- School of Basic Medical Sciences, Chengdu University of Traditional Chinese Medicine, Chengdu, China
Obesity rates are rising globally and have become a major public health issue. Recent research emphasizes the bidirectional communication between gut microbiota and mitochondrial function in obesity development. Gut microbiota regulates energy metabolism through metabolites that impact mitochondrial processes, such as oxidative phosphorylation, biogenesis, and autophagy. In turn, alterations in mitochondrial function impact microbiota homeostasis. Traditional Chinese medicine (TCM), which encompasses TCM formulas and the metabolites of botanical drugs, employs a holistic and integrative approach that shows promise in regulating gut microbiota–mitochondrial crosstalk. This review systematically explores the intricate interactions between gut microbiota and mitochondrial function, underscoring their crosstalk as a critical mechanistic axis in obesity pathogenesis. Furthermore, it highlights the potential of TCM in developing innovative, targeted interventions, paving the way for personalized approaches in obesity treatment through the precise modulation of gut microbiota–mitochondrial interactions, offering more effective and individualized therapeutic options.
1 Introduction
The global incidence of obesity, a multifaceted metabolic disorder, has surged in recent decades, becoming a pressing public health challenge (Welsh et al., 2024). While excessive calorie intake and inactivity are known causes of obesity, attention is shifting toward gut microbiota and mitochondrial interactions (Belda et al., 2022). In a healthy state, the gut microbiota supports host energy metabolism, lipid metabolism, and glucose homeostasis through diverse metabolic activities (Fan and Pedersen, 2021). This microbiota supports the intestinal barrier functionality through the modulation of the host’s cellular processes and immune responses (Leshem et al., 2020). However, a disruption in the gut microbial balance can increase intestinal permeability, thereby allowing bacterial toxins and metabolic products to enter the bloodstream and disrupt the body’s overall metabolic equilibrium (Gasmi et al., 2021). Unfortunately, the exact mechanisms within the intestinal microbial community that trigger these metabolic abnormalities remain poorly understood (Michaudel and Sokol, 2020).
Gut microbiota–mitochondria interactions play a crucial role in various health conditions has become increasingly evident (Ballard and Towarnicki, 2020; Li Y. et al., 2023). By metabolizing dietary elements, gut microbiota generates short-chain fatty acids (SCFAs) and secondary bile acids (SBAs), which influence mitochondrial oxidative phosphorylation (OXPHOS), biogenesis, dynamics, and autophagy mechanisms, thereby significantly affecting energy balance and metabolism (Yoo et al., 2021; Fogelson et al., 2023). As the main cellular powerhouses, mitochondria are pivotal in regulating fat metabolism, thermoregulation, and oxidative balance (Brestoff et al., 2021; Xia et al., 2024). Dysfunctional mitochondria can produce excess reactive oxygen species (ROS) and organic acids, subsequently altering the gut microbiota composition and function, leading to intricate two-way interactions (Singh et al., 2022).
The ancient Chinese medical text Lingshu Jing of the Huangdi Neijing states that obesity is characterized by “people having fat, ointment, and flesh.” It describes obesity as a condition primarily caused by spleen and kidney deficiency and liver qi stagnation, with phlegm, dampness, heat, and blood stasis as its key pathological manifestations. These factors interact with one another, affecting both energy metabolism and material metabolism in the body. Based on this understanding, traditional Chinese medicine (TCM) has been widely applied in the clinical treatment of obesity, demonstrating safe, gentle, and long-lasting effects, and has accumulated extensive experience through long-term practice (Li et al., 2020; Chen Y. K. et al., 2023; Zhang Q. et al., 2023). Rooted in TCM, TCM formulas and metabolites of botanical drugs offer a promising approach to managing obesity by modulating gut microbiota and mitochondrial function. By restoring microbial balance and enhancing mitochondrial efficiency, it addresses key dysfunctions within the gut microbiota–mitochondria axis, providing a novel and integrative approach to targeted obesity interventions.
Although early research has revealed a sophisticated relationship between gut microbiota and mitochondria, the precise mechanisms underlying this interaction in obesity development remain to be fully understood. This review systematically examines the intricate crosstalk between gut microbiota and mitochondria, emphasizing their role as a mechanistic axis in obesity. Furthermore, it explores the potential of TCM-based interventions in modulating this axis, highlighting their prospective applications in precision medicine approaches for obesity treatment.
2 Review methodology
This review conducted a comprehensive literature search across PubMed, Web of Science, ScienceDirect, Google Scholar, and CNKI using a combination of controlled vocabulary and free-text keywords, including “gut microbiota,” “mitochondria,” “obesity,” “metabolic disorders,” “plant metabolites,” “botanical drugs,” and “Traditional Chinese Medicine.” Boolean operators (AND, OR) and database-specific filters were applied to refine results and exclude irrelevant publications. Inclusion criteria encompassed studies investigating gut microbiota-mitochondria interactions in obesity and metabolic disorders using rigorously designed in vivo or in vitro models. Exclusion criteria comprised studies with unclear experimental design, insufficient mechanistic evaluation, inadequate sample sizes, unsupported conclusions, or redundant/duplicate publications.
3 Mitochondrial dysfunction in obesity
Mitochondria generate adenosine triphosphate (ATP) through OXPHOS, providing the necessary energy for cellular activities. Beyond energy production, mitochondria are integral to numerous vital cellular functions, including apoptosis control, calcium homeostasis, lipid metabolism, and thermogenesis. Maintaining mitochondrial homeostasis involves balancing and regulating various processes, including OXPHOS, dynamics, biogenesis, and autophagy, all of which are critical for sustaining energy metabolism. Disruptions in these processes can lead to obesity (Figure 1).
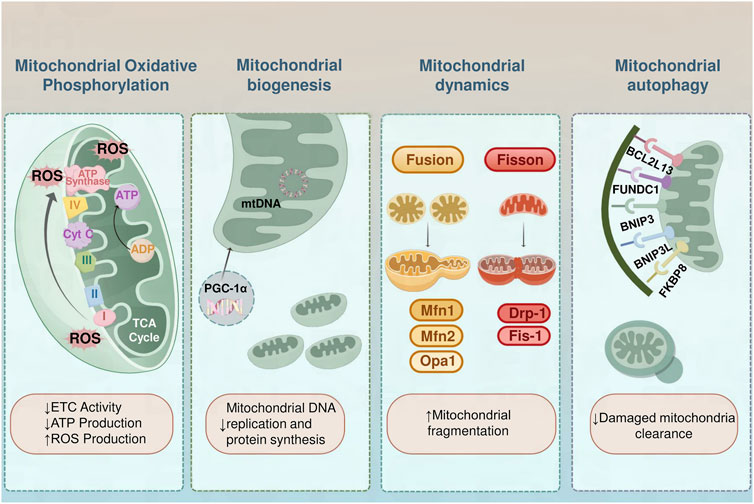
Figure 1. Mitochondrial Dysfunction in Obesity. In individuals with obesity, diminished electron transport chain function results in lower ATP generation and elevated ROS levels, inducing oxidative stress and harm to cells. The process of mitochondrial biogenesis is controlled by PGC-1α, with reduced biogenesis resulting in decreased mitochondrial DNA duplication and protein production. The balance of mitochondrial dynamics is compromised, characterized by enhanced fission facilitated by Drp1 and Fis1, coupled with reduced fusion facilitated by Mfn1/2 and Opa1, leading to a deterioration of network cohesiveness. Mitochondrial autophagy, the process of clearing damaged mitochondria, is impaired, leading to their accumulation. Key mitophagy receptors interacting with the LC3 are essential for the management of mitochondrial quality. BCL2L13 and FUNDC1 bind LC3 to facilitate autophagic clearance, especially under hypoxic conditions. The BNIP3 and BNIP3L possess LC3-interacting region motifs essential for mitochondrial recycling under stressful conditions. FKBP8 also interacts with LC3 to facilitate the clearance of dysfunctional mitochondria.
3.1 Mitochondrial oxidative phosphorylation
Mitochondrial OXPHOS, a key component of cellular respiration, converts chemical energy from nutrients into ATP, the primary energy source for cellular functions (Harrington et al., 2023; Talari et al., 2023). Under normal conditions, electrons move through the electron transport chain sequentially from complexes I to IV, where they ultimately combine with oxygen to form water. As the electrons travel, their movement drives the transfer of protons from the mitochondrial matrix to the intermembrane space. This process creates an electrochemical gradient across the inner mitochondrial membrane. (Pedersen et al., 2022). ATP synthase utilizes this gradient to synthesize ATP from ADP and inorganic phosphate, fueling cellular functions. However, dysfunctional mitochondria impair this process by reducing ATP production and increasing oxidative stress (CA et al., 2021). Obese individuals exhibit significantly reduced activity in complexes I, III, and IV, impairing the effectiveness of the electron transport chain and disrupting ATP production. This dysfunction also increases electron leakage from the chain, leading to the excessive production of ROS (MacLean et al., 2023; Zotta et al., 2024). The accumulation of ROS exacerbates damage to mitochondria and other cell structures, perpetuating a detrimental cycle. In addition, impaired OXPHOS leads to the accumulation of metabolic intermediates, such as lactate, which interferes with normal metabolic processes (Sergio et al., 2021; Abu Shelbayeh et al., 2023).
3.2 Mitochondrial biogenesis
Mitochondrial biogenesis refers to the generation and proliferation of new mitochondria within cells, a process essential for maintaining cellular energy supply and metabolic equilibrium. This process includes the replication of mitochondrial DNA, the formation of mitochondrial membranes, and the synthesis and assembly of mitochondrial proteins (Chen L. et al., 2023; Lapatto et al., 2023; Malik et al., 2023). Overconsumption of nutrients results in elevated free fatty acid levels enhanced mitochondrial ROS generation, compromised mitochondrial performance in fat cells, decreased mitochondrial formation and DNA quantity, and lowered β-oxidation rates. Subsequently, fat cell metabolic processes undergo changes, as evidenced by modifications in fat synthesis, breakdown, fatty acid bonding, and the production of lipocalin by adipocytes. These metabolic shifts lead to reduced insulin responsiveness (Bénédicte A. et al., 2021). Obesity leads to downregulation of the gene expression of mitochondrial respiratory complex components, with the protein peroxisome proliferator-activated receptor γ coactivator 1α (PGC-1α) being a key regulator of mitochondrial biogenesis. PGC-1α stimulates mitochondrial creation by interacting with various nuclear receptors and gene transcription regulators, including peroxisome proliferator-activated receptor γ (PPARγ). However, the expression and function of PGC-1α are significantly impaired in individuals with obesity (Rius-Perez et al., 2020).
3.3 Mitochondrial dynamics
Mitochondrial dynamics are governed by a series of GTPase proteins. Dynamin-related protein 1 (Drp1) is primarily responsible for the division of mitochondrial membranes, while mitochondrial fission protein 1 (Fis1) also plays a crucial role in regulating mitochondrial fragmentation (Duan et al., 2021; Yu et al., 2021; Huan et al., 2023). On the other hand, GTPase optic atrophy 1 (Opa1) is responsible for the fusion of the inner mitochondrial membrane, and mitochondrial fusion proteins 1 (Mfn1) and 2 (Mfn2) control the fusion of the outer mitochondrial membrane (von der Malsburg et al., 2023). In the obese state, mitochondrial division tends to be overactive, leading to mitochondrial fragmentation and dysfunction. Excessive mitochondrial division not only decreases the number of mitochondria but also increases ROS production, triggering a cellular stress response and metabolic dysregulation (Al Ojaimi et al., 2022). Moreover, reduced mitochondrial fusion limits mitochondrial repair and functional optimization, exacerbating obesity-related metabolic issues (Garcia et al., 2022). Changes in the proteins that regulate mitochondrial structure and function are closely linked to obesity-related mitochondrial dysfunction. Specifically, several studies have shown that decreased expression of OPA1 and Mfn2 is linked to impaired mitochondrial functionality in obesity (Mancini et al., 2019; Zheng et al., 2023). Pereira et al. indicated that deletion of the mitochondrial protease OMA1 disrupts OPA1 processing, affecting metabolic balance and leading to increased fat mass and reduced energy expenditure (Pereira et al., 2021). Similarly, Da et al. reported that Mfn2 deficiency can lead to elevated hydrogen peroxide and ROS levels, contributing to mitochondrial dysfunction in liver and muscle tissues (Da Dalt et al., 2024). Further investigations in HFD-fed mice revealed that changes in mitochondrial dynamics—from fusion to increased fission—are associated with respiratory challenges and reduced ATP production in skeletal muscle, strengthening the connection between impaired mitochondrial dynamics and metabolic disturbances in obesity (Yang H.-M. et al., 2023; Kim et al., 2024).
3.4 Mitochondrial autophagy
Mitochondrial autophagy, a crucial process for maintaining mitochondrial quality and function, selectively targets and removes damaged mitochondria. Research has shown that impaired regulation of mitochondrial autophagy in the skeletal muscle of obese individuals leads to a decrease in both the quantity and functionality of mitochondria (Pileggi et al., 2021). Interestingly, while a short-term high-fat diet can trigger mitochondrial autophagy in skeletal muscle, it does not affect mitochondrial respiratory capacity. This phenomenon might be associated with lipid-induced oxidative stress, indicating that mitochondrial autophagy might serve a protective role during the early stages of obesity (Ehrlicher et al., 2021). Additionally, impaired mitochondrial autophagy in adipose tissue may exacerbate the progression of insulin resistance and obesity by influencing the release of adipokines and cytokines (Turchi et al., 2020). Enhanced lipid autophagy and mitochondrial autophagy in brown and beige adipocytes, especially in cold environments, promote adaptive thermogenesis, helping the body cope with cold stimuli (Sakers et al., 2022). Recent studies have also shown that triiodothyronine (T3) modulates mitochondrial homeostasis by inducing lipophagy and mitochondrial autophagy in the liver and skeletal muscle and stimulates thermogenesis (Yau et al., 2021; Hatziagelaki et al., 2022). This mechanism triggers the expression of mitochondrial uncoupling protein 1 (UCP1), enhances autophagy-dependent fatty acid oxidation, and regulates mitochondrial autophagy, functionality, and renewal in brown adipose tissue (BAT) and aging skeletal muscle (Wang et al., 2023). Furthermore, as shown in Table 1, mitochondrial dysfunction presents differently at various stages of obesity.
To address these challenges, antioxidants have been investigated for their potential to reduce oxidative stress and protect mitochondrial function. Among them is mitoquidone (MitoQ), a chemically modified form of coenzyme Q10 that selectively enters mitochondria and accumulates in their inner membrane, thereby safeguarding them from oxidative stress (Chen et al., 2022). Additionally, MitoQ has been shown to normalize metabolic profiles, reduce lipid peroxidation, and restore UCP-2 protein levels to normal values in obese rats (Xu et al., 2021). Research also indicate that MitoQ improves NF-κB activation and mitigates endoplasmic reticulum stress by regulating mitochondrial function (Cojocaru et al., 2023). Water-soluble Szeto-Schiller (SS) peptides, another group of mitochondria-targeted antioxidants, are small molecules that are quickly and selectively taken up into the inner mitochondrial membrane (IMM) across various cell types (Zong et al., 2024). Studies have demonstrated that SS-31 reduces mitochondrial ROS production, suppresses changes in mitochondrial membrane permeability, and safeguards cells from oxidative stress-induced death (Peng et al., 2021; Yang et al., 2021).
4 Gut microbiota and its role in obesity
4.1 Gut microbiota and its metabolites
The gut microbiota consists of many microorganisms, including archaea, protozoa, fungi, viruses, and bacteria, with bacterial species being the most abundant and extensively studied group (Zhang et al., 2022). The major intestinal bacterial phyla are Firmicutes, Bacteroidetes, Actinobacteria, Proteobacteria, Fusobacteria, and Verrucromicrobia, with Firmicutes and Bacteroidetes being 90% of the total bacterial population. Notably, although certain bacteria are less abundant, this does not necessarily indicate a secondary functional role (Fassarella et al., 2021). Different microbial concentration gradients are observed throughout the gut, and understanding this distribution is crucial for investigating the impact of the gut microbiota on the host’s health. The lower microbial density in the upper gastrointestinal tract helps prevent pathogen colonization. In contrast, the more abundant microbiota in the lower intestine facilitates the breakdown of complex carbohydrates and the production of SCFAs, which are metabolic byproducts essential for the host’s energy metabolism and intestinal health (Wen et al., 2022).
The intestinal microbial community begins to form at birth and potentially even earlier in the womb. It is influenced by numerous factors, such as the delivery method, infant nutrition, lifestyle choices, genetic predisposition, medication use, and dietary habits (Valles-Colomer et al., 2023). Typically, the intestinal microbiota attains an adult-like configuration within the first 5 years of life, though it continues to evolve dynamically throughout an individual’s lifetime (Roswall et al., 2021). The symbiotic relationships between humans and their gut microbiota are essential for maintaining health. The intestinal microbiota plays a vital role in various physiological processes, including preserving the integrity of the intestinal barrier, providing against pathogens, and modulating immune responses and diverse metabolic functions (Lee et al., 2022; Loh et al., 2024; Zhu et al., 2024).
Along with the importance of the gut microbiota, the byproducts of microbial metabolism also play crucial roles. The host absorbs and utilizes the gut microbiota metabolites SCFAs, providing additional energy and regulating host metabolic processes through various mechanisms (Xiong et al., 2022). Previous studies have extensively documented key bacteria involved in SCFA production’s metagenomic characteristics and associated pathways (Tsukuda et al., 2021; Frolova et al., 2022).
Currently, Akkermansia muciniphila, Bacteroides spp., Bacteroides vulgatus, Bifidobacterium spp., Lactobacillus spp., and Prevotella spp. are the primary producers of acetate and propionate, while Coprococcus spp., Eubacterium spp., Faecalibacterium prausnitzii, and Roseburia spp. are the main butyrate-producing bacteria (Kim et al., 2020; Lordan et al., 2020). In addition to SCFAs, the gut microbiota can influence host metabolic processes through metabolites such as SBAs, tryptophan metabolites, and hydrogen sulfide (H2S). By metabolizing primary bile acids and producing tryptophan metabolites via the tryptophan metabolic pathway, Bacteroides and Clostridium are converted to SBAs (Matthew F. et al., 2020; Xiaomin S. et al., 2022; Xiaomin T. et al., 2022). Desulfovibrio and Bilophila species produce H2S through cysteine degradation, while Fusobacterium nucleatum generates H2S during digestion (DJ et al., 2021; Matthew W. et al., 2023).
4.2 Gut microbiota and its metabolites in obesity
HFD consumption, lifestyle choices, antibiotics use, and psychological or physical stress are key factors that alter the composition of intestinal microbial and its metabolic products, potentially disrupting gut homeostasis, leading to dysbiosis (IJ et al., 2021; Francois et al., 2022). Extensive research using both animal models and human participants has demonstrated that imbalances in gut microbiota interfere with energy metabolism and lead to obesity (Lee et al., 2020; Wachsmuth et al., 2022; Takeuchi et al., 2023). Certain bacterial species have been identified as either harmful or beneficial in the progression of these conditions. Compared to individuals with normal weight, obese individuals exhibit reduced microbial diversity and richness in their intestines, along with structural changes in the microbiome and a disproportionate Firmicutes-to-Bacteroidetes ratio, typically characterized by an increase in Firmicutes and a decrease in Bacteroidetes (Manor et al., 2020a). Similar findings have been observed in animal studies, where the expression of Lactobacillus and Bifidobacterium, among others, was reduced in the gut of obese mice (Huo et al., 2020; Zhihao L. et al., 2022). Conversely, in cases of obesity induced by excessive fat consumption, elevated levels of bacterial lipopolysaccharide (LPS) have been reported (Ramos-Romero et al., 2020). This condition disrupts intestinal structure, increases intestinal permeability, and allows significant amounts of LPS to enter the bloodstream, triggering chronic inflammatory and accelerating the progression of obesity (Camilleri, 2023; Di Vincenzo et al., 2024).
Given the gut microbiota’s central role in obesity and its associated metabolic dysregulation, targeted modulation of the intestinal ecosystem emerges as a promising therapeutic avenue. The microbiota’s significant adaptability in its makeup and function makes it a promising target for treating and preventing various health conditions. Strategies such as probiotics, prebiotics, or symbiotics are commonly explored. In the field of functional foods and nutritional supplements, Bifidobacterium and Lactobacillus are among the most frequently used probiotics (Sharma et al., 2021). Notably, the South Korean Food and Drug Administration has approved Lactobacillus gasseri BNR17 as a functional component for reducing adipose tissue (Kim et al., 2018). New-generation probiotics, such as F. prausnitzii strains, are also gaining attention, as they are commonly found in the microbiota of healthy individuals. Studies have linked a decline in these strains to a higher likelihood of an increased risk of developing obesity (Kallassy et al., 2023; Yang M. et al., 2023). Faecalibacterium prausnitzii exhibits anti-inflammatory properties, as its supernatant suppresses the NF-κB pathway in vitro and in vivo (Auger et al., 2022). It also synthesizes butyrate and other SCFAs, contributing to its beneficial effects (NM et al., 2017). Prebiotics, defined as nutritional components that undergo selective fermentation, can potentially modify the intestinal microbiota’s composition or functionality, leading to beneficial outcomes for the host’s health (Miyamoto et al., 2023). Most evidence supporting their efficacy stems from studies on dietary constituents, primarily categorized into two chemical groups: inulin-type fructans and galacto-oligosaccharides (GOSs) (Piotr P. et al., 2022). Fibers rich in prebiotics stimulate enteroendocrine L-cell development and elevate the anorexigenic peptides PYY and GLP1 concentrations in both the intestinal lumen and bloodstream, consequently diminishing caloric consumption (Akhlaghi, 2024). Human-based research has demonstrated that consuming diets abundant in prebiotics correlates with decreased food consumption, lowered adipose tissue, and minimized weight increase, particularly among individuals with obesity (Huwiler et al., 2022; Jagielski et al., 2023). Synbiotics, which combine prebiotics and probiotics, offer a promising strategy for addressing imbalances in the intestinal microbiota (Saadati et al., 2024). Dietary symbiotics, incorporating carefully selected bacterial strains—such as L. gasseri variants known for their weight-reducing and anti-inflammatory properties, paired with galactomannan or inulin fibers may provide enhanced benefits for tackling obesity by promoting SCFA production and restructuring the microbiome (Li X. et al., 2023). Studies have shown a significant decrease in obesity among mice on high-fat diets when administered a symbiotic blend of D-allulose and the probiotic strains Lactobacillus sakei and Leuconostoc kimchii (Choi et al., 2018). Moreover, introducing symbiotics to mice early in life modulates their gut microbiota, protecting against diet-related obesity as they age (Kang et al., 2023).
The precise function of gut microbiota in the onset of metabolic disorders remains unclear. Recent research has emphasized the connections between microbiota diversity, composition, and mitochondrial function. Importantly, gut microbiota and their metabolic byproducts significantly regulate different factors, transcriptional coactivators, and enzymatic processes that affect mitochondrial functionality (Qi et al., 2022). In addition, abnormal mitochondrial function may cause excessive ROS release, dysregulation of glucolipid metabolism, and abnormal adipose tissue function, which affect gut microbiota homeostasis and result in bodily imbalance, triggering obesity (Zhu et al., 2023). The following sections will delve into the complex interplay between gut microbiota and mitochondrial operations, exploring potential pathways through which these interactions could be leveraged to develop innovative approaches for obesity management.
5 Gut microbiota–mitochondrial crosstalk in obesity
5.1 Role of mitochondrial energy metabolism in the gut microbiota
Emerging research highlights an interactive relationship between mitochondrial energy metabolism and the gut microbiota. Efficient mitochondrial energy production relies on the integration of fatty acid oxidation, the tricarboxylic acid cycle (TCA cycle), and OXPHOS. OXPHOS utilizes NADH and FADH2, produced via fatty acid oxidation and the TCA cycle, to generate significant amounts of ATP (Guo et al., 2023). Key byproducts of this process are essential in regulating the gut environment and shaping the gut microbiota, thus influencing obesity development (Wan et al., 2020; Bhattacharjee et al., 2023). ROS are produced during mitochondrial OXPHOS. Obese individuals exhibit markedly altered proportions of Bacteroides and Firmicutes in their gut microbiota, which is closely associated with disrupted mitochondrial OXPHOS and ROS production (Jinhua et al., 2022). Interestingly, ROS serves a dual role in intestinal inflammation, functioning as important signaling molecules produced by mitochondria during OXPHOS. Moderate levels of ROS support intestinal homeostasis by regulating cell proliferation, differentiation, and immune responses. Studies have also demonstrated that ROS can activate the Nrf2 signaling pathway, promoting the expression of antioxidant genes and enhancing cellular antioxidant defenses (He et al., 2022).
However, in obese individuals, excessive ROS production leads to oxidative stress, causing damage to proteins, lipids, and DNA (Abdolmaleky and Zhou, 2024). This disrupts intestinal barrier integrity, triggers an inflammatory response, and alters the gut microenvironment, allowing the colonization of harmful bacteria, such as Enterobacteriaceae, while inhibiting the growth of beneficial bacteria like Bifidobacteria (Jeong and Kang, 2023). Organic acids, natural byproducts of the TCA cycle, inhibit the growth of Escherichia coli and Salmonella by lowering intestinal pH, while promoting the proliferation of Lactobacillus and Bifidobacterium. A HFD raises intestinal pH, disrupting gut microbiota equilibrium and affecting the gut’s metabolic state (Guo et al., 2021; Ekkachai et al., 2023). Ketone bodies, including β-hydroxybutyrate (BHB) and acetoacetate, are produced by mitochondria during fatty acid oxidation. These ketones are not only crucial energy metabolites but also important signaling molecules. Metabolism and inflammatory responses in host cells are regulated by BHB through activation of the G protein-coupled receptor GPR109A and suppression of NF-κB and NLRP3 inflammasomes, leading to reduced intestinal inflammation and improved balance of the gut microbiota (Valentina et al., 2022). In an in vitro human colon microbiota model, BHB supplementation increased butyric acid production (Kengo et al., 2020). Furthermore, BHB influences cellular gene expression and metabolic pathways by inhibiting histone deacetylase (HDACs) and modifying SCFA production, which subsequently impacts the host’s energy balance and insulin sensitivity. This process is essential for maintaining the diversity and functionality of the gut microbiota, particularly in metabolic disorders related to obesity (KB et al., 2021).
Moreover, mitochondrial dysfunction exacerbates obesity-related metabolic dysregulation by influencing the gut microbiota through immune and neural pathways. Regarding immune regulation, mitochondrial dysfunction causes the release of mitochondrial DNA into the cytoplasm, where it acts as a pathogen-associated molecular pattern. This mtDNA then interacts with Toll-like receptor 9 (TLR9), triggering a MyD88-dependent signaling cascade that stimulates the production of inflammatory factors, altering the intestinal microenvironment and impacting the composition of the intestinal microbiota (Ren et al., 2020). Additional research has shown that depletion of the mitochondrial membrane potential leads to intracellular potassium ion outflow, subsequently activating various inflammatory vesicles, such as NLRP3. Potassium ion efflux not only activates these inflammatory vesicles but also compromises the integrity of intestinal epithelial cells, impairing gut barrier function and increasing permeability, which further disrupts microbiota homeostasis (Diwakar et al., 2021; Wei et al., 2021; Matthew Y. et al., 2023). Moreover, the state of mitochondrial metabolism significantly affects dendritic cell function. Mitochondrial stress signals can modulate the antigen-presenting function and cytokine secretion of dendritic cells, thereby affecting the immune response in the gut (Adamik et al., 2022). In obesity, mitochondrial stress promotes increased secretion of proinflammatory cytokines by dendritic cells, resulting in an increase in Th17 cells, which are crucial in intestinal inflammation (van der Zande et al., 2023). In terms of neuromodulation, mitochondrial dysfunction disrupts the synthesis and degradation of serotonin and dopamine, key neurotransmitters that regulate intestinal function, affecting intestinal peristalsis and secretion. This in turn alters the composition of the microbiota (Xie et al., 2020). Imbalances in the autonomic nervous system, particularly an underactive or overactive sympathetic and parasympathetic response, also affect the gut microbiota in the obese state. The vagal nerve regulates gut peristalsis, secretion, and barrier function by releasing acetylcholine. In obese individuals, decreased vagal activity leads to increased colonization of E. coli and Salmonella, while inhibiting the growth of Lactobacillus and Bifidobacterium (Bénédicte et al., 2017). Additionally, the enteric nervous system (ENS), often referred to as the “second brain,” directly controls intestinal behavior. Mitochondrial dysfunction in obese individuals impairs the energy-dependent functions of ENS neurons leading to changes in intestinal movement and enzymatic secretion. A decrease in digestive enzyme secretion can lead to incomplete food digestion, increased retention of undigested material in the intestine, and provide nutrients for harmful bacteria. Conversely, excessive secretion may dilute intestinal contents, affecting the growth of beneficial bacteria (Bénédicte N. et al., 2021). In obese individuals, ENS dysfunction also compromises epithelial cell function and reduces tight junction protein expression, enhancing intestinal permeability. This impaired barrier function enables the increased entry of pathogenic bacteria and toxins into the bloodstream, triggering a widespread inflammatory response and further destabilizing the gut microbiota equilibrium (Bénédicte et al., 2020).
In summary, mitochondria regulate the gut microbiota through their metabolic products while maintaining gut microbiota balance by preventing oxidative stress, immune dysregulation, and neuroregulatory imbalances. This underscores the impact of mitochondrial dysfunction on gut microbiota homeostasis and its close association with the development of obesity.
5.2 The role of gut microbiota in mitochondrial energy metabolism
Changes in gut microbiota composition can lead to alterations in environmental metabolites, shedding light on the mechanisms behind obesity development. Extensive studies using germ-free mice that were inoculated with gut microbiota from obese individuals have strongly confirmed the influence of gut microbiota on the host’s energy metabolism, glucose tolerance, and insulin resistance (Diwakar et al., 2022). Additionally, metabolites produced by gut microbiota play a vital role in maintaining homeostasis in the body and affect the progression of obesity through their impact on mitochondrial function. The following section explores the key metabolites derived from gut microbiota that have recently been associated with the advancement of obesity (Figure 2).
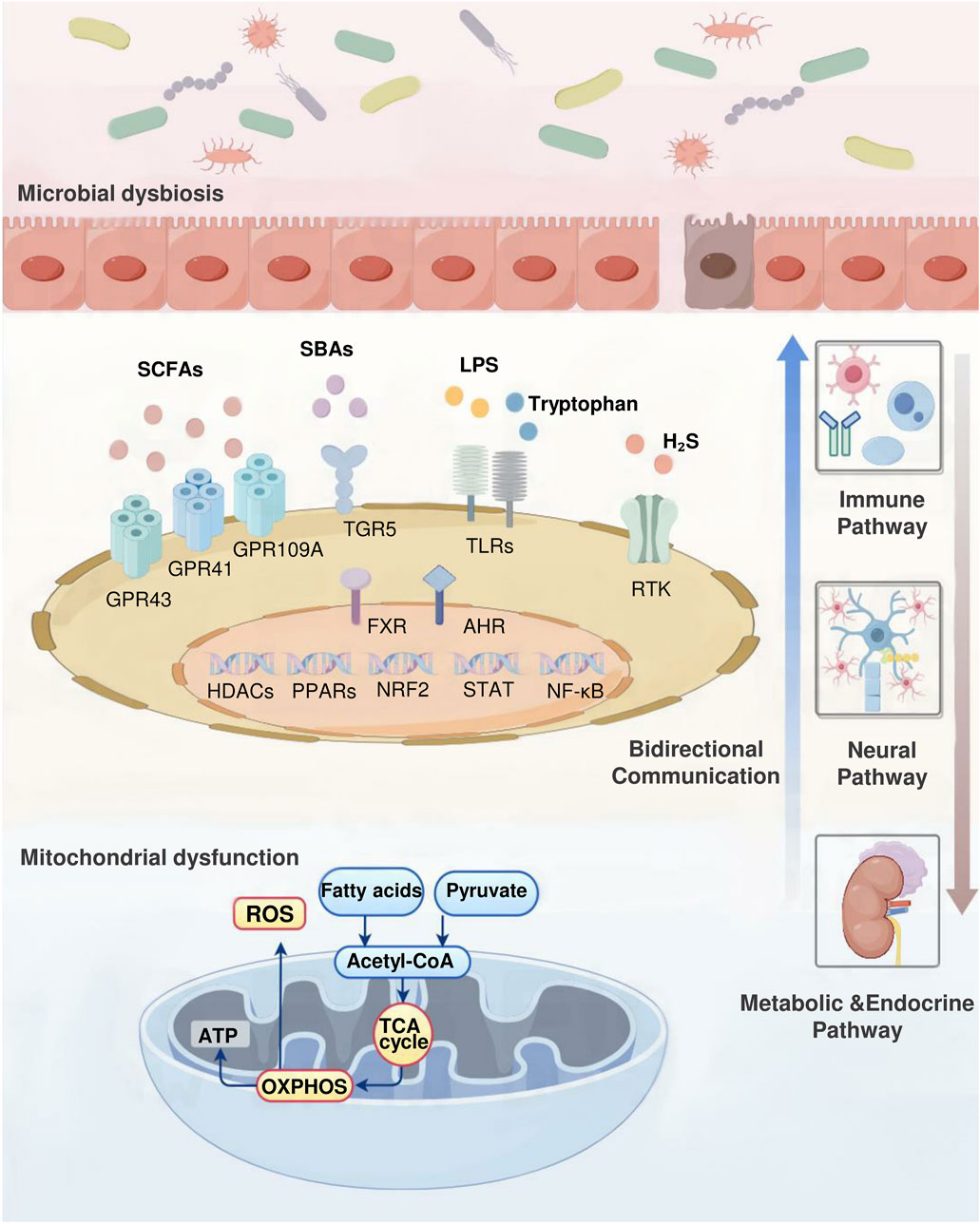
Figure 2. Gut microbiota–mitochondrial crosstalk in obesity. The complex interactions between the gut microbiota and mitochondrial function underscore their key role in regulating obesity. This bidirectional communication occurs through immune, neural, and metabolic-endocrine pathways. Gut microbial metabolites such as SCFAs, SBAs, tryptophan, H2S, and LPS maintain body homeostasis and directly influence the development of obesity by regulating mitochondrial activity. Conversely, mitochondrial energy metabolites such as ROS, organic acids, and ketone bodies alter the intestinal microenvironment, affecting microbial composition. This process involves multiple signaling molecules. Receptors including GPR43, GPR41, GPR109A, FXR, TGR5, TLRs, AHR, RTK. Key transcription factors include Nrf2, NF-κB, STAT, and PPARs. Enzymes including HDACs.
5.2.1 SCFAs
Among the metabolites produced by gut microbiota, SCFAs primarily include acetic, propionic, and butyric acids. Acetic acid regulates adipocytes’ mitochondrial function and fatty acid oxidation by activating the GPR43 receptor. This process increases cAMP production, which, in turn, activates protein kinase A (PKA), influencing fat accumulation and energy metabolism (Manor et al., 2020b). Acetic acid modulates glucose metabolism in obesity-related cell types, including adipocytes and macrophages, via the GPR43 receptor (Li et al., 2024). Propionic acid’s activation of GPR41 and GPR43 receptors triggers the ERK and AMPK signaling cascades, stimulating mitochondrial biogenesis and lipolysis. This process enhances insulin sensitivity, mitigates oxidative stress, and reduces mitochondrial damage, thereby combating the metabolic disorders associated with obesity (Hiroki et al., 2019; Xiaomin W. et al., 2022). Studies have revealed that propionate metabolism involves acetyl coenzyme A synthase short-chain family member 3 (ACSS3) in the IMM. ACSS3 plays a crucial role in propionate metabolism, and ACSS3 deficiencies lead to serum propionic acid accumulation, causing reduced BAT, increased white adipose tissue (WAT), and insulin resistance (Zhihao J. et al., 2022). Research has also shown that propionic acid stimulates thermogenic responses and promotes the browning of adipose tissue through various mechanisms, including the upregulation of PGC-1α expression, a key regulator of mitochondrial biosynthesis (CC et al., 2021). Among the SCFAs, butyric acid improves mitochondrial function through GPR109A receptor activation, AMPK signaling pathway modulation, enhanced mitochondrial autophagy, and attenuation of inflammatory responses (Xaomin et al., 2020). Additionally, research has shown that butyrate enhances UCP1-induced BAT thermogenesis and WAT browning by upregulating PGC-1α expression (YM et al., 2020).
5.2.2 SBAs
In addition to SCFAs, other microbial-derived metabolites are associated with metabolic processes and obesity. SBAs, including deoxycholic acid (DCA) and ursodeoxycholic acid (UDCA), influence mitochondrial energy metabolism primarily through the actions of the farnesoid X receptor (FXR) and the G protein-coupled bile acid receptor 5 (TGR5). FXR reduces hepatic lipid accumulation by suppressing SREBP-1c, regulates hepatic gluconeogenesis by enhancing FGF15/19 and PPARα expression, lowers blood glucose levels, and stimulates mitochondrial biogenesis and fatty acid oxidation. These actions collectively reduce lipid accumulation and enhance insulin sensitivity (JYL et al., 2020; Lulu et al., 2021; Matthew et al., 2021). TGR5, through its regulation of the AMPK signaling pathway, promotes BAT thermogenesis, increases energy expenditure, and enhances mitochondrial OXPHOS (SR et al., 2020).
5.2.3 Tryptophan and H2S
Tryptophan metabolites primarily include indole propionic acid (IPA), indole-3-acetic acid (IAA), kynurenine, and quinolinic acid (QA). IPA promotes fat metabolism and reduces fat accumulation by stimulating the PPARγ-AMPK pathway, which enhances mitochondrial OXPHOS, promotes lipid metabolism, and decreases fat accumulation (Piotr K. et al., 2022). IAA regulates intestinal immune responses, reduces inflammation-related metabolic disorders, and influences mitochondrial biogenesis and autophagy by activating the TLR4-JNK pathway (Ruiz et al., 2020; Chowdhury et al., 2021). The kynurenine pathway produces metabolites that activate the aromatic hydrocarbon receptor, regulate gene expression, influence immune and inflammatory responses, and promote mitochondrial biogenesis (Ren et al., 2022). QA modulates cellular proliferation and metabolic processes by stimulating the mammalian target of rapamycin protein (mTOR) signaling cascade, improving mitochondrial performance, and regulating lipid biosynthesis and energy utilization (Bénédicte H. et al., 2021).
Additionally, hydrogen sulfide (H2S), a metabolite of the intestinal microbiota, affects mitochondrial function. H2S is produced by intestinal anaerobic sulfate-reducing bacteria, and high-fat diets lead to an increase in these bacteria in the gut of obese rats, resulting in elevated H2S production (Francois et al., 2021). H2S comprehensively modulates cellular metabolism, mitochondrial performance, and inflammatory pathways by stimulating the PI3K/Akt and AMPK signaling pathways, while concurrently suppressing the NF-κB pathway (Diwakar et al., 2020; Bénédicte W. et al., 2021; Alex et al., 2022).
5.2.4 Lipopolysaccharides
Under normal physiological conditions, LPS generated from intestinal microbiota metabolism is nonpathogenic. However, prolonged stimulation induces macrophages to secrete inflammatory mediators, triggering ROS generation in the jejunum and ileum. This cascade results in mitochondrial enlargement, reduced membrane potential, structural abnormalities, and impaired functionality of intestinal epithelial cells (Matthew and PY, 2020). Additional research has demonstrated that LPS increases intestinal permeability, allowing more LPS molecules to enter the bloodstream and activate a systemic immune response. In the bloodstream, LPS forms complexes with LPS-binding proteins and CD14, engaging Toll-like receptor 4 (TLR4). This interaction triggers various inflammatory signaling pathways, leading to proinflammatory cytokines like IL-6 and TNF-α secretion (He et al., 2019). These mediators subsequently compromise mitochondrial performance and decrease metabolic energy efficiency (Matthew X. et al., 2020; Zhang X. et al., 2021). Additionally, LPS promotes adipose tissue proliferation and inflammation by activating the JAK/STAT pathway (CC et al., 2019).
6 Therapeutic potential of targeting the gut microbiota and mitochondria from traditional Chinese medicine
Accumulating evidence suggests that therapies targeting the interplay between mitochondria and the microbiota may offer a new approach to treating these diseases or reducing their complications, providing a broader range of effects than targeting mitochondria or the microbiota alone. Recent studies have uncovered multiple molecular mechanisms mediating the gut microbiota and mitochondria interaction, potentially paving the way for the development of precisely targeted therapeutic interventions. Conversely, alterations in mitochondrial activity can influence the microbial community, thereby affecting disease progression and highlighting the gut microbiota and mitochondria bidirectional communication. In this context, TCM formulas and metabolites of botanical drugs which are guided by TCM theory, offer unique advantages (Law et al., 2022; Zhang Q. et al., 2023). These therapeutic providing integrative strategies that align with the complexity of this bidirectional interaction (Figure 3).
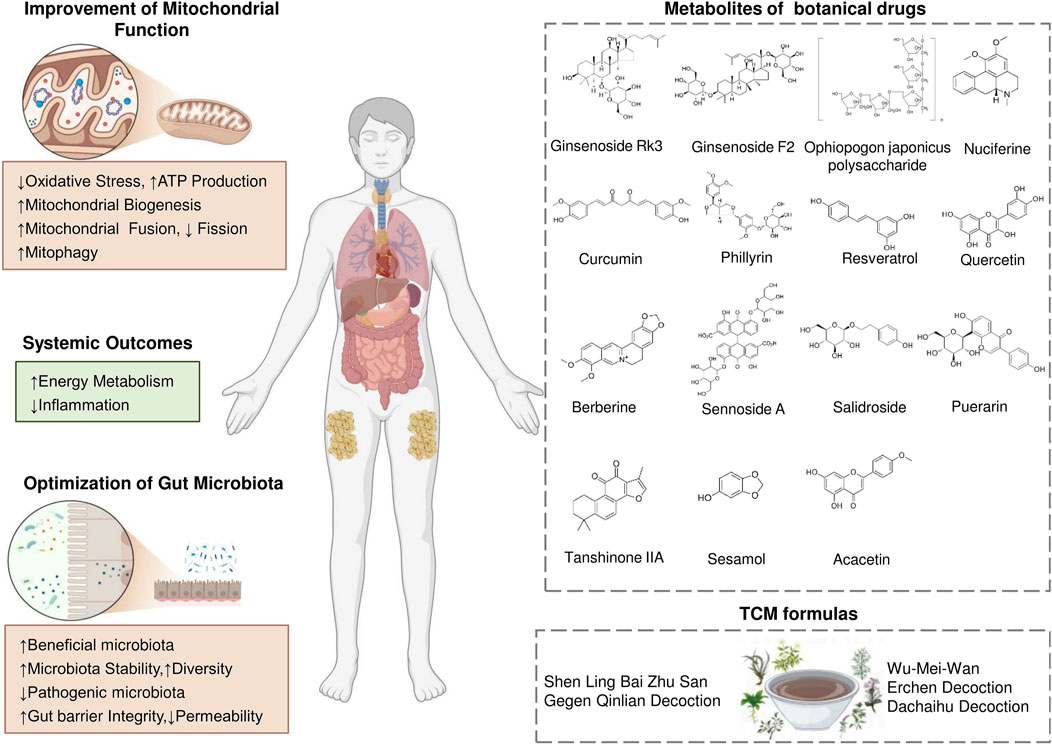
Figure 3. Therapeutic Strategies Targeting the Gut Microbiota–Mitochondrial Crosstalk for Obesity. Traditional Chinese medicine works synergistically to enhance mitochondria-gut crosstalk. This mutual reinforcement between improved mitochondrial function and a healthier gut microbiota leads to better energy metabolism, enhanced insulin sensitivity, and effective inflammation control, offering a comprehensive strategy for obesity management.
Contemporary research has increasingly shown that metabolites of botanical drugs can contribute to obesity prevention and management by influencing mitochondrial energy processes and the gut microbiota (Cheng et al., 2023; Luo et al., 2023; Zhi et al., 2024). The following metabolites of botanical drugs that have demonstrated significant effects in relevant studies are shown in Table 2.
While the therapeutic potential of metabolites of botanical drugs offers a holistic approach to obesity treatment, contemporary research has also highlighted TCM formulas, through their synergistic interactions and multi-target effects (Zhang C. H. et al., 2021). Table 3 summarizes the potential mechanisms of the TCM formulas in detail. Shenling Baizhu San (SLBZS) has the capacity to modulate the proportion of Bacteroidetes to Firmicutes, enhance the presence of beneficial Bifidobacterium, decrease LPS expression, mitigate systemic chronic inflammation, and enhance metabolic efficiency (Yang et al., 2014; Huang et al., 2022). Erchen decoction (ECD) demonstrates notable efficacy in lowering body mass and blood triglyceride concentrations; enhancing gut microbial diversity; and boosting the proportional presence of Lactobacillus, Bifidobacterium, and Butyricicoccus; while diminishing the abundances of Bacteroides, Parabacteroides, and Sediminibacterium (Matthew et al., 2021; Lulu et al., 2023). Subsequent research indicated that ECD facilitated the restoration of compromised membrane potential, and rectified lipid metabolic abnormalities in mice with obesity (Sergio et al., 2018). Dachaihu decoction (DCHD) can notably improve the reduction in the mitochondrial membrane potential and swelling, activate key factors of mitochondrial synthesis, increase mitochondrial function, and balance the abnormalities of energy metabolism in obese rats (Li L. et al., 2021). Furthermore, DCHD enhanced the intestinal microbiota by restoring Lactobacillus, Akkermansia, and Bifidobacterium levels and elevating the proportion of Bacteroidetes to Firmicutes, thus contributing to additional weight reduction (Hussain et al., 2016). Gegen Qinlian decoction (GQD) can decrease the production of LPS, and improve abnormalities in lipid metabolism (Xiong, 2020). Moreover, GQD is capable of suppressing IL-6 expression, alleviating obesity-related inflammation, and modulating energy metabolism through stimulating WAT browning and enhancing caloric expenditure (Zhang X. et al., 2021). Wu-Mei-Wan (WMW) can reduce the body weight of obese mice and decrease the ratio of Firmicutes to Bacteroidetes, thereby adjusting the gut microbiota structure (Nie et al., 2021b). Additionally, WMW treatment resulted in a reduction of white adipocytes, a diminution in lipid droplet quantity, an upregulation of UCP1 expression, an augmentation of mitochondrial count in BAT, and an elevation in energy consumption (Wu et al., 2020).
TCM formulas and metabolites of botanical drugs which are guided by TCM theory targets the bidirectional communication between the gut microbiota and mitochondria, offering a multilevel intervention strategy for obesity treatment. However, further research is needed to elucidate these therapies’ long-term effects and potential side effects across different individuals and clinical settings.
7 Conclusion and prospects
The intricate crosstalk between the gut microbiota and mitochondria is crucial in obesity and related metabolic disorders. The gut microbiota and its metabolites substantially affect host adipogenesis, modulating mitochondrial energy production and metabolism with reciprocal impacts. This bidirectional interaction highlights the gut microbiota’s capacity to influence the host’s metabolic wellbeing, while also indicating that mitochondria, essential cellular structures responsive to internal and external stimuli, reciprocally affect the gut microbiota by modulating mucosal immunity and intestinal barrier. Consequently, this reciprocal relationship between mitochondria and the gut microbiota holds promise for addressing obesity. Given the evolutionary origin of mitochondria as primordial bacterial symbionts, the intricate connection between these organelles and bacteria is understandable. Emerging evidence suggests that TCM formulas and metabolites of botanical drugs could provide novel insights into restoring this gut microbiota–mitochondria axis. This review consolidates findings supporting a connection between gut microbiota imbalances in obesity and mitochondria, offering potential pathways for investigating innovative treatment strategies for obesity.
However, TCM emphasizes individualized treatment, with personalized prescriptions based on the patient’s pathological state of obesity and its comorbidities according to the different stages of development. Most current studies rely on simple obesity or simple metabolic syndrome models, which fail to effectively simulate the complex pathology of obese patients, especially when multiple metabolic complications coexist, and the interactions between the gut microbiota and mitochondrial function may show more complex dynamics. In addition, although studies have proposed that the interaction between gut microbiota and mitochondria plays a key role in the combined effects of obesity onset and progression. Limited comparability of data obtained from different experimental approaches further constrains the integrative interpretation of these findings. Therefore, although formulas and metabolites of botanical drugs have shown potential to alleviate obesity by modulating mitochondria and gut microbiota, their specific mechanisms through gut microbiota-mitochondria interactions still need to be further validated.
Future research should prioritize the development of multifactorial obesity models that more accurately reflect human pathological characteristics, particularly in cases where obesity is accompanied by metabolic comorbidities such as type 2 diabetes, metabolic dysfunction-associated fatty liver disease, and cardiovascular diseases. These models will enable a more precise simulation of clinical conditions, enhancing the translational relevance of research findings and facilitating the optimization of TCM formulations and metabolites of botanical drugs for different pathological states. Furthermore, integrating organoid technology and tissue engineering to establish multi-organ co-culture systems will allow for a more physiologically relevant simulation of systemic interactions, providing a robust platform for investigating the gut microbiota–mitochondria axis and its potential therapeutic mechanisms. Additionally, the combination of synthetic biology and intelligent compounding technologies offers opportunities to optimize drug delivery strategies. Approaches such as nanocarrier systems or pre-drug design can enhance targeting efficiency while reducing off-target effects, thereby providing a strong scientific foundation for the precise intervention of TCM formulations and the metabolites of botanical drugs through modulation of the gut microbiota–mitochondrial crosstalk in obesity and metabolic diseases.
Author contributions
LW: Conceptualization, Writing – original draft. KY: Formal Analysis, Writing – review and editing. JW: Validation, Writing – review and editing. HZ: Methodology, Writing – review and editing. WD: Funding acquisition, Project administration, Supervision, Writing – review and editing.
Funding
The author(s) declare that financial support was received for the research and/or publication of this article. This work was supported by the National Natural Science Foundation of China (No. 82074151); the National Natural Science Foundation of China (No. 82374176); the Sichuan Province Science and Technology Support Program (2023NSFSC1701).
Conflict of interest
The authors declare that the research was conducted in the absence of any commercial or financial relationships that could be construed as a potential conflict of interest.
Generative AI statement
The author(s) declare that no Generative AI was used in the creation of this manuscript.
Publisher’s note
All claims expressed in this article are solely those of the authors and do not necessarily represent those of their affiliated organizations, or those of the publisher, the editors and the reviewers. Any product that may be evaluated in this article, or claim that may be made by its manufacturer, is not guaranteed or endorsed by the publisher.
References
Abdolmaleky, H. M., and Zhou, J. R. (2024). Gut microbiota dysbiosis, oxidative stress, inflammation, and epigenetic alterations in metabolic diseases. Antioxidants 13 (8), 985. doi:10.3390/antiox13080985
Abu Shelbayeh, O., Arroum, T., Morris, S., and Busch, K. B. (2023). PGC-1α is a master regulator of mitochondrial lifecycle and ROS stress response. Antioxidants 12 (5), 1075. doi:10.3390/antiox12051075
Adamik, J., Munson, P. V., Hartmann, F. J., Combes, A. J., Pierre, P., Krummel, M. F., et al. (2022). Distinct metabolic states guide maturation of inflammatory and tolerogenic dendritic cells. Nat. Commun. 13 (1), 5184. doi:10.1038/s41467-022-32849-1
Akhlaghi, M. (2024). The role of dietary fibers in regulating appetite, an overview of mechanisms and weight consequences. Crit. Rev. Food Sci. Nutr. 64 (10), 3139–3150. doi:10.1080/10408398.2022.2130160
Alex, C., Sara, F., Samantha, C., and Badiei, A. (2022). Cystathionine γ-lyase and hydrogen sulfide modulates glucose transporter Glut1 expression via NF-κB and PI3k/Akt in macrophages during inflammation. PLoS One 17 (12), e0278910. doi:10.1371/journal.pone.0278910
Al Ojaimi, M., Salah, A., and El-Hattab, A. W. (2022). Mitochondrial fission and fusion: molecular mechanisms, biological functions, and related disorders. Membranes 12 (9), 893. doi:10.3390/membranes12090893
Arruda, A. P., Pers, B. M., Parlakguel, G., Gueney, E., Inouye, K., and Hotamisligil, G. S. (2014). Chronic enrichment of hepatic endoplasmic reticulum-mitochondria contact leads to mitochondrial dysfunction in obesity. Nat. Med. 20 (12), 1427–1435. doi:10.1038/nm.3735
Auger, S., Kropp, C., Borras-Nogues, E., Chanput, W., Andre-Leroux, G., Gitton-Quent, O., et al. (2022). Intraspecific diversity of microbial anti-inflammatory molecule (MAM) from Faecalibacterium prausnitzii. Int. J. Mol. Sci. 23 (3), 1705. doi:10.3390/ijms23031705
Bai, X., Fu, R. Z., Duan, Z. G., Wang, P., Zhu, C. H., and Fan, D. D. (2021). Ginsenoside Rk3 alleviates gut microbiota dysbiosis and colonic inflammation in antibiotic-treated mice. Food Res. Int. 146, 110465. doi:10.1016/j.foodres.2021.110465
Baldini, F., Fabbri, R., Eberhagen, C., Voci, A., Portincasa, P., Zischka, H., et al. (2021). Adipocyte hypertrophy parallels alterations of mitochondrial status in a cell model for adipose tissue dysfunction in obesity. Life Sci. 265, 118812. doi:10.1016/j.lfs.2020.118812
Ballard, J. W. O., and Towarnicki, S. G. (2020). Mitochondria, the gut microbiome and ROS. Cell. Signal. 75, 109737. doi:10.1016/j.cellsig.2020.109737
Belda, E., Voland, L., Tremaroli, V., Falony, G., Adriouch, S., Assmann, K. E., et al. (2022). Impairment of gut microbial biotin metabolism and host biotin status in severe obesity: effect of biotin and prebiotic supplementation on improved metabolism. Gut 71 (12), 2463–2480. doi:10.1136/gutjnl-2021-325753
Bénédicte, A.-N., S, C.-P., A, V., and Id, O. (2020). Gut microbiota and intestinal trans-epithelial permeability. Int. J. Mol. Sci. 21 (17), 6402. doi:10.3390/ijms21176402
Bénédicte, A., R, S., and Mw, G. (2021a). Adipose tissue and insulin resistance in obese. Biomed. Pharmacother. 137 (1950-6007), 111315. doi:10.1016/j.biopha.2021.111315
Bénédicte, B., V, S., and S, P. (2017). The vagus nerve in the neuro-immune Axis: implications in the pathology of the gastrointestinal tract. Front. Immunol. 2 (8), 1452. doi:10.3389/fimmu.2017.01452
Bénédicte, H., W, Y., L, L., Y, J., X, X., J, H., et al. (2021b). Mammalian target of rapamycin signaling pathway regulates mitochondrial quality control of Brown adipocytes in mice. Front. Physiol. 12, 638352. doi:10.3389/fphys.2021.638352
Bénédicte, N., Id, O., S, K., Id- Orcid, X., Ie, D., Id, O., et al. (2021c). Disorders of the enteric nervous system - a holistic view. Nat. Rev. Gastroenterol. Hepatol. 18 (6), 393–410. doi:10.1038/s41575-020-00385-2
Bénédicte, W., Id, O., Z, S., M, G., L, N., Y, S., et al. (2021d). Hydrogen sulfide protects against IL-1β-induced inflammation and mitochondrial dysfunction-related apoptosis in chondrocytes and ameliorates osteoarthritis. J. Cell Physiol. 236 (6), 4369–4386. doi:10.1002/jcp.30154
Bhattacharjee, P., Carlson, C., Ryan, C., Fadlaoui, A., and Sunny, N. (2023). Impact of sustained de novo lipogenesis on mitochondrial dysfunction and hepatocellular redox during fatty liver disease. Physiology 38. doi:10.1152/physiol.2023.38.S1.5730204
Brestoff, J. R., Wilen, C. B., Moley, J. R., Li, Y., Zou, W., Malvin, N. P., et al. (2021). Intercellular mitochondria transfer to macrophages regulates white adipose tissue homeostasis and is impaired in obesity. Cell Metab. 33 (2), 270–282.e8. doi:10.1016/j.cmet.2020.11.008
Camilleri, M. (2023). Is intestinal permeability increased in obesity? A review including the effects of dietary, pharmacological and surgical interventions on permeability and the microbiome COMMENT. Diabetes Obes. and Metabolism 25 (2), 325–330. doi:10.1111/dom.14899
Ca, P., G, P., Me, H., and Id, O. (2021). The lifecycle of skeletal muscle mitochondria in obesity. Obes. Rev. 22, e13164. doi:10.1111/obr.13164
Cavaliere, G., Cimmino, F., Trinchese, G., Catapano, A., Petrella, L., D'Angelo, M., et al. (2023). From obesity-induced low-grade inflammation to lipotoxicity and mitochondrial dysfunction: altered multi-crosstalk between adipose tissue and metabolically active organs. Antioxidants 12 (6), 1172. doi:10.3390/antiox12061172
Cc, C., Ch, K., Yl, L., and Sh, W. (2021). Corylin reduces obesity and insulin resistance and promotes adipose tissue browning through SIRT-1 and β3-AR activation. Pharmacol. Res. 164, 105291. doi:10.1016/j.phrs.2020.105291
Cc, C., Kc, S., Jf, C., Cm, L., Cm, Y., Ky, H., et al. (2019). Lipopolysaccharide promoted proliferation and adipogenesis of preadipocytes through JAK/STAT and AMPK-regulated cPLA2 expression. Int. J. Med. Sci. 16 (1), 167–179. doi:10.7150/ijms.24068
Chen, D. L., Wang, Y. F., Yang, J. M., Ou, W. Y., Lin, G. R., Zeng, Z., et al. (2024). Shenling Baizhu San ameliorates non-alcoholic fatty liver disease in mice by modulating gut microbiota and metabolites. Front. Pharmacol. 15, 1343755. doi:10.3389/fphar.2024.1343755
Chen, L., Zhou, M., Li, H., Liu, D., Liao, P., Zong, Y., et al. (2023a). Mitochondrial heterogeneity in diseases. Signal Transduct. Target. Ther. 8 (1), 311. doi:10.1038/s41392-023-01546-w
Chen, Y., Yang, F., Chu, Y., Yun, Z., Yan, Y., and Jin, J. (2022). Mitochondrial transplantation: opportunities and challenges in the treatment of obesity, diabetes, and nonalcoholic fatty liver disease. J. Transl. Med. 20 (1), 483. doi:10.1186/s12967-022-03693-0
Chen, Y. K., Liu, T. T., Teia, F. K. F., and Xie, M. Z. (2023b). Exploring the underlying mechanisms of obesity and diabetes and the potential of Traditional Chinese Medicine: an overview of the literature. Front. Endocrinol. 14, 1218880. doi:10.3389/fendo.2023.1218880
Cheng, H., Zhang, D. D., Wu, J., Liu, J., Zhou, Y. C., Tan, Y. Z., et al. (2023). Interactions between gut microbiota and polyphenols: a mechanistic and metabolomic review. Phytomedicine 119, 154979. doi:10.1016/j.phymed.2023.154979
Choi, B.-R., Kwon, E.-Y., Kim, H.-J., and Choi, M.-S. (2018). Role of synbiotics containing D-allulose in the alteration of body fat and hepatic lipids in diet-induced obese mice. Nutrients 10 (11), 1797. doi:10.3390/nu10111797
Chowdhury, M. M. I., Tomii, A., Ishii, K., Tahara, M., Hitsuda, Y., Koto, Y., et al. (2021). TLR4 may be a novel indole-3-acetic acid receptor that is implicated in the regulation of CYP1A1 and TNFα expression depending on the culture stage of Caco-2 cells. Biosci. Biotechnol. Biochem. 85 (9), 2011–2021. doi:10.1093/bbb/zbab128
Cojocaru, K.-A., Luchian, I., Goriuc, A., Antoci, L.-M., Ciobanu, C.-G., Popescu, R., et al. (2023). Mitochondrial dysfunction, oxidative stress, and therapeutic strategies in diabetes, obesity, and cardiovascular disease. Antioxidants 12 (3), 658. doi:10.3390/antiox12030658
Da Dalt, L., Moregola, A., Svecla, M., Pedretti, S., Fantini, F., Ronzio, M., et al. (2024). The inhibition of inner mitochondrial fusion in hepatocytes reduces non-alcoholic fatty liver and improves metabolic profile during obesity by modulating bile acid conjugation. Cardiovasc. Res. 119 (18), 2917–2929. doi:10.1093/cvr/cvad169
Ding, S., Zhuang, Y., Liao, Y., Kang, J., Zhang, L., Shen, J., et al. (2024). Effect of Erchen Decoction on liver mitochondrial function by inhibiting mTORC1/SREBP1/CAV1 pathway in mice with high-fat diet. Zhongguo Zhong Yao Za Zhi 49, 763–769. doi:10.19540/j.cnki.cjcmm.20231103.401
Di Vincenzo, F., Del Gaudio, A., Petito, V., Lopetuso, L. R., and Scaldaferri, F. (2024). Gut microbiota, intestinal permeability, and systemic inflammation: a narrative review. Intern. Emerg. Med. 19 (2), 275–293. doi:10.1007/s11739-023-03374-w
Diwakar, G., P, G., Jh, C., N, K., Hw, C., Bs, J., et al. (2020). AMPK is essential for IL-10 expression and for maintaining balance between inflammatory and cytoprotective signaling. Biochim. Biophys. Acta Gen. Subj. 1864 (8), 129631. doi:10.1016/j.bbagen.2020.129631
Diwakar, L., S, Y., Y, X., L, P., R, Z., Y, Z., et al. (2021). Novel insights and current evidence for mechanisms of atherosclerosis: mitochondrial dynamics as a potential therapeutic target. Front. Cell Dev. Biol. 9, 673839. doi:10.3389/fcell.2021.673839
Diwakar, W., H, W., L, X., and F, H. (2022). Cross-Talk between gut microbiota and adipose tissues in obesity and related metabolic diseases. Front. Endocrinol. (Lausanne) 13, 908868. doi:10.3389/fendo.2022.908868
Dj, B., X, J., M, P., and Ab, H. (2021). The capacity to produce hydrogen sulfide (H(2)S) via cysteine degradation is ubiquitous in the human gut microbiome. Front. Microbiol. 12, 705583. doi:10.3389/fmicb.2021.705583
Dong, Y. Z., Xia, T., Lin, J. B., Wang, L., Song, K., and Zhang, C. X. (2021). Quercetin attenuates high-fat diet-induced excessive fat deposition of spotted seabass lateolabrax maculatus through the regulatory for mitochondria and endoplasmic reticulum. Front. Mar. Sci. 8. doi:10.3389/fmars.2021.746811
Duan, C., Kuang, L., Hong, C., Xiang, X., Liu, J., Li, Q., et al. (2021). Mitochondrial Drp1 recognizes and induces excessive mPTP opening after hypoxia through BAX-PiC and LRRK2-HK2. Cell Death and Dis. 12 (11), 1050. doi:10.1038/s41419-021-04343-x
Ehrlicher, S. E., Stierwalt, H. D., Newsom, S. A., and Robinson, M. M. (2021). Short-term high-fat feeding does not alter mitochondrial lipid respiratory capacity but triggers mitophagy response in skeletal muscle of mice. Front. Endocrinol. 12, 651211. doi:10.3389/fendo.2021.651211
Ekkachai, K., C, C., Id, O., N, L., Id, O., N, M., et al. (2023). Effects of synbiotic lacticaseibacillus paracasei, Bifidobacterium breve, and prebiotics on the growth stimulation of beneficial gut microbiota. Foods 12 (20), 3847. doi:10.3390/foods12203847
Fan, Y., and Pedersen, O. (2021). Gut microbiota in human metabolic health and disease. Nat. Rev. Microbiol. 19 (1), 55–71. doi:10.1038/s41579-020-0433-9
Fang, Z. Z., Wei, L., Lv, Y. P., Wang, T. S., Hamezah, H. S., Han, R. C., et al. (2022). Phillyrin restores metabolic disorders in mice fed with high-fat diet through inhibition of interleukin-6-mediated basal lipolysis. Front. Nutr. 9, 956218. doi:10.3389/fnut.2022.956218
Fassarella, M., Blaak, E. E., Penders, J., Nauta, A., Smidt, H., and Zoetendal, E. G. (2021). Gut microbiome stability and resilience: elucidating the response to perturbations in order to modulate gut health. Gut 70 (3), 595–605. doi:10.1136/gutjnl-2020-321747
Fogelson, K. A., Dorrestein, P. C., Zarrinpar, A., and Knight, R. (2023). The gut microbial bile acid modulation and its relevance to digestive health and diseases. Gastroenterology 164 (7), 1069–1085. doi:10.1053/j.gastro.2023.02.022
François, B., M, A., P, L., E, A., A, L., and E, K. (2021). Production of hydrogen sulfide by the intestinal microbiota and epithelial cells and consequences for the colonic and rectal mucosa. Am. J. Physiol. Gastrointest. Liver Physiol. 320 (2), 125–135. doi:10.1152/ajpgi.00261.2020
François, Z., D, A., Jy, Y., and T, Z. (2022). The gut mycobiome in health, disease, and clinical applications in association with the gut bacterial microbiome assembly. Lancet Microbe 3 (12), 969–983. doi:10.1016/S2666-5247(22)00203-8
Frolova, M. S., Suvorova, I. A., Iablokov, S. N., Petrov, S. N., and Rodionov, D. A. (2022). Genomic reconstruction of short-chain fatty acid production by the human gut microbiota. Front. Mol. Biosci. 9, 949563. doi:10.3389/fmolb.2022.949563
Garcia, D. A., Powers, A. F., Bell, T. A., Guo, S., and Aghajan, M. (2022). Antisense oligonucleotide-mediated silencing of mitochondrial fusion and fission factors modulates mitochondrial dynamics and rescues mitochondrial dysfunction. Nucleic Acid. Ther. 32 (1), 51–65. doi:10.1089/nat.2021.0029
Gasmi, A., Mujawdiya, P. K., Pivina, L., Dosa, A., Semenova, Y., Benahmed, A. G., et al. (2021). Relationship between gut microbiota, gut hyperpermeability and obesity. Curr. Med. Chem. 28 (4), 827–839. doi:10.2174/0929867327666200721160313
Guo, D., He, H., Meng, Y., Luo, S., and Lu, Z. (2023). Determiners of cell fates: the tricarboxylic acid cycle versus the citrate-malate shuttle. Protein and Cell 14 (3), 162–164. doi:10.1093/procel/pwac026
Guo, W., Zhu, S., Li, S., Feng, Y., Wu, H., and Zeng, M. (2021). Microalgae polysaccharides ameliorates obesity in association with modulation of lipid metabolism and gut microbiota in high-fat-diet fed C57BL/6 mice. Int. J. Biol. Macromol. 182, 1371–1383. doi:10.1016/j.ijbiomac.2021.05.067
Harrington, J. S. S., Ryter, S. W. W., Plataki, M., Price, D. R. R., and Choi, A. M. K. (2023). Mitochondria in health, disease, and aging. Physiol. Rev. 103 (4), 2349–2422. doi:10.1152/physrev.00058.2021
Hatziagelaki, E., Paschou, S. A., Schoen, M., Psaltopoulou, T., and Roden, M. (2022). NAFLD and thyroid function: pathophysiological and therapeutic considerations. Trends Endocrinol. Metabolism 33 (11), 755–768. doi:10.1016/j.tem.2022.08.001
He, J., Xiong, X., Yang, H., Li, D., Liu, X., Li, S., et al. (2022). Defined tumor antigen-specific T cells potentiate personalized TCR-T cell therapy and prediction of immunotherapy response. Cell Res. 32 (6), 530–542. doi:10.1038/s41422-022-00627-9
He, W., Rebello, O., Savino, R., Terracciano, R., Schuster-Klein, C., Guardiola, B., et al. (2019). TLR4 triggered complex inflammation in human pancreatic islets. Biochimica Biophysica Acta-Molecular Basis Dis. 1865 (1), 86–97. doi:10.1016/j.bbadis.2018.09.030
Hiroki, Y., M, I., and M, A. (2019). Propionate suppresses hepatic gluconeogenesis via GPR43/AMPK signaling pathway. Arch. Biochem. Biophys. 672, 108057. doi:10.1016/j.abb.2019.07.022
Huan, Y., Hao, G., Shi, Z., Liang, Y., Dong, Y., and Quan, H. (2023). The role of dynamin-related protein 1 in cerebral ischemia/hypoxia injury. Biomed. and Pharmacother. 165, 115247. doi:10.1016/j.biopha.2023.115247
Huang, Y.-H., Wu, Y.-H., Tang, H.-Y., Chen, S.-T., Wang, C.-C., Ho, W.-J., et al. (2022). Gut microbiota and bile acids mediate the clinical benefits of YH1 in male patients with type 2 diabetes mellitus: a pilot observational study. Pharmaceutics 14 (9), 1857. doi:10.3390/pharmaceutics14091857
Huo, Y., Lu, X., Wang, X., Wang, X., Chen, L., Guo, H., et al. (2020). Bifidobacterium animalis subsp. lactis A6 alleviates obesity associated with promoting mitochondrial biogenesis and function of adipose tissue in mice. Molecules 25 (7), 1490. doi:10.3390/molecules25071490
Hussain, A., Yadav, M. K., Bose, S., Wang, J. H., Lim, D., Song, Y. K., et al. (2016). Daesiho-tang is an effective herbal formulation in attenuation of obesity in mice through alteration of gene expression and modulation of intestinal microbiota. Plos One 11 (11), e0165483. doi:10.1371/journal.pone.0165483
Huwiler, V. V., Schonenberger, K. A., Segesser von Brunegg, A., Reber, E., Muhlebach, S., Stanga, Z., et al. (2022). Prolonged isolated soluble dietary fibre supplementation in overweight and obese patients: a systematic review with meta-analysis of randomised controlled trials. Nutrients 14 (13), 2627. doi:10.3390/nu14132627
Ij, M., M, M., Id, O., J, W., Id, O., N, M., et al. (2021). High-fat, western-style diet, systemic inflammation, and gut microbiota: a narrative review. Cells 10 (11), 3164. doi:10.3390/cells10113164
Jagielski, P., Boleslawska, I., Wybranska, I., Przyslawski, J., and Luszczki, E. (2023). Effects of a diet containing sources of prebiotics and probiotics and modification of the gut microbiota on the reduction of body fat. Int. J. Environ. Res. Public Health 20 (2), 1348. doi:10.3390/ijerph20021348
Jeong, S.-R., and Kang, M. (2023). Exploring tumor-immune interactions in Co-culture models of T cells and tumor organoids derived from patients. Int. J. Mol. Sci. 24 (19), 14609. doi:10.3390/ijms241914609
Jinhua, G., Id, O., Y, S., H, Z., M, C., M, G., et al. (2022). Gut microbiota characteristics of people with obesity by meta-analysis of existing datasets. Nutrients 14 (14), 2293. doi:10.3390/nu14142993
Jyl, C., Id, O., and Jm, F. (2020). Bile acid receptors FXR and TGR5 signaling in fatty liver diseases and therapy. Am. J. Physiol. Gastrointest. Liver Physiol. 318 (3), 554–573. doi:10.1152/ajpgi.00223.2019
Kallassy, J., Gagnon, E., Rosenberg, D., Silbart, L. K., and McManus, S. A. (2023). Strains of Faecalibacterium prausnitzii and its extracts reduce blood glucose levels, percent HbA1c, and improve glucose tolerance without causing hypoglycemic side effects in diabetic and prediabetic mice. Bmj Open Diabetes Res. and Care 11 (3), e003101. doi:10.1136/bmjdrc-2022-003101
Kang, Y., Ren, P., Shen, X., Kuang, X., Yang, X., Liu, H., et al. (2023). A newly synbiotic combination alleviates obesity by modulating the gut microbiota-fat Axis and inhibiting the hepatic TLR4/NF-κB signaling pathway. Mol. Nutr. and Food Res. 67 (24), e2300141. doi:10.1002/mnfr.202300141
Kb, K., Cm, G., H, H., Jk, K., Jl, F., P, C., et al. (2021). Ketogenesis impact on liver metabolism revealed by proteomics of lysine β-hydroxybutyrylation. Cell Rep. 36 (5), 109487. doi:10.1016/j.celrep.2021.109487
Kengo, S., D, S., A, H., J, T., and A, K. (2020). In vitro human colonic microbiota utilises D-β-hydroxybutyrate to increase butyrogenesis. Sci. Rep. 10 (1), 8516. doi:10.1038/s41598-020-65561-5
Kim, H., Jeong, Y., Kang, S., You, H. J., and Ji, G. E. (2020). Co-culture with Bifidobacterium catenulatum improves the growth, gut colonization, and butyrate production of Faecalibacterium prausnitzii: in vitro and in vivo studies. Microorganisms 8 (5), 788. doi:10.3390/microorganisms8050788
Kim, J., Yun, J. M., Kim, M. K., Kwon, O., and Cho, B. (2018). Lactobacillus gasseri BNR17 supplementation reduces the visceral fat accumulation and waist circumference in obese adults: a randomized, double-blind, placebo-controlled trial. J. Med. Food 21 (5), 454–461. doi:10.1089/jmf.2017.3937
Kim, M., Paik, J. H., Lee, H., Kim, M. J., Eum, S. M., Kim, S. Y., et al. (2024). Ancistrocladus tectorius extract inhibits obesity by promoting thermogenesis and mitochondrial dynamics in high-fat diet-fed mice. Int. J. Mol. Sci. 25 (7), 3743. doi:10.3390/ijms25073743
Lapatto, H. A. K., Kuusela, M., Heikkinen, A., Muniandy, M., van der Kolk, B. W., Gopalakrishnan, S., et al. (2023). Nicotinamide riboside improves muscle mitochondrial biogenesis, satellite cell differentiation, and gut microbiota in a twin study. Sci. Adv. 9 (2), eadd5163. doi:10.1126/sciadv.add5163
Law, S. K., Wang, Y. P., Lu, X. C., Au, D. C. T., Chow, W. Y. L., Leung, A. W. N., et al. (2022). Chinese medicinal herbs as potential prodrugs for obesity. Front. Pharmacol. 13, 1016004. doi:10.3389/fphar.2022.1016004
Le, J. M., Zhang, X. Y., Jia, W. P., Zhang, Y., Luo, J. T., Sun, Y. N., et al. (2019). Regulation of microbiota-GLP1 axis by sennoside A in diet-induced obese mice. Acta Pharm. Sin. B 9 (4), 758–768. doi:10.1016/j.apsb.2019.01.014
Lee, C. J., Sears, C. L., and Maruthur, N. (2020). Gut microbiome and its role in obesity and insulin resistance. Ann. N. Y. Acad. Sci. 1461 (1), 37–52. doi:10.1111/nyas.14107
Lee, J.-Y., Tsolis, R. M., and Baumler, A. J. (2022). The microbiome and gut homeostasis. Science 377 (6601), 44. doi:10.1126/science.abp9960
Leshem, A., Liwinski, T., and Elinav, E. (2020). Immune-microbiota interplay and colonization resistance in infection. Mol. Cell 78 (4), 597–613. doi:10.1016/j.molcel.2020.03.001
Li, C., Zhang, H. L., and Li, X. H. (2020). The mechanism of traditional Chinese medicine for the treatment of obesity. Diabetes Metabolic Syndrome Obesity-Targets Ther. 13, 3371–3381. doi:10.2147/dmso.S274534
Li, J. J., Li, J. L., Ni, J. J., Zhang, C. B., Jia, J. L., Wu, G. Y., et al. (2022). Berberine relieves metabolic syndrome in mice by inhibiting liver inflammation caused by a high-fat diet and potential association with gut microbiota. Front. Microbiol. 12, 752512. doi:10.3389/fmicb.2021.752512
Li, L., Li, X., Du, X., and Chai, T. (2021a). Da chaihutang protects liver mitochondrial function of nutritionally obese rats via CREB/PGC-1α pathway. Chin. J. Exp. Traditional Med. Formulae 27 (09), 26–31. doi:10.13422/j.cnki.syfjx.20210601
Li, S., You, J. M., Wang, Z. R., Liu, Y., Wang, B., Du, M., et al. (2021b). Curcumin alleviates high-fat diet-induced hepatic steatosis and obesity in association with modulation of gut microbiota in mice. Food Res. Int. 143, 110270. doi:10.1016/j.foodres.2021.110270
Li, X., Hu, S., Yin, J., Peng, X., King, L., Li, L., et al. (2023a). Effect of synbiotic supplementation on immune parameters and gut microbiota in healthy adults: a double-blind randomized controlled trial. Gut Microbes 15 (2), 2247025. doi:10.1080/19490976.2023.2247025
Li, Y., Yang, S., Jin, X., Li, D., Lu, J., Wang, X., et al. (2023b). Mitochondria as novel mediators linking gut microbiota to atherosclerosis that is ameliorated by herbal medicine: a review. Front. Pharmacol. 14, 1082817. doi:10.3389/fphar.2023.1082817
Li, Y. N., Yu, H., Zhen, J. H., Liu, T. G., Gao, F., He, J. Z., et al. (2024). Impacts of fecal microbiota transplantation on the gut microbiota-short-chain fatty acids-GPR43-interleukin-18 pathway in rats subjected to a high-calorie diet. J. Biol. Regul. Homeost. Agents 38 (5), 3861–3872. doi:10.23812/j.biol.regul.homeost.agents.20243805.305
Lin, C., Chen, J. H., Hu, M. M., Zheng, W. Y., Song, Z. Y., and Qin, H. (2021). Sesamol promotes browning of white adipocytes to ameliorate obesity by inducing mitochondrial biogenesis and inhibition mitophagy via β3-AR/PKA signaling pathway. Food and Nutr. Res. 65. doi:10.29219/fnr.v65.7577
Liu, J. X., Cai, J. P., Zhang, N. S., Tai, J. D., Fan, P., Dong, X., et al. (2023). Salidroside protects mice from high-fat diet-induced obesity by modulating the gut microbiota. Int. Immunopharmacol. 120, 110278. doi:10.1016/j.intimp.2023.110278
Loh, J. S., Mak, W. Q., Tan, L. K. S., Ng, C. X., Chan, H. H., Yeow, S. H., et al. (2024). Microbiota-gut-brain axis and its therapeutic applications in neurodegenerative diseases. Signal Transduct. Target. Ther. 9 (1), 37. doi:10.1038/s41392-024-01743-1
Lordan, C., Thapa, D., Ross, R. P., and Cotter, P. D. (2020). Potential for enriching next-generation health-promoting gut bacteria through prebiotics and other dietary components. Gut Microbes 11 (1), 1–20. doi:10.1080/19490976.2019.1613124
Lulu, S., Id, O., J, C., Fj, G., and Id, O. (2021). The role of farnesoid X receptor in metabolic diseases, and gastrointestinal and liver cancer. Nat. Rev. Gastroenterol. Hepatol. 18 (5), 335–347. doi:10.1038/s41575-020-00404-2
Lulu, Z., N, C., L, Z., T, B., W, Z., L, Z., et al. (2023). Erchen Decoction alleviates obesity-related hepatic steatosis via modulating gut microbiota-drived butyric acid contents and promoting fatty acid β-oxidation. J. Ethnopharmacol. 317, 116811. doi:10.1016/j.jep.2023.116811
Luo, M. C., Zheng, Y. H., Tang, S. Y., Gu, L. S., Zhu, Y., Ying, R. T., et al. (2023). Radical oxygen species: an important breakthrough point for botanical drugs to regulate oxidative stress and treat the disorder of glycolipid metabolism. Front. Pharmacol. 14, 1166178. doi:10.3389/fphar.2023.1166178
Ma, L., Zhao, Z. W., Guo, X. M., Li, J., Xu, L., Mei, W. J., et al. (2022). Tanshinone IIA and its derivative activate thermogenesis in adipocytes and induce beiging of white adipose tissue. Mol. Cell. Endocrinol. 544, 111557. doi:10.1016/j.mce.2022.111557
MacLean, A., Legendre, F., and Appanna, V. D. D. (2023). The tricarboxylic acid (TCA) cycle: a malleable metabolic network to counter cellular stress. Crit. Rev. Biochem. Mol. Biol. 58 (1), 81–97. doi:10.1080/10409238.2023.2201945
Malik, N., Ferreira, B. I., Hollstein, P. E., Curtis, S. D., Trefts, E., Novak, S. W., et al. (2023). Induction of lysosomal and mitochondrial biogenesis by AMPK phosphorylation of FNIP1. Science 380 (6642), eabj5559. doi:10.1126/science.abj5559
Mancini, G., Pirruccio, K., Yang, X., Blucher, M., Rodeheffer, M., and Horvath, T. L. (2019). Mitofusin 2 in mature adipocytes controls adiposity and body weight. Cell Rep. 26 (11), 2849–2858. doi:10.1016/j.celrep.2019.02.039
Manor, O., Dai, C. L., Kornilov, S. A., Smith, B., Price, N. D., Lovejoy, J. C., et al. (2020a). Health and disease markers correlate with gut microbiome composition across thousands of people. Nat. Commun. 11 (1), 5206. doi:10.1038/s41467-020-18871-1
Manor, O., Dai, C. L., Kornilov, S. A., Smith, B., Price, N. D., Lovejoy, J. C., et al. (2020b). Health and disease markers correlate with gut microbiome composition across thousands of people. Nat. Commun. 11 (1), 5206. doi:10.1038/s41467-020-18871-1
Matthew, B., Id, O., S, M., A, C., Id, O., C, D. G., et al. (2021). Bile acids activated receptors in inflammatory bowel disease. Cells 10 (6), 1281. doi:10.3390/cells10061281
Matthew, F., Tl, G., M, W., Y, V., Me, M., Lc, B., et al. (2020a). A metabolic pathway for bile acid dehydroxylation by the gut microbiome. Nature 582 (7813), 566–570. doi:10.1038/s41586-020-2396-4
Matthew, S., and Py, v.d.W. (2020). Lipopolysaccharides modulate intestinal epithelial permeability and inflammation in a species-specific manner. Gut Microbes 11 (3), 421–432. doi:10.1080/19490976.2019.1629235
Matthew, W., Z, W., Dj, L., M, G., W, C., and Id, O. (2023a). Fusobacterium nucleatum and its metabolite hydrogen sulfide alter gut microbiota composition and autophagy process and promote colorectal cancer progression. Microbiol. Spectr. 11 (6), e0229223. doi:10.1128/spectrum.02292-23
Matthew, X., L, Z., L, L., M, F., L, H., and Id, O. (2020b). MiR-339 attenuates LPS-induced intestinal epithelial cells inflammatory responses and apoptosis by targeting TLR4. Genes Genomics 42 (9), 1097–1105. doi:10.1007/s13258-020-00977-x
Matthew, Y., Jh, W., Jh, S., D, L., Sn, L., Jg, S., et al. (2023b). Pharmaceutical efficacy of novel human-origin Faecalibacterium prausnitzii strains on high-fat-diet-induced obesity and associated metabolic disorders in mice. Front. Endocrinol. (Lausanne) 14, 1220044. doi:10.3389/fendo.2023.1220044
Michaudel, C., and Sokol, H. (2020). The gut microbiota at the service of immunometabolism. Cell Metab. 32 (4), 514–523. doi:10.1016/j.cmet.2020.09.004
Miyamoto, J., Shimizu, H., Hisa, K., Matsuzaki, C., Inuki, S., Ando, Y., et al. (2023). Host metabolic benefits of prebiotic exopolysaccharides produced by Leuconostoc mesenteroides. Gut Microbes 15 (1), 2161271. doi:10.1080/19490976.2022.2161271
Montgomery, M. K. (2019). Mitochondrial dysfunction and diabetes: is mitochondrial transfer a friend or foe? Biology-Basel 8 (2), 33. doi:10.3390/biology8020033
Nie, K., Zhao, Y., Su, H., Xu, L., Zou, X., Wang, K., et al. (2021a). Effect of Wu-Mei-Wan on gut microbiota in obese mice. Chin. J. Hosp. Pharm. 41 (08), 796–802. doi:10.13286/j.1001-5213.2021.08.05
Nie, K., Zhao, Y., Su, H., Xu, L., Zou, X., Wang, K., et al. (2021b). Effect of Wu-Mei-Wan on gutmicrobiota in obese mice. Chin. J. Hosp. Pharm. 41 (08), 796–802. doi:10.13286/j.1001-5213.2021.08.05
Nm, B., C, M., Cs, d.S., Pb, V. B., F, C., Va, A., et al. (2017). Microbial anti-inflammatory molecule (MAM) from Faecalibacterium prausnitzii shows a protective effect on DNBS and DSS-induced colitis model in mice through inhibition of NF-κB pathway. Front. Microbiol. 8, 114. doi:10.3389/fmicb.2017.00114
Pedersen, J. S., Rygg, M. O., Chrois, K., Sustarsic, E. G., Gerhart-Hines, Z., Albrechtsen, N. J. W., et al. (2022). Influence of NAFLD and bariatric surgery on hepatic and adipose tissue mitochondrial biogenesis and respiration. Nat. Commun. 13 (1), 2931. doi:10.1038/s41467-022-30629-5
Peng, X., Wang, K., Zhang, C., Bao, J.-P., Vlf, C., Gao, J.-W., et al. (2021). The mitochondrial antioxidant SS-31 attenuated lipopolysaccharide-induced apoptosis and pyroptosis of nucleus pulposus cells via scavenging mitochondrial ROS and maintaining the stability of mitochondrial dynamics. Free Radic. Res. 55 (11-12), 1080–1093. doi:10.1080/10715762.2021.2018426
Pereira, R. O., Marti, A., Olvera, A. C., Tadinada, S. M., Bjorkman, S. H., Weatherford, E. T., et al. (2021). OPA1 deletion in brown adipose tissue improves thermoregulation and systemic metabolism via FGF21. Elife 10, e66519. doi:10.7554/eLife.66519
Pileggi, C. A., Parmar, G., and Harper, M.-E. (2021). The lifecycle of skeletal muscle mitochondria in obesity. Obes. Rev. 22 (5), e13164. doi:10.1111/obr.13164
Piotr, K., Id, O., and I, M. (2022a). Biological effects of indole-3-propionic acid, a gut microbiota-derived metabolite, and its precursor tryptophan in mammals' health and disease. Int. J. Mol. Sci. 23 (3), 1222. doi:10.3390/ijms23031222
Piotr, P., F, S., Id, O., C, J., Id, O., K, K., et al. (2022b). Prebiotic oligofructose protects against high-fat diet-induced obesity by changing the gut microbiota, intestinal mucus production, glycosylation and secretion. Gut Microbes 14 (1), 2152307. doi:10.1080/19490976.2022.2152307
Qi, Z., W, X., Q, W., Z, T., Y, W., and W, G. (2022). Gut microbiota-mitochondrial inter-talk in non-alcoholic fatty liver disease. Front. Nutr. 9, 934113. doi:10.3389/fnut.2022.934113
Ramos-Romero, S., Santocildes, G., Pinol-Pinol, D., Roses, C., Pages, T., Hereu, M., et al. (2020). Implication of gut microbiota in the physiology of rats intermittently exposed to cold and hypobaric hypoxia. Plos One 15 (11), e0240686. doi:10.1371/journal.pone.0240686
Ren, J., Zk, H., F, L., S, H., Ss, Z., Wy, G., et al. (2020). Mitophagy-Mediated mtDNA release aggravates stretching-induced inflammation and lung epithelial cell injury via the TLR9/MyD88/NF-κB pathway. Front. Cell Dev. Biol. 8, 819. doi:10.3389/fcell.2020.00819
Ren, R., Id, O., Y, F., P, S., Q, L., C, L., et al. (2022). Kynurenine/aryl hydrocarbon receptor modulates mitochondria-mediated oxidative stress and neuronal apoptosis in experimental intracerebral hemorrhage. Antioxid. Redox Signal 37 (16-18), 1111–1129. doi:10.1089/ars.2021.0215
Rius-Perez, S., Torres-Cuevas, I., Millan, I., Ortega, A. L., and Perez, S. (2020). PGC-1α, inflammation, and oxidative stress: an integrative view in metabolism. Oxidative Med. Cell. Longev. 2020, 1452696. doi:10.1155/2020/1452696
Roswall, J., Olsson, L. M., Kovatcheva-Datchary, P., Nilsson, S., Tremaroli, V., Simon, M.-C., et al. (2021). Developmental trajectory of the healthy human gut microbiota during the first 5 years of life. Cell Host and Microbe 29 (5), 765–776.e3. doi:10.1016/j.chom.2021.02.021
Ruiz, E., Penrose, H. M., Heller, S., Nakhoul, H., Baddoo, M., Flemington, E. F., et al. (2020). Bacterial TLR4 and NOD2 signaling linked to reduced mitochondrial energy function in active inflammatory bowel disease. Gut Microbes 11 (3), 350–363. doi:10.1080/19490976.2019.1611152
Saadati, S., Naseri, K., Asbaghi, O., Yousefi, M., Golalipour, E., and de Courten, B. (2024). Beneficial effects of the probiotics and synbiotics supplementation on anthropometric indices and body composition in adults: a systematic review and meta-analysis. Obes. Rev. 25 (3), e13667. doi:10.1111/obr.13667
Sakers, A., De Siqueira, M. K., Seale, P., and Villanueva, C. J. (2022). Adipose-tissue plasticity in health and disease. Cell 185 (3), 419–446. doi:10.1016/j.cell.2021.12.016
Sergio, D., Id, O., J, K., Id, O., L, T., Id, O., et al. (2018). Erchen decoction ameliorates lipid metabolism by the regulation of the protein CAV-1 and the receptors VLDLR, LDLR, ABCA1, and SRB1 in a high-fat diet rat model. Evid. Based Complement. Altern. Med. 2018, 5309490. doi:10.1155/2018/5309490
Sergio, R.-C., Id, O., Cj, L., M, C., G, P., M, M.-U., et al. (2021). Allostatic hypermetabolic response in PGC1α/β heterozygote mouse despite mitochondrial defects. FASEB J. 35 (9), e21752. doi:10.1096/fj.202100262RR
Sharma, M., Wasan, A., and Sharma, R. K. (2021). Recent developments in probiotics: an emphasis on Bifidobacterium. Food Biosci. 41, 100993. doi:10.1016/j.fbio.2021.100993
Shi, L. L., Li, Y., Wang, Y., and Feng, Y. (2015). MDG-1, an Ophiopogon polysaccharide, regulate gut microbiota in high-fat diet-induced obese C57BL/6 mice. Int. J. Biol. Macromol. 81, 576–583. doi:10.1016/j.ijbiomac.2015.08.057
Singh, V., Ahlawat, S., Mohan, H., Gill, S. S., and Sharma, K. K. (2022). Balancing reactive oxygen species generation by rebooting gut microbiota. J. Appl. Microbiol. 132 (6), 4112–4129. doi:10.1111/jam.15504
SR, S., Y, H., Lp, N., C, T., M, W., Ls, B., et al. (2020). Dysbiosis-induced secondary bile acid deficiency promotes intestinal inflammation. Cell Host Microbe 27 (4), 659–670. doi:10.1016/j.chom.2020.01.021
Su, L. J., Zeng, Y. P., Li, G. K., Chen, J., and Chen, X. Y. (2022). Quercetin improves high-fat diet-induced obesity by modulating gut microbiota and metabolites in C57BL/6J mice. Phytotherapy Res. 36 (12), 4558–4572. doi:10.1002/ptr.7575
Takeuchi, T., Kubota, T., Nakanishi, Y., Tsugawa, H., Suda, W., Kwon, A. T. J., et al. (2023). Gut microbial carbohydrate metabolism contributes to insulin resistance. Nature 621 (7978), 389–395. doi:10.1038/s41586-023-06466-x
Talari, N. K., Mattam, U., Meher, N. K., Paripati, A. K., Mahadev, K., Krishnamoorthy, T., et al. (2023). Lipid-droplet associated mitochondria promote fatty-acid oxidation through a distinct bioenergetic pattern in male Wistar rats. Nat. Commun. 14 (1), 766. doi:10.1038/s41467-023-36432-0
Teodoro, J. S., Duarte, F. V., Gomes, A. P., Varela, A. T., Peixoto, F. M., Rolo, A. P., et al. (2013). Berberine reverts hepatic mitochondrial dysfunction in high-fat fed rats: a possible role for SirT3 activation. Mitochondrion 13 (6), 637–646. doi:10.1016/j.mito.2013.09.002
Tsukuda, N., Yahagi, K., Hara, T., Watanabe, Y., Matsumoto, H., Mori, H., et al. (2021). Key bacterial taxa and metabolic pathways affecting gut short-chain fatty acid profiles in early life. Isme J. 15 (9), 2574–2590. doi:10.1038/s41396-021-00937-7
Turchi, R., Tortolici, F., Guidobaldi, G., Iacovelli, F., Falconi, M., Rufini, S., et al. (2020). Frataxin deficiency induces lipid accumulation and affects thermogenesis in brown adipose tissue. Cell Death and Dis. 11 (1), 51. doi:10.1038/s41419-020-2253-2
Valentina, S., G, C., Nt, I., G, F., G, M., M, M., et al. (2022). Activation of G protein-coupled receptors by ketone bodies: clinical implication of the ketogenic diet in metabolic disorders. Front. Endocrinol. (Lausanne) 13, 972890. doi:10.3389/fendo.2022.972890
Valles-Colomer, M., Blanco-Miguez, A., Manghi, P., Asnicar, F., Dubois, L., Golzato, D., et al. (2023). The person-to-person transmission landscape of the gut and oral microbiomes. Nature 614 (7946), 125–135. doi:10.1038/s41586-022-05620-1
van der Zande, H. J. P., Brombacher, E. C., Lambooij, J. M., Pelgrom, L. R., Zawistowska-Deniziak, A., Patente, T. A., et al. (2023). Dendritic cell-intrinsic LKB1-AMPK/SIK signaling controls metabolic homeostasis by limiting the hepatic Th17 response during obesity. Jci Insight 8 (11), e157948. doi:10.1172/jci.insight.157948
von der Malsburg, A., Sapp, G. M., Zuccaro, K. E., von Appen, A., Moss, F. R., Kalia, R., et al. (2023). Structural mechanism of mitochondrial membrane remodelling by human OPA1. Nature 620 (7976), 1101–1108. doi:10.1038/s41586-023-06441-6
Wachsmuth, H. R., Weninger, S. N., and Duca, F. A. (2022). Role of the gut-brain axis in energy and glucose metabolism. Exp. Mol. Med. 54 (4), 377–392. doi:10.1038/s12276-021-00677-w
Wan, Y., Yuan, J., Li, J., Li, H., Yin, K., Wang, F., et al. (2020). Overweight and underweight status are linked to specific gut microbiota and intestinal tricarboxylic acid cycle intermediates. Clin. Nutr. 39 (10), 3189–3198. doi:10.1016/j.clnu.2020.02.014
Wang, L., Chen, W. X., Tian, Y. J., Duan, X. R., Yuan, Y., Wang, N., et al. (2022). Preventive effects of sesamol on deep-frying oil-induced liver metabolism disorders by altering gut microbiota and protecting gut barrier integrity. Mol. Nutr. and Food Res. 66 (9), e2101122. doi:10.1002/mnfr.202101122
Wang, L., Hu, J., and Zhou, H. (2021). Macrophage and adipocyte mitochondrial dysfunction in obesity-induced metabolic diseases. World J. Mens Health 39 (4), 606–614. doi:10.5534/wjmh.200163
Wang, L., and Hu, K. (2021). Effect of gegen qinlian decoction on lipid metabolism in obese mice and its mechanism. World J. Integr. Traditional West. Med. 16 (07), 1231–1236. doi:10.13935/j.cnki.sjzx.210713
Wang, P., Gao, J. P., Ke, W. X., Wang, J., Li, D. T., Liu, R. L., et al. (2020a). Resveratrol reduces obesity in high-fat diet-fed mice via modulating the composition and metabolic function of the gut microbiota. Free Radic. Biol. Med. 156, 83–98. doi:10.1016/j.freeradbiomed.2020.04.013
Wang, Y., Li, J., Zhang, Z., Wang, R., Bo, H., and Zhang, Y. (2023). Exercise improves the coordination of the mitochondrial unfolded protein response and mitophagy in aging skeletal muscle. Life-Basel 13 (4), 1006. doi:10.3390/life13041006
Wang, Y., Yao, W. F., Li, B., Qian, S. Y., Wei, B. B., Gong, S. Q., et al. (2020b). Nuciferine modulates the gut microbiota and prevents obesity in high-fat diet-fed rats. Exp. Mol. Med. 52 (12), 1959–1975. doi:10.1038/s12276-020-00534-2
Wei, L. Q., Cheong, I. H., Yang, G. H., Li, X. G., Kozlakidis, Z., Ding, L., et al. (2021). The application of high-throughput technologies for the study of microbiome and cancer. Front. Genet. 12, 699793. doi:10.3389/fgene.2021.699793
Welsh, A., Hammad, M., Pina, I. L., and Kulinski, J. (2024). Obesity and cardiovascular health. Eur. J. Prev. Cardiol. 31 (8), 1026–1035. doi:10.1093/eurjpc/zwae025
Wen, L., Xiong, W., Wei, G., Zhang, L., Liu, Y., Zhang, T., et al. (2022). Differential response of ileal and colonic microbiota in rats with high-fat diet-induced atherosclerosis. Int. J. Mol. Sci. 23 (19), 11154. doi:10.3390/ijms231911154
Wu, F., Yang, X. P., Hu, M. L., Shao, Q. Q., Fang, K., Li, J. B., et al. (2020). Wu-Mei-Wan prevents high-fat diet-induced obesity by reducing white adipose tissue and enhancing brown adipose tissue function. Phytomedicine 76, 153258. doi:10.1016/j.phymed.2020.153258
Xia, W., Veeragandham, P., Cao, Y., Xu, Y., Rhyne, T. E., Qian, J., et al. (2024). Obesity causes mitochondrial fragmentation and dysfunction in white adipocytes due to RalA activation. Nat. Metab. 6 (2), 273–289. doi:10.1038/s42255-024-00978-0
Xiaomin, S., Y, G., R, Y., and Id, O. (2022a). Gut microbiota-derived tryptophan metabolites maintain gut and systemic homeostasis. Cells 11 (15), 2296. doi:10.3390/cells11152296
Xiaomin, T., S, M., Y, L., Y, S., K, Z., Q, Z., et al. (2020). Evaluating the activity of sodium butyrate to prevent osteoporosis in rats by promoting osteal GSK-3β/nrf2 signaling and mitochondrial function. J. Agric. Food Chem. 68 (24), 6588–6603. doi:10.1021/acs.jafc.0c01820
Xiaomin, T., Y, W., C, D., X, Z., Y, L., J, Z., et al. (2022b). Tryptophan was metabolized into beneficial metabolites against coronary heart disease or prevented from producing harmful metabolites by the in vitro drug screening model based on Clostridium sporogenes. Front. Microbiol. 13, 1013973. doi:10.3389/fmicb.2022.1013973
Xiaomin, W., M, Z., Jj, L., Q, J., Y, Z., W, L., et al. (2022c). Propionate alleviates fatty acid-induced mitochondrial dysfunction, oxidative stress, and apoptosis by upregulating PPARG coactivator 1 alpha in hepatocytes. J. Dairy Sci. 105 (5), 4581–4592. doi:10.3168/jds.2021-21198
Xie, Y., Wang, C., Zhao, D., Wang, C., and Li, C. (2020). Dietary proteins regulate serotonin biosynthesis and catabolism by specific gut microbes. J. Agric. Food Chem. 68 (21), 5880–5890. doi:10.1021/acs.jafc.0c00832
Xiong, R.-G., Zhou, D.-D., Wu, S.-X., Huang, S.-Y., Saimaiti, A., Yang, Z.-J., et al. (2022). Health benefits and side effects of short-chain fatty acids. Foods 11 (18), 2863. doi:10.3390/foods11182863
Xiong, X. (2020). Gegen Qinlian Decoction formula syndrome and its application in diabetes, hypertension, hyperlipidemia and obesity. Zhongguo Zhong Yao Za Zhi 45 (12), 2760–2764. doi:10.19540/j.cnki.cjcmm.20190829.501
Xu, J., Shen, J., Yuan, R., Jia, B., Zhang, Y., Wang, S., et al. (2021). Mitochondrial targeting therapeutics: promising role of natural products in non-alcoholic fatty liver disease. Front. Pharmacol. 12, 796207. doi:10.3389/fphar.2021.796207
Yang, C. W., Liu, H. M., Chang, Z. Y., Liu, G. H., Chang, H. H., Huang, P. Y., et al. (2024). Puerarin modulates hepatic farnesoid X receptor and gut microbiota in high-fat diet-induced obese mice. Int. J. Mol. Sci. 25 (10), 5274. doi:10.3390/ijms25105274
Yang, D.-Q., Zuo, Q.-N., Wang, T., Xu, D., Lian, L., Gao, L.-J., et al. (2021). Mitochondrial-targeting antioxidant SS-31 suppresses airway inflammation and oxidative stress induced by cigarette smoke. Oxidative Med. Cell. Longev. 2021, 6644238. doi:10.1155/2021/6644238
Yang, H.-M., Kim, J., Shin, D., Kim, J.-Y., You, J., Lee, H.-C., et al. (2023a). Resistin impairs mitochondrial homeostasis via cyclase-associated protein 1-mediated fission, leading to obesity-induced metabolic diseases. Metabolism-Clinical Exp. 138, 155343. doi:10.1016/j.metabol.2022.155343
Yang, M., Wang, J.-H., Shin, J.-H., Lee, D., Lee, S.-N., Seo, J.-G., et al. (2023b). Pharmaceutical efficacy of novel human-origin Faecalibacterium prausnitzii strains on high-fat-diet-induced obesity and associated metabolic disorders in mice. Front. Endocrinol. 14, 1220044. doi:10.3389/fendo.2023.1220044
Yang, Q.-H., Xu, Y.-J., Liu, Y.-Z., Liang, Y.-J., Feng, G.-F., Zhang, Y.-P., et al. (2014). Effects of chaihu-shugan-san and shen-ling-Bai-Zhu-San on p38 MAPK pathway in kupffer cells of nonalcoholic steatohepatitis. Evidence-Based Complementary Altern. Med. 2014, 671013. doi:10.1155/2014/671013
Yau, W. W., Wong, K. A., Zhou, J., Thimmukonda, N. K., Wu, Y., Bay, B.-H., et al. (2021). Chronic cold exposure induces autophagy to promote fatty acid oxidation, mitochondrial turnover, and thermogenesis in brown adipose tissue. Iscience 24 (5), 102434. doi:10.1016/j.isci.2021.102434
Ym, W., Tl, H., C, M., J, Z., and Ny, F. (2020). SIRT1 deacetylates mitochondrial trifunctional enzyme α subunit to inhibit ubiquitylation and decrease insulin resistance. Cell Death Dis. 11 (10), 821. doi:10.1038/s41419-020-03012-9
Yoo, W., Zieba, J. K., Foegeding, N. J., Torres, T. P., Shelton, C. D., Shealy, N. G., et al. (2021). High-fat diet-induced colonocyte dysfunction escalates microbiota-derived trimethylamine N-oxide. Science 373 (6556), 813–818. doi:10.1126/science.aba3683
Yu, Y., Peng, X.-D., Qian, X.-J., Zhang, K.-M., Huang, X., Chen, Y.-H., et al. (2021). Fis1 phosphorylation by Met promotes mitochondrial fission and hepatocellular carcinoma metastasis. Signal Transduct. Target. Ther. 6 (1), 401. doi:10.1038/s41392-021-00790-2
Zhang, C. H., Sheng, J. Q., Xie, W. H., Luo, X. Q., Xue, Y. N., Xu, G. L., et al. (2021a). Mechanism and basis of traditional Chinese medicine against obesity: prevention and treatment strategies. Front. Pharmacol. 12, 615895. doi:10.3389/fphar.2021.615895
Zhang, F., Aschenbrenner, D., Yoo, J. Y., and Zuo, T. (2022). The gut mycobiome in health, disease, and clinical applications in association with the gut bacterial microbiome assembly. Lancet Microbe 3 (12), 969–983. doi:10.1016/s2666-5247(22)00203-8
Zhang, Q., Bai, Y. Y., Wang, W. X., Li, J. J., Zhang, L., Tang, Y. P., et al. (2023a). Role of herbal medicine and gut microbiota in the prevention and treatment of obesity. J. Ethnopharmacol. 305, 116127. doi:10.1016/j.jep.2022.116127
Zhang, W., and Zhong, X. (2020). Exploring the effects of gegen qinlian decoction on glucose and lipid metabolism in high-fat diet-induced obese mice based on gut microbiota. Lishizhen Med. Materia Medica Res. 31 (08), 1855–1858. doi:10.3969/j.issn.1008-0805.2020.08.020
Zhang, X., Xu, W., Xiao, X., Ding, J., Jiang, Y., and Tu, J. (2021b). Molecular mechanism of Gegen Qinlian Decoction in promoting differentiation of brown adipose tissue to improve glucose and lipid metabolism disorders in diabetic rats. Zhongguo Zhong Yao Za Zhi 46 (17), 4462–4470. doi:10.19540/j.cnki.cjcmm.20210524.403
Zhang, Y. A., Huang, Q. Q., Xiong, X. W., Yin, T. T., Chen, S., Yuan, W. W., et al. (2023b). Acacetin alleviates energy metabolism disorder through promoting white fat browning mediated by AC-cAMP pathway. J. Physiology Biochem. 79 (3), 529–541. doi:10.1007/s13105-023-00947-3
Zhao, D. D., Pan, Y. Y., Yu, N., Bai, Y., Ma, R. F., Mo, F. F., et al. (2021a). Curcumin improves adipocytes browning and mitochondrial function in 3T3-L1 cells and obese rodent model. R. Soc. Open Sci. 8 (3), 200974. doi:10.1098/rsos.200974
Zhao, T., Zhan, L. B., Zhou, W., Chen, W. X., Luo, J. T., Zhang, L. J., et al. (2021b). The effects of erchen decoction on gut microbiota and lipid metabolism disorders in zucker diabetic fatty rats. Front. Pharmacol. 12, 647529. doi:10.3389/fphar.2021.647529
Zheng, L. L., Li, B. Y., Yuan, A. L., Bi, S. J., Puscher, H., Liu, C. Q., et al. (2024). TFEB activator tanshinone IIA and derivatives derived from Salvia miltiorrhiza Bge. Attenuate hepatic steatosis and insulin resistance. J. Ethnopharmacol. 335, 118662. doi:10.1016/j.jep.2024.118662
Zheng, P., Ma, W., Gu, Y., Wu, H., Bian, Z., Liu, N., et al. (2023). High-fat diet causes mitochondrial damage and downregulation of mitofusin-2 and optic atrophy-1 in multiple organs. J. Clin. Biochem. Nutr. 73 (1), 61–76. doi:10.3164/jcbn.22-73
Zhi, N. N., Chang, X. W., Wang, X. R., Guo, J., Chen, J., and Gui, S. Y. (2024). Recent advances in the extraction, purification, structural-property correlations, and antiobesity mechanism of traditional Chinese medicine-derived polysaccharides: a review. Front. Nutr. 10, 1341583. doi:10.3389/fnut.2023.1341583
Zhihao, J., Id, O., X, C., J, C., L, Z., Sn, O., et al. (2022a). ACSS3 in brown fat drives propionate catabolism and its deficiency leads to autophagy and systemic metabolic dysfunction. Clin. Transl. Med. 12 (2), e665. doi:10.1002/ctm2.665
Zhihao, L., X, Z., W, W., L, G., C, H., H, S., et al. (2022b). Lactobacillus paracasei 24 attenuates lipid accumulation in high-fat diet-induced obese mice by regulating the gut microbiota. J. Agric. Food Chem. 70 (15), 4631–4643. doi:10.1021/acs.jafc.1c07884
Zhou, J., Zhang, J., Li, J. Y., Guan, Y. Q., Shen, T., Li, F., et al. (2021). Ginsenoside F2 suppresses adipogenesis in 3T3-L1 cells and obesity in mice via the AMPK pathway. J. Agric. Food Chem. 69 (32), 9299–9312. doi:10.1021/acs.jafc.1c03420
Zhu, B., Wang, Y., Ku, L.-T., van Dijk, D., Zhang, L., Hafler, D. A., et al. (2023). scNAT: a deep learning method for integrating paired single-cell RNA and T cell receptor sequencing profiles. Genome Biol. 24 (1), 292. doi:10.1186/s13059-023-03129-y
Zhu, X., Zhao, L., Lei, L., Zhu, Y., Xu, J., and Liu, L. (2024). Fecal microbiota transplantation ameliorates abdominal obesity through inhibiting microbiota-mediated intestinal barrier damage and inflammation in mice. Microbiol. Res. 282, 127654. doi:10.1016/j.micres.2024.127654
Zong, Y., Li, H., Liao, P., Chen, L., Pan, Y., Zheng, Y., et al. (2024). Mitochondrial dysfunction: mechanisms and advances in therapy. Signal Transduct. Target. Ther. 9 (1), 124. doi:10.1038/s41392-024-01839-8
Keywords: gut microbiota, mitochondria, obesity, metabolism, traditional Chinese medicine
Citation: Wen L, Yang K, Wang J, Zhou H and Ding W (2025) Gut microbiota-mitochondrial crosstalk in obesity: novel mechanistic insights and therapeutic strategies with traditional Chinese medicine. Front. Pharmacol. 16:1574887. doi: 10.3389/fphar.2025.1574887
Received: 11 February 2025; Accepted: 24 March 2025;
Published: 22 April 2025.
Edited by:
Yu-Jie Liu, Shanxi University of Chinese Medicine, ChinaReviewed by:
Feng Zhang, Nanjing University of Chinese Medicine, ChinaIngrid Rivera, University of Guadalajara, Mexico
Copyright © 2025 Wen, Yang, Wang, Zhou and Ding. This is an open-access article distributed under the terms of the Creative Commons Attribution License (CC BY). The use, distribution or reproduction in other forums is permitted, provided the original author(s) and the copyright owner(s) are credited and that the original publication in this journal is cited, in accordance with accepted academic practice. No use, distribution or reproduction is permitted which does not comply with these terms.
*Correspondence: Weijun Ding, RGluZ3dlaWp1bkBjZHV0Y20uZWR1LmNu