- 1BioMag Laboratory, HUS Diagnostic Center, Helsinki University Hospital, University of Helsinki and Aalto University School of Science, Helsinki, Finland
- 2Motion Analysis Laboratory, New Children’s Hospital, Helsinki University Hospital and University of Helsinki, Helsinki, Finland
- 3HUS Medical Imaging Center, Clinical Neurophysiology, Clinical Neurosciences, Helsinki University Hospital and University of Helsinki, Helsinki, Finland
- 4Department of Technical Physics, University of Eastern Finland, Kuopio, Finland
- 5Department of Clinical Physiology and Nuclear Medicine, Kuopio University Hospital, Kuopio, Finland
- 6Department of Physical and Rehabilitation Medicine, Helsinki University Hospital and University of Helsinki, Helsinki, Finland
Objective: A novel protocol for paired associative stimulation (PAS), called high PAS, consists of high-intensity transcranial magnetic stimulation (TMS) and high-frequency peripheral nerve stimulation (PNS). High PAS was developed for spinal cord injury rehabilitation and targets plastic changes in stimulated pathways in the corticospinal tract, which improves motor function. As therapy interventions can last many weeks, it is important to fully understand the effects of high PAS, including its effect on the cardiovascular system. Heart rate variability (HRV) has been used to measure changes in both sympathetic and parasympathetic systems.
Methods: We used short-term HRV measurements to evaluate the effects of one 20-min session of high PAS on 17 healthy individuals. HRV was recorded for 5 min before (PRE), during (STIM), immediately after (POST), 30 min after (POST30), and 60 min after (POST60) the stimulation. Five participants repeated the HRV setup with sham stimulation.
Results: A significant decrease in low-frequency (LF) power (n.u.) (p = 0.002), low-frequency to high-frequency (HF) ratio (p = 0.017), in Poincaré plot [the standard deviation of RR intervals perpendicular to (SD1) and along (SD2) the line of identity SD2/SD1 ratio p < 0.001], and an increase in HF power (n.u.) (p = 0.002) were observed between PRE and STIM conditions; these changes were fully reversible immediately after stimulation. PRE to POST by 3% (p = 0.015) and continued to decline until POST60 by 5% (p = 0.011). LF power (ms2) (p = 0.017) and SD2 (p = 0.015) decreased from PRE to STIM and increased from PRE to POST (p = 0.025 and p = 0.017, respectively). The results from sham PAS exhibited a trend similar to active high-PAS stimulation.
Conclusions: High PAS does not have sustained effects during 60-min follow-up on cardiovascular functions, as measured by HRV. None of the short-term results indicates activation of the sympathetic nervous system in healthy individuals. Observed changes in HRV indicate higher parasympathetic activity during stimulation, which is reversible, and is plausibly explained by the fact that the participants spend 20 min without moving, talking, or using phones while being stimulated.
1. Introduction
Paired associative stimulation (PAS) is a non-invasive stimulation method (1) that may have potential for rehabilitation of patients with neurological disorders such as spinal cord injury (SCI) (2–7) and stroke (8, 9). PAS has also been used to investigate the mechanisms of disease and plasticity in Parkinson's disease (10), dystonia (11), and Huntington's disease (12), among others. Conventional PAS combines simultaneous transcranial magnetic stimulation (TMS) over the motor cortex (M1) and peripheral nerve stimulation (PNS) (1). Stimulation pairs are timed to synchronously activate presynaptic and postsynaptic neurons, leading to improved synaptic efficacy and inducing plastic changes in the stimulated pathways such as the corticospinal tract (5, 12, 13). The effects of PAS on the corticospinal tract can be evaluated as changes in motor-evoked potentials (MEPs) (14), which are the responses to motor-cortex stimulation recorded from the corresponding muscles (15).
Our group has developed a novel PAS protocol, called high PAS, which consists of a high-intensity TMS (100% of maximum stimulator output, MSO) and a high-frequency (100 Hz) PNS (5, 16, 17). We developed this protocol for SCI rehabilitation. The protocol is usually applied to a patient for many weeks (2, 4, 5, 18, 19), which highlights the importance of safety issues of the applied stimulation method, especially when the used TMS intensity is considered. Previously, no studies have reported the effects of PAS on cardiac functions or heart rate variability (HRV), which is the fluctuation in time between heartbeats. Single-pulse TMS is safe (20), as no effects on blood pressure and heart rate have been reported (21). However, no studies have reported the effects of single-pulse TMS on HRV (22). Studies that combine TMS with functional magnetic resonance imaging (fMRI) have revealed that a 1-Hz suprathreshold TMS on the primary motor cortex (M1) creates blood-oxygenation-level-dependent (BOLD) activation mainly in the ipsilateral hemisphere (23). Suprathreshold 4-Hz repetitive TMS also showed an increase of BOLD activation in M1 and in ipsilateral corticocortical connections (24).
HRV can be assessed in the time domain and frequency domain and using nonlinear Poincaré plots. In the time domain, the normal-to-normal (NN) intervals between successive ECG QRS complexes are analyzed. Standard deviation of NN intervals (SDNN) reflects the overall variability. In the root mean square of successive differences between RR intervals (RMSSD), the number of intervals greater than 50 ms (NN50) and the proportion of NN50 with the total number of NN intervals (pNN50) reflect beat-to-beat variability (25). An increase in beat-to-beat time-domain variables indicates activation of the parasympathetic nervous system. In certain circumstances, the SDNN may also increase because of the activation of the sympathetic nervous system. In frequency-domain analysis, HRV data are divided into the following three spectral components: very low frequency (VLF, 0–0.04 Hz), LF (0.04–0.15 Hz), and HF (0.15–0.4 Hz) (25). Breathing frequency usually falls under the HF band. Different frequency bands can be assessed as the relationship between sympathetic and vagally controlled LF and vagally controlled HF, calculated as an LF/HF relation or as normalized values expressing LF or HF contribution to total power excluding the VLF component (25). Oscillations in the HF band are driven by the vagus nerve and follow respiratory frequency. The LF component represents mostly sympathetic modulation (26). Therefore, assuming that respiratory frequency is within the HF band, the LF/HF ratio (usually between 1 and 2) and the normalized LF power represent sympathovagal balance (27). The ratio between the Poincaré plot standard deviations SD2 and SD1 (SD2/SD1) is an alternative approach for assessing sympathovagal balance, but without the assumption on respiratory rate (28).
HRV has been used to measure the functions of the autonomic nervous system, which consists of the sympathetic nervous system (responsible for increase in blood pressure and increase in heart rate) and parasympathetic nervous system (responsible for relaxation and slowing heart rate) (29). Cardiac function is controlled by various areas of the central nervous system, such as the anterior insula, hypothalamus, periaqueductal gray matter, parabrachial nucleus, and several regions of the medulla (30). Heart rate is controlled by both sympathetic and parasympathetic nerves at the sinoatrial node. The vagus nerve serves as an inhibitory control, decreasing the heart rate (31). The preganglionic parasympathetic cardiovascular neurons are located in the nucleus ambiguous, and their axons reach through the superior cervical and inferior thoracic rami to the cardiac ganglia (30). Parasympathetic influence inhibits the heart rate, as shown in studies where sympathetic inputs are blocked (25), and leads to a lower heart rate, thus conserving energy at rest (32). Parasympathetic activation is mediated by a synaptic release of acetylcholine with a short latency, whereas sympathetic activation results in a synaptic release of noradrenaline and an increase in heart rate (33).
Decreased HRV is associated with several diseases and all-cause mortality. Lower HRV can be an indicator of insufficient adaptability in the autonomic nervous system (32, 33). This decrease has been observed in variables in both time domain (SDNN) and frequency domain (HF, absolute power) parameters (25). However, simple activities such as talking or reading can affect HRV by altering breathing frequency (34). Normal HRV in healthy individuals is considered to be adaptable and self-regulatory (35), to improve emotion regulation (36), and to be flexible with environmental changes (37). For short-term measurements, a spectral analysis is used as an estimate for autonomic influence on HRV (38). In spectral analysis, HRV can be divided into VLF, LF, and HF components.
The purpose of this study was to identify acute cardiovascular effects, specifically HRV, of one 20-min session of high PAS on healthy participants. We developed a high PAS consisting of a high-intensity TMS and a high-frequency peripheral component for SCI rehabilitation. As stimulation therapies may last for several weeks, it is important to identify any possible unfavorable effects of high PAS on autonomic cardiac functions. The aim of this study was to validate the protocol on healthy individuals before investigating the effects on an SCI population.
2. Materials and methods
2.1. Participants
Seventeen healthy subjects (10 females, seven males, mean age 29 ± 7.8 years) were recruited for this study. Self-reported handedness (15 right, two left) and self-reported footedness (answer to the question “with which leg do you prefer to kick a ball?”; 15 right, two left) were documented. Exclusion criteria were brain pathology, implanted devices, regular medication, neurological diseases, cardiac diseases, psychiatric diseases, drug abuse, and pregnancy. Each participant provided a written informed consent prior to participation. The Medical Ethics Committee of the Helsinki University Hospital approved the study.
2.2. Experimental setup
The experimental session lasted up to 120 min, including 20 min of active stimulation (Figure 1). Prior to the stimulation, the participants were seated for 15 min to ensure that reliable data were generated from the heart rate and HRV without the effects of commute, climbing stairs, or other physical activities. Electrodes for electrocardiogram (ECG) monitor (Bittium Faros 180, Oulu, Finland) and electromyogram (EMG) for HRV and MEP recordings, respectively, were attached. We asked questions about variables that might possibly affect HRV at the beginning of the session (Table 1) and, from the answers to these questions, we confirmed that no confounding factors would cause a change in HRV variables. Five participants drank 1–4 cups of coffee before coming to the laboratory; these participants were habitual coffee drinkers.
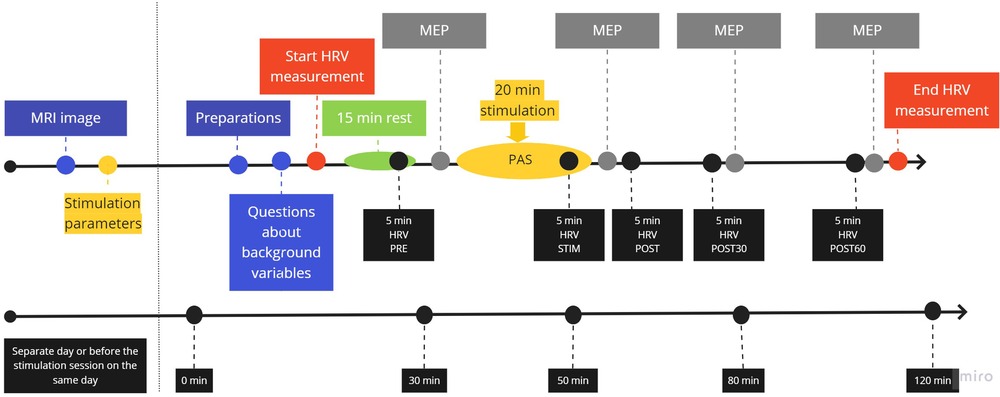
Figure 1. Protocol flowchart. An MRI image was taken and stimulation parameters were determined on a separate day or during the stimulation session before protocol initiation. Preparations included seating the participant comfortably, setting up HRV, attaching stimulation and EMG electrodes, and revising TMS stimulation location in the navigation system.
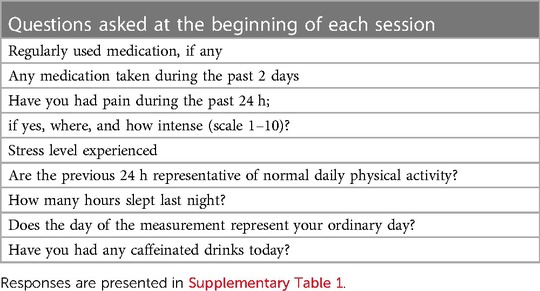
Table 1. List of questions asked in the beginning of each session about variables that might affect HRV measurement.
2.2.1. Transcranial magnetic stimulation
Navigated TMS (eXimia magnetic stimulator, Nexstim Ltd., Helsinki, Finland) was delivered using single pulses at 100% of the maximum stimulator output (MSO), corresponding to 172 V/m ± 2 measured 25 mm below the coil (NextStim NBS manual). Stimulation was performed with a figure-of-eight coil with an outer diameter of 70 mm. Individual stimulation parameters were determined on a different day or on the same day before initiation of the session. First, the stimulation target area for the left abductor hallucis muscle representation area was set using the MRI image and the TMS navigation system. The individual resting motor threshold (RMT) was then determined. MEP recordings were performed at 120% of RMT. MEP amplitude was recorded before, immediately after, and at 30 and 60 min after the stimulation.
2.2.2. Peripheral nerve stimulation
PNS was delivered as trains of six pulses (1-ms biphasic square pulses at 100 Hz). Stimulation (Dantec Keypoint® Natus Medical Incorporated, California, USA) was applied to the tibial nerve, which is behind the left medial malleolus. Stimulation intensity was individually determined during the same session with TMS parameters: the lowest intensity where F-responses were detectable was used for stimulation (39). Local anesthesia using 5% lidocaine/prilocaine (EMLA) ointment was offered to the participants to reduce the effect of the sensation of the pricking skin associated with PNS stimulation (40). Sixteen participants used this ointment.
2.2.3. Paired associative stimulation
We applied high PAS as previously described (5, 41). Presentation® software (Neurobehavioral Systems Inc., Albany, USA) was used to sync TMS and PNS pulses to be delivered with individual interstimulus interval (ISI) (41) once every 5 s. Altogether, 240 PAS sequences were administered during the 20-min stimulation. For five participants, HRV was also recorded during sham PAS, where TMS was delivered at 100% MSO with a 5-cm block between the scull and the coil. PNS electrodes did not deliver current.
2.2.4. Heart rate variability
HRV was measured by using a Bittium Faros 180 (Bittium corporation, Oulu, Finland) device using three ECG electrodes (Blue sensor, Ambu A/S, Ballenrup, Denmark) and a sampling frequency of 1,000 Hz. The ECG electrodes and the recording device were attached at the beginning of the session, recording was done throughout the session, and the device was removed at the end of the session. The following 5-min periods were used for HRV analysis: at the end of the 15-min rest period prior to stimulation (PRE), at the end of the 20-min stimulation period (STIM), immediately after post-stimulation MEP measurement (POST), and immediately before post 30 (POST30) and post 60 (POST60) MEP measurement (Figure 1). A representative 5-min segment from the area of interest without any major noise from movement or MEP stimulation was selected for the HRV analysis after obtaining agreement from two investigators. Thus, the timing of the selected analysis segments might vary by minutes within participants in relation to the protocol. HRV data were analyzed by using Kubios HRV Premium software (Kubios Ltd., Kuopio, Finland). On average, the software used beats correction on 1.2% of the data (maximum 2.8%). The analyzed variables are presented in Table 2.
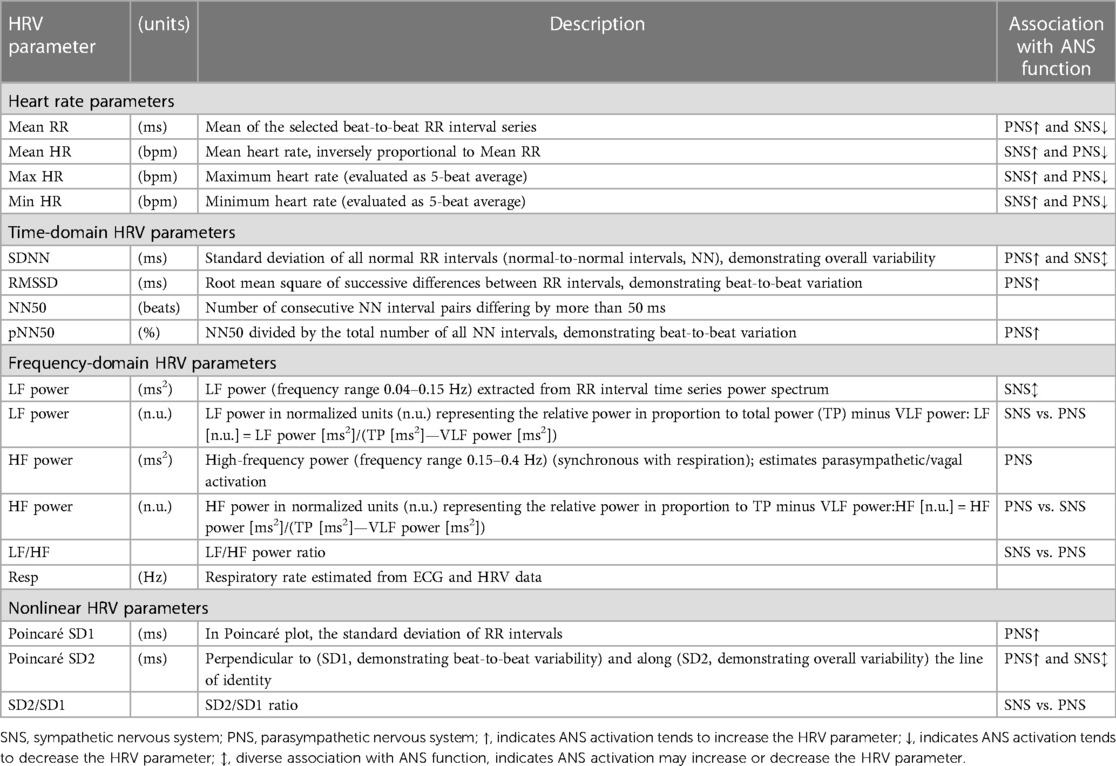
Table 2. Description of heart rate parameters, adopted time-domain, frequency domain, and non-linear HRV parameters and their main association to autonomic nervous system function.
HRV and MEP values were recorded during the 60-min follow-up period at 30 min and at 60 min post stimulation. The participants were asked to sit still, but were allowed to talk, to move a little, and use their phones.
2.3. Statistical analysis
Statistical analysis was performed by using IBM SPSS 27 software. Normality of the data was reviewed by using the Kolmogorov–Smirnov test. For normally distributed data, Levene's test showed that homogeneity was not met and thus the Friedman test and Wilcoxon signed-rank test were used with Bonferroni correction. The effect size was reported as Kendall's W for Friedman test and % change from PRE for the Wilcoxon signed-rank test. We compared the change in HRV variables and MEP amplitudes between PRE and STIM, POST, POST30, and POST60 time points. For determining the sex effect, the Mann–Whitney U test was used and η2 was reported as the effect size. This test was also used for pairwise comparison between PAS and PAS sham sessions.
3. Results
HRV frequency limits were set to LF (0.04–0.15 Hz) and HF (0.15–0.40 Hz). For one participant who had higher respiration frequency, we exceptionally set the higher limit to 0.50 Hz. The data recorded from POST30 min were removed for one participant because his respiration frequency fell under the low-frequency band. One participant had an unpleasant sensation and cold sweat during PAS, but the participant did not want the experiment to be stopped. No other adverse events were observed. The significance level was adjusted with Bonferroni correction to 0.012.
The results obtained from 5-min analysis windows for HRV and MEP measurements from different time points are presented in Table 3. Consistent with our previous work (16, 19, 42), PAS induced significant MEP potentiation (p < 0.001; Kendall's W 0.361). POST, POST30, and POST60 were significantly different from PRE (p < 0.001, p = 0.025, and p = 0.015), respectively. There were no significant differences in mean RR, mean HR, RMSSD, NN50, pNN50, HF power (ms2), resp (Hz), and SD1. Friedman's test showed a significant difference for SDNN (p = 0.003, Kendall's W = 0.234), LF power ms2 (p < 0.001, Kendall's W = 0.379), LF power n.u. (p < 0.001, Kendall's W = 0.305), HF power n.u. (p < 0.001, Kendall's W = 0.291), LF/HF ratio (p < 0.001, Kendall's W = 0.305), SD2 (p < 0.001, Kendall's W = 0.284), and SD2/SD1 ratio (p < 0.001, Kendall's W = 0.388). A further analysis with the Wilcoxon signed-rank test showed significant differences between PRE and STIM on HR max (p = 0.001), LF power (ms2) (p = 0.017), LF power n.u. (p = 0.002), HF power n.u. (p = 0.002), and the SD2/SD1 ratio (p = 0.000) (Table 3). HR min continued to decrease POST (p = 0.015), POST30 (p = 0.028), and POST60 (p = 0.011).
The most pronounced but reversible differences were observed between PRE and STIM on LF power n.u. (p = 0.002) and HF power n.u. (p = 0.002) (Table 3). To assess whether these differences resulted from sitting still and not specifically from PAS, we performed additional sham stimulation in five participants (Figure 2). Although a similar trend was observed for sham stimulation as was the case for active stimulation, because of the smaller number of participants, the changes within the sham session were not significant (Min HR (p = 0.277), LF power n.u. (p = 0.145), and HF power n.u. (p = 0.145). Pairwise comparison with the PAS session was also not significant.
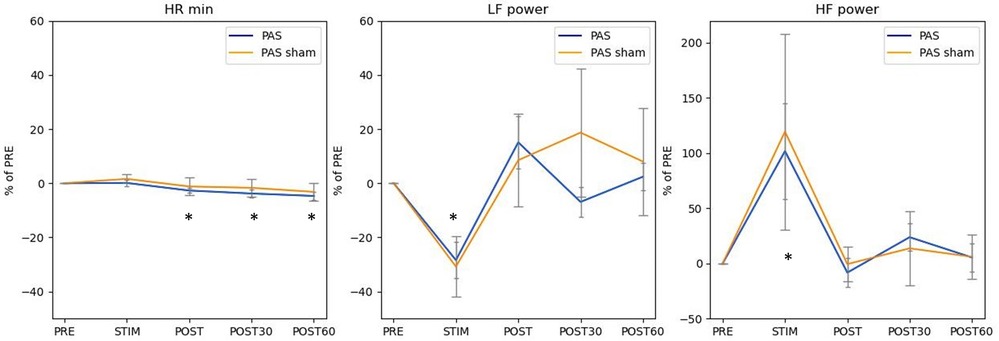
Figure 2. Similar pattern of HR min, LF power (n.u.), and HF power (n.u.) across conditions in both sham (wider error bar caps, n = 5), and active stimulation (n =17). Results are presented as % of PRE condition with standard error of mean. * Statistical difference compared to pre p < 0.05.
The effect of sex on HRV variables is presented in Table 4. Females had an overall higher HR (p < 0.001), a smaller LF power (p < 0.001), and a correspondingly higher HF power (p < 0.001). Females also had a higher respiration frequency (p = 0.007). In the time-domain variables, there were no significant differences between male and female participants. There was a significant difference between males and females at PRE stimulation for LF power (n.u.) (p = 0.013), HF power (n.u.) (p = 0.013), and LF/HF ratio (p = 0.013).
4. Discussion
Our results show that any effect of PAS on HRV is short term, and that the parasympathetic system is transiently activated during the PAS session. Although most changes appeared in the HRV frequency domain, the time domain and nonlinear parameters showed significant changes during and after stimulation compared with PRE. Most of the changes reverted to prestimulation levels immediately after stimulation. HR min continued to decline throughout the observation period up to post 60 min, although the extent of decline was only 5%. PAS did not cause any activation of the sympathetic system.
Our results are similar to that of Yoshida et al. (22). After stimulation, they found that HF power increased after a 25 min supine, indicating activation of the parasympathetic nervous system. They delivered TMS with 0.2 Hz at 90% of the motor threshold, whereas the intensity was maximum stimulator output in our study (22). Other neuromodulation methods, such as anodal transcranial direct current (tDCS), have been shown to reduce the heart rate and modify HRV with an increase in SDNN (43, 44), although there is no consensus in the literature (45). In the frequency domain, an increase in the LF, LF/HF ratio (43), and HF (44) has been observed with anodal tDCS.
Activation of the parasympathetic system may be attributed to 20 min of sitting still and listening to the cyclical sound of TMS. In our previous clinical work, some patients had a tendency to fall asleep during high-PAS treatment (5). The participants were asked to sit still after stimulation, although minimal but little movement (such as changing position, talking, and using a phone) was permitted. Bernardi et al. studied the effects of silent reading, free talking, and controlled breathing on HRV and reported a decrease in RR and an increase in the LF band with silent reading and an increase in both during free talking (34); the effect appeared to be mediated by changes in respiratory frequency. In this study, no changes were observed in respiratory frequency between measurement time points.
Sham control with five participants showed a similar pattern in results, when compared with a regular high-PAS session; a similar significance level as in active treatment was not achieved because of the lower participant number. This indicates that PAS stimulation alone does not plausibly create profound changes in the cardiovascular system. Probable mechanisms for HRV changes that could have activated the parasympathetic nervous system are remaining still for 20 min without talking, using a phone, or moving while listening to the cyclic sound from the stimulator.
There are several confounding factors in HRV measurements that can influence the results. Slow breathing (around six cycles per minute) can increase vagally mediated HRV variables such as RMSSD and LF (46). In our study, no change was observed in breathing frequency between time points. Age, sex, and physical or mental illness may influence HR and HRV (45). Females have a higher heart rate and lower RR interval variability, and in the frequency domain, females have a lower total power (47). In this study, significant differences between male and female participants were found in heart rate parameters and in frequency-domain HRV variables. HRV was not compared between individuals in this study, but changes in HRV were observed over time intraindividually. Five of 17 participants did not remember the instructions to not drink coffee on the morning of the measurement. However, the influence of caffeine on autonomic control of the heart is inconclusive in the literature (48). For example, Rauh et al. have shown that caffeine does not alter HRV in habitual drinkers (49).
High PAS has been developed as a rehabilitation tool for patients with neurological conditions, especially spinal cord injury. In this study, we investigated the effects of high PAS on acute cardiovascular effects in healthy participants. However, more research is needed to determine the relevance of these results in different patient groups.
5. Conclusion
This study revealed that high PAS is safe and does not show any cardiovascular effects over a 60-min follow-up. An increase in the HF band indicates activation of the parasympathetic system during the stimulation, plausibly due to sitting still without any disturbance. The results indicated that HRV parameters return to normal as soon as normal activity is resumed after PAS stimulation. This also indicates that high PAS is not excessively stressful for the participants, which is important and beneficial for its further development as a therapy for neurological patients.
Data availability statement
The raw data supporting the conclusions of this article will be made available by the authors without any undue reservation.
Ethics statement
The studies involving human participants were reviewed and approved by the Helsinki University Hospital Regional Committee on Medical Research Ethics. The patients/participants provided their written informed consent to participate in this study.
Author contributions
PH, EK, MT, and AS contributed to study conception and design. KH and AN performed data collection and organized the database. PH and AN selected representative data for analysis. PH and AS performed statistical analyses. PH wrote the first draft of the manuscript. All authors contributed to the article and approved the submitted version.
Funding
This work was funded by the Sigrid Juselius Foundation (AS), the Academy of Finland (AS) (Grant number 324160), and the Helsinki University Hospital governmental subsidiary funds (AS).
Acknowledgments
We thank David Danger for providing excellent technical assistance (MRI) and Hanna Granroth-Wilding for advice on statistical analysis.
Conflict of interest
The authors declare that the research was conducted in the absence of any commercial or financial relationships that could be construed as a potential conflict of interest.
Publisher's note
All claims expressed in this article are solely those of the authors and do not necessarily represent those of their affiliated organizations, or those of the publisher, the editors and the reviewers. Any product that may be evaluated in this article, or claim that may be made by its manufacturer, is not guaranteed or endorsed by the publisher.
Supplementary material
The Supplementary Material for this article can be found online at: https://www.frontiersin.org/articles/10.3389/fresc.2023.1200958/full#supplementary-material
References
1. Stefan K, Kunesch E, Cohen LG, Benecke R, Classen J. Induction of plasticity in the human motor cortex by paired associative stimulation. Brain. (2000) 123(Pt 3):572–84. doi: 10.1093/brain/123.3.572
2. Rodionov A, Savolainen S, Kirveskari E, Mäkelä JP, Shulga A. Effects of long-term paired associative stimulation on strength of leg muscles and walking in chronic tetraplegia: a proof-of-concept pilot study. Front Neurol. (2020) 11:397. doi: 10.3389/fneur.2020.00397
3. Tolmacheva A, Savolainen S, Kirveskari E, Brandstack N, Mäkelä JP, Shulga A. Paired associative stimulation improves hand function after non-traumatic spinal cord injury: a case series. Clin Neurophysiol Pract. (2019) 4:178–83. doi: 10.1016/j.cnp.2019.07.002
4. Tolmacheva A, Savolainen S, Kirveskari E, Lioumis P, Kuusela L, Brandstack N, et al. Long-term paired associative stimulation enhances motor output of the tetraplegic hand. J Neurotrauma. (2017) 34:2668–74. doi: 10.1089/neu.2017.4996
5. Shulga A, Lioumis P, Kirveskari E, Savolainen S, Mäkelä JP. A novel paired associative stimulation protocol with a high-frequency peripheral component: a review on results in spinal cord injury rehabilitation. Eur J Neurosci. (2021) 53:3242–57. doi: 10.1111/ejn.15191
6. Rodionov A, Savolainen S, Kirveskari E, Mäkelä JP, Shulga A. Restoration of hand function with long-term paired associative stimulation after chronic incomplete tetraplegia: a case study. Spinal Cord Ser Cases. (2019) 5:81. doi: 10.1038/s41394-019-0225-5
7. Bunday KL, Perez MA. Motor recovery after spinal cord injury enhanced by strengthening corticospinal synaptic transmission. Curr Biol. (2012) 22:2355–61. doi: 10.1016/j.cub.2012.10.046
8. Palmer JA, Wolf SL, Borich MR. Paired associative stimulation modulates corticomotor excitability in chronic stroke: a preliminary investigation. Restor Neurol Neurosci. (2018) 36:183–94. doi: 10.3233/RNN-170785
9. Castel-Lacanal E, Marque P, Tardy J, Boissezon X, Guiraud V, Chollet F, et al. Induction of cortical plastic changes in wrist muscles by paired associative stimulation in the recovery phase of stroke patients. Neurorehabil Neural Repair. (2009) 23:366–72. doi: 10.1177/1545968308322841
10. Ueki Y, Mima T, Ali Kotb M, Sawada H, Saiki H, Ikeda A, et al. Altered plasticity of the human motor cortex in Parkinson's disease. Ann Neurol. (2006) 59:60–71. doi: 10.1002/ana.20692
11. Meunier S, Russmann H, Shamim E, Lamy J-C, Hallett M. Plasticity of cortical inhibition in dystonia is impaired after motor learning and paired-associative stimulation. Eur J Neurosci. (2012) 35:975–86. doi: 10.1111/j.1460-9568.2012.08034.x
12. Suppa A, Quartarone A, Siebner H, Chen R, Lazzaro VD, Giudice PD, et al. The associative brain at work: evidence from paired associative stimulation studies in humans. Clin Neurophysiol. (2017) 128:2140–64. S1388-2457(17)30920-3 [pii] 28938144
13. Taylor JL, Martin PG. Voluntary motor output is altered by spike-timing-dependent changes in the human corticospinal pathway. J Neurosci. (2009) 29:11708–16. doi: 10.1523/JNEUROSCI.2217-09.2009
14. Rothwell JC, Hallett M, Berardelli A, Eisen A, Rossini P, Paulus W. Magnetic stimulation: motor evoked potentials. The International Federation of Clinical Neurophysiology. Electroencephalogr Clin Neurophysiol Suppl. (1999) 52:97–103. PMID: 10590980.10590980
15. Bestmann S, Krakauer JW. The uses and interpretations of the motor-evoked potential for understanding behaviour. Exp Brain Res. (2015) 233:679–89. doi: 10.1007/s00221-014-4183-7
16. Tolmacheva A, Mäkelä JP, Shulga A. Increasing the frequency of peripheral component in paired associative stimulation strengthens its efficacy. Sci Rep. (2019) 9:3849. doi: 10.1038/s41598-019-40474-0
17. Shulga A, Zubareva A, Lioumis P, Mäkelä JP. Paired associative stimulation with high-frequency peripheral component leads to enhancement of corticospinal transmission at wide range of interstimulus intervals. Front Hum Neurosci. (2016) 10:470. doi: 10.3389/fnhum.2016.00470
18. Vanhanen J, Parkkonen L, Mäkelä JP, Tolmacheva A, Shulga A, Rodionov A, et al. Effect of long-term paired associative stimulation on the modulation of cortical sensorimotor oscillations after spinal cord injury. Spinal Cord Ser Cases. (2022) 8:38. doi: 10.1038/s41394-022-00506-w
19. Shulga A, Lioumis P, Zubareva A, Brandstack N, Kuusela L, Kirveskari E, et al. Long-term paired associative stimulation can restore voluntary control over paralyzed muscles in incomplete chronic spinal cord injury patients. Spinal Cord Ser Cases. (2016) 2:16016. doi: 10.1038/scsandc.2016.16
20. Anand S, Hotson J. Transcranial magnetic stimulation: neurophysiological applications and safety. Brain Cogn. (2002) 50:366–86. doi: 10.1016/S0278-2626(02)00512-2
21. Chokroverty S, Hening W, Wright D, Walczak T, Goldberg J, Burger R, et al. Magnetic brain stimulation: safety studies. Electroencephalogr Clin Neurophysiol. (1995) 97:36–42. doi: 10.1016/0924-980X(94)00250-B
22. Yoshida T, Yoshino A, Kobayashi Y, Inoue M, Kamakura K, Nomura S. Effects of slow repetitive transcranial magnetic stimulation on heart rate variability according to power spectrum analysis. J Neurol Sci. (2001) 184:77–80. doi: 10.1016/S0022-510X(00)00505-0
23. Bohning D, Shastri A, McConnell K, Nahas Z, Lorberbaum J, Roberts D, et al. A combined TMS/fMRI study of intensity-dependent TMS over motor cortex. Biol Psychiatry. (1999) 45:385–94. doi: 10.1016/S0006-3223(98)00368-0
24. Bestmann S, Baudewig J, Siebner HR, Rothwell JC, Frahm J. Subthreshold high-frequency TMS of human primary motor cortex modulates interconnected frontal motor areas as detected by interleaved fMRI-TMS. NeuroImage. (2003) 20:1685–96. doi: 10.1016/j.neuroimage.2003.07.028
25. Heart rate variability: standards of measurement, physiological interpretation, and clinical use. Task Force of the European Society of Cardiology and the North American Society of Pacing and Electrophysiology. Circulation. (1996) 93:1043–65. doi: 10.1161/01.CIR.93.5.1043
26. Stauss HM. Heart rate variability. Am J Phys Regul Integr Comp Physiol. (2003) 285:R927–31. doi: 10.1152/ajpregu.00452.2003
27. Cygankiewicz I, Zareba W. Heart rate variability. In: Buijs RM, Swaab DF, editors. Handbook of clinical neurology. Amsterdam, The Netherlands: Elsevier B.V. (2013). p. 379–93. doi: 10.1016/B978-0-444-53491-0.00031-6
28. Hsu C-H, Tsai M-Y, Huang G-S, Lin T-C, Chen K-P, Ho S-T, et al. Poincaré plot indexes of heart rate variability detect dynamic autonomic modulation during general anesthesia induction. Acta Anaesthesiol Taiwan. (2012) 50:12–8. doi: 10.1016/j.aat.2012.03.002
29. Ishaque S, Khan N, Krishnan S. Trends in heart-rate variability signal analysis. Front Digit Health. (2021) 3:639444. doi: 10.3389/fdgth.2021.639444
30. Palma J-A, Benarroch EE. Neural control of the heart: recent concepts and clinical correlations. Neurology. (2014) 83:261–71. doi: 10.1212/WNL.0000000000000605
31. Thayer JF. On the importance of inhibition: central and peripheral manifestations of nonlinear inhibitory processes in neural systems. Dose Response. (2006) 4:2–21. doi: 10.2203/dose-response.004.01.002.Thayer
32. Thayer JF, Sternberg E. Beyond heart rate variability: vagal regulation of allostatic systems. Ann N Y Acad Sci. (2006) 1088:361–72. doi: 10.1196/annals.1366.014
33. Pumprla J, Howorka K, Groves D, Chester M, Nolan J. Functional assessment of heart rate variability: physiological basis and practical applications. Int J Cardiol. (2002) 84:1–14. doi: 10.1016/S0167-5273(02)00057-8
34. Bernardi L, Wdowczyk-Szulc J, Valenti C, Castoldi S, Passino C, Spadacini G, et al. Effects of controlled breathing, mental activity and mental stress with or without verbalization on heart rate variability. J Am Coll Cardiol. (2000) 35:1462–9. doi: 10.1016/S0735-1097(00)00595-7
35. Shaffer F, Ginsberg JP. An overview of heart rate variability metrics and norms. Front Public Health. (2017) 5:258. doi: 10.3389/fpubh.2017.00258
36. Smith R, Thayer JF, Khalsa SS, Lane RD. The hierarchical basis of neurovisceral integration. Neurosci Biobehav Rev. (2017) 75:274–96. doi: 10.1016/j.neubiorev.2017.02.003
37. Stange JP, Hamilton JL, Fresco DM, Alloy LB. Flexible parasympathetic responses to sadness facilitate spontaneous affect regulation. Psychophysiol. (2017) 54:1054–69. doi: 10.1111/psyp.12856
38. Malik M, Hnatkova K, Huikuri HV, Lombardi F, Schmidt G, Zabel M. Crosstalk proposal: heart rate variability is a valid measure of cardiac autonomic responsiveness. J Physiol. (2019) 597:2595–8. doi: 10.1113/JP277500
39. Pohjonen M, Nyman A-L, Kirveskari E, Arokoski J, Shulga A. Optimal peripheral nerve stimulation intensity for paired associative stimulation with high-frequency peripheral component in healthy subjects. Sci Rep. (2022) 12:12466. doi: 10.1038/s41598-022-16811-1
40. Gajraj NM, Pennant JH, Watcha MF. Eutectic mixture of local anesthetics (EMLA) cream. Anesth Analg. (1994) 78:574–83. PMID: 7818623.7818623
41. Shulga A, Lioumis P, Kirveskari E, Savolainen S, Makela JP, Ylinen A. The use of F-response in defining interstimulus intervals appropriate for LTP-like plasticity induction in lower limb spinal paired associative stimulation. J Neurosci Methods. (2015) 242C:112–7. doi: 10.1016/j.jneumeth.2015.01.012
42. Mezes M, Havu R, Tolmacheva A, Lioumis P, Makela JP, Shulga A. The impact of TMS and PNS frequencies on MEP potentiation in PAS with high-frequency peripheral component. PLoS One. (2020) 15:e0233999. doi: 10.1371/journal.pone.0233999
43. Gu Z, Chen W, Lu Q, Dai J, Hu S, Xu K, et al. Anodal high-definition transcranial direct current stimulation reduces heart rate and modulates heart-rate variability in healthy young people: a randomized cross-controlled trial. Front Cardiovasc Med. (2022) 9:1070157. doi: 10.3389/fcvm.2022.1070157
44. Petrocchi N, Piccirillo G, Fiorucci C, Moscucci F, Di Iorio C, Mastropietri F, et al. Transcranial direct current stimulation enhances soothing positive affect and vagal tone. Neuropsychologia. (2017) 96:256–61. doi: 10.1016/j.neuropsychologia.2017.01.028
45. Schmaußer M, Hoffmann S, Raab M, Laborde S. The effects of noninvasive brain stimulation on heart rate and heart rate variability: a systematic review and meta-analysis. J Neurosci Res. (2022) 100:1664–94. doi: 10.1002/jnr.25062
46. Laborde S, Allen MS, Borges U, Dosseville F, Hosang TJ, Iskra M, et al. Effects of voluntary slow breathing on heart rate and heart rate variability: a systematic review and a meta-analysis. Neurosci Biobehav Rev. (2022) 138:104711. doi: 10.1016/j.neubiorev.2022.104711
47. Koenig J, Thayer JF. Sex differences in healthy human heart rate variability: a meta-analysis. Neurosci Biobehav Rev. (2016) 64:288–310. doi: 10.1016/j.neubiorev.2016.03.007
48. Porto AA, Benjamim CJR, Gonzaga LA, Luciano de Almeida M, Bueno Júnior CR, Garner DM, et al. Caffeine intake and its influences on heart rate variability recovery in healthy active adults after exercise: a systematic review and meta-analysis. Nutr Metab Cardiovasc Dis. (2022) 32:1071–82. doi: 10.1016/j.numecd.2022.01.015
Keywords: transcranial magnetic stimulation, peripheral nerve stimulation, parasympathetic activity, autonomic nervous system, cardiovascular function
Citation: Haakana P, Holopainen K, Nätkynmäki A, Kirveskari E, Tarvainen MP and Shulga A (2023) The effect of paired associative stimulation with a high-intensity cortical component and a high-frequency peripheral component on heart rate and heart rate variability in healthy subjects. Front. Rehabil. Sci. 4:1200958. doi: 10.3389/fresc.2023.1200958
Received: 5 April 2023; Accepted: 11 July 2023;
Published: 26 July 2023.
Edited by:
Gustavo Balbinot, University Health Network (UHN), CanadaReviewed by:
Shirin Tajali, Toronto Rehabilitation Institute, University Health Network, CanadaLjubica Konstantinovic, University of Belgrade, Serbia
© 2023 Haakana, Holopainen, Nätkynmäki, Kirveskari, Tarvainen and Shulga. This is an open-access article distributed under the terms of the Creative Commons Attribution License (CC BY). The use, distribution or reproduction in other forums is permitted, provided the original author(s) and the copyright owner(s) are credited and that the original publication in this journal is cited, in accordance with accepted academic practice. No use, distribution or reproduction is permitted which does not comply with these terms.
*Correspondence: A. Shulga YW5hc3Rhc2lhLnNodWxnYUBoZWxzaW5raS5maQ==
†These authors have contributed equally to this work