- Cancer Center, Department of Medical Oncology, Zhejiang Provincial People’s Hospital, Affiliated People’s Hospital, Hangzhou Medical College, Hangzhou, Zhejiang, China
Intestinal microbiota is a complex ecosystem of microorganisms that perform diverse metabolic activities to maintain gastrointestinal homeostasis. These microorganisms provide energy and nutrients for growth and reproduction while producing numerous metabolites including lipopolysaccharides (LPS), Bacteroides fragilis toxin (BFT), bile acids (BAs), polyamines (PAs), and short-chain fatty acids (SCFAs). These metabolites are linked to inflammation and various metabolic diseases, such as obesity, type-2 diabetes, non-alcoholic fatty liver disease, cardiometabolic disease, and malnutrition. In addition, they may contribute to tumorigenesis. Evidence suggests that these microbes can increase the susceptibility to certain cancers and affect treatment responses. In this review, we discuss the current knowledge on how the gut microbiome and its metabolites influence tumorigenesis, highlighting the potential molecular mechanisms and prospects for basic and translational research in this emerging field.
1 Introduction
The human microbiota consists of over 100 trillion organisms, including bacteria, viruses, fungi, and protozoans, which primarily reside on the epithelial surfaces of the human body. The human gut provides nutrient-rich and livable conditions for the microbiome. The gut microbiota benefits the human body by producing various metabolites such as short-chain fatty acids (SCFAs) from dietary fiber, synthesizing vitamins B and K, metabolizing compounds such as sterols and xenobiotics, and performing immunoregulatory functions (Rooks and Garrett, 2016). Its role in diseases such as cancer, liver disease, obesity, and neuropsychiatric disorders has been increasingly recognized (Garrett, 2015; Hand et al., 2016). Intestinal microbiota metabolites have been evaluated not only for their impact on the onset and progression of different tumor types but also for their potential as biomarkers and cancer therapies (Yu and Schwabe, 2017; Fernandez et al., 2018).
This review systematically dissects the dual roles of microbiota-derived metabolites in tumorigenesis, focusing on their bidirectional interactions with oncogenic signaling, immune microenvironment, and therapeutic responses (Figure 1). Recent breakthroughs in precision oncology and metabolomics are highlighted to bridge basic science with clinical translation.
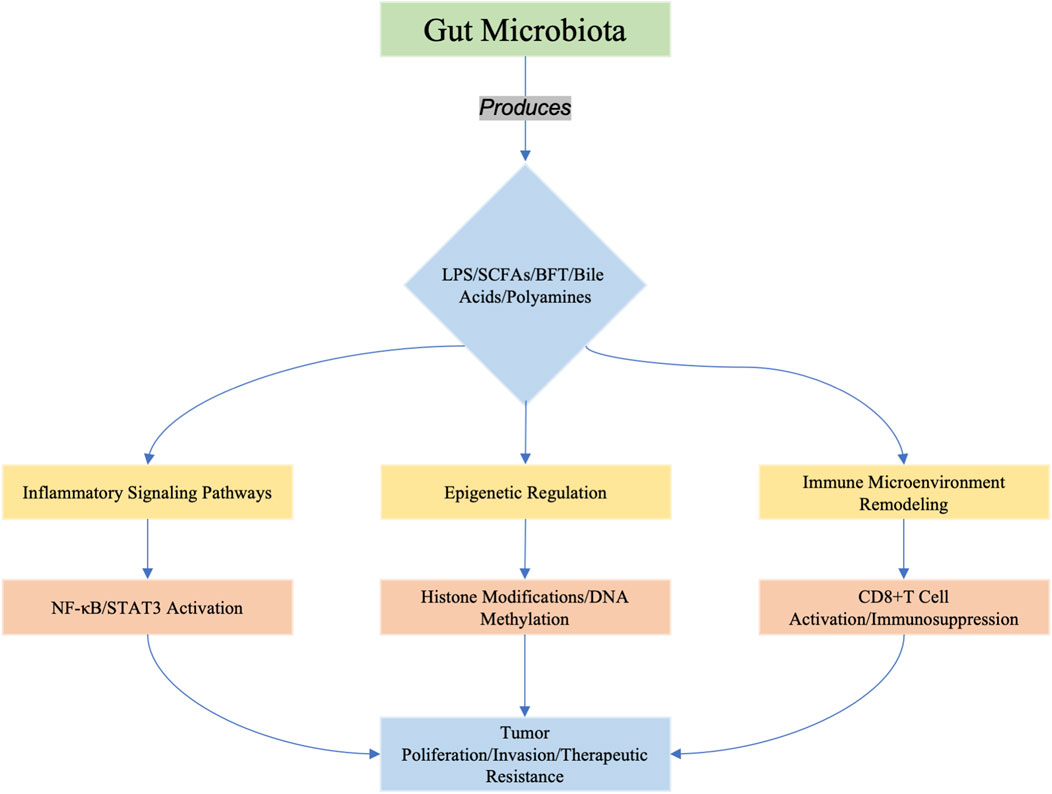
Figure 1. Microbiota-derived metabolites (e.g., LPS, SCFAs, BFT, bile acids, and polyamines) orchestrate tumorigenesis through three interconnected axes: (1) inflammatory signaling (e.g., TLR4/NF-κB activation), (2) epigenetic reprogrammimg (e.g., histone acetylation/DNA methylation), and (3) immune microenvironment modulation (e.g., enhancing immunosurveillance or promoting immunosuppression). These pathways converge to drive tumor cell proliferation, invasion, and resistance to therapy. Recent studies highlight emerging roles of metabolites in shaping cancer stemness (e.g., BFT-induced Notch/β-catenin activation in breast cancer) and metabolic reprogramming (e.g., butyrate-mediated epigenetic regulation in HCC).
2 Microbiota-derived metabolites and oncogenic pathways
2.1 Lipopolysaccharide (LPS)
Gram-negative bacterial LPS is a major component of the outer membrane and plays a key role in host-pathogen interactions with the innate immune system (Maldonado et al., 2016). By activating the transcription factor NF-κB and other cytokines, bacteria-secreting LPS can trigger the host immune response through a cascade of LPS receptors, such as Toll-like receptor 4 (TLR4) and cluster of differentiation 14 (CD14), leading to an inflammatory or immunomodulatory environment. In this study, we focused on the effects and mechanisms of LPS on tumorigenesis.
In colorectal carcinoma (CRC), exposure to LPS increases the expression of CXC chemokine receptor 7 (CXCR7) and enhances the proliferation and migration of SW480 and Colo 205 cells via the TLR4/myeloid differential protein (MD-2) pathway (Xu et al., 2011). Kuo et al. reported that normal human colonocytes are CD14+TLR4−, whereas cancerous tissues are CD14+TLR4+. In the absence of TLR4, LPS mediates colonocyte apoptosis by binding to CD14, which is dependent on CD14-mediated lipid messengers and PKC phosphorylation. In contrast, in CD14+TLR4+ cells, apoptosis can be blocked by competitive antagonism of TLR4 binding to LPS, leading to cancer progression (Kuo et al., 2015). Another study demonstrated that LPS augments VEGF-C secretion to promote cell motility and lymphangiogenesis via TLR4-NF-κB/JNK signaling (Zhu et al., 2016a). LPS can also bind to the gene promoter of VEGFR-3 to facilitate CRC migration and invasion via this signaling axis (Zhu et al., 2016b). Additionally, LPS promotes proliferation by facilitating the mRNA expression of inflammatory cytokines such as COX-2, IL-6, iNOS, and TNF-α (Suh et al., 2019; Hsieh et al., 2016). LPS has emerged as a powerful regulator of CRC tumorigenesis, attracting widespread attention and interest from researchers, leading to the development of countermeasures. In 2018, Song et al. engineered an LPS-targeting fusion protein by loading its coding sequence into a lipid-protamine-DNA (LPD) nanoparticle system for selective expression of the LPS trap protein, blocking LPS inside the tumor. This nanotrapping system significantly relieved the immunosuppressive microenvironment and boosted anti-PD-L1 mAb therapy against CRC tumors (Song et al., 2018). Despite its promise for cancer immunotherapy, the strong proinflammatory properties of LPS result in severe localized and systemic side effects, limiting its administrable dosage and potential for chronic dosing. Boushehri et al. further improved this nanotrapping system and found that size was an important determinant of short-term tolerability, with larger particles being associated with a higher incidence and extent of localized necrosis. In contrast, nanostructure composition predominantly governs long-term systemic tolerability. The higher affinity of LPS molecules for the triglyceride core of the nanoemulsion compared to that of the polymeric matrix significantly improves the tolerability of the former over time (Shetab Boushehri et al., 2019).
In hepatocellular carcinoma (HCC), LPS promotes cell survival, proliferation, invasion, and production of pro-inflammatory mediators, including TNF-α, iNOS, IL-1β, IL-6, CCL-2, CCL-22, vimentin, and epidermal growth factor receptor (EGFR), through the induction of TLR4 signaling (Wang et al., 2013; Lo et al., 2018). Singh et al. found that Nox4 mediates LPS-TLR4 signaling in human hepatoma cells, potentially contributing to LPS-induced liver pathology (Singh et al., 2017). Another study has shown that LPS antagonizes the inhibitory effect of miR-145 via NF-κB p65 activation (Wang RK. et al., 2019). Additionally, LPS was found to enhance HCC migration and invasion by targeting HIF-1 via NF-κB. Thus, we speculated that the LPS-TLR4-NF-κB axis might play a crucial role in HCC tumorigenesis, similar to that in CRC. LPS can also increase IL-1β production via protein kinase R, thereby enhancing HCC proliferation and invasion (Imai et al., 2019). Another key target of LPS is STAT3, which facilitates HCC cell proliferation, migration, and angiogenesis (Wang Z. et al., 2019). A recent study revealed that LPS increases N6-methyladenosine (m6A) methylation of GNAS mRNA, upregulating protein expression and activating STAT3 and IL-6 production (Zhang et al., 2020), thus outlining the GNAS-LPS-STAT3-IL-6 axis in HCC tumorigenesis. Although several studies have reported that LPS promotes tumor development, Honda et al. discovered that LPS-treated human monocytes may effectively suppress tumor invasion and proliferation in hepatic cancers. The co-cultured human monocyte cell line THP-1 and hepatic cancer cell line HepG2 were treated with LPS, resulting in significant suppression of the mRNA expression of monocyte chemotactic protein-1, vascular endothelial growth factor-A, TNF-α, IL-1β, IL-8, NF-κB, RelB, STAT3, IL-10, and transforming growth factor-β in THP-1 cells (Honda and Inagawa, 2016). Similarly, Spirulina-derived LPS was found to manipulate the balance of the IFN-γ-IL-17/IL-23 axis towards IFN-γ production, suppressing HCC progression. The antitumor activity and IFN-γ production were mediated by T cells. In vitro experiments showed that Spirulina LPS impaired the antigen-presenting function, supporting the generation of IL-17-producing cells in a TLR4-dependent manner (Okuyama et al., 2017). This study supports the use of TLR-based immunomodulators in tumor immunotherapy.
In lung cancer (LC), LPS mainly promotes cell proliferation and epithelial-mesenchymal transition (EMT) while attenuates apoptosis (Jiang et al., 2019; Wei et al., 2019). Mechanistically, LPS activates the TLR4 signaling pathway to facilitate immune suppression factors, such as TGF-β, VEGF, and IL-8, aiding the immune escape of cancer cells (Xu et al., 2017). LPS can also bind to CD14 and TLR4, leading to COX-2 activation and subsequent PGE2 release (Hattar et al., 2013). We conclude that CD14/TLR4-dependent COX-2 activation is a crucial step in mediating tumor proliferation in response to LPS. Wang et al. found that LPS activates the NLRP3 inflammasome to promote LC cell proliferation and migration (Wang et al., 2016). Further research demonstrated that LPS could be bound by secretoglobin SCGB3A2 and delivered to the cytosol to activate caspase-11/NLRP3 inflammasome foci formation, thereby decreasing cell proliferation (Yokoyama et al., 2018). Despite this bilateral activation of the inflammasome, LPS is more likely to promote cancer cell proliferation when confined to the cell membrane surface rather than suppressing it.
In breast cancer (BC), LPS facilitates EMT and cell metastasis, partly through the TLR4-Akt-GSK3β-β-catenin signaling pathway (Huang et al., 2013; Hong et al., 2015; Yang et al., 2014; Cho et al., 2015). Specifically, LPS stimulation of the TLR4 pathway in MCF7 and MDA-MB-231 breast cancer cells results in the following: (1) promotes of cell migration, (2) activates of the β-catenin signaling pathway via PI3K/Akt/GSK3β, and (3) enhances transcription of β-catenin target genes, leading to metastasis (Li J. et al., 2017). Additionally, Fried et al. demonstrated that the induction of LPS could mediate BC cell apoptosis in an IFN-β-dependent manner (Fried et al., 2016).
In gastric cancer (GC), LPS binds to CD14, increasing cell viability and inflammatory factor production while inhibiting apoptosis (Li et al., 2015). It also promotes STAT3 phosphorylation, which upregulates MMP7, MMP9, and VEGF expression (Guo et al., 2015). By binding to receptors such as TLR1, TLR4, TLR6, CD14, and MD2, LPS activates the NF-κB and STAT3 signaling pathways, inducing the production of TNF-α, IL-6, IL-1β, IL-8, and CXCR7 (Guo et al., 2015).
In glioma, LPS not only upregulates inflammatory mediators such as IL-8, CXCL8, and IL-1β to support tumorigenesis but also alters the immunophenotype of glioma cells and induces antitumor immunity via TLR4 (Li et al., 2015; Braganhol et al., 2015; Han et al., 2017). Hu et al. reported that LPS induces the Notch signaling pathway, activating TLR4, and reversing tumor differentiation (Han et al., 2017).
Emerging evidence suggests that impaired intestinal barrier function facilitates LPS translocation into systemic circulation. Under pathological conditions such as metabolic syndrome or chemotherapy-induced mucositis, increased gut permeability allows LPS to traverse the intestinal epithelium via paracellular transport or through M-cell mediated transcytosis (Ghosh et al., 2020). Once entering the portal circulation, LPS binds to lipopolysaccharide-binding protein(LBP) and is shuttled to CD14/TLR4 receptors on Kupffer cells, establishing a pro-tumorigenic microenvironment in the liver (Tsukamoto et al., 2018). For extrahepatic tumors, circulating LPS may directly activate TLR4-expressing cancer cells or stromal components. A recent study demonstrated that breast cancer cells exhibit upregulated TLR4 expression during metastasis, enabling LPS to promote epithelial-mesenchymal transition through NF-κB-mediated ZEB1 activation (Jing et al., 2012). These findings establish a gut-liver axis and gut-systemic axis for LPS-mediated oncogenesis beyond intestinal tissues.
Recent studies have highlighted pyroptosis induction in cancer cells as a promising strategy for cancer immunotherapy. The lipopolysaccharide (LPS)-sensitive non-canonical pyroptosis pathway, an essential mechanism for eliminating compromised cells, was leveraged in this study using bacterial outer membrane vesicles (OMVs) as natural LPS delivery vehicles. Engineered OMVs demonstrated remarkable tumor-targeting capability to selectively trigger gasdermin-mediated pyroptosis through caspase-4/5/11 activation. This spatially controlled pyroptosis induction not only enhanced effector T cell infiltration into tumors but also significantly reduced immunosuppressive regulatory T cell populations within the tumor microenvironment. Consequently, OMV-mediated pyroptosis reprogrammed the immunological landscape and achieved potent suppression of tumor progression in multiple murine models. Mechanistically, pyroptotic cell rupture released damage-associated molecular patterns that promoted dendritic cell maturation and antigen cross-presentation. These findings establish OMVs as biocompatible pyroptosis inducers and provide a mechanistic framework for LPS-based antitumor therapies, highlighting the therapeutic potential of harnessing innate immune pathways through bioengineered bacterial derivatives (Chen et al., 2023).
Furthermore, LPS has been reported to facilitate cell proliferation, invasion, and migration in human multiple myeloma, pancreatic cancer, esophageal cancer, melanoma, cervical cancer, bladder cancer, nasopharyngeal carcinoma, ovarian cancer, and prostate cancer (Bao et al., 2011; Ogut et al., 2016). Although some signaling pathways have been explored, more detailed mechanistic research is urgently needed to better understand the effects of microbe-derived LPS on tumorigenesis.
2.2 Short-chain fatty acids (SCFAs)
SCFAs, primarily acetate, propionate, and butyrate, are key metabolites produced from the fermentation of non-digestible carbohydrates (NDC) by the gut microbiota. SCFAs play crucial roles in regulating host metabolism, immune response, cell proliferation, invasion, and apoptosis, generally exerting positive effects. They shape the intestinal microbiota by protecting it and exerting anti-inflammatory functions, which impact intratumoral inflammation (Makki et al., 2018; Morrison and Preston, 2016).
2.2.1 Butyrate
2.2.1.1 Morphology and differentiation
Butyrate initially induced morphological transformations in prostate and hepatoma cell lines (Tsao et al., 1982; Reese et al., 1985). Imbalances between serum lipoprotein-derived and newly synthesized cholesterol can lead to morphological changes in HCC cell lines (Wright et al., 1986). Similarly, butyrate has been shown to alter the morphology of various cancer cell lines (Wright et al., 1986; Nakagawa et al., 2018). One potential mechanism involves butyrate activation of the T-type Ca2+ channel, which upregulates Cav3.2 T-type channel subunits and increases the Ca2+ influx (Weaver et al., 2015).
Butyrate also acts as a differentiation-inducing agent in cancer cell lines, accompanied by increased levels of intestinal alkaline phosphatase (Alpi) and cluster 1 antigen (Hay et al., 1991; Tsukamoto et al., 1991; Ellerhorst et al., 1999; Gillenwater et al., 2000; Perego et al., 2018; Tylichova et al., 2018). Four possible mechanisms for this effect include: 1) Butyrate-induced cell differentiation dependent on diverse patterns of reactive oxygen species (ROS). A dose-dependent increase in ROS was observed in HT29R cells (an HT29-derived human CRC cell line resistant to butyrate-induced differentiation but highly sensitive to cell death), but not in differentiation-positive HT29 cells; in contrast to HT29R, butyrate induced a dose-dependent increase in H2O2 release (Domokos et al., 2010). 2) Butyrate induces differentiation via the PTEN/PI3K/MUC2 axis. In the BGC823 gastric cancer cell line, butyrate treatment significantly suppressed cell proliferation and increased differentiation into intestinal cells, upregulating PTEN and MUC2 levels, while attenuating PI3K expression (Bai et al., 2010). 3) Butyrate alters the subcellular distribution of disaccharidases, enhancing the activity of the soluble (cytoplasmic) fraction and increasing ALK activity (Chung et al., 1985). 4) Butyrate-induced differentiation, marked by an increase Alpi, is mediated by the KLF5 transcription factor. KLF5 is essential for maintaining several regulators of intestinal cell differentiation, such as Elf3, Ascl2, Neurog3, Cdx1, and HNF4α (Shin et al., 2014; Bell et al., 2013). 5) Butyrate-induced differentiation in CRC cell lines is associated with downregulation of CD133 expression and upregulated phosphorylation of Src, along with increased expression of epithelial-to-mesenchymal transition-related genes (Lucchetti et al., 2017; Sgambato et al., 2010).
2.2.1.2 Programmed cell death effects in cancer
There are two types of programmed cell death, Type I (apoptosis) and Type II (autophagy). Butyrate has been shown to inhibit cell proliferation by promoting apoptosis and inducing autophagy in various cancers, thereby killing cancer cells and limiting tumor progression (Wang et al., 1998)- (Donohoe et al., 2011).
The pro-apoptotic effects of butyrate may be attributed to the activation of abnormal signaling pathways, including Wnt, JNK/MAPK, ERK, and AKT/mTOR (Lazarova et al., 2004)- (Huang et al., 2019). Darina et al. demonstrated that butyrate increases gene expression and upregulates Wnt signaling activity, with these effects related to butyrate-induced apoptosis in CRC cells (Bordonaro et al., 2004; Lazarova et al., 2004). Another study suggested that aberrant epigenetic modification of SFRP genes is the main mechanism by which Wnt signaling is activated. Butyrate modulates SFRP1/2 expression through histone modification and promoter demethylation, resulting in anti-tumor effects (Shin et al., 2012). In CRC, butyrate induces apoptosis via activation of the JNK/MAPK signaling pathway and the endoplasmic reticulum (ER) stress response, leading to caspase 3/7 activation and cell death (Fung et al., 2011; Zhang et al., 2010). Butyrate also promotes Syk expression by activating the ERK signaling pathway, which induces CRC apoptosis (Dasgupta et al., 2017). Furthermore, ERK regulates sphingosine kinase 2 export to induce apoptosis. Butyrate can also suppress cell proliferation and migration by regulating endocan expression through the upregulation of the ERK2/MAPK signaling pathway (Zuo et al., 2013). In contrast, Chen et al. used KEGG, Gene Ontology (GO), and Pathway Studio software for data analysis and found that butyrate downregulated most tumor-related signaling pathways (e.g., MAPK, Wnt, insulin, and VEGF pathways) (Chen et al., 2013).
In addition to inducing apoptosis, butyrate promotes autophagy and leads to cell death (Verma et al., 2018). The primary mechanisms include 1) induction of endoplasmic reticulum stress (Zhang et al., 2016) and 2) inhibition of the AKT/mTOR signaling pathway (Pant et al., 2017; Huang et al., 2019; Taylor et al., 2019). Although the precise molecular mechanisms underlying butyrate’s dual role in tumorigenesis and progression remain incompletely elucidated, emerging evidence from recent studies has demonstrated that this short-chain fatty acid can potentiate the therapeutic efficacy of programmed cell death protein 1 (PD-1) inhibitors in colorectal carcinoma through immunomodulatory mechanisms involving enhanced CD8+T lymphocyte infiltration and functional regulation of myeloid-derived suppressor cells (MDSCs) (Zhu et al., 2023). Additionally, a study from last year showed that engineered probiotics delivering butyrate prodrugs suppressed tumor growth in mice by targeting the tumor microenvironment (TME). The probiotics selectively colonized tumors, converting prodrugs into active butyrate that enhances ferroptosis via lipid peroxidation and oxidative stress. Butyrate also inhibited immunosuppressive factors (e.g., PD-L1) and boosted CD8+ T cell infiltration. Combined with ferroptosis inducers, this approach achieved >50% tumor inhibition in pancreatic cancer models while minimizing systemic toxicity. The work highlights engineered probiotics as a precision strategy to modulate TME metabolism and immunity synergistically with existing therapies (Gao et al., 2021).
2.2.1.3 Epigenetic and synergistic therapies
Butyrate is widely recognized for its role in inhibiting cancer cell growth and for acting as a tumor suppressor. Lupton observed that butyrate did not inhibit cell growth when administered to normal colonic epithelium in rodents or to non-cancerous colonocytes in vitro (Lupton, 2004). Unlike most normal tissues, tumor cells often ferment glucose into lactic acid, even when oxygen is sufficient for mitochondrial oxidative phosphorylation, a phenomenon known as the Warburg effect, which mitigates the butyrate paradox. Butyrate stimulates the growth of normal colonocytes by serving as an oxidative energy source but inhibits the growth of cancerous colonocytes by functioning as an histone deacetylase inhibitor (HDACi), promoting histone acetylation through its metabolism to acetyl-CoA (Donohoe et al., 2012).
As an HDACi, butyrate has been reported to induce androgen receptor (AR) expression, thereby inhibiting prostate tumorigenesis by increasing H4 acetylation in the AR promoter region (Paskova et al., 2013; Fialova et al., 2016). This leads to inhibition of the AKT/ERK signaling pathway and upregulation of p21, WAF1/Cip1, Chk1, and Chk2, which contribute to CRC tumorigenesis (Donohoe et al., 2014; Li Q. et al., 2017). Emerging evidence indicates that butyrate exerts inhibitory effects on hepatocellular carcinoma metastasis through epigenetic modulation mechanisms. Specifically, this short-chain fatty acid acts as a HDACi, particularly targeting HDAC3 isoform. The suppression of HDAC3 enzymatic activity subsequently enhances the transcriptional activation of phosphatase and tensin homolog (PTEN), a crucial tumor suppressor gene involved in regulating cell proliferation and metastatic potential. This HDAC3/PTEN regulatory axis has been mechanistically demonstrated to mediate the anti-metastatic properties of butyrate in both in vitro and in vivo models of liver cancer (Eshleman et al., 2024). Carnitine can attenuate butyrate oxidation, diminish its action as an HDACi, and suppress the induction of H3 acetylation by butyrate in CRC cells (Han et al., 2016). Therefore, ensuring that butyrate functions as a HDACi in tumors is crucial for optimizing its antitumor effects, and carnitine may be a promising target.
Although butyrate alone can inhibit tumor cell growth and promote apoptosis, its synergistic effects with other biomolecules or drugs have been proven to be more effective. In CRC, butyrate combines with aspirin, paclitaxel, mitomycin C, diallyl disulfide, docosahexaenoic acid, epigallocatechin gallate, acetylcarnitine, wheat bran, and glycerol to more efficiently inhibit cell proliferation efficiently (Menzel et al., 2002; Rivkin et al., 2014; Koprinarova et al., 2010; Gospodinov et al., 2012; Altonsy and Andrews, 2011; Kolar et al., 2011; Saldanha et al., 2014; Elimrani et al., 2015; Zhao et al., 2019; Lu et al., 2020). In other tumors, combinations of butyrate and artemisinin in lymphoblastoid leukemia (Singh and Lai, 2005), butyrate with N-(4-hydroxyphenyl)-retinamide in prostate cancer (Kuefer et al., 2007), butyrate with N-methyl-N′-nitro-N-nitrosoguanidine in nasopharyngeal carcinoma (Huang et al., 2010), butyrate with cisplatin in bladder cancer (Maruyama et al., 2012), butyrate with vitamin A in breast cancer (Andrade et al., 2012), butyrate with zoledronic acid in Ewing sarcoma (Dos Santos et al., 2014), butyrate with 1′-acetoxychavicol acetate in hepatocellular carcinoma (Kato et al., 2014), butyrate with quercetin in glioblastoma (Taylor et al., 2019), and butyrate with adriamycin in uterine cancer (Yu et al., 2014) have been reported to enhance cancer cell killing. In addition to these molecular compounds or drugs, butyrate can synergize with clinical cancer therapies, such as photodynamic therapy for astrocytoma and boron neutron capture therapy for thyroid carcinoma (Bueno-Carrazco et al., 2012; Perona et al., 2013).
2.2.1.4 Activator of G-protein-coupled receptors
G protein-coupled receptors play a significant role in mediating anti-inflammatory and anti-cancer effects in the gut. Short-chain fatty acids such as butyrate activate GPR109a, thereby promoting anti-cancer effects (Krejner et al., 2018).
GPR109A, a receptor for butyrate, interacts with it to exert anti-cancer effects in CRC and BC (Singh et al., 2014; Elangovan et al., 2014). This mechanism involves two factors: GPR109A also serves as a receptor for niacin produced by the gut microbiota, which suppresses intestinal inflammation and CRC. Butyrate acts as a pharmacological GPR109A agonist, suppressing colitis and colon cancer in a GPR109A-dependent manner (Fielding et al., 2014). Moreover, the binding of butyrate to GPR109A inhibits the IL-6/STAT3 signaling pathway in APC cells and the IL-17/NF-κB signaling pathway in Th17 cells (Chen and Vitetta, 2018), both of which are crucial in promoting inflammation and tumorigenesis.
2.2.2 Propionic acid
While propionic acid has been less studied compared to other microbial metabolites such as butyrate, it exhibits unique health-promoting properties. Propionic acid is a major microbial fermentation metabolite in the human gut and is thought to reduce fat production, serum cholesterol levels, and carcinogenesis in other tissues (Hosseini et al., 2011).
Short-chain fatty acids are the primary products of dietary fiber fermentation in the colon. Studies have shown that feeding animals with fermentable fibers prevents steatosis caused by a high-fat diet (Kim et al., 2014; Zeng et al., 2014). This effect is likely due to propionic acid, as approximately 90% of the propionic acid produced in the colon is absorbed by the liver from the portal vein (Arpaia et al., 2013) and has been shown to alter liver metabolic processes and reduce lipid content. Propionic acid significantly decreased the mRNA levels of fatty acid biosynthesis-related genes (Srebp1c, Fasn, and Elovl6), leading to reduced long-chain fatty acids in the liver (Huang et al., 2020). Although interactions between SCFAs and Tregs (particularly GPR41/43) have been well studied, the effects of SCFAs on cancer cell metabolism and immune evasion remain unclear. Propionic acid upregulates MICA/B surface expression in cancer cells through metabolic pathways that promote synthesis and acylation, highlighting its immunostimulatory potential (Hogh et al., 2020). Tang et al. reported that plasmid transfection increases FFA2 expression in human colon cancer cells, making them more responsive to propionic acid. Bindels et al. proposed that propionic acid production might be a function of gut microbes, contributing to the anti-tumor effects of prebiotic nutrients (Tang et al., 2011; Bindels et al., 2012). Intestinal microbiota-derived propionic acid improved inflammatory markers (TNF-α, IL-6, and Cox2), ATP levels, malondialdehyde levels, and liver histology. The clinical use of triptolide (TP) as a potential drug for treating inflammatory and autoimmune diseases and cancer has been limited by its severe toxicity, particularly liver damage. Modulation of the intestinal microbiota through food, prebiotics, probiotics, or propionic acid supplementation may improve TP toxicity (Huang et al., 2020). Propionic acid regulates CD8+ T cell activation by inhibiting IL-12 secretion by dendritic cells. These findings reveal a novel mechanism by which bacterial fermentation products modulate CD8+ T cell function and may have implications for anticancer immunotherapy (Nastasi et al., 2017).
2.2.3 Acetate
Acetate is a two-carbon monocarboxylic acid and the most produced SCFA, reaching relatively high concentrations in mammalian blood (Hosios and Vander Heiden, 2014). Acetogenic bacteria, such as Blautia hydrogenotrophs, produce acetate from pyruvate via the Wood–Ljungdahl pathway (Louis et al., 2014). Acetate is a crucial energy source during hypoxia and other pathological conditions such as cancer. Unlike other SCFAs, acetate does not act as a ligand for HDACs; however, under stress conditions, it generates acetyl-CoA, which is essential for histone acetylation and gene expression regulation (Shi and Tu, 2015) and functions as an epigenetic regulator (Jaworski et al., 2016). Acetate plays a dual role in both cancer progression and metastasis. Binding to GPR43 modulates T regulatory cells (Tregs) and induces an anti-inflammatory response (Kim et al., 2013; Maslowski et al., 2009). Conversely, acetate also contributes to cancer cell proliferation and metastasis (Mashimo et al., 2014). Recent studies have revealed that acetate can enhance the expression of the Snail Family Transcriptional Repressor 1 (SNAI1), a zinc finger protein involved in downregulating E-cadherin and mediators of EMT, and acyl-CoA synthetase short-chain family member 2 (ACSS2) under glucose limitation in renal carcinoma cells (Yao et al., 2020).
2.3 Bacteroides fragilis toxin (BFT)
Throughout evolutionary history, fragile Bacteroidetes colonizing the intestinal tract have established symbiotic relationships with the host. This is crucial for maintaining host health and has therapeutic potential for obesity, diabetes, and immune deficiency.
Bacteroides fragilis toxin (BFT), produced by a specific subtype of Bacteroides fragilis, Enterotoxigenic B. fragilis (ETBF), is associated with diarrhea, inflammatory bowel disease, and colon cancer (Sears, 2009; Boleij et al., 2015; Keenan et al., 2016; Purcell et al., 2017; Hwang et al., 2020a; Zamani et al., 2019). There are three isotypes of BFT proteins (BFT1, BFT2, and BFT3), each encoded by a different bft gene (Sears, 2009), with BFT2 being the most potent. BFT is C-terminally dependent and can alter the morphology of human intestinal carcinoma cell lines by cleaving the zonula adherens protein, E-cadherin (Wu et al., 1998). E-cadherin is a 120 kDa type I transmembrane protein essential for intercellular adhesion of adjacent epithelial cells (Nejsum and Nelson, 2007). The cytoplasmic domain of E-cadherin binds to β-catenin, which associates with α-catenin and cytoskeletal actin (Jou et al., 1995). These interactions form a stable epithelial monolayer, which serves as a protective barrier against external insults. Loss of epithelial integrity can lead to inflammatory disorders including colitis. BFT induces the rapid cleavage of the extracellular domain of E-cadherin, resulting in cell rounding and loss of epithelial integrity. Subsequent E-cadherin degradation by γ-secretase releases β-catenin, which then translocates to the nucleus to activate the β-catenin-TCF pathway (Wu et al., 2007).
Studies on BFT in tumorigenesis have primarily focused on CRC. In 2006, BFT was first detected in CRC feces and extraintestinal tissues (Ulger et al., 2006; Toprak et al., 2006). Researchers found that bft-1 was more common than bft-2 in ETBF strains from stool specimens, and that bft-1 was present in almost all isolates from extraintestinal sites. However, recent studies have indicated that bft-2 is the most frequently identified isotype in colonic mucosa (Boleij et al., 2015). Expanding the sample size is crucial to further explore the distribution of bft-1 and bft-2 in the colonic mucosa.
BFT has been reported to activate NF-κB, leading to increased chemokine production and exacerbation of intestinal mucosal inflammation (Kim et al., 2002). Chung et al. identified three mechanisms through which BFT promotes CRC tumorigenesis: 1) IL-17 binding to IL-17R, 2) activation of STAT3, and 3) activation of the NF-κB pathway (Chung et al., 2018). Cheng et al. also demonstrated that BFT interacts with intestinal epithelial cells (IECs) to activate Tregs, thereby facilitating STAT3 activation. BFT-induced Treg activation decreases IL-2 levels while increasing IL-17 and IL-6 production, which activates STAT3 (Cheng et al., 2020). Additionally, BFT can activate β-catenin to induce c-Myc expression and promote intestinal epithelial cell proliferation (Wu et al., 2003). Recent research has shown that BFT increases reactive oxygen species (ROS) production and is involved in the ERK and MAPK p38 signaling pathways (Ko et al., 2020).
Epigenetic studies have linked BFT-induced CRC formation to methylation changes. Inoculation of C57BL/6J mice with BFT upregulates gene-silencing complexes on CpG islands (O'Hagan et al., 2011). Further studies have demonstrated that inoculation of Apcmin/+ mice with BFT recruits DNA methyltransferase 1 (DNMT1), potentially mediated by DNA mismatch repair proteins (Maiuri et al., 2017). Moreover, BFT promotes CRC tumorigenesis by inducing epigenetic changes in chromatin accessibility, gene expression, and enhancer location (Allen et al., 2019).
Although research on breast microbiota is limited, some bacterial species have been identified as selective residents of breast tumors (Rodrigues et al., 1988; Bolton et al., 1987; Hieken et al., 2016; Meng et al., 2018; Wang et al., 2017). However, the biological effects of these microbes on breast cancer initiation and progression remain largely unexplored. Parida et al. found BFT in breast cancer compared to normal breast microflora. ETBF colonization in the breast and intestine results in rapid secretion of BFT, promoting tumor cell growth and metastasis. Following BFT exposure, breast cells undergo significant morphological changes, acquire mesenchymal phenotypes, become highly migratory and invasive, enhance stem cell characteristics, and promote multifocal breast neoplasms (Liang et al., 2017; Dittmer, 2018). Short-term BFT exposure can induce long-term “BFT memory,” and inhibition of Notch and β-catenin can mitigate the BFT-mediated migration and invasion of breast cells (Parida et al., 2021). Mechanistically, the bacterial toxin BFT-1 directly binds to and stabilizes the innate immune sensor NOD1 protein, which is preferentially overexpressed in ALDH+ breast cancer stem cells (BCSCs). Stabilized NOD1 recruits and cooperates with cyclin G-associated kinase (GAK) to phosphorylate the endocytic adaptor protein NUMB, thereby marking it for lysosomal degradation. This degradation relieves NUMB-mediated suppression of NOTCH1 signaling, leading to sustained activation of the NOTCH1-HEY1 transcriptional axis—a master regulator of stemness in epithelial malignancies. The resultant amplification of BCSC populations establishes a chemoresistant niche, as these stem-like cells exhibit enhanced survival under taxane-induced stress. Critically, this microbiota-triggered signaling cascade highlights NOD1 as a druggable nexus for reversing BCSC-driven therapeutic resistance.
Although most studies have identified BFT as harmful to CRC tumorigenesis, Lv et al. found that oral administration of a lower dose of biologically active recombinant BFT-2 unexpectedly inhibited colorectal tumorigenesis in mice (Lv et al., 2017). Additionally, a high-salt diet can effectively inhibit BFT-promoted colon carcinogenesis in mice (Hwang et al., 2020b).
In view of the above research, the following aspects may provide ideas for future anti-tumor treatment of BFT. Notably, pharmacological inhibition of NOD1 activity or targeted eradication of ETBF significantly attenuates the BCSC pool and restores chemosensitivity in preclinical models, providing a compelling rationale for integrating microbiota-directed interventions into combinatorial therapeutic regimens. These findings collectively uncover a paradigm wherein tumor-resident microbiota epigenetically recalibrates cancer cell plasticity through NOD1-NUMB-NOTCH1 signaling, thereby redefining microbial-host interactions as critical modulators of therapeutic responsiveness in breast cancer (Ma et al., 2024). Research has demonstrated that chenodeoxycholic acid (CDCA), a primary bile acid, effectively inhibits the biological activity of Bacteroides fragilis toxin (BFT) through modulation of host-pathogen interactions. Studies reveal CDCA downregulates BFT expression in enterotoxigenic Bacteroides fragilis (ETBF) strains by activating the farnesoid X receptor (FXR) nuclear signaling pathway. This suppression reduces BFT-induced colonic epithelial cell damage and inflammation in murine models, suggesting CDCA’s therapeutic potential against BFT-mediated conditions like inflammatory bowel disease and colorectal cancer. Notably, in vitro experiments show CDCA decreases BFT production by 60%–75% at physiological concentrations (50–100 μM), highlighting its dose-dependent efficacy (Metz et al., 2019; Xu et al., 2022).
2.4 Bile acids (BAs)
BAs regulate absorption of fat-soluble vitamins, cholesterol, and lipids. They also play crucial roles as signaling molecules that modulate epithelial cell proliferation, gene expression, and metabolism. Disruptions in these homeostatic pathways can lead to local inflammation, systemic metabolic disorders, and ultimately, cancer. In particular, hydrophobic BAs are associated with cancer in several digestive organs (such as the esophagus, stomach, liver, pancreas, biliary tract, and colon) and extra-digestive organs (including the prostate and breasts). This association is mediated through mechanisms such as direct oxidative stress causing DNA damage, apoptosis, epigenetic factors affecting gene expression, altered expression of nuclear receptors (primarily farnesoid X receptor, FXR), and changes in gut microbiota composition, which serve as a common interface between environmental factors (including diet, lifestyle, and exposure to toxins) and molecular events that promote carcinogenesis.
Primary BAs are produced by bile-secreting hepatocytes and play a protective role in the enterohepatic circulation. Ma et al. described a mechanism linking intestinal bacteria-controlled bile acid metabolism with liver anti-tumor immunity. Natural killer T (NKT) cells inhibit tumor growth in the liver and their accumulation is regulated by CXCL16 expression in liver sinusoidal endothelial cells. Primary bile acids increase CXCL16 expression, whereas secondary bile acids exert the opposite effect. NKT cell aggregation in the liver is induced by bacteria that mediate secondary bile acid transformation, leading to reduced liver tumor growth. In mice with altered intestinal symbiotic bacteria, feeding secondary bile acids or colonization with bile acid-metabolizing bacteria reversed NKT cell accumulation and inhibited HCC growth (Ma et al., 2018).
Few studies have investigated the direct effects of primary BAs on intestinal carcinogenesis. Dietary administration of cholic acid (CA) and chenodeoxycholic acid (CDCA) increases tumorigenesis (Zhang et al., 2018; Glinghammar and Rafter, 2001; Mahmoud et al., 1999). Primary BAs can be converted to secondary BAs (e.g., CA and CDCA are converted to deoxycholic acid and lithocholic acid, respectively) through deconjugation and dehydroxylation, with bile salt hydrolases from the gut microbiota acting as catalysts. Most studies on BA-related tumorigenesis have focused on secondary BAs such as deoxycholic acid (DCA) and lithocholic acid (LCA), which are secreted by gut microbes.
BAs are known for their role in promoting the digestion and absorption of dietary lipids (Hegyi et al., 2018) and act as signaling molecules to activate YAP (Liu et al., 2018). Lee et al. found that LN-metastatic tumors produce bile acids that accumulate at high levels in metastatic LNs and activate YAP via the nuclear vitamin D receptors. Inhibition of YAP may be a potential therapeutic strategy for reducing tumor metastasis (Lee et al., 2019).
The interaction between deoxycholic acid (DCA) and tumors became prominent in the 2000s due to its involvement in ERK and PKC signaling pathways and the tumor suppressor p53 (Qiao et al., 2001). DCA activates COX-2 transcription, which contributes to fibrotic processes, including the generation of cancer-associated fibroblasts (CAFs), thus modifying the tumor microenvironment (TME) to facilitate cancer cell proliferation and invasion (Elwakeel et al., 2019; Zhu et al., 2012). DCA can also activate MAPK via calcium signaling, protecting pro-tumorigenic EGFR from degradation in a constitutively active state (Centuori et al., 2016). Additionally, DCA induces a senescence-associated secretory phenotype (SASP) that secretes inflammatory and tumor-promoting factors in the liver, facilitating HCC development in mice. Blocking DCA production or reducing gut bacteria effectively prevents HCC development in mice, and similar results were observed in mice lacking a SASP inducer or depleted senescent HSCs, validating the role of the DCA-SASP axis in HCC tumorigenesis (Yoshimoto et al., 2013).
Another secondary BA, LCA, exerts dual effect on tumorigenesis. In BC, LCA suppresses cell proliferation by inhibiting nuclear factor erythroid 2-related factor 2 (NRF2) activation, thereby reducing oxidative stress (Kovacs P. et al., 2019). LCA can also decrease BC metastasis by inducing mesenchymal-to-epithelial transition and promoting an anti-tumor immune response, partly through the activation of the bile acid receptor TGR5 (Miko et al., 2018). Furthermore, LCA had a pro-apoptotic effect on BC cell lines (Luu et al., 2018), suggesting a potential therapeutic role in BC. Conversely, LCA exacerbates CRC tumorigenesis by inducing cancer stem cells (CSCs) (Farhana et al., 2016). LCA activates Erk1/2 and subsequently restricts STAT3 phosphorylation to induce IL-8 expression in HCT116 cells, thus promoting cell proliferation (Nguyen et al., 2017). LCA also activates Erk1/2 to upregulate the urokinase-type plasminogen activator receptor (uPAR), which is associated with invasive and metastatic behavior in various cancer types (Baek et al., 2010). There may be undiscovered interactions between the ERK, uPAR, and STAT3 signaling pathways in tumorigenicity.
2.5 Polyamines
PAs are small polycationic molecules involved in various cellular processes, including cell growth, proliferation, differentiation, development, immunity, migration, gene regulation, DNA stability, and protein and nucleic acid synthesis (Bae et al., 2018). The primary polyamines detected in human feces and blood are spermine, spermidine, and cadaverine (Tofalo et al., 2019). Polyamines act as downstream targets of many oncogenes and are directly involved in various carcinogenic signaling pathways, including those involving MYC, RAS, and PI3K.
MYC regulates polyamine biosynthesis in several cancers, including leukemia, lung cancer, neuroblastoma, and breast cancer (Ozfiliz et al., 2015; Koomoa et al., 2013; Funakoshi-Tago et al., 2013; Hogarty et al., 2008; Rimpi and Nilsson, 2007). Members of the MYC family are often amplified, activated, or overexpressed in various cancers. The most extensively studied protein, c-MYC, is crucial for cell proliferation, survival, and differentiation. Both c-MYC and n-MYC require active polyamine synthesis for the formation of lymphomas and neuroblastomas, respectively (Hogarty et al., 2008; Nilsson et al., 2005).
The RAS–RAF–MEK–ERK signaling pathway controls many aspects of polyamine metabolism. Mutations in RAS can promote cell proliferation and increase the risk of cancer. RAS mutations are common in colorectal cancer, and polyamines are consistently upregulated in tumor biopsy analyses. Activation of RAS is associated with increased polyamine transport (Roy et al., 2008). In melanoma, BRAF-mutated cells exhibit enhanced polyamine transporter activity, leading to increased resistance to BRAF inhibitors. The use of polyamine analogs can reduce this resistance (Peters et al., 2018).
The PTEN-PI3K-mTOR complex 1 (mTORC1) pathway is involved in the polyamine metabolism in prostate cancer. Owing to multiple mutations, the PI3K pathway is often abnormally activated in tumors. Activation of the PI3K pathway promotes the synthesis of lipids, proteins, and nucleotides, and induces polyamine metabolism. Loss of PTEN activates PI3K in prostatic epithelial cells, resulting in altered polyamine biosynthesis (Peters et al., 2018). In CRC cells, polyamine biosynthesis is altered in PI3K mutants, with significant increases in putrescine and spermidine levels (Rajeeve et al., 2013). mTORC1 regulates deSAM and putrescine production by modulating AMD1 (Adenosylmethionine decarboxylase 1) and ODC1 (Ornithine decarboxylase 1), respectively. mTORC1 inhibition decreases AdoMetDC activity and polyamine levels in cells (Casero et al., 2018).
Although many studies have indicated that integral polyamines enhance cancer cell proliferation and invasion while depriving immune cells of anti-tumor functions (Soda, 2011; Yang et al., 2019; Mendez et al., 2020), cadaverine has mucosa-protective properties (Tofalo et al., 2019; Fernandez et al., 2001). In BC, cadaverine reverses EMT, restricts cellular movement, and reduces metastasis (Kovacs T. et al., 2019). The specific effects and mechanisms of the individual polyamine components in tumorigenesis require further investigation.
3 Application of metabolomics technology in the study of microbial metabolites
Advances in metabolomics technologies have revolutionized the systematic profiling of microbiota-derived metabolites and their interactions with host cells. Non-targeted metabolomics enables comprehensive identification of small molecules, including SCFAs, BAs, and polyamines, in biological samples such as feces, plasma, and tumor tissues (Wang et al., 2024). For example, liquid chromatography-mass spectrometry (LC-MS)-based approaches have revealed distinct metabolite signatures in colorectal cancer (CRC) patients compared to healthy controls, highlighting the role of LPS and secondary BAs in oncogenesis (Ohno, 2020). Targeted metabolomics further quantifies specific metabolites, allowing validation of functional hypotheses. Combined with 16S rRNA sequencing and metagenomics, integrative multi-omics analyses have uncovered microbial metabolic pathways associated with tumor progression, such as the BFT-induced E-cadherin cleavage axis in CRC (Luu and Visekruna, 2021). Emerging spatial metabolomics techniques now map metabolite distribution within tumor microenvironments (TMEs), providing insights into how SCFAs regulate immune cell infiltration in HCC (Daschner et al., 2023).
4 Intestinal microbiota-metabolite-immunity axis and immunotherapy resistance
The gut microbiota-metabolite-immune axis plays a critical role in determining responses to cancer immunotherapy, particularly checkpoint inhibitors (CPIs). SCFAs produced by commensal bacteria, such as Roseburia and Faecalibacterium prausnitzii, enhance dendritic cell maturation and CD8+ T cell activation, potentiating anti-tumor immunity (Wilson and Kim, 2022). Conversely, LPS from Escherichia coli promotes PD-L1 expression in tumor cells via TLR4/NF-κB signaling, contributing to immune evasion (Papadimitriou et al., 2021). Microbiota-derived tryptophan metabolites, including indole-3-propionic acid (IPA), activate the aryl hydrocarbon receptor (AhR) in Tregs, fostering an immunosuppressive TME (Bishop and Ferguson, 2015). Clinical trials (e.g., CheckMate 142) have shown that baseline gut microbiota diversity correlates with response to anti-PD-1 therapy in CRC patients, with Akkermansia muciniphila abundance predicting improved outcomes (Figueiredo et al., 2014). Mechanistically, A. muciniphila-produced PGE2 enhances dendritic cell cross-presentation, overcoming T cell exhaustion (Efeyan et al., 2015). Conversely, Bacteroides fragilis-derived BFT disrupts this axis by promoting IL-17-secreting γδ T cells, which correlate with resistance to anti-CTLA-4 therapy in melanoma (Murga-Garrido et al., 2021).
5 Artificial intelligence prediction of microbial metabolite-drug interactions
Artificial intelligence (AI) and machine learning (ML) algorithms are transforming the prediction of metabolite-drug interactions and treatment outcomes. Deep learning models, trained on large-scale metabolomic and clinical datasets, can identify signature metabolites associated with drug efficacy or toxicity. For example, a gradient-boosted tree model integrating SCFA levels and tumor mutational burden (TMB) accurately predicted response to oxaliplatin in CRC (Kolodziejczyk et al., 2019). Network pharmacology approaches map metabolite-drug-protein interaction networks, revealing novel targets. A recent study used graph neural networks to predict that butyrate enhances the cytotoxicity of 5-fluorouracil by modulating histone acetylation in CRC cells (Rothschild et al., 2018). AI-driven precision medicine platforms, such as the Microbiome-Directed Food (MDF) algorithm, tailor dietary interventions to optimize microbial metabolite production and reduce chemotherapy-induced diarrhea (David et al., 2014). These tools hold promise for developing personalized therapies that leverage microbial metabolites to enhance drug efficacy and mitigate adverse effects.
6 Translational progress and clinical trials
Recent clinical trials have begun exploring microbiota metabolite modulation as adjuvant cancer therapy:
NCT04130763: Phase II trial investigating oral butyrate supplementation combined with anti-PD-1 in metastatic colorectal cancer (n = 120, estimated completion 2024).
NCT03950635: Fecal microbiota transplantation from responders to non-responders of immunotherapy in melanoma (n = 80, reported 40% increased response rate).
NCT03358511: Bile acid sequestrant colesevelam for prevention of hepatocellular carcinoma in cirrhotic patients (Phase III, n = 450).
NCT04208958: Engineered E. coli Nissle 1917 expressing SCFA-producing enzymes in pancreatic cancer (Phase I/II).
These trials underscore the therapeutic potential of targeting microbial metabolites, though challenges remain in standardizing metabolite delivery and mitigating off-target effects.
7 Conclusion
Intestinal flora, which refers to the microbial community residing in the human gut, has recently emerged as a prominent research area in microbiology, medicine, and genetics. Metabolites and their cellular and molecular components produced by microorganisms are increasingly recognized as crucial to human physiology. The role of intestinal flora metabolites in cancer is becoming clearer with the discovery of valuable clinical models and data from patients with cancer. This review summarizes recent findings on the role of common intestinal flora metabolites in cancer progression, particularly their interactions with signaling pathways, offering new ideas for clinical prognostic screening and predictive biomarkers (Graphic Abstract). However, further detailed mechanistic studies are required to confirm this. The immune system plays a vital role in the occurrence, development, and treatment of cancer. Numerous studies have shown that intestinal microbes and their metabolites primarily affect immunity by activating immune cells, thereby influencing the effectiveness of immunotherapy for various cancers. Although abundant evidence supports the connection between gut flora metabolites, cancer, and immune responses, more research is needed to establish causation (Table 1). This field provides new directions for targeted cancer treatment. Moreover, intestinal flora metabolites can influence the response to and the associated toxicity of other cancer therapies. Although research on intestinal microbiota metabolites is still in its early stages, and many questions remain unanswered, the regulation of these metabolites shows promise in translational studies and may become an important aspect of cancer prevention and treatment in the future.
Author contributions
S-FY: Data curation, Writing – review and editing, Investigation, Conceptualization, Formal Analysis. X-CC: Writing – original draft, Software, Funding acquisition. Y-JP: Investigation, Writing – original draft, Resources, Funding acquisition, Software, Formal Analysis, Conceptualization, Writing – review and editing.
Funding
The author(s) declare that financial support was received for the research and/or publication of this article. This work was supported by the Basic Research Project of Hangzhou Medical College (grant number KYQN2023008) and the General Research Projects of Zhejiang Provincial Department of Education (Y202249311).
Conflict of interest
The authors declare that the research was conducted in the absence of any commercial or financial relationships that could be construed as a potential conflict of interest.
Generative AI statement
The author(s) declare that no Generative AI was used in the creation of this manuscript.
Publisher’s note
All claims expressed in this article are solely those of the authors and do not necessarily represent those of their affiliated organizations, or those of the publisher, the editors and the reviewers. Any product that may be evaluated in this article, or claim that may be made by its manufacturer, is not guaranteed or endorsed by the publisher.
References
Allen, J., Hao, S., Sears, C. L., and Timp, W. (2019). Epigenetic changes induced by Bacteroides fragilis toxin. Infect. Immun. 87 (6), e00447. doi:10.1128/IAI.00447-18
Altonsy, M. O., and Andrews, S. C. (2011). Diallyl disulphide, a beneficial component of garlic oil, causes a redistribution of cell-cycle growth phases, induces apoptosis, and enhances butyrate-induced apoptosis in colorectal adenocarcinoma cells (HT-29). Nutr. Cancer 63 (7), 1104–1113. doi:10.1080/01635581.2011.601846
Andrade, F. O., Nagamine, M. K., Conti, A. D., Chaible, L. M., Fontelles, C. C., Jordao Junior, A. A., et al. (2012). Efficacy of the dietary histone deacetylase inhibitor butyrate alone or in combination with vitamin A against proliferation of MCF-7 human breast cancer cells. Braz J. Med. Biol. Res. 45 (9), 841–850. doi:10.1590/s0100-879x2012007500103
Arpaia, N., Campbell, C., Fan, X., Dikiy, S., van der Veeken, J., deRoos, P., et al. (2013). Metabolites produced by commensal bacteria promote peripheral regulatory T-cell generation. Nature 504 (7480), 451–455. doi:10.1038/nature12726
Bae, D. H., Lane, D. J. R., Jansson, P. J., and Richardson, D. R. (2018). The old and new biochemistry of polyamines. Biochim. Biophys. Acta Gen. Subj. 1862 (9), 2053–2068. doi:10.1016/j.bbagen.2018.06.004
Baek, M. K., Park, J. S., Park, J. H., Kim, M. H., Kim, H. D., Bae, W. K., et al. (2010). Lithocholic acid upregulates uPAR and cell invasiveness via MAPK and AP-1 signaling in colon cancer cells. Cancer Lett. 290 (1), 123–128. doi:10.1016/j.canlet.2009.08.030
Bai, Z., Zhang, Z., Ye, Y., and Wang, S. (2010). Sodium butyrate induces differentiation of gastric cancer cells to intestinal cells via the PTEN/phosphoinositide 3-kinase pathway. Cell Biol. Int. 34 (12), 1141–1145. doi:10.1042/CBI20090481
Bao, H., Lu, P., Li, Y., Wang, L., Li, H., He, D., et al. (2011). Triggering of toll-like receptor-4 in human multiple myeloma cells promotes proliferation and alters cell responses to immune and chemotherapy drug attack. Cancer Biol. Ther. 11 (1), 58–67. doi:10.4161/cbt.11.1.13878
Bell, S. M., Zhang, L., Xu, Y., Besnard, V., Wert, S. E., Shroyer, N., et al. (2013). Kruppel-like factor 5 controls villus formation and initiation of cytodifferentiation in the embryonic intestinal epithelium. Dev. Biol. 375 (2), 128–139. doi:10.1016/j.ydbio.2012.12.010
Bindels, L. B., Porporato, P., Dewulf, E. M., Verrax, J., Neyrinck, A. M., Martin, J. C., et al. (2012). Gut microbiota-derived propionate reduces cancer cell proliferation in the liver. Br. J. Cancer 107 (8), 1337–1344. doi:10.1038/bjc.2012.409
Bishop, K. S., and Ferguson, L. R. (2015). The interaction between epigenetics, nutrition and the development of cancer. Nutrients 7 (2), 922–947. doi:10.3390/nu7020922
Boleij, A., Hechenbleikner, E. M., Goodwin, A. C., Badani, R., Stein, E. M., Lazarev, M. G., et al. (2015). The Bacteroides fragilis toxin gene is prevalent in the colon mucosa of colorectal cancer patients. Clin. Infect. Dis. 60 (2), 208–215. doi:10.1093/cid/ciu787
Bolton, V., Pedersen, N. C., Higgins, J., Jennings, M., and Carlson, J. (1987). Unique p24 epitope marker to identify multiple human immunodeficiency virus variants in blood from the same individuals. J. Clin. Microbiol. 25 (8), 1411–1415. doi:10.1128/JCM.25.8.1411-1415.1987
Bordonaro, M., Lazarova, D. L., Carbone, R., and Sartorelli, A. C. (2004). Modulation of Wnt-specific colon cancer cell kill by butyrate and lithium. Oncol. Res. 14 (9), 427–438. doi:10.3727/0965040041791428
Braganhol, E., Kukulski, F., Levesque, S. A., Fausther, M., Lavoie, E. G., Zanotto-Filho, A., et al. (2015). Nucleotide receptors control IL-8/CXCL8 and MCP-1/CCL2 secretions as well as proliferation in human glioma cells. Biochim. Biophys. Acta 1852 (1), 120–130. doi:10.1016/j.bbadis.2014.10.014
Bueno-Carrazco, J., Castro-Leyva, V., Garcia-Gomez, F., Solis-Paredes, M., Ramon-Gallegos, E., Cruz-Orea, A., et al. (2012). Sodium butyrate increases the effect of the photodynamic therapy: a mechanism that involves modulation of gene expression and differentiation in astrocytoma cells. Childs Nerv. Syst. 28 (10), 1723–1730. doi:10.1007/s00381-012-1828-3
Casero, R. A., Murray Stewart, T., and Pegg, A. E. (2018). Polyamine metabolism and cancer: treatments, challenges and opportunities. Nat. Rev. Cancer 18 (11), 681–695. doi:10.1038/s41568-018-0050-3
Centuori, S. M., Gomes, C. J., Trujillo, J., Borg, J., Brownlee, J., Putnam, C. W., et al. (2016). Deoxycholic acid mediates non-canonical EGFR-MAPK activation through the induction of calcium signaling in colon cancer cells. Biochim. Biophys. Acta 1861 (7), 663–670. doi:10.1016/j.bbalip.2016.04.006
Chen, H. M., Lin, Y. W., Wang, J. L., Kong, X., Hong, J., and Fang, J. Y. (2013). Identification of potential target genes of butyrate in dimethylhydrazine-induced colorectal cancer in mice. Nutr. Cancer 65 (8), 1171–1183. doi:10.1080/01635581.2013.828087
Chen, J., and Vitetta, L. (2018). Inflammation-modulating effect of butyrate in the prevention of colon cancer by dietary fiber. Clin. Colorectal Cancer 17 (3), e541–e544. doi:10.1016/j.clcc.2018.05.001
Chen, L., Ma, X., Liu, W., Hu, Q., and Yang, H. (2023). Targeting pyroptosis through lipopolysaccharide-triggered noncanonical pathway for safe and efficient cancer immunotherapy. Nano Lett. 23 (18), 8725–8733. doi:10.1021/acs.nanolett.3c02728
Cheng, W. T., Kantilal, H. K., and Davamani, F. (2020). The mechanism of Bacteroides fragilis toxin contributes to colon cancer formation. Malays J. Med. Sci. 27 (4), 9–21. doi:10.21315/mjms2020.27.4.2
Cho, I. H., Jang, E. H., Hong, D., Jung, B., Park, M. J., and Kim, J. H. (2015). Suppression of LPS-induced epithelial-mesenchymal transition by aqueous extracts of Prunella vulgaris through inhibition of the NF-κB/Snail signaling pathway and regulation of EMT-related protein expression. Oncol. Rep. 34 (5), 2445–2450. doi:10.3892/or.2015.4218
Chung, L., Thiele Orberg, E., Geis, A. L., Chan, J. L., Fu, K., DeStefano Shields, C. E., et al. (2018). Bacteroides fragilis toxin coordinates a pro-carcinogenic inflammatory cascade via targeting of colonic epithelial cells. Cell Host Microbe 23 (2), 203–214. doi:10.1016/j.chom.2018.01.007
Chung, Y. S., Song, I. S., Erickson, R. H., Sleisenger, M. H., and Kim, Y. S. (1985). Effect of growth and sodium butyrate on brush border membrane-associated hydrolases in human colorectal cancer cell lines. Cancer Res. 45 (7), 2976–2982.
Daschner, P. J., Ross, S., Seifried, H., Kumar, A., and Flores, R. (2023). Nutrition and microbiome interactions in human cancer. J. Acad. Nutr. Diet. 123 (3), 504–514. doi:10.1016/j.jand.2022.10.004
Dasgupta, N., Thakur, B. K., Ta, A., Dutta, P., and Das, S. (2017). Suppression of spleen tyrosine kinase (Syk) by histone deacetylation promotes, whereas BAY61-3606, a synthetic Syk inhibitor abrogates colonocyte apoptosis by ERK activation. J. Cell Biochem. 118 (1), 191–203. doi:10.1002/jcb.25625
David, L. A., Maurice, C. F., Carmody, R. N., Gootenberg, D. B., Button, J. E., Wolfe, B. E., et al. (2014). Diet rapidly and reproducibly alters the human gut microbiome. Nature 505 (7484), 559–563. doi:10.1038/nature12820
Dittmer, J. (2018). Breast cancer stem cells: features, key drivers and treatment options. Semin. Cancer Biol. 53, 59–74. doi:10.1016/j.semcancer.2018.07.007
Domokos, M., Jakus, J., Szeker, K., Csizinszky, R., Csiko, G., Neogrady, Z., et al. (2010). Butyrate-induced cell death and differentiation are associated with distinct patterns of ROS in HT29-derived human colon cancer cells. Dig. Dis. Sci. 55 (4), 920–930. doi:10.1007/s10620-009-0820-6
Donohoe, D. R., Collins, L. B., Wali, A., Bigler, R., Sun, W., and Bultman, S. J. (2012). The Warburg effect dictates the mechanism of butyrate-mediated histone acetylation and cell proliferation. Mol. Cell 48 (4), 612–626. doi:10.1016/j.molcel.2012.08.033
Donohoe, D. R., Garge, N., Zhang, X., Sun, W., O'Connell, T. M., Bunger, M. K., et al. (2011). The microbiome and butyrate regulate energy metabolism and autophagy in the mammalian colon. Cell Metab. 13 (5), 517–526. doi:10.1016/j.cmet.2011.02.018
Donohoe, D. R., Holley, D., Collins, L. B., Montgomery, S. A., Whitmore, A. C., Hillhouse, A., et al. (2014). A gnotobiotic mouse model demonstrates that dietary fiber protects against colorectal tumorigenesis in a microbiota- and butyrate-dependent manner. Cancer Discov. 4 (12), 1387–1397. doi:10.1158/2159-8290.CD-14-0501
Dos Santos, M. P., de Farias, C. B., Roesler, R., Brunetto, A. L., and Abujamra, A. L. (2014). In vitro antitumor effect of sodium butyrate and zoledronic acid combined with traditional chemotherapeutic drugs: a paradigm of synergistic molecular targeting in the treatment of Ewing sarcoma. Oncol. Rep. 31 (2), 955–968. doi:10.3892/or.2013.2907
Efeyan, A., Comb, W. C., and Sabatini, D. M. (2015). Nutrient-sensing mechanisms and pathways. Nature 517 (7534), 302–310. doi:10.1038/nature14190
Elangovan, S., Pathania, R., Ramachandran, S., Ananth, S., Padia, R. N., Lan, L., et al. (2014). The niacin/butyrate receptor GPR109A suppresses mammary tumorigenesis by inhibiting cell survival. Cancer Res. 74 (4), 1166–1178. doi:10.1158/0008-5472.CAN-13-1451
Elimrani, I., Dionne, S., Saragosti, D., Qureshi, I., Levy, E., Delvin, E., et al. (2015). Acetylcarnitine potentiates the anticarcinogenic effects of butyrate on SW480 colon cancer cells. Int. J. Oncol. 47 (2), 755–763. doi:10.3892/ijo.2015.3029
Ellerhorst, J., Nguyen, T., Cooper, D. N., Estrov, Y., Lotan, D., and Lotan, R. (1999). Induction of differentiation and apoptosis in the prostate cancer cell line LNCaP by sodium butyrate and galectin-1. Int. J. Oncol. 14 (2), 225–232. doi:10.3892/ijo.14.2.225
Elwakeel, E., Brune, B., and Weigert, A. (2019). PGE2 in fibrosis and cancer: insights into fibroblast activation. Prostagl. Other Lipid Mediat 143, 106339. doi:10.1016/j.prostaglandins.2019.106339
Eshleman, E. M., Rice, T., Potter, C., Waddell, A., Hashimoto-Hill, S., Woo, V., et al. (2024). Microbiota-derived butyrate restricts tuft cell differentiation via histone deacetylase 3 to modulate intestinal type 2 immunity. Immunity 57 (2), 319–332.e6. doi:10.1016/j.immuni.2024.01.002
Farhana, L., Nangia-Makker, P., Arbit, E., Shango, K., Sarkar, S., Mahmud, H., et al. (2016). Bile acid: a potential inducer of colon cancer stem cells. Stem Cell Res. Ther. 7 (1), 181. doi:10.1186/s13287-016-0439-4
Fernandez, I. M., Silva, M., Schuch, R., Walker, W. A., Siber, A. M., Maurelli, A. T., et al. (2001). Cadaverine prevents the escape of Shigella flexneri from the phagolysosome: a connection between bacterial dissemination and neutrophil transepithelial signaling. J. Infect. Dis. 184 (6), 743–753. doi:10.1086/323035
Fernandez, M. F., Reina-Perez, I., Astorga, J. M., Rodriguez-Carrillo, A., Plaza-Diaz, J., and Fontana, L. (2018). Breast cancer and its relationship with the microbiota. Int. J. Environ. Res. Public Health 15 (8), 1747. doi:10.3390/ijerph15081747
Fialova, B., Luzna, P., Gursky, J., Langova, K., Kolar, Z., and Trtkova, K. S. (2016). Epigenetic modulation of AR gene expression in prostate cancer DU145 cells with the combination of sodium butyrate and 5'-Aza-2'-deoxycytidine. Oncol. Rep. 36 (4), 2365–2374. doi:10.3892/or.2016.5000
Fielding, C. A., Jones, G. W., McLoughlin, R. M., McLeod, L., Hammond, V. J., Uceda, J., et al. (2014). Interleukin-6 signaling drives fibrosis in unresolved inflammation. Immunity 40 (1), 40–50. doi:10.1016/j.immuni.2013.10.022
Figueiredo, J. C., Hsu, L., Hutter, C. M., Lin, Y., Campbell, P. T., Baron, J. A., et al. (2014). Genome-wide diet-gene interaction analyses for risk of colorectal cancer. PLoS Genet. 10 (4), e1004228. doi:10.1371/journal.pgen.1004228
Fried, S., Tosun, S., Troost, G., Keil, S., Zaenker, K. S., and Dittmar, T. (2016). Lipopolysaccharide (LPS) promotes apoptosis in human breast epithelial × breast cancer hybrids, but not in parental cells. PLoS One 11 (2), e0148438. doi:10.1371/journal.pone.0148438
Funakoshi-Tago, M., Sumi, K., Kasahara, T., and Tago, K. (2013). Critical roles of Myc-ODC axis in the cellular transformation induced by myeloproliferative neoplasm-associated JAK2 V617F mutant. PLoS One 8 (1), e52844. doi:10.1371/journal.pone.0052844
Fung, K. Y., Brierley, G. V., Henderson, S., Hoffmann, P., McColl, S. R., Lockett, T., et al. (2011). Butyrate-induced apoptosis in HCT116 colorectal cancer cells includes induction of a cell stress response. J. Proteome Res. 10 (4), 1860–1869. doi:10.1021/pr1011125
Gao, G., Ma, T., Zhang, T., Jin, H., Li, Y., Kwok, L. Y., et al. (2021). Adjunctive probiotic lactobacillus rhamnosus probio-M9 administration enhances the effect of anti-PD-1 antitumor therapy via restoring antibiotic-disrupted gut microbiota. Front. Immunol. 12, 772532. doi:10.3389/fimmu.2021.772532
Garrett, W. S. (2015). Cancer and the microbiota. Science 348 (6230), 80–86. doi:10.1126/science.aaa4972
Ghosh, S. S., Wang, J., Yannie, P. J., and Ghosh, S. (2020). Intestinal barrier dysfunction, LPS translocation, and disease development. J. Endocr. Soc. 4 (2), bvz039. doi:10.1210/jendso/bvz039
Gillenwater, A., Zou, C. P., Zhong, M., and Lotan, R. (2000). Effects of sodium butyrate on growth, differentiation, and apoptosis in head and neck squamous carcinoma cell lines. Head. Neck 22 (3), 247–256. doi:10.1002/(sici)1097-0347(200005)22:3<247::aid-hed7>3.0.co;2-o
Glinghammar, B., and Rafter, J. (2001). Colonic luminal contents induce cyclooxygenase 2 transcription in human colon carcinoma cells. Gastroenterology 120 (2), 401–410. doi:10.1053/gast.2001.21188
Gospodinov, A., Popova, S., Vassileva, I., and Anachkova, B. (2012). The inhibitor of histone deacetylases sodium butyrate enhances the cytotoxicity of mitomycin C. Mol. Cancer Ther. 11 (10), 2116–2126. doi:10.1158/1535-7163.MCT-12-0193
Guo, C., Su, J., Li, Z., Xiao, R., Wen, J., Li, Y., et al. (2015). The G-protein-coupled bile acid receptor Gpbar1 (TGR5) suppresses gastric cancer cell proliferation and migration through antagonizing STAT3 signaling pathway. Oncotarget 6 (33), 34402–34413. doi:10.18632/oncotarget.5353
Han, A., Bennett, N., MacDonald, A., Johnstone, M., Whelan, J., and Donohoe, D. R. (2016). Cellular metabolism and dose reveal carnitine-dependent and -independent mechanisms of butyrate oxidation in colorectal cancer cells. J. Cell Physiol. 231 (8), 1804–1813. doi:10.1002/jcp.25287
Han, S., Wang, C., Qin, X., Xia, J., and Wu, A. (2017). LPS alters the immuno-phenotype of glioma and glioma stem-like cells and induces in vivo antitumor immunity via TLR4. J. Exp. Clin. Cancer Res. 36 (1), 83. doi:10.1186/s13046-017-0552-y
Hand, T. W., Vujkovic-Cvijin, I., Ridaura, V. K., and Belkaid, Y. (2016). Linking the microbiota, chronic disease, and the immune system. Trends Endocrinol. Metab. 27 (12), 831–843. doi:10.1016/j.tem.2016.08.003
Hattar, K., Savai, R., Subtil, F. S., Wilhelm, J., Schmall, A., Lang, D. S., et al. (2013). Endotoxin induces proliferation of NSCLC in vitro and in vivo: role of COX-2 and EGFR activation. Cancer Immunol. Immunother. 62 (2), 309–320. doi:10.1007/s00262-012-1341-2
Hay, F. G., Duncan, L. W., Langdon, S. P., and Leonard, R. C. (1991). Modulation of the cluster 1 and mucin antigens in human small cell lung cancer and other epithelial tumour cell lines after treatment with the differentiation inducing agent, sodium butyrate. Br. J. Cancer Suppl. 14, 33–35.
Hegyi, P., Maleth, J., Walters, J. R., Hofmann, A. F., and Keely, S. J. (2018). Guts and gall: bile acids in regulation of intestinal epithelial function in health and disease. Physiol. Rev. 98 (4), 1983–2023. doi:10.1152/physrev.00054.2017
Hieken, T. J., Chen, J., Hoskin, T. L., Walther-Antonio, M., Johnson, S., Ramaker, S., et al. (2016). The microbiome of aseptically collected human breast tissue in benign and malignant disease. Sci. Rep. 6, 30751. doi:10.1038/srep30751
Hogarty, M. D., Norris, M. D., Davis, K., Liu, X., Evageliou, N. F., Hayes, C. S., et al. (2008). ODC1 is a critical determinant of MYCN oncogenesis and a therapeutic target in neuroblastoma. Cancer Res. 68 (23), 9735–9745. doi:10.1158/0008-5472.CAN-07-6866
Hogh, R. I., Moller, S. H., Jepsen, S. D., Mellergaard, M., Lund, A., Pejtersen, M., et al. (2020). Metabolism of short-chain fatty acid propionate induces surface expression of NKG2D ligands on cancer cells. FASEB J. 34 (11), 15531–15546. doi:10.1096/fj.202000162R
Honda, T., and Inagawa, H. (2016). Gene expression in lipopolysaccharide-treated human monocytes following interaction with hepatic cancer cells. Anticancer Res. 36 (7), 3699–3704.
Hong, D., Jang, S. Y., Jang, E. H., Jung, B., Cho, I. H., Park, M. J., et al. (2015). Shikonin as an inhibitor of the LPS-induced epithelial-to-mesenchymal transition in human breast cancer cells. Int. J. Mol. Med. 36 (6), 1601–1606. doi:10.3892/ijmm.2015.2373
Hosios, A. M., and Vander Heiden, M. G. (2014). Acetate metabolism in cancer cells. Cancer Metab. 2 (1), 27. doi:10.1186/s40170-014-0027-y
Hosseini, E., Grootaert, C., Verstraete, W., and Van de Wiele, T. (2011). Propionate as a health-promoting microbial metabolite in the human gut. Nutr. Rev. 69 (5), 245–258. doi:10.1111/j.1753-4887.2011.00388.x
Hsieh, S. C., Hsieh, W. J., Chiang, A. N., Su, N. W., Yeh, Y. T., and Liao, Y. C. (2016). The methanol-ethyl acetate partitioned fraction from Chinese olive fruits inhibits cancer cell proliferation and tumor growth by promoting apoptosis through the suppression of the NF-κB signaling pathway. Food Funct. 7 (12), 4797–4803. doi:10.1039/c6fo01202g
Huang, J. F., Zhao, Q., Dai, M. Y., Xiao, X. R., Zhang, T., Zhu, W. F., et al. (2020). Gut microbiota protects from triptolide-induced hepatotoxicity: key role of propionate and its downstream signalling events. Pharmacol. Res. 155, 104752. doi:10.1016/j.phrs.2020.104752
Huang, S. Y., Fang, C. Y., Tsai, C. H., Chang, Y., Takada, K., Hsu, T. Y., et al. (2010). N-methyl-N'-nitro-N-nitrosoguanidine induces and cooperates with 12-O-tetradecanoylphorbol-1,3-acetate/sodium butyrate to enhance Epstein-Barr virus reactivation and genome instability in nasopharyngeal carcinoma cells. Chem. Biol. Interact. 188 (3), 623–634. doi:10.1016/j.cbi.2010.09.020
Huang, T., Chen, Z., and Fang, L. (2013). Curcumin inhibits LPS-induced EMT through downregulation of NF-κB-Snail signaling in breast cancer cells. Oncol. Rep. 29 (1), 117–124. doi:10.3892/or.2012.2080
Huang, W., Zeng, C., Liu, J., Yuan, L., Liu, W., Wang, L., et al. (2019). Sodium butyrate induces autophagic apoptosis of nasopharyngeal carcinoma cells by inhibiting AKT/mTOR signaling. Biochem. Biophys. Res. Commun. 514 (1), 64–70. doi:10.1016/j.bbrc.2019.04.111
Hwang, S., Lee, C. G., Jo, M., Park, C. O., Gwon, S. Y., Hwang, S., et al. (2020a). Enterotoxigenic Bacteroides fragilis infection exacerbates tumorigenesis in AOM/DSS mouse model. Int. J. Med. Sci. 17 (2), 145–152. doi:10.7150/ijms.38371
Hwang, S., Yi, H. C., Hwang, S., Jo, M., and Rhee, K. J. (2020b). Dietary salt administration decreases enterotoxigenic Bacteroides fragilis (ETBF)-Promoted tumorigenesis via inhibition of colonic inflammation. Int. J. Mol. Sci. 21 (21), 8034. doi:10.3390/ijms21218034
Imai, Y., Yoshida, O., Watanabe, T., Yukimoto, A., Koizumi, Y., Ikeda, Y., et al. (2019). Stimulated hepatic stellate cell promotes progression of hepatocellular carcinoma due to protein kinase R activation. PLoS One 14 (2), e0212589. doi:10.1371/journal.pone.0212589
Jaworski, D. M., Namboodiri, A. M., and Moffett, J. R. (2016). Acetate as a metabolic and epigenetic modifier of cancer therapy. J. Cell Biochem. 117 (3), 574–588. doi:10.1002/jcb.25305
Jiang, M., Zhou, L. Y., Xu, N., and An, Q. (2019). Hydroxysafflor yellow A inhibited lipopolysaccharide-induced non-small cell lung cancer cell proliferation, migration, and invasion by suppressing the PI3K/AKT/mTOR and ERK/MAPK signaling pathways. Thorac. Cancer 10 (6), 1319–1333. doi:10.1111/1759-7714.13019
Jing, Y. Y., Han, Z. P., Sun, K., Zhang, S. S., Hou, J., Liu, Y., et al. (2012). Toll-like receptor 4 signaling promotes epithelial-mesenchymal transition in human hepatocellular carcinoma induced by lipopolysaccharide. BMC Med. 10, 98. doi:10.1186/1741-7015-10-98
Jou, T. S., Stewart, D. B., Stappert, J., Nelson, W. J., and Marrs, J. A. (1995). Genetic and biochemical dissection of protein linkages in the cadherin-catenin complex. Proc. Natl. Acad. Sci. U. S. A. 92 (11), 5067–5071. doi:10.1073/pnas.92.11.5067
Kato, R., Matsui-Yuasa, I., Azuma, H., and Kojima-Yuasa, A. (2014). The synergistic effect of 1'-acetoxychavicol acetate and sodium butyrate on the death of human hepatocellular carcinoma cells. Chem. Biol. Interact. 212, 1–10. doi:10.1016/j.cbi.2014.01.010
Keenan, J. I., Aitchison, A., Purcell, R. V., Greenlees, R., Pearson, J. F., and Frizelle, F. A. (2016). Screening for enterotoxigenic Bacteroides fragilis in stool samples. Anaerobe 40, 50–53. doi:10.1016/j.anaerobe.2016.05.004
Kim, C. H., Park, J., and Kim, M. (2014). Gut microbiota-derived short-chain Fatty acids, T cells, and inflammation. Immune Netw. 14 (6), 277–288. doi:10.4110/in.2014.14.6.277
Kim, J. M., Cho, S. J., Oh, Y. K., Jung, H. Y., Kim, Y. J., and Kim, N. (2002). Nuclear factor-kappa B activation pathway in intestinal epithelial cells is a major regulator of chemokine gene expression and neutrophil migration induced by Bacteroides fragilis enterotoxin. Clin. Exp. Immunol. 130 (1), 59–66. doi:10.1046/j.1365-2249.2002.01921.x
Kim, M. H., Kang, S. G., Park, J. H., Yanagisawa, M., and Kim, C. H. (2013). Short-chain fatty acids activate GPR41 and GPR43 on intestinal epithelial cells to promote inflammatory responses in mice. Gastroenterology 145 (2), 396–406. doi:10.1053/j.gastro.2013.04.056
Ko, S. H., Jeon, J. I., Woo, H. A., and Kim, J. M. (2020). Bacteroides fragilis enterotoxin upregulates heme oxygenase-1 in dendritic cells via reactive oxygen species-mitogen-activated protein kinase-and Nrf2-dependent pathway. World J. Gastroenterol. 26 (3), 291–306. doi:10.3748/wjg.v26.i3.291
Kolar, S., Barhoumi, R., Jones, C. K., Wesley, J., Lupton, J. R., Fan, Y. Y., et al. (2011). Interactive effects of fatty acid and butyrate-induced mitochondrial Ca²⁺ loading and apoptosis in colonocytes. Cancer 117 (23), 5294–5303. doi:10.1002/cncr.26205
Kolodziejczyk, A. A., Zheng, D., and Elinav, E. (2019). Diet-microbiota interactions and personalized nutrition. Nat. Rev. Microbiol. 17 (12), 742–753. doi:10.1038/s41579-019-0256-8
Koomoa, D. L., Geerts, D., Lange, I., Koster, J., Pegg, A. E., Feith, D. J., et al. (2013). DFMO/eflornithine inhibits migration and invasion downstream of MYCN and involves p27Kip1 activity in neuroblastoma. Int. J. Oncol. 42 (4), 1219–1228. doi:10.3892/ijo.2013.1835
Koprinarova, M., Markovska, P., Iliev, I., Anachkova, B., and Russev, G. (2010). Sodium butyrate enhances the cytotoxic effect of cisplatin by abrogating the cisplatin imposed cell cycle arrest. BMC Mol. Biol. 11, 49. doi:10.1186/1471-2199-11-49
Kovacs, P., Csonka, T., Kovacs, T., Sari, Z., Ujlaki, G., Sipos, A., et al. (2019a). Lithocholic acid, a metabolite of the microbiome, increases oxidative stress in breast cancer. Cancers (Basel) 11 (9), 1255. doi:10.3390/cancers11091255
Kovacs, T., Miko, E., Vida, A., Sebo, E., Toth, J., Csonka, T., et al. (2019b). Cadaverine, a metabolite of the microbiome, reduces breast cancer aggressiveness through trace amino acid receptors. Sci. Rep. 9 (1), 1300. doi:10.1038/s41598-018-37664-7
Krejner, A., Bruhs, A., Mrowietz, U., Wehkamp, U., Schwarz, T., and Schwarz, A. (2018). Decreased expression of G-protein-coupled receptors GPR43 and GPR109a in psoriatic skin can be restored by topical application of sodium butyrate. Arch. Dermatol Res. 310 (9), 751–758. doi:10.1007/s00403-018-1865-1
Kuefer, R., Genze, F., Zugmaier, W., Hautmann, R. E., Rinnab, L., Gschwend, J. E., et al. (2007). Antagonistic effects of sodium butyrate and N-(4-hydroxyphenyl)-retinamide on prostate cancer. Neoplasia 9 (3), 246–253. doi:10.1593/neo.06766
Kuo, W. T., Lee, T. C., Yang, H. Y., Chen, C. Y., Au, Y. C., Lu, Y. Z., et al. (2015). LPS receptor subunits have antagonistic roles in epithelial apoptosis and colonic carcinogenesis. Cell Death Differ. 22 (10), 1590–1604. doi:10.1038/cdd.2014.240
Lazarova, D. L., Bordonaro, M., Carbone, R., and Sartorelli, A. C. (2004). Linear relationship between Wnt activity levels and apoptosis in colorectal carcinoma cells exposed to butyrate. Int. J. Cancer 110 (4), 523–531. doi:10.1002/ijc.20152
Lee, C. K., Jeong, S. H., Jang, C., Bae, H., Kim, Y. H., Park, I., et al. (2019). Tumor metastasis to lymph nodes requires YAP-dependent metabolic adaptation. Science 363 (6427), 644–649. doi:10.1126/science.aav0173
Li, J., Yin, J., Shen, W., Gao, R., Liu, Y., Chen, Y., et al. (2017a). TLR4 promotes breast cancer metastasis via akt/gsk3β/β-catenin pathway upon LPS stimulation. Anat. Rec. Hob. 300 (7), 1219–1229. doi:10.1002/ar.23590
Li, K., Dan, Z., Nie, Y., Hu, X., Gesang, L., Bianba, Z., et al. (2015). CD14 knockdown reduces lipopolysaccharide-induced cell viability and expression of inflammation-associated genes in gastric cancer cells in vitro and in nude mouse xenografts. Mol. Med. Rep. 12 (3), 4332–4339. doi:10.3892/mmr.2015.3924
Li, Q., Ding, C., Meng, T., Lu, W., Liu, W., Hao, H., et al. (2017b). Butyrate suppresses motility of colorectal cancer cells via deactivating Akt/ERK signaling in histone deacetylase dependent manner. J. Pharmacol. Sci. 135 (4), 148–155. doi:10.1016/j.jphs.2017.11.004
Liang, Z. M., Chen, Y., and Luo, M. L. (2017). Targeting stemness: implications for precision medicine in breast cancer. Adv. Exp. Med. Biol. 1026, 147–169. doi:10.1007/978-981-10-6020-5_7
Liu, R., Li, X., Hylemon, P. B., and Zhou, H. (2018). Conjugated bile acids promote invasive growth of esophageal adenocarcinoma cells and cancer stem cell expansion via sphingosine 1-phosphate receptor 2-mediated yes-associated protein activation. Am. J. Pathol. 188 (9), 2042–2058. doi:10.1016/j.ajpath.2018.05.015
Lo, Re O., Panebianco, C., Porto, S., Cervi, C., Rappa, F., Di Biase, S., et al. (2018). Fasting inhibits hepatic stellate cells activation and potentiates anti-cancer activity of Sorafenib in hepatocellular cancer cells. J. Cell Physiol. 233 (2), 1202–1212. doi:10.1002/jcp.25987
Louis, P., Hold, G. L., and Flint, H. J. (2014). The gut microbiota, bacterial metabolites and colorectal cancer. Nat. Rev. Microbiol. 12 (10), 661–672. doi:10.1038/nrmicro3344
Lu, S., Yin, S., Zhao, C., Fan, L., and Hu, H. (2020). Synergistic anti-colon cancer effect of glycyrol and butyrate is associated with the enhanced activation of caspase-3 and structural features of glycyrol. Food Chem. Toxicol. 136, 110952. doi:10.1016/j.fct.2019.110952
Lucchetti, D., Calapa, F., Palmieri, V., Fanali, C., Carbone, F., Papa, A., et al. (2017). Differentiation affects the release of exosomes from colon cancer cells and their ability to modulate the behavior of recipient cells. Am. J. Pathol. 187 (7), 1633–1647. doi:10.1016/j.ajpath.2017.03.015
Lupton, J. R. (2004). Microbial degradation products influence colon cancer risk: the butyrate controversy. J. Nutr. 134 (2), 479–482. doi:10.1093/jn/134.2.479
Luu, M., and Visekruna, A. (2021). Microbial metabolites: novel therapeutic tools for boosting cancer therapies. Trends Cell Biol. 31 (11), 873–875. doi:10.1016/j.tcb.2021.08.005
Luu, T. H., Bard, J. M., Carbonnelle, D., Chaillou, C., Huvelin, J. M., Bobin-Dubigeon, C., et al. (2018). Lithocholic bile acid inhibits lipogenesis and induces apoptosis in breast cancer cells. Cell Oncol. (Dordr) 41 (1), 13–24. doi:10.1007/s13402-017-0353-5
Lv, Y., Ye, T., Wang, H. P., Zhao, J. Y., Chen, W. J., Wang, X., et al. (2017). Suppression of colorectal tumorigenesis by recombinant Bacteroides fragilis enterotoxin-2 in vivo. World J. Gastroenterol. 23 (4), 603–613. doi:10.3748/wjg.v23.i4.603
Ma, C., Han, M., Heinrich, B., Fu, Q., Zhang, Q., Sandhu, M., et al. (2018). Gut microbiome-mediated bile acid metabolism regulates liver cancer via NKT cells. Science 360 (6391), eaan5931. doi:10.1126/science.aan5931
Ma, W., Zhang, L., Chen, W., Chang, Z., Tu, J., Qin, Y., et al. (2024). Microbiota enterotoxigenic Bacteroides fragilis-secreted BFT-1 promotes breast cancer cell stemness and chemoresistance through its functional receptor NOD1. Protein Cell 15 (6), 419–440. doi:10.1093/procel/pwae005
Mahmoud, N. N., Dannenberg, A. J., Bilinski, R. T., Mestre, J. R., Chadburn, A., Churchill, M., et al. (1999). Administration of an unconjugated bile acid increases duodenal tumors in a murine model of familial adenomatous polyposis. Carcinogenesis 20 (2), 299–303. doi:10.1093/carcin/20.2.299
Maiuri, A. R., Peng, M., Podicheti, R., Sriramkumar, S., Kamplain, C. M., Rusch, D. B., et al. (2017). Mismatch repair proteins initiate epigenetic alterations during inflammation-driven tumorigenesis. Cancer Res. 77 (13), 3467–3478. doi:10.1158/0008-5472.CAN-17-0056
Makki, K., Deehan, E. C., Walter, J., and Backhed, F. (2018). The impact of dietary fiber on gut microbiota in host health and disease. Cell Host Microbe 23 (6), 705–715. doi:10.1016/j.chom.2018.05.012
Maldonado, R. F., Sa-Correia, I., and Valvano, M. A. (2016). Lipopolysaccharide modification in Gram-negative bacteria during chronic infection. FEMS Microbiol. Rev. 40 (4), 480–493. doi:10.1093/femsre/fuw007
Maruyama, T., Yamamoto, S., Qiu, J., Ueda, Y., Suzuki, T., Nojima, M., et al. (2012). Apoptosis of bladder cancer by sodium butyrate and cisplatin. J. Infect. Chemother. 18 (3), 288–295. doi:10.1007/s10156-011-0322-2
Mashimo, T., Pichumani, K., Vemireddy, V., Hatanpaa, K. J., Singh, D. K., Sirasanagandla, S., et al. (2014). Acetate is a bioenergetic substrate for human glioblastoma and brain metastases. Cell 159 (7), 1603–1614. doi:10.1016/j.cell.2014.11.025
Maslowski, K. M., Vieira, A. T., Ng, A., Kranich, J., Sierro, F., Yu, D., et al. (2009). Regulation of inflammatory responses by gut microbiota and chemoattractant receptor GPR43. Nature 461 (7268), 1282–1286. doi:10.1038/nature08530
Mendez, R., Kesh, K., Arora, N., Di Martino, L., McAllister, F., Merchant, N., et al. (2020). Microbial dysbiosis and polyamine metabolism as predictive markers for early detection of pancreatic cancer. Carcinogenesis 41 (5), 561–570. doi:10.1093/carcin/bgz116
Meng, S., Chen, B., Yang, J., Wang, J., Zhu, D., Meng, Q., et al. (2018). Study of microbiomes in aseptically collected samples of human breast tissue using needle biopsy and the potential role of in situ tissue microbiomes for promoting malignancy. Front. Oncol. 8, 318. doi:10.3389/fonc.2018.00318
Menzel, T., Schauber, J., Kreth, F., Kudlich, T., Melcher, R., Gostner, A., et al. (2002). Butyrate and aspirin in combination have an enhanced effect on apoptosis in human colorectal cancer cells. Eur. J. Cancer Prev. 11 (3), 271–281. doi:10.1097/00008469-200206000-00011
Metz, P., Tjan, M. J. H., Wu, S., Pervaiz, M., Hermans, S., Shettigar, A., et al. (2019). Drug discovery and repurposing inhibits a major gut pathogen-derived oncogenic toxin. Front. Cell Infect. Microbiol. 9, 364. doi:10.3389/fcimb.2019.00364
Miko, E., Vida, A., Kovacs, T., Ujlaki, G., Trencsenyi, G., Marton, J., et al. (2018). Lithocholic acid, a bacterial metabolite reduces breast cancer cell proliferation and aggressiveness. Biochim. Biophys. Acta Bioenerg. 1859 (9), 958–974. doi:10.1016/j.bbabio.2018.04.002
Morrison, D. J., and Preston, T. (2016). Formation of short chain fatty acids by the gut microbiota and their impact on human metabolism. Gut Microbes 7 (3), 189–200. doi:10.1080/19490976.2015.1134082
Murga-Garrido, S. M., Hong, Q., Cross, T. L., Hutchison, E. R., Han, J., Thomas, S. P., et al. (2021). Gut microbiome variation modulates the effects of dietary fiber on host metabolism. Microbiome 9 (1), 117. doi:10.1186/s40168-021-01061-6
Nakagawa, H., Yui, Y., Sasagawa, S., and Itoh, K. (2018). Evidence for intrathecal sodium butyrate as a novel option for leptomeningeal metastasis. J. Neurooncol 139 (1), 43–50. doi:10.1007/s11060-018-2852-2
Nastasi, C., Fredholm, S., Willerslev-Olsen, A., Hansen, M., Bonefeld, C. M., Geisler, C., et al. (2017). Butyrate and propionate inhibit antigen-specific CD8(+) T cell activation by suppressing IL-12 production by antigen-presenting cells. Sci. Rep. 7 (1), 14516. doi:10.1038/s41598-017-15099-w
Nejsum, L. N., and Nelson, W. J. (2007). A molecular mechanism directly linking E-cadherin adhesion to initiation of epithelial cell surface polarity. J. Cell Biol. 178 (2), 323–335. doi:10.1083/jcb.200705094
Nguyen, T. T., Lian, S., Ung, T. T., Xia, Y., Han, J. Y., and Jung, Y. D. (2017). Lithocholic acid stimulates IL-8 expression in human colorectal cancer cells via activation of erk1/2 MAPK and suppression of STAT3 activity. J. Cell Biochem. 118 (9), 2958–2967. doi:10.1002/jcb.25955
Nilsson, J. A., Keller, U. B., Baudino, T. A., Yang, C., Norton, S., Old, J. A., et al. (2005). Targeting ornithine decarboxylase in Myc-induced lymphomagenesis prevents tumor formation. Cancer Cell 7 (5), 433–444. doi:10.1016/j.ccr.2005.03.036
Ogut, D., Reel, B., Gonen Korkmaz, C., Arun, M. Z., Cilaker Micili, S., and Ergur, B. U. (2016). Doxycycline down-regulates matrix metalloproteinase expression and inhibits NF-κB signaling in LPS-induced PC3 cells. Folia Histochem Cytobiol. 54 (4), 171–180. doi:10.5603/FHC.a2016.0022
O'Hagan, H. M., Wang, W., Sen, S., Destefano Shields, C., Lee, S. S., Zhang, Y. W., et al. (2011). Oxidative damage targets complexes containing DNA methyltransferases, SIRT1, and polycomb members to promoter CpG Islands. Cancer Cell 20 (5), 606–619. doi:10.1016/j.ccr.2011.09.012
Ohno, H. (2020). The impact of metabolites derived from the gut microbiota on immune regulation and diseases. Int. Immunol. 32 (10), 629–636. doi:10.1093/intimm/dxaa041
Okuyama, H., Tominaga, A., Fukuoka, S., Taguchi, T., Kusumoto, Y., and Ono, S. (2017). Spirulina lipopolysaccharides inhibit tumor growth in a Toll-like receptor 4-dependent manner by altering the cytokine milieu from interleukin-17/interleukin-23 to interferon-γ. Oncol. Rep. 37 (2), 684–694. doi:10.3892/or.2017.5346
Ozfiliz, P., Kizilboga, T., Demir, S., Alkurt, G., Palavan-Unsal, N., Arisan, E. D., et al. (2015). Bag-1 promotes cell survival through c-Myc-mediated ODC upregulation that is not preferred under apoptotic stimuli in MCF-7 cells. Cell Biochem. Funct. 33 (5), 293–307. doi:10.1002/cbf.3114
Pant, K., Saraya, A., and Venugopal, S. K. (2017). Oxidative stress plays a key role in butyrate-mediated autophagy via Akt/mTOR pathway in hepatoma cells. Chem. Biol. Interact. 273, 99–106. doi:10.1016/j.cbi.2017.06.001
Papadimitriou, N., Markozannes, G., Kanellopoulou, A., Critselis, E., Alhardan, S., Karafousia, V., et al. (2021). An umbrella review of the evidence associating diet and cancer risk at 11 anatomical sites. Nat. Commun. 12 (1), 4579. doi:10.1038/s41467-021-24861-8
Parida, S., Wu, S., Siddharth, S., Wang, G., Muniraj, N., Nagalingam, A., et al. (2021). A procarcinogenic colon microbe promotes breast tumorigenesis and metastatic progression and concomitantly activates Notch and beta-catenin axes. Cancer Discov. 11 (5), 1138–1157. doi:10.1158/2159-8290.CD-20-0537
Paskova, L., Smesny Trtkova, K., Fialova, B., Benedikova, A., Langova, K., and Kolar, Z. (2013). Different effect of sodium butyrate on cancer and normal prostate cells. Toxicol In Vitro 27 (5), 1489–1495. doi:10.1016/j.tiv.2013.03.002
Perego, S., Sansoni, V., Banfi, G., and Lombardi, G. (2018). Sodium butyrate has anti-proliferative, pro-differentiating, and immunomodulatory effects in osteosarcoma cells and counteracts the TNFα-induced low-grade inflammation. Int. J. Immunopathol. Pharmacol. 32, 394632017752240. doi:10.1177/0394632017752240
Perona, M., Rodriguez, C., Carpano, M., Thomasz, L., Nievas, S., Olivera, M., et al. (2013). Improvement of the boron neutron capture therapy (BNCT) by the previous administration of the histone deacetylase inhibitor sodium butyrate for the treatment of thyroid carcinoma. Radiat. Environ. Biophys. 52 (3), 363–373. doi:10.1007/s00411-013-0470-0
Peters, M. C., Minton, A., Phanstiel Iv, O., and Gilmour, S. K. (2018). A novel polyamine-targeted therapy for BRAF mutant melanoma tumors. Med. Sci. (Basel) 6 (1), 3. doi:10.3390/medsci6010003
Purcell, R. V., Pearson, J., Aitchison, A., Dixon, L., Frizelle, F. A., and Keenan, J. I. (2017). Colonization with enterotoxigenic Bacteroides fragilis is associated with early-stage colorectal neoplasia. PLoS One 12 (2), e0171602. doi:10.1371/journal.pone.0171602
Qiao, D., Gaitonde, S. V., Qi, W., and Martinez, J. D. (2001). Deoxycholic acid suppresses p53 by stimulating proteasome-mediated p53 protein degradation. Carcinogenesis 22 (6), 957–964. doi:10.1093/carcin/22.6.957
Rajeeve, V., Pearce, W., Cascante, M., Vanhaesebroeck, B., and Cutillas, P. R. (2013). Polyamine production is downstream and upstream of oncogenic PI3K signalling and contributes to tumour cell growth. Biochem. J. 450 (3), 619–628. doi:10.1042/BJ20121525
Reese, D. H., Gratzner, H. G., Block, N. L., and Politano, V. A. (1985). Control of growth, morphology, and alkaline phosphatase activity by butyrate and related short-chain fatty acids in the retinoid-responsive 9-1C rat prostatic adenocarcinoma cell. Cancer Res. 45 (5), 2308–2313.
Rimpi, S., and Nilsson, J. A. (2007). Metabolic enzymes regulated by the Myc oncogene are possible targets for chemotherapy or chemoprevention. Biochem. Soc. Trans. 35 (Pt 2), 305–310. doi:10.1042/BST0350305
Rivkin, I., Cohen, K., Bod, T., Argov, M., and Margalit, R. (2014). Cancer cell sensitization and improved treatment efficacy by combined sodium butyrate and paclitaxel formulations is cancer-type specific. Int. J. Pharm. 461 (1-2), 437–447. doi:10.1016/j.ijpharm.2013.12.021
Rodrigues, M., Hidayat, A., and Karesh, J. (1988). Pleomorphic adenocarcinoma of ciliary epithelium simulating an epibulbar tumor. Am. J. Ophthalmol. 106 (5), 595–600. doi:10.1016/0002-9394(88)90593-4
Rooks, M. G., and Garrett, W. S. (2016). Gut microbiota, metabolites and host immunity. Nat. Rev. Immunol. 16 (6), 341–352. doi:10.1038/nri.2016.42
Rothschild, D., Weissbrod, O., Barkan, E., Kurilshikov, A., Korem, T., Zeevi, D., et al. (2018). Environment dominates over host genetics in shaping human gut microbiota. Nature 555 (7695), 210–215. doi:10.1038/nature25973
Roy, U. K., Rial, N. S., Kachel, K. L., and Gerner, E. W. (2008). Activated K-RAS increases polyamine uptake in human colon cancer cells through modulation of caveolar endocytosis. Mol. Carcinog. 47 (7), 538–553. doi:10.1002/mc.20414
Saldanha, S. N., Kala, R., and Tollefsbol, T. O. (2014). Molecular mechanisms for inhibition of colon cancer cells by combined epigenetic-modulating epigallocatechin gallate and sodium butyrate. Exp. Cell Res. 324 (1), 40–53. doi:10.1016/j.yexcr.2014.01.024
Sears, C. L. (2009). Enterotoxigenic Bacteroides fragilis: a rogue among symbiotes. Clin. Microbiol. Rev. 22 (2), 349–369. doi:10.1128/CMR.00053-08
Sgambato, A., Puglisi, M. A., Errico, F., Rafanelli, F., Boninsegna, A., Rettino, A., et al. (2010). Post-translational modulation of CD133 expression during sodium butyrate-induced differentiation of HT29 human colon cancer cells: implications for its detection. J. Cell Physiol. 224 (1), 234–241. doi:10.1002/jcp.22124
Shetab Boushehri, M. A., Yazeji, T., Stein, V., and Lamprecht, A. (2019). Modulation of nanostructure-based lipopolysaccharide active immunotherapy in cancer: size and composition determine short- and long-term tolerability. Mol. Pharm. 16 (11), 4507–4518. doi:10.1021/acs.molpharmaceut.9b00631
Shi, L., and Tu, B. P. (2015). Acetyl-CoA and the regulation of metabolism: mechanisms and consequences. Curr. Opin. Cell Biol. 33, 125–131. doi:10.1016/j.ceb.2015.02.003
Shin, H., Kim, J. H., Lee, Y. S., and Lee, Y. C. (2012). Change in gene expression profiles of secreted frizzled-related proteins (SFRPs) by sodium butyrate in gastric cancers: induction of promoter demethylation and histone modification causing inhibition of Wnt signaling. Int. J. Oncol. 40 (5), 1533–1542. doi:10.3892/ijo.2012.1327
Shin, J., Carr, A., Corner, G. A., Togel, L., Davalos-Salas, M., Tran, H., et al. (2014). The intestinal epithelial cell differentiation marker intestinal alkaline phosphatase (ALPi) is selectively induced by histone deacetylase inhibitors (HDACi) in colon cancer cells in a Kruppel-like factor 5 (KLF5)-dependent manner. J. Biol. Chem. 289 (36), 25306–25316. doi:10.1074/jbc.M114.557546
Singh, A., Koduru, B., Carlisle, C., Akhter, H., Liu, R. M., Schroder, K., et al. (2017). NADPH oxidase 4 modulates hepatic responses to lipopolysaccharide mediated by Toll-like receptor-4. Sci. Rep. 7 (1), 14346. doi:10.1038/s41598-017-14574-8
Singh, N., Gurav, A., Sivaprakasam, S., Brady, E., Padia, R., Shi, H., et al. (2014). Activation of Gpr109a, receptor for niacin and the commensal metabolite butyrate, suppresses colonic inflammation and carcinogenesis. Immunity 40 (1), 128–139. doi:10.1016/j.immuni.2013.12.007
Singh, N. P., and Lai, H. C. (2005). Synergistic cytotoxicity of artemisinin and sodium butyrate on human cancer cells. Anticancer Res. 25 (6B), 4325–4331.
Soda, K. (2011). The mechanisms by which polyamines accelerate tumor spread. J. Exp. Clin. Cancer Res. 30, 95. doi:10.1186/1756-9966-30-95
Song, W., Tiruthani, K., Wang, Y., Shen, L., Hu, M., Dorosheva, O., et al. (2018). Trapping of lipopolysaccharide to promote immunotherapy against colorectal cancer and attenuate liver metastasis. Adv. Mater 30 (52), e1805007. doi:10.1002/adma.201805007
Suh, S. S., Hong, J. M., Kim, E. J., Jung, S. W., Chae, H., Kim, J. E., et al. (2019). Antarctic freshwater microalga, Chloromonas reticulata, suppresses inflammation and carcinogenesis. Int. J. Med. Sci. 16 (2), 189–197. doi:10.7150/ijms.30647
Tang, Y., Chen, Y., Jiang, H., Robbins, G. T., and Nie, D. (2011). G-protein-coupled receptor for short-chain fatty acids suppresses colon cancer. Int. J. Cancer 128 (4), 847–856. doi:10.1002/ijc.25638
Taylor, M. A., Khathayer, F., and Ray, S. K. (2019). Quercetin and sodium butyrate synergistically increase apoptosis in rat C6 and human T98G glioblastoma cells through inhibition of autophagy. Neurochem. Res. 44 (7), 1715–1725. doi:10.1007/s11064-019-02802-8
Tofalo, R., Cocchi, S., and Suzzi, G. (2019). Polyamines and gut microbiota. Front. Nutr. 6, 16. doi:10.3389/fnut.2019.00016
Toprak, N. U., Yagci, A., Gulluoglu, B. M., Akin, M. L., Demirkalem, P., Celenk, T., et al. (2006). A possible role of Bacteroides fragilis enterotoxin in the aetiology of colorectal cancer. Clin. Microbiol. Infect. 12 (8), 782–786. doi:10.1111/j.1469-0691.2006.01494.x
Tsao, D., Morita, A., Bella, A., Luu, P., and Kim, Y. S. (1982). Differential effects of sodium butyrate, dimethyl sulfoxide, and retinoic acid on membrane-associated antigen, enzymes, and glycoproteins of human rectal adenocarcinoma cells. Cancer Res. 42 (3), 1052–1058.
Tsukamoto, A., Kaneko, Y., and Kurokawa, K. (1991). Novobiocin modulates cytokeratin assembly and differentiation of human hepatoma cells induced by butyrate and teleocidin. Cancer Lett. 60 (1), 51–57. doi:10.1016/0304-3835(91)90048-m
Tsukamoto, H., Takeuchi, S., Kubota, K., Kobayashi, Y., Kozakai, S., Ukai, I., et al. (2018). Lipopolysaccharide (LPS)-binding protein stimulates CD14-dependent Toll-like receptor 4 internalization and LPS-induced TBK1-IKKϵ-IRF3 axis activation. J. Biol. Chem. 293 (26), 10186–10201. doi:10.1074/jbc.M117.796631
Tylichova, Z., Slavik, J., Ciganek, M., Ovesna, P., Krcmar, P., Strakova, N., et al. (2018). Butyrate and docosahexaenoic acid interact in alterations of specific lipid classes in differentiating colon cancer cells. J. Cell Biochem. 119 (6), 4664–4679. doi:10.1002/jcb.26641
Ulger, T. N., Rajendram, D., Yagci, A., Gharbia, S., Shah, H. N., Gulluoglu, B. M., et al. (2006). The distribution of the bft alleles among enterotoxigenic Bacteroides fragilis strains from stool specimens and extraintestinal sites. Anaerobe 12 (2), 71–74. doi:10.1016/j.anaerobe.2005.11.001
Verma, S. P., Agarwal, A., and Das, P. (2018). Sodium butyrate induces cell death by autophagy and reactivates a tumor suppressor gene DIRAS1 in renal cell carcinoma cell line UOK146. Vitro Cell Dev. Biol. Anim. 54 (4), 295–303. doi:10.1007/s11626-018-0239-5
Wang, C., Wang, J., Guan, W., and Fei, B. (2024). Impact the impact of gut microbiota on gastric cancer via immune cells: a comprehensive Mendelian randomization study and mediation analysis. Discov. Oncol. 15 (1), 389. doi:10.1007/s12672-024-01285-6
Wang, H., Altemus, J., Niazi, F., Green, H., Calhoun, B. C., Sturgis, C., et al. (2017). Breast tissue, oral and urinary microbiomes in breast cancer. Oncotarget 8 (50), 88122–88138. doi:10.18632/oncotarget.21490
Wang, L., Zhu, R., Huang, Z., Li, H., and Zhu, H. (2013). Lipopolysaccharide-induced toll-like receptor 4 signaling in cancer cells promotes cell survival and proliferation in hepatocellular carcinoma. Dig. Dis. Sci. 58 (8), 2223–2236. doi:10.1007/s10620-013-2745-3
Wang, R. K., Shao, X. M., Yang, J. P., Yan, H. L., and Shao, Y. (2019a). MicroRNA-145 inhibits proliferation and promotes apoptosis of HepG2 cells by targeting ROCK1 through the ROCK1/NF-κB signaling pathway. Eur. Rev. Med. Pharmacol. Sci. 23 (7), 2777–2785. doi:10.26355/eurrev_201904_17551
Wang, X. M., Wang, X., Li, J., and Evers, B. M. (1998). Effects of 5-azacytidine and butyrate on differentiation and apoptosis of hepatic cancer cell lines. Ann. Surg. 227 (6), 922–931. doi:10.1097/00000658-199806000-00016
Wang, Y., Kong, H., Zeng, X., Liu, W., Wang, Z., Yan, X., et al. (2016). Activation of NLRP3 inflammasome enhances the proliferation and migration of A549 lung cancer cells. Oncol. Rep. 35 (4), 2053–2064. doi:10.3892/or.2016.4569
Wang, Z., Yan, M., Li, J., Long, J., Li, Y., and Zhang, H. (2019b). Dual functions of STAT3 in LPS-induced angiogenesis of hepatocellular carcinoma. Biochim. Biophys. Acta Mol. Cell Res. 1866 (4), 566–574. doi:10.1016/j.bbamcr.2018.11.016
Weaver, E. M., Zamora, F. J., Puplampu-Dove, Y. A., Kiessu, E., Hearne, J. L., and Martin-Caraballo, M. (2015). Regulation of T-type calcium channel expression by sodium butyrate in prostate cancer cells. Eur. J. Pharmacol. 749, 20–31. doi:10.1016/j.ejphar.2014.12.021
Wei, S., Wang, K., Huang, X., Tang, W., Zhao, Z., and Zhao, Z. (2019). Knockdown of the lncRNA MALAT1 alleviates lipopolysaccharideinduced A549 cell injury by targeting the miR175p/FOXA1 axis. Mol. Med. Rep. 20 (2), 2021–2029. doi:10.3892/mmr.2019.10392
Wilson, A., and Kim, R. B. (2022). Failure to achieve target drug concentrations during induction and not HLADQA1 *05 carriage is associated with antidrug antibody formation in patients with inflammatory bowel disease: is HLADQA1 *05 gone before it's here? Gastroenterology 163 (4), 1123–1124. doi:10.1053/j.gastro.2022.04.011
Wright, P. S., Dudley, D. T., and Chalkley, R. (1986). Butyrate effects on normal and adapted hepatoma cells: morphological response and implications for vectoral cholesterol transport. Arch. Biochem. Biophys. 248 (1), 243–252. doi:10.1016/0003-9861(86)90422-4
Wu, S., Lim, K. C., Huang, J., Saidi, R. F., and Sears, C. L. (1998). Bacteroides fragilis enterotoxin cleaves the zonula adherens protein, E-cadherin. Proc. Natl. Acad. Sci. U. S. A. 95 (25), 14979–14984. doi:10.1073/pnas.95.25.14979
Wu, S., Morin, P. J., Maouyo, D., and Sears, C. L. (2003). Bacteroides fragilis enterotoxin induces c-Myc expression and cellular proliferation. Gastroenterology 124 (2), 392–400. doi:10.1053/gast.2003.50047
Wu, S., Rhee, K. J., Zhang, M., Franco, A., and Sears, C. L. (2007). Bacteroides fragilis toxin stimulates intestinal epithelial cell shedding and gamma-secretase-dependent E-cadherin cleavage. J. Cell Sci. 120 (Pt 11), 1944–1952. doi:10.1242/jcs.03455
Xu, C., Li, H., Yin, M., Yang, T., An, L., and Yang, G. (2017). Osteopontin is involved in TLR4 pathway contributing to ovarian cancer cell proliferation and metastasis. Oncotarget 8 (58), 98394–98404. doi:10.18632/oncotarget.21844
Xu, H., Wu, Q., Dang, S., Jin, M., Xu, J., Cheng, Y., et al. (2011). Alteration of CXCR7 expression mediated by TLR4 promotes tumor cell proliferation and migration in human colorectal carcinoma. PLoS One 6 (12), e27399. doi:10.1371/journal.pone.0027399
Xu, L., Li, Y., Wei, Z., Bai, R., Gao, G., Sun, W., et al. (2022). Chenodeoxycholic acid (CDCA) promoted intestinal epithelial cell proliferation by regulating cell cycle progression and mitochondrial biogenesis in IPEC-J2 cells. Antioxidants (Basel) 11 (11), 2285. doi:10.3390/antiox11112285
Yang, H., Wang, B., Wang, T., Xu, L., He, C., Wen, H., et al. (2014). Toll-like receptor 4 prompts human breast cancer cells invasiveness via lipopolysaccharide stimulation and is overexpressed in patients with lymph node metastasis. PLoS One 9 (10), e109980. doi:10.1371/journal.pone.0109980
Yang, Y., Misra, B. B., Liang, L., Bi, D., Weng, W., Wu, W., et al. (2019). Integrated microbiome and metabolome analysis reveals a novel interplay between commensal bacteria and metabolites in colorectal cancer. Theranostics 9 (14), 4101–4114. doi:10.7150/thno.35186
Yao, L., Jiang, L., Zhang, F., Li, M., Yang, B., Zhang, F., et al. (2020). Acetate promotes SNAI1 expression by ACSS2-mediated histone acetylation under glucose limitation in renal cell carcinoma cell. Biosci. Rep. 40 (6). doi:10.1042/BSR20200382
Yokoyama, S., Cai, Y., Murata, M., Tomita, T., Yoneda, M., Xu, L., et al. (2018). A novel pathway of LPS uptake through syndecan-1 leading to pyroptotic cell death. Elife 7, e37854. doi:10.7554/eLife.37854
Yoshimoto, S., Loo, T. M., Atarashi, K., Kanda, H., Sato, S., Oyadomari, S., et al. (2013). Obesity-induced gut microbial metabolite promotes liver cancer through senescence secretome. Nature 499 (7456), 97–101. doi:10.1038/nature12347
Yu, L. X., and Schwabe, R. F. (2017). The gut microbiome and liver cancer: mechanisms and clinical translation. Nat. Rev. Gastroenterol. Hepatol. 14 (9), 527–539. doi:10.1038/nrgastro.2017.72
Yu, M., Kong, H., Zhao, Y., Sun, X., Zheng, Z., Yang, C., et al. (2014). Enhancement of adriamycin cytotoxicity by sodium butyrate involves hTERT downmodulation-mediated apoptosis in human uterine cancer cells. Mol. Carcinog. 53 (7), 505–513. doi:10.1002/mc.21998
Zamani, S., Taslimi, R., Sarabi, A., Jasemi, S., Sechi, L. A., and Feizabadi, M. M. (2019). Enterotoxigenic Bacteroides fragilis: a possible etiological candidate for bacterially-induced colorectal precancerous and cancerous lesions. Front. Cell Infect. Microbiol. 9, 449. doi:10.3389/fcimb.2019.00449
Zeng, H., Lazarova, D. L., and Bordonaro, M. (2014). Mechanisms linking dietary fiber, gut microbiota and colon cancer prevention. World J. Gastrointest. Oncol. 6 (2), 41–51. doi:10.4251/wjgo.v6.i2.41
Zhang, B., Xue, H., Wang, W., Chen, T., Su, M., Kang, N., et al. (2020). Comparative proteomic analysis of the brain and colon in three rat models of irritable bowel syndrome. Proteome Sci. 18, 1. doi:10.1186/s12953-020-0157-9
Zhang, J., Yi, M., Zha, L., Chen, S., Li, Z., Li, C., et al. (2016). Sodium butyrate induces endoplasmic reticulum stress and autophagy in colorectal cells: implications for apoptosis. PLoS One 11 (1), e0147218. doi:10.1371/journal.pone.0147218
Zhang, Y., Kang, C., Wang, X. L., Zhou, M., Chen, M. T., Zhu, X. H., et al. (2018). Dietary factors modulate colonic tumorigenesis through the interaction of gut microbiota and host chloride channels. Mol. Nutr. Food Res. 62 (5). doi:10.1002/mnfr.201700554
Zhang, Y., Zhou, L., Bao, Y. L., Wu, Y., Yu, C. L., Huang, Y. X., et al. (2010). Butyrate induces cell apoptosis through activation of JNK MAP kinase pathway in human colon cancer RKO cells. Chem. Biol. Interact. 185 (3), 174–181. doi:10.1016/j.cbi.2010.03.035
Zhao, Y., Shi, L., Hu, C., and Sang, S. (2019). Wheat bran for colon cancer prevention: the synergy between phytochemical alkylresorcinol C21 and intestinal microbial metabolite butyrate. J. Agric. Food Chem. 67 (46), 12761–12769. doi:10.1021/acs.jafc.9b05666
Zhu, G., Huang, Q., Huang, Y., Zheng, W., Hua, J., Yang, S., et al. (2016a). Lipopolysaccharide increases the release of VEGF-C that enhances cell motility and promotes lymphangiogenesis and lymphatic metastasis through the TLR4- NF-κB/JNK pathways in colorectal cancer. Oncotarget 7 (45), 73711–73724. doi:10.18632/oncotarget.12449
Zhu, G., Huang, Q., Zheng, W., Huang, Y., Hua, J., Yang, S., et al. (2016b). LPS upregulated VEGFR-3 expression promote migration and invasion in colorectal cancer via a mechanism of increased NF-κB binding to the promoter of VEGFR-3. Cell Physiol. Biochem. 39 (5), 1665–1678. doi:10.1159/000447868
Zhu, X., Li, K., Liu, G., Wu, R., Zhang, Y., Wang, S., et al. (2023). Microbial metabolite butyrate promotes anti-PD-1 antitumor efficacy by modulating T cell receptor signaling of cytotoxic CD8 T cell. Gut Microbes 15 (2), 2249143. doi:10.1080/19490976.2023.2249143
Zhu, Y., Zhu, M., and Lance, P. (2012). Stromal COX-2 signaling activated by deoxycholic acid mediates proliferation and invasiveness of colorectal epithelial cancer cells. Biochem. Biophys. Res. Commun. 425 (3), 607–612. doi:10.1016/j.bbrc.2012.07.137
Zuo, L., Lu, M., Zhou, Q., Wei, W., and Wang, Y. (2013). Butyrate suppresses proliferation and migration of RKO colon cancer cells though regulating endocan expression by MAPK signaling pathway. Food Chem. Toxicol. 62, 892–900. doi:10.1016/j.fct.2013.10.028
Glossary
Alpi intestinal alkaline phosphatase
AMD1 Adenosylmethionine decarboxylase 1
AR androgen receptor
ACSS acyl-CoA synthetase short-chain family member
BA Bile acid
BC breast cancer
BFT Bacteroides fragilis toxin
CA cholic acid
CAF cancer-associated fibroblast
CDCA chenodeoxycholic acid
CD14 cluster of differentiation 14
CRC colorectal carcinoma
CSCs cancer stem cells
CXCR CXC chemokine receptor
DCA deoxycholic acid
DNMT DNA methyltransferase
EGFR epidermal growth factor receptor
EMT epithelial-mesenchymal transition
ER endoplasmic reticulum
ETBF Enterotoxigenic B. fragilis
GC gastric cancer
HCC hepatocellular carcinoma
HDACi Histone deacetylase inhibitor
IEC intestinal epithelial cell
LCA lithocholic acid
LPD lipid-protamine-DNA
LPS lipopolysaccharide
m6A N6-methyladenosine
NDC non-digestible carbohydrates
NKT Natural killer T
NRF nuclear factor erythroid 2-related factor
ODC Ornithine decarboxylase
NRF nuclear factor erythroid 2-related factor
ROS reactive oxygen species
SASP senescence-associated secretory phenotype
SCFA short-chain fatty acid
SNAI Snail Family Transcriptional Repressor
TLR Toll-like receptor
TME tumor microenvironment
Keywords: microbiota, metabolites, tumorigenesis, signaling/signaling pathways, metabolite
Citation: Yang S-F, Chen X-C and Pan Y-J (2025) Microbiota-derived metabolites in tumorigenesis: mechanistic insights and therapeutic implications. Front. Pharmacol. 16:1598009. doi: 10.3389/fphar.2025.1598009
Received: 22 March 2025; Accepted: 06 May 2025;
Published: 15 May 2025.
Edited by:
Seema Kumari, Dr. B.R. Ambedkar University, IndiaReviewed by:
Miriam Durante, University of Siena, ItalyLukasz Laczmanski, Polish Academy of Sciences, Poland
Copyright © 2025 Yang, Chen and Pan. This is an open-access article distributed under the terms of the Creative Commons Attribution License (CC BY). The use, distribution or reproduction in other forums is permitted, provided the original author(s) and the copyright owner(s) are credited and that the original publication in this journal is cited, in accordance with accepted academic practice. No use, distribution or reproduction is permitted which does not comply with these terms.
*Correspondence: Yao-Jie Pan, cGFueWFvamllQGhtYy5lZHUuY24=
†These authors have contributed equally to this work and share first authorship