- 1Department of Psychiatry, Renmin Hospital of Wuhan University, Wuhan, China
- 2Institute of Neuropsychiatry, Renmin Hospital of Wuhan University, Wuhan, China
Backgrounds: The neural circuit mechanisms underlying depression remain unclear. Recently optogenetics has gradually gained recognition as a novel technique to regulate the activity of neurons with light stimulation. Scientists are now transferring their focus to the function of brain regions and neural circuits in the pathogenic progress of depression. Deciphering the circuitry mechanism of depressive-like behaviors may help us better understand the symptomatology of depression. However, few studies have summarized current progress on optogenetic researches into the neural circuit mechanisms of depressive-like behaviors.
Aims: This review aimed to introduce fundamental characteristics and methodologies of optogenetics, as well as how this technique achieves specific neuronal control with spatial and temporal accuracy. We mainly summarized recent progress in neural circuit discoveries in depressive-like behaviors using optogenetics and exhibited the potential of optogenetics as a tool to investigate the mechanism and possible optimization underlying antidepressant treatment such as ketamine and deep brain stimulation.
Methods: A systematic review of the literature published in English mainly from 2010 to the present in databases was performed. The selected literature is then categorized and summarized according to their neural circuits and depressive-like behaviors.
Conclusions: Many important discoveries have been made utilizing optogenetics. These findings support optogenetics as a powerful and potential tool for studying depression. And our comprehension to the etiology of depression and other psychiatric disorders will also be more thorough with this rapidly developing technique in the near future.
Introduction
Depression is a kind of psychiatric disorder with high prevalence and suicidal incidence. According to the World Health Organization, depression accounts for 10% of worldwide disability caused by non-infectious diseases and turns out to be one of the leading reasons for disability (1, 2). Depression has a complicated etiology that has yet to be fully understood. Current opinions suggest that neuroinflammation, neural plasticity, gut-brain axis and other factors are involved in the process (3–5). Besides, the effects of therapies nowadays still have limitations for major depressive disorder (MDD). Previous research has indicated that the alteration or dysfunction of some brain regions is relevant to the occurrence of depression (6). Thus, insights into the role that neural circuits play in depression may lead us to a better understanding of the etiology, symptoms, and treatment of depression.
Optogenetics, which combines the advantages of genetics and optical methods, is a technology that allows the control of specific neurons in living animals while monitoring their effects on behavior and physiology (7). Scientists have paid much attention to optogenetics in psychiatric disorders, behavior and recognition since opsin was used to trigger neuron activity for the first time by Karl Deisseroth in 2005 (8). For instance, astrocytes play an important role in stress response and inducing depressive-like behavior via glucocorticoid receptors (9). Many studies have used optogenetics to precisely modulate astrocyte activation, investigating its effects in sleep, cognition and other behaviors (10–12). Moreover, optogenetics has also provided us with a more efficient method of learning the effects of neural circuits on psychiatric disease.
This review gives a brief overview of optogenetics as well as recent breakthroughs in this field. The latest progress in learning the mechanisms of depressive-like behaviors utilizing optogenetics will be presented in detail. However, studies on neural circuits and brain regions are numerous and complicated, only those studied thoroughly, such as the medial prefrontal cortex (mPFC), ventral tegmental area (VTA), and hippocampus are included in this review. Finally, the therapeutic potential of optogenetics in clinical practice like ketamine and deep brain stimulation (DBS) will be discussed.
Optogenetics
Optogenetics has become a hot topic in neuroscience during the past decades. With this technology, the microbial opsin gene is sent and integrated, by virus vector or recombinase system, into specific neurons in regions of interest and expresses excitatory or inhibitory opsin. The light with certain wavelength and frequency is then delivered in optic fiber to activate or inhibit target neurons. Thus, we can observe the impact of different brain regions and neural circuits on behavior and psychiatric disorders (13). This innovative technology combines the advantages of both genetic and optical methods: (i) High temporal resolution. Immediate neuronal activity control can be achieved by adjusting light wavelength, frequency and intensity; (ii) High spatial resolution. Light-sensitive opsins expressed in targeted neurons would precisely regulate action potential generation and firing in these cells; (iii) Low damage. Optogenetic technology causes less damage to experimental animals compared with traditional methods like electrical stimulation (14). Here, we mainly introduce the three essential components in optogenetics.
Opsin family
Opsin is a kind of light-sensitive ion channel/ion pump protein that is widely distributed in nature (15). Opsins are divided into two main families: microbial opsins (type I rhodopsin, ion transport proteins) and animal opsins (type II rhodopsin, G protein-coupled receptors, and melanopsin) (16). Microbial have two different types based on the effects: depolarizing microbial opsins (channelrhodopsins, ChR2) and hyperpolarizing microbial opsins (bacteriorhodopsins, BR and halorhodopsins, NpHR). Most optogenetic studies choose ChR2 for cellular activation (17). ChR2 is a non-specific cation channel that is activated by blue light and permits Na+ and Ca2+ to enter cells, depolarizing targeted cells (18). While BR (proton pump activated by green light) and NpHR (chloride pump activated by yellow light) will hyperpolarize neurocytes and inhibit firing after illumination (14).
The past decades have seen great progress in the opsin family to meet different needs in size, kinetic properties and wavelength sensitivity with the development of modern genomics. Faster-deactivating ChR variants, such as ChEAT, reduce extra spikes occurring in response to a single light pulse and evoke sustained spike trains up to at least 200 Hz (19). Another ChR variant ChEF is made by chimeras of the transmembrane domains of ChR1 and ChR2 combined with site-directed mutagenesis. ChEF exhibits a significantly lower inactivation rate during persistent light stimulation. Moreover, point mutation of Ile170 in ChEF to Val has yielded a different variant, named ChIEF, that accelerates the channel closure rate while retaining reduced inactivation (20). ChEAT, ChEF, and ChIEF all allow for more precise temporal control of depolarization and can elicit action potentials that better mimic natural kinetics.
On the contrary, step-function opsin (SFO) is a modified ChR2 variant that can be activated by blue light and turned off by green light. After being exposed to blue light, the SFO keeps opening and maintains the cell depolarized for about 10 min. This method reduces the damage to animals' brains caused by consecutive high-power laser pulses (21). SwiChR, a step-waveform inhibitory ChR, for example, does not hyperpolarize neurons but instead opens up the Cl− channel stably and reversibly. SwiChR has slower kinetics than endogenous GABAA receptors, hence it is more used for long reversible inhibition experiments (22, 23). Investigators have engineered a new type of SFO with a longer spontaneous deactivation time constant approaching 30 min. One of the advantages of having this stabilized SFO (SSFO) is to conduct behavioral protocols in the absence of tethered external light delivery devices. As a result, we can observe animals' activities without affecting their natural behavior (24).
Adjustments are also made in wavelength sensitivity. Red light has longer wavelength and consequently has better tissue penetration compared with blue light, making it easier to optically control deep brain regions. VChR1 is a red-shift channelrhodopsin from Volvox carteri that can drive spiking at 589 nm, with excitation maximum red-shifted ~70 nm compared with ChR2 (25). The red-activatable Channelrhodopsin (ReaChR), a modified VChR1 variant is then developed. ReaChR is optimally excited with light from 590 to 630 nm and offers improved membrane trafficking, higher photocurrents, and faster kinetics compared with VChR1 (26).
Viral vector and recombinase system
As microbial opsins can only produce small photo-currents and neural circuit components are highly diverse, high opsin gene expression in living animal's nervous system, targeting defined cell types or projections and minimizing cytotoxicity are crucial for optogenetics (7). Today, genetic specificity in optogenetics is realized mainly via three strategies, viral vector, recombinase-expressing driver animal lines and anatomical targeting strategies (13). Opsin expression in specific cells is successfully achieved by injecting adeno-associated virus (AAV) vectors or lentivirus under the control of a cell type-specific promoter. Promoter fragments conferring cell type preference are linked to markers like calcium/calmodulin-dependent protein kinase II subunit-α (CaMKIIα), hypocretin, oxytocin, or D2 dopamine receptor (17). However, only sequences that are < ~4 kb, specific, and strong can be packed into viruses, limiting their use in a wide range of cell types.
Utilizing the recombinase system would obviate the limitation and allow for larger promoter fragments. Transgenic mice are injected with recombinase-dependent opsin-expressing viral vector or along with targeted viruses that drive recombinase expression to realize opsin expression in cells of interest (13). This system can provide higher targeted opsin expression selectively in recombinase-expressing cells in the injected brain regions compared with viral vectors (27). For example, using Cre driver lines to comprehensively and selectively label brain-wide connections by layer and projection neuron class, Harris et al. revealed that class-specific connections are organized in a shallow hierarchy within the mouse cortical thalami network (28).
Light delivery and readout equipment
The last essential components in the optogenetic toolbox are light delivery and signal readout devices. In general, a typical device of light delivery contains external light resources [the most popular light source is laser or light-emitting diodes (LEDs)], controller equipment, optical fibers and probes. This set of devices could provide effective optogenetic stimulation that consists of light with sufficient intensity to reach the designated location and enough energy to activate the expression of opsin, triggering a cellular signaling cascade (16). But there are several obvious defects. The physical tethers restrict animals' natural activity and behavior unavoidably, which affects the application and repeatability of the experiments. Optical fiber implantation into certain brain regions by invasive surgical operation causes side effects like brain damage, infection and bleeding. Besides, heat accumulated during illumination also hurts brain cells. The appearance of wireless devices implemented with miniaturized circuitry and specialized antenna designs makes it possible for researchers to observe the behavior, physiology and pathology of animals in a natural condition (29). To reduce injury, microscale light-emitting diode (uLED) arrays and tapered optical fibers have been widely applied in recent years, which meanwhile improve the spatial accuracy of optogenetic stimulation (30, 31).
Whole-cell patch-clamp electrophysiology was developed by Erwin Neher and Bert Sakmann and used for measuring single-channel currents. Although it is the gold standard for monitoring synaptic input and spiking output, it is still difficult to link the resulting data stream to defined cell types in vivo during behavior. This limitation can be circumvented by the integration of patch-clamp techniques with projection-targeted optogenetics (13, 32). Another combination of optogenetics with fast readouts creates the possibility of closed-loop optogenetic interventions, in which photostimulation is guided by real-time readout of ongoing activity. It gives us a chance to begin to understand the casual relationship between neural activity and behavior. Scientists can dynamically control neuronal activity patterns in awake animals and observe the function of neural circuits (16).
Search strategy, study selection, and data extraction
Candidate studies were identified via PubMed and Web of Science by using the following search strategy:
The applied key terms were [(“depression” OR “depressive-like behavior” OR “anhedonia” OR “appetite” OR “social withdrawal” OR “social avoidance”) AND (“optogenetic”) AND (“mice” OR “rats”)] searched for in the topic and/or and other fields in the database. The search was restricted to articles written in English and published between database inception and April 26, 2022. In sum, 706 records were extracted. The set was refined by removing (a) duplicate entries (n = 254). Titles and abstracts were then screened for relevance, removing (b) reviews, meta-analysis, case studies, study protocols and books (n = 143). The full text of the remaining 309 papers was then assessed for eligibility. Subsequently excluding studies that did not deal with (c) depression in the sense of a psychiatric disorder (n= 176), (d) optogenetics (n = 46), (e) tests for depressive-like behavior (n=14). Figure 1 shows the sample development throughout the selection process. The study selection and eligibility screening were conducted according to the preferred reporting items for systematic reviews and meta-analyses (PRISMA) guidelines (33).
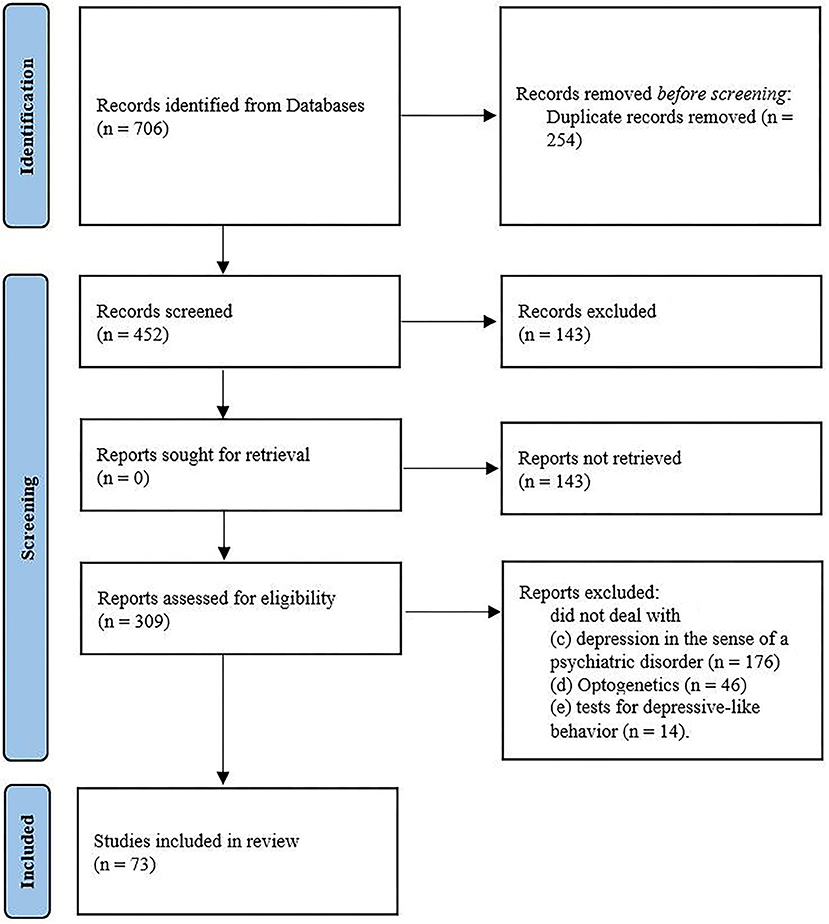
Figure 1. Flow chart of study selection by systematic search process according to the PRISMA group (2020).
Optogenetic findings in depressive-like behaviors
Major depressive disorder (MDD) is a multifaceted illness. MDD consists of a wide range of emotional, cognitive and behavioral symptoms, including core symptoms (anhedonia and depressed mood) as well as other symptoms (appetite changes, social withdrawal, sleep disturbance, worthlessness or self-guilt, and suicidal ideation). We have confirmed that the dysfunctional circuits underlying these symptoms include emotional, cognitive, perceptive and reward systems (34). And symptoms such as anhedonia have been well imitated in animal experiments. However, the existing problem is that symptoms like the feeling of worthless, self-guilt and suicidal ideation cannot be measured quantitively by behavioral tests like sucrose preference test (SPT) or tail suspension test (TST). Therefore, we only discuss publications that deal with anhedonia, appetite change and social avoidance/withdrawal in this section.
Anhedonia
Anhedonia, as one of the core symptoms of depression, is defined as the reduced ability to feel pleasure in normally pleasurable situations (35). Anhedonia is a negative prognostic factor in the therapeutic outcomes of depressed patients (36, 37). Besides improvement of anhedonia significantly correlates with better overall functioning in depressed patients and is a strong prediction for psychiatrical functioning improvement (38, 39), which emphasizes its critical role in depression.
The clinical phenotype anhedonia is now considered to reflect dysfunctional processing of the reward network. Large numbers of reviews have focused on reward circuits in animals and humans (40, 41). The most consistently described reward network is the dopaminergic mesolimbic pathways originating from the VTA and spreading into the nucleus accumbens (NAc) located in the ventral striatum (VS), bed nucleus of the stria terminalis, amygdala, and hippocampus (42).
An animal study investigated the role of VTA in the reward network and found that optical activation of the VTA leads to dopamine release in the NAc and the establishment of conditioned place preference (CPP) (43). With eNpHR3.0 inhibiting DA neurons in VTA, mice show a significant decrease in SPT and also in forced swim test (FST) and TST. Furthermore, phasic illumination of ChR2-VTA DA neurons can reduce immobility time in TST and reverse the anhedonic effect in mice undergoing time-intensive chronic mild stress (CMS) paradigm (44). Another research shows a contradictory result in SPT after phasic firing of VTA DA neurons in mice undergoing subthreshold social defeat paradigm. Phasic photostimulation of VTA DA neurons also induces susceptibility to social defeat stress in mice (45). One possible reason for the bidirectional effects of VTA DA neurons may lie in the different stimulus paradigms. More detailed studies are required to explain the potential mechanisms because of the heterogeneity. Optogenetic activation of DA neurons from VTA projecting to NAc core and shell and infralimbic (IL)-mPFC induces positive reinforcement behavior and intracranial self-stimulation (46). A newly identified subtype of VTA DA neurons molecularly defined by NeuroD6 (NEX1M) also induces significant place preference behavior after illumination in vivo (47). And optical stimulation of D1 neurons in the downstream area NAc enhances CPP for cocaine in mice while activating D2 neurons attenuates this preference (48). Optical stimulation of lateral hypothalamic (LH) orexin/dynorphin inputs in the VTA potentiates mesolimbic dopamine neurotransmission in the NAc core and produces real-time place preference (49). The communication between neural populations within the LH and DA neurons in VTA and NAc is also demonstrated in earlier studies (50–52).
To our knowledge, 60% of efferent projections of the VTA are dopaminergic, and they also contain glutamatergic and GABAergic neurons (53). van Zessen et al. found optical activation of VTA GABA projections to the NAc did not disrupt reward consumption, which is contradictory to the decreased reward consummatory licking with VTA GABA activated during the 5 s period following reward deliver. These findings may imply that activation of VTA GABAergic projections in NAc alone was not sufficient to suppress reward consumption. Interestingly, activation of VTA GABA neurons simultaneously reduced the excitability and activity of VTA DA neurons (54). Photostimulation of vesicular glutamate transporter 2 (VGLUT2) neurons in VTA co-releases GABA/glutamate in projecting regions NAc, VP, and LHb. Nevertheless, the overall effect of the stimulation is serving as a potent reinforcer on positive behavioral tasks (55). Optogenetic activation of a dorsal raphe (DR)-originating pathway reinforces instrumental behavior and establishes conditioned place preferences. Notably, this pathway consists of VGLUT3-containing neurons projecting to VTA dopamine neurons, indicating that the DR-VLGUT3 pathway to VTA utilizes glutamate as a neurotransmitter and is a substrate linking DR to VTA dopaminergic neurons (56). All the discoveries above confirm that GABAergic and glutamatergic neurons engage in reward circuits, and reflect the complicated inner connections between dopaminergic, GABAergic and glutamatergic neurons.
The complexity of VTA in the process of anhedonia outlines its significance as an integral hub for corticolimbic circuitry. Besides, other evidence shows that mPFC, lateral habenula and other regions also contribute to anhedonia (57–59). Undoubtedly, anhedonia-related neural circuits act as critical factors in depression due to their core status in the symptomatology of depression. The function of VTA and other neural circuits in the etiology of depression will be further discussed in the next section.
Appetite change
Appetite change, often accompanied with altered dietary habits and eating behavior, is a common symptom in patients with MDD. About 48% of adult depressed patients are reported to exhibit depression-related appetite decreases, while 35% exhibit depression-related increases in appetite (52, 60).
The hypothalamus has been proved to be the center of appetite regulation and optogenetics gives us a chance to further study how the hypothalamus and other neural circuits regulate appetite. The agouti-related protein (AgRP) neurons are a population of neurons regulating appetite in the arcuate nucleus of hypothalamus (ARH) (61). Another region, the parabrachial nucleus (PBN) also contains subpopulations of neurons that modulate appetite suppression (62). A previous study has shown that ablation of AgRP neurons hyperactivates PBN, causing starvation in adult mice (63). Glutamatergic neurons in the nucleus tractus solitarius (NTS) is then found to be the source of the excitatory inputs to the PBN. Activation of this pathway inhibits feeding, while genetic inactivation of glutamatergic signaling by the NTS onto NMDA-type glutamate receptors in the PBN prevents starvation. Optically suppressing glutamatergic output of the PBN reinstates normal appetite after AgRP neuron ablation (64). Calcitonin gene-related peptide (CGRP)-expressing neuron is another population identified in the outer external lateral subdivision of the PBN which projects to the laterocapsular division of the central nucleus of the amygdala (CeAlc). Optogenetic activation of PBelo CGRP neurons projecting to the CeAlc suppresses appetite. In contrast, photoinhibition of these neurons increases food intake and prevents starvation in AgRP neuron ablated mice (62). Moreover, stimulating AgRP neurons with optogenetic methods can reverse the appetite suppressing effects during chemogenetic-mediated stimulation of CGRP neurons. This result demonstrates that AgRP neurons are sufficient to decrease activity in anorexigenic PBN CGRP neurons, thereby increasing food intake (65).
The pro-opiomelanocortin (POMC)-expressing neurons existing in the ARH also participate in appetite regulation (61). Jeong et al. demonstrated that ARH POMC neurons express capsaicin-sensitive transient receptor potential vanilloid 1 receptor (TRPV1)-like receptor. Selective optogenetic stimulation of TRPV1-like receptor-expressing POMC neurons at 10 Hz for 1 h significantly decreases food intake (66). Besides, optogenetically stimulating ChR2-expressing ARHPOMC reduces short-term food intake via ARHPOMC→ medial amygdala (MeA) pathway (67). From an electrophysiological standpoint, stimulation of AgRP neurons triggers a long-term depression of spontaneous excitatory postsynaptic current (sEPSC) in downstream POMC neurons, which can be enhanced by food deprivation (68). One possible mechanism that underlies the regulatory mechanism of POMC neurons is the rapamycin complex 1 (mTORC1) signaling. Nicolas et al. found inhibiting mTORC1 increases food intake by decreasing POMC neuron activity. They further identified two different POMC cells: POMC/GABAergic neurons activated by rapamycin and POMC/glutamatergic neurons inhibited by rapamycin. Acute inhibition of mTORC1 activity after optogenetic activation of POMC neurons reduced POMC/GABAergic transmission and increased POMC/glutamatergic transmission at the same time. This study indicates the heterogeneity of POMC function relies on mTORC1 signaling, which makes POMC neurons regulate appetite bidirectionally (69).
Apart from AgRP and POMC neurons, other populations in the hypothalamus also contribute to appetite regulation, including a subset of nucleus of the solitary tract neurons containing cholecystokinin (CCKNTS) (70), glutamatergic neurons projecting to PVH in the medial septal complex (MSc) (71), tachykinin-1 expressing neurons (PSTNTac1) in the parasubthalamic nucleus (72), steroidogenic factor 1 (SF1) neurons in the ventromedial hypothalamus (73), and melanin-concentrating hormone-expressing neurons (74). In addition, progress has also been made in some known appetite-regulating factors like neuropeptide Y (NPY) and orexin with optogenetics (75, 76). Many other brain regions are also discovered to engage in appetite change in both clinical and basic experiments (77–80). In conclusion, it is enlightening for us to study appetite change in a hypothalamus-centered direction. Furthermore, we should consider this pathological behavior together with depression in the hope that we better understand this issue in depressed patients.
Social avoidance/social withdrawal
Patients with MDD usually have trouble with normal social behavior, for instance, they may lose interest or pleasure in social activities and interactions, even developing to social withdrawal (or social avoidance). Humans are a gregarious species and interpersonal communication is an indispensable part of people's daily routine, so it is crucial to understand how depression affects their social behavior (81).
Social defeat is one of the most popular paradigms to model stress-induced depression, characterized by the similarities in social behaviors between mice and humans. The test mouse is placed with an aggressive mouse for 10 min every day and will be attacked by the resident one. In the next 10 days, the test mouse is forced to live in visual, olfactive and auditive but not physical contact with the aggressor. After this protocol, the animals display typical depressive-like behaviors, such as social avoidance and increased immobility time in TST and FST (34, 82).
Researchers have revealed how mPFC and its circuits affect social behavior. Earlier experiments showed optogenetic stimulation of glutamatergic or GABAergic neurons in mPFC in mice exposed to social defeat stress all exhibited anti-depressive responses including less social avoidance and improvement in SPT (83). Furthermore, optical activation in left PL- mPFC can reverse social avoidance, while inhibition leads to social avoidance. However, the same effect does not appear when regulating the right PL-mPFC, indicating stress-induced social avoidance is developed due to the dysfunction of the left mPFC (84). At the circuit level, a circuit involving mPFC and basolateral amygdala (BLA) is identified. Activation of descending projections from mPFC→ BLA abolishes social preference and produces behavioral avoidance (85). Interstingly, sub-circuits of mPFC→ BLA pathway exhibit opposite effects. Chemogenetic activation of PL-BLA or inhibition of IL-BLA circuitry impair social behavior in mice (85). Afferent projections to mPFC also shape social avoidance behavior. Inhibiting VTA-NAc and VTA-mPFC projections with optogenetics, scientists have induced resilience and susceptibility to social defeat stress, respectively (45).
The dorsal raphe nucleus (DRN) also contributes to social disorders. Sakurai developed a technology, Capturing Activated Neural Ensembles or CANE. Optogenetic silencing of CANE-captured social fear neurons is sufficient to result in reduced social avoidance (86). Challis et al. combined optogenetics with cFOS mapping and slice electrophysiology and revealed that photoinhibition of the projection from vmPFC to GABAergic neurons in the DRN could prevent the acquisition of social avoidance behavior (87). Another experiment confirmed that optogenetic activation of DA neurons in DRN could increase social preference as well (88). Projections originating from mPFC to different parts of thalamus are also demonstrated to regulate certain aspects of social behavior (89–91).
Optogenetic findings in dysfunctional neural circuits
The mechanisms underlying the pathophysiology and treatment of depression and stress-related disorders remain unclear, but studies in depressed patients and animal models are beginning to yield promising insights. Brain imaging studies have demonstrated altered connectivity and network function in the brains of depressed patients. Thus, an increasing number of studies are focusing on the function and dysfunction of neural circuits and networks in depression (see Figure 2).
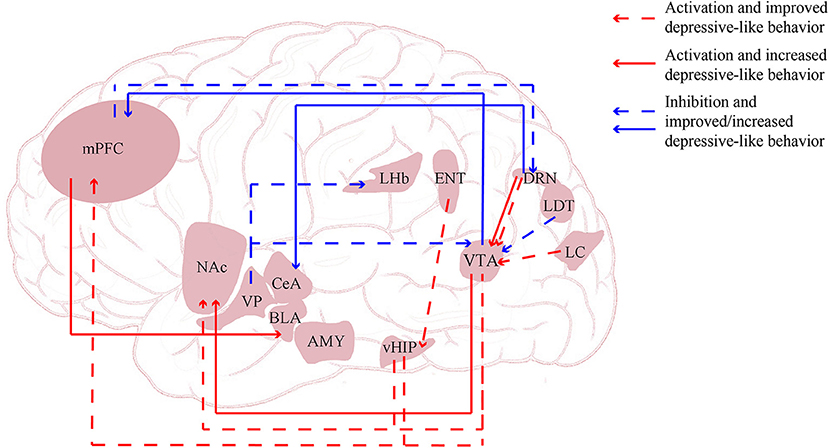
Figure 2. Neural circuits regulated via optogenetics and chegenetics. AMY, amygdala; BLA, basolateral amygdala; CeA, central nucleus of the amygdala; DRN, dorsal raphe nucleus; ENT, entorhinal cortex; LC, locus coeruleus; LDT, lateral dorsal tegmentum; LHb, lateral habenula; mPFC, medial prefrontal cortex; NAc, nucleus accumbens; vHIP, ventral hippocampus; VP, ventral pallidum.
Medial prefrontal cortex
An imaging study of depression revealed robust outcomes regarding prefrontal cortex function, which primarily proved its effect on depression (92). Subsequent experiments have made further explorations. Susceptible mice subjected to chronic social defeat stress (CSDS) show fully restored social interaction times in behavioral tests after in vivo illumination of mPFC (83). Different Paradigm like maternal separation (MS) protocol can affect depression-like phenotypes in mice via mPFC activity (93). Son et al. also found transient anti-depressive effects after optogenetic stimulation of glutamatergic neurons in the mPFC (94). Sirtuin 1 (SIRT1) is a kind of NAD+-dependent deacetylase in forebrain excitatory neurons. Selective deletion of these neurons causes depression-like phenotypes only in male mice, while AAV-Cre-mediated STIR1 knockdown in the mPFC of male mice induced sex-specific depressive-like behaviors (95).
The connections between mPFC with other brain regions also attract great interest (96). Studies on the neurobiological basis of mPFC relevant alterations have focused on glutamatergic, 5-HT neurons as well as inhibitory GABA interneurons (97). The DRN is a crucial node of the widespread network implicated in emotional regulation. Selective optogenetic activation of mPFC cells projecting to the DRN induces a rapid and robust increase in kick frequency during FST, but does not affect non-specific locomotor activity in OFT (98). The mPFC provides a direct monosynaptic, glutamatergic drive to both DRN 5-HT and GABA neurons. Activation of cannabinol (CB) receptors differentially modulates the mPFC inputs onto these cell types in the DRN, finally leading to a robust increase in the synaptic excitatory/inhibitory ratio in DRN 5-HT neurons (99). Srejic et al. inhibited ChR2-5-HT neurons in the DRN with blue light stimulation. Serotonin clearance was significantly faster after 5 min high-frequency stimulation, indicating less release or more effective 5-HT reuptake (100). Another novel pathway that originates from 5-HT in the DRN to somatostatin (SOM)-expressing and non-SOM interneurons in the central nucleus of the amygdala (CeA) in comorbid depressive symptoms (CDS) is identified. Inhibition of the 5-HTDRN→ SOMCeA pathway produces depression-like behavior, and conversely, optogenetic activation of this pathway reduces depression-like behavior. These findings indicate that 5-HTDRN→ SOMCeA might mediate some aspects of CDS (101). These studies imply altered 5-HT activity dynamics in the DRN after mPFC stimulation. Such altered neurotransmitter dynamics and network-based functional framework may help us understand depressive symptoms.
Ventral tegmental area
As discussed in 2.1, VTA DA neurons have been extensively studied for their role in reward circuitry, emphasizing its possible link to depression (102).
Optogenetic induction of phasic firing in VTA DA neurons will rapidly induce a susceptible phenotype in mice undergoing subthreshold social defeat stress (SSDS) (45). Some studies investigate the effects of long-term up-regulation of VTA DA activity. This long-term change leads to antidepressive-like behavior in several experimental paradigms by chronically stimulating VTA DA neurons of unstressed and socially defeated mice (103, 104). The locus coeruleus (LC)→ VTA circuit also mediates homeostatic mechanisms in mesolimbic DA neurons. Stimulation of LC→ VTA neurons can promote a CSDS resilience-like phenotype and reverse hyperactivity of VTA→ NAc DA neurons (105).
Brain-derived neurotrophic factor (BDNF) plays numerous important functions in the brain, one of which is mediating the stress-induced depressive-like behavior via the VTA-NAC pathway. Optogenetic activation of VTA DA neurons projecting to NAc after SSDS paradigm reduces social interaction and increases BDNF levels in the NAc. Both of the effects disappear when BDNF signaling is blocked with the BDNF receptor antagonist tyrosine receptor kinase B (TrkB) or by using genetic BDNF knock-out mice (106). Additionally, the effects of DA VTA→ NAc stimulation during stress exposure can be blocked by infusing D1 receptor antagonist before social interaction testing in the CSDS model, however, not in SSDS. Optogenetic activation of the DA VTA→ NAc pathway after CSDS increases social avoidance without change of DA level in the NAc. Unexpectedly, the effects of social avoidance are blocked through TrkB or genetic BDNF knock-out, suggesting BDNF rather than DA signaling in the VTA→ NAc circuit is crucial for developing depressive symptoms after CSDS (107). DA neurons still work through other pathways to induce hypolocomotion performance. He et al. identified decreased projections from VTA to substantia nigra pars reticulata (SNr) with quantitative whole-brain mapping after CSDS protocol. Subsequent optogenetic stimulation of phasic firing of this projection could significantly increase the locomotor activity of mice. Their data suggests that the VTA-SNr dopaminergic projection involves in CSDS-induced hopolocomotion and provides a potential therapeutic target for MDD (108).
Ventral pallidum (VP) is a relayed nucleus that receives afferent from the NAc and transmits information to downstream targets such as the VTA and LHb (109). PV neurons in VP show increased activity in CSDS-susceptible mice, and silencing them can induce resilience to CSDS. Optogenetic silencing of VP PV neuronal projections to the VTA and LHb influences different depressive-like behavior, respectively. Inhibiting the former pathway exhibits alleviated behavioral despair with reduced immobility in TST, while the same manipulation of the LHb-projecting populations only improves social withdrawal levels in social interaction test (110). The lateral dorsal tegmentum (LDTg) is another essential component in reward responses and also an important input to the VTA (111). CSDS will cause hyperactivity of excitatory neurons projecting to the VTA. While the progression of depressive-like behavior is prevented by chemogenetically inhibiting LDTg neurons before daily defeat paradigm. This protective effect is then shown by inhibiting cholinergic neurons during CSDS, which implies that LDTg cholinergic inputs to the VTA drive maladaptation to social stress (112). Another possible circuit mediating the inhibitory influence is DRN→ VTA. Optogenetic stimulation of 5-HT input to the mesolimbic DA system combined with citalopram produces a synergistic decrease in responding to saccharin, a measure of motivated behavior. However, this inhibitory influence of DRN 5-HT neurons over responding for reward only produces in the VTA, which indicates that the effect is exerted through direct interaction with the mesolimbic DA system at the level of the VTA (113). Undoubtedly, the VTA has connections with many other brain regions that is waiting to be explored.
Hippocampus
An earlier study has reported reduced hippocampal volume in patients with MDD, indicating smaller hippocampal volume might be a trait characteristic for MDD (114). Hippocampus can be divided into three functional segmentation in line with behavioral and anatomical studies including dorsal, intermediate and ventral, among which the ventral hippocampus (vHipp) regulates responses to stress, emotion and affect (115). Mice resilient to CSDS exposure show decreased glutamate release at vHipp-NAc synapses. Low-frequency stimulation to vHipp ChR2-infected mice decreases glutamatergic synaptic transmission at vHipp-NAc synapses. And these mice interact more time with a target mouse. Conversely, up-regulating glutamatergic synaptic transmission in NAc could increase stress susceptibility (116). Therapeutically, the vHipp-mPFC pathway enables an antidepressant response in Wistar-Kyoto (WKY) rats (117). Optogenetic activation of vHipp-mPFC can also mimic the antidepressant-like response to ketamine. Furthermore, optogenetic inactivation of this pathway during FST completely reverses ketamine's antidepressant response, indicating it may be involved in the rapid antidepressant effect of ketamine (118).
Upstream hippocampal circuitry, such as the entorhinal cortex (Ent), shows the potential for MDD treatment. Molecular targeting (Ent-specific knockdown of TRIP8b) and chemogenetic stimulation of Ent neurons induce antidepressive-like effects in mice. Ent stimulation-induced antidepressive-like behavior relies on the generation of new hippocampal neurons, indicating the controlled stimulation of the Ent-hippocampal pathway exerts anti-depressive effects via increased hippocampal neurogenesis (119). Hippocampal engrams represent ensembles of neurons with increased activity after memory formation. Zhang et al. examined social defeat-related hippocampal engrams in susceptible and resilient mice undergoing CSDS paradigm. TetTag mice were also used to label social defeat-related hippocampal ensembles by LacZ. Susceptible mice exhibited higher reactivating of social defeat-related LacZ-labeled cells in both the dorsal and ventral hippocampal CA1 regions compared with resilient and control groups. Activating or inactivating CA1 engram cells with optogenetic approaches enhanced and suppressed social avoidance, respectively. However, increased engram cells could not be found in the dentate gyrus. Taken together, all the evidence suggests that the susceptibility to CSDS is regulated by hippocampal CA1 engrams for negative memory (120). Other brain regions and neural circuits involved in depressive animal models are shown in Table 1.
Discussion
The potential of optogenetics in clinical application
Advances in technology enables us to understand the mechanism of depression and also provides us with new perspectives and insights into its treatment. At present, almost 30% of patients with depression cannot achieve sustained remission with traditional drug treatment, which is defined as treatment-resistant MDD (TRD) (131, 132). But encouragingly, optogenetic approaches have contributed to the development and improvement of depression therapies.
Ketamine, a new fast-acting antidepressant, can exert an antidepressant effect within hours and produce sustained antidepressant actions for a week (133). A previous study has found that inactivating IL-mPFC can completely block the antidepressant effect of ketamine. And optogenetic stimulation of IL-mPFC produces rapid and long-lasting antidepressant effects. These results indicate that the therapeutic action of ketamine may take effect via IL-mPFC (134). Optogenetic disruption of the Drd1 expressing pyramidal cells in mPFC blocks the rapid antidepressant effects of ketamine. Furthermore, this antidepressant response measured by FST is completely blocked by photoinhibition of the mPFC-BLA pathway (135). Carreno et al. found activation of the vHipp-mPFC pathway was necessary and sufficient for the antidepressant response to ketamine and increase in TrkB receptor phosphorylation in the vHipp contributed to ketamine's sustained antidepressant response (118). As a newly discovered fast-acting antidepressant, ketamine does have much to be explored from the perspective of neural circuits utilizing optogenetics.
DBS is another physical therapy evolved to manage patients with TRD (136). The DBS electrodes implanted in the desired target of the brain are connected to a programmable pulse generator. Therapeutically, the generator stimulates neuronal populations and elements to influence local, regional, and up-and downstream projections. However, the identification of the networks and their constituent elements is challenging, and the nature of the network disturbances and their precise location and dynamic properties are also incompletely understood (137).
In some clinical trials, TRD patients receiving DBS treatment show great differences in response rate during the follow-up (138, 139). The reason for these discrepancies may lie in the individual differences in the structure and function of neural circuitry and network. In Papp's study, they used WKY mice, a model resistant to antidepressant treatment, to compare the effects of DBS and optogenetic stimulation on animals' behavior. DBS had minor non-specific effects on exploration and locomotor activity in the novel object recognition (NOR) test. Although optogenetic stimulation of the vHipp-mPFC pathway did not reverse the effect of CMS on impaired NOR, it increased exploratory behavior and locomotor activity in the NOR test. A likely explanation is that DBS excites both intrinsic cells and afferents to PFC in the infected region, whereas optogenetic stimulation of mPFC excites only intrinsic cells (140). We have yielded greater progress by combing DBS with optogenetics in neurological and psychiatric diseases such as Parkinson's Disease and addiction (141–143). But currently, this field in animal models about depression is still blank. Considering the advantages of optogenetics in precise circuit regulation, future researches can seek specific circuits that change in DBS, ultimately reaching the goal of accurate treatment and less damage to the brain.
Methodical limitations
The current generation of optogenetic tools has been adapted to extensive questions in neuroscience and has also been optimized for stronger expression, currents and spectral shifts. Despite its potential and convenience, optogenetics has limitations and challenges to be addressed before further development and use. The main problems include infection, bleeding, and injury to the brain tissue caused by fiber insertion; restriction on animal's activity and natural behavior (16); and heat accumulation during the illumination (144, 145). Fortunately, the appearance of genomics-improved opsin, tapered optical fiber, uLED and wireless technology has improved these problems to a large extent (29–31, 146). We believe ongoing advances in optogenetic toolbox will yield tools that expand the optical control of biochemistry even further.
When it comes to depressive-like behaviors, we mainly summarize recent optogenetic findings in anhedonia, appetite change, and social avoidance. Besides, sleep disturbance is also an unignorable symptom that occurs in up to 70% of MDD patients (147). Although a slew of researches have investigated the relationship between sleep and neural circuits with optogenetics, only a small portion of them consider this issue in the context of depression, which may highlight the importance of considering sleep problems and other symptoms in MDD patients. As previously discussed, symptoms such as the feeling of worthlessness, self-guilt and suicidal ideation can neither be observed in animal models nor be measured quantitatively by behavioral tests, further restricting our steps toward the mechanisms of these depressive-like behaviors. Therefore, we urgently need new tests to detect depressive-like behaviors that have never been measured before to advance our research to new heights.
Future perspective and conclusion
To summarize, our review provide evidence for a complicated neural circuit that has been hypothesized to casually underlie depression. But we had one limitation that we only discussed brain regions and neural circuits that have been studied most in animal models. Due to the complexity of neurocircuitry in depression, the identified circuits in this review are just a subset dissected from the whole brain. There is also emerging evidence to explain the role of regions previously unknown, like medial dorsal thalamus (MDT) and anteroventral subdivision of the bed nuclei of the stria terminalis (avBST) (148, 149). More attention should be attached to these regions, aiming to construct an all-around understanding of our brain networks. Besides, some studies have found stimulation of sub-circuits may produce different effects, reminding us that we should not only concern about the overall effects of neural pathways, but also notice sub-regions/circuits with optogenetics in the future.
In conclusion, this paper reviews the recent progress of optogenetic applications in exploring the etiology of depressive-like behaviors at the circuit level. This unique neuroscience technique has increased our understanding of this complicated psychiatric disorder, and soon it may be useful in improving and optimizing clinical therapy for depression.
Author contributions
SL and GW participated in the design of the study, carried out the data collection, drafted the manuscript, and edited the manuscript. YD, YXia, and YXie helped with the analysis. LX advised on the article ideas and helped to draft the manuscript. All authors read, edited, and approved the final manuscript.
Funding
This study was supported by the National Natural Science Foundation of China (Nos. 81871072 and 82071523) and the Medical Science Advancement Program of Wuhan University (No. TFLC2018001). Design of this study was supported by the Key Research and Development Program of Hubei Province (2020BCA064).
Conflict of interest
The authors declare that the research was conducted in the absence of any commercial or financial relationships that could be construed as a potential conflict of interest.
Publisher's note
All claims expressed in this article are solely those of the authors and do not necessarily represent those of their affiliated organizations, or those of the publisher, the editors and the reviewers. Any product that may be evaluated in this article, or claim that may be made by its manufacturer, is not guaranteed or endorsed by the publisher.
References
1. Cruz-Pereira JS, Rea K, Nolan YM, O'Leary OF, Dinan TG, Cryan JF. Depression's unholy trinity: dysregulated stress, immunity, and the microbiome. Annu Rev Psychol. (2020) 71:49–78. doi: 10.1146/annurev-psych-122216-011613
2. Biselli T, Lange SS, Sablottny L, Steffen J, Walther A. Optogenetic and chemogenetic insights into the neurocircuitry of depression-like behaviour: a systematic review. Eur J Neurosci. (2021) 53:9–38. doi: 10.1111/ejn.14603
3. Kappelmann N, Lewis G, Dantzer R, Jones PB, Khandaker GM. Antidepressant activity of anti-cytokine treatment: a systematic review and meta-analysis of clinical trials of chronic inflammatory conditions. Mol Psychiatry. (2018) 23:335–43. doi: 10.1038/mp.2016.167
4. Lach G, Schellekens H, Dinan TG, Cryan JF. Anxiety, depression, and the microbiome: a role for gut peptides. Neurotherapeutics. (2018) 15:36–59. doi: 10.1007/s13311-017-0585-0
5. Boku S, Nakagawa S, Toda H, Hishimoto A. Neural basis of major depressive disorder: beyond monoamine hypothesis. Psychiatry Clin Neurosci. (2018) 72:3–12. doi: 10.1111/pcn.12604
6. Krishnan V, Nestler EJ. The molecular neurobiology of depression. Nature. (2008) 455:894–902. doi: 10.1038/nature07455
8. Boyden ES, Zhang F, Bamberg E, Nagel G, Deisseroth K. Millisecond-timescale, genetically targeted optical control of neural activity. Nat Neurosci. (2005) 8:1263–8. doi: 10.1038/nn1525
9. Lu CL, Ren J, Mo JW, Fan J, Guo F, Chen LY, et al. Glucocorticoid receptor-dependent astrocytes mediate stress vulnerability. Biol Psychiatry. (2022) 92:204–15. doi: 10.1016/j.biopsych.2021.11.022
10. Pelluru D, Konadhode RR, Bhat NR, Shiromani PJ. Optogenetic stimulation of astrocytes in the posterior hypothalamus increases sleep at night in C57bL/6J mice. Eur J Neurosci. (2016) 43:1298–306. doi: 10.1111/ejn.13074
11. Adamsky A, Kol A, Kreisel T, Doron A, Ozeri-Engelhard N, Melcer T, et al. Astrocytic activation generates de novo neuronal potentiation and memory enhancement. Cell. (2018) 174:59–71.e14. doi: 10.1016/j.cell.2018.05.002
12. Gomez JA, Perkins JM, Beaudoin GM, Cook NB, Quraishi SA, Szoeke EA, et al. Ventral tegmental area astrocytes orchestrate avoidance and approach behavior. Nat Commun. (2019) 10:1455. doi: 10.1038/s41467-019-09131-y
13. Kim CK, Adhikari A, Deisseroth K. Integration of optogenetics with complementary methodologies in systems neuroscience. Nat Rev Neurosci. (2017) 18:222–35. doi: 10.1038/nrn.2017.15
14. Shirai F, Hayashi-Takagi A. Optogenetics: applications in psychiatric research. Psychiatry Clin Neurosci. (2017) 71:363–72. doi: 10.1111/pcn.12516
15. Kuhne J, Vierock J, Tennigkeit SA, Dreier MA, Wietek J, Petersen D, et al. Unifying photocycle model for light adaptation and temporal evolution of cation conductance in channelrhodopsin-2. Proc Natl Acad Sci USA. (2019) 116:9380–9. doi: 10.1073/pnas.1818707116
16. Xu X, Mee T, Jia X. New era of optogenetics: from the central to peripheral nervous system. Crit Rev Biochem Mol Biol. (2020) 55:1–16. doi: 10.1080/10409238.2020.1726279
17. Deisseroth K. Optogenetics: 10 years of microbial opsins in neuroscience. Nat Neurosci. (2015) 18:1213–25. doi: 10.1038/nn.4091
18. Mukerjee S, Lazartigues E. Next-generation tools to study autonomic regulation in vivo. Neurosci Bull. (2019) 35:113–23. doi: 10.1007/s12264-018-0319-2
19. Gunaydin LA, Yizhar O, Berndt A, Sohal VS, Deisseroth K, Hegemann P. Ultrafast optogenetic control. Nat Neurosci. (2010) 13:387–92. doi: 10.1038/nn.2495
20. Lin JY, Lin MZ, Steinbach P, Tsien RY. Characterization of engineered channelrhodopsin variants with improved properties and kinetics. Biophys J. (2009) 96:1803–14. doi: 10.1016/j.bpj.2008.11.034
21. Igarashi H, Ikeda K, Onimaru H, Kaneko R, Koizumi K, Beppu K, et al. Targeted expression of step-function opsins in transgenic rats for optogenetic studies. Sci Rep. (2018) 8:5435. doi: 10.1038/s41598-018-23810-8
22. Berndt A, Lee SY, Wietek J, Ramakrishnan C, Steinberg EE, Rashid AJ, et al. Structural foundations of optogenetics: determinants of channelrhodopsin ion selectivity. Proc Natl Acad Sci USA. (2016) 113:822–9. doi: 10.1073/pnas.1523341113
23. Berndt A, Lee SY, Ramakrishnan C, Deisseroth K. Structure-guided transformation of channelrhodopsin into a light-activated chloride channel. Science. (2014) 344:420–4. doi: 10.1126/science.1252367
24. Fenno L, Yizhar O, Deisseroth K. The development and application of optogenetics. Annu Rev Neurosci. (2011) 34:389–412. doi: 10.1146/annurev-neuro-061010-113817
25. Zhang F, Prigge M, Beyrière F, Tsunoda SP, Mattis J, Yizhar O, et al. Red-shifted optogenetic excitation: a tool for fast neural control derived from Volvox carteri. Nat Neurosci. (2008) 11:631–3. doi: 10.1038/nn.2120
26. Lin JY, Knutsen PM, Muller A, Kleinfeld D, Tsien RY. Reachr: a red-shifted variant of channelrhodopsin enables deep transcranial optogenetic excitation. Nat Neurosci. (2013) 16:1499–508. doi: 10.1038/nn.3502
27. Fenno LE, Mattis J, Ramakrishnan C, Hyun M, Lee SY, He M, et al. Targeting cells with single vectors using multiple-feature boolean logic. Nat Methods. (2014) 11:763–72. doi: 10.1038/nmeth.2996
28. Harris JA, Mihalas S, Hirokawa KE, Whitesell JD, Choi H, Bernard A, et al. Hierarchical organization of cortical and thalamic connectivity. Nature. (2019) 575:195–202. doi: 10.1038/s41586-019-1716-z
29. Gutruf P, Rogers JA. Implantable, wireless device platforms for neuroscience research. Curr Opin Neurobiol. (2018) 50:42–9. doi: 10.1016/j.conb.2017.12.007
30. Pisanello M, Pisano F, Sileo L, Maglie E, Bellistri E, Spagnolo B, et al. Tailoring light delivery for optogenetics by modal demultiplexing in tapered optical fibers. Sci Rep. (2018) 8:4467. doi: 10.1038/s41598-018-22790-z
31. Ji B, Guo Z, Wang M, Yang B, Wang X, Li W, et al. Flexible polyimide-based hybrid opto-electric neural interface with 16 channels of micro-leds and electrodes. Microsyst Nanoeng. (2018) 4:27. doi: 10.1038/s41378-018-0027-0
32. Petersen CCH. Whole-cell recording of neuronal membrane potential during behavior. Neuron. (2017) 95:1266–81. doi: 10.1016/j.neuron.2017.06.049
33. Page MJ, McKenzie JE, Bossuyt PM, Boutron I, Hoffmann TC, Mulrow CD, et al. The Prisma 2020 statement: an updated guideline for reporting systematic reviews. BMJ. (2021) 372:n71. doi: 10.1136/bmj.n71
34. Planchez B, Surget A, Belzung C. Animal models of major depression: drawbacks and challenges. J Neural Transm. (2019) 126:1383–408. doi: 10.1007/s00702-019-02084-y
35. Höflich A, Michenthaler P, Kasper S, Lanzenberger R. Circuit mechanisms of reward, anhedonia, and depression. Int J Neuropsychopharmacol. (2019) 22:105–18. doi: 10.1093/ijnp/pyy081
36. McMakin DL, Olino TM, Porta G, Dietz LJ, Emslie G, Clarke G, et al. Anhedonia predicts poorer recovery among youth with selective serotonin reuptake inhibitor treatment-resistant depression. J Am Acad Child Adolesc Psychiatry. (2012) 51:404–11. doi: 10.1016/j.jaac.2012.01.011
37. Vrieze E, Demyttenaere K, Bruffaerts R, Hermans D, Pizzagalli DA, Sienaert P, et al. Dimensions in major depressive disorder and their relevance for treatment outcome. J Affect Disord. (2014) 155:35–41. doi: 10.1016/j.jad.2013.10.020
38. Llorca P, Gourion D. Management of anhedonia and depressive symptoms in depressed outpatients: benefit for functioning. Eur Psychiatry. (2015) 30:364. doi: 10.1016/S0924-9338(15)31881-2
39. Vinckier F, Gourion D, Mouchabac S. Anhedonia predicts poor psychosocial functioning: results from a large cohort of patients treated for major depressive disorder by general practitioners. Eur Psychiatry. (2017) 44:1–8. doi: 10.1016/j.eurpsy.2017.02.485
40. Keiflin R, Janak PH. Dopamine prediction errors in reward learning and addiction: from theory to neural circuitry. Neuron. (2015) 88:247–63. doi: 10.1016/j.neuron.2015.08.037
41. Russo SJ, Nestler EJ. The brain reward circuitry in mood disorders. Nat Rev Neurosci. (2013) 14:609–25. doi: 10.1038/nrn3381
42. Dunlop BW, Nemeroff CB. The role of dopamine in the pathophysiology of depression. Arch Gen Psychiatry. (2007) 64:327–37. doi: 10.1001/archpsyc.64.3.327
43. Tsai HC, Zhang F, Adamantidis A, Stuber GD, Bonci A, de Lecea L, et al. Phasic firing in dopaminergic neurons is sufficient for behavioral conditioning. Science. (2009) 324:1080–4. doi: 10.1126/science.1168878
44. Tye KM, Mirzabekov JJ, Warden MR, Ferenczi EA, Tsai HC, Finkelstein J, et al. Dopamine neurons modulate neural encoding and expression of depression-related behaviour. Nature. (2013) 493:537–41. doi: 10.1038/nature11740
45. Chaudhury D, Walsh JJ, Friedman AK, Juarez B, Ku SM, Koo JW, et al. Rapid regulation of depression-related behaviours by control of midbrain dopamine neurons. Nature. (2013) 493:532–6. doi: 10.1038/nature11713
46. Han X, Jing MY, Zhao TY, Wu N, Song R, Li J. Role of dopamine projections from ventral tegmental area to nucleus accumbens and medial prefrontal cortex in reinforcement behaviors assessed using optogenetic manipulation. Metab Brain Dis. (2017) 32:1491–502. doi: 10.1007/s11011-017-0023-3
47. Bimpisidis Z, König N, Stagkourakis S, Zell V, Vlcek B, Dumas S, et al. The Neurod6 subtype of VTA neurons contributes to psychostimulant sensitization and behavioral reinforcement. eNeuro. (2019) 6:ENEURO.0066-19.2019. doi: 10.1523/ENEURO.0066-19.2019
48. Soares-Cunha C, Coimbra B, David-Pereira A, Borges S, Pinto L, Costa P, et al. Activation of D2 dopamine receptor-expressing neurons in the nucleus accumbens increases motivation. Nat Commun. (2016) 7:11829. doi: 10.1038/ncomms11829
49. Thomas CS, Mohammadkhani A, Rana M, Qiao M, Baimel C, Borgland SL. Optogenetic stimulation of lateral hypothalamic orexin/dynorphin inputs in the ventral tegmental area potentiates mesolimbic dopamine neurotransmission and promotes reward-seeking behaviours. Neuropsychopharmacology. (2022) 47:728–40. doi: 10.1038/s41386-021-01196-y
50. Nieh EH, Vander Weele CM, Matthews GA, Presbrey KN, Wichmann R, Leppla CA, et al. Inhibitory input from the lateral hypothalamus to the ventral tegmental area disinhibits dopamine neurons and promotes behavioral activation. Neuron. (2016) 90:1286–98. doi: 10.1016/j.neuron.2016.04.035
51. Watabe-Uchida M, Zhu L, Ogawa SK, Vamanrao A, Uchida N. Whole-brain mapping of direct inputs to midbrain dopamine neurons. Neuron. (2012) 74:858–73. doi: 10.1016/j.neuron.2012.03.017
52. Coccurello R. Anhedonia in depression symptomatology: appetite dysregulation and defective brain reward processing. Behav Brain Res. (2019) 372:112041. doi: 10.1016/j.bbr.2019.112041
53. Dobi A, Margolis EB, Wang HL, Harvey BK, Morales M. Glutamatergic and nonglutamatergic neurons of the ventral tegmental area establish local synaptic contacts with dopaminergic and nondopaminergic neurons. J Neurosci. (2010) 30:218–29. doi: 10.1523/JNEUROSCI.3884-09.2010
54. van Zessen R, Phillips JL, Budygin EA, Stuber GD. Activation of VTA gaba neurons disrupts reward consumption. Neuron. (2012) 73:1184–94. doi: 10.1016/j.neuron.2012.02.016
55. Yoo JH, Zell V, Gutierrez-Reed N, Wu J, Ressler R, Shenasa MA, et al. Ventral tegmental area glutamate neurons co-release gaba and promote positive reinforcement. Nat Commun. (2016) 7:13697. doi: 10.1038/ncomms13697
56. Qi J, Zhang S, Wang HL, Wang H, de Jesus Aceves Buendia J, Hoffman AF, et al. A glutamatergic reward input from the dorsal raphe to ventral tegmental area dopamine neurons. Nat Commun. (2014) 5:5390. doi: 10.1038/ncomms6390
57. Ferenczi EA, Zalocusky KA, Liston C, Grosenick L, Warden MR, Amatya D, et al. Prefrontal cortical regulation of brainwide circuit dynamics and reward-related behavior. Science. (2016) 351:aac9698. doi: 10.1126/science.aac9698
58. Zhang T, He K, Bai T, Lv H, Xie X, Nie J, et al. Altered neural activity in the reward-related circuit and executive control network associated with amelioration of anhedonia in major depressive disorder by electroconvulsive therapy. Prog Neuropsychopharmacol Biol Psychiatry. (2021) 109:110193. doi: 10.1016/j.pnpbp.2020.110193
59. Browne CA, Hammack R, Lucki I. Dysregulation of the lateral habenula in major depressive disorder. Front Synaptic Neurosci. (2018) 10:46. doi: 10.3389/fnsyn.2018.00046
60. Maxwell MA, Cole DA. Weight change and appetite disturbance as symptoms of adolescent depression: toward an integrative biopsychosocial model. Clin Psychol Rev. (2009) 29:260–73. doi: 10.1016/j.cpr.2009.01.007
61. Wu Q, Palmiter RD. Gabaergic signaling by agrp neurons prevents anorexia via a melanocortin-independent mechanism. Eur J Pharmacol. (2011) 660:21–7. doi: 10.1016/j.ejphar.2010.10.110
62. Carter ME, Soden ME, Zweifel LS, Palmiter RD. Genetic identification of a neural circuit that suppresses appetite. Nature. (2013) 503:111–4. doi: 10.1038/nature12596
63. Wu Q, Boyle MP, Palmiter RD. Loss of gabaergic signaling by agrp neurons to the parabrachial nucleus leads to starvation. Cell. (2009) 137:1225–34. doi: 10.1016/j.cell.2009.04.022
64. Wu Q, Clark MS, Palmiter RD. Deciphering a neuronal circuit that mediates appetite. Nature. (2012) 483:594–7. doi: 10.1038/nature10899
65. Essner RA, Smith AG, Jamnik AA, Ryba AR, Trutner ZD, Carter ME. Agrp neurons can increase food intake during conditions of appetite suppression and inhibit anorexigenic parabrachial neurons. J Neurosci. (2017) 37:8678–87. doi: 10.1523/JNEUROSCI.0798-17.2017
66. Kwon E, Jo YH. Activation of the ARC(POMC) → mea projection reduces food intake. Front Neural Circuits. (2020) 14:595783. doi: 10.3389/fncir.2020.595783
67. Jeong JH, Lee DK, Liu SM, Chua SC Jr, Schwartz GJ, et al. Activation of temperature-sensitive TRPV1-like receptors in arc pomc neurons reduces food intake. PLoS Biol. (2018) 16:e2004399. doi: 10.1371/journal.pbio.2004399
68. Wang C, Zhou W, He Y, Yang T, Xu P, Yang Y, et al. Agrp neurons trigger long-term potentiation and facilitate food seeking. Transl Psychiatry. (2021) 11:11. doi: 10.1038/s41398-020-01161-1
69. Saucisse N, Mazier W, Simon V, Binder E, Catania C, Bellocchio L, et al. Functional heterogeneity of pomc neurons relies on MTORC1 signaling. Cell Rep. (2021) 37:109800. doi: 10.1016/j.celrep.2021.109800
70. D'Agostino G, Lyons DJ, Cristiano C, Burke LK, Madara JC, Campbell JN, et al. Appetite controlled by a cholecystokinin nucleus of the solitary tract to hypothalamus neurocircuit. Elife. (2016) 5:e12225. doi: 10.7554/eLife.12225
71. Sweeney P, Li C, Yang Y. Appetite suppressive role of medial septal glutamatergic neurons. Proc Natl Acad Sci USA. (2017) 114:13816–21. doi: 10.1073/pnas.1707228114
72. Kim JH, Kromm GH, Barnhill OK, Sperber J, Heuer LB, Loomis S, et al. A discrete parasubthalamic nucleus subpopulation plays a critical role in appetite suppression. Elife. (2022) 11:e75470. doi: 10.7554/eLife.75470
73. Viskaitis P, Irvine EE, Smith MA, Choudhury AI, Alvarez-Curto E, Glegola JA, et al. Modulation of SF1 neuron activity coordinately regulates both feeding behavior and associated emotional states. Cell Rep. (2017) 21:3559–72. doi: 10.1016/j.celrep.2017.11.089
74. Dilsiz P, Aklan I, Sayar Atasoy N, Yavuz Y, Filiz G, Koksalar F, et al. Mch neuron activity is sufficient for reward and reinforces feeding. Neuroendocrinology. (2020) 110:258–70. doi: 10.1159/000501234
75. Zhang L, Hernandez-Sanchez D, Herzog H. Regulation of feeding-related behaviors by arcuate neuropeptide Y neurons. Endocrinology. (2019) 160:1411–20. doi: 10.1210/en.2019-00056
76. Viskaitis P, Arnold M, Garau C, Jensen LT, Fugger L, Peleg-Raibstein D, et al. Ingested non-essential amino acids recruit brain orexin cells to suppress eating in mice. Curr Biol. (2022) 32:1812–21.e4. doi: 10.1016/j.cub.2022.02.067
77. Simmons WK, Burrows K, Avery JA, Kerr KL, Bodurka J, Savage CR, et al. Depression-related increases and decreases in appetite: dissociable patterns of aberrant activity in reward and interoceptive neurocircuitry. Am J Psychiatry. (2016) 173:418–28. doi: 10.1176/appi.ajp.2015.15020162
78. Jennings JH, Kim CK, Marshel JH, Raffiee M, Ye L, Quirin S, et al. Interacting neural ensembles in orbitofrontal cortex for social and feeding behaviour. Nature. (2019) 565:645–9. doi: 10.1038/s41586-018-0866-8
79. Dore R, Krotenko R, Reising JP, Murru L, Sundaram SM, Di Spiezio A, et al. Nesfatin-1 decreases the motivational and rewarding value of food. Neuropsychopharmacology. (2020) 45:1645–55. doi: 10.1038/s41386-020-0682-3
80. Chen L, Lu YP, Chen HY, Huang SN, Guo YR, Zhang JY, et al. Ventral tegmental area gabaergic neurons induce anxiety-like behaviors and promote palatable food intake. Neuropharmacology. (2020) 173:108114. doi: 10.1016/j.neuropharm.2020.108114
81. Hames JL, Hagan CR, Joiner TE. Interpersonal processes in depression. Annu Rev Clin Psychol. (2013) 9:355–77. doi: 10.1146/annurev-clinpsy-050212-185553
82. Gururajan A, Reif A, Cryan JF, Slattery DA. The future of rodent models in depression research. Nat Rev Neurosci. (2019) 20:686–701. doi: 10.1038/s41583-019-0221-6
83. Covington HE 3rd, Lobo MK, Maze I, Vialou V, Hyman JM, Zaman S, et al. Antidepressant effect of optogenetic stimulation of the medial prefrontal cortex. J Neurosci. (2010) 30:16082–90. doi: 10.1523/JNEUROSCI.1731-10.2010
84. Lee E, Hong J, Park YG, Chae S, Kim Y, Kim D. Left brain cortical activity modulates stress effects on social behavior. Sci Rep. (2015) 5:13342. doi: 10.1038/srep13342
85. Huang WC, Zucca A, Levy J, Page DT. Social behavior is modulated by valence-encoding MPFC-amygdala sub-circuitry. Cell Rep. (2020) 32:107899. doi: 10.1016/j.celrep.2020.107899
86. Sakurai K, Zhao S, Takatoh J, Rodriguez E, Lu J, Leavitt AD, et al. Capturing and manipulating activated neuronal ensembles with cane delineates a hypothalamic social-fear circuit. Neuron. (2016) 92:739–53. doi: 10.1016/j.neuron.2016.10.015
87. Challis C, Beck SG, Berton O. Optogenetic modulation of descending prefrontocortical inputs to the dorsal raphe bidirectionally bias socioaffective choices after social defeat. Front Behav Neurosci. (2014) 8:43. doi: 10.3389/fnbeh.2014.00043
88. Matthews GA, Nieh EH, Vander Weele CM, Halbert SA, Pradhan RV, Yosafat AS, et al. Dorsal raphe dopamine neurons represent the experience of social isolation. Cell. (2016) 164:617–31. doi: 10.1016/j.cell.2015.12.040
89. Miller OH, Bruns A, Ammar IB, Mueggler T, Hall BJ. Medial prefrontal cortical nmda receptors regulate depression-like behavior and dictate limbic thalamus innervation. bioRxiv. [Preprint]. (2017) 106419. doi: 10.1101/106419
90. Yamamuro K, Bicks LK, Leventhal MB, Kato D, Im S, Flanigan ME, et al. A prefrontal-paraventricular thalamus circuit requires juvenile social experience to regulate adult sociability in mice. Nat Neurosci. (2020) 23:1240–52. doi: 10.1038/s41593-020-0695-6
91. Biro L, Sipos E, Bruzsik B, Farkas I, Zelena D, Balazsfi D, et al. Task division within the prefrontal cortex: distinct neuron populations selectively control different aspects of aggressive behavior via the hypothalamus. J Neurosci. (2018) 38:4065–75. doi: 10.1523/JNEUROSCI.3234-17.2018
92. Mayberg HS. Targeted electrode-based modulation of neural circuits for depression. J Clin Invest. (2009) 119:717–25. doi: 10.1172/JCI38454
93. Teissier A, Le Magueresse C, Olusakin J, Andrade da Costa BLS, De Stasi AM, Bacci A, et al. Early-life stress impairs postnatal oligodendrogenesis and adult emotional behaviour through activity-dependent mechanisms. Mol Psychiatry. (2020) 25:1159–74. doi: 10.1038/s41380-019-0493-2
94. Son H, Baek JH, Go BS, Jung DH, Sontakke SB, Chung HJ, et al. Glutamine has antidepressive effects through increments of glutamate and glutamine levels and glutamatergic activity in the medial prefrontal cortex. Neuropharmacology. (2018) 143:143–52. doi: 10.1016/j.neuropharm.2018.09.040
95. Lei Y, Wang J, Wang D, Li C, Liu B, Fang X, et al. Sirt1 in forebrain excitatory neurons produces sexually dimorphic effects on depression-related behaviors and modulates neuronal excitability and synaptic transmission in the medial prefrontal cortex. Mol Psychiatry. (2020) 25:1094–111. doi: 10.1038/s41380-019-0352-1
96. Hare BD, Duman RS. Prefrontal cortex circuits in depression and anxiety: contribution of discrete neuronal populations and target regions. Mol Psychiatry. (2020) 25:2742–58. doi: 10.1038/s41380-020-0685-9
97. Duman RS, Sanacora G, Krystal JH. Altered connectivity in depression: gaba and glutamate neurotransmitter deficits and reversal by novel treatments. Neuron. (2019) 102:75–90. doi: 10.1016/j.neuron.2019.03.013
98. Warden MR, Selimbeyoglu A, Mirzabekov JJ, Lo M, Thompson KR, Kim SY, et al. A prefrontal cortex-brainstem neuronal projection that controls response to behavioural challenge. Nature. (2012) 492:428–32. doi: 10.1038/nature11617
99. Geddes SD, Assadzada S, Lemelin D, Sokolovski A, Bergeron R, Haj-Dahmane S, et al. Target-specific modulation of the descending prefrontal cortex inputs to the dorsal raphe nucleus by cannabinoids. Proc Natl Acad Sci USA. (2016) 113:5429–34. doi: 10.1073/pnas.1522754113
100. Srejic LR, Wood KM, Zeqja A, Hashemi P, Hutchison WD. Modulation of serotonin dynamics in the dorsal raphe nucleus via high frequency medial prefrontal cortex stimulation. Neurobiol Dis. (2016) 94:129–38. doi: 10.1016/j.nbd.2016.06.009
101. Zhou W, Jin Y, Meng Q, Zhu X, Bai T, Tian Y, et al. A neural circuit for comorbid depressive symptoms in chronic pain. Nat Neurosci. (2019) 22:1649–58. doi: 10.1038/s41593-019-0468-2
102. Wise RA. Dopamine, learning and motivation. Nat Rev Neurosci. (2004) 5:483–94. doi: 10.1038/nrn1406
103. Sidor MM, Spencer SM, Dzirasa K, Parekh PK, Tye KM, Warden MR, et al. Daytime spikes in dopaminergic activity drive rapid mood-cycling in mice. Mol Psychiatry. (2015) 20:1406–19. doi: 10.1038/mp.2014.167
104. Friedman AK, Walsh JJ, Juarez B, Ku SM, Chaudhury D, Wang J, et al. Enhancing depression mechanisms in midbrain dopamine neurons achieves homeostatic resilience. Science. (2014) 344:313–9. doi: 10.1126/science.1249240
105. Zhang H, Chaudhury D, Nectow AR, Friedman AK, Zhang S, Juarez B, et al. A- and B-adrenergic receptor-mediated mesolimbic homeostatic plasticity confers resilience to social stress in susceptible mice. Biol Psychiatry. (2019) 85:226–36. doi: 10.1016/j.biopsych.2018.08.020
106. Walsh JJ, Friedman AK, Sun H, Heller EA, Ku SM, Juarez B, et al. Stress and CRF gate neural activation of bdnf in the mesolimbic reward pathway. Nat Neurosci. (2014) 17:27–9. doi: 10.1038/nn.3591
107. Wook Koo J, Labonté B, Engmann O, Calipari ES, Juarez B, Lorsch Z, et al. Essential role of mesolimbic brain-derived neurotrophic factor in chronic social stress-induced depressive behaviors. Biol Psychiatry. (2016) 80:469–78. doi: 10.1016/j.biopsych.2015.12.009
108. He F, Zhang P, Zhang Q, Qi G, Cai H, Li T, et al. Dopaminergic projection from ventral tegmental area to substantia nigra pars reticulata mediates chronic social defeat stress-induced hypolocomotion. Mol Neurobiol. (2021) 58:5635–48. doi: 10.1007/s12035-021-02522-7
109. Root DH, Melendez RI, Zaborszky L, Napier TC. The ventral pallidum: subregion-specific functional anatomy and roles in motivated behaviors. Prog Neurobiol. (2015) 130:29–70. doi: 10.1016/j.pneurobio.2015.03.005
110. Knowland D, Lilascharoen V, Pacia CP, Shin S, Wang EH, Lim BK. Distinct ventral pallidal neural populations mediate separate symptoms of depression. Cell. (2017) 170:284–97.e18. doi: 10.1016/j.cell.2017.06.015
111. Lammel S, Lim BK, Ran C, Huang KW, Betley MJ, Tye KM, et al. Input-specific control of reward and aversion in the ventral tegmental area. Nature. (2012) 491:212–7. doi: 10.1038/nature11527
112. Fernandez SP, Broussot L, Marti F, Contesse T, Mouska X, Soiza-Reilly M, et al. Mesopontine cholinergic inputs to midbrain dopamine neurons drive stress-induced depressive-like behaviors. Nat Commun. (2018) 9:4449. doi: 10.1038/s41467-018-06809-7
113. Browne CJ, Abela AR, Chu D, Li Z, Ji X, Lambe EK, et al. Dorsal raphe serotonin neurons inhibit operant responding for reward via inputs to the ventral tegmental area but not the nucleus accumbens: evidence from studies combining optogenetic stimulation and serotonin reuptake inhibition. Neuropsychopharmacology. (2019) 44:793–804. doi: 10.1038/s41386-018-0271-x
114. Neumeister A, Wood S, Bonne O, Nugent AC, Luckenbaugh DA, Young T, et al. Reduced hippocampal volume in unmedicated, remitted patients with major depression versus control subjects. Biol Psychiatry. (2005) 57:935–7. doi: 10.1016/j.biopsych.2005.01.016
115. Fanselow MS, Dong HW. Are the dorsal and ventral hippocampus functionally distinct structures? Neuron. (2010) 65:7–19. doi: 10.1016/j.neuron.2009.11.031
116. Bagot RC, Parise EM, Peña CJ, Zhang HX, Maze I, Chaudhury D, et al. Ventral hippocampal afferents to the nucleus accumbens regulate susceptibility to depression. Nat Commun. (2015) 6:7062. doi: 10.1038/ncomms8062
117. Papp M, Gruca P, Lason M, Litwa E, Solecki W, Willner P. Insufficiency of ventral hippocampus to medial prefrontal cortex transmission explains antidepressant non-response. J Psychopharmacol. (2021) 35:1253–64. doi: 10.1177/02698811211048281
118. Carreno FR, Donegan JJ, Boley AM, Shah A, DeGuzman M, Frazer A, et al. Activation of a ventral hippocampus-medial prefrontal cortex pathway is both necessary and sufficient for an antidepressant response to ketamine. Mol Psychiatry. (2016) 21:1298–308. doi: 10.1038/mp.2015.176
119. Yun S, Reynolds RP, Petrof I, White A, Rivera PD, Segev A, et al. Stimulation of entorhinal cortex-dentate gyrus circuitry is antidepressive. Nat Med. (2018) 24:658–66. doi: 10.1038/s41591-018-0002-1
120. Zhang TR, Larosa A, Di Raddo ME, Wong V, Wong AS, Wong TP. Negative memory engrams in the hippocampus enhance the susceptibility to chronic social defeat stress. J Neurosci. (2019) 39:7576–90. doi: 10.1523/JNEUROSCI.1958-18.2019
121. Vialou V, Bagot RC, Cahill ME, Ferguson D, Robison AJ, Dietz DM, et al. Prefrontal cortical circuit for depression- and anxiety-related behaviors mediated by cholecystokinin: role of Δfosb. J Neurosci. (2014) 34:3878–87. doi: 10.1523/JNEUROSCI.1787-13.2014
122. Teissier A, Chemiakine A, Inbar B, Bagchi S, Ray RS, Palmiter RD, et al. Activity of raphé serotonergic neurons controls emotional behaviors. Cell Rep. (2015) 13:1965–76. doi: 10.1016/j.celrep.2015.10.061
123. Urban DJ, Zhu H, Marcinkiewcz CA, Michaelides M, Oshibuchi H, Rhea D, et al. Elucidation of the behavioral program and neuronal network encoded by dorsal raphe serotonergic neurons. Neuropsychopharmacology. (2016) 41:1404–15. doi: 10.1038/npp.2015.293
124. Nishitani N, Nagayasu K, Asaoka N, Yamashiro M, Andoh C, Nagai Y, et al. Manipulation of dorsal raphe serotonergic neurons modulates active coping to inescapable stress and anxiety-related behaviors in mice and rats. Neuropsychopharmacology. (2019) 44:721–32. doi: 10.1038/s41386-018-0254-y
125. Ohmura Y, Tsutsui-Kimura I, Sasamori H, Nebuka M, Nishitani N, Tanaka KF, et al. Different roles of distinct serotonergic pathways in anxiety-like behavior, antidepressant-like, and anti-impulsive effects. Neuropharmacology. (2020) 167:107703. doi: 10.1016/j.neuropharm.2019.107703
126. Proulx CD, Aronson S, Milivojevic D, Molina C, Loi A, Monk B, et al. A neural pathway controlling motivation to exert effort. Proc Natl Acad Sci USA. (2018) 115:5792–7. doi: 10.1073/pnas.1801837115
127. Tchenio A, Lecca S, Valentinova K, Mameli M. Limiting habenular hyperactivity ameliorates maternal separation-driven depressive-like symptoms. Nat Commun. (2017) 8:1135. doi: 10.1038/s41467-017-01192-1
128. Anderson SA, Michaelides M, Zarnegar P, Ren Y, Fagergren P, Thanos PK, et al. Impaired periamygdaloid-cortex prodynorphin is characteristic of opiate addiction and depression. J Clin Invest. (2013) 123:5334–41. doi: 10.1172/JCI70395
129. Cai YQ, Wang W, Paulucci-Holthauzen A, Pan ZZ. Brain circuits mediating opposing effects on emotion and pain. J Neurosci. (2018) 38:6340–9. doi: 10.1523/JNEUROSCI.2780-17.2018
130. Ramirez S, Liu X, MacDonald CJ, Moffa A, Zhou J, Redondo RL, et al. Activating positive memory engrams suppresses depression-like behaviour. Nature. (2015) 522:335–9. doi: 10.1038/nature14514
131. Rush AJ, Trivedi MH, Wisniewski SR, Nierenberg AA, Stewart JW, Warden D, et al. Acute and longer-term outcomes in depressed outpatients requiring one or several treatment steps: a Star*D report. Am J Psychiatry. (2006) 163:1905–17. doi: 10.1176/ajp.2006.163.11.1905
132. Akil H, Gordon J, Hen R, Javitch J, Mayberg H, McEwen B, et al. Treatment resistant depression: a multi-scale, systems biology approach. Neurosci Biobehav Rev. (2018) 84:272–88. doi: 10.1016/j.neubiorev.2017.08.019
133. Basso L, Bönke L, Aust S, Gärtner M, Heuser-Collier I, Otte C, et al. Antidepressant and neurocognitive effects of serial ketamine administration versus ECT in depressed patients. J Psychiatr Res. (2020) 123:1–8. doi: 10.1016/j.jpsychires.2020.01.002
134. Fuchikami M, Thomas A, Liu R, Wohleb ES, Land BB, DiLeone RJ, et al. Optogenetic stimulation of infralimbic pfc reproduces ketamine's rapid and sustained antidepressant actions. Proc Natl Acad Sci USA. (2015) 112:8106–11. doi: 10.1073/pnas.1414728112
135. Hare BD, Shinohara R, Liu RJ, Pothula S, DiLeone RJ, Duman RS. Optogenetic stimulation of medial prefrontal cortex DRD1 neurons produces rapid and long-lasting antidepressant effects. Nat Commun. (2019) 10:223. doi: 10.1038/s41467-018-08168-9
136. Hariz M. Twenty-five years of deep brain stimulation: celebrations and apprehensions. Mov Disord. (2012) 27:930–3. doi: 10.1002/mds.25007
137. Lozano AM, Lipsman N. Probing and regulating dysfunctional circuits using deep brain stimulation. Neuron. (2013) 77:406–24. doi: 10.1016/j.neuron.2013.01.020
138. Kennedy SH, Giacobbe P, Rizvi SJ, Placenza FM, Nishikawa Y, Mayberg HS, et al. Deep brain stimulation for treatment-resistant depression: follow-up after 3 to 6 years. Am J Psychiatry. (2011) 168:502–10. doi: 10.1176/appi.ajp.2010.10081187
139. Lozano AM, Giacobbe P, Hamani C, Rizvi SJ, Kennedy SH, Kolivakis TT, et al. A multicenter pilot study of subcallosal cingulate area deep brain stimulation for treatment-resistant depression. J Neurosurg. (2012) 116:315–22. doi: 10.3171/2011.10.JNS102122
140. Papp M, Gruca P, Lason M, Litwa E, Solecki W, Willner P. Ampa receptors mediate the pro-cognitive effects of electrical and optogenetic stimulation of the medial prefrontal cortex in antidepressant non-responsive Wistar-Kyoto rats. J Psychopharmacol. (2020) 34:1418–30. doi: 10.1177/0269881120967857
141. Sanders TH, Jaeger D. Optogenetic stimulation of cortico-subthalamic projections is sufficient to ameliorate bradykinesia in 6-ohda lesioned mice. Neurobiol Dis. (2016) 95:225–37. doi: 10.1016/j.nbd.2016.07.021
142. Sanders TH. Stimulation of cortico-subthalamic projections amplifies resting motor circuit activity and leads to increased locomotion in dopamine-depleted mice. Front Integr Neurosci. (2017) 11:24. doi: 10.3389/fnint.2017.00024
143. Creed M, Pascoli VJ, Lüscher C. Addiction therapy. Refining deep brain stimulation to emulate optogenetic treatment of synaptic pathology. Science. (2015) 347:659–64. doi: 10.1126/science.1260776
144. Stujenske JM, Spellman T, Gordon JA. Modeling the spatiotemporal dynamics of light and heat propagation for in vivo optogenetics. Cell Rep. (2015) 12:525–34. doi: 10.1016/j.celrep.2015.06.036
145. Owen SF, Liu MH, Kreitzer AC. Thermal constraints on in vivo optogenetic manipulations. Nat Neurosci. (2019) 22:1061–5. doi: 10.1038/s41593-019-0422-3
146. Sileo L, Bitzenhofer SH, Spagnolo B, Pöpplau JA, Holzhammer T, Pisanello M, et al. Tapered fibers combined with a multi-electrode array for optogenetics in mouse medial prefrontal cortex. Front Neurosci. (2018) 12:771. doi: 10.3389/fnins.2018.00771
147. Medina AB, Lechuga DA, Escandón OS, Moctezuma JV. Update of sleep alterations in depression. Sleep Sci. (2014) 7:165–9. doi: 10.1016/j.slsci.2014.09.015
148. Johnson SB, Emmons EB, Anderson RM, Glanz RM, Romig-Martin SA, Narayanan NS, et al. A basal forebrain site coordinates the modulation of endocrine and behavioral stress responses via divergent neural pathways. J Neurosci. (2016) 36:8687–99. doi: 10.1523/JNEUROSCI.1185-16.2016
Keywords: anhedonia, appetite, depression, neural circuit, optogenetics, social avoidance
Citation: Lin S, Du Y, Xia Y, Xie Y, Xiao L and Wang G (2022) Advances in optogenetic studies of depressive-like behaviors and underlying neural circuit mechanisms. Front. Psychiatry 13:950910. doi: 10.3389/fpsyt.2022.950910
Received: 23 May 2022; Accepted: 17 August 2022;
Published: 08 September 2022.
Edited by:
Kai Zhang, Anhui Medical University, ChinaReviewed by:
Yan Wei, Southwest Medical University, ChinaMariusz Stanisław Wiglusz, Medical University of Gdansk, Poland
Copyright © 2022 Lin, Du, Xia, Xie, Xiao and Wang. This is an open-access article distributed under the terms of the Creative Commons Attribution License (CC BY). The use, distribution or reproduction in other forums is permitted, provided the original author(s) and the copyright owner(s) are credited and that the original publication in this journal is cited, in accordance with accepted academic practice. No use, distribution or reproduction is permitted which does not comply with these terms.
*Correspondence: Gaohua Wang, d2doNjQwMkB3aHUuZWR1LmNu