Increase in Growth and Alteration of C:N Ratios of Avicennia marina and Rhizophora stylosa Subject to Elevated CO2 Concentrations and Longer Tidal Flooding Duration
- 1IMPMC, Institut de Recherche pour le Développement (IRD), UPMC, CNRS, MNHN, Nouméa, New Caledonia
- 2ISEA, EA 7484, BPR4, Université de la Nouvelle-Calédonie, Nouméa, New Caledonia
- 3CNRS, ISTO, Université d'Orléans, BRGM, UMR 7327, Orléans, France
We investigated the effects of elevated CO2 concentrations and longer tidal flooding duration on two-year-old Avicennia marina and Rhizophora stylosa seedlings for a full year. The seedlings were grown in greenhouses for ambient CO2 (400 ppm) and in enclosed CO2-controlled chambers, which were installed inside the greenhouses for elevated CO2 (800 ppm). The tidal flooding duration was set-up according to the species distribution in the intertidal zone in New Caledonia for the controlled treatment and was increased by 1 h 45 min for the experimental treatment. A total of 400 A. marina and 720 R. stylosa were monitored during this experiment. We measured heights and basal diameters of all seedlings every 90 days, and we determined the above and below ground biomass at the end of the experiment. Our results showed that elevated CO2 increased the growth rates of both A. marina and R. stylosa, for which the final biomass was, respectively, 46 and 32% higher than in the ambient CO2 treatment. We suggest that this increase was driven by a stimulation of photosynthesis under elevated CO2, as demonstrated in a previous study. Considering the tidal flooding duration treatment, we observed a contrasted effect between the species. Longer tidal flooding increased the growth of A. marina, whereas it reduced the growth of R. stylosa in comparison to the controlled treatment. This result may be related to the specific ecosystem zonation in this semi-arid climate, which limits water inputs into the Avicennia zone that increases the salt concentration in the soil, whereas Rhizophora is regularly submerged by tides due to its lower position in the intertidal zone. As a result, the combination of both treatments had a positive cumulative effect on the growth of A. marina. Although it was not the case for R. stylosa, the negative effect of longer tidal flooding on this species did not suppress the enhancement of growth resulting from elevated CO2 concentrations. At the end of the experiment, elevated CO2 increased the C:N ratios of the seedlings, thereby producing a more refractory organic matter, which will potentially result in lower decomposition rates and thus may increase carbon accumulation in mangrove soils. These results suggest that future climate changes may enhance the productivity of mangrove seedlings by increasing their growth, which may in turn increase the carbon storage potential of mangroves in their biomass.
Introduction
The steady increase in anthropogenic greenhouse gas emissions since the preindustrial age resulted in a substantial atmospheric CO2 concentrations augmentation, from 280 ppm to over 400 ppm currently (Betts et al., 2016). This increase is strongly inducing global warming (Cook et al., 2016), which, in turn, leads to an augmentation of the global mean sea-level. Projections agree that atmospheric CO2 concentrations will continue to rise in the future, and are predicted to range from 794 to 1,150 ppm by the end of the 21st, depending on simulation models (Collins et al., 2014). As a result, the global mean sea-level is expected to increase from 0.26 to 0.98 m by 2,100 (Church et al., 2013).
Mangroves are forested ecosystem that develop along tropical and subtropical coastlines and are mainly composed of halophytic C3 trees. Due to their high primary productivity (average of 218 ± 72 Tg C yr−1) (Bouillon et al., 2008), mangroves are considered as major ecosystems in the coastal carbon cycle, and have therefore been recently named “blue carbon” sinks (Mcleod et al., 2011). Many studies have investigated the response of C3 terrestrial plants to elevated CO2 concentrations, which mainly results in photosynthesis stimulation and higher productivity (Norby et al., 2005; Prior et al., 2011). However, only a few studies have focused on the response of mangroves to elevated CO2 concentrations. These studies showed that that the response of mangroves plants is similar to other C3 plants with an increase in photosynthesis and productivity (Farnsworth et al., 1996; Ball et al., 1997; McKee and Rooth, 2008; Luo et al., 2010; Reef et al., 2016; Jacotot et al., 2018), highlighting their valuable role in climate change mitigation. However, the effects of elevated CO2 concentrations may be strongly dependent of other biotic and abiotic factors such as temperature, nutrient availability, or flooding (Alongi, 2015).
Due to their position at the interface between land and sea, increase in global mean sea-level may be a major threat to mangroves (Ellison, 2015; Ward et al., 2016). Mangrove ecosystems have the ability to cope with sea-level rise through surface elevation change processes (Krauss et al., 2014), however, this capacity is influenced by several factors (Ward et al., 2016) and may be specific to each mangrove forest. In fact, (Lovelock et al., 2015) reported that 69% of mangroves surveyed in the Indo-Pacific region were not building surface elevation at rates that equal or exceed sea-level rise and, consequently, will experience major changes in their tidal flooding duration if their landward migration is prevented by human activities (Gilman et al., 2008).
A handful studies have examined the response of mangroves plants with climate changes and they mainly focused on elevated CO2 in interaction with salinity (Reef et al., 2015), nutrient availability (McKee and Rooth, 2008; Reef et al., 2016), or species competition (McKee and Rooth, 2008). However, to our knowledge, no studies have focused on the effects of elevated CO2 in interaction with an increase in tidal flooding duration on mangrove seedlings before, thus, the present study is the first to have investigated this interaction.
During one complete year, between 2016 and 2017, we conducted, in New Caledonia, a greenhouses experiment in which 720 R. stylosa and 400 A. marina were grown at two CO2 concentrations (400 vs. 800 ppm) over two tidal flooding durations (one typical of Neo Caledonian mangroves, and a longer one). In a previous paper concerning this experiment (Jacotot et al., 2018), we were mainly interested in the variability of leaf-gas exchange parameters of both species, and our main results were: (i) elevated CO2 concentrations affected seedlings physiology, notably by increasing photosynthesis by more than 37 and 45% for A. marina and R. stylosa, respectively, (ii) higher mean temperature during the warm season further enhanced this photosynthesis increase, and (iii) longer tidal flooding duration slightly reduced photosynthesis but not enough to offset the carbon gain induced by the elevated CO2 concentrations. In the present complementary study, we now focus on seedlings growth and tissues quality (using C:N ratio as a proxy). Measurements presented herein were conducted at the same time, and thus, on the same seedlings as in Jacotot et al. (2018). In the present study, the questions we wanted to answer were: (i) does atmospheric CO2 enrichment, which increased photosynthesis, lead to a stimulation of growth and biomass? (ii) is the growth response to tidal flooding duration reduced due to waterlogging variations? (iii) what will be the effects of these two parameters on the plant tissues quality, and notably on C:N ratios?
Materials and Methods
Greenhouses and Closed Chambers Experiments
Description of the Facility
The study was conducted in New Caledonia (22°13′49″S, 166°31′09″E) from June 2016 to May 2017, a period encompassing the two main seasons (i.e., the cool season from June to November, and the warm season from November to June). Experiments were realized in three semi-open greenhouses (Figure 1a) (12 × 6 m, 6 m height, 72 m2 each), following a split-plot design with CO2 atmospheric concentrations as the whole-plot (Ambient, 400 ppm vs. Elevated, 800 ppm) and tidal flooding duration (TFD) as the split-plot nested within CO2 (Natural vs. Longer). Inside each greenhouse, a circular closed chamber of 36 m2 (2.4 m height) was constructed to maintain elevated atmospheric CO2 concentrations (Figure 1b). To control the tidal duration treatment, custom-designed tidal tables were built (Figure 1b). The tidal tables consisted of a 700 liters polypropylene tank (1 m2 area over 0.7 m height) serving as a water reserve, surmounted by a 300 liters planting tray (1 m2 area over 0.3 m height). Each tidal table worked as an individual unit. Every 2 weeks throughout the studied period, the water reserves were renewed with fresh seawater pumped into the adjacent lagoon (20 m). To simulate high tide periods, an aquarium pump placed in each tank sent the water to the planting trays. During low tide periods, a siphon pipe allowed the planting tray to drain the water back into the reserve. To control the tidal treatment assigned to each tank, the aquarium pumps were connected to three custom-build current controllers (one per greenhouse). The controllers were built from open source Arduino plans freely available under public licenses and were programmed according to the needs of the experiment. Water quality (pH, salinity, DO) in the tanks was checked periodically using YSI probes.
Air temperature and humidity were recorded every 5 min inside the greenhouses and the closed chambers with HOBO sensors (Onset, Cape Cod, Massachusetts, USA). The temperature and humidity could fluctuate naturally inside the greenhouses, however, air cooling units have been installed in the closed chambers to prevent large temperature fluctuations. During the experimental period, average temperatures were, for inside the greenhouses and the closed chambers respectively, 23.1 ± 2.6 and 22.3 ± 3.0°C during the cool season and 26.4 ± 3.2 and 26.7 ± 3.5°C during the warm season. The relative humidity was, for inside the greenhouses and the closed chambers respectively, 85.8 ± 12.6 and 78.4 ± 12.2% during the cool season and 80.7 ± 14.2 and 80.8 ± 12.8% during the warm season.
Plant Materials
A total of 720 R. stylosa and 400 A. marina propagules were collected from multiple trees in the pristine mangrove of Oundjo, in New Caledonia (21°4′8″S, 164°42′51″E). They were collected between February and March 2014, during the optimal fruiting period. They were then individually planted in 2.5-liters polyethylene bags in a 1:1 mixture of mangrove peat and sand and grown for two complete years before being used in the experiment.
The mangrove seedlings were randomly allocated between the tidal tables, with 25 A. marina or 30 R. stylosa on each tidal table, resulting in 100 A. marina (4 tidal tables) and 180 R. stylosa (6 tidal tables) for each of the four treatments (Figure 1b). The tidal tables were then distributed between the three greenhouses as true experimental replicates, and then either inside or outside the closed chambers according to their CO2 treatment. During the experiment, mangrove seedlings were regularly rotated within each tidal table and were rotated three times between the greenhouses to minimize positional effect.
CO2 Enrichment
Pure CO2 was supplied into each of the three closed chambers between 5:00 a.m. to 7:00 p.m. and the concentrations were maintained between 780 and 820 ppm throughout this period. The CO2 concentrations inside the chambers were continuously monitored using CO2 probes connected to a central unit that controlled the entire system (MAXICLIM NG 08/3Z, Anjou Automation). In addition, CO2 concentrations were periodically checked using two different infrared portable CO2 analyzers (a G2131-i CRDS analyzer from Picarro Inc., Santa Clara, USA; and a Li-8100A from LI-COR Biosciences, Lincoln, USA).
Tidal Flooding Duration Treatment
The natural tidal flooding duration (TFD) was set up according to the average flooding duration measured in New Caledonian mangroves for both species (unpublished data). These tidal durations may be different and variable according to the geographical position of the studied mangrove (e.g., Van Loon et al., 2007). In New Caledonia, mangroves develop in semi-arid conditions with a specific zonation of the vegetative species: Rhizophora spp. colonizes the sea side, while Avicennia marina develops at higher elevations. Previous studies have suggested that the main factor controlling this distribution of mangrove species in New Caledonia is soil salinity, which in turn is controlled by the duration of tidal inundation, and thus, by the soil elevation (Marchand et al., 2011b, 2012). As a result, the flooding duration for Rhizophora trees is higher than for Avicennia. Consequently, in the experiment, natural TFD was 3 h 15 min for A. marina and 6 h 00 min for R. stylosa for each high tide. Simulated tidal cycles were semi-diurnal, meaning that there were two high tides each day, separated by a low tide period of 8 h 30 min and 5 h 45 min for A. marina and R. stylosa, respectively.
We hypothesized that an increase in mean sea-level would similarly affect the flooding duration of both species; thus, in the experiment, longer TFD was simulated by increasing the natural times by 1 h 45 min, which corresponded to a high tide of 5 h 00 min and 7 h 45 min for A. marina and R. stylosa, respectively. Consequently, low tides periods were reduced to 6 h 45min and 4 h for A. marina and R. stylosa, respectively. This increase in flooding duration has been randomly defined but remains realistic given the projected sea-level rise at the end of the century. Although we have chosen a similar increase in flooding duration for both species, we are aware that this may be not the case, and that the flooding duration may increase differently in both stands in the natural system. Nevertheless, an increase of 1 h 45 min of tidal duration will have different implications for both species, as it corresponds to an increase of 45% of the natural tidal duration of A. marina, whereas it is only 25% for R. stylosa. During high tide, the water level in the planting trays was identical for all tidal tables, 5 cm above sediment surface to completely submerge the root system.
Measurements
Growth and Biomass
All mangrove seedlings were measured for their height and basal diameter. Height measurements were made along the main axis, from the soil for A. marina, and from the top of the hypocotyl for R. stylosa. Basal diameters were taken at 0.5 cm above these two limitations. The measurements were realized five times during the experimental period: at the beginning, after 90, 180, 260 days, and at the end of the experiment, when the seedlings were three-years old.
Biomass was measured at the beginning and at the end of the experiment. For each species and treatment, 30 seedlings were randomly selected and separated into below (root system) and above (leaves and stems) ground parts. All plant components were then thoroughly washed with deionized water. Then, all plant materials were dried at 60°C during 72 h before being weighted for dry mass (g). Relative growth rates (RGR) were calculated as the difference between final and initial mass over the duration of the experiment. In addition, the leaf mass area (LMA, g cm−2) was calculated as the inverse of the surface leaf area (SLA), which was previously determined on the same trees and during the same experiment (Jacotot et al., 2018).
Carbon and Nitrogen Content
Carbon and nitrogen contents were measured at the end of the experiment. For each species and treatment, 30 individuals were randomly selected and separated in three batches of 10 individuals. Within each batch, all seedlings were separated into leaves, stems and roots. All plants materials were then dried at 60°C for 72 h, and then ground using a ball mill, to finally obtain three batches of leaves, stems and roots for each species and treatment, each one constituted by 10 individuals. Three subsamples (~2 mg) of each lot of leaves, stems and roots were then analyzed for total carbon (C) and nitrogen (N) contents (%) using an elemental analyzer (Integra2, Sercon, UK). All the analyses were performed at the French National Institute for Sustainable Development (IRD) of Noumea, New Caledonia (France). The analytical precision of the analyzer was checked using IAEA-600 caffeine standard (IAEA Nucleus) and was less than 0.3% for C and 0.15% for N. All C:N ratios in this study were calculated on the mass basis.
Statistical Analysis
Final heights, basal diameters, final above and below ground biomasses, below to above ground ratios and C:N ratios were analyzed for significant differences (p < 0.05) between the four treatments. Both species were analyzed independently. We used a Linear Mixed-Effects model (LMER) in which we set the CO2 treatment (whole-plot) and the TFD treatment (subplot) as fixed effects. Multiple pairwise comparisons were then realized using a Tukey test for above and below ground biomasses, below to above ground ratios and C:N ratios, and a Scheffe post-hoc test was used for heights and basal diameters. All statistical analyzes were performed using R software version 3.5.1 (R Development Core Team, 2008). All results in the manuscript are reported by their mean ± standard error (SEM).
Results
Basal Diameters and Heights
Changes in basal diameters and heights of A. marina and R. stylosa subjected to the four different treatments along the year of experiment are presented in Figure 2. The CO2 enrichment resulted in a strong stimulation of seedlings growth from the start of the experiment (Figure 2); however, this effect was significant only 6 months after the beginning of the enrichment. For A. marina, final basal diameters and heights were significantly higher at elevated CO2 than at ambient CO2 concentrations (Figures 2A,C and Table 1), either with normal or longer tidal flooding duration (TFD). In addition, within each CO2 treatment, the longer TFD significantly increased final basal diameters and heights (Figures 2A,C and Table 1). For R. stylosa, elevated CO2 significantly increased final basal diameters and heights for both natural and longer TFD treatments (Figures 2B,D and Table 1); however, no significant effect was observed for longer TFD within each CO2 treatment (Table 1).
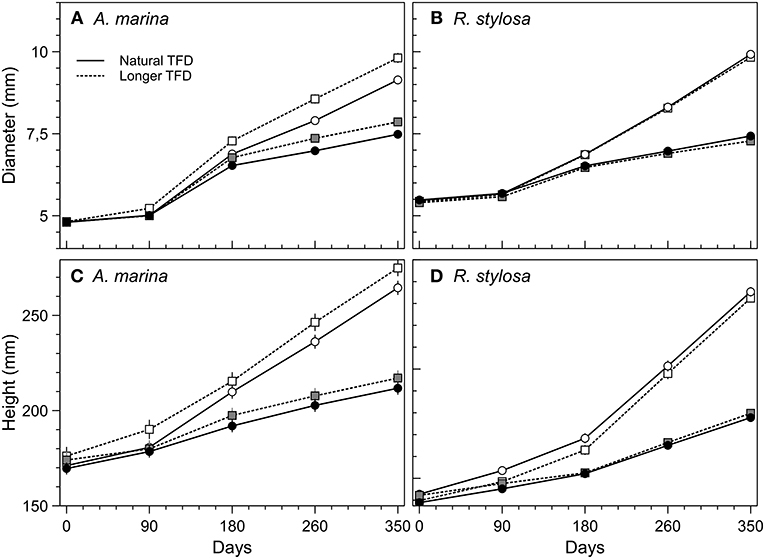
Figure 2. Changes in basal stem diameters and heights of A. marina (A,C) and R. stylosa (B,D) grown in ambient (closed symbols) and elevated (open symbols) CO2, and two lengths of tidal flooding: normal (solid lines) and longer (dotted lines). Values are means ± SEM (A. marina n = 125; R. stylosa n = 180).
Biomass, Below to Above Ground Ratios and Relative Growth Rates
Results of final above and below ground biomass are presented in Figure 3. For A. marina, elevated CO2 significantly increased final above and below ground biomass, either under natural or longer TFD (Figure 2A and Table 1). However, the increase of the above ground biomass was higher (140 and 126% for natural and longer TFD) than the augmentation of the below ground biomass (78 and 74% for natural and longer TFD), which resulted in a significant decrease of the below to above ground ratio (Figure 2C). In addition, no significant effect of the TFD treatment was observed within each CO2 treatment (Figure 2A and Table 1). For R. stylosa, elevated CO2 concentrations also significantly increased the final above and below ground biomass (Figure 3B and Table 1). As for A marina, the increase was higher for the above ground biomass (84 and 78% for natural and longer TFD) in comparison to the below ground biomass (37 and 50% for natural and longer TFD), which resulted in a significant decrease of the below to above ground ratio (Figure 3D). Concerning TFD, a significant reduction was observed for the above ground biomass under elevated CO2 (Figure 3B).
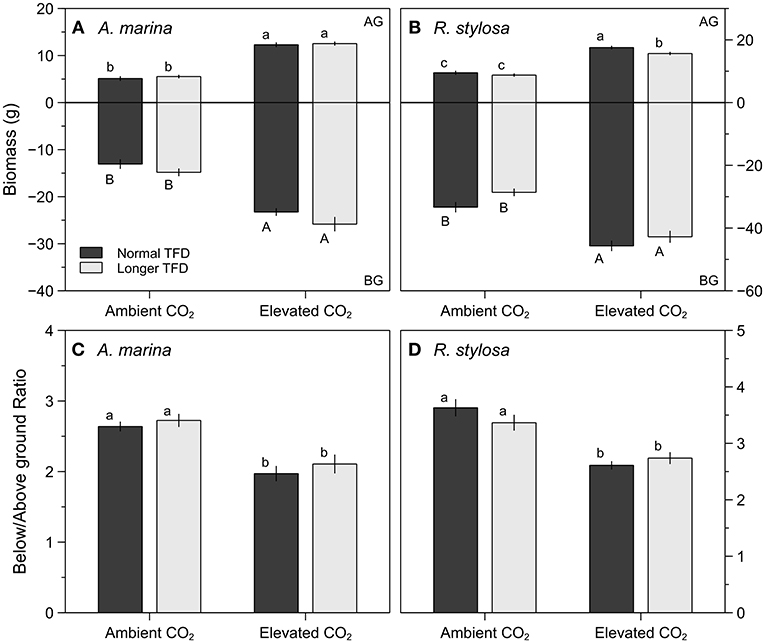
Figure 3. Final above ground (AG) and below ground (BG) biomass (g), and below/above ground biomass ratios of A. marina (A,C) and R. stylosa (B,D) grown in ambient or elevated CO2, and under normal (dark-gray bars) and longer TFD (gray bars), for 12 months. Values are means ± SEM (n = 30). Different letters indicate significant differences (p < 0.05).
Concerning the whole period of the experiment, relative growth rates (RGR) of A. marina were, under ambient CO2 concentrations 18.19 ± 1.33 and 20.36 ± 0.96 g yr−1, and under elevated CO2 concentrations 35.56 ± 0.69 and 38.38 ± 1.46 g yr−1, for natural and longer TFD, respectively. For R. stylosa, RGR were 42.87 ± 1.89 and 37.39 ± 1.33 g yr−1 under ambient CO2 concentrations, and 63.23 ± 1.85 and 58.44 ± 1.99 g yr−1 under elevated CO2 concentrations, for natural and longer TFD, respectively.
C:N Ratios
Results of C:N ratios in the leaves, stems and roots of A. marina and R. stylosa at the end of the experiment are presented in Table 2. Elevated CO2 concentrations significantly increased the C:N ratios in the leaves and roots of A. marina, as well as in the stems and roots of R. stylosa (Tables 1, 2). In addition, TFD significantly decreased the C:N ratios in the stems of both species (Tables 1, 2).
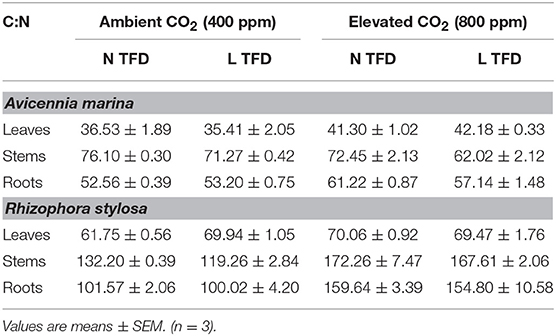
Table 2. C:N ratios in leaves, stems and roots of A. marina and R. stylosa grown in ambient and elevated CO2, and under natural (N) and longer (L) TFD, for 12 months.
Discussion
Elevated CO2 Will Enhance Mangrove Productivity, Carbon Storage, and Seedlings Establishment
Our results clearly confirmed that rise in atmospheric CO2 concentrations would affect the growth of mangrove seedlings. In this experiment, doubling CO2 concentrations for one year resulted in an increase of RGR of more than 89 and 47% for A. marina and R. stylosa, respectively. These strong raises in RGR may be partly related to an increase in the photosynthetic activity of both species under elevated CO2 concentrations. Indeed, the present study was performed concomitantly with another one that focused on the photosynthetic response of the seedlings (Jacotot et al., 2018), and both were conducted during the same time period and on the same seedlings. In this related study, we observed that CO2 enrichment enhanced the mean photosynthetic response of both species (Jacotot et al., 2018). However, a 89% increase in RGR for A. marina and 47% for R. stylosa was surprising as it was much higher than the increase of photosynthesis that rose by more than 37% for A. marina (from 6.25 ± 2.24 to 10.52 ± 3.24 μmol m−2 s−1) and by more than 45% for R. stylosa (from 6.247 ± 1.63 to 11.35 ± 2.91 μmol m−2 s−1). In fact, Kirschbaum (2011) reported a RGR augmentation of 10% for a 30% increase in photosynthesis, which is a reverse ratio in comparison to our study and we should have expected a lower increase in RGR, but no clear explanation was found to clarify this result. However, Kirschbaum (2011) suggested that excess carbohydrates produced by the enhancement of photosynthesis might not be converted into plant tissues due to other resource limitations. In this case, carbohydrates may accumulate in leaves as sugars and starches, resulting in an increase in the leaf mass area ratio (Poorter and Navas, 2003). In fact, a decrease in the leaf mass per area was observed for both species under elevated CO2 concentrations (Jacotot et al., 2018), by more than 33 and 21% for A. marina and R. stylosa, respectively (Figures 5C,D). This development of seedlings leaves suggests that they have not been affected by nutrient limitations during the experiment, which have been sufficiently provided by the frequent water renewal that was operated in the greenhouses.
RGRs were higher during the last 180 days of the experiment than during the first 180 days (Figure 2), i.e., during the warm season rather than during the cool season. Inside the greenhouses, the mean monthly temperature raised from 22.3 to 26.7°C during the warm season. This raise in temperature is thought to be responsible for the increase in productivity of both species. In fact, photosynthetic activity of A. marina and R. stylosa under elevated CO2 increased by 40 and 45% during the warm season, whereas it was only enhanced by 32 and 38%, respectively, during the cool season (Figure 4) (Jacotot et al., 2018), which confirms our hypothesis. Photosynthesis of tropical C3 species, such as A. marina and R. stylosa, can operate between 15 and 45°C, however, there is a temperature optimum for which photosynthesis is maximal. Gilman et al. (2008) reported a temperature optimum for photosynthesis of 28–32°C for mangroves species, but lower temperatures were reported for specific species, such as 24.5°C for Avicennia germinans (Reef et al., 2016), or 26.8°C for A. marina in New Caledonia (Leopold et al., 2016). The temperature reached during the warm season in our study was closer to the temperature optimum for photosynthesis than the one reached during the cold season, therefore, we suggested that seasonal variations in temperature drove the RGRs throughout the year of experiment. With future climate change, the global temperatures are expected to rise by 0.3–4.8°C (Stocker et al., 2013), and our results suggest that elevated CO2 concentrations and increased temperatures could further promote productivity, and thus carbon storage, at least in biomass, of mangrove forests.
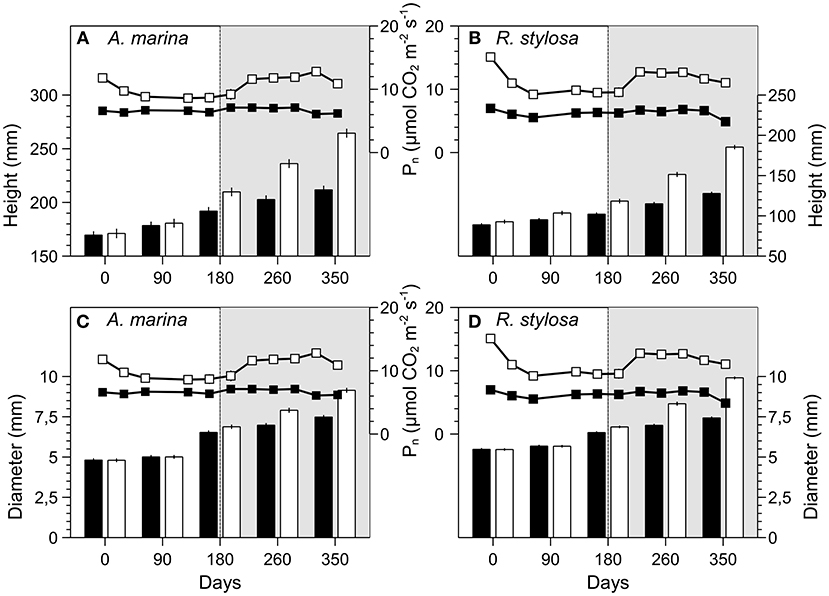
Figure 4. Heights (mm), diameters (mm) and photosynthesis (μmol CO2 m−2 s−1) of A. marina (A,C) and R. stylosa (B,D) grown in ambient (black bars and markers) or elevated CO2 (white bars and markers) for 12 months. Values are means ± SEM (Heights and diameters: A. marina n = 125; R. stylosa n = 180; photosynthesis: n = 30). The white and shaded area correspond respectively to the cool and the warm seasons. Photosynthesis data are from Jacotot et al. (2018).
Elevated CO2 reduced the below to above ground ratio due to a higher increase of the above ground biomass than of the below ground biomass (Figures 3C,D). This allocation of carbon under elevated CO2 with more carbon invested in the above ground parts has previously been observed for A. germinans by Reef et al. (2016). This newly acquired carbon investment reveals a strategy of light capture and photosynthesis optimization that suggests a lack of limitations of the availability of soil resources. This result was consistent with our previous study (Jacotot et al., 2018), in which we observed, for both species, an increase in the specific leaf area with elevated CO2 (Figure 5), which leads to a higher potential for light interception. Although more carbon was invested in above ground parts, a significant increase in the below ground biomass was also observed for both species (Figures 3A,B). This result may lead to several beneficial implications in the response of mangroves ecosystems to climate change: (i) the soil carbon storage potential of the ecosystem may increase, (ii) the increase in root development may facilitate seedlings establishment in intertidal sediments (Balke et al., 2011), which could in turn promote ecosystem expansion, and (iii) the capacity of mangrove ecosystems to face sea-level rise could be improved, as higher root density will increase soil volume and soil organic matter content, two important factors contributing to the vertical accretion of mangrove soils (Cahoon et al., 2006; McKee et al., 2007; McKee, 2011; Krauss et al., 2014, 2017).
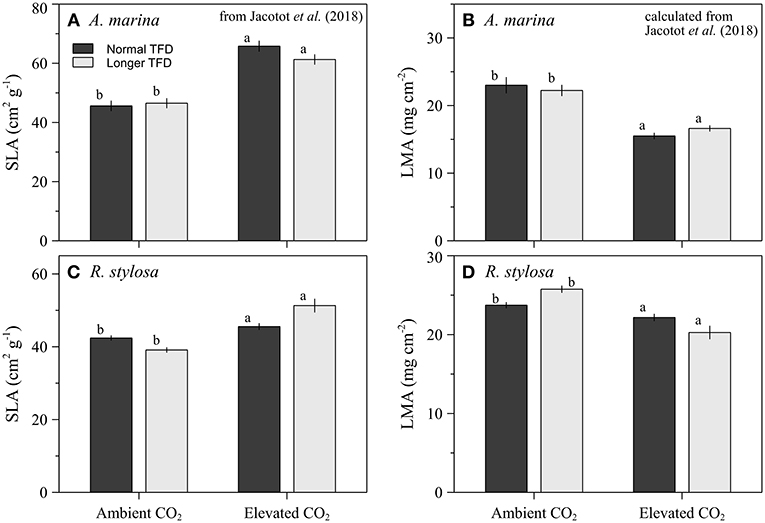
Figure 5. Specific Leaf Area (A,C) and Leaf Mass Area (B,D) of A. marina and R. stylosa grown in ambient or elevated CO2, and under normal (dark-gray bars) and longer TFD (gray bars), for 12 months. Values are means ± SEM (n = 30). Different letters indicate significant differences (p < 0.05). Data are from Jacotot et al. (2018).
In addition to increases in growth and biomass, we also observed a significant change in plant composition, and notably an increase in the C:N ratios in roots and leaves of A. marina and in stems and roots of R. stylosa under elevated CO2 (Tables 1, 2). The effects of elevated CO2 on C:N ratios have been extensively examined in natural ecosystems, however, the available literature shows contrasting results (e.g., Gifford et al., 2000; Luo et al., 2006). C:N ratios may increase, decrease or be unaffected under elevated CO2 but, in general, a 15% of increase is observed (Gifford et al., 2000). In our study, the increase in C:N ratios was mainly due to a decrease in nitrogen content (N) in plant tissues. Such a decrease of N with elevated CO2 has often been observed in terrestrial plants (Cotrufo et al., 1998; Yin, 2002; Ainsworth and Long, 2005; de Graaff et al., 2006), including mangroves (McKee and Rooth, 2008), but remains poorly understood (Taub and Wang, 2008; Lotfiomran et al., 2016). Some authors have shown a general decrease in the specific N uptake rates by the roots under elevated CO2 (McDonald et al., 2002; Taub and Wang, 2008; Lotfiomran et al., 2016). These authors have suggested that this decrease results from both a decrease in N demand by shoots and from a reduction of the soil-root system ability to supply N. They also suggested that the best-supported mechanism for decreased N supply is a decrease in transpiration-driven mass flow of N in soils due to decreased stomatal conductance at elevated CO2. In fact, such a decrease in stomatal conductance has effectively been observed for A. marina and R. stylosa grown under elevated CO2 (Jacotot et al., 2018), which adds to this last hypothesis. Higher C:N ratios may have an important implication, as it may be negatively correlated with lower rates of organic matter decomposition in soils (Hättenschwiler and Gasser, 2005; Zhang et al., 2008; Zimmermann et al., 2009; Jacob et al., 2010), which may lead to a reduction in the greenhouse gas production, and, in turn, to a higher carbon storage capacity. However, this reasoning must be nuanced considering that OM quality is not the only driver of GHG production and emissions in mangrove soils, other parameters influence these biogeochemical processes and will also vary with climate changes and must be taken into account. For example, the increase in soil organic matter decomposition rates with increasing temperature has been widely described in the literature for different ecosystems (Segers, 1998; Fang and Moncrieff, 2001; Fierer et al., 2005; Davidson and Janssens, 2006; Conant et al., 2011; Inglett et al., 2012), including mangroves (Mackey and Smail, 1996; Barroso Matos et al., 2012). Conversely, the increase in tidal flooding duration may lead to a lower renewable of the electron acceptors pool within the soil and to a more anoxic environment, which may limit the organic matter decomposition processes.
Contrasting Effects of the Increase in Tidal Flooding Duration Between A. marina and R. stylosa
According to our initial hypothesis, growth and biomass of R. stylosa were reduced under longer tidal flooding duration (Figures 2B,D, 3B) although this effect was not significant (Table 1). Under longer TFD, basal diameters decreased by 13 and by 5%, and seedlings heights decreased by 6 and 11% for ambient and elevated CO2, respectively. Consequently, final biomass of R. stylosa was reduced by 11 and 4% for ambient and elevated CO2, respectively, in comparison to the controlled TFD treatment. Although we agree that the effect of longer TFD was not significant, we suggest that the reduction in the seedlings growth should not be ignored. We also suggest that the lack of significance in the present work may be due to the limited duration of the experiment and that a longer study could highlight a significant effect of increased TFD on mangrove seedlings. This result may be explained by the 3% reduction in photosynthesis that we observed for R. stylosa seedlings growing under longer tidal duration (Jacotot et al., 2018). However, this slight decrease might be related to other factors. For instance, longer tidal flooding will have probably induced a deficit in oxygen in the soil due to roots and micro-organisms respiration, as well as a reduction of air penetration in the soil from the atmosphere (Naidoo et al., 1997). Although no oxygen measurements have been performed in our experiment, we can expect such a decrease after the longer tidal flooding applied, as it was previously reported in similar experiments (Hovenden et al., 1995; Skelton and Allaway, 1996). In anoxic mangrove sediments, rich in organic matter and in sulfates brought at each tide, organic matter may be oxidized through sulfate reducing processes, leading to sulfide production (Kristensen et al., 2008; Balk et al., 2016). Sulfide compounds, including H2S, are highly toxic to plants (Lamers et al., 2013), and inhibit the growth of mangrove seedlings (Holguin et al., 2001; Lyimo and Mushi, 2005; Reef et al., 2010). The presence of sulfide in the longer TFD treatment was assumed due to the dark color of the sediment and because of the strong rotten egg odor typical of H2S, therefore, we suggest that sulfides were possibly responsible for the seedlings growth inhibition.
In contrast to R. stylosa, final biomass of A. marina increased with longer TFD by 11 and 8% under ambient and elevated CO2 concentrations, respectively (Figures 2A,C, 3A). Natural TFD were based on our field observations and measurements (unpublished data) and were therefore representative of the natural conditions, in which both species develop along the intertidal gradient in New Caledonia, with A. marina growing at a higher elevation than R. stylosa. This position in the intertidal area, combined to the semi-arid climate of New Caledonia, implies A. marina suffer from elevated salt concentrations due to high evaporation processes, low freshwater inputs either by rains or river discharges and low frequency of tidal submersions (Marchand et al., 2011a, 2012). We suggest that longer tidal flooding in our experiment increased water availability that in turn decreased soil salinity, which are two stressing factors affecting growth and biomass, and therefore promoted the growth of A. marina seedlings. With climate changes, due to its positive response to both longer TFD to elevated CO2 concentrations, we can expect that colonization abilities and productivity of A. marina seedlings will be enhanced in the future in this context of a semi-arid climate, where A. marina develops behind Rhizophora spp.
Eventually, to fully understand the response of A. marina and R. stylosa to future climate changes, other parameters should be taken into account such as competition between species (McKee and Rooth, 2008). In New Caledonia, the mangrove ecotone is composed of a succession of different mangrove species, including a saltmarsh C4 grass, Sarcocornia quinqueflora, which competes for suitable spaces for expansion, and future climate may change their specific distribution.
Conclusion
Our results suggest that future climate changes, and specifically the increase in atmospheric CO2 concentrations and tidal flooding duration, will affect A. marina and R. stylosa seedlings physiology. Elevated CO2 concentrations enhanced growth and biomass, with an increase of the relative growth rates of 89% for A. marina and of 47% for R. stylosa, for a 100% increase of atmospheric CO2 concentrations. These results are suggested to be related to photosynthesis enhancement, measured for the same seedlings in a previous study. Elevated CO2 concentrations also increased below-ground biomass, which can lead to soil vertical accretion, thus helping mangroves to face sea-level rise. The increase in below-ground biomass could also favor seedling establishment and mangrove colonization of new available spaces resulting from sea-level rise. Future increase in surface temperature will further stimulate trees development, as seasons affected seedlings productivity with higher relative growth rates during the warmer period. Additionally, C:N ratios of seedlings tissues were increased under elevated CO2, which may lower organic matter degradation in mangrove soils, and thus reduce greenhouse gas emissions while increasing carbon sequestration. When increasing tidal flooding duration, the growth of R. stylosa was slightly reduced, but this effect did not significantly affect the initial enhancement of growth and biomass resulting from elevated CO2 concentrations. Conversely, growth of A. marina was further enhanced by 8% under longer tidal flooding duration in comparison to the control treatment. This result suggests that this species develops in a constraining position along the intertidal gradient in New Caledonia, probably suffering from the lack of water and from high salt concentrations in the soil pore waters. Therefore, the future rise in mean sea level will promote the development of A. marina by a favorable modification of the conditions in the intertidal area under semi-arid climate, which will also affect the recruitment of seedlings and the expansion of mangroves. This study provides complementary information about the productivity of mangrove seedlings with future climate changes. We now suggest that research efforts focus on the impact of climate change on the mineralization processes in mangrove soils to better understand the role of mangroves in climate change mitigation.
Author Contributions
CM, AJ, and MA performed the experimental design. AJ performed data collection and statistical analyses. AJ and CM performed the data interpretation. AJ, CM, and MA wrote the paper.
Conflict of Interest Statement
The authors declare that the research was conducted in the absence of any commercial or financial relationships that could be construed as a potential conflict of interest.
Acknowledgments
This study was funded by the Province Sud of New Caledonia, the City of Mont Dore, KNS Koniambo Nickel SAS and Vale NC. We are very grateful to the IFRECOR Committee for having attributed to this study the IFRECOR Palme National Distinction. We thank Eric Gay, the mayor of the city of Mont-Dore, for his continuous support during the study. We also thank Jacky Mermoud and Diana Burns for their help in managing the facilities. Eventually, we are thankful to Tracy Rolland and Inès Gayral for their help in fieldwork.
References
Ainsworth, E. A., and Long, S. P. (2005). What have we learned from 15 years of free-air CO2 enrichment (FACE)? A meta-analytic review of the responses of photosynthesis, canopy properties and plant production to rising CO2. New Phytol. 165, 351–371. doi: 10.1111/j.1469-8137.2004.01224.x
Alongi, D. M. (2015). The impact of climate change on mangrove forests. Curr. Clim. Change Rep. 1, 30–39. doi: 10.1007/s40641-015-0002-x
Balk, M., Keuskamp, J. A., and Laanbroek, H. J. (2016). Potential for sulfate reduction in mangrove forest soils: comparison between two dominant species of the Americas. Front. Microbiol. 7:1855. doi: 10.3389/fmicb.2016.01855
Balke, T., Bouma, T. J., Horstman, E. M., Webb, E. L., Erftemeijer, P. L., and Herman, P. M. (2011). Windows of opportunity: thresholds to mangrove seedling establishment on tidal flats. Mar. Ecol. Prog. Ser. 440, 1–9. doi: 10.3354/meps09364
Ball, M. C., Cochrane, M. J., and Rawson, H. M. (1997). Growth and water use of the mangroves Rhizophora apiculata and R. stylosa in response to salinity and humidity under ambient and elevated concentrations of atmospheric CO2. Plant Cell Environ. 20, 1158–1166. doi: 10.1046/j.1365-3040.1997.d01-144.x
Barroso Matos, T., Bernini, E., and Rezende, C. E. (2012). Decomposition of mangrove leaves in the estuary of Paraiba do Sul River Rio de Janeiro, Brazil. Lat. Am. J. Aquat. Res. 40, 398–407. doi: 10.3856/vol40-issue2-fulltext-14
Betts, R. A., Jones, C. D., Knight, J. R., Keeling, R. F., and Kennedy, J. J. (2016). El Nino and a record CO2 rise. Nat. Clim. Change 6, 806–810. doi: 10.1038/nclimate3063
Bouillon, S., Borges, A. V., Castañeda-Moya, E., Diele, K., Dittmar, T., Duke, N. C., et al. (2008). Mangrove production and carbon sinks: a revision of global budget estimates. Glob. Biogeochem. Cycles 22, 1–12. doi: 10.1029/2007GB003052
Cahoon, D. R., Hensel, P. F., Spencer, T., Reed, D. J., McKee, K. L., and Saintilan, N. (2006). “Coastal wetland vulnerability to relative sea-level rise: wetland elevation trends and process controls,” in Wetlands and Natural Resource Management Ecological Studies. (Heidelberg: Springer), 271–292.
Church, J. A., Clark, P. U., Cazenave, A., Gregory, J. M., Jevrejeva, S., Levermann, A., et al. (2013). “Sea level change,” in Climate Change 2013: The Physical Science Basis. Contribution of Working Group I to the Fifth Assessment Report of the Intergovernmental Panel on Climate Change, eds T. F. Stocker, D. Qin, G. -K. Plattner, M. Tignor, S. K. Allen, J. Boschung, A. Nauels, Y. Xia, V. Bex, and P.M. Midgley (Cambridge; New York, NY: Cambridge University Press).
Collins, M., Arblaster, J., Dufresne, J.-L., Fichefet, T., Friedlingstein, P., Gao, X., et al. (2014). “Long-term climate change: projections, commitments and irreversibility pages 1029 to 1076,” in Climate Change 2013 - The Physical Science Basis Working Group I Contribution to the Fifth Assessment Report of the Intergovernmental Panel on Climate Change (Cambridge: Cambridge University Press), 1029–1136. doi: 10.1017/CBO9781107415324.024
Conant, R. T., Ryan, M. G., Ågren, G. I., Birge, H. E., Davidson, E. A., Eliasson, P. E., et al. (2011). Temperature and soil organic matter decomposition rates - synthesis of current knowledge and a way forward. Glob. Change Biol. 17, 3392–3404. doi: 10.1111/j.1365-2486.2011.02496.x
Cook, J., Oreskes, N., Doran, P. T., Anderegg, W. R. L., Verheggen, B., Maibach, E. W., et al. (2016). Consensus on consensus: a synthesis of consensus estimates on human-caused global warming. Environ. Res. Lett. 11:048002. doi: 10.1088/1748-9326/11/4/048002
Cotrufo, M. F., Ineson, P., and Scott, A. (1998). Elevated CO2 reduces the nitrogen concentration of plant tissues. Glob. Change Biol. 4, 43–54. doi: 10.1046/j.1365-2486.1998.00101.x
Davidson, E. A., and Janssens, I. A. (2006). Temperature sensitivity of soil carbon decomposition and feedbacks to climate change. Nature 440, 165–173. doi: 10.1038/nature04514
de Graaff, M.-A., van Groenigen, K.-J., Six, J., Hungate, B., and van Kessel, C. (2006). Interactions between plant growth and soil nutrient cycling under elevated CO2. Interactions betwGlob. Change Biol. 12, 2077–2091. doi: 10.1111/j.1365-2486.2006.01240.x
Ellison, J. C. (2015). Vulnerability assessment of mangroves to climate change and sea-level rise impacts. Wetl. Ecol. Manag. 23, 115–137. doi: 10.1007/s11273-014-9397-8
Fang, C., and Moncrieff, J. B. (2001). The dependence of soil CO2 efflux on temperature. Soil Biol. 33, 155–165. doi: 10.1016/S0038-0717(00)00125-5
Farnsworth, E. J., Ellison, A. M., and Gong, W. K. (1996). Elevated CO2 alters anatomy, physiology, growth, and reproduction of red mangrove (Rhizophora mangle L.). Oecologia 108, 599–609. doi: 10.1007/BF00329032
Fierer, N., Craine, J. M., McLauchlan, K., and Schimel, J. P. (2005). Litter quality and the temperature sensitivity of decomposition. Ecology 86, 320–326. doi: 10.1890/04-1254
Gifford, R. M., Barrett, D. J., and Lutze, J. L. (2000). The effects of elevated [CO2] on the C:N and C:P mass ratios of plant tissues. Plant Soil 224, 1–14. doi: 10.1023/A:1004790612630
Gilman, E. L., Ellison, J., Duke, N. C., and Field, C. (2008). Threats to mangroves from climate change and adaptation options: a review. Aquat. Bot. 89, 237–250. doi: 10.1016/j.aquabot.2007.12.009
Hättenschwiler, S., and Gasser, P. (2005). Soil animals alter plant litter diversity effects on decomposition. Proc. Natl. Acad. Sci. U. S. A. 102, 1519–1524. doi: 10.1073/pnas.0404977102
Holguin, G., Vazquez, P., and Bashan, Y. (2001). The role of sediment microorganisms in the productivity, conservation, and rehabilitation of mangrove ecosystems: an overview. Biol. Fertil. Soils 33, 265–278. doi: 10.1007/s003740000319
Hovenden, M. J., Curran, M., Cole, M. A., Goulter, P. F. E., Skelton, N. J., and Allaway, W. G. (1995). Ventilation and respiration in roots of one-year-old seedlings of grey mangrove Avicennia marina (Forsk.) Vierh. Hydrobiologia 295, 23–29. doi: 10.1007/BF00029107
Inglett, K. S., Inglett, P. W., Reddy, K. R., and Osborne, T. Z. (2012). Temperature sensitivity of greenhouse gas production in wetland soils of different vegetation. Biogeochemistry 108, 77–90. doi: 10.1007/s10533-011-9573-3
Jacob, M., Viedenz, K., Polle, A., and Thomas, F. M. (2010). Leaf litter decomposition in temperate deciduous forest stands with a decreasing fraction of beech (Fagus sylvatica). Oecologia 164, 1083–1094. doi: 10.1007/s00442-010-1699-9
Jacotot, A., Marchand, C., Gensous, S., and Allenbach, M. (2018). Effects of elevated atmospheric CO2 and increased tidal flooding on leaf gas-exchange parameters of two common mangrove species: Avicennia marina and Rhizophora stylosa. Photosynth. Res. 138, 249–260. doi: 10.1007/s11120-018-0570-4
Kirschbaum, M. U. (2011). Does enhanced photosynthesis enhance growth? Lessons learned from CO2 enrichment studies. Plant Physiol. 155, 117–124. doi: 10.1104/pp.110.166819
Krauss, K. W., Cormier, N., Osland, M. J., Kirwan, M. L., Stagg, C. L., Nestlerode, J. A., et al. (2017). Created mangrove wetlands store belowground carbon and surface elevation change enables them to adjust to sea-level rise. Sci. Rep. 7:1030. doi: 10.1038/s41598-017-01224-2
Krauss, K. W., McKee, K. L., Lovelock, C. E., Cahoon, D. R., Saintilan, N., Reef, R., et al. (2014). How mangrove forests adjust to rising sea level. New Phytol. 202, 19–34. doi: 10.1111/nph.12605
Kristensen, E., Bouillon, S., Dittmar, T., and Marchand, C. (2008). Organic carbon dynamics in mangrove ecosystems: a review. Aquat. Bot. 89, 201–219. doi: 10.1016/j.aquabot.2007.12.005
Lamers, L. P., Govers, L. L., Janssen, I. C. J. M., Geurts, J. J. M., Van der Welle, M. E. W., Van Katwijk, M. M., et al. (2013). Sulfide as a soil phytotoxin—a review. Front. Plant Sci. 4:268. doi: 10.3389/fpls.2013.00268
Leopold, A., Marchand, C., Renchon, A., Deborde, J., Quiniou, T., and Allenbach, M. (2016). Net ecosystem CO 2 exchange in the “Coeur de Voh” mangrove, New Caledonia: Effects of water stress on mangrove productivity in a semi-arid climate. Agric. For. Meteorol. 223, 217–232. doi: 10.1016/j.agrformet.2016.04.006
Lotfiomran, N., Köhl, M., and Fromm, J. (2016). Interaction effect between elevated CO2 and fertilization on biomass, gas exchange and C/N ratio of European beech (Fagus sylvatica L.). Plants 5:38. doi: 10.3390/plants5030038
Lovelock, C. E., Cahoon, D. R., Friess, D. A., Guntenspergen, G. R., Krauss, K. W., Reef, R., et al. (2015). The vulnerability of Indo-Pacific mangrove forests to sea-level rise. Nature 526, 559–563. doi: 10.1038/nature15538
Luo, Y., Hui, D., and Zhang, D. (2006). Elevated CO2 stimulates net accumulations of carbon and nitrogen in land ecosystems: a meta-analysis. Ecology 87, 53–63. doi: 10.1890/04-1724
Luo, Z., Sun, O. J., Wang, E., Ren, H., and Xu, H. (2010). Modeling productivity in mangrove forests as impacted by effective soil water availability and its sensitivity to climate change using biome-BGC. Ecosystems 13, 949–965. doi: 10.1007/s10021-010-9365-y
Lyimo, T. L., and Mushi, D. (2005). Sulfide concentration and redox potential patterns in mangrove forests of Dar es Salaam: effects on Avicennia Marina and Rhizophora Mucronata seedling establishment. West. Indian Ocean J. Mar. Sci. 4, 163–174. doi: 10.4314/wiojms.v4i2.28485
Mackey, A. P., and Smail, G. (1996). The decomposition of mangrove litter in a subtropical mangrove forest. Hydrobiologia 332, 93–98. doi: 10.1007/BF00016688
Marchand, C., Allenbach, M., and Lallier-Vergès, E. (2011a). Relationships between heavy metals distribution and organic matter cycling in mangrove sediments (Conception Bay, New Caledonia). Geoderma 160, 444–456. doi: 10.1016/j.geoderma.2010.10.015
Marchand, C., Fernandez, J.-M., Moreton, B., Landi, L., Lallier-Vergès, E., and Baltzer, F. (2012). The partitioning of transitional metals (Fe, Mn, Ni, Cr) in mangrove sediments downstream of a ferralitized ultramafic watershed (New Caledonia). Chem. Geol. 300–301, 70–80. doi: 10.1016/j.chemgeo.2012.01.018
Marchand, C., Lallier-Vergès, E., and Allenbach, M. (2011b). Redox conditions and heavy metals distribution in mangrove forests receiving effluents from shrimp farms (Teremba Bay, New Caledonia). J. Soils Sediments 11, 529–541. doi: 10.1007/s11368-010-0330-3
McDonald, E. P., Erickson, J. E., and Kruger, E. L. (2002). Research note: can decreased transpiration limit plant nitrogen acquisition in elevated CO2? Funct. Plant Biol. 29, 1115–1120. doi: 10.1071/FP02007
McKee, K. L. (2011). Biophysical controls on accretion and elevation change in Caribbean mangrove ecosystems. Estuar. Coast. Shelf Sci. 91, 475–483. doi: 10.1016/j.ecss.2010.05.001
McKee, K. L., Cahoon, D. R., and Feller, I. C. (2007). Caribbean mangroves adjust to rising sea level through biotic controls on change in soil elevation. Glob. Ecol. Biogeogr. 16, 545–556. doi: 10.1111/j.1466-8238.2007.00317.x
McKee, K. L., and Rooth, J. E. (2008). Where temperate meets tropical: multi-factorial effects of elevated CO2, nitrogen enrichment, and competition on a mangrove-salt marsh community. Glob. Change Biol. 14, 971–984. doi: 10.1111/j.1365-2486.2008.01547.x
Mcleod, E., Chmura, G. L., Bouillon, S., Salm, R., Björk, M., Duarte, C. M., et al. (2011). A blueprint for blue carbon: toward an improved understanding of the role of vegetated coastal habitats in sequestering CO2. Front. Ecol. Environ. 9, 552–560. doi: 10.1890/110004
Naidoo, G., Rogalla, H., and von Willert, D. J. (1997). Gas exchange responses of a mangrove species, Avicennia marina, to waterlogged and drained conditions. Hydrobiologia 352:39. doi: 10.1023/A:1003088803335
Norby, R. J., DeLucia, E. H., Gielen, B., Calfapietra, C., Giardina, C. P., King, J. S., et al. (2005). Forest response to elevated CO2 is conserved across a broad range of productivity. Proc. Natl. Acad. Sci. U. S. A. 102, 18052–18056. doi: 10.1073/pnas.0509478102
Poorter, H., and Navas, M.-L. (2003). Plant growth and competition at elevated CO2: on winners, losers and functional groups. New Phytol. 157, 175–198. doi: 10.1046/j.1469-8137.2003.00680.x
Prior, S. A., Runion, G. B., Marble, S. C., Rogers, H. H., Gilliam, C. H., and Torbert, H. A. (2011). A review of elevated atmospheric CO2 effects on plant growth and water relations: implications for horticulture. HortScience 46, 158–162. doi: 10.21273/HORTSCI.46.2.158
R Development Core Team, Vienna. (2008). R: A Language and Environment for Statistical Computing. Vienna: R Foundation for Statistical Computing. Available online at: http://www.R-project.org (accessed March 24, 2019).
Reef, R., Feller, I. C., and Lovelock, C. E. (2010). Nutrition of mangroves. Tree Physiol. 30, 1148–1160. doi: 10.1093/treephys/tpq048
Reef, R., Slot, M., Motro, U., Motro, M., Motro, Y., Adame, M. F., et al. (2016). The effects of CO2 and nutrient fertilisation on the growth and temperature response of the mangrove Avicennia germinans. Photosynth. Res. 129, 159–170. doi: 10.1007/s11120-016-0278-2
Reef, R., Winter, K., Morales, J., Adame, M. F., Reef, D. L., and Lovelock, C. E. (2015). The effect of atmospheric carbon dioxide concentrations on the performance of the mangrove Avicennia germinans over a range of salinities. Physiol. Plant. 154, 358–368. doi: 10.1111/ppl.12289
Segers, R. (1998). Methane production and methane consumption: a review of processes underlying wetland methane fluxes. Biogeochemistry 41, 23–51. doi: 10.1023/A:1005929032764
Skelton, N. J., and Allaway, W. G. (1996). Oxygen and pressure changes measured in situ during flooding in roots of the grey mangrove Avicennia marina (Forssk.) Vierh. Aquat. Bot. 54, 165–175. doi: 10.1016/0304-3770(96)01043-1
Stocker, T. F., Qin, D., Plattner, G.-K., Tignor, M., Allen, S. K., Boschung, J., et al. (2013). “IPCC, 2013: climate change 2013: the physical science basis,” in Contribution of Working Group I to the Fifth Assessment Report of the Intergovernmental Panel on Climate Change. Cambridge University Press.
Taub, D. R., and Wang, X. (2008). Why are nitrogen concentrations in plant tissues lower under elevated CO2? A critical examination of the hypotheses. J. Integr. Plant Biol. 50, 1365–1374. doi: 10.1111/j.1744-7909.2008.00754.x
Van Loon, A. F., Dijksma, R., and Van Mensvoort, M. E. F. (2007). Hydrological classification in mangrove areas: a case study in Can Gio, Vietnam. Aquat. Bot. 87, 80–82. doi: 10.1016/j.aquabot.2007.02.001
Ward, R. D., Friess, D. A., Day, R. H., and MacKenzie, R. A. (2016). Impacts of climate change on mangrove ecosystems: a region by region overview. Ecosyst. Health Sustain. 2:e1211. doi: 10.1002/ehs2.1211
Yin, X. (2002). Responses of leaf nitrogen concentration and specific leaf area to atmospheric CO2 enrichment: a retrospective synthesis across 62 species. Glob. Change Biol. 8, 631–642. doi: 10.1046/j.1365-2486.2002.00497.x
Zhang, D., Hui, D., Luo, Y., and Zhou, G. (2008). Rates of litter decomposition in terrestrial ecosystems: global patterns and controlling factors. J. Plant Ecol. 1, 85–93. doi: 10.1093/jpe/rtn002
Keywords: mangrove, elevated CO2, sea-level rise, growth, biomass, climate changes
Citation: Jacotot A, Marchand C and Allenbach M (2019) Increase in Growth and Alteration of C:N Ratios of Avicennia marina and Rhizophora stylosa Subject to Elevated CO2 Concentrations and Longer Tidal Flooding Duration. Front. Ecol. Evol. 7:98. doi: 10.3389/fevo.2019.00098
Received: 04 November 2018; Accepted: 11 March 2019;
Published: 10 April 2019.
Edited by:
Alberto Vieira Borges, University of Liège, BelgiumReviewed by:
Joshua L. Breithaupt, University of Central Florida, United StatesLu Zhai, Los Alamos National Laboratory (DOE), United States
Mohammad Basyuni, University of North Sumatra, Indonesia
Copyright © 2019 Jacotot, Marchand and Allenbach. This is an open-access article distributed under the terms of the Creative Commons Attribution License (CC BY). The use, distribution or reproduction in other forums is permitted, provided the original author(s) and the copyright owner(s) are credited and that the original publication in this journal is cited, in accordance with accepted academic practice. No use, distribution or reproduction is permitted which does not comply with these terms.
*Correspondence: Cyril Marchand, cyril.marchand@unc.nc