy, H. M., Zeidan, A., and Agouni, A. (2019). The role of protein tyrosine phosphatase (ptp)-1b in cardiovascular disease and its interplay with insulin resistance. Biomolecules 9 (7), 286. doi:10.3390/biom9070286
Ariesen, M. J., Algra, A., and Kappelle, L. J. (2006). Antiplatelet drugs in the secondary prevention after stroke: differential efficacy in large versus small vessel disease? A subgroup analysis from esps-2. Stroke 37 (1), 134–138. doi:10.1161/01.STR.0000195045.40409.85
Baeuerle, P. A. (1998). Ikappab-nf-kappab structures: at the interface of inflammation control. Cell 95 (6), 729–731. doi:10.1016/s0092-8674(00)81694-3
Bao, Y., Wang, L., Xu, Y., Yang, Y., Wang, L., Si, S., et al. (2012). Salvianolic acid b inhibits macrophage uptake of modified low density lipoprotein (mldl) in a scavenger receptor cd36-dependent manner. Atherosclerosis 223 (1), 152–159. doi:10.1016/j.atherosclerosis.2012.05.006
Basatemur, G. L., Jorgensen, H. F., Clarke, M. C. H., Bennett, M. R., and Mallat, Z. (2019). Vascular smooth muscle cells in atherosclerosis. Nat. Rev. Cardiol. 16 (12), 727–744. doi:10.1038/s41569-019-0227-9
Benameur, T., Frota Gaban, S. V., Giacomucci, G., Filannino, F. M., Trotta, T., Polito, R., et al. (2023). The effects of curcumin on inflammasome: latest update. Molecules 28 (2), 742. doi:10.3390/molecules28020742
Beutel, O., Maraspini, R., Pombo-Garcia, K., Martin-Lemaitre, C., and Honigmann, A. (2019). Phase separation of zonula occludens proteins drives formation of tight junctions. Cell 179 (4), 923–936. doi:10.1016/j.cell.2019.10.011
Bi, X., Liu, X., Di, L., and Zu, Q. (2016). Improved oral bioavailability using a solid self-microemulsifying drug delivery system containing a multicomponent mixture extracted from salvia miltiorrhiza. Molecules 21 (4), 456. doi:10.3390/molecules21040456
Bosca, L., Zeini, M., Traves, P. G., and Hortelano, S. (2005). Nitric oxide and cell viability in inflammatory cells: a role for no in macrophage function and fate. Toxicology 208 (2), 249–258. doi:10.1016/j.tox.2004.11.035
Brauninger, H., Kruger, S., Bacmeister, L., Nystrom, A., Eyerich, K., Westermann, D., et al. (2023). Matrix metalloproteinases in coronary artery disease and myocardial infarction. Basic Res. Cardiol. 118 (1), 18. doi:10.1007/s00395-023-00987-2
Cainzos-Achirica, M., Glassner, K., Zawahir, H. S., Dey, A. K., Agrawal, T., Quigley, E. M. M., et al. (2020). Inflammatory bowel disease and atherosclerotic cardiovascular disease: jacc review topic of the week. J. Am. Coll. Cardiol. 76 (24), 2895–2905. doi:10.1016/j.jacc.2020.10.027
Chang, B. B., Zhang, L., Cao, W. W., Cao, Y., Yang, W. L., Wang, Y., et al. (2010). Pharmacokinetic interactions induced by content variation of major water-soluble components of danshen preparation in rats. Acta Pharmacol. Sin. 31 (5), 638–646. doi:10.1038/aps.2010.27
Chang, Z., Shi, G., Jin, J., Guo, H., Guo, X., Luo, F., et al. (2013). Dual pi3k/mtor inhibitor nvp-bez235-induced apoptosis of hepatocellular carcinoma cell lines is enhanced by inhibitors of autophagy. Int. J. Mol. Med. 31 (6), 1449–1456. doi:10.3892/ijmm.2013.1351
Chen, C., Ma, J., Xu, Z., Chen, L., Sun, B., Shi, Y., et al. (2022a). Rosmarinic acid inhibits platelet aggregation and neointimal hyperplasia in vivo and vascular smooth muscle cell dedifferentiation, proliferation, and migration in vitro via activation of the keap1-nrf2-are antioxidant system. J. Agric. Food Chem. 70 (24), 7420–7440. doi:10.1021/acs.jafc.2c01176
Chen, C. P., Lin, Y. C., Peng, Y. H., Chen, H. M., Lin, J. T., and Kao, S. H. (2022b). Rosmarinic acid attenuates the lipopolysaccharide-provoked inflammatory response of vascular smooth muscle cell via inhibition of MAPK/NF-κB cascade. Pharmaceuticals (Basel) 15 (4), 437. doi:10.3390/ph15040437
Chen, H. M., Luo, H., Zeng, W. B., Liu, B., Huang, J. C., Liu, M., et al. (2017). Salvianolic acid b attenuates oxidized low-density lipoprotein-induced endothelial cell apoptosis through inhibition of oxidative stress, p53, and caspase-3 pathways. Chin. J. Integr. Med. doi:10.1007/s11655-016-2645-4
Chen, J., Ruan, Z., Lou, H., Yang, D., Shao, R., Xu, Y., et al. (2022c). First-in-human study to investigate the safety and pharmacokinetics of salvianolic acid a and pharmacokinetic simulation using a physiologically based pharmacokinetic model. Front. Pharmacol. 13, 907208. doi:10.3389/fphar.2022.907208
Chen, M., Xiao, J., El-Seedi, H. R., Wozniak, K. S., Daglia, M., Little, P. J., et al. (2024). Kaempferol and atherosclerosis: from mechanism to medicine. Crit. Rev. Food Sci. Nutr. 64 (8), 2157–2175. doi:10.1080/10408398.2022.2121261
Chen, Q., Xu, Q., Zhu, H., Wang, J., Sun, N., Bian, H., et al. (2023). Salvianolic acid b promotes angiogenesis and inhibits cardiomyocyte apoptosis by regulating autophagy in myocardial ischemia. Chin. Med. 18 (1), 155. doi:10.1186/s13020-023-00859-w
Chen, S. C., Lin, Y. L., Huang, B., Wang, D. L., and Cheng, J. J. (2011). Salvianolic acid b suppresses ifn-gamma-induced jak/stat1 activation in endothelial cells. Thromb. Res. 128 (6), 560–564. doi:10.1016/j.thromres.2011.08.032
Chen, Y., Qin, W., Li, L., Wu, P., and Wei, D. (2022d). Mitophagy: critical role in atherosclerosis progression. DNA Cell Biol. 41 (10), 851–860. doi:10.1089/dna.2022.0249
Chen, Y. H., Lin, S. J., Ku, H. H., Shiao, M. S., Lin, F. Y., Chen, J. W., et al. (2001). Salvianolic acid b attenuates vcam-1 and icam-1 expression in tnf-alpha-treated human aortic endothelial cells. J. Cell Biochem. 82 (3), 512–521. doi:10.1002/jcb.1176
Chen, Y. L., Hu, C. S., Lin, F. Y., Chen, Y. H., Sheu, L. M., Ku, H. H., et al. (2006). Salvianolic acid b attenuates cyclooxygenase-2 expression in vitro in lps-treated human aortic smooth muscle cells and in vivo in the apolipoprotein-e-deficient mouse aorta. J. Cell Biochem. 98 (3), 618–631. doi:10.1002/jcb.20793
Cheng, J., Long, J., Zhang, J., Han, L., Hu, Y., Liu, J., et al. (2023). Safety, tolerance, and pharmacokinetics of salvianolic acid b in healthy Chinese volunteers: a randomized, double-blind, placebo-controlled phase 1 clinical trial. Front. Pharmacol. 14, 1146309. doi:10.3389/fphar.2023.1146309
Chook, C. Y. B., Cheung, Y. M., Ma, K. Y., Leung, F. P., Zhu, H., Niu, Q. J., et al. (2023). Physiological concentration of protocatechuic acid directly protects vascular endothelial function against inflammation in diabetes through akt/enos pathway. Front. Nutr. 10, 1060226. doi:10.3389/fnut.2023.1060226
Coppens, M., Eikelboom, J. W., Gustafsson, D., Weitz, J. I., and Hirsh, J. (2012). Translational success stories: development of direct thrombin inhibitors. Circ. Res. 111 (7), 920–929. doi:10.1161/CIRCRESAHA.112.264903
Coughlin, S. R. (2000). Thrombin signalling and protease-activated receptors. Nature 407 (6801), 258–264. doi:10.1038/35025229
Dai, L., Zhou, J., Li, T., Qian, Y., Jin, L., Zhu, C., et al. (2019). Strip2 silencing inhibits vascular smooth muscle cell proliferation and migration via p38-akt-mmp-2 signaling pathway. J. Cell Physiol. 234 (12), 22463–22476. doi:10.1002/jcp.28810
Davi, G., and Patrono, C. (2007). Platelet activation and atherothrombosis. N. Engl. J. Med. 357 (24), 2482–2494. doi:10.1056/NEJMra071014
Davignon, J., and Ganz, P. (2004). Role of endothelial dysfunction in atherosclerosis. Circulation 109 (23 Suppl. 1), III27–32. doi:10.1161/01.CIR.0000131515.03336.f8
Ding, M., Ye, T. X., Zhao, G. R., Yuan, Y. J., and Guo, Z. X. (2005). Aqueous extract of salvia miltiorrhiza attenuates increased endothelial permeability induced by tumor necrosis factor-alpha. Int. Immunopharmacol. 5 (11), 1641–1651. doi:10.1016/j.intimp.2005.05.005
Ding, M., and Yuan, Y. J. (2007). Study on the mechanisms of an extract of salvia miltiorrhiza on the regulation of permeability of endothelial cells exposed to tumour necrosis factor-alpha. J. Pharm. Pharmacol. 59 (7), 1027–1033. doi:10.1211/jpp.59.7.0016
Doran, A. C., Yurdagul, A., and Tabas, I. (2020). Efferocytosis in health and disease. Nat. Rev. Immunol. 20 (4), 254–267. doi:10.1038/s41577-019-0240-6
Duan, H., Zhang, Q., Liu, J., Li, R., Wang, D., Peng, W., et al. (2021). Suppression of apoptosis in vascular endothelial cell, the promising way for natural medicines to treat atherosclerosis. Pharmacol. Res. 168, 105599. doi:10.1016/j.phrs.2021.105599
Duewell, P., Kono, H., Rayner, K. J., Sirois, C. M., Vladimer, G., Bauernfeind, F. G., et al. (2010). Nlrp3 inflammasomes are required for atherogenesis and activated by cholesterol crystals. Nature 464 (7293), 1357–1361. doi:10.1038/nature08938
Emanuel, R., Sergin, I., Bhattacharya, S., Turner, J., Epelman, S., Settembre, C., et al. (2014). Induction of lysosomal biogenesis in atherosclerotic macrophages can rescue lipid-induced lysosomal dysfunction and downstream sequelae. Arterioscler. Thromb. Vasc. Biol. 34 (9), 1942–1952. doi:10.1161/ATVBAHA.114.303342
Endtmann, C., Ebrahimian, T., Czech, T., Arfa, O., Laufs, U., Fritz, M., et al. (2011). Angiotensin ii impairs endothelial progenitor cell number and function in vitro and in vivo: implications for vascular regeneration. Hypertension 58 (3), 394–403. doi:10.1161/HYPERTENSIONAHA.110.169193
Fan, H. Y., Fu, F. H., Yang, M. Y., Xu, H., Zhang, A. H., and Liu, K. (2010). Antiplatelet and antithrombotic activities of salvianolic acid a. Thromb. Res. 126 (1), e17–e22. doi:10.1016/j.thromres.2010.04.006
Farahi, L., Sinha, S. K., and Lusis, A. J. (2021). Roles of macrophages in atherogenesis. Front. Pharmacol. 12, 785220. doi:10.3389/fphar.2021.785220
Gao, Y., Liu, Z., Li, G., Li, C., Li, M., and Li, B. (2009). Acute and subchronic toxicity of danshensu in mice and rats. Toxicol. Mech. Methods 19 (5), 363–368. doi:10.1080/15376510902810672
Gimenez-Bastida, J. A., Gonzalez-Sarrias, A., Vallejo, F., Espin, J. C., and Tomas-Barberan, F. A. (2016). Hesperetin and its sulfate and glucuronide metabolites inhibit tnf-alpha induced human aortic endothelial cell migration and decrease plasminogen activator inhibitor-1 (pai-1) levels. Food Funct. 7 (1), 118–126. doi:10.1039/c5fo00771b
Gong, L., Xu, H., Yuan, H., Wang, L., Yin, X., Fan, M., et al. (2018). Identification of absorbed constituents and evaluation of the pharmacokinetics of main compounds after oral administration of yindanxinnaotong by uplc-q-tof-ms and uplc-qqq-ms. RSC Adv. 8 (28), 15725–15739. doi:10.1039/c7ra12659j
Guo, S., Zhou, Y., and Xie, X. (2022). Resveratrol inhibiting tgf/erk signaling pathway can improve atherosclerosis: backgrounds, mechanisms and effects. Biomed. Pharmacother. 155, 113775. doi:10.1016/j.biopha.2022.113775
Guo, Y., Yang, J. H., Cao, S. D., Gao, C. X., He, Y., Wang, Y., et al. (2021). Effect of main ingredients of danhong injection against oxidative stress induced autophagy injury via mir-19a/sirt1 pathway in endothelial cells. Phytomedicine 83, 153480. doi:10.1016/j.phymed.2021.153480
Gwon, M. H., Im, Y. S., Seo, A. R., Kim, K. Y., Moon, H. R., and Yun, J. M. (2020). Phenethyl isothiocyanate protects against high fat/cholesterol diet-induced obesity and atherosclerosis in c57bl/6 mice. Nutrients 12 (12), 3657. doi:10.3390/nu12123657
Haka, A. S., Singh, R. K., Grosheva, I., Hoffner, H., Capetillo-Zarate, E., Chin, H. F., et al. (2015). Monocyte-derived dendritic cells upregulate extracellular catabolism of aggregated low-density lipoprotein on maturation, leading to foam cell formation. Arterioscler. Thromb. Vasc. Biol. 35 (10), 2092–2103. doi:10.1161/ATVBAHA.115.305843
Han, X., Nieman, M. T., and Kerlin, B. A. (2021). Protease-activated receptors: an illustrated review. Res. Pract. Thromb. Haemost. 5 (1), 17–26. doi:10.1002/rth2.12454
Harel, S., Mayaki, D., Sanchez, V., and Hussain, S. N. A. (2017). Nox2, nox4, and mitochondrial-derived reactive oxygen species contribute to angiopoietin-1 signaling and angiogenic responses in endothelial cells. Vasc. Pharmacol. 92, 22–32. doi:10.1016/j.vph.2017.03.002
He, B., Nie, Q., Wang, F., Han, Y., Yang, B., Sun, M., et al. (2021). Role of pyroptosis in atherosclerosis and its therapeutic implications. J. Cell Physiol. 236 (10), 7159–7175. doi:10.1002/jcp.30366
He, H., Zhang, W., Jiang, L., Tong, X., Zheng, Y., and Xia, Z. (2024). Endothelial cell dysfunction due to molecules secreted by macrophages in sepsis. Biomolecules 14 (8), 980. doi:10.3390/biom14080980
He, M., Huang, T. S., Li, S., Hong, H. C., Chen, Z., Martin, M., et al. (2019). Atheroprotective flow upregulates itpr3 (inositol 1,4,5-trisphosphate receptor 3) in vascular endothelium via klf4 (kruppel-like factor 4)-mediated histone modifications. Arterioscler. Thromb. Vasc. Biol. 39 (5), 902–914. doi:10.1161/ATVBAHA.118.312301
Herrera-Velit, P., Knutson, K. L., and Reiner, N. E. (1997). Phosphatidylinositol 3-kinase-dependent activation of protein kinase c-zeta in bacterial lipopolysaccharide-treated human monocytes. J. Biol. Chem. 272 (26), 16445–16452. doi:10.1074/jbc.272.26.16445
Huang, Z. S., Zeng, C. L., Zhu, L. J., Jiang, L., Li, N., and Hu, H. (2010). Salvianolic acid a inhibits platelet activation and arterial thrombosis via inhibition of phosphoinositide 3-kinase. J. Thromb. Haemost. 8 (6), 1383–1393. doi:10.1111/j.1538-7836.2010.03859.x
Hussain, Y., Abdullah, , Khan, F., Alsharif, K. F., Alzahrani, K. J., Saso, L., et al. (2022). Regulatory effects of curcumin on platelets: an update and future directions. Biomedicines 10 (12), 3180. doi:10.3390/biomedicines10123180
Jeong, S. J., Pise-Masison, C. A., Radonovich, M. F., Park, H. U., and Brady, J. N. (2005). A novel nf-kappab pathway involving ikkbeta and p65/rela ser-536 phosphorylation results in p53 inhibition in the absence of nf-kappab transcriptional activity. J. Biol. Chem. 280 (11), 10326–10332. doi:10.1074/jbc.M412643200
Jia, Q., Zhu, R., Tian, Y., Chen, B., Li, R., Li, L., et al. (2019). Salvia miltiorrhiza in diabetes: a review of its pharmacology, phytochemistry, and safety. Phytomedicine 58, 152871. doi:10.1016/j.phymed.2019.152871
Jiang, J., Zhao, X., Li, X., Wu, S., Yu, S., Lou, Y., et al. (2017). High-throughput determination of sodium danshensu in beagle dogs by the lcms/ms method, employing liquid-liquid extraction based on 96-well format plates. Molecules 22 (5), 667. doi:10.3390/molecules22050667
Joe, Y., Zheng, M., Kim, H. J., Kim, S., Uddin, M. J., Park, C., et al. (2012). Salvianolic acid b exerts vasoprotective effects through the modulation of heme oxygenase-1 and arginase activities. J. Pharmacol. Exp. Ther. 341 (3), 850–858. doi:10.1124/jpet.111.190736
Juan, S. H., Lee, T. S., Tseng, K. W., Liou, J. Y., Shyue, S. K., Wu, K. K., et al. (2001). Adenovirus-mediated heme oxygenase-1 gene transfer inhibits the development of atherosclerosis in apolipoprotein e-deficient mice. Circulation 104 (13), 1519–1525. doi:10.1161/hc3801.095663
Karnewar, S., Pulipaka, S., Katta, S., Panuganti, D., Neeli, P. K., Thennati, R., et al. (2022). Mitochondria-targeted esculetin mitigates atherosclerosis in the setting of aging via the modulation of sirt1-mediated vascular cell senescence and mitochondrial function in apoe(-/-) mice. Atherosclerosis 356, 28–40. doi:10.1016/j.atherosclerosis.2022.07.012
Kashani, A., Phillips, C. O., Foody, J. M., Wang, Y., Mangalmurti, S., Ko, D. T., et al. (2006). Risks associated with statin therapy: a systematic overview of randomized clinical trials. Circulation 114 (25), 2788–2797. doi:10.1161/CIRCULATIONAHA.106.624890
Khoukaz, H. B., Ji, Y., Braet, D. J., Vadali, M., Abdelhamid, A. A., Emal, C. D., et al. (2020). Drug targeting of plasminogen activator inhibitor-1 inhibits metabolic dysfunction and atherosclerosis in a murine model of metabolic syndrome. Arterioscler. Thromb. Vasc. Biol. 40 (6), 1479–1490. doi:10.1161/ATVBAHA.119.313775
Kim, K., Bae, O. N., Lim, K. M., Noh, J. Y., Kang, S., Chung, K. Y., et al. (2012). Novel antiplatelet activity of protocatechuic acid through the inhibition of high shear stress-induced platelet aggregation. J. Pharmacol. Exp. Ther. 343 (3), 704–711. doi:10.1124/jpet.112.198242
Ko, Y. S., Jin, H., Park, S. W., and Kim, H. J. (2020). Salvianolic acid b protects against oxldl-induced endothelial dysfunction under high-glucose conditions by downregulating rock1-mediated mitophagy and apoptosis. Biochem. Pharmacol. 174, 113815. doi:10.1016/j.bcp.2020.113815
Kong, X. L., Lyu, Q., Zhang, Y. Q., Kang, D. F., Li, C., Zhang, L., et al. (2022). Effect of astragaloside iv and salvianolic acid b on antioxidant stress and vascular endothelial protection in the treatment of atherosclerosis based on metabonomics. Chin. J. Nat. Med. 20 (8), 601–613. doi:10.1016/S1875-5364(22)60186-9
Lam, F. F., Yeung, J. H., Chan, K. M., and Or, P. M. (2007). Relaxant effects of danshen aqueous extract and its constituent danshensu on rat coronary artery are mediated by inhibition of calcium channels. Vasc. Pharmacol. 46 (4), 271–277. doi:10.1016/j.vph.2006.10.011
Lam, F. F., Yeung, J. H., Kwan, Y. W., Chan, K. M., and Or, P. M. (2006). Salvianolic acid b, an aqueous component of danshen (salvia miltiorrhiza), relaxes rat coronary artery by inhibition of calcium channels. Eur. J. Pharmacol. 553 (1-3), 240–245. doi:10.1016/j.ejphar.2006.09.030
Lee, H. J., Seo, M., and Lee, E. J. (2014). Salvianolic acid b inhibits atherogenesis of vascular cells through induction of nrf2-dependent heme oxygenase-1. Curr. Med. Chem. 21 (26), 3095–3106. doi:10.2174/0929867321666140601195940
Lertkiatmongkol, P., Liao, D., Mei, H., Hu, Y., and Newman, P. J. (2016). Endothelial functions of platelet/endothelial cell adhesion molecule-1 (cd31). Curr. Opin. Hematol. 23 (3), 253–259. doi:10.1097/MOH.0000000000000239
Li, D., Yang, S., Xing, Y., Pan, L., Zhao, R., Zhao, Y., et al. (2021a). Novel insights and current evidence for mechanisms of atherosclerosis: mitochondrial dynamics as a potential therapeutic target. Front. Cell Dev. Biol. 9, 673839. doi:10.3389/fcell.2021.673839
Li, G., Gao, Y., Li, S., Li, C., Zhu, X., Li, M., et al. (2009). Study on toxicity of danshensu in beagle dogs after 3-month continuous intravenous infusion. Toxicol. Mech. Methods 19 (6-7), 441–446. doi:10.1080/15376510903150623
Li, H., Huang, H., Cui, Y., Li, W., Zhang, S., and Chen, Y. (2021b). Study on the mechanism of capillary leakage caused by hypoxia-inducible factor-1α through inducing high expression of matrix metalloproteinase-9. J. Oncol. 2021, 9130650. doi:10.1155/2021/9130650
Li, H., Xie, Y. H., Yang, Q., Wang, S. W., Zhang, B. L., Wang, J. B., et al. (2012a). Cardioprotective effect of paeonol and danshensu combination on isoproterenol-induced myocardial injury in rats. PLoS ONE 7 (11), e48872. doi:10.1371/journal.pone.0048872
Li, H., Yu, X. H., Ou, X., Ouyang, X. P., and Tang, C. K. (2021c). Hepatic cholesterol transport and its role in non-alcoholic fatty liver disease and atherosclerosis. Prog. Lipid Res. 83, 101109. doi:10.1016/j.plipres.2021.101109
Li, J., Chen, Y. H., Li, L. Z., Wang, F., Song, W., Alolga, R. N., et al. (2021d). Omics and transgenic analyses reveal that salvianolic acid b exhibits its anti-inflammatory effects through inhibiting the mincle-syk-related pathway in macrophages. J. Proteome Res. 20 (7), 3734–3748. doi:10.1021/acs.jproteome.1c00325
Li, J., Liu, S., Li, W., Hu, S., Xiong, J., Shu, X., et al. (2012b). Vascular smooth muscle cell apoptosis promotes transplant arteriosclerosis through inducing the production of SDF-1α. Am. J. Transpl. 12 (8), 2029–2043. doi:10.1111/j.1600-6143.2012.04082.x
Li, L., Xu, T., Du, Y., Pan, D., Wu, W., Zhu, H., et al. (2016). Salvianolic acid A attenuates cell apoptosis, oxidative stress, akt and NF-κB activation in angiotensin-II induced murine peritoneal macrophages. Curr. Pharm. Biotechnol. 17 (3), 283–290. doi:10.2174/138920101703160206150535
Li, M., Zhao, M. Q., Kumar Durairajan, S. S., Xie, L. X., Zhang, H. X., Kum, W. F., et al. (2008). Protective effect of tetramethylpyrazine and salvianolic acid b on apoptosis of rat cerebral microvascular endothelial cell under high shear stress. Clin. Hemorheol. Microcirc. 38 (3), 177–187.
Li, N., Hang, W., Shu, H., Wen, Z., Ceesay, B. M., and Zhou, N. (2022). Salvianolic acid ameliorates pressure overload-induced cardiac endothelial dysfunction via activating hif1[formula: see text]/hsf1/cd31 pathway. Am. J. Chin. Med. 50 (7), 1869–1885. doi:10.1142/S0192415X22500793
Li, Q., Liu, X., Du, Y., Zhang, X., Xiang, P., Chen, G., et al. (2023a). Protocatechuic acid boosts continual efferocytosis in macrophages by derepressing klf4 to transcriptionally activate mertk. Sci. Signal 16 (786), eabn1372. doi:10.1126/scisignal.abn1372
Li, Q., Wei, S., Li, Y., Wu, F., Qin, X., Li, Z., et al. (2023b). Blocking of programmed cell death-ligand 1 (PD-L1) expressed on endothelial cells promoted the recruitment of CD8+IFN-γ+ T cells in atherosclerosis. Inflamm. Res. 72 (4), 783–796. doi:10.1007/s00011-023-01703-5
Li, W., Zhou, H., Chu, Y., Wang, X., Luo, R., Yang, L., et al. (2017). Simultaneous determination and pharmacokinetics of danshensu, protocatechuic aldehyde, 4-hydroxy-3-methyloxyphenyl lactic acid and protocatechuic acid in human plasma by lc-ms/ms after oral administration of compound danshen dripping pills. J. Pharm. Biomed. Anal. 145, 860–864. doi:10.1016/j.jpba.2017.06.014
Li, X., Li, X., Wang, L., Li, Y., Xu, Y., and Xue, M. (2007). Simultaneous determination of danshensu, ferulic acid, cryptotanshinone and tanshinone iia in rabbit plasma by hplc and their pharmacokinetic application in danxiongfang. J. Pharm. Biomed. Anal. 44 (5), 1106–1112. doi:10.1016/j.jpba.2007.04.033
Li, Y., and Liu, L. (2024). Drug-drug interaction between danshensu and irbesartan and its potential mechanism. Xenobiotica 54 (4), 211–216. doi:10.1080/00498254.2024.2338183
Li, Y. J., Duan, C. L., and Liu, J. X. (2014). Salvianolic acid a promotes the acceleration of neovascularization in the ischemic rat myocardium and the functions of endothelial progenitor cells. J. Ethnopharmacol. 151 (1), 218–227. doi:10.1016/j.jep.2013.10.019
Li, Z. M., Xu, S. W., and Liu, P. Q. (2018). Salvia miltiorrhizaburge (danshen): a golden herbal medicine in cardiovascular therapeutics. Acta Pharmacol. Sin. 39 (5), 802–824. doi:10.1038/aps.2017.193
Libby, P., Ridker, P. M., and Hansson, G. K. (2011). Progress and challenges in translating the biology of atherosclerosis. Nature 473 (7347), 317–325. doi:10.1038/nature10146
Lin, M. C., Ou, T. T., Chang, C. H., Chan, K. C., and Wang, C. J. (2015). Protocatechuic acid inhibits oleic acid-induced vascular smooth muscle cell proliferation through activation of amp-activated protein kinase and cell cycle arrest in g0/g1 phase. J. Agric. Food Chem. 63 (1), 235–241. doi:10.1021/jf505303s
Lin, S. J., Lee, I. T., Chen, Y. H., Lin, F. Y., Sheu, L. M., Ku, H. H., et al. (2007). Salvianolic acid b attenuates mmp-2 and mmp-9 expression in vivo in apolipoprotein-e-deficient mouse aorta and in vitro in lps-treated human aortic smooth muscle cells. J. Cell Biochem. 100 (2), 372–384. doi:10.1002/jcb.21042
Lin, Y. K., Chen, Y. J., Li, J. Y., Chen, Y. L., He, D., Zuo, R., et al. (2022). Salvianolic acid a from danhong injection induces vasorelaxation by regulating l-type calcium channel in isolated mouse arteries. J. Ethnopharmacol. 296, 115431. doi:10.1016/j.jep.2022.115431
Ling, W. C., Liu, J., Lau, C. W., Murugan, D. D., Mustafa, M. R., and Huang, Y. (2017). Treatment with salvianolic acid b restores endothelial function in angiotensin ii-induced hypertensive mice. Biochem. Pharmacol. 136, 76–85. doi:10.1016/j.bcp.2017.04.007
Liu, C. D., Liu, N. N., Zhang, S., Ma, G. D., Yang, H. G., Kong, L. L., et al. (2021a). Salvianolic acid a prevented cerebrovascular endothelial injury caused by acute ischemic stroke through inhibiting the src signaling pathway. Acta Pharmacol. Sin. 42 (3), 370–381. doi:10.1038/s41401-020-00568-2
Liu, C. L., Xie, L. X., Li, M., Durairajan, S. S., Goto, S., and Huang, J. D. (2007). Salvianolic acid b inhibits hydrogen peroxide-induced endothelial cell apoptosis through regulating pi3k/akt signaling. PLoS ONE 2 (12), e1321. doi:10.1371/journal.pone.0001321
Liu, H., Ma, S., Xia, H., Lou, H., Zhu, F., and Sun, L. (2018a). Anti-inflammatory activities and potential mechanisms of phenolic acids isolated from salvia miltiorrhiza f. Alba roots in thp-1 macrophages. J. Ethnopharmacol. 222, 201–207. doi:10.1016/j.jep.2018.05.008
Liu, J., Zhang, Y., Qu, D., Zhang, H., Wang, L., Lau, C. W., et al. (2021b). Salvianolic acid b ameliorates vascular endothelial dysfunction through influencing a bone morphogenetic protein 4-ros cycle in diabetic mice. Life Sci. 286, 120039. doi:10.1016/j.lfs.2021.120039
Liu, L., Li, J., Zhang, Y., Zhang, S., Ye, J., Wen, Z., et al. (2014). Salvianolic acid b inhibits platelets as a p2y12 antagonist and pde inhibitor: evidence from clinic to laboratory. Thromb. Res. 134 (4), 866–876. doi:10.1016/j.thromres.2014.07.019
Liu, X., Gao, Z. G., Wu, Y., Stevens, R. C., Jacobson, K. A., and Zhao, S. (2018b). Salvianolic acids from antithrombotic traditional Chinese medicine danshen are antagonists of human p2y(1) and p2y(12) receptors. Sci. Rep. 8 (1), 8084. doi:10.1038/s41598-018-26577-0
Liu, Y., Wang, X., Pang, J., Zhang, H., Luo, J., Qian, X., et al. (2019). Attenuation of atherosclerosis by protocatechuic acid via inhibition of m1 and promotion of m2 macrophage polarization. J. Agric. Food Chem. 67 (3), 807–818. doi:10.1021/acs.jafc.8b05719
Lopes-Bezerra, L. M., and Filler, S. G. (2003). Endothelial cells, tissue factor and infectious diseases. Braz J. Med. Biol. Res. 36 (8), 987–991. doi:10.1590/s0100-879x2003000800004
Luo, X., He, C., Yang, B., Yin, S., and Li, K. (2025). Wtap promotes atherosclerosis by inducing macrophage pyroptosis and m1 polarization through upregulating nlrp3. Appl. Biochem. Biotechnol. doi:10.1007/s12010-024-05106-y
Lv, Y., Jiang, Z., Zhou, W., Yang, H., Jin, G., Wang, D., et al. (2024). Low-shear stress promotes atherosclerosis via inducing endothelial cell pyroptosis mediated by ikkε/STAT1/NLRP3 pathway. Inflammation 47 (3), 1053–1066. doi:10.1007/s10753-023-01960-w
Lv, Y. C., Tang, Y. Y., Peng, J., Zhao, G. J., Yang, J., Yao, F., et al. (2014). Microrna-19b promotes macrophage cholesterol accumulation and aortic atherosclerosis by targeting atp-binding cassette transporter a1. Atherosclerosis 236 (1), 215–226. doi:10.1016/j.atherosclerosis.2014.07.005
Ma, Q., Yang, Q., Chen, J., Yu, C., Zhang, L., Zhou, W., et al. (2020). Salvianolic acid a ameliorates early-stage atherosclerosis development by inhibiting nlrp3 inflammasome activation in zucker diabetic fatty rats. Molecules 25 (5), 1089. doi:10.3390/molecules25051089
Ma, W., Rousseau, Z., Slavkovic, S., Shen, C., Yousef, G. M., and Ni, H. (2022). Doxorubicin-induced platelet activation and clearance relieved by salvianolic acid compound: novel mechanism and potential therapy for chemotherapy-associated thrombosis and thrombocytopenia. Pharmaceuticals (Basel) 15 (12), 1444. doi:10.3390/ph15121444
Ma, Y., and Wang, T. (2007). A solid-phase extraction method for high-performance liquid chromatographic determination of salvianolic acid b in rabbit plasma: application to pharmacokinetic study. Biomed. Chromatogr. 21 (2), 217–220. doi:10.1002/bmc.741
Martinet, W., and De Meyer, G. R. (2009). Autophagy in atherosclerosis: a cell survival and death phenomenon with therapeutic potential. Circ. Res. 104 (3), 304–317. doi:10.1161/CIRCRESAHA.108.188318
Massberg, S., Grahl, L., von Bruehl, M. L., Manukyan, D., Pfeiler, S., Goosmann, C., et al. (2010). Reciprocal coupling of coagulation and innate immunity via neutrophil serine proteases. Nat. Med. 16 (8), 887–896. doi:10.1038/nm.2184
Mauersberger, C., Sager, H. B., Wobst, J., Dang, T. A., Lambrecht, L., Koplev, S., et al. (2022). Loss of soluble guanylyl cyclase in platelets contributes to atherosclerotic plaque formation and vascular inflammation. Nat. Cardiovasc Res. 1 (12), 1174–1186. doi:10.1038/s44161-022-00175-w
Meng, X., Jiang, J., Pan, H., Wu, S., Wang, S., Lou, Y., et al. (2019). Preclinical absorption, distribution, metabolism, and excretion of sodium danshensu, one of the main water-soluble ingredients in salvia miltiorrhiza, in rats. Front. Pharmacol. 10, 554. doi:10.3389/fphar.2019.00554
Moller, W., Heimbeck, I., Hofer, T. P., Khadem Saba, G., Neiswirth, M., Frankenberger, M., et al. (2012). Differential inflammatory response to inhaled lipopolysaccharide targeted either to the airways or the alveoli in man. PLoS ONE 7 (4), e33505. doi:10.1371/journal.pone.0033505
Momtazi-Borojeni, A. A., Abdollahi, E., Nikfar, B., Chaichian, S., and Ekhlasi-Hundrieser, M. (2019). Curcumin as a potential modulator of m1 and m2 macrophages: new insights in atherosclerosis therapy. Heart Fail Rev. 24 (3), 399–409. doi:10.1007/s10741-018-09764-z
Montastruc, J. L. (2023). Rhabdomyolysis and statins: a pharmacovigilance comparative study between statins. Br. J. Clin. Pharmacol. 89 (8), 2636–2638. doi:10.1111/bcp.15757
Moore, K. J., Sheedy, F. J., and Fisher, E. A. (2013). Macrophages in atherosclerosis: a dynamic balance. Nat. Rev. Immunol. 13 (10), 709–721. doi:10.1038/nri3520
Morioka, S., Maueroder, C., and Ravichandran, K. S. (2019). Living on the edge: efferocytosis at the interface of homeostasis and pathology. Immunity 50 (5), 1149–1162. doi:10.1016/j.immuni.2019.04.018
Neves, M. A. D., Ni, T. T., Mackeigan, D. T., Shoara, A. A., Lei, X., Slavkovic, S., et al. (2024). Salvianolic acid b inhibits thrombosis and directly blocks the thrombin catalytic site. Res. Pract. Thromb. Haemost. 8 (4), 102443. doi:10.1016/j.rpth.2024.102443
Ni Gabhann, J., Hams, E., Smith, S., Wynne, C., Byrne, J. C., Brennan, K., et al. (2014). Btk regulates macrophage polarization in response to lipopolysaccharide. PLoS ONE 9 (1), e85834. doi:10.1371/journal.pone.0085834
Nijat, D., Xu, L., Kuang, Y., Yu, R., Zhang, Y., Hasan, A., et al. (2022). A pharmacokinetic-pharmacodynamic study to elucidate the cardiovascular protective constituents in danhong injection. J. Pharm. Biomed. Anal. 219, 114953. doi:10.1016/j.jpba.2022.114953
Niu, W., Wu, F., Cao, W., Chen, Y., Zhang, Y., Chen, Y., et al. (2022). Salvianolic acid b alleviates limb ischemia in mice via promoting sirt1/pi3k/akt pathway-mediated m2 macrophage polarization. Evid. Based Complement. Altern. Med. 2022, 1112394. doi:10.1155/2022/1112394
Nyandwi, J. B., Ko, Y. S., Jin, H., Yun, S. P., Park, S. W., Kang, K. R., et al. (2022). Rosmarinic acid downregulates the oxldl-induced interaction between monocytes and endothelial cells, in addition to monocyte diapedesis, under high glucose conditions. Int. J. Mol. Med. 49 (5), 68. doi:10.3892/ijmm.2022.5125
Nyandwi, J. B., Ko, Y. S., Jin, H., Yun, S. P., Park, S. W., and Kim, H. J. (2021). Rosmarinic acid increases macrophage cholesterol efflux through regulation of abca1 and abcg1 in different mechanisms. Int. J. Mol. Sci. 22 (16), 8791. doi:10.3390/ijms22168791
Orecchioni, M., Kobiyama, K., Winkels, H., Ghosheh, Y., McArdle, S., Mikulski, Z., et al. (2022). Olfactory receptor 2 in vascular macrophages drives atherosclerosis by nlrp3-dependent il-1 production. Science 375 (6577), 214–221. doi:10.1126/science.abg3067
Pan, C. H., Chen, C. W., Sheu, M. J., and Wu, C. H. (2012). Salvianolic acid B inhibits SDF-1α-stimulated cell proliferation and migration of vascular smooth muscle cells by suppressing CXCR4 receptor. Vasc. Pharmacol. 56 (1-2), 98–105. doi:10.1016/j.vph.2011.11.008
Pan, S., White, T. A., Witt, T. A., Chiriac, A., Mueske, C. S., and Simari, R. D. (2009). Vascular-directed tissue factor pathway inhibitor overexpression regulates plasma cholesterol and reduces atherosclerotic plaque development. Circ. Res. 105 (7), 713–720. 8 p following 720. doi:10.1161/CIRCRESAHA.109.195016
Pan, X., Wan, R., Wang, Y., Liu, S., He, Y., Deng, B., et al. (2022). Inhibition of chemically and mechanically activated piezo1 channels as a mechanism for ameliorating atherosclerosis with salvianolic acid b. Br. J. Pharmacol. 179 (14), 3778–3814. doi:10.1111/bph.15826
Paone, S., Baxter, A. A., Hulett, M. D., and Poon, I. K. H. (2019). Endothelial cell apoptosis and the role of endothelial cell-derived extracellular vesicles in the progression of atherosclerosis. Cell Mol. Life Sci. 76 (6), 1093–1106. doi:10.1007/s00018-018-2983-9
Papakyriakopoulou, P., Velidakis, N., Khattab, E., Valsami, G., Korakianitis, I., and Kadoglou, N. P. (2022). Potential pharmaceutical applications of quercetin in cardiovascular diseases. Pharmaceuticals (Basel) 15 (8), 1019. doi:10.3390/ph15081019
Paramel Varghese, G., Folkersen, L., Strawbridge, R. J., Halvorsen, B., Yndestad, A., Ranheim, T., et al. (2016). Nlrp3 inflammasome expression and activation in human atherosclerosis. J. Am. Heart Assoc. 5 (5), e003031. doi:10.1161/JAHA.115.003031
Parsamanesh, N., Asghari, A., Sardari, S., Tasbandi, A., Jamialahmadi, T., Xu, S., et al. (2021). Resveratrol and endothelial function: a literature review. Pharmacol. Res. 170, 105725. doi:10.1016/j.phrs.2021.105725
Pickles, S., Vigie, P., and Youle, R. J. (2018). Mitophagy and quality control mechanisms in mitochondrial maintenance. Curr. Biol. 28 (4), R170-R185–R185. doi:10.1016/j.cub.2018.01.004
Poller, W. C., Nahrendorf, M., and Swirski, F. K. (2020). Hematopoiesis and cardiovascular disease. Circ. Res. 126 (8), 1061–1085. doi:10.1161/CIRCRESAHA.120.315895
Pradhan, A., Bhandari, M., Vishwakarma, P., and Sethi, R. (2024). Clopidogrel resistance and its relevance: current concepts. J. Fam. Med. Prim. Care 13 (6), 2187–2199. doi:10.4103/jfmpc.jfmpc_1473_23
Qi, Q., Hao, K., Li, F. Y., Cao, L. J., Wang, G. J., and Hao, H. P. (2013). The identification and pharmacokinetic studies of metabolites of salvianolic acid b after intravenous administration in rats. Chin. J. Nat. Med. 11 (5), 560–565. doi:10.1016/S1875-5364(13)60101-6
Qin, M., Luo, Y., Meng, X. B., Wang, M., Wang, H. W., Song, S. Y., et al. (2015). Myricitrin attenuates endothelial cell apoptosis to prevent atherosclerosis: an insight into pi3k/akt activation and stat3 signaling pathways. Vasc. Pharmacol. 70, 23–34. doi:10.1016/j.vph.2015.03.002
Qui, Y., Rui, Y. C., Zhang, L., Li, T. J., and Zhang, W. D. (2001). Vegf induced hyperpermeability in bovine aortic endothelial cell and inhibitory effect of salvianolic acid b. Acta Pharmacol. Sin. 22 (2), 117–120.
Ray, R., and Shah, A. M. (2005). Nadph oxidase and endothelial cell function. Clin. Sci. (Lond) 109 (3), 217–226. doi:10.1042/CS20050067
Ren, Y., Tao, S., Zheng, S., Zhao, M., Zhu, Y., Yang, J., et al. (2016). Salvianolic acid b improves vascular endothelial function in diabetic rats with blood glucose fluctuations via suppression of endothelial cell apoptosis. Eur. J. Pharmacol. 791, 308–315. doi:10.1016/j.ejphar.2016.09.014
Schutyser, E., Struyf, S., and Van Damme, J. (2003). The cc chemokine ccl20 and its receptor ccr6. Cytokine Growth Factor Rev. 14 (5), 409–426. doi:10.1016/s1359-6101(03)00049-2
Seneviratne, A., Hulsmans, M., Holvoet, P., and Monaco, C. (2013). Biomechanical factors and macrophages in plaque stability. Cardiovasc Res. 99 (2), 284–293. doi:10.1093/cvr/cvt097
Sergin, I., and Razani, B. (2014). Self-eating in the plaque: what macrophage autophagy reveals about atherosclerosis. Trends Endocrinol. Metab. 25 (5), 225–234. doi:10.1016/j.tem.2014.03.010
Shapira, L., Soskolne, W. A., Houri, Y., Barak, V., Halabi, A., and Stabholz, A. (1996). Protection against endotoxic shock and lipopolysaccharide-induced local inflammation by tetracycline: correlation with inhibition of cytokine secretion. Infect. Immun. 64 (3), 825–828. doi:10.1128/iai.64.3.825-828.1996
Shen, D., Guo, M., Geng, X., Yu, J., Zhang, Z., Lin, J., et al. (2022). Magnesium lithospermate b protects against cisplatin-induced acute kidney injury via alleviating mitochondrial dysfunction. Drug Des. Devel Ther. 16, 2293–2304. doi:10.2147/DDDT.S358830
Shen, L., Chen, W., Ding, J., Shu, G., Chen, M., Zhao, Z., et al. (2023). The role of metabolic reprogramming of oxygen-induced macrophages in the dynamic changes of atherosclerotic plaques. FASEB J. 37 (3), e22791. doi:10.1096/fj.202201486R
Shen, Y., Wang, X., Xu, L., Liu, X., and Chao, R. (2009). Characterization of metabolites in rat plasma after intravenous administration of salvianolic acid a by liquid chromatography/time-of-flight mass spectrometry and liquid chromatography/ion trap mass spectrometry. Rapid Commun. Mass Spectrom. 23 (12), 1810–1816. doi:10.1002/rcm.4078
Shu, H., Peng, Y., Hang, W., Nie, J., Zhou, N., and Wang, D. W. (2022). The role of cd36 in cardiovascular disease. Cardiovasc. Res. 118 (1), 115–129. doi:10.1093/cvr/cvaa319
Siddiqui, M. A., Mukherjee, S., Manivannan, P., and Malathi, K. (2015). Rnase l cleavage products promote switch from autophagy to apoptosis by caspase-mediated cleavage of beclin-1. Int. J. Mol. Sci. 16 (8), 17611–17636. doi:10.3390/ijms160817611
Simion, V., Zhou, H., Haemmig, S., Pierce, J. B., Mendes, S., Tesmenitsky, Y., et al. (2020). A macrophage-specific lncrna regulates apoptosis and atherosclerosis by tethering hur in the nucleus. Nat. Commun. 11 (1), 6135. doi:10.1038/s41467-020-19664-2
Souilhol, C., Harmsen, M. C., Evans, P. C., and Krenning, G. (2018). Endothelial-mesenchymal transition in atherosclerosis. Cardiovasc. Res. 114 (4), 565–577. doi:10.1093/cvr/cvx253
Sun, A., Liu, H., Wang, S., Shi, D., Xu, L., Cheng, Y., et al. (2011). Salvianolic acid B suppresses maturation of human monocyte-derived dendritic cells by activating PPARγ. Br. J. Pharmacol. 164 (8), 2042–2053. doi:10.1111/j.1476-5381.2011.01518.x
Sun, H. J., Zhao, M. X., Ren, X. S., Liu, T. Y., Chen, Q., Li, Y. H., et al. (2016a). Salusin-β promotes vascular smooth muscle cell migration and intimal hyperplasia after vascular injury via ROS/NFκB/MMP-9 pathway. Antioxid. Redox Signal 24 (18), 1045–1057. doi:10.1089/ars.2015.6475
Sun, J., Zhang, L., Song, J., Tian, S., Huang, C., Feng, Z., et al. (2013). Pharmacokinetic study of salvianolic acid a in beagle dog after oral administration by a liquid chromatography-mass spectrometry method: a study on bioavailability and dose proportionality. J. Ethnopharmacol. 148 (2), 617–623. doi:10.1016/j.jep.2013.05.013
Sun, L., Zhao, R., Zhang, L., Zhang, T., Xin, W., Lan, X., et al. (2012). Salvianolic acid a inhibits pdgf-bb induced vascular smooth muscle cell migration and proliferation while does not constrain endothelial cell proliferation and nitric oxide biosynthesis. Molecules 17 (3), 3333–3347. doi:10.3390/molecules17033333
Sun, L., Zhao, R., Zhang, L., Zhang, W., He, G., Yang, S., et al. (2016b). Prevention of vascular smooth muscle cell proliferation and injury-induced neointimal hyperplasia by creb-mediated p21 induction: an insight from a plant polyphenol. Biochem. Pharmacol. 103, 40–52. doi:10.1016/j.bcp.2016.01.015
Sun, M., Ye, Y., Huang, Y., Yin, W., Yu, Z., and Wang, S. (2021). Salvianolic acid b improves autophagic dysfunction and decreases the apoptosis of cholesterol crystal-induced macrophages via inhibiting the akt/mtor signaling pathway. Mol. Med. Rep. 24 (5), 763. doi:10.3892/mmr.2021.12403
Suschek, C. V., Schnorr, O., Hemmrich, K., Aust, O., Klotz, L. O., Sies, H., et al. (2003). Critical role of l-arginine in endothelial cell survival during oxidative stress. Circulation 107 (20), 2607–2614. doi:10.1161/01.CIR.0000066909.13953.F1
Takei, Y., Yamada, M., Saito, K., Kameyama, Y., Aihara, T., Iwasaki, Y., et al. (2024). Endothelium-derived extracellular vesicles expressing intercellular adhesion molecules reflect endothelial permeability and sepsis severity. Anesth. Analg. 139 (2), 385–396. doi:10.1213/ANE.0000000000006988
Tamargo, I. A., Baek, K. I., Kim, Y., Park, C., and Jo, H. (2023). Flow-induced reprogramming of endothelial cells in atherosclerosis. Nat. Rev. Cardiol. 20 (11), 738–753. doi:10.1038/s41569-023-00883-1
Tanase, D. M., Valasciuc, E., Gosav, E. M., Ouatu, A., Buliga-Finis, O. N., Floria, M., et al. (2023). Portrayal of nlrp3 inflammasome in atherosclerosis: current knowledge and therapeutic targets. Int. J. Mol. Sci. 24 (9), 8162. doi:10.3390/ijms24098162
Tang, J., Lobatto, M. E., Hassing, L., van der Staay, S., van Rijs, S. M., Calcagno, C., et al. (2015). Inhibiting macrophage proliferation suppresses atherosclerotic plaque inflammation. Sci. Adv. 1 (3), e1400223. doi:10.1126/sciadv.1400223
Tang, Y., Jacobi, A., Vater, C., Zou, X., and Stiehler, M. (2014). Salvianolic acid b protects human endothelial progenitor cells against oxidative stress-mediated dysfunction by modulating akt/mtor/4ebp1, p38 mapk/atf2, and erk1/2 signaling pathways. Biochem. Pharmacol. 90 (1), 34–49. doi:10.1016/j.bcp.2014.04.008
Tang, Y., Wa, Q., Peng, L., Zheng, Y., Chen, J., Chen, X., et al. (2022). Salvianolic acid b suppresses er stress-induced nlrp3 inflammasome and pyroptosis via the ampk/foxo4 and syndecan-4/rac1 signaling pathways in human endothelial progenitor cells. Oxid. Med. Cell Longev. 2022, 8332825. doi:10.1155/2022/8332825
Teng, F., Yin, Y., Cui, Y., Deng, Y., Li, D., Cho, K., et al. (2016). Salvianolic acid a inhibits endothelial dysfunction and vascular remodeling in spontaneously hypertensive rats. Life Sci. 144, 86–93. doi:10.1016/j.lfs.2015.06.010
Terao, J. (2023). Potential role of quercetin glycosides as anti-atherosclerotic food-derived factors for human health. Antioxidants (Basel) 12 (2), 258. doi:10.3390/antiox12020258
Tulis, D. A., Durante, W., Liu, X., Evans, A. J., Peyton, K. J., and Schafer, A. I. (2001). Adenovirus-mediated heme oxygenase-1 gene delivery inhibits injury-induced vascular neointima formation. Circulation 104 (22), 2710–2715. doi:10.1161/hc4701.099585
Turner, R. M., and Pirmohamed, M. (2019). Statin-related myotoxicity: a comprehensive review of pharmacokinetic, pharmacogenomic and muscle components. J. Clin. Med. 9 (1), 22. doi:10.3390/jcm9010022
Vari, R., Scazzocchio, B., Santangelo, C., Filesi, C., Galvano, F., D'Archivio, M., et al. (2015). Protocatechuic acid prevents oxldl-induced apoptosis by activating jnk/nrf2 survival signals in macrophages. Oxid. Med. Cell Longev. 2015, 351827. doi:10.1155/2015/351827
Wan, R. Z., Zhou, M. J., and Liu, C. X. (2010). The effects of salvianolic acid b from radix salvia miltiorrhizae on the oral pharmacokinetics of metoprolol and metoprolol acid in rats. Phytother. Res. 24 (6), 846–851. doi:10.1002/ptr.3030
Wang, D., Wei, X., Yan, X., Jin, T., and Ling, W. (2010a). Protocatechuic acid, a metabolite of anthocyanins, inhibits monocyte adhesion and reduces atherosclerosis in apolipoprotein e-deficient mice. J. Agric. Food Chem. 58 (24), 12722–12728. doi:10.1021/jf103427j
Wang, J., Uryga, A. K., Reinhold, J., Figg, N., Baker, L., Finigan, A., et al. (2015a). Vascular smooth muscle cell senescence promotes atherosclerosis and features of plaque vulnerability. Circulation 132 (20), 1909–1919. doi:10.1161/CIRCULATIONAHA.115.016457
Wang, L., Bao, Y., Yang, Y., Wu, Y., Chen, X., Si, S., et al. (2010b). Discovery of antagonists for human scavenger receptor cd36 via an elisa-like high-throughput screening assay. J. Biomol. Screen 15 (3), 239–250. doi:10.1177/1087057109359686
Wang, M., Sun, G., Wu, P., Chen, R., Yao, F., Qin, M., et al. (2013). Salvianolic acid b prevents arsenic trioxide-induced cardiotoxicity in vivo and enhances its anticancer activity in vitro. Evid. Based Complement. Altern. Med. 2013, 759483. doi:10.1155/2013/759483
Wang, S. B., Yang, X. Y., Tian, S., Yang, H. G., and Du, G. H. (2009). Effect of salvianolic acid a on vascular reactivity of streptozotocin-induced diabetic rats. Life Sci. 85 (13-14), 499–504. doi:10.1016/j.lfs.2009.07.018
Wang, X., Li, W., Ma, X., Yan, K., Chu, Y., Han, M., et al. (2015b). Identification of a major metabolite of danshensu in rat urine and simultaneous determination of danshensu and its metabolite in plasma: application to a pharmacokinetic study in rats. Drug Test. Anal. 7 (8), 727–736. doi:10.1002/dta.1750
Wang, X., Zhang, M., Zhang, M., Han, Y., Chen, X., Zhao, W., et al. (2022). Salvianolic acid A promotes mitochondrial biogenesis and function via regulating the AMPK/PGC-1α signaling pathway in HUVECs. Exp. Ther. Med. 24 (1), 485. doi:10.3892/etm.2022.11412
Ward, N. C., Watts, G. F., and Eckel, R. H. (2019). Statin toxicity. Circ. Res. 124 (2), 328–350. doi:10.1161/CIRCRESAHA.118.312782
Weber, C., Habenicht, A. J. R., and von Hundelshausen, P. (2023). Novel mechanisms and therapeutic targets in atherosclerosis: inflammation and beyond. Eur. Heart J. 44 (29), 2672–2681. doi:10.1093/eurheartj/ehad304
Wei, X., Zhang, B., Wei, F., Ding, M., Luo, Z., Han, X., et al. (2022). Gegen Qinlian pills alleviate carrageenan-induced thrombosis in mice model by regulating the HMGB1/NF-κB/NLRP3 signaling. Phytomedicine 100, 154083. doi:10.1016/j.phymed.2022.154083
Wen, J. H., and Xiong, Y. Q. (2011). The effect of herbal medicine danshensu and ursolic acid on pharmacokinetics of rosuvastatin in rats. Eur. J. Drug Metab. Pharmacokinet. 36 (4), 205–211. doi:10.1007/s13318-011-0048-7
Whiting, M. J., and Watts, J. M. (1983). Cholesterol crystal formation and growth in model bile solutions. J. Lipid Res. 24 (7), 861–868. doi:10.1016/s0022-2275(20)37931-1
Wilson, H. M. (2022). The intracellular signaling pathways governing macrophage activation and function in human atherosclerosis. Biochem. Soc. Trans. 50 (6), 1673–1682. doi:10.1042/BST20220441
Wolf, D., and Ley, K. (2019). Immunity and inflammation in atherosclerosis. Circ. Res. 124 (2), 315–327. doi:10.1161/CIRCRESAHA.118.313591
Wu, R., Li, Z., Wang, M., Chang, G., Yao, C., and Wang, S. (2017). Paclitaxel-coated versus uncoated balloon angioplasty for femoropopliteal artery in-stent restenosis. Int. J. Surg. 42, 72–82. doi:10.1016/j.ijsu.2017.04.057
Wu, Y. P., Zhao, X. M., Pan, S. D., Guo, D. A., Wei, R., Han, J. J., et al. (2008). Salvianolic acid b inhibits platelet adhesion under conditions of flow by a mechanism involving the collagen receptor alpha2beta1. Thromb. Res. 123 (2), 298–305. doi:10.1016/j.thromres.2008.05.020
Wu, Y. T., Chen, Y. F., Hsieh, Y. J., Jaw, I., Shiao, M. S., and Tsai, T. H. (2006). Bioavailability of salvianolic acid b in conscious and freely moving rats. Int. J. Pharm. 326 (1-2), 25–31. doi:10.1016/j.ijpharm.2006.07.003
Xia, Y., Feng, H., Li, Z. W., Tang, K. X., Gao, H. Q., Wang, W. L., et al. (2020). Low-dose phloretin alleviates diabetic atherosclerosis through endothelial klf2 restoration. Biosci. Biotechnol. Biochem. 84 (4), 815–823. doi:10.1080/09168451.2019.1699396
Xiang, J., Zhang, C., Di, T., Chen, L., Zhao, W., Wei, L., et al. (2022). Salvianolic acid b alleviates diabetic endothelial and mitochondrial dysfunction by down-regulating apoptosis and mitophagy of endothelial cells. Bioengineered 13 (2), 3486–3502. doi:10.1080/21655979.2022.2026552
Xie, D., Song, L., Xiang, D., Gao, X., and Zhao, W. (2023). Salvianolic acid a alleviates atherosclerosis by inhibiting inflammation through trc8-mediated 3-hydroxy-3-methylglutaryl-coenzyme a reductase degradation. Phytomedicine 112, 154694. doi:10.1016/j.phymed.2023.154694
Xu, B., Deng, H., Zhang, X., Luo, J., Zhang, G., Zhang, Z., et al. (2018a). A novel danshensu/tetramethylpyrazine derivative induces vasorelaxation on rat aorta and exerts cardioprotection in dogs. Eur. J. Pharmacol. 818, 158–166. doi:10.1016/j.ejphar.2017.10.034
Xu, J., Wei, K., Zhang, G., Lei, L., Yang, D., Wang, W., et al. (2018b). Ethnopharmacology, phytochemistry, and pharmacology of Chinese salvia species: a review. J. Ethnopharmacol. 225, 18–30. doi:10.1016/j.jep.2018.06.029
Xu, S., Ilyas, I., Little, P. J., Li, H., Kamato, D., Zheng, X., et al. (2021). Endothelial dysfunction in atherosclerotic cardiovascular diseases and beyond: from mechanism to pharmacotherapies. Pharmacol. Rev. 73 (3), 924–967. doi:10.1124/pharmrev.120.000096
Xu, S., Zhong, A., Bu, X., Ma, H., Li, W., Xu, X., et al. (2015). Salvianolic acid b inhibits platelets-mediated inflammatory response in vascular endothelial cells. Thromb. Res. 135 (1), 137–145. doi:10.1016/j.thromres.2014.10.034
Xue, Y., Zhang, L., Zhang, L., Sun, W., Fang, Z., Leng, Y., et al. (2022). Danshensu prevents thrombosis by inhibiting platelet activation via sirt1/ros/mtdna pathways without increasing bleeding risk. Phytomedicine 104, 154271. doi:10.1016/j.phymed.2022.154271
Ya, F., Li, K., Chen, H., Tian, Z., Fan, D., Shi, Y., et al. (2021). Protocatechuic acid protects platelets from apoptosis via inhibiting oxidative stress-mediated PI3K/Akt/GSK3β signaling. Thromb. Haemost. 121 (7), 931–943. doi:10.1055/s-0040-1722621
Yang, K., Luo, Y., Lu, S., Hu, R., Du, Y., Liao, P., et al. (2018). Salvianolic acid b and ginsenoside re synergistically protect against ox-ldl-induced endothelial apoptosis through the antioxidative and antiinflammatory mechanisms. Front. Pharmacol. 9, 662. doi:10.3389/fphar.2018.00662
Yang, M. Y., Song, Z. Y., Gan, H. L., Zheng, M. H., Liu, Q., Meng, X. T., et al. (2022). Non-clinical safety evaluation of salvianolic acid a: acute, 4-week intravenous toxicities and genotoxicity evaluations. BMC Pharmacol. Toxicol. 23 (1), 83. doi:10.1186/s40360-022-00622-1
Yang, S., Liu, L., Meng, L., and Hu, X. (2019). Capsaicin is beneficial to hyperlipidemia, oxidative stress, endothelial dysfunction, and atherosclerosis in Guinea pigs fed on a high-fat diet. Chem. Biol. Interact. 297, 1–7. doi:10.1016/j.cbi.2018.10.006
Yang, X., Pan, W., Xu, G., and Chen, L. (2020a). Mitophagy: a crucial modulator in the pathogenesis of chronic diseases. Clin. Chim. Acta 502, 245–254. doi:10.1016/j.cca.2019.11.008
Yang, X. Y., Qiang, G. F., Zhang, L., Zhu, X. M., Wang, S. B., Sun, L., et al. (2011). Salvianolic acid a protects against vascular endothelial dysfunction in high-fat diet fed and streptozotocin-induced diabetic rats. J. Asian Nat. Prod. Res. 13 (10), 884–894. doi:10.1080/10286020.2011.598457
Yang, Y., Pei, K., Zhang, Q., Wang, D., Feng, H., Du, Z., et al. (2020b). Salvianolic acid b ameliorates atherosclerosis via inhibiting yap/taz/jnk signaling pathway in endothelial cells and pericytes. Biochim. Biophys. Acta Mol. Cell Biol. Lipids 1865 (10), 158779. doi:10.1016/j.bbalip.2020.158779
Yao, Y., Mao, J., Xu, S., Zhao, L., Long, L., Chen, L., et al. (2019). Rosmarinic acid inhibits nicotine-induced c-reactive protein generation by inhibiting nlrp3 inflammasome activation in smooth muscle cells. J. Cell Physiol. 234 (2), 1758–1767. doi:10.1002/jcp.27046
Ye, T., Xiong, D., Li, Y., Gong, S., Zhang, L., Li, B., et al. (2020). Inhibition of nuclear factor kappa b as a mechanism of danshensu during toll-like receptor 2-triggered inflammation in macrophages. Int. Immunopharmacol. 83, 106419. doi:10.1016/j.intimp.2020.106419
Yin, Y., Duan, J., Guo, C., Wei, G., Wang, Y., Guan, Y., et al. (2017). Danshensu accelerates angiogenesis after myocardial infarction in rats and promotes the functions of endothelial progenitor cells through SDF-1α/CXCR4 axis. Eur. J. Pharmacol. 814, 274–282. doi:10.1016/j.ejphar.2017.08.035
Yu, C., Dong, H., Wang, Q., Bai, J., Li, Y. N., Zhao, J. J., et al. (2021). Danshensu attenuates cisplatin-induced nephrotoxicity through activation of Nrf2 pathway and inhibition of NF-κB. Biomed. Pharmacother. 142, 111995. doi:10.1016/j.biopha.2021.111995
Yu, C., Qi, D., Lian, W., Li, Q. Z., Li, H. J., and Fan, H. Y. (2014). Effects of danshensu on platelet aggregation and thrombosis: in vivo arteriovenous shunt and venous thrombosis models in rats. PLoS ONE 9 (11), e110124. doi:10.1371/journal.pone.0110124
Yuan, X., Fei, F., Sun, H., Xiao, C., Zhao, X., Zhang, Y., et al. (2018). Tanshinol borneol ester on nanostructured lipid carriers has longer brain and systemic effector retention and better antioxidant activity in vivo. Int. J. Nanomed. 13, 2265–2274. doi:10.2147/IJN.S159789
Yuan, X., Xiang, Y., Zhu, N., Zhao, X., Ye, S., Zhong, P., et al. (2017). Salvianolic acid a protects against myocardial ischemia/reperfusion injury by reducing platelet activation and inflammation. Exp. Ther. Med. 14 (2), 961–966. doi:10.3892/etm.2017.4619
Yue, J., Li, B., Jing, Q., and Guan, Q. (2015). Salvianolic acid B accelerated ABCA1-dependent cholesterol efflux by targeting PPAR-γ and LXRα. Biochem. Biophys. Res. Commun. 462 (3), 233–238. doi:10.1016/j.bbrc.2015.04.122
Yurdagul, A., Doran, A. C., Cai, B., Fredman, G., and Tabas, I. A. (2017). Mechanisms and consequences of defective efferocytosis in atherosclerosis. Front. Cardiovasc. Med. 4, 86. doi:10.3389/fcvm.2017.00086
Zeng, M., Zhang, X., Lv, N., Wang, L., Suo, Y., Gan, J., et al. (2023). Sodium Danshensu stabilizes atherosclerotic vulnerable plaques by targeting IKKβ mediated inflammation in macrophages. Biomed. Pharmacother. 165, 115153. doi:10.1016/j.biopha.2023.115153
Zhai, J., Qu, X., Zhang, Y., Gao, H., Tao, L., Song, Y., et al. (2019). Salvianolic acid inhibits the effects of high glucose on vascular endothelial dysfunction by modulating the sirt1-enos pathway. J. Biochem. Mol. Toxicol. 33 (2), e22245. doi:10.1002/jbt.22245
Zhang, F., Zhang, Y., Li, X., Zhang, S., Zhu, M., Du, W., et al. (2018). Research on q-markers of qiliqiangxin capsule for chronic heart failure treatment based on pharmacokinetics and pharmacodynamics association. Phytomedicine 44, 220–230. doi:10.1016/j.phymed.2018.03.003
Zhang, M. L., Zhang, M. N., Chen, H., Wang, X., Zhao, K., Li, X., et al. (2024a). Salvianolic acid b alleviates high glucose-induced vascular smooth muscle cell inflammation by upregulating the mir-486a-5p expression. Mediat. Inflamm. 2024, 4121166. doi:10.1155/2024/4121166
Zhang, Q., Liu, J., Duan, H., Li, R., Peng, W., and Wu, C. (2021a). Activation of nrf2/ho-1 signaling: an important molecular mechanism of herbal medicine in the treatment of atherosclerosis via the protection of vascular endothelial cells from oxidative stress. J. Adv. Res. 34, 43–63. doi:10.1016/j.jare.2021.06.023
Zhang, S., Li, L., Chen, W., Xu, S., Feng, X., and Zhang, L. (2021b). Natural products: the role and mechanism in low-density lipoprotein oxidation and atherosclerosis. Phytother. Res. 35 (6), 2945–2967. doi:10.1002/ptr.7002
Zhang, X., Yang, Q., Zhang, R., Zhang, Y., Zeng, W., Yu, Q., et al. (2024b). Sodium danshensu ameliorates cerebral ischemia/reperfusion injury by inhibiting clic4/nlrp3 inflammasome-mediated endothelial cell pyroptosis. Biofactors 50 (1), 74–88. doi:10.1002/biof.1991
Zhang, X. C., Chen, J. Q., and Li, B. (2014). Salvianolic acid a suppresses ccl-20 expression in tnf-alpha-treated macrophages and apoe-deficient mice. J. Cardiovasc. Pharmacol. 64 (4), 318–325. doi:10.1097/FJC.0000000000000117
Zhang, Y., Feng, X., Du, M., Ding, J., and Liu, P. (2022). Salvianolic acid B attenuates the inflammatory response in atherosclerosis by regulating MAPKs/NF-κB signaling pathways in LDLR-/- mice and RAW264.7 cells. Int. J. Immunopathol. Pharmacol. 36, 3946320221079468. doi:10.1177/03946320221079468
Zhang, Y., Yang, M., Ho, N. J., Mok, R. Y., Zhang, Z., Ge, B., et al. (2020). Is it safe to take radix salvia miltiorrhiza - radix pueraria lobate product with warfarin and aspirin? A pilot study in healthy human subjects. J. Ethnopharmacol. 262, 113151. doi:10.1016/j.jep.2020.113151
Zhao, M., Li, F., Jian, Y., Wang, X., Yang, H., Wang, J., et al. (2020). Salvianolic acid b regulates macrophage polarization in ischemic/reperfused hearts by inhibiting mtorc1-induced glycolysis. Eur. J. Pharmacol. 871, 172916. doi:10.1016/j.ejphar.2020.172916
Zhao, X. S., Zheng, B., Wen, Y., Sun, Y., Wen, J. K., and Zhang, X. H. (2019). Salvianolic acid b inhibits ang ii-induced vsmc proliferation in vitro and intimal hyperplasia in vivo by downregulating mir-146a expression. Phytomedicine 58, 152754. doi:10.1016/j.phymed.2018.11.014
Zhao, Y., Shao, C., Zhou, H., Yu, L., Bao, Y., Mao, Q., et al. (2023). Salvianolic acid B inhibits atherosclerosis and TNF-α-induced inflammation by regulating NF-κB/NLRP3 signaling pathway. Phytomedicine 119, 155002. doi:10.1016/j.phymed.2023.155002
Zhaolin, Z., Guohua, L., Shiyuan, W., and Zuo, W. (2019). Role of pyroptosis in cardiovascular disease. Cell Prolif. 52 (2), e12563. doi:10.1111/cpr.12563
Zheng, B., Yin, W. N., Suzuki, T., Zhang, X. H., Zhang, Y., Song, L. L., et al. (2017). Exosome-mediated mir-155 transfer from smooth muscle cells to endothelial cells induces endothelial injury and promotes atherosclerosis. Mol. Ther. 25 (6), 1279–1294. doi:10.1016/j.ymthe.2017.03.031
Zheng, X., Liu, H., Ma, M., Ji, J., Zhu, F., and Sun, L. (2021). Anti-thrombotic activity of phenolic acids obtained from Salvia miltiorrhiza f. alba in TNF-α-stimulated endothelial cells via the NF-κB/JNK/p38 MAPK signaling pathway. Arch. Pharm. Res. 44 (4), 427–438. doi:10.1007/s12272-021-01325-7
Zhong, W., Sun, B., Gao, W., Qin, Y., Zhang, H., Huai, L., et al. (2018). Salvianolic acid a targeting the transgelin-actin complex to enhance vasoconstriction. eBioMedicine 37, 246–258. doi:10.1016/j.ebiom.2018.10.041
Zhou, A. M., Xiang, Y. J., Liu, E. Q., Cai, C. H., Wu, Y. H., Yang, L. B., et al. (2020). Salvianolic acid a inhibits platelet activation and aggregation in patients with type 2 diabetes mellitus. BMC Cardiovasc. Disord. 20 (1), 15. doi:10.1186/s12872-019-01316-z
Zhou, L., Wang, S., Zhang, Z., Lau, B. S., Fung, K. P., Leung, P. C., et al. (2012). Pharmacokinetic and pharmacodynamic interaction of danshen-gegen extract with warfarin and aspirin. J. Ethnopharmacol. 143 (2), 648–655. doi:10.1016/j.jep.2012.07.029
Zhou, L., Zuo, Z., and Chow, M. S. (2005a). Danshen: an overview of its chemistry, pharmacology, pharmacokinetics, and clinical use. J. Clin. Pharmacol. 45 (12), 1345–1359. doi:10.1177/0091270005282630
Zhou, Z., Liu, Y., Miao, A. D., and Wang, S. Q. (2005b). Salvianolic acid b attenuates plasminogen activator inhibitor type 1 production in tnf-alpha treated human umbilical vein endothelial cells. J. Cell Biochem. 96 (1), 109–116. doi:10.1002/jcb.20567
Zhu, J., Chen, H., Le, Y., Guo, J., Liu, Z., Dou, X., et al. (2022). Salvianolic acid a regulates pyroptosis of endothelial cells via directly targeting pkm2 and ameliorates diabetic atherosclerosis. Front. Pharmacol. 13, 1009229. doi:10.3389/fphar.2022.1009229
Zong, Y., Huang, Y., Chen, S., Zhu, M., Chen, Q., Feng, S., et al. (2015). Downregulation of endogenous hydrogen sulfide pathway is involved in mitochondrion-related endothelial cell apoptosis induced by high salt. Oxid. Med. Cell Longev. 2015, 754670. doi:10.1155/2015/754670
Zou, J., Chen, Y., Hoi, M. P. M., Li, J., Wang, T., Zhang, Y., et al. (2018). Discovery of a novel erp57 inhibitor as antiplatelet agent from danshen (salvia miltiorrhiza). Evid. Based Complement. Altern. Med. 2018, 9387568. doi:10.1155/2018/9387568
Keywords: Salvia miltiorrhiza Bunge, salvianolic acid A, salvianolic acid B, danshensu, atherosclerosis
Citation: Liang Q, Liu X, Xu X, Chen Z, Luo T, Su Y, Xie H, Gao H and Xie C (2025) Anti-atherosclerotic effects and molecular targets of salvianolic acids from Salvia miltiorrhiza Bunge. Front. Pharmacol. 16:1574086. doi: 10.3389/fphar.2025.1574086
Received: 10 February 2025; Accepted: 02 April 2025;
Published: 21 May 2025.
Edited by:
Shuai Wang, Liaoning University of Traditional Chinese Medicine, ChinaReviewed by:
Chenghao Fei, Nanjing Agricultural University, ChinaBaonian Liu, Shanghai University of Traditional Chinese Medicine, China
Ke Cai, Nanjing University of Chinese Medicine, China
Copyright © 2025 Liang, Liu, Xu, Chen, Luo, Su, Xie, Gao and Xie. This is an open-access article distributed under the terms of the Creative Commons Attribution License (CC BY). The use, distribution or reproduction in other forums is permitted, provided the original author(s) and the copyright owner(s) are credited and that the original publication in this journal is cited, in accordance with accepted academic practice. No use, distribution or reproduction is permitted which does not comply with these terms.
*Correspondence: Chunguang Xie, eGllY2dAY2R1dGNtLmVkdS5jbg==
†These authors have contributed equally to this work and share first authorship