- Department of Environmental Science and Policy, University of California, Davis, Davis, CA, United States
The metacommunity framework has rapidly become a dominant concept used by ecologists to understand community assembly. By emphasizing extinction-colonization dynamics, dispersal, and species’ niche requirements in determining community structure, metacommunity theory unifies local and regional processes as integral to species distributions across landscapes. Metacommunity structure has traditionally been treated as static. However, habitat characteristics and community composition can shift through time because of factors like seasonal dynamics, ecosystem disturbance, multi-year climate variation (e.g., El Niño Southern Oscillation), and production and emergence of dormant propagules. In most systems, the relevance of such temporal variation for the structure and persistence of metacommunities is an open question that is of potential importance for conservation and management. We evaluate and synthesize the theory and concepts relevant to four major forms of temporal dynamics that are pertinent to metacommunities: disturbance, seasonality, multi-year climate variation, and dormancy. For each type of dynamic we review the theoretical underpinnings and empirical evidence to evaluate how the dynamic drives temporal variation in metacommunity structure. We also consider how major forms of anthropogenic change further influence these patterns. Our survey highlights that seasonal climatic differences can modify the distribution and availability of resources and connectivity, with consequences for species’ use of the landscape and species interactions. Disturbance and multi-year climate cycles can increase the importance of dispersal, but implications for environmental filtering and species interactions remain unresolved. Dormant life stages serve to anchor habitat patches and species pools in space and highlight the importance of dispersal-dormancy tradeoffs and environmental variation for metacommunity structure. Temporal variability in biotic and abiotic conditions associated with these drivers can modify the relative strengths of dispersal, environmental filtering, and species interactions, three biological processes that drive metacommunity structure. Inclusion of spatiotemporal drivers creates patterns of species diversity that differ from traditional metacommunity ideas. We use these insights to highlight research needs, suggest a reconceptualization of metacommunities as undergoing continuous change, and discuss the implications of temporal dynamism for the conservation and management of metacommunities.
Introduction
In their original conception, metacommunities were largely viewed as occupying a temporally invariant stochastic environment. The metacommunity concept depicts idealized types of metacommunity dynamics in such temporally static environments (Leibold et al., 2004; Holyoak et al., 2005). Metapopulations and metacommunities have focused on “ecology at the mesoscale,” reflecting timescales shorter than those required for speciation (<1–100 generations) and spatial scales between those of local populations/communities and biogeography (Holt, 1993). However, systems may have extrinsic temporal dynamics (e.g., Jabot et al., 2020). These may either coincide with timescales over which metapopulation and metacommunity dynamics occur or happen over longer time periods and potentially cause shifts in metacommunity dynamics. Consequently, several forms of extrinsic temporal dynamics are potentially relevant to metacommunities, including disturbance regimes, seasonality, multi-year temporal dynamics such as El Niño cycles, and dormancy. We review relevant theoretical and empirical work that relates to temporal dynamics of metacommunities as well as the consequences of global change for such dynamics. Considering our findings, we make recommendations about how to progress in studying temporal metacommunity dynamics and for the conservation of metacommunities.
A hierarchy of spatial and temporal scales are relevant to how temporal dynamics affect metacommunities and biodiversity (Hart et al., 2017). The original metacommunity paradigm was based on the idea that populations can colonize or go extinct from local habitat patches, or be rescued from extinction by immigration, but the habitat and environment are static (Leibold et al., 2004). This implies a relatively constant resource supply, environmental conditions amenable to individual survival, and patches always present. Such temporal stasis implicitly assumes that there is a fixed per unit time probability of individual birth, death, and movement due to environmental conditions (e.g., Chave, 2004). Another common scenario is that habitat patches are temporary ecosystems that come and go through time, such as tidal pools (Kolasa and Romanuk, 2005), temporary seasonal wetlands (Boudell and Stromberg, 2008), temporary streams (Resh et al., 1988), water-filled tree holes (Ellis et al., 2006), and water-filled pitcher plant leaves (Kneitel and Miller, 2002). Patch formation and destruction might occur stochastically, through external environmental drivers (e.g., seasonal weather, tidal cycles), or through an internal patch process (e.g., succession, aging of individual pitcher plant leaves or tree branches that can hold water). Simplified models typically assume constant probabilities of patch formation and destruction through time (e.g., Hastings, 2003), but patches may have their own temporal dynamics. Temporal environmental variation could cause local extinctions of species or affect population growth rates more generally (Drake and Lodge, 2004), including through seasonal fluctuations, disturbances, or multi-annual climate variation, which we review in this paper.
Temporal variation could be considered in three ways. First, if variation is stochastic and unpredictable but frequent in occurrence, it may be within the norm to which an organism is adapted and, consequently, such organisms may buffer against this variability through existing plasticity (behavior, physiology, etc.) or demographic responses (West-Eberhard, 1989). Conversely, temporally predictable variation (e.g., seasonality) might be overcome by life-history evolution or some form of plasticity in behavior, morphology, or physiology (Boyce, 1979). Disturbance events were originally thought of as unpredictable (Resh et al., 1988), but later were pointed out to be quite predictable in some systems (e.g., Poff, 1992; Tonkin et al., 2017). Recurrent periods of low resources or otherwise stressful conditions within habitat patches that remain in the same places could select for dormancy (McPeek and Kalisz, 1998; Wisnoski et al., 2019) or hibernation (e.g., Bieber et al., 2014). Finally, other temporal variation may be both stochastic and infrequent such as rare, extreme and often large disturbance events including hurricanes, droughts, volcanic eruptions, and some floods or fires (e.g., Foster et al., 1998). Rare events or changes in systems that are observed at a short timescale may appear as shifts in dynamical regimes (Folke et al., 2004), or as transient dynamics, and thus may not be reflective of dynamics that occur over longer timescales (e.g., Hastings, 2004). At spatial scales up to entire continents, organisms may also deal with or benefit from spatially and temporally unpredictable rare events by being extremely mobile and itinerant or nomadic in their movements (Andersson, 1980; Ydenberg, 1987).
We explore how temporal and spatiotemporal variation affect metacommunity dynamics through three separate biological drivers: dispersal, environmental filtering, and species interactions (Biswas and Wagner, 2012; Marrec et al., 2018). Dispersal (in contrast with migration or general movement) is defined as a one-way, permanent movement of individuals from one population to another (Jacobson and Peres-Neto, 2010) and has a well-established role in metacommunity theory (Mouquet and Loreau, 2002). Environmental filtering has been defined as the selection of species that can survive and persist given the environmental conditions at a location (Emerson and Gillespie, 2008; Kraft et al., 2015; Cadotte and Tucker, 2017), and can be understood in a metacommunity context as the environmental conditions that govern species’ occurrence (Biswas and Wagner, 2012). Species interactions in a metacommunity context influence species’ occurrence through interspecific competition and predation (Cottenie, 2005; Biswas and Wagner, 2012), and is an often overlooked but key driver (García-Girón et al., 2020). Thompson et al. (2020) used dispersal, “density-independent responses to abiotic conditions” (instead of environmental filtering) and “density-dependent biotic interactions” (instead of species interactions) as three axes for depicting and modeling metacommunity dynamics, and established overlap with the conceptual paradigms of Leibold et al. (2004): species sorting, mass effects, patch dynamics, and neutral dynamics. Species sorting fits neatly into the structuring mechanism of environmental filtering or strong species interactions for species with low ability to move between patches (Thompson et al., 2020), and spatial niche separation leads to coexistence. Mass effects is the interaction between environmental filtering or species interactions and dispersal, and has most commonly been empirically investigated as an interaction between environmental effects and dispersal or habitat connectivity (Cottenie, 2005). Consequently, mass effects are similar to species sorting but with a stronger role of dispersal (Thompson et al., 2020). Coexistence through patch dynamics involves trade-offs in species traits such as competitive ability and colonization ability (Holyoak et al., 2005), and hence an interaction of species interactions and dispersal (Thompson et al., 2020). Neutral dynamics require localized dispersal and emphasize stochastic demographic processes or individualistic responses to environmental variation (Leibold et al., 2004; Thompson et al., 2020). These paradigms are neither exhaustive (Brown et al., 2017) nor mutually exclusive (Leibold et al., 2004; Winegardner et al., 2012), and empirical studies frequently discover mixed metacommunity structures (Logue et al., 2011).
We discuss the effects of various forms of temporal dynamics on metacommunities by considering biological mechanisms of community structure and species distribution patterns. Our overarching question is how do temporal and spatiotemporal dynamics affect metacommunity structure and dynamics? We consider how disturbance, seasonality, long-term temporal dynamics, and dormancy affect temporal variation in metacommunity structure. More specifically, we explore the influence of these drivers on the relative importance, strength, and interactions among the biological processes of environmental filtering, dispersal, and species interactions. We identify when and describe how these drivers intersect with the traditional metacommunity paradigms identified by Leibold et al. (2004) to link local-scale community assembly processes to regional dynamics. By doing so, we attempt to unify existing theory surrounding the effects of extrinsic temporal dynamics on traditional metacommunity archetypes with a set of biological processes that can be identified and quantified through empirical study. Finally, we discuss how anthropogenic activity and global climate change impact the drivers of spatiotemporal dynamics of metacommunities, highlight management and conservation implications and suggest directions for future research.
Drivers of Temporal Dynamics in Metacommunities
Below, we examine the theoretical underpinnings and empirical evidence that demonstrate the temporally dynamic nature of metacommunities. We describe and summarize the central mechanisms by which dispersal, environmental filtering, and species interactions shift over time and space to structure metacommunities subject to disturbance, seasonality, multi-year climate fluctuations, and dormancy (Table 1). We also highlight empirical examples of studies that best exemplify how the temporal drivers of disturbance, seasonality, multi-year climate fluctuations, and dormancy influence the biological structuring mechanisms of dispersal, environmental filtering, and species interactions (Supplementary Table S1).
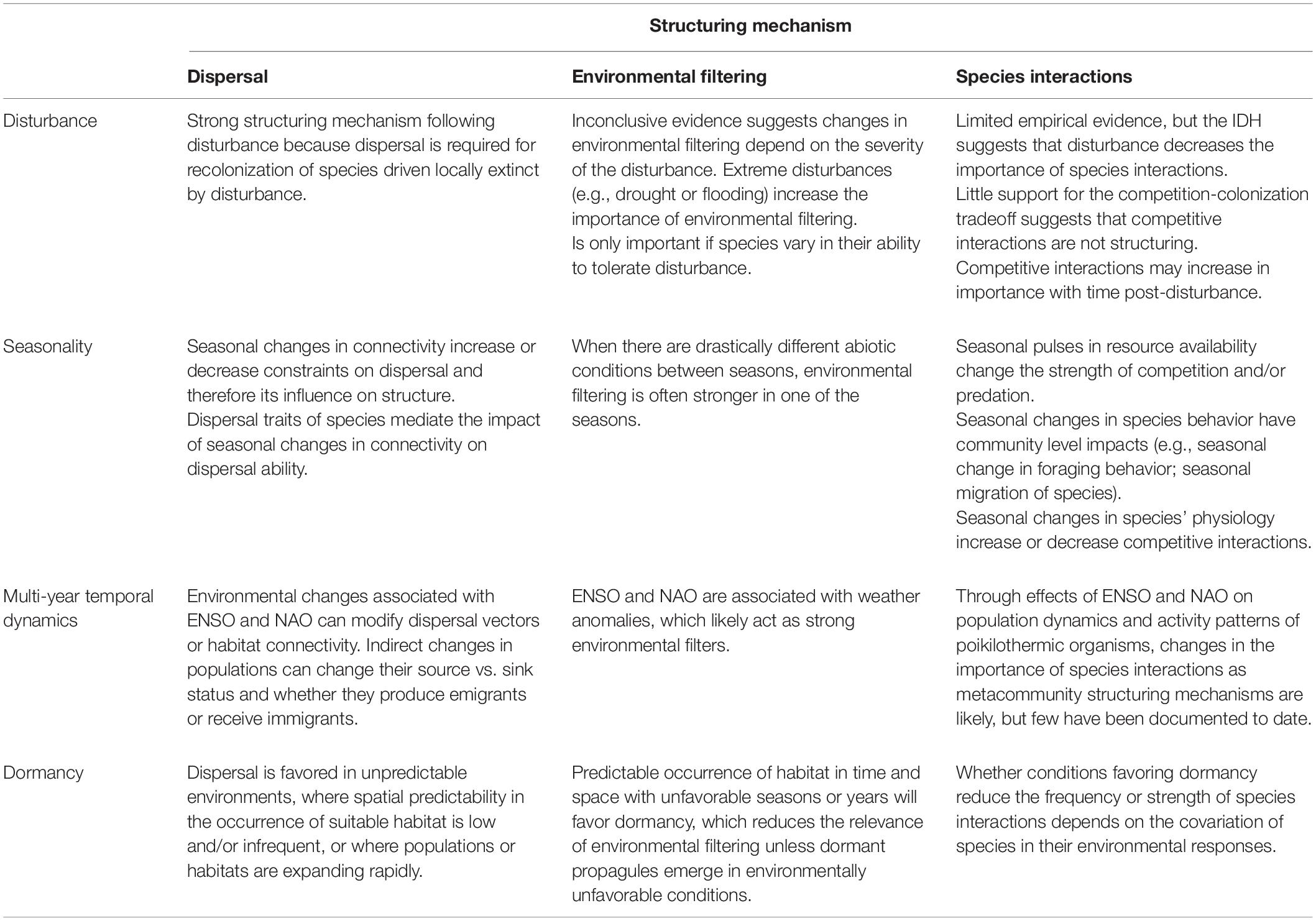
Table 1. Summary of ways in which different temporal drivers influence the biological processes that structure metacommunities.
Disturbance
Disturbance can be defined as any discrete event that disrupts ecosystem, community, or population structure and changes resources, substrate availability, or the physical environment (Petraitis et al., 1989). This definition encompasses lethal and sublethal effects of disturbance but does not include predictable and/or seasonal changes. When applied to metacommunity theory, disturbances create both temporal and spatial variability among habitat patches (Altermatt et al., 2011b). While disturbance theories including the intermediate disturbance hypothesis (IDH, Connell, 1978), the successional mosaic hypothesis (Roxburgh et al., 2004; Battisti et al., 2016), the dynamic equilibrium model (Huston, 1979; Svensson et al., 2012; Battisti et al., 2016), and the subsidy-stress model (Odum et al., 1979; Odum, 1985) discuss how disturbance promotes coexistence, increases diversity, increases productivity, and changes community assembly at a local scale, the role of disturbance on regional metacommunity structure has received little attention (Vanschoenwinkel et al., 2013).
Empirical Evidence
Empirical evidence suggests that disturbance can shift the importance of stochastic vs. deterministic processes in structuring metacommunities by influencing the strength of dispersal, environmental filtering, and species interactions (Table 1). Recolonization of disturbed patches with species from undisturbed patches is a key tenet of disturbance models, and, consequently, dispersal should increase in importance in disturbed metacommunities. The limited body of existing empirical work so far supports this idea, since metacommunity structure after a disturbance is driven by strong mass effects and dispersal between disturbed and non-disturbed patches (Supplementary Table S1; Warren, 1996; Östman et al., 2006; Starzomski and Srivastava, 2007; Altermatt et al., 2011b; Vanschoenwinkel et al., 2013; Ojima and Jiang, 2017; Sarremejane et al., 2018; Cañedo-Argüelles et al., 2020). For example, disturbance in the form of varying rates of pool drying in isolated pools exacerbated existing dispersal limitation (Vanschoenwinkel et al., 2013). Thus, frequently disturbed patches relied on high dispersal rates, which allowed species eliminated by disturbance to recolonize and contribute to maintaining local diversity (Vanschoenwinkel et al., 2013). However, it is challenging to directly measure dispersal in metacommunities and, accordingly, empirical work lags behind theoretical developments (Jacobson and Peres-Neto, 2010). Previous empirical research has mostly been confined to laboratory experiments (e.g., Warren, 1996; Östman et al., 2006; Altermatt et al., 2011b; Ojima and Jiang, 2017) where both dispersal and disturbance can be manipulated, field experiments with aquatic protists and invertebrates (Vanschoenwinkel et al., 2013), or correlative tests of the effects of connectivity (Tornwall et al., 2017; Cañedo-Argüelles et al., 2020). Theoretical work suggests that disturbance results in directionally-biased dispersal, which in turn can reduce species diversity and patch occupancy (Altermatt et al., 2011b). However, contradictory findings regarding the impacts of biased dispersal on metacommunity persistence suggest that disturbance severity could play a key role in the interaction between dispersal and disturbance in metacommunities. For example, biased dispersal from undisturbed to disturbed patches reduced metapopulation viability when disturbances eliminated local populations (Elkin and Possingham, 2008). However, when disturbances reduced population densities, metapopulation viability was highest when there was biased dispersal toward undisturbed patches (Altermatt et al., 2011b). Future work should examine how different disturbance severities interact with dispersal to change metacommunity structure.
Changes to the physical environment are one consequence of disturbance. Accordingly, it makes intuitive sense that the strength of environmental filtering in determining metacommunity structure might increase with disturbance. Theoretical models support this idea, whereby species that persist in heavily disturbed patches have high intrinsic growth rates, are competitively inferior, and exhibit high interaction strengths (Altermatt et al., 2011b). This provides strong evidence for species sorting in the metacommunity. Empirical work with protists and rotifers (Östman et al., 2006; Altermatt et al., 2011a), macroinvertebrates (Chase, 2007; Campbell et al., 2015; Cañedo-Argüelles et al., 2020), and plants (Laliberté et al., 2013) also support that environmental filtering becomes a predominant structuring mechanism in a metacommunity following disturbance (S1). Species with higher intrinsic growth rates are more likely to disperse, demonstrating that certain species succeed with the temporal and spatial variation created by disturbance (Altermatt et al., 2011a). However, there is contradicting evidence with protists (Warren, 1996; Limberger and Wickham, 2012) and invertebrates (Vanschoenwinkel et al., 2013) indicating that disturbance may not increase the importance of environmental filtering. For example, environmental filtering was not a dominant structuring mechanism in an invertebrate community in temporary rock pools, potentially because no disturbance tolerant specialists were present in the metacommunity (Vanschoenwinkel et al., 2013). Therefore, environmental filtering may only be an important structuring mechanism if species vary in their ability to tolerate disturbance. The discrepancy between the relative importance of environmental filtering as a structuring process among similar microcosm experiments suggests that its influence may depend on the severity and scale of disturbance. This is congruent with predictions of the IDH and the dynamic equilibrium model, where disturbance frequency or intensity modulates diversity. Future research is needed to quantify the relationship between disturbance severity and the relative importance of environmental filtering.
The IDH states that high diversity is found at intermediate disturbance rates because disturbance prevents competitive exclusion through a reduction in competition (Connell, 1978). Notwithstanding that the IDH is a mechanism that can alter community composition but lacks a robust mechanism for coexistence (Roxburgh et al., 2004), if the IDH is supported, disturbance in a metacommunity could reduce the importance of competitive interactions and elevate survival and recolonization as stronger drivers of metacommunity structure following disturbance. While some studies at a local scale have supported the competition-colonization trade-off component of the IDH (Caswell and Cohen, 1991; Barradas et al., 1996), the majority of empirical studies fail to find support (Mackey and Currie, 2001). However, Cadotte (2007) suggests that the IDH may still apply at a metacommunity scale. Local disturbance creates spatial heterogeneity and a successional mosaic at a regional scale, favoring certain species at each successional stage. In late-successional, undisturbed patches, strong competitors are expected to be favored suggesting that competitive interactions would be the most important structuring mechanism in that patch. However, in recently disturbed patches, strong colonizers have a higher probability of colonization, so the patch is expected to be structured more by dispersal and less by competitive interactions (Supplementary Table S1; Cadotte, 2007). Thus, in post-disturbance metacommunities, competitive interactions are expected to decrease in importance while dispersal increases. While this theory was supported in a microcosm experiment with protists and rotifers (Cadotte, 2007), there is no additional empirical work that examines species interactions after disturbance in metacommunities.
Overall, current evidence suggests that following a disturbance, dispersal becomes more important in driving metacommunity organization whereas changes in the relative importance of environmental filtering depend on the severity of the disturbance (Figure 1). Although theory suggests that competitive interactions are less important structuring mechanisms in recently disturbed metacommunities, empirical evidence is lacking. While disturbance creates temporally distinct changes – before and after the disturbance event – most metacommunity studies, have examined repeated disturbances but only looked at metacommunity structure at the end of the experiment (e.g., Altermatt et al., 2011b). These studies cannot provide important insight into how the importance of different structuring processes change with time since disturbance or with repeated, predictable disturbance.
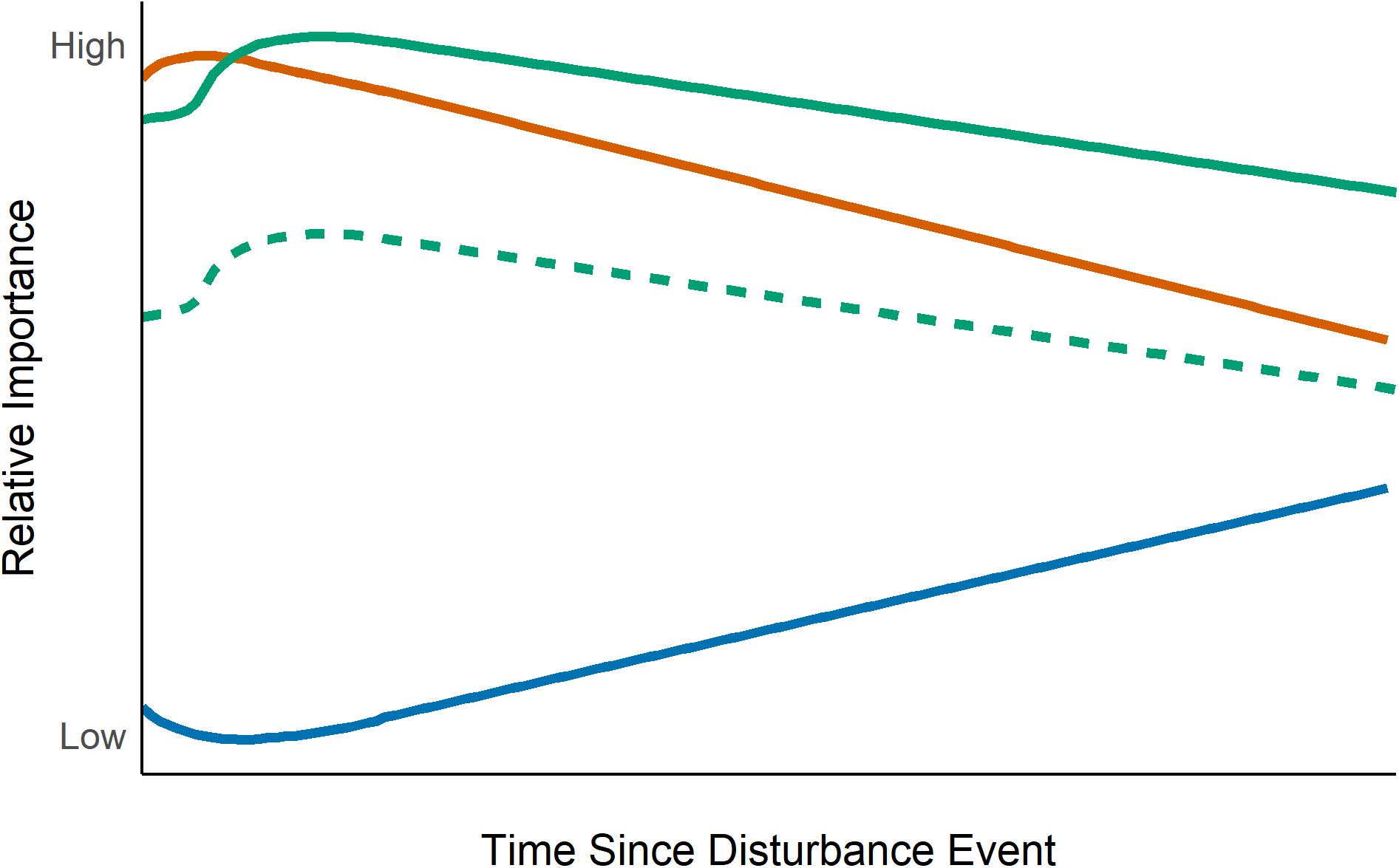
Figure 1. Relative importance of biological processes in structuring metacommunities with increasing time since a disturbance event (at y-axis). The orange line indicates dispersal processes, blue line indicates species interactions, and green lines indicate environmental filtering. Current empirical evidence strongly suggests that the importance of environmental filtering is context dependent. Thus, the solid green line indicates severe disturbances (when disturbed patches become uninhabited) while the dashed green line indicates less severe disturbances (some individuals in each patch remain).
Anthropogenic Global Change, Disturbance and Metacommunities
Global climate change is expected to increase both the frequency and severity of many types of disturbance including fire, insect outbreaks, and pathogens (Seidl et al., 2017). An increase in disturbance suggests metacommunities will be more frequently structured by dispersal. In systems that are further stressed by habitat fragmentation, which reduces connectivity and can limit dispersal (Tischendorf and Fahrig, 2000; Dixo et al., 2009), metacommunity persistence might be at risk. Anthropogenic global change has also altered disturbance regimes. Policies of fire suppression have lengthened the natural fire cycle resulting in fuel accumulation and infrequent but unusually intense fires, and flood control has caused more extreme but infrequent flooding events (Sousa, 1984). In systems adapted to a certain disturbance regime, changes to that regime could result in different structuring forces becoming more important than under historic conditions. Additionally, increasing size and spatial extent of disturbances with climate change may mitigate any potential rescue effects, reducing the importance of dispersal as a structuring mechanism.
Urban environments also present a system to examine how disturbance influences metacommunity structure. Anthropogenic disturbance in urban environments (e.g., construction, pollution, gardening, recreation) is more common than natural disturbance in addition to being more localized and sometimes more severe (Rebele, 1994). In cities, barriers to dispersal are highly prevalent (Blakely et al., 2006; Peralta et al., 2011), urban landscapes create a distinct environmental filter (Swan et al., 2011), and species interactions are changed through high amounts of invasive/non-native species, mesopredator release, decreased predation, and altered competition (Rebele, 1994; McKinney, 2002; Grimm et al., 2008). However, if urbanization itself is considered a long-term disturbance, these observations on dispersal, environmental filtering, and species interactions might be explained by the relative importance of metacommunity structuring processes post-disturbance. Following this reasoning, in urban ecosystems, dispersal and environmental filtering would be important structuring mechanisms while species interactions would be less important. However, there is currently little empirical evidence that examines how the importance of dispersal and environmental filtering differ in urban ecosystems, and the relative importance of species interactions varies across studies (Rodewald et al., 2011; Rivera-López and MacGregor-Fors, 2016). Nevertheless, there is a key difference between urbanization as a disturbance and the types of disturbances previously discussed in this paper. Urbanization can create novel ecosystems which require significantly different conservation and restoration practices to return to pre-disturbed, historic states (Hobbs et al., 2009). Thus, temporal variation in the importance of structuring processes in urban ecosystems is likely to be quite different than that of natural systems.
Theories about disturbance in metacommunities can also be applied to anthropogenic stressors such as eutrophication. The impact of eutrophication on freshwater metacommunity organization is poorly understood (Heino, 2013) but by considering eutrophication as a disturbance, one can predict how shifts in the strength of dispersal, environmental filtering, and species interactions associated with eutrophication may impact metacommunity organization. This knowledge may be useful in guiding conservation efforts toward the amelioration of eutrophication’s most harmful effects. Similar consideration of metacommunity dynamics can help direct conservation efforts regarding other anthropogenic stressors including pesticides, pollution, and salinization.
Seasonality
Most ecological systems experience seasonal oscillations, defined as consistent and recurring annual changes in environmental conditions, including changes in temperature, precipitation, photoperiod, resource availability, and wind. Cyclical changes in abiotic factors result in seasonal resource pulses, which expand temporal niche availability for many species (Tonkin et al., 2017). However, seasonality can also constrain species, acting as an environmental filter when conditions differ from species’ climatic tolerance (Gouveia et al., 2013; Tonkin et al., 2017). Accordingly, seasonality can exert strong controls on biodiversity, and season-diversity relationships are prevalent in many taxa (Mellard et al., 2019).
Empirical Evidence
Despite the recognition that seasonal variation influences community dynamics, seasonality is frequently disregarded in ecological research because it is complex and difficult to investigate (McMeans et al., 2015; White and Hastings, 2018). Similarly, studies of metacommunities have largely ignored seasonality. The shortcomings of excluding seasonality in metacommunity theory are especially salient in highly dynamic systems, where local communities may be affected by intra-annual seasonal changes (Datry et al., 2016; Sarremejane et al., 2017). In such systems, seasonality leads to cyclical fluxes in the drivers of community assembly (e.g., environmental conditions, presence/absence of migrants, resource availability, connectivity) and potentially to subsequent changes in community structure. However, despite the fact that dynamic systems characterized by seasonal change are widespread (e.g., tidal zones, floodplains, intermittent rivers), seasonal variation in metacommunity structure is only recently being explicitly tested (Fernandes et al., 2014; Sarremejane et al., 2017).
Much of the empirical study investigating seasonal changes in metacommunities has focused on intermittent rivers, which provide a strong conceptual basis for understanding the role of seasonality in metacommunity structure because they experience seasonal shifts between aquatic and terrestrial states (Larned et al., 2010). This dynamism is useful for addressing the question of how communities are organized in time and space and which biological processes drive seasonal changes in community structure. For example, in dry phases, flow cessation imposes strong environmental filtering, sorting species such that those adapted to lentic or terrestrial conditions are favored (Datry et al., 2016; Sarremejane et al., 2017). At the start of the wet season, dispersal processes are typically the predominant structuring force since certain species are constrained by dispersal limitation (Datry et al., 2016), ultimately enhancing the effects of patch dynamics in structuring the metacommunity. Later in the wet season, once initial colonization has occurred, species sorting via environmental filtering is expected to again become important with progression toward lentic and terrestrial conditions (Figure 2).
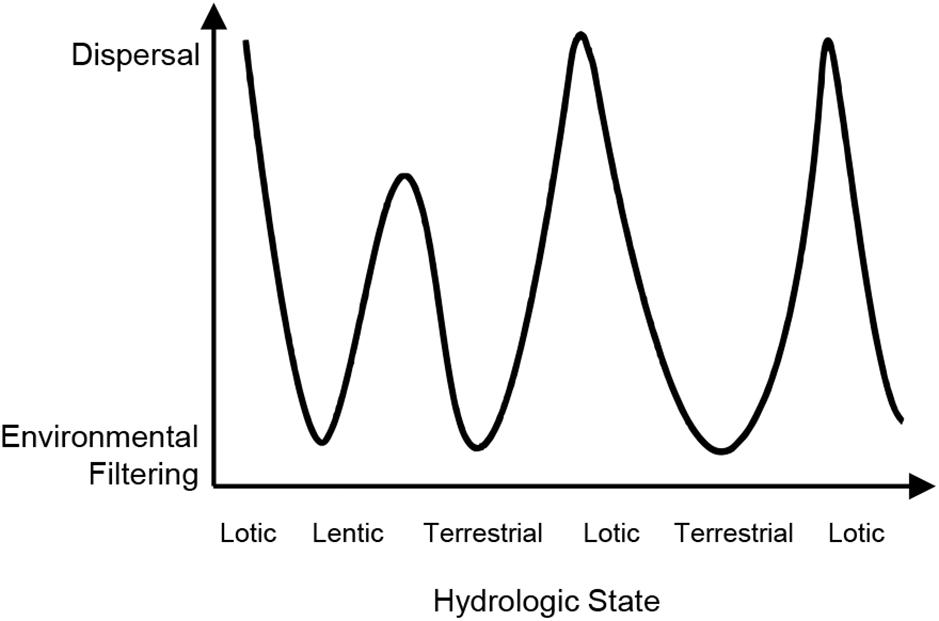
Figure 2. The importance of seasonal shifts in hydrology for environmental filtering and dispersal processes and associated changes in spatial patterns of metacommunity structure (Datry et al., 2016).
Overall, work from intermittent rivers and floodplains indicates that, where there exist significantly different abiotic conditions between seasons, the relative strength of environmental filtering and dispersal as biological processes driving metacommunity structure fluctuate seasonally (Table 1; Fernandes et al., 2014; Datry et al., 2016; Sarremejane et al., 2017). Where specific species are located in time and space is additionally mediated by species traits such as dispersal ability (Csercsa et al., 2019) and ability to cope with environmental conditions (Kraft et al., 2015). Though there is evidence that the biological processes of dispersal and environmental filtering seasonally influence metacommunity structure in terrestrial systems (Delciellos et al., 2018), this line of evidence is less robust. Consequently, further research on seasonally driven temporal variation in metacommunity organization should be conducted in dynamic terrestrial systems.
It is widely acknowledged that local interactions among species impact community composition. However, biotic interactions are not static, and the identity and strength of interspecific interactions can change over time (Saavedra et al., 2016) and space (Ruesink, 1998; Leonard, 2000). Accordingly, seasonal changes in species interactions can drive spatiotemporal changes in metacommunity structure (Table 1). Seasonality mediates species interactions in various ways. Commonly, seasonal pulses in resource availability can strengthen or weaken competition among species, fundamentally altering metacommunity structure (Cisneros et al., 2015). Seasonal resource pulses can also cause predator-prey dynamics to fluctuate if vulnerability of prey species shift seasonally (Owen-Smith, 2008) and/or predators have higher capture rates (Metz et al., 2012). Predation also fluctuates seasonally if predator activity levels are highly seasonal, as is the case in brumating or hibernating species (e.g., Sperry et al., 2008). Ultimately, seasonal variation in the strength of interspecific competition and predation may drive variation in metacommunity structure.
The strength of competition and predation can also fluctuate seasonally in metacommunities that experience migration, a special case of dispersal and movement that is both predictable and seasonal (Dingle, 1996). Because migration is a seasonal phenomenon, migratory species can impact communities in spatiotemporally complex ways (Schlägel et al., 2019). Year-long residents may be exposed to seasonal differences in interspecific competition, predation pressure, food resource availability, and disease as a result of the presence or absence of migrating species (Talbot and Talbot, 1963; Sinclair, 1985; Holdo et al., 2011). These pressures that migrating species impose on year-round residents, by definition, only exist for part of the year. Therefore, the metacommunity impact of these features is likely to fluctuate seasonally in systems where seasonal migration occurs. Competition and predation can also be moderated by seasonal increases or decreases in species’ physiology. For example, greater resource consumption and demands associated with higher summer temperatures and temperature-dependent metabolic rates may increase the role that competitive interactions play in determining metacommunity organization (Ren et al., 2018).
Anthropogenic Global Change, Seasonality, and Metacommunities
Though the above studies suggest that incorporating seasonality into the metacommunity framework may lead to predictable and cyclic patterns in metacommunity organization, several anthropogenic activities that vary temporally may disrupt such patterns. For example, seasonal release of water from dams may alter flood pulses or remove seasonality completely (Junk et al., 1989). High human water use in urban areas can generate dry-season flows (Solins and Cadenasso, 2020), sometimes converting intermittent streams to perennial ones (White and Greer, 2006). Anthropogenic pollution often varies seasonally, with concentrations of pesticides, salts, and other pollutants fluctuating based on seasonal human use. For example, concentrations of the insecticide fipronil in urban residential runoff reach lethal levels for many arthropod species in the spring and summer but decrease in other seasons (Gan et al., 2012). When pesticide use is coupled with elevated water use in urban environments, natural seasonal processes such as stream-drying may be eliminated, leading to seasonal peaks in pesticide concentration in water bodies and posing potential consequences for community structure (White and Greer, 2006; Ricart et al., 2010). Similarly, road deicing salts applied in winter result in seasonal increases in chloride concentrations, with negative impacts on species, communities, and ecosystems (Kaushal et al., 2005; Hintz and Relyea, 2019).
In recent years, the modified timing and enhanced variability of seasonal events brought about by global climate change may create mismatches within and among species and their environments (Parmesan, 2006; Walther, 2010). For example, as the timing of seasonal events shifts, a species may no longer be suited to its environment, increasing the importance of environmental filtering in metacommunity assembly. This has been observed in the phenology of hibernation, where shifts in emergence dates may negatively impact individual fitness or species persistence (Inouye et al., 2000; Lane et al., 2012). Mismatches may also occur among interacting species, such as when reproductive timing and prey availability become asynchronous (Visser et al., 2006; Tylianakis et al., 2008) or when shifts in seasonal migratory patterns create novel species combinations that alter species’ competitive environments (Gilman et al., 2010; Alexander et al., 2015). How these interactions play out in metacommunities and whether they are generalizable is uncertain (Lurgi et al., 2012).
Multi-Year Temporal Dynamics
A large variety of multi-year temporal climate indices capture information about temporal variation in weather (Stenseth et al., 2003), which have many potential and documented effects on metacommunities. Most ecological studies have investigated the effects of two indices on populations and communities, El Niño Southern Oscillation (ENSO) and the North Atlantic Oscillation (NAO) (Glynn, 1988; Post et al., 1997; Lindstrom et al., 2001; Fiedler, 2002; Hurrell et al., 2003; Stenseth et al., 2003). The most obvious biotic events of ENSO and NAO are related to interannual changes in average and extremes of temperature and rainfall, with much local variability in rainfall (Fraedrich and Müller, 1992; Hurrell, 1995; Diaz et al., 2001; Hurrell et al., 2003). ENSO and NAO are marked by their periodicity and occurrence over large spatial scales, and are often more strongly correlated with local population dynamics than are local weather variables (Stenseth et al., 2003; Stenseth and Mysterud, 2005). Of particular relevance to metacommunities, they can also synchronize populations across large spatial scales (Elton, 1924; Lindstrom et al., 2001). Mechanistically, ENSO and NAO can affect population and community dynamics through their influence on local weather, such as temperature, precipitation, and snow (reviewed by Lindstrom et al., 2001). This makes such indices potentially relevant to environmental filtering (e.g., Thompson et al., 2020), and synchrony of local populations, which potentially relates to metacommunity persistence (Gouhier et al., 2010).
Empirical Evidence
Examples of the effects of multi-year climate fluctuations on metacommunities come from rivers, marine and terrestrial systems (Supplementary Table S1). ENSO and NAO produced changes in metacommunity structure through dispersal, environmental filtering and species interactions (Tables 1, 2).
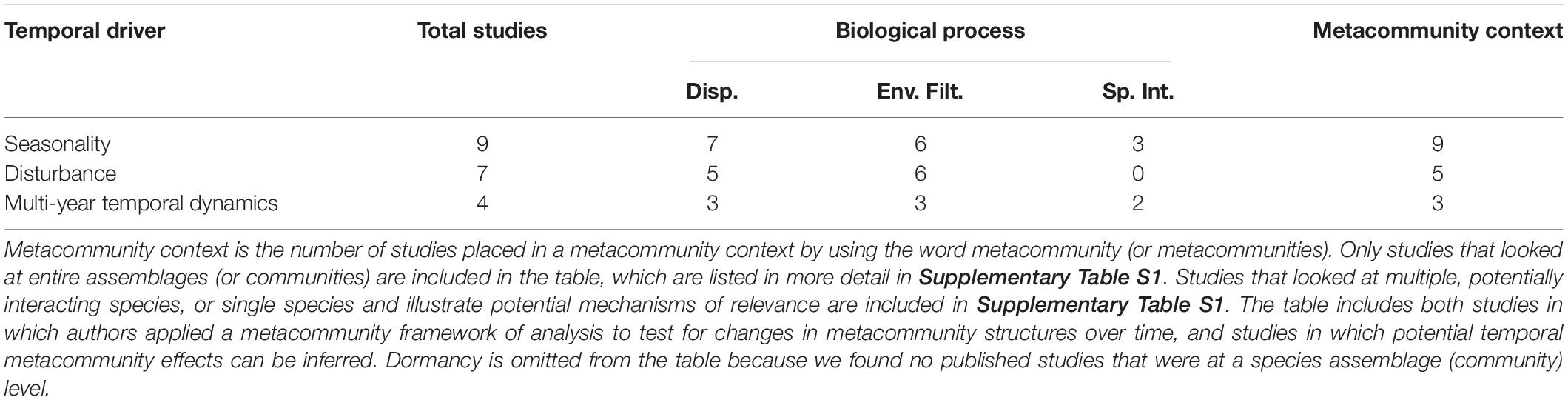
Table 2. Empirical examples linking temporal drivers of metacommunity structure (disturbance, seasonality, multi-year temporal dynamics, and dormancy) to biological processes of dispersal (“Disp.”), environmental filtering (“Env. Filt.”), and species interactions (“Sp. Int.”) that influence metacommunity structure.
In major river systems, El Niño events were associated with flooding, which increased connectivity and reduced spatial heterogeneity (interpatch variation) in biological and limnological conditions (Thomaz et al., 2007). During drying periods that followed river floods, pools became more dissimilar in their conditions (Thomaz et al., 2007), which may increase the potential for environmental filtering and species sorting (Leibold et al., 2004). By contrast, El Niño flood events increased similarity of species composition and environmental conditions among patches (Thomaz et al., 2007). These events may have prevented communities from reaching equilibrium states with local environmental conditions (e.g., Thomaz et al., 2007) and created non-equilibrium metacommunities that do not match any of the four paradigms suggested by Leibold et al. (2004). Likewise, severe flooding in El Niño years made the effects of dispersal stronger than the effects of environmental filtering, and dispersal was the main metacommunity structuring mechanism (Pineda et al., 2019). In marine benthic communities in Chile, El Niño events increased dispersal and mass effects, and also augmented differences in species composition among local communities because many environmental effects of El Niño were localized (Camus, 2008). Overall, ENSO events can increase or decrease dispersal, potentially with opposite events in El Niño and La Niña years. Such effects may extend to other climate indices, since Jeffries (2005) found that interannual variation in dispersal of a Daphnia species was associated with year-to-year variation in NAO.
ENSO and NAO events are important factors in global climate anomalies (Fraedrich and Müller, 1992; Diaz et al., 2001; Jeffries, 2005) that have the potential to act as environmental filters. In the one metacommunity study of environmental filtering, Pineda et al. (2019) partitioned diversity of phytoplankton, ciliates and zooplankton in lakes in a subtropical river system and found varied effects for environmental filtering for different taxonomic groups. For phytoplankton and zooplankton, correlations of environmental variables with species richness and diversity indices were indicative of environmental filtering. However, these environmental filtering effects were not statistically significant for ciliates for unclear reasons. Hence, there is a suggestion that El Niño and La Niña events relate to differences in environmental filtering for some, but not all taxa.
A few studies have documented effects of ENSO or NAO that translate directly to the role of species interactions in structuring metacommunities. The intertidal rocky invertebrate study of Camus (2008) targeted prey species of consumers through gut content analyses, showing changes in species interactions through predation in addition to the changes in dispersal and environmental filtering discussed above. In fishes in the Baltic Sea, winter values of NAO and the Baltic Sea Index (another climate index) were correlated with Atlantic cod recruitment, and the system was modeled as a source-sink system with a food web consisting of cod, herring, and sprat (Lindegren et al., 2014). Higher cod numbers led to greater predation on smaller fish species. In productive winters (higher NAO and Baltic Sea Index values) source to sink dispersal was stronger than in less productive winters (Lindegren et al., 2014). Source-sink dynamics are closely related to mass effects and one could expect periodic fluctuations in the strength of mass effects in such a metacommunity, as well as temporal shifts in the roles of species interactions and dispersal. In a terrestrial system ENSO-associated interannual variation in rainfall altered population dynamics, trophic interactions, and activity patterns of organisms in ways that impact species interactions (Meserve et al., 2003). High rainfall associated with ENSO events in a semiarid thorn scrub community in Chile led to strong bottom-up increase in plants and animals with overall greater importance of biotic interactions in these wet years (Meserve et al., 2003). However, how changes to species interactions facilitated by multi-year climate fluctuations influence temporal patterns of metacommunity structure is not well-documented. Beyond such specific examples, warm periods associated with ENSO events or correlated with NAO are expected to lead to increased predation through changes in activity of poikilothermic organisms (e.g., Tylianakis et al., 2008).
Anthropogenic Global Change, Multi-Year Temporal Dynamics and Metacommunities
Both empirical observations of climate warming during the last 50 years (Zhang et al., 2008) and simulations of climate models project that anthropogenic climate change could produce systematic increases in ENSO strength over many land regions (Fasullo et al., 2018). These increases are expected to heighten interannual variability in the extremes of regional temperature and increase wildfire frequency (Fasullo et al., 2018). The most obvious effect of increased climate forcing is expected to be greater synchronization of community dynamics across space, which can affect metacommunity persistence in theoretical models (e.g., Gouhier et al., 2010). The extent of environmental forcing and resultant environmental filtering could also affect the occurrence of some species (e.g., Thompson et al., 2020). The effect of shifts in multi-year climate cycles on species interactions is harder to predict. If climatic anomalies result in excess resource availability, one could predict rapid increases in local species diversity and abundance, which could produce large temporary increases in the amount of dispersal because of more individuals being present to disperse (density-independent dispersal) and/or density-dependent emigration (Eveleigh et al., 2007). Beyond this, the effects of climate-change driven alteration of ENSO and NAO on the temporal dynamics of metacommunities are unknown.
Dormancy and Dispersal
Dormancy, by allowing species to survive through periods with environmentally unfavorable or low-resource conditions, creates an alternative mechanism to dispersal by which species diversity can be maintained (McPeek and Kalisz, 1998). Consequently, dormancy may limit the roles of environmental filtering and species interactions in metacommunity dynamics. However, if species emerge from dormancy when conditions differ from those they have evolved to tolerate, environmental filtering could increase. Hence, two important questions about the relevance of temporal dynamics to metacommunities are whether community members have dormant life stages (is dormancy possible?), and whether suitable habitat patches are at fixed locations (is dispersal required for survival? McPeek and Kalisz, 1998). Fixed locations would permit non-dispersive life-stages to survive within a location. This would obviate the need for dispersal as a mechanism to maintain diversity and may permit species to be dormant during periods of environmental stress (reducing filtering) or intense competition or predation (limiting the role of species interactions). The temporal storage effect provides a mechanism for such maintenance of diversity (Chesson, 1994). In contrast, suitable patches that are ephemeral in both time and space would create a need for dispersal among temporary ecosystems. If dispersal is necessary, relevant metacommunity structuring mechanisms include not only dispersal but also environmental filtering and species interactions, which may limit patch colonization following local extinction.
Empirical Evidence
There is some empirical evidence for the importance of dormancy vs. dispersal in species occurrence, but it is limited to a few species. For instance, Alexander et al. (2012) studied the importance of local persistence through dormancy vs. regional dispersal in plants. They found that Silene latifolia seed dispersal and landscape connectivity were correlated with local colonization of S. latifolia, which lacks seed banks. However, Helianthus annuus has dormant seeds and its abundance was predicted by local factors such as previous-year local recruitment and local seed-bank size, but not seed dispersal (Alexander et al., 2012); hence in H. annuus, the presence of dormant propagules was the primary determinant of distribution and abundance, above and beyond dispersal and environmental filtering. Scaling up to whole metacommunities, there are expected to be negative correlations between dormancy and dispersal, which therefore trade-off in their importance in adding to species diversity. Dispersal often involves costs and risks, which may select against dispersal. There are costs of developing structures that facilitate dispersal (e.g., winged or fluffy seeds) and risks of mortality or energetic costs during dispersal and of failure to establish in new sites (Bonte et al., 2012). Reality is a bit more complex than this theoretical expectation, and correlations between dormancy and dispersal are often weak or more complex than hypothesized (Buoro and Carlson, 2014).
Wisnoski et al. (2019) explicitly modeled the effects of including dormant propagules into metacommunities. They took a simple model of competitive metacommunities (Shoemaker and Melbourne, 2016) that could represent each of the common metacommunity types (Leibold et al., 2004) and modified it to include transitions in and out of a seed bank. As expected (Bonte et al., 2012; Buoro and Carlson, 2014), when there was a negative dormancy-dispersal correlation, disturbance increased the importance of dormancy in maintaining species diversity (Wisnoski et al., 2019). Other forms of temporal environmental variation beyond disturbance could act in a similar way, and one would expect different species to be active depending on the prevailing environmental conditions. Some conditions promote the emergence of species from seed banks, while others favor species that persist by dispersal (Bonte et al., 2012; Buoro and Carlson, 2014). Therefore, the contribution of dormancy or dispersal to the maintenance of species diversity is expected to negatively covary through time. Conversely, when there is a positive dispersal-dormancy correlation, such as when habitats change rapidly across space and time (Buoro and Carlson, 2014), both dormancy and dispersal contribute to species diversity over relevant periods of time (Wisnoski et al., 2019). In general, positive dormancy-dispersal correlations homogenize species distributions across space. Under these circumstances there would be a breakdown of environmental filtering and species interactions structuring metacommunities and, similarly, a decrease in species sorting and mass effects. Dormancy and dispersal could then potentially contribute to diversity, though the fate of species emerging from dormancy would depend on environmental conditions (environmental filtering). Further, the effects of species interactions could be enhanced by elevated local species diversity resulting from the confluence of immigration by dispersing species and emergence of species from dormancy. Overall, dormant propagules and the direction of dormancy-dispersal correlations alter the extent to which dormancy and dispersal each contribute to metacommunity diversity (Wisnoski et al., 2019). Much remains to explore about such complex effects of the combination of dormancy and dispersal in metacommunities subject to temporal environmental variation.
Anthropogenic Global Change, Dormancy and Dispersal, and Metacommunities
Whether the storage effect as a coexistence mechanism is maintained under climate change is expected to depend on whether or not climate change affects the entire community equally (Rudolf, 2019; Wisnoski et al., 2019). If competing species respond differently to changing weather conditions, then increased variation in environmental conditions due to climate change could promote local coexistence and enhance metacommunity diversity (Rudolf, 2019). If species respond similarly to changing environmental conditions, there may be a loss of local and regional metacommunity diversity through various mechanisms: increased competition due to more species simultaneously consuming resources (Rudolf, 2019); reduced effects of dispersal because populations are similar across space, and mixing homogeneous populations through dispersal has less effect than mixing heterogeneous populations (Wisnoski et al., 2019); and, enhanced environmental filtering if dormant propagules emerge at times when the environment is adverse due to the environmental variability associated with climate change. These mechanisms of biodiversity loss might involve changes in phenology (e.g., Snyder and Adler, 2011), seasonality, or year-to-year differences in activity such as those related to multi-annual climate cycles. Snyder and Adler (2011) suggest that shifts in the germination time in competing plants are a result of climatic shifts in seasonal temperatures that amplify competition. Likewise, Ooi (2012) suggested that seedbanks may become more important because of increased fire frequency under climate change. However, it is also possible that if multiple species shift in the same ways in response to climate change there could be a loss of the role of dormancy and long-lived individuals in buffering populations. This would either reduce overall metacommunity diversity because of stronger environmental filtering and species interactions and/or increase the reliance of surviving species on dispersal.
Discussion
In their original conception, metacommunities were treated as static where populations colonize and go extinct, but the habitat and environment does not vary (Leibold et al., 2004; Holyoak et al., 2005). However, we have demonstrated that metacommunities experience wide-ranging variation across multiple temporal scales that can have broad effects on metacommunity structure. Temporal drivers such as disturbance, seasonality, multi-year dynamics like ENSO and NAO, and dormancy alter the relative importance, strength, and interactions among the biological processes of dispersal, environmental filtering, and species interactions (Table 1). A few patterns are apparent in Table 2. Although our literature survey found six studies of species assemblages in metacommunities subject to disturbance, none of them studied or identified the effects of disturbance on species interactions (compared to 3 of 9 studies on seasonality, and 2 of 4 studies on ENSO or NAO). This is surprising given that species interactions are central to one of the most widely cited ideas about the effects of disturbance on species diversity, the intermediate disturbance hypothesis, which proposes that disturbance will prevent dominant competitors from establishing in all habitat patches. The low frequency of metacommunity studies focusing on the effects of disturbance on species interactions likely reflects that species interactions are difficult to quantify, especially in short post-disturbance periods. Conversely, dispersal and environmental filtering were frequently identified in species assemblage (community-level) studies of metacommunities subject to temporal dynamics including disturbance, seasonality and multi-year climate fluctuations (Table 2). This finding aligns with the expectation that both dispersal and environmental filtering are central to metacommunity dynamics, as reflected in the metacommunity paradigms of Leibold et al. (2004).
Overall, we located few empirical studies on metacommunities and temporal dynamics that drew explicit connections to dispersal, environmental filtering, and/or species interactions. We identified nine studies of whole assemblages in metacommunities for the temporal dynamics of disturbance, nine for seasonality, nine for multi-annual climate fluctuations, and none for the effects of dormancy vs. dispersal (Table 2). Of the studies that focused on assemblages of species in metacommunities subject to the focal types of temporal dynamics, the majority analyzed small-bodied, easily-manipulated, and typically aquatic organisms including algae (1 study), bacteria (1 study), insects (1), macroinvertebrates (9), protists (6), rotifers (4), and zooplankton (1) (Supplementary Table S1). This selectivity broadly parallels studies of metacommunities in general that did not look at temporal dynamics (Logue et al., 2011). Beyond this, only two papers addressed small mammals, one addressed fishes, and one addressed plants. Therefore, studies focusing on more long-lived and larger organisms are needed. Fourteen studies were conducted in freshwater systems, three in marine and five in terrestrial systems. Accordingly, freshwater studies were disproportionately represented (Supplementary Table S1). Terrestrial systems included grasslands, and tropical, temperate, and boreal forests. The distribution of studies across geographical regions generally followed well-known patterns for ecological studies: 7 studies in N America, 6 studies in Europe, 5 studies in S and Central America, 1 study in Asia, and 2 studies in Oceania. Surprisingly, no studies were conducted in Australia or New Zealand and only one took place in Asia (Supplementary Table S1). The only study we located that was relevant to dormancy vs. dispersal focused on the autecology two single plant species in N America and was not specifically conducted in a metacommunity context.
Implications for the Conservation and Management of Metacommunities
Global modification of habitat caused by climate change and anthropogenic disturbance influences species at both local and regional scales. The metacommunity approach can enhance understanding of species persistence under anthropogenic global change by linking community interactions within patches to regional dispersal (Gilbert and O’Connor, 2013). Climate change is expected to intensify the frequency and severity of disturbance regimes (Seidl et al., 2011; Millar and Stephenson, 2015), increase the variability of seasonal events (Von Holle et al., 2010; Feng et al., 2013), and enhance temporal variability in ENSO and NAO (Fasullo et al., 2018). The effects of climate change on coexistence in metacommunities with dormant propagules are expected to vary depending on the extent to which species are correlated in their responses to global environmental change (Rudolf, 2019; Wisnoski et al., 2019). When coupled with anthropogenic modifications to the landscape, these effects of climate change further complicate the ability of researchers to define, predict, and manage patterns of temporal dynamism in metacommunities. Given this uncertainty, the challenge of incorporating metacommunity dynamics into a framework for management in the face of global change is one of the largest faced by ecologists (Urban et al., 2012; Gilbert and O’Connor, 2013).
Understanding of spatial and temporal variation in metacommunities provides insights into strategies for invasive species management. The increased reliance on dispersal after a disturbance combined with the propensity for invasive species to be strong dispersers (Sakai et al., 2001) indicates that lax management of invasive species will be unsuccessful in disturbed ecosystems. However, although efforts to constrain dispersal may help limit invasions, the importance of dispersal for metacommunity persistence in frequently disturbed systems suggests that restricting dispersal may be detrimental to the metacommunity, especially if dispersal among patches (e.g., through corridors) is critical for maintaining metacommunity diversity (Östman et al., 2006). Better understanding of how disturbance theory and metacommunity theory intersect may help guide corridor construction that promotes metacommunity persistence while managing for invasive species. Species invasions may also be influenced by the timing of seasonal activity (Wainwright et al., 2012) and have the potential to alter otherwise predictable temporal patterns in metacommunity structure (Erős et al., 2014). Both insights suggest that phenology, and therefore the temporal dynamics of metacommunities, are important factors to consider for the development of successful restoration strategies and invasive species management. Temporal dynamics also point to the need to consider the presence of dormant or long-lived life stages as traits that are relevant to management strategies. For example, being long-lived or having a seed bank might allow species to become successful invaders, as these individuals may be less vulnerable to stochasticity and better able to cope in novel environments (Sol et al., 2012). Ultimately, where invasive species management is particularly important, better management practices may be developed through the integration of insights learned from the study of metacommunity spatiotemporal dynamics.
Understanding if dispersal or dormancy are required for species coexistence and how they contribute to species diversity is potentially of great importance to conservation (McPeek and Kalisz, 1998). If high temporal predictability is coupled with habitat heterogeneity (low spatial autocorrelation) dispersal is favored over dormancy, whereas high spatial predictability but low temporal predictability favors dormancy over dispersal (Buoro and Carlson, 2014). Such insights are relevant to the construction of permanent (fixed-location) nature reserves, which may favor species exhibiting dormancy traits that can buffer against temporally variable conditions. However, where long-lived individuals have been disproportionately removed, such as the overfishing of older individuals, the buffering effect they provide against temporally variable conditions may be lost (Buoro and Carlson, 2014), and populations may become more vulnerable to large fluctuations and local extinction. In this way, such systems might necessarily switch to become more dependent on dispersal, and trophic interactions (e.g., bycatch) may extend well beyond the target species of fisheries or other harvesting schemes.
Finally, regular seasonal variation in dispersal, environmental filtering, and species interactions underlines the necessity to conduct surveys in all seasons, rather than assuming that the structure observed and management decisions made during one period are applicable to future periods. Recognition that temporal variation in dispersal, environmental filtering, and species interactions exists could improve the accuracy of biomonitoring methods and help guide effective ecosystem management strategies (Cid et al., 2020). Additionally, long-term dynamics such as ENSO and NAO are known to alter metacommunity structure, but the implications of these changes for conservation are unknown because existing studies typically present correlational rather than causational evidence. However, managers should still be aware that conservation strategies may have different impacts along climatic indexes.
Future Research Needs
We have focused on metacommunities at the mesoscale, conceptualizing metacommunities as dynamically fluctuating around some kind of equilibrium set by dispersal, environmental filtering, and species interactions. Indeed, plant metacommunity structure in an English woodland was the same at two points in time, seven decades apart (Keith et al., 2011). However, over longer timescales, metacommunities may not be characterized by such an equilibrial view and may be on a continuous trajectory of change. For example, metacommunities may be undergoing systematic decline (e.g., slow-acting extinction debt that cannot be easily measured in an empirical study) – after all, all species do go extinct eventually. Conversely, metacommunities may be expanding and growing as species continue to spread geographically since the last glacial period, or because of increased primary productivity from anthropogenic fertilization. Just as there are both short-term population studies that investigate demographic processes and long-term studies of population trends, one could envisage short-term studies of metacommunities at the mesoscale and long-term studies as more extensive metacommunity datasets become available. For example, disturbance and seasonality create short-term fluctuations in the structure of metacommunities, but these drivers may operate within multi-year climate cycles and occur in tandem with the removal or emergence of dormant propagules over multi-year periods. This overlap in temporal drivers can shape the trajectory of metacommunities over time in complex ways through synergistic interaction or time-lags (Blanchard et al., 2020). Accordingly, metacommunities are not structurally static at any time scale, but long-term datasets may be required for dynamic patterns to emerge.
There is also a need for models that explicitly include temporal dynamics and evaluate the emergent types of metacommunity dynamics. The framework of dispersal, environmental filtering, and species interactions, or similar axes adopted by Thompson et al. (2020), offers a promising approach for the evaluation of metacommunity structuring mechanisms, with a few caveats. Certainly, the use of the traditional metacommunity paradigms (Leibold et al., 2004) to categorize empirical and model systems can be problematic, as many of these systems do not neatly fit the archetypal types (e.g., Logue et al., 2011; Brown et al., 2017). However, these paradigms do capture both interactions among dispersal and environmental filtering and among dispersal and species interactions in mass effects that could easily be overlooked if we did not use the traditional paradigms. Further, interspecific or individual variation such as competition-colonization trade-offs are essential for patch dynamics, and they could also be passed over by only considering mean or variance of dispersal per species or modeling using dispersal kernels. Studies such as Wisnoski et al. (2019) stand out in reviewing the effect of combining dormancy and dispersal on metacommunity dynamics and structuring mechanisms.
Most work to date investigating the temporal dynamics of metacommunities is theoretical or focuses on invertebrates and protists in highly dynamic aquatic systems (e.g., intermittent rivers, tidal zones). This is especially prevalent in studies that examine the temporal drivers of disturbance and seasonality. Future research that explicitly tests metacommunity organization at different points in time is needed in various taxa, especially large, terrestrial species (Table 3). Further, investigation focusing on these taxonomic groups should explore how temporal changes in species’ physiology (e.g., metabolic rate; Scantlebury et al., 2005; Chamane and Downs, 2009; Lang et al., 2012; McClune et al., 2015) and behavior (e.g., migration, foraging; Ben-David et al., 1997; Schmidt et al., 2008; Davidson et al., 2013) influence metacommunity organization. Additionally, empirical study in more systems will help address questions regarding the generalizability of the temporal patterns identified in simpler, aquatic systems.
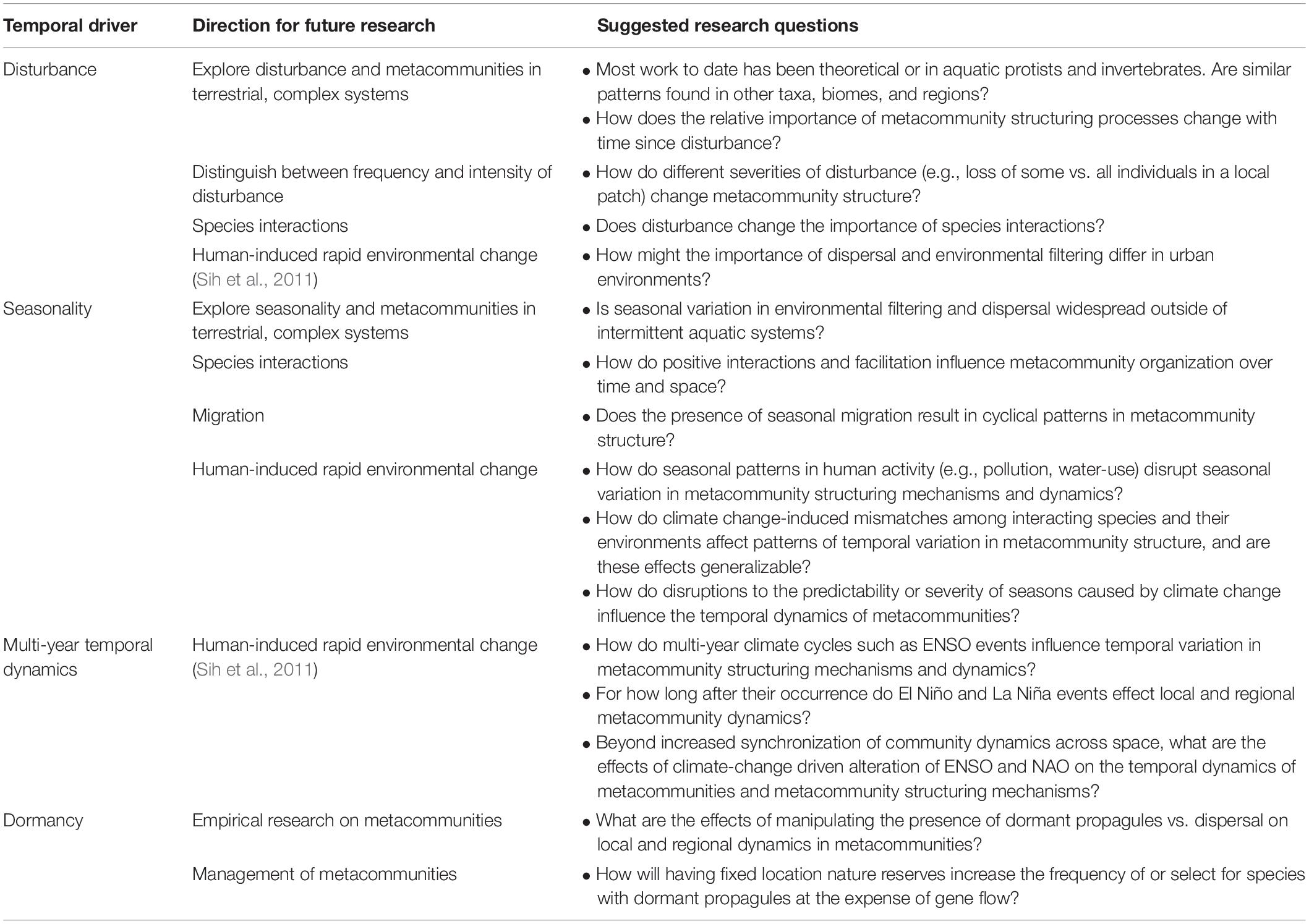
Table 3. Suggested future work to advance thinking about the effects of temporal dynamics on metacommunities.
While many studies identifying temporal variation in metacommunity structure highlight the biological processes of environmental filtering and dispersal, far fewer explicitly test and quantify the importance of species interactions (Supplementary Table S1). However, metacommunity assembly may be primarily driven by biotic interactions (García-Girón et al., 2020). How disturbance may change the importance of species interactions is unknown. Though seasonality is known to alter competition and predation, few studies extend these ideas to quantify structural changes in metacommunities in different seasons (Supplementary Table S1). Further, though facilitation and positive interactions can be important in community assembly (Bruno et al., 2003), they have not typically been included in metacommunity studies (Biswas and Wagner, 2012; but see Hoopes et al., 2005).
Despite mounting evidence that human activity affects both local and regional processes, few studies have attempted to apply metacommunity theory to human-modified landscapes (but see Swan et al., 2011; Johnson et al., 2013), especially in terrestrial systems. Urbanization frequently fragments habitat and impedes connectivity (McKinney, 2002), and, accordingly, theory predicts that dispersal and mass-effects may be especially relevant in human-modified landscapes (Parris, 2006; Johnson et al., 2013). Cities may act as an environmental filter (Swan et al., 2011), eliminating some species (e.g., apex predators) with potential cascading effects on species interactions, but, within a city, successful species may be buffered from environmental extremes (Rodewald and Gehrt, 2014). Accordingly, the environmental conditions associated with seasonal variability may become more subdued and temporal homogenization of metacommunity structure in urban ecosystems may be possible. Alternatively, highly seasonal human behavior exhibited by urban residents (e.g., pesticide use, water use, etc.) may reinstate temporal fluctuations, but these fluctuations would be moderated by anthropogenic activity, therefore linking socioeconomic patterns and processes to ecological theory. Much remains unknown, and further empirical study is needed to bridge metacommunity theory and urbanization research (Table 3).
Finally, global climate change requires scientists to consider how climate impacts on both local and regional dynamics synergistically interact to affect metacommunity persistence (Table 3). Metacommunity theory has been conceptualized without the explicit consideration of changing climate. Acknowledging how temporal drivers such as disturbance, seasonality, multi-year climate fluctuations, and dormancy influence the spatiotemporal dynamics of metacommunities is an important first step to add ecological realism to simplified theory. However, global climate change presents an immense challenge to our ability to extrapolate findings on the spatiotemporal dynamics of metacommunities in a contemporary context. The insights that metacommunity theory has provided on the consistency and patterns of temporal variation in metacommunity structure may not be relevant in a world defined by global climate change. We have suggested various ways in which a changing global climate has the propensity to disrupt predictions about the temporal dynamics of metacommunities – but what exactly these dynamic changes look like is a critical yet unanswered question for the conservation of metacommunities globally.
Author Contributions
All authors contributed equally to literature research and the writing of the manuscript. It was planned, researched, written, and revised together.
Funding
MH was funded by the Hatch Act funding to the University of California at Davis Agricultural Experiment Station.
Conflict of Interest
The authors declare that the research was conducted in the absence of any commercial or financial relationships that could be construed as a potential conflict of interest.
Acknowledgments
We wish to thank two reviewers who provided valuable comments on the manuscript.
Supplementary Material
The Supplementary Material for this article can be found online at: https://www.frontiersin.org/articles/10.3389/fevo.2020.571130/full#supplementary-material
References
Alexander, H. M., Foster, B. L., Ballantyne, F., Collins, C. D., Antonovics, J., and Holt, R. D. (2012). Metapopulations and metacommunities: combining spatial and temporal perspectives in plant ecology. J. Ecol. 100, 88–103. doi: 10.1111/j.1365-2745.2011.01917.x
Alexander, J. M., Diez, J. M., and Levine, J. M. (2015). Novel competitors shape species’ responses to climate change. Nature 525, 515–518. doi: 10.1038/nature14952
Altermatt, F., Bieger, A., Carrara, F., Rinaldo, A., and Holyoak, M. (2011a). Effects of connectivity and recurrent local disturbances on community structure and population density in experimental metacommunities. PLoS One 6:e19525. doi: 10.1371/journal.pone.0019525
Altermatt, F., Schreiber, S., and Holyoak, M. (2011b). Interactive effects of disturbance and dispersal directionality on species richness and composition in metacommunities. Ecology 92, 859–870. doi: 10.1890/10-1095.1
Andersson, M. (1980). Nomadism and site tenacity as alternative reproductive tactics in birds. J. Anim. Ecol. 49, 175–184. doi: 10.2307/4282
Barradas, I., Caswell, H., and Cohen, J. E. (1996). Competition during colonization vs competition after colonization in disturbed environments: a metapopulation approach. Bull. Math. Biol. 58, 1187–1207. doi: 10.1007/BF02458389
Battisti, C., Poeta, G., and Fanelli, G. (2016). An Introduction to Disturbance Ecology: A Road Map for Wildlife Management and Conservation. Berlin: Springer International Publishing. doi: 10.1007/978-3-319-32476-0
Ben-David, M., Flynn, R. W., and Schell, D. M. (1997). Annual and seasonal changes in diets of martens: evidence from stable isotope analysis. Oecologia 111, 280–291. doi: 10.1007/s004420050236
Bieber, C., Lebl, K., Stalder, G., Geiser, F., and Ruf, T. (2014). Body mass dependent use of hibernation: why not prolong the active season, if they can? Funct. Ecol. 28, 167–177. doi: 10.1111/1365-2435.12173
Biswas, S. R., and Wagner, H. H. (2012). Landscape contrast: a solution to hidden assumptions in the metacommunity concept? Landsc. Ecol. 27, 621–631. doi: 10.1007/s10980-012-9732-5
Blakely, T. J., Harding, J. S., Mcintosh, A. R., and Winterbourn, M. J. (2006). Barriers to the recovery of aquatic insect communities in urban streams. Freshw. Biol. 51, 1634–1645. doi: 10.1111/j.1365-2427.2006.01601.x
Blanchard, G., Birnbaum, P., and Munoz, F. (2020). Extinction-immigration dynamics lag behind environmental filtering in shaping the composition of tropical dry forests within a changing landscape. Ecography 43, 869–881. doi: 10.1111/ecog.04870
Bonte, D., Van Dyck, H., Bullock, J. M., Coulon, A., Delgado, M., Gibbs, M., et al. (2012). Costs of dispersal. Biol. Rev. 87, 290–312. doi: 10.1111/j.1469-185X.2011.00201.x
Boudell, J. A., and Stromberg, J. C. (2008). Flood pulsing and metacommunity dynamics in a desert riparian ecosystem. J. Veg. Sci. 19, 373–380. doi: 10.3170/2008-8-18377
Boyce, M. S. (1979). Seasonality and patterns of natural selection for life histories. Am. Nat. 114, 569–583. doi: 10.1086/283503
Brown, B. L., Sokol, E. R., Skelton, J., and Tornwall, B. (2017). Making sense of metacommunities: dispelling the mythology of a metacommunity typology. Oecologia 183, 643–652. doi: 10.1007/s00442-016-3792-1
Bruno, J. F., Stachowicz, J. J., and Bertness, M. D. (2003). Inclusion of facilitation into ecological theory. Trends Ecol. Evol. 18, 119–125. doi: 10.1016/S0169-5347(02)00045-9
Buoro, M., and Carlson, S. M. (2014). Life-history syndromes: integrating dispersal through space and time. Ecol. Lett. 17, 756–767. doi: 10.1111/ele.12275
Cadotte, M. W. (2007). Competition-colonization trade-offs and disturbance effects at multiple scales. Ecology 88, 823–829. doi: 10.1890/06-1117
Cadotte, M. W., and Tucker, C. M. (2017). Should environmental filtering be abandoned? Trends Ecol. Evol. 32, 429–437. doi: 10.1016/j.tree.2017.03.004
Campbell, R. E., Winterbourn, M. J., Cochrane, T. A., and McIntosh, A. R. (2015). Flow-related disturbance creates a gradient of metacommunity types within stream networks. Landsc. Ecol. 30, 667–680. doi: 10.1007/s10980-015-0164-x
Camus, P. A. (2008). Understanding biological impacts of ENSO on the eastern Pacific: an evolving scenario. Int. J. Environ. Health 2, 5–19. doi: 10.1504/IJEnvH.2008.018668
Cañedo-Argüelles, M., Gutiérrez-Cánovas, C., Acosta, R., Castro-López, D., Cid, N., Fortuño, P., et al. (2020). As time goes by: 20 years of changes in the aquatic macroinvertebrate metacommunity of Mediterranean river networks. J. Biogeogr. 47, 1861–1874. doi: 10.1111/jbi.13913
Caswell, H., and Cohen, J. E. (1991). Disturbance, interspecific interaction and diversity in metapopulations. Biol. J. Linn. Soc. 42, 193–218. doi: 10.1111/j.1095-8312.1991.tb00560.x
Chamane, S. C., and Downs, C. T. (2009). Seasonal effects on metabolism and thermoregulation abilities of the red-winged starling (Onychognathus morio). J. Therm. Biol. 34, 337–341. doi: 10.1016/j.jtherbio.2009.06.005
Chase, J. M. (2007). Drought mediates the importance of stochastic community assembly. Proc. Natl. Acad. Sci. U.S.A. 104, 17430–17434. doi: 10.1073/pnas.0704350104
Chave, J. (2004). Neutral theory and community ecology. Ecol. Lett. 7, 241–253. doi: 10.1111/j.1461-0248.2003.00566.x
Chesson, P. (1994). Multispecies competition in variable environments. Theor. Popul. Biol. 45, 227–276. doi: 10.1006/tpbi.1994.1013
Cid, N., Bonada, N., Heino, J., Cañedo-Argüelles, M., Crabot, J., Sarremejane, R., et al. (2020). A metacommunity approach to improve biological assessments in highly dynamic freshwater ecosystems. Bioscience 70, 427–438. doi: 10.1093/biosci/biaa033
Cisneros, L. M., Fagan, M. E., and Willig, M. R. (2015). Season-specific and guild-specific effects of anthropogenic landscape modification on metacommunity structure of tropical bats. J. Anim. Ecol. 84, 373–385. doi: 10.1111/1365-2656.12299
Connell, J. H. (1978). Diversity in tropical rain forests and coral reefs. Science 199, 1302–1310. doi: 10.1126/science.199.4335.1302
Cottenie, K. (2005). Integrating environmental and spatial processes in ecological community dynamics. Ecol. Lett. 8, 1175–1182. doi: 10.1111/j.1461-0248.2005.00820.x
Csercsa, A., Krasznai, -K. E. Á, Várbíró, G., Szivák, I., and Tóth, M. (2019). Seasonal changes in relative contribution of environmental control and spatial structuring on different dispersal groups of stream macroinvertebrates. Hydrobiologia 828, 101–115. doi: 10.1007/s10750-018-3806-6
Datry, T., Bonada, N., and Heino, J. (2016). Towards understanding the organisation of metacommunities in highly dynamic ecological systems. Oikos 125, 149–159. doi: 10.1111/oik.02922
Davidson, Z., Valeix, M., Van Kesteren, F., Loveridge, A. J., Hunt, J. E., Murindagomo, F., et al. (2013). Seasonal diet and prey preference of the African lion in a waterhole-driven semi-arid savanna. PLoS One 8:e55182. doi: 10.1371/journal.pone.0055182
Delciellos, A. C., Borges-Júnior, V. N. T., Prevedello, J. A., Ribeiro, S. E., Braga, C., Vieira, M. V., et al. (2018). Seasonality in metacommunity structure: an empirical test in the Atlantic Forest. Landsc. Ecol. 33, 1769–1783. doi: 10.1007/s10980-018-0701-5
Diaz, H. F., Hoerling, M. P., and Eischeid, J. K. (2001). ENSO variability, teleconnections and climate change. Int. J. Climatol. 21, 1845–1862. doi: 10.1002/joc.631
Dixo, M., Metzger, J. P., Morgante, J. S., and Zamudio, K. R. (2009). Habitat fragmentation reduces genetic diversity and connectivity among toad populations in the Brazilian Atlantic Coastal Forest. Biol. Conserv. 142, 1560–1569. doi: 10.1016/j.biocon.2008.11.016
Drake, J. M., and Lodge, D. M. (2004). Effects of environmental variation on extinction and establishment. Ecol. Lett. 7, 26–30. doi: 10.1046/j.1461-0248.2003.00546.x
Elkin, C. M., and Possingham, H. (2008). The role of landscape-dependent disturbance and dispersal in metapopulation persistence. Am. Nat. 172, 563–575. doi: 10.1086/590962
Ellis, A. M., Lounibos, L. P., and Holyoak, M. (2006). Evaluating the long-term metacommunity dynamics of tree hole mosquitoes. Ecology 87, 2582–2590. doi: 10.1890/0012-9658(2006)87[2582:etlmdo]2.0.co;2
Elton, C. S. (1924). Periodic fluctuations in the numbers of animals: their causes and effects. J. Exp. Biol. 2, 119–163.
Emerson, B. C., and Gillespie, R. G. (2008). Phylogenetic analysis of community assembly and structure over space and time. Trends Ecol. Evol. 23, 619–630. doi: 10.1016/j.tree.2008.07.005
Erős, T., Sály, P., Takács, P., Higgins, C. L., Bíró, P., and Schmera, D. (2014). Quantifying temporal variability in the metacommunity structure of stream fishes: the influence of non-native species and environmental drivers. Hydrobiologia 722, 31–43. doi: 10.1007/s10750-013-1673-8
Eveleigh, E. S., McCann, K. S., McCarthy, P. C., Pollock, S. J., Lucarotti, C. J., Morin, B., et al. (2007). Fluctuations in density of an outbreak species drive diversity cascades in food webs. Proc. Natl. Acad. Sci. U.S.A. 104, 16976–16981. doi: 10.1073/pnas.0704301104
Fasullo, J. T., Otto-Bliesner, B. L., and Stevenson, S. (2018). ENSO’s changing influence on temperature, precipitation, and wildfire in a warming climate. Geophys. Res. Lett. 45, 9216–9225. doi: 10.1029/2018GL079022
Feng, X., Porporato, A., and Rodriguez-Iturbe, I. (2013). Changes in rainfall seasonality in the tropics. Nat. Clim. Chang. 3, 811–815. doi: 10.1038/nclimate1907
Fernandes, I. M., Henriques-Silva, R., Penha, J., Zuanon, J., and Peres-Neto, P. R. (2014). Spatiotemporal dynamics in a seasonal metacommunity structure is predictable: the case of floodplain-fish communities. Ecography 37, 464–475. doi: 10.1111/j.1600-0587.2013.00527.x
Fiedler, P. C. (2002). Environmental change in the eastern tropical Pacific Ocean: review of ENSO and decadal variability. Mar. Ecol. Prog. Ser. 244, 265–283. doi: 10.3354/meps244265
Folke, C., Carpenter, S., Walker, B., Scheffer, M., Elmqvist, T., Gunderson, L., et al. (2004). Regime shifts, resilience, and biodiversity in ecosystem management. Annu. Rev. Ecol. Evol. Syst. 35, 557–581. doi: 10.1146/annurev.ecolsys.35.021103.105711
Foster, D. R., Knight, D. H., and Franklin, J. F. (1998). Landscape patterns and legacies resulting from large, infrequent forest disturbances. Ecosystems 1, 497–510. doi: 10.1007/s100219900046
Fraedrich, K., and Müller, K. (1992). Climate anomalies in Europe associated with ENSO extremes. Int. J. Climatol. 12, 25–31. doi: 10.1002/joc.3370120104
Gan, J., Bondarenko, S., Oki, L., Haver, D., and Li, J. X. (2012). Occurrence of fipronil and its biologically active derivatives in urban residential runoff. Environ. Sci. Technol. 46, 1489–1495. doi: 10.1021/es202904x
García-Girón, J., Heino, J., García-Criado, F., Fernández-Aláez, C., and Alahuhta, J. (2020). Biotic interactions hold the key to understanding metacommunity organisation. Ecography 43, 1180–1190. doi: 10.1111/ecog.05032
Gilbert, B., and O’Connor, M. I. (2013). Climate change and species interactions: beyond local communities. Ann. N. Y. Acad. Sci. 1297, 98–111. doi: 10.1111/nyas.12149
Gilman, S. E., Urban, M. C., Tewksbury, J., Gilchrist, G. W., and Holt, R. D. (2010). A framework for community interactions under climate change. Trends Ecol. Evol. 25, 325–331. doi: 10.1016/j.tree.2010.03.002
Glynn, P. W. (1988). El Nino Southern oscillation 1982-1983: nearshore population, community, and ecosystem responses. Annu. Rev. Ecol. Syst. 19, 309–346. doi: 10.1146/annurev.es.19.110188.001521
Gouhier, T. C., Guichard, F., and Gonzalez, A. (2010). Synchrony and stability of food webs in metacommunities. Am. Nat. 175, E16–E34. doi: 10.1086/649579
Gouveia, S. F., Hortal, J., Cassemiro, F. A. S., Rangel, T. F., and Diniz-Filho, J. A. F. (2013). Nonstationary effects of productivity, seasonality, and historical climate changes on global amphibian diversity. Ecography 36, 104–113. doi: 10.1111/j.1600-0587.2012.07553.x
Grimm, N. B., Faeth, S. H., Golubiewski, N. E., Redman, C. L., Wu, J., Bai, X., et al. (2008). Global change and the ecology of cities. Science 319, 756–760. doi: 10.1126/science.1150195
Hart, S. P., Usinowicz, J., and Levine, J. M. (2017). The spatial scales of species coexistence. Nat. Ecol. Evol. 1, 1066–1073. doi: 10.1038/s41559-017-0230-7
Hastings, A. (2003). Metapopulation persistence with age-dependent disturbance or succession. Science 301, 1525–1526. doi: 10.1126/science.1087570
Hastings, A. (2004). Transients: the key to long-term ecological understanding? Trends Ecol. Evol. 19, 39–45. doi: 10.1016/j.tree.2003.09.007
Heino, J. (2013). The importance of metacommunity ecology for environmental assessment research in the freshwater realm. Biol. Rev. 88, 166–178. doi: 10.1111/j.1469-185X.2012.00244.x
Hintz, W. D., and Relyea, R. A. (2019). A review of the species, community, and ecosystem impacts of road salt salinisation in fresh waters. Freshw. Biol. 64, 1081–1097. doi: 10.1111/fwb.13286
Hobbs, R. J., Higgs, E., and Harris, J. A. (2009). Novel ecosystems: implications for conservation and restoration. Trends Ecol. Evol. 24, 599–605. doi: 10.1016/j.tree.2009.05.012
Holdo, R. M., Holt, R. D., Sinclair, A. R. E., Godley, B. J., and Thirgood, S. (2011). “Migration impacts on communities and ecosystems: empirical evidence and theoretical insights,” in Animal Migration, eds E. J. Milner-Gulland, J. M. Fryxell, and A. R. E. Sinclair (Oxford: Oxford University Press), 130–143. doi: 10.1093/acprof:oso/9780199568994.003.0009
Holt, R. D. (1993). “Ecology at the mesoscale: the influence of regional processes on local communities,” in Species Diversity in Ecological Communities: Historical and Geographical Perspectives, eds R. E. Ricklefs and D. Schluter (Chicago, IL: University of Chicago Press).
Holyoak, M., Leibold, M. A., Holt, R. D., Moquet, N., Hoopes, M. F., and Amarasekare, P. (2005). “Metacommunities: a framework for large-scale community ecology,” in Metacommunities: Spatial Dynamics and Ecological Communities, eds M. Holyoak, M. A. Leibold, and R. D. Holt (Chicago, IL: University of Chicago Press).
Hoopes, M. F., Holt, R. D., and Holyoak, M. (2005). “The effects of spatial processes on two species interactions,” in Metacommunities: Spatial Dynamics and Ecological Communities, eds M. Holyoak, M. A. Leibold, and R. D. Holt (Chicago, IL: The University of Chicago Press), 35–67.
Hurrell, J. W. (1995). Decadal trends in the North Atlantic oscillation: regional temperatures and precipitation. Science 269:676. doi: 10.1126/science.269.5224.676
Hurrell, J. W., Kushnir, Y., Ottersen, G., and Visbeck, M. (eds) (2003). The North Atlantic Oscillation: Climatic Significance and Environmental Impact. New York, NY: John Wiley and Sons. doi: 10.1029/GM134
Huston, M. (1979). A general hypothesis of species diversity. Am. Nat. 113, 81–101. doi: 10.1086/283366
Inouye, D. W., Barr, B., Armitage, K. B., and Inouye, B. D. (2000). Climate change is affecting altitudinal migrants and hibernating species. Proc. Natl. Acad. Sci. U.S.A. 97, 1630–1633. doi: 10.1073/pnas.97.4.1630
Jabot, F., Laroche, F., Massol, F., Arthaud, F., Crabot, J., Dubart, M., et al. (2020). Assessing metacommunity processes through signatures in spatiotemporal turnover of community composition. Ecol. Lett. 23, 1330–1339. doi: 10.1111/ele.13523
Jacobson, B., and Peres-Neto, P. R. (2010). Quantifying and disentangling dispersal in metacommunities: how close have we come? How far is there to go? Landsc. Ecol. 25, 495–507. doi: 10.1007/s10980-009-9442-9
Jeffries, M. (2005). Small ponds and big landscapes: the challenge of invertebrate spatial and temporal dynamics for European pond conservation. Aquat. Conserv. Mar. Freshw. Ecosyst. 15, 541–547. doi: 10.1002/aqc.753
Johnson, P. T. J., Hoverman, J. T., McKenzie, V. J., Blaustein, A. R., and Richgels, K. L. D. (2013). Urbanization and wetland communities: applying metacommunity theory to understand the local and landscape effects. J. Appl. Ecol. 50, 34–42. doi: 10.1111/1365-2664.12022
Junk, W. J., Bayley, P. B., and Sparks, R. E. (1989). The flood pulse concept in river-floodplain systems. Can. Spec. Publ. Fish. Aquat. Sci. 106, 110–127.
Kaushal, S. S., Groffman, P. M., Likens, G. E., Belt, K. T., Stack, W. P., Kelly, V. R., et al. (2005). Increased salinization of fresh water in the northeastern United States. Proc. Natl. Acad. Sci. U.S.A. 102, 13517–13520. doi: 10.1073/pnas.0506414102
Keith, S. A., Newton, A. C., Morecroft, M. D., Golicher, D. J., and Bullock, J. M. (2011). Plant metacommunity structure remains unchanged during biodiversity loss in English woodlands. Oikos 120, 302–310. doi: 10.1111/j.1600-0706.2010.18775.x
Kneitel, J. M., and Miller, T. E. (2002). Resource and top-predator regulation in the pitcher plant (Sarracenia purpurea) inquiline community. Ecology 83, 680–688. doi: 10.1890/0012-9658(2002)083[0680:ratpri]2.0.co;2
Kolasa, J., and Romanuk, T. N. (2005). “Assembly of unequals in the unequal world of a rock pool metacommunity,” in Metacommunities: Spatial Dynamics and Ecological Communities, eds M. Holyoak, M. A. Leibold, and R. D. Holt (Chicago, IL: University of Chicago Press), 212–231.
Kraft, N. J. B., Adler, P. B., Godoy, O., James, E. C., Fuller, S., and Levine, J. M. (2015). Community assembly, coexistence and the environmental filtering metaphor. Funct. Ecol. 29, 592–599. doi: 10.1111/1365-2435.12345
Laliberté, E., Norton, D. A., and Scott, D. (2013). Contrasting effects of productivity and disturbance on plant functional diversity at local and metacommunity scales. J. Veg. Sci. 24, 834–842. doi: 10.1111/jvs.12044
Lane, J. E., Kruuk, L. E. B., Charmantier, A., Murie, J. O., and Dobson, F. S. (2012). Delayed phenology and reduced fitness associated with climate change in a wild hibernator. Nature 489, 554–557. doi: 10.1038/nature11335
Lang, B., Rall, B. C., and Brose, U. (2012). Warming effects on consumption and intraspecific interference competition depend on predator metabolism: temperature effects on interference competition. J. Anim. Ecol. 81, 516–523. doi: 10.1111/j.1365-2656.2011.01931.x
Larned, S. T., Datry, T., Arscott, D. B., and Tockner, K. (2010). Emerging concepts in temporary-river ecology. Freshw. Biol. 55, 717–738. doi: 10.1111/j.1365-2427.2009.02322.x
Leibold, M. A., Holyoak, M., Mouquet, N., Amarasekare, P., Chase, J. M., Hoopes, M. F., et al. (2004). The metacommunity concept: a framework for multi-scale community ecology. Ecol. Lett. 7, 601–613. doi: 10.1111/j.1461-0248.2004.00608.x
Leonard, G. H. (2000). Latitudinal variation in species interactions: a test in the New England rocky intertidal zone. Ecology 81, 1015–1030. doi: 10.1890/0012-9658(2000)081[1015:lvisia]2.0.co;2
Limberger, R., and Wickham, S. A. (2012). Disturbance and diversity at two spatial scales. Oecologia 168, 785–795. doi: 10.1007/s00442-011-2140-8
Lindegren, M., Andersen, K. H., Casini, M., and Neuenfeldt, S. (2014). A metacommunity perspective on source-sink dynamics and management: the Baltic Sea as a case study. Ecol. Appl. 24, 1820–1832. doi: 10.1890/13-0566.1
Lindstrom, J., Ranta, E., Kokko, H., Lundberg, P., and Kaitala, V. (2001). From arctic lemmings to adaptive dynamics: charles Elton’s legacy in population ecology. Biol. Rev. 76, 129–158. doi: 10.1017/S1464793100005637
Logue, J. B., Mouquet, N., Peter, H., and Hillebrand, H. (2011). Empirical approaches to metacommunities: a review and comparison with theory. Trends Ecol. Evol. 26, 482–491. doi: 10.1016/j.tree.2011.04.009
Lurgi, M., López, B. C., and Montoya, J. M. (2012). Novel communities from climate change. Philos. Trans. R. Soc. B Biol. Sci. 367, 2913–2922. doi: 10.1098/rstb.2012.0238
Mackey, R. L., and Currie, D. J. (2001). The diversity-disturbance relationship: is it generally strong and peaked? Ecology 82, 3479–3492. doi: 10.2307/2680166
Marrec, R., Pontbriand-Paré, O., Legault, S., and James, P. M. A. (2018). Spatiotemporal variation in drivers of parasitoid metacommunity structure in continuous forest landscapes. Ecosphere 9:e02075. doi: 10.1002/ecs2.2075
McClune, D. W., Kostka, B., Delahay, R. J., Montgomery, W. I., Marks, N. J., and Scantlebury, D. M. (2015). Winter is coming: seasonal variation in resting metabolic rate of the European badger (Meles meles). PLoS One 10:e0135920. doi: 10.1371/journal.pone.0135920
McKinney, M. L. (2002). Urbanization, biodiversity, and conservation. Bioscience 52, 883–890. doi: 10.1641/0006-3568(2002)052[0883:UBAC]2.0.CO;2
McMeans, B. C., McCann, K. S., Humphries, M., Rooney, N., and Fisk, A. T. (2015). Food web structure in temporally-forced ecosystems. Trends Ecol. Evol. 30, 662–672. doi: 10.1016/j.tree.2015.09.001
McPeek, M. A., and Kalisz, S. (1998). On the joint evolution of dispersal and dormancy in metapopulations. Adv. Limnol. 52, 33–51.
Mellard, J. P., Audoye, P., and Loreau, M. (2019). Seasonal patterns in species diversity across biomes. Ecology 100:e02627. doi: 10.1002/ecy.2627
Meserve, P. L., Kelt, D. A., Milstead, W. B., and Gutiérrez, J. R. (2003). Thirteen years of shifting top-down and bottom-up control. Bioscience 53, 633–646. doi: 10.1641/0006-3568(2003)053[0633:tyosta]2.0.co;2
Metz, M. C., Smith, D. W., Vucetich, J. A., Stahler, D. R., and Peterson, R. O. (2012). Seasonal patterns of predation for gray wolves in the multi-prey system of Yellowstone National Park. J. Anim. Ecol. 81, 553–563. doi: 10.1111/j.1365-2656.2011.01945.x
Millar, C. I., and Stephenson, N. L. (2015). Temperate forest health in an era of emerging megadisturbance. Science 349, 823–826. doi: 10.1126/science.aaa9933
Mouquet, N., and Loreau, M. (2002). Coexistence in metacommunities: the regional similarity hypothesis. Am. Nat. 159, 420–426. doi: 10.1086/338996
Odum, E. P. (1985). Trends expected in stressed ecosystems. Bioscience 35, 419–422. doi: 10.2307/1310021
Odum, E. P., Finn, J. T., and Franz, E. H. (1979). Perturbation theory and the subsidy-stress gradient. Bioscience 29, 349–352. doi: 10.2307/1307690
Ojima, M. N., and Jiang, L. (2017). Interactive effects of disturbance and dispersal on community assembly. Oikos 126, 682–691. doi: 10.1111/oik.03265
Ooi, M. K. J. (2012). Seed bank persistence and climate change. Seed Sci. Res. 22, S53–S60. doi: 10.1017/S0960258511000407
Östman, Ő, Kneitel, J. M., and Chase, J. M. (2006). Disturbance alters habitat isolation’s effect on biodiversity in aquatic microcosms. Oikos 114, 360–366. doi: 10.1111/j.2006.0030-1299.14521.x
Owen-Smith, N. (2008). Changing vulnerability to predation related to season and sex in an African ungulate assemblage. Oikos 117, 602–610. doi: 10.1111/j.0030-1299.2008.16309.x
Parmesan, C. (2006). Ecological and evolutionary responses to recent climate change. Annu. Rev. Ecol. Evol. Syst. 37, 637–669. doi: 10.1146/annurev.ecolsys.37.091305.110100
Parris, K. M. (2006). Urban amphibian assemblages as metacommunities. J. Anim. Ecol. 75, 757–764. doi: 10.1111/j.1365-2656.2006.01096.x
Peralta, G., Fenoglio, M. S., and Salvo, A. (2011). Physical barriers and corridors in urban habitats affect colonisation and parasitism rates of a specialist leaf miner. Ecol. Entomol. 36, 673–679. doi: 10.1111/j.1365-2311.2011.01316.x
Petraitis, P. S., Latham, R. E., and Niesenbaum, R. A. (1989). The maintenance of species diversity by disturbance. Q. Rev. Biol. 64, 393–418. doi: 10.1086/416457
Pineda, A., Peláez, Ó, Dias, J. D., Segovia, B. T., Bonecker, C. C., Velho, L. F. M., et al. (2019). The El Niño Southern Oscillation (ENSO) is the main source of variation for the gamma diversity of plankton communities in subtropical shallow lakes. Aquat. Sci. 81:49. doi: 10.1007/s00027-019-0646-z
Poff, N. L. (1992). Why disturbances can be predictable: a perspective on the definition of disturbance in streams. J. North Am. Benthol. Soc. 11, 86–92. doi: 10.2307/1467885
Post, E., Stenseth, N. C., Langvatn, R., and Fromentin, J.-M. (1997). Global climate change and phenotypic variation among red deer cohorts. Proc. R. Soc. Lond. B Biol. Sci. 264, 1317–1324. doi: 10.1098/rspb.1997.0182
Rebele, F. (1994). Urban ecology and special features of urban ecosystems. Glob. Ecol. Biogeogr. Lett. 4:173. doi: 10.2307/2997649
Ren, L., Song, X., He, D., Wang, J., Tan, M., Xia, X., et al. (2018). Bacterioplankton metacommunity processes across thermal gradients: weaker species sorting but stronger niche segregation in summer than in winter in a subtropical bay. Appl. Environ. Microbiol. 85:e02088-18. doi: 10.1128/AEM.02088-2018
Resh, V. H., Brown, A. V., Covich, A. P., Gurtz, M. E., Li, H. W., Minshall, G. W., et al. (1988). The role of disturbance in stream ecology. J. North Am. Benthol. Soc. 7, 433–455. doi: 10.2307/1467300
Ricart, M., Guasch, H., Barceló, D., Brix, R., Conceição, M. H., Geiszinger, A., et al. (2010). Primary and complex stressors in polluted Mediterranean rivers: pesticide effects on biological communities. J. Hydrol. 383, 52–61. doi: 10.1016/j.jhydrol.2009.08.014
Rivera-López, A., and MacGregor-Fors, I. (2016). Urban predation: a case study assessing artificial nest survival in a neotropical city. Urban Ecosyst. 19, 649–655. doi: 10.1007/s11252-015-0523-z
Rodewald, A. D., and Gehrt, S. D. (2014). “Wildlife population dynamics in urban landscapes,” in Urban Wildlife, eds R. A. McCleery, C. E. Moorman, and M. N. Peterson (Boston, MA: Springer), 117–147. doi: 10.1007/978-1-4899-7500-3_8
Rodewald, A. D., Kearns, L. J., and Shustack, D. P. (2011). Anthropogenic resource subsidies decouple predator-prey relationships. Ecol. Appl. 21, 936–943. doi: 10.1890/10-0863.1
Roxburgh, S. H., Shea, K., and Wilson, J. B. (2004). The intermediate disturbance hypothesis: patch dynamics and mechanisms of species coexistence. Ecology 85, 359–371. doi: 10.1890/03-0266
Rudolf, V. H. W. (2019). The role of seasonal timing and phenological shifts for species coexistence. Ecol. Lett. 22, 1324–1338. doi: 10.1111/ele.13277
Ruesink, J. L. (1998). Variation in per capita interaction strength: thresholds due to nonlinear dynamics and nonequilibrium conditions. Proc. Natl. Acad. Sci. U.S.A. 95, 6843–6847. doi: 10.1073/pnas.95.12.6843
Saavedra, S., Rohr, R. P., Fortuna, M., Selva, N., and Bascompte, J. (2016). Seasonal species interactions minimize the impact of species turnover on the likelihood of community persistence. Ecology 97, 865–873. doi: 10.1890/15-1013.1
Sakai, A. K., Allendorf, F. W., Holt, J. S., Lodge, D. M., Molofsky, J., With, K. A., et al. (2001). The population biology of invasive species. Annu. Rev. Ecol. Syst. 32, 305–332. doi: 10.1146/annurev.ecolsys.32.081501.114037
Sarremejane, R., Cañedo-Argüelles, M., Prat, N., Mykrä, H., Muotka, T., and Bonada, N. (2017). Do metacommunities vary through time? Intermittent rivers as model systems. J. Biogeogr. 44, 2752–2763. doi: 10.1111/jbi.13077
Sarremejane, R., Mykrä, H., Huttunen, K.-L., Mustonen, K.-R., Marttila, H., Paavola, R., et al. (2018). Climate-driven hydrological variability determines inter-annual changes in stream invertebrate community assembly. Oikos 127, 1586–1595. doi: 10.1111/oik.05329
Scantlebury, M., Oosthuizen, M. K., Speakman, J. R., Jackson, C. R., and Bennett, N. C. (2005). Seasonal energetics of the Hottentot golden mole at 1500 m altitude. Physiol. Behav. 84, 739–745. doi: 10.1016/j.physbeh.2005.02.022
Schlägel, U. E., Grimm, V., Blaum, N., Colangeli, P., Dammhahn, M., Eccard, J., et al. (2019). Movement-mediated community assembly and coexistence. EcoEvoRxiv [Preprint], doi: 10.32942/osf.io/d8a4m
Schmidt, N. M., Berg, T. B., Forchhammer, M. C., Hendrichsen, D. K., Kyhn, L. A., Meltofte, H., et al. (2008). Vertebrate predator-prey interactions in a seasonal environment. Adv. Ecol. Res. 40, 345–370. doi: 10.1016/S0065-2504(07)00015-3
Seidl, R., Schelhaas, M.-J., and Lexer, M. J. (2011). Unraveling the drivers of intensifying forest disturbance regimes in Europe. Glob. Change Biol. 17, 2842–2852. doi: 10.1111/j.1365-2486.2011.02452.x
Seidl, R., Thom, D., Kautz, M., Martin-Benito, D., Peltoniemi, M., Vacchiano, G., et al. (2017). Forest disturbances under climate change. Nat. Clim. Chang. 7, 395–402. doi: 10.1038/nclimate3303
Shoemaker, L. G., and Melbourne, B. A. (2016). Linking metacommunity paradigms to spatial coexistence mechanisms. Ecology 97, 2436–2446. doi: 10.1002/ecy.1454
Sih, A., Ferrari, M. C. O., and Harris, D. J. (2011). Evolution and behavioural responses to human-induced rapid environmental change. Evol. Appl. 4, 367–387. doi: 10.1111/j.1752-4571.2010.00166.x
Sinclair, A. R. E. (1985). Does interspecific competition or predation shape the African ungulate community? J. Anim. Ecol. 54:899. doi: 10.2307/4386
Snyder, R. E., and Adler, P. B. (2011). Coexistence and coevolution in fluctuating environments: can the storage effect evolve? Am. Nat. 178, E76–E84. doi: 10.1086/661905
Sol, D., Maspons, J., Vall-llosera, M., Bartomeus, I., García-Peña, G. E., Piñol, J., et al. (2012). Unraveling the life history of successful invaders. Science 337, 580–583. doi: 10.1126/science.1221523
Solins, J. P., and Cadenasso, M. L. (2020). Urban channel incision and stream flow subsidies have contrasting effects on the water status of riparian trees. Urban Ecosyst. 23, 419–430. doi: 10.1007/s11252-020-00926-2
Sousa, W. P. (1984). The role of disturbance in natural communities. Annu. Rev. Ecol. Syst. 15, 353–391. doi: 10.1146/annurev.es.15.110184.002033
Sperry, J. H., Peak, R. G., Cimprich, D. A., and Weatherhead, P. J. (2008). Snake activity affects seasonal variation in nest predation risk for birds. J. Avian Biol. 39, 379–383. doi: 10.1111/j.0908-8857.2008.04451.x
Starzomski, B. M., and Srivastava, D. S. (2007). Landscape geometry determines community response to disturbance. Oikos 116, 690–699. doi: 10.1111/j.0030-1299.2007.15547.x
Stenseth, N. C., and Mysterud, A. (2005). Weather packages: finding the right scale and composition of climate in ecology. J. Anim. Ecol. 74, 1195–1198. doi: 10.1111/j.1365-2656.2005.01005.x
Stenseth, N. C., Ottersen, G., Hurrell, J. W., Mysterud, A., Lima, M., Chan, K., et al. (2003). Review article. Studying climate effects on ecology through the use of climate indices: the North Atlantic Oscillation, El Niño Southern oscillation and beyond. Proc. R. Soc. Lond. B Biol. Sci. 270, 2087–2096. doi: 10.1098/rspb.2003.2415
Svensson, J. R., Lindegarth, M., Jonsson, P. R., and Pavia, H. (2012). Disturbance-diversity models: what do they really predict and how are they tested? Proc. R. Soc. B Biol. Sci. 279, 2163–2170. doi: 10.1098/rspb.2011.2620
Swan, C. M., Pickett, S. T. A., Szlavecz, K., Warren, P., and Willey, K. T. (2011). “Biodiversity and community composition in urban ecosystems: coupled human, spatial, and metacommunity processes,” in Urban Ecology, eds J. H. Breuste, T. Elmqvist, G. Guntenspergen, P. James, and N. E. McIntyre (Oxford: Oxford University Press), 179–186. doi: 10.1093/acprof:oso/9780199563562.003.0021
Talbot, L. M., and Talbot, M. H. (1963). The Wildebeest in Western Masailand, East Africa. Wildl. Monogr. 12, 3–88.
Thomaz, S. M., Bini, L. M., and Bozelli, R. L. (2007). Floods increase similarity among aquatic habitats in river-floodplain systems. Hydrobiologia 579, 1–13. doi: 10.1007/s10750-006-0285-y
Thompson, P. L., Guzman, L. M., De Meester, L., Horváth, Z., Ptacnik, R., Vanschoenwinkel, B., et al. (2020). A process-based metacommunity framework linking local and regional scale community ecology. Ecol. Lett. 23, 1314–1329. doi: 10.1111/ele.13568
Tischendorf, L., and Fahrig, L. (2000). On the usage and measurement of landscape connectivity. Oikos 90, 7–19. doi: 10.1034/j.1600-0706.2000.900102.x
Tonkin, J. D., Bogan, M. T., Bonada, N., Rios-Touma, B., and Lytle, D. A. (2017). Seasonality and predictability shape temporal species diversity. Ecology 98, 1201–1216. doi: 10.1002/ecy.1761
Tornwall, B. M., Swan, C. M., and Brown, B. L. (2017). Manipulation of local environment produces different diversity outcomes depending on location within a river network. Oecologia 184, 663–674. doi: 10.1007/s00442-017-3891-7
Tylianakis, J. M., Didham, R. K., Bascompte, J., and Wardle, D. A. (2008). Global change and species interactions in terrestrial ecosystems. Ecol. Lett. 11, 1351–1363. doi: 10.1111/j.1461-0248.2008.01250.x
Urban, M. C., De Meester, L., Vellend, M., Stoks, R., and Vanoverbeke, J. (2012). A crucial step toward realism: responses to climate change from an evolving metacommunity perspective. Evol. Appl. 5, 154–167. doi: 10.1111/j.1752-4571.2011.00208.x
Vanschoenwinkel, B., Buschke, F., and Brendonck, L. (2013). Disturbance regime alters the impact of dispersal on alpha and beta diversity in a natural metacommunity. Ecology 94, 2547–2557. doi: 10.1890/12-1576.1
Visser, M. E., Holleman, L. J. M., and Gienapp, P. (2006). Shifts in caterpillar biomass phenology due to climate change and its impact on the breeding biology of an insectivorous bird. Oecologia 147, 164–172. doi: 10.1007/s00442-005-0299-6
Von Holle, B., Wei, Y., and Nickerson, D. (2010). Climatic variability leads to later seasonal flowering of Floridian plants. PLoS One 5:e11500. doi: 10.1371/journal.pone.0011500
Wainwright, C. E., Wolkovich, E. M., and Cleland, E. E. (2012). Seasonal priority effects: implications for invasion and restoration in a semi-arid system. J. Appl. Ecol. 49, 234–241. doi: 10.1111/j.1365-2664.2011.02088.x
Walther, G.-R. (2010). Community and ecosystem responses to recent climate change. Philos. Trans. R. Soc. B Biol. Sci. 365, 2019–2024. doi: 10.1098/rstb.2010.0021
Warren, P. H. (1996). Dispersal and destruction in a multiple habitat system: an experimental approach using protist communities. Oikos 77, 317–325. doi: 10.2307/3546071
West-Eberhard, M. J. (1989). Phenotypic plasticity and the origins of diversity. Annu. Rev. Ecol. Syst. 20, 249–278. doi: 10.1146/annurev.es.20.110189.001341
White, E. R., and Hastings, A. (2018). Seasonality in ecology: progress and prospects in theory. PeerJ [Preprints], doi: 10.7287/peerj.preprints.27235v1
White, M. D., and Greer, K. A. (2006). The effects of watershed urbanization on the stream hydrology and riparian vegetation of Los Peñasquitos Creek, California. Landsc. Urban Plan. 74, 125–138. doi: 10.1016/j.landurbplan.2004.11.015
Winegardner, A. K., Jones, B. K., Ng, I. S. Y., Siqueira, T., and Cottenie, K. (2012). The terminology of metacommunity ecology. Trends Ecol. Evol. 27, 253–254. doi: 10.1016/j.tree.2012.01.007
Wisnoski, N. I., Leibold, M. A., and Lennon, J. T. (2019). Dormancy in metacommunities. Am. Nat. 194, 135–151. doi: 10.1086/704168
Ydenberg, R. (1987). Nomadic predators and geographical synchrony in microtine population cycles. Oikos 50, 270–272. doi: 10.2307/3566014
Keywords: environmental filtering, dispersal, species interactions, species diversity, anthropogenic change, species sorting, mass effects
Citation: Holyoak M, Caspi T and Redosh LW (2020) Integrating Disturbance, Seasonality, Multi-Year Temporal Dynamics, and Dormancy Into the Dynamics and Conservation of Metacommunities. Front. Ecol. Evol. 8:571130. doi: 10.3389/fevo.2020.571130
Received: 10 June 2020; Accepted: 14 September 2020;
Published: 02 October 2020.
Edited by:
Miguel Cañedo-Argüelles, University of Barcelona, SpainReviewed by:
Romain Sarremejane, University of California, Berkeley, United StatesJonathan Tonkin, University of Canterbury, New Zealand
Copyright © 2020 Holyoak, Caspi and Redosh. This is an open-access article distributed under the terms of the Creative Commons Attribution License (CC BY). The use, distribution or reproduction in other forums is permitted, provided the original author(s) and the copyright owner(s) are credited and that the original publication in this journal is cited, in accordance with accepted academic practice. No use, distribution or reproduction is permitted which does not comply with these terms.
*Correspondence: Marcel Holyoak, bWFob2x5b2FrQHVjZGF2aXMuZWR1