- 1Unité Mixte de Recherches, CREEC/CANECEV (CREES), MIVEGEC, IRD 224–CNRS 5290–Université de Montpellier, Montpellier, France
- 2Centre for Integrative Ecology, School of Life and Environmental Sciences, Deakin University, Geelong, VIC, Australia
- 3CNRS, UMR 5558, Laboratoire de Biométrie et Biologie Evolutive, Université de Lyon, Université Lyon 1, Villeurbanne, France
- 4Department of Anthropology, University of California, Santa Barbara, Santa Barbara, CA, United States
- 5Institut des Sciences de l’Evolution de Montpellier, Université de Montpellier, Montpellier, France
- 6Institute of Aquatic Ecology, Centre for Ecological Research, Debrecen, Hungary
- 7Evolutionary Ecology Group, Hungarian Department of Biology and Ecology, Babes-Bolyai University, Cluj-Napoca, Romania
- 8Tour du Valat, Institut de Recherche Pour la Conservation des Zones Humides Méditerranéennes, Arles, France
- 9LIENSs,UMR 7266 CNRS-La Rochelle Université, La Rochelle, France
- 10School of Science, Western Sydney University, Penrith, NSW, Australia
- 11Hawkesbury Institute for the Environment, Western Sydney University, Penrith, NSW, Australia
- 12School of Natural Sciences, University of Tasmania, Hobart, TAS, Australia
- 13Fauna Silvestre y Animales de Laboratorio, Departamento de Etología, Facultad de Medicina Veterinaria y Zootecnia, Universidad Nacional Autónoma de México, Mexico City, Mexico
Reproduction is one of the most energetically demanding life-history stages. As a result, breeding individuals often experience trade-offs, where energy is diverted away from maintenance (cell repair, immune function) toward reproduction. While it is increasingly acknowledged that oncogenic processes are omnipresent, evolving and opportunistic entities in the bodies of metazoans, the associations among reproductive activities, energy expenditure, and the dynamics of malignant cells have rarely been studied. Here, we review the diverse ways in which age-specific reproductive performance (e.g., reproductive aging patterns) and cancer risks throughout the life course may be linked via trade-offs or other mechanisms, as well as discuss situations where trade-offs may not exist. We argue that the interactions between host–oncogenic processes should play a significant role in life-history theory, and suggest some avenues for future research.
Introduction
Natural selection shapes organisms to maximize reproductive fitness, and this phenomenon leads to pivotal trade-offs around which all life-history traits ultimately evolve (Williams, 1966; Rose and Mueller, 1993; Roff, 2001; Stearns, 2006; Harshman and Zera, 2007). Exploring the consequences of reproductive trade-offs on organisms has therefore been—and remains—a central topic in evolutionary biology (Schaffer, 1974; Bell, 1980; Gustafsson et al., 1995; Hamel et al., 2010; Schwenke et al., 2016). There are numerous examples showing that the reproductive allocation of a given event correlates negatively with both short-term survival and/or future reproductive performance (Stearns, 2006; Hamel et al., 2010). Similarly, reproductive costs can be paid in the long run, through an earlier and or steeper actuarial and reproductive aging (Lemaître et al., 2015). Trade-offs between reproductive effort and subsequent survival can occur for a variety of reasons, one of them being that reproductive activities are energetically demanding, thus the amount of energy an individual allocates toward reproduction reduces its allocation to health maintenance (Kirkwood, 1977; Maklakov and Immler, 2016). For instance, reproductive activities and allocation to immune function are often mutually constraining: increased reproductive activity may limit immune performance, which in turn can enhance vulnerabilities to infections (Sandland and Minchella, 2003; French et al., 2007; Knowles et al., 2009; Fedorka, 2014; Schwenke et al., 2016). Allocation of resources away from soma to reproduction might also result in a decreased capacity to cope with damage caused by stress and toxicity, and/or be associated with detrimental by-products of metabolism, e.g., the production of damaging reactive oxygen species (Metcalfe and Monaghan, 2013, but see Blagosklonny, 2010). Finally, some of the genes selected for fitness conferring advantages during early life (e.g., increased reproductive output) may also have other functions; for example, carrying alleles that support reproduction may at the same time increase the risk of pathologies later in life (i.e., antagonistic pleiotropy) (Williams, 1957; Leroi et al., 2005; Madimenos, 2015; Austad and Hoffman, 2018; Gunten et al., 2018).
Apart from being a leading cause of human death worldwide, cancer is a biological process that appeared with the evolution of metazoans during the late Precambrian (Domazet-Lošo and Tautz, 2010; Nunney, 2013). Cancer occurs when individual cells become malignant, i.e., lose their normal cooperative behavior, become insensitive to host controls, proliferate in an uncontrolled fashion, and spread from primary tumors to surrounding tissues and then to distant organs (i.e., metastasis), thereby causing morbidity and potentially death (Hanahan and Weinberg, 2011). Chronological age is indisputably the most significant risk factor (in terms of incidence) for developing metastatic cancer, but it is also well established that oncogenic processes frequently exist at sub-clinical levels earlier in life (in humans and other animals, Folkman and Kalluri, 2004; Bissell and Hines, 2011; Madsen et al., 2017), and in fact represent a long continuum between precancerous lesions to invasive forms (Maley et al., 2017). Recent advances in oncology suggest that pathogens, parasites, viruses, or transposable elements may be the most common causes of cancer in wildlife, while second-hand smoke, nutritional challenges, breeding stress, UV radiation, and chemicals in the environment causing somatic mutations may be most important for pets and humans (Aktipis and Nesse, 2013; Giraudeau et al., 2018; Pesavento et al., 2018). Since cancer is observed in almost all branches of multicellular life, from Hydra to whales (Leroi et al., 2003; Aktipis et al., 2015; but see Azpurua and Seluanov, 2013; Fortunato et al., 2021), we hypothesize that cancer should be a major mediator of life-history trade-offs and a contributor to life-history evolution.
The short- and long-term fitness consequences of oncogenic manifestations early in life, at a life-history stage where individuals are expected to maximize their allocation of resources toward growth and reproduction, remain poorly documented (Vittecoq et al., 2013; Thomas et al., 2018). In addition, the precise reasons why oncogenic processes—depending on organs, individuals, and/or species— evolve at variable rates inside the body are not yet well understood, although there is clear evidence that a variety of internal and external parameters are important (e.g., sex, age, immune status, organ ecology and microenvironment, energetic capacity, stress, environment, Tevfik Dorak and Karpuzoglu, 2012; Henry et al., 2015; Thomas et al., 2016; Hochberg and Noble, 2017; DeGregori et al., 2018; Biro et al., 2020; Vibishan and Watve, 2020). Evolutionary ecologists have, until recently, largely neglected the importance of cancer cells for animal ecology (Thomas et al., 2017). There are more than 100 types of cancer (grouped into five major categories: carcinoma, sarcoma, myeloma, leukemia, and lymphoma) that can affect almost every part of the body (Weinberg, 2007). In addition to malignant tumors, multicellular organisms often harbor benign neoplasms (Boutry et al., 2022b). Benign neoplasms have received less attention than malignant tumors because they have less often obvious and serious impacts on the patient/host health, even if noticeable exceptions exist (see Boutry et al., 2022b for a recent synthesis).
Tumoral processes, especially malignant ones, can be detrimental to host fitness by imposing direct costs on it. For instance, malignancies affecting reproductive organs, like cervix, uterus, endometrial, ovary or testicular cancers, can severely compromise their host’s reproductive potential by triggering reproductive aging or inducing infertility (Brinton et al., 2004, 2005; Paduch, 2006; Singh et al., 2011; Hanson et al., 2017). Another well-known direct cost of (notably metastatic) cancers on host fitness is due to their detrimental effect on survival. While the survival cost of cancer is largely documented for human and domestic animals, less information is available for wildlife species. Recent studies from zoo animals showed that cancerous processes are however widespread (see Vincze et al., 2022). The lack of information in the wild is likely due to the fact that tumor-bearing individuals, often in poorer condition than healthy ones, have increased probability to die early, being victims of predation or infections (Boutry et al., 2022a). Thus, cancer is indirectly costly when tumor-bearing individuals are considered within their ecosystems. Although experimental evidence would be welcome, it is also likely that tumor-bearing individuals should be less attractive for sexual partners, and/or will have lower ability to deliver good parental care (Vittecoq et al., 2015).
As with other fitness-reducing factors, evolutionary theory predicts that metazoans are under selective pressure to: (i) avoid the source of malignancies in the first instance, (ii) prevent tumoral progression once initiated, and finally (iii) alleviate the fitness costs if further cancer development is not preventable (Ujvari et al., 2016). These strategies necessarily involve immediate and/or long-term costs that are traded against other functions (Jacqueline et al., 2017a), even if the evolution of cancer defenses is predicted to be variable between species, depending on their ecology. For instance, tumors are common in laboratory mice, but are rarely observed in wild populations. While this difference between natural and artificial environments may in part be due to differences in environmental exposure to cancer inducing agents, it also results from the fact that the average lifespan of a wild mouse (a prey species) is only a few months. While somatic mutations contributing to cancer in laboratory mice may be common, historically, there may have been little selection on immune processes that prevent, or slow cancer because wild mice rarely live long enough to experience tumor formation (Perret et al., 2020).
Thus, cancerous processes, through their direct costs on hosts and/or most often through the costs of host defenses, have the potential to impose life history tradeoffs (Aktipis et al., 2013; Brown and Aktipis, 2015; Muller, 2017). We discuss here the idea that malignant processes are crucial to consider when studying life-history traits because malignant dynamics—and hence their consequences on health and survival—are likely to be linked in multiple ways with the age-specific reproductive activities of organisms (see Figure 1). While there have been many review papers linking host evolutionary ecology to cancer (e.g., Peto’s paradox), to our knowledge, our paper is the first to primarily focus on the associations between cancer and reproduction. This synthesis highlights the underestimated role of oncogenic processes in shaping the multiple facets of the age-specific reproductive biology of multicellular hosts.
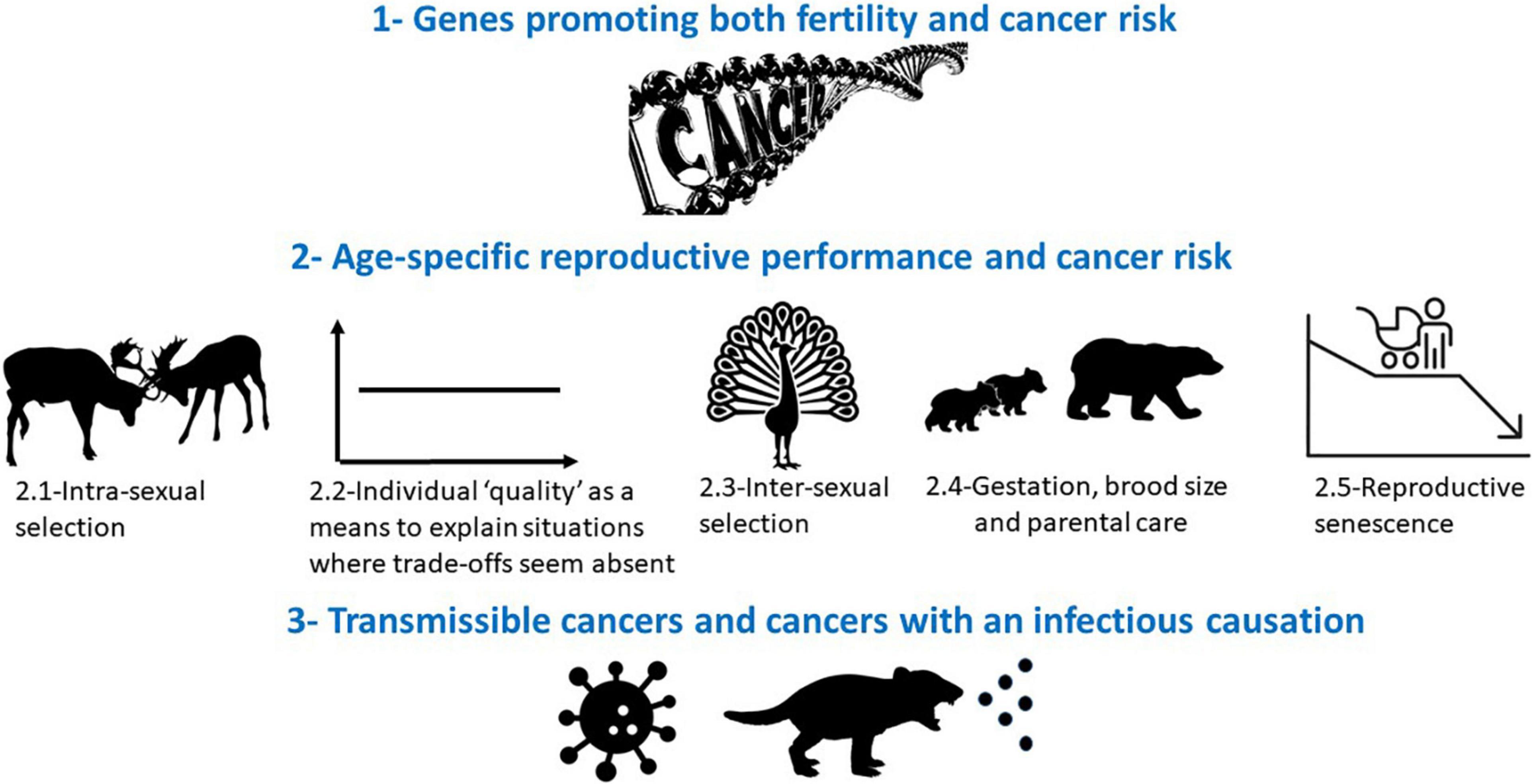
Figure 1. Plan of this synthesis: malignant processes and then consequences on health and survival are linked in multiple ways with the age-specific reproductive activities of organisms.
Genes Promoting Both Fertility and Cancer Risk
One mechanism that can link together reproduction with increased susceptibility to cancer is through genes that have direct effects on both. Like several other biological processes detrimental to health, malignant dynamics can also be embedded in the antagonistic pleiotropy theory (e.g., Crespi and Summers, 2006; Carter and Nguyen, 2011; Jacqueline et al., 2017a; Lemaître et al., 2020a). At the genetic level, for instance, some alleles that increase cancer risk as a secondary effect of their positive roles on reproductive success have been identified; a good example is the Xmrk melanoma-promoting oncogene in the fish Xiphophorus cortezi (Figure 2). Despite significant deleterious cancer-induced effects leading to a shorter lifespan, this oncogenic allele persists in natural populations presumably because it is also associated with a larger body size, which increases the individual’s reproductive success through mate competition. This oncogene is also directly correlated with aggressive behaviors (Fernandez, 2010). In addition, the presence of melanoma on the male’s caudal fin intensifies the spotted caudal melanin pattern, which increases female preference for these mates (Fernandez and Morris, 2008; Summers and Crespi, 2010).
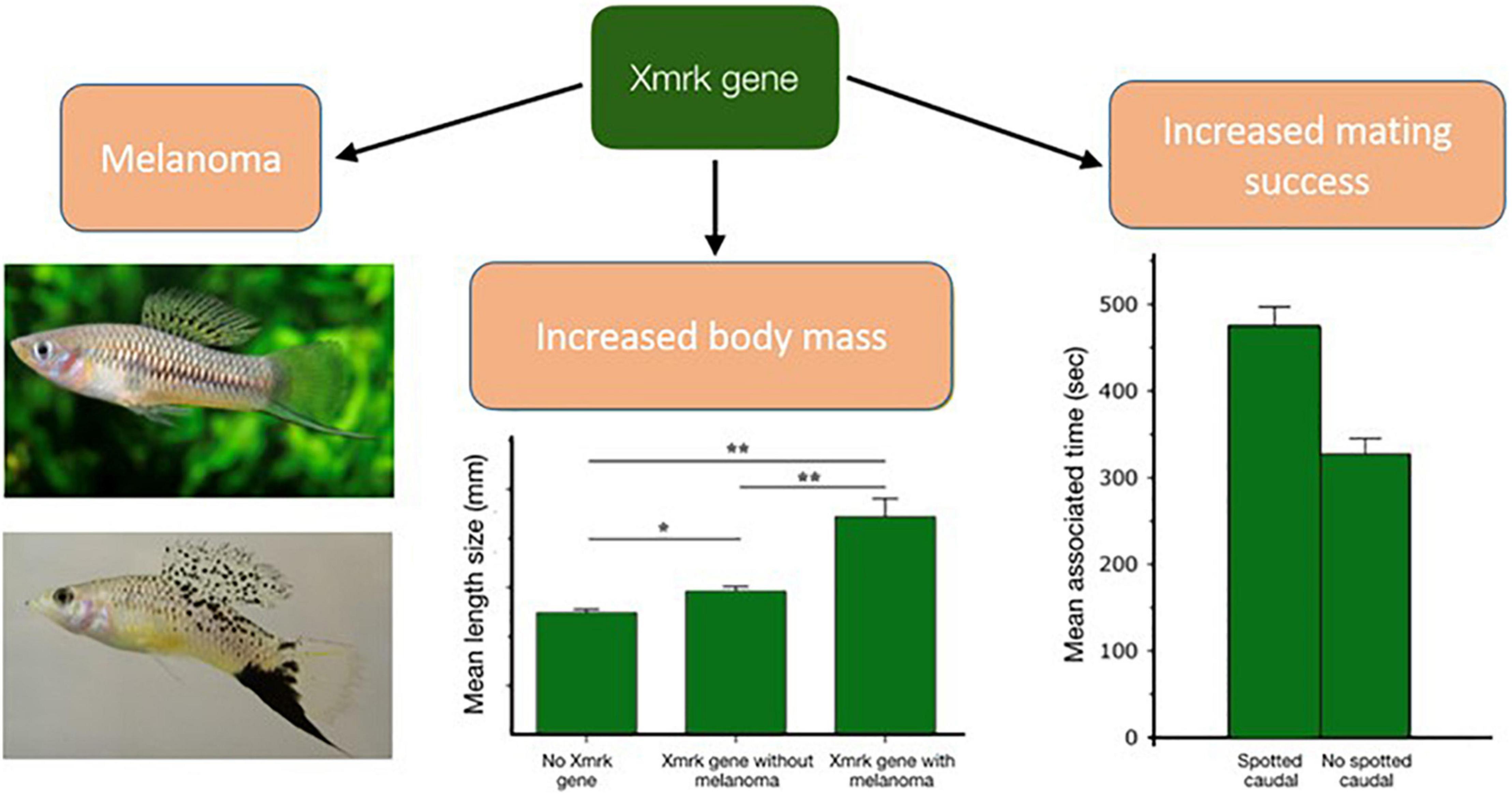
Figure 2. Antagonistic pleiotropy and oncogenic congenital mutations: the Xmrk melanoma-promoting oncogene in the fish Xiphophorus cortezi. Sexual selection processes may sometimes favor cancer promoting oncogene alleles, as this is observed in the fish Xiphophorus developing melanoma (bottom Photo). Despite shorter lifespan due to the deleterious cancer-induced effects, the oncogenic Xmrk allele is not eliminated from natural populations of Xiphophorus, because several associated benefits early in life outweigh late costs (i.e., antagonistic pleiotropy). Xmrk allele is indeed associated to a larger body size and higher aggression, which increases individual’s reproductive success through mate choice and competition for mates. The source and credit for the fish photos: Johnny Jensen (up) and Andre Fernandez (down). Figure modified from Fernandez and Morris (2008). *P < 0.05, **P < 0.01.
Another example concerns mutations on the tumor-suppressing gene BRCA, which is responsible for DNA repair. While women carrying BRCA1/2 mutations have higher cancer incidence and higher mortality, one study reported that BRCA mutation carriers born in Utah prior to 1930 had naturally higher fertility compared to controls (before the advent of modern contraception) (Smith et al., 2012; see however, Oktay et al., 2015; Daum et al., 2018). The precise mechanisms behind this phenomenon are not completely understood, and perhaps are simply the consequence of genetic bottlenecks. However, they could also involve a trade-off between cancer suppression and tolerance for invasive fetal cells (Aktipis, 2020). Placentation in female eutherian mammals, like cancer metastasis, is indeed an invasive process that involves the transplantation of cells into new environments. Females in these species would therefore be potentially more vulnerable to cancer (Kshitiz et al., 2019). This hypothesis seems to be supported at the interspecific level, since species of mammals with more invasive placentation such as felines and canines are also more vulnerable to malignancies (D’Souza and Wagner, 2014). However, Boddy et al. (2020) did not confirm this tendency, and found instead at the interspecific level a positive relationship exists between litter size and prevalence of malignancy. Beyond these examples, the search for positive selection signals on mutations occurring in cancer suppression genes seems to be promising for the identification of candidate genes responsible for both fertility enhancement and cancer risk (Kang and Michalak, 2015).
The fact that cancer genes are not purged by natural selection can also be driven by antagonistic coevolution processes that occur in cases of conflict between evolutionary agents (Graham, 1992; Crespi and Summers, 2006). Some of these conflicts are related to reproductive activities, e.g., parent–offspring conflict, maternal–fetal conflict, sexual conflict, and/or sexual selection (Haig, 2015). The genes involved in such dynamics generate evolutionary disequilibriums, molecular-level arms races, and tugs-of-war over cellular resources, and they may increase susceptibility to cancer as a pleiotropic by-product of their role(s) in antagonistic coevolution (Crespi and Summers, 2006). Situations of sexual conflict, i.e., when the fitness of one sex comes at the expense of the other sex, can occur when the genes involved in rapid cell proliferations—which allow rapid growth or wound healing—are due to sexual selection being more advantageous for fighting males than for females. The optimum trade-off between DNA repair and cell proliferation rate, which also relies on the BRCA1 and BRCA2 alleles (Yoshida and Miki, 2004), is therefore likely to vary between males and females, generating sexually antagonistic selection. Oncogenic consequences for females, such as breast and/or ovarian cancers, could result from a disruption of the trade-off via a BRCA gene mutation in a somatic cell, leading to proliferation with less-effective DNA repair. Total cancer risks arising from perturbations to maternally and paternally opposed growth regulation have yet to be determined (Frank and Crespi, 2011), but exploring this hypothesis with comparative analyses seems promising. In particular, considering species that vary in the intensity of male sexual selection relying on body size and aggressive fights could be of interest since the differential optimum values for the repair–proliferation trade-off between sexes are expected to be somewhat different.
It is also thought that genes contributing to gamete production (rate and/or quality) may use pathways that are highly beneficial for cancer cells able to co-opt or subvert them during somatic evolution. These pathways could allow rapid cell proliferation and/or avoidance of control by tumor suppressors or the immune system (Crespi and Summers, 2006). For example, SPANX genes in primates, which show evidence of strong positive selection, are involved both in spermatogenesis and in melanoma progression by promoting cancer cell growth (Kleene, 2005). More recent studies also support the hypothesis that some genetic pathways of spermatogenesis, whose evolution is governed by responses to sexual selection and intra-sexual conflict, are the same as those used by cancer cells to increase their survival and replication (genes such as BRIP1, BUB1B, KTN1, and RANBP2) (Vicens and Posada, 2018). It has also been suggested that the CAG repeat region of the androgen receptor could be a locus of antagonistic pleiotropy in the context of sexual selection and sexual conflict (Summers and Crespi, 2008). Short repeats are associated with increased fertility at the phenotypic level, but there is also evidence that somatic evolution of malignant cell lineages during cancer progression are often associated with a repeated pattern of shortening of the CAG repeat, a priori because of positive selection among cell lineages.
Age-Specific Reproductive Performance and Cancer Risk
Intra-Sexual Selection
As mentioned before, antagonistic pleiotropy is when one gene has opposite effects on fitness at different ages, such that their effects are beneficial in early life, when natural selection is strong, but harmful at later ages, when selection weakens. In a theoretical study, Boddy et al. (2015) investigated the possible links between cancer risks and the level of intraspecific competition, assuming that an underlying resource-based trade-off between competitiveness and allocation into cancer defenses exists. As has been empirically observed (see Clocchiatti et al., 2016 for an example in humans), the model first showed that cancer prevalence is expected to be lower in females than in males, since mating competition usually has greater reproductive payoffs for males than for females (Clutton-Brock and Vincent, 1991) and also because of the immune-enhancing effect of estrogen (Taneja, 2018; even if the picture is more variable in humans, Mulder and Ross, 2019). Although experimental evidence is currently lacking, the higher male susceptibility is likely to be exacerbated when they grow faster and/or have to develop and maintain extravagant secondary sexual traits. This should theoretically be the case because the required higher cell proliferation itself increases cancer risk (especially in body areas with the greatest cellular divisions, e.g., testes cancer, antleromas). For instance, the insulin/insulin-like growth factor (IGF) signaling pathway to mTOR is essential for the survival and growth of normal cells but also contributes to the genesis and progression of cancer, increasing cell proliferation and anti-apoptosis processes (LeRoith and Roberts, 2003; Floyd et al., 2007). Another reason is because of a diversion of resources from somatic maintenance (e.g., DNA repair or immune defenses). A potential self-maintenance mechanism suffering shortfall could be the antioxidant machinery, when strenuous activity (e.g., mating competition, reproductive effort, maintenance of costly sexual signals) leads to an increased production of reactive oxygen species causing oxidative stress. Even if empirical data have not been always consistent, oxidative stress has been viewed as an important physiological costs of reproduction (Blount et al., 2016; Pap et al., 2018) and it is well known to participate in cancer development by promoting cancer initiation and progression through induced DNA damage, leading to mutagenesis, and by affecting DNA methylation patterns or the expression of oncogenes (Van Remmen et al., 2004; Kreuz and Fischle, 2016). Moreover, as recently highlighted by Ujvari et al. (in press), telomere dynamics could also occupy a pivotal position in these processes. Indeed, allocation toward the maintenance of costly sexual signals often alters oxidative metabolism (Hill, 2014), which can, in turn, provoke telomere erosion (Monaghan and Ozanne, 2018) and influence the emergence of oncogenic mutations. It is predicted that natural selection should favor the expression of secondary sexual traits over the cost of telomere dysfunction until the organism reaches its maximum reproductive potential, even if this is likely to promote malignant progressions beyond this (Taff and Freeman-Gallant, 2017; Pepke and Eisenberg, 2021). In a recent study, Wang et al. (2019) studied the genetic basis of antler regeneration in ruminants; antlers are among the fastest-growing bone in the animal kingdom. In red deer (Cervus elaphus), for instance, antlers regrow annually and reach up to 30 kg, with growth rates of ∼1.7 cm/day, which necessitates a rate of cell proliferation that surpasses classical cancerous tissue growth. Wang et al. (2019) found that the genes underlying the expression of these large sexual ornaments are among those that both promote and suppress cancer (Figure 3). Specifically, there was a higher correlation between the gene expression profiles of antlers and bone cancer such as osteosarcoma than between those of antlers and normal bone tissues, suggesting that antler growth and oncogenesis rely on similar developmental programs. Cancer is typically a pathology arising from perturbations to a precarious balance between strongly opposed growth promoters and growth repressors (Aktipis, 2020). However, these authors discovered that contrary to the situation in osteosarcoma, where neoplasms progress unchecked, antler growth was tightly regulated by the activity of cancer-controlling genes (both tumor-suppressing and tumor-growth-inhibiting genes), suggesting that antler growth is mechanistically equivalent to a controlled form of bone cancer growth. Cervids generally display a very low risk of cancer (Vincze et al., 2022). Ideally, the same genes promoting cancer prevention (tumor-suppressors like BRCA, TP53, BUB1, BUBR1, TGF-βRII, Axin, DPC4, p300, and PPARγ which generally play a role in controlling the cell cycle or DNA repair) would be selected for and likely conserved. However, they would share the same pathway used by cancerous cells because the same genes are used in cell division. Given that antlers are lost every year, we might speculate that this could potentially be a defense mechanism. In line with this hypothesis, a recent comparative analyses of cancer risk across almost 200 species of mammals highlighted that Artiodacyla such as Cervids are much less prone to cancer than other mammalian orders (Vincze et al., 2022).
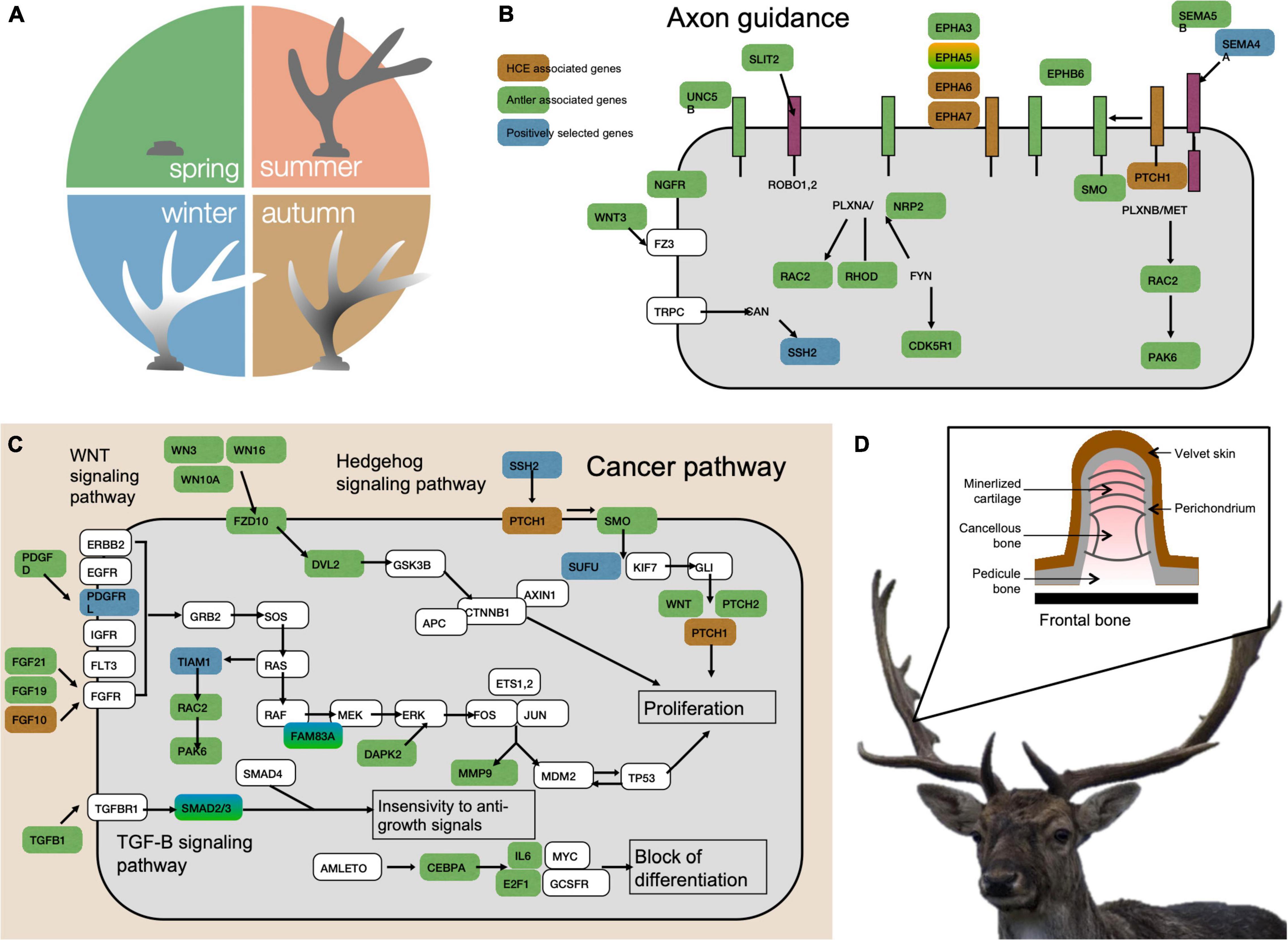
Figure 3. Antler regeneration, neurogenesis and oncogenesis pathways. (A) Annual antler regeneration cycle in red deer. Antlers, after shed in winter regenerate in spring, and then grow most rapidly in summer. In autumn, they calcify and shed their velvet. For (B) (Axon guidance pathways) and (C) (Oncongenesis pathways): blue, genes positively selected in cervids; yellow, cervid-specific HCE associated genes; green, antler-specific expression genes. (D) Antler anatomy. (C) The anatomy of the antler. The source and credit for the red deer: Pixabay. Figure modified from Wang et al. (2019).
The apparent lack of a correlation between sexual ornament size and cancer rate may be explained by mechanisms that offset the increased cancer risk. As possible supportive evidence, albeit in a different ecological context, Thomas et al. (2020) argued that strong ongoing artificial selection in domestic animals has sometimes resulted in extreme phenotypic responses favoring a higher incidence of cancer. However, there is also evidence for effective anti-cancer defenses in several domesticated animals, suggesting that artificial selection may also favor the evolution of compensatory anticancer defenses (see also Ibrahim-Hashim et al., 2020). More research in wild species, especially at the intraspecific level, would be necessary to evaluate how robust and buffered these compensatory protections are. Indeed, because sexual selection processes may be intense in natural populations depending on the ecological contexts, it could be expected that animals benefiting from abundant resources might develop secondary sexual traits whose expression level is higher than that corresponding to their cancer defenses. A rapid evolution in this direction would then drive genotypes away from the optimum, which would result in an increased risk of cancer, at least until the species or population adapts to the changes (Abegglen et al., 2015; Boutry et al., 2020). Such kind of mismatch scenario could potentially be investigated in habitat when wildlife artificially benefits from excessive quantity of food. In humans, it is well established that good nutrition has promoted growth in recent decades, allowing individuals to grow taller (Grasgruber et al., 2014). However, it is also known that cancer risk increases with adult height, at least in part because the number of cells correlates positively with stature, while cancer defenses apparently remain the same (Nunney, 2018). It is unlikely that mechanisms will evolve to prevent large stature because of the sexual selection benefits associated with height in males (Stulp et al., 2013) as well as the reduced risks of obstetric complications in females (Guégan et al., 2000), and also because most oncogenic consequences will occur relatively late in life. However, such processes, at least in wild species, could result in positive feedback cycles in oncogene evolution, leading to improved tumor suppression, greater developmental precision and complexity, and further adaptive changes driving pleiotropic oncogene evolution (Crespi and Summers, 2006). Domestication of animals by humans may provide some support for the hypothesis that a mismatch between cancer risk and cancer defenses may promote malignant proliferation. For example, the artificial selection for size in dogs results in higher incidences of bone cancer in larger breeds compared to smaller ones (Grabovac et al., 2020) because selection for genes responsible for height (and hence a larger number of cells) was not accompanied by selection for more efficient cancer defenses (Thomas et al., 2020). In a related vein, pediatric cancers in humans most often concern three compartments, brain, blood and bone, that have undergone rapid phenotypic changes in their developmental trajectories along the human lineage (Leroi et al., 2003). In current times, the risk of mismatch can also be exacerbated because anthropic activities result in higher pollution by mutagenic substances in ecosystems (Giraudeau et al., 2018) that—all things being equal—lead to unprecedented risks of cell derailment rates in organs that undergo intense cell divisions.
Although potentially specific to certain populations (see for instance Trumble et al., 2014), testosterone in males has frequently been associated not only with a greater investment in mating behavior (Wingfield et al., 1990; Peters et al., 2008; Summers and Crespi, 2008; Dixson, 2015), but also (although still debated, Michaud et al., 2015) with a higher risk of prostate cancer (e.g., Alvarado, 2013 for males in human polygynous societies). This correlation potentially supports the hypothesis of a trade-off, where higher testosterone levels would allow males to engage in more short-term mating with different partners but at the cost of a higher long-term risk of prostate cancer. It is however, difficult to separate hormonal effects and higher exposure to sexually transmitted carcinogenic viruses also affecting prostate tissues, a risk that increases with the number of sexual partners (e.g., Moghoofei et al., 2019). Further research is needed to decipher the interactions between sexual hormones, the immune system, and cancer development in wild species. Promising models could be social species, especially those for which there are differential hormone levels and cancer risks between dominant and subordinate individuals (Jacqueline et al., 2017a).
Individual ‘Quality’ as a Means to Explain Situations Where Trade-Offs Seem Absent
Although trade-offs between reproduction and the ability to ward off cancer are expected based on long-standing life history theory (citations above), we should not always expect that reproductive costs are evident or detected. For example, investment into large antlers by Artiodactyla (discussed above) may not create detectible energy trade-offs and increased cancer risk if dominant individuals monopolize resources prior to breeding as is often the case in some species. That is, some individuals of high ‘quality’ possess abundant energetic resources that permit them to allocate energy to competing demands obscuring trade-offs. Similarly, some of the dramatic anti-cancer adaptations found in domestic animals (Thomas et al., 2020) may be also explained by the fact that abundant food permits simultaneous allocation of energy to reproduction and anti-cancer functions, again obscuring trade-offs. These views are consistent with theory showing that when variation in energy acquisition among individuals is greater than variation in allocation within individuals, competing life history traits will no longer be negatively correlated among individuals (van Noordwijk and de Jong, 1986). Indeed, reviews show energy expenditure on activity, reproduction and maintenance metabolism can often be positively correlated at the among individual level for domestic and laboratory reared animals (Biro and Stamps, 2008, 2010).
Inter-Sexual Selection
In addition to intra-sexual selection, inter-sexual selection through mate choice is central in the theory of sexual selection (Andersson and Simmons, 2006). For many years, it was expected females of many species chose to mate with old rather than young males, because older males often provide better resources (e.g., territories, parental care) and/or pass good genes on to their offspring (Brooks and Kemp, 2001). However, these theoretical predictions are now thoroughly revisited in the light of recent studies demonstrating the under-estimated occurrence of male reproductive aging, as well as the detrimental consequences of this process on female fitness (Lemaître and Gaillard, 2017; Monaghan and Metcalfe, 2019; Monaghan et al., 2020; Segami et al., 2021). Empirical studies suggest that female preference toward young males could be a pervasive mating tactic in the living world (e.g., Verburgt et al., 2011; Vanpé et al., 2019). Here, we propose that mating preferentially with younger males might also be adaptive in terms of a decreased cancer risk in the progeny. One reason is that males accumulate deleterious mutations in their germ-line at an ever-increasing rate as they age (Beck and Promislow, 2007), thereby reducing the quality of genes passed on to the next generation, which potentially favors cancer (e.g., see Choi et al., 2005 for an example that older paternal age increases the risk of breast cancer in female offspring). In addition, in several species – at least in humans and chimpanzees – sperm telomere length is positively correlated with male age, leading to a positive correlation between paternal age at conception and offspring telomere length (Eisenberg and Kuzawa, 2018; Eisenberg, 2019; Eisenberg et al., 2019). Longer telomeres in descendants of old fathers are likely to predispose the novel generation to a higher risk of cancer since cells have a greater chance to accumulate bad mutations before replicative senescence occurs and eliminates them (e.g., Aviv et al., 2017). Theoretical models aiming to predict age-based mating preferences in females based on the benefits-costs balance provided by both young and old partners should now fully consider the risk of cancer in the progeny. For instance, it may remain evolutionarily beneficial for females to produce a progeny whose lifespan will be shortened by cancer in species where old males provide more resources, especially if the cancer-induced death can occur relatively late in the life of offspring (i.e., once most of their reproduction has been achieved). In addition, given the heritability of height (Yang et al., 2010), further studies should also explore the possible oncogenic consequences for tall partner preference in humans.
Gestation, Brood Size, and Parental Care
In numerous species, reproduction is costly for females in terms of energy, nutrients, and metabolic adjustments, which may lead to faster aging and reduced longevity when reproductive effort increases (Westendorp and Kirkwood, 1998; Lemaître et al., 2015; Jasienska, 2020). Physiological consequences of elevated reproductive expenditure are also observed on biological markers of aging. For instance, in species for which males also have a high allocation of resources toward parental care, e.g., common terns (Sterna hirundo), where males are primarily responsible for chick feeding, a negative association between reproductive success and telomere length is observed only in males (Bauch et al., 2016). The direct and/or indirect consequences of these kinds of reproductive costs on oncogenic process dynamics have not yet been fully explored for most species. Pregnancy in women, at least in Western populations, seems to have a dual effect on breast cancer risk (Hsieh et al., 1994). While full-term pregnancies in early life (<30 years) reduce breast cancer risk in the long-term (Albrektsen et al., 2005; Hurt et al., 2006), in the short term they boost the development of oncogene-activated cells into tumors and/or promote a metastatic cascade (Lambe et al., 2002; Lyons et al., 2011), which transiently increases cancer risk. The most common oncogenic progressions observed during pregnancy are malignant melanoma and lymphomas, leukemia, and breast, ovary, cervix, colon, and thyroid cancers (Pavlidis, 2002; Oduncu et al., 2003). The cancer risk is highest within the first 5 years after giving birth, and the risk of breast cancer in parous women is increased for more than 15–20 years compared with nulliparous women (Nichols et al., 2019). Multiple factors are likely responsible for this higher vulnerability to cancer during pregnancy, such as the strategic modulation of the maternal adaptive immune system during the first trimester and a relatively high inflammatory status (Fessler, 2002; Abrams and Miller, 2011; Lyons et al., 2011; but see Hové et al., 2021). The pathophysiology of cancer associated with pregnancy also includes factors like hormonal changes, permeability, and vascularization (D’Souza and Wagner, 2014; Hepner et al., 2019).
Enhanced reproductive effort through increased parental care has often been linked to concomitant or subsequent reduced immune-competence (Nordling et al., 1998), which in return should promote the proliferation of malignant cells (Jacqueline et al., 2017a). However, it seems likely in nature that reduced immunocompetence would first increase the frequency of infections that are detrimental to survival in the short term, long before malignant processes that escape immune-surveillance could have an effect of survival. The fact that detrimental oncogenic consequences in the wild are hidden because of deaths resulting from infectious processes does not mean that they are not significant, nor can we exclude the possibility that oncogenic consequences may interact with infectious dynamics (e.g., see Goldszmid et al., 2014; Jacqueline et al., 2017b,2020). The oncogenic consequences resulting from trade-offs with enhanced parental care, if any, will be mainly observed in parasite- and predator-free environments such as zoos, provided breeding activities continue (see also Tanaka et al., 2020).
While high reproductive investments can potentially result in increased cancer risks for individuals due to immediate trade-offs, it is also expected that natural selection can fix these problems on the long term. For instance, Brown and Aktipis (2015) suggested in a theoretical study that in cases of extended parental care, as well as with cooperative breeding systems, selection can favor cancer suppression into old age. At least for the proof of concept, artificial selection in the context of domestication illustrates that enlarging reproductive efforts may select for compensatory adaptations or additional defenses (Thomas et al., 2020). For example, while the ancestor of domesticated hens may live for 20–30 years and produce only small numbers of fertile eggs during a limited period of the year, strong artificial selection leading to the domesticated jungle fowl hen (Gallus gallus domesticus) has resulted in animals with a short lifespan and prolific daily ovulation and egg laying. Malignant ovarian cancer in domesticated chickens is frequent, but there is a five-fold variability between strains in the incidence of the disease (Johnson and Giles, 2006), suggesting that anticancer responses may have subsequently evolved in certain strains. Similarly, in dairy cows and goats that have been selectively bred for mammary gland growth and milk production, there is paradoxically a low occurrence of mammary tumors compared to domestic carnivores (Munson and Moresco, 2007), suggesting once again that anticancer mechanisms compensating for increased risks of lobular alveolar growth and hence malignancies have been concomitantly and/or subsequently selected. Further studies would be necessary to explore how relevant these phenomena are in wild species currently experiencing alterations in the reproductive biology because of climate change (e.g., Winkler et al., 2002; Pankhurst and Munday, 2011).
Reproductive Aging
The evolutionary ecology literature is replete of studies documenting reproductive aging (i.e., the decline in reproductive performance with increasing age, also coined reproductive aging) in females (see Holmes et al., 2003; Lemaître and Gaillard, 2017 for reviews). The decline in litter size from 10 years of age onward observed in Alpine marmot, Marmota marmota (Berger et al., 2015) constitutes one of the numerous examples of reproductive aging, and many other of reproductive traits (e.g., litter size, birth rate, juvenile survival) have been show to decrease with age. Nowadays, the common view is that female’s reproductive aging is the rule rather than the exception, at least in endotherm vertebrates (more than 60% of the studies species in both birds, Vágási et al., 2021 and mammals, Lemaître et al., 2020b) even if reproductive aging trajectories remain highly variables both within and across species (Reid et al., 2010; Lemaître et al., 2020b). Reproductive aging is obviously particularly pronounced in species displaying menopause (see Péron et al., 2019), a cessation of the reproductive functions that occurs many years before death. In addition, there is now pervasive evidence that reproductive aging is common among males of various species. Indeed, while evidence that male fertilization efficiency decreases with age have been documented for a long time in human (see Johnson et al., 2015) and laboratory rodents (vom Saal et al., 1994), an increasing number of studies are now reporting male reproductive aging in semi-captive or wild populations of animals. Such decline in reproductive performance have been observed using reproductive success metrics (e.g., mating success, Raveh et al., 2010) but also the conspicuousness of secondary sexual traits (e.g., Perrot et al., 2016, or the quality of the ejaculates, Preston et al., 2011). However, while the study of reproductive aging is showing an unprecedent infatuation, the link between both reproductive aging and oncogenic processes are yet to be deciphered.
These two processes, reproductive aging and oncogenic processes, can share similar proximate origins. As emphasized in the sections above, an elevated reproductive expenditure could be responsible for an increased risk of cancer (Boddy et al., 2015). Interestingly, there is compelling evidence that such substantial allocation to reproduction during early-life is also detrimental in terms of an earlier/stronger reproductive aging (e.g., Nussey et al., 2006 and Lemaître et al., 2014, for examples in females and males red deer, Cervus elaphus, respectively) and the physiological pathways that have been suggested to mediate the link between early- and late-life reproductive performance are strikingly similar to the one suggested to be involved in the reproductive effort-cancer trade-offs (e.g., oxidative stress, telomere attrition, see Kalmbach et al., 2015). However, this does not preclude any complex interactions between reproductive aging and oncogenic processes and understanding whether the occurrence of cancer is a cause, a consequence or is – to some extent – independent to the aging process remains a long-standing question (de Maghalaes, 2013; Thomas et al., 2018). Moreover, it is noteworthy that the cancer-reproductive aging dynamic is likely to be different between males and females for which the age-specific cancer rate of reproductive organs show striking differences (see de Maghalaes, 2013).
The various risk of cancers associated to the physiological pathways modulating the reproductive sequence (e.g., pregnancy in mammals) might have constituted a selected pressure on the evolution of reproductive aging patterns. For instance, it has recently been proposed (Thomas et al., 2019a) that menopause in humans (and in a few cetaceans) may have specifically evolved as an anticancer adaptation for some mismatches between the dynamics of oncogenic processes and natural anticancer adaptations that have occurred during recent evolutionary changes (Figure 4). While it is a natural phenomenon that oncogenic processes steadily accumulate in the body with age, pregnancies are unlikely to initiate cancers in an evolutionary stable context. However, mismatches in cancer defense levels can cause oncogenic processes to accumulate at higher rates, enhancing the growth of existing tumors and raising the risk of tipping the balance toward the initiation of uncontrollable metastatic cancers. Thus, after a given age, pregnancy could be associated with a higher probability of premature death due to metastatic cancers. The physiology of fertile women itself is also expected to favor malignant progression because several cancers also depend on hormones (estrogen and progesterone) for their growth (Aktipis et al., 2015; Aktipis, 2016; Atashgaran et al., 2016). Menopause could potentially have evolved as a “natural hormonal therapy” to prevent or alleviate the growth of malignancies before a fatal threshold is reached (Thomas et al., 2019a). If reproduction can contribute to an increase in oncogenic processes later in life, it is however, not the only nor even the most important contributor, and cancers remain common even after menopause and/or in nulliparous women (Gleicher, 2013; Einstein et al., 2015). This emphasizes how cancers must be viewed within multivariate life-history strategies and tradeoffs.
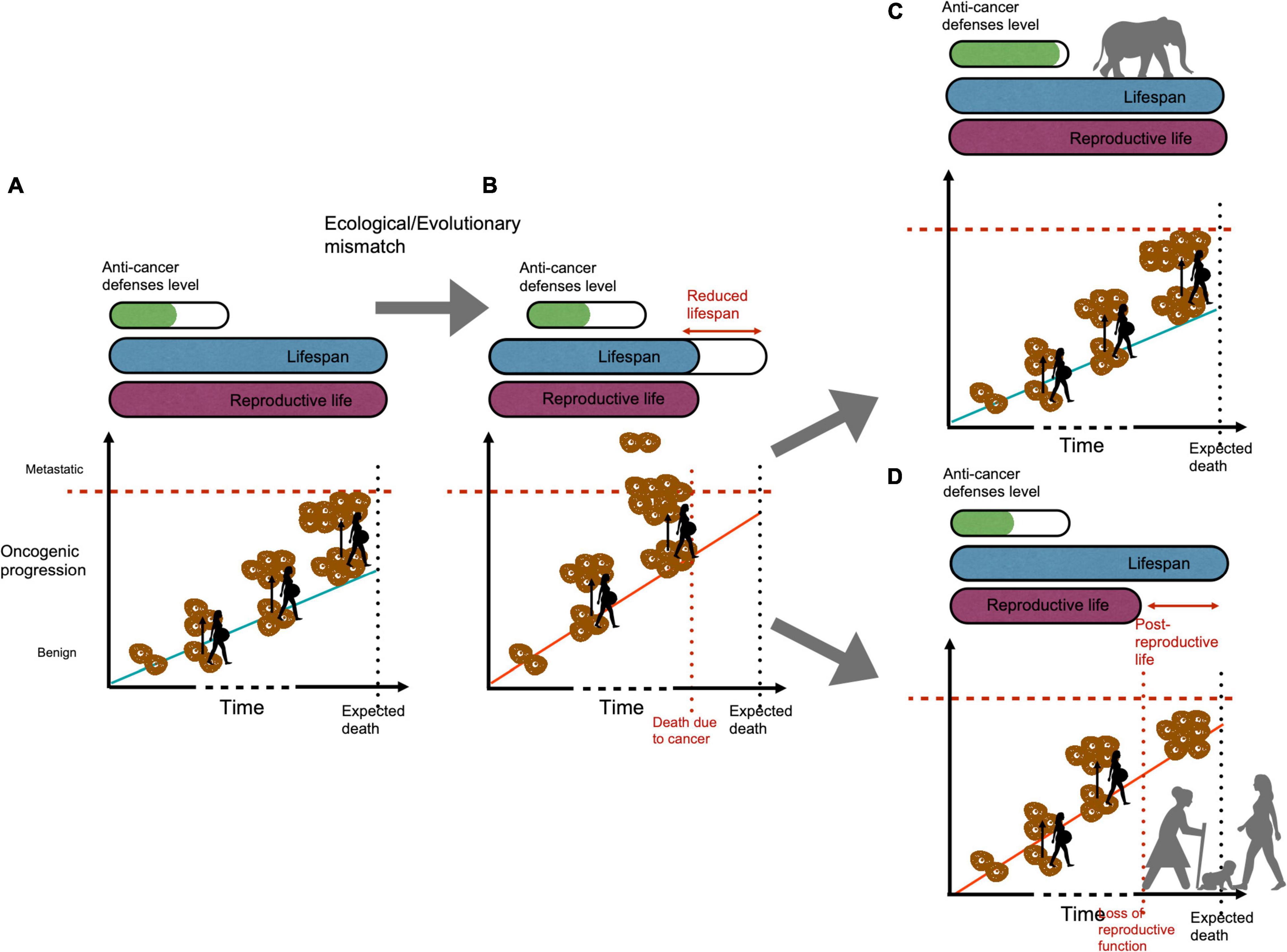
Figure 4. Menopause as a possible (transient) adaptation to cancer risk following an evolutionary mismatch. (A) When cancer defenses are in alignment with malignant risks, oncogenic processes slowly accumulate through time, and even if pregnancy periods exacerbate their dynamics, the risk of metastatic cancers remains low. (B) Following an ecological and/or evolutionary mismatch, cancer defenses may become too weak given the novel cancer risks. Oncogenic processes accumulate rapidly, and this time reproductive episodes exacerbate metastatic cancer risks sooner in life for females, resulting in a short lifespan and a low fitness. From this situation, two evolutionary outcomes are possible, natural selection can (i) favor the evolution of better cancer defenses in these species (i.e., as in large animals such as whales and elephants), coming back to a situation comparable to (A), here (C), or (ii) favor females ceasing their reproduction prematurely to preserve their health and invest their energy/resources for their grandchildren (D). In this later situation, female’s fitness is higher than in (B) because of inclusive fitness. The (D) scenario could in theory be just a transient situation until other cancer defenses are selected (A,C examples). Figure modified from Thomas et al. (2019a).
From this hypothesis, further fascinating directions (still debated in humans, e.g., Saadat, 2010) remain to be explored, such as the influence of offspring sex ratio on cancer risk, for example. All else being equal, sons in sexually dimorphic and polygynous species are often more costly to produce or rear than daughters (Bérubé et al., 1996; Whittingham and Dunn, 2000; Gibson and Mace, 2003; Cameron-MacMillan et al., 2007; Rickard et al., 2007). If male embryos more strongly foster the proliferation of existing malignant cells during pregnancy than female embryos, it could be expected that a sex ratio biased in favor of male progeny could, all else being equal, result in a higher overall maternal cancer risk and/or a higher risk of cancer at a younger age. The literature on this topic remains controversial at the moment (e.g., Hsieh et al., 1999; Wohlfahrt and Melbye, 2000; Saadat, 2010).
In addition, it would be particularly relevant to explore whether some of the mammalian species that show no decline in pregnancy rates with increasing age (e.g., white tailed-deer, Odocoileus virginianus) (DelGiudice et al., 2007) display a higher risk of cancers or have evolved alternative anti-defenses mechanisms to buffer cancer risk. This approach would also be relevant in ectotherm species that often show no decline (or sometimes even an increase) in fertility with age (e.g., Cayuela et al., 2020). Many studies are currently seeking to identify the biological properties enabling many ectotherm species to escape aging (e.g., Hoekstra et al., 2020 in reptiles). It has been notably highlighted that telomere dynamics can be particularly variable in these species (Hoekstra et al., 2020) with an absence of decline in telomere length with age (e.g., Ujvari and Madsen, 2009 in water python, Liasis fuscus). Whether this maintenance of telomere length with age is due to an increased expression of telomerase, which would in turn increased cancer risk is yet to be explored (Olsson et al., 2018) but would constitute a key support for a trade-off between the intensity of reproductive aging and the risk of cancer. Albeit limited, the bunch of empirical evidence compiled so far highlights that the risk of cancer is far from being negligible in ectothermic species such as reptiles (Madsen et al., 2017) which suggest that embracing this research path might be full of promises.
Transmissible Cancers and Cancers With an Infectious Causation
At least for dogs and Tasmanian devils, infection by transmissible malignant cells can be viewed as a cost of reproduction. In the vast majority of cases, cancer is not a contagious disease and malignant cells die with their host. However, in at least nine independent cases, cancer cells have evolved the capacity to be horizontally transmitted: one cancer in dogs, two in Tasmanian devils (Sarcophilus harrisii), and seven in bivalve species (McCallum et al., 2009; Yonemitsu et al., 2019; Dujon et al., 2020; Garcia-Souto et al., 2022). In dogs, canine transmissible venereal tumor (CTVT) is indeed caused by a sexually transmitted cancer cell line that affects dogs worldwide (Strakova and Murchison, 2014). The tumors are usually localized on the external genitalia (penis and foreskin in males and vulva in females), and it spreads between individuals through sexual intercourse and licking and/or biting the affected areas. These transmissible cell lines appeared between 8,000 and 11,000 years ago (Strakova and Murchison, 2015; Ostrander et al., 2016). Presumably as the result of this relatively long co-evolution with its hosts, CTVT rarely metastasizes and even displays a regressive stage (Das and Das, 2000; Martincorena et al., 2017). In Tasmanian devils, malignant cells are responsible for two cancers called devil facial tumor disease (DFTD), and devil facial tumor 2 (DFT2). It is not directly sexually transmitted, rather direct contact through biting is required, which frequently occurs during social interactions linked to reproduction. Infected individuals can still mate but they have reduced survival as a consequence of mating. DFTD was discovered in 1996 in northeastern Tasmania and has evolved into at least five clades (Hawkins et al., 2006; Kwon et al., 2020), whereas the second and independently emerged cancer, DFT2, was discovered in southeastern Tasmania at the d’Entrecasteaux Peninsula in 2014; (Pye et al., 2016; James et al., 2019; Figure 5). DFTD and DFT2 both induce large ulcerating tumors around the face and jaws, and DFT2 neoplasms are also frequently found in other parts of the body (James et al., 2019). DFTD, for which there is now a lot of information, is clearly fatal, with death usually occurring 9–12 months after the appearance of the first lesions with evidence of some individuals surviving up to 2 years (Wells et al., 2019). In only 25 years, the demographic cost of this transmissible cancer has been devastating, posing a serious conservation threat to the species, which is now classified as “Endangered” by the International Union for Conservation of Nature (Hawkins et al., 2008). While natural selection for less aggressive phenotypes could be expected in devils on the long term, it has not yet been observed, presumably because aggression is a trait associated with increased mating and breeding success in this species (Hamede et al., 2013). In evolutionary terms, it remains more beneficial to reproduce at a cost of developing and dying from DFTD than to remain healthy without reproducing. Interestingly, there is increasing evidence of tumor regressions in the wild, suggesting that devils are adapting to DFTD (Margres et al., 2018, 2020), and that a coexistence of devils and this transmissible cancer may be a long-term enzootic outcome (Wells et al., 2019; Hamede et al., 2020).
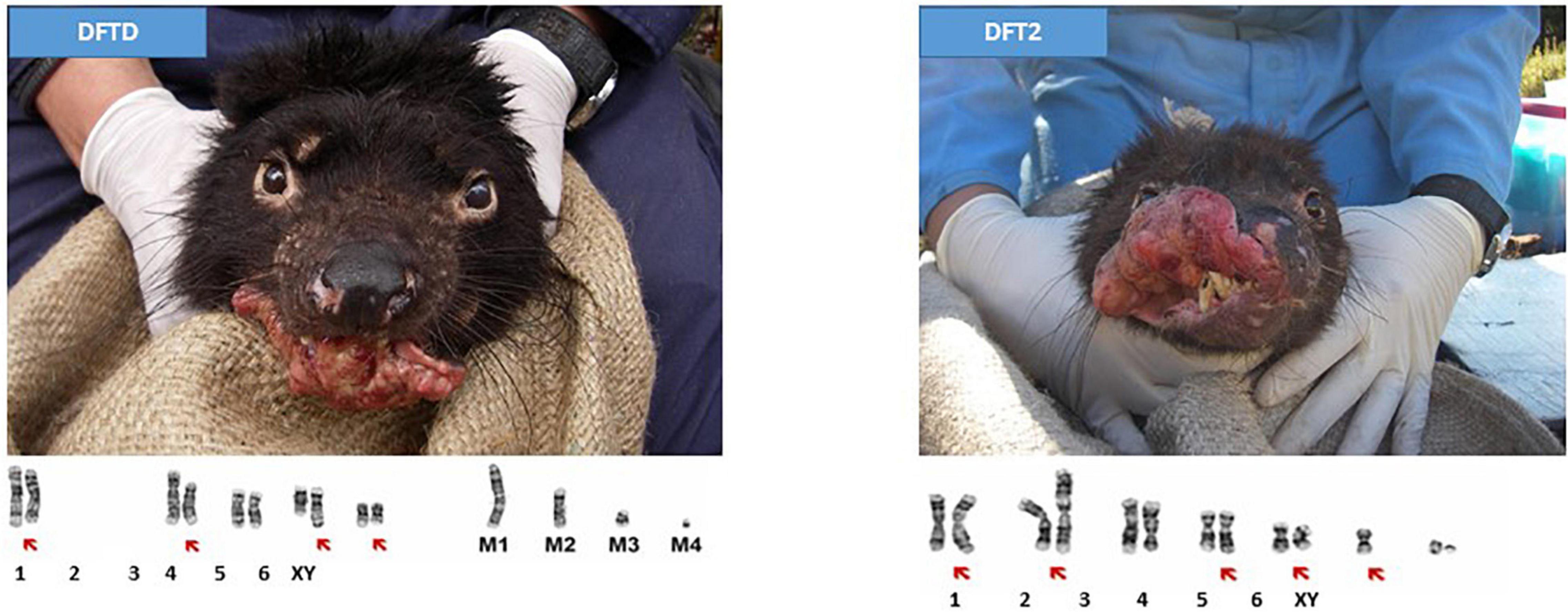
Figure 5. Transmissible cancers in Tasmanian devils. Tasmanian devils with DFTD and DFT2 and the respective tumor karyotypes. Red arrows indicate chromosomes carrying cytogenetic abnormalities. The four marker chromosomes found in DFTD are labeled M1 to M4. Karyotypes from Pye et al. (2016).
Reproduction can also be an opportunity for tumor cells to be vertically transmitted, from parent to offspring. Although rare, transmissions of cancer cells have been observed in humans from mother to fetus (Isoda et al., 2009; Greaves and Hughes, 2018). In addition, neoplastic leukemia cells arising in one monozygotic twin have been shown to transmit to the co-twin via intraplacental anastomoses (Clarkson and Boyse, 1971; Greaves et al., 2003). In the basal metazoan hydra (Hydra oligactis), tumor-bearing individuals (Figure 6) directly transmit tumoral cells to their offspring when reproducing asexually via budding (Domazet-Lošo et al., 2014). Given the omnipresence of oncogenic processes in multicellular organisms as well as the fact that transmissible cancer cells can have dramatic effects on their host’s fitness (e.g., Tasmanian devils), Thomas et al. (2019b) argued that sexual reproduction may have been favored by natural selection over evolutionary time as a radical way to prevent the vertical transmission of cancer cells, since it allows the offspring’s immune system to be more efficient at recognizing and eliminating these cells [but see also Aubier et al. (2020)].
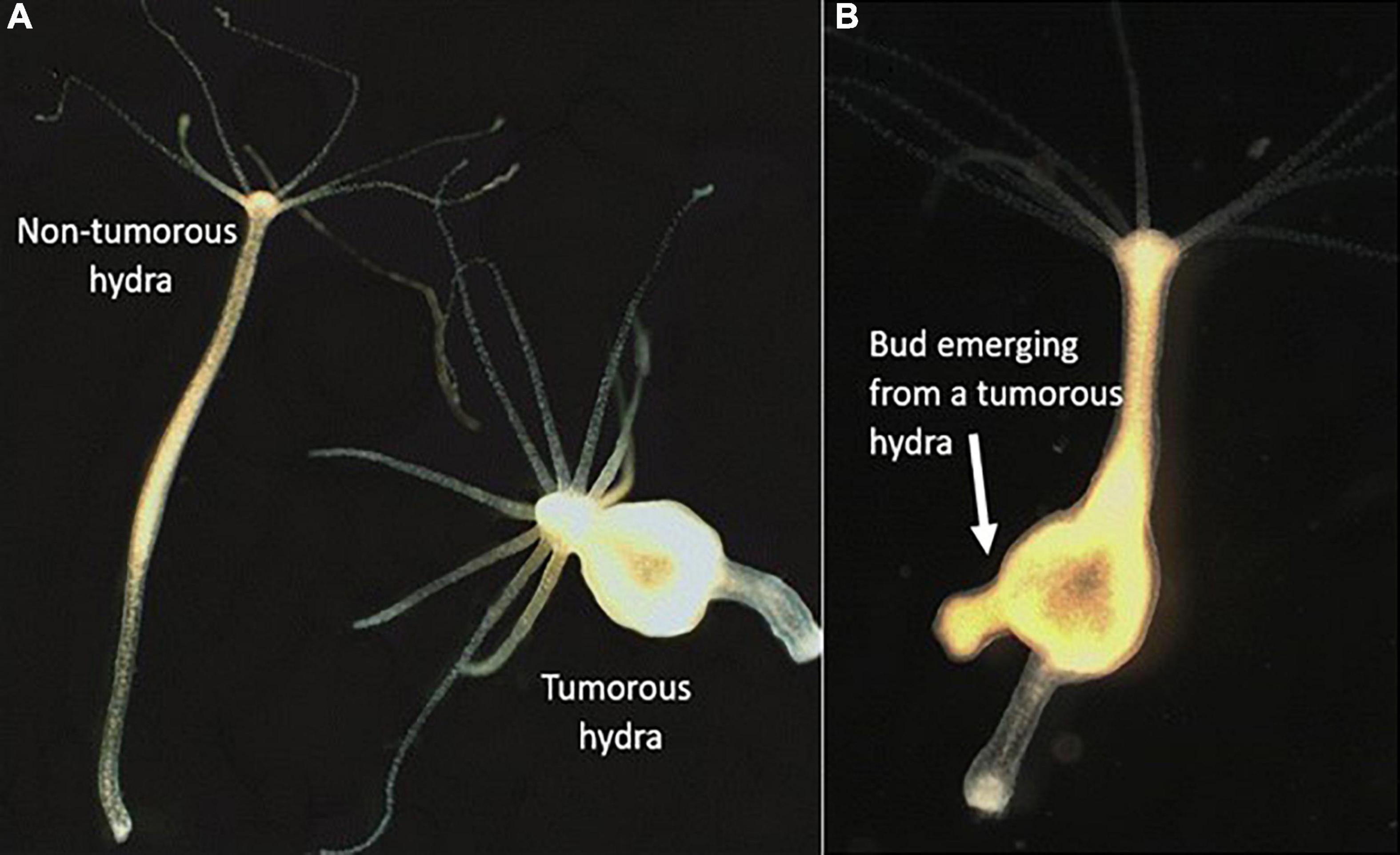
Figure 6. Tumors in Hydra oligactis. (A) Non-tumorous and tumorous hydra. Neoplasia not only severely alter the polyp’s body shape, but tumor-bearing individuals also show a shift in their microbiota and display a higher number of tentacles (Domazet-Lošo et al., 2014; Rathje et al., 2020). (B) Vertical transmission of tumoral cells in Hydra oligactis. After emergence, buds from tumorous hydra are morphologically similar to non-tumorous hydra, but after 4–6 weeks, they develop tumors as their parental polyp (Photo Justine Boutry).
In addition to transmissible malignant cells, several cancers have an infectious causation, and a considerable proportion are transmitted during some step related to reproductive activity. Indeed, pathogens that have been recognized as etiological agents of cancers (e.g., herpes simplex viruses, cytomegalovirus, hepatitis virus, papilloma viruses, human immunodeficiency virus, Eskinazi, 1987) are usually transmitted by genital contact or intimate kissing (Ewald, 2009). For this category of malignancies, cancer risk is not surprisingly associated with increased sexual activity (e.g., Bosch et al., 1996; Hayes et al., 2000; Cooper et al., 2007; Gómez and Santos, 2007).
Conclusion
(1) Reproduction is a central activity for all living organisms, and is also associated with a diversity of costs that need to be considered for a full understanding of the selective landscape in which organisms live and evolve. While decades of research have focused on the identification and implications of these costs, little attention has been devoted to exploring how the schedule of reproductive activities over the life course may also influence malignant cell dynamics and cancer defenses that in turn affect health and survival.
(2) Most ecologists currently consider that reproductive events have little or no impact on oncogenic processes, but this has yet to be properly studied. One must also consider separately the trade-offs that are optimal in a Darwinian logic maximizing reproductive success, from those that are relevant in a public health perspective, for which disease risks occurring after the reproductive period matter too. Further studies would need to explore not only whether there are connections between reproductive costs and the dynamics of malignant processes, but also determine for different species and organs the “shape” of the relationship through time (e.g., linear, exponential, steps with thresholds).
(3) In addition, we need to improve our understanding of the potential interactions between different active evolutionary processes. We can consider birth weight as an example for which it has been established that high values are associated with higher cancer rates later in life. In humans, at least in the past, higher birth weights and/or size have been associated with fitness benefits, especially survival early in life under a wide range of environmental conditions. Certain genes could therefore mediate antagonistic pleiotropy, which is associated with the selection for enhanced birth weight due to survival benefits early in life at the expense of the later increased risk of cancer. Because of the same fitness benefits, this phenomenon could also have a maternal origin, e.g., by modifications to the level of resources available to the fetus during pregnancy and/or by choosing taller men as sexual partners.
(4) Finally, we also need to understand over the long term how these interactions may trigger the evolution of defense mechanisms that prevent and/or alleviate the detrimental effects of malignant cells on the fitness of breeding animals. Whether the directionality of the relationship between reproduction and cancer varies among individuals, populations, or species is poorly understood, but should be explored given that cancer dynamics can sometimes influence reproductive decisions, as is the case in Drosophila (see above sections). Research programs addressing these questions are particularly timely since modifications of environmental conditions by human activities have been associated with both alterations in the reproduction biology and increased cancer rates in wild populations. A better understanding of the dynamic interplay between age-specific reproductive activity and the dynamics of oncogenic processes remains a major goal for diverse research areas such as population dynamics, conservation biology, epidemiology, and public health.
Data Availability Statement
The original contributions presented in the study are included in the article/supplementary material, further inquiries can be directed to the corresponding author.
Author Contributions
All authors listed have made a substantial, direct, and intellectual contribution to the work, and approved it for publication.
Funding
This work was supported by the MAVA Foundation, Rotary Club Les Sables d’Olonne, and by the following grants: ANR TRANSCAN (ANR-18-CE35-0009), ARC Linkage (LP170101105), Deakin SEBE_RGS_2019, and a CNRS International Associated Laboratory Grant. AB was supported by the National Cancer Institute of the National Institutes of Health under Award Number U54CA217376. OV was financed by the János Bolyai Research Scholarship of the Hungarian Academy of Sciences (HAS) and the New National Excellence Programme of the Hungarian Ministry of Innovation and Technology. The funders had no role in study design, data collection and analysis, decision to publish, or manuscript preparation.
Conflict of Interest
The authors declare that the research was conducted in the absence of any commercial or financial relationships that could be construed as a potential conflict of interest.
Publisher’s Note
All claims expressed in this article are solely those of the authors and do not necessarily represent those of their affiliated organizations, or those of the publisher, the editors and the reviewers. Any product that may be evaluated in this article, or claim that may be made by its manufacturer, is not guaranteed or endorsed by the publisher.
Acknowledgments
We would like to thank two referees for very constructive comments on an earlier version of the manuscript.
References
Abegglen, L. M., Caulin, A. F., Chan, A., Lee, K., Robinson, R., Campbell, M. S., et al. (2015). Potential mechanisms for cancer resistance in elephants and comparative cellular response to DNA damage in humans. J. Am. Med. Assoc. 314, 1850–1860. doi: 10.1001/jama.2015.13134
Abrams, E. T., and Miller, E. M. (2011). The roles of the immune system in Women’s reproduction: evolutionary constraints and life history trade-offs. Am. J. Phys. Anthropol. 146(Suppl. 53), 134–154. doi: 10.1002/ajpa.21621
Aktipis, A. (2016). Life history theory and breast cancer risk: methodological and theoretical challenges: response to “Is estrogen receptor negative breast cancer risk associated with a fast life history strategy”? Evol. Med. Public Health 2016, 177–179. doi: 10.1093/EMPH/EOV035
Aktipis, C. A. (2020). The Cheating Cell: How Evolution Helps Us Understand and Treat Cancer. Princeton, NJ: Princeton University Press.
Aktipis, C. A., Boddy, A. M., Gatenby, R. A., Brown, J. S., and Maley, C. C. (2013). Life history trade-offs in cancer evolution. Nat. Rev. Cancer 13, 883–892. doi: 10.1038/nrc3606
Aktipis, C. A., Ellis, B. J., Nishimura, K. K., and Hiatt, R. A. (2015). Modern reproductive patterns associated with estrogen receptor positive but not negative breast cancer susceptibility. Evol. Med. Public Health 2015, 52–74. doi: 10.1093/emph/eou028
Aktipis, C. A., and Nesse, R. M. (2013). Evolutionary foundations for cancer biology. Evol. Appl. 6, 144–159. doi: 10.1111/eva.12034
Albrektsen, G., Heuch, I., Hansen, S., and Kvåle, G. (2005). Breast cancer risk by age at birth, time since birth and time intervals between births: exploring interaction effects. Br. J. Cancer 92, 167–175. doi: 10.1038/sj.bjc.6602302
Alvarado, L. C. (2013). Do evolutionary life-history trade-offs influence prostate cancer risk? A review of population variation in testosterone levels and prostate cancer disparities. Evol. Appl. 6, 117–133. doi: 10.1111/eva.12036
Andersson, M., and Simmons, L. W. (2006). Sexual selection and mate choice. Trends Ecol. Evol. 21, 296–302. doi: 10.1016/j.tree.2006.03.015
Atashgaran, V., Wrin, J., Barry, S. C., Dasari, P., and Ingman, W. V. (2016). Dissecting the biology of menstrual cycle-associated breast cancer risk. Front. Oncol. 6:267. doi: 10.3389/fonc.2016.00267
Aubier, T. G., Galipaud, M., Yagmur, E., and Kokko, H. (2020). Transmissible cancers and the evolution of sex under the Red Queen hypothesis. PLoS Biol. 18:e3000916. doi: 10.1371/journal.pbio.3000916
Austad, S. N., and Hoffman, J. M. (2018). Is antagonistic pleiotropy ubiquitous in aging biology? Evol. Med. Public Health 2018, 287–294. doi: 10.1093/emph/eoy033
Aviv, A., Anderson, J. J., and Shay, J. W. (2017). Mutations, cancer and the telomere length paradox. Trends Cancer 3, 253–258. doi: 10.1016/j.trecan.2017.02.005
Azpurua, J., and Seluanov, A. (2013). Long-lived cancer-resistant rodents as new model species for cancer research. Front. Genet. 3:319. doi: 10.3389/fgene.2012.00319
Bauch, C., Riechert, J., Verhulst, S., and Becker, P. H. (2016). Telomere length reflects reproductive effort indicated by corticosterone levels in a long-lived seabird. Mol. Ecol. 25, 5785–5794. doi: 10.1111/mec.13874
Beck, C. W., and Promislow, D. E. L. (2007). Evolution of female preference for younger males. PLoS One 2:e939. doi: 10.1371/journal.pone.0000939
Bell, G. (1980). The costs of reproduction and their consequences. Am. Nat. 116, 45–76. doi: 10.1086/283611
Berger, V., Lemaître, J.-F., Gaillard, J.-M., and Cohas, A. (2015). How do animals optimize the size–number trade-off when aging? Insights from reproductive senescence patterns in marmots. Ecology 96, 46–53. doi: 10.1890/14-0774.1
Bérubé, C. H., Festa-Bianchet, M., and Jorgenson, J. T. (1996). Reproductive costs of sons and daughters in Rocky Mountain bighorn sheep. Behav. Ecol. 7, 60–68. doi: 10.1093/beheco/7.1.60
Biro, P. A., and Stamps, J. A. (2008). Are animal personality traits linked to life-history productivity? Trends Ecol. Evol. 23, 361–368. doi: 10.1016/j.tree.2008.04.003
Biro, P. A., and Stamps, J. A. (2010). Do consistent individual differences in metabolic rate promote consistent individual differences in behavior? Trends Ecol. Evol. 25, 653–659. doi: 10.1016/j.tree.2010.08.003
Biro, P. A., Thomas, F., Ujvari, B., and Beckmann, C. (2020). Can energetic capacity help explain why physical activity reduces cancer risk? Trends Cancer 6, 829–837. doi: 10.1016/j.trecan.2020.06.001
Bissell, M. J., and Hines, W. C. (2011). Why don’t we get more cancer? A proposed role of the microenvironment in restraining cancer progression. Nat. Med. 17, 320–329. doi: 10.1038/nm.2328
Blagosklonny, M. V. (2010). Revisiting the antagonistic pleiotropy theory of aging: TOR-driven program and quasi-program. Cell Cycle 9, 3151–3156. doi: 10.4161/cc.9.16.13120
Blount, J. D., Vitikainen, E. I. K., Stott, I., and Cant, M. A. (2016). Oxidative shielding and the cost of reproduction. Biol. Rev. 91, 483–497. doi: 10.1111/brv.12179
Boddy, A. M., Abegglen, L. M., Pessier, A. P., Aktipis, A., Schiffman, J. D., Maley, C. C., et al. (2020). Lifetime cancer prevalence and life history traits in mammals. Evol. Med. Public Health 2020, 187–195. doi: 10.1093/EMPH/EOAA015
Boddy, A. M., Kokko, H., Breden, F., Wilkinson, G. S., and Athena Aktipis, C. (2015). Cancer susceptibility and reproductive trade-offs: a model of the evolution of cancer defences. Philos. Trans. R. Soc. B Biol. Sci. 370:20140220. doi: 10.1098/rstb.2014.0220
Bosch, F. X., Castellsagué, X., Muñoz, N., De Sanjosé, S., Ghaffari, A. M., González, L. C., et al. (1996). Male sexual behavior and human papillomavirus DNA: key risk factors for cervical cancer in Spain. J. Natl. Cancer Inst. 88, 1060–1067. doi: 10.1093/jnci/88.15.1060
Boutry, J., Dujon, A. M., Gerard, A.-L., Tissot, S., Macdonald, N., Schultz, A., et al. (2020). Ecological and evolutionary consequences of anticancer adaptations. iScience 23:101716. doi: 10.1016/j.isci.2020.101716
Boutry, J., Tissot, S., Ujvari, B., Capp, J. P., Giraudeau, M., Nedelcu, A. M., et al. (2022b). The evolution and ecology of benign tumors. Biochim. Biophys. Acta Rev. Cancer 1877:188643. doi: 10.1016/j.bbcan.2021.188643
Boutry, J., Mistral, J., Berlioz, L., Klimovich, A., Tökölyi, J., Fontenille, L., et al. (2022a). Tumors (re)shape biotic interactions within ecosystems: experimental evidence from the freshwater cnidarian Hydra. Sci. Total Environ. 803:149923. doi: 10.1016/j.scitotenv.2021.149923
Brinton, L. A., Lamb, E. J., Moghissi, K. S., Scoccia, B., Althuis, M. D., Mabie, J. E., et al. (2004). Ovarian cancer risk associated with varying causes of infertility. Fertil. Steril. 82, 405–414. doi: 10.1016/j.fertnstert.2004.02.109
Brinton, L. A., Westhoff, C. L., Scoccia, B., Lamb, E. J., Althuis, M. D., Mabie, J. E., et al. (2005). Causes of infertility as predictors of subsequent cancer risk. Epidemiology 16, 500–507. doi: 10.1097/01.ede.0000164812.02181.d5
Brooks, R., and Kemp, D. J. (2001). Can older males deliver the good genes? Trends Ecol. Evol. 16, 308–313. doi: 10.1016/S0169-5347(01)02147-4
Brown, J. S., and Aktipis, C. A. (2015). Inclusive fitness effects can select for cancer suppression into old age. Philos. Trans. R. Soc. Lond. B Biol. Sci. 370:20150160. doi: 10.1098/rstb.2015.0160
Cameron-MacMillan, M. L., Walsh, C. J., Wilhelm, S. I., and Storey, A. E. (2007). Male chicks are more costly to rear than females in a monogamous seabird, the common murre. Behav. Ecol. 18, 81–85. doi: 10.1093/beheco/arl048
Carter, A. J. R., and Nguyen, A. Q. (2011). Antagonistic pleiotropy as a widespread mechanism for the maintenance of polymorphic disease alleles. BMC Med. Genet. 12:160. doi: 10.1186/1471-2350-12-160
Cayuela, H., Lemaître, J. F., Rugiero, L., Capula, M., and Luiselli, L. (2020). Asynchrony of actuarial and reproductive senescence: a lesson from an indeterminate grower. Biol. J. Linn. Soc. 131, 667–672. doi: 10.1093/BIOLINNEAN/BLAA127
Choi, J. Y., Lee, K. M., Park, S. K., Noh, D. Y., Ahn, S. H., Yoo, K. Y., et al. (2005). Association of paternal age at birth and the risk of breast cancer in offspring: a case control study. BMC Cancer 5:143. doi: 10.1186/1471-2407-5-143
Clarkson, B., and Boyse, E. (1971). Possible explanation of the high concordance for acute leukæmia in monozygotic twins. Lancet 297, 699–701. doi: 10.1016/S0140-6736(71)92705-X
Clocchiatti, A., Cora, E., Zhang, Y., and Dotto, G. P. (2016). Sexual dimorphism in cancer. Nat. Rev. Cancer 16, 330–339. doi: 10.1038/nrc.2016.30
Clutton-Brock, T. H., and Vincent, A. C. J. (1991). Sexual selection and the potential reproductive rates of males and females. Nature 351, 58–60. doi: 10.1038/351058a0
Cooper, D., Hoffman, M., Carrara, H., Rosenberg, L., Kelly, J., Stander, I., et al. (2007). Determinants of sexual activity and its relation to cervical cancer risk among South African Women. BMC Public Health 7:341. doi: 10.1186/1471-2458-7-341
Crespi, B. J., and Summers, K. (2006). Positive selection in the evolution of cancer. Biol. Rev. Camb. Philos. Soc. 81, 407–424. doi: 10.1017/S1464793106007056
Das, U., and Das, A. K. (2000). Review of canine transmissible venereal sarcoma. Vet. Res. Commun. 24, 545–556. doi: 10.1023/A:1006491918910
Daum, H., Peretz, T., and Laufer, N. (2018). BRCA mutations and reproduction. Fertil. Steril. 109, 33–38. doi: 10.1016/j.fertnstert.2017.12.004
DeGregori, J., Weinberg, R. A., and DeGregori, M. (2018). Adaptive Oncogenesis, A New Understanding of How Cancer Evolve Inside Us. Cambridge, MA: Harvard University Press.
de Maghalaes, J. P. (2013). How ageing processes influence cancer. Nat. Rev. Cancer 13, 357–365. doi: 10.1038/nrc3497
DelGiudice, G. D., Lenarz, M. S., and Powell, M. C. (2007). Age-specific fertility and fecundity in northern free-ranging white-tailed deer: evidence for reproductive senescence? J. Mammal. 88, 427–435. doi: 10.1644/06-MAMM-A-164R.1
Dixson, A. (2015). “Primate sexuality,” in The International Encyclopedia of Human Sexuality, eds P. Whelehan and A. Bolin (Hoboken, NJ: Wiley).
Domazet-Lošo, T., Klimovich, A., Anokhin, B., Anton-Erxleben, F., Hamm, M. J., Lange, C., et al. (2014). Naturally occurring tumours in the basal metazoan Hydra. Nat. Commun. 5:4222. doi: 10.1038/ncomms5222
Domazet-Lošo, T., and Tautz, D. (2010). Phylostratigraphic tracking of cancer genes suggests a link to the emergence of multicellularity in metazoa. BMC Biol. 8:66. doi: 10.1186/1741-7007-8-66
D’Souza, A. W., and Wagner, G. P. (2014). Malignant cancer and invasive placentation: a case for positive pleiotropy between endometrial and malignancy phenotypes. Evol. Med. Public Health 2014, 136–145. doi: 10.1093/emph/eou022
Dujon, A. M., Gatenby, R. A., Bramwell, G., MacDonald, N., Dorhmann, E., Raven, N., et al. (2020). Transmissible cancers in an evolutionary perspective. IScience 23:101269. doi: 10.1016/j.isci.2020.101269
Einstein, M. H., Levine, N. F., and Nevadunsky, N. S. (2015). Menopause and cancers. Endocrinol. Metab. Clin. North Am. 44, 603–617. doi: 10.1016/j.ecl.2015.05.012
Eisenberg, D. T. A. (2019). Paternal age at conception effects on offspring telomere length across species—What explains the variability? PLoS Genet. 15:e1007946. doi: 10.1371/journal.pgen.1007946
Eisenberg, D. T. A., and Kuzawa, C. W. (2018). The paternal age at conception effect on offspring telomere length: mechanistic, comparative and adaptive perspectives. Philos. Trans. R. Soc. B Biol. Sci. 373:20160442. doi: 10.1098/rstb.2016.0442
Eisenberg, D. T. A., Lee, N. R., Rej, P. H., Hayes, M. G., and Kuzawa, C. W. (2019). Older paternal ages and grandpaternal ages at conception predict longer telomeres in human descendants. Proc. R. Soc. B Biol. Sci. 286:20190800. doi: 10.1098/rspb.2019.0800
Eskinazi, D. P. (1987). Oncogenic potential of sexually transmitted viruses with special reference to oral cancer. Oral Surg. Oral Med. Oral Pathol. 64, 35–40. doi: 10.1016/0030-4220(87)90113-7
Ewald, P. W. (2009). Chapter 2 An evolutionary perspective on parasitism as a cause of cancer. Adv. Parasitol. 68, 21–43. doi: 10.1016/S0065-308X(08)00602-7
Fedorka, K. M. (2014). “Reproductive and immune system interactions in the context of life history and sexual selection theory,” in Eco-immunology: Evolutive Aspects and Future Perspectives, eds D. Malagoli and E. Ottaviani (Berlin: Springer Science & Business Media).
Fernandez, A. A. (2010). A cancer-causing gene is positively correlated with male aggression in Xiphophorus cortezi. J. Evol. Biol. 23, 386–396. doi: 10.1111/j.1420-9101.2009.01914.x
Fernandez, A. A., and Morris, M. R. (2008). Mate choice for more melanin as a mechanism to maintain a functional oncogene. Proc. Natl. Acad. Sci. U.S.A. 105, 13503–13507. doi: 10.1073/pnas.0803851105
Fessler, D. M. (2002). Reproductive immunosupression and diet. An evolutionary perspective on pregnancy sickness and meat consumption. Curr. Anthropol. 43, 19–61. doi: 10.1086/324128
Floyd, S., Favre, C., Lasorsa, F. M., Leahy, M., Trigiante, G., Stroebel, P., et al. (2007). The insulin-like growth factor-I-mTOR signaling pathway induces the mitochondrial pyrimidine nucleotide carrier to promote cell growth. Mol. Biol. Cell 18, 3545–3555. doi: 10.1091/mbc.E06-12-1109
Fortunato, A., Fleming, A., Aktipis, A., and Maley, C. C. (2021). Upregulation of DNA repair genes and cell extrusion underpin the remarkable radiation resistance of Trichoplax adhaerens. PLoS Biol. 19:e3001471. doi: 10.1371/journal.pbio.3001471
Frank, S. A., and Crespi, B. J. (2011). Pathology from evolutionary conflict, with a theory of X chromosome versus autosome conflict over sexually antagonistic traits. Proc. Natl. Acad. Sci. U.S.A. 108(Suppl. 2), 10886–10893. doi: 10.1073/pnas.1100921108
French, S. S., DeNardo, D. F., and Moore, M. C. (2007). Trade-offs between the reproductive and immune systems: facultative responses to resources or obligate responses to reproduction? Am. Nat. 170, 79–89. doi: 10.1086/518569
Garcia-Souto, D., Bruzos, A. L., Diaz, S., Rocha, S., Pequeño-Valtierra, A., Roman-Lewis, C. F., et al. (2022). Mitochondrial genome sequencing of marine leukaemias reveals cancer contagion between clam species in the Seas of Southern Europe. Elife 11:e66946. doi: 10.7554/eLife.66946
Gibson, M. A., and Mace, R. (2003). Strong mothers bear more sons in rural ethiopia. Proc. R. Soc. B Biol. Sci. 270(Suppl. 1), S108–S109. doi: 10.1098/rsbl.2003.0031
Giraudeau, M., Sepp, T., Ujvari, B., Ewald, P. W., and Thomas, F. (2018). Human activities might influence oncogenic processes in wild animal populations. Nat. Ecol. Evol. 2, 1065–1070. doi: 10.1038/s41559-018-0558-7
Gleicher, N. (2013). Why are reproductive cancers more common in nulliparous women? Reprod. Biomed. 26, 416–419. doi: 10.1016/j.rbmo.2013.01.007
Goldszmid, R. S., Dzutsev, A., and Trinchieri, G. (2014). Host immune response to infection and cancer: unexpected commonalities. Cell Host Microbe 15, 295–305. doi: 10.1016/j.chom.2014.02.003
Gómez, D. T., and Santos, J. L. (2007). “Human papillomavirus infection and cervical cancer: pathogenesis and epidemiology,” in Communicating Current Research and Educational Topics and Trends in Applied Microbiology, ed. A. M. éndez-Vilas (Badajoz: FORMATEX).
Grabovac, I., Smith, L., Yang, L., Soysal, P., Veronese, N., Turan Isik, A., et al. (2020). The relationship between chronic diseases and number of sexual partners: an exploratory analysis. BMJ Sex. Reprod. Health 46, 100–107. doi: 10.1136/bmjsrh-2019-200352
Grasgruber, P., Cacek, J., Kalina, T., and Sebera, M. (2014). The role of nutrition and genetics as key determinants of the positive height trend. Econ. Hum. Biol. 15, 81–100. doi: 10.1016/j.ehb.2014.07.002
Greaves, M., and Hughes, W. (2018). Cancer cell transmission via the placenta. Evol. Med. Public Health 2018, 106–115. doi: 10.1093/emph/eoy011
Greaves, M. F., Maia, A. T., Wiemels, J. L., and Ford, A. M. (2003). Leukemia in twins: lessons in natural history. Blood 102, 2321–2333. doi: 10.1182/blood-2002-12-3817
Guégan, J. F., Teriokhin, A. T., and Thomas, F. (2000). Human fertility variation, size-related obstetrical performance and the evolution of sexual stature dimorphism. Proc. Biol. Sci. 267, 2529–2535. doi: 10.1098/rspb.2000.1316
Gunten, A. V., Clerc, M. T., Tomar, R., and John-Smith, P. S. (2018). Evolutionary considerations on aging and Alzheimer’s disease. J. Alzheimers Dis. Park. 8:423. doi: 10.4172/2161-0460.1000423
Gustafsson, L., Qvarnström, A., and Sheldon, B. C. (1995). Trade-offs between life-history traits and a secondary sexual character in male collared flycatchers. Nature 375, 311–313. doi: 10.1038/375311a0
Haig, D. (2015). Maternal–fetal conflict, genomic imprinting and mammalian vulnerabilities to cancer. Philos. Trans. R. Soc. B Biol. Sci. 370:20140178. doi: 10.1098/rstb.2014.0178
Hamede, R., Madsen, T., McCallum, H., Storfer, A., Hohenlohe, P. A., Siddle, H., et al. (2020). Darwin, the devil, and the management of transmissible cancers. Conserv. Biol. 35, 748–751. doi: 10.1111/cobi.13644
Hamede, R. K., Mccallum, H., and Jones, M. (2013). Biting injuries and transmission of Tasmanian devil facial tumour disease. J. Anim. Ecol. 82, 182–190. doi: 10.1111/j.1365-2656.2012.02025.x
Hamel, S., Gaillard, J. M., Yoccoz, N. G., Loison, A., Bonenfant, C., and Descamps, S. (2010). Fitness costs of reproduction depend on life speed: empirical evidence from mammalian populations. Ecol. Lett. 13, 915–935. doi: 10.1111/j.1461-0248.2010.01478.x
Hanahan, D., and Weinberg, R. A. (2011). Review hallmarks of cancer: the next generation. Cell 144, 646–674. doi: 10.1016/j.cell.2011.02.013
Hanson, B., Johnstone, E., Dorais, J., Silver, B., Peterson, C. M., and Hotaling, J. (2017). Female infertility, infertility-associated diagnoses, and comorbidities: a review. J. Assist. Reprod. Genet. 34, 167–177. doi: 10.1007/s10815-016-0836-8
Harshman, L. G., and Zera, A. J. (2007). The cost of reproduction: the devil in the details. Trends Ecol. Evol. 22, 80–86. doi: 10.1016/j.tree.2006.10.008
Hawkins, C. E., Baars, C., Hesterman, H., Hocking, G. J., Jones, M. E., Lazenby, B., et al. (2006). Emerging disease and population decline of an island endemic, the Tasmanian devil Sarcophilus harrisii. Biol. Conserv. 131, 307–324. doi: 10.1016/j.biocon.2006.04.010
Hawkins, C. E., McCallum, H., Mooney, N., and Jones, M. (2008). Sarcophilus harrisii. IUCN Red List of Threatened Species. Version 2011.2. Available online at: https://www.environment.gov.au/resource/tasmanian-devil-sarcophilus-harrisii (accessed January 12, 2012).
Hayes, R. B., Pottern, L. M., Strickler, H., Rabkin, C., Pope, V., Swanson, G. M., et al. (2000). Sexual behaviour, STDs and risks for prostate cancer. Br. J. Cancer 82, 718–725. doi: 10.1054/bjoc.1999.0986
Henry, C. J., Casás-Selves, M., Kim, J., Zaberezhnyy, V., Aghili, L., Daniel, A. E., et al. (2015). Aging-associated inflammation promotes selection for adaptive oncogenic events in B cell progenitors. J. Clin. Invest. 125, 4666–4680. doi: 10.1172/JCI83024
Hepner, A., Negrini, D., Hase, E. A., Exman, P., Testa, L., Trinconi, A. F., et al. (2019). Cancer during pregnancy: the oncologist overview. World J. Oncol. 10, 28–34. doi: 10.14740/wjon1177
Hill, G. E. (2014). Cellular respiration: the nexus of stress, condition, and ornamentation. Integr. Compar. Biol. 54, 645–657. doi: 10.1093/icb/icu029
Hochberg, M. E., and Noble, R. J. (2017). A framework for how environment contributes to cancer risk. Ecol. Lett. 20, 117–134. doi: 10.1111/ele.12726
Hoekstra, L. A., Schwartz, T. S., Sparkman, A. M., Miller, D. A. W., and Bronikowski, A. M. (2020). The untapped potential of reptile biodiversity for understanding how and why animals age. Funct. Ecol. 34, 38–54. doi: 10.1111/1365-2435.13450
Holmes, D. J., Thomson, S. L., Wu, J., and Ottinger, M. A. (2003). Reproductive aging in female birds. Exp. Gerontol. 38, 751–756. doi: 10.1016/S0531-5565(03)00103-7
Hové, C., Trumble, B. C., Anderson, A. S., Stieglitz, J., Kaplan, H., Gurven, M. D., et al. (2021). Immune function during pregnancy varies between ecologically distinct populations. Evol. Med. Public Health 2020, 114–128. doi: 10.1093/EMPH/EOAA022
Hsieh, C., Pavia, M., Lambe, M., Lan, S. J., Colditz, G. A., Ekbom, A., et al. (1994). Dual effect of parity on breast cancer risk. Eur. J. Cancer 95, 478–483. doi: 10.1016/0959-8049(94)90125-2
Hsieh, C. C., Wuu, J., Trichopoulos, D., Adami, H. O., and Ekbom, A. (1999). Gender of offspring and maternal breast cancer risk. Int. J. Cancer 81, 335–338. doi: 10.1002/(sici)1097-0215(19990505)81:3<335::aid-ijc4>3.0.co;2-l
Hurt, L. S., Ronsmans, C., and Thomas, S. L. (2006). The effect of number of births on women’s mortality: systematic review of the evidence for women who have completed their childbearing. Popul. Stud. 60, 55–71. doi: 10.1080/00324720500436011
Ibrahim-Hashim, A., Luddy, K., Abrahams, D., Enriquez-Navas, P., Damgaci, S., Yao, J., et al. (2020). Artificial selection for host resistance to tumour growth and subsequent cancer cell adaptations: an evolutionary arms race. Br. J. Cancer 124, 455–465. doi: 10.1038/s41416-020-01110-1
Isoda, T., Ford, A. M., Tomizawa, D., Van Delft, F. W., De Castro, D. G., Mitsuiki, N., et al. (2009). Immunologically silent cancer clone transmission from mother to offspring. Proc. Natl. Acad. Sci. U.S.A. 106, 17882–17885. doi: 10.1073/pnas.0904658106
Jacqueline, C., Biro, P. A., Beckmann, C., Moller, A. P., Renaud, F., Sorci, G., et al. (2017a). Cancer: a disease at the crossroads of trade-offs. Evol. Appl. 10, 215–225. doi: 10.1111/eva.12444
Jacqueline, C., Tasiemski, A., Sorci, G., Ujvari, B., Maachi, F., Missé, D., et al. (2017b). Infections and cancer: the “fifty shades of immunity” hypothesis. BMC Cancer 17:257. doi: 10.1186/s12885-017-3234-4
Jacqueline, C., Parvy, J. P., Rollin, M. L., Faugère, D., Renaud, F., Missé, D., et al. (2020). The role of innate immunity in the protection conferred by a bacterial infection against cancer: study of an invertebrate model. Sci. Rep. 10:10106. doi: 10.1038/s41598-020-66813-0
James, S., Jennings, G., Kwon, Y. M., Stammnitz, M., Fraik, A., Storfer, A., et al. (2019). Tracing the rise of malignant cell lines: distribution, epidemiology and evolutionary interactions of two transmissible cancers in Tasmanian devils. Evol. Appl. 12, 1772–1780. doi: 10.1111/eva.12831
Jasienska, G. (2020). Costs of reproduction and ageing in the human female: reproduction and ageing in women. Philos. Trans. R. Soc. B Biol. Sci. 375:20190615. doi: 10.1098/rstb.2019.0615rstb20190615
Johnson, P. A., and Giles, J. R. (2006). Use of genetic strains of chickens in studies of ovarian cancer. Poult. Sci. 85, 246–250. doi: 10.1093/ps/85.2.246
Johnson, S. L., Dunleavy, J., Gemmell, N. J., and Nakagawa, S. (2015). Consistent age-dependent declines in human semen quality: a systematic review and meta-analysis. Ageing Res. Rev. 19, 22–33. doi: 10.1016/j.arr.2014.10.007
Kalmbach, K. H., Antunes, D. M. F., Kohlrausch, F., and Keefe, D. L. (2015). Telomeres and female reproductive aging. Semin. Reprod. Med. 33, 389–395. doi: 10.1055/s-0035-1567823
Kang, L., and Michalak, P. (2015). The evolution of cancer-related genes in hominoids. J. Mol. Evol. 80, 37–41. doi: 10.1007/s00239-014-9649-5
Kleene, K. C. (2005). Sexual selection, genetic conflict, selfish genes, and the atypical patterns of gene expression in spermatogenic cells. Dev. Biol. 277, 16–26. doi: 10.1016/j.ydbio.2004.09.031
Knowles, S. C. L., Nakagawa, S., and Sheldon, B. C. (2009). Elevated reproductive effort increases blood parasitaemia and decreases immune function in birds: a meta-regression approach. Funct. Ecol. 23, 405–415. doi: 10.1111/j.1365-2435.2008.01507.x
Kreuz, S., and Fischle, W. (2016). Oxidative stress signaling to chromatin in health and disease. Epigenomics 8, 843–862. doi: 10.2217/epi-2016-0002
Kshitiz, Afzal, J., Maziarz, J. D., Hamidzadeh, A., Liang, C., Erkenbrack, E. M., et al. (2019). Evolution of placental invasion and cancer metastasis are causally linked. Nat. Ecol. Evol. 3, 1743–1753. doi: 10.1038/s41559-019-1046-4
Kwon, Y. M., Gori, K., Park, N., Potts, N., Swift, K., Wang, J., et al. (2020). Evolution and lineage dynamics of a transmissible cancer in Tasmanian devils. PLoS Biol. 18:e3000926. doi: 10.1371/journal.pbio.3000926
Lambe, M., Hsieh, C., Trichopoulos, D., Ekbom, A., Pavia, M., and Adami, H.-O. (2002). Transient increase in the risk of breast cancer after giving birth. N. Engl. J. Med. 331, 5–9. doi: 10.1056/nejm199407073310102
Lemaître, J. F., Berger, V., Bonenfant, C., Douhard, M., Gamelon, M., Plard, F., et al. (2015). Early-late life trade-offs and the evolution of ageing in the wild. Proc. R. Soc. B Biol. Sci. 282:20150209. doi: 10.1098/rspb.2015.0209
Lemaître, J. F., and Gaillard, J. M. (2017). Reproductive senescence: new perspectives in the wild. Biol. Rev. 92, 2182–2199. doi: 10.1111/brv.12328
Lemaître, J. F., Gaillard, J. M., Pemberton, J. M., Clutton-Brock, T. H., and Nussey, D. H. (2014). Early life expenditure in sexual competition is associated with increased reproductive senescence in male red deer. Proc. R. Soc. B Biol. Sci. 281:20140792. doi: 10.1098/rspb.2014.0792
Lemaître, J. F., Pavard, S., Giraudeau, M., Vincze, O., Jennings, G., Hamede, R., et al. (2020a). Eco-evolutionary perspectives of the dynamic relationships linking senescence and cancer. Funct. Ecol. 34, 141–152. doi: 10.1111/1365-2435.13394
Lemaître, J. F., Ronget, V., and Gaillard, J. M. (2020b). Female reproductive senescence across mammals: a high diversity of patterns modulated by life history and mating traits. Mech. Ageing Dev. 192:111377. doi: 10.1016/j.mad.2020.111377
Leroi, A. M., Bartke, A., de Benedictis, G., Franceschi, C., Gartner, A., Gonos, E., et al. (2005). What evidence is there for the existence of individual genes with antagonistic pleiotropic effects? Mech. Ageing Dev. 126, 421–429. doi: 10.1016/j.mad.2004.07.012
Leroi, A. M., Koufopanou, V., and Burt, A. (2003). Opinion: cancer selection. Nat. Rev. Cancer 3, 226–231.
LeRoith, D., and Roberts, C. T. (2003). The insulin-like growth factor system and cancer. Cancer Lett. 195, 127–137. doi: 10.1016/S0304-3835(03)00159-9
Lyons, T. R., O’Brien, J., Borges, V. F., Conklin, M. W., Keely, P. J., Eliceiri, K. W., et al. (2011). Postpartum mammary gland involution drives progression of ductal carcinoma in situ through collagen and COX-2. Nat. Med. 17, 1109–1115. doi: 10.1038/nm.2416
Madimenos, F. C. (2015). An evolutionary and life-history perspective on osteoporosis. Annu. Rev. Anthropol. 44, 189–206. doi: 10.1146/annurev-anthro-102214-013954
Madsen, T., Arnal, A., Vittecoq, M., Bernex, F., Abadie, J., Labrut, S., et al. (2017). “Cancer in the animal kingdom,” in Ecology and Evolution of Cancer, eds B. Ujvari, B. Roche, and F. Thomas (Cambridge, MA: Academic Press), 11–46.
Maklakov, A. A., and Immler, S. (2016). The expensive germline and the evolution of ageing. Curr. Biol. 26, R577–R586. doi: 10.1016/j.cub.2016.04.012
Maley, C. C., Aktipis, A., Graham, T. A., Sottoriva, A., Boddy, A. M., Janiszewska, M., et al. (2017). Classifying the evolutionary and ecological features of neoplasms. Nat. Rev. Cancer 17, 605–619. doi: 10.1038/nrc.2017.69
Margres, M. J., Ruiz-Aravena, M., Hamede, R., Chawla, K., Patton, A. H., Lawrance, M. F., et al. (2020). Spontaneous tumor regression in Tasmanian devils associated with RASL11A activation. Genetics 215, 1143–1152. doi: 10.1534/genetics.120.303428
Margres, M. J., Ruiz-Aravena, M., Hamede, R., Jones, M. E., Lawrance, M. F., Hendricks, S. A., et al. (2018). The genomic basis of tumor regression in Tasmanian devils (Sarcophilus harrisii). Genome Biol. Evol. 10, 3012–3025. doi: 10.1093/gbe/evy229
Martincorena, I., Raine, K. M., Gerstung, M., Dawson, K. J., Haase, K., Van Loo, P., et al. (2017). Universal patterns of selection in cancer and somatic tissues. Cell 171, 1029.e21–1041.e21. doi: 10.1016/j.cell.2017.09.042
McCallum, H., Jones, M., Hawkins, C., Hamede, R., Lachish, S., Sinn, D. L., et al. (2009). Transmission dynamics of Tasmanian devil facial tumor disease may lead to disease-induced extinction. Ecology 90, 3379–3392. doi: 10.1890/08-1763.1
Metcalfe, N. B., and Monaghan, P. (2013). Does reproduction cause oxidative stress? An open question. Trends Ecol. Evol. 28, 347–350. doi: 10.1016/j.tree.2013.01.015
Michaud, J. E., Billups, K. L., and Partin, A. W. (2015). Testosterone and prostate cancer: an evidence-based review of pathogenesis and oncologic risk. Ther. Adv. Urol. 7, 378–387. doi: 10.1177/1756287215597633
Moghoofei, M., Keshavarz, M., Ghorbani, S., Babaei, F., Nahand, J. S., Tavakoli, A., et al. (2019). Association between human papillomavirus infection and prostate cancer: a global systematic review and meta-analysis. Asia. Pac. J. Clin. Oncol. 15, e59–e67. doi: 10.1111/ajco.13124
Monaghan, P., Maklakov, A. A., and Metcalfe, N. B. (2020). Intergenerational transfer of ageing: parental age and offspring lifespan. Trends Ecol. Evol. 35, 927–937. doi: 10.1016/j.tree.2020.07.005
Monaghan, P., and Metcalfe, N. B. (2019). The deteriorating soma and the indispensable germline: gamete senescence and offspring fitness. Proc. R. Soc. B Biol. Sci. 286:20192187. doi: 10.1098/rspb.2019.2187
Monaghan, P., and Ozanne, S. E. (2018). Somatic growth and telomere dynamics in vertebrates: relationships, mechanisms and consequences. Philos. Trans. R. Soc. B Biol. Sci. 373:20160446. doi: 10.1098/rstb.2016.0446
Mulder, M. B., and Ross, C. T. (2019). Unpacking mating success and testing Bateman’s principles in a human population. Proc. R. Soc. B Biol. Sci. 286:20191516. doi: 10.1098/rspb.2019.1516
Muller, A. W. J. (2017). Cancer is an adaptation that selects in animals against energy dissipation. Med. Hypotheses. 104, 104–115. doi: 10.1016/j.mehy.2017.05.030
Munson, L., and Moresco, A. (2007). Comparative pathology of mammary gland cancers in domestic and wild animals. Breast Dis. 28, 7–21. doi: 10.3233/BD-2007-28102
Nichols, H. B., Schoemaker, M. J., Cai, J., Xu, J., Wright, L. B., Brook, M. N., et al. (2019). Breast cancer risk after recent childbirth: a pooled analysis of 15 prospective studies. Ann. Intern. Med. 170, 22–30. doi: 10.7326/M18-1323
Nordling, D., Andersson, M., Zohari, S., and Gustafsson, L. (1998). Reproductive effort reduces specific immune response and parasite resistance. Proc. R. Soc. B Biol. Sci. 265, 1291–1298. doi: 10.1098/rspb.1998.0432
Nunney, L. (2013). The real war on cancer: the evolutionary dynamics of cancer suppression. Evol. Appl. 6, 11–19. doi: 10.1111/eva.12018
Nunney, L. (2018). Size matters: height, cell number and a person’s risk of cancer. Proc. R. Soc. B Biol. Sci. 285:20181743. doi: 10.1098/rspb.2018.1743
Nussey, D. H., Kruuk, L. E. B., Donald, A., Fowlie, M., and Clutton-Brock, T. H. (2006). The rate of senescence in maternal performance increases with early-life fecundity in red deer. Ecol. Lett. 9, 1342–1350. doi: 10.1111/j.1461-0248.2006.00989.x
Oduncu, F. S., Kimmig, R., Hepp, H., and Emmerich, B. (2003). Cancer in pregnancy: maternal–fetal confict. J. Cancer Res. Clin. Oncol. 129, 133–146. doi: 10.1007/s00432-002-0406-6
Oktay, K., Turan, V., Titus, S., Stobezki, R., and Liu, L. (2015). BRCA mutations, DNA repair deficiency, and ovarian aging. Biol. Reprod. 93:67. doi: 10.1095/biolreprod.115.132290
Olsson, M., Wapstra, E., and Friesen, C. (2018). Ectothermic telomeres: it’s time they came in from the cold. Philos. Trans. R. Soc. B Biol. Sci. 373:449. doi: 10.1098/rstb.2016.0449
Ostrander, E. A., Davis, B. W., and Ostrander, G. K. (2016). Transmissible tumors: breaking the cancer paradigm. Trends Genet. 32, 1–15. doi: 10.1016/j.tig.2015.10.001
Paduch, D. A. (2006). Testicular cancer and male infertility. Curr. Opin. Urol. 16, 419–427. doi: 10.1097/01.mou.0000250282.37366.d2
Pankhurst, N. W., and Munday, P. L. (2011). Effects of climate change on fish reproduction and early life history stages. Mar. Freshw. Res. 62:1015. doi: 10.1071/MF10269
Pap, P. L., Vincze, O., Fülöp, A., Székely-Béres, O., Pãtraş, L., Pénzes, J., et al. (2018). Oxidative physiology of reproduction in a passerine bird: a field experiment. Behav. Ecol. Sociobiol. 72:18. doi: 10.1007/s00265-017-2434-x
Pavlidis, N. A. (2002). Coexistence of pregnancy and malignancy. Oncologist 7, 279–287. doi: 10.1634/theoncologist.2002-0279
Pepke, M. L., and Eisenberg, D. T. (2021). On the comparative biology of mammalian telomeres: telomere length co-evolves with body mass, lifespan and cancer risk. Mol. Ecol. 1–11. doi: 10.1111/mec.15870
Péron, G., Bonenfant, C., Lemaître, J.-F., Ronget, V., Tidière, M., and Gaillard, J.-M. (2019). Does grandparental care select for a longer lifespan in non-human mammals? Biol. J. Linn. Soc. 128, 360–372.
Perret, C., Gidoin, C., Ujvari, B., Thomas, F., and Roche, B. (2020). Predation shapes the impact of cancer on population dynamics and the evolution of cancer resistance. Evol. Appl. 13, 1733–1744. doi: 10.1111/eva.12951
Perrot, C., Béchet, A., Hanzen, C., Arnaud, A., Pradel, R., and Cézilly, F. (2016). Sexual display complexity varies non-linearly with age and predicts breeding status in greater flamingos. Sci. Rep. 6:36242. doi: 10.1038/srep36242
Pesavento, P. A., Agnew, D., Keel, M. K., and Woolard, K. D. (2018). Cancer in wildlife: patterns of emergence. Nat. Rev. Cancer 18, 646–661. doi: 10.1038/s41568-018-0045-0
Peters, M., Simmons, L. W., and Rhodes, G. (2008). Testosterone is associated with mating success but not attractiveness or masculinity in human males. Anim. Behav. 76, 297–303. doi: 10.1016/j.anbehav.2008.02.008
Preston, B. T., Jalme, M. S., Hingrat, Y., Lacroix, F., and Sorci, G. (2011). Sexually extravagant males age more rapidly. Ecol. Lett. 14, 1017–1024. doi: 10.1111/j.1461-0248.2011.01668.x
Pye, R. J., Pemberton, D., Tovar, C., Tubio, J. M. C., Dun, K. A., Fox, S., et al. (2016). A second transmissible cancer in Tasmanian devils. Proc. Natl. Acad. Sci. U.S.A. 113, 374–379. doi: 10.1073/pnas.1519691113
Rathje, K., Mortzfeld, B., Hoeppner, M. P., Taubenheim, J., Bosch, T. C. G., and Klimovich, A. (2020). Dynamic interactions within the host-associated microbiota cause tumor formation in the basal metazoan Hydra. PLoS Pathog. 16:e1008375. doi: 10.1371/journal.ppat.1008375
Raveh, S., Heg, D., Dobson, F. S., Coltman, D. W., Gorrell, J. C., Balmer, A., et al. (2010). Mating order and reproductive success in male Columbian ground squirrels (Urocitellus columbianus). Behav. Ecol. 21, 537–547. doi: 10.1093/beheco/arq004
Reid, J. M., Bignal, E. M., Bignal, S., McCracken, D. I., Monaghan, P., and Bogdanova, M. I. (2010). Parent age, lifespan and offspring survival: structured variation in life history in a wild population. J. Anim. Ecol. 79, 851–862. doi: 10.1111/j.1365-2656.2010.01669.x
Rickard, I. J., Russell, A. F., and Lummaa, V. (2007). Producing sons reduces lifetime reproductive success of subsequent offspring in pre-industrial Finns. Proc. R. Soc. B Biol. Sci. 274, 2981–2988. doi: 10.1098/rspb.2007.1051
Roff, D. (2001). “Life history, evolution,” in Encyclopedia of Biodiversity, Second Edition, ed. S. A. Levin (Cambridge, MA: Academic Press).
Rose, M. R., and Mueller, L. D. (1993). Stearns, Stephen C., 1992. The evolution of life histories. Oxford University Press, London xii + 249 pp., £16.95. J. Evol. Biol. 6, 304–306. doi: 10.1046/j.1420-9101.1993.6020304.x
Saadat, M. (2010). Offspring sex ratio at birth and maternal breast cancer risk: a case-control study and meta-analysis of literature. EXCLI J. 9, 76–81. doi: 10.17877/DE290R-15808
Sandland, G. J., and Minchella, D. J. (2003). Costs of immune defense: an enigma wrapped in an environmental cloak? Trends Parasitol. 19, 571–574. doi: 10.1016/j.pt.2003.10.006
Schaffer, W. M. (1974). Selection for optimal life histories: the effects of age structure. Ecology 55, 291–303. doi: 10.2307/1935217
Schwenke, R. A., Lazzaro, B. P., and Wolfner, M. F. (2016). Reproduction-immunity trade-offs in insects. Annu. Rev. Entomol. 61, 239–256. doi: 10.1146/annurev-ento-010715-023924
Segami, J. C., Lind, M. I., and Qvarnström, A. (2021). Should females prefer old males? Evol. Lett. 5, 507–520. doi: 10.1002/evl3.250
Singh, S. R., Burnicka-Turek, O., Chauhan, C., and Hou, S. X. (2011). Spermatogonial stem cells, infertility and testicular cancer. J. Cell. Mol. Med. 15, 468–483. doi: 10.1111/j.1582-4934.2010.01242.x
Smith, K. R., Hanson, H. A., Mineau, G. P., and Buys, S. S. (2012). Effects of BRCA1 and BRCA2 mutations on female fertility. Proc. R. Soc. B Biol. Sci. 279, 1389–1395. doi: 10.1098/rspb.2011.1697
Stearns, S. C. (2006). Trade-offs in life-history evolution. Funct. Ecol. 3, 259–268. doi: 10.2307/2389364
Strakova, A., and Murchison, E. P. (2014). The changing global distribution and prevalence of canine transmissible venereal tumour. BMC Vet. Res. 10:168. doi: 10.1186/s12917-014-0168-9
Strakova, A., and Murchison, E. P. (2015). The cancer which survived: insights from the genome of an 11000 year-old cancer. Curr. Opin. Genet. Dev. 30, 49–55. doi: 10.1016/j.gde.2015.03.005
Stulp, G., Buunk, A. P., and Pollet, T. V. (2013). Women want taller men more than men want shorter women. Pers. Individ. Diff. 54, 877–883. doi: 10.1016/j.paid.2012.12.019
Summers, K., and Crespi, B. (2008). The androgen receptor and prostate cancer: a role for sexual selection and sexual conflict? Med. Hypotheses. 70, 435–443. doi: 10.1016/j.mehy.2007.04.044
Summers, K., and Crespi, B. J. (2010). Xmrks the spot: life history tradeoffs, sexual selection and the evolutionary ecology of oncogenesis. Mol. Ecol. 19, 3022–3024. doi: 10.1111/j.1365-294X.2010.04739.x
Taff, C. C., and Freeman-Gallant, C. R. (2017). Sexual signals reflect telomere dynamics in a wild bird. Ecol. Evol. 7, 3436–3442. doi: 10.1002/ece3.2948
Tanaka, M., Yamaguchi, S., and Iwasa, Y. (2020). Enhanced risk of cancer in companion animals as a response to the longevity. Sci. Rep. 10:19508. doi: 10.1038/s41598-020-75684-4
Taneja, V. (2018). Sex hormones determine immune response. Front. Immunol. 9:1931. doi: 10.3389/fimmu.2018.01931
Tevfik Dorak, M., and Karpuzoglu, E. (2012). Gender differences in cancer susceptibility: an inadequately addressed issue. Front. Genet. 3:268. doi: 10.3389/fgene.2012.00268
Thomas, F., Giraudeau, M., Dheilly, N. M., Gouzerh, F., Boutry, J., Beckmann, C., et al. (2020). Rare and unique adaptations to cancer in domesticated species: an untapped resource? Evol. Appl. 13, 1605–1614. doi: 10.1111/eva.12920
Thomas, F., Giraudeau, M., Renaud, F., Ujvari, B., Roche, B., Pujol, P., et al. (2019a). Can postfertile life stages evolve as an anticancer mechanism? PLoS Biol. 17:e3000565. doi: 10.1371/journal.pbio.3000565
Thomas, F., Madsen, T., Giraudeau, M., Misse, D., Hamede, R., Vincze, O., et al. (2019b). Transmissible cancer and the evolution of sex. PLoS Biol. 17:e3000275. doi: 10.1371/journal.pbio.3000275
Thomas, F., Jacqueline, C., Tissot, T., Henard, M., Blanchet, S., Loot, G., et al. (2017). The importance of cancer cells for animal evolutionary ecology. Nat. Ecol. Evol. 1, 1592–1595. doi: 10.1038/s41559-017-0343-z
Thomas, F., Nesse, R. M., Gatenby, R., Gidoin, C., Renaud, F., Roche, B., et al. (2016). Evolutionary ecology of organs: a missing link in cancer development? Trends Cancer 2, 409–415. doi: 10.1016/j.trecan.2016.06.009
Thomas, F., Vavre, F., Tissot, T., Vittecoq, M., Giraudeau, M., Bernex, F., et al. (2018). Cancer is not (only) a senescence problem. Trends Cancer 4, 169–172. doi: 10.1016/j.trecan.2018.01.002
Trumble, B. C., Stieglitz, J., Rodriguez, D. E., Linares, E. C., Kaplan, H. S., and Gurven, M. D. (2014). Challenging the inevitability of prostate enlargement: low levels of benign prostatic hyperplasia among tsimane forager-horticulturalists. J. Gerontol. Ser. A Biol. Sci. Med. Sci. 70, 1262–1268. doi: 10.1093/gerona/glv051
Ujvari, B., Beckmann, C., Biro, P. A., Arnal, A., Tasiemski, A., Massol, F., et al. (2016). Cancer and life-history traits: lessons from host-parasite interactions. Parasitology 143, 533–541. doi: 10.1017/S0031182016000147
Ujvari, B., and Madsen, T. (2009). Short telomeres in hatchling snakes: erythrocyte telomere dynamics and longevity in tropical pythons. PLoS One 4:e7493. doi: 10.1371/journal.pone.0007493
Vágási, C. I., Vincze, O., Lemaître, J. F., Pap, P. L., Ronget, V., and Gaillard, J. M. (2021). Is degree of sociality associated with reproductive senescence? A comparative analysis across birds and mammals. Philos. Trans. R. Soc. B Biol. Sci. 376:20190744. doi: 10.1098/rstb.2019.0744
van Noordwijk, A. J., and de Jong, G. (1986). Acquisition and allocation of resources: their influence on variation in life history tactics. Am. Nat. 128:137. doi: 10.1086/284547
Van Remmen, H., Ikeno, Y., Hamilton, M., Pahlavani, M., Wolf, N., Thorpe, S. R., et al. (2004). Life-long reduction in MnSOD activity results in increased DNA damage and higher incidence of cancer but does not accelerate aging. Physiol. Genomics 16, 29–37. doi: 10.1152/physiolgenomics.00122.2003
Vanpé, C., Gaillard, J. M., Hewison, A. J. M., Quemere, E., Kjellander, P., Pellerin, M., et al. (2019). Old females rarely mate with old males in roe deer, Capreolus capreolus. Biol. J. Linn. Soc. 128, 515–525. doi: 10.1093/biolinnean/blz110
Verburgt, L., Ferreira, M., and Ferguson, J. W. H. (2011). Male field cricket song reflects age, allowing females to prefer young males. Anim. Behav. 81, 19–29. doi: 10.1016/j.anbehav.2010.09.010
Vibishan, B., and Watve, M. G. (2020). Context-dependent selection as the keystone in the somatic evolution of cancer. Sci. Rep. 10:4223. doi: 10.1038/s41598-020-61046-7
Vicens, A., and Posada, D. (2018). Selective pressures on human cancer genes along the evolution of mammals. Genes 9:582. doi: 10.3390/genes9120582
Vincze, O., Colchero, F., Lemaître, J. F., Conde, D. A., Pavard, S., Bieuville, M., et al. (2022). Cancer risk across mammals. Nature 601, 263–267. doi: 10.1038/s41586-021-04224-5
Vittecoq, M., Ducasse, H., Arnal, A., Møller, A. P., Ujvari, B., Jacqueline, C. B., et al. (2015). Animal behaviour and cancer. Anim. Behav. 101, 19–26. doi: 10.1016/j.anbehav.2014.12.001
Vittecoq, M., Roche, B., Daoust, S. P., Ducasse, H., Missé, D., Abadie, J., et al. (2013). Cancer: a missing link in ecosystem functioning? Trends Ecol. Evol. 28, 628–635. doi: 10.1016/j.tree.2013.07.005
vom Saal, F. S., Finch, C. E., and Nelson, J. F. (1994). “Natural history and mechanisms of reproductive aging in humans, laboratory rodents, and other selected vertebrates,” in The Physiology of Reproduction, eds E. Knobil and J. D. Neill (New York, NY: Raven Press), 1213–1314.
Wang, Y., Zhang, C., Wang, N., Li, Z., Heller, R., Liu, R., et al. (2019). Genetic basis of ruminant headgear and rapid antler regeneration. Science 364:eaav6335. doi: 10.1126/science.aav6335
Wells, K., Hamede, R. K., Jones, M. E., Hohenlohe, P. A., Storfer, A., and McCallum, H. I. (2019). Individual and temporal variation in pathogen load predicts long-term impacts of an emerging infectious disease. Ecology 100:e02613. doi: 10.1002/ecy.2613
Westendorp, R. G. J., and Kirkwood, T. B. L. (1998). Human longevity at the cost of reproductive success. Nature 396, 743–746. doi: 10.1038/25519
Whittingham, L. A., and Dunn, P. O. (2000). Offspring sex ratios in tree swallows: females in better condition produce more sons. Mol. Ecol. 9, 1123–1129. doi: 10.1046/j.1365-294X.2000.00980.x
Williams, G. C. (1957). Pleiotropy, natural selection, and the evolution of senescence. Evolution 11, 398–411. doi: 10.2307/2406060
Williams, G. C. (1966). Natural selection, the costs of reproduction, and a refinement of lack’s principle. Am. Nat. 100, 687–690. doi: 10.1086/282461
Wingfield, J. C., Hegner, R. E., Dufty, A. M., and Ball, G. F. (1990). The “challenge hypothesis”: theoretical implications for patterns of testosterone secretion, mating systems, and breeding strategies. Am. Nat. 136, 829–846. doi: 10.1086/285134
Winkler, D. W., Dunn, P. O., and McCulloch, C. E. (2002). Predicting the effects of climate change on avian life-history traits. Proc. Natl. Acad. Sci. U.S.A. 99, 13595–13599. doi: 10.1073/pnas.212251999
Wohlfahrt, J., and Melbye, M. (2000). Gender of offspring and long term maternal breast cancer risk. Br. J. Cancer 82, 1070–1072. doi: 10.1054/bjoc.1999.1044
Yang, J., Benyamin, B., McEvoy, B. P., Gordon, S., Henders, A. K., Nyholt, D. R., et al. (2010). Common SNPs explain a large proportion of the heritability for human height. Nat. Genet. 42, 517–524. doi: 10.1038/ng.608
Yonemitsu, M. A., Giersch, R. M., Polo-Prieto, M., Hammel, M., Simon, A., Cremonte, F., et al. (2019). A single clonal lineage of transmissible cancer identified in two marine mussel species in South America and Europe. eLife 8:e47788. doi: 10.7554/eLife.47788
Keywords: disease, sexual selection, reproduction, reproductive aging, neoplasia, transmissible cancer, senescence
Citation: Dujon AM, Boutry J, Tissot S, Lemaître J-F, Boddy AM, Gérard A-L, Alvergne A, Arnal A, Vincze O, Nicolas D, Giraudeau M, Telonis-Scott M, Schultz A, Pujol P, Biro PA, Beckmann C, Hamede R, Roche B, Ujvari B and Thomas F (2022) Cancer Susceptibility as a Cost of Reproduction and Contributor to Life History Evolution. Front. Ecol. Evol. 10:861103. doi: 10.3389/fevo.2022.861103
Received: 24 January 2022; Accepted: 05 April 2022;
Published: 09 May 2022.
Edited by:
Jerry Husak, University of St. Thomas, United StatesReviewed by:
Wendy Hood, Auburn University, United StatesClay Cressler, University of Nebraska–Lincoln, United States
Copyright © 2022 Dujon, Boutry, Tissot, Lemaître, Boddy, Gérard, Alvergne, Arnal, Vincze, Nicolas, Giraudeau, Telonis-Scott, Schultz, Pujol, Biro, Beckmann, Hamede, Roche, Ujvari and Thomas. This is an open-access article distributed under the terms of the Creative Commons Attribution License (CC BY). The use, distribution or reproduction in other forums is permitted, provided the original author(s) and the copyright owner(s) are credited and that the original publication in this journal is cited, in accordance with accepted academic practice. No use, distribution or reproduction is permitted which does not comply with these terms.
*Correspondence: Frédéric Thomas, ZnJlZGVyaWMudGhvbWFzMkBpcmQuZnI=
†These authors have contributed equally to this work