- 1Chemical Oceanography Unit, FOCUS, University of Liège, Liège, Belgium
- 2Laboratory of Oceanology, FOCUS, University of Liège, Liège, Belgium
- 3Econum, COMPLEXYS Institute, University of Mons, Mons, Belgium
- 4Seaviews, La Ciotat, France
- 5STAtion de REcherches Sous-marines et Océanographiques (STARESO), Calvi, France
- 6Center of Marine Sciences of Algarve, University of Algarve, Faro, Portugal
- 7Eukaryotic Phylogenomics, InBioS–PhytoSYSTEMS, University of Liège, Liège, Belgium
Posidonia oceanica is the only reported seagrass to produce significant amount of dimethylsulfoniopropionate (DMSP). It is also the largest known producer of DMSP among coastal and inter-tidal higher plants. Here, we studied (i) the weekly to seasonal variability and the depth variability of DMSP and its related compound dimethylsulfoxide (DMSO) in P. oceanica leaves of a non-disturbed meadow in Corsica, France, (ii) the weekly to seasonal variability and the depth variability of DMSP to DMSO concentration to assess the potential of the DMSP:DMSO ratio as indicator of stress, and (iii) the relationships between DMSP, DMSO, and the DMSP:DMSO ratio with potential explanatory variables such as light, temperature, photosynthetic activity (effective quantum yield of photosystem II), and leaf size. The overall average concentrations of organosulfured compounds in P. oceanica leaves were 130 ± 39 for DMSP and 4.9 ± 2.1 for DMSO. Concentrations of DMSP and DMSO in P. oceanica were overall distinctly higher and exhibited a wider range of variations than other marine primary producers such as Spartina alterniflora, phytoplankton communities, epilithic Cyanobacteria and macroalgae. Concentrations of both DMSP and DMSO in P. oceanica leaves decreased from a maximum in autumn to a minimum in summer; they changed little with depth. Potential explanatory variables except the leaf size, i.e., the leaf age were little or not related to measured concentrations. To explain the seasonal pattern of decreasing concentrations with leaf aging, we hypothesized two putative protection functions of DMSP in young leaves: antioxidant against reactive oxygen species and predator-deterrent. The similar variation of the two molecule concentrations over time and with depth suggested that DMSO content in P. oceanica leaves results from oxidation of DMSP. The DMSP:DMSO ratio remained constant around a mean value of 29.2 ± 9.0 μmol:μmol for the non-disturbed harvested meadow regardless of the time of the year, the depth or the leaf size. As suggested for the salt march plant S. alterniflora, we hypothesized the DMSP:DMSO ratio could be considered as indicator of stress in seagrasses exposed to environmental or anthropogenic stressors. More research would now be needed to confirm the functions of DMSP and DMSO in seagrasses and how the DMSP:DMSO ratio will vary under various disturbances.
Introduction
The synthesis and metabolism of dimethylsulfoniopropionate (DMSP) has been studied for 70 years (Challenger and Simpson, 1948). DMSP and related sulfonium compounds dimethyl sulfide (DMS) and dimethylsulfoxide (DMSO) constitute an integral part of the marine sulfur cycle and play an important role in the global sulfur budget (Stefels et al., 2007; Asher et al., 2017). DMS, via transfer from the ocean to the atmosphere, could have a cooling effect on climate and could help to compensate for warming from “greenhouse effect” (Lovelock and Maggs, 1972; Charlson et al., 1987). Although this climatic role has been found to be much more complex than originally thought (Quinn and Bates, 2011), it nevertheless explains the interest of research on the production and fate of these organosulfured compounds in the marine environment.
Among the terrestrial and coastal magnoliophytes studied for DMSP production, only few poaceae and asteraceae have a high DMSP content, e.g., Spartina anglica C.E. Hubbard, 1978 (Van Diggelen et al., 1986) and Spartina alterniflora Loisel (Dacey et al., 1994), Saccharum officinarum L., 1753 (Paquet et al., 1994), and Wollastonia biflora (L.) DC., 1836 (Hanson et al., 1994). Recently, Borges and Champenois (2015) added the seagrass Posidonia oceanica (Linnaeus) Delile, 1813 to this short list. This seagrass is endemic to the Mediterranean Sea and forms dense monospecific meadows from the surface to depths of 40–48 m (Boudouresque and Meinesz, 1982; Gobert et al., 2006; Boudouresque et al., 2012). P. oceanica beds are major coastal ecosystems (Gobert et al., 2006; Boudouresque et al., 2012), highly productive (Champenois and Borges, 2019b), provide many goods and services (Campagne et al., 2015; Mtwana Nordlund et al., 2016) and have considerable environmental, financial, and heritage value (Vassallo et al., 2013; Campagne et al., 2015).
To our best knowledge only one previous study investigated the occurrence of DMSP in seagrass species other than P. oceanica (Dacey et al., 1994). In this study, the DMSP content in the epiphytized leaves of the three seagrasses Halodule wrightii Ascherson, 1868, Syringodium filiforme Kützing, 1860, and Thalassia testudinum K.D. Koenig, 1805 was mostly attributed to their epiphytes (Dacey et al., 1994). Recently, Borges and Champenois (2015, 2017) analyzed P. oceanica leaf segments non-epiphytized or carefully cleaned of their epiphytes prior DMSP analysis. In addition epiphyte flora growing on P. oceanica leaves is dominated by red coralline algae (Jacquemart and Demoulin, 2006; Piazzi et al., 2016) that have a very low DMSP content of below 0.1 (Kamenos et al., 2008). For these reasons, it is obvious that DMSP measured in P. oceanica material came from the plant itself. Borges and Champenois (2015) reported depth and seasonal variability of the DMSP content in P. oceanica leaves, suggesting a relationship with irradiance. Because these authors followed the sample preparation protocol used in the vast majority of DMSP-related studies dealing with macroalgae, i.e., performing measurements on oven-dried samples, their work and similar are not quantitative. Indeed, most DMSP is lost and/or transformed into DMSO because of the drying treatment, and fresh or frozen tissues of P. oceanica have a DMSP content orders of magnitude higher (Borges and Champenois, 2017). Consequently, there is a need to investigate again depth and seasonal variations of DMSP content in P. oceanica tissues.
DMSP plays physiological roles in marine autotrophs that has stimulated numerous studies on its production, especially on marine phytoplankton (Challenger and Simpson, 1948; Stefels et al., 2007; Bullock et al., 2017). Briefly, DMSP may act as osmolyte, cryoprotectant, antioxidant, predator deterrent, antibiotic, and overflow metabolite for carbon and nitrogen (reviews in: Otte et al., 2004; Stefels et al., 2007; Van Alstyne, 2008). These functions like, e.g., the role of antioxidant in S. alterniflora (Husband and Kiene, 2007; Husband et al., 2012), remain often hypothetical and still require testing. Coastal higher plants further have to deal with toxic sediment-reduced sulfur (Zheng et al., 2017; Apostolaki et al., 2018) and are colonized by epiphytes (Jackson et al., 2006; Piazzi et al., 2016). In Spartina spp., the production of DMSP could allow the plant to excrete excess sulfur (Van Diggelen et al., 1986; Otte and Morris, 1994). DMSP production as an overflow mechanism for excess reduced sulfur and for excess energy was also hypothesized by Stefels (2000) in microalgae. Regarding an hypothetical role of antifouling agent, it seems ruled out from experimental observations in S. alterniflora (Jackson and Stuckey, 2007), but was reported in the brown algae Fucus vesiculosus Linnaeus, 1753 against bacterial attachment (Saha et al., 2012). Studies are to date still too few to fully elucidate the roles DMSP and its related compounds effectively play, in particular in coastal higher plants.
DMSO is present in phytoplankton (Simó and Vila-Costa, 2006) and in intertidal salt marsh plants of the genus Spartina (Husband et al., 2012; McFarlin and Alber, 2013). DMSO is also present in the seagrass P. oceanica, as reported in the protocol papers of Borges and Champenois (2017) and Champenois and Borges (2019a). In comparison to DMSP and DMS, DMSO has received less attention (Lee et al., 1999) because of the later discovery of particulate DMSO in the late 90s (Simó et al., 1998) and its importance for the sulfur cycle (Green and Hatton, 2014). DMSO is formed through photochemical oxidation of DMS in seawater (Brimblecombe and Shooter, 1986) and through biological oxidation of DMS(P) in cells (Zhang et al., 1991; Simó and Vila-Costa, 2006). The reasons why DMSO is biosynthesized by phytoplankton remain unresolved. Similar to DMSP, DMSO could act as a cryoprotectant, a free-radical scavenger and an osmoregulator (Lee and de Mora, 1999).
The significant correlation between DMSO and DMSP frequently observed in marine phytoplankton indicates that the production of DMSO is closely related to DMSP production (Simó and Vila-Costa, 2006; Hatton and Wilson, 2007). From field (Simó and Vila-Costa, 2006) and experimental (Simó et al., 1998) observations of DMSO and DMSP kinetics in phytoplankton, Simó and Vila-Costa (2006) suggested the ratio of their concentrations would be a rather good indicator for oxidative stress caused by, e.g., nutrient exhaustion and light. Studies on S. alterniflora have further argued the usefulness of this ratio as generic indicator of physiological stress: tissue senescence (Husband and Kiene, 2007) and sudden dieback (McFarlin and Alber, 2013), chemical stress: herbicides (Husband et al., 2012), and mechanical stress: wrack deposition and herbivory (McFarlin and Alber, 2013).
DMSP and its relatives are compounds that clearly deserve attention in higher plants. This consideration, discussed 15 years ago by Otte et al. (2004), is still relevant today. The study of DMSP and DMSO in seagrasses is at its very beginning, the little we currently know being to some extent biased methodologically (DMSP analysis in leaf-epiphyte complex or dried samples; Dacey et al., 1994; Borges and Champenois, 2015). In order to deepen our knowledge on the ecology of these organosulfured compounds in P. oceanica, we investigated (i) the weekly to seasonal variability and the depth variability of DMSP and DMSO in P. oceanica leaves, (ii) the weekly to seasonal variability and the depth variability of DMSP to DMSO concentration to assess the potential of the DMSP:DMSO ratio as indicator of stress, and (iii) the relationships between DMSP, DMSO, and the DMSP:DMSO ratio with potential explanatory variables such as light, temperature, photosynthetic activity (effective quantum yield of photosystem II), and leaf size. To reach these objectives, we monitored during 15 months the DMSP and DMSO content in P. oceanica leaves of a non-disturbed meadow in Corsica, France, in the framework of the STARECAPMED program (Richir et al., 2015).
Materials and Methods
Study Site and Environmental Parameters
The study was conducted between April 2015 and July 2016 in a dense and healthy P. oceanica meadow in the northwestern part of the Revellata Bay in the Gulf of Calvi (Corsica, France; Norie, 1831), close to the STARESO research station (42.580°N, 8.725°E). The Gulf of Calvi has an area of about 22 km2, opens to the Ligurian Sea on the northeast with a border of about 6 km and connects to the deep sea by a canyon. STARESO, with a direct access to the sea, boats and diving facilities enables high-frequency scientific observations in all areas of oceanographic research. The Gulf of Calvi is a “reference site” in a very good state of environmental conservation (Gobert et al., 2009; Lopez y Royo et al., 2010, 2011). The sea level to about 38 m depth benthic ecosystem is dominated by a dense and healthy P. oceanica meadow. The four other main benthic communities in the Gulf are shallow rocky substrates covered by photophilic macroalgae, bare coarse sand, Cymodocea nodosa (Ucria) Ascherson, 1870 meadows and heterogeneous muddy sand rich in debris of P. oceanica leaves, with sciaphilic macroalgae down to 100 m depth (Bay, 1984; Champenois and Borges, 2012; Richir et al., 2015). The study site in the present work was identical to that of Borges and Champenois (2015, 2017).
Water temperature and light intensity are parameters recorded continuously by STARESO with probes and loggers deployed in the water column and the P. oceanica meadow facing the research station, i.e., where the survey was conducted. The collected environmental data was made accessible via the shared database RACE (Binard, 2017). Photosynthetically active radiation (PAR, μmol photons.m−2.s−1) was recorded (10 or 20 min interval) at a depth of 10 m just above the seagrass meadow canopy with an Odyssey logger (Odyssey Submersible Photosynthetic Active Radiation Logger, Dataflow Systems Ltd). The Odyssey logger was calibrated in the air against a LICOR LI-190 quantum sensor (LI-COR Inc.). Temperature (°C) and light intensity (lux, cd.sr.m−2) were recorded (10 or 20 min interval) along the depth gradient of distribution of P. oceanica, i.e., from the sea level to 36 m depth, with Hobo loggers (HOBO Pendant® Temperature/Light Data Logger, Onset Computer Corporation). Five Hobo loggers were deployed along the depth transect at 1, 8, 18, 27, and 34 m depth just above the seagrass bed to record light intensity that reached the meadow canopy. Five supplementary Hobo loggers were deployed along the depth transect at 3, 10, 20, 29, and 36 m depth at the water column-sediment interface to record water temperature within the meadow canopy (and light absorption by the meadow canopy).
Measurements of P. oceanica leaf photosynthetic activity and seagrass sampling were performed around midday between 10:25 and 13:33 (Universal Time; see below section Photosynthetic Activity and Sample Collection). Only the temperature and light data corresponding to this 3-h time window were selected for analysis.
Photosynthetic Activity and Sample Collection
Measurements of P. oceanica leaf photosynthetic activity and seagrass sampling for subsequent biometry and DMSP and DMSO analysis were performed by scuba dives at 10 m depth at a weekly to fortnightly frequency between April 15, 2015 and July 11, 2016. Between June 23, 2015 and July 13, 2015, measurements of leaf photosynthetic activity and seagrass sampling were additionally performed by scuba dives along the depth transect at 3, 10, 15, 20, 25, 29, and 36 m depth (no leaf sampling for biometry at 15 and 25 m depth). Measurements of leaf photosynthetic activity and seagrass sampling were performed on orthotropic shoots (i.e., vertical growth, as opposite to plagiotropic—horizontal—growth; Boudouresque et al., 2012) randomly sampled on a few m2 surfaces.
During each dive, the chlorophyll fluorescence effective quantum yield of photosystem II (Yield of PSII, or ΦPSII) of six P. oceanica shoots was recorded using an underwater fluorometer (DIVING-PAM, Heinz Walz GmbH). ΦPSII is a measure of the effective photochemical efficiency of the plant, in the conditions of light and temperature at the time of measurement (Silva et al., 2009; Murchie and Lawson, 2013). To obtain reliable and comparable data, the protocol was standardized. The measurement of ΦPSII was performed on the convex middle part of the third internal leaf from the inside of the leaf bundle [juveniles leaves—<5 cm long (Giraud, 1979)—excluded], around midday, during cloud free and calm weather days. The measurement of ΦPSII was made on leaves in their natural position in the water column, i.e., at the vertical (measurements are indeed often performed with leaves maintained horizontally, which exposes them de facto to higher light intensities). PAR at half height of the meadow canopy was recorded concomitantly to leaf ΦPSII in the same vertical position with the Fiber Quantum Sensor DIVING-LI of the DIVING-PAM. The calibration of the DIVING-LI was checked in the air before each dive against a LICOR LI-190 quantum sensor. PAR was recorded close to the spot of the sample where fluorescence was recorded using the Universal Sample Holder DIVING-USH.
Of the six leaves analyzed for ΦPSII during each dive, three were sampled for DMSP and DMSO analysis. Sampling was performed by cutting the leaf just above the meristem area with a scissor to ensure its post-regrowth (De los Santos et al., 2016). Six supplementary P. oceanica complete leaf bundles were sampled with the same method for shoot biometry analysis (concomitantly to sampling for DMSP and DMSO analysis). Leaf bundles were clipped with plastic tongs prior cutting to keep the insertion order of the leaves. Sampled third leaves and leaf bundles were brought back to the STARESO facility in opaque plastic bags.
Quickly after the end of the dive, the first 20 photosynthetic cm from the base of P. oceanica third leaves (i.e., first little pigmented cm excluded) sampled for DMSP and DMSO analysis were dissected, then prepared and stored according to the protocol of Borges and Champenois (2017). Briefly, dissected leaf fragments were cleaned of their epiphytes (when present) with a razor blade (Dauby and Poulicek, 1995), conditioned individually in 20 ml glass vials sealed with polytetrafluoroethylene coated silicone septa stopper and frozen at −20°C until DMSP and DMSO analysis. P. oceanica leaf bundles clipped with plastic tongs were stored individually in plastic bags and frozen at −20°C until biometry measurements. Samples were brought back frozen to the home laboratory in Belgium to avoid DMSP loss during transport (Borges and Champenois, 2017). Samples stored in the home laboratory at −20°C were then analyzed for DMSP and DMSO (see section DMSP and DMSO Analysis) and for biometry according to the method of Giraud (1979) for measuring and counting foliar structures of P. oceanica. The third leaf foliar surface (cm2) was calculated as: leaf length x leaf width; the leaf bundle foliar surface was calculated as: (the sum of the lengths of intermediate leaves x mean width of intermediate leaves) + (the sum of the lengths of adult leaves x mean width of adult leaves).
In addition to P. oceanica sampling and for comparison purpose of DMSP and DMSO analysis between benthic primary producers of the Revellata Bay, 29 species of epilithic Cyanobacteria and macroalgae (Ochrophyta, Chlorophyta, and Rhodophyta) were collected by snorkel dives between surface and 8 m depth in May 2016 along the shore close to the STARESO research station. Quickly after the end of the dive, the samples were rinsed with running seawater in the STARESO facility, stored in 50 ml polyethylene bottles and kept frozen (storage, transport; Borges and Champenois, 2017) until analysis. Variable amounts of algae biomass (depending on the expected DMSP content based on literature values) were processed for DMSP and DMSO content (three replicates by species-specific pool sample) in a similar fashion as for P. oceanica leaves (see section DMSP and DMSO Analysis).
DMSP and DMSO Analysis
P. oceanica third leaves sampled for DMSP and DMSO analysis were unfrozen, gently dried of water droplets on absorptive paper and cut in 3 mm2 square fragments. About 20 mg of fresh leaf fragments were put in three different pre-weighted 20 ml glass vials for analysis (three pseudoreplicates by leaf, three leaves by sampling; Borges and Champenois, 2017). DMSP and DMSO concentrations were measured after conversion into DMS with the headspace technique with a gas chromatograph (GC) with a flame photometric detector (FPD) (Agilent 7890A, Agilent Technologies Inc.) according to the method of Champenois and Borges (2019a). In brief, the method consists first in digesting P. oceanica leaf fragments in their 20 ml closed vials with NaOH (6M). In the presence of NaOH, DMSP cleaves quantitatively into DMS and acrylate. The DMS in the vial headspace is measured by GC-FPD. The NaOH digestate is then bubbled with ambient air to evacuate the DMS and acidified with HCl. The DMSO present in the digestate is then reduced by TiCl3 in DMS. The DMS in the vial headspace is again measured by GC-FPD. The GC-FPD peaks of DMS are finally converted into DMSP and DMSO concentrations from a series of standards of known concentrations treated in the same way and at the same time as the samples. Sample DMSP and DMSO concentrations are given in μmol per g of leaf fresh weight (). The ratio of DMSP to DMSO concentration was further computed.
Statistical Analysis
The study had two datasets to analyze: (i) a 15-month time series dataset from a fixed depth (10 m) and (ii) a depth gradient dataset (3–36 m) of variation in environmental (temperature, light) and biological (ΦPSII, foliar surfaces, DMSP and DMSO concentrations, DMSP:DMSO ratio) observations. The statistical analysis of the two datasets was performed in RStudio version 1.1.383 (RStudio Team., 2016). The significance level alpha was set to 1% to reduce Type I error.
Regarding the 15-month survey dataset (see Supplementary Material Table 1), cross-validated smoothing cubic splines (Pope and Gadh, 1988) were fitted to the mean PAR and mean ΦPSII recorded with the DIVING-PAM. Cross-validated smoothing cubic splines were also fitted to P. oceanica third leaf and shoot mean foliar surfaces, and to P. oceanica third leaf mean DMSP concentration, mean DMSO concentration, and mean DMSP:DMSO ratio. DMSP and DMSO concentrations, and to a lesser extend the DMSP:DMSO ratio showed breaks during their seasonal evolution at 10 m depth in late summer and autumn. In P. oceanica, the fall of senescent adult leaves is not a continuous process, most remaining in position until being torn off by autumnal storms (Wittmann, 1984). The potential effect of this non-continuous leaf renewal cycle on the dynamics of leaf DMSP and DMSO concentrations and their ratio was identified using segmented linear regression analysis (Muggeo, 2003; Toms and Lesperance, 2003). This semi parametric procedure is useful for assessing if the relationships between explanatory and response variables can be divided into more intervals. Identified linear segments were tested for model assumptions.
Regarding the depth transect performed between June 23, 2015 and July 13, 2015 (see Supplementary Material Table 2), it included four samplings at 10 m depth (data shared between both the 15-month survey and the depth gradient datasets) and one sampling at, respectively, 3, 15, 20, 25, 29, and 36 m depth. The four samplings at 10 m depth were compared for P. oceanica biological parameters using one-way ANOVA after testing for assumptions. Because none of the parameters displayed differences at 10 m depth over the 3 weeks of the depth gradient sampling (results not shown), we could consider that depths were comparable over this short period. Depths were compared for P. oceanica biological parameters using one-way ANOVAs after testing for assumptions, followed by Tukey's multiple comparison test of means.
Relationships between response variables: DMSP and DMSO concentrations and the DMSP:DMSO ratio and potential explanatory biological (ΦPSII and third leaf foliar surface) and environmental (light and temperature) variables were explored using Spearman's rank correlation coefficient (Pearson, 2001; Schober et al., 2018) and analyzed using Generalized Linear Model (GLM, with gaussian distribution and identity link function; McCullagh and Nedler, 1989). The interpretation of the meaning of the Spearman's rank correlation coefficients was from Fowler and Cohen (1990). From correlation and GLM analyses results (coefficient p-values), we modeled linear relationships between P. oceanica third leaf foliar surface and third leaf DMSP concentration, DMSO concentration and DMSP:DMSO ratio, for 10 m depth data. We further modeled the linear relationship between P. oceanica third leaf DMSP and DMSO concentrations for all 10 m depth survey and depth gradient data, after removing from this global dataset two influential observations measured by Cook's distance (Cook, 1977). GLMs and linear regressions were checked for model assumptions.
Results
Environmental Parameters
PAR and temperature at 10 m depth followed a classic annual sinusoidal cycle (Figure 1A), with about 2-month time lag between seasonal extrema of the two parameters. Summer and winter water temperatures were, on average, about 1.2°C higher than their respective climatological seasonal mean values averaged over the 1981–2018 period (unpubl. data). The autumn-winter period of the survey was further characterized by a low occurrence of storms (Champenois and Borges, 2019b). The low predicted values of the PAR profile within the meadow canopy resulted from their measurement vertical to the surface and the shading effect of the dense seagrass meadow (Figure 1A). PAR within the meadow canopy peaked with time lag late summer, during the period of large P. oceanica leaf decay.
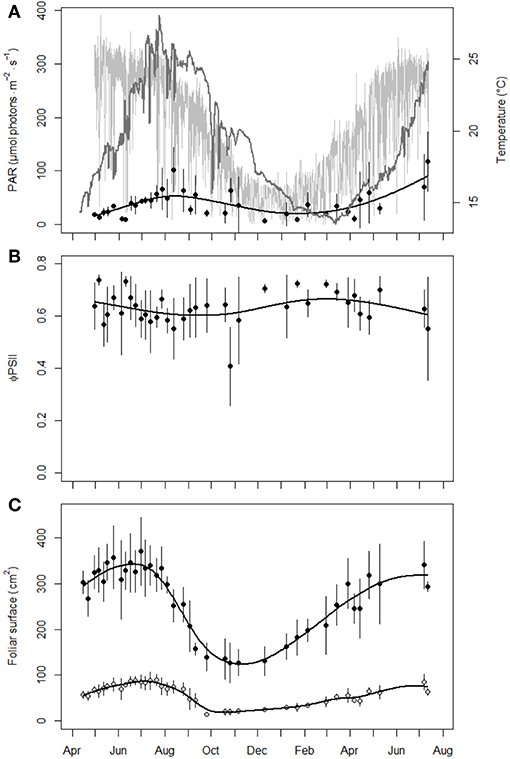
Figure 1. Evolution of environmental and seagrass biological parameters in P. oceanica meadow at 10 m depth over time. (A) Temperature (°C, solid dark gray line) and photosynthetically active radiation (PAR, μmol photons.m−2.s−1) recorded above the meadow canopy (solid light gray line) or within the meadow canopy (dots; mean ± sd, n = 6). (B) Effective quantum yield of photosystem II (ΦPSII) of P. oceanica third leaf (mean ± sd, n = 6). (C) P. oceanica shoot (black dots) and third leaf (gray dots) foliar surfaces (cm2; mean ± sd, n = 6 except February 29, 2016, n = 5). The solid black lines on graphs (A–C) are predictions from cross-validated smoothing cubic spline analyses on within meadow PAR, ΦPSII and foliar surfaces, respectively.
The average lux over the 3-week sampling period along the depth transect decreased exponentially with depth while the average temperature decreased linearly with depth (results of models not shown; Figure 2A). The more marked decrease in temperature below 10 m depth indicated the progressive installation of the summer thermocline. The random pattern of the PAR profile recorded during sampling scuba dives within the meadow canopy illustrated the day-to-day irradiance variability during the 3-week depth gradient survey (Figure 2A). After normalization of mean PAR within the canopy by mean lux of corresponding days above the canopy, a more structured profile of increase of light in the meadow canopy with depth was obvious. The most consistent increase between 20 and 29 m depth resulted from the net reduction of P. oceanica shoot density and biomass (Gobert et al., 2003), which de facto decreased shading in the meadow canopy at depths where light becomes limiting.
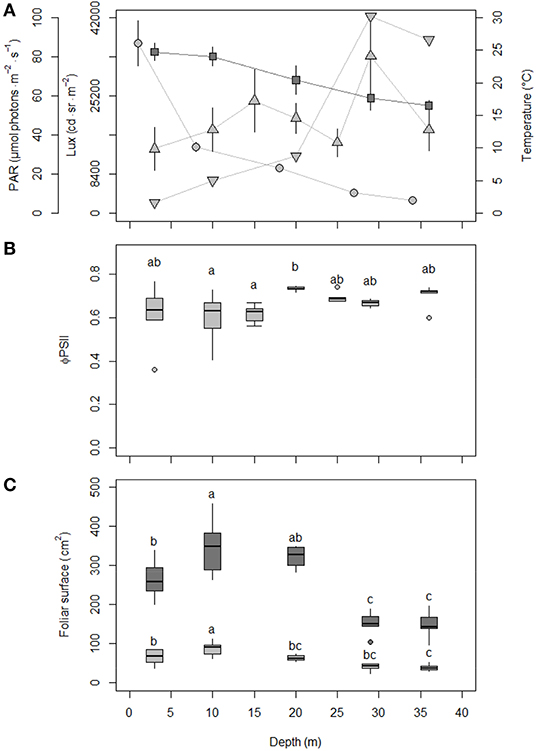
Figure 2. Evolution of environmental and seagrass biological parameters in P. oceanica meadow along the 3–36 m depth transect. (A) Temperature (°C; dark gray squares; mean ± sd, n = 23) and light recorded above the meadow canopy (lux, cd.sr.m−2; light gray dots; mean ± sd, n = 23) or within the meadow canopy (photosynthetically active radiation (PAR), μmol photons.m−2.s−1; light gray triangles; n = 6 at all depths except 10 m, n = 24). For comparison purpose between depths, mean PAR recorded during dives were normalized by mean lux of corresponding days (flipped light gray triangles, no scale). (B) Boxplot of the effective quantum yield of photosystem II (ΦPSII) of P. oceanica third leaf. (C) Boxplot of P. oceanica shoot (dark gray) and third leaf (light gray) foliar surfaces (cm2). Letters on boxplots (B,C) represent significant differences (one-way ANOVA and Tukey test) in mean ΦPSII or mean foliar surfaces between depths, respectively (n = 6 at all depths except 10 m, n = 24).
Effective Quantum Yield of Photosystem II and Biometry
P. oceanica third leaf ΦPSII measured at 10 m depth showed inter-shoot and inter-date variability (Figure 1B). Globally, ΦPSII decreased from late winter to late summer, for an average general value of 0.63 ± 0.06 over the survey period. P. oceanica third leaf ΦPSII changed little with depth (Figure 2B). Mean ΦPSII along the 3-36 m depth gradient was 0.66 ± 0.05, i.e., a value close to the mean annual one of 0.63 ± 0.06 at 10 m depth. Globally, ΦPSII showed lower mean values at lower 3–15 m depths.
P. oceanica shoot and third leaf foliar surfaces displayed at 10 m depth the same classic annual cycle, with summer maxima and autumn minima after the massive decay of senescent and necrotic adult leaves and the renewing of leaf bundles before winter (Figure 1C). Foliar surfaces along the 3–36 m depth gradient were maximal at 10 m depth, decreased close to the surface and with depth and were minimal at 36 m depth (Figure 2C), i.e., at the lower limit of the meadow with the lowest access to light. The highest Spearman's rank correlation coefficient of the analysis of the leaf foliar surface matrices (results of supplementary analyses not detailed) were observed between the shoot and the third leaf (P. oceanica shoots dissected for biometry had in average 6 ± 0 leaves), both at 10 m depth over time (rho = 0.85, p < 0.001) and along the depth gradient (rho = 0.88, p < 0.001). The third leaf from the inside of the leaf bundle (juvenile leaves excluded, see Giraud, 1979) therefore appears to be the best proxy for P. oceanica shoot total foliar surface and justifies, by extension, its election as representative tissue to study the plant biology (similar inference for 15 m depth in: Romero et al., 2007; Luy et al., 2012).
DMSP, DMSO, and DMSP:DMSO Ratio
Mean DMSP concentrations at 10 m depth ranged between 62 ± 17 and 205 ± 58 (reported as preliminary result in Borges and Champenois, 2017), i.e., a ratio of about three between leaf extrema (Figure 3A). The DMSP concentration decreased continuously from autumn to summer, with a clear break in the cycle between the two seasons properly predicted by segmented linear regression analysis unlike spline smoothing (Figure 3A). DMSO differed from DMSP in terms of both absolute concentrations and relative difference between extrema. Mean DMSO concentration at 10 m depth ranged between 1.5 and 8.6 ± 2.0 , i.e., a ratio of about six between leaf extrema (Figure 3B). The DMSO concentration also decreased continuously from autumn to summer, with however a smoother break in the cycle between both seasons (Figure 3B); as a result, both spline model and segmented linear regression adequately predicted the cyclic evolution of the DMSO concentration. The DMSP and DMSO concentrations in P. oceanica leaves at 10 m depth were strongly positively correlated with each other (rho = 0.74, p < 0.001). They were, respectively, strongly (rho = −0.75, p < 0.001) and modestly (rho = −0.55, p < 0.001) negatively correlated with the third leaf foliar surface. Correlations of concentrations were non-significant with ΦPSII and weak to modest with environmental parameters light (rho = −0.59, p < 0.001 for DMSP; rho = −0.38, p = 0.025 for DMSO) and temperature (rho = −0.50, p = 0.002 for DMSP; rho = −0.33, p = 0.041 for DMSO). Confirming the results of the exploratory correlation analyses, results from the GLM analyses showed an effect of the third leaf foliar surface only on DMSP (p = 0.004) and DMSO (p = 0.033) concentrations at 10 m depth. Indeed, DMSP and DMSO concentrations decreased linearly with the increase of the leaf foliar surface (p < 0.001, R2 = 0.53 for DMSP; p < 0.001, R2 = 0.34 for DMSO), i.e., the aging of the leaf (Wittmann et al., 1981; Wittmann and Ott, 1982).
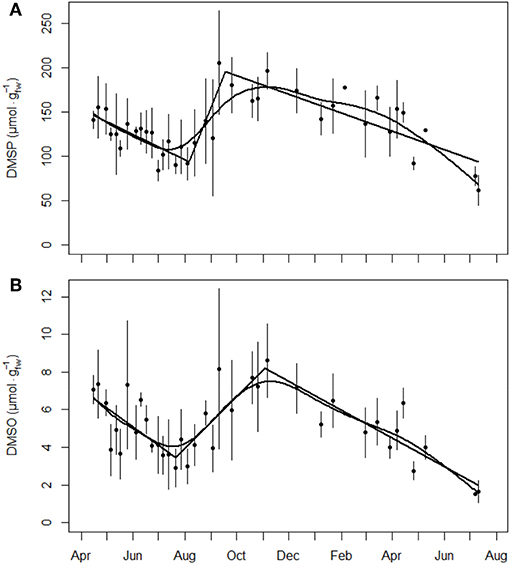
Figure 3. Evolution of (A) the DMSP concentration () and (B) the DMSO concentration () in P. oceanica third leaf at 10 m depth (mean ± sd, n = 3 except February 5, 2016 for DMSP and DMSO and July 7, 2016 for DMSO, n = 2). The solid black lines are predictions from segmented linear regression and cross-validated smoothing cubic spline analyses on leaf concentrations.
Mean DMSP concentrations along the depth gradient ranged from a minimum of 71 ± 21 at 20 m depth to a maximum of 143 ± 26 at 29 m depth. Depth influence on DMSP concentration was not significant at alpha 1% (ANOVA-p = 0.042; Figure 4A). Mean DMSO concentrations remained close to 3.9 along the depth gradient, except for the lower mean value of 1.8 ± 0.2 at 20 m depth (ANOVA-p = 0.080; Figure 4B). The positive correlation between the two molecule concentrations was modest (rho = 0.58, p = 0.001). The DMSP and DMSO concentrations remaining relatively constant with depth, none of the potential explanatory variables that for most showed a clear depth gradient were related to the concentrations of the two molecules, as observed from correlation and GLM analyses. Overall, the general scatterplot of DMSP and DMSO concentrations for all P. oceanica leaves sampled at 10 m depth over time and along the depth transect showed a clear linear relationship between the two compounds (Figure 5).
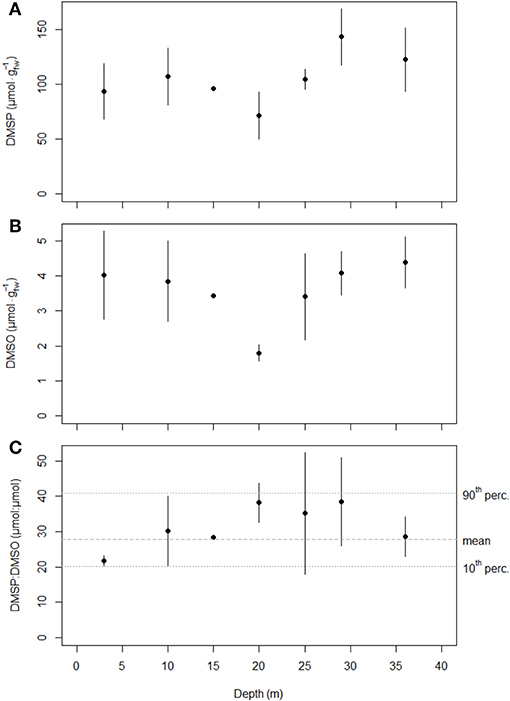
Figure 4. Evolution of (A) the DMSP concentration (), (B) the DMSO concentration () and (C) and the DMSP:DMSO ratio (μmol:μmol) in P. oceanica third leaf along the 3–36 m depth transect (mean ± sd, n = 3 except at depths 15 m, n = 2 and 10 m, n = 12). Tenth and ninetieth percentiles (horizontal dotted gray lines) and the mean ratio value (horizontal dashed gray line) of the interpercentile range for the 10 m depth survey are reported on the DMSP:DMSO ratio graph (C).
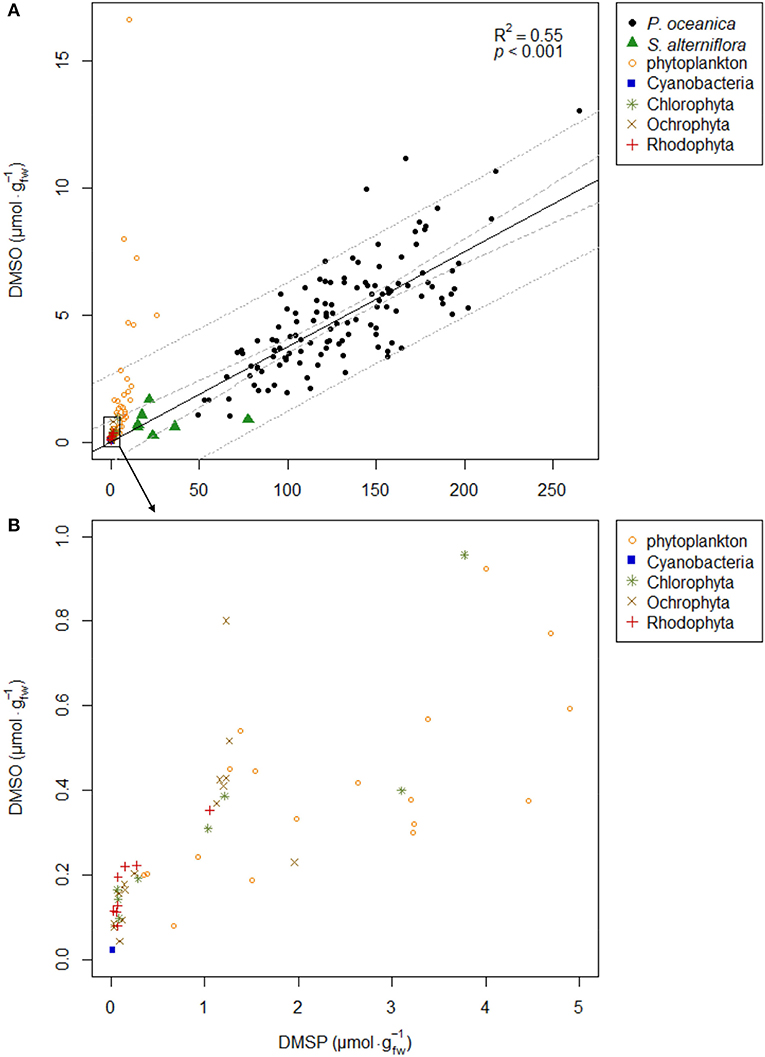
Figure 5. Scatterplot of DMSP and DMSO concentrations () in P. oceanica third leaf, S. alterniflora leaves, epilithic Cyanobacteria, macroalgae (Ochrophyta, Chlorophyta, and Rhodophyta), and marine phytoplankton communities. P. oceanica data are for individual leaf samples, all 10 m depth survey and depth transect observations combined (n = 129). S. alterniflora data are mean values from Husband and Kiene (2007), Husband et al. (2012), and McFarlin and Alber (2013). Epilithic Cyanobacteria and macroalgae data are mean species-specific pool sample values. Phytoplankton data are mean values from Simó and Vila-Costa (2006). Graph (B) is a zoom of graph (A) bottom left area framed by a rectangle. The legends of marine primary producer symbols are given next to the graphs. The solid black line on graph (A) is the linear regression modeling the relationship between P. oceanica DMSP and DMSO concentrations. Model R2 and p-value are shown on the graph. 95% confidence (dashed gray lines) and prediction (dotted gray lines) intervals are plotted.
Mean DMSP:DMSO ratio values at 10 m depth ranged between 20.2 ± 1.0 and 55.9 μmol:μmol, i.e., a ratio of about three between leaf extrema (Figure 6A). All mean DMSP:DMSO ratio values except the maximum were distributed in the 10th−90th interpercentile range of leaf ratio values, around a corresponding mean of 27.7 ± 5.0 μmol:μmol (Figure 6A). Segmented linear regression on the 10th−90th interpercentile range predicted, unlike spline smoothing, a slight cycle break around mid-October when DMSP and DMSO concentrations were at high seasonal levels (Figure 3) and foliar surfaces at their lowest (Figure 1C). October thus corresponded to a new physiological cycle for the seagrass. Accordingly, the evolution of the DMSP:DMSO ratio was bounded to the month of October, regardless of the year. October-bounded DMSP:DMSO leaf ratio values in the 10th−90th interpercentile range showed no significant linear trend (Figure 6B). This was consistent with the absence of significant linear relationship between the DMSP:DMSO ratio and the third leaf foliar surface (p = 0.160, R2 = 0.05), i.e., the leaf age (Wittmann et al., 1981; Wittmann and Ott, 1982).
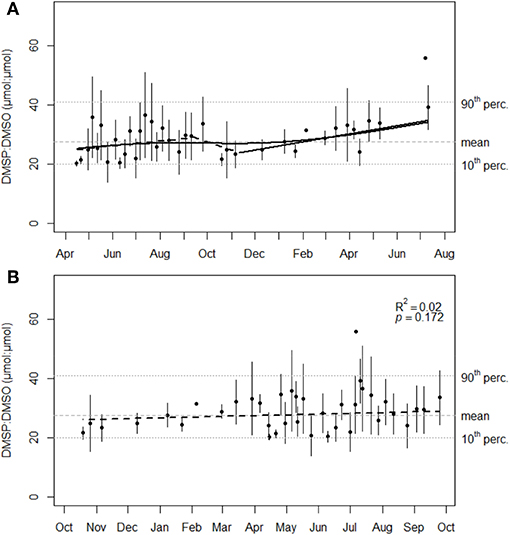
Figure 6. (A) Evolution of the DMSP:DMSO ratio (μmol:μmol) in P. oceanica third leaf at 10 m depth (mean ± sd, n = 3 except February 5, 2016 and July 7, 2016, n = 2). The dashed (non-significant slope at p = 0.01) and solid (significant slope at p = 0.01) segments of the broken black line and the solid black line are predictions from segmented linear regression and cross-validated smoothing cubic spline analyses, respectively, for the leaf ratio values between 10th and 90th percentiles. (B) DMSP:DMSO ratio values (μmol:μmol) bounded to October, regardless of the year. The dashed black line represent the non-significant linear trend of leaf ratio values over the year for data between 10th and 90th percentiles. Model R2 and p-value are shown on the graph. Tenth and ninetieth percentiles (horizontal dotted gray lines) and the mean ratio value (horizontal dashed gray line) of the interpercentile range are reported on both graphs.
Mean DMSP:DMSO ratio values along the depth gradient did not significantly differ between depths (ANOVA-p = 0.356) and were included in the 10 m depth 10th−90th interpercentile range of leaf ratio values (Figures 4C, 6). The DMSP:DMSO ratio general mean of 31.5 ± 6.1 μmol:μmol along the depth gradient was further close to the 10 m depth value of 27.7 ± 5.0 μmol:μmol. From these results, we can reasonably conclude that the DMSP:DMSO ratio in P. oceanica third leaves remained constant regardless of the depth, just as it was over time at 10 m depth. Consequently, none of the potential explanatory variables that for most showed an annual cycle and/or a depth gradient were related to the DMSP:DMSO ratio, as observed from correlation and GLM analyses.
Discussion
DMSP
The maximum mean DMSP concentration measured in this work was 205 ± 58 , for an overall average in P. oceanica leaves of 130 ± 39 . These values are much higher than the maximum mean DMSP concentration of 33.9 μmol per g of oven-dried leaf, i.e., 6.8 (P. oceanica leaves contain about 80% water) reported by Borges and Champenois (2015), and of the same order of magnitude as the DMSP concentrations of fresh and frozen leaf samples reported by Borges and Champenois (2017). The present survey thus confirms the quantitative important role played by P. oceanica as DMSP producer in Mediterranean coastal environments. The DMSP concentration in P. oceanica leaves showed a continuous decrease over the seagrass physiological year. The scientific literature on the seasonal evolution of DMSP in marine species, and more specifically macrophytes is sparse. Husband (2007) reported that DMSP in S. alterniflora seemed to present a general pattern of increasing concentrations through summer to an autumnal maximum followed by a decrease over winter into early spring. Lyons et al. (2010) observed that DMSP content in Codium fragile subsp. fragile (Suringar) Hariot, 1889 off the Atlantic coast of Nova Scotia were highest in boreal spring, lowest in autumn, and negatively related to temperature. These authors further studied experimentally the DMSP production plasticity by C. fragile subsp. fragile. Results indicated it increased with decreasing temperature and with light intensity. Conversely, Karsten et al. (1992) measured a decrease in DMSP concentration in Antarctic green macroalgae acclimated to higher temperatures. Statistical relationships with temperature do not suggest any cryoprotectant function of DMSP in P. oceanica. The species, endemic of the Mediterranean, grows in temperate environmental conditions, must not cope with cold water temperatures (Boudouresque and Meinesz, 1982) but with global warming (Duarte et al., 2018; Darmaraki et al., 2019). According to Sunda et al. (2002), DMSP has the ability to capture free oxygen radicals (known collectively as reactive oxygen species, or ROS) produced especially during the photolysis of water molecules by PSII during photosynthesis (Lesser, 2006). The study did not find any deterministic relationship between light intensity or photosynthetic activity and the DMSP concentration measured in P. oceanica leaves. Our sampling protocol was standardized at around midday, during calm and sunny weather days; this may have partially masked a real effect of light and ΦPSII on leaf DMSP content. We can therefore not confirm or refute a role of DMSP in P. oceanica related to light regime and put forward for macroalgae (Rix et al., 2012) and phytoplankton (Slezak and Herndl, 2003), i.e., to be an antioxidant for ROS and to cope with oxidative stress.
The main significant relationship of DMSP content with potential explanatory variables was with the third leaf foliar surface: the longer, i.e., the older the leaf (Wittmann et al., 1981; Wittmann and Ott, 1982), the less DMSP. DMSP concentration in the first 20 photosynthetic cm of P. oceanica young third leaves was the highest in September, after the decay of senescent leaves and the renewal of the leaf bundle. The plant, with acropetal growth (Boudouresque et al., 2012), will allocate costly defenses to tissues that contribute most to fitness and survival (Rhoades, 1979), that is, the basal segment of newly grown leaves. Two known functions of DMSP production could explain higher concentrations in young growing tissues. First, the protection against grazers. Sarpa salpa (Linnaeus, 1758), the main herbivore on P. oceanica, can either feed on any range of leaf ages or select mid-age leaves (Pinna et al., 2009; Steele et al., 2014). Paracentrotus lividus (Lamarck, 1816), the second most important grazer on that species feeds preferentially on the epiphytes, more abundant on older leaves (Traer, 1979; Tomas et al., 2006). Higher DMSP concentration (or related compounds DMSO, DMS, and acrylic acid) in younger tissues could thus reduce, as repulsive, the grazing pressure (Vergés et al., 2007). Predator deterrence by DMSP and its metabolites was indicated by grazing observations by rats on inner tissues of S. alterniflora stems depleted in DMSP after nitrogen fertilization (Morris et al., 2002; Sundareshwar et al., 2003; Otte et al., 2004), difficulties to experimentally feed rats at high concentrations of DMSP (Nakajima, 1989) and DMSP cleavage to DMS and acrylic acid which deter feeding on algae by some herbivores (Van Alstyne et al., 2001; Van Alstyne and Houser, 2003). Changes in tolerance to grazing and defense allocation against herbivores in aging tissues (Schultz et al., 2013; Martínez-Crego et al., 2015) could then lead to the decrease of the DMSP concentration in P. oceanica leaves over time. Second, the protection against ROS as suggested above. Alcoverro et al. (1998) experimentally reported higher primary production from net oxygen release in younger leaf segments, i.e., close to the base. High productivity implies high rate of photosynthesis that leads unavoidably to the production of ROS (Hajiboland, 2014). We observed no relationship between DMSP concentration and ΦPSII in P. oceanica third leaf. Seagrass canopy photosynthetic response is a function of canopy density and light environment (Hedley et al., 2014). Thus, although P. oceanica younger leaf segments analyzed for DMSP and DMSO have the ability to be more productive, the shading effect of the canopy at low to medium depths and poor light availability deeper where the meadow is sparse are probably unfavorable for the production of ROS at deleterious levels for the plant. The possibility of these two DMSP functions (grazer deterrent and protection against ROS from photosynthesis) remains open and will require further testing. However, considering these two potential functions, knowing the dynamics of the meadow canopy and the physiology of the plant, a potential scheme of DMSP production by P. oceanica leaves can be established. In late summer early autumn, during the main renewal of the leaf bundles, the old senescent leaves are replaced by young productive leaves whose access to light and grazers (Prado et al., 2007; Pinna et al., 2009) is facilitated by the drastic fall of the leaf biomass. The production of DMSP by these new leaves is, from this moment, maximum. The annual cycle of light and the biology of organisms (predation by grazers, plant productivity and growth cycle) evolved toward a linear decrease in DMSP concentration until next summer. This cycle, evident at 10 m, will have to be investigated along depth transects. The absence of difference observed along the unique depth profile performed in this study, a snapshot at the beginning of the summer period when the DMSP content levels were seasonally very low, does not allow emitting any hypotheses.
DMSO
The maximum mean DMSO concentration measured in this work was 8.6 ± 2.0 , for an overall average in P. oceanica leaves of 4.9 ± 2.1 . These values are much higher than for the other coastal higher plant S. alterniflora. Husband and Kiene (2007) and Husband et al. (2012) reported DMSO concentrations ranging from 0.25 to 0.88 (after conversion to fresh weight for Husband et al., 2012 DMSO data—S. alterniflora leaf mean moisture content of 62.5%; Hardisky et al., 1983—since they analyzed fresh samples but published results per g of dry weight), McFarlin and Alber (2013) from 0.57 to 1.66 . The latter argued that differences might result from a high variability of DMSO content across marshes, differences in DMSO to DMS conversion efficiency during analysis (Kiene and Gerard, 1994) and methodological differences, the former estimating DMSO from within the same serum vial (same plant sample) that was used to estimate DMSP, the latter not. Husband and Kiene (2007) collected their samples in summer in Alabama, McFarlin and Alber (2013) in autumn in Georgia. These states are neighbors and sampling locations are at similar latitude. In addition, performing both DMSP and DMSO analyses on the same leaf sample does not lead to any loss of DMSO (Champenois and Borges, 2019a). So presumably, the reasons for the differences observed for S. alterniflora are natural, not methodological. Because Husband (2007) did not measure any consistent seasonal trend of the DMSO concentration in S. alterniflora leaves, differences might indeed result mainly from variability across marshes. In contrast to S. alterniflora, the DMSO concentration in P. oceanica leaves evolved clearly over the seasons (as was for DMSP). The spatial variability across meadows remains however to be investigated, all the current data coming from the same location.
The distribution of DMSO within healthy S. alterniflora tissues and between sampling areas reflected somewhat the distribution of DMSP (Husband and Kiene, 2007; Husband et al., 2012; McFarlin and Alber, 2013). Such a similar distribution between the two molecules within healthy P. oceanica leaves was particularly evident in the present study, both over time and with depth. Some evidences suggested that DMSO production in S. alterniflora leaves could result both from direct oxidation of DMSP and via cleavage of DMSP to DMS and subsequent oxidation of DMS (Dacey et al., 1987; Husband and Kiene, 2007; Husband et al., 2012). Such information on the metabolic pathway of DMSP to DMSO in seagrasses are for the moment missing. However, DMSO and DMSP concentrations in P. oceanica leaves increased concomitantly in late summer when the seagrass leaf bundle was renewed. This period corresponds to maximum potential grazing on shoot inner leaves (Prado et al., 2007; Pinna et al., 2009) and maximum access to light for young productive tissues. Leaf wound and grazing (McDowell et al., 2014a) and photosynthesis (Hajiboland, 2014) lead to the production of ROS, the latter being also predator deterrent (McDowell et al., 2014b). DMSP conversion to DMSO could then be mediated by these ROS because of the hypothesized antioxidant function of DMSP (Sunda et al., 2002; Simó and Vila-Costa, 2006; Husband and Kiene, 2007). Considering the similar distribution of DMSP and DMSO in P. oceanica and a main function of DMSP as antioxidant for ROS, the strong relationship between the two molecules reported in this study is evident. The absence of any other strong relationship with potential explanatory variables except with third leaf foliar surface reinforces the hypothesis of a close link between the concentrations of the two molecules.
Strong relationships between particulate DMSP and DMSO have also been reported within cultured marine phytoplanctonic cells (Hatton and Wilson, 2007) and natural communities in coastal and open surface waters collected in a variety of marine biomes and different seasons (Simó and Vila-Costa, 2006). The scatterplot distribution of DMSP and DMSO for S. alterniflora, for epilithic Cyanobacteria and macroalgae and for most (88%) natural marine phytoplankton communities fall within the 95% prediction interval of the regression line of Figure 5A derived from the P. oceanica data. Their DMSP contents are distinctly lower than in P. oceanica, as well as their DMSO contents. It is particularly the case for epilithic Cyanobacteria and macroalgae (Ochrophyta, Chlorophyta and Rhodophyta) from the Revellata Bay (see Supplementary Material Table 3), with DMSP and DMSO content globally very low compared to other marine primary producers. DMSP and DMSO in macroalgae showed a proportional relationship between their concentrations, all species considered (Figure 5B). The highest DMSP content was reported in the Chlorophyta group, for Ulva intestinalis Linnaeus, 1753 (3.10 ± 0.42 ) and Codium adhaerens C. Agardh, 1822 (3.77 ± 0.34 ) in agreement with Van Alstyne and Puglisi (2007) for Ulva spp. and Lyons et al. (2010) for C. fragile subsp. fragile. DMSO data in macroalgae are currently very scarce. The DMSO content in U. intestinalis (0.40 ± 0.02 ) was in agreement with Deschaseaux et al. (2014) who reported similar value for Ulva lactuca Linnaeus, 1753, but a DMSP content one order of magnitude higher in U. lactuca compared to U. intestinalis. In contrast to S. alterniflora, epilithic Cyanobacteria and macroalgae, some of the average DMSO concentrations of natural marine phytoplankton communities from warm tropical waters are very high (up to 16.67 ). Simó and Vila-Costa (2006) provided two possible explanations for these high DMSO values: first, the dominance of phytoplankton community by nano- and pico-phytoplankton in warmer waters supposedly enriched in DMSO relative of DMSP; second, higher solar irradiance in the sampled warmer waters, and higher DMSO content to scavenge ROS.
DMSP:DMSO Ratio
The monitoring of seagrass biological responses to stressors at the molecular and cellular level is a useful complementary tool in environmental quality evaluation and risk assessment (Ferrat et al., 2003; Hansen, 2003). A multiparametric approach including structural and physiological indicators, indicators of general stress and stress-related specific indicators seems to be required to establish an appreciable ecotoxicological diagnostic using seagrasses (Ferrat et al., 2003; Roca et al., 2016). Considering the source of DMSO in the plant is DMS(P), their ratio represents the degree of oxidation of the DMSP pool (Husband and Kiene, 2007). In our study, DMSO accounted for 3.8 ± 1.0% of DMSP in P. oceanica leaves, a value similar to the 4.0% reported for S. alterniflora leaves from a natural population (Husband and Kiene, 2007). In yellowing and experimentally stressed (application of herbicides) S. alterniflora (Husband and Kiene, 2007; Husband et al., 2012) and in plants collected from areas affected by different disturbances (sudden marsh dieback, horse grazing, littorinid snail grazing, wrack deposition; McFarlin and Alber, 2013), DMSP was converted to its oxidation product DMSO resulting in a higher DMSO:DMSP ratio compared to healthy unstressed plants. These studies on S. alterniflora reported evidences that the DMSO:DMSP ratio could be useful as generic indicator of stress in plants, the response being consistent regardless of disturbance type. For obvious reasons of understanding, in order to work with numbers (not just decimals), for stress to result in a decrease in the value of the indicator for monitoring purpose in the framework of Environmental Directives and as expected by environmental actors and decision-makers, we report the ratio as DMSP:DMSO.
In the present study, the DMSP:DMSO ratio in P. oceanica leaves remained constant over time and with depth. The absence of a seasonal trend of the ratio of the two molecules was also observed in S. alterniflora (Husband, 2007). The primary contributor to the evolution over time of DMSP and DMSO concentrations was the elongation, i.e., the aging (Wittmann et al., 1981; Wittmann and Ott, 1982) of the third leaf over the annual growth cycle of the plant. Regardless of the age of the leaf, the DMSP:DMSO ratio remained constant as indicated by the absence of a significant relationship between the two variables. A generic early warning indicator of stress, not subject to the time of year, the depth, the stage of development of the plant would be a real asset for the monitoring of the quality of coastal waters, especially for diffuse stressors such as pollution that are currently difficult to assess. The main biotic indices based on P. oceanica for assessing the ecological status of Mediterranean coastal waters reported good to high quality index values for the Gulf of Calvi (Gobert et al., 2009; Lopez y Royo et al., 2010, 2011). Here, the DMSP:DMSO ratio remained constant over time and with depth, in a narrow range of values around a mean of 29.2 ± 9.0 μmol:μmol. This constant ratio for a healthy P. oceanica meadow not subject to environmental or direct anthropogenic stressors (except global change) can be considered as reference value for future work (field and laboratory) on the assessment of the DMSP:DMSO ratio as early generic indicator of stress.
Conclusions
P. oceanica leaves are important producers of DMSP and DMSO, their concentrations being overall much higher than phytoplankton, epilithic Cyanobacteria, macroalgae, and saltmarsh plants. Concentrations of the two organosulfured compounds varied with time, probably not with depth. Considering the similar distribution of both molecules, we hypothesized DMSO content results from the oxidation of DMS(P). Their concentration dynamics could be an important homeostatic process involved in the maintenance of an internal steady state of the plant. Of all physiological (photosynthetic activity), biometrical (foliar surface) and environmental (light, temperature) parameters monitored, the annual cycle of the plant aging was the main vector of evolution of leaf DMSP and DMSO concentrations. We hypothesized two protective functions of DMSP to explain higher concentrations in young tissues that contribute most to the plant fitness and survival: antioxidant against ROS and predator-deterrent. Finally, we observed a constant DMSP:DMSO ratio in P. oceanica leaves for the studied non-disturbed meadow. We hypothesized this ratio, constant for unstressed plants, could be useful as early warning indicator of stress in seagrasses independently of the season, the depth or the age of the leaf bundle. In conclusion, the present study deepened our knowledge on the ecology of DMSP and DMSO in P. oceanica and brought new insights on the concentration dynamics of both molecules in coastal ecosystems overall. More research is now needed to confirm the functions of DMSP and DMSO in seagrasses, notably because grazing was not investigated and the relationship with light and photosynthesis was not obvious, and to assess how the DMSP:DMSO ratio would vary under disturbance.
Data Availability Statement
All datasets generated for this study are included in the article/Supplementary Material.
Author Contributions
JR, WC, SG, GL, JS, RS, and AB elaborated the survey protocol. JR and AA performed seagrass sampling and in situ measurements. DS and WC performed algae sampling and DS identified algae species. WC, GE, and AB analyzed samples for DMSP and DMSO. JR, JS, and RS analyzed photosynthetic activity data. SG and AB provided access to tools and instruments and provided fundings. JR processed data and performed statistical analysis. JR, WC, and AB wrote the manuscript. AA, SG, GL, JS, RS, and DS participated to the manuscript revision.
Funding
This work was funded by the Fonds National de la Recherche Scientifique (FNRS) (Fellowship-Grant 1237018F and contract 2.4.637.10), the University of Liège (C-10/78 Fonds Spéciaux) and the Territorial Collectivity of Corsica and the Rhone-Mediterranean and Corsica Water Agency (STARECAPMED—Station of reference and research on change of local and global anthropogenic pressures on Mediterranean ecosystem drifts). The Portuguese Foundation for Science and Technology supported this research through the contract program of DL 57/2016 and project UID/Multi/04326/2019.
Conflict of Interest
AA is at present employed by company Seaviews.
The remaining authors declare that the research was conducted in the absence of any commercial or financial relationships that could be construed as a potential conflict of interest.
The reviewer, MS, is currently co-organizing a Research Topic with three of the authors, JR, SG, and AB, and confirms the absence of any other collaboration.
Acknowledgments
Authors thank Nicolas Cimiterra and Jon Lapeyra Martin for their help during fieldwork and Marianadjah Zemmouri for the analysis of the DMSP and DMSO content of the algae samples. Authors also thank Reviewers for their careful consideration and suggestions to improve the manuscript. JR is a postdoctoral researcher at the FNRS, GL is a research associate at the FNRS, and AB is a research director at the FNRS. This paper has the MARE number MARE392.
Supplementary Material
The Supplementary Material for this article can be found online at: https://www.frontiersin.org/articles/10.3389/fevo.2019.00510/full#supplementary-material
References
Alcoverro, T., Manzanera, M., and Romero, J. (1998). Seasonal and age-dependent variability of Posidonia oceanica (L.) Delile photosynthetic parameters. J. Exp. Mar. Bio. Ecol. 230, 1–13. doi: 10.1016/S0022-0981(98)00022-7
Apostolaki, E. T., Holmer, M., Santinelli, V., and Karakassis, I. (2018). Species-specific response to sulfide intrusion in native and exotic Mediterranean seagrasses under stress. Mar. Environ. Res. 134, 85–95. doi: 10.1016/j.marenvres.2017.12.006
Asher, E., Dacey, J. W., Ianson, D., Peña, A., and Tortell, P. D. (2017). Concentrations and cycling of DMS, DMSP, and DMSO in coastal and offshore waters of the Subarctic Pacific during summer, 2010-2011. J. Geophys. Res. Ocean 122, 3269–3286. doi: 10.1002/2016JC012465
Bay, D. (1984). A field study of the growth dynamics and productivity of Posidonia oceanica in Calvi Bay, Corsica, France. Aquat. Bot. 20, 43–64. doi: 10.1016/0304-3770(84)90026-3
Binard, M. (2017). Composantes météorologiques de la base de données océanographique RACE de STARESO (Baie de Calvi – Corse). Bull. Soc. Géogr. Liège 68, 37–47. doi: 10.25518/0770-7576.4517
Borges, A. V., and Champenois, W. (2015). Seasonal and spatial variability of dimethylsulfoniopropionate (DMSP) in the Mediterranean seagrass Posidonia oceanica. Aquat. Bot. 125, 72–79. doi: 10.1016/j.aquabot.2015.05.008
Borges, A. V., and Champenois, W. (2017). Preservation protocol for dimethylsulfoniopropionate and dimethylsulfoxide analysis in plant material of the Mediterranean seagrass Posidonia oceanica, and re-evaluation of dimethylsulfoniopropionate leaf content. Aquat. Bot. 143, 8–10. doi: 10.1016/j.aquabot.2017.08.004
Boudouresque, C. F., Bernard, G., Bonhomme, P., Charbonnel, E., Meinesz, A., Pergent, G, et al. (2012). Protection Conservation of Posidonia oceanica Meadows. Tunis: RAMOGE RAC/SPA Publisher.
Boudouresque, C. F., and Meinesz, A. (1982). Découverte de l'herbier de posidonie. Cah. Parc. Natl. Port Cros. 4, 1–79.
Brimblecombe, P., and Shooter, D. (1986). Photo-oxidation of dimethylsulphide in aqueous solution. Mar. Chem. 19, 343–353. doi: 10.1016/0304-4203(86)90055-1
Bullock, H. A., Luo, H., and Whitman, W. B. (2017). Evolution of dimethylsulfoniopropionate metabolism in marine phytoplankton and bacteria. Front. Microbiol. 8:637. doi: 10.3389/fmicb.2017.00637
Campagne, C. S., Salles, J. M., Boissery, P., and Deter, J. (2015). The seagrass Posidonia oceanica: ecosystem services identification and economic evaluation of goods and benefits. Mar. Pollut. Bull. 97, 391–400. doi: 10.1016/j.marpolbul.2015.05.061
Challenger, F., and Simpson, M. I. (1948). Studies on biological methylation. Part, X. I. I. A precursor of the dimethyl sulphide evolved by Polysiphonia fastigiata. Dimethyl-2-carboxyethylsulphonium hydroxide and its salts. J. Chem. Soc. 3, 1591–1597. doi: 10.1039/jr9480001591
Champenois, W., and Borges, A. V. (2012). Seasonal and interannual variations of community metabolism rates of a Posidonia oceanica seagrass meadow. Limnol. Ocean 57, 347–361. doi: 10.4319/lo.2012.57.1.0347
Champenois, W., and Borges, A. V. (2019a). Determination of dimethylsulfoniopropionate and dimethylsulfoxide in Posidonia oceanica leaf tissue. MethodsX 6, 56–62. doi: 10.1016/j.mex.2018.12.014
Champenois, W., and Borges, A. V. (2019b). Inter-annual variations over a decade of primary production of the seagrass Posidonia oceanica. Limnol. Oceanogr. 64, 32–45. doi: 10.1002/lno.11017
Charlson, R. J., Lovelock, J. E., Andreae, M. O., and Warren, S. G. (1987). Oceanic phytoplancton, atmoshperic sulphur, cloud albedo and climate. Nature 326, 655–661. doi: 10.1038/326655a0
Cook, R. D. (1977). Detection of influential observation in linear regression. Technometrics 19, 15–18. doi: 10.1080/00401706.1977.10489493
Dacey, J. W. H., King, G. M., and Lobel, P. S. (1994). Herbivory by reef fishes and the production of dimethylsulfide and acrylic acid. Mar. Ecol. Prog. Ser. 112, 67–74. doi: 10.3354/meps112067
Dacey, J. W. H., Wakeham, S. G., and Howes, B. L. (1987). Factors controlling emission of dimethylsulphide from salt marshes. Nature 330, 643–645. doi: 10.1038/330643a0
Darmaraki, S., Somot, S., Sevault, F., Nabat, P., Narvaez, W. D. C., Cavicchia, L., et al. (2019). Future evolution of marine heatwaves in the Mediterranean Sea. Clim. Dyn. 53, 1371–1392. doi: 10.1007/s00382-019-04661-z
Dauby, P., and Poulicek, M. (1995). Methods for removing epiphytes from seagrasses: SEM observations on treated leaves. Aquat. Bot. 52, 217–228. doi: 10.1016/0304-3770(95)00500-5
De los Santos, C. B., Vicencio-Rammsy, B., Lepoint, G., Remy, F., Bouma, T. J., and Gobert, S. (2016). Ontogenic variation and effect of collection procedure on leaf biomechanical properties of Mediterranean seagrass Posidonia oceanica (L.) Delile. Mar. Ecol. 37, 750–759. doi: 10.1111/maec.12340
Deschaseaux, E. S. M., Kiene, R. P., Jones, G. B., Deseo, M. A., Swan, H. B., Oswald, L., et al. (2014). Dimethylsulphoxide (DMSO) in biological samples: A comparison of the TiCl 3 and NaBH 4 reduction methods using headspace analysis. Mar. Chem. 164, 9–15. doi: 10.1016/j.marchem.2014.05.004
Duarte, B., Martins, I., Rosa, R., Matos, A. R., Roleda, M. Y., Reusch, T. B. H., et al. (2018). Climate change impacts on seagrass meadows and macroalgal forests: An integrative perspective on acclimation and adaptation potential. Front. Mar. Sci. 5:190. doi: 10.3389/fmars.2018.00190
Ferrat, L., Pergent-Martini, C., and Roméo, M. (2003). Assessment of the use of biomarkers in aquatic plants for the evaluation of environmental quality: application to seagrasses. Aquat. Toxicol. 65, 187–204. doi: 10.1016/S0166-445X(03)00133-4
Fowler, J., and Cohen, L. (1990). Practical Statistics for Field Biology. Chichester: John Wiley & Sons Ltd.
Giraud, G. (1979). Sur une méthode de mesure et de comptage des structures foliaires de Posidonia oceanica (Linnaeus) Delile. Bull. Mus. Hist. Nat. Marseille 39, 33–39.
Gobert, S., Cambridge, M. L., Velimirov, B., Pergent, G., Lepoint, G., Bouquegneau, J. M., et al. (2006). “Biology of posidonia,” in Seagrasses: Biology, Ecology and Conservation, eds A. W. D. Larkum, R. J. Orth, and C. M. Duarte (Dordrecht: Springer), 387–408.
Gobert, S., Kyramarios, M., Lepoint, G., Pergent-Martini, C., and Bouquegneau, J. (2003). Variations at different spatial scales of Posidonia oceanica (L.) Delile beds; effects on the physico-chemical parameters of the sediment. Oceanol. Acta 26, 199–207. doi: 10.1016/S0399-1784(02)00009-9
Gobert, S., Sartoretto, S., Rico-Raimondino, V., Andral, B., Chery, A., Lejeune, P., et al. (2009). Assessment of the ecological status of Mediterranean French coastal waters as required by the Water Framework Directive using the Posidonia oceanica Rapid Easy Index: PREI. Mar. Pollut. Bull. 58, 1727–1733. doi: 10.1016/j.marpolbul.2009.06.012
Green, T. K., and Hatton, A. D. (2014). The Claw hypothesis: a new perspective on the role of biogenic sulphur in the regulation of global climate. Oceanogr. Mar. Biol. An. Annu. Rev. 52, 315–336. doi: 10.1201/b17143-7
Hajiboland, R. (2014). “Reactive oxygen species and photosynthesis,” in Oxidative Damage to Plants: Antioxidant Networks and Signaling, ed. P. Ahmad (Elsevier Inc.), 1–63. doi: 10.1016/B978-0-12-799963-0.00001-0
Hansen, P. D. (2003). “Biomarkers,” in Bioindicators and Biomonitors, eds B. A. Markert, A. M. Breure, and H. G. Zechmeiste (Elsevier Science Ltd.), 203–220. Available online at: https://www.sciencedirect.com/bookseries/trace-metals-and-other-contaminants-in-the-environment/vol/6
Hanson, A. D., Rivoal, J., Paquet, L., and Cage, D. A. (1994). Biosynthesis of 3-dimethylsulfoniopropionate in Wollastonia biflora (L.) DC. Plant Physiol. 105, 103–110. doi: 10.1104/pp.105.1.103
Hardisky, M. A., Klemas, V., and Smart, R. M. (1983). The influence of soil salinity, growth form, and leaf moisture on the spectral radiance of Spartina alterniflora canopies. Photogramm. Eng. Remote Sens. 49, 77–83.
Hatton, A. D., and Wilson, S. T. (2007). Particulate dimethylsulphoxide and dimethylsulphoniopropionate in phytoplankton cultures and Scottish coastal waters. Aquat. Sci. 69, 330–340. doi: 10.1007/s00027-007-0891-4
Hedley, J. D., McMahon, K., and Fearns, P. (2014). Seagrass canopy photosynthetic response is a function of canopy density and light environment: a model for Amphibolis griffithii. PLoS ONE 9:e111454. doi: 10.1371/journal.pone.0111454
Husband, J., and Kiene, R. (2007). Occurrence of dimethylsulfoxide in leaves, stems, and roots of Spartina alterniflora. Wetlands 27, 224–229. doi: 10.1672/0277-5212(2007)27[224:OODILS]2.0.CO;2
Husband, J. D. (2007). The role of DMSP in oxidative stress protection in Spartina alterniflora Loisel (PhD thesis). University of South Alabama, Mobile, AL, United States.
Husband, J. D., Kiene, R. P., and Sherman, T. D. (2012). Oxidation of dimethylsulfoniopropionate (DMSP) in response to oxidative stress in Spartina alterniflora and protection of a non-DMSP producing grass by exogenous DMSP+acrylate. Environ. Exp. Bot. 79, 44–48. doi: 10.1016/j.envexpbot.2012.01.006
Jackson, G., and Stuckey, J. (2007). Effects of dimethylsulfoniopropionate (DMSP) produced by the cordgrass Spartina alterniflora on its epiphytic algal community. Aquat. Sci. 69, 419–426. doi: 10.1007/s00027-007-0890-5
Jackson, G., Zingmark, R., Lewitus, A. J., Tymowski, R. G., and Stuckey, J. (2006). Spatial and temporal dynamics of epiphytic microalgae on the cordgrass Spartina alterniflora in North Inlet Estuary, South Carolina. Estuaries Coasts 29, 1212–1221. doi: 10.1007/BF02781821
Jacquemart, J., and Demoulin, V. (2006). Inventaire des macroalgues épiphytes des feuilles de Posidonia oceanica (L.) Delile dans la Baie de la Revellata (Calvi, Corse). Lejeunia Rev Bot 181:71. Available online at: https://popups.uliege.be:443/0457-4184/index.php?id=464
Kamenos, N. A., Strong, S. C., Shenoy, D. M., Wilson, S. T., Hatton, A. D., and Geoffrey Moore, P. (2008). Red coralline algae as a source of marine biogenic dimethylsulphoniopropionate. Mar. Ecol. Prog. Ser. 372, 61–66. doi: 10.3354/meps07687
Karsten, U., Wiencke, C., and Kirst, G. O. (1992). Dimethylsulphoniopropionate (DMSP) accumulation in green macroalgae from polar to temperate regions: interactive effects of light versus salinity and light versus temperature. Polar Biol. 12, 603–607. doi: 10.1007/BF00236983
Kiene, R. P., and Gerard, G. (1994). Determination of trace levels of dimethylsulfoxide (DMSO) in seawater and rainwater. Mar. Chem. 47, 1–12. doi: 10.1016/0304-4203(94)90009-4
Lee, P. A., and de Mora, S. J. (1999). Intracellular dimethylsulfoxide (DMSO) in unicellular marine algae: speculations on its origin and possible biological role. J. Phycol. 35, 8–18. doi: 10.1046/j.1529-8817.1999.3510008.x
Lee, P. A., De.Mora, S. J., and Levasseur, M. (1999). A review of dimethylsulfoxide in aquatic environments. Atmos. Ocean 37, 439–456. doi: 10.1080/07055900.1999.9649635
Lesser, M. P. (2006). Oxidative stress in marine environments: biochemistry and physiological ecology. Annu. Rev. Physiol. 68, 253–278. doi: 10.1146/annurev.physiol.68.040104.110001
Lopez y Royo, C., Casazza, G., Pergent-Martini, C., and Pergent, G. (2010). A biotic index using the seagrass Posidonia oceanica (BiPo), to evaluate ecological status of coastal waters. Ecol. Indic. 10, 380–389. doi: 10.1016/j.ecolind.2009.07.005
Lopez y Royo, C., Pergent, G., Alcoverro, T., Buia, M. C., Casazza, G., Martínez-Crego, B., et al. (2011). The seagrass Posidonia oceanica as indicator of coastal water quality : experimental intercalibration of classification systems. Ecol. Indic. 11, 557–563. doi: 10.1016/j.ecolind.2010.07.012
Lovelock, E. J., and Maggs, R. J. (1972). Atmospheric dimethyl sulphide and the natural sulfur cycle. Nature 237, 452–453. doi: 10.1038/237452a0
Luy, N., Gobert, S., Sartoretto, S., Biondo, R., Bouquegneau, J. M., and Richir, J. (2012). Chemical contamination along the Mediterranean French coast using Posidonia oceanica (L.) Delile above-ground tissues: a multiple trace element study. Ecol. Indic. 18, 269–277. doi: 10.1016/j.ecolind.2011.11.005
Lyons, D. A., Scheibling, R. E., and Van Alstyne, K. L. (2010). Spatial and temporal variation in DMSP content in the invasive seaweed Codium fragile ssp. fragile: effects of temperature, light and grazing. Mar. Ecol. Prog. Ser. 417, 51–61. doi: 10.3354/meps08818
Martínez-Crego, B., Arteaga, P., Ueber, A., Engelen, A. H., Santos, R., and Molis, M. (2015). Specificity in mesograzer-induced defences in seagrasses. PLoS ONE 10:e0141219. doi: 10.1371/journal.pone.0141219
McCullagh, P., and Nedler, J. A. (1989). Generalized Linear Models. London: Chapman and Hall/CRC. doi: 10.1007/978-1-4899-3242-6
McDowell, R. E., Amsler, C. D., Dickinson, D. A., McClintock, J. B., and Baker, B. J. (2014a). Reactive oxygen species and the antarctic macroalgal wound response. J. Phycol. 50, 71–80. doi: 10.1111/jpy.12127
McDowell, R. E., Amsler, C. D., McClintock, J. B., and Baker, B. J. (2014b). Reactive oxygen species as a marine grazing defense: H2O2 and wounded Ascoseira mirabilis both inhibit feeding by an amphipod grazer. J. Exp. Mar. Bio. Ecol. 458, 34–38. doi: 10.1016/j.jembe.2014.04.012
McFarlin, C. R., and Alber, M. (2013). Foliar DMSO:DMSP ratio and metal content as indicators of stress in Spartina alterniflora. Mar. Ecol. Prog. Ser. 474, 1–13. doi: 10.3354/meps10184
Morris, J. T., Sundareshwar, P. V., Nietch, C. T., Kjerfve, B., and Cahoon, D. R. (2002). Responses of coastal wetlands to rising sea level. Ecology 83, 2869–2877. doi: 10.1890/0012-9658(2002)083[2869:ROCWTR]2.0.CO;2
Mtwana Nordlund, L., Koch, E. W., Barbier, E. B., and Creed, J. C. (2016). Seagrass ecosystem services and their variability across genera and geographical regions. PLoS ONE 11:e0163091. doi: 10.1371/journal.pone.0163091
Muggeo, V. M. R. (2003). Estimating regression models with unknown break-points. Stat. Med. 22, 3055–3071. doi: 10.1002/sim.1545
Murchie, E. H., and Lawson, T. (2013). Chlorophyll fluorescence analysis: a guide to good practice and understanding some new applications. J. Exp. Bot. 64, 3983–3998. doi: 10.1093/jxb/ert208
Nakajima, K. (1989). Effects of High Concentrations of Dimethylthetin, Dimethyl-b-Propiothetin and Vitamin U on Young Rats. Takarazuka: Memoirs of Koshien University.
Norie, J. W. (Ed.). (1831). “Section, V. I. The Islands of Corsica and Sardinia,” in New Piloting Directions for the Mediterranean Sea, the Adriatic, or Gulf of Venice, the Black Sea, Grecian Archipelago, and the Seas of Marmara and Azof (London: S. M'Dowall, Printer), 72–89.
Otte, M. L., and Morris, J. T. (1994). Dimethylsulphoniopropionate (DMSP) in Spartina alterniflora Loisel. Aquat. Bot. 48, 239–259. doi: 10.1016/0304-3770(94)90018-3
Otte, M. L., Wilson, G., Morris, J. T., and Moran, B. M. (2004). Dimethylsulphoniopropionate (DMSP) and related compounds in higher plants. J. Exp. Bot. 55, 1919–1925. doi: 10.1093/jxb/erh178
Paquet, L., Rathinasabapathi, B., Saini, H., Zamir, L., Gage, D., Huang, Z. H., et al. (1994). Accumulation of the compatible solute 3-dimethylsulfoniopropionate in sugarcane and its relatives, but not other gramineous crops. Aust. J. Plant Physiol. 21, 37–48. doi: 10.1071/PP9940037
Pearson, R. K. (2001). Exploring process data. J. Process Control. 11, 179–194. doi: 10.1016/S0959-1524(00)00046-9
Piazzi, L., Balata, D., and Ceccherelli, G. (2016). Epiphyte assemblages of the Mediterranean seagrass Posidonia oceanica: an overview. Mar. Ecol. 37, 3–41. doi: 10.1111/maec.12331
Pinna, S., Pais, A., Chessa, L., Nicola, S., and Ceccherelli, G. (2009). Leaf partitioning of the seagrass Posidonia oceanica between two herbivores: is Sarpa salpa herbivory underestimated because of Paracentrotus lividus grazing? Estuar. Coast. Shelf Sci. 84, 21–27. doi: 10.1016/j.ecss.2009.05.025
Pope, S. B., and Gadh, R. (1988). Fitting noisy data using cross-validated cubic smoothing splines. Commun. Stat. Simul. Comput. 17, 349–376. doi: 10.1080/03610918808812668
Prado, P., Tomas, F., Alcoverro, T., and Romero, J. (2007). Extensive direct measurements of Posidonia oceanica defoliation confirm the importance of herbivory in temperate seagrass meadows. Mar. Ecol. Prog. Ser. 340, 63–71. doi: 10.3354/meps340063
Quinn, P. K., and Bates, T. S. (2011). The case against climate regulation via oceanic phytoplankton sulphur emissions. Nature 480, 51–56. doi: 10.1038/nature10580
Rhoades, D. F. (1979). “Evolution of plant chemical defense against herbivores,” in Herbivores: Their Interactions With Secondary Plant Metabolites, eds G. A. Rosenthal and D. H. Janzen (New York, NY: Academic Press), 3–54.
Richir, J., Abadie, A., Binard, M., Biondo, R., Borges, A., Cimiterra, N., et al. (2015). STARECAPMED (STAtion of Reference and rEsearch on change of local and global anthropogenic pressures on Mediterranean ecosystems drifts) - Année 2014. Calvi: Rapport de recherches, STARESO.
Rix, L. N., Burdett, H. L., and Kamenos, N. A. (2012). Irradiance-mediated dimethylsulphoniopropionate (DMSP) responses of red coralline algae. Estuar. Coast. Shelf Sci. 96, 268–272. doi: 10.1016/j.ecss.2011.11.022
Roca, G., Alcoverro, T., Krause-Jensen, D., Balsby, T. J. S., van Katwijk, M. M., Marbà, N., et al. (2016). Response of seagrass indicators to shifts in environmental stressors: A global review and management synthesis. Ecol. Indic. 63, 310–323. doi: 10.1016/j.ecolind.2015.12.007
Romero, J., Martínez-Crego, B., Alcoverro, T., and Pérez, M. (2007). A multivariate index based on the seagrass Posidonia oceanica (POMI) to assess ecological status of coastal waters under the water framework directive (WFD). Mar. Pollut. Bull. 55, 196–204. doi: 10.1016/j.marpolbul.2006.08.032
RStudio Team. (2016). RStudio: Integrated Development for R. RStudio, Inc., Boston, MA. Available online at: http://www.rstudio.com/.
Saha, M., Rempt, M., Gebser, B., Grueneberg, J., Pohnert, G., and Weinberger, F. (2012). Dimethylsulphopropionate (DMSP) and proline from the surface of the brown alga Fucus vesiculosus inhibit bacterial attachment. Biofouling 28, 593–604. doi: 10.1080/08927014.2012.698615
Schober, P., Boer, C., and Schwarte, L. A. (2018). Correlation coefficients: Appropriate use and interpretation. Anesth. Analg. 126, 1763–1768. doi: 10.1213/ANE.0000000000002864
Schultz, J. C., Appel, H. M., Ferrieri, A. P., and Arnold, T. M. (2013). Flexible resource allocation during plant defense responses. Front. Plant Sci. 4:324. doi: 10.3389/fpls.2013.00324
Silva, J., Sharon, Y., Santos, R., and Beer, S. (2009). Measuring seagrass photosynthesis: methods and applications. Aquat. Biol. 7, 127–141. doi: 10.3354/ab00173
Simó, R., Hatton, A. D., Malin, G., and Liss, P. S. (1998). Particulate dimethyl sulphoxide in seawater: production by microplankton. Mar. Ecol. Prog. Ser. 167, 291–296. doi: 10.3354/meps167291
Simó, R., and Vila-Costa, M. (2006). Ubiquity of algal dimethylsulfoxide in the surface ocean: geographic and temporal distribution patterns. Mar. Chem. 100, 136–146. doi: 10.1016/j.marchem.2005.11.006
Slezak, D., and Herndl, G. J. (2003). Effects of ultraviolet and visible radiation on the cellular concentrations of dimethylsulfoniopropionate (DMSP) in Emiliania huxleyi (strain L). Mar. Ecol. Prog. Ser. 246, 61–71. doi: 10.3354/meps246061
Steele, L., Darnell, K. M., Cebrián, J., and Sanchez-Lizaso, J. L. (2014). Sarpa salpa herbivory on shallow reaches of Posidonia oceanica beds. Anim. Biodivers. Conserv. 37, 49–57. Available online at: http://abc.museucienciesjournals.cat/volume-37-1-2014-abc/sarpa-salpa-herbivory-on-shallow-reaches-of-posidonia-oceanica-beds-2/?lang=en
Stefels, J. (2000). Physiological aspects of the production and conversion of DMSP in marine algae and higher plants. J. Sea Res. 43, 183–197. doi: 10.1016/S1385-1101(00)00030-7
Stefels, J., Steinke, M., Turner, S., Malin, G., and Belviso, S. (2007). “Environmental constraints on the production removal of the climatically active gas dimethylsulphide (DMS) implications for ecosystem modelling,” in Phaeocystis, Major Link in the Biogeochemical Cycling of Climate-Relevant Elements, eds M. A. van Leeuwe, J. Stefels, S. Belviso, C. Lancelot, P. G. Verity, W. W. C. Gieskes (Dordrecht: Springer), 245–275. doi: 10.1007/978-1-4020-6214-8_18
Sunda, W., Kieber, D. J., Kiene, R. P., and Huntsman, S. (2002). An antioxydant function for DMSP and DMS in marine algae. Lett. Nat. 418, 317–320. doi: 10.1038/nature00851
Sundareshwar, P. V., Morris, J. T., Koepfler, E. K., and Fornwalt, B. (2003). Phosphorus limitation of coastal ecosystem processes. Science 299, 563–565. doi: 10.1126/science.1079100
Tomas, F., Alvarez-Cascos, D., Turon, X., and Romero, J. (2006). Differential element assimilation by sea urchins Paracentrotus lividus in seagrass beds: implications for trophic interactions. Mar. Ecol. Prog. Ser. 306, 125–131. doi: 10.3354/meps306125
Toms, J. D., and Lesperance, M. L. (2003). Piecewise regression: a tool for identifying ecological thresolds. Ecology 84, 2034–2041. doi: 10.1890/02-0472
Traer, K. (1979). “The consumption of Posidonia oceanica Delile by Echinoids at the Isle of Ischia,” in Proceedings of the European Colloquium on Echinoderms, ed. M. Jangoux (Brussels).
Van Alstyne, K. L. (2008). “Ecological and physiological roles of dimethylsulfoniopropionate and its products in marine macroalgae,” in Algal Chemical Ecology, ed. C. D. Amsler (Berlin; Heidelberg: Springer-Verlag), 173–194. doi: 10.1007/978-3-540-74181-7_8
Van Alstyne, K. L., and Houser, L. T. (2003). Dimethylsulfide release during macroinvertebrate grazing and its role as an activated chemical defense. Mar. Ecol. Prog. Ser. 250, 175–181. doi: 10.3354/meps250175
Van Alstyne, K. L., and Puglisi, M. P. (2007). DMSP in marine macroalgae and macroinvertebrates: distribution, function, and ecological impacts. Aquat. Sci. 69, 394–402. doi: 10.1007/s00027-007-0888-z
Van Alstyne, K. L., Wolfe, G. V., Freidenburg, T. L., Neill, A., and Hicken, C. E. (2001). Activated defense systems in marine macroalgae: evidence for an ecological role for DMSP cleavage. Mar. Ecol. Prog. Ser. 213, 53–65. doi: 10.3354/meps213053
Van Diggelen, J., Rozema, J., Dickson, D. M. J., and Broekman, R. (1986). Beta-3-dimethylsulphonioproprionate, proline and quaternary ammonium coumpounds in Spartina anglica in relation to sodium chloride, nitrogen and sulphur. New Phytol. 103, 573–586. doi: 10.1111/j.1469-8137.1986.tb02894.x
Vassallo, P., Paoli, C., Rovere, A., Montefalcone, M., Morri, C., and Bianchi, C. N. (2013). The value of the seagrass Posidonia oceanica: a natural capital assessment. Mar. Pollut. Bull. 75, 157–167. doi: 10.1016/j.marpolbul.2013.07.044
Vergés, A., Becerro, M. A., Alcoverro, T., and Romero, J. (2007). Experimental evidence of chemical deterrence against multiple herbivores in the seagrass Posidonia oceanica. Mar. Ecol. Prog. Ser. 343, 107–114. doi: 10.3354/meps06885
Wittmann, K., Mazzella, L., and Fresi, E. (1981). Age specific patterns of leaf growth: their determination and importance for epiphytic colonization in Posidonia oceanica (L.) Delile. Rapp. Comm. Int. Mer. Mediter. 27, 189–192.
Wittmann, K. J. (1984). Temporal and morphological variations of growth in a natural stand of Posidonia oceanica (L.) Delile. PSZNI Mar. Ecol. 5, 301–316. doi: 10.1111/j.1439-0485.1984.tb00128.x
Wittmann, K. J., and Ott, J. A. (1982). Effects of cropping on growth in the Mediterranean seagrass Posidonia oceanica (L.) Delile. PSZNI Mar. Ecol. 3, 151–159. doi: 10.1111/j.1439-0485.1982.tb00380.x
Zhang, L., Kuniyoshi, I., Hirai, M., and Shoda, M. (1991). Oxidation of dimethyl sulfide by Pseudomonas acidovorans DMR-11 isolated from peat biofilter. Biotechnol. Lett. 13, 223–228. doi: 10.1007/BF01025822
Keywords: Posidonia oceanica, seagrass, organosulfured compounds, dimethylsulfoniopropionate (DMSP), dimethylsulfoxide (DMSO), DMSP:DMSO ratio, ecology, physiology
Citation: Richir J, Champenois W, Engels G, Abadie A, Gobert S, Lepoint G, Silva J, Santos R, Sirjacobs D and Borges AV (2020) A 15-Month Survey of Dimethylsulfoniopropionate and Dimethylsulfoxide Content in Posidonia oceanica. Front. Ecol. Evol. 7:510. doi: 10.3389/fevo.2019.00510
Received: 17 January 2019; Accepted: 16 December 2019;
Published: 31 January 2020.
Edited by:
Mark A. Elgar, The University of Melbourne, AustraliaReviewed by:
Joseph Daniel Husband, Florida State College at Jacksonville, United StatesMartin Wiggers Skov, Bangor University, United Kingdom
Copyright © 2020 Richir, Champenois, Engels, Abadie, Gobert, Lepoint, Silva, Santos, Sirjacobs and Borges. This is an open-access article distributed under the terms of the Creative Commons Attribution License (CC BY). The use, distribution or reproduction in other forums is permitted, provided the original author(s) and the copyright owner(s) are credited and that the original publication in this journal is cited, in accordance with accepted academic practice. No use, distribution or reproduction is permitted which does not comply with these terms.
*Correspondence: Jonathan Richir, am9uYXRoYW4ucmljaGlyQHVsaWVnZS5iZQ==; Alberto V. Borges, YWxiZXJ0by5ib3JnZXNAdWxpZWdlLmJl
†These authors have contributed equally to this work
‡ORCID: Jonathan Richir orcid.org/0000-0001-5890-5724
Willy Champenois orcid.org/0000-0002-4678-175X
Arnaud Abadie orcid.org/0000-0003-2704-8680
Sylvie Gobert orcid.org/0000-0001-6000-0190
Gilles Lepoint orcid.org/0000-0003-4375-0357
João Silva orcid.org/0000-0002-7211-1661
Rui Santos orcid.org/0000-0002-7861-4366
Damien Sirjacobs orcid.org/0000-0002-1770-2063
Alberto V. Borges orcid.org/0000-0002-5434-2247