Driver's Seat: Understanding Divergent Zoochorous Dispersal of Propagules
- 1Institute for Global Food Security, School of Biological Science, Medical Biology Centre, Queen's University Belfast, Belfast, United Kingdom
- 2Queen's Marine Laboratory, Queen's University Belfast, Portaferry, United Kingdom
- 3School of Biological, Earth and Environmental Sciences & Environmental Research Institute, University College Cork, Cork, Ireland
The functional role, relative importance, and the spatial and temporal parameters of different vector species, which underpin the passive dispersal (zoochory) of organisms (or their propagules), are frequently poorly understood. Accordingly, a conceptual framework capable of providing a rigorous and unified assessment for the dispersal capacity of vector species is required. Here, we propose and apply a series of novel metrics, the Dispersal Potential (DP), the Relative Dispersal Potential (RDP), and the Combined Dispersal Potential (CDP), to predict and classify likely dispersal and vector importance. In essence, DP = Np × Tv, whereby Np is the per capita propagule load (e.g., mean, minimum, or maximum abundance) or species richness of propagules carried per individual vector species, while Tv is the total number of possible vectors (e.g., individuals of a single species at a source site, local scale abundances, or entire continental populations). Further, the ratio based metric RDP allows for DP comparison between species, while the CDP accumulates the DP of a variety of vector species. An additional Relative CDP (RCDP) metric facilitates comparison between the CDP for multiple vectors to that of one or more additional vectors. The proposed metrics can also be used to assess intraspecific differences (e.g., ontogeny). Accordingly, we examine a variety of case studies and present calculations to ascertain the usefulness of our proposed metrics. Overall, the metrics can be used to quantify and rank the prominence of different dispersers that facilitate biological connectivity. Finally, we argue that adoption of these metrics and variants thereof, will provide a more realistic measure of species' functional roles than examination of interaction intensities alone, which will enhance understanding of zoochory within and across dispersal networks.
Introduction
Dispersal is an essential ecological process which impacts biodiversity through metacommunity and population dynamics across multiple spatial scales (Leibold et al., 2004; Shurin et al., 2009; Moritz et al., 2013). In particular, dispersal events can impact species establishment, demographic viability, and eco-evolutionary dynamics (Trakhtenbrot et al., 2005; García et al., 2017). Moreover, assisted dispersal away from the natal site can increase species distribution, aid enemy-release, and reduce both inter- and intraspecific competition for resources (Corlett, 2017). The assisted transport of plants and invertebrates by more mobile organisms (zoochory) is considered to be a key mechanism for both long- and short-distance dispersal, and can be essential to the maintenance of connectivity between isolated habitat patches on a metacommunity scale (Nathan, 2006; Viana et al., 2016; van Leeuwen, 2018). However, despite the ecological importance of assisted dispersal, current knowledge of zoochorous interactions within terrestrial (Corlett, 2017; Hämäläinen et al., 2017; Castañeda et al., 2018; Steyaert et al., 2018), and aquatic ecosystems (Tesson et al., 2015; Coughlan et al., 2017a,b; Lovas-Kiss et al., 2018a,b,c), frequently remains limited.
To date, the majority of studies examining animal-mediated dispersal have focused on the transport of fleshy fruits in terrestrial systems (Figure 1; Czarnecka and Kitowski, 2013; Coughlan et al., 2017a; Bartel et al., 2018; Lovas-Kiss et al., 2018c). Despite this, evidence suggests that the assisted dispersal of whole organisms or propagule stages that lack a fleshy fruit, e.g., seeds, spores, eggs, ephippia, gemmules, statoblasts, or cysts, frequently occurs through either endozoochory (internal transport within the digestive system: Pellerin et al., 2016; Lovas-Kiss et al., 2018b,c) or ectozoochory (external adherence; synonyms epizoochorous, exozoochorous: Coughlan et al., 2017a; Lovas-Kiss et al., 2018a). However, whilst frugivory-based dispersal networks have received substantial scientific attention, many basic questions concerning the relative importance of individual frugivorous vector species, propagule survival, and likely dispersal distances, frequently remain unanswered (Corlett, 2017). For example, due to the destruction of seeds or lack of post consumption movements, consumers of fruits may not necessarily act as viable seed dispersers (Simmons et al., 2018). These issues are further magnified in non-frugivorous dispersal networks, such as those operating between isolated aquatic habitats, which have been considerably less studied (Figure 1; Coughlan et al., 2017a,b; Lovas-Kiss et al., 2018a,b,c; van Leeuwen, 2018).
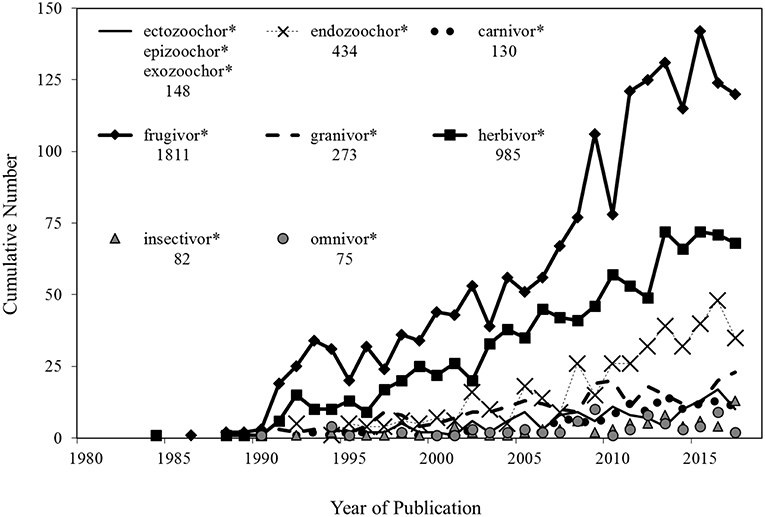
Figure 1. The annual cumulative number of zoochory-related publications cataloged within the on-line scientific database Thomson-Reuters Web of Science, 1st January 1980–31st December 2017. Data were extracted through a Topic Search using the zoochorous and dietary terminologies shown, which were combined with the additional search terms of “seed,” “propagule,” “dispersal,” and “dispersion” to create a search string. For example: (endozoochor*) AND (seed OR propagule) AND (dispersal OR dispersion). The total number of publications for each examined search string are shown. Ectozoochor* was combined with frequently used synonyms, epizoochor*, and exozoochor*.
Although an array of valuable ecosystem services provided by dispersers have been repeatedly documented, the functional role of vector species, even possible keystone dispersers in mutualistic systems, remains poorly studied (Farwig and Berens, 2012; Mello et al., 2015). Moreover, research has revealed that vectors not traditionally associated with the dispersal of certain propagule taxa, frequently facilitate substantial dispersal events (Vanschoenwinkel et al., 2011; Farmer et al., 2017; Hämäläinen et al., 2017; Bartel et al., 2018). Dispersal events can be considered “primary dispersal” when organisms adhere to the external surfaces of vector species, or when dispersers feed directly on seeds, fruits, invertebrates or other propagule structures but fail to digest all of them (Coughlan et al., 2017b; Lovas-Kiss et al., 2018b,c). Additionally “secondary dispersal” can occur when a predator consumes a prey item that has itself already ingested a propagule, resulting in the predator facilitating a transport event (Hämäläinen et al., 2017; van Leeuwen et al., 2017). A predator may also secondarily disperse through the ingestion of propagules found adhering to the external surfaces of prey (Lovas-Kiss et al., 2018a). Thus, animal-mediated dispersal is underpinned by a broad range of specific interactions between disperser and propagule.
Although the number of studies examining zoochory continues to increase (Figure 1), in many cases only the abundance, diversity and subsequent viability of propagules recovered from vectors, i.e., propagule load, are reported (Coughlan et al., 2017a). In a more advanced examination of assisted dispersal, particularly in relation to frugivory research, construction, and assessment of dispersal networks has highlighted a complex web of interactions between propagules and potential dispersers (Simmons et al., 2018; Timóteo et al., 2018). In essence, networks are simplified representations of reality which can be used to assess the overall patterns across a complex web of interaction frequencies (Heleno et al., 2014). Although the proportional interactions of both different vector species and propagules are frequently examined, greater consideration of the relationship between assisted dispersal and the temporal or spatial variation of vectors species abundance is required (Gleditsch et al., 2017; Kleyheeg et al., 2017; Andresen et al., 2018; Steyaert et al., 2018). In particular, taxon-specific dispersal and interaction networks should not be considered in isolation. For example, a poor vector species may have a high proportion of interactions with a specific taxon, yet these interactions may lead to almost zero dispersal (Simmons et al., 2018). The extensiveness and success of dispersal events will depend on both the identities and abundance of vector species (Peredo et al., 2013; Rumeu et al., 2017). Moreover, even poor or infrequent facilitators of assisted dispersal may make substantial contributions to the transport of propagules at high vector densities. Therefore, the number of propagules dispersed by each individual is an important component of the dispersal process (Schupp et al., 2010). In particular, even at low densities, large bodied dispersers can have a profound impact on dispersal networks, which is in part due to either their high per capita ingestion of propagules (Vidal et al., 2013), or the increased surface area for external propagule attachment. Accordingly, as the number of studies of animal-mediated dispersal continue to increase (Figure 1), there is a clear and timely need to begin analyzing dispersal data in a more quantitative manner.
Basic ecological networks facilitate mapping of observed links between vector species and propagule sources. Further development of these networks through the inclusion of a comparable link weight (e.g., interaction frequency or vector biomass) across network matrices has greatly enhanced understandings of dispersal (Vidal et al., 2013; Heleno et al., 2014). However, ecological networks are often devoid of species abundance estimates, combined functional role, vector intraspecific differences, and spatially and temporally resolved network data (Heleno et al., 2014). Despite this, changes in vector species abundance or richness can have a substantial effect on the assisted dispersal of propagules (Pérez-Méndez et al., 2016; Rumeu et al., 2017).
Recently, Comparative Functional Response (CFR) has been proposed as a concept capable of unifying the fragmented discipline of invasion ecology (Dick et al., 2017a,b; Laverty et al., 2017). In essence, the Functional Response (FR; Holling, 1959) can be defined as the relationship between resource availability (e.g., prey density) and per capita consumer uptake (e.g., prey consumption rate; Dick et al., 2014, 2017a,b). Further, in developing this classical ecological metric, to improve understanding of resource and consumer uptake relationships, Dick et al. (2017c) proposed a series of novel metrics known as the Impact Potential (IP) and the Relative Impact Potential (RIP), whereby species CFR data is combined with known abundance data to better estimate likely ecological impact. Abundance data is used as a readily measurable proxy for Numerical Response (NR), which describes the population state of an organism in relation to that of a resource (e.g., prey). Ultimately, the ecological impact of an organism on a resource may be best described as the product of the consumer FR and NR see Dick et al. (2017c). Although originally designed to assess and predict the ecological impacts of existing, emerging, and future invasive species, the concepts of IP and RIP are transferable to other ecological scenarios where taxa utilize available resources in a different manner. For instance, the rate at which propagules are taken-up (e.g., ingested) can vary widely with different potential vector species.
To date, many basic questions concerning the extent to which different vectors facilitate dispersal often remain unanswered. However, if propagules capable of surviving vector-mediated dispersal are considered as an acquirable resource, then the ecological concepts of IP and RIP can be further developed to decipher, compare and contrast the functional role, relative importance, and the spatial and temporal dispersal parameters of different vector species. Here, based on the premise of the IP and RIP metrics, we propose an analogous sequence of conceptual metrics: (1) the Dispersal Potential (DP); (2) the Relative Dispersal Potential (RDP); (3) the Combined Dispersal Potential (CDP), and (4), the Relative Combined Dispersal Potential (RCDP). The usefulness of the proposed metrics is that calculation is straightforward and can be based on readily obtainable data. Furthermore, the calculated metrics will enable improved assessment, prediction and classification for likely propagule dispersal and vector importance, at species, community and population levels.
Metric One: The Dispersal Potential (DP)
If classical FR is considered in the context of assisted dispersal, the relationship between “resource availability” and “per capita consumer uptake” could be considered as a function of the available propagule density and the per capita propagule load carried by a disperser. However, many studies only report data concerning the number of propagules recovered from dispersers. Therefore, firstly, we propose that the basic DP of any vector species can be calculated as:
whereby, Np is the per capita propagule load recovered from a disperser, such as the mean, minimum, or maximum number propagules carried per individual vector species. However, the per capita propagule load (Np) can be used to assess various stages of the dispersal process, such as propagule uptake rates, recovery rates, survival rates (e.g., germination or hatching), or viability rates in relation to post-dispersal growth and establishment. For example, with an uptake rate of 100 seeds by mallard duck, Anas platyrhynchos, Soons et al. (2008) documented maximum recovery and subsequent germination rates of 54 and 78%, respectively. Ultimately, however, only comparison of post-dispersal propagule viability data will provide the most informative assessment. Notably, Np can represent the per capita propagule load in relation to a single taxon or multiple taxa. Moreover, the per capita propagule load can also be substituted with per capita propagule species richness, if assessment of the number of different species being transported by a disperser is required.
As a concept, Tv is considered the total number of possible available vectors, such as the number of individuals of a single species at a source site, their local scale abundance, or an entire continental population. Vector abundance data could be further refined in the context of proportional “seed shadows,” whereby the number of dispersers which relocate prior to or beyond selected distances, e.g., 10 km, are considered for metric compassions. Additionally, in response to data availability, or temporal and spatial changes in vector abundance, Tv can be further presented as the mean (Mv) or minimum (Minv) number of vectors. Although dependent on data availability, estimates of minimum or mean vector abundances may be more attainable than confirmation of the total number of dispersers utilizing an area.
As a worked example of the DP, we will consider the data presented by Reynolds and Cumming (2015), who sampled a variety of waterbird species from two different freshwater sites in South Africa. Not knowing the actual species counts for these sites, we will arbitrarily assume that Reynolds and Cumming (2015) sampled a fifth of the population in all cases. This assumption is used to allow us to present the metric. However, the metric is a “snap-shot” and will always be subject to change in relation to fluctuations of propagule and vector abundances. In their study, 12 resting eggs of Daphnia sp. were recovered from the examination of 60 Egyptian goose, Alopochen aegyptiaca, fecal samples obtained from the first study site, “Barberspan.” Each sample was recovered from a different individual. Accordingly, the DP of any vector species can be calculated as:
In contrast, five resting eggs of Daphnia sp. were recovered from across 55 A. aegyptiaca fecal samples obtained from a second study site, “False Bay,” in which case the calculated DP = 25. Therefore, A. aegyptiaca inhabiting the first sample site have a higher DP for resting eggs of Daphnia. Similarly, 60 yellow-billed duck, Anas undulata, were sampled at site one, with 15 Daphnia eggs being recovered. A resulting DP of 75 was calculated, indicating a marginally higher DP for Daphnia eggs by A. undulata relative to both populations of A. aegyptiaca. Moreover, examination of DP for Daphnia resting eggs in relation to all waterbird species sampled at site one, while retaining our assumption of bird numbers, i.e., one fifth of all birds present were sampled for each species, allows the importance of the vectors to be ranked: A. undulata > A. aegyptiaca > white-faced duck, Dendrocygna viduata > spur winged goose, Plectropterus gambensis > red-billed teal, A. erythrorhyncha (DP scores: 75; 60; 20; 5; 0, respectively).
Similarly, Pellerin et al. (2016) documented the mean (± SE) combined recovery rate of the seeds from three plant species (Plantago media, Prunella vulgaris, and Rubus fruticosus) following gut passage through roe deer, Capreolus capreolus, red deer, Cervus elaphus, and wild boar, Sus scrofa, to be 5.6% (±4%), 13.1% (±4.9%), and 44.1% (±9.5%), respectively. In areas of co-occurrence, such as north-eastern France, C. capreolus, C. elaphus, and S. scrofa, attain calculated mean densities of 0.51 ind. km−2 (range = 0.46–0.55: Richard et al., 2010), 0.96 ind. km−2 (0.69–1.23: Garel et al., 2010; Baltzinger et al., 2016), and 6.46 ind. km−2 (5–7.92: Lang et al., 2000; Baltzinger et al., 2016), respectively. Taking the mean seed recovery rates, and vector species density values, the DP of the dispersers can be ranked as: S. scrofa > C. elaphus > C. capreolus (DP scores: 284.89; 12.58; 2.86).
In an additional example, utilizing the data presented in Soons et al. (2016), we rank the DP of the European breeding populations of several dabbling duck species. Taking the highest estimate of the breeding population and the average number of seeds for all plant species recorded within the diet of each vector species, the DP of the waterbirds is ranked as follows: mallard, A. platyrhynchos > common teal, A. crecca > Eurasian wigeon, A. penelope > northern pintail, A. acuta > gadwall, A. strepera > northern shoveler, A. clypeata (DP scores: 3.27 × 109; 1.75 × 109; 2.24 × 108; 1.84 × 108; 6.14 × 107; 3.12 × 107).
Metric Two: The Relative Dispersal Potential (RDP)
Although the DP can be used to quantify the importance of vector species, the comparative relationship between different vectors also requires consideration. Ranking of vector DP alone does not inform on the extent to which a species likely influences dispersal. However, the RDP is a ratio-based metric that can facilitate the assessment of DP for one vector relative to that of another. Simply, when the calculated value for RDP of Species A is < 1, then its comparative DP is less than that of Species B. However, RDP > 1 indicates a superior DP of Species A to that of Species B. Finally, when RDP = 1, both species have an equal DP. The RDP can be calculated as:
For example, taking the above calculated DP scores for ungulate species, the RDP of S. scrofa (Species A) to that of C. capreolus (Species B) is 99.61, which suggests the DP of S. scrofa is almost 100 times greater than that of C. capreolus. Similarly, for the previously assessed European dabbling duck species, the RDP of A. platyrhynchos (Species A) in comparison to A. crecca (Species B) is 1.87, suggesting the DP of A. platyrhynchos is almost 1.9 times greater than that of A. crecca, at the level of the estimated breeding population. Interestingly, “species” could be replaced with different age cohorts, sample sites, or populations to allow for in-depth examination of comparative DP within and between species, across both community and ecosystem levels. In a more refined hypothetical example, concerning transport distances beyond 1,000 km, we will arbitrarily assume that 20 and 80% of the European breeding populations for A. platyrhynchos and A. crecca populations engage in such movements, respectively. Accordingly, the calculated RDP for A. platyrhynchos (Species A: 20% = 6.54 × 108) and A. crecca (Species B: 80% = 14 × 108) is 0.47. This would indicate that at distances >1,000 km, A. crecca have a predicted DP which is approximately double that of A. platyrhynchos.
Furthermore, an improved understanding of propagule retention/attachment times, their subsequent viability, and vector directional movements will facilitate further refinement of such calculations. For instance, with an average flight speed of 78 and 58 km h−1 (Clausen et al., 2002), flight-times of 13 and 17 h would be required for A. platyrhynchos and A. crecca, respectively, to surpass a distance of 1,000 km. As the probability of seed germination decreases with longer gut retention times (Soons et al., 2008), we will hypothetically assume that seed mixtures transported by A. platyrhynchos and A. crecca for 1,000 km will have successful germination rates of 10 and 5%, respectively. Accordingly, a conceptually more refined RDP for A. platyrhynchos (Species A: 10% = 6.54 × 107) and A. crecca (Species B: 5% = 7 × 107) is 0.934, which would predict an almost equal DP for a distances >1,000 km.
Alternatively, assessment of the RDP can also be performed visually via biplot, as per Figure 2. Using the waterbird species data reported by Reynolds and Cumming (2015) for Daphnia sp. dispersal at first study site, “Barberspan,” we present Np (here the per capita number of propagules each vector species) on the x-axis and Tv (the abundance of each vector species at the study site) on the y-axis. By scanning the biplot from bottom left to top right, we clearly see that while D. viduata has the highest Np, its low Tv means it has only the third highest dispersal potential. While A. undulata and A. aegyptiaca, each with much higher Tv, have the highest and second highest dispersal potentials, respectively. P. gambensis, having a moderate Tv, but a low Np, had the fourth highest dispersal potential. As A. erythrorhyncha was found to have carried zero Daphnia sp. resting eggs by Reynolds and Cumming, and hence having no dispersal potential, this species was omitted from Figure 2.
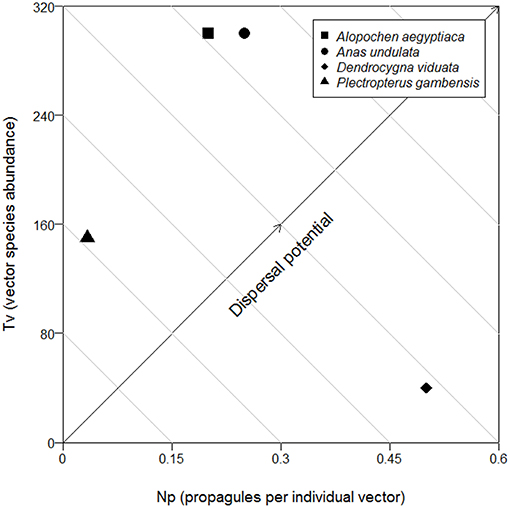
Figure 2. Biplot showing the Relative Dispersal Potential potential of yellow-billed duck, A. undulata; Egyptian goose, A. aegyptiaca; white-faced duck, D. viduata; and spur-winged goose, P. gambensis, with Daphnia sp. propagules. The red-billed teal, A. erythrorhyncha, had zero Daphnia propagules and hence was omitted from the biplot. Dispersal potential increases from bottom left to top right.
Metric 3: The Combined Dispersal Potential (CDP)
Multiple different vectors may contribute to the dispersal process, increasing the DP for a particular propagule type. Accordingly, we propose a third metric, the CDP, whereby the cumulative DP of several vectors may be considered:
As an example, we use the DP calculations derived above for A. undulata, A. aegyptiaca, and D. viduata in relation to Daphnia eggs (see above). The CDP of these three vector species is 155. Similarly, based on our derived DP values concerning the dispersal rate of seed mixtures for three plant species (P. media, P. vulgaris, and R. fruticosus) by multiple ungulate species, the CDP of C. capreolus and C. elaphus is calculated as 15.44. Further, as an additional example, we will consider the data presented by Banha et al. (2016) concerning the mean number of zebra mussel, Dreissena polymorpha, larval stages found adhering to an A. platyrhynchos carcass, an angler's waders and a fishing keep net, following 10 min exposure to infested water. In calculating the DP, due to lack to known abundance data, let us assume that the number of adult A. platyrhynchos will consistently outnumber anglers at an arbitrary ratio of 7.5 to 1 at this site. Accordingly, using an abundance of two anglers, the DP for each vector is calculated as follows: A. platyrhynchos = 120; angler's waders = 36; keep net = 44. When taken together these vectors have a CDP of 200. Secondary dispersal of propagules by additional vectors can be accounted for through addition of the DP for a primary disperser (Species A) and that of the secondary vector (Species B).
Metric Four: Relative Combined Dispersal Potential (RCDP)
Similar to the concept of RDP, we suggest that a ratio-based metric to facilitate comparison of the CDP for multiple vectors to that of one or more others is worthwhile.
Therefore, using our above calculated DP values for the South African waterbirds, a RCDP of 6.77 can be calculated for combined A. undulata and A. aegyptiaca in relation to the DP score of D. viduata. Similarly, an RCDP of 31 can be derived for all three of these species when compared to the DP of P. gambensis. Further, the RCDP of ungulate species C. capreolus and C. elaphus, when compared to S. scrofa, is calculated as 0.054. This indicates that C. capreolus and C. elaphus have a CDP which is equivalent to 5.4% of the DP shown by S. scrofa. Moreover, as a final example, the CDP of both angling equipment items relative to the DP of A. platyrhynchos (see above), results in a RCDP of 0.666. This suggests the CDP of the combined angling equipment is approximately two thirds of the DP predicted for A. platyrhynchos at the examined abundances. Additionally, RCDP could also be used to compare the DP of biodiverse systems, with numerous vector species present, to the DP of systems dominated by a single or relatively few vector species. Further, the impacts of range shifts, invasive species and defaunation, on vector assemblages and the subsequent dispersal of propagules, can also be explored in relation to the spread, introduction or loss of dispersers.
Discussion and Synthesis
Here, we have proposed a series of novel metrics to predict and classify likely dispersal and vector importance. The proposed metrics offer a basic straightforward assessment of dispersal, with potential for more in-depth network analysis concerning the relative importance of vector species. In addition to vector species abundances, the per capita effect of dispersers may also be assessed through use of other proxies, such as density or vector biomass (Dick et al., 2017c; Dickey et al., 2018). However, these calculations are context dependant on disperser and propagule interactions underpinned by both biotic and abiotic conditions. For instance, in certain scenarios “Vector Species A” may have a higher DP in some ecosystems than “Vector Species B,” but in other systems this may be completely different. In addition, initial contact between disperser and propagule, particularly for more incidental vector species such as carnivorous secondary dispersers, may be a matter of coincidence and a rare event. Ultimately, the availability of propagules and their rate of uptake by vector species will vary. However, differential seasonal DP calculations reflective of both propagule and vector availability can be examined through the RDP, the CDP, and the RCDP.
So far, we have shown that the DP metrics can be used to quantitatively assess and predict likely dispersal, and classify vector importance. In the examples provided, differential dispersal of propagules of a single taxon or multiple taxa has been examined in relation to the calculated DP for various vector species, which allows for an improved understanding of disperser functional roles. However, the proposed analogous series of metrics can also be used to examine DP in the context of differential dispersal of multiple propagule combinations transported by one or more dispersers. For example, following the ingestion of plant seeds by adult A. platyrhynchos, Soons et al. (2008) observed mean germination rates of 32 and 8% for Lycopus europaeus and Lythrum salicaria, respectively. Although unknown, let us assume that smaller juvenile A. platyrhynchos will facilitate greater germination rates of these plants due to shorter gut retention times, in the arbitrary order of 64 and 16%, respectively. Accordingly, in the context of 10 adult and 10 juvenile A. platyrhynchos, which have each consumed exactly 100 seeds of each plant species, we would expect a germination based DP of: L. europaeus by juvenile mallard > L. europaeus by adult mallard > L. salicaria by juvenile mallard > L. salicaria by adult mallard (DP scores = 640 > 320 > 160 > 80, respectively). As before, variation of the in-field per capita propagule load (Np) and vector abundances (Tv) will alter these outputs. Yet, as highlighted, assessment of DP in relation to different propagules carried by a single disperser, a vector species, or numerous combinations of both propagules, and dispersers can be performed. Thus, the metrics can facilitate an improved understanding of biological connectivity within meta-community dynamics.
Overall, the final fate of propagules is not a simple function of the per capita acquired propagule load and vector abundances. Propagule retention or adherence times, propagule release, subsequent viability, disperser distances traveled, failure to relocate, and suitability of new environments will also influence dispersal success (Vidal et al., 2013; Coughlan et al., 2017a,b). Therefore, the proposed DP metrics always have to be considered in the context of the data used in the calculation. Currently, a variety of studies have documented the abundance and richness of propagules carried by different vector species, and increasingly, quantitative data detailing propagule survival, and viability rates is being reported (e.g., Farmer et al., 2017; Bartel et al., 2018; Lovas-Kiss et al., 2018a,b,c). These are mostly recovered from coat or feather brushings, and regurgitated pellets, fecal or lower intestine gut samples. Although one vector species may carry more propagules than another different vector species, the relative abundance of vectors appears to be rarely considered. Our proposed metrics provide a blend of individual and population-level effects, which could be further refined beyond the concept of dispersal “potential” by reducing the knowledge gap between potential and realized dispersal, through uncertainty analysis techniques and further integration of factors such as post-dispersal viability, vector movements, and frequency of time spent by vector within habitat patches suitable for dispersed propagules. In particular, as briefly highlighted through an RDP example, these metrics could be used to assess the number of potential dispersal events across distance gradients, i.e., within different spatial gradients of seed (propagule) shadows. Equally, the propagule shadow created by multiple vector species across such spatial and temporal gradients can also be considered with the CDP.
Although vector species assemblages and their proportionality of interactions with propagules are frequently assessed in the context of ecological networks, the relative importance of individual species or functional groups at the community and population scale remain inadequately defined (Farwig and Berens, 2012; Vidal et al., 2013; Hämäläinen et al., 2017). For example, through a bipartite network analysis of recovered scat samples, Peredo et al. (2013) documented that red fox Vulpes vulpes facilitated a slightly higher proportion of Rubus sp. dispersal than wild boar S. scrofa, in relation to the total number of seeds recovered. However, the mean number (± SE) of Rubus seeds per scat sample was higher for S. scrofa (984.3 ± 299.2) than for V. vulpes (444.2 ± 90.7). Therefore, if the number of co-existing boars outnumbers those of territorial V. vulpes within a shared range, then RDP analysis would indicate that S. scrofa has a higher DP. Additionally, a single vector species may facilitate dispersal across multiple ecological networks, e.g., S. scrofa can disperse propagules originating from both terrestrial and aquatic systems (Vanschoenwinkel et al., 2008; Peredo et al., 2013). As generalists, high abundances of omnivorous S. scrofa may provide for greater levels of realized dispersal, in comparison to lower abundances of more specialist propagule consumers. Accordingly, the relative importance of S. scrofa within and across dispersal networks could be assessed with DP based metrics.
Even within relatively well-studied mutualistic frugivorous networks, interactions are generally sampled by recording the number of foraging visits by potential dispersers to source plants, with interaction frequency taken as a proxy for vector species abundances (Simmons et al., 2018). Often, subsequent calculations rely on the assumption that similar propagule uptake rates occur with each visit (Schupp et al., 2010). However, this assumption is likely less reliable for chance ectozoochorous dispersal, and also fails to account for opportunistic feeding, gorging, selectivity, and prey switching. Although dispersers can visit propagule source sites multiple times, with increased frequency of visitation likely resulting in greater occurrence of dispersal events, this detailed data is not always available for understudied systems. Accordingly, the proposed metrics are basic and require minimal data, as more complex metrics such as the Seed Disperser Effectiveness (SDE) as proposed by Schupp et al. (2010), cannot always be calculated from available data. The proposed series of DP metrics estimate likely disperser functional roles based on the result of a single source site visitation, when the per capita consumer uptake of propagules has occurred. However, multiplication of DP by the number of visits, or accumulative CDP and RCDP calculations, can be used to account for differential dispersal caused by visitation events. Nevertheless, we also suggest that the SDE metric could be considered in the context of the relative role played by multiple vector species. For example, calculations of: (1) Relative SDE (RSDE); (2) Combined SDE (CSDE); and (3), the Relative Combined SDE (RCSDE), could greatly enhance understanding of dispersal facilitated by different vector groups. Assessment of relative dispersal potential could also be examined for the propagule Ingestion and Excretion equations derived by (Kleyheeg et al., 2017).
Although further development and validation will be required, the proposed metrics represent a novel starting point for greater consideration of disperser functional roles across spatial and temporal gradients. As the relative capacity for assisted dispersal will differ between vectors, these metrics provide a means to quantify and rank the importance of different dispersers. This will facilitate an improved assessment of the prominence of different dispersers for the maintenance of connectivity, both within and between ecosystems. Furthermore, in studies of dispersal networks, the inclusion of these metrics will provide a more realistic measure of the functional role of different species than through examination of interaction intensities alone, since the metrics can account for vector abundance. Finally, incorporation of vector life history data, such as fecundity and lifespan (see Dickey et al., 2018), and spatial or temporal changes in interaction opportunities, such as shifts in vector diets in response to propagule availability (e.g., seasonal changes) or preferential interaction with invasive species by dispersers (Green, 2016; van Leeuwen, 2018), will present a more realistic representation of dispersal with increased prediction power.
Author Contributions
NC conceived and designed the study. JWD, RC, TK, MJ, and JTD assisted in developing the study. All authors contributed to the writing of the manuscript, which was led by NC.
Conflict of Interest Statement
The authors declare that the research was conducted in the absence of any commercial or financial relationships that could be construed as a potential conflict of interest.
Acknowledgments
NC and JTD are supported by the Irish Environmental Protection Agency research grant 2015-NC-MS-4. JWD acknowledges support from Queen's University Belfast and Inland Fisheries Ireland. RC is supported by the Department for the Economy, Northern Ireland. JTD also acknowledges funding received from Natural Environment Research Council. We particularly wish to thank Casper H.A. van Leeuwen and our two reviewers for helpful comments.
References
Andresen, E., Arroyo-Rodríguez, V., and Ramos-Robles, M. (2018). Primate seed dispersal: old and new challenges. Int. J. Primatol. 39, 443–465. doi: 10.1007/s10764-018-0024-z
Baltzinger, M., Mårell, A., Archaux, F., Pérot, T., Leterme, F., and Deconchat, M. (2016). Overabundant ungulates in French sologne? Increasing red deer and wild boar pressure may not threaten woodland birds in mature forest stands. Basic Appl. Ecol. 17, 552–563. doi: 10.1016/j.baae.2016.04.005
Banha, F., Gimeno, I., Lanao, M., Touya, V., Durán, C., Peribáñez, M., et al. (2016). The role of waterfowl and fishing gear on zebra mussel larvae dispersal. Biol. Invas. 18, 115–125. doi: 10.1007/s10530-015-0995-z
Bartel, R. D., Sheppard, J. L., Lovas-Kiss, A., and Green, A. J. (2018). Endozoochory by mallards in New Zealand: what seeds are dispersed and how far? PeerJ 6:e4811. doi: 10.7717/peerj.4811
Castañeda, I., Fedriani, J. M., and Delibes, M. (2018). Potential of red deer (Cervus elaphus) to disperse viable seeds by spitting them from the cud. Mamm. Biol. 90, 89–91. doi: 10.1016/j.mambio.2017.10.004
Clausen, P., Nolet, B. A., Fox, A. D., and Klaassen, M. (2002). Long-distance endozoochorous dispersal of submerged macrophyte seeds by migratory waterbirds in northern Europe – a critical review of possibilities and limitations. Acta Oecol. 23, 191–203. doi: 10.1016/S1146-609X(02)01150-5
Corlett, R. T. (2017). Frugivory and seed dispersal by vertebrates in tropical and subtropical Asia: an update. Glob. Ecol. Con. 11, 1–22. doi: 10.1016/j.gecco.2017.04.007
Coughlan, N. E., Kelly, T. C., Davenport, J., and Jansen, M. A. K. (2017a). Up, up and away: bird-mediated ectozoochorous dispersal between aquatic environments. Freshw. Biol. 62, 631–648. doi: 10.1111/fwb.12894
Coughlan, N. E., Stevens, A. L., Kelly, T. C., Dick, J. T. A., and Jansen, M. A. K. (2017b). Zoochorous dispersal of freshwater bivalves: an overlooked vector in biological invasions? Knowl. Manag. Aquat. Ecosyst. 418:42. doi: 10.1051/kmae/2017037
Czarnecka, J., and Kitowski, I. (2013). Rook spring seed dispersal in the agricultural landscape – frugivory, granivory or accidental transport? Folia Geobot. 48, 55–73. doi: 10.1007/s12224-012-9134-0
Dick, J. T. A., Alexander, M. E., Jeschke, J. M., Ricciardi, A., MacIsaac, H. J., Robinson, T. B., et al. (2014). Advancing impact prediction and hypothesis testing in invasion ecology using a comparative functional response approach. Biol. Invas. 16, 735–753. doi: 10.1007/s10530-013-0550-8
Dick, J. T. A., Alexander, M. E., Ricciardi, A., Laverty, C., Downey, P. O., Xu, M., et al. (2017a). Functional responses can unify invasion ecology. Biol. Invas. 19, 1667–1672. doi: 10.1007/s10530-016-1355-3
Dick, J. T. A., Alexander, M. E., Ricciardi, A., Laverty, C., Downey, P. O., Xu, M., et al. (2017b). Fictional responses from Vonesh et al. Biol. Invas. 19, 1677–1678. doi: 10.1007/s10530-016-1360-6
Dick, J. T. A., Laverty, C., Lennon, J. J., Barrios-O'Neill, D., Mensink, P. J., Britton, R., et al. (2017c). Invader relative impact potential: a new metric to understand and predict the ecological impacts of existing, emerging and future invasive alien species. J. Appl. Ecol. 54, 1259–1267. doi: 10.1111/1365-2664.12849
Dickey, J. W. E., Cuthbert, R. N., Rea, M., Laverty, C., Crane, K., Briski, E., et al. (2018). Assessing the relative potential ecological impacts and invasion risks of emerging invasive species. Neobiota 40, 1–24. doi: 10.3897/neobiota.40.28519
Farmer, J. A., Webb, E. B., Pierce, R. A., and Bradley, K. W. (2017). Evaluating the potential for weed seed dispersal based on waterfowl consumption and seed viability. Pest Manag. Sci. 73, 2592–2603. doi: 10.1002/ps.4710
Farwig, N., and Berens, D. G. (2012). Imagine a world without seed dispersers: a review of threats, consequences and future directions. Basic Appl. Ecol. 13, 109–115. doi: 10.1016/j.baae.2012.02.006
García, C., Klein, E. K., and Jordano, P. (2017). Dispersal processes driving plant movement: challenges for understanding and predicting range shifts in a changing world. J. Ecol. 105, 1–5. doi: 10.1111/1365-2745.12705
Garel, M., Bonenfant, C., Hamann, J.-L., Klein, F., and Gaillard, J.-M. (2010). Are abundance indices derived from spotlight counts reliable to monitor red deer Cervus elaphus populations? Wildl. Biol. 16, 77–84. doi: 10.2981/09-022
Gleditsch, J. M., Hruska, A. M., and Foster, J. T. (2017). Connecting resource tracking by frugivores to temporal variation in seed dispersal networks. Front. Ecol. Evol. 5:98. doi: 10.3389/fevo.2017.00098
Green, A. J. (2016). The importance of waterbirds as an overlooked pathway of invasion for alien species. Divers. Distrib. 22, 239–247. doi: 10.1111/ddi.12392
Hämäläinen, A., Broadley, K., Droghini, A., Haines, J. A., Lamb, C. T., Boutin, S., et al. (2017). The ecological significance of secondary seed dispersal by carnivores. Ecosphere 8:e01685. doi: 10.1002/ecs2.1685
Heleno, R., García, C., Jordano, P., Traveset, A., Gómez, J. M., Blüthgen, N., et al. (2014). Ecological networks: delving into the architecture of biodiversity. Biol. Lett. 10:20131000. doi: 10.1098/rsbl.2013.1000
Holling, C. S. (1959). Some characteristics of simple types of predation and parasitism. Can. Entomol. 91, 385–398. doi: 10.4039/Ent91385-7
Kleyheeg, E., Treep, J., Jager, M., Nolet, B. A., and Soons, M. B. (2017). Seed dispersal distributions resulting from landscape-dependent daily movement behaviour of a key vector species, Anas Platyrhynchos. J. Ecol. 105, 1279–1289. doi: 10.1111/1365-2745.12738
Lang, S., Pesson, B., Klein, F., and Schreiber, A. (2000). Wildlife genetics and disease: allozyme evolution in the wild boar (Sus scrofa) caused by a swine fever epidemy. Genet. Sel. Evol. 32, 303–310. doi: 10.1186/1297-9686-32-3-303
Laverty, C., Green, K. D., Dick, J. T. A., Barrios-O'Neill, D., Mensink, P. J., Médoc, V., et al. (2017). Assessing the ecological impacts of invasive species based on their functional responses and abundances. Biol. Invasions 19, 1653–1665. doi: 10.1007/s10530-017-1378-4
Leibold, M. A., Holyoak, M., Mouquet, N., Amarasekare, P., Chase, J. M., Hoopes, M. F., et al. (2004). The metacommunity concept: a framework for multi-scale community ecology. Ecol. Lett. 7, 601–613. doi: 10.1111/j.1461-0248.2004.00608.x
Lovas-Kiss, Á., Sánchez, M. I., Molnár, A., Valls, L., Armengol, X., Mesquita-Joanes, F., et al. (2018a). Crayfish invasion facilitates dispersal of plants and invertebrates by gulls. Freshw. Biol. 63, 392–404. doi: 10.1111/fwb.13080
Lovas-Kiss, Á., Sanchez, M. I., Wilkinson, D., Coughlan, N., Alves, J., and Green, A. J. (2018b). Shorebirds as important vectors for plant dispersal in Europe. Ecography. doi: 10.1111/ecog.04065. [Epub ahead of print].
Lovas-Kiss, Á., Vizi, B., Vincze, O., Molnár, V. A., and Green, A. J. (2018c). Endozoochory of aquatic ferns and angiosperms by mallards in Central Europe. J. Ecol. 106, 1714–1723. doi: 10.1111/1365-2745.12913
Mello, M. A. R., Rodrigues, F. A., Costa, L. D. F., Kissling, W. D., Şekercioğlu, Ç. H., Marquitti, F. M. D., and Kalko, E. K. V. (2015). Keystone species in seed dispersal networks are mainly determined by dietary specialization. Oikos 124, 1031–1039. doi: 10.1111/oik.01613
Moritz, C., Meynard, C. N., Devictor, V., Guizien, K., Labrune, C., Guarini, J.-M., et al. (2013). Disentangling the role of connectivity, environmental filtering, and spatial structure on metacommunity dynamics. Oikos 122, 1401–1410. doi: 10.1111/j.1600-0706.2013.00377.x
Nathan, R. (2006). Long-distance dispersal of plants. Science 313, 786–788. doi: 10.1126/science.1124975
Pellerin, M., Picard, M., Saïd, S., Baubet, E., and Baltzinger, C. (2016). Complementary endozoochorous longdistance seed dispersal by three native herbivorous ungulates in Europe. Basic Appl. Ecol. 17, 321–332. doi: 10.1016/j.baae.2016.01.005
Peredo, A., Martinez, D., Rodriguez-Perez, J., and García, D. (2013). Mammalian seed dispersal in Cantabrian woodland pastures: network structure and response to forest loss. Basic Appl. Ecol. 14, 378–386. doi: 10.1016/j.baae.2013.05.003
Pérez-Méndez, N., Jordano, P., and Valido, A. (2016). Persisting in defaunated landscapes: reduced plant population connectivity after seed dispersal col-lapse. Ecology 6, 1707–1719. doi: 10.1111/1365-2745.1284
Reynolds, C., and Cumming, G. S. (2015). The role of waterbirds in the dispersal of freshwater cladocera and bryozoa in southern Africa. Afr. Zool. 50, 307–311. doi: 10.1080/15627020.2015.1108164
Richard, E., Gaillard, J.-M., Saïd, S., Hamann, J.-L., and Klein, F. (2010). High red deer density depresses body mass of roe deer fawns. Oecologia 163, 91–97. doi: 10.1007/s00442-009-1538-z
Rumeu, B., Devoto, M., Traveset, A., Olesen, J. M., Vargas, P., Nogales, M., et al. (2017). Predicting the consequences of disperser extinction: richness matters the most when abundance is low. Funct. Ecol. 31, 1910–1920. doi: 10.1111/1365-2435.12897
Schupp, E. W., Jordano, P., and Gómez, J. M. (2010). Seed dispersal effectiveness revisited: a conceptual review. New Phytol. 188, 333–353. doi: 10.1111/j.1469-8137.2010.03402.x
Shurin, J. B., Cottenie, K., and Hillebrand, H. (2009). Spatial autocorrelation and dispersal limitation in freshwater organisms. Oecologia 159, 151–159. doi: 10.1007/s00442-008-1174-z
Simmons, B. I., Sutherland, W. J., Dicks, L. V., Albrecht, J., Farwig, N., García, D., et al. (2018). Moving from frugivory to seed dispersal: incorporating the functional outcomes of interactions in plant–frugivore networks. J. Anim. Ecol. 87, 995–1007. doi: 10.1111/1365-2656.12831
Soons, M. B., Brochet, A. L., Kleyheeg, E., and Green, A. J. (2016). Seed dispersal by dabbling ducks: an overlooked dispersal pathway for a broad spectrum of plant species. J. Ecol. 104, 443–455. doi: 10.1111/1365-2745.12531
Soons, M. B., Van der Vlugt, C., Van Lith, B., Heil, G. W., and Klaassen, M. (2008). Small seed size increases the potential for dispersal of wetland plants by ducks. J. Ecol. 96, 619–627. doi: 10.1111/j.1365-2745.2008.01372.x
Steyaert, S. M. J. G., Frank, S. C., Puliti, S., Badia, R., Arnberg, M. P., Beardsley, J., et al. (2018). Special delivery: scavengers direct seed dispersal towards ungulate carcasses. Biol. Lett. 14:20180388. doi: 10.1098/rsbl.2018.0388
Tesson, S. V. M., Okamura, B., Dudaniec, R. Y., Vyverman, W., Londahl, J., Rushing, C., et al. (2015). Integrating microorganism and macroorganism dispersal: modes, techniques and challenges with particular focus on co-dispersal. Ecoscience 22, 109–124. doi: 10.1080/11956860.2016.1148458
Timóteo, S., Correia, M., Rodríguez-Echeverría, F. H., and Heleno, R. (2018). Multilayer networks reveal the spatial structure of seed-dispersal interactions across the great rift landscapes. Nat. Commun. 9:140. doi: 10.1038/s41467-017-02658-y
Trakhtenbrot, A., Nathan, R., Perry, G., and Richardson, D. M. (2005). The importance of long-distance dispersal in biodiversity conservation. Divers. Distrib. 11, 173–181. doi: 10.1111/j.1366-9516.2005.00156.x
van Leeuwen, C. H. A. (2018). Internal and external dispersal of plants by animals: an aquatic perspective on alien interference. Front. Plant Sci. 9:153. doi: 10.3389/fpls.2018.00153
van Leeuwen, C. H. A., Lovas-Kiss, Á., Ovegård, M., and Green, A. J. (2017). Great cormorants reveal overlooked secondary dispersal of plants and invertebrates by piscivorous waterbirds. Biol. Lett. 13:20170406. doi: 10.1098/rsbl.2017.0406
Vanschoenwinkel, B., Waterkeyn, A., Nhiwatiwa, T., Pinceel, T., Spooren, E., Geerts, A., et al. (2011). Passive external transport of freshwater invertebrates by elephant and other mud-wallowing mammals in an African savannah habitat. Freshw. Biol. 5, 1606–1619. doi: 10.1111/j.1365-2427.2011.02600.x
Vanschoenwinkel, B., Waterkeyn, A., Vandecaetsbeek, T., Pineau, O., Grillas, P., and Brendonck, L. (2008). Dispersal of freshwater invertebrates by large terrestrial mammals: a case study with wild boar (Sus scrofa) in Mediterranean wetlands. Freshw. Biol. 53, 2264–2273. doi: 10.1111/j.1365-2427.2008.02071.x
Viana, D. S., Gangoso, L., Bouten, W., and Figuerola, J. (2016). Overseas seed dispersal by migratory birds. Proc. R. Soc. B 283:20152406. doi: 10.1098/rspb.2015.2406
Keywords: ectozoochory, endozoochory, frugivory, propagules, dispersal networks, secondary dispersal, vector
Citation: Coughlan NE, Dickey JWE, Cuthbert RN, Kelly TC, Jansen MAK and Dick JTA (2019) Driver's Seat: Understanding Divergent Zoochorous Dispersal of Propagules. Front. Ecol. Evol. 7:70. doi: 10.3389/fevo.2019.00070
Received: 30 September 2018; Accepted: 25 February 2019;
Published: 19 March 2019.
Edited by:
Casper H. A. Van Leeuwen, Netherlands Institute of Ecology (NIOO-KNAW), NetherlandsReviewed by:
Duarte S. Viana, German Center for Integrative Biodiversity Research, GermanyJelle Treep, Utrecht University, Netherlands
Copyright © 2019 Coughlan, Dickey, Cuthbert, Kelly, Jansen and Dick. This is an open-access article distributed under the terms of the Creative Commons Attribution License (CC BY). The use, distribution or reproduction in other forums is permitted, provided the original author(s) and the copyright owner(s) are credited and that the original publication in this journal is cited, in accordance with accepted academic practice. No use, distribution or reproduction is permitted which does not comply with these terms.
*Correspondence: Neil E. Coughlan, neil.coughlan.zoology@gmail.com